- 1School of Food and Bioengineering, Institute for Advanced Study, Chengdu University, Chengdu, China
- 2School of Preclinical Medicine, Chengdu University, Chengdu, China
- 3Antibiotics Research and Re-Evaluation Key Laboratory of Sichuan Province, Sichuan Industrial Institute of Antibiotics, Chengdu University, Chengdu, China
Polysaccharides are one of the important components of the human diet, offering a wide range of biological activities, especially in improving inflammation in the digestive system and addressing metabolic diseases. Among all the reported bioactivities of polysaccharides, their regulation effects on intestinal function and bile acids (BAs) are gradually attracting the attention of more researchers. Bile acids, the main components of intestinal lipid digestive fluid, are also key signal factors for metabolic homeostasis and impact overall health. Polysaccharides usually interact directly or indirectly with the gut and gut microbiota to participate in the regulation process of reabsorption, metabolism, and excretion of bile acids in humans, thereby exerting their role in the intervention of human diseases. In this review, we comprehensively reviewed the effects of bioactive polysaccharides on the regulation of intestinal function and bile acids. The chemical structures, bioactivities, and potential mechanisms for their activities were also reviewed. This study aimed to provide a comprehensive reference for future research on the activities and mechanisms of polysaccharides, as well as to offer important strategies and insights for the development of bioactive polysaccharides to prevent inflammatory and metabolic diseases.
Introduction
Polysaccharides, a type of polymer formed by aldoses or ketoses with glycosidic bonds, are often composed of more than 10 monosaccharides arranged in chains and branch shapes, and monosaccharide compositions and structures are complex and diverse. Polysaccharides are widely distributed in nature and have great development and application potential. They are one of the important components of fruits, grains, and vegetables. The biological activities of polysaccharides include lowering blood sugar (1), anti-obesity (2), liver protection (3), regulating immunity (4), anti-oxidation (5), and anti-tumor effects (6). Hence, research interest in these kinds of polymers has been rapidly increasing in recent years.
The intestine plays an important role in digestion and absorption and is also a barrier that prevents harmful substances from entering the bloodstream. Intestinal lymphocytes often secrete inflammatory mediators and cytokines to regulate the intestinal immune environment (7). In addition, the intestinal barrier function often affects intestinal-liver metabolism. When the intestinal barrier function is changed, it can easily induce obesity (8), type 2 diabetes (9), asthma (10), rheumatoid arthritis (11), and other diseases in humans. Metabolites in the intestine (such as intestinal D-lactate) can enter the bloodstream through damaged intestinal mucosa and participate in regulating various metabolic reactions in the body, serving as an important biological barrier for intestinal anti-infection. Bile acids (BAs) play an important role in maintaining the homeostasis of the bile pool (12), improving metabolic inflammation (13), and providing neuroprotection (14). Meanwhile, the Farnesoid X receptor (FXR) is a bile acid-activated nuclear receptor mainly expressed in the liver and intestine. Upon stimulation by specific bile acids for the FXR, key genes involved in bile acid synthesis, transport, and reabsorption metabolism are regulated and participate in the regulation of carbohydrate and lipid metabolism (15). Hence, it seems that targeting the regulation of intestinal function and bile acid metabolism might be valuable for the treatment of various diseases. Therefore, focusing on the regulatory effects of bioactive polysaccharides on intestinal function and bile acid metabolism is important for future research.
For most orally administrated polysaccharides, they are firstly and widely exposed to the gastrointestinal tract. Therefore, their biological activities in regulating intestinal function may involve the following aspects: (1) polysaccharides may promote the proliferation of beneficial bacteria and/or inhibit the growth of pathogenic bacteria, thereby affecting the metabolism of drugs and food by gut microbiota and presenting various biological activities (16). (2) Polysaccharides may enhance intestinal barrier function and present activities in regulating immune, anti-inflammatory, and other related biological functions (17). (3) Polysaccharides may regulate the digestion and absorption of food by affecting the activity of intestinal digestive enzymes, which may be beneficial for relieving constipation and other related diseases (18).
Apart from the interactions between polysaccharides and the gut, the regulation of the bile acid pool by polysaccharides also has some effects on human health. Bile acids, an important component of bile, are often considered to promote the digestion and absorption of lipids and fat-soluble vitamins in the intestine. Furthermore, evidence also demonstrates that these components are important metabolic regulatory signal factors in the human body, widely involved in the metabolism of glucose, lipids, and energy (19). Three pathways may be involved in the connections between the biological activities of polysaccharides and bile acids: 1) polysaccharides may inhibit the absorption of fat, cholesterol, and bile acids in the intestine through physical or chemical combinations, and thus the excretion of fat, cholesterol, and bile acids is increased, which leads to an increase in the content of blood lipids and a reduction in the risk of cardiovascular diseases. 2) Polysaccharides directly combine with bile acids to inhibit their reabsorption, increase their excretion, regulate bile acids in other ways, and exert biological activities, such as hypoglycemic, hypolipidemic, and hepatoprotective. 3) Polysaccharides regulate the expression of bile acid synthase, metabolic enzymes, and transporters by activating a variety of nuclear receptors in the intestines. The changes in bile acids in the intestine contribute significantly to the changes in gut microbiota structure and, in turn, affect the metabolism of bile acids at all levels. The regulatory effects of bioactive polysaccharides on intestinal function and bile acids are briefly listed in Table 1.
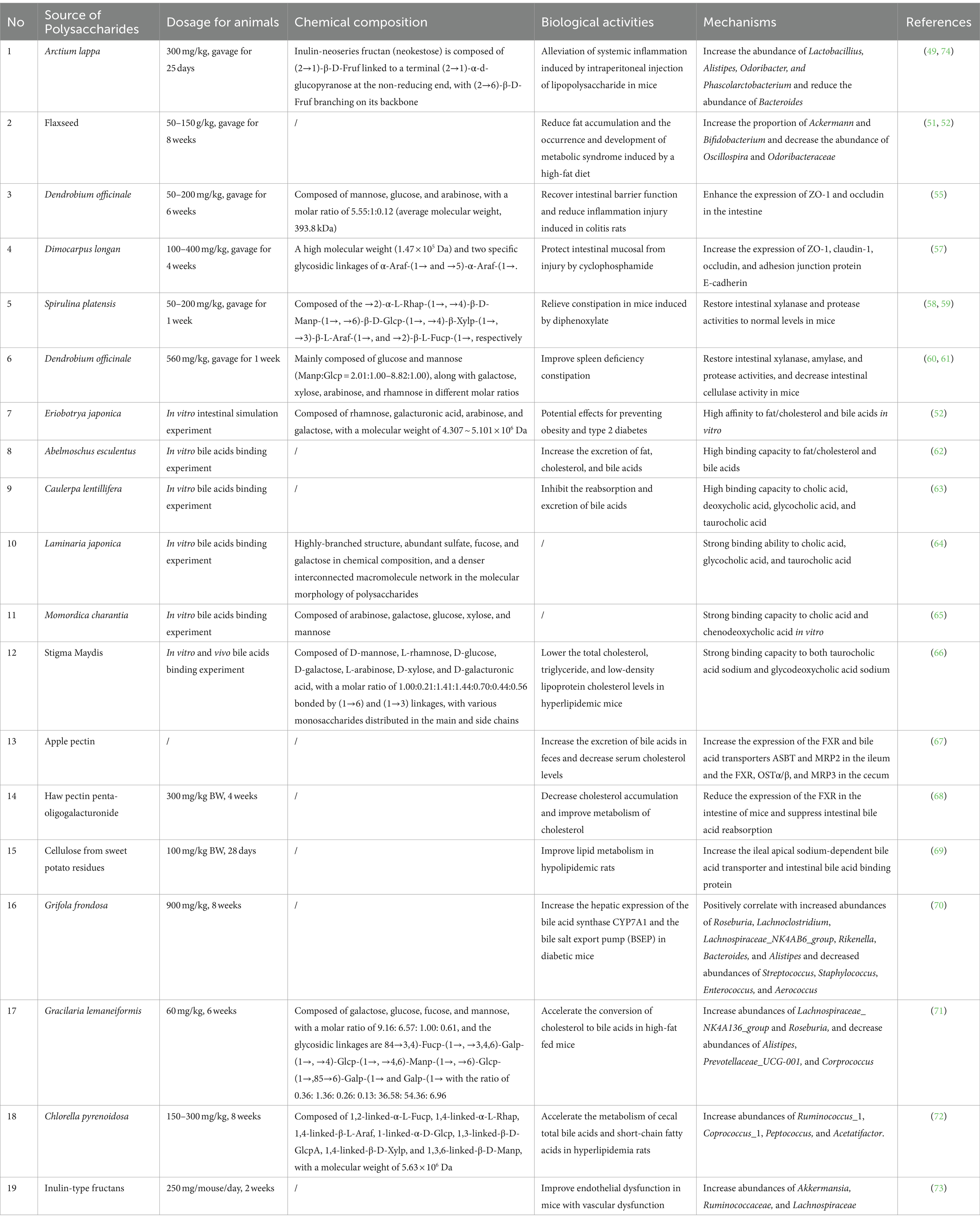
Table 1. Summary of the regulatory effects of bioactive polysaccharides on intestinal function and bile acids.
In this review, the recent research progress on the regulatory effects of bioactive polysaccharides on intestinal function and bile acids is comprehensively summarized. Focusing on the research progress of polysaccharides in regulating bile acid synthesis, reabsorption, metabolism, and excretion through intestinal action is expected to provide a foundational basis for studying the regulatory mechanisms by which polysaccharides exert various bioactivities in the human body.
Regulation of intestinal function by gut mucosa and microbiota
The intestinal mucosa consists of gut microbiota, the mucus layer, intestinal epithelial cells, and immune barriers in the gut, which act between the gut and the external environment and work together to prevent harmful bacteria, toxins, and other external stimuli from entering the gut (20). The highly glycosylated, polymerized mucins on the surface of epithelial cells serve as the first line of defense against physical and chemical damage in the gut. These mucins are rich in serine and threonine protein backbone structure and are linked with multiple O-linked oligosaccharide side chains (21, 22). Intestinal goblet cells not only secrete mucins to create a mechanical protective barrier for intestinal homeostasis but also contribute to the intestinal immune system barrier, which helps maintain intestinal health (23). In addition, some studies have also found that changes in the structure and function of mucins are closely related to the onset and progression of intestinal inflammation and other intestinal diseases (24).
The gut microbiome plays an important role in the development of pathogen resistance, mucosal immune responses, and nutrient metabolism. This is partly due to the interaction between the microbiota and the composition of the mucus layer and intestinal epithelial cells following damage to the mucus. The outer permeable mucus layer is considered the natural habitat of many symbionts because they use exposed mucins as attachment sites for nutritional support and bacterial adhesions (25). Some enzymes produced by gut bacteria are associated with the digestion of various glycans from the mucus and fibers from the host’s diet. The mucus regulatory effects of certain commensals, probiotics, especially Lactobacillus and Bifidobacterium strains, and probiotic mixtures have been demonstrated in animal models of IBD, reproductive disorders, diet-induced obesity, malnutrition, and aging (26–29). Taken together, these results suggest that regulating intestinal mucosal barrier function and gut microbiota may provide benefits for the prevention and treatment of a variety of diseases. Therefore, the regulatory effects of polysaccharides on intestinal mucosal function and gut microbiota are gradually becoming a focus of research for many researchers.
Mechanisms of bile acids in biological activity in humans
As one of the important steroid molecules, bile acids (BAs) are synthesized by cholesterol metabolism in the liver. In the human body, cholesterol is often converted into cholic acid (CA) and chenodeoxycholic acid (CDCA) through two pathways—classical and alternative pathways. Subsequently, CA and CDCA are acylated with glycine or taurine in the liver to form conjugated bile salts, which are stored in the gallbladder and then released from the bile duct into the duodenum. Cholesterol 7α-hydroxylase (cytochrome P450 family 7 subfamily A member 1, CYP7A1) and mitochondrial sterol 27 hydroxylase (cytochrome P450 family 27 subfamily A member 1, CYP27A1) are key enzymes involved in the two pathways of bile acid synthesis (30). After being discharged into the intestine with bile, a portion of primary bile acids is reabsorbed by intestinal epithelial cells and returned to the liver through the portal vein, while another portion is metabolized into secondary bile acids (including lithocholic acid and deoxycholic acid) by intestinal microbiota. In the intestine, the conjugated BAs undergo uncoupling, oxidation, isomerization, and dehydroxylation reactions to convert primary BAs into secondary BAs (31). During the process of hepatic intestinal circulation, approximately 95% of BAs are reabsorbed and transported from the intestine to the liver through specific transporters at the end of the ileum. Then, the free BAs are recombined with taurine or glycine and released into the intestine through bile ducts.
Bile acids are produced through cholesterol catabolism and play an important role in maintaining whole-body cholesterol homeostasis (32). Cholic acid (CA) accounts for approximately 31% of total human bile acids. Evidence from modern pharmacological studies demonstrates that CA acts as a signaling molecule to bind to bile acid receptors, regulate bile acid metabolism, and restore bile acid homeostasis (33). CA activates the PPARα -UGT pathway and compensates for the loss of bile acid transport and synthesis function in SHP knockout mice, ultimately helping to maintain overall intestinal structure and bile acid homeostasis (34).
At the same time, CA can also act as a regulator of glucose, lipids, and energy metabolism to improve metabolic-related inflammation (35). Bile acids regulate a wide range of complex symbiotic metabolic networks by activating various bile acid receptors, including glucose, lipids, steroids, and metabolism, as well as regulating energy homeostasis, thus profoundly affecting the host’s metabolism and immune function (36). The fibroblast growth factor 21 (FGF21) pathway is considered an important pathway for regulating glucose and lipid metabolism. Acetyl CoA carboxylase-1 (ACC1) and hormone-sensitive lipase (HSL) are key enzymes that control the supply of long-chain fatty acid precursors for liver triglyceride (TG) synthesis. On the one hand, CA can reduce the activity of HSL and ACC1 through the FGF21 pathway and ultimately play a role in regulating glucose and lipid metabolism. On the other hand, CA reduces the expression of MIP-1 α, IL-10, and IL-8, upregulates the expression of PPARα, and downregulates the expression of LXRα to exert their anti-inflammatory effects (37–39).
In addition, CA exerts its novel activities by reducing oxidative stress in brain cells and playing a neuroprotective role in brain injury due to its good blood–brain barrier permeability and antioxidant properties (14). Defects or mutations in key enzyme genes of bile acid metabolism often cause disorders of bile acid anabolism. Then, they affect the body’s bile acid metabolism homeostasis and lead to the occurrence of some diseases, such as Zellweger spectrum disorders and cerebrotendinous xanthomatosis (40). CA can interact with the peripheral anion sites of acetylcholinesterase to inhibit its activity and reduce the deposition of amyloid plaques, thus playing a therapeutic role in AD (41). In addition, CA plays a neuroprotective role by promoting the release of brain-derived neurotrophic factor (BDNF), which is beneficial for activating the BDNF Trkb—PI3K/Akt and BDNF Trkb MAPK/ERK signaling pathways, reducing cell damage caused by oxidative stress, and inhibiting cell apoptosis (42).
Metabolism of bile acids by gut microbiota
Gut microbiota is considered one of the important organs of the human body. It is widely involved in the metabolism of bile acids. It is manifested in the role of modifying the chemical structure of bile acid, reabsorption, and detoxification. The mechanisms of gut microbiota in the metabolism of bile acids mainly include early dissociation, dehydroxylation, dehydrogenation, and desulfurization. Firstly, primary bile acids derived from the host are early dissociated by bile salt hydrolases (BSHs), which are mainly found in lactic acid bacteria, Clostridium, Bifidobacterium, Enterococcus, Listeria, and Clostridium (43). Secondly, Clostridium is involved in the 7α-dehydroxylation of bile acids, which converts primary bile acids into secondary bile acids (44). 3) Three kinds of hydroxysteroid dehydrogenases (HSDHs)—3α-HSDH, 7α-HSDH, and 12α-HSDH—expressed by gut microbiota are involved in bile acid hydroxyl oxidation and specific epimerization to convert hydrophobic toxic bile acids into non-toxic water-soluble ursodeoxycholic acid (45). 3α-HSDH is expressed by Clostridium perfringens, Peptostreptococcus, Pseudomonas, and others. 7α -HSDH is widely found in Bacteroides, Clostridium, Escherichia coli, and Ruminococcus. 12α-HSDH is mainly found in Clostridium (46). 4) Some gut microbiota, such as Clostridium S2, produce sulfatase to increase the desulfurization of bile acids and promote the reabsorption of bile acids (46).
The roles of polysaccharides in the regulation of the intestines
Regulatory effects of polysaccharides on gut microbiota
Gut microbiota is considered one of the important components that participates in regulating certain biological activities in humans through different pathways (47). Polysaccharides often serve as important energy sources for gut microbiota and are thus involved in regulating its composition and function. Gut microbiota often encodes a variety of carbohydrate-activated enzymes to catalyze the degradation of polysaccharides into short-chain fatty acids, such as acetic acid, propionic acid, and butyric acid, thereby providing necessary energy for gut microbiota (48). Research demonstrates that polysaccharides from Arctium lappa (300 mg/kg, gavage for 25 days) significantly increase the abundance of Lactobacillius, Alistipes, Odoribacter, and Phascolarctobacterium and reduce the abundance of Bacteroides. This induces a significant increase in the content of short-chain fatty acids in the intestine and alleviates systemic inflammation caused by intraperitoneal injection of lipopolysaccharide in mice (49). The chemical structure of the polysaccharides used in this experiment is reported to be a type of inulin-neoseries fructan (neokestose), which consists of (2→1)-β-D-Fruf linked to a terminal (2→1)-α-d-glucopyranose at the non-reducing end, with (2→6)-β-D-Fruf branching on its backbone (50). Yang et al. conducted a study on the effect of flaxseed polysaccharides (50–150 g/kg, gavage for 8 weeks) in mice with metabolic syndrome induced by a high-fat diet. They found that the proportion of Ackermann and Bifidobacterium increased with the decreased abundance of Oscillospira and Odoribacteraceae. This is considered to be beneficial for reducing fat accumulation and the occurrence and development of metabolic syndrome (51, 52). The chemical composition of the polysaccharides in this experiment is not reported.
The effect of polysaccharides on intestinal barrier function
Tight junctions between cells play an important role in maintaining the integrity of the intestinal barrier and function by regulating paracellular transport. Intercellular tight junction-related proteins mainly include ZO family proteins, occludin, claudins, immunoglobulin superfamily proteins, and others (53, 54). A previous study investigated the effect of Dendrobium officinale polysaccharides (DOPSs; 50–200 mg/kg, gavage for 6 weeks) on colitis in rats induced by DSS. The study found that DOPSs enhance the expression of ZO-1 and occludin in the intestine, therefore effectively recovering intestinal barrier function and reducing inflammation caused by colitis in the rats (55). The monosaccharide composition of the DOPSs used in the experiment was composed of mannose, glucose, and arabinose, with a molar ratio of 5.55:1:0.12 (average molecular weight 393.8 kDa) (56). In another study, it was demonstrated that longan pulp acidic polysaccharides (LPIa, LPIIa, and LPIIIa; 100–400 mg/kg, gavage for 4 weeks) could increase the expression of ZO-1, claudin-1, occludin, and the adhesion junction protein E-cadherin to protect the intestinal mucosa from being injured by cyclophosphamide (57). Among all the reported polysaccharides, it is noted that LPIa has specific structure characteristics, including a porous surface structure, a high molecular weight (1.47 × 105 Da), and two specific glycosidic linkages of α-Araf-(1→ and →5)-α-Araf-(1→. It is deduced that the special structural features of LPIa significantly contribute to the protection of the intestinal barrier (57).
Regulatory effect of polysaccharides on intestinal digestive enzyme activity
Polysaccharides also play a key role in regulating the activity of intestinal digestive enzymes, optimizing the intestinal environment, and improving intestinal diseases, which influence the digestion and absorption of food in humans. It was reported that constipation in mice induced by diphenoxylate was significantly relieved by the administration of polysaccharides from Spirulina platensis (PSP; 50–200 mg/kg, gavage for 1 week) through the restoration of intestinal xylanase and protease activities to normal levels (58). The chemical composition of PSP in this article is not presented. In another study, it was reported that PSP is composed of →2)-α-L-Rhap-(1→, →4)-β-D-Manp-(1→, →6)-β-D-Glcp-(1→, →4)-β-Xylp-(1→, →3)-β-L-Araf-(1→, and →2)-β-L-Fucp-(1→, (59). In addition, a study demonstrated that polysaccharides from Dendrobium officinale (560 mg/kg, gavage for 1 week) are effective in restoring intestinal xylanase, amylase, and protease activities and in decreasing intestinal cellulase activity in mice with spleen deficiency constipation (60). The core structure of polysaccharides from Dendrobium officinale is composed mainly of glucose and mannose (Manp:Glcp = 2.01:1.00–8.82:1.00), along with galactose, xylose, arabinose, and rhamnose in varying molar ratios (61). Taken together, these results indicate that the regulation of intestinal digestive enzyme activity is also closely related to the regulation of gut microbiota.
The interactions between polysaccharides and bile acids
Polysaccharides often exert their regulatory effects on human physiological functions and for the treatment of diseases by regulating intestinal function, including gut microbiota, intestinal physiological barriers, and the intestinal digestive enzymes system. In addition, polysaccharides are involved in regulating the metabolism of bile acids, thereby indirectly contributing to the regulation of human physiological functions and the treatment of some diseases. The regulation of bile acids by polysaccharides is mainly through three pathways. Firstly, polysaccharides directly bind some bile acids, which leads to a decrease in reabsorption and an increase in the excretion of bile acids. Secondly, polysaccharides directly regulate the expression of intestinal transporters, thereby affecting the reabsorption and excretion of bile acids in the intestines. Lastly, the composition and structure of gut microbiota involved in the activities of synthesis, reabsorption, metabolism, and excretion of bile acids are regulated by polysaccharides. The interactions between polysaccharides and bile acids in regulating human physiological functions and treating diseases are summarized as follows.
Polysaccharides directly bind to fats, cholesterol, and bile acids
Polysaccharides often play an important role in the regulation of lipid metabolism and energy consumption by directly binding to fats, cholesterol, and bile acids in the intestine. Hence, it is considered that some polysaccharides are effective in the regulation of diseases related to metabolism, such as diabetes and obesity. In an in vitro intestinal simulation experiment, research showed that pectic polysaccharides from loquat (Eriobotrya japonica) leaves exhibit a higher affinity to fat/cholesterol and bile acids compared to carboxymethyl cellulose and cholestyramine, which may be developed as food for the prevention and treatment of obesity and type 2 diabetes (52). The major monosaccharide components of the polysaccharides from loquat leaves are rhamnose, galacturonic acid, arabinose, and galactose. In addition, the novel binding capacity of the polysaccharides from loquat leaves to fat/cholesterol and bile acids is correlated with their molecular weights (4.307 ~ 5.101 × 106 Da), molecular weight distributions, and degree of esterification (52). In the same experiment, it was also found that polysaccharides from okra (Abelmoschus esculentus) exhibit an obvious binding capacity to fat/cholesterol and bile acids, which may be useful for increasing the excretion of fat, cholesterol, and bile acids (62). In addition, polysaccharides often directly bind bile acids to inhibit the reabsorption and excretion of bile acids. The bile acid-binding capacities of polysaccharides from Caulerpa lentillifera were investigated, and it was found that the binding ability of the sub-fraction WCLP-70 to cholic acid, deoxycholic acid, glycocholic acid, and taurocholic acid is 68.1, 36.1, 74.9, and 72.3%, respectively (63). Researchers have also found that crude polysaccharides from Laminaria japonica (LP-A8) present a strong binding ability to cholic acid, glycocholic acid, and taurocholic acid, with binding values of 68.29, 81.99, and 161.72%, respectively. It is deduced that the highly-branched structure, abundant sulfate, fucose, and galactose in the chemical composition, and the denser interconnected macromolecule network in the molecular morphology of the polysaccharides (LP-A8) contribute significantly to its bile acid-binding capacity (64). Polysaccharides from Momordica charantia L., which are mainly composed of arabinose, galactose, glucose, xylose, and mannose, also exhibit a strong binding capacity to cholic acid and chenodeoxycholic acid in vitro (65). In addition, research has demonstrated that the stigma maydis polysaccharide (SMP-1) exhibits a strong binding capacity to both taurocholic acid sodium (65.96–83.64%) and glycodeoxycholic acid sodium (71.76–103.50%) in vitro, while also lowering total cholesterol, triglyceride, and low-density lipoprotein cholesterol levels in hyperlipidemic mice (66). Chemical structure analysis has shown that the SMP-1 is composed of D-mannose, L-rhamnose, D-glucose, D-galactose, L-arabinose, D-xylose, and D-galacturonic acid, with a molar ratio of 1.00:0.21:1.41:1.44:0.70:0.44:0.56. These components are bonded by (1→6) and (1→3) linkages, with various monosaccharides distributed in the main and side chains (66). Taken together, these results suggest that although various polysaccharides have been tested for their bile acid-binding capacities in vitro in previous studies, it should be noted that their actual binding abilities to bile acids in vivo have been scarcely investigated. The real mechanisms underlying the anti-hyperlipidemic effects through direct binding to bile acids in vivo should be further explored.
Polysaccharides regulate bile acids by modulating intestinal transporters
Apart from directly binding to bile acids, polysaccharides also participate in regulating the expression of some intestinal transporters to facilitate the reabsorption and excretion of bile acids. It has been found that the expression of the FXR and bile acid transporters ASBT and MRP2 in the ileum, as well as the FXR, OSTα/β, and MRP3 in the cecum, are significantly increased by the daily consumption of apple pectin for piglets (67). This leads to an increased amount of excreted bile acids in feces and a decreased serum cholesterol level. In another in vivo experiment, it was noted that cholesterol accumulation was significantly decreased, along with improved cholesterol metabolism, by daily administration of Haw pectin penta-oligogalacturonide (300 mg/kg BW, 4 weeks) to mice fed with a high-cholesterol diet. A mechanism study reported that Haw pectin penta-oligogalacturonide treatment significantly reduces the expression of the FXR in the intestine of mice and suppresses intestinal bile acid reabsorption (68). It has also been found cellulose from sweet potato residues (100 mg/kg BW, 28 days) is beneficial for lipid metabolism in rats, inhibiting body weight gain, food intake, plasma lipids, and hepatic lipids. Mechanisms investigation has demonstrated that the ileal apical sodium-dependent bile acid transporter and intestinal bile acid-binding protein are increased by treatment with this substance in hypolipidemic rats (69).
Polysaccharides regulate the metabolism of bile acids by modulating gut microbiota
In fact, gut microbiota is widely involved in the metabolism of numerous nutrients in humans and plays an important role in the entire process of life. In addition to affecting the expression of receptors related to intestinal bile acid metabolism, orally administrated polysaccharides also widely interfere with gut microbiota to regulate bile acid metabolism. It was reported that polysaccharides from Grifola frondosa (900 mg/kg, 8 weeks) significantly increased the hepatic expression of the bile acid synthase CYP7A1 and the bile salt export pump (BSEP) in diabetic mice, which is beneficial for the enhancement of bile acids (BAs) synthesis and excretion in the liver. A correlation analysis indicated that Roseburia, Lachnoclostridium, Lachnospiraceae_NK4AB6_group, Rikenella, Bacteroides, and Alistipes positively correlate to the mRNA levels of CYP7A1 and BSEP, while Streptococcus, Staphylococcus, Enterococcus, and Aerococcus negatively correlate with BSEP mRNA levels (70). Huang et al. found that sulfated polysaccharides from Gracilaria lemaneiformis (GLP, 60 mg/kg, 6 weeks) significantly accelerated the conversion of cholesterol to bile acids by promoting the expression of LxRα and CYP7A1 genes in high-fat fed mice. It was found that the serum concentration of chenodeoxycholic acid and deoxycholic acid is negatively correlated with the relative abundances of Alistipes, Prevotellaceae_UCG-001, and Corprococcus, while ursodeoxycholic acid and tauroursodeoxycholic acid are positively correlated with the relative abundances of Lachnospiraceae_NK4A136_group and Roseburia. The chemical composition of the polysaccharides in the experiment was reported to be composed of galactose, glucose, fucose, and mannose, with a molar ratio of 9.16: 6.57: 1.00: 0.61. The glycosidic linkages of GLP are as follows: 84→3,4)-Fucp-(1→, →3,4,6)-Galp-(1→, →4)-Glcp-(1→, →4,6)-Manp-(1→, →6)-Glcp-(1→,85→6)-Galp-(1→ and Galp-(1→ with the ratio of 0.36: 1.36: 0.26: 0.13: 36.58: 54.36: 6.96 (71).
Polysaccharides from green microalga Chlorella pyrenoidosa (150–300 mg/kg, 8 weeks) were also reported to accelerate the metabolism of cecal total bile acids and short-chain fatty acids in hyperlipidemia rats. The results indicated that total bile acids in the rat cecum are positively correlated with Ruminococcus_1, Coprococcus_1, Peptococcus, and Acetatifactor. The chemical structure of polysaccharides from green microalga Chlorella pyrenoidosa is identified as follows: the main glycosidic bonds include 1,2-linked-α-L-Fucp, 1,4-linked-α-L-Rhap, 1,4-linked-β-L-Araf, 1-linked-α-D-Glcp, 1,3-linked-β-D-GlcpA, 1,4-linked-β-D-Xylp, and 1,3,6-linked-β-D-Manp, with a molecular weight of 5.63 × 106 Da (72). Catry et al. reported that inulin-type fructan treatment (250 mg/mouse/day, 2 weeks) successfully replenished the abundance of Akkermansia and decreased the abundance of bacterial taxa involved in secondary BA synthesis in mice with vascular dysfunction. It was found that the content of cholic acid and chenodeoxycholic acid (primary bile acid) in the blood and cecum of mice were increased, while the content of lithocholic acid and deoxycholic acid (secondary bile acid) was decreased, which facilitated the improvement of endothelial dysfunction. Meanwhile, lithocholic acid and deoxycholic acid were positively correlated with the abundances of Ruminococcaceae and Lachnospiraceae (73). A summary of the mechanisms of bioactive polysaccharides in the regulation of intestinal function and bile acids for the treatment of various diseases is presented in Figure 1.
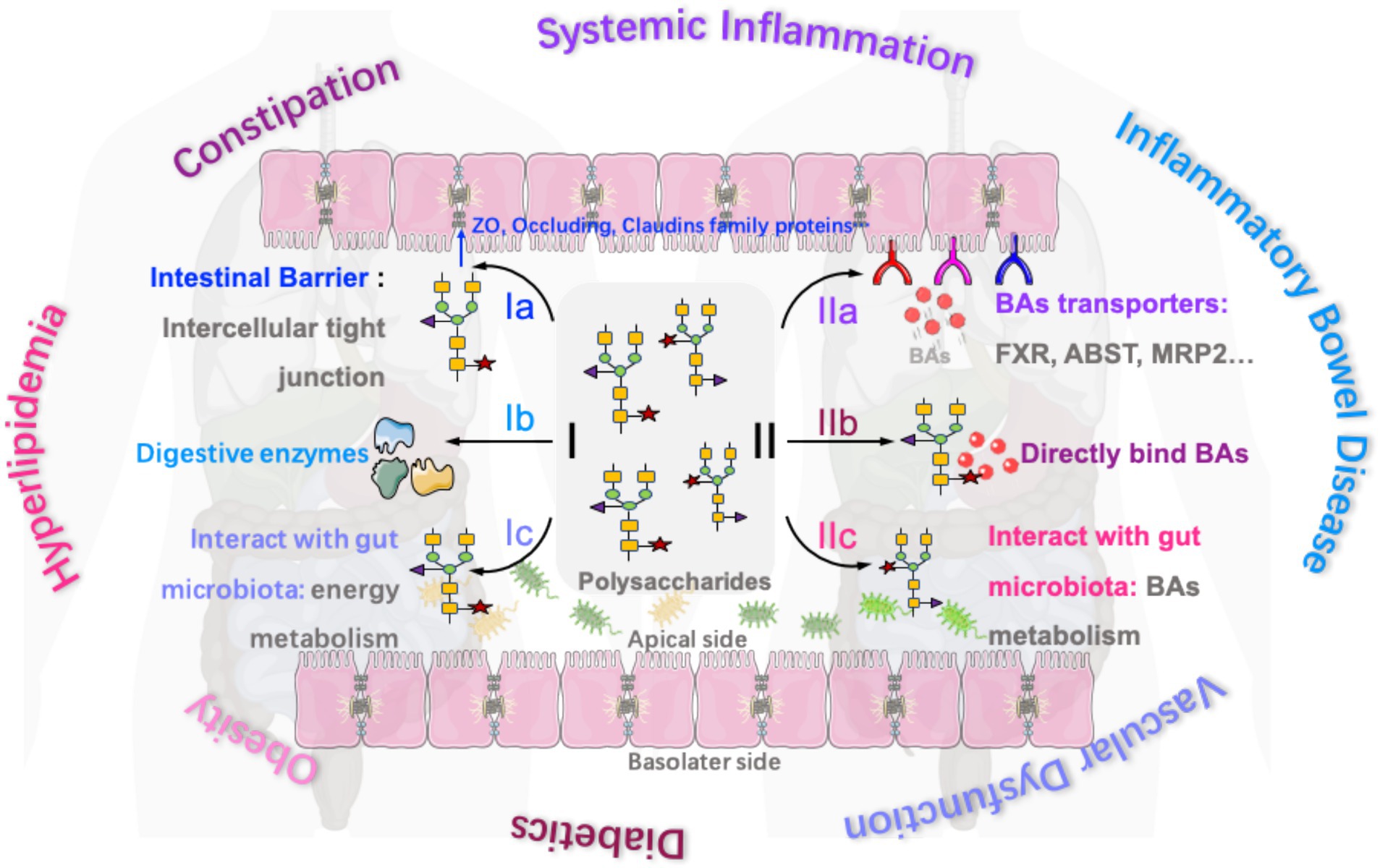
Figure 1. Mechanisms of bioactive polysaccharides in the regulation of intestinal function and bile acids for the treatment of various diseases (Ia, polysaccharides regulate the expression of intercellular tight junction proteins in the intestines for treating inflammatory bowel disease and constipation; Ib, polysaccharides regulate the production of some digestive enzymes in the intestines for treating constipation and aiding nutrient digestion; Ic, polysaccharides interact with gut microbiota for regulating energy metabolism and treating hyperlipidemia and obesity; IIa, polysaccharides regulate the expression of some bile acids transporters (such as FXR, ABST, and MRP2) to regulate bile acids resorption in the intestine for treating systemic inflammation, obesity, and diabetes; IIb, some polysaccharides also directly bind to certain bile acids to inhibit bile acids resorption for treating hyperlipidemia and obesity; and IIc, polysaccharides interact with gut microbiota for regulating bile acid metabolism and treating diabetics and obesity).
From previous studies, we have found that polysaccharides effectively regulate gut microbiota and bile acid metabolism, thus playing a therapeutic role in some diseases. However, further research should focus more on the crosstalk between orally administrated polysaccharides, gut microbiota, and bile acid metabolism. Whether polysaccharides directly regulate the composition or enzyme activity of gut microbiota, thereby indirectly affecting the metabolism of bile acids; whether polysaccharides affect the composition or content of intestinal bile acids, thereby indirectly regulating the composition and biological activity of gut microbiota; or whether polysaccharides participate in more complex regulatory processes— these questions still need further investigation. Further and more in-depth research on bioactive polysaccharides should be conducted to investigate the exact chemical composition of polysaccharides and their corresponding activities in the regulation of intestine function and bile acid metabolism. Answering these kinds of questions will be more conducive to the development of bioactive polysaccharides for human health.
Conclusion
Polysaccharides regulate bile acid synthesis, reabsorption, metabolism, and excretion through the intestine, which can provide new insights for the development and mechanistic research of polysaccharide-based drugs for the treatment of diseases caused by abnormal bile acid metabolism, such as hyperlipidemia, diabetes, cardiovascular diseases, and non-alcoholic fatty liver diseases, as well as for formulating new targeted treatment strategies. The structure of polysaccharides is complex due to the differences in monosaccharide composition, glycosidic bonds, and degree of polymerization. The structure of flora is easily altered by external or internal factors, and a variety of substances can be obtained after the polysaccharide is degraded by carbohydrate-active enzymes. The dynamic changes of the gut-bile acid-host axis are complex. People’s understanding of the degradation mechanism of polysaccharides, the end products of bacterial metabolism, the regulation of microbiota structure, and the impact of metabolites on the body is still limited. The mechanisms by which polysaccharides regulate bile acids require further research to be fully understood.
Author contributions
AW: Conceptualization, Writing – original draft, Writing – review & editing. WX: Writing – review & editing. JL: Writing – review & editing. YH: Writing – review & editing. LZ: Writing – review & editing. YL: Writing – review & editing.
Funding
The author(s) declare that financial support was received for the research, authorship, and/or publication of this article. This work was financially supported by the Key Scientific Research Fund Project of Department of Science and Technology of Sichuan Province (No. 2024YFFK0179) and the Scientific and Technological Projects for Distinguished Young Scholars of Sichuan Province (2024NSFJQ0058).
Conflict of interest
The authors declare that the research was conducted in the absence of any commercial or financial relationships that could be construed as a potential conflict of interest.
Publisher’s note
All claims expressed in this article are solely those of the authors and do not necessarily represent those of their affiliated organizations, or those of the publisher, the editors and the reviewers. Any product that may be evaluated in this article, or claim that may be made by its manufacturer, is not guaranteed or endorsed by the publisher.
References
1. Luo, Y, Peng, B, Wei, W, Tian, X, and Wu, Z. Antioxidant and anti-diabetic activities of polysaccharides from guava leaves. Molecules. (2019) 24:1343. doi: 10.3390/molecules24071343
2. Wu, TR, Lin, CS, Chang, CJ, Lin, TL, Martel, J, Ko, YF, et al. Gut commensal Parabacteroides goldsteinii plays a predominant role in the anti-obesity effects of polysaccharides isolated from Hirsutella sinensis. Gut. (2019) 68:248–62. doi: 10.1136/gutjnl-2017-315458
3. Yuan, R, Tao, X, Liang, S, Pan, Y, He, L, Sun, J, et al. Protective effect of acidic polysaccharide from Schisandra chinensis on acute ethanol-induced liver injury through reducing CYP2E1-dependent oxidative stress. Biomed Pharm. (2018) 99:537–42. doi: 10.1016/j.biopha.2018.01.079
4. Shan, C, Sun, B, Dalloul, RA, Zhai, Z, Sun, P, Li, M, et al. Effect of the oral administration of astragalus polysaccharides on jejunum mucosal immunity in chickens vaccinated against Newcastle disease. Microb Pathog. (2019) 135:103621. doi: 10.1016/j.micpath.2019.103621
5. Pak, U, Cheng, H, Liu, X, Wang, Y, Ho, C, Ri, H, et al. Structural characterization and anti-oxidation activity of pectic polysaccharides from Swertia mileensis. Int J Biol Macromol. (2023) 248:125896. doi: 10.1016/j.ijbiomac.2023.125896
6. Li, W, Hu, X, Wang, S, Jiao, Z, Sun, T, Liu, T, et al. Characterization and anti-tumor bioactivity of astragalus polysaccharides by immunomodulation. Int J Biol Macromol. (2020) 145:985–97. doi: 10.1016/j.ijbiomac.2019.09.189
7. Wong, CK, Yusta, B, Koehler, JA, Baggio, LL, McLean, BA, Matthews, D, et al. Divergent roles for the gut intraepithelial lymphocyte GLP-1R in control of metabolism, microbiota, and T cell-induced inflammation. Cell Metab. (2022) 34:1514–1531.e7. doi: 10.1016/j.cmet.2022.08.003
8. Shemtov, SJ, Emani, R, Bielska, O, Covarrubias, AJ, Verdin, E, Andersen, JK, et al. The intestinal immune system and gut barrier function in obesity and ageing. FEBS J. (2023) 290:4163–86. doi: 10.1111/febs.16558
9. Odenwald, MA, and Turner, JR. The intestinal epithelial barrier: a therapeutic target? Nat Rev Gastroenterol Hepatol. (2017) 14:9–21. doi: 10.1038/nrgastro.2016.169
10. Niewiem, M, and Grzybowska-Chlebowczyk, U. Intestinal barrier permeability in allergic diseases. Nutrients. (2022) 14:1893. doi: 10.3390/nu14091893
11. Li, J, Jia, N, Cui, M, Li, Y, Li, X, and Chu, X. The intestinal mucosal barrier - a key player in rheumatoid arthritis? Clin Anat. (2023) 36:977–85. doi: 10.1002/ca.24055
12. Jia, W, Li, Y, Cheung, KCP, and Zheng, X. Bile acid signaling in the regulation of whole body metabolic and immunological homeostasis. Sci China Life Sci. (2024) 67:865–78. doi: 10.1007/s11427-023-2353-0
13. McGlone, ER, and Bloom, SR. Bile acids and the metabolic syndrome. Ann Clin Biochem. (2019) 56:326–37. doi: 10.1177/0004563218817798
14. Grant, SM, and DeMorrow, S. Bile acid signaling in neurodegenerative and neurological disorders. Int J Mol Sci. (2020) 21:5982. doi: 10.3390/ijms21175982
15. Jiang, L, Zhang, H, Xiao, D, Wei, H, and Chen, Y. Farnesoid X receptor (FXR): structures and ligands. Comput Struct Biotechnol J. (2021) 19:2148–59. doi: 10.1016/j.csbj.2021.04.029
16. Alexander, JL, Wilson, ID, Teare, J, Marchesi, JR, Nicholson, JK, and Kinross, JM. Gut microbiota modulation of chemotherapy efficacy and toxicity. Nat Rev Gastroenterol Hepatol. (2017) 14:356–65. doi: 10.1038/nrgastro.2017.20
17. Cao, J, Zhang, H, Yang, Z, Zhao, J, and Ma, H. Effect of dehydroepiandrosterone on the immune response and gut microbiota in dextran sulfate sodium-induced colitis mice. Mol Immunol. (2020) 118:60–72. doi: 10.1016/j.molimm.2019.12.008
18. Zhu, Y, Wang, D, Zhou, S, and Zhou, T. Hypoglycemic effects of Gynura divaricata (L.) DC polysaccharide and action mechanisms via modulation of gut microbiota in diabetic mice. J Agric Food Chem. (2024) 72:9893–905. doi: 10.1021/acs.jafc.4c00626
19. Wang, W, Cheng, Z, Wang, Y, Dai, Y, Zhang, X, and Hu, S. Role of bile acids in bariatric surgery. Front Physiol. (2019) 10:374. doi: 10.3389/fphys.2019.00374
20. Rohr, MW, Narasimhulu, CA, Rudeski-Rohr, TA, and Parthasarathy, S. Negative effects of a high-fat diet on intestinal permeability: a review. Adv Nut. (2020) 11:77–91. doi: 10.1093/advances/nmz061
21. Hollingsworth, MA, and Swanson, BJ. Mucins in cancer: protection and control of the cell surface. Nat Rev Cancer. (2004) 4:45–60. doi: 10.1038/nrc1251
22. Andrianifahanana, M, Moniaux, N, and Batra, SK. Regulation of mucin expression: mechanistic aspects and implications for cancer and inflammatory diseases. Biochim Biophys Acta. (2006) 1765:189–222. doi: 10.1016/j.bbcan.2006.01.002
23. Pelaseyed, T, Bergström, JH, Gustafsson, JK, Ermund, A, Birchenough, GM, Schütte, A, et al. The mucus and mucins of the goblet cells and enterocytes provide the first defense line of the gastrointestinal tract and interact with the immune system. Immunol Rev. (2014) 260:8–20. doi: 10.1111/imr.12182
24. Sheng, YH, Hasnain, SZ, Florin, TH, and McGuckin, MA. Mucins in inflammatory bowel diseases and colorectal cancer. J Gastroenterol Hepatol. (2012) 27:28–38. doi: 10.1111/j.1440-1746.2011.06909.x
25. Johansson, ME, and Hansson, GC. Immunological aspects of intestinal mucus and mucins. Nat Rev Immunol. (2016) 16:639–49. doi: 10.1038/nri.2016.88
26. Laval, L, Martin, R, Natividad, JN, Chain, F, Miquel, S, Desclée de Maredsous, C, et al. Lactobacillus rhamnosus CNCM I-3690 and the commensal bacterium Faecalibacterium prausnitzii A2-165 exhibit similar protective effects to induced barrier hyper-permeability in mice. Gut Microbes. (2015) 6:1–9. doi: 10.4161/19490976.2014.990784
27. Ahl, D, Liu, H, Schreiber, O, Roos, S, Phillipson, M, and Holm, L. Lactobacillus reuteri increases mucus thickness and ameliorates dextran sulphate sodium-induced colitis in mice. Acta Physiol (Oxford). (2016) 217:300–10. doi: 10.1111/apha.12695
28. Garg, S, Singh, TP, and Malik, RK. In vivo implications of potential probiotic Lactobacillus reuteri LR6 on the gut and immunological parameters as an adjuvant against protein energy malnutrition. Probiot Antimicrob Prot. (2020) 12:517–34. doi: 10.1007/s12602-019-09563-4
29. van der Lugt, B, van Beek, AA, Aalvink, S, Meijer, B, Sovran, B, Vermeij, WP, et al. Akkermansia muciniphila ameliorates the age-related decline in colonic mucus thickness and attenuates immune activation in accelerated aging Ercc1 (−/Δ7) mice. Immun Ageing. (2019) 16:6. doi: 10.1186/s12979-019-0145-z
30. Quinn, RA, Melnik, AV, Vrbanac, A, Fu, T, Patras, KA, Christy, MP, et al. Global chemical effects of the microbiome include new bile-acid conjugations. Nature. (2020) 579:123–9. doi: 10.1038/s41586-020-2047-9
31. Chiang, JYL, and Ferrell, JM. Bile acid metabolism in liver pathobiology. Gene Expr. (2018) 18:71–87. doi: 10.3727/105221618X15156018385515
32. Chiang, JYL, and Ferrell, JM. Bile acid receptors FXR and TGR5 signaling in fatty liver diseases and therapy. Am J Physiol Gastrointest Liver Physiol. (2020) 318:G554–g573. doi: 10.1152/ajpgi.00223.2019
33. Choudhuri, S, and Klaassen, CD. Molecular regulation of bile acid homeostasis. Drug Metab Dispos. (2022) 50:425–55. doi: 10.1124/dmd.121.000643
34. Nguyen, JT, Riessen, R, Zhang, T, Kieffer, C, and Anakk, S. Deletion of intestinal SHP impairs short-term response to cholic acid challenge in male mice. Endocrinology. (2021) 162:63. doi: 10.1210/endocr/bqab063
35. Guo, C, Xie, S, Chi, Z, Zhang, J, Liu, Y, Zhang, L, et al. Bile acids control inflammation and metabolic disorder through inhibition of NLRP3 Inflammasome. Immunity. (2016) 45:802–16. doi: 10.1016/j.immuni.2016.09.008
36. Cai, J, Rimal, B, Jiang, C, Chiang, JYL, and Patterson, AD. Bile acid metabolism and signaling, the microbiota, and metabolic disease. Pharmacol Ther. (2022) 237:108238. doi: 10.1016/j.pharmthera.2022.108238
37. Ippagunta, SM, Kharitonenkov, A, Adams, AC, and Hillgartner, FB. Cholic acid supplementation of a high-fat obesogenic diet suppresses hepatic triacylglycerol accumulation in mice via a fibroblast growth factor 21-dependent mechanism. J Nutr. (2018) 148:510–7. doi: 10.1093/jn/nxy022
38. Cao, Y, Araki, M, Nakagawa, Y, Deisen, L, Lundsgaard, A, Kanta, JM, et al. Dietary medium-chain fatty acids reduce hepatic fat accumulation via activation of a CREBH-FGF21 axis. Molecular Metabol. (2024) 87:101991. doi: 10.1016/j.molmet.2024.101991
39. Uppal, H, Saini, SP, Moschetta, A, Mu, Y, Zhou, J, Gong, H, et al. Activation of LXRs prevents bile acid toxicity and cholestasis in female mice. Hepatology. (2007) 45:422–32. doi: 10.1002/hep.21494
40. Sundaram, SS, Bove, KE, Lovell, MA, and Sokol, RJ. Mechanisms of disease: inborn errors of bile acid synthesis. Nat Clin Pract Gastroenterol Hepatol. (2008) 5:456–68. doi: 10.1038/ncpgasthep1179
41. Sadeghi, L, Yekta, R, and Dehghan, G. The inhibitory effects of bile acids on catalytic and non-catalytic functions of acetylcholinesterase as a therapeutic target in Alzheimer's disease. Acta Neurobiol Exp. (2020) 80:108–16. doi: 10.21307/ane-2020-011
42. Li, C, Wang, X, Yan, J, Cheng, F, Ma, X, Chen, C, et al. Cholic acid protects in vitro neurovascular units against oxygen and glucose deprivation-induced injury through the BDNF-TrkB signaling pathway. Oxidative Med Cell Longev. (2020) 2020:1201624–14. doi: 10.1155/2020/1201624
43. Cai, J, Sun, L, and Gonzalez, FJ. Gut microbiota-derived bile acids in intestinal immunity, inflammation, and tumorigenesis. Cell Host Microbe. (2022) 30:289–300. doi: 10.1016/j.chom.2022.02.004
44. Wang, M, Osborn, LJ, Jain, S, Meng, X, Weakley, A, Yan, J, et al. Strain dropouts reveal interactions that govern the metabolic output of the gut microbiome. Cell. (2023) 186:2839–2852.e21. doi: 10.1016/j.cell.2023.05.037
45. Doden, HL, and Ridlon, JM. Microbial Hydroxysteroid dehydrogenases: from alpha to omega. Microorganisms. (2021) 9:469. doi: 10.3390/microorganisms9030469
46. Ridlon, JM, Kang, DJ, and Hylemon, PB. Bile salt biotransformations by human intestinal bacteria. J Lipid Res. (2006) 47:241–59. doi: 10.1194/jlr.R500013-JLR200
47. Adak, A, and Khan, MR. An insight into gut microbiota and its functionalities. Cell Mole Life Sci. (2019) 76:473–93. doi: 10.1007/s00018-018-2943-4
48. Holscher, HD . Dietary fiber and prebiotics and the gastrointestinal microbiota. Gut Microbes. (2017) 8:172–84. doi: 10.1080/19490976.2017.1290756
49. Zhang, N, Wang, Y, Kan, J, Wu, X, Zhang, X, Tang, S, et al. In vivo and in vitro anti-inflammatory effects of water-soluble polysaccharide from Arctium lappa. Int J Biol Macromol. (2019) 135:717–24. doi: 10.1016/j.ijbiomac.2019.05.171
50. Wang, Y, Zhang, N, Kan, J, Zhang, X, Wu, X, Sun, R, et al. Structural characterization of water-soluble polysaccharide from Arctium lappa and its effects on colitis mice. Carbohydr Polym. (2019) 213:89–99. doi: 10.1016/j.carbpol.2019.02.090
51. Yang, C, Xu, Z, Deng, Q, Huang, Q, Wang, X, and Huang, F. Beneficial effects of flaxseed polysaccharides on metabolic syndrome via gut microbiota in high-fat diet fed mice. Food Res Int. (2020) 131:108994. doi: 10.1016/j.foodres.2020.108994
52. Fu, Y, Yuan, Q, Lin, S, Liu, W, Du, G, Zhao, L, et al. Physicochemical characteristics and biological activities of polysaccharides from the leaves of different loquat (Eriobotrya japonica) cultivars. Int J Biol Macromol. (2019) 135:274–81. doi: 10.1016/j.ijbiomac.2019.05.157
53. Buckley, A, and Turner, JR. Cell biology of tight junction barrier regulation and mucosal disease. Cold Spring Harb Perspect Biol. (2018) 10:10. doi: 10.1101/cshperspect.a029314
54. Suzuki, T . Regulation of the intestinal barrier by nutrients: the role of tight junctions. Anim Sci J. (2020) 91:e13357. doi: 10.1111/asj.13357
55. Liang, J, Li, H, Chen, J, He, L, Du, X, Zhou, L, et al. Dendrobium officinale polysaccharides alleviate colon tumorigenesis via restoring intestinal barrier function and enhancing anti-tumor immune response. Pharmacol Res. (2019) 148:104417. doi: 10.1016/j.phrs.2019.104417
56. Liang, J, Chen, S, Chen, J, Lin, J, Xiong, Q, Yang, Y, et al. Therapeutic roles of polysaccharides from Dendrobium Officinaleon colitis and its underlying mechanisms. Carbohydr Polym. (2018) 185:159–68. doi: 10.1016/j.carbpol.2018.01.013
57. Bai, Y, Huang, F, Zhang, R, Dong, L, Jia, X, Liu, L, et al. Longan pulp polysaccharides relieve intestinal injury in vivo and in vitro by promoting tight junction expression. Carbohydr Polym. (2020) 229:115475. doi: 10.1016/j.carbpol.2019.115475
58. Cheng, Y, Ma, H, Mao, X, Zhu, X, Ge, B, Li, R, et al. Regulation of Spirulina polysaccharide on intestinal enzyme activity and microbial Flora in constipation mice. Acta Laser Biool Sinica. (2019) 28:563–70.
59. Chen, Y, Wan, X, Wu, D, Ouyang, Y, Gao, L, Chen, Z, et al. Characterization of the structure and analysis of the anti-oxidant effect of microalga Spirulina platensis polysaccharide on Caenorhabditis elegans mediated by modulating microRNAs and gut microbiota. Int J Biol Macromol. (2020) 163:2295–305. doi: 10.1016/j.ijbiomac.2020.09.041
60. Long, C, He, L, Guo, Y, Liu, Y, Yu, Z, Ren, T, et al. Effects of Dendrobium candidum polysaccharide on immunity, intestinal microbiota and enzyme activity in mice with spleen deficiency constipation. Nat Prod Res Dev. (2017) 29:1020–4.
61. Chen, WH, Wu, JJ, Li, XF, Lu, JM, Wu, W, Sun, YQ, et al. Isolation, structural properties, bioactivities of polysaccharides from Dendrobium officinale Kimura et. Migo: a review. Int J Biol Macromol. (2021) 184:1000–13. doi: 10.1016/j.ijbiomac.2021.06.156
62. Yuan, Q, He, Y, Xiang, PY, Huang, YJ, Cao, ZW, Shen, SW, et al. Influences of different drying methods on the structural characteristics and multiple bioactivities of polysaccharides from okra (Abelmoschus esculentus). Int J Biol Macromol. (2020) 147:1053–63. doi: 10.1016/j.ijbiomac.2019.10.073
63. Long, H, Gu, X, Zhou, N, Zhu, Z, Wang, C, Liu, X, et al. Physicochemical characterization and bile acid-binding capacity of water-extract polysaccharides fractionated by stepwise ethanol precipitation from Caulerpa lentillifera. Int J Biol Macromol. (2020) 150:654–61. doi: 10.1016/j.ijbiomac.2020.02.121
64. Gao, J, Lin, L, Sun, B, and Zhao, M. Comparison study on polysaccharide fractions from Laminaria japonica: structural characterization and bile acid binding capacity. J Agric Food Chem. (2017) 65:9790–8. doi: 10.1021/acs.jafc.7b04033
65. Yan, JK, Wu, LX, Qiao, ZR, Cai, WD, and Ma, H. Effect of different drying methods on the product quality and bioactive polysaccharides of bitter gourd (Momordica charantia L.) slices. Food Chem. (2019) 271:588–96. doi: 10.1016/j.foodchem.2018.08.012
66. Deng, W, Yang, X, Zhu, Y, Yu, J, and Xu, X. Structural characterization and hypolipidemic activities of purified stigma maydis polysaccharides. Food Sci Nutr. (2019) 7:2674–83. doi: 10.1002/fsn3.1123
67. Fang, W, Zhang, L, Meng, Q, Wu, W, Lee, YK, Xie, J, et al. Effects of dietary pectin on the profile and transport of intestinal bile acids in young pigs. J Anim Sci. (2018) 96:4743–54. doi: 10.1093/jas/sky327
68. Zhu, R, Hou, Y, Sun, Y, Li, T, Fan, J, Chen, G, et al. Pectin Penta-Oligogalacturonide suppresses intestinal bile acids absorption and downregulates the FXR-FGF15 Axis in high-cholesterol fed mice. Lipids. (2017) 52:489–98. doi: 10.1007/s11745-017-4258-x
69. Lu, H, Gui, Y, Guo, T, Wang, Q, and Liu, X. Effect of the particle size of cellulose from sweet potato residues on lipid metabolism and cecal conditions in ovariectomized rats. Food Funct. (2015) 6:1185–93. doi: 10.1039/C4FO00799A
70. Guo, WL, Deng, JC, Pan, YY, Xu, JX, Hong, JL, Shi, FF, et al. Hypoglycemic and hypolipidemic activities of Grifola frondosa polysaccharides and their relationships with the modulation of intestinal microflora in diabetic mice induced by high-fat diet and streptozotocin. Int J Biol Macromol. (2020) 153:1231–40. doi: 10.1016/j.ijbiomac.2019.10.253
71. Huang, S, Pang, D, Li, X, You, L, Zhao, Z, Cheung, PC, et al. A sulfated polysaccharide from Gracilaria lemaneiformis regulates cholesterol and bile acid metabolism in high-fat diet mice. Food Funct. (2019) 10:3224–36. doi: 10.1039/C9FO00263D
72. Wan, XZ, Ai, C, Chen, YH, Gao, XX, Zhong, RT, Liu, B, et al. Physicochemical characterization of a polysaccharide from green microalga Chlorella pyrenoidosa and its Hypolipidemic activity via gut microbiota regulation in rats. J Agric Food Chem. (2020) 68:1186–97. doi: 10.1021/acs.jafc.9b06282
73. Catry, E, Bindels, LB, Tailleux, A, Lestavel, S, Neyrinck, AM, Goossens, JF, et al. Targeting the gut microbiota with inulin-type fructans: preclinical demonstration of a novel approach in the management of endothelial dysfunction. Gut. (2018) 67:271–83. doi: 10.1136/gutjnl-2016-313316
Keywords: polysaccharides, bile acids, metabolic diseases, inflammation, gut microbiota, intestinal function
Citation: Wang A, Xiong W, Li J, Hu Y, Zou L and Liu Y (2024) The regulatory effects of bioactive polysaccharides on intestinal function and bile acids: chemical structures, bioactivities, and mechanisms. Front. Nutr. 11:1495993. doi: 10.3389/fnut.2024.1495993
Edited by:
Jian-lin Wu, Macau University of Science and Technology, Macao, SAR ChinaReviewed by:
Xi Liang, Qingdao University, ChinaQi Wang, Yibin Vocational and Technical College, China
Copyright © 2024 Wang, Xiong, Li, Hu, Zou and Liu. This is an open-access article distributed under the terms of the Creative Commons Attribution License (CC BY). The use, distribution or reproduction in other forums is permitted, provided the original author(s) and the copyright owner(s) are credited and that the original publication in this journal is cited, in accordance with accepted academic practice. No use, distribution or reproduction is permitted which does not comply with these terms.
*Correspondence: Ying Liu, eWluZ2xpdTIwMjExMkAxNjMuY29t
†These authors have contributed equally to this work