- 1Department of Nutrition Science, College of Health and Human Sciences, Purdue University, West Lafayette, IN, United States
- 2Department of Medicine, Johns Hopkins School of Medicine, Baltimore, MD, United States
- 3Department of Pharmacology and Molecular Sciences, Johns Hopkins School of Medicine, Baltimore, MD, United States
- 4Department of Psychiatry, Johns Hopkins School of Medicine, Baltimore, MD, United States
- 5Institute of Medicine, University of Maine, Orono, ME, United States
- 6Department of Pediatrics, UMass Chan Medical School, Worcester, MA, United States
- 7Center on Aging and the Life Course, Purdue University, West Lafayette, IN, United States
Neuroinflammation in response to environmental stressors is an important common pathway in a number of neurological and psychiatric disorders. Responses to immune-mediated stress can lead to epigenetic changes and the development of neuropsychiatric disorders. Isothiocyanates (ITC) have shown promise in combating oxidative stress and inflammation in the nervous system as well as organ systems. While sulforaphane from broccoli is the most widely studied ITC for biomedical applications, ITC and their precursor glucosinolates are found in many species of cruciferous and other vegetables including moringa. In this review, we examine both clinical and pre-clinical studies of ITC on the amelioration of neuropsychiatric disorders (neurodevelopmental, neurodegenerative, and other) from 2018 to the present, including documentation of protocols for several ongoing clinical studies. During this time, there have been 16 clinical studies (9 randomized controlled trials), most of which reported on the effect of sulforaphane on autism spectrum disorder and schizophrenia. We also review over 80 preclinical studies examining ITC treatment of brain-related dysfunctions and disorders. The evidence to date reveals ITC have great potential for treating these conditions with minimal toxicity. The authors call for well-designed clinical trials to further the translation of these potent phytochemicals into therapeutic practice.
1 Introduction
Isothiocyanates (ITC) found in cruciferous and related vegetables (e.g., broccoli and cabbage), exhibit diverse properties such as antibacterial, antifungal, antioxidant, and cytoprotective effects. They are produced as a result of enzymatic activity of myrosinase on glucosinolates (ITC precursors) present in plant cell vacuoles (Figure 1). Myrosinase is compartmentalized in cells of the same plant tissues and released upon cell lysis (e.g., chewing or wounding) (1). Additionally, microbiota in the gastrointestinal tracts of animals also contribute to this conversion. However, intraindividual differences in microbial conversion vary greatly, resulting in highly variable bioavailability (2). The formation of isothiocyanates from glucosinolates is also influenced by several other factors (3), including pH value, ferrous ions, and specifier proteins such as epithiospecifier protein (ESP), nitrile-specifier protein (NSP), and thiocyanate-forming protein (TFP). ESP, NFP and TFP can lead to the production of epithionitriles or nitriles upon the hydrolysis of glucosinolates in plant tissues (4). Additionally, glucosinolates undergo enzymatic conversion to amines (3, 5). The incomplete or variable conversion of glucosinolates to isothiocyanates may account for differing effects observed in various studies. Additional factors include genetic variability in the glutathione S-transferase M1 gene that could affect the metabolism of SF (6).
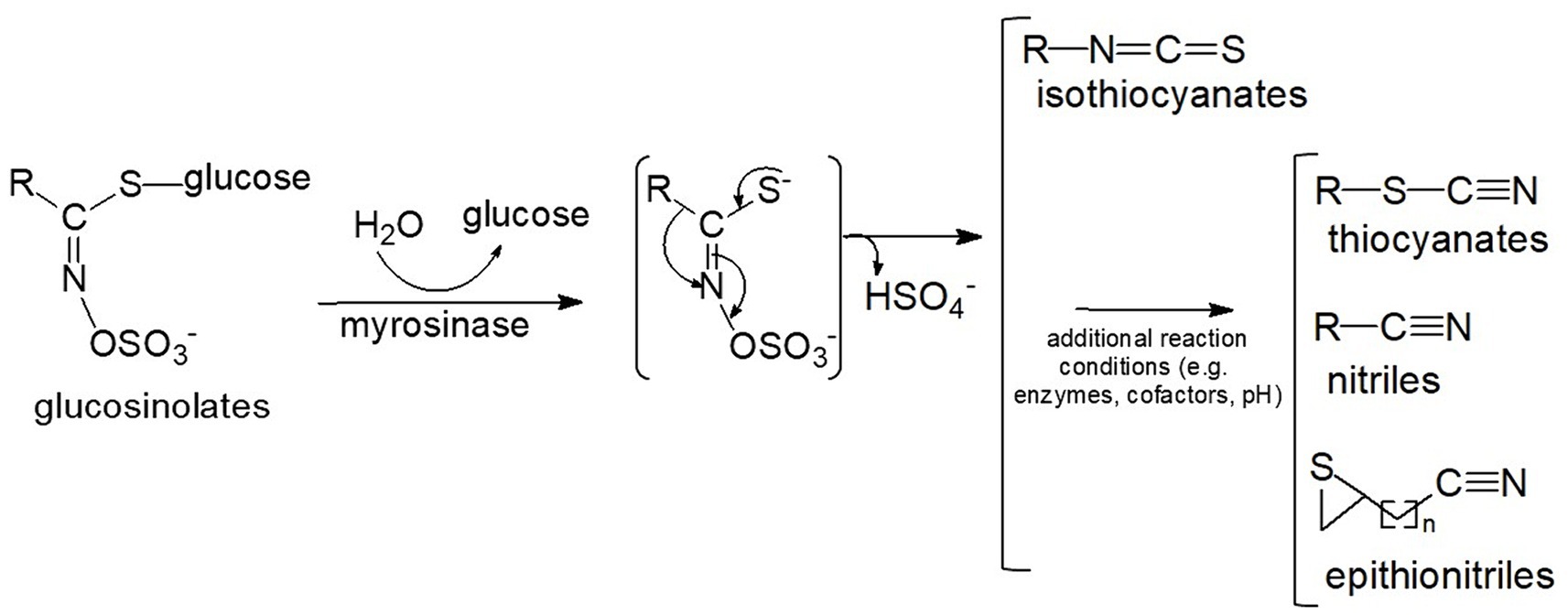
Figure 1. Conversion of glucosinolates to isothiocyanates and other reaction products. The myrosinase reaction produces an unstable intermediate which then non-enzymatically rearranges to isothiocyanates, or, in the presence of additional enzymes, cofactors and favorable conditions (e.g., low pH) can form a variety of alternative end-products including thiocyanates, nitriles, and epithionitriles (8).
Sulforaphane (SF), derived from its glucosinolate precursor, glucoraphanin, is produced in greatest relative abundance in the cruciferous species Brassica oleracea var. italica (broccoli) as well as in a rangeland weed (Cardaria draba), some red cabbage (B. oleracea var. capitata) and red kale (B. oleracea var. capitata) sprouts. Sulforaphane stands out as a highly studied and promising therapeutic agent currently undergoing preclinical and clinical evaluation of a multitude of diseases and disorders (7). Apart from cruciferous vegetables, which are most familiar to human consumers, 15 other plant families produce over 120 different glucosinolates (8–10). A noteworthy example includes the tropical tree vegetable, Moringa oleifera, which is gaining wide recognition for its exceptional nutrient profile along with its cytoprotective properties; these properties are attributable in part to its primary ITC, moringin, derived from the glucosinolate glucomoringin (11). While there are documented toxic effects associated with the overconsumption of cruciferous and related vegetables, such as cabbages rich in indoles and thiocyanates and the presence of plant-derived toxins, they have been safely consumed by many people across the globe as part of a well-balanced diet (12).
Chronic oxidative stress, along with neuroinflammation, plays a central role in the pathogenesis of numerous neuropsychiatric disorders. For instance, in several clinical and preclinical studies, SF counteracts several molecular abnormalities associated with autism spectrum disorder (ASD) that address reduced antioxidant capacity, enhanced oxidative stress, deficient glutathione synthesis, mitochondrial dysfunction, increased lipid peroxidation, and neuroinflammation (2). SF’s ability to readily cross the blood–brain barrier likely enables it to exert its clinical neurological effects (1).
A key mechanism of SF involves the activation of the nuclear transcription factor Nrf2 (nuclear factor erythroid 2–related factor 2), which orchestrates a suite of cytoprotective responses. SF binds with Keap1 (Kelch-like ECH-associated protein 1), causing its dissociation from Nrf2. Nrf2 is then free to translocate into the nucleus and induce the transcription of phase 2 detoxification enzyme genes (2). SF also induces anti-inflammatory, heat shock-response (HSR)-inducing, and histone deacetylase (HDAC) inhibiting responses, contributing to its multifaceted cytoprotective effects (2, 13).
Evidence for the effects of ITC from plants other than broccoli, such as Moringa oleifera and Raphanus sativus (radish), on chronic diseases including cancer, neurologic disorders, and cardiovascular diseases has been reviewed (14–25). We seek herein to review the effects of ITC and ITC-rich plants on brain-related disorders. We include reports on both clinical and pre-clinical studies demonstrating the potential of ITC to ameliorate these disorders. Our focus is on studies published from 2018 to 2023, and serves as an update to our previous review conducted in 2018, though we do reference some studies prior to this period to provide context (26).
2 Neurodevelopmental disorders
Recent clinical and preclinical studies have explored interventions in neurodevelopmental conditions using ITC. These studies mark the initial attempts to address disorders with disparate causes and pathophysiology through targeting potentially common pathways with these phytochemicals.
2.1 Autism spectrum disorder
Autism spectrum disorder (ASD) is a complex and heterogeneous neurodevelopmental disorder characterized by deficits in social communication and restricted, repetitive behaviors (27). The current prevalence of ASD among 8-year-old children in the US is 1 in 36 (28), with over 600,000 new cases diagnosed annually worldwide (29). Currently, there are no FDA-approved pharmacological treatments for the core features of ASD. In this review, we report 9 clinical studies from 2014 to 2023, including 6 randomized controlled trials (RCTs), 1 non-randomized single arm clinical trial, and 1 case study related to the benefits of ITC on ASD (Table 1). We also report findings of ITC treatment in five preclinical studies, including 4 in vivo and 1 in vitro models of ASD.
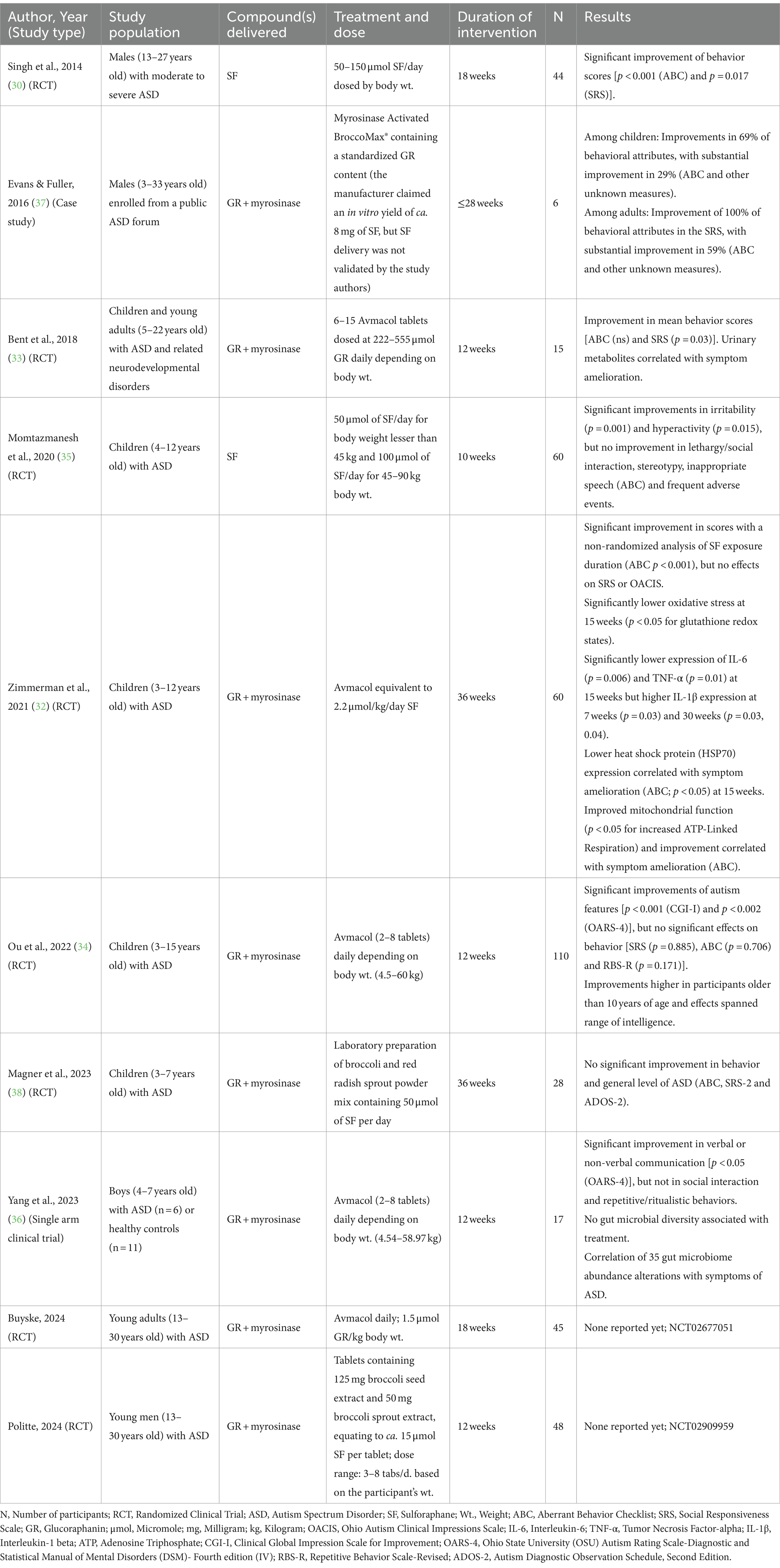
Table 1. Clinical studies reported the effects of sulforaphane or glucoraphanin treatments on behavioral and health outcomes in individuals with ASD.
2.1.1 Clinical studies
The first randomized controlled trial conducted in 2014 provided compelling evidence of the therapeutic potential of SF in the management of core features of ASD (NCT01474993) (30). Over an 18-week period, SF treatment (2.2 μmol/kg/day SF) significantly improved social interaction, reduced aberrant behavior, and enhanced verbal communication in autistic males aged 13–27 years. These effects returned to near baseline after a 4-week washout period. Further, responders who continued to consume dietary supplements containing glucoraphanin (GR), the glucosinolate precursor of SF reported sustained benefits for 3 years (31).
Since this first RCT, several clinical studies have attempted to replicate these findings. While the methodology has varied, most studies reported positive results though improvements were not as profound as in the initial study (32–37). The same group conducted another RCT in children aged 3–13 years, using GR plus myrosinase (2.2 μmol/kg/day SF), which showed mixed results (32). While clinical ratings did not significantly differ between the intervention and placebo groups using the clinician-rated Ohio Autism Clinical Impressions Scale (OACIS), there were significant improvements in caregiver-reported measures of aberrant behaviors (ABC-2). In addition, reductions in inflammatory biomarkers (TNF-α, IL-6, IL-1β) and improvements in glutathione redox status and mitochondrial function were apparent in the intervention group compared to the placebo group. Another study tested an adjuvant treatment of SF (50 or 100 μmol of SF/day depending on body weight) with risperidone and found children in the treatment group exhibited greater improvements in irritability and hyperactivity compared to the placebo group (35). A single-arm clinical trial found minimal amelioration in both ASD-related behaviors and fecal microbiome diversity with 2–8 GR plus myrosinase tablets/day depending on body weight (36), and one study found no difference in behavioral measures between treatment and placebo groups with 50 μmol/day (38).
In evaluating the mixed findings, we observed that the two studies that showed greatest improvements delivered SF itself (the plant-derived ITC, not a synthetic version, in a supplement matrix that represented some subset of the original plant extract with its attendant phytochemical diversity) while all other studies relied on the combination of a plant extract rich in GR and co-delivering active plant-derived myrosinase (30, 35). While one of these studies used SF as an adjunctive therapy, we posit that the method of delivery, SF, may be the key to minimizing differences in bioavailability and, consequently, achieving significant behavioral improvements. In the study that did not show any difference in behavior (30), SF (45.1 μmol) may have been inactivated due to high temperatures involved in its preparation. The pharmacokinetics of ITC production from glucosinolates in the gastrointestinal system, vs. delivery of pre-formed SF, are not fully understood, but what is currently known gives credence to this conjecture. The metabolic conversion of GR to SF may vary both developmentally and between individuals. At this writing (spring 2024) there are two ongoing studies using GR + myrosinase that are yet to report their findings on treatment in individuals with ASD (NCT02677051, NCT02909959; Table 1).
2.1.2 Pre-clinical studies
Interventions including oral SF-rich and glucoraphanin-rich preparations, as well as those of the plant maca (Lepidium meyenii), have shown promising outcomes in improving autism-associated outcomes. For example, SF treatment (282 μmol/kg/day for 7 days) reduced self-grooming/marble-burying behavior, improved social interaction, decreased T helper 17 (Th17) immune responses and oxidative stress, and increased enzymatic antioxidant defenses in an autism mouse model (39). In another study of a maternal immune activation (MIA) mouse model, GR intake (0.1% GR food pellets) of dams during pregnancy and lactation prevented cognitive and social interaction deficits in juvenile offspring and cognitive deficits in adult offspring (40). Moreover, oral administration of supplements containing GR and active myrosinase (2.2 μmol SF/kg/day for 14 days) increased mRNA levels of cytoprotective enzymes and heat shock proteins while decreasing mRNA levels of pro-inflammatory markers in peripheral blood mononuclear cells (PBMC) of patients with ASD (41). A study focusing on Nrf2 activation demonstrated SF (5 μmol incubated overnight) ameliorated inflammation and nitrative stress in ASD monocytes induced by LPS (lipopolysaccharide) (42). Finally, supplementation with maca (5 g/kg/day for 14 days)—an uncommon vegetable in the US, but one with high levels of glucosinolates—improved social deficits by upregulating oxytocinergic pathways in a valproic acid mouse model of ASD (43).
2.2 Schizophrenia
Schizophrenia is a progressive neurodevelopmental disorder with disruptions in cognitive functions, perceptual understanding, and executive function. Antipsychotic drugs are commonly used in the management of schizophrenia and can have long-term side effects. There have been four clinical studies—1 of which was an RCT—and 2 in vivo preclinical studies on schizophrenia and ITC treatment. Early intervention efforts for prodromal symptoms are being explored in patients with schizophrenia, including the incorporation of ITC like SF in the diet (44).
2.2.1 Clinical studies
Several clinical studies have reported on the effects of SF treatment in schizophrenia (Table 2). In an open-phase trial, participants with schizophrenia (N = 7) displayed significantly enhanced accuracy in the One Card Learning Task after 8 weeks of GR (68.5 μmol/day) administration (45). Similarly, another open-label study in this patient population (N = 45) demonstrated that a higher dose of GR + myrosinase (507.4 μmol SF/day) for 24 weeks resulted in significantly increased superoxide dismutase activity and HsCRP levels and a significant decrease in Positive and Negative Syndrome Scale (PANSS) negative subscale scores (46). Investigators aiming to study schizophrenia first conducted a study in healthy volunteers (N = 9) and demonstrated elevated blood glutathione levels after a week of daily SF (100 μmol/day for 7 days) consumption (47). The investigators also measured brain metabolites through magnetic resonance spectroscopy (MRS) scanning and reported a significant increase in glutathione concentration in the hippocampus. However, a randomized controlled trial (N = 58) found no differences between GR + myrosinase (100 μmol SF per day for 16 weeks) treatment and placebo groups in PANSS scores and cognitive functioning among individuals with schizophrenia (48). An ongoing RCT (N = 480) in China is exploring add-on treatments of minocycline (200 mg) and GR (68.5 μmol SF/day for 8 weeks) with antipsychotics to patients with schizophrenia who were non-responsive to the antipsychotic drugs alone (NCT03451734) (49).
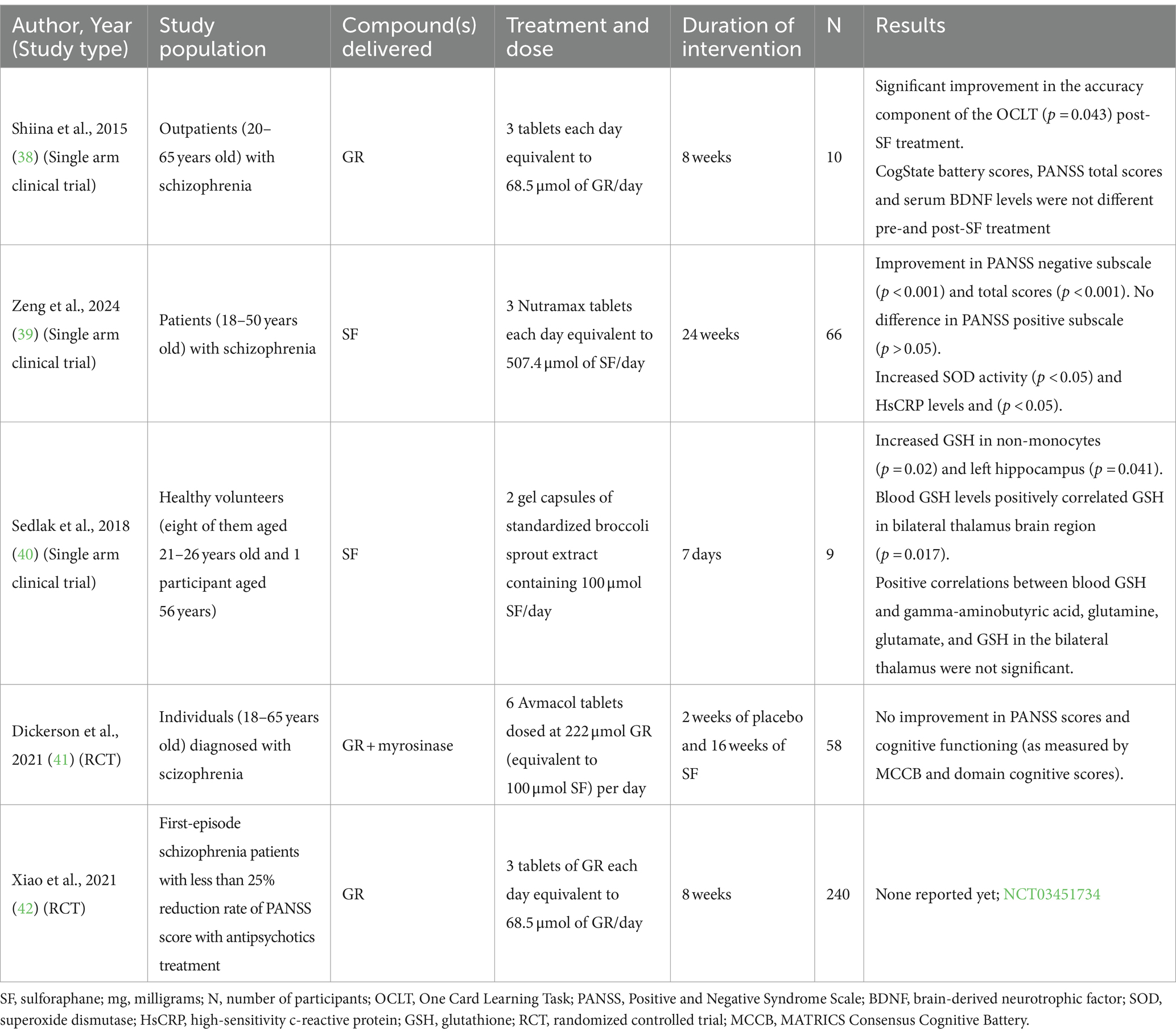
Table 2. Clinical studies reported the effects of sulforaphane or glucoraphanin treatments on behavioral and health outcomes in individuals with schizophrenia.
2.2.2 Pre-clinical studies
Preclinical studies have provided further insights into the potential benefits of SF in schizophrenia. For example, offspring from pregnant mouse dams subjected to maternal immune activation, fed with a GR-rich diet (~2.3 μmol/kg), showed protection from cognitive defects (50). Similarly, mice repeatedly exposed to phencyclidine demonstrated attenuated cognitive deficits when pretreated with a GR-containing diet (~2.3 μmol/kg) (51).
2.3 Cerebral palsy
Cerebral palsy is a neurological disorder characterized by difficulties in movement, balance, and posture due to weakness or impairment in muscle control and is the most common motor disability in childhood. It is usually associated with pre-or perinatal brain injury or maldevelopment. One in vivo study of cerebral palsy with SF treatment has been published. In this preclinical study, rat offspring were exposed to prolonged intrauterine ischemia in utero. Offspring born to dams given a diet supplemented with broccoli sprouts (200 mg/day from the beginning of the third trimester to postnatal day 14) had a reduced likelihood of neurocognitive impairment compared to those born to dams that did not receive the treatment (52).
2.4 Fetal alcohol syndrome disorders
Fetal alcohol syndrome disorder (FASD) encompasses a spectrum of conditions resulting from prenatal alcohol exposure, often presenting as a combination of physical, behavioral, and learning difficulties. We have identified two in vivo models and one in vitro experiment examining the effects of SF on mechanisms involved in FASD, including cell death and oxidative stress. Mouse embryos exposed to ethanol in vivo and treated with SF (1 μM for 24 h) displayed reduced apoptosis in neural crest cells compared to untreated exposed mice (53). The reported preventive mechanism of SF against ethanol-induced apoptosis was inhibition of HDAC and the subsequent elevation of histone acetylation in the cells (53). Inhibition of HDAC causes histone hyperacetylation, resulting in neuroprotective effects (54). Furthermore, pharmacological blockade of catalase or Nrf2 activity intensified ethanol intoxication in mice, and 28.2 μM /kg/day SF (a potent Nrf2 inducer) treatment for 5 days, attenuated these effects (55), leading the study’s authors to suggest that Nrf2 activation may serve as a potential therapeutic strategy for preventing acute alcoholism by regulating catalase-mediated ethanol oxidation.
2.5 Fetal hypoxic–ischemic brain injury
Fetal hypoxic–ischemic encephalopathy (HIE) due to placental insufficiency is characterized by low blood and oxygen supply to the fetus. This can result in both intrauterine growth restriction and brain injury. Despite the severity of this condition, research on potential interventions remains limited. One in vitro study highlighted the potential efficacy of SF in mitigating the effects of placental insufficiency on neuronal cells (56). In this study, the dose response of SF (0–200 μM) within different brain cell types was examined. A significant protective effect of SF was documented at a concentration of 2.5 μM in astrocytes and co-cultures of multiple cell types but not in neurons alone. Toxicity was not observed until concentrations reached ≥100 μM in astrocytes and ≥ 50 μM in co-cultures under oxygen/glucose deprived conditions.
3 Neurodegenerative disorders
Since oxidative stress and inflammation play a large role in the pathophysiology of neurodegenerative disorders, the ITC SF and moringin, among other Nrf2 inducers, have garnered attention for their potential to alleviate various symptoms. These disorders include Alzheimer’s disease, Parkinson’s disease, amyotrophic lateral sclerosis, Huntington’s disease, and multiple sclerosis, among others (57). While there are only a couple of clinical studies examining the effects of ITC in neurodegenerative disorders, several preclinical studies support the benefits of these phytochemicals (58).
3.1 Alzheimer’s disease
Alzheimer’s Disease (AD), the most common form of dementia, is estimated to affect 6.93 million people in the U.S. as of 2024 and is projected to reach 13.85 million by 2060 (59). ITC has shown promise in AD by preventing tau and amyloid-beta (Aβ) accumulation, two notable markers of AD. Abnormal tau builds up in memory-related brain regions, while Aβ forms plaques between neurons (60). To date, there have been 15 in vivo, 6 in vitro, and 1 in silico AD models studied with ITC.
SF has shown promising effects in mitigating AD pathology in several preclinical studies. Both mouse primary cortical neurons (10 or 20 μM for 3 or 6 h) and a triple-transgenic AD mouse model treated with SF (56.4 or 282 μmol/kg/day for 6 days/week for 8 weeks) exhibited enhanced brain-derived neurotropic factor (BDNF) expression, crucial for neuronal survival and synaptic plasticity, suggesting a potential epigenetic mechanism for SF’s neuroprotective effects (61). Additionally, SF administered at 28.2 μmol/kg/day for 4 months (62), 141 μmol/kg/day for 80 days (63), or 141 μmol/kg/day for 5 months (64) ameliorated spatial cognitive impairment, reduced Aβ plaques, and regulated specific HDACs, resulting in diminished plaque burden in other AD mouse models (62–64). Changes in memory consolidation and spatial learning were also induced by SF (5 μM) in adult mice (65). A mechanistic study revealed that SF (56.4 or 282 μmol/kg/day for 6 days/week for 8 weeks) cleared Aβ and tau accumulation by increasing levels of a heat shock protein (HSP70) and co-chaperone (CHIP), and mitigated memory deficits in a triple transgenic mouse model of AD (66). SF (141 or 282 μmol/kg/day for 4 months) has also been shown to protect against Aβ-induced neurotoxicity in primary mouse neurons and suppress tau protein phosphorylation in a transgenic AD mouse model (67). Moreover, the prophylactic mechanisms of SF (141 mg/kg/day for 2 weeks) have been shown to protect against LPS-induced prefrontal cortex-related recognition memory impairment in mice by improving recognition memory and reducing neuroinflammation, oxidative stress, neurodegeneration, Aβ accumulation, and microglial activation (68).
Molecular mechanisms underlying the neuroprotective effects of ITC have also been studied in vitro. SF (1.25 or 2.5 μM treated for 48 h) upregulated Nrf2 expression and facilitated Nrf2 nuclear translocation by reducing DNA methylation of the Nrf2 promoter in a cellular AD model (69). Furthermore, hydrogen sulfide (H2S)-releasing hybrids combining rivastigmine with either SF or its metabolite erucin [4-(methylthio) butyl ITC; 5 μmol treated for 24 h] exhibited protective effects against LPS-induced microglia inflammation and increased the expression of antioxidant proteins like glutathione in human neuronal (SH-SY5Y) cells (70). Examining yet another mechanism, SF (0.03 to 3 μM for 60 min) demonstrated six-fold greater potency against β-site amyloid precursor protein cleaving enzyme 1 (BACE1), a rate-limiting enzyme in Aβ production, as compared to resveratrol and quercetin in silico (71). Other preclinical investigations demonstrated positive benefits of SF on AD by attenuating Aβ oligomers-mediated reduction of phagocytic activity (5 μM for 24 h) (72), reducing streptozotocin-induced cognitive deficits (141 or 282 μmol/kg/day for 6 weeks) (73), decreasing neuroinflammation and inhibiting tau phosphorylation (1 or 2 μM for 1 h and stimulated with LPS for 23 h) (73), and reducing Aβ production (226 μmol/kg three times a week for 4 weeks) (74) in in vivo and in vitro AD models.
In addition to SF, other ITC and ITC-rich plants have also demonstrated consistent benefits in ameliorating AD-related pathology. Rats supplemented with Moringa peregrina (150 mg/kg/day for 2 months), rich in the ITC moringin, exhibited significant enhancement in short-term and long-term memories, along with increased BDNF, glutathione, and glutathione peroxidase (GPx), and decreased oxidized glutathione in the hippocampus (75). Leaf extracts of the widely consumed Moringa oleifera (400 mg/kg/day for 4 months) improved AD-related pathology, reduced Aβ burden, and enhanced synaptic plasticity in mice (76). Similar findings were observed in rats along with reduced homocysteine-induced tau hyperphosphorylation with a Moringa oleifera dose of 200 or 400 mg/kg/day for 14 days (77). Additionally, supplementation with Moringa oleifera (1, 5% or 10% in diet for 7 or 14 days) in mice mitigated scopolamine-induced spatial memory deficits, restored cholinergic transmission, and maintained neuronal integrity (78). Pretreatment with moringin conjugated with α-cyclodextrin (0.5 μM for 24 h) in an AD cell model reduced gene expression related to autophagy and mitophagy (79). Finally, a study of mice treated with 6-MSITC treatment [6-(methylsulfinyl) hexyl isothiocyanate; 24.3 μmol/kg/day for 10 days] from Wasabia japonica, or wasabi, demonstrated attenuated neuroinflammation, memory impairments, inhibited apoptosis, and oxidative stress (80).
3.2 Parkinson’s disease
Parkinson’s disease (PD), characterized by dopaminergic neuron loss, involves neuroinflammation, oxidative stress, mitochondrial dysfunction, and protein aggregation. One clinical study has been reported on the effects of sulforaphane on PD, while preclinical investigations examining the effects of ITCs on PD include 10 in vivo and three in vitro studies.
3.2.1 Clinical studies
A self-experimented clinical study was conducted in individuals diagnosed with Parkinson’s disease using broccoli seed tea (25–45 μmol SF/day for at least 4 weeks, with weekly administration in the first week and up to twice per week thereafter) rich in SF intended to induce Nrf2 (81). Although statistical significance is uncertain as p-values were not provided, the findings revealed the tea reduced non-motor symptoms, including fatigue, constipation, and urinary urgency, while not affecting motor symptoms (Table 3). Currently, there is an ongoing phase 2 placebo-controlled randomized clinical trial (NCT05084365) examining the effect of SF on PD in 100 participants.
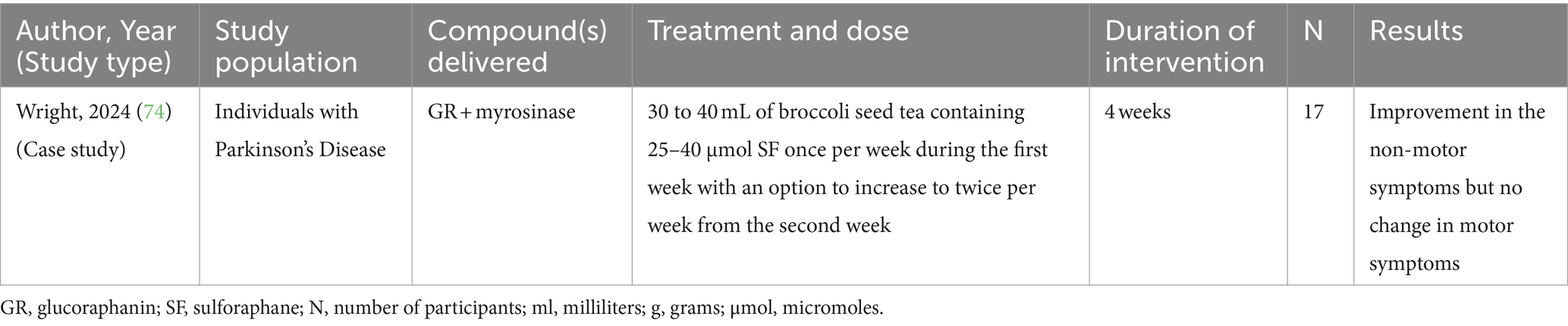
Table 3. A clinical study reported the effects of glucoraphanin + myrosinase compound on non-motor symptoms in individuals with PD.
3.2.2 Preclinical studies
Several animal studies have also outlined the neuroprotective effects of SF in PD. One study demonstrated that SF treatment (0.05 μM for 24 h) reduced dopamine-induced cell death and restored mitochondrial membrane potential in a knockout mouse model (82). In another PD mouse model, SF (282 μmol/kg once in 2 days for 60 days) inhibited rotenone-induced deficiencies in locomotor activity and dopaminergic neuronal loss (83). Further, in MPTP (1-methyl-4-phenyl-1,2,3,6-tetrahydropyridine)-treated mice, SF treatment (56.4 μmol/kg/day for 10 days) attenuated dopaminergic neurotoxicity by activating BDNF and suppressing methyl CpG-binding protein 2 (MeCP2) (84) and activated Nrf2 (1 μM SF) (85). Sustained activation of this transcription factor led to a reduction of abnormal α-synuclein expression (1 μmol SF) and decrease in dopaminergic neuron degeneration in the substantia nigra pars compacta (0.1% GR for 30 days) (78). Further, GR intake (0.1% GR diet for 28 days) protected against the reduction in dopamine transporter density in mice treated with MPTP, indicating its potential in preserving dopaminergic function (86). SF and erucin (from Eruca sativa or arugula) at 5 μmol/kg for 4 weeks exhibited neuroprotective effects by protecting against neuronal death and increasing glutathione and Nrf2 levels in a 6-hyzdroxydopamine (6-OHDA) PD mouse model (87). Finally, two synthetic ITC protected dopaminergic neurons and prevented motor deficits associated with PD in mice (88, 89).
Three in vitro studies were also identified, providing mechanistic insights into the neuroprotective effects of SF. The first showed that SF treatment (56.4 μmol/kg/day for 10 days) upregulated Nrf2 and BDNF while downregulating MeCP2 in SH-SY5Y cells (84). A second study reported that compared to erucin, SF (5 μmol/kg for 4 weeks) exhibited greater Nrf2 activation, glutathione levels, and resistance to 6-OHDA-induced apoptosis in SH-SY5Y cells (87). The third study revealed that Nrf2 activation by SF (1 μmol SF) suppressed CCAAT/enhancer-binding protein β (C/EBPβ) transcription in SH-SY5Y cells treated with MPP+ (1-methyl-4-phenylpyridinium) (85). SF also reduced α-synuclein aggregation in HEK293-α-Syn-YFP cells treated with preformed fibrils. These findings collectively draw attention to the role of SF in antioxidant defense, neurotrophic support, and neuroprotection.
Additional studies have highlighted the neuroprotective effects of other natural compounds, such as benzyl ITC and indole-3-carbinol (not an ITC, but derived from indole glucosinolates), in preclinical models of PD. Benzyl ITC (67.1 μmol/kg/day for 12 weeks) enhanced the activity and expression of an endogenous antioxidant, GST-π (glutathione S-transferase pi), in Wistar rats with zinc-induced Parkinsonism and ameliorated neurological deficits, neurodegenerative markers, and oxidative stress (90). Similarly, indole-3-carbinol (680 μmol/kg/day) amended striatal dopamine levels and neurodegeneration, and prevented motor dysfunctions in rotenone-induced PD in male albino rats (91).
3.3 Multiple sclerosis
Multiple sclerosis (MS) is an inflammation-mediated demyelinating disease of the human central nervous system, with neurodegeneration being the primary cause of irreversible neurological disability. A total of 5 in vivo studies have demonstrated the benefits of ITC in MS.
The experimental mouse models of autoimmune encephalomyelitis (EAE) and cuprizone (a copper-chelator causing demyelination) are the most commonly employed animal models used to study different aspects of MS pathology. In one study using the EAE model, invesigators demonstrated that SF (282 μmol/kg/day for 14 days before EAE induction and 28 days after EAE induction) had anti-inflammatory effects, improved clinical scores in behavioral study, and inhibited demyelination in the spinal cords (92). Four additional studies have reported protective effects of Moringa oleifera (or its ITC moringin) in animal models of MS. First, mice receiving moringin pretreatment (16.4 μmol/kg glucomoringin +5 μL myrosinase daily for a week before EAE induction and 28 days after EAE induction) exhibited increased expression of Nrf2, reduced cell apoptosis, and suppressed aberrant Wnt-β-catenin signaling in EAE mice (93). Another study demonstrated that Moringa oleifera (1.875 mg/mL for 5 weeks) reversed cuprizone-induced neuropathological deficits by mitigating oxidative stress, memory decline, and cortico-hippocampal neuronal deficits in Wistar rats (94). Moreover, Moringa oleifera leaf extract (1.875 mg/mL/day for 5 weeks) ameliorated cuprizone-induced weight loss and histological alterations in the hippocampal CA3 region in female Wistar rats (95). Co-administration of cuprizone with Moringa oleifera (1.875 mg/mL/day for 5 weeks) resulted in improved cellular assortment and delineated cytoarchitectural manifestations in cuprizone-treated rats (95). Finally, a topical treatment of 2% moringin cream twice per day alleviated neuropathic pain in the EAE model by attenuating proinflammatory cytokines (interleukin-17 and interferon-γ) while increasing the expression of the anti-inflammatory cytokine interleukin-10 (IL-10) (96).
3.4 Amyotrophic lateral sclerosis
Amyotrophic lateral sclerosis (ALS) is a progressive neurodegenerative disease characterized by the degeneration and death of motor neurons, leading to the inability to control muscle movement. One in vivo study has examined the effect of glucomoringin on ALS. In a transgenic rat model, daily treatment with glucomoringin (16.4 μmol/kg) + myrosinase (20 μL/rat) for 2 weeks prior to disease onset demonstrated protective effects, as indicated by measuring inflammatory and apoptotic markers, and it inhibited motor neuron degradation, a key to ALS pathophysiology (97).
3.5 Huntington’s disease
Huntington’s disease, an autosomal dominant neurodegenerative disorder, involves motor dysfunction, psychiatric issues, and cognitive decline. Mitochondrial dysfunction is a molecular feature implicated in most neurologic disorders, including Huntington’s disease. One in vivo study showed that SF (two doses of 28.2 μmol/kg/day) restored mitochondrial function against quinolinic acid-induced damage in an experimental rodent model of Huntington’s disease (98).
3.6 Friedreich’s ataxia
Friedreich’s ataxia (FRDA) is a progressive neurodegenerative disease characterized by oxidative stress and mitochondrial dysfunction with decreased expression of the mitochondrial protein frataxin (FXN). Two preclinical studies, both conducted in vitro, have examined the effects of SF in FRDA fibroblasts. First, treatment with SF (5 μM for 24 h) increased frataxin protein expression, promoted axonal re-growth in frataxin-silenced motor neurons and restored Nrf2 transcriptional activity in fibroblasts from patients with FRDA (99). In the second study, there was increased expression of FXN and Nrf2 target genes and increased glutathione concentration in SF (10 μM for 24 h) treated FRDA fibroblasts compared to untreated cells (100).
3.7 Fragile X-associated tremor/ataxia syndrome
Fragile X-Associated Tremor/Ataxia Syndrome (FXTAS), typically caused by a premutation in the FMR1 (fragile X messenger ribonucleoprotein 1) gene, is a nervous system disorder in older adults characterized by tremors, ataxia, memory issues, and mood disorders. One clinical study (Table 4) and one in vitro study have demonstrated the effects of SF on FXTAS. The clinical study was a 24-week open-label trial in 11 adults aged 60–88 years with FXTAS (101). SF (100 μmol SF/day) improved spatial working memory but did not improve other clinical outcomes, molecular changes in mitochondria-derived vesicles, or bioenergetics in PBMCs (101). The in vitro study was conducted in FRDA fibroblasts in which SF treatment (5 μmol for 72–96 h) that resulted in improvements in deficient pathways associated with FXTAS in both Nrf-2-dependent and independent manners (102). This was observed in primary fibroblasts from FXTAS-affected subjects ranging from stages 2.5 to 5. These improvements included the modulation of oxidative stress, enhancement of mitochondrial function, promotion of DNA repair, and increased clearance of damaged organelles and macromolecules through parkin-ubiquitin proteasomal mechanisms (102).
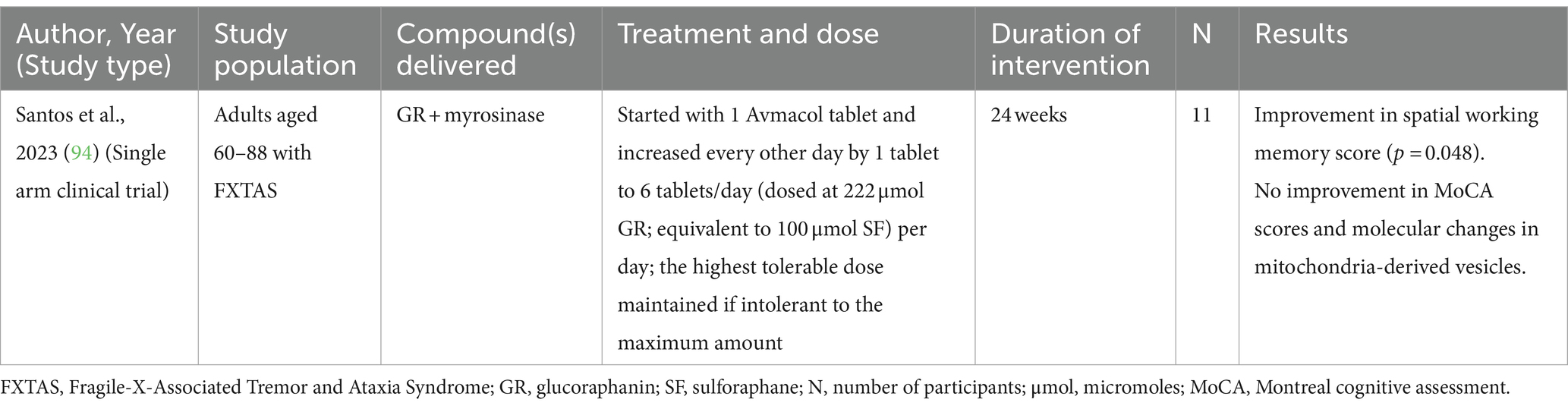
Table 4. A clinical study reported the effects of glucoraphanin + myrosinase compound on cognitive outcomes in individuals with FXTAS.
4 Other brain-related disorders
Neurodevelopmental and neurodegenerative conditions share common pathological mechanisms, encompassing neuronal cell death, microglial activation, neuroinflammation, disrupted redox homeostasis, mitochondrial dysfunction, and synaptic dysfunction. There are other brain-related disorders and conditions that also share these mechanisms, including spinal cord injury, traumatic brain injury, diabetes-induced cognitive decline, depression, and anxiety. There is both clinical and preclinical evidence of amelioration of symptoms with ITC treatment for these conditions.
4.1 Spinal cord injury
Spinal cord injury (SCI) is marked by neurologic dysfunction and neuronal death resulting from inflammatory and oxidative insults. Seven in vivo studies with ITC treatment for SCI have been identified.
SF has shown notable effects in mitigating inflammation and oxidative stress associated with SCI in mouse models. SF treatment (56.4 μmol/kg for 11 days) increased the expression of Nrf2 and phase 2 cytoprotective enzymes while inhibiting markers induced by inflammation in two mouse models when inflammatory pain was induced in the spinal cord using complete Freund’s adjuvant (103). Beyond its anti-inflammatory effects, SF enhanced the antinociceptive actions of morphine, increasing the effectiveness of pain alleviation compared to morphine alone (103). Similarly, rats subjected to mild thoracic SCI had significantly greater levels of Nrf2 and glutamate-cysteine ligase, decreased levels of inflammatory cytokines and spinal cord lesion volumes, and improved coordination after SCI with an SF dose of 28.2 μmol/kg/day for 3 days (104). SF (28.2 μmol/kg an hour after SCI induction) activation of Nrf2 post-SCI improved locomotor function and reduced inflammatory damage, neuron death, and spinal cord edema, while neurological deficits were more severe and the protective effect of SF was not observed in Nrf2 deficient mice (105). SF (282 μmol/kg at 10 min and 72 h after SCI contusion) in a rat model of contusion SCI upregulated antioxidant responses, reduced inflammatory cytokine mRNA levels, improved hindlimb locomotor function, and increased serotonergic axons caudal to the site of lesion (106). Further, histological examination of spinal cord sections revealed improvements in demyelination and apoptosis, but not inflammation as compared to the vehicle group with SFX-01 (10, 50 or 300 mg/kg twice a day for 3 weeks), a stabilized form of SF, treatment in EAE mice (107).
In addition to SF, moringin has shown promising anti-inflammatory and anti-apoptotic effects in the context of SCI. Pretreatment of gingival mesenchymal stem cells (GMSCs) with moringin (0.05 μM 1 h post-SCI) in a mouse model of SCI demonstrated anti-inflammatory and anti-apoptotic effects (108). Moringin-treated GMSCs reduced inflammatory marker levels, restored spinal cord morphology, increased expression of anti-apoptotic marker B-cell lymphoma 2 (Bcl-2) and decreased expressions of apoptotic markers such as Bcl-2-associated X protein (Bax) and caspases 3 and 9 (108). Similarly, post-injury administration of moringin (16.4 μmol glucomoringin/kg + 5 μL myrosinase/day for 7 days before SCI and continued until sacrifice) in mice subjected to SCI through the application of vascular clips showed protective effects against secondary damage by reducing oxidative stress, inflammation, and apoptosis (109).
4.2 Traumatic brain injury
Traumatic brain injury (TBI), often resulting from blunt force, shares pathophysiological similarities with stroke-induced cerebral ischemia, involving cellular damage from excitotoxicity, oxidative stress, apoptosis, and inflammation. Preclinical studies including eight in vivo and one in vitro experiments have been identified to examine the effects of ITC on traumatic brain injury.
SF has consistently demonstrated protective effects against cerebral ischemia/reperfusion (CIR) injury and associated inflammation in rat models. Two rat studies using a CIR model found SF (28.2 μmol or 56.4 μmol /kg 1 h at the beginning of the injury; 56.4, 112.8 or 225.6 μmol /kg/day for 14 days) had congruent protective effects against CIR-induced damage and inflammation (110, 111). Additionally, SF demonstrated protective effects in rodent models of vascular cognitive impairment induced by ischemia at 56.4 μmol/kg twice a week (112), subarachnoid hemorrhage at 282 μmol/kg every 24 h (113), and intracerebral hemorrhage at 5.64 μmol/kg twice a day (114), with upregulation of Nrf2 and reduced inflammation reported in the latter two.
Moreover, studies have investigated the potential synergistic effects of SF with other compounds in mitigating neuroinflammation and oxidative stress associated with traumatic brain injury. An in vivo study in a rat model of traumatic brain injury demonstrated that a combination therapy of N-acetylcysteine and SF yielded modest improvements in motor movement and coordination (115). However, there were no significant effects on composite neuromotor score or cortical lesion area (115). Yet, an in vitro combination therapy with N-acetylcysteine and SF (5 and 10 μM) significantly reduced neuroinflammation and nitrite levels while improving neuronal viability (115).
Similarly, moringin treatment (~8 μmol glucomoringin/ml plus 30 μL enzyme 15 min after beginning of ischemia and daily) of rats with CIR injury prevented CIR-induced damage and decreased inflammatory and oxidative mediators that exacerbate disease progression (116). Further, allyl isothiocyanate (AITC; 101 μmol/kg immediately after TBI induction) administered immediately after traumatic brain injury in mice significantly reduced infarct volume and serum IgG extravasation, revealing an improvement in blood–brain barrier permeability (117). AITC also decreased proinflammatory cytokines while increasing Nrf2, neural outgrowth and regeneration marker growth-associated protein 43 (GAP43), and neurogenesis-associated neural cell adhesion molecule levels (117).
4.3 Diabetes-related cognitive decline
Compelling evidence establishes a correlation and potential pathophysiological connection between type 2 diabetes (T2D) and cognitive dysfunction, leading to an increased risk of AD (118). The implicated mechanisms include defects in insulin signaling, neuroinflammation, and mitochondrial metabolism, among others (118). Both SF and moringin have been studied for their potential to attenuate diabetic complications including cognitive decline (119, 120). Eight in vivo and two in vitro experiments have been identified that demonstrate the effect of these ITC on diabetes-related cognitive decline.
Several studies have investigated the potential neuroprotective effects of SF in various models of diabetes-induced cognitive impairment. In a streptozotocin-induced diabetic rat model, SF (141 μmol/kg/day for 14 days) supplementation yielded preventive effects against learning and memory impairment (121). The rats also showcased attenuated decline in memory, protection against hippocampal neuron apoptosis, decreased caspase-3 expression, and increased expression of an antiapoptotic marker, MCL-1 (myeloid leukemia cell differentiation protein). Similarly, in a type 2 diabetes mellitus mouse model, SF (5.64 μmol/kg/day for 28 days) administration mitigated cognitive decline and reduced pathological hallmarks of AD, such as Aβ-oligomers, Aβ 1–42 plaques, and phospho-tau (122). SF activated Nrf2-regulated antioxidant defenses, enhancing the expression of NrF2 downstream genes HO-1 and NQO1. The study also reported reduced reactive oxygen/nitrogen species in the mice brains and mitigated cognitive decline (122). Additionally, SF (5.64 μmol/kg/day for 15 days) demonstrated therapeutic effects in a rat model induced with nicotinamide and streptozotocin by restoring insulin levels, normalizing motor coordination, improving sensory responses, and ameliorating histopathological changes (123). One study also examined a combination therapy with ulinastatin (10,000 U/kg/day for 26 days) and SF (141 μmol/kg/day for 26 days), which attenuated streptozotocin-induced diabetes and vascular dementia in rats, improving behavioral, endothelial, and biochemical parameters (124).
One in vitro study showed that a combination of SF (20 μmoL/L) and vitamin E (200 μg/mL alpha-tocopherol) protected against glucotoxic damages on neuronal structure and function in the nematode Caenorhabditis elegans, a model organism used to study the effects of high glucose on neuronal function (125). The duo also prevented reactive oxygen species and methylglyoxal-derived advanced glycation end products formation under hyperglycemic conditions (125). In both in vitro (0.5 μM for 24 h) and in vivo (141 μmol/kg/day for 14 days) settings, SF mitigated high glucose-induced apoptosis by inhibiting the upregulation of apoptotic markers and the downregulation of antiapoptotic maker Bcl-2 protein in hippocampal neurons exposed to high glucose (126).
Moringa oleifera has also been shown to provide neuroprotection against diabetes-induced cognitive dysfunction. In one study, Moringa oleifera leaves added to the diet (0.5 g, 1.0 g and 2.0 g per day for 30 days) reduced brain cholinergic enzymes (AChE, BChE), glycemic index, and lipid profile (TC, TG, LDL-C), while elevating antioxidant enzymes (SOD, CAT) in rats compared to controls not fed the leaves (127). In another study, the plant’s leaves (2.0, 4.0 and 8.0 g/kg/day for 8 weeks) exhibited protective effects on cognitive dysfunction and hippocampal neuron apoptosis in streptozotocin-induced diabetic rats (128). Furthermore, hyperglycemic rats supplemented with Moringa oleifera leaves or seeds (2 and 4% of the diet for 14 days) exhibited elevated levels of various antioxidant enzymes and glutathione, concomitantly decreasing biomarkers associated with hyperglycemia-induced cognitive decline (129).
4.4 Anxiety and depression
Mice subjected to acute or chronic stress often exhibit depressive and anxiety-like behaviors, paralleled by immune dysregulation. One RCT and 13 in vivo with ITC treatment have been identified for anxiety and depression.
4.4.1 Clinical study
There has been one randomized, double-blind, placebo-controlled clinical trial on the antidepressant effects of SF (N = 66) (130). In patients with a history of cardiac interventions, 169.2 μmol/day SF for 6 weeks alleviated mild to moderate depression, leading to greater improvements in the Hamilton Rating Scale for Depression (HAM-D) compared to placebo (p < 0.001). Participants on SF demonstrated greater response to treatment rates at the end of the trial compared to those on placebo (30% vs. 6.67%, p < 0.05) (Table 5).
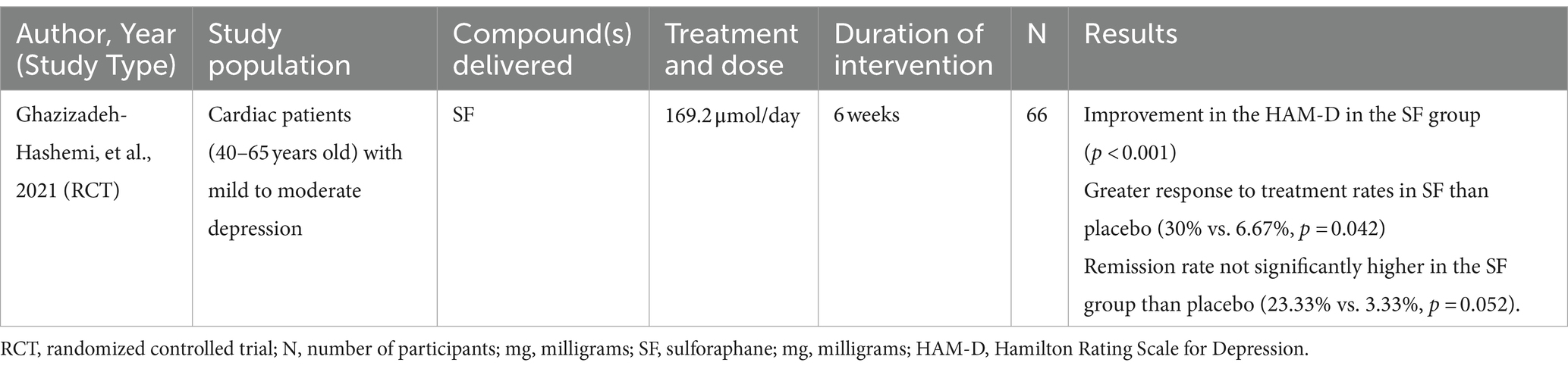
Table 5. A clinical study reported the effects of sulforaphane on depression outcomes in cardiac patients with mild to moderate depression.
4.4.2 Preclinical studies
Preclinical studies have further elucidated the potential mechanisms underlying the antidepressant and anxiolytic effects of SF. SF treatment (56.4 μmol/kg/day for 14 days) significantly reversed anxiety-like behaviors in acutely and chronically stressed mice, reducing serum corticosterone, adrenocorticotropic hormone, interleukin-6, and TNF-α in chronically stressed mice (131). SF (16.92, 56.4 and 169.2 μmol/kg) also combated inflammation-induced depression-like behavior in mice, reducing immobility time and restoring dendritic changes induced by LPS administration (132). Similarly, chronic administration of 56.4 μmol/kg SF for 15 days alleviated anxiety-like behavior and produced antidepressant effects in neuropathic mice, as evidenced by behavioral tests in neuropathic mice (133). Moreover, SF (28.2 μmol/kg/day for 7 days) reversed Aβ-oligomer-induced depressive-like behavior in rats, protecting the serotonergic system and mitigating depressive-like behavior (134).
In one study, GR administration (114.17 μmol/kg) brought about antidepressant effects showcased through reduction in immobility frequency in the forced swimming test (FST) and restoring serotonin (5-HT) levels in soluble Aβ 1–42 (a 42-residue form of Aβ) treated rats (135). Further, treating mice with dietary GR (0.1% GR food pellets) during juvenile and adolescent stages has been shown to prevent LPS-induced depression-like behaviors in adulthood (132, 136).
Moringa oleifera has also demonstrated promising anxiolytic and antidepressant effects in animal models. For example, ITC-rich extracts of Moringa oleifera leaf (500 mg/kg administered 30 min prior to tests) induced anxiolytic effects in Swiss mice in light–dark and open field tests (OFT) (137). Additionally, treatment with Moringa oleifera seed oil (1 or 2 mL/kg for 23 days) decreased anxiety-and depression-like behaviors, measured using the OFT and FST in the water-immersion restraint stress mouse model. (138). And, pretreatment with Moringa oleifera leaf extract (400 mg/kg/day for 14 days) produced anxiolytic effects in both FST and tail suspension test (TST) in the hepatic encephalopathy mouse model (139).
Other ITC-rich plants have also been studied for their antidepressant and anxiolytic properties. Ethanolic and methanolic extracts of Camelina sativa var. Madalina (5 g/kg), rich in sinapine, glucosinolates, and flavonol glycosides, brought about anxiolytic and antidepressant effects in stress-induced irritable bowel syndrome mouse models (140). Isatis tinctoria leaf extract (50, 100 or 500 mg/kg) reversed stress-induced anxiety-like behavior and regulation of neurooxidative, neuronitrosative, and neuroimmune pathways in mice (141). Further, Raphanus caudatus (250 and 500 mg/kg), a radish variety, significantly reduced anxiety-like behavior in mice, comparable to diazepam, a pharmaceutical anxiolytic drug (142). And, indole-3-carbinol (408 μmol/kg for 10 days), an ITC usually found in cabbage, broccoli, and collard greens, prevented chronic social defeat stress (CSDS)-induced behavioral abnormalities associated with depression in mice, though it did not affect behavioral abnormalities related to anxiety (143).
5 Adverse effects
Progoitrin and indole glucosinolates in Brassica vegetables can degrade to goitrin in the presence of myrosinase, potentially decreasing thyroid hormone production (144–146). While a clinical study found that a single 25 mg or 50 mg dose of goitrin inhibited thyroid radioiodine uptake, two 10 mg doses did not have this effect (146, 147). Vegetables with low progoitrin levels, such as commercial broccoli and kale, pose minimal risk to iodine uptake. A recent systematic review of 123 studies suggests that regular consumption of Brassica vegetables does not pose a risk of adverse effects, particularly with adequate iodine intake (148).
Some ITCs demonstrate a hormetic effect, offering cytoprotective benefits at low doses and cytotoxic, antitumor properties at higher doses (149, 150). A few studies have shown that SF increases reactive oxygen species (ROS) generation and DNA double-strand breaks (DSB) in cancer cells in a dose-and time-dependent manner (151, 152). While these effects reduce cancer cell survival, normal cells like human dermal fibroblasts and gastric mucosal cells exhibit higher viability and faster DNA repair when exposed to similar doses, suggesting that SF could be an effective cancer therapeutic without harming normal cells (153, 154).
Ingestion of isothiocyanate-containing preparations may cause adverse effects. Higher doses of SF can cause a harsh, burning sensation in the back of the throat, which may deter some from consuming broccoli or sulforaphane preparations (155, 156). Strategies to mask flavors of brocolli extracts and moringa teas have been studied (157, 158). Some individuals may experience gastrointestinal discomfort when consuming SF in large quantities (155). Gradually increasing the dose may help mitigate these effects, allowing the gut to adapt over time (159).
6 Alternative treatments
Bioactive compounds, along with plant sources beyond cruciferous vegetables and dietary nutrients, offer potential alternative approaches for managing neurologic disorders (160–162). Among these, polyphenols (163), flavonoids (164) and certain herbs (165) along with other phytonutrients (166) have demonstrated therapeutic benefits in neurologic disorders. Curcumin is one of the most extensively studied compounds (167, 168), with other common treatments including quercetin (169), resveratrol (170) and luteolin (171). Despite the potential of dietary nutrients like vitamin D and omega-3 fatty acids for neuroprotection (172), findings from studies have yielded mixed results without consistent beneficial effects (173, 174). Exploring the effect of nutraceuticals as treatments for neurologic disorders remains a significant area of interest.
7 Methods
All research articles were retrieved from PubMed and Scopus. The search criteria consisted of the following terms: (“glucosinolate” OR “glucoraphanin” OR “sulforaphane” OR “isothiocyanate” OR “moringin”) AND (“brain” OR “mental” OR “cognit*” OR “neuro*” OR “psych*”) NOT (FITC OR SITS OR “fluorescein isothiocyanate” OR RITC OR “rhodamine B isothiocyanate” OR “fentanyl isothiocyanate” OR “di-o-tolyl-guanidine-isothiocyanate” OR “isothiocyanate labelled” OR IFN OR “rhodamine isothiocyanate” OR LHRH OR “affinity labeled”) for articles published from November 2018 through May 2024. Since this review extends the previous review published in 2018, literature from that review is included along with additional relevant clinical trials that were omitted in the original publication. After 404 duplicates were removed, 1,108 abstracts were screened by two independent reviewers, and 293 full texts were reviewed. A total of 106 articles (clinical and preclinical studies) were included. Criteria for inclusion were original research articles focusing on isothiocyanates derived from natural plant sources and their effects on neuropsychiatric disorders and other brain-related conditions. Studies focusing on other phytochemicals like flavonoids, alkaloids, and polyphenols, and non-brain-related outcomes, were excluded.
8 Conclusion
We illustrate that cruciferous and related vegetables (or supplements rich in glucosinolates or ITC) can contribute to a dietary strategy for combating neuropsychiatric disorders and improving the quality of life. A few studies have also reported their effects as comparable to pharmaceutical treatments with fewer side effects. While these plants contain various phytochemicals, it is the potent and highly bioavailable compounds within the glucosinolate/myrosinase/ITC system that uniquely contribute to the highly potent indirect antioxidant properties of these vegetables. However, other phytochemicals in these plants, including phenols and flavonoids, have also been shown to be beneficial for brain-related outcomes (175). It may therefore be more valuable to consume the vegetable as a whole (or as sprouts) or as water extracted vegetable supplements rather than those containing only a high refined glucosinolate or isothiocyanate.
Through our comprehensive search, we found around 11 preclinical studies on the effects of ITCs from other cruciferous vegetables including maca, radish, and wasabi on neuropsychiatric disorders. A realm of opportunity remains to explore the potential benefits of cabbage, turnips, mustard and other cruciferous vegetables on neuroinflammation and outcomes related to neuropsychiatric disorders.
While this review updates our review published 6 years ago (26), it does not delve into the extensive epidemiological evidence supporting all the benefits of ITC. Furthermore, it is essential to note that there is a plethora of literature highlighting their other properties (155). The comprehensive understanding of the underlying mechanisms now sheds light on why this protection extends across a broad spectrum of chronic conditions, encompassing both neurodevelopmental and neurodegenerative origins. This emphasizes the potential of cruciferous and other ITC-containing vegetables as a safe and effective dietary intervention for enhancing brain health and mitigating the risk and effects of various brain-related disorders. Given the increasing number of in vitro and animal studies that show the metabolic and behavioral benefits and safety of ITC, it is now important that clinical trials be expanded to facilitate their application in patients with disorders of the nervous system.
Author contributions
MR: Writing – original draft, Writing – review & editing. JF: Conceptualization, Writing – review & editing. AZ: Conceptualization, Writing – review & editing. XZ: Writing – review & editing. AP: Writing – review & editing, Conceptualization, Resources, Supervision, Writing – original draft.
Funding
The author(s) declare that no financial support was received for the research, authorship, and/or publication of this article.
Conflict of interest
The authors declare that the research was conducted in the absence of any commercial or financial relationships that could be construed as a potential conflict of interest.
One of us (JWF) has consulted for both food and supplement companies in the past year.
Publisher’s note
All claims expressed in this article are solely those of the authors and do not necessarily represent those of their affiliated organizations, or those of the publisher, the editors and the reviewers. Any product that may be evaluated in this article, or claim that may be made by its manufacturer, is not guaranteed or endorsed by the publisher.
References
1. Parchem, K, Piekarska, A, and Bartoszek, A. Enzymatic activities behind degradation of glucosinolates In: CM Galanakis, editor. Glucosinolates: Properties, recovery, and applications. United Kingdom: Academic Press (2020). 79–106.
2. Liu, H, Talalay, P, and Fahey, JW. Biomarker-guided strategy for treatment of autism Spectrum disorder (ASD). CNS Neurol Disord Drug Targets. (2016) 15:602–13. doi: 10.2174/1871527315666160413120414
3. Bones, AM, and Rossiter, JT. The enzymic and chemically induced decomposition of glucosinolates. Phytochemistry. (2006) 67:1053–67. doi: 10.1016/j.phytochem.2006.02.024
4. Wittstock, U, and Burow, M. Tipping the scales ‐ specifier proteins in Glucosinolate hydrolysis. IUBMB Life. (2007) 59:744–51. doi: 10.1080/15216540701736277
5. Ettlinger, MG, and Lundeen, AJ. The structures of sinigrin and sinalbin; an enzymatic rearrangement. J Am Chem Soc. (1956) 78:4172–3. doi: 10.1021/ja01597a090
6. Gasper, AV, al-janobi, A, Smith, JA, Bacon, JR, Fortun, P, Atherton, C, et al. Glutathione S-transferase M1 polymorphism and metabolism of sulforaphane from standard and high-glucosinolate broccoli. Am J Clin Nutr. (2005) 82:1283–91. doi: 10.1093/ajcn/82.6.1283
7. Fahey, JW, Olson, ME, Stephenson, KK, Wade, KL, Chodur, GM, Odee, D, et al. The diversity of chemoprotective glucosinolates in Moringaceae (Moringa spp.). Sci Rep. (2018) 8:7994. doi: 10.1038/s41598-018-26058-4
8. Fahey, JW, Zalcmann, AT, and Talalay, P. The chemical diversity and distribution of glucosinolates and isothiocyanates among plants. Phytochemistry. (2001) 56:5–51. doi: 10.1016/S0031-9422(00)00316-2
9. Blažević, I, Montaut, S, Burčul, F, Olsen, CE, Burow, M, Rollin, P, et al. Glucosinolate structural diversity, identification, chemical synthesis and metabolism in plants. Phytochemistry. (2020) 169:112100. doi: 10.1016/j.phytochem.2019.112100
10. Agerbirk, N, and Olsen, CE. Glucosinolate structures in evolution. Phytochemistry. (2012) 77:16–45. doi: 10.1016/j.phytochem.2012.02.005
11. Fahey, JW, Zhang, Y, and Talalay, P. Broccoli sprouts: an exceptionally rich source of inducers of enzymes that protect against chemical carcinogens. Proc Natl Acad Sci USA. (1997) 94:10367–72. doi: 10.1073/pnas.94.19.10367
12. Cancer IAfRo. Cruciferous vegetables, isothiocyanates and indoles. Lyon, France: IARC Press (2004).
13. Ho, E, Clarke, JD, and Dashwood, RH. Dietary sulforaphane, a histone deacetylase inhibitor for cancer prevention. J Nutr. (2009) 139:2393–6. doi: 10.3945/jn.109.113332
14. Li, N, Wu, X, Zhuang, W, Wu, C, Rao, Z, du, L, et al. Cruciferous vegetable and isothiocyanate intake and multiple health outcomes. Food Chem. (2022) 375:131816. doi: 10.1016/j.foodchem.2021.131816
15. Kim, J. Pre-clinical neuroprotective evidences and plausible mechanisms of sulforaphane in Alzheimer's disease. Int J Mol Sci. (2021) 22:2929. doi: 10.3390/ijms22062929
16. Schepici, G, Bramanti, P, and Mazzon, E. Efficacy of sulforaphane in neurodegenerative diseases. Int J Mol Sci. (2020) 21:8637. doi: 10.3390/ijms21228637
17. Jaafaru, MS, Abd Karim, NA, Enas, ME, Rollin, P, Mazzon, E, and Abdull Razis, AF. Protective effect of glucosinolates hydrolytic products in neurodegenerative diseases (NDDs). Nutrients. (2018) 10:580. doi: 10.3390/nu10050580
18. Kou, X, Li, B, Olayanju, JB, Drake, JM, and Chen, N. Nutraceutical or pharmacological potential of Moringa oleifera lam. Nutrients. (2018) 10:343. doi: 10.3390/nu10030343
19. Agrawal, S, Yallatikar, T, and Gurjar, P. Brassica Nigra: Ethopharmacological review of a routinely used condiment. Curr Drug Discov Technol. (2019) 16:40–7. doi: 10.2174/1570163815666180308143400
20. Venditti, A, and Bianco, A. Sulfur-containing secondary metabolites as neuroprotective agents. Curr Med Chem. (2020) 27:4421–36. doi: 10.2174/0929867325666180912105036
21. Connolly, EL, Sim, M, Travica, N, Marx, W, Beasy, G, Lynch, GS, et al. Glucosinolates from cruciferous vegetables and their potential role in chronic disease: investigating the preclinical and clinical evidence. Front Pharmacol. (2021) 12:767975. doi: 10.3389/fphar.2021.767975
22. Wu, YY, Xu, YM, and Lau, ATY. Anti-cancer and medicinal potentials of Moringa isothiocyanate. Molecules. (2021) 26:7512. doi: 10.3390/molecules26247512
23. Gao, Q, Wei, Z, Liu, Y, Wang, F, Zhang, S, Serrano, C, et al. Characterization, large-scale HSCCC separation and neuroprotective effects of polyphenols from Moringa oleifera leaves. Molecules. (2022) 27:27. doi: 10.3390/molecules27030678
24. Kamal, RM, Abdull Razis, AF, Mohd Sukri, NS, Perimal, EK, Ahmad, H, Patrick, R, et al. Beneficial health effects of glucosinolates-derived isothiocyanates on cardiovascular and neurodegenerative diseases. Molecules. (2022) 27:27. doi: 10.3390/molecules27030624
25. Mundkar, M, Bijalwan, A, Soni, D, and Kumar, P. Neuroprotective potential of Moringa oleifera mediated by NF-kB/Nrf2/HO-1 signaling pathway: a review. J Food Biochem. (2022) 46:e14451. doi: 10.1111/jfbc.14451
26. Panjwani, AA, Liu, H, and Fahey, JW. Crucifers and related vegetables and supplements for neurologic disorders: what is the evidence? Curr Opin Clin Nutr Metab Care. (2018) 21:451–7. doi: 10.1097/MCO.0000000000000511
27. Autism spectrum disorder (ASD). (2024). Centers for disease control and prevention. Available from: https://www.cdc.gov/ncbddd/autism/facts.html#:~:text=people%20without%20ASD.-,Signs%20and%20Symptoms,can%20make%20life%20very%20challenging (Accessed February 04, 2024).
28. CDC. (2023). Autism prevalence higher, according to data from 11 ADDM communities. Available from: https://www.cdc.gov/media/releases/2023/p0323-autism.html (Accessed February 04, 2024).
29. Solmi, M, Song, M, Yon, DK, Lee, SW, Fombonne, E, Kim, MS, et al. Incidence, prevalence, and global burden of autism spectrum disorder from 1990 to 2019 across 204 countries. Mol Psychiatry. (2022) 27:4172–80. doi: 10.1038/s41380-022-01630-7
30. Singh, K, Connors, SL, Macklin, EA, Smith, KD, Fahey, JW, Talalay, P, et al. Sulforaphane treatment of autism spectrum disorder (ASD). Proc Natl Acad Sci USA. (2014) 111:15550–5. doi: 10.1073/pnas.1416940111
31. Lynch, R, Diggins, EL, Connors, SL, Zimmerman, AW, Singh, K, Liu, H, et al. Sulforaphane from broccoli reduces symptoms of autism: a follow-up case series from a randomized double-blind study. Glob Adv Health Med. (2017) 6:2164957X1773582. doi: 10.1177/2164957X17735826
32. Zimmerman, AW, Singh, K, Connors, SL, Liu, H, Panjwani, AA, Lee, LC, et al. Randomized controlled trial of sulforaphane and metabolite discovery in children with autism spectrum disorder. Mol Autism. (2021) 12:38. doi: 10.1186/s13229-021-00447-5
33. Bent, S, Lawton, B, Warren, T, Widjaja, F, Dang, K, Fahey, JW, et al. Identification of urinary metabolites that correlate with clinical improvements in children with autism treated with sulforaphane from broccoli. Mol Autism. (2018) 9:35. doi: 10.1186/s13229-018-0218-4
34. Ou, J, Smith, RC, Tobe, RH, Lin, J, Arriaza, J, Fahey, JW, et al. Efficacy of sulforaphane in treatment of children with autism spectrum disorder: a randomized double-blind placebo-controlled multi-center trial. J Autism Dev Disord. (2022) 54:628–41. doi: 10.1007/s10803-022-05784-9
35. Momtazmanesh, S, Amirimoghaddam-Yazdi, Z, Moghaddam, HS, Mohammadi, MR, and Akhondzadeh, S. Sulforaphane as an adjunctive treatment for irritability in children with autism spectrum disorder: a randomized, double-blind, placebo-controlled clinical trial. Psychiatry Clin Neurosci. (2020) 74:398–405. doi: 10.1111/pcn.13016
36. Yang, J, He, L, Dai, S, Zheng, H, Cui, X, Ou, J, et al. Therapeutic efficacy of sulforaphane in autism spectrum disorders and its association with gut microbiota: animal model and human longitudinal studies. Front Nutr. (2023) 10:1294057. doi: 10.3389/fnut.2023.1294057
37. Evans, S, and Fuller, DJ. Initial outcomes from an autism treatment demonstration. Clin Med Invest. (2016) 1:16–9. doi: 10.15761/CMI.1000103
38. Magner, M, Thorová, K, Župová, V, Houška, M, Švandová, I, Novotná, P, et al. Sulforaphane treatment in children with autism: a prospective randomized double-blind study. Nutrients. (2023) 15:718. doi: 10.3390/nu15030718
39. Nadeem, A, Ahmad, SF, al-Harbi, NO, Attia, SM, Bakheet, SA, Ibrahim, KE, et al. Nrf2 activator, sulforaphane ameliorates autism-like symptoms through suppression of Th17 related signaling and rectification of oxidant-antioxidant imbalance in periphery and brain of BTBR T+tf/J mice. Behav Brain Res. (2019) 364:213–24. doi: 10.1016/j.bbr.2019.02.031
40. Fujita, Y, Fujita, A, Ishima, T, Hirai, A, Suzuki, S, Suganuma, H, et al. Dietary intake of glucoraphanin during pregnancy and lactation prevents the behavioral abnormalities in the offspring after maternal immune activation. Neuropsychopharmacol Rep. (2020) 40:268–74. doi: 10.1002/npr2.12112
41. Liu, H, Zimmerman, AW, Singh, K, Connors, SL, Diggins, E, Stephenson, KK, et al. Biomarker exploration in human peripheral blood mononuclear cells for monitoring sulforaphane treatment responses in autism spectrum disorder. Sci Rep. (2020) 10:5822. doi: 10.1038/s41598-020-62714-4
42. Nadeem, A, Ahmad, SF, al-Ayadhi, LY, Attia, SM, al-Harbi, NO, Alzahrani, KS, et al. Differential regulation of Nrf2 is linked to elevated inflammation and nitrative stress in monocytes of children with autism. Psychoneuroendocrinology. (2020) 113:104554. doi: 10.1016/j.psyneuen.2019.104554
43. Fu, P, Luo, S, Liu, Z, Furuhara, K, Tsuji, T, Higashida, H, et al. Oral supplementation with maca improves social recognition deficits in the valproic acid animal model of autism spectrum disorder. Brain Sci. (2023) 13:316. doi: 10.3390/brainsci13020316
44. Hashimoto, K. Recent advances in the early intervention in schizophrenia: future direction from preclinical findings. Curr Psychiatry Rep. (2019) 21:75. doi: 10.1007/s11920-019-1063-7
45. Shiina, A, Kanahara, N, Sasaki, T, Oda, Y, Hashimoto, T, Hasegawa, T, et al. An open study of sulforaphane-rich broccoli sprout extract in patients with schizophrenia. Clin Psychopharmacol Neurosci. (2015) 13:62–7. doi: 10.9758/cpn.2015.13.1.62
46. Zeng, J, Zhang, W, Lu, X, Zhou, H, Huang, J, Xu, Z, et al. The association of SOD and HsCRP with the efficacy of sulforaphane in schizophrenia patients with residual negative symptoms. Eur Arch Psychiatry Clin Neurosci. (2023) 274:1083–92. doi: 10.1007/s00406-023-01679-7
47. Sedlak, TW, Nucifora, LG, Koga, M, Shaffer, LS, Higgs, C, Tanaka, T, et al. Sulforaphane augments glutathione and influences brain metabolites in human subjects: a clinical pilot study. Mol Neuropsychiatry. (2018) 3:214–22. doi: 10.1159/000487639
48. Dickerson, F, Origoni, A, Katsafanas, E, Squire, A, Newman, T, Fahey, J, et al. Randomized controlled trial of an adjunctive sulforaphane nutraceutical in schizophrenia. Schizophr Res. (2021) 231:142–4. doi: 10.1016/j.schres.2021.03.018
49. Xiao, J, Huang, J, Long, Y, Wang, X, Wang, Y, Yang, Y, et al. Optimizing and individualizing the pharmacological treatment of first-episode schizophrenic patients: study protocol for a multicenter clinical trial. Front Psychol. (2021) 12:611070. doi: 10.3389/fpsyt.2021.611070
50. Matsuura, A, Ishima, T, Fujita, Y, Iwayama, Y, Hasegawa, S, Kawahara-Miki, R, et al. Dietary glucoraphanin prevents the onset of psychosis in the adult offspring after maternal immune activation. Sci Rep. (2018) 8:2158. doi: 10.1038/s41598-018-20538-3
51. Shirai, Y, Fujita, Y, Hashimoto, R, Ohi, K, Yamamori, H, Yasuda, Y, et al. Dietary intake of sulforaphane-rich broccoli sprout extracts during juvenile and adolescence can prevent phencyclidine-induced cognitive deficits at adulthood. PLoS One. (2015) 10:e0127244. doi: 10.1371/journal.pone.0127244
52. Black, AM, Armstrong, EA, Scott, O, Juurlink, BJH, and Yager, JY. Broccoli sprout supplementation during pregnancy prevents brain injury in the newborn rat following placental insufficiency. Behav Brain Res. (2015) 291:289–98. doi: 10.1016/j.bbr.2015.05.033
53. Yuan, F, Chen, X, Liu, J, Feng, W, Cai, L, Wu, X, et al. Sulforaphane restores acetyl-histone H3 binding to Bcl-2 promoter and prevents apoptosis in ethanol-exposed neural crest cells and mouse embryos. Exp Neurol. (2018) 300:60–6. doi: 10.1016/j.expneurol.2017.10.020
54. Shukla, S, and Tekwani, BL. Histone deacetylases inhibitors in neurodegenerative diseases, neuroprotection and neuronal differentiation. Front Pharmacol. (2020) 11:537. doi: 10.3389/fphar.2020.00537
55. Xu, JF, Lu, JJ, Cao, Y, Wang, W, Li, HH, Chen, JG, et al. Sulforaphane alleviates ethanol-mediated central inhibition and reverses chronic stress-induced aggravation of acute alcoholism via targeting Nrf2-regulated catalase expression. Neuropharmacology. (2020) 176:108235. doi: 10.1016/j.neuropharm.2020.108235
56. Ladak, Z, Garcia, E, Yoon, J, Landry, T, Armstrong, EA, Yager, JY, et al. Sulforaphane (SFA) protects neuronal cells from oxygen & glucose deprivation (OGD). PLoS One. (2021) 16:e0248777. doi: 10.1371/journal.pone.0248777
57. Liddell, JR. Are astrocytes the predominant cell type for activation of nrf2 in aging and neurodegeneration? Antioxidants (Basel). (2017) 6:65. doi: 10.3390/antiox6030065
58. Giacoppo, S, Galuppo, M, Montaut, S, Iori, R, Rollin, P, Bramanti, P, et al. An overview on neuroprotective effects of isothiocyanates for the treatment of neurodegenerative diseases. Fitoterapia. (2015) 106:12–21. doi: 10.1016/j.fitote.2015.08.001
59. Rajan, KB, Weuve, J, Barnes, LL, McAninch, EA, Wilson, RS, and Evans, DA. Population estimate of people with clinical Alzheimer's disease and mild cognitive impairment in the United States (2020-2060). Alzheimers Dement. (2021) 17:1966–75. doi: 10.1002/alz.12362
60. Rajmohan, R, and Reddy, PH. Amyloid-beta and phosphorylated tau accumulations cause abnormalities at synapses of Alzheimer's disease neurons. J Alzheimers Dis. (2017) 57:975–99. doi: 10.3233/JAD-160612
61. Kim, J, Lee, S, Choi, BR, Yang, H, Hwang, Y, Park, JH, et al. Sulforaphane epigenetically enhances neuronal BDNF expression and TrkB signaling pathways. Mol Nutr Food Res. (2017) 61:1600194. doi: 10.1002/mnfr.201600194
62. Hou, TT, Yang, HY, Wang, W, Wu, QQ, Tian, YR, and Jia, JP. Sulforaphane inhibits the generation of amyloid-β oligomer and promotes spatial learning and memory in Alzheimer's disease (ps1v97l) transgenic mice. J Alzheimers Dis. (2018) 62:1803–13. doi: 10.3233/JAD-171110
63. Zhang, R, Miao, QW, Zhu, CX, Zhao, Y, Liu, L, Yang, J, et al. Sulforaphane ameliorates neurobehavioral deficits and protects the brain from amyloid β deposits and peroxidation in mice with Alzheimer-like lesions. Am J Alzheimers Dis Other Dement. (2015) 30:183–91. doi: 10.1177/1533317514542645
64. Zhang, J, Zhang, R, Zhan, Z, Li, X, Zhou, F, Xing, A, et al. Beneficial effects of sulforaphane treatment in Alzheimer's disease may be mediated through reduced HDAC 1/3 and increased P75NTR expression. Front Aging Neurosci. (2017) 9:121. doi: 10.3389/fnagi.2017.00121
65. Sunkaria, A, Bhardwaj, S, Yadav, A, Halder, A, and Sandhir, R. Sulforaphane attenuates postnatal proteasome inhibition and improves spatial learning in adult mice. J Nutr Biochem. (2017) 51:69–79. doi: 10.1016/j.jnutbio.2017.09.016
66. Lee, S, Choi, BR, Kim, J, LaFerla, FM, Park, JHY, Han, JS, et al. Sulforaphane upregulates the heat shock protein co-chaperone chip and clears amyloid-β and tau in a mouse model of Alzheimer's disease. Mol Nutr Food Res. (2018) 62:e1800240. doi: 10.1002/mnfr.201800240
67. Yang, W, Xu, QQ, Yuan, Q, Xian, YF, and Lin, ZX. Sulforaphene, a CDK5 inhibitor, attenuates cognitive deficits in a transgenic mouse model of Alzheimer's disease via reducing Aβ deposition, tau hyperphosphorylation and synaptic dysfunction. Int Immunopharmacol. (2023) 114:109504. doi: 10.1016/j.intimp.2022.109504
68. Alzahrani, NA, Bahaidrah, KA, Mansouri, RA, Aldhahri, RS, Abd El-Aziz, GS, and Alghamdi, BS. Possible prophylactic effects of sulforaphane on LPS-induced recognition memory impairment mediated by regulating oxidative stress and neuroinflammatory proteins in the prefrontal cortex region of the brain. Biomedicine. (2024) 12:1107. doi: 10.3390/biomedicines12051107
69. Zhao, F, Zhang, J, and Chang, N. Epigenetic modification of Nrf2 by sulforaphane increases the antioxidative and anti-inflammatory capacity in a cellular model of Alzheimer's disease. Eur J Pharmacol. (2018) 824:1–10. doi: 10.1016/j.ejphar.2018.01.046
70. Sestito, S, Pruccoli, L, Runfola, M, Citi, V, Martelli, A, Saccomanni, G, et al. Design and synthesis of H(2) S-donor hybrids: a new treatment for Alzheimer's disease? Eur J Med Chem. (2019) 184:111745. doi: 10.1016/j.ejmech.2019.111745
71. Youn, K, Yoon, JH, Lee, N, Lim, G, Lee, J, Sang, S, et al. Discovery of sulforaphane as a potent BACE1 inhibitor based on kinetics and computational studies. Nutrients. (2020) 12:3026. doi: 10.3390/nu12103026
72. Chilakala, RR, Manchikalapudi, AL, Kumar, A, and Sunkaria, A. Sulforaphane attenuates Aβ oligomers mediated decrease in phagocytic activity of microglial cells. Neuroscience. (2020) 429:225–34. doi: 10.1016/j.neuroscience.2020.01.002
73. Yang, W, Liu, Y, Xu, QQ, Xian, YF, and Lin, ZX. Sulforaphene ameliorates neuroinflammation and hyperphosphorylated tau protein via regulating the PI3K/akt/GSK-3β pathway in experimental models of Alzheimer's disease. Oxidative Med Cell Longev. (2020) 2020:1–17. doi: 10.1155/2020/4754195
74. Zhang, S, Zhao, J, Bai, Z, Luo, L, Wu, F, Li, B, et al. Sulforaphane inhibits the production of Aβ partially through the activation of Nrf2-regulated oxidative stress. Food Funct. (2021) 12:11482–90. doi: 10.1039/D1FO02651H
75. Alzoubi, KH, Rawashdeh, NQ, Khabour OFEl-Elimat, T, Albataineh, H, and Al-Zghool, HM. Evaluation of the effect of Moringa peregrina extract on learning and memory: role of oxidative stress. J Mol Neurosci. (2017) 63:355–63. doi: 10.1007/s12031-017-0986-x
76. Mahaman, YAR, Feng, J, Huang, F, Salissou, MTM, Wang, J, Liu, R, et al. Moringa oleifera alleviates Aβ burden and improves synaptic plasticity and cognitive impairments in APP/PS1 mice. Nutrients. (2022) 14:4284. doi: 10.3390/nu14204284
77. Mahaman, YAR, Huang, F, Wu, M, Wang, Y, Wei, Z, Bao, J, et al. Moringa oleifera alleviates homocysteine-induced alzheimer's disease-like pathology and cognitive impairments. J Alzheimers Dis. (2018) 63:1141–59. doi: 10.3233/JAD-180091
78. Onasanwo, SA, Adamaigbo, VO, Adebayo, OG, and Eleazer, SE. Moringa oleifera-supplemented diet protect against cortico-hippocampal neuronal degeneration in scopolamine-induced spatial memory deficit in mice: role of oxido-inflammatory and cholinergic neurotransmission pathway. Metab Brain Dis. (2021) 36:2445–60. doi: 10.1007/s11011-021-00855-9
79. Silvestro, S, Chiricosta, L, Gugliandolo, A, Iori, R, Rollin, P, Perenzoni, D, et al. The moringin/α-CD pretreatment induces neuroprotection in an in vitro model of Alzheimer's disease: a transcriptomic study. Curr Issues Mol Biol. (2021) 43:197–214. doi: 10.3390/cimb43010017
80. Morroni, F, Sita, G, Graziosi, A, Turrini, E, Fimognari, C, Tarozzi, A, et al. Protective effects of 6-(methylsulfinyl)hexyl isothiocyanate on Aβ(1-42)-induced cognitive deficit, oxidative stress, inflammation, and apoptosis in mice. Int J Mol Sci. (2018) 19:2083. doi: 10.3390/ijms19072083
81. Wright, AF. The redox stress test: a novel technique reveals oxidative stress in Parkinson’s disease. Medical Research Archives. (2024) 12. doi: 10.18103/mra.v12i1.4955
82. Dinkova-Kostova, AT, Baird, L, Holmström, KM, Meyer, CJ, and Abramov, AY. The spatiotemporal regulation of the Keap1-Nrf2 pathway and its importance in cellular bioenergetics. Biochem Soc Trans. (2015) 43:602–10. doi: 10.1042/BST20150003
83. Zhou, Q, Chen, B, Wang, X, Wu, L, Yang, Y, Cheng, X, et al. Sulforaphane protects against rotenone-induced neurotoxicity in vivo: involvement of the mTOR, Nrf2, and autophagy pathways. Sci Rep. (2016) 6:32206. doi: 10.1038/srep32206
84. Cao, Q, Zou, Q, Zhao, X, Zhang, Y, Qu, Y, Wang, N, et al. Regulation of BDNF transcription by Nrf2 and MeCP2 ameliorates MPTP-induced neurotoxicity. Cell Death Dis. (2022) 8:267. doi: 10.1038/s41420-022-01063-9
85. Lin, Z, Huang, L, Cao, Q, Luo, H, Yao, W, and Zhang, JC. Inhibition of abnormal C/EBPβ/α-Syn signaling pathway through activation of Nrf2 ameliorates Parkinson's disease-like pathology. Aging Cell. (2023) 22:e13958. doi: 10.1111/acel.13958
86. Pu, Y, Qu, Y, Chang, L, Wang, SM, Zhang, K, Ushida, Y, et al. Dietary intake of glucoraphanin prevents the reduction of dopamine transporter in the mouse striatum after repeated administration of MPTP. Neuropsychopharmacol Rep. (2019) 39:247–51. doi: 10.1002/npr2.12060
87. Morroni, F, Sita, G, Djemil, A, D’Amico, M, Pruccoli, L, Cantelli-Forti, G, et al. Comparison of adaptive neuroprotective mechanisms of sulforaphane and its interconversion product erucin in in vitro and in vivo models of Parkinson's disease. J Agric Food Chem. (2018) 66:856–65. doi: 10.1021/acs.jafc.7b04641
88. Lee, JA, Son, HJ, Kim, JH, Park, KD, Shin, N, Kim, HR, et al. A novel synthetic isothiocyanate ITC-57 displays antioxidant, anti-inflammatory, and neuroprotective properties in a mouse Parkinson's disease model. Free Radic Res. (2016) 50:1188–99. doi: 10.1080/10715762.2016.1223293
89. Lee, JA, Son, HJ, Park, KD, Han, SH, Shin, N, Kim, JH, et al. A novel compound ITC-3 activates the Nrf2 signaling and provides neuroprotection in parkinson's disease models. Neurotox Res. (2015) 28:332–45. doi: 10.1007/s12640-015-9550-z
90. Chauhan, AK, Mittra, N, Singh, BK, and Singh, C. Inhibition of glutathione S-transferase-pi triggers c-Jun N-terminal kinase-dependent neuronal death in Zn-induced parkinsonism. Mol Cell Biochem. (2019) 452:95–104. doi: 10.1007/s11010-018-3415-8
91. Mohamad, KA, El-Naga, RN, and Wahdan, SA. Neuroprotective effects of indole-3-carbinol on the rotenone rat model of Parkinson's disease: impact of the SIRT1-AMPK signaling pathway. Toxicol Appl Pharmacol. (2022) 435:115853. doi: 10.1016/j.taap.2021.115853
92. Yoo, IH, Kim, MJ, Kim, J, Sung, JJ, Park, ST, and Ahn, SW. The anti-inflammatory effect of sulforaphane in mice with experimental autoimmune encephalomyelitis. J Korean Med Sci. (2019) 34:e197. doi: 10.3346/jkms.2019.34.e197
93. Giacoppo, S, Soundara Rajan, T, De Nicola, GR, Iori, R, Bramanti, P, and Mazzon, E. Moringin activates Wnt canonical pathway by inhibiting GSK3β in a mouse model of experimental autoimmune encephalomyelitis. Drug Des Devel Ther. (2016) 10:3291–304. doi: 10.2147/DDDT.S110514
94. Omotoso, GO, Gbadamosi, IT, Afolabi, TT, Abdulwahab, AB, and Akinlolu, AA. Ameliorative effects of Moringa on cuprizone-induced memory decline in rat model of multiple sclerosis. Anat Cell Biol. (2018) 51:119–27. doi: 10.5115/acb.2018.51.2.119
95. Omotoso, GO, Kolo, RM, Afolabi, T, Jaji-Sulaimon, R, and Gbadamosi, IT. Moringa oleifera ameliorates histomorphological changes associated with cuprizone neurotoxicity in the hippocampal cornu ammonis (CA) 3 region. Niger J Physiol Sci. (2018) 33:95–9.
96. Giacoppo, S, Iori, R, Bramanti, P, and Mazzon, E. Topical moringin-cream relieves neuropathic pain by suppression of inflammatory pathway and voltage-gated ion channels in murine model of multiple sclerosis. Mol Pain. (2017) 13:174480691772431. doi: 10.1177/1744806917724318
97. Galuppo, M, Giacoppo, S, Iori, R, De Nicola, GR, Bramanti, P, and Mazzon, E. Administration of 4-(α-L-rhamnosyloxy)-benzyl isothiocyanate delays disease phenotype in SOD1(G93A) rats: a transgenic model of amyotrophic lateral sclerosis. Biomed Res Int. (2015) 2015:259417:1–12. doi: 10.1155/2015/259417
98. Luis-García, ER, Limón-Pacheco, JH, Serrano-García, N, Hernández-Pérez, AD, Pedraza-Chaverri, J, and Orozco-Ibarra, M. Sulforaphane prevents quinolinic acid-induced mitochondrial dysfunction in rat striatum. J Biochem Mol Toxicol. (2017) 31. doi: 10.1002/jbt.21837
99. Petrillo, S, Piermarini, E, Pastore, A, Vasco, G, Schirinzi, T, Carrozzo, R, et al. Nrf2-inducers counteract neurodegeneration in Frataxin-silenced motor neurons: disclosing new therapeutic targets for Friedreich's Ataxia. Int J Mol Sci. (2017) 18:2173. doi: 10.3390/ijms18102173
100. Petrillo, S, D'Amico, J, La Rosa, P, Bertini, ES, and Piemonte, F. Targeting Nrf2 for the treatment of Friedreich's Ataxia: a comparison among drugs. Int J Mol Sci. (2019) 20:5211. doi: 10.3390/ijms20205211
101. Santos, E, Clark, C, Biag, HMB, Tang, SJ, Kim, K, Ponzini, MD, et al. Open-label sulforaphane trial in FMR1 premutation carriers with fragile-x-associated tremor and ataxia syndrome (FXTAS). Cells. (2023) 12:2773. doi: 10.3390/cells12242773
102. Napoli, E, Flores, A, Mansuri, Y, Hagerman, RJ, and Giulivi, C. Sulforaphane improves mitochondrial metabolism in fibroblasts from patients with fragile X-associated tremor and ataxia syndrome. Neurobiol Dis. (2021) 157:105427. doi: 10.1016/j.nbd.2021.105427
103. Redondo, A, Chamorro, PAF, Riego, G, Leánez, S, and Pol, O. Treatment with sulforaphane produces antinociception and improves morphine effects during inflammatory pain in mice. J Pharmacol Exp Ther. (2017) 363:293–302. doi: 10.1124/jpet.117.244376
104. Wang, X, de Rivero Vaccari, JP, Wang, H, Diaz, P, German, R, Marcillo, AE, et al. Activation of the nuclear factor E2-related factor 2/antioxidant response element pathway is neuroprotective after spinal cord injury. J Neurotrauma. (2012) 29:936–45. doi: 10.1089/neu.2011.1922
105. Mao, L, Wang, H, Wang, X, Liao, H, and Zhao, X. Transcription factor Nrf2 protects the spinal cord from inflammation produced by spinal cord injury. J Surg Res. (2011) 170:e105–15. doi: 10.1016/j.jss.2011.05.049
106. Benedict, AL, Mountney, A, Hurtado, A, Bryan, KE, Schnaar, RL, Dinkova-Kostova, AT, et al. Neuroprotective effects of sulforaphane after contusive spinal cord injury. J Neurotrauma. (2012) 29:2576–86. doi: 10.1089/neu.2012.2474
107. Galea, I, Copple, IM, Howat, DW, and Franklin, S. SFX-01 reduces residual disability after experimental autoimmune encephalomyelitis. Mult Scler Relat Disord. (2019) 30:257–61. doi: 10.1016/j.msard.2019.02.027
108. Mammana, S, Gugliandolo, A, Cavalli, E, Diomede, F, Iori, R, Zappacosta, R, et al. Human gingival mesenchymal stem cells pretreated with vesicular moringin nanostructures as a new therapeutic approach in a mouse model of spinal cord injury. J Tissue Eng Regen Med. (2019) 13:1109–21. doi: 10.1002/term.2857
109. Giacoppo, S, Galuppo, M, De Nicola, GR, Iori, R, Bramanti, P, and Mazzon, E. 4(α-l-rhamnosyloxy)-benzyl isothiocyanate, a bioactive phytochemical that attenuates secondary damage in an experimental model of spinal cord injury. Bioorg Med Chem. (2015) 23:80–8. doi: 10.1016/j.bmc.2014.11.022
110. Yu, C, He, Q, Zheng, J, Li, LY, Hou, YH, and Song, FZ. Sulforaphane improves outcomes and slows cerebral ischemic/reperfusion injury via inhibition of NLRP3 inflammasome activation in rats. Int Immunopharmacol. (2017) 45:74–8. doi: 10.1016/j.intimp.2017.01.034
111. Ma, LL, Xing, GP, Yu, Y, Liang, H, Yu, TX, Zheng, WH, et al. Sulforaphane exerts neuroprotective effects via suppression of the inflammatory response in a rat model of focal cerebral ischemia. Int J Clin Exp Med. (2015) 8:17811–7.
112. Mao, L, Yang, T, Li, X, Lei, X, Sun, Y, Zhao, Y, et al. Protective effects of sulforaphane in experimental vascular cognitive impairment: contribution of the Nrf2 pathway. J Cereb Blood Flow Metab. (2019) 39:352–66. doi: 10.1177/0271678X18764083
113. Zhao, X, Wen, L, Dong, M, and Lu, X. Sulforaphane activates the cerebral vascular Nrf2-ARE pathway and suppresses inflammation to attenuate cerebral vasospasm in rat with subarachnoid hemorrhage. Brain Res. (2016) 1653:1–7. doi: 10.1016/j.brainres.2016.09.035
114. Yin, XP, Chen, ZY, Zhou, J, Wu, D, and Bao, B. Mechanisms underlying the perifocal neuroprotective effect of the Nrf2-ARE signaling pathway after intracranial hemorrhage. Drug Des Devel Ther. (2015) 9:5973–86. doi: 10.2147/DDDT.S79399
115. Kyyriäinen, J, Kajevu, N, Bañuelos, I, Lara, L, Lipponen, A, Balosso, S, et al. Targeting oxidative stress with antioxidant duotherapy after experimental traumatic brain injury. Int J Mol Sci. (2021) 22:10555. doi: 10.3390/ijms221910555
116. Galuppo, M, Giacoppo, S, Iori, R, De Nicola, GR, Milardi, D, Bramanti, P, et al. 4(α-l-rhamnosyloxy)-benzyl isothiocyanate, a bioactive phytochemical that defends cerebral tissue and prevents severe damage induced by focal ischemia/reperfusion. J Biol Regul Homeost Agents. (2015) 29:343–56.
117. Caglayan, B, Kilic, E, Dalay, A, Altunay, S, Tuzcu, M, Erten, F, et al. Allyl isothiocyanate attenuates oxidative stress and inflammation by modulating Nrf2/HO-1 and NF-κB pathways in traumatic brain injury in mice. Mol Biol Rep. (2019) 46:241–50. doi: 10.1007/s11033-018-4465-4
118. Riederer, P, Korczyn, AD, Ali, SS, Bajenaru, O, Choi, MS, Chopp, M, et al. The diabetic brain and cognition. J Neural Transm (Vienna). (2017) 124:1431–54. doi: 10.1007/s00702-017-1763-2
119. Sajja, RK, Prasad, S, Tang, S, Kaisar, MA, and Cucullo, L. Blood-brain barrier disruption in diabetic mice is linked to Nrf2 signaling deficits: role of ABCB10? Neurosci Lett. (2017) 653:152–8. doi: 10.1016/j.neulet.2017.05.059
120. Jiménez-Osorio, AS, González-Reyes, S, and Pedraza-Chaverri, J. Natural Nrf2 activators in diabetes. Clin Chim Acta. (2015) 448:182–92. doi: 10.1016/j.cca.2015.07.009
121. Wang, G, Fang, H, Zhen, Y, Xu, G, Tian, J, Zhang, Y, et al. Sulforaphane prevents neuronal apoptosis and memory impairment in diabetic rats. Cell Physiol Biochem. (2016) 39:901–7. doi: 10.1159/000447799
122. Pu, D, Zhao, Y, Chen, J, sun, Y, Lv, A, Zhu, S, et al. Protective effects of sulforaphane on cognitive impairments and ad-like lesions in diabetic mice are associated with the upregulation of Nrf2 transcription activity. Neuroscience. (2018) 381:35–45. doi: 10.1016/j.neuroscience.2018.04.017
123. Moustafa, PE, Abdelkader, NF, El Awdan, SA, El-Shabrawy, OA, and Zaki, HF. Extracellular matrix remodeling and modulation of inflammation and oxidative stress by sulforaphane in experimental diabetic peripheral neuropathy. Inflammation. (2018) 41:1460–76. doi: 10.1007/s10753-018-0792-9
124. Sharma, P, Kaushik, P, Jain, S, Sharma, BM, Awasthi, R, Kulkarni, GT, et al. Efficacy of ulinastatin and sulforaphane alone or in combination in rat model of streptozotocin diabetes induced vascular dementia. Clin Psychopharmacol Neurosci. (2021) 19:470–89. doi: 10.9758/cpn.2021.19.3.470
125. Schlotterer, A, Masri, B, Humpert, M, Krämer, BK, Hammes, HP, and Morcos, M. Sulforaphane and vitamin E protect from glucotoxic neurodegeneration and lifespan reduction in C. elegans. Exp Clin Endocrinol Diabetes. (2021) 129:887–94. doi: 10.1055/a-1158-9248
126. Tang, L, Ren, X, Han, Y, Chen, L, Meng, X, Zhang, C, et al. Sulforaphane attenuates apoptosis of hippocampal neurons induced by high glucose via regulating endoplasmic reticulum. Neurochem Int. (2020) 136:104728. doi: 10.1016/j.neuint.2020.104728
127. Ademosun, AO, Oboh, G, and Ajeigbe, OF. Influence of Moringa (Moringa oleifera) enriched ice creams on rats' brain: exploring the redox and cholinergic systems. Curr Res Food Sci. (2022) 5:366–73. doi: 10.1016/j.crfs.2022.01.021
128. Sun, Y, Zhao, C, Liu, YY, Sui, YL, and Zhu, JZ. Effects of spicy leaves on cognitive function and apoptosis of hippocampal neurons in diabetic rats. Zhongguo Ying Yong Sheng Li Xue Za Zhi. (2021) 37:638–43. doi: 10.12047/j.cjap.6123.2021.070
129. Oboh, G, Oyeleye, SI, Akintemi, OA, and Olasehinde, TA. Moringa oleifera supplemented diet modulates nootropic-related biomolecules in the brain of STZ-induced diabetic rats treated with acarbose. Metab Brain Dis. (2018) 33:457–66. doi: 10.1007/s11011-018-0198-2
130. Ghazizadeh-Hashemi, F, Bagheri, S, Ashraf-Ganjouei, A, Moradi, K, Shahmansouri, N, Mehrpooya, M, et al. Efficacy and safety of sulforaphane for treatment of mild to moderate depression in patients with history of cardiac interventions: a randomized, double-blind, placebo-controlled clinical trial. Psychiatry Clin Neurosci. (2021) 75:250–5. doi: 10.1111/pcn.13276
131. Wu, S, Gao, Q, Zhao, P, Gao, Y, Xi, Y, Wang, X, et al. Sulforaphane produces antidepressant-and anxiolytic-like effects in adult mice. Behav Brain Res. (2016) 301:55–62. doi: 10.1016/j.bbr.2015.12.030
132. Zhang, JC, Yao, W, Dong, C, Yang, C, Ren, Q, Ma, M, et al. Prophylactic effects of sulforaphane on depression-like behavior and dendritic changes in mice after inflammation. J Nutr Biochem. (2017) 39:134–44. doi: 10.1016/j.jnutbio.2016.10.004
133. Ferreira-Chamorro, P, Redondo, A, Riego, G, Leánez, S, and Pol, O. Sulforaphane inhibited the nociceptive responses, anxiety-and depressive-like behaviors associated with neuropathic pain and improved the anti-allodynic effects of morphine in mice. Front Pharmacol. (2018) 9:1332. doi: 10.3389/fphar.2018.01332
134. Wang, W, Wei, C, Quan, M, Li, T, and Jia, J. Sulforaphane reverses the amyloid-β oligomers induced depressive-like behavior. J Alzheimers Dis. (2020) 78:127–37. doi: 10.3233/JAD-200397
135. Tucci, P, Bove, M, Sikora, V, Dimonte, S, Morgese, MG, Schiavone, S, et al. Glucoraphanin triggers rapid antidepressant responses in a rat model of beta amyloid-induced depressive-like behaviour. Pharmaceuticals (Basel). (2022) 15:1054. doi: 10.3390/ph15091054
136. Yao, W, Zhang, JC, Ishima, T, Dong, C, Yang, C, Ren, Q, et al. Role of Keap1-Nrf2 signaling in depression and dietary intake of glucoraphanin confers stress resilience in mice. Sci Rep. (2016) 6:30659. doi: 10.1038/srep30659
137. Islam, MT, Martins, N, Imran, M, Hameed, A, Ali, SW, Salehi, B, et al. Anxiolytic-like effects of Moringa oleifera in Swiss mice. Cell Mol Biol (Noisy-le-Grand). (2020) 66:73–7. doi: 10.14715/cmb/2020.66.4.12
138. Purwoningsih, E, Arozal, W, Lee, HJ, Barinda, AJ, Sani, Y, and Munim, A. The oil formulation derived from Moringa oleifera seeds ameliorates behavioral abnormalities in water-immersion restraint stress mouse model. J Exp Pharmacol. (2022) 14:395–407. doi: 10.2147/JEP.S386745
139. Mahmoud, MS, El-Kott, AF, AlGwaiz, HIM, and Fathy, SM. Protective effect of Moringa oleifera lam. Leaf extract against oxidative stress, inflammation, depression, and apoptosis in a mouse model of hepatic encephalopathy. Environ Sci Pollut Res Int. (2022) 29:83783–96. doi: 10.1007/s11356-022-21453-x
140. Cojocariu, RO, Balmus, IM, Lefter, R, Hritcu, L, Ababei, DC, Ciobica, A, et al. Camelina sativa methanolic and ethanolic extract potential in alleviating oxidative stress, memory deficits, and affective impairments in stress exposure-based irritable bowel syndrome mouse models. Oxidative Med Cell Longev. (2020) 2020:1–20. doi: 10.1155/2020/9510305
141. Nicosia, N, Kwiecień, I, Mazurek, J, Mika, K, Bednarski, M, Miceli, N, et al. Hydroalcoholic leaf extract of Isatis tinctoria L. via antioxidative and anti-inflammatory effects reduces stress-induced behavioral and cellular disorders in mice. Oxidative Med Cell Longev. (2022) 2022:1–18. doi: 10.1155/2022/3567879
142. Siddiq, A, and Younus, I. The radish, Raphanus sativus L. Var. caudatus reduces anxiety-like behavior in mice. Metab Brain Dis. (2018) 33:1255–60. doi: 10.1007/s11011-018-0240-4
143. Pan, S, Ma, Y, Yang, R, Lu, X, You, Q, Ye, T, et al. Indole-3-carbinol selectively prevents chronic stress-induced depression-but not anxiety-like behaviors via suppressing pro-inflammatory cytokine production and oxido-nitrosative stress in the brain. Front Pharmacol. (2022) 13:829966. doi: 10.3389/fphar.2022.829966
144. Latté, KP, Appel, KE, and Lampen, A. Health benefits and possible risks of broccoli - an overview. Food Chem Toxicol. (2011) 49:3287–309. doi: 10.1016/j.fct.2011.08.019
145. Vanderpas, J. Nutritional epidemiology and thyroid hormone metabolism. Annu Rev Nutr. (2006) 26:293–322. doi: 10.1146/annurev.nutr.26.010506.103810
146. Felker, P, Bunch, R, and Leung, AM. Concentrations of thiocyanate and goitrin in human plasma, their precursor concentrations in Brassica vegetables, and associated potential risk for hypothyroidism. Nutr Rev. (2016) 74:248–58. doi: 10.1093/nutrit/nuv110
147. Langer, P, Michajlovskij, N, Sedlák, J, and Kutka, M. Studies on the antithyroid activity of naturally occurring L-5-vinyl-2-thiooxazolidone in man. Endokrinologie. (1971) 57:225–9.
148. Galanty, A, Grudzińska, M, Paździora, W, Służały, P, and Paśko, P. Do Brassica vegetables affect thyroid function?- a comprehensive systematic review. Int J Mol Sci. (2024) 25:3988. doi: 10.3390/ijms25073988
149. Zanichelli, F, Capasso, S, Cipollaro, M, Pagnotta, E, Cartenì, M, Casale, F, et al. Dose-dependent effects of R-sulforaphane isothiocyanate on the biology of human mesenchymal stem cells, at dietary amounts, it promotes cell proliferation and reduces senescence and apoptosis, while at anti-cancer drug doses, it has a cytotoxic effect. Age (Dordr). (2012) 34:281–93. doi: 10.1007/s11357-011-9231-7
150. Zanichelli, F, Capasso, S, di Bernardo, G, Cipollaro, M, Pagnotta, E, Cartenì, M, et al. Low concentrations of isothiocyanates protect mesenchymal stem cells from oxidative injuries, while high concentrations exacerbate DNA damage. Apoptosis. (2012) 17:964–74. doi: 10.1007/s10495-012-0740-3
151. Sestili, P, Paolillo, M, Lenzi, M, Colombo, E, Vallorani, L, Casadei, L, et al. Sulforaphane induces DNA single strand breaks in cultured human cells. Mutat Res. (2010) 689:65–73. doi: 10.1016/j.mrfmmm.2010.05.003
152. Sekine-Suzuki, E, Yu, D, Kubota, N, Okayasu, R, and Anzai, K. Sulforaphane induces DNA double strand breaks predominantly repaired by homologous recombination pathway in human cancer cells. Biochem Biophys Res Commun. (2008) 377:341–5. doi: 10.1016/j.bbrc.2008.09.150
153. Xie, J, Yang, MR, Hu, X, Hong, ZS, Bai, YY, Sheng, J, et al. Isothiocyanate quinazolinone derivatives inhibit U251 glioma cell proliferation through cell cycle regulation and apoptosis induction. Int J Mol Sci. (2023) 24:11376. doi: 10.3390/ijms241411376
154. Hać, A, Brokowska, J, Rintz, E, Bartkowski, M, Węgrzyn, G, and Herman-Antosiewicz, A. Mechanism of selective anticancer activity of isothiocyanates relies on differences in DNA damage repair between cancer and healthy cells. Eur J Nutr. (2020) 59:1421–32. doi: 10.1007/s00394-019-01995-6
155. Yagishita, Y, Fahey, JW, Dinkova-Kostova, AT, and Kensler, TW. Broccoli or sulforaphane: is it the source or dose that matters? Molecules. (2019) 24:3593. doi: 10.3390/molecules24193593
156. Lüthy, B, and Matile, P. The mustard oil bomb: rectified analysis of the subcellular organisation of the myrosinase system. Biochem Physiol Pflanz. (1984) 179:5–12. doi: 10.1016/S0015-3796(84)80059-1
157. Bierwirth, JE, Oftedal, KN, Civille, GV, and Fahey, JW. Flavor misattribution: a novel approach to improving compliance and blinding in food-based clinical interventions. NFS J. (2015) 1:24–30. doi: 10.1016/j.nfs.2015.07.001
158. Fahey, JWWK, Stephenson, KK, Shi, Y, Liu, H, Panjwani, AA, Warrick, CR, et al. A strategy to deliver precise oral doses of the glucosinolates or isothiocyanates from moringa oleifera leaves for use in clinical studies. Nutrients. (2019) 11:1547. doi: 10.3390/nu11071547
159. Houghton, CA. The rationale for sulforaphane favourably influencing gut homeostasis and gut-organ dysfunction: a clinician's hypothesis. Int J Mol Sci. (2023) 24:13448. doi: 10.3390/ijms241713448
160. Grosso, C, Santos, M, and Barroso, MF. From plants to psycho-neurology: unravelling the therapeutic benefits of bioactive compounds in brain disorders. Antioxidants (Basel). (2023) 12:1603. doi: 10.3390/antiox12081603
161. Amro, MS, Teoh, SL, Norzana, AG, and Srijit, D. The potential role of herbal products in the treatment of Parkinson's disease. Clin Ter. (2018) 169:e23–33. doi: 10.7417/T.2018.2050
162. Makkar, R, Behl, T, Bungau, S, Zengin, G, Mehta, V, Kumar, A, et al. Nutraceuticals in neurological disorders. Int J Mol Sci. (2020) 21:4424. doi: 10.3390/ijms21124424
163. Arias-Sánchez, RA, Torner, L, and Fenton, NB. Polyphenols and neurodegenerative diseases: potential effects and mechanisms of neuroprotection. Molecules. (2023) 28:5415. doi: 10.3390/molecules28145415
164. Minocha, T, Birla, H, Obaid, AA, Rai, V, Sushma, P, Shivamallu, C, et al. Flavonoids as promising neuroprotectants and their therapeutic potential against Alzheimer's disease. Oxidative Med Cell Longev. (2022) 2022:1–13. doi: 10.1155/2022/6038996
165. Iriti, M, Vitalini, S, Fico, G, and Faoro, F. Neuroprotective herbs and foods from different traditional medicines and diets. Molecules. (2010) 15:3517–55. doi: 10.3390/molecules15053517
166. Murugesan, V, Govindraj, R, Amarnath Satheesh, M, and Rajagopal, S. Phytonutrients in neurological disorders In: S Rajagopal, S Ramachandran, G Sundararaman, and S Gadde Venkata, editors. Role of nutrients in neurological disorders. Singapore: springer Singapore (2022). 3–15.
167. Garodia, P, Hegde, M, Kunnumakkara, AB, and Aggarwal, BB. Curcumin, inflammation, and neurological disorders: how are they linked? Integr Med Res. (2023) 12:100968. doi: 10.1016/j.imr.2023.100968
168. Joshi, P, Bisht, A, Joshi, S, Semwal, D, Nema, NK, Dwivedi, J, et al. Ameliorating potential of curcumin and its analogue in central nervous system disorders and related conditions: a review of molecular pathways. Phytother Res. (2022) 36:3143–80. doi: 10.1002/ptr.7522
169. Chiang, MC, Tsai, TY, and Wang, CJ. The potential benefits of quercetin for brain health: a review of anti-inflammatory and neuroprotective mechanisms. Int J Mol Sci. (2023) 24:6328. doi: 10.3390/ijms24076328
170. Yadav, E, Yadav, P, Khan, MMU, Singh, H, and Verma, A. Resveratrol: a potential therapeutic natural polyphenol for neurodegenerative diseases associated with mitochondrial dysfunction. Front Pharmacol. (2022) 13:922232. doi: 10.3389/fphar.2022.922232
171. Kempuraj, D, Thangavel, R, Kempuraj, DD, Ahmed, ME, Selvakumar, GP, Raikwar, SP, et al. Neuroprotective effects of flavone luteolin in neuroinflammation and neurotrauma. Biofactors. (2021) 47:190–7. doi: 10.1002/biof.1687
172. Patrick, RP, and Ames, BN. Vitamin D and the omega-3 fatty acids control serotonin synthesis and action, part 2: relevance for ADHD, bipolar disorder, schizophrenia, and impulsive behavior. FASEB J. (2015) 29:2207–22. doi: 10.1096/fj.14-268342
173. Okereke, OI, Vyas, CM, Mischoulon, D, Chang, G, Cook, NR, Weinberg, A, et al. Effect of long-term supplementation with marine omega-3 fatty acids vs placebo on risk of depression or clinically relevant depressive symptoms and on change in mood scores: a randomized clinical trial. JAMA. (2021) 326:2385–94. doi: 10.1001/jama.2021.21187
174. Kang, JH, Vyas, CM, Okereke, OI, Ogata, S, Albert, M, Lee, IM, et al. Effect of vitamin D on cognitive decline: results from two ancillary studies of the VITAL randomized trial. Sci Rep. (2021) 11:23253. doi: 10.1038/s41598-021-02485-8
Keywords: sulforaphane, glucosinolate, cruciferous, neurodevelopmental, neurodegenerative, neuroinflammation
Citation: Ramakrishnan M, Fahey JW, Zimmerman AW, Zhou X and Panjwani AA (2024) The role of isothiocyanate-rich plants and supplements in neuropsychiatric disorders: a review and update. Front. Nutr. 11:1448130. doi: 10.3389/fnut.2024.1448130
Edited by:
Amanda N. Carey, Simmons University, United StatesReviewed by:
Jong-Sang Kim, Kyungpook National University, Republic of KoreaFranziska S. Hanschen, Leibniz Institute of Vegetable and Ornamental Crops, Germany
Copyright © 2024 Ramakrishnan, Fahey, Zimmerman, Zhou and Panjwani. This is an open-access article distributed under the terms of the Creative Commons Attribution License (CC BY). The use, distribution or reproduction in other forums is permitted, provided the original author(s) and the copyright owner(s) are credited and that the original publication in this journal is cited, in accordance with accepted academic practice. No use, distribution or reproduction is permitted which does not comply with these terms.
*Correspondence: Anita A. Panjwani, YXBhbmp3YW5AcHVyZHVlLmVkdQ==