- RAMSES Laboratory, Rizzoli RIT-Research, Innovation & Technology Department, Istituto di Ricerca Codivilla Putti, IRCCS Istituto Ortopedico Rizzoli, Bologna, Italy
Nutraceuticals are gaining popularity as they can contribute to bone health by delaying the onset or slowing down the progression of pathological bone loss. Osteoporosis’s bone loss is a concern for older adults and a crucial aspect of aging. Maintaining healthy bones is the key to living a full and active life. Our review explores the current knowledge on the role of nutraceuticals in preventing osteoporosis by focusing on three main aspects. First, we provide an overview of osteoporosis. Second, we discuss the latest findings on natural nutraceuticals and their efficacy in reducing bone loss, emphasizing clinical trials. Third, we conduct a structured analysis to evaluate nutraceuticals’ pros and cons and identify translational gaps. In conclusion, we must address several challenges to consolidate our knowledge, better support clinicians in their prescriptions, and provide people with more reliable nutritional recommendations to help them lead healthier lives.
1 Introduction
Osteoporosis is a common, debilitating, chronic, progressive, systemic, and metabolic skeletal disease. Bone loss is not just a concern for older adults but a crucial aspect of aging. Maintaining healthy bones is the key to living a full and active life (1–3).
Osteoporotic patient management carries significant health, social, and economic burdens on countries with an increase in the population’s average age. Financial costs are direct, especially concerning treating osteoporotic fractures, and indirect, such as functional disability and loss of productivity. Bone loss prevention can significantly diminish the health care costs and improve the quality of life (4). Available therapeutic options are effective but may present side effects, which can cause discontinuing therapy (5). Moreover, a part of the high-risk fracture population does not get proper care due to cultural and socio-economic factors and a recent diffused skepticism towards official medicine (6).
Several compounds that fall under the definition of nutraceuticals can complement pharmacological treatments aimed at increasing bone mineral density and preventing bone tissue loss (7, 8). Our literature review will highlight the latest developments in natural nutraceuticals that promote bone health and prevent bone loss. Lastly, we will analyze nutraceutical pros and cons, with a focus on their pros and cons.
2 Osteopenia and osteoporosis
2.1 Bone
Bone is a mineralized connective tissue exerting essential functions: muscle attachment and locomotion, soft tissue support, vital organ protection, bone marrow harboring, calcium (Ca) and phosphate (H3PO4) storage, and homeostasis (9). Mature bone tissue includes an abundant calcified extracellular matrix (ECM) that supports and interacts with the resident cell types contributing to bone development and maintenance: osteoblasts, osteocytes, osteoclasts, and bone lining cells.
Mesenchymal stem cell (MSC)-derived osteoblasts serve for bone formation; osteocytes are terminally differentiated mature osteoblasts embedded in the mineralized bone, no longer involved in bone formation, but helping control the remodeling process. Osteoclasts, derived from hematopoietic precursors, degrade mineralized ECM (bone resorption). Bone lining cells are quiescent osteoblasts localized at bone surfaces, where bone resorption and bone formation do not take place (9).
Besides cells, the ECM comprises a nanostructured organic phase rich in collagen (mainly type I collagen, mucopolysaccharides, and water), which provides flexibility and tensile strength, and an inorganic mineral phase presenting nanocrystalline hydroxyapatite (Ca and H3PO4), which ensures bone mechanical rigidity and compressive strength (10–12).
2.2 Bone remodeling
Resorption and formation are the two main phases of bone remodeling involving mechanical and chemical signals together with systemic and local endogenous factors that can act synergically or antagonistically. Dis-regulations within this complicated functional network can handle pathological conditions or diseases and thus be the target of the treatment (13, 14).
The bone remodeling cycle comprises five steps: activation, resorption, reversal, formation, and termination/quiescence. Osteocytes detect bone matrix damage and communicate with the other cells through their network of dendrites to start bone remodeling process (16, 17). During activation, osteoclast precursors differentiate towards mature osteoclasts, which attach to the ECM. This way, osteoclasts expose the bone surface and isolate the resorbing compartment from the surrounding bone (15). During resorption, osteoclasts degrade the bone ECM and, in the end, undergo programmed cell death (18). Osteoclasts may either secrete cytokines or act via a regulatory surface receptor to release osteogenic signals to prompt bone formation (19, 20). The reversal phase switches to osteoblast-mediated bone formation, promoting new bone deposition. In the formation phase, pre-osteoblasts continue their commitment toward differentiated cells, secreting molecules to aid bone formation. In the termination/quiescence phase, remodeling process concludes with an equal amount of resorbed and newly formed bone (21, 22). After mineralization, osteoblasts may undergo apoptosis, become bone-lining cells, or differentiate into osteocytes. The bone surface undergoes a new cycle.
2.3 Risk factors
Risk factors can be unmodifiable or modifiable or associated with concomitant diseases or the use of specific drugs that can cause bone loss (23).
The main non-modifiable risk factors are: (i) age, osteoporosis is a typical disease of aging; (ii) gender, women are more susceptible to osteoporosis than men due to a lighter and thinner skeleton (23); (iii) menopause, the sharp decline in estrogen levels after menopause and longer average lifespan contribute to increased risk; (iv) genetics, numerous loci are associated with bone mineral density (BMD), and others are related to bone shape, geometry, and microarchitecture (24); (v) ethnicity, osteoporosis prevails in Caucasian and Asian populations than in African and Hispanic ones.
The main modifiable risk factors concern healthy lifestyle habits and often have essential consequences on the gut microbiota (GM) (25). Other modifiable risk factors are: (i) insufficient Ca consumption and hypovitaminosis D that can lead to secondary hyperparathyroidism, which increases bone resorption (26); (ii) low intakes of fruits and vegetables can deprive the body of essential nutrients like magnesium (Mg), potassium (K), and vitamin K; (iii) consuming excessive proteins, sodium, and caffeine can cause Ca loss through urine; (iv) excessive alcohol consumption can reduce new bone formation and increase the risk of fractures; (v) tobacco smoke affects the receptor activator of nuclear factor kappa-light-chain-enhancer of activated B cells (NF-kB) and RANK-RANKL-OPG pathway, directly and indirectly influencing BMD and intestinal microbiota (27); (vi) being excessively thin and having a slight build can increase the likelihood of osteoporosis. Osteoblasts produce receptor activator of nuclear factor kappa beta RANKL, and its binding to RANK supports osteoclastogenesis. Osteoblasts also secrete OPG, which competes with RANK for RANKL, inhibiting osteoclastogenesis. A sedentary life facilitates bone loss, the opposite of low-to-moderate physical activity (28); the skeleton reacts to the reduction of the forces applied by the muscles on the bone, reducing its mineralization and weakening; on the contrary, even moderate but regular physical activity helps maintain bone density. Comorbidities may affect the osteoporosis course and increase the risk of multiple fractures: many diseases or disorders can increase the risk of osteoporosis, directly or indirectly. Moreover, they require the use of specific drugs, which in turn negatively affect the skeleton or imply inactivity or reduced mobility (29). Further, air pollution is associated with decreased bone mass (30).
2.4 Epidemiology and etiology
Osteopenia and osteoporosis occur when BMD decreases due to an osteo-metabolic imbalance that disrupts the microarchitecture of bone tissue (31). The third decade of life marks the peak of bone mass, and the exact age varies with gender and skeletal site. After peaking, both sexes undergo a decline in bone mass, and the loss can reach pathological levels, like during menopause in women.
Osteopenia indicates a BMD value lower than the average reference but not low enough to reach the diagnostic criteria of osteoporosis (32). Osteoporosis affects approximately 200 million women worldwide, often resulting in painful fractures (33). A high fracture risk involves about 23 million people in the European Union (34).
Primary osteoporosis forms are related to female post-menopausal state (Type I) due to estrogen deficiency or advancing age (Type II) in men and women. Secondary osteoporosis derives from pre- or co-existing pathologies, medical conditions, or medications interfering with physiological bone formation (35).
The term “fragility fractures” indicates all the fractures resulting from low-level or low-energy trauma (e.g., a fall from standing height or less), which are generally considered the clinical outcome of osteoporosis (36). The most relevant consequence is that a patient may be at high risk of experiencing a secondary fracture in the first 2 years after the initial fracture. The severe complications include increasing morbidity and mortality risks (37, 38).
2.5 Diagnosis and therapeutical approaches
According to the World Health Organization’s (WHO) indications, osteopenia diagnosis relies mainly on BMD evaluation by densitometric investigation. The dual-energy x-ray absorptiometry (DEXA) technique at the lumbar spine, proximal femur, or total hip is recognized as a standard diagnostic criterion (39).
However, screening programs with standard DEXA in women over 50 are primarily available in Western countries (40); in men, a diagnosis is usually made only when a fracture occurs.
Other imaging techniques are quantitative computerized tomography, quantitative ultrasound, and conventional radiology (41, 42).
The first approach for treating osteoporosis is correcting or eliminating the “modifiable risk” factors. A dietary intake or supplementation with vitamin D and Ca is usually suggested (43) as a prerequisite for drug treatment.
Current pharmacological therapies pertain to either antiresorptive or osteoanabolic drugs or are dual-acting. Antiresorptive therapies, incrementing bone mass by inhibiting bone resorption, encompass bisphosphonates (the most extensively used osteoporosis drug), monoclonal antibody denosumab, estrogens, and selective estrogen receptor modulators (SERMs). Osteoanabolic therapies aim to increase bone mass by stimulating bone formation. The most frequent approach relies on parathyroid hormone (PTH) activating effects: Teriparatide is a recombinant human PTH and Abaloparatide is a synthetic analog acting like teriparatide. Romosozumab is a monoclonal antibody hindering sclerostin, a significant inhibitor of bone formation (44).
Therapies for osteoporosis can exert several adverse effects, such as gastrointestinal irritation, musculoskeletal discomfort, and bone pain. However, rare but severe adverse effects can occur upon long-term exposure or high doses, like osteonecrosis of the jaw or atypical femoral fractures (45).
3 Nutraceuticals for bone health
The concept of health has evolved to emphasize a decreased risk of developing diseases rather than being a illness-free state. This shift has also brought attention to the protective and preventive role of healthy nutrition, conveying confidence in the benefits of nutraceuticals and increasing the demand and supply (46–51).
The term “nutraceutical” is a blend of “nutrient,” which refers to nourishing food, and “pharmaceutical,” which signifies medical drugs. Nutraceuticals offer health benefits that go beyond their traditional nutrients, such as preventing pathological conditions or in addition to conventional drugs (52). On the other hand, dietary supplements are products specifically formulated to enhance the diet by providing a concentrated or extracted form of nutrients (53).
Nutraceuticals are a vast, non-uniform, and ever-expanding group. They can be natural/traditional (directly derived from natural sources) or unnatural/non-traditional (artificially synthesized through agricultural breeding or biotechnology) (53).
Six main nutraceutical groups are beneficial for bone health: minerals, herbs, phytochemicals, dairy products, probiotics and prebiotics, dietary lipids, and melatonin (Figure 1). In the following paragraphs, we will resume the updated existing literature (54).
3.1 Minerals
This group includes essential human nutrients, except for boron (B).
3.1.1 Calcium
The 90% of Ca is in bones and teeth as salt. This mineral is crucial for the growth and development of the skeleton, bone mineralization, and achieves peak BMD during adolescence (55). Ca also plays a crucial role in enhancing the effectiveness of bisphosphonates (56). Oxalic and phytic acids in some foods and high sodium consumption can hinder Ca absorption (57).
Ca is key in bone regeneration pathways, including Wingless (Wnt) and β-catenin. Wnts can mediate antagonism of β-catenin signaling through Ca-dependent or independent mechanisms. Additionally, Ca participates in the crosstalk between β-catenin-dependent and independent pathways (58).
3.1.2.Magnesium
About 60% of the human body’s Mg remains in the bones. Like Ca, it can be mobilized by increased intestinal absorption to maintain serum levels, which is helpful for several body functions. Mg is essential for energy metabolism as it binds ATP and is a cofactor for many enzymes; its positive charge plays a vital role in membrane stabilization. It contrasts the acidic environment of inflammation and protects the skeleton from releasing inflammatory cytokines that stimulate bone loss (59). However, an excessive intake may interfere with the formation of hydroxyapatite crystals due to competition with Ca, consequently reducing bone mass. Conversely, Mg deficiency enhances osteoclast and reduces osteoblast activity, decreases bone stiffness, weakens the apatite crystal structure, and interferes with vitamin D and parathyroid hormone (PTH) and, consequently, Ca homeostasis (60, 61).
Like Ca, Mg can activate the β-catenin pathway and the Wnt signaling of human bone marrow MSCs (BMSCs), promoting bone regeneration. Moreover, by regulating RANK and RANKL, Mg supplementation could prevent bone resorption while promoting bone formation (58).
3.1.3 Phosphorus
Phosphorus (P) is predominantly found in bones and teeth in the hydroxyapatite form (mostly Ca hydroxyapatite), which is crucial for bone mineralization. P can be found as PO43−, which regulates bone cell cycle and metabolism and alters signal transduction pathways and gene expression.
High PO43− levels, in concomitance with low Ca intake, induce an increase of PTH, which, in turn, mobilizes Ca from bones (62). Excessive PO43− intake or hyperphosphatemia can lead to ectopic calcification and has been linked to various health issues, including bone disorders. Therefore, maintaining an appropriate PO43− balance in the body is crucial for overall bone health (58).
3.1.4 Potassium
Potassium (K) balances the endogenous acids produced by acid-generating foods like meat, maintaining an alkaline environment. This mechanism helps preserve bone Ca, which is available for mobilization to maintain normal pH. Therefore, consuming foods that are rich in K can help prevent Ca loss from the bone (62).
K-rich diets have been associated with a lower risk of osteoporosis. K-rich foods, such as fruits and vegetables, have an alkalizing effect: bones can neutralize blood acidosis conditions by releasing Ca, potentially reducing bone density. K supplementation improves Ca retention, decreasing bone resorption. High extra-cellular K levels activate the K channels in osteoblasts and osteoclasts, specifically the inwardly rectifying K and voltage-gated K channels. Osteoblasts undergo membrane hyperpolarization, triggering Ca influx and leading toward bone mineralization. On the contrary, osteoclasts are inhibited in their activity (63). Optimal K levels are necessary for collagen production and converting vitamin D to its active form is essential for Ca absorption and bone remodeling (58).
3.1.5 Iron
Iron (Fe) is an enzyme cofactor that helps modulate bone metabolism (62). In most cases, Fe ions are present in the insoluble oxide form that can be changed to a soluble form suitable for body functions. This conversion generates free radicals (64). Excess Fe or reactive oxygen species (ROS) has two effects on bone: they can either activate osteoclast or inhibit osteoblast activity. The first effect occurs through enhanced RANKL activation, suggesting an impact on Wnt signaling. The second is through the mitigation of the differentiation process conducted by bone morphogenic proteins (BMP) and Wnt, which trigger the Runt-related transcription factor 2 (Runx2) and xx (Osx) (58).
3.1.6 Zinc
Zinc (Zn) activates osteoblasts, favoring collagen synthesis and alkaline phosphatase activity. It can influence β-catenin signaling, evidenced by activating the Wnt pathway components Axin2 and LRP5. The osteoclast gene RANKL is also upregulated, suggesting Zn’s ability to regulate osteoclastogenesis (65). At least 20% of people are at risk of Zn deficiency, which correlates to osteoporosis (66).
3.1.7 Boron
Boron (B) is present in high concentrations in bone. It has beneficial effects in combination with Ca, Mg, and vitamin D. It boosts the half-life of vitamin D and estrogen, preserving them and preventing Ca loss and bone demineralization (67). Deficiency of B is associated with osteoporosis, possibly by modulating the Wnt/β-catenin pathway (58).
3.1.8 Copper
Copper (Cu) is involved in collagen fibril formation, angiogenesis, and osteogenic differentiation (62, 68, 69). It is a cofactor for antioxidant enzymes and destroys free radicals (70).
Cu has been found to play a role in activating the β-catenin signaling pathway and in down-regulating Wnt signaling (58).
3.2 Herbs and natural phytochemicals
Phytochemicals are chemical bioactive components of nutrient plants that may provide desirable health benefits beyond nutrition. They can reduce the risk of osteoporosis. Phytochemicals include several compounds: terpenoids, polyphenols, alkaloids, organosulfur compounds (OSCs), and phytosterols (71).
3.2.1 Curcumin
Curcuma longa commonly known as turmeric, noted for its coloring, flavoring and digestive properties, was originally used as a spice in India.
Curcumin (Cur), a polyphenolic chemical constituent derived from turmeric, can help prevent bone loss by reducing NF-κB, Wnt/β-catenin, RANKL, and tumor necrosis factor-alpha (TNF-α) (72–74), and enhancing the differentiation of osteoblasts from adipose tissue-derived human MSCs through inhibition of Wnt/β-catenin signaling (75).
Cur is a powerful antioxidant that prevents bone weakening by removing harmful molecules and reducing cell death. It achieves this by activating protein kinase B (Akt) to reduce the activity of glycogen synthase kinase-3 beta (Gsk3β), reducing the activity of a critical protective protein, nuclear factor-like 2 (Nrf2) (75).
Cur plays a role in bone resorption by blocking the differentiation of osteoclast precursors into osteoclasts by inhibiting the production of the chemokine CCL3. Cur inhibits osteoclast differentiation markers, including cathepsin K, matrix metalloprotein-9 (MMP-9), and MMP-13, by upregulating miR-365 expression.
Cur exerts immunomodulatory effects on macrophages by inhibiting inflammatory responses, reducing the release of inflammatory factors, and preventing osteoclast formation by improving Akt/NF-κB/NFATc1 signaling (76–81).
3.2.2 Alfalfa
Medicago sativa L. is a native plant of Eurasia that contains ipriflavone, which inhibits bone resorption, enhances osteoblast proliferation, induces estrogen-mediated calcitonin secretion, and strengthens estrogen-protecting action on bones (82, 83).
3.2.3 Red clover
Red clover is the extract from Trifolium pratense L. It contains genistein and diadzein, phytoestrogens with structural similarity to estrogen, thus preventing bone loss (84, 85).
3.2.4 Equisetum
Also known as horsetail, equisetum is a plant that grows naturally in the northern hemisphere. It contains silica, flavonoids, and triterpenoids, all beneficial for bone health (86, 87).
3.2.5 Citrus fruits
Citrus fruits contain carbohydrates, fiber, minerals, vitamins A, E, and B, and antioxidants such as flavonoids, vitamin C, phenolic compounds, and terpenoids (88).
Compared to other fruits, they are valuable sources of Ca (88–90). Two flavonoids, hesperidin and its aglycone, hesperetin, have protective roles in the osteogenesis of MSCs (90–95). In an ovariectomized mouse model of osteoporosis, hesperetin improved bone volume ratio and bone thickness and decreased trabecular separation (95). Liu et al. (96) also reported the resorption effect of hesperetin. The authors attributed these effects to the elimination of ROS and inhibition of NF-κB and mitogen-activated protein kinase (MAPK) signaling pathways. Further, authors found that hesperetin reduced trabecular bone loss in lipopolysaccharide-induced osteoporotic mice (96).
3.2.6 Alliaceae and Brassicaceae
Naturally derived organic sulfur compounds (OSCs) are molecules containing sulfur, predominantly found in edible plants belonging to the Allium and Brassica. OSCs promote cell proliferation and viability of MSCs while inhibiting the proliferation and viability of monocytes. OSCs promote osteogenic differentiation and bone formation, inhibit at different stages osteoclast differentiation, reduce bone erosion, and inhibit the viability of osteocytes. Additionally, certain OSCs, such as allicin, allyl sulfide, sulforaphane, and glucoraphanin, are known to modulate bone processes (97).
Polysulfides in Alliaceae, glucosinolates, and isothiocyanates in Brassicaceae have anti-inflammatory, antioxidant, vasorelaxant, and hypolipemic potential. These compounds can induce osteoblast and reduce osteoclast activities by releasing hydrogen sulfide, a gasotransmitter (97).
Flavonoids and organo-sulfur conjugates are present in onion (Allium cepa L.), which offers numerous bone health benefits (98), including inhibiting bone resorption (99, 100) and positively modulating BMD in peri- and postmenopausal women (101).
When administered in tablet form over 30 days, garlic (Allium sativum) can decrease oxidative stress in menopausal women, which may reduce osteoporosis onset and progression (102). Garlic and leek can also suppress bone resorption (103, 104).
3.2.7 Green tea
Green tea is a beverage derived from dried leaves of Camellia sinensis that contains catechins, like epicatechin and epigallocatechin (105). Epigallocatechin—gallate can suppress osteoclast formation and bone resorption by inducing osteoclast cell death, suppressing MMP-9 in osteoblast, inhibiting interleukin-6 (IL-6), suppressing p44/p42 mitogen-activated protein, and down-regulating RANKL-induced expression. At the same time, epigallocatechin-3-gallate can reduce TNF-α and IL-6, promoting osteoblast survival and positively acting on bone formation (99, 104).
3.2.8 Herba Epimedii
Herba Epimedii is a traditional Chinese medicine that contains icariin. This flavonoid glycoside can arrest inflammatory bone loss by inhibiting osteoclastogenesis-induced lipopolysaccharide, TNF-α, and IL-6 and producing type-2 cyclooxygenase and prostaglandin E2 (106).
3.2.9 Berries
Berries contain vitamins A, B9, C, E, and K, minerals, and carotenoids, and are rich in fumaric and citric acid. Berries possess antioxidant (107) and anti-inflammatory properties and can improve osteoblasts differentiation. For example, raspberry trans-retinoid acid and ketones stimulate osteoblast differentiation by improving osteocalcin expression in stem cell cultures.
In addition, berries contain phenolics like flavonoids (anthocyanins, flavonols, and flavanols), phenolic acids, proanthocyanidins, ellagitannins, gallotannins, and stilbenoids. Anthocyanins seem to inhibit RANKL and osteoclastogenesis (108).
3.2.10 Dried plums
Dried plums contain carbohydrates, vitamins A, B, and K, Ca, K, Mg, boron, selenium, dietary fibers, and polyphenols, such as chlorogenic acid, rutin, and proanthocyanidin. Polyphenols decrease bone resorption, acting on RANKL signaling (109). Administering dried plums to postmenopausal females increased insulin-like growth factor 1 (IGF-1) and bone-specific alkaline phosphatase (BSAP) levels (110).
3.2.11 Resveratrol
Resveratrol (RSV) is a polyphenol in red wine, nuts, grapes, and cranberries.
RSV functions as both anabolic and antiresorptive agent. Indeed, RSV is a SIRT1 activator or directly acts on Runx2 and Osx to regulate osteoblast differentiation; it also activates Runx2 and OSX expressions, RANKL/OPG, NFACT1, and NF-kB expressions to regulate osteoclast differentiation. In addition, it displays antioxidant and anti-inflammatory features (111, 112).
These dual actions of RSV on osteoblast and osteoclast are beneficial in maintaining balance in bone remodeling, a critical endpoint in the management of osteoporosis (113).
3.3 Dairy products
Milk and milk-derived products contain Ca, lipids, proteins, K, sodium, Zn, P, and vitamins A and B2, valuable substances for bone loss prevention. Functional milk contains compounds like casein phosphopeptide, milk basic protein (MBP), and lactoferrin, enhancing osteoblast proliferation and differentiation while preventing osteoclast formation (114–116). Oliveira et al. studied the effect of drinking water supplemented with milk extracellular vesicles (mEVs) on mice with diet-induced obesity and on OVX mice. Mice from both models showed a systemic and local decrease in the RANKL/OPG and were protected from bone loss and osteoclastogenesis. In vitro experiments corroborated those results (117).
Fermented dairy products, including yogurt, soft cheese, and Kefir, positively influence bone growth and homeostasis through different mechanisms involving intake of essential nutrients such as Ca P, protein, and potentially pre-and probiotics (118). Kefir is formed when milk is fermented by lactic acid bacteria and yeasts enclosed in a protein-and-polysaccharide matrix. Fermentation can negatively affect IGF-I due to lactic acid bacteria, which can utilize IGF-I or IGF-binding protein complex as their nutrition source (119). Dahiya et al. observed that kefir supplementation in OVX mice caused increased BMD (120).
Cheese is an excellent source of Ca, vitamin D, and high-quality proteins such as casein and whey, which contain all the essential amino acids except methionine and cysteine (121). These nutrients are essential for bone structure and maintenance. Parmigiano Reggiano cheese is considered a “functional food” for bone health and osteoporosis prevention due to its high-biological value proteins and easily accessible Ca (122). Swiss cheese contains H3PO4 and protein in large amounts. Gouda and Brie cheeses contain vitamin K2, which is associated with improved Ca metabolism and acts as a cofactor in glutamic acid carboxylation, essential for controlling bone metabolism (121).
3.4 Probiotics and prebiotics
Gut microbiota (GM) is a complex microflora consisting of millions of microbes in humans. Under physiological conditions, GM lives in a balanced state known as eubiosis, forming multidirectional connections with other organs such as the brain, gut, and bone axis while interacting with many pathways (123, 124).
Changes in GM can cause dysbiosis, impacting on bone remodeling and metabolism (125). This process can occur directly through extracellular vesicles (EVs), short-chain fatty acids (SCFAs), polyamines, and hydrogen sulfide or indirectly by interacting with immune cells or hormones (126). The GM may regulate bone metabolism by interacting with the Wnt/β-catenin signaling pathway, which relies on Ca. Differences in GM compositions have been detected in women with normal BMD, osteopenia, or osteoporosis (127).
3.4.1 Probiotics
Probiotics are beneficial bacteria present in GM (128, 129), such as Lactobacillus, Bifidobacterium, Escherichia coli, Enterococcus, Bacillus subtilis, and Saccharomyces species. By impacting the RANKL/RANK/OPG pathway, they can favor bone anabolism and decrease bone loss caused by estrogen deficiency (130). Lactobacillus reuteri administration prevented type-1 diabetes-induced osteoporosis by inhibiting TNF-mediated suppression of Wnt10b. It enhanced bone density by increasing osteoclast activity or restoring Wnt10b suppression in mice with glucocorticoid-induced osteoporosis. Wnt10b can increase osteoblastogenesis by downregulating the expression of PPAR-γ (124, 127).
3.4.2 Prebiotics
Prebiotics are non-digestible food ingredients that can promote bacteria growth and activity by acting as a substrate of selective fermentation from the intestinal microflora. In most cases, they are products of the enzymatic conversion of sugars. Among them, non-digestible oligosaccharides (NDOs) like lactulose, galactooligosaccharides (GOS), fructooligosaccharides (FOS), oligofructose, inulin, lactose derivatives, xylooligosaccharides (XOSs), and soluble corn fiber (SCF) can enhance mineral absorption and reduce bone resorption. Resistant starches are a dietary fiber subgroup promoting soya isoflavone production and increasing the ratio of Bifidobacteria, Lactobacillus species, and Bacteroides. These starches reduce bone loss in OVX mice, decrease inflammation, and interfere with the RANKL/OPG pathway. The prebiotics share the GM’s ability to convert to SCFAs, including acetate, propionate, and butyrate. They can affect Ca and Mg intestinal absorption in animals and humans. The process occurs by lowering the cecal pH and BMD (123, 131–135).
3.4.3 Synbiotics
Synbiotics combine probiotics and prebiotics in synergy and enhance their effects and characteristics. In OVX rats, FOS and soy isoflavone mixture increased bone trabecular microarchitectural properties (136); FOS combined with dried plum fraction in soy-based diets resulted in greater whole-body BMD (137). FOS and Bifidobacterium longum increased the Ca, Mg, and P content of bone and bone-breaking force in rats (138). Further, combining Bifidobacterium longum, and GOS augmented Ca, Mg, and P bioavailability and hind limb bone mineral content (139, 140).
3.4.4 Postbiotics
Postbiotics have recently been defined as bioactive compounds produced by food-grade microorganisms during fermentation (141). Postbiotics is an umbrella term that includes metabolites, SCFAs, microbial cell fractions, functional proteins, extracellular polymeric substances (EPSs), cell lysates, teichoic acid, peptidoglycan-derived muropeptides, and pili-type structures, which may be used to promote health by modulating the GM. Only a few recent studies have shown how postbiotic administration ameliorates osteopenia and osteoporosis (142). In OVX rats, the Bacillus coagulans-derived-postbiotics positively impacted on BMD (143). In a murine model of postmenopausal-related osteoporosis, the postbiotic Lactobacillus curvatus 38-CS affected RANKL-induced osteoclast differentiation and bone loss by downregulating the TRAF6/NF-κB/MAPKs axis (144).
3.5 Dietary lipids
Dietary lipids enclose saturated (SAFAs), monounsaturated (MUFAs), and polyunsaturated (PUFAs) fatty acids based on the double bond number and common chain length.
3.5.1 SAFA
Animal-based food is the primary source of nutrition for SAFAs.
High-fat diets (HFD), particularly those rich in saturated fatty acids (SAFA-HFD), can harm bone health. These diets can lead to an imbalance between bone resorption and formation, as well as an increase in DNA damage, potentially contributing to bone loss (145).
Oxidative damage is responsible for high osteocyte apoptosis in SAFA-HFD-fed animals compared to those fed a standard diet; micropetrosis and the canalicular system disruption cause this, leading to bone fragility. Moreover, SAFA-HFD strongly inhibits osteoclast apoptosis in IL-6-deficient mouse models. Consequently, survival of osteoclasts and apoptosis of bone-forming cells may lead to osteoporosis (145).
3.5.2 MUFA
MUFAs are abundant in nuts, avocados, olive oil, and canola (rapeseed). Olive oil, one of the essential foods in the Mediterranean diet, contains MUFA in the form of oleic acid. In extra virgin olive oil (EVOO), phenolic compounds are also present, which have a beneficial role. Fat from meat also contains MUFAs and SAFAs but is not recommended as a good source of MUFAs (146).
3.5.3 PUFA
PUFAs enclose the omega-3 (n-3) mainly derived from α-linolenic acid (ALA) and omega-6 (n-6) groups, primarily synthesized from linoleic acid (LA) (147). Humans do not synthesize ALA and LA, so all the sources are exogenous (148). The n-6 PUFAs are in vegetable oils such as sunflower, corn, cottonseed, and safflower. In contrast, the n-3 PUFAs, such as eicosapentaenoic (EPA) and docosahexaenoic (DHA) acids are found in fish and fish oil. PUFAs are beneficial for bone health, primarily ALA: n-3 PUFAs supplementation decreases bone resorption (measured as CTX marker) and increases BMD (149).
To enhance bone health in advanced age, dietary oils rich in the n-6 fatty acid gamma-linolenic acid (GLA) can be combined with the n-3 PUFA EPA to reduce PGE2 synthesis and enhance PGE1 production, which has anti-inflammatory effects. Decreasing the amount of n-6 PUFAs allows the manipulation of endogenous prostaglandin synthesis (145).
In animals fed with PUFA-rich diets, n-6 and n-3 PUFA may interact with PPAR-γ to inhibit osteoblast differentiation and promote the adipocyte one (145).
Animals fed with MUFA-rich diets had superior BMD values to those fed n-6 PUFA-rich diets. However, PUFA-rich diets were more beneficial to bone than SAFA-rich diets (145).
PUFA or HFD-SAFA-rich diets increase ROS production in growing mice compared to other unsaturated fat alimentations. This activates osteoclasts and inhibits osteoblast maturation through NF-κB, enhancing adipogenesis (145).
3.6 Melatonin
Melatonin (Mel) is a bioamine (N-acetyl-5-methoxytryptamine) secreted and released by the pineal gland at night. It controls circadian rhythms, body temperature, reproduction, immune and cardiovascular systems, energy, and bone metabolism. Mel predominantly acts through its cognate receptor, Mel receptor 2 (MT2R), expressed on MSCs, osteoblasts, and osteoclasts (150).
Mel has been shown to have an anti-osteoporotic effect since it induces beneficial effects on bone tissue. Altered levels of Mel are associated with the occurrence and development of osteoporosis in postmenopausal women (151).
Mel is an inducer of osteoblast proliferation and differentiation, mineralized ECM formation, and cortical bone formation (152). In Wistar rats, it improved bone mass, volume, and stiffness and increased skeletal strength compared to the control group (153). Mel promotes osteogenic differentiation by multiple mechanisms, including receptor-mediated signaling to stimulate osteogenic genes, support BMP signaling, upregulate OPG, and downregulate PPARγ expression. These effects are achieved by MT2R-dependent signaling involving the classical signaling molecules and antioxidant effects of the hormone and RNAs. As a result, Mel stimulates osteoblast formation and downregulates osteoclast and adipocyte formation (150, 151). In a rat model, Mel inverted the decrease in the osteogenic differentiation ability of bone marrow MSCs, a condition usually associated with osteoporosis. Additionally, it reversed TNF-’s anti-osteogenic differentiation and inflammation in BMSCs by suppressing the NF-kB signaling pathways (154).
Mel can enhance the osteogenic differentiation of BMSCs and retards bone loss through the HGF/PTEN/Wnt/β-catenin axis in OVX mice. Additionally, HGF diminished the expression of PTEN, resulting in an activated Wnt/β-catenin pathway both in vitro and in vivo (155).
Mel promoted BMSC-mediated osteogenesis-angiogenesis coupling in OVX rats with tibia defects as an osteoporotic model. Mel also promoted angiogenesis by upregulating VEGF levels and enhanced the expression of the typical osteogenesis and angiogenesis-related markers compared to the untreated group (156).
Mel suppressed the osteoclastogenic RANKL and upregulated the anti-osteoclastogenic RANKL decoy receptor OPG in osteoblasts, exerting an anti-resorptive effect. Thus, Mel antagonizes the osteoclastogenic function, also inhibiting the SIRT signaling that acts as a suppressor of the NF-κB signaling pathway that, on the contrary, leads to enhanced osteoclastogenesis. In addition, Mel inhibits the osteoclastogenic differentiation of hematopoietic stem cells and protects bone marrow cells exposed to a cytotoxic drug, aracytin. However, the cellular source of Mel in bone marrow is unknown (157).
In a rat model for glucocorticoid induced osteoporosis, high doses of dexamethasone caused BMSCs to undergo ferroptosis, a programmed cell death that depends on Fe. The prevention of osteoporosis was demonstrated by early Mel inhibition of the ferroptosis pathway. Indeed, Mel was able to block the phosphatidylinositol 3-kinase (PI3K), protein kinase B (AKT), and mammalian target of the rapamycin (mTOR) axis that controls bone metabolism (158).
In the type 2 diabetic osteoporotic mouse model, high glucose triggers ferroptosis by increasing ROS, lipid peroxidation, and glutathione depletion. However, administration of Mel can inhibit ferroptosis by triggering the nuclear factor erythroid 2-related factor 2 (Nrf2) and heme oxygenase-1 (HO-1) signaling pathway. This helps improve the osteogenic capacity of osteoblasts. These findings have been confirmed through in vitro experiments (159).
In osteoclasts, Mel inhibits differentiation and function by suppressing RANKL-induced ROS production by inhibiting NF-κB activation. It attenuates the damage induced by oxidative stress and inflammation on osteoblasts and prevents osteolysis from ROS and inflammatory factors. Mitochondria is the central organelle that generates free radicals and oxidative stress, contributing to aging-related diseases, including osteoporosis. Moreover, mitochondrial dysfunction inhibits osteogenesis and favors osteoclastic function, contributing to bone loss during aging. Mel could serve as an endogenous mitochondria-targeted antioxidant to diminish oxidative stress in bone cells more efficiently, thereby preventing bone loss (160).
4 Legislation framework
An internationally recognized definition of nutraceuticals is absent and, consequently, different definitions have emerged over the years, generating confusion. Nutraceuticals are regulated as food category in some countries, focusing on safety and labeling (161). However, they do not always have a specific definition, and, in some countries, supplements, herbal products, pre-and pro-biotics, and functional and fortified foods are not classified as nutraceuticals (Table 1).
For a detailed update on the regulatory framework and policies concerning nutraceuticals in the global markets, see Chopra’s et al. (162) review.
Nutraceuticals require a sharp classification and shared regulation to protect the category, safeguard consumers’ health, and avoid counterfeits.
5 Clinical trials
Clinical trials are crucial for understanding the dosage, mechanism of action, and side effects of nutraceuticals in managing osteoporosis. Therefore, we conducted a literature research through the PubMed database, with the CLINICAL TRIAL filter and the keywords “type/name of the nutraceutical,” “osteopenia,” “osteoporosis,” “bone loss,” and “bone fragility.” We considered papers published from 2000 until today and written in English. We excluded one paper written in Chinese and one in Russian. We also evaluated previous reviews on this topic.
The trials we found did not cover all nutraceuticals, and the data obtained so far needs to be consolidated. Our research led us to diverse studies, revealing homogeneities and heterogeneities (Supplementary Tables S1–S6). We found some homogeneity in the chosen population, such as gender (female), age (advancing years), and hormonal status (post-menopausal). On the other hand, we encountered much heterogeneity due to variations in sample sizes, methodologies, doses, administration lengths, outcomes, adverse effects, and follow-up (163–177). We found three studies on dosage effects (178–180).
A study evaluating Ca supplementation’s long-term effects (5 years) revealed that the bone benefits did not persist once supplements were stopped (181). Men with primary osteoporosis administered vitamin D or Ca supplements reported transient beneficial effects (182). Those studies highlight the need for further research on the long-term effects of nutraceuticals, which could significantly impact osteoporosis management.
Measuring the effects of nutraceuticals on bone health has been challenging, leading various clinical trials to utilize the 41Ca methodology. The reason for choosing this approach was that traditional analytical methods were often unable to detect the small effects of those compounds on bone (166, 183).
Some studies we found on probiotics showed beneficial effects on bone, as confirmed by other reviews (149). However, according to the systematic review by Billington et al., some RCTs had a high risk of bias based on the Cochrane Risk of Bias 2 Tool. Furthermore, the effects of probiotics were inconsistent (184). We also found studies evaluating n-3 fatty acids but reporting no significant beneficial effects on bone health (185–187).
The side effect issue still needs to be addressed. Some of the reported publications did not indicate any side effects, but it is unclear if they were not evaluated or if there were none.
Finally, we must acknowledge that a few authors question the practicality and feasibility of using randomized clinical trials (RCTs) when studying nutraceuticals (188–194). The multifunctional nature of nutraceuticals can make it hard to estimate their effects and choose the appropriate time points and biomarkers (195). Patients enrolled in a clinical trial for nutraceuticals must maintain specific healthy lifestyle habits and diet regimen not influencing data; this, in turn, can generate a high dropout rate. Another concern is the statistical analysis method generally used in RCTs, which may require some adjustments (196). Some nutrition and nutraceutical studies have employed the N-of-1 study design, which may address their heterogeneity and crossover features, including carry-over effects (197–201).
6 Discussion
We have utilized the “strengths, weaknesses, opportunities, threats (SWOT)” analysis to identify and discuss the advantages and disadvantages of nutraceuticals in preventing bone loss and osteoporosis, consider internal and external variables, and define goals to achieve and future directions (Figure 2). Strengths are internal factors that can be exploited, whereas weaknesses are internal limitations that must be addressed. Opportunities are external possibilities that can be capitalized on, while threats are external issues that need to be evaluated and faced.
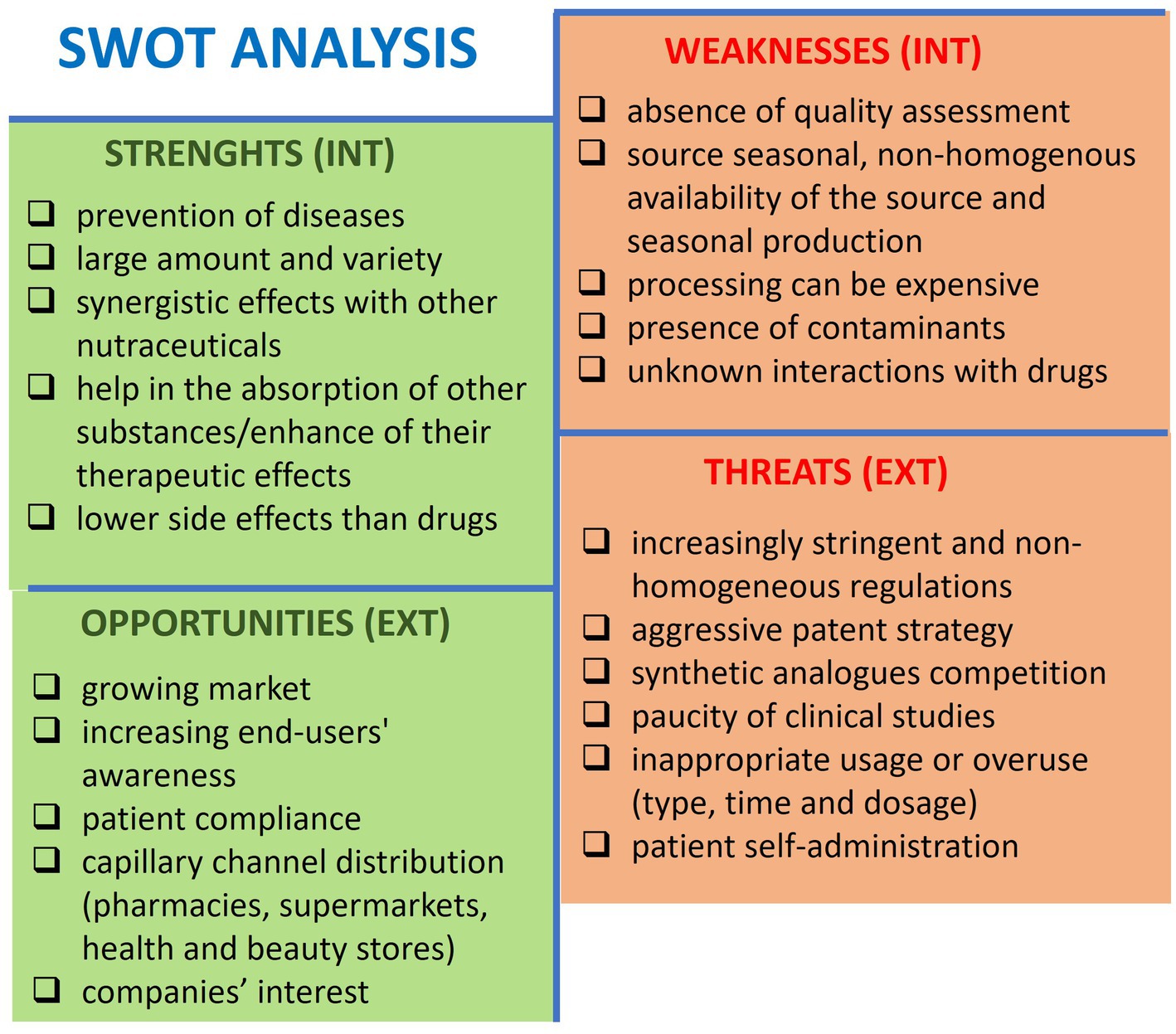
Figure 2. SWOT analysis showing the critical aspects of nutraceuticals for preventing bone loss and osteoporosis. INT, internal context; EXT, external context.
6.1 Strengths
Nutraceuticals exist in various forms, such as tablets, capsules, liquids, and powders, which make them easy to consume. They have few side effects (202), becoming promising for prevention. They can also be combined with other nutraceuticals, supplements, or drugs to improve or enhance their therapeutic effects or absorption (54, 203).
6.2 Weakness
Nutraceuticals may be available in different countries, considering seasonal variations and crop yield fluctuations, leading to potential supply shortages or price fluctuations. Moreover, the manufacturing process can be complex, time-consuming, and expensive, and it can carry contaminants. Indeed, nutraceuticals can become contaminated during any production stage with drugs, pesticides, mycotoxins, heavy metals, metalloids, and radioactivity. This can lead to declining quality standards and safety; informing the population and healthcare professionals about such issues is essential (204). Consolidating data on the potential interference of nutraceuticals with other drugs is necessary to avoid undesired changes and side effects in absorption, bioavailability, transport, metabolism, and elimination (205).
6.3 Opportunities
The global nutraceuticals market is constantly increasing, and academia and sector companies are allocating more resources to Research & Development. The diversity of nutraceuticals allows for a wide distribution range, including hypermarkets, supermarkets, pharmacies, and online sales channels. Consumers are more aware of the potential of natural-based alternatives and personalized nutrition that they prefer over synthetic pharmaceutics, which are perceived to carry side effects.
6.4 Threats
The regulation of nutraceuticals varies across different countries and is not consistent worldwide. Differently from drugs, nutraceuticals are subject to less strict production requirements, which can lead to a lack of safety and effectiveness data (206).
There is no proper knowledge about the dosage and intake regimen to avoid inappropriate use or overuse, which can lead to toxic effects and health complications (202, 207).
Natural ingredients that are beneficial to health cannot be patented.
Furthermore, synthetic food supplements such as vitamins, minerals, amino acids, and antioxidants pose significant competition.
7 Conclusions and perspectives
Along with behavioral habits, nutraceuticals can help prevent osteoporosis and promote overall well-being in individual with increased risk for severe illness. This potential warrants further research and exploration in nutrition and osteoporosis management.
Our intent to stay abreast of ongoing investigations led us to the ClinicalTrials.gov website (February 2nd, 2024) with the RECRUITING filter. Our search, using keywords such as nutraceutical name (in the field: other terms), bone loss, bone fragility, osteopenia, and osteoporosis (in the field: condition/disease), revealed that while some efforts are underway, there is still a significant gap to bridge (Table 2). The need for more comprehensive and evidence-based data on their mechanisms of action, effects, safety, appropriate dosage, potential adverse effects, and interactions with other compounds is paramount. Defining the safety profile is crucial since nutraceuticals are not subject to reporting adverse reactions to competent bodies.
Author contributions
LR: Conceptualization, Data curation, Formal analysis, Investigation, Methodology, Software, Visualization, Writing – original draft. GB: Conceptualization, Data curation, Formal analysis, Investigation, Methodology, Software, Visualization, Writing – original draft. FG: Writing – review & editing. GD: Writing – review & editing. LG: Writing – review & editing. BG: Conceptualization, Funding acquisition, Project administration, Supervision, Writing – review & editing.
Funding
The author(s) declare that no financial support was received for the research, authorship, and/or publication of this article.
Acknowledgments
The authors thank Patrizia Rappini for her assistance in preparing the manuscript.
Conflict of interest
The authors declare that the research was conducted in the absence of any commercial or financial relationships that could be construed as a potential conflict of interest.
The author(s) declared that they were an editorial board member of Frontiers, at the time of submission. This had no impact on the peer review process and the final decision.
Publisher’s note
All claims expressed in this article are solely those of the authors and do not necessarily represent those of their affiliated organizations, or those of the publisher, the editors and the reviewers. Any product that may be evaluated in this article, or claim that may be made by its manufacturer, is not guaranteed or endorsed by the publisher.
Supplementary material
The Supplementary material for this article can be found online at: https://www.frontiersin.org/articles/10.3389/fnut.2024.1445955/full#supplementary-material
References
1. Albrecht, BM, Stalling, I, Foettinger, L, Recke, C, and Bammann, K. Adherence to lifestyle recommendations for bone health in older adults with and without osteoporosis: cross-sectional results of the outdoor active study. Nutrients. (2022) 14:2463. doi: 10.3390/nu14122463
2. Zamudio-Rodríguez, A, Letenneur, L, Féart, C, Avila-Funes, JA, Amieva, H, and Pérès, K. The disability process: is there a place for frailty? Age Ageing. (2020) 49:764–70. doi: 10.1093/ageing/afaa031
3. Cristea, M, Noja, GG, Stefea, P, and Sala, AL. The impact of population aging and public health support on EU labor markets. Int J Environ Res Public Health. (2020) 17:1439. doi: 10.3390/ijerph17041439
4. Kemmak, AR, Rezapour, A, Jahangiri, R, Nikjoo, S, Farabi, H, and Soleimanpour, S. Economic burden of osteoporosis in the world: a systematic review. Med J Islam Repub Iran. (2020) 34:154. doi: 10.34171/mjiri.34.154
5. Lindsay, BR, Olufade, T, Bauer, J, Babrowicz, J, and Hahn, R. Patient-reported barriers to osteoporosis therapy. Arch Osteoporos. (2016) 11:19. doi: 10.1007/s11657-016-0272-5
6. Valentin, G, Ravn, MB, Jensen, EK, Friis, K, Bhimjiyani, A, Ben-Shlomo, Y, et al. Socio-economic inequalities in fragility fracture incidence: a systematic review and meta-analysis of 61 observational studies. Osteoporos Int. (2021) 32:2433–48. doi: 10.1007/s00198-021-06038-7
7. Hejazi, J, Davoodi, A, Khosravi, M, Sedaghat, M, Abedi, V, Hosseinverdi, S, et al. Nutrition and osteoporosis prevention and treatment. Biomed Res Ther. (2020) 7:3709–20. doi: 10.15419/bmrat.v7i4.598
8. Pandey, MK, Gupta, SC, Karelia, D, Gilhooley, PJ, Shakibaei, M, and Aggarwal, BB. Dietary nutraceuticals as backbone for bone health. Biotechnol Adv. (2018) 36:1633–48. doi: 10.1016/j.biotechadv.2018.03.014
9. Florencio-Silva, R, da Silva Sasso, GR, Sasso-Cerri, E, Simões, MJ, and Cerri, PS. Biology of bone tissue: structure, function, and factors that influence bone cells. Biomed Res Int. (2015) 2015:421746. doi: 10.1155/2015/421746
10. Mackie, EJ, Tatarczuch, L, and Mirams, M. The skeleton: a multi-functional complex organ. The growth plate chondrocyte and endochondral ossification. J Endocrinol. (2011) 211:109–21. doi: 10.1530/JOE-11-0048
11. Yang, G, Zhu, L, Hou, N, Lan, Y, Wu, XM, Zhou, B, et al. Osteogenic fate of hypertrophic chondrocytes. Cell Res. (2014) 24:1266–9. doi: 10.1038/cr.2014.111
12. Zhang, W, Zhu, Y, Li, J, Guo, Q, Peng, J, Liu, S, et al. Cell-derived extracellular matrix: basic characteristics and current applications in orthopedic tissue engineering. Tissue Eng Part B Rev. (2016) 22:193–207. doi: 10.1089/ten.TEB.2015.0290
13. Bentolila, V, Boyce, TM, Fyhrie, DP, Drumb, R, Skerry, TM, and Schaffler, MB. Intracortical remodeling in adult rat long bones after fatigue loading. Bone. (1998) 23:275–81. doi: 10.1016/S8756-3282(98)00104-5
14. Delaisse, JM, Andersen, TL, Kristensen, HB, Jensen, PR, Andreasen, CM, and Søe, K. Re-thinking the bone remodeling cycle mechanism and the origin of bone loss. Bone. (2020) 141:115628. doi: 10.1016/j.bone.2020.115628
15. Hauge, EM, Qvesel, D, Eriksen, EF, Mosekilde, L, and Melsen, F. Cancellous bone remodeling occurs in specialized compartments lined by cells expressing osteoblastic markers. J Bone Miner Res. (2001) 16:1575–82. doi: 10.1359/jbmr.2001.16.9.1575
16. Atkins, GJ, and Findlay, DM. Osteocyte regulation of bone mineral: a little give and take. Osteoporos Int. (2012) 23:2067–79. doi: 10.1007/s00198-012-1915-z
17. Dallas, SL, Prideaux, M, and Bonewald, LF. The osteocyte: an endocrine cell … and more. Endocr Rev. (2013) 34:658–90. doi: 10.1210/er.2012-1026
18. Soysa, NS, and Alles, N. Positive and negative regulators of osteoclast apoptosis. Bone Rep. (2019) 11:100225. doi: 10.1016/j.bonr.2019.100225
19. Matsuo, K, and Otaki, N. Bone cell interactions through Eph/ephrin: bone modeling, remodeling and associated diseases. Cell Adh Migr. (2012) 6:148–56. doi: 10.4161/cam.20888
20. Sims, NA, and Martin, TJ. Coupling signals between the osteoclast and osteoblast: how are messages transmitted between these temporary visitors to the bone surface? Front Endocrinol. (2015) 6:41. doi: 10.3389/fendo.2015.00041
21. Cui, L, Houston, DA, Farquharson, C, and MacRae, VE. Characterisation of matrix vesicles in skeletal and soft tissue mineralisation. Bone. (2016) 87:147–58. doi: 10.1016/j.bone.2016.04.007
22. Anderson, HC, Garimella, RG, and Tague, SE. The role of matrix vesicles in growth plate development and biomineralization. Front Biosci. (2005) 10:822–37. doi: 10.2741/1576
23. Pouresmaeili, F, Kamalidehghan, B, Kamarehei, M, and Goh, YM. A comprehensive overview on osteoporosis and its risk factors. Ther Clin Risk Manag. (2018) 14:2029–49. doi: 10.2147/TCRM.S138000
24. Trajanoska, K, and Rivadeneira, F. The genetic architecture of osteoporosis and fracture risk. Bone. (2019) 126:2–10. doi: 10.1016/j.bone.2019.04.005
25. Lyu, Z, Hu, Y, Guo, Y, and Liu, D. Modulation of bone remodeling by the gut microbiota: a new therapy for osteoporosis. Bone Res. (2023) 11:31. doi: 10.1038/s41413-023-00264-x
26. Catharine, RA, Taylor, LC, Yaktine, LA, and Del Valle, BHInstitute of Medicine (US) Committee to Review Dietary Reference Intakes for Vitamin D and Calcium. Dietary reference intakes for calcium and vitamin D. Washington, DC: National Academies Press (2011). 1115 p.
27. Ratajczak, AE, Szymczak-Tomczak, A, Rychter, AM, Zawada, A, Dobrowolska, A, and Krela-Kaźmierczak, I. Impact of cigarette smoking on the risk of osteoporosis in inflammatory bowel diseases. J Clin Med. (2021) 10:1515. doi: 10.3390/jcm10071515
28. Papadopoulou, SK, Papadimitriou, K, Voulgaridou, G, Georgaki, E, Tsotidou, E, Zantidou, O, et al. Exercise and nutrition impact on osteoporosis and sarcopenia—the incidence of osteosarcopenia: a narrative review. Nutrients. (2021) 13:4499. doi: 10.3390/nu13124499
29. David, C, Confavreux, CB, Mehsen, N, Paccou, J, Leboime, A, and Legrand, E. Severity of osteoporosis: what is the impact of co-morbidities? Joint Bone Spine. (2010) 77:S103–6. doi: 10.1016/S1297-319X(10)70003-8
30. Snega Priya, P, Pratiksha Nandhini, P, and Arockiaraj, J. A comprehensive review on environmental pollutants and osteoporosis: insights into molecular pathways. Environ Res. (2023) 237:117103. doi: 10.1016/j.envres.2023.117103
31. Srivastava, RK, Sapra, L, and Mishra, PK. Osteometabolism: metabolic alterations in bone pathologies. Cells. (2022) 11:3943. doi: 10.3390/cells11233943
32. Varacallo, MA, and Fox, EJ. Osteoporosis and its complications. Med Clin North Am. (2014) 98:817–31. doi: 10.1016/j.mcna.2014.03.007
33. Shen, Y, Huang, X, Wu, J, Lin, X, Zhou, X, Zhu, Z, et al. The global burden of osteoporosis, low bone mass, and its related fracture in 204 countries and territories, 1990–2019. Front Endocrinol. (2022) 13:882241. doi: 10.3389/fendo.2022.882241
34. Kanis, JA, Norton, N, Harvey, NC, Jacobson, T, Johansson, H, Lorentzon, M, et al. SCOPE 2021: a new scorecard for osteoporosis in Europe. Arch Osteoporos. (2021) 16:82. doi: 10.1007/s11657-020-00871-9
35. Feng, X, and McDonald, JM. Disorders of bone remodeling. Annu Rev Pathol. (2011) 6:121–45. doi: 10.1146/annurev-pathol-011110-130203
36. LeBoff, MS, Greenspan, SL, Insogna, KL, Lewiecki, EM, Saag, KG, Singer, AJ, et al. The clinician’s guide to prevention and treatment of osteoporosis. Osteoporos Int. (2022) 33:2049–102. doi: 10.1007/s00198-021-05900-y
37. Maghbooli, Z, Hossein-Nezhad, A, Jafarpour, M, Noursaadat, S, Ramezani, M, Hashemian, R, et al. Direct costs of osteoporosis-related hip fractures: protocol for a cross-sectional analysis of a national database. BMJ Open. (2017) 7:e014898. doi: 10.1136/bmjopen-2016-014898
38. Adami, G, Fassio, A, Gatti, D, Viapiana, O, Benini, C, Danila, MI, et al. Osteoporosis in 10 years time: a glimpse into the future of osteoporosis. Ther Adv Musculoskelet Dis. (2022) 14:1759720X221083541. doi: 10.1177/1759720X221083541
39. Kanis, JA . Assessment of fracture risk and its application to screening for postmenopausal osteoporosis: synopsis of a WHO report. WHO Study Group. Osteoporosis Int. (1994) 4:368–81. doi: 10.1007/BF01622200
40. Khashayar, P, Taheri, E, Adib, G, Zakraoui, L, and Larijani, B. Osteoporosis strategic plan for the Middle East and North Africa region. Arch Osteoporos. (2019) 14:20. doi: 10.1007/s11657-019-0567-4
41. Nuti, R, Brandi, ML, Checchia, G, Di Munno, O, Dominguez, L, Falaschi, P, et al. Guidelines for the management of osteoporosis and fragility fractures. Intern Emerg Med. (2019) 14:85–102. doi: 10.1007/s11739-018-1874-2
42. McCloskey, EV, Johansson, H, Oden, A, and Kanis, JA. From relative risk to absolute fracture risk calculation: the FRAX algorithm. Curr Osteoporos Rep. (2009) 7:77–83. doi: 10.1007/s11914-009-0013-4
43. Sunyecz, JA . The use of calcium and vitamin D in the management of osteoporosis. Ther Clin Risk Manag. (2008) 4:827–36. doi: 10.2147/tcrm.s3552
44. Foessl, I, Dimai, HP, and Obermayer-Pietsch, B. Long-term and sequential treatment for osteoporosis. Nat Rev Endocrinol. (2023) 19:520–33. doi: 10.1038/s41574-023-00866-9
45. Rizzoli, R, Reginster, JY, Boonen, S, Bréart, G, Diez-Perez, A, Felsenberg, D, et al. Adverse reactions and drug-drug interactions in the management of women with postmenopausal osteoporosis. Calcif Tissue Int. (2011) 89:91–104. doi: 10.1007/s00223-011-9499-8
46. Riso, P, and Soldati, L. Food ingredients and supplements: is this the future? J Transl Med. (2012) 10:227. doi: 10.1186/1479-5876-10-227
47. Fenech, M, El-Sohemy, A, Cahill, L, Ferguson, LR, French, TAC, Tai, ES, et al. Nutrigenetics and nutrigenomics: viewpoints on the current status and applications in nutrition research and practice. J Nutrigenet Nutrigenomics. (2011) 4:69–89. doi: 10.1159/000327772
48. da Costa, JP . A current look at nutraceuticals—key concepts and future prospects. Trends Food Sci Technol. (2017) 62:68–78. doi: 10.1016/j.tifs.2017.02.010
49. Elkhalifa, AEO, Alshammari, E, Adnan, M, Alcantara, JC, Awadelkareem, AM, Eltoum, NE, et al. Okra (Abelmoschus esculentus) as a potential dietary medicine with nutraceutical importance for sustainable health applications. Molecules. (2021) 26:696. doi: 10.3390/molecules26030696
50. Chanda, S, Tiwari, RK, Kumar, A, and Singh, K. Nutraceuticals inspiring the current therapy for lifestyle diseases. Adv Pharmacol Sci. (2019) 2019:6908716. doi: 10.1155/2019/6908716
51. Scicchitano, P, Cameli, M, Maiello, M, Modesti, PA, Muiesan, ML, Novo, S, et al. Nutraceuticals and dyslipidaemia: beyond the common therapeutics. J Funct Foods. (2014) 6:11–32. doi: 10.1016/j.jff.2013.12.006
52. Santini, A, and Novellino, E. Nutraceuticals—shedding light on the grey area between pharmaceuticals and food. Expert Rev Clin Pharmacol. (2018) 11:545–7. doi: 10.1080/17512433.2018.1464911
53. Nwosu, OK, and Ubaoji, KI. Nutraceuticals: history, classification and market demand In: Functional foods and nutraceuticals. Cham: Springer (2020). 13–22.
54. Rajput, R, Wairkar, S, and Gaud, R. Nutraceuticals for better management of osteoporosis: an overview. J Funct Foods. (2018) 47:480–90. doi: 10.1016/j.jff.2018.06.013
55. Rizzoli, R . Dairy products and bone health. Aging Clin Exp Res. (2022) 34:9–24. doi: 10.1007/s40520-021-01970-4
56. Ringe, JD, van der Geest, SAP, and Möller, G. Importance of calcium co-medication in bisphosphonate therapy of osteoporosis an approach to improving correct intake and drug adherence. Drugs Aging. (2006) 23:569–78. doi: 10.2165/00002512-200623070-00003
57. Karpouzos, A, Diamantis, E, Farmaki, P, Savvanis, S, and Troupis, T. Nutritional aspects of bone health and fracture healing. J Osteoporos. (2017) 2017:4218472. doi: 10.1155/2017/4218472
58. Su, Y, Cappock, M, Dobres, S, Kucine, AJ, Waltzer, WC, and Zhu, D. Supplemental mineral ions for bone regeneration and osteoporosis treatment. Eng Regen. (2023) 4:170–82. doi: 10.1016/j.engreg.2023.02.003
59. Rondanelli, M, Faliva, MA, Tartara, A, Gasparri, C, Perna, S, Infantino, V, et al. An update on magnesium and bone health. Biometals. (2021) 34:715–36. doi: 10.1007/s10534-021-00305-0
60. Capozzi, A, Scambia, G, and Lello, S. Calcium, vitamin D, vitamin K2, and magnesium supplementation and skeletal health. Maturitas. (2020) 140:55–63. doi: 10.1016/j.maturitas.2020.05.020
61. Castiglioni, S, Cazzaniga, A, Albisetti, W, and Maier, JAM. Magnesium and osteoporosis: current state of knowledge and future research directions. Nutrients. (2013) 5:3022–33. doi: 10.3390/nu5083022
62. Palacios, C . The role of nutrients in bone health, from A to Z. Crit Rev Food Sci Nutr. (2006) 46:621–8. doi: 10.1080/10408390500466174
63. Singh, W, and Kushwaha, P. Potassium: a frontier in osteoporosis. Horm Metab Res. (2024) 56:329–40. doi: 10.1055/a-2254-8533
64. Balogh, E, Paragh, G, and Jeney, V. Influence of iron on bone homeostasis. Pharmaceuticals. (2018) 11:107. doi: 10.3390/ph11040107
65. Wang, S, Gu, R, Wang, F, Zhao, X, Yang, F, Xu, Y, et al. 3D-printed PCL/Zn scaffolds for bone regeneration with a dose-dependent effect on osteogenesis and osteoclastogenesis. Mater Today Bio. (2022) 13:100202. doi: 10.1016/j.mtbio.2021.100202
66. Sandstead, HH, and Freeland-Graves, JH. Dietary phytate, zinc and hidden zinc deficiency. J Trace Elem Med Biol. (2014) 28:414–7. doi: 10.1016/j.jtemb.2014.08.011
68. Mutlu, M, Argun, M, Kilic, E, Saraymen, R, and Yazar, AS. Magnesium, zinc and copper status in osteoporotic, osteopenic and normal post-menopausal women. J Int Med Res. (2007) 35. doi: 10.1177/147323000703500514
69. Zofkova, I, Davis, M, and Blahos, J. Trace elements have beneficial, as well as detrimental effects on bone homeostasis. Physiol Res. (2017) 66:391–402. doi: 10.33549/physiolres.933454
70. Klevay, LM . Is the Western diet adequate in copper? J Trace Elem Med Biol. (2011) 25:204–12. doi: 10.1016/j.jtemb.2011.08.146
71. Hanga-Farcaș, A, Miere, F, Filip, GA, Clichici, S, Fritea, L, Vicaș, LG, et al. Phytochemical compounds involved in the bone regeneration process and their innovative administration: a systematic review. Plants. (2023) 12:2055. doi: 10.3390/plants12102055
72. Kim, JH, Gupta, SC, Park, B, Yadav, VR, and Aggarwal, BB. Turmeric (Curcuma longa) inhibits inflammatory nuclear factor (NF)-κB and NF-κB-regulated gene products and induces death receptors leading to suppressed proliferation, induced chemosensitization, and suppressed osteoclastogenesis. Mol Nutr Food Res. (2012) 56:454–65. doi: 10.1002/mnfr.201100270
73. von Metzler, I, Krebbel, H, Kuckelkorn, U, Heider, U, Jakob, C, Kaiser, M, et al. Curcumin diminishes human osteoclastogenesis by inhibition of the signalosome-associated IκB kinase. J Cancer Res Clin Oncol. (2009) 135:173–9. doi: 10.1007/s00432-008-0461-8
74. Bharti, AC, Takada, Y, and Aggarwal, BB. Curcumin (diferuloylmethane) inhibits receptor activator of NF-B ligand-induced NF-B activation in osteoclast precursors and suppresses osteoclastogenesis 1. J Immunol. (2004) 172:5940. doi: 10.4049/jimmunol.172.10.5940
75. Wang, N, Wang, F, Gao, Y, Yin, P, Pan, C, Liu, W, et al. Curcumin protects human adipose-derived mesenchymal stem cells against oxidative stress-induced inhibition of osteogenesis. J Pharmacol Sci. (2016) 132:192–200. doi: 10.1016/j.jphs.2016.10.005
76. French, DL, Muir, JM, and Webber, CE. The ovariectomized, mature rat model of postmenopausal osteoporosis: an assessment of the bone sparing effects of curcumin. Phytomedicine. (2008) 15:1069–78. doi: 10.1016/j.phymed.2008.06.007
77. Kim, WK, Ke, K, Sul, OJ, Kim, HJ, Kim, SH, Lee, MH, et al. Curcumin protects against ovariectomy-induced bone loss and decreases osteoclastogenesis. J Cell Biochem. (2011) 112:3159–66. doi: 10.1002/jcb.23242
78. Cho, DC, Kim, KT, Jeon, Y, and Sung, JK. A synergistic bone sparing effect of curcumin and alendronate in ovariectomized rat. Acta Neurochir. (2012) 154:2215–23. doi: 10.1007/s00701-012-1516-9
79. Hussan, F, Ibraheem, NG, Kamarudin, TA, Shuid, AN, Soelaiman, IN, and Othman, F. Curcumin protects against ovariectomy-induced bone changes in rat model. Evid Based Complement Alternat Med. (2012) 2012:174916. doi: 10.1155/2012/174916
80. Jiang, Q, Lei, YH, Krishnadath, DC, Zhu, BY, and Zhou, XW. Curcumin regulates EZH2/Wnt/β-catenin pathway in the mandible and femur of ovariectomized osteoporosis rats. Kaohsiung J Med Sci. (2021) 37:513–9. doi: 10.1002/kjm2.12346
81. Cho, DC, Jung, HS, Kim, KT, Jeon, Y, Sung, JK, and Hwang, JH. Therapeutic advantages of treatment of high-dose curcumin in the ovariectomized rat. J Korean Neurosurg Soc. (2013) 54:461–6. doi: 10.3340/jkns.2013.54.6.461
82. Alexandersen, P . Ipriflavone in the treatment of postmenopausal osteoporosis: a randomized controlled trial. JAMA. (2001) 285:1482. doi: 10.1001/jama.285.11.1482
83. Yao, J, Zhang, J, and Hou, JF. Effects of ipriflavone on caged layer bone metabolism in vitro and in vivo. Poult Sci. (2007) 86:503–7. doi: 10.1093/ps/86.3.503
84. Occhiuto, F, De Pasquale, R, Guglielmo, G, Palumbo, DR, Zangla, G, Samperi, S, et al. Effects of phytoestrogenic isoflavones from red clover (Trifolium pratense L.) on experimental osteoporosis. Phytother Res. (2007) 21:130–4. doi: 10.1002/ptr.2037
85. Sarao, L, Kaur, S, Malik, T, and Singh, A. Chapter 19 - Genistein and daidzein In: Nutraceuticals and health care. J Kour and GA Nayik editors. Academic Press (2022) 331–41.
86. Costa-Rodrigues, J, Carmo, SC, Silva, JC, and Fernandes, MHR. Inhibition of human in vitro osteoclastogenesis by Equisetum arvense. Cell Prolif. (2012) 45:566–76. doi: 10.1111/j.1365-2184.2012.00848.x
87. Kim, TH, Jung, JW, Ha, BG, Hong, JM, Park, EK, Kim, HJ, et al. The effects of luteolin on osteoclast differentiation, function in vitro and ovariectomy-induced bone loss. J Nutr Biochem. (2011) 22:8–15. doi: 10.1016/j.jnutbio.2009.11.002
88. Uthman, A, and Garba, Y. Citrus mineral nutrition and health benefits: a review In: Citrus research—horticultural and human health aspects : IntechOpen (2023) Available at: www.intechopen.com
89. Saini, RK, Ranjit, A, Sharma, K, Prasad, P, Shang, X, Gowda, KGM, et al. Bioactive compounds of citrus fruits: a review of composition and health benefits of carotenoids, flavonoids, limonoids, and terpenes. Antioxidants. (2022) 11:239. doi: 10.3390/antiox11020239
90. Zhang, J, Liu, Z, Luo, Y, Li, X, Huang, G, Chen, H, et al. The role of flavonoids in the osteogenic differentiation of mesenchymal stem cells. Front Pharmacol. (2022) 13:849513. doi: 10.3389/fphar.2022.849513
91. Parhiz, H, Roohbakhsh, A, Soltani, F, Rezaee, R, and Iranshahi, M. Antioxidant and anti-inflammatory properties of the citrus flavonoids hesperidin and hesperetin: an updated review of their molecular mechanisms and experimental models. Phytother Res. (2015) 29:323–31. doi: 10.1002/ptr.5256
92. Liu, L, Zheng, J, Yang, YZ, Ni, L, Chen, H, and Yu, D. Hesperetin alleviated glucocorticoid-induced inhibition of osteogenic differentiation of BMSCs through regulating the ERK signaling pathway. Med Mol Morphol. (2021) 54:1–7. doi: 10.1007/s00795-020-00251-9
93. Ortiz, AC, SOM, F, CHB, R, Bellini, MZ, ESBM, P, JPG, P, et al. Therapeutic effects of citrus flavonoids neohesperidin, hesperidin and its aglycone, hesperetin on bone health. Biomolecules. (2022) 23:626. doi: 10.3390/biom12050626
94. Wen, CY, Jun, ZW, Gu, W, Sun, J, Qiang, LZ, and Xen, W. Neohesperidin promotes the osteogenic differentiation of bone mesenchymal stem cells by activating the Wnt/β-catenin signaling pathway. J Orthop Surg Res. (2021) 16:334. doi: 10.1186/s13018-021-02761-3
95. Zhang, Q, Tang, X, Liu, Z, Song, X, Peng, D, Zhu, W, et al. Hesperetin prevents bone resorption by inhibiting RANKL-induced osteoclastogenesis and Jnk mediated Irf-3/c-Jun activation. Front Pharmacol. (2018) 9:1527. doi: 10.3389/fphar.2018.01527
96. Liu, H, Dong, Y, Gao, Y, Zhao, L, Cai, C, Qi, D, et al. Hesperetin suppresses RANKL-induced osteoclastogenesis and ameliorates lipopolysaccharide-induced bone loss. J Cell Physiol. (2019) 234:11009–22. doi: 10.1002/jcp.27924
97. Gambari, L, Grigolo, B, and Grassi, F. Dietary organosulfur compounds: emerging players in the regulation of bone homeostasis by plant-derived molecules. Front Endocrinol. (2022) 13:937956. doi: 10.3389/fendo.2022.937956
98. Zhao, XX, Lin, FJ, Li, H, Bin, LH, Wu, DT, Geng, F, et al. Recent advances in bioactive compounds, health functions, and safety concerns of onion (Allium cepa L.). Front Nutr. (2021) 8:669805. doi: 10.3389/fnut.2021.669805
99. Wong, RWK, and Rabie, ABM. Effect of quercetin on preosteoblasts and bone defects. Open Orthop J. (2008) 2. doi: 10.2174/1874325000802010027
100. Tsuji, M, Yamamoto, H, Sato, T, Mizuha, Y, Kawai, Y, Taketani, Y, et al. Dietary quercetin inhibits bone loss without effect on the uterus in ovariectomized mice. J Bone Miner Metab. (2009) 27:673–81. doi: 10.1007/s00774-009-0088-0
101. Suleria, HAR, Butt, MS, Anjum, FM, Saeed, F, and Khalid, N. Onion: nature protection against physiological threats. Crit Rev Food Sci Nutr. (2015) 55:50–66. doi: 10.1080/10408398.2011.646364
102. Ahmadian, F, Mozaffari-Khosravi, H, Azaraein, MH, Faraji, R, and Zavar-Reza, J. The effect of consumption of garlic tablet on proteins oxidation biomarkers in postmenopausal osteoporotic women: a randomized clinical trial. Electron Physician. (2017) 9:5670–5. doi: 10.19082/5670
103. Weaver, CM, Alekel, DL, Ward, WE, and Ronis, MJ. Flavonoid intake and bone health. J Nutr Gerontol Geriatr. (2012) 31:239–53. doi: 10.1080/21551197.2012.698220
104. Shen, CL, Chyu, MC, and Wang, JS. Tea and bone health: steps forward in translational nutrition. Am J Clin Nutr. (2013) 98:1694S–9S. doi: 10.3945/ajcn.113.058255
105. Ko, CH, Lau, KM, Choy, WY, and Leung, PC. Effects of tea catechins, epigallocatechin, gallocatechin, and gallocatechin gallate, on bone metabolism. J Agric Food Chem. (2009) 57:7293–7. doi: 10.1021/jf901545u
106. Hsieh, TP, Sheu, SY, Sun, JS, and Chen, MH. Icariin inhibits osteoclast differentiation and bone resorption by suppression of MAPKs/NF-κB regulated HIF-1α and PGE2 synthesis. Phytomedicine. (2011) 18:176–85. doi: 10.1016/j.phymed.2010.04.003
107. Perut, F, Roncuzzi, L, Avnet, S, Massa, A, Zini, N, Sabbadini, S, et al. Strawberry-derived exosome-like nanoparticles prevent oxidative stress in human mesenchymal stromal cells. Biomolecules. (2021) 11:1–14. doi: 10.3390/biom11010087
108. Davicco, MJ, Wittrant, Y, and Coxam, V. Berries, their micronutrients and bone health. Curr Opin Clin Nutr Metab Care. (2016) 19:453–7. doi: 10.1097/MCO.0000000000000324
109. Jabeen, Q, and Aslam, N. The pharmacological activities of prunes: the dried plums. J Med Plant Res. (2011) 5:1508–11. Available at: http://www.academicjournals.org/JMPR
110. Arjmandi, BH, Khalil, DA, Lucas, EA, Georgis, A, Stoecker, BJ, Hardin, C, et al. Dried plums improve indices of bone formation in postmenopausal women. J Womens Health Gend Based Med. (2002) 11:61. doi: 10.1089/152460902753473471
111. Tou, JC . Resveratrol supplementation affects bone acquisition and osteoporosis: pre-clinical evidence toward translational diet therapy. Biochim Biophys Acta. (2014) 1852:1186–94. doi: 10.1016/j.bbadis.2014.10.003
112. Lin, Q, Huang, YM, Xiao, BX, and Ren, GF. Effects of resveratrol on bone mineral density in ovarectomized rats. Int J Biomed Sci. (2005) 1:76–81.
113. Ahmad Hairi, H, Jayusman, PA, and Shuid, AN. Revisiting resveratrol as an osteoprotective agent: molecular evidence from in vivo and in vitro studies. Biomedicines. (2023) 11:1453. doi: 10.3390/biomedicines11051453
114. Toba, Y, Takada, Y, Matsuoka, Y, Morita, Y, Motouri, M, Hirai, T, et al. Milk basic protein promotes bone formation and suppresses bone resorption in healthy adult men. Biosci Biotechnol Biochem. (2001) 65:1353–7. doi: 10.1271/bbb.65.1353
115. Aoe, S, Toba, Y, Ichi, YJ, Kawakami, H, Yahiro, M, Kumegawa, M, et al. Controlled trial of the effects of milk basic protein (MBP) supplementation on bone metabolism in healthy adult women. Biosci Biotechnol Biochem. (2001) 65:913–8. doi: 10.1271/bbb.65.913
116. Ratajczak, AE, Zawada, A, Rychter, AM, Dobrowolska, A, and Krela-Kaźmierczak, I. Milk and dairy products: good or bad for human bone? Practical dietary recommendations for the prevention and management of osteoporosis. Nutrients. (2021) 13:1329. doi: 10.3390/nu13041329
117. Oliveira, MC, Pieters, BCH, Guimarães, PB, Duffles, LF, Heredia, JE, Silveira, ALM, et al. Bovine milk extracellular vesicles are osteoprotective by increasing osteocyte numbers and targeting RANKL/OPG system in experimental models of bone loss. Front Bioeng Biotechnol. (2020) 8:891. doi: 10.3389/fbioe.2020.00891
118. Rizzoli, R, and Biver, E. Effects of fermented milk products on bone. Calcif Tissue Int. (2018) 102:489–500. doi: 10.1007/s00223-017-0317-9
119. Kang, SH, Kim, JU, Imm, JY, Oh, S, and Kim, SH. The effects of dairy processes and storage on insulin-like growth factor-I (IGF-I) content in milk and in model IGF-I-fortified dairy products. J Dairy Sci. (2006) 89:402–9. doi: 10.3168/jds.S0022-0302(06)72104-X
120. Dahiya, D, and Nigam, PS. Therapeutic and dietary support for gastrointestinal tract using kefir as a nutraceutical beverage: dairy-milk-based or plant-sourced kefir probiotic products for vegan and lactose-intolerant populations. Fermentation. (2023) 9:388. doi: 10.3390/fermentation9040388
121. Sohail, Z, Khan, N, Moazzam, M, Mujahid, S, Sindhu, AT, Khan, H, et al. Perspective chapter: beyond delicious-the hidden functional benefits of cheese In: Recent trends on cheese as functional food with great nutritive and health benefits food science and nutrition : IntechOpen Available at: www.intechopen.com
122. Pampaloni, B, Bartolini, E, and Brandi, ML. Parmigiano Reggiano cheese and bone health. Clin Cases Miner Bone Metab. (2011) 8:33–6.
123. Zhang, YW, Cao, MM, Li, YJ, Dai, GC, Lu, PP, Zhang, M, et al. The regulative effect and repercussion of probiotics and prebiotics on osteoporosis: involvement of brain-gut-bone axis. Crit Rev Food Sci Nutr. (2023) 63:7510–28. doi: 10.1080/10408398.2022.2047005
124. de Sire, A, de Sire, R, Curci, C, Castiglione, F, and Wahli, W. Role of dietary supplements and probiotics in modulating microbiota and bone health: The gut-bone axis. Cells. (2022) 11:743. doi: 10.3390/cells11040743
125. Afzaal, M, Saeed, F, Shah, YA, Hussain, M, Rabail, R, Socol, CT, et al. Human gut microbiota in health and disease: unveiling the relationship. Front Microbiol. (2022) 13:999001. doi: 10.3389/fmicb.2022.999001
126. Catinean, A, Neag, MA, Muntean, DM, Bocsan, IC, and Buzoianu, AD. An overview on the interplay between nutraceuticals and gut microbiota. PeerJ. (2018) 6:e4465. doi: 10.7717/peerj.4465
127. Das, M, Cronin, O, Keohane, DM, Cormac, EM, Nugent, H, Nugent, M, et al. Gut microbiota alterations associated with reduced bone mineral density in older adults. Rheumatology. (2019) 58:2295–304. doi: 10.1093/rheumatology/kez302
128. Parvaneh, K, Jamaluddin, R, Karimi, G, and Erfani, R. Effect of probiotics supplementation on bone mineral content and bone mass density. Sci World J. (2014) 2014:595962. doi: 10.1155/2014/595962
129. Chen, Z, Cai, Z, Zhuang, P, Li, F, Cui, W, and Li, Z. Living probiotic biomaterials for osteoporosis therapy. Biomed Technol. (2023) 1:52–64. doi: 10.1016/j.bmt.2022.11.007
130. Lilian Ilesanmi-Oyelere, B, and Cathorina, KM. Prebiotics, probiotics and synbiotic for bone health In: Prebiotics and probiotics-from food to health : IntechOpen (2022)
131. Scholz-Ahrens, KE, Schaafsma, G, van den Heuvel, EG, and Schrezenmeir, J. Effects of prebiotics on mineral metabolism. Am J Clin Nutr. (2001) 73:459s–64s. doi: 10.1093/ajcn/73.2.459s
132. Whisner, CM, and Castillo, LF. Prebiotics, bone and mineral metabolism. Calcif Tissue Int. (2018) 102:443–79. doi: 10.1007/s00223-017-0339-3
133. Weaver, CM, Martin, BR, Story, JA, Hutchinson, I, and Sanders, L. Novel fibers increase bone calcium content and strength beyond efficiency of large intestine fermentation. J Agric Food Chem. (2010) 58:8952–7. doi: 10.1021/jf904086d
134. Seijo, M, Bryk, G, Zeni Coronel, M, Bonanno, M, Río, ME, Martín, P, et al. Effect of adding a galacto-oligosaccharides/fructo-oligosaccharides (GOS/FOS®) mixture to a normal and low calcium diet, on calcium absorption and bone health in ovariectomy-induced osteopenic rats. Calcif Tissue Int. (2019) 104:301–12. doi: 10.1007/s00223-018-0490-5
135. Karakan, T, Tuohy, KM, and Janssen-van Solingen, G. Low-dose lactulose as a prebiotic for improved gut health and enhanced mineral absorption. Front Nutr. (2021) 8:672925. doi: 10.3389/fnut.2021.672925
136. Devareddy, L, Khalil, DA, Korlagunta, K, Hooshmand, S, Bellmer, DD, and Arjmandi, BH. The effects of fructo-oligosaccharides in combination with soy protein on bone in osteopenic ovariectomized rats. Menopause. (2006) 13:692–9. doi: 10.1097/01.gme.0000195372.74944.71
137. Arjmandi, BH, Johnson, CD, Lucas, EA, Hooshmand, S, Campbell, S, and Akhter, MP. Addition of fructooligosaccharides and dried plum to soy-based diets reverses bone loss in the ovariectomized rat. Evid Based Complement Alternat Med. (2011) 2011:836267. doi: 10.1093/ecam/nen050
138. Rodrigues, FC, Castro, ASB, Rodrigues, VC, Fernandes, SA, Fontes, EAF, De Oliveira, TT, et al. Yacon flour and bifidobacterium longum modulate bone health in rats. J Med Food. (2012) 15:664–70. doi: 10.1089/jmf.2011.0296
139. Pérez-Conesa, D, López, G, Abellán, P, and Ros, G. Bioavailability of calcium, magnesium and phosphorus in rats fed probiotic, prebiotic and synbiotic powder follow-up infant formulas and their effect on physiological and nutritional parameters. J Sci Food Agric. (2006) 86:2327–36. doi: 10.1002/jsfa.2618
140. Pérez-Conesa, D, López, G, and Ros, G. Effects of probiotic, prebiotic and synbiotic follow-up infant formulas on large intestine morphology and bone mineralisation in rats. J Sci Food Agric. (2007) 87:1059–68. doi: 10.1002/jsfa.2812
141. Ma, L, Tu, H, and Chen, T. Postbiotics in human health: a narrative review. Nutrients. (2023) 15:291. doi: 10.3390/nu15020291
142. Bhardwaj, A, Sapra, L, Tiwari, A, Mishra, PK, Sharma, S, and Srivastava, RK. “Osteomicrobiology”: the nexus between bone and bugs. Front Microbiol. (2022) 12:812466. doi: 10.3389/fmicb.2021.812466
143. Wegh, CAM, Geerlings, SY, Knol, J, Roeselers, G, and Belzer, C. Postbiotics and their potential applications in early life nutrition and beyond. Int J Mol Sci. (2019) 20:4673. doi: 10.3390/ijms20194673
144. Jang, AR, Park, JS, Kim, DK, Park, JY, Ahn, JH, Kim, DY, et al. Cell-free culture supernatant of Lactobacillus curvatus Wikim38 inhibits RANKL-induced osteoclast differentiation and ameliorates bone loss in ovariectomized mice. Lett Appl Microbiol. (2021) 73:383–91. doi: 10.1111/lam.13525
145. Romero-Márquez, JM, Varela-López, A, Navarro-Hortal, MD, Badillo-Carrasco, A, Forbes-Hernández, TY, Giampieri, F, et al. Molecular interactions between dietary lipids and bone tissue during aging. Int J Mol Sci. (2021) 22:6473. doi: 10.3390/ijms22126473
146. Zong, G, Li, Y, Sampson, L, Dougherty, LW, Willett, WC, Wanders, AJ, et al. Monounsaturated fats from plant and animal sources in relation to risk of coronary heart disease among US men and women. Am J Clin Nutr. (2018) 107:445–53. doi: 10.1093/ajcn/nqx0004
147. Catalá, A . Five decades with polyunsaturated fatty acids: chemical synthesis, enzymatic formation, lipid peroxidation and its biological effects. J Lipids. (2013) 2013:1–19. doi: 10.1155/2013/710290
148. Murff, HJ, and Edwards, TL. Endogenous production of long-chain polyunsaturated fatty acids and metabolic disease risk. Curr Cardiovasc Risk Rep. (2014) 8:418. doi: 10.1007/s12170-014-0418-1
149. Dou, Y, Wang, Y, Chen, Z, Yu, X, and Ma, D. Effect of n-3 polyunsaturated fatty acid on bone health: a systematic review and meta-analysis of randomized controlled trials. Food Sci Nutr. (2022) 10:145–54. doi: 10.1002/fsn3.2655
150. Zhao, Y, Shao, G, Liu, X, and Li, Z. Assessment of the therapeutic potential of melatonin for the treatment of osteoporosis through a narrative review of its signaling and preclinical and clinical studies. Front Pharmacol. (2022) 13:866625. doi: 10.3389/fphar.2022.866625
151. Yang, K, Qiu, X, Cao, L, and Qiu, S. The role of melatonin in the development of postmenopausal osteoporosis. Front Pharmacol. (2022) 13:975181. doi: 10.3389/fphar.2022.975181
152. Satomura, K, Tobiume, S, Tokuyama, R, Yamasaki, Y, Kudoh, K, Maeda, E, et al. Melatonin at pharmacological doses enhances human osteoblastic differentiation in vitro and promotes mouse cortical bone formation in vivo. J Pineal Res. (2007) 42:231–9. doi: 10.1111/j.1600-079X.2006.00410.x
153. Tresguerres, IF, Tamimi, F, Eimar, H, Barralet, JE, Prieto, S, Torres, J, et al. Melatonin dietary supplement as an anti-aging therapy for age-related bone loss. Rejuvenation Res. (2014) 17:341–6. doi: 10.1089/rej.2013.1542
154. Hu, Y, Xiong, Y, Zha, K, Tao, R, Chen, L, Xue, H, et al. Melatonin promotes BMSCs osteoblastic differentiation and relieves inflammation by suppressing the NF-κ B pathways. Stem Cells Int. (2023) 2023:7638842. doi: 10.1155/2023/7638842
155. Zhang, J, Jia, G, Xue, P, and Li, Z. Melatonin restores osteoporosis-impaired osteogenic potential of bone marrow mesenchymal stem cells and alleviates bone loss through the HGF/PTEN/Wnt/β-catenin axis. Ther Adv Chronic Dis. (2021) 12:204062232199568. doi: 10.1177/2040622321995685
156. Zheng, S, Zhou, C, Yang, H, Li, J, Feng, Z, Liao, L, et al. Melatonin accelerates osteoporotic bone defect repair by promoting osteogenesis–angiogenesis coupling. Front Endocrinol. (2022) 13:826660. doi: 10.3389/fendo.2022.826660
157. Eslami, B, Zhou, S, Van Eekeren, I, Leboff, MS, and Glowacki, J. Reduced osteoclastogenesis and RANKL expression in marrow from women taking alendronate. Calcif Tissue Int. (2011) 88:272–80. doi: 10.1007/s00223-011-9473-5
158. Li, M, Yang, N, Hao, L, Zhou, W, Li, L, Liu, L, et al. Melatonin inhibits the ferroptosis pathway in rat bone marrow mesenchymal stem cells by activating the PI3K/AKT/mTOR signaling axis to attenuate steroid-induced osteoporosis. Oxid Med Cell Longev. (2022) 2022:8223737. doi: 10.1155/2022/7264649
159. Ma, H, Wang, X, Zhang, W, Li, H, Zhao, W, Sun, J, et al. Melatonin suppresses ferroptosis induced by high glucose via activation of the Nrf2/HO-1 signaling pathway in type 2 diabetic osteoporosis. Oxid Med Cell Longev. (2020) 2020:9067610. doi: 10.1155/2020/9067610
160. Purushothaman, A, Sheeja, AA, and Janardanan, D. Hydroxyl radical scavenging activity of melatonin and its related indolamines. Free Radic Res. (2020) 54:373–83. doi: 10.1080/10715762.2020.1774575
161. Santini, A, Cammarata, SM, Capone, G, Ianaro, A, Tenore, GC, Pani, L, et al. Nutraceuticals: opening the debate for a regulatory framework. Br J Clin Pharmacol. (2018) 84:659–72. doi: 10.1111/bcp.13496
162. Chopra, AS, Lordan, R, Horbańczuk, OK, Atanasov, AG, Chopra, I, Horbańczuk, JO, et al. The current use and evolving landscape of nutraceuticals. Pharmacol Res. (2022) 175:106001. doi: 10.1016/j.phrs.2021.106001
163. Norman, M, Lambert, T, Thybo, CB, Lykkeboe, S, Rasmussen, LM, Frette, X, et al. Combined bioavailable isoflavones and probiotics improve bone status and estrogen metabolism in postmenopausal osteopenic women: a randomized controlled trial. Am J Clin Nutr. (2017) 106:909–29. doi: 10.3945/ajcn.117.153353
164. Squadrito, F, Imbalzano, E, Rottura, M, Arcoraci, V, Pallio, G, Catalano, A, et al. Effects of genistein aglycone in glucocorticoid induced osteoporosis: a randomized clinical trial in comparison with alendronate. Biomed Pharmacother. (2023) 163:114821. doi: 10.1016/j.biopha.2023.114821
165. Knudson Schult, TM, Ensrud, KE, Blackwell, T, Ettinger, B, Wallace, R, and Tice, JA. Effect of isoflavones on lipids and bone turnover markers in menopausal women. Maturitas. (2004) 48:209–18. doi: 10.1016/j.maturitas.2003.09.027
166. Martin, BR, McCabe, GP, McCabe, L, Jackson, GS, Horcajada, MN, Offord-Cavin, E, et al. Effect of hesperidin with and without a calcium (calcilock) supplement on bone health in postmenopausal women. J Clin Endocrinol Metab. (2016) 101:923–7. doi: 10.1210/jc.2015-3767
167. Mahdaviroshan, M, Golzarand, M, Taramsari, MR, and Mahdaviroshan, M. Effect of zinc supplementation on serum zinc and calcium levels in postmenopausal osteoporotic women in Tabriz, Islamic Republic of Iran. East Mediterr Health J. (2013) 19:271–5. Available at: http://www.ncbi.nlm.nih.gov/pubmed/23879079
168. Nielsen, FH, Lukaski, HC, Johnson, LK, and Roughead, ZK. Reported zinc, but not copper, intakes influence whole-body bone density, mineral content and T score responses to zinc and copper supplementation in healthy postmenopausal women. Br J Nutr. (2011) 106:1872–9. doi: 10.1017/S0007114511002352
169. Aydin, H, Deyneli, O, Yavuz, D, Gözü, H, Mutlu, N, Kaygusuz, I, et al. Short-term oral magnesium supplementation suppresses bone turnover in postmenopausal osteoporotic women. Biol Trace Elem Res. (2010) 133:136–43. doi: 10.1007/s12011-009-8416-8
170. Granchi, D, Caudarella, R, Ripamonti, C, Spinnato, P, Bazzocchi, A, Massa, A, et al. Potassium citrate supplementation decreases the biochemical markers of bone loss in a group of osteopenic women: the results of a randomized, double-blind, placebo-controlled pilot study. Nutrients. (2018) 10:1293. doi: 10.3390/nu10091293
171. Jehle, S, Zanetti, A, Muser, J, Hulter, HN, and Krapf, R. Partial neutralization of the acidogenic western diet with potassium citrate increases bone mass in postmenopausal women with osteopenia. J Am Soc Nephrol. (2006) 17:3213–22. doi: 10.1681/ASN.2006030233
172. Gregory, NS, Kumar, R, Stein, EM, Alexander, E, Christos, P, Bockman, RS, et al. Potassium citrate decreases bone resorption in postmenopausal women with osteopenia: a randomized, double-blind clinical trial. Endocr Pract. (2015) 21:1380–6. doi: 10.4158/EP15738.OR
173. Heaney, RP, Recker, RR, Watson, P, and Lappe, JM. Phosphate and carbonate salts of calcium support robust bone building in osteoporosis. Am J Clin Nutr. (2010) 92:101–5. doi: 10.3945/ajcn.2009.29085
174. Elam, ML, Johnson, SA, Hooshmand, S, Feresin, RG, Payton, ME, Gu, J, et al. A calcium-collagen chelate dietary supplement attenuates bone loss in postmenopausal women with osteopenia: a randomized controlled trial. J Med Food. (2015) 18:324–31. doi: 10.1089/jmf.2014.0100
175. Prince, RL, Devine, A, Dhaliwal, SS, and Dick, IM. Effects of calcium supplementation on clinical fracture and bone structure results of a 5-year, double-blind, placebo-controlled trial in elderly women. Arch Intern Med. (2006) 166:869–75. doi: 10.1001/archinte.166.8.869
176. Albertazzi, P, Steel, SA, Howarth, EM, and Purdie, DW. Comparison of the effects of two different types of calcium supplementation on markers of bone metabolism in a postmenopausal osteopenic population with low calcium intake: a double-blind placebo-controlled trial. Climacteric. (2004) 7:33–40. doi: 10.1080/13697130310001651454
177. Ciria-Recasens, M, Blanch-Rubió, J, Coll-Batet, M, Del Pilar, L-PM, Díez-Perez, A, Carbonell-Abelló, J, et al. Comparison of the effects of ossein-hydroxyapatite complex and calcium carbonate on bone metabolism in women with senile osteoporosis: a randomized, open-label, parallel-group, controlled, prospective study. Clin Drug Investig. (2011) 31:817–24. doi: 10.1007/BF03256920
178. Hooshmand, S, Kern, M, Metti, D, Shamloufard, P, Chai, SC, Johnson, SA, et al. The effect of two doses of dried plum on bone density and bone biomarkers in osteopenic postmenopausal women: a randomized, controlled trial. Osteoporos Int. (2016) 27:2271–9. doi: 10.1007/s00198-016-3524-8
179. Amstrup, AK, Sikjaer, T, Heickendorff, L, Mosekilde, L, and Rejnmark, L. Melatonin improves bone mineral density at the femoral neck in postmenopausal women with osteopenia: a randomized controlled trial. J Pineal Res. (2015) 59:221–9. doi: 10.1111/jpi.12252
180. Hodges, JK, Maiz, M, Cao, S, Lachcik, PJ, Peacock, M, McCabe, GP, et al. Moderate consumption of freeze-dried blueberry powder increased net bone calcium retention compared with no treatment in healthy postmenopausal women: a randomized crossover trial. Am J Clin Nutr. (2023) 118:382–90. doi: 10.1016/j.ajcnut.2023.05.033
181. Radford, LT, Bolland, MJ, Mason, B, Horne, A, Gamble, GD, Grey, A, et al. The Auckland calcium study: 5-year post-trial follow-up. Osteoporos Int. (2014) 25:297–304. doi: 10.1007/s00198-013-2526-z
182. Ebeling, PR, Wark, JD, Yeung, S, Poon, C, Salehi, N, Nicholson, GC, et al. Effects of calcitriol or calcium on bone mineral density, bone turnover, and fractures in men with primary osteoporosis: a two-year randomized, double blind, double placebo study. J Clin Endocrinol Metab. (2001) 86:4098–103. doi: 10.1210/jcem.86.9.7847
183. Weaver, CM, Martin, BR, Jackson, GS, McCabe, GP, Nolan, JR, McCabe, LD, et al. Antiresorptive effects of phytoestrogen supplements compared with estradiol or risedronate in postmenopausal women using 41Ca methodology. J Clin Endocrinol Metab. (2009) 94:3798–805. doi: 10.1210/jc.2009-0332
184. Billington, EO, Mahajan, A, Benham, JL, and Raman, M. Effects of probiotics on bone mineral density and bone turnover: a systematic review. Crit Rev Food Sci Nutr. (2023) 63:4141–52. doi: 10.1080/10408398.2021.1998760
185. Sharif, PS, Asalforoush, M, Ameri, F, Larijani, B, and Abdollahi, M. The effect of n-3 fatty acids on bone biomarkers in Iranian postmenopausal osteoporotic women: a randomized clinical trial. Age. (2010) 32:179–86. doi: 10.1007/s11357-009-9122-3
186. Vanlint, SJ, and Ried, K. Efficacy and tolerability of calcium, vitamin D and a plant-based omega-3 oil for osteopenia: a pilot RCT. Maturitas. (2012) 71:44–8. doi: 10.1016/j.maturitas.2011.10.004
187. García-Gavilán, JF, Bulló, M, Canudas, S, Martínez-González, MA, Estruch, R, Giardina, S, et al. Extra virgin olive oil consumption reduces the risk of osteoporotic fractures in the PREDIMED trial. Clin Nutr. (2018) 37:329–35. doi: 10.1016/j.clnu.2016.12.030
188. Evans, M, Lewis, ED, Antony, JM, Crowley, DC, Guthrie, N, and Blumberg, JB. Breaking new frontiers: assessment and re-evaluation of clinical trial design for nutraceuticals. Front Nutr. (2022) 9:958753. doi: 10.3389/fnut.2022.958753
189. Durazzo, A, Lucarini, M, and Santini, A. Nutraceuticals in human health. Foods. (2020) 9:370. doi: 10.3390/foods9030370
190. Goodman, GE, Thornquist, MD, Balmes, J, Cullen, MR, Meykens, FL, Omenn, GS, et al. The beta-carotene and retinol efficacy trial: incidence of lung cancer and cardiovascular disease mortality during 6-year follow-up after stopping β-carotene and retinol supplements. J Natl Cancer Inst. (2004) 96:1743–50. doi: 10.1093/jnci/djh320
191. Bonds, DE, Harrington, M, Worrall, BB, Bertoni, AG, Eaton, CB, Hsia, J, et al. Effect of long-chain ω-3 fatty acids and lutein + zeaxanthin supplements on cardiovascular outcomes: results of the age-related eye disease study 2 (AREDS2) randomized clinical trial. JAMA Intern Med. (2014) 174:763–71. doi: 10.1001/jamainternmed.2014.328
192. Lippman, SM, Klein, EA, Goodman, PJ, Lucia, MS, Thompson, IM, Ford, LG, et al. Effect of selenium and vitamin E on risk of prostate cancer and other cancers: the selenium and vitamin E cancer prevention trial (SELECT). JAMA. (2009) 301:39–51. doi: 10.1001/jama.2008.864
193. Manson, JE, Cook, NR, Lee, IM, Christen, W, Bassuk, SS, Mora, S, et al. Vitamin D supplements and prevention of cancer and cardiovascular disease. N Engl J Med. (2019) 380:33–44. doi: 10.1056/NEJMoa1809944
194. Sesso, HD, Rist, PM, Aragaki, AK, Rautiainen, S, Johnson, LG, Friedenberg, G, et al. Effect of cocoa flavanol supplementation for the prevention of cardiovascular disease events: the COcoa Supplement and Multivitamin Outcomes Study (COSMOS) randomized clinical trial. Am J Clin Nutr. (2022) 115:1501–10. doi: 10.1093/ajcn/nqac056
195. Saldanha, LG . US Food and Drug Administration regulations governing label claims for food products, including probiotics. Clin Infect Dis. (2008) 46:S144–51. doi: 10.1086/523328
196. Nuzzo, R . Scientific method: statistical errors. Nature. (2014) 506:150–2. doi: 10.1038/506150a
197. Kaput, J . Developing the pathway to personalized health: the potential of N-of-1 studies for personalizing nutrition. J Nutr. (2021) 151:2863–4. doi: 10.1093/jn/nxab243
198. Ma, Y, Fu, Y, Tian, Y, Gou, W, Miao, Z, Yang, M, et al. Individual postprandial glycemic responses to diet in n-of-1 trials: Westlake N-of-1 trials for macronutrient intake (WE-MACNUTR). J Nutr. (2021) 151:3158–67. doi: 10.1093/jn/nxab227
199. Potter, T, Vieira, R, and De Roos, B. Perspective: application of N-of-1 methods in personalized nutrition research. Adv Nutr. (2021) 12:579–89. doi: 10.1093/advances/nmaa173
200. Soldevila-Domenech, N, Boronat, A, Langohr, K, and de la Torre, R. N-of-1 clinical trials in nutritional interventions directed at improving cognitive function. Front Nutr. (2019) 6:110. doi: 10.3389/fnut.2019.00110
201. Tian, Y, Ma, Y, Fu, Y, and Zheng, JS. Application of n-of-1 clinical trials in personalized nutrition research: a trial protocol for Westlake N-of-1 trials for macronutrient intake (WE-MACNUTR). Curr Dev Nutr. (2020) 4:nzaa143. doi: 10.1093/cdn/nzaa143
202. Alali, M, Alqubaisy, M, Aljaafari, MN, Alali, AO, Baqais, L, Molouki, A, et al. Nutraceuticals: transformation of conventional foods into health promoters/disease preventers and safety considerations. Molecules. (2021) 26:2540. doi: 10.3390/molecules26092540
203. Godad, A, Ansari, A, Bhatia, N, Ali, A, Zine, S, and Doshi, G. Drug nutraceutical interactions In: Industrial application of functional foods, ingredients and nutraceuticals : Elsevier (2023). 663–723. Available at: https://linkinghub.elsevier.com/retrieve/pii/B9780128243121000200
204. Gil, F, Hernández, AF, and Martín-Domingo, MC. Toxic contamination of nutraceuticals and food ingredients In: Nutraceuticals: efficacy, safety and toxicity : Elsevier (2016). 825–37. Available at: https://linkinghub.elsevier.com/retrieve/pii/B9780128021477000589
205. Anadón, A, Martínez-Larrañaga, MR, Ares, I, and Martínez, MA. Interactions between nutraceuticals/nutrients and therapeutic drugs In: Nutraceuticals: efficacy, safety and toxicity : Elsevier (2016). 855–74. Available at: https://linkinghub.elsevier.com/retrieve/pii/B9780128021477000607
206. Lockwood, GB . The quality of commercially available nutraceutical supplements and food sources. J Pharm Pharmacol. (2011) 63:3–10. doi: 10.1111/j.2042-7158.2010.01159.x
207. Ronis, MJJ, Pedersen, KB, and Watt, J. Adverse effects of nutraceuticals and dietary supplements. Annu Rev Pharmacol Toxicol. (2018) 58:583–601. doi: 10.1146/annurev-pharmtox-010617-052844
208. Paller, CJ, Denmeade, SR, and Carducci, MA. Challenges of conducting clinical trials of natural products to combat cancer. Clin Adv Hematol Oncol. (2016) 14:447–55. Available at: http://www.ncbi.nlm.nih.gov/pubmed/27379814.
209. Tapsell, LC . Evidence for health claims: a perspective from the Australia-New Zealand region. J Nutr. (2008) 138:1206S–9S. doi: 10.1093/jn/138.6.1206S
Keywords: osteopenia, osteoporosis, nutraceuticals, prevention, lifestyle, bone health
Citation: Roseti L, Borciani G, Grassi F, Desando G, Gambari L and Grigolo B (2024) Nutraceuticals in osteoporosis prevention. Front. Nutr. 11:1445955. doi: 10.3389/fnut.2024.1445955
Edited by:
Malgorzata Ziarno, Warsaw University of Life Sciences, PolandReviewed by:
Elvy Suhana Mohd Ramli, National University of Malaysia, MalaysiaStefano Gonnelli, University of Siena, Italy
Copyright © 2024 Roseti, Borciani, Grassi, Desando, Gambari and Grigolo. This is an open-access article distributed under the terms of the Creative Commons Attribution License (CC BY). The use, distribution or reproduction in other forums is permitted, provided the original author(s) and the copyright owner(s) are credited and that the original publication in this journal is cited, in accordance with accepted academic practice. No use, distribution or reproduction is permitted which does not comply with these terms.
*Correspondence: Giorgia Borciani, Z2lvcmdpYS5ib3JjaWFuaUBpb3IuaXQ=