- 1Department of Human Health and Nutritional Sciences, University of Guelph, Guelph, ON, Canada
- 2Department of Family Medicine, McMaster University, Hamilton, ON, Canada
- 3Massachusetts General Hospital and Harvard Medical School, Boston, MA, United States
- 4Omega-3 and Global Health Institute, Boston, MA, United States
Purpose: Repeated mild traumatic brain injuries (mTBI) are a continuing healthcare concern worldwide, given its potential for enduring adverse neurodegenerative conditions. Past research suggests a potential protective effect of n-3 polyunsaturated fatty acids (PUFA) in experimental models of mTBI. The aim of this study was to investigate whether the neuroprotective benefits of n-3 PUFA persist following repetitive weight drop injury (WDI).
Methods: Male fat-1 mice (n = 12), able to endogenously convert n-6 PUFA to n-3 PUFA, and their wild type (WT) counterparts (n = 12) were maintained on a 10% w/w safflower diet. At 9–10 weeks of age, both groups received one mild low-impact WDI on the closed cranium daily, for three consecutive days. Following each WDI, time to righting reflex and seeking behaviour were measured. Neurological recovery, cognitive, motor, and neurobehavioural outcomes were assessed using the Neurological Severity Score (NSS) over 7 days (168 h) post-last WDI. Brains were assessed for cerebral microhemorrhages by Prussian blue and cellular damage by glial fibrillary acidic protein (GFAP) staining.
Results: Fat-1 mice exhibited significantly faster righting reflex and seeking behaviour time, and lower mean NSS scores and at all post-WDI time points (p ≤ 0.05) compared to WT mice. Immunohistochemistry showed no significant difference in presence of cerebral microhemorrhage however, fat-1 mice had significantly lower GFAP staining in comparison to WT mice (p ≤ 0.05).
Conclusion: n-3 PUFA is effective in restoring cognitive, motor, and behavioural function after repetitive WDI, which may be mediated through reduced cellular damage of the brain.
1 Introduction
Mild traumatic brain injury (mTBI) stands out as a prevalent neurological disorder and as the predominant form of traumatic brain injury (TBI), consisting of 70–90% of brain injuries (1). While frequently used interchangeably, concussions are classified as a subgroup within mTBI; nevertheless, it is important to note that mTBI does not always manifest as a concussion (2, 3). The defining features of mTBI involve a non-life-threatening impact, either direct or indirect, to the head, lacking any indication of skull fracture, alongside a Glasgow Coma Scale score ranging from 13 to 15 (2, 4, 5). The manifestations of mTBI encompass a diverse array of symptoms, spanning in severity from headaches, sleep disturbances, challenges in memory retention, to persistent cognitive impairments (6). While the symptoms of acute mTBI often subside after several months, the increased likelihood of developing permanent sequelae such as long-term cognitive and neurobehavioural impairments, is observed with repetitive mild insults (6, 7). Thus, given the potential cumulative effects of such exposures, there is rising concern about the long-term adverse consequences associated with repeated mTBI and to sub-concussive impacts, where no apparent signs or symptoms are evident following a head impact (7, 8).
Across Canada and the United States, epidemiological studies report an annual incidence of mTBI ranging between 200,000 and 1.6–3.8 million, respectively (9, 10). However, in reality, estimates of incidence are projected to be much higher due to underreporting and lack of acknowledgement by individuals regarding the severity of the injury (11). Consequently, it is important to understand both the acute response profile following an mTBI and long-term risk in affected individuals. Until recently, a significant portion of research directed towards mitigating the pathophysiological response to injury has concentrated on pharmaceutical interventions post-injury. However, there is no substantiated evidence supporting the efficacy of pharmacological therapy in reducing recovery duration or offering neuroprotection before the occurrence of an impact (12, 13). Acknowledging the inherent constraints in existing treatment methods and recognizing the necessity for proactive preventive measures, research efforts have expanded towards strategies involving nutraceuticals and nutritional supplementation (14).
Previous studies indicate that n-3 polyunsaturated fatty acids (n-3 PUFA), such as eicosapentaenoic acid (EPA) and docosahexaenoic acid (DHA) and, might prove beneficial in protecting the brain against mTBI (15). The essential and major dietary form of n-3 PUFA is alpha-linolenic acid (ALA) (16). Although EPA and DHA are downstream products metabolized from ALA (17), conversion rates are said to be below 1%, with EPA conversion being higher than DHA (18, 19). Thus, obtaining EPA and DHA through marine-based dietary sources is more efficient (16). DHA composes over 90% of the n-3 PUFA content and approximately 10–20% of the total lipids in the human brain (19, 20). As a result, DHA plays a vital role as a structural component in plasma membranes, contributes to neuronal signaling, and shares anti-inflammatory properties with EPA (17).
Prior research conducted by our lab demonstrated in the fat-1 mouse model, which is capable of de novo synthesis of n-3 PUFA from n-6 PUFA (21), has shown improvements in functional outcomes after a single mild weight drop injury (WDI) (15). This initial study was a proof of concept study to establish a model that could later be used to further study the effects of n-3 PUFA and conditions such as multiple repeated mTBI observed in football, rugby, and hockey. Therefore, in the present study, we aimed to ascertain whether the effects of n-3 PUFA persist in the context of repetitive mTBI using the novel fat-1 transgenic mouse model. The fat-1 mouse is capable of synthesizing n-3 from a single diet enriched in n-6 PUFA. Building upon the findings observed in our previous study involving a single WDI, wherein significant neurological recovery was evident in fat-1 mice, we hypothesized that fat-1 mice will continue to display better outcomes after three consecutive WDI.
2 Materials and methods
2.1 Animals, housing, and experimental design
All procedures related to the care, handling, and experimentation of animals were carried out in accordance with and approval from the Animal Care and Use Committee of the University of Guelph (AUP #4207). Male C57BL/6 fat-1 mice were bred with C57/Bl6 female mice, acquired from Charles River, to produce fat-1 and WT progeny. Post-weaning at 3 weeks, offspring were fed a diet consisting of 10% w/w safflower (D04092701, Research Diets, New Brunswick, NJ, United States) and given distilled water ad libitum. Housing conditions included group accommodations of 3–4 mice per cage. The environment was regulated to maintain a temperature range of 22–24°C, and 12 h light/dark cycle, with experimental testing occurring exclusively in the light periods. When mice reached 9 to 10 weeks of age, male fat-1 and WT mice (n = 24), were exposed to one WDI, for three consecutive days. After the third WDI, mice were monitored for a span of 1 week (i.e., 168 h), during which their motor, cognitive, and neurobehavioural functions were evaluated before being euthanized for tissue collection. WT control and fat-1 control (n = 24), underwent all experimental procedures with the exclusion of the repetitive WDI.
2.2 Inducing three consecutive mild traumatic brain injury
Mice were exposed to a singular WDI for three consecutive days as established by Flierl et al. (22). Anesthesia induction involved the administration of 4% Baxter isoflurane (Cat #19476, CMDV, St. Hyacinthe, Quebec) vaporized in oxygen. Upon achieving the surgical plane (between 45 and 105 s), mice were removed from the anesthetic chamber and placed within a nose cone. The isoflurane concentration was reduced to a maintenance level of 1.5% until pedal reflexes ceased, totaling a maximum of 4 min under anesthesia. Anesthesia was terminated just before initiating the WDI. The induction of a single concussive impact included placing the anesthetized mouse chest-down on a slit-piece of aluminum foil, positioned 10 cm above a foam cushion and secured by a Plexiglass apparatus. The alignment ensured that a 100 g steel weight (1.3 cm × 28 cm) fell directly onto the dorsal surface of the scalp, midline between the bregma and lambda. Concussion administration involved rapidly elevating the weight using a nylon fly fishing line. Subsequently, the weight was released vertically from a height of 163 cm through a PVC guide tube (20 mm × 163 cm), delivering a force of impact clinically relevant to closed cranium scenarios (15). This single WDI design was repeated at the same time on the following 2 days, resulting in a total of three mTBI on successive days.
2.3 Time to first movement – righting reflex and seeking behaviour
Following all three WDI, each mouse was promptly relocated to a containment enclosure and positioned on their dorsal side. Subsequently, the righting reflex duration was assessed, measuring the time required for the mouse to reorient itself, transitioning from dorsal to ventral positioning. A secondary time was recorded to gauge the seeking behaviour of the mouse. The time to first movements were evaluated as an indicator of neurological recovery, akin to the righting reflex parameter assessed in the study by Kane et al. (23).
2.4 Neurological severity score
Mice were evaluated for both functional recovery and the extent of mTBI induced by the weight drop apparatus through the Neurological Severity Score (NSS). The NSS is a comprehensive assessment with a set of clinical criteria, established in 2009 by Flierl et al. (22) and encompasses ten distinct clinical tasks that assesses motor, cognitive, and neurobehavioural performance (Table 1). For each of the ten tasks, a score of one point is awarded for the inability to perform the task. A maximal NSS score of 10 indicates an inability to fulfill all tasks, reflecting severe neurological dysfunction, while a score of zero denotes unimpaired neurological function. NSS assessments were carried out on both fat-1 and WT mice at 1 h following each WDI. Following the 3rd WDI, NSS assessments were evaluated specifically at 1, 4, 24, 48, 72, and 168 h.
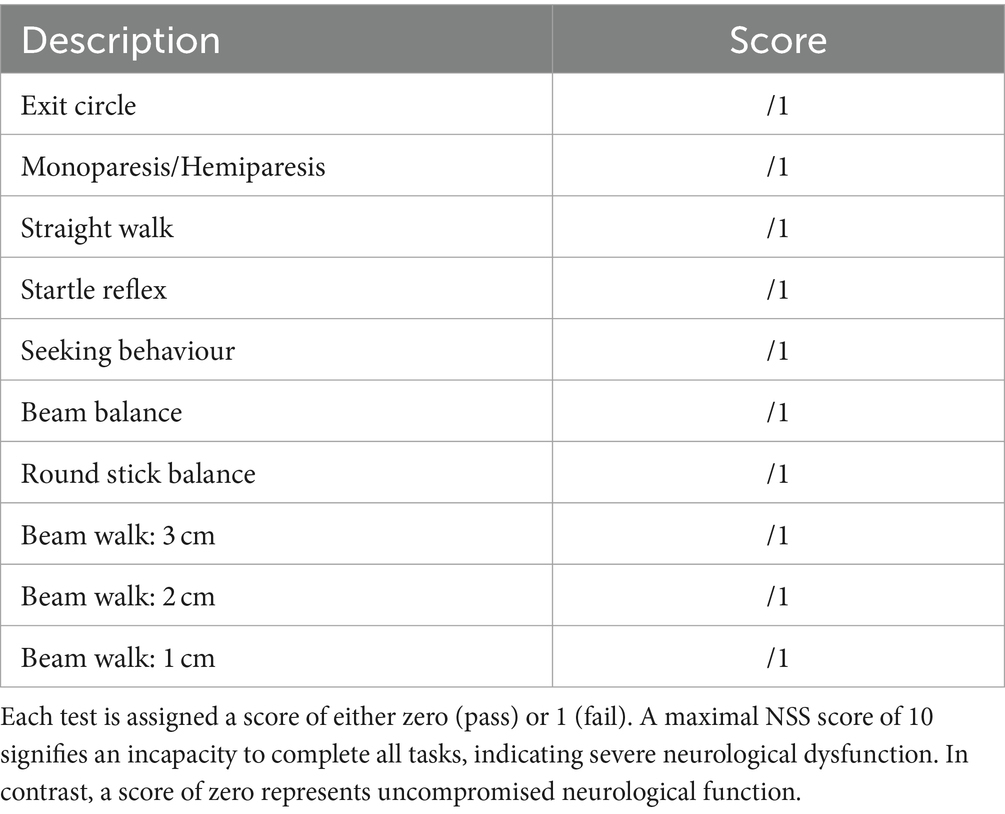
Table 1. Neurological severity score (NSS) tasks as described by Flierl et al. (22).
2.5 GFAP and Prussian blue staining for detection of inflammatory cytokines and cerebral microhemorrhages
Prussian blue (PB) staining for cerebral microhemorrhages and the presence of cellular damage by glial fibrillary acidic protein (GFAP) staining were evaluated 168 h after the last WDI. Brain hemispheres were fixed in 4% formaldehyde and processed in a tissue processor overnight for immunohistochemistry staining. The brain tissues were embedded in a paraffin block, and 5 μm sections were sliced along the dorsoventral plane using a Leica RM2235 microtome. The first 50 μm of brain sections were discarded to reach a point of visibility. Two brain slices each were used to analyze PB (55–60 μm) and GFAP (65–70 μm) appearance. These free-floating tissue sections were then fixed onto Superfrost Plus slides (Cat#12-550-15, Fisher Scientific, Pittsburgh, PA).
Following embedding and slicing of brain tissue, sections intended for PB staining were deparaffinized and hydrated using distilled water before submersion in a solution comprising equal parts of 20% hydrochloric acid (Cat #A144-500, Fisher Chemical) and 10% potassium hexacyanoferrate (II) trihydrate (Cat #P3289-5G, Sigma-Aldrich Canada Co., Oakville, ON) for a duration of 20 min. The sections were then rinsed in 2 successive changes of water and subsequently counterstained with nuclear fast red (Cat #R5463200-500A, Ricca Chemical Company) for 5 min, followed by rinsing in 3 successive changes of water (15). Sections intended for GFAP staining was processed using the Proteintech IHCeasy GFAP Ready-To-Use IHC Kit (Cat #KHC0002). The staining procedure for slides designated for GFAP followed the protocol provided by Proteintech.
After processing for each respective stain of either PB or GFAP, all sections underwent a sequence of baths in the following sequence: dehydration in 95% isopropanol (Cat #HC5001GAL, Fisherbrand™ HistoPrep™); 2 changes in isopropanol for dehydration; and 3 changes of xylene (Cat #HC7001GAL, Fisherbrand™ HistoPrep™), each for a duration of 2 min. Following, sections were mounted with Cytoseal XYL (Cat #8312-4, Epredia, Kalamazoo, MI) and cover slipped (Cat #12-541-023CA, Fisher Scientific, Pittsburgh, PA).
Two independent observers scored PB and GFAP staining of the entire brain slice using a Nikon Eclipse TS100 microscope at 20X and 40X original magnification, respectively. Scores were averaged based on both independent observers. The average number of PB-positive clusters for each brain section was computed following the method described by Fisher et al. (24). Scoring of GFAP staining was based on the stain density of the brain section, which was adapted from the method by Luijerink et al. (25) to allow for greater discrimination between lightly stained sections across a broader range of scores. Intensity of the stain was scored between 0 and 4, with 0 being the least intense and 4 being the most intense.
2.6 Statistical analysis
Statistical analysis was conducted using SAS version 9.4 (SAS Institute, Cary, NC, United States). Initially, a two-way analysis of variance (ANOVA) was conducted. In the absence of a significant interaction effect in the number of mTBI, a one-way ANOVA analysis was conducted on the 1 h NSS, righting reflex, and seeking behaviour measurements. Subsequently, Tukey’s Honest Significant Difference (HSD) post-hoc test was used to determine differences between means across each group. NSS was analyzed by repeated measures analysis, followed by a one-way ANOVA with Tukey’s HSD post-hoc test following the 3rd WDI, to detect differences between each group over the 168 h NSS evaluation period. A two-tailed t-test was conducted to identify differences in PB and GFAP staining in WT and fat-1 mouse brains. Significance was set at p ≤ 0.05 for all analyses.
3 Results
3.1 Time to first movement – righting reflex and seeking behaviour
Fat-1 mice had significantly faster times to recover the righting reflex (Figure 1A; WT, n = 12, 3,211 ± 282 s; fat-1, n = 12, 84. ± 47 s) and seeking behaviour (Figure 1B; WT, n = 12, 1,082 ± 480 s; fat-1, n = 12, 271 ± 167 s) times following each exposure to the WDI (p ≤ 0.05), in comparison to WT mice. Fat-1 mice were not different in comparison to WT control mice groups (p ≥ 0.05).
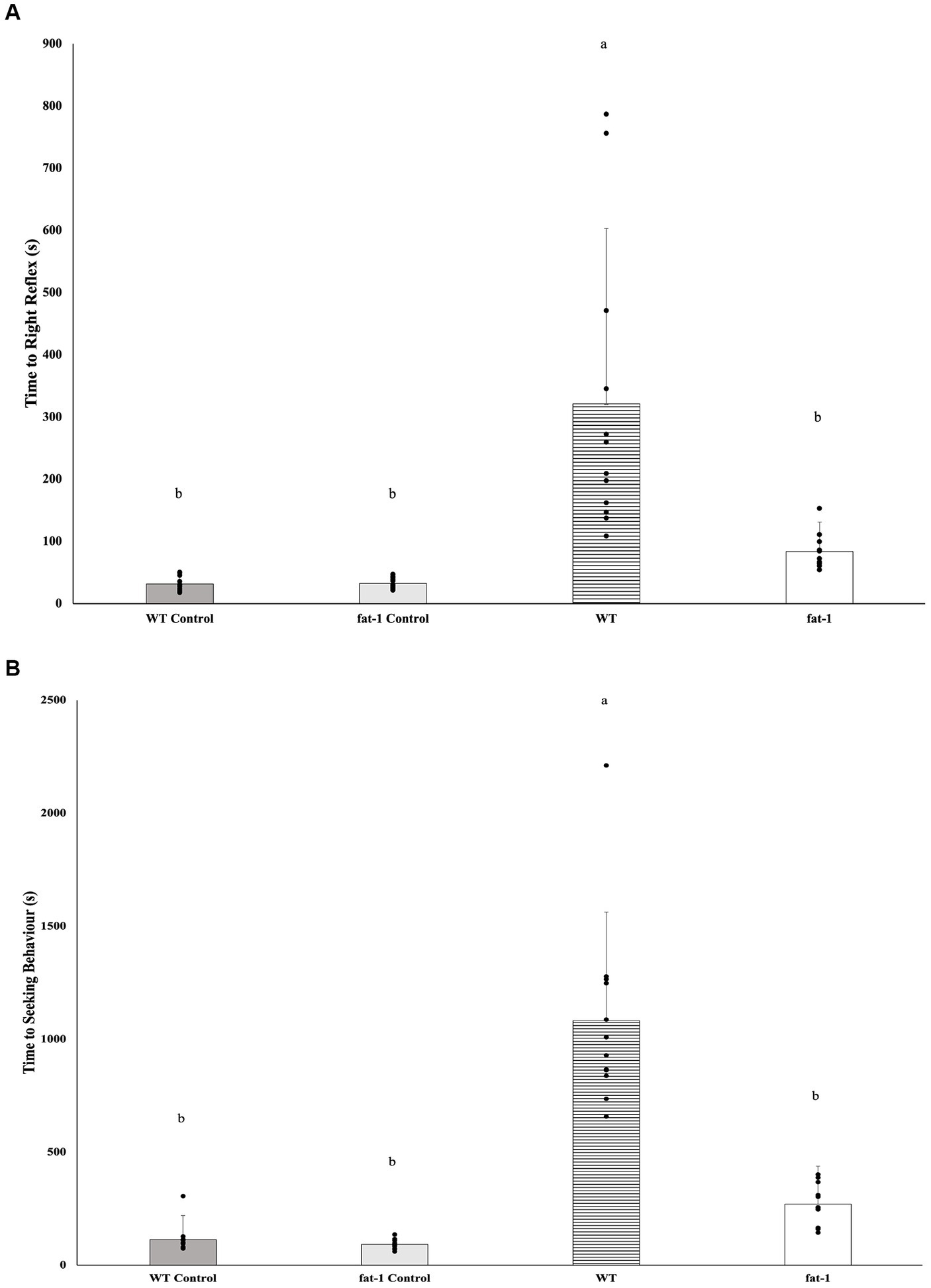
Figure 1. (A) Average righting reflex times and (B) average time to showing seeking behaviour after three-repeated weight drop injury (WDI) wherein a lower time reflects greater neurological restoration (n = 12/group); data analyzed by one-way ANOVA followed by Tukey’s Honest Significant Difference (HSD) post-hoc test. Bars with different letters are significantly different (p ≤ 0.05) according to Tukey’s HSD. Data are presented as mean values ± SD.
3.2 Neurological severity score
Fat-1 mice had significantly lower mean NSS scores (p ≤ 0.05) at 1 h following each WDI in comparison to WT mice (Figure 2). Fat-1 mice also had significantly lower mean NSS scores (p ≤ 0.05) at all time points post-last WDI; the precise timing of when these differences became significant was at the 48 h time point (Figure 3). Both fat-1 and WT mice demonstrated continuous enhancements in NSS scores over time. In fat-1 mice, a progressive decrease in mean NSS score can be seen through the 1 h and 168 h timepoints (mean values ± SD, 1.00 ± 0.74 and 0.08 ± 0.29, respectively). In WT mice, a similar trend can be seen following the 24 h timepoint. However, throughout the evaluation period, the mean NSS score stayed higher for WT mice compared to fat-1 counterparts. The one-way ANOVA with Tukey HSD post-hoc revealed that the WT group was significantly different (p ≤ 0.05) at all time points. Beginning at the 24 h NSS timepoint, fat-1 mice showed no significant difference with WT control mice (Figure 3).
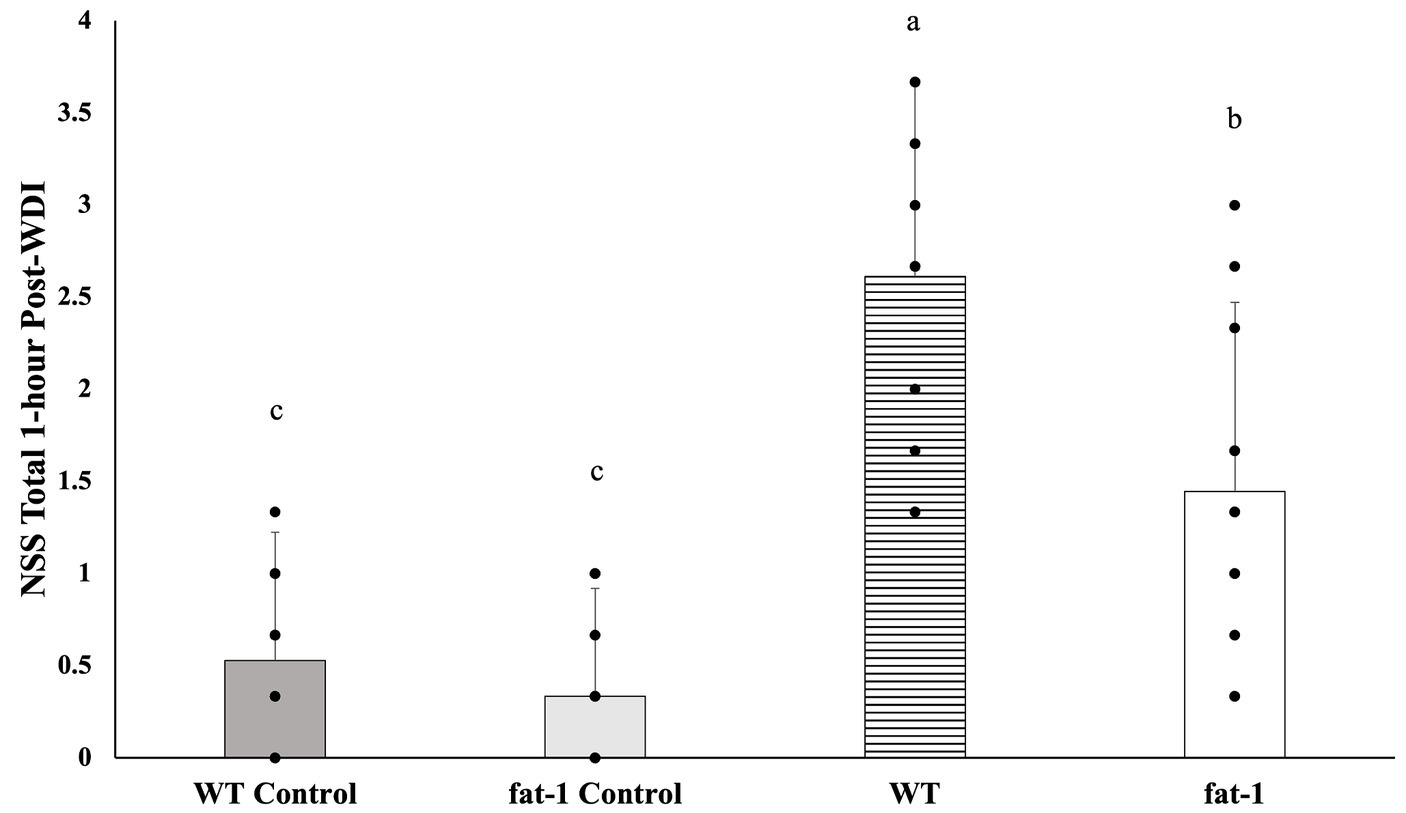
Figure 2. NSS for mice at the 1 h time point following each WDI. n = 12 for all groups; wildtype (WT); fat-1; WT control and fat-1 control. Data analyzed by one-way ANOVA with Tukey’s HSD post-hoc test. Bars with different letters are significantly different according to Tukey’s HSD (p ≤ 0.05). Data are presented as mean values ± SD.
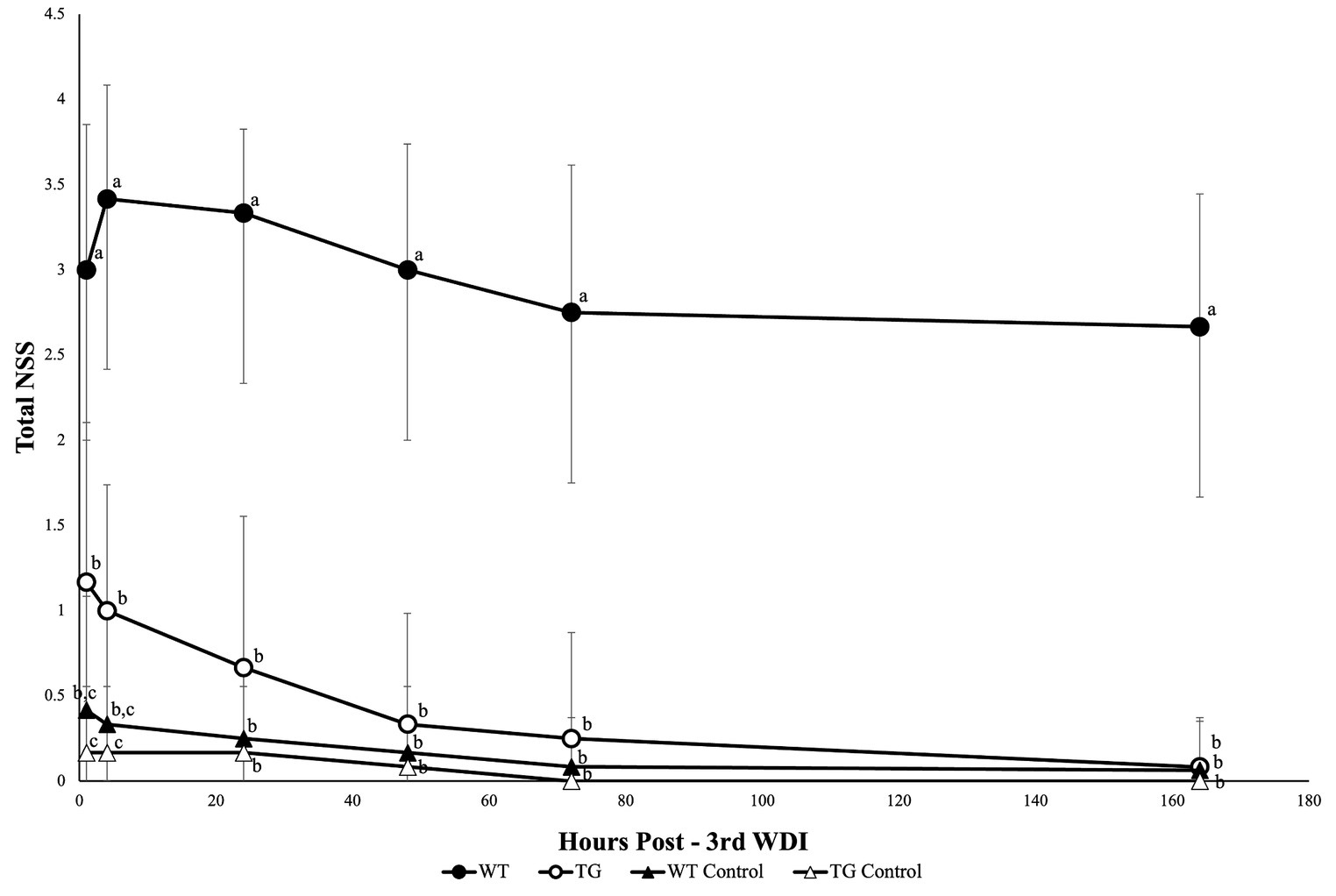
Figure 3. NSS for mice at the 1, 4, 24, 48, 72, and 168 h timepoints following the last WDI. n = 12/group; wildtype (WT); fat-1; WT control and fat-1 control. Repeated measures analysis showed a significant difference in NSS (p ≤ 0.05) between groups at all timepoints. Subsequently, a one-way ANOVA with Tukey’s HSD post-hoc analysis was conducted at each timepoint to determine differences between groups. Different letters at each time point are significantly different according to Tukey’s HSD (p ≤ 0.05). Data are presented as mean values ± SD.
3.3 GFAP and cerebral microhemorrhage
No statistical differences (p ≥ 0.05) were found in the average number of microhemorrhage clusters through PB staining between fat-1 and WT groups (Figures 4, 5). However, fat-1 mice had significantly less GFAP staining when compared to WT mice at the 168 h timepoint post-last WDI (Figures 6, 7). Analysis of WT control and fat-1 control mice found no detectable appearance of staining, resulting in a mean score of zero.
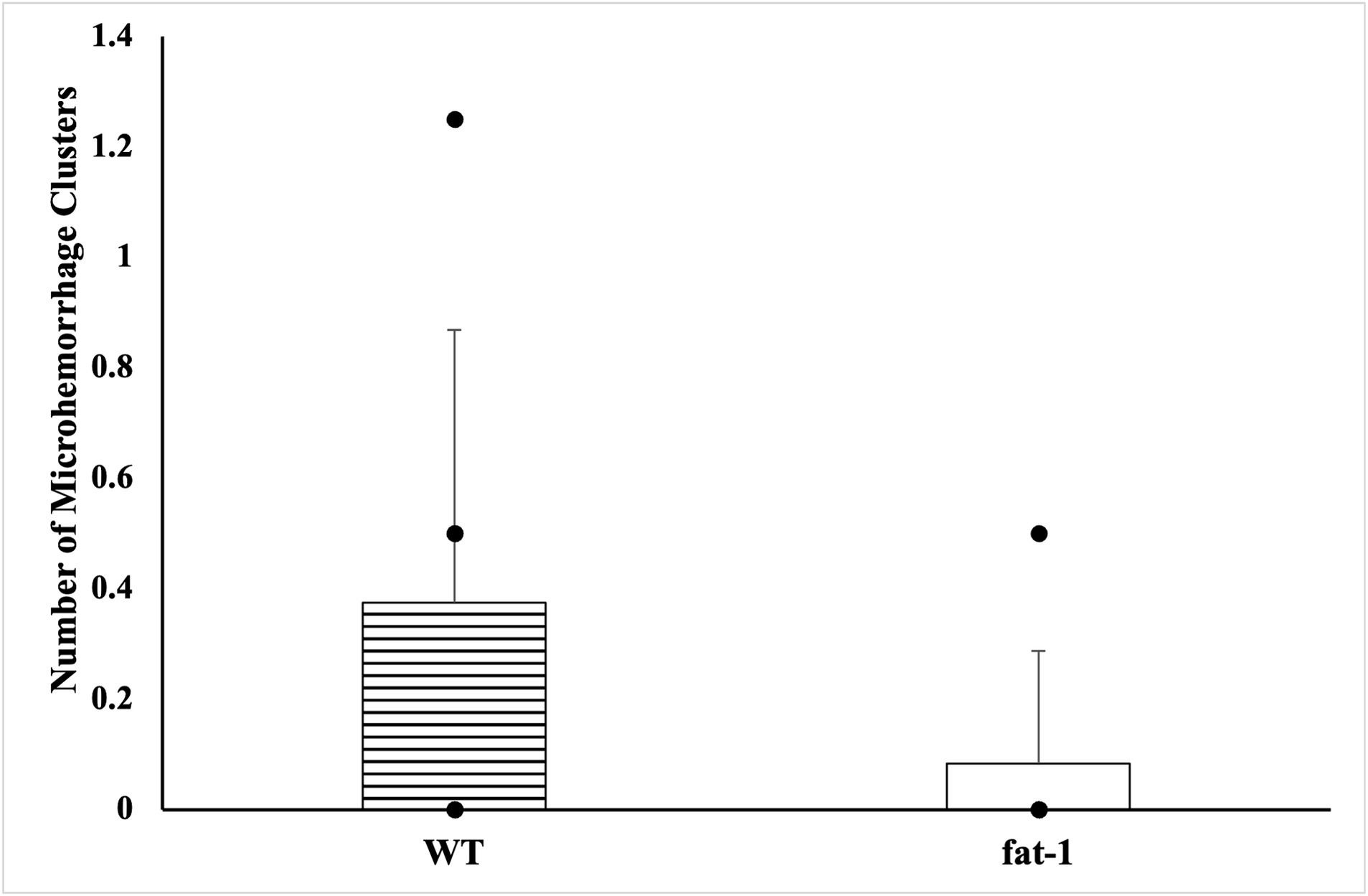
Figure 4. Appearance of cerebral microhemorrhage clusters by Prussian Blue staining for mice at the 168 h timepoint following the last WDI [n = 6/group; wildtype (WT); fat-1; WT control and fat-1 control]. WT control and fat-1 control had no detectable staining and therefore not shown. * denotes a significant difference (p ≤ 0.05) by two-tailed t-test. Data are presented as mean values ± SD.
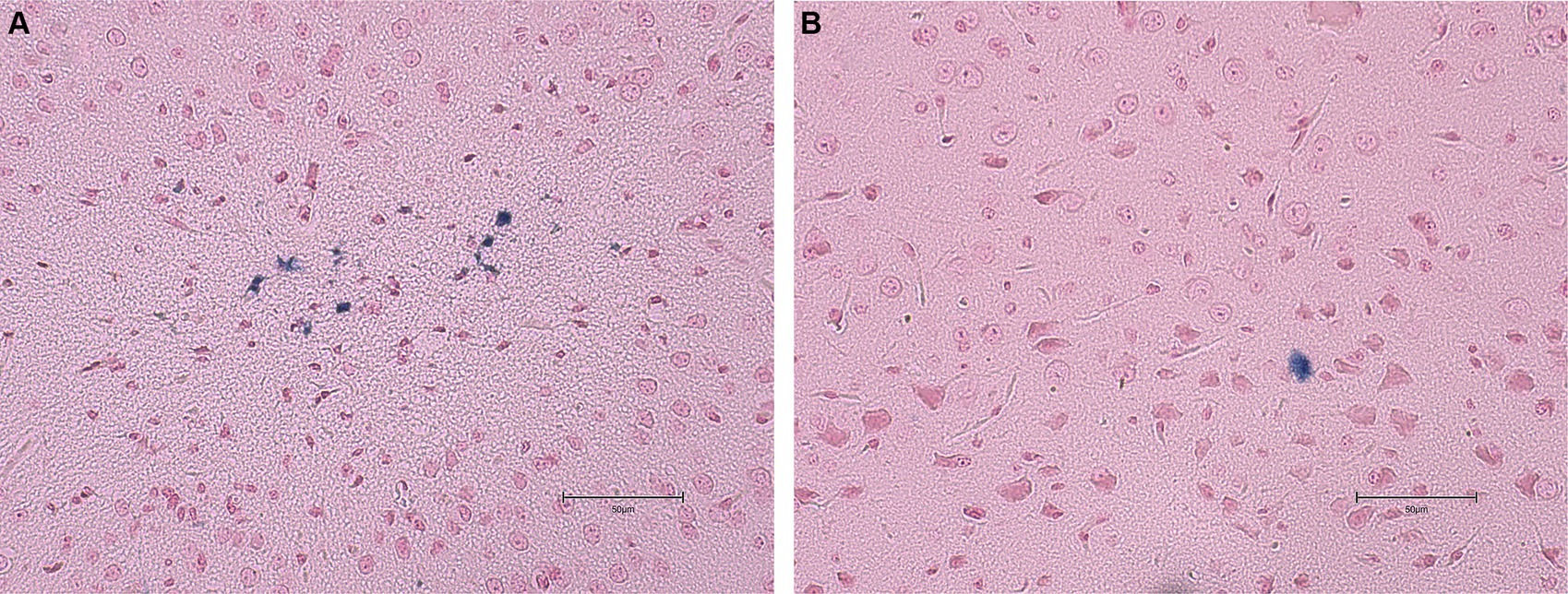
Figure 5. Images of the dorsoventral brain sections of WT and fat-1 mice stained with Prussian blue 168 h post 3rd WDI. Positive Prussian blue granules are indicative of microhemorrhage. (A) WT; (B) fat-1.
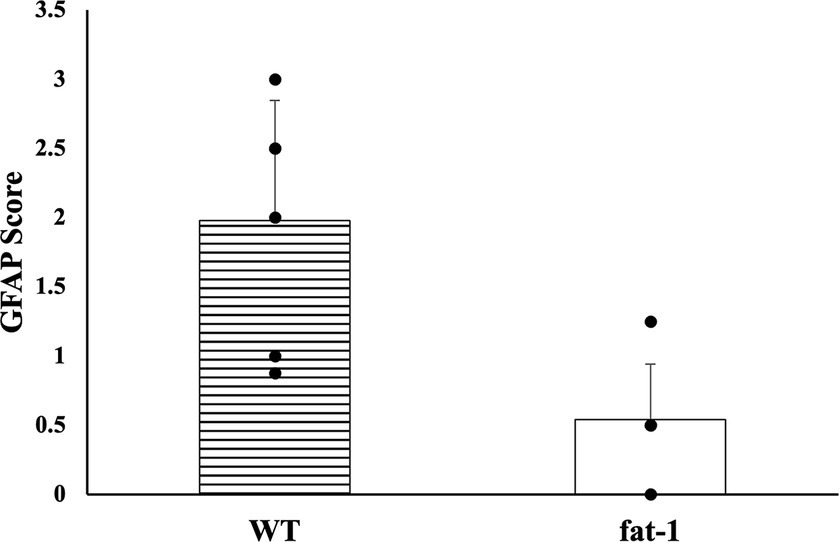
Figure 6. GFAP density for mice at the 168 h timepoint following the last WDI [n = 6/group; wildtype (WT); fat-1; WT control and fat-1 control]. WT control and fat-1 control had no detectable staining and therefore not shown. * denotes a significant difference (p ≤ 0.05) by two-tailed t-test. Data are presented as mean values ± SD.
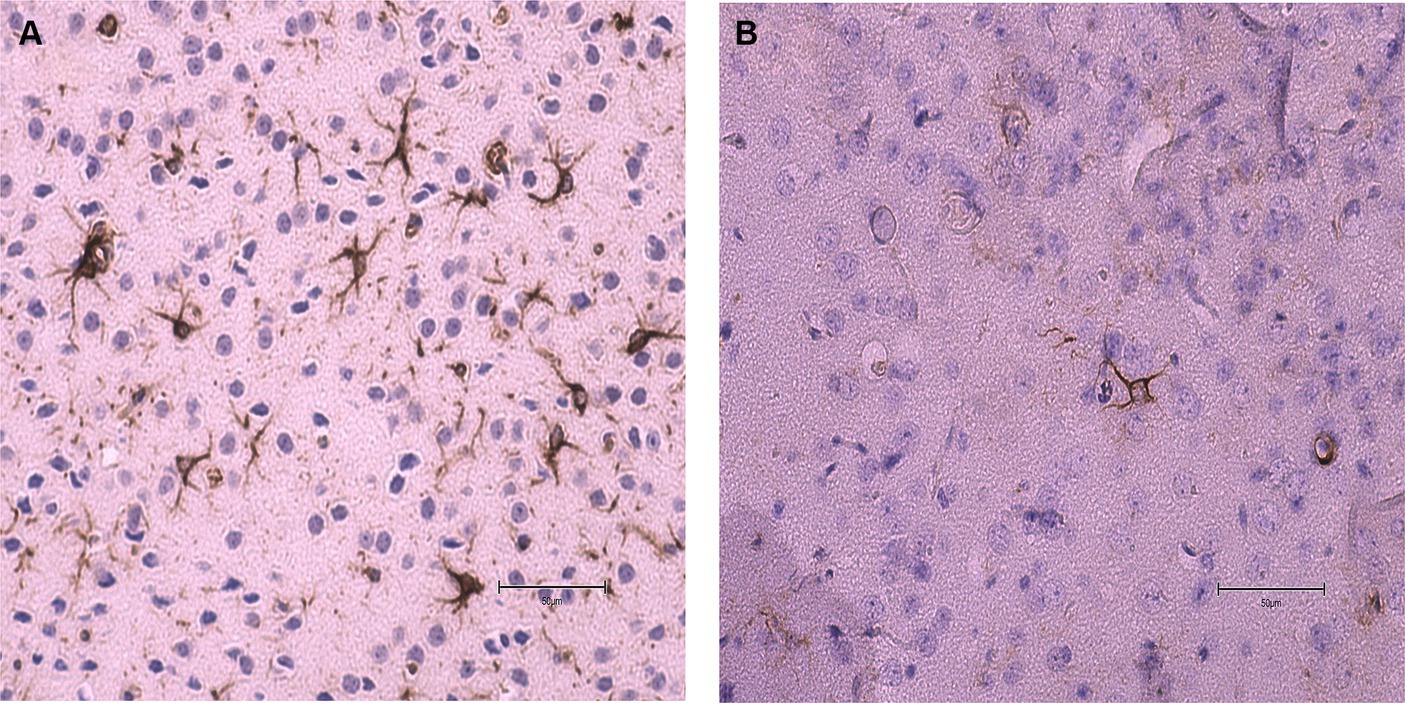
Figure 7. Images of the dorsoventral brain sections of WT and fat-1 mice stained with GFAP 168 h post 3rd WDI. Dark brown astrocyte appearance is indicative of positive GFAP staining. (A) WT; (B) fat-1.
4 Discussion
This study showed the potential and causal protective benefit of n-3 PUFA in a model of repeated mTBI in fat-1 mice. We demonstrated that n-3 PUFA effectively improves neurological recovery, restores cognitive, motor, and behavioural function caused by repetitive WDI. In tandem, lower levels of GFAP, a cellular marker of damage, was observed in fat-1 mice.
4.1 Weight drop injury model
Repeated mTBI in humans has been associated with chronic neurodegeneration (7, 26, 27), whereas isolated incidents may pose little to no harm long-term (28). Following previous work conducted by our lab investigating the effect of n-3 PUFA on a single mTBI (15), the present study sought to determine whether the mitigative effects seen through the previous model would continue with repeated WDI. The model utilized in this study overcomes the intrinsic limitations apparent in other animal models. Studying the pathology resulting from mTBI necessitates a model that integrates forces acting on the brain, aligning with clinical relevance to human injuries (29–31). This includes both linear and rotational acceleration and deceleration forces, leading to diffuse damage rather than focal damage (29, 32, 33). Furthermore, previous examination of biomechanics in head impacts occurring during athletic activities have revealed that the primary factors contributing to mTBI are high-velocity impacts and swift acceleration of the head (29, 32–34). Earlier models including cortical controlled impact (CCI), fluid-percussion injury, and other fixed-head weight drop models are intricate, involve applying a direct load onto the brain, and are expensive methods that do not effectively replicate the required rapid alterations in head acceleration (35–41). These models were initially designed to simulate moderate or severe cases of TBI. Subsequently, studies on mTBI have adjusted these protocols by reducing impact velocity, depth, or duration however, the biochemical limitations of these models do not replicate the outcomes of mTBI in athletic situations (42). Recently, various models incorporating these biomechanics have been developed, diverging from previous traditional models that cause localized damage characteristic of moderate or severe TBI. These models include modified weight drop (MWD), lateral impact (LI), and modified CCI (43). In these newer adaptations of traditional models, subtle adjustments allow for strategies for contacting the head and inducing inertial force, mimicking the conditions associated with mTBI occurring in athletes (43).
In the current study, a mouse model of mTBI was employed, closely replicating the acceleration forces and biomechanical characteristics of head impacts in humans. This was achieved by administering a weight drop impact to the closed cranium of an unrestrained subject, allowing for rapid acceleration of the head and torso, as described by Flierl et al. (22). The current model minimizes the confounding effects on mTBI outcomes associated with extended exposure to anesthetic agents, as it only requires light anesthesia. Since no surgical alterations were required, the process was streamlined, making it both simple and cost-effective. Taking into account these factors and the minor impairments resulting from the impact, it can be contended that this model generates effects comparable to the mechanistic features of human mTBI (21, 35, 44). This, in turn, allows for the investigation and understanding of the neurobiological and neurobehavioural outcomes associated with repetitive mTBI.
4.2 Fat-1 mouse model
In the present study, the utilization of the genetically altered fat-1 mouse provides the means to investigate the preventive effects of n-3 PUFA in alleviating the pathological sequence that follows a mTBI, all while minimizing the impact of potential confounding factors. Conventionally, dietary supplementation is used to alter the nutrient composition of tissue in nutritional animal studies (21). While it is a broadly acknowledged approach to investigating the effects of nutrients on various physiological processes and pathological conditions, this method introduces various confounding variables. The inherent variations between diets in nutritional studies can lead to inconsistent or conflicting results, especially in studies within the field of lipid nutrition, particularly n-3 PUFA (45). The most challenging aspect of dietary fat studies conducted on an isocaloric basis is ascertaining whether effects are due to the presence of absence of the fatty acid being manipulated. Thus, in the case of studies comparing n-6 and n-3 PUFA, it is not possible to identify the causal relationship between the fatty acid being manipulated and study outcomes. Also, these diets test varying n-3/n-6 ratios by manipulating levels of n-3 PUFA from varying sources of fish oils and plant seed or vegetable oils. Therefore, these oils may also contain traces of additional bioactive compounds (21). Moreover, it is widely acknowledged that PUFA are prone to oxidation, thus antioxidants must be added for storage (46). Hence, numerous variables stemming from diet supplementation have the potential to introduce confounding effects on the profiles of fatty acids and study outcomes.
Our earlier study employed the genetically engineered fat-1 mouse model to explore how n-3 PUFA can act preventively in reducing the pathological sequence that follows one mTBI (15). In the current study, the fat-1 mouse model was utilized once again, to reaffirm the causal relationship with repetitive mTBI. The fat-1 transgenic mouse expresses the C. elegans fat-1 gene encoding an n-3 fatty acid desaturase which allows for endogenous conversion of n-6 PUFA to n-3 PUFA (47, 48). Tissues of transgenic fat-1 mice have decreased levels of n-6 PUFA, such as linoleic acid (LA; 18:2n6) and arachidonic acid (AA; 20:4n6), resulting in n-6: n-3 PUFA ratios approaching 1 (49). Previous investigations within our laboratory analyzed the complete brain fatty acid composition in fat-1 and WT mice, showing significantly higher levels of EPA and DHA in fat-1 mice (15). Furthermore, transgenic fat-1 mice exhibit the capability to convert n-6 PUFA to n-3 PUFA from the embryonic stage (49). This eliminates the need for the prolonged and costly feeding periods typically required to alter tissue nutrient profiles in traditional dietary supplementation studies. Thus, this model offers an ideal alternative method to dietary studies to investigate the prophylactic function of n-3 PUFA in mTBI.
4.3 Neurological severity score – cognitive, behavioural, and motor function
NSS in this study was consistently lower all post-WDI timepoints, in fat-1 compared to WT mice. The initial increase in NSS at the earliest timepoint of 1 h, reflected immediate response to the initial injury. Characterization of neurological recovery by NSS at later times post-injury is highly feasibility in the mouse model. In contrast, detecting mTBI or repetitive mTBI in mouse or humans poses a heightened challenge due to its inherent complexity. Common imaging methods such as CT and MRI, for the most part, offer limited assistance in assessing and managing mTBI (50). However, there is promise in more advanced and specialized techniques such as diffusion tensor imaging (51, 52). Selecting suitable neurological assessments is essential in evaluating the impact on both the behavioural and neuroanatomical facets of recovery. These tests must have the capability to identify clinically significant alterations in behaviour that align with underlying biological changes (53). The NSS has undergone prior validation and is recognized as a highly dependable prognostic tool (22, 54). This practical assessment has several properties making it applicable in neurological evaluation as it is easily administered and evaluates various deficits encompassing cognitive, behavioural, and motor aspects (54, 55). Moreover, it exhibits a strong correlation with the severity of brain damage and has been found to align consistently with recovery (22). Thus, different from the rotarod or wire grip test where the focus is typically on one aspect of injury outcome, the NSS provides a comprehensive and reliable method to evaluate the overall neurological status of animals. Its simplicity and accessibility make it stand out as a valuable tool for assessing a wide range of physiological functions.
Previous studies by others investigating the relationship between supplemental n-3 PUFA and TBI also utilized the NSS to study the neuroprotective potential of n-3 PUFA against TBI. The findings revealed that n-3 PUFA can improve the neurological function, as well as learning and memory ability in animals following TBI (56–58). Similarly, in this study, improvements in NSS among fat-1 mice can be attributed to motor, cognitive, and behavioural function as it comprises tasks that incorporate all these domains. The coordination of movement is facilitated by an intricate network of neural pathways within the brain that travels through various brain structures and the spinal cord, ultimately terminating in skeletal muscle (35, 59). These interconnected regions of cerebral circuitry collaborate to transmit signals through the spinal cord, ultimately regulating motor control through skeletal muscle activity. Motor deficits can result from disturbances caused by brain injury in any or all segments of these neural pathways. Therefore, improvements in motor impairments might be the driving force behind swift reductions in mean NSS noted in fat-1 mice compared to WT mice following repeated WDI. Future investigations should strive to clarify the precise mechanisms through which n-3 PUFA may alleviate motor deficits. However, recent literature suggests that n-3 PUFA have the ability to enhance information processing and allocated resources more effectively for task demands by improving cortical networking (60).
One significant outcome of mTBI is the impairment of neuronal structures, with a particular focus on axonal damage, leading to potential neuronal apoptosis (61, 62). Such disturbances in the expression of various molecular systems crucial for cognitive function are evident after mTBI. The structural harm to the axon leads to multiple biochemical changes that can impact the uncontrolled release of neurotransmitters following the primary axonal damage, as well as the regulation of genes association with neuronal function (63–65). There are various potential mechanisms to elucidate how elevated levels of circulating n-3 PUFA can counteract the harmful impacts of mTBI (66). One specific mechanism involves the release of the neurotransmitter dopamine, which holds significant roles in learning, memory, and emotion. Fluctuations in dopamine release might be a contributing factor to cognitive and behavioural deficits observed following mTBI. A substantial rise in dopamine levels in the CNS leads to various outcomes, including heightened oxidative stress and the activation of inflammatory signals (63, 67). Conversely, a reduction in dopamine, commonly observed in chronic TBI cases, results in different consequences, such as impairments in memory, attention, and cognitive function. Hence, regardless of fluctuations of dopamine levels increase or decrease dramatically, dopamine can induce notable cellular dysfunction (68). As a result, fluctuations in dopamine levels and associated modifications in dopaminergic systems may exert a profound influence on functional outcomes in cognition and behaviour (68). Previous research indicates that n-3 PUFA can restore dopamine release following brain injury (66). N-3 PUFA protects the brain by minimizing axonal structural damage and neuronal apoptosis while also contributing to the restoration of specific protective mediators, thereby decreasing injury-induced dysfunction (3, 69). Therefore, higher levels of n-3 PUFA observed in fat-1 mice may have imparted neuroprotective qualities, potentially preserving cognitive and behavioural function. This preservation could explain the overall lower mean NSS scores observed in fat-1 mice compared to WT mice. However, future experiments exploring the mechanisms underlying neuronal apoptosis and dopamine regulation are required.
4.4 Righting reflex and seeking behaviour
In both assessments evaluating the time to display the righting reflex and seeking behaviour, fat-1 mice subjected to mTBI consistently demonstrated faster times when compared to WT mice. These measurements indicate injury severity (70) thus, the faster times measured suggest n-3 PUFA continues to enhance neurological recovery post-concussion, even after multiple WDI. This is likely attributed to the neuroprotective and neurorestorative qualities associated with n-3 PUFA (71).
In our previous study investigating the mitigative effects of n-3 PUFA on a single mTBI, we measured “time to first movement” as an assessment parameter (15). This metric was employed to evaluate the protective capabilities of n-3 PUFA on neurological recovery. In a prior investigation conducted by Kane et al. (23), the indication of recovery through a righting reflex was assessed as an indicator of neurological restoration. Following the WDI, mice were positioned on their backs in a sanitized cage. Subsequently, the righting reflex was defined as the time it took for the injured mice to naturally revert to a prone position following the injury and/or anesthesia (23). Our investigation adopted a slightly adjusted methodology. This involved incorporating two key metrics: the time taken to reorient themselves rotating from a dorsal to a ventral position (prone position) and the time required to exhibit novelty seeking behaviour. The former metric resembles the “time to first movement” parameter utilized in our previous study following WDI, serving as indicators of neurological recovery. The addition of the seeking behaviour measurement was introduced to assess the restoration of both motor and cognitive functions.
4.5 Cerebral microhemorrhage and GFAP
This study employed two approaches to assess brain injury by measuring the prevalence of cerebral microhemorrhage defined by PB and the presence of GFAP. Cerebral microhemorrhages result from bleeding in damaged small cerebral arteries, arterioles, and capillaries, indicating substantial damage in specific anatomical regions and cumulative effects of lesions (72). However, intracranial cerebral microhemorrhages are less common in cases of mTBI (73). Consequently, as mTBI triggers a neuroinflammatory response leading to elevated levels of inflammatory cytokines and axonal injury, measurements of GFAP would be more suggestive of mTBI (74).
The results from the immunohistochemical analysis in this study revealed that, at the 168 h timepoint after the last WDI, there were no significant differences in the prevalence of cerebral microhemorrhages between fat-1 mice and WT mice. This aligns with prior research suggesting that cerebral microhemorrhages are not a prominent feature of mTBI and do not serve as a significant predictor for cognitive outcomes and post-injury symptoms (75). Additionally, although cerebral microhemorrhages may be present in all severities of TBI, a study by Griffin et al. in 2019 found that microhemorrhages were detected in only 27% of individuals with mTBI, 47% with moderate TBI, and 58% with severe TBI (76). Cerebral microhemorrhages provide a broad overview of injury however, markers of inflammation can elucidate the underlying biological alterations that take place following mTBI.
In this study, the density and intensity of GFAP was significantly lower in fat-1 mice in comparison to their WT counterparts. GFAP, a cytoskeletal protein specific to astrocytes (77, 78), is a well-established, indicator of glial damage in various traumatic neurological disorders (79–82). Previous studies have consistently documented significant elevated serum levels of GFAP, a well-established marker of glial damage, following TBI. These findings show apparent associations with the severity of injury and subsequent outcomes thus, marking it as a possible diagnostic biomarker (81, 83). While GFAP serves as an indicator of injury in varying severities of TBI, previous studies also revealed that GFAP levels can be identified in serum within 1 hour of injury, reaching their peak at 20 h post-injury, gradually decrease over the subsequent 72 h, and be detectible at 7 days (77); thus, could attribute to lower concentrations of GFAP observed.
Exploring the efficacy of n-3 PUFA in guarding against cerebral microhemorrhages may be more pertinent in the context of moderate to severe TBI, rather than mTBI, as examined in this study. Nevertheless, investigating the hallmark features of mTBI, such as the presence of inflammatory cytokines, provides important specific insights into the injury-specific mechanisms inherent in mTBI.
5 Limitations and future directions
This study has provided important insights into the utility of n-3 PUFA in repetitive mTBI. Nevertheless, the current study has several limitations. First, the model used in this study is subject to some variability in impact site than models using a fixed-head model. This may result in variability in outcomes; however, this compromise allows freedom of head movement and rapid acceleration that more closely reflects most human mTBI, and therefore gives more clinically relevant outcomes. Second, another limitation of the study is that only male mice were used and thus sex differences were not examined and future research including females are needed. Lastly, immunohistochemistry was conducted at 168 h post-last WDI. It would be valuable for future studies to sample inflammatory cytokines at earlier timepoints.
6 Conclusion
Given the high prevalence of repetitive mTBI and its significant morbidity potential, it is important to address improved treatment, amelioration, or even prevention of the cumulative effects of repetitive mTBI (44, 84). This study was the first to assess the mitigative effects of n-3 PUFA on repetitive mTBI through a genetic approach, utilizing the fat-1 mouse model. The results from this study demonstrate that n-3 PUFA have the potential to mitigate the motor, neurological, cognitive, and behavioural impairments arising from repetitive concussive injury over 1 week. This work establishes the preventative and protective role of n-3 PUFA in support of brain health. This work has direct clinical relevance and supports the need for the inclusion of n-3 PUFA in the diet as a protective measure for the brain, whether in the context of sports injuries or daily living. However, additional investigation to elucidate the molecular mechanisms governing the action of n-3 PUFA and exploring potential sex-based differences in the utilization of n-3 PUFA as part of protective measures against mTBI is needed.
Data availability statement
The raw data supporting the conclusions of this article will be made available by the authors, without undue reservation.
Ethics statement
The animal study was approved by Animal Care and Use Committee of the University of Guelph. The study was conducted in accordance with the local legislation and institutional requirements.
Author contributions
JL: Data curation, Formal analysis, Investigation, Visualization, Writing – original draft, Writing – review & editing. CL: Writing – review & editing. J-DL: Writing – review & editing, Methodology. LH: Writing – review & editing, Methodology, Project administration, Supervision. MM: Writing – review & editing, Funding acquisition. JK: Writing – review & editing, Conceptualization, Data curation, Formal analysis, Funding acquisition, Investigation, Methodology, Project administration, Resources, Software, Supervision, Validation, Visualization. LR: Writing – review & editing, Conceptualization, Funding acquisition, Methodology, Project administration, Resources, Supervision. DM: Conceptualization, Writing – review & editing, Funding acquisition, Methodology, Project administration, Resources, Supervision.
Funding
The author(s) declare financial support was received for the research, authorship, and/or publication of this article. Funding for this project was provided by the Natural Sciences and Engineering Research Council of Canada (NSERC) to DM.
Conflict of interest
The authors declare that the research was conducted in the absence of any commercial or financial relationships that could be construed as a potential conflict of interest.
Publisher’s note
All claims expressed in this article are solely those of the authors and do not necessarily represent those of their affiliated organizations, or those of the publisher, the editors and the reviewers. Any product that may be evaluated in this article, or claim that may be made by its manufacturer, is not guaranteed or endorsed by the publisher.
Abbreviations
ALA, alpha-linolenic acid; ANOVA, analysis of variance; CCI, cortical controlled impact; CNS, central nervous system; CT, computed tomography; DHA, docosahexaenoic acid; EPA, eicosapentaenoic acid; GFAP, glial fibrillary acidic protein; HSD, honestly significant difference; LI, lateral impact; MRI, magnetic resonance imaging; mTBI, mild traumatic brain injury; MWD, modified weight drop; NSS, Neurological Severity Score; PUFA, Polyunsaturated fatty acids Standard deviation; TBI, traumatic brain injury; WDI, weight drop injury; WT, wild type
References
1. Vos, PE, Alekseenko, Y, Battistin, L, Ehler, E, Gerstenbrand, F, Muresanu, DF, et al. Mild traumatic brain injury. Eur J Neurol. (2012) 19:191–8. doi: 10.1111/j.1468-1331.2011.03581.x
2. McCrory, P, Meeuwisse, W, Dvořák, J, Aubry, M, Bailes, J, Broglio, S, et al. Consensus statement on concussion in sport-the 5th international conference on concussion in sport held in Berlin, October 2016. Br J Sports Med. (2017) 51:838–47. doi: 10.1136/BJSPORTS-2017-097699
3. Mayer, AR, Quinn, DK, and Master, CL. The spectrum of mild traumatic brain injury: a review. Neurology. (2017) 89:623–32. doi: 10.1212/WNL.0000000000004214
4. Harmon, KG, Drezner, JA, Gammons, M, Guskiewicz, KM, Halstead, M, Herring, SA, et al. American medical Society for Sports Medicine position statement: concussion in sport. Br J Sports Med. (2013) 47:15–26. doi: 10.1136/BJSPORTS-2012-091941
5. Joseph, B, Pandit, V, Aziz, H, Kulvatunyou, N, Zangbar, B, Green, DJ, et al. Mild traumatic brain injury defined by Glasgow coma scale: is it really mild? Brain Inj. (2015) 29:11–6. doi: 10.3109/02699052.2014.945959
6. Grandhi, R, Tavakoli, S, Ortega, C, and Simmonds, MJ. A review of chronic pain and cognitive, mood, and motor dysfunction following mild traumatic brain injury: complex, comorbid, and/or overlapping conditions? Brain Sci. (2017) 7:160. doi: 10.3390/brainsci7120160
7. Daneshvar, DH, Nowinski, CJ, Mckee, AC, and Cantu, RC. The epidemiology of sport-related concussion. Clin Sports Med. (2011) 30:1–17. doi: 10.1016/J.CSM.2010.08.006
8. Guskiewicz, KM, McCrea, M, Marshall, SW, Cantu, RC, Randolph, C, Barr, W, et al. Cumulative effects associated with recurrent concussion in collegiate football players: the NCAA concussion study. JAMA. (2003) 290:2549–55. doi: 10.1001/JAMA.290.19.2549
9. Public Health Agency of Canada. Injury in review, 2020 edition: spotlight on traumatic brain injuries across the life course. Available at https://www.canada.ca/en/public-health/services/injury-prevention/canadian-hospitals-injury-reporting-prevention-program/injury-reports/2020-spotlight-traumatic-brain-injuries-life-course.html (Accessed December 2, 2023).
10. Langlois, JA, Rutland-Brown, W, and Wald, MM. The epidemiology and impact of traumatic brain injury: a brief overview. J Head Trauma Rehabil. (2006) 21:375–8. doi: 10.1097/00001199-200609000-00001
11. LaRoche, AA, Nelson, LD, Connelly, PK, Walter, KD, and McCrea, MA. Sport-related concussion reporting and state legislative effects. Clin J Sport Med. (2016) 26:33–9. doi: 10.1097/JSM.0000000000000192
12. Xiong, Y, Mahmood, A, and Chopp, M. Emerging treatments for traumatic brain injury. Expert Opin Emerg Drugs. (2009) 14:67–84. doi: 10.1517/14728210902769601
13. Petraglia, AL, Maroon, JC, and Bailes, JE. From the field of play to the field of combat: a review of the pharmacological management of concussion. Neurosurgery. (2012) 70:1520–33. doi: 10.1227/NEU.0b013e31824cebe8
14. Walrand, S, Gaulmin, R, Aubin, R, Sapin, V, Coste, A, and Abbot, M. Nutritional factors in sport-related concussion. Neurochirurgie. (2021) 67:255–8. doi: 10.1016/J.NEUCHI.2021.02.001
15. Lecques, JD, Kerr, BJK, Hillyer, LM, Kang, JX, Robinson, LE, and Ma, DWL. N-3 polyunsaturated fatty acids ameliorate neurobehavioral outcomes post-mild traumatic brain injury in the fat-1 mouse model. Nutrients. (2021) 13:4092. doi: 10.3390/NU13114092
16. Richard, C, and Calder, PC. Docosahexaenoic Acid. Adv Nutr. (2016) 7:1139–41. doi: 10.3945/AN.116.012963
17. Dinicolantonio, JJ, and O’keefe, JH. The importance of marine omega-3s for brain development and the prevention and treatment of behavior, mood, and other brain disorders. Nutrients. (2020) 12:2333. doi: 10.3390/NU12082333
18. Hussein, N, Ah-Sing, E, Wilkinson, P, Leach, C, Griffin, BA, and Millward, DJ. Long-chain conversion of [13C] linoleic acid and α-linolenic acid in response to marked changes in their dietary intake in men. J Lipid Res. (2005) 46:269–80. doi: 10.1194/jlr.M400225-JLR200
19. Weiser, MJ, Butt, CM, and Mohajeri, MH. Docosahexaenoic acid and cognition throughout the lifespan. Nutrients. (2016) 8:99. doi: 10.3390/NU8020099
20. Miller, SM, Zynda, AJ, Sabatino, MJ, Jo, C, Ellis, HB, and Dimeff, RJ. A pilot randomized controlled trial of docosahexaenoic acid for the treatment of sport-related concussion in adolescents. Clin Pediatr (Phila). (2022) 61:785–94. doi: 10.1177/00099228221101726
21. Kang, JX, Wang, J, Wu, L, and Kang, ZB. Fat-1 mice convert n-6 to n-3 fatty acids. Nature. (2004) 427:504–4. doi: 10.1038/427504a
22. Flierl, MA, Stahel, PF, Beauchamp, KM, Morgan, SJ, Smith, WR, and Shohami, E. Mouse closed head injury model induced by a weight-drop device. Nat Protoc. (2009) 4:1328–37. doi: 10.1038/nprot.2009.148
23. Kane, MJ, Angoa Pérez, M, Briggs, DI, Viano, DC, Kreipke, CW, Kuhn, DM, et al. A mouse model of human repetitive mild traumatic brain injury. J Neurosci Methods. (2012) 203:41–9. doi: 10.1016/j.jneumeth.2011.09.003
24. Fisher, M, Vasilevko, V, Passos, GF, Ventura, C, Quiring, D, and Cribbs, DH. Therapeutic modulation of cerebral microhemorrhage in a mouse model of cerebral amyloid angiopathy. Stroke. (2011) 42:3300–3. doi: 10.1161/STROKEAHA.111.626655
25. Luijerink, L, Rodriguez, M, and Machaalani, R. Quantifying GFAP immunohistochemistry in the brain – introduction of the reactivity score (R-score) and how it compares to other methodologies. J Neurosci Methods. (2024) 402:110025. doi: 10.1016/J.JNEUMETH.2023.110025
26. Stern, RA, Riley, DO, Daneshvar, DH, Nowinski, CJ, Cantu, RC, and McKee, AC. Long-term consequences of repetitive brain trauma: chronic traumatic encephalopathy. PM R. (2011) 3:460–7. doi: 10.1016/J.PMRJ.2011.08.008
27. Weber, JT. Experimental models of repetitive brain injuries. Prog Brain Res. (2007) 161:253–61. doi: 10.1016/S0079-6123(06)61018-2
28. McCrory, P, Meeuwisse, W, Aubry, M, Cantu, B, Dvorak, J, Echemendia, R, et al. Consensus statement on concussion in sport-the 4th international conference on concussion in sport held in Zurich, November 2012. J Sci Med Sport. (2013) 16:178–89. doi: 10.1016/J.JSAMS.2013.02.009
29. Guskiewicz, KM, Marshall, SW, Bailes, J, Mccrea, M, Harding, HP, Matthews, A, et al. Recurrent concussion and risk of depression in retired professional football players. Med Sci Sports Exerc. (2007) 39:903–9. doi: 10.1249/mss.0b013e3180383da5
30. Viano, DC, and Pellman, EJ. Concussion in professional football: biomechanics of the striking player – part 8. Neurosurgery. (2005) 56:266–80. doi: 10.1227/01.neu.0000150035.54230.3C
31. Kerr, ZY, Marshall, SW, Harding, HP, and Guskiewicz, KM. Nine-year risk of depression diagnosis increases with increasing self-reported concussions in retired professional football players. Am J Sports Med. (2012) 40:2206–12. doi: 10.1177/0363546512456193
32. Pellman, EJ, Viano, DC, Tucker, AM, Casson, IR, Waeckerle, JF, Maroon, JC, et al. Concussion in professional football: reconstruction of game impacts and injuries. Neurosurgery. (2003) 53:799–814. doi: 10.1227/01.NEU.0000083559.68424.3F
33. Urban, JE, Davenport, EM, Golman, AJ, Maldjian, JA, Whitlow, CT, Powers, AK, et al. Head impact exposure in youth football: high school ages 14 to 18 years and cumulative impact analysis. Ann Biomed Eng. (2013) 41:2474–87. doi: 10.1007/S10439-013-0861-Z
34. Viano, DC, Hamberger, A, Bolouri, H, and Säljö, A. Concussion in professional football: animal model of brain injury – part 15. Neurosurgery. (2009) 64:1162–73. doi: 10.1227/01.NEU.0000345863.99099.C7
35. Xiong, Y, Mahmood, A, and Chopp, M. Animal models of traumatic brain injury. Nat Rev Neurosci. (2013) 14:128–42. doi: 10.1038/NRN3407
36. Feeney, DM, Boyeson, MG, Linn, RT, Murray, HM, and Dail, WG. Responses to cortical injury: i. methodology and local effects of contusions in the rat. Brain Res. (1981) 211:67–77. doi: 10.1016/0006-8993(81)90067-6
37. Marmarou, A, Abd-elfattah Foda, MA, Van Den Brink, W, Campbell, J, Kita, H, Demetriadou, K, et al. A new model of diffuse brain injury in rats part I: pathophysiology and biomechanics. J Neurosurg. (1994) 80:291–300. doi: 10.3171/jns.1994.80.2.0291
38. Chen, Y, Constantin, S, Trembovler, V, Weinstock, M, and Shohami, E. An experimental model of closed head injury in mice: pathophysiology, histopathology, and cognitive deficits. J Neurotrauma. (1996) 13:557–68. doi: 10.1089/neu.1996.13.557
39. Stahel, PF, Shohami, T, Younis, M, Kariya, K, Otto, VI, Lenzlinger, PM, et al. Experimental closed head injury: analysis of neurological outcome, blood-brain barrier dysfunction, intracranial neutrophil infiltration, and neuronal cell death in mice deficient in genes for pro-inflammatory cytokines. J Cereb Blood Flow Metab. (2000) 20:369–80. doi: 10.1097/00004647-200002000-00019
40. Thompson, HJ, Lifshitz, J, Marklund, N, Grady, MS, Graham, DI, Hovda, DA, et al. Lateral fluid percussion brain injury: a 15-year review and evaluation. J Neurotrauma. (2005) 22:42–75. doi: 10.1089/neu.2005.22.42
41. Lighthall, JW. Controlled cortical impact: a new experimental brain injury model. J Neurotrauma. (1988) 5:1–15. doi: 10.1089/neu.1988.5.1
42. Guskiewicz, KM, and Mihalik, JP. Biomechanics of sport concussion: quest for the elusive injury threshold. Exerc Sport Sci Rev. (2011) 39:4–11. doi: 10.1097/JES.0b013e318201f53e
43. Hiskens, MI, Angoa-Pérez, M, Schneiders, AG, Vella, RK, and Fenning, AS. Modeling sports-related mild traumatic brain injury in animals—a systematic review. J Neurosci Res. (2019) 97:1194–222. doi: 10.1002/JNR.24472
44. Cancelliere, C, Cassidy, JD, Côté, P, Hincapié, CA, Hartvigsen, J, Carroll, LJ, et al. Protocol for a systematic review of prognosis after mild traumatic brain injury: an update of the WHO collaborating Centre task force findings. Syst Rev. (2012) 1:1–6. doi: 10.1186/2046-4053-1-17/tables/1
45. Balk, EM, Horsley, TA, Newberry, SJ, Lichtenstein, AH, Yetley, EA, Schachter, HM, et al. A collaborative effort to apply the evidence-based review process to the field of nutrition: challenges, benefits, and lessons learned. Am J Clin Nutr. (2007) 85:1448–56. doi: 10.1093/AJCN/85.6.1448
46. Albert, BB, Cameron-Smith, D, Hofman, PL, and Cutfield, WS. Oxidation of marine omega-3 supplements and human health. Biomed Res Int. (2013) 2013:464921. doi: 10.1155/2013/464921
47. Spychalla, JP, Kinney, AJ, and Browse, J. Identification of an animal ω-3 fatty acid desaturase by heterologous expression in Arabidopsis. Proc Natl Acad Sci USA. (1997) 94:1142–7. doi: 10.1073/pnas.94.4.1142
48. Kang, ZB, Ge, Y, Chen, Z, Cluette-Brown, J, Laposata, M, Leaf, A, et al. Adenoviral gene transfer of Caenorhabditis elegans n−3 fatty acid desaturase optimizes fatty acid composition in mammalian cells. Proc Natl Acad Sci USA. (2001) 98:4050–4. doi: 10.1073/PNAS.061040198
49. Kang, JX. Fat-1 transgenic mice: a new model for omega-3 research. Prostaglandins Leukot Essent Fatty Acids. (2007) 77:263–7. doi: 10.1016/J.PLEFA.2007.10.010
50. Van Boven, RW, Harrington, GS, Hackney, DB, Ebel, A, Gauger, G, Douglas Bremner, J, et al. Advances in neuroimaging of traumatic brain injury and posttraumatic stress disorder. J Rehabil Res Dev. (2009) 46:717–56. doi: 10.1682/JRRD.2008.12.0161
51. Levin, HS, Wilde, E, Troyanskaya, M, Petersen, NJ, Scheibel, R, Newsome, M, et al. Diffusion tensor imaging of mild to moderate blast-related traumatic brain injury and its sequelae. J Neurotrauma. (2010) 27:683–94. doi: 10.1089/NEU.2009.1073
52. Bennett, RE, Mac, DCL, and Brody, DL. Diffusion tensor imaging detects axonal injury in a mouse model of repetitive closed-skull traumatic brain injury. Neurosci Lett. (2012) 513:160–5. doi: 10.1016/j.neulet.2012.02.024
53. Yarnell, AM, Barry, ES, Mountney, A, Shear, D, Tortella, F, and Grunberg, NE. The revised neurobehavioral severity scale (NSS-R) for rodents. Curr Protoc Neurosci. (2016) 75:9.52.1–9.52.16. doi: 10.1002/cpns.10
54. Tsenter, J, Beni-Adani, L, Assaf, Y, Alexandrovich, AG, Trembovler, V, and Shohami, E. Dynamic changes in the recovery after traumatic brain injury in mice: effect of injury severity on T2-weighted MRI abnormalities, and motor and cognitive functions. J Neurotrauma. (2008) 25:324–33. doi: 10.1089/neu.2007.0452
55. Wu, A, Ying, Z, and Gomez-Pinilla, F. Dietary strategy to repair plasma membrane after brain trauma: implications for plasticity and cognition. Neurorehabil Neural Repair. (2014) 28:75–84. doi: 10.1177/1545968313498650
56. Chen, X, Pan, Z, Fang, Z, Lin, W, Wu, S, and Yang, F. Omega-3 polyunsaturated fatty acid attenuates traumatic brain injury-induced neuronal apoptosis by inducing autophagy through the upregulation of SIRT1-mediated deacetylation of Beclin-1. J Neuroinflammation. (2018) 15:1–15. doi: 10.1186/S12974-018-1345-8
57. Chen, X, Chen, C, Fan, S, Wu, S, Yang, F, Fang, Z, et al. Omega-3 polyunsaturated fatty acid attenuates the inflammatory response by modulating microglia polarization through SIRT1-mediated deacetylation of the HMGB1/NF-ΚB pathway following experimental traumatic brain injury. J Neuroinflammation. (2018) 15:1–15. doi: 10.1186/S12974-018-1151-3
58. Zhu, W, Ding, Y, Kong, W, Li, T, and Chen, H. Docosahexaenoic acid (DHA) provides neuroprotection in traumatic brain injury models via activating Nrf2-ARE signaling. Inflammation. (2018) 41:1182–93. doi: 10.1007/s10753-018-0765-z
59. Fujimoto, ST, Longhi, L, Saatman, KE, and Mcintosh, TK. Motor and cognitive function evaluation following experimental traumatic brain injury. Neurosci Biobehav Rev. (2004) 28:365–78. doi: 10.1016/j.neubiorev.2004.06.002
60. Dighriri, IM, Alsubaie, AM, Hakami, FM, Hamithi, DM, Alshekh, MM, Khobrani, FA, et al. Effects of omega-3 polyunsaturated fatty acids on brain functions: a systematic review. Cureus. (2022) 14:e30091. doi: 10.7759/cureus.30091
61. Danielli, E, Simard, N, DeMatteo, CA, Kumbhare, D, Ulmer, S, and Noseworthy, MD. A review of brain regions and associated post-concussion symptoms. Front Neurol. (2023) 14:1136367. doi: 10.3389/fneur.2023.1136367
62. Giza, CC, and Hovda, DA. The neurometabolic cascade of concussion. J Athl Train. (2001) 36:228–35. doi: 10.1227/NEU.0000000000000505
63. Bailes, JE, and Mills, JD. Docosahexaenoic acid reduces traumatic axonal injury in a rodent head injury model. J Neurotrauma. (2010) 27:1617–24. doi: 10.1089/NEU.2009.1239
64. Mills, JD, Bailes, JE, Sedney, CL, Hutchins, H, and Sears, B. Omega-3 fatty acid supplementation and reduction of traumatic axonal injury in a rodent head injury model: laboratory investigation. J Neurosurg. (2011) 114:77–84. doi: 10.3171/2010.5.JNS08914
65. Wang, T, Van, KC, Gavitt, BJ, Grayson, JK, Lu, YC, Lyeth, BG, et al. Effect of fish oil supplementation in a rat model of multiple mild traumatic brain injuries. Restor Neurol Neurosci. (2013) 31:647–59. doi: 10.3233/RNN-130316
66. Shin, SS, and Dixon, CE. Oral fish oil restores striatal dopamine release after traumatic brain injury. Neurosci Lett. (2011) 496:168–71. doi: 10.1016/j.neulet.2011.04.009
67. Chen, YH, Huang, EYK, Kuo, TT, Miller, J, Chiang, YH, and Hoffer, BJ. Impact of traumatic brain injury on dopaminergic transmission. Cell Transplant. (2017) 26:1156–68. doi: 10.1177/0963689717714105
68. Lan, Y-L, Li, S, Lou, J-C, Ma, X-C, and Zhang, B. The potential roles of dopamine in traumatic brain injury: a preclinical and clinical update. Am J Transl Res. (2019) 11:2616–31.
69. Barrett, EC, McBurney, MI, and Ciappio, ED. ω-3 fatty acid supplementation as a potential therapeutic aid for the recovery from mild traumatic brain injury/concussion. Adv Nutr. (2014) 5:268–77. doi: 10.3945/AN.113.005280
70. Berman, R, Spencer, H, Boese, M, Kim, S, Radford, K, and Choi, K. Loss of consciousness and righting reflex following traumatic brain injury: predictors of post-injury symptom development (a narrative review). Brain Sci. (2023) 13:750. doi: 10.3390/brainsci13050750
71. Wang, H, Hasadsri, L, Wang, BH, Lee, JV, Erdman, JW, Llano, DA, et al. Omega-3 fatty acids as a putative treatment for traumatic brain injury. J Neurotrauma. (2013) 30:897–906. doi: 10.1089/NEU.2012.2672
72. Toth, L, Czigler, A, Horvath, P, Szarka, N, Kornyei, B, Toth, A, et al. The effect of mild traumatic brain injury on cerebral microbleeds in aging. Front Aging Neurosci. (2021) 13:717391. doi: 10.3389/fnagi.2021.717391
73. Kushner, D. Mild traumatic brain injury: toward understanding manifestations and treatment. Arch Intern Med. (1998) 158:1617–24. doi: 10.1001/ARCHINTE.158.15.1617
74. Malik, S, Alnaji, O, Malik, M, Gambale, T, Farrokhyar, F, and Rathbone, MP. Inflammatory cytokines associated with mild traumatic brain injury and clinical outcomes: a systematic review and meta-analysis. Front Neurol. (2023) 14:1123407. doi: 10.3389/fneur.2023.1123407
75. Huovinen, A, Marinkovic, I, Isokuortti, H, Korvenoja, A, Mäki, K, Nybo, T, et al. Traumatic microbleeds in mild traumatic brain injury are not associated with delayed return to work or persisting post-concussion symptoms. J Neurotrauma. (2021) 38:2400–6. doi: 10.1089/NEU.2021.0055
76. Griffin, AD, Turtzo, LC, Parikh, GY, Tolpygo, A, Lodato, Z, Moses, AD, et al. Traumatic microbleeds suggest vascular injury and predict disability in traumatic brain injury. Brain. (2019) 142:3550–64. doi: 10.1093/brain/awz290
77. Papa, L, Brophy, GM, Welch, RD, Lewis, LM, Braga, CF, Tan, CN, et al. Time course and diagnostic accuracy of glial and neuronal blood biomarkers GFAP and UCH-L1 in a large cohort of trauma patients with and without mild traumatic brain injury. JAMA Neurol. (2016) 73:551–60. doi: 10.1001/jamaneurol.2016.0039
78. Bazarian, JJ, Biberthaler, P, Welch, RD, Lewis, LM, Barzo, P, Bogner-Flatz, V, et al. Serum GFAP and UCH-L1 for prediction of absence of intracranial injuries on head CT (ALERT-TBI): a multicentre observational study. Lancet Neurol. (2018) 17:782–9. doi: 10.1016/S1474-4422(18)30231-X
79. Bogoslovsky, T, Wilson, D, Chen, Y, Hanlon, D, Gill, J, Jeromin, A, et al. Increases of plasma levels of glial fibrillary acidic protein, tau, and amyloid β up to 90 days after traumatic brain injury. J Neurotrauma. (2017) 34:66–73. doi: 10.1089/NEU.2015.4333
80. Gill, J, Latour, L, Diaz-Arrastia, R, Motamedi, V, Turtzo, C, Shahim, P, et al. Glial fibrillary acidic protein elevations relate to neuroimaging abnormalities after mild TBI. Neurology. (2018) 91:e1385–9. doi: 10.1212/WNL.0000000000006321
81. Kulbe, JR, Jain, S, Nelson, LD, Korley, FK, Mukherjee, P, Sun, X, et al. Association of day-of-injury plasma glial fibrillary acidic protein concentration and six-month posttraumatic stress disorder in patients with mild traumatic brain injury. Neuropsychopharmacology. (2022) 47:2300–8. doi: 10.1038/s41386-022-01359-5
82. Metting, Z, Wilczak, N, Rodiger, LA, Schaaf, JM, and Van Der Naalt, J. GFAP and S100B in the acute phase of mild traumatic brain injury. Neurology. (2012) 78:1428–33. doi: 10.1212/WNL.0B013E318253D5C7
83. McKee, AC, Stein, TD, Kiernan, PT, and Alvarez, VE. The neuropathology of chronic traumatic encephalopathy. Brain Pathol. (2015) 25:350–64. doi: 10.1111/BPA.12248
Keywords: repetitive, mild traumatic brain injury, omega 3 (n-3) polyunsaturated fatty acids, concussion, inflammation
Citation: Lau JS, Lust CAC, Lecques J-D, Hillyer LM, Mountjoy M, Kang JX, Robinson LE and Ma DWL (2024) n-3 PUFA ameliorate functional outcomes following repetitive mTBI in the fat-1 mouse model. Front. Nutr. 11:1410884. doi: 10.3389/fnut.2024.1410884
Edited by:
Amanda N. Carey, Simmons University, United StatesReviewed by:
Zhen Yu, Jiangsu University of Science and Technology, ChinaJean-Christophe Delpech, INRAE Nouvelle-Aquitaine Bordeaux, France
Copyright © 2024 Lau, Lust, Lecques, Hillyer, Mountjoy, Kang, Robinson and Ma. This is an open-access article distributed under the terms of the Creative Commons Attribution License (CC BY). The use, distribution or reproduction in other forums is permitted, provided the original author(s) and the copyright owner(s) are credited and that the original publication in this journal is cited, in accordance with accepted academic practice. No use, distribution or reproduction is permitted which does not comply with these terms.
*Correspondence: David W. L. Ma, ZGF2aWRtYUB1b2d1ZWxwaC5jYQ==