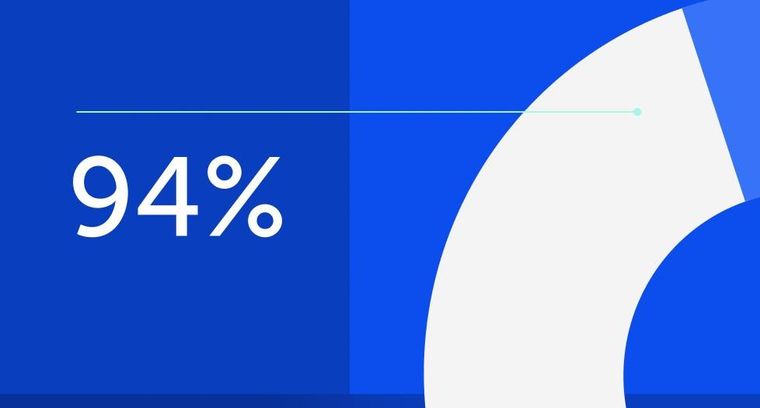
94% of researchers rate our articles as excellent or good
Learn more about the work of our research integrity team to safeguard the quality of each article we publish.
Find out more
MINI REVIEW article
Front. Nutr., 19 April 2024
Sec. Clinical Nutrition
Volume 11 - 2024 | https://doi.org/10.3389/fnut.2024.1385591
This article is part of the Research TopicMicronutrients, Immunity and InfectionView all 27 articles
Zinc (Zn) is a vital micronutrient that strengthens the immune system, aids cellular activities, and treats infectious diseases. A deficiency in Zn can lead to an imbalance in the immune system. This imbalance is particularly evident in severe deficiency cases, where there is a high susceptibility to various viral infections, including COVID-19 caused by SARS-CoV-2. This review article examines the nutritional roles of Zn in human health, the maintenance of Zn concentration, and Zn uptake. As Zn is an essential trace element that plays a critical role in the immune system and is necessary for immune cell function and cell signaling, the roles of Zn in the human immune system, immune cells, interleukins, and its role in SARS-CoV-2 infection are further discussed. In summary, this review paper encapsulates the nutritional role of Zn in the human immune system, with the hope of providing specific insights into Zn research.
The nutritional importance of trace metal has been known for a long time, but in the last decades its importance in immune modulation has arisen. The nutritional roles of trace metals are significant, not only as constituent components of many metal proteins and metalloenzymes, but also as essential micronutrients (1). They are required for various body functions and the well-being of the immune system (2). Balanced levels of trace metals are essential for maintaining immunity and are crucial in the prevention and management of viral infections (3). Several trace metals, such as Zn, are essential for the normal functioning of the immune system and immune-cell homeostasis. Increasing the Zn concentration can efficiently inhibit virus replication in host cells, thus exhibiting antiviral activity (4). Zn deficiency in humans is an emerging global health issue. Deficiencies of Zn often coexist with infectious diseases and exhibit complex interactions (5). Furthermore, Zn supplementation has shown a positive impact on enhancing immunity in viral infections (6). This trace metal has immunomodulatory functions, influencing the susceptibility, course, and outcome of a variety of viral infections (7). Given the current COVID-19 pandemic, where no effective preventive or curative medicine is available, a healthy immune system is one of our most important defenses.
In recent years, serum trace metal levels have been frequently reported as reliable markers for diagnosing various infectious diseases, such as COVID-19 (8). An exuberant innate immunoinflammatory response is a hallmark of severe COVID-19 with the cytokine storm, hyperinflammation, and multiorgan failure, which are correlated with elevated blood levels of proinflammatory cytokines and chemokines, e.g., IL-1β, IL-2, IL-4, IL-6, IL-8, IL-10, IL-17, IFN-γ, and TNF-α (9). SARS-CoV-2 is a pathogenic coronavirus of COVID-19, which caused a pandemic of acute respiratory disease (10). The cellular anti-SARS-CoV-2 response starts from NK cells through cytokine production. Subsequently, T cells destroy infected cells, whereas B cells to produce antibodies (11). The structural or non-structural SARS-CoV-2 proteins can elicit a host immune response. Among them, the S protein of SARS-CoV-2 contains a receptor-binding domain that serves as the critical target for antiviral compounds. B cells elicit an early response against the nucleocapsid protein of SARS-CoV-2, while antibodies against S protein could be detected after 4–8 days from the appearance of initial symptoms (12). This article reviews the nutritional roles of Zn on the immune system and SARS-CoV-2 infections, highlighting the importance of this trace metal in not only optimizing the immune response to infections but also in understanding its role in COVID-19 therapeutic approaches.
In the adult human body, approximately 2–3 g of Zn is present (13). The reference range for serum Zn concentration in healthy individuals is between 60 and 120 μg/dL, although there may be slight regional or national variations (14, 15). For example, the reference range for Zn concentration in the human body in Bangladesh is 60–120 μg/dL (16), in Japan is ≥80 μg/dL (17), and in U.S. is 80–120 μg/dL (18). In medical testing, serum Zn levels below 60 μg/dL are indicative of inadequate Zn status (19). This inadequate Zn status is associated with a wide variety of systemic disorders, including cardiovascular impairment, musculoskeletal dysfunctions, and oromaxillary diseases (20). The human body requires daily adequate amounts of Zn, which it obtains from food or supplements. The jejunum and ileum are the primary organs for Zn absorption (Figure 1). Approximately 25–66% of consumed Zn is absorbed from the jejunum and ileum, and then distributed throughout the body (in tissues, cells, and fluids). The distribution of Zn varies across different tissues within the human body (21). The highest content of Zn is found in skeletal muscles (57%), bones (29%), and skin (6%) (22). Over 95% of total body Zn is present in the intracellular compartments, bound to intracellular proteins and cell membranes (Figure 1) (23).
Zn plays a crucial role in various processes within the human body, functioning in two states: Zn-finger transcription factors and Zn enzymes. It is involved in the synthesis of numerous proteins, as all Zn-finger transcription factors require Zn for gene expression regulation (24). Additionally, Zn serves as an activator for more than 300 Zn enzymes and participates in all biochemical reactions dependent on these enzymes (25). These reactions encompass growth, development, neurological behavior, immune system functionality, catalytic functions, acceleration of chemical reactions, modulation of neuronal communication, maintenance of cell membrane integrity and tissue balance, protein and DNA synthesis, wound healing, and cell signaling and division (26, 27). Zn also possesses antioxidant and anti-inflammatory properties that prevent damage to cells (28). Clinically, Zn supplementation is used for treating several infectious diseases, such as diarrhea, malaria, and COVID-19 (29). Of particular interest to many researchers is the fact that Zn is a nutrient required for maintaining a healthy sense of taste and smell (30).
Zn homeostasis is primarily maintained through the gastrointestinal system, which manages the absorption of exogenous Zn and the excretion of endogenous Zn (31). The primary site for the absorption of exogenous Zn in humans is believed to be the proximal small bowel, specifically the distal duodenum or proximal jejunum (32). The absorption of Zn involves several steps. In the stomach, the strong acidic pH liberates Zn from salts, converting it into an ionic form. These Zn ions then travel to the small intestine. At the intestinal brush border membrane, Zn is absorbed from the lumen into the enterocytes. The Zn is then excreted at the basolateral side of the enterocytes, releasing Zn ions into the portal blood, which distributes the ions throughout the body (33, 34). To maintain homeostatic balance in the body, Zn is excreted or eliminated through the kidneys, skin, and intestines when necessary (35). After circulating in the body, Zn is excreted into the small intestinal lumen along with pancreatic secretions and bile (36). Most of the Zn is reabsorbed by the duodenum and proximal jejunum, and the remaining portion is excreted in the feces (37). A significant source of fecal Zn is the pancreatic and biliary secretion of Zn-containing enzymes (38). In addition to inadequate consumption of Zn, reduced intestinal absorption is a common cause of Zn inadequacy (39). The amount of Zn excreted in the feces can vary between 0.8 and 2.7 mg per day. The daily urinary Zn excretion is approximately 0.5 mg.
Certain food-derived compounds, such as Phytic acid and Casein, can interfere with the absorption of Zn. Phytic acid, a unique natural substance found in plant seeds (present in cereals, corn, and rice), can bind to certain dietary minerals including Iron, Zn, Manganese, and to a lesser extent, Calcium (40). This binding can slow their absorption from consumed meals (41). Casein, which makes up about 80% of the total protein in cow’s milk, also exerts a modest inhibitory effect on Zn absorption (42, 43). Therefore, the consumption of cow’s milk can potentially lead to Zn deficiency. The binding of Zn to casein is pH-dependent. At slightly alkaline pH, 1 mg of casein binds 8.4 μg of Zn (44). In addition, some trace elements also affect the absorption of Zn. For instance, iron supplementation can negatively influence Zn absorption. Supplements containing 25 mg of iron or more can reduce Zn absorption and plasma Zn concentrations. This is because Zn and Iron, both positively charged ions, compete with each other for intestinal absorption (45).
Copper and Zn are antagonists, competing for the GABA (A) receptor sites in the body (46). This implies that an excess of either mineral can cause and mask a deficiency of the other. High dietary Zn intakes depress copper absorption, increase copper sequestration in the mucosal cell bound to metallothioneins, and increase fecal excretion of copper (46). Conversely, Zn-deficient animals have shown increased copper absorption, and high dietary copper can depress Zn absorption (47). According to research, the ideal Zn to copper ratio is between 8:1 and 15:1 (48). Maintaining this ratio is crucial for good health and various physiological functions. An excess of copper, coupled with a lack of Zn, may predispose an individual to oxidative stress and trigger the inflammatory process (49). Disruption of this balance can lead to several health conditions. For instance, copper toxicity can cause symptoms such as diarrhea, headaches, and in severe cases, kidney failure (50). On the other hand, Zn deficiency can result in growth retardation, hypogonadism, immune dysfunction, and cognitive impairment (51).
Zn plays a crucial role in the absorption of certain vitamins. It facilitates the absorption of vitamin A, vitamin E, and folate1 (52). Zn is a component of the retinol-binding protein, which is necessary for transporting vitamin A in the blood (53). Additionally, Zn is required for the enzyme that converts retinol (vitamin A) to retina (54). The exact mechanism by which a deficiency in Zn affects the absorption of vitamin E and folate is not entirely clear. However, it is known to involve the loss of Zn enzyme function. Special attention should be given to the balance of intracellular and extracellular Zn concentrations. Cellular Zn homeostasis is primarily maintained by the Zn importers family, ZnT (SLC30), which allows Zn to accumulate in the cytosol, and by the Zn exporters’ family, ZIP (SLC39) (55). The main controllers of intracellular Zn concentration are the ZnT importers, ZIP exporters, and intracellular binding proteins known as metallothioneins (55).
The recommended daily intake of Zn varies across countries. The World Health Organization (WHO) recommends a dietary Zn intake of 9.8 mg/day for women and 14 mg/day for men, both aged between 19 and 65 years (56). In the U.S., the current recommended daily value (DV) for Zn is 8 mg for women and 11 mg for adult men (57). The requirements increase slightly during pregnancy and lactation. In the UK, the reference nutrient intake is 4–7 mg/d for females and 5.5–9.5 mg/d for males (Food Standards Agency) (58). In Australia, the current Recommended Dietary Intake (RDI) for Zn is 14 mg/day for men and 8 mg/day for women (19+ years), with an upper limit of 40 mg/day (59). In Germany, the recommended intake values for Zn range from 7 mg/day to 16 mg/day for adults, including pregnant and lactating women, depending on sex and dietary phytate intake (60). The Ministry of Health, Labor and Welfare in Japan recommends a standard Zn intake of 10 mg per day for men and 8 mg per day for women (61). According to the Chinese dietary guidelines, the recommended daily Zn intake for adults over 18 years old is 7.5 mg for women and 12.5 mg for men, with tolerable upper intake levels of 40 mg (62).
A Zn deficiency can lead to small stature, mild anemia, and impaired wound healing. Good sources of Zn are meats, whole grains, and legumes. The Recommended Dietary Allowance (RDA) is 8 mg/d for women and 11 mg/d for adult men (63). The amount of Zn provided by the U.S. food supply has varied between 11 and 13 mg/day/person since the beginning of the 20 century. Currently, it is estimated that the U.S. food supply provides 12.3 mg Zn per person per day (64). While red meat is a particularly good source, all kinds of meat, including beef, lamb, and pork, contain Zn (65). According to the information provided by U.S. Department of Agriculture,1 a 100-gram (3.5-ounce) serving of raw ground beef contains 4.79 mg of Zn. This is approximately 44% of the DV for males and 60% of the DV for females. Shellfish are healthy, low-calorie sources of Zn. Oysters, in particular, contain high amounts, with six medium oysters providing 33 mg, or 300% of the DV for males and 413% of the DV for females. Legumes, such as chickpeas, lentils, and beans, contain substantial amounts of Zn. Table 1 lists the 10 foods with the highest Zn content.
Zn is a member of the transition metals family, characterized by low ionization energies and a broad spectrum of oxidation states, or positively charged forms (66). It plays a significant role in regulating cytokine expression and suppressing inflammation. Zn is necessary for the activation of antioxidant enzymes that scavenge reactive oxygen species (ROS), thereby reducing oxidative stress (67). Dysregulated Zn homeostasis can impair overall immune function, leading to increased susceptibility to infection (68). Zn has several mechanisms for combating pathogens. In the body, Zn generally exists as positively charged ions. These Zn ions act as chemo-attractants, drawing in pathogens for phagocytosis and killing them through the production of ROS (69). One such mechanism involves Zn oxide nanoparticles, which induce the generation of abundant ROS, including singlet oxygen species (1O2), within cells. An elevated level of ROS triggers an antioxidant cellular response and mitochondrial dysfunctions (70). Interestingly, researchers have discovered that Zn can ‘starve’ Streptococcus pneumoniae by inhibiting their uptake of manganese, a metal essential for the protein transporter that Streptococcus pneumoniae requires to invade and cause disease in humans (71).
Zn plays a vital role in many immune processes as a catalyst, structural element, and regulatory ion (72). The immune system, being highly proliferative, is particularly susceptible to Zn deficiency (73). Here’s a summary of how Zn impacts the immune system: (1) Zn helps control infections by moderating the immune response, thus preventing inflammation that can be damaging and even deadly (74); (2) Zn is essential for the development of a specialized type of immune cell and stimulates a critical immune organ to regenerate after damage (75); (3) Zn is necessary for immune cell function and cell signaling. A deficiency can lead to a weakened immune response (76). For instance, Zn is crucial for the normal development and function of cells mediating innate immunity, such as neutrophils and natural killer cells (77). Zn is also required for DNA synthesis, RNA transcription, cell division, and cell activation (78). In the absence of adequate levels of Zn, programmed cell death (apoptosis) is potentiated (79).
Zn boosts the immune system and combats viruses by enhancing the actions of immune cells such as Neutrophils, B cells, NK cells, and T cells (80). Neutrophils, a type of white blood cell, aid the body in fighting off infections by engulfing and destroying invading pathogens (81). Zn plays a pivotal role in the development and activation of neutrophils (82). A study has shown that Zn deficiency leads to increased neutrophil transmigration and impairs the ability of neutrophils to phagocytose and produce ROS (83). Research also indicates that Zn supplementation can help reduce neutrophil recruitment and activity, thereby helping to prevent lung injury (84). B cells are antigen-presenting cells that produce antibodies and cytokines, represent immunological memory, and even appear to have regulatory and suppressing functions in inflammation (85). Significantly elevated Zn levels have been observed in activated B cells (86). Existing research confirms that Zn deficiency leads to a reduction in B cells, affecting the development of immature and pre-mature B cells and impacting antibody production (87). Low levels of cytoplasmic Zn in deficient B cells were associated with reduced cell signaling during crucial stages in B cell development (88). Zn also modulates the response of NK cells, with a decreased recognition and stimulation of their MHC-class I expression (89). Furthermore, Zn supplementation increases the differentiation of CD34+ cells toward NK cells as well as their cytotoxicity (Figure 2) (90).
T cells, a crucial type of white blood cells, play a central role in the adaptive immune response (91). The impact of Zn on T cells has been confirmed experimentally. Individuals with low Zn levels have few, if any, T cells to fight infections. This may be because Zn deficiency leads to thymic atrophy and subsequent T-cell lymphopenia (92). Moreover, Zn deficiency negatively affects the growth and function of T cells and various aspects of innate and adaptive immunity, including T cell lymphopoiesis and antibody-mediated immune defense (93). Animal studies have also corroborated this conclusion. It has been demonstrated that Zn enhances the regeneration of the thymus in mice, the organ where T cells develop, and aids in immune-cell recovery post bone marrow transplant (94). According to a study, a lack of Zn during T cell maturation results in a 50% reduction in the transition from “potential” pre-T-cells to “effective” T cells. This is associated with an increased apoptosis of pre-T-cells (Figure 2) (95). Some researchers hypothesize that Zn interacts with protein kinase C and the lymphocyte protein tyrosine kinase. The dysfunction of these two kinases, which are involved in mature T-cell activation, is a reason that Zn deficiency leads to a reduced T cell count (96). Similarly, Zn deficiency results in a decreased ratio of type 1 to type 2 T-helper cells, with reduced production of T-helper type 1 cytokines like interferon-gamma (IFNG), and compromised T-cell mediated immune defense (97). Zn supplementation has been shown to enhance immunity and effectively downregulate chronic inflammatory responses (98).
One of the important functions of Zn ions is to serve as second messengers. They act as intracellular signaling molecules, capable of communicating between cells, converting extracellular signals to intracellular ones, and regulating the activation of interleukins (99). Zn can induce monocytes to produce pro-inflammatory cytokines such as interleukin-1 (IL-1), interleukin-6 (IL-6), and interleukin-8 (IL-8) (100). The IL-1 family, a group of 11 cytokines, plays a central role in the regulation of immune and inflammatory responses to infections (101). Zn has been found to reduce IL-1 dependent T cell stimulation by inhibiting the IL-1 receptor-associated kinase-1 (102). IL-1β is a major cytokine involved in monocyte activation and the activation of proinflammatory signaling pathways (103). Cytoplasmic Zn promotes IL-1β production and subsequently inhibits inflammation, depending on the transcription factor NF-κB, an inflammatory activating factor (104). However, a previous study has shown that Zn depletion in macrophages induces the activation of proteases that cleave pro-IL-1β, leading to an increased release of active IL-1β (105). These studies suggest a complex correlation between Zn and IL-1.
Interleukin-2 (IL-2), which is produced by T cells during an immune response, is essential for the growth, proliferation, and differentiation of naive T cells into effector T cells (106). The level of Zn has a positive correlation with IL-2. Zn enhances the proliferation of T cells in response to IL-2, as well as the production of IL-2 by T cells (107). A previous study evaluated the circulating cytokines and Zn status, showing that reduced circulating Zn correlates with increased levels of IL-6 and IL-8 (108). The proliferative response of T and B lymphocytes following IL-6 and IL-8 stimulation increases in Zn deficiency, while it adversely influences IL-4 signaling, leading to an impairment of the immune system (109). Research has found that Zn deficiency decreases the production of TH1 cytokines (IFN-γ, IL-2, and TNF-α), whereas the TH2 response (IL-4, IL-6, and IL-10) is less affected. This results in an imbalance between TH1 and TH2 subsets, which is restored when Zn reaches physiological levels (110). The proinflammatory TH17 cells are also negatively affected by Zn deficiency. The development of TH17 cells is critically controlled by IL-6-induced STAT3 activation during chronic inflammation (111). Zn suppresses TH17 development by attenuating this activation (112).
The effects of Zn on animals are diverse and significant. One study conducted on swine discovered that dietary supplementation of Zn in an organic form influenced the expression of inflammatory molecules within the intestine. This organic form of Zn resulted in a reduced level of the pro-inflammatory cytokine IL-18. This finding suggests that organic Zn sources could enhance the health and immune response of animals under stress by altering gene expression in the intestine (113). Another study explored the impact of Zn deficiency in rats. The researchers found that a lack of Zn exacerbated the inflammatory response, leading to hemolytic anemia and splenomegaly. Notably, the administration of IL-4 and Zn supplementation were found to reverse the hemolytic anemia and splenomegaly induced by Zn deficiency (114). In a separate study using a mouse model of allergic inflammation, it was found that Zn exhibited anti-inflammatory effects (115).
Zn deficiency has a broad impact on human health, leading to dysfunctions in both innate and adaptive immunity. This can result in diminished lytic activity, impaired signaling, altered cytokine production, and reduced proliferation. Consequently, individuals deficient in Zn are more susceptible to infectious diseases (72). Zn supplementation in the elderly has been found to decrease the incidence of infections, reduce oxidative stress, and lower the generation of inflammatory cytokines (116). A sensitivity analysis revealed that Zn supplementation decreases IL-6 levels and increases IL-2 levels (117). In an experimental model of human Zn deficiency, there was a reported decrease in thymulin activity in Th1 cells, a reduction in IL-2 and IFN-gamma genes, and diminished activity of natural killer cells (NK) and T cytotoxic T cells (118). These findings underscore the vital role of Zn in regulating interleukin expression and immune function. However, the exact mechanisms and pathways involved are complex and may vary depending on the specific context and conditions.
Clinically, COVID-19 patients have shown an imbalance in Zn levels. Low Zn levels appear to be common in these patients (119). In a previous study, all COVID-19 patients (33 out of 33) were found to be Zn deficient, with a mean serum level of 6.9 ± 1.1 μmoL/L, which is well below the Zn deficiency cutoff value of 10.7 μmoL/L (120). This Zn deficiency observed in COVID-19 patients may be an acute phase reaction to SARS-CoV-2 infection (121). Compared to other interventions, those who received a combination of Zn and vitamin C were found to mount a greater antibody response. This suggests that oral Zn and vitamin C treatment could stimulate antibody production following SARS-CoV-2 infection (122). Zn therapy is considered logical in COVID-19 due to the inhibitory effect of Zn on viral replication (123). A study found that the combination of doxycycline and Zn has a protective effect in COVID-19 patients (124). Interestingly, Zn therapy plays a significant role in shortening the duration of smell recovery in these patients (125). It’s important to note that administering high-dose Zn appears to be safe, feasible, and associated with minimal peripheral infusion site irritation in COVID-19 patients (126).
Although case studies may not hold the same universal significance as group studies, they offer specific parameters, treatment plans, and treatment outcomes, which make them quite intriguing. Elanjian et al. reported three cases of COVID-19 patients who received Zn supplementation, with experiencing hypoglycemia (127). Rosenberg et al. reported a case of copper deficiency, which was caused by Zn supplementation in the context of COVID-19. This deficiency presented very similarly to myelodysplastic syndrome (128). Farolfi et al. reported a case of a 98-year-old patient who received vitamin D and adjuvant dietary supplements (quercetin, vitamin C, Zn, and vitamin K2) at home. The patient fully recovered, suggesting that careful home assistance under strict medical supervision can be successful, even in very old subjects with comorbidities (129). Ahmed et al. reported a case of a 48-year-old Hispanic female patient with COVID-19, who presented with severe isolated thrombocytopenia. She was treated with intravenous immunoglobulin, prednisone, rituximab, vitamin C, and Zn, and achieving hemodynamic stability (130).
Multiple pieces of evidence indicate that Zn plays a significant role in the treatment of patients with COVID-19 and a deficiency in Zn is associated with increased severity of COVID-19. However, the exact mechanism by which Zn inhibits the virus, particularly SARS-CoV-2, remains unclear. We propose the following mechanisms: (1) Zn is known to impair the replication of several RNA viruses including poliovirus, influenza virus, and SARS-CoV-2, by inhibiting the main protease, which is crucial for the virus’s replication (131); (2) Zn is an essential trace element with potent immunoregulatory and antiviral properties (132). This suggests that Zn could inhibit inflammation and alleviate oxidative stress by inducing the aforementioned interleukin. (3) Zn is used by SARS-CoV-2 as an essential step in preparation for entering and invading cells. It latches onto the exterior of potential host cells. During this process, Zn is strongly implicated in inhibiting the virus’s binding to angiotensin-converting enzyme-2 (ACE2) receptors on the cell membrane, thus mitigating the attack by those virus particles that do manage to enter host cells (133). Supplemental Zn could replenish Zn in ACE2, stabilize the ACE2 axis, and prevent disruption of the renin-angiotensin system (134). Moreover, Zn minimizes the activity of Sirtuin-1 (SIRT-1), which regulates ACE-2 expression and could potentially block virus entry (135).
This review article explores the multifaceted roles of Zn in immune system functions. The dynamic equilibrium of Zn in the human body is crucial for maintaining normal immune cell function and response by inducing monocytes to produce pro-inflammatory cytokines. Given its significant role in the immune system, Zn has demonstrated positive effects and potential therapeutic benefits in the treatment of COVID-19. However, current research on the function of Zn in the immune system and its therapeutic impact on viral infections remains somewhat superficial. These research primary focus on the changes in Zn concentration in virus-infected patients, the correlation between Zn and pro-inflammatory cytokines, or the role of Zn in inhibiting virus replication or preventing virus entry into cells. Zn, serving as a structural or catalytic cofactor, fulfills various biological functions through Zn enzymes or Zn finger proteins. For instance, our recent research discovered that the Zn finger protein ZBTB34 can bind to telomere DNA, regulate telomere length, and is associated with the onset of liver cancer (136, 137). The biological functions of Zn in the immune system, such as stimulating the expression of pro-inflammatory cytokine genes, are achieved through these Zn enzymes and finger proteins. Regrettably, the functions of over 300 Zn enzymes and hundreds of Zn finger proteins are not well-understood at present. Future research should prioritize the study of Zn enzymes and Zn finger proteins, which will undoubtedly unveil more biological functions of Zn in the immune system.
DJ: Conceptualization, Writing – original draft. XW: Investigation, Writing – original draft. YH: Investigation, Writing – original draft. LZ: Investigation, Writing – original draft. HLu: Investigation, Writing – original draft. JL: Investigation, Writing – original draft. YW: Investigation, Writing – original draft. ZL: Conceptualization, Funding acquisition, Supervision, Writing – review & editing. HLi: Funding acquisition, Supervision, Writing – review & editing.
The author(s) declare that financial support was received for the research, authorship, and/or publication of this article. The study was supported by the National Natural Science Foundation of China (No. 32260175, 82160313); Guangxi Natural Science Foundation (No. 2020GXNSFDA297027, 2018GXNSFAA281048); Guilin Science and Technology Development Program (Grant No. 2020011202); Guangxi Science and Technology Base and Talent Project (No. AD19110161); Guangxi Medical and Health Key Discipline Construction Project.
The authors declare that the research was conducted in the absence of any commercial or financial relationships that could be construed as a potential conflict of interest.
All claims expressed in this article are solely those of the authors and do not necessarily represent those of their affiliated organizations, or those of the publisher, the editors and the reviewers. Any product that may be evaluated in this article, or claim that may be made by its manufacturer, is not guaranteed or endorsed by the publisher.
1. Jomova, K, Makova, M, Alomar, SY, Alwasel, SH, Nepovimova, E, Kuca, K, et al. Essential metals in health and disease. Chem Biol Interact. (2022) 367:110173. doi: 10.1016/j.cbi.2022.110173
2. Munteanu, C, and Schwartz, B. The relationship between nutrition and the immune system. Front Nutr. (2022) 9:1082500. doi: 10.3389/fnut.2022.1082500
3. Rashed, MN. The role of trace elements on hepatitis virus infections: a review. J Trace Elem Med Biol. (2011) 25:181–7. doi: 10.1016/j.jtemb.2011.07.001
4. te Velthuis, AJ, van den Worm, SH, Sims, AC, Baric, RS, Snijder, EJ, and van Hemert, MJ. Zn (2+) inhibits coronavirus and arterivirus RNA polymerase activity in vitro and zinc ionophores block the replication of these viruses in cell culture. PLoS Pathog. (2010) 6:e1001176. doi: 10.1371/journal.ppat.1001176
5. Pvsn, KK, Tomo, S, Purohit, P, Sankanagoudar, S, Charan, J, Purohit, A, et al. Comparative analysis of serum zinc, copper and magnesium level and their relations in association with severity and mortality in SARS-CoV-2 patients. Biol Trace Elem Res. (2023) 201:23–30. doi: 10.1007/s12011-022-03124-7
6. Girodon, F, Galan, P, Monget, AL, Boutron-Ruault, MC, Brunet-Lecomte, P, Preziosi, P, et al. Impact of trace elements and vitamin supplementation on immunity and infections in institutionalized elderly patients: a randomized controlled trial. MIN. VIT. AOX. Geriatric network. Arch Intern Med. (1999) 159:748–54. doi: 10.1001/archinte.159.7.748
7. Jayawardena, R, Sooriyaarachchi, P, Chourdakis, M, Jeewandara, C, and Ranasinghe, P. Enhancing immunity in viral infections, with special emphasis on COVID-19: a review. Diabetes Metab Syndr. (2020) 14:367–82. doi: 10.1016/j.dsx.2020.04.015
8. Skalny, AV, Timashev, PS, Aschner, M, Aaseth, J, Chernova, LN, Belyaev, VE, et al. Serum zinc, copper, and other biometals are associated with COVID-19 severity markers. Meta. (2021) 11:244. doi: 10.3390/metabo11040244
9. Kamińska, D, Dęborska-Materkowska, D, Kościelska-Kasprzak, K, Mazanowska, O, Remiorz, A, Poznański, P, et al. Immunity after COVID-19 recovery and vaccination: similarities and differences. Vaccines (Basel). (2022) 10:1068. doi: 10.3390/vaccines10071068
10. Hu, B, Guo, H, Zhou, P, and Shi, ZL. Characteristics of SARS-CoV-2 and COVID-19. Nat Rev Microbiol. (2021) 19:141–54. doi: 10.1038/s41579-020-00459-7
11. Gallardo-Zapata, J, and Maldonado-Bernal, C. Natural killer cell exhaustion in SARS-CoV-2 infection. Innate Immun. (2022) 28:189–98. doi: 10.1177/17534259221077750
12. Shah, VK, Firmal, P, Alam, A, Ganguly, D, and Chattopadhyay, S. Overview of immune response during SARS-CoV-2 infection: lessons from the past. Front Immunol. (2020) 11:1949. doi: 10.3389/fimmu.2020.01949
13. Kaur, K, Gupta, R, Saraf, SA, and Saraf, SK. Zinc: the metal of life. Compr Rev Food Sci Food Saf. (2014) 13:358–76. doi: 10.1111/1541-4337.12067
14. Frydrych, A, Krośniak, M, and Jurowski, K. The role of chosen essential elements (Zn, cu, se, Fe, Mn) in food for special medical purposes (FSMPs) dedicated to oncology patients-critical review: state-of-the-art. Nutrients. (2023) 15:1012. doi: 10.3390/nu15041012
15. Andrew, D, Gail, R, Morag, B, and Kishor, R. Recommended reference intervals for copper and zinc in serum using the US National Health and nutrition examination surveys (NHANES) data. Clin Chim Acta. (2023) 546:117397. doi: 10.1016/j.cca.2023.117397
16. Barman, N, Salwa, M, Ghosh, D, Rahman, MW, Uddin, MN, and Haque, MA. Reference value for serum zinc level of adult population in Bangladesh. EJIFCC. (2020) 31:117–24.
17. Yokokawa, H, Morita, Y, Hamada, I, Ohta, Y, Fukui, N, Makino, N, et al. Demographic and clinical characteristics of patients with zinc deficiency: analysis of a nationwide Japanese medical claims database. Sci Rep. (2024) 14:2791. doi: 10.1038/s41598-024-53202-0
18. Hedera, P, Fink, JK, Bockenstedt, PL, and Brewer, GJ. Myelopolyneuropathy and pancytopenia due to copper deficiency and high zinc levels of unknown origin: further support for existence of a new zinc overload syndrome. Arch Neurol. (2003) 60:1303–6. doi: 10.1001/archneur.60.9.1303
19. Takic, M, Zekovic, M, Terzic, B, Stojsavljevic, A, Mijuskovic, M, Radjen, S, et al. Zinc deficiency, plasma fatty acid profile and desaturase activities in hemodialysis patients: is supplementation necessary? Front Nutr. (2021) 8:700450. doi: 10.3389/fnut.2021.700450
20. Uwitonze, AM, Ojeh, N, Murererehe, J, Atfi, A, and Razzaque, MS. Zinc adequacy is essential for the maintenance of optimal Oral health. Nutrients. (2020) 12:949. doi: 10.3390/nu12040949
21. Razzaque, MS. COVID-19 pandemic: can zinc supplementation provide an additional shield against the infection? Comput Struct Biotechnol J. (2021) 19:1371–8. doi: 10.1016/j.csbj.2021.02.015
22. Jackson, MJ. Physiology of zinc: general aspects In: CF Mills, editor. Zinc in human biology. ILSI human nutrition reviews. London: Springer (1989)
23. Cummings, JE, and Kovacic, JP. The ubiquitous role of zinc in health and disease. J Vet Emerg Crit Care (San Antonio). (2009) 19:215–40. doi: 10.1111/j.1476-4431.2009.00418.x
24. Bird, AJ, McCall, K, Kramer, M, Blankman, E, Winge, DR, and Eide, DJ. Zinc fingers can act as Zn2+ sensors to regulate transcriptional activation domain function. EMBO J. (2003) 22:5137–46. doi: 10.1093/emboj/cdg484
25. Cheng, Y, and Chen, H. Aberrance of zinc metalloenzymes-induced human diseases and its potential mechanisms. Nutrients. (2021) 13:4456. doi: 10.3390/nu13124456
26. Costa, MI, Sarmento-Ribeiro, AB, and Gonçalves, AC. Zinc: from biological functions to therapeutic potential. Int J Mol Sci. (2023) 24:4822. doi: 10.3390/ijms24054822
27. Wessels, I, Maywald, M, and Rink, L. Zinc as a gatekeeper of immune function. Nutrients. (2017) 9:1286. doi: 10.3390/nu9121286
28. Prasad, AS. Zinc is an antioxidant and anti-inflammatory agent: its role in human health. Front Nutr. (2014) 1:14. doi: 10.3389/fnut.2014.00014
29. Kim, B, and Lee, WW. Regulatory role of zinc in immune cell signaling. Mol Cells. (2021) 44:335–41. doi: 10.14348/molcells.2021.0061
30. Mozaffar, B, Ardavani, A, Muzafar, H, and Idris, I. The effectiveness of zinc supplementation in taste disorder treatment: a systematic review and meta-analysis of randomized controlled trials. J Nutr Metab. (2023) 2023:1–13. doi: 10.1155/2023/6711071
31. Cousins, RJ. Gastrointestinal factors influencing zinc absorption and homeostasis. Int J Vitam Nutr Res. (2010) 80:243–8. doi: 10.1024/0300-9831/a000030
32. Lee, HH, Prasad, AS, Brewer, GJ, and Owyang, C. Zinc absorption in human small intestine. Am J Phys. (1989) 256:G87–91. doi: 10.1152/ajpgi.1989.256.1.G87
33. Chan, LN, and Mike, LA. The science and practice of micronutrient supplementations in nutritional anemia: an evidence-based review. JPEN J Parenter Enteral Nutr. (2014) 38:656–72. doi: 10.1177/0148607114533726
34. Wang, X, and Zhou, B. Dietary zinc absorption: a play of zips and ZnTs in the gut. IUBMB Life. (2010) 62:176–82. doi: 10.1002/iub.291
35. Livingstone, C. Zinc: physiology, deficiency, and parenteral nutrition. Nutr Clin Pract. (2015) 30:371–82. doi: 10.1177/0884533615570376
36. Kondaiah, P, Yaduvanshi, PS, Sharp, PA, and Pullakhandam, R. Iron and zinc homeostasis and interactions: does enteric zinc excretion cross-talk with intestinal iron absorption? Nutrients. (2019) 11:1885. doi: 10.3390/nu11081885
37. Foulkes, EC, and Voner, C. Effects of Zn status, bile and other endogenous factors on jejunal cd absorption. Toxicology. (1981) 22:115–22. doi: 10.1016/0300-483x(81)90111-6
38. Holodova, M, Cobanova, K, Sefcikova, Z, Barszcz, M, Tuśnio, A, Taciak, M, et al. Dietary zinc and fibre source can influence the mineral and antioxidant status of piglets. Animals (Basel). (2019) 9:497. doi: 10.3390/ani9080497
39. Wapnir, RA. Zinc deficiency, malnutrition and the gastrointestinal tract. J Nutr. (2000) 130:1388S–92S. doi: 10.1093/jn/130.5.1388S
40. Kumar, A, Singh, B, Raigond, P, Sahu, C, Mishra, UN, Sharma, S, et al. Phytic acid: blessing in disguise, a prime compound required for both plant and human nutrition. Food Res Int. (2021) 142:110193. doi: 10.1016/j.foodres.2021.110193
41. Castro-Alba, V, Lazarte, CE, Bergenståhl, B, and Granfeldt, Y. Phytate, iron, zinc, and calcium content of common Bolivian foods and their estimated mineral bioavailability. Food Sci Nutr. (2019) 7:2854–65. doi: 10.1002/fsn3.1127
42. Lönnerdal, B. Dietary factors influencing zinc absorption. J Nutr. (2000) 130:1378S–83S. doi: 10.1093/jn/130.5.1378S
43. Coulon, JB, Hurtaud, C, Remond, B, and Verite, R. Factors contributing to variation in the proportion of casein in cows’ milk true protein: a review of recent INRA experiments. J Dairy Res. (1998) 65:375–87. doi: 10.1017/s0022029998002866
44. Harzer, G, and Kauer, H. Binding of zinc to casein. Am J Clin Nutr. (1982) 35:973–80. doi: 10.1093/ajcn/35.5.981
45. Whittaker, P. Iron and zinc interactions in humans. Am J Clin Nutr. (1998) 68:442S–6S. doi: 10.1093/ajcn/68.2.442S
46. Sharonova, IN, Vorobjev, VS, and Haas, HL. Interaction between copper and zinc at GABA (a) receptors in acutely isolated cerebellar Purkinje cells of the rat. Br J Pharmacol. (2000) 130:851–6. doi: 10.1038/sj.bjp.0703392
47. Hoffman, HN 2nd, Phyliky, RL, and Fleming, CR. Zinc-induced copper deficiency. Gastroenterology. (1988) 94:508–12. doi: 10.1016/0016-5085(88)90445-3
48. Rębacz-Maron, E, Baranowska-Bosiacka, I, Gutowska, I, and Chlubek, D. Blood pressure and levels of Fe, ca, mg, Zn, cu, Na and K in the hair of young Bantu men from Tanzania. Biol Trace Elem Res. (2013) 151:350–9. doi: 10.1007/s12011-012-9578-3
49. Guo, CH, Chen, PC, Yeh, MS, Hsiung, DY, and Wang, CL. Cu/Zn ratios are associated with nutritional status, oxidative stress, inflammation, and immune abnormalities in patients on peritoneal dialysis. Clin Biochem. (2011) 44:275–80. doi: 10.1016/j.clinbiochem.2010.12.017
50. Park, KS, Kwon, JH, Park, SH, Ha, W, Lee, J, An, HC, et al. Acute copper sulfate poisoning resulting from dermal absorption. Am J Ind Med. (2018) 61:783–8. doi: 10.1002/ajim.22892
51. Prasad, AS. Impact of the discovery of human zinc deficiency on health. J Am Coll Nutr. (2009) 28:257–65. doi: 10.1080/07315724.2009.10719780
52. Degerud, EM, Manger, MS, Strand, TA, and Dierkes, J. Bioavailability of iron, vitamin a, zinc, and folic acid when added to condiments and seasonings. Ann N Y Acad Sci. (2015) 1357:29–42. doi: 10.1111/nyas.12947
53. Christian, P, and West, KP. Interactions between zinc and vitamin a: an update. Am J Clin Nutr. (1998) 68:435S–41S. doi: 10.1093/ajcn/68.2.435S
54. Ugarte, M, and Osborne, NN. Zinc in the retina. Prog Neurobiol. (2001) 64:219–49. doi: 10.1016/s0301-0082(00)00057-5
55. Schweigel-Röntgen, M. The families of zinc (SLC30 and SLC39) and copper (SLC31) transporters. Curr Top Membr. (2014) 73:321–55. doi: 10.1016/B978-0-12-800223-0.00009-8
56. Gibson, RS, King, JC, and Lowe, N. A review of dietary zinc recommendations. Food Nutr Bull. (2016) 37:443–60. doi: 10.1177/0379572116652252
57. Maret, W, and Sandstead, HH. Zinc requirements and the risks and benefits of zinc supplementation. J Trace Elem Med Biol. (2006) 20:3–18. doi: 10.1016/j.jtemb.2006.01.006
58. Duncan, A, Dean, P, Simm, M, O’Reilly, DS, and Kinsella, J. Zinc supplementation in intensive care: results of a UK survey. J Crit Care. (2012) 27:102.e1–6. doi: 10.1016/j.jcrc.2011.07.083
59. Day, KJ, Adamski, MM, Dordevic, AL, and Murgia, C. Genetic variations as modifying factors to dietary zinc requirements-a systematic review. Nutrients. (2017) 9:148. doi: 10.3390/nu9020148
60. Haase, H, Ellinger, S, Linseisen, J, Neuhäuser-Berthold, M, and Richter, M German Nutrition Society (DGE). Revised D-A-CH-reference values for the intake of zinc. J Trace Elem Med Biol. (2020) 61:126536. doi: 10.1016/j.jtemb.2020.126536
61. Kodama, H, Tanaka, M, Naito, Y, Katayama, K, and Moriyama, M. Japan’s practical guidelines for zinc deficiency with a particular focus on taste disorders, inflammatory bowel disease, and liver cirrhosis. Int J Mol Sci. (2020) 21:2941. doi: 10.3390/ijms21082941
62. Xing, B, Yu, J, Liu, Y, He, S, Chen, X, Li, Z, et al. High dietary zinc intake is associated with shorter leukocyte telomere length, mediated by tumor necrosis factor-α: a study of China adults. J Nutr Health Aging. (2023) 27:904–10. doi: 10.1007/s12603-023-1992-z
63. Moser-Veillon, PB. Zinc: consumption patterns and dietary recommendations. J Am Diet Assoc. (1990) 90:1089–93. doi: 10.1016/S0002-8223(21)01706-5
64. Schifferle, RE. Nutrition and periodontal disease. Dent Clin N Am. (2005) 49:595–610, vii. doi: 10.1016/j.cden.2005.03.008
65. Hazell, T. Iron and zinc compounds in the muscle meats of beef, lamb, pork and chicken. J Sci Food Agric. (1982) 33:1049–56. doi: 10.1002/jsfa.2740331017
66. Krężel, A, and Maret, W. The biological inorganic chemistry of zinc ions. Arch Biochem Biophys. (2016) 611:3–19. doi: 10.1016/j.abb.2016.04.010
67. Prasad, AS. Clinical, immunological, anti-inflammatory and antioxidant roles of zinc. Exp Gerontol. (2008) 43:370–7. doi: 10.1016/j.exger.2007.10.013
68. Gao, H, Dai, W, Zhao, L, Min, J, and Wang, F. The role of zinc and zinc homeostasis in macrophage function. J Immunol Res. (2018) 2018:1–11. doi: 10.1155/2018/6872621
69. Shen, C, James, SA, de Jonge, MD, Turney, TW, Wright, PF, and Feltis, BN. Relating cytotoxicity, zinc ions, and reactive oxygen in ZnO nanoparticle-exposed human immune cells. Toxicol Sci. (2013) 136:120–30. doi: 10.1093/toxsci/kft187
70. Sharma, V, Anderson, D, and Dhawan, A. Zinc oxide nanoparticles induce oxidative DNA damage and ROS-triggered mitochondria mediated apoptosis in human liver cells (hep G2). Apoptosis. (2012) 17:852–70. doi: 10.1007/s10495-012-0705-6
71. Eijkelkamp, BA, Morey, JR, Ween, MP, Ong, CL, McEwan, AG, Paton, JC, et al. Extracellular zinc competitively inhibits manganese uptake and compromises oxidative stress management in Streptococcus pneumoniae. PLoS One. (2014) 9:e89427. doi: 10.1371/journal.pone.0089427
72. Haase, H, and Rink, L. Zinc signals and immune function. Biofactors. (2014) 40:27–40. doi: 10.1002/biof.1114
73. Fraker, PJ, and King, LE. Reprogramming of the immune system during zinc deficiency. Annu Rev Nutr. (2004) 24:277–98. doi: 10.1146/annurev.nutr.24.012003.132454
74. Vasto, S, Mocchegiani, E, Malavolta, M, Cuppari, I, Listì, F, Nuzzo, D, et al. Zinc and inflammatory/immune response in aging. Ann N Y Acad Sci. (2007) 1100:111–22. doi: 10.1196/annals.1395.009
75. Prasad, AS. Zinc in human health: effect of zinc on immune cells. Mol Med. (2008) 14:353–7. doi: 10.2119/2008-00033
76. Hojyo, S, and Fukada, T. Roles of zinc signaling in the immune system. J Immunol Res. (2016) 2016:1–21. doi: 10.1155/2016/6762343
77. Maares, M, and Haase, H. Zinc and immunity: An essential interrelation. Arch Biochem Biophys. (2016) 611:58–65. doi: 10.1016/j.abb.2016.03.022
78. Mac Donald, RS. The role of zinc in growth and cell proliferation. J Nutr. (2000) 130:1500S–8S. doi: 10.1093/jn/130.5.1500S
79. Meerarani, P, Ramadass, P, Toborek, M, Bauer, HC, Bauer, H, and Hennig, B. Zinc protects against apoptosis of endothelial cells induced by linoleic acid and tumor necrosis factor alpha. Am J Clin Nutr. (2000) 71:81–7. doi: 10.1093/ajcn/71.1.81
80. Shankar, AH, and Prasad, AS. Zinc and immune function: the biological basis of altered resistance to infection. Am J Clin Nutr. (1998) 68:447S–63S. doi: 10.1093/ajcn/68.2.447S
81. Döhrmann, S, Cole, JN, and Nizet, V. Conquering Neutrophils. PLoS Pathog. (2016) 12:e1005682. doi: 10.1371/journal.ppat.1005682
82. Wang, H, Lv, G, Lian, S, Wang, J, and Wu, R. Effect of copper, zinc, and selenium on the migration of bovine neutrophils. Vet Sci. (2021) 8:281. doi: 10.3390/vetsci8110281
83. Moroni, F, Di Paolo, ML, Rigo, A, Cipriano, C, Giacconi, R, Recchioni, R, et al. Interrelationship among neutrophil efficiency, inflammation, antioxidant activity and zinc pool in very old age. Biogerontology. (2005) 6:271–81. doi: 10.1007/s10522-005-2625-0
84. Wessels, I, Pupke, JT, von Trotha, KT, Gombert, A, Himmelsbach, A, Fischer, HJ, et al. Zinc supplementation ameliorates lung injury by reducing neutrophil recruitment and activity. Thorax. (2020) 75:253–61. doi: 10.1136/thoraxjnl-2019-213357
85. Chekol Abebe, E, Asmamaw Dejenie, T, Mengie Ayele, T, Dagnew Baye, N, Agegnehu Teshome, A, and Tilahun, MZ. The role of regulatory B cells in health and diseases: a systemic review. J Inflamm Res. (2021) 14:75–84. doi: 10.2147/JIR.S286426
86. Ollig, J, Kloubert, V, Taylor, KM, and Rink, L. B cell activation and proliferation increase intracellular zinc levels. J Nutr Biochem. (2019) 64:72–9. doi: 10.1016/j.jnutbio.2018.10.008
87. Ibs, KH, and Rink, L. Zinc-altered immune function. J Nutr. (2003) 133:1452S–6S. doi: 10.1093/jn/133.5.1452S
88. Anzilotti, C, Swan, DJ, Boisson, B, Deobagkar-Lele, M, Oliveira, C, Chabosseau, P, et al. An essential role for the Zn2+ transporter ZIP7 in B cell development. Nat Immunol. (2019) 20:350–61. doi: 10.1038/s41590-018-0295-8
89. Mocchegiani, E, Giacconi, R, Cipriano, C, and Malavolta, M. NK and NKT cells in aging and longevity: role of zinc and metallothioneins. J Clin Immunol. (2009) 29:416–25. doi: 10.1007/s10875-009-9298-4
90. Muzzioli, M, Stecconi, R, Moresi, R, and Provinciali, M. Zinc improves the development of human CD34+ cell progenitors towards NK cells and increases the expression of GATA-3 transcription factor in young and old ages. Biogerontology. (2009) 10:593–604. doi: 10.1007/s10522-008-9201-3
91. O’Garra, A, and Vieira, P. Regulatory T cells and mechanisms of immune system control. Nat Med. (2004) 10:801–5. doi: 10.1038/nm0804-801
92. Perkey, E, and Maillard, I. Zinc: a damage signal promoting thymic repair. Blood. (2022) 139:3569–70. doi: 10.1182/blood.2022016333
93. John, E, Laskow, TC, Buchser, WJ, Pitt, BR, Basse, PH, Butterfield, LH, et al. Zinc in innate and adaptive tumor immunity. J Transl Med. (2010) 8:118. doi: 10.1186/1479-5876-8-118
94. Savino, W, and Dardenne, M. Nutritional imbalances and infections affect the thymus: consequences on T-cell-mediated immune responses. Proc Nutr Soc. (2010) 69:636–43. doi: 10.1017/S0029665110002545
95. Zhang, X, Hou, Y, Huang, Y, Chen, W, and Zhang, H. Interplay between zinc and cell proliferation and implications for the growth of livestock. J Anim Physiol Anim Nutr (Berl). (2023) 107:1402–18. doi: 10.1111/jpn.13851
96. Hönscheid, A, Rink, L, and Haase, H. T-lymphocytes: a target for stimulatory and inhibitory effects of zinc ions. Endocr Metab Immune Disord Drug Targets. (2009) 9:132–44. doi: 10.2174/187153009788452390
97. Metz, CH, Schröder, AK, Overbeck, S, Kahmann, L, Plümäkers, B, and Rink, L. T-helper type 1 cytokine release is enhanced by in vitro zinc supplementation due to increased natural killer cells. Nutrition. (2007) 23:157–63. doi: 10.1016/j.nut.2006.10.007
98. Haase, H, and Rink, L. The immune system and the impact of zinc during aging. Immun Ageing. (2009) 6:9. doi: 10.1186/1742-4933-6-9
99. Yamasaki, S, Sakata-Sogawa, K, Hasegawa, A, Suzuki, T, Kabu, K, Sato, E, et al. Zinc is a novel intracellular second messenger. J Cell Biol. (2007) 177:637–45. doi: 10.1083/jcb.200702081
100. Bao, B, Prasad, AS, Beck, FW, and Godmere, M. Zinc modulates mRNA levels of cytokines. Am J Physiol Endocrinol Metab. (2003) 285:E1095–102. doi: 10.1152/ajpendo.00545.2002
101. Dinarello, CA. Overview of the IL-1 family in innate inflammation and acquired immunity. Immunol Rev. (2018) 281:8–27. doi: 10.1111/imr.12621
102. Wellinghausen, N, Martin, M, and Rink, L. Zinc inhibits interleukin-1-dependent T cell stimulation. Eur J Immunol. (1997) 27:2529–35. doi: 10.1002/eji.1830271010
103. Netea, MG, Nold-Petry, CA, Nold, MF, Joosten, LA, Opitz, B, van der Meer, JH, et al. Differential requirement for the activation of the inflammasome for processing and release of IL-1beta in monocytes and macrophages. Blood. (2009) 113:2324–35. doi: 10.1182/blood-2008-03-146720
104. Kim, B, Kim, HY, Yoon, BR, Yeo, J, In Jung, J, Yu, KS, et al. Cytoplasmic zinc promotes IL-1β production by monocytes and macrophages through mTORC1-induced glycolysis in rheumatoid arthritis. Sci Signal. (2022) 15:eabi 7400. doi: 10.1126/scisignal.abi7400
105. Summersgill, H, England, H, Lopez-Castejon, G, Lawrence, CB, Luheshi, NM, Pahle, J, et al. Zinc depletion regulates the processing and secretion of IL-1β. Cell Death Dis. (2014) 5:e 1040. doi: 10.1038/cddis.2013.547
106. Bachmann, MF, and Oxenius, A. Interleukin 2: from immunostimulation to immunoregulation and back again. EMBO Rep. (2007) 8:1142–8. doi: 10.1038/sj.embor.7401099
107. Tanaka, Y, Shiozawa, S, Morimoto, I, and Fujita, T. Role of zinc in interleukin 2 (IL-2)-mediated T-cell activation. Scand J Immunol. (1990) 31:547–52. doi: 10.1111/j.1365-3083.1990.tb02805.x
108. Golovine, K, Uzzo, RG, Makhov, P, Crispen, PL, Kunkle, D, and Kolenko, VM. Depletion of intracellular zinc increases expression of tumorigenic cytokines VEGF, IL-6 and IL-8 in prostate cancer cells via NF-kappa B-dependent pathway. Prostate. (2008) 68:1443–9. doi: 10.1002/pros.20810
109. Bonaventura, P, Benedetti, G, Albarède, F, and Miossec, P. Zinc and its role in immunity and inflammation. Autoimmun Rev. (2015) 14:277–85. doi: 10.1016/j.autrev.2014.11.008
110. Prasad, AS. Effects of zinc deficiency on Th1 and Th2 cytokine shifts. J Infect Dis. (2000) 182:S62–8. doi: 10.1086/315916
111. Harbour, SN, DiToro, DF, Witte, SJ, Zindl, CL, Gao, M, Schoeb, TR, et al. TH17 cells require ongoing classic IL-6 receptor signaling to retain transcriptional and functional identity. Sci Immunol. (2020) 5:eaaw 2262. doi: 10.1126/sciimmunol.aaw2262
112. Kitabayashi, C, Fukada, T, Kanamoto, M, Ohashi, W, Hojyo, S, Atsumi, T, et al. Zinc suppresses Th17 development via inhibition of STAT3 activation. Int Immunol. (2010) 22:375–86. doi: 10.1093/intimm/dxq017
113. Medida, RL, Sharma, AK, Guo, Y, Johnston, LJ, Urriola, PE, Gomez, A, et al. Dietary zinc supplemented in organic form affects the expression of inflammatory molecules in swine intestine. Animals (Basel). (2023) 13:2519. doi: 10.3390/ani13152519
114. Kido, T, Hachisuka, E, Suka, M, and Yanagisawa, H. Interleukin-4 administration or zinc supplementation is effective in preventing zinc deficiency-induced hemolytic anemia and splenomegaly. Biol Trace Elem Res. (2021) 199:668–81. doi: 10.1007/s12011-020-02172-1
115. Lang, C, Murgia, C, Leong, M, Tan, LW, Perozzi, G, Knight, D, et al. Anti-inflammatory effects of zinc and alterations in zinc transporter mRNA in mouse models of allergic inflammation. Am J Physiol Lung Cell Mol Physiol. (2007) 292:L577–84. doi: 10.1152/ajplung.00280.2006
116. Prasad, AS, Beck, FW, Bao, B, Fitzgerald, JT, Snell, DC, Steinberg, JD, et al. Zinc supplementation decreases incidence of infections in the elderly: effect of zinc on generation of cytokines and oxidative stress. Am J Clin Nutr. (2007) 85:837–44. doi: 10.1093/ajcn/85.3.837
117. Faghfouri, AH, Baradaran, B, Khabbazi, A, Khaje Bishak, Y, Zarezadeh, M, Tavakoli-Rouzbehani, OM, et al. Profiling inflammatory cytokines following zinc supplementation: a systematic review and meta-analysis of controlled trials. Br J Nutr. (2021) 126:1441–50. doi: 10.1017/S0007114521000192
118. Prasad, AS. Lessons learned from experimental human model of zinc deficiency. J Immunol Res. (2020) 2020:1–12. doi: 10.1155/2020/9207279
119. Wessels, I, Rolles, B, Slusarenko, AJ, and Rink, L. Zinc deficiency as a possible risk factor for increased susceptibility and severe progression of Corona virus disease 19. Br J Nutr. (2022) 127:214–32. doi: 10.1017/S0007114521000738
120. Patel, O, Chinni, V, El-Khoury, J, Perera, M, Neto, AS, McDonald, C, et al. A pilot double-blind safety and feasibility randomized controlled trial of high-dose intravenous zinc in hospitalized COVID-19 patients. J Med Virol. (2021) 93:3261–7. doi: 10.1002/jmv.26895
121. Ivanova, ID, Pal, A, Simonelli, I, Atanasova, B, Ventriglia, M, Rongioletti, M, et al. Evaluation of zinc, copper, and cu: Zn ratio in serum, and their implications in the course of COVID-19. J Trace Elem Med Biol. (2022) 71:126944. doi: 10.1016/j.jtemb.2022.126944
122. Quek, AML, Ooi, DSQ, Teng, O, Chan, CY, Ng, GJL, Ng, MY, et al. Zinc and vitamin C intake increases spike and neutralising antibody production following SARS-CoV-2 infection. Clin Transl Med. (2022) 12:e731. doi: 10.1002/ctm2.731
123. Vogel-González, M, Talló-Parra, M, Herrera-Fernández, V, Pérez-Vilaró, G, Chillón, M, Nogués, X, et al. Low zinc levels at admission associates with poor clinical outcomes in SARS-CoV-2 infection. Nutrients. (2021) 13:562. doi: 10.3390/nu13020562
124. Stambouli, N, Driss, A, Gargouri, F, Bahrini, K, Arfaoui, B, Abid, R, et al. Gharsallah H; of “OD-doxy-PNV-COVID-19 trial”. COVID-19 prophylaxis with doxycycline and zinc in health care workers: a prospective, randomized, double-blind clinical trial. Int J Infect Dis. (2022) 122:553–8. doi: 10.1016/j.ijid.2022.06.016
125. Abdelmaksoud, AA, Ghweil, AA, Hassan, MH, Rashad, A, Khodeary, A, Aref, ZF, et al. Olfactory disturbances as presenting manifestation among Egyptian patients with COVID-19: possible role of zinc. Biol Trace Elem Res. (2021) 199:4101–8. doi: 10.1007/s12011-020-02546-5
126. Rahman, MT, and Idid, SZ. Can Zn be a critical element in COVID-19 treatment? Biol Trace Elem Res. (2021) 199:550–8. doi: 10.1007/s12011-020-02194-9
127. Elanjian, AI, Elder, A, and Hazin, R. Hypoglycemia induced by zinc supplementation for COVID-19 prophylaxis: a case series. Cureus. (2023) 15:e38828. doi: 10.7759/cureus.38828
128. Rosenberg, M, Thapa, S, Jeurkar, C, and Kasner, M. Copper deficiency mimicking myelodysplastic syndrome: zinc supplementation in the setting of COVID-19. Case Rep Oncol. (2023) 16:55–61. doi: 10.1159/000528899
129. Farolfi, F, Cavazza, S, Mangiagalli, A, and Cavanna, L. A 98-year-old male with paroxysmal atrial fibrillation treated for COVID-19 at home. Cureus. (2022) 14:e30653. doi: 10.7759/cureus.30653
130. Ahmed, N, Asreb, A, Chofor, R, and Melese, A. Treatment of severe immune thrombocytopenic purpura associated with COVID-19. Am J Case Rep. (2021) 22:e932557. doi: 10.12659/AJCR.932557
131. Read, SA, Obeid, S, Ahlenstiel, C, and Ahlenstiel, G. The role of zinc in antiviral immunity. Adv Nutr. (2019) 10:696–710. doi: 10.1093/advances/nmz013
132. Hirano, T, Murakami, M, Fukada, T, Nishida, K, Yamasaki, S, and Suzuki, T. Roles of zinc and zinc signaling in immunity: zinc as an intracellular signaling molecule. Adv Immunol. (2008) 97:149–76. doi: 10.1016/S0065-2776(08)00003-5
133. Gouda, AS, Adbelruhman, FG, Elbendary, RN, Alharbi, FA, Alhamrani, SQ, and Mégarbane, B. A comprehensive insight into the role of zinc deficiency in the renin-angiotensin and kinin-kallikrein system dysfunctions in COVID-19 patients. Saudi J Biol Sci. (2021) 28:3540–7. doi: 10.1016/j.sjbs.2021.03.027
134. Salgo, MP. COVID-19: zinc and angiotensin-converting enzyme 2 (ACE2) deficiencies as determinants of risk and severity of disease: a narrative review. Infect Dis Ther. (2021) 10:1215–25. doi: 10.1007/s40121-021-00478-8
135. Rosenkranz, E, Metz, CH, Maywald, M, Hilgers, RD, Weßels, I, Senff, T, et al. Zinc supplementation induces regulatory T cells by inhibition of Sirt-1 deacetylase in mixed lymphocyte cultures. Mol Nutr Food Res. (2016) 60:661–71. doi: 10.1002/mnfr.201500524
136. Liu, Z, Wei, X, Gao, Y, Gao, X, Li, X, Zhong, Y, et al. Zbtb 34 promotes embryonic stem cell proliferation by elongating telomere length. Aging (Albany NY). (2022) 14:7126–36. doi: 10.18632/aging.204285
Keywords: zinc, nutrition, immune cells, interleukin, COVID-19
Citation: Jin D, Wei X, He Y, Zhong L, Lu H, Lan J, Wei Y, Liu Z and Liu H (2024) The nutritional roles of zinc for immune system and COVID-19 patients. Front. Nutr. 11:1385591. doi: 10.3389/fnut.2024.1385591
Received: 13 February 2024; Accepted: 09 April 2024;
Published: 19 April 2024.
Edited by:
Philip Calder, University of Southampton, United KingdomReviewed by:
Aneta Kopeć, University of Agriculture in Krakow, PolandCopyright © 2024 Jin, Wei, He, Zhong, Lu, Lan, Wei, Liu and Liu. This is an open-access article distributed under the terms of the Creative Commons Attribution License (CC BY). The use, distribution or reproduction in other forums is permitted, provided the original author(s) and the copyright owner(s) are credited and that the original publication in this journal is cited, in accordance with accepted academic practice. No use, distribution or reproduction is permitted which does not comply with these terms.
*Correspondence: Zheng Liu, emxpdTExMTFAMTYzLmNvbQ==; Hongbo Liu, aGJsaXVAZ2xtYy5lZHUuY24=
Disclaimer: All claims expressed in this article are solely those of the authors and do not necessarily represent those of their affiliated organizations, or those of the publisher, the editors and the reviewers. Any product that may be evaluated in this article or claim that may be made by its manufacturer is not guaranteed or endorsed by the publisher.
Research integrity at Frontiers
Learn more about the work of our research integrity team to safeguard the quality of each article we publish.