- 1State Key Laboratory of Animal Nutrition and Feeding, China Agricultural University, Beijing, China
- 2Beijing Advanced Innovation Center for Food Nutrition and Human Health, China Agricultural University, Beijing, China
Intestine is responsible for nutrients absorption and plays a key role in defending against various dietary allergens, antigens, toxins, and pathogens. Accumulating evidence reported a critical role of intestine in maintaining animal and human health. Since the use of antibiotics as growth promoters in animal feed has been restricted in many countries, alternatives to antibiotics have been globally investigated, and polysaccharides are considered as environmentally friendly and promising alternatives to improve intestinal health, which has become a research hotspot due to its antibiotic substitution effect. Astragalus polysaccharide (APS), a biological macromolecule, is extracted from astragalus and has been reported to exhibit complex biological activities involved in intestinal barrier integrity maintenance, intestinal microbiota regulation, short-chain fatty acids (SCFAs) production, and immune response regulation, which are critical for intestine health. The biological activity of APS is related to its chemical structure. In this review, we outlined the source and structure of APS, highlighted recent findings on the regulation of APS on physical barrier, biochemical barrier, immunological barrier, and immune response as well as the latest progress of APS as an antibiotic substitute in animal production. We hope this review could provide scientific basis and new insights for the application of APS in nutrition, clinical medicine and health by understanding particular effects of APS on intestine health, anti-inflammation, and animal production.
1 Introduction
Intestine, one part of the digestive tract, is not only responsible for digestion and absorption of dietary nutrients, but also plays a key role in immune homeostasis, protecting the body from various dietary allergens, antigens, toxins, and pathogens. Nowadays, accumulating evidence reported a critical role of intestine health in animal and human health maintenance (1). Indeed, intestinal homeostasis disorder may result in impaired morphology and function of intestine, reduced digestion and absorption capacity, increased diarrhea rate, reduced feed intake, and growth retardation (2–4). Hence, intestinal health status is crucial for an optimal production result and sustainable animal production (5). With raise public attention to intestinal health, intestine health has become a hot research topic in recent years (6). Intestinal barrier (physical barrier, biochemical barrier, and immunological barrier) composed of epithelial cells, microbiota, immune cells, and their secretions is key for intestinal health and involved in protecting body from the penetration of harmful entities (e.g., microorganisms, luminal antigens, and luminal proinflammatory factors) to maintain a stable internal environment (7). Moreover, dysfunction of intestinal barrier is believed to contribute to a broad range of diseases, such as inflammatory bowel disease (IBD), colorectal cancer, chronic liver disease, type 1 diabetes, and obesity (8, 9).
Since the use of antibiotics as growth promoters in animal feed has been restricted in many countries, accumulating evidence reported that probiotics, vitamins, minerals, amino acids, or plant extracts, etc. have been used to regulate intestinal barrier. Notably, plant extracts are natural, and multi-compounds products formed through an extraction and separation process from plant and exert positive effects on the integrity of the intestinal barrier with high efficiency and no residue. Astragalus comes from a type of leguminous herb and known as Huang Qi in China. Astragalus have been widely used to replenish qi by soaking into the water in folk. Astragalus supplements contains polysaccharides, saponins, flavonoids, and etc. (10). Among these biologically active ingredients, Astragalus polysaccharide (APS), a water-soluble heteropolysaccharide extracted from the stem or dried root of astragalus, is the most abundant and important active substance in Astragalus (11). Chemical composition analysis indicates that APS is mainly composed of glucose, galactose, rhamnose, mannose, xylose, arabinose, glucuronic acid, and galacturonic acid (12). Growing pharmacological and clinical trials had shown that APS is used to protect and support the immune system (13). In addition, APS exerts anti-oxidation, anti-aging, anti-fibrosis, anti-tumor, antiviral and antibacterial, blood sugar reduction, blood lipid reduction, anti-fibrosis, and radiation protection effects (14). Studies showed that traditional Chinese medicine (TCM) have applied as effective method to modify intestinal dysfunction, regulate structure and function of gut microbiota, reduce inflammation response, as well as cell repair intestinal barrier. Likewise, emerging evidence focused on the regulation of intestinal health by APS have indicated the beneficial effects and underlying mechanisms involved in intestinal barrier maintenance, intestinal microbiota regulation, immune response, and redox homeostasis (15–17).
In this review, we firstly outlined the source and structure of APS. And then, we highlighted recent advancements on APS as a potential therapeutic intervention for intestinal disease associated with intestinal barrier dysfunction, intestinal microbiota disorder, intestinal inflammatory response, as well as intestinal oxidative stress response. We hope this review could provide scientific basis and new insights for the application of APS in nutrition, clinical medicine, and health by understanding particular effects of APS on intestine homeostasis and immune response.
2 Characteristics of Astragalus polysaccharide
Polysaccharides are polymers constituted of more than 10 monosaccharides with condensation reaction (18, 19). Polysaccharides are widely present in plants, algae, animals and microorganisms (bacteria, fungi, and yeasts) and generally obtained from plants through extraction, separation, and purification (20). Growing evidences revealed that polysaccharides possess complex biological activities and a variety of biological functions involved in antioxidant, antitumor, antiviral, immune regulation activities and so on (21). Of note, polysaccharides are widely present in TCM and considered as one of the important bioactive ingredients in TCM (22). Additionally, polysaccharides can be divided into homopolysaccharides and heteropolysaccharides according to the composition of monosaccharides. Homopolysaccharides refer to polysaccharides composed of only one monosaccharide, while heteropolysaccharides are polysaccharides composed of two or more monosaccharides. APS, a water-soluble heteropolysaccharide, is extracted from a common Chines herbal plant (Astragalus membranaceus) and considered as important bioactive components of Astragalus membranaceus (23).
Astragalus polysaccharide process complex biological activities including anti-inflammation, antioxidant, antiviral, anticancer, and immune functions (16, 24–27) and are applied as an additive with non-toxic side effects, low cost, and no residue. Indeed, polysaccharides are macromolecules with the chemical structures (primary, secondary, tertiary, and quaternary structures) which contribute to its biological activities (28, 29). Nevertheless, polysaccharide structural analysis indicated that polysaccharide structures are very complex and comprehensive structures were characterized by monosaccharide composition, Fourier transform infrared and nuclear magnetic resonance spectroscopy (NMR) analysis (29, 30). Although APS is formed by galactose, glucose, mannose, rhamnose, xylose, arabinose, glucuronic acid, and galacturonic acid with condensation reaction (12), but content and monosaccharide compositions of APS obtained from different original materials, different areas, and different extraction methods contribute to differentiated health benefits. The biological activities of APS are related to their chemical structure. Extraction, separation, and purification are essential steps for the APS structure determination, and then structural analysis performed by high performance liquid chromatography (HPLC), NMR, and other methods (12). To date, water extraction, enzymatic hydrolysis extraction, ultrasonic wave extraction, and microwave-assisted extraction are commonly used methods in APS extraction (31). A previous study reported that APS extracted by hot water was performed NMR analysis, structural analysis suggested that APS is a kind of glucan, the main chain is connected by α-1,4-glycoside bonds and the branch chain is α-1,6-glycoside bonds (12, 32). Nevertheless, four APSs were obtained with an ethanol precipitation procedure. Molecular weight and monosaccharide composition analysis indicated that ASP1 with molecular weight 257.7 kDa is consisted of glucose, ASP2 with molecular weight 40.1 kDa is consisted of arabinose, ASP3 with molecular weight 15.3 kDa is consisted of rhamnose, glucose, and galactose, and ASP4 with molecular weight 3.2 kDa is consisted of galactose and arabinose (33). Furthermore, HPLC method was performed to define the monosaccharide composition of APS, results indicated that APS was composed of fucose, arabinose, galactose, glucose, and xylose with molar ratios of 0.01:0.06:0.20:1.00:0.06 (34). Simultaneously, structure of APS determined by gas chromatography (GC) and Fourier transmission-infrared spectroscopy (FT-IR) suggested that ASP was composed of arabinose, mannose, glucose, mannose, and galactose and with a ratio of 0.0992:1.26:1.00:0.015 (35). In addition, a literature reported that monosaccharide formation of APS was defined by HPLC and other methods, and analysis showed that APS-I with molecular weight 1699.1 kDa was composed of arabinose and glucose (1:3.45) and APS-II with molecular weight 1197.6 kDa was composed of rhamnose, arabinose and glucose (1:6.25:17.86) (36).
3 Effects of Astragalus polysaccharide on intestinal barrier
Intestinal barrier composed of physical barrier, biochemical barrier, and immunological barrier plays a key role in preventing the passage of harmful or unwanted substances from entering the internal environment which is a crucial for humans and animals health (37–39). Dysfunction of intestinal barrier function would lead to intestinal diseases such as enteritis, IBD, celiac disease, irritable bowel syndrome (IBS), and colorectal cancer (40–42). A variety of factors, such as food antigens, pathogenic organisms, and toxins, have been reported to disorder intestinal barrier integrity, which in turn lead to a reduced animal growth performance and animal production quality (43). Schematic representation for effects of Astragalus polysaccharide on the intestinal health was presented in Figure 1.
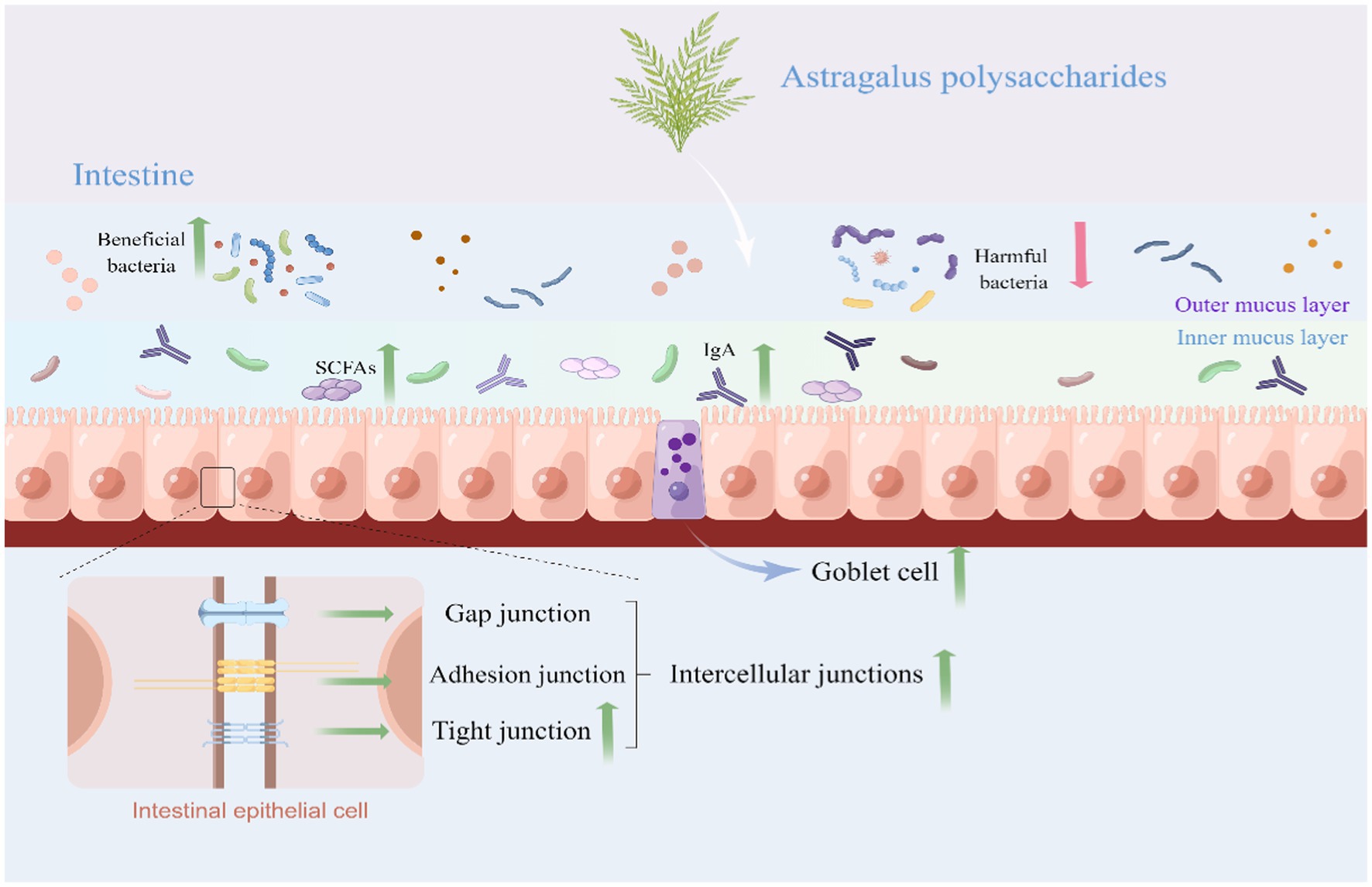
Figure 1. Schematic representation for effects of Astragalus polysaccharide on the intestinal health (By Figdraw). APS could protect the intestine from damage by upregulating the intestinal tight junction protein and enhancing goblet cell number. APS improve the intestinal immune response mainly by regulating sIgA secretion, cytokines production, and immune cells proliferation and differentiation. APS protects the intestinal biochemical barrier by regulating composition and structure of gut microbiota and SCFAs levels. APS, Astragalus polysaccharide; SCFAs, Short-chain fatty acids; and sIgA, Secretory immunoglobulin A.
3.1 Astragalus polysaccharide improves the intestinal physical barrier
The intestinal physical barrier is a single-cell layer composed of intestinal epithelial cells, intercellular junctions, and intestinal mucosa, which separates the intestinal luminal contents from the internal milieu (44, 45). Furthermore, the functionally specialized epithelial cells contain enterocytes, Paneth cells, goblet cells, tuft cells, enteroendocrine cells, and microfold cells (46, 47). Enterocytes account for >80% of epithelial cells are specialized to absorb and export luminal nutrients (48). Goblet cells secrete mucins to protect mucous membranes, enteroendocrine cells secrete peptide hormones and Paneth cells produce a number of antimicrobial peptides (49, 50). Additionally, intercellular junctional complexes including tight junctions (TJ), adherens junctions, gap junctions, and desmosomes provide contact or tightly bound between neighboring cells and play a critical role in the regulation of paracellular permeability and epithelial barrier integrity (42, 51). Notably, TJ composed of Occludin, Claudins, and Zonula occludens have been extensively studied and are responsible for intestinal barrier function (52). Accumulating studies suggested that TJ are crucial for the maintenance of epithelial barrier integrity by selectively transporting essential molecules and preventing harmful substances from entering into the internal environment (42, 53, 54).
A variety of factors, such as reactive oxygen species (ROS), infection, cytokines, and pathogens, have been reported to disorder intestinal physical barrier. Oxidative stress occurs when an imbalance emerged between the ROS production and antioxidant system, which induced intestinal cells apoptosis and disturbed abundance and distribution of TJ proteins resulting in damage of the epithelial barrier (55–57). Furthermore, ROS significantly enhanced crypt depth (CD) and reduced villus height (VH) of the intestine and ratio of villus height/crypt depth (VH/CD) (58). Emerging studies had shown that APS could inhibit inflammation, repair the integrity of intestinal barrier, and ultimately improve digestion and absorption of nutrients. Indeed, a previous study demonstrated that lipopolysaccharide (LPS)-challenged weaned piglets supplemented with 800 mg/kg APS enhanced superoxide dismutase (SOD) and total antioxidant capacity (T-AOC) in serum, and enhanced abundance of claudin and occluding in the jejunum (44). Similarly, BALB/c mice exposed to Salmonella typhimurium (S. t.). supplemented with 200 mg/kg APS improved intestinal barrier through enhancing mRNA expression of ZO-1, Occludin, and Claudin-1 in jejunum and attenuating inflammation response (59). Furthermore, immunosuppressed broilers supplemented with 900 mg/kg γ-irradiation APS alleviated cyclophosphamide (CPM)-induced intestinal mucosa damage and increased jejunal goblet cell number (60). Collectively, these studies have shown that APS could reduce the epithelial cells loss caused by inflammation and repair the integrity of intestinal barrier.
3.2 Astragalus polysaccharide improves the intestinal biochemical barrier
Intestinal microbial barrier refers to commensal microorganisms (bacteria, archaea, fungi, and viruses) colonized in the digestive tracts and have been reported to play potential role in protecting against external stimuli, modulating immunity, modulating the metabolism of lipids and bile acids as well as neuromodulation (38, 61–63). In general, gut bacteria mostly account for gut microorganisms and members of Firmicutes, Bacteroidetes, Actinobacteria, Proteobacteria, Fusobacteria, and Verrucomicrobia have been identified as major bacterial phylum of gut microbiota (64, 65). Mounting studies suggested that intestinal microbiota maintain a symbiotic relationship with the host (66) and play a critical role in animal health maintenance and the pathogenesis of intestinal diseases, such as IBS, IBD, celiac disease, and colorectal cancer (67). Growing evidence demonstrated that gut microbiota not only provide essential capacities for the metabolism of nutrients, but also participate in regulating the integrity and function of the intestinal barrier in a homeostatic balance (68, 69). Therefore, gut microbiota homeostasis is vital for intestinal barrier integrity and the underlying mechanism are largely unknown (70–72). Gut microbiota provide essential capacities for the fermentation of non-digestible substrates like dietary fibers and endogenous intestinal endogenous intestinal mucus (73). Emerging evidence indicated that APS might improve intestinal barrier through promoting colonization of good bacteria, preventing pathogenic bacteria from invasion and growth, eliminating ROS and attenuating intestinal inflammation (56, 74, 75). A previous study found that dietary diet supplemented with 220 mg/kg APS enhanced the abundance of beneficial bacteria numbers (Lactobacilli and Bifidobacteria) and reduced E. coli abundance in the gut (76). Similarly, broiler supplemented with 900 mg/kg γ-irradiation APS reduced abundance of Bacteroides, Faecalibacterium, and Butyricicoccus, enhanced the abundance of Ruminococcaceae UCG-014, Negativibacillus, Shuttleworthia, Sellimonas, and Mollicutes RF39_norank, and elevated butyrate concentration in cecum (77). Moreover, alcoholic fatty liver disease (AFLD) mice were given 22 mg/kg corresponding polysaccharide solution (APS with polysaccharide content of 62.73%) markedly enhanced Bacteroides S24-7 abundance, reduced abundance of Clostridiales and Lachnospiraceae, and reduced ratio of Firmicutes to Bacteroidetes (78), and ultimately elevated the abundance of beneficial bacteria and reduced the abundance of pathogenic bacteria.
Short-chain fatty acids (SCFAs) are organic linear carboxylic acids with two to six carbon atoms and are mainly produced by the gut microbiota via the fermentation of complex carbohydrates and fibers (79). Natural polysaccharides are favorable for the production of SCFAs (80, 81). Mounting evidence indicated that SCFAs play crucial roles in maintenance of intestinal health including regulation of intestinal barrier, intestinal epithelial cell growth and function, and inflammatory response modulation (82–84). SCFAs are mainly composed of acetic acid, propionic acid, and butyric acid, the main of which is butyric acid, which consumes oxygen, creating an anaerobic condition for the intestinal tract and prevent aerobic pathogens from invasion in the gut. Correspondingly, reduced SCFAs level in gut contributed to the enhanced intestinal permeability and intestinal diseases (85). APS as a natural polysaccharide has been reported to enhance SCFAs levels and modulate composition and function of gut microbiota (17). Type II diabetes mice supplemented with 600 mg/kg APS significantly enhanced fecal SCFAs level, G-protein-coupled receptor 41/43 expression, and TJ proteins (Occudin and ZO-1) abundance (86). Furthermore, APS enhanced secretion of glucagon-like peptide-1 (GLP-1) in serum and improved intestinal microbial barrier, resulting in alleviation of diabetes symptoms in mice. Similarly, a basal diet with 800 mg/kg APS markedly enhanced the level of acetic acid, propionic acid, isobutyric acid, and butyrate in colon and enhanced the colonic microbial population and diversity (87). In addition, the literature also suggested that APS (200 mg/kg) attenuated the intestinal injury caused by necrotic enteritis in broiler through enhancing the concentrations of propionic acid, butyric acid, isobutyric acid, and hexanoic acid in the ileum (88). Collectively, APS exerts intestinal barrier protection through promoting the growth of beneficial microbiota, inhibiting colonization of pathogenic bacteria, and elevating level of SCFAs. The effects of APS on the intestinal biochemical barrier can be seen in Figure 1.
3.3 Astragalus polysaccharide improves the intestinal immunological barrier
Intestine is not only responsible for nutrient digestion and absorption, but is also the largest immune organ comprised around 80% immune cells in the body (89, 90). The intestinal immune barrier is mainly composed of secretory immunoglobulin A (sIgA), gut associated lymphoid tissue (GALT), cytokines and other immune-producing substances, which play a key role in fighting against pathogens or toxins (90, 91). sIgA constitutively localize in mucosal secretions and serve as the first line of defense in blocking microorganisms from attaching to, colonizing and invading epithelial cells (92). Moreover, mounting evidence demonstrated that sIgA play a crucial role in regulating TJ proteins expression, shaping commensal microbiota composition, and maintaining epithelial barrier integrity and immune homeostasis (92–94). GALT include Peyer’s patches (PPs), numerous isolated lymphoid follicles (ILF), mesenteric lymph nodes (MLN) and diffuse GALT would modulate cytokine/chemokine production and immune cell function (91, 95). Additionally, excessive cytokine production aggravates intestine inflammation and intestinal barrier impairment (96). Interestingly, accumulating evidence reported that APS exerts the potentiality to promote the activities of immune cells (e.g., macrophages, natural killer cells, dendritic cells, T lymphocytes, B lymphocytes, and microglia) and regulates the production of cytokines and chemokines (16, 97, 98). A previous study was conducted to examine the effects of APS (in ovo injection) on number of immune cells, sIgA, and intestinal immune-related genes expression in broiler chickens. Results found that APS injection at 2 or 4 mg in ovo significantly enhanced VH and VH/CD ratio, increased IgA+ cells population and sIgA content, and enhanced mRNA expressions of interleukin (IL)-2, IL-4, interferon gamma (IFN-γ), and Toll-like receptor (TLR)-4 (99). Similarly, in ovo administration of Newcastle disease vaccine (NDV) conjugated with APS exerts beneficial effects on the intestinal mucosal immunity of chicks through enhancing the levels of slgA and the abundance of IgA+ cells in duodenal lamina propria and villi when compared with NDV treatment alone (100). Furthermore, broilers supplemented with 300 mg/kg APS improved the intestinal mucosal immune barrier function of broilers by enhancing mRNA expression of Occludin, Claudin-1, ZO-1, and MUC2 in small intestine, and improved growth by enhancing concentration of immunoglobulins (Ig) A, IgM and IgG, and lowing concentrations of TNF-α, IL-1β, IL-6, and diamine oxidase (DAO) in serum (74). In addition, broilers administrated with 0.5, 1, or 2 mg APS in 0.5 mL saline enhanced VH/CD ratio, IgA+ cells population, sIgA levels when compared with vaccinated control group and one non-vaccinated negative control group (101) (Table 1).
4 Anti-inflammatory properties of APS
Currently, APS derived from natural sources has been reported to play an important role in regulating inflammatory response (106, 107). TLRs and NoD-like receptors (NLRP) have been reported to play important role in pro-inflammatory cytokines expressions regulation (108). Emerging evidence revealed that APS could inhibit the expression of NOD-like receptor thermal protein domain associated protein 3 (NLRP3) inflammasome in colon tissue, and reduced production of IL-18 and IL-1β (109). Interestingly, a previous study revealed that APS alleviated inflammatory damage of the BPD cell model through inhibiting the activation of nuclear factor-κB (NF-κB) and reducing mRNA and protein expression levels of IL-8 and intercellular adhesion molecule 1 (ICAM-1) in bronchopulmonary dysplasia (BPD) (110). It is speculated that APS may be a safe alternative to glucocorticoid in the treatment of BPD. Furthermore, a rat model of pulmonary arterial hypertension (PAH) induced by injection of monocrotaline, which was intraperitoneally injected with APS (200 mg/kg, once every 2 days) for 2 weeks leading to reduced mRNA expression of pro-inflammatory mediators TNF-α, IL-1β, and IL-6 and inflammation alleviation (111). Correspondingly, APS could inhibit the activation of phosphorylation level of IκBα, thereby inhibiting the NF-κB signaling pathway and improving PAH induced by monocrotaline. IBD, a kind of intestinal disease, has become a global burden with rapidly increasing incidence and prevalence in both industrialized countries and developing countries (112–114). Although the etiology of IBD is still unknown, previous reports had shown that the imbalanced production of pro-inflammatory cytokines and anti-inflammatory cytokines contributed to intestinal tissue damage (115). Intriguingly, dextran sulfate sodium (DSS)-induced mice colitis daily intraperitoneal injection with 0.5 mL of APS (200 mg/kg) for 3 days remarkedly reduced phosphorylation level of NF-κB and downregulated the mRNA expression of TNF-α, IL-1β, IL-6, and IL-17 (116). These results demonstrated that APS function as a natural active ingredient to treat ulcerative colitis. In a LPS-challenged Caco-2 cells model, APS addition (100 or 200 μg/mL) in cell culture remarkedly downregulated mRNA expression of TNF-α, IL-1β, and IL-8 in a dose manner (117). Consequently, result indicated that APS exerts anti-inflammatory properties on LPS-infected Caco-2 cells and is regarded as a preventive therapy for LPS induced intestinal cells damage. In addition to APS, honey-processed APS (HAPS) is a product that Radix Astragalus mixed with honey, which exhibits better efficacy pharmacological activity (118). Correspondingly, HAPS alleviated LPS-induced inflammatory responses in RAW264.7 cells by significantly reducing NO concentration and the expression of TNF-α, IFN-γ, IL-1β, and IL-22. These results indicated that anti-inflammatory activities of HAPS were more effective than those of APS (119). Furthermore, LPS-induced inflammatory lung injury mice orally administrated with 200 mg/kg APS for 14 consecutive days significantly reduced neutrophilic infiltration, phosphorylated NF-κB expression level and relative expressions of ICAM-1, Il-1β, Il-6, and TNF-α (120). Taken together, APS functions as an anti-inflammatory agent in animals and exerts its anti-inflammation mainly by inhibiting NF-κB signaling pathways and reducing expression of pro-inflammatory cytokines.
5 Effects of APS on animal production
The efficiency of animal production is closely related with the economic benefits of animal husbandry (121). Nowadays, the general use of antibiotics in animal feed is banned, alternatives to antibiotics are urgently needed in animal agriculture (122). Emerging evidence demonstrated that some polysaccharides could function as antibiotics alternatives to inhibit pathogens colonization and promote animal growth performance (123, 124). APS is a kind of polysaccharides and has been investigated for its effects on animal growth performance (74). A study reported that APS enhanced average daily gain (ADG) and feed conversion rate (FCR) through improving VH and VH/CD ratio, reducing immunological stress, as well as enhancing the intestinal barrier function in LPS-challenged piglets (125). Furthermore, 0.1% APS supplementation improved ADG and F/G ratio, enhanced apparent ileal digestibility (AID), and the contents of most essential amino acids and non-essential amino acids in the serum in early-weaned piglets (126). In addition, in ovo injection of 2 mg APS per egg significantly enhanced VH and the ratio of VH/CD and improved intestinal morphology and development of chicks, resulting in marked increase on average daily feed intake (ADFI), body weight (BW), and FCR of layer chicks (127). Interestingly, recent studies revealed that APS could regulate lipid metabolism and adipogenesis (128). A previous study reported that in ovo injection of 4.5 mg APS enhanced carcass percentage, reduced abdominal fat, as well as reduced educed triglycerides, total cholesterol, low-density lipoproteins, and very low-density lipoproteins in the plasma of broilers (129). When compared with control group, APS treatment improved meat quality and feed conversion rate by reducing fat metabolism. This result is attributed to induced expression of amylase by restraining the activity of other intestinal digestive enzymes (130). Furthermore, young broilers supplemented with 1 g/kg APS markedly enhanced BW and reduced FCR by enhancing activities of lipase, amylase and protease (131). In addition, 10 g/kg APS addition significantly enhanced BW, improved the intestinal morphology, enhanced VH and the ratio of VH/CD in jejunum, as well as reduced CD of the duodenum (132). In addition to the application of APS on livestock (pigs or broilers), APS has been reported to play potential role on the growth and development of fish (133). Sun and coauthors demonstrated that turbot (Scophthalmus maximus L.) supplemented with 150 mg/kg APS remarkably enhanced final body weight (FBW), specific growth rate (SGR), weight gain (WG), and ADFI by improving the activity of digestive enzymes (134). Consistently, crucian carps orally administrated with 100 mg/kg APS markedly enhanced body weight gain rate (BVGR), SGR and reduced the FCR (135). Additionally, 30 g/kg APS addition exerted beneficial effects on body protein composition, body weight gain, feed efficiency of white shrimp, and lipid metabolism (Litopenaeus vannamei) (136). Moreover, 0.01% APS in the diet of zebrafish significantly upregulated TJ protein 1b and Occludin1, improved intestinal permeability and promoted intestinal health. Furthermore, APS supplementation enhanced BW and reduced FCR (136).
Collectively, the improvement of APS on growth performance is attributed to intestinal villus morphology improvement, intestinal digestion and absorption as well as digestion enzymes activities improvement. Main functions of APS on animal growth performance were displayed in Table 2.
6 Conclusion
Astragalus polysaccharide is a natural bioactive component and possesses a variety of biological activities involved in anti-oxidation, anti-aging, anti-fibrosis, anti-tumor, antiviral and antibacterial, blood sugar reduction, blood lipid reduction, anti-fibrosis, and radiation protection effects. Furthermore, emerging evidence demonstrated that APS plays a potential role in intestinal epithelial barrier integrity maintenance, intestinal microbiota regulation, immune response, and redox homeostasis, which are critical for intestinal health, immune response, and animal’s growth performance. It can provide reference for further study on the effect of APS on intestinal barrier. In addition, it can be seen from the above numerous reports that Astragalus polysaccharide is widely used in the intestine, and there will be some breakthroughs in the future. On the one hand, origin, extraction, separation and purification methods contribute to the activities of APS, thus these processes are needed to be improved. On the other hand, the biological activities of APS are related to their chemical structure. APS is formed by galactose, glucose, mannose, rhamnose, xylose, arabinose, glucuronic acid, and galacturonic acid with condensation reaction, but accurate molecular structures of APS are largely unknown. Additionally, further understanding the regulatory role of APS in the interaction between microbiota and intestinal barrier are needed. In this context, analysis structure and further understanding the regulatory mechanical role of APS are crucial for the application of APS on intestinal health. APS might be a therapeutic intervention for intestinal disease. Therefore, APS will be a potential research object with broad prospects.
Author contributions
HL: Data curation, Resources, Writing – original draft. ST: Resources, Writing – original draft. YaW: Resources, Writing – original draft. JZ: Resources, Writing – original draft. CY: Resources, Writing – original draft. YiW: Resources, Writing – original draft. NL: Funding acquisition, Supervision, Writing – original draft, Writing – review & editing. YQ: Funding acquisition, Resources, Writing – review & editing.
Funding
The author(s) declare that financial support was received for the research, authorship, and/or publication of this article. This work was supported by the National Key Research and Development Program of China (2023YFD1302005), National Natural Science Foundation of China (No. 32000082), Earmarked Fund for Modern Agro-Industry Technology Research System (CARS-43), China Postdoctoral Science Foundation (No. 2022M713405), and the 2115 Talent Program of China Agricultural University.
Conflict of interest
The authors declare that the research was conducted in the absence of any commercial or financial relationships that could be construed as a potential conflict of interest.
Publisher’s note
All claims expressed in this article are solely those of the authors and do not necessarily represent those of their affiliated organizations, or those of the publisher, the editors and the reviewers. Any product that may be evaluated in this article, or claim that may be made by its manufacturer, is not guaranteed or endorsed by the publisher.
References
1. Tang, X, Xiong, K, Fang, R, and Li, M. Weaning stress and intestinal health of piglets: a review. Front Immunol. (2022) 13:1042778. doi: 10.3389/fimmu.2022.1042778
2. Feng, P, Ye, Z, Kakade, A, Virk, AK, Li, X, and Liu, P. A review on gut remediation of selected environmental contaminants: possible roles of probiotics and gut microbiota. Nutrients. (2018) 11:22. doi: 10.3390/nu11010022
3. Gou, HZ, Zhang, YL, Ren, LF, Li, ZJ, and Zhang, L. How do intestinal probiotics restore the intestinal barrier? Front Microbiol. (2022) 13:929346. doi: 10.3389/fmicb.2022.929346
4. Wen, C, Guo, Q, Wang, W, Duan, Y, Zhang, L, Li, J, et al. Taurine alleviates intestinal injury by mediating tight junction barriers in Diquat-challenged piglet models. Front Physiol. (2020) 11:449. doi: 10.3389/fphys.2020.00449
5. Celi, P, Cowieson, AJ, Fru-Nji, F, Steinert, RE, Kluenter, AM, and Verlhac, V. Gastrointestinal functionality in animal nutrition and health: new opportunities for sustainable animal production. Anim Feed Sci Technol. (2017) 234:88–100. doi: 10.1016/j.anifeedsci.2017.09.012
6. de Vos, WM, Tilg, H, Van Hul, M, and Cani, PD. Gut microbiome and health: mechanistic insights. Gut. (2022) 71:1020–32. doi: 10.1136/gutjnl-2021-326789
7. Che, Q, Luo, T, Shi, J, He, Y, and Xu, DL. Mechanisms by which traditional Chinese medicines influence the intestinal flora and intestinal barrier. Front Cell Infect Microbiol. (2022) 12:863779. doi: 10.3389/fcimb.2022.863779
8. Farhadi, A, Banan, A, Fields, J, and Keshavarzian, A. Intestinal barrier: an interface between health and disease. J Gastroenterol Hepatol. (2003) 18:479–97. doi: 10.1046/j.1440-1746.2003.03032.x
9. Vancamelbeke, M, and Vermeire, S. The intestinal barrier: a fundamental role in health and disease. Expert Rev Gastroenterol Hepatol. (2017) 11:821–34. doi: 10.1080/17474124.2017.1343143
10. Li, X, Guo, X, Sha, M, Gao, W, and Li, X. Combining network pharmacology with chromatographic fingerprinting and multicomponent quantitative analysis for the quality evaluation of Astragali Radix. Biomed Chromatogr. (2022b) 36:e5319. doi: 10.1002/bmc.5319
11. Chen, Z, Liu, L, Gao, C, Chen, W, Vong, CT, Yao, P, et al. Astragali Radix (Huangqi): a promising edible immunomodulatory herbal medicine. J Ethnopharmacol. (2020) 258:112895. doi: 10.1016/j.jep.2020.112895
12. Wang, J, Jia, J, Song, L, Gong, X, Xu, J, Yang, M, et al. Extraction, structure, and pharmacological activities of Astragalus polysaccharides. Appl Sci. (2018) 9:122. doi: 10.3390/app9010122
13. Tang, Z, and Huang, G. Extraction, structure, and activity of polysaccharide from Radix astragali. Biomed Pharmacother. (2022) 150:113015. doi: 10.1016/j.biopha.2022.113015
14. Zheng, Y, Ren, W, Zhang, L, Zhang, Y, Liu, D, and Liu, Y. A review of the pharmacological action of Astragalus polysaccharide. Front Pharmacol. (2020) 11:349. doi: 10.3389/fphar.2020.00349
15. Auyeung, KK, Han, QB, and Ko, JK. Astragalus membranaceus: a review of its protection against inflammation and gastrointestinal cancers. Am J Chin Med. (2016) 44:1–22. doi: 10.1142/S0192415X16500014
16. Li, CX, Liu, Y, Zhang, YZ, Li, JC, and Lai, J. Astragalus polysaccharide: a review of its immunomodulatory effect. Arch Pharm Res. (2022a) 45:367–89. doi: 10.1007/s12272-022-01393-3
17. Wei, X, Xin, J, Chen, W, Wang, J, Lv, Y, Wei, Y, et al. Astragalus polysaccharide ameliorated complex factor-induced chronic fatigue syndrome by modulating the gut microbiota and metabolites in mice. Biomed Pharmacother. (2023) 163:114862. doi: 10.1016/j.biopha.2023.114862
18. Shi, L. Bioactivities, isolation and purification methods of polysaccharides from natural products: a review. Int J Biol Macromol. (2016) 92:37–48. doi: 10.1016/j.ijbiomac.2016.06.100
19. Zhou, Y, Chen, X, Chen, T, and Chen, X. A review of the antibacterial activity and mechanisms of plant polysaccharides. Trends Food Sci Technol. (2022) 123:264–80. doi: 10.1016/j.tifs.2022.03.020
20. Albuquerque, PBS, de Oliveira, WF, Dos Santos Silva, PM, Dos Santos Correia, MT, Kennedy, JF, and Coelho, L. Skincare application of medicinal plant polysaccharides—a review. Carbohydr Polym. (2022) 277:118824. doi: 10.1016/j.carbpol.2021.118824
21. Mohammed, ASA, Naveed, M, and Jost, N. Polysaccharides; classification, chemical properties, and future perspective applications in Fields of pharmacology and biological medicine (a review of current applications and upcoming potentialities). J Polym Environ. (2021) 29:2359–71. doi: 10.1007/s10924-021-02052-2
22. Yue, B, Zong, G, Tao, R, Wei, Z, and Lu, Y. Crosstalk between traditional Chinese medicine-derived polysaccharides and the gut microbiota: a new perspective to understand traditional Chinese medicine. Phytother Res. (2022) 36:4125–38. doi: 10.1002/ptr.7607
23. Song, J, Chen, Y, He, D, Tan, W, Lv, F, Liang, B, et al. Astragalus polysaccharide promotes Adriamycin-induced apoptosis in gastric cancer cells. Cancer Manag Res. (2020) 12:2405–14. doi: 10.2147/CMAR.S237146
24. Harikrishnan, R, Devi, G, Doan, HV, Tapingkae, W, Balasundaram, C, Arockiaraj, J, et al. Changes in immune genes expression, immune response, digestive enzymes -antioxidant status, and growth of catla (Catla catla) fed with Astragalus polysaccharides against edwardsiellosis disease. Fish Shellfish Immunol. (2022) 121:418–36. doi: 10.1016/j.fsi.2022.01.022
25. Li, W, Hu, X, Wang, S, Wang, H, Parungao, R, Wang, Y, et al. Detection and evaluation of anti-Cancer efficiency of astragalus polysaccharide via a tissue engineered tumor model. Macromol Biosci. (2018a) 18:e1800223. doi: 10.1002/mabi.201800223
26. Long, H, Lin, H, Zheng, P, Hou, L, Zhang, M, Lin, S, et al. WTAP mediates the anti-inflammatory effect of Astragalus mongholicus polysaccharide on THP-1 macrophages. Front Pharmacol. (2022) 13:1023878. doi: 10.3389/fphar.2022.1023878
27. Zhang, P, Liu, X, Liu, H, Wang, W, Liu, X, Li, X, et al. Astragalus polysaccharides inhibit avian infectious bronchitis virus infection by regulating viral replication. Microb Pathog. (2018) 114:124–8. doi: 10.1016/j.micpath.2017.11.026
28. Guru, PR, Kar, RK, Nayak, AK, and Mohapatra, S. A comprehensive review on pharmaceutical uses of plant-derived biopolysaccharides. Int J Biol Macromol. (2023) 233:123454. doi: 10.1016/j.ijbiomac.2023.123454
29. Zeng, P, Li, J, Chen, Y, and Zhang, L. The structures and biological functions of polysaccharides from traditional Chinese herbs. Prog Mol Biol Transl Sci. (2019) 163:423–44. doi: 10.1016/bs.pmbts.2019.03.003
30. Huang, X, Ai, C, Yao, H, Zhao, C, Xiang, C, Hong, T, et al. Guideline for the extraction, isolation, purification, and structural characterization of polysaccharides from natural resources. eFood. (2022) 3:e37. doi: 10.1002/efd2.37
31. Shang, H, Chen, S, Li, R, Zhou, H, Wu, H, and Song, H. Influences of extraction methods on physicochemical characteristics and activities of Astragalus cicer L. polysaccharides. Process Biochem. (2018) 73:220–7. doi: 10.1016/j.procbio.2018.07.016
32. Sg, L, and Yq, Z. Characterization and renal protective effect of a polysaccharide from Astragalus membranaceus. Carbohydr Polym. (2009) 78:343–8. doi: 10.1016/j.carbpol.2009.04.013
33. Jiang, Y, Qi, X, Gao, K, Liu, W, Li, N, Cheng, N, et al. Relationship between molecular weight, monosaccharide composition and immunobiologic activity of Astragalus polysaccharides. Glycoconj J. (2016) 33:755–61. doi: 10.1007/s10719-016-9669-z
34. Liu, AJ, Yu, J, Ji, HY, Zhang, HC, Zhang, Y, and Liu, HP. Extraction of a novel cold-water-soluble polysaccharide from Astragalus membranaceus and its antitumor and immunological activities. Molecules. (2017) 23:62. doi: 10.3390/molecules23010062
35. Yan, H, Xie, Y, Sun, S, Sun, X, Ren, F, Shi, Q, et al. Chemical analysis of Astragalus mongholicus polysaccharides and antioxidant activity of the polysaccharides. Carbohydr Polym. (2010) 82:636–40. doi: 10.1016/j.carbpol.2010.05.026
36. Xu, DJ, Xia, Q, Wang, JJ, and Wang, PP. Molecular weight and monosaccharide composition of Astragalus polysaccharides. Molecules. (2008) 13:2408–15. doi: 10.3390/molecules13102408
37. Liu, N, Ma, X, Luo, X, Zhang, Y, He, Y, Dai, Z, et al. L-glutamine attenuates apoptosis in porcine enterocytes by regulating glutathione-related redox homeostasis. J Nutr. (2018) 148:526–34. doi: 10.1093/jn/nxx062
38. Ma, J, Piao, X, Mahfuz, S, Long, S, and Wang, J. The interaction among gut microbes, the intestinal barrier and short chain fatty acids. Anim Nutr. (2022) 9:159–74. doi: 10.1016/j.aninu.2021.09.012
39. Wang, B, Wu, G, Zhou, Z, Dai, Z, Sun, Y, Ji, Y, et al. Glutamine and intestinal barrier function. Amino Acids. (2015a) 47:2143–54. doi: 10.1007/s00726-014-1773-4
40. Camilleri, M, Madsen, K, Spiller, R, Greenwood-Van Meerveld, B, and Verne, GN. Intestinal barrier function in health and gastrointestinal disease. Neurogastroenterol Motil. (2012) 24:503–12. doi: 10.1111/j.1365-2982.2012.01921.x
41. Stolfi, C, Maresca, C, Monteleone, G, and Laudisi, F. Implication of intestinal barrier dysfunction in gut dysbiosis and diseases. Biomedicine. (2022) 10:289. doi: 10.3390/biomedicines10020289
42. Wang, Y, Hong, C, Wu, Z, Li, S, Xia, Y, Liang, Y, et al. Resveratrol in intestinal health and disease: focusing on intestinal barrier. Front Nutr. (2022) 9:848400. doi: 10.3389/fnut.2022.848400
43. Di Tommaso, N, Gasbarrini, A, and Ponziani, FR. Intestinal barrier in human health and disease. Int J Environ Res Public Health. (2021) 18:12836. doi: 10.3390/ijerph182312836
44. Guo, S, Xing, Y, Xu, Y, Jin, X, Yan, S, and Shi, B. Progress of studies on plant-derived polysaccharides affecting intestinal barrier function in poultry. Animals. (2022) 12:3205. doi: 10.3390/ani12223205
45. He, W, Wang, Y, Wang, P, and Wang, F. Intestinal barrier dysfunction in severe burn injury. Burns Trauma. (2019) 7:24. doi: 10.1186/s41038-019-0162-3
46. Chelakkot, C, Ghim, J, and Ryu, SH. Mechanisms regulating intestinal barrier integrity and its pathological implications. Exp Mol Med. (2018) 50:1–9. doi: 10.1038/s12276-018-0126-x
47. Vereecke, L, Beyaert, R, and van Loo, G. Enterocyte death and intestinal barrier maintenance in homeostasis and disease. Trends Mol Med. (2011) 17:584–93. doi: 10.1016/j.molmed.2011.05.011
48. Hohman, LS, and Osborne, LC. A gut-centric view of aging: do intestinal epithelial cells contribute to age-associated microbiota changes, inflammaging, and immunosenescence? Aging Cell. (2022) 21:e13700. doi: 10.1111/acel.13700
49. Allaire, JM, Crowley, SM, Law, HT, Chang, SY, Ko, HJ, and Vallance, BA. The intestinal epithelium: central coordinator of mucosal immunity. Trends Immunol. (2018) 39:677–96. doi: 10.1016/j.it.2018.04.002
50. Menard, S, Lacroix-Lamande, S, Ehrhardt, K, Yan, J, Grassl, GA, and Wiedemann, A. Cross-talk between the intestinal epithelium and Salmonella Typhimurium. Front Microbiol. (2022) 13:906238. doi: 10.3389/fmicb.2022.906238
51. Chen, S, Zhang, C, He, B, He, R, Xu, L, and Zhang, S. The role of lncRNAs in regulating the intestinal mucosal mechanical barrier. Biomed Res Int. (2021b) 2021:2294942. doi: 10.1155/2021/2294942
52. Citi, S. Intestinal barriers protect against disease. Science. (2018) 359:1097–8. doi: 10.1126/science.aat0835
53. Gao, Y, Meng, L, Liu, H, Wang, J, and Zheng, N. The compromised intestinal barrier induced by mycotoxins. Toxins. (2020) 12:619. doi: 10.3390/toxins12100619
54. Xu, QR, Du, XH, Huang, TT, Zheng, YC, Li, YL, Huang, DY, et al. Role of cell-cell junctions in oesophageal squamous cell carcinoma. Biomol Ther. (2022) 12:1378. doi: 10.3390/biom12101378
55. Chen, L, Chu, H, Hu, L, Li, Z, Yang, L, and Hou, X. The role of NADPH oxidase 1 in alcohol-induced oxidative stress injury of intestinal epithelial cells. Cell Biol Toxicol. (2022a) 39:2345–64. doi: 10.1007/s10565-022-09725-1
56. Chen, X, Chen, C, and Fu, X. Hypoglycemic effect of the polysaccharides from Astragalus membranaceus on type 2 diabetic mice based on the "gut microbiota-mucosal barrier". Food Funct. (2022c) 13:10121–33. doi: 10.1039/d2fo02300h
57. Sharma, A, Lee, J, Fonseca, AG, Moshensky, A, Kothari, T, Sayed, IM, et al. E-cigarettes compromise the gut barrier and trigger inflammation. iScience. (2021) 24:102035. doi: 10.1016/j.isci.2021.102035
58. Toschi, A, Piva, A, and Grilli, E. Phenol-rich botanicals modulate oxidative stress and epithelial integrity in intestinal epithelial cells. Animals. (2022) 12:2188. doi: 10.3390/ani12172188
59. Dong, N, Li, X, Xue, C, Wang, C, Xu, X, Bi, C, et al. Astragalus polysaccharides attenuated inflammation and balanced the gut microflora in mice challenged with Salmonella typhimurium. Int Immunopharmacol. (2019) 74:105681. doi: 10.1016/j.intimp.2019.105681
60. Li, S, Wang, XF, Ren, LN, Li, JL, Zhu, XD, Xing, T, et al. Protective effects of gamma-irradiated Astragalus polysaccharides on intestinal development and mucosal immune function of immunosuppressed broilers. Poult Sci. (2019) 98:6400–10. doi: 10.3382/ps/pez478
61. Thursby, E, and Juge, N. Introduction to the human gut microbiota. Biochem J. (2017) 474:1823–36. doi: 10.1042/BCJ20160510
62. Zhang, R, Zhang, L, Li, P, Pang, K, Liu, H, and Tian, L. Epithelial barrier in the nasal mucosa, related risk factors and diseases. Int Arch Allergy Immunol. (2023) 184:481–501. doi: 10.1159/000528969
63. Zhao, H, He, M, Zhang, M, Sun, Q, Zeng, S, Chen, L, et al. Colorectal cancer, gut microbiota and traditional Chinese medicine: a systematic review. Am J Chin Med. (2021) 49:805–28. doi: 10.1142/s0192415x21500385
64. Putignani, L, Del Chierico, F, Petrucca, A, Vernocchi, P, and Dallapiccola, B. The human gut microbiota: a dynamic interplay with the host from birth to senescence settled during childhood. Pediatr Res. (2014) 76:2–10. doi: 10.1038/pr.2014.49
65. Theriot, CM, and Young, VB. Interactions between the gastrointestinal microbiome and Clostridium difficile. Ann Rev Microbiol. (2015) 69:445–61. doi: 10.1146/annurev-micro-091014-104115
66. Zhang, H, Xu, Z, Chen, W, Huang, F, Chen, S, Wang, X, et al. Algal oil alleviates antibiotic-induced intestinal inflammation by regulating gut microbiota and repairing intestinal barrier. Front Nutr. (2022a) 9:1081717. doi: 10.3389/fnut.2022.1081717
67. Fehily, SR, Basnayake, C, Wright, EK, and Kamm, MA. The gut microbiota and gut disease. Intern Med J. (2021) 51:1594–604. doi: 10.1111/imj.15520
68. Jandhyala, SM, Talukdar, R, Subramanyam, C, Vuyyuru, H, Sasikala, M, and Nageshwar Reddy, D. Role of the normal gut microbiota. World J Gastroenterol. (2015) 21:8787–803. doi: 10.3748/wjg.v21.i29.8787
69. Takiishi, T, Fenero, CIM, and Camara, NOS. Intestinal barrier and gut microbiota: shaping our immune responses throughout life. Tissue Barriers. (2017) 5:e1373208. doi: 10.1080/21688370.2017.1373208
70. Pontarollo, G, Kollar, B, Mann, A, Khuu, MP, Kiouptsi, K, Bayer, F, et al. Commensal bacteria weaken the intestinal barrier by suppressing epithelial neuropilin-1 and hedgehog signaling. Nat Metab. (2023) 5:1174–87. doi: 10.1038/s42255-023-00828-5
71. Shin, W, and Kim, HJ. Intestinal barrier dysfunction orchestrates the onset of inflammatory host-microbiome cross-talk in a human gut inflammation-on-a-chip. Proc Natl Acad Sci USA. (2018) 115:E10539–47. doi: 10.1073/pnas.1810819115
72. Sun, X, Cui, Y, Su, Y, Gao, Z, Diao, X, Li, J, et al. Dietary fiber ameliorates lipopolysaccharide-induced intestinal barrier function damage in piglets by modulation of intestinal microbiome. mSystems. (2021) 6:2379–5077. doi: 10.1128/mSystems.01374-20
73. Valdes, AM, Walter, J, Segal, E, and Spector, TD. Role of the gut microbiota in nutrition and health. BMJ. (2018) 361:k2179. doi: 10.1136/bmj.k2179
74. Qiao, Y, Liu, C, Guo, Y, Zhang, W, Guo, W, Oleksandr, K, et al. Polysaccharides derived from Astragalus membranaceus and Glycyrrhiza uralensis improve growth performance of broilers by enhancing intestinal health and modulating gut microbiota. Poult Sci. (2022) 101:101905. doi: 10.1016/j.psj.2022.101905
75. Su, M, Tang, T, Tang, W, Long, Y, Wang, L, and Liu, M. Astragalus improves intestinal barrier function and immunity by acting on intestinal microbiota to treat T2DM: a research review. Front Immunol. (2023) 14:1243834. doi: 10.3389/fimmu.2023.1243834
76. Li, SP, Zhao, XJ, and Wang, JY. Synergy of Astragalus polysaccharides and probiotics (Lactobacillus and Bacillus cereus) on immunity and intestinal microbiota in chicks. Poult Sci. (2009) 88:519–25. doi: 10.3382/ps.2008-00365
77. Liu, YS, Li, S, Wang, XF, Xing, T, Li, JL, Zhu, XD, et al. Microbiota populations and short-chain fatty acids production in cecum of immunosuppressed broilers consuming diets containing gamma-irradiated Astragalus polysaccharides. Poult Sci. (2021b) 100:273–82. doi: 10.1016/j.psj.2020.09.089
78. Liu, J, Kong, L, Shao, M, Sun, C, Li, C, Wang, Y, et al. Seabuckthorn polysaccharide combined with astragalus polysaccharide ameliorate alcoholic fatty liver by regulating intestinal flora. Front Endocrinol. (2022) 13:1018557. doi: 10.3389/fendo.2022.1018557
79. Shahab, RL, Brethauer, S, Davey, MP, Smith, AG, Vignolini, S, Luterbacher, JS, et al. A heterogeneous microbial consortium producing short-chain fatty acids from lignocellulose. Science. (2020) 369:eabb1214. doi: 10.1126/science.abb1214
80. Tang, C, Ding, R, Sun, J, Liu, J, Kan, J, and Jin, C. The impacts of natural polysaccharides on intestinal microbiota and immune responses—a review. Food Funct. (2019) 10:2290–312. doi: 10.1039/c8fo01946k
81. Yu, S, Sun, Y, Shao, X, Zhou, Y, Yu, Y, Kuai, X, et al. Leaky gut in IBD: intestinal barrier-gut microbiota interaction. J Microbiol Biotechnol. (2022a) 32:825–34. doi: 10.4014/jmb.2203.03022
82. Barbara, G, Barbaro, MR, Fuschi, D, Palombo, M, Falangone, F, Cremon, C, et al. Inflammatory and microbiota-related regulation of the intestinal epithelial barrier. Front Nutr. (2021) 8:718356. doi: 10.3389/fnut.2021.718356
83. Blaak, EE, Canfora, EE, Theis, S, Frost, G, Groen, AK, Mithieux, G, et al. Short chain fatty acids in human gut and metabolic health. Benefic Microbes. (2020) 11:411–55. doi: 10.3920/BM2020.0057
84. Deleu, S, Machiels, K, Raes, J, Verbeke, K, and Vermeire, S. Short chain fatty acids and its producing organisms: an overlooked therapy for IBD? EBioMedicine. (2021) 66:103293. doi: 10.1016/j.ebiom.2021.103293
85. Chen, SJ, Chen, CC, Liao, HY, Lin, YT, Wu, YW, Liou, JM, et al. Association of Fecal and Plasma Levels of short-chain fatty acids with gut microbiota and clinical severity in patients with Parkinson disease. Neurology. (2022b) 98:e848–58. doi: 10.1212/WNL.0000000000013225
86. Song, Q, Cheng, SW, Li, D, Cheng, H, Lai, YS, Han, Q, et al. Gut microbiota mediated hypoglycemic effect of Astragalus membranaceus polysaccharides in db/db mice. Front Pharmacol. (2022b) 13:1043527. doi: 10.3389/fphar.2022.1043527
87. Yang, CM, Han, QJ, Wang, KL, Xu, YL, Lan, JH, and Cao, GT. Astragalus and ginseng polysaccharides improve developmental, intestinal morphological, and immune functional characters of weaned piglets. Front Physiol. (2019) 10:418. doi: 10.3389/fphys.2019.00418
88. Song, B, Li, P, Yan, S, Liu, Y, Gao, M, Lv, H, et al. Effects of dietary Astragalus polysaccharide supplementation on the Th17/Treg balance and the gut microbiota of broiler chickens challenged with necrotic enteritis. Front Immunol. (2022a) 13:781934. doi: 10.3389/fimmu.2022.781934
89. Rivera, CA, and Lennon-Dumenil, AM. Gut immune cells and intestinal niche imprinting. Semin Cell Dev Biol. (2023) 150-151:50–7. doi: 10.1016/j.semcdb.2023.01.006
90. Zundler, S, Gunther, C, Kremer, AE, Zaiss, MM, Rothhammer, V, and Neurath, MF. Gut immune cell trafficking: inter-organ communication and immune-mediated inflammation. Nat Rev Gastroenterol Hepatol. (2023) 20:50–64. doi: 10.1038/s41575-022-00663-1
91. Chen, S, He, R, He, B, Xu, L, and Zhang, S. Potential roles of Exosomal lncRNAs in the intestinal mucosal immune barrier. J Immunol Res. (2021a) 2021:7183136. doi: 10.1155/2021/7183136
92. Beller, A, Kruglov, A, Durek, P, von Goetze, V, Werner, K, Heinz, GA, et al. Specific microbiota enhances intestinal IgA levels by inducing TGF-beta in T follicular helper cells of Peyer's patches in mice. Eur J Immunol. (2020) 50:783–94. doi: 10.1002/eji.201948474
93. Liu, N, Feng, G, Zhang, X, Hu, Q, Sun, S, Sun, J, et al. The functional role of lactoferrin in intestine mucosal immune system and inflammatory bowel disease. Front Nutr. (2021a) 8:759507. doi: 10.3389/fnut.2021.759507
94. Mantis, NJ, Rol, N, and Corthesy, B. Secretory IgA's complex roles in immunity and mucosal homeostasis in the gut. Mucosal Immunol. (2011) 4:603–11. doi: 10.1038/mi.2011.41
95. Alexander, DB, Iigo, M, Hamano, H, Kozu, T, Saito, Y, Saito, D, et al. An ancillary study of participants in a randomized, placebo-controlled trial suggests that ingestion of bovine lactoferrin promotes expression of interferon alpha in the human colon. J Funct Foods. (2014) 10:305–17. doi: 10.1016/j.jff.2014.06.028
96. Deng, F, Peng, L, Li, Z, Tan, G, Liang, E, Chen, S, et al. YAP triggers the Wnt/beta-catenin signalling pathway and promotes enterocyte self-renewal, regeneration and tumorigenesis after DSS-induced injury. Cell Death Dis. (2018) 9:153. doi: 10.1038/s41419-017-0244-8
97. Xu, Q, Cheng, W, Wei, J, Ou, Y, Xiao, X, and Jia, Y. Synergist for antitumor therapy: Astragalus polysaccharides acting on immune microenvironment. Discov Oncol. (2023) 14:179. doi: 10.1007/s12672-023-00798-w
98. Xue, X, and Falcon, DM. The role of immune cells and cytokines in intestinal wound healing. Int J Mol Sci. (2019) 20:6097. doi: 10.3390/ijms20236097
99. Yang, SB, Qin, YJ, Ma, X, Luan, WM, Sun, P, Ju, AQ, et al. Effects of in ovo injection of Astragalus polysaccharide on the intestinal development and mucosal immunity in broiler chickens. Front Vet Sci. (2021) 8:738816. doi: 10.3389/fvets.2021.738816
100. Xue, L, Zhang, Y, Wang, D, Luan, W, and Yang, S. Effect of in ovo administration of Newcastle disease vaccine conjugated with Astragalus polysaccharide on growth performance, intestinal development, and mucosal immunity in broiler chickens. J Anim Physiol Anim Nutr. (2022) 107:897–906. doi: 10.1111/jpn.13771
101. Shan, C, Sun, B, Dalloul, RA, Zhai, Z, Sun, P, Li, M, et al. Effect of the oral administration of astragalus polysaccharides on jejunum mucosal immunity in chickens vaccinated against Newcastle disease. Microb Pathog. (2019) 135:103621. doi: 10.1016/j.micpath.2019.103621
102. Wang, X, Li, Y, Shen, J, Wang, S, Yao, J, and Yang, X. Effect of Astragalus polysaccharide and its sulfated derivative on growth performance and immune condition of lipopolysaccharide-treated broilers. Int J Biol Macromol. (2015b) 76:188–94. doi: 10.1016/j.ijbiomac.2015.02.040
103. Jin, Q, Cheng, L, Zhu, Y, Zhao, X, Zhang, W, Gao, X, et al. Immune-related effects of compound astragalus polysaccharide and sulfated epimedium polysaccharide on newborn piglets. Anim Biotechnol. (2021) 34:508–19. doi: 10.1080/10495398.2021.1979022
104. Liao, L, Li, J, Li, J, Huang, Y, and Wu, Y. Effects of Astragalus polysaccharides on intestinal morphology and intestinal immune cells of Muscovy ducklings infected with Muscovy duck reovirus. Poult Sci. (2021) 100:64–72. doi: 10.1016/j.psj.2020.10.021
105. Wang, Q, Wang, XF, Xing, T, Li, JL, Zhu, XD, Zhang, L, et al. The combined impact of xylo-oligosaccharides and gamma-irradiated Astragalus polysaccharides on growth performance and intestinal mucosal barrier function of broilers. Poult Sci. (2021) 100:100909. doi: 10.1016/j.psj.2020.11.075
106. Ren, Q, Zhao, S, Ren, C, and Ma, Z. Astragalus polysaccharide alleviates LPS-induced inflammation injury by regulating miR-127 in H9c2 cardiomyoblasts. Int J Immunopathol Pharmacol. (2018) 32:2058–7384. doi: 10.1177/2058738418759180
107. Zhou, L, Liu, Z, Wang, Z, Yu, S, Long, T, Zhou, X, et al. Astragalus polysaccharides exerts immunomodulatory effects via TLR4-mediated MyD88-dependent signaling pathway in vitro and in vivo. Sci Rep. (2017) 7:44822. doi: 10.1038/srep44822
108. Fukata, M, Vamadevan, AS, and Abreu, MT. Toll-like receptors (TLRs) and nod-like receptors (NLRs) in inflammatory disorders. Semin Immunol. (2009) 21:242–53. doi: 10.1016/j.smim.2009.06.005
109. Tian, Z, Liu, Y, Yang, B, Zhang, J, He, H, Ge, H, et al. Astagalus polysaccharide attenuates murine colitis through inhibiton of the NLRP3 inflammasome. Planta Med. (2017) 83:70–7. doi: 10.1055/s-0042-108589
110. Huang, WM, Liang, YQ, Tang, LJ, Ding, Y, and Wang, XH. Antioxidant and anti-inflammatory effects of Astragalus polysaccharide on EA.hy926 cells. Exp Ther Med. (2013) 6:199–203. doi: 10.3892/etm.2013.1074
111. Yuan, LB, Hua, CY, Gao, S, Yin, YL, Dai, M, Meng, HY, et al. Astragalus polysaccharides attenuate monocrotaline-induced pulmonary arterial hypertension in rats. Am J Chin Med. (2017) 45:773–89. doi: 10.1142/S0192415X17500410
112. Khatri, V, and Kalyanasundaram, R. Therapeutic implications of inflammasome in inflammatory bowel disease. FASEB J. (2021) 35:e21439. doi: 10.1096/fj.202002622R
113. Zhang, S, Jin, W, Zhang, W, Ren, F, Wang, P, and Liu, N. Pea albumin attenuates dextran sulfate sodium-induced colitis by regulating NF-kappaB signaling and the intestinal microbiota in mice. Nutrients. (2022b) 14:3611. doi: 10.3390/nu14173611
114. Zhang, Y, Si, X, Yang, L, Wang, H, Sun, Y, and Liu, N. Association between intestinal microbiota and inflammatory bowel disease. Anim Model Exp Med. (2022c) 5:311–22. doi: 10.1002/ame2.12255
115. Marafini, I, Sedda, S, Dinallo, V, and Monteleone, G. Inflammatory cytokines: from discoveries to therapies in IBD. Expert Opin Biol Ther. (2019) 19:1207–17. doi: 10.1080/14712598.2019.1652267
116. Lv, J, Zhang, Y, Tian, Z, Liu, F, Shi, Y, Liu, Y, et al. Astragalus polysaccharides protect against dextran sulfate sodium-induced colitis by inhibiting NF-kappacapital VE, Cyrillic activation. Int J Biol Macromol. (2017) 98:723–9. doi: 10.1016/j.ijbiomac.2017.02.024
117. Wang, X, Li, Y, Yang, X, and Yao, J. Astragalus polysaccharide reduces inflammatory response by decreasing permeability of LPS-infected Caco2 cells. Int J Biol Macromol. (2013) 61:347–52. doi: 10.1016/j.ijbiomac.2013.07.013
118. Wu, J, Li, C, Bai, L, Wu, J, Bo, R, Ye, M, et al. Structural differences of polysaccharides from Astragalus before and after honey processing and their effects on colitis mice. Int J Biol Macromol. (2021) 182:815–24. doi: 10.1016/j.ijbiomac.2021.04.055
119. Liao, J, Li, C, Huang, J, Liu, W, Chen, H, Liao, S, et al. Structure characterization of honey-processed Astragalus polysaccharides and its anti-inflammatory activity in vitro. Molecules. (2018) 23:168. doi: 10.3390/molecules23010168
120. Ming, K, Zhuang, S, Ma, N, Nan, S, Li, Q, Ding, M, et al. Astragalus polysaccharides alleviates lipopolysaccharides-induced inflammatory lung injury by altering intestinal microbiota in mice. Front Microbiol. (2022) 13:1033875. doi: 10.3389/fmicb.2022.1033875
121. Zhu, C, Yao, J, Zhu, M, Zhu, C, Yuan, L, Li, Z, et al. A meta-analysis of Lactobacillus-based probiotics for growth performance and intestinal morphology in piglets. Front Vet Sci. (2022) 9:1045965. doi: 10.3389/fvets.2022.1045965
122. Allen, HK, Levine, UY, Looft, T, Bandrick, M, and Casey, TA. Treatment, promotion, commotion: antibiotic alternatives in food-producing animals. Trends Microbiol. (2013) 21:114–9. doi: 10.1016/j.tim.2012.11.001
123. Menchicchi, B, Hensel, A, and Goycoolea, FM. Polysaccharides as bacterial antiadhesive agents and "smart" constituents for improved drug delivery systems against Helicobacter pylori infection. Curr Pharm Des. (2015) 21:4888–906. doi: 10.2174/1381612821666150820104028
124. Rocha, GA, and Ferreira, RB. Antimicrobial polysaccharides obtained from natural sources. Future Microbiol. (2022) 17:701–16. doi: 10.2217/fmb-2021-0257
125. Wang, K, Zhang, H, Han, Q, Lan, J, Chen, G, Cao, G, et al. Effects of astragalus and ginseng polysaccharides on growth performance, immune function and intestinal barrier in weaned piglets challenged with lipopolysaccharide. J Anim Physiol Anim Nutr. (2020) 104:1096–105. doi: 10.1111/jpn.13244
126. Yin, FG, Liu, YL, Yin, YL, Kong, XF, Huang, RL, Li, TJ, et al. Dietary supplementation with Astragalus polysaccharide enhances ileal digestibilities and serum concentrations of amino acids in early weaned piglets. Amino Acids. (2009) 37:263–70. doi: 10.1007/s00726-008-0142-6
127. Duan, AY, Ju, AQ, Zhang, YN, Qin, YJ, Xue, LG, Ma, X, et al. The effects of in Ovo injection of Synbiotics on the early growth performance and intestinal health of chicks. Front Vet Sci. (2021) 8:658301. doi: 10.3389/fvets.2021.658301
128. Ma, D, Wu, T, Qu, Y, Yang, J, Cai, L, Li, X, et al. Astragalus polysaccharide prevents heart failure-induced cachexia by alleviating excessive adipose expenditure in white and brown adipose tissue. Lipids Health Dis. (2023) 22:9. doi: 10.1186/s12944-022-01770-3
129. El-Fakhrany, HH, Ibrahim, ZA, Ashour, EA, Osman, A, and Alagawany, M. Effects of in ovo injection of Astragalus kahericus polysaccharide on early growth, carcass weights and blood metabolites in broiler chickens. Anim Biotechnol. (2022) 33:1639–45. doi: 10.1080/10495398.2021.1924763
130. Ma, Y, Liu, C, Qu, D, Chen, Y, Huang, M, and Liu, Y. Antibacterial evaluation of sliver nanoparticles synthesized by polysaccharides from Astragalus membranaceus roots. Biomed Pharmacother. (2017) 89:351–7. doi: 10.1016/j.biopha.2017.02.009
131. Wu, S. Effect of dietary Astragalus membranaceus polysaccharide on the growth performance and immunity of juvenile broilers. Poult Sci. (2018) 97:3489–93. doi: 10.3382/ps/pey220
132. Li, Y, Lei, X, Guo, W, Wu, S, Duan, Y, Yang, X, et al. Transgenerational endotoxin tolerance-like effect caused by paternal dietary Astragalus polysaccharides in broilers' jejunum. Int J Biol Macromol. (2018b) 111:769–79. doi: 10.1016/j.ijbiomac.2018.01.095
133. Yu, W, Yang, Y, Zhou, Q, Huang, X, Huang, Z, Li, T, et al. Effects of dietary Astragalus polysaccharides on growth, health and resistance to Vibrio harveyi of Lates calcarifer. Int J Biol Macromol. (2022b) 207:850–8. doi: 10.1016/j.ijbiomac.2022.03.176
134. Sun, Y, Wang, X, Zhou, H, Mai, K, and He, G. Dietary Astragalus polysaccharides ameliorates the growth performance, antioxidant capacity and immune responses in turbot (Scophthalmus maximus L.). Fish Shellfish Immunol. (2020) 99:603–8. doi: 10.1016/j.fsi.2020.02.056
135. Wu, S. Dietary Astragalus membranaceus polysaccharide ameliorates the growth performance and innate immunity of juvenile crucian carp (Carassius auratus). Int J Biol Macromol. (2020) 149:877–81. doi: 10.1016/j.ijbiomac.2020.02.005
136. Pu, Y, and Wu, S. The growth performance, body composition and nonspecific immunity of white shrimps (Litopenaeus vannamei) affected by dietary Astragalus membranaceus polysaccharide. Int J Biol Macromol. (2022) 209:162–5. doi: 10.1016/j.ijbiomac.2022.04.010
137. Li, Y, Ran, C, Wei, K, Xie, Y, Xie, M, Zhou, W, et al. The effect of Astragalus polysaccharide on growth, gut and liver health, and anti-viral immunity of zebrafish. Aquaculture. (2021) 540:736677. doi: 10.1016/j.aquaculture.2021.736677
Glossary
Keywords: anti-inflammation, Astragalus polysaccharide, intestinal barrier, microbiota, short-chain fatty acids
Citation: Liang H, Tao S, Wang Y, Zhao J, Yan C, Wu Y, Liu N and Qin Y (2024) Astragalus polysaccharide: implication for intestinal barrier, anti-inflammation, and animal production. Front. Nutr. 11:1364739. doi: 10.3389/fnut.2024.1364739
Edited by:
Julio Plaza-Diaz, Children's Hospital of Eastern Ontario (CHEO), CanadaReviewed by:
Youyou Lu, Huazhong Agricultural University, ChinaKit Leong Cheong, Guangdong Ocean University, China
Copyright © 2024 Liang, Tao, Wang, Zhao, Yan, Wu, Liu and Qin. This is an open-access article distributed under the terms of the Creative Commons Attribution License (CC BY). The use, distribution or reproduction in other forums is permitted, provided the original author(s) and the copyright owner(s) are credited and that the original publication in this journal is cited, in accordance with accepted academic practice. No use, distribution or reproduction is permitted which does not comply with these terms.
*Correspondence: Ning Liu, ningliu007@hotmail.com; Yinghe Qin, qinyinghe@cau.edu.cn
†These authors have contributed equally to this work