- College of Life Science and Technology, Xinjiang University, Urumqi, China
This study employed mixed bacterial strains to ferment seabuckthorn seed meal into peptides, and conducted a comprehensive evaluation of the growth adaptive conditions, molecular weight distribution, volatile compounds, and in vitro hypoglycemic activity required for fermentation. Results showed that when the amount of maltose was 1.1% and MgSO4·7H2O was added at 0.15 g/L, the peptide yield reached 43.85% with a mixed fermentation of Lactobacillus fermentum, Bacillus subtilis, Lactobacillus casei, Lactobacillus rhamnosus, and Lactobacillus acidophilus. Components with a molecular weight below 1 kDa were found to be more effective in inhibiting the activity of α-amylase and α-glucosidase, with the identified sequence being FYLPKM. Finally, SPME/GC–MS results showed that 86 volatile components were detected during the fermentation of seabuckthorn seed meal, including 22 alcohols, 9 acids, 7 ketones, 14 alkanes, 20 esters, and 14 other compounds. With prolonged fermentation time, the content of acids and esters increased significantly.
1 Introduction
Sea buckthorn (Hippophae rhamnoides L.) is a small deciduous tree or shrub in the family Elaeagnaceae, also known as the vinegar tree fruit. It is rich in nutritional value and is used as a medicinal and edible plant (1). The stem, leaves, berries, and seeds of sea buckthorn all contain abundant vitamins, fatty acids, amino acids, flavonoids, superoxide, and other bioactive substances. These bioactive substances have significant effects such as lowering blood pressure, anti-inflammatory, anti-diabetic, and preventing cardiovascular diseases (2). Sea buckthorn seed meal is a by-product of extracting oil from sea buckthorn seeds. It has a protein content of over 35% and a complete range of amino acids, making it an important source of complete protein (3). However, sea buckthorn seed meal is usually used as animal feed or directly discarded, leading to serious waste of protein resources.
Bioactive peptides are generally released from proteins through proteolysis or fermentation, and they have broad biological activities and pharmacological properties, including anti-tumor activity, improvement of memory, and regulation of metabolic disorders (4). Therefore, they have broad application prospects in health products, food preservation, and pharmaceutical engineering (5). From the perspective of sustainable development and utilization of biological resources, the protein resources in sea buckthorn seed meal have enormous potential in the production of bioactive peptides.
Currently, the main methods for preparing bioactive peptides include enzymatic hydrolysis, microbial fermentation, chemical hydrolysis, and genetic engineering (6). Most peptides are prepared by enzymatic hydrolysis, and there is limited research on the direct fermentation for peptide production. Enzymatic hydrolysis has strong specificity and high extraction rate, but it is costly and not suitable for large-scale production. However, microbial fermentation can effectively address this issue. Fermentation has low costs, can convert substrates into beneficial bioactive substances, and allows for the production of unique products (7). Research have shown that Lactobacillus thermophilus can be used for fermentative production of whey protein peptides, which exhibit high ACE inhibition and antioxidant activity (8). Bacillus fermentation of coffee grounds can increase the content of protein hydrolysates, leading to a significant increase in soluble protein and small peptide content (9). Lactobacillus plantarum fermentation of almonds can degrade the molecular weight of almond proteins, altering the external morphology of the proteins and impacting their immunoreactivity (10). Additionally, microbial fermentation not only preserves nutritional components but also generates new volatile compounds through microbial metabolism, enhancing the aroma of the product (11). Different strains have different effects on the bioactivity and flavor of the fermentation substrate. For example, Lactococcus lactis can better utilize carbon sources for proliferation, increase the production of organic acids, and effectively improve the bitterness and astringency of sea buckthorn juice (12). Lactobacillus plantarum can synthesize extracellular polysaccharides with blood glucose-lowering activity through its glycoside hydrolase activity (13). Furthermore, compared to single-strain fermentation, mixed-strain fermentation can not only increase the variety and content of volatile flavor compounds but also enhance the antioxidant activity of the fermentation substrate (14). However, bioactive peptides as a functional food have not been well commercialized yet. Therefore, the fermentation-based preparation of sea buckthorn seed meal peptides and exploration of changes in volatile compounds and in vitro hypoglycemic activity in fermented peptide solutions are of significant importance for the reutilization of sea buckthorn seed meal and its future food applications. This study employed a mixed-strain fermentation method and screened out 5 strains with the best fermentation effect using peptide yield to degrade protein from sea buckthorn seed meal. The peptide molecular weight and amino acid content of samples at different fermentation times were detected and analyzed to determine the optimal conditions for fermentation peptide preparation. Then, we investigated the inhibitory activity of components with different molecular weights on α-amylase and α-glucosidase, and sequenced the best components. Finally, HS-SPME-GC–MS was used to detect the dynamic changes in volatile compounds during the fermentation process of sea buckthorn seed meal, providing a standard for its edibility, contributing to reducing waste of sea buckthorn protein resources and increasing the added value of the products.
2 Materials and methods
2.1 Materials and instruments
The sea buckthorn seed meal (protein: 45%) was obtained from Xinjiang, China, and all chemicals had analytical purity or better. The water bath and incubator were purchased from Beijing Yong Guangming Medical Instrument Co. (Beijing, China) and Shanghai Qixin Scientific Instrument Co. (Shanghai, China), respectively. The UV–Vis spectrophotometer and electrophoresis instrument were obtained from Mpoda Instruments Ltd. (Shanghai, China) and Beijing Liuyi Instrument Factory (Beijing, China), respectively. The enzyme labeler and centrifuge were purchased from Molecular Devices (California, United States) and Jiangsu Jinyi Instrument Technology Co. (Jiangsu, China), respectively. The microscope was from Motic (Fujian, China). Amylase (3,800 U/g) and lipase (100,000 U/g) were obtained from Beijing Aobo Star Biotechnology Co. (Beijing, China) and Shandong Longke Keto Enzyme Preparation Co. (Shandong, China), respectively. Ten species of commercial strains Lactobacillus delbrueckii subsp. Bulgaricus CICC6098 (L. bulgaricus), Bifidobacterium animalis subsp. lactis CICC21712 (B. lactis), Lacticaseibacillus paracasei CICC6237 (L. paracasei), Lactococcus lactis subsp. Lactis CICC23196 (L. lactis), Lactobacillus acidophilus CICC6086 (L. acidophilus), Limosilactobacillus fermentum CICC25124 (L. fermentum), Limosilactobacillus reuteri CICC6123 (L. reuteri), Lacticaseibacillus case CICC6114 (L. case), Lactipalntibacillus plantarum CICC25125 (L. plantarum), Bacillus subtilis CICC24713 (B. subtilis) were provided by the China Industrial Microbial Strain Preservation and Management Centre (Beijing, China).
2.2 Selection of culture media
The sea buckthorn seed meal had a material-to-water ratio of 1:34 (W/V). The bacterial density was used as an indicator. The activated 1 × 107 CFU/mL L. casei was inoculated in the aqueous solution of the sea buckthorn seed meal, followed by the addition of 2% glucose, maltose, sucrose, lactose, and starch as carbon sources to the medium for the one-factor experiments on the basis of MRS to determine the carbon source, and 0.5, 0.8, 1.1, 1.4, 1.7, and 2% of the carbon sources for the one-factor experiments. Factorial experiment. Based on the previous step, different concentrations of FeSO4·7H2O, ZnSO4·7H2O, CuSO4·5H2O, MgSO4·H2O and NaCl trace elements were added to determine the fermentation conditions of the sea buckthorn seed meal in one-way experiments.
2.3 Preparation of the fermented peptide
The aqueous solution of the sea buckthorn seed meal was pre-cooked with water for 30 min at 85°C and cooled. Based on the weight of the sea buckthorn seed meal powder, the reaction was terminated by adding 0.2% α-amylase and 0.4% lipase for 5 h in a water bath at 50°C. The reaction was then inactivated at 85°C for 30 min. After inactivation of the reaction, the sea buckthorn seed meal solution was cooled, and based on an initial bacterial density of 1 × 107 CFU/mL, 10 fermentation strains were separately added to the seed meal solution. Following inoculation, the aqueous solution of the sea buckthorn seed meal was fermented statically at 37°C. The peptide content was detected every 4 h, and the fermentation was terminated when the peptide content was stable.
2.4 Measurement of the peptide content
According to the method of Yan et al. (15), 1 mL sample solution was collected. Then, 1 mL of 15% (W/W) trichloroacetic acid (TCA) aqueous solution was added to the solution, mixed, left to stand for 8 min, and centrifuged at 4,000 r/min for 10 min. Subsequently, 1 mL of the supernatant was collected and 4 mL of bis(ureido)urea reagent was added to the supernatant. Similarly, the solution was mixed and left to stand for 25 min. At the same time, l mL of water and 4 mL of bis(ureido)urea reagent were mixed as a blank. Absorbance values were determined at 540 nm. The peptide concentration C (mg/mL) in the hydrolysate was calculated using a standard regression equation, and the peptide yield was the percentage of soluble peptides obtained through fermentation in the total protein.
2.5 Determination of protein hydrolysis
Following the determination of the fermentation time for each strain, the time point with the highest peptide yield was selected, and the fermentation residue was dried. And the nitrogen content was detected using the Kjeldahl method GB5009.5-2016. The protein conversion coefficient was 6.25, and the hydrolysis degree of the sea buckthorn seed meal fermented by different strains was calculated as follows:
Note: N1 is nitrogen in the fermentation residue, g; N is total nitrogen in the feedstock, g.
2.6 Unified design to determine the proportion of mixed bacteria
Based on the experimental results of the peptide content and hydrolysis degree, 5 dominant strains were selected from among the 10 fermentation strains for mixed fermentation. The optimization experiments were designed using a uniform design table with 5 dominant strains, 10 factors as levels, 19 h as fermentation time and peptide yield as the response value. Quadratic polynomial stepwise regression analyses were conducted using SPSS 20.0 software to obtain quadratic polynomial regression equations. The programmed solver function in Excel was then used to solve the problem and determine the optimal fermentation inoculation ratio.
2.7 Sodium dodecyl sulfate-polyacrylamide gel electrophoresis
We then conducted SDS-PAGE analyses of sea buckthorn seed meal solutions fermented at different times by mixed bacteria and the optimal fermentation time of the samples was preliminarily determined using the electrophoretic conditions of Fan et al. (16).
2.8 Molecular weight distribution
The main operating parameters were as follows:
Column: TSKgel 2000 SWXL 300 mm × 7.8 mm; mobile phase: acetonitrile/water/trifluoroacetic acid, 45/55/0.1 (V/V); detection: UV 220 nm; flow rate: 0.5 mL/min; column temperature: 30°C.
Sample preparation: 100 mg of the sample was placed in a 10-mL volumetric flask, diluted proportionally with the mobile phase, and filtered using a 0.45-μm microfilm.
Sample solutions with different fermentation times were analyzed under the aforementioned chromatographic conditions, and the data were processed using GPC software to obtain the peptide phases of the samples, and paired molecular mass distributions and their distribution ranges.
2.9 Detection of amino acid content
First, 2 mL of each sample was added to a 10-mL glass tube. Then, 2 mL of concentrated hydrochloric acid (containing 1% phenol) was added to the tube, followed by the addition of nitrogen for 1 min. The bottle was sealed, and hydrolysis was allowed to occur at 110°C for 22 h. The solution was removed, cooled, and diluted with water to 50 mL. Then, 1 mL of the solution was blown dry at 95°C under a nitrogen atmosphere, and 1 mL of 0.01 M HCl was accurately added to dissolve the mixture and measured over a membrane.
Mobile phase A: 40 mM sodium dihydrogen phosphate (pH 7.8); mobile phase B: acetonitrile/methanol/water = 45/45/10.
An Agilent transparent ODS column (5 μL, 4.0 mm × 250 mm) was used to detect the amino acid content. The gradient elution was performed using the following elution program: 0 min 0% B; 23 min 57% B; 27 min 100% B; 40 min 0% B. The flow rate of the mobile phase was 1.0 mL/min, and detection was performed using an ultraviolet detector (VWD). The wavelength: 338 nm, proline: 266 nm. The amino acid content was quantified using the external standard method.
2.10 Isolation of peptides from the sea buckthorn seed meal
The samples were initially purified using macroporous resin DA201-C, and the fermentation broth was ultrafiltered using ultrafiltration centrifuge tubes with cut-off values of 1 and 3 kDa, respectively, to obtain peptides of 0–1, 1–3, and >3 kDa. These peptides were then lyophilized and stored at −80°C until use.
2.11 Detection of α-glucosidase and α-amylase inhibition rates
According to the method of Sadeghi et al. (17), with modification, 30 μL of 5 mg/mL sample solutions of different molecular weights were taken and mixed with 30 μL of α-glucosidase (1 U, dissolved in 0.1 mol/L PBS, pH 6.8). Then, 120 μL of phosphate buffer was added, and the reaction mixture was incubated in a 96-well plate for 25 min at 37°C. Subsequently, 60 μL of pNPG solution was added. The reaction was terminated by adding 70 μL of sodium carbonate solution (0.8 mol/L). The absorbance of the sample was measured at 405 nm. Acarbose was used as a positive control, and an equal volume of phosphate buffer (0.1 mol/L, pH 6.8) was used as a control instead of α-glucosidase solution.
Note: A1: absorbance of sample; A2: absorbance of sample control; A3: absorbance of the blank group; A4: absorbance of the blank control.
Referring to and modifying the method of Huang et al. (18). 10 μL of α-amylase solution (10 U/mL) was mixed with 10 μL of sample solution of different molecular sizes. The mixture was incubated at 37°C for 10 min in a water bath, followed by the addition of 500 μL of 0.9% soluble starch solution. The mixture was then incubated at 37°C for 15 min, and 500 μL of DNS reagent was added and placed in a boiling water bath for 8 min to stop the reaction. After cooling, 4 mL of distilled water was added for dilution. Then, 250 μL was transferred to a 96-well plate, and the absorbance was measured at a wavelength of 540 nm. Acarbose was used as a positive control, and the α-amylase enzyme inhibition rate was calculated according to Formula (1).
2.12 Sequence identification of peptides
According to the method of Heymich et al. (19), with modifications, the amino acid sequences of the peptides were determined using LC–MS (Thermo Fisher Scientific, United States). A C18 (Acclaim PepMap RSLC, 75 μm × 25 cm, 2 μm, 100 A) reverse-phase chromatography column was used, with the mobile phase B increasing from 5 to 38% within 30 min. Mass spectrometry was performed using the ThermoFisher Q Exactive system (ThermoFisher, United States) combined with a nanospray Nano Flex ion source (ThermoFisher, USA), with a voltage of 1.9 kV, a heating temperature of 275°C, a scan range of 100–1,500 m/z, and a collision energy set at 28 eV. Data processing and analysis were conducted using PEAKS Studio 8.5 software (Bioinformatics Solutions Inc., Waterloo, Canada). The biological activity of the peptides was predicted using the online analysis tool.1 Additionally, the online analysis tool2 was used to analyze the predicted toxicity, hydrophilicity, and hydrophobicity of the peptides.
2.13 Analysis of volatile compounds
Referring to and modifying the method of Shi et al. (20). The internal standard was decanol diluted 1,000 times, and samples at different fermentation times were processed. Thermo Fisher Scientific (Waltham, Massachusetts, United States) GC–MS instrument was used to detect volatile compounds in sea buckthorn seed meal. Helium was used as the carrier gas with a flow rate of 1 mL/min, an initial temperature of 40°C, and a hold time of 2 min. The temperature was then increased by 3°C/min to 150°C and held for 4 min, followed by an increase of 10°C/min to 230°C and held for 4 min. The volatile compounds were separated on an HP-5MS chromatographic column (30 m × 250 m × 0.25 um). The ion source temperature was 230°C, and the electron energy was 70 ev. Data were collected at a scan rate of 1/scan within the range of 40–500 m/z.
2.14 Statistical analysis
All results are presented as mean ± standard deviation (S.D.). Statistical significance (p < 0.05; Duncan test) was determined using SPSS 20.0 software (SPSS Science, Chicago, Michigan, United States). Graphs were created using Origin 2021 software (OriginLab, Northampton, MA, United States).
3 Results and discussion
3.1 Colony density
The protein content is relatively high in seabuckthorn seed meal, and microbial growth and metabolism are slow. Therefore, some nutrients need to be supplemented to promote microbial growth. Carbon is a nutritional factor necessary for microbial growth and reproduction. It can regulate the growth and metabolism of microbes and their metabolic properties. It has a direct impact on the fermentation process (21). Among the 5 carbon sources, maltose exerted the most significant effect on the growth and reproduction of L. case (Figure 1A). After the addition of different maltose concentrations, the colony density first increased gradually and reached the maximum amount of maltose added was 1.1% (Figure 1B). Then, the colony density exhibited a decreasing trend. Therefore, the optimal amount of the carbon source was 1.1%.
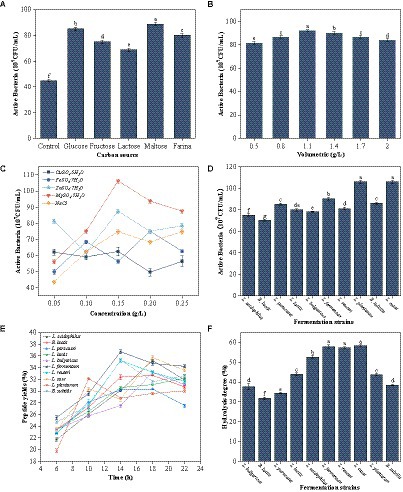
Figure 1. Screening of fermentation strains. (A) Effect of different types of carbon sources on viable counts of L. casei. (B) Effect of different mass concentrations of maltose on viable counts of L. casei. (C) Effect of different mass concentrations of Cu2+, Fe2+, Zn2+, Mg2+ and Nacl on viable counts of L. casei. (D) Colony densities of different strains of bacteria after media optimisation. (E) Changes in peptide yield (%) during fermentation of sea buckthorn seed meal by different strains. (F) Results of hydrolysis degree (%) in fermentation residues of different strains (these values are expressed as mean of three replicates ± standard deviation (SD), lower case letters represent significance, p < 0.05).
Trace elements promote microbial growth, enhance probiotics activity, change their physicochemical properties, and are a constituent of certain active substances (22). The promoting effect of adding NaCl, Cu2+, Zn2+, and Fe2+ on L. case growth and reproduction was not obvious (Figure 1C). By contrast, the promoting effect of MgSO4·7H2O addition on L. case growth was obvious. The colony density reached the highest when the amount of trace elements (e.g., MgSO4·7H2O) added was 0.15 g/L (Figure 1C) Therefore, the optimal concentration of trace elements to be added was 0.15 g/L.
Additionally, under the same conditions, the colony density of nine other fermentation strains was measured (Figure 1D). It was observed that after the addition of carbon sources and trace elements, the colony density of these 9 strains was all above 6.5 × 108 CFU/mL, indicating that their growth and reproduction were promoted to varying degrees under these conditions.
3.2 Determination of peptide content
Different fermentation strains had different fermentation effects (Figure 1E). L. fermentum had the best fermentation effect among the 10 strains, with the highest polypeptide production rate being 36.75% ± 0.1%. L. paracasei had the worst fermentation effect, with the polypeptide production rate being 30.34% ± 0.03%. Most strains exhibited a trend of increase in the polypeptide production rate and then a decrease in the rate with an increase in fermentation time. The fermentation trend of L. casei, L. plantarum, and B. subtilis fluctuated, exhibiting an increase, then a decrease, followed by a slow increase (Figure 1E). However, the polypeptide production rate did not exceed the peak value in the subsequent fermentation time. The rate of peptide production from L. lactis exhibited a trend of slow increase, but the peptide yield was low. The peptide yield of the sea buckthorn seed meal solution fermented by L. fermentum, L. reuteri, and B. subtilis reached a peak at 14 h. A flat or decreasing trend was noted after the peak was attained. By contrast, the polypeptide production rate of the sea buckthorn seed meal solution fermented by L. acidophilus, B. lactis, L. paracasei, L. bulgaricus, and L. casei increased gradually as the fermentation time was prolonged, and reached the peak value at 18 h. The polypeptide production rate gradually decreased after 18 h, possibly because different strains of microorganisms metabolize and secrete different proteases, which act on the different cleavage sites of the proteins present in the sea buckthorn seed meal, producing peptide fragments with different molecular weights and sizes (23). Thus, the optimal fermentation times are different for different strains and would produce the highest peptide yield. By analyzing the data, we noted that L. fermentum, L. casei, and L. acidophilus produced the best polypeptide yield. The optimal fermentation time was between 14 and 19 h.
3.3 Measurement of protein hydrolysis
The fermentation residue at the highest peptide yield during fermentation by the 10 strains was used to determine nitrogen content and thus verify the hydrolysis degree of the sea buckthorn seed meal protein during fermentation. The hydrolysis degree of the sea buckthorn seed meal fermentation broths was >30% in all cases (Figure 1F). Ma et al. (24) performed microbial fermentation of chickpea proteins and examined their nitrogen content after fermentation. The hydrolysis degree was >60% in all cases. This phenomenon may be a result of the high protein hydrolysis capacity of lactic acid bacteria, which have a wide range of adaptability to various environments, inducing secondary protein hydrolysis and enriching them with bioactive peptides. Hou et al., revealed that fermentation can increase the degree of protein hydrolysis, and fermented proteins have better bioactivity (25). Based on the peptide yield and the hydrolysis degree, 5 dominant strains were screened, namely L. fermentum, B. subtilis, L. casei, L. reuteri, and L. acidophilus.
3.4 Homogeneous design determines the proportion of strains
Mixed fermentation has better growth dynamic characteristics, which can improve the quality of the fermented material and reduce the off-flavor (26). According to Peng et al. (27) mixed fermentation can enrich the taste of soymilk and improve the flavor characteristics of soymilk. The proteins in soymilk can be quickly and effectively hydrolyzed into peptides and amino acids to improve their nutritional value. Therefore, the uniform design experiment was used in this study and the results are presented in Table 1. The peptide production rate was used as the response value and the fermentation time was 19 h to determine the ratio between different bacterial strains. A quadratic polynomial stepwise regression equation was established as follows:
The regression equation was R2 = 0.99. The final optimal mix of bacteria was noted to be L. fermentum 25.53%, B. subtilis 28.36%, L. casei 21.27%, L. reuteri 17.73%, and L. acidophilus 7.11%. The predicted peptide yield at this point was 47.44%. A validation test conducted based on the predicted optimal conditions provided a peptide yield of 43.85% after the fermentation of the sea buckthorn seed meal. This indicates that the equation can predict the optimal ratio well, which confirms the validity of the model.
3.5 SDS-PAGE
The peptide solution of the sea buckthorn seed meal fermented for different fermentation times was analyzed through SDS-PAGE. The mixed strain fermentation is favorable for the degradation of large-molecular-weight proteins (Figure 2A). The mixed strains can degrade large-molecular-weight proteins into small-molecule peptides and increase the peptide yield. This result is in agreement with Wang et al. (28) who used mixed strains of bacteria to ferment soybean meal to improve its nutritional value. The molecular weight of most proteins in the raw material was distributed in the range of 15 and 130 kDa (Figure 2A). As the fermentation time was prolonged, the large molecules of proteins in the fermentation broth were gradually degraded to <10 kDa. At 10–16 h of fermentation, lactic acid bacteria degraded the macromolecular proteins in the raw material to <15 kDa. However, two shallow bands were still seen above and below 25 and 35 kDa, respectively, indicating that the macromolecular proteins were not completely degraded. After 18–24 h of fermentation, at >10 kDa, the bands were no longer seen on the electrophoresis graph. This indicates that the large molecules of protein were degraded. The bands at approximately 10 kDa were also relatively shallow, which indicated that the mixed strain fermentation had a good decomposition effect on the sea buckthorn seed meal protein. We preliminarily concluded that from 18 h onwards, the macromolecular proteins in the raw material were almost completely degraded to <10 kDa. Thus, the optimal fermentation time of sea buckthorn seed meal peptides was further analyzed.
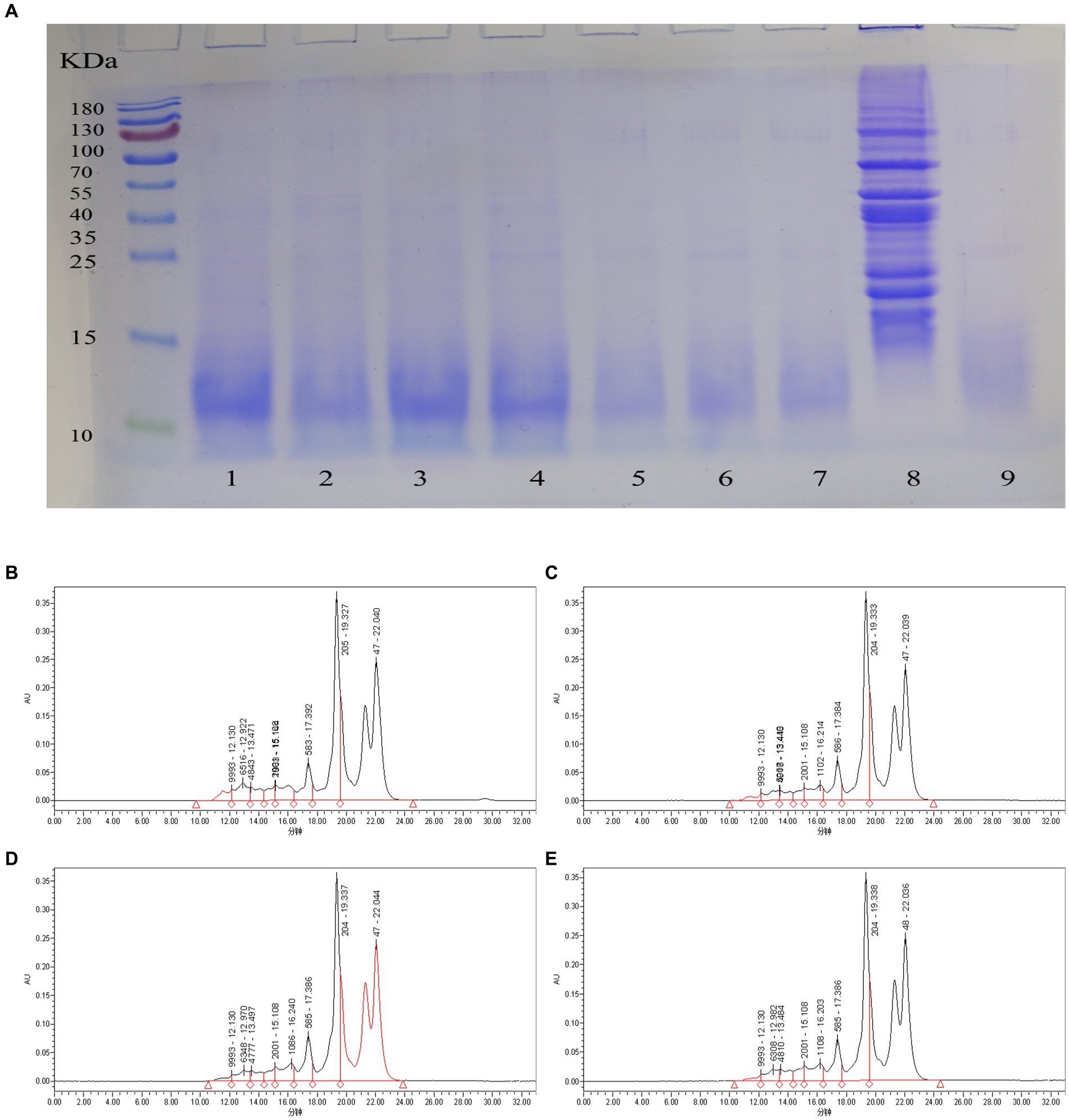
Figure 2. Degree of protein degradation of sea buckthorn seed meal at different times. (A) SDS-PAGE of different fermentation times. 1: 10 h, 2: 12 h, 3: 14 h, 4: 16, 5: 18 h, 6: 20 h, 7: 22 h, 8: Raw material, 9: 24 h; (B) Molecular weight distribution of peptides at 17 h; (C) Molecular weight distribution of peptides at 18 h; (D) Molecular weight distribution of peptides at 19 h; (E) Molecular weight distribution of peptides at 20 h.
3.6 Molecular weight distribution
The molecular weight distributions of sea buckthorn seed meal peptides at different fermentation times were relatively similar (Table 2). Most of them were distributed in the <1 kDa range. The >10 kDa fraction was degraded to 2.3% at 17 h (Figure 2B), and as the fermentation time was prolonged, the fraction was reduced to 0.91% at 20 h (Figure 2E) This indicates that large-molecule proteins are gradually degraded into small-molecule peptides during fermentation. The 3–10 kDa fraction was decreased to 5.26% at 18 h (Figure 2C), and then, a small recovery to 5.97% was noted at 20 h. The 1–3 kDa fraction decreased to 6.7% at 18 h, and then gradually increased to 7.43% at 20 h, which may be the result of a decrease in the 1–10 kDa fraction to 7.43%. This result was possibly observed because, with the extension of fermentation time, the mixed strains continued to degrade proteins >10 kDa and those in the 3–10 kDa fraction. This increased the content of proteins <3 kDa to more than 90% (Figure 2D). The <1 kDa fraction increased to a maximum value of 86.82% at 18 h, followed by a small decrease to 85.69% by 20 h. This indicates that fermentation had successfully degraded most large proteins into small peptides or amino acids. According to studies, peptides with small molecules are more chemically stable and have better biological activities (29).
3.7 Amino acids content
The amino acid content exhibited a trend of increase and then decrease with fermentation. The highest total amino acid content was 1.963 mg/mL at 19 h, and a small decrease was observed at 20 h. The decrease was observed possibly because lactic acid bacteria require nutritional factors, such as amino acids, minerals, and vitamins, in a nutrient-rich environment to grow and reproduce (30). Essential amino acids and hydrophobic amino acids reached maximum values of 0.533 and 0.334 mg/mL, respectively, at this time. Among the types of amino acids obtained from the sea buckthorn seed meal, the contents of Glu and Arg were relatively high, with their contents being >0.2 mg/mL. The content of Asp., Ser, and Lys was approximately 0.1 mg/mL. Glu contributes to the synthesis of erythrocytes, proteins, and other amino acids in humans. It can help activate the mind and improve the nutritional environment of brain cells (31). Arg is one of the medicinal amino acid compositions. It has various roles. In addition to being a component of protein synthesis, it can also promote hormone secretion and insulin production and enhance cellular immune function (32). Asp can promote the synthesis of urea from oxygen and carbon dioxide, delay bone damage, eliminate fatigue, protect the liver, and play a role in the lungs to adjust the respiratory effect. Different types of amino acids have different biological effects (33). The presence of these valuable amino acids is one of the crucial reasons for the high value attached to the sea buckthorn seed meal peptide. Thus, 19 h is the best fermentation time for the sea buckthorn seed meal peptide (Table 3).
3.8 Detection of inhibition rates of different molecular weight fractions and amino acid sequencing
In vitro hypoglycemic activity of the sea buckthorn seed peptides of <1 kDa, 1–3 kDa, and >3 kDa fractions was detected through ultrafiltration. At 5 mg/mL, the <1 kDa fraction of the sea buckthorn seed meal peptide exhibited the best inhibitory activity against α-glucosidase and α-amylase, which were 49.87% ± 0.37 and 55.56% ± 0.51%, respectively (Figures 3A,B), followed by the peptides of 1–3 kDa fractions. Subsequently, inhibition rate tests were conducted on different concentrations of <1 kDa components. The IC50 values for α-glucosidase and α-amylase of the <1 kDa components were found to be 5.04 mg/mL and 4.65 mg/mL, respectively (Figures 3C,D). α-Glucosidase catalyzes carbohydrate digestion. Hydrolysis of both starch and disaccharides produces glucose, which causes an increase in blood glucose levels (34). α-Amylase catalyzes the hydrolysis of starch, glycogen, and α-1,4-glycosidic bonds to monosaccharides (35). The <1 kDa fraction inhibited these two enzymes possibly because hydrophobic amino acids in the sea buckthorn seed meal peptides are the most abundant by 19 h of fermentation. The catalytic effect of the enzyme is affected by the binding site of the enzyme or that of the substrate (36). Obaroakpo et al., found that the higher the content of hydrophobic amino acids, the higher the α-glucosidase inhibitory activity of the protein in the fermented quinoa protein drink. This provides a basis for the development of novel hypoglycemic drugs (37). In addition, the contents of glutamic acid, arginine, lysine, serine, and glycine in the sea buckthorn seed meal peptide were higher. This may be among the reasons for the inhibitory effect noted on these two enzymes.
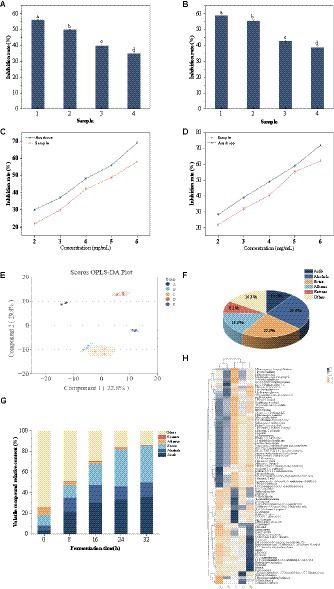
Figure 3. Volatile compound dynamics and in vitro hypoglycaemic activity. (A,B) Inhibition of α-glucosidase and α-amylase by different fractions at a concentration of 5.0 mg/mL. (1) acarbose, (2) peptide of <1 kDa fraction, (3) peptide of 1–3 kDa fraction, (4) peptide of >3 kDa fraction, (A) α-glucosidase, (B) α-amylase. (C) Determination of α-glucosidase inhibition by different concentrations of >1 kDa fractions. (D) Determination of α-amylase inhibition by different concentrations of >1 kDa fractions. (E) OPLS-DS score plots. (A) 0 h, (B) 8 h, (C) 16 h, (D) 24 h, (E) 32 h; (F) Plot of the percentage of volatile compounds species; (G) Column stacking plot of the relative percentage of volatile compounds; (H) Hierarchical cluster analysis of volatile compounds. Rows: different fermentation times, columns: volatile compounds [these values are expressed as mean of three replicates ± standard deviation (SD), lower case letters represent significance, p < 0.05].
The determination results of <1 kDa sea buckthorn seed meal peptides were compared with the sea buckthorn NCBI database, identifying 18 peptide sequences. The total number of amino acids in these peptides ranged from 5 to 9 (Table 4), and all peptides had a molecular weight below 1 kDa, with hydrophobicity ranging from 29 to 80%. Moreover, peptides with a molecular weight below 600 Da exhibited relatively higher hydrophobicity. These results indicate that the selected peptide segments all contain hydrophobic amino acids, demonstrating a certain correlation between hydrophobic amino acids and hypoglycemic activity. Based on the predicted hydrophobicity and biological activity, the peptides FLVAG and FYLPKM were preliminarily selected. These two peptides exhibited superior hydrophobicity and biological activity compared to the other peptides. Studies have shown that amino acid sequences containing leucine, proline, tyrosine, and valine can participate in blood sugar control, enhancing the hypoglycemic activity of peptides (38). Considering these factors, we chose the peptide FYLPKM for further research, as it may be an advantageous peptide for hypoglycemic activity.
3.9 Detection of volatile compounds with different fermentation time
Lactic acid fermentation can increase the flavor compounds, produce organic acids, and enhance the nutritional and sensory properties (e.g., antioxidant capacity, color) of the substrate (39). OPLS-DA was used to analyze the volatile components of sea buckthorn seed meal peptide solution at fermentation times of 0 h, 8 h, 16 h, 24 h, and 32 h. As shown in Figure 3E, the model had good explanatory and predictive capabilities with Q2 = 0.908, p < 0.005 and R2Y = 0.981, p < 0.005. Based on VIP > 1, 86 volatile compounds were screened out (Supplementary Table S1), including 9 acids, 22 alcohols, 20 esters, 14 alkanes, 7 ketones, and 14 other compounds. The proportions of each compound are shown in Figure 3F.
The relative content of volatile compounds in sea buckthorn seed meal varied at different fermentation stages. With the extension of fermentation time, the relative content of volatile flavor compounds increased, consistent with the results of Suo et al. (40). Mixed-strain fermentation activated the hexose kinase pathway of the phosphogluconate pathway, leading to an increase in acid content. Acetyl-CoA and alcohols produced esters under the action of esterase or acyltransferase (41). The relative content of acids and esters increased significantly from 3 and 10% to 33 and 36% (0–24 h), respectively. It is worth noting that the relative content of acetic acid significantly increased from 0 to 0.057 μg/mL (0–24 h), contributing greatly to the aroma of sea buckthorn seed meal peptide solution (Figure 3G). In addition, Hexanoic acid, 2-phenylethyl ester and Formic acid, heptyl ester imparted fruity and rose aromas to the solution (42). Importantly, the relative content of acids and esters remained at 36% until the end of fermentation, which may play a positive role in the aroma characteristics of mixed-strain fermented sea buckthorn seed meal peptide solution.
The relative content of alcohol substances increased from 5 to 17% (0–16 h). This is because the fermentation of the strain causes the protein in the raw material to break down into amino acids, which then undergo deamination and decarboxylation reactions to generate alcohol substances (43). The production of alcohol substances such as 1-Heptanol, 1-Hexanol, 1-Hexanol, 2-ethyl- increased the floral and fruity aroma. Subsequently, some alcohols and carboxylic acids react to form esters, mostly in the form of methyl esters (44). In our study, we also found a significant increase in the relative content of Damascenone and Ionone, which impart fruity, sweet, woody, and floral notes to the seabuckthorn seed meal peptide liquid (45). They may have played a positive role in enhancing the overall sensory properties of seabuckthorn seed meal. The results of hierarchical cluster analysis (HCA) are consistent with the changes in the above-mentioned volatile compounds (Figure 3H).
4 Conclusion
This study prepared seabuckthorn seed meal peptide through mixed fermentation, increasing the utilization value of seabuckthorn seed meal. The research indicates that maltose and MgSO4·7H2O supplemented the necessary nutrients for fermentation. When the fermentation time was 19 h, a mixed fermentation of L. fermentum, B. subtilis, L. case, L. reuteri, and L. acidophilus degraded 43.85% of the protein in seabuckthorn seed meal into small molecule peptides. At this time, the peptide molecular weight was mainly distributed below 3 kDa, and the amino acid content reached its maximum value, demonstrating that mixed strain fermentation is beneficial for the degradation of seabuckthorn protein. At a peptide concentration of 5 mg/mL, peptides with a molecular weight of <1 kDa showed the best inhibition activity against α-amylase and α-glucosidase, possibly due to the amino acid sequence of FYLPKM. Additionally, during the fermentation process, the content of acids and esters significantly increased from 3 and 10% to 33 and 36% (0–24 h). This change had a certain positive effect on the aroma characteristics of the mixed fermentation seabuckthorn seed meal peptide liquid and provided a theoretical basis for the application of seabuckthorn seed meal peptide in food. Subsequent work may focus on the synthesis, functional verification, hypoglycemic mechanism, and peptide beverage research of sea buckthorn seed meal peptides.
Data availability statement
The original contributions presented in the study are included in the article/Supplementary material, further inquiries can be directed to the corresponding author.
Author contributions
JY: Formal analysis, Methodology, Project administration, Writing – original draft, Writing – review & editing. JH: Writing – review & editing, Writing – original draft. AA: Writing – review & editing. YM: Data curation, Methodology, Writing – original draft. XY: Writing – review & editing. XL: Data curation, Writing – original draft. MZ: Writing – review & editing. LW: Funding acquisition, Writing – review & editing.
Funding
The author(s) declare financial support was received for the research, authorship, and/or publication of this article. This work was supported by The Key Technology Research and Development Program of Autonomous Region (No. 2022A02009-3).
Acknowledgments
I would like to thank LW for his help in the research process and to my classmates and others for their help in the experimental process.
Conflict of interest
The authors declare that the research was conducted in the absence of any commercial or financial relationships that could be construed as a potential conflict of interest.
Publisher’s note
All claims expressed in this article are solely those of the authors and do not necessarily represent those of their affiliated organizations, or those of the publisher, the editors and the reviewers. Any product that may be evaluated in this article, or claim that may be made by its manufacturer, is not guaranteed or endorsed by the publisher.
Supplementary material
The Supplementary material for this article can be found online at: https://www.frontiersin.org/articles/10.3389/fnut.2024.1355116/full#supplementary-material
Footnotes
References
1. Ma, Y, Yao, J, Zhou, L, Zhao, M, Wang, W, Liu, J, et al. Comprehensive untargeted lipidomic analysis of sea buckthorn using UHPLC-HR-AM/MS/MS combined with principal component analysis. Food Chem. (2024) 430:136964. doi: 10.1016/j.foodchem.2023.136964
2. Chen, A, Feng, X, Dorjsuren, B, Chimedtseren, C, Damda, T-A, and Zhang, C. Traditional food, modern food and nutritional value of sea buckthorn (Hippophae rhamnoides L.): a review. J Fut Foods. (2023) 3:191–205. doi: 10.1016/j.jfutfo.2023.02.001
3. Lin, J, Xiang, H, Sun-Waterhouse, D, Cui, C, and Wang, W. Deep eutectic solvents and alkaline extraction of protein from seabuckthorn seed meal: a comparison study. Food Sci Human Wellness. (2022) 11:1028–35. doi: 10.1016/j.fshw.2022.03.019
4. Liu, C, Zhang, Z, Shang, Y, Li, S, Xia, J, Tian, Y, et al. Progress in the preparation, identification and biological activity of walnut peptides. J Fut Foods. (2024) 4:205–20. doi: 10.1016/j.jfutfo.2023.07.003
5. Kumar, M, Hasan, M, Choyal, P, Tomar, M, Gupta, OP, Sasi, M, et al. Cottonseed feedstock as a source of plant-based protein and bioactive peptides: evidence based on biofunctionalities and industrial applications. Food Hydrocoll. (2022) 131:107776. doi: 10.1016/j.foodhyd.2022.107776
6. Du, Z, and Li, Y. Review and perspective on bioactive peptides: a roadmap for research, development, and future opportunities. J Agricult Food Res. (2022) 9:100353. doi: 10.1016/j.jafr.2022.100353
7. Wang, Y, Cao, K, Li, H, Sun, H, and Liu, X. Improvement of active peptide yield, antioxidant activity and anti-aging capacity of rapeseed meal fermented with YY-112 pure fermentation and co-fermentation. Food Biosci. (2022) 49:101938. doi: 10.1016/j.fbio.2022.101938
8. Solieri, L, Valentini, M, Cattivelli, A, Sola, L, Helal, A, Martini, S, et al. Fermentation of whey protein concentrate by Streptococcus thermophilus strains releases peptides with biological activities. Process Biochem. (2022) 121:590–600. doi: 10.1016/j.procbio.2022.08.003
9. Ramírez, K, Pineda-Hidalgo, KV, and Rochín-Medina, JJ. Fermentation of spent coffee grounds by Bacillus clausii induces release of potentially bioactive peptides. LWT-Food Sci Technol. (2021) 138:110685. doi: 10.1016/j.lwt.2020.110685
10. Tang, X, Chen, J, Jiang, B, Zhu, Q, and Zhang, R. Effects of Lactiplantibacillus plantarum fermentation on hydrolysis and immunoreactivity of Siberian apricot (Prunus sibirica L.) kernel. Food. Bioscience. (2023) 53:102585. doi: 10.1016/j.fbio.2023.102585
11. Lan, L, Wang, J, Wang, S, He, Q, Wei, R, Sun, Z, et al. Correlation between microbial community succession and flavor substances during fermentation of Yongchuan Douchi. Food Biosci. (2023) 56:103192. doi: 10.1016/j.fbio.2023.103192
12. Liu, Y, Sheng, J, Li, J, Zhang, P, Tang, F, and Shan, C. Influence of lactic acid bacteria on physicochemical indexes, sensory and flavor characteristics of fermented sea buckthorn juice. Food Biosci. (2022) 46:101519. doi: 10.1016/j.fbio.2021.101519
13. Tang, T, Li, Q, Huang, Z, Wu, Y, Yan, B, Zhao, J, et al. Evaluation of Shandong pancake with sourdough fermentation on the alleviation of type 2 diabetes symptoms in mice. J Funct Foods. (2022) 90:104952. doi: 10.1016/j.jff.2022.104952
14. Fang, L, Wang, W, Dou, Z, Chen, J, Meng, Y, Cai, L, et al. Effects of mixed fermentation of different lactic acid bacteria and yeast on phytic acid degradation and flavor compounds in sourdough. LWT-Food Sci Technol. (2023) 174:114438. doi: 10.1016/j.lwt.2023.114438
15. Yan, F, Wang, Q, Teng, J, Wu, F, and He, Z. Preparation process optimization and evaluation of bioactive peptides from Carya cathayensis Sarg meal. Curr Res Food Sci. (2023) 6:100408. doi: 10.1016/j.crfs.2022.100408
16. Fan, X, Ma, X, Maimaitiyiming, R, Aihaiti, A, Yang, J, Li, X, et al. Study on the preparation process of quinoa anti-hypertensive peptide and its stability. Front Nutr. (2023) 9:1119042. doi: 10.3389/fnut.2022.1119042
17. Sadeghi, M, Miroliaei, M, Ghanadian, M, Szumny, A, and Rahimmalek, M. Exploring the inhibitory properties of biflavonoids on α-glucosidase; computational and experimental approaches. Int J Biol Macromol. (2023) 253:127380. doi: 10.1016/j.ijbiomac.2023.127380
18. Huang, Y, Richardson, SJ, Brennan, CS, and Kasapis, S. Mechanistic insights into α-amylase inhibition, binding affinity and structural changes upon interaction with gallic acid. Food Hydrocoll. (2023) 148:109467. doi: 10.1016/j.foodhyd.2023.109467
19. Heymich, M-L, Friedlein, U, Trollmann, M, Schwaiger, K, Böckmann, RA, and Pischetsrieder, M. Generation of antimicrobial peptides Leg1 and Leg2 from chickpea storage protein, active against food spoilage bacteria and foodborne pathogens. Food Chem. (2021) 347:128917. doi: 10.1016/j.foodchem.2020.128917
20. Shi, F, Wang, L, and Li, S. Enhancement in the physicochemical properties, antioxidant activity, volatile compounds, and non-volatile compounds of watermelon juices through Lactobacillus plantarum JHT78 fermentation. Food Chem. (2023) 420:136146. doi: 10.1016/j.foodchem.2023.136146
21. Ghazanfari, N, Fallah, S, Vasiee, A, and Tabatabaei, YF. Optimization of fermentation culture medium containing food waste for l-glutamate production using native lactic acid bacteria and comparison with industrial strain. LWT-Food Sci Technol. (2023) 184:114871. doi: 10.1016/j.lwt.2023.114871
22. Norouzi, S, Daneshyar, M, Farhoomand, P, Tukmechi, A, and Tellez-Isaiasc, G. In vitro evaluation of probiotic properties and selenium bioaccumulation of lactic acid bacteria isolated from poultry gastrointestinal, as an organic selenium source. Res Vet Sci. (2023) 162:104934. doi: 10.1016/j.rvsc.2023.06.012
23. Guo, Q, Chen, P, and Chen, X. Bioactive peptides derived from fermented foods: preparation and biological activities. J Funct Foods. (2023) 101:105422. doi: 10.1016/j.jff.2023.105422
24. Ma, X, Fan, X, Wang, D, Li, X, Wang, X, Yang, J, et al. Study on preparation of chickpea peptide and its effect on blood glucose. Front Nutr. (2022) 9:988628. doi: 10.3389/fnut.2022.988628
25. Hou, D, Feng, Q, Niu, Z, Wang, L, Yan, Z, and Zhou, S. Promising mung bean proteins and peptides: a comprehensive review of preparation technologies, biological activities, and their potential applications. Food Biosci. (2023) 55:102972. doi: 10.1016/j.fbio.2023.102972
26. Lan, T, Lv, X, Zhao, Q, Lei, Y, Gao, C, Yuan, Q, et al. Optimization of strains for fermentation of kiwifruit juice and effects of mono- and mixed culture fermentation on its sensory and aroma profiles. Food Chem X. (2023) 17:100595. doi: 10.1016/j.fochx.2023.100595
27. Peng, X, Liao, Y, Ren, K, Liu, Y, Wang, M, Yu, A, et al. Fermentation performance, nutrient composition, and flavor volatiles in soy milk after mixed culture fermentation. Process Biochem. (2022) 121:286–97. doi: 10.1016/j.procbio.2022.07.018
28. Wang, C, Qiu, X, Hou, R, Liu, J, Li, L, and Mao, X. Improvement of soybean meal quality by one-step fermentation with mixed-culture based on protease activity. Innovative Food Sci Emerg Technol. (2023) 85:103311. doi: 10.1016/j.ifset.2023.103311
29. Zielińska, E, Karaś, M, Baraniak, B, and Jakubczyk, A. Evaluation of ACE, α-glucosidase, and lipase inhibitory activities of peptides obtained by in vitro digestion of selected species of edible insects. Eur Food Res Technol. (2020) 246:1361–9. doi: 10.1007/s00217-020-03495-y
30. Domínguez-Gutiérrez, GA, Perraud-Gaime, I, Escalona-Buendía, H, Durand, N, Champion-Martínez, EI, Fernández-Soto, RR, et al. Inhibition of aspergillus carbonarius growth and Ochratoxin a production using lactic acid bacteria cultivated in an optimized medium. Int J Food Microbiol. (2023) 404:110320. doi: 10.1016/j.ijfoodmicro.2023.110320
31. R. S. SWarke, VG, Mahajan, GB, and Annapure, US. Effect of amino acids on growth, elemental content, functional groups, and essential oils composition on hydroponically cultivated coriander under different conditions. Ind Crop Prod. (2023) 197:116577. doi: 10.1016/j.indcrop.2023.116577
32. Mossmann, D, Müller, C, Park, S, Ryback, B, Colombi, M, Ritter, N, et al. Arginine reprograms metabolism in liver cancer via RBM39. Cell. (2023) 186:5068–5083.e23. doi: 10.1016/j.cell.2023.09.011
33. Zhang, X, Su, M, Du, J, Zhou, H, Li, X, Zhang, M, et al. Analysis of the free amino acid content and profile of 129 peach (Prunus persica (L.) Batsch) germplasms using LC-MS/MS without derivatization. J Food Compos Anal. (2022) 114:104811. doi: 10.1016/j.jfca.2022.104811
34. Liu, H, Li, Z, Xia, X, Zhang, R, Wang, W, and Xiang, X. Chemical profile of phenolic extracts from rapeseed meal and inhibitory effects on α-glucosidase: UPLC-MS/MS analysis, multispectral approaches, molecular simulation and ADMET analysis. Food Res Int. (2023) 174:113517. doi: 10.1016/j.foodres.2023.113517
35. Rupolo, PE, Monteiro, DP, Ribeiro, TP, De Azevedo, LB, Gregory, CR, Careli, PS, et al. Effects of supplementation of α-amylase alone in a model of growing and finishing pigs fed metabolizable energy-reduced diets. Livest Sci. (2023) 278:105361. doi: 10.1016/j.livsci.2023.105361
36. Qiao, H, Bi, X, Zhang, Y, Liu, M, Zu, S, Jia, N, et al. Enzymic polypeptide antioxidant activity and inhibitory activity on α-glucosidase and α-amylase from Paeonia ostii cake. Ind Crop Prod. (2020) 146:112158. doi: 10.1016/j.indcrop.2020.112158
37. Obaroakpo, JU, Liu, L, Zhang, S, Lu, J, Pang, X, and Lv, J. α-Glucosidase and ACE dual inhibitory protein hydrolysates and peptide fractions of sprouted quinoa yoghurt beverages inoculated with Lactobacillus casei. Food Chem. (2019) 299:124985. doi: 10.1016/j.foodchem.2019.124985
38. Wang, X, Fan, Y, Xu, F, Xie, J, Gao, X, Li, L, et al. Characterization of the structure, stability, and activity of hypoglycemic peptides from Moringa oleifera seed protein hydrolysates. Food Funct. (2022) 13:3481–94. doi: 10.1039/D1FO03413H
39. Xing, Y, Qiu, Y, Yue, T, Yi, R, Xu, Q, Rao, Y, et al. Correlation between microbial communities and volatile flavor compounds in the fermentation of new pickle fermentation broth. Food Biosci. (2023) 56:103167. doi: 10.1016/j.fbio.2023.103167
40. Suo, B, Chen, X, and Wang, Y. Recent research advances of lactic acid bacteria in sourdough: origin, diversity, and function. Curr Opin Food Sci. (2021) 37:66–75. doi: 10.1016/j.cofs.2020.09.007
41. Li, T, Jiang, T, Liu, N, Wu, C, Xu, H, and Lei, H. Biotransformation of phenolic profiles and improvement of antioxidant capacities in jujube juice by select lactic acid bacteria. Food Chem. (2021) 339:127859. doi: 10.1016/j.foodchem.2020.127859
42. Yang, Y, Cui, J, Jiang, Z, and Zhao, X. GC × GC-ToF-MS combined with multivariate statistical methods to explore the effects of L. paracasei fermentation on bread flavor characteristics. Food Chem. (2024) 435:137643. doi: 10.1016/j.foodchem.2023.137643
43. Bai, J, He, L, Zhang, J, Gu, X, Wu, B, Wang, A, et al. Influences of Lactiplantibacillus plantarum and Saccharomyces cerevisiae fermentation on the nutritional components, flavor property and lipid-lowering effect of highland barley. J Fut Foods. (2024) 4:258–66. doi: 10.1016/j.jfutfo.2023.07.008
44. Tian, H, Xiong, J, Yu, H, Chen, C, and Lou, X. Flavor optimization in dairy fermentation: from strain screening and metabolic diversity to aroma regulation. Trends Food Sci Technol. (2023) 141:104194. doi: 10.1016/j.tifs.2023.104194
45. Fei, Z, Xie, D, Wang, M, Zhang, Y, Zhang, H, Du, Q, et al. Enhanced biotransformation of bioactive components and volatile compounds of bamboo (Phyllostachys glauca McClure) leaf juice fermented by probiotic Streptococcus thermophiles. LWT-Food Sci Technol. (2023) 173:114363. doi: 10.1016/j.lwt.2022.114363
Keywords: sea buckthorn seed meal, fermentation, volatile compounds, peptides, hypoglycemic
Citation: Yang J, Hong J, Aihaiti A, Mu Y, Yin X, Zhang M, Liu X and Wang L (2024) Preparation of sea buckthorn (Hippophae rhamnoides L.) seed meal peptide by mixed fermentation and its effect on volatile compounds and hypoglycemia. Front. Nutr. 11:1355116. doi: 10.3389/fnut.2024.1355116
Edited by:
Zhiyang Du, Jilin University, ChinaReviewed by:
Long Ding, Northwest A&F University, ChinaHefei Zhao, University of California, Davis, United States
Copyright © 2024 Yang, Hong, Aihaiti, Mu, Yin, Zhang, Liu and Wang. This is an open-access article distributed under the terms of the Creative Commons Attribution License (CC BY). The use, distribution or reproduction in other forums is permitted, provided the original author(s) and the copyright owner(s) are credited and that the original publication in this journal is cited, in accordance with accepted academic practice. No use, distribution or reproduction is permitted which does not comply with these terms.
*Correspondence: Liang Wang, V0wxMzkwNTkzNzg2QDE2My5jb20=
†These authors have contributed equally to this work