- 1College of Food and Health, Zhejiang Agriculture and Forestry University, Hangzhou, China
- 2School of Biological and Chemical Engineering, Zhejiang University of Science and Technology, Hangzhou, China
- 3Department of Anesthesiology, Sir Run Run Shaw Hospital, School of Medicine, Zhejiang University, Hangzhou, China
- 4College of Biology and Environmental Engineering, Zhejiang Shuren University, Hangzhou, China
- 5Institute of Quality and Standard for Agriculture Products, Zhejiang Academy of Agricultural Science, Hangzhou, China
The severe acute respiratory syndrome coronavirus type 2 (SARS-CoV-2), which causes the coronavirus disease 2019 (COVID-19), is spreading worldwide. Although the COVID-19 epidemic has passed its peak of transmission, the harm it has caused deserves our attention. Scientists are striving to develop medications that can effectively treat COVID-19 symptoms without causing any adverse reactions. SARS-CoV-2 inhibitory peptides derived from animal proteins have a wide range of functional activities in addition to safety. Identifying animal protein sources is crucial to obtaining SARS-CoV-2 inhibitory peptides from animal sources. This review aims to reveal the mechanisms of action of these peptides on SARS-CoV-2 and the possibility of animal proteins as a material source of SARS-CoV-2 inhibitory peptides. Also, it introduces the utilization of computer-aided design methods, phage display, and drug delivery strategies in the research on SARS-CoV-2 inhibitor peptides from animal proteins. In order to identify new antiviral peptides and boost their efficiency, we recommend investigating the interaction between SARS-CoV-2 inhibitory peptides from animal protein sources and non-structural proteins (Nsps) using a variety of technologies, including computer-aided drug approaches, phage display techniques, and drug delivery techniques. This article provides useful information for the development of novel anti-COVID-19 drugs.
1 Introduction
In 2020, a global outbreak of coronavirus disease 2019 (COVID-19) posed a severe threat to human life and health. The pathogen has been identified as severe acute respiratory syndrome coronavirus type 2 (SARS-CoV-2), and the receptor for this pathogen has been found to be angiotensin-converting enzyme 2 (ACE2) (1). In addition, there are other factors associated with SARS-CoV-2 infection. Researchers have adopted various methods to prevent and treat COVID-19, such as by blocking the binding of ACE2 to SARS-CoV-2, or by preventing the entry of SARS-CoV-2 into the body (2). New strains of SARS-CoV-2 have appeared, and the virus is spreading more quickly. There is an urgent need for highly effective and safe antiviral medications to stop the virus from spreading and to speed up the recovery process. The development of antiviral medications against COVID-19 is also particularly important to addressing vaccine escape and treating symptoms caused by new strains. Currently available anti-COVID-19 drugs show significant efficacy, but their accessibility and price pose challenges to widespread adoption.
Bioactive peptides are small fragments of isolated proteins with potent biological effects (3), and they typically contain 2–20 amino acids (4). Bioactive peptides offer a multitude of benefits for the human body, including anti-hypertensive, anti-SARS-CoV-2 (5), anti-inflammatory (6), anti-bacterial (7, 8), anti-diabetic (9), and anti-cancer (10). Also, bioactive peptides can protect humans from SARS-CoV-2 infection (11). Animal proteins, which contain all the nine essential amino acids, can provide nutrition essential for the human body (12). Functional peptides from animal protein sources are peptides derived from animal proteins, such as muscle protein, egg protein, and milk protein. Functional peptides of animal origin have several advantages. First, they do not have any side effects; second, they do not lead to drug resistance; third, they can be easily absorbed by the human body; and fourth, they have a high level of safety; fifth, they exhibit unique biological activities. Plant-based protein ingredients often contain allergenic proteins and other anti-nutritional factors, so that may not be suitable for pregnant women, children, and allergy sufferers (13).
Animal protein-derived SARS-CoV-2 inhibitory peptides have been shown to act on ACE2 and receptor-binding domain (RBD). IRW, an animal protein-derived peptide, may inhibit the interaction of spike (S) protein of SARS-CoV-2 with ACE2 and have greater contact with RBD. Cellular assays have demonstrated that IRW can inhibit the expression of SARS-CoV-2 S protein and ACE2 (14). With the characteristics of designability, strong stability, small volume, and easy absorption, peptides from animal proteins can be combined with nanomaterials for targeted therapies. Thanks to these characteristics, peptides from animal proteins have played an important role in preventing and treating COVID-19. Zhao found that a human-derived peptide 4H30 defends against SARS-CoV-2 by preventing its entry, membrane fusion, and release. Animal experiments showed that 4H30 can decrease the concentration of the virus in vivo (15). Many other animal protein-derived peptides can also protect against SARS-CoV-2 besides 4H30 (16). However, most of the peptides currently used for SARS-CoV-2 prevention are of synthetic origin, leaving substantial room for the application and development of animal protein-derived peptides. Safety, designability, and high efficiency make animal protein-derived peptides a potential material for formulated foods that serve particular medical purposes. In comparison to SARS-CoV-2 inhibitors of other sources, the number of known SARS-CoV-2 inhibitory peptides from animal proteins is too small. The source materials with the greatest potential to produce SARS-CoV-2 inhibitory peptides from animal proteins have not been reported. Peptides have a short half-life, so it is important to maintain their effective concentration. The development of technology has made it feasible to combine computational biology and phage display methods with the exploration of novel potent SARS-CoV-2 inhibitory peptides from animal proteins. Additionally, the drug distribution strategy can be used to guarantee the concentration of those peptides in vivo.
This review aims to reveal the mechanisms of action of these animal protein-derived peptides on SARS-CoV-2 and explore the possibilities of using animal proteins as a source of SARS-CoV-2 inhibitory peptides. Also, the review introduces the application of computer-aided design methods, phage display, and drug delivery strategies in identifying or using SARS-CoV-2 inhibitory peptides from animal proteins.
2 Severe acute respiratory syndrome coronavirus type 2
COVID-19 showed high levels of lethality and infectivity at first. Its lethality has been decreasing as the virus mutates. Researchers have successfully determined that SARS-CoV-2 is the primary cause (17, 18). Patients experience variable degrees of discomfort within 6 months following acute COVID-19 infection (19).
2.1 The structure of SARS-CoV-2
SARS-CoV-2 consists of four structural proteins: membrane protein (M), spike protein (S), nucleocapsid protein (N), and envelope protein (E) (Figure 1A) (20). S1 and S2 subunits make up the S protein, and ACE2 functions as a functional receptor by binding to the S1 subunit (Figure 1B) (1, 21). The carboxypeptidase structural domain located at the extracellular N-terminus of ACE2 can interact with SARS-CoV-2 (22). The four unique structural domains of the S1 subunit are the receptor-binding domain (RBD), two carboxy-terminal (C-terminal) structural domains (structural domain 1 and structural domain 2), and the amino-terminal (N-terminal) structural domain (23). The S2 subunit initiates membrane fusion and releases the viral RNA into the host cytoplasm (24).
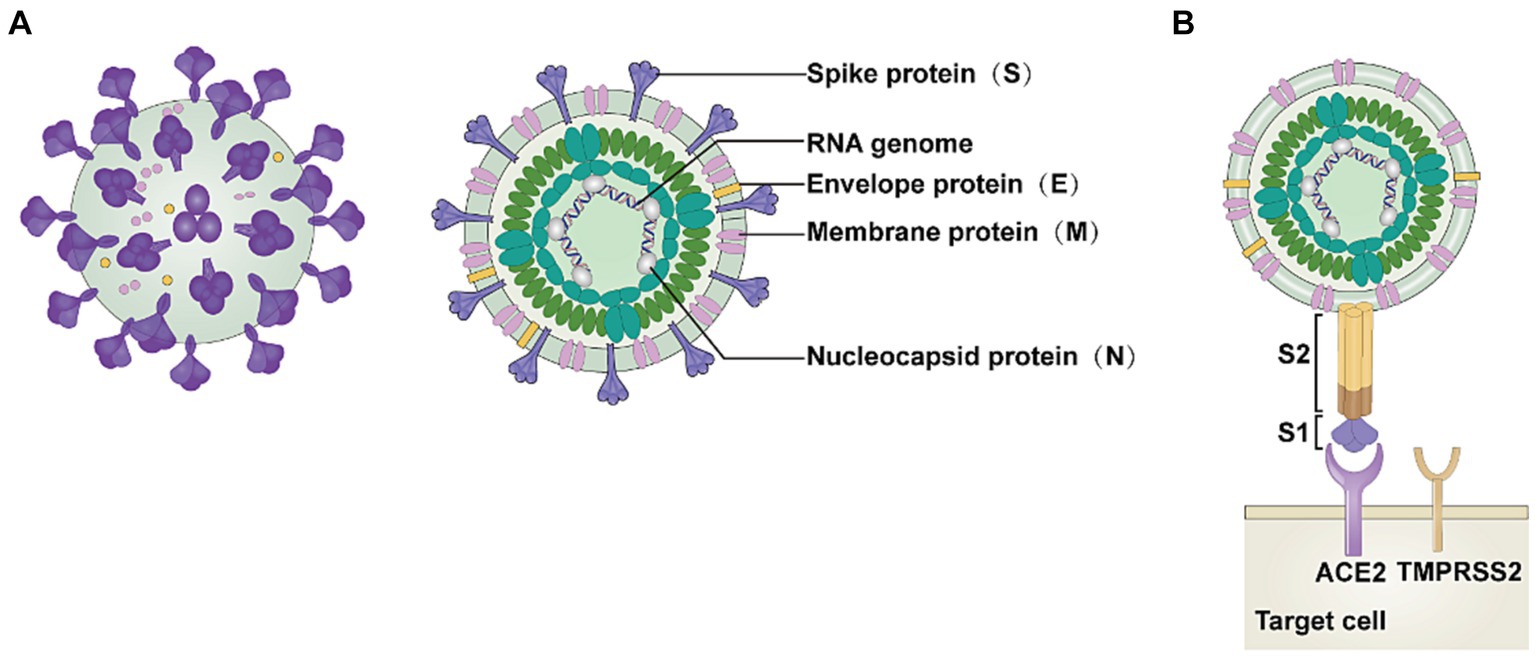
Figure 1. Structure of SARS-CoV-2. (A) The severe acute respiratory syndrome coronavirus 2 (SARS-CoV-2) virion consists of the following structural proteins: membrane protein (M), spike protein (S), nucleocapsid protein (N), and envelope protein (E). (B) The S protein attaches to the host cell receptor angiotensin-converting enzyme 2 (ACE2) using the S1 domain. This image refers to the cited literature, with some adjustments (20).
In addition to the structural proteins mentioned above, SARS-CoV-2 also contains non-structural proteins (Nsps). The open reading frame 1a/1ab (ORF1a/ORF1ab), which makes up the great majority of the genetic information in the SARS-CoV-2 genome, can be translated into two polyproteins pp1a and pp1ab (25). pp1ab, which is involved in viral genome replication and production, can be broken down by viral proteases like main protease/3 chymotrypsin-like protease (Mpro/3CLpro; Mpro is also known as 3CLpro) and papain-like protease (PLpro) into Nsps with different functions. There are a total of 16 types of Nsps, Nsp1-16. Nsp12, and cofactors Nsp7/8 facilitate genome replication and transcription (26).
2.2 Pathogenesis of SARS-CoV-2
The SARS-CoV-2 complex enters cells through two main pathways: endocytosis and cell membrane fusion (Figure 2) (23). The transmembrane serine proteinase 2 (TMPRSS2) enables the separation of the S1 and S2 subunits (16). When little or no TMPRSS2 is present, SARS-CoV-2 enters host cells by endocytosis following endosomal acidification (27). Conversely, the SARS-CoV-2 complex enters the host cell by cell membrane fusion in the presence of a significant amount of TMPRSS2 (28).
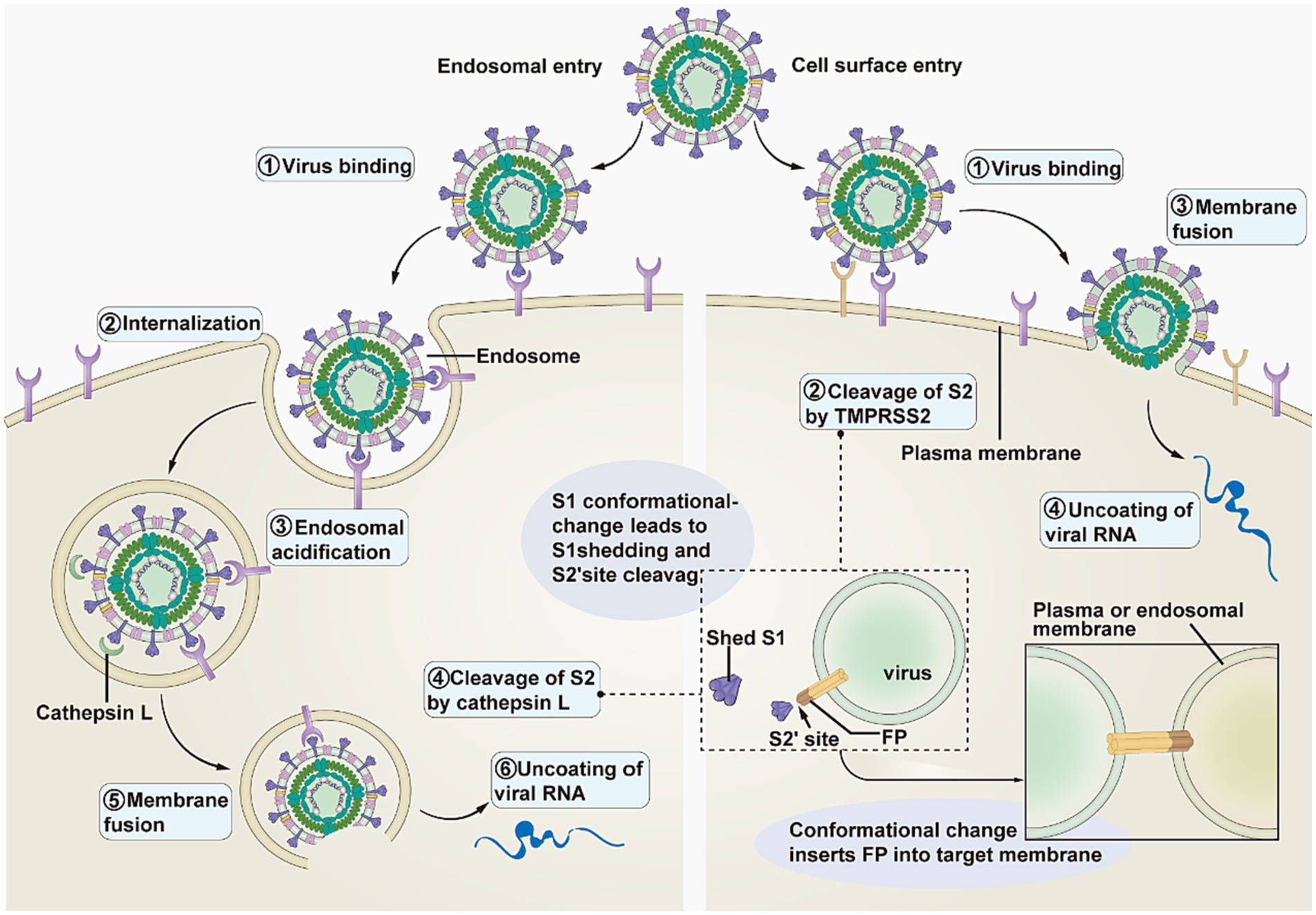
Figure 2. Pathways of SARS-CoV-2 entering the host cell. This image refers to Figure 3 in the cited literature, with some adjustments (23).
In addition to the involvement in viral replication, Nsps also help SARS-CoV-2 evade host defenses (Figure 3). In the initial phase, SARS-CoV-2 enters the host cell (30). Subsequently, Nsps act as mediators in the second phase. The structural changes that Nsp3 and Nsp4 cause in the endoplasmic reticulum (ER) membrane lead to the creation of double-membrane vesicles and convoluted membranes, which are components of replication organelles (RO). To permit lipid flow, Nsp6 creates a molecular rope that resembles a zipper between the RO and the ER. In the third stage, double-stranded RNA (dsRNA) completes viral methylation through Nsp12, Nsp13, Nsp16, Nsp14, and Nsp10 (31). In order to facilitate the viral replication process without being recognized by the host, negative-sense single-stranded RNA (-ssRNA) and dsRNA are isolated from the RO. In the fourth stage, as replication intensifies, viral RNA accumulates outside the RO and is subsequently masked and/or minimized by the N protein and/or Nsp15 (29).
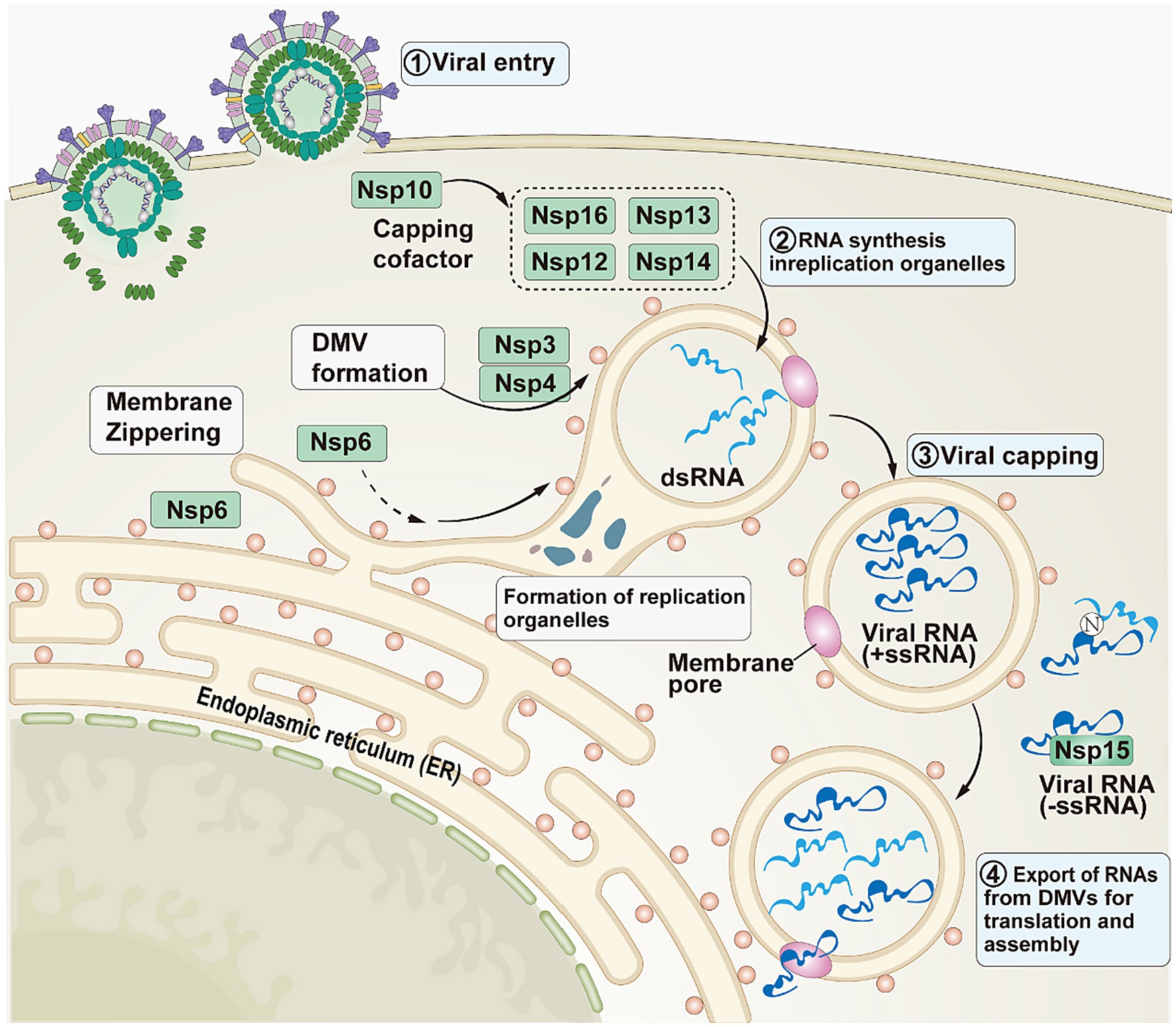
Figure 3. The role played by Nsps in SARS-CoV-2. This image refers to Figure 1 in the cited literature, with some adjustments (29).
Therefore, ACE2 (32), S proteins (33), N proteins, TMPRSS2 (34), RBD, PLpro, Mpro/3CLpro (35), and Nsps are all key to blocking SARS-CoV-2.
3 Potential of bioactive peptides in COVID-19 treatment
Bioactive peptides have been found to play a significant role in inhibiting the activity of SARS-CoV-2. Research literature on SARS-CoV-2 has highlighted the potential inhibitory effects of whey protein peptides (36), peanut protein peptides (37), and soy cheese peptides (38). These peptides with antiviral properties offer a safer method to achieve patients’ nutritional balance and to combat viral attacks. Furthermore, these discoveries serve as a fundamental basis for the design and synthesis of antiviral drugs using peptides from animal proteins.
3.1 Antiviral pathways of bioactive peptides
Peptides hold great promise for the development of anti-COVID-19 drugs. Antiviral peptides can be developed based on existing knowledge of the structures and targets of viral proteins. Based on the known structure of viruses and process of infection, researchers have divided virus combating strategies into the following three categories: interfering with the binding of viral proteins to host receptors to block viral entry; blocking viral replication; and inhibiting the assembly and release of viral particles (Figure 4). (1) Interfering with the binding of viral proteins to host receptors. Peptides play a role in the prevention of COVID-19 by interfering with the binding of the SARS-CoV-2 S protein to ACE2. Curreli et al. (39) designed four peptides to block the binding of SARS-CoV-2 to ACE2 to prevent COVID-19 infection. He et al. (40) designed 15 peptides that effectively blocked the binding of ACE2 to RBD, thereby significantly reducing infection with COVID-19. (2) Blocking virus replication. The peptides stop viral replication in the body by blocking the action of PLpro and Mpro, which are involved in mediating viral replication. Liu et al. (41) described a novel peptide-drug conjugate derived from the PLpro-specific substrate LRGG, which has demonstrated its antiviral properties in cell experiments. (3) Preventing the assembly and discharge of viral particles. Peptides treat COVID-19 by inhibiting the activity of TMPRSS2, which encourages the assembly and release of viral particles. Shapira et al. (42) found that the peptidomimetic drug Ms-Gln-Phe-Arg-kbt inhibits TMPRSS2 activity by 83%. The effectiveness of N-0385 has been demonstrated in both cell and animal experiments.
3.2 Peptide vaccines
Peptides have been used in the production of vaccines. Human phase I trials have demonstrated the effectiveness of CoVac-1, a peptide-based vaccine that stimulates T-cell responses to offer long-term immunity after infection (43). Shen et al. (44) constructed an anti-COVID-19 peptide vaccine that induces human immunoglobulin G response to the RBD of SARS-CoV-2 and enhances immune responses in mice. To reduce disease severity, Eszter et al. (45) investigated T-cell-based peptide vaccines against two novel SARS-CoV-2 variants.
4 SARS-CoV-2 inhibitory peptides from animal protein sources
Animal products such as eggs, milk, and meat have a higher protein content and greater health benefits compared with plant-based foods. Animal peptides can be better absorbed by the human body because they have a lower molecular weight than proteins. Animal protein-derived peptides have a variety of characteristics, such as antiviral, anti-inflammatory, and antioxidant. At a time when SARS-CoV-2 poses a serious threat to human health, those peptides have become a focus in the search for a strong SARS-CoV-2 inhibitor that is free of side effects. We have summarized the SARS-CoV-2 inhibitory peptides of animal origin in Table 1.
4.1 Milk
Milk contains abundant superior proteins, fats, minerals (calcium, magnesium, and selenium), and vitamins (riboflavin, vitamin B12, and pantothenic acid). Mammals can get both nutrients and immunity from milk. Food undergoes drastic changes during fermentation. Milk fermented with L. paracasei has proven to have an ACE2 inhibitory effect (55), and it also has an anti-inflammatory effect. However, the study did not clarify the mechanism of action of peptides against SARS-CoV-2.
Milk’s whey protein contains high levels of mucin, lactoferrin, and α-lactalbumin. It was discovered that α-lactalbumin stops the S protein of SARS-CoV-2 from attaching to ACE2, therefore preventing the virus from entering the host (46). Lactoferrin and α-lactalbumin have demonstrated significant efficacy in preventing infection with SARS-CoV-2. The antiviral properties of whey proteins have attracted researchers’ attention (56). β-lactoglobulin-derived animal protein peptides are a type of lactoferrin. Çakır et al. (47) utilized in silico approaches to demonstrate β-lactoglobulin-derived peptides ALMPHIR and IPAVFK can bind to Mpro. It was found that ALMPHIR and IPAVFK may interfere with viral protease and viral binding. Therefore, β-lactoglobulin derived peptides may have antiviral properties. In conclusion, additional antiviral peptides may exist in lactoferrin and α-lactalbumin in milk. According to these results, breast milk is recommended for the development of anti-COVID-19 medications. Meanwhile, these studies have contributed to an in-depth investigation of SARS-CoV-2.
The information above indicates that milk-derived peptides are effective in stopping SARS-CoV-2 from invading the human body. In addition to mucin, lactoferrin, and α-lactalbumin, peptides derived from β-lactoglobulin from animal protein sources have also been found to exhibit anti-SARS-CoV-2 properties. Thus, it is considered that peptides prepared from other breast milk proteins may have similar characteristics. Milk’s strong nutritional value improves human health while its antiviral properties provide immune protection. However, people who are prone to allergies should still be cautious of milk consumption because it contains several allergens. Notably, when those allergens undergo enzymolysis or other degradation processes, they are much less likely to trigger allergic reactions. Utilizing milk as the primary animal protein source for the development of a SARS-CoV-2 inhibitory peptide is advantageous.
4.2 Venom
Approximately 15% of known creatures on earth are venomous. Venom contains peptides and enzymes that can help fight cancer and alleviate autoimmune illnesses (57). Venom serves as a source of new molecules that can be used for drug discovery and development, and other physiological purposes (58). In the process of studying snake venom-derived peptides, it was found that they contain substances that can fight against SARS-CoV-2. Eberle et al. (48) used crotamine derivative L-peptides (L-CDP) derived from rattlesnake venom to attack SARS-CoV-2. Cell experiments showed that its inhibition rate against SARS-CoV-2 was 80% when the concentration of CDP1 and CDP7 was lower than 2 μM. It has been found that both CDP1 and CDP7 possess the ability to combat SARS-CoV-2. However, their mechanism of action is still unclear.
In addition to blocking the pathway of viral entry, affecting the replication and transcription of the virus is also one of the strategies adopted to prepare antiviral inhibitors. Enayathullah et al. (49) found that bee venom peptide can effectively reduce SARS-CoV-2 activity, with a LogEC50 value of 2.826 corresponding to 0.656 μg. Proteomic analysis showed that when treated with bee venom peptide, SARS-CoV-2 infected cells downregulate S protein and ORF1ab. ORF1a/ORF1ab are associated with viral proteases that mediate intracellular replication and transcription after SARS-CoV-2 enters the body. Overall, the anti-viral properties of bee venom peptides have been verified.
Venom peptides have two distinct effects: hindering the multiplication of viruses that have successfully infected the host organism, and preventing SARS-CoV-2 from attaching to host receptors. Venom peptides are very attractive material candidates for therapeutic medicines because of their unique selectivity, stability, and exceptional potency in regulating their molecular targets (59). Technological advances like high-throughput screening (60) methods and computational approaches have facilitated the development of tools used to simulate the interaction between venom peptides and their molecular targets. Thanks to those technological advances, the process of identifying specific venom peptides is now less expensive and more predictable, and the research on venom peptides and their antiviral capabilities have been promoted. In the future, a significant number of venom peptides are expected to be discovered.
4.3 Fish
According to recent studies, the protein content of most fish exceeds 15%, or 30–50% of the daily protein intake required for humans. Fish provides 75% of the animal protein intake each day (61). Fish that live in cold water have high concentrations of essential amino acids, including arginine, glycine, and lysine. Those essential amino acids can be easily absorbed by humans and are used to cure and prevent diseases (62). Many studies have been conducted on the functional activities of fish-derived peptides, such as anti-bacterial (63), blood pressure-lowering, and anti-bacterial (64). Nevertheless, research on the anti-SARS-CoV-2 effect of fish-derived peptides has been rarely reported.
In recent years, the COVID-19 epidemic has prompted more researchers to conduct research on the anti-SARS-CoV-2 effect of fish-derived peptides. Researchers have found antiviral peptides in tuna bones. Yu et al. (50) found that when combined with Mpro, the peptide E-M extracted from tuna protein hydrolysate can prevent the polyprotein cleavage of SARS-CoV-2. Also, they found that E-M competes with SARS-CoV-2 for ACE2 binding and shows high binding stability. E-M prevents SARS-CoV-2 from binding to ACE2, thus preventing the invasion of SARS-CoV-2 and achieving an antiviral effect.
PTR (PTR = 372.2115 Da), one of the four antiviral peptides discovered by Yathisha et al. (51), exhibits the greatest efficacy. Through molecular docking and molecular dynamics techniques, it was found that PTR from ribbonfish binds to SARS-CoV-2 3CLpro by establishing hydrogen bonds with residues Lys5, Gln127, Lys137, and Gly138. When comparing the binding energies, it was found that the binding ability of PTR was consistently higher than that of remdesivir in all cases. This observation indicates that PTR possesses potential antiviral properties. Nevertheless, studies on cells and animals have not confirmed the precise mechanism of action.
Fish processing generates a large amount of waste like fish skin, bones, and offal, which accounts for more than 60% of the biomass of processed fish (65). Waste accumulation leads to environmental contamination; therefore, waste management is important for not only better resource use and greater economic value of fish but also sustainable economic growth. The finding that animal protein-derived peptides from tuna and ribbonfish can suppress SARS-CoV-2 suggests that fish is a potential raw material for the preparation of antiviral peptides. The high nutritional content and other functional properties of fish-derived peptides make them suitable candidates for nutritional supplements with antiviral properties. Products derived from fish may exhibit an inherent odor, commonly known as a fishy smell. However, this issue can be effectively addressed through the deodorization process, which involves the combined use of physical, chemical, and biological techniques (66). However, fish-derived antiviral peptides are yet to be validated in vivo.
4.4 Other sources
Defensin peptide is one of the anti-microbial peptides found in the epithelial cells of vertebrates (67). Although defensin peptides can be classified as antimicrobial peptides, they also possess extensive antiviral properties (68).
Several attempts have been made to investigate the possibility of producing antiviral peptides by using positively charged defensin peptides from animal protein sources. In a study conducted by Zhao et al. (52), researchers examined the inhibitory effects of a modified positively charged mouse β-defensin-4 peptide (P9R) on the replication of SARS-CoV-2. P9R was found to be able to bind directly to the virus and thus exert inhibitory effects on the virus. Positive charges can inhibit endosomal acidification. Consequently, P9R effectively prevented endosomal acidification of the virus in the host, which prevented viral multiplication. Based on the research result, Zhao et al. then conducted a more intensive study on defensin peptides in another study. They found that modified frog-defensin-derived basic peptide (FBP) produced similar results, that is, modified FBP was found to prevent viral replication in the lungs of hamsters infected with SARS-CoV-2 (53).
The experiments showed that the introduction of positive charges inhibits acidification in the host body, and that animal protein-derived peptides bind to SARS-CoV-2. In this way, SARS-CoV-2 entry into the host cell by endocytosis is prevented, thereby mitigating the risk of infection. Modified positively charged functional peptides of animal origin block the way of viral entry into the human body to prevent SARS-CoV-2 infection, which demonstrates the role of animal protein-derived peptides in the field of antivirals. They act as an inhibitor to interfere with the viral invasion mechanism. The interaction between SARS-CoV-2 and peptides from animal protein sources can be identified and validated using fluorescence staining microscopy alone; however, a detailed examination of the specific binding sites of these peptides and SARS-CoV-2 is needed.
Moreover, it has been found that human nasal, upper respiratory, and oral mucosa contain the host defense peptide human β-defensin 2 (hBD-2), which possesses antiviral characteristics. Through molecular docking studies, Zhang (54) found that at a concentration of 12.8 μM, the recombinant hBD-2 exhibited an inhibition rate of 80% on the S protein. This suggests that hBD-2 can play an important role in the fight against COVID-19. If the expression or structure of hBD-2 is modified by added compounds, it is expected to exhibit greater antiviral efficacy. hBD-2 can be administered from the respiratory tract to block SARS-CoV-2 and is expected to become an antiviral agent.
In summary, a variety of animal-derived defensins have anti-SARS-CoV-2 effects. Defensins derived from animals are unique from the perspective of virus binding to receptor proteins. Moreover, they are distinct in that they can inhibit endosomal acidification to stop the virus from entering the body by altering the intracellular pH through the introduction of peptides and positive charges. The broad-spectrum antiviral action of defensins challenges the established knowledge of antimicrobial peptides, thereby opening up new research possibilities. Peptides from animal proteins have a wide variety of functional characteristics. Many researchers are interested in their possible anti-SARS-CoV-2 effect.
According to most recently published literature, few raw materials that can be used to prepare SARS-CoV-2 inhibitory peptides have been identified. As far as we know, the most affordable, accessible, and safe ingredient is milk. Milk-derived SARS-CoV-2 inhibitory peptides have been validated by cellular tests and has passed molecular docking predictions, thereby offering some theoretical support. Milk may be an important material source for the preparation of SARS-CoV-2 inhibitory peptides from animal protein sources. A small number of animal protein sources are available for the preparation of SARS-CoV-2 inhibitory peptides so far. Fewer studies have investigated the inhibition of viral replication; most literature focuses on blocking ACE2 or stopping ACE2 from binding to RBD. No research has been done on the relationship between animal protein-derived SARS-CoV-2 inhibitory peptides and Nsps. Nsps not only contribute to the spread of viruses but also enable viruses to evade the immune system, which needs to be tackled with in the battle against SARS-CoV-2. Research on SARS-CoV-2 inhibitory peptides of animal origin has been included in some papers on animal studies, cell analysis, bioavailability, and simulated digestion. Additionally, the relationship between Nsps and SARS-CoV-2 inhibitory peptides is unclear. Overall, SARS-CoV-2 inhibitory peptides derived from animal proteins have not been thoroughly investigated.
More in-depth research places new and higher demands on cost, raw materials, time, and error toleration. By using computational biology, the search for SARS-CoV-2 inhibitory peptides of animal origin can be carried out on a broader basis and from a higher perspective. Meanwhile, it helps reduce waste of time, money, and raw materials and increase error toleration.
5 Development of computational biology for SARS-CoV-2 inhibitory peptides from animal protein sources
Research on animal protein derived peptides involves preparation, extraction, separation, purification, and finally in vitro and in vivo experiments to verify the mechanism of action. With the development of science and technology, most of the results mentioned above can be obtained by employing computational biology based on known peptide sequences, enzymes, etc.
5.1 Computer-aided drug design methods
Computer-aided drug design applies computer software and chemical simulation techniques to predict drug-target interactions, discover novel drugs, and evaluate drug safety (69). As a computer-aided drug design approach, the bioinformatics approach can be used to perform virtual digestion and virtual screening, so as to obtain the target peptide, predict its efficacy, and search for the site of action. The overall workflow of computer-aided drug design in the research on SARS-CoV-2 inhibitory peptides of animal origin is as follows (70).
5.1.1 Bioinformatics approaches
Currently, there are different kinds of peptide databases, such as the antihypertensive peptide database AHTPDB (71), the bioactive peptide database BIOPEP-UWM (72), the food protein database FeptideDB (73), the milk database MBPDB and MilkAMP (74), the fermented food database FermFooDB (75), and the insect antimicrobial peptides database APD3 (76). These databases offer useful tools and reliable sources of information for subsequent prediction of the physicochemical characteristics of peptides. Nevertheless, some of the most recently identified peptides might not be included in the databases due to information incompleteness. The creation of those databases facilitates subsequent molecular modeling and quantitative structure–activity relationships (QSAR) modeling.
Computer-simulated digestion can simulate the digestive reactions of peptides. Simulated digestion predicts theoretically generated peptides based on the potential biological activity of peptides released from proteins in the presence of specific enzymes. First, the sequences of the proteins involved are obtained from Universal Protein (Uniprot) and National Center for Biotechnology Information (NCBI). Next, software programs or web servers, like PeptideCutter, FeptideDB, Peptide Cutter, and BIOPEP-UWM, are used to simulate digestion. Villadóniga et al. (77) used computer-simulated gastrointestinal digestion to determine the stability of peptides produced from enzymatically digested α-lactalbumin in the gastrointestinal tract. However, computer simulation is still not perfect. Computer simulation outcomes may differ from the results obtained from real tests. Computer simulation represents ideal settings. In real tests, some temperature and pH values will change since real test conditions are not exactly the same as simulation conditions.
In addition to simulating gastrointestinal digestion, Web servers can be used to predict absorption, distribution, metabolism, excretion, and toxicity (ADMET). Web servers include Discovery Studio (DS) 2017 (78), admetSAR (79), etc. Chen et al. (78) isolated and purified peptides from eggs and then used databases to screen for ACE-inhibitory peptides. ADMET prediction was then performed using admetSAR to select peptides that met the ADMET criteria for further study. Bioinformatics approaches can be used to investigate the properties of novel bioactive peptides. PeptideRanker scores and ranks bioactive peptides based on their binding structure–activity relationships, with the score ranging from 0 to 0.8. Specifically, 0 represents the lowest bioactivity while 0.8 is the highest. For example, Wei et al. (80) scored 16 functional peptides found in fermented cheese using PeptideRanker, and all scored higher than 0.4. Relative molecular mass, isoelectric point, net charge, hydrophobicity index, enzyme-cleaved electron protein, and other parameters can be calculated using Expasy-PI/MW, PepDraw (81), and ExPASy-Peptidecutter (82). Peptide targets can be predicted using SwissTarget Prediction (83).
Virtual screening methods mainly fall into two categories: the ligand-based approach and the structure-based approach. Ligand-based methods, such as the QSAR method, focus on peptides that are already known to be biologically active. On the other hand, structure-based methods, like molecular docking, rely on the knowledge of the ligand structure and the receptor structure (70).
5.1.2 Quantitative structure–activity relationships
Computer-aided drug design involves the utilization of quantitative structure–activity relationships (QSAR). QSAR correlates predictions based on chemical data and linear or nonlinear correlations between the observed values of molecular properties or biological activities and the calculated values of molecular structures (84).
The science of teaching computers to think and behave like humans, known as artificial intelligence (AI), is to help computers learn automatically based on real-world facts and knowledge (85). The key to realizing AI is machine learning (ML), which is a data-accessing subset of AI. ML uses data access and trend analysis to create actionable insights. Moreover, ML involves techniques like random forests, support vector machines, deep learning, neural networks, matrix, etc. (86).
Conventional QSAR models are limited to a single kind of prediction. These days, QSAR models are being used in combination with AI approaches. To improve prediction performance, most QSAR models combine multitasking templates with AI technologies. Their neural network-based techniques can simultaneously predict related targets in the same or different species as well as the same target under diverse experimental conditions (87). Grant (88) used neural networks in conjunction with cryo-electron microscopy pictures to capture molecular motions and comprehend the dynamics of proteins. Utilizing ML scoring matrices, comparative sequencing, and phylogenetic trees, four SARS-CoV-2 inhibitory peptides were found to block Mpro activity (89). Elend et al. (90) improved the quantity and caliber of Mpro inhibitors found through SARS-CoV-2 screening by training a neural network model and evolutionary algorithms on a library of drug-like chemicals.
5.1.3 Molecular docking and molecular dynamics simulation
Molecular docking criteria for the evaluation of ligand-receptor binding modes are based on conformational sampling and high/low binding affinity scoring functions (91). During docking, a superior ligand is selected by sampling various locations, orientations, and conformations of ligands related to the receptor. Moreover, ligand-receptor binding free energy is another factor for judgment. Though it is not entirely clear how to obtain specific bioactivity values from the association constants (Ka) and dissociation constants (Kd), the binding energy produced out of molecular docking is directly related to bioactivity values (92). This issue is compensated by molecular dynamics simulation. Based on a quantum chemical approach, molecular dynamics simulation explicitly deals with all or some of the electrons at the quantum mechanical level, thereby determining energies and molecular geometries of high precision. Molecular dynamics simulation of the ligand-receptor relationship is the last hurdle in the screening process (93). Available tools are Auto Dock, Dockey, Openeye, Mosoft, etc. (94). Gromacs and VMD are also used in molecular dynamics simulation (95).
Angiotensin-converting enzyme 2 is one of the most commonly used targets in SARS-CoV-2 inhibitory peptide studies. ACE2 causes blood pressure to drop (96). SARS-CoV-2 infection requires the binding of S protein to ACE2 in the cell membrane. ACE2 acts as a cellular receptor for viral entry (97). Cao et al. (98) used human ACE2 residues, rotamer interaction field docking, and large in silico miniprotein libraries to designed LCB1 and LCB3. They characterized the LCB1-LCB3 binding structure and the RBD-LCB3 binding structure by cryo-electron microscopy. And the force of action of LCB1 and LCB3 is similar to that of ACE2. The IC50 of LCB1 and LCB3 against SARS-CoV-2 was 23.540 and 48.100 pM, respectively. LCB1 and LCB3 were found to be six times more effective against SARS-CoV-2 than common antibodies. Miniature protein conjugates can be used to produce spray and aerosol agents, which can be added to existing delivery methods. Gouda et al. (99) and Basit et al. (100) designed an ACE2-derived peptide that binds well to SARS-CoV-2 in molecular docking, with a binding energy of 154.5 and 13.2 kcal/mol, respectively. Based on the human ACE2 sequence and the RBD region of the S protein of SARS-CoV-2 or SARS-CoV, Chen et al. (101) created a series of peptides, two of which performed well: AYp28 (KKKKKKKVEGFNCYFPLQS) and AYn1 (KKKKKKDKFNHEAEDLFY). In their computational simulations of peptide-protein interactions, the two peptides demonstrated a strong binding affinity to S protein and ACE2. The AYp28 peptide was shown to bind to ACE2 in cellular experiments, which resulted in a drop in intracellular levels of markers such as TMPRSS. Experiments on animals have also shown that AYp28 and AYn1 can inhibit viral invasion. These four studies suggest that ACE2-derived peptide mimics are an established approach against SARS-CoV-2 and provide new opportunities for COVID-19 treatment.
Mpro is one of the key enzymes associated with viral gene expression and replication, making it a target of anti-SARS-CoV-2 drugs (102). Hemmati and Tabein (103) obtained two peptides as potent agents: RKGCPPH derived from the desert locust, and NPCACFRNY derived from the assassin bug. They bind more tightly and more stably to MPro. The binding sites of the two antiviral peptidesto MPro are similar to those of lopinavir and ritonavir, two effective anti-SARS-CoV-2 medications. It was reported that four SARS-CoV-2 inhibitory peptides derived from antimicrobial peptides bind to Domain 2 and Domain 3 of SARS-CoV-2 MPro (104). These findings suggest that antiviral peptides targeting Mpro can also be screened using molecular docking and molecular dynamics simulation.
The genes encoding the 5′ proximal two-thirds of the SARS-CoV-2 RNA genome are translated into pp1a and pp1ab. These polyproteins are cleaved by MPro and PLPro to form 16 non-structural proteins (Nsp1-16) (105), Nsps are important for viral replication. Alomair et al. (106) used neural networks and molecular dynamics simulation to predict the effect of phosphorylation on Nsps. Root mean square fluctuation values showed that phosphorylation has an impact on Nsp1-6 and Nsp16. Residues Gly281, Lys430, Pro434, and Phy 437 on Nps13 structural domain 1A and Tyr457, Asp458, and Lys460 on Nps13 structural domain 2A were identified by Raubenolt et al. (107) as potential active sites for the Nps13 binding pocket. Finding these Nsps active sites makes it easier to find and study SARS-CoV-2 inhibitory peptides against Nsps targets in the future. Currently, there are a limited number of studies on animal protein peptides related to MPro and Nsps; more articles focus on the mechanism of Nsps in the process of SRAS-CoV-2 invasion into body. Nsps inhibitory peptides will become a major hotspot in the field of bioactive peptide research. With the advancement of technology, the size and diversity of data have increased dramatically, and more convenient and accurate research methods are imminent. The emergence of bioinformatics has expanded the database and laid the foundation for computer-aided drug design. The development of AI has continued to advance, contributing to the development of computer-aided drug design. Several articles have mentioned the use of AI in combination with molecular docking, molecular dynamics simulation, and QASR to improve the speed and quality of SARS-CoV-2 inhibitory peptides screening.
Computer-aided drug design offers a time-saving alternative to conventional techniques for research on antiviral peptides. With scientific and technological advancements, it is expected to be increasingly applied in experimental research. Nevertheless, researchers should possess specialized expertise and adhere to rigorous experimental designs, so as to validate the outcomes obtained through bioinformatics approaches. Notably, the assessment of novel potential drugs should not solely rely on simulation results. Bioinformatics approaches are capable of effective antiviral peptide screening, clarifying the mechanism of action, and intelligently designing food-derived peptides suitable for nutraceutical, cosmeceutical, and pharmaceutical use.
6 Phage display
Phage display was invented in 1981 (108). It is a type of genetic modification technique, in which genetic modification of phages enables the target gene to bind to the phage shell protein, so that the corresponding fragment can be expressed on the phage surface when the phage is infected (109). Researchers found that phages have several advantages. For example, phage genome can accommodate larger exogenous DNA fragments; the transformation of phage is high; phage genome is convenient for DNA recombination and genetic manipulation; the expression level of phage fusion proteins is easy to control and regulate; and phages are genetically stable (110). With the development and wide application of phage display, a phage display library has been established, paving the way for subsequent research and experiments. These advantages make phage display a widely used tool in bioengineering research, such as screening of ligands, development of new drugs, vaccine design, disease diagnosis, and delivery of targeted drugs (111). Since the COVID-19 outbreak, researchers have been actively searching for anti-SARS-CoV-2 vaccines. Phages play an important role in related studies. Gaynor et al. (112) utilized phage display library screening to discover small-molecule inhibitors. They found a multivalent bicyclic peptide that acts on the S protein of SARS-CoV-2. A multivalent bicyclic peptide is a thioether-bonded bicyclic peptide formed by three cysteines that are cyclized in situ pairing with an amido-PEG10-triazolyl linker. Multivalent bicyclic peptides inhibit SARS-CoV-2 replication and cell–cell fusion, as demonstrated in animal studies. Pang et al. (113). used a phage display approach that combined non-amplification panning and single-amplification panning to identify the phage displaying the cyclic heptapeptide ACLDWLFNSC. This phage binds specifically to the S protein of SARS-CoV-2 and is highly stable.
Phage display can reveal the linkage between a molecule and the DNA sequence encoding the gene of the displayed molecule, and determine the amino acid sequence of the binding agent produced by the inserted fragment. These advantages contribute to the application of phage display in various fields, ranging from material utilization to diagnostics to the development of novel therapeutic drugs.
7 Drug delivery methods for SARS-CoV-2 inhibitory peptides from animal protein sources
Animal protein-derived SARS-CoV-2 inhibitory peptides have anti-COVID-19 effects. However, some drawbacks remain. Animal protein-derived SARS-CoV-2 inhibitory peptides have a short half-life. This is mostly due to the fact that enzymes can easily hydrolyze peptides (114). Enzymes in the oral cavity and gastrointestinal tract readily break down bioactive peptides when they are administered orally (115). The drug distribution method makes up for this deficiency. Bioactive peptides can be delivered using a range of techniques, including intramuscular, subcutaneous, and intravenous injections (116). However, these delivery routes cannot guarantee that the bioactive peptides can reach the designated site. Therefore, various carriers have been created to facilitate the effective delivery of bioactive peptides. The drug delivery system based on those carriers can reduce the side effect on healthy cells, increase stability, extend the half-life of bioactive peptides, maintain a steady and effective blood concentration of bioactive peptides, and guarantee that the drug is concentrated in the target tissue (117).
An artificial cell membrane polymer is a self-assembling nanoscale vesicle that can be efficiently absorbed by dendritic cells. Containing medications and antibodies, an artificial cell membrane polymer enables delivery to certain tissue targets (118). The medication in a polymer induces the body to modify the immune response, so as to provide the desired immunological impact when it is absorbed by the dendritic cells. Polypeptide vaccines are a good fit for this delivery method. In addition, there are other novel carriers. Biodegradable polymer micro-cells are stable, biocompatible, non-toxic, biodegradable, and injectable. Also, they can continuously provide encapsulated antigen by delaying its release (119). However, the organic compounds used to create biodegradable mono-polymer microspheres may have an adverse effect on the activity of bioactive peptides. In pharmacodynamic simulations and cell experiments for SARS-CoV-2 infection, supermolecular polymer nanoparticle hydrogel have been found to perform well. It can enhance the stability and biocompatibility of protein medications in the body (120). However, the hydrogel and the cell have an erratic osmotic relation, thereby degrading the protein and releasing the enclosed medicine. Lungs are most severely affected by SARS-CoV-2, primarily due to respiratory infection. Additionally, there is a novel method of preventing the virus from entering the lungs through the nose. LCB1, which is a SARS-CoV-2 inhibitor, can bond with trehalose and L-leucine to form ultra-thin particles. This mode of delivery enables SARS-CoV-2 inhibitory peptides to directly act on target organs without destroying the polymeric structure, contributing to the development of potential anti-SARS-CoV-2 drugs (121). It has been demonstrated that LCB1 peptides in dry powder form are effective in fighting the SARS-CoV-2 Delta variant but ineffective in combating the Omicron strain.
The effectiveness of the delivery of SARS-CoV-2 inhibitory peptides from animal protein sources is significantly influenced by the carrier’s stability and inclusivity. The application of bioactive peptides is further expanded by injectable vectors. However, different carriers have different drawbacks. The key to developing new drugs is to find appropriate carriers for those SARS-CoV-2 inhibitory peptides. Future research is likely to focus heavily on combining carriers with SARS-CoV-2 inhibitory peptides of animal origin.
8 Application prospects and challenges of SARS-CoV-2 inhibitory peptides from animal protein sources
Few papers published so far have focused on SARS-CoV-2 inhibitory peptides produced from animal proteins, leaving great room for future research on these peptides.
Researchers in the fields of health care and skin care have taken notice of the broad activity of animal protein-derived peptides (122). There is great potential for anti-SARS-CoV-2 pharmacological developments. The pharmaceutical industry is interested in further research on SARS-CoV-2 inhibitory peptides because of their non-toxicity, low cost, and targeting properties (123). SARS-CoV-2 inhibitory peptides derived from animal proteins are potential candidates for COVID-19 medications (15). Besides, the peptides’ nutrition and additional properties, including anti-inflammatory, antibacterial, and antioxidant properties, benefit patients’ quicker recovery, and dietary replenishment.
At the moment, milk, venom, and fish are the primary material sources of SARS-CoV-2 inhibitory peptides of animal origin. SARS-CoV-2 inhibitory peptides from milk sources are more effective and can prevent the main target from SARS-CoV-2 infection. The primary sites of SARS-CoV-2 infection in the human body should be studied in research on novel SARS-CoV-2 inhibitory peptides from animal protein sources. Additionally, more research should be conducted on the mechanism and activity of SARS-CoV-2 inhibitory peptides. The conventional approach to identifying SARS-CoV-2 inhibitory peptides from animal proteins is expensive and time-consuming. Phage display technology and computer-aided drug design can save time, but they still require experimental verification, and the validity of experimental outcomes should be considered (124). Peptide databases are the foundation of computer-aided drug discovery, and related research is based on increasing the quantity of peptides and expanding related data in these databases. Few articles have addressed the need for animal and clinical trials to confirm the safety of polypeptide-based agents in humans before incorporating SARS-CoV-2 inhibitory peptides of animal origin into drug therapies. The animal proteins used to create SARS-CoV-2 inhibitory peptides can be readily degraded by enzymes, which prevents polypeptides from accumulating in the human body and smoothly reaching the intended target position, affecting the medication’s impact. Encapsulated peptides not only show greater stability by adherence to carriers but also increases the efficiency of injectable delivery compared with the conventional oral administration method. Nevertheless, further experimental research is necessary to identify vectors that interact appropriately with polypeptides (125). The difficulties in the identification, preparation, delivery, and validation of SARS-CoV-2 inhibitory peptides of animal origin are yet to be solved.
9 Conclusion
This review includes a thorough analysis of the SARS-CoV-2 infection mechanism, the use of animal proteins as a raw material for the preparation of SARS-CoV-2 inhibitory peptides, computer-aided design, phage display technology, and drug administration methods. Multiple intervention targets that could be used to slow the spread of SARS-CoV-2 have been found by researchers, including Nsps, ACE2, and SARS-CoV-2 proteases like MPro and PLPro. Nonetheless, the research on the relationship between Nsps and inhibitory peptides produced from animal proteins is insufficient.
Animal protein-derived SARS-CoV-2 inhibitory peptides can be prepared using fish, venom, and milk. It was found that milk has the greatest potential to produce SARS-CoV-2 inhibitory peptides. Using computer-aided drug design technologies, new SARS-CoV-2 inhibitory peptides of animal origin can be tested, and the relationship between SARS-CoV-2 and the inhibitory peptides can be examined. The combination of AI and QSAR analysis has great potential to accelerate the identification of novel SARS-CoV-2 inhibitory peptides. These techniques can theoretically facilitate the discovery of SARS-CoV-2 inhibitory peptides made from animal proteins. New peptides that can inhibit SARS-CoV-2 can be found using the phage display method. Drug delivery techniques contribute to effective in vivo distribution of SARS-CoV-2 inhibitory peptides derived from animal proteins. These techniques have allowed for continuous optimization and development of SARS-CoV-2 inhibitory peptides of animal origin, opening up new possibilities for COVID-19 research and development. These results suggest that peptides made from animal proteins have great potential for protecting human health. Peptide safety and efficacy are expected to be enhanced in the near future with the development of drug delivery techniques.
The paper puts forward some suggestions for future research on animal protein-derived SARS-CoV-2 inhibitory peptides: (1) The interaction between Nsps and SARS-CoV-2 inhibitory peptides of animal origin should be investigated. (2) The viability of combining different AI techniques with computer-aided drug design should be examined. (3) The phage display technology should be used to investigate SARS-CoV-2 inhibitory peptides from animal protein sources. (4) Appropriate vectors for animal protein-derived SARS-CoV-2 inhibitory peptides should be identified for drug development.
Author contributions
XK: Writing – original draft, Writing – review & editing. WW: Writing – original draft, Writing – review & editing. YiZ: Writing – original draft, Writing – review & editing. NW: Writing – original draft, Writing – review & editing. KB: Writing – original draft, Writing – review & editing. YW: Writing – original draft, Writing – review & editing. QQ: Writing – original draft, Writing – review & editing. YuZ: Writing – original draft, Writing – review & editing. XL: Writing – original draft, Writing – review & editing. JX: Writing – original draft, Writing – review & editing.
Funding
The author(s) declare financial support was received for the research, authorship, and/or publication of this article. This study was financially supported by Zhejiang Provincial Natural Sciences Foundation of China under Grant (No. LZ22C200006), Top young talents of the ten thousand talents program of Zhejiang Province (ZJWR0308016), and Zhejiang Basic Public Welfare Research Project (No. LGN22C200024).
Acknowledgments
We would like to thank all contributors of the current study for their concepts, ideas, contribution, and provision.
Conflict of interest
The authors declare that the research was conducted in the absence of any commercial or financial relationships that could be construed as a potential conflict of interest.
Publisher’s note
All claims expressed in this article are solely those of the authors and do not necessarily represent those of their affiliated organizations, or those of the publisher, the editors and the reviewers. Any product that may be evaluated in this article, or claim that may be made by its manufacturer, is not guaranteed or endorsed by the publisher.
References
1. Hoffmann, M , Kleine-Weber, H , Schroeder, S , Kruger, N , Herrler, T , Erichsen, S, et al. SARS-CoV-2 cell entry depends on ACE2 and TMPRSS2 and is blocked by a clinically proven protease inhibitor. Cell. (2020) 181:271–280.e8. doi: 10.1016/j.cell.2020.02.052
2. Rani, P , Kapoor, B , Gulati, M , Atanasov, AG , Alzahrani, Q , and Gupta, R . Antimicrobial peptides: a plausible approach for COVID-19 treatment. Expert Opin Drug Discov. (2022) 17:473–87. doi: 10.1080/17460441.2022.2050693
3. Kehinde, BA , and Sharma, P . Recently isolated antidiabetic hydrolysates and peptides from multiple food sources: a review. Crit Rev Food Sci Nutr. (2018) 60:322–40. doi: 10.1080/10408398.2018.1528206
4. Chen, J , Yu, X , Chen, Q , Wu, Q , and He, Q . Screening and mechanisms of novel angiotensin-I-converting enzyme inhibitory peptides from rabbit meat proteins: a combined in silico and in vitro study. Food Chem. (2022) 370:131070. doi: 10.1016/j.foodchem.2021.131070
5. Kim, D , Kim, J , An, S , Kim, M , Baek, K , Kang, BM, et al. In vitro and in vivo suppression of SARS-CoV-2 replication by a modified, short, cell-penetrating peptide targeting the C-terminal domain of the viral spike protein. J Med Virol. (2023) 95:e28626. doi: 10.1002/jmv.28626
6. Sangtanoo, P , Srimongkol, P , Saisavoey, T , Reamtong, O , and Karnchanatat, A . Anti-inflammatory action of two novel peptides derived from peanut worms (Sipunculus nudus) in lipopolysaccharide-induced RAW264.7 macrophages. Food Funct. (2020) 11:552–60. doi: 10.1039/c9fo02178g
7. Taylor, G , Frommherz, Y , Katikaridis, P , Layer, D , Sinning, I , Carroni, M, et al. Antibacterial peptide CyclomarinA creates toxicity by deregulating the Mycobacterium tuberculosis ClpC1-ClpP1P2 protease. J Biol Chem. (2022) 298:102202. doi: 10.1016/j.jbc.2022.102202
8. Pierre, JF , Peters, BM , La Torre, D , Sidebottom, AM , Tao, Y , Zhu, X, et al. Peptide YY: a Paneth cell antimicrobial peptide that maintains Candida gut commensalism. Science. (2023) 381:502–8. doi: 10.1126/science.abq3178
9. Lafferty, RA , Flatt, PR , and Irwin, N . Emerging therapeutic potential for peptide YY for obesity-diabetes. Peptides. (2018) 100:269–74. doi: 10.1016/j.peptides.2017.11.005
10. Deng, Z , Yang, Z , and Peng, J . Role of bioactive peptides derived from food proteins in programmed cell death to treat inflammatory diseases and cancer. Crit Rev Food Sci Nutr. (2023) 63:3664–82. doi: 10.1080/10408398.2021.1992606
11. Acquah, C , Chan, YW , Pan, S , Agyei, D , and Udenigwe, CC . Structure-informed separation of bioactive peptides. J Food Biochem. (2019) 43:e12765. doi: 10.1111/jfbc.12765
12. Cao, C , Xiao, Z , Ge, C , and Wu, Y . Animal by-products collagen and derived peptide, as important components of innovative sustainable food systems—a comprehensive review. Crit Rev Food Sci Nutr. (2022) 62:8703–27. doi: 10.1080/10408398.2021.1931807
13. Hou, Y , Wu, Z , Dai, Z , Wang, G , and Wu, G . Protein hydrolysates in animal nutrition: industrial production, bioactive peptides, and functional significance. J Anim Sci Biotechnol. (2017) 8:24. doi: 10.1186/s40104-017-0153-9
14. Bhullar, KS , Nael, MA , Elokely, KM , Drews, SJ , and Wu, J . Structurally modified bioactive peptide inhibits SARS-CoV-2 lentiviral particles expression. Pharmaceutics. (2022) 14:2045. doi: 10.3390/pharmaceutics14102045
15. Zhao, H , To, KKW , Lam, H , Zhang, C , Peng, Z , Meng, X, et al. A trifunctional peptide broadly inhibits SARS-CoV-2 Delta and omicron variants in hamsters. Cell. Discovery. (2022) 8:62. doi: 10.1038/s41421-022-00428-9
16. Zhao, W , Li, X , Yu, Z , Wu, S , Ding, L , and Liu, J . Identification of lactoferrin-derived peptides as potential inhibitors against the main protease of SARS-CoV-2. LWT-Food Sci Technol. (2022) 154:112684. doi: 10.1016/j.lwt.2021.112684
17. Oudit, GY , Wang, K , Viveiros, A , Kellner, MJ , and Penninger, JM . Angiotensin-converting enzyme 2-at the heart of the COVID-19 pandemic. Cell. (2023) 186:906–22. doi: 10.1016/j.cell.2023.01.039
18. Gheblawi, M , Wang, K , Viveiros, A , Nguyen, Q , Zhong, JC , Turner, AJ, et al. Angiotensin-converting enzyme 2: SARS-CoV-2 receptor and regulator of the renin-angiotensin system: celebrating the 20th anniversary of the discovery of ACE2. Circ Res. (2020) 126:1456–74. doi: 10.1161/CIRCRESAHA.120.317015
19. Varga, Z , Flammer, AJ , Steiger, P , Haberecker, M , Andermatt, R , Zinkernagel, AS, et al. Endothelial cell infection and endotheliitis in COVID-19. Circ Res. (2020) 395:1417–8. doi: 10.1016/S0140-6736(20)30937-5
20. Lamers, MM , and Haagmans, BL . SARS-CoV-2 pathogenesis. Nat Rev Microbiol. (2022) 20:270–84. doi: 10.1038/s41579-022-00713-0
21. Wu, F , Zhao, S , Yu, B , Chen, YM , Wang, W , Song, ZG, et al. A new coronavirus associated with human respiratory disease in China. Nature. (2020) 579:265–9. doi: 10.1038/s41586-020-2008-3
22. Yadav, R , Hasan, S , Mahato, S , Celik, I , Mary, YS , Kumar, A, et al. Molecular docking, DFT analysis, and dynamics simulation of natural bioactive compounds targeting ACE2 and TMPRSS2 dual binding sites of spike protein of SARS CoV-2. J Mol Liq. (2021) 342:116942. doi: 10.1016/j.molliq.2021.116942
23. Jackson, CB , Farzan, M , Chen, B , and Choe, H . Mechanisms of SARS-CoV-2 entry into cells. Nat Rev Mol Cell Biol. (2022) 23:3–20. doi: 10.1038/s41580-021-00418-x
24. Cecconi, M , Forni, G , and Mantovani, A . Ten things we learned about COVID-19. Intensive Care Med. (2020) 46:1590–3. doi: 10.1007/s00134-020-06140-0
25. Finkel, Y , Mizrahi, O , Nachshon, A , Weingarten-Gabbay, S , Morgenstern, D , Yahalom-Ronen, Y, et al. The coding capacity of SARS-CoV-2. Nature. (2020) 589:125–30. doi: 10.1038/s41586-020-2739-1
26. Sun, C , Xie, C , Bu, G-L , Zhong, L-Y , and Zeng, M-S . Molecular characteristics, immune evasion, and impact of SARS-CoV-2 variants. Signal transduction and targeted. Therapy. (2022) 7:202. doi: 10.1038/s41392-022-01039-2
27. Schütz, D , Ruiz-Blanco, YB , Münch, J , Kirchhoff, F , Sanchez-Garcia, E , and Müller, JA . Peptide and peptide-based inhibitors of SARS-CoV-2 entry. Adv Drug Deliv Rev. (2020) 167:47–65. doi: 10.1016/j.addr.2020.11.007
28. Mao, B , Le-Trilling, VTK , Wang, K , Mennerich, D , Hu, J , Zhao, Z, et al. Obatoclax inhibits SARS-CoV-2 entry by altered endosomal acidification and impaired cathepsin and furin activity in vitro. Emerg Microb Infect. (2022) 11:483–97. doi: 10.1080/22221751.2022.2026739
29. Minkoff, JM , and tenOever, B . Innate immune evasion strategies of SARS-CoV-2. Nat Rev Microbiol. (2023) 21:178–94. doi: 10.1038/s41579-022-00839-1
30. Bian, J , and Li, Z . Angiotensin-converting enzyme 2 (ACE2): SARS-CoV-2 receptor and RAS modulator. Acta Pharm Sin B. (2021) 11:1–12. doi: 10.1016/j.apsb.2020.10.006
31. Carabelli, AM , Peacock, TP , Thorne, LG , Harvey, WT , Hughes, J , de Silva, TI, et al. SARS-CoV-2 variant biology: immune escape, transmission and fitness. Nat Rev Microbiol. (2023) 21:162–77. doi: 10.1038/s41579-022-00841-7
32. Monteil, V , Kwon, H , Prado, P , Hagelkruys, A , Wimmer, RA , Stahl, M, et al. Inhibition of SARS-CoV-2 infections in engineered human tissues using clinical-grade soluble human ACE2. Cell. (2020) 181:905–913.e7. doi: 10.1016/j.cell.2020.04.004
33. Yang, P-P , Ye, X-W , Liu, M-Q , Yang, J-X , Feng, X-L , Li, Y-J, et al. Entangling of peptide nanofibers reduces the invasiveness of SARS-CoV-2. Adv Healthc Mater. (2023) 12:2300673. doi: 10.1002/adhm.202300673
34. Keller, C , Böttcher-Friebertshäuser, E , and Lohoff, M . TMPRSS2, a novel host-directed drug target against SARS-CoV-2. Signal transduction and targeted. Therapy. (2022) 7:251. doi: 10.1038/s41392-022-01084-x
35. Yuan, S , Gao, X , Tang, K , Cai, J-P , Hu, M , Luo, P, et al. Targeting papain-like protease for broad-spectrum coronavirus inhibition. Protein Cell. (2022) 13:940–53. doi: 10.1007/s13238-022-00909-3
36. Chamata, Y , Jackson, KG , Watson, KA , and Jauregi, P . Whey-derived peptides at the heart of the COVID-19 pandemic. Int J Mol Sci. (2021) 22:1–20.. doi: 10.3390/ijms222111662
37. Zhao, W , Xu, G , Yu, Z , Li, J , and Liu, J . Identification of nut protein-derived peptides against SARS-CoV-2 spike protein and main protease. Comput Biol Med. (2021) 138:104937. doi: 10.1016/j.compbiomed.2021.104937
38. Chourasia, R , Padhi, S , Chiring Phukon, L , Abedin, MM , Singh, SP , and Rai, AK . A potential peptide from soy cheese produced using Lactobacillus delbrueckii WS4 for effective inhibition of SARS-CoV-2 Main protease and S1 glycoprotein. Front Mol Biosci. (2020) 7:601753. doi: 10.3389/fmolb.2020.601753
39. Curreli, F , Victor, SMB , Ahmed, S , Drelich, A , Tong, X , Tseng, C-TK, et al. Stapled peptides based on human angiotensin-converting enzyme 2 (ACE2) potently inhibit SARS-CoV-2 infection in vitro. MBio. (2020) 11:e02451–20. doi: 10.1128/mBio.02451-20
40. He, M , Wang, Y , Huang, S , Zhao, N , Cheng, M , and Zhang, X . Computational exploration of natural peptides targeting ACE2. J Biomol Struct Dyn. (2022) 40:8018–29. doi: 10.1080/07391102.2021.1905555
41. Liu, N , Zhang, Y , Lei, Y , Wang, R , Zhan, M , Liu, J, et al. Design and evaluation of a novel peptide–drug conjugate covalently targeting SARS-CoV-2 papain-like protease. J Med Chem. (2022) 65:876–84. doi: 10.1021/acs.jmedchem.1c02022
42. Shapira, T , Monreal, IA , Dion, SP , Buchholz, DW , Imbiakha, B , Olmstead, AD, et al. A TMPRSS2 inhibitor acts as a pan-SARS-CoV-2 prophylactic and therapeutic. Nature. (2022) 605:340–8. doi: 10.1038/s41586-022-04661-w
43. Heitmann, JS , Bilich, T , Tandler, C , Nelde, A , Maringer, Y , Marconato, M, et al. A COVID-19 peptide vaccine for the induction of SARS-CoV-2 T cell immunity. Nature. (2022) 601:617–22. doi: 10.1038/s41586-021-04232-5
44. Shen, F , Xiong, Z , Wu, Y , Peng, R , Wang, Y , Sun, L, et al. Precise epitope organization with self-adjuvant framework nucleic acid for efficient COVID-19 peptide vaccine construction. Angew Chem Int Ed. (2023) 62:e202301147. doi: 10.1002/anie.202301147
45. Somogyi, E , Kremlitzka, M , Csiszovszki, Z , Molnár, L , Lőrincz, O , Tóth, J, et al. T cell immunity ameliorates COVID-19 disease severity and provides post-exposure prophylaxis after peptide-vaccination, in Syrian hamsters. Front Immunol. (2023) 14:1111629. doi: 10.3389/fimmu.2023.1111629
46. Lai, X , Yu, Y , Xian, W , Ye, F , Ju, X , Luo, Y, et al. Identified human breast milk compositions effectively inhibit SARS-CoV-2 and variants infection and replication. iScience. (2022) 25:104136. doi: 10.1016/j.isci.2022.104136
47. Çakır, B , Okuyan, B , Şener, G , and Tunali-Akbay, T . Investigation of beta-lactoglobulin derived bioactive peptides against SARS-CoV-2 (COVID-19): in silico analysis. Eur J Pharmacol. (2021) 891:173781. doi: 10.1016/j.ejphar.2020.173781
48. Eberle, RJ , Gering, I , Tusche, M , Ostermann, PN , Müller, L , Adams, O, et al. Design of D-amino acids SARS-CoV-2 Main protease inhibitors using the cationic peptide from rattlesnake venom as a scaffold. Pharmaceuticals. (2022) 15:1–20. doi: 10.3390/ph15050540
49. Enayathullah, MG , Parekh, Y , Banu, S , Ram, S , Nagaraj, R , Kumar, BK, et al. Gramicidin S and melittin: potential anti-viral therapeutic peptides to treat SARS-CoV-2 infection. Sci Rep. (2022) 12:3446. doi: 10.1038/s41598-022-07341-x
50. Yu, Z , Kan, R , Ji, H , Wu, S , Zhao, W , Shuian, D, et al. Identification of tuna protein-derived peptides as potent SARS-CoV-2 inhibitors via molecular docking and molecular dynamic simulation. Food Chem. (2021) 342:128366. doi: 10.1016/j.foodchem.2020.128366
51. Yathisha, UG , Srinivasa, MG , Siddappa BC, R , P Mandal, S , Dixit, SR , Pujar, GV, et al. Isolation and characterization of ACE-I inhibitory peptides from ribbonfish for a potential inhibitor of the main protease of SARS-CoV-2: An in silico analysis. Proteins Struct, Funct, Bioinformatics. (2022) 90:982–92. doi: 10.1002/prot.26291
52. Zhao, H , To, KKW , Sze, K-H , Yung, TT-M , Bian, M , Lam, H, et al. A broad-spectrum virus- and host-targeting peptide against respiratory viruses including influenza virus and SARS-CoV-2. Nat Commun. (2020) 11:4252. doi: 10.1038/s41467-020-17986-9
53. Zhao, H , Meng, X , Peng, Z , Lam, H , Zhang, C , Zhou, X, et al. Fusion-inhibition peptide broadly inhibits influenza virus and SARS-CoV-2, including Delta and omicron variants. Emerg Microb Infect. (2022) 11:926–37. doi: 10.1080/22221751.2022.2051753
54. Zhang, L , Ghosh, SK , Basavarajappa, SC , Chen, Y , Shrestha, P , Penfield, J, et al. HBD-2 binds SARS-CoV-2 RBD and blocks viral entry: strategy to combat COVID-19. iScience. (2022) 25:103856. doi: 10.1016/j.isci.2022.103856
55. Paparo, L , Bruno, C , Ferrucci, V , Punto, E , Viscardi, M , Fusco, G, et al. Protective effects elicited by cow milk fermented with L. paracasei CBAL74 against SARS-CoV-2 infection in human enterocytes. J Funct Foods. (2021) 87:104787. doi: 10.1016/j.jff.2021.104787
56. Gallo, V , Giansanti, F , Arienzo, A , and Antonini, G . Antiviral properties of whey proteins and their activity against SARS-CoV-2 infection. J Funct Foods. (2022) 89:104932. doi: 10.1016/j.jff.2022.104932
57. Khalil, A , Elesawy, BH , Ali, TM , and Ahmed, OM . Bee Venom: From Venom to Drug. Molecules. (2021) 26:4941. doi: 10.3390/molecules26164941
58. Lau, M-T , Manion, J , Littleboy, JB , Oyston, L , Khuong, TM , Wang, Q-P, et al. Molecular dissection of box jellyfish venom cytotoxicity highlights an effective venom antidote. Nat Commun. (2019) 10:1655. doi: 10.1038/s41467-019-09681-1
59. Holford, M , Daly, M , King, GF , and Norton, RS . Venoms to the rescue. Science. (2018) 361:842–4. doi: 10.1126/science.aau7761
60. Slagboom, J , Derks, RJE , Sadighi, R , Somsen, GW , Ulens, C , Casewell, NR, et al. High-throughput Venomics. J Proteome Res. (2023) 22:1734–46. doi: 10.1021/acs.jproteome.2c00780
61. Maulu, S , Nawanzi, K , Abdel-Tawwab, M , and Khalil, HS . Fish nutritional value as an approach to Children's nutrition. Front Nutr. (2021) 8:780844. doi: 10.3389/fnut.2021.780844
62. Mohanty, BP , Mahanty, A , Ganguly, S , Mitra, T , Karunakaran, D , and Anandan, R . Nutritional composition of food fishes and their importance in providing food and nutritional security. Food Chem. (2019) 293:561–70. doi: 10.1016/j.foodchem.2017.11.039
63. Abachi, S , Pilon, G , Marette, A , Bazinet, L , and Beaulieu, L . Beneficial effects of fish and fish peptides on main metabolic syndrome associated risk factors: diabetes, obesity and lipemia. Crit Rev Food Sci Nutr. (2023) 63:7896–944. doi: 10.1080/10408398.2022.2052261
64. Valero, Y , Saraiva-Fraga, M , Costas, B , and Guardiola, FA . Antimicrobial peptides from fish: beyond the fight against pathogens. Rev Aquac. (2020) 12:224–53. doi: 10.1111/raq.12314
65. Halim, NRA , Yusof, HM , and Sarbon, NM . Functional and bioactive properties of fish protein hydolysates and peptides: a comprehensive review. Trends Food Sci Technol. (2016) 51:24–33. doi: 10.1016/j.tifs.2016.02.007
66. Wang, X , Le, B , Zhang, N , Bak, KH , Zhang, Y , and Fu, Y . Off-flavour compounds in collagen peptides from fish: formation, detection and removal. Int J Food Sci Technol. (2023) 58:1543–63. doi: 10.1111/ijfs.15962
67. Ganz, T . Defensins: antimicrobial peptides of innate immunity. Nat Rev Immunol. (2003) 3:710–20. doi: 10.1038/nri1180
68. Solanki, SS , Singh, P , Kashyap, P , Sansi, MS , and Ali, SA . Promising role of defensins peptides as therapeutics to combat against viral infection. Microb Pathog. (2021) 155:104930. doi: 10.1016/j.micpath.2021.104930
70. Du, Z , Comer, J , and Li, Y . Bioinformatics approaches to discovering food-derived bioactive peptides: reviews and perspectives. TrAC Trends Anal Chem. (2023) 162:117051. doi: 10.1016/j.trac.2023.117051
71. Kęska, P , Gustaw, W , and Stadnik, J . Trends in in silico approaches to the prediction of biologically active peptides in meat and meat products as an important factor for preventing food-related chronic diseases. Appl Sci. (2021) 11:1–15. doi: 10.3390/app112311236
72. Minkiewicz, P , Iwaniak, A , and Darewicz, M . BIOPEP-UWM database of bioactive peptides: current opportunities. Int J Mol Sci. (2019) 20:1–23. doi: 10.3390/ijms20235978
73. Panyayai, T , Ngamphiw, C , Tongsima, S , Mhuantong, W , Limsripraphan, W , Choowongkomon, K, et al. FeptideDB: a web application for new bioactive peptides from food protein. Heliyon. (2019) 5:e02076. doi: 10.1016/j.heliyon.2019.e02076
74. Nielsen, SD , Purup, S , and Larsen, LB . Effect of casein hydrolysates on intestinal cell migration and their peptide profiles by LC-ESI/MS/MS. Food Secur. (2019) 8:1–11. doi: 10.3390/foods8030091
75. Peredo-Lovillo, A , Hernandez-Mendoza, A , Vallejo-Cordoba, B , and Romero-Luna, HE . Conventional and in silico approaches to select promising food-derived bioactive peptides: a review. Food Chem X. (2022) 13:100183. doi: 10.1016/j.fochx.2021.100183
76. Wang, G , Li, X , and Wang, Z . APD3: the antimicrobial peptide database as a tool for research and education. Nucleic Acids Res. (2015) 44:D1087–93. doi: 10.1093/nar/gkv1278
77. Villadóniga, C , and Cantera, AMB . New ACE-inhibitory peptides derived from α-lactalbumin produced by hydrolysis with Bromelia antiacantha peptidases. Biocatal Agric. Biotechnology. (2019) 20:101258. doi: 10.1016/j.bcab.2019.101258
78. Zhao, W , Zhang, D , Yu, Z , Ding, L , and Liu, J . Novel membrane peptidase inhibitory peptides with activity against angiotensin converting enzyme and dipeptidyl peptidase IV identified from hen eggs. J Funct Foods. (2020) 64:103649. doi: 10.1016/j.jff.2019.103649
79. Yang, H , Lou, C , Sun, L , Li, J , Cai, Y , Wang, Z, et al. admetSAR 2.0: web-service for prediction and optimization of chemical ADMET properties. Bioinformatics. (2019) 35:1067–9. doi: 10.1093/bioinformatics/bty707
80. Wei, G , Zhao, Q , Wang, D , Fan, Y , Shi, Y , and Huang, A . Novel ACE inhibitory, antioxidant and α-glucosidase inhibitory peptides identified from fermented rubing cheese through peptidomic and molecular docking. LWT-food. Sci Technol. (2022) 159:113196. doi: 10.1016/j.lwt.2022.113196
81. Maaroufi, H , Potvin, M , Cusson, M , and Levesque, RC . Novel antimicrobial anionic cecropins from the spruce budworm feature a poly-L-aspartic acid C-terminus. Proteins. (2021) 89:1205–15. doi: 10.1002/prot.26142
82. Jakubczyk, A , Karas, M , Rybczynska-Tkaczyk, K , Zielinska, E , and Zielinski, D . Current trends of bioactive peptides-new sources and therapeutic effect. Food Secur. (2020) 9:1–28. doi: 10.3390/foods9070846
83. Daina, A , Michielin, O , and Zoete, V . SwissTargetPrediction: updated data and new features for efficient prediction of protein targets of small molecules. Nucleic Acids Res. (2019) 47:W357–64. doi: 10.1093/nar/gkz382
84. Muratov, EN , Bajorath, J , Sheridan, RP , Tetko, IV , Filimonov, D , Poroikov, V, et al. QSAR without borders. Chem Soc Rev. (2020) 49:3525–64. doi: 10.1039/d0cs00098a
85. Gopinath, N . Artificial intelligence: potential tool to subside SARS-CoV-2 pandemic. Process Biochem. (2021) 110:94–9. doi: 10.1016/j.procbio.2021.08.001
86. Janiesch, C , Zschech, P , and Heinrich, K . Machine learning and deep learning. Electron Mark. (2021) 31:685–95. doi: 10.1007/s12525-021-00475-2
87. Chellappa, R , and Theodoridis, S . Schaik Av. advances in machine learning and deep neural networks. Proc IEEE. (2021) 109:607–11. doi: 10.1109/JPROC.2021.3072172
88. Grant, T . Neural networks learn the motions of molecular machines. Nat Methods. (2021) 18:869–71. doi: 10.1038/s41592-021-01235-y
89. Kabra, R , and Singh, S . Evolutionary artificial intelligence based peptide discoveries for effective Covid-19 therapeutics. Biochim Biophys Acta Mol Basis Dis. (2021) 1867:165978. doi: 10.1016/j.bbadis.2020.165978
90. Elend, L , Jacobsen, L , Cofala, T , Prellberg, J , Teusch, T , Kramer, O, et al. Design of SARS-CoV-2 Main protease inhibitors using artificial intelligence and molecular dynamic simulations. Molecules. (2022) 27:1–25. doi: 10.3390/molecules27134020
91. Zhou, J , Yang, Z , He, Y , Ji, J , Lin, Q , and Li, J . A novel molecular docking program based on a multi-swarm competitive algorithm. Swarm Evol Comput. (2023) 78:101292. doi: 10.1016/j.swevo.2023.101292
92. Lin, R , Zhang, J , Xu, R , Yuan, C , Guo, L , Liu, P, et al. Developments in molecular docking technologies for application of polysaccharide-based materials: a review. Crit Rev Food Sci Nutr. (2023):1–13. doi: 10.1080/10408398.2023.2200833
93. Damjanovic, J , Miao, J , Huang, H , and Lin, Y-S . Elucidating solution structures of cyclic peptides using molecular dynamics simulations. Chem Rev. (2021) 121:2292–324. doi: 10.1021/acs.chemrev.0c01087
94. Du, L , Geng, C , Zeng, Q , Huang, T , Tang, J , Chu, Y, et al. Dockey: a modern integrated tool for large-scale molecular docking and virtual screening. Brief Bioinform. (2023) 24:1–7. doi: 10.1093/bib/bbad047
95. Deganutti, G , Liang, Y-L , Zhang, X , Khoshouei, M , Clydesdale, L , Belousoff, MJ, et al. Dynamics of GLP-1R peptide agonist engagement are correlated with kinetics of G protein activation. Nat Commun. (2022) 13:1–18. doi: 10.1038/s41467-021-27760-0
96. Jiang, J , Li, MY , Wu, XY , Ying, YL , Han, HX , and Long, YT . Protein nanopore reveals the renin-angiotensin system crosstalk with single-amino-acid resolution. Nat Chem. (2023) 15:578–86. doi: 10.1038/s41557-023-01139-8
97. Tanzadehpanah, H , Lotfian, E , Avan, A , Saki, S , Nobari, S , Mahmoodian, R, et al. Role of SARS-COV-2 and ACE2 in the pathophysiology of peripheral vascular diseases. Biomed Pharmacother. (2023) 166:115321. doi: 10.1016/j.biopha.2023.115321
98. Cao, L , Goreshnik, I , Coventry, B , Case, JB , Miller, L , Kozodoy, L, et al. De novo design of picomolar SARS-CoV-2 miniprotein inhibitors. Science. (2020) 370:426–31. doi: 10.1126/science.abd9909
99. Paul, S , Nadendla, S , and Sobhia, ME . Identification of potential ACE2-derived peptide mimetics in SARS-CoV-2 omicron variant therapeutics using computational approaches. J Phys Chem Lett. (2022) 13:7420–8. doi: 10.1021/acs.jpclett.2c01155
100. Basit, A , Karim, AM , Asif, M , Ali, T , Lee, JH , Jeon, JH, et al. Designing short peptides to block the interaction of SARS-CoV-2 and human ACE2 for COVID-19 therapeutics. Front Pharmacol. (2021) 12:731828. doi: 10.3389/fphar.2021.731828
101. Chen, J , Li, S , Lei, Z , Tang, Q , Mo, L , Zhao, X, et al. Inhibition of SARS-CoV-2 pseudovirus invasion by ACE2 protecting and spike neutralizing peptides: An alternative approach to COVID19 prevention and therapy. Int J Biol Sci. (2021) 17:2957–69. doi: 10.7150/ijbs.61476
102. Yang, H , Yang, M , Ding, Y , Liu, Y , Lou, Z , Zhou, Z, et al. The crystal structures of severe acute respiratory syndrome virus main protease and its complex with an inhibitor. Proc Natl Acad Sci USA. (2003) 100:13190–5. doi: 10.1073/pnas.1835675100
103. Hemmati, SA , and Tabein, S . Insect protease inhibitors; promising inhibitory compounds against SARS-CoV-2 main protease. Comput Biol Med. (2022) 142:105228. doi: 10.1016/j.compbiomed.2022.105228
104. Mahmud, S , Biswas, S , Kumar Paul, G , Mita, MA , Afrose, S , Robiul Hasan, M, et al. Antiviral peptides against the main protease of SARS-CoV-2: a molecular docking and dynamics study. Arab J Chem. (2021) 14:103315. doi: 10.1016/j.arabjc.2021.103315
105. Park, GJ , Osinski, A , Hernandez, G , Eitson, JL , Majumdar, A , Tonelli, M, et al. The mechanism of RNA capping by SARS-CoV-2. Nature. (2022) 609:793–800. doi: 10.1038/s41586-022-05185-z
106. Alomair, L , Mustafa, S , Jafri, MS , Alharbi, W , Aljouie, A , Almsned, F, et al. Molecular dynamics simulations to decipher the role of phosphorylation of SARS-CoV-2 nonstructural proteins (nsps) in viral replication. Viruses. (2022) 14:2436. doi: 10.3390/v14112436
107. Raubenolt, BA , Islam, NN , Summa, CM , and Rick, SW . Molecular dynamics simulations of the flexibility and inhibition of SARS-CoV-2 NSP 13 helicase. J Mol Graph Model. (2022) 112:108122. doi: 10.1016/j.jmgm.2022.108122
108. Smith, GP . Filamentous fusion phage: novel expression vectors that display cloned antigens on the Virion surface. Science. (1985) 228:1315–7. doi: 10.1126/science.4001944
109. Pierzynowska, K , Morcinek-Orłowska, J , Gaffke, L , Jaroszewicz, W , Skowron, PM , and Węgrzyn, G . Applications of the phage display technology in molecular biology, biotechnology and medicine. Crit Rev Microbiol. (2023):1–41. doi: 10.1080/1040841X.2023.2219741
110. Qi, H , Lu, H , Qiu, H-J , Petrenko, V , and Liu, A . Phagemid vectors for phage display: properties, characteristics and construction. J Mol Biol. (2012) 417:129–43. doi: 10.1016/j.jmb.2012.01.038
111. Osbourn, JK . Making the most of phage display. Trends Genet. (2001) 17:359. doi: 10.1016/S0168-9525(01)02271-5
112. Gaynor, KU , Vaysburd, M , Harman, MAJ , Albecka, A , Jeffrey, P , Beswick, P, et al. Multivalent bicyclic peptides are an effective antiviral modality that can potently inhibit SARS-CoV-2. Nat Commun. (2023) 14:3583. doi: 10.1038/s41467-023-39158-1
113. Pang, S , Yu, H , Zhang, Y , Jiao, Y , Zheng, Z , Wang, M, et al. Bioscreening specific peptide-expressing phage and its application in sensitive dual-mode immunoassay of SARS-CoV-2 spike antigen. Talanta. (2024) 266:125093. doi: 10.1016/j.talanta.2023.125093
114. Essa, RZ , Wu, Y-s , Batumalaie, K , Sekar, M , and Poh, C-l . Antiviral peptides against SARS-CoV-2: therapeutic targets, mechanistic antiviral activity, and efficient delivery. Pharmacol Rep. (2022) 74:1166–81. doi: 10.1007/s43440-022-00432-6
115. Pandya, AK , and Patravale, VB . Computational avenues in oral protein and peptide therapeutics. Drug Discov Today. (2021) 26:1510–20. doi: 10.1016/j.drudis.2021.03.003
116. Zhang, Y , Zhang, H , Ghosh, D , and Williams, RO . Just how prevalent are peptide therapeutic products? Int J Pharm. (2020) 587:119491. doi: 10.1016/j.ijpharm.2020.119491
117. Li, C , Wang, J , Wang, Y , Gao, H , Wei, G , Huang, Y, et al. Recent progress in drug delivery. Acta Pharm Sin B. (2019) 9:1145–62. doi: 10.1016/j.apsb.2019.08.003
118. Lam, JH , Khan, AK , Cornell, TA , Chia, TW , Dress, RJ , Yeow, WWW, et al. Polymersomes as stable Nanocarriers for a highly immunogenic and durable SARS-CoV-2 spike protein subunit vaccine. ACS Nano. (2021) 15:15754–70. doi: 10.1021/acsnano.1c01243
119. Shahjin, F , Patel, M , Machhi, J , Cohen, JD , Nayan, MU , Yeapuri, P, et al. Multipolymer microsphere delivery of SARS-CoV-2 antigens. Acta Biomater. (2023) 158:493–509. doi: 10.1016/j.actbio.2022.12.043
120. Kasse, CM , Yu, AC , Powell, AE , Roth, GA , Liong, CS , Jons, CK, et al. Subcutaneous delivery of an antibody against SARS-CoV-2 from a supramolecular hydrogel depot. Biomater Sci. (2023) 11:2065–79. doi: 10.1039/D2BM00819J
121. Glieca, S , Cavazzini, D , Levati, E , Garrapa, V , Bolchi, A , Franceschi, V, et al. A dry powder formulation for peripheral lung delivery and absorption of an anti-SARS-CoV-2 ACE2 decoy polypeptide. Eur J Pharm Sci. (2023) 191:106609. doi: 10.1016/j.ejps.2023.106609
122. Veiga, E , Ferreira, L , Correia, M , Pires, PC , Hameed, H , Araújo, ARTS, et al. Anti-aging peptides for advanced skincare: focus on nanodelivery systems. J Drug Delivery Sci Technol. (2023) 89:105087. doi: 10.1016/j.jddst.2023.105087
123. Wang, G , Yang, M-L , Duan, Z-L , Liu, F-L , Jin, L , Long, C-B, et al. Dalbavancin binds ACE2 to block its interaction with SARS-CoV-2 spike protein and is effective in inhibiting SARS-CoV-2 infection in animal models. Cell Res. (2021) 31:17–24. doi: 10.1038/s41422-020-00450-0
124. Zabo, S , and Lobb, KA . In silico substrate-binding profiling for SARS-CoV-2 Main protease (Mpro) using Hexapeptide substrates. Viruses. (2023) 15:1480. doi: 10.3390/v15071480
Keywords: efficacy, mechanisms, peptides derived from animal proteins, SARS-CoV-2, computer-aided design methods, drug delivery strategies
Citation: Kong X, Wang W, Zhong Y, Wang N, Bai K, Wu Y, Qi Q, Zhang Y, Liu X and Xie J (2024) Recent advances in the exploration and discovery of SARS-CoV-2 inhibitory peptides from edible animal proteins. Front. Nutr. 11:1346510. doi: 10.3389/fnut.2024.1346510
Edited by:
Nemat Ali, King Saud University, Saudi ArabiaCopyright © 2024 Kong, Wang, Zhong, Wang, Bai, Wu, Qi, Zhang, Liu and Xie. This is an open-access article distributed under the terms of the Creative Commons Attribution License (CC BY). The use, distribution or reproduction in other forums is permitted, provided the original author(s) and the copyright owner(s) are credited and that the original publication in this journal is cited, in accordance with accepted academic practice. No use, distribution or reproduction is permitted which does not comply with these terms.
*Correspondence: Wei Wang, d2FuZ3dlaTUyMjgzNDVAenVzdC5lZHUuY24=; Xingquan Liu, bGl1eHFAemFmdS5lZHUuY24=; Nan Wang, WGlhb2ZlaXl1MDcwOEAxNjMuY29t; Junran Xie, eGllanVucmFuQHpqdS5lZHUuY24=
†These authors have contributed equally to this work