- Division of Bioorganic Chemistry, School of Pharmacy, Saarland University, Saarbruecken, Germany
Bioconversion of organic waste requires the development and application of rather simple, yet robust technologies capable of transferring biomass into energy and sustainable materials for the future. Food waste plays a significant role in this process as its valorisation reduces waste and at the same time avoids additional exploitation of primary resources. Nonetheless, to literally become “litterate”. extensive research into such robust large-scale methods is required. Here, we highlight some promising avenues and materials which fulfill these “waste to value” requirements, from various types of food waste as sustainable sources for biogas, bioethanol and biodiesel to fertilizers and antioxidants from grape pomace, from old-fashioned fermentation to the magic of anaerobic digestion.
1 Introduction
In retrospect, future generations may look back at the first half of the 21st Century as a watershed, not only in politics, but also in economy and especially in the way we allocate and manage our limited resources and generate energy (1). If the present years turn out to be a truly epochal change, as some have already claimed, or just as another small milestone in a slow yet continuous transformation toward a carbon neutral and fairer world, needs to be seen (2). In any case, change is on the horizon and there is a rising demand – and interest – in new technologies and their various practical implementations (2, 3).
Our economic activities and practices during the last decades have indeed placed tremendous pressure on the ecological boundaries of planet Earth and are now threatening to ravage nature’s delicate balances and eco-systems. The constant drive toward growth and economic efficiency at the cost of society and the environment must be reconsidered (4, 5). As of 2022, the global estimate of atmospheric CO2 is around 414 ppm which exceeds the proposed planetary limit by over 60 ppm already. Fertilizers rich in nitrogen and phosphorous are being employed with amounts double what the soil, surface water and various species can tolerate (4, 5). It is expected that more than 100 species are being extinct every day and one should embrace the fact that we ourselves are also just such a species and we certainly can be healthier and living better within a healthy environment (4, 5).
When considering sustainability of our limited natural resources, several strategies come to mind, such as the 5Rs, i.e., refuse, reuse, refine, recycle or revaluate. We believe this list can be extended further to even 10 such Rs, including remove, reduce, repair, replace and rally (6–9). It is, for instance, possible to reduce dependence of or to even entirely refuse a plastic bag at the supermarket or to reuse it a couple of times for different purposes (10, 11). The plastic bag can be even removed and replaced with a more sustainable alternative such as a cotton bag which can be repaired and rallied for as an item worth revaluation. It is also possible to refine petrol engines so they consume less gasoline (12, 13). Some plastics may even be recycled whereas others, such as yogurt beakers, can be decorated with a lick of paint and thus re-valued as decorative flowerpots (14).
Yogurt beakers besides, however, one major source of potential value comes from waste itself, especially in form of wasted food and food waste. This material is of particular interest, since it is organic, renewable and available in large quantities at low or no costs.
Indeed, food waste may originate at any point during the complex modern food production system stretching from the “farm to the fork” (15). Whether at production, processing, distribution, retail or consumption, unused leftover materials are not only considered loss and waste, they also pose a serious ecological hazard. The composition of food waste varies depending on the source and can be categorized as pre-consumer and post-consumer food wastes (16). The pre-consumer food waste usually contains higher amounts of fruit and vegetable (48%), root and tubers (26%), cereals (14%) and lower amounts of dairy (6%), meat (3%), oil (2%) and fish (1%) based on dry weight. The post-consumer food waste primarily comprises of cereals (52%), fruits and vegetables (21%), dairy (12%), lower content of meat (6%), roots and tubers (6%), oil (2%) and fish (1%) of the dry weight (16). Similarly, the content of nutritional substances such as carbohydrate, fat, protein, starch, hemicellulose and non-nutritional products such as lignin also varies not only for pre- and post-consumer food wastes but also for different types of wastes within these two categories (16). Pre-consumer apple waste, for instance, contains carbohydrates (48.1%), hemicellulose (24.4%), lignin (23.5%) and cellulose (7.2%) and the pre-consumer grape waste contains hemicellulose (30.3%), starch (21.0%), lignin (17.4%) and proteins (6.1%) (16, 17). The post-consumer food waste varies for its nutritional composition. Kitchen garbage comprises of fats (18.0%), carbohydrate (16.0%) and proteins (15.6%) (16, 18). Moreover, post-consumer used frying oil comprises of carbohydrates (28.4%), proteins (21.6%), fats (19.4%) and cellulose (3.9%) (16, 19). Post-consumer food industry waste such as grape pomace primarily comprises of proteins (43–75%) and lipids (6–15%) (16, 20).
According to the Food and Agriculture Organization (FAO), more than 17% of the total food produced globally becomes waste each year. In 2022, 2% were lost in retail, 5% from food services, and 11% were wasted by households (21). It is important to mention that the size of population is a crucial determinant which influences the volume of food waste generated within a country. Nations with larger populations tend to produce higher amounts of food waste (22, 23). In 2023, India and China, represent the world’s most populous countries with approximately 1,428,627,663 and 1,425,671,352 inhabitants, respectively. China’s annual food waste amounted to a staggering 91.65 million metric tons, while India generated approximately 68.76 million metric tons for 2020 (22, 23). Conversely, Germany, with a population of 83,294,633, produced a comparatively lesser amount of, approximately 6.26 million metric tons of food waste in 2020 (22, 23).
In Germany, household food waste alone currently amounts to a staggering 75 kg per adult per year, often to the delight of Rattus norvegicus, the brown rat (24). Indeed, food waste undermines the sustainability of our food systems as all the resources invested into producing this food, such as land, energy, and water, are in one way or another “wasted” (25, 26). Furthermore, the disposal of food waste in landfills or through incineration, besides attracting vermin, also contributes to greenhouse gas emissions by 8%, thereby exacerbating climate change (25, 27).
In contrast, bioconversion can be used to give such waste a renewed purpose, for instance to produce biofuels, fertilizers, animal feed, and nutraceuticals (25, 27). This approach offers a sustainable means to utilize food waste and reduce its environmental impact (28). It, therefore, hardly comes as a surprise that a future circular economy probably has to be based to a large extent on renewable materials and energies, among which organic natural materials are likely to play a dominant role (29–31). Apart from especially cultivating plants for energy and materials, which requires land, energy, effort and labor, organic waste - a cornucopia of resources if treated properly and with respect - only needs to be collected (32). Not surprisingly, our attitudes about traditional waste are currently undergoing major changes. To put it simple: Waste is simply a valuable resource at the wrong place, at the wrong time and in the wrong hands (33–35). It is time to close the circle with the missing stretch from “the fork to farm”.
With this new attitude in hand, novel research topics and strategies have come into view in order to unlock the potential of such biological waste-resources as part of a future circular bioeconomy (33, 35). In this review, we shall showcase a few rather stimulating projects aiming to unlock the potential of organic food waste for new sources of (old) materials. The overarching attraction of these strategies results from a combination of (a) harvesting raw materials, (b) efficient waste management and (c) local economic circles. Rather than presenting a comprehensive review, though, we shall focus on a few selected and indeed elegant examples which we consider equally instructive and stimulating (36).
2 Aerobic decomposition (composting, vermicomposting and insect based-bio conversion)
As the litter rat has already been mentioned, the compost heap is the first port of call when it comes to processing food waste. Aerobic decomposition is an eco-friendly process to convert organic waste into fertilizers for soil. This method requires specific conditions such as an amenable carbon to nitrogen ratio (the ideal C to N ratio ranges from 25:1 to 40:1), moisture content (40–60%), aeration, pH, the mix of feedstock materials and, of course, microorganisms (37).
Composting can be divided into three main phases. The first phase, known as the mesophilic phase, is carried out by mesophilic microorganisms such as bacteria (Pseudomonas and actinobacteria) and fungi (Penicillium and Aspergillus) (38–40). In this phase, the microorganisms break down the organic compounds such as sugars and starch into simpler molecules with production of carbon dioxide (CO2) and water. The mesophilic phase lasts for 2 days. The second phase is the thermophilic phase, which occurs as the temperature rises to reach 55°C to 77°C due to bacterial and fungal activity. Decomposition is now facilitated by thermophilic microorganisms such as Thermus aquaticus and Streptomyces coelicolor (41). Here the thermophilic bacteria break down the more complex organic compounds such as fats, cellulose and proteins to produce carbon dioxide (CO2) and water. This phase lasts for a few weeks to several months. Third, follows the maturation phase, which is characterized by a drop in the temperature, with mesophilic bacteria such as Actinobacteria and fungi such as Mucor and Trichoderma dominating the process (38–40). In this phase, after the waste pile cools down and mesophilic microorganisms become active again, the remaining organic matter continues to break down until the pile stabilizes. Then, the compost matures and over time becomes similar to the normal traditional soil. This phase lasts for several months (42). Subsequently, the resulting compost requires a pathogen removal process such as exposure to direct sunlight for drying, steam treatment and pasteurization (at around 60°C to 71°C) (43). The pathogen removal step is important to minimize the risk of spreading various plant and animal diseases, such as histoplasmosis, aspergillosis, tetanus, paronychia, and hypersensitivity pneumonitis (Farmer’s lung). On the other hand, several studies have affirmed a natural antimicrobial inhibitory effect of compost products, e.g., tea compost, against certain phyto-pathogens, such as Pythium debaryanum, Rhizoctonia species, and Fusarium oxysporum (40). In any case, it is actually counterproductive to try to sterilize food waste prior to composting, as has been suggested in the past, especially because of Aspergillus.
Besides producing a valuable organic material which nowadays replaces peat in gardening and agriculture, composting additionally benefits the environment by reducing residential food waste and lowering greenhouse gas emissions. As for the “logistics”, composting can be achieved easily in a highly decentralized fashion, for instance at home, and thus does not require collection and transportation of materials, although collections and centralized facilities are also very common, especially in urban areas. Curiously, some German cities, such as Sankt Ingbert, even employ professional “compost inspectors” who will check your personal heap and either approve it or force you to take part in the city’s centralized “green bin” collection system (44–46). Indeed, different composting methods for organic waste are amenable, such as aerated static pile composting, in-vessel composting and mound bed composting (the famous Germanic “Hügelkultur”) (47–50). Figure 1 briefly illustrates the composting process (44–46).
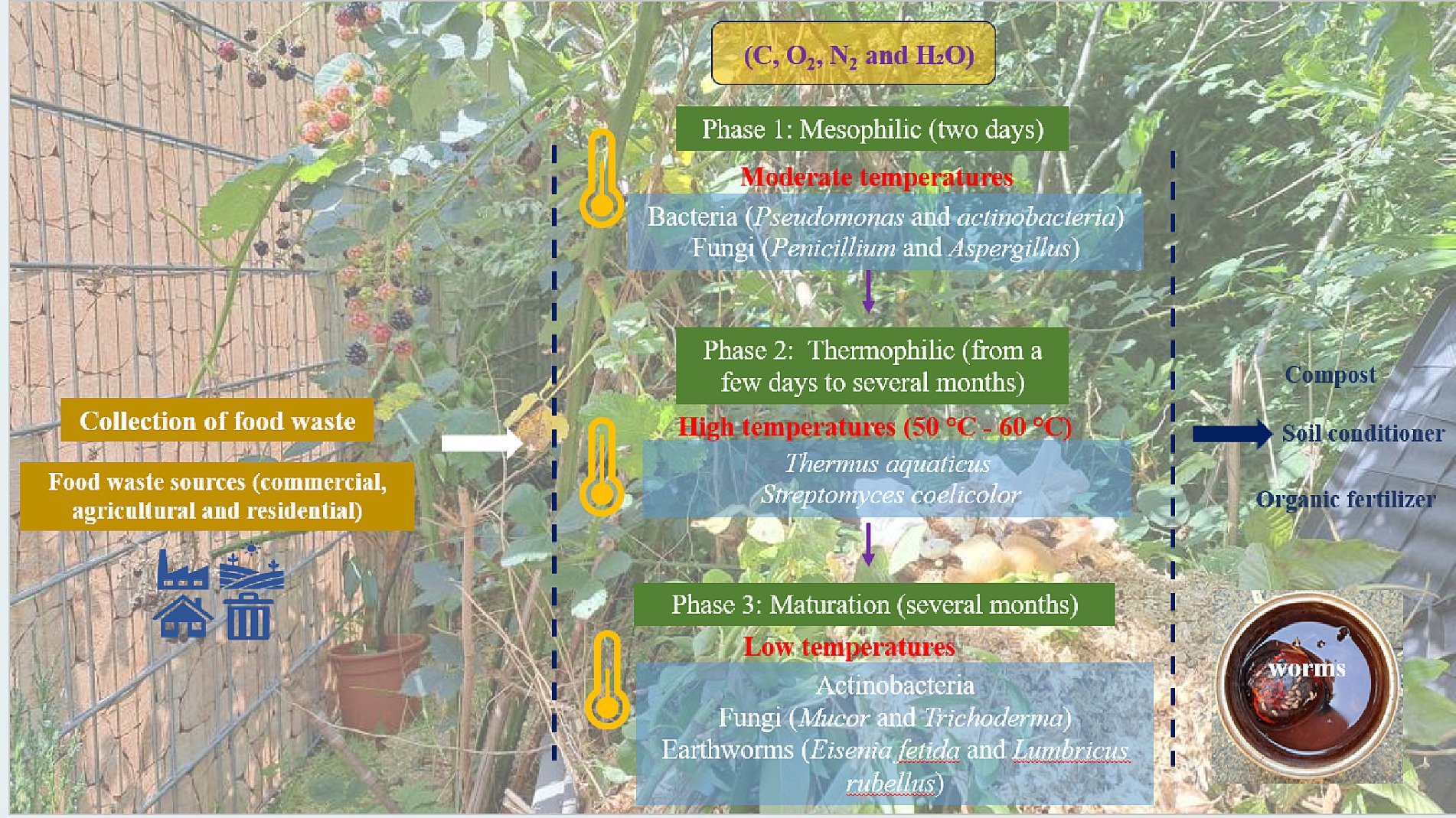
Figure 1. Phases of composting including the relevant microorganisms: mesophilic phase, thermophilic phase and the final maturation phase to produce soil compost, conditioner and fertilizers. Photographs were created by © 2024 Elizabeth Jacob.
Another type of composting is known as “vermicomposting” by using earth worms to convert food waste into soil fertilizer. In this composting method the commercial, agricultural and residential food waste are passed through the worm-gut to form a nutrient rich vermicompost (51). The vermicompost - or worm castings - can be easily implemented indoors, in small batches with less nitrogen loss, and even in the cold weather. It is the richest nutrient fertilizer known to gardeners (52). People who are interested in growing their own vegetables are becoming interested in earthworm castings (52, 53). After the vermicompost or worm castings feed on the food waste, the worms produce particles which improve the soil properties and increase the water retention within the soil. The vermicompost contains phosphorous (1.8–2.2%), potassium (1.0–1.5%), nitrogen (1.5–2.2%) and micronutrients such as iron, magnesium, sulfur, zinc, calcium and sodium. More details on vermi-worm-compost are presented in Table 1 (51, 62). It is important to mention that well-managed vermicomposting systems are also aerobic and no worms are being hurt, thus vermicomposting can create a sustainable environment for earthworms to thrive and continue their life cycle.
Notably, vermicomposting differs from normal composting in several ways. The temperature in vermicomposting, for instance, ranges from 10°C to just 32°C and the process is faster than microbial composting because the food waste passes through the earthworm gut, where a significant but not fully understood transformation takes place, enriching the resulting earthworm castings (worm manure) with plant growth regulators (51, 54, 55). Furthermore, earthworms produce a richer kind of compost, and thus are capable of transforming the food garbage into “gardeners’ gold” (51, 63).
The quantity of CO2 released from composting and vermicomposting varies for different waste systems as well as the organisms involved. In terms of aerobic digestion, anaerobic digestion and vermicomposting, CO2 constitutes the largest portion of greenhouse gas emissions that is 63.6, 80.9 and 78.3%, respectively (64–66). The main differences between composting and vermicomposting are also summarized in Table 1.
Amazingly, besides bacteria, fungi and worms, even insects can be utilized to convert food waste into soil fertilizers (67). Larvae of more than 2,000 species of insects participate in such conversions and include, for instance, the larvae of black soldier flies (Hermetia illucens), two-spotted crickets (Gryllus bimaculatus), desert locusts (Schistocerca gregaria), house flies (Musca domestica), Cambodian field crickets (Teleogryllus testaceus), yellow mealworms (Tenebrio molitor) and others (see Figure 1, insert) (68–72). Insects are employed already in such processes for a number of advantages which range from being cost effective to the possibility of reducing waste odors compared to other methods (73–75).
The process of insect bioconversion begins with the collection of food waste from residential areas, farms, or restaurants. Subsequently, the collected waste is placed into certain reactors such as bins, cages, vertical farming systems, insect bags and sleeves, modular systems or insect trays (76). After that, it is exposed to species such as the black soldier fly (BSF) larvae, which can devour fruits, vegetables, and various organic waste into soil fertilizers. The larvae consume the organic waste as part of their natural feeding source, converting waste into a biomass after breaking down the organic materials within their digestive systems (75, 76). Once the insects have completed their life cycles and reached their desired stage of development the biomass can then be collected and used as nutrient-rich soil amendment and fertilizer (77). The biomass produced by such insects generally is rich in nutrients such as N, P, K, Ca, Mg, S, as well as microelements, like Mn, Na, B, Zn, Fe, and Cu (75). It is important to mention that after producing the fertilizers, the insects are not intentionally killed and they continue their life cycles. Different insect species can be used but BSF is the most common choice due to its ability to reduce organic waste biomass by 60%, transforming it into nutrient-rich biomass (69, 75). This method is environmentally friendly and sustainable as it helps reduce waste volume and greenhouse gas emissions. Also, BSF, two-spotted crickets or desert locust larvae have the capability to produce high-quality fertilizers known as frass fertilizers (70). These insect-driven processes could yield a variety of beneficial materials besides fertilizers, such as precursors of bioplastics (67, 74). Table 2 provides a more comprehensive list of insect species suitable for bioconversion of food waste.
In this context, one may also mention that in the past, food waste has been fed to many higher animals, from rabbits to pigs and cows, and that in some instances, these animals have become seriously ill. The transmission of “Mad Cow Disease”, for instance, has been due to cows being fed meat-based products. Thus employing higher animals rather than microbes, worms and larvae for such bioconversions may be a considerably more controversial issue.
3 Anaerobic digestion
Aerobic digestion is an energy intensive process which comsumes a lot of energy and air to produce CO2 instead of methane. As far as the energy balance is concerned, it is therefore often more economical to digest anaerobically. Here, organic waste from the food industry, such as fruits and vegetable waste, rice and maize straw and coffee husk, form the basis for bio-methanation thanks to their intrinsic methanation potential (84, 85). In fact, anaerobic digestion (AD) is one of the most common industrial-scale processes to convert food waste into renewable materials using “biological” means, in contrast, for instance, to pyrolysis. AD encompasses several phases: the process starts with preparing the feedstock where food waste is collected and sorted to remove the non-biodegradable materials. Then, the selected food waste is added into a large tank called “anaerobic digester” in the absence of dioxygen (86).
Once inside the digester, the digestion process begins through microbial activity of specialized bacterial communities which break down food waste in successive, different phases. In the first phase, hydrolysis occurs, facilitated by hydrolytic bacteria such as Bacteriodes, Clostridium, and Acetivibrio (86, 87). These bacteria break down food waste into its basic building blocks, e.g., amino acids, sugars, alcohols, and fatty acids at a pH of around 6.5 to 7.5. In the second phase, called acidogenesis, acidogenic bacteria such as Enterobacterium, Acetobacterium, and Eubacterium transform the hydrolysed materials into intermediate products, such as acetate, propionate, ethanol, lactate, and volatile fatty acids at pH ranging from 5.5 to 6.5 (86, 88). In the third acetogenesis phase, acetogenic bacteria such as Syntrophomonas wolinii and Syntrophomonas wolfeii utilize acidogenic products to produce acetic acid (CH3COOH), carbon dioxide (CO2) and hydrogen (H2) at a pH ranging from 6.2 to 6.8 (86, 89). Eventually, acetoclastic methanogens such as Methanosarcina barkeri, Methanosarcina frisius, and Methanobacterium formicicum come into play and convert acetate to methane (CH4), CO2 and water (H2O) (86, 90). Additionally, hydrogenotrophic methanogens such as Methanobacterium arbophilicum and Methanothermus fervidus convert H2 and CO2 into predominantly CH4, thus contributing further to the production of CH4 and scavenging some of the CO2 at a pH ranging from 6.5 to 7.5 (86, 90). Biogas produced from AD comprises of 50–75% CH4 and 50–25% CO2 concentration. The flow of materials and different stages of AD are presented in Figure 2 (91).
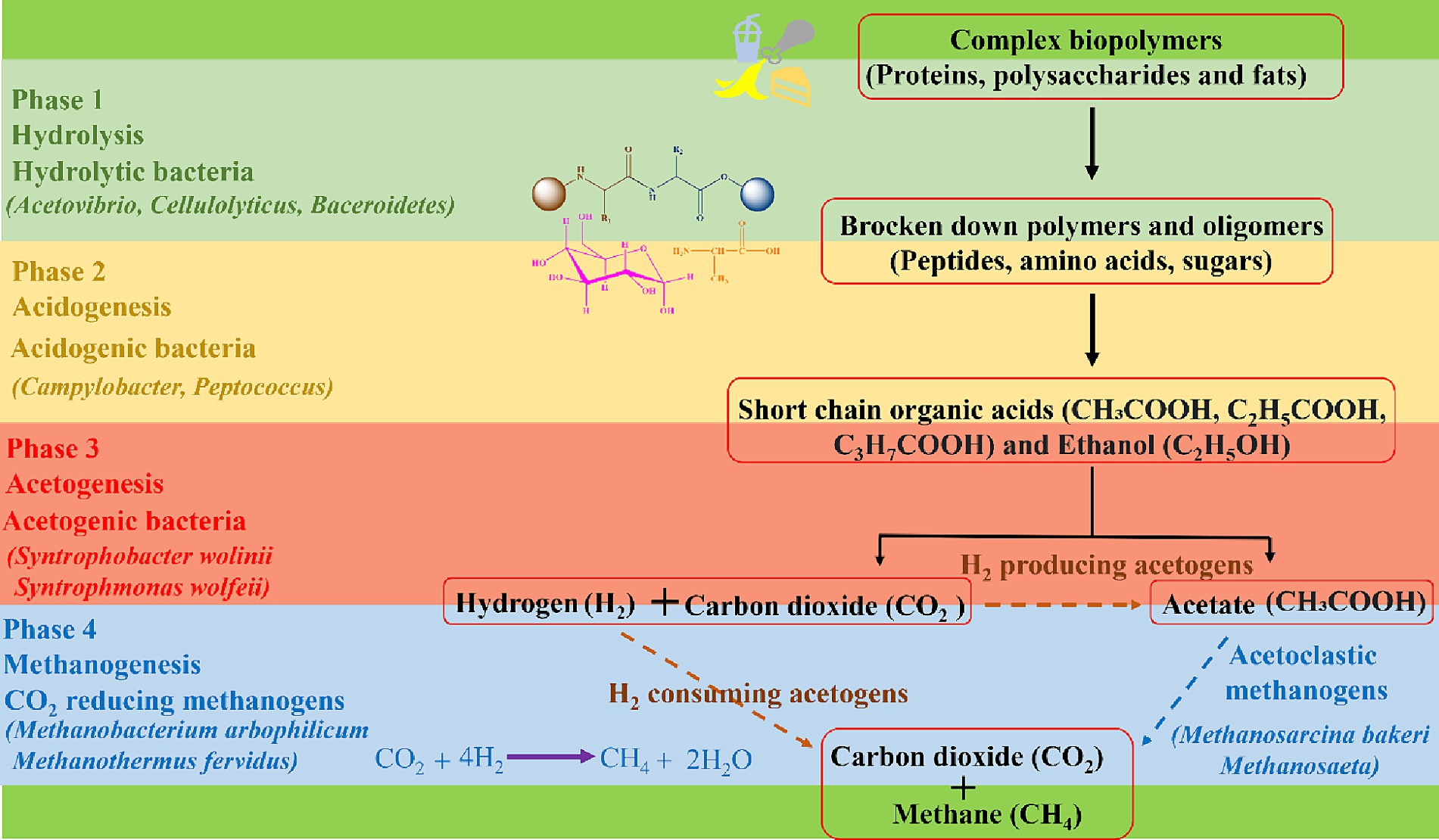
Figure 2. The complex biopolymers undergo Anaerobic Digestion (AD) which involves a series of biological processes in the presence of microorganisms to produce biogas.
The choice of the most suitable microorganisms is important for successful AD and lends itself to further research and development. Interesting examples of such anaerobic bacterial species employed in AD for biogas production include Clostridium kluyveri, Methanosaeta harundinacea, and Propionibacterium propionicus, among approximately a thousand other species (86, 92). Notably, certain bacteria, such as Selenomonas ruminantium, Rhodopseudomonas palustris P4, Citrobacter spp. y19, and Clostridium butyricum, are capable of producing “green” H2 in place of CH4, which can be utilized directly or converted chemically into biogas (93). Indeed, the topic of fermentative H2 production has gained significant attention recently not only in research but also in politics and legislation as part of the “net zero” strategy of switching the traditional carbon-based to a new hydrogen-fuelled economy (92, 94–96). As for numbers, Germany is one of the leading biogas producers globally with 28.8 million m3 of raw biogas generated and fed into the gas grid annually which is indeed a step into the right direction considering the huge demand and usage of more than 77 billion m3 in 2022. According to the International Energy Agency (IEA), China has established the highest number of biogas plants (more than 100,000 plants), followed by Germany (more than10,000 plants). In comparison, there were less than 350 Anaerobic Digesters operating in the United States in 2022 (97, 98). AD is, however, associated with a few disadvantages, such as the emission of significant amounts of CH4 and the release of odors during production. Moreover, AD necessitates a careful equilibrium between the energy consumed during the operating process and the energy gained (45, 86, 99, 100). The costs associated with the AD can vary significantly depending on several factors such as the feedstock type, the size of the AD system-whether it’s a small-scale on-farm digester or a large municipal facility- and the use of mesophilic or thermophilic digestion equipment and infrastructure required for the transportation of the biogas produced may contribute additional costs (86, 99, 101, 102). Furthermore, the cost of land and infrastructure for AD should also be considered (86, 101). The total capital cost for the AD in Tollenaar Holsteins Dairy, California, United States was US$1.7 million, with annual operating and maintenance costs of 50,000 USD (103). Butler Farms, Texas, USA implemented a lagoon AD with costs ranging from 550,000 USD to 650,000 USD with an annual maintenance cost of 25,000 USD (103). Fair Oaks Dairy Indiana, United States, employed a mixed-plug flow AD costing 12 million USD with estimated annual operating and maintenance costs of around 600,000 USD (103).
In sharp contrast to aerobic digestion, which focusses on the production of compost and thus ignores the effluvium, the products of AD can be divided into two main categories, biogas and digestate. Biogas can be utilized directly, for instance for heating, as vehicle fuel or to generate electricity. Digestate, on the other hand, is still rich in nutrients and can be converted further to biofertilizers for agricultural use (104). Compared to composting, AD entails higher costs, both in construction and operation, yet has the benefit of producing clean energy and heat, providing increased value to EU countries that may import most or all of their fossil fuels (105, 106). Recent advancement in the field of AD includes, anaerobic co-digestion (AcoD), where different organic waste products can be combined and blended with various other substrates. Additionally, soft magnetic ferrite serves as an effective and economical AD additive which not only promotes biomethane production but also recovers the materials and microorganisms (107, 108). Moreover, decentralized AD which operates in smaller-scale facilities gets rid of food waste generated from restaurants, cafeterias, cooked food centers or households (109).
3.1 Digestate
After the completion of the AD process, the remaining materials from each step are collected together as a whole digestate and exploited as a fertilizer (110, 111). Digestates can originate from different sources, such as maize, clover, corn, coffee, tea, fruits, stems, bakery waste, and sugar industrial waste. They are generally rich in potassium, ammonium and phosphorus (111). Phosphorus is found in food waste such as grains, dairy, and meat. Similarly, nitrogen is also generated during the AD process from proteins and other organic compounds, and it is converted into ammonia (NH3) (112, 113). A relevant example of a digestate rich in phosphorous and nitrogen is the one generated from algal biomass. Such digestate contains around 390 mg/L of total phosphorous and 2,940 mg/L ammonia-nitrogen among other elements such as sodium and potassium (113, 114).
Such digestate is used primarily as a soil fertilizer and in hydroponic systems in greenhouses (111). It has been reported that applying the digestate in bubble-insulated greenhouses leads to higher yields of vegetables, such as lettuce, cape gooseberries, tomatoes, cucumbers, mushrooms (such as Agaricus subrufescens and Agaricus arvensis), and herbs (such as thyme, dill, parsley, basil, coriander, and melissa) (115). Moreover, the utilization of AD digestate as a fertilizer has demonstrated its ability to induce resistance against plant diseases and soil-borne pathogens, such as Fusarium oxysporum f. spp. Spinaciae, Ralstonia spp. and Phytophthora spp. (116–118). The activity against these soil-borne pathogens comes from the content of antagonistic microorganisms within the AD digestate. For example, Bacillus spp. promotes the forming of rhizobacteria due to biotic stress-tolerant spores. Also, Trichoderma fungus in AD digestate is reported to suppress a variety of plant pathogenic fungi, such as Sclerotinia sclerotiorum, Pythium ultimum and Botrytis cinerea by colonizing the plant roots and releasing enzymes that degrade fungal cell walls of their competitors (119, 120). This protective activity of the anaerobically produced digestate stands in contrast to the aerobically generated compost, which needs to be sterilized prior to use as the microorganisms contained therein may pose a danger to plants and animals (see section 2).
4 Biofuels
Besides the rather crude methods of aerobic or anaerobic digestion of such “mixed” food waste, one may also envisage a more refined collection and thus more directed processing of certain food (waste) items, such as sugars and fats. Indeed, expired or used cooking oils have a long tradition of being used as fuels, with some diesel-powered busses already in the 1980s smelling distinctively of French Fries or Fish & Chips on the go. Today, the large-scale production of “biofuels” such as biodiesel from plant-based oils and bioethanol from sugars is rather common and increasing steadily. Then again, given that agriculture has the potential to provide us with renewable materials year after year, it is not surprising that energy crops, rather than food waste, are used as primary source of biofuels, which is highly controversial from an ecological perspective. Indeed, such “energy plants” are increasingly cultivated in fields where they replace crops traditionally used for animal and human consumption (37). Various examples of energy plants exist, including sugarcane, sugar beets, sunflowers, soybeans, and corn (32).
As for biodiesel production, fats and oils, such as algae oil, cooking waste oil, and plant-based oils (among them soybean oil, palm oil, sunflower oil, peanut oil, Jatropha and rapeseed oil) can be hydrolysed and re-esterified through a process known as “transesterification.” As part of the transesterification process, the oils or fats react with an alcohol (mostly methanol) in the presence of a suitable catalyst to form esters and glycerol (121, 122). This process is also exemplified in Figure 3 (123, 124). Essentially, transesterification entails the (bio-)chemical hydrolysis of triglycerides into free fatty acids (FFA) and glycerol, followed by separation of glycerol and esterification of the FFAs with methanol to yield liquid fatty acid esters with high energy density, low melting (−35°C) and high boiling (192°C) points. These esters may serve as biodiesel. The glycerol obtained as a side product in this process is not wasted either – as a “natural product” it finds applications in moisturizers, soaps, cosmetics, and medicines. Microorganisms involved in this biodiesel production process are highly methanol resistant or genetically modified to sustain the harsh chemical conditions and include bacteria such as Stenotrophomonas maltophilia D18, Lysinibacillus fusiformis B23, Acinetobacter junii C69, Acinetobacter pitti C95, Rhodococcus opacus pd630, Pseudomonas citronellolis, and Escherichia coli, as well as yeast species such as Naganishia liquefaciens and Rhodosporidium toruloides (125–127). It is important to mention that not all types of waste oil need transesterification before being used as a replacement for diesel fuel. Vegetable oils and cooking oils from restaurants or households need transesterification to be converted into biodiesel. The insect oils which are produced through insect-based bioconversion, in contrast, can be used as a replacement for diesel directly, yet with challenges related to cold weather and emissions of pollutants. In general, the total production costs for a gallon of biodiesel can vary from country to country, for instance in 2014 it ranged from 5.53 USD to 6.38 USD in Texas, United States (128). As of April 2023, the price of biodiesel in Germany was 1,471 USD per ton (129). Besides production costs, one should also consider the competition for feedstock resources. This competition, if not managed properly can lead to several adverse consequences (130, 131). The exploitation of edible oils for the production of biodiesel can trigger the “food vs. fuel” debate, diverting valuable agricultural resources from food production to biofuel production which may potentially impact the food prices (132, 133). Moreover, the production of biodiesel is a resource-intensive process which demands excessive amounts of water, energy, and agricultural inputs such as fertilizers and pesticides (130, 131). Biodiesel production offers several advantages, such as reduced greenhouse gas emissions and renewable energy sources, yet one should also consider the competition for feedstock resources and the related challenges to understand the overall situation (130, 131).
Another example of biofuel which can be produced from food waste is bioethanol, in which sugars, especially single sugars, play a vital role. This process typically involves fermentation, relying on microorganisms such as yeasts (Saccharomyces cerevisiae and Issatchenkia orientalis), bacteria (Thermoanaerobacter mathranii, Zymomonas mobilis, and Geobacillus thermoglucosidasius), fungi (Zygomycetes and Mucor indicus), and even algae, such as Chlorella vulgaris (37, 134–137). In practice, bioethanol production from food waste involves four main steps. Firstly, the food waste needs to be collected, sorted and pretreated by heating, grinding or shredding to break down complex carbohydrates (136). Secondly, hydrolysis starts to break down complex carbohydrates, mostly cellulose or starch, into simpler sugars, such as xylose or glucose. Thirdly, the fermentation of hydrolysed sugars into ethanol and CO2 takes place by microorganisms in the absence of dioxygen as part of an anaerobic fermentation. After fermentation, the ethanol is separated from the fermentation broth via distillation (134).
The duration and yield of such a bioethanol production process depend on the type of food waste and microorganisms involved. Vegetable and fruit waste, such as apple pomace, potato and tomato has been reported to yield 149.9 g/L, 7.6 g/L and 23.7 g/L of ethanol, respectively, when fermented in the presence of Saccharomyces cerevisiae. Dairy products such as cheese whey yield 8 g/L ethanol when digested by Kluyveromyces marxianus (138). The individual bioethanol production phases and more details about the bioethanol yields from different food waste samples are summarized in Figure 4 (138).
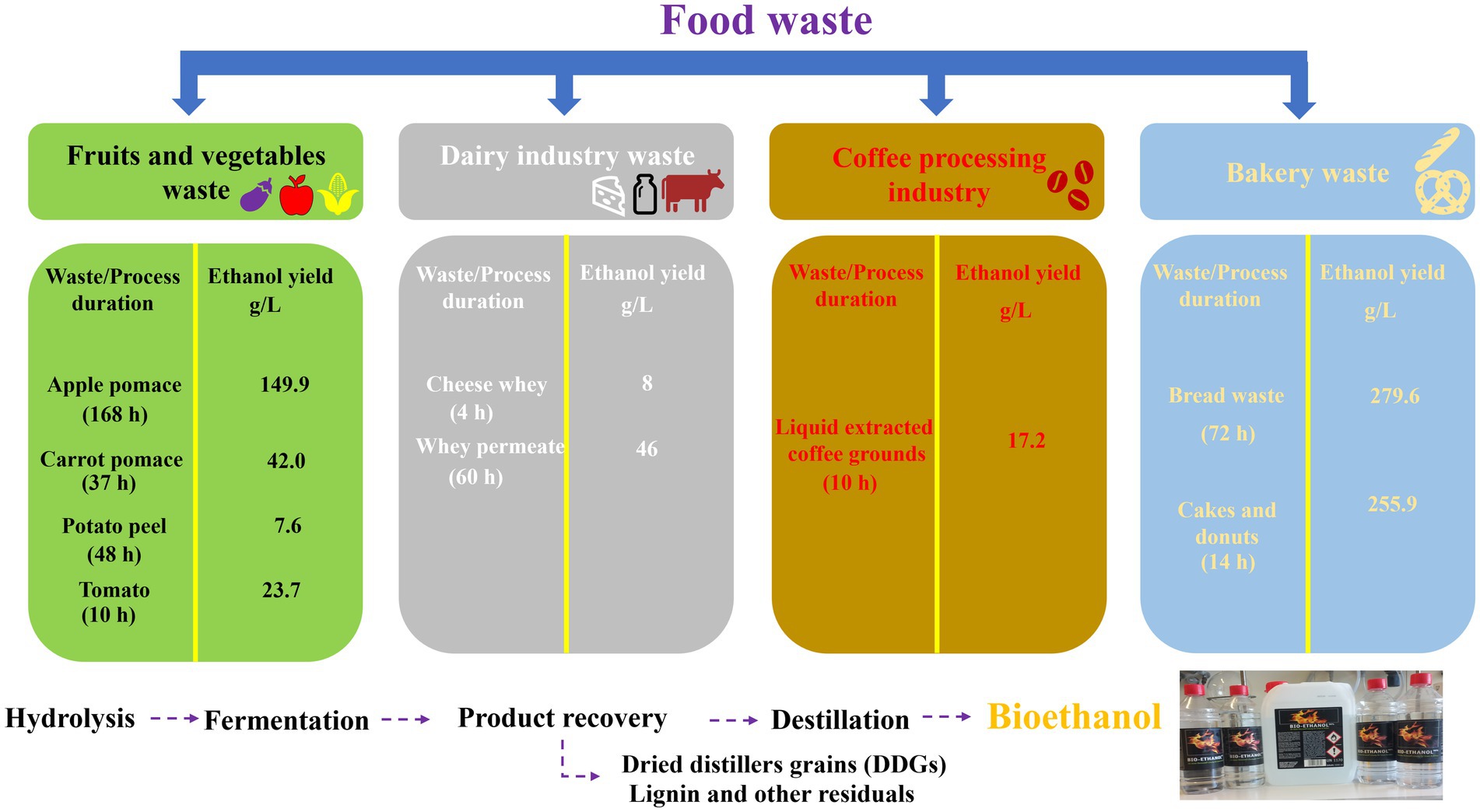
Figure 4. Production of bioethanol from different waste products varies not only in the time required for fermentation but also the yield obtained.
In Germany, bioethanol in its pure form is not suitable as petrol-substitute and can only be added to conventional oil-based gasoline at concentrations up to 5% (E5) or 10% (E10) and quite recently even 20% (E20) (139, 140). Brazil has been a pioneer in the use of bioethanol as a fuel source. The “Proalcool” Program, which started in the 1970s, was a key factor in the country’s success in transitioning from fossil fuel to bioethanol (141–143).
Nonetheless, there is also a rather heated ecological debate regarding such renewable “power plants”. On the one side, these plants need to be cultivated and therefore take up valuable agricultural space which is no longer available for the plantation of other plants, for instance wheat (144). In fact, the production of such energy plants in ecologically sensitive regions, for instance in the rainforests, adds to these arguments and issues which need to be resolved first and before such biofuels from cultivars rather than waste can be considered as truly sustainable (144). In addition, the process of bioethanol production is itself energy intensive, especially the distillation step, and encompasses the formation of CO2. Indeed, neither biodiesel nor bioethanol, if used in a combustion engine, can be considered as entirely “clean” as burning them in an engine still produces environmental pollutants such as sooth and nitric oxides (NOx). Although bioethanol may be used in fuel cells, this technology is still in its infancy. At the same time, bioethanol production poses food security risks, especially in low and middle-income countries. It is essential to strike a balance between utilizing food waste and addressing the global population’s food needs (145, 146). The costs of bioethanol production depend on the type of biomass used, but in general, they range from 0.20 to 0.30 USD per liter (134). Recently, genetic engineering strategies have been employed for the enhancement of biofuel production. Biodiesels, bioalcohols and isoprenoid-based biofuels are, nowadays, obtained by exploiting different genetically engineered yeasts, such as S. cerevisiae XUSE, S. cerevisiae SXA-R2P-E, S. cervisiae M1: kurdriavzevii NUPHS33, S. cerevisiae YG5C423, Yarrowia lipolytica, Cryptococcus curvatus and Rhodotorula toruloides (147–152).
5 Waste into value: upcycling of industrial food waste (wine, beer, bagasse and beet pulp)
Admittedly, some of the recent examples have been less appetizing, especially for the ones of us who are not yet quite “litterate” or dedicated litter rats yet. They may also have raised the question if initially unwanted biological side products necessarily have to end up in the litter bin or have to be used for applications which are less valuable than the ones associated with the products they are derived from (107, 108). Indeed, in order to fuel the circle of bioeconomy from a truly economical perspective, one may wish to derive at applications for waste - or products from waste - which would be as or even more valuable than the ones of the material itself. In fact, such an increase in value should provide the financial impetus needed for companies to engage in such bio-economic circles (153, 154). Here, re-validation, such as upcycling and bio-valorization, come into play. As the neighbors tends to say: “Are you eventually removing the weed around your house?” – “Not quite, I am collecting my smoke”. At closer inspection, and besides weed(s), a surprising spectrum of organic by-products from food production may be valorized, such as grape seeds, brewed coffee waste, tomato stems, berries, fruits, potatoes, walnut shells and peels (155, 156).
The first and perhaps the most prominent example of industrial food waste is grape pomace which comprises of seeds, stalks and skin and is a high-quality residue of the wine industry laced with flavonoids, anthocyanins and (poly-)phenols (157, 158). The grape pomace contains a higher amount of dietary fibers (19–38%), sugars (12–33%), proteins (8.49–10.32%) and lower amounts of pectin (3.68–29.20%), catechin (150.16 mg) and anthocyanin (84.40-131 mg) per 100 g of pomace based on dry weight (20, 155, 159–161).
The whole grape pomace can be used or up-cycled to produce energy and various products, such as ruminant feed, biofertilizer, biopolymers, composites, and even a basis for mushroom cultivation through microbial processing, as summarized in Figure 5 (20, 162).
Grape seeds constitute a major part of the grape pomace and for many centuries have been discarded as part of low-value fertilizer (20, 162). Today, the seeds are collected, cleaned and pressed to produce a highly valuable grape seed oil. The increased interest toward grape seeds is primarily due to their high content of phytochemicals, such as phytosterols, carotenoids, vitamin E, unsaturated fatty acids, phenolic compounds (hydroxybenzoic and cinnamic acid derivatives, gallic acid, kaempferol, quercetin, catechin, epicatechin, gallocatechin and procyanidin dimers) (163, 164). Moreover, this oil is rich in beta-sitosterol, which has the potential to reduce cholesterol levels and possibly even benign prostatic hyperplasia (BPH, resulting in so-called ewer urinating in men) (165, 166). As for economics, the oil is sold at prices considerably higher than sunflower oil and perhaps even fetches more than the wines the grapes had been processed for initially (20, 161, 163). These days a bottle of reasonable wine in Germany goes for 4 €/L whereas a bottle of grapeseed oil easily catches 8 €/L. (167, 168)
Interestingly, the oil cake from de-oiled grape seeds is no waste either – it may be processed further to grape seed flour as depicted in Figure 5. Again, this flour by itself is a highly valuable product, not only economically, also nutritionally (163, 169, 170). Moreover, the antioxidant and anti-inflammatory properties of de-oiled grape seeds and grape seed flour make them a popular ingredient in cosmetics and personal care products (171). They are often used in the production of anti-aging and skin brightening products (171, 172). Intriguingly, de-oiled grapeseed meal, which is the by-product obtained after extraction of oil, is often also used as a source of protein and dietary fiber in animal feed (171). Besides improving the nutritional value of various food products, the de-oiled grape seeds are often added as a natural preservative to dairy products, processed meats and oils (173–176), thanks to the presence of astringent polyphenolic substances. As for the economics of grape seed flour, 1 kg goes for a staggering 20 € these days.
Brewers spent grain (BSG) is another by-product of the food / beverage industry formed during the process of brewing. The production of 100 L of beer generates 20 kg of BSG which primarily comprises of lignocellulose fibers (around 70% dry weight basis) but is also rich in nutrients such as proteins (20% on a dry weight basis), minerals and vitamins (177). Today 70% of BSG is exploited as feed for animal such as cattle, chicken and poultry, 10% for the production of biogas, and the rest goes to landfills (178) (Figure 6).
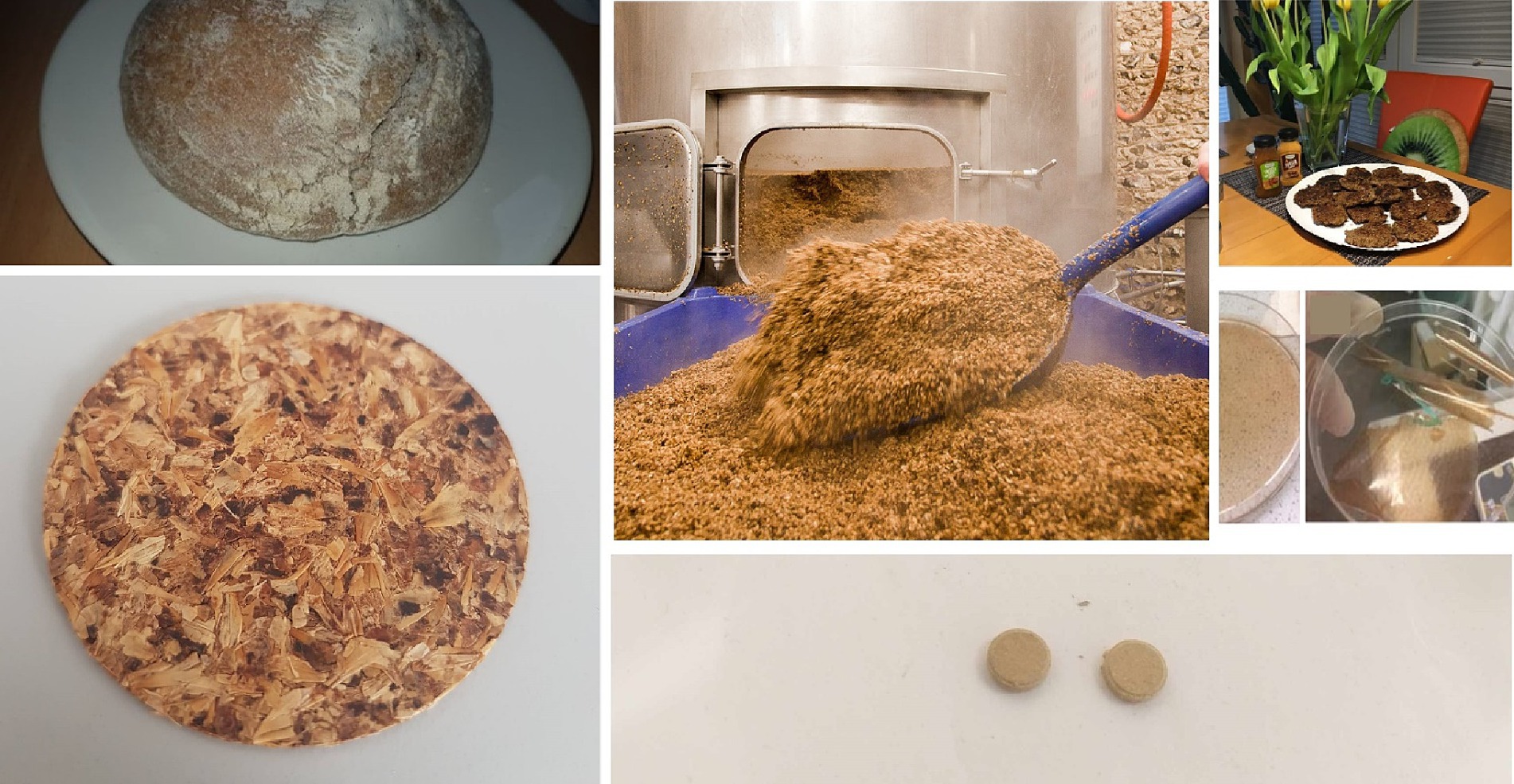
Figure 6. Brewer’s spent grain (BSG, by Homburger Brauhaus, photo generated by the authors) can be employed to produce bread, vegetarian patty or even bioplastics. It can also be pressed to produce sheets or tablets thanks to the presence of lignin which serves as a natural binder.
Considering these low-value applications, if any, BSG represents another fine example of value gone to waste (179). Indeed, BSG or what is called now occasionally SuperGrain+ (SG+) contains considerable amounts of nutritionally valuable components, such as proteins, fibers and phenolic compounds, which in turn may benefit in some health conditions, such as heart diseases, hypercholesterolemia and cancer (180). Today, it is attractive to process BSG further to produce BSG flour and use it in baking and cooking, in vegan patties and in bread, as a powerful source of dietary fiber and provider of proteins and polyphenols, and as a regulator of the gut microbiota (181).
As for applications outside nutrition, and besides being used as a low-value fuel in biogas plants, BSG can also be processed further to various novel materials, such as solid plates and even bioplastics. BSG plates, for instance, are formed as a result of high temperature and pressure treatment and due to the “melting” and resolidifying of the lignin “glue” (12–28 % w/w) (179). These plates are not only 100% “bio” yet also 100% biodegradable (181). The formation of polymers / plastics, on the other hand, is considerably more complicated and involves acid treatment, similar to the one used to form bioplastics from banana peels (182, 183).
Once again, valorized BSG may be or become more valuable economically and also socially and ecologically than the beer produced in the first instance. And although this is indeed a matter of taste, it shows that such strategies of “turning waste into value” by re-validation may provide the necessary incentives for closing the relevant processing circles in an economically viable fashion turn out to be attractive for local companies, including bakeries, mills and breweries (181). As mentioned before, there is no waste, only material at the wrong place, wrong time and in the wrong hands.
Another example of the valorization of industrial waste are sugar beets which are frequently used to extract sugar, especially in colder climates such as Russia, Germany and the United States, producing 41.2, 31.9 and 8.1 million tonnes of sugar beets per year, respectively (184–186). The production of sugar from beets results in by-products known as Sugar Beet Pulp (SBP). Sugar Beet Pulp Pellets (SBPP), derived from these bulbs, serve as popular livestock feed, suitable for animals such as horses, cattle and sheep (187, 188). The dried form of SBPP, known as “shreds” can be stored for several years without expiring. SBPP is a valuable source of nutrients due to its carbohydrate, fiber and protein content (187, 188). The main carbohydrates present in SBP are sucrose (10% of dry matter) and polysaccharides, including cellulose (22–40%), galactan, araban (24–32%), and pectin (24–32%) (187, 188).
Besides the beverage industry which, not surprisingly, produces millions of tons of by-products every year, there are also other examples of - more limited - food waste turned into value. In fact, some of these materials feed the field of natural cosmetics. Spent coffee grounds (SCG), for instance, is rich in chlorogenic acid and polyhydroxy-alkanoates and apart from being used traditionally in home gardening and against slugs has recently found its way into cosmetics. In this context, it is used as a source of antioxidants or for anti-cellulite activity (189, 190). SCG has also turned into an ingredient of specific hair shampoos as it is still rich in caffeine and thus may be useful in the treatment of androgenetic alopecia. There are even certain bakery products, such as biscuits, which contain SCG (190, 191). Indeed, as for pomace and BSG, SCG has plenty of potential to be used in various ways other than just for slugs in home gardening (192).
The same considerations also apply to the peels of many fruits, especially citrus fruits, such as oranges and lemons. Their peels are increasingly being used in household products, such as cleaning liquids (193). Here, the etherical oils and flavors contained in these peels are of particular importance as they endow these products with natural cleansing properties and fragrances. Notably, these peels also represent a valuable raw material in the field of cosmetics and, if cultivated free of pesticides, can even be processed further by the food industry itself, for instance for the production of jams and marmalades. Again, some of these applications, especially in the field of nutrition, provide such peels with a value which may even exceed the one of the fruits and juices derived from them, such as the famous coarse cut English Orange Marmalade (193, 194). Indeed, one does not have to be a Peelite to admire the use of such peels in exquisite marmalades, cakes, teas and, of course, in cosmetics – and the zero-waste approach that goes with it. Alternatively, the citrus essential oils (CEOs) derived from such peels contain substances such as terpenoids and phenols and thus offer broad-spectrum insecticidal, antifungal and antibacterial properties (195).
Similar opportunities arise for nutshells, which can be processed to abrasive materials, or for ordinary corncobs, which after removing the grains still have a value once dried and milled, for instance as adsorbents (196). Even stale bread can be considered as a valuable raw material and turned into drinks such as Kvass via fermentation by lactic acid bacteria (LAB) (197, 198). Such bacteria can also offer a pickling condition necessary for the manufacture of pickles, green olives, sauerkraut, sausages, buttermilk, yogurt, and some cheeses. Important members include Streptococcus, Lactobacillus, and Pediococcus (197, 199). Another example, LAB such as Lactiplantibacillus plantarum and Lactobacillus acidophilus bacteria can be used to prepare the fermented Agri-waste for animal feed, often referred to as “silage” (200). Corn silage production requires the waste of corn, i.e., stalks, leaves and the remaining parts of the cobs and LAB such as, Lactiplantibacillus plantarum, Lactobacillus buchneri and Weissella hellenica which may produce a high-quality animal feed able to improve animal physical performance (200, 201).
Eventually, even eggshells may be converted into value-added products. Due to their high content of calcium carbonate (CaCO3), eggshells in the form of “eggshell powder” are utilized as calcium supplements for humans. Additionally, their potassium and magnesium content makes them suitable as plant fertilizers (202). Moreover, eggshells can serve as animal feed supplements for livestock. More recently, eggshells have also been employed in natural filters designed to remove impurities and contaminants from waste water (77, 202, 203).
6 Bioconversion vs. traditional disposal methods
The previous sections have shown that there are many avenues available to deal with litter, from simple composting in the backyard to sophisticated valorization in shampoos. Notably, the ecological as well as economic impact of these different methods may differ significantly depending on the circumstances. It may be more reasonable, for instance, to simply compost the odd SCG in your garden if you live in a remote village, whereas a highly frequented coffee shop in the center of Paris would probably prefer to donate or even sell the same “waste” to the cosmetics industry – unless, of course, there is a bakery nearby.
In any case, the enormous amounts of food waste produced globally should not simply end up in landfills or incinerators, as they still often do. On the one hand, disposing of food waste in landfills or through incineration has detrimental consequences for the environment (153–155). It leads to greenhouse gas emissions, especially CO2 and CH4, increased demand for landfill capacity, which affects ecosystems and nearby populations, and contamination of ground- and surface water. The ash generated from incinerating waste and the leachate from landfilling are documented to contain toxic residues eventually worsening air and water pollution and resulting in loss of biodiversity. In contrast, utilizing bioconversion as an alternative method may reduce the negative environmental consequences and extract the value of otherwise hazardous pollutants (202). The food waste management methods, benefits and their impacts on the environment are summarized in Table 3.
Various strategies should therefore be implemented to minimize food waste, such as food sharing and donations, “lick your plate with pride” campaigns and maximizing recycling and upcycling of food waste wherever and whenever possible. By adopting these alternative approaches, individually or in concert, the environmental impact of food waste disposed in landfills or incinerated may be minimized, thereby contributing to more sustainable waste management practices (202, 214, 215).
7 Future trends in waste management
An enormous amount of food, i.e., around 2.5 billion tons, which accounts for almost one third of the food produced annually, goes to the bin. The economic value of this wasted food amounts to approximately 230 billion USD, according to a report by the Boston Consulting Group (BCG) (216). At the same time, one-third of the world’s population is facing the challenge of insufficient food, particularly in developing countries, impacting around 320 million people according to the United Nations. Food waste levels are expected to increase by another third by 2030 (216). In order to counteract the global impacts of food waste, sustainable food waste management approaches have to be adopted. First and foremost important strategies would require waste reduction at the place of origin not only through improved management but also via bio-valorization. Additionally, implementation of advanced recycling and upcycling methods which transform food waste into valuable products, such as bioplastics, fertilizers, and biofuels are equally important (217).
Sustainable practices, such as composting, anaerobic digestion and insect-based bioconversion of food waste-to-energy are slowly yet gradually evolving and need to be integrated into waste management. Composting is a traditional method, which is becoming more popular not only due to its simplicity but also its ability to produce nutrient-rich soil (44, 45). In contrast, anaerobic digestion is gaining attraction for its capacity to generate biogas for energy production and decreasing waste (101). Insect biorefinery is also another very important emerging tool in the context of the circular bioeconomy since this refinery is able to transform organic waste material to value-added products such as biofertilizers, animal feeds, edible foods, biopolymers, bio-enzymes and biodiesel (76, 218). Furthermore, recent developments in the field of enzyme technology are focused at breaking down the food waste at the molecular level to obtain valuable products (219, 220). Another innovative approach involves utilizing food waste as culture medium to grow algae which provides a sustainable source of biomass for various applications (221, 222). A few strategies involve a combination of techniques for the management or upcycling of organic waste products. Palm bunches have been exploited as biodiesel feedstocks through integrated solid-state and submerged fermentations by fungal co-cultures (223). This technique employs a combination of solid-state and submerged fermentations, with multiple types of fungi working together (fungal co-cultures) for the production of biodiesel (223). Moreover, the extraction of valuable nutrients from organic waste employing supercritical fluids is also an important technique although it may not be cost effective (224). In summary, the future of food waste management is likely to involve a combination of these methods, which will be contingent on local regulations and specific waste streams. The primary focus will revolve around adopting a comprehensive approach that incorporates technological innovation, raises consumer awareness and corporate social responsibility, promotes consumer behavior change and gives more value to surplus “saved” food (225, 226). The Food Recovery Hierarchy outlines actions which organizations, individuals and families can take to prevent and manage wasted food, as summarized in the Figure 7.
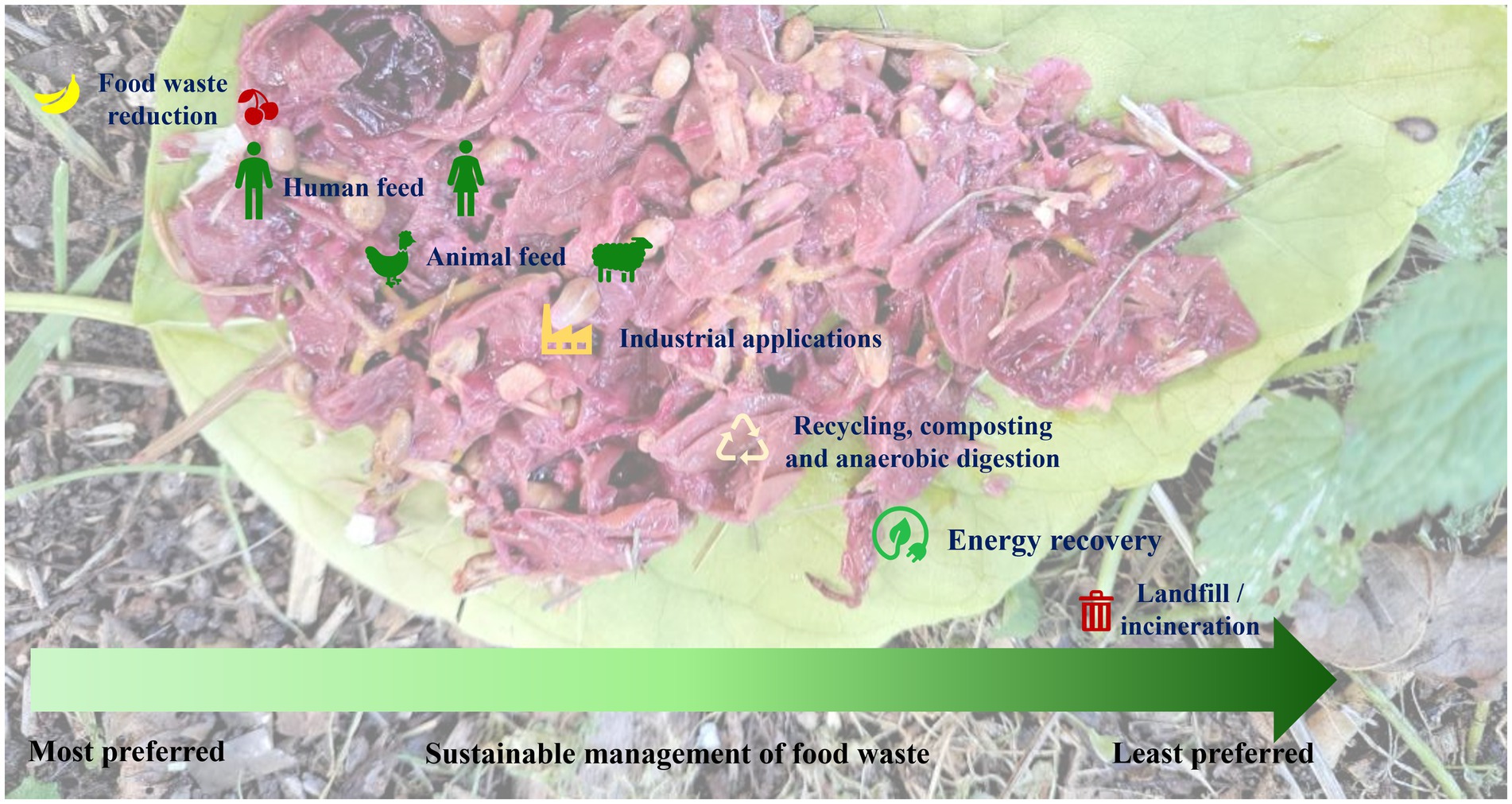
Figure 7. The Food Recovery Hierarchy enlists the actions which can be carried out by the organizations, individuals, and families for the sustainable management of food waste. The highest priority has been assigned to feed humans and animals, followed by industrial applications, recycling, AD and energy recovery. Landfill and/or incineration have been assigned as the least preferred actions.
8 Conclusion
As part of this manuscript, we have aimed to showcase a selection of modern chemical, biochemical and (micro-)biological techniques which may eventually enable us to turn organic by-products or “waste” into valuable (raw) materials and energy. Indeed, valorization of biological (waste) materials currently appears as one of the most promising avenues to limit waste on the one hand and to avoid depletion of natural resources on the other. As one important pillar of a future circular bioeconomy, the “waste into value” concept promises carbon neutrality and also local employment. Rather surprisingly, the underlying strategies of bio-valorization are often based on traditional, robust methods and techniques and therefore may have disappointed the ones expecting high-tech molecular or microbiology. Still, the projects presented are not trivial and often do require modern (bio-)chemistry and analysis to enable a safe, large-scale handling, production, use and, in some cases, consumption.
In future, microbiology, together with molecular biology is likely to improve the fermentation processes mentioned here considerably, for instance in the fields of biofuels and biogas. It may become possible to produce H2 rather than CH4 via (an)aerobic digestion, thus departing further from carbon-based materials and fuels. Then again, some of these biofuels, such as CH4 and bioethanol and most recently ammonia (NH3), are easier to store and handle compared to electricity or H2, and thus may eventually become amenable to uses in low-emission fuel cells rather than combustion engines.
At the same time, more selective collection and processing methods are likely to increase the yields and thus also value of bio-derived products, such as “green” urea and phosphate from human and animal excrements. Here, methods to collect and refine the waste produced by farm animals more efficiently could be a first step, as it may be easier to implement logistically than returning to the good old family bog hole in the garden.
No doubt, even if driving a honey wagon around town may not be your dream job of the future, new innovations in the field of household waste, from peels to coffee, may indeed provide fascinating opportunities. Citrus peels can already be processed on a small scale and with the help of vinegar to cleaning liquids at home, and decentralized local fermenters and production facilities are springing up and into action even in the smallest villages, such as in Kirkel-Altstadt, Saarland, Germany, where the foundation stone for a new biogas plant running on horse manure and hay has just been laid, and which may soon supply heat and electricity - and odor - for about 300 households (227).
In most cases, a biochemist or biologist is at hand to supervise and control such faculties and to ensure that, literally, no shit happens, from the anecdotal detonation of bog holes to a large-scale escape of BSF. Maybe this simply underlines the fact that numerous challenges for modern biochemistry await us outside the Noble-Prize winning fields of biotechnology and medicine, and despite their low-key, down-to-earth and hands-on applications, these research and development projects are certainly not litter and definitely not for the bin.
Author contributions
AR: Data curation, Investigation, Formal analysis, Writing – review & editing. ET: Data curation, Formal analysis, Writing – review & editing. AG: Data curation, Formal analysis, Writing – review & editing. AA: Data curation, Formal analysis, Writing – review & editing. MN: Conceptualization, Data curation, Formal analysis, Supervision, Validation, Writing – review & editing. CJ: Conceptualization, Supervision, Validation, Writing – original draft, Writing – review & editing.
Funding
The author(s) declare financial support was received for the research, authorship, and/or publication of this article. The authors would like to acknowledge the financial support of the University of Saarland, Saarbuecken, Germany), the “Landesforschungsfoerderungs programm” of the State of Saarland (Grant No. WT/2-LFFP 16/01) and the INTERREG VA GR program (BIOVAL, Grant No. 4-09-21).
Acknowledgments
We would like to thank Sodomir Popojuk and all the “Litter rats” and colleagues from Pharmasophy, the Academiacs International Network (www.academiacs.eu, accessed on 7th of March 2023) as well as GENAWIF – Society for Natural Compound- and Drug Research for their helpful discussions and advice. We further extend our gratitude to the kind staff at Homburger Brewery, Reformhaus Escher Saarbruecken, and BioFrischMarkt GmbH, Saarbruecken. We would also like to acknowledge Elizabeth Jacob for providing the original photographs used for the preparation of this review article. Please contact Claus Jacob for permission to re-use photographs.
Conflict of interest
The authors declare that the research was conducted in the absence of any commercial or financial relationships that could be construed as a potential conflict of interest.
Publisher’s note
All claims expressed in this article are solely those of the authors and do not necessarily represent those of their affiliated organizations, or those of the publisher, the editors and the reviewers. Any product that may be evaluated in this article, or claim that may be made by its manufacturer, is not guaranteed or endorsed by the publisher.
References
1. Raworth, K . Doughnut economics: Seven ways to think like a 21st-century economist. Chelsea, Vermont: Chelsea Green Publishing (2017).
2. Fritsche, U, Brunori, G, Chiaramonti, D, Galanakis, C, Hellweg, S, Matthews, RAPC, et al. Future transitions for the bioeconomy towards sustainable development and a climate-neutral economy–knowledge synthesis final report. (2020). Publications Office of the European Union, Luxembourg, 10: 667966.
3. Fritsche, U, Brunori, G, Chiaramonti, D, Galanakis, C, Matthews, R, and Panoutsou, C. Future transitions for the bioeconomy towards sustainable development and a climate-neutral economy-foresight scenarios for the EU bioeconomy in 2050. (2021).
4. Steffen, W, Richardson, K, Rockström, J, Cornell, S, Fetzer, I, Bennett, E, et al. Science supplementary material planetary boundaries. Science Express (2015).
5. Schoenmaker, D . A framework for sustainable finance. SSRN Electron J. (2018). doi: 10.2139/ssrn.3125351
6. Hedstrom, GS . Sustainability: What it is and how to measure it. Berlin, Germany: Walter de Gruyter GmbH and Co KG (2018).
8. Orensanz, JM, Parma, AM, Jerez, G, Barahona, N, Montecinos, M, and Elias, I. What are the key elements for the sustainability of. Bull Mar Sci. (2005) 76:527–56.
9. The University of Tennessee . What are the 5 R’s of Sustainability?. Office of Sustainability. (2023). Available at: https://sustainability.utk.edu/2020/01/10/what-are-the-5-rs-of-sustainability/ (Accessed March 02, 2023).
10. Hunt, K . Here’s how many times you need to reuse your reusable grocery bags. CNN. (2023). Available at: https://www.cnn.com/2022/12/13/world/cotton-tote-vs-plastic-bags-environment-climate-cost-scn/index.html (Accessed March 02, 2023).
11. Blog . Save plastic when shopping bring! (2023). Available at: https://www.getbring.com/blog-posts/save-plastic-when-shopping (Accessed March 02, 2023).
13. CarsDirect . How to reduce fuel consumption. (2023). Available at: https://www.carsdirect.com/car-buying/10-ways-to-lower-engine-fuel-consumption (Accessed March 02, 2023).
14. Osborne, H . The reusable suspects. The Guardian, (2007). Available at: https://www.theguardian.com/environment/2007/mar/05/practicaladvice (Accessed March 02, 2023).
15. ScienceDirect . Food waste management, valorization, and sustainability in the food industry - ScienceDirect. (2023). Available at: https://www.sciencedirect.com/science/article/abs/pii/B9780128205631000081 (Accessed July 16, 2023).
16. Carmona-Cabello, M, Garcia, IL, Leiva-Candia, D, and Dorado, MP. Valorization of food waste based on its composition through the concept of biorefinery. Curr Opin Green Sustain Chem. (2018) 14:67–79. doi: 10.1016/j.cogsc.2018.06.011
17. Van Dyk, JS, Gama, R, Morrison, D, Swart, S, and Pletschke, BI. Food processing waste: problems, current management and prospects for utilisation of the lignocellulose component through enzyme synergistic degradation. Renew Sust Energ Rev. (2013) 26:521–31. doi: 10.1016/j.rser.2013.06.016
18. Wang, Q, Ma, H, Xu, W, Gong, L, Zhang, W, and Zou, D. Ethanol production from kitchen garbage using response surface methodology. Biochem Eng J. (2008) 39:604–10. doi: 10.1016/j.bej.2007.12.018
19. Kobayashi, T, Xu, K-Q, Li, Y-Y, and Inamori, Y. Evaluation of hydrogen and methane production from municipal solid wastes with different compositions of fat, protein, cellulosic materials and the other carbohydrates. Int J Hydrog Energy. (2012) 37:15711–8. doi: 10.1016/j.ijhydene.2012.05.044
20. Sirohi, R, Tarafdar, A, Singh, S, Negi, T, Gaur, VK, Gnansounou, E, et al. Green processing and biotechnological potential of grape pomace: current trends and opportunities for sustainable biorefinery. Bioresour Technol. (2020) 314:123771. doi: 10.1016/j.biortech.2020.123771
21. Food and Agriculture Organization . International day food loss and waste, technical platform on the measurement and reduction of food loss and waste, Food and agriculture Organization of the United Nations. FoodLossWaste. (2023). Available at: https://www.fao.org/platform-food-loss-waste/flw-events/international-day-food-loss-and-waste/en (Accessed July 9, 2023).
22. Statista . Annual food waste by select country worldwide. (2023). Available at: https://www.statista.com/statistics/933083/food-waste-of-selected-countries/ (Accessed Nov 14, 2023).
23. Worldometer . Population by Country (2023). Available at: https://www.worldometers.info/world-population/population-by-country/ (Accessed Nov. 14, 2023).
24. Deutschland.de. Appreciating Food . (2021). Available at: https://www.deutschland.de/en/topic/environment/food-waste-in-germany-and-worldwide#:~:text=In%20Germany%2C%20we%20estimate%20that,estimated%20at%201.3%20billion%20tonnes (Accessed July 16, 2023).
25. Goossens, Y, Wegner, A, and Schmidt, T. Sustainability assessment of food waste prevention measures: review of existing evaluation practices. Front Sustain Food Syst. (2019) 3:90. doi: 10.3389/fsufs.2019.00090
26. Ishangulyyev, R, Kim, S, and Lee, SH. Understanding food loss and waste–why are we losing and wasting food? Food Secur. (2019) 8:297. doi: 10.3390/foods8080297
27. Isah, S, and Ozbay, G. Valorization of food loss and wastes: feedstocks for biofuels and valuable chemicals. Front Sustain Food Syst. (2020) 4:82. doi: 10.3389/fsufs.2020.00082
28. Vågsholm, I, Arzoomand, NS, and Boqvist, S. Food security, safety, and sustainability–getting the trade-offs right. Front Sustain Food Syst. (2020) 4:16. doi: 10.3389/fsufs.2020.00016
29. Camara, C . How renewable materials can power a circular economy. The European Files. (2023). Available at: https://www.europeanfiles.eu/environment/how-renewable-materials-can-power-a-circular-economy (Accessed Mar 2, 2023).
30. Harris, S, Staffas, L, Rydberg, T, and Eriksson, E. Renewable Materials in the Circular Economy (2018).
31. Brunklaus, B, and Riise, E. Bio-based materials within the circular economy: opportunities and challenges In: E Benetto, K Gericke, and M Guiton, editors. Designing sustainable technologies, products and policies: From science to innovation. Cham: Springer International Publishing (2018). 43–7.
32. Koçar, G, and Civaş, N. An overview of biofuels from energy crops: current status and future prospects. Renew Sust Energ Rev. (2013) 28:900–16. doi: 10.1016/j.rser.2013.08.022
33. Mbogo, G . We need to change our attitude on waste. Kenya association of manufacturers. (2023). Available at: https://kam.co.ke/need-change-attitude-waste/ (Accessed Mar 2, 2023).
34. Global Opportunity Explorer . Changing attitudes with improved waste management. (2023). Available at: https://goexplorer.org/changing-attitudes-with-improved-waste-management/ (Accessed Mar 02, 2023).
35. Purcell, M, and Magette, WL. Attitudes and behaviour towards waste management in the Dublin, Ireland region. Waste Manag. (2010) 30:1997–2006. doi: 10.1016/j.wasman.2010.02.021
36. Bioeconomy in Germany - BMBF,” Bundesministerium für Bildung und Forschung - BMBF (2024). Available at: https://www.bmbf.de/SharedDocs/Publikationen/de/bmbf/FS/31106_Biooekonomie_in_Deutschland_en.html
37. Sridhar, A, Kapoor, A, Senthil Kumar, P, Ponnuchamy, M, Balasubramanian, S, and Prabhakar, S. Conversion of food waste to energy: a focus on sustainability and life cycle assessment. Fuel. (2021) 302:121069. doi: 10.1016/j.fuel.2021.121069
38. Zhang, Y, Chen, M, Guo, J, Liu, N, Yi, W, Yuan, Z, et al. Study on dynamic changes of microbial community and lignocellulose transformation mechanism during green waste composting. Eng Life Sci. (2022) 22:376–90. doi: 10.1002/elsc.202100102
39. Jurado, M, López, MJ, Suárez-Estrella, F, Vargas-García, MC, López-González, JA, and Moreno, J. Exploiting composting biodiversity: study of the persistent and biotechnologically relevant microorganisms from lignocellulose-based composting. Bioresour Technol. (2014) 162:283–93. doi: 10.1016/j.biortech.2014.03.145
40. Pfaltzgraff, LA, Bruyn, MD, Cooper, EC, Budarin, V, and Clark, JH. Food waste biomass: a resource for high-value chemicals. Green Chem. (2013) 15:307–14. doi: 10.1039/C2GC36978H
41. Meena, AL, Karwal, M, Dutta, D, and Mishra, RP. Composting: phases and factors responsible for efficient and improved composting. Agric Food. (2021) 1:85–90. doi: 10.13140/RG.2.2.13546.95689
42. Chew, KW, Chia, SR, Yen, H-W, Nomanbhay, S, Ho, Y-C, and Show, PL. Transformation of biomass waste into sustainable organic fertilizers. Sustain For. (2019) 11:2266. doi: 10.3390/su11082266
43. van Loenen, MCA, Turbett, Y, Mullins, CE, Feilden, NEH, Wilson, MJ, Leifert, C, et al. Low temperature–short duration steaming of soil kills soil-borne pathogens, nematode pests and weeds. Eur J Plant Pathol. (2003) 109:993–1002. doi: 10.1023/B:EJPP.0000003830.49949.34
44. Awasthi, SK, Sarsaiya, S, Awasthi, MK, Liu, T, Zhao, J, Kumar, S, et al. Changes in global trends in food waste composting: research challenges and opportunities. Bioresour Technol. (2020) 299:122555. doi: 10.1016/j.biortech.2019.122555
45. Cerda, A, Artola, A, Font, X, Barrena, R, Gea, T, and Sánchez, A. Composting of food wastes: status and challenges. Bioresour Technol. (2018) 248:57–67. doi: 10.1016/j.biortech.2017.06.133
46. Margo . Compost and fertilizer: how to use them in the garden. HomeBiogas. (2023). Available at: https://www.homebiogas.com/blog/compost-and-fertilizer/ (Accessed Jul 10, 2023).
47. Baghlani, AW . Sustainable agriculture and farming Logen Robinson. Sustainable agriculture and farming, (2022). Available at: https://www.academia.edu/87842402/Sustainable_Agriculture_and_Farming_Logen_Robinson (Accessed Oct 3, 2023).
48. Hugelkultur: the ultimate raised garden bed. (2023). Available at: https://richsoil.com/hugelkultur/ (Accessed Oct 3, 2023).
49. The art and science of making a Hugelkultur bed - transforming Woody debris into a garden resource - the permaculture research institute. (2023). Available at: https://web.archive.org/web/20151105061510/http://permaculture.org.au/2010/08/03/the-art-and-science-of-making-a-hugelkultur-bed-transforming-woody-debris-into-a-garden-resource/ (Accessed Oct 3, 2023).
50. Rynk, R, Van de Kamp, M, Willson, GB, Singley, ME, Richard, TL, Kolega, JJ, et al. On-farm composting handbook. Ithaca: Riley-Robb Hall (1992).
51. Adhikary, S . Vermicompost, the story of organic gold: a review. Agric Sci. (2012) 3:110. doi: 10.4236/as.2012.37110
52. Vite Greenhouses . Worm castings–Nature’s finest fertilizer! (2023). Available at: https://www.vitegreenhouses.com/wormcastings.asp (Accessed Aug 20, 2023).
53. Worm castings: what are the benefits of adding in Lawn & Garden? (2023). Available at: https://pinchofseeds.com/earthworm-castings/ (Accessed Aug 20, 2023).
54. Dominguez, J, Edwards, CA, and Subler, S. A comparison of vermicomposting and composting. Biocycle. (1997) 38:57–9.
55. Tognetti, C, Laos, F, Mazzarino, MJ, and Hernandez, MT. Composting vs. vermicomposting: a comparison of end product quality. Compost Sci Util. (2005) 13:6–13. doi: 10.1080/1065657X.2005.10702212
56. Hanc, A, Dume, B, and Hrebeckova, T. Differences of enzymatic activity during composting and vermicomposting of sewage sludge mixed with straw pellets. Front Microbiol. (2023) 18:1107. doi: 10.3389/fmicb.2021.801107/full
57. Management of urban solid waste: vermicomposting a sustainable option - ScienceDirect. (2023). Available at: https://www.sciencedirect.com/science/article/abs/pii/S0921344911000371 (Accessed Aug 18, 2023)
58. Difference between compost and Vermicompost. GeeksforGeeks. (2023). Available at: https://www.geeksforgeeks.org/difference-between-compost-and-vermicompost/ (Accessed Oct 09, 2023).
59. Wani, S., Chander, G., and Challagulla, V. Vermicomposting: recycling wastes into valuable manure for sustained crop intensification in the semi-arid tropics, pp. 123–151. (2015).
60. Fornes, F, Mendoza-Hernández, D, García-de-la-Fuente, R, Abad, M, and Belda, RM. Composting versus vermicomposting: a comparative study of organic matter evolution through straight and combined processes. Bioresour Technol. (2012) 118:296–305. doi: 10.1016/j.biortech.2012.05.028
61. Kokhia, M . Composting: advantages and disadvantages. 24th Proceedings of the Institute of Zoology, pp. 133–140. (2015).
62. Appelhof, M, and Olszewski, J. Worms Eat My Garbage, 35th anniversary edition: How to set up and maintain a worm composting system: Compost food waste, produce fertilizer for houseplants and garden, and educate your kids and family. Ontario: Storey Publishing (2017).
63. Worm castings vs compost (which is better, Vermicompost or regular compost?). (2023). Available at: https://helpmecompost.com/compost/basics/worm-castings-vs-compost/ (Accessed Aug 18, 2023).
64. Yang, F, Li, G, Zang, B, and Zhang, Z. The maturity and CH4, N2O, NH3 emissions from vermicomposting with agricultural waste. Compost Sci Util. (2017) 25:262–71. doi: 10.1080/1065657X.2017.1329037
65. Yasmin, N, Jamuda, M, Panda, AK, Samal, K, and Nayak, JK. Emission of greenhouse gases (GHGs) during composting and vermicomposting: measurement, mitigation, and perspectives. Energy Nexus. (2022) 7:100092. doi: 10.1016/j.nexus.2022.100092
66. Vergara, SE, and Silver, WL. Greenhouse gas emissions from windrow composting of organic wastes: patterns and emissions factors. Environ Res Lett. (2019) 14:124027. doi: 10.1088/1748-9326/ab5262
67. Ojha, S, Bußler, S, and Schlüter, OK. Food waste valorisation and circular economy concepts in insect production and processing. Waste Manag. (2020) 118:600–9. doi: 10.1016/j.wasman.2020.09.010
68. Nutrient composition of insects and their potential application in food and feed in Europe. (2023). Available at: https://www.semanticscholar.org/paper/Nutrient-composition-of-insects-and-their-potential-Rumpold-Schl%C3%BCter/4003d3ba1d834b61ba47cd91713076e2b364b0c0 (Accessed Jul 10, 2023).
69. Beesigamukama, D, Subramanian, S, and Tanga, CM. Nutrient quality and maturity status of frass fertilizer from nine edible insects. Sci Rep. (2022) 12:7182. doi: 10.1038/s41598-022-11336-z
70. Varelas, V . Food wastes as a potential new source for edible insect mass production for food and feed: a review. Fermentation. (2019) 5:81. doi: 10.3390/fermentation5030081
71. Insect mass production technologies. (2023). Available at: https://www.semanticscholar.org/paper/Insect-Mass-Production-Technologies-Ortiz-Ruiz/5daf188fcbca7d2fdc6591156c33347bea199bcf (Accessed Jul 10, 2023).
72. Bhatia, DR . Observations on the biology of the desert locust (Schistocerca gregaria Forsk.) in Sind-Rajputana Desert area. I. Thepreferred food plants of the locust. Indian J Entomol. (1940) 2:11.
73. Mannaa, M, Mansour, A, Park, I, Lee, D-W, and Seo, Y-S. Insect-based Agri-food waste valorization: agricultural applications and roles of insect gut microbiota. Environ Sci Ecotechnol. (2023) 17:100287. doi: 10.1016/j.ese.2023.100287
74. Pleissner, D, and Smetana, S. Estimation of the economy of heterotrophic microalgae-and insect-based food waste utilization processes. Waste Manag. (2020) 102:198–203. doi: 10.1016/j.wasman.2019.10.031
75. Ravi, HK, Degrou, A, Costil, J, Trespeuch, C, Chemat, F, and Vian, MA. Larvae mediated valorization of industrial, agriculture and food wastes: biorefinery concept through bioconversion, processes, procedures, and products. PRO. (2020) 8:857. doi: 10.3390/pr8070857
76. Fowles, TM, and Nansen, C. Insect-based bioconversion: value from food waste In: E Närvänen, N Mesiranta, M Mattila, and A Heikkinen, editors. Food waste management: Solving the wicked problem. Cham: Springer International Publishing (2020). 321–46.
77. Beesigamukama, D, Mochoge, B, Korir, NK, Fiaboe, KKM, Nakimbugwe, D, Khamis, FM, et al. Low-cost technology for recycling agro-industrial waste into nutrient-rich organic fertilizer using black soldier fly. Waste Manag. (2021) 119:183–94. doi: 10.1016/j.wasman.2020.09.043
78. Zheng, L, Hou, Y, Li, W, Yang, S, Li, Q, and Yu, Z. Biodiesel production from rice straw and restaurant waste employing black soldier fly assisted by microbes. Energy. (2012) 47:225–9. doi: 10.1016/j.energy.2012.09.006
79. Manurung, R, Supriatna, A, Esyanthi, RR, and Putra, RE. Bioconversion of rice straw waste by black soldier fly larvae (Hermetia illucens L.): optimal feed rate for biomass production. J Entomol Zool Stud. (2016) 4:1036–41.
80. Almqvist, R . Fly larvae composting of fibrous food industry waste. (2021). Available at: https://stud.epsilon.slu.se/16540/ (Accessed Nov 14, 2023).
81. Niu, Y, Zheng, D, Yao, B, Cai, Z, Zhao, Z, Wu, S, et al. A novel bioconversion for value-added products from food waste using Musca domestica. Waste Manag. (2017) 61:455–60. doi: 10.1016/j.wasman.2016.10.054
82. Miech, P, Berggren, Å, Lindberg, JE, Chhay, T, Khieu, B, and Jansson, A. Growth and survival of reared Cambodian field crickets (Teleogryllus testaceus) fed weeds, agricultural and food industry by-products. J Insects Food Feed. (2016) 2:285–92. doi: 10.3920/JIFF2016.0028
83. Wang, Y-S, and Shelomi, M. Review of black soldier fly (Hermetia illucens) as animal feed and human food. Food Secur. (2017) 6:91. doi: 10.3390/foods6100091
84. Muthu, P, Muniappan, G, and Jeyakumar, RB. Efficacious utilization of food waste for bioenergy generation through the anaerobic digestion method. PRO. (2023) 11:702. doi: 10.3390/pr11030702
85. Appels, L, Baeyens, J, Degrève, J, and Dewil, R. Principles and potential of the anaerobic digestion of waste-activated sludge. Prog Energy Combust Sci. (2008) 34:755–81. doi: 10.1016/j.pecs.2008.06.002
86. Goswami, R, Chattopadhyay, P, Shome, A, Banerjee, SN, Chakraborty, AK, Mathew, AK, et al. An overview of physico-chemical mechanisms of biogas production by microbial communities: a step towards sustainable waste management. 3 Biotech. (2016) 6:72. doi: 10.1007/s13205-016-0395-9
87. Demirel, B, and Scherer, P. The roles of acetotrophic and hydrogenotrophic methanogens during anaerobic conversion of biomass to methane: a review. Rev Environ Sci Biotechnol. (2008) 7:173–90. doi: 10.1007/s11157-008-9131-1
88. Moestedt, J, Müller, B, Nagavara Nagaraj, Y, and Schnürer, A. Acetate and lactate production during two-stage anaerobic digestion of food waste driven by Lactobacillus and Aeriscardovia. Front Energy Res. (2020) 8:105. doi: 10.3389/fenrg.2020.00105
89. MJ, MI, Struchtemeyer, CG, Sieber, J, Mouttaki, H, Stams, AJ, Schink, B, et al. Physiology, ecology, phylogeny, and genomics of microorganisms capable of syntrophic metabolism. Ann N Y Acad Sci. (2023) 1125:58–72. doi: 10.1196/annals.1419.005
90. Godon, JJ, Zumstein, E, Dabert, P, Habouzit, F, and Moletta, R. Molecular microbial diversity of an anaerobic digestor as determined by small-subunit rDNA sequence analysis. Appl Environ Microbiol. (1997) 63:2802–13. doi: 10.1128/aem.63.7.2802-2813.1997
92. Scheifinger, C, Linehan, B, and Wolin, M. H2 production by Selenomonas ruminantium in the absence and presence of methanogenic Bacteria. Appl Microbiol. (1975) 29:480–3. doi: 10.1128/AEM.29.4.480-483.1975
93. Soni, SK . Microbes: a source of energy for 21st century. New Delhi: New India Publishing (2007).
94. Nath, K, and Das, D. Improvement of fermentative hydrogen production: various approaches. Appl Microbiol Biotechnol. (2004) 65:520–9. doi: 10.1007/s00253-004-1644-0
95. Oh, Y-K, Park, M, Seol, E-H, Lee, S-J, and Park, S. Isolation of hydrogen-producing bacteria from granular sludge of an upflow anaerobic sludge blanket reactor. Biotechnol Bioprocess Eng. (2003) 8:54–7. doi: 10.1007/BF02932899
96. Oh, Y-K, Seol, E-H, Lee, EY, and Park, S. Fermentative hydrogen production by a new chemoheterotrophic bacterium Rhodopseudomonas palustris P4. Int J Hydrog Energy. (2002) 27:1373–9. doi: 10.1016/S0360-3199(02)00100-3
97. Comparison of anaerobic digestion in the EU vs. the U.S. - part one. Waste360. (2023). Available at: https://www.waste360.com/anaerobic-digestion/comparison-anaerobic-digestion-eu-vs-us-part-one (Accessed Aug 18, 2023).
98. Anaerobic digestion Germany biogas industry sells AD technology abroad. (2023). Available at: https://blog.anaerobic-digestion.com/anaerobic-digestion-germany/ (Accessed Aug 18, 2023).
99. Morales-Polo, C, Cledera-Castro, MDM, and Moratilla Soria, BY. Reviewing the anaerobic digestion of food waste: from waste generation and anaerobic process to its perspectives. Appl Sci. (2018) 8:1804. doi: 10.3390/app8101804
100. Xu, F, Li, Y, Ge, X, Yang, L, and Li, Y. Anaerobic digestion of food waste–challenges and opportunities. Bioresour Technol. (2018) 247:1047–58. doi: 10.1016/j.biortech.2017.09.020
101. Arsova, L . Anaerobic digestion of food waste: current status, problems and an alternative product. Department of earth and environmental engineering foundation of engineering and applied science Columbia University, (2010). Available at: https://www.researchgate.net/profile/Kartik-Chandran/publication/265220417_Anaerobic_digestion_of_food_waste_Current_status_problems_and_an_alternative_product/links/55ca803908aea2d9bdcc01ed/Anaerobic-digestion-of-food-waste-Current-status-problems-and-an-alternative-product.pdf (Accessed Nov 02, 2023).
102. Hegde, G, and Pullammanappallil, P. Comparison of thermophilic and mesophilic one-stage, batch, high-solids anaerobic digestion. Environ Technol. (2007) 28:361–9. doi: 10.1080/09593332808618797
103. Funding Digestion . (2023). Available at: https://www.epa.gov/sites/default/files/2014-12/documents/funding_digestion.pdf (Accessed Nov 02, 2023).
104. Wambugu, CW, Rene, ER, van de Vossenberg, J, Dupont, C, and van Hullebusch, ED. Role of biochar in anaerobic digestion based biorefinery for food waste. Front Energy Res. (2019) 7:14. doi: 10.3389/fenrg.2019.00014
105. Zulkepli, NE, Muis, ZA, Mahmood, NAN, Hashim, H, and Ho, WS. Cost benefit analysis of composting and anaerobic digestion in a community: a review. Chem Eng Trans. (2017) 56:1777–82. doi: 10.3303/CET1756297
106. Everything, FD . Integrating anaerobic digestion with composting. Biocycle. (2014) 55:32. doi: 10.1016/j.biteb.2023.101642
107. Zhang, F, Qin, Y, Zhao, C, and Wu, W. Soft magnetic ferrite for enhanced anaerobic digestion of food waste: effects on methane production and magnetic recovery. Bioresour Technol. (2023) 387:129601. doi: 10.1016/j.biortech.2023.129601
108. Zhang, H, Fu, Z, Guan, D, Zhao, J, Wang, Y, Zhang, Q, et al. A comprehensive review on food waste anaerobic co-digestion: current situation and research prospect. Process Saf Environ Prot. (2023) 179:546–58. doi: 10.1016/j.psep.2023.09.030
109. Tiong, YW, Sharma, P, Tian, H, Tsui, T-H, Lam, HT, and Tong, YW. Startup performance and microbial communities of a decentralized anaerobic digestion of food waste. Chemosphere. (2023) 318:137937. doi: 10.1016/j.chemosphere.2023.137937
110. Samoraj, M, Mironiuk, M, Izydorczyk, G, Witek-Krowiak, A, Szopa, D, Moustakas, K, et al. The challenges and perspectives for anaerobic digestion of animal waste and fertilizer application of the digestate. Chemosphere. (2022) 295:133799. doi: 10.1016/j.chemosphere.2022.133799
111. Möller, K, and Müller, T. Effects of anaerobic digestion on digestate nutrient availability and crop growth: a review. Eng Life Sci. (2012) 12:242–57. doi: 10.1002/elsc.201100085
112. Holm-Nielsen, JB, Al Seadi, T, and Oleskowicz-Popiel, P. The future of anaerobic digestion and biogas utilization. Bioresour Technol. (2009) 100:5478–84. doi: 10.1016/j.biortech.2008.12.046
113. Collet: life-cycle assessment of microalgae culture. (2023). Available at: https://scholar.google.com/scholar_lookup?title=Life-cycle%20assessment%20of%20microalgae%20culture%20coupled%20to%20bigas%20production&author=P.%20Collet&publication_year=2010&pages=207-214 (Accessed Jul 16, 2023).
114. De Schamphelaire, L, and Verstraete, W. Revival of the biological sunlight-to-biogas energy conversion system. Biotechnol Bioeng. (2009) 103:296–304. doi: 10.1002/bit.22257
115. Stoknes, K, Scholwin, F, Krzesiński, W, Wojciechowska, E, and Jasińska, A. Efficiency of a novel ‘food to waste to food’ system including anaerobic digestion of food waste and cultivation of vegetables on digestate in a bubble-insulated greenhouse. Waste Manag. (2016) 56:466–76. doi: 10.1016/j.wasman.2016.06.027
116. Digestate System - FarmWater. (2023). Available at: https://www.farmwater.co.uk/our-systems/digestate-system/ (Accessed Jul. 09, 2023).
117. Cao, Y, Chang, Z, Wang, J, Ma, Y, Yang, H, and Fu, G. Potential use of anaerobically digested manure slurry to suppress Phytophthora root rot of chilli pepper. Sci Hortic. (2023) 168:124–31. doi: 10.1016/j.scienta.2013.11.004
118. Amari, M, Toyota, K, Islam, M, Masuda, K, Kuroda, T, and Watanabe, A. Effect of the addition of anaerobically digested slurry to soil and hydroponics on soil-borne plant diseases. Soil Microorg. (2008) 62:106–13. doi: 10.18946/jssm.62.2_106
119. Brotman, Y, Landau, U, Cuadros-Inostroza, Á, Takayuki, T, Fernie, AR, Chet, I, et al. Trichoderma-plant root colonization: escaping early plant defense responses and activation of the antioxidant machinery for saline stress tolerance. PLoS Pathog. (2013) 9:e1003221. doi: 10.1371/journal.ppat.1003221
120. Morán-Diez, ME, Martínez de Alba, ÁE, Rubio, MB, Hermosa, R, and Monte, E. Trichoderma and the plant heritable priming responses. J Fungi (Basel). (2021) 7:318. doi: 10.3390/jof7040318
121. Singh, G, Jeyaseelan, C, Bandyopadhyay, KK, and Paul, D. Comparative analysis of biodiesel produced by acidic transesterification of lipid extracted from oleaginous yeast Rhodosporidium toruloides. 3 Biotech. (2018) 8:434. doi: 10.1007/s13205-018-1467-9
122. Zeng, J, Zeng, H, and Wang, Z. Review on technology of making biofuel from food waste. Int J Energy Res. (2022) 46:10301–19. doi: 10.1002/er.7868
123. Fukuda, H, Kondo, A, and Noda, H. Biodiesel fuel production by transesterification of oils. J Biosci Bioeng. (2001) 92:405–16. doi: 10.1016/S1389-1723(01)80288-7
124. Rashid, U, and Anwar, F. Production of biodiesel through optimized alkaline-catalyzed transesterification of rapeseed oil. Fuel. (2008) 87:265–73. doi: 10.1016/j.fuel.2007.05.003
125. Elgharbawy, AS, Sadik, WA, Sadek, OM, and Kasaby, MA. A review on biodiesel feedstocks and production technologies. J Chilean Chem Soc. (2023) 10:5098. doi: 10.4067/S0717-97072021000105098
126. Tan, Z, Chen, G, Zhao, Y, Shi, H, Wang, S, Bilal, M, et al. Digging and identification of novel microorganisms from the soil environments with high methanol-tolerant lipase production for biodiesel preparation. Environ Res. (2022) 212:113570. doi: 10.1016/j.envres.2022.113570
127. Lin, H, Wang, Q, Shen, Q, Zhan, J, and Zhao, Y. Genetic engineering of microorganisms for biodiesel production. Bioengineered. (2013) 4:292–304. doi: 10.4161/bioe.23114
128. Illukpitiya, P, and de Koff, JP. Economics of small-scale biodiesel production. (2014). Available at: https://digitalscholarship.tnstate.edu/cgi/viewcontent.cgi?article=1050&context=extension (Accessed Nov 02, 2023).
129. IndexBox . Biodiesel Price in Germany - 2023 - Charts and Tables. (2023). Available at: https://www.indexbox.io/search/biodiesel-price-germany/ (Accessed Nov 02, 2023).
130. Borugadda, VB, and Goud, VV. Biodiesel production from renewable feedstocks: status and opportunities. Renew Sust Energ Rev. (2012) 16:4763–84. doi: 10.1016/j.rser.2012.04.010
131. Anuar, MR, and Abdullah, AZ. Challenges in biodiesel industry with regards to feedstock, environmental, social and sustainability issues: a critical review. Renew Sust Energ Rev. (2016) 58:208–23. doi: 10.1016/j.rser.2015.12.296
132. Ajanovic, A . Biofuels versus food production: does biofuels production increase food prices? Energy. (2011) 36:2070–6. doi: 10.1016/j.energy.2010.05.019
133. Naylor, RL, and Higgins, MM. The rise in global biodiesel production: implications for food security. Glob Food Sec. (2018) 16:75–84. doi: 10.1016/j.gfs.2017.10.004
134. Bušić, A, Marđetko, N, Kundas, S, Morzak, G, Belskaya, H, Ivančić Šantek, M, et al. Bioethanol production from renewable raw materials and its separation and purification: a review. Food Technol Biotechnol. (2018) 56:289–311. doi: 10.17113/ftb.56.03.18.5546
135. Salman, J . Bioethanol production from green alga Chlorella vulgaris under different concentrations of nitrogen. Asian J Nat Appl Sci. (2014) 3:27–36.
136. Zabed, H, Sahu, JN, Suely, A, Boyce, AN, and Faruq, G. Bioethanol production from renewable sources: current perspectives and technological progress. Renew Sust Energ Rev. (2017) 71:475–501. doi: 10.1016/j.rser.2016.12.076
137. Diaz, CJ, Douglas, KJ, Kang, K, Kolarik, AL, Malinovski, R, Torres-Tiji, Y, et al. Developing algae as a sustainable food source. Front Nutr. (2023) 9:1029841. doi: 10.3389/fnut.2022.1029841
138. Gavahian, M, Munekata, PES, Eş, I, Lorenzo, JM, Khaneghah, AM, and Barba, FJ. Emerging techniques in bioethanol production: from distillation to waste valorization. Green Chem. (2019) 21:1171–85. doi: 10.1039/C8GC02698J
139. Mojarro, N . Ethanol and oil firms–the beginning of a new role for alternative fuels? Oxford: Oxford Institute for Energy Studies (2014).
140. SS SWR . Deutschlands erste Tankstelle bietet E20-Benzin an. (2023). Available at: https://www.tagesschau.de/wirtschaft/verbraucher/e20-tankstelle-100.html (Accessed: Nov 9, 2023)
141. Rosillo-Calle, F, and Cortez, LA. Towards ProAlcool II—a review of the Brazilian bioethanol programme. Biomass Bioenergy. (1998) 14:115–24. doi: 10.1016/S0961-9534(97)10020-4
142. Matsuoka, S, Ferro, J, and Arruda, P. The Brazilian experience of sugarcane ethanol industry. In Vitro Cell Dev Biol Plant. (2009) 45:372–81. doi: 10.1007/s11627-009-9220-z
143. Hira, A, and de Oliveira, LG. No substitute for oil? How Brazil developed its ethanol industry. Energy Policy. (2009) 37:2450–6. doi: 10.1016/j.enpol.2009.02.037
145. Gunatilake, HM, and Abeygunawardena, P. Energy security, food security, and economics of sugarcane bioethanol in India. Asian Development Bank economics working paper series, no. 255 (2011). Available at: https://papers.ssrn.com/sol3/papers.cfm?abstract_id=1869325 (Accessed Nov 02, 2023).
146. Koizumi, T . Biofuels and food security. Renew Sust Energ Rev. (2015) 52:829–41. doi: 10.1016/j.rser.2015.06.041
147. Maurya, R, Gohil, N, Nixon, S, Kumar, N, Noronha, SB, Dhali, D, et al. Rewiring of metabolic pathways in yeasts for sustainable production of biofuels. Bioresour Technol. (2023) 372:128668. doi: 10.1016/j.biortech.2023.128668
148. Cordova, LT, Butler, J, and Alper, HS. Direct production of fatty alcohols from glucose using engineered strains of Yarrowia lipolytica. Metab Eng Commun. (2020) 10:e00105. doi: 10.1016/j.mec.2019.e00105
149. Annamalai, N, Sivakumar, N, and Oleskowicz-Popiel, P. Enhanced production of microbial lipids from waste office paper by the oleaginous yeast Cryptococcus curvatus. Fuel. (2018) 217:420–6. doi: 10.1016/j.fuel.2017.12.108
150. d’Espaux, L, Ghosh, A, Runguphan, W, Wehrs, M, Xu, F, Konzock, O, et al. Engineering high-level production of fatty alcohols by Saccharomyces cerevisiae from lignocellulosic feedstocks. Metab Eng. (2017) 42:115–25. doi: 10.1016/j.ymben.2017.06.004
151. Kong, M, Li, X, Li, T, Zhao, X, Jin, M, Zhou, X, et al. Overexpressing CCW12 in Saccharomyces cerevisiae enables highly efficient ethanol production from lignocellulose hydrolysates. Bioresour Technol. (2021) 337:125487. doi: 10.1016/j.biortech.2021.125487
152. Liu, Z, Moradi, H, Shi, S, and Darvishi, F. Yeasts as microbial cell factories for sustainable production of biofuels. Renew Sust Energ Rev. (2021) 143:110907. doi: 10.1016/j.rser.2021.110907
153. Chhandama, MVL, Chetia, AC, Satyan, KB, Supongsenla Ao, JV, Ruatpuia, JVL, and Rokhum, SL. Valorisation of food waste to sustainable energy and other value-added products: a review. Bioresource Technol Rep. (2022) 17:100945. doi: 10.1016/j.biteb.2022.100945
154. Karmee, SK, and Lin, CSK. Valorisation of food waste to biofuel: current trends and technological challenges. Sustain Chem Process. (2014) 2:22. doi: 10.1186/s40508-014-0022-1
155. Bordiga, M, Travaglia, F, and Locatelli, M. Valorisation of grape pomace: an approach that is increasingly reaching its maturity – a review. Int J Food Sci Technol. (2019) 54:933–42. doi: 10.1111/ijfs.14118
156. Miladinović, MR, Zdujić, MV, Veljović, DN, Krstić, JB, Banković-Ilić, IB, Veljković, VB, et al. Valorization of walnut shell ash as a catalyst for biodiesel production. Renew Energy. (2020) 147:1033–43. doi: 10.1016/j.renene.2019.09.056
157. Zhu, F, Du, B, Zheng, L, and Li, J. Advance on the bioactivity and potential applications of dietary fibre from grape pomace. Food Chem. (2015) 186:207–12. doi: 10.1016/j.foodchem.2014.07.057
158. Caponio, GR, Noviello, M, Calabrese, FM, Gambacorta, G, Giannelli, G, and De Angelis, M. Effects of grape pomace polyphenols and in vitro gastrointestinal digestion on antimicrobial activity: recovery of bioactive compounds. Antioxidants. (2022) 11:567. doi: 10.3390/antiox11030567
159. Guo, L, Yang, ZY, Tang, RC, and Yuan, HB. Preliminary studies on the application of grape seed extract in the dyeing and functional modification of cotton fabric. Biomolecules. (2020) 10:220. doi: 10.3390/biom10020220
160. Sousa, EC, Uchôa-Thomaz, AMA, Carioca, JOB, Morais, SM, Lima, A, Martins, CG, et al. Chemical composition and bioactive compounds of grape pomace (Vitis vinifera L.), Benitaka variety, grown in the semiarid region of Northeast Brazil. Food Sci Technol. (2014) 34:135–42. doi: 10.1590/S0101-20612014000100020
161. Yu, J, and Ahmedna, M. Functional components of grape pomace: their composition, biological properties and potential applications. Int J Food Sci Technol. (2013) 48:221–37. doi: 10.1111/j.1365-2621.2012.03197.x
162. Ilyas, T, Chowdhary, P, Chaurasia, D, Gnansounou, E, Pandey, A, and Chaturvedi, P. Sustainable green processing of grape pomace for the production of value-added products: An overview. Environ Technol Innov. (2021) 23:101592. doi: 10.1016/j.eti.2021.101592
163. Lucarini, M, Durazzo, A, Romani, A, Campo, M, Lombardi-Boccia, G, and Cecchini, F. Bio-based compounds from grape seeds: a biorefinery approach. Molecules. (2018) 23:1888. doi: 10.3390/molecules23081888
164. Yang, C, Shang, K, Lin, C, Wang, C, Shi, X, Wang, H, et al. Processing technologies, phytochemical constituents, and biological activities of grape seed oil (GSO): a review. Trends Food Sci Technol. (2021) 116:1074–83. doi: 10.1016/j.tifs.2021.09.011
165. Kim, T-H, Lim, H-J, Kim, M-S, and Lee, MS. Dietary supplements for benign prostatic hyperplasia: an overview of systematic reviews. Maturitas. (2012) 73:180–5. doi: 10.1016/j.maturitas.2012.07.007
166. Wilt, T, Ishani, A, MacDonald, R, Stark, G, Mulrow, C, and Lau, J. Beta-sitosterols for benign prostatic hyperplasia. Cochrane Database Syst Rev. (2000) 1999:CD001043. doi: 10.1002/14651858.CD001043
167. Page analysis » Selina Wamucii. Selina Wamucii. (2023). Available at: https://www.selinawamucii.com/insights/prices/germany/wine/ (Accessed Mar 03, 2023).
168. Purchase price grapeseed oil + advantages and disadvantages. (2023). Available at: https://aradbranding.com/en/grapeseed-oil-benefits-for-body/ (Accessed Mar 3, 2023).
169. Hegedüs, I, Andreidesz, K, Szentpéteri, JL, Kaleta, Z, Szabó, L, Szigeti, K, et al. The utilization of physiologically active molecular components of grape seeds and grape Marc. Int J Mol Sci. (2022) 23:1165. doi: 10.3390/ijms231911165
170. Elkatry, HO, Ahmed, AR, El-Beltagi, HS, Mohamed, HI, and Eshak, NS. Biological activities of grape seed by-products and their potential use as natural sources of food additives in the production of Balady bread. Food Secur. (2022) 11:1948. doi: 10.3390/foods11131948
171. Bogoeva, AL, Durakova, AG, Pavlov, AI, Yanakieva, VB, Vrancheva, RZ, Bozadzhiev, BV, et al. Antioxidant activity and storage regime of defatted grape seeds flour. Wine Stud. (2017) 6:6695. doi: 10.4081/ws.2017.6695
172. 10 grapeseed oil benefits for skin 100% PURE. (2023). Available at: https://www.100percentpure.com/blogs/feed/10-benefits-of-grapeseed-oil-for-skin (Accessed Mar 03, 2023).
173. Sindhu, R, Gnansounou, E, Rebello, S, Binod, P, Varjani, S, Thakur, IS, et al. Conversion of food and kitchen waste to value-added products. J Environ Manag. (2019) 241:619–30. doi: 10.1016/j.jenvman.2019.02.053
174. Thorat, ID, Jagtap, DD, Mohapatra, D, Joshi, DC, Sutar, RF, and Kapdi, SS. Antioxidants, their properties, uses in food products and their legal implications. Int J Food Stud. (2013) 2:13. doi: 10.7455/ijfs/2.1.2013.a7
175. Lombardi, A, Campo, M, Vignolini, P, Papalini, M, Pizzetti, M, and Bernini, R. Phenolic-rich extracts from circular economy: chemical profile and activity against filamentous Fungi and dermatophytes. Molecules. (2023) 28:4374. doi: 10.3390/molecules28114374
176. Helkar, PB, Sahoo, AK, and Patil, NJ. Review: food industry by-products used as a functional food ingredients. Int J Waste Resources. (2016) 6:1–6. doi: 10.4172/2252-5211.1000248
177. Jackowski, M, Niedźwiecki, Ł, Jagiełło, K, Uchańska, O, and Trusek, A. Brewer’s spent grains—valuable beer industry by-product. Biomol Ther. (2020) 10:669. doi: 10.3390/biom10121669
178. Mitri, S, Salameh, SJ, Khelfa, A, Leonard, E, Maroun, RG, Louka, N, et al. Valorization of brewers’ spent grains: pretreatments and fermentation, a review. Fermentation. (2022) 8:50. doi: 10.3390/fermentation8020050
179. Lynch, KM, Steffen, EJ, and Arendt, EK. Brewers’ spent grain: a review with an emphasis on food and health. J Inst Brew. (2016) 122:553–68. doi: 10.1002/jib.363
180. Merten, D, Erman, L, Marabelli, GP, Leners, B, Ney, Y, Nasim, MJ, et al. Potential health effects of brewers’ spent grain as a functional food ingredient assessed by markers of oxidative stress and inflammation following gastro-intestinal digestion and in a cell model of the small intestine. Food Funct. (2022) 13:5327–42. doi: 10.1039/d1fo03090f
181. Salman, W, Ney, Y, Nasim, J, Bohn, T, Mitschang, P, and Jacob, C. Turning apparent waste into new value: up-cycling strategies exemplified by Brewer’s spent grains (BSG). Curr Nutraceuticals. (2020) 1:6–13. doi: 10.2174/2665978601666200220100600
182. Taddele, A . Synthesis, process parameters optimization and characterization of Banana Peel based bio-plastic. PhD Thesis, Addis Ababa university Addis Ababa. (2019).
184. Russia’s 2022 sugar beet production seen up 5% - producers’ group. (2022). Available at: https://www.nasdaq.com/articles/russias-2022-sugar-beet-production-seen-up-5-producers-group (Accessed Aug 18, 2023).
185. Germany sugar beet production, 1961-2022. Knoema. (2023). Available at: https://knoema.com//atlas/Germany/topics/Agriculture/Crops-Production-Quantity-tonnes/Sugar-beet-production (Accessed Aug 18, 2023).
186. About the U.S. sugar industry the sugar association. (2023). Available at: https://www.sugar.org/about/us-industry/ (Accessed Aug 18, 2023).
187. Sugar beet pulp as a source of valuable biotechnological products. (2023). Available at: https://www.bing.com/search?pglt=41&q=Sugar+Beet+Pulp+as+a+Source+of+Valuable+Biotechnological+Products+-+ScienceDirect&cvid=ca7d287e1cb34016b0f50954c748e864&aqs=edge.69i57j69i64.644j0j1&FORM=ANNTA1&PC=LCTS (Accessed July 10, 2023).
188. Ptak, M, Skowrońska, A, Pińkowska, H, and Krzywonos, M. Sugar beet pulp in the context of developing the concept of circular bioeconomy. Energies. (2021) 15:175. doi: 10.3390/en15010175
189. Reddy, CSK, Ghai, R, Rashmi, R, and Kalia, VC. Polyhydroxyalkanoates: an overview. Bioresour Technol. (2003) 87:137–46. doi: 10.1016/s0960-8524(02)00212-2
190. Herman, A, and Herman, AP. Caffeine’s mechanisms of action and its cosmetic use. Skin Pharmacol Physiol. (2013) 26:8–14. doi: 10.1159/000343174
191. Martinez-Saez, N, García, AT, Pérez, ID, Rebollo-Hernanz, M, Mesías, M, Morales, FJ, et al. Use of spent coffee grounds as food ingredient in bakery products. Food Chem. (2017) 216:114–22. doi: 10.1016/j.foodchem.2016.07.173
192. Mata, TM, Martins, AA, and Caetano, NS. Bio-refinery approach for spent coffee grounds valorization. Bioresour Technol. (2018) 247:1077–84. doi: 10.1016/j.biortech.2017.09.106
193. Lu, Q, Huang, N, Peng, Y, Zhu, C, and Pan, S. Peel oils from three Citrus species: volatile constituents, antioxidant activities and related contributions of individual components. J Food Sci Technol. (2019) 56:4492–502. doi: 10.1007/s13197-019-03937-w
194. Razola-Díaz, MC, Guerra-Hernández, EJ, García-Villanova, B, and Verardo, V. Recent developments in extraction and encapsulation techniques of orange essential oil. Food Chem. (2021) 354:129575. doi: 10.1016/j.foodchem.2021.129575
195. Zhang, W, Liu, D, Fu, X, Xiong, C, and Nie, Q. Peel essential oil composition and antibacterial activities of Citrus x sinensis L. Osbeck ‘Tarocco’ and Citrus reticulata Blanco. Horticulturae. (2022) 8:793. doi: 10.3390/horticulturae8090793
196. Zhou, C, and Wang, Y. Recent progress in the conversion of biomass wastes into functional materials for value-added applications. Sci Technol Adv Mater. (2020) 21:787–804. doi: 10.1080/14686996.2020.1848213
197. Carr, FJ, Chill, D, and Maida, N. The lactic acid Bacteria: a literature survey. Crit Rev Microbiol. (2002) 28:281–370. doi: 10.1080/1040-840291046759
198. Teuber, M . Lactic acid Bacteria. Biotechnology. (2008) 1:325–66. doi: 10.1002/9783527620999.ch10
199. Stiles, ME . Biopreservation by lactic acid bacteria. Antonie Van Leeuwenhoek. (1996) 70:331–45. doi: 10.1007/BF00395940
200. Jiang, F, Cheng, HJ, Liu, D, Wei, C, An, WJ, Wang, YF, et al. Treatment of whole-plant corn silage with lactic acid Bacteria and organic acid enhances quality by elevating acid content, reducing pH, and inhibiting undesirable microorganisms. Front Microbiol. (2020) 11:88. doi: 10.3389/fmicb.2020.593088
201. Okoye, CO, Wang, Y, Gao, L, Wu, Y, Li, X, Sun, J, et al. The performance of lactic acid bacteria in silage production: a review of modern biotechnology for silage improvement. Microbiol Res. (2023) 266:127212. doi: 10.1016/j.micres.2022.127212
202. Baláž, M, Boldyreva, EV, Rybin, D, Pavlović, S, Rodríguez-Padrón, D, Mudrinić, T, et al. State-of-the-art of eggshell waste in materials science: recent advances in catalysis, pharmaceutical applications, and Mechanochemistry. Front Bioeng Biotechnol. (2021) 8:567. doi: 10.3389/fbioe.2020.612567
203. Carvalho, J, Araujo, J, and Castro, F. Alternative low-cost adsorbent for water and wastewater decontamination derived from eggshell waste: An overview. Waste Biomass Valor. (2011) 2:157–67. doi: 10.1007/s12649-010-9058-y
204. Lopes, C, Herva, M, García-Diéguez, C, and Roca, E. Valorization of organic wastes as fertilizer: environmental concerns of composting anaerobic digestion technologies. Organic fertilizers–Types, production and environmental impact, pp. 97–137 (2012).
205. Chew, KR, Leong, HY, Khoo, KS, Vo, DVN, Anjum, H, Chang, CK, et al. Effects of anaerobic digestion of food waste on biogas production and environmental impacts: a review. Environ Chem Lett. (2021) 19:2921–39. doi: 10.1007/s10311-021-01220-z
206. Slorach, PC, Jeswani, HK, Cuéllar-Franca, R, and Azapagic, A. Environmental sustainability of anaerobic digestion of household food waste. J Environ Manag. (2019) 236:798–814. doi: 10.1016/j.jenvman.2019.02.001
207. Singh, A, Singhania, RR, Soam, S, Chen, CW, Haldar, D, Varjani, S, et al. Production of bioethanol from food waste: status and perspectives. Bioresour Technol. (2022) 360:127651. doi: 10.1016/j.biortech.2022.127651
208. Karmee, SK . Liquid biofuels from food waste: current trends, prospect and limitation. Renew Sust Energ Rev. (2016) 53:945–53. doi: 10.1016/j.rser.2015.09.041
209. Katami, T, Yasuhara, A, and Shibamoto, T. Formation of dioxins from incineration of foods found in domestic Garbage. Environ Sci Technol. (2004) 38:1062–5. doi: 10.1021/es030606y
210. Tait, PW, Brew, J, Che, A, Costanzo, A, Danyluk, A, Davis, M, et al. The health impacts of waste incineration: a systematic review. Aust N Z J Public Health. (2020) 44:40–8. doi: 10.1111/1753-6405.12939
211. Yang, N, Zhang, H, Chen, M, Shao, L-M, and He, P-J. Greenhouse gas emissions from MSW incineration in China: impacts of waste characteristics and energy recovery. Waste Manag. (2012) 32:2552–60. doi: 10.1016/j.wasman.2012.06.008
212. Behera, SK, Park, JM, Kim, KH, and Park, H-S. Methane production from food waste leachate in laboratory-scale simulated landfill. Waste Manag. (2010) 30:1502–8. doi: 10.1016/j.wasman.2010.02.028
213. Assamoi, B, and Lawryshyn, Y. The environmental comparison of landfilling vs. incineration of MSW accounting for waste diversion. Waste Manag. (2012) 32:1019–30. doi: 10.1016/j.wasman.2011.10.023
214. Mondello, G, Salomone, R, Ioppolo, G, Saija, G, Sparacia, S, and Lucchetti, MC. Comparative LCA of alternative scenarios for waste treatment: the case of food waste production by the mass-retail sector. Sustain For. (2017) 9:827. doi: 10.3390/su9050827
215. Sharma, P, Bano, A, Singh, SP, Atkinson, JD, Lam, SS, Iqbal, HMN, et al. Biotransformation of food waste into biogas and hydrogen fuel–a review. Int J Hydrog Energy. (2022) 52:46–60. doi: 10.1016/j.ijhydene.2022.08.081
216. Global food waste in 2023. (2023). Available at: https://greenly.earth/en-us/blog/ecology-news/global-food-waste-in-2022 (Accessed Nov 5, 2023).
217. O’Connor, J, Hoang, SA, Bradney, L, Dutta, S, Xiong, X, Tsang, DCW, et al. A review on the valorisation of food waste as a nutrient source and soil amendment. Environ Pollut. (2021) 272:115985. doi: 10.1016/j.envpol.2020.115985
218. Kee, PE, Cheng, YS, Chang, JS, Yim, HS, Tan, JCY, Lam, SS, et al. Insect biorefinery: a circular economy concept for biowaste conversion to value-added products. Environ Res. (2023) 221:115284. doi: 10.1016/j.envres.2023.115284
219. Maitan-Alfenas, GP, Visser, EM, and Guimarães, VM. Enzymatic hydrolysis of lignocellulosic biomass: converting food waste in valuable products. Curr Opin Food Sci. (2015) 1:44–9. doi: 10.1016/j.cofs.2014.10.001
220. Teigiserova, DA, Hamelin, L, and Thomsen, M. Review of high-value food waste and food residues biorefineries with focus on unavoidable wastes from processing. Resour Conserv Recycl. (2019) 149:413–26. doi: 10.1016/j.resconrec.2019.05.003
221. Pleissner, D, and Lin, CSK. Valorisation of food waste in biotechnological processes. Sustain Chem Process. (2013) 1:21. doi: 10.1186/2043-7129-1-21
222. Kumar, Y, Kaur, S, Kheto, A, Munshi, M, Sarkar, A, Om Pandey, H, et al. Cultivation of microalgae on food waste: recent advances and way forward. Bioresour Technol. (2022) 363:127834. doi: 10.1016/j.biortech.2022.127834
223. Intasit, R, Cheirsilp, B, Louhasakul, Y, and Thongchul, N. Enhanced biovalorization of palm biomass wastes as biodiesel feedstocks through integrated solid-state and submerged fermentations by fungal co-cultures. Bioresour Technol. (2023) 380:129105. doi: 10.1016/j.biortech.2023.129105
224. Ray, A, Dubey, KK, Marathe, SJ, and Singhal, R. Supercritical fluid extraction of bioactives from fruit waste and its therapeutic potential. Food Biosci. (2023) 52:102418. doi: 10.1016/j.fbio.2023.102418
225. Mourad, M . Recycling, recovering and preventing ‘food waste’: competing solutions for food systems sustainability in the United States and France. J Clean Prod. (2016) 126:461–77. doi: 10.1016/j.jclepro.2016.03.084
226. Pingault, N, Caron, P, Kalafatic, C, Allahoury, A, Fresco, LO, Kennedy, E, et al. Nutrition and food systems. A report by the High-Level Panel of Experts on Food Security and Nutrition of the Committee on World Food Security.
Keywords: food biomass, anaerobic digestion, composting of food waste, insect-based-conversion, circular economy, sustainability, biofuels
Citation: Razouk A, Tiganescu E, von Glahn AJ, Abdin AY, Nasim MJ and Jacob C (2024) The future in the litter bin – bioconversion of food waste as driver of a circular bioeconomy. Front. Nutr. 11:1325190. doi: 10.3389/fnut.2024.1325190
Edited by:
Sanjay Kumar Singh Patel, Konkuk University, Republic of KoreaReviewed by:
Liana Claudia Salanta, University of Agricultural Sciences and Veterinary Medicine Cluj-Napoca, RomaniaCunsheng Zhang, Jiangsu University, China
Copyright © 2024 Razouk, Tiganescu, von Glahn, Abdin, Nasim and Jacob. This is an open-access article distributed under the terms of the Creative Commons Attribution License (CC BY). The use, distribution or reproduction in other forums is permitted, provided the original author(s) and the copyright owner(s) are credited and that the original publication in this journal is cited, in accordance with accepted academic practice. No use, distribution or reproduction is permitted which does not comply with these terms.
*Correspondence: Muhammad Jawad Nasim, jawad.nasim@uni-saarland.de; Claus Jacob, c.jacob@mx.uni-saarland.de