- 1Department of Radiation Oncology, First Affiliated Hospital of Guangxi Medical University, Nanning, China
- 2Key Laboratory of Early Prevention and Treatment for Regional High Frequency Tumor (Guangxi Medical University), Ministry of Education, Nanning, China
- 3Department of Otolaryngology-Head and Neck Surgery, First Affiliated Hospital of Guangxi Medical University, Nanning, China
- 4Guangxi Zhuang Autonomous Region Institute of Product Quality Inspection (GXQT), Nanning, China
- 5The First School of Clinical Medicine, Guangxi Medical University, Nanning, China
- 6Life Science Institute, Guangxi Medical University, Nanning, China
Dietary fibers (DFs) and their metabolites attract significant attention in research on health and disease, attributing to their effects on regulating metabolism, proliferation, inflammation, and immunity. When fermented by gut microbiota, DFs mainly produce short-chain fatty acids (SCFAs), such as acetic acid, propionic acid, and butyric acid. As the essential nutrients for intestinal epithelial cells, SCFAs maintain intestinal homeostasis and play essential roles in a wide range of biological functions. SCFAs have been found to inhibit histone deacetylase, activate G protein-coupled receptors, and modulate the immune response, which impacts cancer and anti-cancer treatment. Notably, while extensive studies have illuminated the roles of SCFAs in colorectal cancer development, progression, and treatment outcomes, limited evidence is available for other types of cancers. This restricts our understanding of the complex mechanisms and clinical applications of SCFAs in tumors outside the intestinal tract. In this study, we provide a comprehensive summary of the latest evidence on the roles and mechanisms of SCFAs, with a focus on butyric acid and propionic acid, derived from microbial fermentation of DFs in cancer. Additionally, we recapitulate the clinical applications of SCFAs in cancer treatments and offer our perspectives on the challenges, limitations, and prospects of utilizing SCFAs in cancer research and therapy.
Introduction
Recent research has highlighted the significant impact of dietary fibers (DFs) on human health (1, 2) influencing the risk of chronic diseases, such as cancer, obesity, type 2 diabetes, and cardiovascular diseases (3, 4). DFs encompass soluble and insoluble fibers, which are a group of carbohydrates that cannot be digested or absorbed in the small intestine (3, 5). Soluble fibers, including oligo galactose, oligofructose, inulin, β-glucan, resistant starch, and pectin, are widely recognized as prebiotics (6). When fermented by gut microbiota, soluble fibers mainly produce short-chain fatty acids (SCFAs), such as acetic acid, propionic acid, and butyric acid (7).
SCFAs, which serve as essential nutrients for colonocytes and gut microbes, play a crucial role in maintaining intestinal and systemic homeostasis, impacting lipid and glucose metabolism, cell proliferation, inflammation, and immune system functionality (7, 8). In particular, the roles of butyric acid and propionic acid have been extensively investigated, revealing their contributions to health and diseases, including human cancers. It is suggested that butyric acid and propionic acid act as histone deacetylase inhibitors (HDACIs) to epigenetic modulate gene expression, influencing cell growth, proliferation, and apoptosis (9–12); act as ligands for G protein-coupled receptors (GPCRs), regulating cell proliferation, apoptosis, and immune response (11, 13); furthermore, they exhibit anti-inflammatory and immunomodulatory effects by regulating inflammatory factors and cytokines and promoting the differentiation and migration of immune cells (10, 11, 14–16).
Notably, while extensive studies have illuminated the roles and applications of SCFAs in colorectal cancer (CRC) (17–22), limited evidence is available for other types of cancers. This restricts our understanding of the roles of SCFAs in tumors outside the intestinal tract and the complex mechanisms underlying the regulation of the tumor-immune microenvironment (TIME). In this study, we provide a comprehensive summary of the latest evidence on the roles and mechanisms of SCFAs, with a focus on butyric acid and propionic acid, derived from microbial fermentation of DFs in cancer. Additionally, we recapitulate the clinical applications of SCFAs in cancer treatments and offer our perspectives on the challenges, limitations, and prospects for utilizing SCFAs in cancer research and therapy.
The roles and mechanisms of SCFAs in cancer
Functioning as epigenetic modificators
SCFAs as HDACIs play a crucial role in the epigenetic regulation of gene expression, influencing cell survival, proliferation, and differentiation (23, 24). Numerous in vitro studies have demonstrated that SCFAs presented HDACI activities in various cancer cell lines, including (9, 25–27) breast (28), gastric (29), and cervical cancer (30). SCFAs have been shown to inhibit cell proliferation, induce cell cycle arrest at G0/G1 or G2/M phase, trigger apoptosis mediated via the mitochondrial pathway, promote autophagy, and increase the accumulation of reactive oxygen species (ROS). In a study of BALB/c nude mouse model with HCT-116 cells inoculation by Ma et al. (31), sitosterols feeding elevated diversity of gut microbiota, increased levels of SCFAs in fecal samples, and restrained CRC cell growth. The study further revealed that SCFAs induced tumor apoptosis through the PI3K/Akt pathway and altered the expression levels of apoptosis-related proteins, such as Bad, Bcl-xl, and cytochrome C (31). Hence, SCFAs by acting as HDACI show potential as attractive targets for developing novel therapeutic strategies, as discussed in Section 3.
Acting as G protein-coupled receptor ligands
SCFAs are natural ligands for the G protein-coupled receptors (GPCRs), including GPR43 (also termed free fatty acid receptor, FFAR2), GPR41 (also termed FFAR3), and GPR109A (13, 32). In colon cancer cells, by combining these receptors, SCFAs inhibit cell proliferation, induce apoptosis, and cycle arrest via the NF-κB, MAPK, ERK1/2, PI3K, and Wnt signaling pathways (13, 32). For instance, SCFAs induced cell proliferation inhibition, apoptosis, and invasion inhibition, mediated by GPR43 in colon cancer cells (9, 33), HeLa cells (34), BaF3 leukemia cells (35), and breast cancer cells (36). Propionate and butyrate are high-affinity ligands for GPR43, dual-coupled to the pertussis-sensitive Gαi/o and Gq protein, and reduce cAMP levels (37). Similarly, Yonezawa found that both GPR41 and GPR43 were expressed in breast cancer cell lines; while combining with SCFAs, they raised intracellular concentration of Ca2+ and activated the p38 MAPK pathway, thereby inhibiting cell proliferation (38) (Table 1). In an intestinal cancer model, Kim et al. (40) observed that the SCFA-GPR43 axis suppresses the Th17-driven inflammatory response and intestinal carcinogenesis. In addition, GPR109A binds only to butyrate and reduces cAMP through Gαi/o proteins (37). GPR109A mediated butyrate anti-cancer activity in colon cancer cell lines by inhibiting the activation of NF-kB, downregulating anti-apoptotic genes, and upregulating pro-apoptotic genes (9, 41). Moreover, propionate and butyrate could activate GPR41 which was coupled through Gαi/o proteins to reduce cAMP (37), increase the intracellular concentration of Ca2+, and inhibit the MAPK signaling pathway to lower the invasion of breast cancer cells (36).
Regulating TIME
SCFAs play essential roles in the host immune system, such as influencing the differentiation of myeloid and lymphocytes (42–44). SCFAs exert their immunomodulatory effects through two primary mechanisms: acting as HDACIs and interacting with GPCRs (43) (Figure 1). For example, in vitro and in vivo investigations involving C57BL/6 mice, various gene-deficient mouse models [Rag1(–/–), GPR41(–/–), GPR43(–/–), IL-10(–/–)] and T cell lines (CD4 +, CD8 +) showed that SCFAs promoted the differentiation of naive T cells into effector cells (Th1 or Th17) or regulatory T cells (Tregs). This regulation influences the production of IL-17, IFN-γ, and IL-10, thereby affecting immunity or immune tolerance (45). Additionally, SCFAs regulate the MAPK signaling pathways (ERK, JNK, and p38) to modulate immune and endothelial cells, leading to the suppression of inflammation and tumors (16). SCFAs have been observed to suppress inflammatory cytokines IL-1β, IL-2, IL-3, IL-5, IL-6, IL-8, IL-12, IL-17, IL-21, IL-23, TNF-α, TNF-β, NOS, and COX2, while increasing the expression of anti-inflammatory cytokines IL-10 and IL-18. This reduction in inflammation contributes to the suppression of CRC development (9, 11, 16, 22, 32). Furthermore, SCFAs affected both innate and adaptive immune responses by stimulating B cells to secrete intestinal immunoglobulin A (IgA) (13, 46, 47). Notably, a study by Luu et al. (48) demonstrated that SCFAs enhanced the secretion of cytokines (including IL-2, TNF-α, and IFN-γ) by modulating CD8+ T cells, thereby improving cancer immunotherapy.
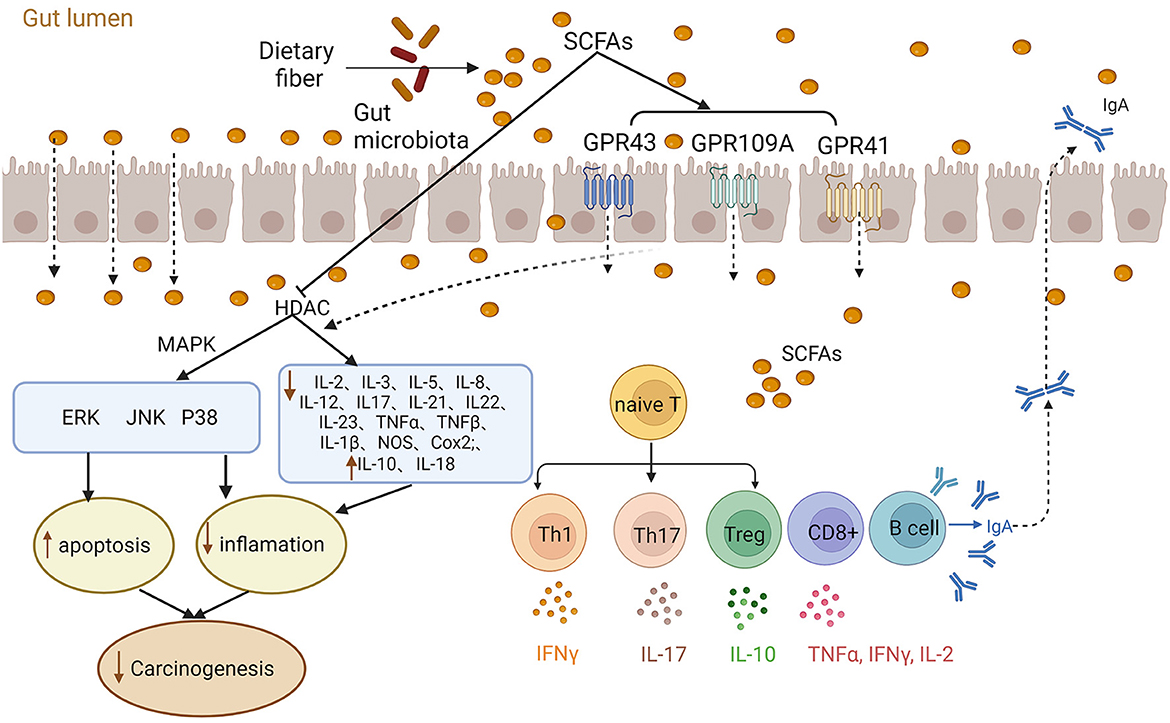
Figure 1. Role of short-chain fatty acids (SCFAs) in inflammation and immunity. The gut microbiota ferments dietary fibers producing SCFA that exert their effects through various mechanisms. SCFAs can act as histone deacetylase inhibitors (HDACIs) and activate G protein-coupled receptors (GPCRs) to modulate cellular responses. For instance, SCFAs regulate MAPK signaling pathways (ERK, JNK, and p38) and stimulate the release of inflammatory factors, contributing to inflammation and carcinogenesis. Additionally, SCFAs play a vital role in immune regulation by promoting the differentiation of naive T cells into effector cells and regulatory T cells (Tregs). Moreover, SCFAs influence cytokines release, impacting intestinal homeostasis and immunity.
Carcinogenic effects of SCFAs
While SCFAs have commonly been recognized as tumor-suppressive metabolites, it is noteworthy that under certain conditions, SCFAs can promote tumorigenesis (49–51). Matsushita et al. (52) conducted research using prostate-specific Pten knockout mice (Pb-Creþ; Ptenfl/fl) and prostate cancer cell lines (DU145, 22Rv1) to demonstrate that SCFAs supplementation promoted prostate carcinogenesis by increasing insulin-like growth factor-1 production. Another study reported that long-term consumption of fiber-enriched foods in dysbiosis mice resulted in hepatocellular carcinoma (HCC) (53). In addition, a mouse model with colon cancer driven by mutations in the mismatch repair gene Msh2 and Apc gene showed that butyrate promoted the development of CRC (54). Okumura et al. (55) have currently described that the overgrowth of Porphyromonas species in an ApcΔ14/+ mouse model is casually related to colorectal cancer due to butyrate-engaged senescence. Notably, scientists have long debated these opposing observations and dubbed the phenomenon “butyrate paradox” (51). Given the “Warburg effect” (56), it has been widely accepted that butyrate provides energy to normal cells to promote cell growth. In contrast, cancerous cells instead relied on aerobic glycolysis; therefore, butyrate accumulated and functioned as an HDACI to halt cell cycle progression. Surprisingly, growing evidence in colon cancer cells showed that butyrate could directly combine and change the metabolic enzymes, leading to an anti-tumor effect without following the “Warburg effect” (57–59). Moreover, GPR41 could decrease butyrate-induced histone acetylation and negatively regulate butyric-induced anti-proliferative and apoptosis (39). Thus, it would be narrow to define butyrate or SCFAs simply as onco-metabolites or tumor-suppressive metabolites, given their complex effects that are waiting for exploration.
Advancements of gut microbiota-derived SCFAs in cancer treatment
SCFAs gained attention in the 1980s when butyrate was reported to modulate the malignant biological behavior of cultured colon cancer cells (60, 61). Sodium butyrate has been shown to inhibit the growth of hepatocellular carcinoma (HCC) cells in both in vitro using the HuH-7 human HCC cell line and in vivo utilizing an HCC tumor-bearing mice model (62). These inhibitory effects are likely mediated by a p21-dependent mechanism. In addition, sodium butyrate has demonstrated the ability to hinder the G1-S transition of human glioma cells, as evidenced by increased expression of p21 and cyclin D1, and reduced phosphorylation of pRb (63). It has also been found to impede cell proliferation in the MCF-7 human breast cancer cell line, reflected by increased expression levels of p21WAF1 and RARβ (64). Moreover, sodium butyrate induced AMPK-mTOR-mediated autophagy and ROS-mediated apoptosis of bladder cancer cells (T24, 5637, and SV-HUC-1 bladder cancer cell lines) (65), induced DAPK-mediated apoptosis in human gastric cancer cell lines (AGS, Kato III, etc.) (66), and triggered mitochondrial-mediated apoptosis in colon cancer cell line (Caco-2 cell line) (67). However, the translation of SCFAs to clinical applications has been impeded by their low concentration in peripheral blood and rapid plasma clearance (68), which will be further discussed in Section 4. Two decades later, with the iteration of sequencing technology, the association between gut microbiota-derived SCFAs and their role as anti-cancer agents once again captured scientists' attention for SCFAs as anti-cancer agents.
The investigations of the association between SCFAs and cancers fell into several research modes as follows:
1) In vitro studies. Nakkarach et al. (69) isolated the bacterial strain (Escherichia coli KUB-36) from fecal samples collected from healthy individuals which demonstrated the highest production of SCFAs. The researchers applied the metabolites and individual SCFA to various tumor cell lines, including breast cancer, colorectal cancer, and leukemia. Remarkably, all treatments exhibited inhibitory effects on tumor cell growth, with breast cancer cells showing the greatest sensitivity to the treatments (69). Additionally, Zheng et al. indicated that secretions of C. butyricum induced cytotoxic effects on CRC cells, including human CRC cell lines HCT116 and HT29, as well as the mouse CRC cell line CT26. However, the subsequent addition of butyrate kinase inhibitors impaired the cytotoxic effects specifically in CT26 cells, providing strong evidence that the anti-cancer effect of C. butyricum was mainly attributed to the secretion of butyrate (70).
2) In vivo studies. In a recent study, it was demonstrated that the concentration of intestinal SCFAs concentration in mice with HCC can be increased by administering a probiotic mixture named Prohep. Prohep, composed of Lactobacillus rhamnosus GG, Escherichia coli Nissle 1917, and VSL#3, was found to confer tumor suppression effect. This effect was associated with alterations in the composition and diversity of gut microbiota and an increase in SCFA-producing bacteria in the group of mice treated with the probiotic mixture (71). The intervention with Prohep appeared to be relevant to the downregulation of IL-17, the reduction of Th17 polarization, and the differentiation of Treg/Tr1 (72). In another study, the effect of SCFAs on extra-intestinal tumor progression was investigated in a mouse model of lung metastasis from melanoma. Supplementation with VSL#3, a registered probiotic formula consisting of eight different strains of probiotic bacteria, resulted in an increased amount of propionate and butyrate in plasma and fecal samples. Subsequent analysis showed that these SCFAs significantly decreased the volume of tumors, possibly by recruiting Th17 cells to the lung tissue through the chemokine ligand 20/chemokine receptor 6 axis (73).
3) Multi-omics analysis. Multi-omics analyses have emerged as novel approaches, integrating metagenomic, transcriptomic, proteomic, metabolomic, and lipidomic analysis. These comprehensive investigations shed further light on the host's response to probiotics at multiple levels (74). For instance, in a mouse model of HCC treated with probiotics, researchers utilized metagenomic analysis to identify altered pathways and corresponding biological functions (71). Notably, they observed significant changes in pathways involved in SCFAs synthesis within tumor cells. Furthermore, applying metabolomic analysis provides valuable insights into the modulation of metabolite profiles following probiotic intervention (75). In a study that combined metagenomics and metabolomics (using gas chromatography-mass spectrometry, GC-MS), researchers screened for phages associated with CRC promotion (mainly Fusobacterium nucleatum) and inhibition (mainly Clostridium butyricum). Through gene ontology enrichment analysis, differentially expressed genes were found to be enriched in apoptosis and autophagy, uncovering the potential mechanism. Additionally, GC-MS analysis of C. butyricum's secretome revealed that butyrate played a prominent role in the cytotoxic effects on CRC cells (70).
While many studies regarding SCFAs in cancer management provided valuable insights into their potential effects and mechanisms, these preclinical studies were limited in vitro and in vivo. It is essential to conduct well-designed clinical trials (including double-blinded or triple-blinded studies) to further investigate the efficacy and safety of SCFAs in human subjects.
SCFAs combined with chemotherapy and radiotherapy
Recently, SCFAs have been studied as a sensitizer for radiotherapy and chemotherapy. Sodium butyrate combined with cisplatin has been described to promote apoptosis in different tumor cells, such as gastric cancer (76) and cervical cancer (77) in vitro and in vivo. In the tumor-bearing mouse model of gastric cancer, butyrate plus cisplatin inhibited tumor growth via the mitochondrial apoptosis-related pathway, surpassing other groups with monotherapy (76). The combination of butyrate and cisplatin has been reported in the cervical cancer model (Hela and Siha cell lines and tumor cell-inoculation mice) that inhibited cell migration and invasion by blocking the nuclear conversion of β-catenin, reversing epithelial–mesenchymal transition, upregulating the expression of E-cadherin and downregulating matrix metalloproteinase (MMP)2, MMP7, and MMP9 (77). In addition, Park et al. (78) investigated the effects of radiotherapy combined with butyrate, propionate, and acetate in organoids. Among them, butyrate showed radio-sensibilization and weak toxicity to normal mucosa and inhibited the proliferation of organoids. Data on the safety and efficacy of the combination in animal studies and clinical trials are yet to come.
SCFAs combined with immunotherapy
Several studies focused on patients with different types of cancer receiving immune checkpoint inhibition (ICI) therapy and collected patients' fecal samples (20, 79, 80). They suggested that the concentration of SCFAs in fecal samples might be associated with the efficacy of anti-programmed cell death protein 1 (PD-1) and anti-programmed death-ligand 1 (PD-L1) immunotherapy. These findings prompt that gut microbiota links to ICI therapeutic efficacy through SCFAs, which show the potential to be a response marker. Animal studies found that SCFAs had diverse effects on different ICI therapies (81, 82). In a CRC mouse model, researchers found that the dietary supplement of pectin increased butyrate production in the gut, promoted T-cell infiltration, and enhanced the anti-cancer effect of anti-PD-1 drugs in CRC mice (81). Another mouse model CRC/fibrosarcoma reported that butyrate restrained anti-CTLA-4 response through downregulating CD80/CD86 on dendritic cells and Inducible costimulatory on T cells and preventing the accumulation of tumor-specific T cells, memory T cells, and IL-2 (82).
SCFAs in the comprehensive management of cancer
SCFAs have therapeutic potential in treating intestinal inflammation induced by chemotherapy or radiotherapy. They reconstruct the intestinal epithelium barrier and regulate intestinal immunomodulatory function (83). In addition, direct administration of SCFA-producing bacteria (probiotics) can restore intestinal ecology and inhibit the secretion of proinflammatory cytokines (84). In the perioperative management of resectable tumors, the application of SCFA-producing bacteria (probiotics) could decrease the incidence of postoperative complications (85, 86). For CRC patients, adding butyrate before the operation helps to improve the integrity of the intestinal barrier (87).
SCFAs from dietary fibers supplementation in cancer treatment
Numerous studies support the health-promoting effects of DFs from daily food (88, 89), including the anti-tumor effect. Pectin and inulin have been reported to enhance the immune response to tumors in mouse models. Pectin supplementation was associated with an improved response to immunotherapy in mice with CRC (81). Another study suggested a potential link between SCFAs derived from inulin fermentation and the anti-tumor activity of ICIs (90). Nevertheless, pectin has been shown to accelerate carcinogenesis in Apc-deficient mice (91), while dietary inulin supplementation may induce gut microbiota-dependent hepatocellular carcinoma (53). In addition to animal experiments, clinical research has indicated that adequate DFs intake can improve the prognosis of cancer patients. A cross-section study revealed that sufficient DFs intake was associated with significantly improved PFS and response to ICIs in melanoma patients, compared to a combination of DFs and probiotics (92). However, the study did not find a significant association between DF proportions and the SCFA levels in the gut. Furthermore, SCFAs play a critical role in the health-promoting effect of vegetarian and Mediterranean diets, which are characterized by high DF content (93–95). Nevertheless, the absence of relevant cohort studies makes it uncertain whether cancer patients can benefit from these dietary patterns. These findings highlight the need to carefully evaluate the potential benefits of DFs in future studies, considering their potential risks.
Challenges and limitations
Challenges as a therapeutic approach for cancer
The anti-cancer drug usually requires a comprehensive understanding of its pharmacology, toxicology, and high specificity on its target molecules. SCFAs have been found ambiguity effects on tumor progression: suppression and promotion, which challenges the further application of SCFAs in anti-cancer treatment. Donohoe et al. (96) reported decreased production of butyrate and increased butyrate nuclear accumulation in a microbiota- and butyrate-dependent mouse model with colon tumor cells. These phenomena were associated with enhanced apoptosis and reduced proliferation in tumors. Another mouse model with colon cancer driven by mutations in the mismatch repair genes Msh2 and Apc showed that butyrate drove the hyperproliferation of Msh2-deficient epithelial cells and promoted the development of CRC (54). Noteworthy, tumor genetics and butyrate concentrations were considered the key factors that led to the opposite effects of SCFAs on carcinogenesis between these investigations mentioned above (49, 50). So far, the questions about which are the responsible mutations and what is the cut-off concentration still need to be answered. It indicates that researchers should be aware that SCFAs may play more complex and comprehensive roles in cancer than we used to understand. Thus, we urge that more efforts be put into unraveling the spectrum of SCFAs' biological effects on cancer.
Limitations of distribution and plasma clearance
SCFAs serve as the primary energy source for intestinal epithelial cells; therefore the systemic absorption of butyrate is low (51). Their concentrations significantly differ between enteral and abenteric environments (butyrate concentration is 29 μM in portal vein vs. 4 μM in peripheral circulation) (68, 97). To engage their anti-tumor effects, SCFAs shall maintain different effective concentrations continuously in a patient's circulation given cancer types. For example, butyrate concentration in circulation should reach at least 0.5 mM to induce tumor cell differentiation in CRC (98) and breast cancer (28), However, butyrate at the concentration of 0.5 mM did not significantly affect the gastric cancer cell viability in vitro experiments (76). In addition, butyrate has a rapid plasma clearance in the human body with only a 6 min half-life. Once absorbed, SCFAs are transported to the liver via portal circulation and become the substrate for longer-chain fatty acids (51). Researchers reported that the peak concentration of butyrate in plasma among patients with acute leukemia was merely 0.05 mM by intravenous infusion (99). The insufficient concentration and short half-life of SCFAs in human circulation challenge their application. Current efforts have been made to innovate drug administration and explore stable derivatives:
1) Drug administration. Oral administration of solid lipid nanoparticles (SLN) (100) is an attempt to deliver butyrate across the intestinal barrier to target organs using a sustained-release drug delivery system. SLN is not absorbed by the gastrointestinal tract and cannot pass through the blood–brain barrier. Cholesteryl-butyrate SLN has been confirmed to increase the stability and efficacy of butyrate in a mouse glioma model (100).
2) Stable derivatives. Researchers tried to use prodrugs of SCFAs [Trybutirin (101), phenylbutyrate (102), and pivaloyloxymethyl butyrate (Pivanex, AN-9) (103, 104)] and explore their effects on tumors [leukemia (102), non-small cell lung cancer (104), and prostate cancer (105)]. These prodrugs had not only similar effects as butyrate in inducing apoptosis (101) and anti-angiogenesis effects (106) but also longer half-life and higher stable plasma concentrations (107). Notably, the doses were still insufficient to exert consistent anti-tumor effects (108).
To sum up, exploring various local delivery methods (such as enema, nasal spray, aerosol inhalation, intravaginal administration, and bladder irrigation) or developing new drug delivery systems may be the direction of future translational research.
Conclusion and future perspectives
Although astounding clinical successes in anti-cancer treatments have been achieved, cancer remains the second leading cause of death worldwide and dramatically affects the quality of life of cancer survivors. In the present review, we summarize advancements in the roles of the microbial fermentation of DFs-derived SCFAs in cancer and recapitulate the up-to-date evidence on the applications of SCFAs in cancer treatment. Additionally, we notice that SCFAs present the potential to mediate a wide range of biological effects beyond function as HDACIs, GPCRs, and TIME modulators, resulting in both tumor suppression and promotion. It highlighted the challenges of applying prebiotics, probiotics, and microbial metabolites to a therapeutic modality for cancer. We urge more effort to be put into unraveling the spectrum of SCFAs' biological effects and their functional organizing network, which is the prerequisite for better management of cancer.
Moreover, SCFAs might influence carcinogenesis and inflammation similarly in other regions beyond the gut, such as the reproductive tract, respiratory tract, and urinary tract. A fiber-rich diet can increase the production of SCFAs by altering the composition, diversity, and abundance of the microbiome to promote health. Hence, we might regulate SCFAs by prebiotics or probiotics to alter the commensal microbiome and modulate the desirable concentration of SCFAs in particular regions. To test these hypotheses, future investigations are warranted to explore the associations between commensal microbiota and its metabolites in various body sites and various types of cancer, consequently developing novel therapeutic approaches for improving prognosis and quality of life among cancer patients.
Author contributions
All authors listed have made a substantial, direct, and intellectual contribution to the work and approved it for publication.
Funding
This work was supported by grants from the National Natural Science Foundation of China (U22A20322 to ZZ), the National Natural Science Youth Foundation of China (82202939 to TH), and China Postdoctoral Science Foundation (2022MD723765 to TH).
Conflict of interest
The authors declare that the research was conducted in the absence of any commercial or financial relationships that could be construed as a potential conflict of interest.
Publisher's note
All claims expressed in this article are solely those of the authors and do not necessarily represent those of their affiliated organizations, or those of the publisher, the editors and the reviewers. Any product that may be evaluated in this article, or claim that may be made by its manufacturer, is not guaranteed or endorsed by the publisher.
References
1. Barber TM, Kabisch S, Pfeiffer AFH, Weickert MO. The health benefits of dietary fibre. Nutrients. (2020) 12:209. doi: 10.3390/nu12103209
2. Mathers JC. Dietary fibre and health: the story so far. Proc Nutr Soc. (2023) 82:120–9. doi: 10.1017/S0029665123002215
3. Shah BR, Li B, Al Sabbah H, Xu W, Mráz J. Effects of prebiotic dietary fibers and probiotics on human health: with special focus on recent advancement in their encapsulated formulations. Trends Food Sci Technol. (2020) 102:178–92. doi: 10.1016/j.tifs.2020.06.010
4. Rezende ESV, Lima GC, Naves MMV. Dietary fibers as beneficial microbiota modulators: a proposed classification by prebiotic categories. Nutrition. (2021) 89:111217. doi: 10.1016/j.nut.2021.111217
5. Makki K, Deehan EC, Walter J, Bäckhed F. The impact of dietary fiber on gut microbiota in host health and disease. Cell Host Microbe. (2018) 23:705–15. doi: 10.1016/j.chom.2018.05.012
6. Lattimer JM, Haub MD. Effects of dietary fiber and its components on metabolic health. Nutrients. (2010) 2:1266–89. doi: 10.3390/nu2121266
7. Sivaprakasam S, Prasad PD, Singh N. Benefits of short-chain fatty acids and their receptors in inflammation and carcinogenesis. Pharmacol Ther. (2016) 164:144–51. doi: 10.1016/j.pharmthera.2016.04.007
8. Campos-Perez W, Martinez-Lopez E. Effects of short chain fatty acids on metabolic and inflammatory processes in human health. Biochim Biophys Mol Cell Biol Lipids. (2021) 1866:158900. doi: 10.1016/j.bbalip.2021.158900
9. Fung KY, Cosgrove L, Lockett T, Head R, Topping DL. A review of the potential mechanisms for the lowering of colorectal oncogenesis by butyrate. Br J Nutr. (2012) 108:820–31. doi: 10.1017/S0007114512001948
10. Mirzaei R, Afaghi A, Babakhani S, Sohrabi MR, Hosseini-Fard SR, Babolhavaeji K, et al. Role of microbiota-derived short-chain fatty acids in cancer development and prevention. Biomed Pharmacother. (2021) 139:111619. doi: 10.1016/j.biopha.2021.111619
11. Fattahi Y, Heidari HR, Khosroushahi AY. Review of short-chain fatty acids effects on the immune system and cancer. Food Biosci. (2020) 38:100793. doi: 10.1016/j.fbio.2020.100793
12. Davie JR. Inhibition of histone deacetylase activity by butyrate. J Nutr. (2003) 133:2485s−93s. doi: 10.1093/jn/133.7.2485S
13. Carretta MD, Quiroga J, López R, Hidalgo MA, Burgos RA. Participation of short-chain fatty acids and their receptors in gut inflammation and colon cancer. Front Physiol. (2021) 12:662739. doi: 10.3389/fphys.2021.662739
14. Thiruvengadam M, Subramanian U, Venkidasamy B, Thirupathi P, Samynathan R, Shariati MA, et al. Emerging role of nutritional short-chain fatty acids (SCFAS) against cancer via modulation of hematopoiesis. Crit Rev Food Sci Nutr. (2021) 63:827–44. doi: 10.1080/10408398.2021.1954874
15. Arpaia N, Campbell C, Fan X, Dikiy S, van der Veeken J, deRoos P, et al. Metabolites produced by commensal bacteria promote peripheral regulatory T-cell generation. Nature. (2013) 504:451–5. doi: 10.1038/nature12726
16. Li M, van Esch B, Wagenaar GTM, Garssen J, Folkerts G, Henricks PAJ. Pro- and anti-inflammatory effects of short chain fatty acids on immune and endothelial cells. Eur J Pharmacol. (2018) 831:52–9. doi: 10.1016/j.ejphar.2018.05.003
17. Whitehead RH, Young GP, Bhathal PS. Effects of short chain fatty acids on a new human colon carcinoma cell line (LIM1215). Gut. (1986) 27:1457–63. doi: 10.1136/gut.27.12.1457
18. Wilson AJ, Gibson PR. Short-chain fatty acids promote the migration of colonic epithelial cells in vitro. Gastroenterology. (1997) 113:487–96. doi: 10.1053/gast.1997.v113.pm9247468
19. Roy S, Trinchieri G. Microbiota: a key orchestrator of cancer therapy. Nat Rev Cancer. (2017) 17:271–85. doi: 10.1038/nrc.2017.13
20. Nomura M, Nagatomo R, Doi K, Shimizu J, Baba K, Saito T, et al. Association of short-chain fatty acids in the gut microbiome with clinical response to treatment with nivolumab or pembrolizumab in patients with solid cancer tumors. JAMA Netw Open. (2020) 3:e202895. doi: 10.1001/jamanetworkopen.2020.2895
21. Li J, Zhang AH, Wu FF, Wang XJ. Alterations in the gut microbiota and their metabolites in colorectal cancer: recent progress and future prospects. Front Oncol. (2022) 12:841552. doi: 10.3389/fonc.2022.841552
22. Kazmierczak-Siedlecka K, Marano L, Merola E, Roviello F, Połom K. Sodium butyrate in both prevention and supportive treatment of colorectal cancer. Front Cell Infect Microbiol. (2022) 12:1023806. doi: 10.3389/fcimb.2022.1023806
23. Steliou K, Boosalis MS, Perrine SP, Sangerman J, Faller DV. Butyrate histone deacetylase inhibitors. Biores Open Access. (2012) 1:192–8. doi: 10.1089/biores.2012.0223
24. Manal M, Chandrasekar MJ, Gomathi Priya J, Nanjan MJ. Inhibitors of histone deacetylase as antitumor agents: a critical review. Bioorg Chem. (2016) 67:18–42. doi: 10.1016/j.bioorg.2016.05.005
25. Archer SY, Meng S, Shei A, Hodin RA. P21(Waf1) is required for butyrate-mediated growth inhibition of human colon cancer cells. Proc Natl Acad Sci U S A. (1998) 95:6791–6. doi: 10.1073/pnas.95.12.6791
26. Blottière HM, Buecher B, Galmiche JP, Cherbut C. Molecular analysis of the effect of short-chain fatty acids on intestinal cell proliferation. Proc Nutr Soc. (2003) 62:101–6. doi: 10.1079/PNS2002215
27. Tang Y, Chen Y, Jiang H, Nie D. The role of short-chain fatty acids in orchestrating two types of programmed cell death in colon cancer. Autophagy. (2011) 7:235–7. doi: 10.4161/auto.7.2.14277
28. Salimi V, Shahsavari Z, Safizadeh B, Hosseini A, Khademian N, Tavakoli-Yaraki M. Sodium butyrate promotes apoptosis in breast cancer cells through reactive oxygen species (ROS) formation and mitochondrial impairment. Lipids Health Dis. (2017) 16:208. doi: 10.1186/s12944-017-0593-4
29. Matthews GM, Howarth GS, Butler RN. Short-chain fatty acid modulation of apoptosis in the kato iii human gastric carcinoma cell line. Cancer Biol Ther. (2007) 6:1051–7. doi: 10.4161/cbt.6.7.4318
30. Pham CH, Lee JE, Yu J, Lee SH, Yu KR, Hong J, et al. Anticancer effects of propionic acid inducing cell death in cervical cancer cells. Molecules. (2021) 26:951. doi: 10.3390/molecules26164951
31. Ma H, Yu Y, Wang M, Li Z, Xu H, Tian C, et al. Correlation between microbes and colorectal cancer: tumor apoptosis is induced by sitosterols through promoting gut microbiota to produce short-chain fatty acids. Apoptosis. (2019) 24:168–83. doi: 10.1007/s10495-018-1500-9
32. Moniri NH, Farah Q. Short-chain free-fatty acid g protein-coupled receptors in colon cancer. Biochem Pharmacol. (2021) 186:114483. doi: 10.1016/j.bcp.2021.114483
33. Tang Y, Chen Y, Jiang H, Robbins GT, Nie D. G-protein-coupled receptor for short-chain fatty acids suppresses colon cancer. Int J Cancer. (2011) 128:847–56. doi: 10.1002/ijc.25638
34. Matsuya-Ogawa M, Shibata T, Itoh H, Murakami H, Yaguchi C, Sugihara K, et al. Oncoprotective effects of short-chain fatty acids on uterine cervical neoplasia. Nutr Cancer. (2019) 71:312–9. doi: 10.1080/01635581.2019.1578388
35. Bindels LB, Porporato P, Dewulf EM, Verrax J, Neyrinck AM, Martin JC, et al. Gut microbiota-derived propionate reduces cancer cell proliferation in the liver. Br J Cancer. (2012) 107:1337–44. doi: 10.1038/bjc.2012.409
36. Thirunavukkarasan M, Wang C, Rao A, Hind T, Teo YR, Siddiquee AA, et al. Short-chain fatty acid receptors inhibit invasive phenotypes in breast cancer cells. PLoS ONE. (2017) 12:e0186334. doi: 10.1371/journal.pone.0186334
37. Kimura I, Ichimura A, Ohue-Kitano R, Igarashi M. Free fatty acid receptors in health and disease. Physiol Rev. (2020) 100:171–210. doi: 10.1152/physrev.00041.2018
38. Yonezawa T, Kobayashi Y, Obara Y. Short-chain fatty acids induce acute phosphorylation of the p38 mitogen-activated protein kinase/heat shock protein 27 pathway via GPR43 in the MCF-7 human breast cancer cell line. Cell Signal. (2007) 19:185–93. doi: 10.1016/j.cellsig.2006.06.004
39. Wu J, Zhou Z, Hu Y, Dong S. Butyrate-induced GPR41 activation inhibits histone acetylation and cell growth. J Genet Genomics. (2012) 39:375–84. doi: 10.1016/j.jgg.2012.05.008
40. Kim M, Friesen L, Park J, Kim HM, Kim CH. Microbial metabolites, short-chain fatty acids, restrain tissue bacterial load, chronic inflammation, and associated cancer in the colon of mice. Eur J Immunol. (2018) 48:1235–47. doi: 10.1002/eji.201747122
41. Thangaraju M, Cresci GA, Liu K, Ananth S, Gnanaprakasam JP, Browning DD, et al. Gpr109a is a G-protein-coupled receptor for the bacterial fermentation product butyrate and functions as a tumor suppressor in colon. Cancer Res. (2009) 69:2826–32. doi: 10.1158/0008-5472.CAN-08-4466
42. Kim CH, Park J, Kim M. Gut microbiota-derived short-chain fatty acids, T cells, and inflammation. Immune Netw. (2014) 14:277–88. doi: 10.4110/in.2014.14.6.277
43. Corrêa-Oliveira R, Fachi JL, Vieira A, Sato FT, Vinolo MA. Regulation of immune cell function by short-chain fatty acids. Clin Transl Immunol. (2016) 5:e73. doi: 10.1038/cti.2016.17
44. Rangan P, Mondino A. Microbial short-chain fatty acids: a strategy to tune adoptive T cell therapy. J Immunother Cancer. (2022) 10:147. doi: 10.1136/jitc-2021-004147
45. Park J, Kim M, Kang SG, Jannasch AH, Cooper B, Patterson J, et al. Short-chain fatty acids induce both effector and regulatory T cells by suppression of histone deacetylases and regulation of the mTOR-S6K pathway. Mucosal Immunol. (2015) 8:80–93. doi: 10.1038/mi.2014.44
46. Wu W, Sun M, Chen F, Cao AT, Liu H, Zhao Y, et al. Microbiota metabolite short-chain fatty acid acetate promotes intestinal IgA response to microbiota which is mediated by GPR43. Mucosal Immunol. (2017) 10:946–56. doi: 10.1038/mi.2016.114
47. Kim M, Qie Y, Park J, Kim CH. Gut microbial metabolites fuel host antibody responses. Cell Host Microbe. (2016) 20:202–14. doi: 10.1016/j.chom.2016.07.001
48. Luu M, Riester Z, Baldrich A, Reichardt N, Yuille S, Busetti A, et al. Microbial short-chain fatty acids modulate CD8(+) T cell responses and improve adoptive immunotherapy for cancer. Nat Commun. (2021) 12:4077. doi: 10.1038/s41467-021-24331-1
50. Bultman SJ, Jobin C. Microbial-derived butyrate: an oncometabolite or tumor-suppressive metabolite? Cell Host Microbe. (2014) 16:143–5. doi: 10.1016/j.chom.2014.07.011
51. Salvi PS, Cowles RA. Butyrate and the intestinal epithelium: modulation of proliferation and inflammation in homeostasis and disease. Cells. (2021) 10:775. doi: 10.3390/cells10071775
52. Matsushita M, Fujita K, Hayashi T, Kayama H, Motooka D, Hase H, et al. Gut microbiota-derived short-chain fatty acids promote prostate cancer growth via IGF1 signaling. Cancer Res. (2021) 81:4014–26. doi: 10.1158/0008-5472.CAN-20-4090
53. Singh V, Yeoh BS, Chassaing B, Xiao X, Saha P, Aguilera Olvera R, et al. Dysregulated microbial fermentation of soluble fiber induces cholestatic liver cancer. Cell. (2018) 175:679–94.e22. doi: 10.1016/j.cell.2018.09.004
54. Belcheva A, Irrazabal T, Robertson SJ, Streutker C, Maughan H, Rubino S, et al. Gut microbial metabolism drives transformation of MSH2-deficient colon epithelial cells. Cell. (2014) 158:288–99. doi: 10.1016/j.cell.2014.04.051
55. Okumura S, Konishi Y, Narukawa M, Sugiura Y, Yoshimoto S, Arai Y, et al. Gut bacteria identified in colorectal cancer patients promote tumourigenesis via butyrate secretion. Nat Commun. (2021) 12:5674. doi: 10.1038/s41467-021-25965-x
56. Donohoe DR, Collins LB, Wali A, Bigler R, Sun W, Bultman SJ. The warburg effect dictates the mechanism of butyrate-mediated histone acetylation and cell proliferation. Mol Cell. (2012) 48:612–26. doi: 10.1016/j.molcel.2012.08.033
57. Blouin JM, Penot G, Collinet M, Nacfer M, Forest C, Laurent-Puig P, et al. Butyrate elicits a metabolic switch in human colon cancer cells by targeting the pyruvate dehydrogenase complex. Int J Cancer. (2011) 128:2591–601. doi: 10.1002/ijc.25599
58. Li Q, Cao L, Tian Y, Zhang P, Ding C, Lu W, et al. Butyrate suppresses the proliferation of colorectal cancer cells via targeting pyruvate kinase M2 and metabolic reprogramming. Mol Cell Proteomics. (2018) 17:1531–45. doi: 10.1074/mcp.RA118.000752
59. Geng HW, Yin FY, Zhang ZF, Gong X, Yang Y. Butyrate suppresses glucose metabolism of colorectal cancer cells via GPR109a-AKT signaling pathway and enhances chemotherapy. Front Mol Biosci. (2021) 8:634874. doi: 10.3389/fmolb.2021.634874
60. Dexter DL, Lev R, McKendall GR, Mitchell P, Calabres P. Sodium butyrate-induced alteration of growth properties and glycogen levels in cultured human colon carcinoma cells. Histochem J. (1984) 16:137–49. doi: 10.1007/BF01003545
61. Jacobs LR. Effect of dietary fiber on colonic cell proliferation and its relationship to colon carcinogenesis. Prevent Med. (1987) 16:566–71. doi: 10.1016/0091-7435(87)90073-9
62. Yamamoto H, Fujimoto J, Okamoto E, Furuyama J, Tamaoki T, Hashimoto-Tamaoki T. Suppression of growth of hepatocellular carcinoma by sodium butyrate in vitro and in vivo. Int J Cancer. (1998) 76:897–902.
63. Ito N, Sawa H, Nagane M, Noguchi A, Hara M, Saito I. Inhibitory effects of sodium butyrate on proliferation and invasiveness of human glioma cells. Neurosurgery. (2001) 49:430–6; discussion 6–7. doi: 10.1227/00006123-200108000-00031
64. Andrade FO, Nagamine MK, Conti AD, Chaible LM, Fontelles CC, Jordão Junior AA, et al. Efficacy of the dietary histone deacetylase inhibitor butyrate alone or in combination with vitamin a against proliferation of MCF-7 human breast cancer cells. Brazil J Med Biol Res. (2012) 45:841–50. doi: 10.1590/S0100-879X2012007500103
65. Wang F, Wu H, Fan M, Yu R, Zhang Y, Liu J, et al. Sodium butyrate inhibits migration and induces AMPK-mTOR pathway-dependent autophagy and ROS-mediated apoptosis via the miR-139-5p/Bmi-1 axis in human bladder cancer cells. FASEB J. (2020) 34:4266–82. doi: 10.1096/fj.201902626R
66. Shin H, Lee YS, Lee YC. Sodium butyrate-induced DAPK-mediated apoptosis in human gastric cancer cells. Oncol Rep. (2012) 27:1111–5. doi: 10.3892/or.2011.1585
67. Ruemmele FM, Schwartz S, Seidman EG, Dionne S, Levy E, Lentze MJ. Butyrate induced Caco-2 cell apoptosis is mediated via the mitochondrial pathway. Gut. (2003) 52:94–100. doi: 10.1136/gut.52.1.94
68. Bridgeman SC, Northrop W, Melton PE, Ellison GC, Newsholme P, Mamotte CDS. Butyrate generated by gut microbiota and its therapeutic role in metabolic syndrome. Pharmacol Res. (2020) 160:105174. doi: 10.1016/j.phrs.2020.105174
69. Nakkarach A, Foo HL, Song AA, Mutalib NEA, Nitisinprasert S, Withayagiat U. Anti-cancer and anti-inflammatory effects elicited by short chain fatty acids produced by Escherichia coli isolated from healthy human gut microbiota. Microb Cell Fact. (2021) 20:36. doi: 10.1186/s12934-020-01477-z
70. Zheng DW, Dong X, Pan P, Chen KW, Fan JX, Cheng SX, et al. Phage-guided modulation of the gut microbiota of mouse models of colorectal cancer augments their responses to chemotherapy. Nat Biomed Eng. (2019) 3:717–28. doi: 10.1038/s41551-019-0423-2
71. Li J, Sung CY, Lee N, Ni Y, Pihlajamäki J, Panagiotou G, et al. Probiotics modulated gut microbiota suppresses hepatocellular carcinoma growth in mice. Proc Natl Acad Sci U S A. (2016) 113:E1306–15. doi: 10.1073/pnas.1518189113
72. Marques C, Oliveira CS, Alves S, Chaves SR, Coutinho OP, Côrte-Real M, et al. Acetate-induced apoptosis in colorectal carcinoma cells involves lysosomal membrane permeabilization and cathepsin D release. Cell Death Dis. (2013) 4:e507. doi: 10.1038/cddis.2013.29
73. Chen L, Zhou X, Wang Y, Wang D, Ke Y, Zeng X. Propionate and butyrate produced by gut microbiota after probiotic supplementation attenuate lung metastasis of melanoma cells in mice. Mol Nutr Food Res. (2021) 65:e2100096. doi: 10.1002/mnfr.202100096
74. Kwoji ID, Aiyegoro OA, Okpeku M, Adeleke MA. ‘Multi-Omics' data integration: applications in probiotics studies. NPJ Sci Food. (2023) 7:25. doi: 10.1038/s41538-023-00199-x
75. Kiousi DE, Rathosi M, Tsifintaris M, Chondrou P, Galanis A. Pro-biomics: omics technologies to unravel the role of probiotics in health and disease. Adv. Nutr. (2021) 12:1802–20. doi: 10.1093/advances/nmab014
76. Li Y, He P, Liu Y, Qi M, Dong W. Combining sodium butyrate with cisplatin increases the apoptosis of gastric cancer in vivo and in vitro via the mitochondrial apoptosis pathway. Front Pharmacol. (2021) 12:708093. doi: 10.3389/fphar.2021.708093
77. Chu H, Sun X, Wang J, Lei K, Shan Z, Zhao C, et al. Synergistic effects of sodium butyrate and cisplatin against cervical carcinoma in vitro and in vivo. Front Oncol. (2022) 12:999667. doi: 10.3389/fonc.2022.999667
78. Park M, Kwon J, Shin HJ, Moon SM, Kim SB, Shin US, et al. Butyrate enhances the efficacy of radiotherapy via FOXO3A in colorectal cancer patient-derived organoids. Int J Oncol. (2020) 57:1307–18. doi: 10.3892/ijo.2020.5132
79. Martini G, Ciardiello D, Dallio M, Famiglietti V, Esposito L, Corte CMD, et al. Gut microbiota correlates with antitumor activity in patients with mCRC and NSCLC treated with cetuximab plus avelumab. Int J Cancer. (2022) 151:473–80. doi: 10.1002/ijc.34033
80. Peng Z, Cheng S, Kou Y, Wang Z, Jin R, Hu H, et al. The gut microbiome is associated with clinical response to anti-PD-1/PD-L1 immunotherapy in gastrointestinal cancer. Cancer Immunol Res. (2020) 8:1251–61. doi: 10.1158/2326-6066.CIR-19-1014
81. Zhang SL, Mao YQ, Zhang ZY, Li ZM, Kong CY, Chen HL, et al. Pectin supplement significantly enhanced the anti-PD-1 efficacy in tumor-bearing mice humanized with gut microbiota from patients with colorectal cancer. Theranostics. (2021) 11:4155–70. doi: 10.7150/thno.54476
82. Coutzac C, Jouniaux JM, Paci A, Schmidt J, Mallardo D, Seck A, et al. Systemic short chain fatty acids limit antitumor effect of CTLA-4 blockade in hosts with cancer. Nat Commun. (2020) 11:2168. doi: 10.1038/s41467-020-16079-x
83. Tian T, Zhao Y, Yang Y, Wang T, Jin S, Guo J, et al. The protective role of short-chain fatty acids acting as signal molecules in chemotherapy- or radiation-induced intestinal inflammation. Am J Cancer Res. (2020) 10:3508–31.
84. Zhao R, Wang Y, Huang Y, Cui Y, Xia L, Rao Z, et al. Effects of fiber and probiotics on diarrhea associated with enteral nutrition in gastric cancer patients: a prospective randomized and controlled trial. Medicine. (2017) 96:e8418. doi: 10.1097/MD.0000000000008418
85. Liu Z, Qin H, Yang Z, Xia Y, Liu W, Yang J, et al. Randomised clinical trial: the effects of perioperative probiotic treatment on barrier function and post-operative infectious complications in colorectal cancer surgery - a double-blind study. Aliment Pharmacol Ther. (2011) 33:50–63. doi: 10.1111/j.1365-2036.2010.04492.x
86. Gianotti L, Morelli L, Galbiati F, Rocchetti S, Coppola S, Beneduce A, et al. A randomized double-blind trial on perioperative administration of probiotics in colorectal cancer patients. World J Gastroenterol. (2010) 16:167–75. doi: 10.3748/wjg.v16.i2.167
87. Kazmierczak-Siedlecka K, Skonieczna-Zydecka K, Palma J, Sobocki BK, Swierblewski M, Siedlecka-Kroplewska K, et al. Microbiota-derived metabolites in colorectal cancer patients in preoperative period. Eur Rev Med Pharmacol Sci. (2023) 27:1443–9. doi: 10.26355/eurrev_202302_31384
88. Gill SK, Rossi M, Bajka B, Whelan K. Dietary fibre in gastrointestinal health and disease. Nat Rev Gastroenterol Hepatol. (2021) 18:101–16. doi: 10.1038/s41575-020-00375-4
89. Guan ZW, Yu EZ, Feng Q. Soluble dietary fiber, one of the most important nutrients for the gut microbiota. Molecules. (2021) 26:802. doi: 10.3390/molecules26226802
90. Han K, Nam J, Xu J, Sun X, Huang X, Animasahun O, et al. Generation of systemic antitumour immunity via the in situ modulation of the gut microbiome by an orally administered inulin gel. Nat Biomed Eng. (2021) 5:1377–88. doi: 10.1038/s41551-021-00749-2
91. Jacobasch G, Dongowski G, Florian S, Müller-Schmehl K, Raab B, Schmiedl D. Pectin does not inhibit intestinal carcinogenesis in APC-deficient min/+ mice. J Agric Food Chem. (2008) 56:1501–10. doi: 10.1021/jf070872l
92. Spencer CN, McQuade JL, Gopalakrishnan V, McCulloch JA, Vetizou M, Cogdill AP, et al. Dietary fiber and probiotics influence the gut microbiome and melanoma immunotherapy response. Science. (2021) 374:1632–40. doi: 10.1126/science.aaz7015
93. Szczyrek M, Bitkowska P, Chunowski P, Czuchryta P, Krawczyk P, Milanowski J. Diet, microbiome, and cancer immunotherapy-a comprehensive review. Nutrients. (2021) 13:217. doi: 10.3390/nu13072217
94. Zhang C, Björkman A, Cai K, Liu G, Wang C, Li Y, et al. Impact of a 3-months vegetarian diet on the gut microbiota and immune repertoire. Front Immunol. (2018) 9:908. doi: 10.3389/fimmu.2018.00908
95. Seethaler B, Nguyen NK, Basrai M, Kiechle M, Walter J, Delzenne NM, et al. Short-chain fatty acids are key mediators of the favorable effects of the mediterranean diet on intestinal barrier integrity: data from the randomized controlled libre trial. Am J Clin Nutr. (2022) 116:928–42. doi: 10.1093/ajcn/nqac175
96. Donohoe DR, Holley D, Collins LB, Montgomery SA, Whitmore AC, Hillhouse A, et al. A gnotobiotic mouse model demonstrates that dietary fiber protects against colorectal tumorigenesis in a microbiota- and butyrate-dependent manner. Cancer Discov. (2014) 4:1387–97. doi: 10.1158/2159-8290.CD-14-0501
97. Morrison DJ, Preston T. Formation of short chain fatty acids by the gut microbiota and their impact on human metabolism. Gut Microbes. (2016) 7:189–200. doi: 10.1080/19490976.2015.1134082
98. Scheppach W, Bartram HP, Richter F. Role of short-chain fatty acids in the prevention of colorectal cancer. Eur J Cancer. (1995) 31a:1077–80. doi: 10.1016/0959-8049(95)00165-F
99. Miller AA, Kurschel E, Osieka R, Schmidt CG. Clinical pharmacology of sodium butyrate in patients with acute leukemia. Eur J Cancer Clin Oncol. (1987) 23:1283–7. doi: 10.1016/0277-5379(87)90109-X
100. Brioschi A, Zara GP, Calderoni S, Gasco MR, Mauro A. Cholesterylbutyrate solid lipid nanoparticles as a butyric acid prodrug. Molecules. (2008) 13:230–54. doi: 10.3390/molecules13020230
101. Maier S, Reich E, Martin R, Bachem M, Altug V, Hautmann RE, et al. Tributyrin induces differentiation, growth arrest and apoptosis in androgen-sensitive and androgen-resistant human prostate cancer cell lines. Int J Cancer. (2000) 88:245–51.
102. Gore SD, Weng LJ, Zhai S, Figg WD, Donehower RC, Dover GJ, et al. Impact of the putative differentiating agent sodium phenylbutyrate on myelodysplastic syndromes and acute myeloid leukemia. Clin Cancer Res. (2001) 7:2330–9.
103. Patnaik A, Rowinsky EK, Villalona MA, Hammond LA, Britten CD, Siu LL, et al. A phase I study of pivaloyloxymethyl butyrate, a prodrug of the differentiating agent butyric acid, in patients with advanced solid malignancies. Clin Cancer Res. (2002) 8:2142–8.
104. Reid T, Valone F, Lipera W, Irwin D, Paroly W, Natale R, et al. Phase II trial of the histone deacetylase inhibitor pivaloyloxymethyl butyrate (pivanex, AN-9) in advanced non-small cell lung cancer. Lung Cancer. (2004) 45:381–6. doi: 10.1016/j.lungcan.2004.03.002
105. Kuefer R, Hofer MD, Altug V, Zorn C, Genze F, Kunzi-Rapp K, et al. Sodium butyrate and tributyrin induce in vivo growth inhibition and apoptosis in human prostate cancer. Br J Cancer. (2004) 90:535–41. doi: 10.1038/sj.bjc.6601510
106. Giermasz A, Nowis D, Jalili A, Basak G, Marczak M, Makowski M, et al. Antitumor activity of tributyrin in murine melanoma model. Cancer Lett. (2001) 164:143–8. doi: 10.1016/S0304-3835(01)00375-5
107. Newmark HL, Young CW. Butyrate and phenylacetate as differentiating agents: practical problems and opportunities. J Cell Biochem Suppl. (1995) 22:247–53. doi: 10.1002/jcb.240590831
Keywords: dietary fiber, gut microbiota, short-chain fatty acids, cancer, immunotherapy
Citation: Li Y, Huang Y, Liang H, Wang W, Li B, Liu T, Huang Y, Zhang Z, Qin Y, Zhou X, Wang R and Huang T (2023) The roles and applications of short-chain fatty acids derived from microbial fermentation of dietary fibers in human cancer. Front. Nutr. 10:1243390. doi: 10.3389/fnut.2023.1243390
Received: 20 June 2023; Accepted: 21 July 2023;
Published: 08 August 2023.
Edited by:
Junrui Cheng, Ingredion Incorporated, United StatesReviewed by:
Karolina Kazmierczak-Siedlecka, Medical University of Gdansk, PolandMara Roxana Rubinstein, CONICET Institute for Biomedical Research (BIOMED), Argentina
Copyright © 2023 Li, Huang, Liang, Wang, Li, Liu, Huang, Zhang, Qin, Zhou, Wang and Huang. This is an open-access article distributed under the terms of the Creative Commons Attribution License (CC BY). The use, distribution or reproduction in other forums is permitted, provided the original author(s) and the copyright owner(s) are credited and that the original publication in this journal is cited, in accordance with accepted academic practice. No use, distribution or reproduction is permitted which does not comply with these terms.
*Correspondence: Tingting Huang, dGluZ3RpbmdodWFuZzE5ODYmI3gwMDA0MDtnbWFpbC5jb20=; Rensheng Wang, MTM4MDc4MDYwMDgmI3gwMDA0MDsxNjMuY29t
†These authors have contributed equally to this work