- 1Department of Agricultural Sciences, University of Naples Federico II, Naples, Italy
- 2Department of Biology, University of Naples Federico II, Naples, Italy
- 3NBFC, National Biodiversity Future Center, Palermo, Italy
- 4Department of Bio-Agrofood Science, Institute for the Animal Production System in the Mediterranean Environment, National Research Council Naples (CNR-ISPAAM), Naples, Italy
Introduction: Microencapsulation of probiotic bacteria is an efficient and innovative new technique aimed at preserving bacterial survival in the hostile conditions of the gastrointestinal tract. However, understanding whether a microcapsule preserves the effectiveness of the bacterium contained within it is of fundamental importance.
Methods: Male Wistar rats aged 90 days were fed a control diet or a Western diet for 8 weeks, with rats fed the Western diet divided into three groups: one receiving the diet only (W), the second group receiving the Western diet and free L. reuteri DSM 17938 (WR), and the third group receiving the Western diet and microencapsulated L. reuteri DSM 17938 (WRM). After 8 weeks of treatment, gut microbiota composition was evaluated, together with occludin, one of the tight junction proteins, in the ileum and the colon. Markers of inflammation were also quantified in the portal plasma, ileum, and colon, as well as markers for gut redox homeostasis.
Results: The Western diet negatively influenced the intestinal microbiota, with no significant effect caused by supplementation with free and microencapsulated L. reuteri. However, L. reuteri, in both forms, effectively preserved the integrity of the intestinal barrier, thus protecting enterocytes from the development of inflammation and oxidative stress.
Conclusion: From these whole data, it emerges that L. reuteri DSM 17938 can be an effective probiotic in preventing the unhealthy consequences of the Western diet, especially in the gut, and that microencapsulation preserves the probiotic effects, thus opening the formulation of new preparations to be able to improve gut function independent of dietary habits.
1. Introduction
The human environment, behaviors, and lifestyle have all dramatically changed in the last 50 years. Unhealthy dietary patterns and sedentary behavior are risk factors for major chronic diseases (1), becoming a major public health concern due to their global prevalence (2). In this context, the Western diet, in particular, is considered a major risk factor (3) due to its high content of energy, fat, and sugar. As a matter of fact, this diet model seems to exert a negative effect on the richness and function of gut microbiota, leading to a significant decrease in the number of commensal bacteria and reducing microbial diversity (4, 5). Moreover, the shift in microbiota composition can, in turn, lead to inflammation, impairment of gut function, and alteration of the immune system in this organ (4, 6).
Physical, psychological, pharmaceutical, and dietary therapies have been proposed to manage gut dysfunction (7). Among these therapies, the administration of probiotics as potential biotherapeutics has become of central interest, considering their ability, when given in sufficient amounts, to be beneficial to the host by improving gut microbial balance (8) and modulating their interaction with intestinal cells, with positive effects on the health of the whole organism (9). However, since the physiological effects attributed to probiotics are highly strain-specific, their selection may be critical for their functional efficacy (10). Therefore, each strain may work through different mechanisms of action and have a specific genotype/phenotype accordingly, and these mechanisms are not deeply and entirely understood yet (11).
A major selection criterion for a probiotic is its capacity to pass through the human gastrointestinal tract as undamaged as possible to reach the gut and exert its activity (12, 13). Indeed, the insufficient viability and survival of probiotics remain pivotal in supplements and foods (13). Constructing a probiotic release system is extremely complex due to the diverse range of conditions in the human digestive tract. However, this diversity also presents an opportunity to develop a customized system that specifically targets the intended gut site (14).
The bacteria's survivability can be improved by the microencapsulation technique. The microencapsulation matrix is designed to resist the gastrointestinal acid condition, and the preparation routine must be delicate to not harm the cells within it. Furthermore, the polymer utilized must be non-cytotoxic and non-antimicrobial to ensure that neither the host nor the bacteria are damaged (15). This technique has been successfully applied in other oral delivery applications, proving how the specialized ultrathin semipermeable polymer membranes that constitute the microcapsule can protect live bacterial cells (16). Limosilactobacillus reuteri DSM 17938 (L. reuteri), known as Lactobacillus reuteri before the changes in the taxonomy of the Lactobacillus genus, deeply revised in 2020 (17), is a probiotic strain that can colonize several human body sites, such as the gastrointestinal tract, the skin, breast milk, and the urinary tract. Several studies reported in the literature showed that L. reuteri administration can be beneficial to human health and disease (18). In particular, it is a probiotic well recognized for its useful effects in pediatric gastrointestinal diseases, especially in children (19, 20) and in cases of constipation in adults (21), but, to date, only two studies have investigated its effect in the context of obesity and metabolic syndrome (22, 23).
The overall aim of this study has been to evaluate the effects of free or microencapsulated probiotic Limosilactobacillus reuteri DSM 17938 on the intestinal microbiota and the gut metabolic alteration induced by a Western diet that combines the consumption of a high saturated fat diet with high fructose intake, perfectly simulating the current dietary habits in Western countries. In particular, we focused on the integrity of the intestinal barrier, gut inflammation, oxidative stress, and enzymes playing a critical role in redox homeostasis and lipopolysaccharide (LPS) detoxification.
2. Materials and methods
2.1. Cultivation and microencapsulation of Limosilactobacillus reuteri DSM 17938
Limosilactobacillus reuteri DSM 17938 was kindly provided by BioGaia (Noos S.r.l.; BioGaia AB, Stockholm, Sweden). It was cultured in MRS broth (OXOID Ltd., Basingstoke, Hampshire, England) at 37°C, checked for purity, and kept on MRS Agar (OXOID Ltd., Basingstoke, Hampshire, England). The free and microencapsulated cells were cultured in aerobic conditions and counted routinely on MRS agar at 37°C for 48 h. The encapsulator B-395 Pro was equipped with a 120-mm nozzle and a syringe pump (BÜCHI Labortechnik, Flawil, Switzerland) to microencapsulate bacterial cells. The number of microencapsulated cells was determined according to De Prisco et al. (24), and the entrapment efficiency was calculated using the formula:
where N is the average number of CFU per microcapsule, Nd is the cell count (CFU/ml) of disrupted microcapsules, and Ni is the cell count of integer microcapsules. Each colony is derived from a single integer microcapsule; therefore, Ni's measure unit is microcapsules/ml (25).
2.2. Animals and treatments
All animal experiments were authorized by the Italian Health Ministry (137/2022-PR) and approved by the “Comitato Etico-Scientifico per la Sperimentazione Animale” of the University of Naples “Federico II.” The procedures used in this work adhere to the animal ethics principles and regulations of the Italian Health Ministry. The authors ensured that all steps were taken to minimize the pain and suffering of the animals.
Male Wistar rats (Charles River, Calco, Lecco, Italy) aged 90 days were caged in a temperature-controlled room (23 ± 1°C) with a 12-h light/dark cycle (06.30–18.30 h).
Rats were divided into four groups (each composed of 8 rats) and treated for 8 weeks with a control diet (C group) or with a high-fat, high-fructose diet (W, WR, and WRM groups). In addition, WR rats daily received 0.1 mL of a 10% sucrose solution containing 108 CFU of L. reuteri, WRM rats daily received 0.1 mL of a 10% sucrose solution containing 108 CFU of microencapsulated L. reuteri, and C and W rats received the same amount of sucrose solution without probiotics. Sucrose solution with or without probiotics was presented by an operator every day at the same hour through a needless syringe and voluntarily consumed by rats. We also calculated that the quantity of alginate per dose is 6 mg. The two diets are shown in Supplementary Table S1. During the treatment, body weight and food intake were monitored, and the feces were collected daily. The results are reported in Supplementary Figure S1. After 8 weeks on the diet, the rats were euthanized, and portal blood, colon, and ileum were taken and frozen at −80°C for further analysis.
2.3. DNA extraction, high-throughput sequencing, and bioinformatic analysis
Fresh fecal samples of 32 rats (8 for each of the four groups) were collected after 8 weeks of treatment. The DNeasy PowerSoil Pro Kit (Qiagen, Hilden, Germany) was used to extract total DNA according to the manufacturer's instructions and quantified using the NanoDrop spectrophotometer. Bacterial diversity was determined by high-throughput sequencing (HTS) of the amplicons from the V4-V3 region of the 16S rRNA gene (~460 bp). PCR and bioinformatic analysis were carried out as previously reported (26–28).
2.4. Markers of inflammation
Portal plasma samples were obtained from portal blood collected in tubes containing EDTA at the time of sacrifice. These samples were then centrifuged for 15 min at 1400 x g and stored at −20°C.
LPS in portal plasma was measured using a protocol based on a Limulus amoebocyte lysate (LAL) extract (ThermoFisher Scientific, Rockford, IL, USA) in accordance with the manufacturer's instructions.
Portal plasma concentrations of tumor necrosis factor-alpha (TNF-α), interleukin-6 (IL-6), and interleukin-10 (IL-10) were assessed using an enzyme-linked immunosorbent assay (R&D Systems, Minneapolis, MN, USA), specific for rats, which was in accordance with the kit instructions.
Intestinal alkaline phosphatase (IAP) activity was measured in the ileum and the colon. The ileum and colon tissues for the analysis were prepared according to Kaliannan et al. (29), and IAP activity was determined following the reported protocol (29), with the addition of the selective IAP inhibitor phenylalanine (10 mM) to subtract the result with phenylalanine from the result without phenylalanine (30). The specific activity of the enzyme is expressed as picomoles of pNPP hydrolyzed/min/μg of protein. A protein assay reagent from Fisher Scientific determined the protein concentration in each sample.
2.5. Oxidative stress parameters in the ileum and colon
To measure oxidative stress markers, homogenates from the colon and ileum were made in a 50 mM phosphate buffer with a pH of 7.0 (1:50 w/v).
Nitro-tyrosine (N-Tyr) levels were determined by ELISA, as described previously (31, 32).
According to Fernandes et al. (33), the thiobarbituric acid assay has been used to measure lipid peroxidation.
Superoxide dismutase (SOD) activity was assessed by following the decrease in the reduction rate of cytochrome c by superoxide radicals in a buffer with 20 mM cytochrome c, 0.1 mM xanthine, 0.01 units of xanthine oxidase, 50 mM KH2PO4, pH 7.8, as reported in a previous study (34).
Catalase activity was determined in a 50-mM phosphate buffer, pH 7.0, with 0.25% Triton X-100 and 10 mM H2O2 by following the decay of H2O2 at 240 nm, as reported in a previous study (35).
Glutathione reductase (GR) activity was measured following the decrease of NADPH absorbance at 340 nm, as described in a previous study (36).
NADPH oxidase activity was assayed by monitoring the change in absorbance at 340 nM, as previously reported (37).
2.6. Immunofluorescence analysis
Sections of the colon and ileum from all the groups were paraffin-embedded and stained with an occludin-specific monoclonal antibody (Invitrogen, Carlsbad, CA, USA) as a marker of gut barrier integrity. Subsequently, the slides were stained with DAPI (Sigma Aldrich, Saint Louis, MO, USA). The images were captured and visualized using a Zeiss Confocal Microscope LSM 700 at 40× magnification, and three random fields/section per rat were analyzed using ImageJ software (National Institutes of Health, Bethesda, MD, USA).
2.7. Western blot quantifications of TLR4 and p-NFkB in the ileum and colon
A Western Blot analysis was carried out on protein extracts from the colon and ileum to detect toll-like receptor-4 (TLR4) and phospho-nuclear factor kB (p-NFkB) signals as markers of inflammation, as previously described (38). A list of antibodies with their source and catalog number is presented in Supplementary Table S2.
2.8. Statistical analysis
To evaluate the presence of significant differences in the abundance of taxa between the four groups of rats, the pairwise Wilcoxon-Mann–Whitney test (“pairwise.Wilcox.test” function in package “base” was used). A p < 0.05 was considered statistically significant.
The animal physiological data were expressed as mean values ± SEM. GraphPad Prism 6 (GraphPad Software, San Diego, CA, USA) was utilized to check the normal distribution of the raw data and to perform a one-way ANOVA. In all analyses, a p < 0.05 (probability < 5%) was considered statistically significant.
3. Results
3.1. EE and cell survival
We calculated an EE of 97% and an average CFU/microcapsule of 100. The results of cell counting showed a cell load of microcapsules of 2.0 × 108 CFU/ml, and this value remained constant during the storage of the microcapsules in Ringer at 4°C for more than 14 days. However, we replaced the microcapsules weekly with a fresh preparation to ensure a dose of 108 CFU of L. reuteri.
3.2. Intestinal microbiota composition
To profile the effects of diet and L. reuteri supplementation on microbiota composition, we performed 16S rRNA gene sequencing of rat fecal samples collected at 8 weeks. The Western diet dramatically changed gut microbiota composition. The heat map in Figure 1 shows the average abundance of the most abundant taxa in C, W, WR, and WRM rats. Prevotella and Clostridiales levels decreased in western diet-fed rats (W), as well as in the rats fed the Western diet and treated with free (WR) and microencapsulated L. reuteri (WRM) groups compared to control rats (C) (P < 0.05), while Blautia, Ruminococcaceae, Lachnospiraceae, and Akkermansia muciniphila increased in all the groups consuming the Western diet compared to the C groups (P < 0.05). However, we did not observe a shift in the overall microbiota composition due to L. reuteri treatment. It is important to underline that we exclude any effect of the microencapsulating agent that is well known to be undigestible and not absorbable from the human intestine (39). Furthermore, it was previously ascertained that the scarce effect of alginate on mice was at doses much higher than those we used (40), and the alginate capsules proved to be highly biocompatible not only for short periods (i.e., 1 month) but also in the long term, as evidenced by the absence of any significant biological response up to 2 years after implantation in rats (41).
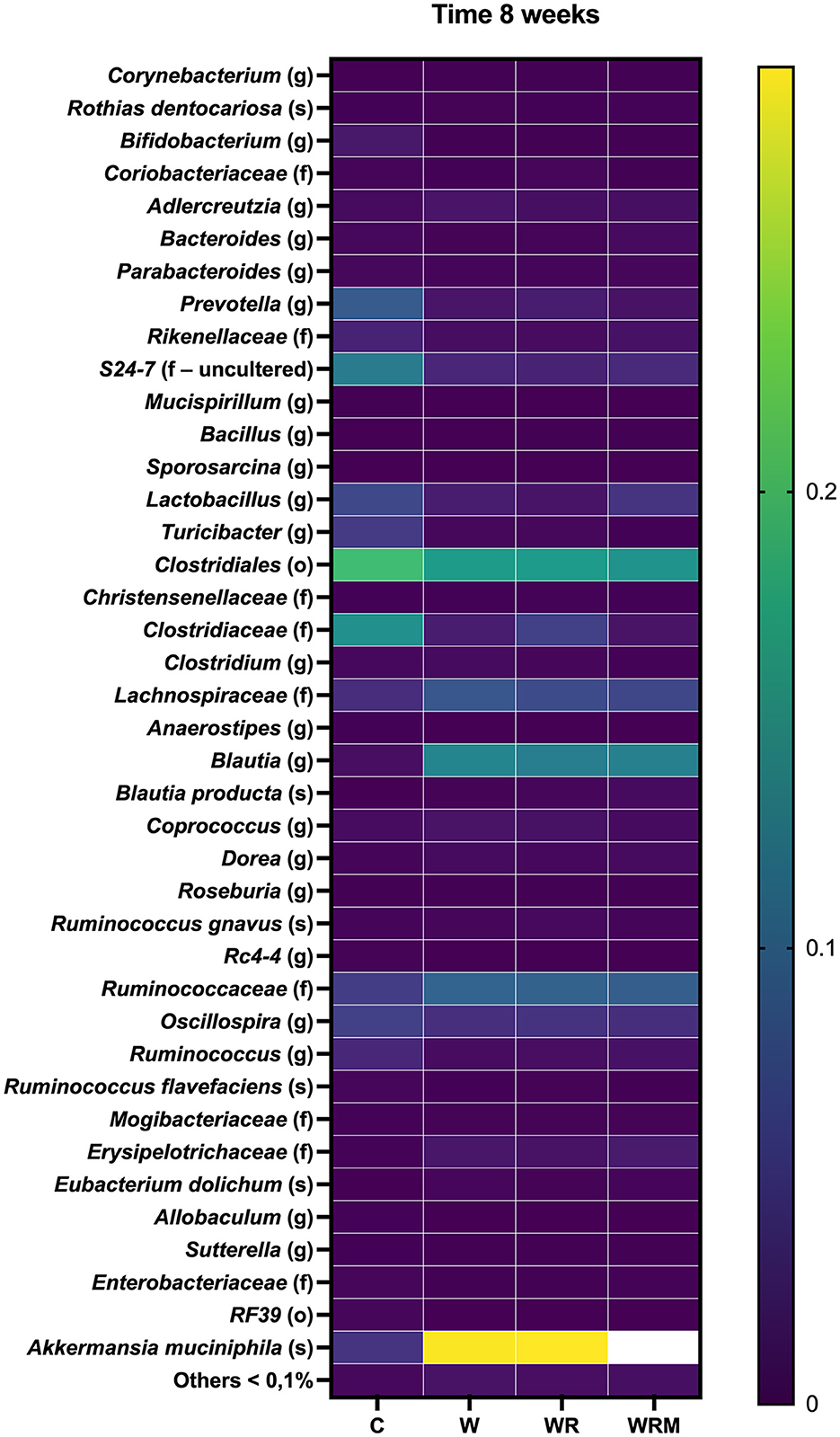
Figure 1. Heatmap showing average abundance (%) of the most abundant taxa (>0.1% abundance) in the gut microbiota of C, W, WR, and WRM rats. The values are the means of eight different rats.
3.3. Tight junction protein content and portal plasma inflammation
Tight junction occludin protein content was significantly lower in the ileum (Figure 2) and the colon (Figure 3) of W rats. In contrast, there was a significantly higher occludin content in WR and WRM rats (Figures 2, 3) in the ileum and colon compared to W rats, with even higher levels in WRM rats compared to C.
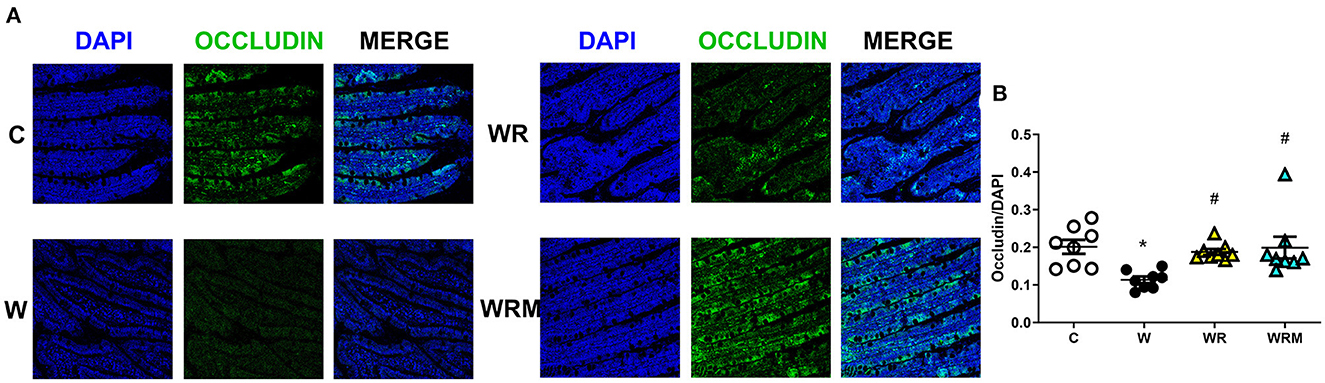
Figure 2. Immunofluorescence quantification of occludin (magnification 40x, scalebar = 50 μm) in the ileum (B) with representative images (A). Values are expressed as the means ± SEM of eight rats. *P < 0.05, compared to C; #P < 0.05, compared to W (one-way ANOVA followed by a Bonferroni post-test).
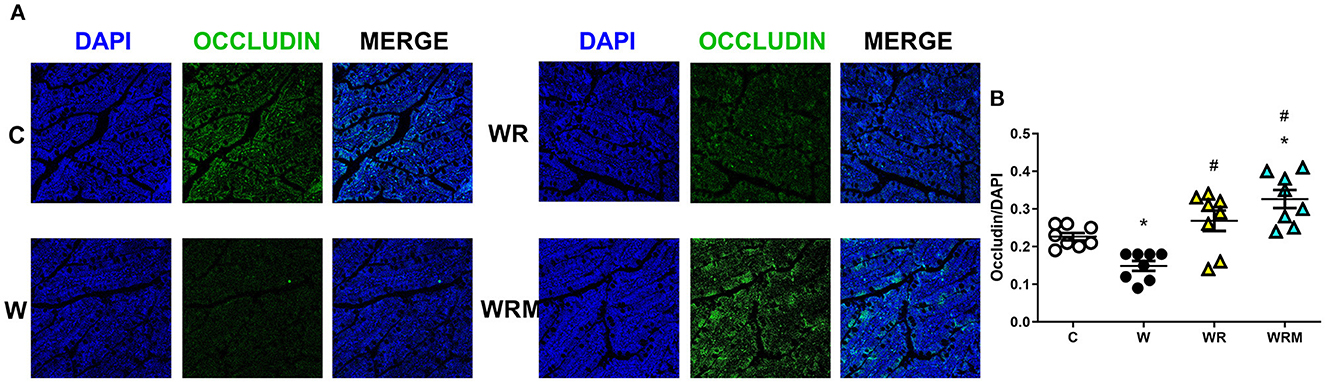
Figure 3. Immunofluorescence quantification of occludin (magnification 40x, scalebar = 50 μm) in colon (B) with representative images (A). Values are expressed as the means ± SEM of eight rats. *P < 0.05, compared to C; #P < 0.05, compared to W (one-way ANOVA followed by a Bonferroni post-test).
The altered integrity of the gut barrier induces the development of the so-called “leaky gut” (42), determining the uncontrolled passage of substances, such as LPS, in the portal plasma. We found increased levels of LPS in the portal plasma of W rats (Figure 4A), associated with the increase of proinflammatory IL-6 (Figure 4C). These parameters were comparable to control rats in WR rats, while in WRM rats, they were even significantly improved compared to C (Figures 4A, C). No changes were found in the levels of TNF-α, a proinflammatory cytokine, and IL-10, an anti-inflammatory one, in the four groups of rats (Figures 4B, D).
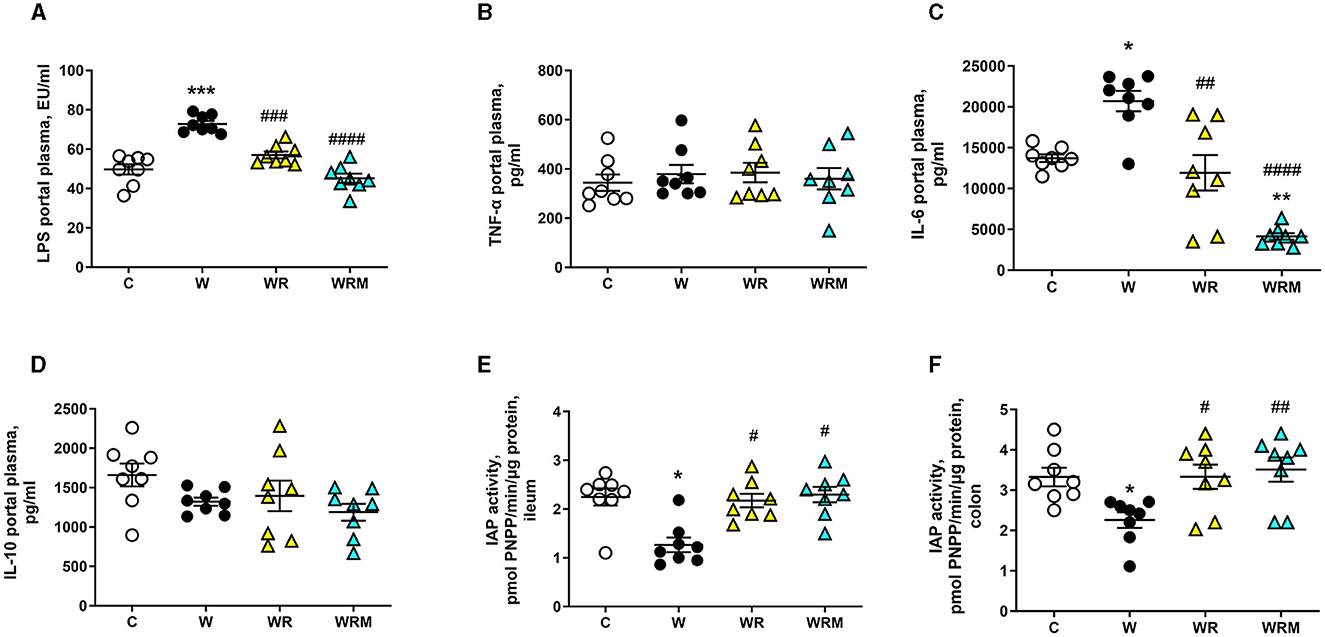
Figure 4. Portal plasma levels of lipopolysaccharides (LPS) (A), TNF-α (B), IL-6 (C), and IL-10 (D) and intestinal alkaline phosphatase (IAP) (E, F). Values are expressed as the means ± SEM of eight rats. *P < 0.05, **P < 0.01, ***P < 0.001 compared to (C); #P < 0.05, ##P < 0.01, ###P < 0.001, ####P < 0.0001 compared to W (one-way ANOVA followed by Bonferroni post-test).
IAP activity has been evaluated as an anti-inflammatory marker, considering its ability to detoxify LPS and other bacterial toxins. In agreement with the results obtained on portal LPS levels, IAP activity was found to be significantly lower in the ileum and colon of W rats compared to the control group, while it was unaltered in the above tissues of WR and WRM rats (Figures 4E, F).
3.4. Modulation of the inflammatory response in the ileum and colon
TLR4 protein content in the ileum and colon was assessed as a marker of inflammation and the degree of activation of the transcription factor NFκB. In the ileum and colon, the Western diet caused an increase in TLR4 levels (Figure 5A), together with a higher degree of phosphorylation and, hence, the activation of NFκB (Figure 5B). All the above increases were prevented by the administration of L. reuteri, both free and microencapsulated.
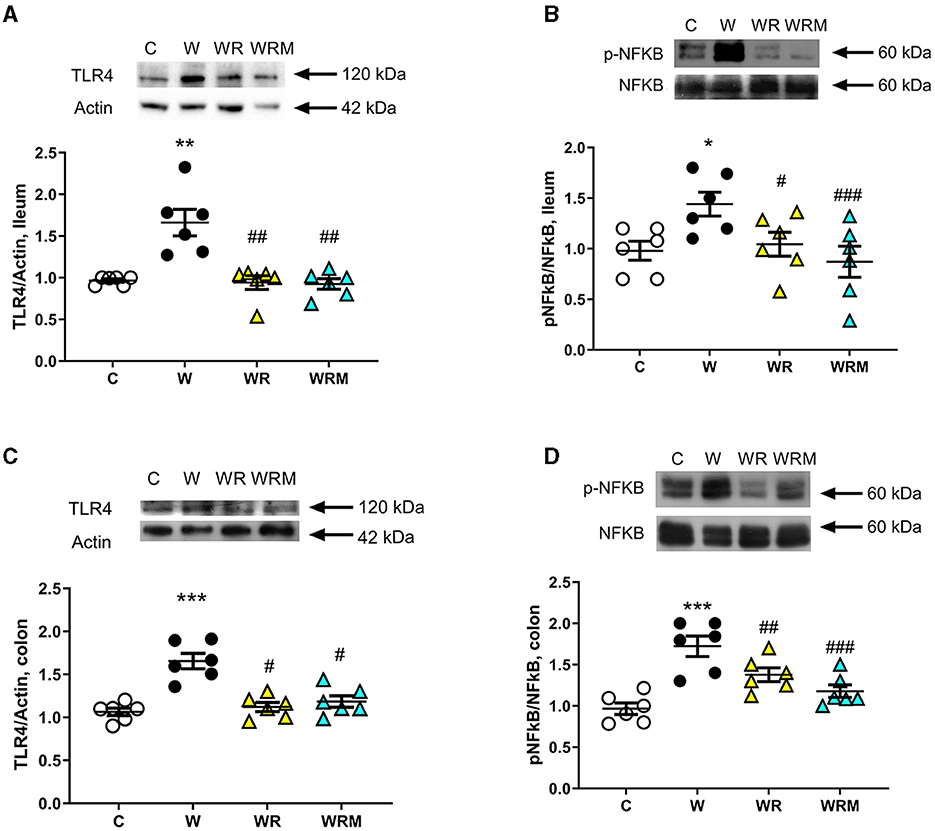
Figure 5. The protein content of toll-like receptor 4 (TLR4) and the degree of phosphorylation of nuclear factor kB (NFkB) (with representative blots, normalized to controls) in the ileum (A, B) and colon (C, D). Values are expressed as the means ± SEM of six rats. *P < 0.05, **P < 0.01, ***P < 0.001, compared to (C); #P < 0.05, ##P < 0.01, ###P < 0.001 compared to W (one-way ANOVA followed by Bonferroni post-test).
3.5. Ileum and colon oxidative stress
The gut inflammatory status is strictly related to oxidative stress in the enterocyte (43). Therefore, we evaluated, in both the ileum and colon, the oxidative stress induced by the Western diet by measuring lipid peroxidation and N-Tyr as markers of damage to lipids and proteins, respectively, and NADPH oxidase activity as a source of reactive oxygen species (ROS) and the activity of GSR, catalase, SOD, as antioxidant enzymes.
In particular, we observed significantly higher levels of TBARS and N-Tyr in the ileal cells of rats fed the Western diet (Figures 6A, B), together with an increase in NADPH oxidase activity (Figure 6C) and a decrease in GSR, catalase, and SOD activities (Figures 6D–F). Interestingly, the administration of both free and microencapsulated L. reuteri prevented the onset of oxidative damage since TBARS, N-Tyr levels, and NADPH oxidase activity were comparable to the control rats (Figures 6A–C). In agreement with these results, the analysis of SOD, catalase, and GSR (Figures 6D–F) activities in the ileum showed an increase in WR and WRM rats compared to W rats, confirming the beneficial effect of L. reuteri, both free and microencapsulated, in protecting this tissue from oxidative stress.
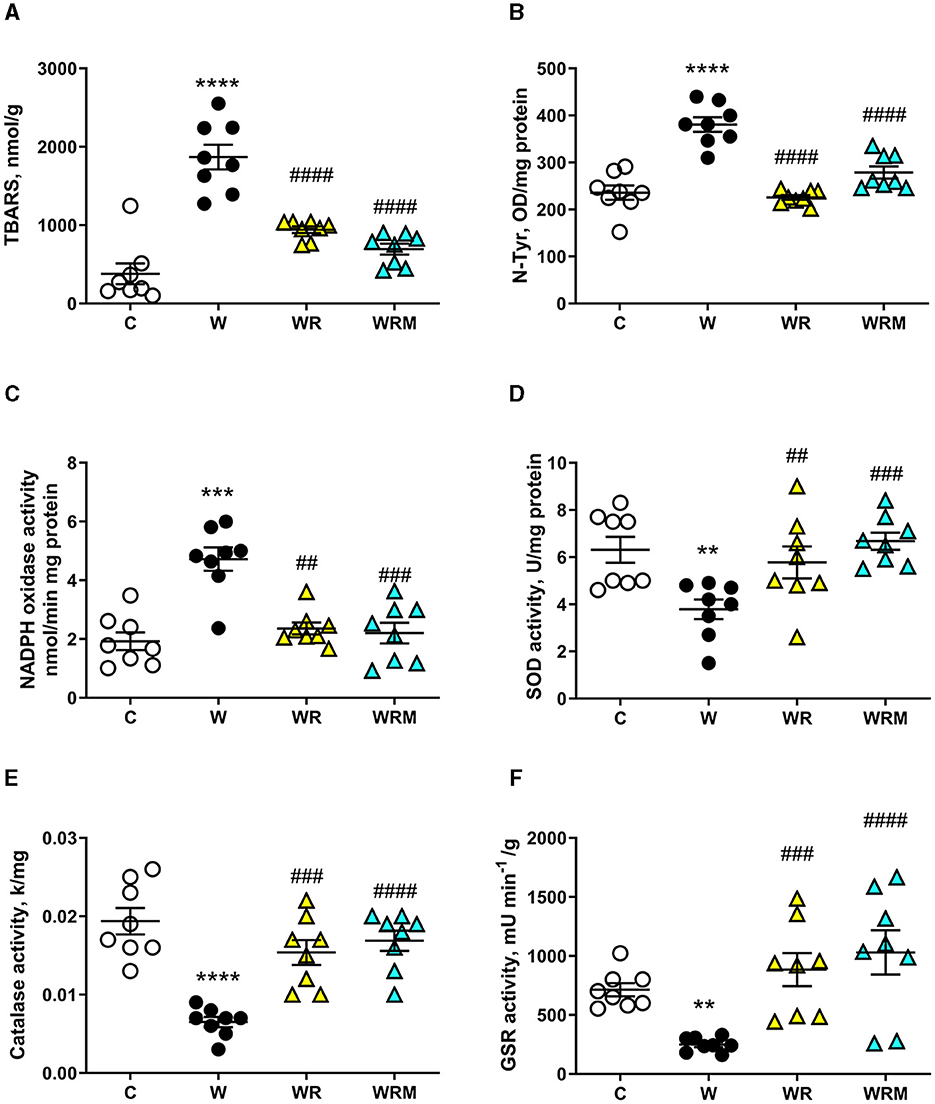
Figure 6. Thiobarbituric acid reactive substances (TBARS) (A) and N-Tyr (B) levels, NADPH oxidase (C), superoxide dismutase (SOD) activity (D), catalase (E), and glutathione reductase (GSR) (F) activities in the ileum. Values are expressed as the means ± SEM of eight rats. **P < 0.01, ***P < 0.001, ****P < 0.0001 compared to (C), ##P < 0.01, ###P < 0.001, ####P < 0.0001 compared to W (one-way ANOVA, followed by Bonferroni's post-test).
A slightly different pattern was found in the colon. Indeed, while N-Tyr levels and the activity of GSR and NADPH oxidase were positively influenced by treatment with both free and microencapsulated L. reuteri (Figures 7B, C, F), the activities of catalase and SOD remained significantly lower (Figures 7D, E) and the levels of TBARS remained significantly increased (Figure 7A) in WR rats. Therefore, in the colon, it seems that the free bacterium is less efficient than in the ileum, while it retains its efficacy in the microencapsulated form even in this intestinal area.
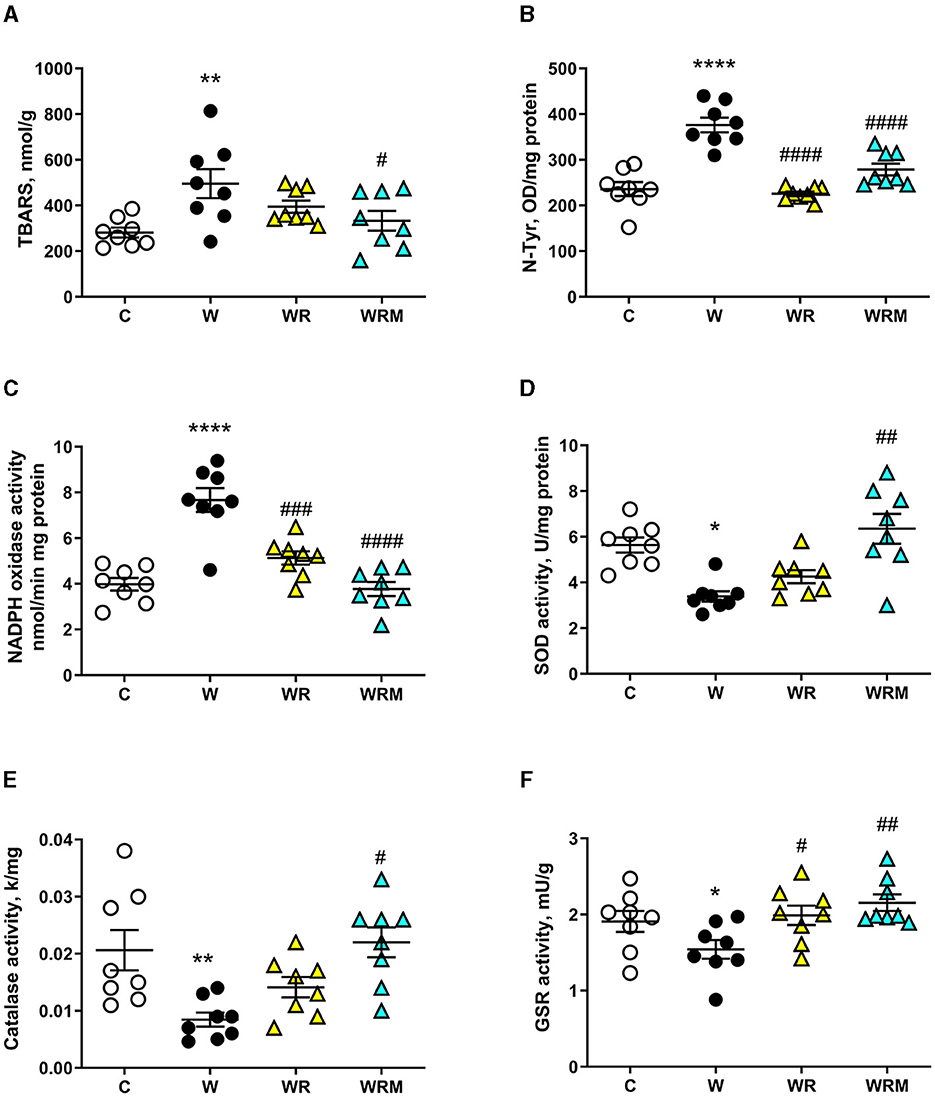
Figure 7. Thiobarbituric acid reactive substances (TBARS) (A) and N-Tyr (B) levels, NADPH oxidase (C), superoxide dismutase (SOD) activity (D), catalase (E), and glutathione reductase (GSR) (F) activities in the colon. Values are expressed as the means ± SEM of eight rats. *P < 0.05, **P < 0.01, ****P < 0.0001 compared to (C); #P < 0.05, ##P < 0.01, ###P < 0.001, ####P < 0.0001 compared to W (one-way ANOVA, followed by Bonferroni's post-test).
4. Discussion
In this study, we examined the effect of Limosilactobacillus reuteri DSM 17938 on contrasting Western diet-induced gut metabolic disturbances and clarified whether its microencapsulation would maintain unaltered or even enhance its beneficial effect.
The Western diet is widely recognized to cause gut dysbiosis, inflammation, and metabolic endotoxemia. In agreement with this, we highlighted a strong effect of the Western diet on gut microbiota composition, including a decrease in Prevotella, which is typically associated with high-fiber diets (44), and an increase in Blautia, Ruminococcaceae, Lachnospiraceae, and Akkermansia muciniphila, as previously found by other authors (45). Moreover, our results proved that supplementation with L. reuteri DSM 17938 cannot control the consequences of the Western diet on gut microbiota deviation. We found no significant differences in any of the taxa (P > 0.05) among the W, WR, and WRM groups, which is in agreement with previous studies (23, 46). Although we analyzed fecal rather than cecal microbiota, it has been shown that the fecal microbiome represents a good non-invasive proxy of the cecal microbiome, making it suitable for detecting biologically relevant patterns (47).
Western diet-induced dysbiosis is associated with many abnormalities. One of the most relevant is the disruption of the gut barrier, whose main function is to provide a physical barrier to counteract the possible bacterial incursion from the lumen of the gut (48). Moreover, several studies have shown that high-fat diet administration can alter intestinal permeability and induce endotoxemia (49, 50). We also previously demonstrated that even 3 weeks (short term) of a high fructose diet in young rats can lead to gut barrier dysfunction (38). In this study, it was demonstrated that the consumption of a Western diet over a period of 8 weeks resulted in both gut dysbiosis and a change in the permeability of the gut barrier's mechanical line of defense, as indicated by a reduction in ileum and colon occludin levels. Occludin is a crucial functional element of tight junctions, which highly regulate the movement of substances through the paracellular space and prevent uncontrolled leakage (51).
As a consequence, we found high portal plasma levels of bacterial LPS, a powerful player in the activation of the inflammatory response (52), in rats fed with the Western diet. This is congruent with some previous studies showing an increase in plasma LPS upon consumption of high-fat (53) and high-fructose diets (50). Moreover, LPS, in turn, can further impair gut permeability through the downregulation of occludin itself and induce the establishment of the “leaky gut” (54). What is interesting to notice here is that the administration of L. reuteri prevented the disruption of the gut barrier in WR rats and even improved occludin expression on the intestinal barrier in the colon of WRM rats. This result shows that the free L. reuteri supplementation is efficient in avoiding the Western diet's negative effect on intestinal barrier integrity, while the microencapsulated form of L. reuteri exhibits a stronger protective effect, even increasing the integrity of the intestinal barrier, compared to C rats. These effects on the gut barrier exert an effect on the passage of LPS in WR rats, whose portal levels are comparable to those of C rats and are lower in WRM rats compared to controls. The results obtained with the free form of L. reuteri are in agreement with studies demonstrating that treatment with different strains of L. reuteri leads to a higher expression of tight junction proteins in intestinal epithelial cells (55–57), consequently inhibiting the passage of LPS through the gut barrier.
Although microencapsulation is not a novel technique, vibrating technology has been introduced in the last few years. Compared to others, the main advantages of this technology are that the process is performed at room temperature, the resulting microcapsules are very homogenous in size, shape, and number of entrapped bacterial cells, and the efficiency of encapsulation is very high. In addition, the viability of the culture is not affected by the microencapsulation process, and all the microcapsules have the same behavior in terms of bacterial activity. A previous study (24) demonstrated the stability of the carrier and the encapsulated probiotic strain during the storage of microcapsules.
Although it is acknowledged that a diet rich in fat causes metabolic endotoxemia in humans and animals (53, 58), the underlying molecular mechanisms are not fully understood yet. In this context, a role has been proposed for the enzyme IAP, which is an enzyme produced exclusively in the intestine that belongs to the alkaline phosphatase family (59). IAP can prevent the recognition of LPS from its receptor TLR4, removing a phosphate group from the LPS lipid A moiety, thus detoxifying it (60). In addition, IAP can upregulate the expression of the tight junction proteins, preserving intestinal barrier integrity and function (61). Moreover, in intestinal inflammatory conditions such as IBD (inflammatory bowel disease) and T2-DM (type 2 diabetes mellitus), the endogenous IAP levels have been shown to be extremely low (62, 63). Therefore, we investigated whether the increased LPS portal levels elicited by the Western diet were correlated with changes in IAP activity. Our present results support the involvement of IAP activity since W rats exhibited lower levels of IAP activity both in the ileum and the colon, while the administration of free or microencapsulated L. reuteri completely reversed the above decrease.
LPS in portal plasma is recognized by the TLR4 receptor and activates a signal cascade that involves the NFkB pathway (64). Concurrently, we found an increase in the ileal and colonic TLR4, whose expression on intestinal epithelial cells has shown a proinflammatory role, recognizing specifically the LPS (65) and NFkB involved in the intestinal dysfunctional states (66). This, in turn, implies a higher gene expression of TNF-α (67) and IL-6 (68) and a consequent inflammatory status. Indeed, an increase in the inflammatory cytokine IL-6 was detected in the portal plasma of W rats compared to controls, but quite surprisingly, no differences were found in TNF-α levels among C, W, WR, and WRM rats. This result can be explained by considering that increased IL-6 levels in humans have been shown to inhibit endotoxin-induced TNF-α production (69). Moreover, attenuated TNF-α production has been shown after IL-6 treatment in plasmacytoid dendritic cells in rheumatoid arthritis (70).
Moreover, in agreement with us, unchanged TNF-α levels were found by Bedoui and colleagues in male rats fed for 10 weeks with a high-fat diet (71), and by Semeraro and colleagues in female rats fed for 10 months with a high-fat diet (72). These changes suggest additional damage to intestinal cell function and structure. It is known that IL-6 and NFkB have a key role in the regulation of tight junction integrity (73, 74). Moreover, in the rats that were fed the Western diet with the free probiotic L. reuteri administration, IL-6 levels, TLR4, and p-NFkB were comparable to the levels found in the C rats, confirming the hypothesis that the supplementation of a probiotic in parallel with the Western diet protects against the development of inflammation. Again, the microencapsulated L. reuteri exhibits a more powerful anti-inflammatory activity, with IL-6 levels lower than those found in C rats.
Oxidative stress development is often associated with intestinal inflammation (75), and several studies show a close interaction between oxidative stress and gut microbiome alterations (76). In agreement, we demonstrated that the Western diet induces oxidative damage to both proteins and lipids in the ileum and colon due to an imbalance between ROS production and the functioning of the antioxidant defense system. Indeed, we found an increase in the ROS-producing NADPH oxidase activity coupled with a decrease in the antioxidant enzymes SOD, catalase, and GSR in Western diet-fed rats compared to the control rats both in the ileum and the colon. In the ileum, we also demonstrated the efficiency of L. reuteri in preventing oxidative stress, acting on the inhibition of ROS production, and stimulating antioxidant defense, with only a marginal effect on the microcapsule. However, in the colon, we evidenced a strong improvement of the microcapsule in the action of the probiotic bacterium. Although we found a decrease in ROS production in WR rats, the lack of effect on catalase and SOD activities resulted in increased TBARS levels, while in WRM rats, both ROS production and antioxidant enzymes were comparable to those found in control rats. Therefore, we can speculate that the microcapsule enhances the resistance of probiotics to the acid environment in the stomach, which is severely fatal to the majority of bacteria and the bile acids and digestive enzymes in the small intestine, thus increasing the survival rate and viability and ensuring that a higher number of viable probiotics arrive in the colon, thus allowing greater effectiveness of the probiotic bacterium in this district. Previous studies performed with microencapsulated Lactobacillus salivarious Li01 and Pediococcus pentosaceus Li05 showed an enhancement of storage viability and delivery to gut microbiota (77, 78). In fact, in conjunction with its well-established role as a mere physical barrier to harsh gut conditions, microencapsulation was recently found to enhance the stress resistance of bacterial cells through the mediation of quorum-sensing activity (79). Moreover, considering that the colon has the largest bacterial density (1011 to 1012 CFU/ml), probiotics will find a high colonization resistance from commensal bacteria (80). Hence, the protection provided by the microcapsule is even more important in guaranteeing the release of a greater number of bacteria that can colonize the colon.
The results obtained in this study demonstrate, for the first time, the efficiency of Limosilactobacillus reuteri DSM 17938 treatment in improving the metabolic alterations induced in the gut by the Western diet. Indeed, the fact that L. reuteri safeguards intestinal permeability plays an important role in reducing low-grade inflammation induced by elevated lipopolysaccharide levels and oxidative stress in enterocytes. Moreover, our data also demonstrate how microencapsulation not only does not alter the effectiveness of the bacterium but even improves it, especially in the colon (Figure 8). From these whole data, it emerges that L. reuteri DSM 17938 can be an effective probiotic in preventing the unhealthy consequences of the Western diet, especially in the gut, and that microencapsulation preserves the probiotic effects, thus opening up the formulation of new preparations that can improve gut function independent of dietary habits.
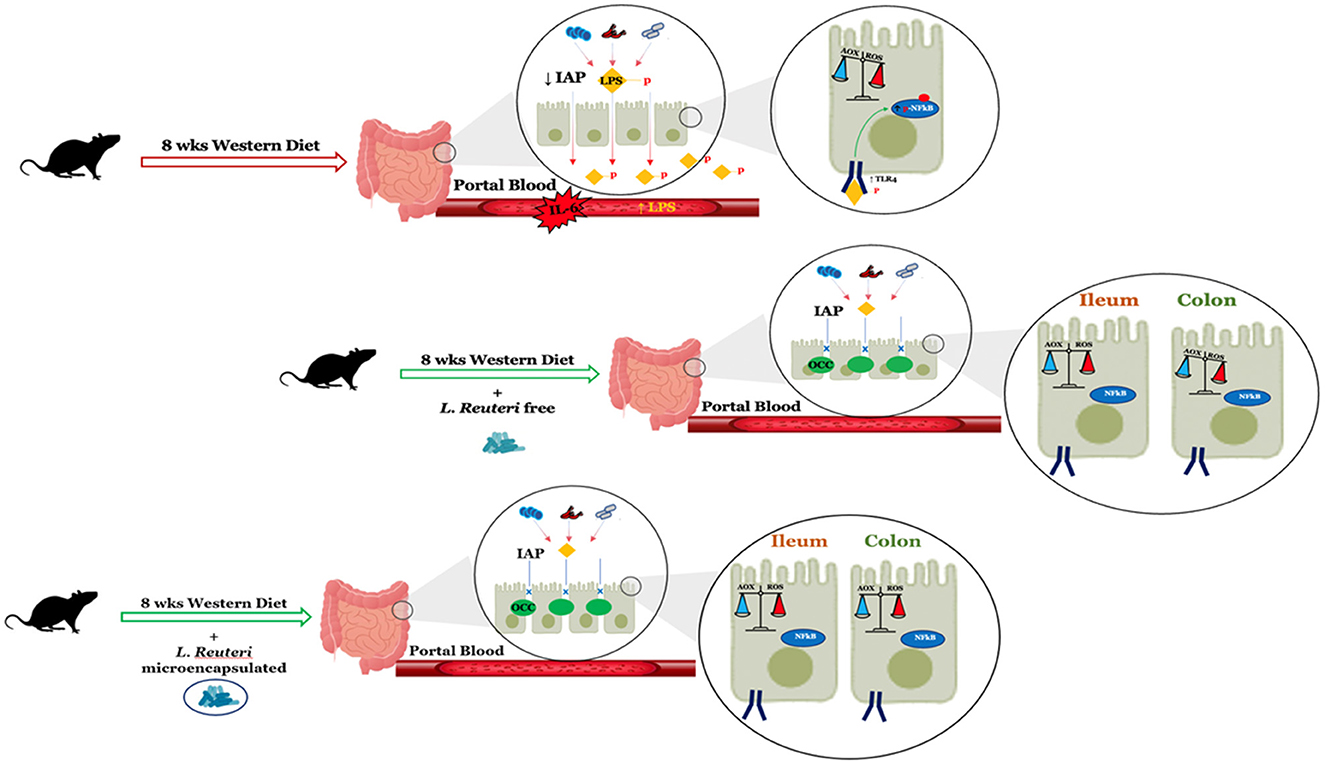
Figure 8. Summary of the changes in intestinal function after 8 weeks of a Western diet with or without L. reuteri free or microencapsulated supplementation in adult rats. LPS, lipopolysaccharide; IAP, intestinal alkaline phosphatase; OCC, occludin.
Data availability statement
The original contributions presented in the study are included in the article/Supplementary material, further inquiries can be directed to the corresponding author.
Author contributions
GM, SI, and AM: conceptualization and supervision. JA, SI, and AM: data curation. SI, AM, and GM: funding acquisition. JA, AD, VB, CG, GS, FD, RC, and MS: investigation. JA, SI, GM, and AM: writing—original draft. JA, AD, VB, CG, GS, FD, RC, MS, LC, GM, SI, and AM: writing—review and editing. All authors contributed to the article and approved the submitted version.
Funding
This study was supported by a grant from the University of Naples Federico II, Ricerca Dip 2021. Project funded under the National Recovery and Resilience Plan (NRRP), Mission 4 Component 2 Investment 1.3—Call for proposals No. 341 of 15 March 2022 of Italian Ministry of University and Research funded by the European Union—NextGenerationEU; Project code PE00000003, Concession Decree No. 1550 of 11 October 2022 adopted by the Italian Ministry of University and Research, CUP D93C22000890001, Project title ON Foods—Research and innovation network on food and nutrition Sustainability, Safety and Security—Working ON Foods.
Acknowledgments
The authors wish to thank Dr. Emilia de Santis for the skillful management of the animal house.
Conflict of interest
The authors declare that the research was conducted in the absence of any commercial or financial relationships that could be construed as a potential conflict of interest.
Publisher's note
All claims expressed in this article are solely those of the authors and do not necessarily represent those of their affiliated organizations, or those of the publisher, the editors and the reviewers. Any product that may be evaluated in this article, or claim that may be made by its manufacturer, is not guaranteed or endorsed by the publisher.
Supplementary material
The Supplementary Material for this article can be found online at: https://www.frontiersin.org/articles/10.3389/fnut.2023.1236417/full#supplementary-material
References
1. Zimmet P, Magliano D, Matsuzawa Y, Alberti G, Shaw J. The metabolic syndrome: a global public health problem and a new definition. J Atheroscler Thromb. (2005) 12:295–300. doi: 10.5551/jat.12.295
2. Andersen CJ, Murphy KE, Fernandez ML. Impact of obesity and metabolic syndrome on immunity. Adv Nutr. (2016) 7:66–75. doi: 10.3945/an.115.010207
3. Rakhra V, Galappaththy SL, Bulchandani S, Cabandugama PK. Obesity and the western diet: how we got here. Mo Med. (2020) 117:536–8.
4. Agus A, Denizot J, Thévenot J, Martinez-Medina M, Massier S, Sauvanet P. Western diet induces a shift in microbiota composition enhancing susceptibility to adherent-invasive E. coli infection and intestinal inflammation. Sci Rep. (2016) 6:19032. doi: 10.1038/srep19032
5. Las Heras V, Melgar S, MacSharry J, Gahan CGM. The influence of the western diet on microbiota and gastrointestinal immunity. Annu Rev Food Sci Technol. (2022) 13:489–512. doi: 10.1146/annurev-food-052720-011032
6. Liu TC, Kern JT, Jain U, Sonnek NM, Xiong S, Simpson KF, et al. Western diet induces Paneth cell defects through microbiome alterations and farnesoid X receptor and type I interferon activation. Cell Host Microbe. (2021) 29:988–1001. doi: 10.1016/j.chom.2021.04.004
7. Hachuła M, Kosowski M, Zielańska K, Basiak M, Okopień B. The impact of various methods of obesity treatment on the quality of life and mental health-a narrative review. Int J Environ Res Public Health. (2023) 20:2122. doi: 10.3390/ijerph20032122
8. Hill C, Guarner F, Reid G, Glenn GR, Mereinster DJ, Pot B. The international scientific association for probiotics and prebiotics consensus statement on the scope and appropriate use of the term probiotic. Nat Rev Gastroenterol Hepatol. (2014) 11:506–14. doi: 10.1038/nrgastro.2014.66
9. Fan Y, Pedersen O. Gut microbiota in human metabolic health and disease. Nat Rev Microbiol. (2021) 19:55–71. doi: 10.1038/s41579-020-0433-9
10. Bubnov RV, Babenko LP, Lazarenko LM, Mokrozub VV, Spivak MY. Specific properties of probiotic strains: relevance and benefits for the host. EPMA J. (2018) 9:205–23. doi: 10.1007/s13167-018-0132-z
11. Salman MK, Mauriello G. Special issue “probiotics and their metabolism”: editorial. Microorganisms. (2023) 11:687. doi: 10.3390/microorganisms11030687
12. Han S, Lu Y, Xie J, Fei Y, Zheng G, Wang Z. Probiotic gastrointestinal transit and colonization after oral administration: a long journey. Front Cell Infect Microbiol. (2021) 11:609722. doi: 10.3389/fcimb.2021.609722
13. Avdeeva LV, Osadchaia AI, Kharkhota MA. Influence of lactitol and lactulose on adhesion properties of Bacillus subtilis probiotic strains. Mikrobiol Z. (2012) 74:22–5.
14. Cook MT, Tzortzis G, Charalampopoulos D, Khutoryanskiy VV. Microencapsulation of probiotics for gastrointestinal delivery. J Control Release. (2012) 162:56–67. doi: 10.1016/j.jconrel.2012.06.003
15. Mandal S, Hati S, Puniya AK, Khamrui K, Singh K. Enhancement of survival of alginate-encapsulated Lactobacillus casei NCDC 298. J Sci Food Agric. (2014) 94:1994–2001. doi: 10.1002/jsfa.6514
16. Ainsley Reid A, Vuillemard JC, Britten M, Arcand Y, Farnworth E, Champagne CP. Microentrapment of probiotic bacteria in a Ca(2+)-induced whey protein gel and effects on their viability in a dynamic gastrointestinal model. J Microencapsul. (2005) 22:603–19. doi: 10.1080/02652040500162840
17. Zheng J, Wittouck S, Salvetti E, Franz CMAP, Harris HMB, Mattarelli P, et al. taxonomic note on the genus Lactobacillus: description of 23 novel genera, emended description of the genus Lactobacillus Beijerinck 1901, and union of Lactobacillaceae and Leuconostocaceae. Int J Syst Evol Microbiol. (2020) 70:2782–858. doi: 10.1099/ijsem.0.004107
18. Abuqwider J, Altamimi M, Mauriello G. Limosilactobacillus reuteri in health and disease. Microorganisms. (2022) 10:522. doi: 10.3390/microorganisms10030522
19. Kubota M, Ito K, Tomimoto K, Kanazaki M, Tsukiyama K, Kubota A. Lactobacillus reuteri DSM 17938 and magnesium oxide in children with functional chronic constipation: a double-blind and randomized clinical trial. Nutrients. (2020) 12:225. doi: 10.3390/nu12010225
20. Kołodziej M, Szajewska H. Lactobacillus reuteri DSM 17938 in the prevention of antibiotic-associated diarrhoea in children: a randomized clinical trial. Clin Microbiol Infect. (2019) 25:699–704. doi: 10.1016/j.cmi.2018.08.017
21. Ojetti V, Ianiro G, Tortora A, D'Angelo G, Di Rienzo TA, Bibbò S. The effect of Lactobacillus reuteri supplementation in adults with chronic functional constipation: a randomized, double-blind, placebo-controlled trial. J Gastrointestin Liver Dis. (2014) 23:387–91. doi: 10.15403/jgld.2014.1121.234.elr
22. Seif El-Din SH, Salem MB, El-Lakkany NM, Hammam OA, Nasr SM, Okasha H. Early intervention with probiotics and metformin alleviates liver injury in NAFLD rats via targeting gut microbiota dysbiosis and p-AKT/mTOR/LC-3II pathways. Hum Exp Toxicol. (2021) 40:1496–509. doi: 10.1177/0960327121999445
23. Mobini R, Tremaroli V, Ståhlman M, Karlsson F, Levin M, Ljungberg M. Metabolic effects of Lactobacillus reuteri DSM 17938 in people with type 2 diabetes: a randomized controlled trial. Diabetes Obes Metab. (2017) 19:579–89. doi: 10.1111/dom.12861
24. De Prisco A, Maresca D, Ongeng D, Mauriello G. Microencapsulation by vibrating technology of the probiotic strain Lactobacillus reuteri DSM 17938 to enhance its survival in foods and in gastrintestinal environment. LWT Food Sci Technol. (2015) 61:452–62. doi: 10.1016/j.lwt.2014.12.011
25. Graff S, Hussain S, Chaumeil JC, Charrueau C. Increased intestinal delivery of viable Saccharomyces boulardii by encapsulation in microspheres. Pharm Res. (2008) 25:1290–6. doi: 10.1007/s11095-007-9528-5
26. Klindworth A, Pruesse E, Schweer T, Peplies J, Quast C, Horn M. Evaluation of general 16S ribosomal RNA gene PCR primers for classical and next-generation sequencing-based diversity studies. Nucleic Acids Res. (2013) 41:e1. doi: 10.1093/nar/gks808
27. Caporaso JG, Kuczynski J, Stombaugh J, Bittinger K, Bushman FD, Costello EK, et al. allows analysis of high-throughput community sequencing data. Nat Methods. (2010) 7:335–6. doi: 10.1038/nmeth.f.303
28. McDonald D, Price MN, Goodrich J, Nawrocki EP, DeSantis TZ, Probst A. An improved Greengenes taxonomy with explicit ranks for ecological and evolutionary analyses of bacteria and archaea. ISME J. (2012) 6:610–8. doi: 10.1038/ismej.2011.139
29. Kaliannan K, Hamarneh SR, Economopoulos KP, Nasrin Alam S, Moaven O, Patel P. Intestinal alkaline phosphatase prevents metabolic syndrome in mice. Proc Natl Acad Sci U S A. (2013) 110:7003–8. doi: 10.1073/pnas.1220180110
30. Liu Y, Cavallaro PM, Kim BM, Liu T, Wang H, Kühn F, et al. role for intestinal alkaline phosphatase in preventing liver fibrosis. Theranostics. (2021) 11:14–26. doi: 10.7150/thno.48468
31. Crescenzo R, Spagnuolo MS, Cancelliere R, Iannotta L, Mazzoli A, Gatto C. Effect of initial aging and high-fat/high-fructose diet on mitochondrial bioenergetics and oxidative status in rat brain. Mol Neurobiol. (2019) 56:7651–63. doi: 10.1007/s12035-019-1617-z
32. Spagnuolo MS, Mollica MP, Maresca B, Cavaliere G, Cefaliello C, Trinchese G. High fat diet and inflammation - modulation of haptoglobin level in rat brain. Front Cell Neurosci. (2015) 9:479. doi: 10.3389/fncel.2015.00479
33. Fernandes MA, Custódio JB, Santos MS, Moreno AJ, Vicente JA. Tetrandrine concentrations not affecting oxidative phosphorylation protect rat liver mitochondria from oxidative stress. Mitochondrion. (2006) 6:176–85. doi: 10.1016/j.mito.2006.06.002
35. Maehly AC, Chance B. The assay of catalases and peroxidases. Meth Biochem Anal. (1954) 1:357–424. doi: 10.1002/9780470110171.ch14
37. Bettaieb A, Vazquez Prieto MA, Rodriguez Lanzi C, Miatello RM, Haj FG. (-)-Epicatechin mitigates high-fructose-associated insulin resistance by modulating redox signaling and endoplasmic reticulum stress. Free Radic Biol Med. (2014) 72:247–56. doi: 10.1016/j.freeradbiomed.2014.04.011
38. Mazzoli A, Gatto C, Crescenzo R, Spagnuolo MS, Nazzaro M, Iossa S. Gut and liver metabolic responses to dietary fructose - are they reversible or persistent after switching to a healthy diet? Food Funct. (2021) 12:7557–68. doi: 10.1039/D1FO00983D
39. Younes M, Aggett P, Aguilar F, Crebelli R, Filipič M, Frutos MJ. Re-evaluation of alginic acid and its sodium, potassium, ammonium and calcium salts (E 400-E 404) as food additives. EFSA J. (2017) 15:e05049. doi: 10.2903/j.efsa.2017.5049
40. Al-Najjar MAA, Athamneh T, AbuTayeh R, Basheti I, Leopold C, Gurikov P. Evaluation of the orally administered calcium alginate aerogel on the changes of gut microbiota and hepatic and renal function of Wistar rats. PLoS ONE. (2021) 16:e0247633. doi: 10.1371/journal.pone.0247633
41. de Vos P, van Hoogmoed CG, van Zanten J, Netter S, Strubbe JH, Busscher HJ. Long-term biocompatibility, chemistry, and function of microencapsulated pancreatic islets. Biomaterials. (2003) 24:305–12. doi: 10.1016/S0142-9612(02)00319-8
42. Camilleri M, Vella A. What to do about the leaky gut. Gut. (2022) 71:424–35. doi: 10.1136/gutjnl-2021-325428
43. Malesza IJ, Malesza M, Walkowiak J, Mussin N, Walkowiak D, Aringazina R. High-fat, western-style diet, systemic inflammation, and gut microbiota: a narrative review. Cells. (2021) 10:3164. doi: 10.3390/cells10113164
44. Prasoodanan PKV, Sharma AK, Mahajan S, Dhakan DB, Maji A, Scaria J. Western and non-western gut microbiomes reveal new roles of Prevotella in carbohydrate metabolism and mouth-gut axis. NPJ Biofilms Microbiomes. (2021) 7:77. doi: 10.1038/s41522-021-00248-x
45. Tan R, Dong H, Chen Z, Jin M, Yin J, Li H. Intestinal microbiota mediates high-fructose and high-fat diets to induce chronic intestinal inflammation. Front Cell Infect Microbiol. (2021) 11:654074. doi: 10.3389/fcimb.2021.654074
46. Simon MC, Strassburger K, Nowotny B, Kolb H, Nowotny P, Burkart V. Intake of Lactobacillus reuteri improves incretin and insulin secretion in glucose-tolerant humans: a proof of concept. Diabetes Care. (2015) 38:1827–34. doi: 10.2337/dc14-2690
47. CíŽková, D., Dureje, L., Piálek, J. Experimental validation of small mammal gut microbiota sampling from faeces and from the caecum after death. Heredity. (2021) 127:141–50. doi: 10.1038/s41437-021-00445-6
48. Ghosh S, Whitley CS, Haribabu B, Jala VR. Regulation of intestinal barrier function by microbial metabolites. Cell Mol Gastroenterol Hepatol. (2021) 11:1463–82. doi: 10.1016/j.jcmgh.2021.02.007
49. Moreira AP, Texeira TF, Ferreira AB, Peluzio MC, Alfenas Rde C. Influence of a high-fat diet on gut microbiota, intestinal permeability and metabolic endotoxaemia. Br J Nutr. (2012) 108:801–9. doi: 10.1017/S0007114512001213
50. Murakami Y, Tanabe S, Suzuki T. High-fat diet-induced intestinal hyperpermeability is associated with increased bile acids in the large intestine of mice. J Food Sci. (2016) 81:H216–22. doi: 10.1111/1750-3841.13166
51. Assimakopoulos SF, Triantos C, Maroulis I, Gogos C. The role of the gut barrier function in health and disease. Gastroenterology Res. (2018) 11:261–3. doi: 10.14740/gr1053w
52. Ngkelo A, Meja K, Yeadon M, Adcock I, Kirkham PA. LPS induced inflammatory responses in human peripheral blood mononuclear cells is mediated through NOX4 and Giα dependent PI-3kinase signalling. J Inflamm. (2012) 9:1. doi: 10.1186/1476-9255-9-1
53. Cani PD, Amar J, Iglesias MA, Poggi M, Knauf C, Bastelica D. Metabolic endotoxemia initiates obesity and insulin resistance. Diabetes. (2007) 56:1761–72. doi: 10.2337/db06-1491
54. Wu J, He C, Bu J, Luo Y, Yang S, Ye C. Betaine attenuates LPS-induced downregulation of Occludin and Claudin-1 and restores intestinal barrier function. BMC Vet Res. (2020) 16:75. doi: 10.1186/s12917-020-02298-3
55. Cui Y, Liu L, Dou X, Wang C, Zhang W, Gao K. Lactobacillus reuteri ZJ617 maintains intestinal integrity via regulating tight junction, autophagy and apoptosis in mice challenged with lipopolysaccharide. Oncotarget. (2017) 8:77489–99. doi: 10.18632/oncotarget.20536
56. Karimi S, Jonsson H, Lundh T, Roos S. Lactobacillus reuteri strains protect epithelial barrier integrity of IPEC-J2 monolayers from the detrimental effect of enterotoxigenic Escherichia coli. Physiol Rep. (2018) 6:e13514. doi: 10.14814/phy2.13514
57. Schepper JD, Collins FL, Rios-Arce ND, Raehtz S, Schaefer L, Gardinier JD. Probiotic Lactobacillus reuteri prevents postantibiotic bone loss by reducing intestinal dysbiosis and preventing barrier disruption. J Bone Miner Res. (2019) 34:681–98. doi: 10.1002/jbmr.3635
58. Pendyala S, Walker JM, Holt PR, A. high-fat diet is associated with endotoxemia that originates from the gut. Gastroenterology. (2012) 142:1100–1. doi: 10.1053/j.gastro.2012.01.034
59. Bilski J, Mazur-Bialy A, Wojcik D, Zahradnik-Bilska J, Brzozowski B, Magierowski M. The role of intestinal alkaline phosphatase in inflammatory disorders of gastrointestinal tract. Mediators Inflamm. (2017) 2017:9074601. doi: 10.1155/2017/9074601
60. Soares JB, Pimentel-Nunes P, Roncon-Albuquerque R, Leite-Moreira A. The role of lipopolysaccharide/toll-like receptor 4 signaling in chronic liver diseases. Hepatol Int. (2010) 4:659–72. doi: 10.1007/s12072-010-9219-x
61. Liu W, Hu D, Huo H, Zhang W, Adiliaghdam F, Morrison S. Intestinal alkaline phosphatase regulates tight junction protein levels. J Am Coll Surg. (2016) 222:1009–17. doi: 10.1016/j.jamcollsurg.2015.12.006
62. Tuin A, Poelstra K, de Jager-Krikken A, Bok L, Raaben W, Velders MP. Role of alkaline phosphatase in colitis in man and rats. Gut. (2009) 58:379–87. doi: 10.1136/gut.2007.128868
63. Hamarneh SR, Kim BM, Kaliannan K, Morrison SA, Tantillo TJ, Tao Q. Intestinal alkaline phosphatase attenuates alcohol-induced hepatosteatosis in mice. Dig Dis Sci. (2017) 62:2021–34. doi: 10.1007/s10620-017-4576-0
64. Park BS, Lee JO. Recognition of lipopolysaccharide pattern by TLR4 complexes. Exp Mol Med. (2013) 45:e66. doi: 10.1038/emm.2013.97
65. Qi X, Qin L, Du R, Chen Y, Lei M, Deng M. Lipopolysaccharide upregulated intestinal epithelial cell expression of Fn14 and activation of Fn14 signaling amplify intestinal TLR4-mediated inflammation. Front Cell Infect Microbiol. (2017) 7:315. doi: 10.3389/fcimb.2017.00315
66. Yu C, Wang D, Yang Z, Wang T. Pharmacological effects of polyphenol phytochemicals on the intestinal inflammation via targeting TLR4/NF-κB signaling pathway. Int J Mol Sci. (2022) 23:6939. doi: 10.3390/ijms23136939
67. Shames BD, Selzman CH, Pulido EJ, Meng X, Meldrum DR, McIntyre J. LPS-Induced NF-kappaB activation and TNF-alpha release in human monocytes are protein tyrosine kinase dependent and protein kinase C independent. J Surg Res. (1999) 83:69–74. doi: 10.1006/jsre.1998.5564
68. Medzhitov R, Horng T. Transcriptional control of the inflammatory response. Nat Rev Immunol. (2009) 9:692–703. doi: 10.1038/nri2634
69. Starkie R, Ostrowski SR, Jauffred S, Febbraio M, Pedersen BK. Exercise and IL-6 infusion inhibit endotoxin-induced TNF-alpha production in humans. FASEB J. (2003) 17:884–6. doi: 10.1096/fj.02-0670fje
70. Papadaki G, Goutakoli P, Tiniakou I, Grün JR, Grützkau A, Pavlopoulos GA. IL-6 signaling attenuates TNF-α production by plasmacytoid dendritic cells in rheumatoid arthritis. J Immunol. (2022) 209:1906–17. doi: 10.4049/jimmunol.2100882
71. Bedoui S, Velkoska E, Bozinovski S, Jones JE, Anderson GP, Morris MJ. Unaltered TNF-alpha production by macrophages and monocytes in diet-induced obesity in the rat. J Inflamm. (2005) 2:2. doi: 10.1186/1476-9255-2-2
72. Semeraro MD, Almer G, Kaiser M, Zelzer S, Meinitzer A, Scharnagl H. The effects of long-term moderate exercise and Western-type diet on oxidative/nitrosative stress, serum lipids and cytokines in female Sprague Dawley rats. Eur J Nutr. (2022) 61:255–68. doi: 10.1007/s00394-021-02639-4
73. Guo Y, Wang B, Wang T, Gao L, Yang ZJ, Wang FF. Biological characteristics of IL-6 and related intestinal diseases. Int J Biol Sci. (2021) 17:204–19. doi: 10.7150/ijbs.51362
74. Wang F, Graham WV, Wang Y, Witkowski ED, Schwarz BT, Turner JR. Interferon-gamma and tumor necrosis factor-alpha synergize to induce intestinal epithelial barrier dysfunction by upregulating myosin light chain kinase expression. Am J Pathol. (2005) 166:409–19. doi: 10.1016/S0002-9440(10)62264-X
75. Kim YJ, Kim EH, Hahm KB. Oxidative stress in inflammation-based gastrointestinal tract diseases: challenges and opportunities. J Gastroenterol Hepatol. (2012) 27:1004–10. doi: 10.1111/j.1440-1746.2012.07108.x
76. Gyuraszova M, Kovalcikova A, Gardlik R. Association between oxidative status and the composition of intestinal microbiota along the gastrointestinal tract. Med Hypotheses. (2017) 103:81–5. doi: 10.1016/j.mehy.2017.04.011
77. Yao MF, Li B, Ye HW, Huang WH, Luo QX, Xiao H. Enhanced viability of probiotics (Pediococcus pentosaceus Li05) by encapsulation in microgels doped with inorganic nanoparticles. Food Hydrocoll. (2018) 83:246–52. doi: 10.1016/j.foodhyd.2018.05.024
78. Yao M, Xie J, Du H, McClements DJ, Xiao H, Li L. Progress in microencapsulation of probiotics: a review. Compr Rev Food Sci Food Saf. (2020) 19:857–74. doi: 10.1111/1541-4337.12532
79. Li C, Gao M, Zheng G, Ma X, Liu X, Yu W. Enhanced quorum sensing capacity via regulating microenvironment to facilitate stress resistance of probiotic in alginate-based microcapsules. Int J Biol Macromol. (2023) 225:605–14. doi: 10.1016/j.ijbiomac.2022.11.119
Keywords: gut, microbiota, microencapsulation, inflammation, oxidative stress
Citation: Abuqwider J, Di Porzio A, Barrella V, Gatto C, Sequino G, De Filippis F, Crescenzo R, Spagnuolo MS, Cigliano L, Mauriello G, Iossa S and Mazzoli A (2023) Limosilactobacillus reuteri DSM 17938 reverses gut metabolic dysfunction induced by Western diet in adult rats. Front. Nutr. 10:1236417. doi: 10.3389/fnut.2023.1236417
Received: 07 June 2023; Accepted: 18 September 2023;
Published: 16 October 2023.
Edited by:
Nabil Bosco, Lesaffre Group, FranceCopyright © 2023 Abuqwider, Di Porzio, Barrella, Gatto, Sequino, De Filippis, Crescenzo, Spagnuolo, Cigliano, Mauriello, Iossa and Mazzoli. This is an open-access article distributed under the terms of the Creative Commons Attribution License (CC BY). The use, distribution or reproduction in other forums is permitted, provided the original author(s) and the copyright owner(s) are credited and that the original publication in this journal is cited, in accordance with accepted academic practice. No use, distribution or reproduction is permitted which does not comply with these terms.
*Correspondence: Arianna Mazzoli, YXJpYW5uYS5tYXp6b2xpQHVuaW5hLml0