- 1School of Public Health, Lanzhou University, Lanzhou, China
- 2Institute of Food and Nutrition Development, Ministry of Agriculture and Rural Affairs, Beijing, China
The gut microbiome is a complex biological community that deeply affects various aspects of human health, including dietary intake, disease progression, drug metabolism, and immune system regulation. Edible mushroom polysaccharides (EMPs) are bioactive fibers derived from mushrooms that possess a range of beneficial properties, including anti-tumor, antioxidant, antiviral, hypoglycemic, and immunomodulatory effects. Studies have demonstrated that EMPs are resistant to human digestive enzymes and serve as a crucial source of energy for the gut microbiome, promoting the growth of beneficial bacteria. EMPs also positively impact human health by modulating the composition of the gut microbiome. This review discusses the extraction and purification processes of EMPs, their potential to improve health conditions by regulating the composition of the gut microbiome, and their application prospects. Furthermore, this paper provides valuable guidance and recommendations for future studies on EMPs consumption in disease management.
1. Introduction
The gut microenvironment consists of physicochemical conditions and the gut microbiome, which are interdependent and both are critical to body function. Its essential role in supporting overall health stems from its involvement in metabolic regulation, signaling pathways, synthesis of crucial nutrients, and maturation of immune cells, among other functions (1, 2). However, dysbiosis of the gut microbiota can result from external factors such as alterations in the environment, diet, or the use of antibiotics and other medications. This condition, also referred to as intestinal ecological dysbiosis or gut dysbiosis, disrupts the normal balance of microorganisms in the gut, leading to a loss of immune balance and the development of diseases. The three main types of gut microbiota dysbiosis are disproportionate, translocation, and autoinfection. Typically, the gut microbiota and the host are interdependent and mutually regulated, establishing a dynamic equilibrium. However, any changes to the internal or external environment can disrupt this balance, leading to dysbiosis of the gut flora, as depicted in Figure 1. Multiple studies have demonstrated that disruptions in the intestinal ecosystem are linked to various gastrointestinal and extraintestinal metabolic disorders, including but not limited to obesity, type 2 diabetes, cardiovascular disease, and cancer (3–6). Among external factors, diet plays a significant role in inducing short-term shifts in the gut microbiome. Both digestible and non-digestible carbohydrates, proteins, lipids, polyphenols, probiotics, and prebiotics can affect gut ecology. Therefore, it is evident that diet can exert control over the gut microbiota to improve overall health (7, 8).
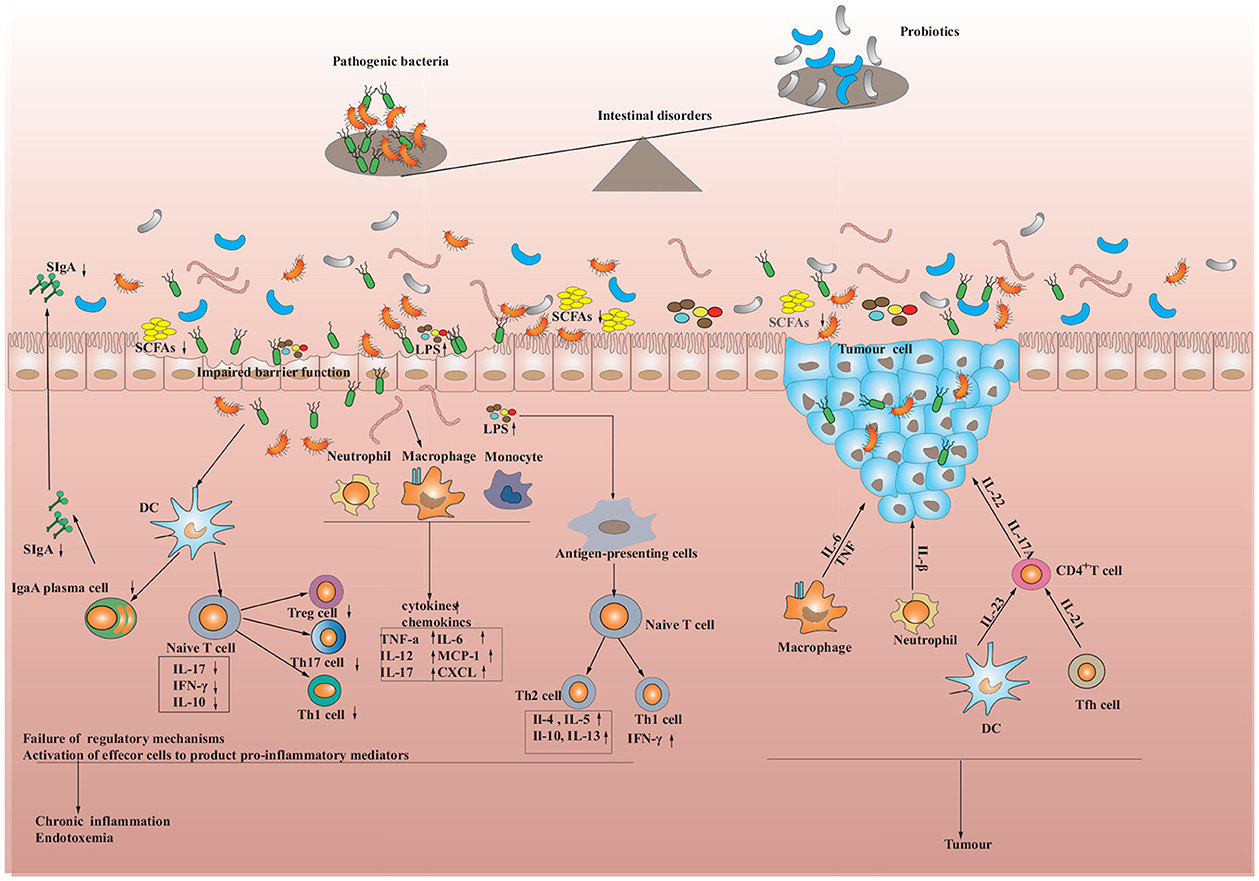
Figure 1. Intestinal dysbiosis and the immune response in the intestine. When pathogens are encountered, dendritic cells capture the pathogens' antigens through epithelial cells, which are then processed. The processed antigens are presented to T cells and trigger their activation. Activated T cells stimulate the secretion of cytokines by epithelial cells, which in turn increases the production of antimicrobial peptides. Meanwhile, T cells and dendritic cells can also activate B cells, transforming them into plasma cells that produce Immunoglobulin A (IgA), which as a consequence provides further resistance against pathogen invasion. However, if the immune cells continue to activate or the pathogens persist in stimulating the immune system, the functioning of regulatory cells may be suppressed, leading to chronic inflammation and the development of metabolic diseases, inflammatory bowel diseases (IBD), and tumors.
Mushrooms, which are macroscopic fruiting bodies produced by basidiomycete and ascomycete fungi, have gained a diverse range of reputations throughout history. Ancient Egyptians regarded them as “sons of the gods”, while ancient Chinese believed they were the “elixir of life” (9, 10). Currently, various species of mushrooms are commonly consumed, including for example Grifola frondosa, Hericium erinaceus, Flammulina velutipes, Auricularia auricula, Lentinula edodes, and Ganoderma lucidum. Around the world at least 2,786 mushroom species are consumed in 99 countries (11). Mushrooms are a rich source of essential nutrients such as proteins, carbohydrates, fats, dietary fiber, and vitamins (12). In addition to their nutritional value, various bioactive compounds can be found in mushrooms, including β-glucans, polysaccharide-protein complexes, lectins, polysaccharide-peptides, terpenoids, sterols, alkaloids, and phenolic compounds (13–15). Polysaccharides, in particular, have garnered significant attention due to their notable physiological effects, including immunomodulatory, antioxidant, antitumor, antiviral, anti-carcinogenic, and anti-inflammatory activities (16, 17). Currently, the interaction between edible mushroom polysaccharides (EMPs) and the intestinal microbiota, as well as the development of related dietary interventions, are heavily researched topics in the international arena. As such, this review offers a thorough exploration of the extraction and purification of EMPs, their potential for regulating intestinal microbiota and promoting health, and their promising prospects for applications.
2. EMPs: extraction, purification, and characterization
EMPs, which are polar macromolecular compounds, can be obtained through a process involving isolation, extraction, and purification. Initially, the dried mushroom is ground, and terpenes, phenols, and lipids are removed using alcohol or organic solvents. Crude EMPs are then extracted with hot water, and ethanol precipitation, deproteinization, dialysis, and fractionation are used for purification (Figure 2). However, traditional processes are hindered by high costs, environmental pollution, potential degradation and condensation of active ingredients, and time consumption. To overcome these limitations, advanced techniques such as microwave-assisted extraction, enzymatic-assisted extraction, ultrasound-assisted extraction, high pressure-assisted extraction, pulsed electric-assisted extraction, and pressurized hot water extraction have been developed (2). These techniques differ in several aspects (Tables 1, 2).
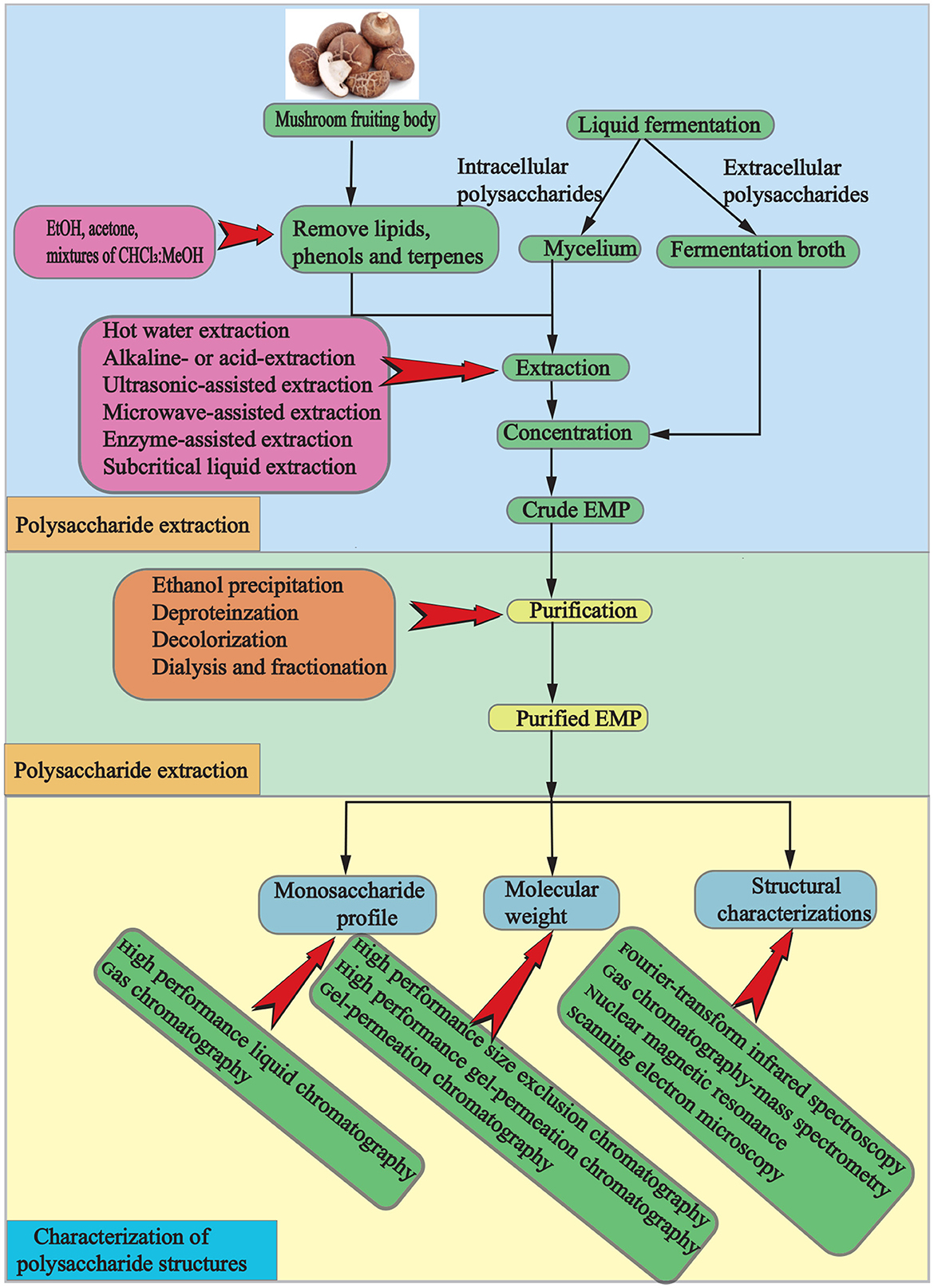
Figure 2. A schematic representation of the process for extracting, purifying, and characterizing polysaccharides from mushrooms.
The extraction method and purification parameters can significantly influence the interpretation of biological activity of polysaccharides (18). Hence, it is crucial to carefully consider these factors, such as volume, solvent type, pH, time, and temperature, when extracting polysaccharides to preserve their biological activity and achieve the desired extraction yield. Additionally, combining different extraction procedures may also help to improve recovery and maintain the bioactivity of EMPs. Kinetic models and mathematical tools can also be utilized to forecast the yield of EMPs under specific conditions (32). For example, In a study on Agrocybe aegerita polysaccharides, the response surface methodology (RSM) was used to optimize the accelerated solvent extraction parameters, and the results showed that the optimal conditions were water as extraction solvent, extraction temperature of 71°C, extraction time of 6.5 min, number of cycles of 3, extraction pressure of 10 MPa, and extraction yield of 19.77% (33).
Typically, raw EMPs often contain various “contaminants,” including proteins, inorganic salts, lignin, amino acids, and other substances (34). Therefore, a purification process is necessary to obtain pure EMPs. One common approach for removing proteins from raw EMPs is to use the Sevag method, trichloroacetic acid method, or enzymatic hydrolysis method (35). However, the Sevag method can be time-consuming and complicated, so a combination of enzymatic hydrolysis and Sevag methods is often used to minimize the loss of polysaccharides. After protein removal, activated carbon, macroporous resin, or hydrogen peroxide can be used to decolorize the raw polysaccharide extracts. However, as the extracts are rich in negative ions such as ketones, phenols, and quinones, decolorization using the activated carbon method may not be effective. In contrast, the hydrogen peroxide method is effective in decolorizing negative ions (36). Recently, adsorption resins or ion exchange resins have become popular for decolorization due to their stable characteristic group structure and high decolorization rate (34). To analyze the molecular weight, monosaccharide composition, and glycosidic bond configuration of polysaccharides, further separation and purification of raw EMPs through ion exchange chromatography and gel chromatography is necessary to obtain homogeneous polysaccharides based on the purification process described above (37).
EMPs are categorized into primary and advanced structures (34, 38, 39). The primary structure focuses on the components of the main chain and branching chains, whereas the advanced structure encompasses the conformation between the main chains and non-covalent bonding between EMPs. The chemical characterization of polysaccharides is critical, as their activity is closely tied to their structure. Currently, structural characterization of EMPs mainly involves analysis of their primary structure, such as total sugar content, molecular weight size and distribution, monosaccharide composition and molar ratio, and glycosidic bond type (40–43). Methods for determining EMPs content include the anthrone-sulfuric acid method, phenol-sulfuric acid method, colorimetric method, high-performance liquid chromatography (HPLC) method, enzymatic method, DNS reduction method, and ion exchange chromatography (44). Research has indicated that immune cells display distinct affinities toward high molecular weights, resulting in varied therapeutic outcomes (45). Hence, exploring the size of molecular weight in polysaccharides derived from mushrooms is imperative. High-performance liquid chromatography (HPLC), high-performance gel filtration chromatography (HPGFC), high-performance gel chromatography (HPGPC), polyacrylamide gel electrophoresis, and ultrafiltration retention method are used to determine molecular weight (18, 46, 47), while gas chromatography (GC), high-performance liquid chromatography (HPLC), and capillary electrophoresis are applied to identify monosaccharide composition (48). The advanced structure encompasses supramolecular structure, solution conformation, reticular structure, and is analyzed through X-ray diffraction, fluorescence correlation spectroscopy, molecular modeling, and atomic force microscopy (49, 50). The structure of hydrolyzed and derivatized EMPs can be characterized through high-performance liquid chromatography (HPLC), infrared spectroscopy (IR), gas chromatography-mass spectrometry (GC-MS), 1 hydrogen-nuclear magnetic resonance (1H-NMR), and atomic force microscopy (AFM) (18, 51). EMPs sugar residue amount and composition, glycosidic bond type, anomeric carbon configuration, attachment sites, and sequence can be expressed through 1D and 2D nuclear magnetic resonance (NMR) spectroscopy (52, 53). At this point, there are limited studies on the structural characterization of EMPs. However, the determination of polysaccharide structure remains an ongoing area of exploration. The methods currently available are not fully developed and have several drawbacks, such as the high molecular weight of polysaccharides, difficulties in UV absorption, and limited control over qualitative and quantitative analysis after purification. These limitations hinder the advancement and utilization of EMPs to a certain extent.
3. Regulatory effects of EMPs on intestinal bacteria
3.1. Enzymes from intestinal bacteria hydrolyze EMPs
The degradation of EMPs in the colon is facilitated by a group of enzymes known as carbohydrate-active enzymes (CAZymes) (54). While the human genome encodes only 8–17 GH enzymes with limited capability to digest complex carbohydrates, more than 10,000 CAZymes that can break down complex carbohydrates have been identified in 177 reference bacterial genomes (55). This indicates that the gut microbiota is responsible for the degradation of various polysaccharides, including β-glucans, into monosaccharides or oligosaccharides, which can modulate the intestinal microbiota and promote the growth of probiotics (56, 57). Numerous studies have shown that most EMPs are fermented by the intestinal microbiota, resulting in the generation of short-chain fatty acids (SCFAs) and alteration of the microbiota composition (Table 3). For instance, after simulated digestion, the Pleurotus eryngii polysaccharide (PEP) remained structurally intact with maintained molecular weight and was effectively utilized and broken down by the intestinal microbiota through fermentation, leading to the production of various SCFAs (62). Conversely, the molecular weight of Oudemansiella radicata polysaccharides (ORP) decreased during simulated digestion, but its overall structure remained intact. Additionally, no free monosaccharides were detected, implying that ORP is indigestible (64).
3.2. EMPs impact the composition of the gut microbiota community
EMPs, which are difficult to digest and absorb, can function as a carbon source for the intestinal microbiota. This, in turn, can enhance human intestinal health and preserve physiological activity by promoting diversity and regulating the composition of gut microbiota. Research has demonstrated that the protective effects of EMPs on intestinal microbiota regulation are diverse. In terms of gut microbiota composition, The intake of EMPs can stimulate the growth of beneficial bacteria while suppressing the harmful ones, which contributes to a more balanced microbiota composition (68). In terms of gut microbiota function, EMPs can enhance CAZymes activity, increase SCFAs production, reduce proinflammatory factor expression, and boost tight junction protein expression, thereby promoting the overall intestinal health of the organism (69). In vitro fermentation studies have shown that Pleurotus eryngii polysaccharide (PEP) altered gut microbiota composition by boosting Firmicutes and reducing Proteobacteria and Bacteroidetes (62). Tremella fuciformi polysaccharide was found to be significantly consumed by the colonic microbiota in human feces, stimulating the growth of Phascolarctobacterium, Bacteroides, and Lachnoclostridium (61). Meanwhile, Cyclocybe cylindracea, Pleurotus eryngii, and Pleurotus ostreatus polysaccharides positively impacted gut microbiota composition, increasing populations of F. prausnitzii and Bifidobacteriumspp (70). Flammulina velutipes polysaccharides elevated the Bacteroidetes to Firmicutes ratio and fostered the growth of beneficial gut microbiota, particularly Bifidobacterium and Bacteroides (71). Although in vitro studies are confined to specific types of gut microbiota bacteria, it is noteworthy that EMPs have a considerable influence on the diversity, richness, and overall composition of the gut microbiota. Additionally, EMPs from various sources have different impacts on gut microbiota modulation, which could be due to structural variations in the EMPs (72, 73).
Besides, research from both animal models and clinical trials has shown that the consumption of EMPs can modify the composition of the gut microbiota. Animal studies have demonstrated that Agrocybe cylindracea Polysaccharides can favorably modify the gut microbiota and related metabolites, reducing levels of Desulfovibrio and increasing abundance of Parabacteroides to prevent diet-induced obesity in mice (74). Tremella fuciformis polysaccharide (TPs) can substantially increase the diversity of the gut microbiota and restore the relative abundance of certain bacterial species, such as Odoribacter, Lactobacillus, Marinifilaceae, and Ruminococcaceae. This finding suggests that TPs may exert a protective effect on dextran sulfate sodium (DSS)-induced colitis in mice (75). Furthermore, in a clinical trial, a β-D-glucan-enriched extract derived from Lentinula edodes was found to raise the relative abundance of Ruminococcaceae UCG-014, Erysipelotrichaceae UCG-003, Akkermansia, and Subdoligranulum, thereby influencing lipid metabolism (76).
In summary, the supplementation of EMPs can alter the composition of the gut microbiota, enhance diversity and richness, and raise the abundance of various species, all of which have important health implications. Additionally, EMPs can restrict the growth of harmful microorganisms. There are several reasons behind these effects. Firstly, gut microbiota ferment EMPs into short-chain fatty acids (SCFAs) as end-products, which lower the intestinal pH and provide a more favorable environment for the growth of beneficial bacteria (66, 76). For instance, Firmicutes are more tolerant to weakly acidic environments than Bacteroidetes (77). Microorganisms specializing in polysaccharide degradation become dominant by breaking down polysaccharides (78). Moreover, some microorganisms can use the metabolites produced during the degradation of EMPs by other microorganisms as a carbon and energy source to promote their growth (79). For example, multi-species symbiotic cross-feeding can occur between Enterobacteriaceae and Bacteroidales spp., leading to synergistic development of mixed communities in vitro (80). Ruminococcus bromii can produce fermentation products by fermenting resistant starch to support the growth of other symbiotic bacteria (81). Overall, while it is evident that EMPs play a role in regulating gut microecology, the precise mechanisms by which they affect microbial growth and the pathways involved are still unclear and require further investigation. In addition, mushrooms may be a source of toxic heavy metals that may have adverse health effects (82). For example, the report on heavy metal concentrations in wild edible mushrooms in Yunnan Province (China) showed that heavy metal contamination in wild edible mushrooms is a serious problem (83). More studies are recommended to elucidate possible procedures for the accumulation of toxic heavy metals in edible mushrooms.
3.3. EMPs influence SCFAs production
The gut microbiota can produce energy for its growth through the fermentation of EMPs. This process generates a mixture of gases, including CO2, methane, and hydrogen, as well as short-chain fatty acids (SCFAs) such as acetic, propionate, and butyrate. Studies have demonstrated that SCFAs can reach other tissues and organs through the circulatory system, contributing to various health-promoting effects. These molecules modulate the intestinal immune system to maintain homeostasis and regulate the production of hormones such as Glucagon Peptide 1 (GLP-1) and insulin, through their interactions with L-endocrine cells and small intestine endothelial cells (84). SCFAs also activate G-protein-coupled receptor (GPCR) signaling pathways, inducing the transcription of factors such as hypoxia-inducible factor 1 (HIF-1), signal transducer and activator of transcription 3 (STAT3), and specificity protein 1 (SP1), thereby enhancing epithelial barrier function, boosting the production of antimicrobial peptides, and reducing inflammation (85). Furthermore, they reduce the activity of histone acetyltransferases and histone deacetylases, which control gene expression, and prevent the maturation of monocytes into dendritic cells and macrophages, along with their production of inflammatory cytokines (86). SCFAs have also been shown to act as ligands for the free fatty acid receptor (FFAR), influencing the host's immunological activity (87). Therefore, SCFAs have a broad range of effects on host metabolism, proliferation, and differentiation, serving as signaling molecules.
Studies have shown that SCFAs are the primary byproducts of gut microbiota fermentation of EMPs and play a critical role in maintaining overall health. For instance, polysaccharides derived from Flammulina velutipes have been shown to increase the levels of acetic acid, propionic acid, and butyric acid, which can enhance the immunity of immunocompromised mice (88). The polysaccharides from Ganoderma lucidum have also been observed to increase the concentration of SCFAs in human gastrointestinal simulations, specifically acetic acid, propionic acid, and n-butyrate (89). Furthermore, polysaccharides from Sparassis crispa have been found to regulate blood sugar levels through modulation of SCFAs concentration (90). Therefore, the health benefits of EMPs can be attributed to the type and amount of SCFAs produced in the gut.
3.4. Effect of molecular weight, polysaccharide composition, and structure of EMPs on intestinal microbiota
The structural features of EMPs have significant impacts on their biological activities and their regulatory effects on the gut microbiota (Table 4). Specifically, the molecular weight of EMPs can affect their absorption and metabolism in the gut. Research has shown that EMPs with lower molecular weights are more readily metabolized by gut microbiota, leading to higher production of short-chain fatty acids (SCFAs), such as propionate, butyrate, and acetate, while EMPs with higher molecular weights are less readily metabolized, resulting in lower SCFA production (108–110). For example, low molecular weight polysaccharides (<10 kDa) from Ganoderma lucidum have better fermentation and higher gas production ability, stimulating the growth of intestinal bacteria quickly. On the other hand, high molecular weight polysaccharides (>100 kDa) are more difficult to be fermented by intestinal bacteria and have a longer residence time in the intestines, resulting in a more prolonged effect on the intestinal microbiota (110). Furthermore, the monosaccharide composition of EMPs also plays a crucial role in regulating the gut microbiota. For instance, glucose, xylose, and galactose are among the most common monosaccharides found in EMPs, with glucose being one of the most prevalent. Glucose has been found to promote the growth and metabolism of gut microbiota, thereby maintaining gut health (79). Research has indicated that glucose can stimulate the metabolic activity of gut microbiota, increase the growth of beneficial bacteria, and promote the production of metabolites, thereby enhancing the immune function of the gut and protecting the gut mucosa (111). Although xylose and galactose are present in relatively low amounts in EMPs, their effects on the gut microbiota cannot be ignored. Xylose can promote the growth of probiotics and protect the gut mucosa by increasing the production of beneficial metabolites (112). Galactose, on the other hand, can inhibit the growth of harmful bacteria, reducing the production of harmful metabolites and benefiting gut health (113). For example, A polysaccharide from Pleurotus eryngii, rich in glucose (78.32%), galactose (8.47%), and mannose (9.43%), promoted the relative abundance of Firmicutes and reduced that of Bacteroidetes and Proteobacteria, while increasing the production of acetic and propionic acids (62). A polysaccharide from Hericium erinaceus, composed of fructose, mannose, glucose and galactose, enhanced the abundance of SCFA-producing bacteria (58). In addition, the length and branching patterns of EMPs can also affect their degradation and absorption in the gut (2). Studies have indicated that shorter chain lengths and fewer branching patterns can enhance the absorption and utilization of EMPs in the gut, resulting in better regulatory effects (18). Additionally, the length and branching patterns of EMPs can also influence their metabolites in the gut, which in turn affect the growth and metabolism of gut microbiota (114). Zhang et al. obtained Sparassis crispa polysaccharides with main chain structures of (1 → 6)-α-D-Galp, (1 → 6)-β-D-Glcp and (1 → 3)-β-D-Glcp and side chain structures of (1 → 4)-β-D-Glcp, (1 → 3)-β-D-Glcp, T-α-L-Fucp and T-β-D-Glcp can promote the production of short-chain fatty acids and the abundance of probiotics, such as Dialister and Megasphaera (63, 98). Therefore, in order to fully realize the regulatory effects of EMPs, further research is needed to explore the relationships between their structural features and gut microbiota metabolism, as well as the impacts of different mushroom species and polysaccharide sources on their regulatory effects. This will provide a more robust scientific basis for the application of EMPs.
4. EMPs regulate intestinal ecological disorders and improve host health
Research has demonstrated that EMPs can have substantial positive effects on blood glucose, body weight, insulin resistance, inflammation, endotoxemia, and gut ecological dysregulation, while preserving the integrity of the gut barrier. For intestinal dysbiosis, EMPs promote the proliferation of beneficial bacteria, inhibit the proliferation of pathogenic bacteria, and increase the concentration of SCFAs in the intestine (2). Beneficial bacteria, such as Lactobacillus, Bifidobacteria, Streptococcus, and Bacillus coagulans, establish a symbiotic relationship with the host and constitute the primary bio-antagonistic and bio-barrier microbiota. They perform essential immune, metabolic, and nutritional functions within the host (115). On the other hand, harmful bacteria, including Shigella flexneri, Citrobacter rodentium, Listeria monocytogenes, and Salmonella enterica, are typically non-pathogenic to the host under normal circumstances. However, if their populations exceed a certain threshold, they can instigate infections and result in disease (116). As a result, EMPs can be considered a nutritious and potentially beneficial bioactive food source for promoting overall health, as summarized in Figure 3 and Tables 5, 6.
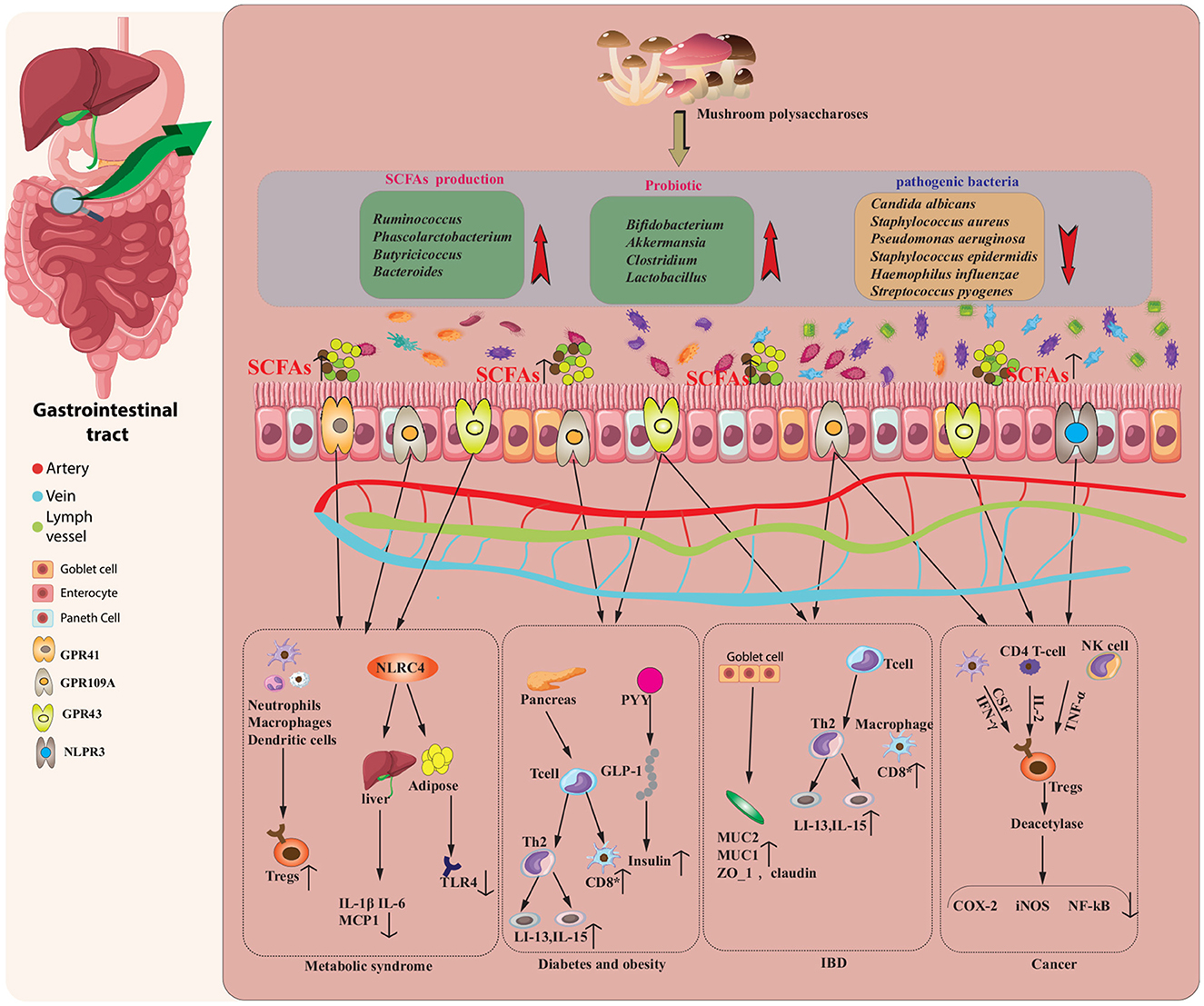
Figure 3. EMPs modulate the composition and function of the intestinal microbiota, as well as its derived short-chain fatty acids (SCFAs), to potentially treat various diseases, including inflammatory metabolic syndrome, diabetes, obesity, bowel disease (IBD), and cancer. EMPs enhance the levels of SCFAs and stimulate the proliferation of probiotics in the gut. These SCFAs modulate immune cells, inhibit pro-inflammatory mediators, and induce anti-inflammatory molecules, ultimately ameliorating metabolic syndrome. The secretion of PYY and GLP-1 and the expansion of regulatory T cells (Tregs) mediated by probiotics contribute to the attenuation of diabetes and obesity, while the adherence of probiotics to the intestinal mucus layer and the induction of anti-inflammatory factors help relieve the symptoms of IBD. Moreover, SCFAs interact with specific receptors to downregulate the expression of pro-inflammatory signaling molecules, resulting in anticancer effects.
4.1. Metabolic disorders
Metabolic diseases encompass a range of conditions affecting the metabolism of the human body. Research on metabolic syndrome populations and animal models has revealed that gut ecological dysbiosis often results in a decrease in the abundance of probiotics, an increase in opportunistic and pathogenic bacteria, and a reduction in microbial diversity (135). Imbalances in the intestinal ecosystem can cause a reduction in the expression of tight junction proteins in the intestine, resulting in an increase of intestinal permeability, impaired intestinal integrity, and the release of bacterial debris and endotoxins from the gut into the bloodstream. This triggers the activation of the NF-κB and NLRP3 signaling pathways in inflammatory vesicles, resulting in elevated levels of pro-inflammatory factors such as IL-1, IL-6, and TNF-α, ultimately leading to chronic low-grade inflammation throughout the body (136). Additionally, activation of the NF-κB signaling pathway increases the levels of protein phosphatase 1B (PTP1B), which dephosphorylates the insulin receptor or its substrate, hindering the insulin receptor's ability to bind with insulin, and causing insulin resistance (137). Dysbiosis in the intestinal tract also impairs the synthesis of vitamins K and B, folic acid, neurotransmitters, SCFAs, and other bioactive components by gut microbes (138). Therefore, imbalances in the gut microbiota are a key contributor to the onset and expression of metabolic disorders.
Studies have indicated that the consumption of EMPs has a positive impact on metabolic disorders. In animal models, such as dietary-induced obese mice, a polysaccharide from Ganoderma lucidum (BSGLP) containing (1 → 3)-β-D-Glcp, (1 → 3,6)-β-D-Glcp, (1 → 6)-β-D-Glcp, and terminal-β-D-Glcp moieties has been demonstrated to restore balance to the gut microbiota and mitigate dysbiosis, leading to a reduction in fat accumulation and a decrease in inflammation (69). Similar results have been observed with Dictyophora indusiata polysaccharides consisting of glucose 59.84%, mannose 23.55% and galactose 12.95% which mitigated hepatic steatosis, hyperlipidemia, hyperglycemia, and LPS-induced inflammation in mice with high-fat diet-induced obesity (DIO) by reducing the abundance of Bacilli, Gammaproteobacteria, and increasing abundance of Bacteroidiaas (139). Supplementation with Cordyceps militaris Polysaccharides containing 1, 3-branched-galactomannoglucan with a linear backbone of (1 → 4)-linked α-D-Glcp in mice on a high-fat diet led to reduced body weight, pro-inflammatory cytokine levels, and fat accumulation, as well as improved glucose tolerance and intestinal barrier function. This was shown to be a result of reversing intestinal dysbiosis, as evidenced by an increased population of Alloprevotella, Butyricimonas, Parabacteroides, and Alistipes (122). Supplementation with Ganoderma lucidum polysaccharides consisting of arabinose, galactose, glucose, xylose, mannose, ribose, and rhamnose with molar percentages of 5.32%, 5.47%, 57.63%, 0.84%, 25.41%, 1.95%, and 3.38%, respectively, and molecular weight of 15900 Da improved insulin secretion and repaired islet cells, stimulated glycogen synthesis and storage in the liver, and enhanced antioxidant enzyme activities and insulin sensitivity in diabetic mice induced by a high-fat diet (HFD) and streptozotocin (STZ) by elevating the abundance of Lactobacillus, Bacteroides, and Ruminococcaceae (130). The polysaccharide from Ganoderma lucidum with a molecular weight of 13.7 kDa, mannose, glucose, galactose, rhamnose, and arabinose in a molar ratio of 3.16:16.17:3.74:1.65:1 effectively reduced fasting blood glucose and insulin levels and improved gut microbiome imbalances by decreasing the levels of Aerococcus, Corynebacterium, Proteus, and Ruminococcus (140). Polysaccharide extracted from Phellinus linteus with the backbone of → 3)-β-d-Glcp-(1 → and → 6)-β-d-Glcp-(1 → has been shown to effectively reduce fasting blood glucose levels in mice. This is achieved by altering liver phospholipid metabolism and improving insulin signaling, as well as increasing the abundance of Porphyromonas (141). In general, EMPs have the potential to regulate gut microbiota by promoting the growth of beneficial bacteria and inhibiting that of harmful bacteria, which could improve gut health and prevent or treat metabolic disorders.
4.2. Inflammatory bowel disease
Inflammatory bowel disease (IBD), encompassing ulcerative colitis (UC) and Crohn's disease (CD), is marked by persistent inflammation in the gastrointestinal tract (142). Symptoms of IBD include diarrhea, bloating, abdominal pain, blood in stools, weight loss, and discomfort. Research suggests that IBD arises from a genetically susceptible individual's misguided inflammatory response to the gut microbiome (143). The most prevalent imbalance in the intestinal flora among IBD patients is a decrease in Bifidobacterium adolescentis, prausnitzii, Dialister invisus, Faecalibacterium and an unnamed member of Clostridium cluster XIVa, and an increase in Ruminococcus gnavus (144, 145). In addition to intestinal ecological dysbiosis, metabolites derived from gut bacteria, such as short-chain fatty acids (SCFAs), bile acids (BAs) and tryptophan metabolites, also play a crucial role in the development and progression of IBD. The latest extensive and comprehensive study found that gut microbiome metabolites impact host immunity, mucosal health, homeostasis, and energy metabolism (146). As such, the composition and function of the intestinal microbiota are crucial factors that impact the progression of IBD.
Medical treatments for IBD mainly aim to control inflammation and prevent the progression of the disease. There are three main types of conventional drugs that are commonly used in IBD treatment, including aminosalicylic acid derivatives, glucocorticoids, and immunosuppressive drugs (147). However, prolonged use of these drugs can lead to reduced immunity, and increase the risk of electrolyte imbalances, peptic ulcers, cataracts, and osteoporosis (148). Recent studies have shown that EMPs can improve intestinal health with minimal adverse effects. EMPs, as a natural immunomodulator, are effective in treating and preventing IBD by regulating gut bacteria and upregulating the expression of tight junction proteins (TJs), such as junctional adhesion molecules, cytosolic scaffold proteins, occludin, and intracellular zonulae occludens like ZO-1. For instance, high doses of polysaccharides from Tremella fuciformis have the potential to improve gut health by increasing the diversity of the gut microbiome, restoring the relative abundance of specific bacteria such as Helicobacter, Odoribacter, Lactobacillus, Marinifilaceae, and Ruminococcaceae, and activating Foxp3+ T cells to produce anti-inflammatory cytokines. This was observed in mice with colitis induced by dextran sulfate sodium, and the treatment showed minimal side effects (75). A polysaccharide from Flammulina velutipes has been shown to alleviate colitis by controlling colonic microbial dysbiosis, increasing short-chain fatty acids, and inhibiting the TLR4-NF-κB signaling pathway (133). The polysaccharide from Dictyophora indusiata consisting of 59.84% glucose, 23.55% mannose, and 12.95% galactose has been shown to improve gut microbiota composition and intestinal barrier function by increasing the expression of mucins and tight junction proteins, suppressing harmful bacteria such as Gammaproteobacteria, Proteobacteria, Bacteroidaceae, Bacteroides, and Enterobacteriaceae, and enhancing beneficial bacteria such as Lactobacillus acidophilus (149). Polysaccharides isolated from sporoderm-removed fragments of Ganoderma lucidum consisting of arabinose (4.19%), mannose (15.69%), glucose (78.15%), and galactose (1.97%) have been shown to mitigate colitis induced by Azoxymethane/Dextran Sodium Sulfate by improving gut dysbiosis, increasing short-chain fatty acid production, and suppressing TLR4/MyD88/NF-κB signaling to alleviate endotoxemia (134). Yu et al. (150) demonstrated that polysaccharides from Porphyra haitanensis improved the integrity of the colonic mucosal barrier by upregulating tight junction proteins, augmenting the mucus layer and its secretion, and regulating the gut microbial community, enriching beneficial bacteria such as Bacteroides, Muribaculum, and Lactobacillus species, thus mitigating DSS-induced colonic injury. In general, EMPs may alleviate IBD by regulating gut microbiota and repairing the damaged intestinal barrier, creating an environment conducive to the growth of probiotics and suppressing potentially pathogenic bacteria, and modulating TLR4 and NF-κB signaling pathways to reduce intestinal inflammation.
4.3. Tumor/Cancer
Microbial commensal dysbiosis, responsible for 20% of tumorigenesis and a greater number of cancers, has been linked to microbial pathogens (151). In a healthy gut microbiome, many probiotics and other resident bacteria secrete molecules to fight tumor growth and prevent tumorigenesis. However, when the intestinal flora is dysbiotic, certain bacterial pathogens can grow and proliferate, secreting toxins such as CagA protein from Helicobacter pylori, adhesin A (FadA) from Fusobacterium nucleatum, and metalloproteinase toxin (MP toxin) from Bacteroides fragilis, which interfere with the development of the host cell and eventually lead to the onset of cancer (152). A recent study has shown that lung cancer patients experience a significant shift in gut microbiota composition compared to healthy individuals, with pathogens increasing and some probiotic bacteria decreasing (153). Additionally, certain bacteria can also interfere with the host's hormone metabolism. Intestinal dysbiosis also increases the relative abundance of Clostridium leptum and Clostridium coccoides, leading to increased secretion of bacterial β-glucuronidase enzymes, which promotes cell proliferation in organs such as the endometrium and breast and may contribute to the development of breast cancer (152, 154). During dysbiosis, several microbiota subpopulations may proliferate, producing high quantities of toxins that cause inflammation and cancer.
According to research in vivo and clinical tests, consuming EMPs may be a viable and efficient method for cancer prevention and treatment (155). EMPs can induce the proliferation of beneficial bacteria in the gut, thus increasing the ability to combat carcinogens and reducing the nourishment of harmful bacteria. This reduces the amount of harmful substances in the gut, reducing cellular damage and preventing intestinal cancer. In addition, EMPs have been shown to enhance the immune system's activity, which can lead to the inhibition of cancer cell growth. For instance, A polysaccharide extracted from the mushroom Pleurotus ostreatus has been demonstrated to decrease tumor cell metastasis and increase survival in mice with H22 malignant ascites. This effect was achieved by downregulating the expression of genes such as Stat3 and Foxp3, as well as releasing immunological factors including TNFα, INFγ, and IL-2 (156). Polysaccharides from Ganoderma lucidum have been found to be more effective in alleviating colorectal cancer symptoms than guar gum, as they increase the prevalence of Akkermansia, colon length, and downregulate rectal cancer-related genes (157). Ganoderma lucidum polysaccharides have demonstrated cancer-preventive and therapeutic functions by dynamically modulating the gut microbiota and host immune responses (158). Studies have also shown that sporoderm-broken fragments of (G. lucidum) polysaccharides (BSGLP) were more effective in reducing gastric cancer cell survival than removed fragments of G. lucidum (RSGLP). RSGLP induced apoptosis in AGS cells by dramatically increasing cleaved-PARP and decreasing Bcl-2 and pro-caspase-3 expression levels, while enhancing the expression of LC3-II and p62, indicating autophagy was induced and the autophagic flow was disrupted in AGS cells (159). A recent study discovered that polysaccharides from the Sporoderm-Breaking Spore of Ganoderma lucidum with a molecular weight of 3659 Da can serve as a natural adjuvant in breast cancer treatment, boosting the number of cytotoxic T cells and helper T cells. The gut microbiota is also affected, with increased levels of Firmicutes and Proteobacteria and reduced levels of Actinobacteria, Bacteroidetes, and Cyanobacteria, leading to improved symptoms (160). Additionally, a polysaccharide derived from the spore of Ganoderma lucidum when combined with Paclitaxel can act as an adjuvant against Pertussis toxin in breast cancer treatment. This restores gut microbiota dysbiosis, increasing levels of Ruminococcus and Bacteroides while reducing the presence of cancer-risk genera such as Odoribacter and Desulfovibrio (161). These studies suggest that EMPs can inhibit tumor proliferation by remodeling the gut microbiota, but the anti-tumor effect of EMPs in regulating intestinal microbiota has been only demonstrated in some EMPs.
4.4. Other beneficial effects
In-depth research has revealed that EMPs offer a range of health benefits, including prevention of cardiovascular disease, liver protection, and improvement of neurological symptoms. For instance, treatment with Auricularia auricula polysaccharides with monosaccharides composed of glucose (72%), mannose (8%), xylose (10%), and fucose (10%) and containing a pyranose ring has been found to enhance cardiac function due to its potent antioxidant properties (162). Studies have demonstrated that polysaccharides from Agrocybe aegerita composed of arabinose, mannose, Galactose, and glucose display potential anti-aging, antioxidant, and organ-protective effects on the liver, brain, and kidney against D-gal-induced aging toxicity, slowing the aging process (163). The polysaccharide of Hypsizygus ulmarius containing galactose (44.24%), glucose (34.27%), mannose (15.61%) along with the small amount of xylose (3.33%), fucose (1.33%), and rhamnose (1.20%) has been shown to have a significant hepatoprotective effect against acute alcoholic liver damage in rats, as it protects the biological system from oxidative stress (26). Ganoderma lucidum polysaccharides with a molecular weight of 15.0 kDa have been found to reduce cognitive impairments in transgenic Alzheimer's disease (AD) mice by boosting neural progenitor cell proliferation (164). The polysaccharide-peptide complex of Cordyceps militaris consisting of mannose, glucose, and galactose with a molar ratio of 2.0:11.4:1 has been shown to regulate the lncRNA-miRNA-mRNA axis, thereby improving atherosclerosis (165). In conclusion, EMPs possess multiple bioactivities and play a positive role in maintaining bodily health.
5. Application prospect of EMPs
Due to their potential health benefits, EMPs can be utilized as additives in various food products, including bread, biscuits, and fruit juice, to enhance their nutritional value and health-promoting properties. For example, incorporating polysaccharides from Lentinula edodes into bread and rice muffins has been shown to improve their texture and nutritional quality (166, 167). Similarly, Helvella leucopus polysaccharides have been added to a compound beverage to enhance its antioxidant activity (168). In addition, the inclusion of 0.1–0.5% polysaccharides from Lentinula edodes, Pleurotus eryngii, and Flammulina velutipes in yogurt has been found to help probiotics survive, maintaining at least 107 CFU/ml at 4°C (169).
EMPs have also been utilized as dietary supplements to boost immunity and alleviate fatigue, with extracts of Ganoderma lucidum polysaccharides being a common ingredient (170). Furthermore, extracts of Lentinus edodes polysaccharides have been utilized in functional foods to aid in regulating blood glucose levels and preventing diabetes (171). Additionally, due to their antioxidant and anti-aging properties, EMPs have been utilized in cosmetics and personal care products (172). For example, Tremella fuciformis polysaccharides can protect skin cells against damage caused by ultraviolet (UV) radiation by decreasing the generation of reactive oxygen species and inhibiting the expression of matrix metalloproteinases. Moreover, this polysaccharides have demonstrated the potential to enhance skin hydration and elasticity, while diminishing the appearance of wrinkles triggered by UV exposure (173).
In addition, EMPs can be blended with other materials to create a composite material with excellent strength and toughness, which can be utilized in the production of food packaging materials. For example, blending mushroom polysaccharides with cellulose nanofibers to create a film can improve the film's tensile strength and elongation at break, while also decreasing its water vapor and oxygen permeability. As a result, the film exhibits superior moisture resistance and oxygen barrier properties (174).
Although EMPs have many potential health benefits and are widely used as a functional food ingredient, dietary supplement, pharmaceutical, and cosmetic products such as Immune Assist 24/7™, active hexose correlated compound (AHCC®), and MaitakeGold 404®, safety considerations should also be taken into account (175–177). The safety of EMPs depends on the source of mushrooms, the extraction method, and the purity of the polysaccharides. Some mushrooms may contain harmful compounds, such as heavy metals and mycotoxins, that can cause health problems if consumed in large amounts. Therefore, it is important to use mushrooms from reliable sources and to use safe extraction methods to obtain high-quality polysaccharides. In addition, the biological activity of EMPs are mainly revealed through in vitro and animal model experiments, and there is a lack of clinical trials to ensure that they do not cause harmful side effects in humans (178, 179). For example, the AHCC® trial in patients resulted in mild gastrointestinal distress, headache, fatigue and foot cramps in 12% of the total patients (180). Therefore, further research is needed to explore the full potential of EMPs and to develop safe and effective applications in food and health products.
6. Conclusions and future perspectives
Through continuous investigation of the effects of EMPs on regulating the gut microbiome, mounting evidence suggests their potential to enhance human wellbeing. This review provides a comprehensive analysis of the methods used for extraction and purification of EMPs and the correlation between gut microbiome and disease. We also explore the interplay between EMPs and the gut microbiome, emphasizing their ability to preserve microbial equilibrium, bolster gut lining integrity, enhance short-chain fatty acid production, and modulate cellular signaling pathways for metabolic homeostasis and immune system reactivity. Despite the demonstrated benefits of EMPs as a natural form of treatment through regulation of the gut microbiome, there is still room for improvement in future studies.
With an emphasis on enhancing the structural analysis of EMPs and their relationship with the intestinal microbiota, there is a growing interest in investigating the impact of EMPs on gut health. The varying molecular mass and length of different EMPs play a crucial role in determining the recognition and digestion process of polysaccharides by gut bacteria. In recent years, there has been a growing emphasis on comprehending the structural-activity relationship of EMPs and their function in modulating the gut microbiota. However, there is still much to be understood regarding the relationship between the molecular weight, monosaccharide content, glycoside binding mechanism, and advanced structure of polysaccharides, and their effect on the gut microecology. Further validation is needed to clarify these relationships.
Conduct Further Clinical Trials for Safe and Effective Dosages of EMPs: Although both in vitro and in vivo animal studies have demonstrated the gut microbiota-modulating effects of EMPs, specific differences between humans and animals call for more clinical trials to be conducted. This will enable the development of tailored therapies that take into account the unique circumstances of individual patients and ensure safe and effective dosages of EMPs.
Conduct further research into the exact role of EMPs using omics technologies, and continue to investigate the specific degradation pathways of gut microbiota for EMPs, to provide a foundational understanding for personalized gut microbiota nutrition.
In summary, EMPs have rich nutritional value and medicinal value and have a wide application prospect in food, medicine, and cosmetics. Basic applied research on the healthcare value of EMPs should be further strengthened, as well as product development and standard setting in the fields of food, healthcare products, cosmetics, etc., to enhance human health or well-being.
Author contributions
QLZ and YJ: conceptualization and writing original draft. QZ, HP, DLi, DLo, and XH: methodology. ZT and YZ: investigation and supervision. YZ: resources. LS, QZ, HP, DLi, DLo, and XH: writing—review and editing. All authors have read and agreed to the published version of the manuscript.
Funding
This research was funded by the Fundamental Research Funds for the Central Universities (Lanzhou, China; project numbers: lzujbky-2021-ct20) and the Young Science and Technology Talents Lifting Project of Gansu Association of Science and Technology (Lanzhou, China; project number: GXH-20200626-05).
Conflict of interest
The authors declare that the research was conducted in the absence of any commercial or financial relationships that could be construed as a potential conflict of interest.
Publisher's note
All claims expressed in this article are solely those of the authors and do not necessarily represent those of their affiliated organizations, or those of the publisher, the editors and the reviewers. Any product that may be evaluated in this article, or claim that may be made by its manufacturer, is not guaranteed or endorsed by the publisher.
References
1. Rajakovich LJ, Balskus EP. Metabolic functions of the human gut microbiota: the role of metalloenzymes. Nat Prod Rep. (2019) 36:593–625. doi: 10.1039/C8NP00074C
2. Yin C, Noratto GD, Fan X, Chen Z, Yao F, Shi D, et al. The impact of mushroom polysaccharides on gut microbiota and its beneficial effects to host: a review. Carbohydr Polym. (2020) 250:116942. doi: 10.1016/j.carbpol.2020.116942
3. Noye Tuplin EW, Alukic E, Lowry DE, Chleilat F, Wang W, Cho NA, et al. Dietary fiber combinations to mitigate the metabolic, microbial, and cognitive imbalances resulting from diet-induced obesity in rats. FASEB J. (2022) 36:e22269. doi: 10.1096/fj.202101750R
4. Hur KY, Lee MS. Gut microbiota and metabolic disorders. Diabetes Metab J. (2015) 39:198–203. doi: 10.4093/dmj.2015.39.3.198
5. Sanz Y, Santacruz A, Gauffin P. Gut microbiota in obesity and metabolic disorders. Proc Nutr Soc. (2010) 69:434–41. doi: 10.1017/S0029665110001813
6. Boulangé CL, Neves AL, Chilloux J, Nicholson JK, Dumas ME. Impact of the gut microbiota on inflammation, obesity, and metabolic disease. Genome Med. (2016) 8:1–12. doi: 10.1186/s13073-016-0303-2
7. Ramos S, Martin MA. Impact of diet on gut microbiota. Curr Opin Food Sci. (2021) 37:83–90. doi: 10.1016/j.cofs.2020.09.006
8. Shen T-C. Diet and gut microbiota in health and disease. Intestinal Microb. (2017) 88:117–26. doi: 10.1159/000455220
10. Chang S, Buswell J. Mushroom nutriceuticals. World J Microbiol Biotechnol. (1996) 12:473–6. doi: 10.1007/BF00419460
11. Li H, Tian Y, Menolli N, Ye L, Karunarathna SC, Perez-Moreno J. Reviewing the world's edible mushroom species: A new evidence-based classification system. Food Sci Food Safety. (2021) 20:1982–2014. doi: 10.1111/1541-4337.12708
12. Maity P, Sen IK, Chakraborty I, Mondal S, Bar H, Bhanja SK, et al. Biologically active polysaccharide from edible mushrooms: a review. Int J Biol Macromol. (2021) 172:408–17. doi: 10.1016/j.ijbiomac.2021.01.081
13. Perez-Moreno J, Martinez-Reyes M. Edible ectomycorrhizal mushrooms: biofactories for sustainable development. Biosyst Eng. (2014) XXI:151–233. doi: 10.1007/978-3-319-03880-3_6
14. Sharma C, Bhardwaj N, Sharma A, Tuli HS, Batra P, Beniwal V, et al. Bioactive metabolites of Ganoderma lucidum: factors, mechanism and broad spectrum therapeutic potential. J Herb Med. (2019) 17:100268. doi: 10.1016/j.hermed.2019.100268
15. Bains A, Chawla P, Kaur S, Najda A, Fogarasi M, Fogarasi S. Bioactives from mushroom: health attributes and food industry applications. Materials. (2021) 14:7640. doi: 10.3390/ma14247640
16. Rathore H, Prasad S, Sharma S. Mushroom nutraceuticals for improved nutrition and better human health: a review. PharmaNutrition. (2017) 5:35–46. doi: 10.1016/j.phanu.2017.02.001
17. Niego AG, Rapior S, Thongklang N, Raspé O, Jaidee W, Lumyong S, et al. Macrofungi as a nutraceutical source: promising bioactive compounds and market value. J Fungi. (2021) 7:397. doi: 10.3390/jof7050397
18. Huang Y, Chen H, Zhang K, Lu Y, Wu Q, Chen J, et al. Extraction, purification, structural characterization, and gut microbiota relationship of polysaccharides: a review. Int J Biol Macromol. (2022) 6:49. doi: 10.1016/j.ijbiomac.2022.06.049
19. Leong YK, Yang FC, Chang JS. Extraction of polysaccharides from edible mushrooms: Emerging technologies and recent advances. Carbohydr Polym. (2021) 251:117006. doi: 10.1016/j.carbpol.2020.117006
20. Sangthong S, Pintathong P, Pongsua P, Jirarat A, Chaiwut P. Polysaccharides from Volvariella volvacea mushroom: extraction, biological activities and cosmetic efficacy. J Fungi. (2022) 8:572. doi: 10.3390/jof8060572
21. Rizkyana AD, Ho TC, Roy VC, Park JS, Kiddane AT, Kim GD, et al. Sulfation and characterization of polysaccharides from Oyster mushroom (Pleurotus ostreatus) extracted using subcritical water. J Supercrit Fluids. (2022) 179:105412. doi: 10.1016/j.supflu.2021.105412
22. Guo XY, Guo HB, Wu SY, Yu XD. Extraction, bioactive composition, and antioxidant activity of polysaccharides from the mushroom Rugiboletus extremiorientalis. Starch-Stärke. (2022) 74:2100248. doi: 10.1002/star.202100248
23. Badshah SL, Riaz A, Muhammad A, Tel Çayan G, Çayan F, Emin Duru M, et al. Isolation, characterization, and medicinal potential of polysaccharides of Morchella esculenta. Molecules. (2021) 26:1459. doi: 10.3390/molecules26051459
24. Zhang X, Cai Z, Mao H, Hu P, Li X. Isolation and structure elucidation of polysaccharides from fruiting bodies of mushroom Coriolus versicolor and evaluation of their immunomodulatory effects. Int J Biol Macromol. (2021) 166:1387–95. doi: 10.1016/j.ijbiomac.2020.11.018
25. Abreu H, Zavadinack M, Smiderle FR, Cipriani TR, Cordeiro LM, Iacomini M. Polysaccharides from Pleurotus eryngii: selective extraction methodologies and their modulatory effects on THP-1 macrophages. Carbohydr Polym. (2021) 252:117177. doi: 10.1016/j.carbpol.2020.117177
26. Govindan S, Jayabal A, Shanmugam J, Ramani P. Antioxidant and hepatoprotective effects of Hypsizygus ulmarius polysaccharide on alcoholic liver injury in rats. Food Sci Hum Wellness. (2021) 10:523–35. doi: 10.1016/j.fshw.2021.04.015
27. Li F, Jin Y, Wang J, Xu H. Structure identification of two polysaccharides from Morchella sextelata with antioxidant activity. Foods. (2022) 11:982. doi: 10.3390/foods11070982
28. He P, Zhang A, Zhang F, Linhardt RJ, Sun P. Structure and bioactivity of a polysaccharide containing uronic acid from Polyporus umbellatus sclerotia. Carbohydr Polym. (2016) 152:222–30. doi: 10.1016/j.carbpol.2016.07.010
29. Zhang J, Wen C, Gu J, Ji C, Duan Y, Zhang H. Effects of subcritical water extraction microenvironment on the structure and biological activities of polysaccharides from Lentinus edodes. Int J Biol Macromol. (2019) 123:1002–11. doi: 10.1016/j.ijbiomac.2018.11.194
30. Liu C, Sun Y, Mao Q, Guo X, Li P, Liu Y, et al. Characteristics and antitumor activity of Morchella esculenta polysaccharide extracted by pulsed electric field. Int J Mol Sci. (2016) 17:986. doi: 10.3390/ijms17060986
31. Zheng Y, Li S, Li C, Shao Y, Chen A. Aqueous two-phase extraction, antioxidant and renal protective effects of polysaccharides from spores of Cordyceps cicadae. Processes. (2022) 10:348. doi: 10.3390/pr10020348
32. Yi Y, Xu W, Wang HX, Huang F, Wang LM. Natural polysaccharides experience physiochemical and functional changes during preparation: a review. Carbohydr Polym. (2020) 234:115896. doi: 10.1016/j.carbpol.2020.115896
33. Du B, Peng F, Xie Y, Wang H, Wu J, Liu C, et al. Optimization extraction and antioxidant activity of crude polysaccharide from chestnut mushroom (agrocybe aegerita) by accelerated solvent extraction combined with response surface methodology (ase-rsm). Molecules. (2022) 27:2380. doi: 10.3390/molecules27082380
34. Ren Y, Bai Y, Zhang Z, Cai W, Del Rio Flores A. The preparation and structure analysis methods of natural polysaccharides of plants and fungi: a review of recent development. Molecules. (2019) 24:3122. doi: 10.3390/molecules24173122
35. Wang J, Wang L, Zhou H, Liang XD, Zhang MT, Tang YX, et al. The isolation, structural features and biological activities of polysaccharide from Ligusticum chuanxiong: a review. Carbohydr Polym. (2021) 2021:118971. doi: 10.1016/j.carbpol.2021.118971
36. Shao L, Sun Y, Liang J, Li M, Li X. Decolorization affects the structural characteristics and antioxidant activity of polysaccharides from Thesium chinense Turcz: comparison of activated carbon and hydrogen peroxide decolorization. Int J Biol Macromol. (2020) 155:1084–91. doi: 10.1016/j.ijbiomac.2019.11.074
37. Xu J, Zhang J, Sang Y, Wei Y, Chen X, Wang Y, et al. Polysaccharides from medicine and food homology materials: a review on their extraction, purification, structure, and biological activities. Molecules. (2022) 27:3215. doi: 10.3390/molecules27103215
38. Yu M, Cao Y, Xin G, Ji C, Gao S, Zou X, et al. Advances in study on structure of polysaccharide. In: International Symposium on Mechanical Engineering and Material Science (ismems-16). Amsterdam: Atlantis Press. (2016). p. 227–33.
39. Diener M, Adamcik J, Sanchez-Ferrer A, Jaedig F, Schefer L, Mezzenga R. Primary, secondary, tertiary and quaternary structure levels in linear polysaccharides: from random coil, to single helix to supramolecular assembly. Biomacromolecules. (2019) 20:1731–9. doi: 10.1021/acs.biomac.9b00087
40. Li QZ, Wu D, Zhou S, Liu YF, Li ZP, Feng J, et al. Structure elucidation of a bioactive polysaccharide from fruiting bodies of Hericium erinaceus in different maturation stages. Carbohydrate Polym. (2016) 144:196–204. doi: 10.1016/j.carbpol.2016.02.051
41. Xu D, Wang H, Zheng W, Gao Y, Wang M, Zhang Y, et al. Charaterization and immunomodulatory activities of polysaccharide isolated from Pleurotus eryngii. Int J Biol Macromol. (2016) 92:30–6. doi: 10.1016/j.ijbiomac.2016.07.016
42. Román Y, Iacomini M, Sassaki GL, Cipriani TR. Optimization of chemical sulfation, structural characterization and anticoagulant activity of Agaricus bisporus fucogalactan. Carbohydr Polym. (2016) 146:345–52. doi: 10.1016/j.carbpol.2016.03.061
43. Zeng P, Li J, Chen Y, Zhang L. The structures and biological functions of polysaccharides from traditional Chinese herbs. Prog Mol Biol Transl Sci. (2019) 163:423–44. doi: 10.1016/bs.pmbts.2019.03.003
44. Kurzyna-Szklarek M, Cybulska J, Zdunek A. Analysis of the chemical composition of natural carbohydrates–an overview of methods. Food Chem. (2022) 2022:133466. doi: 10.1016/j.foodchem.2022.133466
45. El Enshasy HA, Hatti-Kaul R. Mushroom immunomodulators: unique molecules with unlimited applications. Trends Biotechnol. (2013) 31:668–77. doi: 10.1016/j.tibtech.2013.09.003
46. Guo Q, Liang S, Xiao Z, Ge C. Research progress on extraction technology and biological activity of polysaccharides from Edible Fungi: a review. Food Rev Int. (2022) 2022:1–32. doi: 10.1080/87559129.2022.2039182
47. Behlke J, Kleinpeter E, Kraft R, Laßmann G, Pfeil W, Rohde K, et al. Methoden in der Proteinanalytik. Berlin: Springer-Verlag. (2013).
48. Gerwig GJ. Monosaccharide composition analysis. In: The Art of Carbohydrate Analysis. Berlin: Springer (2021). p. 127–56.
49. Du B, Nie S, Peng F, Yang Y, Xu B. A narrative review on conformational structure characterization of natural polysaccharides. Food Front. (2022) 2022:fft2.150. doi: 10.1002/fft2.150
50. Chakraborty I, Rongpipi S, Govindaraju I, Mal SS, Gomez EW, Gomez ED, et al. An insight into microscopy and analytical techniques for morphological, structural, chemical, and thermal characterization of cellulose. Microsc Res Techniq. (2022) 2022:jemt.24057. doi: 10.1002/jemt.24057
51. Chen B, Qiao Y, Wang X, Zhang Y, Fu L. Extraction, structural characterization, biological functions, and application of rice bran polysaccharides: a review. Foods. (2023) 12:639. doi: 10.3390/foods12030639
52. Gong P, Wang S, Liu M, Chen F, Yang W, Chang X, et al. Extraction methods, chemical characterizations and biological activities of mushroom polysaccharides: a mini-review. Carbohydr Res. (2020) 494:108037. doi: 10.1016/j.carres.2020.108037
53. Hatzakis E. Nuclear magnetic resonance (NMR) spectroscopy in food science: a comprehensive review. Comprehens Rev Food Sci Food Saf . (2019) 18:189–220. doi: 10.1111/1541-4337.12408
54. Cantarel BL, Lombard V, Henrissat B. Complex carbohydrate utilization by the healthy human microbiome. PLoS ONE. (2012) 7:e28742. doi: 10.1371/journal.pone.0028742
55. El Kaoutari A, Armougom F, Raoult D, Henrissat B. Le microbiote intestinal et la digestion des polysaccharides. Medicine. (2014) 30:259–65. doi: 10.1051/medsci/20143003013
56. Kaoutari AE, Armougom F, Gordon JI, Raoult D, Henrissat B. The abundance and variety of carbohydrate-active enzymes in the human gut microbiota. Nat Rev Microbiol. (2013) 11:497–504. doi: 10.1038/nrmicro3050
57. Grondin JM, Tamura K, Déjean G, Abbott DW, Brumer H. Polysaccharide utilization loci: fueling microbial communities. J Bacteriol. (2017) 199:e00860–16. doi: 10.1128/JB.00860-16
58. Tian B, Geng Y, Xu T, Zou X, Mao R, Pi X, et al. Digestive characteristics of Hericium erinaceus polysaccharides and their positive effects on fecal microbiota of male and female volunteers during in vitro fermentation. Front Nutr. (2022) 9:858585. doi: 10.3389/fnut.2022.858585
59. Guo D, Lei J, He C, Peng Z, Liu R, Pan X, et al. In vitro digestion and fermentation by human fecal microbiota of polysaccharides from Clitocybe squamulose. Int J Biol Macromol. (2022) 208:343–55. doi: 10.1016/j.ijbiomac.2022.03.126
60. Zhou R, Wang Y, Li C, Jia S, Shi Y, Tang Y, et al. A preliminary study on preparation, characterization, and prebiotic activity of a polysaccharide from the edible mushroom Ramaria flava. J Food Biochem. (2022) 2022:e14371. doi: 10.1111/jfbc.14371
61. Wu DT, An LY, Liu W, Hu YC, Wang SP, Zou L. In vitro fecal fermentation properties of polysaccharides from Tremella fuciformis and related modulation effects on gut microbiota. Food Res Int. (2022) 156:111185. doi: 10.1016/j.foodres.2022.111185
62. Ma G, Xu Q, Du H, Kimatu BM, Su A, Yang W, et al. Characterization of polysaccharide from Pleurotus eryngii during simulated gastrointestinal digestion and fermentation. Food Chem. (2022) 370:131303. doi: 10.1016/j.foodchem.2021.131303
63. Zhang W, Hu B, Liu C, Hua H, Guo Y, Cheng Y, et al. Comprehensive analysis of Sparassis crispa polysaccharide characteristics during the in vitro digestion and fermentation model. Food Res Int. (2022) 154:111005. doi: 10.1016/j.foodres.2022.111005
64. Liu Y, Li Y, Ke Y, Li C, Zhang Z, Wu Y, et al. In vitro saliva-gastrointestinal digestion and fecal fermentation of Oudemansiella radicata polysaccharides reveal its digestion profile and effect on the modulation of the gut microbiota. Carbohydr Polym. (2021) 251:117041. doi: 10.1016/j.carbpol.2020.117041
65. Yang K, Zhang Y, Cai M, Guan R, Neng J, Pi X, et al. In vitro prebiotic activities of oligosaccharides from the by-products in Ganoderma lucidum spore polysaccharide extraction. RSC Adv. (2020) 10:14794–802. doi: 10.1039/C9RA10798C
66. Xue Z, Ma Q, Chen Y, Lu Y, Wang Y, Jia Y, et al. Structure characterization of soluble dietary fiber fractions from mushroom Lentinula edodes (Berk.) Pegler and the effects on fermentation and human gut microbiota in vitro. Food Res Int. (2020) 129:108870. doi: 10.1016/j.foodres.2019.108870
67. Yang L, Kang X, Dong W, Wang L, Liu S, Zhong X, et al. Prebiotic properties of Ganoderma lucidum polysaccharides with special enrichment of Bacteroides ovatus and B. uniformis in vitro. J Funct Foods. (2022) 92:105069. doi: 10.1016/j.jff.2022.105069
68. Li Q, Li L, Li Q, Wang J, Nie S, Xie M. Influence of natural polysaccharides on intestinal microbiota in inflammatory bowel diseases: an overview. Foods. (2022) 11:1084. doi: 10.3390/foods11081084
69. Sang T, Guo C, Guo D, Wu J, Wang Y, Wang Y, et al. Suppression of obesity and inflammation by polysaccharide from sporoderm-broken spore of Ganoderma lucidum via gut microbiota regulation. Carbohydr Polym. (2021) 256:117594. doi: 10.1016/j.carbpol.2020.117594
70. Mitsou EK, Saxami G, Stamoulou E, Kerezoudi E, Terzi E, Koutrotsios G, et al. Effects of rich in β-glucans edible mushrooms on aging gut microbiota characteristics: an in vitro study. Molecules. (2020) 25:2806. doi: 10.3390/molecules25122806
71. Su A, Ma G, Xie M, Ji Y, Li X, Zhao L, et al. Characteristic of polysaccharides from Flammulina velutipes in vitro digestion under salivary, simulated gastric and small intestinal conditions and fermentation by human gut microbiota. Int J Food Sci Technol. (2019) 54:2277–87. doi: 10.1111/ijfs.14142
72. Zhang H, Jiang F, Zhang J, Wang W, Li L, Yan J. Modulatory effects of polysaccharides from plants, marine algae and edible mushrooms on gut microbiota and related health benefits: a review. Int J Biol Macromol. (2022) 204:169–92. doi: 10.1016/j.ijbiomac.2022.01.166
73. Li M, Yu L, Zhao J, Zhang H, Chen W, Zhai Q, et al. Role of dietary edible mushrooms in the modulation of gut microbiota. J Funct Foods. (2021) 83:104538. doi: 10.1016/j.jff.2021.104538
74. Zhu Z, Huang R, Huang A, Wang J, Liu W, Wu S, et al. Polysaccharide from Agrocybe cylindracea prevents diet-induced obesity through inhibiting inflammation mediated by gut microbiota and associated metabolites. Int J Biol Macromol. (2022) 209:1430–8. doi: 10.1016/j.ijbiomac.2022.04.107
75. Xu Y, Xie L, Zhang Z, Zhang W, Tang J, He X, et al. Tremella fuciformis polysaccharides inhibited colonic inflammation in dextran sulfate sodium-treated mice via foxp3+ t cells, gut microbiota, and bacterial metabolites. Front Immunol. (2021) 12:648162. doi: 10.3389/fimmu.2021.648162
76. Morales D, Shetty SA, López-Plaza B, Gómez-Candela C, Smidt H, Marín FR, et al. Modulation of human intestinal microbiota in a clinical trial by consumption of a β-d-glucan-enriched extract obtained from Lentinula edodes. Eur J Nutr. (2021) 60:3249–65. doi: 10.1007/s00394-021-02504-4
77. Duncan SH, Louis P, Thomson JM, Flint HJ. The role of pH in determining the species composition of the human colonic microbiota. Environ Microbiol. (2009) 11:2112–22. doi: 10.1111/j.1462-2920.2009.01931.x
78. Ma G, Du H, Hu Q, Yang W, Pei F, Xiao H. Health benefits of edible mushroom polysaccharides and associated gut microbiota regulation. Crit Rev Food Sci Nutr. (2021) 2021:1903385. doi: 10.1080/10408398.2021.1903385
79. Cockburn DW, Koropatkin NM. Polysaccharide degradation by the intestinal microbiota and its influence on human health and disease. J Mol Biol. (2016) 428:3230–52. doi: 10.1016/j.jmb.2016.06.021
80. Huus K, Hoang T, Creus-Cuadros A, Cirstea M, Vogt S, Knuff-Janzen K, et al. Cross-feeding between intestinal pathobionts promotes their overgrowth during undernutrition. Nat Commun. (2021) 12:1–14. doi: 10.1038/s41467-021-27191-x
81. Ze X, Duncan SH, Louis P, Flint HJ. Ruminococcus bromii is a keystone species for the degradation of resistant starch in the human colon. ISME J. (2012) 6:1535–43. doi: 10.1038/ismej.2012.4
82. Dowlati M, Sobhi HR, Esrafili A, FarzadKia M, Yeganeh M. Heavy metals content in edible mushrooms: A systematic review, meta-analysis and health risk assessment. Trends Food Sci Technol. (2021) 109:527–35. doi: 10.1016/j.tifs.2021.01.064
83. Liu B, Huang Q, Cai H, Guo X, Wang T, Gui M. Study of heavy metal concentrations in wild edible mushrooms in yunnan province, china. Food Chem. (2021) (2015) 188:294–300. doi: 10.1016/j.foodchem.2015.05.010
84. Kaji I, Karaki Si, Kuwahara A. Short-chain fatty acid receptor and its contribution to glucagon-like peptide-1 release. Digestion. (2014) 89:31–6. doi: 10.1159/000356211
85. He J, Zhang P, Shen L, Niu L, Tan Y, Chen L, et al. Short-chain fatty acids and their association with signalling pathways in inflammation, glucose and lipid metabolism. Int J Mol Sci. (2020) 21:6356. doi: 10.3390/ijms21176356
86. Martin-Gallausiaux C, Marinelli L, Blottière HM, Larraufie P, Lapaque N. SCFA: mechanisms and functional importance in the gut. Proc Nutr Soc. (2021) 80:37–49. doi: 10.1017/S0029665120006916
87. Yamamoto I, Kawasumi K, Ohkusu-Tsukada K, Arai T. Molecular characterization of free fatty acid receptors FFAR2 and FFAR3 in the domestic cat. Vet Med Sci. (2021) 7:77–85. doi: 10.1002/vms3.356
88. Cunningham A, Stephens J, Harris D. Gut microbiota influence in type 2 diabetes mellitus (T2DM). Gut Pathog. (2021) 13:1–13. doi: 10.1186/s13099-021-00446-0
89. Cai M, Mu H, Xing H, Li Z, Xu J, Liu W, et al. In vitro gastrointestinal digestion and fermentation properties of Ganoderma lucidum spore powders and their extracts. LWT. (2021) 135:110235. doi: 10.1016/j.lwt.2020.110235
90. Liu My, Yun Sj, Cao Jl, Cheng F, Chang Mc, Meng Jl, et al. The fermentation characteristics of Sparassis crispa polysaccharides and their effects on the intestinal microbes in mice. Chem Biol Technol Agri. (2021) 8:1–15. doi: 10.1186/s40538-021-00225-8
91. Duan H, Li J, Fan L. Agaricus bisporus polysaccharides ameliorates behavioural deficits in D-galactose-induced aging mice: mediated by gut microbiota. Foods. (2023) 12:424. doi: 10.3390/foods12020424
92. He J, Zhang A, Ru Q, Dong D, Sun P. Structural characterization of a water-soluble polysaccharide from the fruiting bodies of Agaricus bisporus. Int J Mol Sci. (2014) 15:787–97. doi: 10.3390/ijms15010787
93. Wang WH, Zhang JS, Feng T, Deng J, Lin CC, Fan H, et al. Structural elucidation of a polysaccharide from Flammulina velutipes and its immunomodulation activities on mouse B lymphocytes. Sci Rep. (2018) 8:3120. doi: 10.1038/s41598-018-21375-0
94. Hao R, Zhou X, Zhao X, Lv X, Zhu X, Gao N, et al. Flammulina velutipes polysaccharide counteracts cadmium-induced gut injury in mice via modulating gut inflammation, gut microbiota and intestinal barrier. Sci Tot Environ. (2023) 877:162910. doi: 10.1016/j.scitotenv.2023.162910
95. Guo WL, Deng JC, Pan YY, Xu JX, Hong JL, Shi FF, et al. Hypoglycemic and hypolipidemic activities of Grifola frondosa polysaccharides and their relationships with the modulation of intestinal microflora in diabetic mice induced by high-fat diet and streptozotocin. Int J Biol Macromol. (2020) 153:1231–40. doi: 10.1016/j.ijbiomac.2019.10.253
96. Bie N, Han L, Wang Y, Wang X, Wang C. A polysaccharide from Grifola frondosa fruit body induces HT-29 cells apoptosis by PI3K/AKT-MAPKs and NF-κB-pathway. Int J Biol Macromol. (2020) 147:79–88. doi: 10.1016/j.ijbiomac.2020.01.062
97. Zhang W, Guo Y, Cheng Y, Zhao W, Zheng Y, Qian H. Ultrasonic-assisted enzymatic extraction of Sparassis crispa polysaccharides possessing protective ability against H2O2-induced oxidative damage in mouse hippocampal HT22 cells. RSC Adv. (2020) 10:22164–75. doi: 10.1039/D0RA01581D
98. Zhang W, Hu B, Han M, Guo Y, Cheng Y, Qian H. Purification, structural characterization and neuroprotective effect of a neutral polysaccharide from Sparassis crispa. Int J Biol Macromol. (2022) 201:389–99. doi: 10.1016/j.ijbiomac.2021.12.165
99. Xu X, Zhang X. Lentinula edodes-derived polysaccharide alters the spatial structure of gut microbiota in mice. PLoS ONE. (2015) 10:e0115037. doi: 10.1371/journal.pone.0115037
100. Sheng K, Wang C, Chen B, Kang M, Wang M, Liu K, et al. Recent advances in polysaccharides from Lentinus edodes (Berk.): Isolation, structures and bioactivities. Food Chem. (2021) 358:129883. doi: 10.1016/j.foodchem.2021.129883
101. Wang XY, Zhang Dd, Yin JY, Nie SP, Xie MY. Recent developments in Hericium erinaceus polysaccharides: extraction, purification, structural characteristics and biological activities. Crit Rev Food Sci Nutr. (2019) 59:S96–S115. doi: 10.1080/10408398.2018.1521370
102. Han ZH, Ye JM, Wang GF. Evaluation of in vivo antioxidant activity of Hericium erinaceus polysaccharides. Int J Biol Macromol. (2013) 52:66–71. doi: 10.1016/j.ijbiomac.2012.09.009
103. Gong M, Zhang H, Wu D, Zhang Z, Zhang J, Bao D, et al. Key metabolism pathways and regulatory mechanisms of high polysaccharide yielding in Hericium erinaceus. BMC Genom. (2021) 22:1–19. doi: 10.1186/s12864-021-07480-x
104. Pan M, Kong F, Xing L, Yao L, Li Y, Liu Y, et al. The structural characterization and immunomodulatory activity of polysaccharides from Pleurotus abieticola fruiting bodies. Nutrients. (2022) 14:4410. doi: 10.3390/nu14204410
105. Wang T, Han J, Dai H, Sun J, Ren J, Wang W, et al. Polysaccharides from Lyophyllum decastes reduce obesity by altering gut microbiota and increasing energy expenditure. Carbohydr Polym. (2022) 295:119862. doi: 10.1016/j.carbpol.2022.119862
106. Kanwal S, Joseph TP, Owusu L, Xiaomeng R, Meiqi L, Yi X. A polysaccharide isolated from Dictyophora indusiata promotes recovery from antibiotic-driven intestinal dysbiosis and improves gut epithelial barrier function in a mouse model. Nutrients. (2018) 10:1003. doi: 10.3390/nu10081003
107. Zhang Y, Lei Y, Qi S, Fan M, Zheng S, Huang Q, et al. Ultrasonic-microwave-assisted extraction for enhancing antioxidant activity of Dictyophora indusiata polysaccharides: the difference mechanisms between single and combined assisted extraction. Ultrason Sonochem. (2023) 95:106356. doi: 10.1016/j.ultsonch.2023.106356
108. Li QY, Dou ZM, Chen C, Jiang YM, Yang B, Fu X. Study on the effect of molecular weight on the gut microbiota fermentation properties of blackberry polysaccharides in vitro. J Agric Food Chem. (2022) 70:11245–57. doi: 10.1021/acs.jafc.2c03091
109. Fu X, Liu Z, Zhu C, Mou H, Kong Q. Nondigestible carbohydrates, butyrate, and butyrate-producing bacteria. Crit Rev Food Sci Nutr. (2019) 59:S130–52. doi: 10.1080/10408398.2018.1542587
110. Mao YH, Song AX, Li LQ, Siu KC, Yao ZP, Wu JY. Effects of exopolysaccharide fractions with different molecular weights and compositions on fecal microflora during in vitro fermentation. Int J Biol Macromol. (2020) 144:76–84. doi: 10.1016/j.ijbiomac.2019.12.072
111. Jayachandran M, Xiao J, Xu B. A critical review on health promoting benefits of edible mushrooms through gut microbiota. Int J Mol Sci. (2017) 18:1934. doi: 10.3390/ijms18091934
112. Canaviri-Paz P, Oscarsson E, Kjellström A, Olsson H, Jois C, Håkansson Å. Effects on microbiota composition after consumption of quinoa beverage fermented by a novel xylose-metabolizing L. plantarum strain. Nutrients. (2021) 13:3318. doi: 10.3390/nu13103318
113. Ryu EJ, An SJ, Sim J, Sim J, Lee J, Choi BK. Use of D-galactose to regulate biofilm growth of oral streptococci. Arch Oral Biol. (2020) 111:104666. doi: 10.1016/j.archoralbio.2020.104666
114. Rastall RA, Diez-Municio M, Forssten S, Hamaker B, Meynier A, Moreno FJ, et al. Structure and function of non-digestible carbohydrates in the gut microbiome. Benef Microbes. (2022) 13:95–168. doi: 10.3920/BM2021.0090
115. Fijan S. Probiotics and their Antimicrobial Effect. (2023). doi: 10.3390/books978-3-0365-6932-1
116. Shastry RP, Rekha P. Bacterial cross talk with gut microbiome and its implications: a short review. Folia Microbiol. (2021) 66:15–24. doi: 10.1007/s12223-020-00821-5
117. Li L, Guo Y, Huang Q, Shi X, Liu Q, Wang F, et al. GPP (composition of Ganoderma lucidum polysaccharides and Polyporus umbellatus polysaccharides) protects against DSS-induced murine colitis by enhancing immune function and regulating intestinal flora. Food Sci Hum Wellness. (2022) 11:795–805. doi: 10.1016/j.fshw.2022.03.010
118. Abdureyim Z, Wang L, Tao Q, Xu J, Yimiti D, Gao Q. Bachu mushroom polysaccharide alleviates colonic injury by modulating the gut microbiota. Comput Math Methods Med. (2022) 2022:1353724. doi: 10.1155/2022/1353724
119. Hu Y, Xu J, Sheng Y, Liu J, Li H, Guo M, et al. Pleurotus ostreatus ameliorates obesity by modulating the gut microbiota in obese mice induced by high-fat diet. Nutrients. (2022) 14:1868. doi: 10.3390/nu14091868
120. Yu M, Yue J, Hui N, Zhi Y, Hayat K, Yang X, et al. Anti-hyperlipidemia and gut microbiota community regulation effects of selenium-rich cordyceps militaris polysaccharides on the high-fat diet-fed mice model. Foods. (2021) 10:2252. doi: 10.3390/foods10102252
121. Lee BH, Chen CH, Hsu YY, Chuang PT, Shih MK, Hsu WH. Polysaccharides obtained from Cordyceps militaris alleviate hyperglycemia by regulating gut microbiota in mice fed a high-fat/sucrose diet. Foods. (2021) 10:1870. doi: 10.3390/foods10081870
122. Huang R, Zhu Z, Wu S, Wang J, Chen M, Liu W, et al. Polysaccharides from Cordyceps militaris prevent obesity in association with modulating gut microbiota and metabolites in high-fat diet-fed mice. Food Res Int. (2022) 157:111197. doi: 10.1016/j.foodres.2022.111197
123. Nakahara D, Nan C, Mori K, Hanayama M, Kikuchi H, Hirai S, et al. Effect of mushroom polysaccharides from Pleurotus eryngii on obesity and gut microbiota in mice fed a high-fat diet. Eur J Nutr. (2020) 59:3231–44. doi: 10.1007/s00394-019-02162-7
124. Lv XC, Guo WL, Li L, Yu XD, Liu B. Polysaccharide peptides from Ganoderma lucidum ameliorate lipid metabolic disorders and gut microbiota dysbiosis in high-fat diet-fed rats. J Funct Foods. (2019) 57:48–58. doi: 10.1016/j.jff.2019.03.043
125. Li L, Guo WL, Zhang W, Xu JX, Qian M, Bai WD, et al. Grifola frondosa polysaccharides ameliorate lipid metabolic disorders and gut microbiota dysbiosis in high-fat diet fed rats. Food Funct. (2019) 10:2560–72. doi: 10.1039/C9FO00075E
126. Hu R, Guo W, Huang Z, Li L, Liu B, Lv X. Extracts of Ganoderma lucidum attenuate lipid metabolism and modulate gut microbiota in high-fat diet fed rats. J Funct Foods. (2018) 46:403–12. doi: 10.1016/j.jff.2018.05.020
127. Zhu L, Ye C, Hu B, Xia H, Bian Q, Liu Y, et al. Regulation of gut microbiota and intestinal metabolites by Poria cocos oligosaccharides improves glycolipid metabolism disturbance in high-fat diet-fed mice. J Nutr Biochem. (2022) 107:109019. doi: 10.1016/j.jnutbio.2022.109019
128. Zhang T, Zhao W, Xie B, Liu H. Effects of Auricularia auricula and its polysaccharide on diet-induced hyperlipidemia rats by modulating gut microbiota. J Funct Foods. (2020) 72:104038. doi: 10.1016/j.jff.2020.104038
129. Chen Y, Liu D, Wang D, Lai S, Zhong R, Liu Y, et al. Hypoglycemic activity and gut microbiota regulation of a novel polysaccharide from Grifola frondosa in type 2 diabetic mice. Food Chem Toxicol. (2019) 126:295–302. doi: 10.1016/j.fct.2019.02.034
130. Shao W, Xiao C, Yong T, Zhang Y, Hu H, Xie T, et al. A polysaccharide isolated from Ganoderma lucidum ameliorates hyperglycemia through modulating gut microbiota in type 2 diabetic mice. Int J Biol Macromol. (2022) 197:23–38. doi: 10.1016/j.ijbiomac.2021.12.034
131. Yang R, Li Y, Mehmood S, Yan C, Huang Y, Cai J, et al. Polysaccharides from Armillariella tabescens mycelia ameliorate renal damage in type 2 diabetic mice. Int J Biol Macromol. (2020) 162:1682–91. doi: 10.1016/j.ijbiomac.2020.08.006
132. Zhao D, Dai W, Tao H, Zhuang W, Qu M, Chang YN. Polysaccharide isolated from Auricularia auricular-judae (Bull.) prevents dextran sulfate sodium-induced colitis in mice through modulating the composition of the gut microbiota. J Food Sci. (2020) 85:2943–51. doi: 10.1111/1750-3841.15319
133. Zhang R, Yuan S, Ye J, Wang X, Zhang X, Shen J, et al. Polysaccharide from flammuliana velutipes improves colitis via regulation of colonic microbial dysbiosis and inflammatory responses. Int J Biol Macromol. (2020) 149:1252–61. doi: 10.1016/j.ijbiomac.2020.02.044
134. Guo C, Guo D, Fang L, Sang T, Wu J, Guo C, et al. Ganoderma lucidum polysaccharide modulates gut microbiota and immune cell function to inhibit inflammation and tumorigenesis in colon. Carbohydr Polym. (2021) 267:118231. doi: 10.1016/j.carbpol.2021.118231
135. Stephens RW, Arhire L, Covasa M. Gut microbiota: from microorganisms to metabolic organ influencing obesity. Obesity. (2018) 26:801–9. doi: 10.1002/oby.22179
136. Li X, Watanabe K, Kimura I. Gut microbiota dysbiosis drives and implies novel therapeutic strategies for diabetes mellitus and related metabolic diseases. Front Immunol. (2017) 8:1882. doi: 10.3389/fimmu.2017.01882
137. Pardo V, González-Rodríguez Á, Guijas C, Balsinde J, Valverde ÁM. Opposite cross-talk by oleate and palmitate on insulin signaling in hepatocytes through macrophage activation. J Biol Chem. (2015) 290:11663–77. doi: 10.1074/jbc.M115.649483
138. Tian T, Zhao Y, Yang Y, Wang T, Jin S, Guo J, et al. The protective role of short-chain fatty acids acting as signal molecules in chemotherapy-or radiation-induced intestinal inflammation. Am J Cancer Res. (2020) 10:3508.
139. Kanwal S, Aliya S, Xin Y. Anti-obesity effect of dictyophora indusiata mushroom polysaccharide (dip) in high fat diet-induced obesity via regulating inflammatory cascades and intestinal microbiome. Front Endocrinol. (2020) 11:558874. doi: 10.3389/fendo.2020.558874
140. Chen M, Xiao D, Liu W, Song Y, Zou B, Li L, et al. Intake of Ganoderma lucidum polysaccharides reverses the disturbed gut microbiota and metabolism in type 2 diabetic rats. Int J Biol Macromol. (2020) 155:890–902. doi: 10.1016/j.ijbiomac.2019.11.047
141. Feng H, Zhang S, Wan JMF, Gui L, Ruan M, Li N, et al. Polysaccharides extracted from Phellinus linteus ameliorate high-fat high-fructose diet induced insulin resistance in mice. Carbohydr Polym. (2018) 200:144–53. doi: 10.1016/j.carbpol.2018.07.086
142. Seyedian SS, Nokhostin F, Malamir MD. A review of the diagnosis, prevention, and treatment methods of inflammatory bowel disease. J Med Life. (2019) 12:113. doi: 10.25122/jml-2018-0075
143. Ramos GP, Papadakis KA. Mechanisms of disease: inflammatory bowel diseases. In: Mayo Clinic Proceedings. Vol. 94. Amsterdam: Elsevier (2019). p. 155–65.
144. Joossens M, Huys G, Cnockaert M, De Preter V, Verbeke K, Rutgeerts P, et al. Dysbiosis of the faecal microbiota in patients with Crohn's disease and their unaffected relatives. Gut. (2011) 60:631–7. doi: 10.1136/gut.2010.223263
145. Khan I, Ullah N, Zha L, Bai Y, Khan A, Zhao T, et al. Alteration of gut microbiota in inflammatory bowel disease (IBD): cause or consequence? IBD treatment targeting the gut microbiome. Pathogens. (2019) 8:126. doi: 10.3390/pathogens8030126
146. Franzosa E, Sirota-Madi A, Avila-Pacheco J, Fornelos N, Haiser H, Reinker S, et al. Gut microbiome structure and metabolic activity in inflammatory bowel disease. Nat Microbiol. (2019) 19:5. doi: 10.1038/s41564-019-0442-5
147. Yang W, Zhao P, Li X, Guo L, Gao W. The potential roles of natural plant polysaccharides in inflammatory bowel disease: a review. Carbohydr Polym. (2022) 277:118821. doi: 10.1016/j.carbpol.2021.118821
148. Shen X, Wan Q, Zhao R, Wu Y, Wang Y, Cui Y, et al. Inflammatory bowel diseases and the risk of adverse health outcomes: Umbrella review of meta-analyses of observational studies. Digest Liver Dis. (2021) 53:809–16. doi: 10.1016/j.dld.2021.01.018
149. Kanwal S, Joseph TP, Aliya S, Song S, Saleem MZ, Nisar MA, et al. Attenuation of DSS induced colitis by Dictyophora indusiata polysaccharide (DIP) via modulation of gut microbiota and inflammatory related signaling pathways. J Funct Foods. (2020) 64:103641. doi: 10.1016/j.jff.2019.103641
150. Yu B, Wang M, Teng B, Veeraperumal S, Cheung PCK, Zhong S, et al. Partially acid-hydrolyzed porphyran improved dextran sulfate sodium-induced acute colitis by modulation of gut microbiota and enhancing the mucosal barrier. J Agri Food Chem. (2023) 2023:8564. doi: 10.1021/acs.jafc.2c08564
151. Sheflin AM, Whitney AK, Weir TL. Cancer-promoting effects of microbial dysbiosis. Curr Oncol Rep. (2014) 16:1–9. doi: 10.1007/s11912-014-0406-0
152. Vivarelli S, Salemi R, Candido S, Falzone L, Santagati M, Stefani S, et al. Gut microbiota and cancer: from pathogenesis to therapy. Cancers. (2019) 11:38. doi: 10.3390/cancers11010038
153. Zheng Y, Fang Z, Xue Y, Zhang J, Zhu J, Gao R, et al. Specific gut microbiome signature predicts the early-stage lung cancer. Gut Microbes. (2020) 11:1030–42. doi: 10.1080/19490976.2020.1737487
154. Fernández MF, Reina-Pérez I, Astorga JM, Rodríguez-Carrillo A, Plaza-Díaz J, Fontana L. Breast cancer and its relationship with the microbiota. Int J Environ Res Public Health. (2018) 15:1747. doi: 10.3390/ijerph15081747
155. Pandya U, Dhuldhaj U, Sahay NS. Bioactive mushroom polysaccharides as antitumor: an overview. Nat Prod Res. (2019) 33:2668–80. doi: 10.1080/14786419.2018.1466129
156. Khinsar KH, Abdul S, Hussain A, Ud Din R, Lei L, Cao J, et al. Anti-tumor effect of polysaccharide from Pleurotus ostreatus on H22 mouse Hepatoma ascites in-vivo and hepatocellular carcinoma in-vitro model. AMB Expr. (2021) 11:1–15. doi: 10.1186/s13568-021-01314-5
157. Luo J, Li T, Xie J, Guo H, Liu L, Zhang G, et al. Guar gum different from Ganoderma lucidum polysaccharide in alleviating colorectal cancer based on omics analysis. Food Funct. (2020) 11:572–84. doi: 10.1039/C9FO02786F
158. Khan I, Huang G, Li Xa, Liao W, Leong WK, Xia W, et al. Mushroom polysaccharides and jiaogulan saponins exert cancer preventive effects by shaping the gut microbiota and microenvironment in ApcMin/+ mice. Pharmacol Res. (2019) 148:104448. doi: 10.1016/j.phrs.2019.104448
159. Zhong J, Fang L, Chen R, Xu J, Guo D, Guo C, et al. Polysaccharides from sporoderm-removed spores of Ganoderma lucidum induce apoptosis in human gastric cancer cells via disruption of autophagic flux. Oncol Lett. (2021) 21:1–12. doi: 10.3892/ol.2021.12686
160. Su J, Su L, Li D, Shuai O, Zhang Y, Liang H, et al. Ganoderma lucidum antitumor activity of extract from the sporoderm-breaking spore of: restoration on exhausted cytotoxic T cell with gut microbiota remodeling. Front Immunol. (2018) 9:1765. doi: 10.3389/fimmu.2018.01765
161. Su J, Li D, Chen Q, Li M, Su L, Luo T, et al. Anti-breast cancer enhancement of a polysaccharide from spore of Ganoderma lucidum with paclitaxel: suppression on tumor metabolism with gut microbiota reshaping. Front Microbiol. (2018) 9:3099. doi: 10.3389/fmicb.2018.03099
162. Wu Q, Tan Z, Liu H, Gao L, Wu S, Luo J, et al. Chemical characterization of Auricularia auricula polysaccharides and its pharmacological effect on heart antioxidant enzyme activities and left ventricular function in aged mice. Int J Biol Macromol. (2010) 46:284–8. doi: 10.1016/j.ijbiomac.2010.01.016
163. Jing H, Zhang Q, Liu M, Zhang J, Zhang C, Li S, et al. Polysaccharides with antioxidative and antiaging activities from enzymatic-extractable mycelium by Agrocybe aegerita (Brig.) sing. Evid Based Complement Alternat Med. (2018) 2018:1584647. doi: 10.1155/2018/1584647
164. Ahmad MF. Ganoderma lucidum: Persuasive biologically active constituents and their health endorsement. Biomed Pharmacother. (2018) 107:507–19. doi: 10.1016/j.biopha.2018.08.036
165. Li Y, Miao M, Yin F, Shen N, Yu WQ, Guo SD. The polysaccharide–peptide complex from mushroom Cordyceps militaris ameliorates atherosclerosis by modulating the lncRNA–miRNA–mRNA axis. Food Funct. (2022) 13:3185–97. doi: 10.1039/D1FO03285B
166. Olawuyi IF, Lee WY. Quality and antioxidant properties of functional rice muffins enriched with shiitake mushroom and carrot pomace. Int J Food Sci Technol. (2019) 54:2321–8. doi: 10.1111/ijfs.14155
167. Lu X, Brennan MA, Serventi L, Brennan CS. Incorporation of mushroom powder into bread dough-effects on dough rheology and bread properties. Cereal Chem. (2018) 95:418–27. doi: 10.1002/cche.10043
168. XuJie H, Na Z, SuYing X, ShuGang L, BaoQiu Y. Extraction of BaChu mushroom polysaccharides and preparation of a compound beverage. Carbohydr Polym. (2008) 73:289–94. doi: 10.1016/j.carbpol.2007.11.033
169. Chou WT, Sheih IC, Fang TJ. The applications of polysaccharides from various mushroom wastes as prebiotics in different systems. J Food Sci. (2013) 78:M1041–8. doi: 10.1111/1750-3841.12160
170. Rossi P, Buonocore D, Altobelli E, Brandalise F, Cesaroni V, Iozzi D, et al. Improving training condition assessment in endurance cyclists: effects of Ganoderma lucidum and Ophiocordyceps sinensis dietary supplementation. Evid Based Complement Alternat Med. (2014) 2014:979613. doi: 10.1155/2014/979613
171. Ahmad I, Arif M, Mimi X, Zhang J, Ding Y, Lyu F. Therapeutic values and nutraceutical properties of shiitake mushroom (Lentinula edodes): a review. Trends Food Sci Technol. (2023) 3:7. doi: 10.1016/j.tifs.2023.03.007
172. Visvanathan S, Krishnamoorthy R, Sabesan GS. Fungal cosmetics: mushrooms in beauty care and the new age of natural cosmetics. In: Applied Mycology: Entrepreneurship with Fungi. Berlin: Springer (2022). p. 1–37.
173. Wen L, Gao Q, Ma Cw, Ge Y, You L, Liu RH, et al. Effect of polysaccharides from Tremella fuciformis on UV-induced photoaging. J Funct Foods. (2016) 20:400–10. doi: 10.1016/j.jff.2015.11.014
174. Fang D, Deng Z, Jung J, Hu Q, Zhao Y. Mushroom polysaccharides-incorporated cellulose nanofiber films with improved mechanical, moisture barrier, and antioxidant properties. J Appl Polym Sci. (2018) 135:46166. doi: 10.1002/app.46166
175. Teplyakova TV, Gashnikova N. Medicinal mushrooms in complex treatment of human immunodeficiency virus infection. Int J Med Mushrooms. (2019) 21:35. doi: 10.1615/IntJMedMushrooms.2019030175
176. Shin MS, Park H-J, Maeda T, Nishioka H, Fujii H, Kang I, et al. The effects of ahccR, a standardized extract of cultured lentinura edodes mycelia, on natural killer and t cells in health and disease: reviews on human and animal studies. J Immunology Res. (2019) 2019:1. doi: 10.1155/2019/3758576
177. Vetvicka V, Vetvickova J. Immune-enhancing effects of maitake (grifola frondosa) and shiitake (lentinula edodes) extracts. Annals Transl Med. (2014) 2:1.
178. Sharma VK, Liu X, Oyarzun DA, Abdel-Azeem AM, Atanasov AG, Hesham AE-L, et al. Microbial polysaccharides: An emerging family of natural biomaterials for cancer therapy and diagnostics. Seminars in Cancer Biology. (Amsterdam: Elsevier). (2022) 706–31. doi: 10.1016/j.semcancer.2021.05.021
179. Qiang M, Cai P, Ao M, Li X, Chen Z, Yu L. Polysaccharides from chinese materia medica: Perspective towards cancer management. Int J Biol Macromol. (2022) 224:496–509. doi: 10.1016/j.ijbiomac.2022.10.139
Keywords: edible mushroom polysaccharides, gut microbiota, host health, short chain fatty acids, beneficial effects
Citation: Zhao Q, Jiang Y, Zhao Q, Patrick Manzi H, Su L, Liu D, Huang X, Long D, Tang Z and Zhang Y (2023) The benefits of edible mushroom polysaccharides for health and their influence on gut microbiota: a review. Front. Nutr. 10:1213010. doi: 10.3389/fnut.2023.1213010
Received: 27 April 2023; Accepted: 20 June 2023;
Published: 06 July 2023.
Edited by:
Fuqiang Yu, Chinese Academy of Sciences (CAS), ChinaReviewed by:
Kit Leong Cheong, Guangdong Ocean University, ChinaJesus Perez-Moreno, Colegio de Postgraduados (COLPOS), Mexico
Copyright © 2023 Zhao, Jiang, Zhao, Patrick Manzi, Su, Liu, Huang, Long, Tang and Zhang. This is an open-access article distributed under the terms of the Creative Commons Attribution License (CC BY). The use, distribution or reproduction in other forums is permitted, provided the original author(s) and the copyright owner(s) are credited and that the original publication in this journal is cited, in accordance with accepted academic practice. No use, distribution or reproduction is permitted which does not comply with these terms.
*Correspondence: Zhenchuang Tang, tangzhenchuang@caas.cn; Ying Zhang, yingz@lzu.edu.cn