- 1The Key Laboratory of Geriatrics, Beijing Institute of Geriatrics, Institute of Geriatric Medicine, Chinese Academy of Medical Sciences, Beijing Hospital/National Center of Gerontology of National Health Commission, Beijing, China
- 2General Surgery Department, The First People’s Hospital of Tai’an City, Tai’an, China
- 3Department of Nutrition, The First Medical Center, Chinese PLA General Hospital, Beijing, China
- 4National Clinical Research Center for Geriatric Diseases, Chinese PLA General Hospital, Beijing, China
Aging is the most important factor contributing to cardiovascular diseases (CVDs), and the incidence and severity of cardiovascular events tend to increase with age. Currently, CVD is the leading cause of death in the global population. In-depth analysis of the mechanisms and interventions of cardiovascular aging and related diseases is an important basis for achieving healthy aging. Tea polyphenols (TPs) are the general term for the polyhydroxy compounds contained in tea leaves, whose main components are catechins, flavonoids, flavonols, anthocyanins, phenolic acids, condensed phenolic acids and polymeric phenols. Among them, catechins are the main components of TPs. In this article, we provide a detailed review of the classification and composition of teas, as well as an overview of the causes of aging-related CVDs. Then, we focus on ten aspects of the effects of TPs, including anti-hypertension, lipid-lowering effects, anti-oxidation, anti-inflammation, anti-proliferation, anti-angiogenesis, anti-atherosclerosis, recovery of endothelial function, anti-thrombosis, myocardial protective effect, to improve CVDs and the detailed molecular mechanisms.
1. Introduction
With the advent of an aging society, aging-related issues are becoming a growing concern (1). Aging-related diseases have also become the most common diseases among middle-aged and elderly people (1, 2). Cardiovascular disease (CVD) is closely related to aging and is a serious threat to the lives and health of middle-aged and elderly people (3). CVD is a complex disease that involves multiple environmental and genetic factors, particularly atherosclerosis (AS), which mainly affects the large and middle arteries (3). This disease is characterized by lesions in the affected arteries starting from the intima, followed by a combination of lesions, including lipid accumulation, fibrous tissue proliferation and calcification, along with degenerative changes in the middle layers of the arteries (4). The secondary lesions of AS include lipid deposition, intimal thickening, thrombosis, inflammatory cell infiltration, subintimal inflammation, vessel wall remodeling, neovascularization, plaque rupture, intraplaque hemorrhage, plaque rupture and local thrombosis, which eventually cause narrowing or blockage of the vascular lesion, resulting in ischemic injury to the affected organs (4). AS and related diseases, such as coronary heart disease (CHD) and myocardial infarction, have become serious threats to human health and have become the leading causes of high morbidity, disability and mortality worldwide (5). Hence, there is a growing interest in exploring new ways to prevent and treat aging-related CVD.
With aging, cardiomyocytes gradually develop physiological changes such as hypertrophy, senescence, lipofuscin aggregation, fibrosis, and apoptosis, which lead to cardiac hypertrophy and heart failure (6). Vascular endothelial cells, smooth muscle cells and extracellular matrix gradually change, resulting in reduced endothelial function, a thickened intima, vascular sclerosis, increased arterial pressure, reduced number of capillaries and decreased permeability, which further cause tissue ischemia and hypoxia, oxidative stress damage and necrosis (7, 8). Eventually, this causes AS, CHD, and atherosclerotic occlusive disease (8, 9). In addition, during aging, physiological changes in glucose and lipid metabolism also occur, resulting in diabetes, hyperlipidemia, and metabolic syndrome, which in turn damage cardiovascular function and can lead to diseases such as diabetic heart disease (10, 11). Therefore, blocking these mechanisms may be a therapeutic strategy to resist aging-related CVD.
Tea is the second most widely consumed beverage after water and has been consumed for thousands of years in China (12). Tea is the dried young leaves or leaf buds of Camelliasinenis o. Ktze., a plant in the Camelliaceae family, and is used as a drink with great nutritional, health and medicinal value (13, 14). Depending on the degree of fermentation, tea is divided into six main categories: white (not fermented), green (not fermented), yellow (slightly fermented), oolong (deeply fermented), black (deeply fermented), and dark (deeply fermented) (15, 16). Tea is rich in many biologically active components, such as polyphenols, pigments, polysaccharides, alkaloids, free amino acids and saponins (15–17). Although tea contains several chemicals, tea polyphenols (TPs) play a major role in promoting health, and green tea contains far more polyphenols than other teas (12, 18–20). A large amount of evidence confirms that TPs are effective antioxidants with anti-inflammatory, antiradiation, and antiaging properties that can prevent CVD (20, 21).
The treatment methods for CVDs are mainly divided into two categories: (1) drug conservative treatment; (2) active surgical treatment. No matter which treatment method is used, it needs to be carried out on the basis of improving lifestyle, such as a light diet, rest, physical exercise, controlling weight, blood pressure, blood sugar, blood lipids, quitting smoking, and limiting alcohol. These are important lifestyle improvement measures to reduce the risk of CVDs recurrence. Compared to these traditional treatment methods, tea is widely recognized as a healthy beverage, and multiple studies have confirmed that drinking tea regularly can reduce the risk of CVDs (22–24). This may be related to various components in tea, and TPs have the effects of lowering blood lipids, antioxidation, and inhibiting thrombosis. There have been many reports describing the role of TPs in the prevention and treatment of CVDs (25–27). These articles focus on a particular component of tea or emphasize a particular CVD. In this article, we provide a detailed review of the classification and composition of teas and an overview of the causes of aging-related CVDs; then, we focus on ten aspects of the effects of TPs to improve CVD and the detailed molecular mechanisms.
2. Physicochemical properties and composition of TPs
Many bioactive TPs have been identified in dry tea leaves, including flavonols, flavonoids, anthocyanins, and phenolic acids (23, 24, 28, 29). Flavanols are the main components of TPs, and flavanols are dominated by catechins and their derivatives (15, 16). According to their chemical structure, catechins can be divided into four main types: (-)-epigallocatechin-3-gallate (EGCG), (-)-epigallocatechin (EGC), (-)-epicatechin-3-gallate (ECG) and (-)-epicatechin (EC) (18). EGCG is the most physiologically active substance among catechols, accounting for approximately 50%–70%, and the physiological effects of green tea are mainly exerted by EGCG (18). Unlike green tea, oolong and black teas are fermented, and their catechins are oxidized to theaflavins (including four isomers: theaflavin, theaflavin-3-gallate, theaflavin-3′-gallate and theaflavin-3,3′-gallate) (Table 1) (18). These theophyllins exert cardiovascular protective effects, but their antioxidant activity may be lower than that of catechins (18).
3. Traditional risk factors for CVD
3.1. Abnormal blood pressure
Hypertension is a chronic CVD (32). A study showed that for every 10 mmHg reduction in systolic blood pressure, the risk of major cardiovascular events (e.g., coronary heart disease, stroke, heart failure) is significantly reduced (33). Therefore, effective control of blood pressure can reduce the incidence of cardiovascular-related diseases, morbidity and mortality (34). In addition, prehypertension is already prevalent and accompanied by increased aortic stiffness, impaired elasticity, decreased cardiac function, and diminished insulin resistance (34). Hence, hypertension is not only a chronic form of CVD but also worsens the morbidity and mortality of major CVD (35).
3.2. Abnormal metabolic indices
3.2.1. Abnormal glucose metabolism
Abnormal fasting plasma glucose (FPG) increases the risk of CVD (36). Abnormal glucose metabolism, especially hyperglycemia, leads to oxidative stress, microvascular damage, vascular tone and endothelial damage, as well as platelet aggregation and embolism (37, 38). In addition, hyperglycemia induces certain inflammatory factors [tumor necrosis factor-α (TNF-α), interleukin-6 (IL-6), C-reactive protein (CRP), etc.] and inflammatory reactions, all of which cause varying degrees of damage to cardiomyocytes, blood vessels, and even the heart (39).
3.2.2. Dyslipidemia
Lipids are the general term for neutral fats [triacylglycerols (TG) and total cholesterol (TC)] and lipids in plasma (phospholipids, glycolipids, sterols, and steroids), which are essential for the basic metabolism of living cells (40). Among them, cholesterol [low density lipoprotein (LDL) and high density lipoprotein (HDL)] and TG are closely related to the development of atherosclerosis (AS) (40, 41). Studies have shown that for every 1 mmol/L reduction in LDL-C, the risk of CVD is reduced by 21% to 24% (41, 42).
3.3. Poor lifestyle habits
3.3.1. Smoking
It is estimated that tobacco use causes approximately 10% of CVD worldwide (43–45). Tobacco contains approximately 4,000 chemicals, of which nicotine, carbon monoxide and other components stimulate blood pressure, lead to coronary AS, increase blood and platelet viscosity, reduce the ability to dissolve blood clots and oxygen-carrying capacity of hemoglobin, and even induce ventricular fibrillation, increasing the incidence of cardiovascular events (46, 47).
3.3.2. Alcohol consumption
Many studies now indicate that small amounts of alcohol consumption can moderately reduce the risk of myocardial infarction (48, 49). However, the effects of heavy alcohol use on exacerbating CVD cannot be ignored. Both long-term heavy drinking and occasional heavy drinking can, to varying degrees, decrease HDL-C, increase plasma viscosity and fibrinogen concentration, cause platelet aggregation, impair endothelial function, increase inflammatory responses, increase heart rate, and inhibit cardiac contractile function, thereby increasing the incidence of CVD, morbidity and mortality (50, 51).
3.3.3. Diet
The structure, quantity, and type of diet can also influence the occurrence of cardiovascular events (52, 53). For example, a high-salt diet can exacerbate vasoconstriction, leading to elevated blood pressure and plasma cholesterol and contributing to the development of AS (52–55). Sugar can increase blood viscosity and slow blood flow, which, combined with damage to the vascular endothelium, causes the generation of a large number of atherosclerotic plaques that block blood vessels and trigger the occurrence of acute cardiovascular events (56, 57). A high-fat diet can cause obesity or overweight, leading to metabolic disorders such as hyperlipidemia, hypertension, and other CVDs (58, 59).
3.3.4. Sleep and mental factors
It is reported that in patients with insomnia, serum HDL is low, while TG level is high (60, 61). In addition, CVDs are closely related to psychological conditions such as depression, chronic psychological stress, post traumatic stress disorder (PTSD), and anxiety (62).
3.4. Others
Numerous epidemiological studies have shown that sex, age, and family history influence the incidence and mortality rates of CVD (63, 64). With increasing age, the onset of various metabolic diseases, and the reduction in the body’s immune system, CVD increases each year (65). Moreover, the prevalence and mortality rates are higher in men than in women, especially in premenopausal women (66). Postmenopausal women lack the protective mechanisms of a specific physiological period, and with the decrease in estrogen levels, the metabolism of the body changes, leading to an increase in the incidence of CVD (65, 67).
4. Molecular mechanism of the cardioprotective effect of TPs
As a natural polyphenol complex, TPs are characterized by their simple availability and wide range of biological effects (68, 69). In recent years, TPs have been shown to have good preventive and curative effects against AS, thrombosis, myocarditis, coronary artery disease, antiarrhythmia and myocardial ischemia/reperfusion (I/R) injury (70). Studies have shown that the cardioprotective effects of TPs are closely related to their antioxidant, anti-inflammatory, and blood viscosity-altering characteristics (68, 70). Here, we have reviewed the relevant literature and summarized ten mechanisms of TPs associated with protection against CVD (anti-hypertension, lipid-lowering effects, anti-oxidation, anti-inflammation, anti-proliferation, anti-angiogenesis, anti-AS, recovery of endothelial function, anti-thrombosis, myocardial protective effect),. Undoubtedly, TPs can significantly reduce the risk of CVDs by reducing the factors related to CVDs.
4.1. Hypotensive effects
Hypertension is a major risk factor for CVD and a common disease with a high incidence worldwide that is characterized by elevated arterial pressure (71). At present, there are many drugs that treat hypertension and can effectively lower blood pressure but have large side effects and fluctuate greatly while lowering blood pressure (72, 73). Therefore, the screening of functional food factors with antihypertensive effects is critical for the prevention and treatment of hypertension. One of the pathogeneses of hypertension is elevated levels of renin, angiotensin, and aldosterone, and so patients with hypertension will experience high renin in their bodies (74). Aqueous extracts of fermented oolong and black teas strongly inhibit renin (74). In addition, supplementation with white, black and green teas in obese mice prevented the development of hypertension (75). Further analysis revealed that this antihypertensive effect was mainly associated with increased expression of antioxidant enzymes induced by TPs such as gallic acid, xanthine and flavan-3-ol (75). In a randomized, double-blind, controlled crossover study, black tea intake increased functionally active circulating angiogenic cells compared to placebo, thereby greatly offsetting the reduction in blood flow-mediated dilation due to fat intake (76). In two epidemiological studies [ATTICA and MEDiterranean ISlands (MEDIS)], green tea is rich in high levels of catechins (e.g., EGCG) compared to black tea and, therefore, significantly reduces the likelihood of hypertension in adults aged 50 years and older (77). In addition, tannins in tea have been shown to have a hypotensive effect on rats (78). Gao et al. (52, 53) found that green tea had an antihypertensive effect on hypertension induced by a high salt diet in aged male rats, and its main mechanism of action included inhibiting the activity of the renin-angiotensin II-aldosterone system, altering the expression of sodium-potassium pumps in heart, kidney and aortic tissues and increasing the synthesis of nitric oxide in endothelial cells.
4.2. Lipid-lowering effects
Hyperlipidemia is an important factor that induces CVD. An increase in LDL-C and a decrease in HDL-C in serum can cause arterial endothelial cell damage, increase permeability and accelerate LDL-C deposition in the subendothelium of blood vessels (79). In recent years, a large number of studies have shown that TPs can significantly reduce serum TC, TG, and LDL-C levels and increase HDL-C levels in patients with hyperlipidemia, which can protect vascular endothelial function (79). For example, serum levels of cholesterol, LDL and TG were reduced and HDL was significantly increased in experimental rats fed a high-cholesterol diet after the administration of beverages containing theaflavin and theaflavin (80). Results from a clinical trial of tea drinking habits and HDL in Chinese adults found that in people aged 60 years or older, serum HDL concentrations decreased more slowly in tea drinkers compared to non-tea drinkers, suggesting a significant association between tea consumption and HDL-C (81). In a randomized, controlled trial, ingestion of GTC for 4 consecutive weeks significantly reduced fasting serum TG levels (82). TPs have been widely demonstrated to improve lipid metabolism abnormalities by modulating gut microbial species and functions. Ma et al. (83) found that different doses of TPs could regulate intestinal redox status and the intestinal microbiota through different patterns, thus improving the disorders of lipid metabolism induced by a high-fat diet (HFD). Wang et al. (84) found that green tea leaf powder could reshape the intestinal microbiota in the cecum of mice and increase satiety hormone secretion, there by reducing lipid metabolism disorders in mice fed a HFD. Conversely, excessive intake of TPs reduced their beneficial effects on intestinal health (83). Moreover, TPs were effective in reducing leptin in rat serum and inhibiting fatty acid uptake, thereby improving lipid and antioxidant levels (85). It is worth noting that the lipid-lowering effect of black teas (such as Liubao and Pu′er teas) is increased significantly after fermentation compared to that of the raw material, probably due to the significant increase in browning and gallic acid in the tea leaves after fermentation (86).
4.3. Inhibiting oxidation
Oxidative stress is present throughout the pathology of AS, and another important effect of TPs is their antioxidant properties (87). Due to the number and structure of phenolic hydroxyl groups, catecholates and theaflavins are excellent electron donors and effective free radical scavengers (87). In vitro, the antioxidant effects of catechols and theaflavins against human LDL oxidation were similar, and the antioxidant capacity of polyphenols was in the following order: TF3 > ECG ≥ TF2B ≥ TF2A ≥ TF1 ≥ EC > EGC (88). In addition, after drinking 600 mL of green tea daily for 4 weeks, plasma levels of oxidized LDL (ox-LDL) were reduced in smokers (89). The inhibition of ROS-producing enzymes by TPs may also enhance their antioxidant effects. Both catechols and TFs inhibit the expression of inducible NO synthase (iNOS). Another physiological source of ROS occurs during the oxidation of hypoxanthine and xanthine to uric acid (87, 90). This reaction is catalyzed by xanthine oxidase, which has now been shown to be inhibited by catechol and theaflavin. Several studies have shown that catechol induces a variety of enzymes involved in cellular antioxidant defense mechanisms (87, 90, 91). Negishi et al. (91) found that oral administration of TPs for 2 weeks induced peroxidase in the aorta in spontaneously hypertensive rats. In endothelial cells, EGCG significantly induced subtilisin oxygenase-1 through activation of AKT and Nrf2, resulting in significant protection against hydroperoxide-regulated oxidative stress (87, 90). In vitro, TPs ameliorated heat stress injury in cardiomyocytes by upregulating Keap1-Nrf2-ARE signaling to enhance its antioxidant capacity and inducing the expression of heat shock proteins (69). Moreover, in Wistar rats, TPs attenuated the HFD-induced increase in intima-media thickness and significantly inhibited vascular oxidative damage (92). In addition, TPs can inhibit the oxidation of lipoproteins in vivo. In a clinical study, urinary levels of 4-O-methylglutamic acid were significantly increased after subjects took green and black tea, suggesting that intake of TPs could inhibit LDL oxidation in vivo (93). Besides, in a randomized, placebo-controlled, double-blind, crossover trial, green tea extract was ingested, with EGCG and EGC as the main components. Both of them rapidly bind LDL particles and reduce the degree of oxidation of LDL, thereby reducing the risk of AS associated with oxidative stress (94).
4.4. Inhibiting proliferation
The proliferation and migration of vascular smooth muscle cells (VSMCs) play key roles in the formation and development of AS, postvalvular restenosis and graft vascular lesions (95). In vivo and in vitro experiments showed that catechols inhibited VSMC proliferation and migration (95). Among catechols, EGC, ECG and EGCG were significantly more effective than catechins and epicatechins in preventing proliferation (95). Kim et al. (96) found that EGCG blocked the transition of VSMCs from G1 to S phase by initiating the expression of p21/WAF1, which in turn inhibited NF-kappaB and AP-1-mediated VSMC proliferation. Additionally, the antiproliferative effects of TPs include interactions with growth factors involved in the proliferation and migration of VSMCs, such as fibroblast growth factor (bFGF) (97, 98). EGCG also significantly inhibits c-Jun nuclear translocation and AP-1 binding activity and reduces iNOS expression (99). Moreover, TPs can interact with the matrix metalloprotein (MMP) system, which contributes to the migration, proliferation, and neointima formation of VSMCs after vascular injury (100). In a rat model of carotid artery injury, catechins reduced MMP-2 activity by upregulating matrix metalloproteinase (MMP)-2 and TIMP-2, thereby inhibiting neointimal proliferation and improving vascular remodeling (100). Furthermore, in a carotid artery injury model, EGCG reduced VSMC proliferation by inhibiting extracellular signal-regulated kinase (ERK), but c-jun and p38 signaling was not affected (101). Moreover, EGCG was shown to inhibit the expression of apoptosis-related proteins and attenuate apoptosis in VSMCs induced by H2O2 (102).
4.5. Anti-inflammation
Acute and chronic inflammation plays a key role in the development of CVD (103, 104). TPs can modulate immune responses and have potential anti-inflammatory activity. For example, in rats fed an atherosclerotic diet, the administration of 0.2% green tea extract (Polyphenon®) resulted in a significant reduction in serum inflammatory markers (CRP) (103). A clinical study showed that consistent use of green tea or green tea extract significantly reduced serum amyloid alpha, which is an important CVD risk factor, in obese individuals with metabolic syndrome (105). In another randomized, double-blind trial, long-term black tea consumption reduced platelet activation and lowered plasma CRP levels in healthy men, leading to long-term cardiovascular health maintenance (106). Moreover, in female rats with chronic inflammation, supplementation with TPs suppressed the innate immune response to chronic inflammation, thereby alleviating the development of myocardial fibrosis (107). In the early stages of atherosclerosis, leukocytes adhere to vascular endothelial cells and gradually migrate to the vessel wall. EGCG significantly reduced the migration of neutrophils to the endothelial cell monolayer by inhibiting chemokine production (108). In vitro experiments revealed that EGCG treatment inhibited TNF-α-induced adhesion of THP-1 cells to human umbilical vein endothelial cells (109). Moreover, EGCG reduced the expression of intracellular adhesion molecule 1, which affected the adhesion and migration of peripheral blood monocytes and CD8+ T cells (110). In RAW264.7 macrophages, EGCG inhibited NF-κB activation and reduced lipopolysaccharide (LPS)-induced TNFα production in a dose-dependent manner (111). In obese mice fed a HFD, TPs reduced the serum levels of TNFα, IL-1β and IL-6 by inhibiting the activation of NF-κB (28). Lu′an GuaPian tea, which is a green tea, is rich in kaempferol-3-O-rutinoside (KR), which can protect against cardiovascular disease by inhibiting TLR4/MyD88/NF-κB signaling and protect against myocardial injury (112). In addition, endothelial cells control vascular tone and permeability and are important for maintaining vascular homeostasis (113). Reddy et al. (113) found that EGCG reduced inflammation and decreased vasodilation by inhibiting the NF-κB pathway, thereby protecting against endothelial dysfunction and delaying the onset of CVD. In addition to the NF-κB signaling pathway, TPs improved the species abundance of the intestinal microbiota in the cecum, thereby improving the intestinal inflammatory response (114). Additionally, TPs could increase the expression of intestinal tight junction proteins to maintain the integrity of the intestinal barrier, thereby improving intestinal flora dysbiosis and reducing systemic inflammatory responses in obese mice (28, 115).
4.6. Improving the vascular endothelium function
The pathophysiological features of the cardiovascular system are characterized by a decrease in protective vasoactive substances in the endothelium, which is called endothelial dysfunction (43, 44). Numerous studies have shown that TPs improve endothelial cell function, lower blood pressure and have vasodilatory effects (116–118). For example, in obese prehypertensive women, short-term daily intake of GTE could improve endothelial function (119). Excessive accumulation of ROS is one of the important causal factors leading to endothelial cell dysfunction and hypertension (120). In bovine carotid artery endothelial cells (BCAECs), TPs could inhibit ROS production by reducing nicotinamide adenine dinucleotide phosphate (NADPH) expression, thereby alleviating angiotensin (Ang) II-induced endothelial cell hyperpermeability and possibly preventing the development of CVD (120). Moreover, in endothelial cells, TPs can bind endothelial extracellular superoxide dismutase (eEC-SOD) to inhibit LDL oxidation and thus counteract atherosclerosis (121). Endothelial nitric oxide synthase (eNOS) is a source of nitric oxide in endothelial cells and plays an important role in maintaining the function of endothelial cells (122). Caveolin-1 (Cav-1) is a negative regulator of eNOS that can affect cardiovascular function in multiple ways (123). Liu et al. (123) found that in BCAECs, TPs activated ERK1/2 and inhibited p38MAPK signaling in a dose-dependent manner, downregulating Cav-1 expression and thereby protecting endothelial cells. In addition, TPs can reduce the expression and secretion of plasminogen activator inhibitor-1 (PAI-1), a regulator that plays a key role in AS and hypertensive disease, in endothelial cells in a time-and dose-dependent manner, contributing to cardiovascular protection (123). In isolated rat mesenteric arteries, (-)-epicatechin increased NO concentrations in the vasculature and promoted vasodilation by activating iberiotoxin-sensitive K+ channels (116). A clinical study showed that acute black tea intake could activate NO production in endothelial cells, thereby reducing the risk of CVD (124). Kim et al. (125) found that EGCG increased LC3-II production and autophagosome formation in primary bovine aortic endothelial cells (BAECs), thereby reducing lipid accumulation and improving the development of CVD.
4.7. Inhibiting angiogenesis
Angiogenesis is an important pathological cause of the development of CVD (126). For instance, myocardial infarction (MI) is mainly associated with partial or complete occlusion of microvessels at the site of the lesion (126). Myocardial ischemia–reperfusion mainly refers to the production of necrotic material by ischemic cells when a patient has a myocardial infarction (127). After revascularization, blood passes through the necrotic myocardium in a short time to create reperfusion damage and increase cellular necrosis, which aggravates the symptoms of infarction and leads to malignant arrhythmias (127). To combat these conditions, restoring blood supply to the infarcted area can reduce cardiac remodeling and improve myocardial function (126). Vascular endothelial growth factor (VEGF), a homodimeric vasoactive glycoprotein, is a key regulator of angiogenesis. VEGF levels are significantly elevated in the serum of patients with different CVDs and are often associated with a poor prognosis (126). A growing number of studies have shown that TPs can protect against CVD by suppressing VEGF-mediated angiogenesis. In HUVECs, EGCG blocks the formation of the vascular endothelial growth factor receptor 2 complex, which in turn inhibits VEGF-mediated angiogenesis (128, 129). In a high-cholesterol diet male New Zealand White rabbit atherosclerosis model, green tea consumption significantly reduced VEGF expression in foam cells and smooth muscle cells, and it is hypothesized that green tea may slow the progression of atherosclerosis by reducing VEGF-induced angiogenesis (128). EGCG also inhibits angiogenesis by reducing the expression of the angiogenic factor bFGF (basic fibroblast growth factor) (130). After EGCG pretreatment, endothelial cells could induce the expression of membrane-type-1 matrix metalloproteinase (MT1-MMP), which promoted endothelial cell migration, and Cav-1, which caused tube formation, was significantly decreased, suggesting that EGCG inhibits angiogenesis (131).
4.8. Antiatherosclerosis
AS is the underlying cause of CVD (132). The development of AS has been associated with multiple molecular mechanisms, including endothelial dysfunction, inflammation, oxidative stress, and dysfunctional lipid metabolism (132). The protective effect of TPs on AS has been widely reported (133, 134). For example, a clinical study from Japan showed that patients who consumed >3 cups of green tea/day had a lower prevalence of coronary artery disease (CAD) than those who consumed <1 cup/day, suggesting that green tea intake may help improve coronary artery atherosclerosis in the Japanese population (135). TPs inhibit oxLDL production and thus IKB kinase (IKK)-mediated NF-κB activation in a dose-dependent manner and reduce the production of the proinflammatory cytokine TNF-α (134). In a mouse model of AS, EGCG reduced proinflammatory genes and increased antioxidant protein expression in the mouse aorta, and serum C-reactive protein, monocyte chelator protein-1 and ox-LDL were significantly decreased after EGCG treatment (133). Theaflavins in tea not only reduced the concentrations of F(2)-isoprostane, vascular superoxide, vascular leukotriene B(4) and plasma-SP-selectin in the aorta but also enhanced eNOS activity, thereby improving NO bioavailability to alleviate the development of AS in apolipoprotein E-deficient (ApoE−/−) mice (136). Changes in the gut microbiota are also closely associated with the development of AS (137). Liao et al. (137) found that TPs promoted the proliferation of intestinal bifidobacteria in ApoE−/− mice, thereby reducing total cholesterol and LDL cholesterol levels and reducing HFD-induced AS plaques. In addition, TPs increased the expression of autophagic markers (such as LC3, Beclin1 and p62) in the vascular wall of mice, ameliorated lipid metabolism disorders and inhibited AS plaque formation (138).
4.9. Inhibiting thrombosis
Platelet activation and subsequent thromboembolism are important pathophysiological mechanisms of ischemic CVD (139). The antithrombotic effect of green tea catechins is achieved mainly through the inhibition of platelet aggregation (140). EGCG has been reported to exert its inhibitory effect on platelet viability through several mechanisms: the inhibition of collagen-mediated phospholipase (PL) Cgamma2, blockade of protein tyrosine phosphorylation, and the enhancement of Ca2(+)-ATPase activity, thereby reducing platelet aggregation and alleviating atherothrombosis (140). In addition, GTC did not alter anticoagulant activity but mainly altered antiplatelet activity to exert antithrombotic effects in human platelet aggregation assays induced by ADP, collagen, epinephrine, and the calcium ion polymer A23187 in vitro (141). EGCG has also been shown to stimulate tyrosine phosphorylation of platelet-associated proteins (e.g., Syk and SLP-76) and reduce the phosphorylation levels of focal adhesion kinases, thereby improving platelet aggregation (142). Moreover, Kang et al. (143) found that catechol modulates the reduction in intracellular calcium levels in platelets, which led to Ca2+-ATPase activation and the inhibition of IP3 production, thereby inhibiting fibrinogen-GPIIb/IIIb binding and reducing platelet aggregation. Inflammatory and oxidative responses caused by endothelial cell injury play equally important roles in thrombosis (144). A recent study showed that EGCG combined with warfarin significantly reduced thrombus weight in a rat model of deep vein thrombosis (144). Further in vitro studies showed that the combination of EGCG and warfarin protected HUVECs from oxidative stress and prevented apoptosis, and the specific mechanism involved the inhibition of HIF-1α-mediated activation of PI3K/AKT and ERK1/2 signaling (144).
4.10. Myocardial protective effects
Ischemia is an extremely common pathological process in myocardial lesions (145). The protective effect of TPs against myocardial injury may be due to their ability to inhibit oxidative stress associated with ischemic injury (145). For example, in a cardiac hypertrophy model in rats established by abdominal aortic constriction (AC), myocardial tissue had increased malondialdehyde (MDA) levels and decreased superoxide dismutase (SOD) activity (146). In contrast, after EGCG treatment, the MDA levels in myocardial tissue decreased, and SOD activity increased. These results suggest that EGCG ameliorates myocardial injury in rats by inhibiting oxidative stress (146). In a rat model of diabetic cardiomyopathy, TPs significantly improved myocardial function in rats, and cardiomyocyte disorders and hypertrophy were significantly improved (147). An in-depth study revealed that TPs significantly upregulated LC3-II/I and Beclin-1 expression and reduced SQSTM1/p62 expression in rat myocardial tissue (147). In addition, ingestion of TPs significantly alleviated heat stress injury in hen cardiomyocytes at 38°C, as evidenced by the downregulation of myocardial injury-related indicators (LDH, CK, CK-MB and TNF-α), and the mechanism mainly involved Keap1-Nrf2-ARE and heat shock protein (Hsp)-related heat stress responses (69). Interestingly, a recent study showed that despite the low plasma concentration of polyphenols, polyphenols were transported to the arterial intima at pH 7.4 in the form of bound lipoproteins, and polyphenol levels were significantly elevated in endothelial cells and macrophages (148). Thereafter, such high local concentrations of polyphenols protect the heart through direct antioxidant effects (148). In addition, TPs alleviate myocardial fibrosis in female rats by attenuating chronic inflammation and suppressing innate immune responses (149).
Overall, TPs improve aging-related CVDs in the following five ways (Figure 1, Table 2): (1) TPs cause activation of autophagic flux; (2) TPs inhibit ox-LDL-mediated NF-κB, ERK1/2, p38MAPK, and JNK-induced inflammatory responses; (3) TPs activate the NRF2-mediated antioxidant signaling pathway; 4; (4) TPs improve vascular endothelial cell function via PI3K/AKT/eNOS pathway; (5) TPs inhibit VEGF-mediated angiogenesis. By modulating these molecular mechanisms, TPs can improve aging-related CVDs.
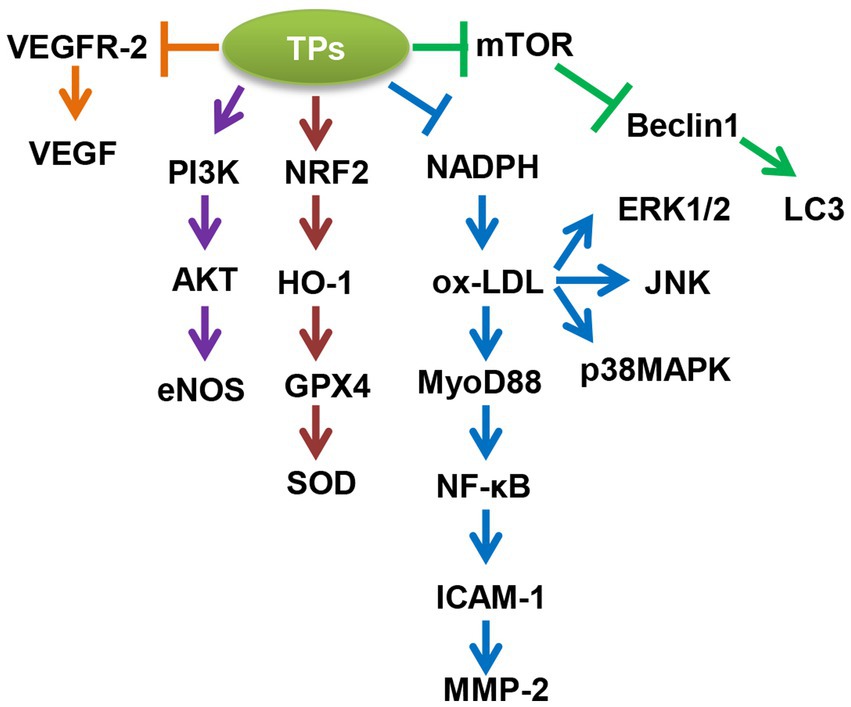
Figure 1. Molecular mechanism of tea polyphenols (TPs) to improve aging-related cardiovascular diseases (CVDs). AKT, AKT serine/threonine kinase 1; eNOS, nitric oxide synthase 3; ERK1/2, mitogen-activated protein kinase 1; GPX4, glutathione peroxidase 4; HO-1, heme oxygenase 1; ICAM-1, intercellular adhesion molecule 1; JNK, mitogen-activated protein kinase 8; LC3, microtubule associated protein 1 light chain 3; MMP-2, matrix metallopeptidase 2; mTOR, mechanistic target of rapamycin kinase; MyD88, MYD88 innate immune signal transduction adaptor; NADPH, 2,4-dienoyl-CoA reductase 1; NF-κB, nuclear factor kappa B subunit 1; NRF2, NFE2 like bZIP transcription factor 2; ox-LDL, oxidized low density lipoprotein; p38MAPK, mitogen-activated protein kinase 14; PI3K, phosphatidylinositol-4,5-bisphosphate 3-kinase catalytic subunit beta; SOD, superoxide dismutase 1; VEGF, vascular endothelial growth factor A; VEGFR-2, kinase insert domain receptor.
5. Conclusion
Natural substances originating from natural food and plants are of great interest due to their low toxicity, low cost and easy availability. However, the underlying physiological mechanisms of these substances are not fully understood, especially with respect to the cardiovascular system.
The pathophysiological process of CVD is multifactorial and can be affected by tea components in several processes: anti-hypertension, lipid-lowering effects, anti-oxidation, anti-inflammation, anti-proliferation, anti-angiogenesis, anti-AS, recovery of endothelial function, anti-thrombosis, myocardial protective effect (Figure 2). However, a large number of unresolved issues exist that limit the clinical use of TPs. The debated issues are mainly related to dose, specificity, potency, feasibility and short-or long-term side effects in humans. Although naturally occurring polyphenols are generally considered pharmacologically safe, it is also important to note the presence of deleterious effects of these compounds in the body, which are largely dependent on their distribution in the body and the type of cells on which they act. In addition, the bioavailability of TPs is relatively low when administered orally, and the effective transport of TPs to target organs is an important issue (156). Moreover, some components of tea polyphenols can also interact with nutrients in the body as well as conventional drugs, which are also potential safety issues (156). To address these issues, animal experiments, large cohort studies and human intervention trials are very necessary in the future.
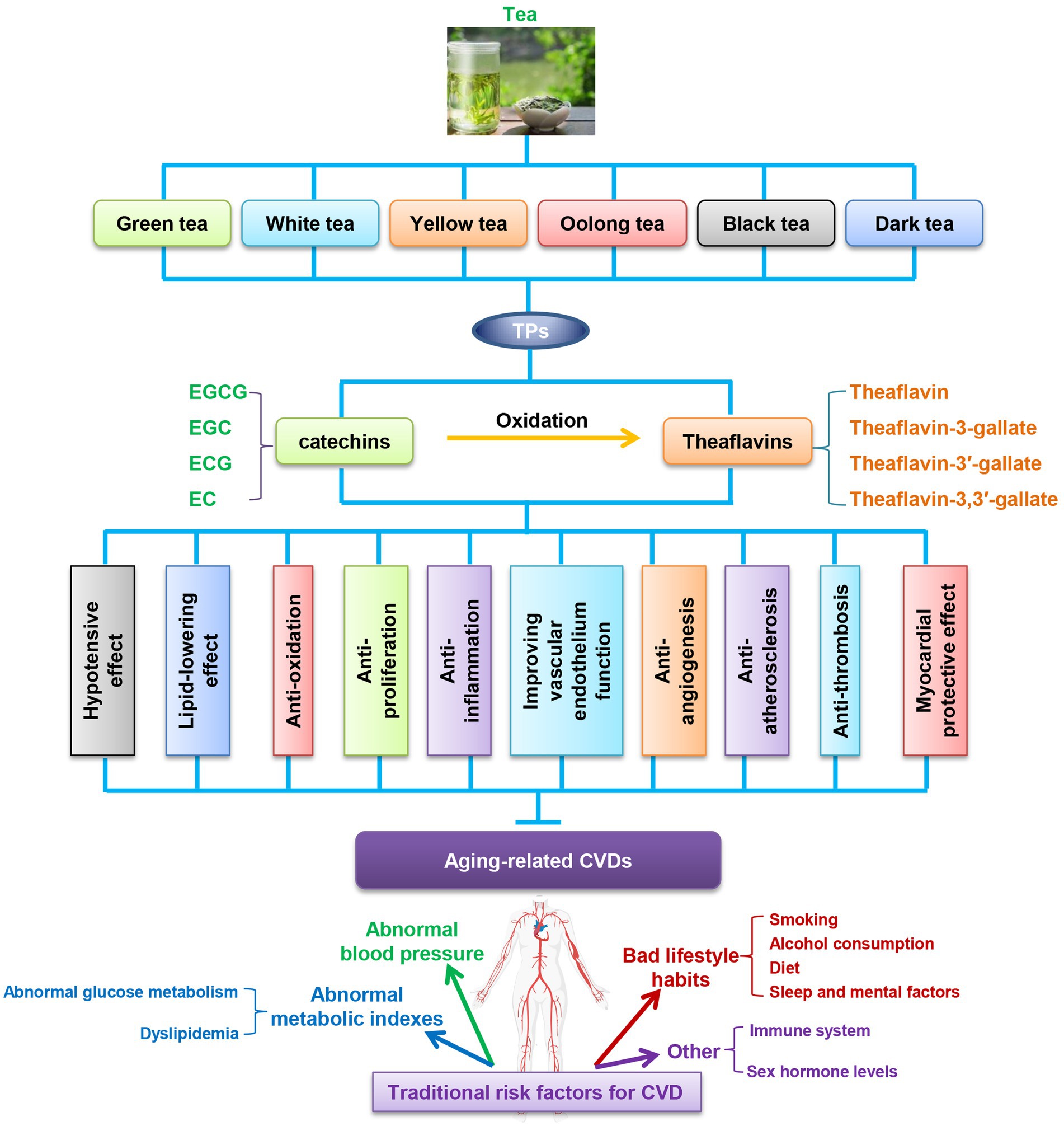
Figure 2. TPs improve aging related-CVDs in ten ways. CVDs, cardiovascular diseases; EC, (-)-epicatechin; ECG, (-)-epicatechin-3-gallate; EGC, (-)-epigallocatechin; EGCG, (-)-epigallocatechin-3-gallate; TPs, tea polyphenols.
In conclusion, a growing body of data suggests that TPs have an important role in the prevention and treatment of CVD by interfering with multiple signal transduction pathways. However, the specific molecular roles of TPs in various cells need to be studied in great depth.
Author contributions
JG, YaL, and YiL wrote the paper. KL collected the references. All authors contributed to the article and approved the submitted version.
Funding
This work is supported by funds from the National Natural Science Foundation of China (82271597), the Beijing Hospital Nova Project (BJ-2020-086), and the Chinese Academy of Medical Sciences Innovation Fund for Medical Sciences (2021-I2M-1-050).
Conflict of interest
The authors declare that the research was conducted in the absence of any commercial or financial relationships that could be construed as a potential conflict of interest.
Publisher’s note
All claims expressed in this article are solely those of the authors and do not necessarily represent those of their affiliated organizations, or those of the publisher, the editors and the reviewers. Any product that may be evaluated in this article, or claim that may be made by its manufacturer, is not guaranteed or endorsed by the publisher.
References
1. Aguilar, VM, Paul, A, Lazarko, D, and Levitan, I. Paradigms of endothelial stiffening in cardiovascular disease and vascular aging. Front Physiol. (2022) 13:1081119. doi: 10.3389/fphys.2022.1081119
2. Appiah, D, Luitel, S, Fuentes, N, and Nwabuo, CC. Perceived neighborhood social cohesion and the 10-year risk of cardiovascular disease in low-and middle-income countries: the World Health Organization study on global aging and adult health. Health Place. (2022) 77:102895. doi: 10.1016/j.healthplace.2022.102895
3. Budhathoki, S, Graham, C, Sethu, P, and Kannappan, R. Engineered aging cardiac tissue Chip model for studying cardiovascular disease. Cells Tissues Organs. (2022) 211:348–59. doi: 10.1159/000516954
4. Cao, Q, Li, M, Wang, T, Chen, Y, Dai, M, Zhang, D, et al. Association of early and supernormal vascular aging categories with cardiovascular disease in the Chinese population. Front Cardiovasc Med. (2022) 9:895792. doi: 10.3389/fcvm.2022.895792
5. Liberale, L, Badimon, L, Montecucco, F, Luscher, TF, Libby, P, and Camici, GG. Inflammation, aging, and cardiovascular disease: JACC review topic of the week. J Am Coll Cardiol. (2022) 79:837–47. doi: 10.1016/j.jacc.2021.12.017
6. Mathews, L, Han, D, Evans, MK, Zonderman, AB, Ndumele, CE, and Crews, DC. Prevalence of guideline-directed medical therapy for cardiovascular disease among Baltimore city adults in the healthy aging in neighborhoods of diversity across the life span (HANDLS) study. J Racial Ethn Health Disparities. (2022) 9:538–45. doi: 10.1007/s40615-021-00984-y
7. Montegut, L, Joseph, A, Chen, H, Abdellatif, M, Ruckenstuhl, C, Martins, I, et al. DBI/ACBP is a targetable autophagy checkpoint involved in aging and cardiovascular disease. Autophagy. (2022) 29:1–4. doi: 10.1080/15548627.2022.2160565
8. Moturi, S, Ghosh-Choudhary, SK, and Finkel, T. Cardiovascular disease and the biology of aging. J Mol Cell Cardiol. (2022) 167:109–17. doi: 10.1016/j.yjmcc.2022.04.005
9. Natarajan, P . Genomic aging, clonal hematopoiesis, and cardiovascular disease. Arterioscler Thromb Vasc Biol. (2023) 43:3–14. doi: 10.1161/ATVBAHA.122.318181
10. Puspitasari, YM, Ministrini, S, Schwarz, L, Karch, C, Liberale, L, and Camici, GG. Modern concepts in cardiovascular disease: inflamm-aging. Front Cell Dev Biol. (2022) 10:882211. doi: 10.3389/fcell.2022.882211
11. Sudre, CH, Moriconi, S, Rehwald, R, Smith, L, Tillin, T, Barnes, J, et al. Accelerated vascular aging: ethnic differences in basilar artery length and diameter, and its association with cardiovascular risk factors and cerebral small vessel disease. Front Cardiovasc Med. (2022) 9:939680. doi: 10.3389/fcvm.2022.939680
12. Quintero-Borregales, LM, Vergara-Rubio, A, Santos, A, Fama, L, and Goyanes, S. Black tea extracts/polyvinyl alcohol active nanofibers electrospun mats with sustained release of polyphenols for food packaging applications. Polymers. (2023) 15:1311. doi: 10.3390/polym15051311
13. Chen, Z, Shi, Z, and Meng, Z. Development and characterization of antioxidant-fortified oleogels by encapsulating hydrophilic tea polyphenols. Food Chem. (2023) 414:135664. doi: 10.1016/j.foodchem.2023.135664
14. Chu, C, Wang, X, Deng, Y, Ma, Y, Zou, C, Yang, M, et al. Discrimination of Chinese green tea according to tea polyphenols using fluorescence sensor array based on Tb (III) and Eu (III) doped Zr (IV) metal-organic frameworks. Spectrochim Acta A. (2023) 292:122380. doi: 10.1016/j.saa.2023.122380
15. Tang, GY, Meng, X, Gan, RY, Zhao, CN, Liu, Q, Feng, YB, et al. Health functions and related molecular mechanisms of tea components: an update review. Int J Mol Sci. (2019) 20:6196. doi: 10.3390/ijms20246196
16. Tang, GY, Zhao, CN, Xu, XY, Gan, RY, Cao, SY, Liu, Q, et al. Phytochemical composition and antioxidant capacity of 30 Chinese teas. Antioxidants. (2019) 8:180. doi: 10.3390/antiox8060180
17. Zhao, CN, Tang, GY, Cao, SY, Xu, XY, Gan, RY, Liu, Q, et al. Phenolic profiles and antioxidant activities of 30 tea infusions from green, black, oolong, white, yellow and dark teas. Antioxidants. (2019) 8:215. doi: 10.3390/antiox8070215
18. Khan, N, and Mukhtar, H. Tea polyphenols in promotion of human health. Nutrients. (2018) 11:39. doi: 10.3390/nu11010039
19. Liu, W, Ma, C, Li, HY, Yuan, SS, and Li, KJ. Tea polyphenols reduce inflammation of orbital fibroblasts in Graves’ Ophthalmopathy via the NF-kappaB/NLRP3 pathway. Curr Med Sci. (2023) 43:123–9. doi: 10.1007/s11596-023-2708-7
20. Zhang, W, Jiang, H, Rhim, JW, Cao, J, and Jiang, W. Tea polyphenols (TP): a promising natural additive for the manufacture of multifunctional active food packaging films. Crit Rev Food Sci Nutr. (2023) 63:288–301. doi: 10.1080/10408398.2021.1946007
21. Yu, Y, Song, J, Liu, X, Chen, B, Zhang, C, and Zhang, S. Tea polyphenols and catechins postpone evolution of antibiotic resistance genes and alter microbial community under stress of tetracycline. Ecotoxicol Environ Saf. (2023) 253:114675. doi: 10.1016/j.ecoenv.2023.114675
22. Keller, A, and Wallace, TC. Tea intake and cardiovascular disease: an umbrella review. Ann Med. (2021) 53:929–44. doi: 10.1080/07853890.2021.1933164
23. Zheng, H, Lin, F, Xin, N, Yang, L, and Zhu, P. Association of coffee, tea, and caffeine consumption with all-cause risk and specific mortality for cardiovascular disease patients. Front Nutr. (2022) 9:842856. doi: 10.3389/fnut.2022.842856
24. Zheng, K, Chen, Z, Fu, Y, Chen, L, Zhu, X, Chen, X, et al. Effect of tea polyphenols on the storage stability of non-fermented frozen dough: protein structures and state of water. Foods. (2022) 12:80. doi: 10.3390/foods12010080
25. Al Hroob, AM, Abukhalil, MH, Hussein, OE, and Mahmoud, AM. Pathophysiological mechanisms of diabetic cardiomyopathy and the therapeutic potential of epigallocatechin-3-gallate. Biomed Pharmacother. (2019) 109:2155–72. doi: 10.1016/j.biopha.2018.11.086
26. Hodgson, JM, Woodman, RJ, Puddey, IB, Mulder, T, Fuchs, D, and Croft, KD. Short-term effects of polyphenol-rich black tea on blood pressure in men and women. Food Funct. (2013) 4:111–5. doi: 10.1039/C2FO30186E
27. Khan, J, Deb, PK, Priya, S, Medina, KD, Devi, R, Walode, SG, et al. Dietary flavonoids: cardioprotective potential with antioxidant effects and their pharmacokinetic toxicological and therapeutic concerns. Molecules. (2021) 26:4021. doi: 10.3390/molecules26134021
28. Ye, Y, Warusawitharana, H, Zhao, H, Liu, Z, Li, B, Wu, Y, et al. Tea polyphenols attenuates inflammation via reducing lipopolysaccharides level and inhibiting TLR4/NF-kappaB pathway in obese mice. Plant Foods Hum Nutr. (2022) 77:105–11. doi: 10.1007/s11130-021-00937-0
29. Zhao, YQ, Jia, WB, Liao, SY, Xiang, L, Chen, W, Zou, Y, et al. Dietary assessment of ochratoxin a in Chinese dark tea and inhibitory effects of tea polyphenols on ochratoxigenic Aspergillus niger. Front Microbiol. (2022) 13:1073950. doi: 10.3389/fmicb.2022.1073950
30. Wu, D, Chen, R, Zhang, W, Lai, X, Sun, L, Li, Q, et al. Tea and its components reduce the production of uric acid by inhibiting xanthine oxidase. Food Nutr Res. (2022) 66:10. doi: 10.29219/fnr.v66.8239
31. Yao, Y, Chen, H, Chen, L, Ju, SY, Yang, H, Zeng, Y, et al. Type of tea consumption and depressive symptoms in Chinese older adults. BMC Geriatr. (2021) 21:331. doi: 10.1186/s12877-021-02203-z
32. Crea, F . Physical exercise, inflammation, and hypertension: how to improve cardiovascular prevention. Eur Heart J. (2022) 43:4763–6. doi: 10.1093/eurheartj/ehac695
33. Ettehad, D, Emdin, CA, Kiran, A, Anderson, SG, Callender, T, Emberson, J, et al. Blood pressure lowering for prevention of cardiovascular disease and death: a systematic review and meta-analysis. Lancet. (2016) 387:957–67. doi: 10.1016/S0140-6736(15)01225-8
34. Al, GH, Gotzinger, F, Bohm, M, and Mahfoud, F. Arterial hypertension—clinical trials update 2021. Nutr Metab Cardiovasc Dis. (2022) 32:21–31. doi: 10.1016/j.numecd.2021.09.007
35. Kim, Y, and Lee, S. Prevalence and risk factors associated with prehypertension by gender and age in a Korean population in the KNHANES 2010-2012. Iran J Public Health. (2015) 44:1594–602.
36. Esteghamati, A, Zandieh, A, Hafezi-Nejad, N, Sheikhbahaei, S, Abbasi, M, Gouya, MM, et al. Revising the fasting glucose threshold for detection of cardiovascular risk factors: analysing two representative population-based studies of more than 50,000 Iranians in 3 years: the national survey of risk factors for non-communicable diseases of Iran. Ann Hum Biol. (2015) 42:150–8. doi: 10.3109/03014460.2014.932011
37. Vignini, A, Moroni, C, Nanetti, L, Raffaelli, F, Cester, A, Gabrielli, O, et al. Alterations of platelet biochemical and functional properties in newly diagnosed type 1 diabetes: a role in cardiovascular risk? Diabetes Metab Res Rev. (2011) 27:277–85. doi: 10.1002/dmrr.1173
38. Watanabe, K, Thandavarayan, RA, Harima, M, Sari, FR, Gurusamy, N, Veeraveedu, PT, et al. Role of differential signaling pathways and oxidative stress in diabetic cardiomyopathy. Curr Cardiol Rev. (2010) 6:280–90. doi: 10.2174/157340310793566145
39. Siervo, M, Corander, M, Stranges, S, and Bluck, L. Post-challenge hyperglycaemia, nitric oxide production and endothelial dysfunction: the putative role of asymmetric dimethylarginine (ADMA). Nutr Metab Cardiovasc Dis. (2011) 21:1–10. doi: 10.1016/j.numecd.2010.10.003
40. Pirillo, A, Casula, M, Olmastroni, E, Norata, GD, and Catapano, AL. Global epidemiology of dyslipidaemias. Nat Rev Cardiol. (2021) 18:689–700. doi: 10.1038/s41569-021-00541-4
41. Atar, D, Jukema, JW, Molemans, B, Taub, PR, Goto, S, Mach, F, et al. New cardiovascular prevention guidelines: how to optimally manage dyslipidaemia and cardiovascular risk in 2021 in patients needing secondary prevention? Atherosclerosis. (2021) 319:51–61. doi: 10.1016/j.atherosclerosis.2020.12.013
42. Cholesterol Treatment Trialists’ (CTT) CollaborationFulcher, J, O'Connell, R, Voysey, M, Emberson, J, Blackwell, L, et al. Efficacy and safety of LDL-lowering therapy among men and women: meta-analysis of individual data from 174,000 participants in 27 randomised trials. Lancet. (2015) 385:1397–405. doi: 10.1016/S0140-6736(14)61368-4
43. Wang, P, Huang, Y, Ren, J, Rong, Y, Fan, L, Zhang, P, et al. Large-leaf yellow tea attenuates high glucose-induced vascular endothelial cell injury by up-regulating autophagy and down-regulating oxidative stress. Food Funct. (2022) 13:1890–905. doi: 10.1039/D1FO03405G
44. Wang, X, Dong, JY, Cui, R, Muraki, I, Shirai, K, Yamagishi, K, et al. Smoking cessation, weight gain and risk of cardiovascular disease. Heart. (2022) 108:375–81. doi: 10.1136/heartjnl-2021-318972
45. Zhang, WT, Liu, Z, Zhu, BC, Cui, ZY, Huang, C, Wang, XJ, et al. Effects of tobacco smoking on cardiovascular disease in patients with systemic lupus erythematosus: a systematic review and meta-analysis. Front Immunol. (2022) 13:967506. doi: 10.3389/fimmu.2022.967506
46. Duncan, MS, Freiberg, MS, Greevy, RA Jr, Kundu, S, Vasan, RS, and Tindle, HA. Association of smoking cessation with subsequent risk of cardiovascular disease. JAMA. (2019) 322:642–50. doi: 10.1001/jama.2019.10298
47. Kubozono, T, Miyata, M, Ueyama, K, Hamasaki, S, Kusano, K, Kubozono, O, et al. Acute and chronic effects of smoking on arterial stiffness. Circ J. (2011) 75:698–702. doi: 10.1253/circj.CJ-10-0552
48. Roerecke, M . Alcohol’s impact on the cardiovascular system. Nutrients. (2021) 13:3419. doi: 10.3390/nu13103419
49. Ronksley, PE, Brien, SE, Turner, BJ, Mukamal, KJ, and Ghali, WA. Association of alcohol consumption with selected cardiovascular disease outcomes: a systematic review and meta-analysis. BMJ. (2011) 342:d671. doi: 10.1136/bmj.d671
50. Minzer, S, Losno, RA, and Casas, R. The effect of alcohol on cardiovascular risk factors: is there new information? Nutrients. (2020) 12:912. doi: 10.3390/nu12040912
51. Rubin, E . To drink or not to drink: that is the question. Alcohol Clin Exp Res. (2014) 38:2889–92. doi: 10.1111/acer.12585
52. Gao, J, Akbari, A, and Wang, T. Green tea could improve elderly hypertension by modulating arterial stiffness, the activity of the renin/angiotensin/aldosterone axis, and the sodium-potassium pumps in old male rats. J Food Biochem. (2022) 46:e14398. doi: 10.1111/jfbc.14398
53. Gao, P, You, M, Li, L, Zhang, Q, Fang, X, Wei, X, et al. Salt-induced hepatic inflammatory memory contributes to cardiovascular damage through epigenetic modulation of SIRT3. Circulation. (2022) 145:375–91. doi: 10.1161/CIRCULATIONAHA.121.055600
54. Chrysant, SG . Effects of high salt intake on blood pressure and cardiovascular disease: the role of COX inhibitors. Clin Cardiol. (2016) 39:240–2. doi: 10.1002/clc.22536
55. Pradhan, I, Zeldin, DC, Ledent, C, Mustafa, JS, Falck, JR, and Nayeem, MA. High salt diet exacerbates vascular contraction in the absence of adenosine A2A receptor. J Cardiovasc Pharmacol. (2014) 63:385–94. doi: 10.1097/FJC.0000000000000058
56. Ganguli, D, Das, N, Saha, I, Biswas, P, Datta, S, Mukhopadhyay, B, et al. Major dietary patterns and their associations with cardiovascular risk factors among women in West Bengal. India Br J Nutr. (2011) 105:1520–9. doi: 10.1017/S0007114510005131
57. Malik, VS, and Hu, FB. The role of sugar-sweetened beverages in the global epidemics of obesity and chronic diseases. Nat Rev Endocrinol. (2022) 18:205–18. doi: 10.1038/s41574-021-00627-6
58. Aoqui, C, Chmielewski, S, Scherer, E, Eissler, R, Sollinger, D, Heid, I, et al. Microvascular dysfunction in the course of metabolic syndrome induced by high-fat diet. Cardiovasc Diabetol. (2014) 13:31. doi: 10.1186/1475-2840-13-31
59. Feriani, A, Bizzarri, M, Tir, M, Aldawood, N, Alobaid, H, Allagui, MS, et al. High-fat diet-induced aggravation of cardiovascular impairment in permethrin-treated Wistar rats. Ecotoxicol Environ Saf. (2021) 222:112461. doi: 10.1016/j.ecoenv.2021.112461
60. Bastien, CH, Vallieres, A, and Morin, CM. Validation of the insomnia severity index as an outcome measure for insomnia research. Sleep Med. (2001) 2:297–307. doi: 10.1016/S1389-9457(00)00065-4
61. Syauqy, A, Hsu, CY, Rau, HH, Kurniawan, AL, and Chao, JC. Association of sleep duration and insomnia symptoms with components of metabolic syndrome and inflammation in middle-aged and older adults with metabolic syndrome in Taiwan. Nutrients. (2019) 11:1848. doi: 10.3390/nu11081848
62. Cohen, BE, Edmondson, D, and Kronish, IM. State of the art review: depression, stress, anxiety, and cardiovascular disease. Am J Hypertens. (2015) 28:1295–302. doi: 10.1093/ajh/hpv047
63. Imes, CC, Lewis, FM, Austin, MA, and Dougherty, CM. My family medical history and me: feasibility results of a cardiovascular risk reduction intervention. Public Health Nurs. (2015) 32:246–55. doi: 10.1111/phn.12130
64. Zheng, X, Dreyer, RP, Hu, S, Spatz, ES, Masoudi, FA, Spertus, JA, et al. Age-specific gender differences in early mortality following ST-segment elevation myocardial infarction in China. Heart. (2015) 101:349–55. doi: 10.1136/heartjnl-2014-306456
65. Kane, AE, and Howlett, SE. Differences in cardiovascular aging in men and women. Adv Exp Med Biol. (2018) 1065:389–411. doi: 10.1007/978-3-319-77932-4_25
66. Soriano-Maldonado, A, Aparicio, VA, Felix-Redondo, FJ, and Fernandez-Berges, D. Severity of obesity and cardiometabolic risk factors in adults: sex differences and role of physical activity. The HERMEX study. Int J Cardiol. (2016) 223:352–9. doi: 10.1016/j.ijcard.2016.07.253
67. Bassig, BA, Dean, HH, Shu, XO, Vermeulen, R, Chen, BE, Katki, HA, et al. Ischaemic heart disease and stroke mortality by specific coal type among non-smoking women with substantial indoor air pollution exposure in China. Int J Epidemiol. (2020) 49:56–68. doi: 10.1093/ije/dyz158
68. Sang, S . Impacts of biotransformation on the health benefits of tea polyphenols. J Nutr Sci Vitaminol. (2022) 68:S124–5. doi: 10.3177/jnsv.68.S124
69. Yin, B, Lian, R, Li, Z, Liu, Y, Yang, S, Huang, Z, et al. Tea polyphenols enhanced the antioxidant capacity and induced Hsps to relieve heat stress injury. Oxidative Med Cell Longev. (2021) 2021:9615429. doi: 10.1155/2021/9615429
70. Pan, SY, Nie, Q, Tai, HC, Song, XL, Tong, YF, Zhang, LJ, et al. Tea and tea drinking: China’s outstanding contributions to the mankind. Chin Med. (2022) 17:27. doi: 10.1186/s13020-022-00571-1
71. Serban, C, Sahebkar, A, Ursoniu, S, Andrica, F, and Banach, M. Effect of sour tea (Hibiscus sabdariffa L.) on arterial hypertension: a systematic review and meta-analysis of randomized controlled trials. J Hypertens. (2015) 33:1119–27. doi: 10.1097/HJH.0000000000000585
72. Mahdavi-Roshan, M, Salari, A, Ghorbani, Z, and Ashouri, A. The effects of regular consumption of green or black tea beverage on blood pressure in those with elevated blood pressure or hypertension: a systematic review and meta-analysis. Complement Ther Med. (2020) 51:102430. doi: 10.1016/j.ctim.2020.102430
73. Teramoto, M, Yamagishi, K, Muraki, I, Tamakoshi, A, and Iso, H. Coffee and green tea consumption and cardiovascular disease mortality among people with and without hypertension. J Am Heart Assoc. (2023) 12:e026477. doi: 10.1161/JAHA.122.026477
74. Li, F, Ohnishi-Kameyama, M, Takahashi, Y, and Yamaki, K. Tea polyphenols as novel and potent inhibitory substances against renin activity. J Agric Food Chem. (2013) 61:9697–704. doi: 10.1021/jf403710b
75. de la Fuente Munoz, M, de la Fuente Fernandez, M, Roman-Carmena, M, Iglesias de la Cruz, MDC, Amor, S, Martorell, P, et al. Supplementation with two new standardized tea extracts prevents the development of hypertension in mice with metabolic syndrome. Antioxidants. (2022) 11:1573. doi: 10.3390/antiox11081573
76. Grassi, D, Draijer, R, Schalkwijk, C, Desideri, G, D'Angeli, A, Francavilla, S, et al. Black tea increases circulating endothelial progenitor cells and improves flow mediated dilatation counteracting deleterious effects from a fat load in hypertensive patients: a randomized controlled study. Nutrients. (2016) 8:727. doi: 10.3390/nu8110727
77. Naumovski, N, Foscolou, A, D’Cunha, NM, Tyrovolas, S, Chrysohoou, C, Sidossis, LS, et al. The association between green and black tea consumption on successful aging: a combined analysis of the ATTICA and MEDiterranean ISlands (MEDIS) epidemiological studies. Molecules. (2019) 24:1862. doi: 10.3390/molecules24101862
78. Turgut Coşan, D, Saydam, F, Ozbayer, C, Doganer, F, Soyocak, A, Gunes, HV, et al. Impact of tannic acid on blood pressure, oxidative stress and urinary parameters in L-NNA-induced hypertensive rats. Cytotechnology. (2015) 67:97–105. doi: 10.1007/s10616-013-9661-4
79. Chatree, S, Sitticharoon, C, Maikaew, P, Pongwattanapakin, K, Keadkraichaiwat, I, Churintaraphan, M, et al. Epigallocatechin gallate decreases plasma triglyceride, blood pressure, and serum kisspeptin in obese human subjects. Exp Biol Med (Maywood). (2021) 246:163–76. doi: 10.1177/1535370220962708
80. Imran, A, Butt, MS, Arshad, MS, Arshad, MU, Saeed, F, Sohaib, M, et al. Exploring the potential of black tea based flavonoids against hyperlipidemia related disorders. Lipids Health Dis. (2018) 17:57. doi: 10.1186/s12944-018-0688-6
81. Huang, S, Li, J, Wu, Y, Ranjbar, S, Xing, A, Zhao, H, et al. Tea consumption and longitudinal change in high-density lipoprotein cholesterol concentration in Chinese adults. J Am Heart Assoc. (2018) 7:e008814. doi: 10.1161/JAHA.118.008814
82. Katada, S, Oishi, S, Yanagawa, K, Ishii, S, Oki, M, Matsui, Y, et al. Concomitant use of tea catechins affects absorption and serum triglyceride-lowering effects of monoglucosyl hesperidin. Food Funct. (2021) 12:9339–46. doi: 10.1039/D1FO01917A
83. Ma, H, Zhang, B, Hu, Y, Wang, J, Liu, J, Qin, R, et al. Correlation analysis of intestinal redox state with the gut microbiota reveals the positive intervention of tea polyphenols on hyperlipidemia in high fat diet fed mice. J Agric Food Chem. (2019) 67:7325–35. doi: 10.1021/acs.jafc.9b02211
84. Wang, J, Li, P, Liu, S, Zhang, B, Hu, Y, Ma, H, et al. Green tea leaf powder prevents dyslipidemia in high-fat diet-fed mice by modulating gut microbiota. Food Nutr Res. (2020) 64:10. doi: 10.29219/fnr.v64.3672
85. Xu, Y, Zhang, M, Wu, T, Dai, S, Xu, J, and Zhou, Z. The anti-obesity effect of green tea polysaccharides, polyphenols and caffeine in rats fed with a high-fat diet. Food Funct. (2015) 6:297–304. doi: 10.1039/c4fo00970c
86. Ma, W, Shi, Y, Yang, G, Shi, J, Ji, J, Zhang, Y, et al. Hypolipidaemic and antioxidant effects of various Chinese dark tea extracts obtained from the same raw material and their main chemical components. Food Chem. (2022) 375:131877. doi: 10.1016/j.foodchem.2021.131877
87. Yu, J, Li, W, Xiao, X, Huang, Q, Yu, J, Yang, Y, et al. (-)-Epicatechin gallate blocks the development of atherosclerosis by regulating oxidative stress in vivo and in vitro. Food Funct. (2021) 12:8715–27. doi: 10.1039/D1FO00846C
88. Leung, LK, Su, Y, Chen, R, Zhang, Z, Huang, Y, and Chen, ZY. Theaflavins in black tea and catechins in green tea are equally effective antioxidants. J Nutr. (2001) 131:2248–51. doi: 10.1093/jn/131.9.2248
89. Lee, W, Min, WK, Chun, S, Lee, YW, Park, H, Lee, DH, et al. Long-term effects of green tea ingestion on atherosclerotic biological markers in smokers. Clin Biochem. (2005) 38:84–7. doi: 10.1016/j.clinbiochem.2004.09.024
90. Wu, CC, Hsu, MC, Hsieh, CW, Lin, JB, Lai, PH, and Wung, BS. Upregulation of heme oxygenase-1 by Epigallocatechin-3-gallate via the phosphatidylinositol 3-kinase/Akt and ERK pathways. Life Sci. (2006) 78:2889–97. doi: 10.1016/j.lfs.2005.11.013
91. Negishi, H, Xu, JW, Ikeda, K, Njelekela, M, Nara, Y, and Yamori, Y. Black and green tea polyphenols attenuate blood pressure increases in stroke-prone spontaneously hypertensive rats. J Nutr. (2004) 134:38–42. doi: 10.1093/jn/134.1.38
92. Xiao, XT, He, SQ, Wu, NN, Lin, XC, Zhao, J, and Tian, C. Green tea polyphenols prevent early vascular aging induced by high-fat diet via promoting autophagy in young adult rats. Curr Med Sci. (2022) 42:981–90. doi: 10.1007/s11596-022-2604-6
93. Hodgson, JM, Puddey, IB, Croft, KD, Burke, V, Mori, TA, Caccetta, RA, et al. Acute effects of ingestion of black and green tea on lipoprotein oxidation. Am J Clin Nutr. (2000) 71:1103–7. doi: 10.1093/ajcn/71.5.1103
94. Suzuki-Sugihara, N, Kishimoto, Y, Saita, E, Taguchi, C, Kobayashi, M, Ichitani, M, et al. Green tea catechins prevent low-density lipoprotein oxidation via their accumulation in low-density lipoprotein particles in humans. Nutr Res. (2016) 36:16–23. doi: 10.1016/j.nutres.2015.10.012
95. Zhan, XL, Yang, XH, Gu, YH, Guo, LL, and Jin, HM. Epigallocatechin gallate protects against homocysteine-induced vascular smooth muscle cell proliferation. Mol Cell Biochem. (2018) 439:131–40. doi: 10.1007/s11010-017-3142-6
96. Kim, CH, and Moon, SK. Epigallocatechin-3-gallate causes the p21/WAF1-mediated G(1)-phase arrest of cell cycle and inhibits matrix metalloproteinase-9 expression in TNF-alpha-induced vascular smooth muscle cells. Arch Biochem Biophys. (2005) 435:264–72. doi: 10.1016/j.abb.2004.12.022
97. Sukhthankar, M, Yamaguchi, K, Lee, SH, McEntee, MF, Eling, TE, Hara, Y, et al. A green tea component suppresses posttranslational expression of basic fibroblast growth factor in colorectal cancer. Gastroenterology. (2008) 134:1972–80. doi: 10.1053/j.gastro.2008.02.095
98. Xu, S, Chang, L, Hu, Y, Zhao, X, Huang, S, Chen, Z, et al. Tea polyphenol modified, photothermal responsive and ROS generative black phosphorus quantum dots as nanoplatforms for promoting MRSA infected wounds healing in diabetic rats. J Nanobiotechnology. (2021) 19:362. doi: 10.1186/s12951-021-01106-w
99. Zhang, N, Weber, A, Li, B, Lyons, R, Contag, PR, Purchio, AF, et al. An inducible nitric oxide synthase-luciferase reporter system for in vivo testing of anti-inflammatory compounds in transgenic mice. J Immunol. (2003) 170:6307–19. doi: 10.4049/jimmunol.170.12.6307
100. Cheng, XW, Kuzuya, M, Sasaki, T, Kanda, S, Tamaya-Mori, N, Koike, T, et al. Green tea catechins inhibit neointimal hyperplasia in a rat carotid arterial injury model by TIMP-2 overexpression. Cardiovasc Res. (2004) 62:594–602. doi: 10.1016/j.cardiores.2004.01.023
101. Orozco-Sevilla, V, Naftalovich, R, Hoffmann, T, London, D, Czernizer, E, Yang, C, et al. Epigallocatechin-3-gallate is a potent phytochemical inhibitor of intimal hyperplasia in the wire-injured carotid artery. J Vasc Surg. (2013) 58:1360–5. doi: 10.1016/j.jvs.2012.11.090
102. Yan, X, Li, Y, Yu, H, Wang, W, Wu, C, Yang, Y, et al. Epigallocatechin-3-gallate inhibits H2O2-induced apoptosis in mouse vascular smooth muscle cells via 67kD Laminin receptor. Sci Rep. (2017) 7:7774. doi: 10.1038/s41598-017-08301-6
103. Bornhoeft, J, Castaneda, D, Nemoseck, T, Wang, P, Henning, SM, and Hong, MY. The protective effects of green tea polyphenols: lipid profile, inflammation, and antioxidant capacity in rats fed an atherogenic diet and dextran sodium sulfate. J Med Food. (2012) 15:726–32. doi: 10.1089/jmf.2011.0258
104. Roh, E, Kim, JE, Kwon, JY, Park, JS, Bode, AM, Dong, Z, et al. Molecular mechanisms of green tea polyphenols with protective effects against skin photoaging. Crit Rev Food Sci Nutr. (2017) 57:1631–7. doi: 10.1080/10408398.2014.1003365
105. Basu, A, Du, M, Sanchez, K, Leyva, MJ, Betts, NM, Blevins, S, et al. Green tea minimally affects biomarkers of inflammation in obese subjects with metabolic syndrome. Nutrition. (2011) 27:206–13. doi: 10.1016/j.nut.2010.01.015
106. Steptoe, A, Gibson, EL, Vuononvirta, R, Hamer, M, Wardle, J, Rycroft, JA, et al. The effects of chronic tea intake on platelet activation and inflammation: a double-blind placebo controlled trial. Atherosclerosis. (2007) 193:277–82. doi: 10.1016/j.atherosclerosis.2006.08.054
107. Shen, CL, Samathanam, C, Graham, S, Dagda, RY, Chyu, MC, and Dunn, DM. Green tea polyphenols and 1-alpha-OH-vitamin D3 attenuate chronic inflammation-induced myocardial fibrosis in female rats. J Med Food. (2012) 15:269–77. doi: 10.1089/jmf.2011.0163
108. Hofbauer, R, Frass, M, Gmeiner, B, Handler, S, Speiser, W, and Kapiotis, S. The green tea extract epigallocatechin gallate is able to reduce neutrophil transmigration through monolayers of endothelial cells. Wien Klin Wochenschr. (1999) 111:278–82.
109. Ludwig, A, Lorenz, M, Grimbo, N, Steinle, F, Meiners, S, Bartsch, C, et al. The tea flavonoid epigallocatechin-3-gallate reduces cytokine-induced VCAM-1 expression and monocyte adhesion to endothelial cells. Biochem Biophys Res Commun. (2004) 316:659–65. doi: 10.1016/j.bbrc.2004.02.099
110. Kawai, K, Tsuno, NH, Kitayama, J, Okaji, Y, Yazawa, K, Asakage, M, et al. Epigallocatechin gallate attenuates adhesion and migration of CD8+ T cells by binding to CD11b. J Allergy Clin Immunol. (2004) 113:1211–7. doi: 10.1016/j.jaci.2004.02.044
111. Yang, F, de Villiers, WJ, CJ, MC, and Varilek, GW. Green tea polyphenols block endotoxin-induced tumor necrosis factor-production and lethality in a murine model. J Nutr. (1998) 128:2334–40. doi: 10.1093/jn/128.12.2334
112. Hua, F, Zhou, P, Liu, PP, and Bao, GH. Rat plasma protein binding of kaempferol-3-O-rutinoside from Lu’an GuaPian tea and its anti-inflammatory mechanism for cardiovascular protection. J Food Biochem. (2021) 45:e13749. doi: 10.1111/jfbc.13749
113. Reddy, AT, Lakshmi, SP, Maruthi, PE, Varadacharyulu, NC, and Kodidhela, LD. Epigallocatechin gallate suppresses inflammation in human coronary artery endothelial cells by inhibiting NF-kappaB. Life Sci. (2020) 258:118136. doi: 10.1016/j.lfs.2020.118136
114. Chen, PY, Li, S, Koh, YC, Wu, JC, Yang, MJ, Ho, CT, et al. Oolong tea extract and citrus peel polymethoxyflavones reduce transformation of l-carnitine to trimethylamine-N-oxide and decrease vascular inflammation in l-carnitine feeding mice. J Agric Food Chem. (2019) 67:7869–79. doi: 10.1021/acs.jafc.9b03092
115. Li, Y, Rahman, SU, Huang, Y, Zhang, Y, Ming, P, Zhu, L, et al. Green tea polyphenols decrease weight gain, ameliorate alteration of gut microbiota, and mitigate intestinal inflammation in canines with high-fat-diet-induced obesity. J Nutr Biochem. (2020) 78:108324. doi: 10.1016/j.jnutbio.2019.108324
116. Huang, Y, Chan, NW, Lau, CW, Yao, XQ, Chan, FL, and Chen, ZY. Involvement of endothelium/nitric oxide in vasorelaxation induced by purified green tea (-)epicatechin. Biochim Biophys Acta. (1999) 1427:322–8. doi: 10.1016/s0304-4165(99)00034-3
117. Huang, Y, Zhang, A, Lau, CW, and Chen, ZY. Vasorelaxant effects of purified green tea epicatechin derivatives in rat mesenteric artery. Life Sci. (1998) 63:275–83. doi: 10.1016/S0024-3205(98)00273-2
118. Papparella, I, Ceolotto, G, Montemurro, D, Antonello, M, Garbisa, S, Rossi, G, et al. Green tea attenuates angiotensin II-induced cardiac hypertrophy in rats by modulating reactive oxygen species production and the Src/epidermal growth factor receptor/Akt signaling pathway. J Nutr. (2008) 138:1596–601. doi: 10.1093/jn/138.9.1596
119. Nogueira, LP, Nogueira Neto, JF, Klein, MR, and Sanjuliani, AF. Short-term effects of green tea on blood pressure, endothelial function, and metabolic profile in obese Prehypertensive women: a crossover randomized clinical trial. J Am Coll Nutr. (2017) 36:108–15. doi: 10.1080/07315724.2016.1194236
120. Ying, CJ, Xu, JW, Ikeda, K, Takahashi, K, Nara, Y, and Yamori, Y. Tea polyphenols regulate nicotinamide adenine dinucleotide phosphate oxidase subunit expression and ameliorate angiotensin II-induced hyperpermeability in endothelial cells. Hypertens Res. (2003) 26:823–8. doi: 10.1291/hypres.26.823
121. Uto-Kondo, H, Ayaori, M, Kishimoto, Y, Adachi, T, Takiguchi, S, Yakushiji, E, et al. Consumption of polyphenol-rich juar tea increases endothelium-bound extracellular superoxide dismutase levels in men with metabolic syndrome: link with LDL oxidizability. Int J Food Sci Nutr. (2013) 64:407–14. doi: 10.3109/09637486.2012.759185
122. Tenopoulou, M, and Doulias, PT. Endothelial nitric oxide synthase-derived nitric oxide in the regulation of metabolism. F1000Res. (2020) 9:F1000. doi: 10.12688/f1000research.19998.1
123. Liu, J, Ying, C, Meng, Y, Yi, W, Fan, Z, Zuo, X, et al. Green tea polyphenols inhibit plasminogen activator inhibitor-1 expression and secretion in endothelial cells. Blood Coagul Fibrinolysis. (2009) 20:552–7. doi: 10.1097/MBC.0b013e32832e05f0
124. Woodward, KA, Hopkins, ND, Draijer, R, de Graaf, Y, Low, DA, and DHJ, T. Acute black tea consumption improves cutaneous vascular function in healthy middle-aged humans. Clin Nutr. (2018) 37:242–9. doi: 10.1016/j.clnu.2016.12.013
125. Kim, HS, Montana, V, Jang, HJ, Parpura, V, and Kim, JA. Epigallocatechin gallate (EGCG) stimulates autophagy in vascular endothelial cells: a potential role for reducing lipid accumulation. J Biol Chem. (2013) 288:22693–705. doi: 10.1074/jbc.M113.477505
126. Braile, M, Marcella, S, Cristinziano, L, Galdiero, MR, Modestino, L, Ferrara, AL, et al. VEGF-A in cardiomyocytes and heart diseases. Int J Mol Sci. (2020) 21:5294. doi: 10.3390/ijms21155294
127. Rahbarghazi, A, Siahkouhian, M, Rahbarghazi, R, Ahmadi, M, Bolboli, L, Keyhanmanesh, R, et al. Role of melatonin in the angiogenesis potential; highlights on the cardiovascular disease. J Inflamm. (2021) 18:4. doi: 10.1186/s12950-021-00269-5
128. Kavantzas, N, Chatziioannou, A, Yanni, AE, Tsakayannis, D, Balafoutas, D, Agrogiannis, G, et al. Effect of green tea on angiogenesis and severity of atherosclerosis in cholesterol-fed rabbit. Vasc Pharmacol. (2006) 44:461–3. doi: 10.1016/j.vph.2006.03.008
129. Rodriguez, SK, Guo, W, Liu, L, Band, MA, Paulson, EK, and Meydani, M. Green tea catechin, epigallocatechin-3-gallate, inhibits vascular endothelial growth factor angiogenic signaling by disrupting the formation of a receptor complex. Int J Cancer. (2006) 118:1635–44. doi: 10.1002/ijc.21545
130. Sartippour, MR, Heber, D, Zhang, L, Beatty, P, Elashoff, D, Elashoff, R, et al. Inhibition of fibroblast growth factors by green tea. Int J Oncol. (2002) 21:487–91.
131. Annabi, B, Lee, YT, Martel, C, Pilorget, A, Bahary, JP, and Beliveau, R. Radiation induced-tubulogenesis in endothelial cells is antagonized by the antiangiogenic properties of green tea polyphenol (-) epigallocatechin-3-gallate. Cancer Biol Ther. (2003) 2:642–9.
132. Poznyak, AV, Litvinova, L, Poggio, P, Moschetta, D, Sukhorukov, VN, and Orekhov, AN. From diabetes to atherosclerosis: potential of metformin for management of cardiovascular disease. Int J Mol Sci. (2022) 23:9738. doi: 10.3390/ijms23179738
133. Cai, Y, Kurita-Ochiai, T, Hashizume, T, and Yamamoto, M. Green tea epigallocatechin-3-gallate attenuates Porphyromonas gingivalis-induced atherosclerosis. Pathog Dis. (2013) 67:76–83. doi: 10.1111/2049-632X.12001
134. Wahyudi, S, and Sargowo, D. Green tea polyphenols inhibit oxidized LDL-induced NF-KB activation in human umbilical vein endothelial cells. Acta Med Indones. (2007) 39:66–70.
135. Kishimoto, Y, Saita, E, Taguchi, C, Aoyama, M, Ikegami, Y, Ohmori, R, et al. Associations between green tea consumption and coffee consumption and the prevalence of coronary artery disease. J Nutr Sci Vitaminol. (2020) 66:237–45. doi: 10.3177/jnsv.66.237
136. Loke, WM, Proudfoot, JM, Hodgson, JM, McKinley, AJ, Hime, N, Magat, M, et al. Specific dietary polyphenols attenuate atherosclerosis in apolipoprotein E-knockout mice by alleviating inflammation and endothelial dysfunction. Arterioscler Thromb Vasc Biol. (2010) 30:749–57. doi: 10.1161/ATVBAHA.109.199687
137. Liao, ZL, Zeng, BH, Wang, W, Li, GH, Wu, F, Wang, L, et al. Impact of the consumption of tea polyphenols on early atherosclerotic lesion formation and intestinal Bifidobacteria in high-fat-fed ApoE−/− mice. Front Nutr. (2016) 3:42. doi: 10.3389/fnut.2016.00042
138. Ding, S, Jiang, J, Yu, P, Zhang, G, Zhang, G, and Liu, X. Green tea polyphenol treatment attenuates atherosclerosis in high-fat diet-fed apolipoprotein E-knockout mice via alleviating dyslipidemia and up-regulating autophagy. PLoS One. (2017) 12:e0181666. doi: 10.1371/journal.pone.0181666
139. Alkarithi, G, Duval, C, Shi, Y, Macrae, FL, and Ariens, RAS. Thrombus structural composition in cardiovascular disease. Arterioscler Thromb Vasc Biol. (2021) 41:2370–83. doi: 10.1161/ATVBAHA.120.315754
140. Jin, YR, Im, JH, Park, ES, Cho, MR, Han, XH, Lee, JJ, et al. Antiplatelet activity of epigallocatechin gallate is mediated by the inhibition of PLCgamma2 phosphorylation, elevation of PGD2 production, and maintaining calcium-ATPase activity. J Cardiovasc Pharmacol. (2008) 51:45–54. doi: 10.1097/FJC.0b013e31815ab4b6
141. Kang, WS, Lim, IH, Yuk, DY, Chung, KH, Park, JB, Yoo, HS, et al. Antithrombotic activities of green tea catechins and (-)-epigallocatechin gallate. Thromb Res. (1999) 96:229–37. doi: 10.1016/S0049-3848(99)00104-8
142. Lill, G, Voit, S, Schror, K, and Weber, AA. Complex effects of different green tea catechins on human platelets. FEBS Lett. (2003) 546:265–70. doi: 10.1016/S0014-5793(03)00599-4
143. Kang, WS, Chung, KH, Chung, JH, Lee, JY, Park, JB, Zhang, YH, et al. Antiplatelet activity of green tea catechins is mediated by inhibition of cytoplasmic calcium increase. J Cardiovasc Pharmacol. (2001) 38:875–84. doi: 10.1097/00005344-200112000-00009
144. Li, Y, Ge, JP, Ma, K, Yin, YY, He, J, and Gu, JP. The combination of EGCG with warfarin reduces deep vein thrombosis in rabbits through modulating HIF-1alpha and VEGF via the PI3K/AKT and ERK1/2 signaling pathways. Chin J Nat Med. (2022) 20:679–90. doi: 10.1016/S1875-5364(22)60172-9
145. Hsieh, SR, Cheng, WC, Su, YM, Chiu, CH, and Liou, YM. Molecular targets for anti-oxidative protection of green tea polyphenols against myocardial ischemic injury. Biomedicine. (2014) 4:23. doi: 10.7603/s40681-014-0023-0
146. Sheng, R, Gu, ZL, and Xie, ML. Epigallocatechin gallate, the major component of polyphenols in green tea, inhibits telomere attrition mediated cardiomyocyte apoptosis in cardiac hypertrophy. Int J Cardiol. (2013) 162:199–209. doi: 10.1016/j.ijcard.2011.07.083
147. Zhou, H, Chen, Y, Huang, SW, Hu, PF, and Tang, LJ. Regulation of autophagy by tea polyphenols in diabetic cardiomyopathy. J Zhejiang Univ Sci B. (2018) 19:333–41. doi: 10.1631/jzus.B1700415
148. Tung, WC, Rizzo, B, Dabbagh, Y, Saraswat, S, Romanczyk, M, Codorniu-Hernandez, E, et al. Polyphenols bind to low density lipoprotein at biologically relevant concentrations that are protective for heart disease. Arch Biochem Biophys. (2020) 694:108589. doi: 10.1016/j.abb.2020.108589
149. Shen, CL, Samathanam, C, Tatum, OL, Graham, S, Tubb, C, Cao, JJ, et al. Green tea polyphenols avert chronic inflammation-induced myocardial fibrosis of female rats. Inflamm Res. (2011) 60:665–72. doi: 10.1007/s00011-011-0320-y
150. Li, W, Yu, J, Xiao, X, Li, W, Zang, L, Han, T, et al. The inhibitory effect of (-)-epicatechin gallate on the proliferation and migration of vascular smooth muscle cells weakens and stabilizes atherosclerosis. Eur J Pharmacol. (2021) 891:173761. doi: 10.1016/j.ejphar.2020.173761
151. Locher, R, Emmanuele, L, Suter, PM, Vetter, W, and Barton, M. Green tea polyphenols inhibit human vascular smooth muscle cell proliferation stimulated by native low-density lipoprotein. Eur J Pharmacol. (2002) 434:1–7. doi: 10.1016/S0014-2999(01)01535-7
152. Meng, J, Chen, Y, Wang, J, Qiu, J, Chang, C, Bi, F, et al. EGCG protects vascular endothelial cells from oxidative stress-induced damage by targeting the autophagy-dependent PI3K-AKT-mTOR pathway. Ann Transl Med. (2020) 8:200. doi: 10.21037/atm.2020.01.92
153. Zhang, Z, and Zhang, D. (-)-Epigallocatechin-3-gallate inhibits eNOS uncoupling and alleviates high glucose-induced dysfunction and apoptosis of human umbilical vein endothelial cells by PI3K/AKT/eNOS pathway. Diabetes Metab Syndr Obes. (2020) 13:2495–504. doi: 10.2147/DMSO.S260901
154. Liu, S, Sun, Z, Chu, P, Li, H, Ahsan, A, Zhou, Z, et al. EGCG protects against homocysteine-induced human umbilical vein endothelial cells apoptosis by modulating mitochondrial-dependent apoptotic signaling and PI3K/Akt/eNOS signaling pathways. Apoptosis. (2017) 22:672–80. doi: 10.1007/s10495-017-1360-8
155. Moyle, CW, Cerezo, AB, Winterbone, MS, Hollands, WJ, Alexeev, Y, Needs, PW, et al. Potent inhibition of VEGFR-2 activation by tight binding of green tea epigallocatechin gallate and apple procyanidins to VEGF: relevance to angiogenesis. Mol Nutr Food Res. (2015) 59:401–12. doi: 10.1002/mnfr.201400478
Keywords: aging, cardiovascular diseases, tea polyphenols, prevention, treatment
Citation: Guo J, Li K, Lin Y and Liu Y (2023) Protective effects and molecular mechanisms of tea polyphenols on cardiovascular diseases. Front. Nutr. 10:1202378. doi: 10.3389/fnut.2023.1202378
Edited by:
Peng An, China Agricultural University, ChinaReviewed by:
Guangshan Zhao, Henan Agricultural University, ChinaShuhong Dai, Shenzhen Polytechnic, China
Wuli Zhao, Chinese Academy of Medical Sciences and Peking Union Medical College, China
Copyright © 2023 Guo, Li, Lin and Liu. This is an open-access article distributed under the terms of the Creative Commons Attribution License (CC BY). The use, distribution or reproduction in other forums is permitted, provided the original author(s) and the copyright owner(s) are credited and that the original publication in this journal is cited, in accordance with accepted academic practice. No use, distribution or reproduction is permitted which does not comply with these terms.
*Correspondence: Yajun Lin, bGlueWFqdW4yMDAwQDEyNi5jb20=; Yinghua Liu, bGl1eWluZ2h1YTc3QDE2My5jb20=