- 1Department of Medical Emergencies, School of Nursing, Social Development and Health Promotion Research Center, Gonabad University of Medical Sciences, Gonabad, Iran
- 2Research Center for Biochemistry and Nutrition in Metabolic Diseases, Institute for Basic Sciences, Kashan University of Medical Sciences, Kashan, Iran
- 3Student Research Committee, Kashan University of Medical Sciences, Kashan, Iran
- 4Laser Research Centre, University of Johannesburg, Doornfontein, South Africa
- 5Social Determinants of Health (SDH) Research Center, Kashan University of Medical Sciences, Kashan, Iran
Nanoplastics (NPs) and microplastics (MPs) made of polystyrene (PS) can be toxic to humans, especially by ingestion of plastic particles. These substances are often introduced into the gastrointestinal tract, where they can cause several adverse effects, including disturbances in intestinal flora, mutagenicity, cytotoxicity, reproductive toxicity, neurotoxicity, and exacerbated oxidative stress. Although there are widespread reports of the protective effects of probiotics on the harm caused by chemical contaminants, limited information is available on how these organisms may protect against PS toxicity in either humans or animals. The protective effects of probiotics can be seen in organs, such as the gastrointestinal tract, reproductive tract, and even the brain. It has been shown that both MPs and NPs could induce microbial dysbiosis in the gut, nose and lungs, and probiotic bacteria could be considered for both prevention and treatment. Furthermore, the improvement in gut dysbiosis and intestinal leakage after probiotics consumption may reduce inflammatory biomarkers and avoid unnecessary activation of the immune system. Herein, we show probiotics may overcome the toxicity of polystyrene nanoplastics and microplastics in humans, although some studies are required before any clinical recommendations can be made.
1. Introduction
The global use of plastics is increasing annually, and it has been predicted that plastic manufacturing may rise from 368 million tons in 2019 to 33 billion tons by 2050 (1, 2).
The widespread use of plastics in fields such as industrial production, medical manufacturing, construction, and agriculture can be attributed to their unique properties, such as chemical stability, light weight, wear resistance, and corrosion resistance. The accumulation of plastic aggregates highly resistant to degradation is an important global environmental issue because of their stable crystal structure and high molecular weight (2). Plastic particles smaller than 5 mm are defined as microplastics (MPs) while those smaller than 0.1 μm as nanoplastics (NPs) (2).
Polystyrene (PS) is a synthetic aromatic hydrocarbon polymer formed by polymerizing the styrene monomer (vinylbenzene), which is synthesized from benzene and ethylene followed by dehydrogenation of ethylbenzene. PS is a thermoplastic polymer with good transparency, long-lasting stability, and is easy to paint (3). It is a solid material extensively used to fabricate consumer products like toys, CDs, and toothbrushes, as well as to make Styrofoam. PS has limited elasticity and is melt-formed or expanded. Styrofoam has been extensively employed to fabricate food containers such as plates, trays, and cups, and is also used in a variety of packaging products, clips, toys, and office equipment (4).
PS MPs can be formed in the environment by photooxidative and mechanical degradation processes, although more study is needed on the mechanisms involved. Degradation of PS products such as disposable plates, coffee cup lids, and foams has recently been simulated by using UV exposure (5) or by mechanical stress in the marine environment (6). Ekvall et al. (7) observed that the mechanical degradation of expanded foam as well as coffee cups and lids could produce small PS particles (MPs as well as NPs) (6).
The relatively easy absorption of these MPs and NPs from the gastrointestinal (GI) tract makes them a matter of concern (2, 8). MPs as well as NPs may be incorporated into many food products due to their widespread distribution and broad bioavailability in most terrestrial and aquatic environments. According to reports, there are a variety of ways that plastic MPs as well as NPs can be introduced into the human food chain, including the consumption of various food products obtained from exposed livestock, or the various stages of food processing involving plastic wrapping and packaging (9–11). Different foods (like honey or sea food) as well as beverages (like beer) have been found to be contaminated by MPs as well as NPs (12–16).
The routes by which these particles enter the body involve the mouth, esophagus, stomach, and intestines. This means the initial toxic effects are often observed in the GI tract. Some of the reported side effects include, disruption of the normal intestinal flora, decreased intestinal mucosal secretion, damage to the intestinal mucosal epithelium, and impaired metabolism of fatty acids and amino acids leading to the excessive accumulation of lipids (17). The chronic ingestion of MPs and NPs can negatively affect the gut barrier function. Previous studies reported that MPs and NPs could induce microbial dysbiosis in the gut (18, 19), or nose and lungs (20). NPs increased mRNA and protein levels of interleukin (IL)-8, IL-10, IL-1β and TNF-α in the gut of zebrafish to a greater extent than MPs, indicating that the NPs may have a more serious effect on gut microbial dysbiosis and inflammation (19). Demonstrated that oral administration of these MPs and NPs decreased beneficial intestinal microbes with known tight junction-promoting functionality, suggesting an important indirect toxic effect on the gut microbiota as well as NP-induced gut barrier dysfunction. In a mouse study, showed that MPs had a stronger effect on microbial dysbiosis in the lungs compared to NPs (18). Abnormal levels of Staphylococcus spp. in the nose, as well as Roseburia, Eggerthella and Corynebacterium spp. in the lungs were observed in both MP and NP exposed mice. These bacteria could be potential biomarkers of MP and NP-induced airway dysbiosis in mice (20).
The permissible range for chronic exposure to styrene has been set at 300 ppm (i.e., 1,000 mg/m3) by the Environmental Protection Agency (EPA). The FDA stated that the admissible daily intake (ADI) of styrene should be limited to 90,000 micrograms per person per day (4, 21).
One study developed a probabilistic model of lifelong exposure to plastic MPs for children and adults. In this physiology-based pharmacokinetic sub-model, the routes of administration were considered as intestinal absorption from food or by inhalation, while biliary excretion, and exposure to plastic-related chemicals was also included. The goal of this model was to simulate the concentration of MPs in the intestine, feces, and body tissues, while the stool samples provided validation versus empirical data. The median dose of MPs was calculated to be 583 ng per person per day in adults, and 184 ng per person per day in children. This dose could result in irreversible accumulation of particles in body tissues up to 6.4 ng per person for children under 18 years of age, and up to 40.7 ng per person for adults up to 70 years of age. They confirmed the agreement between the concentrations of the simulated MPs in the feces with the empirical data. The final analysis found there was a small share of MPs within the total chemical intake due to adsorption from food or MPs swallowed from 9 consumption simulations based on two-phase adsorption kinetics, reversibility, and specific size (22).
The goal of the current review was therefore to summarize the absorption routes of PS MPs as well as NPs contaminating the food chain, their existing levels in foods, and possible side effects on human health. We further survey how probiotics could function to protect against these adverse effects in humans, and finally provide relevant suggestions for future work (Figure 1).
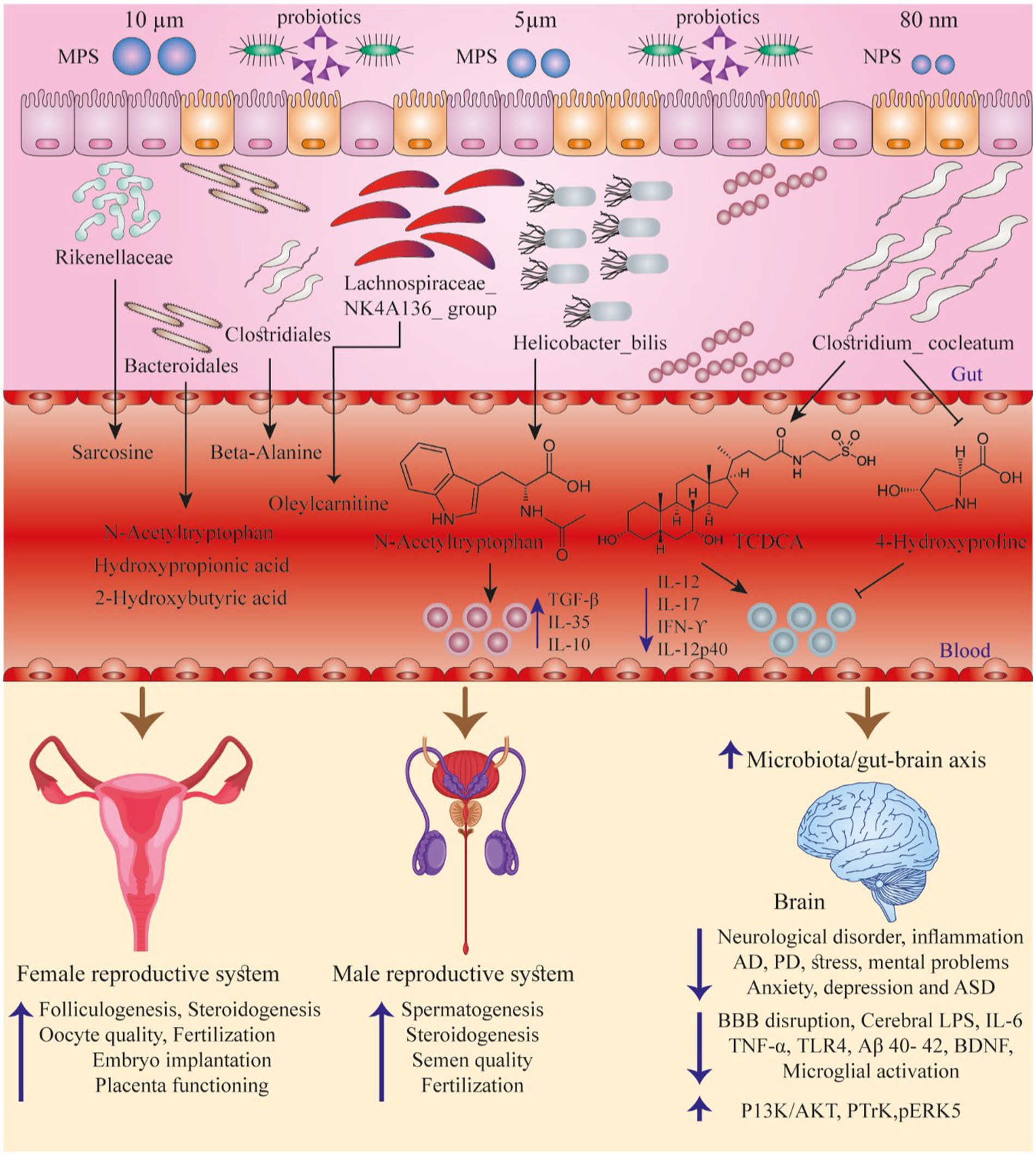
Figure 1. Summarizes some studies discussing the interactions between PS particles, GI normal flora, and the function of various organs. Adapted from (23).
2. Literature searching and data acquisition
In this review, we searched for reports of the toxic hazard effects of PS MPs or NPs in vitro and in vivo. In addition, we investigated the detoxification activity of probiotics on chemical food contaminants, with an emphasis on the protective effects against PS MPs and NPs. We searched the electronic databases Web of Science, PubMed, EMBASE, CINAHL and Google Scholar from 2015 to 2023. The inclusion criteria were as follows: studies on the toxicity of PS MPs and NPs in various cell and animal models; protective effects of probiotic bacteria; suggested mechanisms of action. The exclusion criteria were as follows: studies written in a language other than English; studies did not focus on the toxicity of PS MPs and NPs; studies that did not discuss the relationship between the protective effects of probiotic bacteria against chemical contaminants. Considering that there was no study discussing the protective effect of probiotics specifically against the toxicity of PS MPs and NPs, several studies on the mechanism of detoxification by probiotics on various chemical food contaminants were included.
3. Oral ingestion, cellular internalization and diffusion of PS MPs and NPs
Oral ingestion of PS MPs as well as NPs has been identified as the main way these particles enter the human body (24). We found a few studies that examined the toxicity of PS NPs in humans, even though research has shown that plastic MPs are regularly ingested via drink and food products by the majority of the population in advanced countries (10, 25). Another study evaluated human colectomy samples for the presence of MPs, and detected the presence of MPs in all 11 samples with a mean value of 331 particles per sample or 28.1 ± 15.4 particles per gram of tissue, showing that MPs were ubiquitous within the human colon (26).
There is a very low probability of paracellular penetration of the MPs in the intestine, because the size of the biological pores in the narrow junction channels is only about 1.5 nm (27). NPs probably penetrate via the lymphatic tissue and in particular enter the microfold (M) cells of Peyer’s patches via phagocytosis (28). In one study, peritoneal macrophages in mice were able to phagocytose polystyrene and polymethacrylate particles of 1, 5, and 12 μm in size. They also confirmed the uptake of only 0.04–0.3% of these particles from the intestines of exposed mice (29, 30).
One important question is whether the NPs can penetrate the intestinal epithelium, which could result in systemic exposure in humans. Multiple in vivo as well as in vitro studies have been performed to investigate how PS NPs behave in a variety of animal models. Credible evidence has shown that when consumed orally, PS NPs may be 10 to 100 times more bioavailable than MPs (31, 32). Whether or not there is a correlation between absorption, size, and the structure of NPs is still controversial (1, 32).
Wang et al. (33) investigated the toxicity of PS NPs on the renal tubular epithelial cell line HKC and the human liver cell line HL-7702 using integrated proteomic and metabolomic analysis. Most of the differentially expressed proteins and metabolites were involved in a variety of metabolic pathways, for example, glycolysis, citrate cycle, oxidative phosphorylation, and amino acid metabolism, suggesting that PS NPs could disturb the global metabolism in human cells. The altered energy metabolism induced by PS NPs was confirmed by further studies. Moreover, mTORC1 signaling, a central regulator of cellular metabolism, was inhibited after nanoplastic exposure, likely serving as a link between lysosome dysfunction and metabolic disturbances (33). Cortés et al. (34) showed that PS NPs had toxic effects on human intestinal epithelial Caco-2 cells. According to their results, after the cells were exposed to PS NPs, cytotoxicity and ROS were increased, along with genotoxicity mediated by DNA oxidative damage, and an increase in the expression of stress-related genes.
Moreover when the PS NPs are swallowed and reach the gastrointestinal lumen, they can be mechanically or chemically degraded thus altering their rate of adsorption. The interaction of nanoparticles with different molecules such as carbohydrates, proteins, water, lipids, nucleic acids and ions within the GI tract according to the (35) may produce NPs that are surrounded by a set of proteins called a “protein corona” (36). The PS NPs may then become coated with protein coronas with different complex shapes or chemical properties (37). Walczak et al. (32) showed that the protein-based corona underwent changes when subjected to a laboratory model of human digestive fluid, resulting in further morphological variation in the NPs. Therefore, ingested PS NPs, depending on their size and physicochemical properties, could potentially translocate across the intestinal barrier (32). Furthermore, the NPs may interact with dispersed organic matter or metal nanoparticles present in the marine environment, which could then affect their aggregation and deposition in the ocean, and thus their uptake by various types of seafood (37).
Active endocytosis and passive diffusion are the two main mechanisms by which plastic particles can be taken up into cells (38–40). Endocytosis includes phagocytosis and pinocytosis, e.g., clathrin-mediated macropinocytosis, caveolin-mediated pinocytosis, as well as pinocytosis independent of clathrin/caveolin (41). Macrophages, neutrophils, monocytes, and dendritic cells are designed to carry out phagocytosis, i.e., professional phagocytes (42). Endothelial cells may internalize 40 nm PS NPs through caveolin-mediated as well as clathrin-mediated endocytosis (43). Reportedly, the endocytosis of PS particles with different sizes of 20, 120, 190, and 200 nm occurred via the clathrin-mediated pathway in canine renal cells, lung epithelial cells, and murine melanoma cells (44–46).
Another study reported that clathrin-mediated endocytosis was responsible for the uptake of particles smaller than 200 nm, while caveolin-mediated endocytosis occurred for particles bigger than 500 nm (46). However, porcine endothelial cells used macropinocytosis (independent of caveolae or clathrin) for the internalization of PS NPs smaller than 100 nm, (47), while Caco-2 cells (human colorectal carcinoma) adsorbed these particles by micropinocytosis (48). The MPs larger than 1 μm were not taken up by non-phagocytic eukaryotic cells through endocytosis, but were taken up by macrophages through micropinocytosis or phagocytosis (43, 46). Thus, the size of the PS particles and the cell type determine the type of endocytosis employed for cell uptake. The particle size may also be correlated with the rate of cell uptake, because the smaller the particle, the faster the rate of cell uptake (46).
Passive diffusion appears to be the pathway of choice for internalization of carboxylate as well as amidine-modified PS particles of 20 nm and 120 nm diameter into alveolar epithelial cells (49). The mechanisms of cellular excretion of these particles may also be divided into active and passive pathways (38, 50). If the particles enter the cell passively, they may then enter the lysosomes and undergo active exocytosis in an energy-consuming process, or may exit the cell passively without any energy expenditure. If the particles enter the cell through endocytosis they are automatically routed to lysosomes, and will be excreted from the cells by active exocytosis (51). In conclusion, the particular route of cell uptake for PS particles will also affect their excretion mechanisms.
4. Toxic effects of PS particles
Generally speaking, the cellular effects of PS particles will differ according to their size and concentration, as well as the type of organism or cells that are exposed to the PS particles, and the duration of exposure (2, 52). In the nervous system of mice, PS particles (MPs or NPs) may alter the level of various neurotransmitters (catecholamines, acetylcholine, and serotonin) which could then affect stress responses and behavior (52, 53). These PS particles could also increase the level of nitric oxide (NO), acetylcholine (by inhibiting acetylcholine esterase), and thiobarbituric acid reactive substances (TBARS) (52), which are a marker of oxidative damage (54). Increased acetylcholine and oxidative damage could explain the memory deficits observed in mice exposed to PS particles (54).
With reference to possible genotoxic effects, it was reported that when Ctenopharyngodon idella (grass carp) were exposed to PS NPs, DNA molecules were damaged either by strand breaks or cross-links with other molecules. The DNA damage could be caused either by direct interaction between the particles and the DNA, or by disturbances in cholesterol metabolism, which then produces free radicals (52).
Some evidence for PS MP-mediated mutagenesis was provided by the detection of nuclear contracted erythrocytes, dual-nucleated erythrocytes, kidney-shaped displaced nuclei, micronuclei, nuclear vacuoles and notched nuclei. In addition, cytotoxic effects were related to the MP shape and size (52). Mutagenicity as well as the formation of oxygen free radicals caused by PS NPs has also been reported in HCT116 colorectal cancer cells (55).
Mice with experimental colitis exposed to PS MPs showed an increased inflammatory response (56). The proinflammatory cytokine levels were significantly increased by PS MPs, which in turn could cause abnormal lipid metabolism and elevated triglyceride levels. These changes could promote monocyte recruitment into tissues and a stronger inflammatory response, i.e., formation of a vicious cycle (56, 57). The elevated triglycerides may be taken up by hepatocytes, resulting in hepatitis, or the PS MPs may directly find their way into the liver tissue to cause hepatitis (56).
Ding et al. treated mice with PS NPs for 3 days, and identified their presence in intestinal, gastric, as well as hepatic cells. In a mechanistic study, they used GES-1 cells (gastric epithelial cells), and found that PS NPs could increase the formation of reactive oxygen species (ROS), which in turn inhibited growth and caused apoptosis in these cells. They proposed that these particles could interfere with the barrier function of the epithelium in the GI tract (58).
Pan et al. treated mice with PS MPs for 90 days to assess their hepatotoxic effects. They found that PS MPs could activate PERK (protein kinase RNA-like endoplasmic reticulum kinase in murine hepatocytes to increase ER stress and induce apoptosis). The fact that PERK knockdown inhibited the induction of apoptosis confirmed this finding (59).
Murine models were also designed to evaluate the toxic effects of PS MPs in the germinal organs, i.e., ovaries (60) and testes (60, 61). These researchers observed that different cell types in these organs adsorbed the particles with a consequent increase in oxidative damage, which in turn activated signaling cascades, such as NLRP3/Caspase-I or Nrf2/HO-1/NF-kB (60) to induce apoptosis.
Shengchen et al. (62) examined how PS MPs would affect the injury response of murine rhabdomyocytes and their ability to repair muscle damage. Again, it was observed that these particles increased oxidative damage in the cells, and inhibited rhabdomyocyte proliferation, as well as their ability to repair the muscle tissue. Furthermore, the PS MPs prevented MAPK from being phosphorylated, while activating NF-κB, which induced the myocytes to differentiate into adipocytes.
In fish exposed to MPs, hematological parameters can be used as sensitive indicators (63). The hematopoietic system of mammals has also been studied, where it was found that PS NPs accumulated in the bone marrow of mice and altered cellular functions (31, 64). Mice treated with PS particles showed a lower number of white blood cells, but a higher number of thrombocytes (64, 65). In vitro, it was shown that PS NPs increased oxidative stress as well as DNA damage in human lymphocytes (66).
Table 1 lists some published studies on the toxicity of PS particles in vivo as well as in vitro.
5. Probiotics and their protective effects
Probiotics are living microbial supplements which can be consumed by the host. They have beneficial effects on the host by promoting a better microbial balance within the gut and by modulating the host immune system (84–87). These microorganisms, specifically lactic acid bacteria (LAB), have been reported to control hypertension, modify lipid profiles and hyperglycemia, and also suppress oxidative damage (88, 89).
Probiotic microorganisms, whether they be the normal flora of the GI tract, or are consumed as microbial supplements may also interact with PS particles to modify their toxic effects on different tissues (90, 91). With regard to the hematopoietic system, a relationship between the intestinal microflora and hematological disorders has been observed in mammals (92). Excessive antibiotic administration can paradoxically cause bacterial infections in the gut (Clostridium difficile), which can also cause neutropenia, thrombocytopenia, and pancytopenia, demonstrating how various microorganisms can affect the interactions between the gut and the hematopoietic system (93, 94). However, the precise mechanisms explaining why probiotics could modify PS hematotoxicity remain elusive (23). One probable explanation may be that probiotics can produce specific small molecule metabolites, or else drive signaling cascades, which help the organism to boost its immune response or to suppress inappropriate inflammatory responses (95–98).
5.1. Mechanism of the protective effects of probiotics against damage by toxic materials
The introduction of probiotic bacteria (including LAB strains) into the GI tract can eliminate or decrease the toxic effects of heavy metals or toxic fungi (99). Bacterial molecules like mannan oligosaccharides or peptidoglycans present in the cell wall of these organisms can enable the cells to bind to these toxins (99–101). Some probiotic bacteria and yeasts, including L. rhamnosus, L. plantarum, and Saccharomyces cerevisiae, are able to adsorb various heavy metals like lead, cadmium, copper, as well as mercury (102–104). Probiotics can also bind to molecules and toxins like benzo[a]pyrene (105), mycotoxins (106), bisphenol A (BPA), or phthalates (100).
Some probiotics were even able to degrade the common plastic ingredient, bisphenol A (107, 108). Given the ability of these microorganisms to affect the toxicodynamics of different endocrine-disrupting chemicals, the use of probiotic supplementation for improving the microbiome could be an effective intervention to counter different toxins (100, 109). In diabetic patients, it was observed that probiotics could decrease the level of systemic inflammation (110) and also control hyperglycemia (88, 111). It should be noted that compared to single-strain probiotics, polybiotics (mixed probiotics) have a greater ability to bind to toxins (112, 113). Another factor that may affect the toxin-binding ability of the probiotics is the pH of the GI tract. For example, the biosorptive ability of L. plantarum P1 was observed to be higher in an alkaline environment, probably because the cell wall structure is more stable at a higher pH (114).
The natural gut microbiota is composed of beneficial as well as pathogenic bacterial species, and when the pathogenic species dominate over the beneficial species, this is called gut dysbiosis. Dysbiosis in the gut has been associated with worse gastrointestinal damage after oral exposure to MPs and NPs (18, 115). Microbial dysbiosis leads to a more pronounced inflammatory immune response, increases oxidative damage, and stimulates the progression of cancer (116). Several studies have reported the use of probiotic strains to modulate the gut microbiome and normalize immune responses for the prevention and management of intestinal dysbiosis (Table 2).
Clinical trials have also highlighted the efficacy of probiotic strains in reducing the side effects of microbial dysbiosis caused by various diseases or medical treatments (124–126). Showed that consumption of a complex mixture of probiotics (Bifidobacterium infantis, Lactobacillus acidophilus, Enterococcus faecalis, and Bacillus cereus) significantly restored the gut and oral microbial diversity(125). Probiotics comsumption increased the prevalence of Holdemanella, Enterococcus, and Coprococcus_2 species, while it decreased Fusobacterium, Eubacterium_ruminantium_group, Ruminococcus_1, and Parasutterella in the human gut. The use of probiotics can improve the gut microbial population, increase mucus-secretion, and prevent the destruction of tight junction proteins by decreasing the level of lipopolysaccharides (LPS). Furthermore, the decrease in gut dysbiosis and intestinal leakage after probiotics therapy could reduce the secretion of inflammatory biomarkers and blunt any unnecessary activation of the immune system (127).
5.2. Mechanism of the protective effects of probiotics against organ damage
The consumption of beneficial probiotics can affect body weight, glucose and lipid metabolism, resulting in less systemic inflammation as well as improved insulin sensitivity (100, 128).
Baralić et al. treated mice with a combination of BPA and phthalates (toxins often occurring in the human daily diet) and simultaneously administered polybiotics (mainly different species of Lactobacillus) to examine how these probiotics would modify the toxicity of these compounds. The probiotics showed a beneficial effect on the endocrine system, as well as hepatic, renal, and splenic tissues. Furthermore, it was reported that at the cellular level probiotics may have anti-oxidant properties (100).
With regard to the biosorptive ability or toxin binding, this ability of probiotics has been repeatedly confirmed by different studies, and was found to be due to the binding properties of specific protein and polysaccharide structures present in their cell walls (121, 129–131).
With regard to hepatoprotective effects, S. boulardii was able to improve liver function as well reduce liver damage caused by hepatic steatosis, liver fibrosis, or infections (132). As mentioned above, the hepatoprotective effects of probiotics were discussed by Baralić et al. (109).
Lactobacillus spp. and S. boulardii can act as antioxidants by preventing lipids from being peroxidized, as well as by activating antioxidant enzymes (133). S. boulardii was able to improve the tissue antioxidant status and inhibit neutrophil recruitment, thereby reducing intestinal damage and associated hepatitis (134). A reduction of oxidative damage in hepatocytes by probiotics was also reported by Oumeddour et al. (135).
In another study, mice received oral L. rhamnosus GG over 13 weeks, after which they were protected from insulin resistance as well as showing less adiposity in mesenteric adipose tissue and the liver. However, they also found increased fatty acid oxidation in muscles and liver, a reduction in liver gluconeogenesis, increased muscle expression of GLUT4, and increased white adipose tissue secretion of adiponectin (136). There was a reduction in hepatic total lipid levels and lower liver weight in mice with leptin-resistant obesity and type 2 diabetes, following daily oral gavage over 4 weeks with S. boulardii (137).
A murine model of colitis was employed by Kim et al. to test the effects of L. plantarum CBT. They found systemic anti-inflammatory effects and an improved immunomodulatory function, predominantly in lymphocytes (138).
The kidney protective effects of probiotics were reported in a study by Baralić et al. (109). They found a lower serum urea level as well as less nephronic damage in pathologic assessments of tissue from mice treated with probiotics (109). The nephroprotective effects of these microorganisms against mercury toxicity were also reported by Majlesi et al. (139).
5.3. Probiotics and neurotoxicity
In a study by Alipour Nosrani et al. (140) polybiotics (consisting of B. bifidum, L. acidophilus, L. fermentum and L. reuteri) were administered to patients with Parkinson’s disease. They found improvements in cognitive function, rotational motor responses, less nerve injury and lipid peroxidation.
Cheon et al. cultured SH-SY5Y cells (neuroblastoma cell line) to assess the neuroprotective effects of three strains of Lactobacillus. MPP+ (1-methyl-4-phenylpyridinium) was used as a neuotoxin to induce Parkinson’s disease-like neuronal damage. In general, the probiotic treatment was able to inhibit cellular death induced by MPP+, and at the molecular level, it upregulated brain-derived neurotrophic factor (BDNF) (141).
5.4. Probiotics and reproductive toxicity
One study combined probiotics (L. salivarius, L. brevis, L. planarum) in the form of a human vaginal tablet to treat bacterial vaginosis. They also investigated the protective effects of this probiotic preparation on the peroxidation of sperm lipids, which resulted in maintainance of sperm vitality and motility (142). Dardmeh et al. (143) recently suggested that probiotics could be an option to reduce the adverse consequences of obesity on the quality of semen. The effect of probiotic L. rhamnosus was evaluated on lipid status, testicular weight, sperm kinetics and relevant hormones like LH, FSH, as well as testosterone in mice. The direct effect was to improve sperm maturation and spermatogenesis, and the indirect effect was to eliminate obesity-related complications.
Another recent study examined the effect of probiotics on relieving the inflammation caused by polycystic ovary syndrome (PCOS). PCOS is a treatable endocrine disorder, associated with ovarian dysfunction, excessive secretion of testosterone, increased inflammation, and consequent infertility. The beneficial effects of Lactobacillus in PCOS patients, included reduction of inflammation, as demonstrated by lower IL-6 and higher IL-10 levels, which resulted in improved fertility (144).
5.5. Mechanism of protective effects of probiotics against mutagenicity and cytotoxicity
The carcinogenicity and genotoxicity of heterocyclic aromatic amines could be decreased by probiotics, owing to their ability to bind to toxins (122). One study demonstrated that L. rhamnosus GG was able to absorb 61.0% of aflatoxin B1 (AFB1) administered to Caco-2 cells, after one hour of incubation at 37°C. The cells exposed to AFB1 alone underwent DNA fragmentation, but not when co-incubated with the probiotic strain (145). In the human hepatoma cell line (HepG2), there was a significant decrease in the generation of micronuclei mediated by patulin and ochratoxin A in the presence of B. animalis VM12 as well as L. acidophilus VM20 (146). In another study using Caco-2 cells, L. acidophilus NCFM was able to inhibit DNA damage and cell death induced by phthalate, mainly by altering expression levels of genes related to P53, MAPK and mTOR pathways (122).
6. Conclusion
The broad use of plastics and their products are steadily increasing today. Due to various benefits, such as low cost and suitable physicochemical properties, plastics are being used in many industrial products involved in construction, packaging, and transport. This wide use of plastics has led to the contamination of all possible natural environments, i.e., water, soil, and air, with microplastic or nanoplastic particles as well as bulk plastic. These plastics (especially PS) undergo degradation in these environments to produce particles with micro and nano sizes, which may find their way into the body of different organisms, including humans. They have been proved to have toxic effects on many different lifeforms. Hence, finding or developing new platforms/procedures for reducing the toxicity of nanoplastics and microplastics are needed.
According to studies on toxicity effects of MPS/NPS on gut microbiota, we suggest that major mechanisms that may be recruited by probiotics against MPS/NPS, are enhancement of the intestinal epithelial barrier, production of various cytokines and chemokines from dendritic cells, lymphocytes, macrophages, mast cells, granulocytes, and intestinal epithelial cells, and IgA-producing cells and consequent IgA secretion, colonization and normalization of perturbed intestinal microbial communities (147, 148). In addition, probiotics production of volatile fatty acids, namely, short-chain fatty acids and branched-chain fatty acids, increase of anti-inflammatory effects, e.g., through alterations of TLR4/TLR2/MyD88/NF𝂻 signaling and alterations in the NLRP3 inflammasome, which play a role in the maintenance of energy homeostasis and regulation of functionality in peripheral tissues. Probiotic metabolites are also able to interact with the brain-gut axis and enhancement of the CNS homeostasis. In this regard, it has been suggested that the use of probiotics might reduce the toxicity of these materials in humans; a claim that needs further evaluation.
Author contributions
JB and ZB: investigation, validation, writing-review, and editing. RS: investigation, project administration, validation, writing-original draft, writing-review, and editing. MH: writing-review and editing. All authors contributed to the article and approved the submitted version.
Conflict of interest
The authors declare that the research was conducted in the absence of any commercial or financial relationships that could be construed as a potential conflict of interest.
Publisher’s note
All claims expressed in this article are solely those of the authors and do not necessarily represent those of their affiliated organizations, or those of the publisher, the editors and the reviewers. Any product that may be evaluated in this article, or claim that may be made by its manufacturer, is not guaranteed or endorsed by the publisher.
References
1. Yee, MSL , Hii, LW , Looi, CK , Lim, WM , Wong, SF , Kok, YY, et al. Impact of microplastics and nanoplastics on human health. Nano. (2021) 11:496. doi: 10.3390/nano11020496
2. Yin, K , Wang, Y , Zhao, H , Wang, D , Guo, M , Mu, M, et al. A comparative review of microplastics and nanoplastics: toxicity hazards on digestive, reproductive and nervous system. Sci Total Environ. (2021) 774:145758. doi: 10.1016/j.scitotenv.2021.145758
3. Wünsch, JR . Polystyrene: synthesis, production and applications. Shawbury, Shrewsbury, Shropshire, UK: Rapra Technology Ltd (2000).
4. Kik, K , Bukowska, B , and Sicińska, P . Polystyrene nanoparticles: sources, occurrence in the environment, distribution in tissues, accumulation and toxicity to various organisms. Environ Pollut. (2020) 262:114297. doi: 10.1016/j.envpol.2020.114297
5. Lambert, S , and Wagner, M . Characterisation of nanoplastics during the degradation of polystyrene. Chemosphere. (2016) 145:265–8. doi: 10.1016/j.chemosphere.2015.11.078
6. Efimova, I , Bagaeva, M , Bagaev, A , Kileso, A , and Chubarenko, IP . Secondary microplastics generation in the sea swash zone with coarse bottom sediments: laboratory experiments. Front Mar Sci. (2018) 5:313. doi: 10.3389/fmars.2018.00313
7. Ekvall, MT , Lundqvist, M , Kelpsiene, E , Šileikis, E , Gunnarsson, SB , and Cedervall, T . Nanoplastics formed during the mechanical breakdown of daily-use polystyrene products. Nanoscale Adv. (2019) 1:1055–61. doi: 10.1039/C8NA00210J
8. Chen, Q , Zhang, H , Allgeier, A , Zhou, Q , Ouellet, JD , Crawford, SE, et al. Marine microplastics bound dioxin-like chemicals: model explanation and risk assessment. J Hazard Mater. (2019) 364:82–90. doi: 10.1016/j.jhazmat.2018.10.032
9. Karami, A , Golieskardi, A , Choo, CK , Larat, V , Galloway, TS , and Salamatinia, B . The presence of microplastics in commercial salts from different countries. Sci Rep. (2017) 7:1–11. doi: 10.1038/srep46173
10. Mason, SA , Welch, VG , and Neratko, J . Synthetic polymer contamination in bottled water. Front Chem. (2018) 6:407. doi: 10.3389/fchem.2018.00407
11. Santillo, D , Miller, K , and Johnston, P . Microplastics as contaminants in commercially important seafood species. Integr Environ Assess Manag. (2017) 13:516–21. doi: 10.1002/ieam.1909
12. Devriese, LI , Van der Meulen, MD , Maes, T , Bekaert, K , Paul-Pont, I , Frère, L, et al. Microplastic contamination in brown shrimp (Crangon crangon, Linnaeus 1758) from coastal waters of the southern North Sea and channel area. Mar Pollut Bull. (2015) 98:179–87. doi: 10.1016/j.marpolbul.2015.06.051
13. Li, J , Yang, D , Li, L , Jabeen, K , and Shi, H . Microplastics in commercial bivalves from China. Environ Pollut. (2015) 207:190–5. doi: 10.1016/j.envpol.2015.09.018
14. Liebezeit, G , and Liebezeit, E . Non-pollen particulates in honey and sugar. New York, USA: Food Addit Contam Part A. (2013) 30:2136–40. doi: 10.1080/19440049.2013.843025
15. Neves, D , Sobral, P , Ferreira, JL , and Pereira, T . Ingestion of microplastics by commercial fish off the Portuguese coast. Mar Pollut Bull. (2015) 101:119–26. doi: 10.1016/j.marpolbul.2015.11.008
16. Yang, D , Shi, H , Li, L , Li, J , Jabeen, K , and Kolandhasamy, P . Microplastic pollution in table salts from China. Environ Sci Technol. (2015) 49:13622–7. doi: 10.1021/acs.est.5b03163
17. Fackelmann, G , and Sommer, S . Microplastics and the gut microbiome: how chronically exposed species may suffer from gut dysbiosis. Mar Pollut Bull. (2019) 143:193–203. doi: 10.1016/j.marpolbul.2019.04.030
18. Qiao, J , Chen, R , Wang, M , Bai, R , Cui, X , Liu, Y, et al. Perturbation of gut microbiota plays an important role in micro/nanoplastics-induced gut barrier dysfunction. Nanoscale. (2021) 13:8806–16. doi: 10.1039/D1NR00038A
19. Xie, S , Zhou, A , Wei, T , Li, S , Yang, B , Xu, G, et al. Nanoplastics induce more serious microbiota dysbiosis and inflammation in the gut of adult zebrafish than microplastics. Bull Environ Contam Toxicol. (2021) 107:640–50. doi: 10.1007/s00128-021-03348-8
20. Zha, H , Xia, J , Li, S , Lv, J , Zhuge, A , Tang, R, et al. Airborne polystyrene microplastics and nanoplastics induce nasal and lung microbial dysbiosis in mice. Chemosphere. (2023) 310:136764. doi: 10.1016/j.chemosphere.2022.136764
21. United States Food and Drug Administration . The safety of styrene-based polymers for food-contact use 2013. Washington, DC: American Chemistry Council (2015).
22. Mohamed Nor, NH , Kooi, M , Diepens, NJ , and Koelmans, AA . Lifetime accumulation of microplastic in children and adults. Environ Sci Technol. (2021) 55:5084–96. doi: 10.1021/acs.est.0c07384
23. Jing, J , Zhang, L , Han, L , Wang, J , Zhang, W , Liu, Z, et al. Polystyrene micro−/nanoplastics induced hematopoietic damages via the crosstalk of gut microbiota, metabolites, and cytokines. Environ Int. (2022) 161:107131. doi: 10.1016/j.envint.2022.107131
24. Lee, KW , Shim, WJ , Kwon, OY , and Kang, JH . Size-dependent effects of micro polystyrene particles in the marine copepod Tigriopus japonicus. Environ Sci Technol. (2013) 47:11278–83. doi: 10.1021/es401932b
25. Ge, H , Yan, Y , Di Wu, YH , and Tian, F . Potential role of LINC00996 in colorectal cancer: a study based on data mining and bioinformatics. OncoTargets Ther. (2018) 11:4845–55. doi: 10.2147/OTT.S173225
26. Ibrahim, YS , Tuan Anuar, S , Azmi, AA , Wan Mohd Khalik, WMA , Lehata, S , Hamzah, SR, et al. Detection of microplastics in human colectomy specimens. J Gastroenterol Hepatol. (2021) 5:116–21. doi: 10.1002/jgh3.12457
27. Alberts, B , Johnson, A , Lewis, J , Raff, M , Roberts, K , and Walter, P . Molecular biology of the cell. 4th ed. New York: Garland Science (2002). 1463 p.
28. Bergmann, M , Gutow, L , and Klages, M . Marine anthropogenic litter Springer Nature (2015). 447 p.
29. Carr, KE , Smyth, SH , McCullough, MT , Morris, JF , and Moyes, SM . Morphological aspects of interactions between microparticles and mammalian cells: intestinal uptake and onward movement. J Histochem Cytochem. (2012) 46:185–252. doi: 10.1016/j.proghi.2011.11.001
30. Tomazic-Jezic, VJ , Merritt, K , and Umbreit, TH . Significance of the type and the size of biomaterial particles on phagocytosis and tissue distribution. J Biomed Mater Res. (2001) 55:523–9. doi: 10.1002/1097-4636(20010615)55:4<523::AID-JBM1045>3.0.CO;2-G
31. Jani, P , Halbert, GW , Langridge, J , and Florence, AT . Nanoparticle uptake by the rat gastrointestinal mucosa: quantitation and particle size dependency. J Pharm Pharmacol. (1990) 42:821–6. doi: 10.1111/j.2042-7158.1990.tb07033.x
32. Walczak, AP , Kramer, E , Hendriksen, PJ , Tromp, P , Helsper, JP , van der Zande, M, et al. Translocation of differently sized and charged polystyrene nanoparticles in in vitro intestinal cell models of increasing complexity. Nanotoxicology. (2015) 9:453–61. doi: 10.3109/17435390.2014.944599
33. Wang, Q , Huang, F , Liang, K , Niu, W , Duan, X , Jia, X, et al. Polystyrene nanoplastics affect digestive function and growth in juvenile groupers. Sci Total Environ. (2022) 808:152098. doi: 10.1016/j.scitotenv.2021.152098
34. Cortés, C , Domenech, J , Salazar, M , Pastor, S , Marcos, R , and Hernández, A . Nanoplastics as a potential environmental health factor: effects of polystyrene nanoparticles on human intestinal epithelial Caco-2 cells. Environ Sci Nano. (2020) 7:272–85. doi: 10.1039/C9EN00523D
35. EFSA Panel on Contaminants in the Food Chain (CONTAM) . Presence of microplastics and nanoplastics in food, with particular focus on seafood. EFSA J. (2016) 14:e04501. doi: 10.2903/j.efsa.2016.4501
36. Lundqvist, M , Stigler, J , Elia, G , Lynch, I , Cedervall, T , and Dawson, KA . Nanoparticle size and surface properties determine the protein corona with possible implications for biological impacts. Proc Natl Acad Sci. (2008) 105:14265–70. doi: 10.1073/pnas.0805135105
37. Tenzer, S , Docter, D , Kuharev, J , Musyanovych, A , Fetz, V , Hecht, R, et al. Rapid formation of plasma protein corona critically affects nanoparticle pathophysiology. Nat Nanotechnol. (2013) 8:772–81. doi: 10.1038/nnano.2013.181
38. Fiorentino, I , Gualtieri, R , Barbato, V , Mollo, V , Braun, S , Angrisani, A, et al. Energy independent uptake and release of polystyrene nanoparticles in primary mammalian cell cultures. Exp Cell Res. (2015) 330:240–7. doi: 10.1016/j.yexcr.2014.09.017
39. Lesniak, A , Salvati, A , Santos-Martinez, MJ , Radomski, MW , Dawson, KA , and Åberg, C . Nanoparticle adhesion to the cell membrane and its effect on nanoparticle uptake efficiency. J Am Chem Soc. (2013) 135:1438–44. doi: 10.1021/ja309812z
40. Wang, T , Bai, J , Jiang, X , and Nienhaus, GU . Cellular uptake of nanoparticles by membrane penetration: a study combining confocal microscopy with FTIR Spectroelectrochemistry. ACS Nano. (2012) 6:1251–9. doi: 10.1021/nn203892h
41. Zhao, J , and Stenzel, MH . Entry of nanoparticles into cells: the importance of nanoparticle properties. Polym Chem. (2018) 9:259–72. doi: 10.1039/C7PY01603D
42. Li, L , Sun, S , Tan, L , Wang, Y , Wang, L , Zhang, Z, et al. Polystyrene nanoparticles reduced ROS and Inhibited Ferroptosis by triggering lysosome stress and TFEB nucleus translocation in a size-dependent manner. Nano Lett. (2019) 19:7781–92. doi: 10.1021/acs.nanolett.9b02795
43. Kuhn, DA , Vanhecke, D , Michen, B , Blank, F , Gehr, P , Petri-Fink, A, et al. Different endocytotic uptake mechanisms for nanoparticles in epithelial cells and macrophages. Beilstein J Nanotechnol. (2014) 5:1625–36. doi: 10.3762/bjnano.5.174
44. Fazlollahi, F , Angelow, S , Yacobi, NR , Marchelletta, R , Yu, ASL , Hamm-Alvarez, SF, et al. Polystyrene nanoparticle trafficking across MDCK-II. Nanomed. (2011) 7:588–94.
45. Ji, Y , Wang, Y , Shen, D , Kang, Q , and Chen, L . Mucin corona delays intracellular trafficking and alleviates cytotoxicity of nanoplastic-benzopyrene combined contaminant. J Hazard Mater. (2021) 406:124306. doi: 10.1016/j.jhazmat.2020.124306
46. Rejman, J , Oberle, V , Zuhorn, IS , and Hoekstra, D . Size-dependent internalization of particles via the pathways of clathrin- and caveolae-mediated endocytosis. Biochem J. (2004) 377:159–69. doi: 10.1042/bj20031253
47. Guarnieri, D , Guaccio, A , Fusco, S , and Netti, PA . Effect of serum proteins on polystyrene nanoparticle uptake and intracellular trafficking in endothelial cells. J Nanopart Res. (2011) 13:4295–309. doi: 10.1007/s11051-011-0375-2
48. Reinholz, J , Diesler, C , Schöttler, S , Kokkinopoulou, M , Ritz, S , Landfester, K, et al. Protein machineries defining pathways of nanocarrier exocytosis and transcytosis. Acta Biomater. (2018) 71:432–43. doi: 10.1016/j.actbio.2018.03.006
49. Yacobi, NR , Malmstadt, N , Fazlollahi, F , DeMaio, L , Marchelletta, R , Hamm-Alvarez, SF, et al. Mechanisms of alveolar epithelial translocation of a defined population of nanoparticles. Am J Respir Cell Mol. (2010) 42:604–14. doi: 10.1165/rcmb.2009-0138OC
50. Behzadi, S , Serpooshan, V , Tao, W , Hamaly, MA , Alkawareek, MY , Dreaden, EC, et al. Cellular uptake of nanoparticles: journey inside the cell. Chem Soc Rev. (2017) 46:4218–44. doi: 10.1039/C6CS00636A
51. Sakhtianchi, R , Minchin, RF , Lee, K-B , Alkilany, AM , Serpooshan, V , and Mahmoudi, M . Exocytosis of nanoparticles from cells: role in cellular retention and toxicity. Adv Colloid Interf Sci. (2013) 201-202:18–29. doi: 10.1016/j.cis.2013.10.013
52. Estrela, FN , Batista Guimarães, AT , Silva, FG , Marinho da Luz, T , Silva, AM , Pereira, PS, et al. Effects of polystyrene nanoplastics on Ctenopharyngodon idella (grass carp) after individual and combined exposure with zinc oxide nanoparticles. J Hazard Mater. (2021) 403:123879. doi: 10.1016/j.jhazmat.2020.123879
53. Kaur, S , Dhiman, AK , Kaur, S , Narang, V , Gupta, SK , and Sood, N . Sweet’s syndrome: disease spectrum from an Indian perspective. Int J Res. (2017) 3:411.
54. Broadbent, NJ , Gaskin, S , Squire, LR , and Clark, RE . Object recognition memory and the rodent hippocampus. Learn Mem. (2010) 17:5–11. doi: 10.1101/lm.1650110
55. Vecchiotti, G , Colafarina, S , Aloisi, M , Zarivi, O , Di Carlo, P , and Poma, A . Genotoxicity and oxidative stress induction by polystyrene nanoparticles in the colorectal cancer cell line HCT116. PLoS One. (2021) 16:e0255120. doi: 10.1371/journal.pone.0255120
56. Zheng, H , Wang, J , Wei, X , Chang, L , and Liu, S . Proinflammatory properties and lipid disturbance of polystyrene microplastics in the livers of mice with acute colitis. Sci Total Environ. (2021) 750:143085. doi: 10.1016/j.scitotenv.2020.143085
57. Cole, JE , Kassiteridi, C , and Monaco, C . Toll-like receptors in atherosclerosis: a ‘Pandora's box’ of advances and controversies. Trends Pharmacol Sci. (2013) 34:629–36. doi: 10.1016/j.tips.2013.09.008
58. Ding, Y , Zhang, R , Li, B , Du, Y , Li, J , Tong, X, et al. Tissue distribution of polystyrene nanoplastics in mice and their entry, transport, and cytotoxicity to GES-1 cells. Environ Pollut. (2021) 280:116974. doi: 10.1016/j.envpol.2021.116974
59. Pan, L , Yu, D , Zhang, Y , Zhu, C , Yin, Q , Hu, Y, et al. Polystyrene microplastics-triggered mitophagy and oxidative burst via activation of PERK pathway. Sci Total Environ. (2021) 781:146753. doi: 10.1016/j.scitotenv.2021.146753
60. Hou, J , Lei, Z , Cui, L , Hou, Y , Yang, L , An, R, et al. Polystyrene microplastics lead to pyroptosis and apoptosis of ovarian granulosa cells via NLRP3/Caspase-1 signaling pathway in rats. Ecotoxicol Environ Saf. (2021) 212:112012. doi: 10.1016/j.ecoenv.2021.112012
61. Jin, H , Ma, T , Sha, X , Liu, Z , Zhou, Y , Meng, X, et al. Polystyrene microplastics induced male reproductive toxicity in mice. J Hazard Mater. (2021) 401:123430. doi: 10.1016/j.jhazmat.2020.123430
62. Shengchen, W , Jing, L , Yujie, Y , Yue, W , and Shiwen, X . Polystyrene microplastics-induced ROS overproduction disrupts the skeletal muscle regeneration by converting myoblasts into adipocytes. J Hazard Mater. (2021) 417:125962. doi: 10.1016/j.jhazmat.2021.125962
63. Scanes, E , Wood, H , and Ross, P . Microplastics detected in haemolymph of the Sydney rock oyster Saccostrea glomerata. Mar Pollut Bull. (2019) 149:110537. doi: 10.1016/j.marpolbul.2019.110537
64. Sun, R , Xu, K , Yu, L , Pu, Y , Xiong, F , He, Y, et al. Preliminary study on impacts of polystyrene microplastics on the hematological system and gene expression in bone marrow cells of mice. Ecotoxicol Environ Saf. (2021) 218:112296. doi: 10.1016/j.ecoenv.2021.112296
65. Xu, D , Ma, Y , Han, X , and Chen, Y . Systematic toxicity evaluation of polystyrene nanoplastics on mice and molecular mechanism investigation about their internalization into Caco-2 cells. J Hazard Mater. (2021) 417:126092. doi: 10.1016/j.jhazmat.2021.126092
66. Rubio, L , Barguilla, I , Domenech, J , Marcos, R , and Hernández, A . Biological effects, including oxidative stress and genotoxic damage, of polystyrene nanoparticles in different human hematopoietic cell lines. J Hazard Mater. (2020) 398:122900. doi: 10.1016/j.jhazmat.2020.122900
67. Pei, X , Heng, X , and Chu, W . Polystyrene nano/microplastics induce microbiota dysbiosis, oxidative damage, and innate immune disruption in zebrafish. Microb Pathog. (2022) 163:105387. doi: 10.1016/j.micpath.2021.105387
68. Zhang, F , Li, D , Yang, Y , Zhang, H , Zhu, J , Liu, J, et al. Combined effects of polystyrene microplastics and copper on antioxidant capacity, immune response and intestinal microbiota of Nile tilapia (Oreochromis niloticus). Sci Total Environ. (2022) 808:152099. doi: 10.1016/j.scitotenv.2021.152099
69. Liu, S , Li, H , Wang, J , Wu, B , and Guo, X . Polystyrene microplastics aggravate inflammatory damage in mice with intestinal immune imbalance. Sci Total Environ. (2022) 833:155198. doi: 10.1016/j.scitotenv.2022.155198
70. Guo, X , Lv, M , Li, J , Ding, J , Wang, Y , Fu, L, et al. The distinct toxicity effects between commercial and realistic polystyrene microplastics on microbiome and histopathology of gut in zebrafish. J Hazard Mater. (2022) 434:128874. doi: 10.1016/j.jhazmat.2022.128874
71. Guimarães, ATB , Estrela, FN , Pereira, PS , de Andrade Vieira, JE , de Lima Rodrigues, AS , Silva, FG, et al. Toxicity of polystyrene nanoplastics in Ctenopharyngodon idella juveniles: a genotoxic, mutagenic and cytotoxic perspective. Sci Total Environ. (2021) 752:141937. doi: 10.1016/j.scitotenv.2020.141937
72. Liang, B , Zhong, Y , Huang, Y , Lin, X , Liu, J , Lin, L, et al. Underestimated health risks: polystyrene micro-and nanoplastics jointly induce intestinal barrier dysfunction by ROS-mediated epithelial cell apoptosis. Part Fibre Toxicol. (2021) 18:1–19. doi: 10.1186/s12989-021-00414-1
73. Liu, S , Wu, X , Gu, W , Yu, J , and Wu, B . Influence of the digestive process on intestinal toxicity of polystyrene microplastics as determined by in vitro Caco-2 models. Chemosphere. (2020) 256:127204. doi: 10.1016/j.chemosphere.2020.127204
74. Heinlaan, M , Kasemets, K , Aruoja, V , Blinova, I , Bondarenko, O , Lukjanova, A, et al. Hazard evaluation of polystyrene nanoplastic with nine bioassays did not show particle-specific acute toxicity. Sci Total Environ. (2020) 707:136073. doi: 10.1016/j.scitotenv.2019.136073
75. He, Y , Li, J , Chen, J , Miao, X , Li, G , He, Q, et al. Cytotoxic effects of polystyrene nanoplastics with different surface functionalization on human HepG2 cells. Sci Total Environ. (2020) 723:138180. doi: 10.1016/j.scitotenv.2020.138180
76. Choi, D , Bang, J , Kim, T , Oh, Y , Hwang, Y , and Hong, J . In vitro chemical and physical toxicities of polystyrene microfragments in human-derived cells. J Hazard Mater. (2020) 400:123308. doi: 10.1016/j.jhazmat.2020.123308
77. Xie, X , Deng, T , Duan, J , Xie, J , Yuan, J , and Chen, M . Exposure to polystyrene microplastics causes reproductive toxicity through oxidative stress and activation of the p38 MAPK signaling pathway. Ecotoxicol Environ Saf. (2020) 190:110133. doi: 10.1016/j.ecoenv.2019.110133
78. Hwang, J , Choi, D , Han, S , Jung, SY , Choi, J , and Hong, J . Potential toxicity of polystyrene microplastic particles. Sci Rep. (2020) 10:1–12. doi: 10.1038/s41598-020-64464-9
79. Yang, Y-F , Chen, C-Y , Lu, T-H , and Liao, C-M . Toxicity-based toxicokinetic/toxicodynamic assessment for bioaccumulation of polystyrene microplastics in mice. J Hazard Mater. (2019) 366:703–13. doi: 10.1016/j.jhazmat.2018.12.048
80. Stock, V , Böhmert, L , Lisicki, E , Block, R , Cara-Carmona, J , Pack, LK, et al. Uptake and effects of orally ingested polystyrene microplastic particles in vitro and in vivo. Arch Toxicol. (2019) 93:1817–33. doi: 10.1007/s00204-019-02478-7
81. Rafiee, M , Dargahi, L , Eslami, A , Beirami, E , Jahangiri-Rad, M , Sabour, S, et al. Neurobehavioral assessment of rats exposed to pristine polystyrene nanoplastics upon oral exposure. Chemosphere. (2018) 193:745–53. doi: 10.1016/j.chemosphere.2017.11.076
82. Sussarellu, R , Suquet, M , Thomas, Y , Lambert, C , Fabioux, C , Pernet, MEJ, et al. Oyster reproduction is affected by exposure to polystyrene microplastics. Proc Natl Acad Sci. (2016) 113:2430–5. doi: 10.1073/pnas.1519019113
83. Forte, M , Iachetta, G , Tussellino, M , Carotenuto, R , Prisco, M , De Falco, M, et al. Polystyrene nanoparticles internalization in human gastric adenocarcinoma cells. Toxicol In Vitro. (2016) 31:126–36. doi: 10.1016/j.tiv.2015.11.006
84. Arani, NM , Emam-Djomeh, Z , Asemi, Z , Tavakolipour, H , Chaleshtori, RS , and Alizadeh, S-A . Physiochemical and microbial properties of honey containing heat-resistant Bacillus coagulans T11. Food Measure. (2019) 13:1917–23. doi: 10.1007/s11694-019-00110-x
85. Chaleshtori, RS , Jadi, Z , Arani, NM , and Arian, A . Survey of physiochemical and microbial properties of functional beverage of date juice and Salix aegyptiaca L. distillate contained Bacillus coagulans. J Appl Microbiol Food Ind. (2021) 6:41–53.
86. Davoodvandi, A , Fallahi, F , Tamtaji, OR , Tajiknia, V , Banikazemi, Z , Fathizadeh, H, et al. An update on the effects of probiotics on gastrointestinal cancers. Front Pharmacol. (2021) 12:680400. doi: 10.3389/fphar.2021.680400
87. Davoodvandi, A , Marzban, H , Goleij, P , Sahebkar, A , Morshedi, K , Rezaei, S, et al. Effects of therapeutic probiotics on modulation of microRNAs. Cell Commun Signal. (2021) 19:4. doi: 10.1186/s12964-020-00668-w
88. Arani, NM , Emam-Djomeh, Z , Tavakolipour, H , Sharafati-Chaleshtori, R , Soleimani, A , and Asemi, Z . The effects of probiotic honey consumption on metabolic status in patients with diabetic nephropathy: a randomized, double-blind, controlled trial. Probiotics Antimicrob Proteins. (2019) 11:1195–201. doi: 10.1007/s12602-018-9468-x
89. Zhu, YT , Lai, JH , Liao, XD , and Liu, SL . Screening of lactic acid bacteria strains for their ability to bind phthalate monoesters in vitro and the binding characteristics. Food Control. (2018) 90:364–71. doi: 10.1016/j.foodcont.2018.02.013
90. Jin, Y , Lu, L , Tu, W , Luo, T , and Fu, Z . Impacts of polystyrene microplastic on the gut barrier, microbiota and metabolism of mice. Sci Total Environ. (2019) 649:308–17. doi: 10.1016/j.scitotenv.2018.08.353
91. Li, B , Ding, Y , Cheng, X , Sheng, D , Xu, Z , Rong, Q, et al. Polyethylene microplastics affect the distribution of gut microbiota and inflammation development in mice. Chemosphere. (2020) 244:125492. doi: 10.1016/j.chemosphere.2019.125492
92. Yan, H , Baldridge, MT , and King, KY . Hematopoiesis and the bacterial microbiome. Blood. (2018) 132:559–64. doi: 10.1182/blood-2018-02-832519
93. Andersohn, F , Konzen, C , and Garbe, E . Systematic review: agranulocytosis induced by nonchemotherapy drugs. Ann Intern Med. (2007) 146:657–65. doi: 10.7326/0003-4819-146-9-200705010-00009
94. Furtek, KJ , Kubiak, DW , Barra, M , Varughese, CA , Ashbaugh, CD , and Koo, S . High incidence of neutropenia in patients with prolonged ceftaroline exposure. J Antimicrob Chemother. (2016) 71:2010–3. doi: 10.1093/jac/dkw062
95. Anand, S , Kaur, H , and Mande, SS . Comparative in silico analysis of butyrate production pathways in gut commensals and pathogens. Front Microbiol. (2016) 7:1945. doi: 10.3389/fmicb.2016.01945
97. Hooper, LV , Midtvedt, T , and Gordon, JI . How host-microbial interactions shape the nutrient environment of the mammalian intestine. Annu Rev Nutr. (2002) 22:283–307. doi: 10.1146/annurev.nutr.22.011602.092259
98. Rooks, MG , and Garrett, WS . Gut microbiota, metabolites and host immunity. Nat Rev Immunol. (2016) 16:341–52. doi: 10.1038/nri.2016.42
99. Lili, Z , Hongfei, Z , Shoukat, S , Xiaochen, Z , and Bolin, Z . Screening lactic acid bacteria strains with ability to bind di-n-butyl phthalate via Turbiscan technique. Antonie Van Leeuwenhoek. (2017) 110:759–69. doi: 10.1007/s10482-017-0846-2
100. Baralić, K , Živančević, K , Jorgovanović, D , Javorac, D , Radovanović, J, et al. Probiotic reduced the impact of phthalates and bisphenol a mixture on type 2 diabetes mellitus development: merging bioinformatics with in vivo analysis. Food Chem Toxicol. (2021) 154:112325. doi: 10.1016/j.fct.2021.112325
101. Yang, Y , and Pei, J . Isolation and characterization of an Enterococcus strain from Chinese sauerkraut with potential for lead removal. Eur Food Res Technol. (2020) 246:2055–64. doi: 10.1007/s00217-020-03555-3
102. Alcántara, C , Crespo, A , Solís, C , Devesa, V , Vélez, D , Monedero, V, et al. Lipoteichoic acid depletion in Lactobacillus impacts cell morphology and stress response but does not abolish mercury surface binding. Benef Microbes. (2020) 11:791–802. doi: 10.3920/BM2019.0184
103. Do Nascimento, JM , de Oliveira, JD , Rizzo, ACL , and Leite, SGF . Biosorption cu (II) by the yeast Saccharomyces cerevisiae. Biotechnol Rep. (2019) 21:e00315. doi: 10.1016/j.btre.2019.e00315
104. Huang, H , Jia, Q , Jing, W , Dahms, H-U , and Wang, L . Screening strains for microbial biosorption technology of cadmium. Chemosphere. (2020) 251:126428. doi: 10.1016/j.chemosphere.2020.126428
105. Zhao, H , Zhou, F , Qi, Y , Dziugan, P , Bai, F , Walczak, P, et al. Screening of Lactobacillus strains for their ability to bind benzo(a)pyrene and the mechanism of the process. Food Chem Toxicol. (2013) 59:67–71. doi: 10.1016/j.fct.2013.05.040
106. Karazhiyan, H , Mehraban, SM , Karazhyan, R , Mehrzad, A , and Haghighi, E . Ability of different treatments of Saccharomyces cerevisiae to surface bind aflatoxin M1 in yoghurt. JAST. (2016) 18:1489–98.
107. Ju, J , Shen, L , Xie, Y , Yu, H , Guo, Y , Cheng, Y, et al. Degradation potential of bisphenol a by Lactobacillus reuteri. LWT. (2019) 106:7–14. doi: 10.1016/j.lwt.2019.02.022
108. Kyrila, G , Katsoulas, A , Schoretsaniti, V , Rigopoulos, A , Rizou, E , Doulgeridou, S, et al. Bisphenol a removal and degradation pathways in microorganisms with probiotic properties. J Hazard Mater. (2021) 413:125363. doi: 10.1016/j.jhazmat.2021.125363
109. Baralić, K , Živančević, K , Javorac, D , Buha Djordjevic, A , Anđelković, M , Jorgovanović, D, et al. Multi-strain probiotic ameliorated toxic effects of phthalates and bisphenol a mixture in Wistar rats. Food Chem Toxicol. (2020) 143:111540. doi: 10.1016/j.fct.2020.111540
110. Alokail, MS , Sabico, S , Al-Saleh, Y , Al-Daghri, NM , Alkharfy, KM , Vanhoutte, PM, et al. Effects of probiotics in patients with diabetes mellitus type 2: study protocol for a randomized, double-blind, placebo-controlled trial. Trials. (2013) 14:195. doi: 10.1186/1745-6215-14-195
111. Zhang, Q , Wu, Y , and Fei, X . Effect of probiotics on glucose metabolism in patients with type 2 diabetes mellitus: a meta-analysis of randomized controlled trials. Medicina. (2016) 52:28–34. doi: 10.1016/j.medici.2015.11.008
112. Bagherzadeh Kasmani, F , and Mehri, M . Effects of a multi-strain probiotics against aflatoxicosis in growing Japanese quails. Livest Sci. (2015) 177:110–6. doi: 10.1016/j.livsci.2015.04.018
113. Hamad, GM , Taha, TH , Hafez, EE , Ali, SH , and El Sohaimy, SA . Supplementation of Cerelac baby food with yeast–probiotic cocktail strains induces high potential for aflatoxin detoxification both in vitro and in vivo in mother and baby albino rats. J Sci Food Agric. (2018) 98:707–18. doi: 10.1002/jsfa.8518
114. Shi, X , Hu, C , Cai, S , Tao, X , Zhou, Y , Smidt, H, et al. Protective effects of Lactobacillus plantarum strain P1 against toxicity of the environmental oestrogen di-n-butyl phthalate in rats. Benef Microbes. (2020) 11:803–13. doi: 10.3920/BM2019.0181
115. Sarkar, SR , Mazumder, PM , and Banerjee, S . Probiotics protect against gut dysbiosis associated decline in learning and memory. J Neuroimmunol. (2020) 348:577390. doi: 10.1016/j.jneuroim.2020.577390
116. Singh, D , Khan, MA , and Siddique, HR . Therapeutic implications of probiotics in microbiota dysbiosis: a special reference to the liver and oral cancers. Life Sci. (2021) 285:120008. doi: 10.1016/j.lfs.2021.120008
117. Liew, WP , Sabran, MR , Than, LT , and Abd-Ghani, F . Metagenomic and proteomic approaches in elucidating aflatoxin B1 detoxification mechanisms of probiotic Lactobacillus casei Shirota towards intestine. Food Chem Toxicol. (2022) 160:112808. doi: 10.1016/j.fct.2022.112808
118. Wu, J , Tian, X , Xu, X , Gu, X , Kong, J , and Guo, T . Engineered probiotic Lactococcus lactis for lycopene production against ROS stress in intestinal epithelial cells. ACS Synth Biol. (2022) 11:1568–76. doi: 10.1021/acssynbio.1c00639
119. Yousefi, M , Khorshidian, N , and Hosseini, H . The ability of probiotic Lactobacillus strains in removal of benzo[a]pyrene: a response surface methodology study. Probiotics Antimicrob Proteins. (2022) 14:464–75. doi: 10.1007/s12602-021-09810-7
120. Khalafalla, MM , Zayed, NF , Amer, AA , Soliman, AA , Zaineldin, AI , Gewaily, MS, et al. Dietary Lactobacillus acidophilus ATCC 4356 relieves the impacts of aflatoxin B1 toxicity on the growth performance, Hepatorenal functions, and Antioxidative capacity of Thinlip Grey mullet (Liza ramada)(Risso 1826). Probiotics Antimicrob Proteins. (2022) 14:189–203. doi: 10.1007/s12602-021-09888-z
121. Fan, YH , Shen, YL , Lin, ZW , Zhou, Y , and Ye, BC . Key role of exopolysaccharide on di-butyl phthalate adsorbing by Lactobacillus plantarum CGMCC18980. Appl Microbiol Biotechnol. (2021) 105:2587–95. doi: 10.1007/s00253-021-11145-w
122. Zhao, L , Wei, J , Pan, X , Jie, Y , Zhu, B , Zhao, H, et al. Critical analysis of peptidoglycan structure of Lactobacillus acidophilus for phthalate removal. Chemosphere. (2021) 282:130982. doi: 10.1016/j.chemosphere.2021.130982
123. Karamese, M , Aydin, H , Gelen, V , Sengul, E , and Karamese, SA . The antiinflammatory, anti-oxidant and protective effects of a probiotic mixture on organ toxicity in a rat model. Future Microbiol. (2020) 15:401–12. doi: 10.2217/fmb-2020-0005
124. Hung, YP , Lee, CC , Lee, JC , Tsai, PJ , and Ko, WC . Gut dysbiosis during COVID-19 and potential effect of probiotics. Microorganisms. (2021) 9:1605. doi: 10.3390/microorganisms9081605
125. Lin, B , Zhao, F , Liu, Y , Wu, X , Feng, J , Jin, X, et al. Randomized clinical trial: probiotics alleviated Oral-gut microbiota Dysbiosis and thyroid hormone withdrawal-related complications in thyroid Cancer patients before radioiodine therapy following thyroidectomy. Front Endocrinol. (2022) 13:286. doi: 10.3389/fendo.2022.834674
126. Wasser, CI , Mercieca, EC , Kong, G , Hannan, AJ , Allford, B , McKeown, SJ, et al. A randomized controlled trial of probiotics targeting gut Dysbiosis in Huntington's disease. J Huntingtons Dis. (2023) 12:43–55. doi: 10.3233/JHD-220556
127. Cristofori, F , Dargenio, VN , Dargenio, C , Miniello, VL , Barone, M , and Francavilla, R . Anti-inflammatory and immunomodulatory effects of probiotics in gut inflammation: a door to the body. Front Immunol. (2021) 12:578386. doi: 10.3389/fimmu.2021.578386
128. Kobyliak, N , Conte, C , Cammarota, G , Haley, AP , Styriak, I , Gaspar, L, et al. Probiotics in prevention and treatment of obesity: a critical view. Nutr Metab. (2016) 13:14. doi: 10.1186/s12986-016-0067-0
129. Hatab, S , Yue, T , and Mohamad, O . Removal of patulin from apple juice using inactivated lactic acid bacteria. J Appl Microbiol. (2012) 112:892–9. doi: 10.1111/j.1365-2672.2012.05279.x
130. Zhao, L , Li, X , Yang, Q , Zhuang, D , Pan, X , and Li, L . Adsorption kinetics and mechanism of di-n-butyl phthalate by Leuconostoc mesenteroides. Food Sci Nutr. (2020) 8:6153–63. doi: 10.1002/fsn3.1908
131. Zoghi, A , Khosravi-Darani, K , and Sohrabvandi, S . Surface binding of toxins and heavy metals by probiotics. Mini Rev Med Chem. (2014) 14:84–98. doi: 10.2174/1389557513666131211105554
132. Yu, L , Zhao, XK , Cheng, ML , Yang, GZ , Wang, B , Liu, HJ, et al. Saccharomyces boulardii administration changes gut microbiota and attenuates D-galactosamine-induced liver injury. Sci Rep. (2017) 7:1–7.
133. Tong, Y , Hua, X , Zhao, W , Liu, D , Zhang, J , Zhang, W, et al. Protective effects of Lactobacillus plantarum CCFM436 against acute manganese toxicity in mice. Food Biosci. (2020) 35:100583. doi: 10.1016/j.fbio.2020.100583
134. Duman, DG , Kumral, ZNÖ , Ercan, F , Deniz, M , Can, G , and Yeğen, BÇ . Saccharomyces boulardii ameliorates clarithromycin-and methotrexate-induced intestinal and hepatic injury in rats. Br J Nutr. (2013) 110:493–9. doi: 10.1017/S000711451200517X
135. Oumeddour, A , Zaroure, D , Haroune, R , Zaimeche, R , Riane, K , Sifour, M, et al. Protective effects of Propolis and probiotic Lactobacillus acidophilus against carbon tetrachloride-induced hepatotoxicity in rats. Pharm Sci. (2019) 25:190–7. doi: 10.15171/PS.2019.36
136. Kim, SW , Park, KY , Kim, B , Kim, E , and Hyun, CK . Lactobacillus rhamnosus GG improves insulin sensitivity and reduces adiposity in high-fat diet-fed mice through enhancement of adiponectin production. Biochem Biophys Res Commun. (2013) 431:258–63. doi: 10.1016/j.bbrc.2012.12.121
137. Everard, A , Matamoros, S , Geurts, L , Delzenne, NM , and Cani, PD . Saccharomyces boulardii administration changes gut microbiota and reduces hepatic steatosis, low-grade inflammation, and fat mass in obese and type 2 diabetic db/db mice. MBio. (2014) 5:e01011–4. doi: 10.1128/mBio.01011-14
138. Kim, DH , Kim, S , Ahn, JB , Kim, JH , Ma, HW , Seo, DH, et al. Lactobacillus plantarum CBT LP3 ameliorates colitis via modulating T cells in mice. Int J Med Microbiol. (2020) 310:151391. doi: 10.1016/j.ijmm.2020.151391
139. Majlesi, M , Shekarforoush, SS , Ghaisari, HR , Nazifi, S , Sajedianfard, J , and Eskandari, MH . Effect of probiotic Bacillus coagulans and Lactobacillus plantarum on alleviation of mercury toxicity in rat. Probiotics Antimicrob. (2017) 9:300–9. doi: 10.1007/s12602-016-9250-x
140. Alipour Nosrani, E , Tamtaji, OR , Alibolandi, Z , Sarkar, P , Ghazanfari, M , Azami Tameh, A, et al. Neuroprotective effects of probiotics bacteria on animal model of Parkinson’s disease induced by 6-hydroxydopamine: a behavioral, biochemical, and histological study. J Immunoassay Immunochem. (2021) 42:106–20. doi: 10.1080/15321819.2020.1833917
141. Cheon, MJ , Lim, SM , Lee, NK , and Paik, HD . Probiotic properties and neuroprotective effects of Lactobacillus buchneri KU200793 isolated from Korean fermented foods. Int J Mol Sci. (2020) 21:1227. doi: 10.3390/ijms21041227
142. Barbonetti, A , Cinque, B , Vassallo, MRC , Mineo, S , Francavilla, S , Cifone, MG, et al. Effect of vaginal probiotic lactobacilli on in vitro–induced sperm lipid peroxidation and its impact on sperm motility and viability. Fertil Steril. (2011) 95:2485–8. doi: 10.1016/j.fertnstert.2011.03.066
143. Dardmeh, F , Alipour, H , Gazerani, P , van der Horst, G , Brandsborg, E , and Nielsen, HI . Lactobacillus rhamnosus PB01 (DSM 14870) supplementation affects markers of sperm kinematic parameters in a diet-induced obesity mice model. PLoS One. (2017) 12:e0185964. doi: 10.1371/journal.pone.0185964
144. Ghanei, N , Rezaei, N , Amiri, GA , Zayeri, F , Makki, G , and Nasseri, E . The probiotic supplementation reduced inflammation in polycystic ovary syndrome: a randomized, double-blind, placebo-controlled trial. J Funct Foods. (2018) 42:306–11. doi: 10.1016/j.jff.2017.12.047
145. Gratz, S , Wu, Q , El-Nezami, H , Juvonen, R , Mykkänen, H , and Turner, P . Lactobacillus rhamnosus strain GG reduces aflatoxin B1 transport, metabolism, and toxicity in Caco-2 cells. Appl Environ Microbiol. (2007) 73:3958–64. doi: 10.1128/AEM.02944-06
146. Fuchs, S , Sontag, G , Stidl, R , Ehrlich, V , Kundi, M , and Knasmüller, S . Detoxification of patulin and ochratoxin a, two abundant mycotoxins, by lactic acid bacteria. Food Chem Toxicol. (2008) 46:1398–407. doi: 10.1016/j.fct.2007.10.008
147. Hemarajata, P , and Versalovic, J . Effects of probiotics on gut microbiota: mechanisms of intestinal immunomodulation and neuromodulation. Ther Adv Gastroenterol. (2013) 6:39–51. doi: 10.1177/1756283X12459294
Keywords: probiotics, nanoplastics, microplastics, polystyrene, toxicity, gut microflora
Citation: Bazeli J, Banikazemi Z, Hamblin MR and Sharafati Chaleshtori R (2023) Could probiotics protect against human toxicity caused by polystyrene nanoplastics and microplastics? Front. Nutr. 10:1186724. doi: 10.3389/fnut.2023.1186724
Edited by:
Miguel Angelo Faria, LAQV Network of Chemistry and Technology, PortugalReviewed by:
Prabhakar Mishra, REVA University, IndiaArmindo Melo, National Institute of Health Dr. Ricardo Jorge (INSA), Portugal
Copyright © 2023 Bazeli, Banikazemi, Hamblin and Sharafati Chaleshtori. This is an open-access article distributed under the terms of the Creative Commons Attribution License (CC BY). The use, distribution or reproduction in other forums is permitted, provided the original author(s) and the copyright owner(s) are credited and that the original publication in this journal is cited, in accordance with accepted academic practice. No use, distribution or reproduction is permitted which does not comply with these terms.
*Correspondence: Reza Sharafati Chaleshtori, c2hhcmFmYXRpLnJlemFAZ21haWwuY29t