- 1Graduate School of Regional Innovation Studies, Mie University, Tsu, Mie, Japan
- 2Mie University Zebrafish Research Center, Mie University, Tsu, Mie, Japan
- 3Department of Marine Biology, Faculty of Marine Science and Oceanography, Khorramshahr University of Marine Science and Technology, Khorramshahr, Iran
- 4Konan Chemical Manufacturing Co., Ltd., Yokkaichi, Mie, Japan
- 5Department of Integrative Pharmacology, Mie University Graduate School of Medicine, Tsu, Mie, Japan
- 6Department of Bioinformatics, Mie University Advanced Science Research Promotion Center, Tsu, Mie, Japan
Metabolic syndrome comprises a group of conditions that collectively increase the risk of abdominal obesity, diabetes, atherosclerosis, cardiovascular diseases, and cancer. Gut microbiota is involved in the pathogenesis of metabolic syndrome, and microbial diversity and function are strongly affected by diet. In recent years, epidemiological evidence has shown that the dietary intake of seaweed can prevent metabolic syndrome via gut microbiota modulation. In this review, we summarize the current in vivo studies that have reported the prevention and treatment of metabolic syndrome via seaweed-derived components by regulating the gut microbiota and the production of short-chain fatty acids. Among the surveyed related articles, animal studies revealed that these bioactive components mainly modulate the gut microbiota by reversing the Firmicutes/Bacteroidetes ratio, increasing the relative abundance of beneficial bacteria, such as Bacteroides, Akkermansia, Lactobacillus, or decreasing the abundance of harmful bacteria, such as Lachnospiraceae, Desulfovibrio, Lachnoclostridium. The regulated microbiota is thought to affect host health by improving gut barrier functions, reducing LPS-induced inflammation or oxidative stress, and increasing bile acid production. Furthermore, these compounds increase the production of short-chain fatty acids and influence glucose and lipid metabolism. Thus, the interaction between the gut microbiota and seaweed-derived bioactive components plays a critical regulatory role in human health, and these compounds have the potential to be used for drug development. However, further animal studies and human clinical trials are required to confirm the functional roles and mechanisms of these components in balancing the gut microbiota and managing host health.
1. Introduction
Metabolic syndrome comprises a cluster of metabolic disorders associated with abdominal obesity, diabetes, hypertension, hyperlipidemia, hyperglycemia, atherosclerosis, cancer, and cardiovascular disease (1). The increasing prevalence of metabolic syndrome worldwide is developing into a severe health problem and economic burden. The bulk of modern lifestyles, such as increased high-caloric intake and decreased physical activity, result in a high incidence of metabolic syndrome. Patients with metabolic syndrome should first be consulted for lifestyle modifications to reduce the risk of developing metabolic syndrome, in the light of which, diet and exercise modifications, weight loss, and smoking cessation have been emphasized. However, once lifestyle interventions fail to control disease progression, drug therapy is required to direct the risk factors. Current drug therapies for metabolic syndrome mainly focus on treating dysglycemia, dyslipidemia, and hypertension separately. However, several adverse side effects of these medications have been reported, including depressed mood and anxiety (2). Therefore, the interest in natural products as potential treatments with minimal side effects has increased. Instead of calorie or single-nutrient restrictions, functional food-based dietary interventions are recommended to prevent or treat metabolic syndrome. Seaweeds (also known as macroalgae) and their bioactive components have recently attracted attention as potential functional foods for managing metabolic syndrome in humans (3).
Seaweeds comprise more than 25,000 species (4) and are essential for marine ecosystems. They are crucial blue carbon sinks for a sustainable economy, and their wide variety, abundance, and fast proliferation make them attractive future bioresources (5). Edible seaweeds have been consumed as vegetables in East Asian countries for several thousand years, and their ability to improve human health has recently been recognized in the Western world (6). Seaweeds can be classified into three major groups based on the thallus pigment color: brown (Phaeophyceae), red (Rhodophyta), and green (Chlorophyta) (6, 7). All groups are rich in multiple valuable macro-and micronutrients, including carbohydrates, proteins, polyunsaturated fatty acids (PUFAs), dietary fiber, vitamins, minerals, and bioactive compounds (8, 9). Polysaccharides are the major components of seaweed biomass, and they account for up to 76% of the dry weight of certain species (10). Phenolic compounds and proteins derived from seaweeds have been widely studied as potential functional compounds associated with antioxidant, antibacterial, antiviral, and antifungal properties (11). Therefore, seaweeds are considered aquatic plant-based proteins for sustainable nutrition (12) and can provide a potential source of probiotics (13, 14).
The human body contains at least 100 trillion microbial cells, of which 95% are harbored by the human gastrointestinal tract (15). Microorganisms that inhabit the human gut include bacteria, viruses, archaea, fungi, and protozoa, collectively termed the gut microbiota (16). The gut microbiota compiles complex ecosystems in which microorganisms interact with both each other and the host, thereby exerting a profound influence on human health and physiology (17, 18). The gut microbiota can be considered a “microbial organ” that contributes to host physiological processes, including energy harvesting and storage, metabolism (such as the fermentation of dietary fiber or host-derived glycans) (19), the management of the stability of the human intestinal microenvironment (20), and is closely related to the development and regulation of the host immune system (21). Additionally, short-chain fatty acids (SCFAs), the main end products of gut microbial fermentation of indigestible foods, are crucial for intestinal health and are involved in crosstalk between the gut and peripheral tissues (22, 23). Furthermore, key metabolites produced by the gut microbiota play critical roles and interact with several vital metabolic pathways related to incretin production, insulin signaling, and inflammation (24). In recent years, it has been demonstrated that dysbiosis of the gut microbiota, which indicates an alteration in its composition, is closely associated with the development of several diseases (25), such as metabolic syndrome (26–28), inflammatory bowel disease (29–32), and central nervous system-related disorders (33–35).
In this review, we provide an up-to-date summary of the current state of knowledge regarding bioactive compounds derived from seaweeds and how these compounds prevent or treat metabolic syndrome and related diseases by modulating the gut microbiota. Finally, future perspectives on seaweed and gut microbiota are discussed.
2. Methodology
Systemic literature was obtained through an advanced search of Web of Science interfaces (last accessed on June 10, 2022). The detailed steps of the literature search are shown in Figure 1. Peer-reviewed scientific articles were collected using the following keywords: “Fucoidan, “Alginate,” “Laminarin” or “Laminaran,” “Agar,” “Porphyran,” “Carrageenan,” “Floridean starch,” “Floridoside,” “Ulvan,” “Rhamnan sulfate,” “Bromophenol,” “Phlorotannin,” “Flavonoid,” “Proteins,” “Peptides,” “Carotenoids,” “PUFAs,” “Obesity,” “Insulin resistance,” “Hyperglycemia,” “Diabetes,” “Hyperlipidemia,” “Hypertension,” “Non-alcoholic fatty liver disease (NAFLD),” “Non-alcoholic steatohepatitis (NASH),” “Atherosclerosis,” “Cardiovascular disease,” “Cancer,” “Immune disorders,” “Gut microbiota,” “Organic acid,” and “Short-chain fatty acid.” For compounds not specifically found in seaweed, such as “Agar,” “Bromophenol,” “Flavonoid,” “Peptides,” “Proteins,” “Carotenoids,” and “PUFAs” were searched simultaneously with “seaweed” or “marine algae.” In addition, purified bioactive fractions were not included in the search. Only research articles published in English were included.
Initially, we collected papers using the plural forms of different words, such as the names of 17 “seaweed or marine algae-derived compounds” combined with 12 “metabolic syndrome-related diseases,” respectively. Compounds were ranked sequentially based on the number of relevant publications for each disease (Supplementary Figure S1). Furthermore, 136 papers were collected when filtered using a combination of three factors: the “compound’s name” AND “metabolic syndrome-related diseases’ name” AND “gut microbiota or organic acid or SCFAs” (Figure 2). Fucoidan was found to be the most prevalent seaweed-derived component investigated, with 58 articles revealing its potential ability to prevent or treat metabolic syndrome-related diseases by modulating the gut microbiota. Among the 12 diseases searched for, the highest number of publications (25) related to “obesity,” and no published reports for “hypertension” included the relationship between fucoidan and gut microbiota. Alginate is also a well-studied compound and 52 articles were found pertaining to it. However, articles on “NAFLD/NASH,” “Immune disease,” and “Atherosclerosis” that included the relationship between alginate and gut microbiota have yet to be published. There were comparatively few related publications on carrageenan (n = 12), laminarin (n = 7), porphyran (n = 3), bromophenol (n = 2), peptides (n = 1), rhamnan sulfate (n = 1), carotenoids (n = 1), and phlorotannin (n = 1). Notably, most of the articles included in this study were published within the last 5 years, indicating that the health benefits of seaweed-derived components via regulation of the gut microbiota have recently attracted research attention. In addition, this study found no reports on seaweed-derived PUFAs, agar, floridean starch or floridoside, and ulvan; therefore, future studies are required. This section briefly summarizes the representative in vivo studies of seaweed-derived components that affect metabolic syndrome-related diseases via gut microbiota modulation.
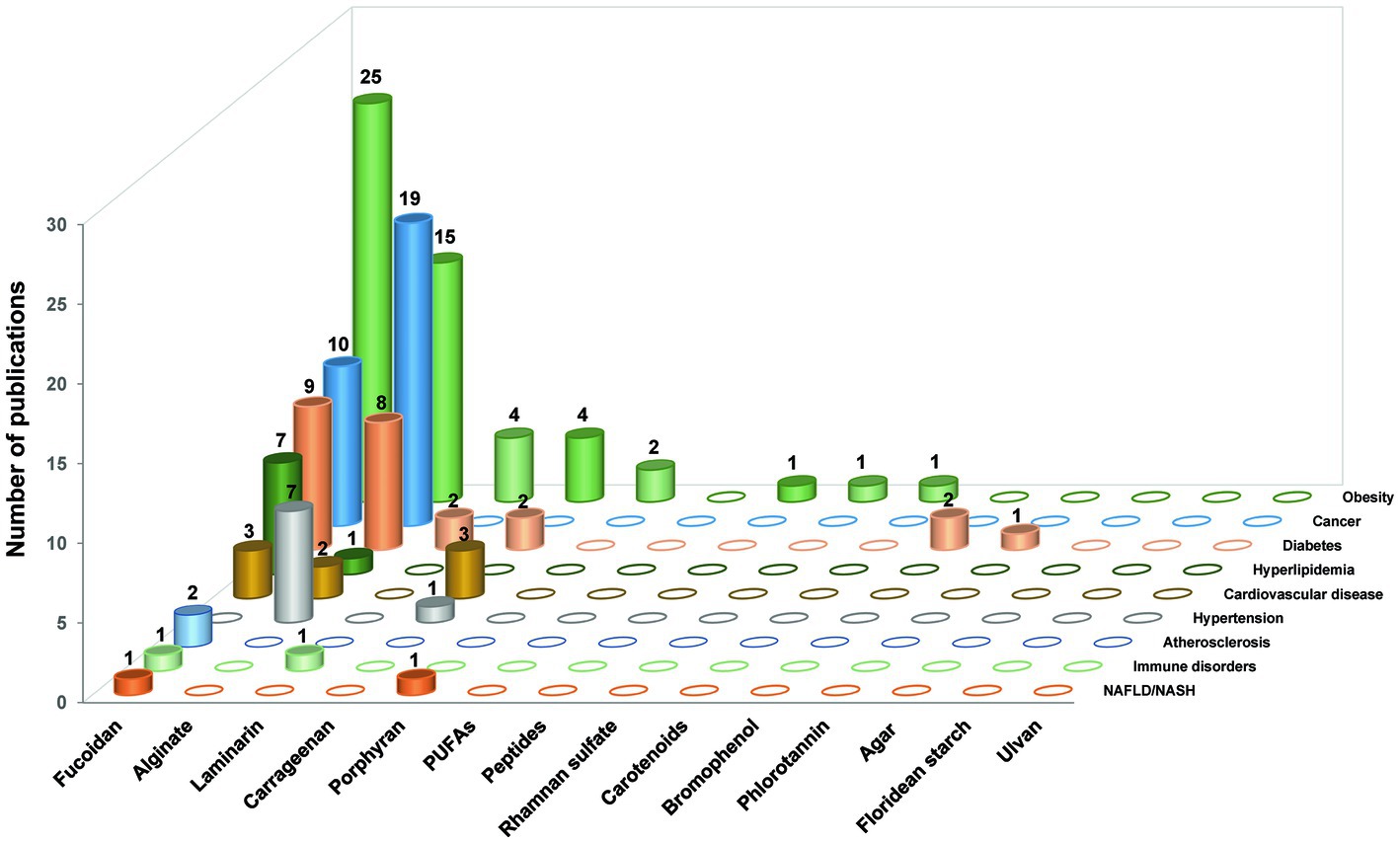
Figure 2. The number of research publications based on the relationship between gut microbiota, different seaweed-derived components, and different types of metabolic syndrome-related diseases. A total of 136 articles were sourced.
3. Seaweed components
Seaweeds contain nutritional elements such as carbohydrates, proteins, lipids, vitamins, and minerals, and the proportional contents of the elements derived depend on the habitat of the particular seaweed, the season in which it was harvested (6), and the extraction and purification methods employed (36, 37). The major bioactive compounds in seaweeds that are beneficial to human health comprise polysaccharides, polyphenols, proteins, peptides, and phytochemicals. In this section, we briefly summarize representative components that promote human health and/or ameliorate diseases. Details of the major seaweed-derived bioactive compounds, including seaweed source, characteristics, and biological activities, are listed in Table 1.
3.1. Polysaccharides
In seaweed, polysaccharides and polycarbohydrates are biomacromolecules composed of repeating monosaccharide units linked by glycosidic bonds. The relevant taxonomies of polysaccharides differ between seaweeds: fucoidans, alginates, and laminarins are predominantly found in brown seaweeds; agars, porphyrins, carrageenan, and floridean starch in red seaweeds; and rhamnan sulfate and ulvan are found in green seaweeds (38, 39).
Fucoidans comprise a family of polymeric molecules derived from sulfated polysaccharides consisting of l-fucose (6-deoxy-l-galactose) (40). They are primarily produced by brown algae and, to a lesser extent, by other marine aquatics such as sea cucumbers (41). Fucoidan provides an outer cell wall structure and a hydrophilic coating to prevent seaweed from drying during low tides (42). In general, fucoidans have long, simple structures based on their fucose and sulfate groups. Fucoidans are usually formed by monosaccharides, such as mannose, galactose, glucose, xylose, uronic acids, acetyl groups, and proteins (43), and some fucoidans contain monosaccharides with alternating α (1 → 3) and α (1 → 4) bonds (44). Over the past several decades, fucoidan has been shown to have anticancer, antidiabetic, anti-hyperlipidemic, antioxidant, anti-inflammatory, anticoagulant, antithrombotic, and antiviral activities (45). Recently, the prebiotic effects of fucoidans have gained attention in both in vitro and in vivo studies (46).
Alginate is a water-soluble linear polysaccharide containing linear copolymers composed of blocks of (1,4)-linked β-D-mannuronate (M) and-l-guluronate (G) (47). Alginate is the most abundant polysaccharide naturally present in the cell walls of brown seaweeds (48) and is a naturally occurring anionic polymer. They have a variety of biochemical and biomedical applications, such as wound healing, drug delivery, cell culture, and tissue engineering (blood vessels, bones, cartilage, muscles, nerves, pancreas, and liver), owing to their biocompatibility, low toxicity, low cost, and mild gelation caused by the addition of divalent cations such as Ca2+ (49, 50). Hydrogels are composed of hydrophilic polymers with a high water content and three-dimensional cross-linked networks. Depolymerized alginate, also known as alginate oligosaccharide, is widely used in the food and pharmaceutical industries. Alginate oligosaccharides have received attention for their biological functions, such as decreasing the risk of cardiovascular disease in addition to having anti-inflammatory, antitumor, and antioxidant activities (51).
Laminarin, or laminaran, is a type of β-(1 → 3)-glucan containing β-(1 → 6)-linked branches (52). It is mainly found as a storage polysaccharide (up to 35% of the dry weight) in brown algae. In Asian countries, laminarin is formulated for use as a food, medicine, and dietary supplement (53), and it provides a wide range of bioactivities with anticancer, anti-metastatic, and antioxidant properties (46), making it increasingly attractive as an essential nutritious source or functional food.
Agar is a galactose-based, heterogeneous polysaccharide derived from the cell walls of red algae. It is a thermo-reversible gelling agent composed of 70% agarose and 30% agaropectin polymers. Agarose (or agaran) is a gel-forming component with a linear chain of 3-O-substituted β-d-galactopyranosyl units linked by (1 → 4) chains to 3,6-anhydro-α-l-galactopyranosyl units (54). Agaropectin is a branched, non-gelling component of agar that contains the D- and L-isomers of galactose. Agar is best known as a growth medium to identify and enumerate microorganisms (55). The properties of agar include anti-pathogenic activity (56), maintenance of cellular ionic equilibrium (57), and protection from extreme salinity, pH, temperature, and desiccation (58). Agars are used as phycocolloids in diverse industries such as food, pharmaceuticals, cosmetics, medicine, and biotechnology (59).
Porphyran is a water-soluble polysaccharide within the algal cell wall and intercellular space and is the main component of porphyrin. A typical porphyran structure consists of a linear skeleton of alternating 1,3-linked β-D-galactosyl units (G) and 1,4-linked L-residues (60). The key roles of porphyrans in functional foods, cosmetics, and pharmaceuticals are well established. This polysaccharide exhibits many biological properties, including antioxidant, anti-inflammatory, immunomodulatory, anticancer, anti-aging, anti-allergenic, anti-hyperglycemic, and anti-hyperlipidemic effects (61).
Carrageenans are linear hydrophilic sulfated polysaccharides of the galactan family extracted from various genera of red algae. Carrageenans contain 15–40% ester sulfate, and they are formed by alternating units of d-galactose and 3,6-anhydro-galactose (3,6-AG) linked together by α-1,3 and β-1,4-glycosidic bonds. Carrageenans are classified into λ-, κ-, ι, β, μ-, ν-, and θ-carrageenan, depending on the repeating disaccharide units, all of which contain 22–35% sulfate groups. Due to their fundamental structure, carrageenans exhibit various physicochemical properties and are used for gelling, solubility, electronegativity, and synergistic effects (62). Gel formation from carrageenan is extensively used in the food, pharmaceutical, and bioengineering industries (63). Carrageenans have been reported to exhibit antiviral (64), anti-tumor (65), anti-endotoxic (66), and immunomodulatory activities (67).
Floridean starch and floridoside are the main storage carbohydrates of red algae. Floridean starch comprises a semi-crystalline polysaccharide that is similar to starch, but in green algae, differing from land plants due to its amylopectin-like glucan and amylose contents (68). In addition to serving as an effective photosynthetic substrate for red algae, floridean starch provides an efficient method for producing hydroxymethylfurfural (69). Floridoside (α-d-galactopyranosyl-(1,2)-glycerol), a natural galactosyl glycerol, is the main soluble photosynthetic molecule synthesized in the cytoplasm of red algae and has antioxidant properties (70).
Rhamnan sulfate (RS) and ulvans are sulfated polysaccharides extracted from green algae. They are sometimes categorized into the same group; however, there are clear differences between them with respect to their sugar compositions and main-chain structures (71). RS is obtained from the cell walls of the green alga Monostroma sp. and contains varied amounts of l-rhamnose and d-glucose, respectively (72). RS is composed of octa-saccharide repeating units with a linear chain of α-1,3-linked l-rhamnose attached to α-1,2-linked branched chains, to which several units are bound to macromolecular polysaccharides with molecular weights ranging from tens of thousands to millions (71). RS has therapeutic effects in metabolic syndrome-related disorders and provides antioxidative, anticoagulant, anti-inflammatory, and antitumor benefits (73, 74). Furthermore, the RS from M. nitidum has antiviral effects against several viruses (75), especially the wild-type SARS-CoV-2 and the delta variant (76). Ulvan is mainly obtained from Ulva sp., and it is primarily composed of l-rhamnose, d-xylose, d-glucose, iduronic acid, and d-glucuronic acid (77). Similar to RS, ulvan is also a potential candidate with anti-inflammatory, antioxidant, antibacterial, antiviral, antiherpetic, anticancer, biomedical, and pharmacological activities (78).
3.2. Polyphenols
Polyphenols are a group of heterogeneous compounds that contain a multitude of phenolic structures, ranging from simple molecules to highly polymerized compounds, where each phenolic compound contains one or more hydroxyl groups. Seaweeds have been found to contain several polyphenolic compounds (5–30% of the dry algal mass), including catechins, flavonols, and phlorotannins. Green and red algae contain the highest percentages of phenolic compounds such as phenolic acids, flavonoids, and bromophenols, whereas brown algae have the highest concentration of phlorotannin polyphenols (79). The three main types of chemically diverse polyphenols found in seaweeds are phlorotannins, bromophenols, and flavonoids.
Phlorotannins are polyphenolic derivatives found primarily in brown seaweeds (5–12% of the dry algal mass), and they are structurally distinct from tannins produced by terrestrial plants. These compounds are composed of polymeric chains of basic phloroglucinol residues (1,3,5-trihydroxybenzene) connected by C–C or C–O–C interactions. Phlorotannins have been found to have neuroprotective, antidiabetic, anticancer, antioxidant, anti-inflammatory, antihypertensive, hepatoprotective, and antimicrobial properties (80).
Bromophenols (BPs) are polyphenolic compounds with one or more benzene rings, hydroxyl substituents, bromine, and other groups in their structure (81). Marine BPs are conventional secondary metabolites biosynthesized by bromoperoxidases, bromases, laccases, hydrogen peroxide, and bromide (82). BPs exhibit diverse biological activities, including antioxidant (83), antiradical (84), anticancer (85), antimicrobial (86), anti-diabetic (87), anti-obesity (88), and anti-inflammatory properties (89).
Flavonoids are hydroxylated polyphenolic compounds with various structures that are found as aglycones or glycosides in many fruits and vegetables. Seaweeds such as Ulva clathrata also have high flavonoid content (90). With respect to their chemical structure, flavonoids consist of 15 carbons and include phenyl-benzo-γ-pyrans (C6-C3-C6), known as nucleus flava, comprising two phenyl rings (A and B) linked by a heterocyclic ring C (pyran). Flavonoids in brown algae have been reported to exhibit anti-allergenic activities (91).
3.3. Phytochemicals
Phytochemicals are plant-based chemicals produced via both primary and secondary metabolic pathways. Phytochemicals serve as antioxidant compounds but are not considered essential nutrients. There exists evidence of their beneficial health effects, such as those pertaining to carotenoids and PUFAs.
Carotenoids are terpenoid pigments that contain linear C40 polyene chains. They are abundant in seaweeds, are generally found in chloroplasts, and function in photosynthesis (92). Beta-carotene (β-carotene), lutein, zeaxanthin, astaxanthin, and fucoxanthin are essential carotenoids. Among these, β-carotene is one of the most abundant carotenoids in seaweeds and is a primary source of vitamin A. The powerful antioxidant properties of β-carotene make it widely used in photoprotection (93). Zeaxanthin is a dihydroxy-form derivative of β-carotene, whereas lutein is a dihydroxy-form derivative of α-carotene. In addition to being major components of macular pigments in the retina (eyesight), lutein and zeaxanthin are essential for eyesight and the prevention of strokes and lung cancer (94). Astaxanthin is a red fat-soluble pigment without pro-vitamin A properties, but has been shown to have antioxidant, anti-inflammatory, and immune-enhancing biological activities in humans and animals (95, 96). Fucoxanthins are among the most abundant carotenoids in seaweeds and are mainly found in brown seaweeds and microalgae. Owing to its potent health benefits, fucoxanthin has been the focus of much attention due to its ability to prevent cancers through its antioxidant activity and apoptosis-inducing action (97).
Polyunsaturated fatty acids (PUFAs) are fatty acids with more than two double bonds in their backbones. Many brown seaweeds possess high levels of total lipids in their dry weights (ranging from 1 to 10% per dry weight). These seaweeds are an immense source of omega-3 PUFAs (such as EPA and stearidonic acid [18,4n–3)], whereas omega-6 PUFAs are predominantly arachidonic acid (ARA, 20:4n–6) (98). These nutrients play crucial roles in brain development, cognition, prevention of neurodegeneration, regulation of inflammatory and immune responses, and prevention of many diseases, including cardiovascular diseases, cancer, and diabetes (99).
3.4. Proteins derived from seaweeds
Seaweed proteins contain several amino acids, including glycine, arginine, alanine, and glutamic acid. The protein content varies from 10 to 40% per dry weight, depending on the species and season. In general, the content was low for brown seaweeds (3% ± 15% per dry weight), moderate for green algae (9% ± 26% per dry weight), and high for red seaweeds (with a maximum of almost 50% of dry weight). The two functionally active proteins in seaweeds are lectins and phycobiliproteins (8). Lectins are glycoproteins of non-immune origin that bind to carbohydrates and are associated with many biological processes, such as intercellular communication and red blood cell agglutination (100). They have been detected in several seaweed species and possess antibacterial (101), anti-inflammatory (102), antiviral, and anticancer properties (103). The phycobiliprotein family of fluorescent proteins found in red seaweeds is relatively stable, highly soluble, and exhibits strong absorption in the visible light spectrum. Three major categories of phycobiliproteins (phycocyanins, allophycocyanins, and phycoerythrins) are the major light-harvesting pigments in red seaweeds and are regularly used as fluorescent probes in scientific experiments (104).
3.5. Peptides derived from seaweeds
Marine bioactive peptides are typically composed of approximately 3–40 amino acids, and their activities are determined by their amino acid sequences and composition. These amino acids are not active within the sequence of the parent protein but can be released during gastrointestinal digestion, food processing, and fermentation. Aspartic and glutamic acids are the predominant amino acids found in seaweeds. Recently, seaweed-derived peptides have been widely investigated in the nutraceutical and pharmaceutical industries because of their health benefits. Seaweed-derived bioactive peptides were detected to be involved in various biological functions, including antioxidant (105, 106), anticancer (107, 108), antihypertensive (109), and anti-atherosclerotic effects (110). Bioactive peptides are believed to positively affect the body’s ability to function and thus influence human health (111–113).
4. Impact of seaweed-derived components on metabolic syndrome via regulation of gut microbiota or organic acid
4.1. Obesity and hyperlipidemia
The dietary intake of seaweeds or seaweed-derived bioactive molecules has been shown to reduce the prevalence of (or alleviate) certain chronic diseases, including obesity and obesity-induced hyperlipidemia. Representative studies related to the potential of seaweed-derived bioactive compounds in relieving obesity via modulation of gut microbiota are listed in Table 2.
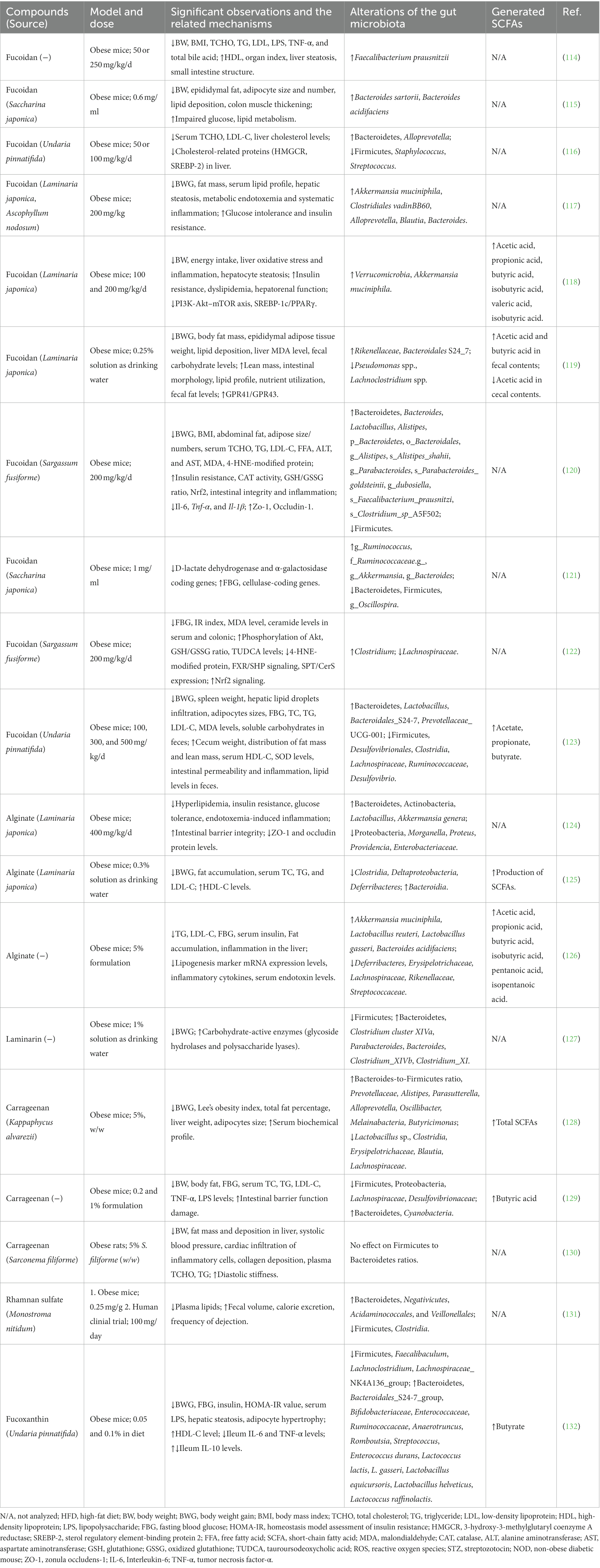
Table 2. Effects of seaweed-derived compounds on obesity or hyperlipidemia through gut microbiota regulation.
4.1.1. Polysaccharides against obesity via modulating gut microbiota
In vivo studies have revealed the benefits of fucoidan in a high-fat diet (HFD)-induced obesity mouse model, including a reduction in body weight gain or BMI, improved lipid profiles in the serum and liver, decreased lipid deposition in adipocytes and hepatocytes, and suppressed adipocyte hypertrophy (Table 2). The mechanisms of action of fucoidans in the prevention and treatment of obesity include decreased energy intake, regulation of lipid metabolism by affecting lipid absorption, and improved anti-oxidant capacity (99). In addition, the gut microbiota has attracted attention as a crucial mechanism involved in the fucoidan-induced alleviation of obesity.
The gut microbiota is mainly composed of two dominant bacterial phyla, Firmicutes and Bacteroidetes, which represent 90% of gut bacteria (133). Firmicutes contain numerous carbohydrate metabolism enzymes that contribute to macronutrient metabolism and allow greater energy absorption (134), whereas Bacteroidetes are responsible for the degradation of many complex glycans. Therefore, Bacteroidetes is positively correlated with obesity, but Firmicutes is negatively correlated, and previous studies have found that obese individuals have an increased Firmicutes/Bacteroidetes ratio (135). Fucoidan administration to HFD-induced obese mice resulted in a reversed Firmicutes/Bacteroidetes ratio compared with that in HFD-fed mice (116, 120, 121, 123). Therefore, the anti-obesity effect of fucoidan may be partially related to alterations in the Firmicutes/Bacteroidetes ratio. At the genus level, fucoidan improves the bacterial proportions of Lactobacillus, Faecalibacterium, Blautia, Bacteroides, Alistipes, Ruminococcus, and Alloprevotella, which are beneficial bacteria negatively correlated with obesity (116, 117, 120, 121, 123). In addition, the abundances of Firmicutes, Staphylococcus, Streptococcus, Pseudomonas spp., Lachnoclostridium spp., g_Oscillospira, Lachnospiraceae, Desulfovibrionales, and Clostridia, which are positively correlated with obesity, were significantly decreased by fucoidan administration in HFD mice (114, 116, 119, 121, 123). A notable bacterial genus, Akkermansia, has been reported to be negatively correlated with obesity (136). Oral administration of Akkermansia reverses HFD-induced metabolic syndrome, indicating its potential use for treatment by modulating the gut microbiota (137). Several studies have reported that fucoidan has powerful effects on increasing the abundance of Akkermansia, which may also partly explain the beneficial effect of fucoidan in modulating this gut microbe (117, 118, 121). In addition, fucoidan alleviates gut dysbiosis, consequently improving nutritional utilization by decreasing total fecal carbohydrates and increasing fecal fat levels (119, 123). The intestinal contents of HFD-fed mice showed that fucoidan treatment significantly increased SCFAs such as acetic acid, propionic acid, butyric acid, isobutyric acid, valeric acid, and isobutyric acid (118, 119, 123), which greatly contributed to the control of body weight, glucose homeostasis, and insulin sensitivity (22). Notably, fucoidan treatment ameliorates HFD-induced intestinal structural damage, including thickened colon muscle tissue, decreased cecal weight, shortened colon length, and impaired colon mucosa structure (115, 119, 123). This therapeutic effect may be caused by regulated beneficial bacteria (Bacteroides, Lactobacillus, and Akkermansia) that are positively related to intestinal integrity and inflammation (120).
Alginate also has a strong effect on the gut microbiota of HFD-induced obese mice. Li et al. reported that HFD-induced obese mice fed unsaturated alginate oligosaccharides (UAOS; 400 mg/kg/day) for 7 weeks showed attenuated obesity-related metabolic abnormalities, such as hyperlipidemia, insulin resistance, and low-grade inflammation, via modulation of the gut microbiota (124). UAOS treatment partially reversed HFD-induced gut dysbiosis by increasing the abundance of Bacteroidetes and Actinobacteria and decreasing the abundance of Proteobacteria. In contrast, the abundance of Morganella, Proteus, Providencia, and Enterobacteriaceae was significantly reduced. Interestingly, UAOS treatment selectively increased the abundance of beneficial intestinal bacteria (Lactobacillus and Akkermansia) and decreased the abundance of inflammatory bacteria (Parabacteroides). Another study involving HFD-induced obese mice showed that low-molecular-weight alginate improved body weight gain, fat accumulation, and hyperlipidemia by modulating the gut microbiota, increasing the number of beneficial bacteria (Bacteroides), and decreasing the number of harmful bacteria (Lachnospiraceae) in the gut of alginate-treated obese mice (125). The increased production of SCFAs showed that low-molecular-weight alginates benefit host health through SCFA-mediated pathways. Wang et al. also demonstrated that the supplementation of HFD-induced obese mice with alginate oligosaccharides significantly ameliorated metabolic disorders by promoting the growth of Akkermansia muciniphila, Lactobacillus reuteri, and Lactobacillus gasseri. These bacteria exhibit multiple correlations with metabolic traits, including negative correlations with total cholesterol (TC), LDL-C, triglyceride (TG) levels, fasting blood glucose (FBG), and serum endotoxins (126).
4.1.2. Other anti-obesity seaweed-derived compounds via modulation of gut microbiota
The anti-obesity effects of other bioactive compounds derived from seaweeds, such as laminarin, carrageenan, porphyran, rhamnan sulfate, fucoxanthin, and bromophenol, are also related to gut microbiota modulation. Nguyen et al. found that laminarin treatment in HFD mice decreased body weight gain with an improved Firmicutes/Bacteroidetes ratio, enriched beneficial bacteria (Clostridium cluster XIVa, Parabacteroides, and Bacteroides), and reduced the abundance of potentially pathogenic bacteria (Clostridium_XIVb and Clostridium_XI). A high abundance of carbohydrate-active enzymes (glycoside hydrolases and polysaccharide lyases) has also been reported (127).
Two studies found that κ-carrageenan relieved HFD-induced body weight gain, hyperlipidemia, and body fat accumulation in mice (128, 129). Gut microbiota profiling analysis revealed that the Firmicutes to Proteobacteria ratio was restored compared to that in obese mice. There was an increased abundance of Prevotellaceae and the genera Alistipes and Bacteroidetes, which negatively correlated with hepatic and serum lipid profiles, and a decreased abundance of Blautia, Lachnospiraceae, and Erysipelotrichaceae, which positively correlated with body weight, FBG, and serum lipid profiles.
RS was also found to regulate the gut microbiota during the treatment of metabolic syndrome. Shimada et al. reported that RS administration in HFD-fed mice significantly increased fecal volume and calorie excretion (131). The latter authors further performed a clinical trial in which RS (100 mg/day) was administered to subjects with infrequent defecation (3–5 times/week) in a double-blind, placebo-controlled manner. After 2 weeks of consumption, the subjects showed increased dejection frequency without changes in body weight and blood lipid levels. Furthermore, the gut microbiota exhibited an increased Bacteroidetes-to-Firmicutes ratio. Decreased proportions of Clostridia and increased proportions of Negativicutes, Acidaminococcales, and Veillonellales were observed. Clostridia are positively associated with constipation by producing medium-length fatty acids that increase water absorption and dry feces (138). Additionally, increased Negativicutes and Acidaminococcales have been found to be positively related to the alleviation of constipation, and Negativicutes increase during Bifidobacterium-based probiotic treatment for constipation (139). The main metabolite of Acidaminococcus is acetic acid, which promotes intestinal peristalsis and relieves constipation (140).
Overall, the bioactive compounds of seaweeds described above may comprise promising anti-obesity agents that act via modulation of the gut microbiota. Although PUFAs (141), phlorotannin (79), agar (142), and ulvan (143) have anti-metabolic syndrome functions, few studies have investigated their effects on the gut microbiota.
4.2. Diabetes
It is well known that people with metabolic syndrome have a significantly higher risk of developing type 2 diabetes mellitus (T2DM), which is associated with insulin resistance, of which obesity is a major contributor. Previous studies have shown a significant effect of using seaweeds to treat diabetes, which is achieved by regulating the gut microbiota. The representative studies are listed in Table 3.
4.2.1. Effect of polysaccharides against diabetes via gut microbiota
In HFD and streptozotocin (STZ)-induced T2DM mouse experiments, fucoidan effectively decreased FBG levels and improved glucose tolerance (122, 144, 145). The potential mechanism underlying the amelioration of T2DM by fucoidan is thought to involve the enrichment of benign microbes (Bacteroides, Faecalibacterium, and Blautia) and a decrease in the abundance of Proteobacteria (especially Desulfovibrio). Desulfovibrio is a sulfate-reducing bacterium and opportunistic pathogen associated with inflammatory diseases (150). Wu et al. (144) further analyzed the correlation between Desulfovibrio and metabolic parameters and found that Desulfovibrio was positively correlated with FBG levels and that the decreased abundance of Desulfovibrio by fucoidan may contribute to improving glucose metabolism. Fucoidan treatment was also found to increase SCFA production and promote a relatively high abundance of SCFA-producing bacteria such as Blautia, Faecalibacterium, and Alloprevotella. UAOS also improved obesity-induced insulin resistance and increased serum insulin levels in diabetic mice (124, 126). UAOS treatment significantly increases the abundance of Acetatifactor, which is positively associated with glucose-insulin homeostasis (124). Anaerotruncus, Tyzzerella, Acetatifactor, and Intestinimonas were decreased by alginate oligosaccharide, and their reductions showed positive and negative relationships with FBG and serum insulin, respectively (126). The growth of Akkermansia. muciniphila also improved, showing a positive correlation with serum insulin (151). These results suggest the potential use of alginate oligosaccharides as novel prebiotic agents for the treatment of diabetes and related metabolic diseases.
Wang et al. reported that dietary supplementation of κ-carrageenan in HFD-induced obese mice significantly decreased FBG levels and effectively increased glucose tolerance (129). The relative abundances of Firmicutes, Proteobacteria, Lachnospiraceae, and Desulfovibrionaceae were decreased by κ-carrageenan, and these microbes showed a positive relationship with FBG. However, a recent study found that carrageenan did not affect glycemic control in HFD- and STZ-induced type 2 diabetic rat models (147). Porphyrans have also been found to regulate the gut microbiota during the treatment of metabolic syndrome. He et al. found that Pyropia yezoensis porphyran ameliorated the circulating sugar content in high-sucrose-fed Drosophila melanogaster larvae (148). The abundance of bacteria causing metabolic abnormalities (Escherichia, Shigella, and Fusobacterium) was decreased, whereas the abundance of beneficial bacteria (Bacillus and Akkermansia) increased. However, further mammalian experiments and clinical trials are needed to confirm the effects of carrageenan and porphyran against diabetes by modulating the gut microbiota.
4.2.2. Effect of polyphenols against diabetes via alteration of gut microbiota
Recently, a natural bromophenol (3-bromo-4,5-bis(2,3-dibromo-4,5-dihydroxybenzyl)-1,2-benzenediol; BDB) isolated from the marine red algae, Rhodomela confervoides, was found to alleviate T2DM in diabetic BKS db mice (149). The antidiabetic effect of BDB is closely related to the modulating structure of the gut microbiota, including the increased abundance of short-chain fatty acid (SCFA)-producing bacteria Lachnospiraceae and Bacteroides and the elevation of Akkermansia spp. to enhance glucose homeostasis. Interestingly, BDB alleviated metabolic disorders in T2DM mice by promoting propanoate metabolism and inhibiting starch, sucrose, amino sugars, and nucleotide sugar metabolism.
4.3. Cancer
Although cancer has traditionally been considered a growth disorder, recent evidence suggests that it should be considered a metabolic disease. When tumors grow, they alter their metabolic programs to meet and even exceed the bioenergetic and biosynthetic demands of continued abnormal cell growth. The cancer-promoting effects of gut microbiota dysbiosis have been extensively investigated in recent years (152). In addition, the gut microbiota has been linked to cancer and has been shown to modulate the effects of anticancer drugs.
We found fucoidan to be the most studied seaweed, having been the focus of investigations since the 1980s. It has been found to both decrease the viability of a wide range of cancer cell lines in vitro and to inhibit tumor growth and metastasis in vivo (153). Ten studies have elucidated the anticancer effects of fucoidan by regulating gut microecology. Xue et al. investigated the effects of fucoidans derived from Fucus vesiculosus in a 1,2-dimethylhydrazine-induced colorectal cancer rat model (154). Dietary fucoidan significantly reduces tumor incidence, decreases tumor weight, and increases tumor cell apoptosis. 16S rDNA high-throughput sequencing revealed that gut microbial dysbiosis in the cancer group resulted in an increased Bacteroidetes/Firmicutes ratio. However, fucoidan administration ameliorated this disorder, decreased the abundance of Prevotella (an opportunistic pathogen), and increased the abundance of Alloprevotella (an anti-inflammatory bacterium). The production of SCFAs (especially butyric and caproic acids) increased significantly in the fucoidan-treated groups. Another previous study demonstrated the potential of fucoidan as a gut microbiota modulator for breast cancer prevention (155). Oral administration of fucoidan to breast cancer model rats significantly ameliorated the damaged mucosal morphology of the jejunal tissue by enhancing the expression of tight junction proteins, including ZO-1, occludin, Claudin-1, and Claudin-8. Interestingly, the Bacteroidetes/Firmicutes ratio increased in the intestines of fucoidan-treated cancer model rats. In this example, the abundance of Prevotella increased, which may have contributed to increased SCFAs concentrations. Additionally, fucoidan decreased the abundance of Sutterella, which produces endotoxins that are harmful to animals. Therefore, fucoidan may prevent tumorigenesis or suppress tumor growth by regulating gut microecology. However, more evidence (such as that from clinical trials) is needed to further elucidate the relationship between fucoidan and different types of cancers via gut microbiota regulation.
Alginate has shown considerable activity against murine tumors, such as sarcoma 180 (156); however, studies on the relationship between the antitumor activity of alginate and gut microbiota are lacking. Instead, we found two studies that examined the effects of alginate oligosaccharide (AOS) on the adverse effects of chemotherapy with anticancer drugs such as busulfan. Zhang et al. (157) reported that fecal microbiota transplantation from AOS-treated mice to busulfan-treated ICR mice abolished busulfan-induced small intestinal mucositis and gut microbiota dysbiosis. The ratio of Bacteroidetes/Firmicutes improved, and the abundance of beneficial microbes, such as Leuconostocaceae and Lactobacillales, increased to assist in blood metabolome recovery. In another study, AOS increased murine sperm concentration and motility, and rescued busulfan-disrupted spermatogenesis (132). These results indicate that alginate can be used to prevent small intestinal mucositis and improve fertility in patients undergoing chemotherapy.
4.4. Hypertension
Hypertension is a common disease associated with a high risk of cardiovascular mortality, and often occurs in tandem with metabolic disturbances, specifically dyslipidemia (158). Sodium alginate oligosaccharides have been reported to attenuate several types of hypertension in rat models, such as Dahl salt-sensitive hypertension, spontaneous hypertension, and pulmonary hypertension (159–162). However, compared with the number of reports on the use of seaweed and seaweed-derived components against hypertension, few studies have investigated the relationship between these components and the related gut microbiota. Han et al. found that the oral administration of potassium alginate oligosaccharides (PAO) significantly decreased systolic blood pressure and mean arterial pressure in spontaneously hypertensive rats (163). The relative abundances of Phascolarctobacterium and Prevotella_9 were markedly decreased to nearly zero by PAO and were positively correlated with systolic blood pressure. In addition, lactic acid levels increased with a decrease in the abundance of Lactobacillus, which may be correlated with changes in systolic blood pressure. Carrageenans from the red seaweed Sarconema filiforme were also found to decrease high systolic blood pressure induced by HFD in rats (130), while there was an increase in the relative abundance of the family Ruminococcaceae UCG-014 (belonging to the phylum Firmicutes), which was positively correlated with systolic blood pressure.
4.5. Immune disorders
Any immune system disorder causes the body’s defense mechanisms to malfunction or become disabled. Immune disorders are characterized by inflammatory symptoms. Several studies have investigated the immunomodulatory and regulatory effects of bioactive seaweed-derived compounds (164). Administration of fucoidan extracted from Okinawa mozuku (Cladosiphon okamuranus) to healthy adult zebrafish significantly altered the gut microbiota composition by increasing the relative abundance of Comamonadaceae (AB076847) and Rhizobiaceae (Shinella granuli), which was accompanied by decreased expression levels of the pro-inflammatory cytokine il1b (165). Tang et al. investigated the effects of fucoidan obtained from Laminaria japonica on the immune response and intestinal microflora in a mouse model of immunosuppressant-induced immune disorder (166). Fucoidan treatment significantly enhanced the spleen and thymus indices, relieved the cellular immune response by promoting splenic lymphocyte proliferation, and ameliorated immunosuppression in mice by enhancing cytokine and IgG production. The regulated gut microflora composition included a remarkably decreased abundance of Lactobacillaceae, Bacilli, and Lactobacillus, which was positively correlated with pro-inflammatory cytokine levels, such as IL-6 and TNF-α. Moreover, the abundance of Alistipes significantly increased, which was negatively correlated with MDA levels and positively correlated with SOD and GSH-Px activities. In addition, the disturbance in five gut microbiota species, Erysipelotrichia, Turicibacter, Romboutsia, Peptostreptococcaceae, and Faecalibaculum, was significantly reversed by fucoidan treatment. Another previous study revealed that fucoidan treatment in a mouse model of psoriasis (a chronic autoimmune inflammatory disease) significantly alleviated symptoms and decreased facial scratching (167). Analysis of fecal microbiota revealed that fucoidan increased the relative abundance of Bacteroidetes and decreased that of Firmicutes. Two Clostridiales families, Lachnospiraceae and Ruminococcaceae, were reduced, which are related to pro-inflammatory cytokine secretion and autoimmune disorder induction. The increased relative abundance of Desulfovibrionaceae and Bacteroidetes acidifaciens induced by fucoidan may be associated with the anti-inflammatory effect noted in the improvements in psoriasis symptoms and may promote the expression of secreted IgA in the large intestine to rearrange the intestinal environment by regulating the immune response. Therefore, fucoidan has the potential to be used as an immunoregulatory adjuvant to improve immunosuppression.
The intestinal tract is the major organ involved in immune and inflammatory reactions, and the gut microbiota adversely affected by metabolic syndrome can be reversed using seaweed and seaweed-derived components, such as fucoidan, alginate, and fucoxanthin (117, 118, 120, 126, 168). The enhanced abundance of beneficial bacteria (e.g., Lactobacillaceae and Roseburia) may contribute to the immunoregulatory effects of these bioactive compounds. Fucoxanthin was found to reduce the secretion of pro-inflammatory cytokines (TNF-α and IL-6) and increase IL-10 levels in the ileum of HFD-induced obese mice owing to the decreased abundance of Faecalibaculum, which was proven to be positively correlated with LPS and TNF-α and negatively correlated with IL-10 (132). Although we found no publications focusing on the effects of ulvan on immune disorders via the regulation of the gut microbiota, an in vivo study revealed that feeding ulvan extracted from Ulva ohnoi to normal mice for 28 days positively modulated the gut microbiota (169). The bacterial classes Bacteroidia, Bacilli, Clostridia, and Verrucomicrobia, which are probiotics that assist in maintaining the intestinal barrier, were significantly increased in the fecal samples of ulvan-fed mice. An increased relative abundance of Lachnospiraceae (class Clostridia) has been observed, and reduced levels of these bacteria have been linked to inflammatory bowel disease and chronic gastrointestinal tract infections (170).
In summary, the bioactive compounds present in seaweeds may serve as functional molecules that regulate and modulate the human immune system. However, few such studies have been conducted to date, and further in vivo research and clinical trials are required to better understand their bioactivities.
4.6. Atherosclerosis and cardiovascular disease
Metabolic syndrome-induced atherogenic dyslipidemia, particularly hypertriglyceridemia and reduced HDL levels, is commonly associated with chronic inflammation and endothelial dysfunction, which subsequently accelerates atherosclerosis and increases cardiovascular risk (171). We found 11 publications that reported the anti-atherosclerosis or anti-cardiovascular disease effects of seaweed-derived components (nine and two publications, respectively). Of these, carrageenan appeared in three search results, but the focus of the associated papers was unrelated to the purpose of this study. Carrageenan was used to induce rodent-specific acute inflammation models, such as pleurisy or thrombosis; however, humans do not experience this phenomenon. Therefore, we did not discuss carrageenan further in this section.
Fucoidan from Sargassum fusiforme was reported to ameliorate pathological injury in the cardiac tissue of HFD- and STZ-induced mice, with an increase in enriched benign microbes, including Bacteroides, Faecalibacterium, and Blautia (144). The oral administration of PAO prevents heart failure and microbiome alterations in spontaneously hypertensive rats (163). There was an increase in microbial diversity and a decrease in the Firmicutes to Bacteroidetes ratio via reductions in the abundance of Prevotella and Phascolarctobacterium. The authors also found that Prevotella and Phascolarctobacterium were positively correlated with LPS, a mechanistic biomarker of cardiovascular disease (172). These findings indicate the potential of fucoidan and alginate in preventing the development of atherosclerosis or cardiovascular disease by improving gut dysbiosis.
4.7. Non-alcoholic fatty liver disease and non-alcoholic steatohepatitis
Non-alcoholic fatty liver disease results from the accumulation of fat in the liver, with two hallmarks of inflammation and liver damage (173). Although previous studies have found that seaweed-derived components reduce the incidence of NAFLD/NASH, only two previous studies focused on the role of gut microbiota. For example, Wang et al. reported that porphyran-derived oligosaccharides from Porphyra yezoensis administered to mice with HFD-induced NAFLD markedly reduced hepatic oxidative stress and lipid accumulation and relieved hepatic fibrosis (174). An increased abundance of Akkermansia and a decreased abundance of Helicobacter were observed. Akkermansia is considered to be a next-generation beneficial microbe for its effects on reversing obesity, inflammation, metabolic endotoxemia, and insulin resistance, whereas Helicobacter has been proven to be positively associated with NAFLD with respect to causing inflammation and hepatocyte ballooning. Another study revealed that treatment with the whole macroalga Laminaria japonica prevented HFD-induced NAFLD in a rat model (175). Although this study did not evaluate single compounds, consumption of whole seaweed ameliorated HFD-induced NAFLD by restoring gut microbiota dysbiosis.
5. Conclusion and future perspectives
Studies have demonstrated that seaweeds and their bioactive components are potential foods that can be used to manage human metabolic syndrome, and that they have positive effects on obesity, hyperglycemia, hyperlipidemia, and hypertension. Animal experiments have shown that seaweed-derived bioactive components can decrease the risk of metabolic syndrome-related diseases by reducing the Firmicutes/Bacteroidetes ratio, increasing the relative abundance of beneficial bacteria, such as Bacteroides, Akkermansia, Lactobacillus, or decreasing the abundance of harmful bacteria, such as Lachnospiraceae, Desulfovibrio, Lachnoclostridium. The proposed mechanisms for the observed effects are summarized in Figure 3. Briefly, the regulated gut microbiota affects the generation of SCFAs and influences glucose and lipid metabolism. The intestinal structure can be improved by inhibiting intestinal inflammation and alleviating intestinal integrity. Altered microbiota inhibits endotoxin-induced inflammation and oxidative stress. Increased bile acid production may also be associated with improved glucose homeostasis and lipid metabolism. Thus, the interaction between the gut microbiota and seaweed-derived bioactive components plays a critical regulatory role in human health, and these compounds can be used as potential materials for drug development.
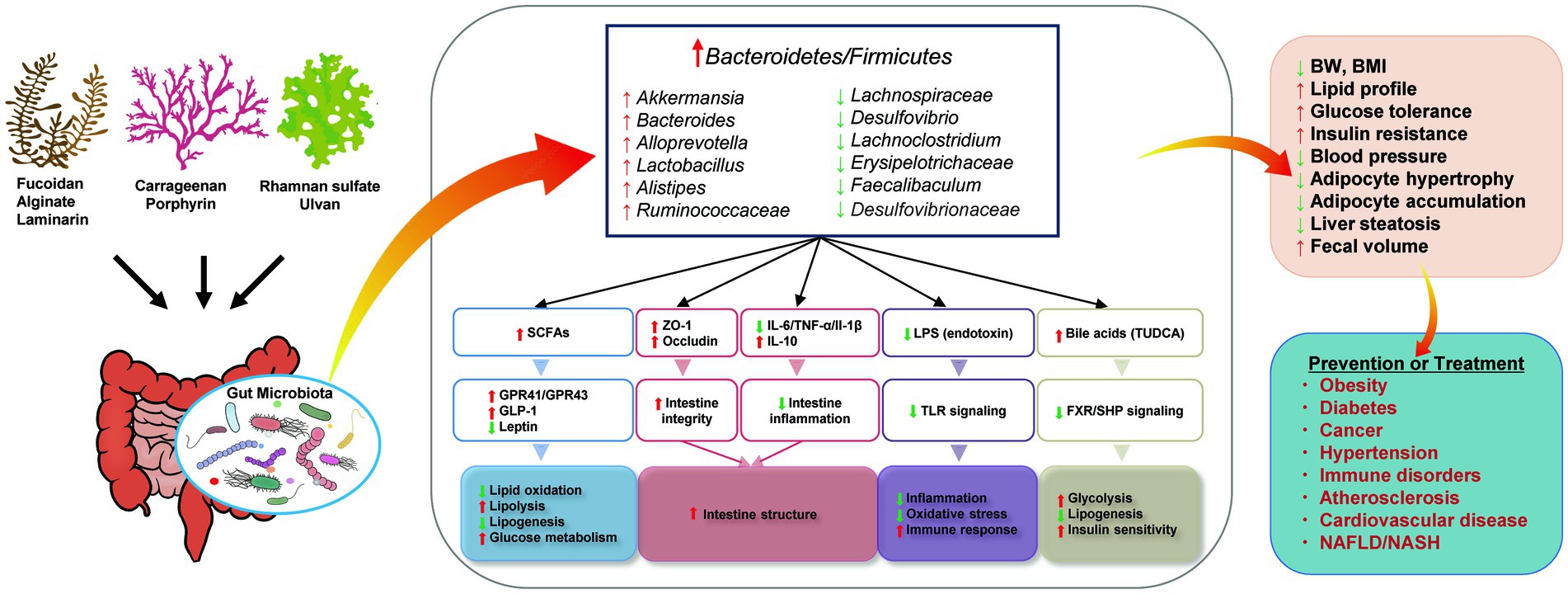
Figure 3. Proposed mechanisms for the effects of seaweed-derived bioactive components on improving metabolic syndrome via regulating gut microbiota. Illustrations were provided by Illust AC (https://www.ac-illust.com) with no copyright issue.
However, most of the published articles we collected focused on fucoidan and alginate, and very few papers have reported how other compounds affect gut microbiota and diseases. For example, in addition to fucoidan and alginate, other seaweed-derived compounds, such as rhamnan sulfate, have the potential to prevent or treat metabolic syndrome-related diseases by improving gut dysbiosis. Therefore, more in vitro and in vivo data are required to understand the functional roles and mechanisms of these components in balancing the gut microbiota and managing host health. In addition, only one clinical trial revealed a relationship between seaweed-derived biocomponents and modulated gut microbiota to alleviate metabolic syndrome-related diseases. However, further clinical trials are required to verify the therapeutic efficacy of these compounds. Although comparatively few studies have been conducted on whole algae, those that exist have shown promising results, such as prebiotic effects (176, 177). Therefore, whole seaweed consumption for the prevention and treatment of metabolic syndrome via the modulation of the gut microbiota needs to be investigated further. This reduces the economic and environmental costs of bioactive compound extraction.
Author contributions
LZ, YS, MT, and NN: conceptualization. LZ: investigation. LZ and MB: writing—original draft preparation. LZ, YS, and MT: writing—review and editing. NN: supervision, project administration, and funding acquisition. All authors contributed to the article and approved the submitted version.
Funding
This study received research grants from the chemical company Konan Chemical Manufacturing Co., Ltd.
Acknowledgments
The authors thank Ms. Takako Taguchi, Ms. Chikako Araki, and Mrs. Yukako Demiya for secretarial assistance.
Conflict of interest
MT is an employee by Konan Chemical Manufacturing Co., Ltd. NN is a board member of Konan Chemical Manufacturing Co., Ltd.
The authors declare that this study received funding from Konan Chemical Manufacturing Co., Ltd. The funder had the following involvement in the study: manuscript preparation and decision to publish.
Publisher’s note
All claims expressed in this article are solely those of the authors and do not necessarily represent those of their affiliated organizations, or those of the publisher, the editors and the reviewers. Any product that may be evaluated in this article, or claim that may be made by its manufacturer, is not guaranteed or endorsed by the publisher.
Supplementary material
The Supplementary material for this article can be found online at: https://www.frontiersin.org/articles/10.3389/fnut.2023.1173225/full#supplementary-material
References
1. Swarup, S, Goyal, A, Grigorova, Y, and Zeltser, R. Metabolic syndrome. Statpearls Treasure Island (FL). (2022) Available at: https://www.ncbi.nlm.nih.gov/books/NBK459248/
2. Bessesen, DH. Update on obesity. J Clin Endocrinol Metab. (2008) 93:2027–34. doi: 10.1210/jc.2008-0520
3. Gabbia, D, and De Martin, S. Brown seaweeds for the management of metabolic syndrome and associated diseases. Molecules. (2020) 25:4182. doi: 10.3390/molecules25184182
4. Santos, SA, Vilela, C, Freire, CS, Abreu, MH, Rocha, SM, and Silvestre, AJ. Chlorophyta and Rhodophyta macroalgae: a source of health promoting phytochemicals. Food Chem. (2015) 183:122–8. doi: 10.1016/j.foodchem.2015.03.006
5. Yong, WTL, Thien, VY, Rupert, R, and Rodrigues, KF. Seaweed: a potential climate change solution. Renew Sust Energ Rev. (2022) 159:112222. doi: 10.1016/j.rser.2022.112222
6. Holdt, SL, and Kraan, S. Bioactive compounds in seaweed: functional food applications and legislation. J Appl Phycol. (2011) 23:543–97. doi: 10.1007/s10811-010-9632-5
7. Mohamed, S, Hashim, SN, and Rahman, HA. Seaweeds: a sustainable functional food for complementary and alternative therapy. Trends Food Sci Tech. (2012) 23:83–96. doi: 10.1016/j.tifs.2011.09.001
8. Fleurence, J, Morançais, M, and Dumay, J. 9–seaweed proteins In: RY Yada, editor. Proteins in Food Processing. 2nd ed. Sawston, Cambridge: Woodhead Publishing (2018). 245–62.
9. Lomartire, S, and Goncalves, AMM. An overview of potential seaweed-derived bioactive compounds for pharmaceutical applications. Mar Drugs. (2022) 20:141. doi: 10.3390/md20020141
10. Charoensiddhi, S, Conlon, MA, Franco, CMM, and Zhang, W. The development of seaweed-derived bioactive compounds for use as prebiotics and Nutraceuticals using enzyme technologies. Trends Food Sci Tech. (2017) 70:20–33. doi: 10.1016/j.tifs.2017.10.002
11. El-Beltagi, HS, Mohamed, AA, Mohamed, HI, Ramadan, KMA, Barqawi, AA, and Mansour, AT. Phytochemical and potential properties of seaweeds and their recent applications: a review. Mar Drugs. (2022) 20:342. doi: 10.3390/md20060342
12. Raja, K, Kadirvel, V, and Subramaniyan, T. Seaweeds, an aquatic plant-based protein for sustainable nutrition–a review. Future Foods. (2022):5. doi: 10.1016/j.fufo.2022.100142
13. Lopez-Santamarina, A, Miranda, JM, Mondragon, ADC, Lamas, A, Cardelle-Cobas, A, Franco, CM, et al. Potential use of marine seaweeds as prebiotics: a review. Molecules. (2020) 25:1004. doi: 10.3390/molecules25041004
14. Cherry, P, Yadav, S, Strain, CR, Allsopp, PJ, McSorley, EM, Ross, RP, et al. Prebiotics from seaweeds: an ocean of opportunity? Mar Drugs. (2019) 17:327. doi: 10.3390/md17060327
15. Altves, S, Yildiz, HK, and Vural, HC. Interaction of the microbiota with the human body in health and diseases. Biosci Microbiota Food Health. (2020) 39:23–32. doi: 10.12938/bmfh.19-023
16. Barko, PC, McMichael, MA, Swanson, KS, and Williams, DA. The gastrointestinal microbiome: a review. J Vet Intern Med. (2018) 32:9–25. doi: 10.1111/jvim.14875
17. Ding, RX, Goh, WR, Wu, RN, Yue, XQ, Luo, X, Khine, WWT, et al. Revisit gut microbiota and its impact on human health and disease. J Food Drug Anal. (2019) 27:623–31. doi: 10.1016/j.jfda.2018.12.012
18. Zhang, YJ, Li, S, Gan, RY, Zhou, T, Xu, DP, and Li, HB. Impacts of gut bacteria on human health and diseases. Int J Mol Sci. (2015) 16:7493–519. doi: 10.3390/ijms16047493
19. Gill, SR, Pop, M, Deboy, RT, Eckburg, PB, Turnbaugh, PJ, Samuel, BS, et al. Metagenomic analysis of the human distal gut microbiome. Science. (2006) 312:1355–9. doi: 10.1126/science.1124234
20. Uchiyama, K, Naito, Y, and Takagi, T. Intestinal microbiome as a novel therapeutic target for local and systemic inflammation. Pharmacol Therapeut. (2019) 199:164–72. doi: 10.1016/j.pharmthera.2019.03.006
21. Levy, M, Blacher, E, and Elinav, E. Microbiome, metabolites and host immunity. Curr Opin Microbiol. (2017) 35:8–15. doi: 10.1016/j.mib.2016.10.003
22. Canfora, EE, Jocken, JW, and Blaak, EE. Short-chain fatty acids in control of body weight and insulin sensitivity. Nat Rev Endocrinol. (2015) 11:577–91. doi: 10.1038/nrendo.2015.128
23. Xiong, RG, Zhou, DD, Wu, SX, Huang, SY, Saimaiti, A, Yang, ZJ, et al. Health benefits and side effects of short-chain fatty acids. Foods. (2022) 11:2863. doi: 10.3390/foods11182863
24. Baothman, OA, Zamzami, MA, Taher, I, Abubaker, J, and Abu-Farha, M. The role of gut microbiota in the development of obesity and diabetes. Lipids Health Dis. (2016) 15:108. doi: 10.1186/s12944-016-0278-4
25. Carding, S, Verbeke, K, Vipond, DT, Corfe, BM, and Owen, LJ. Dysbiosis of the gut microbiota in disease. Microb Ecol Health Dis. (2015) 26:26191. doi: 10.3402/mehd.v26.26191
26. Santos-Marcos, JA, Perez-Jimenez, F, and Camargo, A. The role of diet and intestinal microbiota in the development of metabolic syndrome. J Nutr Biochem. (2019) 70:1–27. doi: 10.1016/j.jnutbio.2019.03.017
27. Aron-Wisnewsky, J, Vigliotti, C, Witjes, J, Le, P, Holleboom, AG, Verheij, J, et al. Gut microbiota and human Nafld: disentangling microbial signatures from metabolic disorders. Nat Rev Gastroenterol Hepatol. (2020) 17:279–97. doi: 10.1038/s41575-020-0269-9
28. Carrizales-Sanchez, AK, Garcia-Cayuela, T, Hernandez-Brenes, C, and Senes-Guerrero, C. Gut microbiota associations with metabolic syndrome and relevance of its study in pediatric subjects. Gut Microbes. (2021) 13:1960135. doi: 10.1080/19490976.2021.1960135
29. Khan, I, Ullah, N, Zha, L, Bai, Y, Khan, A, Zhao, T, et al. Alteration of gut microbiota in inflammatory bowel disease (Ibd): cause or consequence? Ibd treatment targeting the gut microbiome. Pathogens. (2019) 8:126. doi: 10.3390/pathogens8030126
30. Aggeletopoulou, I, Konstantakis, C, Assimakopoulos, SF, and Triantos, C. The role of the gut microbiota in the treatment of inflammatory bowel diseases. Microb Pathog. (2019) 137:103774. doi: 10.1016/j.micpath.2019.103774
31. Knox, NC, Forbes, JD, Peterson, CL, Van Domselaar, G, and Bernstein, CN. The gut microbiome in inflammatory bowel disease: lessons learned from other immune-mediated inflammatory diseases. Am J Gastroenterol. (2019) 114:1051–70. doi: 10.14309/ajg.0000000000000305
32. Lavelle, A, and Sokol, H. Gut microbiota-derived metabolites as key actors in inflammatory bowel disease. Nat Rev Gastroenterol Hepatol. (2020) 17:223–37. doi: 10.1038/s41575-019-0258-z
33. Pietrucci, D, Cerroni, R, Unida, V, Farcomeni, A, Pierantozzi, M, Mercuri, NB, et al. Dysbiosis of gut microbiota in a selected population of Parkinson's patients. Parkinsonism Relat Disord. (2019) 65:124–30. doi: 10.1016/j.parkreldis.2019.06.003
34. Kandpal, M, Indari, O, Baral, B, Jakhmola, S, Tiwari, D, Bhandari, V, et al. Dysbiosis of gut microbiota from the perspective of the gut&Ndash;brain Axis: role in the provocation of neurological disorders. Meta. (2022) 12:1064. doi: 10.3390/metabo12111064
35. Shabbir, U, Arshad, MS, Sameen, A, and Oh, D-H. Crosstalk between gut and brain in Alzheimer’s disease: the role of gut microbiota modulation strategies. Nutrients. (2021) 13:690. doi: 10.3390/nu13020690
36. Zhang, H, Jiang, F, Zhang, J, Wang, W, Li, L, and Yan, J. Modulatory effects of polysaccharides from plants, marine algae and edible mushrooms on gut microbiota and related health benefits: a review. Int J Biol Macromol. (2022) 204:169–92. doi: 10.1016/j.ijbiomac.2022.01.166
37. Wassie, T, Niu, K, Xie, C, Wang, H, and Xin, W. Extraction techniques, biological activities and health benefits of marine algae Enteromorpha Prolifera polysaccharide. Front Nutr. (2021) 8:747928. doi: 10.3389/fnut.2021.747928
38. Priyan Shanura Fernando, I, Kim, KN, Kim, D, and Jeon, YJ. Algal polysaccharides: potential bioactive substances for Cosmeceutical applications. Crit Rev Biotechnol. (2018):1–15. doi: 10.1080/07388551.2018.1503995
39. Olsson, J, Toth, GB, and Albers, E. Biochemical composition of red, green and Brown seaweeds on the Swedish west coast. J Appl Phycol. (2020) 32:3305–17. doi: 10.1007/s10811-020-02145-w
40. Ale, MT, Mikkelsen, JD, and Meyer, AS. Important determinants for Fucoidan bioactivity: a critical review of structure-function relations and extraction methods for Fucose-containing sulfated polysaccharides from Brown seaweeds. Mar Drugs. (2011) 9:2106–30. doi: 10.3390/md9102106
41. Oh, G-W, Ko, S-C, Lee, DH, Heo, S-J, and Jung, W-K. Biological activities and biomedical potential of sea cucumber (Stichopus Japonicus): a review. Fish Aquatic Sci. (2017) 20:28. doi: 10.1186/s41240-017-0071-y
42. Berteau, O, and Mulloy, B. Sulfated Fucans, fresh perspectives: structures, functions, and biological properties of sulfated Fucans and an overview of enzymes active toward this class of polysaccharide. Glycobiology. (2003) 13:29R–40R. doi: 10.1093/glycob/cwg058
43. Li, B, Lu, F, Wei, XJ, and Zhao, RX. Fucoidan: structure and bioactivity. Molecules. (2008) 13:1671–95. doi: 10.3390/molecules13081671
44. Badrinathan, S, Shiju, TM, Christa, ASS, Arya, R, and Pragasam, V. Purification and structural characterization of sulfated polysaccharide from Sargassum Myriocystum and its efficacy in scavenging free radicals. Indian J Pharm Sci. (2012) 74:549–55. doi: 10.4103/0250-474X.110600
45. Luthuli, S, Wu, SY, Cheng, Y, Zheng, XL, Wu, MJ, and Tong, HB. Therapeutic effects of Fucoidan: a review on recent studies. Mar Drugs. (2019) 17:487. doi: 10.3390/md17090487
46. Shannon, E, Conlon, M, and Hayes, M. Seaweed components as potential modulators of the gut microbiota. Mar Drugs. (2021) 19:358. doi: 10.3390/md19070358
47. Lee, KY, and Mooney, DJ. Alginate: properties and biomedical applications. Prog Polym Sci. (2012) 37:106–26. doi: 10.1016/j.progpolymsci.2011.06.003
48. Ravanal, MC, Sharma, S, Gimpel, J, Reveco-Urzua, FE, Overland, M, Horn, SJ, et al. The role of alginate Lyases in the enzymatic Saccharification of Brown macroalgae, Macrocystis Pyrifera and Saccharina Latissima. Algal Res. (2017) 26:287–93. doi: 10.1016/j.algal.2017.08.012
49. Becker, TA, Kipke, DR, and Brandon, T. Calcium alginate gel: a biocompatible and mechanically stable polymer for endovascular embolization. J Biomed Mater Res. (2001) 54:76–86. doi: 10.1002/1097-4636(200101)54:1<76::aid-jbm9>3.0.co;2-v
50. Jain, D, and Bar-Shalom, D. Alginate drug delivery systems: application in context of pharmaceutical and biomedical research. Drug Dev Ind Pharm. (2014) 40:1576–84. doi: 10.3109/03639045.2014.917657
51. Liu, J, Yang, S, Li, X, Yan, Q, Reaney, MJT, and Jiang, Z. Alginate oligosaccharides: production, biological activities, and potential applications. Compr Rev Food Sci Food Saf. (2019) 18:1859–81. doi: 10.1111/1541-4337.12494
52. Rioux, LE, Turgeon, SL, and Beaulieu, M. Structural characterization of Laminaran and Galactofucan extracted from the brown seaweed Saccharina Longicruris. Phytochemistry. (2010) 71:1586–95. doi: 10.1016/j.phytochem.2010.05.021
53. Tanna, B, and Mishra, A. Nutraceutical potential of seaweed polysaccharides: structure, bioactivity, safety, and toxicity. Compr Rev Food Sci Food Saf. (2019) 18:817–31. doi: 10.1111/1541-4337.12441
54. Zeece, M. Chapter seven–food additives In:. Introduction to the Chemistry of Food. Cambridge, Massachusetts: Academic Press (2020). 251–311.
55. Bonnet, M, Lagier, JC, Raoult, D, and Khelaifia, S. Bacterial culture through selective and non-selective conditions: the evolution of culture media in clinical microbiology. New Microbes New Infections. (2020) 34:100622. doi: 10.1016/j.nmni.2019.100622
56. Lahaye, M, and Rochas, C. Chemical structure and Physico-chemical properties of agar. Hydrobiologia. (1991) 221:137–48. doi: 10.1007/BF00028370
57. Percival, E. The polysaccharides of green, red and Brown seaweeds: their basic structure, biosynthesis and function. Brit Phycol J. (1979) 14:103–17. doi: 10.1080/00071617900650121
58. Deniaud-Bouet, E, Kervarec, N, Michel, G, Tonon, T, Kloareg, B, and Herve, C. Chemical and enzymatic fractionation of cell walls from Fucales: insights into the structure of the extracellular matrix of Brown algae. Ann Bot. (2014) 114:1203–16. doi: 10.1093/aob/mcu096
59. Lee, WK, Lim, YY, Leow, AT, Namasivayam, P, Ong Abdullah, J, and Ho, CL. Biosynthesis of agar in red seaweeds: a review. Carbohydr Polym. (2017) 164:23–30. doi: 10.1016/j.carbpol.2017.01.078
60. Zhang, Q, Li, N, Liu, X, Zhao, Z, Li, Z, and Xu, Z. The structure of a sulfated Galactan from Porphyra Haitanensis and its in vivo antioxidant activity. Carbohydr Res. (2004) 339:105–11. doi: 10.1016/j.carres.2003.09.015
61. Qiu, YJ, Jiang, H, Fu, LL, Ci, FF, and Mao, XZ. Porphyran and Oligo-Porphyran originating from red algae Porphyra: preparation, biological activities, and potential applications. Food Chem. (2021) 349:129209. doi: 10.1016/j.foodchem.2021.129209
62. Li, L, Ni, R, Shao, Y, and Mao, S. Carrageenan and its applications in drug delivery. Carbohydr Polym. (2014) 103:1–11. doi: 10.1016/j.carbpol.2013.12.008
63. Zia, KM, Tabasum, S, Nasif, M, Sultan, N, Aslam, N, Noreen, A, et al. A review on synthesis, properties and applications of natural polymer based carrageenan blends and composites. Int J Biol Macromol. (2017) 96:282–301. doi: 10.1016/j.ijbiomac.2016.11.095
64. Diogo, JV, Novo, SG, González, MJ, Ciancia, M, and Bratanich, AC. Antiviral activity of lambda-carrageenan prepared from red seaweed (Gigartina Skottsbergii) against Bohv-1 and Suhv-1. Res Vet Sci. (2015) 98:142–4. doi: 10.1016/j.rvsc.2014.11.010
65. Luo, M, Shao, B, Nie, W, Wei, X-W, Li, Y-L, Wang, B-L, et al. Antitumor and adjuvant activity of Λ-carrageenan by stimulating immune response in cancer immunotherapy. Sci Rep. (2015) 5:11062. doi: 10.1038/srep11062
66. Solov'eva, T, Davydova, V, Krasikova, I, and Yermak, I. Marine compounds with therapeutic potential in gram-negative sepsis. Mar Drugs. (2013) 11:2216–29. doi: 10.3390/md11062216
67. Yermak, IM, Barabanova, AO, Aminin, DL, Davydova, VN, Sokolova, EV, Solov’eva, TF, et al. Effects of structural peculiarities of Carrageenans on their Immunomodulatory and anticoagulant activities. Carbohydr Polym. (2012) 87:713–20. doi: 10.1016/j.carbpol.2011.08.053
68. Rioux, L-E, and Turgeon, SL. Chapter 7–seaweed carbohydrates In: BK Tiwari and DJ Troy, editors. Seaweed sustainability. San Diego: Academic Press (2015). 141–92.
69. Bonilla, J, Paiano, RB, Lourenco, RV, Bittante, AMQB, and Sobral, PJA. Biodegradation of films based on natural and synthetic biopolymers using an aquatic system from active sludge. J Polym Environ. (2021) 29:1380–95. doi: 10.1007/s10924-020-01962-x
70. Niu, TT, Fu, GQ, Zhou, JW, Han, H, Chen, JJ, Wu, W, et al. Floridoside exhibits antioxidant properties by activating Ho-1 expression via P38/Erk Mapk pathway. Mar Drugs. (2020) 18:105. doi: 10.3390/md18020105
71. Masakuni Tako, YY, Teruya, T, and Uechi, S. Structure-function relationship of Rhamnan sulfate isolated from commercially cultured edible green seaweed, Monostroma Nitidum. Am J Appl Chem. (2017) 5:38–44. doi: 10.11648/j.ajac.20170502.13
72. Lee, JB, Yamagaki, T, Maeda, M, and Nakanishi, H. Rhamnan sulfate from cell walls of Monostroma Latissimum. Phytochemistry. (1998) 48:921–5. doi: 10.1016/S0031-9422(97)00927-8
73. Zang, L, Shimada, Y, Tanaka, T, and Nishimura, N. Rhamnan Sulphate from Monostroma Nitidum attenuates hepatic Steatosis by suppressing Lipogenesis in a diet-induced obesity Zebrafish model. J Funct Foods. (2015) 17:364–70. doi: 10.1016/j.jff.2015.05.041
74. Suzuki, K, and Terasawa, M. Biological activities of Rhamnan sulfate extract from the green algae Monostroma Nitidum (Hitoegusa). Mar Drugs. (2020) 18:228. doi: 10.3390/md18040228
75. Terasawa, M, Hayashi, K, Lee, JB, Nishiura, K, Matsuda, K, Hayashi, T, et al. Anti-influenza a virus activity of Rhamnan sulfate from green algae Monostroma Nitidum in mice with Normal and compromised immunity. Mar Drugs. (2020) 18:254. doi: 10.3390/md18050254
76. Song, YF, He, P, Rodrigues, AL, Datta, P, Tandon, R, Bates, JT, et al. Anti-Sars-Cov-2 activity of Rhamnan sulfate from Monostroma Nitidum. Mar Drugs. (2021) 19:685. doi: 10.3390/md19120685
77. Robic, A, Bertrand, D, Sassi, JF, Lerat, Y, and Lahaye, M. Determination of the chemical composition of Ulvan, a Cell Wall polysaccharide from Ulva Spp. (Ulvales, Chlorophyta) by Ft-Ir and Chemometrics. J Appl Phycol. (2009) 21:451–6. doi: 10.1007/s10811-008-9390-9
78. Cindana Mo’o, FR, Wilar, G, Devkota, HP, and Wathoni, N. Ulvan, a polysaccharide from macroalga Ulva Sp.: a review of chemistry, biological activities and potential for food and biomedical applications. Appl Sci. (2020) 10:5488. doi: 10.3390/app10165488
79. Chen, L, Liu, R, He, X, Pei, SJ, and Li, D. Effects of Brown seaweed polyphenols, a class of Phlorotannins, on metabolic disorders via regulation of fat function. Food Funct. (2021) 12:2378–88. doi: 10.1039/d0fo02886j
80. Shrestha, S, Zhang, W, and Smid, SD. Phlorotannins: a review on biosynthesis, chemistry and bioactivity. Food Biosci. (2021):39. doi: 10.1016/j.fbio.2020.100832
81. Liu, M, Hansen, PE, and Lin, X. Bromophenols in marine algae and their bioactivities. Mar Drugs. (2011) 9:1273–92. doi: 10.3390/md9071273
82. Dong, H, Dong, ST, Hansen, PE, Stagos, D, Lin, XK, and Liu, M. Progress of Bromophenols in marine algae from 2011 to 2020: structure, bioactivities, and applications. Mar Drugs. (2020) 18:411. doi: 10.3390/md18080411
83. Öztaşkın, N, Kaya, R, Maraş, A, Şahin, E, Gülcin, İ, and Göksu, S. Synthesis and characterization of novel Bromophenols: determination of their anticholinergic, antidiabetic and antioxidant activities. Bioorg Chem. (2019) 87:91–102. doi: 10.1016/j.bioorg.2019.03.010
84. Duan, X-J, Li, X-M, and Wang, B-G. Highly brominated mono- and Bis-phenols from the marine red alga Symphyocladia Latiuscula with radical-scavenging activity. J Nat Prod. (2007) 70:1210–3. doi: 10.1021/np070061b
85. Guo, C, Wang, L, Zhao, Y, Jiang, B, Luo, J, and Shi, D. Bos-93, a novel Bromophenol derivative, induces apoptosis and autophagy in human A549 lung cancer cells via Pi3k/Akt/Mtor and Mapk signaling pathway. Exp Ther Med. (2019) 17:3848–58. doi: 10.3892/etm.2019.7402
86. Colon, M, Guevara, P, Gerwick, WH, and Ballantine, D. 5'-Hydroxyisoavrainvilleol, a new Diphenylmethane derivative from the tropical green alga Avrainvillea Nigricans. J Nat Prod. (1987) 50:368–74. doi: 10.1021/np50051a005
87. Luo, J, Jiang, B, Li, C, Jia, X, and Shi, D. Cyc27 synthetic derivative of Bromophenol from red alga Rhodomela Confervoides: anti-diabetic effects of sensitizing insulin signaling pathways and modulating Rna splicing-associated Rbps. Mar Drugs. (2019) 17:49. doi: 10.3390/md17010049
88. Ko, S-C, Ding, Y, Kim, J, Ye, B-R, Kim, E-A, Jung, W-K, et al. Bromophenol (5-Bromo-3,4-Dihydroxybenzaldehyde) isolated from red alga Polysiphonia Morrowii inhibits Adipogenesis by regulating expression of Adipogenic transcription factors and amp-activated protein kinase activation in 3t3-L1 adipocytes. Phytother Res. (2019) 33:737–44. doi: 10.1002/ptr.6266
89. Rocha, DHA, Pinto, DCGA, and Silva, AMS. Macroalgae specialized metabolites: evidence for their anti-inflammatory health benefits. Mar Drugs. (2022) 20:789. doi: 10.3390/md20120789
90. Farasat, M, Khavari-Nejad, RA, Nabavi, SM, and Namjooyan, F. Antioxidant activity, Total Phenolics and flavonoid contents of some edible green seaweeds from northern coasts of the Persian Gulf. Iran J Pharm Res. (2014) 13:163–70. doi: 10.11648/j.ajac.20170502.13
91. Sugiura, Y, Katsuzaki, H, Imai, K, and Amano, H. The anti-allergic and anti-inflammatory effects of Phlorotannins from the edible Brown Algae, Eckloniasp. andEiseniasp. Nat Prod Commun. (2021) 16:1934578X2110609. doi: 10.1177/1934578x211060924
92. Rehman, A, Tong, QY, Jafari, SM, Assadpour, E, Shehzad, Q, Aadil, RM, et al. Carotenoid-loaded Nanocarriers: a comprehensive review. Adv Colloid Interfac. (2020):275. doi: 10.1016/j.cis.2019.102048
93. Boominathan, M, and Mahesh, A. Seaweed carotenoids for cancer therapeutics In: S-K Kim, editor. Handbook of Anticancer Drugs From Marine Origin. Cham: Springer International Publishing (2015). 185–203.
94. Mares, J. Lutein and Zeaxanthin isomers in eye health and disease. Annu Rev Nutr. (2016) 36:571–602. doi: 10.1146/annurev-nutr-071715-051110
95. Davinelli, S, Nielsen, ME, and Scapagnini, G. Astaxanthin in skin health, repair, and disease: a comprehensive review. Nutrients. (2018) 10:522. doi: 10.3390/nu10040522
96. Galasso, C, Orefice, I, Pellone, P, Cirino, P, Miele, R, Ianora, A, et al. On the neuroprotective role of astaxanthin: new perspectives? Mar Drugs. (2018) 16:247. doi: 10.3390/md16080247
97. Meresse, S, Fodil, M, Fleury, F, and Chenais, B. Fucoxanthin, a marine-derived carotenoid from Brown seaweeds and microalgae: a promising bioactive compound for cancer therapy. Int J Mol Sci. (2020) 21:9273. doi: 10.3390/ijms21239273
98. Miyashita, K, Mikami, N, and Hosokawa, M. Chemical and nutritional characteristics of Brown seaweed lipids: a review. J Funct Foods. (2013) 5:1507–17. doi: 10.1016/j.jff.2013.09.019
99. Djuricic, I, and Calder, PC. Beneficial outcomes of Omega-6 and Omega-3 polyunsaturated fatty acids on human health: an update for 2021. Nutrients. (2021) 13:2421. doi: 10.3390/nu13072421
100. Singh, RS, and Walia, AK. Lectins from red algae and their biomedical potential. J Appl Phycol. (2018) 30:1833–58. doi: 10.1007/s10811-017-1338-5
101. Holanda, ML, Melo, VMM, Silva, LMCM, Amorim, RCN, Pereira, MG, and Benevides, NMB. Differential activity of a Lectin from Solieria Filiformis against human pathogenic bacteria. Braz J Med Biol Res. (2005) 38:1769–73. doi: 10.1590/S0100-879x2005001200005
102. Mesquita, JX, de Brito, TV, Fontenelle, TPC, Damasceno, ROS, de Souza, MHLP, de Souza Lopes, JL, et al. Lectin from red algae Amansia Multifida Lamouroux: extraction, characterization and anti-inflammatory activity. Int J Biol Macromol. (2021) 170:532–9. doi: 10.1016/j.ijbiomac.2020.12.203
103. Barre, A, Simplicien, M, Benoist, H, Van Damme, EJM, and Rouge, P. Mannose-specific Lectins from marine algae: diverse structural scaffolds associated to common Virucidal and anti-cancer properties. Mar Drugs. (2019) 17:440. doi: 10.3390/md17080440
104. Moya, R, Norris, AC, Kondo, T, and Schlau-Cohen, GS. Observation of robust energy transfer in the photosynthetic protein allophycocyanin using single-molecule pump-probe spectroscopy. Nat Chem. (2022) 14:153–9. doi: 10.1038/s41557-021-00841-9
105. Sheih, IC, Wu, TK, and Fang, TJ. Antioxidant properties of a new antioxidative peptide from algae protein waste hydrolysate in different oxidation systems. Bioresour Technol. (2009) 100:3419–25. doi: 10.1016/j.biortech.2009.02.014
106. Sun, KL, Gao, M, Wang, YZ, Li, XR, Wang, P, and Wang, B. Antioxidant peptides from protein Hydrolysate of marine red algae Eucheuma Cottonii: preparation, identification, and Cytoprotective mechanisms on H2o2 oxidative damaged Huvecs. Front Microbiol. (2022) 13:791248. doi: 10.3389/fmicb.2022.791248
107. Wang, X, and Zhang, X. Separation, antitumor activities, and encapsulation of polypeptide from chlorella Pyrenoidosa. Biotechnol Prog. (2013) 29:681–7. doi: 10.1002/btpr.1725
108. Zhang, B, and Zhang, X. Separation and Nanoencapsulation of antitumor polypeptide from Spirulina Platensis. Biotechnol Prog. (2013) 29:1230–8. doi: 10.1002/btpr.1769
109. Li, Z, He, Y, He, H, Zhou, W, Li, M, Lu, A, et al. Purification identification and function analysis of ace inhibitory peptide from Ulva Prolifera protein. Food Chem. (2023) 401:134127. doi: 10.1016/j.foodchem.2022.134127
110. Vo, TS, and Kim, SK. Down-regulation of histamine-induced endothelial cell activation as potential anti-atherosclerotic activity of peptides from Spirulina Maxima. Eur J Pharm Sci. (2013) 50:198–207. doi: 10.1016/j.ejps.2013.07.001
111. Admassu, H, Gasmalla, MAA, Yang, RJ, and Zhao, W. Bioactive peptides derived from seaweed protein and their health benefits: antihypertensive, antioxidant, and Antidiabetic properties. J Food Sci. (2018) 83:6–16. doi: 10.1111/1750-3841.14011
112. Fan, XD, Bai, L, Zhu, L, Yang, L, and Zhang, XW. Marine algae-derived bioactive peptides for human nutrition and health. J Agr Food Chem. (2014) 62:9211–22. doi: 10.1021/jf502420h
113. O’Connor, J, Garcia-Vaquero, M, Meaney, S, and Tiwari, BK. Bioactive peptides from algae: traditional and novel generation strategies, structure-function relationships, and bioinformatics as predictive tools for bioactivity. Mar Drugs. (2022) 20:317. doi: 10.3390/md20050317
114. Huang, J, Huang, J, Li, Y, Lv, H, Yin, T, Fan, S, et al. Fucoidan protects against high-fat diet-induced obesity and modulates gut microbiota in Institute of Cancer Research mice. J Med Food. (2021) 24:1058–67. doi: 10.1089/jmf.2021.K.0030
115. Wei, B, Zhang, B, Du, AQ, Zhou, ZY, Lu, DZ, Zhu, ZH, et al. Saccharina japonica Fucan suppresses high fat diet-induced obesity and enriches Fucoidan-degrading gut bacteria. Carbohydr Polym. (2022):290. doi: 10.1016/j.carbpol.2022.119411
116. Liu, M, Ma, L, Chen, QZ, Zhang, PY, Chen, C, Jia, LL, et al. Fucoidan alleviates dyslipidemia and modulates gut microbiota in high-fat diet-induced mice. J Funct Foods. (2018) 48:220–7. doi: 10.1016/j.jff.2018.07.006
117. Shang, QS, Song, GR, Zhang, MF, Shi, JJ, Xu, CY, Hao, JJ, et al. Dietary Fucoidan improves metabolic syndrome in association with increased Akkermansia population in the gut microbiota of high-fat diet-fed mice. J Funct Foods. (2017) 28:138–46. doi: 10.1016/j.jff.2016.11.002
118. Deng, ZZ, Wu, N, Wang, J, Geng, LH, Yue, Y, Wang, FH, et al. Low molecular weight Fucoidan fraction Lf2 improves metabolic syndrome via up-regulating Pi3k-Akt-Mtor Axis and increasing the abundance of Akkermansia Muciniphila in the gut microbiota. Int J Biol Macromol. (2021) 193:789–98. doi: 10.1016/j.ijbiomac.2021.10.188
119. Duan, MM, Sun, XN, Ma, N, Liu, YL, Luo, TR, Song, S, et al. Polysaccharides from Laminaria Japonica alleviated metabolic syndrome in Balb/C mice by normalizing the gut microbiota. Int J Biol Macromol. (2019) 121:996–1004. doi: 10.1016/j.ijbiomac.2018.10.087
120. Zhang, Y, Zuo, JH, Yan, LP, Cheng, Y, Li, QJ, Wu, SY, et al. Sargassum Fusiforme Fucoidan alleviates high-fat diet-induced obesity and insulin resistance associated with the improvement of hepatic oxidative stress and gut microbiota profile. J Agr Food Chem. (2020) 68:10626–38. doi: 10.1021/acs.jafc.0c02555
121. Ke, SZ, Yu, YL, Xu, QL, Zhang, B, Wang, SJ, Jin, WH, et al. Composition-activity relationships of polysaccharides from Saccharina japonica in regulating gut microbiota in short-term high-fat diet-fed mice. J Agr Food Chem. (2021) 69:11121–30. doi: 10.1021/acs.jafc.1c04490
122. Zhang, Y, Liu, J, Mao, G, Zuo, J, Li, S, Yang, Y, et al. Sargassum Fusiforme Fucoidan alleviates diet-induced insulin resistance by inhibiting Colon-derived Ceramide biosynthesis. Food Funct. (2021) 12:8440–53. doi: 10.1039/d1fo01272j
123. Jiang, PR, Zheng, WY, Sun, XN, Jiang, GP, Wu, S, Xu, YX, et al. Sulfated polysaccharides from Undaria Pinnatifida improved high fat diet-induced metabolic syndrome, gut microbiota Dysbiosis and inflammation in Balb/C mice. Int J Biol Macromol. (2021) 167:1587–97. doi: 10.1016/j.ijbiomac.2020.11.116
124. Li, SY, Wang, LN, Liu, B, and He, NN. Unsaturated alginate oligosaccharides attenuated obesity-related metabolic abnormalities by modulating gut microbiota in high-fat-diet mice. Food Funct. (2020) 11:4773–84. doi: 10.1039/c9fo02857a
125. Zheng, WY, Duan, MM, Jia, JH, Song, S, and Ai, CQ. Low-molecular alginate improved diet-induced obesity and metabolic syndrome through modulating the gut microbiota in Balb/C mice. Int J Biol Macromol. (2021) 187:811–20. doi: 10.1016/j.ijbiomac.2021.08.003
126. Wang, YT, Li, LL, Ye, CQ, Yuan, JY, and Qin, S. Alginate oligosaccharide improves lipid metabolism and inflammation by modulating gut microbiota in high-fat diet fed mice. Appl Microbiol Biot. (2020) 104:3541–54. doi: 10.1007/s00253-020-10449-7
127. Nguyen, SG, Kim, J, Guevarra, RB, Lee, JH, Kim, E, Kim, SI, et al. Laminarin favorably modulates gut microbiota in mice fed a high-fat diet. Food Funct. (2016) 7:4193–201. doi: 10.1039/c6fo00929h
128. Chin, YX, Mi, Y, Cao, WX, Lim, PE, Xue, CH, and Tang, QJ. A pilot study on anti-obesity mechanisms of Kappaphycus Alvarezii: the role of native kappa-carrageenan and the leftover sans-carrageenan fraction. Nutrients. (2019) 11:1133. doi: 10.3390/nu11051133
129. Qiong, W. Effect of Κ-carrageenan on Glucolipid metabolism and gut microbiota in high-fat diet-fed mice. J Funct Foods. (2021) 86:104707–2021. doi: 10.1016/j.jff.2021.104707
130. du Preez, R, Paul, N, Mouatt, P, Majzoub, ME, Thomas, T, Panchal, SK, et al. Carrageenans from the red seaweed Sarconema Filiforme attenuate symptoms of diet-induced metabolic syndrome in rats. Mar Drugs. (2020) 18:97. doi: 10.3390/md18020097
131. Shimada, Y, Terasawa, M, Okazaki, F, Nakayama, H, Zang, L, Nishiura, K, et al. Rhamnan Sulphate from green algae Monostroma Nitidum improves constipation with gut microbiome alteration in double-blind placebo-controlled trial. Sci Rep. (2021) 11:13384. doi: 10.1038/s41598-021-92459-7
132. Zhao, Y, Zhang, PF, Ge, W, Feng, YN, Li, L, Sun, ZY, et al. Alginate oligosaccharides improve germ cell development and testicular microenvironment to rescue Busulfan disrupted spermatogenesis. Theranostics. (2020) 10:3308–24. doi: 10.7150/thno.43189
133. Eckburg, PB, Bik, EM, Bernstein, CN, Purdom, E, Dethlefsen, L, Sargent, M, et al. Diversity of the human intestinal microbial Flora. Science. (2005) 308:1635–8. doi: 10.1126/science.1110591
134. Ibrahim, M, and Anishetty, S. A meta-Metabolome network of carbohydrate metabolism: interactions between gut microbiota and host. Biochem Bioph Res Co. (2012) 428:278–84. doi: 10.1016/j.bbrc.2012.10.045
135. Crovesy, L, Masterson, D, and Rosado, EL. Profile of the gut microbiota of adults with obesity: a systematic review. Eur J Clin Nutr. (2020) 74:1251–62. doi: 10.1038/s41430-020-0607-6
136. Belzer, C, and de Vos, WM. Microbes inside--from diversity to function: the case of Akkermansia. ISME J. (2012) 6:1449–58. doi: 10.1038/ismej.2012.6
137. Everard, A, Belzer, C, Geurts, L, Ouwerkerk, JP, Druart, C, Bindels, LB, et al. Cross-talk between Akkermansia Muciniphila and intestinal epithelium controls diet-induced obesity. Proc Natl Acad Sci U S A. (2013) 110:9066–71. doi: 10.1073/pnas.1219451110
138. Johnson, S. Clostridial Constipation’s broad pathology. Med Hypotheses. (2001) 56:532–6. doi: 10.1054/mehy.2000.1304
139. Naumova, N, Alikina, T, Tupikin, A, Kalmykova, A, Soldatova, G, Vlassov, V, et al. Human gut microbiome response to short-term Bifidobacterium-based probiotic treatment. Indian J Microbiol. (2020) 60:451–7. doi: 10.1007/s12088-020-00888-1
140. Tian, Y, Zuo, L, Guo, Q, Li, J, Hu, ZY, Zhao, K, et al. Potential role of fecal microbiota in patients with constipation. Ther Adv Gastroenter. (2020):13. doi: 10.1177/1756284820968423
141. Patterson, E, Wall, R, Fitzgerald, GF, Ross, RP, and Stanton, C. Health implications of high dietary Omega-6 polyunsaturated fatty acids. J Nutr Metab. (2012) 2012:539426. doi: 10.1155/2012/539426
142. Lee, MR, Kim, JE, Jin, YJ, Roh, YJ, Seol, A, Song, HJ, et al. Anti-obesity effects of agar (Gelidium Amansii)-derived oligosaccharides in high-fat diet-treated C57bl/6n mice due to differential regulations of lipogenesis and lipolysis. Biosci Biotech Bioch. (2022) 86:1648–57. doi: 10.1093/bbb/zbac159
143. Chi, Y, Wu, Z, Du, C, Zhang, M, Wang, X, Xie, A, et al. Regulatory effects mediated by Ulvan oligosaccharide and its zinc complex on lipid metabolism in high-fat diet-fed mice. Carbohydr Polym. (2023) 300:120249. doi: 10.1016/j.carbpol.2022.120249
144. Wu, Q, Wu, S, Cheng, Y, Zhang, Z, Mao, G, Li, S, et al. Sargassum Fusiforme Fucoidan modifies gut microbiota and intestinal metabolites during alleviation of hyperglycemia in type 2 diabetic mice. Food Funct. (2021) 12:3572–85. doi: 10.1039/d0fo03329d
145. Cheng, Y, Sibusiso, L, Hou, LF, Jiang, HJ, Chen, PC, Zhang, X, et al. Sargassum Fusiforme Fucoidan modifies the gut microbiota during alleviation of Streptozotocin-induced hyperglycemia in mice. Int J Biol Macromol. (2019) 131:1162–70. doi: 10.1016/j.ijbiomac.2019.04.040
146. Xue, M, Liang, H, Ji, X, Liu, Y, Ge, Y, Hou, L, et al. Fucoidan prevent murine autoimmune diabetes via suppression Tlr4-signaling pathways, regulation dc/Treg induced immune tolerance and improving gut microecology. Nutr Metab (Lond). (2019) 16:87. doi: 10.1186/s12986-019-0392-1
147. Nie, Q, Hu, J, Gao, H, Li, M, Sun, Y, Chen, H, et al. Bioactive dietary fibers selectively promote gut microbiota to exert antidiabetic effects. J Agric Food Chem. (2021) 69:7000–15. doi: 10.1021/acs.jafc.1c01465
148. He, D, Wu, Q, Lu, C, Wu, J, Chen, P, Wu, M, et al. Pyropia Yezoensis Porphyran alleviates metabolic disorders via modulating gut microbiota in high-sucrose-fed drosophila melanogaster. J Sci Food Agric. (2022) 102:4802–12. doi: 10.1002/jsfa.11843
149. Zhang, L, Luo, J, Li, X, Guo, S, and Shi, D. 16s Rrna sequencing and Metagenomics study of gut microbiota: implications of Bdb on type 2 diabetes mellitus. Mar Drugs. (2020) 18:469. doi: 10.3390/md18090469
150. Weglarz, L, Dzierzewicz, Z, Skop, B, Orchel, A, Parfiniewicz, B, Wisniowska, B, et al. Desulfovibrio Desulfuricans lipopolysaccharides induce endothelial cell Il-6 and Il-8 secretion and E-Selectin and Vcam-1 expression. Cell Mol Biol Lett. (2003) 8:991–1003.
151. Feng, W, Hu, Y, An, N, Feng, Z, Liu, J, Mou, J, et al. Alginate oligosaccharide alleviates Monocrotaline-induced pulmonary hypertension via anti-oxidant and anti-inflammation pathways in rats. Int Heart J. (2020) 61:160–8. doi: 10.1536/ihj.19-096
152. Sheflin, AM, Whitney, AK, and Weir, TL. Cancer-promoting effects of microbial Dysbiosis. Curr Oncol Rep. (2014) 16:406. doi: 10.1007/S11912-014-0406-0
153. Lin, Y, Qi, X, Liu, H, Xue, K, Xu, S, and Tian, Z. The anti-cancer effects of Fucoidan: a review of both in vivo and in vitro investigations. Cancer Cell Int. (2020) 20:154. doi: 10.1186/s12935-020-01233-8
154. Xue, M, Liang, H, Ji, X, Zhou, Z, Liu, Y, Sun, T, et al. Effects of Fucoidan on gut Flora and Tumor prevention in 1,2-Dimethylhydrazine-induced colorectal carcinogenesis. J Nutr Biochem. (2020) 82:108396. doi: 10.1016/j.jnutbio.2020.108396
155. Xue, M, Ji, X, Liang, H, Liu, Y, Wang, B, Sun, L, et al. The effect of Fucoidan on Intestinal Flora and Intestinal barrier function in rats with breast cancer. Food Funct. (2018) 9:1214–23. doi: 10.1039/c7fo01677h
156. de Sousa, APA, Torres, MR, Pessoa, C, de Moraes, MO, Rocha, FD, Alves, APNN, et al. In vivo growth-inhibition of sarcoma 180 tumor by alginates from brown seaweed Sargassum Vulgare. Carbohydr Polym. (2007) 69:7–13. doi: 10.1016/j.carbpol.2006.08.018
157. Zhang, PF, Liu, J, Xiong, BH, Zhang, C, Kang, BN, Gao, YS, et al. Microbiota from alginate oligosaccharide-dosed mice successfully mitigated small intestinal Mucositis. Microbiome. (2020) 8:112. doi: 10.1186/s40168-020-00886-x
158. Rajeshwari, T, Raja, B, Manivannan, J, Silambarasan, T, and Dhanalakshmi, T. Valproic acid prevents the deregulation of lipid metabolism and renal renin-angiotensin system in L-name induced nitric oxide deficient hypertensive rats. Environ Toxicol Pharmacol. (2014) 37:936–45. doi: 10.1016/j.etap.2014.02.008
159. Terakado, S, Ueno, M, Tamura, Y, Toda, N, Yoshinaga, M, Otsuka, K, et al. Sodium alginate oligosaccharides attenuate hypertension and associated kidney damage in Dahl salt-sensitive rats fed a high-salt diet. Clin Exp Hypertens. (2012) 34:99–106. doi: 10.3109/10641963.2011.618196
160. Ueno, M, Tamura, Y, Toda, N, Yoshinaga, M, Terakado, S, Otsuka, K, et al. Sodium alginate oligosaccharides attenuate hypertension in spontaneously hypertensive rats fed a low-salt diet. Clin Exp Hypertens. (2012) 34:305–10. doi: 10.3109/10641963.2011.577484
161. Moriya, C, Shida, Y, Yamane, Y, Miyamoto, Y, Kimura, M, Huse, N, et al. Subcutaneous Administration of Sodium Alginate Oligosaccharides Prevents Salt-Induced Hypertension in Dahl salt-sensitive rats. Clin Exp Hypertens. (2013) 35:607–13. doi: 10.3109/10641963.2013.776568
162. Hu, Y, Feng, Z, Feng, W, Hu, T, Guan, H, and Mao, Y. Aos ameliorates Monocrotaline-induced pulmonary hypertension by restraining the activation of P-Selectin/P38mapk/Nf-Κb pathway in rats. Biomed Pharmacother. (2019) 109:1319–26. doi: 10.1016/j.biopha.2018.10.109
163. Han, ZL, Chen, M, Fu, XD, Yang, M, Hrmova, M, Zhao, YH, et al. Potassium alginate oligosaccharides Alter gut microbiota, and have potential to prevent the development of hypertension and heart failure in spontaneously hypertensive rats. Int J Mol Sci. (2021) 22:9823. doi: 10.3390/ijms22189823
164. Xing, M, Cao, Q, Wang, Y, Xiao, H, Zhao, J, Zhang, Q, et al. Advances in research on the bioactivity of alginate oligosaccharides. Mar Drugs. (2020) 18:144. doi: 10.3390/md18030144
165. Ikeda-Ohtsubo, W, López Nadal, A, Zaccaria, E, Iha, M, Kitazawa, H, Kleerebezem, M, et al. Intestinal microbiota and immune modulation in Zebrafish by Fucoidan from Okinawa Mozuku (Cladosiphon Okamuranus). Front Nutr. (2020) 7:67. doi: 10.3389/fnut.2020.00067
166. Tang, Y, Pu, Q, Zhao, Q, Zhou, Y, Jiang, X, and Han, T. Effects of Fucoidan isolated from Laminaria Japonica on immune response and gut microbiota in cyclophosphamide-treated mice. Front Immunol. (2022) 13:916618. doi: 10.3389/fimmu.2022.916618
167. Takahashi, M, Takahashi, K, Abe, S, Yamada, K, Suzuki, M, Masahisa, M, et al. Improvement of psoriasis by alteration of the gut environment by Oral Administration of Fucoidan from Cladosiphon Okamuranus. Mar Drugs. (2020) 18:154. doi: 10.3390/md18030154
168. Sun, X, Zhao, H, Liu, Z, Sun, X, Zhang, D, Wang, S, et al. Modulation of gut microbiota by Fucoxanthin during alleviation of obesity in high-fat diet-fed mice. J Agric Food Chem. (2020) 68:5118–28. doi: 10.1021/acs.jafc.0c01467
169. Pratap, K, Majzoub, ME, Taki, AC, Hernandez, SM, Magnusson, M, Glasson, CRK, et al. The algal polysaccharide Ulvan and carotenoid Astaxanthin both positively modulate gut microbiota in mice. Foods. (2022) 11:565. doi: 10.3390/foods11040565
170. Vacca, M, Celano, G, Calabrese, FM, Portincasa, P, Gobbetti, M, and De Angelis, M. The controversial role of human gut Lachnospiraceae. Microorganisms. (2020) 8:573. doi: 10.3390/microorganisms8040573
171. McCracken, E, Monaghan, M, and Sreenivasan, S. Pathophysiology of the metabolic syndrome. Clin Dermatol. (2018) 36:14–20. doi: 10.1016/j.clindermatol.2017.09.004
172. Wang, Z, and Zhao, Y. Gut microbiota derived metabolites in cardiovascular health and disease. Protein Cell. (2018) 9:416–31. doi: 10.1007/s13238-018-0549-0
173. Michelotti, GA, Machado, MV, and Diehl, AM. Nafld, Nash and liver cancer. Nat Rev Gastroenterol Hepatol. (2013) 10:656–65. doi: 10.1038/nrgastro.2013.183
174. Wang, X, Liu, D, Wang, Z, Cai, C, Jiang, H, and Yu, G. Porphyran-derived oligosaccharides alleviate Nafld and related Cecal microbiota Dysbiosis in mice. FASEB J. (2021) 35:e21458. doi: 10.1096/fj.202000763RRR
175. Zhang, Q, Fan, XY, Guo, WL, Cao, YJ, Lin, YC, Cheng, WJ, et al. The protective mechanisms of macroalgae Laminaria Japonica consumption against lipid metabolism disorders in high-fat diet-induced Hyperlipidemic rats. Food Funct. (2020) 11:3256–70. doi: 10.1039/d0fo00065e
176. Lopez-Santamarina, A, Cardelle-Cobas, A, Del Carmen, MA, Sinisterra-Loaiza, L, Miranda, JM, and Cepeda, A. Evaluation of the potential prebiotic effect of Himanthalia Elongata, an Atlantic Brown seaweed, in an in vitro model of the human distal Colon. Food Res Int. (2022) 156:111156. doi: 10.1016/j.foodres.2022.111156
Keywords: seaweed, gut microbiota, short-chain fatty acids, obesity, diabetes, metabolic syndrome
Citation: Zang L, Baharlooeian M, Terasawa M, Shimada Y and Nishimura N (2023) Beneficial effects of seaweed-derived components on metabolic syndrome via gut microbiota modulation. Front. Nutr. 10:1173225. doi: 10.3389/fnut.2023.1173225
Edited by:
Jose Manuel Miranda, University of Santiago de Compostela, SpainReviewed by:
Aroa Lopez Santamarina, University of Santiago de Compostela, SpainGeorge Grant, University of Aberdeen, United Kingdom
Bin Wang, Zhejiang Ocean University, China
Copyright © 2023 Zang, Baharlooeian, Terasawa, Shimada and Nishimura. This is an open-access article distributed under the terms of the Creative Commons Attribution License (CC BY). The use, distribution or reproduction in other forums is permitted, provided the original author(s) and the copyright owner(s) are credited and that the original publication in this journal is cited, in accordance with accepted academic practice. No use, distribution or reproduction is permitted which does not comply with these terms.
*Correspondence: Liqing Zang, bGlxaW5nQG1lZC5taWUtdS5hYy5qcA==