- 1REQUIMTE, LAQV, ICBAS, Instituto de Ciências Biomédicas Abel Salazar, Universidade do Porto, Porto, Portugal
- 2REQUIMTE, LAQV, Instituto Superior de Engenharia do Porto, Instituto Politécnico do Porto, Porto, Portugal
- 3CIIMAR, Centro Interdisciplinar de Investigação Marinha e Ambiental, Terminal de Cruzeiros de Leixões, Matosinhos, Portugal
- 4ICBAS, Instituto de Ciências Biomédicas Abel Salazar, Universidade do Porto, Porto, Portugal
- 5REQUIMTE, LAQV, Departamento de Ciências Químicas, Laboratório de Química Aplicada, Faculdade de Farmácia, Universidade do Porto, Porto, Portugal
- 6ALGAplus-Produção e Comercialização de Algas e seus Derivados, Lda, PCI, Ílhavo, Portugal
- 7ALLMICROALGAE—Natural Products, Pataias, Portugal
Algae can leverage aquaculture sustainability and improve the nutritional and functional value of fish for human consumption, but may pose challenges to carnivorous fish. This study aimed to evaluate the potential of a commercial blend of macroalgae (Ulva sp. and Gracilaria gracilis) and microalgae (Chlorella vulgaris and Nannochloropsis oceanica) in a plant-based diet up to 6% (dry matter basis) on digestibility, gut integrity, nutrient utilization, growth performance, and muscle nutritional value of European seabass juveniles. Fish (11.3 ± 2.70 g) were fed with isoproteic, isolipidic, and isoenergetic diets: (i) a commercial-type plant-based diet with moderate fishmeal (125 g kg−1 DM basis) and without algae blend (control diet; Algae0), (ii) the control diet with 2% algae blend (Algae2), (iii) the control diet with 4% algae blend (Algae4), and (iv) the control diet with 6% algae blend (Algae6) for 12 weeks. The digestibility of experimental diets was assessed in a parallel study after 20 days. Results showed that most nutrients and energy apparent digestibility coefficients were promoted by algae blend supplementation, with a concomitant increase in lipid and energy retention efficiencies. Growth performance was significantly promoted by the algae blend, the final body weight of fish fed Algae6 being 70% higher than that of fish fed Algae0 after 12 weeks, reflecting up to 20% higher feed intake of algae-fed fish and the enhanced anterior intestinal absorption area (up to 45%). Whole-body and muscle lipid contents were increased with dietary algae supplementation levels by up to 1.79 and 1.74 folds in Algae 6 compared to Algae0, respectively. Even though the proportion of polyunsaturated fatty acids was reduced, the content of EPA and DHA in the muscle of algae-fed fish increased by nearly 43% compared to Algae0. The skin and filet color of juvenile European seabass were significantly affected by the dietary inclusion of the algae blend, but changes were small in the case of muscle, meeting the preference of consumers. Overall results highlight the beneficial effects of the commercial algae blend (Algaessence®) supplementation in plant-based diets for European seabass juveniles, but feeding trials up to commercial-size fish are needed to fully assess its potential.
1. Introduction
The quest for healthy and nutritious food is one of the main concerns of the Food and Agriculture Organization (FAO) of the United Nations to address food security and the growing demand for animal protein sources by the world's growing population, expected to reach 9.7 billion by 2050 (1). According to the latest report, severe food insecurity in 2021 increased compared to 2020 (2), mainly due to the long-lasting effects of the COVID-19 pandemic. A trend that may continue as a result of the ongoing war and the political, economic, and financial uncertainty. Indeed, FAO anticipates that 670 million people may suffer from hunger by 2030, a figure similar to 2015 when the Sustainable Development Goals of the 2030 Agenda was launched (2). It is thus of paramount importance to produce high-quality foods to address food insecurity.
Aquaculture, the fastest-growing sector of the food industry, has the potential to contribute to food security and meet the nutritional requirements of the world's growing population (3). To address the growing demand for aquafeeds and the limited supply of fishmeal and fish oil, fewer marine sources and more plant sources are currently used in nutrient-based formulations that not only meet fish nutrient and energy requirements but also attend to their nutraceutical and functional properties (4, 5). These modern formulations of aquafeed pose challenges to fish, in particular to carnivorous species such as European seabass (Dicentrarchus labrax L.), a marine species of high commercial importance in European aquaculture, particularly in the Mediterranean region (6). Compared to marine sources, land plant sources have lower digestibility, are deficient in essential amino acids, such as lysine and methionine, and have a lipid profile rich in n-6 and completely lacking n-3 long-chain polyunsaturated fatty acids (LC-PUFA) (7). The search for alternative, locally produced, and more sustainable ingredients to be included in modern aquafeed formulations is of paramount importance to reduce or replace traditional marine sources, as well as plant ingredients with a high environmental footprint, nutrient imbalance, or antinutritional compounds or that are used for terrestrial animal feeding or human consumption (8). By addressing aquaculture sustainability and improving the nutritional and functional value of fish for human consumption, the search for alternative aquafeed ingredients fits into the One Health concept and the goal to achieve the best health outcomes for fish, consumers, and the environment.
Most research on alternative aquafeed ingredients has focused on alternative plants, rendered animal and aquaculture by-products, insects, single-cell organisms, and algae (8–10). Algae, including microalgae and macroalgae, are of particular interest due to their high growth rates and biomass productivity, low environmental footprint, and non-competition with other cultures for arable land, being even able to grow in waste water (11). In addition, algae are valuable sources of macro- and micro-nutrients and bioactive compounds (12–14), with levels varying with species and within species with abiotic and biotic growth conditions (15, 16). Microalgae contain all essential amino acids, and some species are rich sources of protein and lipids, with marine species being particularly rich in n-3 LC-PUFA, such as eicosapentaenoic acid (EPA; C20:5 n-3) and docosahexaenoic acid (DHA; C22:6 n-3) (17–19) with important health-promoting properties (20). On the contrary, macroalgae species have lower protein content with a less balanced amino acids profile, in particular, brown macroalgae, and are poor sources of lipids, although marine species have a fairly high proportion of LC-PUFA (16, 21). Both microalgae and macroalgae are good sources of complex polysaccharides, pigments, and organic minerals with potential health-promoting effects such as prebiotic, immunomodulatory, and antioxidant activities (12, 14, 22, 23). Although algae lack lignin and are poor in hemicellulose (24, 25), the complex structural polysaccharides of eukaryotic microalgae and macroalgae may reduce the digestibility and availability of macro- and micronutrients for carnivorous fish (16, 18, 26). In face of their nutritional value, the potential of micro- and macroalgae as alternative aquafeeds has been suggested for fingerlings and fish diets, including the replacement of traditional (fishmeal) and current (plant-based) protein sources (10, 18, 25) and lipid sources such as fish oil (27). In addition, algae are sustainable and valuable sources of bioactive compounds, in particular, n-3 LC-PUFA, that are essential for aquafeed formulations (12, 28). Although their high production costs are still a bottleneck for algae use in fish diets as ingredients, it is anticipated that in the near future this limitation can be overcome (25).
In this context, several studies have evaluated the inclusion of individual species of microalgae or macroalgae in carnivorous fish diets, namely Atlantic salmon (Salmo salar) (29), European seabass (30–32), turbot (Scophthalmus maximus) (33), barramundi (Lates calcarifer) (34), meager (Argyrosomus regius) (35), Persian sturgeon (Acipenser persicus) (36), and Senegalese sole (Solea senegalensis) (37). In most studies, growth or feed efficiency was not impaired at dietary inclusion levels up to 10% (dry matter, DM, basis), but in some cases, decreased nutrient and energy digestibility was reported (13, 26). At higher inclusion levels, growth performance, feed utilization, and digestibility of diets were reduced (38–41). Available data support species-specific and dose-dependent effects of dietary algae inclusion (42) and highlight the need for further studies.
Chlorella spp. and Nannochloropsis spp. are among the most produced microalgae species in Europe (82 and 21 tons DM year−1, respectively) (43). Chlorella vulgaris is a rich source of protein (51.5–67.7% DM basis) (18), with a high content of essential amino acids, particularly arginine, lysine, and leucine (44), while N. oceanica is an oleaginous microalga with the ability to accumulate EPA, a health-promoting n-3 LC-PUFA (20). Although produced on a small scale at the European level, the production of macroalgae species native to the Mediterranean, such as Ulva spp. and Gracilaria spp., has a considerable expression in Portugal (43). These macroalgae species are rich sources of polysaccharides (30–75% DM basis) (16), including sulfated polysaccharides, such as ulvan in Ulva spp. and carrageenan and agar in Gracilaria spp. (45), with prebiotic, immunomodulatory, and antioxidant properties (46–48).
Although the combination of algae species has been suggested to improve the nutritional and functional value of diets (49), few studies have evaluated the supplementation of mixtures of inclusion of microalgae or macroalgae species in European seabass diets (50–53), and only one study has evaluated the combination of one micro- and one macroalgae species in an in vivo trial with D. labrax (54). We hypothesize that the combination of several species of micro- and macroalgae can exert strong synergistic effects and improve the nutritional and functional value of modern plant source-based aquafeeds. Thus, the present study aimed to evaluate the effects of dietary inclusion of an algae blend composed of two macroalgae (Ulva sp. and Gracilaria gracilis) and two microalgae (Chlorella vulgaris and Nannochloropsis oceanica) on digestibility, gut integrity, nutrient utilization, growth performance, and muscle quality of European seabass juveniles.
2. Materials and methods
Two trials were conducted at the Fish Culture Experimental Unit of CIIMAR (Matosinhos, Portugal) to evaluate the potential of the algae blend as a novel feed for European seabass juveniles: a digestibility trial and a growth trial. All procedures with animals were reviewed and approved by the Animal Welfare and Ethics Body of CIIMAR (ORBEA-CIIMAR 06-2016), licenced by the Portuguese Veterynary Authority (1005/92, DGAV-Portugal), and carried out by trained researchers accredited in laboratory animal science following FELASA C recommendations. The experiments were conducted in strict compliance with European Union guidelines on the protection of animals for scientific purposes (Directive 2010/63/EU).
2.1. Fish
European seabass (D. labrax) juveniles were obtained from a commercial fish farm (Acuinuga, S.L., La Coruña, Spain), transported to CIIMAR facilities, and kept in quarantine for 3 weeks. During this period, the fish were fed a commercial diet (49% crude protein, CP, and 20% ether extract, EE, DM basis; AQUASOJA, Sorgal, S.A., Ovar, Portugal). After acclimation, the fish were fasted for 24 h, anesthetized (60 μl L−1 of 2-phenoxyethanol, Sigma-Aldrich, St. Louis, MO, USA), and individually weighted (g) and measured (total length, cm). Homogeneous groups of fish were then distributed among the tanks used for the digestibility and growth trials.
2.2. Algae blend and experimental diets
The algae blend used in this study, composed of two macroalgae species (Ulva sp. and G. gracilis) and two microalgae species (C. vulgaris and N. oceanica), is a commercial product (Algaessence® feed) produced by ALGAplus (Ílhavo, Portugal) and Allmicroalgae (Pataias, Portugal). The blend was supplied as a spray-dried power in sealed bags protected from light.
Four isoproteic (527 g kg−1 DM basis), isolipidic (153 g kg−1 DM basis), and isoenergetic (21.7 MJ kg−1 DM basis) diets were formulated according to the nutritional requirements of European seabass juveniles (55) and considering current trends in commercial aquafeeds of high vegetable protein sources (c.a., 700 g kg−1 DM basis) and moderate fishmeal (125 g kg−1 DM basis) inclusion level. The algae blend was included in the experimental diets at expense of wheat gluten and whole peas, with levels of fishmeal and fish oil held constant. The experimental diets were as follows: i) a commercial plant protein-based diet without algae blend inclusion (control diet; Algae0), ii) the control diet with 2% algae blend inclusion (Algae2), iii) the control diet with 4% algae blend inclusion (Algae4), and iv) the control diet with 6% algae blend inclusion (Algae6). Yttrium oxide (Y2O3, 0.2 g kg−1, DM basis) was included in all diets as an inert marker for determining the apparent digestibility coefficients. Diets were manufactured and extruded by SPAROS Lda. (Olhão, Portugal), using a pilot-scale twin-screw extruder (CLEXTRAL BC45, Firminy, France). The pellets (2.0 mm) were dried in a convection oven (OP 750-UF, LTE Scientific, Oldham, UK), and the fish oil was added by vacuum coating (Pegasus PG-10VCLAB, DINNISSEN, Sevenum, Netherlands). The diets were stored at 4°C until use. The ingredients and chemical composition of the experimental diets are shown in Table 1 and Supplementary Table S1.
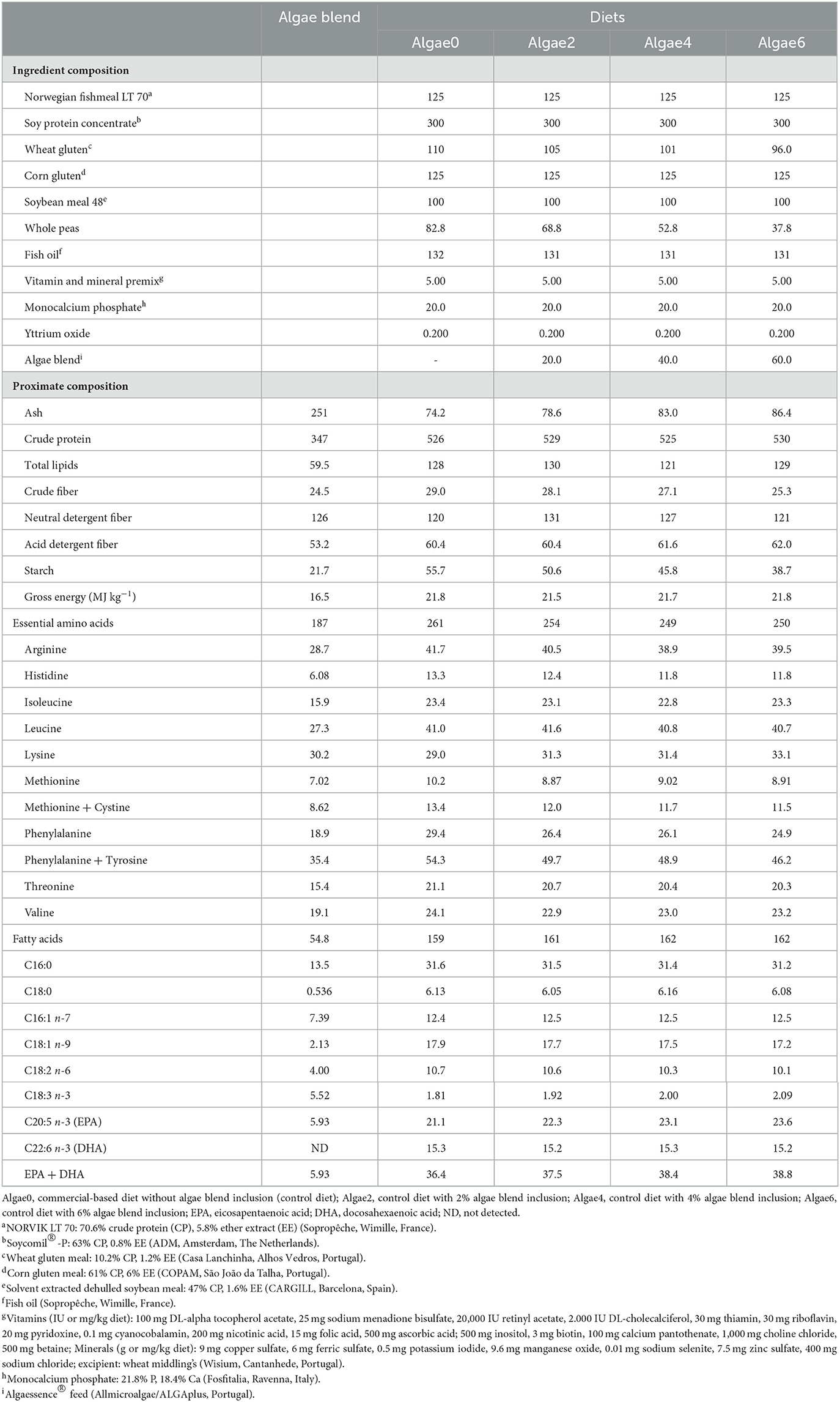
Table 1. Ingredient composition (g kg−1, as is) and proximate composition, essential amino acids, and selected fatty acids content (g kg−1, dry matter) of the algae blend and experimental diets.
2.2.1. Proximate analysis
The algae blend and the ground (1-mm) experimental diets were homogenized, and their proximate composition was analyzed in duplicate according to official methods (56). Samples were analyzed for DM (ID 934.01), ash (ID 942.05), and nitrogen (N) (ID 990.03) contents. Crude protein was calculated as N x 6.25 (ID 990.03). Gross energy (GE) was determined using an adiabatic bomb calorimeter (Werke C2000, IKA, Staufen, Germany). The starch content was analyzed in 0.5-mm ground samples (57). Crude fiber (CF; ID 962.09), neutral detergent fiber (NDF), and acid detergent fiber (ADF) (58, 59) of the algae blend and diets were also determined. Due to the small size of the microalgae species present in the blend (<25 μm diameter), the filtration step to determine the fiber content (CF, NDF, and ADF) was modified by replacing the P2 crucibles (porosity 40–100 μm) with glass microfiber filter (Whatman GF/A, 1.6 μm porosity, Merck KGaA, Darmstadt, Germany). Fiber fractions were expressed exclusive of residual ash. Analyses were run in duplicate.
2.2.2. Lipids and fatty acids analyses
The total lipids were quantified following the method of Folch et al. (60), modified by using dichloromethane:methanol (2:1) instead of trichloromethane:methanol (2:1). The fatty acids were transesterified to fatty acid methyl esters by acid-catalyzed methylation (61). Non-adecanoic acid (C19:0, Matreya LLC, State College, PA, USA) was added as an internal standard. Fatty acid methyl esters were analyzed by gas chromatography, using a Shimadzu GC-2010 Plus gas chromatograph (Shimadzu Europe GmbH, Duisburg, Germany) equipped with a capillary column (Omegawax 250, 30 m × 0.25 mm × 0.25 μm; Supelco, Bellefonte, PA, USA) and a flame-ionization detector. The carrier gas was helium at 1.30 ml min−1, with a split ratio of 1:100, and the injection volume was 1.0 μl. The initial column temperature of 150°C was held for 7 min, increased at 3°C min−1 to 170°C and held for 25 min, and then increased at 3°C min−1 to 220°C and held for 30 min. The injector and detector temperatures were 250 and 260°C, respectively. Fatty acids were identified by comparing retention times with those of commercially available standards (Supelco 37 Component FAME Mix, BAME Mix, PUFA No.1, PUFA No.2, PUFA No.3, Sigma-Aldrich Co. LLC; GLC-110 Mixture, Matreya LLC) and quantified by using the internal standard (C19:0). Analyses were run in duplicate.
2.2.3. Amino acids analysis
The amino acid content of the algae blend and experimental diets were determined after hydrolysis with 6 M HCl at 116°C, for 48 h, followed by pre-column derivatization with 6-aminoquinolyl-N-hydroxysuccinimidyl carbamate (Waters AccQ Fluor Reagent; Waters, Milford, MA, USA) as described by Aragão et al. (62), using norvaline (Waters) as an internal standard. Amino acids were analyzed by ultra-high-performance liquid chromatography on a Waters reverse phase amino acid analysis system and identified by comparison of retention times of commercial standard mixtures (Waters) and pure standards (Sigma-Aldrich Co. LLC). Data were acquired and analyzed using the EMPOWER software (Waters). The analysis was run in duplicate.
2.2.4. Element analysis
Macro and trace elements of algae blend and diets were determined after mineralization in a Milestone (Sorisole, Italy) MLS 1200 Mega high-performance microwave digestion unit (63). Samples were analyzed by inductively coupled plasma-mass spectrometry (ICP-MS; Thermo Fisher Scientific iCAP Q ICP-MS instrument, Waltham, MA, USA) and flame atomic absorption spectrometry (FAAS; PerkinElmer AAnalyst 200 FAAS instrument, Waltham). The calibration standards for FAAS were prepared from single-element standard stock solutions (Fluka, Buchs, Switzerland) by appropriate dilution with HNO3 0.2% (v/v). For ICP-MS determinations, internal standards and tuning solutions were prepared by appropriate dilution of the following solutions: periodic table mix 3 for ICP-MS (TraceCERT®, Sigma-Aldrich) containing 10 mg L−1 of 16 elements (Sc, Y, La, Ce, Pr, Nd, Sm, Eu, Gd, Tb, Dy, Ho, Er, Tm, Yb, and Lu in 5% HNO3) and a custom solution (SCP Science, Quebec, Canada) with 1 mg L−1 of Ba, Bi, Ce, Co, In, Li, and U in a 5% HNO3 + 0.5% HCl, respectively. The iodine content of the algae blend and diets were also determined. After alkaline extraction with tetramethylammonium hydroxide (TMAH, Sigma-Aldrich Co. LLC) at a high temperature (90 ± 3 °C) for 3 h, iodine was analyzed by ICP-MS, and the concentration was calculated based on external calibration with iodine standards prepared in 0.5% (v/v) TMAH (64). The analyses were carried out in triplicate.
2.3. Digestibility trial
From an initial lot of European seabass juveniles, six homogeneous groups of 30 fish (14.1 ± 6.36 g of body weight, BW) were randomly allocated to 50 L fiberglass tanks with individual feces sedimentation columns, in a Guelph system as described by Cho and Slinger (65). Fish were adapted to the new conditions (21.5 ± 0.27°C of water temperature, 35.7 ± 1.15‰ of salinity, 2 L min−1 of flow rate, and 12 h of light/dark photoperiod) for 19 days. During this period, fish were fed a commercial diet (49% CP and 20% EE, DM basis; AQUASOJA, Sorgal, S.A.). After acclimatization, the digestibility of the experimental diets was assessed in two runs of 20 days; 7 days for adaptation to each experimental diet and 13 days for total feces collection. The experimental diets were hand-fed until apparent satiation two times a day (9:00 and 17:00 h), 7 days a week. During the feces collection period, the tank and the sedimentation column were thoroughly cleaned after 30 min of feeding to ensure that all uneaten feed was removed. Feces were collected from the sedimentation column two times a day, before feeding, centrifuged at 3,000 x g for 5 min at 4°C to eliminate the excess water, and stored at −20°C until further analysis. At the end of the first run, the fish were fasted for 24 h for gut evacuation. After this period, the second run of the digestibility trial began. Diets were randomly distributed by the tanks in the first run and caution was taken to ensure that diets were not allocated to the same tank in the second run. By the end of the digestibility trial, all experimental diets were run in triplicate.
Before further analysis, feces were pooled per tank (n = 3), freeze-dried, and sieved. Feces samples were homogenized and analyzed for DM, ash, N (CP calculated as N x 6.25), starch, GE, total lipids, fatty acids, and minerals content according to the methodologies described for the algae blend and experimental diets.
2.4. Growth trial
From the initial lot, 12 groups of 46 fish (11.3 ± 2.70 g of BW and 10.5 ± 1.04 cm total length) were randomly allocated to 160 L fiberglass tanks within a saltwater recirculation system. The fish were adapted to the new conditions (20.9 ± 0.43°C of water temperature, 35.8 ± 1.29‰ of salinity, 10 L min−1 of flow rate, and 12 h of light/dark photoperiod) for 20 days. After acclimation, the experimental diets were randomly assigned to triplicate groups of fish, which were fed by automatic feeders until apparent satiation three times a day, at 9:00, 13:00, and 17:00 h, 7 days a week. The amount of feed supplied to each tank was adjusted according to the presence or absence of feed in the tank (66). Water quality parameters (pH, nitrogenous compounds, and dissolved O2) were monitored daily and maintained within recommended levels for marine fish species (67). The growth trial lasted 12 weeks. Fish were bulk weighed after 5 weeks to monitor weight gain and feed consumption.
Before starting the trial, six fish from the initial lot were sacrificed by anesthetic overdose (1.5 ml L−1 of 2-phenoxyethanol, Sigma-Aldrich) and stored at −20°C until whole-body composition analysis. At the end of the growth trial (12 weeks), all fish were fasted for 24 h, anesthetized (60 μl L−1 of 2-phenoxyethanol, Sigma-Aldrich), and individually weighted (g) and measured (total length, cm). Six fish per tank were sacrificed by anesthetic overdose (1.5 ml L−1 of 2-phenoxyethanol, Sigma-Aldrich) for further whole-body composition analysis, and the remaining fish were sacrificed by a sharp blow to the head. The viscera and liver of 12 fish per tank were weighted (g) to determine the viscerosomatic and hepatosomatic indices. Six fish per tank were collected for skin, muscle, and intestine analysis. Left dorsal skin and muscle were collected and color was immediately assessed. Then, the instrumental texture of the muscle was determined. The right dorsal muscle was collected, snap frozen, and kept at −80°C until nutritional value analysis (DM, CP, total lipids, and fatty acids profile). A section of ~0.5 cm of the anterior intestine (after the pyloric ceca) was collected, rinsed, and fixed in a 10% neutral-buffered formalin for 24 h, and then transferred to 70% ethanol until histomorphological evaluation.
2.5. Intestine histomorphology evaluation
Fixed samples of the anterior intestine from three fish per tank (nine fish per diet) were selected for histological analysis and embedded in paraffin. The embedded tissues were cut into 3 μm sections by a semiautomated rotary microtome (Leica RM 2245, Leica Biosystems, Nussloch, Germany). For quantitative analysis, sections were stained with specific Alcian blue/PAS (pH 2.5) and observed under a light microscope (Olympus BX51; Olympus, Tokyo, Japan) with a camera (Olympus DP50; Olympus). In each section of the samples, cross-sectional perimeter (mm), muscularis externa thickness (μm), submucosa width (μm), lamina propria width (μm), absorption area (mm2), and villus length and width (μm) were measured, and neutral (magenta) and acid (blue) goblet cells were counted using imaging software (Olympus cellSens Dimension Desktop; Olympus). For muscularis externa thickness and submucosa and lamina propria width, eight points of each section were measured, and the average value was calculated. Fold's length was measured in the eight highest folds from the folding tip to the bottom, following the curves of the fold. Goblet cell counts were expressed per villus area.
Intestinal integrity was evaluated using a semi-quantitative analysis: cross-sectional intestinal sections were stained with hematoxylin/eosin and a range of tissue scores set from 1 (normal tissue) to 5 (highly altered) of submucosa and lamina propria cellularity, mucosal folds, inflammatory infiltrates, and enterocytes nucleolus position (68, 69).
2.6. Whole-body composition
Before analysis, fish collected for whole-body composition were pooled per tank (n = 12), minced frozen in a commercial meat grinder, and freeze-dried. Whole-fish samples were homogenized and analyzed for DM [ID 934.01, (56)], ash [ID 942.05, (56)], EE [ID 920.39, (56)], and N [ID 990.03, (56)] contents. Crude protein was calculated as N x 6.25, and GE content was determined using an adiabatic bomb calorimeter. All analyses were run in duplicate.
2.7. Muscle nutritional value
Dorsal muscle samples were freeze-dried and pooled per two individuals per tank (n = 9). Muscle samples were homogenized and analyzed for DM and N contents (56), with CP being calculated as N x 6.25. Total lipids of muscle samples were extracted with dichloromethane:methanol (2:1) and determined gravimetrically (60). Fatty acids methyl esters were prepared by direct acid-catalyzed transmethylation (61) and analyzed by gas chromatography as described for the algae blend and experimental diets. The analyses were performed in duplicate.
2.8. Skin and muscle color
Skin and muscle color was assessed using a CR-400 Chroma Meter (Konica Minolta Inc., Osaka, Japan) with an aperture of 8 mm, at the CIE D65 standard illuminant. The color was expressed in CIELAB coordinates, where L* measures the degree of lightness (on a scale of 0 to 100, from black to white), a* the degree of redness/greenness (+ red and – green), and b* the degree of yellowness/blueness (+ yellow and – blue). The colorimeter was calibrated against a white plate reference standard (L* = 98.0; a* = 0.3; b* = 2.4; Minolta Co. Ltd.). Color measurements were made by leaning the colorimeter on the surface of the skin and muscle, at three points per fish (54, 66). After flashing, the reflected light values were saved, and the Hue angle (h* = tan−1 b* / a*) and Chroma (C* = (a*2 + b*2)1/2) values were calculated.
2.9. Muscle texture
Instrumental dorsal muscle texture was determined using a TA.XT Plus Texture Analyzer equipped with a 2.0-mm diameter probe (Stable Micro Systems, Surrey, UK). The texture profile was obtained in a sequence of two compressions, with a 5-kg load cell, a constant speed of 1.0 mm s−1, and a penetration depth of 4.0 mm. Compressions were made 5 s apart at three points of the thickest part of each filet. Texture data were analyzed using Exponent v6 software (Stable Micro Systems), and the texture parameters hardness (N), adhesiveness (J), chewiness (J), springiness, cohesiveness, and resilience were determined (54, 66).
2.10. Calculations
Growth performance parameters were calculated based on BW and body length, as follows:
Feed efficiency parameters were calculated based on feed intake corrected for the number of fish lost due to mortality and/or sampling, as follows:
The apparent digestibility coefficients (ADC) of the experimental diets were calculated based on the amount of yttrium oxide in diets and feces as proposed by Maynard et al. (70):
The nutritional quality indices of lipids in juvenile European seabass filets were calculated according to Chen and Liu (71) as follows:
2.11. Statistical analysis
Data were analyzed using the general linear model (GLM) procedure of SPSS (2009; IBM SPSS statistics V26; IBM, Armonk, NY, USA). The model included the fixed effect of diet (Algae0, Algae2, Algae4, and Algae6) and the random residual error. When statistical differences were observed, multiple comparisons of means were performed using the post hoc HSD Tukey test. Effects were considered significant when p < 0.05 and a trend when 0.05 ≤ p ≤ 0.10.
3. Results
3.1. Algae blend and experimental diets
The algae blend had moderate CP (347 g kg−1 DM) and NDF (126 g kg−1 DM) contents, low starch (21.7 g kg−1 DM) and GE (16.5 MJ kg−1 DM) contents, and high ash content (251 g kg−1 DM; Table 1). All amino acids considered essential for European seabass were found in the algae blend (Table 1). Lysine was the most abundant essential amino acid (30.2 g kg−1 DM), followed by arginine and leucine (28.7 and 27.3 g kg−1 DM, respectively), while methionine and histidine (7.02 and 6.08 g kg−1 DM, respectively) were the least abundant. The fatty acids profile of the algae blend was highly unsaturated (Table 1), comprising 37.9% PUFA and 26.8% MUFA. However, the blend had only moderate content of the essential highly unsaturated fatty acid EPA (5.93 g kg−1 DM) and no DHA was detected. The algae blend proved to be a good source of macro and trace elements, particularly magnesium, potassium, sodium, aluminum, boron, chromium, copper, iodine, iron, manganese, strontium, and zinc (Supplementary Table S1).
Although experimental diets were formulated to be isoproteic, isolipidic, and isoenergetic, the essential amino acids content was slightly decreased by algae blend inclusion, reflecting its lower content of arginine, histidine, methionine, phenylalanine, and threonine (Table 1). Conversely, the lysine content increased with the inclusion levels of the algae blend. The fatty acids content was globally similar among diets with algae blend inclusion leading to a small decrease in MUFA and an increase in PUFA content (Table 1). The content of magnesium, phosphorus, potassium, sodium, aluminum, cobalt, iron, lithium, manganese, and zinc increased in the diets with algae blend inclusion, while the content of cadmium, chromium, copper, molybdenum, nickel, and selenium decreased (Supplementary Table S1).
3.2. Digestibility
The ADC of the nutrients and energy of the experimental diets were greatly affected by algae blend supplementation (p < 0.05; Table 2). ADC of DM was 12% higher in Algae4 and Algae6 (72.2 and 73.0%) compared to Algae0 (64.8%); Algae2 did not differ from other diets. Likewise, the algae blend inclusion promoted the ADC of CP and GE, the highest values being found with Algae4 and Algae6 and the lowest with Algae0; Algae2 was similar to other diets. Organic matter and total lipids ADC increased by 9% and 8%, respectively, with algae blend supplementation compared to control (0%), with no differences being observed among inclusion levels.
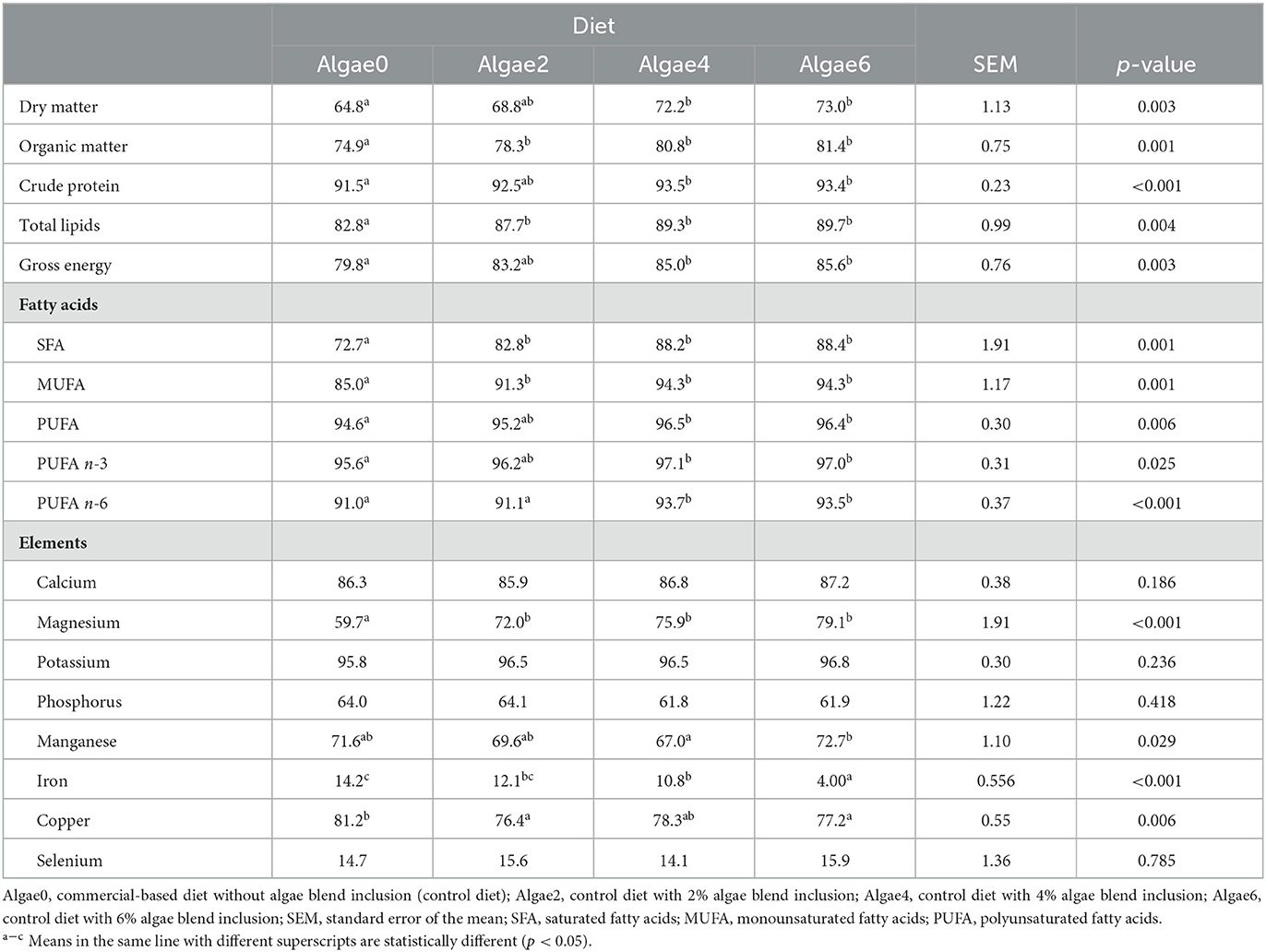
Table 2. Apparent digestibility coefficients (%) of nutrients, energy, and minerals of the experimental diets fed to European seabass juveniles.
The ADC of most individual fatty acids was affected by dietary algae blend inclusion (p < 0.05; Supplementary Table S2), except C10:0, C17:1 n-7, C20:3 n-6, and C22:4 n-6 (p > 0.05). In general, the ADC of individual even-chain fatty acids (ECFA), odd-chain fatty acids (OCFA), branched-chain fatty acids (BCFA), and MUFA were promoted (p < 0.05) in diets with algae supplementation, with no differences among inclusion levels. The exceptions were C22:0, which was the highest with Algae6, and C13:0 and C20:1 n-11, which were the highest with Algae4 and Algae6 compared to Algae0. Effects on individual PUFA ADC were also marked (p < 0.05) but less consistent. ADC of C18:2 n-6 and C20:2 n-6 were the highest with Algae4 and Algae6 compared to Algae0 and Algae2, and ADC of C18:3 n-3 was the lowest and the highest with 2% and 4% algae inclusion, respectively. The ADC of C18:3 n-6 was the lowest with Algae0 and the highest with Algae4, while C20:4 n-6 was the lowest with Algae6 and the highest with Algae0; Algae2 and Algae4 not differing from the other levels. C18:4 n-3, C20:3 n-3, C20:4 n-3, C21:5 n-3, and DHA ADC were the highest with Algae4 and Algae6 compared to the control; Algae2 was similar to other levels. In addition, algae blend dietary inclusion also increased the ADC of total ECFA by 19%, OCFA by 20%, BCFA by 15%, total SFA by 19%, and MUFA by 10% compared to Algae0 (p < 0.05), with no differences being observed among inclusion levels. Algae4 and Algae6 improved the ADC of total PUFA n-3 by 1% and total PUFA by 2% compared to Algae0, and of PUFA n-6 by 2% compared to Algae0 and Algae2 (p < 0.05).
The ADC of calcium, potassium, phosphorus, zinc, and selenium were not affected by the inclusion of the algae blend (p >0.05; Table 2). Magnesium ADC was increased by 27% with algae blend supplementation compared to Algae0, with no differences being observed among inclusion levels. The manganese ADC was the lowest with Algae4 and the highest with Algae6; Algae0 and Algae2 not differing from the other diets. Copper ADC was reduced with algae supplementation; the lowest values were found with Algae2 and Algae6 and the highest with Algae0; Algae4 not differing from the other diets. Similarly, iron ADC decreased with increasing levels of the algae blend, with Algae6 being 72% lower than Algae0 (Table 2).
The effects of algae blend inclusion on N, lipid, and energy balances of European seabass juveniles are shown in Table 3. Digestible N, lipid, energy intake, and lipid gain gradually increased with algae blend inclusion (p < 0.05). Nitrogen and energy gains were promoted by the algae blend (p < 0.05), but no differences were observed among inclusion levels. Nitrogen retention efficiency was not affected by algae supplementation, while lipid and energy retention efficiencies were increased (p < 0.05), regardless of the inclusion level. Fecal N losses were the lowest with Algae4 and the highest with Algae0, while non-fecal N losses increased with the algae blend inclusion levels (p < 0.05). Fecal and non-fecal lipid losses were promoted by algae blend supplementation (p < 0.05), with no differences being observed among inclusion levels. Non-fecal energy losses were the highest with Algae6 compared to Algae0 (p < 0.05). Metabolizable energy gradually increased with algae blend supplementation levels (p < 0.05), with Algae6 being 30.7% higher than Algae0. Total heat loss was not affected by the dietary algae blend (p > 0.05).
3.3. Growth performance and feed utilization
Most growth performance and feed utilization parameters were affected by algae blend feeding (p < 0.05; Table 4). Final fish BW and length were improved, with the BW of fish fed Algae6 being 70% higher than that of fish fed Algae0. Algae blend supplementation promoted DGI, VSI, and HIS compared to the control (0%), but no differences were observed among inclusion levels. The lowest FCR was observed with 2% and 4% of algae blend and the highest with Algae0. The PER was the highest with Algae4, not differing from Algae2 and Algae 6. The algae blend also improved the condition factor, which was highest at the 4% and 6% inclusion levels.
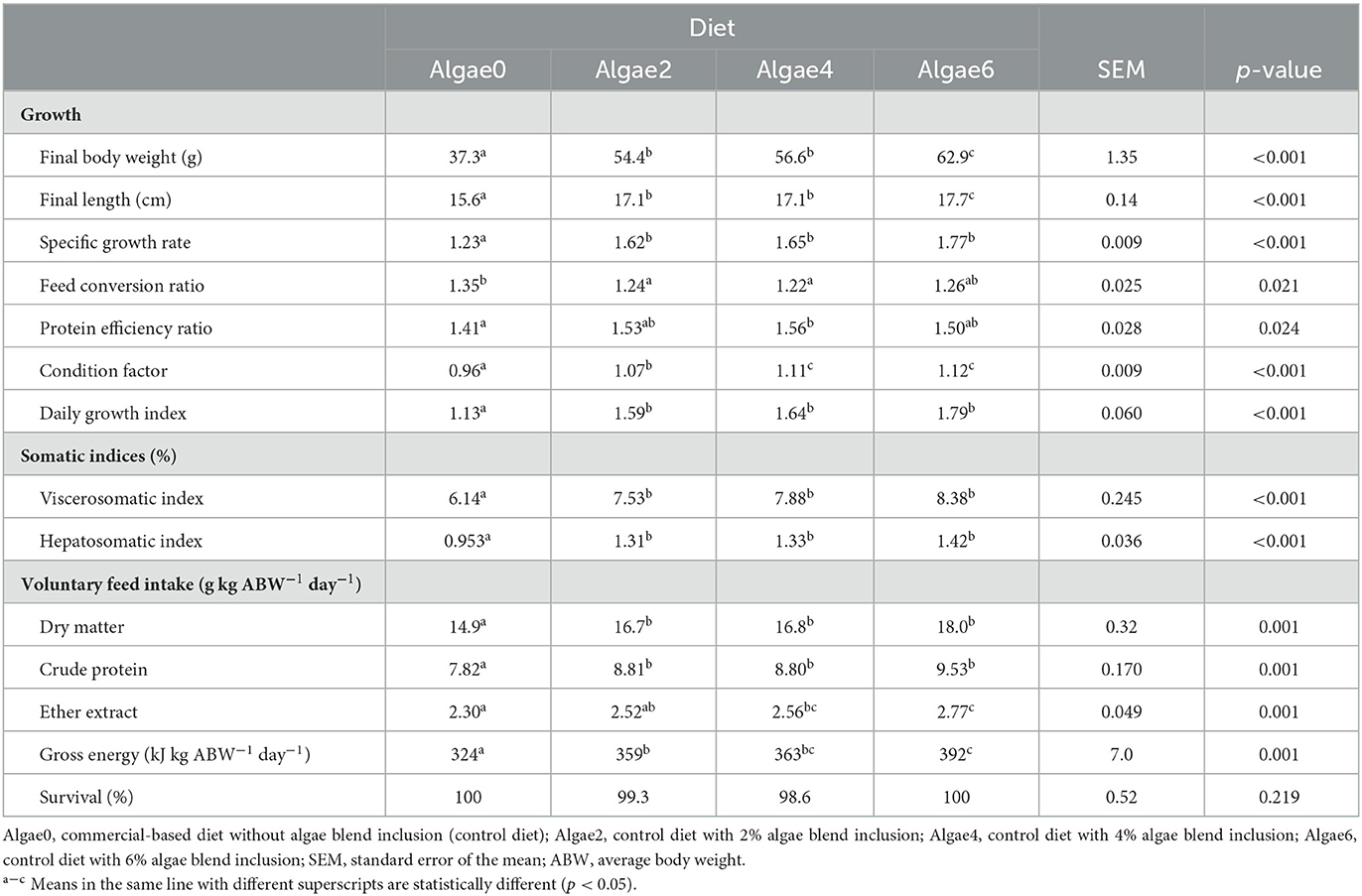
Table 4. Growth performance and feed utilization of European seabass juveniles fed the experimental diets.
Feed intake of DM and CP was promoted in diets with algae supplementation, with no differences among inclusion levels. Feed intake of EE and GE was the highest at 6% and 4% algae inclusion, the latter not differing from 2% (Table 4).
The survival rate was not affected by algae blend supplementation (p > 0.05; Table 4).
3.4. Histomorphology of anterior intestine
The overall integrity of the anterior intestine was well preserved in all fish, but algae blend supplementation had a significant impact on its morphology (p < 0.05; Figure 1, Table 5).
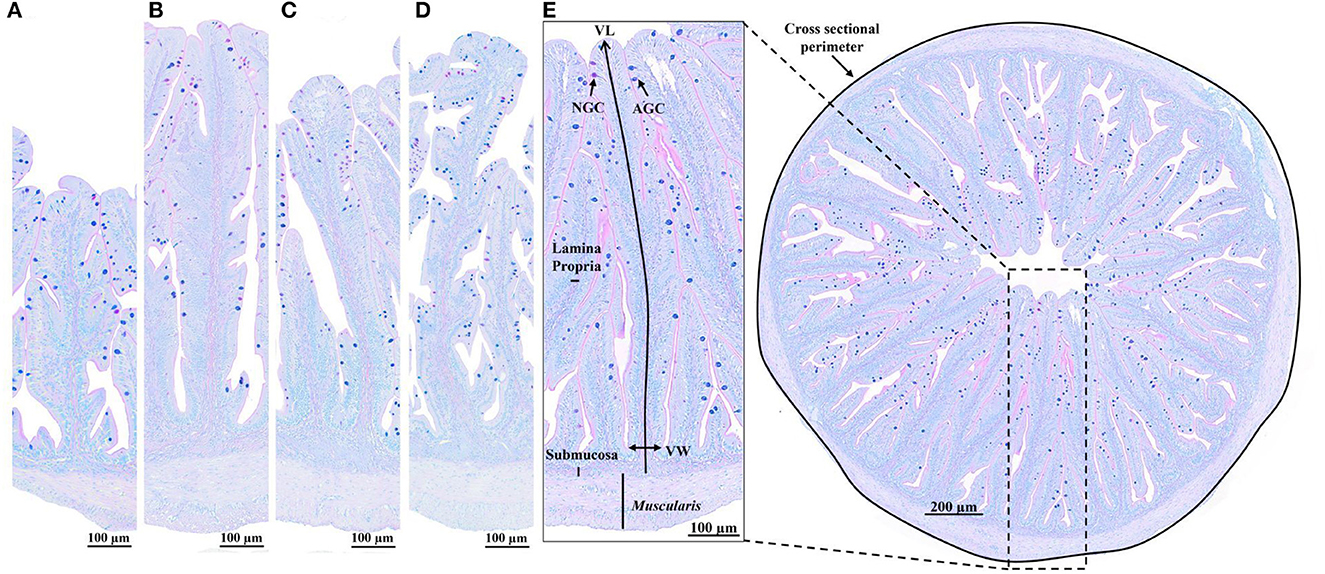
Figure 1. Histological sections (Alcian blue/PAS staining, pH = 2.5) of the anterior intestine of European seabass fed (A) commercial-based diet without algae blend inclusion (control diet; Algae0), (B) control diet with 2% algae blend inclusion (Algae2), (C) control diet with 4% algae blend inclusion (Algae4), and (D) control diet with 6% algae blend inclusion (Algae6). (E) Measured parameters: cross-sectional perimeter, villus length (VL), villus width (VW), muscularis, submucosa and lamina propria width, acid goblet cells (AGC, blue), and neutral goblet cells (NGC, pink).
Most quantitative parameters were affected by the dietary inclusion of algae (p < 0.05), except for muscularis thickness, number of acid goblet cells, and number of goblet cells per villus area (p > 0.05). The cross-sectional perimeter, the submucosa width, and the villus length increased with algae inclusion levels, being the highest in fish fed Algae4 and Algae6 and the lowest in fish fed Algae0 and Algae2 for submucosa width; Algae2 was similar to other inclusion levels for cross-sectional perimeter and villus length. Compared to Algae0, lamina propria width was the highest in fish fed Algae4 while the highest absorption area was observed in fish fed Algae6. Goblet cell counts were the highest in fish fed Algae2 and Algae6.
Submucosal and lamina propria cellularity, mucosal folds, inflammatory infiltrates, and enterocyte nucleolus position ranged from normal to slightly altered tissue, not being significantly affected by algae blend inclusion (p > 0.05).
3.5. Whole-body composition
After 12 weeks of feeding, whole-body moisture and EE gradually decreased and increased, respectively, with the inclusion levels of the algae blend (p < 0.05; Table 6). Whole-body GE content of fish fed algae-supplemented diets was enhanced; no differences were observed among inclusion levels (p < 0.05). Ash and CP contents were not affected (p > 0.05).
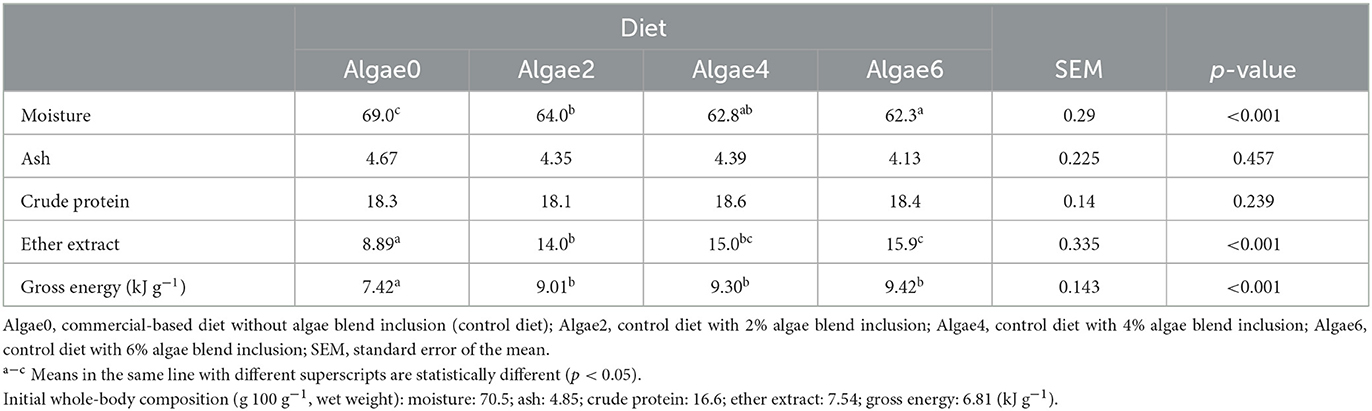
Table 6. Whole-body composition (g 100 g−1, wet weight) of European seabass juveniles fed the experimental diets.
3.6. Muscle composition
Muscle moisture content decreased whereas total lipids and fatty acids content increased with algae supplementation compared to control (p < 0.05; Table 7), with no differences observed among levels. Algae supplementation did not affect muscle CP content (p > 0.05).
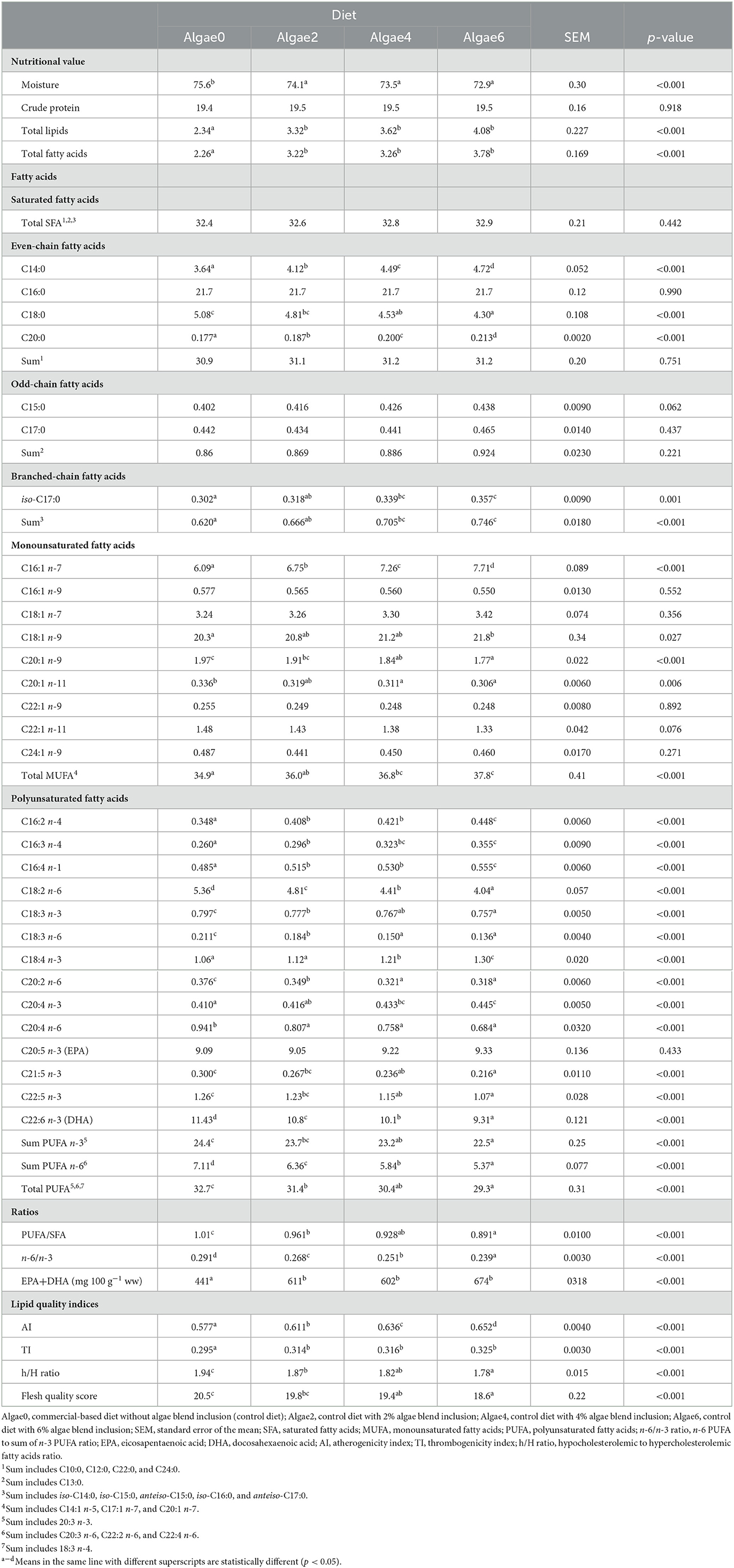
Table 7. Muscle nutritional value (g 100 g−1 wet weight, ww), fatty acids profile (g 100 g−1 total fatty acids), and lipid quality indices of European seabass juveniles fed the experimental diets.
The muscle fatty acids profile was greatly affected by algae blend supplementation (p < 0.05; Table 7). Total SFA remained unchanged, reflecting the effect on C16:0 (p > 0.05). Total MUFA, C16:1 n-7, and C18:1 n-9 proportions were the highest in fish fed with 6% algae blend and the lowest in those fed control (0%) diets. Conversely, C20:1 n-9 and C20:1 n-11 decreased with algae blend inclusion compared to Algae0. Dietary inclusion of the algae blend decreased the proportions of all individual n-6 PUFA, leading to a decrease in total n-6 PUFA by 24.5%. Effects on n-3 PUFA differed among individual fatty acids. Dietary inclusion of the algae blend increased C18:4 n-3 and C20:4 n-3 and decreased C21:5 n-3, C22:5 n-3, and DHA proportions in muscle. Muscle EPA remained unchanged (p > 0.05). Overall, the total n-3 PUFA proportion gradually decreased with algae supplementation, with Algae6 being 7.8% lower than Algae0. The observed decrease in PUFA n-6 and n-3 led to a decrease in total PUFA and n-6/n-3 ratio with algae blend inclusion levels.
Although the PUFA proportions decreased with the algae blend inclusion, the higher lipid and fatty acids content of algae blend-fed European seabass muscle led to an increase in the essential fatty acids (EPA + DHA) content by nearly 43%; no differences were found among inclusion levels.
Algae blend supplementation increased the thrombogenicity and the atherogenicity indices (p < 0.05; Table 7); no differences were observed among levels in the former, while a gradual increase with increasing algae inclusion levels was observed in the latter. The h/H ratio and flesh quality score decreased with algae inclusion levels (p < 0.05), in fish fed Algae0 being the highest and in those fed Algae6 being the lowest.
3.7. Color of skin and muscle, and texture of muscle
Dorsal skin color parameters were affected by algae blend feeding (p < 0.05; Table 8). Skin brightness (L*) was the highest in control fish and the lowest in those fed Algae2, with Algae4 and Algae6 not differing from Algae0 and Algae2. The algae blend reduced the greenness (a*) of the skin compared to the control, while Alage4 and Algae6 increased the yellowness (b*) compared to Algae2, although not differing from Algae0. Chroma (C*) was the highest in the skin of fish fed Algae4 and the lowest in those fed Algae2. The skin hue angle (h) of fish fed Algae4 and Algae6 was lower compared to those fed Algae0 and Algae2, reflecting a less greenish skin tone.
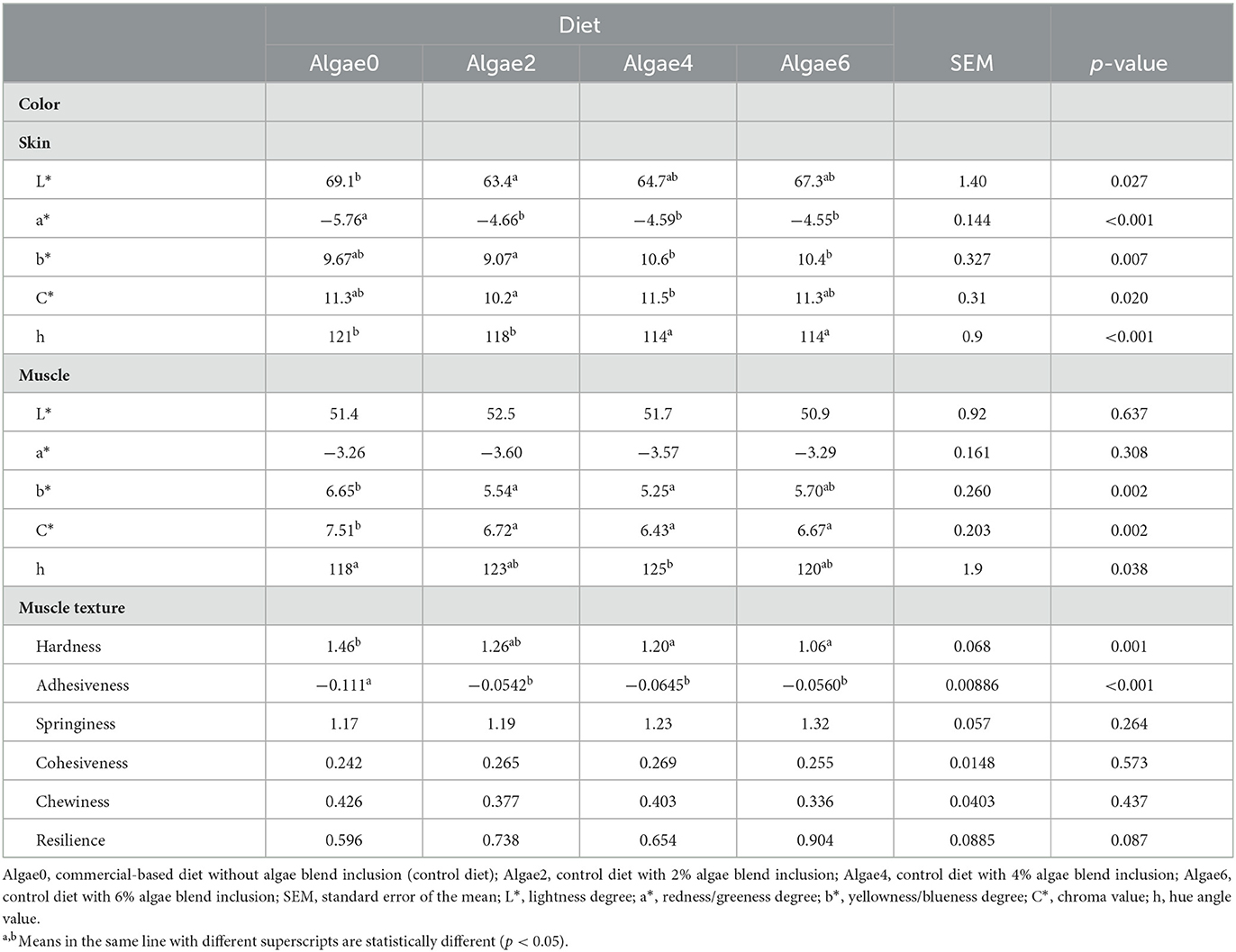
Table 8. Skin and muscle color and muscle texture of European seabass juveniles fed the experimental diets.
Muscle brightness (L*) and greenness (a*) were not affected by algae blend inclusion levels, while yellowness (b*) was the highest in fish fed Algae0 and the lowest in those fed Algae2 and Algae4 (Table 7); Algae6 fish did not differ from other diets. Compared to the control, algae blend inclusion reduced muscle chroma (C*), with no differences among inclusion levels. Conversely, the hue angle (h) was increased by algae feeding, with the highest value found in the muscle of fish fed Algae4 (more greenish) and the lowest in that of fish fed Algae0 (Table 8).
Regarding texture (Table 8), muscle hardness was the lowest in fish fed Algae4 and Algae6 and the highest in those fed the control diet (Algae0); Algae2 did not differ from the other levels. Muscle adhesiveness increased in fish fed algae blend, regardless of supplementation level. Muscle springiness, cohesiveness, and chewiness were not affected by algae supplementation. A trend toward increased muscle resilience with algae supplementation was observed.
4. Discussion
To ensure aquaculture sustainability, challenges related to aquafeed formulation and ingredient selection must be addressed, in line with the circular economy, the Blue Growth strategy of the European Union, and the Sustainable Development Goals of the 2030 Agenda. In recent years, algae have emerged as alternative aquafeed ingredients due to their nutritional and functional values and lower environmental footprint (13, 72), particularly macroalgae produced in integrated multi-trophic aquaculture (IMTA) systems and microalgae produced locally. Although dietary supplementation of micro- or macroalgae species as sustainable alternative aquafeeds to fishmeal and fish oil (13, 73, 74) or plant source ingredients (34, 75) has been assessed, the synergetic effects of the blend of micro- and macroalgae remain largely unexploited. The present study addressed this gap and unveiled the potential of dietary supplementation of a commercial blend of macro- (Ulva sp. and G. gracilis) and microalgae (C. vulgaris and N. oceanica) species up to 6% (DM basis) in digestibility, growth performance, and muscle nutritional value and quality of European seabass juveniles.
The functional potential of this algae blend has recently been suggested based on its chemical composition and bacteriostatic and bactericidal activities evidenced in vitro against some of the most common fish pathogenic bacteria (23). To the best of our knowledge, no other study has yet evaluated an algae blend composed of these four species in an in vivo study. The composition of macro- and microalgae is known to vary between species and within species with biotic and abiotic growth factors (76). Nonetheless, the overall proximate composition and amino acids content of the blend previously analyzed (23) are in broad agreement with the present results. The most relevant differences were in the polysaccharide content and the fatty acids content and profile. The commercial blend used in the present study had lower polysaccharides content (148 vs. 341 g kg−1) and total FA content (54.9 vs. 79.0 g kg−1), but higher PUFA n-3 (22.0 vs. 17.6% total FA) and EPA (10.8 vs. 6.91% total FA) proportions than that previously reported (23). The high content of macro (e.g., magnesium, potassium, and sodium) and trace elements (e.g., iron, manganese, and zinc) reported here further support the interest in the algae blend as functional aquafeed ingredients. However, the levels of toxic elements such as aluminum, arsenic, and copper may limit the inclusion level of the algae blend in fish diets.
In the present study, the commercial blend of Ulva sp., G. gracilis, C. vulgaris, and N. oceanica replaced protein-rich plant ingredients and had a positive effect if included up to 6% (DM basis): growth performance, feed intake, utilization efficiency, and body composition of D. labrax juveniles were enhanced compared to those fed the control diet (Algae0), a commercial-type formulated plant-based diet with moderate (125 g kg−1 DM basis) inclusion of fishmeal. These remarkable results were mostly unexpected as most studies evaluating dietary inclusion of mixtures of macroalgae species, microalgae species, or a combination of both have reported neutral to negative effects on growth performance and feed utilization in carnivorous fish. Indeed, the mixture of red macroalgae species (Pyropia columbina and Gracilaria chilensis) included up to 1.0% in diets for Atlantic salmon (77) and Fucus sp., Gracilaria sp., and Ulva sp. at 7.5% in European seabass (31, 52, 78) diets did not affect growth performance and feed intake. Similarly, no effect on growth performance and feed utilization was observed on meager fed 10% Nannochloropsis gaditana, Tisochrysis lutea, Rhodomonas lens, and Isochrysis galbana (79), and red sea bream fed 25% Nannochloropsis sp. and Schizochytrium sp. or 45% Nannochloropsis sp., Chlorella sp., and Schizochytrium sp. (80). On the other hand, the inclusion of Nannochloropsis sp. and Isochrysis sp. up to 11.9% reduced the feed intake and growth performance of Atlantic cod (81) and Schizochytrium limacinum and N. oceanica supplementation up to 17% reduced rainbow trout growth performance (82). The negative impact of microalgae blends was suggested to be due to their low palatability (81). The combination of macroalgae (G. gracilis) and microalgae (N. oceanica) species included at 30% did not affect European seabass growth performance or feed utilization (54).
Effects of dietary algae inclusion are species-specific with ideal inclusion levels varying with algae species and fish species (42, 72). In general, carnivorous fish, such as European seabass, digest algae recalcitrant cell walls more poorly than herbivorous fish due to the shorter intestine, the main organ for digestion and absorption (83). However, in the present study, DM, OM, CP, total lipids, GE, and most FA ADC were higher in diets with algae blend supplementation. These results contrast with the consistently reduced digestibility reported in the literature with algae inclusion, which has been attributed to algae cell walls complex polysaccharides that can resist enzymatic degradation in the stomach and small intestine of monogastric animals (84) and reduce the availability of intracellular nutrients (29, 54, 85, 86), namely ulvans in Ulva sp., carrageenans in Gracilaria sp. (16), cellulosic polymers and glucosamine, a chitin-like glycan, in Chlorella sp. (87), and algaenans (outer layer) and cellulosic polymers (inner layer) in Nannochloropsis sp. (88). We hypothesize that the ADC improvement observed with algae blend inclusion may be related to the plant-based reference diet used in this study. Experimental diets were formulated to include the algae blend at the expense of wheat gluten and whole peas, keeping constant the fishmeal and fish oil levels across diets. Whole peas were the main ingredient replaced by the algae blend (11% in Algae2, 24% in Algae4, and 32% in Algae 6). Peas (Pisum sativum) are moderate sources of protein (c.a. 22% DM basis) with low sulfur-containing amino acids, high polysaccharides, and low lipid content, but also contain antinutritional factors such as tannins, phytic acid, saponins, and trypsin inhibitor activities (89). Thus, we hypothesize that the lower digestibility observed in the control group (Algae0) with a higher pea content may be due to antinutritional factors, which may have affected the digestion and absorption of nutrients, and consequently growth performance and body composition of D. labrax juveniles. Gouveia and Davies (90) found that whole pea meal inclusion at 20% and 40% (DM basis) had no negative effect on palatability, feed intake, and growth performance of European seabass juveniles, but reduced carbohydrates and energy digestibility. The lower digestibility may result from the complex matrix of highly digestible non-structural polysaccharides (starch) and low digestible structural polysaccharides (fiber) of whole peas, which was suggested to limit the nutrient digestion and assimilation in rainbow trout (91). Moreover, the processing of whole peas, as peeling and extrusion, can reduce or even eliminate the antinutritional factors that may compromise feed intake and growth of fish (92).
The morphological structure of the intestine is considered a biomarker of the nutritional and physiological status, with changes related to altered nutrient digestibility (93). In the present study, the algae blend promoted villus length and width and anterior intestinal absorption area, which suggests an enhanced ability to absorb nutrients. This can at least partially explain the greater digestibility of algae-supplemented diets and consequent better feed utilization and growth of D. labrax juveniles. In contrast, previous studies reported no effects or even a reduction of intestinal area, or villus length and width in carnivorous fish fed diets supplemented with individual Ulva sp., Gracilaria sp., Chlorella sp., or Nannochloropsis sp. (41, 54, 94, 95), or their mixtures (54, 79, 80). Fish fed the control diet (Algae0) had the lowest number of goblet cells. These mucin-producing cells produce gel-like layers that protect epithelial mucosa, facilitate digesta transport, and protect against bacterial invasion (96). Two main subtypes of mucins are produced along the gastrointestinal tract, neutral, and acidic mucins; the former is related to digestive and absorptive processes (97) and the latter to protection against bacterial translocation (96). The tendency for neutral mucins to increase further support enhanced nutrient digestion and absorption in fish fed diets supplemented with algae blend compared to the control diet. Algae blend did not affect submucosa and lamina propria cellularity and inflammatory infiltrates, while increased submucosa and lamina propria width compared to fish fed with no algae. Further studies are needed to assess the algae blend impact on posterior intestine morphology that best relates to inflammatory processes and microbiota abundance and diversity.
Algae blend dietary inclusion had no negative impact on fish protein retention efficiency or whole-body and muscle protein content. Conversely, lipid and energy retention efficiency were promoted, which was reflected in a higher whole-body lipid and energy content of fish fed algae blend. The most marked effect of algae blend supplementation was observed on body lipid content, which gradually increased with algae inclusion levels compared to the control. Lipid metabolism, including whole body lipid deposition and partitioning pattern, of carnivorous fish has been shown to be related to dietary energy intake and affected by dietary protein sources (marine vs. vegetable) that regulate lipogenic enzyme expressions and activities (98–100). In our study, a general increase in lipid deposition was observed in a fish fed algae blend for 12 weeks, with increased body and muscle lipid content and HSI. These findings are in line with the observed improvement in the digestibility of algae blend-supplemented diets and suggest the absence of bioactive compounds with lipotropic activity in the algae blend, thus contrasting with the findings of Tulli et al. (101) and suggestion of the presence of algae bioactive compounds with lipotropic activity.
Fish is the most important source of n-3 LC-PUFA EPA and DHA in the human diet (102). Although European seabass, like other marine finfish species, has the enzymatic ability for endogenous LC-PUFA biosynthesis, the low activity of enzymes involved in the desaturation/elongation pathway hampers the production of EPA and DHA from the C18 fatty acid precursor (α-linolenic acid; C18:3 n-3) at rates that meet physiological demands (103, 104). Thus, marine fish depend on dietary supplies of EPA and DHA to fulfill their essential n-3 PUFA requirements (102). Algae, particularly microalgae, may constitute alternative sustainable sources of n-3 LC-PUFA, although marked differences in the fatty acids profile are found among and within algae species (105). In addition, we hypothesize that the bioactive compounds present in algae can modulate the lipid metabolism of fish fed algae-supplemented diets, and prevent dietary fatty acids oxidation, with a putative impact on fish nutritional value and consumers' health.
In the present study, the experimental diets were formulated to include graded levels of algae blend at a constant fish oil content, thus ensuring high levels (>30 g kg−1 DM basis) of EPA and DHA. Dietary algae blend inclusion was found to alter the dorsal muscle fatty acids profile, mainly by promoting MUFA and reducing PUFA proportion while total SFA proportion remained unaffected. As the fatty acids profile and content in European seabass muscle have been reported to reflect dietary fatty acids (106–108), a stepwise increase in α-linolenic (C18:3 n-3), stearidonic (C18:4 n-3), arachidonic (C20:4 n-6), EPA, docosapentaenoic (C22:5 n-3) acids, and total n-3 PUFA and a decrease in linoleic acid (C18:2 n-6), DHA, total n-6 PUFA, and n-6/n-3 ratio were expected in the muscle mirroring the diet fatty acids profile. However, only linoleic acid, DHA, and total PUFA n-6 proportion and n-6/n-3 ratio followed the expected pattern, while most individual n-3 PUFA and all n-6 PUFA proportion decreased in the dorsal muscle. These results may suggest a potential for the algae blend to modulate the lipid metabolism of D. labrax juveniles through selective retention or catabolism of specific fatty acids. The observed decrease in linoleic and α-linolenic acids proportions in the muscle of fish fed algae supplemented diets is in agreement with previous reports in microalgae-supplemented diets for rainbow trout (82) and turbot (33), suggesting that these C18 PUFA may have been selectively catabolized. On the other hand, dietary algae blend supplementation up to 6% had no negative effect on muscle EPA, which may suggest a preferential deposition and retention of this essential fatty acid in the muscle of European seabass juveniles. The concomitant effect of the algae blend on muscle EPA and DHA proportion contrasts with previous studies that reported DHA selective deposition and retention in the flesh of marine fish species, including European seabass (51, 107), as a result of the high specificity transferases and low catabolism of DHA, whereas EPA is often selectively catabolized by β-oxidation (82, 107). The observed modifications in the muscle fatty acids profile may be due to the high PUFA content of experimental diets provided by fish oil and algae lipids, which may have partially suppressed de novo fatty acid synthesis (109) and thus affected the lipid metabolism of juvenile D. labrax. Further studies focused on intermediary metabolism are needed to clarify this point. Of particular importance is that the algae blend improved overall muscle fatty acid retention in these fish, resulting in an increased EPA and DHA (EPA+DHA) content (mg g−1 wet weight) to values well above the recommended 500 mg EPA+DHA per day to prevent coronary heart disease (110). Consumers would have to ingest 113 g of Algae0 fed or only 74.2 g of Algae6 fed European seabass filets. Seabass muscle lipid quality indices provide additional information on the effects of dietary algae blends on the flesh's nutritional and functional value. Atherogenicity and thrombogenicity indices are related to the risk of atherosclerosis and thrombosis, and the h/H ratio to cholesterol metabolism; lower indices and higher ratio relating to coronary health promotion (71, 111). Although dietary algae blend supplementation promoted AI and TI and reduced h/H ratio of seabass muscle, all values obtained were within the range considered to exert potential cardiovascular promoting effects (71, 111, 112). But a longer-term study should be carried out until the fish reach a commercial size to confirm the full potential of the algal blend in aquafeeds.
The skin color of the fish is of utmost importance for consumer acceptance (113). European seabass is appreciated by consumers for its white flesh, mild flavor, and low-fat content (114). In the present study, dietary algae blend supplementation altered the skin pigmentation of juvenile European seabass to a darker and less greenish color than observed in fish fed Algae0. While significant, the changes in skin pigmentation were small and mostly imperceptible to the naked eye. However, our results contrast with a more greenish skin of European seabass fed T. suecica (101) and Isochrysis sp. (108), and with the absence of effects of G. vermicullophyla. and N. oceanica supplementation, individually or as a mixture (54). In the present study, the dorsal filet color of fish fed control diet was more yellowish than those fed algae blend diets. This result agrees with the observation of Grigorakis (112) that a higher lipid content promotes a whiter color, as the muscle lipid content increased by 41.9%, 54.7%, and 66.0% with 2%, 4%, and 6% algae blend inclusion, respectively. The less yellowish color observed here may suggest an enhanced acceptance of European seabass juveniles fed up to 6% algae blend inclusion by consumers. However, these results were obtained in juvenile fish, and further studies are needed to assess the effects on pigmentation of commercial-sized fish and on consumers preference.
The fish texture is an important attribute for assessing quality, freshness, and palatability (115). A firmer texture is preferred for consumers and industry as it is considered an indicator of freshness (116), and soft filets pose difficulties to the fish processing industry (117). Algae blend supplementation reduced muscle hardness and improved adhesiveness of European seabass juveniles, compared to fish fed Algae0. These results suggest that the algae blend diminished the texture of seabass muscle, by presenting softer traits. The softer texture of algae-fed seabass muscle was associated with lower moisture and higher lipid content. The intramuscular lipid content is considered to enhance the fish flavor and provide a smoother, juicier mouthfeel, thereby improving muscle juiciness (112). Improved juiciness may counteract the softer texture of algae blend-fed European sea juveniles. Thus, longer feeding trials and a sensory panel evaluation should be carried out to fully assess the impact of algae blend supplementation on texture traits.
5. Conclusion
Supplementation of Ulva sp., G. gracilis, C. vulgaris, and N. oceanica blend up to 6% to a commercial-type plant-based diet significantly improved the digestibility and feed utilization of diets as well as anterior intestine absorption area, feed intake, and growth performance of European seabass juveniles. Muscle nutritional value and quality were also improved by algae blend supplementation. Of particular importance is the increase in essential fatty acids (EPA+DHA) content, which allows for achieving daily intake recommendations for EPA and DHA even with lower consumption of fish.
Data availability statement
The original contributions presented in the study are included in the article/Supplementary material, further inquiries can be directed to the corresponding author.
Ethics statement
The animal study was reviewed and approved by Animal Welfare and Ethics Body of CIIMAR (ORBEA-CIIMAR 06-2016) and licensed by the Portuguese Veterinary Authority (1005/92, DGAV-Portugal).
Author contributions
MM, LV, AF, HA, and JS conceived and designed the study. CM, OP, TS, and MF conducted the research and performed the analyses. CD-M, AC, AA, AF, LV, and MM participated in the study coordination. CM and MM drafted the manuscript. All authors contributed to the manuscript revision, and read and approved the submitted version.
Funding
This work received financial support from PT National Funds (FCT/MCTES, Fundação para a Ciência e Tecnologia and Ministério da Ciência, Tecnologia e Ensino Superior) through the projects UIDB/50006/2020 | UIDP/50006/2020 (REQUIMTE) and UIDB/04423/2020 | UIDP/04423/2020 (CIIMAR) and from ALGAVALOR project (POCI-01-0247-FEDER-035234; LISBOA-01-0247-FEDER-035234; and ALG-01-0247-FEDER-035234) funded by FEDER and Portugal2020 through COMPETE2020. PhD fellowships of CM (PD/BDE/150585/2020) and MF (SFRH/BD/144843/2019) are acknowledged to FCT, SANFEED Doctoral Programme, ALGAplus, and Allmicroalgae, and FCT/FSE. MM also thanks FCT for funding through program DL 57/2016—Norma transitória (SFRH/BPD/70176/2010).
Acknowledgments
The authors thank Sílvia Azevedo (ICBAS-UP) for the valuable technical assistance.
Conflict of interest
HA and JS are employed by the companies ALGAplus and Allmicroalgae, respectively, and CM grant is partly financed by ALGAplus and Allmicroalgae.
The remaining authors declare that the research was conducted in the absence of any commercial or financial relationships that could be construed as a potential conflict of interest.
Publisher's note
All claims expressed in this article are solely those of the authors and do not necessarily represent those of their affiliated organizations, or those of the publisher, the editors and the reviewers. Any product that may be evaluated in this article, or claim that may be made by its manufacturer, is not guaranteed or endorsed by the publisher.
Supplementary material
The Supplementary Material for this article can be found online at: https://www.frontiersin.org/articles/10.3389/fnut.2023.1165343/full#supplementary-material
References
1. United Nations. World Population Prospects 2019: Highlights. NY, USA: United Nations (2019). Contract No.: ST/ESA/SER.A/423.
2. FAO IFAD UNICEF WFP WHO. The State of Food Security and Nutrition in the World 2022. Repurposing Food and Agricultural Policies to Make Healthy Diets More Affordable. Rome: FAO (2022).
3. FAO. The State of World Fisheries and Aquaculture 2022. Towards Blue Transformation. Rome: FAO (2022).
4. Rombenso A, Araujo B, Li E. Recent advances in fish nutrition: Insights on the nutritional implications of modern formulations. Animals. (2022) 12:1705. doi: 10.3390/ani12131705
5. Turchini GM, Trushenski JT, Glencross BD. Thoughts for the future of aquaculture nutrition: Realigning perspectives to reflect contemporary issues related to judicious use of marine resources in aquafeeds. N Am J Aquac. (2019) 81:13–39. doi: 10.1002/naaq.10067
6. Kousoulaki K, Sæther B-S, Albrektsen S, Noble C. Review on European sea bass (Dicentrarchus labrax, Linnaeus, 1,758) nutrition and feed management: a practical guide for optimizing feed formulation and farming protocols. Aquac Nutr. (2015) 21:129–51. doi: 10.1111/anu.12233
7. Ghosh K, Ray AK. Aquafeed formulation using plant feedstuffs: Prospective application of fish-gut microorganisms and microbial biotechnology. In: Grumezescu AM, Holban AM, editors. Soft Chemistry and Food Fermentation. Academic Press (2017). p. (109-44. doi: 10.1016/B978-0-12-811412-4.00005-9
8. Petereit J, Hoerterer C, Bischoff-Lang AA, Conceição LEC, Pereira G, Johansen J, et al. Adult European seabass (Dicentrarchus labrax) perform well on alternative circular-economy-driven feed formulations. Sustainability. (2022) 14:7279. doi: 10.3390/su14127279
9. Albrektsen S, Kortet R, Skov PV, Ytteborg E, Gitlesen S, Kleinegris D, et al. Future feed resources in sustainable salmonid production: a review. Rev Aquac. (2022) 14:1790–812. doi: 10.1111/raq.12673
10. Aragão C, Gonçalves AT, Costas B, Azeredo R, Xavier MJ, Engrola S. Alternative proteins for fish diets: Implications beyond growth. Animals. (2022) 12:1211. doi: 10.3390/ani12091211
11. Hull-Cantillo M, Lay M, Rosentrater K. Agriculture waste bioremediation with algae and potential for methane production. In: Shah M, Rodriguez-Couto S, De La Cruz CBV, Biswas J, editors. An Integration of Phycoremediation Processes in Wastewater Treatment: Elsevier (2022). p. 419-50. doi: 10.1016/B978-0-12-823499-0.00015-8
12. Wells ML, Potin P, Craigie JS, Raven JA, Merchant SS, Helliwell KE, et al. Algae as nutritional and functional food sources: revisiting our understanding. J Appl Phycol. (2017) 29:949–82. doi: 10.1007/s10811-016-0974-5
13. Wan AHL, Davies SJ, Soler-Vila A, Fitzgerald R, Johnson MP. Macroalgae as a sustainable aquafeed ingredient. Rev Aquac. (2019) 11:458–92. doi: 10.1111/raq.12241
14. Nagarajan D, Varjani S, Lee D-J, Chang J-S. Sustainable aquaculture and animal feed from microalgae—Nutritive value and techno-functional components. Renew Sust Energ Rev. (2021) 150:111549. doi: 10.1016/j.rser.2021.111549
15. Spolaore P, Joannis-Cassan C, Duran E, Isambert A. Commercial applications of microalgae. J Biosci Bioeng. (2006) 101:87–96. doi: 10.1263/jbb.101.87
16. Øverland M, Mydland LT, Skrede A. Marine macroalgae as sources of protein and bioactive compounds in feed for monogastric animals. J Sci Food Agric. (2019) 99:13–24. doi: 10.1002/jsfa.9143
17. Saadaoui I, Rasheed R, Aguilar A, Cherif M, Al Jabri H, Sayadi S, et al. Microalgal-based feed: Promising alternative feedstocks for livestock and poultry production. J Anim Sci Biotechnol. (2021) 12:76. doi: 10.1186/s40104-021-00593-z
18. Valente LMP, Cabrita ARJ, Maia MRG, Valente IM, Engrola S, Fonseca AJM, et al. Microalgae as feed ingredients for livestock production and aquaculture. In: Galanakis CM, editor. Microalgae. Academic Press (2021). p. (239-312. doi: 10.1016/B978-0-12-821218-9.00009-8
19. Shekarabi SPH, Mehrgan MS, Razi N, Sabzi S. Biochemical composition and fatty acid profile of the marine microalga Isochrysis galbana dried with different methods. J Microbiol Biotechnol Food Sci. (2019) 2021:521–4. doi: 10.15414/jmbfs.2019/20.9.3.521-524
20. Swanson D, Block R, Mousa SA. Omega-3 fatty acids EPA and DHA: Health benefits throughout life. Adv Nutr. (2012) 3:1–7. doi: 10.3945/an.111.000893
21. Makkar HPS, Tran G, Heuzé V, Giger-Reverdin S, Lessire M, Lebas F, et al. Seaweeds for livestock diets: a review. Anim Feed Sci Technol. (2016) 212:1–17. doi: 10.1016/j.anifeedsci.2015.09.018
22. Patel AK, Singhania RR, Awasthi MK, Varjani S, Bhatia SK, Tsai M-L, et al. Emerging prospects of macro-and microalgae as prebiotic. Microb Cell Factories. (2021) 20:112. doi: 10.1186/s12934-021-01601-7
23. Ferreira M, Teixeira C, Abreu H, Silva J, Costas B, Kiron V, et al. Nutritional value, antimicrobial and antioxidant activities of micro- and macroalgae, single or blended, unravel their potential use for aquafeeds. J Appl Phycol. (2021) 33:3507–18. doi: 10.1007/s10811-021-02549-2
24. Niccolai A, Chini Zittelli G, Rodolfi L, Biondi N, Tredici MR. Microalgae of interest as food source: Biochemical composition and digestibility. Algal Res. (2019) 42:101617. doi: 10.1016/j.algal.2019.101617
25. Nagappan S, Das P, AbdulQuadir M, Thaher M, Khan S, Mahata C, et al. Potential of microalgae as a sustainable feed ingredient for aquaculture. J Biotechnol. (2021) 341:1–20. doi: 10.1016/j.jbiotec.2021.09.003
26. Annamalai SN, Das P, Thaher MIA, Abdul Quadir M, Khan S, Mahata C, et al. Nutrients and energy digestibility of microalgal biomass for fish feed applications. Sustainability. (2021) 13:13211. doi: 10.3390/su132313211
27. Cottrell RS, Blanchard JL, Halpern BS, Metian M, Froehlich HE. Global adoption of novel aquaculture feeds could substantially reduce forage fish demand by 2030. Nature Food. (2020) 1:301–8. doi: 10.1038/s43016-020-0078-x
28. Adarme-Vega TC, Thomas-Hall SR, Schenk PM. Towards sustainable sources for omega-3 fatty acids production. Curr Opin Biotechnol. (2014) 26:14–8. doi: 10.1016/j.copbio.2013.08.003
29. Gong Y, Sørensen SL, Dahle D, Nadanasabesan N, Dias J, Valente LMP, et al. Approaches to improve utilization of Nannochloropsis oceanica in plant-based feeds for Atlantic salmon. Aquaculture. (2020) 522:735122. doi: 10.1016/j.aquaculture.2020.735122
30. Valente LMP, Custódio M, Batista S, Fernandes H, Kiron V. Defatted microalgae (Nannochloropsis sp) from biorefinery as a potential feed protein source to replace fishmeal in European sea bass diets. Fish Physiol Biochem. (2019) 45:1067–81. doi: 10.1007/s10695-019-00621-w
31. Passos R, Correia AP, Pires D, Pires P, Ferreira I, Simões M, et al. Potential use of macroalgae Gracilaria gracilis in diets for European seabass (Dicentrarchus labrax): health benefits from a sustainable source. Fish Shellfish Immunol. (2021) 119:105–13. doi: 10.1016/j.fsi.2021.09.033
32. Peixoto MJ, Magnoni L, Gonçalves JFM, Twijnstra RH, Kijjoa A, Pereira R, et al. Effects of dietary supplementation of Gracilaria sp. extracts on fillet quality, oxidative stress, and immune responses in European seabass (Dicentrarchus labrax). J Appl Phycol. (2019) 31:761–70. doi: 10.1007/s10811-018-1519-x
33. Qiao H, Hu D, Ma J, Wang X, Wu H, Wang J. Feeding effects of the microalga Nannochloropsis sp on juvenile turbot (Scophthalmus maximus L). Algal Res. (2019) 41:101540. doi: 10.1016/j.algal.2019.101540
34. Morshedi V, Nafisi Bahabadi M, Sotoudeh E, Azodi M, Hafezieh M. Nutritional evaluation of Gracilaria pulvinata as partial substitute with fish meal in practical diets of barramundi (Lates calcarifer). J Appl Phycol. (2018) 30:619–28. doi: 10.1007/s10811-017-1199-y
35. Peixoto MJ, Salas-Leitón E, Brito F, Pereira LF, Svendsen JC, Baptista T, et al. Effects of dietary Gracilaria sp and Alaria sp supplementation on growth performance, metabolic rates and health in meagre (Argyrosomus regius) subjected to pathogen infection. J Appl Phycol. (2017) 29:433–47. doi: 10.1007/s10811-016-0917-1
36. Adel M, Omidi AH, Dawood MAO, Karimi B, Shekarabi SPH. Dietary Gracilaria persica mediated the growth performance, fillet colouration, and immune response of Persian sturgeon (Acipenser persicus). Aquaculture. (2021) 530:735950. doi: 10.1016/j.aquaculture.2020.735950
37. Moutinho S, Linares F, Rodríguez JL, Sousa V, Valente LMP. Inclusion of 10% seaweed meal in diets for juvenile and on-growing life stages of Senegalese sole (Solea senegalensis). J Appl Phycol. (2018) 30:3589–601. doi: 10.1007/s10811-018-1482-6
38. Sotoudeh E, Jafari M. Effects of dietary supplementation with red seaweed, Gracilaria pygmaea, on growth, carcass composition and hematology of juvenile rainbow trout, Oncorhynchus mykiss. Aquac Int. (2017) 25:1857–67. doi: 10.1007/s10499-017-0158-6
39. Shpigel M, Guttman L, Shauli L, Odintsov V, Ben-Ezra D, Harpaz S. Ulva lactuca from an Integrated Multi-Trophic Aquaculture (IMTA) biofilter system as a protein supplement in gilthead seabream (Sparus aurata) diet. Aquaculture. (2017) 481:112–8. doi: 10.1016/j.aquaculture.2017.08.006
40. Nakagawa H, Kasahara S, Sugiyama T. Effect of Ulva meal supplementation on lipid metabolism of black sea bream, Acanthopagrus schlegeli (Bleeker). Aquaculture. (1987) 62:109–21. doi: 10.1016/0044-8486(87)90315-2
41. Batista S, Pintado M, Marques A, Abreu H, Silva JL, Jessen F, et al. Use of technological processing of seaweed and microalgae as strategy to improve their apparent digestibility coefficients in European seabass (Dicentrarchus labrax) juveniles. J Appl Phycol. (2020) 32:3429–46. doi: 10.1007/s10811-020-02185-2
42. Norambuena F, Hermon K, Skrzypczyk V, Emery JA, Sharon Y, Beard A, et al. Algae in fish feed: Performances and fatty acid metabolism in juvenile Atlantic salmon. PLoS ONE. (2015) 10:e0124042. doi: 10.1371/journal.pone.0124042
43. Araújo R, Calderón F, López J, Azevedo I, Bruhn A, Fluch S, et al. Current status of the algae production industry in Europe: an emerging sector of the blue bioeconomy. Front Mar Sci. (2021) 7:626389. doi: 10.3389/fmars.2020.626389
44. Cabrita ARJ, Guilherme-Fernandes J, Valente IM, Almeida A, Lima SAC, Fonseca AJM, et al. Nutritional composition and untargeted metabolomics reveal the potential of Tetradesmus obliquus, Chlorella vulgaris and Nannochloropsis oceanica as valuable nutrient sources for dogs. Animals. (2022) 12:2643. doi: 10.3390/ani12192643
45. Silva A, Silva SA, Carpena M, Garcia-Oliveira P, Gullón P, Barroso MF, et al. Macroalgae as a source of valuable antimicrobial compounds: Extraction and applications. Antibiotics. (2020) 9:642. doi: 10.3390/antibiotics9100642
46. Leiro JM, Castro R, Arranz JA, Lamas J. Immunomodulating activities of acidic sulphated polysaccharides obtained from the seaweed Ulva rigida C. Agardh Int Immunopharmacol. (2007) 7:879–88. doi: 10.1016/j.intimp.2007.02.007
47. Olasehinde TA, Mabinya LV, Olaniran AO, Okoh AI. Chemical characterization of sulfated polysaccharides from Gracilaria gracilis and Ulva lactuca and their radical scavenging, metal chelating, and cholinesterase inhibitory activities. Int J Food Prop. (2019) 22:100–10. doi: 10.1080/10942912.2019.1573831
48. de Jesus Raposo M, de Morais A, de Morais R. Emergent sources of prebiotics: seaweeds and microalgae. Mar Drugs. (2016) 14:27. doi: 10.3390/md14020027
49. Hemaiswarya S, Raja R, Ravi Kumar R, Ganesan V, Anbazhagan C. Microalgae: a sustainable feed source for aquaculture. World J Microbiol Biotechnol. (2011) 27:1737–46. doi: 10.1007/s11274-010-0632-z
50. Hasanein SS, Saleh NE, El-Sayed HS, Helal AM. The effect of dietary supplementation of Spirulina platensis and Chlorella vulgaris algae on the growth and disease resistance of the sea bass (Dicentrarchus labrax). Egypt J Aquat Biol Fish. (2018) 22:249–62. doi: 10.21608/ejabf.2018.17160
51. Cardinaletti G, Messina M, Bruno M, Tulli F, Poli BM, Giorgi G, et al. Effects of graded levels of a blend of Tisochrysis lutea and Tetraselmis suecica dried biomass on growth and muscle tissue composition of European sea bass (Dicentrarchus labrax) fed diets low in fish meal and oil. Aquaculture. (2018) 485:173–82. doi: 10.1016/j.aquaculture.2017.11.049
52. Peixoto MJ, Svendsen JC, Malte H, Pereira LF, Carvalho P, Pereira R, et al. Diets supplemented with seaweed affect metabolic rate, innate immune, and antioxidant responses, but not individual growth rate in European seabass (Dicentrarchus labrax). J Appl Phycol. (2016) 28:2061–71. doi: 10.1007/s10811-015-0736-9
53. Lobo G, Pereira LF, Gonçalves JFM, Peixoto MJ, Ozório ROA. Effect of dietary seaweed supplementation on growth performance, antioxidant and immune responses in European seabass (Dicentrarchus labrax) subjected to rearing temperature and salinity oscillations. Int Aquat Res. (2018) 10:321–31. doi: 10.1007/s40071-018-0208-3
54. Batista S, Pereira R, Oliveira B, Baião LF, Jessen F, Tulli F, et al. Exploring the potential of seaweed Gracilaria gracilis and microalga Nannochloropsis oceanica, single or blended, as natural dietary ingredients for European seabass Dicentrarchus labrax. J Appl Phycol. (2020) 32:2041–59. doi: 10.1007/s10811-020-02118-z
55. NRC. Nutrient Requirements of Fish and Shrimp. Washington, DC: The National Academies Press (2011). 392 p.
56. AOAC. Official Methods of Analysis. 17th ed. Gaithersburg, MD, USA: Association of Official Analytical Chemists (2000).
57. Salomonsson AC, Theander O, Westerlund E. Chemical characterization of some Swedish cereal whole meal and bran fractions. Swed J Agr Res. (1984) 14:111–7.
58. Van Soest PJ, Robertson JB, Lewis BA. Methods for dietary fiber, neutral detergent fiber, and nonstarch polysaccharides in relation to animal nutrition. J Dairy Sci. (1991) 74:3583–97. doi: 10.3168/jds.S0022-0302(91)78551-2
59. Robertson JB, Van Soest PJ. The Analysis of Dietary Fiber in Food. James WPT, Teander O, editors. New York: Marcel Dekker Inc. (1981). 123-58 p.
60. Folch J, Lees M, Stanley GHS, A. simple method for the isolation and purification of total lipides from animal tissues. J Biol Chem. (1957) 226:497–509. doi: 10.1016/S0021-9258(18)64849-5
61. Lepage G, Roy CC. Direct transesterification of all classes of lipids in a one-step reaction. J Lipid Res. (1986) 27:114–20. doi: 10.1016/S0022-2275(20)38861-1
62. Aragão C, Cabano M, Colen R, Fuentes J, Dias J. Alternative formulations for gilthead seabream diets: Towards a more sustainable production. Aquac Nutr. (2020) 26:444–55. doi: 10.1111/anu.13007
63. Cabrita ARJ, Maia MRG, Oliveira HM, Sousa-Pinto I, Almeida AA, Pinto E, et al. Tracing seaweeds as mineral sources for farm-animals. J Appl Phycol. (2016) 28:3135–50. doi: 10.1007/s10811-016-0839-y
64. Pinto E, Ramos P, Vital C, Santos A, Almeida A. Iodine levels in different regions of the human brain. J Trace Elem Med Biol. (2020) 62:126579. doi: 10.1016/j.jtemb.2020.126579
65. Cho CY, Slinger SJ. Apparent digestibility measurement in feedstuffs for rainbow trout. In: Halver JE, Tiews KZ, editors. Finfish Nutrition and Fishfeed Technology. II. Berlin: Heenemann Verlagsgesellschaft (1979). p. 239-49.
66. Basto A, Calduch-Giner J, Oliveira B, Petit L, Sá T, Maia MRG, et al. The use of defatted Tenebrio molitor larvae meal as a main protein source is supported in European sea bass (Dicentrarchus labrax) by data on growth performance, lipid metabolism, and flesh quality. Front Physiol. (2021) 12:659567. doi: 10.3389/fphys.2021.659567
67. Blancheton JP. Developments in recirculation systems for Mediterranean fish species. Aquac Eng. (2000) 22:17–31. doi: 10.1016/S0144-8609(00)00030-3
68. Penn MH, Bendiksen EÅ, Campbell P, Krogdahl Å. High level of dietary pea protein concentrate induces enteropathy in Atlantic salmon (Salmo salar L). Aquaculture. (2011) 310:267–73. doi: 10.1016/j.aquaculture.2010.10.040
69. Booman M, Forster I, Vederas JC, Groman DB, Jones SRM. Soybean meal-induced enteritis in Atlantic salmon (Salmo salar) and Chinook salmon (Oncorhynchus tshawytscha) but not in pink salmon (O. gorbuscha) Aquaculture. (2018) 483:238–43. doi: 10.1016/j.aquaculture.2017.10.025
71. Chen J, Liu H. Nutritional indices for assessing fatty acids: a mini-review. Int J Mol Sci. (2020) 21:5695. doi: 10.3390/ijms21165695
72. Shah MR, Lutzu GA, Alam A, Sarker P, Chowdhury MAK, Parsaeimehr A, et al. Microalgae in aquafeeds for a sustainable aquaculture industry. J Appl Phycol. (2018) 30:197–213. doi: 10.1007/s10811-017-1234-z
73. Sagaram US, Gaikwad MS, Nandru R, Dasgupta S. Microalgae as feed ingredients: recent developments on their role in immunomodulation and gut microbiota of aquaculture species. FEMS Microbiol Lett. (2021) 368:71. doi: 10.1093/femsle/fnab071
74. Chen F, Leng Y, Lu Q, Zhou W. The application of microalgae biomass and bio-products as aquafeed for aquaculture. Algal Res. (2021) 60:102541. doi: 10.1016/j.algal.2021.102541
75. Grammes F, Reveco FE, Romarheim OH, Landsverk T, Mydland LT, Øverland M. Candida utilis and Chlorella vulgaris counteract intestinal inflammation in Atlantic salmon (Salmo salar L). PLoS ONE. (2013) 8:e83213. doi: 10.1371/journal.pone.0083213
76. Hua K, Cobcroft JM, Cole A, Condon K, Jerry DR, Mangott A, et al. The future of aquatic protein: implications for protein sources in aquaculture diets. One Earth. (2019) 1:316–29. doi: 10.1016/j.oneear.2019.10.018
77. Lozano Muñoz I, Wacyk J, Perez C, Carrasco J, Martin MC-S. Diets enriched in red seaweed (Pyropia columbina and Gracilaria chilensis) cryo concentrates modulate the immune-relevant gene encoding the Mx antiviral protein in salmon (Salmo salar) white blood cells. J Appl Phycol. (2019) 31:1415–24. doi: 10.1007/s10811-018-1595-y
78. Peixoto MJ, Salas-Leitón E, Pereira LF, Queiroz A, Magalhães F, Pereira R, et al. Role of dietary seaweed supplementation on growth performance, digestive capacity and immune and stress responsiveness in European seabass (Dicentrarchus labrax). Aquac Rep. (2016) 3:189–97. doi: 10.1016/j.aqrep.2016.03.005
79. Estévez A, Blanco B, Fernández L, Ferreira M, Soula M. Effects of alternative and sustainable ingredients, insect meal, microalgae and protein and lipid from tuna cooking water, on meagre (Argyrosomus regius) growth, food conversion and muscle and liver composition. Aquaculture. (2022) 548:737549. doi: 10.1016/j.aquaculture.2021.737549
80. Seong T, Uno Y, Kitagima R, Kabeya N, Haga Y, Satoh S. Microalgae as main ingredient for fish feed: non-fish meal and non-fish oil diet development for red sea bream, Pagrus major, by blending of microalgae Nannochloropsis, Chlorella, and Schizochytrium. Aquac Res. (2021) 52:6025–36. doi: 10.1111/are.15463
81. Walker AB, Berlinsky DL. Effects of partial replacement of fish meal protein by microalgae on growth, feed intake, and body composition of Atlantic cod. N Am J Aquac. (2011) 73:76–83. doi: 10.1080/15222055.2010.549030
82. Serrano E, Simpfendorfer R, Medina A, Sandoval C, Martínez A, Morales R, et al. Partially replacing fish oil with microalgae (Schizochytrium limacinum and Nannochloropsis oceanica) in diets for rainbow trout (Oncorhynchus mykiss) reared in saltwater with reference to growth performance, muscle fatty acid composition and liver ultrastructure. Aquac Res. (2021) 52:4401–13. doi: 10.1111/are.15279
83. Ray AK, Ringø E. The Gastrointestinal Tract of Fish. Aquaculture Nutrition: Gut Health, Probiotics and Prebiotics. Chichester: John Wiley & Sons, Ltd (2014). p. 1–13. doi: 10.1002/9781118897263.ch1
84. Zheng L-X, Chen X-Q, Cheong K-L. Current trends in marine algae polysaccharides: the digestive tract, microbial catabolism, and prebiotic potential. Int J Biol Macromol. (2020) 151:344–54. doi: 10.1016/j.ijbiomac.2020.02.168
85. Cerri R, Niccolai A, Cardinaletti G, Tulli F, Mina F, Daniso E, et al. Chemical composition and apparent digestibility of a panel of dried microalgae and cyanobacteria biomasses in rainbow trout (Oncorhynchus mykiss). Aquaculture. (2021) 544:737075. doi: 10.1016/j.aquaculture.2021.737075
86. Sørensen M, Gong YY, Bjarnason F, Vasanth GK, Dahle D, Huntley M, et al. Nannochloropsis oceania-derived defatted meal as an alternative to fishmeal in Atlantic salmon feeds. PLoS ONE. (2017) 12:907. doi: 10.1371/journal.pone.0179907
87. Ferreira AS, Ferreira SS, Correia A, Vilanova M, Silva TH, Coimbra MA, et al. Reserve, structural and extracellular polysaccharides of Chlorella vulgaris: a holistic approach. Algal Res. (2020) 45:101757. doi: 10.1016/j.algal.2019.101757
88. Scholz MJ, Weiss TL, Jinkerson RE, Jing J, Roth R, Goodenough U, et al. Ultrastructure and composition of the Nannochloropsis gaditana cell wall. Eukaryot Cell. (2014) 13:1450–64. doi: 10.1128/EC.00183-14
89. Khan TN, Croser JS. Pea | Overview. In: Wrigley C, editor. Encyclopedia of Grain Science. Oxford: Elsevier (2004). p. 418-27. doi: 10.1016/B0-12-765490-9/00126-9
90. Gouveia A, Davies SJ. Preliminary nutritional evaluation of pea seed meal (Pisum sativum) for juvenile European sea bass (Dicentrarchus labrax). Aquaculture. (1998) 166:311–20. doi: 10.1016/S0044-8486(98)00292-0
91. Davies SJ. The role of dietary fibre in fish nutrition. In: Muir JF, Roberts RJ, editors. Recent Advances in Aquaculture. Volume 2. Boston, MA: Springer US (1985). p. 219-49. doi: 10.1007/978-1-4684-8736-7_6
92. Burel C, Boujard T, Tulli F, Kaushik SJ. Digestibility of extruded peas, extruded lupin, and rapeseed meal in rainbow trout (Oncorhynchus mykiss) and turbot (Psetta maxima). Aquaculture. (2000) 188:285–98. doi: 10.1016/S0044-8486(00)00337-9
93. Gisbert E, Ortiz-Delgado JB, Sarasquete C. Nutritional cellular biomarkers in early life stages of fish. Histol Histopathol. (2008) 23:1525–39. doi: 10.14670/HH-23.1525
94. Tibbetts SM, Mann J, Dumas A. Apparent digestibility of nutrients, energy, essential amino acids and fatty acids of juvenile Atlantic salmon (Salmo salar L) diets containing whole-cell or cell-ruptured Chlorella vulgaris meals at five dietary inclusion levels. Aquaculture. (2017) 481:25–39. doi: 10.1016/j.aquaculture.2017.08.018
95. Pascon G, Messina M, Petit L, Valente LMP, Oliveira B, Przybyla C, et al. Potential application and beneficial effects of a marine microalgal biomass produced in a high-rate algal pond (HRAP) in diets of European sea bass, Dicentrarchus labrax. Environ Sci Pollut Res. (2021) 28:62185–99. doi: 10.1007/s11356-021-14927-x
96. Deplancke B, Gaskins HR. Microbial modulation of innate defense: Goblet cells and the intestinal mucus layer. Am J Clin Nutr. (2001) 73:1131S−41S. doi: 10.1093/ajcn/73.6.1131S
97. Grau A, Crespo S, Sarasquete MC, de Canales MLG. The digestive tract of the amberjack Seriola dumerili, Risso: a light and scanning electron microscope study. J Fish Biol. (1992) 41:287–303. doi: 10.1111/j.1095-8649.1992.tb02658.x
98. Dias J, Alvarez MJ, Arzel J, Corraze G, Diez A, Bautista JM, et al. Dietary protein source affects lipid metabolism in the European seabass (Dicentrarchus labrax). Comp Biochem Physiol Part A Mol Integr Physiol. (2005) 142:19–31. doi: 10.1016/j.cbpb.2005.07.005
99. Messina M, Piccolo G, Tulli F, Messina CM, Cardinaletti G, Tibaldi E. Lipid composition and metabolism of European sea bass (Dicentrarchus labrax L.) fed diets containing wheat gluten and legume meals as substitutes for fish meal. Aquaculture. (2013) 376:6–14. doi: 10.1016/j.aquaculture.2012.11.005
100. De Francesco M, Parisi G, Pérez-Sánchez J, Gómez-Réqueni P, Médale F, Kaushik SJ, et al. Effect of high-level fish meal replacement by plant proteins in gilthead sea bream (Sparus aurata) on growth and body/fillet quality traits. Aquac Nutr. (2007) 13:361–72. doi: 10.1111/j.1365-2095.2007.00485.x
101. Tulli F, Chini Zittelli G, Giorgi G, Poli BM, Tibaldi E, Tredici MR. Effect of the inclusion of dried Tetraselmis suecica on growth, feed utilization, and fillet composition of European sea bass juveniles fed organic diets. J Aquat Food Prod Technol. (2012) 21:188–97. doi: 10.1080/10498850.2012.664803
102. Tocher DR, Betancor MB, Sprague M, Olsen RE, Napier JA. Omega-3 long-chain polyunsaturatedfatty acids, EPA and DHA: bridging the gap between supply and demand. Nutrients. (2019) 11:89. doi: 10.3390/nu11010089
103. Tocher DR. Metabolism and functions of lipids and fatty acids in teleost fish. Rev Fish Sci. (2003) 11:107–84. doi: 10.1080/713610925
104. González-Rovira A, Mourente G, Zheng X, Tocher DR, Pendón C. Molecular and functional characterization and expression analysis of a Δ6 fatty acyl desaturase cDNA of European Sea Bass (Dicentrarchus labrax L). Aquaculture. (2009) 298:90–100. doi: 10.1016/j.aquaculture.2009.10.012
105. Maltsev Y, Maltseva K. Fatty acids of microalgae: diversity and applications. Rev Environ Sci Biotechnol. (2021) 20:515–47. doi: 10.1007/s11157-021-09571-3
106. Izquierdo MS, Obach A, Arantzamendi L, Montero D, Robaina L, Rosenlund G. Dietary lipid sources for seabream and seabass: Growth performance, tissue composition and flesh quality. Aquac Nutr. (2003) 9:397–407. doi: 10.1046/j.1365-2095.2003.00270.x
107. Mourente G, Good JE, Bell JG. Partial substitution of fish oil with rapeseed, linseed and olive oils in diets for European sea bass (Dicentrarchus labrax L): effects on flesh fatty acid composition, plasma prostaglandins E2 and F2α, immune function and effectiveness of a fish oil finishing diet. Aquac Nutr. (2005) 11:25–40. doi: 10.1111/j.1365-2095.2004.00320.x
108. Tibaldi E, Zittelli GC, Parisi G, Bruno M, Giorgi G, Tulli F, et al. Growth performance and quality traits of European sea bass (D. labrax) fed diets including increasing levels of freeze-dried Isochrysis sp (T-ISO) biomass as a source of protein and bat-3 long chain PUFA in partial substitution of fish derivatives. Aquaculture. (2015) 440:60–8. doi: 10.1016/j.aquaculture.2015.02.002
109. Jensen-Urstad AP, Semenkovich CF. Fatty acid synthase and liver triglyceride metabolism: housekeeper or messenger? Biochim Biophys Acta. (2012) 1821:747–53. doi: 10.1016/j.bbalip.2011.09.017
110. EFSA. Scientific opinion on dietary reference values for fats, including saturated fatty acids, polyunsaturated fatty acids, monounsaturated fatty acids, trans fatty acids, and cholesterol. EFSA J. (2010) 8:1–107. doi: 10.2903/j.efsa.2010.1461
111. Santos-Silva J, Bessa RJB, Santos-Silva F. Effect of genotype, feeding system and slaughter weight on the quality of light lambs: II. Fatty acid composition of meat. Livest Prod Sci. (2002) 77:187–94. doi: 10.1016/S0301-6226(02)00059-3
112. Grigorakis K. Compositional and organoleptic quality of farmed and wild gilthead sea bream (Sparus aurata) and sea bass (Dicentrarchus labrax) and factors affecting it: a review. Aquaculture. (2007) 272:55–75. doi: 10.1016/j.aquaculture.2007.04.062
113. Arechavala-Lopez P, Fernandez-Jover D, Black KD, Ladoukakis E, Bayle-Sempere JT, Sanchez-Jerez P, et al. Differentiating the wild or farmed origin of Mediterranean fish: a review of tools for sea bream and sea bass. Rev Aquac. (2013) 5:137–57. doi: 10.1111/raq.12006
114. Mokrani D, Oumouna M, Cuesta A. Fish farming conditions affect to European sea bass (Dicentrarchus labrax L) quality and shelf life during storage in ice. Aquaculture. (2018) 490:120–4. doi: 10.1016/j.aquaculture.2018.02.032
115. Cheng J-H, Sun D-W, Han Z, Zeng X-A. Texture and structure measurements and analyses for evaluation of fish and fillet freshness quality: a review. Compr Rev Food Sci Food Saf. (2014) 13:52–61. doi: 10.1111/1541-4337.12043
116. Isaksson T, Swensen LP, Taylor RG, Fjæra SO, Skjervold PO. Non-destructive texture analysis of farmed Atlantic salmon using visual/near-infrared reflectance spectroscopy. J Sci Food Agric. (2002) 82:53–60. doi: 10.1002/jsfa.997
Keywords: algae blend, digestibility, growth performance, gut integrity, functional value, microalgae, muscle quality, seaweed
Citation: Mota CSC, Pinto O, Sá T, Ferreira M, Delerue-Matos C, Cabrita ARJ, Almeida A, Abreu H, Silva J, Fonseca AJM, Valente LMP and Maia MRG (2023) A commercial blend of macroalgae and microalgae promotes digestibility, growth performance, and muscle nutritional value of European seabass (Dicentrarchus labrax L.) juveniles. Front. Nutr. 10:1165343. doi: 10.3389/fnut.2023.1165343
Received: 14 February 2023; Accepted: 23 March 2023;
Published: 17 April 2023.
Edited by:
Miroslava Rossenova Atanassova, Møreforsking AS, NorwayReviewed by:
Seyed Pezhman Hosseini Shekarabi, Islamic Azad University, IranChang'An Wang, Chinese Academy of Fishery Sciences, China
Copyright © 2023 Mota, Pinto, Sá, Ferreira, Delerue-Matos, Cabrita, Almeida, Abreu, Silva, Fonseca, Valente and Maia. This is an open-access article distributed under the terms of the Creative Commons Attribution License (CC BY). The use, distribution or reproduction in other forums is permitted, provided the original author(s) and the copyright owner(s) are credited and that the original publication in this journal is cited, in accordance with accepted academic practice. No use, distribution or reproduction is permitted which does not comply with these terms.
*Correspondence: Margarida R. G. Maia, bXJtYWlhJiN4MDAwNDA7aWNiYXMudXAucHQ=