- NutriNeurO, UMR 1286, Bordeaux INP, INRAE, University of Bordeaux, Bordeaux, France
Nutrition is now well recognized to be an environmental factor which positively or negatively influences the risk to develop neurological and psychiatric disorders. The gut microbiota has recently been shown to be an important actor mediating the relationship between environmental factors, including nutrition, and brain function. While its composition has been widely studied and associated with the risk of brain diseases, the mechanisms underlying the relationship between the gut and brain diseases remain to be explored. The wide range of bioactive molecules produced by the gut microbiota, called gut-derived metabolites (GDM), represent new players in the gut to brain interactions and become interesting target to promote brain health. The aim of this narrative review is to highlight some GDMs of interest that are produced in response to healthy food consumption and to summarize what is known about their potential effects on brain function. Overall, GDMs represent future useful biomarkers for the development of personalized nutrition. Indeed, their quantification after nutritional interventions is a useful tool to determine individuals’ ability to produce microbiota-derived bioactive compounds upon consumption of specific food or nutrients. Moreover, GDMs represent also a new therapeutic approach to counteract the lack of response to conventional nutritional interventions.
1. Introduction
The influence of dietary habits on the incidence of non-communicable brain diseases such as psychiatric and neurodegenerative disorders, has been extensively reported by observational studies in humans (1–3). Regular consumption of foods rich in saturated fats and/or carbohydrates as well as ultra-processed foods and meat has been highlighted as potentially harmful for brain health. On the other side, regular consumption of vegetables, fruits and/or fish characteristic of Mediterranean (Medi-diet), “Dietary Approaches to Stopping Hypertension” (DASH) or Mediterranean-DASH Intervention for Neurodegenerative Delay (MIND) diets, has been associated with beneficial effects (4–6). The positive effects of these diets on the brain could be due to the neurobiological effects of specific nutrients/micronutrients contained in these foods. Several clinical and preclinical studies indicate that n-3 polyunsaturated fatty acids (PUFAs), polyphenols, and dietary fibers, which are present in fatty fish, fruits, and vegetables, have neuroprotective effects (7–9). In addition, B vitamins which are present in green leafy vegetables (an important component of the MIND diet), have been associated with a lower risk of dementia or aging-related brain atrophy (10, 11). Overall, insufficient vitamins intake has been associated with increased risk for neuropsychiatric disorders or cognitive decline (12–14). However, the neurobiological mechanisms underlying the individual and/or added effects of these nutrients/micronutrients are still poorly understood, making translation to humans difficult. The clinical trials aiming at using dietary intervention (food or nutrients/micronutrients alone or in combination) in the management of brain diseases shows that there are substantial variations in the response of individuals to these dietary interventions (15). Here, it is considered that a responder to a dietary intervention is characterized by the improvement of mental health and/or brain functioning. This improvement is biologically defined by a specific GDM/CM profile in responder that is distinct to the one of non-responders who do not have brain health improvement. Individual response to food is influenced by physiological factors, such as gender and age, by metabolic factors such as weight status and exercise, and by genetics. It is important to mention that other behaviors, such as the practice of physical activity and contextual factors, are also key in this diversity of response (16, 17). However, these aspects have not been really taken into account for the understanding of the relationship between diet and brain health which is crucial to establish dietary recommendations adapted to the individuals. A novel important actor participating to the individual response to food is the gut microbiota. Indeed, the latter turns out to be not only influenced by diet but also to influence host’s health (18, 19). Recent reports indicate that the interaction between the intestinal microbiota and the individual’s physiology could possibly influence brain health (20). These nutrition-microbiota-host physiology interactions lead to the production of a wide range of molecules that reach the different organs through the bloodstream including the brain. Thus, distinct individual neurobiological response to food could depend on the ability to produce these gut-derived molecules.
The circulating metabolome contains molecules directly derived from food (minerals, some vitamins) and from the digestion of such food by the gut microbiota. These latter are termed gut-derived metabolites (GDM) and include several type of molecules like bioactive lipids, bile acids, short-chain fatty acids (SCFA), phenolic compounds and neurotransmitters (21). These GDM can give rise to phase II metabolites or co-metabolites (CM) under the metabolization of host enzymes like glucuronidation, sulfatation, methylation. 58% of blood metabolites variability can be explained by the gut microbiota composition (22). Bar et al. (23), have elegantly shown that gut microbiota composition, dietary habits as well as the clinical profile are major factors influencing the circulating metabolome. Deciphering the respective contribution of nutrition, gut microbiota and the subsequent metabolic and inflammatory conditions on brain health is crucial to adapt nutrition-based interventions to individuals. Recent clinical studies addressed this important question. Indeed, the potential causal link between gut microbiota disturbances and neurodevelopmental disorders (like autism) (24–26) has been challenged by a recent study from Yap et al. (27). In this study conducted in autistic children, authors revealed that the restricted diet adopted by the patients, rather than a shift in gut microbiota composition, is predictive of the symptoms (27). This illustrate the importance of considering not only gut microbiota composition but also dietary habits as well as clinical profile and the GDM/CM circulating signature of patients suffering of brain diseases. This is crucial to design efficient nutritional or gut microbiota targeting interventions.
The aim of this scoping review is to summarize current knowledge on the potential role of GDM and CM as biological mediators of the effect of nutrition and gut microbiota on brain health. Indeed, we bring in this review a collection of selected data from the literature to feed the new concept that a part of the effect of nutrition on brain function relies on these bioactive compounds. In addition, we bring new angles of data interpretation to feed the concept that molecular mechanisms underlying the inter-individual differences in the response towards nutrition-based interventions at the behavioral level. First, we will present some diets and food components that have been shown to be protective against brain disorders. Then, we will discuss the possible role of GDM and CM in mediating the beneficial effects of above-mentioned diets on brain function.
2. Diets promoting brain health
Several types of healthy diets have been associated with the promotion of brain health and the protection toward neuropsychiatric and neurological diseases. These diets include plant-based diet, the Medi-diet, the DASH and the diet. These diets follow some of the world health organization (WHO) eating guidelines that advice to increase consumption of vegetables, fruits, whole grain and dietary fibers while decreasing consumptions of fats, sugars and salt (28). The Medi and MIND diets have been shown to improve cognition and morphological brain parameters, such as cortical thickness and white matter integrity in healthy elderly or inferior frontal gyrus surface in obese women (29–31). In normal aging process, the consumption of green Medi-diet, which consist in a Medi-diet coupled to a supplementation on two sources polyphenols (green tea and Wolffia globosa duckweed also called “Mankai”) has been shown to slow the age-related brain atrophy (32). Greater adherence to a dietary pattern consistent with a plant-based diet was related to better performance on all cognitive tasks in older adults (33). The anti-inflammatory and antioxidant properties of some foods present in these diets have been proposed to be the biological contributors of their brain health-promoting effects (3, 33, 34). In addition to its pro-cognitive activity, the Medi-diet is associated with a decreased risk of developing mood disorder in adults and elderly as shown by several observational studies (35–41). Moreover, the Medi-diet alleviated depression risks or symptoms in two interventional studies (42, 43) while another intervention based on dietary advice resulting in increased consumption of fibers and polyunsaturated fatty acids (PUFAs) led to a decrease in perceived stress (44). Of note, two studies highlighted that the protective role of Medi-diet against depression is noticeable in cross sectional studies while it is less clear in longitudinal studies warranting more studies to understand the short vs long-term impact of Medi-diet adherence (45, 46). From all the aforementioned studies it appears that diet quality (intake of fruits, vegetables, fish, whole grain) may have a protective role towards neurological and psychiatric disorders (47, 48).
However, the large variability in the response towards nutritional interventions is a potential limitation in the use of dietary interventions to protect and/or counteract brain disorders. It is therefore crucial to better understand the parameters which define the response of individual as it could on several parameters that remain poorly characterized. The gut microbiota function (i.e.:, its ability to process foods and to produce a wide range of bioactive molecules) is an interesting target to better understand this variability. Even if out of the scope of this review several other parameters can be important in the inter-individual variability towards nutritional interventions. They include genetic factors (49), metabolic health or underlying conditions like diabetes or kidney diseases (50–52). The production of GDM or CM from several types of nutrients highlighted in the previous part has been shown to be largely variable between individuals (53–56). This is notably the case for the production of the bioactive derivatives of polyphenols (53, 57, 58). For example, the association between coffee consumption and a lower dementia risk in elderly is affected by inter-individual differences in coffee metabolism (49), which has been shown to be influenced by gut microbiota composition and activity (59). Also urolithin A, a GDM from polyphenols, is produced by less than half of those consuming ellagic acid found in berries and walnuts (54). In response to Flavan-3-ol consumption (enriched in coffee and cocoa), three different populations or “metabotypes” have been reported based on the presence of the metabolites trihydroxyphenyl-γ-valerolactones, dihydroxyphenyl-γ-valerolactones (DPVL) or hydroxyphenylpropionic acids (60, 61). The pivotal role of the gut microbiota in these metabotypes has been highlighted and some bacteria has been found to be responsible for the ability to produce these polyphenol-derived GDM (62). Inter-individual differences in the response toward other food or nutrients of special interest for brain health has been described. For example, the prebiotic effect of different dietary fibers, measured by assessing the changes in the production of the short chain fatty acids (SCFAs), is controlled by the gut microbiota and the habitual dietary fiber intake (63). Moreover, the emotional improvements in response to dietary fiber intervention in obese subjects has been shown to be associated with differences in the prior gut microbiota composition (15) further illustrating the difficulties to predict the response to a dietary intervention. One actual challenge in human nutrition is to identify relevant biomarkers predictive of a positive response to nutritional intervention for the promotion of brain health (Figure 1). Besides this objective, the use of GDM or CM to overcome the inter-individual variability in nutritional intervention is appealing. Indeed, these compounds, alone and in combination, can be responsible for beneficial effects of healthy food in preventing neuropsychiatric and neurological illnesses. Thus, observational studies help to identify potential GDM or CM candidates while preclinical and clinical intervention allow to test their potential protective effects in different neuropathological conditions.
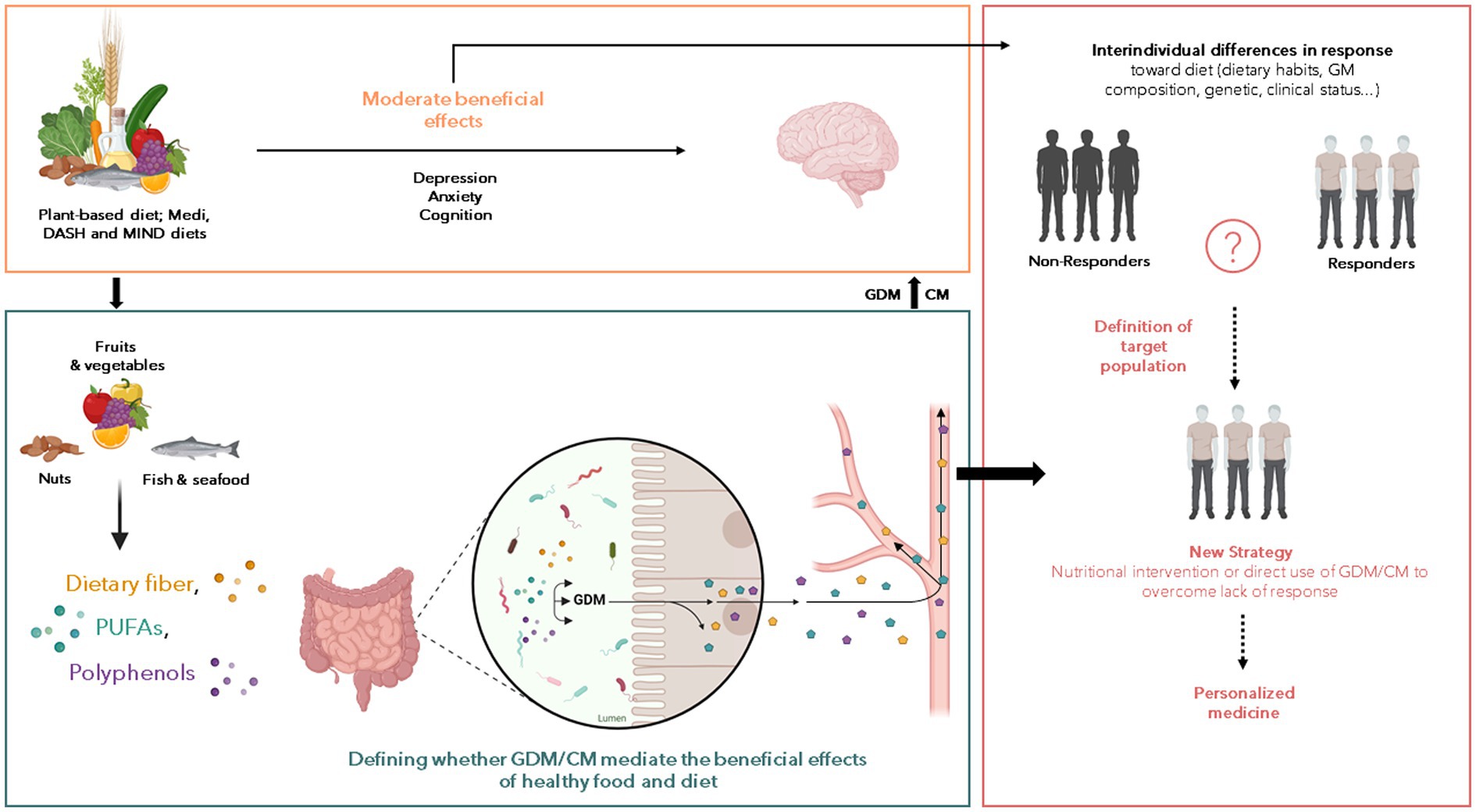
Figure 1. Potential of gut-derived metabolites (GDM) and co-metabolites (CM) to improve the effect of nutritional intervention on the neurological and psychiatric disorders. Numerous reports of associations between nutrition and beneficial or harmful consequences on the brain have been published over the last decades but the nutritional interventions displayed limited effects. Several factors interact with each other’s to influence the response toward food intervention including the gut microbiota composition, genetic and clinical status and we postulate that the GDM and CM production from food can be a pivotal factor explaining such differences. Thus, the understanding of factors that characterize the positive response toward nutritional intervention is crucial to (1) decipher the role of GDM and CM in the beneficial effect of healthy food on brain health, and (2) to define population to target for nutritional intervention aiming to promote brain health. The so-called “non-responders” could benefit from intervention based on GDM or CM. These approaches can be combined with medications, pre or probiotics to achieve a synergistic effect. But the main goal here is to being able to understand and bypass the “resistance” to nutritional intervention to define personalized strategy according to clinical profile of subjects.
3. Gut-derived metabolites as mediators of healthy food effects on the brain
In this section, GDM and CM produced in response to fibers, polyphenols and PUFAs and their brain effects will be presented (Table 1). The focus on these GDM and CM relies on the fact that their parent nutrients are enriched in fruits, vegetables or fish which consumption is associated with beneficial effects on brain pathological processes both in preclinical and clinical settings (7, 125, 126). GDM that are not derived from the aforementioned nutrients will not be included in this review despite potent action on brain. GDM that are not derived from the aforementioned nutrients will not be included in this review despite potential effect on brain functions. For example, in the context of aging and dementia it has been shown that disturbances of the vasculature and BBB integrity can promote pathological processes (127). Recently, trimethylamine N-oxide (TMAO) or the p-cresol glucuronide, two GDM originating from choline and tyrosine respectively, has been shown to promote BBB function (128, 129) while at the opposite the p-cresol was associated with several detrimental effects such as inducing autistic-like behavior or impairing synaptic function in preclinical models (130, 131).
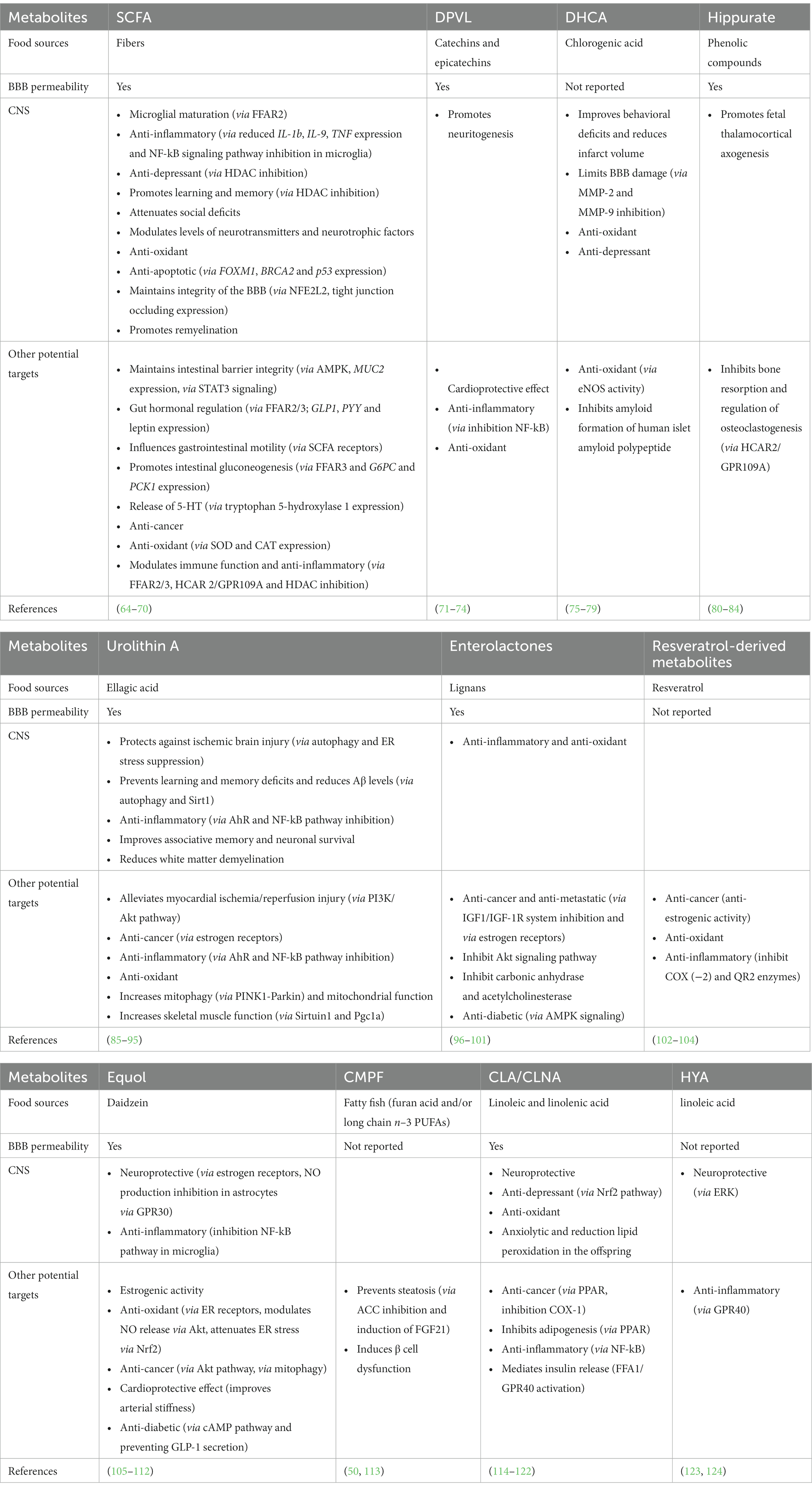
Table 1. Gut-derived metabolites, their precursors and their effects both in the CNS and at the periphery.
Dietary fibers are complex carbohydrates found in fruits, vegetables, legumes and whole grain. The soluble fibers are not digested by human enzymes but are fermented by the gut microbiota (132). Observational studies have shown that dietary fiber intake is associated with better cognitive performance in children aged 7–9 years old (133) and in elderly (134, 135). High consumers of fibers have a lower risk to develop anxiety, psychological distress or depression (7, 136). Dietary fibers intervention with inulin (found notably in onion, chicory, banana, garlic, Jerusalem artichoke and leek) in alcohol use disorder patients improves sociability score and increases circulating levels of brain derived neurotrophic factor (BDNF) during withdrawal (137). The gut microbiota fermentation of soluble dietary fiber stimulates the production of SCFAs that reach the bloodstream and influence the function of several organs (64). If SCFA can cross the blood brain barrier when administered systemically in different animal models, the brain uptake in humans seems to be minimal (64). In rodents, a fiber-deprived diet impairs cognition and SCFA blood levels (138). Moreover, the genetic ablation of GPR 41 and 43 which are SCFA receptors leads to cognitive alteration (138). In a mice model of Alzheimer’s disease (AD), fructans stimulates the release of SCFA and improves cognition, an effect lost upon antibiotic treatment (139). Besides inulin and fructans, other fibers like pectins (found notably in apple) or arabinoxylan (found in cereal) has been found to improve cognition and depressive-like behavior in rodents (140, 141). The SCFAs propionate and butyrate alter astrocyte metabolism which could be involved in cognitive alteration (139). SCFA dietary supplementation also attenuates anhedonia, intestinal permeability and stress responsiveness in chronically stressed mice (142). In mice, inulin-rich diet and butyrate protect against aging-associated brain inflammation (143). SCFAs are essential for the maturation of microglia, which are brain resident immune cells (67). In particular, acetate improves microglia transcriptomic signature and mitochondrial activity that is altered in germ-free mice (66). This was associated with a lower engulfment of amyloid deposits in a mice model of AD (66). In healthy men, supplementation with SCFA attenuates the cortisol response to psychosocial stress (144). Levels of SCFA actually reaching the brain are quite low (64), thus further studies clarifying the mechanisms through which SCFAs modulate neurobiological processes are required. Moreover, comparison of the effectiveness of the different type of soluble fiber in counteracting emotion or cognitive disturbances in preclinical or clinical settings would be interesting. It could be useful to decipher the mechanisms through which dietary fiber may improve brain health besides their stimulating effect on SCFA production.
Medi-diet or MIND diets are particularly rich in fruits, vegetables, olive oil, wine which are particularly rich in polyphenols. Numerous clinical studies and meta-analysis report that polyphenols and polyphenol rich-food dietary interventions have both beneficial effects on cognition and/or emotional symptoms in healthy or diseased (obese, depressed, cognitively impaired population) cohorts (9, 145–155). Despite the efficiency of polyphenols on several psychological and cognitive dimensions, more work is needed to precisely determine how it affects brain activity (156). Interestingly, dietary polyphenols are poorly absorbed and are metabolized through a collaboration between host and its gut microbiota improving their absorption and their ability to cross the blood–brain barrier thus resulting in an increased bioactivity (BBB) (99, 157, 158). Of note, the structure of the polyphenols and especially the degree of polymerization has been shown to modulate the production of GDM from polyphenols (159).
Polyphenols encompass two families (flavonoids and non-flavonoids) including several subgroups such as anthocyanins, flavanols, phenolic acids or lignans among others (58). Dietary supplementation containing high levels of flavonoids improves cognitive performances and increases serum BDNF in adult men aged from 26 to 70 years old and aged women (160). Interestingly, polyphenols are potent modulators of the gut microbiota composition. In turn, microbiota is essential to metabolize polyphenolic compounds into bioactive molecules (161). Actually, polyphenols are poorly absorbed in the upper part of the gastrointestinal tract and 90% reach the colon where the gut microbiota metabolizes them (162, 163). Polyphenols affect gut microbiota composition through their prebiotic effect. They stimulate the growth of health-promoting bacteria such as Akkermansia muciniphila, Faecalibacterium prausnitzii, Bifidobacteria and Lactobacilli while they inhibit the growth of bacteria with reported detrimental effects through antimicrobial activity, leading to the concept of “duplibiotics” (58). Catechins and epicatechins, which are flavanols enriched in cocoa and tea are metabolized by the gut microbiota (161, 164) into dihydroxyphenyl-γ-valerolactones (DPVL) which ultimately reach the brain and stimulate neuritogenesis (71, 73). The chlorogenic acid, one of the main bioactive compounds of coffee, is converted into caffeic acid which is absorbed or metabolized into dihydrocaffeic acid (DHCA) by gut bacteria (59). DHCA improves behavioral deficits and reduces infarct volume in the brain of rat with cerebral ischemia (72). It also decreases the production of interleukin (IL)-6 and counteracts depressive-like behavior in a mice model of stress (79). Hippurate is a CM product of phenolic compound first metabolized into benzoate by gut microbiota and further conjugated to glycine in the liver (165). Hippurate levels increases with intake of fruit and vegetable (165–168) and gut microbiota diversity (167). In a mice model of obesity, its peripheral administration improves metabolic health (169). It has been reported that hippurate reaches the brain (81, 82) and promotes neuritogenesis in the thalamo-cortical pathway during development (82). As previously reported, urolithin A is produced by gut bacteria from hydrolysable tannin, especially gut-derived ellagic acid (91, 170). Pomegranate, which is particularly rich in ellagic acid has been shown to be protective in several rodent models of neurodegeneration (171). Urolithin A is among the pomegranate metabolites with the highest ability to cross the BBB, making this GDM a good candidate for pomegranate’ neuroprotective effects (95). In addition, urolithin A alleviates neuroinflammation in vitro (89), in rodent models of AD and multiple sclerosis (91, 92, 170) and modulates microglia phagocytosis activity and mitophagy as well as AMP-activated protein kinase (AMPK) and the nuclear factor-kappa B (NFκB) signaling (88). Urolithin A activity is mediated by its binding to Aryl Hydrocarbon Receptor (AhR) (88). The enterolactones are produced through the metabolization of lignans by gut bacteria including Ruminococcus species (172). These compounds exert anticholinesterase activity and thus have been proposed as candidates to tackle neurodegenerative disorders like AD (172). Even if the molecular mechanisms remain unknown, preclinical findings have shown that enterolactones are neuroprotective in AD and Parkinson disease (PD) animal models (98, 173). Dihydroresveratrol (DHR), lunularin (LUN) and 3,4′–dihydroxy-trans-stilbene have been identified as gut microbiota-derived metabolites of resveratrol (53) with some having more potent anti-inflammatory effects than resveratrol (102). Interestingly, these GDM and the resveratrol itself can be transported within extracellular vesicles which represent a very interesting pathway to study in order to better understand how GDM and CM can influence the brain (62). The isoflavones like daidzein is metabolized by gut bacteria into equol that exhibits, as its precursor, neuroprotective effects in vitro possibly through its ability to activate oestrogen receptors (106, 174). Overall, a large amount of polyphenols-derived GDM and CM deserve more attention as their bioactive properties can be responsible for the widely recognized health-promoting effects of polyphenols (58, 175). Regarding the neurobiological/behavioral response toward food intervention, taking into account the interindividual differences in both gut microbiota and host metabolism of nutrients like polyphenols is crucial. Indeed, individual may not be able to produce polyphenols-derived metabolites for several reasons (lacking the appropriate bacteria or disturbances in phase II metabolites producing enzymes) which may introduce a bias in the interpretation of the efficacy of nutritional intervention. Of note, the structure of the polyphenols and especially the degree of polymerization has been shown to modulate the production of GDM from polyphenols which underline the importance of the food sources and the choice of the compounds selected in nutritional intervention (159). Thus, studying GDM and CM would allow to select individuals that may benefit from the nutritional supplementation, to select the better compounds and to adapt the strategy by using symbiotic or postbiotic approach in individual who are not able to produce the GDM/CM of interest.
Several type of GDM and CM can be produced upon n-3 PUFA consumption (113, 124, 176). The 3-carboxy-4-methyl-5-propyl-2-furanpropanoic acid (CMPF) is a GDM produced in response to fatty fish intake or dietary supplementation (113, 177, 178). This GDM has been associated with a slower cognitive decline in middle-aged men (179). Its bioactive potential has been shown especially in the context of steatosis where it mediates the beneficial effects of n–3 PUFA (113). The gut microbiota also produces some conjugated compounds like the conjugated linoleic acid (CLA) or the conjugated linolenic acid (CLNA) from n–6 and n–3 PUFA precursors, respectively, (176). When administered through the diet, CLA alleviates several markers of brain ageing in a mice model of lupus (114, 115). As a result, CLA improves synaptic markers and BDNF decrease as well as acetylcholine esterase activity and oxidative stress in the brain of aged mice (114). CLA administered to mice during pregnancy and lactation triggers anxiolytic and antioxidant effects in offspring (119). Of note, the brain effect of CNLA can involve changes in the level of long-chain PUFAs in the brain which is known to influence several neurobiological mechanisms (8, 182–185). Indeed, several studies have shown that these metabolites can change the level of long-chain PUFA in the liver and in the brain (114, 118). Finally, the 10-hydroxy-cis-12-octadecenoic acid (HYA) which is produced by some Lactobacillus has been shown to protect from obesity related alterations like insulin-resistance and adipose tissue inflammation (124). It also attenuates neuroinflammatory processes by inhibiting ERK phosphorylation in activated microglia in vitro (123).
4. Concluding remarks and future challenges
Although the molecular mechanisms are largely unexplained, several GDM/CM seem to be neuroactive compounds. Thus, exploring further their role in the crosstalk between nutrition, gut microbiota and brain function may help to improve nutritional intervention by giving new possibilities to intervene in people who are not able to respond to food or nutrients-based supplementation. Several challenges arise from the complex relationship between the GDM/CM and nutrition, gut microbiota, clinical status as well as their pleiotropic effect. Indeed, GDM/CM production is controlled by several parameters (22, 23). A first challenge is therefore to unravel the mechanisms through which GDM and CM affect brain function. As discussed in this review, understanding the mechanisms involved in the inter-individual differences in the ability to produce GDM/CM in humans will allow to better select the target population that would benefit from nutritional intervention. For this, it is necessary to study the production of GDM/CM of interest in response to dietary intake of specific healthy food (nuts, fish, dark leaf vegetables …) or a specific precursor (lignans, tannins, fibers, PUFA) in healthy and diseased populations. To confirm that GDM/CM may mediate the effect of nutrition and gut microbiota on brain function, more mechanistic studies are needed. They should aim at (1) decipher how GDM/CM reach the brain, (2) test the specificity of the effect of these molecules on different brain structures and brain cell types, and (3) elucidate their receptors and molecular targets. To achieve these goals, it is necessary to combine dietary habits assessments and circulating GDM/CM measurements especially in clinical studies. The use of predictive models may help to select a pool of metabolites associated with beneficial effects on neurobiological process or behavior rather than a single GDM. It would allow to design innovative intervention with postbiotics alone or in combination which efficiency could then be tested in preclinical models. In vitro, the use of fermentation models (159, 184) can help to decipher the bacteria and the molecular machinery involved in the production of GDM.
Author contributions
QL, LP-P, and SL wrote or contribute to the writing of the manuscript. QL created the figures and LP-P created the table. All authors contributed to the article and approved the submitted version.
Funding
SL was supported by the Chaire d’excellence Région Nouvelle Aquitaine ExoMarquAge (13059720–13062120), the Région Nouvelle Aquitaine Autisme (2019-1R3M08 AUTISME), the JPND SOLID (ANR-21-JPW2-0004-05), the RRI Food4BrainHealth, INRAE. QL and SL were supported by the ERA-NET NEURON 2019 Translational Biomarkers Grant Gut2Behave (ANR-19-NEUR-0003-03).
Acknowledgments
We thank INRAE and the University of Bordeaux for their support.
Conflict of interest
The authors declare that the research was conducted in the absence of any commercial or financial relationships that could be construed as a potential conflict of interest.
Publisher’s note
All claims expressed in this article are solely those of the authors and do not necessarily represent those of their affiliated organizations, or those of the publisher, the editors and the reviewers. Any product that may be evaluated in this article, or claim that may be made by its manufacturer, is not guaranteed or endorsed by the publisher.
References
1. Scarmeas, N, Anastasiou, CA, and Yannakoulia, M. Nutrition and prevention of cognitive impairment. Lancet Neurol. (2018) 17:1006–15. doi: 10.1016/S1474-4422(18)30338-7
2. Samieri, C, Yassine, HN, Melo van Lent, D, Lefèvre-Arbogast, S, van de Rest, O, Bowman, GL, et al. Personalized nutrition for dementia prevention. Alzheimers Dement. (2022) 18:1424–37. doi: 10.1002/alz.12486
3. Marx, W, Lane, M, Hockey, M, Aslam, H, Berk, M, Walder, K, et al. Diet and depression: exploring the biological mechanisms of action. Mol Psychiatry. (2021) 26:134–50. doi: 10.1038/s41380-020-00925-x
4. Muth, AK, and Park, SQ. The impact of dietary macronutrient intake on cognitive function and the brain. Clin Nutr. (2021) 40:3999–4010. doi: 10.1016/j.clnu.2021.04.043
5. Melo, HM, Santos, LE, and Ferreira, ST. Diet-derived fatty acids, brain inflammation, and mental health. Front Neurosci. (2019) 13:265. doi: 10.3389/fnins.2019.00265
6. Lane, MM, Gamage, E, Travica, N, Dissanayaka, T, Ashtree, DN, Gauci, S, et al. Ultra-processed food consumption and mental health: a systematic review and Meta-analysis of observational studies. Nutrients. (2022) 14:2568. doi: 10.3390/nu14132568
7. Saghafian, F, Sharif, N, Saneei, P, Keshteli, AH, Hosseinzadeh-Attar, MJ, Afshar, H, et al. Consumption of dietary Fiber in relation to psychological disorders in adults. Front. Psychiatry [Internet]. (2021):12. doi: 10.3389/fpsyt.2021.587468
8. Bazinet, RP, and Layé, S. Polyunsaturated fatty acids and their metabolites in brain function and disease. Nat Rev Neurosci. (2014) 15:771–85. doi: 10.1038/nrn3820
9. Bayes, J, Schloss, J, and Sibbritt, D. Effects of polyphenols in a Mediterranean diet on symptoms of depression: a systematic literature review. Adv Nutr. (2020) 11:602–15. doi: 10.1093/advances/nmz117
10. Lefèvre-Arbogast, S, Féart, C, Dartigues, JF, Helmer, C, Letenneur, L, and Samieri, C. Dietary B vitamins and a 10-year risk of dementia in older persons. Nutrients. (2016) 8:761. doi: 10.3390/nu8120761
11. Douaud, G, Refsum, H, de Jager, CA, Jacoby, RE, Nichols, T, Smith, SM, et al. Preventing Alzheimer’s disease-related gray matter atrophy by B-vitamin treatment. Proc Natl Acad Sci. (2013) 110:9523–8. doi: 10.1073/pnas.1301816110
12. Zhang, Y, Ding, J, and Liang, J. Associations of dietary vitamin a and Beta-carotene intake with depression. A Meta-analysis of observational studies. Front Nutr. (2022) 9:9. doi: 10.3389/fnut.2022.881139
13. Amadieu, C, Lefèvre-Arbogast, S, Delcourt, C, Dartigues, JF, Helmer, C, Féart, C, et al. Nutrient biomarker patterns and long-term risk of dementia in older adults. Alzheimers Dement. (2017) 13:1125–32. doi: 10.1016/j.jalz.2017.01.025
14. Parker, GB, Brotchie, H, and Graham, RK. Vitamin D and depression. J Affect Disord. (2017) 208:56–61. doi: 10.1016/j.jad.2016.08.082
15. Leyrolle, Q, Cserjesi, R, Mulders, M, Zamariola, G, Hiel, S, Gianfrancesco, MA, et al. Prebiotic effect on mood in obese patients is determined by the initial gut microbiota composition: a randomized, controlled trial. Brain Behav Immun. (2021) 94:289–98. doi: 10.1016/j.bbi.2021.01.014
16. Schneider, S, Diehl, K, Görig, T, Schilling, L, De Bock, F, Hoffmann, K, et al. Contextual influences on physical activity and eating habits -options for action on the community level. BMC Public Health. (2017) 17:760. doi: 10.1186/s12889-017-4790-x
17. Swindle, T, Bellows, LL, Mitchell, V, Johnson, SL, Shakya, S, Zhang, D, et al. Predictors of sustainment of two distinct nutrition and physical activity programs in early care and education. Front Health Serv. (2022) 2:2. doi: 10.3389/frhs.2022.1010305
18. Valdes, AM, Walter, J, Segal, E, and Spector, TD. Role of the gut microbiota in nutrition and health. BMJ. (2018) 361:k2179. doi: 10.1136/bmj.k2179
19. de Vos, WM, Tilg, H, Hul, MV, and Cani, PD. Gut microbiome and health: mechanistic insights. Gut. (2022) 71:1020–32. doi: 10.1136/gutjnl-2021-326789
20. Cryan, JF, O’Riordan, KJ, Cowan, CSM, Sandhu, KV, Bastiaanssen, TFS, Boehme, M, et al. The microbiota-gut-brain Axis. Physiol Rev. (2019) 99:1877–2013. doi: 10.1152/physrev.00018.2018
21. Ahmed, H, Leyrolle, Q, Koistinen, V, Kärkkäinen, O, Layé, S, Delzenne, N, et al. Microbiota-derived metabolites as drivers of gut-brain communication. Gut Microbes. (2022) 14:2102878. doi: 10.1080/19490976.2022.2102878
22. Dekkers, KF, Sayols-Baixeras, S, Baldanzi, G, Nowak, C, Hammar, U, Nguyen, D, et al. An online atlas of human plasma metabolite signatures of gut microbiome composition. Nat Commun. (2022) 13:5370. doi: 10.1038/s41467-022-33050-0
23. Bar, N, Korem, T, Weissbrod, O, Zeevi, D, Rothschild, D, Leviatan, S, et al. A reference map of potential determinants for the human serum metabolome. Nature. (2020) 588:135–40. doi: 10.1038/s41586-020-2896-2
24. Sharon, G, Cruz, NJ, Kang, DW, Gandal, MJ, Wang, B, Kim, YM, et al. Human gut microbiota from autism Spectrum disorder promote behavioral symptoms in mice. Cells. (2019) 177:1600–1618.e17. doi: 10.1016/j.cell.2019.05.004
25. Sgritta, M, Dooling, SW, Buffington, SA, Momin, EN, Francis, MB, Britton, RA, et al. Mechanisms underlying microbial-mediated changes in social behavior in mouse models of autism Spectrum disorder. Neuron. (2019) 101:246–259.e6. doi: 10.1016/j.neuron.2018.11.018
26. Needham, BD, Funabashi, M, Adame, MD, Wang, Z, Boktor, JC, Haney, J, et al. A gut-derived metabolite alters brain activity and anxiety behaviour in mice. Nature. (2022) 602:647–53. doi: 10.1038/s41586-022-04396-8
27. Yap, CX, Henders, AK, Alvares, GA, Wood, DLA, Krause, L, Tyson, GW, et al. Autism-related dietary preferences mediate autism-gut microbiome associations. Cells. (2021) 184:5916–5931.e17. doi: 10.1016/j.cell.2021.10.015
28. Morris, MC, Tangney, CC, Wang, Y, Sacks, FM, Barnes, LL, Bennett, DA, et al. MIND diet slows cognitive decline with aging. Alzheimers Dement. (2015) 11:1015–22. doi: 10.1016/j.jalz.2015.04.011
29. Mosconi, L, Murray, J, Tsui, WH, Li, Y, Davies, M, Williams, S, et al. Mediterranean diet and magnetic resonance imaging-assessed brain atrophy in cognitively Normal individuals at risk for Alzheimer’s disease. J Prev Alzheimers Dis. (2014) 1:23–32.
30. Pelletier, A, Barul, C, Féart, C, Helmer, C, Bernard, C, Periot, O, et al. Mediterranean diet and preserved brain structural connectivity in older subjects. Alzheimers Dement. (2015) 11:1023–31. doi: 10.1016/j.jalz.2015.06.1888
31. Arjmand, G, Abbas-Zadeh, M, and Eftekhari, MH. Effect of MIND diet intervention on cognitive performance and brain structure in healthy obese women: a randomized controlled trial. Sci Rep. (2022) 12:2871. doi: 10.1038/s41598-021-04258-9
32. Kaplan, A, Zelicha, H, Yaskolka Meir, A, Rinott, E, Tsaban, G, Levakov, G, et al. The effect of a high-polyphenol Mediterranean diet (green-MED) combined with physical activity on age-related brain atrophy: the dietary intervention randomized controlled trial polyphenols unprocessed study (DIRECT PLUS). Am J Clin Nutr. (2022) 115:1270–81. doi: 10.1093/ajcn/nqac001
33. Ramey, MM, Shields, GS, and Yonelinas, AP. Markers of a plant-based diet relate to memory and executive function in older adults. Nutr Neurosci. (2022) 25:276–85. doi: 10.1080/1028415X.2020.1751506
34. Firth, J, Stubbs, B, Teasdale, SB, Ward, PB, Veronese, N, Shivappa, N, et al. Diet as a hot topic in psychiatry: a population-scale study of nutritional intake and inflammatory potential in severe mental illness. World Psychiatry. (2018) 17:365–7. doi: 10.1002/wps.20571
35. Mantzorou, M, Vadikolias, K, Pavlidou, E, Tryfonos, C, Vasios, G, Serdari, A, et al. Mediterranean diet adherence is associated with better cognitive status and less depressive symptoms in a Greek elderly population. Aging Clin Exp Res. (2021) 33:1033–40. doi: 10.1007/s40520-020-01608-x
36. Fresán, U, Bes-Rastrollo, M, Segovia-Siapco, G, Sanchez-Villegas, A, Lahortiga, F, de la Rosa, PA, et al. Does the MIND diet decrease depression risk? A comparison with Mediterranean diet in the SUN cohort. Eur J Nutr. (2019) 58:1271–82. doi: 10.1007/s00394-018-1653-x
37. Pagliai, G, Sofi, F, Vannetti, F, Caiani, S, Pasquini, G, Molino Lova, R, et al. Mediterranean diet, food consumption and risk of late-life depression: the Mugello study. J Nutr Health Aging. (2018) 22:569–74. doi: 10.1007/s12603-018-1019-3
38. Yin, W, Löf, M, Chen, R, Hultman, CM, Fang, F, and Sandin, S. Mediterranean diet and depression: a population-based cohort study. Int J Behav Nutr Phys Act. (2021) 18:153. doi: 10.1186/s12966-021-01227-3
39. Oddo, VM, Welke, L, McLeod, A, Pezley, L, Xia, Y, Maki, P, et al. Adherence to a Mediterranean diet is associated with lower depressive symptoms among U.S. adults. Nutrients. (2022) 14:278. doi: 10.3390/nu14020278
40. Lassale, C, Batty, GD, Baghdadli, A, Jacka, F, Sánchez-Villegas, A, Kivimäki, M, et al. Healthy dietary indices and risk of depressive outcomes: a systematic review and meta-analysis of observational studies. Mol Psychiatry. (2019) 24:965–86. doi: 10.1038/s41380-018-0237-8
41. Madani, S, Ahmadi, A, Shoaei-Jouneghani, F, Moazen, M, and Sasani, N. The relationship between the Mediterranean diet and Axis I disorders: a systematic review of observational studies. Food Sci Nutr. (2022) 10:3241–58. doi: 10.1002/fsn3.2950
42. Parletta, N, Zarnowiecki, D, Cho, J, Wilson, A, Bogomolova, S, Villani, A, et al. A Mediterranean-style dietary intervention supplemented with fish oil improves diet quality and mental health in people with depression: a randomized controlled trial (HELFIMED). Nutr Neurosci. (2019) 22:474–87. doi: 10.1080/1028415X.2017.1411320
43. Francis, HM, Stevenson, RJ, Chambers, JR, Gupta, D, Newey, B, and Lim, CK. A brief diet intervention can reduce symptoms of depression in young adults—a randomised controlled trial. PLoS One. (2019) 14:e0222768. doi: 10.1371/journal.pone.0222768
44. Berding, K, Bastiaanssen, TFS, Moloney, GM, Boscaini, S, Strain, CR, Anesi, A, et al. Feed your microbes to deal with stress: a psychobiotic diet impacts microbial stability and perceived stress in a healthy adult population. Mol Psychiatry. (2022) 28:601–10. doi: 10.1038/s41380-022-01817-y
45. Sahasrabudhe, N, Soo Lee, J, Zhang, X, Scott, T, Punnett, L, Tucker, KL, et al. Adherence to Mediterranean diet and depressive symptomatology among Boston area Puerto Ricans. J Gerontol A. (2022) 78:258–66. doi: 10.1093/gerona/glac057
46. Shafiei, F, Salari-Moghaddam, A, Larijani, B, and Esmaillzadeh, A. Adherence to the Mediterranean diet and risk of depression: a systematic review and updated meta-analysis of observational studies. Nutr Rev. (2019) 77:230–9. doi: 10.1093/nutrit/nuy070
47. Grajek, M, Krupa-Kotara, K, Białek-Dratwa, A, Sobczyk, K, Grot, M, Kowalski, O, et al. Nutrition and mental health: a review of current knowledge about the impact of diet on mental health. Front Nutr. (2022) 9:9. doi: 10.3389/fnut.2022.943998
48. To JShao, ZY, Gandawidjaja, M, Tabibi, T, Grysman, N, and Grossberg, GT. Comparison of the impact of the Mediterranean diet, anti-inflammatory diet, seventh-day adventist diet, and ketogenic diet relative to cognition and cognitive decline. Curr Nutr Rep. (2022) 11:161–71. doi: 10.1007/s13668-022-00407-2
49. Lefèvre-Arbogast, S, Dartigues, JF, Tzourio, C, Berr, C, Helmer, C, and Samieri, C. Gene-diet interaction and dementia risk: the example of coffee and CYP1A2 gene. Alzheimers Dement. (2020) 16:e039850. doi: 10.1002/alz.039850
50. Prentice, KJ, Luu, L, Allister, EM, Liu, Y, Jun, LS, Sloop, KW, et al. The furan fatty acid metabolite CMPF is elevated in diabetes and induces β cell dysfunction. Cell Metab. (2014) 19:653–66. doi: 10.1016/j.cmet.2014.03.008
51. Poesen, R, Claes, K, Evenepoel, P, de Loor, H, Augustijns, P, Kuypers, D, et al. Microbiota-derived Phenylacetylglutamine associates with overall mortality and cardiovascular disease in patients with CKD. J Am Soc Nephrol. (2016) 27:3479–87. doi: 10.1681/ASN.2015121302
52. Morand, C, De Roos, B, Garcia-Conesa, MT, Gibney, ER, Landberg, R, Manach, C, et al. Why interindividual variation in response to consumption of plant food bioactives matters for future personalised nutrition. Proc Nutr Soc. (2020) 79:225–35. doi: 10.1017/S0029665120000014
53. Cortés-Martín, A, Selma, MV, Tomás-Barberán, FA, González-Sarrías, A, and Espín, JC. Where to look into the puzzle of polyphenols and health? The Postbiotics and gut microbiota associated with human Metabotypes. Mol Nutr Food Res. (2020) 64:e1900952. doi: 10.1002/mnfr.201900952
54. Singh, A, D’Amico, D, Andreux, PA, Dunngalvin, G, Kern, T, Blanco-Bose, W, et al. Direct supplementation with Urolithin a overcomes limitations of dietary exposure and gut microbiome variability in healthy adults to achieve consistent levels across the population. Eur J Clin Nutr. (2022) 76:297–308. doi: 10.1038/s41430-021-00950-1
55. Serini, S, and Calviello, G. Omega-3 PUFA responders and non-responders and the prevention of lipid Dysmetabolism and related diseases. Nutrients. (2020) 12:1363. doi: 10.3390/nu12051363
56. Milder, IEJ, Kuijsten, A, Arts, ICW, Feskens, EJM, Kampman, E, Hollman, PCH, et al. Relation between plasma Enterodiol and Enterolactone and dietary intake of Lignans in a Dutch endoscopy-based population. J Nutr. (2007) 137:1266–71. doi: 10.1093/jn/137.5.1266
57. Narduzzi, L, Agulló, V, Favari, C, Tosi, N, Mignogna, C, Crozier, A, et al. (poly)phenolic compounds and gut microbiome: new opportunities for personalized nutrition. Microbiome Res Reports. (2022) 1:16. doi: 10.20517/mrr.2022.06
58. Rodríguez-Daza, MC, Pulido-Mateos, EC, Lupien-Meilleur, J, Guyonnet, D, Desjardins, Y, and Roy, D. Polyphenol-mediated gut microbiota modulation: toward prebiotics and further. Front Nutr. (2021) 8:689456. doi: 10.3389/fnut.2021.689456
59. Kerimi, A, Kraut, NU, da Encarnacao, JA, and Williamson, G. The gut microbiome drives inter- and intra-individual differences in metabolism of bioactive small molecules. Sci Rep. (2020) 10:19590. doi: 10.1038/s41598-020-76558-5
60. Mena, P, Ludwig, IA, Tomatis, VB, Acharjee, A, Calani, L, Rosi, A, et al. Inter-individual variability in the production of flavan-3-ol colonic metabolites: preliminary elucidation of urinary metabotypes. Eur J Nutr. (2019) 58:1529–43. doi: 10.1007/s00394-018-1683-4
61. Li, Q, Stautemas, J, Omondi Onyango, S, De Mey, M, Duchi, D, Tuenter, E, et al. Human gut microbiota stratified by (+)-catechin metabolism dynamics reveals colon region-dependent metabolic profile. Food Chem. (2023) 408:135203. doi: 10.1016/j.foodchem.2022.135203
62. Iglesias-Aguirre, CE, Ávila-Gálvez, MÁ, de Las, L, Hazas, MC, Dávalos, A, and Espín, JC. Exosome-containing extracellular vesicles contribute to the transport of resveratrol metabolites in the bloodstream: a human pharmacokinetic study. Nutrients. (2022) 14:3632. doi: 10.3390/nu14173632
63. Holmes, ZC, Villa, MM, Durand, HK, Jiang, S, Dallow, EP, Petrone, BL, et al. Microbiota responses to different prebiotics are conserved within individuals and associated with habitual fiber intake. Microbiome. (2022) 10:114. doi: 10.1186/s40168-022-01307-x
64. Dalile, B, Van Oudenhove, L, Vervliet, B, and Verbeke, K. The role of short-chain fatty acids in microbiota-gut-brain communication. Nat Rev Gastroenterol Hepatol. (2019) 16:461–78. doi: 10.1038/s41575-019-0157-3
65. Dong, Y, and Cui, C. The role of short-chain fatty acids in central nervous system diseases. Mol Cell Biochem. (2022) 477:2595–607. doi: 10.1007/s11010-022-04471-8
66. Erny, D, Dokalis, N, Mezö, C, Castoldi, A, Mossad, O, Staszewski, O, et al. Microbiota-derived acetate enables the metabolic fitness of the brain innate immune system during health and disease. Cell Metab. (2021) 33:2260–2276.e7. doi: 10.1016/j.cmet.2021.10.010
67. Erny, D, Hrabě de Angelis, AL, Jaitin, D, Wieghofer, P, Staszewski, O, David, E, et al. Host microbiota constantly control maturation and function of microglia in the CNS. Nat Neurosci. (2015) 18:965–77. doi: 10.1038/nn.4030
68. Mirzaei, R, Bouzari, B, Hosseini-Fard, SR, Mazaheri, M, Ahmadyousefi, Y, Abdi, M, et al. Role of microbiota-derived short-chain fatty acids in nervous system disorders. Biomed Pharmacother. (2021) 139:111661. doi: 10.1016/j.biopha.2021.111661
69. O’Riordan, KJ, Collins, MK, Moloney, GM, Knox, EG, Aburto, MR, Fülling, C, et al. Short chain fatty acids: microbial metabolites for gut-brain axis signalling. Mol Cell Endocrinol. (2022) 546:111572. doi: 10.1016/j.mce.2022.111572
70. Silva, YP, Bernardi, A, and Frozza, RL. The role of short-Chain fatty acids from gut microbiota in gut-brain communication. Front Endocrinol. (2020) 11:11. doi: 10.3389/fendo.2020.00025
71. Angelino, D, Carregosa, D, Domenech-Coca, C, Savi, M, Figueira, I, Brindani, N, et al. 5-(Hydroxyphenyl)-γ-Valerolactone-sulfate, a key microbial metabolite of Flavan-3-ols, is able to reach the brain: evidence from different in silico, in vitro and in vivo experimental models. Nutrients. (2019) 11:2678. doi: 10.3390/nu11112678
72. Lee, CC, Kim, JH, Kim, JS, Oh, YS, Han, SM, Yoon Park, JH, et al. 5-(3′,4′-Dihydroxyphenyl-γ-valerolactone), a major microbial metabolite of Proanthocyanidin, attenuates THP-1 monocyte-endothelial adhesion. Int J Mol Sci. (2017) 18:1363. doi: 10.3390/ijms18071363
73. Unno, K, Pervin, M, Nakagawa, A, Iguchi, K, Hara, A, Takagaki, A, et al. Blood-brain barrier permeability of green tea Catechin metabolites and their Neuritogenic activity in human neuroblastoma SH-SY5Y cells. Mol Nutr Food Res. (2017) 61:2595–607. doi: 10.1002/mnfr.201700294
74. Wu, L, Zhang, QL, Zhang, XY, Lv, C, Li, J, Yuan, Y, et al. Pharmacokinetics and blood-brain barrier penetration of (+)-catechin and (−)-epicatechin in rats by microdialysis sampling coupled to high-performance liquid chromatography with chemiluminescence detection. J Agric Food Chem. (2012) 60:9377–83. doi: 10.1021/jf301787f
75. Cheng, B, Liu, X, Gong, H, Huang, L, Chen, H, Zhang, X, et al. Coffee components inhibit amyloid formation of human islet amyloid polypeptide in vitro: possible link between coffee consumption and diabetes mellitus. J Agric Food Chem. (2011) 59:13147–55. doi: 10.1021/jf201702h
76. Huang, J, de Paulis, T, and May, JM. Antioxidant effects of dihydrocaffeic acid in human EA.hy926 endothelial cells. J Nutr Biochem. (2004) 15:722–9. doi: 10.1016/j.jnutbio.2004.07.002
77. Lee, K, Lee, BJ, and Bu, Y. Protective effects of Dihydrocaffeic acid, a coffee component metabolite, on a focal cerebral ischemia rat model. Molecules. (2015) 20:11930–40. doi: 10.3390/molecules200711930
78. Verzelloni, E, Pellacani, C, Tagliazucchi, D, Tagliaferri, S, Calani, L, Costa, LG, et al. Antiglycative and neuroprotective activity of colon-derived polyphenol catabolites. Mol Nutr Food Res. (2011) 55:S35–43. doi: 10.1002/mnfr.201000525
79. Wang, J, Hodes, GE, Zhang, H, Zhang, S, Zhao, W, Golden, SA, et al. Epigenetic modulation of inflammation and synaptic plasticity promotes resilience against stress in mice. Nat Commun. (2018) 9:477. doi: 10.1038/s41467-017-02794-5
80. Chen, JR, Zhao, H, Wankhade, UD, Chintapalli, SV, Li, C, Gai, D, et al. GPR109A mediates the effects of hippuric acid on regulating osteoclastogenesis and bone resorption in mice. Commun Biol. (2021) 4:1–12. doi: 10.1038/s42003-020-01564-2
81. Swann, JR, Spitzer, SO, and Diaz, HR. Developmental signatures of microbiota-derived metabolites in the mouse brain. Meta. (2020) 10:E172. doi: 10.3390/metabo10050172
82. Vuong, HE, Pronovost, GN, Williams, DW, Coley, EJL, Siegler, EL, Qiu, A, et al. The maternal microbiome modulates fetal neurodevelopment in mice. Nature. (2020) 586:281–6. doi: 10.1038/s41586-020-2745-3
83. Margalef, M, Pons, Z, Bravo, FI, Muguerza, B, and Arola-Arnal, A. Tissue distribution of rat flavanol metabolites at different doses. J Nutr Biochem. (2015) 26:987–95. doi: 10.1016/j.jnutbio.2015.04.006
84. Carecho, R, Carregosa, D, and Dos Santos, CN. Low molecular weight (poly)phenol metabolites across the blood-brain barrier: the underexplored journey. Brain Plast. (2021) 6:193–214. doi: 10.3233/BPL-200099
85. Ahsan, A, Zheng, Y, Wu, X, Tang, W, Liu, M, Ma, S, et al. Urolithin A-activated autophagy but not mitophagy protects against ischemic neuronal injury by inhibiting ER stress in vitro and in vivo. CNS Neurosci Ther. (2019) 25:976–86. doi: 10.1111/cns.13136
86. Al-Harbi, SA, Abdulrahman, AO, Zamzami, MA, and Khan, MI. Urolithins: the gut based polyphenol metabolites of Ellagitannins in Cancer prevention, a review. Front Nutr. (2021) 8:647582. doi: 10.3389/fnut.2021.647582
87. Cho, SI, Jo, ER, and Song, H. Urolithin a attenuates auditory cell senescence by activating mitophagy. Sci Rep. (2022) 12:7704. doi: 10.1038/s41598-022-11894-2
88. D’Amico, D, Andreux, PA, Valdés, P, Singh, A, Rinsch, C, and Auwerx, J. Impact of the natural compound Urolithin a on health, disease, and aging. Trends Mol Med. (2021) 27:687–99. doi: 10.1016/j.molmed.2021.04.009
89. DaSilva, NA, Nahar, PP, Ma, H, Eid, A, Wei, Z, Meschwitz, S, et al. Pomegranate ellagitannin-gut microbial-derived metabolites, urolithins, inhibit neuroinflammation in vitro. Nutr Neurosci. (2019) 22:185–95. doi: 10.1080/1028415X.2017.1360558
90. Ghosh, N, Das, A, Biswas, N, Gnyawali, S, Singh, K, Gorain, M, et al. Urolithin a augments angiogenic pathways in skeletal muscle by bolstering NAD+ and SIRT1. Sci Rep. (2020) 10:20184. doi: 10.1038/s41598-020-76564-7
91. Gong, Z, Huang, J, Xu, B, Ou, Z, Zhang, L, Lin, X, et al. Urolithin a attenuates memory impairment and neuroinflammation in APP/PS1 mice. J Neuroinflammation. (2019) 16:62. doi: 10.1186/s12974-019-1450-3
92. Shen, PX, Li, X, Deng, SY, Zhao, L, Zhang, YY, Deng, X, et al. Urolithin a ameliorates experimental autoimmune encephalomyelitis by targeting aryl hydrocarbon receptor. EBioMedicine. (2021) 64:103227. doi: 10.1016/j.ebiom.2021.103227
93. Tang, L, Mo, Y, Li, Y, Zhong, Y, He, S, Zhang, Y, et al. Urolithin a alleviates myocardial ischemia/reperfusion injury via PI3K/Akt pathway. Biochem Biophys Res Commun. (2017) 486:774–80. doi: 10.1016/j.bbrc.2017.03.119
94. Toney, AM, Fox, D, Chaidez, V, Ramer-Tait, AE, and Chung, S. Immunomodulatory role of Urolithin a on metabolic diseases. Biomedicine. (2021) 9:192. doi: 10.3390/biomedicines9020192
95. Yuan, T, Ma, H, Liu, W, Niesen, DB, Shah, N, Crews, R, et al. Pomegranate’s neuroprotective effects against Alzheimer’s disease are mediated by Urolithins, its Ellagitannin-gut microbial derived metabolites. ACS Chem Neurosci. (2016) 7:26–33. doi: 10.1021/acschemneuro.5b00260
96. Chen, LH, Fang, J, Sun, Z, Li, H, Wu, Y, Demark-Wahnefried, W, et al. Enterolactone inhibits insulin-like growth Factor-1 receptor signaling in human prostatic carcinoma PC-3 cells. J Nutr. (2009) 139:653–9. doi: 10.3945/jn.108.101832
97. Damdimopoulou, P, Nurmi, T, Salminen, A, Damdimopoulos, AE, Kotka, M, van der Saag, P, et al. A single dose of enterolactone activates estrogen signaling and regulates expression of circadian clock genes in mice. J Nutr. (2011) 141:1583–9. doi: 10.3945/jn.111.140277
98. Giuliano, C, Siani, F, Mus, L, Ghezzi, C, Cerri, S, Pacchetti, B, et al. Neuroprotective effects of lignan 7-hydroxymatairesinol (HMR/lignan) in a rodent model of Parkinson’s disease. Nutrition. (2020) 69:110494. doi: 10.1016/j.nut.2019.04.006
99. Johnson, SL, Kirk, RD, DaSilva, NA, Ma, H, Seeram, NP, and Bertin, MJ. Polyphenol microbial metabolites exhibit gut and blood–brain barrier permeability and protect murine microglia against LPS-induced inflammation. Meta. (2019) 9:78. doi: 10.3390/metabo9040078
100. Mali, AV, Padhye, SB, Anant, S, Hegde, MV, and Kadam, SS. Anticancer and antimetastatic potential of enterolactone: clinical, preclinical and mechanistic perspectives. Eur J Pharmacol. (2019) 852:107–24. doi: 10.1016/j.ejphar.2019.02.022
101. Zhou, F, Furuhashi, K, Son, MJ, Toyozaki, M, Yoshizawa, F, Miura, Y, et al. Antidiabetic effect of enterolactone in cultured muscle cells and in type 2 diabetic model db/db mice. Cytotechnology. (2017) 69:493–502. doi: 10.1007/s10616-016-9965-2
102. Li, F, Han, Y, Wu, X, Cao, X, Gao, Z, Sun, Y, et al. Gut microbiota-derived resveratrol metabolites, Dihydroresveratrol and Lunularin, significantly contribute to the biological activities of resveratrol. Front Nutr. (2022) 9:9. doi: 10.3389/fnut.2022.912591
103. Ruotolo, R, Calani, L, Fietta, E, Brighenti, F, Crozier, A, Meda, C, et al. Anti-estrogenic activity of a human resveratrol metabolite. Nutr Metab Cardiovasc Dis. (2013) 23:1086–92. doi: 10.1016/j.numecd.2013.01.002
104. Springer, M, and Moco, S. Resveratrol and its human metabolites—effects on metabolic health and obesity. Nutrients. (2019) 11:143. doi: 10.3390/nu11010143
105. Horiuchi, H, Usami, A, Shirai, R, Harada, N, Ikushiro, S, Sakaki, T, et al. S-Equol activates cAMP signaling at the plasma membrane of INS-1 pancreatic β-cells and protects against Streptozotocin-induced hyperglycemia by increasing β-cell function in male mice. J Nutr. (2017) 147:1631–9. doi: 10.3945/jn.117.250860
106. Mayo, B, Vázquez, L, and Flórez, AB. Equol: a bacterial metabolite from the Daidzein Isoflavone and its presumed beneficial health effects. Nutrients. (2019) 11:2231. doi: 10.3390/nu11092231
107. Moriyama, M, Hashimoto, A, Satoh, H, Kawabe, K, Ogawa, M, Takano, K, et al. S-Equol, a major Isoflavone from soybean, inhibits nitric oxide production in lipopolysaccharide-stimulated rat astrocytes partially via the GPR30-mediated pathway. Int J Inflam. (2018) 2018:8496973. doi: 10.1155/2018/8496973
108. Muthyala, RS, Ju, YH, Sheng, S, Williams, LD, Doerge, DR, Katzenellenbogen, BS, et al. Equol, a natural estrogenic metabolite from soy isoflavones: convenient preparation and resolution of R- and S-equols and their differing binding and biological activity through estrogen receptors alpha and beta. Bioorg Med Chem. (2004) 12:1559–67. doi: 10.1016/j.bmc.2003.11.035
109. Sekikawa, A, Wharton, W, Butts, B, Veliky, CV, Garfein, J, Li, J, et al. Potential protective mechanisms of S-equal, a metabolite of soy Isoflavone by the gut microbiome, on cognitive decline and dementia. Int J Mol Sci. (2022) 23:11921. doi: 10.3390/ijms231911921
110. Subedi, L, Ji, E, Shin, D, Jin, J, Yeo, JH, and Kim, SY. Equol, a dietary Daidzein gut metabolite attenuates microglial activation and potentiates neuroprotection in vitro. Nutrients. (2017) 9:207. doi: 10.3390/nu9030207
111. Usui, T, Tochiya, M, Sasaki, Y, Muranaka, K, Yamakage, H, Himeno, A, et al. Effects of natural S-equal supplements on overweight or obesity and metabolic syndrome in the Japanese, based on sex and equal status. Clin Endocrinol. (2013) 78:365–72. doi: 10.1111/j.1365-2265.2012.04400.x
112. Yang, Z, Zhao, Y, Yao, Y, Li, J, Wang, W, and Wu, X. Equol induces mitochondria-dependent apoptosis in human gastric Cancer cells via the sustained activation of ERK1/2 pathway. Mol Cells. (2016) 39:742–9. doi: 10.14348/molcells.2016.0162
113. Prentice, KJ, Wendell, SG, Liu, Y, Eversley, JA, Salvatore, SR, Mohan, H, et al. CMPF, a metabolite formed upon prescription Omega-3-acid ethyl Ester supplementation, prevents and reverses steatosis. EBioMedicine. (2018) 27:200–13. doi: 10.1016/j.ebiom.2017.12.019
114. Cigliano, L, Spagnuolo, MS, Boscaino, F, Ferrandino, I, Monaco, A, Capriello, T, et al. Dietary supplementation with fish oil or conjugated linoleic acid relieves depression markers in mice by modulation of the Nrf2 pathway. Mol Nutr Food Res. (2019) 63:1900243. doi: 10.1002/mnfr.201900243
115. Monaco, A, Ferrandino, I, Boscaino, F, Cocca, E, Cigliano, L, Maurano, F, et al. Conjugated linoleic acid prevents age-dependent neurodegeneration in a mouse model of neuropsychiatric lupus via the activation of an adaptive response[S]. J Lipid Res. (2018) 59:48–57. doi: 10.1194/jlr.M079400
116. Dachev, M, Bryndová, J, Jakubek, M, Moučka, Z, and Urban, M. The effects of conjugated linoleic acids on cancer. PRO. (2021) 9:454. doi: 10.3390/pr9030454
117. Kennedy, A, Martinez, K, Schmidt, S, Mandrup, S, LaPoint, K, and McIntosh, M. Antiobesity mechanisms of action of conjugated linoleic acid. J Nutr Biochem. (2010) 21:171–9. doi: 10.1016/j.jnutbio.2009.08.003
118. Murru, E, Carta, G, Manca, C, Sogos, V, Pistis, M, Melis, M, et al. Conjugated linoleic acid and brain metabolism: a possible anti-Neuroinflammatory role mediated by PPARα activation. Front Pharmacol. (2020) 11:587140. doi: 10.3389/fphar.2020.587140
119. Queiroz, MP, Lima, MS, de Melo, MFFT, Bertozzo, CCMS, de Araújo, DF, Guerra, GCB, et al. Maternal suppplementation with conjugated linoleic acid reduce anxiety and lipid peroxidation in the offspring brain. J Affect Disord. (2019) 243:75–82. doi: 10.1016/j.jad.2018.09.020
120. Schmidt, J, Liebscher, K, Merten, N, Grundmann, M, Mielenz, M, Sauerwein, H, et al. Conjugated linoleic acids mediate insulin release through islet G protein-coupled receptor FFA1/GPR40. J Biol Chem. (2011) 286:11890–4. doi: 10.1074/jbc.C110.200477
121. Yuan, GF, Chen, XE, and Li, D. Conjugated linolenic acids and their bioactivities: a review. Food Funct. (2014) 5:1360–8. doi: 10.1039/c4fo00037d
122. Miller, JR, Siripurkpong, P, Hawes, J, Majdalawieh, A, Ro, HS, and McLeod, RS. The trans-10, cis-12 isomer of conjugated linoleic acid decreases adiponectin assembly by PPARgamma-dependent and PPARgamma-independent mechanisms. J Lipid Res. (2008) 49:550–62. doi: 10.1194/jlr.M700275-JLR200
123. Ikeguchi, S, Izumi, Y, Kitamura, N, Kishino, S, Ogawa, J, Akaike, A, et al. Inhibitory effect of the gut microbial linoleic acid metabolites, 10-oxo-trans-11-octadecenoic acid and 10-hydroxy-cis-12-octadecenoic acid, on BV-2 microglial cell activation. J Pharmacol Sci. (2018) 138:9–15. doi: 10.1016/j.jphs.2018.06.015
124. Miyamoto, J, Igarashi, M, Watanabe, K, Karaki, SI, Mukouyama, H, Kishino, S, et al. Gut microbiota confers host resistance to obesity by metabolizing dietary polyunsaturated fatty acids. Nat Commun. (2019) 10:4007. doi: 10.1038/s41467-019-11978-0
125. Layé, S, Nadjar, A, Joffre, C, and Bazinet, RP. Anti-inflammatory effects of Omega-3 fatty acids in the brain: physiological mechanisms and relevance to pharmacology. Pharmacol Rev. (2018) 70:12–38. doi: 10.1124/pr.117.014092
126. D’Angelo, S. Current evidence on the effect of dietary polyphenols intake on brain health. Curr Nutr Food Sci. (2020) 16:1170–82. doi: 10.2174/1573401316999200714160126
127. Hussain, B, Fang, C, and Chang, J. Blood–brain barrier breakdown: an emerging biomarker of cognitive impairment in Normal aging and dementia. Front Neurosci. (2021) 15:15. doi: 10.1016/j.jad.2018.09.020
128. Hoyles, L, Pontifex, MG, Rodriguez-Ramiro, I, Anis-Alavi, MA, Jelane, KS, Snelling, T, et al. Regulation of blood-brain barrier integrity by microbiome-associated methylamines and cognition by trimethylamine N-oxide. Microbiome. (2021) 9:235. doi: 10.1186/s40168-021-01181-z
129. Stachulski, AV, Knausenberger, TBA, Shah, SN, Hoyles, L, and McArthur, S. A host–gut microbial co-metabolite of aromatic amino acids, p -cresol glucuronide, promotes blood–brain barrier integrity in vivo [internet]. Syst Biol. (2022) 5:1360–8. doi: 10.1101/2022.01.11.475932
130. Guzmán-Salas, S, Weber, A, Malci, A, Lin, X, Herrera-Molina, R, Cerpa, W, et al. The metabolite p-cresol impairs dendritic development, synaptogenesis, and synapse function in hippocampal neurons: implications for autism spectrum disorder. J Neurochem. (2022) 161:335–49. doi: 10.1111/jnc.15604
131. Bermudez-Martin, P, Becker, JAJ, Caramello, N, Fernandez, SP, Costa-Campos, R, Canaguier, J, et al. The microbial metabolite p-cresol induces autistic-like behaviors in mice by remodeling the gut microbiota. Microbiome. (2021) 9:157. doi: 10.1186/s40168-021-01103-z
132. Ye, S, Shah, BR, Li, J, Liang, H, Zhan, F, Geng, F, et al. A critical review on interplay between dietary fibers and gut microbiota. Trends Food Sci Technol. (2022) 124:237–49. doi: 10.1016/j.tifs.2022.04.010
133. Khan, NA, Raine, LB, Drollette, ES, Scudder, MR, Kramer, AF, and Hillman, CH. Dietary fiber is positively associated with cognitive control among prepubertal children. J Nutr. (2015) 145:143–9. doi: 10.3945/jn.114.198457
134. Prokopidis, K, Giannos, P, Ispoglou, T, Witard, OC, and Isanejad, M. Dietary Fiber intake is associated with cognitive function in older adults: data from the National Health and nutrition examination survey. Am J Med. (2022) 135:e257–62. doi: 10.1016/j.amjmed.2022.03.022
135. Sun, W, Li, S, Chen, C, Lu, Z, and Zhang, D. Dietary fiber intake is positively related with cognitive function in US older adults. J Funct Foods. (2022) 90:104986. doi: 10.1016/j.jff.2022.104986
136. Fatahi, S, Matin, SS, Sohouli, MH, Găman, MA, Raee, P, Olang, B, et al. Association of dietary fiber and depression symptom: a systematic review and meta-analysis of observational studies. Complement Ther Med. (2021) 56:102621. doi: 10.1016/j.ctim.2020.102621
137. Amadieu, C, Coste, V, Neyrinck, AM, Thijssen, V, Leyrolle, Q, Bindels, LB, et al. Restoring an adequate dietary fiber intake by inulin supplementation: a pilot study showing an impact on gut microbiota and sociability in alcohol use disorder patients. Gut Microbes. (2022) 14:2007042. doi: 10.1080/19490976.2021.2007042
138. Shi, H, Ge, X, Ma, X, Zheng, M, Cui, X, Pan, W, et al. A fiber-deprived diet causes cognitive impairment and hippocampal microglia-mediated synaptic loss through the gut microbiota and metabolites. Microbiome. (2021) 9:223. doi: 10.1186/s40168-021-01172-0
139. Cuervo-Zanatta, D, Syeda, T, Sánchez-Valle, V, Irene-Fierro, M, Torres-Aguilar, P, Torres-Ramos, MA, et al. Dietary Fiber modulates the release of gut bacterial products preventing cognitive decline in an Alzheimer’s mouse model. Cell Mol Neurobiol. (2023) 43:1595–618. doi: 10.1007/s10571-022-01268-7
140. Paderin, NM, and Popov, SV. The effect of dietary pectins on object recognition memory, depression-like behaviour, and il-6 in mouse hippocampi. J Funct Foods. (2018) 43:131–8. doi: 10.1016/j.jff.2018.02.015
141. Kim, CY, Lee, GY, Park, GH, Lee, J, and Jang, JH. Protective effect of Arabinoxylan against scopolamine-induced learning and memory impairment. Biomol Ther (Seoul). (2014) 22:467–73. doi: 10.4062/biomolther.2014.063
142. van de Wouw, M, Boehme, M, Lyte, JM, Wiley, N, Strain, C, O’Sullivan, O, et al. Short-chain fatty acids: microbial metabolites that alleviate stress-induced brain-gut axis alterations. J Physiol. (2018) 596:4923–44. doi: 10.1113/JP276431
143. Matt, SM, Allen, JM, Lawson, MA, Mailing, LJ, Woods, JA, and Johnson, RW. Butyrate and dietary soluble Fiber improve Neuroinflammation associated with aging in mice. Front Immunol. (2018) 9:1832. doi: 10.3389/fimmu.2018.01832
144. Dalile, B, Vervliet, B, Bergonzelli, G, Verbeke, K, and Van Oudenhove, L. Colon-delivered short-chain fatty acids attenuate the cortisol response to psychosocial stress in healthy men: a randomized, placebo-controlled trial. Neuropsychopharmacology. (2020) 45:2257–66. doi: 10.1038/s41386-020-0732-x
145. Martín, MA, Goya, L, and de Pascual-Teresa, S. Effect of cocoa and cocoa products on cognitive performance in young adults. Nutrients. (2020) 12:3691. doi: 10.3390/nu12123691
146. Mastroiacovo, D, Kwik-Uribe, C, Grassi, D, Necozione, S, Raffaele, A, Pistacchio, L, et al. Cocoa flavanol consumption improves cognitive function, blood pressure control, and metabolic profile in elderly subjects: the cocoa, cognition, and aging (CoCoA) study--a randomized controlled trial. Am J Clin Nutr. (2015) 101:538–48. doi: 10.3945/ajcn.114.092189
147. Sokolov, AN, Pavlova, MA, Klosterhalfen, S, and Enck, P. Chocolate and the brain: neurobiological impact of cocoa flavanols on cognition and behavior. Neurosci Biobehav Rev. (2013) 37:2445–53. doi: 10.1016/j.neubiorev.2013.06.013
148. Bensalem, J, Dudonné, S, Gaudout, D, Servant, L, Calon, F, Desjardins, Y, et al. Polyphenol-rich extract from grape and blueberry attenuates cognitive decline and improves neuronal function in aged mice. J Nutr Sci. (2018) 7:e19. doi: 10.1017/jns.2018.10
149. Fusar-Poli, L, Gabbiadini, A, Ciancio, A, Vozza, L, Signorelli, MS, and Aguglia, E. The effect of cocoa-rich products on depression, anxiety, and mood: a systematic review and meta-analysis. Crit Rev Food Sci Nutr. (2022) 62:7905–16. doi: 10.1080/10408398.2021.1920570
150. Lucas, L, Russell, A, and Keast, R. Molecular mechanisms of inflammation. Anti-inflammatory benefits of virgin olive oil and the phenolic compound oleocanthal. Curr Pharm Des. (2011) 17:754–68. doi: 10.2174/138161211795428911
151. Low, DY, Lefèvre-Arbogast, S, González-Domínguez, R, Urpi-Sarda, M, Micheau, P, Petera, M, et al. Diet-related metabolites associated with cognitive decline revealed by untargeted metabolomics in a prospective cohort. Mol Nutr Food Res. (2019) 63:e1900177. doi: 10.1002/mnfr.201900177
152. González-Domínguez, R, Castellano-Escuder, P, Carmona, F, Lefèvre-Arbogast, S, Low, DY, Du Preez, A, et al. Food and microbiota metabolites associate with cognitive decline in older subjects: a 12-year prospective study. Mol Nutr Food Res. (2021) 65:e2100606. doi: 10.1002/mnfr.202100606
153. Zhang, Y, Yang, H, Li, S, Li, W, and Wang, Y. Consumption of coffee and tea and risk of developing stroke, dementia, and poststroke dementia: a cohort study in the UK biobank. PLoS Med. (2021) 18:e1003830. doi: 10.1371/journal.pmed.1003830
154. Lin, K, Li, Y, Toit, ED, Wendt, L, and Sun, J. Effects of polyphenol supplementations on improving depression, anxiety, and quality of life in patients with depression. FPSYT. (2021) 12:12. doi: 10.3389/fpsyt.2021.765485
155. Rodrigo-Gonzalo, MJ, González-Manzano, S, Mendez-Sánchez, R, Santos-Buelga, C, and Recio-Rodríguez, JI. Effect of polyphenolic complements on cognitive function in the elderly: a systematic review. Antioxidants (Basel). (2022) 11:1549. doi: 10.3390/antiox11081549
156. Lamport, DJ, and Williams, CM. Polyphenols and cognition in humans: an overview of current evidence from recent systematic reviews and Meta-analyses. Brain Plast. 6:139–53. doi: 10.3233/BPL-200111
157. Luca, SV, Macovei, I, Bujor, A, Miron, A, Skalicka-Woźniak, K, Aprotosoaie, AC, et al. Bioactivity of dietary polyphenols: the role of metabolites. Crit Rev Food Sci Nutr. (2020) 60:626–59. doi: 10.1080/10408398.2018.1546669
158. Carregosa, D, Carecho, R, Figueira, I, and Santos, N. Low-molecular weight metabolites from polyphenols as effectors for attenuating Neuroinflammation. J Agric Food Chem. (2020) 68:1790–807. doi: 10.1021/acs.jafc.9b02155
159. Di Pede, G, Bresciani, L, Brighenti, F, Clifford, MN, Crozier, A, Del Rio, D, et al. In vitro Faecal fermentation of monomeric and oligomeric Flavan-3-ols: catabolic pathways and stoichiometry. Mol Nutr Food Res. (2022) 66:2101090. doi: 10.1002/mnfr.202101090
160. Neshatdoust, S, Saunders, C, Castle, SM, Vauzour, D, Williams, C, Butler, L, et al. High-flavonoid intake induces cognitive improvements linked to changes in serum brain-derived neurotrophic factor: two randomised, controlled trials. Nutr Healthy Aging. 4:81–93. doi: 10.3233/NHA-1615
161. Sorrenti, V, Ali, S, Mancin, L, Davinelli, S, Paoli, A, and Scapagnini, G. Cocoa polyphenols and gut microbiota interplay: bioavailability, prebiotic effect, and impact on human health. Nutrients. (2020) 12:1908. doi: 10.3390/nu12071908
162. Duda-Chodak, A, Tarko, T, Satora, P, and Sroka, P. Interaction of dietary compounds, especially polyphenols, with the intestinal microbiota: a review. Eur J Nutr. (2015) 54:325–41. doi: 10.1007/s00394-015-0852-y
163. Braune, A, and Blaut, M. Bacterial species involved in the conversion of dietary flavonoids in the human gut. Gut Microbes. (2016) 7:216–34. doi: 10.1080/19490976.2016.1158395
164. Mayorga-Gross, AL, and Esquivel, P. Impact of cocoa products intake on plasma and urine metabolites: a review of targeted and non-targeted studies in humans. Nutrients. (2019) 11:1163. doi: 10.3390/nu11051163
165. Brachem, C, Oluwagbemigun, K, Langenau, J, Weinhold, L, Alexy, U, Schmid, M, et al. Exploring the association between habitual food intake and the urine and blood metabolome in adolescents and young adults: a cohort study. Mol Nutr Food Res. (2022) 66:2200023. doi: 10.1002/mnfr.202200023
166. Guthrie, L, Spencer, SP, Perelman, D, Van Treuren, W, Han, S, Yu, FB, et al. Impact of a 7-day homogeneous diet on interpersonal variation in human gut microbiomes and metabolomes. Cell Host Microbe. (2022) 30:863–874.e4. doi: 10.1016/j.chom.2022.05.003
167. Pallister, T, Jackson, MA, Martin, TC, Zierer, J, Jennings, A, Mohney, RP, et al. Hippurate as a metabolomic marker of gut microbiome diversity: modulation by diet and relationship to metabolic syndrome. Sci Rep. (2017) 7:13670. doi: 10.1038/s41598-017-13722-4
168. Garcia-Perez, I, Posma, JM, Gibson, R, Chambers, ES, Hansen, TH, Vestergaard, H, et al. Objective assessment of dietary patterns by use of metabolic phenotyping: a randomised, controlled, crossover trial. Lancet Diabetes Endocrinol. (2017) 5:184–95. doi: 10.1016/S2213-8587(16)30419-3
169. Brial, F, Chilloux, J, Nielsen, T, Vieira-Silva, S, Falony, G, Andrikopoulos, P, et al. Human and preclinical studies of the host–gut microbiome co-metabolite hippurate as a marker and mediator of metabolic health. Gut. (2021) 70:2105–14. doi: 10.1136/gutjnl-2020-323314
170. Fang, EF, Hou, Y, Palikaras, K, Adriaanse, BA, Kerr, JS, Yang, B, et al. Mitophagy inhibits amyloid-β and tau pathology and reverses cognitive deficits in models of Alzheimer’s disease. Nat Neurosci. (2019) 22:401–12. doi: 10.1038/s41593-018-0332-9
171. Mehdi, A, Lamiae, B, Samira, B, Ramchoun, M, Abdelouahed, K, Tamas, F, et al. Pomegranate (Punica granatum L.) attenuates Neuroinflammation involved in neurodegenerative diseases. Foods. (2022) 11:2105–14. doi: 10.3390/foods11172570
172. Reddy, VP, Aryal, P, Robinson, S, Rafiu, R, Obrenovich, M, and Perry, G. Polyphenols in Alzheimer’s disease and in the gut–brain axis. Microorganisms. (2020) 8:199. doi: 10.3390/microorganisms8020199
173. Wei, M, Liu, Y, Pi, Z, Li, S, Hu, M, He, Y, et al. Systematically characterize the anti-Alzheimer’s disease mechanism of Lignans from S. chinensis based on in-vivo ingredient analysis and target-network pharmacology strategy by UHPLC−Q-TOF-MS. Molecules. (2019) 24. doi: 10.3390/molecules24071203
174. Johnson, SL, Park, HY, Vattem, DA, Grammas, P, Ma, H, and Seeram, NP. Equol, a blood-brain barrier permeable gut microbial metabolite of dietary Isoflavone Daidzein, exhibits neuroprotective effects against neurotoxins induced toxicity in human neuroblastoma SH-SY5Y cells and Caenorhabditis elegans. Plant Foods Hum Nutr. (2020) 75:512–7. doi: 10.1007/s11130-020-00840-0
175. Koudoufio, M, Desjardins, Y, Feldman, F, Spahis, S, Delvin, E, and Levy, E. Insight into polyphenol and gut microbiota crosstalk: are their metabolites the key to understand protective effects against metabolic disorders? Antioxidants. (2020) 9:982. doi: 10.3390/antiox9100982
176. Druart, C, Bindels, LB, Schmaltz, R, Neyrinck, AM, Cani, PD, Walter, J, et al. Ability of the gut microbiota to produce PUFA-derived bacterial metabolites: proof of concept in germ-free versus conventionalized mice. Mol Nutr Food Res. (2015) 59:1603–13. doi: 10.1002/mnfr.201500014
177. Lankinen, MA, Hanhineva, K, Kolehmainen, M, Lehtonen, M, Auriola, S, Mykkänen, H, et al. CMPF does not associate with impaired glucose metabolism in individuals with features of metabolic syndrome. PLoS One. (2015) 10:e0124379. doi: 10.1371/journal.pone.0124379
178. Xyda, SE, Vuckovic, I, Petterson, XM, Dasari, S, Lalia, AZ, Parvizi, M, et al. Distinct influence of Omega-3 fatty acids on the plasma metabolome of healthy older adults. J Gerontol A Biol Sci Med Sci. (2020) 75:875–84. doi: 10.1093/gerona/glz141
179. Huo, Z, Rana, BK, Elman, JA, Dong, R, Engelman, CD, Johnson, SC, et al. Metabolic profiling of cognitive aging in midlife. Front Aging Neurosci. (2020) 12:12. doi: 10.3389/fnagi.2020.555850
180. Madore, C, Leyrolle, Q, Lacabanne, C, Benmamar-Badel, A, Joffre, C, Nadjar, A, et al. Neuroinflammation in autism: plausible role of maternal inflammation, dietary omega 3, and microbiota. Neural Plast. (2016) 2016:3597209. doi: 10.1155/2016/3597209
181. Madore, C, Leyrolle, Q, Morel, L, Rossitto, M, Greenhalgh, AD, Delpech, JC, et al. Essential omega-3 fatty acids tune microglial phagocytosis of synaptic elements in the mouse developing brain. Nat Commun. (2020) 11:6133. doi: 10.1038/s41467-020-19861-z
182. Leyrolle, Q, Decoeur, F, Briere, G, Amadieu, C, Quadros, AR, Voytyuk, I, et al. Maternal dietary omega-3 deficiency worsens the deleterious effects of prenatal inflammation on the gut-brain axis in the offspring across lifetime. Neuropsychopharmacology. (2021) 46:579–602. doi: 10.1038/s41386-020-00793-7
183. Leyrolle, Q, Decoeur, F, Dejean, C, Brière, G, Leon, S, Bakoyiannis, I, et al. N-3 PUFA deficiency disrupts oligodendrocyte maturation and myelin integrity during brain development. Glia. (2022) 70:50–70. doi: 10.1002/glia.24088
184. Roussel, C, Anunciação Braga Guebara, S, Plante, PL, Desjardins, Y, Di Marzo, V, and Silvestri, C. Short-term supplementation with ω-3 polyunsaturated fatty acids modulates primarily mucolytic species from the gut luminal mucin niche in a human fermentation system. Gut Microbes. 14:2120344. doi: 10.1080/19490976.2022.2120344
185. Healey, G, Murphy, R, Butts, C, Brough, L, Whelan, K, and Coad, J. Habitual dietary fibre intake influences gut microbiota response to an insulin-type fructan prebiotic: a randomised, double-blind, placebo-controlled, cross-over, human intervention study. Br J Nutr. (2018) 119:176–89. doi: 10.1017/S0007114517003440
Keywords: nutrition, gut-brain axis, gut-derived metabolites, behavior, fibers, polyphenols, polyunsaturated fatty acids, postbiotic
Citation: Leyrolle Q, Prado-Perez L and Layé S (2023) The gut-derived metabolites as mediators of the effect of healthy nutrition on the brain. Front. Nutr. 10:1155533. doi: 10.3389/fnut.2023.1155533
Edited by:
Alexandre Benani, Centre National de la Recherche Scientifique (CNRS), FranceReviewed by:
Cécilia Samieri, INSERM U1219 Bordeaux Population Health Centre Recherche, FranceSofia Cussotto, Université Paris-Saclay, France
Yves Desjardins, Laval University, Canada
Tatjana Rundek, University of Miami, United States
Copyright © 2023 Leyrolle, Prado-Perez and Layé. This is an open-access article distributed under the terms of the Creative Commons Attribution License (CC BY). The use, distribution or reproduction in other forums is permitted, provided the original author(s) and the copyright owner(s) are credited and that the original publication in this journal is cited, in accordance with accepted academic practice. No use, distribution or reproduction is permitted which does not comply with these terms.
*Correspondence: Quentin Leyrolle, cXVlbnRpbi5sZXlyb2xsZUBpbnJhZS5mcg==