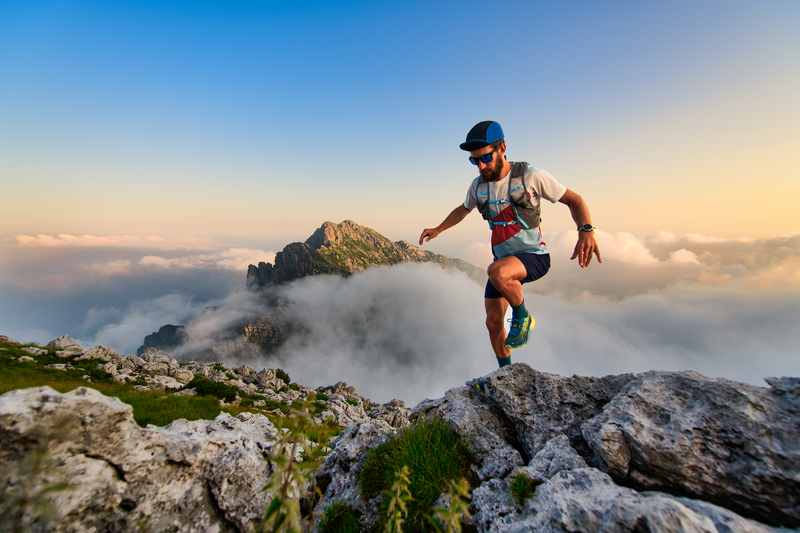
95% of researchers rate our articles as excellent or good
Learn more about the work of our research integrity team to safeguard the quality of each article we publish.
Find out more
REVIEW article
Front. Nutr. , 25 September 2023
Sec. Nutrition and Metabolism
Volume 10 - 2023 | https://doi.org/10.3389/fnut.2023.1126272
This article is part of the Research Topic Foods, Dietary Supplements, and Herbal Products Treating the Diseases of the 21st Century: Moving from Traditional to Scientific Research View all 11 articles
Introduction: Long used in traditional medicine, Nigella sativa (NS; Ranunculaceae) has shown significant efficacy as an adjuvant therapy for diabetes mellitus (DM) management by improving glucose tolerance, decreasing hepatic gluconeogenesis, normalizing blood sugar and lipid imbalance, and stimulating insulin secretion from pancreatic cells. In this review, the pharmacological and pharmacokinetic properties of NS as a herbal diabetes medication are examined in depth, demonstrating how it counteracts oxidative stress and the onset and progression of DM.
Methods: This literature review drew on databases such as Google Scholar and PubMed and various gray literature sources using search terms like the etiology of diabetes, conventional versus herbal therapy, subclinical pharmacology, pharmacokinetics, physiology, behavior, and clinical outcomes.
Results: The efficiency and safety of NS in diabetes, notably its thymoquinone (TQ) rich volatile oil, have drawn great attention from researchers in recent years; the specific therapeutic dose has eluded determination so far. TQ has anti-diabetic, anti-inflammatory, antioxidant, and immunomodulatory properties but has not proved druggable. DM’s intimate link with oxidative stress, makes NS therapy relevant since it is a potent antioxidant that energizes the cell’s endogenous arsenal of antioxidant enzymes. NS attenuates insulin resistance, enhances insulin signaling, suppresses cyclooxygenase-2, upregulates insulin-like growth factor-1, and prevents endothelial dysfunction in DM.
Conclusion: The interaction of NS with mainstream drugs, gut microbiota, and probiotics opens new possibilities for innovative therapies. Despite its strong potential to treat DM, NS and TQ must be examined in more inclusive clinical studies targeting underrepresented patient populations.
DM is an ancient scourge, with evidence of its description first appearing in pre-biblical medical writings (1). It’s complicated pathophysiology consists of metabolic derangements marked by chronically elevated blood glucose, resulting from abnormalities in insulin action, secretion, or both (2–4). The name itself was coined in the 2nd century CE derived from the Greek word “diabaínein” which means “a passing through,” alluding to the disease’s generally profuse urination. The term “mellitus” was added much later in the 16th century because of the sugar found in the urine of people with diabetes (5, 6). In addition to its effect on sugar regulation, DM negatively impacts many important physiological processes, including fat and protein metabolism (2). Chronic hyperglycemia characteristic of DM correlates with long-term damage, dysfunction, and failure of multiple organs, including the eyes, kidneys, nerves, heart, and blood vessels.
The categorization of DM has long baffled medical professionals. Numerous classifications have been proposed, including the now-defunct insulin-dependent (ID) and non-insulin-dependent (NID) types of DM, which have been replaced with a nomenclature more consistent with the disease’s accepted etiology (7). DM is currently characterized clinically into four primary categories and two subtypes (8). Type 2 diabetes mellitus (T2DM) is the most prevalent worldwide, with estimates ranging from 85 to 95%, followed by type 1 (9, 10). The gestational and other diabetes types are rarer and entail a broader range of causes, such as pancreatitis, genetic defects, and endocrinopathies (2). Type 1 DM (T1DM) is distinguishable from T2DM because the former is an autoimmune disorder where pancreatic beta-cells are destroyed, while the latter is characterized by progressively dysfunctional glucose regulation attributable to a combination of insulin resistance and pancreatic beta-cell destruction (11, 12). T2DM and prediabetes are often associated with a broader disorder known as “metabolic syndrome” (13). Differentiating between these various diabetes types is challenging since more than one type can manifest in a single patient (13), prompting calls for a revision of how the disease is classified (2).
Diabetes mellitus (DM) morbidity and mortality have become serious worldwide health concerns in both developed and developing nations (14, 15) straining the world economy (16). Recent controversial claims argue that the disease burden has shifted to developing countries, exacerbating the problem (17, 18) because developing countries already house 79 percent of the world’s diabetes population (19), and the majority comprises of young people belonging to the lowest socioeconomic strata (20). Public health organizations tasked with epidemiological monitoring of DM have struggled to explain prediabetes’s soaring frequency and incidence and the seeming failure to diagnose the condition globally (21). Diabetes is a prime example of the so-called “over-nutrition disease” that is often connected with a surfeit of nutrients and dietary richness (22, 23). “Extra-nutritional” variables, such as the usage of bisphenol A (BPA) in food processing and packaging, also contribute to the spread of diabetes and accompanying comorbidities (24). Societies that have recently transitioned to modern lifestyles have been particularly hard hit by T2DM (14). Asians appear more vulnerable to T2DM, including those in Pakistan, where its incidence has exceeded projections (25). The entire subcontinent of South Asia has been labeled the “diabetes capital of the world” (26). Current estimates indicate the number of DM sufferers globally at 451 million; this figure is projected to rise to 693 million by 2045 (27). Additionally, a whopping 374 million are estimated to be prediabetic, a physiological state that usually leads to full-blown T2DM, a number that is projected to climb by an additional 200 million in the next decades (28). Due to their inadequate healthcare infrastructure, developing nations such as Pakistan will be the hardest hit by these dismal projections (25); thus, traditional medicine will assume even greater importance for the general population in the coming years (14, 29).
The deteriorating situation has spurred several recommendations, including from the WHO (30–32), to investigate the use of plant-based therapies for DM in conjunction with conventional treatments to sustainably and affordably combat the DM epidemic (14, 30, 33). The inability of Western medicine to produce a treatment for DM is another major factor driving the demand for novel alternative medication. Allopathy relies on managing DM with oral hypoglycemic and hypolipidemic drugs with suboptimal therapeutic outcomes and potentially severe side effects (15, 34). The four most common oral hypoglycemics, sulfonylureas/insulinotropics, biguanides, α-glucosidase inhibitors, and thiazolidinediones, have demonstrated efficacy but also safety concerns (35). Natural plant-derived compounds are being increasingly investigated as alternatives to synthetic antioxidants to safely treat numerous oxidative stress-related diseases and conditions (36). Due to their greater molecular variety compared to synthetics (37), medicinal plants have given many therapeutic compounds for treating human ailments, including antidiabetics (38–40).
A comprehensive literature search was conducted with publicly available web-based search engines and databases, PubMed, Scopus, ScienceDirect, Web of Science, Google Scholar, and other sources (R&D reports, graduate theses, and dissertations). The search was based on keyword combinations such as DM and etiology, oxidative stress and DM, herbal medicine and DM, challenges of conventional diabetes treatment, herbal medicine and DM, N. sativa and black seed oil, N. sativa and DM, N. sativa and phytoconstituents, thymoquinone and mechanism of action, antioxidant activity, gut microbiota, and DM. Research and review articles published in English from 1985 to 2023 were included. We also checked the references cited in the retrieved articles and reviews to avoid missing pertinent studies. The research articles were managed using EndNote software, version X9 (Thomson Reuters). Conference proceedings or abstracts, non-original research such as letters, protocols, editorials, commentaries, duplicated literature, clinical trials lacking robust controls, and papers dealing with homeopathic agents were excluded. A total of 481 relevant articles were found, which were exhaustively examined. An attempt was made to include literature from the disparate disciplines of human physiology, plant taxonomy, microbiology, and pharmacochemistry related to NS and its therapeutic potential in DM. The review incorporates the findings of 16 clinical trials.
The rebirth of interest in traditional herbal therapy is due, in part, to the progressively dwindling returns of the reductionist paradigm of drug development prevalent in the industry (25, 41, 42). A notable example is the ineffectiveness of conventional medications in treating chronic diabetes and their inability to address insulin sensitivity and secretion at the same time (43). Even metformin (metf), the US Food and Drug Administration’s (FDA) recommended front-line medicine for DM (44), is devoid of contraindications (45, 46), yet it fails to exert its therapeutic effect due to patient noncompliance (47). Herbal products, as part of the broader notion of integrative medicine, can be used to supplement standard allopathy or to completely replace it, a concept known as complementary and alternative medicine (CAM) (48). CAM is more commonly used in patients with chronic DM, with most patients preferring to supplement rather than replace their orthodox drug regimens (49). The holistic approach inherent in herbal medication gradually strengthens the body’s healing abilities and can better be described as preventive rather than curative.
Herbal therapy is based on the utilization of multi-ingredient formulations to achieve a combinatorial impact, with surprising effectiveness when compared to modern pharmaceutics, which is overwhelmingly centered on using single target molecules for treating complicated chronic condition like diabetes (50–52). According to WHO, 80 percent of the world’s population still relies on traditional medicine for healthcare (53), making it a legitimate element of the global healthcare network (54). Interestingly, many of the drugs used in modern medicine have botanical origins. The primary antidiabetic drug Metf was originally obtained from the French lilac Galega officinalis (44, 55). Certain plant materials that are rich in antioxidants, have been found effective in treating diabetes (56–58). A considerable body of evidence underscores the importance of herbal medicines in the treatment of diabetes (59), particularly those classified as spices (60–63). However, due to a lack of compelling evidence, many in the medical community remain skeptical of the utility of herbal medicine for DM treatment (64). This has prompted efforts to better understand the safety and efficacy of CAM products, practices, and interactions, often using radical trans-disciplinary approaches such as reverse pharmacology (49, 65).
It is worth noting that simply consuming antioxidant rich plants foods cannot be considered herbal therapy because the antioxidant molecules are enmeshed in the food matrix, where dosage and bioavailability can be problematic. Individual plant-derived antioxidants such as vitamins and polyphenols for diabetes treatment, on the other hand, have only partially succeeded due to stability issues and differences in the physiologies of lab animals compared to humans. Despite these challenges, it has been demonstrated that combining different antioxidants can have a synergistic effect, and such formulations are becoming increasingly popular (66). Because of the complexities of diabetes, where oxidative stress is so deeply intertwined with multiple metabolic pathways, therapies including herbal ones that have the twin capacity of antioxidant renewal and ROS route blocking would have the best chance of success (67, 68). Many front-line contemporary drugs currently used for diabetes treatment, such as thiazolidinediones, metf, and glucagon-like peptide-1 (GLP-1) agonists, owe their effectiveness to antioxidant activity and glucose-lowering capability (66).
NS is an erect, annual flowering herb 20–90 cm tall, is one of the 20 species belonging to the genus Nigella L. (Family Ranunculaceae, Order Ranunculales, Class Magnoliopsida, Division Tracheophyta, Kingdom Plantae), accorded the taxonomic serial number of 506,592 by Integrated Taxonomic Information System (ITIS) (69), all the species withing this genus having utility as either food or medicine (70). The black tint of its discoid seeds occurs once they are exposed to air and is the source of many of its colloquial names “Black cumin” (71), “Black caraway” (72) “Alkamoun Alaswad” (73), or Black seed (74). Pertinent is the distinction between black cumin and black caraway from true cumin and true caraway, the latter two being the seeds of Cuminum cyminium L., and Carum carvi L, respectively, belonging to family Umbellifera (72). Despite the taxonomic impasse of dividing the Nigellae tribe into genera or sections, the consensus splits it into three genera, of which only Nigella is found in South Asia. Komaroffia and Garidella, on the other hand, are common throughout southern Europe and central Asia (74). NS stands out from the other Nigella spp. because its seed’s volatile oils are particularly rich in TQ (75), making it a promising candidate for both traditional, modern evidence-based phytomedicine with preventive and therapeutic potential (76–79). It remains one of the most widely researched medicinal herbs (80) and the one most frequently cited throughout medical history as the ultimate “cure-all” (81), having been described by the Greeks, the Bible (82), and in traditional Arab and Islamic medicine (TAIM) where it is exalted as a prophetic medicine (83). Besides having culinary value (84), its seeds and oil are believed to have holistic medicinal properties and are used in many ancient medicinal schemas such as Ayurvedic, Siddha, Unani, Chinese, and Islamic (76, 85–86). NS and its oil have been labeled GRAS (generally regarded as safe) by the USFDA for use as a spice (87) but given only a qualified GRAS approval for use as a dietary supplement (88). It is one of the most frequently purchased herbal supplements in many of the world’s leading markets (89). The Aegean and Irano-Turanian regions have been considered the evolutionary fountainhead of NS (90); however, a broader nativity claim is also made that includes North Africa and southwest Asia. Egypt produces the best commercial quality though it is also found growing wild in the Middle East, sub-continent, and Mediterranean countries (90, 91). India is its largest global producer (92). NS has an extensive array of pharmacological activities against various ailments and is considered a sacred herb in various religions, and a native, health-promoting plant in traditional medicine (78). In recent decades, researchers have found that NS has anti-inflammatory, and hepato-, neuro-, and gastro-protective effects (76, 78). It has also been substantiated that NS and its chemical constituents exert a nephroprotective effect by normalizing kidney function and reversing tubular damage along with suppression of biochemical alterations (93, 94).
NS seeds (NSS) have been the focus of study since the latter part of the 19th century (73) as they are the principal source of the herb’s bioactive components, and their volatile oil consists mainly of alkaloids, terpenes, and phenolics (90, 95). However, volatile oils comprise only 0.4–2.5% of the total seed content (96), the fixed oil being the principal part at 36–38% (87). The most active constituent is TQ (97, 98), discovered in 1963 (75). TQ makes up 18 to 57% of the volatile oil (96, 99), with the exact composition depending on species, seed chemotype, and oil extraction method (90, 100). The TQ content in NS oil (NSO) can be as low as 0.05 mg/ml to as high as 7.2 mg/ml (101). Some traditional processing practices employ solvents to effectively remove the TQ from NSS in an attempt to make them safer for people with specific health concerns (102). Other volatiles of interest are thymohydroquinone, pinene, p-cymene, and dithymoquinone. In addition to TQ, other potent radical scavengers include dithymoquinone, trans-anethole, thymol, and carvacrol, except dithymoquinone (90). In addition to these, carvacrol (5–12%), 4-terpinol (2–6%), and thymol are also present (83). NSS also contains limonene, citronellol, and two types of alkaloids, isoquinoline (nigellimin and nigellimin N-oxide) and pyrazole (nigelliden and nigellicin) (78, 87). TQ is present in several plant families besides the Ranunculaceae, but NS stands out as its richest source (103, 104), a claim worth investigating since members of the convergently co-evolved family Lamiaceae have shown amounts of TQ in their floral parts far exceeding those of NS (104, 105).
The essential oil of NSS is also high in polyunsaturated fatty acids, linoleic acid (50–55%), eicosanoic acid (4%), and monounsaturated fatty acids such as oleic (20%). NSS contains substantial phenolic compounds such as salicylic acid, quercetin, tocopherols, and phytosterols such as β-sitosterol (90).
Oxidative stress (OS) is a central concept in biology and medicine. Since its introduction in 1985, it has evolved from a simple imbalance in oxidative species in cells and tissues (106) to a much more complex interaction between reactive oxygen species (ROS) and receptors, signaling pathways, and antioxidant defenses. It incorporates a loss of homeostasis in a cell’s many redox-driven physiological processes, defined as “an imbalance between oxidants and antioxidants in favor of the oxidants, leading to a disruption of redox signaling and molecular damage” (107). Depending on the degree of oxidative stress, it can be either harmful (distress) or helpful (eustress) (108). The pivotal role of redox control in cellular functions describing redox homeostasis as “aurea mediocritas” the golden mean of healthy life (109) has led many researchers to view T2DM as a redox disease (110).
OS is brought about by two broad classes of molecules, reactive oxygen species (ROS), such as H2O2, ·OH, and O2-and reactive nitrogen species (RNS), such as NO and NO2. These are naturally generated as byproducts of metabolic activities (111), but their accumulation can damage biological macromolecules like proteins, lipids, and nucleic acids (112). Under normal circumstances, with a robust antioxidant system, these molecules do not constitute a threat; only when the antioxidant response is compromised or oxidant levels rise too rapidly do they cause oxidative damage leading to diseases such as T2DM (113, 115). Free radicals are species containing one or more unpaired electrons, and this incomplete electron shell accounts for their high reactivity (116, 117). The most common free radical is superoxide anion (O2−.) (118), generated by the action of nicotinamide adenine dinucleotide phosphate (NADPH) oxidase (119). The most unstable and destructive is the hydroxyl radical (·OH) formed by the reaction of H2O2 with metal ions (Fenton reaction) which damages lipids through peroxidation, triggering a chain of adverse events (113).
Peroxynitrite is a potent pro-oxidant (114) implicated as the causative agent of the cardiovascular endothelial dysfunction seen in T2DM (120). ROS can be generated via many pathways within cells; however, metabolic activities occurring in the mitochondria and endoplasmic reticulum and enzymes such as nicotinamide adenine dinucleotide phosphate (NADPH) oxidase are the usual causes associated with OS-mediated onset of T2DM (66) (Figure 1). However, it has been argued that terms like total antioxidant capacity (TAC) and reactive oxygen species (ROS) are too general and prone to misinterpretation when applied to the complex system of redox and oxidative components inherent in OS. Careful identification of the individual oxidant and antioxidant moieties and their behaviors allows for a better understanding of the mechanics of OS (121, 122).
Figure 1. Various cellular pathways involved in hypoglycemia mediated ROS generation, mitochondrial damage, and cell death.
The nexus of OS with diabetes has remained a matter of scientific debate since the 1980s (123), their association being explicitly propounded in the “common soil” hypothesis (124). OS plays a primary role in the pathogenesis of DM (125, 126) as manifested through many enzymatic, non-enzymatic, and mitochondrial mechanisms (127). Free radicals and peroxides are produced in large quantities in T2DM via glucose oxidation and non-enzymatic protein glycation, which can overwhelm antioxidant defense mechanisms leading to cellular damage (119), a phenomenon termed “hyperglycemic or metabolic memory” (128). Auto-oxidation of glucose generates hydroxyl radicals, while membrane-associated xanthine oxidase and nitric oxide synthase generate free radicals, ROS, and RNS (129). Accumulating these oxidants can result in the formation of lipid peroxidation products such as the highly hazardous malondialdehyde (MDA) and acrolein generated by free radical-driven peroxidation of polyunsaturated fatty acids like arachidonic and linoleic acids (113). Heightened MDA levels are typical of diseases with an OS component (130). Although ROS and free radicals are involved in the proinflammatory cytokine-mediated beta-cell injury, not all free radicals generated are implicated in their destruction, as in the NO case (131). High ROS levels also reduce the bioavailability of nitric oxide (NO) generated by endothelial cells, whose multifunctional signaling role is crucial to vascular integrity (132). The proper functioning of cellular antioxidant systems is the mainstay in limiting oxidative damage (129), especially in the context of DM (133).
DM harms the tissues by increasing the non-enzymatic formation of advanced glycation end products (AGEs) through the Maillard reaction and glucose auto-oxidation, leading to loss of protein function. The binding of AGEs to the receptor for AGE (RAGE) induces NADPH oxidase-1 to produce more ROS (134, 135) in addition to activating atherogenesis-promoting signal transduction mechanisms (136) like mitogen-activated protein kinase (MAPK) that further magnifies the inhibition of NO formation by AGEs (137). AGE-induced oxidative stress and the ensuing diabetic progression ultimately depend on the cellular balance between RAGE and AGER1; AGER1 binds and degrades AGEs while RAGE promotes oxidative stress and has a freer rein in chronic diabetes since AGER1 removal of AGE is suppressed (138).
Recent research suggests that mitochondria unavoidably generate significant ROS through oxidative phosphorylation (139). The ROS and RNS generated in mitochondria pass out into the cellular milieu, harming cytoplasmic organelles and damaging macromolecules (140), making DNA more vulnerable to mutagenesis (141). Critical cellular homeostases pathways such as autophagy and mitophagy that clear the cell of damaged macromolecules and organelles such as mitochondria and endoplasmic reticula appear less active in people with diabetes, although the mechanism is unknown (66). The diametrically opposed concept of mitohormesis proposes a dual dose-dependent action of ROS species, wherein, when produced by mitochondria in typically “small” amounts, they have a salubrious instead of a noxious effect. Some researchers proposed that DM may be a consequence of slowed-down mitochondrial machinery, which can restore health if restored to normal levels of superoxide production (142, 143). Intriguingly, a mitohormetic effect in animals has been demonstrated for the front-line antidiabetic metf (144). However, ambiguities remain since the studies did not consider the damaging effects of reductive stress that precedes oxidative stress in hyperglycemia on mitochondria and malfunctioning feedback mechanisms (142). Nonetheless, the importance of reducing the physiological factors that cause oxidative stress in conjunction with the use of natural antioxidant products, known as the “optimal redox” (OptRedox) approach, is being studied as a public health policy to stem the rising incidence of T2DM in global populations, particularly those of the very young (145). Instead of the common “detect and treat” medical practice of redox medicine, a more targeted use of inhibitors and agonists impacting oxidative stress linked biochemical pathways is hypothesized as a more successful therapeutic approach, while supporting empirical proof is currently a pipedream (108).
ROS levels rise throughout the progression of diabetes, and they are known to be implicated in the destruction of beta cells. TQ’s lack of effect on the transcription factor nuclear factor kappa-B (NF-κB), whose activation by OS is a prelude to diabetes, has not lessened its value as a potential therapeutic (146). It is still a powerful inhibitor of the inflammatory pathways that underpin autoimmune illnesses like T1DM, particularly those involving MAPKs (147, 148) and several in vivo and in vitro studies substantiate the antidiabetic efficacy of NS (38, 149–153). In comparison to fixed oil, the essential oil of NS is thought to have more effective antioxidant activity (154), and if stored appropriately, the antioxidant capacity of the NS oil rises over time despite having a lower TQ content than when it was first extracted (101). Both the NS volatile oil and its principal bioactive ingredient, TQ, are known to improve hyperglycemia and hyperlipidemia (83). In animal studies, NSO’s anti-hyperglycemic effect was equivalent to, if not superior to, metf, the principal hypoglycemic medicine now in use (155). Because this was a one-time trial, larger-scale research using more NS parameters may provide a clearer picture. NS has been linked to pancreatic islet regeneration, and the antidiabetic mechanism maybe due to NS’s ability to increase insulin secretion by boosting β-cell proliferation (156–158). In addition, NS extracts can decrease the body’s inflammatory and OS markers (159) as well as boost skeletal muscle glucose uptake and adenosine monophosphate-activated protein kinase (AMPK) activity (160). NS and TQ repress gluconeogenesis in the liver (161) by explicitly targeting the enzymes glucose-6-phosphatase and fructose-1, 6-biphosphatase (162) and also retard glucose absorption in the alimentary tract while enhancing glucose tolerance in rats (163). Lowering increased glucose uptake in diabetics by inhibiting the intestinal glucose transporter, sodium-glucose linked transporter 1 (SLGT1) through bioactive compounds represents a promising target for novel drug development (164). Controlling the postprandial glycemic spike in T2DM patients by blocking the digestive enzymes α-glucosidase and α-amylase with NS as opposed to clinical drugs that tax the gut physiology is also a feasible strategy (165). The feeding of NS extract decreased lipid peroxidation and increased antioxidant enzymes such as Superoxide dismutase (SOD), Catalase (CAT), and Glutathione peroxidase (GPx) in the organs of rats with chemically-induced diabetes (166, 167). The antidiabetic benefit of ground NSS via an antioxidant-based mechanism has been proven in large-scale clinical trials involving T2DM patients (168, 169). However, the same could not be said of NSO in such trials (170, 171) even though NSO supplementation causes a marked reduction in oxidative stress in healthy individuals (172). A host of confounding factors related to intervention methodology and the quality of the tested herbal product have been cited as the cause of this inconsistency (173). In a first-of-its-kind clinical trial comparing NS as monotherapy to metf in the treatment of diabetic patients, the former failed to achieve therapeutic outcomes (174). It could that the NS quantity was subtherapeutic (1,350 mg/day) since studies suggest that NS doses of less than 2 g are clinically inconsequential (168). On the basis of a meta-analysis of relevant clinical trials, the general consensus is that NS supplementation is an effective treatment for T2DM (175). Chronic T2DM marked by hyperglycemia-mediated insulin resistance remains a therapeutic challenge and the ameliorative impact of TQ has just lately come to light (176). TQ can also improve levels of insulin receptors improving insulin action, low levels of which are the cause of insulin resistance and type 2 DM (177). TQ can dramatically reverse the diabetes associated drop in Glut-2 levels (177), a transporter protein responsible for glucose transfer between the liver and blood and its reabsorption by the kidneys (178). By suppressing oxidative stress, reducing low-density lipoprotein (LDL), raising high-density lipoprotein (HDL-C), and lowering total blood cholesterol, TQ also mitigates cardiovascular complications such as atherosclerosis that accompany the course of diabetes (162, 179, 180).
It has been discovered that all forms of NS, including oil, water extracts, dried and crushed seed portions, show substantial hypoglycemic potential, particularly those based on aqueous extraction (181). Since this form of extraction gives the lowest TQ content (182), it implies the presence of active compounds in NS seeds other than TQ, of which there are over a hundred, many of which are unknown (183), but which may be equally beneficial in diabetes management (184). Multiple clinical trials (Table 1) and related studies (Table 2) evaluating different oral quantities advised for NSS, oils, and TQ have demonstrated that NS ingestion does not result in acute or chronic toxicity (219, 220) and is deemed safe among the hundreds of candidate medicinal plants and the oral antidiabetics currently available (76). However, few significant clinical trials on the safety and efficacy of NS have been conducted, highlighting the need for additional study (221). Some trial results, such as the one evaluating the suitability of NS supplementation for diabetic patients undergoing hemodialysis, have not yet been published (222). Even if unfavorable reactions in human subjects are uncommon, it is prudent to proceed with caution and prudence. There is some evidence linking NSS and essential oil to negative health effects in laboratory animals (223, 224). Consumption of NSS has been associated with inhibition of the drug-detoxifying enzymes cytochrome P450 2D6 (CYP2D6) and cytochrome P450 3A4 (CYP3A4), which raises the specter of unforeseen drug–drug interactions and prescription drug toxicity (225). Reaction to these enzymes is a key aspect of the protocols followed by worldwide drug regulatory authorities, including the FDA, when reviewing innovative drug candidates (226). TQ inhibits CYP2C19 and CYP3A4 in vitro; the latter is involved in the biotransformation of various oral antidiabetic drugs, a concern which must be investigated through in vivo studies (227).
Due to the features of several new medications, the necessity for hepatoprotection is becoming an increasing concern (228). Acute hepatotoxicity can lead to liver cancer (229), and almost half of drug-induced hepatoxicity cases were attributed to acetaminophen/paracetamol overdosing (230). The liver is the primary site of drug detoxification and is particularly vulnerable to OS (231). NS, because of its protective activity against an array of natural and synthetic toxins (232), including xenobiotics (233), and because of its relative safety and potent antioxidant and anti-inflammatory effects, is ideal for reducing the side effects of neoadjuvant therapy of the cancerous liver before ablative surgeries (228). NSS extracts stabilized lipopolysaccharide-induced hepatotoxicity by normalizing levels of aspartate aminotransferase (AST), Alanine aminotransferase (ALT), and Alkaline phosphatase (ALP) (234). Similarly, NSO effectively raised antioxidant enzyme levels and improved liver function in malathion-induced liver dysfunction (235) and hypervitaminosis (236). TQ also normalized hepatic OS and lowered cholesterol levels, which were elevated due to a cholesterol-rich diet (237). Research has demonstrated that TQ can normalize the amounts of the liver enzymes such as oxidized glutathione (GSSG), SOD, and MDA and enhance reduced glutathione (GSH), essentially protecting against oxidant damage to the liver (238). NSS also augmented the hepatoprotective effect by enhancing CAT, and GPx activity (215, 239). Liver damage due to therapeutic drug overdose is a growing health concern (240). Presently, N-acetylcysteine is the sole clinical intervention for acetaminophen overdose, but its drawbacks such as poor bioavailability, high costs, and side effects warrant exploration for a new, natural curative (221, 241–243). Acetaminophen (APAP)-related hepatotoxicity was effectively countered in experimental rats receiving a combination of α-Lipoic acid (ALA) and TQ as assessed by a decrease in ALT and ALP function and down-regulation of cyclooxygenase-2 (COX-2) and vascular endothelial growth factor (VEGFR1, flt-1) expression (216). Another study suggested that TQ’s mechanism for attenuating APAP-induced acute liver injury involved inhibition of the entire MAPK family with simultaneous activation of the AMPK pathway (221). High doses of NS supplements over prolonged periods can reduce ALP levels substantially, but because of the imprecision of NS’s dose, duration and effect on liver parameters, its approval as a treatment for liver ailments has remained elusive (244).
Since the 1990s, the notion of antioxidants has been in the public eye, and their role in disease prevention a subject of interest. They have been defined as “any substance able to eliminate ROS and derivatives (RNS, or reactive sulfur species, RSS), directly or indirectly, acting as an antioxidant defense regulator, or reactive species production inhibitor” (245). Using antioxidants to complement diabetes therapy has gained much prominence, and many compounds of plant origin and vitamins have been scrutinized as possible candidates, each with its challenges and shortcomings (246–249). NS essential oil has been markedly better at radical scavenging than many commercially available synthetic antioxidants (250). Recent work has shown that TQ, carvacrol, t-anethole, 4-terpineol, tannins, flavonoids, and alkaloids contribute to the radical scavenging properties of NSS with TQ and nigellone accounting for the majority of the activity (251). NS sustains the cellular microenvironment by increasing the body’s antioxidant enzymes, SOD, GPx, and CAT, and enhancing ROS scavenging capacity (169) by increasing vitamin C and E levels (252). In addition to TQ, NS contains other antioxidants such as flavonoids, phenolics, ascorbic acids, and tocopherols (253). Because of its antioxidant capacity, TQ decreases tissue MDA levels, prevents DNA damage, reduces mitochondrial vacuolization and fragmentation, and maintains pancreatic β-cell integrity (252). Lipid peroxidation is a marker of significant stress, and TQ can reduce lipid peroxidation by its robust scavenging of ROS (254). TQ can be reduced to thymohydroquinone under normal intracellular physiological conditions, and to the pro-oxidant semiquinone under pathological conditions with excess of metal ions (255, 256). Based on examination of its molecular structure, the claim that reduced thymohydroquinone possesses any radical scavenging activity, let alone exceeding that of TQ, has been questioned (257). The non-enzymatic binding of TQ with intracellular antioxidants, GSH, NADH, and NADPH results in moieties whose scavenging potency far exceeds that of the free TQ and is at par with Trolox, a powerful antioxidant and vitamin E analog (258, 259). TQ can also be reduced to dihydro-thymoquinone by DT diaphorase, which induces oxidative stress from ROS, causing cytotoxicity and DNA damage (100). Dihydro-thymoquinone becomes a concern in specific circumstances, such as cancer, where DT diaphorase levels are elevated, or in animal trials where large quantities of TQ are administered (260). TQ’s lipophilic properties are similar to those of the mitochondrial electron transporter ubiquinone, and more research is needed to determine the precise link between thymohydroquinone and mitochondria (261).
NS and TQ improve insulin sensitivity by increasing MAPK pathway activation, muscle GLUT-4 levels, which helps to gradually normalize glycemia (Figure 2). TQ also in a dose-dependent manner inhibits COX and lipoxygenase (LOX) enzyme activities, consequently blocking the synthesis of inflammatory mediators, prostaglandins, thromboxane, and leukotrienes and reducing joint inflammation (262). TQ by suppressing pro-inflammatory cytokines, interleukin-1β (IL-1β), and tumor necrosis factor-alpha (TNF-α), as well as interferon-gamma (IFN-γ), and interleukin-6 (IL-6), mitigates disease severity. TQ also inhibits NO production from activated cells and macrophages, increasing inflammatory responses and promoting apoptosis (254). The antioxidant properties of NS and TQ were responsible for reversing streptozotocin-induced modifications in creatine kinase-MB and brain monoamines (263). It has been demonstrated that NS promotes AMPK in the liver and muscles, resulting in antioxidant and health-protective effects. Synthetic AGE inhibitors have largely failed to combat hyperglycemic and OS-related AGEs due to side effects, hence attention has switched to natural plant-based extracts (264). NS represents a promising natural candidate since both TQ and NSS extract have been found to inhibit AGE formation in vitro (265, 266). However, the NS-mediated AGE inhibition mechanism remains largely unknown (267). Recent computational studies have made significant advancements in our mechanistic knowledge of TQ’s anti-glycation action on the eye lens crystallin proteins and its therapeutic promise in reducing DM-related ocular cataract (268). Furthermore, nothing is known about how NS and TQ interact with novel AGE forms such as melibiose-derived (MAGE), which is detected in high amounts in diabetics with microangiopathy (269). Because of their antioxidant content, both NSS and its alcohol or aqueous extract have effectively reduced diabetes-induced cytotoxicity in vitro (270, 271) and diabetic lab animals (272). However, it must be borne in mind that solvent extracts of Nigella seeds carry an array of antioxidants that can complicate establishing causality (90). Exogenous antioxidant supplementation for diabetic patients has some advantages, but the strategy was initially disregarded due to worries about a lack of clinical evidence and potential side effects (273), and it is still not used as a clinical strategy today (274), despite positive results from clinical trials using antioxidant supplementation for particular diabetic complications (274–276). It is broadly understood that any potential new antioxidant therapy for DM must target the disease and simultaneously effectively prevent the associated vascular complications (247).
Using the isolated and purified active principle of medicinal plants instead of the crude extract is advantageous because it circumvents the variability in the content of the bioactive compound in the natural materials and losses due to processing and preparation. Avoiding potential interactions among the constituents using a purified preparation allows for more accurate and reproducible dosage and better analytical assaying of safety and efficacy (36, 277). Several compounds derived from NS have therapeutic value, but none comes close to TQ as the main bioactive component with the most diverse pharmacological benefits (83, 278). It has been argued that the effectiveness of NS fractions in lowering glycemia and serum lipid levels might be a function of their TQ content, with the volatile oil outperforming the others (175). TQ is a monoterpene benzoquinone compound synthesized in plants from γ-terpinene during secondary metabolism (279). Reportedly first isolated from NSS in the 1960s (73, 280), it is a yellow crystalline substance chemically known as 2-isopropyl-5-methylbenzo 1,4 quinone having a molar mass of 164.20 g/mol with a molecular formula of C10H12O2 (281) and CASRN: 490–91. Its tautomerism, where only the keto form of the molecule is thought to be pharmacologically active (162, 281), is disputed, and the reduced form of TQ has been misinterpreted as the enol form (282).
TQ has been shown in animal studies to effectively treat OS-related diseases with few or no side effects (36). It has been shown to have antioxidant, anti-inflammatory, antineoplastic, antimicrobial, analgesic, hypoglycemic, antihypertensive, and hepatoprotective properties (162, 254, 283, 284). TQ’s oxidant-scavenging prowess has been ascribed to molecular quinone and the ease with which it passes through cell membranes to reach intracellular targets (285). TQ represents a relatively new class of compounds with antioxidant ability in CH bonds rather than phenolic OH groups (171, 286). The TQ molecule has specific CH groups whose bond dissociation values of the hydrogen atom transfer mechanism impart a free radical-based antioxidant activity comparable to potent antioxidants like ascorbic and gallic acids (171).
Depending on the cellular and physiological milieu, TQ can undergo both enzymatic and non-enzymatic redox reactions to generate either pro-oxidants (semiquinone) or antioxidants (thymohydroquinone); the former is associated with ROS generation, while the latter exert radical-scavenging activity (278). Animal studies have shown that TQ synergizes with metf to markedly reduce serum glucose, HbA1c, MDA, and TAC levels, which neither of them could do as well individually, substantiating its combinatorial role in conventional drug therapy (177). However, clinical trials using such combinations have reported minor health concerns that merit further investigation (287).
TQ has significant pharmacological and pharmacokinetic potential to be a strong drug candidate, as reflected by its compliance with Lipinski’s “rule of five” (176, 288). One of the main problems in testing TQ in clinical trials has been a lack of standardized protocols to ensure uniform quality and dosage (182) (Table 1). Its high hydrophobicity, time-dependent aqueous solubility, aversion to alkaline pH, and significant photo-and thermolability have challenged pharmaceutical formulations (289). It is also poorly bioavailable and vulnerable to transformation by liver enzymes upon oral intake, which necessitated the development of a version encapsulated in nanoparticles that have proven more effective than the non-encapsulated natural TQ as an anti-glycemic drug (103, 205, 290, 291). Recently nanosuspensions and gold nanoparticles phyto-formulated using NSS extract have demonstrated significant antioxidant and antidiabetic activity (292, 293). In addition, an array of nanotechnological carriers have come to the fore which could potentially overcome the poor solubility and bioavailability of orally administered TQ (280, 294), delivering a high payload of TQ via the oral route by mixing it with relatively non-toxic solvents like DMSO is also an option, and not just limited to diabetes treatment (295). Recently, synthetic analogs of TQ have come to the fore with greater efficacy and safety than the natural version, but these have been chiefly used against cancer and other diseases and not for diabetes treatment (284, 296, 297). TQ’s chemical and biological transformation has yielded derivatives with enhanced antioxidant potential (298, 299). Given the limited supply of natural TQ (NS being the primary source), escalating future needs may have to be supplied through synthetic versions. The performance of synthetic TQ analogs, which surpass the natural version in oncology experiments, is very encouraging, but whether this holds true for diabetes remains to be seen (75).
Although the high levels of TQ used in animal research have raised some safety concerns, its usage in mixtures by people for more than a millennium has been relatively incident-free (100) (Table 2). TQ’s non-toxicity and safety makes it ideal for consideration as a pharmacological agent with substantial therapeutic and commercial potential (296). Increased oxidant levels and lipid peroxidation are hallmarks of diabetes (300), and TQ can directly scavenge ROS such as superoxide (O2−), hydroxyl radicals (OH−) and hydrogen peroxide (H2O2) that cause OS (87) (Figure 3). Its ability to quench free radicals matches that of SOD (179, 301), although it is not so effective against hydroxyl and 2,2′-diphenyl-p-picrylhydrazyl (DPPH) radicals (302, 305). TQ has been shown to suppress lipid peroxidation, reduce intracellular MDA (302, 303), and enhance antioxidant defenses by non-enzymatically augmenting the activity of antioxidant enzymes (304). Several studies have demonstrated that TQ increased the level and activity of both the primary antioxidant enzymes, SOD, CAT, and glutathione S-transferase (GST), and the secondary antioxidant enzymes like glutathione reductase (GR) and GPx (303, 305, 306). Its ability to react in vivo with such antioxidant enzymes, especially GSH, and form more potent quenching moieties that replenish and eventually replace the endogenous antioxidant system is critical in fighting OS-mediated pathogenesis (285). TQ is also able to check the auto-oxidation of glucose to prevent the runaway generation of NFκ-B-mediated ROS and proinflammatory cytokines typical of DM onset (307). Because of TQ’s propensity to react with thiol-rich proteins and modulate powerful antioxidant enzymes like GSH, it is bundled with an exclusive group of therapeutic drugs called Michael reaction acceptors (308) that are associated with safeguarding overall cellular health (239, 309) and is a natural activator of Nrf2 signaling. In the presence of OS, Nrf2 induced many of the cell’s antioxidant enzymes while simultaneously repressing NF-κB, IL-1β, IL-6, TNK-z, COX-2, iNOS, TGF-β1, and NOX4, which decreased inflammation, DNA and mitochondrial damage (310). The possibility of using TQ to augment the new diabetic therapy based on the gut hormone incretin has gained much currency because of its few side effects compared to conventional drugs and the absence of any other herbal derivatives that demonstrably modulate incretin (GLP-1) (248, 311, 312).
NS produces ample amounts of TQ, but problems with the preparation of NS extract, non-standardization of testing parameters, disease severity, and duration of NS dosing could account for the conflicting claims where clinical trials have failed to find a role for NS in lowering MDA levels. Variable TQ content in commercially available NS products is another confusing issue in their varied efficacy, with requests for regulating them for TQ content (313, 314). A new study suggests that a TQ content of 30 mg per day be tested in a therapeutic context (314). Significant diversity in NSO chemotypes is also a concern, as those from Turkey and Egypt are classified as TQ phenotypes with the highest TQ content, whereas others, such as those from the subcontinent, are more mixed (p-cymene/TQ), and some may have phenylpropanoids instead of TQ, the so-called trans-anethole chemotype (315, 316). Similarly, care needs to be exercised when interpreting NS’s in vivo antioxidant efficacy based on TAC measurements since it is an in vitro assay that measures only non-enzymatic antioxidant capacity, whereas NS has both (107, 253, 317).
Genotype and environment both play a role in the etiology of T2DM (318, 319), and there has been strong interest in establishing the genetic triggers of OS in diabetes (276).
NS has potent antidiabetic activity, as reflected by the up-regulation of several essential genes, such as insulin-like growth factor-1 and IGF-1. IGF-1 is widely present in mammalian tissues, and its functions include regulating metabolism and enhancing tissue development and growth (320). IGF-1 manifests its effects by binding specific receptors on target cells, stimulating glucose uptake, lowering blood glucose, and improving insulin sensitivity (321). Its mechanism of action appears independent of insulin receptor activation, but NSS has been shown to induce hypoglycemia by upregulating the IGF-1 gene and reducing DM-induced OS (322). IGF-1 improves insulin resistance in T2DM and in patients with more severe insulin resistance, where clinical trials have demonstrated the potential utility of IGF-1 in ameliorating clinical symptoms (157, 323, 324).
In diabetes, loss of insulin responsiveness can occur because some elements of insulin signaling pathways, such as insulin receptors, are disrupted. NSO upregulates insulin-signaling pathways and augments the expression of insulin-like growth factor-1 (IGF-1), inducing the signaling molecule, protein kinase B (Akt), and activating glucose transporter-4 (GLUT4). GLUT4 is then translocated to the plasma membrane, where it imports glucose into the cell (325). Dysfunctional GLUT-4 has been linked to insulin resistance (326). Thus, NS can decrease insulin resistance by improving tissue sensitivity to insulin action (327, 328), presumably in concert with its suppression of insulin clearance via inhibition of insulin-degrading enzymes (IDEs). Greater insulin sensitivity is also linked to NSO’s ability to lower triglyceride levels (329).
Endothelial dysfunction is described as an “impairment of the ability of the endothelium to maintain vascular homeostasis” properly and is the principal underlying reason for DM-associated vascular pathologies (330). DM is associated with reduced expression and activity of endothelial nitric oxide synthase (eNOS), which is central to maintaining cardiovascular tone and function (149, 331). TQ improves endothelial function by inhibiting OS and stabilizing the renin-angiotensin (RAS) system (332). The proliferation and migration of vascular smooth muscle cells (VSMCs) is a characteristic of endothelial dysfunction in diabetes, and inhibitory drugs are highly sought after. Animal studies show TQ’s antiproliferative and anti-migratory effect on VSMCs through the AMPK/ Peroxisome proliferator-activated receptor gamma PPARγ/ Peroxisome proliferator-activated receptor-gamma coactivator (PGC-1α) pathway (333). TQ reduces vascular inflammation by repressing the expression of vascular endothelial growth factor (VEGF) and monocyte chemo-attractant protein-1 (MCP-1), besides lowering levels of cytokines IL-6 and IL-8 in human vascular endothelial cells (HUVECs) (334). TQ has been shown to reverse endothelial dysfunction by increasing NO generation and bioavailability. Vascular cell adhesion protein-1 (VCAM-1) is involved in the adhesion of lymphocytes, eosinophils, and basophils to the vascular endothelium and is an essential mediator in developing atherosclerosis and DM. VCAM-1 can recruit monocytes to the sites of atherosclerotic lesions, initiating and developing vascular inflammation (335, 336). Vcam-1 gene expression was upregulated in the aortic tissue of diabetic rats, while NS seeds significantly reduced vcam-1 gene expression in the aorta, potentially reducing vascular inflammation and restoring endothelial function (149). TQ interfered with TNF-α signaling to modulate IL-6 and IL-8 expression and downregulated IL-8 and ICAM-1/VCAM-1 expression in rheumatoid arthritis (337). TQ also downregulated toll-like receptor-2 (TLR-2) and-4, which are crucial to the vascular inflammation of diabetic microangiopathy (338, 339), underscoring its potential for controlling and managing DM.
The LOX-1 LDL receptor is an essential element in the progression of atherosclerosis through its intimate relationship with CV dysfunction and DM pathogenesis. Experiments with diabetic rats showed upregulation of LOX-1 expression in the vascular endothelium of the aorta. LDL uptake by the LOX-1 receptor triggers many pathophysiological changes, such as decreased eNOS activity and stimulation of adhesion molecule expression (340, 341). NSS extract inhibited lox-1 gene expression in aortic tissue (342). LDL binding to the LOX-1 receptor increased OS, decreased NO production, potentiated superoxide generation, and activated NF-kB-all of which exacerbate DM pathogenesis. Additional studies on human aortic endothelial cells demonstrated that high glucose levels increased lox-1 gene expression; thus, down-regulating LOX-1 using NSS extract could potentially suppress the pathophysiological processes related to LOX-1 (343, 344), restore normal endothelial function and decrease vascular complications in DM (149).
COX-2 continues to be a target for repression because of its central role in inducing the inflammatory processes associated with diabetes and metabolic syndrome (345, 346). The classical non-steroidal inflammatory drugs (NSAIDs) such as salicylates and the newer, more selective COXIBs (an NSAID sub-category of COX-2 inhibitors) have been used, but their side effects have initiated a search for safer, natural COX inhibitors (221). Culinary spices, such as NS, have garnered much attention because of their anti-inflammatory potential (347). Among the NS-derived benzoquinones, both TQ and its partially reduced form, hydroquinone, stand out as potent inhibitors of COX-2, while thymol selectively inhibited COX-1 (221). TQ demonstrated efficacy against autoimmune diseases, including T1DM, by repressing COX, LOX, cytokines, lipid peroxidation, and IL-1. ROS activates the Nuclear factor kappa-light-chain-enhancer of activated B cells (NF-κB) (348), increasing DM-related inflammation (349, 350). Many inflammatory response factors such as adhesion molecules, inflammatory enzymes, proinflammatory cytokines, and chemokines are products of genes regulated by NF-κB (351, 352), including the inflammatory COX-2. COX-2 is a multiplex enzyme with cyclooxygenase and peroxidase activities that generate ROS and cause OS (353, 354). NS and TQ treatment of streptozotocin (STZ)-induced diabetic rats significantly suppressed the COX-2 expression in pancreatic tissue. The treatments that decreased COX-2 mRNA also reduced pancreatic tissue lipid peroxidation and MDA levels and increased SOD activity (355).
The link between gut microbiota and the onset and incidence of type 1 and type 2 DM has been unequivocally established, triggered by the leaky gut condition arising from altered gut microbiota (356, 357). The microbial population in the gut is complex, unique to the individual, and plays a vital role in the body’s physiological and metabolic processes. Extensive studies of the gut bacterial community have revealed species predominantly belonging to the phyla, Firmicutes, Bacteroidetes, Actinobacteria, Proteobacteria, and Verrucomicrobia (10, 357). Gut bacteria are vulnerable to diet and stress (357), and a dysbiotic population contributes to the onset of T2DM through an altered synthesis of short-chain fatty acids, gut hormone levels, bile acids, and branched-chain amino acid metabolism (358). Metf, the mainstream glucose-lowering medication for DM, ameliorates gut dysbiosis (359, 360). Many treatments exist for diabetes, but its complexity has made curing it a challenge (202, 357), emphasizing the need for testing novel therapeutic approaches (358). Modulating the gut microbiota through pre-and pro-biotics for the complementary treatment and management of T2DM has recently gained much currency (361, 362). Several recent reviews have focused on the potential of next-generation probiotic candidates as adjuncts to conventional probiotics such as lactobacilli and bifidobacteria to offset the harmful consequences of DM (363). The antioxidant activity of a probiotic Lactobacillus species was identified as the reason for its hypoglycemic effect (364). Combining probiotics with existing allopathic antidiabetic medications and plant-based nutraceuticals is an exciting research avenue (357, 365). Co-administering NS with probiotics has yielded fruitful results in treating unrelated human disorders (366). One proposed mechanism of the antibacterial effect of NS and TQ against a variety of Gram-positive and Gram-negative bacteria is by targeting them with ROS while at the same time protecting host tissues from OS through antioxidants (284) and selectively allowing probiotic LAB species to flourish (367). This activity holds promise for T2DM patients once their gut becomes populated with opportunistic pathogens such as Clostridium spp., E. coli, and betaproteobacteria (368). Reversing diabetes-related gut dysbiosis through plant extracts such as those from NSS is of great scientific interest (202). High doses of NSS polysaccharides have been used to promote Bacteroidetes over Firmicutes to alleviate T2DM (202), and the use of beneficial yeast strains like Saccharomyces boulardii is also promising (369).
This review (1985 to January 2022) discusses the latest findings on diabetic pathogenesis and its treatment, emphasizing the herb Nigella sativa and its active constituent, thymoquinone. An obvious outcome of this narrative is that the different experimental designs used by various investigators mean that conclusions about its interventional potential should be interpreted with care. Despite many publications, the medically relevant dosage of TQ and NS supplements remains contentious. The multi-modal nature of NS fractions and TQ is minutely examined for their antidiabetic effects and verified in the lab, and clinical trials make their therapeutic potential as a complementary medication patently clear. However, the pharmacokinetic interaction of NS with conventional drugs raises some concerns which need addressing. Nevertheless, the review clarifies the need to assess NS and its products in clinical trials using diabetic patients with reasonable glycemic control but vascular complications.
AS: co-developed the concept, data collection, analysis, and preliminary draft writing. AZ: co-developed the concept, data collection and analysis, and improved and finalized the manuscript. HA: validated the data analysis. NK: did part of the data collection and analysis. All authors contributed to the article and approved the submitted version.
The authors would like to acknowledge the assistance of Ms. Ghazal Aziz, PhD scholar, NIBGE-C PIEAS with and data sorting and organization.
The authors declare that the research was conducted in the absence of any commercial or financial relationships that could be construed as a potential conflict of interest.
All claims expressed in this article are solely those of the authors and do not necessarily represent those of their affiliated organizations, or those of the publisher, the editors and the reviewers. Any product that may be evaluated in this article, or claim that may be made by its manufacturer, is not guaranteed or endorsed by the publisher.
1. Dupras, TL, Williams, LJ, Willems, H, and Peeters, C. Pathological skeletal remains from ancient Egypt: the earliest case of diabetes mellitus? Pract Diab Int. (2010) 27:358–363a. doi: 10.1002/pdi.1523
2. Genuth, SM, Palmer, JP, and Nathan, DM. Classification and diagnosis of diabetes. 3rd ed. Bethesda (MD): National Institute of Diabetes and Digestive and Kidney Diseases (US) (2018).
3. Kerner, W, and Brückel, J. Definition, classification and diagnosis of diabetes mellitus. Exp Clin Endocrinol Diabetes. (2014) 122:384–6. doi: 10.1055/s-0034-1366278
4. Petersmann, A, Nauck, M, Müller-Wieland, D, Kerner, W, Müller, UA, Landgraf, R, et al. Definition, classification and diagnosis of diabetes mellitus. Exp Clin Endocrinol Diabetes. (2018) 126:406–10. doi: 10.1055/a-0584-6223
5. Laios, K, Karamanou, M, Saridaki, Z, and Androutsos, G. Aretaeus of Cappadocia and the first description of diabetes. Hormones. (2012) 11:109–13. doi: 10.1007/BF03401545
6. Rydén, L, and Lindsten, J. The history of the Nobel prize for the discovery of insulin. Diabetes Res Clin Pract. (2021) 175:108819. doi: 10.1016/j.diabres.2021.108819
7. Gavin, JR, Albert, KGMM, Davidson, MB, DeFronzo, RA, Drash, Allan, Gabbe., Steven G, Genuth., Saul, Harris, Maureen, I, et al. Report of the expert committee on the diagnosis and classification of diabetes mellitus. Diabetes Care. (2003) 26:s5–s20. doi: 10.2337/diacare.26.2007.S5
8. ADA. Diagnosis and classification of diabetes mellitus. Diabetes Care. (2013) 36:S67–74. doi: 10.2337/dc13-S067
9. CDC (2020). National diabetes statistics report, 2020, Centers for Disease Control and Prevention, US Dept of health and human services, Atlanta, GA, CS 314227-A, 1–32
10. Yuan, T, Yang, T, Chen, H, Fu, D, Hu, Y, Wang, J, et al. New insights into oxidative stress and inflammation during diabetes mellitus-accelerated atherosclerosis. Redox Biol. (2019) 20:247–60. doi: 10.1016/j.redox.2018.09.025
11. Blair, M. Diabetes Mellitus Review. Urol Nurs. (2016) 36:27–36. doi: 10.7257/1053-816X.2016.36.1.27
12. Piero, MN, Nzaro, GM, and Njagi, JM. Diabetes mellitus – a devastating metabolic disorder. Asian J Biomed Pharm Sci. (2014) 4:1–7. doi: 10.15272/ajbps.v4i40.645
13. Punthakee, Z, Goldenberg, R, and Katz, P. Definition, classification and diagnosis of diabetes, prediabetes and metabolic syndrome. Can J Diabetes. (2018) 42:S10–5. doi: 10.1016/j.jcjd.2017.10.003
14. Ozturk, M, Altay, V, Latiff, A, Ziaee, MA, Choudhry, MI, Shaheen, F, et al. A comparative analysis of the medicinal plants used for diabetes mellitus in the traditional medicine in Turkey, Pakistan, and Malaysia In: MH Ozturk and R Khalid, editors. Plant and human health. Cham: Springer (2018). 409–61. doi: 10.1007/978-3-319-93997-1_11
15. Zarvandi, M, Rakhshandeh, H, Abazari, M, Shafiee-Nick, R, and Ghorbani, A. Safety and efficacy of a polyherbal formulation for the management of dyslipidemia and hyperglycemia in patients with advanced-stage of type-2 diabetes. Biomed Pharmacother. (2017) 89:69–75. doi: 10.1016/j.biopha.2017.02.016
16. Williams, R, Karuranga, S, Malanda, B, Saeedi, P, Basit, A, Besançon, S, et al. Global and regional estimates and projections of diabetes-related health expenditure: results from the international diabetes federation diabetes atlas, 9th edition. Diabetes Res Clin Pract. (2020) 162:108072. doi: 10.1016/j.diabres.2020.108142
17. Ali, MK, Seiglie, JA, and Narayan, KMV. Progress in diabetes prevention or epidemiology-or both, or neither? Lancet Diabetes Endocrinol. (2021) 9:190–1. doi: 10.1016/S2213-8587(20)30433-2
18. Magliano, DJ, Chen, L, Islam, RM, Carstensen, B, Gregg, EW, Pavkov, ME, et al. Trends in the incidence of diagnosed diabetes: a multicountry analysis of aggregate data from 22 million diagnoses in high-income and middle-income settings. Lancet Diab Endocrinol. (2021) 9:203–11. doi: 10.1016/S2213-8587(20)30402-2
20. Marshall, SM. A life course perspective on diabetes: developmental origins and beyond. Diabetologia. (2019) 62:1737–9. doi: 10.1007/s00125-019-4954-6
21. Gregg, EW, and Bracco, P. The dynamics of diabetes prevalence, morbidity, and mortality In: SJ R, editor. The diabetes textbook. Switzerland: Springer, Cham (2019). 11–21.
22. Dhana, K, Nano, J, Ligthart, S, Peeters, A, Hofman, A, Nusselder, W, et al. Obesity and life expectancy with and without diabetes in adults aged 55 years and older in the Netherlands: a prospective cohort study. PLoS Med. (2016) 13:1–13. doi: 10.1371/journal.pmed.1002086
23. Mastorci, F, Vassalle, C, Chatzianagnostou, K, Marabotti, C, Siddiqui, K, Eba, AO, et al. Undernutrition and overnutrition burden for diseases in developing countries: the role of oxidative stress biomarkers to assess disease risk and interventional strategies. Antioxidants. (2017) 6:1–10. doi: 10.3390/antiox6020041
24. Fadishei, M, Rahbardar, G, Mahboobeh, I, Mohsen, M, Ahmad, R, Marjan, B, et al. Effects of Nigella sativa oil and thymoquinone against bisphenol A-induced metabolic disorder in rats. Phytother Res. (2021) 35:2005–24. doi: 10.1002/ptr.6944
25. Aamir, AH, Ul-Haq, Z, Mahar, SA, Qureshi, FM, Ahmad, I, Jawa, A, et al. Diabetes prevalence survey of Pakistan (DPS-PAK): prevalence of type 2 diabetes mellitus and prediabetes using HbA1c: a population-based survey from Pakistan. BMJ Open. (2019) 9:e025300–9. doi: 10.1136/bmjopen-2018-025300
26. Joshi, SR, and Parikh, RM. India--diabetes capital of the world: now heading towards hypertension. J Assoc Physicians India. (2007) 55:323–4.
27. Cho, NH, Shaw, JE, Karuranga, S, Huang, Y, da Rocha Fernandes, JD, Ohlrogge, AW, et al. IDF diabetes atlas: global estimates of diabetes prevalence for 2017 and projections for 2045. Diabetes Res Clin Pract. (2018) 138:271–81. doi: 10.1016/j.diabres.2018.02.023
28. Saeedi, P, Petersohn, I, Salpea, P, Malanda, B, Karuranga, S, Unwin, N, et al. [withdrawn] global and regional diabetes prevalence estimates for 2019 and projections for 2030 and 2045: results from the international diabetes federation diabetes atlas, 9th edition. Diabetes Res Clin Pract. (2019) 157:107843. doi: 10.1016/j.diabres.2019.107932
29. Shaikh, BT, and Hatcher, J. Complementary and alternative medicine in Pakistan: prospects and limitations. Evid Based Complement Alternat Med. (2005) 2:401707. doi: 10.1093/ecam/neh088
30. WHO (1985). Diabetes mellitus: report of a WHO study group, WHO, Geneva, Switzerland, 727, 1–113
31. WHO (2000). General guidelines for methodologies on research and evaluation of traditional medicine, WHO-UNO, Geneva, Switzerland, WHO/EDM/TRM/2000.1, 1–74
32. WHO. WHO traditional medicine strategy: 2014–2023. Hong Kong SAR China: World Health Organization (2013).
33. Marles, RJ, and Farnsworth, NR. Antidiabetic plants and their active constituents. Phytomedicine. (1995) 2:137–89. doi: 10.1016/S0944-7113(11)80059-0
34. Yu, A, Adelson, D, and Mills, D. Chinese herbal medicine versus other interventions in the treatment of type 2 diabetes: a systematic review of randomized controlled trials. J Evid Based Integrative Med. (2018) 23:2515690X1878151–10. doi: 10.1177/2515690X18781519
35. Srinivasan, K, and Ramarao, P. Animal model in type 2 diabetes research: an overview. Indian J Med Res. (2007) 125:451.
36. Sarkar, C, Jamaddar, S, Islam, T, Mondal, M, Islam, MT, and Mubarak, MS. Therapeutic perspectives of the black cumin component thymoquinone: a review. Food Funct. (2021) 12:6167–213. doi: 10.1039/D1FO00401H
37. Attiq, A, Jalil, J, Husain, K, and Ahmad, W. Raging the war against inflammation with natural products. Front Pharmacol. (2018) 9:1–27.
38. Ghorbani, A. Best herbs for managing diabetes: a review of clinical studies. Braz J Pharm Sci. (2013) 49:413–22. doi: 10.1590/S1984-82502013000300003
39. Ghorbani, A. Phytotherapy for diabetic dyslipidemia: evidence from clinical trials. Clin Lipidol. (2013) 8:311–9. doi: 10.2217/clp.13.26
40. Patel, DK, Kumar, R, Laloo, D, and Hemalatha, S. Diabetes mellitus: an overview on its pharmacological aspects and reported medicinal plants having antidiabetic activity. Asian Pac J Trop Biomed. (2012) 2:411–20. doi: 10.1016/S2221-1691(12)60067-7
41. Duval, MX. The inadequacy of the reductionist approach in discovering new therapeutic agents against complex diseases. Exp Biol Med. (2018) 243:1004–13. doi: 10.1177/1535370218794365
42. Munos, B. Lessons from 60 years of pharmaceutical innovation. Nat Rev Drug Discov. (2009) 8:959–68. doi: 10.1038/nrd2961
43. Akter, D, Rokeya, CA, Tahsin, MDR, Haque, E, Sultana, A, Kabir, S, et al. An assessment of source and dose-dependent diabetes ameliorating activity of ethanolic extract of Nigella sativa on Alloxan-induced diabetic rat model. J Clin Mol Endocrinol. (2021) 4:6–13. doi: 10.22259/2638-4981.0401002
44. Bailey, CJ. Metformin: historical overview. Diabetologia. (2017) 60:1566–76. doi: 10.1007/s00125-017-4318-z
45. DeFronzo, R, Fleming, GA, Chen, K, and Bicsak, TA. Metformin-associated lactic acidosis: current perspectives on causes and risk. Metab Clin Exp. (2016) 65:20–9. doi: 10.1016/j.metabol.2015.10.014
46. Florez, H, Luo, J, Castillo-Florez, S, Mitsi, G, Hanna, J, Tamariz, L, et al. Impact of metformin-induced gastrointestinal symptoms on quality of life and adherence in patients with type 2 diabetes. Postgrad Med. (2010) 122:112–20. doi: 10.3810/pgm.2010.03.2128
47. Tang, Y, Weiss, T, Liu, J, Rajpathak, S, and Khunti, K. Metformin adherence and discontinuation among patients with type 2 diabetes: a retrospective cohort study. J Clin Transl Endocrinol. (2020) 20:100225. doi: 10.1016/j.jcte.2020.100225
48. Moquin, B, Blackman, MR, Mitty, E, and Flores, S. Complementary and alternative medicine (CAM). Geriatr Nurs. (2009) 30:196–203. doi: 10.1016/j.gerinurse.2009.03.002
49. Alzahrani, AS, Price, MJ, Greenfield, SM, and Paudyal, V. Global prevalence and types of complementary and alternative medicines use amongst adults with diabetes: systematic review and meta-analysis. Eur J Clin Pharmacol. (2021) 77:1259–74. doi: 10.1007/s00228-021-03097-x
50. Kiyohara, H, Matsumoto, T, and Yamada, H. Combination effects of herbs in a multi-herbal formula: expression of Juzen-taiho-to's Immuno-modulatory activity on the intestinal immune system. Evid Based Complement Alternat Med. (2004) 1:363262. doi: 10.1093/ecam/neh004
51. Li, F-S, and Weng, J-K. Demystifying traditional herbal medicine with modern approach. Nat Plants. (2017) 3:17109. doi: 10.1038/nplants.2017.109
52. Pasalar, M. Persian medicine as a holistic therapeutic approach. Curr Drug Discov Technol. (2021) 18:159–9. doi: 10.2174/157016381802210127102842
53. Parasuraman, S, Thing, GS, and Dhanaraj, SA. Polyherbal formulation: concept of ayurveda. Pharmacogn Rev. (2014) 8:73–80. doi: 10.4103/0973-7847.134229
54. Burton, A, Smith, M, and Falkenberg, T. Building WHO's global strategy for traditional medicine. Europ J Integrative Med. (2015) 7:13–5. doi: 10.1016/j.eujim.2014.12.007
55. Bailey, CJ, and Day, C. Metformin: its botanical background. Pract Diab Int. (2004) 21:115–7. doi: 10.1002/pdi.606
56. Cooper, AJM, Sharp, SJ, Luben, RN, Khaw, KT, Wareham, NJ, and Forouhi, NG. The association between a biomarker score for fruit and vegetable intake and incident type 2 diabetes: the EPIC-Norfolk study. Eur J Clin Nutr. (2015) 69:449–54. doi: 10.1038/ejcn.2014.246
57. Cory, H, Passarelli, S, Szeto, J, Tamez, M, and Mattei, J. The role of polyphenols in human health and food systems: a Mini-review. Front Nutr. (2018) 5:87. doi: 10.3389/fnut.2018.00087
58. Langhans, W. Food components in health promotion and disease prevention. J Agric Food Chem. (2018) 66:2287–94. doi: 10.1021/acs.jafc.7b02121
59. Pang, G-M, Li, F-X, Yan, Y, Zhang, Y, Kong, L-L, Zhu, P, et al. Herbal medicine in the treatment of patients with type 2 diabetes mellitus. Chin Med J. (2019) 132:78–85. doi: 10.1097/CM9.0000000000000006
60. Bi, X, Lim, J, and Henry, CJ. Spices in the management of diabetes mellitus. Food Chem. (2017) 217:281–93. doi: 10.1016/j.foodchem.2016.08.111
61. Kelble, A. Spices and type 2 diabetes. Nut Food Sci. (2005) 35:81–7. doi: 10.1108/00346650510585868
62. Rajput, DS, Dash, DK, Sahu, AK, Mishra, K, Kashyap, P, and Mishra, SP. Brief update on Indian herbs and spices used for diabetes in rural area of Chhattisgarh. Int J Pharm Chem Anal. (2017) 4:1–4.
63. Sanlier, N, and Gencer, F. Role of spices in the treatment of diabetes mellitus: a minireview. Trends Food Sci Technol. (2020) 99:441–9. doi: 10.1016/j.tifs.2020.03.018
64. ADA. Standards of medical Care in Diabetes—2014. Diabetes Care. (2013) 37:S14–80. doi: 10.2337/dc14-S014
65. Surh, Y-J. Reverse pharmacology applicable for botanical drug development - inspiration from the legacy of traditional wisdom. J Tradit Complement Med. (2011) 1:5–7. doi: 10.1016/S2225-4110(16)30051-7
66. Burgos-Morón, E, Abad-Jiménez, Z, de Marañón, M, Aranzazu, I, Francesca, E-L, Irene, L-D, et al. Relationship between oxidative stress, ER stress, and inflammation in type 2 diabetes: the Battle continues. J Clin Med. (2019) 8:1385. doi: 10.3390/jcm8091385
67. Aloud, AA, Veeramani, C, Govindasamy, C, Alsaif, MA, and Al-Numair, KS. Galangin, a natural flavonoid reduces mitochondrial oxidative damage in Streptozotocin-induced diabetic rats. Redox Rep. (2018) 23:29–34. doi: 10.1080/13510002.2017.1365224
68. Cripps, MJ, Hanna, K, Lavilla, C, Sayers, SR, Caton, PW, Sims, C, et al. Carnosine scavenging of glucolipotoxic free radicals enhances insulin secretion and glucose uptake. Sci Rep. (2017) 7:13313. doi: 10.1038/s41598-017-13649-w
69. Sanower, HM, Sharfaraz, A, Dutta, A, Ahsan, A, Anwarul, MM, Ahmed, IA, et al. A review of ethnobotany, phytochemistry, antimicrobial pharmacology and toxicology of Nigella sativa L. Biomed Pharmacother. (2021) 143:112182–25. doi: 10.1016/j.biopha.2021.112182
70. Niu, Y, Zhou, L, Meng, L, Chen, S, Ma, C, Liu, Z, et al. Recent progress on chemical constituents and pharmacological effects of the genus Nigella. Evid Based Complement Alternat Med. (2020) 2020:1–15. doi: 10.1155/2020/6756835
71. Zhao, J, Xu, F, Huang, H, Gu, Z, Wang, L, Tan, W, et al. Evaluation on anti-inflammatory, analgesic, antitumor, and antioxidant potential of total saponins from Nigella glandulifera seeds. Evid Based Complement Alternat Med. (2013) 2013:1–8. doi: 10.1155/2013/827230
72. Burdock, GA. Assessment of black cumin (Nigella sativa L.) as a food ingredient and putative therapeutic agent. Regul Toxicol Pharmacol. (2022) 128:105088. doi: 10.1016/j.yrtph.2021.105088
73. El-Tahir, H, El-Din, K, and Bakeet, DM. The black seed Nigella sativa Linnaeus - a mine for multi cures: a plea for urgent clinical evaluation of its volatile oil. J Taibah Univ Med Sci. (2006) 1:1–19. doi: 10.1016/S1658-3612(06)70003-8
74. Aydın, U, Zübeyde, D, and Ali, A. Numerical analyses of seed morphology and its taxonomic significance in the tribe Nigelleae (Ranunculaceae). Nord J Bot. (2019) 37:njb.02323. doi: 10.1111/njb.02323
75. Edris, AE. Thymoquinone: chemistry and functionality In: MF Ramadan, editor. Black cumin (Nigella sativa) seeds: Chemistry, technology, functionality, and applications. Cham, Switzerland: Springer (2021). 81–95.
76. Ahmad, A, Husain, A, Mujeeb, M, Khan, SA, Najmi, AK, Siddique, NA, et al. A review on therapeutic potential of Nigella sativa: a miracle herb. Asian Pac J Trop Biomed. (2013) 3:337–52. doi: 10.1016/S2221-1691(13)60075-1
77. Majeed, A, Muhammad, Z, Ahmad, H, Rehmanullah, H, Sikandar, SS, Inayat, N, et al. Nigella sativa L.: uses in traditional and contemporary medicines – an overview. Acta Ecol Sin. (2021) 41:253–8. doi: 10.1016/j.chnaes.2020.02.001
78. Tembhurne, SV, Feroz, S, More, BH, and Sakarkar, DM. A review on therapeutic potential of Nigella sativa (kalonji) seeds. J Med Plants Res. (2014) 8:167–77. doi: 10.5897/JMPR10.737
79. Yimer, EM, Tuem, KB, Karim, A, Ur-Rehman, N, and Anwar, F. Nigella sativa L. (black cumin): a promising natural remedy for wide range of illnesses. Evid Based Complement Alternat Med. (2019) 2019:1–16. doi: 10.1155/2019/1528635
80. Goleva, TN, Rogov, AG, Korshunova, GA, Trendeleva, TA, Mamaev, DV, Aliverdieva, DA, et al. SkQThy, a novel and promising mitochondria-targeted antioxidant. Mitochondrion. (2019) 49:206–16. doi: 10.1016/j.mito.2019.09.001
81. Butt, MS, and Sultan, MT. Nigella sativa: reduces the risk of various maladies. Crit Rev Food Sci Nutr. (2010) 50:654–65. doi: 10.1080/10408390902768797
82. Mohammad, HE-M, El-Sayed, H, and Osman,. Morphological characters of Nigella sativa In: M, F.R.,editor. Black cumin (Nigella sativa) seeds: Chemistry, technology, functionality, and applications. Food bioactive ingredients. Cham., Switzerland: Springer (2021). 23–9. doi: 10.1007/978-3-030-48798-0_3
83. Ahmad, MDF, Ahmad, FA, Ashraf, SA, Saad, HH, Wahab, S, Khan, MI, et al. An updated knowledge of black seed (Nigella sativa Linn.): review of phytochemical constituents and pharmacological properties. J Herb Med. (2021) 25:100404–10. doi: 10.1016/j.hermed.2020.100404
84. Al-Oqail, MM, Al-Sheddi, ES, Al-Massarani, SM, Siddiqui, MA, Ahmad, J, Musarrat, J, et al. Nigella sativa seed oil suppresses cell proliferation and induces ROS dependent mitochondrial apoptosis through p53 pathway in hepatocellular carcinoma cells. S Afr J Bot. (2017) 112:70–8. doi: 10.1016/j.sajb.2017.05.019
85. Al-Saleh, IA, Billedo, G, and El-Doush, II. Levels of selenium, dl-α-tocopherol, dl-γ-tocopherol, all-trans-retinol, thymoquinone and thymol in different brands of Nigella sativa seeds. J Food Compos Anal. (2006) 19:167–75. doi: 10.1016/j.jfca.2005.04.011
86. Randhawa, MA, and Alghamdi, MS. Anticancer activity of Nigella sativa (black seed) — a review. Am J Chin Med. (2011) 39:1075–91. doi: 10.1142/S0192415X1100941X
87. FDA. § 182.10 spices and other natural seasonings and flavorings. Code of Federal Regulations In: Administration, F.A.D. Washington DC: US Government Printing Office (2016). 474–5.
88. FDA. Current good manufacturing practice in manufacturing, packaging, labeling, or holding operations for dietary supplements. Final Rule Fed Regist. (2007) 72:34751–958.
89. Williamson, EM, Liu, X, and Izzo, AA. Trends in use, pharmacology, and clinical applications of emerging herbal nutraceuticals. Br J Pharmacol. (2020) 177:1227–40. doi: 10.1111/bph.14943
90. Salehi, B, Quispe, C, Imran, M, Ul-Haq, I, Živković, J, Abu-Reidah, IM, et al. Nigella plants – traditional uses, bioactive phytoconstituents, preclinical and clinical studies. Front Pharmacol. (2021) 12:1–26. doi: 10.3389/fphar.2021.625386
92. Omar, B, Nusrat, J, Gousia, G, Naik, HR, Hussain, SZ, Monika, R, et al. Food applications of Nigella sativa seeds In: MF Ramadan, editor. Black cumin (Nigella sativa) seeds: Chemistry, technology, functionality, and applications. Switzerland: Springer, Cham (2021). 191–208.
93. Alsuhaibani, AMA. Effect of Nigella sativa against cisplatin induced nephrotoxicity in rats. Ital J Food Saf. (2018) 7:7242–2. doi: 10.4081/ijfs.2018.7242
94. Rana, MA, Arshad, MN, Siddiqui, SS, and Nasiruddin, M. Study of effect of Nigella sativa on prevention of nephrotoxicity induced by colistin in experimental animals. Int J Basic Clin Pharmacol. (2019) 8:306–11. doi: 10.18203/2319-2003.ijbcp20190151
95. Mahmoudvand, H, Sepahvand, A, Jahanbakhsh, S, Ezatpour, B, and Ayatollahi Mousavi, SA. Evaluation of antifungal activities of the essential oil and various extracts of Nigella sativa and its main component, thymoquinone against pathogenic dermatophyte strains. J Mycol Méd. (2014) 24:e155–61. doi: 10.1016/j.mycmed.2014.06.048
96. Rahman, MT. Potential benefits of combination of Nigella sativa and Zn supplements to treat COVID-19. J Herb Med. (2020) 23:100382. doi: 10.1016/j.hermed.2020.100382
97. Hossen, MJ, Yang, WS, Kim, D, Aravinthan, A, Kim, J-H, and Cho, JY. Thymoquinone: an IRAK1 inhibitor with in vivo and in vitro anti-inflammatory activities. Sci Rep. (2017) 7:42995. doi: 10.1038/srep42995
98. Mostofa, AGM, Kamal, HM, Basak, D, Sayeed, B, and Shahdaat, M. Thymoquinone as a potential adjuvant therapy for Cancer treatment: evidence from preclinical studies. Front Pharmacol. (2017) 8:295. doi: 10.3389/fphar.2017.00295
99. Herlina, A, Arifin, S, Kurniawati, A, and Faridah, DN. Changes of thymoquinone, thymol, and malondialdehyde content of black cumin (Nigella sativa L.) in response to Indonesia tropical altitude variation. HAYATI. J Biosci. (2017) 24:156–61. doi: 10.1016/j.hjb.2017.08.004
100. Mashayekhi-Sardoo, H, Rezaee, R, and Karimi, G. An overview of in vivo toxicological profile of thymoquinone. Toxin Rev. (2020) 39:115–22. doi: 10.1080/15569543.2018.1514637
101. Bordoni, L, Fedeli, D, Nasuti, C, Maggi, F, Papa, F, Wabitsch, M, et al. Antioxidant and anti-inflammatory properties of Nigella sativa oil in human pre-adipocytes. Antioxidants. (2019) 8:1–12. doi: 10.3390/antiox8020051
102. Ghourchian, A, Hajimehdipoor, H, Ara, L, Choopani, R, Kamalinejad, M, Salimzadeh, A, et al. Essential oil and fixed oil content of Nigella sativa after a traditional medicine processing-a comparative study. Biol Forum. (2016) 8:120–5.
103. Ali, A, Warsi, MH, Ahmad, W, Amir, M, Ahmad, N, Ali, A, et al. Thymoquinone: therapeutic potential and molecular targets In: DK Mahapatra, SG Talele, and AK Haghi, editors. Applied pharmaceutical science and microbiology: Novel green chemistry methods and natural products. Canada: Apple Academic Press (2020). 195–212.
104. Botnick, I, Xue, W, Bar, E, Ibdah, M, Schwartz, A, Joel, DM, et al. Distribution of primary and specialized metabolites in Nigella sativa seeds, a spice with vast traditional and historical uses. Molecules. (2012) 17:10159–77. doi: 10.3390/molecules170910159
105. Taborsky, J, Kunt, M, Kloucek, P, Lachman, J, Zeleny, V, and Kokoska, L. Identification of potential sources of thymoquinone and related compounds in Asteraceae, Cupressaceae, Lamiaceae, and Ranunculaceae families. Cent Eur J Chem. (2012) 10:1899–906. doi: 10.2478/s11532-012-0114-2
106. Sies, H. Hydroperoxides and thiol oxidants in the study of oxidative stress in intact cells and organs In: H Sies, editor. Oxidative stress. London, UK: Academic Press (1985). 73–90.
107. Sies, H. Oxidative stress: concept and some practical aspects. Antioxidants. (2020) 9:1–6. doi: 10.3390/antiox9090852
108. Lichtenberg, D, Pinchuk, I, Yonassi, E, Weber, D, and Grune, T. Oxidative stress is a concept, not an indication for selective antioxidant treatment. Antioxidants. (2023) 12:1–10. doi: 10.3390/antiox12061188
109. Ursini, F, Maiorino, M, and Forman, HJ. Redox homeostasis: the Golden mean of healthy living. Redox Biol. (2016) 8:205–15. doi: 10.1016/j.redox.2016.01.010
110. Watson, JD. Type 2 diabetes as a redox disease. Lancet. (2014) 383:841–3. doi: 10.1016/S0140-6736(13)62365-X
111. Pizzino, G, Irrera, N, Cucinotta, M, Pallio, G, Mannino, F, Arcoraci, V, et al. Oxidative stress: harms and benefits for human health. Oxidative Med Cell Longev. (2017) 2017:8416763. doi: 10.1155/2017/8416763
112. Wu, JQ, Kosten, TR, and Zhang, XY. Free radicals, antioxidant defense systems, and schizophrenia. Prog Neuro-Psychopharmacol Biol Psychiatry. (2013) 46:200–6. doi: 10.1016/j.pnpbp.2013.02.015
113. Ito, F, Sono, Y, and Ito, T. Measurement and clinical significance of lipid peroxidation as a biomarker of oxidative stress: oxidative stress in diabetes, atherosclerosis, and chronic inflammation. Antioxidants. (2019) 8:72. doi: 10.3390/antiox8030072
114. Radi, R. Oxygen radicals, nitric oxide, and peroxynitrite: Redox pathways in molecular medicine. Proceedings of the National Academy of Sciences. (2018) 115:5839–5848.
115. Zhang, P, Li, T, Wu, X, Nice, EC, Huang, C, and Zhang, Y. Oxidative stress and diabetes: Antioxidative strategies. Front Med. (2020) 14:583–600. doi: 10.1007/s11684-019-0729-1
116. Hasanuzzaman, Mirza, Bhuyan, M.H.M. Borhannuddin, Zulfiqar, Faisal, Raza, Ali, Mohsin, Sayed Mohammad, Mahmud, JubayerAl, et al. (2020). Reactive oxygen species and antioxidant defense in plants under abiotic stress: revisiting the crucial role of a universal defense regulator. Antioxidants 9, 1–52, doi: 10.3390/antiox9080681
117. Lawson, M, Jomova, K, Poprac, P, Kuča, K, Musílek, K, and Valko, M. Free radicals and antioxidants in human disease In: KHL Al-Gubory and Ismail, editors. Nutritional antioxidant therapies: Treatments and perspectives. Switzerland: Springer, Cham (2017). 283–305.
118. Collin, F. Chemical basis of reactive oxygen species reactivity and involvement in neurodegenerative diseases. Int J Mol Sci. (2019) 20:2407. doi: 10.3390/ijms20102407
119. Rehman, K, Akash, MS, and Hamid,. Mechanism of generation of oxidative stress and pathophysiology of type 2 diabetes mellitus: how are they interlinked? J Cell Biochem. (2017) 118:3577–85. doi: 10.1002/jcb.26097
120. Mahdi, A, Tengbom, J, Alvarsson, M, Wernly, B, Zhou, Z, and Pernow, J. Red blood cell peroxynitrite causes endothelial dysfunction in type 2 diabetes mellitus via arginase. Cells. (2020) 9:1–11.
121. Sies, H. Oxidative stress: a concept in redox biology and medicine. Redox Biol. (2015) 4:180–3. doi: 10.1016/j.redox.2015.01.002
122. Sies, H, Berndt, C, and Jones, DP. Oxidative stress. Annu Rev Biochem. (2017) 86:715–48. doi: 10.1146/annurev-biochem-061516-045037
123. Baynes, JW. Role of oxidative stress in development of complications in diabetes. Diabetes. (1991) 40:405–12. doi: 10.2337/diab.40.4.405
124. Ceriello, A, and Motz, E. Is oxidative stress the pathogenic mechanism underlying insulin resistance, diabetes, and cardiovascular disease? The common soil hypothesis revisited. Arterioscler Thromb Vasc Biol. (2004) 24:816–23. doi: 10.1161/01.ATV.0000122852.22604.78
125. Evans, JL, Goldfine, ID, Maddux, BA, and Grodsky, GM. Oxidative stress and stress-activated signaling pathways: a unifying hypothesis of type 2 diabetes. Endocr Rev. (2002) 23:599–622. doi: 10.1210/er.2001-0039
126. Unuofin, JO, and Lebelo, SL. Antioxidant effects and mechanisms of medicinal plants and their bioactive compounds for the prevention and treatment of type 2 diabetes: an updated review. Oxidative Med Cell Longev. (2020) 2020:1–36. doi: 10.1155/2020/1356893
127. Bandeira, DM, Suziy, DF, Lucas, JS, Guedes, DS, Glaucevane, R, Luíza, A, et al. Oxidative stress as an underlying contributor in the development of chronic complications in diabetes mellitus. Int J Mol Sci. (2013) 14:3265–84. doi: 10.3390/ijms14023265
128. Testa, R, Bonfigli, AR, Prattichizzo, F, Sala, L, Lucia, DN, Valeria, C, et al. The “metabolic memory” theory and the early treatment of hyperglycemia in prevention of diabetic complications. Nutrients. (2017) 9:1–9. doi: 10.3390/nu9050437
129. Khan, AN, Khan, RA, Ahmad, M, and Mushtaq, N. Role of antioxidant in oxidative stress and diabetes mellitus. J Pharm Phytochemistry. (2015) 3:217–20.
130. Zorawar, S, Indrakaran, PK, Pramjit, S, and Rupinder, K. Use of malondialdehyde as a biomarker for assessing oxidative stress in different disease pathologies: a review. Iran J Public Health. (2015) 43:7–16.
131. Arif, S, Moore, F, Marks, K, Bouckenooghe, T, Dayan, CM, Planas, R, et al. Peripheral and islet interleukin-17 pathway activation characterizes human autoimmune diabetes and promotes cytokine-mediated β-cell death. Diabetes. (2011) 60:2112–9. doi: 10.2337/db10-1643
132. Ting, HH, Timimi, FK, Boles, KS, Creager, SJ, Ganz, P, and Creager, MA. Vitamin C improves endothelium-dependent vasodilation in patients with non-insulin-dependent diabetes mellitus. J Clin Invest. (1996) 97:22–8. doi: 10.1172/JCI118394
133. Pasupuleti, VR, Arigela, CS, Gan, SH, Salam, SK, Nainamohamed, K, Thevan, K, et al. A review on oxidative stress, diabetic complications, and the roles of honey polyphenols. Oxidative Med Cell Longev. (2020) 2020:8878172. doi: 10.1155/2020/8878172
134. Chen, J, Jing, J, Yu, S, Song, M, Tan, H, Cui, B, et al. Advanced glycation endproducts induce apoptosis of endothelial progenitor cells by activating receptor RAGE and NADPH oxidase/JNK signaling axis. Am J Transl Res. (2016) 8:2169–78.
135. Giacco, F, Brownlee, M, and Schmidt, AM. Oxidative stress and diabetic complications. Circ Res. (2010) 107:1058–70. doi: 10.1161/CIRCRESAHA.110.223545
136. Bongarzone, S, Savickas, V, Luzi, F, and Gee, AD. Targeting the receptor for advanced glycation endproducts (RAGE): a medicinal chemistry perspective. J Med Chem. (2017) 60:7213–32. doi: 10.1021/acs.jmedchem.7b00058
137. Shen, C, Li, Q, Zhang, YC, Ma, G, Feng, Y, Zhu, Q, et al. Advanced glycation endproducts increase EPC apoptosis and decrease nitric oxide release via MAPK pathways. Biomed Pharmacother. (2010) 64:35–43. doi: 10.1016/j.biopha.2009.03.002
138. Vlassara, H, and Uribarri, J. Advanced glycation end products (age) and diabetes: cause, effect, or both? Curr Diab Rep. (2013) 14:1–10. doi: 10.1007/s11892-013-0453-1
139. Mailloux, RJ. An update on mitochondrial reactive oxygen species production. Antioxidants. (2020) 9:1–14. doi: 10.3390/antiox9060472
140. Ceriello, A, and Testa, R. Antioxidant anti-inflammatory treatment in type 2 diabetes. Diabetes Care. (2009) 32:S232–6. doi: 10.2337/dc09-S316
141. Blasiak, J, Arabski, M, Krupa, R, Wozniak, K, Zadrozny, M, Kasznicki, J, et al. DNA damage and repair in type 2 diabetes mellitus. Mutation Res. (2004) 554:297–304. doi: 10.1016/j.mrfmmm.2004.05.011
142. Kumar, A, Yerra, VG, and Malik, RA. Mitochondrial hormesis and diabetic complications. Diabetes. (2015) 64:663–72. doi: 10.2337/db14-0874
143. Ristow, M. Unraveling the truth about antioxidants: Mitohormesis explains ROS-induced health benefits. Nat Med. (2014) 20:709–11. doi: 10.1038/nm.3624
144. Haes, Wouter De, Frooninckx, Lotte, Assche, Roel Van, Smolders, Arne, Depuydt, Geert, Billen, Johan, et al., (2014). Metformin promotes lifespan through mitohormesis via the peroxiredoxin PRDX-2. Proc Natl Acad Sci 111, E2501–E2509, doi: 10.1073/pnas.1321776111
145. Tuell, DS, Los, EA, Ford, GA, and Stone, WL. The role of natural antioxidant products that optimize redox status in the prevention and management of type 2 diabetes. Antioxidants. (2023) 12:1–19. doi: 10.3390/antiox12061139
146. Usta, A, and Dede, S. The effect of thymoquinone on nuclear factor kappa b levels and oxidative dna damage on experimental diabetic rats. Pharmacogn Mag. (2017) 13:S458–61. doi: 10.4103/pm.pm_134_17
147. Pai, S, and Thomas, R. Immune deficiency or hyperactivity-Nf-κb illuminates autoimmunity. J Autoimmun. (2008) 31:245–51. doi: 10.1016/j.jaut.2008.04.012
148. Pannu, A, Goyal, RK, Ojha, S, and Nandave, M. Therapeutic potential of thymoquinone in treatment of rheumatoid arthritis and related autoimmune diseases In: RR Watson and VR Preedy, editors. Bioactive food as dietary interventions for arthritis and related inflammatory diseases academic press-Elsevier. USA: CA (2019). 575–87. doi: 10.1016/B978-0-12-813820-5.00033-7
149. Abbasnezhad, A, Niazmand, S, Mahmoudabady, M, Rezaee, SA, Soukhtanloo, M, Mosallanejad, R, et al. Nigella sativa L. seed regulated eNOS, VCAM-1 and LOX-1 genes expression and improved vasoreactivity in aorta of diabetic rat. J Ethnopharmacol. (2019) 228:142–7. doi: 10.1016/j.jep.2018.09.021
150. Abdelrazek, HMA, Kilany, OE, Muhammad, AA, Tag, HM, and Abdelazim, AM. Black seed thymoquinone improved insulin secretion, hepatic glycogen storage, and oxidative stress in Streptozotocin-induced diabetic male Wistar rats. Oxidative Med Cell Longev. (2018) 2018:1–10. doi: 10.1155/2018/8104165
151. Askari, G, Rouhani, MH, Ghaedi, E, Ghavami, A, Nouri, M, and Mohammadi, H. Effect of Nigella sativa (black seed) supplementation on glycemic control: a systematic review and meta-analysis of clinical trials. Phytother Res. (2019) 33:1341–52. doi: 10.1002/ptr.6337
152. Hassan, NM, El-Ameen, MM, Elhassan, T, Ibrahim, S, Abdelwahab, A, Khalid, F, et al. Anti-diabetic properties of thymoquinone is unassociated with glycogen phosphorylase inhibition. Pharm J. (2015) 7:406–10. doi: 10.5530/pj.2015.6.16
153. Pelegrin, S, Galtier, F, Chalançon, A, Gagnol, J-P, Barbanel, A-M, Pélissier, Y, et al. Effects of Nigella sativa seeds (black cumin) on insulin secretion and lipid profile: a pilot study in healthy volunteers. Br J Clin Pharmacol. (2019) 85:1607–11. doi: 10.1111/bcp.13922
154. Sultan, MT, Butt, MS, Karim, R, Zia-Ul-Haq, M, Batool, R, Ahmad, S, et al. Nigella sativa fixed and essential oil supplementation modulates hyperglycemia and allied complications in Streptozotocin-induced diabetes mellitus. Evid Based Complement Alternat Med. (2014) 2014:1–8. doi: 10.1155/2014/826380
155. Sadiq, Neeraj, Subhani, Ghulam, Fatima, Syeda Ayesha, Nadeem, M., Zafer, Shaima, and Mohsin, M., (2021). Antidiabetic effect of Nigella sativa compared with metformin on blood glucose levels in streptozotocin induced diabetic albino wistar rats 10(4), 7.
156. Alimohammadi, S, Hobbenaghi, R, Javanbakht, J, Kheradmand, D, Mortezaee, R, Tavakoli, M, et al. RETRACTED ARTICLE: protective and antidiabetic effects of extract from Nigella sativa on blood glucose concentrations against Streptozotocin (STZ)-induced diabetic in rats: an experimental study with histopathological evaluation. Diagn Pathol. (2013) 8:1–7. doi: 10.1186/1746-1596-8-137
157. Kanter, M, Akpolat, M, and Aktas, C. Protective effects of the volatile oil of Nigella sativa seeds on β-cell damage in streptozotocin-induced diabetic rats: a light and electron microscopic study. J Mol Histol. (2009) 40:379–85. doi: 10.1007/s10735-009-9251-0
158. Mansi, KMS. Effects of oral administration of water extract of Nigella sativa on serum concentrations of insulin and testosterone in alloxan-induced diabetic rats. Pak J Biol Sci. (2005) 8:1152–6. doi: 10.3923/pjbs.2005.1152.1156
159. Montazeri, RS, Fatahi, S, Sohouli, MH, Abu-Zaid, A, Santos, HO, Găman, M-A, et al. The effect of nigella sativa on biomarkers of inflammation and oxidative stress: a systematic review and meta-analysis of randomized controlled trials. J Food Biochem. (2021) 45:e13625. doi: 10.1111/jfbc.13625
160. Benhaddou-Andaloussi, A, Martineau, LC, Spoor, D, Vuong, T, Leduc, C, Joly, E, et al. Antidiabetic activity of Nigella sativa. Seed extract in cultured pancreatic β-cells, skeletal muscle cells, and adipocytes. Pharm Biol. (2008) 46:96–104. doi: 10.1080/13880200701734810
161. Fararh, K, Atoji, Y, Shimizu, Y, and Takewaki, T. Isulinotropic properties of Nigella sativa oil in Streptozotocin plus nicotinamide diabetic hamster. Res Vet Sci. (2002) 73:279–82. doi: 10.1016/S0034-5288(02)00108-X
162. Farkhondeh, T, Samarghandian, S, and Borji, A. An overview on cardioprotective and anti-diabetic effects of thymoquinone. Asian Pac J Trop Med. (2017) 10:849–54. doi: 10.1016/j.apjtm.2017.08.020
163. Meddah, B, Ducroc, R, Faouzi, EA, Moulay, E, Bruno, M, Lahcen, B-A, et al. Nigella sativa inhibits intestinal glucose absorption and improves glucose tolerance in rats. J Ethnopharmacol. (2009) 121:419–24. doi: 10.1016/j.jep.2008.10.040
164. Rtibi, K, Selmi, S, Balti, R, Marzouki, L, and Sebai, H. Natural bioactive compounds with small-bowel glucose/anti-absorption and sugar digestion enzymes’ inhibition actions: new strategy to relieve hyperglycemia and diabetes. Recent Adv Biol Med. (2019) 5:1–3. doi: 10.18639/RABM.2019.879443
165. Tiji, S, Bouhrim, M, Addi, M, Drouet, S, Lorenzo, JM, Hano, C, et al. Linking the phytochemicals and the α-glucosidase and α-amylase enzyme inhibitory effects of Nigella sativa seed extracts. Foods. (2021) 10:1–19.
166. Wafai, A, and Rana, J. Nigella sativa and thymoquinone suppress cyclooxygenase-2 and oxidative stress in pancreatic tissue of Streptozotocin-induced diabetic rats. Pancreas. (2013) 42:841–9. doi: 10.1097/MPA.0b013e318279ac1c
167. Nehar, S, Rani, P, and Kumar, C. Evaluation of genoprotective and antioxidative potentiality of ethanolic extract of N. sativa seed in Streptozotocin induced diabetic albino rats. Vegetos. (2021) 34:453–9. doi: 10.1007/s42535-021-00201-5
168. Bamosa, Abdullah O., Kaatabi, Huda, Lebdaa, Fatma M., Elq, Abdul-MuhssenAl, and Al-Sultanb, Ali, (2010). Effect of Nigella sativa seeds on the glycemic control of patients with type 2 diabetes mellitus. Indian J Physiol Pharmacol 54, 344–354
169. Kaatabi, H, Bamosa, AO, Badar, A, Al-Elq, A, Abou-Hozaifa, B, Lebda, F, et al. Nigella sativa improves glycemic control and ameliorates oxidative stress in patients with type 2 diabetes mellitus: placebo controlled participant blinded clinical trial. PLoS One. (2015) 10:1–15.
170. Heshmati, J, Namazi, N, Memarzadeh, M-R, Taghizadeh, M, and Kolahdooz, F. Nigella sativa oil affects glucose metabolism and lipid concentrations in patients with type 2 diabetes: a randomized, double-blind, placebo-controlled trial. Food Res Int. (2015) 70:87–93. doi: 10.1016/j.foodres.2015.01.030
171. Hossen, J, Ali, MA, and Reza, S. Theoretical investigations on the antioxidant potential of a non-phenolic compound thymoquinone: a DFT approach. J Mol Model. (2021) 27:173. doi: 10.1007/s00894-021-04795-0
172. Abdollahi, N, Nadjarzadeh, A, Salehi-Abargouei, A, Fallahzadeh, H, Razmpoosh, E, Lorzaedeh, E, et al. The effect of Nigella sativa on TAC and MDA in obese and overweight women: secondary analysis of a crossover, double blind, randomized clinical trial. J Diabetes Metab Disord. (2022) 21:171–9. doi: 10.1007/s40200-021-00954-5
173. Mohit, M, Farrokhzad, A, Faraji, SN, Heidarzadeh-Esfahani, N, and Kafeshani, M. Effect of Nigella sativa L. supplementation on inflammatory and oxidative stress indicators: a systematic review and meta-analysis of controlled clinical trials. Complement Ther Med. (2020) 54:102535–10. doi: 10.1016/j.ctim.2020.102535
174. Moustafa, HA, Mohamed, EW, Mohamed, L, Halawa, MR, Sabri, NA, El-Bahy, AZ, et al. Effect of Nigella Sativa oil versus metformin on glycemic control and biochemical parameters of newly diagnosed type 2 diabetes mellitus patients. Endocrine. (2019) 65:286–94. doi: 10.1007/s12020-019-01963-4
175. Daryabeygi-Khotbehsara, R, Golzarand, M, Ghaffari, MP, and Djafarian, K. Nigella sativa improves glucose homeostasis and serum lipids in type 2 diabetes: a systematic review and meta-analysis. Complement Ther Med. (2017) 35:6–13. doi: 10.1016/j.ctim.2017.08.016
176. Alshahrani, S, Anwer, T, Alam, MF, Ahmed, RA, Khan, G, Sivakumar, SM, et al. Effect of thymoquinone on high fat diet and STZ-induced experimental type 2 diabetes: a mechanistic insight by in vivo and in silico studies. J Food Biochem. (2021) 45:e13807. doi: 10.1111/jfbc.13807
177. El-Aarag, B, Hussein, W, Ibrahim, W, and Zahran, M. Thymoquinone improves anti-diabetic activity of metformin in streptozotocin-induced diabetic male rats. J Diabetes Metab. (2017) 8:780
178. Freitas, HS, Schaan, BD’A, Seraphim, PM, Nunes, MT, and Machado, UF. Acute and short-term insulin-induced molecular adaptations of GLUT2 gene expression in the renal cortex of diabetic rats. Mol Cell Endocrinol. (2005) 237:49–57. doi: 10.1016/j.mce.2005.03.005
179. Nader, MA, El-Agamy, DS, and Suddek, GM. Protective effects of propolis and thymoquinone on development of atherosclerosis in cholesterol-fed rabbits. Arch Pharm Res. (2010) 33:637–43. doi: 10.1007/s12272-010-0420-1
180. Ragheb, A, Elbarbry, F, Prasad, K, Mohamed, A, Ahmed, MS, and Shoker, A. Attenuation of the development of hypercholesterolemic atherosclerosis by thymoquinone. Int J Angiol. (2008) 17:186–92. doi: 10.1055/s-0031-1278307
181. Bensiameur-Touati, K, Kacimi, G, Haffaf, E-M, Berdja, S, and Aouichat-Bouguerra, S. In vivo subacute toxicity and antidiabetic effect of aqueous extract of Nigella sativa. Evid Based Complement Alternat Med. (2017) 2017:1–13. doi: 10.1155/2017/8427034
182. Iqbal, MS, Ahmad, A, and Pandey, B. Solvent based optimization for extraction and stability of thymoquinone from Nigella sativa Linn. And its quantification using RP-HPLC. Physiol Mol Biol Plants. (2018) 24:1209–19. doi: 10.1007/s12298-018-0593-5
183. Salem, ML. Immunomodulatory and therapeutic properties of the Nigella sativa L. seed. Int Immunopharmacol. (2005) 5:1749–70. doi: 10.1016/j.intimp.2005.06.008
184. Hamdan, A, Idrus, H, Ruszymah, M, and Helmy, M. Effects of Nigella Sativa on Type-2 diabetes mellitus: a systematic review. Int J Environ Res Public Health. (2019) 16:4911. doi: 10.3390/ijerph16244911
185. El-Shamy, KA, Mosa, MMA, El-Nabarawy, SK, and El-Qattan, GM. Effect of Nigella sativa tea in type 2-diabetic patients as regards glucose homeostasis, liver and kidney functions. J Appl Sci Res. (2011) 7:2524–34.
186. Memon, AR, Shah, SS, Memon, AR, and Naqvi, SHR. Effect of combination of Nigella sativa and Trigonella foenum-graecum with glibenclamide on serum triglyceride, HDL and creatinine levels in type-2 diabetes mellitus patients. Pak J Pharmacol. (2012) 29:1–6.
187. Hadi, S, Daryabeygi-Khotbehsara, R, Mirmiran, P, McVicar, J, Hadi, V, Soleimani, D, et al. Effect of Nigella sativa oil extract on cardiometabolic risk factors in type 2 diabetes: a randomized, double-blind, placebo-controlled clinical trial. Phytother Res. (2021) 35:3747–55. doi: 10.1002/ptr.6990
188. Najmi, A, Haque, SF, Naseeruddin, M, and Khan, RA. Effect of Nigella sativa oil on various clinical and biochemical parameters of metabolic syndrome. Int J Diabetes Metabol. (2008) 16:85–7.
189. Bilal, A, Masud, T, Uppal, AM, and Naveed, AK. Effects of Nigella sativa oil on some blood parameters in type 2 diabetes mellitus patients. Asian J Chem. (2009) 21:5373–81.
190. Bilal, A, Masud, T, and Uppal, AM. BS5-5 black seed (Nigella sativa) regulates glucose, insulin level and lipid profile in patients with type 2 diabetes. Diabetes Res Clin Pract. (2008) 79:S19–20. doi: 10.1016/S0168-8227(08)70696-X
191. Allah, HA, Khalaf, M, Allah, G, Elkarim, A, and Abdrabo, A. Biochemical effect of Nigella sativa (black cumin) on glucose and lipid profile among sudanese diabetic patients. Open Access Libr J. (2021) 8:1–8. doi: 10.4236/oalib.1107500
192. Hosseini, MS, Mirkarimi, SA, Amini, M, Mohtashami, R, Kianbakht, S, and Fallah, HH. Effects of Nigella sativa L. seed oil in type ii diabetic patients: a randomized, double-blind, placebo - controlled clinical trial. J Med Plants. (2013) 12:93–9.
193. Mostafa, TM, Hegazy, SK, Elnaidany, SS, Shehabeldin, WA, and Sawan, ES. Nigella sativa as a promising intervention for metabolic and inflammatory disorders in obese prediabetic subjects: a comparative study of Nigella sativa versus both lifestyle modification and metformin. J Diabetes Complicat. (2021) 35:107947–10. doi: 10.1016/j.jdiacomp.2021.107947
194. Amin, F, Islam, N, Anila, N, and Gilani, AH. Clinical efficacy of the co-administration of turmeric and black seeds (Kalongi) in metabolic syndrome – a double blind randomized controlled trial – TAK-MetS trial. Complement Ther Med. (2015) 23:165–74. doi: 10.1016/j.ctim.2015.01.008
195. Najmi, A, Nasiruddin, M, Khan, RA, and Haque, S. Therapeutic effect of Nigella sativa in patients of poor glycemic control. Asian J Pharm Clin Res. (2012) 5:224–8.
196. Al-amri, AM, and Bamosa, AO. Phase I safety and clinical activity study of thymoquinone in patients with advanced refractory malignant disease. Shiraz E-Med J. (2009) 10:107–11.
197. Thomas, JV, Mohan, ME, Prabhakaran, P, Das, S, Syam, M, and Krishnakumar, BIM. A phase I clinical trial to evaluate the safety of thymoquinone-rich black cumin oil (BlaQmax®) on healthy subjects: randomized, double-blinded, placebo-controlled prospective study. Toxicol Rep. (2022) 9:999–1007. doi: 10.1016/j.toxrep.2022.04.020
198. Atta, MS, Almadaly, EA, El-Far, AH, Saleh, RM, Assar, DH, Jaouni, A, et al. Thymoquinone defeats diabetes-induced testicular damage in rats targeting antioxidant, inflammatory and aromatase expression. Int J Mol Sci. (2017) 18:1–15. doi: 10.3390/ijms18050919
199. Aboul-Mahasen, L, and Abdulrahman Alshali, R. The possible protective effects of virgin olive oil and Nigella sativa seeds on the biochemical and histopathological changes in pancreas of hyperlipidaemic rats. Folia Morphol (Warsz). (2019) 78:762–72. doi: 10.5603/FM.a2019.0017
200. El-Aarag, B, Hussein, W, Ibrahim, W, and Zahran, M. Thymoquinone improves anti-diabetic activity of metformin in Streptozotocin-induced diabetic male rats. J Diab Metabol. (2017) 8:1–8. doi: 10.4172/2155-6156.1000780
201. Sadiq, N, Subhani, G, Fatima, SA, Nadeem, M, Zafer, S, and Mohsin, M. Antidiabetic effect of Nigella sativa compared with metformin on blood glucose levels in Streptozotocin induced diabetic albino Wistar rats. Int J Basic Clin Pharmacol. (2021) 10:361–7. doi: 10.18203/2319-2003.ijbcp20211016
202. Dong, J, Liang, Q, Niu, Y, Jiang, S, Zhou, L, Wang, J, et al. Effects of Nigella sativa seed polysaccharides on type 2 diabetic mice and gut microbiota. Int J Biol Macromol. (2020) 159:725–38. doi: 10.1016/j.ijbiomac.2020.05.042
203. Faisal, L, Mohamed, A-M, Hafez, A-M, Alsharidah, AS, Mobark, MA, Abdellatif, AAH, et al. Thymoquinone lowers blood glucose and reduces oxidative stress in a rat model of diabetes. Molecules. (2021) 26:1–13.
204. Safhi, MM, Qumayri, HM, Masmali, AUM, Siddiqui, R, Alam, MF, Khan, G, et al. Thymoquinone and fluoxetine alleviate depression via attenuating oxidative damage and inflammatory markers in type-2 diabetic rats. Arch Physiol Biochem. (2019) 125:150–5. doi: 10.1080/13813455.2018.1443141
205. Rani, R, Dahiya, S, Dhingra, D, Dilbaghi, N, Kim, K-H, and Kumar, S. Improvement of antihyperglycemic activity of nano-thymoquinone in rat model of type-2 diabetes. Chem Biol Interact. (2018) 295:119–32. doi: 10.1016/j.cbi.2018.02.006
206. Rani, R, Dahiya, S, Dhingra, D, Dilbaghi, N, Kaushik, A, Kim, K-H, et al. Antidiabetic activity enhancement in Streptozotocin + nicotinamide-induced diabetic rats through combinational polymeric nanoformulation. Int J Nanomedicine. (2019) 14:4383–95. doi: 10.2147/IJN.S205319
207. Lee, SP, Kuo, FY, Cheng, J-T, and Wu, MC. Thymoquinone activates imidazoline receptor to enhance glucagon-like peptide-1 secretion in diabetic rats. Arch Med Sci. (2019). doi: 10.5114/aoms.2019.86938
208. Hannan, JMA, Ansari, P, Haque, A, Sanju, A, Huzaifa, A, Rahman, A, et al. Nigella sativa stimulates insulin secretion from isolated rat islets and inhibits the digestion and absorption of (CH2O)n in the gut. Biosci Rep. (2019) 39:1–10. doi: 10.1042/BSR20190723
209. Sri, Sutrisna Em, and Tanti, Aziza; Wahyun, (2022). Potency of Nigella sativa Linn. Seed as antidiabetic (preclinical study). Res J Pharm Technol 15, 381–384, doi: 10.52711/0974-360X.2022.00062
210. Amatu Chisom, S, Nwaka Chinyere, S, and Nwaka Andrew, C. Evaluation of the effect of black seed (Nigella sativa) on oxidative stress markers of Alloxan–induced diabetic wistar rat. IAA J Biol Sci. (2022) 8:164–77.
211. Akhtar, MT, Ilyas, HF, Shaukat, UA, Qadir, R, Masood, S, Batool, S, et al. Comparative study of hypoglycaemic and antioxidant potential of methanolic seed extract and oil of Nigella sativa on alloxanized diabetic rabbits. Pak J Pharm Sci. (2022) 35:1755–60.
212. Khan, SS, and Zaidi, KU. Protective effect of Nigella sativa seed extract and its bioactive compound Thymoquinone on Streptozotocin-induced diabetic rats. Cardiovasc Hematol Agents Med Chem. (2022). doi: 10.2174/1871525721666221221161742
213. Tariq, SM, Khan, K, Sadiq, MM, Pooja, S, Suyog, S, and Devendra, SK. Nigella Sativa’s effect on biochemical as well as anthropometric parameters in diabetic rats on high fat diet. J Med Sci Health. (2023) 9:16–22. doi: 10.46347/jmsh.v9i1.21.253
214. Ayaz, H, Kaya, S, Seker, U, and Nergiz, Y. Comparison of the anti-diabetic and nephroprotective activities of vitamin E, metformin, and Nigella sativa oil on kidney in experimental diabetic rats. Iran J Basic Med Sci. (2023) 26:395–9. doi: 10.22038/IJBMS.2023.68051.14876
215. Erisgin, Z, Atasever, M, Cetinkaya, K, Dizakar, A, Özen, S, Omeroglu, S, et al. Protective effects of Nigella sativa oil against carboplatin-induced liver damage in rats. Biomed Pharmacother. (2019) 110:742–7. doi: 10.1016/j.biopha.2018.12.037
216. Al-Rasheed, M, Nawal Fadda, L, Al-Rasheed, NM, Hasan, IH, Hanaa, MA, Al-Fayez, M, et al. Hepatoprotective role of α-lipoic acid and thymoquinone in acetaminophen-induced liver injury: Down-regulation of COX-2 and flt-1 expression. Braz Arch Biol Technol. (2017) 60:1–12. doi: 10.1590/1678-4324-2017160703
217. Pop, RM, Sabin, O, Suciu, Ș, Vesa, SC, Socaci, SA, Chedea, VS, et al. Nigella sativa’s anti-inflammatory and antioxidative effects in experimental inflammation. Antioxidants. (2020) 9:1–13. doi: 10.3390/antiox9100921
218. Ramalingam, K, Kuttan, R, Sivadasan, SD, Ittiyavirah, S, and Madhavamenon, KI. Safety assessment of thymoquinone rich black cumin (Nigella sativa) oil (BlaQmax®): acute and sub chronic toxicity studies. J Nutr Food Sci. (2021) 11:1–8.
219. Gholamnezhad, Z, Havakhah, S, and Boskabady, MH. Preclinical and clinical effects of Nigella sativa and its constituent, thymoquinone: a review. J Ethnopharmacol. (2016) 190:372–86. doi: 10.1016/j.jep.2016.06.061
220. Tavakkoli, A, Mahdian, V, Razavi, BM, and Hosseinzadeh, H. Review on clinical trials of black seed (Nigella sativa) and its active constituent, thymoquinone. J Pharm. (2017) 20:179–93. doi: 10.3831/KPI.2017.20.021
221. Cui, B-W, Bai, T, Yang, Y, Zhang, Y, Jiang, M, Yang, H-X, et al. Thymoquinone attenuates acetaminophen overdose-induced acute liver injury and inflammation via regulation of JNK and AMPK signaling pathway. Am J Chin Med. (2019) 47:577–94. doi: 10.1142/S0192415X19500307
222. Rahmani, A, Maleki, V, Niknafs, B, Tavakoli-Rouzbehani, OM, and Tarighat-Esfanjani, A. Effect of Nigella sativa supplementation on kidney function, glycemic control, oxidative stress, inflammation, quality of life, and depression in diabetic hemodialysis patients: study protocol for a double-blind, randomized controlled trial. Trials. (2022) 23:1–9. doi: 10.1186/s13063-021-05917-y
223. Asgary, S, Najafi, S, Ghannadi, A, Dashti, G, and Helalat, A. Efficiency of black cumin seeds on hematological factors in normal and hypercholesterolemic rabbits. ARYA Atheroscler. (2012) 7:146–50.
224. Tahir, E, Kamal, EH, Ashour, MMS, and Al-Harbi, MM. The respiratory effects of the volatile oil of the black seed (Nigella sativa) in guinea-pigs: elucidation of the mechanism(s) of action. Gen Pharmacol Vasc S. (1993) 24:1115–22. doi: 10.1016/0306-3623(93)90358-5
225. Akbar, S. “Nigella sativa” (black seeds): panacea or hyperbole?: a critical review of experimental and clinical observations. Aus J Herb Naturopath Med. (2018) 30:157–72.
226. Hermann, R, and von Richter, O. Clinical evidence of herbal drugs as perpetrators of pharmacokinetic drug interactions. Planta Med. (2012) 78:1458–77. doi: 10.1055/s-0032-1315117
227. Elbarbry, F, Ung, A, and Abdelkawy, K. Studying the inhibitory effect of quercetin and thymoquinone on human cytochrome P450 enzyme activities. Pharmacogn Mag. (2018) 13:S895–9. doi: 10.4103/0973-1296.224342
228. Tekbas, A, Huebner, J, Settmacher, U, and Dahmen, U. Plants and surgery: the protective effects of thymoquinone on hepatic injury—a systematic review of in vivo studies. Int J Mol Sci. (2018) 19:1–26.
229. Saadia, M, Sher, M, Bashir, S, Murtaza, MA, Shah, A, and Khan, MA. Comparative hepatoprotective effect of Nigella sativa pre-and post-treatment to rabbits. Pak J Pharm Sci. (2019) 32:205–12.
230. Maes, M, Vinken, M, and Jaeschke, H. Experimental models of hepatotoxicity related to acute liver failure. Toxicol Appl Pharmacol. (2016) 290:86–97. doi: 10.1016/j.taap.2015.11.016
231. Mollazadeh, H, and Hosseinzadeh, H. The protective effect of Nigella sativa against liver injury: a review. Iran J Basic Med Sci. (2014) 17:958–66.
232. Tavakkoli, A, Ahmadi, A, Razavi, BM, and Hosseinzadeh, H. Black seed (Nigella Sativa) and its constituent thymoquinone as an antidote or a protective agent against natural or chemical toxicities. Iran J Pharm Res. (2017) 16:2–23.
233. Ostadpoor, M, and Gholami-Ahangaran, M. A review on hepatoprotective effects of Nigella sativa L. J Med Herb. (2021) 12:49–54.
234. Saleem, AM, Gani, S, and Ahmed, J. Evaluation of hepatoprotective effect of Nigella sativa L. Int J Pharm Pharm Sci. (2013) 5:428–30.
235. El-Gharieb, MA, El-Masry, TA, Emara, AM, and Hashem, MA. Potential hepatoprotective effects of vitamin E and Nigella sativa oil on hepatotoxicity induced by chronic exposure to malathion in human and male albino rats. Toxicol Environ Chem. (2010) 92:391–407. doi: 10.1080/02772240902955719
236. Al-Suhaimi, EA. Hepatoprotective and immunological functions of Nigella sativa seed oil against hypervitaminosis a in adult male rats. Int J Vitam Nutr Res. (2012) 82:288–97. doi: 10.1024/0300-9831/a000121
237. Attia, A, Ragheb, A, Sylwestrowicz, T, and Shoker, A. Attenuation of high cholesterol-induced oxidative stress in rabbit liver by thymoquinone. Eur J Gastroenterol Hepatol. (2010) 22:826–34. doi: 10.1097/MEG.0b013e328336000d
238. Parthasarathy, M, and Sabina, EP. The potential effect of phytochemicals and herbal plant remedies for treating drug-induced hepatotoxicity: a review. Mol Biol Rep. (2021) 48:4767–88. doi: 10.1007/s11033-021-06444-4
239. Khan, A, Chen, HC, Tania, M, and Zhang, DZ. Anticancer activities of Nigella sativa (black cumin). Afr J Tradit Complement Altern Med. (2011) 8:226–32. doi: 10.4314/ajtcam.v8i5S.10
240. Ke, L, Lu, C, Shen, R, Lu, T, Ma, B, and Hua, Y. Knowledge mapping of drug-induced liver injury: a scientometric investigation (2010-2019). Front Pharmacol. (2020) 11:842–2. doi: 10.3389/fphar.2020.00842
241. Blackford, MG, Felter, T, Gothard, MD, and Reed, MD. Assessment of the clinical use of intravenous and oral n-acetylcysteine in the treatment of acute acetaminophen poisoning in children: a retrospective review. Clin Ther. (2011) 33:1322–30. doi: 10.1016/j.clinthera.2011.08.005
242. Dawson, AH, Henry, DA, and McEwen, J. Adverse reactions to N-acetylcysteine during treatment for paracetamol poisoning. Med J Aust. (1989) 150:329–31. doi: 10.5694/j.1326-5377.1989.tb136496.x
243. Waring, WS. Novel acetylcysteine regimens for treatment of paracetamol overdose. Therap Adv Drug Safety. (2012) 3:305–15. doi: 10.1177/2042098612464265
244. Razmpoosh, E, Safi, S, Abdollahi, N, Nadjarzadeh, A, Nazari, M, Fallahzadeh, H, et al. The effect of Nigella sativa on the measures of liver and kidney parameters: a systematic review and meta-analysis of randomized-controlled trials. Pharmacol Res. (2020) 156:104767. doi: 10.1016/j.phrs.2020.104767
245. Salehi, B, Martorell, M, Arbiser, JL, Sureda, A, Martins, N, Maurya, PK, et al. Antioxidants: positive or negative actors? Biomol Ther. (2018) 8:1–11. doi: 10.3390/biom8040124
246. Balbi, ME, Tonin, FS, Mendes, AM, Borba, HH, Wiens, A, Fernandez-Llimos, F, et al. Antioxidant effects of vitamins in type 2 diabetes: a meta-analysis of randomized controlled trials. Diabetol Metab Syndr. (2018) 10:1–12. doi: 10.1186/s13098-018-0318-5
247. Beeraka, MN, Tomilova, KI, Batrak, AG, Zhaburina, VM, Nikolenko, NV, Sinelnikov, YM, et al. Recent insights into the nutritional antioxidant therapy in prevention and treatment of diabetic vascular complications: a comprehensive review. Curr Med Chem. (2021) 28:1–16.
248. El-Abhar, HS, and Schaalan, MF. Phytotherapy in diabetes: review on potential mechanistic perspectives. World J Diabetes. (2014) 5:176–97. doi: 10.4239/wjd.v5.i2.176
249. Nasri, H, Shirzad, H, Baradaran, A, and Rafieian-Kopaei, M. Antioxidant plants and diabetes mellitus. J Res Med Sci. (2015) 20:491–502. doi: 10.4103/1735-1995.163977
250. Singh, G, Marimuthu, P, de Heluani, CS, and Catalan, C. Chemical constituents and antimicrobial and antioxidant potentials of essential oil and acetone extract of Nigella sativa seeds. J Sci Food Agric. (2005) 85:2297–306. doi: 10.1002/jsfa.2255
251. Aydin, E, and Kart, A. Health promoting activities of Nigella sativa seeds In: M, F.R., editor. Black cumin (Nigella sativa) seeds: Chemistry, technology, functionality, and applications. Cham. Switzerland: Springer (2021). 153–77.
252. Sankaranarayanan, C, and Pari, L. Thymoquinone ameliorates chemical induced oxidative stress and β-cell damage in experimental hyperglycemic rats. Chem Biol Interact. (2011) 190:148–54. doi: 10.1016/j.cbi.2011.02.029
253. Ardiana, M, Pikir, BS, Santoso, A, Hermawan, HO, and Al-Farabi, MJ. Effect of Nigella sativa supplementation on oxidative stress and antioxidant parameters: a meta-analysis of randomized controlled trials. Sci World J. (2020) 2020:1–7. doi: 10.1155/2020/2390706
254. Gupta, B, Ghosh, KK, and Gupta, RC. Chapter 39 - Thymoquinone In: RC Gupta, editor. Nutraceuticals. Boston: Academic Press (2016). 541–50.
255. Woo, CC, Kumar, AP, Sethi, G, and Tan, KHB. Thymoquinone: potential cure for inflammatory disorders and cancer. Biochem Pharmacol. (2012) 83:443–51. doi: 10.1016/j.bcp.2011.09.029
256. Zubair, H, Khan, HY, Sohail, A, Azim, S, Ullah, MF, Ahmad, A, et al. Redox cycling of endogenous copper by thymoquinone leads to ROS-mediated DNA breakage and consequent cell death: putative anticancer mechanism of antioxidants. Cell Death Dis. (2013) 4:e660–28. doi: 10.1038/cddis.2013.172
257. Sakib, R, Caruso, F, Aktar, S, Belli, S, Kaur, S, Hernandez, M, et al. Antioxidant properties of Thymoquinone, Thymohydroquinone and black cumin (Nigella sativa L.) seed oil: scavenging of superoxide radical studied using cyclic voltammetry, DFT and single crystal X-ray diffraction. Antioxidants. (2023) 12:1–17. doi: 10.3390/antiox12030607
258. Armutcu, F, Akyol, S, and Akyol, O. The interaction of glutathione and thymoquinone and their antioxidant properties. Electronic journal of. Gen Med. (2018) 15:1–8. doi: 10.29333/ejgm/89493
259. Lúcio, M, Nunes, C, Gaspar, D, Ferreira, H, Lima, JLFC, and Reis, S. Antioxidant activity of vitamin e and trolox: understanding of the factors that govern lipid peroxidation studies in vitro. Food Biophysics. (2009) 4:312–20. doi: 10.1007/s11483-009-9129-4
260. Khader, M, Bresgen, N, and Eckl, PM. In vitro toxicological properties of thymoquinone. Food Chem Toxicol. (2009) 47:129–33. doi: 10.1016/j.fct.2008.10.019
261. Staniek, K, and Gille, L. Is thymoquinone an antioxidant? BMC Pharmacol. (2010) 10:A9. doi: 10.1186/1471-2210-10-S1-A9
262. Mansour, M, and Tornhamre, S. Inhibition of 5-lipoxygenase and leukotriene C4 synthase in human blood cells by thymoquinone. J Enzyme Inhib Med Chem. (2004) 19:431–6. doi: 10.1080/14756360400002072
263. Hamdy, NM, and Taha, RA. Effects of Nigella sativa oil and thymoquinone on oxidative stress and neuropathy in Streptozotocin-induced diabetic rats. Pharmacology. (2009) 84:127–34. doi: 10.1159/000234466
264. Sharma, C, Kaur, A, Thind, SS, Singh, B, and Raina, S. Advanced glycation end-products (AGEs): an emerging concern for processed food industries. J Food Sci Technol. (2015) 52:7561–76. doi: 10.1007/s13197-015-1851-y
265. Losso, JN, Bawadi, HA, and Chintalapati, M. Inhibition of the formation of advanced glycation end products by thymoquinone. Food Chem. (2011) 128:55–61. doi: 10.1016/j.foodchem.2011.02.076
266. Pandey, R, Kumar, D, and Ahmad, A. Nigella sativa seed extracts prevent the glycation of protein and DNA. Curr Persp Med Arom Plants. (2018) 1:1–7.
267. Dinesh, K, and Ahmad, A. Antiglycation and antiaggregation potential of thymoquinone. NVEO J. (2019) 6:25–33.
268. Amiruddin Hashmi, M, Kausar, T, Khan, A, Masood, Y, and Hina,. Assessing the inhibition of glycation of ζ-crystallin by thymoquinone: a mechanistic approach using experimental and computational methods. J Mol Liq. (2023) 380:121750. doi: 10.1016/j.molliq.2023.121750
269. Bronowicka-Szydełko, A, Krzystek-Korpacka, M, Gacka, M, Pietkiewicz, J, Jakobsche-Policht, U, and Gamian, A. Association of novel advanced glycation end-product (AGE10) with complications of diabetes as measured by enzyme-linked immunosorbent assay. Journal of. Clin Med. (2021) 10:1–20. doi: 10.3390/jcm10194499
270. Babazadeh, B, Sadeghnia, HR, Kapurchal, S, Elham, P, Heydar, N, Sima, T-N, et al. Protective effect of Nigella sativa and thymoquinone on serum/glucose deprivation-induced DNA damage in PC12 cells. Avicenna J Phytomed. (2012) 2:125–32.
271. Mousavi, SH, Tayarani-Najaran, Z, Asghari, M, and Sadeghnia, HR. Protective effect of Nigella sativa extract and thymoquinone on serum/glucose deprivation-induced PC12 cells death. Cell Mol Neurobiol. (2010) 30:591–8. doi: 10.1007/s10571-009-9484-1
272. Mahmoud, A, Abdel-Salam, B, and Gomaa, W. Protective effect of Nigella Sativa in alloxan induced diabetic rats. Bull Egypt Soc Physiol Sci. (2010) 30:221–32. doi: 10.21608/besps.2010.36175
273. Golbidi, S, Alireza Ebadi, S, and Laher, I. Antioxidants in the treatment of diabetes. Curr Diabetes Rev. (2011) 7:106–25. doi: 10.2174/157339911794940729
274. Thakur, P, Kumar, A, and Kumar, A. Targeting oxidative stress through antioxidants in diabetes mellitus. J Drug Target. (2018) 26:766–76. doi: 10.1080/1061186X.2017.1419478
275. Mahmoodnia, L, Aghadavod, E, Beigrezaei, S, and Rafieian-Kopaei, M. An update on diabetic kidney disease, oxidative stress and antioxidant agents. J Renal Inj Prev. (2017) 6:153–7. doi: 10.15171/jrip.2017.30
276. Sanz-González, SM, García-Medina, JJ, Zanón-Moreno, V, López-Gálvez, MI, Galarreta-Mira, D, Duarte, L, et al. Clinical and molecular-genetic insights into the role of oxidative stress in diabetic retinopathy: antioxidant strategies and future avenues. Antioxidants. (2020) 9:1101. doi: 10.3390/antiox9111101
277. Colegate, S.M, and Molyneux, R.J, (2008). An introduction and overview,in: S.M. Colegate and R.J. Molyneux (Eds.), Bioactive natural products. Detection, isolation, and structural determination. CRC Press, Boca Raton FL, USA, pp. 1–9, doi: 10.1201/9781420006889.ch1
278. Gomathinayagam, R, Ha, JH, Jayaraman, M, Song, YS, Isidoro, C, and Dhanasekaran, DN. Chemopreventive and anticancer effects of thymoquinone: cellular and molecular targets. J Cancer Prev. (2020) 25:136–51. doi: 10.15430/JCP.2020.25.3.136
279. Ahmad, A, Mishra, RK, Vyawahare, A, Kumar, A, Rehman, MU, Qamar, W, et al. Thymoquinone (2-Isopropyl-5-methyl-1, 4-benzoquinone) as a chemopreventive/anticancer agent: chemistry and biological effects. Saudi Pharm J. (2019) 27:1113–26. doi: 10.1016/j.jsps.2019.09.008
280. Malik, S, Singh, A, Negi, P, and Kapoor, VK. Thymoquinone: a small molecule from nature with high therapeutic potential. Drug Discov Today. (2021) 26:2716–25. doi: 10.1016/j.drudis.2021.07.013
281. Venkatachallam, T, Kumar, S, Pattekhan, H, Divakar, S, and Kadimi, US. Chemical composition of Nigella sativa L. seed extracts obtained by supercritical carbon dioxide. J Food Sci Technol. (2010) 47:598–605. doi: 10.1007/s13197-010-0109-y
282. Arroo, RRJ, and Alfa, HH. Chemical properties of thymoquinone, a monoterpene isolated from the seeds of Nigella sativa Linn. Pharmacol Res. (2018) 133:151. doi: 10.1016/j.phrs.2018.05.003
283. Farkhondeh, T, Samarghandian, S, Shahri, AM, and Samini, F. The neuroprotective effects of thymoquinone: a review. Dose-Response. (2018) 16:155932581876145–11. doi: 10.1177/1559325818761455
284. Mekhemar, M, Hassan, Y, and Dörfer, C. Nigella sativa and thymoquinone: a natural blessing for periodontal therapy. Antioxidants. (2020) 9:1–20.
285. Darakhshan, S, Pour, B, Ali, HC, Abasalt, S, and Sajjad,. Thymoquinone and its therapeutic potentials. Pharmacol Res. (2015) 95-96:138–58. doi: 10.1016/j.phrs.2015.03.011
286. Ngo, TC, Dao, DQ, Thong, NM, and Nam, PC. Insight into the antioxidant properties of non-phenolic terpenoids contained in essential oils extracted from the buds of Cleistocalyx operculatus: a DFT study. RSC Adv. (2016) 6:30824–34. doi: 10.1039/C6RA02683D
287. Ali, SM, Chen, P, Sheikh, S, Ahmad, A, Ahmad, M, Paithankar, M, et al. Thymoquinone with metformin decreases fasting, post prandial glucose, and HbA1c in type 2 diabetic patients. Drug Res. (2021) 71:302–6. doi: 10.1055/a-1388-5415
288. Harvey, AL, Edrada-Ebel, RA, and Quinn, RJ. The re-emergence of natural products for drug discovery in the genomics era. Nat Rev Drug Discov. (2015) 14:111–29. doi: 10.1038/nrd4510
289. Salmani, JMM, Asghar, S, Lv, H, and Zhou, J. Aqueous solubility and degradation kinetics of the phytochemical anticancer thymoquinone; probing the effects of solvents, pH and light. Molecules. (2014) 19:5925–39. doi: 10.3390/molecules19055925
290. El-Far, AH, Jaouni, A, Soad, K, Li, W, and Mousa, SA. Protective roles of thymoquinone nanoformulations: potential nanonutraceuticals in human diseases. Nutrients. (2018) 10:1–12.
291. Jain, A, Dhruw, L, Sinha, P, Pradhan, A, Sharma, R, and Gupta, B. Thymoquinone In: RC Gupta, R Lall, and A Srivastava, editors. Nutraceuticals academic press-Elsevier. USA: CA (2021). 891–901.
292. Ali, T, Hussain, F, Naeem, M, Khan, A, and Al-Harrasi, A. Nanotechnology approach for exploring the enhanced bioactivities and biochemical characterization of freshly prepared Nigella sativa L. nanosuspensions and their phytochemical profile. Front Bioeng Biotechnol. (2022) 10:1–15. doi: 10.3389/fbioe.2022.888177
293. Veeramani, S, Narayanan, AP, Yuvaraj, K, Sivaramakrishnan, R, Pugazhendhi, A, Rishivarathan, I, et al. Nigella sativa flavonoids surface coated gold NPs (au-NPs) enhancing antioxidant and anti-diabetic activity. Process Biochem. (2022) 114:193–202. doi: 10.1016/j.procbio.2021.01.004
294. Ahmad, A, Raish, M, Alkharfy, KM, Alsarra, IA, Khan, A, Ahad, A, et al. Solubility, solubility parameters and solution thermodynamics of thymoquinone in different mono solvents. J Mol Liq. (2018) 272:912–8. doi: 10.1016/j.molliq.2018.10.104
295. Nili-Ahmadabadi, A, Alibolandi, P, Ranjbar, A, Mousavi, L, Nili-Ahmadabadi, H, Larki-Harchegani, A, et al. Thymoquinone attenuates hepatotoxicity and oxidative damage caused by diazinon: an in vivo study. Res Pharm Sci. (2018) 13:500–8. doi: 10.4103/1735-5362.245962
296. Goyal, SN, Prajapati, CP, Gore, PR, Patil, CR, Mahajan, UB, Sharma, C, et al. Therapeutic potential and pharmaceutical development of thymoquinone: a multitargeted molecule of natural origin. Front Pharmacol. (2017) 8:1–19. doi: 10.3389/fphar.2017.00656
297. Johnson-Ajinwo, OR, Ullah, I, Mbye, H, Richardson, A, Horrocks, P, and Li, W-W. The synthesis and evaluation of thymoquinone analogues as anti-ovarian cancer and antimalarial agents. Bioorg Med Chem Lett. (2018) 28:1219–22. doi: 10.1016/j.bmcl.2018.02.051
298. Iqbal, MS, Jafri, A, Arshad, M, and Ansari, MI. Stress response due to sodium azide treatment inside Nigella sativa L. plant and its effect on antioxidative property. Biocatalysis and agricultural. Biotechnology. (2019) 19:101171. doi: 10.1016/j.bcab.2019.101171
299. Yasin, MM, Shakya, A, Al-Bakain, R, Haroon, MH, and Choudhary, MI. New monoterpenoid by biotransformation of thymoquinone using aspergillus Niger. Bioorg Chem. (2018) 80:212–5. doi: 10.1016/j.bioorg.2018.06.019
300. Kutan Fenercioglu, A, Saler, T, Genc, E, Sabuncu, H, and Altuntas, Y. The effects of polyphenol-containing antioxidants on oxidative stress and lipid peroxidation in type 2 diabetes mellitus without complications. J Endocrinol Investig. (2010) 33:118–24. doi: 10.1007/BF03346565
301. Khalife, KH, and Lupidi, G. Reduction of hypervalent states of myoglobin and hemoglobin to their ferrous forms by thymoquinone: the role of GSH, NADH and NADPH. Biochim Biophys Acta Gen Subj. (2008) 1780:627–37. doi: 10.1016/j.bbagen.2007.12.006
302. Badary, OA, Taha, RA, El-Din, G, Ayman, M, and Abdel-Wahab, MH. Thymoquinone is a potent superoxide anion scavenger. Drug Chem Toxicol. (2003) 26:87–98. doi: 10.1081/DCT-120020404
303. Ali, MDY, Akter, Z, Mei, Z, Zheng, M, Tania, M, and Asaduzzaman, KM. Thymoquinone in autoimmune diseases: therapeutic potential and molecular mechanisms. Biomed Pharmacother. (2021) 134:111157–12. doi: 10.1016/j.biopha.2020.111157
304. Khalife, KH, and Lupidi, G. Nonenzymatic reduction of thymoquinone in physiological conditions. Free Radic Res. (2007) 41:153–61. doi: 10.1080/10715760600978815
305. Ebru, U, Burak, U, Yusuf, S, Reyhan, B, Arif, K, Faruk, TH, et al. Cardioprotective effects of Nigella sativa oil on cyclosporine a-induced cardiotoxicity in rats. Basic Clin Pharmacol Toxicol. (2008) 103:574–80. doi: 10.1111/j.1742-7843.2008.00313.x
306. Ismail, M, Al-Naqeep, G, and Chan, KW. Nigella sativa thymoquinone-rich fraction greatly improves plasma antioxidant capacity and expression of antioxidant genes in hypercholesterolemic rats. Free Radic Biol Med. (2010) 48:664–72. doi: 10.1016/j.freeradbiomed.2009.12.002
307. Bule, M, Nikfar, S, Amini, M, and Abdollahi, M. The antidiabetic effect of thymoquinone: a systematic review and meta-analysis of animal studies. Food Res Int. (2020) 127:108736. doi: 10.1016/j.foodres.2019.108736
308. Maucher, IV, Rühl, M, Kretschmer, SBM, Hofmann, B, Kühn, B, Fettel, J, et al. Michael acceptor containing drugs are a novel class of 5-lipoxygenase inhibitor targeting the surface cysteines C416 and C418. Biochem Pharmacol. (2017) 125:55–74. doi: 10.1016/j.bcp.2016.11.004
309. Khan, M. Chemical composition and medicinal properties of Nigella sativa Linn. Inflammopharmacology. (1999) 7:15–35. doi: 10.1007/s10787-999-0023-y
310. Talebi, M, Talebi, M, Farkhondeh, T, and Samarghandian, S. Biological and therapeutic activities of thymoquinone: focus on the Nrf2 signaling pathway. Phytother Res. (2021) 35:1739–53. doi: 10.1002/ptr.6905
311. Drucker, DJ, Sherman, SI, Gorelick, FS, Bergenstal, RM, Sherwin, RS, and Buse, JB. Incretin-based therapies for the treatment of type 2 diabetes: evaluation of the risks and benefits. Diabetes Care. (2010) 33:428–33. doi: 10.2337/dc09-1499
312. Giorgino, F, Penfornis, A, Pechtner, V, Gentilella, R, and Corcos, A. Adherence to antihyperglycemic medications and glucagon-like peptide 1-receptor agonists in type 2 diabetes: clinical consequences and strategies for improvement. Patient Prefer Adher. (2018) 12:707–19. doi: 10.2147/PPA.S151736
313. Alkhatib, H, Mawazi, SM, Al-Mahmood, SM, Abdullah, Z, Ahmad, D, and Almonem, A. Thymoquinone content in marketed black seed oil in Malaysia. J Pharm Bioallied Sci. (2020) 12:284–8. doi: 10.4103/jpbs.JPBS_208_20
314. Khaikin, E, Chrubasik-Hausmann, S, Kaya, S, and Zimmermann, BF. Screening of Thymoquinone content in commercial Nigella sativa products to identify a promising and safe study medication. Nutrients. (2022) 14:1–7. doi: 10.3390/nu14173501
315. Abedi, A-S, Rismanchi, M, Shahdoostkhany, M, Mohammadi, A, and Mortazavian, AM. Microwave-assisted extraction of Nigella sativa L. essential oil and evaluation of its antioxidant activity. J Food Sci Technol. (2017) 54:3779–90. doi: 10.1007/s13197-017-2718-1
316. Piras, A, Rosa, A, Marongiu, B, Porcedda, S, Falconieri, D, Dessì, MA, et al. Chemical composition and in vitro bioactivity of the volatile and fixed oils of Nigella sativa L. extracted by supercritical carbon dioxide. Ind Crop Prod. (2013) 46:317–23. doi: 10.1016/j.indcrop.2013.02.013
317. Bartosz, G. Non-enzymatic antioxidant capacity assays: limitations of use in biomedicine. Free Radic Res. (2010) 44:711–20. doi: 10.3109/10715761003758114
318. Himanshu, D, Ali, W, and Wamique, M. Type 2 diabetes mellitus: pathogenesis and genetic diagnosis. J Diabetes Metab Disord. (2020) 19:1959–66. doi: 10.1007/s40200-020-00641-x
319. Mambiya, M, Shang, M, Wang, Y, Li, Q, Liu, S, Yang, L, et al. The play of genes and non-genetic factors on type 2 diabetes. Front Public Health. (2019) 7:1–8. doi: 10.3389/fpubh.2019.00349
320. Balbaa, M, El-Zeftawy, M, Ghareeb, D, Taha, N, and Mandour, AW. Nigella sativa relieves the altered insulin receptor signaling in Streptozotocin-induced diabetic rats fed with a high-fat diet. Oxidative Med Cell Longev. (2016) 2016:1–16. doi: 10.1155/2016/2492107
321. Rosenzweig, SA. The continuing evolution of insulin-like growth factor signaling. F1000Res. (2020) 9:1–8. doi: 10.12688/f1000research.22198.1
322. El-Bahr, SM, and El-Sabagh, IM. Hepatic gene expression of insulin like growth factor and selected antioxidants in diabetic rats treated with turmeric or black cumin seed. Biotechnol J Int. (2014) 4:778–93. doi: 10.9734/BBJ/2014/11121
323. Huang, Z, Huang, L, Waters, MJ, and Chen, C. Insulin and growth hormone balance: implications for obesity. Trends Endocrinol Metab. (2020) 31:642–54. doi: 10.1016/j.tem.2020.04.005
324. Pari, L, and Sankaranarayanan, C. Beneficial effects of thymoquinone on hepatic key enzymes in Streptozotocin–nicotinamide induced diabetic rats. Life Sci. (2009) 85:830–4. doi: 10.1016/j.lfs.2009.10.021
325. Yaribeygi, H, Sathyapalan, T, Atkin, SL, and Sahebkar, A. Molecular mechanisms linking oxidative stress and diabetes mellitus. Oxidative Med Cell Longev. (2020) 2020:1–13. doi: 10.1155/2020/8609213
326. James, DE, and Piper, RC. Insulin resistance, diabetes, and the insulin-regulated trafficking of GLUT-4. J Cell Biol. (1994) 126:1123–6. doi: 10.1083/jcb.126.5.1123
327. Li, J, Bai, L, Wei, F, Zhao, J, Wang, D, Xiao, Y, et al. Therapeutic mechanisms of herbal medicines against insulin resistance: a review. Front Pharmacol. (2019) 10:661. doi: 10.3389/fphar.2019.00661
328. Song, F, Jia, W, Yao, Y, Hu, Y, Lei, L, Lin, J, et al. Oxidative stress, antioxidant status and DNA damage in patients with impaired glucose regulation and newly diagnosed type 2 diabetes. Clin Sci. (2007) 112:599–606. doi: 10.1042/CS20060323
329. Elseweidy, MM, Amin, RS, Atteia, HH, and Aly, MA. Nigella sativa oil and chromium picolinate ameliorate fructose-induced hyperinsulinemia by enhancing insulin signaling and suppressing insulin-degrading enzyme in male rats. Biol Trace Elem Res. (2018) 184:119–26. doi: 10.1007/s12011-017-1167-z
330. Widlansky, ME, Gokce, N, Keaney, JF, and Vita, JA. The clinical implications of endothelial dysfunction. J Am Coll Cardiol. (2003) 42:1149–60. doi: 10.1016/S0735-1097(03)00994-X
331. Adela, R, Nethi, SK, Bagul, PK, Barui, AK, Mattapally, S, Kuncha, M, et al. Hyperglycaemia enhances nitric oxide production in diabetes: a study from south Indian patients. PLoS One. (2015) 10:1–17. doi: 10.1371/journal.pone.0125270
332. Idris-Khodja, N, and Schini-Kerth, V. Thymoquinone improves aging-related endothelial dysfunction in the rat mesenteric artery. Naunyn Schmiedeberg's Arch Pharmacol. (2012) 385:749–58. doi: 10.1007/s00210-012-0749-8
333. Pei, X, Li, X, Chen, H, Han, Y, and Fan, Y. Thymoquinone inhibits angiotensin II-induced proliferation and migration of vascular smooth muscle cells through the AMPK/PPARγ/PGC-1α pathway. DNA Cell Biol. (2016) 35:426–33. doi: 10.1089/dna.2016.3262
334. Jason, A, Samuel, G, Nurudeen, H, Keith, M, and Cathryn, EW. Nigella sativa extract and thymoquinone regulate inflammatory cytokine and tet-2 expression in endothelial cells. Artery Res. (2019) 25:157–63. doi: 10.2991/artres.k.191114.002
335. El-Agamy, DS, and Nader, MA. Attenuation of oxidative stress-induced vascular endothelial dysfunction by thymoquinone. Exp Biol Med. (2012) 237:1032–8. doi: 10.1258/ebm.2012.012107
336. Gustavsson, C, Agardh, C-D, Zetterqvist, AV, Nilsson, J, Agardh, E, and Gomez Maria, F. Vascular cellular adhesion molecule-1 (VCAM-1) expression in mice retinal vessels is affected by both hyperglycemia and hyperlipidemia. PLoS ONE. (2010) 5:1–12.
337. Umar, S, Hedaya, O, Agere, S, and Ahmed, S. Thymoquinone inhibits TNF-α-induced pro-inflammatory mediators in rheumatoid arthritis synovial fibroblasts in vitro. The FASEB Journal. (2015) 29:393–398.
338. Arjumand, S, Shahzad, M, Shabbir, A, and Yousaf, MZ. Thymoquinone attenuates rheumatoid arthritis by downregulating TLR2, TLR4, TNF-α, IL-1, and NFκB expression levels. Biomed Pharmacother. (2019) 111:958–63. doi: 10.1016/j.biopha.2019.01.006
339. Mudaliar, H, Pollock, C, Ma, J, Wu, H, Chadban, S, and Panchapakesan, U. The role of TLR2 and 4-mediated inflammatory pathways in endothelial cells exposed to high glucose. PLoS One. (2014) 9:e108844. doi: 10.1371/journal.pone.0108844
340. Barreto, J, Karathanasis, SK, Remaley, A, and Sposito, AC. Role of LOX-1 (lectin-like oxidized low-density lipoprotein receptor 1) as a cardiovascular risk predictor. Arterioscler Thromb Vasc Biol. (2021) 41:153–66. doi: 10.1161/ATVBAHA.120.315421
341. Singh, P, Goncalves, I, Tengryd, C, Nitulescu, M, Persson, AF, To, Fong, et al. Reduced oxidized LDL in T2D plaques is associated with a greater statin usage but not with future cardiovascular events. Cardiovasc Diabetol. (2020) 19:1–12. doi: 10.1186/s12933-020-01189-z
342. Yan, M, Mehta, JL, Zhang, W, and Hu, C. LOX-1, oxidative stress and inflammation: a novel mechanism for diabetic cardiovascular complications. Cardiovasc Drugs Ther. (2011) 25:451. doi: 10.1007/s10557-011-6342-4
343. Akhmedov, A, Sawamura, T, Chen, C-H, Kraler, S, Vdovenko, D, and Lüscher, TF. Lectin-like oxidized low-density lipoprotein receptor-1 (LOX-1): a crucial driver of atherosclerotic cardiovascular disease. Eur Heart J. (2020) 42:1797–807. doi: 10.1093/eurheartj/ehaa770
344. Kattoor, AJ, Goel, A, and Mehta, JL. LOX-1: regulation, signaling and its role in atherosclerosis. Antioxidants. (2019) 8:218. doi: 10.3390/antiox8070218
345. Chan, P-C, Liao, M-T, and Hsieh, P-S. The dualistic effect of COX-2-mediated signaling in obesity and insulin resistance. Int J Mol Sci. (2019) 20:3115. doi: 10.3390/ijms20133115
346. Jiahua, C, and Jinping, J. Natural COX-2 inhibitors as promising anti-inflammatory agents: an update. Curr Med Chem. (2021) 28:3622–46. doi: 10.2174/0929867327999200917150939
347. Srinivasan, K. Anti-inflammatory influences of culinary spices and their bioactives. Food Rev Int. (2020) 38:1–17. doi: 10.1080/87559129.2020.1839761
348. Pirot, P, Cardozo, AK, and Eizirik, DL. Mediators and mechanisms of pancreatic beta-cell death in type 1 diabetes. Arquivos Brasileiros Endocrinol Metabol. (2008) 52:156–65. doi: 10.1590/S0004-27302008000200003
349. Chow, YY, and Chin, K-Y. The role of inflammation in the pathogenesis of osteoarthritis. Mediat Inflamm. (2020) 2020:1–19. doi: 10.1155/2020/8293921
350. Gelfo, V, Romaniello, D, Mazzeschi, M, Sgarzi, M, Grilli, G, Morselli, A, et al. Roles of IL-1 in cancer: from tumor progression to resistance to targeted therapies. Int J Mol Sci. (2020) 21:1–14.
351. Hariharan, A, Hakeem, AR, Radhakrishnan, S, Reddy, MS, and Rela, M. The role and therapeutic potential of NF-kappa-Β pathway in severe COVID-19 patients. Inflammopharmacology. (2021) 29:91–100. doi: 10.1007/s10787-020-00773-9
352. Xiao, K, Liu, C, Tu, Z, Xu, Q, Chen, S, Zhang, Y, et al. Activation of the NF-κB and MAPK signaling pathways contributes to the inflammatory responses, but not cell injury, in IPEC-1 cells challenged with hydrogen peroxide. Oxidative Med Cell Longev. (2020) 2020:1–14. doi: 10.1155/2020/5803639
353. Moon, H, White, AC, and Borowsky, AD. New insights into the functions of cox-2 in skin and esophageal malignancies. Exp Mol Med. (2020) 52:538–47. doi: 10.1038/s12276-020-0412-2
354. Sheng, J, Sun, H, Yu, F-B, Li, B, Zhang, Y, and Zhu, Y-T. The role of cyclooxygenase-2 in colorectal cancer. Int J Med Sci. (2020) 17:1095–101. doi: 10.7150/ijms.44439
355. Zarghi, A, and Arfaei, S. Selective COX-2 inhibitors: a review of their structure-activity relationships. Iran J Pharm Res. (2011) 10:655–83.
356. Davis-Richardson, AG, and Triplett, EW. A model for the role of gut bacteria in the development of autoimmunity for type 1 diabetes. Diabetologia. (2015) 58:1386–93. doi: 10.1007/s00125-015-3614-8
357. Khursheed, R, Singh, SK, Wadhwa, S, Kapoor, B, Gulati, M, Kumar, R, et al. Treatment strategies against diabetes: success so far and challenges ahead. Eur J Pharmacol. (2019) 862:172625–37. doi: 10.1016/j.ejphar.2019.172625
358. Utzschneider, KM, Kratz, M, Damman, CJ, and Hullarg, M. Mechanisms linking the gut microbiome and glucose metabolism. J Clin Endocrinol Metab. (2016) 101:1445–54. doi: 10.1210/jc.2015-4251
359. Lee, H, Ko, GP, and Griffiths, MW. Effect of metformin on metabolic improvement and gut microbiota. Appl Environ Microbiol. (2014) 80:5935–43. doi: 10.1128/AEM.01357-14
360. Montandon, SA, and Jornayvaz, FR. Effects of antidiabetic drugs on gut microbiota composition. Genes. (2017) 8:1–12. doi: 10.3390/genes8100250
361. Brunkwall, L, and Orho-Melander, M. The gut microbiome as a target for prevention and treatment of hyperglycaemia in type 2 diabetes: from current human evidence to future possibilities. Diabetologia. (2017) 60:943–51. doi: 10.1007/s00125-017-4278-3
362. Kocsis, T, Molnár, B, Németh, D, Hegyi, P, Szakács, Z, Bálint, A, et al. Probiotics have beneficial metabolic effects in patients with type 2 diabetes mellitus: a meta-analysis of randomized clinical trials. Sci Rep. (2020) 10:1–14. doi: 10.1038/s41598-020-68440-1
363. Sun, Z, Sun, X, Li, J, Li, Z, Hu, Q, Li, L, et al. Using probiotics for type 2 diabetes mellitus intervention: advances, questions, and potential. Crit Rev Food Sci Nutr. (2020) 60:670–83. doi: 10.1080/10408398.2018.1547268
364. Ardeshirlarijani, E, Tabatabaei-Malazy, O, Mohseni, S, Qorbani, M, Larijani, B, and Baradar Jalili, R. Effect of probiotics supplementation on glucose and oxidative stress in type 2 diabetes mellitus: a meta-analysis of randomized trials. DARU J Pharm Sci. (2019) 27:827–37. doi: 10.1007/s40199-019-00302-2
365. George, R, Kerry, G, Das, U, Golla, M, del Pilar, R-T, HanSeung, S, et al. Engineered probiotic and prebiotic nutraceutical supplementations in combating non-communicable disorders: a review. Curr Pharm Biotechnol. (2020) 21:1–26.
366. Tahmasebi, S, Oryan, S, Mohajerani, HR, Akbari, N, and Palizvan, MR. Probiotics and Nigella sativa extract supplementation improved behavioral and electrophysiological effects of PTZ-induced chemical kindling in rats. Epilepsy Behav. (2020) 104:106897–8. doi: 10.1016/j.yebeh.2019.106897
367. Rostami, H, Hamedi, H, and Ghaderi, M. Viability of commercial probiotic cultures in cottage cheese containing black cumin seed. J Food Measure Charact. (2018) 12:1648–53. doi: 10.1007/s11694-018-9780-x
368. Salgaço, MK, Oliveira, LG, Segura, C, Nobre, G, Bianchi, F, and Sivieri, K. Relationship between gut microbiota, probiotics, and type 2 diabetes mellitus. Appl Microbiol Biotechnol. (2019) 103:9229–38. doi: 10.1007/s00253-019-10156-y
369. Everard, A, Matamoros, S, Geurts, L, Delzenne, NM, Cani, PD, and Blaser, MJ. Saccharomyces boulardii administration changes gut microbiota and reduces hepatic steatosis, low-grade inflammation, and fat mass in obese and type 2 diabetic db/db mice. MBio. (2014) 5:e01011–4. doi: 10.1128/mBio.01011-14
Keywords: diabetes mellitus, Nigella sativa , antioxidant, thymoquinone, anti-glycemic, gut microbiota
Citation: Shaukat A, Zaidi A, Anwar H and Kizilbash N (2023) Mechanism of the antidiabetic action of Nigella sativa and Thymoquinone: a review. Front. Nutr. 10:1126272. doi: 10.3389/fnut.2023.1126272
Received: 17 December 2022; Accepted: 27 July 2023;
Published: 25 September 2023.
Edited by:
Ana Sanches Silva, Instituto Nacional Investigaciao Agraria e Veterinaria (INIAV), PortugalReviewed by:
Ajmer Singh Grewal, Guru Gobind Singh College of Pharmacy, IndiaCopyright © 2023 Shaukat, Zaidi, Anwar and Kizilbash. This is an open-access article distributed under the terms of the Creative Commons Attribution License (CC BY). The use, distribution or reproduction in other forums is permitted, provided the original author(s) and the copyright owner(s) are credited and that the original publication in this journal is cited, in accordance with accepted academic practice. No use, distribution or reproduction is permitted which does not comply with these terms.
*Correspondence: Arsalan Zaidi, YXpsYW56YWlkaUB5YWhvby5jb20=
Disclaimer: All claims expressed in this article are solely those of the authors and do not necessarily represent those of their affiliated organizations, or those of the publisher, the editors and the reviewers. Any product that may be evaluated in this article or claim that may be made by its manufacturer is not guaranteed or endorsed by the publisher.
Research integrity at Frontiers
Learn more about the work of our research integrity team to safeguard the quality of each article we publish.