- 1College of Agriculture and Forestry Science and Technology, Hebei North University, Hebei Key Laboratory of Quality and Safety Analysis-Testing for Agro-Products and Food, Zhangjiakou, China
- 2State Key Laboratory of Vegetable Biobreeding, Institute of Vegetables and Flowers, Chinese Academy of Agricultural Sciences, Key Laboratory of Vegetables Quality and Safety Control, Ministry of Agriculture and Rural Affairs of China, Beijing, China
- 3College of Horticulture, Northeast Agricultural University, Harbin, Heilongjiang, China
- 4Internal Trade Food Science Research Institute Co., Ltd, Beijing, China
At present, nano-carrier materials with antibacterial activity are of great significance. Due to the widespread resistance of many pathogenic microorganisms, it has seriously threatened human health. The natural antimicrobial substances extracted from fruits and vegetables can significantly improve their stability combined with nano-carrier materials. The resistance of pathogenic microorganisms will be substantially reduced, greatly enhancing the effect of active antimicrobial substances. Nanotechnology has excellent research prospects in the food industry, antibacterial preservation, food additives, food packaging, and other fields. This paper introduces nano-carrier materials and preparation techniques for loading and encapsulating active antibacterial substances in detail by constructing a nano-release system for active antibacterial substances. The antibacterial effect can be achieved by protecting them from adverse external conditions and destroying the membrane of pathogenic microorganisms. The mechanism of the slow release of the bacteriostatic active substance is also described. The mechanism of carrier loading and release is mainly through non-covalent forces between the bacteriostatic active substance and the carrier material, such as hydrogen bonding, π-π stacking, van der Waals forces, electrostatic interactions, etc., as well as the loading and adsorption of the bacteriostatic active substance by the chemical assembly. Finally, its wide application in food and medicine is introduced. It is hoped to provide a theoretical basis and technical support for the efficient utilization and product development of bacteriostatic active substances.
1. Introduction
The rapid emergence of antibiotic-resistant pathogens seriously threatens human health (1). Therefore, developing natural, efficient, low-toxic antibacterial products has become a research hotspot. Natural antibacterial active substances are essential resources to maintain human health. There are three main natural antibacterial active substances: natural plant antibacterial agents (carvacrol, eucalyptol, thymol, etc.) (2, 3). Natural animal antimicrobials (high molecular carbohydrates, natural peptides, and amino acids) (4, 5) and natural mineral antimicrobials (clay, etc.) (6). Unlike chemical antimicrobials, which are widely used, natural products are the primary source of bioactive substances. Due to their structural characteristics and chemical heterogeneity, they can play a crucial role in disease treatment and drug innovation (7, 8), and have superior performance in the food industry and packaging.
Antibacterial active substances (such as anthocyanins, sulforaphane, etc.) extracted from vegetables generally have good antibacterial, fresh-keeping, anti-oxidation, anti-cancer, anti-inflammatory, and other active functions, and are widely used in food and pharmaceutical industries (9, 10). Among them, since the active substances extracted from vegetables have the characteristics of environmental protection, no pollution, no residue, low toxicity, and side effects, antibacterial and antioxidants have become the research focus of researchers in recent years. Therefore, a large number of active substances have been developed and applied in the fields of food preservation, natural preservatives (11), and anti-inflammatory and bacteriostatic (12). Nevertheless, the existing active substances and active substance products have certain disadvantages, such as unstable product properties, easy decomposition, and poor water solubility, resulting in the practical application effect is not outstanding.
Meanwhile, with growing interest in nanotechnology, researchers are eager to use nanotechnology to design drug-release delivery systems that limit, protect, and specifically release functional bioactive substances, thereby synergistically improving the antibacterial effect of active substances (Figure 1). In particular, nanotechnology-based slow-release systems for biological ingredients nanocarriers have nanoscale dimensions, generally between 50 and 300 nm compared with traditional pharmaceutical formulations. They can also control the kinetics of the reaction. This will facilitate the application of nano-delivery systems in various fields such as medicine, agriculture, and food (13). In this paper, firstly, the carrier materials and encapsulation techniques of different nano-release systems were briefly introduced. Secondly, the release and action mechanism of different nanocarriers and their applications in bacteriostasis and antioxidant preservation fields were reviewed. Finally, the problems and future challenges in the delivery of nanocarriers were discussed. This review aims to highlight the applications of nano systems in functional bioactive substances and their potential opportunities and challenges.
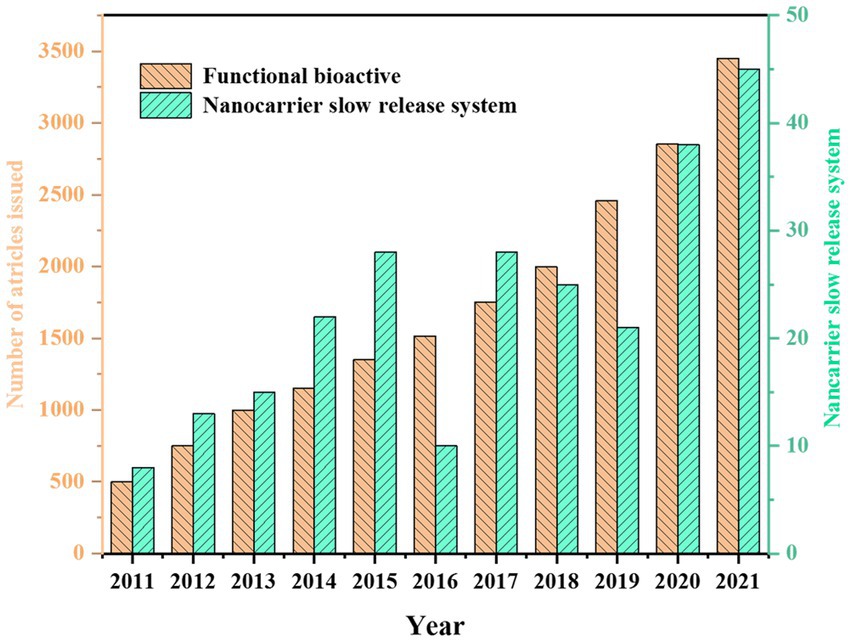
Figure 1. Number of publications for the term Functional bioactive and Nanocarrier slow-release system in the last decades (data collected from the Web of Science).
2. Construction of nano slow-release system
Stability is critical to ensuring bioactive compounds, but most bioactive compounds exhibit poor stability. In this case, bioactive compounds can be loaded by selecting a suitable nanocarrier slow-release system that allows them to be transported to specific receptors to exert their efficacy (14). In addition, nanoencapsulation techniques can be used as a means to control stability and solubility to ensure the perfect release of bioactive components. Figure 2 shows the construction of a nano slow-release system.
2.1. Nano-encapsulation materials
Nano-encapsulated materials for the antibacterial active substances can be divided into three main categories: inorganic nanocarrier materials, organic nanocarrier materials, and hybridized nanomaterials. Inorganic nanomaterials mostly refer to metallic materials, such as carbon nanomaterials, gold and silver nanoparticles, quantum dots, and other materials. Organic nanomaterials refer to various proteins and polysaccharides, such as wheat alcohol-soluble protein, corn alcohol-soluble protein, modified starch, pectin, and other substances. In contrast, a hybrid nanomaterial is a mixture of two nano-or molecular-level components. In nature, it is usually a mixture of an inorganic and an organic substance. So, they differ from the usual sense of a mixture in that the usual mixture is of macroscopic magnitude (microns or millimeters). Mixing at the microscopic level can result in a homogeneous mixture of substances that will exhibit properties intermediate to the two components or even some new properties. Therefore, the choice of each type of nanocarrier is limited by its bioavailability, ease of application, biodistribution, food matrix compatibility, biodegradability, and biocompatibility (15). Thus, the different antibacterial active substances can be encapsulated by choosing suitable nanomaterials according to the above properties.
2.1.1. Inorganic nanocarriers
Inorganic nanomaterials are a class of nanoparticles with various morphologies and particle sizes ranging from 1 to 100 nm that can be synthesized by physical or chemical methods. Inorganic nanomaterials are not only easy for surface modification but also can be bound to drug molecules in different ways. For example, electrostatic interactions, hydrophobic interactions, covalent bonding of enzyme-sensitive groups, etc. This leads to responsive release, making them ideal for drug delivery. Compared with other nanomaterials, inorganic nanomaterials have the advantages of easy preparation, high drug loading rate, controllable shape and size, good biocompatibility, and easy surface modification for drug delivery (12, 16). Therefore, it is possible to achieve an enhanced antibacterial effect.
2.1.1.1. Carbon nanotubes
The unique biological and physicochemical properties of carbon nanotubes (CNT) make them an ideal nanocarrier material for the biologically antibacterial active substance. It is a tubular hollow structure containing sheets of graphene rolled together at discrete and specific angles. Depending on the number of graphene sheets rolled together, carbon nanotubes are classified as single-walled and multi-walled carbon nanotubes. These tubes can have a cross-sectional diameter of 0.4–100 nm, while the length of the tube extends thousands of times the diameter. In nano-delivery systems, such carbon nanotubes have a high aspect ratio, ultra-light specific surface area, nano-needle-like structure, and a wide range of application prospects (17). The structural stability, flexibility and surface modification of functionalized nanotubes make them good carriers of active substances. Under this concept, functionalized carbon nanotubes are widely used to encapsulate or connect antibacterial active substances. The carbon nanotubes also have superior antibacterial properties (18). To enhance the antibacterial activity of multi-walled carbon nanotube (MWCNT), modification of MWCNT is necessary. Chen Yuan et al. (19) applied a nanohybrid comprising silver nanoparticles within dendritic poly(amidoamine) dendrimer-modified MWCNT as an antimicrobial solution against Gram-negative and Gram-positive bacteria.
Hence Ghahremani was inspired by mussels to design a novel nanocarrier consisting of polydopamine (PDA), chitosan (CH) and zinc cations modified on the surface of multi-walled carbon nanotubes (MWCNT) (20). PDA, CH and Zn2+ were found to be successfully modified on the oxidized multi-walled carbon nanotube (OMWCNT) surface employing characterization. The material was incorporated into an epoxy coating to form a nanocomposite that exhibited superior barrier capabilities and provided stable corrosion inhibition for nearly 9 weeks.
2.1.1.2. Au nanoparticles
Au nanoparticle (AuNP) carriers construct an effective delivery medium that can be applied in different fields, and gold nanoparticles have different anisotropic properties such as nanostars, nanorods, nanocages, nanoshells, and nano prisms (21–23). Among the many properties of gold nanocarriers, their optical properties are the primary factor that attracts them to biomedical and food applications. It enables the attachment of different biomolecules to gold nanoparticles. Such as enzymes, carbohydrates, fluorophores, peptides, proteins and genes. This enables efficient intracellular transport of molecules to overcome relevant barriers (24).
Au nanoparticles revealed a noteworthy antimicrobial effect against Gram-positive and Gram-negative bacterial strains. Usually, the negatively charged surface of the bacterial cell wall is attracted to the positively charged nanoparticles due to the electrostatic force of the interaction (25). In contrast, gold nanoparticles create strong bonds with the bacterial cell membrane, leading to the rupture of the cell wall and cell membrane, which leads to the disruption of biological processes. The bonds formed between metal ions and biomolecules are reported to be indeterminate and gold nanoparticles exhibit broad-spectrum activity (26, 27).
The advantages of synthesizing gold nanoparticles from active substances extracted from vegetables are not limited to the reduction of environmental toxicity, the production of nanoparticles in large quantities, the simplicity of reducing metal salts, the rapidity, the economic efficiency, the safety of clinical studies, and the nanoparticles are other advantages associated with this synthetic method. Aderonke used active substances extracted from vegetables in combination with gold nanoparticles to prepare a nanoparticle with good antibacterial effect. The percentage inhibition zones of the synthesized gold nanoparticles against the tested fungi and bacteria ranged from 30 to 66% and 40 to 54%, respectively (27).
2.1.1.3. Quantum dots
Quantum dots (QD) include atoms in the II-VI (Se, Zn, Te, Cd) or III-V (in, As, P) elemental groups of the periodic table. They are colloidal nanocrystals and energy donors. The size of the quantum dots changes the luminescence between the UV–NIR region, i.e., smaller quantum dots (2 nm) fluoresce blue and larger ones (5 nm) fluoresce red (28). This optical property with long-time light emission and less photobleaching makes it superior to other organic dyes, which allows it to be used for cell imaging. Normally, the toxic cadmium in Cd Se quantum dots is encapsulated within a ZnS shell to protect it from toxicity. This enhances the accumulation of nanoparticles at the desired vascular sites. These quantum dots are also considered to be efficient delivery and reporting systems.
2.1.2. Organic nanocarriers
2.1.2.1. Polysaccharides
Chitosan is a polycationic polymer derivative of chitin, prepared by alkaline deacetylation of chitin. Besides cellulose, chitosan is the most abundant biopolymer existing in nature, and its structure consists of-d-glucosamine deacetylation unit and n -acetyl- d -glucosamine acetylation unit linked by β- (1, 4) glycosidic bond. Chitosan and its nanoparticles have good bioactivity and loading capacity and have been gaining more and more attention in pharmaceutical and functional food applications (29), where it has been found that the nanoparticles not only have good loading capacity for antibacterial active substance but also have outstanding slow-release properties (30).
2.1.2.2. Starches
Starch has been widely used in food, textile and pharmaceutical fields under its wide source, low cost and high biocompatibility (31, 32). However, natural starch has a large relative molecular weight, poor enzymatic resistance, and does not contain hydrophobic groups, so direct preparation of nanocarriers from natural starch for functional factor encapsulation has a limited application. The starch structure can be easily modified and its molecular fine structure can be targeted and regulated according to the properties of functional factors to prepare starch nanocarriers to achieve effective encapsulation. The starch nanocarriers can be prepared for the purpose of effective embedding. Starch nanocarriers are generally in the form of starch nanoparticles, starch nanocrystals (33), starch nanofibers (34), and nanostructured starch. The starch nanocarrier forms generally include starch nanoparticles, starch nanocrystals, starch nanofibers and nanostructured starch.
2.1.2.3. Protein
Protein is a biological macromolecule with multiple moieties and is characterized by a variety of chemical and physical spatial structures that can interact with a variety of food functional factors. Therefore, proteins are excellent carriers for the delivery of food active ingredients. Therefore, proteins are excellent carriers for the delivery of food active ingredients (such as curcumin), and they are also hot materials for the research of food colloidal delivery systems in recent years. The most widely used protein nano-delivery systems are animal proteins, plant proteins, and protein peptides.
2.1.3. Hybrid nanocarriers
A hybridized nanocarrier is a nanocarrier that combines two or more organic nanocarriers and inorganic nanocarriers together or separately. It includes organic–inorganic, inorganic–inorganic, and multi-component. For example, lipid-polymer hybridization, ceramic-polymer hybridization, etc. combine two nanoparticles and the nanocarrier will have the dual properties of both nanoparticles, thus improving its performance many times.
2.1.3.1. Metal–organic framework material
Metal–organic framework materials (MOFs) are crystalline materials with a three-dimensional mesh structure formed by inorganic substituents (metal clusters, metal ions, central chains) and organic ligands, through coordination bonds. MOFs are an important component of hybrid nanomaterials. The most commonly used MOFs material sofistitute Lavoisier frameworks (MILs series), isoreticular metal–organic frameworks (IRMOFs series), zeolite imidazole frameworks (ZIFs series), etc. As Figure 3 shows the typical material diagram of different kinds of MOFs. Compared with traditional porous materials such as activated carbon (AC), silica gel and molecular sieve, MOF materials have the advantages of high porosity, high adsorption capacity, large specific surface area, and high modifiability. In recent years, MOF materials have been used in drug encapsulation (36), biocatalysis (37), antibacterial preservation (38), etc. MOF materials can meet the requirements of high biocompatibility, degree of dispersion and targeting required for food biomedicine through surface modification, synthesis methods, ligand changes, etc.
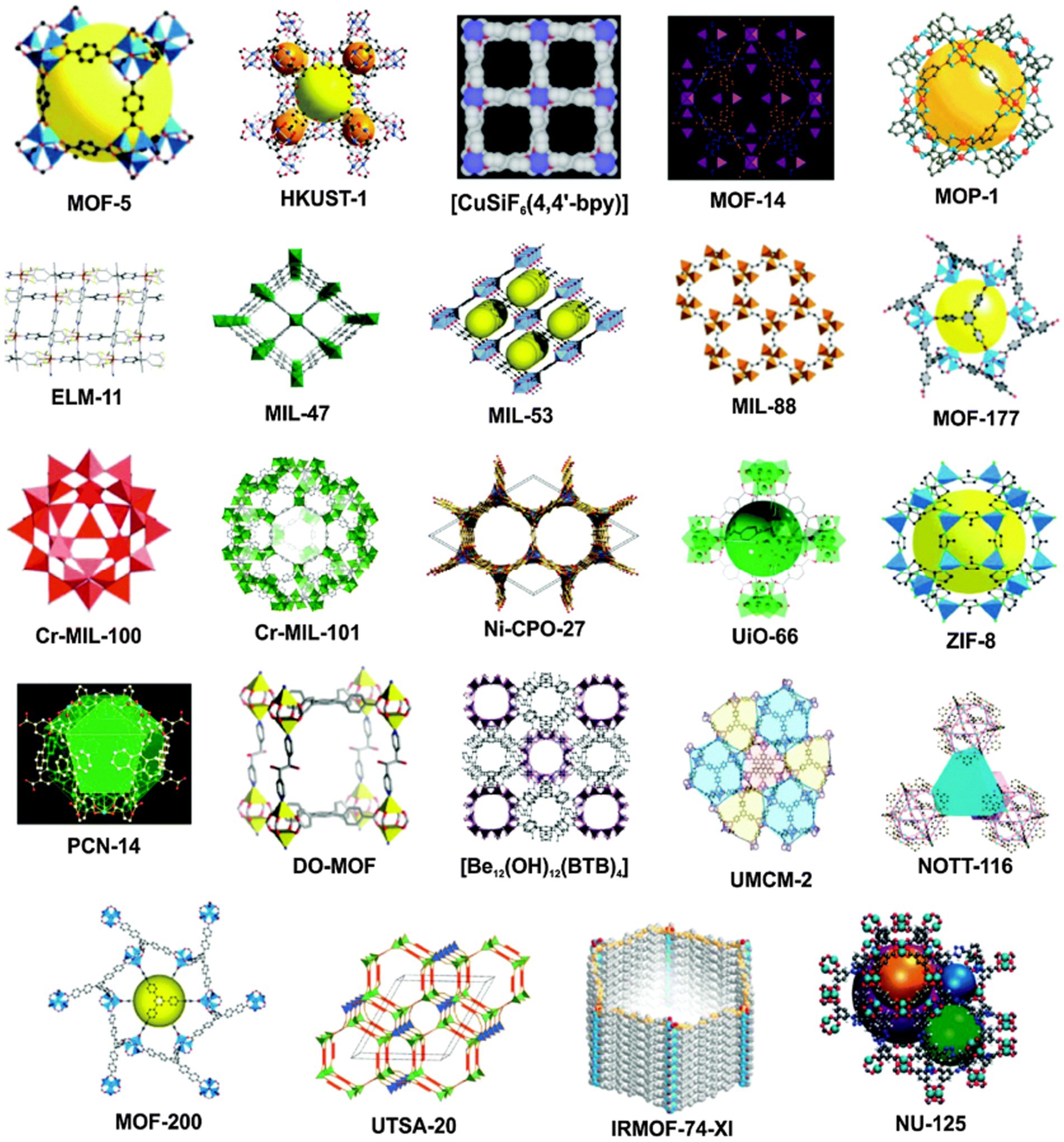
Figure 3. Typical examples of different types of porous MOFs structures (35).
For example, Zeinab combined curcumin with MOF materials to construct cyclodextrin-metal organic backbone nanomaterials (CD-MOFs). The stability of curcumin was improved by three orders of magnitude and exhibited superior active functions (39). Xiao ligated HKUST-1 with folic acid to make a hydrogel wound excipient, which played a better bactericidal effect and promoted wound healing. In summary, MOF materials are promising and valuable for research in food biomedical applications, and their versatile structures and multiple applications provide new ideas for solving some problems (40).
2.1.3.2. Hydrogel
Hydrogels are three-dimensional solid networks made of physically or chemically cross-linked hydrophilic polymeric structures that can entangle large amounts of water or other biological fluids within their networks (41). The formation of hydrogel nanoparticles involves the self-assembly between different charged polymers through electrostatic interactions. In their production process, the liquid phase is gelled by temperature regulation, cross-linking agents or acidification or the addition of multivalent ions. They can encapsulate hydrophilic and lipophilic bioactive, prevent degradation and target release efficiently.
Nano-hydrogels have an extremely important role in the field of drug slow release, specifically in reducing the concentration fluctuation of drug solution and releasing the loaded drug smoothly. Adila prepared a carvacrol-containing hydrogel (GG-Carv), the combination of which promotes bioavailability and delivery while maximizing the antibacterial properties of carvacrol (42). Table 1 shows the Comparison and application of different nanomaterials.
2.2. Nano-packaging technology
2.2.1. Top-down methods
2.2.1.1. Emulsification method
Emulsification is the process of uniformly dispersing one liquid in very small droplets in another liquid that is immiscible (or partially immiscible) with each other. The organic polymer solution is homogenized with the aqueous phase and the solvent is then evaporated, leading to the precipitation of polymer molecules and the formation of nanospheres. This process of emulsification leads to nano emulsions loaded with active substances.
The polymer solution containing biological compounds is emulsified in the aqueous phase and then the solvent that previously dissolved the polymer is vaporized to produce nanospheres of precipitated polymer. The advantage of this method is that it is suitable for both hydrophilic and hydrophobic compounds with good solubilization ability, while the disadvantage is that this process requires a large amount of surfactant (62, 63). It provides a higher loading capacity for lipophilic compounds. Hydrophilic antibacterial active substances are poorly trapped and more difficult to scale up (64).
2.2.1.2. Extrusion gelation method
Squeeze gelation is a process in which a biopolymer solution is passed through a nozzle into a gelling environment. On a small scale, the biopolymer solution is loaded into a syringe and passed through a needle into the gel state to form a gel. The technique is also suitable for industrial scale and is a gentle and convenient method for the encapsulation of hydrophilic and hydrophobic compounds (65).
Zeng introduced catechins (CC) and proanthocyanidins (PC) into rice starch gels by extrusion gelation to explore the effects of polyphenol molecules on the structure of starch gel systems in hot extrusion 3D printing (66). Owing to the strong intermolecular interactions between polyphenol molecules and starch chains, the starch gel network structure was partially disrupted, leading to a decrease in viscosity and thus improving the extrudability of the starch gel.
2.2.1.3. Nano spray drying method
Spray drying is one of the most popular embedding techniques for a variety of health food products. It can be used directly for hydrophilic ingredients or indirectly for oleophilic ingredients. This technique is beneficial for many applications because it converts the liquid feed into a dry powder, which usually has higher storage stability and is also easier to transport and store (67). In brief, the process of spray drying is that the fluid material is first pumped through the atomizer, which breaks it down into a stream of fine droplets, which are then rapidly dried by the heated gas flowing through the chamber, where the droplets dry and fall to the bottom of the chamber in a few seconds, and the resulting powder is drawn into the cyclone, which is connected to an outlet fan, and the dried air is passed through a filter to separate the very fine powder, which is then removed from the entire embedding process is completed with the discharge from the spray dryer. The main challenge of this technique is the viscosity of the biopolymer suspension, which may clog the atomizer and reduce the encapsulation yield (68). Application of modified chitosan (e.g., glycol chitosan) has been proposed to obtain better nanoparticles by this method (69).
2.2.2. Bottom-up method
2.2.2.1. Supercritical fluid technology
Supercritical fluid technology is a new type of nanocarrier preparation technology. A supercritical fluid is a special state of fluid formed when the material is above its critical temperature and critical pressure (70). Carbon dioxide, nitrogen, methane, water, and other common gases and liquids can be used as supercritical fluids, and in the industrial application of supercritical fluid technology, the most used is supercritical carbon dioxide (SC-CO2). SC-CO2 is a new solvent with broad industrial application prospects (71). In addition, SC-CO2 can be used not only as a solvent, antisolvent, and solute but also in applications in drug delivery, such as the formulation of polymeric nanocarriers in combination with different drug molecules. With its tunability above the critical pressure and temperature, it provides control over particle size, particle morphology, and drug loading (72).
Curcumin is an excellent antibacterial active substance and its antibacterial effect can be improved by combining it with different nanoparticles. Xie (73) prepared curcumin nanoparticles by supercritical CO2, and this nano preparation This nanoparticle preparation showed higher solubility and enhanced antibacterial, antioxidant, and anticancer effects with a minimum inhibitory concentration (MIC) 50% lower than that of free curcumin solution.
2.2.2.2. Nanoprecipitation
Nanoprecipitation (FNP) relies on the spontaneous emulsification of the organic internal phase of the polymer or bioactive compound and organic solvent, accommodating the external phase that then enters the aqueous phase (74). The nanoprecipitation method prepares nanoparticles by controlled mixing of solute solution and non-solvent with rapid reaction and is suitable for the preparation of a wide range of nanoparticles. Ahmed (75) used FNP to encapsulate β-carotene in NPs via a food-grade protein (sodium caseinate). FNP showed significant advantages in controlling NP size and increasing β-carotene loading and stability. The internal structure of NPs can be tuned by the flow rate. The loose structure is accompanied by a high release of β-carotene, while the compact structure is accompanied by a slight release.
2.2.2.3. Sequential deposition
Sequential Deposition is the sequential processing of the donor and acceptor layers, thus allowing, on the one hand, the two active components to be individually regulated and optimized; on the other hand, weakening the interactions between the two components and reducing the complexity of the film formation kinetics, thus providing new opportunities for reducing production requirements. Widely used in the development of multilayer nanocarriers, with low cost, easy adoption, simple assembly techniques and better protection of lipophilic compounds (76).
Multilayer coconut oil–water emulsions containing curcumin were prepared and stabilized using a layer-by-layer technique assembled using multilayer gelatin, gum Arabic and tannic acid. Thus, the ability to control interfacial film properties by combining electrostatic, hydrophobic and hydrogen-bonding interactions may be able to control the physical stability, payload retention and accessibility of multilayer emulsions.
3. Loading and release mechanism of nano-delivery
The nano slow-release system can be used as a carrier to load antibacterial active substances. After reaching the corresponding target environment, there can be different release rates depending on the type or ratio of the carrier material. By adjusting the carrier material’s type or ratio, the bioactive substances’ release rate can be controlled, thus producing a nano slow-release system with targeting and slow-release characteristics.
There are two main purposes for carrier loading of the antibacterial active substance: one is to improve the situation of poor water solubility and low bioavailability of functional and active substances, and the other is to achieve a slow-release effect and improve the therapeutic effect of functional and active substances. The slow-release refers to the slow release of the drug at a predetermined rate by maintaining a constant drug concentration level for a specific time and system, reducing fluctuations in the drug concentration in the body to reduce the side effects of the drug. This slow-release system ensures that the antibacterial active substance is maintained in the effective concentration range for extended periods. The loading and release mechanism of the carrier is mainly through non-covalent forces between the antibacterial active substance and the carrier material, such as hydrogen bonding, π-π stacking, van der Waals forces, electrostatic interactions, etc., as well as the loading and adsorption of the antibacterial active substance by the chemical assembly.
3.1. Covalent bonding
Nano slow-release systems can incorporate high concentrations of therapeutic agents through the presence of appropriate functional groups on their surfaces. The drug is covalently coupled to the nanocarrier due to the presence of a large number of functional groups on the surface of the nanocarrier. The release of the drug after binding occurs through enzymatic cleavage or chemical breakage of readily hydrolyzable bonds. The nanocarrier-drug coupling slowly diffuses into the cell membrane, resulting in a specific and controlled release of the drug to the target site. This covalent coupling allows stable nanocarrier systems to be used for targeted drug delivery. For instance, cross-linking and reduction of ab-binding activity can be minimized by reacting with dimethyl maleic anhydride (DMMA) and preventing them from participating in aminolysis coupling reactions. At the end of the conjugation reaction, the protecting group can be removed from the final conjugate by simply lightly acidifying the solution. By using a polymer carrier with only one reactive group at the end of the polymer chain, branching during the conjugation reaction can be avoided (Figure 4).
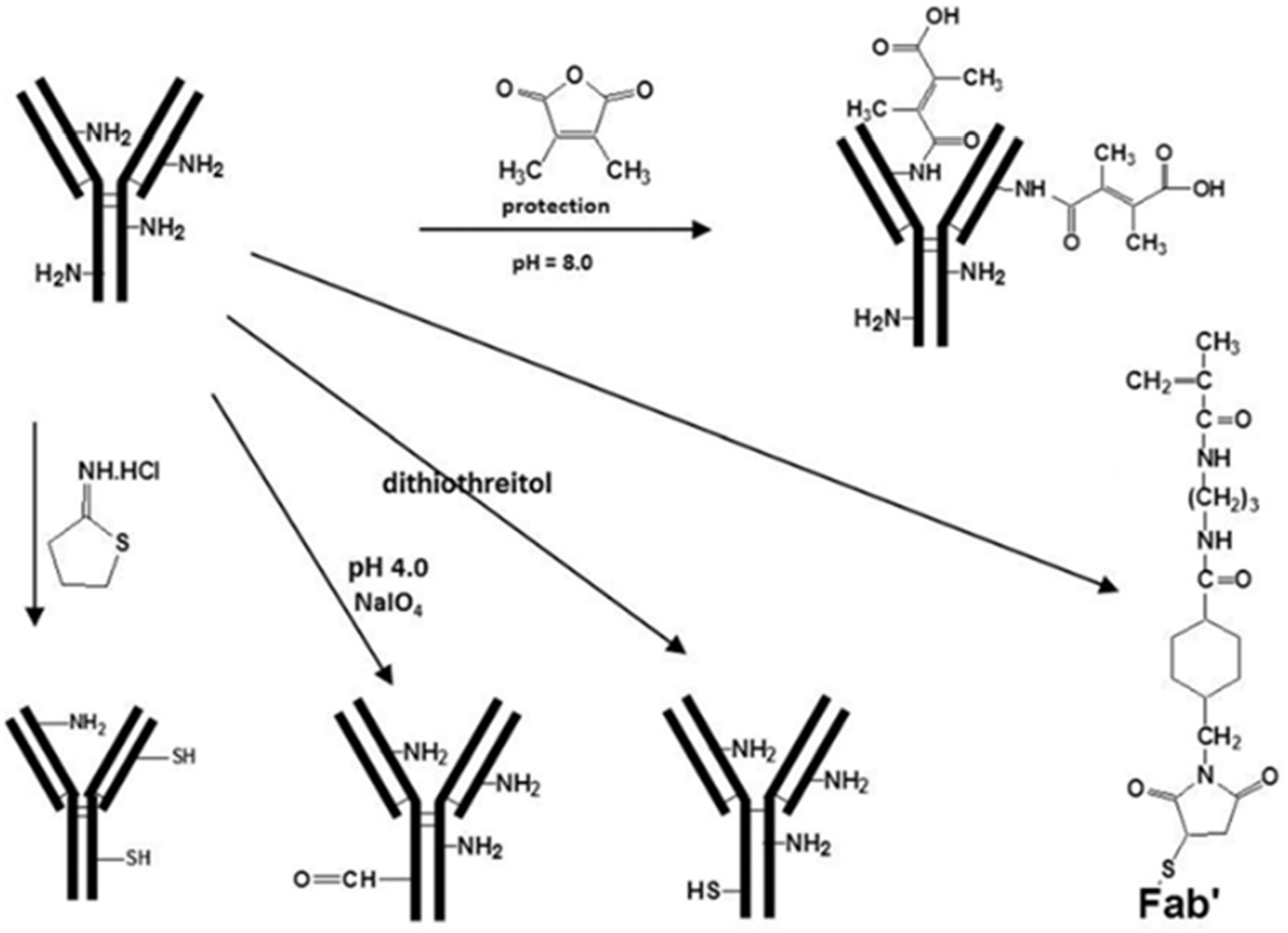
Figure 4. Structural modifications for covalent binding reactions of polymer precursors (77).
3.2. Encapsulation
Encapsulation is a strategy for loading therapeutic drugs into nanocarrier systems. The hollow space within the nanocarrier is capable of adequately encapsulating the drug molecules. Nanocarriers such as polymeric nanocarriers, β-cyclodextrins, nanocapsules, dendrimers, etc. can effectively encapsulate drugs within their hollow cavities (78, 79). The hydrophobic nature of the inner cavity allows the incorporation of more hydrophobic drugs in the nanocarrier through hydrophobic interactions or hydrogen bonding. This encapsulation can also occur through physical interactions. The carotenoid-rich oil recovered by Chuyen from the rind of gac fruit, a waste product of gac fruit processing, has been encapsulated using a spray dryer with a mixture of whey protein concentrate and gum Arabic. As shown in Figure 5. The encapsulation of the carotenoids by spray drying technology significantly improved the stability of the carotenoids and extended the storage period, while also providing them with a slow and controlled release effect (81). In the case of liposomes, encapsulation occurs through active and passive drug loading. The release of the drug takes place through pH-tendency neutralization or hydrolysis, thiolysis and thermal decomposition mechanisms (81).
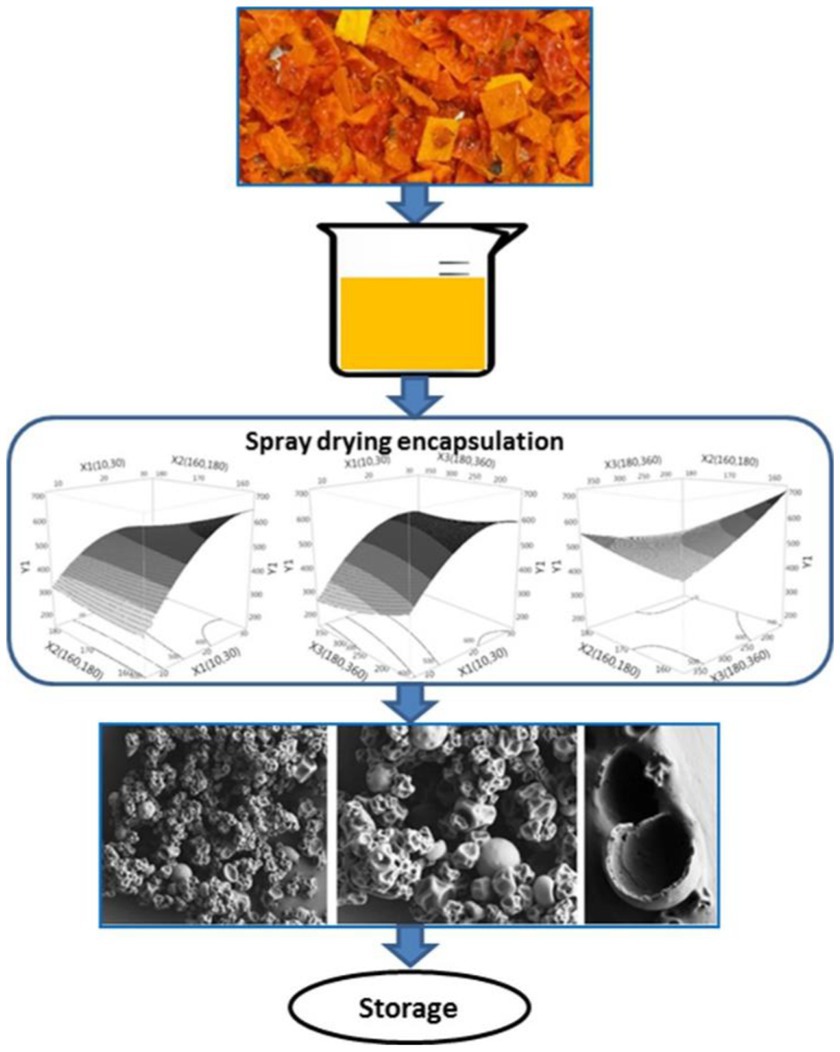
Figure 5. Encapsulation process of carotenoids (80).
3.3. Electrostatic interactions
Electrostatic interactions are the most direct loading strategy, while the adsorption of active substance molecules depends mainly on the surface potential of the nanocarrier material and the type of active substance structure. In general, the adsorption behavior of common nanocarriers is mainly influenced by the pH value. When the solution pH is low, i.e., the solution is acidic, a large number of hydrated hydrogen ions in the solution will compete with the metal ions on the surface of the material for available adsorption sites, and the positively charged surface of the nanocarrier material at this time does not have the electrostatic gravitational force and cannot load the functional active substance well into the pore structure of the nanocarrier material. On the contrary, when the solution pH is high, electrostatic attraction exists between the active substance and the metal ions in the pores of the carrier material (Figure 6).
Nanocarriers with functional groups such as carboxyl and amine groups increase the solubility of hydrophobic drugs, and these high-density functional groups enable electrostatic interactions between antibacterial active substance and nanocarrier materials, which in turn encapsulate them in the nanomaterials to form a nano-delivery system. Certain NSAIDs, such as indomethacin, ciprofloxacin, diflunisal, and ibuprofen, are effectively integrated within the nanocarriers through electrostatic interactions, which greatly improves the therapeutic efficacy of these drugs (82).
Furthermore, nanocarriers with functional groups such as carboxyl and amine groups can increase the solubility of bioactive substances in solvents. These high-density functional groups can be efficiently integrated within the nanocarriers through electrostatic interactions. For example, polymeric nanoparticles with a bilayer load representative flavonoids into deblock polymeric nanoparticle carriers (NPC) DDS with a cationic corona and hydrophobic core. It can improve the problems of poor water solubility and low bioavailability of flavonoids for clinical applications (Figure 7) (83).
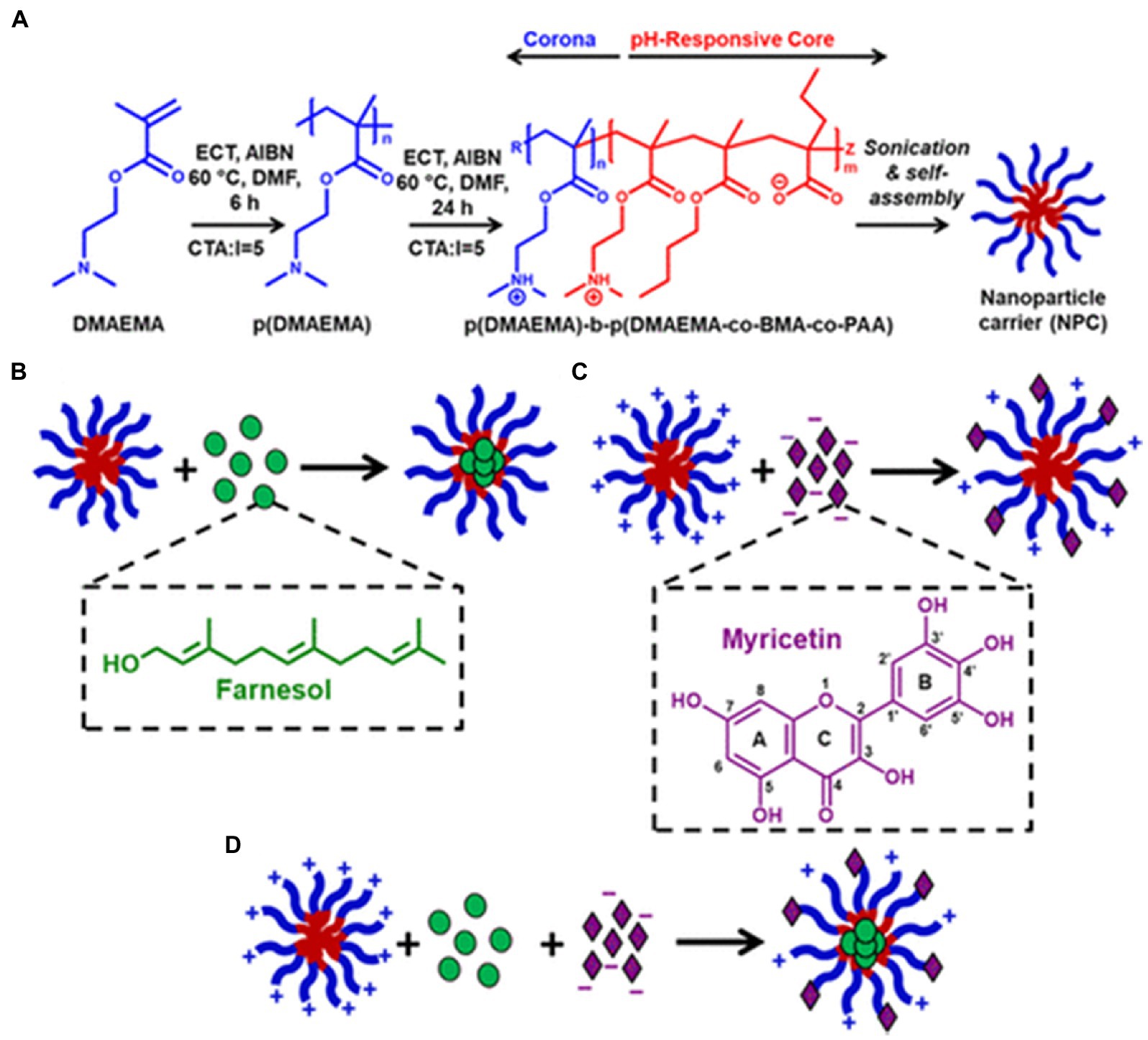
Figure 7. Cationic NPCs hypothesized to coload hydrophobic drugs and flavonoids using different mechanisms. (A) Scheme showing the cationic NPC polymer synthesis process, diblock composition, and micelle self-assembly in aqueous conditions; (B) Cartoon illustrating the known mechanism of hydrophobic drug (e.g., farnesol) loading within the NPC hydrophobic core; (C) Cartoon illustrating the hypothesized mechanism of flavonoid (e.g., myricetin) loading with cationic NPCs via an electrostatic interaction; (D) Cartoon illustrating the proposed mechanism of coloading NPCs with farnesol and myricetin. (A, B) Reproduced with permission from (27).
3.4. Hydrogen bonding
Hydrogen bonding is a special type of inter- or intra-molecular interaction force. A hydrogen atom is bonded covalently to an atom X, which has a large electronegativity. If it is close to an atom Y with a large electronegativity and small radius (O F N, etc.), a special intermolecular or intramolecular interaction in the form of X-H.Y is generated between X and Y using hydrogen as a medium, called hydrogen bonding. Hydrogen bonding can combine antibacterial active substance with poor water solubility with macromolecular nanocarriers. β-carotene (Car) has a wide range of physiological effects, but its insolubility in water leads to low bioavailability. Therefore, hydroxyl and carboxyl groups on the surface of carotene can be used to combine with organic functional groups on the surface of oleanolic acid (OA) to form hydrogen bonds (84). As shown in Figure 8, this is a dynamic process of self-assembly of Car and OA hydrogen bonds. Before assembly, both the OA and Car were free to disperse in DCM (Figure 8B). After 1,000 ps simulation, Car was successfully encapsulated (Figure 8C), and two types of hydrogen bonds with a length of 3.562 and 3.347 Å were observed (as shown in red circles in Figure 8D), corresponding to the –C––O···H–O– and –O–Ḥ··O–H, respectively. Therefore, it could be assumed that the self-assembly of OA was driven by hydrogen bonding.
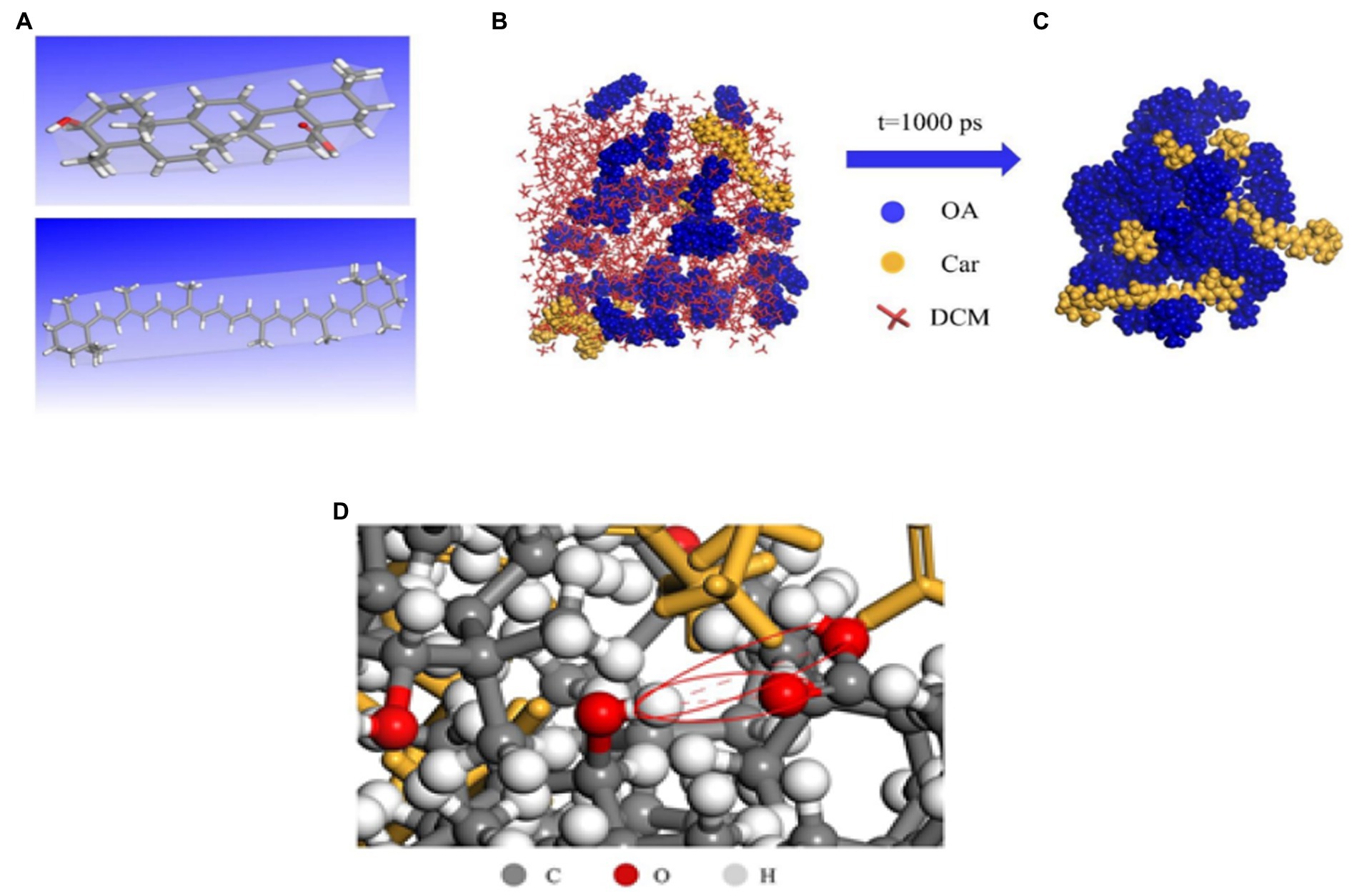
Figure 8. Molecular dynamics (MD) simulation of Car/OA NPs. The optimal geometry of OA (A, top), Car (A, bottom); OA and Car dispersed in DCM before simulation (B); and Schematic diagram of Car/OA NPs after MD simulation for 1,000 ps (C); Hydrogen bonding in Car/OA NPs (D) (84).
4. Application of nano slow-release system
Various bioactive compounds are usually poorly stable, water-soluble, and have toxic effects that make it difficult to perform their potential active functions. These drawbacks can be addressed by nano-release systems (e.g., nanoparticles and nano-emulsions) to improve stability and water solubility and mitigate toxicity. Loading and transporting antibacterial active substances through nano-delivery systems can be applied to antibacterial preservation, food packaging, the food industry, etc., greatly expanding the range of applications of the antibacterial active substance (Figure 9) (86).
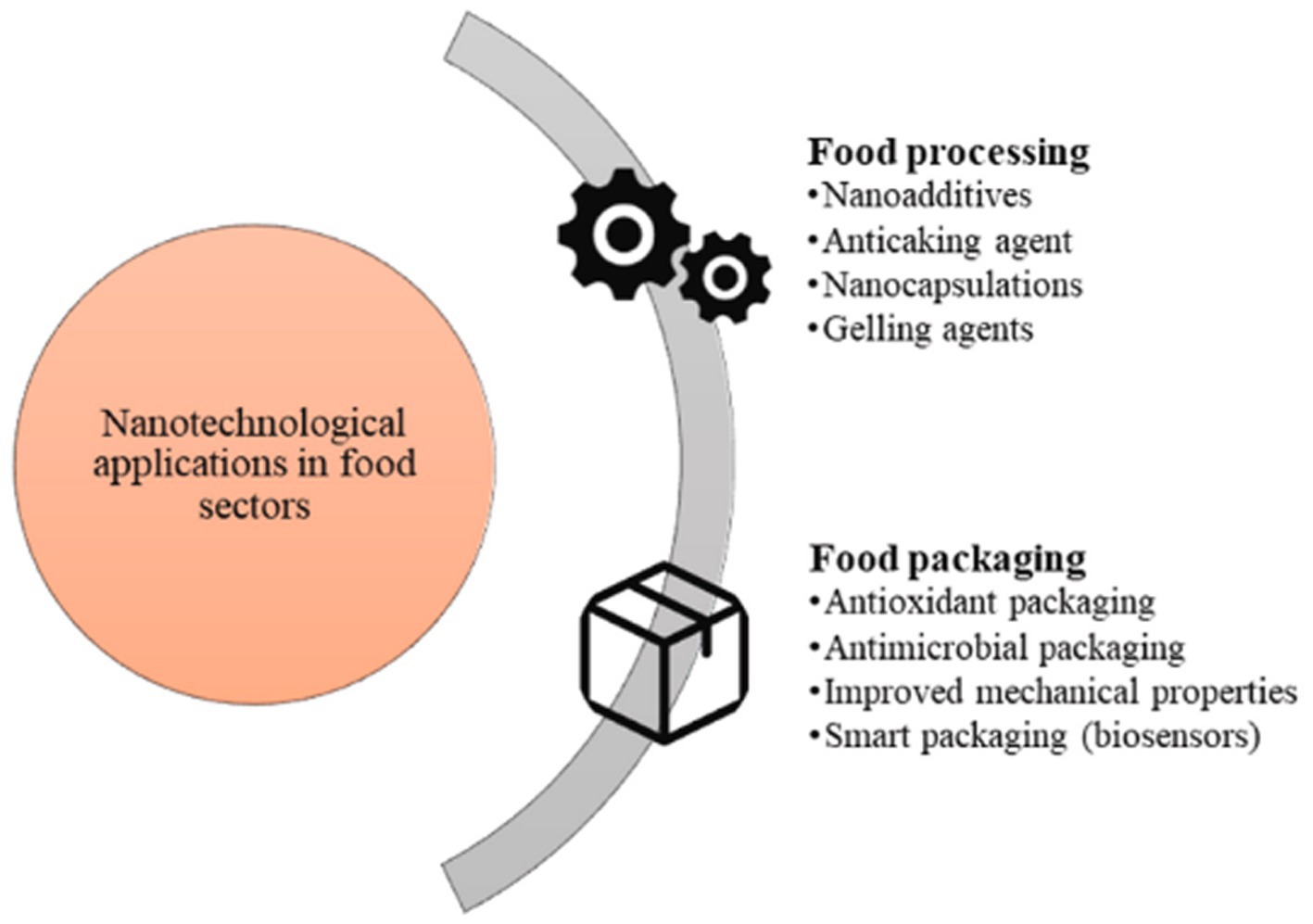
Figure 9. Nanotechnology in Food (85).
4.1. Application of nano slow-release system in bacterial inhibition and preservation
A natural antibacterial active substances such as curcumin, quercetin, and sulforaphane have superior antibacterial properties and can be applied to food preservation for both antiseptic purposes and without any toxic side effects to the human body. However, the most urgent problem in the field of food preservation is that these antibacterial active substances react with food components, resulting in a decrease in the efficiency of their application in food matrices. Therefore, an antibacterial active substance with antibacterial properties can be encapsulated into nanomaterials to achieve sustained release, prevent undesirable interactions and provide high stability and effective antibacterial activity during food storage (87, 88).
Wang Y et al. (89) studied the antibacterial activity of curcumin nano-capsules using gelatin and porous starch against a variety of foodborne pathogenic and spoilage bacteria such as Gram-negative bacteria (Escherichia coli and Yersinia pestis), Gram-positive bacteria (Staphylococcus aureus, Bacillus subtilis and Bacillus cereus) and fungi (Aspergillus niger, Penicillium nodosum and Saccharomyces cerevisiae). Due to the structural differences in cell membranes, the inhibition efficiency of curcumin was different for different strains of bacteria, and curcumin nanocarriers showed better inhibition for fungi than bacteria and higher inhibition for Gram-positive bacteria than Gram-negative bacteria. This study illustrates that the nano slow-release system can maintain the antibacterial properties of curcumin well, which provides a theoretical basis for the application of curcumin nanocarriers in practical food products.
4.1.1. Application in fruits and vegetables
Fruits and vegetables in the post-harvest storage and transportation process due to temperature, humidity and other external conditions susceptible to oxidation, browning, weight loss and other physiological and biochemical changes, seriously affecting the quality of fruits and vegetables and shelf life (90). In particular, microbial contamination caused by spoilage will not only cause losses but more serious is likely to cause food-borne diseases and health hazards (91).
Therefore, it is possible to combine active substances with nanomaterials to make nanofilms, nano coatings, nano packaging materials and other products. It provides effective help for the further preservation of fruits and vegetables. It can greatly extend the shelf life, and preservation period. Deka et al. (92) prepared sodium chitosan phosphate nanoparticles (CPN) containing curcumin and measured the average particle size of CPN and curcumin-loaded CPN to be 53 nm and 91 nm, respectively, and found in practical applications in fruits and vegetables that curcumin nano preparations released more under acidic pH conditions than under normal pH conditions, while curcumin at trace amounts (0.5 mg/ml) had a higher effect on The curcumin nanoparticles showed inhibitory activity against Gram-positive and Gram-negative bacteria as well as fungi, and the nanoparticles were effective in retaining freshness in foods where acidity increases with time. The antibacterial properties and stability of curcumin were maintained after complexation, and the combination of curcumin and nanomaterials produced an antibacterial agent that is less likely to develop resistance both in application and preservation, which increases the potential of curcumin applications.
4.1.2. Application in dairy products
Antioxidant compounds such as flavonoids are the most important plant active compounds and they have significant health-promoting effects. Direct addition of flavonoids to food, especially to foods such as beverages and dairy products, can lead to undesirable taste and color, while encapsulation with nanomaterials can reduce the loss of bioactive compounds, enhance their dispersion in aqueous solutions, and improve their oral bioavailability. Concerned scholars have added curcumin to milk to reduce the oxidative effect of lipids in milk. Hee prepared a curcumin nanoemulsion (Cur-Nes) that could be added to milk to reduce lipid oxidation, and its free radical scavenging activity did not vary significantly with water content but increased significantly with surfactant concentration (93). In vitro lipid, digestibility tests revealed that high surfactant concentration promoted the dissolution of curcumin in the oil phase and increased the slow-release effect. This resulted in increased antioxidant activity, delayed lipid degradation and improved milk quality.
Colloidal formulations containing curcumin are generally available in micelles and hydrogels, and the use of colloidal formulations containing curcumin can be equally effective in increasing its solubility, and stability. The use of colloidal formulations containing curcumin is also effective in increasing its solubility, stability, and slow-release properties, as well as its functionality and antioxidant and antimicrobial activities in food products (94). Esmaili et al. (95) proposed a scheme to load curcumin with protein as a colloidal carrier using proteins from camel milk to make micelles containing curcumin and measured that the solubility of curcumin loading in the colloid increased by at least The solubility of curcumin loading in colloids was measured to be increased by at least 2,500-fold, and it also exhibited more antioxidant properties than free curcumin.
4.2. Application in the biomedical field
Some wound dressings currently in use have interesting characteristics such as excellent porosity, good water absorption, moderate water vapor transmission, high drug loading efficiency, and good ability to provide a moist environment, but they are limited in terms of antimicrobial properties (96). They do not protect wounds from microbial invasion, leading to exposure to microbial infection and causing delays in the wound-healing process. In addition, some wound dressings contain synthetic antibiotics that can cause undesirable side effects to the patient. Natural active substances exhibit unique characteristics such as good biocompatibility and reduced toxicity (97). Curcumin is one such natural compound that has demonstrated several biological activities such as anticancer, antibacterial and antioxidant properties. Its good antibacterial and antioxidant activity make it beneficial in the treatment of wounds. Selenia (98) developed a novel wound dressing comprising curcumin-in-liposomes-in-chitosan hydrogel. The prolonged retention time of curcumin is assured by chitosan hydrogel and sustained delivery provided by liposomes-in-hydrogel. The antibacterial ability of curcumin is greatly improved, which provides rapid wound healing.
5. Conclusion and prospects
With the improvement of people’s living standards and more and more attention to food health and safety, the demand for food is also increasing to “green” and “natural” change. On the other hand, with the abuse of chemical pesticides, pathogenic microorganisms develop resistance to drugs, endangering the environment and human health. Therefore, it has become a new direction of food safety research to find new natural antibacterial active substances with application value. This paper reviews the construction of a nano-sustained-release system for active antibacterial substances, from the selection of nanomaterials to the innovation of preparation technology. Different nano-carrier materials are selected for loading and encapsulation according to different application ways of active antibacterial substances. Food-grade antibacterial preservation packaging mainly uses green nanocarrier materials such as polysaccharides, porous starch, and protein, which can significantly improve the stability of active antibacterial substances and import safety to ensure food safety. However, fruit and vegetable pathogenic microorganisms can use inorganic and mixed nanocarrier materials. Compared with simple antibacterial substances based on the specificity and specificity of pathogenic microorganisms, there is almost no resistance to nanocarrier materials. Moreover, the nanocarrier materials of the bacteriostatic active substances can gradually destroy the bacterial membrane of bacteria and achieve an excellent bacterial inhibition effect.
Although the research on using nanotechnology to deliver active antibacterial substances has been very extensive, there are still some problems in its practical application based on the instability of active antibacterial substances and the safety of nanocarrier materials. First, the current assessment methods for antibacterial preservation are limited, mainly E. coli and S. aureus, and some indicators of antioxidant tests. Less information is related to fungi, viruses, and other dangerous bacteria. Secondly, the targeted modification of active antibacterial substances based on delivery systems is still challenging to achieve scale-up production and commercial application in practice, mainly because the materials’ low loading, high cost, and potential toxicity restrict the scale-up production. At the same time, the existing preparation process and encapsulation technology methods have particular contingency and irreducibility and lack uniform quality control standards and clinical trial systems.
Therefore, more research is needed to gain a deeper understanding of the interactions between these nanomaterials and active antibacterial substances and to continuously explore and optimize the preparation process of nanomaterials to accelerate the development of more economical, stable, high-utilization, slow-release nanomaterials. It is necessary to strengthen the research on the mechanism of assembly and release of bioactive substances in nanocarriers to clarify the chemical interaction between the substances and carriers. To enhance the research on the assembly and release mechanism of bioactive substances in nanocarriers, clarify the chemical interaction between the substances and the carrier, establish an ideal drug delivery method, and provide a theoretical basis for the prediction and development of the subsequent formulation of drug delivery carriers. This review will inspire researchers to develop and synthesize nano-delivery platforms and technological approaches with various properties and functions to broaden their application range in agriculture, food, and medicine.
Author contributions
JC and MG: conceptualization and writing – original draft. JW: resources. YL and JL: methodology and formal analysis. XZ and YP: visualization. GC and XH: project administration. DX: funding acquisition. GL: writing – review and editing. All authors contributed to the article and approved the submitted version.
Funding
This work was supported by National Key Research and Development Program of China (2022YFF0606800), China Agriculture Research System of MOF and MARA (CARS-23-E03), and the Risk Assessment on Vegetable Products(GJFP20210201).
Conflict of interest
JL was employed by the company Internal Trade Food Science Research Institute Co., Ltd.
The remaining authors declare that the research was conducted in the absence of any commercial or financial relationships that could be construed as a potential conflict of interest.
Publisher’s note
All claims expressed in this article are solely those of the authors and do not necessarily represent those of their affiliated organizations, or those of the publisher, the editors and the reviewers. Any product that may be evaluated in this article, or claim that may be made by its manufacturer, is not guaranteed or endorsed by the publisher.
References
1. Imran, M, Das, KR, and Naik, MM. Co-selection of multi-antibiotic resistance in bacterial pathogens in metal and microplastic contaminated environments: an emerging health threat. Chemosphere. (2019) 215:846–57. doi: 10.1016/j.chemosphere.2018.10.114
2. Sharma, A, Flores-Vallejo, RDC, Cardoso-Taketa, A, and Villarreal, ML. Antibacterial activities of medicinal plants used in Mexican traditional medicine. J Ethnopharmacol. (2017) 208:264–329. doi: 10.1016/j.jep.2016.04.045
3. Mathabe, MC, Nikolova, RV, Lall, N, and Nyazema, NZ. Antibacterial activities of medicinal plants used for the treatment of diarrhoea in Limpopo Province. South Africa J Ethnopharmacol. (2006) 105:286–93. doi: 10.1016/j.jep.2006.01.029
4. Hye-Ra Lee, D-GY, Kim, HK, Sohnd, JW, Kim, MJ, Park, JK, Lee, GY, et al. Romo1-derived antimicrobial peptide is a new antimicrobial agent against multidrug-resistant bacteria in a murine model of sepsis. MBio. (2020) 11:e03258–19. doi: 10.1128/mbio.03258-19
5. Park, SI, Daeschel, MA, and Zhao, Y. Functional properties of antimicrobial lysozyme-chitosan composite films. J Food Sci. (2004) 69:M215–21. doi: 10.1111/j.1365-2621.2004.tb09890.x
6. Gomes, CF, Gomes, JH, and da Silva, EF. Bacteriostatic and bactericidal clays: an overview. Environ Geochem Health. (2020) 42:3507–27. doi: 10.1007/s10653-020-00628-w
7. Chorianopoulos, N, Epameinontas Evergetis, AM, Kalpoutzakis, E, and Asah, G-JN. Characterization of the essential oil volatiles of Satureja thymbra and Satureja parnassica: influence of harvesting time and antimicrobial activity. J Agric Food Chem. (2006) 54:3139–45. doi: 10.1021/jf053183n
8. Querido, MM, Paulo, I, Hariharakrishnan, S, Rocha, D, Barbosa, N, Galhano Dos Santos, R, et al. Self-disinfecting paints with the natural antimicrobial substances: colophony and curcumin. Antibiotics (Basel). (2021) 10:1351–65. doi: 10.3390/antibiotics10111351
9. Quinto, EJ, Caro, I, Villalobos-Delgado, LH, Mateo, J, De-Mateo-Silleras, B, and Redondo-Del-Rio, MP. Food safety through natural antimicrobials. Antibiotics (Basel). (2019) 8:208. doi: 10.3390/antibiotics8040208
10. Su, R, Bai, X, Liu, X, Song, L, Liu, X, Zhan, X, et al. Antibacterial mechanism of eugenol against Shigella sonnei and its antibacterial application in lettuce juice. Foodborne Pathog Dis. (2022) 19:779–86. doi: 10.1089/fpd.2022.0046
11. Wang, Y-F, Shao, J-J, Zhou, C-H, Zhang, D-L, Bie, X-M, Lv, F-X, et al. Food preservation effects of curcumin microcapsules. Food Control. (2012) 27:113–7. doi: 10.1016/j.foodcont.2012.03.008
12. Quichaba, MB, Moreira, TFM, de Oliveira, A, de Carvalho, AS, de Menezes, JL, Gonçalves, OH, et al. Biopreservatives against foodborne bacteria:combined effect of nisin and nanoncapsulated curcumin and co encapsulation of nisin and curcumin. J Food Sci Technol. (2022). doi: 10.1007/s13197-022-05641-8
13. Rashidinejad, A, Boostani, S, Babazadeh, A, Rehman, A, Rezaei, A, Akbari-Alavijeh, S, et al. Opportunities and challenges for the nanodelivery of green tea catechins in functional foods. Food Res Int. (2021) 142:110186. doi: 10.1016/j.foodres.2021.110186
14. Zhang, H, Feng, H, Ling, J, Ouyang, XK, and Song, X. Enhancing the stability of zein/fucoidan composite nanoparticles with calcium ions for quercetin delivery. Int J Biol Macromol. (2021) 193:2070–8. doi: 10.1016/j.ijbiomac.2021.11.039
15. Rashidi, L. Different nano-delivery systems for delivery of nutraceuticals. Food Biosci. (2021) 43:101258. doi: 10.1016/j.fbio.2021.101258
16. Chen, S, Hao, X, Liang, X, Zhang, Q, Zhang, C, Zhou, G, et al. Inorganic nanomaterials as carriers for drug delivery. J Biomed Nanotechnol. (2016) 12:1–27. doi: 10.1166/jbn.2016.2122
17. Niezabitowska, E, Smith, J, Prestly, MR, Akhtar, R, von Aulock, FW, Lavallee, Y, et al. Facile production of nanocomposites of carbon nanotubes and polycaprolactone with high aspect ratios with potential applications in drug delivery. RSC Adv. (2018) 8:16444–54. doi: 10.1039/C7RA13553J
18. Chad, D, Vecitis, KRZ, Kang, S, and Elimelech, M. Electronic-structure-dependent bacterial cytotoxicity of single-walled carbon nanotubes. ACS Nano. (2010) 4:5471–9. doi: 10.1021/nn101558x
19. Wei Yuan, GJ, Che, J, Qi, X, Rong, X, Chang, MW, Chen, Y, et al. Deposition of silver nanoparticles on multiwalled carbon nanotubes grafted with Hyperbranched poly(amidoamine) and their antimicrobial effects. J Phys Chem C. (2008) 112:18754–9. doi: 10.1021/jp807133j
20. Ghahremani, P, Mostafatabar, AH, Bahlakeh, G, and Ramezanzadeh, B. Rational design of a novel multi-functional carbon-based nano-carrier based on multi-walled-CNT-oxide/polydopamine/chitosan for epoxy composite with robust pH-sensitive active anti-corrosion properties. Carbon. (2022) 189:113–41. doi: 10.1016/j.carbon.2021.11.067
21. Thakor, AS, Jokerst, J, Zavaleta, C, Massoud, TF, and Gambhir, SS. Gold nanoparticles: a revival in precious metal administration to patients. Nano Lett. (2011) 11:4029–36. doi: 10.1021/nl202559p
22. Ling, L, Huang, XY, and Zhang, WX. Enrichment of precious metals from wastewater with Core-Shell nanoparticles of iron. Adv Mater. (2018) 30:e1705703. doi: 10.1002/adma.201705703
23. Attia, YA, Vázquez-Vázquez, C, Blanco, MC, Buceta, D, and López-Quintela, MA. Gold nanorod synthesis catalysed by au clusters. Faraday Discuss. (2016) 191:205–13. doi: 10.1039/C6FD00015K
24. Ahmad, B, Hafeez, N, Bashir, S, Rauf, A, and Mujeeb, UR. Phytofabricated gold nanoparticles and their biomedical applications. Biomed Pharmacother. (2017) 89:414–25. doi: 10.1016/j.biopha.2017.02.058
25. Pajerski, W, Ochonska, D, Brzychczy-Wloch, M, Indyka, P, Jarosz, M, Golda-Cepa, M, et al. Attachment efficiency of gold nanoparticles by gram-positive and gram-negative bacterial strains governed by surface charges. J Nanopart Res. (2019) 21:186. doi: 10.1007/s11051-019-4617-z
26. Yuan, P, Ding, X, Yang, YY, and Xu, QH. Metal nanoparticles for diagnosis and therapy of bacterial infection. Adv Healthc Mater. (2018) 7:e1701392. doi: 10.1002/adhm.201701392
27. Folorunso, A, Akintelu, S, Oyebamiji, AK, Ajayi, S, Abiola, B, Abdusalam, I, et al. Biosynthesis, characterization and antimicrobial activity of gold nanoparticles from leaf extracts of Annona muricata. J Nanostructure Chem. (2019) 9:111–7. doi: 10.1007/s40097-019-0301-1
28. Marcel, MM, Gin, P, and Shimon Weiss, A. Paul Alivisatos semiconductor nanocrystals as fluorescent biological labels. Science. (1998) 281:2013–16. doi: 10.1126/science.281.5385.2013
29. Yang, J, Lu, H, Li, M, Liu, J, Zhang, S, Xiong, L, et al. Development of chitosan-sodium phytate nanoparticles as a potent antibacterial agent. Carbohydr Polym. (2017) 178:311–21. doi: 10.1016/j.carbpol.2017.09.053
30. Zhang, R, Li, Q, Yang, L, Dwibedi, V, Ge, Y, Zhang, D, et al. The antibacterial activity and antibacterial mechanism of the tea polyphenol liposomes/lysozyme–chitosan gradual sustained release composite coating. Int J Food Sci Technol. (2022) 57:3691–701. doi: 10.1111/ijfs.15694
31. Tibolla, H, Feltre, G, Sartori, T, Czaikoski, A, Pelissari, FM, Menegalli, FC, et al. Shelf life of cashew nut kernels packed in banana starch-based nanocomposites. Int J Food Sci Technol. (2020) 56:3682–90. doi: 10.1111/ijfs.14920
32. Tibolla, H, Czaikoski, A, Pelissari, FM, Menegalli, FC, and Cunha, RL. Starch-based nanocomposites with cellulose nanofibers obtained from chemical and mechanical treatments. Int J Biol Macromol. (2020) 161:132–46. doi: 10.1016/j.ijbiomac.2020.05.194
33. Mohammad Amini, A, and Razavi, SMA. A fast and efficient approach to prepare starch nanocrystals from normal corn starch. Food Hydrocoll. (2016) 57:132–8. doi: 10.1016/j.foodhyd.2016.01.022
34. Wang, H, Kong, L, and Ziegler, GR. Fabrication of starch - Nanocellulose composite fibers by electrospinning. Food Hydrocoll. (2019) 90:90–8. doi: 10.1016/j.foodhyd.2018.11.047
35. Adesina Adegoke, K, Samuel Agboola, O, Ogunmodede, J, Oluyomi Araoye, A, and Solomon, BO. Metal-organic frameworks as adsorbents for sequestering organic pollutants from wastewater. Mater Chem Phys. (2020) 253:123246. doi: 10.1016/j.matchemphys.2020.123246
36. Abedi, M, Abolmaali, SS, Heidari, R, Mohammadi Samani, S, and Tamaddon, AM. Hierarchical mesoporous zinc-imidazole dicarboxylic acid MOFs: surfactant-directed synthesis, pH-responsive degradation, and drug delivery. Int J Pharm. (2021) 602:120685. doi: 10.1016/j.ijpharm.2021.120685
37. Zhu, J, Li, J, Chu, B, Liu, S, Fu, S, Qin, Q, et al. Excitation of catalytic performance on MOFs derivative carrier by residual carbon for low-temperature NH3-SCR reaction. Molecular. Catalysis. (2023) 535:112859–869. doi: 10.1016/j.mcat.2022.112859
38. Li, H, Zhang, Y, Zhang, Y, Wei, F, Deng, Y, Lin, Z, et al. Hybridization of carboxymethyl chitosan with bimetallic MOFs to construct renewable metal ion "warehouses" with rapid sterilization and long-term antibacterial effects. Carbohydr Polym. (2023) 301:120317. doi: 10.1016/j.carbpol.2022.120317
39. Moussa, Z, Hmadeh, M, Abiad, MG, Dib, OH, and Patra, D. Encapsulation of curcumin in cyclodextrin-metal organic frameworks: dissociation of loaded CD-MOFs enhances stability of curcumin. Food Chem. (2016) 212:485–94. doi: 10.1016/j.foodchem.2016.06.013
40. Xiao, J, Zhu, Y, Huddleston, S, Li, P, Xiao, B, Farha, OK, et al. Copper metal-organic framework nanoparticles stabilized with folic acid improve wound healing in diabetes. ACS Nano. (2018) 12:1023–32. doi: 10.1021/acsnano.7b01850
41. Iemma, F, Spizzirri, UG, Puoci, F, Muzzalupo, R, Trombino, S, Cassano, R, et al. pH-sensitive hydrogels based on bovine serum albumin for oral drug delivery. Int J Pharm. (2006) 312:151–7. doi: 10.1016/j.ijpharm.2006.01.010
42. Jaafar, AM, Hasnu, N, Zainal, Z, Masarudin, MJ, Md Ajat, MM, Aung, MM, et al. Preparation, characterisation and antibacterial activity of Carvacrol encapsulated in Gellan gum hydrogel. Polymers (Basel). (2021) 13:153. doi: 10.3390/polym13234153
43. Singh, G, Nenavathu, BP, Imtiyaz, K, Moshahid, A, and Rizvi, M. Fabrication of chlorambucil loaded graphene- oxide nanocarrier and its application for improved antitumor activity. Biomed Pharmacother. (2020) 129:110443. doi: 10.1016/j.biopha.2020.110443
44. Serafín, V, Valverde, A, Martínez-García, G, Martínez-Periñán, E, Comba, F, Garranzo-Asensio, M, et al. Graphene quantum dots-functionalized multi-walled carbon nanotubes as nanocarriers in electrochemical immunosensing. Determination of IL-13 receptor α2 in colorectal cells and tumor tissues with different metastatic potential. Sensors Actuators B Chem. (2019) 284:711–22. doi: 10.1016/j.snb.2019.01.012
45. Chen, P, Wang, Z, Zong, S, Zhu, D, Chen, H, Zhang, Y, et al. pH-sensitive nanocarrier based on gold/silver core–shell nanoparticles decorated multi-walled carbon manotubes for tracing drug release in living cells. Biosens Bioelectron. (2016) 75:446–51. doi: 10.1016/j.bios.2015.09.002
46. Baghbanbashi, M, Pazuki, G, and Khoee, S. One pot silica nanoparticle modification and doxorubicin encapsulation as pH-responsive Nanocarriers, applying PEG/lysine aqueous two phase system. J Mol Liq. (2022) 349:118472. doi: 10.1016/j.molliq.2022.118472
47. Taheri-Kafrani, A, Shirzadfar, H, Abbasi Kajani, A, Kudhair, BK, Jasim Mohammed, L, Mohammadi, S, et al. Functionalized graphene oxide/Fe3O4 nanocomposite: A biocompatible and robust nanocarrier for targeted delivery and release of anticancer agents. J Biotechnol. (2021) 331:26–36. doi: 10.1016/j.jbiotec.2021.03.005
48. Pan, G, Jia, T-t, Huang, Q-x, Qiu, Y-y, Xu, J, Yin, P-h, et al. Mesoporous silica nanoparticles (MSNs)-based organic/inorganic hybrid nanocarriers loading 5-fluorouracil for the treatment of colon cancer with improved anticancer efficacy. Colloids Surf B: Biointerfaces. (2017) 159:375–85. doi: 10.1016/j.colsurfb.2017.08.013
49. Su, S, Lin, L, Li, H, Wen, X, Yan, R, and Tao, C. Preparation and properties study of F-SiO2@MPDA-AuNPs drug nanocarriers. Microporous Mesoporous Mater. (2022) 330:111571. doi: 10.1016/j.micromeso.2021.111571
50. Min, K, Yoo, S, Han, MS, and Tae, G. Effective and prolonged targeting of a nanocarrier to the inflammation site by functionalization with ZnBPMP and chitosan. Mater Sci Eng C. (2021) 131:112521. doi: 10.1016/j.msec.2021.112521
51. Lacatusu, I, Badea, N, Badea, G, Mihaila, M, Ott, C, Stan, R, et al. Advanced bioactive lipid nanocarriers loaded with natural and synthetic anti-inflammatory actives. Chem Eng Sci. (2019) 200:113–26. doi: 10.1016/j.ces.2019.01.044
52. Yang, R, Zuo, P, Zhang, M, Meng, D, Wang, B, and Zhen, T. Transglutaminase induced oligochitosan glycosylation of ferritin as a novel nanocarrier for food bioactive molecules. Food Hydrocoll. (2019) 94:500–9. doi: 10.1016/j.foodhyd.2019.03.049
53. De Paz, E, Martín, Á, Estrella, A, Rodríguez-Rojo, S, et al. Formulation of β-carotene by precipitation from pressurized ethyl acetate-on-water emulsions for application as natural colorant - ScienceDirect. Food Hydrocoll. (2012) 26:17–27. doi: 10.1016/j.foodhyd.2011.02.031
54. Rajabi, H, Jafari, SM, Rajabzadeh, G, Sarfarazi, M, and Sedaghati, S. Chitosan-gum Arabic complex nanocarriers for encapsulation of saffron bioactive components. Colloids Surf A Physicochem Eng Asp. (2019) 578:123644. doi: 10.1016/j.colsurfa.2019.123644
55. Lacatusu, I, Niculae, G, Badea, N, Stan, R, Popa, O, Oprea, O, et al. Design of soft lipid nanocarriers based on bioactive vegetable oils with multiple health benefits. Chem Eng J. (2014) 246:311–21. doi: 10.1016/j.cej.2014.02.041
56. Salem, A, Ramadan, AR, and Shoeib, T. Entrapment of β-carotene and zinc in whey protein nanoparticles using the pH cycle method: evidence of sustained release delivery in intestinal and gastric fluids. Food Biosci. (2018) 26:161–8. doi: 10.1016/j.fbio.2018.10.002
57. Liu, Q, Jing, Y, Han, C, Zhang, H, and Tian, Y. Encapsulation of curcumin in zein/ caseinate/sodium alginate nanoparticles with improved physicochemical and controlled release properties. Food Hydrocoll. (2019) 93:432–42. doi: 10.1016/j.foodhyd.2019.02.003
58. Chang, C, Xue, J, Wang, T, et al. Pectin coating improves physicochemical properties of caseinate/zein nanoparticles as oral delivery vehicles for curcumin. Food Hydrocoll. (2017) 70:143–51. doi: 10.1016/j.foodhyd.2017.03.033
59. Kang, P, and Zhong, Q. Low energy, organic solvent-free co-assembly of zein and caseinate to prepare stable dispersions. Food Hydrocoll. (2016) 52:600–6. doi: 10.1016/j.foodhyd.2015.08.014
60. Xiong-Fei, Z, Xiaofeng, M, Ting, H, et al. Inorganic salts induce thermally reversible and anti-freezing cellulose hydrogels. Angew Chem. (2019) 131:7444–8. doi: 10.1002/ange.201902578
61. Ahn, J, Ryu, J, Song, G, Whang, M, and Kim, J. Network structure and enzymatic degradation of chitosan hydrogels determined by crosslinking methods. Carbohydr Polym. (2019) 217:160–7. doi: 10.1016/j.carbpol.2019.04.055
62. Rehman, A, Ahmad, T, Aadil, RM, Spotti, MJ, and Tong, Q. Pectin polymers as wall materials for the nano-encapsulation of bioactive compounds. Trends Food Sci Technol. (2019) 90:35–46. doi: 10.1016/j.tifs.2019.05.015
63. Gaber Ahmed, GH, Fernández-González, A, and Díaz García, ME. Nano-encapsulation of grape and apple pomace phenolic extract in chitosan and soy protein via nanoemulsification. Food Hydrocoll. (2020) 108:105806. doi: 10.1016/j.foodhyd.2020.105806
64. Reis, CP, Neufeld, RJ, Ribeiro, AJ, Veiga, F, and Nanoencapsulation, I. Methods for preparation of drug-loaded polymeric nanoparticles. Nanomedicine. (2006) 2:8–21. doi: 10.1016/j.nano.2005.12.003
65. Cardenas-Vasquez, ED, Smith, KM, Doolan, TJ, and Hsiao, LC. Shear-induced microstructural variations in Nanoemulsion-laden Organohydrogel fibers. ACS Applied Polymer Materials. (2019) 2:594–603. doi: 10.1021/acsapm.9b00979
66. Zeng, X, Li, T, Zhu, J, Chen, L, and Zheng, B. Printability improvement of rice starch gel via catechin and procyanidin in hot extrusion 3D printing. Food Hydrocoll. (2021) 121:106997. doi: 10.1016/j.foodhyd.2021.106997
67. Assadpour, E, and Jafari, SM. Advances in spray-drying encapsulation of food bioactive ingredients: from microcapsules to Nanocapsules. Annu Rev Food Sci Technol. (2019) 10:103–31. doi: 10.1146/annurev-food-032818-121641
68. Jafari, SM, Ghalegi Ghalenoei, M, and Dehnad, D. Influence of spray drying on water solubility index, apparent density, and anthocyanin content of pomegranate juice powder. Powder Technol. (2017) 311:59–65. doi: 10.1016/j.powtec.2017.01.070
69. Mf, A, Nm, B, and Mc, C. Nanoencapsulation of food ingredients using carbohydrate based delivery systems - ScienceDirect. Trends Food Sci Technol. (2014) 39:18–39. doi: 10.1016/j.tifs.2014.06.007
70. Park, H, Kim, JS, Kim, S, Ha, ES, Kim, MS, and Hwang, SJ. Pharmaceutical applications of supercritical fluid extraction of emulsions for micro-/nanoparticle formation. Pharmaceutics. (2021) 13:928. doi: 10.3390/pharmaceutics13111928
71. Ubeyitogullari, A, and Ciftci, ON. A novel and green nanoparticle formation approach to forming low-crystallinity curcumin nanoparticles to improve curcumin's bioaccessibility. Sci Rep. (2019) 9:19112. doi: 10.1038/s41598-019-55619-4
72. Gangapurwala, G, Vollrath, A, De San, LA, and Schubert, US. PLA/PLGA-based drug delivery systems produced with supercritical CO2-A green future for particle formulation? Pharmaceutics. (2020) 12:1118. doi: 10.3390/pharmaceutics12111118
73. Xie, M, Fan, D, Zhao, Z, Li, Z, Li, G, Chen, Y, et al. Nano-curcumin prepared via supercritical: Improved anti-bacterial, anti-oxidant and anti-cancer efficacy. Int J Pharm. (2015) 496:732–40. doi: 10.1016/j.ijpharm.2015.11.016
74. Hari, N, Francis, S, Nair, AR, and Nair, AJ. Synthesis, characterization and biological evaluation of chitosan film incorporated with β-carotene loaded starch nanocrystals. Food Packag Shelf Life. (2018) 16:69–76. doi: 10.1016/j.fpsl.2018.02.003
75. Ahmed Bhutto, R, Fu, Z, Wang, M, Yu, J, Zhao, F, Khanal, S, et al. Facile controlling internal structure of β-carotene-loaded protein nanoparticles by flash nanoprecipitation. Mater Lett. (2021) 304:130523. doi: 10.1016/j.matlet.2021.130523
76. Pavlitschek, T, Gretz, M, and Plank, J. Microcapsules prepared from a polycondensate-based cement dispersant via layer-by-layer self-assembly on melamine-formaldehyde core templates. J Appl Polym Sci. (2013) 127:3705–11. doi: 10.1002/app.37981
77. Ulbrich, K, Hola, K, Subr, V, Bakandritsos, A, Tucek, J, and Zboril, R. Targeted drug delivery with polymers and magnetic nanoparticles: covalent and noncovalent approaches, release control, and clinical studies. Chem Rev. (2016) 116:5338–431. doi: 10.1021/acs.chemrev.5b00589
78. Alvarez-Roman, R, Naik, A, Kalia, YN, Guy, RH, and Fessi, H. Skin penetration and distribution of polymeric nanoparticles. J Control Release. (2004) 99:53–62. doi: 10.1016/j.jconrel.2004.06.015
79. Arpicco, S, Battaglia, L, Brusa, P, Cavalli, R, Chirio, D, Dosio, F, et al. Recent studies on the delivery of hydrophilic drugs in nanoparticulate systems. Journal of Drug Delivery Science and Technology. (2016) 32:298–312. doi: 10.1016/j.jddst.2015.09.004
80. Chuyen, HV, Roach, PD, Golding, JB, Parks, SE, and Nguyen, MH. Encapsulation of carotenoid-rich oil from Gac peel: optimisation of the encapsulating process using a spray drier and the storage stability of encapsulated powder. Powder Technol. (2019) 344:373–9. doi: 10.1016/j.powtec.2018.12.012
81. Patil, CD, Borase, HP, Suryawanshi, RK, and Patil, SV. Trypsin inactivation by latex fabricated gold nanoparticles: A new strategy towards insect control. Enzym Microb Technol. (2016) 92:18–25. doi: 10.1016/j.enzmictec.2016.06.005
82. Huang, D, Zhou, Y, Xiang, Y, Shu, M, Chen, H, Yang, B, et al. Polyurethane/doxorubicin nanoparticles based on electrostatic interactions as pH-sensitive drug delivery carriers. Polym Int. (2018) 67:1186–93. doi: 10.1002/pi.5618
83. Sims, KR, He, B, Koo, H, and Benoit, DSW. Electrostatic interactions enable nanoparticle delivery of the flavonoid Myricetin. ACS Omega. (2020) 5:12649–59. doi: 10.1021/acsomega.9b04101
84. Liu, S, Zhang, J, Fu, R, Feng, H, Chu, Y, Huang, D, et al. Improved stability and aqueous solubility of beta-carotene via encapsulation in self-assembled bioactive oleanolic acid nanoparticles. Food Chem. (2022) 373:131498. doi: 10.1016/j.foodchem.2021.131498
85. Bashir, O, Bhat, SA, Basharat, A, Qamar, M, Qamar, SA, Bilal, M, et al. Nano-engineered materials for sensing food pollutants: technological advancements and safety issues. Chemosphere. (2022) 292:133320. doi: 10.1016/j.chemosphere.2021.133320
86. Gonçalves, RFS, Martins, JT, Duarte, CMM, Vicente, AA, and Pinheiro, AC. Advances in nutraceutical delivery systems: from formulation design for bioavailability enhancement to efficacy and safety evaluation. Trends Food Sci Technol. (2018) 78:270–91. doi: 10.1016/j.tifs.2018.06.011
87. Bahrami, A, Delshadi, R, Jafari, SM, and Williams, L. Nanoencapsulated nisin: an engineered natural antimicrobial system for the food industry. Trends Food Sci Technol. (2019) 94:20–31. doi: 10.1016/j.tifs.2019.10.002
88. Sharma, S, Barkauskaite, S, Jaiswal, AK, and Jaiswal, S. Essential oils as additives in active food packaging. Food Chem. (2021) 343:128403. doi: 10.1016/j.foodchem.2020.128403
89. Wang, Y, Lu, Z, Wu, H, and Lv, F. Study on the antibiotic activity of microcapsule curcumin against foodborne pathogens. Int J Food Microbiol. (2009) 136:71–4. doi: 10.1016/j.ijfoodmicro.2009.09.001
90. de Oliveira Filho, JG, Bertolo, MRV, da Costa, BS, Malafatti, JOD, Bertazzo, GB, Colacique, MN, et al. Recent advances in the application of nanotechnology to reduce fruit and vegetable losses during post-harvest. Braz J Phys. (2022) 52:126–17. doi: 10.1007/s13538-022-01132-5
91. Giannakourou, MC, and Tsironi, TN. Application of processing and packaging hurdles for fresh-cut fruits and vegetables preservation. Foods. (2021) 10:830. doi: 10.3390/foods10040830
92. Deka, C, Aidew, L, Devi, N, Buragohain, AK, and Kakati, DK. Synthesis of curcumin-loaded chitosan phosphate nanoparticle and study of its cytotoxicity and antimicrobial activity. J Biomater Sci Polym Ed. (2016) 27:1659–73. doi: 10.1080/09205063.2016.1226051
93. Hee Joung Joung, M-JC, Kim, JT, Park, SH, Park, HJ, and Shin, GH. Development of food-grade curcumin nanoemulsion and its potential application to food beverage system: antioxidant property and in vitro digestion. J Food Sci. (2016) 81:N745–53. doi: 10.1111/1750-3841.13224
94. Huang, F, Gao, Y, Zhang, Y, Cheng, T, Ou, H, Yang, L, et al. Silver-decorated polymeric micelles combined with curcumin for enhanced antibacterial activity. ACS Appl Mater Interfaces. (2017) 9:16880–9. doi: 10.1021/acsami.7b03347
95. Esmaili, M, Ghaffari, SM, Moosavi-Movahedi, Z, Atri, MS, Sharifizadeh, A, Farhadi, M, et al. Beta casein-micelle as a nano vehicle for solubility enhancement of curcumin; food industry application. LWT Food Sci Technol. (2011) 44:2166–72. doi: 10.1016/j.lwt.2011.05.023
96. Liang, Y, He, J, and Guo, B. Functional hydrogels as wound dressing to enhance wound healing. ACS Nano. (2021) 15:12687–722. doi: 10.1021/acsnano.1c04206
97. El-Wakil, NA, Hassan, EA, Hassan, ML, and Abd El-Salam, SS. Bacterial cellulose/phytochemical's extracts biocomposites for potential active wound dressings. Environ Sci Pollut Res Int. (2019) 26:26529–41. doi: 10.1007/s11356-019-05776-w
Keywords: nanocarriers, encapsulation technology, assembly/release mechanism, bacteriostatic, antibacterial active substances
Citation: Cao J, Gao M, Wang J, Liu Y, Zhang X, Ping Y, Liu J, Chen G, Xu D, Huang X and Liu G (2023) Construction of nano slow-release systems for antibacterial active substances and its applications: A comprehensive review. Front. Nutr. 10:1109204. doi: 10.3389/fnut.2023.1109204
Edited by:
Ricardo Calhelha, Centro de Investigação de Montanha (CIMO), PortugalReviewed by:
Josiana Vaz, Centro de Investigação de Montanha (CIMO), PortugalKhashayar Sarabandi, Zahedan University of Medical Sciences, Iran
Copyright © 2023 Cao, Gao, Wang, Liu, Zhang, Ping, Liu, Chen, Xu, Huang and Liu. This is an open-access article distributed under the terms of the Creative Commons Attribution License (CC BY). The use, distribution or reproduction in other forums is permitted, provided the original author(s) and the copyright owner(s) are credited and that the original publication in this journal is cited, in accordance with accepted academic practice. No use, distribution or reproduction is permitted which does not comply with these terms.
*Correspondence: Jian Wang, ✉ eHVhbnl1YW5qaWFuMDIyOEAxMjYuY29t; Donghui Xu, ✉ eHVkb25naHVpQGNhYXMuY24=; Guangyang Liu, ✉ bGl1Z3Vhbmd5YW5nQGNhYXMuY24=
†These authors have contributed equally to this work