- 1Department of Nutrition and Food Hygiene, School of Public Health, Institute of Nutrition, Fudan University, Shanghai, China
- 2Department of Pediatric Surgery, Guangzhou Women and Children’s Medical Center, Guangzhou Medical University, Guangzhou, China
Diet dictates nutrient availability in the tumor microenvironment, thus affecting tumor metabolic activity and growth. Intrinsically, tumors develop unique metabolic features and are sensitive to environmental nutrient concentrations. Tumor-driven nutrient dependencies provide opportunities to control tumor growth by nutritional restriction or supplementation. This review summarized the existing data on nutrition and pediatric cancers after systematically searching articles up to 2023 from four databases (PubMed, Web of Science, Scopus, and Ovid MEDLINE). Epidemiological studies linked malnutrition with advanced disease stages and poor clinical outcomes in pediatric cancer patients. Experimental studies identified several nutrient dependencies (i.e., amino acids, lipids, vitamins, etc.) in major pediatric cancer types. Dietary modifications such as calorie restriction, ketogenic diet, and nutrient restriction/supplementation supported pediatric cancer treatment, but studies remain limited. Future research should expand epidemiological studies through data sharing and multi-institutional collaborations and continue to discover critical and novel nutrient dependencies to find optimal nutritional approaches for pediatric cancer patients.
Introduction
Childhood cancers represent the leading cause of disease-related mortality in childhood (1). Major childhood cancer types include leukemias (i.e., acute lymphoblastic leukemia ALL, acute myeloid leukemia AML), lymphomas (i.e., Hodgkin lymphoma HL, non-Hodgkin lymphoma NHL), brain and spinal cord tumors (i.e., glioblastoma GBM, medulloblastoma MB), peripheral nervous system tumors (i.e., neuroblastoma NB), renal cancers (i.e., Wilms tumor WT), liver cancers (i.e., hepatoblastoma HB), eye cancers (i.e., retinoblastoma RB), bone cancers (i.e., osteosarcoma OS and Ewing sarcoma ES), and soft tissue sarcomas (i.e., rhabdomyosarcoma RMS). The mutational burden in most childhood cancers is substantially lower than that in adult cancers (2, 3). Instead, fusion oncoproteins and epigenetic dysregulations frequently occur in childhood cancers (1, 4). For example, the EWS-FLI1 fusion protein plays a central role in the pathogenesis of ES (4). Oncohistones and aberrant DNA methylations have been identified in pediatric brain tumors (5–7). In addition, copy number alterations such as MYCN amplification occurs in many pediatric cancer types, such as NB (8), MB (9), WT (10), RB (11), and RMS (12).
Malnutrition (undernutrition and overnutrition) problems are increasing worldwide (13), raising concerns about the relationship between nutrition and childhood cancers (Table 1). Nutrient deficiency and obesity at diagnosis are associated with poor clinical outcomes in childhood cancers (34, 45, 77–79). Maternal nutritional status also links to the risk of developing hematopoietic and solid childhood tumors (17–21). Overall, the current epidemiological studies are limited. This is partly due to a lower incidence of childhood cancers and little nutritional evaluation at diagnosis (80). On the other side of the coin, multi-omics technology corroborating with basic and translational cancer research sheds light on discovering new metabolic dependencies of pediatric cancers. Like adult cancers, aggressive pediatric tumors require specific lipids, amino acids, carbohydrates, vitamins, and minerals for survival (63, 81, 82). The most vulnerable metabolite is determined under a particular context of cancer and is associated with the tumor microenvironment (83). Finding critical nutrient dependencies for each cancer type will aid in developing optimal treatment regimens.
Here, we summarize recent findings on the associations between nutrition and pediatric cancers, nutritional dependencies under different tumor contexts, and dietary approaches during pediatric cancer treatment. Much remains to be uncovered in pediatric cancers compared to adult cancers. Thus, we also discuss the current challenges and research gaps in the field and point to interesting future directions. The ultimate goal is finding optimal and precise nutritional strategies to improve patient survival and quality of life.
The link between nutrition and childhood cancers
The State of Food Security and Nutrition in the World reported that 9.8 percent of the global population (768 million) were undernourished in 2021 (13). Infants and children are more susceptible populations due to the high demand for energy and essential nutrients, especially for cancer patients. In a prospective study of 1,787 newly diagnosed pediatric patients, 18% had moderate nutritional depletion, and 45–59% were severely depleted (34). Another study showed that 50% of children with stage IV NB (high-risk patients) were undernourished at diagnosis (45). Undernourished children abandoned therapy more frequently, resulting in inferior event-free survival (34). Protein-energy malnutrition, a specific undernutrition defined as an energy deficit due to a lack of macronutrients, is commonly seen in leukemias and solid tumors (14, 15). Apart from the macronutrients, deficiencies of micronutrients such as magnesium, zinc, selenium, vitamin D, and vitamin B12 were reported in pediatric cancer patients (Table 1) (16, 36, 73, 84). There is a lack of standard clinical practice guidelines for monitoring the nutritional status of children with cancer. Therefore, a systematic comparison of different evaluation methods and longitudinal nutritional assessment throughout diagnosis and treatment is urgently needed.
The number of overweight and obese individuals (85) and the cancer risk (16–18) have increased over the years. A recent study of 640 pediatric ALL patients found that 27% were overweight/obese, and 79% exceeded the dietary reference amount (79). Obese pediatric acute leukemia patients had a higher mortality risk than non-obese patients (77, 78). In recent years, the consumption of food-added sugars has also increased dramatically. High sugar consumption was associated with increased incidences of multiple adult malignancies, such as pancreatic and endometrial cancers (86, 87). However, whether sugar contributes to pediatric cancers is poorly understood.
Maternal obesity and diabetes also increase the risk of childhood cancers. Children born to mothers with a body mass index of ≥40 had a 57% higher leukemia risk (18). Maternal diabetes was associated with an increased risk of childhood cancers, particularly ALL, and medications reduced the risk of offspring childhood cancers (17). Additionally, maternal diets correlate with the risk of childhood cancers. Maternal consumption of vegetables and fruits before or during pregnancy was inversely associated with offspring ALL and AML incidence (19, 20, 28). In contrast, consuming flavonoid-rich foods may interfere with DNA topoisomerase II and increase the risk of AML (28). Maternal folate fortification correlated with a reduced risk of ALL, NB, and WT (21, 46, 66). Additionally, a maternal diet rich in yellow-orange vegetables, fresh fish, and grains decreased the risk of childhood brain tumors, whereas a maternal diet rich in cured meats, eggs/dairy, oil products, non-chocolate candy, and chili increased the risk (41).
The current epidemiological studies remain limited, particularly for rare diseases. Future efforts should increase subjects through data sharing and multi-institutional collaborations.
Nutrient dependencies in childhood cancers
Metabolic reprogramming has emerged as an essential cancer hallmark (88). Mutation of tumor suppressors and activation of oncogenic signaling make tumor cells promote the synthesis and uptake of nutrients for survival (89), thus enhancing tumor dependency on certain nutrients (81) (Table 1).
Amino acids
Amino acids are the building blocks of proteins. They also regulate the redox state and contribute to epigenetic and immune responses linked to tumorigenesis and metastasis (90). Therefore, tumors present a heightened amino acid dependence.
Glutamine, the most abundant amino acid in serum, is surprisingly depleted in developing cancers (91). Glutamine supported childhood AML and ALL survival and contributed to adipocyte-induced cell resistance to asparaginase (22, 29). Inhibition of glucose metabolism or Akt signaling also activated glutamine metabolism in GBM (37). In NB, the oncogenic driver MYCN promoted glutamine uptake and catabolism (47, 48). Similarly, high MYC-expressing atypical teratoid/rhabdoid tumors demonstrated higher glutamine metabolism activity compared to low MYC-expressing tumors (92). TAp73, which is frequently overexpressed in human tumors, sustained a subset of MB growth and proliferation by upregulating glutamine metabolism (42).
Another critical amino acid pathway is the serine-glycine-one-carbon (SGOC) metabolism. SGOC incorporates serine–glycine biosynthesis, one-carbon metabolism, and purine nucleotide biosynthesis in a positive feedback loop, generating diverse metabolites (93). In NB, high expression of an SGOC gene signature (49) or glycine decarboxylase (50), the enzyme which catalyzes glycine breakdown to produce one-carbon metabolism intermediate 5,10-methylene-tetrahydrofolate, was identified in MYCN-amplified patients and was associated with advanced disease stage and poor prognosis. In OS, the rate-limiting enzyme in serine biosynthesis 3-phosphoglycerate dehydrogenase is inversely correlated with patient survival (94). In ES, two methylenetetrahydrofolate dehydrogenase genes (MTHFD2 and MTHFD1L) were upregulated by EWS-FLI1, and high expressions were linked with high-risk disease and poor survival (74).
Arginine is a semi-essential amino acid and an intermediate in many biological pathways, such as the urea cycle and tricarboxylic acid cycle (95). AML depends on arginine, as depletion of intracellular arginine (via a pegylated arginine deiminase ADI-PEG20) and extracellular arginine (via a pegylated human recombinant arginase BCT-100) decreased proliferation of AML (23, 30). Depletion of arginine in combination with chemotherapy cytarabine exerted greater efficacy compared to single therapy in AML and ALL. Still, resistance eventually occurred (30, 96), likely due to compensatory activation of endogenous production of arginine (23, 97). Therefore, additional metabolic dependencies in AML must be targeted.
Asparagine is a nonessential amino acid that facilitates glycoprotein synthesis and the uptake of extracellular amino acids such as arginine, histidine, and serine (98). Asparagine presents a potential nutrient dependency in leukemias as these tumors lack asparagine synthetase (24, 99). Deprivation of exogenous asparagine by asparaginase resulted in a remission rate of >90% in children with ALL (24).
Polyamines are active organic compounds with at least two amino groups (100). They can be synthesized by ornithine decarboxylase (ODC1), the rate-limiting enzyme in polyamine synthesis (100), and imported by transporters such as SLC3A2 (53). Polyamines are frequently deregulated in cancer because they involve fundamental processes related to cell growth and survival (100). For example, putrescine, spermidine, and spermine levels were elevated in children with leukemias (101). MYCN directly increased polyamine synthesis in NB and promoted NB tumor growth by upregulating ODC1 and SLC3A2 (51–53).
Glycolysis and oxidative phosphorylation
As discovered by Otto Warburg in the 1920s, cancer cells exhibit an increased dependence on glycolysis, preferentially catalyzing the conversion of glucose to lactate in the presence of oxygen (102). Despite a relatively low mutational burden, pediatric cancers exhibit aberrant expressions of key glycolytic enzymes, suggesting an increased dependence on glycolysis. Expression of hexokinase (HK1/2), which converts glucose to glucose-6-phosphate, was raised in several pediatric cancers and high HK expression predicted poor prognosis [i.e., metastatic NB (54), the SHH subtype of MB (43), diffuse large B-cell lymphoma (35) and OS (71)]. High expression of lactate dehydrogenase (LDHA/B) that converts pyruvate to lactate is linked to poor prognosis in NB, ES, and HB (55, 69, 75). Additionally, glucose transporter GLUT1 was highly expressed in ALL (25), NB (56), and WT (67), and GLUT3 upregulated in HB (69).
More recent work has demonstrated that mitochondrial respiration also plays a significant role in tumor growth and survival (103). WT and NB exhibited heterogeneity in mitochondrial phenotypes and energy metabolism (57, 58, 68). Stromal regions of WT showed reduced mtDNA copy number, whereas the epithelial and blastemal regions were normal (68). MYCN-amplified NB demonstrated higher mitochondrial activity than non-MYCN-amplified NB (57, 58). The pyruvate dehydrogenase kinase 1 (PDK1) phosphorylates pyruvate dehydrogenase and in turn, lowers its activity, which reduces the conversion of pyruvate to acetyl-CoA. PDK1 was activated in AML, GBM, and RB, and its inhibition blocked cell proliferation and restored sensitivity to chemotherapy (31, 38, 65).
Lipids
Lipids are also critical for cancer cell proliferation by serving as membrane components, providing energy sources, maintaining redox homeostasis, and acting as signaling molecules (104). Several pediatric cancers heavily rely on lipids for survival. For example, fatty acid transport (via fatty acid transporter SLC27A2), biosynthesis (via fatty acid synthase FASN and stearoyl-CoA desaturase SCD), and oxidation (via carnitine palmitoyltransferase 1 CPT1) were activated in MYCN-amplified NB (58–61). Inhibition of fatty acid oxidation by malonyl-CoA decarboxylase inhibitor prohibited RMS growth, and knockdown of fatty acid metabolism regulator sterol regulatory element-binding protein-1c (SREBP-1c) suppressed HB, suggesting a dependency on lipid metabolism (70, 76). Lipids such as phosphatidylinositol, sphingolipids, free cholesterol, and monounsaturated fatty acids were increased in isocitrate dehydrogenases mutant AML cells (32). Moreover, AML blasts activated adipocyte lipolysis, thus allowing fatty acids to be transferred from adipocytes to blasts (33). A recent study identified the mevalonate pathway as a novel vulnerability in early T-cell ALL (26). Inhibition of 3-hydroxy-3-methylglutaryl-CoA reductase, the rate-limiting enzyme in the mevalonate pathway, significantly blocked T-cell ALL growth (26). Besides, smoothened-activating sterol lipids such as cholesterol and 7-keto-cholesterol sustained oncogenic Hedgehog signaling in MB (44).
Vitamins and minerals
Besides the macronutrients, vitamins and minerals are essential as substrates and cofactors for critical metabolic processes (105). Pediatric cancer patients were disproportionately vitamin D deficient (27, 36, 82), suggesting sequestration of this vitamin by tumor cells. Vitamin B12 has also been identified as a pediatric cancer dependency, specifically in NB cells, such that B12 depletion induced cell-cycle arrest and neuronal differentiation (62).
Among minerals, iron serves as a significant dependency across pediatric cancers. Iron depletion by iron chelator deferoxamine or sodium ascorbate has demonstrated anti-proliferative activity in NB (63, 64). However, exogenous iron exposure induced ferroptosis in malignant brain tumors and NB (106–108), indicating that tight control of iron levels is required for cancer cell survival.
Dietary modifications in pediatric cancers
Inhibitors used to disrupt active cancer metabolism have been extensively summarized in many reviews (81, 109–111). Nevertheless, a majority of drugs fail to enter clinical trials. Compensatory activation of other metabolic routes or uptake of the source metabolite reduces the anti-tumor effects (59, 112). Moreover, the specificity of the metabolic inhibitors to tumor cells and their potential toxicities remain a question. Therefore, supportive approaches such as dietary modifications should be considered during cancer treatment. Dietary composition determines nutrient availability in the microenvironment of cells in the body (113). Accumulating evidence suggests that dietary modifications, including calorie and nutrient restriction/supplementation, reprogram tumor metabolic activity and produce shifts in proliferation rate and drug sensitivity (114, 115). Herein, this review will focus on dietary modifications applied to pediatric cancers.
Calorie restriction and ketogenic diet
Calorie restriction emphasizes a chronic 20–30% reduction of the standard calorie intake (116). This approach reduces tumor growth in several adult tumor models, including breast cancer, pancreatic cancer, and lymphoma (117–119). Interestingly, calorie restriction also inhibited tumor growth in neuroblast mouse xenograft models, although its molecular mechanism remains unknown (120). However, it remains a concern to use calorie restriction during childhood, given the risk of malnutrition and disrupted endocrine function (121).
Consequently, researchers have sought a safer approach that sustains overall calorie intake but modifies the diet composition. The ketogenic diet was introduced to meet such demand. Ketogenic diets have normal calorie, low-carbohydrate but high-fat content, leading to increased ketone bodies in plasma (122). The ketogenic diet showed anticancer effects in preclinical models of NB and GBM (39, 120, 123). Researchers further identified that a medium-chain fatty acid-containing ketogenic diet was more effective than a long-chain fatty acid-containing diet (120, 123). It remains uncertain whether ketogenic diet can be applied to all cancer types.
Nutrient restriction
Germline mutations in the methionine synthase gene have been associated with childhood leukemia risk (124), and methionine depletion augmented the anticancer activities of chemotherapeutics against pediatric sarcoma cells in vitro (125). However, it remains unknown whether methionine dependence is a broader feature across pediatric cancers and whether this dependency can be effectively exploited against tumors in children. Besides methionine, serine deprivation has also been shown to induce oxidative stress and prohibit tumor growth in adult cancer models (126, 127). Given that NB and ES showed active serine metabolism (50, 74), it will be interesting to determine whether serine restriction is effective in treating those cancers.
Besides amino acids, restriction of vitamins can be used in tumors that rely on those vitamins for survival. Restriction of vitamin B9 (folate) and B12 (cobalamin) together with other methyl donors (methionine and choline) inhibited one-carbon metabolism and protected against adenoma development (128). Restriction of minerals like iron may selectively target cancer cells (63, 64). However, care must be taken to avoid restriction toxicity since pediatric cancer patients are frequently vitamin deficient already (82, 129).
Nutrient supplementation
Omega-3 fatty acids exert anti-inflammatory and anti-tumor effects (130). Docosahexaenoic acid (DHA) and eicosapentaenoic acid (EPA) slowed MB tumor growth by alleviating the inflammatory tumor microenvironment (131). MYCN-amplified NB contained lower DHA levels than non-MYCN-amplified NB. DHA supplementation delayed the progression of NB in cell line-derived mouse xenograft models (132). However, another study using TH-MYCN transgenic NB model did not observe significant DHA effects (133). More studies and standard treatment strategies are needed to evaluate the efficacy of DHA supplementation in NB.
Folate and vitamin B12 deficiency was found in ALL patients who showed anemia on maintenance therapy (134). Supplementation of these deficient micronutrients significantly alleviated anemia. Supplementation of vitamin K2 and D3 improved bone mineral density in ALL patients during intensive steroid therapy (135). Further research is needed to comprehensively characterize micronutrient status before and after treatment and to precisely monitor the effects of micronutrient supplementation on patients’ health conditions.
Cachexia is a complex syndrome presenting with decreased food intake, weight loss, muscle and adipose tissue wasting, and hormonal/metabolic abnormalities (136). Muscle wasting is associated with reduced protein synthesis and increased protein degradation (137). Oral supplementation of a mixture of amino acids partially reversed cachexia in patients with advanced solid tumors (138). Similarly, a diet enriched in leucine or branch-chain amino acids stimulated muscle protein synthesis and alleviated cachexia (139, 140). However, most studies were conducted on adult cancer patients. Further investigations into the effects of cachexia on pediatric tumor development and strategies for its reversal through dietary modifications are urgently needed.
Challenges for nutritional interventions and future directions
Studies on dietary modifications for adult cancer treatment have provided insights into pediatric cancer interventions. Nevertheless, there is still a long way to go. First, additional studies are needed to understand specific nutrient dependencies in different pediatric cancers and to evaluate the efficacies of various dietary interventions. Second, because dietary modifications induce systemic responses that impact both tumor and tumor microenvironment, a holistic understanding of how the nutrient restriction or supplementation affects tumor and tumor microenvironment such as anti-/pro-tumor immune response, stromal cell activity, angiogenesis, and whole-body homeostasis is highly demanded. Third, the duration and start time for nutritional therapy as well as the long-term toxicity from nutrient deprivation or supplementation should be evaluated. Fourth, it should be emphasized that no treatment works for all types of cancers. For example, histidine supplementation made leukemia xenografted tumors more sensitive to methotrexate (141), whereas histidine depletion in a Drosophila model selectively limited the growth of MYC-dependent neural tumors (142). Thus, specific tumor context needs to be mentioned when advertising nutritional therapies.
Conclusion
Compared to adult cancers, the understanding of crosstalks between nutrition and pediatric cancers remains poor, thus providing tremendous opportunities for studying how nutrition can help prevent and treat childhood cancers (Figure 1). Large-scale epidemiological studies of pediatric cancers could uncover additional relationships between nutrients and pediatric cancers. Moreover, omics technology combined with mechanistic studies can reveal novel nutrient dependencies. This will further guide preclinical and clinical nutritional interventions to optimize therapeutic strategies. This process can be applied to populations, subgroups, and individuals. Each patient’s genetic information may guide the discovery of personalized nutrient dependencies and nutritional interventions. There is still much to learn, but additional studies will enlighten the future by overlapping nutrition and pediatric cancer fields.
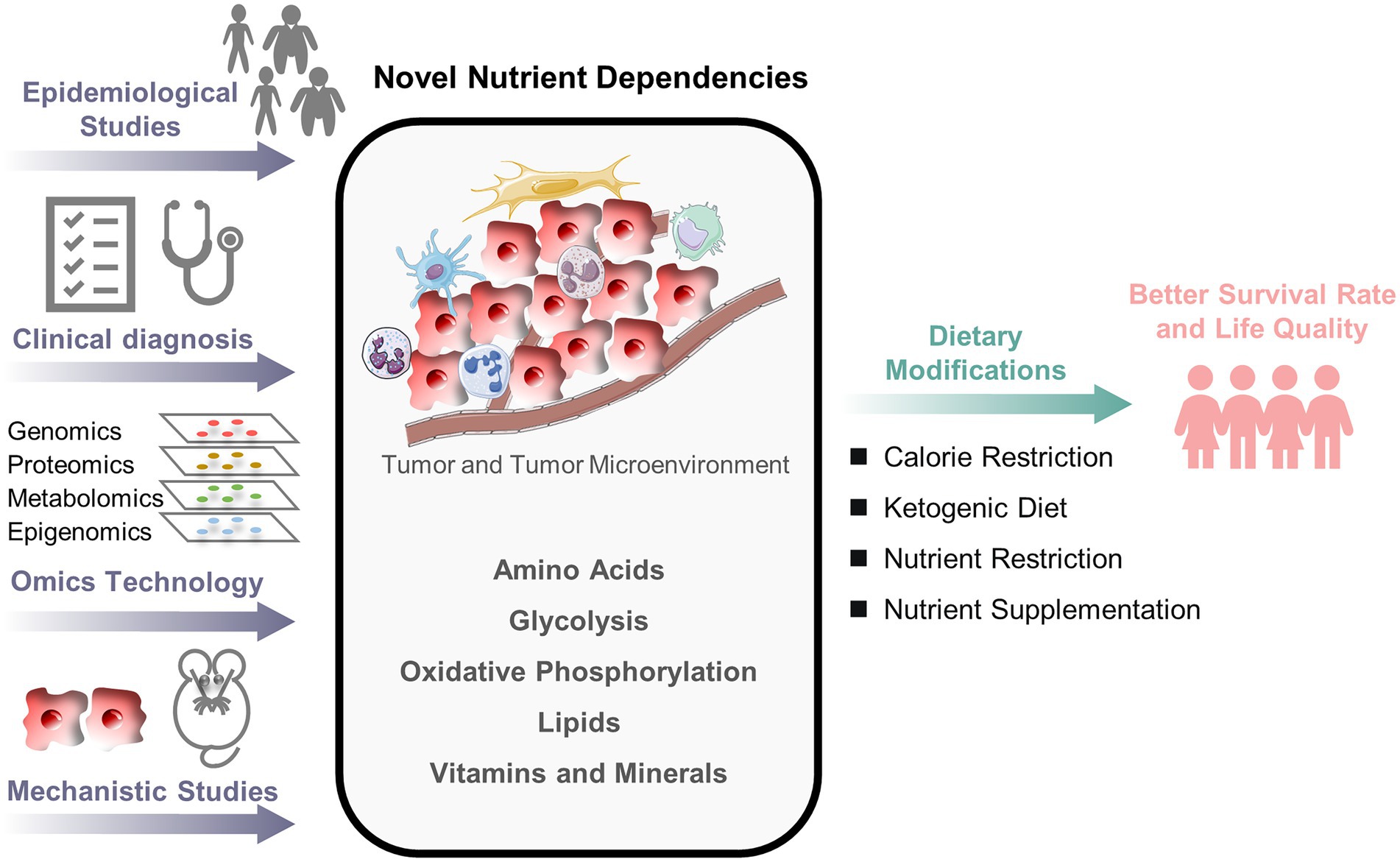
Figure 1. An outlook of nutritional research in pediatric cancers. The crosstalks between nutrition and pediatric cancers remain poorly understood. A combined approach of epidemiological investigation, clinical diagnosis, omics technology, and mechanistic study is necessary to understand better how nutrients alter cancer fate and to develop the optimal nutritional strategy for pediatric cancer patients.
Author contributions
LT and KW wrote the manuscript and prepared the figure. LT and TY conceived and organized the structure of the review. KW, TY, YZ, XG, and LT reviewed the manuscript. LT revised the manuscript and supervised the entire writing process. All authors approved the final manuscript before submission and publication.
Funding
This work was supported by Start-up Funding from Fudan University (to LT) and Sub-project of the Shanghai Local High-Level University Construction Program from Shanghai Municipal Education Commission (to LT).
Acknowledgments
We thank Nathan Drolet for assisting with the language editing of this manuscript.
Conflict of interest
The authors declare that the research was conducted in the absence of any commercial or financial relationships that could be construed as a potential conflict of interest.
Publisher’s note
All claims expressed in this article are solely those of the authors and do not necessarily represent those of their affiliated organizations, or those of the publisher, the editors and the reviewers. Any product that may be evaluated in this article, or claim that may be made by its manufacturer, is not guaranteed or endorsed by the publisher.
Abbreviations
ALL, acute lymphoblastic leukemia; AML, acute myeloid leukemia; CPT1, carnitine palmitoyltransferase 1; DHA, docosahexaenoic acid; EPA, eicosapentaenoic acid; ES, Ewing sarcoma; FASN, fatty acid synthase; GBM, glioblastoma; GLUT, glucose transporter; HB, hepatoblastoma; HK, hexokinase; HL, Hodgkin lymphoma; LDHA, lactate dehydrogenase; MB, medulloblastoma; MTHFD, methylenetetrahydrofolate dehydrogenase; NB, neuroblastoma; NHL, non-Hodgkin lymphoma; ODC1, ornithine decarboxylase; OS, osteosarcoma; PDK, pyruvate dehydrogenase kinase; RB, retinoblastoma; RMS, rhabdomyosarcoma; SCD, stearoyl-CoA desaturase; SGOC, serine-glycine-one-carbon; SLC27A2, fatty acid transporter; SLC3A2, Polyamine transporter; SREBP-1c, sterol regulatory element-binding protein-1c; WT, Wilms tumor.
References
1. Filbin, M, and Monje, M. Developmental origins and emerging therapeutic opportunities for childhood cancer. Nat Med. (2019) 25:367–76. doi: 10.1038/s41591-019-0383-9
2. Grobner, SN, Worst, BC, Weischenfeldt, J, Buchhalter, I, Kleinheinz, K, Rudneva, VA, et al. The landscape of genomic alterations across childhood cancers. Nature. (2018) 555:321–7. doi: 10.1038/s41586-018-0167-2
3. Ma, X, Liu, Y, Liu, Y, Alexandrov, LB, Edmonson, MN, Gawad, C, et al. Pan-cancer genome and transcriptome analyses of 1,699 paediatric leukaemias and solid tumours. Nature. (2018) 555:371–6. doi: 10.1038/nature25795
4. Tanaka, K, Iwakuma, T, Harimaya, K, Sato, H, and Iwamoto, Y. EWS-Fli1 antisense oligodeoxynucleotide inhibits proliferation of human Ewing's sarcoma and primitive neuroectodermal tumor cells. J Clin Invest. (1997) 99:239–47. doi: 10.1172/JCI119152
5. Schwartzentruber, J, Korshunov, A, Liu, XY, Jones, DT, Pfaff, E, Jacob, K, et al. Driver mutations in histone H3.3 and chromatin remodelling genes in paediatric glioblastoma. Nature. (2012) 482:226–31. doi: 10.1038/nature10833
6. Bender, S, Tang, Y, Lindroth, AM, Hovestadt, V, Jones, DT, Kool, M, et al. Reduced H3K27me3 and DNA hypomethylation are major drivers of gene expression in K27M mutant pediatric high-grade gliomas. Cancer Cell. (2013) 24:660–72. doi: 10.1016/j.ccr.2013.10.006
7. Bayliss, J, Mukherjee, P, Lu, C, Jain, SU, Chung, C, Martinez, D, et al. Lowered H3K27me3 and DNA hypomethylation define poorly prognostic pediatric posterior fossa ependymomas. Sci Transl Med. (2016) 8:366ra161. doi: 10.1126/scitranslmed.aah6904
8. Weiss, WA, Aldape, K, Mohapatra, G, Feuerstein, BG, and Bishop, JM. Targeted expression of MYCN causes neuroblastoma in transgenic mice. EMBO J. (1997) 16:2985–95. doi: 10.1093/emboj/16.11.2985
9. Aldosari, N, Bigner, SH, Burger, PC, Becker, L, Kepner, JL, Friedman, HS, et al. MYCC and MYCN oncogene amplification in medulloblastoma. A fluorescence in situ hybridization study on paraffin sections from the Children's oncology group. Arch Pathol Lab Med. (2002) 126:540–4. doi: 10.5858/2002-126-0540-MAMOAI
10. Williams, RD, Al-Saadi, R, Natrajan, R, Mackay, A, Chagtai, T, Little, S, et al. Molecular profiling reveals frequent gain of MYCN and anaplasia-specific loss of 4q and 14q in Wilms tumor. Genes Chromosomes Cancer. (2011) 50:982–95. doi: 10.1002/gcc.20907
11. Lee, WH, Murphree, AL, and Benedict, WF. Expression and amplification of the N-myc gene in primary retinoblastoma. Nature. (1984) 309:458–60. doi: 10.1038/309458a0
12. Driman, D, Thorner, PS, Greenberg, ML, Chilton-MacNeill, S, and Squire, J. MYCN gene amplification in rhabdomyosarcoma. Cancer. (1994) 73:2231–7. doi: 10.1002/1097-0142(19940415)73:8<2231::AID-CNCR2820730832>3.0.CO;2-E
13. FAO I, UNICEF, WFP and WHO. The State of Food Security and Nutrition in the World 2022. Repurposing Food and Agricultural Policies to Make Healthy Diets More Affordable. Rome: FAO (2022).
14. Yu, LC, Kuvibidila, S, Ducos, R, and Warrier, RP. Nutritional status of children with leukemia. Med Pediatr Oncol. (1994) 22:73–7. doi: 10.1002/mpo.2950220202
15. Revuelta Iniesta, R, Paciarotti, I, Davidson, I, McKenzie, JM, Brougham, MFH, and Wilson, DC. Nutritional status of children and adolescents with cancer in Scotland: a prospective cohort study. Clin Nutr ESPEN. (2019) 32:96–106. doi: 10.1016/j.clnesp.2019.04.006
16. Sahin, G, Ertem, U, Duru, F, Birgen, D, and Yuksek, N. High prevelance of chronic magnesium deficiency in T cell lymphoblastic leukemia and chronic zinc deficiency in children with acute lymphoblastic leukemia and malignant lymphoma. Leuk Lymphoma. (2000) 39:555–62. doi: 10.3109/10428190009113385
17. Seppala, LK, Vettenranta, K, Pitkaniemi, J, Hirvonen, E, Leinonen, MK, and Madanat-Harjuoja, LM. Maternal diabetes and risk of childhood cancer in the offspring. Int J Cancer. (2020) 147:662–8. doi: 10.1002/ijc.32757
18. Stacy, SL, Buchanich, JM, Ma, ZQ, Mair, C, Robertson, L, Sharma, RK, et al. Maternal obesity, birth size, and risk of childhood cancer development. Am J Epidemiol. (2019) 188:1503–11. doi: 10.1093/aje/kwz118
19. Abiri, B, Kelishadi, R, Sadeghi, H, and Azizi-Soleiman, F. Effects of maternal diet during pregnancy on the risk of childhood acute lymphoblastic leukemia: a systematic review. Nutr Cancer. (2016) 68:1065–72. doi: 10.1080/01635581.2016.1206581
20. Kwan, ML, Jensen, CD, Block, G, Hudes, ML, Chu, LW, and Buffler, PA. Maternal diet and risk of childhood acute lymphoblastic leukemia. Public Health Rep. (2009) 124:503–14. doi: 10.1177/003335490912400407
21. Metayer, C, Milne, E, Dockerty, JD, Clavel, J, Pombo-de-Oliveira, MS, Wesseling, C, et al. Maternal supplementation with folic acid and other vitamins and risk of leukemia in offspring: a Childhood Leukemia International Consortium study. Epidemiology. (2014) 25:811–22.
22. Ehsanipour, EA, Sheng, X, Behan, JW, Wang, X, Butturini, A, Avramis, VI, et al. Adipocytes cause leukemia cell resistance to L-asparaginase via release of glutamine. Cancer Res. (2013) 73:2998–3006.
23. Miraki-Moud, F, Ghazaly, E, Ariza-McNaughton, L, Hodby, KA, Clear, A, Anjos-Afonso, F, et al. Arginine deprivation using pegylated arginine deiminase has activity against primary acute myeloid leukemia cells in vivo. Blood. (2015) 125:4060–8. doi: 10.1182/blood-2014-10-608133
24. Story, MD, Voehringer, DW, Stephens, LC, and Meyn, RE. L-asparaginase kills lymphoma cells by apoptosis. Cancer Chemother Pharmacol. (1993) 32:129–33. doi: 10.1007/BF00685615
25. Liu, T, Kishton, RJ, Macintyre, AN, Gerriets, VA, Xiang, H, Liu, X, et al. Glucose transporter 1-mediated glucose uptake is limiting for B-cell acute lymphoblastic leukemia anabolic metabolism and resistance to apoptosis. Cell Death Dis. (2014) 5:e1470. doi: 10.1038/cddis.2014.431
26. Rashkovan, M, Albero, R, Gianni, F, Perez-Duran, P, Miller, HI, Mackey, AL, et al. Intracellular cholesterol pools regulate oncogenic signaling and epigenetic circuitries in early T-cell precursor acute lymphoblastic leukemia. Cancer Discov. (2022) 12:856–71. doi: 10.1158/2159-8290.CD-21-0551
27. Oosterom, N, Dirks, NF, Heil, SG, de Jonge, R, Tissing, WJE, Pieters, R, et al. A decrease in vitamin D levels is associated with methotrexate-induced oral mucositis in children with acute lymphoblastic leukemia. Support Care Cancer. (2019) 27:183–90. doi: 10.1007/s00520-018-4312-0
28. Spector, LG, Xie, Y, Robison, LL, Heerema, NA, Hilden, JM, Lange, B, et al. Maternal diet and infant leukemia: the DNA topoisomerase II inhibitor hypothesis: a report from the children's oncology group. Cancer Epidemiol Biomarkers Prev. (2005) 14:651–5. doi: 10.1158/1055-9965.EPI-04-0602
29. Matre, P, Velez, J, Jacamo, R, Qi, Y, Su, X, Cai, T, et al. Inhibiting glutaminase in acute myeloid leukemia: metabolic dependency of selected AML subtypes. Oncotarget. (2016) 7:79722–35.
30. Mussai, F, Egan, S, Higginbotham-Jones, J, Perry, T, Beggs, A, Odintsova, E, et al. Arginine dependence of acute myeloid leukemia blast proliferation: a novel therapeutic target. Blood. (2015) 125:2386–96. doi: 10.1182/blood-2014-09-600643
31. Qin, L, Tian, Y, Yu, Z, Shi, D, Wang, J, Zhang, C, et al. Targeting PDK1 with dichloroacetophenone to inhibit acute myeloid leukemia (AML) cell growth. Oncotarget. (2016) 7:1395–407. doi: 10.18632/oncotarget.6366
32. Stuani, L, Riols, F, Millard, P, Sabatier, M, Batut, A, Saland, E, et al. Stable isotope labeling highlights enhanced fatty acid and lipid metabolism in human acute myeloid leukemia. Int J Mol Sci. (2018) 19:3325. doi: 10.3390/ijms19113325
33. Shafat, MS, Oellerich, T, Mohr, S, Robinson, SD, Edwards, DR, Marlein, CR, et al. Leukemic blasts program bone marrow adipocytes to generate a protumoral microenvironment. Blood. (2017) 129:1320–32. doi: 10.1182/blood-2016-08-734798
34. Sala, A, Rossi, E, Antillon, F, Molina, AL, de Maselli, T, Bonilla, M, et al. Nutritional status at diagnosis is related to clinical outcomes in children and adolescents with cancer: a perspective from Central America. Eur J Cancer. (2012) 48:243–52. doi: 10.1016/j.ejca.2011.06.006
35. Gu, JJ, Singh, A, Xue, K, Mavis, C, Barth, M, Yanamadala, V, et al. Up-regulation of hexokinase II contributes to rituximab-chemotherapy resistance and is a clinically relevant target for therapeutic development. Oncotarget. (2018) 9:4020–33. doi: 10.18632/oncotarget.23425
36. Rosen, GP, Beebe, KL, and Shaibi, GQ. Vitamin D levels differ by cancer diagnosis and decline over time in survivors of childhood cancer. Pediatr Blood Cancer. (2013) 60:949–52. doi: 10.1002/pbc.24349
37. Yang, C, Sudderth, J, Dang, T, Bachoo, RM, McDonald, JG, and DeBerardinis, RJ. Glioblastoma cells require glutamate dehydrogenase to survive impairments of glucose metabolism or Akt signaling. Cancer Res. (2009) 69:7986–93. doi: 10.1158/0008-5472.CAN-09-2266
38. Damanskiene, E, Balnyte, I, Valanciute, A, Lesauskaite, V, Alonso, MM, and Stakisaitis, D. The comparative experimental study of sodium and magnesium dichloroacetate effects on pediatric PBT24 and SF8628 cell glioblastoma tumors using a chicken embryo chorioallantoic membrane model and on cells in vitro. Int J Mol Sci. (2022) 23:10455. doi: 10.3390/ijms231810455
39. Vallejo, FA, Shah, SS, de Cordoba, N, Walters, WM, Prince, J, Khatib, Z, et al. The contribution of ketone bodies to glycolytic inhibition for the treatment of adult and pediatric glioblastoma. J Neuro Oncol. (2020) 147:317–26. doi: 10.1007/s11060-020-03431-w
40. Bunin, GR, Kushi, LH, Gallagher, PR, Rorke-Adams, LB, McBride, ML, and Cnaan, A. Maternal diet during pregnancy and its association with medulloblastoma in children: a children's oncology group study (United States). Cancer Causes Control. (2005) 16:877–91. doi: 10.1007/s10552-005-3144-7
41. Pogoda, JM, Preston-Martin, S, Howe, G, Lubin, F, Mueller, BA, Holly, EA, et al. An international case-control study of maternal diet during pregnancy and childhood brain tumor risk: a histology-specific analysis by food group. Ann Epidemiol. (2009) 19:148–60. doi: 10.1016/j.annepidem.2008.12.011
42. Niklison-Chirou, MV, Erngren, I, Engskog, M, Haglof, J, Picard, D, Remke, M, et al. TAp73 is a marker of glutamine addiction in medulloblastoma. Genes Dev. (2017) 31:1738–53. doi: 10.1101/gad.302349.117
43. Bhatia, B, Potts, CR, Guldal, C, Choi, S, Korshunov, A, Pfister, S, et al. Hedgehog-mediated regulation of PPARgamma controls metabolic patterns in neural precursors and shh-driven medulloblastoma. Acta Neuropathol. (2012) 123:587–600. doi: 10.1007/s00401-012-0968-6
44. Daggubati, V, Hochstelter, J, Bommireddy, A, Choudhury, A, Krup, AL, Kaur, P, et al. Smoothened-activating lipids drive resistance to CDK4/6 inhibition in hedgehog-associated medulloblastoma cells and preclinical models. J Clin Invest. (2021) 131:e141171. doi: 10.1172/JCI141171
45. Green, GJ, Weitzman, SS, and Pencharz, PB. Resting energy expenditure in children newly diagnosed with stage IV neuroblastoma. Pediatr Res. (2008) 63:332–6. doi: 10.1203/PDR.0b013e318163a2d4
46. French, AE, Grant, R, Weitzman, S, Ray, JG, Vermeulen, MJ, Sung, L, et al. Folic acid food fortification is associated with a decline in neuroblastoma. Clin Pharmacol Ther. (2003) 74:288–94. doi: 10.1016/S0009-9236(03)00200-5
47. Wang, T, Liu, L, Chen, X, Shen, Y, Lian, G, Shah, N, et al. MYCN drives glutaminolysis in neuroblastoma and confers sensitivity to an ROS augmenting agent. Cell Death Dis. (2018) 9:220. doi: 10.1038/s41419-018-0295-5
48. Ren, P, Yue, M, Xiao, D, Xiu, R, Gan, L, Liu, H, et al. ATF4 and N-Myc coordinate glutamine metabolism in MYCN-amplified neuroblastoma cells through ASCT2 activation. J Pathol. (2015) 235:90–100. doi: 10.1002/path.4429
49. Xia, Y, Ye, B, Ding, J, Yu, Y, Alptekin, A, Thangaraju, M, et al. Metabolic reprogramming by MYCN confers dependence on the serine-glycine-one-carbon biosynthetic pathway. Cancer Res. (2019) 79:3837–50. doi: 10.1158/0008-5472.CAN-18-3541
50. Alptekin, A, Ye, B, Yu, Y, Poole, CJ, van Riggelen, J, Zha, Y, et al. Glycine decarboxylase is a transcriptional target of MYCN required for neuroblastoma cell proliferation and tumorigenicity. Oncogene. (2019) 38:7504–20. doi: 10.1038/s41388-019-0967-3
51. Hogarty, MD, Norris, MD, Davis, K, Liu, X, Evageliou, NF, Hayes, CS, et al. ODC1 is a critical determinant of MYCN oncogenesis and a therapeutic target in neuroblastoma. Cancer Res. (2008) 68:9735–45. doi: 10.1158/0008-5472.CAN-07-6866
52. Evageliou, NF, Haber, M, Vu, A, Laetsch, TW, Murray, J, Gamble, LD, et al. Polyamine antagonist therapies inhibit neuroblastoma initiation and progression. Clin Cancer Res. (2016) 22:4391–404. doi: 10.1158/1078-0432.CCR-15-2539
53. Gamble, LD, Purgato, S, Murray, J, Xiao, L, Yu, DMT, Hanssen, KM, et al. Inhibition of polyamine synthesis and uptake reduces tumor progression and prolongs survival in mouse models of neuroblastoma. Sci Transl Med. (2019) 11:eaau1099. doi: 10.1126/scitranslmed.aau1099
54. Botzer, LE, Maman, S, Sagi-Assif, O, Meshel, T, Nevo, I, Yron, I, et al. Hexokinase 2 is a determinant of neuroblastoma metastasis. Br J Cancer. (2016) 114:759–66. doi: 10.1038/bjc.2016.26
55. Dorneburg, C, Fischer, M, Barth, TFE, Mueller-Klieser, W, Hero, B, Gecht, J, et al. LDHA in neuroblastoma is associated with poor outcome and its depletion decreases neuroblastoma growth independent of aerobic glycolysis. Clinical Cancer Res. (2018) 24:5772–83. doi: 10.1158/1078-0432.CCR-17-2578
56. Matsushita, K, Uchida, K, Saigusa, S, Ide, S, Hashimoto, K, Koike, Y, et al. Glycolysis inhibitors as a potential therapeutic option to treat aggressive neuroblastoma expressing GLUT1. J Pediatr Surg. (2012) 47:1323–30. doi: 10.1016/j.jpedsurg.2011.12.007
57. Feichtinger, RG, Zimmermann, F, Mayr, JA, Neureiter, D, Hauser-Kronberger, C, Schilling, FH, et al. Low aerobic mitochondrial energy metabolism in poorly-or undifferentiated neuroblastoma. BMC Cancer. (2010) 10:149. doi: 10.1186/1471-2407-10-149
58. Oliynyk, G, Ruiz-Perez, MV, Sainero-Alcolado, L, Dzieran, J, Zirath, H, Gallart-Ayala, H, et al. MYCN-enhanced oxidative and glycolytic metabolism reveals vulnerabilities for targeting neuroblastoma. iScience. (2019) 21:188–204. doi: 10.1016/j.isci.2019.10.020
59. Tao, L, Mohammad, MA, Milazzo, G, Moreno-Smith, M, Patel, TD, Zorman, B, et al. MYCN-driven fatty acid uptake is a metabolic vulnerability in neuroblastoma. Nat Commun. (2022) 13:3728. doi: 10.1038/s41467-022-31331-2
60. Moreno-Smith, M, Milazzo, G, Tao, L, Fekry, B, Zhu, B, Mohammad, MA, et al. Restoration of the molecular clock is tumor suppressive in neuroblastoma. Nat Commun. (2021) 12:4006. doi: 10.1038/s41467-021-24196-4
61. Ruiz-Perez, MV, Sainero-Alcolado, L, Oliynyk, G, Matuschek, I, Balboni, N, Ubhayasekera, S, et al. Inhibition of fatty acid synthesis induces differentiation and reduces tumor burden in childhood neuroblastoma. iScience. (2021) 24:102128. doi: 10.1016/j.isci.2021.102128
62. Battaglia-Hsu, SF, Akchiche, N, Noel, N, Alberto, JM, Jeannesson, E, Orozco-Barrios, CE, et al. Vitamin B12 deficiency reduces proliferation and promotes differentiation of neuroblastoma cells and up-regulates PP2A, proNGF, and TACE. Proc Natl Acad Sci U S A. (2009) 106:21930–5. doi: 10.1073/pnas.0811794106
63. Wijesinghe, TP, Dharmasivam, M, Dai, CC, and Richardson, DR. Innovative therapies for neuroblastoma: the surprisingly potent role of iron chelation in up-regulating metastasis and tumor suppressors and down-regulating the key oncogene. N-myc Pharmacol. Res. (2021) 173:105889. doi: 10.1016/j.phrs.2021.105889
64. Carosio, R, Zuccari, G, Orienti, I, Mangraviti, S, and Montaldo, PG. Sodium ascorbate induces apoptosis in neuroblastoma cell lines by interfering with iron uptake. Mol Cancer. (2007) 6:55. doi: 10.1186/1476-4598-6-55
65. Sradhanjali, S, Tripathy, D, Rath, S, Mittal, R, and Reddy, MM. Overexpression of pyruvate dehydrogenase kinase 1 in retinoblastoma: a potential therapeutic opportunity for targeting vitreous seeds and hypoxic regions. PLoS One. (2017) 12:e0177744. doi: 10.1371/journal.pone.0177744
66. Linabery, AM, Johnson, KJ, and Ross, JA. Childhood cancer incidence trends in association with US folic acid fortification (1986-2008). Pediatrics. (2012) 129:1125–33. doi: 10.1542/peds.2011-3418
67. Rakheja, D, Khokhar, S, Mitui, M, and Cost, NG. Immunohistochemical expression of GLUT1 and its correlation with unfavorable histology and TP53 codon 72 polymorphism in Wilms tumors. Pediatric Dev Pathol. (2012) 15:286–92. doi: 10.2350/12-01-1151-OA.1
68. Feichtinger, RG, Neureiter, D, Royer-Pokora, B, Mayr, JA, Zimmermann, FA, Jones, N, et al. Heterogeneity of mitochondrial energy metabolism in classical triphasic Wilms' tumor. Front Biosci. (2011) 3:187–93. doi: 10.2741/e232
69. Crippa, S, Ancey, PB, Vazquez, J, Angelino, P, Rougemont, AL, Guettier, C, et al. Mutant CTNNB1 and histological heterogeneity define metabolic subtypes of hepatoblastoma. EMBO Mol Med. (2017) 9:1589–604. doi: 10.15252/emmm.201707814
70. Hu, Y, Zai, H, Jiang, W, Ou, Z, Yao, Y, and Zhu, Q. The mutual inhibition of FoxO1 and SREBP-1c regulated the progression of hepatoblastoma by regulating fatty acid metabolism. Mediat Inflamm. (2021) 2021:5754592. doi: 10.1155/2021/5754592
71. Zhuo, B, Li, Y, Li, Z, Qin, H, Sun, Q, Zhang, F, et al. PI3K/Akt signaling mediated Hexokinase-2 expression inhibits cell apoptosis and promotes tumor growth in pediatric osteosarcoma. Biochem Biophys Res Commun. (2015) 464:401–6. doi: 10.1016/j.bbrc.2015.06.092
72. Qian, H, Lei, T, Hu, Y, and Lei, P. Expression of lipid-metabolism genes is correlated with immune microenvironment and predicts prognosis in osteosarcoma. Front Cell Dev Biol. (2021) 9:673827. doi: 10.3389/fcell.2021.673827
73. Chanihoon, GQ, Afridi, HI, Kazi, TG, Talpur, FN, and Baig, JA. Evaluation of zinc and cadmium levels in the biological samples of Ewing sarcomas patients and healthy subjects, clin chim acta 522 (2021)1-7. Clin. Chim. Acta. (2021) 522:1–7. doi: 10.1016/j.cca.2022.01.011
74. Sen, N, Cross, AM, Lorenzi, PL, Khan, J, Gryder, BE, Kim, S, et al. EWS-FLI1 reprograms the metabolism of Ewing sarcoma cells via positive regulation of glutamine import and serine-glycine biosynthesis. Mol Carcinog. (2018) 57:1342–57. doi: 10.1002/mc.22849
75. Yeung, C, Gibson, AE, Issaq, SH, Oshima, N, Baumgart, JT, Edessa, LD, et al. Targeting glycolysis through inhibition of lactate dehydrogenase impairs tumor growth in preclinical models of Ewing sarcoma. Cancer Res. (2019) 79:5060–73. doi: 10.1158/0008-5472.CAN-19-0217
76. Miyagaki, S, Kikuchi, K, Mori, J, Lopaschuk, GD, Iehara, T, and Hosoi, H. Inhibition of lipid metabolism exerts antitumor effects on rhabdomyosarcoma. Cancer Med. (2021) 10:6442–55. doi: 10.1002/cam4.4185
77. Amankwah, EK, Saenz, AM, Hale, GA, and Brown, PA. Association between body mass index at diagnosis and pediatric leukemia mortality and relapse: a systematic review and meta-analysis. Leuk Lymphoma. (2016) 57:1140–8. doi: 10.3109/10428194.2015.1076815
78. Orgel, E, Genkinger, JM, Aggarwal, D, Sung, L, Nieder, M, and Ladas, EJ. Association of body mass index and survival in pediatric leukemia: a meta-analysis. Am J Clin Nutr. (2016) 103:808–17. doi: 10.3945/ajcn.115.124586
79. Ladas, EJ, Orjuela, M, Stevenson, K, Cole, PD, Lin, M, Athale, UH, et al. Dietary intake and childhood leukemia: the diet and acute lymphoblastic leukemia treatment (DALLT) cohort study. Nutrition. (2016) 32:1103–1109.e1. doi: 10.1016/j.nut.2016.03.014
80. Johnston, WT, Erdmann, F, Newton, R, Steliarova-Foucher, E, Schuz, J, and Roman, E. Childhood cancer: estimating regional and global incidence. Cancer Epidemiol. (2021) 71:101662. doi: 10.1016/j.canep.2019.101662
81. Issaq, SH, and Heske, CM. Targeting metabolic dependencies in pediatric cancer. Curr Opin Pediatr. (2020) 32:26–34. doi: 10.1097/MOP.0000000000000853
82. Revuelta Iniesta, R, Rush, R, Paciarotti, I, Rhatigan, EB, Brougham, FHM, McKenzie, JM, et al. Systematic review and meta-analysis: prevalence and possible causes of vitamin D deficiency and insufficiency in pediatric cancer patients. Clin Nutr. (2016) 35:95–108. doi: 10.1016/j.clnu.2014.12.023
83. Balkwill, FR, Capasso, M, and Hagemann, T. The tumor microenvironment at a glance. J Cell Sci. (2012) 125:5591–6. doi: 10.1242/jcs.116392
84. Ganguly, S, Srivastava, R, Agarwala, S, Dwivedi, S, Bansal, PG, Gonmei, Z, et al. Prevalence of micronutrient deficiency and its impact on the outcome of childhood cancer: a prospective cohort study. Clin Nutr. (2022) 41:1501–11. doi: 10.1016/j.clnu.2022.05.010
85. Sahoo, K, Sahoo, B, Choudhury, AK, Sofi, NY, Kumar, R, and Bhadoria, AS. Childhood obesity: causes and consequences. J Family Med Prim Care. (2015) 4:187–92. doi: 10.4103/2249-4863.154628
86. Larsson, SC, Bergkvist, L, and Wolk, A. Consumption of sugar and sugar-sweetened foods and the risk of pancreatic cancer in a prospective study. Am J Clin Nutr. (2006) 84:1171–6. doi: 10.1093/ajcn/84.5.1171
87. Friberg, E, Wallin, A, and Wolk, A. Sucrose, high-sugar foods, and risk of endometrial cancer--a population-based cohort study. Cancer Epidemiol Biomarkers Pre. (2011) 20:1831–7. doi: 10.1158/1055-9965.EPI-11-0402
88. Hanahan, D, and Weinberg, RA. Hallmarks of cancer: the next generation. Cells. (2011) 144:646–74. doi: 10.1016/j.cell.2011.02.013
89. DeBerardinis, RJ, and Chandel, NS. Fundamentals of cancer metabolism. Sci Adv. (2016) 2:e1600200. doi: 10.1126/sciadv.1600200
90. Lieu, EL, Nguyen, T, Rhyne, S, and Kim, J. Amino acids in cancer. Exp Mol Med. (2020) 52:15–30. doi: 10.1038/s12276-020-0375-3
91. Souba, WW. Glutamine and cancer. Ann Surg. (1993) 218:715–28. doi: 10.1097/00000658-199312000-00004
92. Wang, SZ, Poore, B, Alt, J, Price, A, Allen, SJ, Hanaford, AR, et al. Unbiased metabolic profiling predicts sensitivity of high MYC-expressing atypical teratoid/rhabdoid tumors to glutamine inhibition with 6-Diazo-5-Oxo-L-Norleucine. Clin Cancer Res. (2019) 25:5925–36. doi: 10.1158/1078-0432.CCR-19-0189
93. Zhao, E, Hou, J, and Cui, H. Serine-glycine-one-carbon metabolism: vulnerabilities in MYCN-amplified neuroblastoma. Oncogenesis. (2020) 9:14. doi: 10.1038/s41389-020-0200-9
94. Rathore, R, Caldwell, KE, Schutt, C, Brashears, CB, Prudner, BC, Ehrhardt, WR, et al. Metabolic compensation activates pro-survival mTORC1 signaling upon 3-phosphoglycerate dehydrogenase inhibition in osteosarcoma. Cell Rep. (2021) 34:108678. doi: 10.1016/j.celrep.2020.108678
95. Patil, MD, Bhaumik, J, Babykutty, S, Banerjee, UC, and Fukumura, D. Arginine dependence of tumor cells: targeting a chink in cancer's armor. Oncogene. (2016) 35:4957–72. doi: 10.1038/onc.2016.37
96. Hernandez, CP, Morrow, K, Lopez-Barcons, LA, Zabaleta, J, Sierra, R, Velasco, C, et al. Pegylated arginase I: a potential therapeutic approach in T-ALL. Blood. (2010) 115:5214–21. doi: 10.1182/blood-2009-12-258822
97. Tsai, HJ, Jiang, SS, Hung, WC, Borthakur, G, Lin, SF, Pemmaraju, N, et al. A phase II study of arginine deiminase (ADI-PEG20) in relapsed/refractory or poor-risk acute myeloid leukemia patients. Sci Rep. (2017) 7:11253. doi: 10.1038/s41598-017-10542-4
98. Krall, AS, Xu, S, Graeber, TG, Braas, D, and Christofk, HR. Asparagine promotes cancer cell proliferation through use as an amino acid exchange factor. Nat Commun. (2016) 7:11457. doi: 10.1038/ncomms11457
99. Chiu, M, Taurino, G, Bianchi, MG, Kilberg, MS, and Bussolati, O. Asparagine synthetase in cancer: beyond acute lymphoblastic leukemia. Front Oncol. (2019) 9:1480. doi: 10.3389/fonc.2019.01480
100. Casero, RA Jr, Murray Stewart, T, and Pegg, AE. Polyamine metabolism and cancer: treatments, challenges and opportunities. Nat Rev Cancer. (2018) 18:681–95. doi: 10.1038/s41568-018-0050-3
101. Rennert, O, Miale, T, Shukla, J, Lawson, D, and Frias, J. Polyamine concentrations in bone marrow aspirates of children with leukemia and other malignancies. Blood. (1976) 47:695–701.
102. Warburg, O, Wind, F, and Negelein, E. The metabolism of tumors in the body. J Gen Physiol. (1927) 8:519–30. doi: 10.1085/jgp.8.6.519
103. Martinez-Reyes, I, Diebold, LP, Kong, H, Schieber, M, Huang, H, Hensley, CT, et al. TCA cycle and mitochondrial membrane potential are necessary for diverse biological functions. Mol Cell. (2016) 61:199–209. doi: 10.1016/j.molcel.2015.12.002
104. Snaebjornsson, MT, Janaki-Raman, S, and Schulze, A. Greasing the wheels of the cancer machine: the role of lipid metabolism in cancer. Cell Metab. (2020) 31:62–76. doi: 10.1016/j.cmet.2019.11.010
105. Hathcock, JN. Vitamins and minerals: efficacy and safety. Am J Clin Nutr. (1997) 66:427–37. doi: 10.1093/ajcn/66.2.427
106. Zhao, J, Wang, Y, Tao, L, and Chen, L. Iron transporters and ferroptosis in malignant brain tumors. Front Oncol. (2022) 12:861834. doi: 10.3389/fonc.2022.861834
107. Floros, KV, Cai, J, Jacob, S, Kurupi, R, Fairchild, CK, Shende, M, et al. MYCN-amplified neuroblastoma is addicted to iron and vulnerable to inhibition of the system xc−/glutathione axis. Cancer Res. (2021) 81:1896–908. doi: 10.1158/0008-5472.CAN-20-1641
108. Lu, Y, Yang, Q, Su, Y, Ji, Y, Li, G, Yang, X, et al. MYCN mediates TFRC-dependent ferroptosis and reveals vulnerabilities in neuroblastoma. Cell Death Dis. (2021) 12:511. doi: 10.1038/s41419-021-03790-w
109. Martinez-Outschoorn, UE, Peiris-Pages, M, Pestell, RG, Sotgia, F, and Lisanti, MP. Cancer metabolism: a therapeutic perspective. Nat Rev Clin Oncol. (2017) 14:11–31. doi: 10.1038/nrclinonc.2016.60
110. Farhadi, P, Yarani, R, Dokaneheifard, S, and Mansouri, K. The emerging role of targeting cancer metabolism for cancer therapy. Tumour Biol. (2020) 42:1010428320965284. doi: 10.1177/1010428320965284
111. Stine, ZE, Schug, ZT, Salvino, JM, and Dang, CV. Targeting cancer metabolism in the era of precision oncology. Nat Rev Drug Discov. (2022) 21:141–62. doi: 10.1038/s41573-021-00339-6
112. Sabnis, HS, Bradley, HL, Tripathi, S, Yu, WM, Tse, W, Qu, CK, et al. Synergistic cell death in FLT3-ITD positive acute myeloid leukemia by combined treatment with metformin and 6-benzylthioinosine. Leuk Res. (2016) 50:132–40. doi: 10.1016/j.leukres.2016.10.004
113. Sullivan, MR, Danai, LV, Lewis, CA, Chan, SH, Gui, DY, Kunchok, T, et al. Quantification of microenvironmental metabolites in murine cancers reveals determinants of tumor nutrient availability. Elife. (2019) 8:e44235. doi: 10.7554/eLife.44235
114. Kanarek, N, Petrova, B, and Sabatini, DM. Dietary modifications for enhanced cancer therapy. Nature. (2020) 579:507–17. doi: 10.1038/s41586-020-2124-0
115. Tajan, M, and Vousden, KH. Dietary approaches to cancer therapy. Cancer Cell. (2020) 37:767–85. doi: 10.1016/j.ccell.2020.04.005
116. Holloszy, JO, and Fontana, L. Caloric restriction in humans. Exp Gerontol. (2007) 42:709–12. doi: 10.1016/j.exger.2007.03.009
117. Berrigan, D, Perkins, SN, Haines, DC, and Hursting, SD. Adult-onset calorie restriction and fasting delay spontaneous tumorigenesis in p53-deficient mice. Carcinogenesis. (2002) 23:817–22. doi: 10.1093/carcin/23.5.817
118. Fernandes, G, Chandrasekar, B, Troyer, DA, Venkatraman, JT, and Good, RA. Dietary lipids and calorie restriction affect mammary tumor incidence and gene expression in mouse mammary tumor virus/v-Ha-ras transgenic mice. Proc Natl Acad Sci U S A. (1995) 92:6494–8. doi: 10.1073/pnas.92.14.6494
119. Harvey, AE, Lashinger, LM, Hays, D, Harrison, LM, Lewis, K, Fischer, SM, et al. Calorie restriction decreases murine and human pancreatic tumor cell growth, nuclear factor-kappaB activation, and inflammation-related gene expression in an insulin-like growth factor-1-dependent manner. PLoS One. (2014) 9:e94151. doi: 10.1371/journal.pone.0094151
120. Morscher, RJ, Aminzadeh-Gohari, S, Feichtinger, RG, Mayr, JA, Lang, R, Neureiter, D, et al. Inhibition of neuroblastoma tumor growth by ketogenic diet and/or calorie restriction in a CD1-Nu mouse model. PLoS One. (2015) 10:e0129802. doi: 10.1371/journal.pone.0129802
121. Chen, JH, Cottrell, EC, and Ozanne, SE. Early growth and ageing. Nestle Nutr Workshop Ser Pediatr Program. (2010) 65:41–50; discussion-4. doi: 10.1159/000281144
122. De Feyter, HM, Behar, KL, Rao, JU, Madden-Hennessey, K, Ip, KL, Hyder, F, et al. A ketogenic diet increases transport and oxidation of ketone bodies in RG2 and 9L gliomas without affecting tumor growth. Neuro Oncology. (2016) 18:1079–87. doi: 10.1093/neuonc/now088
123. Aminzadeh-Gohari, S, Feichtinger, RG, Vidali, S, Locker, F, Rutherford, T, O'Donnel, M, et al. A ketogenic diet supplemented with medium-chain triglycerides enhances the anti-tumor and anti-angiogenic efficacy of chemotherapy on neuroblastoma xenografts in a CD1-nu mouse model. Oncotarget. (2017) 8:64728–44. doi: 10.18632/oncotarget.20041
124. Ma, LM, Yang, HP, Yang, XW, and Ruan, LH. Methionine synthase A2756G polymorphism influences pediatric acute lymphoblastic leukemia risk: a meta-analysis. Biosci Rep. (2019) 39:BSR20181770. doi: 10.1042/BSR20181770
125. Stern, PH, and Hoffman, RM. Enhanced in vitro selective toxicity of chemotherapeutic agents for human cancer cells based on a metabolic defect. J Natl Cancer Inst. (1986) 76:629–39. doi: 10.1093/jnci/76.4.629
126. Maddocks, OD, Berkers, CR, Mason, SM, Zheng, L, Blyth, K, Gottlieb, E, et al. Serine starvation induces stress and p53-dependent metabolic remodelling in cancer cells. Nature. (2013) 493:542–6. doi: 10.1038/nature11743
127. Maddocks, ODK, Athineos, D, Cheung, EC, Lee, P, Zhang, T, van den Broek, NJF, et al. Modulating the therapeutic response of tumours to dietary serine and glycine starvation. Nature. (2017) 544:372–6. doi: 10.1038/nature23471
128. Hanley, MP, Kadaveru, K, Perret, C, Giardina, C, and Rosenberg, DW. Dietary methyl donor depletion suppresses intestinal adenoma development. Cancer Prev Res. (2016) 9:812–20. doi: 10.1158/1940-6207.CAPR-16-0042
129. Muggeo, P, Muggeo, VMR, Giordano, P, Delvecchio, M, Altomare, M, Novielli, C, et al. Cardiovascular dysfunction and vitamin D status in childhood acute lymphoblastic leukemia survivors. World J Pediatr. (2019) 15:465–70. doi: 10.1007/s12519-019-00258-y
130. Wei, L, Wu, Z, and Chen, YQ. Multi-targeted therapy of cancer by omega-3 fatty acids-an update. Cancer Lett. (2022) 526:193–204. doi: 10.1016/j.canlet.2021.11.023
131. Ljungblad, L, Bergqvist, F, Tummler, C, Madawala, S, Olsen, TK, Andonova, T, et al. Omega-3 fatty acids decrease CRYAB, production of oncogenic prostaglandin E2 and suppress tumor growth in medulloblastoma. Life Sci. (2022) 295:120394. doi: 10.1016/j.lfs.2022.120394
132. Gleissman, H, Segerstrom, L, Hamberg, M, Ponthan, F, Lindskog, M, Johnsen, JI, et al. Omega-3 fatty acid supplementation delays the progression of neuroblastoma in vivo. Int J Cancer. (2011) 128:1703–11. doi: 10.1002/ijc.25473
133. Ljungblad, L. Omega-3 Fatty Acids in the Treatment of Childhood Cancer. Stockholm: Karolinska Institute (2022).
134. Pattnaik, J, Kayal, S, Dubashi, B, Basu, D, Vinod, KV, Nandeesha, H, et al. Profile of anemia in acute lymphoblastic leukemia patients on maintenance therapy and the effect of micronutrient supplementation. Support Care Cancer. (2020) 28:731–8. doi: 10.1007/s00520-019-04862-6
135. Solmaz, I, Ozdemir, MA, Unal, E, Abdurrezzak, U, Muhtaroglu, S, and Karakukcu, M. Effect of vitamin K2 and vitamin D3 on bone mineral density in children with acute lymphoblastic leukemia: a prospective cohort study. J Pediatr Endocrinol Metab. (2021) 34:441–7. doi: 10.1515/jpem-2020-0637
136. Baracos, VE, Martin, L, Korc, M, Guttridge, DC, and Fearon, KCH. Cancer-associated cachexia. Nat Rev Dis Primers. (2018) 4:17105. doi: 10.1038/nrdp.2017.105
137. Argiles, JM. Cancer-associated malnutrition. Eur J Oncol Nurs. (2005) 9:S39–50. doi: 10.1016/j.ejon.2005.09.006
138. May, PE, Barber, A, D'Olimpio, JT, Hourihane, A, and Abumrad, NN. Reversal of cancer-related wasting using oral supplementation with a combination of beta-hydroxy-beta-methylbutyrate, arginine, and glutamine. Am J Surg. (2002) 183:471–9. doi: 10.1016/S0002-9610(02)00823-1
139. Deutz, NE, Safar, A, Schutzler, S, Memelink, R, Ferrando, A, Spencer, H, et al. Muscle protein synthesis in cancer patients can be stimulated with a specially formulated medical food. Clin Nutr. (2011) 30:759–68. doi: 10.1016/j.clnu.2011.05.008
140. Hunter, DC, Weintraub, M, Blackburn, GL, and Bistrian, BR. Branched chain amino acids as the protein component of parenteral nutrition in cancer cachexia. Br J Surg. (1989) 76:149–53. doi: 10.1002/bjs.1800760215
141. Kanarek, N, Keys, HR, Cantor, JR, Lewis, CA, Chan, SH, Kunchok, T, et al. Histidine catabolism is a major determinant of methotrexate sensitivity. Nature. (2018) 559:632–6. doi: 10.1038/s41586-018-0316-7
Keywords: malnutrition, pediatric cancer, nutrient dependency, dietary modifications, precision nutrition
Citation: Wang K, Yang T, Zhang Y, Gao X and Tao L (2023) The opportunities and challenges for nutritional intervention in childhood cancers. Front. Nutr. 10:1091067. doi: 10.3389/fnut.2023.1091067
Edited by:
Ling Zhao, The University of Tennessee, Knoxville, United StatesReviewed by:
Guoxun Chen, The University of Tennessee, Knoxville, United StatesJin-Rong Zhou, Harvard Medical School, United States
Copyright © 2023 Wang, Yang, Zhang, Gao and Tao. This is an open-access article distributed under the terms of the Creative Commons Attribution License (CC BY). The use, distribution or reproduction in other forums is permitted, provided the original author(s) and the copyright owner(s) are credited and that the original publication in this journal is cited, in accordance with accepted academic practice. No use, distribution or reproduction is permitted which does not comply with these terms.
*Correspondence: Ling Tao, bGluZ190YW9AZnVkYW4uZWR1LmNu