- 1Key Lab of Medical Molecular Cell Biology of Shanxi Province, Institutes of Biomedical Sciences, Shanxi University, Taiyuan, China
- 2The Provincial Key Laboratories for Prevention and Treatment of Major Infectious Diseases Shanxi, Institutes of Biomedical Sciences, Shanxi University, Taiyuan, China
- 3Department of Internal Medicine, Fourth People's Hospital of Taiyuan, Taiyuan, China
- 4Central Laboratory, Shanxi Provincial People's Hospital, Taiyuan, China
- 5State Key Laboratory for Managing Biotic and Chemical Threats to the Quality and Safety of Agro-Products, Institute of Food Research, Zhejiang Academy of Agricultural Sciences, Hangzhou, China
Introduction: Obesity is a health issue worldwide. This study aimed to evaluate the beneficial effects of Pediococcus pentococcus PR-1 on the modulating of gut microbiota, inflammation and lipid metabolism in high-fat-diet (HFD)-fed zebrafish.
Methods: Adult zebrafish were fed a commercial (C), high fat (H, 25% fat), probiotic (P, 106 CFU/g), or high fat with probiotic (HP) diets twice daily for 5 weeks. Gut microbiota were analysed using 16S rRNA gene sequencing. Gene expressions of intestinal cytokine, intestinal TJ protein, and liver lipid metabolism were analysed by quantitative real-time polymerase chain reaction. Biochemical and histological analysis were also performed.
Results and discussion: P. pentosaceus PR-1 reduced body weight and BMI, indicating its anti-obesity effect. The 16S rRNA sequencing results showed HFD induced a distinct gut microbiota structure from C group, which was restored by probiotic. P. pentosaceus PR-1 improved gut health by decreasing the abundance of Ralstonia and Aeromonas which were increased induced by HFD. Moreover, probiotic restored abundance of Fusobacteria, Cetobacterium and Plesiomonas, which were decreased in HFD-fed zebrafish. The results of quantitative real-time polymerase chain reaction showed probiotic suppressed HFD-induced inflammation by decreasing the expressions of IL-1b and IL-6. Levels of hepatic TNF-α, IL-1ß, and IL-6 were reduced by probiotic in HFD-fed zebrafish. Probiotic also ameliorated gut barrier function by increasing the expressions of occludin, Claudin-1, and ZO-1. Probiotic exerted anti-adipogenic activity through regulating the expressions of SREBP1, FAS and LEPTIN. Levels of hepatic triglyceride, total cholesterol, low density lipoprotein were also reduced by probiotic. Histological analysis showed probiotic alleviated liver steatosis and injury induced by HFD. P. pentosaceus PR-1 might be useful as a dietary health supplement, especially for reducing obesity.
1. Introduction
There has been an increasing prevalence of overweight and obesity during the last 50 years, which are characterized as excessive fat accumulation and low-grade systemic inflammatory state (1). Obesity is mainly caused by long-term imbalance between energy intake and energy expenditure, and would increase the risk of a serious chronic diseases including, diabetes, cardiovascular diseases, and cancer (2). Epidemiological and animal studies have shown that high-fat diet (HFD) is associated with body weight gain and would promote obesity (3, 4).
Several studies have identified the alterations in the gut microbiota, immune response and metabolic functions induced by HFD and obesity (4–6). The increased ratio of Firmicutes/Bacteroidetes is one of primary changes induced by a consumption of HFD in human and animal studies (7). Velázquez et al. (8) found long-term HFD in mice could lead to pathophysiological and microbial changes that observed in non-alcoholic fatty liver disease patients. The increased relative abundance of Firmicutes phylum, Adercreutzia, Coprococcus, Dorea, and Ruminococcus species, and decreased relative abundance of Turicibacter and Anaeroplasma were observed in HFD mice. Moreover, a higher ratio of Firmicutes/Bacteroidetes was found in HFD mice (8). Microbiota changes contributing to the increase of Firmicutes/Bacteroidetes ratio may arise from the increases in Erysipelotrichales, Bacilli, and Clostridiales (belonging to Firmicutes phylum) (9). The increased abundance of Dorea and Ruminococcus (belonging to Firmicutes phylum) also contribute to the increased ratio of Firmicutes/Bacteroidetes (8–10). A consumption of HFD could also lead to the increased abundance of Proteobacteria, which could promote intestinal inflammation and induce a mucosal proinflammatory immune response (11). The decrease in Prevotellaceae and Rikenellaceae abundance is also observed in HFD-fed rats (12, 13). In addition, HFD often drive a decrease in abundance of Bifidobacterium spp., which is considered to be beneficial to host health and negatively correlated with metabolic endotoxemia (14). Besides, HFD could also directly or indirectly perturb the gut barrier function and increase the intestinal permeability through suppressing the expression of intestinal tight junction (TJ) proteins, such as occludin, claudin, and zona occludens (ZO) (15–17). A consumption of HFD could also disrupt immune homeostasis and promote intestinal inflammation, with increased levels of proinflammatory cytokines tumor necrosis factor α (TNF-α), interleukins (IL)-1β, and IL-6 (2, 18, 19).
Probiotics are live microorganisms, conferring beneficial effects to host health when administered in adequate amounts (20, 21). Several studies have shown that probiotics could positively modulate the gut microbial composition, immune, and lipid metabolism markers and following a HFD (22–26). Pediococcus pentosaceus, one type of LAB, has attracted much attention in recent years. P. pentosaceus LI05, isolated from a heathy volunteer fecal sample, could protect against Clostridium difficile infection (27–29). In addition, P. pentosaceus has been shown to reduce oxidative stress (30), alleviate constipation (31), liver (32, 33) and reproductive system injury (34), and improve hyperlipidaemia (35). P. pentosaceus PP04 isolated from the Northeast pickled cabbage could ameliorate hyperlipidemia by the AMPK signaling pathway and improve oxidative stress in HFD mice (35). In addition, PP04 could improve gut barrier integrity by stimulating the expressions of TJ proteins, subsequently decreasing levels of hepatic lipopolysaccharides, alanine aminotransferase, and aspartate aminotransferase in HFD mice (35). Moreover, PP04 could relive intestinal inflammation by the NF-κB/Nrf2 signaling pathway, and restore the gut microbiota homeostasis in HFD mice (36). A clinical trial found that heat-killed P. pentosaceus LP28 showed an antiobesity effect and reduced BMI, body fat and waist circumference. P. pentococcus PR-1 is a probiotic strain that was isolated from an adult fecal sample in our laboratory. It was found to be acid and bile resistant, and its metabolites showed antioxidant, and antibacterial properties (37). However, the functional effect of P. pentosaceus PR-1 has not previously been investigated as probiotics in in vivo studies of HFD-induced obesity.
Several studies have found zebrafish serve as a useful animal model of human HFD-induced obesity for research into the alterations of gut microbiota community and inflammation driven by HFD due to its similarity of organs to humans, the availability of a complete genome sequence, and its highly conserved physiological pathways (2, 38–43). Although zebrafish is dominated by Proteobacteria, mice and human are dominated by Firmicutes and Bacteroidetes, the response to microbiota colonization are similar (44).
The current study aimed to investigate the influence of P. pentosaceus PR-1 isolated from human feces on gut microbiota composition, intestinal permeability and inflammation, and lipid metabolism in HFD-fed zebrafish.
2. Materials and methods
2.1. Chemicals
Bacterial media were obtained from Oxoid Ltd. (Basingstoke, UK). Unless stated differently, chemicals were obtained from Sangon Biotech (Shanghai, China). Polymerase Chain Reaction (PCR) primers were synthetized from Sangon Biotech (Shanghai, China).
2.2. Probiotic strain
P. pentosaceus PR-1 (Genebank ID: MW800163) was a potential probiotic isolated from healthy adult feces and stored in our laboratory. It was cultured in de Man Rogosa and Sharpe (MRS) broth/agar at 37°C. The cells were harvested from 1 L of 24 h culture of P. pentosaceus PR-1 by centrifuge at 5,000 × g, 20 min, 4°C. Next, the cells were washed twice with sterile PBS and resuspended in skim milk medium contained 10% skim milk and 5% sucrose (cryoprotectant). The suspension was frozen at −20°C for 4 h and at −80°C overnight, and then transferred into a Modulyo bench top freeze dryer (Edwards, UK; −45°C, 48 h, 10−1 torr) for lyophilization. Upon completion, the lyophilized probiotic strain was retrieved and stored at 4°C for further use.
2.3. Zebrafish care and diets
Three-month-old adult zebrafish (Danio rerio) were kept in a zebrafish breeding recirculating system under a 14-h light−10-h dark cycle at 28°C. The zebrafish were fed twice daily at 09:00 a.m. and 17:00 p.m. with a commercial diet (Tetra Bits Complete) according to standard protocol (45). One week before the experiment, the male zebrafish were selected for the treatments to avoid the sex differences in metabolism. Four experimental groups (30 zebrafish per group) were evaluated: control (C), high fat (H), probiotic (P), and HFD supplemented with probiotic (HP). Zebrafish of each group were kept in a 4 L tank. C group were fed with a commercial diet (Tetra Bits Complete), and the commercial diet was prepared in Milli-Q water (1 g in 100 mL water) and autoclaved. H group were fed with HFD, which consisted of a commercial diet supplemented with 25% (w/w) of lard. The lard was melted and added to the liquid control diet previously to the sterilization. P group were fed with a commercial diet supplemented with probiotic. Lyophilized probiotic P. pentosaceus PR-1 was added to the sterilized liquid control diet at a final concentration of 106 CFU/g. For HP group, P. pentosaceus PR-1 (106 CFU/g) were added to the HFD. During experiment, zebrafish were fed with the diets at a ratio of 4% of their average body weight twice daily at 09:00 a.m. and 17:00 p.m. during the feeding period, and water was changed daily at the end of the day. Five zebrafish were collected randomly form each group and weighted every week. Meanwhile, the body length (mm) was also recorded and the body mass index (BMI, body weight/body length2) was calculated as described (38). After 5 weeks, the zebrafish were anesthetized using an ice bath and sacrificed for specimens. This study was performed in strict accordance with the Laboratory Animal Guideline for Ethical Review of Animal Welfare (GB/T 35892-2018, China) and was approved by the Experimental Animal Welfare and Ethics Committee of the Shanxi University (SXULL2019004).
2.4. Gut microbiota analysis
Gut microbiota were analyzed using 16S rRNA gene sequencing. Intestinal content samples were collected at 4–6 h post the last feeding. The intestinal contents of three zebrafish were pooled as one sample and total intestinal bacterial DNA was extracted by using TIANamp Bacteria DNA Kit (TianGen, Beijing, China) according to the instruction of manufacturer. Universal primers 343F (5′-TACGGRAGGCAGCAG-3′) and 798R (5′-AGGGTATCTAATCCT-3′) were used to amplify the V3–V4 regions of 16S rRNA genes. The high-throughput 16S rRNA gene sequencing of the samples was performed by commercial company Personalbio Co., Ltd. (Shanghai, China). Sequencing was conducted on a MiSeq PE300 platform (Illumina) using the 2 × 250 bp paired-end protocol by Personalbio. A sample barcode was contained in the reverse primer, and both primers were linked with Illumina sequencing adapters. After sequencing the raw data were saved in the FASTQ format. With application of sliding window trimming method and Trimmomatic software (version 0.35), the sequence data were scanned to discard sequences with quality score below 20 and shorter than 50 bp. Afterwards, Flash software (version 1.2.11) and split_libraries software (version 1.8.0) in QIIME was used to assemble the paired-end reads and obtain clean tags. The chimeras in clean tags were removed by UCHIME (version 2.4.2) software and valid tags were obtained. Then Vsearch (version 2.4.2) software was used to form operational taxonomic units (OTUs) with 97% similarity cutoff, and sequence with the highest abundance was selected as the representative of this OTU. RDP classifier Naive Bayesian was used to blast representative reads against Silva database (version 123), and annotation of OTUs was confirmed.
2.5. Bioinformatics analysis
Analysis of sequencing data analyses were mainly performed using QIIME2 2019.4 and R packages (v.3.2.0). The alpha diversity and beta diversity based on Bray–Curtis metrics was analyzed with QIIME2 following the standard protocol (46). Taxa abundances at the at the genus levels were statistically compared among groups by MetagenomeSeq and visualized as Manhattan plots. Linear discriminant analysis (LDA) coupled with effect size measurements (LEfSe) were performed to obtain the important indicator taxa with significant changes in relative abundance among groups. Microbial functions were predicted using PICRUSt2 (47) against KEGG (https://www.kegg.jp/), and Metacyc (https://metacyc.org/) databases using default parameters.
2.6. Quantitative real-time polymerase chain reaction analysis
After 24 h fast, six fish from each tank were randomly sampled for gene expression analysis of intestinal cytokine, intestinal TJ protein, and liver lipid metabolism. Total RNA from intestine and liver were extracted using TRIzol method. One microgram RNA from each sample was transformed into cDNA. SYBR Green SuperReal PreMix Plus (TianGen, Beijing, China) was used for qRT-PCR with cDNA as template. ß-actin was used as the reference gene to normalize the mRNA levels of the target gene, and then the fold changes were calculated by 2−ΔΔCt method. The detailed methods were described as previously (48). The primer sequences were listed in Table 1.
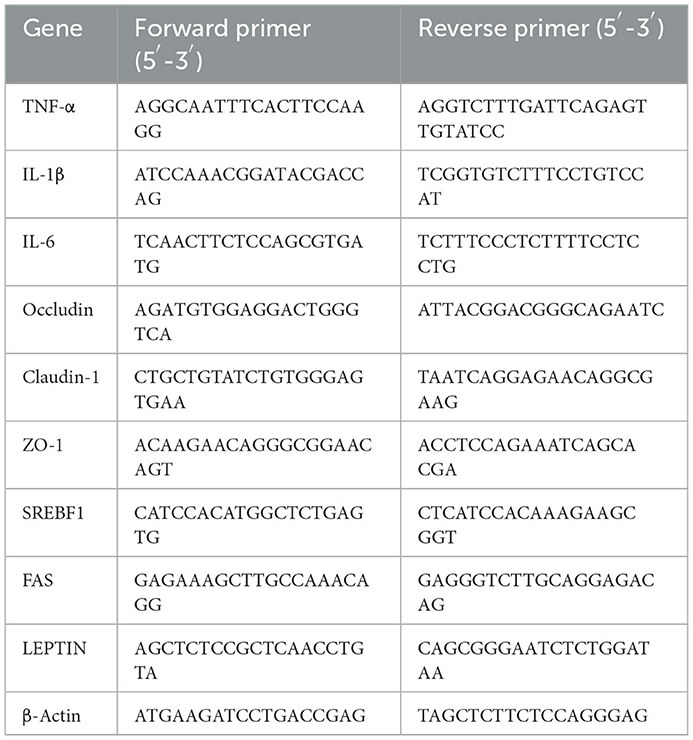
Table 1. The primer sequences for quantitative (qPCR) analysis of intestinal inflammation, tight junction proteins, and lipid metabolism-related genes.
2.7. Biochemical analysis
The liver tissue of three zebrafish were pooled as one sample, homogenized in ice-cold PBS, and centrifuged at 13,000 × g, 10 min, 4°C, giving totally three mixed samples for biochemical analysis. Supernatants were used to determine concentrations of triglyceride, total cholesterol, low density lipoprotein (LDL) using commercial kits (Nanjing Jiancheng Technology, China), and TNF-α, IL-1ß, IL-6 using ELISA kits (Shanghai Qingqi Biotechnology, China), following the manufacturer's instructions. The samples were tested in triplicate, and the average values was used as the result.
2.8. Histological analysis
The liver tissues were collected from three fish per treatment. The samples were rinsed with sterilized PBS and fixed in 4% paraformaldehyde in PBS, then embedded in paraffin, sectioned, and stained with hematoxylin and eosin (H&E). Images were obtained using microscope (Leica DMIL-LED, Germany).
2.9. Statistical analysis
Statistical analysis was carried out by using SPSS 21.0 statistical software. The results are expressed as mean ± standard deviation (SD) and were analyzed by one-way ANOVA. Significant differences were assessed by post-hoc Tukey HSD (Honestly Significant Difference) test. A value of p < 0.05 indicates there was a significant difference.
3. Results
3.1. P. pentosaceus PR-1 suppresses HFD-induced obesity in zebrafish
This study aimed to explore the beneficial effects of P. pentosaceus PR-1 in HFD-fed zebrafish, and alterations in body weight and BMI were measured. As shown in Figure 1, zebrafish in all groups have gained weight significantly compared to their baseline weights after 5 weeks. The H group had a significantly higher body weight gain (22.14%) than the C group (p < 0.05; Figures 1A, B). P. pentosaceus PR-1 could attenuate zebrafish body weight gain; the body weight of HP group was 12.22% higher than that of C group after 5 week feeding (Figures 1A, B). Similarly, at the end of the experiment, H group showed the highest BMI (2.780 mg/mm2) compared to the other three groups, and P. pentosaceus PR-1 could attenuate zebrafish BMI (Figure 1C). The results indicated that the obesity model in zebrafish was successfully established following 5 week feeding of HFD. Moreover, the results indicated that the probiotic strain P. pentosaceus PR-1 has an anti-obesity activity due to their suppressing effects on body weight gain and BMI in HFD-fed zebrafish.
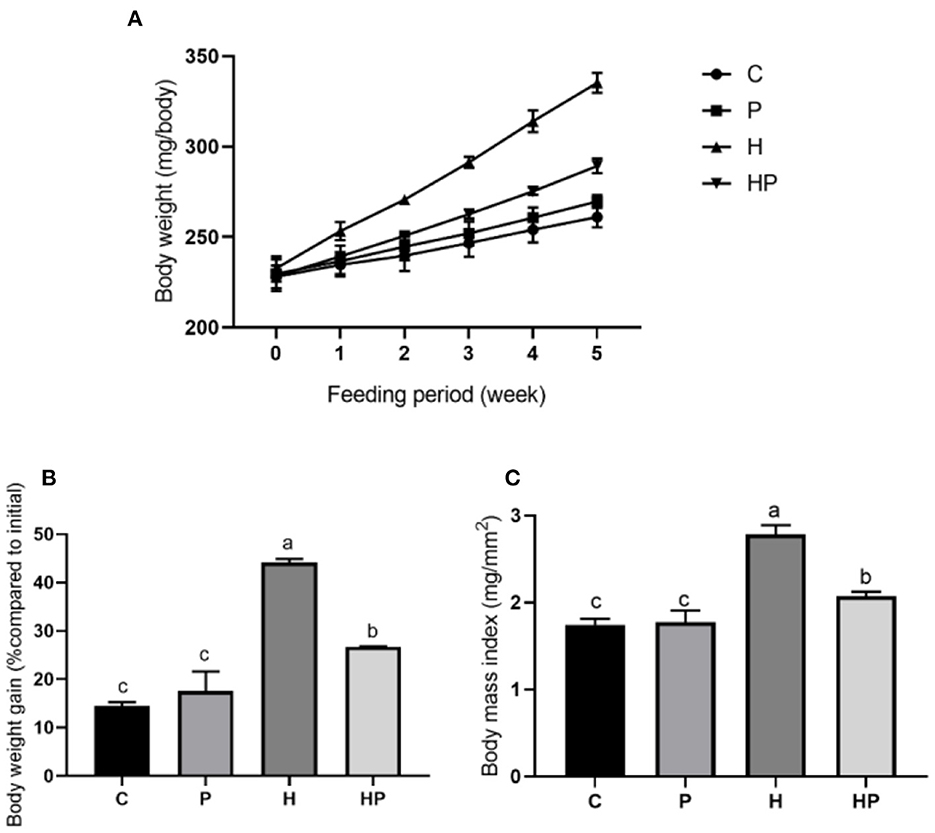
Figure 1. Changes in body weight and BMI in zebrafish on the different diets. (A, B) Changes in body weight during 5 weeks. (C) BMI in adult zebrafish after 5 weeks.
3.2. P. pentosaceus PR-1 modulates the gut microbiota of HFD-fed zebrafish
The alpha diversity of different groups was assessed using Chao 1, Shannon index, Simpson index, and observed richness (Figures 2A–H). Probiotic administration increased alpha diversity with higher levels of four indexes, but not significant compared to C group. In addition, the alpha diversity analysis revealed that the microbiota of H group had significantly higher levels of Shannon and Simpson indexes compared to C group (Figures 2E, F), and P. pentosaceus PR-1 supplementation induced significantly decrease in Shannon and Simpson indexes in zebrafish fed with HFD (Figures 2G, H). We then analyzed beta diversity by Principal coordinates analysis (PCoA) (Bray-Curtis dissimilarity distance) (Figures 3A–C). Samples representing zebrafish fed with a normal diet and HFD showed distinct clustering pattern on the PCoA score plot (Figures 3A, B), suggesting differences in the gut microbiota structure between C and H groups. Anosim analysis showed that there was difference between the C and H groups (Bray-Curtis: P = 0.065; Supplementary Table 1). In addition, probiotic supplementation would restore the microbiota structure in HFD-fed zebrafish to some extent (Figure 3A), suggesting the ability of probiotic to restore the gut microbiota diversity, which was disrupted by HFD. Anosim analysis showed that there was significant difference between the H and HP groups (Bray-Curtis: P = 0.016; Supplementary Table 2).
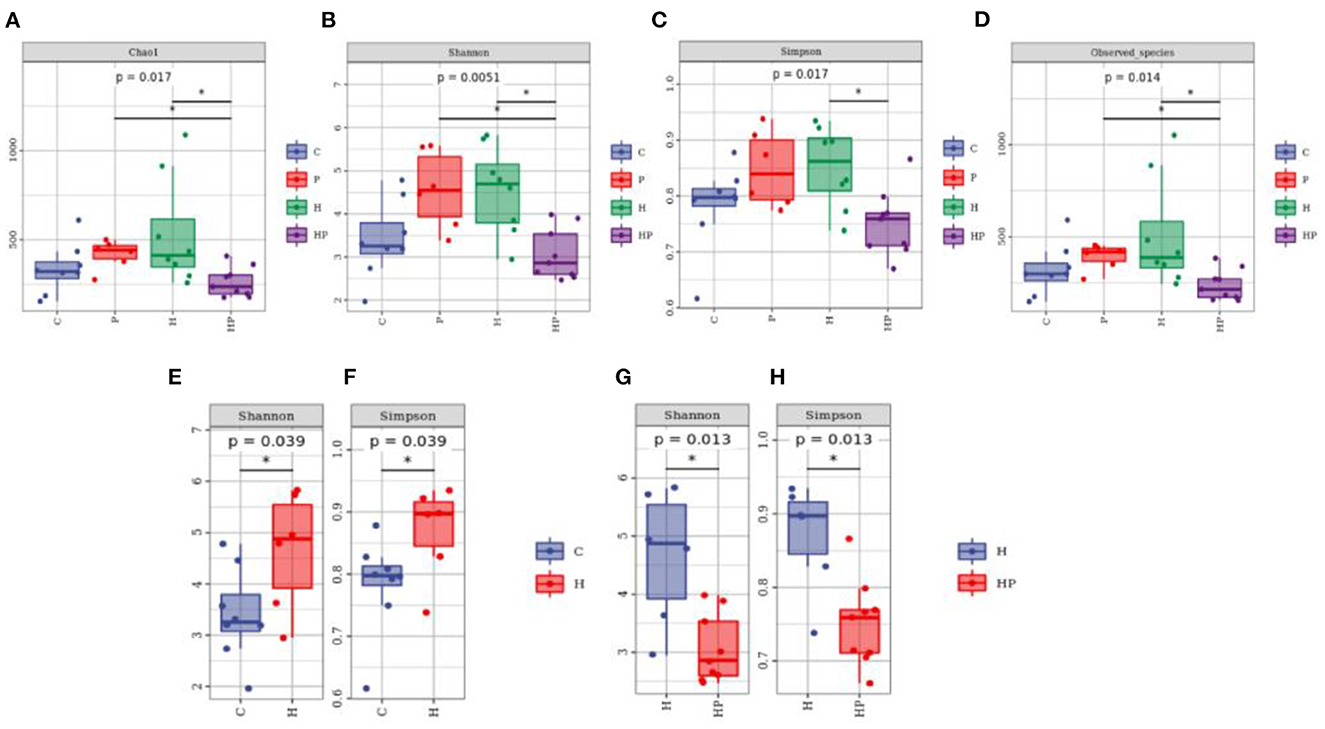
Figure 2. Boxplots of alpha diversity indices of gut microbiota community based on 16S rRNA sequencing. (A–D) Gut microbial alpha diversity of C, P, H and HP groups based on (A) Chao1, (B) Shannon, (C) Simpson, and (D) Observed species. All data are normalized to C group (100%) (*p < 0.05). (E, F) Gut microbial alpha diversity of C and H groups based on (E) Shannon, and (F) Simpson. All data are normalized to C group (100%) (*p < 0.05). (G, H) Gut microbial alpha diversity of H and HP groups based on (G) Shannon and (H) Simpson. All data are normalized to H group (100%) (*p < 0.05).
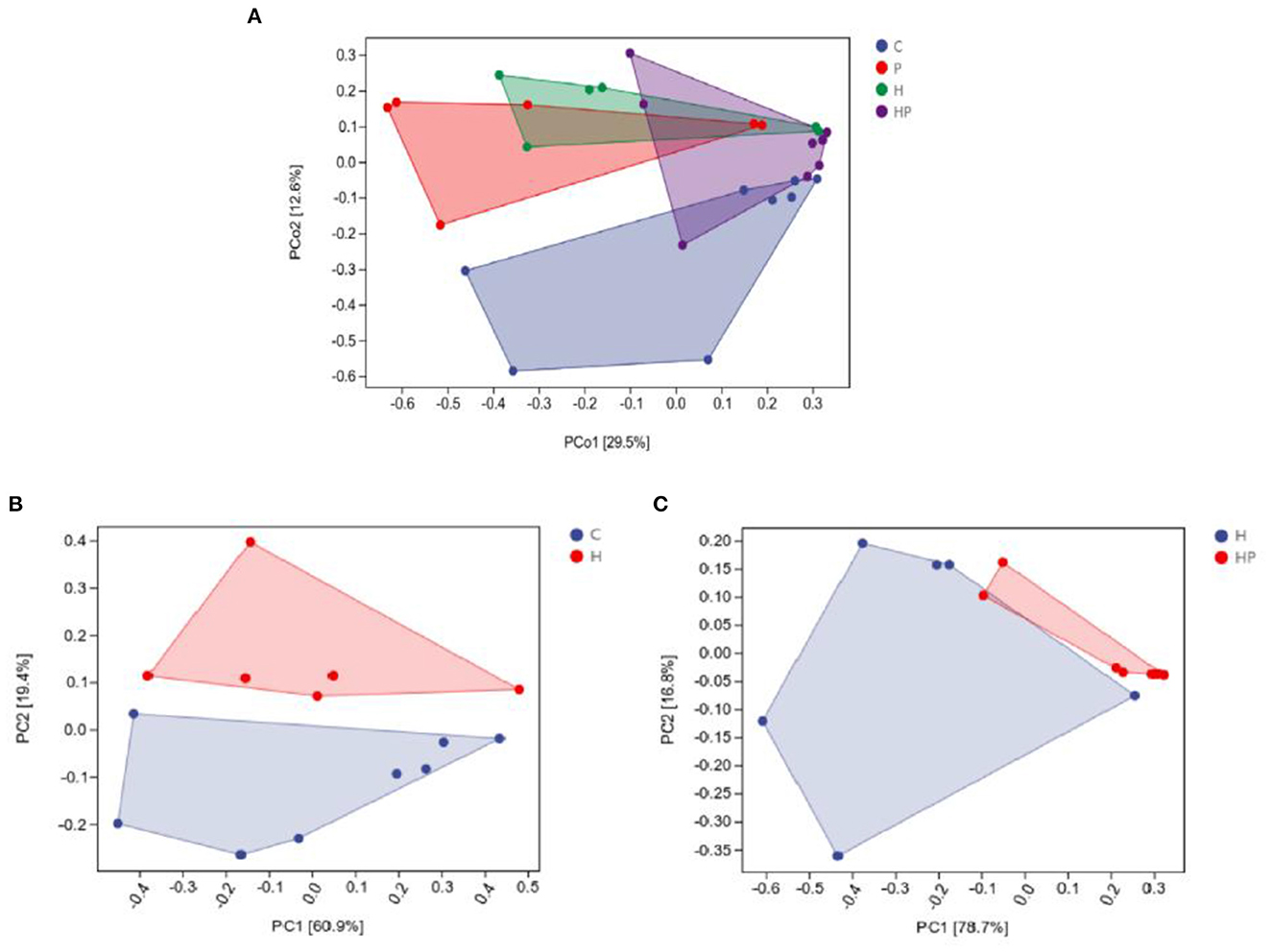
Figure 3. Principal coordinate analysis (Bray-Curtis dissimilarity) score plot of gut microbiota of the zebrafish fed on four different groups (A), on C and H group (B), and on H and HP group (C).
At the phylum level, Proteobacteria and Fusobacteria were dominated in the intestine, with a total proportion of over 80%. P. pentosaceus PR-1 supplementation group showed higher relative abundance of Fusobacteria than that of H group. The relative abundance of Proteobacteria and Tenericutes was decreased in probiotic supplementation group compared to H group (Figure 4A). At genus level, HFD induced an increased in the relative abundance of Ralstonia, Aeromonas, Sphingomonas and a decrease in the relative abundance of Cetobacterium, Plesiomonas. The zebrafish fed with probiotic had the highest relative abundance of Pediococcus. More importantly, the probiotic supplementation in HFD-fed zebrafish increased and restored the relative abundance of Cetobacterium and Plesiomonas. The proportions of Ralstonia, Aeromonas, Sphingomonas were decreased in HP group compared to H group (Figure 4B). MetagenoeSeq analysis was used to identify the phyla and genera that were significantly upregulated between the C group and H group, and between H group and HP group. Compared to C group, HFD induced significant upregulation of Pseudonocardia, Staphylococcus, and Aeromonas in H group (Supplementary Figure 1A). Compared to H group, probiotic supplementation significantly upregulated the abundance of Pediococcus and Cetobacterium (Supplementary Figure 1B).
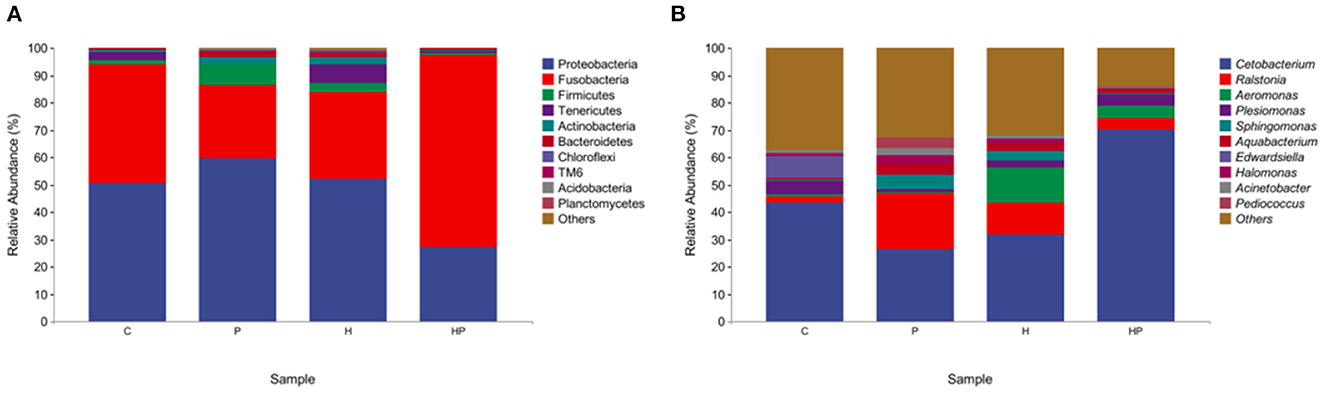
Figure 4. The gut microbiota of zebrafish in C, P, H, and HP groups. Relative abundance at phylum (A) and genus (B) level of the gut microbiota.
LEfSe analysis was used to explore the discriminative biomarker taxa in each group (Figure 5). In LDA score histogram, different stable taxa in different groups are marked with different colors, and the higher the LDA score value, the deeper the impact degree. According to Figure 5A, there were 5, 29, 7, and 26 different bacteria taxa in group C, H, HP and P, respectively. Compared to C group, Pseudonocardia, Staphylococcus, Aeromonas, and Streptococcus were enriched in H group, represented as the biomarker species induced by HFD (Supplementary Figure 2A). Cetobacterium and Pediococcus were enriched in HP and P groups, respectively. Compared to H group, Cetobacterium and Pediococcus were enriched in HP group, represented as the biomarker species induced by probiotic (Supplementary Figure 2B). In cladogram, the points scattering from the inside out represent the level of taxa from kingdom to genus (Figure 5B). The blue, red, green, and purple points represent the biomarker microbiota in group C, P, H, and HP, respectively. The biomarker genus of group HP mainly belonged to Fusobacteria phylum, and those of group H belonged to Gammaproteobacteria and Staphylococcaceae (Figure 5B).
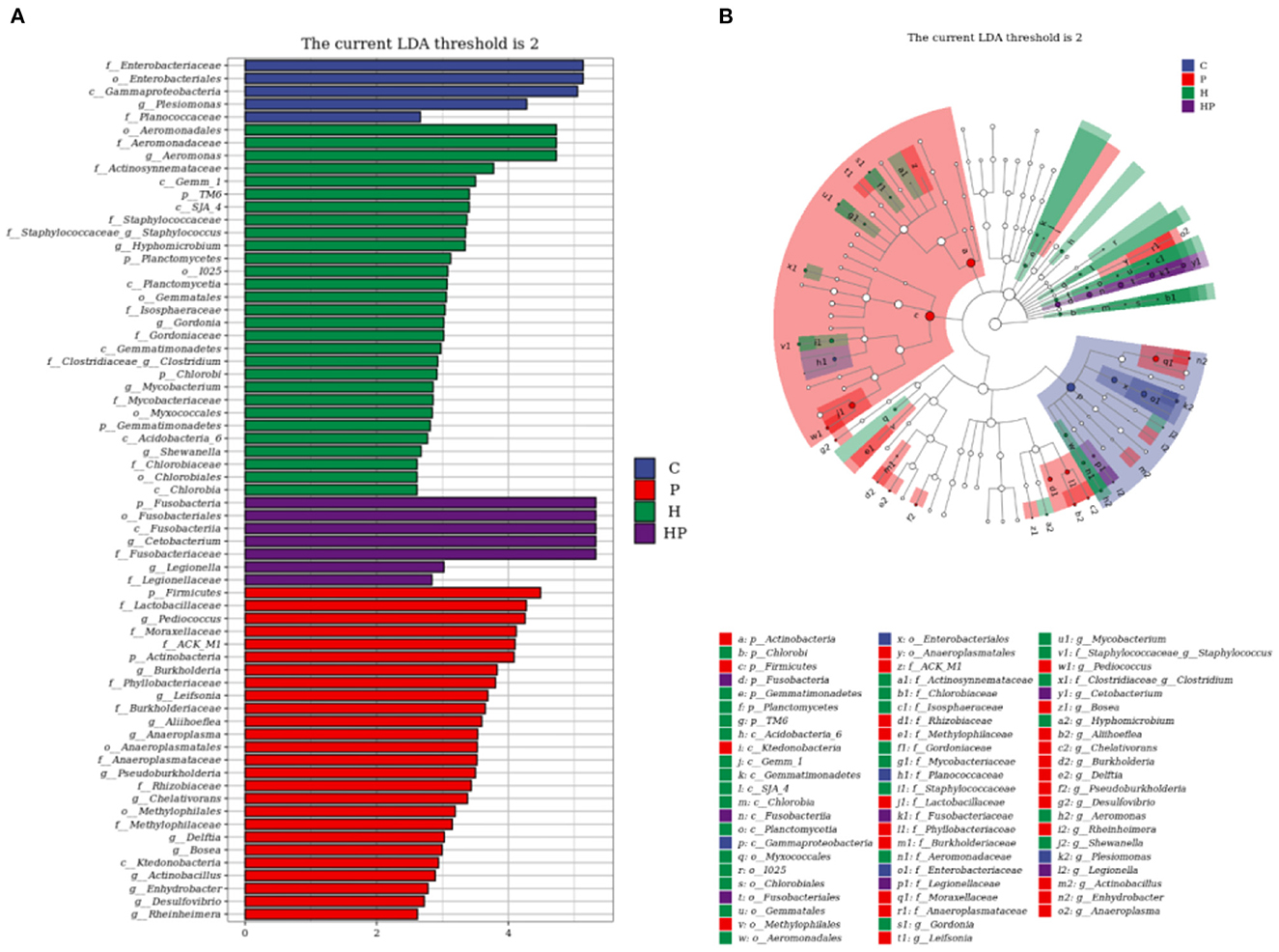
Figure 5. LEfSe analysis explored the discriminative microbiota in group C, P, H, and HP, respectively. (A) The LDA score histogram; (B) the cladogram. p < 0.05 was used as a threshold for LEfSe analysis.
While studying microbial ecology, the functional potential of microbiota was also investigated. PICRUSt2 software predicts sample functional abundances based on the abundance of labeled gene sequences in the samples. All samples were predicted through the KEGG, and Metacyc databases. We observed the most enrichment in the metabolism of carbohydrates, amino acids, cofactors, and vitamins (Figure 6A). In addition, we found that cofactors, the prosthetic group, electron carriers, vitamin biosynthesis, nucleoside and nucleotide biosynthesis, and amino acid biosynthesis were abundant (Figure 6B). Besides, there were significant differences in pathways were detected between groups. According to results of predicted from KEGG databases, compared to H group, HP significantly downregulated photosynthesis—antenna proteins (ko00196, P = 0.000472), sesquiterpenoid biosynthesis (ko00909, P = 3.23 × 10−8), and mRNA surveillance pathway (ko03015, P = 6.67 × 10−6; Figure 6C). According to results of predicted from Metacyc databases, compared to C group, H significantly upregulated glycolysis V (Pyrococcus) (P341-PWY, P = 9.25 × 10−5), and superpathway of mycolyl-arabinogalactan-peptidoglycan complex biosynthesis (PWY-6404, P = 4.45 × 10−9; Figure 6D). Compared to H group, HP significantly downregulated sitosterol degradation to androstenedione (PWY-6948, P = 1.85 × 10−8), and superpathway of mycolyl-arabinogalactan-peptidoglycan complex biosynthesis (PWY-6404, P = 1.62 × 10−14; Figure 6E).
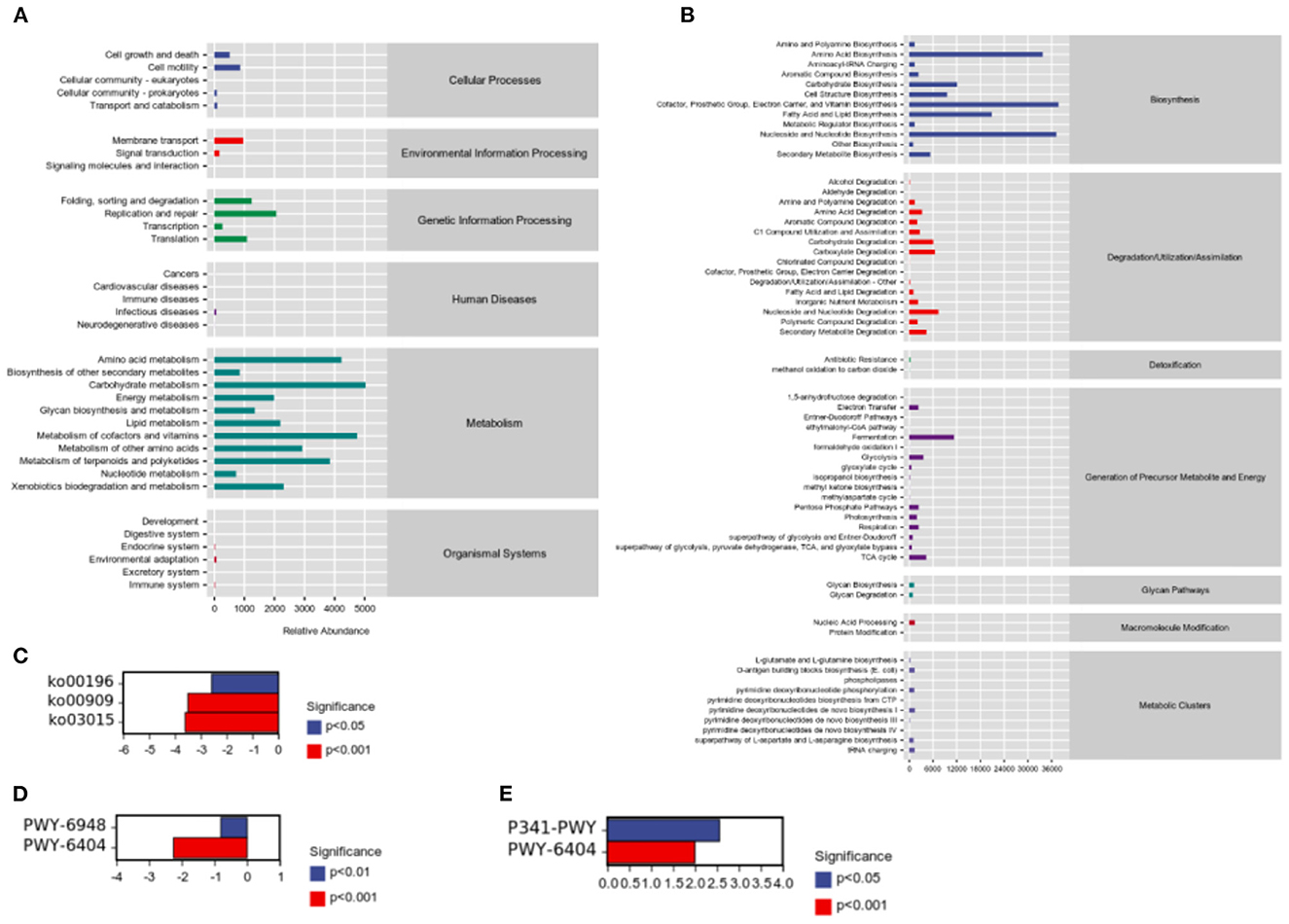
Figure 6. Sample functional abundances predicted by PICRUSt2 based on the abundance of labeled gene sequences in the samples. (A) KEGG databases. (B) Metacyc databases. (C) Significant pathway analysis between H and HP groups based on KEGG databases. (D) Significant pathway analysis between C and H groups based on Metacyc databases. (E) Significant pathway analysis between H and HP groups based on Metacyc databases.
3.3. P. pentosaceus PR-1 regulated intestinal inflammation, gut permeability, and lipid metabolism in HFD-fed zebrafish
Considering the influence of inflammation on the level of adipokine, the relative expressions of inflammatory genes encoding TNF-α and interleukins (IL-1β, IL-6) in all treatment groups were evaluated. As shown in Figures 7A–C, HFD increased all tested genes encoding pro-inflammatory cytokines compared to the C group. Probiotic supplementation could significantly down-regulate the expression of IL-1β and IL-6 (Figures 7B, C). In addition, the hepatic levels of pro-inflammatory cytokines were shown in Figures 8A–C. Compared to the C group, the concentrations of TNF-α, IL-1ß, and IL-6 were significantly elevated in zebrafish fed with HFD. The probiotic supplementation decreased the hepatic concentrations of TNF-α, IL-1ß, and IL-6 in HF group. The results indicate that P. pentosaceus PR-1 attenuated intestinal inflammation induced by HFD.
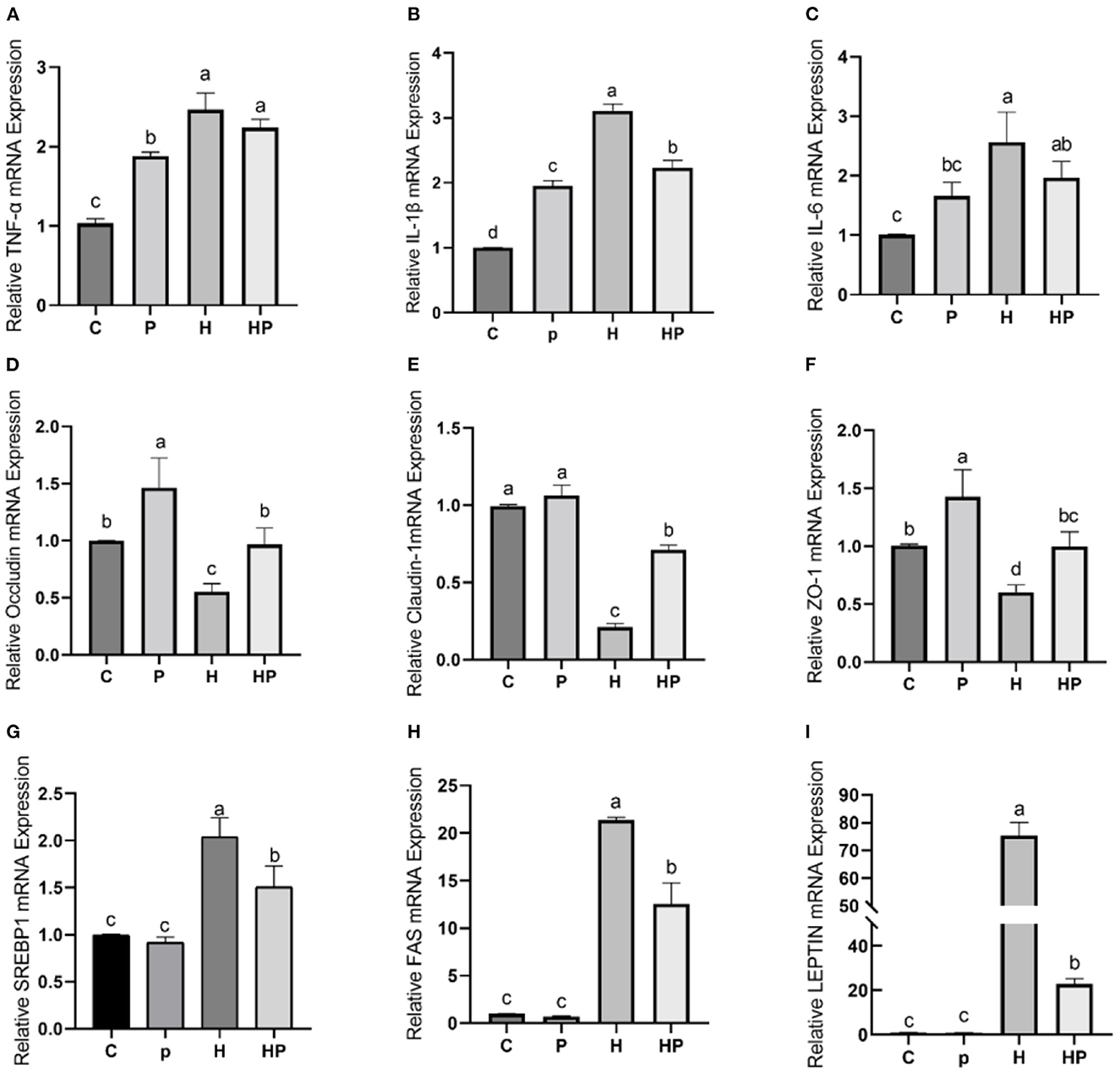
Figure 7. Effect of P. pentosaceus PR-1 on intestinal inflammation, gut permeability, and lipid metabolism in HFD-fed zebrafish. (A–C) The total RNA was extracted from the intestine, and the relative mRNA expression levels of TNF-α (A), IL-1ß (B), and IL-6 (C) were determined by qRT-PCR. (D–F) The total RNA was extracted from the intestine, and the relative mRNA expression levels of Occludin (D), Claudin-1 (E), and ZO-1 (F) were determined by qRT-PCR. (G, H) The total RNA was extracted from the liver, and the relative mRNA expression levels of SREBP1 (G), FAS (H), and LEPTIN (I) were determined by qRT-PCR. Values were represented as the mean ± S.D. (n = 6). Values with different superscript letters are significantly different (p < 0.05).
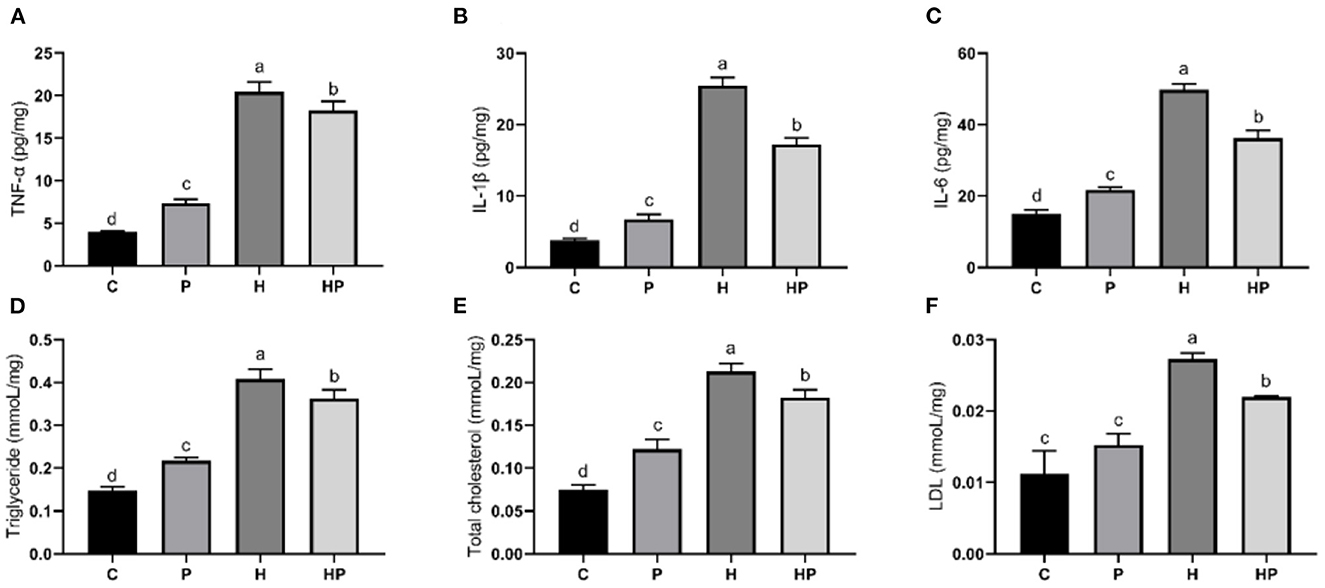
Figure 8. Effect of P. pentosaceus PR-1 on liver pro-inflammatory cytokines (A–C) and biochemical parameters (D–F) in HFD-fed zebrafish. (A) Hepatic concentration of tumor necrosis factor α (TNF-α). (B) Hepatic concentration of interleukin-1ß (IL-1ß). (C) Hepatic concentration of interleukin-6 (IL-6). (D) Hepatic level of triglyceride. (E) Hepatic level of total cholesterol. (F) Hepatic level of low-density lipoprotein (LDL). Values were represented as the mean ± S.D. (n = 3). Values with different superscript letters are significantly different (p < 0.05).
The results of qRT-PCR also showed that HFD dramatically decreased relative expression of all tested genes encoding TJ proteins, including Occludin, Claudin-1, and Zonula occludens-1 (ZO-1) compared to the C group (Figures 7D–F). Notably, P. pentosaceus PR-1 supplementation could restore their expressions in HFD-fed zebrafish (Figures 7D–F).
Furthermore, the regulatory effects of P. pentosaceus PR-1 on lipid metabolism were also investigated. Elevated levels of triglyceride, total cholesterol and LDL were observed in zebrafish fed with HFD (Figures 8D–F). Probiotic supplementation significantly reduced the hepatic triglyceride, total cholesterol, and LDL compared with those in the HF group, whereas did not return their levels to normal compared to C group (Figures 8D–F). In addition, the relative expressions of genes encoding lipid metabolism markers, such as sterol regulatory element binding proteins (SREBP1), fatty acid synthase (FAS) and leptin (LEPTIN) in all treatment groups were evaluated by qRT-PCR. As shown in Figures 7G–I, the relative expressions of SREBP1, FAS, and LEPTIN were significantly higher in HFD-fed zebrafish compared to zebrafish in group C, P, and HP. Moreover, HFD mostly strongly stimulated the expressions of LEPTIN. Probiotic supplementation significantly inhibited the expressions of SREBP1, FAS, and LEPTIN which were induced by HFD, suggesting the anti-adipogenic activity of P. pentosaceus PR-1.
3.4. Effect of P. pentosaceus PR-1 on liver injury in HFD-fed zebrafish
It could be observed from the H&E staining of the liver sections (Figure 9) that the boundary was blurred and the integrity of the hepatic cell was damaged in H group, indicating the liver injury in HFD-fed zebrafish. More importantly, HFD detrimentally contributed to substantial accumulation of fat in the liver in H group, whereas probiotic could effectively attenuate the fat accumulation caused by HFD (Figure 9), indicating that P. pentosaceus PR-1 supplementation ameliorated liver injury caused by HFD.
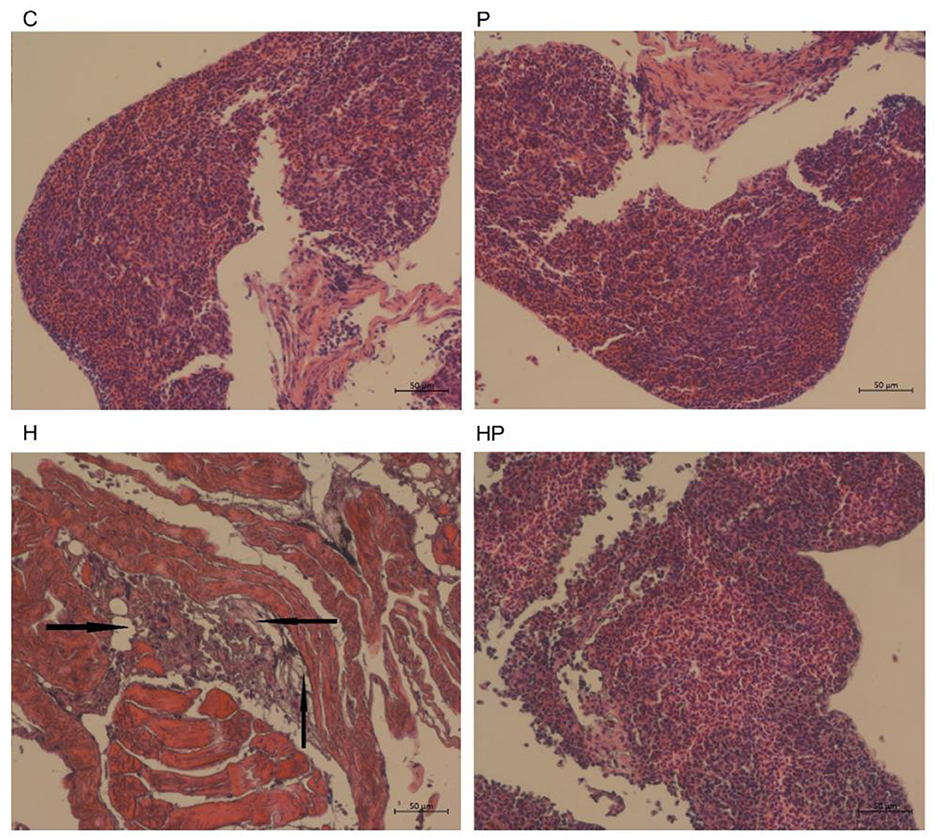
Figure 9. Histological changes of liver sections measured by H&E staining (scale bar is 50 μm). The black arrows indicate extensive hydropic degeneration of hepatocytes characterized by swollen, pale, vacuolated cytoplasm.
4. Discussion
Previous studies showed that probiotics could prevent and ameliorate obesity through regulating microbiota composition, suppressing metabolic inflammation and improving lipid metabolism (22–26). Recently, P.pentosaceus, one type of LAB, has drawn much attention due to its antimicrobial and antioxidant activities, immunoregulatory activity and therapeutic effects on colitis, constipation, liver injury, and hyperlipidaemia (49–52). However, only a few works have investigated its anti-obesity effect. Zebrafish have similar morphology, physiology, and functions with mammals and have been considered as a useful in vivo model for HFD-induced obesity research (2, 38–43). Although the temperature of zebrafish intestine is not optimum for the growth of probiotics such as Lactobacillus sp., Bacillus sp., Bifidobacterium sp., and Pediococcus, a rising number of studies have applied zebrafish as model to investigate their potentials. Bacillus coagulans 09·712 and Lactobacillus plantarum 08·923 showed robust zebrafish gut adhesion capability, and played immunoregulatory and immunoprotective roles in effective stimulation of anti-inflammatory response and barrier regeneration within the mucosa to protect zebrafish against infection (53). Bifidobacterium lactis BL-99 also could promote the intestinal integrity, improved the histopathology of the adult zebrafish intestinal inflammation, increased the goblet cell numbers, and recover microbiota metabolism to maintain intestinal health (54). Besides, several studies have found Pediococcus could exert beneficial effects in zebrafish (55–58). A previous study found P. pentosaceus YC enhanced host resistance against Aeromonas hydrophila infection through increasing intestinal butyrate, IL-1β production, and raising intestinal neutrophil level (50). Therefore, P. pentosaceus could exert beneficial effect in zebrafish, subsequently the current study investigated the beneficial effects of P.pentosaceus PR-1 in a HFD-induced obesity zebrafish model.
Here, we found that probiotic supplementation could ameliorate HFD-induced obesity, reflected by the decreased body weight gain and BMI in HP group compared with H group. This was in agreement with a clinical trial, that P. pentosaceus LP28 displayed an anti-obesity effect through decreasing BMI, body fat and waist circumference of subjects (59).
Previous studies showed that HFD could detrimentally influence gut health by disrupting gut microbiota homeostasis (2, 41). In the present study, HFD induced a distinct gut microbiota structure from C group, which was restored by P. pentosaceus PR-1 supplementation. Proteobacteria and Fusobacteria have been reported to be the predominant phyla in zebrafish intestine (48). Compared with the H group, there was an increased abundance of Fusobacteria, and decreased abundance of Proteobacteria and Tenericutes in P. pentosaceus PR-1 supplementation groups. Besides, HFD detrimentally affected intestinal health, through inducing an increased in the relative abundance of Ralstonia and Aeromonas in the current study. Ralstonia have been shown to cause infections, even more serious, such as osteomyelitis and meningitis (60). Aeromonas are widely distributed in aquatic environment and becoming important pathogens which is associated with enteric and extraintestinal infections, such as skin and soft-tissue infections, and lower respiratory tract/urinary tract infections (50). P. pentosaceus PR-1 could improve gut health by decreasing the relative abundance of Ralstonia and Aeromonas. Moreover, P. pentosaceus PR-1 supplementation restored abundance of Cetobacterium and Plesiomonas, which were decreased in HFD-fed zebrafish. Increased proportions of Fusobacteria/Cetobacterium were proved to maintain intestinal health of zebrafish through elevating the efficiency of dietary carbohydrate utilization and regulating of glucose metabolism (61, 62). Cetobacterium is dominant in the intestines of freshwater and marine fish, and could be applied as potential probiotics in aquaculture (63), due to its ability to improve gut and liver health through decreasing gut inflammation, lipid deposition in liver and serum levels of aspartate aminotransferase and alanine aminotransferase (63), to improve glucose homeostasis through increasing insulin expression (61). Metabolites of Cetobacterium such as short-chain fatty acids including acetate, propionate and butyrate and vitamin B12 are dominant in fish intestines and beneficial to fish health (64). The results suggested that P. pentosaceus PR-1 improved intestinal health of HFD-fed zebrafish through modulating gut microbiota. In addition, the functional potential of microbiota was also investigated by PICRUSt2 software. According to the results, probiotic administration would inhibit sitosterol degradation. A few studies have found the anti-obesity effect of sitosterol. Sitosterol could lower cholesterol levels and alleviate HFD-induced non-alcoholic fatty liver disease through ameliorating levels of hepatic total lipids, triacylglycerols, cholesterol and liver histopathology, decreasing levels of intestinal bile acids, and increasing the expression of genes involved in lipid metabolism, including HMGCoAR, ABCG5, peroxisome proliferator-activated receptor-α (PPAR-α), and decreasing the expression of CD36 (65). In addition, sitosterol could also reduce inflammatory stress leading to decreased levels of TNF-α, IL-1β, IL-6, and modulate microbiota structure in sheep (66). Therefore, the beneficial effects of P. pentosaceus PR-1 in zebrafish may stem from the microbiota metabolites, such as sitosterol. Metabonomics profile would be determined in future to investigate the effects of microbiota metabolites induced by P. pentosaceus PR-1 administration, and to further clarify the mechanisms of action of P. pentosaceus PR-1 through combination analysis of microbial-omics and metabonomics.
In vivo studies reported that HFD would lead to inflammation mediated by IL-1β activation via Toll-like receptor 4/NF-κB pathway, and increase expressions of MCP-1, TNF-α, IL-1β, IL-6, MyD88, and NF-κB (2, 36, 48, 67). Consistently, in this study, HFD significantly upregulated hepatic levels and gene expressions of pro-inflammatory cytokines TNF-α, IL-1β, and IL-6, suggesting that HFD induced intestinal inflammation. The hepatic levels and expressions of IL-1β and IL-6 were significantly reduced with a probiotic supplementation, indicating the suppressing inflammation role of P. pentosaceus PR-1. The immunoregulatory effect of P. pentosaceus was also proved in a murine study that P. pentosaceus PP04 relieved the HFD-caused gut inflammation by inhibiting NF-κB/Nrf2 pathway and expressions of TNF-α, IL-1β, IL-6 (36). In the current study, P. pentosaceus PR-1 may exert anti-inflammatory effects through restoring the abundance of Cetobacterium. Previous studies have found Cetobacterium could decrease gut and liver inflammation though stimulating production of butyrate which could inhibit NF-κB, and subsequently lead to decreased expression of pro-inflammatory cytokine genes, including TNFα, IL-1β (63, 68). In addition, P. pentosaceus PR-1 may reduce intestinal inflammation through inhibiting the degradation of sitosterol (66). Subsequently, western blot would be performed to determine the alterations in inflammation pathways, including Toll-like receptor 4/NF-κB and NF-κB/Nrf2, induced by P. pentosaceus PR-1.
Moreover, previous studies showed HFD could detrimentally affect gut health by inducing intestinal injury (48, 56). Intestinal TJ proteins, including occludin, claudin, and ZO-1 play an important role in maintaining gut permeability and barrier function (48). Previous studies reported that as the TJ expression levels decreased induced by HFD, the gut permeability increased, which lead to increased level of serum endotoxemia and impaired immune response (42, 48). We investigated whether probiotic P. pentosaceus PR-1 could improve gut barrier function in HFD-fed zebrafish. The results suggested that P. pentosaceus PR-1 supplementation restored the expressions of occludin, claudin, and ZO-1, indicating that P. pentosaceus PR-1 exerted benefits to gut integrity and gut health which were impaired by HFD. This was similar to an in vivo study, which reported that the expressions of TJ were significantly up-regulated in obesity C57BL/6N mice model induced by HFD (36). The protective effects of P. pentosaceus PR-1 on gut barrier function may be associated with its stimulation effect on activity of Cetobacterium, which have been found to up-regulate expression of claudin occluding and tight junction protein 2a, playing important role in tight junction assembling and integrity maintaining (68). In addition, Cetobacterium could up-regulate the expression of hypoxia-inducible factor-1α, which protects gut barrier function through inhibiting oxidative stress, down-regulating intestinal inflammation, and maintaining intestinal microbiota homeostasis (69, 70). To date, several signaling pathways have been found to be involved in regulation of intestinal barrier function, including the protein kinase C (PKC), PKA, PKG, protein phosphatases PP1, PP2A, PP2B, Rho, myosin light chain kinase, mitogen-activated protein kinase, phosphatidylinositol 3-kinase/Akt, and Wnt/β-catenin pathways (71, 72). Subsequently, western blot and immunofluorescence analysis would be performed to investigate the effects and mechanisms of P. pentosaceus PR-1 on levels of intestinal TJ proteins.
SREBP1 is a transcription factor that stimulates hepatic fatty acid biosynthesis via upregulation of lipogenesisrelated genes, such as FAS (35). The uncontrolled activation of SREBP-1c is associated with hepatosteatosis, insulin resistance and pro-inflammatory signaling cascade (73). Circulating leptin levels are related to body fat mass. The binding of leptin to its hypothalamic receptor regulates food intake and caused the suppression of fat accumulation and reduction in body weight (57). However, HFD-induce obesity is associated with leptin resistance, which is characterized by high circulating leptin levels (57). Our study found HFD-fed zebrafish significantly up-regulated expression of SREBP1, FAS, and LEPTIN, with the strongest up-regulatory effect on Leptin. P. pentosaceus PR-1 supplementation exerted anti-adipogenic activity in HFD-fed zebrafish through regulating adipogenesis-related genes. A murine study also reported a similar finding with P. pentosaceus PP04 supplementation to an HFD-induced hyperlipidemia mice model (36). Moreover, we observed that HFD induced liver steatosis and injury in zebrafish, which was obviously reversed by P. pentosaceus PR-1 supplementation. The liver plays critical role in nutrients metabolism including fat, and fatty acids which would be stored at triglyceride when exceed the removal capacity of liver (74). Therefore, HFD would lead to cholesterol accumulation in liver, and levels of hepatic cholesterol could be used to indicate the cholesterol absorption. The current study observed the hepatic levels of triglyceride and cholesterol was elevated by HFD and down-regulated by probiotic supplementation, indicating that probiotic could attenuate lipid accumulation and abnormalities in liver. A higher level of LDL is associated with the incidence and severity of diabetes, non-alcoholic fatty liver disease, cardiovascular disease (75). Down-regulatory effect of probiotic administration on LDL was observed in this study, suggesting the beneficial effect of probiotic on lipid homeostasis of liver. The liver protective and anti-obesity effect of P. pentosaceus PR-1 may result from the increased abundance of Cetobacterium and its metabolites such as short chain fatty acids, which could inhibit the formation of lipid droplets and the expression of lipogenesis genes, and stimulate the expression of lipolysis genes (63, 76). Besides, several studies have explored that probiotics could regulate lipid metabolism through several signaling pathways including PPAR-α, PPAR-γ, carnitine palmitoyltransferase 1, lipoprotein lipase, and CCAAT enhancer-binding protein-α, which are involved in regulation of oxidative respiration, fatty acid β-oxidation, adipocyte proliferation, and differentiation (77, 78). Subsequently, to further clarify the liver protective and anti-obesity effect of P. pentosaceus PR-1, signaling pathway expression levels involved in lipid metabolism would be investigated in the future.
5. Conclusion
This study is the first to report that P. pentosaceus PR-1 improves intestinal health in zebrafish. P. pentosaceus PR-1 regulated the gut microbiota, suppressed intestinal inflammation, improved gut barrier function, inhibited adipogenesis. Since zebrafish has become a well-established vertebrate model, our findings suggest the application of P. pentosaceus PR-1 for improving lipid metabolic disorder. Although the beneficial effects of P. pentosaceus PR-1 on intestinal health of zebrafish were observed, the current study did not proof the underlying mechanisms. Therefore, it would be of significant importance to further explore the mechanisms of action of P. pentosaceus PR-1 in depth.
Data availability statement
The original contributions presented in the study are publicly available. This data can be found at: https://www.ncbi.nlm.nih.gov/bioproject/PRJNA899980.
Ethics statement
The animal study was reviewed and approved by Experimental Animal Welfare and Ethics Committee of the Shanxi University.
Author contributions
YL designed the study. DZ and JL performed most of the experiments. XS and FG performed statistical analysis. YL wrote the manuscript. HD and LD conducted microbiota analysis. YL and XW contributed to data organization or analysis. YL and CW supervised the study. All authors contributed to comments and revision on the manuscript and approved the final manuscript.
Funding
This research was funded by the Fundamental Research Program of Shanxi Province (20210302124187) and the Program of Introducing Talents of Discipline to Universities (D21004).
Conflict of interest
The authors declare that the research was conducted in the absence of any commercial or financial relationships that could be construed as a potential conflict of interest.
Publisher's note
All claims expressed in this article are solely those of the authors and do not necessarily represent those of their affiliated organizations, or those of the publisher, the editors and the reviewers. Any product that may be evaluated in this article, or claim that may be made by its manufacturer, is not guaranteed or endorsed by the publisher.
Supplementary material
The Supplementary Material for this article can be found online at: https://www.frontiersin.org/articles/10.3389/fnut.2023.1087703/full#supplementary-material
Supplementary Figure 1. Manhattan map of differentially abundant amplicon sequence variants (ASVs) based on metagenomeSeq analysis. The plot is ordered by taxonomy line and the colors correspond to different phyla. The y-axis displays the negative log of the p-value, and higher values indicate increased statistical significance. Each dot or circle in the coordinate system represents one ASVs, and the size indicates its relative abundance. The dotted line separates the significant difference (above) from the insignificant ASVs. Significantly different points are marked with colors, and insignificant ones are gray circles. Significantly upward alterations are displayed with colored solid dots; the color of the dots suggests phylum name of x-axis. (A) H group compared to C group. (B) HP group compared to H group.
Supplementary Figure 2. LEfSe analysis explored the discriminative microbiota between C and H groups (A), and discriminative microbiota between H and HP groups (B), p < 0.05 was used as a threshold for LEfSe analysis.
Supplementary Table 1. Anosim (comparing C and H group).
Supplementary Table 2. Anosim (comparing H and HP group).
References
1. Lin X, Li H. Obesity: epidemiology, pathophysiology, and therapeutics. Front Endocrinol. (2021) 12:706978. doi: 10.3389/fendo.2021.706978
2. Arias-Jayo N, Abecia L, Alonso-Sáez L, Ramirez-Garcia A, Rodriguez A, Pardo MA. High-fat diet consumption induces microbiota dysbiosis and intestinal inflammation in zebrafish. Microb Ecol. (2018) 76:1089–101. doi: 10.1007/s00248-018-1198-9
3. Golay A, Bobbioni E. The role of dietary fat in obesity. Int J Obes Relat Metab Disord. (1997) 21 (Suppl. 3):S2–11.
4. de Moura EDM, Dos Reis SA, da Conceição LL, Sediyama C, Pereira SS, de Oliveira LL, et al. Diet-induced obesity in animal models: points to consider and influence on metabolic markers. Diabetol Metab Syndr. (2021) 13:32. doi: 10.1186/s13098-021-00647-2
5. Guo J, Han X, Tan H, Huang W, You Y, Zhan J. Blueberry extract improves obesity through regulation of the gut microbiota and bile acids via pathways involving FXR and TGR5. iScience. (2019) 19:676–90. doi: 10.1016/j.isci.2019.08.020
6. Du H, Zhao A, Wang Q, Yang X. Supplementation of inulin with various degree of polymerization ameliorates liver injury and gut microbiota dysbiosis in high fat-fed obese mice. J Agric Food Chem. (2020) 68:779–87. doi: 10.1021/acs.jafc.9b06571
7. Malesza IJ, Malesza M, Walkowiak J, Mussin N, Walkowiak D, Aringazina R, et al. High-fat, western-style diet, systemic inflammation, and gut microbiota: a narrative review. Cells. (2021) 10:3164. doi: 10.3390/cells10113164
8. Velázquez KT, Enos RT, Bader JE, Sougiannis AT, Carson MS, Chatzistamou I, et al. Prolonged high-fat-diet feeding promotes non-alcoholic fatty liver disease and alters gut microbiota in mice. World J Hepatol. (2019) 11:619–37. doi: 10.4254/wjh.v11.i8.619
9. Velasquez MT. Altered gut microbiota: a link between diet and the metabolic syndrome. Metab Syndr Relat Disord. (2018) 16:321–8. doi: 10.1089/met.2017.0163
10. Jiao N, Baker SS, Nugent CA, Tsompana M, Cai L, Wang Y, et al. Gut microbiome may contribute to insulin resistance and systemic inflammation in obese rodents: a meta-analysis. Physiol Genomics. (2018) 50:244–54. doi: 10.1152/physiolgenomics.00114.2017
11. Shin NR, Whon TW, Bae JW. Proteobacteria: microbial signature of dysbiosis in gut microbiota. Trends Biotechnol. (2015) 33:496–503. doi: 10.1016/j.tibtech.2015.06.011
12. Sen T, Cawthon CR, Ihde BT, Hajnal A, DiLorenzo PM, de La Serre CB, et al. Diet-driven microbiota dysbiosis is associated with vagal remodeling and obesity. Physiol Behav. (2017) 173:305–17. doi: 10.1016/j.physbeh.2017.02.027
13. Vaughn AC, Cooper EM, DiLorenzo PM, O'Loughlin LJ, Konkel ME, Peters JH, et al. Energy-dense diet triggers changes in gut microbiota, reorganization of gut-brain vagal communication and increases body fat accumulation. Acta Neurobiol Exp. (2017) 77:18–30. doi: 10.21307/ane-2017-033
14. Heinritz SN, Weiss E, Eklund M, Aumiller T, Heyer CM, Messner S, et al. Impact of a high-fat or high-fiber diet on intestinal microbiota and metabolic markers in a pig model. Nutrients. (2016) 8:317. doi: 10.3390/nu8050317
15. Rohr MW, Narasimhulu CA, Rudeski-Rohr TA, Parthasarathy S. Negative effects of a high-fat diet on intestinal permeability: a review. Adv Nutr. (2020) 11:77–91. doi: 10.1093/advances/nmz061
16. Duan R, Huang K, Guan X, Li S, Xia J, Shen M, et al. Tectorigenin ameliorated high-fat diet-induced nonalcoholic fatty liver disease through anti-inflammation and modulating gut microbiota in mice. Food Chem Toxicol. (2022) 164:112948. doi: 10.1016/j.fct.2022.112948
17. Lu JF, Zhu MQ, Zhang H, Liu H, Xia B, Wang YL, et al. Neohesperidin attenuates obesity by altering the composition of the gut microbiota in high-fat diet-fed mice. FASEB J. (2020) 34:12053–71. doi: 10.1096/fj.201903102RR
18. Lee S, Keirsey KI, Kirkland R, Grunewald ZI, Fischer JG, de La Serre CB. Blueberry supplementation influences the gut microbiota, inflammation, and insulin resistance in high-fat-diet-fed rats. J Nutr. (2018) 148:209–19. doi: 10.1093/jn/nxx027
19. Fang W, Xue H, Chen X, Chen K, Ling W. Supplementation with sodium butyrate modulates the composition of the gut microbiota and ameliorates high-fat diet-induced obesity in mice. J Nutr. (2019) 149:747–54. doi: 10.1093/jn/nxy324
20. Hill C, Guarner F, Reid G, Gibson GR, Merenstein DJ, Pot B, et al. Expert consensus document. The international scientific association for probiotics and prebiotics consensus statement on the scope and appropriate use of the term probiotic. Nat Rev Gastroenterol Hepatol. (2014) 11:506–14. doi: 10.1038/nrgastro.2014.66
21. Araya M, Morelli L, Reid G, Sanders M, Stanton C, Pineiro M, et al. Guidelines for the Evaluation of Probiotics in Food. Joint FAO/WHO Working Group Report on Drafting Guidelines for the Evaluation of Probiotics in Food. London (2002).
22. Núñez IN, Galdeano CM, de LeBlanc Ade M, Perdigón G. Evaluation of immune response, microbiota, and blood markers after probiotic bacteria administration in obese mice induced by a high-fat diet. Nutrition. (2014) 30:1423–32. doi: 10.1016/j.nut.2014.03.025
23. Fabersani E, Marquez A, Russo M, Ross R, Torres S, Fontana C, et al. Lactic acid bacteria strains differently modulate gut microbiota and metabolic and immunological parameters in high-fat diet-fed mice. Front Nutr. (2021) 8:718564. doi: 10.3389/fnut.2021.718564
24. Ahmadi S, Wang S, Nagpal R, Wang B, Jain S, Razazan A, et al. A human-origin probiotic cocktail ameliorates aging-related leaky gut and inflammation via modulating the microbiota/taurine/tight junction axis. JCI Insight. (2020) 5:e132055. doi: 10.1172/jci.insight.132055
25. Tarrah A, Dos Santos Cruz BC, Sousa Dias R, da Silva Duarte V, Pakroo S, Licursi de Oliveira L, et al. Lactobacillus paracasei DTA81, a cholesterol-lowering strain having immunomodulatory activity, reveals gut microbiota regulation capability in BALB/c mice receiving high-fat diet. J Appl Microbiol. (2021) 131:1942–57. doi: 10.1111/jam.15058
26. Wang B, Kong Q, Cui S, Li X, Gu Z, Zhao J, et al. Bifidobacterium adolescentis isolated from different hosts modifies the intestinal microbiota and displays differential metabolic and immunomodulatory properties in mice fed a high-fat diet. Nutrients. (2021) 13:1017. doi: 10.3390/nu13031017
27. Xu Q, Gu S, Chen Y, Quan J, Lv L, Chen D, et al. Protective effect of Pediococcus pentosaceus LI05 against Clostridium difficile infection in a mouse model. Front Microbiol. (2018) 9:2396. doi: 10.3389/fmicb.2018.02396
28. Bian X, Yang L, Wu W, Lv L, Jiang X, Wang Q, et al. Pediococcus pentosaceus LI05 alleviates DSS-induced colitis by modulating immunological profiles, the gut microbiota and short-chain fatty acid levels in a mouse model. Microb Biotechnol. (2020) 13:1228–44. doi: 10.1111/1751-7915.13583
29. Xie J, Yao M, Lu Y, Yu M, Han S. Impact of encapsulating a probiotic (Pediococcus pentosaceus Li05) within gastro-responsive microgels on Clostridium difficile infections. Food Funct. (2021) 12:3180–90. doi: 10.1039/D0FO03235B
30. Hao L, Cheng Y, Su W, Wang C, Lu Z, Jin M, et al. Pediococcus pentosaceus ZJUAF-4 relieves oxidative stress and restores the gut microbiota in diquat-induced intestinal injury. Appl Microbiol Biotechnol. (2021) 105:1657–68. doi: 10.1007/s00253-021-11111-6
31. Huang J, Li S, Wang Q, Guan X, Qian L, Li J, et al. Pediococcus pentosaceus B49 from human colostrum ameliorates constipation in mice. Food Funct. (2020) 11:5607–20. doi: 10.1039/D0FO00208A
32. Dubey V, Mishra AK, Ghosh AR. Probiotic Pediococcus pentosaceus GS4 shields brush border membrane and alleviates liver toxicity imposed by chronic cadmium exposure in Swiss albino mice. J Appl Microbiol. (2019) 126:1233–44. doi: 10.1111/jam.14195
33. Jiang XW, Li YT, Ye JZ, Lv LX, Yang LY, Bian XY, et al. New strain of Pediococcus pentosaceus alleviates ethanol-induced liver injury by modulating the gut microbiota and short-chain fatty acid metabolism. World J Gastroenterol. (2020) 26:6224–40. doi: 10.3748/wjg.v26.i40.6224
34. Yang S, Gong P, Pan J, Wang N, Tong J, Wang M, et al. Pediococcus pentosaceus xy46 can absorb zearalenone and alleviate its toxicity to the reproductive systems of male mice. Microorganisms. (2019) 7:266. doi: 10.3390/microorganisms7080266
35. Wang Y, You Y, Tian Y, Sun H, Li X, Wang X, et al. Pediococcus pentosaceus PP04 ameliorates high-fat diet-induced hyperlipidemia by regulating lipid metabolism in C57BL/6N mice. J Agric Food Chem. (2020) 68:15154–63. doi: 10.1021/acs.jafc.0c05060
36. Wang Y, Tian Y, Zhang N, Li X, Wang X, Wang W, et al. Pediococcus pentosaceus PP04 improves high-fat diet-induced liver injury by the modulation of gut inflammation and intestinal microbiota in C57BL/6N mice. Food Funct. (2021) 12:6851–62. doi: 10.1039/D1FO00857A
37. Liu Y, Sun X, Zhang J, Gao F, Yu L, Dong L, et al. Isolation and characterisation of pulsatilla radix-utilising bacteria Pediococcus pentosaceus PR-1 from human faeces. FEMS Microbiol Lett. (2022) 369:fnac089. doi: 10.1093/femsle/fnac089
38. Tran VC, Cho SY, Kwon J, Kim D. Alginate oligosaccharide (AOS) improves immuno-metabolic systems by inhibiting STOML2 overexpression in high-fat-diet-induced obese zebrafish. Food Funct. (2019) 10:4636–48. doi: 10.1039/C9FO00982E
39. Falcinelli S, Rodiles A, Hatef A, Picchietti S, Cossignani L, Merrifield DL, et al. Dietary lipid content reorganizes gut microbiota and probiotic L. rhamnosus attenuates obesity and enhances catabolic hormonal milieu in zebrafish. Sci Rep. (2017) 7:5512. doi: 10.1038/s41598-017-05147-w
40. Navarro-Barrón E, Hernández C, Llera-Herrera R, García-Gasca A, Gómez-Gil B. Overfeeding a high-fat diet promotes sex-specific alterations on the gut microbiota of the zebrafish (Danio rerio). Zebrafish. (2019) 16:268–79. doi: 10.1089/zeb.2018.1648
41. Qiao F, Tan F, Li LY, Lv HB, Chen L, Du ZY, et al. Alteration and the function of intestinal microbiota in high-fat-diet- or genetics-induced lipid accumulation. Front Microbiol. (2021) 12:741616. doi: 10.3389/fmicb.2021.741616
42. Zhang FL, Yang YL, Zhang Z, Yao YY, Xia R, Gao CC, et al. Surface-displayed Amuc_1100 from Akkermansia muciniphila on Lactococcus lactis ZHY1 improves hepatic steatosis and intestinal health in high-fat-fed zebrafish. Front Nutr. (2021) 8:726108. doi: 10.3389/fnut.2021.726108
43. Xiang JY, Chi YY, Han JX, Kong P, Liang Z, Wang D, et al. Litchi chinensis seed prevents obesity and modulates the gut microbiota and mycobiota compositions in high-fat diet-induced obese zebrafish. Food Funct. (2022) 13:2832–45. doi: 10.1039/D1FO03991A
44. López Nadal A, Ikeda-Ohtsubo W, Sipkema D, Peggs D, McGurk C, Forlenza M, et al. Feed, microbiota, and gut immunity: using the zebrafish model to understand fish health. Front Immunol. (2020) 11:114. doi: 10.3389/fimmu.2020.00114
45. Dai W, Wang K, Zheng X, Chen X, Zhang W, Zhang Y, et al. High fat plus high cholesterol diet lead to hepatic steatosis in zebrafish larvae: a novel model for screening anti-hepatic steatosis drugs. Nutr Metab. (2015) 12:42. doi: 10.1186/s12986-015-0036-z
46. Bolyen E, Rideout JR, Dillon MR, Bokulich NA, Abnet CC, Al-Ghalith GA, et al. Reproducible, interactive, scalable and extensible microbiome data science using QIIME 2. Nat Biotechnol. (2019) 37:852–7. doi: 10.1038/s41587-019-0209-9
47. Douglas GM, Maffei VJ, Zaneveld JR, Yurgel SN, Brown JR, Taylor CM, et al. PICRUSt2 for prediction of metagenome functions. Nat Biotechnol. (2020) 38:685–8. doi: 10.1038/s41587-020-0548-6
48. Wang A, Meng D, Hao Q, Xia R, Zhang Q, Ran C, et al. Effect of supplementation of solid-state fermentation product of Bacillus subtilis HGcc-1 to high-fat diet on growth, hepatic lipid metabolism, epidermal mucus, gut and liver health and gut microbiota of zebrafish. Aquaculture. (2022) 560:738542. doi: 10.1016/j.aquaculture.2022.738542
49. Jiang S, Cai L, Lv L, Li L. Pediococcus pentosaceus, a future additive or probiotic candidate. Microb Cell Fact. (2021) 20:45. doi: 10.1186/s12934-021-01537-y
50. Shan C, Li M, Liu Z, Xu R, Qiao F, Du ZY, et al. Pediococcus pentosaceus enhances host resistance against pathogen by increasing IL-1β production: understanding probiotic effectiveness and administration duration. Front Immunol. (2021) 12:766401. doi: 10.3389/fimmu.2021.766401
51. Yang SJ, Kim KT, Kim TY, Paik HD. Probiotic properties and antioxidant activities of Pediococcus pentosaceus SC28 and Levilactobacillus brevis KU15151 in fermented black gamju. Foods. (2020) 9:1154. doi: 10.3390/foods9091154
52. Dong F, Xiao F, Li X, Li Y, Wang X, Yu G, et al. Pediococcus pentosaceus CECT 8330 protects DSS-induced colitis and regulates the intestinal microbiota and immune responses in mice. J Transl Med. (2022) 20:33. doi: 10.1186/s12967-022-03235-8
53. Wang Y, Ren Z, Fu L, Su X. Two highly adhesive lactic acid bacteria strains are protective in zebrafish infected with Aeromonas hydrophila by evocation of gut mucosal immunity. J Appl Microbiol. (2016) 120:441–51. doi: 10.1111/jam.13002
54. Chen M, Liu C, Dai M, Wang Q, Li C, Hung W. Bifidobacterium lactis BL-99 modulates intestinal inflammation and functions in zebrafish models. PLoS One. (2022) 17:e0262942. doi: 10.1371/journal.pone.0262942
55. Arani MM, Salati AP, Keyvanshokooh S, Safari O. The effect of Pediococcus acidilactici on mucosal immune responses, growth, and reproductive performance in zebrafish (Danio rerio). Fish Physiol Biochem. (2021) 47:153–62. doi: 10.1007/s10695-020-00903-8
56. Ahmadifar E, Dawood MAO, Moghadam MS, Shahrestanaki AH, Van Doan H, Saad AH, et al. The effect of Pediococcus acidilactici MA 18/5M on immune responses and mRNA levels of growth, antioxidant and immune-related genes in zebrafish (Danio rerio). Aquacult Rep. (2020) 17:100374. doi: 10.1016/j.aqrep.2020.100374
57. Mohammadi Arani M, Salati AP, Safari O, Keyvanshokooh S. Dietary supplementation effects of Pediococcus acidilactici as probiotic on growth performance, digestive enzyme activities and immunity response in zebrafish (Danio rerio). Aquacult Nutr. (2019) 25:854–61. doi: 10.1111/anu.12904
58. Lim FT, Lim SM, Ramasamy K. Cholesterol lowering by Pediococcus acidilactici LAB4 and Lactobacillus plantarum LAB12 in adult zebrafish is associated with improved memory and involves an interplay between npc1l1 and abca1. Food Funct. (2017) 8:2817–28. doi: 10.1039/C7FO00764G
59. Higashikawa F, Noda M, Awaya T, Danshiitsoodol N, Matoba Y, Kumagai T, et al. Antiobesity effect of Pediococcus pentosaceus LP28 on overweight subjects: a randomized, double-blind, placebo-controlled clinical trial. Eur J Clin Nutr. (2016) 70:582–7. doi: 10.1038/ejcn.2016.17
60. Nasir N, Sayeed MA, Jamil B. Ralstonia pickettii bacteremia: an emerging infection in a tertiary care hospital setting. Cureus. (2019) 11:e5084. doi: 10.7759/cureus.5084
61. Wang A, Zhang Z, Ding Q, Yang Y, Bindelle J, Ran C, et al. Intestinal cetobacterium and acetate modify glucose homeostasis via parasympathetic activation in zebrafish. Gut Microbes. (2021) 13:1–15. doi: 10.1080/19490976.2021.1900996
62. Zhang Z, Ran C, Ding QW, Liu HL, Xie MX, Yang YL, et al. Ability of prebiotic polysaccharides to activate a HIF1α-antimicrobial peptide axis determines liver injury risk in zebrafish. Commun Biol. (2019) 2:274. doi: 10.1038/s42003-019-0526-z
63. Xie M, Zhou W, Xie Y, Li Y, Zhang Z, Yang Y, et al. Effects of Cetobacterium somerae fermentation product on gut and liver health of common carp (Cyprinus carpio) fed diet supplemented with ultra-micro ground mixed plant proteins. Aquaculture. (2021) 543:736943. doi: 10.1016/j.aquaculture.2021.736943
64. Xie M, Xie Y, Li Y, Zhou W, Zhang Z, Yang Y, et al. Stabilized fermentation product of Cetobacterium somerae improves gut and liver health and antiviral immunity of zebrafish. Fish Shellfish Immunol. (2022) 120:56–66. doi: 10.1016/j.fsi.2021.11.017
65. Feng S, Dai Z, Liu AB, Huang J, Narsipur N, Guo G, et al. Intake of stigmasterol and β-sitosterol alters lipid metabolism and alleviates NAFLD in mice fed a high-fat western-style diet. Biochim Biophys Acta Mol Cell Biol Lipids. (2018) 1863:1274–84. doi: 10.1016/j.bbalip.2018.08.004
66. Xia G, Sun J, Fan Y, Zhao F, Ahmed G, Jin Y, et al. β-sitosterol attenuates high grain diet-induced inflammatory stress and modifies rumen fermentation and microbiota in sheep. Animals. (2020) 10:171. doi: 10.3390/ani10010171
67. Kim KA, Gu W, Lee IA, Joh EH, Kim DH. High fat diet-induced gut microbiota exacerbates inflammation and obesity in mice via the TLR4 signaling pathway. PLoS ONE. (2012) 7:e47713. doi: 10.1371/journal.pone.0047713
68. Zhou W, Xie M, Xie Y, Liang H, Li M, Ran C, et al. Effect of dietary supplementation of Cetobacterium somerae XMX-1 fermentation product on gut and liver health and resistance against bacterial infection of the genetically improved farmed tilapia (GIFT, Oreochromis niloticus). Fish Shellfish Immunol. (2022) 124:332–42. doi: 10.1016/j.fsi.2022.04.019
69. Lei X, Teng W, Fan Y, Zhu Y, Yao L, Li Y, et al. The protective effects of HIF-1α activation on sepsis induced intestinal mucosal barrier injury in rats model of sepsis. PLoS ONE. (2022) 17:e0268445. doi: 10.1371/journal.pone.0268445
70. Shao T, Zhao C, Li F, Gu Z, Liu L, Zhang L, et al. Intestinal HIF-1α deletion exacerbates alcoholic liver disease by inducing intestinal dysbiosis and barrier dysfunction. J Hepatol. (2018) 69:886–95. doi: 10.1016/j.jhep.2018.05.021
71. Cong X, Kong W. Endothelial tight junctions and their regulatory signaling pathways in vascular homeostasis and disease. Cell Signal. (2020) 66:109485. doi: 10.1016/j.cellsig.2019.109485
72. González-Mariscal L, Tapia R, Chamorro D. Crosstalk of tight junction components with signaling pathways. Biochim Biophys Acta. (2008) 1778:729–56. doi: 10.1016/j.bbamem.2007.08.018
73. Zeng SJ, Chen YZ, Wei CX, Tan LH, Li C, Zhang YJ, et al. Protective effects of polysaccharide from Artocarpus heterophyllus Lam. (jackfruit) pulp on non-alcoholic fatty liver disease in high-fat diet rats via PPAR and AMPK signaling pathways. J Funct Foods. (2022) 95:105195. doi: 10.1016/j.jff.2022.105195
74. Keles U, Ow JR, Kuentzel KB, Zhao LN, Kaldis P. Liver-derived metabolites as signaling molecules in fatty liver disease. Cell Mol Life Sci. (2022) 80:4. doi: 10.1007/s00018-022-04658-8
75. Matsuzaka T, Shimano H. New perspective on type 2 diabetes, dyslipidemia and non-alcoholic fatty liver disease. J Diabetes Investig. (2020) 11:532–4. doi: 10.1111/jdi.13258
76. He J, Zhang P, Shen L, Niu L, Tan Y, Chen L, et al. Short-chain fatty aids and their association with signalling pathways in inflammation, glucose and lipid metabolism. Int J Mol Sci. (2020) 21:6356. doi: 10.3390/ijms21176356
77. Zhu K, Tan F, Mu J, Yi R, Zhou X, Zhao X. Anti-obesity effects of Lactobacillus fermentum CQPC05 isolated from Sichuan pickle in high-fat diet-induced obese mice through PPAR-α signaling pathway. Microorganisms. (2019) 7:194. doi: 10.3390/microorganisms7070194
Keywords: probiotic, gut microbiota, inflammation, high fat diet, zebrafish
Citation: Liu Y, Zhu D, Liu J, Sun X, Gao F, Duan H, Dong L, Wang X and Wu C (2023) Pediococcus pentosaceus PR-1 modulates high-fat-died-induced alterations in gut microbiota, inflammation, and lipid metabolism in zebrafish. Front. Nutr. 10:1087703. doi: 10.3389/fnut.2023.1087703
Received: 02 November 2022; Accepted: 16 January 2023;
Published: 01 February 2023.
Edited by:
Hengyi Xu, Nanchang University, ChinaReviewed by:
Ahmad Ud Din, Sichuan University, ChinaMinhao Xie, Nanjing University of Finance and Economics, China
Copyright © 2023 Liu, Zhu, Liu, Sun, Gao, Duan, Dong, Wang and Wu. This is an open-access article distributed under the terms of the Creative Commons Attribution License (CC BY). The use, distribution or reproduction in other forums is permitted, provided the original author(s) and the copyright owner(s) are credited and that the original publication in this journal is cited, in accordance with accepted academic practice. No use, distribution or reproduction is permitted which does not comply with these terms.
*Correspondence: Yue Liu, eXVlbGl1QHN4dS5lZHUuY24=; Changxin Wu,
Y3h3MjBAc3h1LmVkdS5jbg==
†These authors have contributed equally to this work