- 1Medical Biology Research Center (MBRC), Kermanshah University of Medical Sciences, Kermanshah, Iran
- 2Department of Biotechnology, Faculty of Agricultural and Natural Sciences, Imam Khomeini International University (IKIU), Qazvin, Iran
The future GCC-connected environmental risk factors expedited the progression of nCDs. Indeed, the emergence of AFs is becoming a global food security concern. AFs are lethal carcinogenic mycotoxins, causing damage to the liver, kidney, and gastrointestinal organs. Long-term exposure to AFs leads to liver cancer. Almost a variety of food commodities, crops, spices, herbaceous materials, nuts, and processed foods can be contaminated with AFs. In this regard, the primary sections of this review aim to cover influencing factors in the occurrence of AFs, the role of AFs in progression of nCDs, links between GCC/nCDs and exposure to AFs, frequency of AFs-based academic investigations, and world distribution of AFs. Next, the current trends in the application of PPs to alleviate AFs toxicity are discussed. Nearly, more than 20,000 published records indexed in scientific databases have been screened to find recent trends on AFs and application of PPs in AFs therapy. Accordingly, shifts in world climate, improper infrastructures for production/storage of food commodities, inconsistency of global polices on AFs permissible concentration in food/feed, and lack of the public awareness are accounting for a considerable proportion of AFs damages. AFs exhibited their toxic effects by triggering the progression of inflammation and oxidative/nitrosative stress, in turn, leading to the onset of nCDs. PPs could decrease AFs-associated oxidative stress, genotoxic, mutagenic, and carcinogenic effects by improving cellular antioxidant balance, regulation of signaling pathways, alleviating inflammatory responses, and modification of gene expression profile in a dose/time-reliant fashion. The administration of PPs alone displayed lower biological properties compared to co-treatment of these metabolites with AFs. This issue might highlight the therapeutic application of PPs than their preventative content. Flavonoids such as quercetin and oxidized tea phenolics, curcumin and resveratrol were the most studied anti-AFs PPs. Our literature review clearly disclosed that considering PPs in antioxidant therapies to alleviate complications of AFs requires improvement in their bioavailability, pharmacokinetics, tissue clearance, and off-target mode of action. Due to the emergencies in the elimination of AFs in food/feedstuffs, further large-scale clinical assessment of PPs to decrease the consequences of AFs is highly required.
Prologue
Nowadays, the estimations predict that the global demand for intensified food production has been increased, and the statistics are expected to be doubled by 2050 (1, 2). Providing safe foods to nurture the world population requires a significant improvement in crop cultivation systems, plant breeding techniques, and the development of climate-smart crops (3). GCC and environmental forces are two determinant factors to influence crops' sustainable growth. GCC influences crop production practices in sophisticated modes (3, 4). Numerous studies have been addressed the direct and indirect effects of GCC on world food demand and agricultural systems (5, 6). Accordingly, modification of cultivation systems and increasing the susceptibility of crops to future climate are typical direct effects of climate change. From a large-scale perspective, affecting the world economy, food demand, and distributions of incomes are indirect effects of climate change on world societies (4).
Studies have shown that changes in climate humidity, temperature, and precipitation patterns are associated with the outbreak of some invasive fungal pathogens (7). The uncontrolled growth of these fungi affects the quality and quantity of crop yield, stored foodstuffs, grains, processed foods, and herbaceous products (8). Studies highlighted the possible health risks linked to fungal toxins to the human body (8). The APF including Aspergillus, Fusarium, and Penicillium, are well-known mycotoxigenic fungal species with potentially fatal effects on human and animal health (9). The occurrence of AFs in food commodities depends on environmental factors such as air temperature, humidity, CO2 levels, pH, susceptibility of foods to contaminations, and improper harvest and storage of food products (10, 11). According to scientific reports, nearly 25% of global food supplies are contaminated with AFs, making them a serious issue of concern for world nations' health (12). AFs are health hazardous environmental risk factors in the onset of liver cancer, kidney failure, and gastrointestinal problems (13), in turn, their health consequences depend on duration of exposure and enzymatic/genetic alterations in target organs (14).
Poverty, hunger and contaminated foods are the foremost health risk factors to threaten human life. Numerous studies suggested that mycotoxins, including aflatoxins, ergot alkaloids, ochratoxins, trichothecenes, zearalenone, and fumonisins, are among the most lethal naturally occurring toxins (15, 16). Characterization and elimination of mycotoxins in food/feed items require specific technical and analytical methods (17). Developed countries implemented strict regulatory gates to control and monitor the occurrence of AFs in import/export sites to reduce the health complications of these toxins (18). In contrast, many people in low-income countries are at risk of long-term exposure to AFs, resultantly this might increase the progression of different cancers in these locations (19).
Studies have shown that natural products (e.g., PPs, berberine, plant extracts, polysaccharides, etc.) can reduce the toxicity and production of AFs (20, 21). PPs are a large heterogeneous group of secondary plant metabolites, display a wide range of biological activities, and are substantially studied for their anticancer activities (22–24). The regular consumption of PPs is associated with lower risks for developing cardiovascular diseases, obesity, DM, cancer, stroke, and AD (23–26), though this finding requires further well-designed clinical validations. Global interest in PPs studies to alleviate AFs health consequences has been increased during the past decades. Preliminary studies showed that PPs can directly and/or indirectly alter the possible toxicity effects of AFs in complicated ways (27, 28). However, the molecular mechanisms underlying PPs effects on AFs toxicity in the human body are still not understood comprehensively, and scientific efforts are ongoing to find out the health-promoting content of PPs against AFs.
In this regard, this review aims to summarize recent findings on AFs and the application of PPs in alleviating AFs end effects. Due to the strategic roles of antioxidant phytochemicals in preventing human chronic diseases (29), and beneficial effects of PPs in the cornerstone of therapeutic programs (30, 31), we have followed five goals herein: (1) providing a comprehensive insight on the association of GCC and the occurrence of AFs; (2) characterizing the most potent anti-AFs phenolics; (3) role of AFs in the onset of nCDs; (4) understanding anti-AFs mechanism of actions of PPs; and (5) addressing the current gaps regarding the large-scale application of PPs for clinical applications.
Literature search strategy
Scientific databases including Scopus, PubMed, Google Patents, and Scholar were separately searched to find relevant papers using keywords such as “aflatoxin or human diseases,” “aflatoxin B1/B2/G1/G2/M1,” “aflatoxin and GMO,” “aflatoxin and diabetes,” “aflatoxin and cancer,” “aflatoxin B1 or chronic diseases,” “aflatoxin or transcriptome,” “aflatoxin and/or Alzheimer's,” “aflatoxin and flavonoids,” “aflatoxin or polyphenols,” “aflatoxin and/or stilbenes,” “aflatoxin and curcumin,” “aflatoxin and/or epigenetic,” “aflatoxin and/or plant extract,” “aflatoxin and/or crops,” “aflatoxin M1 and/or foodstuff,” aflatoxin and/or spices,” “aflatoxin and climate change,” “aflatoxin and/or temperature,” “aflatoxin and/or CO2 levels.”
The outputs of searches were used to generate bibliometric network using VOSviewer software (32). More than 20,000 papers (published from 1990 to 2021) were appeared in search outputs. To unify the results, we compared the outcomes together, thus the redundant papers with similar title or content were deleted from search outputs. To interpret the statistical output of literature searches, we used Scopus data to illustrate the relevant graphs. We also used Cytoscape (ClueGo module) software (33) to construct simplified protein-protein interaction networks where it was needed. Because the exact binding modes of all AFB1 metabolites into human serum albumin (HSA) were not available in the literature, we used AutoDock Vina tools (34) to generate the expected interactions. The Protein-Imager software (35) was recruited to inspire some of graphical illustrations.
General overview of AFs chemistry
Fungal AFs including B1(AFB1), B2(AFB2), M1(AFM1), G1(AFG1), and G2(AFG2) are well-known AFs in contaminated crops, foods, dairy products, herbal materials, spices, and processed foods (19, 36). AFs showed mutagenic, carcinogenic, hepatogenic, teratogenic, and immunosuppressive toxicological properties. The toxic properties of AFs depend on alteration of enzymatic activity, modification of gene expression patterns, epigenetics changes, and dysregulation of signaling pathways (11–13, 37, 38).
Chemically, AFs can be classified into two main groups including difuran-coumarin lactones (AFG1/2) and difuran-cyclo-pentanones (AFB1/2 and AFM1/2) (39, 40). These fungal toxins displayed a “CHO” molecular formula with a different number of H/O atoms. The molecular weight of AFs ranges from 312 to 330 g·mol−1. AFs displayed a colorful fluorescent pattern under the UV light. In this respect, AFB1/2 showed a blue color while AFGs displayed green color (39, 40) (Figure 1). To date, more than 20 different AFs have been identified with moderate to high toxicity effects on the human body (40). The evidence suggests that AFs are potent carcinogenic toxins with potential side effects on human liver organs and tissues (37).
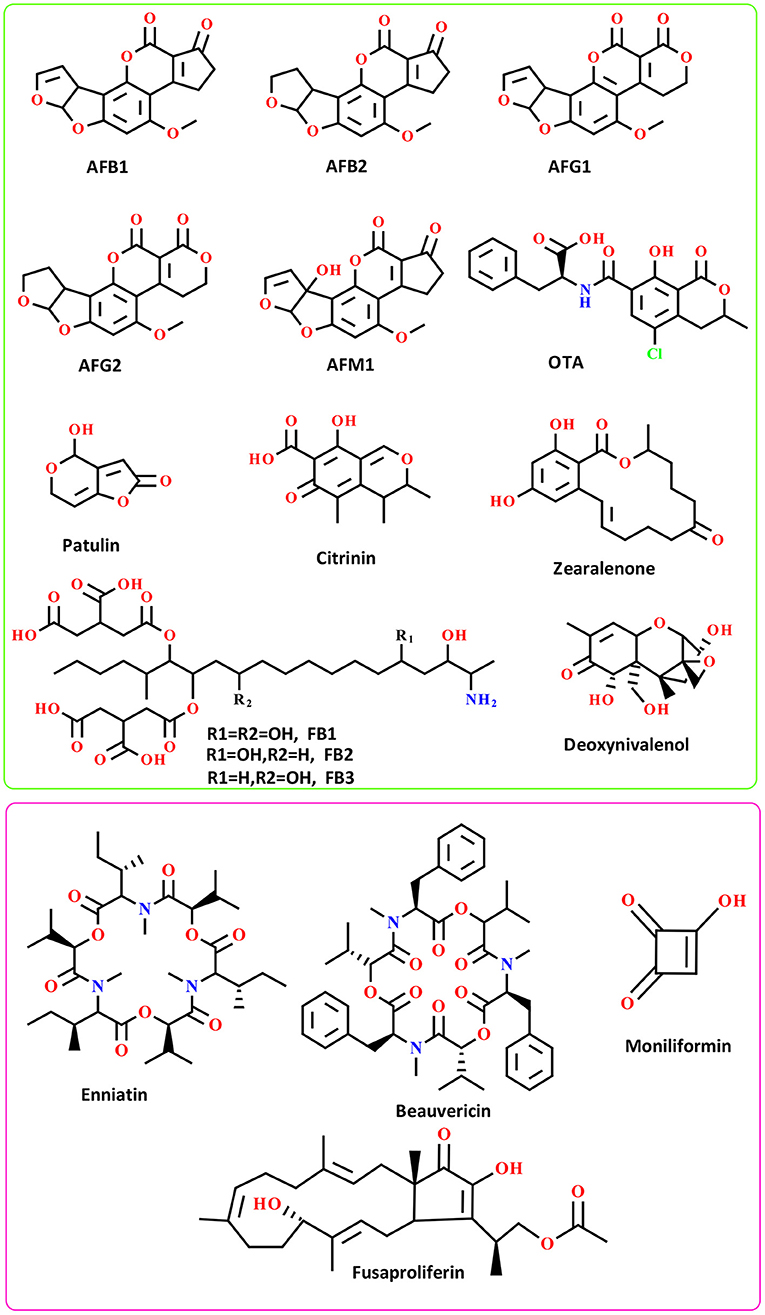
Figure 1. The chemical structure of well-known AFs (green box) and emerging mycotoxins (pink box); FB: Fumonisins B.
The laboratory analysis of AFs identified several metabolized AFs derivatives that rarely found in the human body (40). The most abundant AFs derivatives found in human and animal bodies are AFM1/2 metabolites-the derivatives of AFB1 metabolism in the liver (41). These AFs are widely found in milk and milk-based products, and recently received much attention from the literature due to their potential health problems for consumers of dairy products (41). Studies have shown that AFs are thermostable substances, therefore elevated temperature might not destroy these mycotoxins (40). The toxicity content of AFs is in the order of AFB1 > AFG1 > AFB2 > AFG2 (42). These mycotoxins have been considered as group I highly carcinogenic substances by IARC (40, 43). No only structural features of well-known AFs, but also the chemistry of emerging toxins mainly produced by Fusarium species should be taken into account because of their potential to outbreak and influence the future food safety and security (44). For more details, the chemistry and distribution of emerging mycotoxins have been reviewed by Gruber-Dorninger and colleagues (45).
The upcoming GCC and future AFs contamination risks
The GCC is becoming a serious concern, and being the future driver of food safety and security (46). The expanded worldwide industrial activities and the increasing rate of world population are the two of the most crucial components of countries' climate modification (47–49). The outbreak of invasive plant pests/pathogens, soil erosion, drought, erratic rainfall patterns, GW, salinity, crop cultivation failure, shortage of irrigation water, and reduction in the fertility of arable soils are the foremost consequences of the GCC (47, 48, 50, 51).
Studies disclosed that GCC induces the occurrence of plant fungal pathogens by providing appropriate environmental requirements for their growth and development (52, 53). While elevated temperatures and irregular precipitation patterns can significantly reduce crop yield, the growing evidence suggests that these climatic consequences also triggered the production of AFs at the beginning of crops growing seasons (53). The emerging evidence postulates that under at least +2 to +5°C climate temperature increase, maize and wheat crops might prone to higher levels of AFs contamination (46). Studies also demonstrated that APF tolerated a wide range of temperatures, resultantly enabled these fungi to grow easily in the production/storage sites of crops (54).
The GCC might support the growth and development of APF in crop production sites (7, 55). The overgrowth of these fungi leads to a rapid expansion of spores in the environment, increases AFs contaminants level, ultimately leading to health impacts on consumers (7, 54). Due to the increased universal demand for food, the GCC intensifies crop cultivation and production, in turn, might lead to the establishment of single-crop cultivation systems (56). Additionally, underestimating of crop rotation and plantation of susceptible crops may also increase the risk of future AFs occurrence (57).
Different strategies have been applied to address the GCC in advanced and developing countries (3, 54). These policies mainly focused on improving breeding strategies (3) and recruiting integrated and novel methods to control plant pathogens (58). In contrast, farming systems in undeveloped countries are almost entirely influenced by GCC (1). On such occasions, traditional methods of crop cultivation, harvest, and storage have mainly been followed by local farmers, these may in turn be leading to the exacerbation of APF outbreak (59). Although a variety of sophisticated methods have been employed in developed countries for integrated control of mycotoxin-producing fungi in food and feed (54, 60), the outcomes, however, suggested that these methods were rarely successful to completely reduce the occurrence of AFs (18, 61).
The emerging reports on the GCC demonstrated that GW has begun to occur but the empirical data on how GW might affect crops yield is markedly overlooked (62). Strategically, GW and GCC might alter the distribution of plant pests and diseases, resulted in a significant damage to crop production. The literature suggests that pest-infested crops are prone to AFs contaminants (63). Therefore, predicting the exact roles of GCC on the prevalence of AFs relies the development of accurate models to estimate the future damages of AFs under GCC (64). Presently, mechanistic (65), empirical, and hybrid models in predicting future economic costs of AFs occurrence have been developed in Australia, the USA and some European countries (64) while the lack of comprehensive predictive models in less developed countries (e.g., Africa, Middle-East, Latin America) might decrease the effectiveness of such estimations in preventing future AFs production (64, 66).
Therefore, recruiting predictive models to simulate the occurrence of AFs requires an in-depth knowledge of future AFP-GCC interactions (67) to know where and how these mycotoxins will be emerged in the target production/storage sites (64, 66), which future crops are more susceptible to AFs, and ultimately which future country-specific regulations must be taken into consideration in order to better elimination of AFs production. Indeed, in addressing modeling of future APF-GCC interactions, it is also meritorious to highlight this note that the contemporaneous predictive studies have been validated in limited geographical regions, therefore it is imperative to conduct large-scale multinational investigations for better understanding of GCC impacts on future APF mycoflora and global pattern of AFs distribution (68).
In this regard, Yu et al. reported that global temperature modification has an impact on the prevalence of AFs contaminants (62). According to the given model for simulation of AFs occurrence based on corn phenology, it is believed that some corn grown US states will experience an increased level of AFs occurrence by 2031–2040 (62). On the contrary, this estimation also postulated that under elevated temperatures AFs might be inactivated, therefore, some the US counties might experience lower level of AFs occurrence (62). Correspondingly, other outcomes also suggested that water stress and elevated temperatures are two determinant factors in changing the relative expression pattern of structural genes (aflD, aflR) involved in the production of AFB1, leading to higher occurrence of this carcinogenic mycotoxin (67).
Scientists recruited high-throughput multi-omics technologies to assess the impact of GCC on the production of AFB1 (69). The outcomes unraveled that the elevated CO2 levels, as a consequence of GW, might alter aflR gene expression in AFB1 biosynthetic pathway (69). In another study, researchers simulated climate change condition to investigate how it might influence the growth of A. carbonarius and OTA production under elevated temperature/CO2 levels (70). The results surprisingly displayed that the interaction between elevated CO2 levels and temperature lead to the up-regulation of velvet complex regulatory elements and OTA biosynthetic genes in A. carbonarius. This finding suggests that elevated CO2/temperature levels are two quintessential factors in increasing the risk of OTA contaminants in grape-based products (70). The outcomes from a similar study also demonstrated that changes in temperature/CO2 levels in stored coffee beans and coffee-based media attributed to OTA production in A. westerdijkiae compared to A. carbonarius (71).
In another study, it was also reported that the GCC-associated factors have differential impacts on AFB1 production in pistachio nuts (7). In a case study conducted on maize grown in Eastern Europe using different climatological models, the estimations predicted that climate change can lead to a probable increase in the occurrence of maize AFB1 and cow's milk AFM1 (72). Other studies on the effects of GW on the occurrence of AFB1 and trichothecenes mycotoxins in wheat and maize crops also suggested that the prevalence of these hazardous mycotoxins is expected to increase as consequences of the future GCC (46, 68). Although little is understood on the effects of future GCC on mycotoxigenic fungi growth and mycotoxins production, developing accurate predictive models to characterize mechanistic interactions of APF with climatological factors will provide a ground for better controlling of these fungi (68).
It is estimated that economic losses due to the occurrence of AFs are between $500 million to $1.6 billion for maize, peanuts and other crops in the USA (73, 74). These obvious economic costs, however, are associated with GCC and its impact on AFs production (73). Another pivotal issue, that is crops grown in low and northern latitudes might negatively or positively deal with future GCC (44). In this regard, the evidence suggests that low latitude regions will be suffered from consistent and negative consequences of GCC compared to the northern regions where its effects may be positive or negative (44).
Considering the relationship between GCC and increased global demand for food, this might threaten the production of certain crops such as maize using the available infrastructures. In this respect, the occurrence of AFs and other mycotoxins is expected to increase on such occasions (75). In spite of the fact that the universal temperature may be rising above the optimum condition for APF, it is pivotal to consider the threat posed by emerging thermotolerant fungi that can produce novel health hazardous toxins (76). Therefore, smart crop breeding for developing resistance against both GCC/GW and APF might be considered as an alternative scenario in managing the reduction of AFs occurrence in food and feed (75).
In addition to financial losses to animal and agricultural commodities, AFs-contaminated foods are leading to significant clinical costs due to the side effects of long-term exposure to these toxins for the human body (18). However, decreasing milk production, reducing of crops quality, weakening animal immune system, and many other demerits are few examples of AFs financial burden for animal and crop products (18). Various chemical and biological methods have been suggested in controlling AFs-producing molds (60, 77, 78), nevertheless, the evidence suggests that there has been no efficient method to completely eliminate these molds (60, 78, 79). It should be expected that the universal quantity/distribution of AFs will probably be grown under GCC/GW. Therefore, due to the upcoming future GCC, systematic modification of crop cultivation practices, applying crop rotation, developing climate-smart crops, set limits to APF growth in production sites, modernizing crop storage facilities, utilizing modern crop irrigation systems, and increasing public awareness about the association of climate change and AFs risks are influential scenarios to reduce the occurrence of AFs under modified future climate (Figure 2).
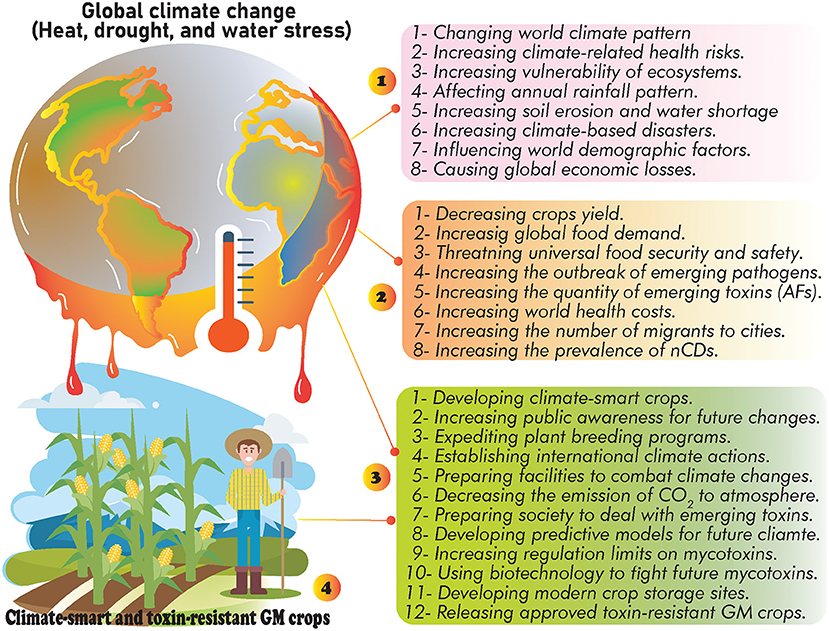
Figure 2. The possible effects of GCC: (1) general side effects of GCC, (2) consequences of GCC on food security and public health, (3) the suggested strategies to deal with future GCC, and (4) developing climate-smart crops as alternative way to decrease the occurrence of AFs and neutralize the consequences of future GCC.
Studies have also highlighted the role of GCC in the prevalence of nCDs such as cancer, MetSys, stroke, chronic respiratory disorders, and cardiovascular diseases (80, 81). In this regard, several investigations purported that the future GW/GCC will highly increase exposure levels to GCC-associated health hazardous risk factors (49, 66, 68, 72), consequently leading to higher rates of global deaths (49, 81). As discussed, AFs exposure will probably be growing in the upcoming years owing to a significant modification in countries' climate patterns (82), nevertheless, the current estimations require further validations to address all gaps and challenges in preparing world communities for future changes (68).
The co-occurrence of GCC-associated risk factors offering synergistic effects on human health and the onset of nCDs (83, 84). Therefore, due to the complexity of interactions between GCC/exposure to AFs (and other emerging mycotoxins) (85), and the onset of nCDs (86), it is obligatory to establish country-specific regulations to deal with the upcoming challenges (64) and decrease the global burden of incurable human diseases. While only 2% of global health funds allocated to treat these diseases, the estimations predicted that the number of people affected by nCDs have dramatically been increased over the past decades (81, 87). Therefore, due to the lack of specific international leadership to combat nCDs (81), the increased exposure to AFs and other emerging mycotoxins under countries' climate change will worsen global health status, particularly in low and middle-income countries (88, 89). In this regard, to decrease the economic costs of AFs exposure and the progression of nCDs, the possible threats of future GCC should be taken into account in alleviating the health consequences of AFs risks.
Increasing world studies on AFs
According to the Scopus statistics (https://www.scopus.com/), the global studies on AFs have markedly increased during the past few decades. The literature mining of scientific databases showed that more than 20,000 papers have been published on different classes of AFs from 1990 to 2021. As depicted in Figure 3, the frequencies of studies on different types of AFs metabolites displayed that most of these studies had targeted AFs metabolites such as AFB1/2, AFG1/2, and AFM1. Interestingly, the number of studies on AFM2 was lower than other AFs metabolites during the investigated timeline, and these investigations have increased from 2008 to 2021. Accordingly, a large proportion of scientific studies on AFs were conducted in field of agriculture and biological sciences, followed by biochemistry, medicine, and chemistry, respectively. These frequencies of studies on AFs displayed that these mycotoxins received much attention from academia and clinical sectors due to their health hazardous risks.
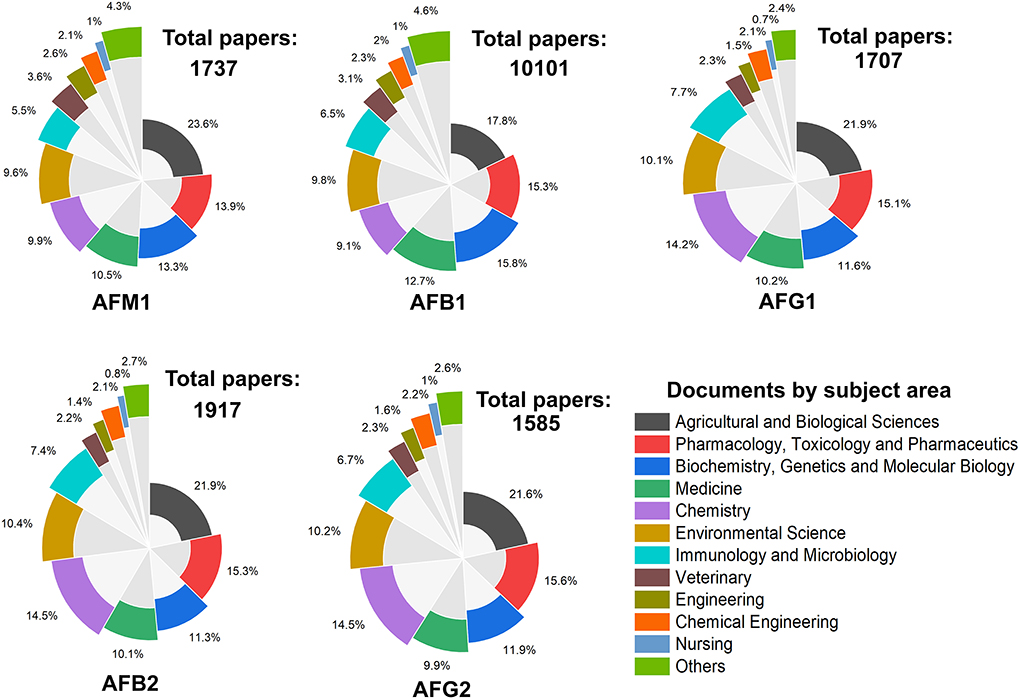
Figure 3. The frequency of studies on AFs metabolites from 1990 to 2021. Each plot represents the number of papers published on searched keywords and the percentage of documents per subject area.
Evaluating the searched papers to highlight the top countries for research on AFs showed that the USA, China, and India were occupied the top ranks for studies on AFB1. In the case of AFM1, the USA, China and Iran were the three top countries to publish academic investigations on this aflatoxin. Indeed, China, the USA, and Brazil published many articles on AFG1 and ranked first to third countries to conduct research in this area. Interestingly, these results are in consistent with previous outcomes on the frequency of global studies on AFs in which the USA, China, and India were the top publishing sources from 1998 to 2017 (90). These outcomes together suggest that the global frequency of scientific studies on AFs has markedly extended over the past few years. Evaluating and monitoring academic publications on AFs can support researchers to identify the critical gaps in these studies for improving current regulatory policies, local and international awareness programs and making political decisions to protect target consumers from complications of AFs. Figure 4 shows a detailed representation on the proportion of the top 10 countries to conduct studies on different classes of AFs metabolites.
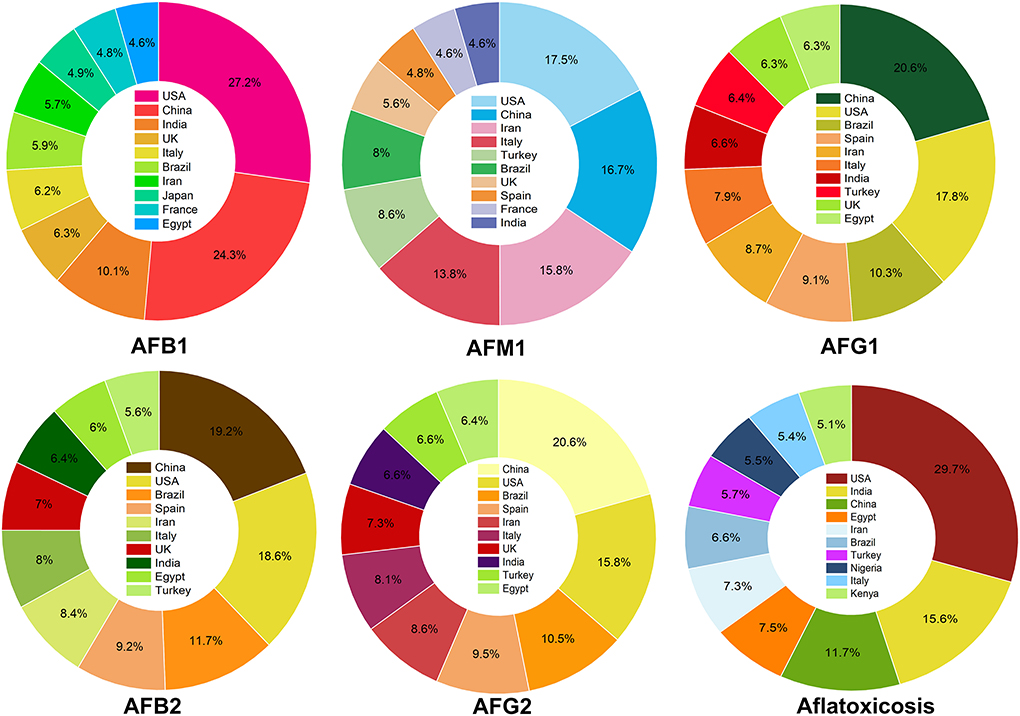
Figure 4. The proportion of academic investigations on AFs. For each class of AFs metabolites, the percentage of published studies has shown for the top 10 countries.
The review of literature unraveled that the conducted studies on AFs can be specified in different clusters. The majority of these studies targeted technical procedures for detecting and monitoring AFs in food sources, crops, spices, dairy products, nuts, and herbaceous products. Additionally, research interest in characterization of AFs in vegetable oils has been increased over the past 5 years. For example, a recent meta-analysis disclosed that vegetable oils such as sesame oil showed a differential AFs contamination (91). In another study, vegetable oil samples such as coconut oil was contaminated with different AFB1 concentrations (92). This indicates that consumption of such oil samples may pose serious health risks.
Analytical methods such as HPLC, ELISA, TLC, HPTLC, UHPLC, mass spectroscopy, and immunoassays were the most frequent procedures used for diagnosing the AFs contaminants in foods/feeds. Although the basic concept of these methods was previously reviewed in several studies (93–95), however, a fast and accurate method to characterize AFs in suspected sources has not been reported. Miklós et al. reviewed recent trends in developing accurate analytical/immunological measurements to identify AFs in different food and feed items (94). According to their finding, ELISA and LFIA are two promising methods to quantify the minimum concentrations of AFs in food or feed items. Indeed, IAC-clean up followed by HPLC-FLD is another accurate system for AFs measurements (94).
Due to the co-occurrence of mycotoxins in food/feed items, the currently applied diagnostic methods might not appropriately detect different types of mycotoxins in evaluated items, therefore, the application of LC-MS/MS technique has been received much attention from academia owing to its ability in multiplex identification of AFs (94). As shown in Figure 5, a considerable number of studies also highlighted the carcinogenic effects of AFs and their role in the development of human cancers such as liver and gastrointestinal tumors. Studies on biological and chemical control of APF were also frequent among screened investigations.
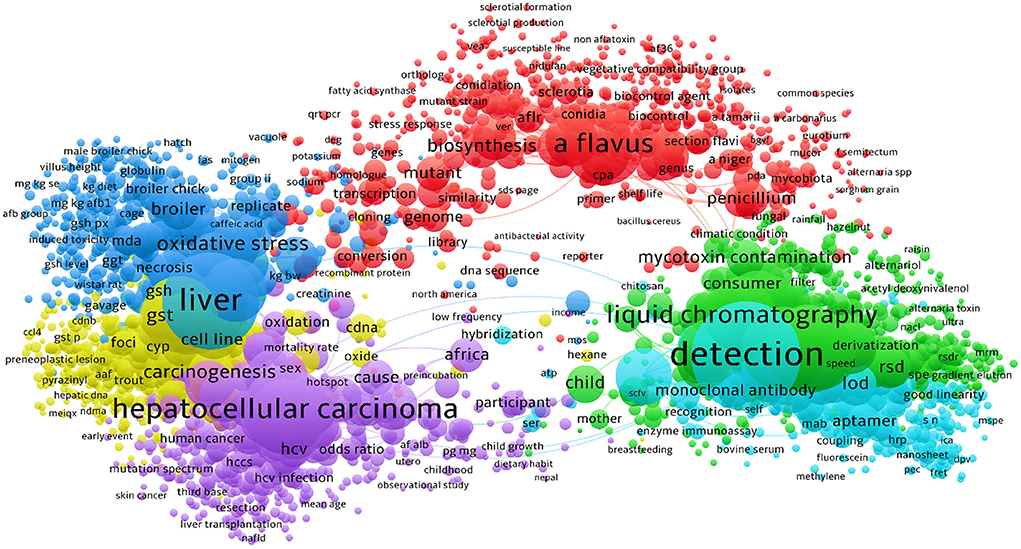
Figure 5. The bibliometric network of studies on AFs from 1990 to 2021 in scientific databases. As detailed in this figure, the majority of studies have been targeted the technical methods to identify the AFs contaminants in suspected samples. Research on carcinogenic effects of AFs was also occupied a large proportion of studies in this field.
We also found that studies on the effects of AFs on human and animal reproduction systems were increased during the past two decades. This is an interesting topic because the evidence suggests that AFs have negative impacts on reproductive organs (96). Studies on the toxicity of AFs for animals and chickens were also increased up to 5–10 folds over the past few years. More interestingly, the frequency of intervention studies using synthetic and/or natural compounds to alleviate the complications of AFs were also grown during the past decade. According to these statistical data, AFB1 and AFM1 were the most studied AFs during the past decades, though studies on AFB2, AFG1/2, and AFM2 were remarkably increased in the same time. Figure 5 highlights the major clusters for studies on AFs using searched keywords in scientific databases.
Literature mining using available scientific resources has also manifested that the risk assessment investigations for characterization of AFs contaminants were dramatically increased. As depicted in Figure 6, the detailed bibliometric networks of studies on AFs unraveled that the carcinogenic effects of AFB1 in inducing hepatocellular carcinoma were significantly investigated. Interestingly, the outcomes showed that the application of PPs (e.g., curcumin and resveratrol) to alleviate health-related complications of AFs has increased during the last 10 years due to the health-promoting effects of these natural metabolites. In this regard, studies suggested that antioxidant therapy might ameliorate the hepatotoxicity of AFs metabolites, leading to lower health risks for cancer development (97). As it is discussed in the next sections, antioxidants are critical chemical agents provided the human body with ability to protect both lipid and protein elements from free radicals and oxidative agents (98). Therefore, considering antioxidants as promising agents in the prevention of AFs complications might decrease the deterioration of hepatic cells and prevent the development of liver cancer, though this claim requires further clinical assessment. Based on the data discussed in this section, it can be concluded that in which fields AFs have considerably studied and where they have been ruled out in scientific investigations. Because AFs are emerging health hazardous threats to the human society, studies on the complications of these fungal toxins, developing reliable medicines to decrease the toxicity of AFs and integrated management of AFs in production/storage sites should be increased to mitigate the quantity of AFs in food/feed items.
Prevalence of mycotoxins in different geographic regions
As discussed in the previous sections, the worldwide occurrence of AFs depends on several factors such as climate, the availability of standard crop/food storage facilities, the awareness of local farmers, food processing methods, post-harvest contaminations, and temperature and moisture of post-harvest storage sites. Of all, air humidity and temperature are two determinant factors to enhance the emergence of APF (99, 100). In an interesting study, the worldwide occurrence of several mycotoxins in feed has been summarized (101). Accordingly, in some geographic regions, the average concentrations of mycotoxin contaminants in feed are considerably higher than other ones owing to the imbalance distribution of these toxins. For instance, the highest median concentration of AFB1 was reported for Sub-Saharan Africa (23 μg/kg), South Asia (20 μg/kg), Southeast Asia (10 μg/kg), and East Asia (10 μg/k) (101). Fumonisins (B1, B2, and B3) are other mycotoxins that pose high health risks to the human societies. The highest median concentration of fumonisins was reported for South America (1390 μg/kg), Central America (929 μg/kg), and Sub-Saharan Africa (789 μg/kg) (101). Compared to other mycotoxins, fumonisins showed a broader geographic occurrence. In contrast, OTA displayed a domineering abundancy ratio in Central Asia (22 μg/kg) and South America (17 μg/kg), respectively (101). Figure 7 represents the worldwide median concentration of five mycotoxins found in feed in different geographical regions based on information adopted from Gruber-Dorninger et al. (101).
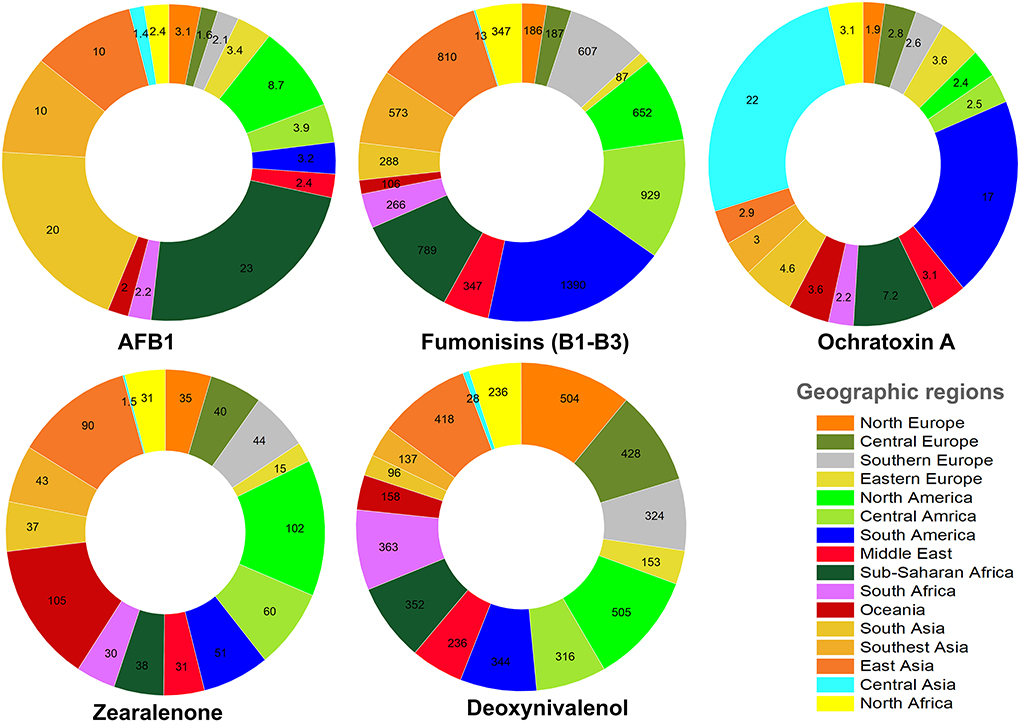
Figure 7. The median concentration (μg/kg) of AFB1 and other mycotoxins in feed from different geographic areas.
Other studies have also highlighted the observed level of AFs in different geographical regions (102, 103). The results showed that the occurrence of AFs in various foods, cereals, nuts, oilseeds and processed foods is inevitable and these sources showed a differential level of contaminations with AFs derivatives (102). According to these outcomes, the prevalence of AFs in each region might be affected by local climate and the abundancy of crops found in these regions (103, 104). Therefore, it can be concluded that AFs are almost not equally distributed in production/storage sites. Therefore, the construction of predictive models for the occurrence of AFs during specific seasons is helpful in identifying the contaminated food/feed items to prevent the circulation of AFs in local and international food chains (105, 106).
AFs contaminants in cereals and nuts
Over the past decades, studies on various food/feed items to trace the fingerprint of AFs contaminants have been increased (13). Cereals are most commonly cultivated in the world, supporting human societies in reaching essential nutrients in their diet (10, 107). Studies reported that AFs occurrence in cereals is becoming a serious worldwide concern (11). The formation of AFs in cereals and cereals-based processed products depends on several factors such as fungal genotype, processing methods (drying, milling, blending, chemical additives), and environmental factors such as oxygen level, environmental pH, field temperature and humidity content (11).
A comprehensive analysis of different cereals samples using published records in GEMS/Food database showed that around 12.7% of all samples were positive for contamination with at least one of AFs (10). Correspondingly, rice, sorghum, and maize samples possessed a higher level of AFs (10). Other investigations also suggested that the highest level of AFs was detected in maize in the concentration of 3,760 μg/kg, which extremely exceeded from the USA and EU permissible standards (108). Among cereals, rice is surprisingly a susceptible crop prone to AFs pollutants (103, 104, 109). After rice, corn and sorghum are prone to AFs contaminants (109). The evidence suggests that AFB1 is the main AFs found in cereals (11).
Another study on 108 Brazilian wheat and wheat by-products samples disclosed that 30.6% of studied samples were positive for at least contamination with one of the AFs in which AFB1 was the most dominant fungal mycotoxin in these samples (110). The highest contamination levels were observed for wheat grains, followed by the barn, whole and refined flour (110). Presently in EU and other countries, only limited concentrations of AFs are allowed to be found in food products (36). In this regard, the allowed concentration of AFB1 and total AFs for nuts and cereals in the EU is 2 and 4 μg/kg, respectively (109). In cereals, applying inappropriate drying methods allowed for maintaining higher humidity content in these crops, leading to a higher ratio of AFs-contaminated crops (13). Recent studies on contaminated cereals demonstrated that differential concentrations of AFs are found in cereals grown in different countries (109). However, nuts, groundnuts, and cereals are prone to AFB1 contaminations under field and non-standard storage whenever temperature, humidity, and field soil are suitable for APF growth (13, 111).
A recent study on the prevalence of AFs in nuts from different origins demonstrated that peanuts from Argentina, Congo, Nigeria, and South-western Uganda were differentially contaminated with AFB1 (112). Accordingly, the average concentrations of AFB1 in peanuts from these countries were 530, 163.22, 110.95, and 103.10 μg/kg, respectively (112). Countries such as Taiwan, Morocco, and Iran ranked as first to third countries for contamination of pistachio with AFs (112). The maximum average concentration of AFB1 in almond, hazelnut, walnut, and Brazilian nut samples was observed for countries such as Cyprus, Italy, Morocco, and Brazil (112). Other interesting studies have also reviewed the contamination of Iranian pistachio with AFB1 using different procedures (113). Accordingly, the outcomes suggested that there has been differential AFB1 concentrations in this nut. Based on these results, around 37% of studies reported AFB1 contaminations in the concentration of ≥10 μg/kg, 35% of studies reported ≤ 10 μg/kg, and 28% of studies reported ≤ 5 μg/kg, respectively (113).
In another interesting study, Bui-Klimke et al. also analyzed the global regulations on prevalence of AFs in pistachio samples and reported that pistachio nuts are accounting for substantial quantity of dietary AFs (114). Accordingly, estimations showed that contaminated pistachio nuts remarkably affected the global market of this valuable nut and ignoring the presence of AFs in these samples will increase the health risks of these mycotoxins for target exported/imported locations (114, 115). Presently, global markets between Asia and other world countries have been spectacularly increased (116). Due to the higher rates of AFs occurrence in pistachio samples (114), top producers of this nut should develop modernized infrastructures for drying and processing pistachio to eliminate the expected level of AFs. Increasing monitoring gates in import/export gates of target consumers of pistachio can help to early detection of AFs sources and prevention of APF growth.
Walnut kernel and oil are two important products worldwide. Studies have shown that walnut kernel and oil have an interesting metabolic profile which in turn can be considered as a source of antioxidants and mineral elements (117, 118). Recent findings on the contamination of Iranian walnuts with AFs showed that nearly half of these samples were contaminated with AFB1 in concentrations of 0.8–14.5 μg/kg, respectively (119). Nuts such as walnut maintain remarkable moisture in their structure, leading to providing a favorable environment for APF growth (119). The grown fungi inside walnuts significantly affect these tree nuts quality and destroy their taste and flavor. Applying modern drying technologies to process, drying and shipping walnut and other nuts can extraordinarily decline the occurrence of AFs in such nuts. The prevalence of AFs in salt-roasted nuts is also becoming an emerging concern. In this regard, Ostadrahimi et al. (120) reported that salt-roasted pistachio and peanuts possessed a differential concentration of AFs. Correspondingly, the observed average AFs concentration in these samples was about 19.88 μg/kg in comparison to pure nuts (6.51 μg/kg), respectively (120). These outcomes indicate that processing of economic nuts should be carefully conducted because the occurrence of AFs after nut preparing steps for increasing their taste and flavor is inevitable; therefore, it can pose health risks to target consumers.
Considering the world climate zones map (Supplementary Figure S1), it can be said that the higher prevalence of AFB1 in peanuts harvested from different geographic regions may be related to the type and dominancy of countries' climate. The literature suggested that the moisture content above 17% and warmer temperatures (above 24°C) are effective in inducing the formation of AFs in corn and feed (121, 122). Indeed, the review of literature manifested that due to the higher moisture content of nuts, these products are the main susceptible foodstuffs for AFs contaminations (123). Therefore, in addition to local climatological factors, standard storage of nuts and decreasing the moisture content of these products before entering storage sites and local/global markets can dominantly affect the prevalence of AFB1 in such foodstuffs.
AFs in animal products
A majority of AFB1/2 concentrations in contaminated crops have entered the animal feed network and metabolized to AFM1 (109). In this regard, the evidence suggests that nearly 1% of AFB1 metabolized into AFM1 in dairy cows (124). About 1–3% ingested AFB1 and metabolized AFM1 are excreted by feces and urine (109). However, the remained AFM1 level in the animal body will later enter the human food chain through dairy products. Due to the considerable affinity of AFM1 to dairy proteins (125), it seems that in dairy products with higher protein content (e.g., cheese), the occurrence of AFM1 is more probable compared to other AFs (109, 125). Generally, the occurrence of AFM1 in milk, cheeses, butter, and yogurt is surprisingly high; however, the final concentration of AFM1 in these products depends on processing methods and the quality of animal feed (109, 126).
Today, the presence of AFM1 is becoming a global concern (127), as discussed in previous sections, the frequency of studies on AFM1 has been dramatically increased over the past decades. A recent meta-analysis indicated that the prevalence of this mycotoxin among dairy products is averagely between 40 and 60% which has seriously been considered a biological threat to public health (128). Although the toxicity of AFM1, a hydroxylated derivative of AFB1, is relatively lower than other types of AFs (129), the available evidence indicates that the long-term exposure to AFM1 might be effective in the onset of liver cancer (39, 128). Animals supplemented with AFs-contaminated feed are major sources of AFM1. Because dairy products are unique sources of proteins, vitamins, and calcium, they are becoming the principal part of the human diet (127). Therefore, AFM1 contaminants in these products pose a threat to public health (128), and regular monitoring measurements should be conducted to diagnose target AFs in dairy products. However, to eliminate the AFM1 in animal products, increasing public awareness and regular monitoring of dairy products can be helpful (130).
Scientific studies also reported that the occurrence of AFs contaminants in livestock meat products is probable (131–133). Accordingly, AFs such as AFB1/B2 and AFG1/G2 with different concentrations occurred in meat-based foods (132–134). In an interesting study on meat products collected in Riyadh, Saudi Arabia, incredibly 37.5% of gathered samples were contaminated with AFs, and 4% of samples have exceeded from permissible standards (the acceptable Saudi limit: 20 μg/kg). Correspondingly, AFB1 and AFG1 were the most commonly identified AFs, followed by AFB2, respectively (133). In another study, the occurrence of AFs in meat products such as basterma, sausage, kofta, and luncheon was investigated, and the results unraveled that AFB1/2 occurred in higher concentrations compared to AFG1/G2 (132). Investigations on domestic fowls feeding diets containing AFs also displayed that the accumulation of AFs in their liver is higher than other organs (135). Indeed, the outcomes showed that the highest abundancy of AFB1 was observed for tissues of quails compared to other birds (135).
In another study conducted on meat, milk, and eggs samples, collected in Jordan, the outcomes indicated that the samples were contaminated with AFB1/2, AFG1/G2, and AFM1/AFM2 (136). In milk samples, however, the highest concentration of AFM1 was exceeded from EU standard for liquid milk (50 ng/L) (136). These outcomes suggested that the proportion of AFs contaminants in meat samples depends on several factors, including meat processing methods, post-storage contaminations, and non-standard transportation and shipment facilities of meat products to local and international markets.
Presently, the maximum permissible AFs concentration in animal feeds is 5 μg/kg based on EU limits (137). Therefore, preparing animal feeds from credited sources, improving of the storage condition of livestock inputs (137), improving the quality and accuracy of AFs detection systems (138), improving of animal feed manufacturing procedures (139), and regular monitoring of animal feeds to identify the source of AFs contaminants are possible strategies to reduce the concentration of AF pollutants in dairy products. Governments must seriously deal with providers of livestock feeds that might supply contaminated animal inputs to dairy farms by imposing strict limitations on their business to prevent further consequences of AFs. It is now well-documented that AFB1 was widely found in contaminated livestock products fed on contaminated forage and grain (140). Therefore, due to health threats of fungal toxins to public health, dairy products should be repeatedly monitored to decrease the quantity of AFs contaminants. Such strategies will later decrease the health consequences of AFs and help consumers to reach safe and AFs-free dairy items.
In practice, however, characterization of AFs-contaminated animal feeds is difficult because the available techniques require allocating sufficient time and financial support for early detection of different types of AFs metabolites (137–139). Governments should support researchers in developing accurate, fast and low-cost measuring systems to abate AFs metabolites from suspected feeding resources. As discussed in the previous sections, large quantities of scientific studies have been conducted on measuring processes of AFs diagnosing systems. Expanding academic studies without practical innovations cannot alone help the elimination of AFs in animal feeds; therefore, efforts to convert the results of academic investigations into touchable outcomes should be highly followed to recruit the power of science in controlling health hazardous mycotoxins. As we discussed later, AFs are major environmental risks factors in developing nCDs. Therefore, elimination of these fungal toxins in animal feeds can primarily decrease their occurrence in human food chain.
AFs contaminants in spices and medicinal herbs
Spices are interesting food additives that constructed a valuable financial global market for spice-producing countries (141, 142). Presently, countries such as India, China, Nigeria, Indonesia, Thailand, Vietnam, Bangladesh, Nepal, Ethiopia, and Turkey occupy the first to tenth ranks of the top spice-producing countries (143). Estimations indicated that the demand for fresh, powdered, and processed spices have been increased over the past decades due to multi-functional applicability of these products for various purposes such as traditional medicine, cooking, etc. (142, 144). Interestingly, AFs contaminants also occur in spices with higher content of moisture. Since 2002, the EU has implemented rigorous regulatory policies to identify AFs in spices (36, 144). Accordingly, the permissible concentration of AFB1 and total AFs in spices has been reported up to 5 and 10 μg/kg, respectively (36). Studies proclaimed that well-distinguished spices such as pepper, ground red pepper, paprika, curcumin (or turmeric), chili, nutmeg, and ginger are the susceptible natural food additives prone to AFs pollutants (36, 109).
The evidence also suggests that the highest permissible AFs limits for all foods in the USA are 20 μg/kg (145). In Croatia, the permissible AFB1 and total AFs levels for spices are 30 and 15 μg/kg, while in Bulgaria, the accepted limits are in the concentrations of 2 and 5 μg/kg (144). Iran also follows the EU regulation on spices and the permitted maximum levels of AFB1, and total AFs range in the concentrations of 5 and 10 μg/kg (144). In this respect, several studies comprehensively reviewed the occurrence of AFs in spices (144, 146, 147). The outcomes of these scientific investigations demonstrated that the occurrence of AFs in spices depend on the type and processing methods of spices (144, 146, 147). Compared to cereals, and edible nuts, spices and plant-based food additives are highly prone to maintain higher humidity levels in their structure (148).
On such occasions, opportunist APF can easily grow among stored spices to decrease the quality, taste, fragrance, color and marketability of these popular plant-based food additives (149). In Asian countries, in particular Iran and India, food spices (or additives) are indispensable parts of daily cooking and different forms of spices including raw and processed stuffs can be purchased from local providers. Due to bulk production of spices, these products short immediately after harvest would send for local and international markets. Recent studies on herbal products and spices of different locations of Iran indicated that AFB1 is the most prevalent AFs among these spices and nearly 100% of analyzed red pepper samples were contaminated with AFs (148).
In another interesting study, different samples of commercial spices in Iran has been analyzed using HPLC method to identify the quantity and abundance of culprit AFs (149). According to these results, spices such as cinnamon, turmeric, black and red pepper diagnosed with different concentrations of AFs (149). Similar to the previous results (148), AFB1 was the most domineering AFs among analyzed spices, though different concentrations of AFB2, AFG1/2 were also observed among the evaluated samples (149). These results are in agreement with previous studies that confirmed herbal spices are remarkably prone to AFs contaminants (150, 151).
Monitoring of spices marketed in Africa also showed that the Ethiopian ground red pepper was extremely contaminated with AFB1 in a dose of 250–525 μg/kg (152). Simultaneous investigations on Iranian and Indian spices to detect AFs contaminants purported that spices from these origins were differentially contaminated with AFB1 in a concentration of 63.16–626.81 ng/kg (Iranian samples) and 31.15–245.94 ng/kg (Indian samples), respectively (153). More interestingly, the outcome of this investigation demonstrated that contamination of studied samples was not exceeded from EU standard concentration of AFB1 in spices (5 μg/kg) (153), though AFs metabolites were characterized in the monitored samples. In another study in Turkey, 93 spices and 37 medicinal herbs were evaluated to identify hazardous AFs derivatives. The results manifested that AFB1 was domineering fungal metabolite in nearly 32 herbs and 58 spice samples (154). Resultantly, the maximum concentration of AFB1 was found in cinnamon at the concentration of 53 μg/kg so that the measured concentrations in these samples were obviously exceeded from EU permissible values (154).
Evaluation of marketed spices in Doha, Qatar, showed that Aspergillus and Penicillium spp. were the most prevalent fungi in these samples (155). Interestingly, this investigation demonstrated that five spices, including turmeric, black paper, chili, tandoori and garam masala, were contaminated with AFB1 (155). For the first four samples, the detected AFB1 concentrations have deviated from EU standards (155). Indeed, according to the outcomes of a recent meta-analysis on the occurrence of AFB1 in red pepper, the prevalence of AFB1 among studied samples was 50.8%, respectively (156). This study reported that the minimum and maximum concentrations of AFB1 were detected in Korean and Turkey samples in the concentration of 0.14 and 31.13 mg/kg, respectively (156).
The growing body of evidence suggests health promoting medicinal plants are also prone to AFs contaminants because of their ability to support AFs-fungi growth (12, 157). In an interesting investigation the results displayed that AFB1 metabolite was found among medicinal plants with a significant prevalence percentage (12). The results also confirmed that other AFs metabolites, including AFB2 and AFG1/2, were also characterized in herbal supplies (12). These outcomes are in agreement with other studies that confirmed that AFs are commonly found among medicinal plants in different concentrations (157, 158). More interestingly, herbal products not only hosted AFs metabolites, but also various scientific reports confirmed that OTA is another hazardous mycotoxin found in these herbal stuffs (159). In some studies that revolved around the occurrence of AFs in medicinal plants, spices and herb-teas incongruous results come to view in which spices were contaminated with AFs while medicinal plant samples from tropical countries were free of these hazardous fungal metabolites (36). Evaluation of medicinal plants fungal flora also showed that Aspergillus species were the most superior fungal strains isolated from target medicinal herbs (160).
Monitoring of medicinal herbs in Thailand for AFs contaminants showed that these fungal toxins in the range of 1.7–14.3 ng/g have been occurred in these samples and AFB1 was abundantly detected in the evaluated herbal products (161). Although medicinal herbs are at risk of AFs pollutants, however, it is trustworthy to note that these herbal supplies have the ability to produce specific metabolites to detoxify AFs metabolites. In this regard, in vitro studies have shown that aqueous extracts of medicinal herbs such as Centella asiatica, Hybanthus enneaspermus and Eclipta prostrata displayed nearly 70% degradation of AFB1 (162). Meanwhile, it can be said that the concentration of characterized AFs in medicinal plants and herbal supplies depends on the type of plants, herbal processing methods, storage condition, variation of grown mycotoxigenic fungal strains, temperature and humidity of storage sites, secondary contaminations during herbal supplies storage, and the infestation of pests and plant pathogens to stored herbal products (163).
Contaminated herbal products in local markets are potential health risks to consumers because there are no accurate scrutinizing systems to monitor local herbal suppliers and identify contaminated commodities (164). The local and international markets of medicinal herbs are flourishing yearly, and it is now valued at more than 100 billion dollars (165). Therefore, by regular risk assessment of spices and herbal supplies as well as increasing the number of monitoring gates for local and international markets and also by increasing the quality of processed spices and herbal products and suitable storage and packaging of these supplies, official health and agricultural organizations can significantly mitigate the prevalence of AFs contaminations in this industry (144).
As shown in Figure 8, many herbal products and spices are marketed outdoors in local markets, which in turn might lead to post-harvest contaminations. Because herbal and spice providers might be not cautious enough to identify the source of AFs contaminants; sequentially, AFs-contaminated products may be intentionally or inadvertently passed to consumers, eventually leading to the progression of nCDs. Therefore, increasing the awareness of herbal farmers, manufacturers, and consumers is an effective strategy to eliminate AFs in such herbaceous products (166).
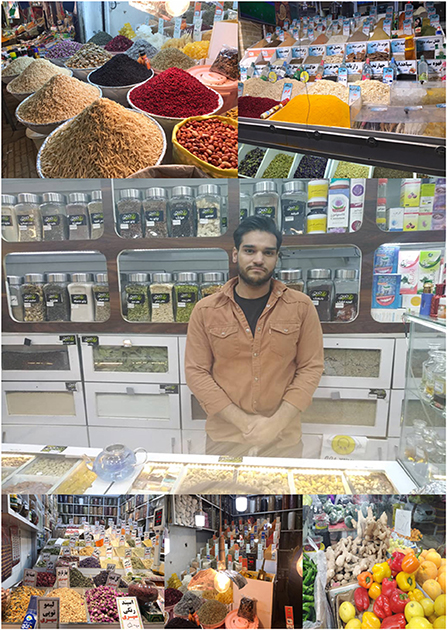
Figure 8. Local herbal and spices markets in Iran. Consumers should carefully check the flavor and taste of purchased spices and herbal products to reduce the health risks of AFs. The color of AF-contaminated spices, in some cases, changed significantly, and characterization of contaminations and spoiled materials is easy. In the case of invisible AFs contaminants, preparing regular samples from local herbal and spice markets will decrease the occurrence of these carcinogenic mycotoxins.
As discussed in previous sections, like many other foodstuffs, spices and herbal products were prone to AFs contaminants (148). In some cases, however, the occurrence of AFs is not in detectable concentrations. Additionally, due to the increasing demand for herbal spices and plant-based food additives (142), many national and international suppliers adhered to spice markets, leading to intensified cultivation and extensive processing of these products. The lack of fundamental infrastructures and suitability of climate factors enhanced the growth of APF, consequently leading to an increased level of detectable AFs contaminants. Therefore, to mitigate the total level of AFs in spices, medicinal herbs, and other popular plant-based food additives, local and international herbal markets, however, should be constantly monitored for detection of AFs metabolites (167, 168). Keeping in mind the most popular quote perhaps assigned to Hippocrates (400 BC) “let food be thy medicine and medicine be thy food” (169), healthy foods are indispensable parts of our dietetic regime and are complement to modern pharmacology (169). Therefore, preparing foodstuffs from mycotoxin-free sources not only improve our lifestyle, but also can decrease the progression of nCDs and improve the quality of daily diet.
In this regard, to bring concentration of AFs in spices and herbal products down, taking precautionary actions, such as raising public awareness, might support consumers to purchase healthy and AFs-free products (170, 171). According to scientific data, AFs contaminants in herbal products used in traditional medicine mainly occur in two stages during drying/processing and storage of target herbs/spices (172–174). Therefore, the lack of strict regulations on herbal ingredients used in traditional medicine might increase the occurrence of AFs in these health-promoting products (174). It is pivotal to implement rigorous regulations on the production, processing, packaging, manufacturing and exporting/importing of herbal products that are prone to AFs contaminations. Improving packaging systems (108) and developing standard infrastructures for the distribution, storage, and transportation of medicinal herbs and spices can also help consumers to use more safer products (175, 176). Providing safety guidelines for preparing herbal products and spices helps the public to broaden their knowledge about the consequences of AFs and associated health complications; therefore, leading to increasing demands for mycotoxin-free commodities and a healthy lifestyle. In the next sections, we will discuss the circulation of AFs in the human body and major AFs health consequences reported in the literature. This helps readers to a better understanding of AFs biological properties and their role in developing human chronic diseases.
Interactions of AFs and human serum albumin protein
Studies have shown that AFs metabolites are prone to bind human serum albumin (HSA) (177). HSA is one of the most prevalent proteins in human blood plasma (178). HSA is a globular protein produced in the liver and constructed from a monomeric structure with several subdomains (179, 180). HSA functions as a carrier in the human body to transport fatty acids, drugs, hormones, and other biomolecules (178). This multifunctional, negatively charged, and non-glycosylated protein also participates in the regulation of plasma osmotic pressure. HSA is formed from 585 amino acids, and its 3D crystallographic structure is well-documented (179).
Structurally, HSA spatial conformation is formed by a heart-shape molecule, possesses three helical domains (I, II, and III), and is divided into A and B subdomains (IA, IIA, IIIA and IB, IIB, and IIIB) (178, 179, 181) (Figure 10). In the structure of HSA, there are two distinct binding sites, including Sudlow's I and II, each prone to bind different types of chemical agents (178, 182). Generally, negatively charged large heterocycles bind to site I, whereas small molecules prefer to interact with site II (182). The decreased concentration of HSA in blood plasma is associated with AD (183), cancer, obesity, diabetes, heart failure, stroke, and venous thromboembolism (178, 184).
HSA plays a critical role in the tissue distribution of AFs metabolites (185). To date, only few studies have been conducted on the possible interaction of AFs and HSA binding sites. The available evidence suggests that AFs could non-covalently bind to HSA binding cavities (185). Evaluating the binding mode of chemical ligands to target receptors helps researchers to characterize the molecular behavior of these molecules in vivo (186). To understand how AFs metabolites might interact with HSA, we computationally investigated the binding affinity of AFs and AF-derived metabolites and HSA binding sites. As explained, only few studies are available to show the exact binding mode of well-known AFs to HAS (185). Therefore, to broaden the literature consistency on this topic, as part of this review 14 AFs metabolites, including AFB1/2, AFG1/2, AFM1/2, AFB2a/G2a, AFP1, AFH1, aflatoxicol, AFB1exo-8,9-Epoxide-GSH, AFB1exo-8,9-Epoxide, and aflatoxin-N7-guanine have been structurally prepared and docked into HSA using supervised and blind docking protocols (187, 188). The docking results for the interaction of AFs metabolites and HSA binding site I showed that docked AFs possessed different binding affinities to interact with HSA site I residues. The calculated binding energies for these metabolites ranged from −6.2 to −9.5 kcal·mol−1, respectively (Figure 9). AFB1exo-8,9-Epoxide, AFB1, aflatoxicol, AFG1/2, and AFM1 significantly formed H-bonds and Van der Waals forces to interact with HSA binding site I.
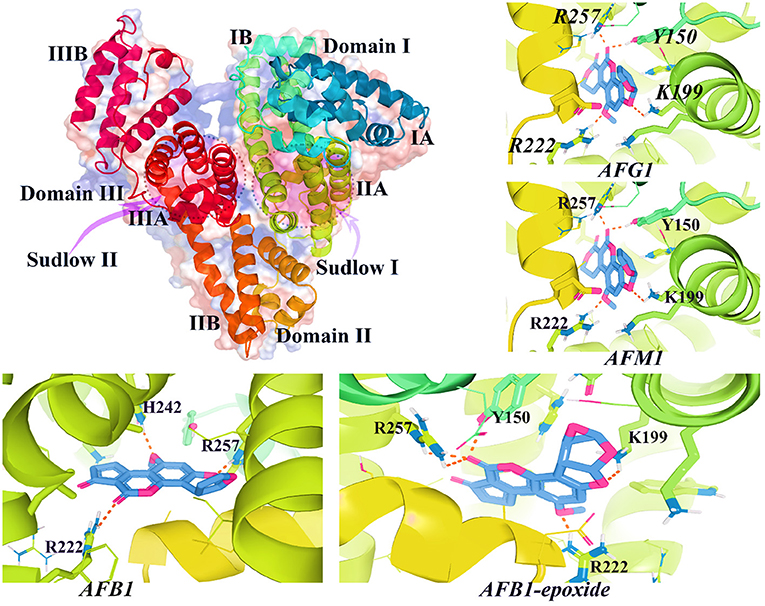
Figure 9. The 3D structure of HAS (PDB id: 1AO6) and interacted AFs metabolites with HSA binding site I. As shown in this figure, AFs metabolites bind to critical active sites of HSA by forming H-bonds and other relevant chemical bonds.
Interestingly, docking results demonstrated that AFs metabolites such as aflatoxicol, AFB1, and AFB1-exo-8,9-epoxide, might also interact with HSA subdomain IB. Previous studies suggested that AFB1/2, AFG1, and AFM1 mainly interacted with HSA binding site I (185), but the binding affinities of the remaining AFs metabolites are not comprehensively investigated in the literature. In the case of other mycotoxins such as zearalenone, the binding mode inhibitory assays showed that this fungal toxin could strongly bind to a non-conventional binding cavity between Sudlow's site I and II (189). More interestingly, OTA has two binding sites in the structure of HSA with different binding constants so that the highest binding affinity for this toxin was observed for subdomain IIA HSA protein (190). Other interesting experimental studies also confirmed that AFB1 is mainly bound to HSA in binding site I located in subdomain IIA with a binding affinity around 104 M−1 (177, 191). Similarly, the results of spectroscopic and computational assays also determined that AFB1 and AFG1 also interacted with subdomain IB residues in HSA (192). AFB1 also displayed a similar binding affinity to interact with bovine serum albumin (BSA) binding site I with a binding constant of nearly 4.20 × 104 M−1 (193). These outcomes together demonstrated the precise interaction of AFs and HSA, leading to a better understanding of toxicokinetic properties of these mycotoxins. Therefore, displacement of HSA-AFs complexes has been suggested as a therapeutic strategy to diminish the affinity of these mycotoxins to HSA and decrease the tissue delivery and uptake of AFs (177).
Decreasing the affinity of HSA to AFs with chemical compounds sharing similar binding patterns with higher affinities in comparison to AFB1 might bring down the toxicity of this mycotoxin for the human body (193). Studies have shown that natural products such as PPs interfere with the interaction between AFB1 and HSA and reduce the transportation of AFs to delivery locations (177, 194). More interestingly, scientific outcomes reported that administration of vitamins A and E could reduce carcinogenic properties of AFs in studied animals (195–197), though controversial results on the protective roles of vitamin E in cancer therapies have been reported (198). These findings are in agreement with previous outcomes demonstrated that exposure to AFs metabolites is associated with plasma micronutrient deficiencies (199). However, mycotoxin metabolites could bind to HSA (189, 193); therefore, these toxins are easily transported to different parts of the human body and causing chronic health consequences (189). In the next section, we explain how metabolized AFs derivatives are accounting for prevalence of nCDs. The discussed nCDs have been selected based on the frequency of conducted studies on each field of interest.
May AFs promote the onset of diabetes mellitus?
DM is a chronic metabolic disease mainly characterized by elevated blood glucose level and insulin deficiency (200, 201). More than 422 million people are expected to suffer from DM in which the number of DM affected people in low-income countries has steadily grown during the past decades (202). To date, various subtypes of DM have been identified by which scientists can treat affected individuals through observed symptoms. Generally, type 1 DM (T1D) and type 2 DM (T2D) are the two the most prevalent subtypes of DM, leading to thousands of deaths yearly. T2D is responsible for more than 95% of all diabetic cases, while T1D only represents 5% of diabetic individuals. T1D is more prevalent among juvenile people and is significantly dependent on insulin deficiency (188, 203). In contrast, T2D is insulin-free DM, by which affected people suffer from elevated blood glucose and associated complications (203).
The emergence of modern drug design technologies leads to the development of potent anti-diabetic drugs. Different anti-diabetic medicines with specific molecular targets have presently been introduced into global markets (200). However, these drugs could not entirely suppress the complications of DM (200, 201), in turn, leading to an increased economic healthcare cost that allocated on caring for DM-affected people.
Recently, the role of exposome measurements has been highlighted in the progression of DM (204, 205). Exposome-associated factors can be divided into external and internal factors. External factors are features that directly linked to nearby environment such as pollutants, chemical materials, lifestyle and dietary regimes (205). Instead, internal factors are accounting for epigenetics alterations, gut microbiota and relevant molecular processes (204). This ongoing paradigm helps to understand how and where exposure to environmental factors lead to the progression of MetSys and other human diseases (204).
As a complicated metabolic disorder, DM progression depends on various factors (206–208). By considering exposome-associated factors in the development of DM, it is worthy to note that the adopting of a healthy lifestyle can decrease the incidence of this metabolic disorder (206). Environmental factors such as exposure to hazardous chemical agents (209) and toxins might increase the onset of DM (210). Indeed, the complex interaction between environmental and genetic risk factors might worsen the health complications of DM (206, 211). Biological toxins might act as health hazardous diabetogenic agents to disrupt normal function of the human body in controlling blood sugar levels and associated signaling pathways (212).
In this regard, evidence-based studies imparted that long-term exposure to particular types of AFs, such as AFM1, might increase health risk factors for developing T2D and other metabolic disorders (213). Interestingly, long-term exposure to AFB1 increased liver injuries in mice, disrupting blood glucose levels, insulin sensitivity, and a high chance of inducing liver cancer (39). Recent studies have shown that type 1 diabetic mice exposed to AFB1 showed a significant reduction in MUP1 levels, in turn, indicated an elevated blood glucose level and decreased insulin sensitivity (214). Molecular mechanisms underlying the diabetogenic effects of AFs are not completely understood, however recent investigations reported that AFs metabolites, in particular AFB1, might influence the regulatory switches of specific signaling pathways, genes, transcription factors, and receptors such as IGF2 and IGF1 receptor IGF-IR (215). In this regard, the evidence suggests that the increased level of IGF2 expression in pancreatic islets is associated with the onset of DM and dysfunction of β-cells (216).
The overexpression of IGF2 affects the functionality of β-cells, leading to chronic endoplasmic reticulum stress and dysfunction of pancreatic islets (216). Indeed, the evidence also suggests that IGF1 plays a critical role in DM by lowering blood glucose levels and insulin secretion (217). Therefore, the interaction of AFs with such molecular targets might negatively cause molecular abnormalities, which later lead to the development of DM. Additionally, hepatorenal injuries, lipid peroxidation, DNA damage, oxidative stress, and inflammation are other symptoms of animal models exposed to AFB1 metabolite (218).
In an interesting study (219), it has shown that the long-term exposure to mycotoxins was significantly associated with DM development in affected rats (219). In this finding, OTA could remarkably increase blood glucose levels, cause damage to pancreatic islets, and decrease insulin secretion (219). The cross-talks between the progression of MetSys and prevalence of HCC have been widely investigated (220, 221). The evidence suggests that MetSys might be connected to the progression of cancer (221). Yesheng et al. meta-analysis (221) reported a possible link between MetSys and pathogenesis of HCC among Euro-US societies, though there has not been association between HCC and MetSys clinicopathological feature (221).
In another study, Marchioro et al. (222) reported that in broilers chickens supplemented with a mixture of AFs (B1/2-G1/2) in the concentrations of 0.7–2.8 mg/kg for 42 days, chickens' performance features and enzymatic activity of pancreas have notably been altered (222). The outcomes imparted that long-term chronic exposure to AFs mixture increased the activity of pancreatic α-amylase and lipase while trypsin levels has been affected by the maximum concentration of AFs mixture (2.8 mg/kg) (222). The literature has disclosed that AFs altered the accumulation of lipids droplets and lipoproteins in addition to the dysregulation of lipid metabolism-related genes (CHO, TAG, PHOL, MDA, Lipc, Lcat, Scarb1, etc.) (223, 224). The evidence imparted that the dysregulation of fatty acids, cholesterol, and other health affecting lipids biosynthesis and metabolism is accounted for the progression of DM (225). Therefore, exposure to AFs in the dose-dependent fashion might contribute to the development of DM and cardiovascular diseases via alteration in the body lipids metabolism pathways (223, 226), though this claim requires future confirmation.
In rats exposed to penitrem A, a highly toxic mycotoxin from Aspergillus genus, a considerable diabetogenic properties has been observed (227). In this regard, chronic exposure to OTA (45 μg/daily diet) for 6–24 weeks caused a significant decrease in insulin levels and increase in blood glucose and glucagon levels (227). The observed diabetogenic activity of OTA is attributed to its impact on degeneration of pancreatic Langerhans islets (227). The elevated diabetogenic effect of mycotoxins in combination with chemical agents such as insecticides has also been investigated (228). Correspondingly, the outcomes displayed a remarkable synergistic interaction between mycotoxins and chemical agents in the onset of DM by increasing blood glucose and dysregulation of liver enzymes (228).
In a cross-sectional study conducted on Guatemalan participants, the outcomes manifested a significant association between AFB1-albumin adduct levels and pathogenesis of DM (229). Additionally, there was no significant association between AFB1-adducts and the progression of other metabolic diseases such as central obesity, obesity, non-alcoholic fatty liver diseases (229). This result, however, was aligning with previous animal-based studies that confirmed the association between exposure to mycotoxins and the onset/progression of DM (219). The evidence imparts that fungal toxins may increase the susceptibility to the onset of MetSys; therefore, well-designed human-based studies are needed to show how mycotoxins and AFs may contribute to the progression of MetSys (213, 230).
AFs and pathogenesis of Alzheimer's disease
According to WHO statistics (231), there are more than 50 million AD-affected people worldwide such the statistics that have projected to increase by 2050 (187, 232). This prevailing neurodegenerative disorder is chiefly characterized by a remarkable decline in thinking, memorial dysfunction, unpredictable behaviors, language problems, and cognitive impairments, in turn, sequentially causes significant damage to the brain cells (231–233). Accordingly, the lesion of brain cells, the accumulation of amyloid plaques, neurofibrillary tangles, oxidative stress, NIF, and synaptic dysfunction are typical clinical symptoms of AD (187, 232, 234).
Different hypotheses have been postulated for the progression of AD; however, it is not completely clear which of molecular switches drives the inception of AD to cause obvious damages to the brain (234, 235). Scientists suggested that environmental and genetic risk factors, exposure to chemical pollutants, heavy metals, mycotoxins, lifestyle, age, infections, cardiovascular dysfunctions, T2D, cellular senescence, and head injuries may play a critical role in pathogenesis of AD (232, 236–238). Studies have shown that the AFs metabolites can alter various brain enzymatic actions, leading to AD development. For instance, in rodent models, exposure to AFB1 could significantly decrease the activity of brain protein kinases (239). The SH-SY5Y human neuroblastoma cell lines exposed to 100 and 50 μM AFB1 and FB1 mycotoxins for 24 h, manifested a significant increase in ROS formation, though the trace of endoplasmic reticulum stress was not observed (240). On the contrary, in adult male rats treated with 25 μg/kg/week AFB1 for 8 weeks, AFB1 could trigger obvious neurotoxicity, inflammatory responses, oxidative stress and, anxiety and depression-like behaviors (241). The finding showed that AFB1 supplementation was linked to a reduction in the activity of GSH, GST, SOD, and GSH-Px enzymes and increased MDA, IL-1 and TNF-α levels in right region of cerebral tissues (241).
The AFB1 also negatively influenced the distribution of astrocytes in rats' cerebral cortex and hippocampus (242). The effects of AFs on the BBB were also investigated such that the outcomes showed that AFs (in particular AFB1) could alter mitochondrial gene expression profile in the human BBB cells model (243). More interestingly, AFB1 could inhibit the electron transport chain function, affect ATP synthesis and dysregulate key genes in mitochondria (243), leading to genetic mutations and DNA damage (244).
The AFB1-NIF is attributed to the interaction of AFB1 metabolized derivatives with neuroinflammatory signaling pathways (245). It is now well-established that neuroinflammation promotes the pathogenesis of AD and other neurodegenerative diseases (246). The molecular mechanisms underlying neuroinflammation have partially been investigated, however, little is known on how exposure to mycotoxins may have impact on the incidence of AFB1-NIF (247).
To elucidate the AFB1-NIF mechanism of action, it is important to take this question into consideration how AFB1 metabolites may alter the NIF signaling pathways? Briefly, the activation of microglial cells elevates glial neuroimmune responses (248, 249). Next, CNS-related genes might be up- and/or down-regulated, sequentially resulted in the reactivity of astrocytes and expression of pro-inflammatory molecules such as IL-1/1β/6, INF-γ, and TNF-α (248). Activation of these neuroinflammatory signaling mediators will increase the ROS/RNS levels in the brain, leading to a significant oxidative/nitrosative stress and neuronal damage (246, 250).
Studies have proven that TLRs, MAPK, MyD88, CxCR4, PI3K/AKT, mTOR, COX-2, iNOS, Nrf2, HO-1, γ-enolase, STAT, AMPK, JAK, and NF-κB signaling pathways are major components of NIF (248, 251, 252). The alteration of kynurenine/tryptophan ratio (253), dysregulation of intracellular protein kinases (PKs) (254), the loss of neuronal integrity (255), and dysregulation of neurotransmitters signaling circuits are other pivotal components of NIF in response to brain abnormalities (256). The evidence introduced thus far supports the scenario that AFB1 enhances the secretion of pro-inflammatory cytokines such as TNF-α and IL-6 in CNS-derived cells, leading to the promotion of immune responses and significant oxidative stress in the CNS (247). These elevated level of neuroimmune reactions and activated signaling pathways in astrocytes and glial cells have been reported as consequences of AFs (257). Interestingly, low and high-dose exposure to AFs might alter the activity of brain signaling cascades based on exposure time duration and toxicodynamic properties of culprit AFs (258).
The acute exposure to AFs could notably affect the expression of genes and enzymatic activation in the brain. In rats, acute treatment with AFB1 influenced the activation of protein kinase C by phosphorylation of Ser957 position in the cerebral cortex (259). CCK is another critical protein kinase in the brain accounted for pathogenesis of AD (260). Studies have revealed that the functionality of brain cells depends on ATP molecules produced by CCK (245). Blocking CCK activity is associated with energy depletion in the brain, which can lead to significant oxidative stress and brain abnormalities. AFB1 inhibited the CCK enzyme to decrease ATP metabolism and trigger oxidative stress in the brain (245, 261).
Park et al. reported that AFB1 decreased human astrocyte cell proliferation by arresting cell cycle, sequentially induced the mitochondrial dysfunction and apoptosis of astrocytes (262). Accordingly, the interesting part of this finding that is AFB1 dysregulated calcium hemostasis and increased ROS formation, leading to neurotoxic effects on astrocytes cells in vitro and in vivo (262). In female Wistar rats given 15.75 μg/kg/orally for 8 weeks, the outcomes suggested that AFB1 decreased the distribution of astrocytes in frontal cortex without effect on neuronal numbers (242). In contrast, AFB1 increased neuronal number and decreased astrocyte distribution percentage in the hippocampal CA1 subfield. Importantly, the withdrawal of AFB1 restored the observed changes in rat brain (242).
In the support of these outcomes, Alsayyah et al. reported that the severity of chronic neurodegenerative effects of exposure to AFB1 is associated with astrocyte immune responses and alteration of brain enzymes (239). Accordingly, chronic exposure to AFB1 altered the activity of antioxidant enzymes (GPX, CAT, SOD, GSH), increased the activity of AP and LDH, and decreased CK activity (239). The observed AP and LDH increased activity are attributed to neuronal death, astrocytes damage, and necrosis (239). This finding also indicated that the chronic side effect of long-term exposure to AFB1 depends on the passed quantity of these toxin from the BBB and duration of exposure (239). In another study, animals feed with 5 ml AFB1 for 8 weeks also showed noxious neuronal degenerative changes in cerebral cortex (263). Another animal-based investigation also manifested that AFB1 increased the activity of AChE and ADA enzymes (264). The up-regulation of these enzymes might be responsible for elevated level of inflammatory responses due to tissue damage. Additionally, AChE and ADA may be contributed to clinical signs of apathy because of their participation in neuromodulation and neurotransmission (264).
By the way of illustration, the evidence suggests that AFB1 triggered acute neurodegenerative consequences in the CNS, induced encephalopathy by influencing glutamate neurotransmitters, increased ATP depletion, modified brain catalase, SOD, MDA levels, and GST concentrations (245). What is outstanding in these outcomes is that differential exposure to AFB1 induces oxidative stress (265, 266) and neuronal damage in the brain (245). Additionally, exposure to AFB1 is associated with reduction in CNS phagocytic ability, increased levels nitrosative stress, increased expression of cytokines (TNF-α and IL-1β/6/8/10) (267), induced microglia cell apoptosis, dysregulation of p-NF-κB signaling pathway (268), significant alteration in brain integrity, substantial DNA damage, S-phase cell cycle arrest (269), and other neuroimmunotoxic complications (245). AFM1 also could degrade the BBB structure by influencing astrocytes, vascular endothelial and microglia cells to trigger remarkable neurotoxicity in the brain (262, 270). These data indicated that AFB1-NIF affected brain enzymatic and none-enzymatic reactions as well as other CNS molecular components, ultimately leading to the onset of AD.
As an epidemiological standpoint, the clinical framework of AD pathogenesis and exposure to AFs has still not transparent, consequently further well-supervised trials should be conducted to know how do exactly these mycotoxins contributed to molecular dysfunctionalities in the brain (245). As explained earlier, the neurotoxicity and side effects of AFs in the brain have been documented through in vitro and animal studies, therefore, for cautionary reasons the elimination of these mycotoxins in food/feed should be repeatedly followed to reduce their clinical end effects.
AFs and the onset of cancer
Cancer is one of the most lethal chronic diseases, leading to millions of deaths yearly (271, 272). According to global statistics (271, 273), the prevalence of cancers is remarkably increased during the past decades (271, 273). Still, an efficient and safe medicine for the treatment of cancer has not been introduced (274). Among all influencing environmental and genetic risk factors, the carcinogenic effects of fungal toxins in contaminated foods raised global concerns about the prevalence of cancer (271). As discussed, studies have reported that AFs are toxic, carcinogenic substances (154). These fungal metabolites disrupt the normal activity of signaling pathways, gene expression, and enzymatic activities in the human body. The evidence suggests that long-term exposure to high concentrations of AFs remarkably influences liver and kidney function (19). Briefly, AFs affect the expression level of many genes involved in phase I and II metabolism in the human body (275). Compared to other xenobiotics, the highly liposoluble AFs are rapidly absorbed at the site of exposure (276). Studies have shown that respiratory tracts and gastrointestinal organs are two major sites for the absorption of AFs into the body (276, 277).
AFs affinity to carrier proteins in the body helps these carcinogens to enter the blood and circulate around tissues and organs (191, 194). Highly toxic and reactive AFs metabolites bind to DNA to form AFs-DNA adducts (278, 279). Studies have reported that the interaction of AFs and DNA causes significant damage to DNA and associated biological processes such as transcription and chromatin packaging (280). Binding AFs to DNA and key enzymes in the liver and other organs can induce cancer in different ways. Studies suggest that occurring mutation in specific sites of DNA and proteins is linked to the pathogenesis of cancer (281).
The formation of AFs-DNA adducts can affect the topology of packaged DNA and DNA conformation (276, 278). The exo-8, 9-epoxide metabolites of AFB1 are surprisingly prone to construct DNA adducts. This AFB1 metabolite is highly reactive, and its genotoxic effects have been well-documented (276, 278). Aflatoxin-N7-guanine metabolite also binds to the DNA, induces transversion mutations (pyrimidine ⇆ purine), affects the expression of tumor suppressor proteins and transcription factors, and ultimately dysregulates cell cycle events (276, 278). Acute exposure to AFs pollutants disrupts the fundamental function of genes involved in the glutathione pathway (282, 283). Studies reported that AFs could dysregulate the cellular levels of PKC, PKA, p53, CDK, NF-κB, Bcl2, CKI, and cyclins (276). Disruption of mitochondrial function, ATP synthesis, and mitochondrial gene expression profile have been observed as side effects of AFs in the animal and human bodies (276).
The growing evidence suggests that AFs also influence the expression of xenobiotics metabolism genes, leading to upregulation of CYP3A4 and pregnane X receptor (PXR) (275). In this regard, scientific outcomes have proven that the activation of PXR upregulates phase I and II metabolism genes and proteins such as CYP2B6, CYP2C9, CYP3A4, CYP3A7, UGT, GST, and SULT enzymes (275, 284). The dysregulation of MDR1 and OATP2 genes after activation of PXR has also been reported in several studies (275, 285). Studies reported that in primary liver cancer and cirrhosis, these genes are significantly overexpressed, leading to highlighting their critical role in the pathogenesis of cancer (286, 287). Considerable number of scientific investigations also demonstrated that exposure to AFB1 could disrupt the expression of ERK, PKC-β, COX-2, caspases3/7/9, ASK1, SAPK, STAT3, E2FA, MYC, Bax/Bak, PUMA, CDKN1A, p21, DNA/RNA polymerases, PLK, MAPK, and TRPs signaling pathways (288–291).
Additionally, continuous exposure to AFB1 promotes epigenetic modifications in liver cells (292). As detailed in the literature, AFB1 triggered various epigenetic alterations such as increased levels of aberrant DNA methylation, histone post-translational modifications, and up/down-regulation of non-coding RNAs (ncRNAs) and transcription factors (TFs) (293). AFB1-induced epigenetic drivers in the liver cells are associated with the development of hepatocellular carcinoma (14). Both AFB1-Lysine-protein and AFB1-DNA adducts inhibited the fundamental molecular process of infected cells by preventing transcription/translation of target genes. AFB1-based epigenetic alterations potentially increased the level of genomic mutations, inhibited the interaction TFs-gene-promoter complexes, modified the normal pattern of ncRNAs expression (e.g., miR34a/21/221 and lncRNA-H19), and altered signaling pathways (14, 293).
The evidence suggests that AFs strengthen the consequences of hepatocellular carcinoma risk factors such as DM, obesity, over-drinking alcohol, and viral infections (HBV, HCV) to influence the onset and progression of this catastrophic diseases (294). On the other hands, studies proven that AFB1/M1 end effects are not limited to liver cells so that it was shown that these AFs could also influence the metabolic profile of kidney (295). For example, in CD-1 mice co-treated with AFB1+AFM1 (0.5 mg/kg + 3.5 mg/kg) for 35 days, the results manifested that AFB1/M1 promoted the onset of oxidative stress in mice kidney, altered proline dehydrogenase and L-proline levels, sequentially induced upstream apoptosis, in turn, leading to kidney damage (295).
Evaluation of PPI networks of genes/proteins involved in AFs and xenobiotics metabolism unraveled that these genes (or proteins) constructed a network of interactions with various key proteins in cancer-linked signaling pathways. Up or down-regulation of these genes provides a ground for toxicity of AFs. In the human body, genes including CYP1A2, CYP3A4, CYP2A13, GSTT1, GSTM1, EPHX1, AKR7A2, and AKR7A3 are driving AFs metabolism (296). The upregulation of these genes affects the expression of upstream/downstream genes, leading to a remarkable disturbance of cancer-associated signaling pathways (296) (Supplementary Figure S2). Studies have also shown that genetic polymorphisms in the structure of dominant genes involved in AFs metabolism might increase the risk of developing cancer (297).
Due to the presence of complex interactions between genes, proteins, and transcription factors in the human body, modification of gene expression levels after exposure to AFs can surprisingly affect the genotoxic, immunosuppressive, and mutagenic properties of these fungal metabolites (278). However, our literature searches showed that AFs interfere with DNA integrity, increase the secretion of pro-inflammatory factors, cytokines, and chemokines, inhibit DNA repair mechanisms, induce genomic instability, increase lipid peroxidation, induce DNA damage, cause tissue necrosis and organ failure, and dysregulate innate and adaptive immunity (290, 298–300). Reactive metabolites generated from AFs metabolism are highly toxic for body tissues, leading to obvious oxidative stress and the accumulation of ROS/RNS radicals (301).
Indeed, DNA-AFs structures can inhibit protein synthesis by disrupting the interaction of transcription factors and polymerase enzymes with DAN or increasing the occurrence of mutation in promoters and coding sequences (290). Considering the PPI networks, it is trustworthy to note that the dysregulation of the AFB1 metabolism network might be associated with disruption of gene expression networks in hepatocarcinoma, colorectal, pancreatic, melanoma, thyroid, bladder, and other types of cancers (Supplementary Figure S3). It seems that chronic exposure to AFs expedites a wide range of molecular irregularities in cells, ultimately leading to the pathogenesis of cancer, and more probably other nCDs.
Cross-links between exposure to AFs and the onset of NCDs
According to WHO statistics (302), nCDs are accounted for more than 41 million deaths yearly, in turn, 77% all deaths occurred in low or middle-income countries (302). Environmental and genetic risk factors, such as exposure to biological toxins, pesticides, air pollutants, smoking, alcohol, unhealthy diet, cholesterol, obesity, physical inactivity, mental stress, work tension, elevated blood glucose, and blood pressure are common factors in triggering nCDs (303–306). Studies manifested that withdrawal of environmental risks factors and decreasing the exposure rate to health hazardous substances are effective strategies in preventing nCDs (306).
In the case of AFs, however, it is worthy to point out this fact that the elimination of these mycotoxins in food/feed is impossible, leading to the continuous existence of these risk factors in the environment. As evidenced in the previous sections, exposure to AFs is associated with development of liver cancer, though its role in the progression of MetSys and neurodegenerative diseases has not extensively been investigated. Therefore, this part of our review, by focusing on data obtained from high-tech omics-assisted outputs, describes how AFs may alter biological networks and/or gene expression profile being effective in the onset and development of nCDs.
Integrated transcriptomics and metabolomics analyses conducted on male Fischer rats given 0.25–1.5 mg/kg/b.w. AFB1 for 7 days reported that exposure to low-high concentrations of AFB1 is associated with dysregulation of tumor suppressor genes (at least 27 critical genes were up/down-regulated), antioxidant enzymes, cyclins, cyclin-dependent kinases, cytokine receptors, and inflammatory signaling pathways (307). According to this finding, acute exposure to AFB1 resulted in p53-induced oxidative damage, dysregulation of gluconeogenesis, and lipid metabolism, in turn, leading to hepatotoxicity of AFB1 (307).
In ducklings exposed to 0–40 μg/kg/b.w. AFB1 for 2 weeks, the RNAseq data disclosed that at least 749 transcripts responded to chronic exposure to AFB1 (308). Interestingly, these genes were critical components of phase I/II metabolism (CYP1A5, CYP2H1, CYP2K1, CYP2F3, etc), antioxidant enzymes (GST1/3/K1), fatty acid metabolism (ACAA1, ACOX1, ACAT1, FADS1, FASN, HADH, etc), apoptotic genes (CASP3, CBR1, CCBL1, PPIF, KRT18, etc), protein kinases (PLK2), oxidative responsive genes (AKR1A1, AR, AO, FMO3, GPX4, NQO1, TXN, TDO2, etc), cell cycles and cancer-associated genes (PRELID1, PLK2, UGT1A8, MDM2) (308). This study manifested that phase II detoxification enzymes such as GST1, GSTK1, GST3 were up-regulated under chronic exposure to AFB1 (308), though previous studies found that these genes were down-regulated or not significantly influenced due to the difference between animal models selected for omics-based investigations (308, 309).
In Wistar male rats received 100–200 μg/kg intraperitoneal AFB1 for 4 weeks, the high-throughput gene expression analyses showed that exposure to AFB1 altered gene and lncRNAs expression (310). According to this finding, the identified differentially expressed lncRNAs were associated with upregulation of genes involved in cancer, apoptosis, DNA repair, and cell cycle arrest (310). Because several genes such as Bcl2, MAPK8, and NFKB1 were up-regulated after exposure to AFB1, therefore, it has suggested that apoptotic-associated responses to AFB1 exposure played a critical role in hepatotoxicity of this mycotoxin (310). Similarly, in another omics-assisted study on chickens, the outcomes showed that exposure to AFB1 dysregulated genes involved in apoptosis and lipid metabolism in liver (311). This finding highlighted that Bcl-6 gene was down-regulated whereas PPARG was up-regulated, in turn, might be leading to hepatic fat deposition and hepatocellular apoptosis (311).
The whole transcriptome of BFH12 (bovine fetal hepatocyte cell line) exposed to 0.9–3.6 μM AFB1 for 48 h displayed that AFB1 significantly dysregulated the expression of genes involved in inflammatory responses, apoptosis, oxidative stress, cancer and xenobiotics metabolism (312). Indeed, this investigation disclosed that exposure to low-high concentrations of AFB1 markedly influenced the activation of TLR2, p38β MAPK, AP-1 and NF-κB signaling pathway and pro-inflammatory cytokines (312). This outcome is important because a large quantity of studies published on toxicity of AFB1 for the animal and human bodies addressed oxidative stress and inflammatory responses as health consequences of this carcinogenic mycotoxin.
Bao et al. (313) reported that in Caco-2 cell lines exposed to 0.0005–4 μg/ml AFM1 for 48 h, totally 165 genes were down-regulated after exposure to AFM1. This finding demonstrated that exposure to AFM1 is associated with dysregulation of CDK1, AMPK, SOS1/Akt signaling pathways that are involved in cell cycle arrest (313). The metabolomics and transcriptomics analyses of mice liver and serum showed that co-exposure to AFM1 + OTA (3.5 mg/kg/b.w. for 35 days) significantly affected the phase I metabolism enzymes (ALT, AST, glutamyltransferase) by increasing their levels (314). Additionally, due to synergistic side effects of AFM1 + OTA, the accumulation of lipid droplets and liver steatosis were observed in co-treated groups (314). The metabolome profile of groups co-exposed to AFM1 + OTA disclosed that in liver and serum lysophosphatidylcholines levels were significantly increased (314). In another transcriptomics study conducted on wild and domesticated turkey exposed to 0.015–0.32 mg/kg AFB1, a significant dysregulation in phase I and II metabolism, inflammatory responses, and apoptotic genes was observed, in turn, provided another evidence for side effects of AFs on cellular and molecular targets (315).
As evidenced in these findings, chronic and acute exposure to AFs lead to remarkable alterations in cellular and molecular pathways. Based upon the given standpoints in the previous sections, it is important to note that continuous exposure to low-high doses of AFs certainly promotes the onset and progression of nCDs in societies with higher risks to encounter AFs in food/feed. These studies also addressed the role of inflammation, apoptosis and oxidative stress in progress of AFs-induced consequences, which in turn, can be considered as a scenario to characterize the susceptible signaling pathways and enzymes to develop future clinical management strategies.
On the other hand, the growing body of evidence postulates that the public health impact of climate change is negative, might leading to expedite the onset of nCDs (316). Climate change alone is one of the most important drivers of AFs production under future conditions (72). Other climate change associated environmental risk factors such as improper storage of crops, infestation of secondary pests/pathogens, humidity and temperature can strengthen the production of AFs and other carcinogenic mycotoxins (53, 62, 72). Therefore, more contaminated foodstuffs will be entered local and international food chains, in turn, probably leading to increased risk of nCDs (305). The high-throughput outcomes of multi-omics-based data disclosed that AFs mainly have impact on the inflammatory responses and antioxidant defensive system. In HCC, however, dysregulation of inflammatory responses and antioxidant enzymes promoted the onset of hepatocarcinoma cancer (317–319), though these factors function in corporation with a complex network of protein-gene interactions. However, for other nCDs such as DM and AD, the mechanism underlying inflammatory responses, antioxidant enzymes, and cellular signaling cascades is markedly a sophisticated process and dysregulation of these pathways has a significant negative effect on the progression of diseases. Therefore, elimination of AFs risk factors in food/feed might, at least, provide a ground to decline the onset of nCDs. Figure 10 represents a cross-link between exposure to AFs and the onset of nCDs. An exciting point should be highlighted that is nCDs such as cancer, AD, and DM shared a commonality in the occurrence of oxidative/nitrosative stress and inflammatory responses. As shown in Figure 10, the onset of inflammation is the core component of these nCDs, in turn, leading to cellular apoptosis, dysregulation of enzymatic activity and ultimately the severity of nCDs pathogenesis.
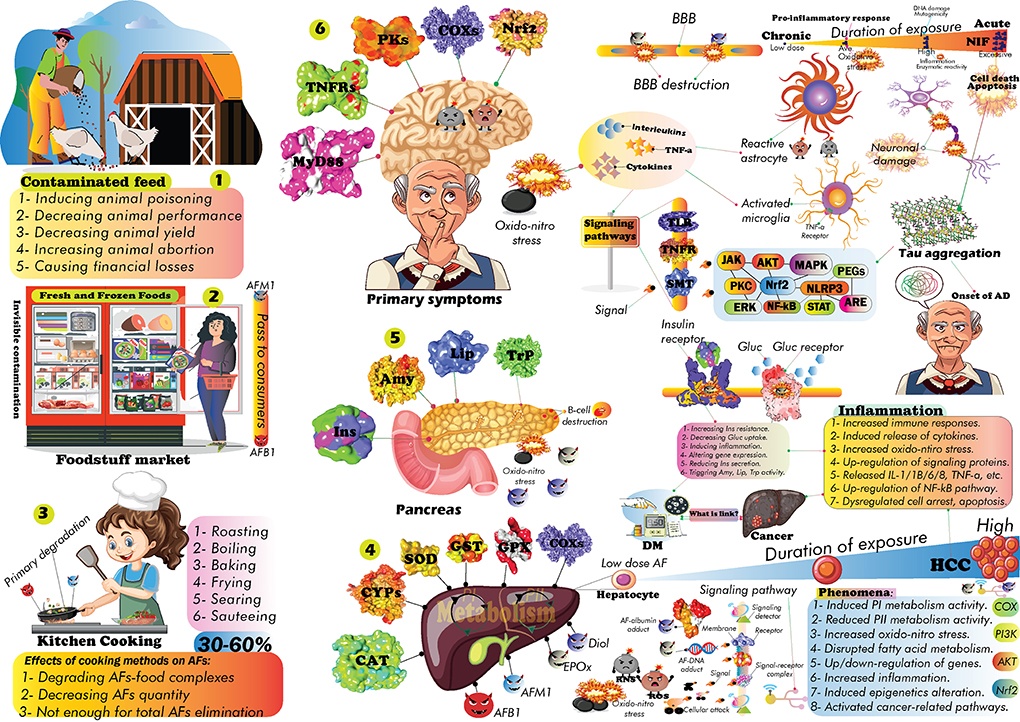
Figure 10. The cross-links between exposure to AFs and the progression of nCDs. Contaminated feed caused damages to animal farms and produced products will pass to the consumers (Steps 1–2). Food processing methods can effectively be used to eliminate nearly 30–60% of total AFs based on the cooking method (Step 3). The rest of AFs residues in food will later affect critical organs to trigger the onset of nCDs (Steps 4–6). Duration of AFs exposure plays a critical role in the pathogenesis of AFs side effects by affecting signaling pathways, triggering inflammation and oxido-nitro stress.
Phytometabolites interventions in AFs-induced health problems
According to FDA guidelines (320, 321), there has been no medicine to prevent the poisoning of AFs, though several protective interventional substances (e.g., NovaSil clay, chlorophyllin, oltipraz, sulforaphane, and tea PPs) have been addressed (322). Safe withdrawal of AFs, using modern agricultural practices, improving food processing methods, and preventing the consumption of contaminated foods have been reported as scenarios to reduce AFs quantity in food commodities (320–322). Recent findings suggested that the supportive and symptomatic care is a reliable health management strategy to reduce the poisonous effects of AFs (323). Correspondingly, using specific carbohydrate-rich and protein-restricted diets followed by administration of vitamins (e.g., B and K) can be helpful in the suitable prevention of AFs end effects (323). However, preventative methods should be cost-effective, and available for all individuals. By considering the link between climate change, onset of nCDs, and AFs health complications, it can be said that phytochemicals and plant-based products are contemporary solution in preventing the poisonous effects of AFs.
It is now well-documented that plant secondary metabolites are health promising compounds in the cornerstone of human diseases prevention (324). Medicinal herbaceous metabolites are ubiquitously found in fruits, vegetable, and even inedible plant materials (324). Efforts to reduce the complications of AFs demonstrated that plant-based products such as extracts, teas, essential oils, pure metabolites can decrease the toxicity of AFs by maintaining cellular normal function (324, 325). These herbaceous materials not only display health promoting properties in detoxification of AFs, but also prevent the formation of AFs in producing molds (326). Considering the link between exposure to chronic/acute doses of AFs and progression of nCDs, and also paying attention to this fact that there is no antidote to treat AFs poisonous effects (323), screening natural products pools to identify anti-AFs substances can alternatively help scientists in developing practical medicines against these biological carcinogens (20). Recently, the potential benefits of plant extracts and naturally occurring phytochemicals in mitigating mycotoxins consequences has been partially reviewed in the literature (324). Accordingly, herbaceous products could show rigorous antifungal activities to improve enzymatic function in degrading AFs (324) and enhancing their metabolism.
Despite the effectiveness of phytochemicals in reducing the severity of AFs side effects, there have been no comprehensive clinical studies to use these herbaceous metabolites for detoxifying of AFs. However, among natural products PPs are presently taken into consideration to detoxify AFs, and their health benefits expedited scientific investigations in this respect (191, 327–336). The popularity of PPs can be attributed to their spectacular antioxidant and biological properties (337, 338). According to our literature searches, hundreds of studies have been conducted on anti-AFs properties of PPs to show how these phytochemicals might ameliorate the toxicity of AFs in the animal body (339, 340). Previous studies substantially confirmed the health promoting impacts of PPs in alleviating MetSys (341), neurodegenerative (342) and chronic diseases (343).
General overview of PPs chemistry
PPs are a diverse category of plant secondary metabolites, displayed health-promoting properties, and are marketed as dietary supplements (338, 344, 345). Originally, PPs are defensive metabolites in plants secreted in response to abiotic and biotic tensions (346). It is now well established that the regular consumption of PPs and/or PPs-rich foods are linked to a healthy lifestyle (347–349). To date, the chemical structure of more than 8,000 PPs has been characterized, and the emerging evidence suggests that global demands for PPs-rich foods (or supplements) have increased over the past decades (344, 347).
PPs could scavenge free toxic radicals and display potential antioxidant activity (350). Evidence-based data reported that PPs showed no toxicity effects on the human body (338, 350). These metabolites mainly function as potent anti-bacterial, antiviral, anti-diabetic, anti-cancer agents, and are modulators of molecular signaling pathways (351, 352). A plethora of studies has been conducted on PPs to modify their structure in developing reliable medicines for treatment of human diseases (353). After ingestion of PPs-rich foods, these metabolites are metabolized in liver, enter the blood circulation system and are transported to different parts of the human body (345).
The chemical backbone of PPs consists of at least one aromatic ring and several OH groups. In this regard, PPs can be classified into two main groups, including flavonoids and non-flavonoid metabolites (346). Simple PPs mainly shared C1–C6 and C3–C6 backbones. These PPs have a low molecular weight and are widely biosynthesized in flowering plants. Instead, flavonoids share a C6–C3–C6 backbone, and two benzene rings are existed in their structure (354). Flavonoids are among the most abundant and well-studied PPs, displayed potential antioxidant and health-promising effects. This class of PPs divided into several subgroups with distinct biological and chemical features (355).
Studies have shown that the biological activity of flavonoids is associated with the substitution of functional chemical moieties on their backbone (356). In nature, flavonoids and other PPs mainly occur in the form of O-glycosylated metabolites. These highly hydroxylated PPs are prone to other chemical modifications such as methylation and acetylation (347). The review of the literature showed that the majority of studies on PPs targeted O-glycosylated PPs, while the biological properties of C-glycosylated PPs have remained relatively unclear (357, 358). Stilbenes, lignans, curcuminoids, coumarins, and xanthones are other classes of non-flavonoid metabolites with potential biological activities (345, 347, 359). Figure 11 summarizes relevant information on PPs.
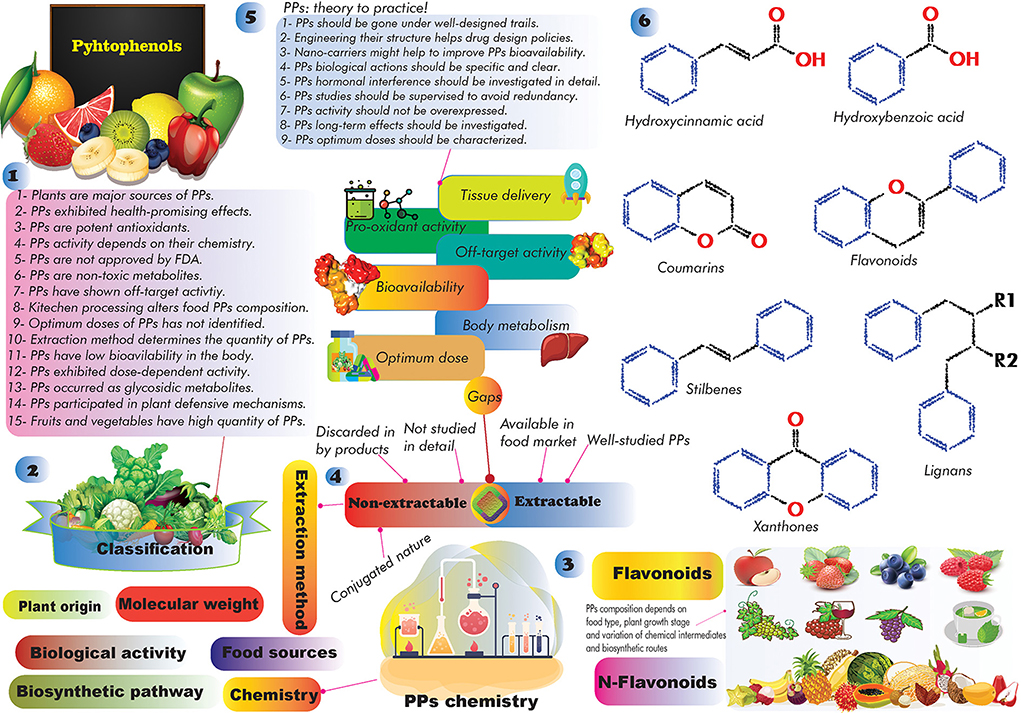
Figure 11. A quick glance at PPs. (1) general facts about PPs, (2–4) PPs classification systems and relevant gaps, (5) PPs ignored facts, (6) the core backbone of well-known PPs. R1 and R2 represent the chemical moieties prone to bind these positions.
Using modern biotechnology for ME of PBPs
The advancement of biotechnological methods supports researchers to integrate new genes, and other interested molecular components into target organisms for improving their qualitative and quantitative traits (360, 361). Genetically modified organisms have a particular gene expression profile in which genes might be silenced or over-expressed to obtain the desired traits (362–365). In this regard, ME of PBPs received much attention from academic and industrial sectors due to health benefits of PPs (366). Today, sufficient pieces of information about PBPs are available to introduce their biosynthetic genes into new hosts for overproduction of these highly valuable metabolites (360, 366).
The ME of PBPs can be performed in different ways, including overexpression of PPs biosynthetic structural genes and transcription factors, up and down-regulation of genes/enzymes involved in the biosynthesis of certain PPs, silencing of specific metabolic routes in phenylpropanoid pathway, down-regulation of PPs biosynthesis structural genes using interference miRNAs, and enhancing the production of intermediary substrates of phenylpropanoid enzymes (365–369).
In this regard, several lines of evidence suggest that the metabolic engineering of PBPs could enhance crop resistance against plant pathogens (369, 370). In an interesting study, ME of PBPs in soybean hairy roots influenced root resistance to fungal pathogens (370). Additionally, scientific outcomes also reported that the presence of gallic acid and hydrolysable tannins in the pellicle tissues of walnuts were accounting for prevention of AFs biosynthesis (371). Biofortification lignin biosynthesis in plants has been considered as a defensive strategy in the retardation of pathogen entrance and growth (372). For example, the overexpression of OsWRKY89 gene in GM rice plants has shown to influence resistance to rice blast by modulating the biosynthesis of PPs and increasing the lignification process of GM lines (373).
The overexpression of PAL gene in GM tobacco plants could enhance their resistance against Phytophthora parasitica and Cercospora nicotinae fungal pathogens (372, 374). In this respect, studies manifested that transformation of other key regulators of phenylpropanoid pathway along with PAL gene might increase phenolic content (in particular rutin and chlorogenic acid) of GM plants compared to wild-type lines (375). These outcomes, as documented in the literature, indicated that production of PPs-rich GM plants not only improved plant resistance to fungal pathogens, but also enhanced their antioxidant properties (372). Correspondingly, engineering of flavonoids pathways in flax plants has shown to increase the accumulation of fatty acids in GM flax seeds and oil (376). This outcome has shown that the overproduction of flavonoids resulted in the prevention of lipid oxidation during seed development and maturation and increased its antioxidant properties for biomedical applications (376). Multi-level engineering of tomato plants by targeting specific transcription factors (e.g., AtMYB12) has been increased the fruit dry weight, carbon metabolisms, and improved the functionality of shikimic acid and phenylalanine pathways (377). This outcome indicated that the accumulation of phenylpropanoid bioactive metabolites not only improved the quality of engineered tomato plants, but also provided a ground for biofortification of healthy foods enriched in health promoting secondary metabolites (377).
The overexpression of maize Lc gene in transgenic apples spectacularly increased the production and accumulation of anthocyanins and falvan-3-ols resulted in a higher resistance to Erwinia amylovora bacterium and Venturia inaequalis fungus (378). In another line of research, the cloning of Solanum sogarandinum 7-O-glycomethyltransferase enzyme in transgenic flax plants significantly increased the accumulation and stability of flavonoid glycosides (anthocyanidins and flavonols), leading to a higher resistance to the fungus Fusarium and improved antioxidant and oil content of GM flax (379). Further information regarding scientific investigations on ME of plants PBPs can be obtained in the available literature reviews (366, 367). Indeed, Microbial production of PPs using GM bacterial strains have also resulted in significant over-production of these health promising metabolites (360, 380) which can be used to expand clinical and experimental studies on PPs.
The available evidence suggests that such biotechnological methods not only increased the quantity and availability of PPs for industrial, food and medicinal purposes (360, 381, 382), but also expedited the number of scientific investigations conducted on PPs to find the most potent metabolites for large-scale applications (361). The available literature suggests that there have been no comprehensive studies in the literature to engineer crops PBPs against APF. Therefore, developing highly PPs-rich GM plants among susceptible crops to AFs using modern biotechnological and breeding procedures might be considered as alternative mycotoxin management strategy to decrease the global spread of AFs. Although presently GM crops are not universally popular due to the rumors revolved around (383, 384), nevertheless, GMOs are part of hundreds of suggestions to eliminate AFs in food/feed. Additionally, the ME of PPs biosynthetic pathways might increase the long-term resistance of recombinant plants to invasive fungal pathogens and decrease the application of chemical fungicides, though this benefit will require further large-scale investigations to confirm its efficacy against crop fungal pathogen damages.
PPs as modulators of critical signaling pathways
As anticancer agents, PPs could modulate signaling pathways involved in cancer by up and down-regulation of gene expression and suppression of the release of inflammatory factors (385, 386). Experimental assays also unraveled that PPs could inhibit cancerous tumor growth by triggering apoptosis and blocking signaling pathways involved in tumor cell angiogenesis (387). The antioxidant content of PPs also support these metabolites in decreasing ROS/RNS levels in cancerous cells (388). Indeed, PPs affect the cancerous cells gene expression profile, leading to suppressing cancerous cell development and metastasis (389, 390). The growing body of evidence suggests that PPs have impacts on DNA methylation and epigenetic modifications associated with progress of cancer (390). PPs also inhibited the activity of DNA methyltransferases, leading to significant changes in the methylation pattern of specific genes involved in various types of cancers (391). PPs could also modify the expression of microRNAs involved in the regulation of cancer metastasis pathways (392).
Proteins such as G protein-coupled receptors, PI3K, AKT, MMPS, EGFR, VEGF, ERK, STAT3, p53, FOX, JNK, caspases, JAK, PKC, FGF, Nrf2, ALK, ROS1, mTOR, and MAPK are pivotal derivers in the pathogenesis of human cancers (386, 392). In a dose-dependent fashion, PPs could modulate the activity of these proteins, leading to inhibition of primary phases of tumor development (386, 393). Blocking and/or modulating the secretion of cancer-induced pro-inflammatory mediators is another health-promising effect of PPs (394). Inhibition of cancerous cell proliferation (395), inducing apoptosis (387), and suppressing cell cycle events are promising strategies in the cornerstone of cancer therapies. PPs have been shown to regulate these critical processes in cancerous cells through a range of molecular mechanisms (396). Anticancer activity of PPs in clinical trials and animal studies has also substantially studied (397–400).
As evidenced in the literature, as anti-inflammatory phytochemicals certain PPs (e.g., flavonoids, stilbenes, curcuminoids) could ameliorate the consequences of neuroinflammation (248). These phytochemicals principally interacted with pivotal neuro-inflammatory signaling waterfalls, improved brain enzymatic activity, decreased nitrosative stress and RNS formation, improved brain antioxidant defense, regulated of pro-inflammatory-related gene expression, restored the activity of astrocytes and microglial cells, modulated brain transcription factors expression, alleviated COXs expression, enhanced expression of anti-inflammatory genes, and protected brain neuronal cells (248, 401, 402).
As anti-diabetic phytochemicals, PPs showed a broad-spectrum of biological activities to alleviate the complications of DM (403, 404). In this regard, several studies generally attributed the anti-diabetic potential of PPs to their capability to reduce blood glucose level, improve insulin sensitivity and secretion, alleviate oxidative/nitrosative stress, inhibit carbohydrate digestive enzymes, alleviate β-cells apoptosis, ameliorated lipogenesis, alleviate glucogenolysis and gluconeogenesis, up- and down-regulate of DM-associated genes, and modulate signaling pathways (NF-κB, ERK, PPAR, AMPK, cytokines, protein tyrosine phosphatases, glucose transporter receptors, hepatic enzymes, tyrosine kinases, insulin receptors) (403–406).
Biochemical and metabolic factors, including concentrations of PPs, duration of PPs administration, PPs metabolism and post-metabolism modifications, interaction with intestine metabolites/enzymes, interaction with gut microbiota, gastrointestinal uptake, and their bioavailability the body tissues affect the molecular effects of PPs. The following sections summarized the recent trends on the application of PPs in prevention of AFs consequences.
PPs mechanism of actions for improving AFs complications
As documented in the literature, PPs exhibited anti-fungal activity against various types fungal pathogens (407). Respectively, Ahmed and colleagues comprehensively reviewed the inhibitory profile of PPs in preventing AFs production (407). This study, however, focused on PPs mechanism of actions to inhibit AFs formation, and highlighted the associated food safety issues (407). Indeed, another review by Fan et al. summarized recent updates on the application of phytochemicals in detoxifying AFB1-induced hepatotoxicity (21). This study also included PPs as possible candidates to ameliorate AFB1 side effects, however, the study mainly discussed anti-AFB1 mechanisms of different phytochemicals (21). There have not been similar comprehensive review studies on anti-AFs activity of PPs in the literature until the time we prepared this review. Therefore, to increase our current knowledge of anti-AFB1 properties of PPs, in this section we provided an in-depth insight into PPs/PPs-rich extracts mechanism of actions in alleviating health hazardous effects of AFs, in particular AFB1, by summarizing recent trends obtained from animal-based and in vitro studies.
Due to multiscale biological activity of PPs, scientific investigations promoted these naturally occurring metabolites in preventing AFs complications (324, 408). Studies suggested that PPs can directly or indirectly affect the metabolism of AFs, leading to significant reduction of AFs toxicity (409–414). These interesting results purported that PPs substantially interfered with the formation of AFs-HSA complex (191), reduced the construction of AFs-DNA adducts (415), regulated AFs-induced inflammation (416), and also improved detoxification of AFs in liver (417). Bearing this fabulous quote “All things are poison, and nothing is without poison; the dosage alone makes it so a thing is not a poison,” credited to Paracelsus (418) in mind, it can be said that only particular doses of PPs might suppress the onset of AFs-induced metabolic and chronic disorders. Therefore, understanding the biological properties of PPs after their metabolism and tissue intake in the body can supposedly help to identify the exact behavior of these metabolites in AFs therapy scenarios. In this regard, there are hundreds of studies confirmed that PPs could alleviate the end effects of AFs-induced complications, as detailed in the following paragraphs.
In rats fed with 72 μg/kg/b.w. AFB1 and 100 mg/kg/b.w. PPs-rich leaf extract of artichoke (Cynara scolymus L) (PLEA) for 42 days, the outcome manifested that the PLEA promoted partial neuroprotective properties. Accordingly, PLEA down-regulated total plasma lipids/LDL/VLDL, and simultaneously increased HDL levels (419). Although supplementation of PLEA alone had no effects on TNF-α, TIMP3 and IDO concentrations in the brain of rats, however, the results displayed that when rats supplemented with PLEA + AFB1 the total concentrations of these biomarkers relatively decreased but still was not meaningful compared to control group (419). Co-treatment of rats with PLEA +AFB1 was also ameliorated the oxidative stress in the brain of rats, and improved antioxidant enzymes. It seems that the joint administration of PLEA + AFB1 could alleviate oxidative stress in the brain and improve the histological effects of AFB1 on the brain (419).
Similarly, quercetin (30 mg/kg) showed significant neuroprotective effects in Balb/c mice co-administrated with AFB1 (0.75 mg/kg/b.w.) (328). Quercetin reduced TNF-α and IL-1β levels, increased GSH, CAT, and SOD levels, and prevented memory impairment in mice exposed to chronic levels of AFB1 (328). In rats co-supplemented with 80 μg/kg/b.w. AFB1 and 300 m/kg/b.w. PPs-rich ethanolic extract of Chelidonium majus (PEEC), the outcome showed that PEEC alleviated the neurochemical biomarkers (420). In AFB1-treated group, rats showed a significant increase in TNF-α, IL-1β and CD4, AChE, dopamine, and caspase 3 levels whereas co-treatment of PEEC (not PEEC alone) significantly alleviated the increased levels of studied neurochemical markers in rats' cortex and hippocampus areas, and improved the activity of antioxidant enzymes (GSH, SOD, CAT, GPx) (420).
As evidenced in the literature, gallic acid (GAc) abated the health consequences of exposure to AFs. In a study conducted by Owumi et al. (421), the evidence suggested that gallic acid exhibited potential anti-inflammatory and antioxidant activity in rats co-treated with 75 μg/kg AFB1 and 20–40 mg/kg GAc for 28 days. GAc exhibited its antioxidant activity by increasing GSH, SOD, CAT, GPx levels (421). This compound improved antioxidant balance in testes, hypothalamus, and epididymis of rats. The outcome also unraveled that GAc decreased lipid peroxidation, and oxidative/nitrosative stress (421). Correspondingly, GAc alleviated apoptosis mediators in rats by reducing IL-1β, TNF-α, nitric oxide levels, and suppressing myeloperoxidase (421). This observation proven that GAc could ameliorate AFB1-induced oxido-inflammatory responses in reproduction system (421). In Swiss male albino rats co-supplemented with 750–1,000 μg/kg/b.w./day AFB1 and 2 mg/0.2 ml olive oil/day, the outcomes showed that curcumin could ameliorate the AFB1-toxic effects in reproduction system of rats by improving caput and cauda epididymis weight and enzymatic activity (422). In a similar study, co-treatment of curcumin and AFB1 displayed that curcumin alleviated AFB1-induced toxic effects in rats' reproduction system by improving semen parameters such as sperm quality and quantity, mobility, viability and other sperm relevant features (423). In another study, the whole transcriptome analysis of BFH12 cell lines co-treated with curcumin and AFB1, the finding purported that curcumin abated the inflammatory responses and improved antioxidant enzymes in AFB1-treated cells (424).
In a dose-dependent manner, phenolic metabolites such as ellagic acid improved the activity of endogenous antioxidant enzymes, prevented DNA damage and exhibited antimutagenic properties in animal models exposed to AFB1 (324). Caffeic acid in the concentration of 40 mg/kg exhibited protective effects in reproduction system of male rats exposed to 50 μg/kg/b.w. AFB1 through modulation of antioxidant enzymes, apoptosis, and inflammatory factors (425). Apigeninidin-rich extracts of Sorghum bicolor L. Moench (ASBEs) including ASBE-05/06/07 modulated inflammation and apoptosis mechanisms in kidney and liver of rats exposed to 50 μg/kg doses of AFB1 (426). ASBE-06 with IC50 = 6.5 μg/ml suppressed lung cancer cell lines. Correspondingly, ASBEs were also modulated the expression of STAT3 and caspase 3 proteins and displayed protective role against oxidative and nitrosative stress (426). In an interesting study, total flavonoids extract of Rhizoma Drynariae in the concentration of 125 mg/kg inhibited AFB1-induced apoptosis and regulated the expression of PI3K, AKT, Bax and Bcl2 in broilers chickens (427). In rats fed with 400 mg/kg/b.w. AFB1, oxidized tea phenolic compounds in the concentration of 100 μg/kg directly bound to AFB1, lowered its plasma level, and increased AFB1 fecal excretion (27).
Quercetin in the concentration of 50 and 100 mg/kg/b.w. displayed protective hepatocellular effects in liver of rats received 1.4 mg/kg/b.w. AFs-containing diet (428). This finding is in agreement with previous outcomes that examined the efficacy of different doses of quercetin (15–45 mg/kg/b.w.) in prevention hepatic damage of AFs in mice (327). Quercetin also showed protective role against DNA damage when HepG2 cells treated with 5 μg/ml quercetin and 1 μM AFB1 for 2 h, leading to a significant decrease in DNA damage from nearly 60–32% (21, 333). In an interesting investigation, chicks exposed to 5 mg/kg AFB1 displayed an alteration in the activity of AST, ALT, nitric oxide synthase, COX-2, caspase1/3/11, antioxidant enzymes, and pro-inflammatory factors such as TNF-α, IL-1β/6 (429). Morin, a flavonol derivative, in a dose-dependent manner (20–80 mg/kg) ameliorated inflammatory responses, restored AFB1-induced liver and kidney damages by modulation of gene expression and prevention of hepatocyte disruption in AFB1 fed chicks (429).
Kolaviron, a bioflavonoid extracted from Garcinia kola in mice administrated with 100 and 200 mg/kg of this compound and 2 mg/kg AFB1 for 4 weeks significantly reduced the AFB1-induced genotoxicity and oxidative stress (430). This bioflavonoid not only abated the total level of AFB1-DNA adducts, but also decreased the AFB1-induced GGT, AST, and ALT activity by 72, 62 and 56% (430). In adult rats treated with either 10 mg/kg/b.w. quercetin nanoparticles (QNPs) or quercetin and 80 μg/kg/b.w. AFB1, the outcomes have shown that QNPs showed significant anti-aflatoxigenic properties compared to pure quercetin (330). In this regard, QNPs (52.70 nm size) significantly reduced ROS formation, AST/ALT and alkaline phosphatase levels, improved cell viability, glutathione level, and mitochondrial function, and decreased lipid peroxidation (330). This outcomes suggested that Nano-formulation of PPs such as quercetin strengthened their hepatoprotective properties to alleviate the compilations of AFs (330). In rats administrated with subcutaneous 25 mg/kg/b.w. ternatin, a tetramethoxyflavone extracted form Egletes viscosa, and 1 mg/kg/b.w. for 72 h, the finding suggested that this bioflavonoid significantly reduced AFB1-induced AST/ALT levels, modulated MDA level, and displayed chemoprotective effects against liver injury (431). Ternatin markedly inhibited lipid peroxidation, bile-duct proliferation and hepatic necrosis as vitamin E did (431). In another study, the outcomes showed that ternatin decreased plasma liver GSH quantity, alleviated liver oxidative stress and glycemic state, but had no effects on liver regeneration (432).
As discussed formerly, AFs displayed a potent binding affinity to human HSA (191) as well as BSA (193). PPs such as resveratrol could compete with AFs to bind to critical active sites of HSA by which showed an influential effect to decrease the bioavailability of AFB1 and displace the stability of AFB1-HSA complex (177). Studies demonstrated that flavonoids generally displayed a moderate binding potency to HSA, however, flavones and flavonols disclosed a higher tendency to interact with HSA (433). Chemical modification (e.g., sulfation and glycosylation) of flavonoids backbone might also be effective in lowering or increasing their binding affinity to HSA (433). In a dose-time dependent manner, green tea PPs (GTPs) modulated the AFB1 metabolism (by inhibiting of phase I and inducing of phase II metabolism), and decreased the formation of AFB1-HSA adducts (408). Additionally, GTPs in the concentration of 500 and 1,000 mg significantly increased the excretion of AFB1–mercapturic acid, the metabolized by-product of AFB1-8,9-epoxide, into urine which indicated a significant modification in the activity of GSTs (408). The outcome of this clinical trial was also displayed that there was no significant change in urine AFM1 levels, however, this finding strongly supports the protective roles of GTPs in regulating AFB1 metabolism and detoxification (408).
Notably, AFB1 induced hepatocellular pyroptosis (434), and caused critical impairment of liver KCs (435). In mice treated with 1 mg/kg/b.w. AFB1 for 4 weeks, the outcomes showed that AFB1 activated NLRP3 inflammasome and inflammatory infiltration, up-regulated COX-2, enhanced the secretion of IL-1β, and activated KCs, leading to inflammatory-induced liver injury (434). The flavonoid silibinin displayed hepatoprotective activity via selective modulation of certain pathways in activated KCs isolated form rat liver (436). Accordingly, silibinin inhibited nitrosative and oxidative stress in a dose-dependent fashion (IC50 = 80 μM/L). While it has not inhibited prostaglandin E2, silibinin was effective in inhibiting of leukotriene B4 (IC50 = 15 μM/L) and 5-lipooxygenase pathway (436). Curcumin was also found to be functional in preventing hepatic pyroptosis and oxidative stress (416). In this respect, in mice given oral curcumin (100–200 mg/kg) and AFB1 (0.75 mg/kg) for 30 days, the outcome showed that this metabolite mitigated AFB1-induced inflammatory liver injury by inhibiting of NLRP3 inflammasome activation, enhancing phase II AFs detoxifying metabolism, up-regulating of Nrf2 signaling pathway, and preventing the release of pro-inflammatory IL-1β/18 (416).
An increasing volume of evidence suggests that flavonoid subclasses mainly attenuated toxin-induced liver injury by regulating MAPK/NF-κB, CYPs, TLRs, c-JNK/ERK, cytokines/chemokines, Nrf2/CYP2E1, Bcl2/AKT/caspases signaling cascades, preventing oxidative stress, and enhancing antioxidant enzymes (437). AFs has shown to induce the expression of CYPs genes (438). Studies also proven that PPs prone to interaction with CYPs isoforms, which in turn can decrease the biotransformation of AFs after ingestion (439). Generally, mechanism of AFs-induced liver injury is a sophisticated process. The accumulating body of evidence suggests that the liver toxicity of AFs is mainly associated with oxidative/nitrosative stress, cellular apoptosis, mitochondrial dysfunction, lipid/protein peroxidation, construction of AFs-DNA adducts, DNA damage, induction of genomic mutation, inhibition of tumor suppressor proteins, up-regulation of gene expression, induction of inflammatory signaling pathways (21, 440).
In this regard, robinetin and other polyhxdroxy flavonols inhibited microsome-assisted formation of AFB1-DNA adducts (334). Mutually, in Wistar male albino rats exposed to 2 mg/kg/b.w. AFB1 for 6 weeks, administration of 25 mg/kg/b.w. silymarin (or silibinin) could decrease lipid peroxidation and improve the activity of antioxidant enzymes of liver (up to 44–100%) and kidney (up to 82–100%) (441). It could also protect liver from the altered levels of DNA, RNA, glycogen and cholesterol by 70–100%, and hepatic GSH up to 25–37%, respectively (441). Grape seed proanthocyanidins (GSPAs) also showed protective role against AFB1-induced DNA damage. In male Swiss albino rats received 0.5–1 mg/kg AFB1 for 2 days and 100–200 mg/kg/day GSPAs, the outcome suggested GSPAs modulated the expression of Ogg1, Parp1, and p53 genes involved in DNA repair (442).
PPs-rich cocoa extract has also been tested to investigate its anti-AFs properties (443). The outcomes suggested that it was not effective against AFB1 but it significantly reduced the ROS formation and increased cell viability in cells treated with AFB1 alone or mixture of AFB1 + OTA (443). On the contrary, flavonoids-rich fractions prepared from Rhus verniciflua Stokes (FRVs) displayed both in vitro and in vivo chemoprotective against AFB1-induced liver injury. FRVs remarkably decreased ROS formation and MDA level and improved cell viability in HepG2 cells (444). Correspondingly, oral administration of FRVs suppressed AFB1-increased serum level of ALT, lactate dehydrogenase and alkaline phosphatase. FRVs improved glutathione balance and superoxide dismutase activity in AFB1-adminstarted mice liver (444). Indeed, FRVs increased IgA and IgG titers in mice serum. Form this outcome, it can be concluded that FRVs increased the formation of AFB1-GSH complex and restored antioxidant defense (444). In male Wistar rats treated with 100 and 250 mg/kg ginger PPs-rich extract (GPE) and 200 μg/kg AFB1 on the basis of daily, the results showed that GPE could significantly reduce liver damage, AFB1-induced toxicity, and showed remarkable hepatoprotective properties (445). Additionally, it was observed that GPE could improve the activity of endogenous antioxidant enzymes, up-regulate Nrf2/HO-1 pathway, and reduce lipid peroxidation (445). In piglets fed with 8% PPs-rich grape seed extract (PGSE) and 320 μg/kg AFB1 for 30 days, the outcome showed that lower concentration of PGSE has a low to moderate impact on oxidative stress and inflammation in piglet spleen, suggesting that greater concentration of PGSE is required for better alleviation of AFB1 toxicity (446). The flavone aglycone diosmetin displayed anti-OTA activity in MDCK kidney cells by regulation of cellular ATP levels (447).
By-products of palm oil industry such as PPs-rich palm kernel cake (PPKC) also alleviated AFB1-induced cell damage and showed hepatoprotective effects in chicken hepatocytes (448). It is believed that the molecular mechanisms underlying anti-AFB1 properties of PPKC are associated with prevention of lipid peroxidation, modulation of pro-inflammatory and apoptosis genes, and improving the activity of antioxidant enzymes (448). A study has displayed that in rats administrated with 250 mg/kg Korean red ginseng (Panax ginseng) extract (KRGE) and 150 μg/kg AFB1, KRGE could alleviate the adverse effects of AFB1-induced inflammation and hepatotoxic effects (449). Accordingly, KRGE improved serum biomarkers and antioxidant enzymes and prevented apoptosis in hepatocytes and liver inflammation (449). The recent studies have shown that P. ginseng comprised various types of PPs in which ferulic acid, rutin, chlorogenic acid, gentisic acid, p-/m-coumaric acid, catechin, and kaempferol were the foremost domineering phenolic metabolites in different tissues of this plant (450, 451).
Grapefruit juice extract in the concentration of 100 mg/kg has displayed protective effects against AFB1-induced liver DNA damage in F344 rats treated with 5 mg/kg AFB1 by gavage (452). Correspondingly, the administrated extract remarkably reduced hepatic CYP3A content but had no effects on CYP1A and CYP2C quantities (452). Studies confirmed that flavonoids (in particular naringin a flavanone-7-O-glycoside), vitamin C and other organic acids are major metabolic components of this extract (453), accounting for its antioxidant and biological properties (454). In rats treated with different concentration of olive cake PPs-rich extracts (OCPEs) (0.2–0.5 ml), its nanoparticle-based formulation and 22 μg/kg AFB1 for 4 weeks, OCPEs and its nano-formulation improved the neurotoxicity of AFB1 in rats brain (455). In male Wistar rats given 20 g/kg basal diet bee pollen (BP) and 3 mg/kg basal diet AFs for 30 days, the BP could ameliorate the toxicity of AFs by increasing the proliferation of lymphocytes (456). Although this observed benefit of BP was attributed to minerals, vitamins, and amino acids, however, PPs are also accounting for 1.6% total BP metabolites (456), which in turn might affect the anti-AFs properties of BP. The evidence also suggests that honeybee propolis, a resinous mixture of phytochemicals such as flavonoids and non-flavonoid metabolites, vitamins B/C/E, amino acids and other aromatic metabolites (457) could alleviate the toxicity of AFB1 by improving the activity of cytochrome P450 in honeybees (458). In male rats received 50 mg/kg/b.w. Iraqi propolis and 0.025 mg/kg/b.w. AFB1, the propolis has found to be effective in the restoring AFB1-induced gastrointestinal damages (459).
In mice orally administrated with different concentration of AFB1 and 2% aqueous black tea extract (ABTE) (instead of drinking water) for 30 days, the outcomes showed that ABTE ameliorated the AFB1-induced lipid peroxidation in mice liver by increasing the activity of enzymatic and non-enzymatic antioxidant contents (460). The observed benefit is accounted for the fact that ABTE is a PPs-rich fraction which exhibited significant antioxidant activities (460). Similarly, in mice co-administrated with low/high doses of ABTE and AFB1, supplementation of AFB1 resulted in significant reduction of DNA, RNA, glycogen and protein contents, and increased phospholipase activity and cholesterol content (461). In this regard, the ABTE co-administration displayed a protective role in mice liver against AFB1-induced biochemical changes (461).
The co-supplementation of 2% ABTE, 200 μg/kg/b.w. curcumin in rats given 750 μg/kg/b.w. for 90 days, the outcomes confirmed that the co-administration of ABTE-curcumin displayed synergistic effects in alleviating AFB1-induced liver damages in rats (462). Correspondingly, ABTE-curcumin could improve liver architecture, activity of antioxidant enzymes, lipid profile (in particular lowering cholesterol content) and liver biomarkers (462). In rabbits treated with 5 g/kg/b.w. coumarin and 0.25 mg/kg/b.w. AFB1, coumarin improved body weight and carcass gains, and reduced the toxicity of AFB1-induced complications (463). As detailed, PPs could exhibit their anti-AFs activity in a concentration-dependent manner, thus optimization of PPs concentration for treatment of AFs therapies is the most pivotal step in experimental assays regarding this field. These outcomes together confirmed that these plant metabolites are promising substances to reduce the health consequences of AFs. Table 1 provides detailed information on the mechanism of action of anti-AFs properties of studied PPs.
As detailed in Table 1, PPs ameliorate AFs toxicity in different ways. Accordingly, the anti-AFs activity of PPs mainly contributed to preventing oxidative stress and inflammatory responses, inhibiting mutations in DNA, regulating signaling cascades, modulating phase I and II metabolism enzymes, improving cellular antioxidant balance, and interfering interaction of AFs and HSA. These data showed that flavonoids, in particular oxidized tea PPs, were the most studied PPs in the prevention of AFs toxicity. Indeed, the anti-AFs activities of resveratrol and curcumin were also highly investigated. Interestingly, different classes of PPs exhibited a wide range of heterogenous biological properties against toxicity of AFs. Our review clearly disclosed that PPs targeted the core pathways (inflammation-based responses) in the pathogenesis of AFs. This interaction is important because not only in the onset of cancer, but also inflammation (in particular NIF) play a critical role in in the progression of neurodegenerative disorders and MetSys (248, 250, 256). Therefore, as modulator secondary metabolites, PPs have the potential to maintain the normal status of cell by regulating the onset of inflammation-assisted signaling pathways and preventing the development of nCDs (338).
PPs-rich extracts for inhibiting AFs production
PPs also prevent the formation of AFs in target fungi (A. flavus and A. parasiticus strains) by modulating fungal transcription factors activity (513, 514). For example, water-soluble and methanol extract of peanut tannins were also inhibited A. parasiticus growth and impaired the formation of AFs in a dose-dependent manner (515). Studies have also shown that the type of PPs extraction methods might affect antifungal activity of these metabolites. In this regard, solid-phase extraction of PPs-rich citrus peel extract displayed up to 40% antifungal properties against A. flavus compared to crude extracts (516). Correspondingly, 300–400 mg/ml mandarin PPs-rich extract is enough to completely inhibit A. flavus growth depending on extraction method and applied solvents (516).
PPs-rich methanolic extract of Zanthoxylum bungeanum (a traditional Chinese food additive) with the IC50 2–4 μg/ml significantly repressed the AFB1 biosynthetic pathway (517). The omics-based analysis of this extract unraveled that it could show the anti-aflatoxigenic properties by down-regulating of the global regulators of AFB1 biosynthesis such as velvet complex proteins, Medusa and brlA genes, and GPCR/oxylipin-based signaling cascade (517). Intriguingly, PPs-rich olive processing wastes (POPWs) also showed differential anti-aflatoxigenic properties in a dose-dependent manner to inhibit the growth of APF (518). Of all POPWs, olive pomace extract displayed a higher anti-aflatoxigenic properties in comparison to olive leave and pomace olive oil extracts, respectively (518). 5′-hydroxy-auraptene, a coumarin derivative isolated from Lotus lalambensis, in the concentration of 40 μg/ml prevented the AFB1 production and exhibited potential antifungal activity against A. flavus by down-regulation of genes involved in different phases of AFB1 biosynthetic pathway and inhibition of conidial germination of this fungus by 60% (519). The outcomes also suggested that 5′-hydroxy-auraptene disrupted the structure of mycelia sugar units and up-regulated stress mediated transcription factors (atfA and atfB) up to 2 and 2.5 folds (519).
Buckwheat hull PPs-rich extracts (BHPEs) displayed a dose-dependent inhibitory profile against A. flavus growth and AFB1 biosynthesis (520). According to this outcome the higher concentration of BHPE was accounting for longer inhibition of AFB1 formation (520). In another study, the pau ferro (Libidibia ferrea), a Brazilian medicinal plant, ethanolic fruit extract has found to be effective in the inhibition of A. parasiticus growth (521). PPs-rich Cistus incanus L. methanolic extract in the concentration of 0.2 g/ml significantly decreased the formation of AFB1 production from 72.5 to 90.1% and inhibited A. parasiticus growth (522). The essential oil of C. ladanifer, another species of Cistus genus, has also found to be effective in the prevention of A. flavus growth by suppressing AFB1 production, and inhibiting the fungus ergosterol biosynthetic pathway with MIC value of 0.6 μl/ml, respectively (523).
An increasing trend of evidence purported that glycosidic and aglycone derivatives of flavonoids and non-flavonoids in a dose-dependent manner suppressed the production of AFB1 and other mycotoxins by targeting the critical routes in biosynthetic pathways of toxicogenic fungi (407). For instance, compound of interest, quercetin also disrupted AFs-producing fungal proliferation in addition to blocking the formation of AFs (28). Similarly, Green tea PPs in the concentration of 70 mg/ml suppressed the formation of AFs without side effects on the mycelial growth of APF (524). Phenolics also showed potential inhibitory profile against the production of other mycotoxins. In an interesting study, Boonmee and colleagues reported that simple hydroxycinnamic acid derivative, ferulic acid inhibited the production of OTA in A. westerdijkiae and P. verrucosum by 35 and 75% (525). Synthetic derivatives of flavonoids such as 5,6-dihydroxy-flavone and 5,6-dihydroxy-7-methoxy-flavone, in the concentration of 25 and 50 μg/ml, have significantly decreased the production of OTA in A. carbonarius after 8-day incubation (526). Similar to these outcomes, Romero et al. reported that caffeic acid, rutin and quercetin in the concentration of 250 mg/L remarkably decreased the production of OTA in A. carbonarius (527). Indeed, higher concentrations of these PPs (500 mg/L) completely inhibited the growth of OTA-producing fugus (527). The aqueous seed extract of Trachyspermum ammi also showed beneficial effects in degrading AFB1 mycotoxin. The phytochemical analyses revealed that T. ammi has different types of metabolites such as PPs, alkaloids, tannins, and other well-known natural substances (528).
Olive mill wastewater (OMWW) pure PPs such as caffeic acid, hydroxytyrosol, tyrosol and verbascoside also showed a decrease of nearly 99% in AFB1 production but had not influenced A. flavus growth (512). Accordingly, OMWW extract in the concentration of 15% was also decreased the formation of AFB1 ranged from 88 to 100%, respectively (512). In a dose-dependent manner, flavonoids-rich spent coffee grounds extract (PSCGE) has also found to be effective in degrading AFs (B1/2-G1/2) and OTA in vitro (529). The PSCGE was remarkably decreased the growth of toxicogenic fungi such as A. flavus and A. ochraceus as well as Fusarium species (529). These outcomes demonstrated that food wastes/residues have considerable level of health promoting metabolites; alternatively, can be used as potent inhibitors of APF and the production of AFB1 and other mycotoxins (529). Therefore, it should be noted that these waste by-products are trustworthy candidates to develop and formulate modified extracts with added values as anti-fungal agents.
Compared to PPs-rich extracts, alkaloids-rich extracts (ALEs) showed potential inhibitory properties in detoxifying of AFB1. In this respect, vasaka (Adhatoda vasica Nees) leaf ALEs displayed functionality to degrade AFB1 up to ≥98% after 24 h incubation at 37°C (530). The amide alkaloid piperlongumine isolated from Piper longum L. in the concentration of 0.2%w/v inhibited the biosynthesis of AFB1 in A. flavus up to 96% (531). Another piperidine alkaloid, piperoctadecalidine isolated from P. longum displayed 100% inhibitory profile against biosynthesis of AFB1 in the concentration of 0.7%, respectively (531). Other relevant studies also reported that ALEs are promising anti-fungal agents to prevent the formation of AFB1 and APF growth (532). It seems that the anti-aflatoxigenic property of alkaloids is dose-dependent, and the chemical variation of alkaloids might determine their inhibitory profile. In this regard, it can be said that both alkaloids/PPs-rich extracts and pure metabolites are influential compounds in the prevention of AFs; however, their chemistry and concentration are two determinant factors in defining their inhibitory/biological profile. In modern food industries, however, these metabolites are promising candidates to develop antifungal agents. These results together permit to harness phytochemicals for dealing with health hazardous mycotoxins.
PPs-AFs related patents
Chemical fungicides (ChFs) are presently recruited for large-scale inhibition of AFs production and APF growth (533). However, as detailed in the literature, long-term application of ChFs may lead to ChFs-resistant APF (533) and the onset of health threatening symptoms (534). In this regard, there are only few registered patents publicly available to use PPs as candidate inhibitors of AFs biosynthesis. These innovations developed specific products or GM plants to prevent the spread of AFs-contaminated foods/crops. In an interesting patent, engineered transgenic plants with elevated levels polyphenol oxidase/gallic acid content showed resistance to A. flavus and AFs production (535). In another patent issued by Xiang et al., tea PPs in the concentrations of 0.2–1% were effective in the reduction of AFs biosynthesis by 21–81% (536). Dacheng et al. developed an anti-mycotoxigenic feed additive for cattle in which tea PPs (1–5 parts of whole patent formulation) have been used as main ingredients of this product (537). In another patent, a preparation method for constructing catechin nanoparticles has been suggested that could decrease the bioavailability of AFB1 and prevent hepatic injury (538). Despite the scarcity of patents on PPs for inhibition of AFs production, however, in the light of discussed materials herein further formulation of PPs can be crafted to develop potent anti-AFs products.
Current status and future perspectives
Increasing knowledge on the antioxidant content of natural products and vitamins leads to developing “antioxidant therapy” to relieve human diseases (539), though there have been doubts in this field (540, 541). Studies have shown that the combination of PPs and vitamins enhances their antioxidant activity (539). It is now proven that cancer, MetSys and neurodegenerative diseases are associated with a higher level of oxidative/nitrosative stress and ROS/RNS production (30, 542–544). In this regard, natural antioxidants are primary defensive agents in preventing early phases of cancer, AD and DM progression (30, 388, 545). Accordingly, PPs received a huge volume of attention due to their antioxidant properties and becoming the relevant metabolites in antioxidant therapy programs (546). Presently a considerable number of PPs in the form of antioxidant supplements, cosmetic and food additive products, sanitary agents, antibacterial products, and pain relievers are available in global markets for non-clinical applications (338).
Regarding PPs applications in alleviating AFs-induced health challenges, there are several key points should be highlighted before consideration of these metabolites for large-scale studies. First, despite considerable studies conducted on PPs, the optimum doses of phenolic compounds for amelioration of AFs side effects have not yet determined. This case causes a significant variability in observed biological effects assigned to PPs. For example, studies have shown that the anticancer activity of PPs is dose-dependent, in which PPs shared different IC50 and Ki values for inhibition of target enzymes (547). On the other hand, the intake of high concentration of PPs might show adverse effects on kidney and thyroid hormones level, as reported in animal models (548, 549) and argued in the literature (540).
Second, standardization of PPs mode of action toward cellular receptors requires sufficient data generated from clinical trials and large-scale studies (546). For instance, the anticancer activity of PPs depends on their chemical structure, doses and subtypes of cancers (399). Third, as detailed in Table 1, anti-AFs properties of PPs are not generally focused on specific pathways, though the majority of studies confirmed that these metabolites function through suppressing of AFs-induced oxidative stress. This demonstrated that PPs might show off-target effects to interact with several different receptors in the body. Fourth, the pro-oxidant activity of PPs is another concern (547, 550) might delay the recruitment of these metabolites against AFs. On the other hand, some phenolic compounds such as daidzein and genistein exhibited controversial effects on the pathogenesis of hormone-associated cancers (399). Such incongruous results have still not been reported for other phenolic categories, and it is now believed that PPs displayed their health-promising effects by interacting with various receptors and signaling pathways (551). In this respect, the emerging scientific investigations reported that supplementation of antioxidants (vitamin E and N-acetylcysteine) in mice increased the progression of lung cancer by inducing P53-assisted oxidative stress (198). This finding suggests that excessive supplementation of antioxidants might increase the progression of nCDs, therefore, to avoid further complications, and for cautionary reasons, the consumption of antioxidants should be followed by considering optimum doses under strictly controlled condition. Therefore, recruiting PPs or PPs-rich extracts in anti-AFs therapies requires a deep insight into their bioavailability, on-target mode of action, pharmacokinetics, drug interventions, and chemical stability in the human body (188, 547).
Another worthy point that should be addressed that is PPs-rich waste products (e.g., OMWW) disclosed potential inhibitory activity against AFB1 formation (512). Such products have a certain group of PPs, called non-extractable PPs (NEPPs) (552, 553), in turn, their biological activities have not extensively been investigated due to limitations in extraction methods or insolubility and polymeric essence of these metabolites (554). Studies have shown that the optimization of PPs extraction methods using innovative technologies reduced required time and energy to elucidate PPs, and improved the quantity of achievable phenolics metabolites (555). These innovations help researcher to access the whole PPs profile of herbaceous materials to determine their biological activities. In this regard, we previously reviewed the valorization methods of OMWW PPs extraction to highlight the biological benefits of these phenolics that widely released into the nearby environment (556). Similar studies also suggested that such NEPPs from waste products have a remarkable antioxidant content, resultantly may exhibit health promoting effects in the cornerstone of the human diseases prevention (557).
On the other hand, the current knowledge of PPs is mainly associated with extractable O-glycosidic derivatives. The evidence purported that C-glycosidic PPs such as schaftoside derivatives exhibited potential biological activity to enhance crop resistance against pests (558). Therefore, such metabolites can also be used to inhibit the growth of APF or prevent the production of AFs.
Additionally, the current findings on anti-AFs activity of PPs obtained from animal models (rodents and chicken) and experimental assays, in turn, requiring further validations. Future studies on how PPs might interact with nCDs-associated molecular receptors and signaling pathways (in particular inflammation) and their exact mode of action in relieving AFs end effects should be conducted to confirm their efficacy and safety for medical management of mycotoxins. Figure 12 summarized the current findings of this study.
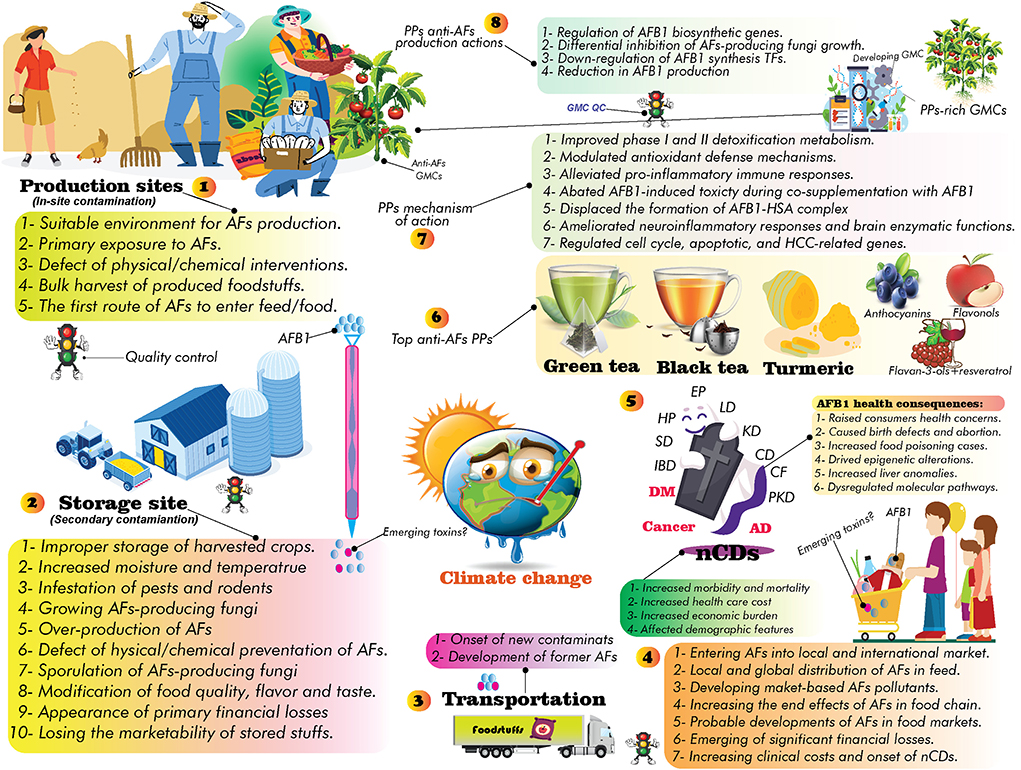
Figure 12. The findings of this study at a quick glance. AFs in production/storage sites caused damage to target products and passed to consumers, leading to the progression of nCDs (Steps 1–5). Certain classes of PPs alleviated the side effects of AFs by modulating several critical signaling pathways involved in the pathogenesis of AFs. Indeed, these compounds could inhibit the formation of AFs by regulating expression of critical genes involved in biosynthetic pathways of AFs (Steps 6–8). Climate change markedly affects the occurrence of AFs, therefore, developing PPs-rich GM plants might be considered as a strategic policy to decrease the quantity of these mycotoxins. GMCs, genetically modified crops; PKD, Parkinson's disease; EP, epilepsy; IBD, inflammatory bowel disease; CD, cardiovascular diseases; KD, kidney diseases; LD, liver diseases; HP, hemophilia; SD, seizure disorder; CF, cystic fibrosis.
Concluding remarks
Our literature review clearly manifested that shifts in world climate can influence the distribution/quantity of AFs and prevalence of nCDs. Additionally, the triangle of GCC/exposure to AFs/progression of nCDs has significant complications for human health, in turn, can increase the economic costs to countries health care system. It is important to conduct more large-scale and long-term investigations in susceptible countries to GCC (in particular low and middle-income regions) to predict future threats for designing effective preventative policies and health risk assessments. Various types of food/feed items have been identified with exceeded level of AFs contaminants, resultantly this phenomenon might bring countries more deaths during the upcoming years owing to the onset of nCDs complications. The co-occurrence of other GCC-associated risk factors may expedite the progression of nCDs, though this claim requires further approvement to know how synergistic effects of environmental health hazardous risks will threaten human health and lifestyle.
Countries implemented rigorous regulatory measurements to monitor and diagnosis of AFs in contaminated foods/feeds. However, the literature indicates that the current regulatory settings should immediately be revisited due to the advent of AFs and other emerging mycotoxins in various food commodities (559, 560). AFs decreased the quality and marketability of food/feed products, therefore causing damages to countries food safety and economy (561). In this regard, increasing public awareness, in particular among farmers and local food/feed producers, plays a critical role in elimination of AFs and preparing countries local society to deal with complications of GCC and health threatening environmental risk factors.
Owing to the role of AFs in triggering immunosuppression (37), interfering with protein metabolism and micronutrient deficiency (276), and reducing antibody production (562), specific diagnostic/predictive biomarkers should be characterized in early detection of AFs-induced health challenges to a better management of clinical symptoms. On the contrary, PPs promoted initiate immune responses by activating certain signaling pathways (563). This indicates that dietetic intervention using PPs is an effective way to modulate immune responses to alleviate the toxicity of AFs, though current PPs gaps for clinical applications should be addressed in detail. In neurodegenerative diseases, the accumulation of aggregated proteins leads to the progression of these disorders (30, 564). PPs have also shown regulatory effects in the modulation of protein metabolism and activation of protein degrading systems to prevent the accumulation of misfolded proteins (564). Having such biological properties enabled PPs to combat AFs health problems.
As discussed, no antidote has been introduced to alleviate the toxicity of AFs and current management scenarios of AFs complications are based on the removal of these mycotoxins in foods. Indeed, the frequency of international studies on AFs has spectacularly surged up in the past years in which the USA, China, India occupied the top ranks of studies in this field. Bibliometric analysis of the literature displayed that the majority of studies conducted on AFs were focused on carcinogenic, properties and detection methods of these toxins, though association of exposure to AFs and the onset of DM and AD has been remarkably taken into consideration. This indicates that long-term exposure to AFs evoked multidimensional health challenges in addition to their potential in inducing HCC.
The literature reviewed herein suggested that the quest for characterization of natural inhibitors of AFs is becoming a global trend in food safety field. According to our literature review, phenolics and PPs-rich extracts are promising AFs detoxifying products. Green and black tea, turmeric, anthocyanins and flavonols were the most studied phenolics metabolites to detoxify AFs complications in animal and in vitro studies. Despite the lack of enough clinical data on the effectiveness of PPs in preventing AFs consequences, the available data displayed that PPs showed a heterogenous biological activities in preventing the side effects of AFs by targeting several different molecular receptors. PPs reviewed in this paper can be used for decontamination of AFs (and possibly other emerging mycotoxins) either in the human/animal body or production/storage sites after considering safety cautions.
As discussed, due to the negative effects of nCDs and GCC on world economy, future studies should seek to develop strategies that may improve the bioavailability, mode of action, and pharmacokinetics properties of PPs in the cornerstone of AFs-induced nCDs treatment. Coupling antioxidant-assisted interventions with conventional physio-chemical removal procedures of AFs in food/feed items and developing GM PPs-rich crops are highly recommended to decrease the quantity of AFs to lowest concentrations and improve lifestyle and longevity of affected individuals. It is also treasured to address this point that regular consumption of PPs or PPs-rich functional foods might help in early preventing of AFs-induced nCDs, though this finding requires further clinical assessments.
Author contributions
HR designed the study, wrote the first draft, and prepared the graphical illustrations. HR and FN conducted a literature search. RK revised the draft. HR, FN, and RK revised the final draft. All authors contributed to the article and approved the submitted version.
Acknowledgments
The authors would like to thank Iran National Science Foundation (PN: 4004326) in providing scientific support to complete this project.
Conflict of interest
The authors declare that the research was conducted in the absence of any commercial or financial relationships that could be construed as a potential conflict of interest.
Publisher's note
All claims expressed in this article are solely those of the authors and do not necessarily represent those of their affiliated organizations, or those of the publisher, the editors and the reviewers. Any product that may be evaluated in this article, or claim that may be made by its manufacturer, is not guaranteed or endorsed by the publisher.
Supplementary material
The Supplementary Material for this article can be found online at: https://www.frontiersin.org/articles/10.3389/fnut.2022.981984/full#supplementary-material
Abbreviations
PPs, phytophenolics or polyphenols; HSA, human serum albumin; AD, Alzheimer's disease; DM, diabetes mellitus; nCDs, non-communicable diseases; AFs, aflatoxins; GMO, Genetically modified organisms; GM, genetically modified; N-flavonoids, non-flavonoids; SOD, superoxide dismutase; OTA, Ochratoxin A, T1D, type 1 DM; T2D, type 2 DM; EU, European union; US, United states; IARC, international agency for research on cancer; ROS, reactive oxygen species; RNS, reactive nitrogen species; BBB, the blood brain barrier; CNS, central nervous system; NIF, neuroinflammation; AFB1-NIF, AFB1-induced neuroinflammation; IL, interleukins; TNF, tumor necrosis factor; IGF2, insulin-like growth factor-2; IGF-IR, IGF1 receptor; HCC, hepatocellular carcinoma; MetSys, metabolic syndromes; WHO, world health organization; GSH, glutathione; GST, glutathione-S-transferase; GSH-Px, Glutathione peroxidase; MDA, Malondialdehyde; AST, aspartate aminotransferase; ALT, alanine aminotransferase; GGT, gamma glutamyl-transferase; KCs, Kupffer cells; INF-γ, Interferon gamma; TLRs, toll-like receptors; MAPK, mitogen-activated protein kinase; MyD88, myeloid differentiation primary response 88; CxCR4, C-X-C chemokine receptor type 4; PI3K, phosphoinositide 3-kinases; AKT, protein kinase B; COX-2, prostaglandin-endoperoxide synthase 2; iNOS, inducible nitric oxide synthase; mTOR, mammalian target of rapamycin; Nrf2, nuclear factor erythroid 2–related factor 2; HO-1, Heme oxygenase-1; STAT, signal transducer and activator of transcription; AMPK, 5’ AMP-activated protein kinase, ; JAK, Janus tyrosine kinase; NF-κB, Nuclear factor kappa B; CCK, cerebral creatine kinase; PKC, protein kinase C; CAT, catalase; LDH, lactate dehydrogenase; TNFRs, TNF-α receptors; CK, creatine kinase; AP, acid phosphatase; AChE, acetylcholinesterase; ADA, adenosine deaminase; p-NF-κB, phosphorylated NF-κB; CYP, Cytochromes P450; HBC/V, hepatitis B/C virus; PPI, protein-protein interaction; HPLC, High-performance liquid chromatography; IAC, immunoaffinity chromatography; LC-MS/MS, Liquid Chromatography with tandem mass spectrometry; HPLC/FLD, HPLC with fluorescence detector; LFIA, Lateral flow immunoassay; ME, metabolic engineering; PBPs, PPs biosynthetic pathways; PAL, phenylalanine ammonia lyase; MMPs, Matrix metalloproteinases; EGFR, epidermal growth factor receptor; ERK, Extracellular signal-regulated kinase; FOX, fork-head box; ALK, Anaplastic lymphoma kinase; JNK, c-Jun N-terminal kinase; VEGF, Vascular endothelial growth factor; ROS1, Proto-oncogene tyrosine-protein kinase; PPAR, peroxisome proliferator-activated receptors; IDO, Indoleamine 2, 3 dioxygenase; TIMP3, TIMP Metallopeptidase Inhibitor 3; PDK, Phosphoinositide-dependent Protein Kinase; MUP1, major urinary protein 1; GCC, Global climate change; GW, global warming; APF, AFs-producing fungi.
References
1. Hertel TW, Rosch SD. Climate change, agriculture, and poverty. Appl Econ Perspect Policy. (2010) 32:355–85. doi: 10.1093/aepp/ppq016
2. Keating BA, Herrero M, Carberry PS, Gardner J, Cole MB. Food wedges: framing the global food demand and supply challenge towards 2050. Glob Food Sec. (2014) 3:125–32. doi: 10.1016/j.gfs.2014.08.004
3. Hickey LT, N Hafeez A, Robinson H, Jackson SA, Leal-Bertioli S, Tester M, et al. Breeding crops to feed 10 billion. Nat Biotechnol. (2019) 37:744–54. doi: 10.1038/s41587-019-0152-9
4. Schmidhuber J, Tubiello FN. Global food security under climate change. Proc Natl Acad Sci USA. (2007) 104:19703–19708. doi: 10.1073/pnas.0701976104
5. Smith P, Gregory PJ. Climate change and sustainable food production. Proc Nutr Soc. (2013) 72:21–8. doi: 10.1017/S0029665112002832
6. Wheeler T, Von Braun J. Climate change impacts on global food security. Science. (2013) 341:508–13. doi: 10.1126/science.1239402
7. Baazeem A, Rodriguez A, Medina A, Magan N. Impacts of climate change interacting abiotic factors on growth, aflD and aflR gene expression and aflatoxin B1 production by Aspergillus flavus strains in vitro and on pistachio nuts. Toxins. (2021) 13:385. doi: 10.3390/toxins13060385
8. Adeyeye SAO. Aflatoxigenic fungi and mycotoxins in food: a review. Crit Rev Food Sci Nutr. (2020) 60:709–721. doi: 10.1080/10408398.2018.1548429
9. Levin RE. PCR detection of aflatoxin producing fungi and its limitations. Int J Food Mircob. (2012) 156:1–6. doi: 10.1016/j.ijfoodmicro.2012.03.001
10. Andrade P, Caldas E. Aflatoxins in cereals: worldwide occurrence and dietary risk assessment. World Mycotoxin J. (2015) 8:415–31. doi: 10.3920/WMJ2014.1847
11. Mousavi Khaneghah A, Eş I, Raeisi S, Fakhri Y. Aflatoxins in cereals: state of the art. J Food Saf . (2018) 38:e12532. doi: 10.1111/jfs.12532
12. Lee SD, Yu IS, Jung K, Kim YS. Incidence and level of aflatoxins contamination in medicinal plants in Korea. Mycobiology. (2014) 42:339–45. doi: 10.5941/MYCO.2014.42.4.339
13. Mahato DK, Lee KE, Kamle M, Devi S, Dewangan KN, Kumar P, et al. Aflatoxins in food and feed: an overview on prevalence, detection and control strategies. Front Microbiol. (2019) 10:2266. doi: 10.3389/fmicb.2019.02266
14. Mungamuri SK, Mavuduru VA. Role of epigenetic alterations in aflatoxin-induced hepatocellular carcinoma. Liver Cancer Int. (2020) 1:41–50. doi: 10.1002/lci2.20
15. Freire F, da Rocha MEB. Impact of mycotoxins on human health. In:Mérillon J-M, Ramawat KG, , editors. Fungal Metabolites. India:Springer (2017). p. 239–61. doi: 10.1007/978-3-319-25001-4_21
16. Vieira T, Cunha S, Casal S. Mycotoxins in coffee. In:Preedy VR, , editor. Coffee in Health and Disease Prevention. London: Elsevier, Academic Press (2015). p. 225–33. doi: 10.1016/B978-0-12-409517-5.00025-5
17. Buszewska-Forajta M. Mycotoxins, invisible danger of feedstuff with toxic effect on animals. Toxicon. (2020) 182:34–53. doi: 10.1016/j.toxicon.2020.04.101
18. Negash D. A review of aflatoxin: occurrence, prevention, and gaps in both food and feed safety. J Appl Microb Res. (2018) 1:35–43. doi: 10.31031/NTNF.2018.01.000511
19. Sowley ENK. Aflatoxins: a silent threat in developing countries. Afr J Biotechnol. (2016) 15:1864–70. doi: 10.5897/AJB2016.15305
20. Tian F, Chun HS. Natural products for preventing and controlling aflatoxin contamination of food. In:Abdulra'Uf L, , editor. Aflatoxin-Control, Analysis, Detection Health Risks. Croatia: InTechOpen (2017). p. 13–44. doi: 10.5772/intechopen.68413
21. Fan T, Xie Y, Ma W. Research progress on the protection and detoxification of phytochemicals against aflatoxin B1-Induced liver toxicity. Toxicon. (2021) 195:58–68. doi: 10.1016/j.toxicon.2021.03.007
22. Boccellino M, D'Angelo S. Anti-obesity effects of polyphenol intake: current status and future possibilities. Int J Mol Sci. (2020) 21:5642. doi: 10.3390/ijms21165642
23. Luca SV, Macovei I, Bujor A, Miron A, Skalicka-Wozniak K, Aprotosoaie AC, et al. Bioactivity of dietary polyphenols: the role of metabolites. Crit Rev Food Sci Nutr. (2020) 60:626–59. doi: 10.1080/10408398.2018.1546669
24. Mutha RE, Tatiya AU, Surana SJ. Flavonoids as natural phenolic compounds and their role in therapeutics: an overview. Future J Pharm Sci. (2021) 7:1–13. doi: 10.1186/s43094-020-00161-8
25. Zeb A. Concept, mechanism, and applications of phenolic antioxidants in foods. J Food Biochem. (2020) 44:e13394. doi: 10.1111/jfbc.13394
26. Marino M, Del Bo' C, Martini D, Porrini M, Riso P. A review of registered clinical trials on dietary (poly) phenols: past efforts and possible future directions. Foods. (2020) 9:1606. doi: 10.3390/foods9111606
27. Lu H, Liu F, Zhu Q, Zhang M, Li T, Chen J, et al. Aflatoxin B1 can be complexed with oxidised tea polyphenols and the absorption of the complexed aflatoxin B1 is inhibited in rats. J Sci Food Agric. (2017) 97:1910–5. doi: 10.1002/jsfa.7994
28. Zhou W, Hu L-B, Zhao Y, Wang MYZ. Inhibition of fungal aflatoxin B1 biosynthesis by diverse botanically-derived polyphenols. Trop J Pharm Res. (2015) 14:605–9. doi: 10.4314/tjpr.v14i4.7
29. Zhang Y-J, Gan R-Y, Li S, Zhou Y-N, Xu D-P, Li H-B, et al. Antioxidant phytochemicals for the prevention and treatment of chronic diseases. Molecules. (2015) 20:21138–56. doi: 10.3390/molecules201219753
30. Bukhari SNA. Dietary polyphenols as therapeutic intervention for Alzheimer's disease: a mechanistic insight. Antioxidants. (2022) 11:554. doi: 10.3390/antiox11030554
31. Mehrabi M, Karami F, Siah M, Esmaeili S, Khodarahmi R. Is curcumin an active suicidal antioxidant only in the aqueous environments? J Iran Chem Soc. (2022) 19:3441–50. doi: 10.1007/s13738-022-02538-3
32. Van Eck N, Waltman L. Software survey: VOSviewer, a computer program for bibliometric mapping. Scientometrics. (2010) 84:523–38. doi: 10.1007/s11192-009-0146-3
33. Bindea G, Mlecnik B, Hackl H, Charoentong P, Tosolini M, Kirilovsky A, et al. ClueGO: a Cytoscape plug-in to decipher functionally grouped gene ontology and pathway annotation networks. Bioinformatics. (2009) 25:1091–3. doi: 10.1093/bioinformatics/btp101
34. Trott O, Olson AJ. AutoDock Vina: improving the speed and accuracy of docking with a new scoring function, efficient optimization, and multithreading. J Comput Chem. (2010) 31:455–61. doi: 10.1002/jcc.21334
35. Tomasello G, Armenia I, Molla G. The Protein Imager: a full-featured online molecular viewer interface with server-side HQ-rendering capabilities. Bioinformatics. (2020) 36:2909–11. doi: 10.1093/bioinformatics/btaa009
36. Romagnoli B, Menna V, Gruppioni N, Bergamini C. Aflatoxins in spices, aromatic herbs, herb-teas and medicinal plants marketed in Italy. Food Control. (2007) 18:697–701. doi: 10.1016/j.foodcont.2006.02.020
37. Pickova D, Ostry V, Toman J, Malir F. Aflatoxins: history, significant milestones, recent data on their toxicity and ways to mitigation. Toxins. (2021) 13:399. doi: 10.3390/toxins13060399
38. Khlangwiset P, Shephard GS, Wu F. Aflatoxins and growth impairment: a review. Crit Rev Toxicol. (2011) 41:740–55. doi: 10.3109/10408444.2011.575766
39. Marchese S, Polo A, Ariano A, Velotto S, Costantini S, Severino L. Aflatoxin B1 and M1: Biological properties and their involvement in cancer development. Toxins. (2018) 10:214. doi: 10.3390/toxins10060214
40. Hakeem KR, Oliveira CA, Ismail A. Aflatoxins in Food: A Recent Perspective. Switzerland: Springer Nature (2022).
41. Prandini A, Tansini G, Sigolo S, Filippi L, Laporta M, Piva G. On the occurrence of aflatoxin M1 in milk and dairy products. Food Chem Toxicol. (2009) 47:984–91. doi: 10.1016/j.fct.2007.10.005
42. Kumar P, Mahato DK, Kamle M, Mohanta TK, Kang SG. Aflatoxins: a global concern for food safety, human health and their management. Front Microbiol. (2017) 7:2170. doi: 10.3389/fmicb.2016.02170
43. Ostry V, Malir F, Toman J, Grosse Y. Mycotoxins as human carcinogens-the IARC Monographs classification. Mycotoxin Res. (2017) 33:65–73. doi: 10.1007/s12550-016-0265-7
44. Medina Á, González-Jartín JM, Sainz MJ. Impact of global warming on mycotoxins. Curr Opin Food Sci. (2017) 18:76–81. doi: 10.1016/j.cofs.2017.11.009
45. Gruber-Dorninger C, Novak B, Nagl V, Berthiller F. Emerging mycotoxins: beyond traditionally determined food contaminants. J Agric Food Chem. (2017) 65:7052–70. doi: 10.1021/acs.jafc.6b03413
46. Battilani P, Toscano P, der Fels-Klerx V, Moretti A, Camardo Leggieri M, Brera C, et al. Aflatoxin B1 contamination in maize in Europe increases due to climate change. Sci Rep. (2016) 6:1–7. doi: 10.1038/srep24328
47. Maja MM, Ayano SF. The impact of population growth on natural resources and farmers' capacity to adapt to climate change in low-income countries. Earth Syst Environ. (2021) 5:271–83. doi: 10.1007/s41748-021-00209-6
48. Lyon C, Saupe EE, Smith CJ, Hill DJ, Beckerman AP, Stringer LC, et al. Climate change research and action must look beyond 2100. Glob Change Biol. (2022) 28:349–61. doi: 10.1111/gcb.15871
49. Romanello M, McGushin A, Di Napoli C, Drummond P, Hughes N, Jamart L, et al. The 2021 report of the Lancet Countdown on health and climate change: code red for a healthy future. Lancet. (2021) 398:1619–62. doi: 10.1016/S0140-6736(21)01787-6
50. Shahzad A, Ullah S, Dar AA, Sardar MF, Mehmood T, Tufail MA, et al. Nexus on climate change: agriculture and possible solution to cope future climate change stresses. Environ Sci Pollut Res. (2021) 28:14211–32. doi: 10.1007/s11356-021-12649-8
51. Al-Tawaha ARM, Günal H, Kreček J, Zamfir RHC, Patel H, Vyas R, et al. Soil fertility decline under climate change. In:Shah Fahad OS, Saud S, Wang D, Wu C, Adnan M, Turan V, , editors. Sustainable Soil and Land Management and Climate Change. Boca Raton, Florida: CRC Press (2021). p. 127–45.
52. Nnadi NE, Carter DA. Climate change and the emergence of fungal pathogens. PLoS Pathogens. (2021) 17:e1009503. doi: 10.1371/journal.ppat.1009503
53. Warnatzsch EA, Reay DS, Camardo Leggieri M, Battilani P. Climate change impact on aflatoxin contamination risk in Malawi's maize crops. Front Sustain Food Syst. (2020) 4:591792. doi: 10.3389/fsufs.2020.591792
54. Valencia-Quintana R, Milić M, Jakšić D, Šegvić Klarić M, et al. Environment changes, aflatoxins, and health issues, a review. Int J Environ Res Public Health. (2020) 17:7850. doi: 10.3390/ijerph17217850
55. Leggieri MC, Toscano P, Battilani P. Predicted aflatoxin b1 increase in Europe due to climate change: actions and reactions at global level. Toxins. (2021) 13:292. doi: 10.3390/toxins13040292
56. Raven PH, Wagner DL. Agricultural intensification and climate change are rapidly decreasing insect biodiversity. Proc Natl Acad Sci USA. (2021) 118:e2002548117. doi: 10.1073/pnas.2002548117
57. Xu F, Baker R, Whitaker T, Luo H, Zhao Y, Stevenson A, et al. Review of good agricultural practices for smallholder maize farmers to minimise aflatoxin contamination. World Mycotoxin J. (2021) 15:171–86. doi: 10.3920/WMJ2021.2685
58. Civantos E, Thuiller W, Maiorano L, Guisan A, Araújo MB. Potential impacts of climate change on ecosystem services in Europe: the case of pest control by vertebrates. Bioscience. (2012) 62:658–66. doi: 10.1525/bio.2012.62.7.8
59. Phan LTK, Tran TM, De Boevre M, Jacxsens L, Eeckhout M, De Saeger S. Impact of season, region, and traditional agricultural practices on aflatoxins and fumonisins contamination in the rice chain in the Mekong Delta, Vietnam. Toxins. (2021) 13:667. doi: 10.3390/toxins13090667
60. Sipos P, Peles F, Brassó DL, Béri B, Pusztahelyi T, Pócsi I, Gyori Z. Physical and chemical methods for reduction in aflatoxin content of feed and food. Toxins. (2021) 13:204. doi: 10.3390/toxins13030204
61. Cotty PJ, Probst C, Jaime-Garcia R. Etiology and management of aflatoxin contamination. In:Leslie JF, Bandyopadhyay R, Visconti A, , editors. Mycotoxins: Detection Methods, Management, Public Health and Agricultural Trade. Wallingford: CABI (2008). p. 287–99. doi: 10.1079/9781845930820.0287
62. Yu J, Hennessy DA, Tack J, Wu F. Climate change will increase aflatoxin presence in US Corn. Environ Res Lett. (2022) 17:054017. doi: 10.1088/1748-9326/ac6435
63. Doster MA, Michailides TJ. Relationship between shell discoloration of pistachio nuts and incidence of fungal decay and insect infestation. Plant Dis. (1999) 83:259–64. doi: 10.1094/PDIS.1999.83.3.259
64. Nji QN, Babalola OO, Mwanza M. Aflatoxins in maize: can their occurrence be effectively managed in Africa in the face of climate change and food insecurity? Toxins. (2022) 14:574. doi: 10.3390/toxins14080574
65. Kaminiaris MD, Camardo Leggieri M, Tsitsigiannis DI, Battilani P. AFLA-pistachio: development of a mechanistic model to predict the aflatoxin contamination of pistachio nuts. Toxins. (2020) 12:445. doi: 10.3390/toxins12070445
66. Paterson RRM, Lima N. Further mycotoxin effects from climate change. Food Res Int. (2011) 44:2555–66. doi: 10.1016/j.foodres.2011.05.038
67. Medina A, Rodriguez A, Magan N. Effect of climate change on Aspergillus flavus and aflatoxin B1 production. Front Microbiol. (2014) 5:348. doi: 10.3389/fmicb.2014.00348
68. Van der Fels-Klerx H, Liu C, Battilani P. Modelling climate change impacts on mycotoxin contamination. World Mycotoxin J. (2016) 9:717–26. doi: 10.3920/WMJ2016.2066
69. Gilbert M, Mack B, Payne G, Bhatnagar D. Use of functional genomics to assess the climate change impact on Aspergillus flavus and aflatoxin production. World Mycotoxin J. (2016) 9:665–72. doi: 10.3920/WMJ2016.2049
70. Cervini C, Verheecke-Vaessen C, Ferrara M, García-Cela E, Magistà D, Medina A, et al. Interacting climate change factors (CO2 and temperature cycles) effects on growth, secondary metabolite gene expression and phenotypic ochratoxin A production by Aspergillus carbonarius strains on a grape-based matrix. Fungal Biol. (2021) 125:115–22. doi: 10.1016/j.funbio.2019.11.001
71. Akbar A, Medina A, Magan N. Impact of interacting climate change factors on growth and ochratoxin A production by Aspergillus section Circumdati and Nigri species on coffee. World Mycotoxin J. (2016) 9:863–74. doi: 10.3920/WMJ2016.2041
72. Van der Fels-Klerx H, Vermeulen L, Gavai A, Liu C. Climate change impacts on aflatoxin B1 in maize and aflatoxin M1 in milk: a case study of maize grown in Eastern Europe and imported to the Netherlands. PLoS ONE. (2019) 14:e0218956. doi: 10.1371/journal.pone.0218956
73. Gilbert MK, Medina A, Mack BM, Lebar MD, Rodríguez A, Bhatnagar D, et al. Carbon dioxide mediates the response to temperature and water activity levels in Aspergillus flavus during infection of maize kernels. Toxins. (2017) 10:5. doi: 10.3390/toxins10010005
74. Mitchell NJ, Bowers E, Hurburgh C, Wu F. Potential economic losses to the US corn industry from aflatoxin contamination. Food Addit Contam A. (2016) 33:540–50. doi: 10.1080/19440049.2016.1138545
75. Miedaner T, Juroszek P. Global warming and increasing maize cultivation demand comprehensive efforts in disease and insect resistance breeding in north-western Europe. Plant Pathol. (2021) 70:1032–46. doi: 10.1111/ppa.13365
76. Paterson RRM, Lima N. Ecology and biotechnology of thermophilic fungi on crops under global warming. In: Fungi in Extreme Environments: Ecological Role and Biotechnological Significance. Switzerland: Springer (2019). p. 81–96. doi: 10.1007/978-3-030-19030-9_5
77. Wu Y, Cheng J-H, Sun D-W. Blocking and degradation of aflatoxins by cold plasma treatments: applications and mechanisms. Trends Food Sci Technol. (2021) 109:647–61. doi: 10.1016/j.tifs.2021.01.053
78. Peng Z, Chen L, Zhu Y, Huang Y, Hu X, Wu Q, et al. Current major degradation methods for aflatoxins: a review. Trends Food Sci Technol. (2018) 80:155–66. doi: 10.1016/j.tifs.2018.08.009
79. Samarajeewa U, Sen A, Cohen M, Wei C. Detoxification of aflatoxins in foods and feeds by physical and chemical methods. J Food Prot. (1990) 53:489–501. doi: 10.4315/0362-028X-53.6.489
80. Beaglehole R, Bonita R, Horton R, Adams C, Alleyne G, Asaria P, et al. Priority actions for the non-communicable disease crisis. Lancet. (2011) 377:1438–47. doi: 10.1016/S0140-6736(11)60393-0
81. Nugent R, Fottrell E. Non-communicable diseases and climate change: linked global emergencies. Lancet. (2019) 394:622–3. doi: 10.1016/S0140-6736(19)31762-3
82. Assunção R, Martins C, Viegas S, Viegas C, Jakobsen LS, Pires S, et al. Climate change and the health impact of aflatoxins exposure in Portugal-an overview. Food Addit Contam A. (2018) 35:1610–21. doi: 10.1080/19440049.2018.1447691
83. Anenberg SC, Haines S, Wang E, Nassikas N, Kinney PL. Synergistic health effects of air pollution, temperature, and pollen exposure: a systematic review of epidemiological evidence. Environ Health. (2020) 19:1–19. doi: 10.1186/s12940-020-00681-z
84. Sillmann J, Aunan K, Emberson L, Büker P, Van Oort B, O'Neill C, et al. Combined impacts of climate and air pollution on human health and agricultural productivity. Environ Res Lett. (2021) 16:093004. doi: 10.1088/1748-9326/ac1df8
85. Paterson RRM, Lima N. How will climate change affect mycotoxins in food? Food Res Int. (2010) 43:1902–14. doi: 10.1016/j.foodres.2009.07.010
86. Savage A, McIver L, Schubert L. the nexus of climate change, food and nutrition security and diet-related non-communicable diseases in Pacific Island Countries and Territories. Clim Dev. (2020) 12:120–33. doi: 10.1080/17565529.2019.1605284
87. Owen J, Reisin E. Non-communicable disease: A welcome and long needed addition to the WHO's 2012 World Heath Statistics. Curr Hypertens Rep. (2012) 14:475–7. doi: 10.1007/s11906-012-0303-6
88. Rasheed H, Xu Y, Kimanya ME, Pan X, Li Z, Zou X, et al. Estimating the health burden of aflatoxin attributable stunting among children in low income countries of Africa. Sci Rep. (2021) 11:1–11. doi: 10.1038/s41598-020-80356-4
89. Ahlberg S, Grace D, Kiarie G, Kirino Y, Lindahl J. A risk assessment of aflatoxin M1 exposure in low and mid-income dairy consumers in Kenya. Toxins. (2018) 10:348. doi: 10.3390/toxins10090348
90. Zyoud SH. Global scientific trends on aflatoxin research during 1998–2017: a bibliometric and visualized study. J. Occup. Med. Toxicol. (2019) 14:1–11. doi: 10.1186/s12995-019-0248-7
91. Einolghozati M, Talebi-Ghane E, Ranjbar A, Mehri F. Concentration of aflatoxins in edible vegetable oils: a systematic meta-analysis review. Eur Food Res Technol. (2021) 247:2887–97. doi: 10.1007/s00217-021-03844-5
92. Karunarathna NB, Fernando CJ, Munasinghe D, Fernando R. Occurrence of aflatoxins in edible vegetable oils in Sri Lanka. Food Control. (2019) 101:97–103. doi: 10.1016/j.foodcont.2019.02.017
93. Wacoo AP, Wendiro D, Vuzi PC, Hawumba JF. Methods for detection of aflatoxins in agricultural food crops. J Appl Chem. (2014) 2014:706291. doi: 10.1155/2014/706291
94. Miklós G, Angeli C, Ambrus Á, Nagy A, Kardos V, Zentai A, et al. Detection of aflatoxins in different matrices and food-chain positions. Front Microbiol. (2020) 11:1916. doi: 10.3389/fmicb.2020.01916
95. Espinosa-Calderón A, Contreras-Medina LM, Muñoz-Huerta RF, Millán-Almaraz JR, González RGG, Torres-Pacheco I. Methods for detection and quantification of aflatoxins. In: Aflatoxins: Detection, Measurement and Control. New York, NY: InTech (2011). p. 109–28. doi: 10.5772/28439
96. Shuaib FM, Ehiri J, Abdullahi A, Williams JH, Jolly PE. Reproductive health effects of aflatoxins: a review of the literature. Reprod Toxicol. (2010) 29:262–70. doi: 10.1016/j.reprotox.2009.12.005
97. Saad-Hussein A, Moubarz G, Mohgah SA, Wafaa GS, Aya HM. Role of antioxidant supplementation in oxidant/antioxidant status and hepatotoxic effects due to aflatoxin B1 in wheat miller workers. J Complement Integr Med. (2019) 16:1–4. doi: 10.1515/jcim-2018-0218
98. Yadav A, Kumari R, Yadav A, Mishra J, Srivatva S, Prabha S. Antioxidants and its functions in human body-a review. Res Environ Life Sci. (2016) 9:1328–31.
99. Villers P. Aflatoxins and safe storage. Front Microbiol. (2014) 5:158. doi: 10.3389/fmicb.2014.00158
100. Pratiwi C, Rahayu WP, Lioe HN, Herawati D, Broto W, Ambarwati S. The effect of temperature and relative humidity for Aspergillus flavus BIO 2237 growth and aflatoxin production on soybeans. Int Food Res J. (2015) 22:82–87.
101. Gruber-Dorninger C, Jenkins T, Schatzmayr G. Global mycotoxin occurrence in feed: a ten-year survey. Toxins. (2019) 11:375. doi: 10.3390/toxins11070375
102. Jallow A, Xie H, Tang X, Qi Z, Li P. Worldwide aflatoxin contamination of agricultural products and foods: from occurrence to control. Compr Rev Food Sci. (2021) 20:2332–81. doi: 10.1111/1541-4337.12734
103. Ali N. Aflatoxins in rice: worldwide occurrence and public health perspectives. Toxicol Rep. (2019) 6:1188–97. doi: 10.1016/j.toxrep.2019.11.007
104. Aydin A, Aksu H, Gunsen U. Mycotoxin levels and incidence of mould in Turkish rice. Environ Monit Assess. (2011) 178:271–80. doi: 10.1007/s10661-010-1688-9
105. Ojiambo PS, Battilani P, Cary JW, Blum BH, Carbone I. Cultural and genetic approaches to manage aflatoxin contamination: recent insights provide opportunities for improved control. Phytopathology. (2018) 108:1024–37. doi: 10.1094/PHYTO-04-18-0134-RVW
106. Battilani P, Leggieri MC. Predictive modelling of aflatoxin contamination to support maize chain management. World Mycotoxin J. (2015) 8:161–70. doi: 10.3920/WMJ2014.1740
107. Gil A, Ortega RM, Maldonado J. Wholegrain cereals and bread: a duet of the Mediterranean diet for the prevention of chronic diseases. Public Health Nutr. (2011) 14:2316–22. doi: 10.1017/S1368980011002576
108. Kaale L, Kimanya M, Macha I, Mlalila N. Aflatoxin contamination and recommendations to improve its control: a review. World Mycotoxin J. (2021) 14:27–40. doi: 10.3920/WMJ2020.2599
109. Filazi A, Sireli UT. Occurrence of aflatoxins in food. In:Razzaghi-Abyaneh M, , editors. Aflatoxins-Recent Advances and Future Prospects. New York: InTechOpen (2013). p. 143–70. doi: 10.5772/51031
110. Trombete FM, de Ávila Moraes D, Porto YD, Santos TB, Direito GM, Fraga ME, et al. Determination of aflatoxins in wheat and wheat by-products intended for human consumption, marketed in Rio de Janeiro, Brazil. J Food Nutr Res. (2014) 2:671–4. doi: 10.12691/jfnr-2-10-3
111. Achaglinkame MA, Opoku N, Amagloh FK. Aflatoxin contamination in cereals and legumes to reconsider usage as complementary food ingredients for Ghanaian infants: a review. J Nutr Intermed Metab. (2017) 10:1–7. doi: 10.1016/j.jnim.2017.09.001
112. Ebrahimi A, Emadi A, Arabameri M, Jayedi A, Abdolshahi A, Yancheshmeh BS, et al. The prevalence of aflatoxins in different nut samples: a global systematic review and probabilistic risk assessment. AIMS Agric Food. (2022) 7:130–48. doi: 10.3934/agrfood.2022009
113. Mohsen M, Hamid T, Foroud B, Fernandes OCA, Humberto CC, Mousavi KA. Aflatoxin B1 in the Iranian pistachio nut and decontamination methods: a systematic review. Qual Assur Saf Crops Foods. (2020) 12:15–25. doi: 10.15586/qas.v12i4.784
114. Bui-Klimke TR, Guclu H, Kensler TW, Yuan J-M, Wu F. Aflatoxin regulations and global pistachio trade: insights from social network analysis. PLoS ONE. (2014) 9:e92149. doi: 10.1371/journal.pone.0092149
115. WHO and FAO. Evaluation of Certain Food Additives and Contaminants: Sixty-Eighth Report of the Joint FAO/WHO Expert Committee on Food Additives. Geneva, Switzerland: WHO/FAO (2007).
116. Chizari A, Sadafi Abkenar S. Impact of price and non-price factors on the Iranian Pistachios Market. J Agric Sci Technol. (2020) 22:1415–30.
117. Trandafir I, Cosmulescu S, Botu M, Nour V. Antioxidant activity, and phenolic and mineral contents of the walnut kernel (Juglans regia L.) as a function of the pellicle color. Fruits. (2016) 71:177–84. doi: 10.1051/fruits/2016006
118. Gharibzahedi SMT, Mousavi SM, Hamedi M, Rezaei K, Khodaiyan F. Evaluation of physicochemical properties and antioxidant activities of Persian walnut oil obtained by several extraction methods. Ind Crops Prod. (2013) 45:133–40. doi: 10.1016/j.indcrop.2012.11.040
119. Taghizadeh SF, Rezaee R, Badibostan H, Karimi G. Aflatoxin B1 in walnuts: a probabilistic cancer risk assessment for Iranians. Toxicol Environ Chem. (2020) 102:506–19. doi: 10.1080/02772248.2020.1791868
120. Ostadrahimi A, Ashrafnejad F, Kazemi A, Sargheini N, Mahdavi R, Farshchian M, et al. Aflatoxin in raw and salt-roasted nuts (pistachios, peanuts and walnuts) sold in markets of Tabriz, Iran. Jundishapur J Microbiol. (2014) 7:e8674. doi: 10.5812/jjm.8674
121. Trenk HL, Hartman PA. Effects of moisture content and temperature on aflatoxin production in corn. Appl Microbiol. (1970) 19:781–4. doi: 10.1128/am.19.5.781-784.1970
122. Moreno Romo MA, Ramos Cartagena MC, Rodriguez Ferri EF, Suárez Fernández G. Minimal moisture content for growth and aflatoxin production by Aspergillus parasiticus in mixed feeds. Mycopathologia. (1986) 95:145–8. doi: 10.1007/BF00437118
123. Arrus K, Blank G, Abramson D, Clear R, Holley R. Aflatoxin production by Aspergillus flavus in Brazil nuts. J Stored Prod Res. (2005) 41:513–27. doi: 10.1016/j.jspr.2004.07.005
124. Min L, Fink-Gremmels J, Li D, Tong X, Tang J, Nan X, et al. An overview of aflatoxin B1 biotransformation and aflatoxin M1 secretion in lactating dairy cows. Anim Nutr. (2021) 7:42–8. doi: 10.1016/j.aninu.2020.11.002
125. Barbiroli A, Bonomi F, Benedetti S, Mannino S, Monti L, Cattaneo T, et al. Binding of aflatoxin M1 to different protein fractions in ovine and caprine milk. J Dairy Sci. (2007) 90:532–40. doi: 10.3168/jds.S0022-0302(07)71536-9
126. Mohammed S, Munissi JJ, Nyandoro SS. Aflatoxin M1 in raw milk and aflatoxin B1 in feed from household cows in Singida, Tanzania. Food Addit Contam B. (2016) 9:85–90. doi: 10.1080/19393210.2015.1137361
127. Omar SS. Aflatoxin M1 levels in raw milk, pasteurised milk and infant formula. Italian J Food Saf. (2016) 5:5788. doi: 10.4081/ijfs.2016.5788
128. Salari N, Kazeminia M, Vaisi-Raygani A, Jalali R, Mohammadi M. Aflatoxin M1 in milk worldwide from 1988 to 2020: a systematic review and meta-analysis. J Food Qual. (2020) 2020:8862738. doi: 10.1155/2020/8862738
129. Neal G, Eaton D, Judah D, Verma A. Metabolism and toxicity of aflatoxins M1and B1in human-derivedin VitroSystems. Toxicol Appl Pharmacol. (1998) 151:152–8. doi: 10.1006/taap.1998.8440
130. Gebreegziabher T, Dean M, Elias E, Tsegaye W, Stoecker BJ. Urinary aflatoxin M1 concentration and its determinants in school-age children in southern Ethiopia. Nutrients. (2022) 14:2580. doi: 10.3390/nu14132580
131. Markov K, Pleadin J, Bevardi M, Vahčić N, Sokolić-Mihalak D, Frece J. Natural occurrence of aflatoxin B1, ochratoxin A and citrinin in Croatian fermented meat products. Food Control. (2013) 34:312–7. doi: 10.1016/j.foodcont.2013.05.002
132. Shaltout FA, Amin RA, Nassif MZ, Abd-Elwahab SA. Detection of aflatoxins in some meat products. Benha Vet Med J. (2014) 27:368–74.
133. Elzupir AO, Abdulkhair BY. Health risk from aflatoxins in processed meat products in Riyadh, KSA. Toxicon. (2020) 181:1–5. doi: 10.1016/j.toxicon.2020.04.092
134. Aziz NH, Youssef YA. Occurrence of aflatoxins and aflatoxin-producing moulds in fresh and processed meat in Egypt. Food Addit Contam. (1991) 8:321–31. doi: 10.1080/02652039109373981
135. Bintvihok A, Thiengnin S, Doi K, Kumagai S. Residues of aflatoxins in the liver, muscle and eggs of domestic fowls. J Vet Med Sci. (2002) 64:1037–9. doi: 10.1292/jvms.64.1037
136. Herzallah SM. Determination of aflatoxins in eggs, milk, meat and meat products using HPLC fluorescent and UV detectors. Food Chem. (2009) 114:1141–6. doi: 10.1016/j.foodchem.2008.10.077
137. Beheshti HR, Asadi M. Aflatoxins in animal feed in Iran. Food Addit Contam A. (2014) 7:40–2. doi: 10.1080/19393210.2013.834485
138. Arroyo-Manzanares N, Huertas-Pérez JF, Garcia-Campana AM, Gámiz-Gracia L. Aflatoxins in animal feeds: a straightforward and cost-effective analytical method. Food Control. (2015) 54:74–8. doi: 10.1016/j.foodcont.2015.01.027
139. Alvarado AM, Zamora-Sanabria R, Granados-Chinchilla F. A focus on aflatoxins in feedstuffs: levels of contamination, prevalence, control strategies, and impacts on animal health. In: Aflatoxin-Control, Analysis, Detection and Health Risks. London: IntechOpen (2017). p. 116–52. doi: 10.5772/intechopen.69468
140. Scaglioni PT, Becker-Algeri T, Drunkler D, Badiale-Furlong E. Aflatoxin B1 and M1 in milk. Anal Chim Acta. (2014) 829:68–74. doi: 10.1016/j.aca.2014.04.036
141. García-Casal MN, Peña-Rosas JP, Malavé HG. Sauces, spices, condiments: definitions, potential benefits, consumption patterns, global markets. Ann N Y Acad Sci. (2016) 1379:3–16. doi: 10.1111/nyas.13045
142. Nguyen L, Duong LT, Mentreddy RS. The US import demand for spices and herbs by differentiated sources. J Appl Res Med Aromat Plants. (2019) 12:13–20. doi: 10.1016/j.jarmap.2018.12.001
143. FAO. Top Countries in Spices Production. Available online at: https://www.nationmaster.com/nmx/ranking/spices-production (accessed June 19, 2022).
144. Ahmed F, Asghar MA. Aflatoxins occurrence in spices. In:Abdulra'Uf L, , editor. Aflatoxins-Occurrence, Detoxification, Determination and Health Risks. Crotia: IntechOpen (2021). doi: 10.5772/intechopen.96793
145. Wu L, Li J, Li Y, Li T, He Q, Tang Y, et al. Aflatoxin B1, zearalenone and deoxynivalenol in feed ingredients and complete feed from different Province in China. J Anim Sci Biotechnol. (2016) 7:1–10. doi: 10.1186/s40104-016-0122-8
146. Llewellyn GC, Mooney RL, Cheatle TF, Flannigan B. Mycotoxin contamination of spices-an update. Int Biodeterior Biodegradation. (1992) 29:111–21. doi: 10.1016/0964-8305(92)90011-C
147. Pickova D, Ostry V, Malir J, Toman J, Malir F. A review on mycotoxins and microfungi in spices in the light of the last five years. Toxins. (2020) 12:789. doi: 10.3390/toxins12120789
148. Khazaeli P, Mehrabani M, Heidari MR, Asadikaram G, Najafi ML. Prevalence of aflatoxin contamination in herbs and spices in different regions of Iran. Iran J Public Health. (2017) 46:1540.
149. Jalili M. Natural occurrence of aflatoxins contamination in commercial spices in Iran. Iran J Health Saf Environ. (2016) 3:513–17. doi: 10.18869/acadpub.nfsr.3.3.25
150. Elshafie AE, Al-Rashdi TA, Al-Bahry S, Bakheit CS. Fungi and aflatoxins associated with spices in the Sultanate of Oman. Mycopathologia. (2002) 155:155–60. doi: 10.1023/A:1020427527963
151. Martins ML, Martins HM, Bernardo F. Aflatoxins in spices marketed in Portugal. Food Addit Contam. (2001) 18:315–9. doi: 10.1080/02652030120041
152. Fufa H, Urga K. Screening of aflatoxins in Shiro and ground red pepper in Addis Ababa. Ethiop Med J. (1996) 34:243–9.
153. Mozaffari Nejad AS, Sabouri Ghannad M, Kamkar A. Determination of aflatoxin B1 levels in Iranian and Indian spices by ELISA method. Toxin Rev. (2014) 33:151–4. doi: 10.3109/15569543.2014.942319
154. Tosun H, Arslan R. Determination of aflatoxin B1 levels in organic spices and herbs. Sci World J. (2013) 2013:874093. doi: 10.1155/2013/874093
155. Hammami W, Fiori S, Al Thani R, Kali NA, Balmas V, Migheli Q, et al. Fungal and aflatoxin contamination of marketed spices. Food Control. (2014) 37:177–81. doi: 10.1016/j.foodcont.2013.09.027
156. Sevdin S, Çelik E, Çömçe AN, Batar N, Özdemir AA. A Systematic meta-analysis of Aflatoxin B1 presence in red pepper. Avrupa Bilim ve Teknoloji Dergisi. (2021) 32:1162–7. doi: 10.31590/ejosat.1045487
157. Siddique NA, Mujeeb M, Ahmad S, Panda BP, Makhmoor M. Determination of aflatoxins in medicinal plants by high-performance liquid chromatography-tandem mass spectrometry. J Pharm Pharm Sci. (2013) 16:321–30. doi: 10.18433/J3MK6P
158. Reif K, Metzger W. Determination of aflatoxins in medicinal herbs and plant extracts. J Chromatogr A. (1995) 692:131–6. doi: 10.1016/0021-9673(94)00841-V
159. Ałtyn I, Twaruzek M. Mycotoxin contamination concerns of herbs and medicinal plants. Toxins. (2020) 12:182. doi: 10.3390/toxins12030182
160. Abeywickrama K, Bean GA. Toxigenic Aspergillus flavus and aflatoxins in Sri Lankan medicinal plant material. Mycopathologia. (1991) 113:187–90. doi: 10.1007/BF00436129
161. Tassaneeyakul W, Razzazi-Fazeli E, Porasuphatana S, Bohm J. Contamination of aflatoxins in herbal medicinal products in Thailand. Mycopathologia. (2004) 158:239–44. doi: 10.1023/B:MYCO.0000041892.26907.b4
162. Al-Owaisi A, Al-Sadi AM, Al-Sabahi JN, Sathish Babu S, Al-Harrasi MMA, Hashil Al-Mahmooli I, et al. In vitro detoxification of aflatoxin B1 by aqueous extracts of medicinal herbs. All Life. (2022) 15:314–24. doi: 10.1080/26895293.2022.2049900
163. Kumar A, Pathak H, Bhadauria S, Sudan J. Aflatoxin contamination in food crops: causes, detection, and management: a review. Food Prod Process Nutr. (2021) 3:1–9. doi: 10.1186/s43014-021-00064-y
164. Katerere D, Stockenström S, Thembo K, Rheeder J, Shephard G, Vismer H. A preliminary survey of mycological and fumonisin and aflatoxin contamination of African traditional herbal medicines sold in South Africa. Hum Exp Toxicol. (2008) 27:793–8. doi: 10.1177/0960327108099535
165. Sofowora A, Ogunbodede E, Onayade A. The role and place of medicinal plants in the strategies for disease prevention. Afr J Tradit Complement Altern Med. (2013) 10:210–29. doi: 10.4314/ajtcam.v10i5.2
166. Chien M-Y, Yang C-M, Huang C-MH. Investigation of aflatoxins contamination in herbal materia medica in a Taiwan pharmaceutical factory. J Food Drug Anal. (2018) 26:1154–9. doi: 10.1016/j.jfda.2018.01.016
167. Cho S-H, Lee C-H, Jang, M-R, Lee S-M, Choi I-S, et al. Aflatoxins contamination in spices and processed spice products commercialized in Korea. Food Chem. (2008) 107:1283–8. doi: 10.1016/j.foodchem.2007.08.049
168. Tansakul N, Limsuwan S, Böhm J, Hollmann M, Razzazi-Fazeli E. Aflatoxins in selected Thai commodities. Food Addit Contam B. (2013) 6:254–9. doi: 10.1080/19393210.2013.812148
169. Witkamp RF, van Norren K. Let thy food be thy medicine…. when possible. Eur J Pharmacol. (2018) 836:102–14. doi: 10.1016/j.ejphar.2018.06.026
170. Mutegi C, Cotty P, Bandyopadhyay R. Prevalence and mitigation of aflatoxins in Kenya (1960-to date). World Mycotoxin J. (2018) 11:341. doi: 10.3920/WMJ2018.2362
171. Lee HS, Nguyen-Viet H, Lindahl J, Thanh HM, Khanh TN, Hien L, et al. A survey of aflatoxin B1 in maize and awareness of aflatoxins in Vietnam. World Mycotoxin J. (2017) 10:195–202. doi: 10.3920/WMJ2016.2144
172. Ezekwesili-Ofili J, Onyemelukwe N, Agwaga P, Orji I. The bioload and aflatoxin content of herbal medicines from selected states in Nigeria. Afr J Tradit Complement Altern Med. (2014) 11:143–7. doi: 10.4314/ajtcam.v11i3.21
173. Shim W-B, Kim K, Ofori JA, Chung, Y-C. Occurrence of aflatoxins in herbal medicine distributed in South Korea. J Food Prot. (2012) 75:1991–9. doi: 10.4315/0362-028X.JFP-12-190
174. Ali N, Hashim N, Saad B, Safan K, Nakajima M, Yoshizawa T. Evaluation of a method to determine the natural occurrence of aflatoxins in commercial traditional herbal medicines from Malaysia and Indonesia. Food Chem Toxicol. (2005) 43:1763–72. doi: 10.1016/j.fct.2005.05.019
175. Bradford KJ, Dahal P, Van Asbrouck J, Kunusoth K, Bello P, Thompson J, et al. The dry chain: Reducing postharvest losses and improving food safety in humid climates. Trends Food Sci Technol. (2020) 71:375–89. doi: 10.1016/B978-0-12-817121-9.00017-6
176. Omara T, Kiprop AK, Wangila P, Wacoo AP, Kagoya S, Nteziyaremye P, et al. The scourge of aflatoxins in Kenya: a 60-year review (1960 to 2020). J Food Qual. (2021) 2021:1–31. doi: 10.1155/2021/8899839
177. Qureshi MA, Javed S. Investigating binding dynamics of trans resveratrol to HSA for an efficient displacement of aflatoxin B1 using spectroscopy and molecular simulation. Sci Rep. (2022) 12:1–17. doi: 10.1038/s41598-022-06375-5
178. Fanali G, Di Masi A, Trezza V, Marino M, Fasano M, Ascenzi P. Human serum albumin: from bench to bedside. Mol Asp Med. (2012) 33:209–90. doi: 10.1016/j.mam.2011.12.002
179. Shahlaei M, Rahimi B, Ashrafi-Kooshk MR, Sadrjavadi K, Khodarahmi R. Probing of possible olanzapine binding site on human serum albumin: combination of spectroscopic methods and molecular dynamics simulation. J Lumin. (2015) 158:91–8. doi: 10.1016/j.jlumin.2014.09.027
180. Kragh-Hansen U. Structure and ligand binding properties of human serum albumin. Dan Med Bull. (1990) 37:57–84.
181. Mishra V, Heath RJ. Structural and biochemical features of human serum albumin essential for eukaryotic cell culture. Int J Mol Sci. (2021) 22:8411. doi: 10.3390/ijms22168411
182. Murray C, Hartshorn M. New applications for structure-based drug design. In:Triggle J, , editors. Comprehensive Medicinal Chemistry II. New York: Elseiver (2007). p. 775–806. doi: 10.1016/B0-08-045044-X/00277-7
183. Ezra A, Rabinovich-Nikitin I, Rabinovich-Toidman P, Solomon B. Multifunctional effects of human serum albumin toward neuroprotection in Alzheimer Disease. In:Gozes I, , editor. Neuroprotection in Alzheimer's Disease. New York: Elsevier. (2017). p. 217–38. doi: 10.1016/B978-0-12-803690-7.00011-9
184. Arques S. Human serum albumin in cardiovascular diseases. Eur J Intern Med. (2018) 52:8–12. doi: 10.1016/j.ejim.2018.04.014
185. Poór M, Bálint M, Hetényi C, Godér B, Kunsági-Máté S, Koszegi T, et al. Investigation of non-covalent interactions of aflatoxins (B1, B2, G1, G2, and M1) with serum albumin. Toxins. (2017) 9:339. doi: 10.3390/toxins9110339
186. Sadeghi M, Miroliaei M, Ghanadian M. Inhibitory effect of flavonoid glycosides on digestive enzymes: in silico, in vitro, and in vivo studies. Int. J. Biol. Macromol. (2022) 217:714–30. doi: 10.1016/j.ijbiomac.2022.07.086
187. Rasouli H, Hosseini-Ghazvini SMB, Adibi H, Khodarahmi R. Differential α-amylase/α-glucosidase inhibitory activities of plant-derived phenolic compounds: a virtual screening perspective for the treatment of obesity and diabetes. Food Funct. (2017) 8:1942–54. doi: 10.1039/C7FO00220C
188. Rasouli H, Hosseini Ghazvini SMB, Yarani R, Altintaş A, Jooneghani SGN, Ramalho TC. Deciphering inhibitory activity of flavonoids against tau protein kinases: a coupled molecular docking and quantum chemical study. J Biomol Struct Dyn. (2022) 40:411–24. doi: 10.1080/07391102.2020.1814868
189. Poór M, Kunsági-Máté S, Bálint M, Hetényi C, Gerner Z, Lemli B. Interaction of mycotoxin zearalenone with human serum albumin. J Photochem Photobiol B Biol. (2017) 170:16–24. doi: 10.1016/j.jphotobiol.2017.03.016
190. Il'ichev YV, Perry JL, Simon JD. Interaction of ochratoxin A with human serum albumin. Preferential binding of the dianion pH effects. J Phys Chem B. (2002) 106:452–9. doi: 10.1021/jp012314u
191. Tan H, Chen L, Ma L, Liu S, Zhou H, Zhang Y, et al. Fluorescence spectroscopic investigation of competitive interactions between quercetin and aflatoxin B1 for binding to human serum albumin. Toxins. (2019) 11:214. doi: 10.3390/toxins11040214
192. Bagheri M, Fatemi MH. Fluorescence spectroscopy, molecular docking and molecular dynamic simulation studies of HSA-Aflatoxin B1 and G1 interactions. J Lumin. (2018) 202:345–53. doi: 10.1016/j.jlumin.2018.05.066
193. Qureshi MA, Javed S. Aflatoxin B1 induced structural and conformational changes in bovine serum albumin: a multispectroscopic and circular dichroism-based study. ACS Omega. (2021) 6:18054–64. doi: 10.1021/acsomega.1c01799
194. Fan T, Xie Y, Sun S. Interference of anthocyanin extracted from black soybean coats on aflatoxin B1-human serum albumin in the binding process. Food Addit Contam. (2021) 38:1571–82. doi: 10.1080/19440049.2021.1930198
195. Verma R, Nair A. Vitamin E ameliorates aflatoxin-induced biochemical changes in the testis of mice. Asian J Androl. (2001) 3:305–10.
196. Nyandieka H, Wakhisi J. The impact of vitamins A, C, E, and selenium compound on prevention of liver cancer in rats. East Afr Med J. (1993) 70:151–3.
197. Nyandieka H, Wakhis J, Kilonzo M. Association of reduction of AFB1-induced liver tumours by antioxidants with increased activity of microsomal enzymes. Indian J Med Res. (1990) 92:332–6.
198. Sayin VI, Ibrahim MX, Larsson E, Nilsson JA, Lindahl P, Bergo MO. Antioxidants accelerate lung cancer progression in mice. Sci Transl Med. (2014) 6:221ra15. doi: 10.1126/scitranslmed.3007653
199. Obuseh FA, Jolly PE, Jiang Y, Shuaib FM, Waterbor J, Ellis WO, et al. Aflatoxin B1 albumin adducts in plasma and aflatoxin M1 in urine are associated with plasma concentrations of vitamins A and E. Int J Vitam Nutr Res. (2010) 80:355. doi: 10.1024/0300-9831/a000021
200. Rasouli H, Yarani R, Pociot F, Popović-Djordjević, J. Anti-diabetic potential of plant alkaloids: revisiting current findings and future perspectives. Pharmacol Res. (2020) 155:104723. doi: 10.1016/j.phrs.2020.104723
201. Tapan BA, Gupta A, Albratty M, Najmi A, Meraya AM, et al. Alkaloidal phytoconstituents for diabetes management: exploring the unrevealed potential. Molecules. (2022) 27:1–25. doi: 10.3390/molecules27185851
202. WHO. Diabetes Mellitus Factsheet. Available online at: https://www.who.int/health-topics/diabetes#tab=tab_1 (accessed June 14, 2022).
203. Lee HJ. Introduction to Type 2 diabetes mellitus. In:Davies TF, , editors. A Case-Based Guide to Clinical Endocrinology. Switzerland: Springer (2022). p. 351–2. doi: 10.1007/978-3-030-84367-0_38
204. Beulens JW, Pinho MG, Abreu TC, den Braver NR, Lam TM, Huss A, et al. Environmental risk factors of type 2 diabetes-an exposome approach. Diabetologia. (2021) 65:1–12. doi: 10.1007/s00125-021-05618-w
205. Misra BB, Misra A. The chemical exposome of type 2 diabetes mellitus: opportunities and challenges in the omics era. Diabetes Metab Syndr. (2020) 14:23–38. doi: 10.1016/j.dsx.2019.12.001
206. Dendup T, Feng X, Clingan S, Astell-Burt T. Environmental risk factors for developing type 2 diabetes mellitus: a systematic review. Int J Environ Res. (2018) 15:78. doi: 10.3390/ijerph15010078
207. Rewers M, Ludvigsson J. Environmental risk factors for type 1 diabetes. Lancet. (2016) 387:2340–8. doi: 10.1016/S0140-6736(16)30507-4
208. Raman PG. Environmental factors in causation of diabetes mellitus. In:Larramendy ML, Soloneski S, , editors. Environmental Health Risk-Hazardous Factors to Living Species. New York: IntechOpen (2016). doi: 10.5772/62543
209. Liu W, Wang B, Yang S, Xu T, Yu L, Wang X, et al. Associations of propylene oxide exposure with fasting plasma glucose and diabetes: roles of oxidative DNA damage and lipid peroxidation. Environ Pollut. (2022) 292:118453. doi: 10.1016/j.envpol.2021.118453
210. Parker VG, Mayo RM, Logan BN, Holder BJ, Smart PT. Toxins and diabetes mellitus: an environmental connection? Diabetes Spectr. (2002) 15:109–2. doi: 10.2337/diaspect.15.2.109
211. Kido Y. Gene-environment interaction in type 2 diabetes. Diabetol Int. (2017) 8:7–13. doi: 10.1007/s13340-016-0299-2
213. Akash MSH, Qader A, Rehman K. Biochemical investigation of human exposure to aflatoxin M1 and its association with risk factors of diabetes mellitus. Environ Sci Pollut Res Int. (2021) 28:62907–18. doi: 10.1007/s11356-021-14871-w
214. Tsai F-J, Chen S-Y, Liu Y-C, Chen C-J. The comparison of CHCA solvent compositions for improving LC-MALDI performance and its application to study the impact of aflatoxin B1 on the liver proteome of diabetes mellitus type 1 mice. PLoS ONE. (2017) 12:e0181423. doi: 10.1371/journal.pone.0181423
215. Ma Y, Kong Q, Hua H, Luo T, Jiang Y. Aflatoxin B1 up-regulates insulin receptor substrate 2 and stimulates hepatoma cell migration. PLoS ONE. (2012) 7:e47961. doi: 10.1371/journal.pone.0047961
216. Casellas A, Mallol C, Salavert A, Jimenez V, Garcia M, Agudo J, et al. Insulin-like growth factor 2 overexpression induces β-cell dysfunction and increases beta-cell susceptibility to damage. J Biol Chem. (2015) 290:16772–85. doi: 10.1074/jbc.M115.642041
217. Garcia-Rubi E, Calles-Escandón J. Insulin resistance and type 2 diabetes mellitus: its relationship with the β3-adrenergic receptor. Arch Med Res. (1999) 30:459–64. doi: 10.1016/S0188-4409(99)00077-6
218. Aleissa MS, Alkahtani S, Abd Eldaim MA, Ahmed AM, Bungău SG, Almutairi B, et al. Fucoidan ameliorates oxidative stress, inflammation, DNA damage, and hepatorenal injuries in diabetic rats intoxicated with aflatoxin B1. Oxid Med Cell Longev. (2020) 2020:9316751. doi: 10.1155/2020/9316751
219. Mor F, Sengul O, Topsakal S, Kilic MA, Ozmen O. Diabetogenic effects of ochratoxin A in female rats. Toxins. (2017) 9:144. doi: 10.3390/toxins9040144
220. Siegel AB, Zhu AX. Metabolic syndrome and hepatocellular carcinoma: two growing epidemics with a potential link. Cancer. (2009) 115:5651–61. doi: 10.1002/cncr.24687
221. Li Y, Shi J, Liu X, Deng Q, Huang Y, Yang Z. Metabolic syndrome relates to high risk in hepatocellular carcinoma: a meta-analysis. Discov. Med. (2018) 26:185–96.
222. Marchioro A, Mallmann A, Diel A, Dilkin P, Rauber R, Blazquez F, et al. Effects of aflatoxins on performance and exocrine pancreas of broiler chickens. Avian Dis. (2013) 57:280–4. doi: 10.1637/10426-101712-Reg.1
223. Rotimi OA, Rotimi SO, Duru CU, Ebebeinwe OJ, Abiodun AO, Oyeniyi BO, et al. Acute aflatoxin B1-Induced hepatotoxicity alters gene expression and disrupts lipid and lipoprotein metabolism in rats. Toxicol Rep. (2017) 4:408–14. doi: 10.1016/j.toxrep.2017.07.006
224. Ugbaja RN, Okedairo OM, Oloyede AR, Ugwor EI, Akinloye DI, Ojo OP, et al. Probiotics consortium synergistically ameliorates aflatoxin B1-induced disruptions in lipid metabolism of female albino rats. Toxicon. (2020) 186:109–19. doi: 10.1016/j.toxicon.2020.08.007
225. Busik JV. Lipid metabolism dysregulation in diabetic retinopathy. J Lipid Res. (2021) 62, 1–12. doi: 10.1194/jlr.TR120000981
226. Rotimi OA, Onuzulu CD, Dewald AL, Ehlinger J, Adelani IB, Olasehinde OE, et al. Early life exposure to aflatoxin B1 in rats: alterations in lipids, hormones, and DNA methylation among the offspring. Int J Environ Res Public Health. (2021) 18:589. doi: 10.3390/ijerph18020589
227. Suseela R, Shanmugasundaram E, Shanmugasundaram K. Effect of Penitrem A on glucose tolerance studied in rats. Curr Sci. (1986) 55:98–100.
228. Kumar SN, Gopal A, Telang KPS, Bastia B, Jain AK. Toxic manifestation of endosulfan and ochratoxin-A in adult male rats. MOJ Toxicol. (2015) 1:96–101. doi: 10.15406/mojt.2015.01.00012
229. Alvarez CS, Rivera-Andrade A, Kroker-Lobos MF, Florio AA, Smith JW, Egner PA, et al. Associations between aflatoxin B1-albumin adduct levels with metabolic conditions in Guatemala: a cross-sectional study. Health Sci Rep. (2022) 5:e495. doi: 10.1002/hsr2.495
230. Sabir S, Rehman K, Fiayyaz F, Kamal S, Akash MSH. Role of aflatoxins as EDCs in metabolic disorders. In:Hamid Akash MS, Hashmi MZ, Rehman K, , editors. Endocrine Disrupting Chemicals-induced Metabolic Disorders and Treatment Strategies. Switzerland: Springer (2021). p. 381–9. doi: 10.1007/978-3-030-45923-9_23
231. WHO. Dementia Statistics. Available online at: https://www.who.int/news-room/fact-sheets/detail/dementia (accessed August 24, 2022).
232. Breijyeh Z, Karaman R. Comprehensive review on Alzheimer's disease: causes and treatment. Molecules. (2020) 25:5789. doi: 10.3390/molecules25245789
233. Lyketsos CG, Carrillo MC, Ryan JM, Khachaturian AS, Trzepacz P, Amatniek J, et al. Neuropsychiatric symptoms in Alzheimer's disease. Alzheimers Dement. (2011) 7:532–9. doi: 10.1016/j.jalz.2011.05.2410
234. Armstrong RA. What causes Alzheimer's disease? Folia Neuropathol. (2013) 51:169–88. doi: 10.5114/fn.2013.37702
235. Rudge JDA. A new hypothesis for Alzheimer's disease: the lipid invasion model. J Alzheimers Dis. (2022). doi: 10.3233/ADR-210299. [Epub ahead of print].
236. James BD, Bennett DA. Causes and patterns of dementia: an update in the era of redefining Alzheimer's disease. Annu Rev Public Health. (2019) 40:65–84. doi: 10.1146/annurev-publhealth-040218-043758
237. Arce-López B, Alvarez-Erviti L, De Santis B, Izco M, López-Calvo S, Marzo-Sola ME, et al. Biomonitoring of mycotoxins in plasma of patients with Alzheimer's and Parkinson's disease. Toxins. (2021) 13:477. doi: 10.3390/toxins13070477
238. Guerrero A, De Strooper B, Arancibia-Cárcamo IL. Cellular senescence at the crossroads of inflammation and Alzheimer's disease. Trends Neurosci. (2021) 44:714–727. doi: 10.1016/j.tins.2021.06.007
239. Alsayyah A, ElMazoudy R, Al-Namshan M, Al-Jafary M, Alaqeel N. Chronic neurodegeneration by aflatoxin B1 depends on alterations of brain enzyme activity and immunoexpression of astrocyte in male rats. Ecotoxicol Environ Saf. (2019) 182:109407. doi: 10.1016/j.ecoenv.2019.109407
240. Kara M, Öztaş, E. In-vitro evaluation of aflatoxin-B1 and fumonisin-B1 cellular stress effects on SH-SY5Y cell line. Med Sci. (2020) 9:1–4. doi: 10.5455/medscience.2019.08.9206
241. Sahin GA, Karabulut D, Unal G, Sayan M, Sahin H. Effects of probiotic supplementation on very low dose AFB1-induced neurotoxicity in adult male rats. Life Sci. (2022) 306:120798. doi: 10.1016/j.lfs.2022.120798
242. Bahey NG, Abd Elaziz HO, Gadalla KKES. Toxic effect of aflatoxin B1 and the role of recovery on the rat cerebral cortex and hippocampus. Tissue Cell. (2015) 47:559–66. doi: 10.1016/j.tice.2015.09.001
243. Alonso-Garrido M, Tedeschi P, Maietti A, Font G, Marchetti N, Manyes L. Mitochondrial transcriptional study of the effect of aflatoxins, enniatins and carotenoids in vitro in a blood brain barrier model. Food Chem Toxicol. (2020) 137:111077. doi: 10.1016/j.fct.2019.111077
244. Sharma AC, Proshad R, Kormoker T, Islam MS, Chandra K. A review on aflatoxins in stored grain food, their sources, mechanisms and possible health hazard. Arch Agric Environ Sci. (2018) 3:416–423. doi: 10.26832/24566632.2018.0304015
245. Richard SA, Manaphraim NY, Kortei NK. The novel neurotoxic and neuroimmunotoxic capabilities of aflatoxin B1 on the nervous system: a review. Adv BioSci Clin Med. (2020) 8:1–8. doi: 10.7575/aiac.abcmed.v.8n.3p.1
246. Hampel H, Caraci F, Cuello AC, Caruso G, Nisticò R, Corbo M, et al. A path toward precision medicine for neuroinflammatory mechanisms in Alzheimer's disease. Front Immunol. (2020) 11:456. doi: 10.3389/fimmu.2020.00456
247. Mehrzad J, Malvandi AM, Alipour M, Hosseinkhani S. Environmentally relevant level of aflatoxin B1 elicits toxic pro-inflammatory response in murine CNS-derived cells. Toxicol Lett. (2017) 279:96–106. doi: 10.1016/j.toxlet.2017.07.902
248. Kaur N, Chugh H, Sakharkar MK, Dhawan U, Chidambaram SB, Chandra R. Neuroinflammation mechanisms and phytotherapeutic intervention: a systematic review. ACS Chem Neurosci. (2020) 11:3707–31. doi: 10.1021/acschemneuro.0c00427
249. Chantong B, Nusuetrong P. Possible involvement of glucocorticoids in mycotoxin-induced neuroinflammation. J Appl Anim Sci. (2015) 8:17–28.
250. Ljubisavljevic S, Stojanovic I. Neuroinflammation and demyelination from the point of nitrosative stress as a new target for neuroprotection. Rev Neurosci. (2015) 26:49–73. doi: 10.1515/revneuro-2014-0060
251. Liu X-J, Liu T, Chen G, Wang B, Yu, L, Yin C, et al. TLR signaling adaptor protein MyD88 in primary sensory neurons contributes to persistent inflammatory and neuropathic pain and neuroinflammation. Sci Rep. (2016) 6:1–11. doi: 10.1038/srep28188
252. Luo X, Wang X, Xia Z, Chung SK, Cheung CW. CXCL12/CXCR4 axis: an emerging neuromodulator in pathological pain. Rev Neurosci. (2016) 27:83–92. doi: 10.1515/revneuro-2015-0016
253. Yan J, Kuzhiumparambil U, Bandodkar A, Bandodkar S, Dale RC, Fu S. Cerebrospinal fluid metabolites in tryptophan-kynurenine and nitric oxide pathways: biomarkers for acute neuroinflammation. Dev Med Child Neurol. (2021) 63:552–9. doi: 10.1111/dmcn.14774
254. Lee S-H, Suk K. Emerging roles of protein kinases in microglia-mediated neuroinflammation. Biochem Pharmacol. (2017) 146:1–9. doi: 10.1016/j.bcp.2017.06.137
255. Rama Rao KV, Kielian T. Neuron-astrocyte interactions in neurodegenerative diseases: role of neuroinflammation. Clin Exp Neuroimmunol. (2015) 6:245–63. doi: 10.1111/cen3.12237
256. Rhie SJ, Jung E-Y, Shim I. The role of neuroinflammation on pathogenesis of affective disorders. J Exerc Rehabil. (2020) 16:2–9. doi: 10.12965/jer.2040016.008
257. Pei X, Zhang W, Jiang H, Liu D, Liu X, Li L, et al. Food-origin mycotoxin-induced neurotoxicity: intend to break the rules of neuroglia cells. Oxid Med Cell Longev. (2021) 2021:9967334. doi: 10.1155/2021/9967334
258. Shahba S, Mehrzad J, Malvandi AM. Neuroimmune disruptions from naturally occurring levels of mycotoxins. Environ Sci Pollut Res. (2021) 28:32156–76. doi: 10.1007/s11356-021-14146-4
259. Souto NS, Claudia Monteiro Braga A, Lutchemeyer de Freitas M, Rechia Fighera M, Royes LFF, Schneider Oliveira M, et al. Aflatoxin B1 reduces non-enzymatic antioxidant defenses and increases protein kinase C activation in the cerebral cortex of young rats. Nutr Neurosci. (2018) 21:268–75. doi: 10.1080/1028415X.2017.1278837
260. David S, Shoemaker M, Haley BE. Abnormal properties of creatine kinase in Alzheimer's disease brain: correlation of reduced enzyme activity and active site photolabeling with aberrant cytosol-membrane partitioning. Mol Brain Res. (1998) 54:276–87. doi: 10.1016/S0169-328X(97)00343-4
261. Souza CF, Baldissera MD, Zeppenfeld CC, Descovi S, Stefani LM, Baldisserotto B, et al. Oxidative stress mediated the inhibition of cerebral creatine kinase activity in silver catfish fed with aflatoxin B1-contaminated diet. Fish Physiol Biochem. (2019) 45:63–70. doi: 10.1007/s10695-018-0534-9
262. Park S, Lee J-Y, You S, Song G, Lim W. Neurotoxic effects of aflatoxin B1 on human astrocytes in vitro and on glial cell development in zebrafish in vivo. J Hazard Mater. (2020) 386:121639. doi: 10.1016/j.jhazmat.2019.121639
263. Laag EM, Abd Elaziz HO. Effect of aflatoxin-B1 on rat cerebellar cortex: light and electron microscopic study. Egypt J Histol. (2013) 36:601–10. doi: 10.1097/01.EHX.0000432619.75801.15
264. da Silva AS, Santurio JM, Roza LF, Bottari NB, Galli GM, Morsch VM, et al. Aflatoxins produced by Aspergillus parasiticus present in the diet of quails increase the activities of cholinesterase and adenosine deaminase. Microb Pathog. (2017) 107:309–12. doi: 10.1016/j.micpath.2017.03.041
265. Da Silva E, Bracarense AP, Oswald IP. Mycotoxins and oxidative stress: where are we? World Mycotoxin J. (2018) 11:113–34. doi: 10.3920/WMJ2017.2267
266. Malvandi AM, Shahba S, Mehrzad J, Lombardi G. Metabolic disruption by naturally occurring mycotoxins in circulation: a focus on vascular and bone homeostasis dysfunction. Front Nutr. (2022) 9:915681. doi: 10.3389/fnut.2022.915681
267. Mehrzad J. Environmentally relevant level of aflatoxin B1 and the role of (non) oxidative immuno-/neurodysregulation and toxicity. In:Preedy V. B. P. a. V. R., , editors. Toxicology. London: Elsevier (2021). p. 165–79. doi: 10.1016/B978-0-12-819092-0.00018-2
268. Zhou Y, Wang S, Luo H, Xu F, Liang J, Ma C, et al. Aflatoxin B1 induces microglia cells apoptosis mediated by oxidative stress through NF-κB signaling pathway in mice spinal cords. Environ Toxicol Pharmacol. (2022) 90:103794. doi: 10.1016/j.etap.2021.103794
269. Huang B, Chen Q, Wang L, Gao X, Zhu W, Mu P, et al. Aflatoxin B1 induces neurotoxicity through reactive oxygen species generation, DNA damage, apoptosis, and S-phase cell cycle arrest. Int J Mol Sci. (2020) 21:6517. doi: 10.3390/ijms21186517
270. Zhang J, Su D, Liu Q, Yuan Q, Ouyang Z, Wei Y, et al. Gasdermin D-mediated microglial pyroptosis exacerbates neurotoxicity of aflatoxins B1 and M1 in mouse primary microglia and neuronal cultures. Neurotoxicology. (2022) 91:305–20. doi: 10.1016/j.neuro.2022.06.003
271. Sung H, Ferlay J, Siegel RL, Laversanne M, Soerjomataram I, Jemal A, et al. Global cancer statistics 2020: GLOBOCAN estimates of incidence and mortality worldwide for 36 cancers in 185 countries. CA Cancer J Clin. (2021) 71:209–49. doi: 10.3322/caac.21660
272. Siegel RL, Miller KD, Jemal A. Cancer statistics, 2019. CA Cancer J Clin. (2019) 69:7–34. doi: 10.3322/caac.21551
273. Bray F, Ferlay J, Soerjomataram I, Siegel RL, Torre LA, Jemal A. Global cancer statistics 2018: GLOBOCAN estimates of incidence and mortality worldwide for 36 cancers in 185 countries. CA Cancer J. Clin. (2018) 68:394–424. doi: 10.3322/caac.21492
274. Pignatti F, Wilking U, Postmus D, Wilking N, Delgado J, Bergh J. The value of anticancer drugs-a regulatory view. Nat Rev Clin Oncol. (2022) 19:207–15. doi: 10.1038/s41571-021-00584-z
275. Ratajewski M, Walczak-Drzewiecka A, Sałkowska A, Dastych J. Aflatoxins upregulate CYP3A4 mRNA expression in a process that involves the PXR transcription factor. Toxicol Lett. (2011) 205:146–53. doi: 10.1016/j.toxlet.2011.05.1034
276. Bbosa GS, Kitya D, Lubega A, Ogwal-Okeng J, Anokbonggo WW, Kyegombe DB. Review of the biological and health effects of aflatoxins on body organs and body systems. In:Razzaghi-Abyaneh M, , editors. Aflatoxins-Recent Advances and Future Prospects, Volume 12. London: InTechOpen (2013). p. 239–65.
278. Gramantieri L, Gnudi F, Vasuri F, Mandrioli D, Fornari F, Tovoli F, et al. Aflatoxin B1 DNA-adducts in hepatocellular carcinoma from a low exposure area. Nutrients. (2022) 14:1652. doi: 10.3390/nu14081652
279. Swetha C, Girish BP. An overview on aflatoxin B1 induced initiation and progression of hepatocellular carcinoma. In:Nagaraju GP, Peela S, , editors. Theranostics and Precision Medicine for the Management of Hepatocellular Carcinoma, Vol 1. London: Elsevier, Academic Press (2022). p. 73–9. doi: 10.1016/B978-0-323-98806-3.00016-7
280. Poirier MC. DNA adducts as exposure biomarkers and indicators of cancer risk. Environ Health Perspect. (1997) 105(Suppl 4):907–12. doi: 10.1289/ehp.97105s4907
281. Bressac B, Puisieux A, Kew M, Volkmann M, Bozcall S, Mura JB, et al. p53 mutation in hepatocellular carcinoma after aflatoxin exposure. Lancet. (1991) 338:1356–9. doi: 10.1016/0140-6736(91)92236-U
282. Balogh K, Kövesi B, Zándoki E, Kulcsár S, Ancsin Z, Erdélyi M, et al. Effect of sterigmatocystin or aflatoxin contaminated feed on lipid peroxidation and glutathione redox system and expression of glutathione redox system regulatory genes in broiler chicken. Antioxidants. (2019) 8:201. doi: 10.3390/antiox8070201
283. Wang J, Lin L, Jiang Q, Huang W, Liu N. Effect of supplemental lactic acid bacteria on growth performance, glutathione turnover and aflatoxin B1 removal in lambs. Czech J Anim Sci. (2019) 64:272–78. doi: 10.17221/5/2019-CJAS
284. Lehmann JM, McKee DD, Watson MA, Willson TM, Moore JT, Kliewer SA. The human orphan nuclear receptor PXR is activated by compounds that regulate CYP3A4 gene expression and cause drug interactions. J Clin Investig. (1998) 102:1016–23. doi: 10.1172/JCI3703
285. Frank C, Makkonen H, Dunlop TW, Matilainen M, Väisänen S, Carlberg C. Identification of pregnane X receptor binding sites in the regulatory regions of genes involved in bile acid homeostasis. J Mol Biol. (2005) 346:505–19. doi: 10.1016/j.jmb.2004.12.003
286. Chenivesse X, Franco D, Bréchot C. MDR1 (multidrug resistance) gene expression in human primary liver cancer and cirrhosis. J Hepatol. (1993) 18:168–72. doi: 10.1016/S0168-8278(05)80243-0
287. Cui Y, König J, Nies AT, Pfannschmidt M, Hergt M, Franke WW, et al. Detection of the human organic anion transporters SLC21A6 (OATP2) and SLC21A8 (OATP8) in liver and hepatocellular carcinoma. Lab Invest. (2003) 83:527–38. doi: 10.1097/01.LAB.0000065015.02412.48
288. Zhu Y, Tan YQ, Leung LK. Aflatoxin B1 disrupts transient receptor potential channel activity and increases COX-2 expression in JEG-3 placental cells. Chem Biol Interact. (2016) 260:84–90. doi: 10.1016/j.cbi.2016.11.002
289. Ghufran M, Soni P, Kanade SR. Aflatoxin-induced upregulation of protein arginine methyltransferase 5 is mediated by protein kinase C and extracellular signal-regulated kinase. Cell Biol Toxicol. (2019) 35:67–80. doi: 10.1007/s10565-018-9439-8
290. Benkerroum N. Chronic and acute toxicities of aflatoxins: Mechanisms of action. Int J Environ Res Public Health. (2020) 17:423. doi: 10.3390/ijerph17020423
291. Owumi SE, Irozuru CE, Arunsi UO, Oyelere AK. Caffeic acid protects against DNA damage, oxidative and inflammatory mediated toxicities, and upregulated caspases activation in the hepatorenal system of rats treated with aflatoxin B1. Toxicon. (2022) 207:1–12. doi: 10.1016/j.toxicon.2021.12.021
292. Dai Y, Huang K, Zhang B, Zhu L, Xu W. Aflatoxin B1-induced epigenetic alterations: an overview. Food Chem Toxicol. (2017) 109:683–9. doi: 10.1016/j.fct.2017.06.034
293. Ferreira RG, Cardoso MV, De Souza Furtado KM, Espíndola KMM, Amorim RP, Monteiro MC. Epigenetic alterations caused by aflatoxin b1: a public health risk in the induction of hepatocellular carcinoma. Transl Res. (2019) 204:51–71. doi: 10.1016/j.trsl.2018.09.001
294. Herceg Z, Paliwal A. Epigenetic mechanisms in hepatocellular carcinoma: how environmental factors influence the epigenome. Mutat Res Rev Mutat. (2011) 727:55–61. doi: 10.1016/j.mrrev.2011.04.001
295. Li H, Xing L, Zhang M, Wang J, Zheng N. The toxic effects of aflatoxin B1 and aflatoxin M1 on kidney through regulating L-proline and downstream apoptosis. Biomed Res. Int. (2018) 2018:9074861. doi: 10.1155/2018/9074861
296. Deng J, Zhao L, Zhang, N-Y, Karrow NA, Krumm CS, Sun L-H. Aflatoxin B1 metabolism: Regulation by phase I and II metabolizing enzymes and chemoprotective agents. Mutat Res Rev Mutat. (2018) 778:79–89. doi: 10.1016/j.mrrev.2018.10.002
297. Eom S-Y, Yim D-H, Zhang Y, Yun, K, Moon SI, et al. Dietary aflatoxin B1 intake, genetic polymorphisms of CYP1A2, CYP2E1, EPHX1, GSTM1, and GSTT1, and gastric cancer risk in Korean. Cancer Causes Control. (2013) 24:1963–72. doi: 10.1007/s10552-013-0272-3
298. Engin AB, Engin A. DNA damage checkpoint response to aflatoxin B1. Environ Toxicol Pharm. (2019) 65:90–6. doi: 10.1016/j.etap.2018.12.006
299. Bedard LL, Massey TE. Aflatoxin B1-induced DNA damage and its repair. Cancer Lett. (2006) 241:174–83. doi: 10.1016/j.canlet.2005.11.018
300. Komsky-Elbaz A, Saktsier M, Roth Z. Aflatoxin B1 impairs sperm quality and fertilization competence. Toxicology. (2018) 393:42–50. doi: 10.1016/j.tox.2017.11.007
301. Marin DE, Taranu I. Overview on aflatoxins and oxidative stress. Toxin Rev. (2012) 31:32–43. doi: 10.3109/15569543.2012.730092
302. WHO. Noncommunicable Diseases. Available online at: https://www.who.int/news-room/fact-sheets/detail/noncommunicable-diseases (accessed September 12, 2022).
303. Azadnajafabad S, Mohammadi E, Aminorroaya A, Fattahi N, Rezaei S, Haghshenas R, et al. Non-communicable diseases' risk factors in Iran; a review of the present status and action plans. J Diabetes Metab Disord. (2021) 2021:1–9. doi: 10.1007/s40200-020-00709-8
304. WHO. Noncommunicable Diseases: Risk Factors. Available online at: https://www.who.int/data/gho/data/themes/topics/topic-details/GHO/ncd-risk-factors (accessed September 12, 2022).
305. Ladeira C, Frazzoli C, Orisakwe OE. Engaging one health for non-communicable diseases in Africa: perspective for mycotoxins. Front Public Health. (2017) 5:266. doi: 10.3389/fpubh.2017.00266
306. Dang HV, Nguyen LT, Tran HT, Nguyen HT, Dang AK, Ly VD, et al. Risk factors for non-communicable diseases in Vietnam: a focus on pesticides. Front Environ Sci. (2017) 5:58. doi: 10.3389/fenvs.2017.00058
307. Lu X, Hu B, Shao L, Tian Y, Jin T, Jin Y, et al. Integrated analysis of transcriptomics and metabonomics profiles in aflatoxin B1-induced hepatotoxicity in rat. Food Chem Toxicol. (2013) 55:444–55. doi: 10.1016/j.fct.2013.01.020
308. Zhang N-Y, Qi M, Gao X, Zhao L, Liu J, Song W-J, et al. Response of the hepatic transcriptome to aflatoxin B1 in ducklings. Toxicon. (2016) 111:69–76. doi: 10.1016/j.toxicon.2015.12.022
309. Yarru L, Settivari R, Antoniou E, Ledoux D, Rottinghaus G. Toxicological and gene expression analysis of the impact of aflatoxin B1 on hepatic function of male broiler chicks. Poult Sci. (2009) 88:360–71. doi: 10.3382/ps.2008-00258
310. Shi J, He J, Lin J, Sun X, Sun F, Ou C, et al. Distinct response of the hepatic transcriptome to Aflatoxin B1 induced hepatocellular carcinogenesis and resistance in rats. Sci Rep. (2016) 6:1–9. doi: 10.1038/srep31898
311. Liu X, Kumar Mishra S, Wang T, Xu Z, Zhao X, Wang Y, et al. AFB1 induced transcriptional regulation related to apoptosis and lipid metabolism in liver of chicken. Toxins. (2020) 12:290. doi: 10.3390/toxins12050290
312. Iori S, Pauletto M, Bassan I, Bonsembiante F, Gelain ME, Bardhi A, et al. Deepening the whole transcriptomics of bovine liver cells exposed to AFB1: a spotlight on toll-like receptor 2. Toxins. (2022) 14:504. doi: 10.3390/toxins14070504
313. Bao X, Li S, Gao Y, Wang J, Zheng N. Transcriptome analysis revealed that aflatoxin M1 could cause cell cycle arrest in differentiated Caco-2 cells. Toxicol In Vitro. (2019) 59:35–43. doi: 10.1016/j.tiv.2019.03.035
314. Gao Y-N, Wu C-Q, Wang J-Q. Metabolomic analysis reveals the mechanisms of hepatotoxicity induced by aflatoxin M1 and ochratoxin A. Toxins. (2022) 14:141. doi: 10.3390/toxins14020141
315. Reed KM, Mendoza KM, Abrahante JE, Coulombe RA. Comparative response of the hepatic transcriptomes of domesticated and wild turkey to aflatoxin B1. Toxins. (2018) 10:42. doi: 10.3390/toxins10010042
316. Kjellstrom T, Butler AJ, Lucas RM, Bonita R. Public health impact of global heating due to climate change: potential effects on chronic non-communicable diseases. Int J Public Health. (2010) 55:97–103. doi: 10.1007/s00038-009-0090-2
317. Del Campo JA, Gallego P, Grande L. Role of inflammatory response in liver diseases: Therapeutic strategies. World J Hepatol. (2018) 10:1–7. doi: 10.4254/wjh.v10.i1.1
318. Weiskirchen R, Tacke F. Cellular and molecular functions of hepatic stellate cells in inflammatory responses and liver immunology. Hepatobiliary Surg Nutr. (2014) 3:344. doi: 10.3978/j.issn.2304-3881.2014.11.03
319. Fehér J, Lengyel G. Silymarin in the prevention and treatment of liver diseases and primary liver cancer. Curr Pharm Biotechnol. (2012) 13:210–17. doi: 10.2174/138920112798868818
320. FDA. Aflatoxin Poisoning in Pets. Available online at: https://www.fda.gov/animal-veterinary/animal-health-literacy/aflatoxin-poisoning-pets#:~:text=There%20is%20no%20antidote%20for,imbalances%20in%20the%20pet's%20system (accessed August 25, 2022).
321. FDA. Mycotoxins: Toxins Found in Food Infected by Certain Molds or Fungi. Available online at: https://www.fda.gov/food/natural-toxins-food/mycotoxins (accessed August 25, 2022).
322. Groopman JD, Kensler TW, Wild CP. Protective interventions to prevent aflatoxin-induced carcinogenesis in developing countries. Annu Rev Public Health. (2008) 29:187–203. doi: 10.1146/annurev.publhealth.29.020907.090859
323. Dhakal A, Sbar E. Aflatoxin Toxicity. Available online at: https://www.ncbi.nlm.nih.gov/books/NBK557781/ (accessed August 23, 2021).
324. Makhuvele R, Naidu K, Gbashi S, Thipe VC, Adebo OA, Njobeh PB. The use of plant extracts and their phytochemicals for control of toxigenic fungi and mycotoxins. Heliyon. (2020) 6:e05291. doi: 10.1016/j.heliyon.2020.e05291
325. Jha A, Krithika R, Manjeet D, Verma RJ. Protective effect of black tea infusion on aflatoxin-induced hepatotoxicity in mice. J Clin Exp Hepatol. (2013) 3:29–36. doi: 10.1016/j.jceh.2012.12.003
326. Reddy K, Reddy C, Muralidharan K. Potential of botanicals and biocontrol agents on growth and aflatoxin production by Aspergillus flavus infecting rice grains. Food Control. (2009) 20:173–8. doi: 10.1016/j.foodcont.2008.03.009
327. Choi K-C, Chung W-T, Kwon J-K, Jang Y-S, Park S-M, et al. Inhibitory effects of quercetin on aflatoxin B1-induced hepatic damage in mice. Food Chem Toxicol. (2010) 48:2747–53. doi: 10.1016/j.fct.2010.07.001
328. Gugliandolo E, Peritore AF, D'Amico R, Licata P, Crupi R. Evaluation of neuroprotective effects of quercetin against aflatoxin B1-intoxicated mice. Animals. (2020) 10:898. doi: 10.3390/ani10050898
329. Wang X, Li L, Zhang G. Quercetin protects the buffalo rat liver (BRL-3A) cells from aflatoxin B1-induced cytotoxicity via activation of Nrf2-ARE pathway. World Mycotoxin J. (2020) 13:299–312. doi: 10.3920/WMJ2019.2465
330. Eftekhari A, Ahmadian E, Panahi-Azar V, Hosseini H, Tabibiazar M, Maleki Dizaj S. Hepatoprotective and free radical scavenging actions of quercetin nanoparticles on aflatoxin B1-induced liver damage: in vitro/in vivo studies. Artif Cells Nanomed Biotechnol. (2018) 46:411–20. doi: 10.1080/21691401.2017.1315427
331. Yang D, Wang T, Long M, Li P. Quercetin: its main pharmacological activity and potential application in clinical medicine. Oxid Med Cell Longev. (2020) 2020:8825387. doi: 10.1155/2020/8825387
332. Buening MK, Chang RL, Huang M-T, Fortner JG, Wood AW. Activation and inhibition of benzo (a) pyrene and aflatoxin B1 metabolism in human liver microsomes by naturally occurring flavonoids. Cancer Res. (1981) 41:67–72.
333. Barcelos GR, Grotto D, Angeli JPF, Serpeloni JM, Rocha BA, Bastos JK, et al. Evaluation of antigenotoxic effects of plant flavonoids quercetin and rutin on HepG2 cells. Phytother Res. (2011) 25:1381–8. doi: 10.1002/ptr.3436
334. Bhattacharya R, Firozi P. Effect of plant flavonoids on microsome catalyzed reactions of aflatoxin B1 leading to activation and DNA adduct formation. Cancer Lett. (1988) 39:85–91. doi: 10.1016/0304-3835(88)90043-2
335. Schwartz A, Rate W. Inhibition of aflatoxin B1-induced cytotoxicity and binding to DNA in cultured rat liver cells by naturally occurring flavones. J Environ Pathol Toxicol. (1979) 2:1021–8.
336. Mistry K, Krishna M, Bhattacharya R. Modulation of aflatoxin B1 activated protein kinase C by phenolic compounds. Cancer Lett. (1997) 121:99–104. doi: 10.1016/S0304-3835(97)00337-6
337. Phan ADT, Zhang J, Seididamyeh M, Srivarathan S, Netzel ME, Sivakumar D, et al. Hydrolysable tannins, physicochemical properties, and antioxidant property of wild-harvested Terminalia ferdinandiana (exell) fruit at different maturity stages. Front Nutr. (2022) 9:961679. doi: 10.3389/fnut.2022.961679
338. Rasouli H, Mazinani MH, Haghbeen K. Benefits and challenges of olive biophenols: a perspective. In:Preedy VR, Watson RR, , editors. Olives and Olive Oil in Health and Disease Prevention. London: Elsevier (2021). p. 489–503. doi: 10.1016/B978-0-12-819528-4.00045-6
339. Simonetti G, Brasili E, Pasqua G. Antifungal activity of phenolic and polyphenolic compounds from different matrices of Vitis vinifera L. against human pathogens. Molecules. (2020) 25:3748. doi: 10.3390/molecules25163748
340. Vestergaard M, Ingmer H. Antibacterial and antifungal properties of resveratrol. Int J Antimicrob Agents. (2019) 53:716–23. doi: 10.1016/j.ijantimicag.2019.02.015
341. Shahwan M, Alhumaydhi F, Ashraf GM, Hasan PM, Shamsi A. Role of polyphenols in combating Type 2 Diabetes and insulin resistance. Int J Biol Macromol. (2022) 206:567–79. doi: 10.1016/j.ijbiomac.2022.03.004
342. Moradi SZ, Jalili F, Farhadian N, Joshi T, Wang M, Zou L, et al. Polyphenols and neurodegenerative diseases: focus on neuronal regeneration. Crit Rev Food Sci Nutr. (2022) 62:3421–36. doi: 10.1080/10408398.2020.1865870
343. Echeverría F, Valenzuela R. Polyphenols in the prevention and treatment of non-communicable diseases. Curr Med Chem. (2022) 29:1027–8. doi: 10.2174/092986732906220221095655
344. Das M, Devi KP, Belwal T, Devkota HP, Tewari D, Sahebnasagh A, et al. Harnessing polyphenol power by targeting eNOS for vascular diseases. Crit Rev Food Sci Nutr. (2021) 46:1–26. doi: 10.1080/10408398.2021.1971153
345. Rasouli H, Farzaei MH, Khodarahmi R. Polyphenols and their benefits: a review. Int J Food Prop. (2017). 20(Suppl. 2):1700–41. doi: 10.1080/10942912.2017.1354017
346. Rasouli H, Farzaei MH, Mansouri K, Mohammadzadeh S, Khodarahmi R. Plant cell cancer: may natural phenolic compounds prevent onset and development of plant cell malignancy? A literature review. Molecules. (2016) 21:1104. doi: 10.3390/molecules21091104
347. Rasouli H, Hosseini-Ghazvini SM-B, Khodarahmi R. Therapeutic potentials of the most studied flavonoids: highlighting antibacterial and antidiabetic functionalities. Stud Nat Prod Chem. (2019) 60:85–122. doi: 10.1016/B978-0-444-64181-6.00003-6
348. Khan H, Sureda A, Belwal T, Çetinkaya S, Süntar I, et al. Polyphenols in the treatment of autoimmune diseases. Autoimmun Rev. (2019) 18:647–57. doi: 10.1016/j.autrev.2019.05.001
349. Yeung AWK, Tzvetkov NT, El-Demerdash A, Horbanczuk OK, Das N, Pirgozliev V, et al. Apple polyphenols in human and animal health. Anim Sci Pap. (2021) 39:105–18.
350. Yeung AWK, Tzvetkov NT, El-Tawil OS, Bungǎu SG, Abdel-Daim MM, Atanasov AG. Antioxidants: scientific literature landscape analysis. Oxid Med Cell Longev. (2019) 2019:1–12. doi: 10.31193/ssap.01.9787509752807
351. El Gharras H. Polyphenols: food sources, properties and applications-a review. Int J Food Sci Technol. (2009) 44:2512–8. doi: 10.1111/j.1365-2621.2009.02077.x
352. Mitra S, Tareq AM, Das R, Emran TB, Nainu F, Chakraborty AJ, et al. Polyphenols: a first evidence in the synergism and bioactivities. Food Rev Int. (2022) 36, 1–23. doi: 10.1080/87559129.2022.2026376
353. Cuccioloni M, Mozzicafreddo M, Bonfili L, Cecarini V, Eleuteri AM, Angeletti M. Natural occurring polyphenols as template for drug design. Focus on serine proteases. Chem Biol Drug Des. (2009) 74:1–15. doi: 10.1111/j.1747-0285.2009.00836.x
354. Tsao R. Chemistry and biochemistry of dietary polyphenols. Nutrients. (2010) 2:1231–46. doi: 10.3390/nu2121231
355. Tapas AR, Sakarkar D, Kakde R. Flavonoids as nutraceuticals: a review. Trop J Pharm Res. (2008) 7:1089–99. doi: 10.4314/tjpr.v7i3.14693
356. Agrawal A. Pharmacological activities of flavonoids: a review. Int J Pharm Sci Nanotechnol. (2011) 4:1394–8. doi: 10.37285/ijpsn.2011.4.2.3
357. Girish T, Kumar KA, Rao UP. C-Glycosylated flavonoids from black gram husk: protection against DNA and erythrocytes from oxidative damage and their cytotoxic effect on HeLa cells. Toxicol Rep. (2016) 3:652–63. doi: 10.1016/j.toxrep.2016.08.006
358. Barros L, Duenas M, Dias MI, Sousa MJ, Santos-Buelga C, Ferreira IC. Phenolic profiles of in vivo and in vitro grown Coriandrum sativum L. Food Chem. (2012) 132:841–8. doi: 10.1016/j.foodchem.2011.11.048
359. Tsopmo A, Awah FM, Kuete V. Lignans and stilbenes from African medicinal plants. In:Kuete V, , editors. Medicinal Plant Research in Africa. London: Elsevier (2013). p. 435–78. doi: 10.1016/B978-0-12-405927-6.00012-6
360. Pandey RP, Parajuli P, Koffas MA, Sohng JK. Microbial production of natural and non-natural flavonoids: pathway engineering, directed evolution and systems/synthetic biology. Biotechnol Adv. (2016) 34:634–62. doi: 10.1016/j.biotechadv.2016.02.012
361. Fangchuan D, Fangkai Z, Feifei C, Anming W, Qiuyan W, Xiaopu Y, et al. Advances in microbial heterologous production of flavonoids. Afr J Microbiol Res. (2011) 5:2566–74. doi: 10.5897/AJMR11.394
362. Iravani S, Varma RS. Biofactories: engineered nanoparticles via genetically engineered organisms. Green Chem. (2019) 21:4583–603. doi: 10.1039/C9GC01759C
363. Dennis MB. Welfare issues of genetically modified animals. ILAR J. (2002) 43:100–9. doi: 10.1093/ilar.43.2.100
364. Hug K. Genetically modified organisms: do the benefits outweigh the risks? Medicina. (2008) 44:87. doi: 10.3390/medicina44020012
365. Tak H, Negi S, Ganapathi T. Overexpression of MusaMYB31, a R2R3 type MYB transcription factor gene indicate its role as a negative regulator of lignin biosynthesis in banana. PLoS ONE. (2017) 12:e0172695. doi: 10.1371/journal.pone.0172695
366. Wang Y, Chen S, Yu O. Metabolic engineering of flavonoids in plants and microorganisms. Appl Microbiol Biotechnol. (2011) 91:949–56. doi: 10.1007/s00253-011-3449-2
367. Wang Y, Li P, Yao L, Shang Y, Liu S, Meng J, Zhang S, Li H. Advances in the application of biosynthesis and metabolic engineering of flavonoids in plants. Biol Plant. (2022) 66:163–71. doi: 10.32615/bp.2022.014
368. Shafrin F, Das SS, Sanan-Mishra N, Khan H. Artificial miRNA-mediated down-regulation of two monolignoid biosynthetic genes (C3H and F5H) cause reduction in lignin content in jute. Plant Mol Biol. (2015) 89:511–27. doi: 10.1007/s11103-015-0385-z
369. Ranjan A, Westrick NM, Jain S, Piotrowski JS, Ranjan M, Kessens R, et al. Resistance against Sclerotinia sclerotiorum in soybean involves a reprogramming of the phenylpropanoid pathway and up-regulation of antifungal activity targeting ergosterol biosynthesis. Plant Biotechnol J. (2019) 17:1567–81. doi: 10.1111/pbi.13082
370. Lozovaya VV, Lygin AV, Zernova OV, Ulanov AV, Li S, Hartman GL, et al. Modification of phenolic metabolism in soybean hairy roots through down regulation of chalcone synthase or isoflavone synthase. Planta. (2007) 225:665–79. doi: 10.1007/s00425-006-0368-z
371. Rajasekaran K, Cary J, Cleveland T. Prevention of preharvest aflatoxin contamination through genetic engineering of crops. Mycotoxin Res. (2006) 22:118–24. doi: 10.1007/BF02956775
372. Wally O, Punja ZK. Genetic engineering for increasing fungal and bacterial disease resistance in crop plants. GM Crops. (2010) 1:199–206. doi: 10.4161/gmcr.1.4.13225
373. Wang H, Hao J, Chen X, Hao Z, Wang X, Lou Y, et al. Overexpression of rice WRKY89 enhances ultraviolet B tolerance and disease resistance in rice plants. Plant Mol Biol. (2007) 65:799–815. doi: 10.1007/s11103-007-9244-x
374. Shadle GL, Wesley SV, Korth KL, Chen F, Lamb C, Dixon RA. Phenylpropanoid compounds and disease resistance in transgenic tobacco with altered expression of L-phenylalanine ammonia-lyase. Phytochemistry. (2003) 64:153–61. doi: 10.1016/S0031-9422(03)00151-1
375. Chang J, Luo J, He G. Regulation of polyphenols accumulation by combined overexpression/silencing key enzymes of phyenylpropanoid pathway. Acta Biochim Biophys Sin. (2009) 41:123–30. doi: 10.1093/abbs/gmn014
376. Zuk M, Kulma A, Dymińska L, Szołtysek K, Prescha A, Hanuza J, Szopa J. Flavonoid engineering of flax potentiate its biotechnological application. BMC Biotechnol. (2011) 11:10. doi: 10.1186/1472-6750-11-10
377. Zhang Y, Butelli E, Alseekh S, Tohge T, Rallapalli G, Luo J, et al. Multi-level engineering facilitates the production of phenylpropanoid compounds in tomato. Nat Commun. (2015) 6:1–11. doi: 10.1038/ncomms9635
378. Flachowsky H, Szankowski I, Fischer TC, Richter K, Peil A, Höfer M, et al. Transgenic apple plants overexpressing the Lc gene of maize show an altered growth habit and increased resistance to apple scab and fire blight. Planta. (2010) 231:623–35. doi: 10.1007/s00425-009-1074-4
379. Lorenc-Kukuła K, Zuk M, Kulma A, Czemplik M, Kostyn K, Skala J, et al. Engineering flax with the GT family 1 Solanum sogarandinum glycosyltransferase SsGT1 confers increased resistance to Fusarium infection. J Agric Food Chem. (2009) 57:6698–705. doi: 10.1021/jf900833k
380. Shah FLA, Ramzi AB, Baharum SN, Noor NM, Goh H-H. Recent advancement of engineering microbial hosts for the biotechnological production of flavonoids. Mol Biol Rep. (2019) 46:6647–59. doi: 10.1007/s11033-019-05066-1
381. De Bruyn F, Van Brempt M, Maertens J, Van Bellegem W, Duchi D, De Mey M. Metabolic engineering of Escherichia coli into a versatile glycosylation platform: production of bio-active quercetin glycosides. Microb Cell Fact. (2015) 14:1–12. doi: 10.1186/s12934-015-0326-1
382. Kallscheuer N, Vogt M, Marienhagen J. A novel synthetic pathway enables microbial production of polyphenols independent from the endogenous aromatic amino acid metabolism. ACS Synth. Biol. (2017) 6:410–5. doi: 10.1021/acssynbio.6b00291
383. Jiang S, Fang W. Misinformation and disinformation in science: examining the social diffusion of rumours about GMOs. Cult Sci. (2019) 2:327–40. doi: 10.1177/209660831900200407
384. Yamaguchi T. Controversy over genetically modified crops in India: discursive strategies and social identities of farmers. Discourse Stud. (2007) 9:87–107. doi: 10.1177/1461445607072107
385. Farha AK, Gan R-Y, Li H-B, Wu T, Atanasov AG, Gul K, et al. The anticancer potential of the dietary polyphenol rutin: current status, challenges, and perspectives. Crit Rev Food Sci Nutr. (2022) 62:832–59. doi: 10.1080/10408398.2020.1829541
386. Nabavi SF, Atanasov AG, Khan H, Barreca D, Trombetta D, Testai L, et al. Targeting ubiquitin-proteasome pathway by natural, in particular polyphenols, anticancer agents: lessons learned from clinical trials. Cancer Lett. (2018) 434:101–13. doi: 10.1016/j.canlet.2018.07.018
387. Szliszka E, Krol W. The role of dietary polyphenols in tumor necrosis factor-related apoptosis inducing ligand (TRAIL)-induced apoptosis for cancer chemoprevention. Eur J Cancer Prev. (2011) 20:63–9. doi: 10.1097/CEJ.0b013e32833ecc48
388. Rasouli H, Norooznezhad AH, Rashidi T, Hoseinkhani Z, Mahnam A, Tarlan M, et al. Comparative in vitro/theoretical studies on the anti-angiogenic activity of date pollen hydro-alcoholic extract: highlighting the important roles of its hot polyphenols. Bioimpacts. (2018) 8:281. doi: 10.15171/bi.2018.31
389. Fantini M, Benvenuto M, Masuelli L, Frajese GV, Tresoldi I, Modesti A, et al. In vitro and in vivo antitumoral effects of combinations of polyphenols, or polyphenols and anticancer drugs: perspectives on cancer treatment. Int J Mol Sci. (2015) 16:9236–82. doi: 10.3390/ijms16059236
390. Paluszczak J, Krajka-Kuzniak V, Baer-Dubowska W. The effect of dietary polyphenols on the epigenetic regulation of gene expression in MCF7 breast cancer cells. Toxicol Lett. (2010) 192:119–25. doi: 10.1016/j.toxlet.2009.10.010
391. Fang M, Chen D, Yang CS. Dietary polyphenols may affect DNA methylation. J Nutr. (2007) 137:223S−8S. doi: 10.1093/jn/137.1.223S
392. Briguglio G, Costa C, Pollicino M, Giambo F, Catania S, Fenga C. Polyphenols in cancer prevention: new insights. Int J Funct Nutr. (2020) 1:1–10. doi: 10.3892/ijfn.2020.9
393. Sajadimajd S, Bahramsoltani R, Iranpanah A, Patra JK, Das G, Gouda S, et al. Advances on natural polyphenols as anticancer agents for skin cancer. Pharmacol Res. (2020) 151:104584. doi: 10.1016/j.phrs.2019.104584
394. Chojnacka K, Lewandowska U. Inhibition of pro-inflammatory cytokine secretion by polyphenol-rich extracts in macrophages via NF-κB pathway. Food Rev Int. (2022) 36:1–20. doi: 10.1080/87559129.2022.2071936
395. Avtanski D, Poretsky L. Phyto-polyphenols as potential inhibitors of breast cancer metastasis. Mol Med. (2018) 24:1–17. doi: 10.1186/s10020-018-0032-7
396. Schoene NW, Kelly MA, Polansky MM, Anderson RA. Water-soluble polymeric polyphenols from cinnamon inhibit proliferation and alter cell cycle distribution patterns of hematologic tumor cell lines. Cancer Lett. (2005) 230:134–40. doi: 10.1016/j.canlet.2004.12.039
397. Colomer R, Sarrats A, Lupu R, Puig T. Natural polyphenols and their synthetic analogs as emerging anticancer agents. Curr Drug Targets. (2017) 18:147–59. doi: 10.2174/1389450117666160112113930
398. Alam MN, Almoyad M, Huq F. Polyphenols in colorectal cancer: current state of knowledge including clinical trials and molecular mechanism of action. Biomed Res. Int. (2018) 2018:4154185. doi: 10.1155/2018/4154185
399. Zhou Y, Zheng J, Li Y, Xu D-P, Li S-M, Li H-B. Natural polyphenols for prevention and treatment of cancer. Nutrients. (2016) 8:515. doi: 10.3390/nu8080515
400. Lambert JD, Hong J, Yang, G, Liao J, Yang CS. Inhibition of carcinogenesis by polyphenols: evidence from laboratory investigations. Am J Clin Nutr. (2005) 81:284S−91S. doi: 10.1093/ajcn/81.1.284S
401. Vauzour D. Dietary polyphenols as modulators of brain functions: biological actions and molecular mechanisms underpinning their beneficial effects. Oxid Med Cell Longev. (2012) 2012:1–12. doi: 10.1155/2012/914273
402. Tayab MA, Islam MN, Chowdhury KAA, Tasnim FM. Targeting neuroinflammation by polyphenols: a promising therapeutic approach against inflammation-associated depression. Biomed Pharmacother. (2022) 147:112668. doi: 10.1016/j.biopha.2022.112668
403. Vinayagam R, Xu B. Antidiabetic properties of dietary flavonoids: a cellular mechanism review. Nutr Metab. (2015) 12:1–20. doi: 10.1186/s12986-015-0057-7
404. Xiao J. Recent advances in dietary flavonoids for management of type 2 diabetes. Curr Opin Food Sci. (2022) 44:100806. doi: 10.1016/j.cofs.2022.01.002
405. Al-Ishaq RK, Abotaleb M, Kubatka P, Kajo K, Büsselberg D. Flavonoids and their anti-diabetic effects: cellular mechanisms and effects to improve blood sugar levels. Biomolecules. (2019) 9:430. doi: 10.3390/biom9090430
406. Shi J, Yang G, You Q, Sun S, Chen R, Lin Z, et al. Updates on the chemistry, processing characteristics, and utilization of tea flavonoids in last two decades (2001-2021). Crit Rev Food Sci Nutr. (2021) 61:1–28. doi: 10.1080/10408398.2021.2007353
407. Ahmed OS, Tardif C, Rouger C, Atanasova V, Richard-Forget F, Waffo-Téguo P. Naturally occurring phenolic compounds as promising antimycotoxin agents: where are we now? Compr Rev Food Sci Food Saf. (2022) 21:1161–97. doi: 10.1111/1541-4337.12891
408. Tang L, Tang M, Xu L, Luo H, Huang T, Yu J, et al. Modulation of aflatoxin biomarkers in human blood and urine by green tea polyphenols intervention. Carcinogenesis. (2008) 29:411–7. doi: 10.1093/carcin/bgn008
409. Conney A, Buening M, Pantuck E, Pantuck C, Fortner J, Anderson K, et al. Regulation of human drug metabolism by dietary factors. Ciba Found Symp. (1980) 76:147–67. doi: 10.1002/9780470720592.ch9
410. Choi JS, Park KY, Moon SH, Rhee SH, Young HS. Antimutagenic effect of plant flavonoids in theSalmonella assay system. Arch Pharm Res. (1994) 17:71–5. doi: 10.1007/BF02974226
411. Singh C, Prakash C, Mishra P, Tiwari KN, Mishra SK, More RS, et al. Hepatoprotective efficacy of Premna integrifolia L. leaves against aflatoxin B1-induced toxicity in mice. Toxicon. (2019) 166:88–100. doi: 10.1016/j.toxicon.2019.05.014
412. Bhouri W, Sghaier MB, Kilani S, Bouhlel I, Dijoux-Franca, M-G, et al. Evaluation of antioxidant and antigenotoxic activity of two flavonoids from Rhamnus alaternus L.(Rhamnaceae): Kaempferol 3-O-β-isorhamninoside and rhamnocitrin 3-O-β-isorhamninoside. Food Chem Toxicol. (2011) 49:1167–73. doi: 10.1016/j.fct.2011.02.011
413. Peter F, Kim D-H. In vitro inhibition of dihydropyridine oxidation and aflatoxin B1 activation in human liver microsomes by naringenin and other flavonoids. Carcinogenesis. (1990) 11:2275–9. doi: 10.1093/carcin/11.12.2275
414. Francis A, Shetty T, Bhattacharya R. Modifying role of dietary factors on the mutagenicity of aflatoxin B1: in vitro effect of plant flavonoids. Mutat Res. (1989) 222:393–401. doi: 10.1016/0165-1218(89)90114-6
415. Kensler TW, Egner PA, Trush MA, Bueding E, Groopman JD. Modification of aflatoxin B 1 binding to DNA in vivo in rats fed phenolic antioxidants, ethoxyquin and a dithiothione. Carcinogenesis. (1985) 6:759–63. doi: 10.1093/carcin/6.5.759
416. Wang Y, Liu F, Liu M, Zhou X, Wang M, Cao K, et al. Curcumin mitigates aflatoxin B1-induced liver injury via regulating the NLRP3 inflammasome and Nrf2 signaling pathway. Food Chem. Toxicol. (2022) 161:112823. doi: 10.1016/j.fct.2022.112823
417. El-Agamy DS. Comparative effects of curcumin and resveratrol on aflatoxin B1-induced liver injury in rats. Arch Toxicol. (2010) 84:389–96. doi: 10.1007/s00204-010-0511-2
418. Stumpf WE. The dose makes the medicine. Drug Discov Today. (2006) 11:550–5. doi: 10.1016/j.drudis.2006.04.012
419. Ibrahim EA, Yousef MI, Ghareeb DA, Augustyniak M, Giesy JP, Aboul-Soud MA, et al. Artichoke leaf extract-mediated neuroprotection against effects of aflatoxin in male rats. Biomed Res Int. (2022) 2022:4421828. doi: 10.1155/2022/4421828
420. Kasem R. Preventive Efficiency of chelidonium majus ethanolic extract against aflatoxin B 1 induced neurochemical deteriorations in rats. Pak J Biol Sci. (2022) 25:234–44. doi: 10.3923/pjbs.2022.234.244
421. Owumi SE, Adedara IA, Akomolafe AP, Farombi EO, Oyelere AK. Gallic acid enhances reproductive function by modulating oxido-inflammatory and apoptosis mediators in rats exposed to aflatoxin-B1. Exp Biol Med. (2020) 245:1016–28. doi: 10.1177/1535370220936206
422. Verma RJ, Mathuria N. Curcumin ameliorates aflatoxin-induced changes in caput and cauda epididymis of mice. Int J Fertil Steril. (2010) 4:17–22. doi: 10.22074/ijfs.2010.45816
423. Mathuria N, Verma RJ. Curcumin ameliorates aflatoxin-induced toxicity in mice spermatozoa. Fertil Steril. (2008) 90:775–80. doi: 10.1016/j.fertnstert.2007.07.1300
424. Pauletto M, Giantin M, Tolosi R, Bassan I, Barbarossa A, Zaghini A, et al. Curcumin mitigates AFB1-induced hepatic toxicity by triggering cattle antioxidant and anti-inflammatory pathways: a whole transcriptomic in vitro study. Antioxidants. (2020) 9:1059. doi: 10.3390/antiox9111059
425. Owumi SE, Irozuru CE, Arunsi UO, Faleke HO, Oyelere AK. Caffeic acid mitigates aflatoxin B1-mediated toxicity in the male rat reproductive system by modulating inflammatory and apoptotic responses, testicular function, and the redox-regulatory systems. J Food Biochem. (2022) 46:e14090. doi: 10.1111/jfbc.14090
426. Owumi SE, Kazeem AI, Wu B, Ishokare LO, Arunsi UO, Oyelere AK. Apigeninidin-rich Sorghum bicolor (L. Moench) extracts suppress A549 cells proliferation and ameliorate toxicity of aflatoxin B1-mediated liver and kidney derangement in rats. Sci Rep. (2022) 12:1–19. doi: 10.1038/s41598-022-10926-1
427. Lin L, Fu P, Chen N, Gao N, Cao Q, Yue K, et al. Total flavonoids of Rhizoma Drynariae protect hepatocytes against aflatoxin B1-induced oxidative stress and apoptosis in broiler chickens. Ecotoxicol Environ Saf. (2022) 230:113148. doi: 10.1016/j.ecoenv.2021.113148
428. El-Nekeety AA, Abdel-Azeim SH, Hassan AM, Hassan NS, Aly SE, Abdel-Wahhab MA. Quercetin inhibits the cytotoxicity and oxidative stress in liver of rats fed aflatoxin-contaminated diet. Toxicol Rep. (2014) 1:319–29. doi: 10.1016/j.toxrep.2014.05.014
429. Gao X, Xu J, Jiang L, Liu W, Hong H, Qian Y, et al. Morin alleviates aflatoxin B1-induced liver and kidney injury by inhibiting heterophil extracellular traps release, oxidative stress and inflammatory responses in chicks. Poult Sci. (2021) 100:101513. doi: 10.1016/j.psj.2021.101513
430. Farombi E, Adepoju B, Ola-Davies O, Emerole G. Chemoprevention of aflatoxin B1-induced genotoxicity and hepatic oxidative damage in rats by kolaviron, a natural biflavonoid of Garcinia kola seeds. Eur J Cancer Prev. (2005) 14:207–14. doi: 10.1097/00008469-200506000-00003
431. Souza M, Tome A, Rao V. Inhibition by the bioflavonoid ternatin of aflatoxin B1-induced lipid peroxidation in rat liver. J Pharm Pharmacol. (1999) 51:125–9. doi: 10.1211/0022357991772222
432. Melo JUS, Melo RB, Santos JMV, Campos Júnior MM, Guimarães SB, Vasconcelos PRL. Effects of bioflavonoid ternatin on liver regeneration and oxidative stress in rats. Acta Cir Bras. (2013) 28:435–40. doi: 10.1590/S0102-86502013000600006
433. Dufour C, Dangles O. Flavonoid-serum albumin complexation: determination of binding constants and binding sites by fluorescence spectroscopy. Biochim Biophys Acta Gen Subj. (2005) 1721:164–73. doi: 10.1016/j.bbagen.2004.10.013
434. Zhang L-Y, Zhan D-L, Chen Y-Y, He C-Y, Lin Y, Lin Y-C, et al. Aflatoxin B1 enhances pyroptosis of hepatocytes and activation of Kupffer cells to promote liver inflammatory injury via dephosphorylation of cyclooxygenase-2: an in vitro, ex vivo and in vivo study. Arch Toxicol. (2019) 93:3305–20. doi: 10.1007/s00204-019-02572-w
435. Cusumano V, Costa G, Trifiletti R, Merendino R, Mancuso G. Functional impairment of rat Kupffer cells induced by aflatoxin B 1 and its metabolites. FEMS Immunol Med Microbiol. (1995) 10:151–5. doi: 10.1111/j.1574-695X.1995.tb00025.x
436. Dehmlow C, Erhard J, de Groot H. Inhibition of Kupffer cell functions as an explanation for the hepatoprotective properties of silibinin. Hepatology. (1996) 23:749–54. doi: 10.1002/hep.510230415
437. Li S, Tan HY, Wang N, Cheung F, Hong M, Feng Y. The potential and action mechanism of polyphenols in the treatment of liver diseases. Oxid Med Cell Longev. (2018) 2018:1–26. doi: 10.31193/ssap.isbn.9787520118873
438. Hua Z, Liu R, Chen Y, Liu G, Li C, Song Y, et al. Contamination of aflatoxins induces severe hepatotoxicity through multiple mechanisms. Front Pharmacol. (2021) 11:605823. doi: 10.3389/fphar.2020.605823
439. Firozi P, Bhattacharya R. Effects of natural polyphenols on aflatoxin B1 activation in a reconstituted microsomal monooxygenase system. J Biochem Toxicol. (1995) 10:25–31.
440. Dey DK, Chang SN, Kang SC. The inflammation response and risk associated with aflatoxin B1 contamination was minimized by insect peptide CopA3 treatment and act towards the beneficial health outcomes. Environ Pollut. (2021) 268:115713. doi: 10.1016/j.envpol.2020.115713
441. Rastogi R, Srivastava AK, Rastogi AK. Long term effect of aflatoxin B1 on lipid peroxidation in rat liver and kidney: effect of picroliv and silymarin. Phytother Res. (2001) 15:307–10. doi: 10.1002/ptr.722
442. Bakheet SA, Alhuraishi AM, Al-Harbi NO, Al-Hosaini KA, Al-Sharary SD, Attia MM, et al. Alleviation of aflatoxin B1-induced genomic damage by proanthocyanidins via modulation of DNA repair. J Biochem Mol Toxicol. (2016) 30:559–66. doi: 10.1002/jbt.21823
443. Corcuera L-A, Amézqueta S, Arbillaga L, Vettorazzi A, Touriño S. A polyphenol-enriched cocoa extract reduces free radicals produced by mycotoxins. Food Chem Toxicol. (2012) 50:989–95. doi: 10.1016/j.fct.2011.11.052
444. Choi KC, Chung WT, Kwon JK, Jang YS, Yu JY, Park SM, et al. Chemoprevention of a flavonoid fraction from Rhus verniciflua Stokes on aflatoxin B1-induced hepatic damage in mice. J Appl Toxicol. (2011) 31:150–6. doi: 10.1002/jat.1575
445. Vipin A, Rao R, Kurrey NK, KA AA, Venkateswaran G. Protective effects of phenolics rich extract of ginger against Aflatoxin B1-induced oxidative stress and hepatotoxicity. Biomed Pharmacother. (2017) 91:415–24. doi: 10.1016/j.biopha.2017.04.107
446. Marin DE, Bulgaru CV, Palade ML, Pistol GC, Gras MA, Taranu I. Effect of the grape seed meal administration on inflammation and oxidative stress in the spleen of piglets fed aflatoxin B1. Arch Zootech. (2019) 22:22–31.
447. Poór M, Veres B, Jakus PB, Antus C, Montskó G, Zrínyi Z, et al. Flavonoid diosmetin increases ATP levels in kidney cells and relieves ATP depleting effect of ochratoxin A. J Photochem Photobiol B Biol. (2014) 132:1–9. doi: 10.1016/j.jphotobiol.2014.01.016
448. Oskoueian E, Abdullah N, Zulkifli I, Ebrahimi M, Karimi E, Goh YM, et al. Cytoprotective effect of palm kernel cake phenolics against aflatoxin B1-induced cell damage and its underlying mechanism of action. BMC Complement Altern Med. (2015) 15:392. doi: 10.1186/s12906-015-0921-z
449. Kim Y-S, Kim Y-H, Noh J-R, Park J-H, Son H-Y. Protective effect of Korean red ginseng against aflatoxin B1-induced hepatotoxicity in rat. J Ginseng Res. (2011) 35:243. doi: 10.5142/jgr.2011.35.2.243
450. Kim J-S. Investigation of phenolic, flavonoid, and vitamin contents in different parts of Korean ginseng (Panax ginseng CA Meyer). Prev Nutr Food Sci. (2016) 21:263. doi: 10.3746/pnf.2016.21.3.263
451. Malathy R, Prabakaran M, Kalaiselvi K, Chung I-M, Kim H. Comparative polyphenol composition, antioxidant and anticorrosion properties in various parts of panax ginseng extracted in different solvents. Appl Sci. (2020) 11:93. doi: 10.3390/app11010093
452. Miyata M, Takano H, Guo LQ, Nagata K, Yamazoe Y. Grapefruit juice intake does not enhance but rather protects against aflatoxin B 1-induced liver DNA damage through a reduction in hepatic CYP3A activity. Carcinogenesis. (2004) 25:203–09. doi: 10.1093/carcin/bgg194
453. Ivanova N, Khomich L, Perova I, Eller K. Grapefruit juice nutritional profile. Vopr Pitan. (2018) 87:85–94. doi: 10.24411/0042-8833-2018-10057
454. Bacanli M, Başaran AA, Başaran N. The major flavonoid of grapefruit: naringin. In:Watson RR, Preedy VR, Zibadi S, , editors. Polyphenols: Prevention and Treatment of Human Disease. New York: Elsevier (2018). p. 37–44. doi: 10.1016/B978-0-12-813008-7.00004-7
455. Mohamed SS, Mohamed SR, Abou-Arab AA, Naguib KM, Helmy MH, Owiss NA. Comparison study on native olive waste extract and its nano-particles effect on oxidative stress induced by aflatoxin B1 in rat brain. Int J Curr Microbiol App Sci. (2014) 3:141–52.
456. Khalifa SA, Elashal MH, Yosri N, Du M, Musharraf SG, Nahar L, et al. Bee pollen: current status and therapeutic potential. Nutrients. (2021) 13:1876. doi: 10.3390/nu13061876
457. Ahangari Z, Naseri M, Vatandoost F. Propolis: chemical composition and its applications in endodontics. Iran Endod J. (2018) 13:285. doi: 10.22037/iej.v13i3.20994
458. Niu G, Johnson RM, Berenbaum MR. Toxicity of mycotoxins to honeybees and its amelioration by propolis. Apidologie. (2011) 42:79–87. doi: 10.1051/apido/2010039
459. Alqayim MA, Shehab AA. Iraqi Propolis effective in avoiding deviations of gut rat's homeostasis exposed to AFB-1. J Kerbala Agric. Sci. (2017) 4:150–63.
460. Choudhary A, Verma R. Ameliorative effects of black tea extract on aflatoxin-induced lipid peroxidation in the liver of mice. Food Chem Toxicol. (2005) 43:99–104. doi: 10.1016/j.fct.2004.08.016
461. Jha A, Shah K, Verma RJ. Aflatoxin-induced biochemical changes in liver of mice and its mitigation by black tea extract. Acta Pol. Pharm. (2012) 69:851–7.
462. Alm-Eldeen AA, Mona MH, Shati AA, El-Mekkawy HI. Synergistic effect of black tea and curcumin in improving the hepatotoxicity induced by aflatoxin B1 in rats. Toxicol Ind Health. (2015) 31:1269–80. doi: 10.1177/0748233713491807
463. Hassan AA, Hafsa SHA, Elghandour MM, Reddy PRK, Monroy JC, Salem AZ. Dietary Supplementation with sodium bentonite and coumarin alleviates the toxicity of aflatoxin B1 in rabbits. Toxicon. (2019) 171:35–42. doi: 10.1016/j.toxicon.2019.09.014
464. Ghadiri S, Spalenza V, Dellafiora L, Badino P, Barbarossa A, Dall'Asta C, et al. Modulation of aflatoxin B1 cytotoxicity and aflatoxin M1 synthesis by natural antioxidants in a bovine mammary epithelial cell line. Toxicol In Vitro. (2019) 57:174–83. doi: 10.1016/j.tiv.2019.03.002
465. Webster R, Gawde M, Bhattacharya R. Protective effect of rutin, a flavonol glycoside, on the carcinogen-induced DNA damage and repair enzymes in rats. Cancer Lett. (1996) 109:185–91. doi: 10.1016/S0304-3835(96)04443-6
466. Snijman PW, Swanevelder S, Joubert E, Green IR, Gelderblom WC. The antimutagenic activity of the major flavonoids of rooibos (Aspalathus linearis): some dose-response effects on mutagen activation-flavonoid interactions. Mutat Res. (2007) 631:111–23. doi: 10.1016/j.mrgentox.2007.03.009
467. Maurya BK, Trigun SK. Fisetin modulates antioxidant enzymes and inflammatory factors to inhibit aflatoxin-B1 induced hepatocellular carcinoma in rats. Oxid Med Cell Longev. (2016) 2016:1–10. doi: 10.1155/2016/1972793
468. Maurya BK, Trigun SK. Fisetin attenuates AKT associated growth promoting events in aflatoxinb1 induced hepatocellular carcinoma. Anticancer Agents Med Chem. (2018) 18:1885–91. doi: 10.2174/1871520618666171229223335
469. Park K-Y, Jung G-O, Lee K-T, Kim G-T, Jung H-J, Park J, et al. Antimutagenic activity of flavonoids from the heartwood of Rhus verniciflua. J Ethnopharmacol. (2004) 90:73–9. doi: 10.1016/j.jep.2003.09.043
470. Resende FA, da Silva Almeida CP, Vilegas W, Varanda EA. Differences in the hydroxylation pattern of flavonoids alter their chemoprotective effect against direct-and indirect-acting mutagens. Food Chem. (2014) 155:251–5. doi: 10.1016/j.foodchem.2014.01.071
471. Lee S-E, Campbell BC, Molyneux RJ, Hasegawa S, Lee S. Inhibitory effects of naturally occurring compounds on aflatoxin B1 biotransformation. J Agric Food Chem. (2001) 49:5171–7. doi: 10.1021/jf010454v
472. Aboobaker V, Balgi A, Bhattacharya R. In vivo effect of dietary factors on the molecular action of aflatoxin B1: role of non-nutrient phenolic compounds on the catalytic activity of liver fractions. In Vivo. (1994) 8:1095–8.
473. Bouhlel I, Limem I, Skandrani I, Nefatti A, Ghedira K, Dijoux-Franca MG, et al. Assessment of isorhamnetin 3-O-neohesperidoside from Acacia salicina: protective effects toward oxidation damage and genotoxicity induced by aflatoxin B1 and nifuroxazide. J Appl Toxicol. (2010) 30:551–8. doi: 10.1002/jat.1525
474. Nones J, Nones J, Trentin AG. Flavonoid hesperidin protects neural crest cells from death caused by aflatoxin B1. Cell Biol Int. (2013) 37:181–6. doi: 10.1002/cbin.10015
475. Guengerich FP, Shimada T, Yun C-H, Yamazaki H, Raney KD. Interactions of ingested food, beverage, and tobacco components involving human cytochrome P4501A2, 2A6, 2E1, and 3A4 enzymes. Environ. Health Perspect. (1994) 102(Suppl 9):49–53. doi: 10.1289/ehp.94102s949
476. Wang W, Liu Q, Liang X, Kang Q, Wang Z. Protective role of naringin loaded solid nanoparticles against aflatoxin B1 induced hepatocellular carcinoma. Chem Biol Interact. (2022) 351:109711. doi: 10.1016/j.cbi.2021.109711
477. Park K-Y, Jung K-O, Rhee S-H. Antimutagenic effects of doenjang (Korean fermented soypaste) and its active compounds. Mutat Res. (2003) 523:43–53. doi: 10.1016/S0027-5107(02)00320-2
478. Miyazawa M, Hisama M. Antimutagenic activity of flavonoids from Chrysanthemum morifolium. Biosci Biotechnol Biochem. (2003) 67:2091–99. doi: 10.1271/bbb.67.2091
479. Nakasugi T, Nakashima M, Komai K. Antimutagens in Gaiyou (Artemisia a rgyi Levl. et Vant.). J Agric Food Chem. (2000) 48:3256–66. doi: 10.1021/jf9906679
480. Ribeiro DL, Cilião HL, Specian AFL, Serpeloni JM, Souza MF, Tangerina MMP, et al. Chemical and biological characterisation of Machaerium hirtum (Vell.) Stellfeld: absence of cytotoxicity and mutagenicity and possible chemopreventive potential. Mutagenesis. (2016) 31:147–60. doi: 10.1093/mutage/gev066
481. Rajput SA, Shaukat A, Wu K, Rajput IR, Baloch DM, Akhtar RW, et al. Luteolin alleviates aflatoxinB1-induced apoptosis and oxidative stress in the liver of mice through activation of Nrf2 signaling pathway. Antioxidants. (2021) 10:1268. doi: 10.3390/antiox10081268
482. De Oliveira APS, De Sousa JF, Da Silva MA, Hilário F, Resende FA, De Camargo MS, et al. Estrogenic and chemopreventive activities of xanthones and flavones of Syngonanthus (Eriocaulaceae). Steroids. (2013) 78:1053–63. doi: 10.1016/j.steroids.2013.07.002
483. Nixon JE, Hendricks JD, Pawlowski NE, Pereira CB, Sinnhuber RO, Bailey GS. Inhibition of aflatoxin B 1 carcinogenesis in rainbow trout by flavone and indole compounds. Carcinogenesis. (1984) 5:615–9. doi: 10.1093/carcin/5.5.615
484. Lake BG, Beamand JA, Tredger JM, Barton PT, Renwick AB, Price RJ. Inhibition of xenobiotic-induced genotoxicity in cultured precision-cut human and rat liver slices. Mutat Res. (1999) 440:91–100. doi: 10.1016/S1383-5718(99)00010-8
485. Muto S, Fujita K, Yamazaki Y, Kamataki T. Inhibition by green tea catechins of metabolic activation of procarcinogens by human cytochrome P450. Mutat Res. (2001) 479:197–206. doi: 10.1016/S0027-5107(01)00204-4
486. Cardador-Martinez A, Castano-Tostado E, Loarca-Pina G. Antimutagenic activity of natural phenolic compounds present in the common bean (Phaseolus vulgaris) against aflatoxin B 1. Food Addit Contam A. (2002) 19:62–9. doi: 10.1080/02652030110062110
487. Prasanna H, Lotlikar P, Hacobian N, Ho L, Magee P. Effect of (+)-catechin, dimethyl sulfoxide and ethanol on the microsome-mediated metabolism of two hepatocarcinogens, N-nitrosodimethylamine and aflatoxin B1. IARC Sci Publ. (1987) 84:175–7.
488. Costa S, Utan A, Cervellati R, Speroni E, Guerra M. Catechins: natural free-radical scavengers against ochratoxin A-induced cell damage in a pig kidney cell line (LLC-PK1). Food Chem Toxicol. (2007) 45:1910–7. doi: 10.1016/j.fct.2007.04.008
489. Popescu RG, Bulgaru C, Untea A, Vlassa M, Filip M, Hermenean A, et al. The effectiveness of dietary byproduct antioxidants on induced CYP genes expression and histological alteration in piglets liver and kidney fed with aflatoxin B1 and Ochratoxin A. Toxins. (2021) 13:148. doi: 10.3390/toxins13020148
490. Wang ZY, Das M, Bickers DR, Mukhtar H. Interaction of epicatechins derived from green tea with rat hepatic cytochrome P-450. Drug Metab Dispos. (1988) 16:98–103.
491. Xu D, Peng S, Guo R, Yao L, Mo H, Li H, et al. EGCG Alleviates oxidative stress and inhibits aflatoxin B1 biosynthesis via MAPK signaling pathway. Toxins. (2021) 13:693. doi: 10.3390/toxins13100693
492. Guerra M, Galvano F, Bonsi L, Speroni E, Costa S, Renzulli C, et al. Cyanidin-3-O-β-glucopyranoside, a natural free-radical scavenger against aflatoxin B1-and ochratoxin A-induced cell damage in a human hepatoma cell line (Hep G2) and a human colonic adenocarcinoma cell line (CaCo-2). Br J Nutr. (2005) 94:211–220. doi: 10.1079/BJN20051425
493. Singto T, Tassaneeyakul W, Porasuphatana S. Protective effects of purple waxy corn on aflatoxin B1-induced oxidative stress and micronucleus in HepG2 Cells. Indian J Pharm Sci. (2020) 82:506–13. doi: 10.36468/pharmaceutical-sciences.674
494. Charoensin S, Taya S, Wongpornchai S, Wongpoomchai R. Assessment of genotoxicity and antigenotoxicity of an aqueous extract of Cleistocalyx nervosum var. paniala in in vitro and in vivo models. Interdiscip Toxicol. (2012) 5:201–6. doi: 10.2478/v10102-012-0033-2
495. Norton RA. Inhibition of aflatoxin B1 biosynthesis in Aspergillus flavus by anthocyanidins and related flavonoids. J Agric Food Chem. (1999) 47:1230–5. doi: 10.1021/jf980995t
496. Punvittayagul C, Sringarm K, Chaiyasut C, Wongpoomchai R. Mutagenicity and antimutagenicity of hydrophilic and lipophilic extracts of Thai northern purple rice. Asian Pac J Cancer Prev. (2014) 15:9517–22. doi: 10.7314/APJCP.2014.15.21.9517
497. Sharath Babu G, Anand T, Ilaiyaraja N, Khanum F, Gopalan N. Pelargonidin modulates Keap1/Nrf2 pathway gene expression and ameliorates citrinin-induced oxidative stress in HepG2 cells. Front Pharmacol. (2017) 8:868. doi: 10.3389/fphar.2017.00868
498. Escribano-Bailón MT, Santos-Buelga C, Rivas-Gonzalo JC. Anthocyanins in cereals. J Chromatogr A. (2004) 1054:129–41. doi: 10.1016/j.chroma.2004.08.152
499. Hitoshi Nagashima KN. Krishan Datt Sharma, Tetsuhisa Goto, Investigation of the effects of anthocyanins on rubratoxin B-treated HL60 cells. Mycotoxins. (2004) 54:27–32. doi: 10.2520/myco.54.27
500. Righetti L, Bhandari DR, Rolli E, Tortorella S, Bruni R, Dall'Asta C, et al. Unveiling the spatial distribution of aflatoxin B1 and plant defense metabolites in maize using AP-SMALDI mass spectrometry imaging. Plant J. (2021) 106:185–99. doi: 10.1111/tpj.15158
501. Chen P-N, Chu S-C, Chiou H-L, Yang S-F, Hsieh Y-S. Cyanidin 3-glucoside and peonidin 3-glucoside inhibit tumor cell growth and induce apoptosis in vitro and suppress tumor growth in vivo. Nutr Cancer. (2005) 53:232–43. doi: 10.1207/s15327914nc5302_12
502. Muhammad I, Wang X, Li S, Li R, Zhang X. Curcumin confers hepatoprotection against AFB1-induced toxicity via activating autophagy and ameliorating inflammation involving Nrf2/HO-1 signaling pathway. Mol Biol Rep. (2018) 45:1775–85. doi: 10.1007/s11033-018-4323-4
503. Wu J, Gan Z, Zhuo R, Zhang L, Wang T, Zhong X. Resveratrol attenuates aflatoxin B1-induced ROS formation and increase of m6A RNA Methylation. Animals. (2020) 10:677. doi: 10.3390/ani10040677
504. Zhou Y, Jin Y, Yu H, Shan A, Shen J, Zhou C, et al. Resveratrol inhibits aflatoxin B1-induced oxidative stress and apoptosis in bovine mammary epithelial cells and is involved the Nrf2 signaling pathway. Toxicon. (2019) 164:10–15. doi: 10.1016/j.toxicon.2019.03.022
505. Pauletto M, Giantin M, Tolosi R, Bassan I, Barbarossa A, Zaghini A, et al. Discovering the protective effects of resveratrol on aflatoxin B1-induced toxicity: a whole transcriptomic study in a bovine hepatocyte cell line. Antioxidants. (2021) 10:1225. doi: 10.3390/antiox10081225
506. Türkez H, Sişman T. The genoprotective activity of resveratrol on aflatoxin B1-induced DNA damage in human lymphocytes in vitro. Toxicol Ind Health. (2012) 28:474–80. doi: 10.1177/0748233711414614
507. Gündüz A, Yalçin E, Çavuşoglu K. Combined toxic effects of aflatoxin B2 and the protective role of resveratrol in Swiss albino mice. Sci Rep. (2021) 11:1–14. doi: 10.1038/s41598-021-95879-7
508. Rawat D, Chhonker SK, Naik RA, Koiri RK. Modulation of antioxidant enzymes, SIRT1 and NF-κB by resveratrol and nicotinamide in alcohol-aflatoxin B1-induced hepatocellular carcinoma. J Biochem Mol Toxicol. (2021) 35:e22625. doi: 10.1002/jbt.22625
509. Wang H, Lei Y, Yan L, Cheng K, Dai X, Wan L, et al. Deep sequencing analysis of transcriptomes in Aspergillus flavus in response to resveratrol. BMC Microbiol. (2015) 15:182. doi: 10.1186/s12866-015-0513-6
510. Yang H, Wang Y, Yu C, Jiao Y, Zhang R, Jin S, et al. Dietary resveratrol alleviates AFB1-induced ileum damage in ducks via the Nrf2 and NF-κB/NLRP3 signaling pathways and CYP1A1/2 expressions. Agriculture. (2022) 12:54. doi: 10.3390/agriculture12010054
511. Kolesarova A, Capcarova M, Maruniakova N, Lukac N, Ciereszko RE, Sirotkin AV. Resveratrol inhibits reproductive toxicity induced by deoxynivalenol. J Environ Sci Health A. (2012) 47:1329–34. doi: 10.1080/10934529.2012.672144
512. Bavaro S, D'Antuono I, Cozzi G, Haidukowski M, Cardinali A, Logrieco A. Inhibition of aflatoxin B1 production by verbascoside and other olive polyphenols. World Mycotoxin J. (2016) 9:545–53. doi: 10.3920/WMJ2015.2018
513. Zhao X, Zhi Q-Q, Li J-Y, Keller NP. The antioxidant gallic acid inhibits aflatoxin formation in Aspergillus flavus by modulating transcription factors FarB and CreA. Toxins. (2018) 10:270. doi: 10.3390/toxins10070270
514. Sobolev V, Walk T, Arias R, Massa A, Lamb M. Inhibition of aflatoxin formation in aspergillus species by peanut (Arachis hypogaea) seed stilbenoids in the course of peanut-fungus interaction. J Agric Food Chem. (2019) 67:6212–21. doi: 10.1021/acs.jafc.9b01969
515. Azaizeh HA, Pettit RE, Sarr BA, Phillips T. Effect of peanut tannin extracts on growth of Aspergillus parasiticus and aflatoxin production. Mycopathologia. (1990) 110:125–32. doi: 10.1007/BF00437535
516. Liu Y, Benohoud M, Yamdeu JHG, Gong YY, Orfila C. Green extraction of polyphenols from citrus peel by-products and their antifungal activity against Aspergillus flavus. Food Chem X. (2021) 12:100144. doi: 10.1016/j.fochx.2021.100144
517. Abbas A, Wright CW, El-Sawi N, Yli-Mattila T, Malinen AM. A methanolic extract of Zanthoxylum bungeanum modulates secondary metabolism regulator genes in Aspergillus flavus and shuts down aflatoxin production. Sci Rep. (2022) 12:1–13. doi: 10.1038/s41598-022-09913-3
518. Abdel-Razek AG, Badr AN, Shehata MG. Characterization of olive oil by-products: Antioxidant activity, its ability to reduce aflatoxigenic fungi hazard and its aflatoxins. Annu Res Rev Biol. (2017) 14:1–14. doi: 10.9734/ARRB/2017/35065
519. Ali EM, Alkuwayti MA, Aldayel MF, Abdallah BM. Coumarin derivative, 5′-hydroxy-auraptene, extracted from Lotus lalambensis, displays antifungal and anti-aflatoxigenic activities against Aspergillus flavus. J King Saud Univ Sci. (2021) 33:101216. doi: 10.1016/j.jksus.2020.10.013
520. Nobili C, De Acutis A, Reverberi M, Bello C, Leone GP, Palumbo D, et al. Buckwheat hull extracts inhibit Aspergillus flavus growth and AFB1 biosynthesis. Front Microbiol. (2019) 10:1997. doi: 10.3389/fmicb.2019.01997
521. Costa LM, Guilhon-Simplicio F, Souza TP. Libidibia ferrea (Mart. ex Tul.) LP Queiroz var. ferrea: pharmacological, phytochemical and botanical aspects. Int J Pharm Pharm. (2015) 4:48–53.
522. Kalli V, Kollia E, Roidaki A, Proestos C, Markaki P. Cistus incanus L. extract inhibits aflatoxin B1 production by Aspergillus parasiticus in macadamia nuts. Ind Crops Prod. (2018) 111:63–8. doi: 10.1016/j.indcrop.2017.10.003
523. Zalegh I, Akssira M, Bourhia M, Mellouki F, Rhallabi N, Salamatullah AM, et al. A review on Cistus sp.: phytochemical and antimicrobial activities. Plants. (2021) 10:1214. doi: 10.3390/plants10061214
524. Gomaa OM, Abou El Nour S. Aflatoxin inhibition in Aspergillus flavus for bioremediation purposes. Ann Microbiol. (2014) 64:975–82. doi: 10.1007/s13213-013-0732-8
525. Boonmee S, Atanasova V, Chéreau S, Marchegay G, Hyde KD, Richard-Forget F. Efficiency of hydroxycinnamic phenolic acids to inhibit the production of ochratoxin A by Aspergillus westerdijkiae and Penicillium verrucosum. Int J Mol Sci. (2020) 21:8548. doi: 10.3390/ijms21228548
526. Ricelli A, De Angelis M, Primitivo L, Righi G, Sappino C, Antonioletti R. Role of some food-grade synthesized flavonoids on the control of Ochratoxin A in Aspergillus carbonarius. Molecules. (2019) 24:2553. doi: 10.3390/molecules24142553
527. Romero SM, Alberto MR, Manca de Nadra MC, Vaamonde G. Inhibition of growth and ochratoxin A biosynthesis in Aspergillus carbonarius by flavonoid and nonflavonoid compounds. Mycotoxin Res. (2009) 25:165–170. doi: 10.1007/s12550-009-0026-y
528. Iram W, Anjum T, Iqbal M, Ghaffar A, Abbas M. Structural elucidation and toxicity assessment of degraded products of aflatoxin B1 and B2 by aqueous extracts of Trachyspermum ammi. Front Microbiol. (2016) 7:346. doi: 10.3389/fmicb.2016.00346
529. Badr AN, El-Attar MM, Ali HS, Elkhadragy MF, Yehia HM, Farouk A. Spent coffee grounds valorization as bioactive phenolic source acquired antifungal, anti-mycotoxigenic, and anti-cytotoxic activities. Toxins. (2022) 14:109. doi: 10.3390/toxins14020109
530. Vijayanandraj S, Brinda R, Kannan K, Adhithya R, Vinothini S, Senthil K, et al. Detoxification of aflatoxin B1 by an aqueous extract from leaves of Adhatoda vasica Nees. Microbiol Res. (2014) 169:294–300. doi: 10.1016/j.micres.2013.07.008
531. Lee S-E, Mahoney N-E, Campbell B-C. Inhibition of aflatoxin B1 biosynthesis by piperlongumine isolated from Piper longum L. J Microbiol Biotechnol. (2002) 12:679–82.
532. Ponzilacqua B, Rottinghaus G, Landers B, Oliveira C. Effects of medicinal herb and Brazilian traditional plant extracts on in vitro mycotoxin decontamination. Food Control. (2019) 100:24–7. doi: 10.1016/j.foodcont.2019.01.009
533. Shakeel Q, Lyu A, Zhang J, Wu M, Li G, Hsiang T, et al. Biocontrol of Aspergillus flavus on peanut kernels using Streptomyces yanglinensis 3-10. Front Microbiol. (2018) 9:1049. doi: 10.3389/fmicb.2018.01049
534. Gupta PK. Toxicity of fungicides. In:Gupta RC, , editor. Veterinary toxicology. USA: Elsevier (2018). p. 569–80. doi: 10.1016/B978-0-12-811410-0.00045-3
535. Dandekar A, Muitr RM, Plants with Elevated Levels of Gallic Acid/Polyphenol Oxidase Methods of Generating Such Plants. U.S. Patent No 7, 449,617. Oakland, CA: The Regents of the University of California (2004). Available online at: https://patentimages.storage.googleapis.com/ea/ca/7d/f9597417872ce1/US7449617.pdf (accessed September 12, 2022).
536. Fang X, Jianliang Z, Yanshan S, Suzhen L, Hongxiang Y, Wei L, Application of Tea Polyphenol in Biological Prevention Control of Aflatoxin. China Patent No 103210921. Guangzhou: South China Agricultural University (2014). Available online at: https://patentimages.storage.googleapis.com/f4/ba/b8/76c367f1400d67/CN103210921A.pdf (accessed September 12, 2022).
537. Wu D, Jinfeng L, Yongpeng Y, Chuntian Z, Anti-Mycotoxin Feed Additive for Cattle. China Patent No 104068217. Guangzhou: Guangzhou Chengnuo Biological Science & Technology Co Ltd (2014). Available online at: https://patentimages.storage.googleapis.com/b4/98/46/20130dd452c8c1/CN104068217A.pdf (accessed September 12, 2022).
538. Xiao Y, Hua Y, Mingrong Q, Rui L, Xiaofeng J, Xiaoli WJ, Reduce the Preparation Method of the Catechin Nanoparticle of Aflatoxin Bioavilability. China Patent No 106074494. Zhejiang: Zhejiang Academy of Agricultural Sciences (2016). Available online at: https://patentimages.storage.googleapis.com/71/cf/d2/9c0a707d2ce5f6/CN106074494A.pdf (accessed September 12, 2022).
539. Sorriento D, De Luca N, Trimarco B, Iaccarino G. The antioxidant therapy: new insights in the treatment of hypertension. Front Physiol. (2018) 9:258. doi: 10.3389/fphys.2018.00258
540. Skibola CF, Smith MT. Potential health impacts of excessive flavonoid intake. Free Radic Biol Med. (2000) 29:375–83. doi: 10.1016/S0891-5849(00)00304-X
541. Halliwell B. Dietary polyphenols: good, bad, or indifferent for your health? Cardiovasc Res. (2007) 73:341–7. doi: 10.1016/j.cardiores.2006.10.004
542. Sosa V, Moliné T, Somoza R, Paciucci R, Kondoh H, LLeonart ME. Oxidative stress and cancer: an overview. Ageing Res Rev. (2013) 12:376–90. doi: 10.1016/j.arr.2012.10.004
543. Hayes JD, Dinkova-Kostova AT, Tew KD. Oxidative stress in cancer. Cancer Cell. (2020) 38:167–97. doi: 10.1016/j.ccell.2020.06.001
544. Bhatti JS, Sehrawat A, Mishra J, Sidhu IS, Navik U, Khullar N, et al. Oxidative stress in the pathophysiology of type 2 diabetes and related complications: current therapeutics strategies and future perspectives. Free Radic Biol Med. (2022) 184:114–34. doi: 10.1016/j.freeradbiomed.2022.03.019
545. Gholamian-Dehkordi N, Luther T, Asadi-Samani M, Mahmoudian-Sani MR. An overview on natural antioxidants for oxidative stress reduction in cancers; a systematic review. Immunopathol Persa. (2017) 3:e12. doi: 10.15171/ipp.2017.04
546. Russo GL, Tedesco I, Spagnuolo C, Russo M. In antioxidant polyphenols in cancer treatment: friend, foe or foil? Semin Cancer Biol. (2017) 2017:1–13. doi: 10.1016/j.semcancer.2017.05.005
547. Yang CS, Landau JM, Huang M-T, Newmark HL. Inhibition of carcinogenesis by dietary polyphenolic compounds. Annu Rev Nutr. (2001) 21:381–406. doi: 10.1146/annurev.nutr.21.1.381
548. Mennen LI, Walker R, Bennetau-Pelissero C, Scalbert A. Risks and safety of polyphenol consumption. Am J Clin Nutr. (2005) 81:326S−9S. doi: 10.1093/ajcn/81.1.326S
549. Cory H, Passarelli S, Szeto J, Tamez M, Mattei J. The role of polyphenols in human health and food systems: a mini-review. Front Nutr. (2018) 5:87. doi: 10.3389/fnut.2018.00087
550. León-González AJ, Auger C, Schini-Kerth VB. Pro-oxidant activity of polyphenols and its implication on cancer chemoprevention and chemotherapy. Biochem Pharmacol. (2015) 98:371–80. doi: 10.1016/j.bcp.2015.07.017
551. Hu Z, Li M, Cao Y, Akan OD, Guo T, Luo F. Targeting AMPK signaling by dietary polyphenols in cancer prevention. Mol Nutr Food Res. (2022) 66:2100732. doi: 10.1002/mnfr.202100732
552. Bermúdez-Oria A, Rodríguez-Juan E, Rodríguez-Gutiérrez G, Fernández-Prior Á, Fernández-Bolaños J. Effect of the olive oil extraction process on the formation of complex pectin-polyphenols and their antioxidant and antiproliferative activities. Antioxidants. (2021) 10:1858. doi: 10.3390/antiox10121858
553. Pérez-Jiménez J, Díaz-Rubio ME, Saura-Calixto F. Obtainment and characterization of a potential functional ingredient from olive. Int J Food Sci Nutr. (2015) 66:749–54. doi: 10.3109/09637486.2015.1095863
554. Saura-Calixto F. The story of the introduction of non-extractable polyphenols into polyphenol research: origin, development and perspectives. In:Fulgencio S-C, Jara P-J, , editors. Non-extractable Polyphenols Carotenoids: Importance in Human Nutrition Health. (2018). London: Royal Society Chemistry Publishing UK.
555. Clodoveo ML, Crupi P, Corbo F. Optimization of a green extraction of polyphenols from sweet cherry (Prunus avium L.) pulp. Processes. (2022) 10:1657. doi: 10.3390/pr10081657
556. Mohammadnejad P, Haghbeen K, Rasouli H. Treatment and valorization of olive mill wastewater. In:Watson V. R. P. a. R. R., , editors. Olives and Olive Oil in Health and Disease Prevention. London: Elsevier (2021). p. 505–19. doi: 10.1016/B978-0-12-819528-4.00058-4
557. Speroni CS, Stiebe J, Guerra DR, Bender ABB, Ballus CA, dos Santos DR, et al. Micronization and granulometric fractionation improve polyphenol content and antioxidant capacity of olive pomace. Ind Crops Prod. (2019) 137:347–55. doi: 10.1016/j.indcrop.2019.05.005
558. Hao P-Y, Feng Y-L, Zhou Y-S, Li H-L, Ma Y, Ye C-L, Schaftoside Schaftoside interacts with NlCDK1 protein: a mechanism of rice resistance to brown planthopper Nilaparvata lugens. Front Plant Sci. (2018) 9:710. doi: 10.3389/fpls.2018.00710
559. Wu F, Stacy SL, Kensler TW. Global risk assessment of aflatoxins in maize and peanuts: are regulatory standards adequately protective? Toxicol Sci. (2013) 135:251–9. doi: 10.1093/toxsci/kft132
560. Keyser JC, Sela S. Innovations in Food Trade: Rethinking Aflatoxin Management in East Africa. Washington, DC: World Bank (2020). doi: 10.1596/34846
561. Ortega-Beltran A, Bandyopadhyay R. Contributions of integrated aflatoxin management strategies to achieve the sustainable development goals in various African countries. Glob Food Sec. (2021) 30:100559. doi: 10.1016/j.gfs.2021.100559
562. Gong YY, Watson S, Routledge MN. Aflatoxin exposure and associated human health effects, a review of epidemiological studies. Food Saf. (2016) 4:14–27. doi: 10.14252/foodsafetyfscj.2015026
563. Ding S, Jiang H, Fang J. Regulation of immune function by polyphenols. J Immunol Res. (2018) 2018:1264074. doi: 10.1155/2018/1264074
Keywords: Climate change, Aflatoxin, Phytophenolics, Cancer, Diabetes, Alzheimer's disease, Oxidative stress, Inflammation
Citation: Rasouli H, Nayeri FD and Khodarahmi R (2022) May phytophenolics alleviate aflatoxins-induced health challenges? A holistic insight on current landscape and future prospects. Front. Nutr. 9:981984. doi: 10.3389/fnut.2022.981984
Received: 30 June 2022; Accepted: 26 September 2022;
Published: 28 October 2022.
Edited by:
Pasquale Crupi, Council for Agricultural and Economics Research (CREA), ItalyReviewed by:
Charles Odilichukwu R. Okpala, Wroclaw University of Environmental and Life Sciences, PolandMohd Redzwan Sabran, Putra Malaysia University, Malaysia
Copyright © 2022 Rasouli, Nayeri and Khodarahmi. This is an open-access article distributed under the terms of the Creative Commons Attribution License (CC BY). The use, distribution or reproduction in other forums is permitted, provided the original author(s) and the copyright owner(s) are credited and that the original publication in this journal is cited, in accordance with accepted academic practice. No use, distribution or reproduction is permitted which does not comply with these terms.
*Correspondence: Hassan Rasouli, SDNuLnJhc291bGlAZ21haWwuY29t; Reza Khodarahmi, ZHJyZXpha2hvZGFyYWhtaUBnbWFpbC5jb20=