- 1Jiangsu Key Laboratory for Food Quality and Safety-State Key Laboratory Cultivation Base, Ministry of Science and Technology, Institute of Food Safety and Nutrition, Jiangsu Academy of Agricultural Sciences, Nanjing, China
- 2Chemical Engineering Laboratory, Synthetic Biology and Intelligent Control Unit, Guangdong Technion Isreal Institute of Technology, Shantou, China
- 3Key Laboratory for Quality Evaluation and Health Benefit of Agro-Products of Ministry of Agriculture and Rural Affairs, Key Laboratory for Quality and Safety Risk Assessment of Agro-Products Storage and Preservation of Ministry of Agriculture and Rural Affairs, College of Biosystems Engineering and Food Science, Zhejiang University, Hangzhou, China
Fermented soybean products are favorite foods worldwide because of their nutritional value and health effects. In this study, solid-state fermentation (SSF) of soybeans with Rhizopus oligosporus RT-3 was performed to investigate its nutraceutical potential. A rich enzyme system was released during SSF. Proteins were effectively transformed into small peptides and amino acids. The small peptide content increased by 13.64 times after SSF for 60 h. The antioxidant activity of soybeans was enhanced due to the release of phenolic compounds. The soluble phenolic content increased from 2.55 to 9.28 gallic acid equivalent (GAE) mg/g after SSF for 60 h and exhibited high correlations with microbial enzyme activities during SSF. The potential metabolic pathways being triggered during SSF indicated that the improved nutritional composition of soybean attributed to the biochemical reactions catalyzed by microbial enzymes. These findings demonstrated that SSF could evidently improve the nutritional value and prebiotic potential of soybeans.
Introduction
Soybean has been cultivated for thousands of years in Asia, and its products are popular worldwide due to their abundant nutrition (1, 2). Proteins occupy approximately 40% of the dry weight (DW) of soybean making it an ideal material for various delicious protein foods with low cost compared with animal proteins (3). Soybean foods are considered promising substitutes of milk products for lactose-intolerant populations because of their free lactose property (4). The dietary consumption of soybean products shows health-promoting benefits, such as anti-diabetic, anticancer, anti-inflammatory, and anti-cardiovascular effects (1). At present, a wide range of soybean products have been made and the variety is still increasing.
Solid-state fermentation (SSF) with microorganism is an economically viable method used to biotransform food materials for nutritional enrichment, and serves a key role in forming new nutritional substances, including pigments, organic acids, and flavors (5). SSF with Monascus anka promotes the liberation of phenolics and enhances the antioxidant capacity of oats (6). SSF with Saccharomyces cerevisiae effectively improves the nutritional profiles of maize flour by decreasing antinutritional factors and increasing protein digestibility (7). In soybean food production, SSF is also used to produce microbial pigments, improve the levels of free amino acids, reduce the content of antinutritional factors, and enhance antioxidant ability (8). The content and composition of volatile compounds in tofu are significantly increased after fermentation with Mucor spp. (3). The co-SSF of soybean with Actinomucor elegans and R. oligosporus is effective in the hydrolysis of antinutritional factors and reduction of immunoreactivity (9). Tempeh is a traditional soybean food produced by SSF with strains of Rhizopus species, such as R. oligosporus, Rhizopus arrhizus, and Rhizopus stolonifer. During SSF, soybeans are covered with a thick white mycelium and the biomacromolecules are hydrolyzed into small-molecule nutrients with high bioavailability by fungal enzymes, thus improving the nutritional value and functional properties of soybeans (10). Thus far, studies on fermented soybean predominantly focus on flavor components, protein hydrolysis, and health-promoting effects, but little information is available on variations of microbial enzyme activities, their effects on small molecule nutrient production, and analysis of the potential biochemical reactions involved in the SSF of soybeans.
In this study, the variations of microbial enzyme activities, such as carbohydrate-hydrolyzing enzymes, esterase, and protease, derived from R. oligosporus RT-3 during SSF were studied. The protein degradation, small peptide production, phenolic content, and metabolite compositions were investigated. Afterward, the relationships among enzyme activities, small peptide content, phenolic content, and antioxidant activities were analyzed. Metabolites were finally tracked to their biosynthesis pathways to interpret the potential biochemical reactions during SSF.
Materials and methods
Materials and strain
The high purity (≥97%) standards, including ascorbic acid, vanillic acid, syringic acid, epicatechin, daidzin, glycitin, ferulic acid, genistin, daidzein, glycitein, quercetin, and genistein were purchased from Yuanye Bio-Technology Co., Ltd. (Shanghai, China). Other reagents of analytical grade. Soybeans (Liaodou, yellow seed coat, mainly planted in northern China, weigh 21.6 g of one hundred seeds) were obtained from a local market. R. oligosporus RT-3 isolated, screened and identified from Tempeh in our laboratory was used for soybean fermentation (11, 12). The strain was cultivated on potato dextrose agar at 30°C for 3 days. Then, spores produced were scraped in water (1 × 107 spores/mL).
Solid-state fermentation of soybeans
One kilogram soybeans were cleaned and then soaked in distilled water for 12 h. Subsequently, they were peeled and sterilized at 105°C for 0.5 h. After cooling to room temperature, 2 mL spore suspension (1 × 107 spores/mL) was inoculated to 100 g soybeans. Soybeans were cultured in a constant temperature incubator at 30°C for 60 h. The fermented soybeans (FS) were sampled at 0, 12, 24, 36, 48, and 60 h. Wet samples were stored at −80°C for further study. The unfermented soybeans (US, 0 h) were used as control.
Microbial enzymes
The enzymes released by R. oligosporus RT-3 during SSF were prepared in accordance with the report of Bei et al. (13). Briefly, 2 g wet sample was firstly ground using a mortar and then mixed with 10 mL citrate buffer (pH 5.5). The flasks were shaken at 30°C for 0.5 h to extract microbial enzymes. Subsequently, the mixture was centrifuged at 12,000 × g and 4°C for 0.5 h. The supernatant obtained was used as crude enzyme solution. The results of enzyme activities were expressed as per gram DW.
α-Amylase and protease activities
α-Amylase and protease activities were measured in line with previous report (14). For α-amylase, 2 mL properly diluted enzyme solution was mixed with 1 mL acetate buffer (0.1 M, pH 4.5) and 1 mL of 1% (w/v) soluble starch at 37°C for 1 h. Then, the glucose content was determined using the 3,5-dinitrosalicylic acid (DNS) method. One unit of α-amylase was defined as the amount required to produce 1 μmol glucose per min. For protease, 1 mL enzyme solution was mixed with 1 mL casein solution (1%, w/v) with shaking at 40°C for 1 h and added with 10% trichloroacetic acid (2 mL) to stop the reaction. The mixture was centrifuged at 10,000 × g for 20 min. The absorbance was measured at 280 nm. One unit of protease was defined as the amount required to produce 1 μg tyrosine per min.
β-Glucosidase and esterase activities
The determination of β-Glucosidase and esterase activities were concurred with previous report (15, 16). For β-glucosidase, 100 μL properly diluted sample was mixed with 900 μL citrate buffer (50 mM, pH 4.8) containing p-nitrobenzene-β-D glucoside (5 mM) at 50°C for 10 min. Two milliliters of 1.0 M Na2CO3 was added to stop the reaction. For esterase, 50 μL enzyme solution was mixed with 50 μL p-nitrophenyl acetate (150 mM) and 2.9 mL Tris-HCl buffer (9.2 mM, pH 7.5) at 25°C for 4 min. One unit of β-glucosidase and esterase was defined as the amount required to produce 1 μmol p-nitrophenol per min.
Endoglucanase and exoglucanase activities
The endoglucanase and exoglucanase activities were measured according to the report of Ang et al. (17). For endoglucanase, 0.5 mL enzyme solution was mixed with 0.5 mL acetate buffer (0.05 M, pH 5.0) containing 2% (w/v) carboxymethylcellulose at 50°C for 0.5 h. For exoglucanase, 0.5 mL enzyme solution was mixed with 1 mL acetate buffer (0.05 M, pH 5.0) containing 50 mg WhatmanNo.1 filter paper at 50°C for 1 h. The glucose content was determined using the DNS method. One unit of endoglucanase and exoglucanase was defined as the amount required to produce 1 μmol glucose per min.
Phytase activity
The phytase activity was measured based on the report of Acuña et al. (18). Briefly, 100 μL enzyme solution was mixed with 2.7 mL Tris-HCl (pH 7.0) containing 2.5 mM sodium phytate at 37°C for 0.5 h, and the mixture was added with 11.5 mL acetone–sulfuric acid–ammonium molybdate mixture (2:1:1). Eight milliliters of 1 M citric acid solution was used to stop the reaction. The mixture was centrifuged at 12,000 × g for 20 min. The absorbance of supernatant was read at 355 nm. One unit of phytase was defined as the amount required to produce 1 μmol inorganic phosphorus per min.
Scanning electron microscope analysis
Soybeans were fixed in 2.5% (v/v) glutaraldehyde solution for 12 h. Then, soybeans were washed three times using distilled water and freeze-dried under −50°C. Afterward, soybeans were sputter-coated with platinum and then observed using SEM (EVO-LS10, Carl Zeiss AG, Germany).
Soluble protein analysis
The soluble protein was obtained in accordance with the report of Huang et al. (9). The freeze-dried samples were ground into powder and prepared in Tris-HCl (50 mM, pH 8.2). The mixture was shaken at room temperature for 1 h and then centrifuged at 12,000 × g for 20 min. The protein content in the supernatant was determined using a bicinchoninic acid (BCA) assay kit. The supernatant was mixed with sample buffer solution and heated at 100°C for 5 min. Sodium dodecyl sulfate–polyacrylamide gel electrophoresis (SDS-PAGE) was performed using 4% stacking gel at 60 V for 0.5 h and 12% separating gel at 120 V for 1.5 h. The result was visualized using gel imaging systems (BIO-RADXR, Bio-Rad Laboratories, United States).
Small peptide analysis
The small peptide content was analyzed in accordance with the report of Muhialdin et al. (19). The freeze-dried sample was prepared in water (1:10, w/v) and shaken for 1 h. After centrifugation at 12,000 × g for 20 min, the supernatant was filtered using ultrafiltration membrane (10 kDa, Millipore, United States). Subsequently, 100 μL filtrate was added to 7.5 mL reaction reagent for 2 min. The reaction reagent was composed of sodium tetraborate (25 mL, 50 mM), SDS (2.5 mL, 20%), o-phthaldialdehyde (40 mg), and β-mercaptoethanol (100 μL). The absorbance was read at 340 nm.
The peptide composition was analyzed using high-performance liquid chromatography (HPLC) equipped with the ZORBAX Eclipse Plus C18 reversed-phase analytical column (4.60 mm × 250 mm, 5 μm, Agilent) and diode array detector (G1315D) in accordance with the report of Rui et al. (20). Mobile phases were composed of water containing 0.1% trifluoroacetic acid (solvent A) and acetonitrile containing 0.1% trifluoroacetic acid (solvent B). Elution was carried out as follows: 0–20 min, 5–35% solvent B; 20–30 min, 35% solvent B. Twenty microliters sample was injected into the column. The flow rate and detection wavelength were 0.7 mL/min and 280 nm, respectively. The peptides in the sample were preliminarily analyzed according to retention time and peak area.
Phenolic compound analysis
Soluble (free) phenolics (SPs) were extracted in accordance with the report of Bei et al. (13). Briefly, 6 g freeze-dried sample was added to 80% methanol. The mixture was subjected to ultrasonic treatment at 45°C for 1 h. After centrifugation at 12,000 × g for 20 min, the residue was treated with 80% methanol again. The combined supernatant was extracted with hexane to eliminate lipids followed by ethyl acetate extraction. The organic phase was concentrated with a rotary evaporator until dryness and then dissolved in 10 mL of 80% methanol for determination of the SP content (SPC).
Insoluble (bound) phenolics were commonly liberated by alkaline hydrolysis as previously described (13, 21, 22). The residue left after SP extraction was treated with 2 mol/L NaOH for 4 h. Subsequently, pH was regulated to 2.0. After centrifugation, the supernatant was extracted using the same method as the soluble fraction. The ethyl acetate fraction obtained was dissolved in 4 mL of 80% methanol for determination of the insoluble phenolic content (IPC). The phenolic content was measured using Folin-Ciocalteu method on the basis of the report of Xiao et al. (11).
The phenolic composition was analyzed using HPLC equipped with the ZORBAX Eclipse Plus C18 reversed-phase analytical column (4.60 mm × 250 mm, 5 μm, Agilent) and diode array detector (G1315D). The SP and IP fractions dissolved in 80% methanol above were filtered using a 0.22 μm syringe filter before HPLC analysis. The mobile phase was composed of water containing 0.4% acetic acid (solvent A) and acetonitrile (solvent B). Elution was carried out as follows: 9–75% solvent A for 0–40 min, 75–65% solvent A for 40–45 min, 65–50% solvent A for 45–50 min, and 50–95% solvent A for 50–55 min. Flow rate and detection wavelength were 0.8 mL/min and 280 nm, respectively. The phenolics in the sample were identified and quantified by their standards according to retention time and peak area.
Antioxidant activity analysis
The IP and SP fractions at different fermentation time (0, 12, 24, 36, 48, and 60 h) were freeze-dried under −50°C and then dissolved in 80% methanol. The antioxidant activities of fermented soybean, including DPPH radical scavenging activity, ABTS radical cation scavenging activity, reducing power, and ferric reducing antioxidant power, were measured in accordance with the report of Yin et al. (23). For DPPH radical scavenging activity, 1 mL sample was mixed with 1 mL DPPH solution (0.2 mM) for 30 min and then the absorbance was read at 517 nm. For ABTS radical cation scavenging activity, ABTS.+ was prepared with the reaction of ABTS solution (7 mM) with K2S2O8 solution (2.45 mM). The mother liquor was diluted to 0.70 (OD734 nm). One milliliter sample was mixed with 4 mL ABTS.+ solution for 6 min and then the absorbance at 734 nm was read. For reducing power, 1 mL sample was mixed with 5 mL 0.2 M PBS (pH 6.6) and 5 mL 1% potassium ferricyanide at 50°C for 20 min. Trichloroacetic acid (5 mL, 10%, w/v) was added. Then, the mixture was centrifuged at 420 × g for 10 min. The supernatant (5 mL) was mixed with 1 mL ferric chloride (0.1%, w/v) for 10 min. The absorbance was measured at 700 nm. For ferric reducing antioxidant power, the reaction reagent was composed of 1 mL 10 mM TPTZ (in 40 mM hydrochloric acid), 1 mL 20 mM ferric chloride and 10 mL 0.3 M acetate buffer (pH 3.6). Two milliliters were mixed with 10 mL reaction reagent at 37°C for 20 min. The absorbance was measured at 593 nm.
Metabolite profile analysis
The metabolite profile was analyzed in accordance with the report of Yin et al. (24). Briefly, 1 g freeze-dried sample was mixed with 5 mL reagent containing chloroform, methanol, and water (1:2.5:1, v/v/v) and supplemented with 40 μg/mL ribitol (internal standard). After centrifugation at 500 × g for 20 min, 2 mL supernatant was dried using a termovap sample concentrator and then supplemented with 50 μL of pyridine containing 20 mg/mL methoxyamine hydrochloride at 37°C for 60 min. Subsequently, 200 μL N-methyl-N-(trimethylsilyl)-trifluoroacetamide (MSTFA) containing 1% trimethylchlorosilane (TMCS) was added and the tube was kept under 70°C for 0.5 h. The supernatant obtained after centrifugation was analyzed using gas chromatography–mass spectrometry (GC-MS, TRACE 1300, flame ionization detector, 30 m × 250 μm × 0.25 μm elasticity quartz capillary, Thermo Fisher Scientific, United States). The operation conditions were as follows: stationary phase, hexane; purge flow rate of the helium carrier gas, 5 mL/min; inlet temperature, 250°C; MS transfer line temperature, 280°C; ion source temperature, 300°C; scan masses, m/z 40–600. The temperature programming of GC oven was shown as follows: 40°C, 0–1 min; 40–280°C, 1–40 min; and 280°C, 40–50 min. The metabolites (identity ≥ 80%) were analyzed by comparing the generated ion fragments in NIST mass spectral library (version 2.3, build 2017, prepared by NIST mass spectrometry data center). Five replicates were conducted.
Statistical analysis
Data were expressed as an average of at least three replicates. One-way ANOVA followed by Duncan’s post–hoc multiple comparisons were performed in SPSS. p < 0.05 indicated significance. Also, bivariate correlations were performed to obtain the Pearson correlation coefficient (PCC) in SPSS.
Results
Trends of enzyme activities during solid-state fermentation
As shown in Supplementary Figure 1, the filamentous fungi R. oligosporus RT-3 grew well on soybeans accompanied by the formation of a large number of white hyphae during SSF. An abundant enzyme system released by microorganisms is the material basis for their growth and proliferation (25, 26). Therefore, the changing trends of several key enzymes released by R. oligosporus RT-3 were analyzed. As shown in Figure 1A, a rapid increase of the α-amylase activity was observed during the whole SSF. The enzyme activities of cellulases, including endoglucanase and exoglucanase, increased and peaked at 48 h and then suffered a slight reduction. The β-glucosidase exhibited a trend similar to cellulase, which increased before 36 h and then slightly decreased. Similar to α-amylase, esterase and protease increased continuously during the whole fermentation. Additionally, SEM analysis indicated that relatively large voids were produced after SSF, which might be due to the degradation of biomacromolecules induced by fungal enzymes released from R. oligosporus RT-3 (Figure 1B).
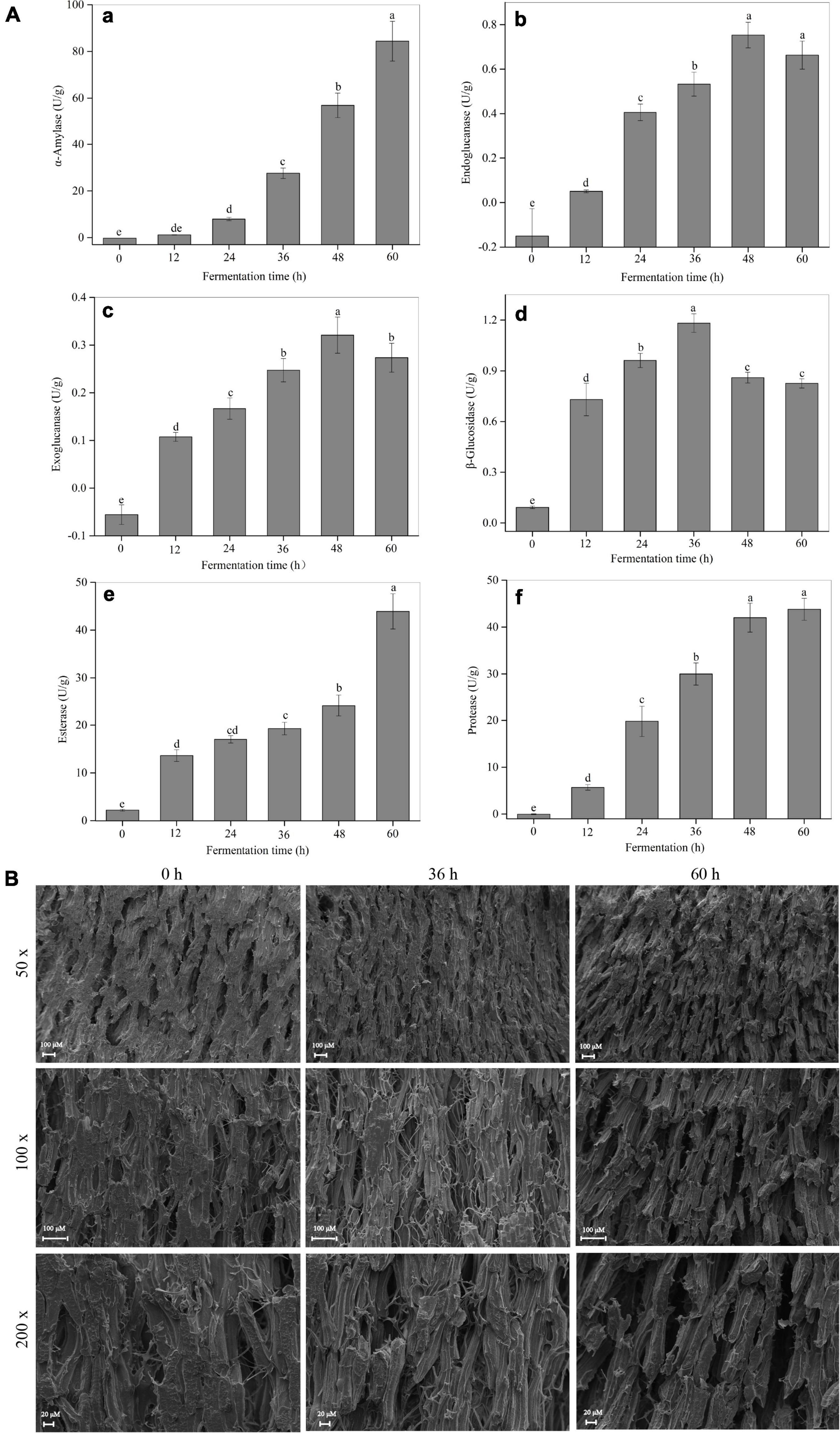
Figure 1. Changes of microbial enzyme activities and microstructure observation during SSF. (A) a, α-amylase; b, endoglucanase; c, exoglucanase; d, β-glucosidase; e, esterase; f, protease. (B) Microstructure. p < 0.05 was considered as the significant level. The small letters were used to show the significant difference between samples at different fermentation time.
Effects of solid-state fermentation on soluble proteins and small peptides
As shown in Figure 2A and Supplementary Figure 2, SSF with R. oligosporus RT-3 made a significant difference on the content and composition of soluble proteins in soybeans. The soluble protein content exhibited an evident increase with the extension of fermentation time. In the control (0 h), several bands, such as α′ and β subunits of β-conglycinin, and acidic and basic subunits of glycinin were observed at molecular mass ranging from 20 to 150 kDa (9). However, the protein bands except some small proteins with a molecular mass less than 20 kDa almost completely disappeared after SSF for 36 h, indicating that macromolecular proteins were degraded into small soluble proteins, peptides or amino acids during SSF.
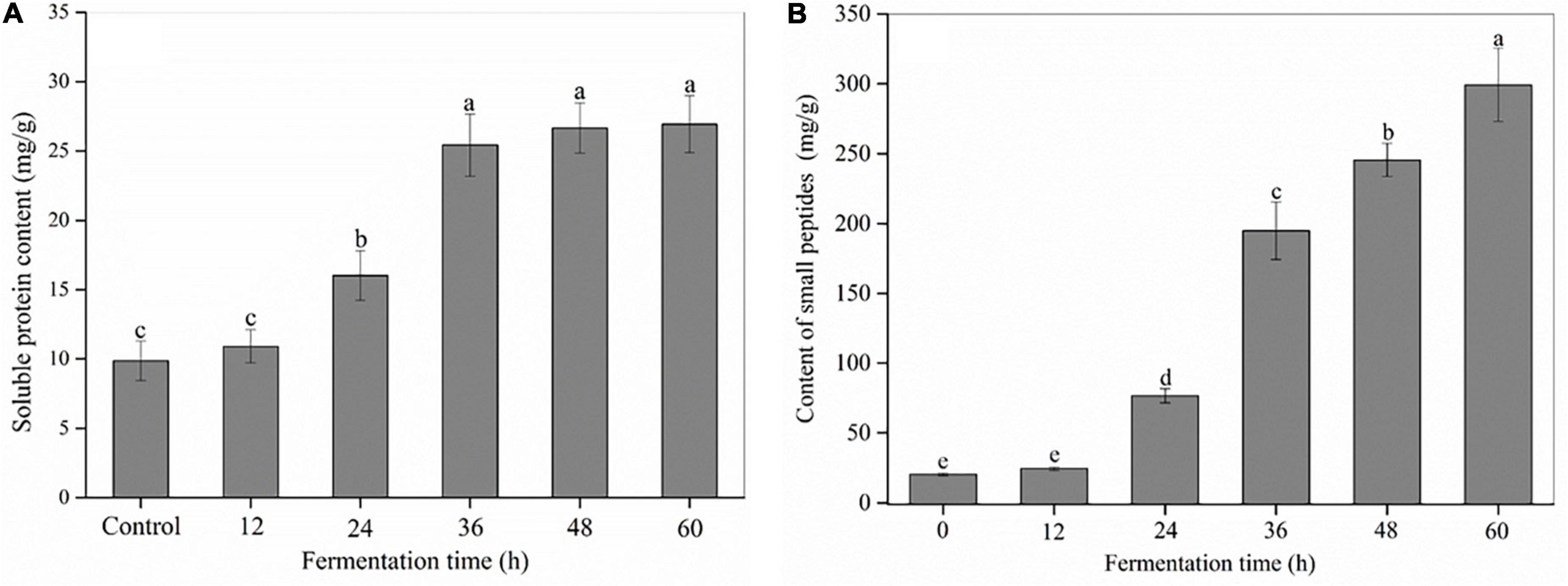
Figure 2. The content of soluble proteins and small peptides in soybeans during the SSF. (A) Soluble proteins; (B) small peptides. p < 0.05 was considered as the significant level. The small letters were used to show the significant difference between samples at different fermentation time.
Small peptides refer to peptides with molecular weight less than 10 kDa (27). As shown in Figure 2B, R. oligosporus RT-3 apparently increased the total content of small peptides in soybeans during SSF. For example, the content of small peptides at 60 h was 14.64 times of that in the control. The increase in the total content of small peptides was due to the protease released by R. oligosporus RT-3 during SSF, which was further confirmed by the high PCC (R2 = 0.974, p < 0.01) between protease and the total content of small peptides. HPLC showed that approximately 90 small peptide peaks were found in US and FS (Supplementary Figures 3A–F). The areas of 11 major peaks at retention time ranging from 3 to 20 min were significantly changed during SSF. The fractions eluted at early retention times were considered peptides with low molecular weight and high hydrophilicity (20). For R. oligosporus RT-3 fermentation, most high peaks were presented at retention times before 10 min. Furthermore, the production of relatively large and increased hydrophobic peptides (peaks 10 and 11) was observed.
Trends of phenolic content and composition during solid-state fermentation
As shown in Supplementary Figure 4, SPC showed a slight increase from 0 to 24 h, a rapid increase from 24 to 36 h, and then a slight increase again from 36 to 60 h. For example, SPC reached 9.28 mg gallic acid equivalent (GAE)/g in FS at 60 h, which was 3.64 times of control. IPC increased from 0 to 24 h and then significantly decreased from 24 to 60 h. Furthermore, HPLC indicated that SSF with R. oligosporus RT-3 changed the phenolic composition of soybeans (Supplementary Figure 5 and Table 1). For SPs, the contents of vanillic and ferulic acids increased first and then decreased during SSF. The contents of epicatechin, daidzein, glycitein, and genistein exhibited a continuous increase during SSF. However, continuous decreases in the contents of syringic acid, daidzin, glycitin, and genistin were observed. For insoluble phenolics (IPs), the contents of vanillic acid, syringic acid, daidzin, ferulic acid, genistin, glycitein, and quercetin increased first and then decreased during SSF. The contents of epicatechin, glycitin, and genistein showed continuous increases. However, daidzein showed a continuous decrease during SSF. Besides, soy isoflavones were effectively transformed into glycosides after SSF. Notably, the insoluble forms of syringic, vanillic, and ferulic acids, respectively, occupied 82.57, 78.77, and 71.04% of their total contents (soluble + insoluble forms) in US, and this finding was in line with the report of Adom and Liu (28). The relationships between enzyme activities and phenolic contents were further analyzed. As shown in Supplementary Table 1, enzyme activities, i.e., α-amylase (R2 = 0.938, p < 0.01), showed high correlations with SPC. A similar result was also observed on the content of the total phenolics (SPC + IPC), demonstrating that the enzymes released by R. oligosporus RT-3 during SSF significantly improved the liberation of phenolic compounds.
Trends of antioxidant activities of soybeans during solid-state fermentation
As shown in Figure 3A, the reducing power of soybeans for SPs evidently increased during SSF. For example, the reducing power of soybeans at 60 h increased by 2.81 times of US. The trends of antioxidant activity of soybeans in other assays showed a similar result with the reducing power for SP fraction (Figures 3B–D). Additionally, the antioxidant activity of SP was much higher than that of IP. However, the antioxidant activity for IP fraction in four antioxidant assays increased first, peaked at 36 h, and then decreased rapidly. Furthermore, as shown in Supplementary Table 2, high correlations (e.g., R2 = 0.991 for reducing power, p < 0.01) were observed between antioxidant activities and SPC, indicating that the antioxidant activities of soybean were predominantly derived from the high content of phenolic compounds especially soluble fractions.
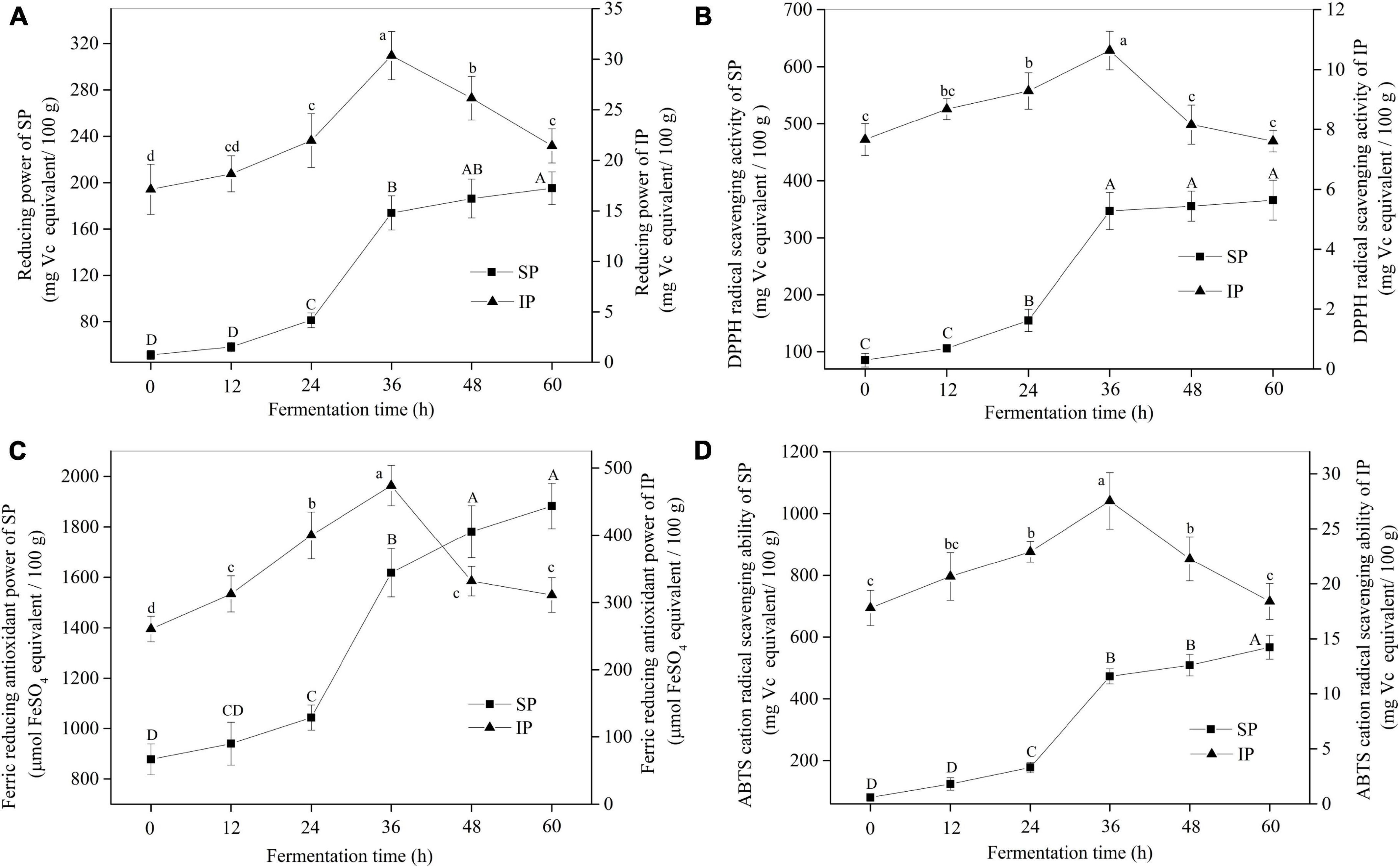
Figure 3. The antioxidant activities of soybeans during SSF. (A) Reducing power; (B) DPPH radical scavenging activity; (C) ferric reducing antioxidant power; (D) ABTS cation radical scavenging ability. p < 0.05 was considered as the significant level. The small letters were used to show the significant difference between samples at different fermentation time for IP. The capital letters indicated the significant difference between samples at different fermentation time for SP. SP, soluble phenolics; IP, insoluble phenolics.
Metabolite profile analysis
The metabolite profiles of US and FS at 36 h were analyzed using GC-MS (Table 2). A total of 38 kinds of metabolites were identified in soybeans. The principal component analysis (PCA) was used to analyze the changes in metabolite profiles. As shown in Figure 4A, the principal components PC1 and PC2 contributed to 73 and 14.6% of the total variance in metabolite compositions, respectively. For PC1, a significant difference was observed between US and FS, demonstrating that the nutritional compositions of soybeans were apparently altered by R. oligosporus RT-3 fermentation. For the small component PC2, results indicated that the nutritional composition of FS only had a slight similarity with that of US. The results of PCA biplot were further confirmed by the analysis of a cluster dendrogram (Figure 4B).
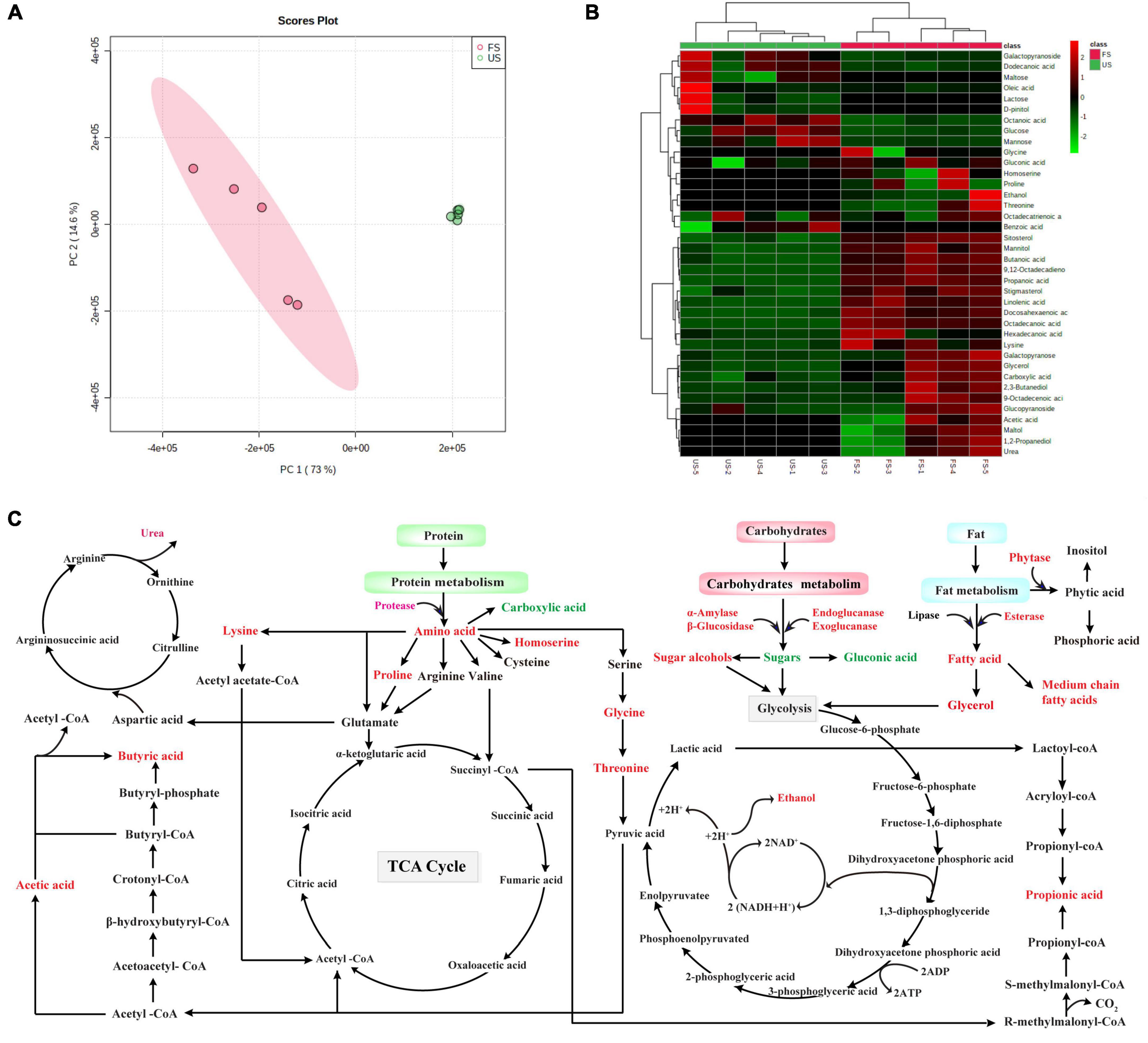
Figure 4. The analysis of metabolite profiles in US and FS and the potential metabolic pathways involved during SSF. (A) PCA biplot; (B) heatmap dendrogram; (C) metabolic pathways involved during SSF. Upregulated metabolites: red color; Downregulated metabolites, green color; Undetected metabolites, black color. US, unfermented soybean; FS, fermented soybean.
Metabolites could be classified into parent categories (Table 2), which were composed of carbohydrates, alcohols, organic acids, fatty acids, amino acids, and a miscellaneous group. Most of carbohydrates, including monosaccharides (glucose and mannose) and disaccharides (lactose and maltose) and their derivatives (galactopyranoside), apparently decreased after SSF. The total level of carbohydrates significantly decreased from 44.21 to 1.99% after fermentation. The decreases in carbohydrate levels indicated that they were used as energy and carbon sources for the growth of R. oligosporus RT-3 during SSF. Additionally, some carbohydrates might be converted into alcohols, and this phenomenon was further verified by the increased levels of maltol, glycerol, ethanol, and 1,2-propanediol. The level of mannitol in FS suffered a decrease after fermentation. Several fatty acids were identified in US and FS. The total level of fatty acids, including saturated and unsaturated medium-chain fatty acids, increased by 1.37 times that of US after SSF. Prominent increases in the contents of octadecanoic and 9,12-octadecadienoic acids were obtained after SSF. The level of 9,12-octadecadienoic acid in FS significantly increased by 14.85 times that of US. The levels of oleic and octanoic acids suffered decreases after SSF. Only 1 kind of free amino acids was detected in US. However, the species of amino acids increased from 1 to 5 in FS after fermentation. All levels of free amino acids except lysine in soybeans were significantly increased after SSF, indicating that R. oligosporus RT-3 fermentation significantly improved the release of amino acids in soybeans, which was in line with the trend of small peptides above. SSF with R. oligosporus RT-3 also significantly increased the total levels of organic acids in soybeans. The levels of short-chain fatty acids (SCFAs), including acetic, propanoic, and butanoic acids, exhibited apparent increases after SSF, which might be due to the biotransformation of dietary fiber in soybeans by R. oligosporus RT-3 (29). The level of gluconic acid evidently decreased after SSF with R. oligosporus RT-3. However, the level of benzoic acid significantly (p < 0.05) decreased, suggesting that it was degraded by R. oligosporus RT-3 during SSF (30). Furthermore, SSF with R. oligosporus RT-3 increased the level of urea and decreased the levels of stigmasterol and β-sitosterol.
Discussion
Soybeans and their products have been a crucial component of our daily diet due to their abundant nutritional value, such as high contents of proteins and unsaturated fatty acids (2, 3). Fermented soybean-based foods are popular worldwide because of their delicious taste and health-promoting effects. SSF with edible fungi is considered as an economically biological enzymatic hydrolysis process that is commonly used in the soybean industry. In the present study, a rich enzyme system composed of α-amylase, esterase, cellulase, protease, and β-glucosidase was secreted into soybeans by R. oligosporus RT-3 during SSF. These enzymes could hydrolyze various biological macromolecules, such as proteins, fats, and carbohydrates, into small-molecule nutrients with relatively high bioavailability and produce secondary metabolites with functional properties (5). This hydrolysis of microbial enzymes was also confirmed by the changes in soybean microstructure during SSF, wherein an increasingly loose spatial structure was observed by SEM.
Proteins occupy approximately 40% of the DW of soybeans, making soybeans as an ideal protein source for human. SSF with R. oligosporus RT-3 significantly affected their contents and compositions. During SSF, the macromolecular proteins in soybean were effectively hydrolyzed by the protease released from R. oligosporus RT-3. Soybean is listed as one of the “big eight” food allergens that can lead to allergic reaction in about 5–8% of children and 2% of adults (31). Glycinin (including acidic and basic subunits) and β-conglycinin (including α′, α, and β subunits), major allergenic proteins (9), were highly degraded in the present study. This phenomenon suggested that the immunoreactivity of soybeans might be reduced by SSF with R. oligosporus RT-3. The gradual increase in the content of small peptides indicated that most soybean proteins were degraded into small peptides and free amino acids during SSF, and this finding was further confirmed by the correlation analysis between protease activities and small peptide content. However, the HPLC analysis at 280 nm in this study could only determine the peptides containing aromatic amino acids, which limited the integrity of the composition analysis of small peptides. In the next step, we will use other methods to further analyze the information of small peptides. Reports have proved that the small peptides generated by in vitro enzymatic hydrolysis and fermentation are beneficially associated with a multitude of metabolic activities, such as reducing blood pressure and cholesterol, inhibiting of the bile acid reabsorption in the intestinal tract and platelet aggregation as well as anticancer, antioxidative, antimicrobial, and immunoregulatory activities (1, 32). In addition, the content of free essential amino acids, which cannot be synthesized in human body and served as important component in cell, significantly increased after fermentation (Table 2). The increased contents of small peptides and essential amino acids induced by SSF with R. oligosporus RT-3 might improve the prebiotic potential of soybeans. Therefore, SSF with R. oligosporus RT-3 can be a feasible strategy to increase the bioavailability of soybean proteins and health-promoting potential.
Phenolic compounds have been paid considerable attention because of their health-promoting effects, which predominantly exist in insoluble forms, and are covalently bound to the structural components of cell wall, such as cellulose, hemicellulose, lignin, pectin, and proteins (33). The absorption of bound phenolics in the gastrointestinal tract is dependent on the release of sugar moiety. In this work, we found that SSF with R. oligosporus RT-3 effectively improved the release of phenolic compounds in soybeans by increasing the content of SPs. As reported by Bei et al. (13), SSF with M. anka evidently enhanced the phenolic content of oat powder especially the ferulic and vanillic acids. Razak et al. (34) also found that SSF with R. oligosporus and M. purpureus apparently improved the release of phenolic compounds, such as ferulic, sinapic, vanillic, caffeic, syringic, and 4-hydroxybenzoic acids, in rice bran. Interestingly, the IPC increased before 36 h and then decreased, which was possibly because the strong covalent bonds formed by soybean components, like protein and cellulose, with insoluble phenolic compounds were weakened by the hydrolytic enzymes derived from R. oligosporus RT-3 during the early stage of SSF, making their extraction easier than those in the control, and later liberated or degraded (35). Additionally, isoflavones, including glycoside (daidzin, genistin, and glycitin) and aglycone (daidzein, genistein, and glycitein) forms, are the major phenolic compounds found in soybeans. Isoflavones predominantly occur as glycoside in US. Reports showed that unfermented soybean foods commonly have a higher level of glycoside isoflavones than aglycones. The former is considered to exhibit a lower bioactivity than the latter because the aglycone form is more easily absorbed by the gastrointestinal tract. Thus, the potential bioactivities of isoflavones are suppressed by their conjugated glycosides (36). However, during SSF with R. oligosporus RT-3, glycoside isoflavones were effectively biotransformed into their corresponding aglycones. As reported by Zheng et al. (37), enzymes, such as cellulase and amylase, secreted by microorganisms during fermentation are demonstrated to destroy the cell walls of cereals and transform the IPs into free form, thus improving the release of phenolic compounds. The PPC between SPC and enzyme activities during SSF indicated that the enzymes secreted by R. oligosporus RT-3 were deeply involved in the liberation of phenolic compounds. The carboxylic groups in the phenolic compound structure can link with biomacromolecules, such as sugar and proteins, by ester bonds (33), which can be broken by carbohydrate-hydrolyzing enzymes, and esterase released by R. oligosporus RT-3 during SSF. Furthermore, the destruction of the original structure of soybeans by enzymatic hydrolysis made the extraction of phenolic compounds easy. Therefore, the increase in the phenolic content of soybeans was predominantly due to the rich enzyme system secreted by R. oligosporus RT-3. Reports have shown that soybean polyphenols especially isoflavones are important phenolic sources in our daily diet, which are beneficial to human health by preventing several diseases, including cerebral ischemia, cancer, and hyperlipidemia and regarding chronic diseases, such as myocardial infarction, insulin resistance, stroke, and systemic inflammation (1, 38). Hence, the increased content of available phenolics induced by SSF could further improve the prebiotic potential of soybeans. The most outstanding bioactivity of phenolic compounds is the antioxidant activity for the aromatic phenolic ring in their structure, which displays free radical-scavenging capacity (25). Different antioxidant compounds in foods may work against oxides through different mechanisms (39). Hence, four assays, including DPPH radical scavenging activity, ABTS radical cation scavenging activity, reducing power, and ferric reducing antioxidant power were used to evaluate the antioxidant activities of soybeans in the present study. The antioxidant activities of soybeans significantly increased during SSF and high correlations were obtained between antioxidant activities and phenolic contents, indicating that the antioxidant activity of soybeans was predominantly derived from their high content of phenolic compounds. Previous studies have also reported that SSF with filamentous fungi evidently improves the phenolic content and the antioxidant ability of scavenging free radical (6, 8, 34). Additionally, the phenolic extracts were not purified in this study. Hence, other compounds like antioxidant peptides might also contribute to the antioxidant capacity of soybeans as previous report (40). Free radical can oxidize proteins, lipids, and DNA; affect mitochondrial function; activate caspase-3; destroy mitochondrial function; and promote apoptosis, consequently causing several diseases such as neurodegenerative disease (23). The fermented soybean with higher free radical scavenging activity could effectively attenuate oxidative stress in our body, thus exhibiting health-promoting potential.
Although many SSF-based studies are conducted on soybeans and their products, a gap still exists in understanding the biochemical pathways involved in SSF. The metabolomic analysis of US and FS were further performed using GC-MS. Figure 4 exhibited the links within these pathways, which were possibly triggered during the SSF of soybeans. Long-chain polymeric carbohydrates were degraded into small-molecule sugars by enzymes, including α-amylase, β-glucosidase, endoglucanase, and exoglucanase, derived from R. oligosporus RT-3 and finally entered the glycolysis pathway and tricarboxylic acid cycle (TCA) or biotransformed into sugar alcohols to provide energy and carbon source for the growth of R. oligosporus RT-3 during SSF. The increase in ethanol could apparently improve the aroma of soybeans. Also, the dietary fiber conversion by R. oligosporus RT-3 resulted in the synthesis of three major SCFAs, acetic, propionic, and butyric acids. As reported by Koh et al. (41), acetic acid may be derived from pyruvate via acetyl-CoA or via the Wood-Ljungdahl pathway. Propionic acid can be produced from phosphoenolpyruvate via the succinate or the acrylate pathway, wherein lactic acid is converted into propionic acid. Butyrate can be formed from two molecules of acetyl-CoA followed by the synthesis of acetoacetyl-CoA, β-hydroxybutyryl-CoA, crotonyl-CoA, butyryl-CoA, and butyryl-phosphate. SSF with R. oligosporus RT-3 significantly increased the contents of SCFAs, including acetic, propionic, and butyric acids. As reported by Mirzaei et al. (42), SCFAs are generated as waste product for microorganisms and are necessary for balancing redox state in anaerobic conditions. However, SCFAs display health-promoting effects on host cellular activities, including differentiation, immune modulation, inflammation, and energy metabolism. Hence, the significant increase in the contents of SCFAs after SSF could improve the bioactive potential of soybeans. Long-chain lipids were hydrolyzed into saturated and unsaturated medium-chain fatty acids by the lipases derived from R. oligosporus RT-3. According to a consistency of bulk experimental data and epidemiologic studies, the dietary consumption of essential polyunsaturated fatty acids can decrease the risk for urolithiasis, chronic inflammation, and urinary tract tumor (43). Some free fatty acids can serve as flavors or flavor precursors to enhance the quality of soybeans (44). Proteins were hydrolyzed into their constituent small peptides and amino acids by proteases derived from R. oligosporus RT-3, which could assist the absorption of amino acids in the gut and attenuate bitterness by the hydrolysis of soy proteins from the C- or N-terminus. Also, some amino acids might be synthesized by glycolysis or transamination, degraded via a range of catalyzing reactions, and entered the TCA or urea cycle. Phytic acid, one of the major antinutritional factors in soybean-based foods, is originally synthesized to store phosphorus in plant seeds. Metal cations, such as Mg2+, Ca2+, Zn2+, and Fe2+, can be attracted and chelated by the negative charge produced by phytic acid, thereby forming insoluble phytates, which make it hard for monogastric animals to absorb divalent cations (45). In the present study, a high level of phytase activity released from R. oligosporus RT-3 was observed during SSF, which indicated that phytic acid in soybeans might be hydrolyzed during SSF (Supplementary Figure 6). As described above, macromolecular components in soybean were effectively degraded into small molecular nutrients that are easily absorbed, and some active substances, such as essential amino acids, small peptides, phenolic compounds, and SCFAs, were released or produced during SSF with R. oligosporus RT-3, indicating that SSF with R. oligosporus RT-3 significantly improved the nutrient compositions and bioactive potentials of soybeans.
Conclusion
This work revealed that R. oligosporus RT-3 secreted a rich enzyme system, such as protease, esterase and carbohydrate hydrolase, into soybeans during SSF. The biomacromolecules, such as proteins, fats and carbohydrates in soybeans, were hydrolyzed into small-molecule nutrients, such as small peptides, free amino acids, medium-chain fatty acids, and simple sugars, which were easier to be digested in human gastrointestinal tract. IPs were effectively transformed into SPs by SSF with R. oligosporus RT-3, thus increasing the antioxidant activities of soybeans. Soy isoflavones were also transformed into glycosides after SSF. The dietary fiber conversion by R. oligosporus RT-3 resulted in the synthesis of three major SCFAs, acetic, propionic, and butyric acids. The potential metabolic pathways being triggered during SSF demonstrated that the improved nutrient profile and prebiotic potential of soybeans attributed to the biochemical reactions catalyzed by microbial enzymes. Our findings provided a strong support for the add value of SSF on soybeans.
Data availability statement
The raw data supporting the conclusions of this article will be made available by the authors, without undue reservation.
Author contributions
RW and BL: resources, conceptualization, data curation, funding acquisition, and writing-review and editing. YZ: investigation, methodology, formal analysis, writing-original draft, and visualization. RCW, FA, LJ, HW, TH, and XL: methodology and writing-review and editing. All authors contributed to the article and approved the submitted version.
Funding
This work was supported by the National key R&D projects of China (Grant No. 2021YFD1600100), the National Natural Science Foundation of China (Grant Nos. 32072179 and 31772091), and the Postdoctoral Science Foundation of Jiangsu Province (Grant No. 2021K484C).
Acknowledgments
We thank Ning Wang and Wei Han in Central Laboratory in Jiangsu Academy of Agricultural Sciences for their help in this work.
Conflict of interest
The authors declare that the research was conducted in the absence of any commercial or financial relationships that could be construed as a potential conflict of interest.
Publisher’s note
All claims expressed in this article are solely those of the authors and do not necessarily represent those of their affiliated organizations, or those of the publisher, the editors and the reviewers. Any product that may be evaluated in this article, or claim that may be made by its manufacturer, is not guaranteed or endorsed by the publisher.
Supplementary material
The Supplementary Material for this article can be found online at: https://www.frontiersin.org/articles/10.3389/fnut.2022.972860/full#supplementary-material
Abbreviations
ABTS, 2,2-azinobis(3-ethylbenzothiazoline-6-sulfonic acid) diammonium salt; BCA, bicinchoninic acid; DNS, 3,5-dinitrosalicylic acid; DPPH, 2,2-diphenyl-1-picrylhydrazyl; DW, dry weight; FS, fermented soybean; GAE, gallic acid equivalent; GC-MS, gas chromatography-mass spectrometry; HPLC, high-performance liquid chromatography; IPs, insoluble phenolics; IPC, insoluble phenolic content; MSTFA, N-methyl -N-(trimethylsilyl)-trifluoroacetamide; PCC, Pearson correlation coefficient; PCA, principal component analysis; SCFAs, short-chain fatty acids; SDS-PAGE, sodium dodecyl sulfate-polyacrylamide gel electrophoresis; SEM, scanning electron microscope; SPs, soluble phenolics; SPC, soluble phenolic content; SSF, solid-state fermentation; TCA, tricarboxylic acid cycle; TMCS, trimethylchlorosilane; TPTZ, 2,4,6-tris(2-pyridyl)-s-triazine; US, unfermented soybean; Vc, ascorbic acid.
References
1. Cai JS, Feng JY, Ni ZJ, Ma RH, Thakur K, Wang S, et al. An update on the nutritional, functional, sensory characteristics of soy products, and applications of new processing strategies. Trends Food Sci Technol. (2021) 112:676–89. doi: 10.1016/j.tifs.2021.04.039
2. Chen XF, Lu YL, Zhao AQ, Wu YM, Zhang YY, Yang XB. Quantitative analyses for several nutrients and volatile components during fermentation of soybean by Bacillus subtilis natto. Food Chem. (2021) 2021:131725. doi: 10.1016/j.foodchem.2021.131725
3. Yan S, Dong D. Main parameters and the dynamics of volatile compounds during the fermentation of Chinese Mao-tofu from Huangshan region. Food Sci Biotechnol. (2019) 28:1315–25. doi: 10.1007/s10068-019-00581-0
4. Sartang MM, Mazloomi SM, Tanideh N, Zadeh AR. The effects of probiotic soymilk fortified with omega-3 on blood glucose, lipid profile, haematological and oxidative stress, and inflammatory parameters in streptozotocin nicotinamide-induced diabetic rats. J Diabetes Res. (2015) 2015:1–9. doi: 10.1155/2015/696372
5. Soccol CR, da Costa ESF, Letti LAJ, Karp SG, Woiciechowski AL, de Souza Vandenberghe LP. Recent developments and innovations in solid state fermentation. Biotechnol Res Innov. (2017) 1:52–71. doi: 10.1016/j.biori.2017.01.002
6. Bei Q, Liu Y, Wang L, Chen G, Wu Z. Improving free, conjugated, and bound phenolic fractions in fermented oats (Avena sativa L.) with Monascus anka and their antioxidant activity. J Funct Foods. (2017) 32:185–94. doi: 10.1016/j.jff.2017.02.028
7. Terefe ZK, Omwamba MN, Nduko JM. Effect of solid state fermentation on proximate composition, antinutritional factors and in vitro protein digestibility of maize flour. Food Sci Nutr. (2021) 9:6343–52. doi: 10.1002/fsn3.2599
8. Handa C, Lima F, Guelfi M, Fernandes M, Georgetti S, Ida E. Parameters of the fermentation of soybean flour by Monascus purpureus or Aspergillus oryzae on the production of bioactive compounds and antioxidant activity. Food Chem. (2019) 271:274–83. doi: 10.1016/j.foodchem.2018.07.188
9. Huang L, Wang C, Zhang Y, Chen X, Huang Z, Xing G, et al. Degradation of anti-nutritional factors and reduction of immunoreactivity of tempeh by co-fermentation with Rhizopus oligosporus RT-3 and Actinomucor elegans DCY-1. Int J Food Sci Technol. (2019) 54:1836–48. doi: 10.1111/ijfs.14085
10. Huang L, Huang Z, Zhang Y, Zhou S, Dong M. Impact of tempeh flour on the rheology of wheat flour dough and bread staling. LWT Food Sci Technol. (2019) 111:694–702. doi: 10.1016/j.lwt.2019.04.004
11. Xiao Y, Fan J, Chen Y, Rui X, Zhang Q, Dong M. Enhanced total phenolic and isoflavone aglycone content, antioxidant activity and DNA damage protection of soybeans processed by solid state fermentation with Rhizopus oligosporus RT-3. RSC Adv. (2016) 6:29741–56. doi: 10.1039/c6ra00074f
12. Jing H, Dong M. Isolation, screening and identification of a thermo-tolerable tempe-producing strain RT-3. J Nanjing Agric Univ. (1992) 15:97–101.
13. Bei Q, Chen G, Lu F, Wu S, Wu Z. Enzymatic action mechanism of phenolic mobilization in oats (Avena sativa L.) during solid-state fermentation with Monascus anka. Food Chem. (2018) 245:297–304. doi: 10.1016/j.foodchem.2017.10.086
14. Elkhalifa AEO, Bernhardt R. Influence of grain germination on functional properties of sorghum flour. Food Chem. (2010) 121:387–92. doi: 10.1016/j.foodchem.2009.12.041
15. Hamza M, Khoufi S, Sayadi S. Fungal enzymes as a powerful tool to release antioxidants from olive mill wastewater. Food Chem. (2012) 131:1430–6. doi: 10.1016/j.foodchem.2011.10.019
16. Mackness MI, Walker CH, Rowlands DG, Price NR. Esterase activity in homogenates of three strains of the rust red flour beetle Tribolium castaneum (Herbst). Comp Biochem Physiol. (1983) 74:65–8. doi: 10.1016/0742-8413(83)90150-0
17. Ang SK, Shaza EM, Adibah Y, Suraini AA, Madihah MS. Production of cellulases and xylanase by Aspergillus fumigatus SK1 using untreated oil palm trunk through solid state fermentation. Process Biochem. (2013) 48:1293–302. doi: 10.1016/j.procbio.2013.06.019
18. Acuña JJ, Jorquera MA, Martínez OA, Menezes-Blackburn D, Ferńandez MT, Marschner P, et al. Indole acetic acid and phytase activity produced by rhizosphere bacilli as affected by pH and metals. J Soil Sci Plant Nutr. (2011) 11:1–12. doi: 10.4067/S0718-95162011000300001
19. Muhialdin BJ, Hassan Z, Bakar FA, Saari N. Identification of antifungal peptides produced by Lactobacillus plantarum IS10 grown in the MRS broth. Food Control. (2016) 59:27–30. doi: 10.1016/j.foodcont.2015.05.022
20. Rui X, Wen D, Li W, Chen X, Jiang M, Dong M. Enrichment of ACE inhibitory peptides in navy bean (Phaseolus vulgaris) using lactic acid bacteria. Food Funct. (2015) 6:622–9. doi: 10.1039/C4FO00730A
21. Zhang MW, Zhang RF, Zhang FX, Liu RH. Phenolic profiles and antioxidant activity of black rice bran of different commercially available varieties. J Agric Food Chem. (2010) 58:7580–7. doi: 10.1021/jf1007665
22. Verma B, Hucl P, Chibbar RN. Phenolic acid composition and antioxidant capacity of acid and alkali hydrolysed wheat bran fractions. Food Chem. (2009) 116:947–54. doi: 10.1016/j.foodchem.2009.03.060
23. Yin L, Zhang Y, Wang L, Wu H, Azi F, Tekliye M, et al. Neuroprotective potency of a soy whey fermented by Cordyceps militaris SN-18 against hydrogen peroxide-induced oxidative injury in PC12 cells. Eur J Nutr. (2021) 61:779–92. doi: 10.1007/s00394-021-02679-w
24. Yin L, Zhang Y, Wu H, Wang Z, Dai Y, Zhou J, et al. Improvement of the phenolic content, antioxidant activity, and nutritional quality of tofu fermented with Actinomucor elegans. LWT-Food Sci Technol. (2020) 133:110087. doi: 10.1016/j.lwt.2020.110087
25. Chen Y, Wang Y, Chen J, Tang H, Wang C, Li Z, et al. Bioprocessing of soybeans (Glycine max L.) by solid-state fermentation with Eurotium cristatum YL-1 improves total phenolic content, isoflavone aglycones, and antioxidant activity. RSC Adv. (2020) 10:16928–41. doi: 10.1039/C9RA10344A
26. Xiao Y, Wu X, Yao X, Chen Y, Wang Y. Metabolite profiling, antioxidant and α-glucosidase inhibitory activities of buckwheat processed by solid-state fermentation with Eurotium cristatum YL-1. Food Res Int. (2021) 143:110262. doi: 10.1016/j.foodres.2021.110262
27. Reynal SM, Ipharraguerre IR, Liñeiro M, Brito AF, Broderick GA, Clark JH. Omasal flow of soluble proteins, peptides, and free amino acids in dairy cows fed diets supplemented with proteins of varying ruminal degradabilities. J Dairy Sci. (2007) 90:1887–903. doi: 10.3168/jds.2006-158
28. Adom KK, Liu RH. Antioxidant activity of grains. J Agric Food Chem. (2002) 50:6182–7. doi: 10.1021/jf0205099
29. Liu Z, Li L, Ma S, Ye J, Zhang H, Li Y, et al. High-dietary fiber intake alleviates antenatal obesity-induced postpartum depression: roles of gut microbiota and microbial metabolite short-chain fatty acid involved. J Agric Food Chem. (2020) 68:13697–710. doi: 10.1021/acs.jafc.0c04290
30. Wang JY, Zhou L, Chen B, Sun S, Zhang W, Li M, et al. A functional 4-hydroxybenzoate degradation pathway in the phytopathogen Xanthomonas campestris is required for full pathogenicity. Sci Rep. (2015) 5:18456. doi: 10.1038/srep18456
31. Meinlschmidt P, Schweiggert-Weisz U, Brode V, Eisner P. Enzyme assisted degradation of potential soy protein allergens with special emphasis on the technofunctionality and the avoidance of a bitter taste formation. LWT Food Sci Technol. (2016) 68:707–16. doi: 10.1016/j.lwt.2016.01.023
32. Kim IS, Yang WS, Kim CH. Beneficial effects of soybean-derived bioactive peptides. Int J Mol Sci. (2021) 22:8570. doi: 10.3390/ijms22168570
33. Alara OR, Abdurahman NH, Ukaegbu CI. Extraction of phenolic compounds: a review. Curr Res Food Sci. (2021) 4:200–14. doi: 10.1016/j.crfs.2021.03.011
34. Razak DLA, Rashid NYA, Jamaluddin A, Sharifudin SA, Long K. Enhancement of phenolic acid content and antioxidant activity of rice bran fermented with Rhizopus oligosporus and Monascus purpureus. Biocatal Agric Biotechnol. (2015) 4:33–8. doi: 10.1016/j.bcab.2014.11.003
35. Jakobek L. Interactions of polyphenols with carbohydrates, lipids and proteins. Food Chem. (2015) 175:556–67. doi: 10.1016/j.foodchem.2014.12.013
36. Hwang CE, Kim SC, Lee HY, Suh HK, Cho KM, Lee JH. Enhancement of isoflavone aglycone, amino acid, and CLA contents in fermented soybean yogurts using different strains: screening of antioxidant and digestive enzyme inhibition properties. Food Chem. (2021) 340:128199. doi: 10.1016/j.foodchem.2020.128199
37. Zheng HZ, Hwang IW, Chung SK. Enhancing polyphenol extraction from unripe apples by carbohydrate-hydrolyzing enzymes. J Zhejiang Univ Sci B. (2009) 10:912. doi: 10.1631/jzus.B0920186
38. Fraga CG, Croft KD, Kennedy DO, Tomás-Barberán FA. The effects of polyphenols and other bioactives on human health. Food and Funct. (2019) 10:514–28. doi: 10.1039/c8fo01997e
39. Shahidi F, Zhong Y. Measurement of antioxidant activity. J Funct Foods. (2015) 18:757–81. doi: 10.1016/j.jff.2015.01.047
40. Sanjukta S, Padhi S, Sarkar P, Singh SP, Rai AK. Production, characterization and molecular docking of antioxidant peptides from peptidome of kinema fermented with proteolytic Bacillus spp. Food Res Int. (2021) 141:110161. doi: 10.1016/j.foodres.2021.110161
41. Koh A, De Vadder F, Kovatcheva-Datchary P, Bäckhed F. From dietary fiber to host physiology: short-chain fatty acids as key bacterial metabolites. Cell. (2016) 165:1332–45. doi: 10.1016/j.cell.2016.05.041
42. Mirzaei R, Dehkhodaie E, Bouzari B, Rahimi M, Gholestani A, Hosseini-Fard SR, et al. Dual role of microbiota-derived short-chain fatty acids on host and pathogen. Biomed Pharmacother. (2021) 26:112352. doi: 10.1016/j.biopha.2021.112352
43. Eynard AR, Navarro A. Crosstalk among dietary polyunsaturated fatty acids, urolithiasis, chronic inflammation, and urinary tract tumor risk. Nutrition. (2013) 29:930–8. doi: 10.1016/j.nut.2012.12.014
44. Esteban-Torres M, Mancheño JM, de las Rivas B, Muñoz R. Characterization of a halotolerant lipase from the lactic acid bacteria Lactobacillus plantarum useful in food fermentations. LWT Food Sci Technol. (2015) 60:246–52. doi: 10.1016/j.lwt.2014.05.063
Keywords: nutraceutical potential, enzyme, antioxidant activity, small peptide, soluble phenolic, metabolic pathway
Citation: Zhang Y, Wei R, Azi F, Jiao L, Wang H, He T, Liu X, Wang R and Lu B (2022) Solid-state fermentation with Rhizopus oligosporus RT-3 enhanced the nutritional properties of soybeans. Front. Nutr. 9:972860. doi: 10.3389/fnut.2022.972860
Received: 19 June 2022; Accepted: 13 July 2022;
Published: 08 September 2022.
Edited by:
Phisit Seesuriyachan, Chiang Mai University, ThailandReviewed by:
Yu Xiao, Hunan Agricultural University, ChinaUtoomporn Surayot, Chiang Mai University, Thailand
Nattakorn Kuncharoen, Kasetsart University, Thailand
Copyright © 2022 Zhang, Wei, Azi, Jiao, Wang, He, Liu, Wang and Lu. This is an open-access article distributed under the terms of the Creative Commons Attribution License (CC BY). The use, distribution or reproduction in other forums is permitted, provided the original author(s) and the copyright owner(s) are credited and that the original publication in this journal is cited, in accordance with accepted academic practice. No use, distribution or reproduction is permitted which does not comply with these terms.
*Correspondence: Ran Wang, cmFud2FuZ0BqYWFzLmFjLmNu; Baiyi Lu, YnlsdUB6anUuZWR1LmNu