- Laboratory of Nutrition and Development, Key Laboratory of Major Diseases in Children, Ministry of Education, Beijing Pediatric Research Institute, Beijing Children’s Hospital, Capital Medical University, National Center for Children’s Health, Beijing, China
Recent studies demonstrate that paternal nutrition prior to conception may determine offspring development and health through epigenetic modification. This study aims to investigate the effects of paternal supplementation of n-3 polyunsaturated fatty acids (n-3 PUFAs) on the brain development and function, and associated gene imprinting in the offspring. Three to four-week-old male C57BL/6J mice (founder) were fed with an n-3 PUFA-deficient diet (n-3 D), and two n-3 PUFA supplementation diets – a normal n-3 PUFA content diet (n-3 N) and a high n-3 PUFA content diet (n-3 H) for 12 weeks. Then they were mated to 10-week-old virgin female C57BL/6J mice to generate the offspring. The results showed that paternal n-3 PUFA supplementation in preconception reduced the anxiety- and depressive-like behavior, and improved sociability, learning and memory in the offspring, along with increased synaptic number, upregulated expressions of neuron specific enolase, myelin basic protein, glial fibrillary acidic protein, brain-derived neurotrophic factor in the hippocampus and cerebral cortex, and altered expressions of genes associated with mitochondria biogenesis, fusion, fission and autophagy. Furthermore, with paternal n-3 PUFA supplementation, the expression of imprinted gene Snrpn was downregulated both in testes of the founder mice and their offspring, but upregulated in the cerebral cortex and hippocampus, with altered DNA methylation in its differentially methylated region. The data suggest that higher paternal intake of n-3 PUFAs in preconception may help to maintain optimal brain development and function in the offspring, and further raise the possibility of paternal nutritional intervention for mental health issues in subsequent generations.
Introduction
N-3 polyunsaturated fatty acids (n-3 PUFAs) are paramount for human health, and their functional roles in cardiovascular system, brain development and function, immune response, allergy, and particular physiological states (e.g., pregnancy, prematurity, infancy), have been focused during the past decades (1, 2). N-3 PUFAs are components of cellular membranes and the precursors of several metabolites with different beneficial effects on cell membrane fluidity, neuronal growth and differentiation, intracellular signaling and gene expression, inflammation and oxidation (3, 4). However, the modern western diet has greatly reduced the intake of n-3 PUFAs with an increase of n-6 PUFAs, resulting in the ratio of n-6/n-3 PUFAs at 20–30:1 which is much higher than that at 1–2:1 in the Paleolithic diet (5, 6). Growing evidence suggests that this altered dietary n-6/n-3 PUFAs is closely associated with chronic non-communicable diseases, including cardiovascular diseases, diabetes, obesity, as well as neurodevelopmental diseases (e.g., autism, attention deficit and hyperactivity disorder, and schizophrenia) in children, and degenerative neurological diseases in the elderly, and that the ratio of n-6 to n-3 PUFAs in diets at 1–2:1 should be the target ratio for health (7, 8).
The fetal programming hypothesis and thereafter the development origins of health and disease hypothesis indicate that nutrition and other environmental factors in early life determine the offspring phenotype and health in later life (9, 10). Both animal and human studies have demonstrated that maternal dietary n-3 PUFA deficiency during pregnancy and lactation impairs learning and memory in adult offspring (11, 12), and a high level of seafood intake or supplementation of docosahexaenoic acid (DHA, C22:6n-3) during pregnancy and lactation can effectively improve the psychological, language learning and intellectual development levels in early childhood (13, 14), although some inconsistent findings exist owing to multiple factors, such as differences in body n-3 PUFA baseline, quantity and duration of supplementation, interference from harmful chemicals or substances in seafood, and/or micronutrient (iron, iodine, zinc, etc.) deficiency (15–20).
During the past decade, a substantial number of studies have strengthened the paternal origins of health and disease paradigm, which stresses the need for more research on the role of the father in the transmission of acquired environmental messages from his environment to his offspring (21, 22). Mammalian spermatozoa are rich in PUFAs, particularly DHA, which are important for spermatogenesis with higher sperm motility and concentration, and normal morphology (23, 24). The reduction of n-3 PUFA intake in modern population leads to obstacles to spermatogenesis and maturation, resulting in the decline of male fertility, which has become a global problem in reproduction (25, 26). However, there is lack of relevant research on whether paternal n-3 PUFA status in preconception impacts on offspring development and health, including brain development and function.
The mechanisms through which parental nutrition determines offspring health have been extensively investigated, but they are still not completely understood. The contribution of maternal n-3 PUFAs to offspring brain development has been considered to be associated with several pathways, including enhancement of prenatal and postnatal DHA accretion in offspring brain, and epigenetic and non-epigenetic regulation on the expression of genes associated with neuronal growth and differentiation, protection against neuroinflammation, oxidation and apoptosis, etc. (4, 27, 28). Additionally, maternal feeding of DHA exerts preventive effects on prenatal stress-induced brain dysfunction through modulating metabolism of mitochondria (29), which plays a decisive role in brain development by providing energy for cell proliferation and differentiation, and synaptogenesis (30, 31). Being different from the direct interaction between the mother and offspring by nutrient exchange during prenatal and postnatal periods, the sperm- and seminal plasma-specific mechanisms connect paternal nutrition with the offspring development and health, as well as the maternal health (32). Gene imprinting, one class of epigenetics, is particularly relevant to early life and transgenerational effects since imprints are established in the germline, maintained during the preimplantation reprogramming phase, and then passed on through the somatic cell lineages impacting on genome function and gene expression (33, 34). Imprints are particularly promising candidates in brain research as they are known to be important for neurogenesis, brain function and behavior (33–36).
Therefore, we hypothesized that paternal higher n-3 PUFA intake in preconception might produce positive influences on brain development and function in the offspring through altering mitochondria metabolism and associated gene imprinting. In this study, using a mouse model that was received feeding intervention, the impact of preconception n-3 PUFA status in the father on the brain function (anxiety-like behaviors, depression-like behaviors, and memory) and histological changes were determined in the offspring. Furthermore, changes in offspring brain mitochondria metabolism, and expressions of imprinted genes associated with brain development in the testis and brain were investigated.
Materials and methods
Diets
Three types of diets with n-3 PUFA deficiency (n-3 D), normal n-3 PUFA content (n-3 N), or high n-3 PUFA content (n-3 H) were designed and manufactured by modifying the oil type in the AIN-93G diet as our previously published (37). The lard oil and sunflower oil were added in the n-3 D diet to produce n-3 PUFA deficiency with an n-6/n-3 PUFA ratio at 47.2:1; whereas the flaxseed oil and fish oil mixed with the lard oil and sunflower oil were added to the n-3 N and n-3 H diets to yield two different n-6/n-3 PUFA ratios at 4.3:1 and 1.5:1, respectively, which represent the current recommendation (4–10:1) and the dietary ratio for our ancestors, containing both very long-chain n-3 PUFAs, eicosapentaenoic acid (EPA; C20:5n-3), DHA and their precursor α-linolenic acid (ALA, C18:3n-3) (5–7). The AIN-93G growing diet and AIN-93M mature diet were used for maternal feeding during pregnancy and lactation, and offspring pup’s feeding after weaning, respectively. Details for the diet formula and fatty acid compositions are shown in Table 1. All the diets were prepared by the Beijing Huafukang Bioscience Co. Inc. (Beijing, China) and were sterilized with γ-irradiation 25 kGy and stored at −20°C before use.
Animals
Three- to four-week-old male C57BL/6J mice were purchased from the Gempharmatech Co., Ltd (Nanjing, China) and were housed at the animal facilities with SPF-grade condition in the National Institute of Occupational Health and Poison Control, China CDC. Following one week of recovery from transportation, the mice were randomly classified into three groups (n = 12 in each group) and fed with one of the n-3 D, n-3 N and n-3 H diets, respectively. All the mice were free access to water and food under the condition of a 12-h light/12-h dark cycle and cycles of air ventilation. After 12 weeks of feeding intervention, the founder male mice were mated with 10-week-old virgin female mice (1 male for 2 females per cage) and fed the AIN-93G diet (H10293G), which lasted for the pregnancy and lactation of the mating female mice. A 12-week feeding intervention for the founder male mice was set up to ensure optimal models of n-3 PUFA deficiency and supplementation, owing to that 3–6 months are needed for tissue saturation of EPA and DHA concentrations with fish oil supplementation (38–40).
After weaning at 3 weeks of age, the offspring mice from the three groups were fed the AIN-93M diet which lasted for 6 weeks. At the end of experiments, examination of the brain function (anxiety-like behaviors, depression-like behaviors, and memory) was conducted in some offspring mice (n = 5 in each group for the same sex). To avoid bias due to behavior tests, the other offspring mice (n = 8 in each group for the same sex) in a fasted state were used for determination of gene expression and DNA methylation. Each offspring mouse selected in each paternal diet group was from separate litters to avoid being born from the same father. The mice were euthanized by intraperitoneal injection of an overdose of Avertin (2,2,2-tribromoethanol) (500 mg/kg) (T-4840-2, Sigma-Aldrich Chemie GmbH, Steinheim, Germany) for anesthesia followed by decapitation. The testis, cerebral cortex and hippocampus were immediately dissected free of surrounding tissue, removed and frozen in liquid N2 and then transferred to −80°C for gene expression analysis. Meanwhile, tissues of the cerebral cortex and hippocampus from mice for the brain function experiment were immediately fixed by immersion in 10% paraformaldehyde and 2.5% glutaraldehyde, respectively, for immunohistochemical determination and transmission electron microscopy analysis. After mating, the founder male mice were also euthanized and testes were collected, frozen in liquid N2 and then transferred to −80°C for later use.
All experiments complied with the ARRIVE guidelines as well as the Guide for the Care and Use of Laboratory Animals in China. All procedures were conducted in accordance with the Animals (Scientific Procedures) 1986 Act (UK) (amended 2013) and approved by the Ethic Committee of the National Institute of Occupational Health and Poison Control, China CDC (No. EAWE-2021-06).
Fatty acid analysis
Fatty acid analysis in diets and tissues was conducted by gas chromatography on Agilent 6890N GC equipped with a flame ionization detector (FID) and injector, using the method of fatty acid methyl esters (FAMEs). Diets and tissues of testis and brain were homogenized using a tissue disrupter in 0.9% sodium chloride solution. Preparation of FAMEs from tissue homogenates was performed according to a modified Lepage method based on our previously published (37). The quantity of each fatty acid was expressed as the percent (%) (wt/wt) of total fatty acids.
Assessment of sperm counting and vitality
During the process of tissue collection, the cauda epididymidis of founder male mice was dissected, punctured and incubated in the prepared HEPES buffer for sperm to swim out. The supernatant was removed, centrifugated (3,000g for 5 min), washed twice in buffer PBS (41). The sperm preparations were assayed for sperm count and vitality assessment using the hemocytometer under the microscope.
Behavioral experiments
Offspring mice aged 9 weeks were subjected to a series of behavioral determination. Five days before the experiment conduction, all mice were kept in the specific room to adapt to the testing environment. All tests were performed between 9 am and 5 pm. Three tests were used to determine anxiety- and depressive-like behavior. The open-field test (OFT) was conducted by placing mice in the open field (L × W × H; 50 cm × 50 cm × 30 cm) individually and allowed 5 min of free movement, and the time spent in the center area was recorded. Light/dark test (LDT) was performed according to the procedure described by Heredia (42). The rectangular box (L × W × H; 50 cm × 30 cm × 30 cm) comprised two compartments, painted black (dark compartment) and another white (light compartment), which separated by a polymeric methyl methacrylate with a centrally-positioned 7.5 × 7.5 cm opening at floor level. Mice were individually placed in the center of the dark compartment and allowed 5 min to explore the apparatus. The inter-compartmental transitions and time spent in the dark compartment were evaluated. Sucrose preference test (SPT) was performed as the described method (43). Before the test, the mice were housed in the cage with two bottles of sucrose water [2% (w/v)] to acclimate for 24 h. Then, one bottle of sucrose water was replaced by tap water for 24 h, alternating the positions of two bottles every 6 h to eliminate the possibility of side or position preference. The sucrose preference (SP) value was calculated as follows: SP (%) = sucrose intake (g)/[sucrose intake (g) + water intake (g)] × 100%.
The three-chamber test (TCT) was used for sociability assessment as described by Liu (44). Briefly, the mouse was first habituated to the empty box (L × W × H; 50 cm × 30 cm × 30 cm) with three equally sized, interconnected chambers (left, center, right) for 5 min. During the second 5-min, the tested mouse could interact either with an empty wire cup or the other wire cup contained a stranger. The time spent interacting (sniffing, crawling upon) with the two cups was recorded. Sociability index was calculated as follows: (time spent with stranger- time spent with empty cup)/(time spent with stranger + time spent with empty cup).
The novel object recognition (NOR) is an efficient method to test learning and memory in mice (45). The mouse was placed in the middle of the rectangular arena (L × W × H; 50 cm × 30 cm × 30 cm) and allowed to freely explore for 5 min and then removed out of the arena. Then two identical objects were placed in the central symmetrical positions of the arena. The mouse was again placed in the center of the arena, freely exploring the two objects for 5 min, the mouse was transported to the holding cage. One hour later, one of the training objects was replaced with a novel object, and the mouse was allowed to freely explore for 5 min. The discrimination index was expressed as the time spent exploring the novel object minus the time spent exploring the familiar object, divided by total exploration time, reflecting the preference for new objects.
Histological examination
Immunohistochemical analysis was performed on neuron specific enolase (NSE), myelin basic protein (MBP) and glial fibrillary acidic protein (GFAP), which are specific biomarkers for neuronal cell bodies, mature myelinated oligodendrocytes and astrocytes, respectively (46–48). Formaldehyde fixed brains were treated with 70% ethanol and xylene, embedded in paraffin, sectioned at 5 mm on the coronal plane, and air dried. At the level of anterior thalamus and hippocampus, samples were taken continuously through the cerebral hemisphere. The slices were dewaxed, rehydrated in ethanol, and then incubated in 0.01 mol/L sodium citrate (pH 6.0) at 98°C for 10 minutes. After cooling, the slices were washed with 0.3% PBS Triton, incubated with 3% hydrogen peroxide for 10 minutes at room temperature, and then washed with water. Sections were incubated overnight at 4°C against antibodies for NSE (1:500) (GB11376-1), GFAP (1:1200) (GB12096), or MBP (1:200) (GB11226). After the slides were washed in PBS, incubated with goat anti-rabbit IgG HRP, and finally stained with 3,3-diaminobenzidine (DAB) (DAB chromogenic kit, G1211). Slides incubated without the addition of primary antibody were used as negative control. All antibodies and reagents were purchased from Servicebio technology Co., Ltd. (Wuhan, China). Morphometric analysis was performed on Image Pro Plus 6.0 system (media cybernetics. USA) to measure the mean optical density (OD) of NSE positive neurons, GFAP positive astrocytes and MBP positive myelinated oligodendrocytes in the cerebral cortex and hippocampus.
Structure changes in mitochondria and synapses were determined by transmission electron microscopy according to description by Rybka (49). Briefly, fresh cerebral cortex and hippocampus were fixed in glutaraldehyde (2.5%). After washed by phosphate buffer (0.1 M, pH 7.4), the slices were postfixed in 1% osmium tetroxide for 2 h. Then, they were rinsed, dehydrated, saturated and embedded in mixtures of acetone and SPI-Pon 812 resin (SPI-Chem, USA). Ultrathin slices were sectioned and poststained with uranyl acetate and lead citrate in the avoidance of carbon dioxide, and then washed with ultrapure water and dried. Imaging was done with a HT7800/HT7700 transmission electron microscope (Hitachi, Tokyo, Japan).
Ribonucleic acid isolation and qRT-pCR
Total RNA in tissues was extracted using the RNAiso Plus (TaKaRa, Kusatsu, Japan) and complementary DNA was prepared from the total RNA using the All-in-One First-Strand cDNA Synthesis SuperMix for qPCR (OneStep gDNA Removal) (TransGen Biotech, Beijing, China) according to the procedures provided by the manufacturer. The mRNA expression of targeted genes was measured by real-time qPCR with a CFX96 Touch™ Real-Time PCR Detection System (Bio-Rad) using Top Green qPCR SuperMix (Trans Gen), with the thermocycle program consisting of an initial hot start cycle at 95°C for 30 s, followed by 40 cycles at 95°C for 5 s, 60°C for 15 s, and 72°C for 10 s. Based on their involvement in neural development, neuronal apoptosis, synaptic transmission, and neuropsychiartric disorders, a total of nine imprinted genes, Zac1, Ube3a, Peg1, Igf2 (Peg2), Peg3, Snrpn (Peg4), Ndn, Kcnk9 and RasGrf1 (34), and brain-derived neurotrophic factor (Bdnf), were included in the present study. The primer sequences can be found in Supplementary Table 1.
Analysis of mitochondria deoxyribonucleic acid copy number
Total DNA in the offspring brain was extracted with the Animal Tissue DNA Kit (catalogue no.3101250; Simegen Biotechnology Co., Ltd.). Mitochondria DNA (mtDNA) was amplified using primers specific for the mitochondrial cytochrome oxidase subunits I (CoxI) gene. Nuclear DNA was amplified using primers specific for the 18S rRNA gene. Primer sequences can be found in Supplementary Table 1. The RT-PCR was performed on individual DNAs by using CFX96 Touch™ Real-Time PCR Detection System (Bio-Rad). The relative number of mtDNA copies (mtDNA-CN) was calculated as the normalized ratio of CoxI/18S rRNA gene.
Deoxyribonucleic acid bisulphite conversion and sequencing
DNA methylation in differentially methylated region 1 (DMR1) of the Snrpn was determined by bisulphite sequencing. Briefly, bisulfite conversion of purified DNA of the testis and brain was treated with sodium bisulfite to convert the unmethylated cytosine into uracil using the EZ DNA Methylation Kit (catalogue no. D5002; Zymo Research). Converted DNA was amplified by nested PCR, and the PCR products were sequenced directly. The methylation fraction was calculated from the amplitude of cytosine and thymine within each CpG dinucleotide [C/(C + T)]. The assays were performed in triplicate. The primers used and annealing temperature are shown in Supplementary Table 1.
Statistical analysis
One-way analysis of variance (ANOVA) was used to compare means in different groups using SPSS 21.0, except for body weight analysis with repeated measures ANOVA. The Kolmogorov–Smirnov test was used to evaluate whether the data is normally distributed. Following ANOVA, a post hoc test was conducted using either Bonferroni test or Dunnett’s T3 test for data lacking homogeneity of variance. For data with the non-normal distribution Kruskal Wallis test was used. P < 0.05 was considered be statistically significant in differences.
Results
Effects of paternal n-3 PUFA supplementation on testis fatty acid composition and sperm vitality in founder
As shown in Table 2, in founder male mice, testis DHA and total n-3 PUFAs were increased with both the n-3 N and n-3 H diet feeding, compared to the n-3 D diet feeding. Consistently, the sperm vitality was significantly increased by the n-3 N diet (84.10 ± 3.78%) and the n-3 H diet (82.02 ± 2.78%), compared with the n-3 D diet (66.62 ± 4.93%). Also, the sperm count was increased by the n-3 N and n-3 H diet (4.76 × 106/L and 4.78 × 106/L sperm preparations), compared to the n-3 D diet (3.51 × 106/L sperm preparations).
Effects of paternal n-3 PUFA supplementation on weight and fatty acid composition of the brain in offspring
As shown in Figure 1, The paternal n-3 N or n-3 H diet increased the hippocampus weight in the offspring, compared to the paternal n-3 D diet, with no effects on the body weight and the whole brain weight either in males or females. The n-3 PUFA content in the offspring brain was shown no differences among the three groups (Table 2).
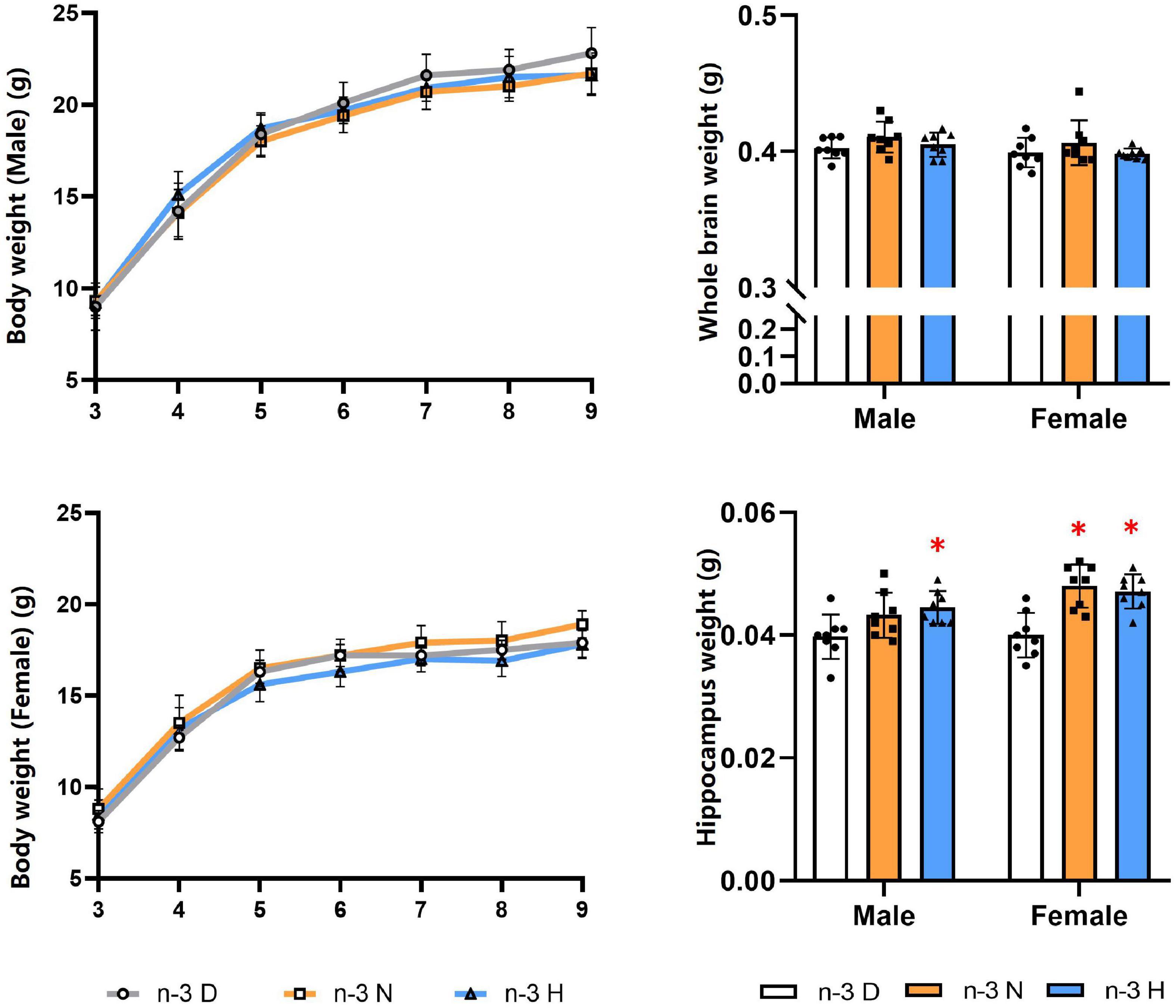
Figure 1. Effects of paternal n-3 PUFA supplementation on body and brain weight in offspring. Three to four-week-old male C57BL/6J mice were fed with a n-3 D, n-3 N and n-3 H diet for 12 weeks, and then mated to 10-week-old virgin female C57BL/6J mice to generate the offspring. Male and female offspring body weight were monitored weekly (n = 13 per diet intervention on each sex). After sacrificed at the end of experiment, brains were dissected and weighed (n = 8 per diet intervention on each sex). Values are means ± SD. *Compared to paternal n-3 D diet group within the same sex, P < 0.05; #Compared to paternal n-3 N diet group within the same sex, P < 0.05.
Effects of paternal n-3 PUFA supplementation on behavior and cognition in offspring
The behavioral and cognitive experiments showed that offspring mice from the paternal n-3 H diet group had more time spent in central area in the OFT than those from the paternal n-3 D diet group. Also, they had shorter time spent in the dark section but higher number of inter-compartmental transitions in the LDT. The SPT indicated that offspring mice from the paternal n-3 N or n-3 H diet group had increased SP value. Results from the TCT exhibited that the sociability index, reflecting the length of interaction with social partner, was increased in offspring mice from the paternal n-3 N or n-3 H diet group, compared with the paternal n-3 D diet group. The changes in tests of the OFT, LDT, TCT and SPT were similar between offspring males and females. Furthermore, the NOR discrimination index was enhanced by both the paternal n-3 N diet and n-3 H diet in males instead of females in the offspring (Figure 2).
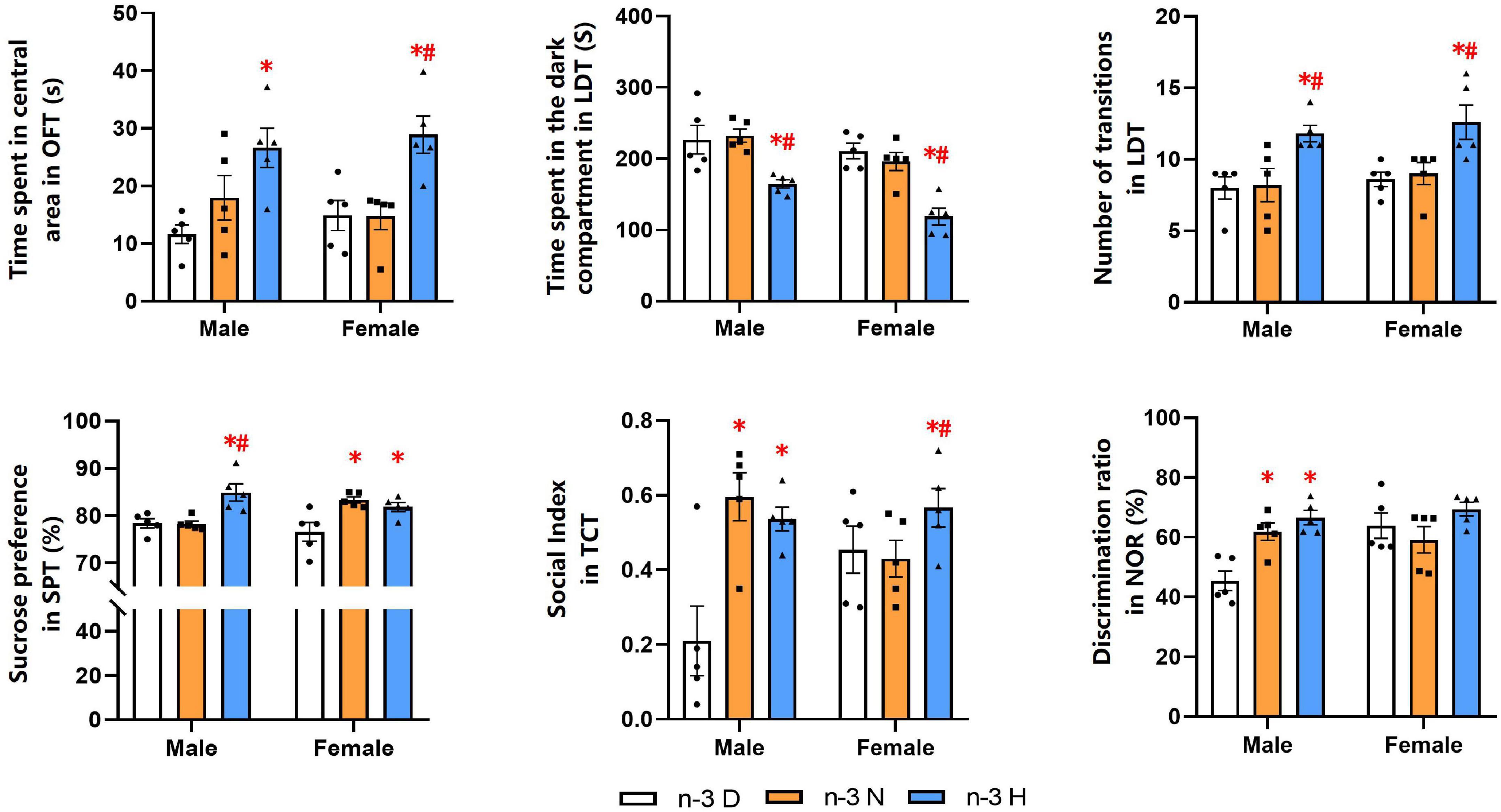
Figure 2. Paternal n-3 PUFA supplementation impacts behaviors and cognition in offspring. Offspring mice aged 9 weeks were subjected to a series of behavioral experiments. Time spent in central area in OFT was used to determine level of tension; Time spent in the dark compartment and the number transitions in LDT indicated anxiety behavior; Sucrose preference index in SPT was used to assess the core symptoms of depression (anhedonia); Social index in TCT represented sociability. Discrimination ratio in NOR was used to test learning and memory. Values are means ± SEM, n = 5 per diet intervention on each sex. *Compared to paternal n-3 D diet group within the same sex, P < 0.05; #Compared to paternal n-3 N diet group within the same sex, P < 0.05.
Effects of paternal n-3 PUFA supplementation on brain histology in offspring
Immunohistochemical analyses on brain NSE, GFAP and MBP in offspring were demonstrated in Figure 3. Compared to the paternal n-3 D diet group, males from the paternal n-3 H diet group had an increase in the average optical density (OD) on area of NSE-positive neurons in the cerebral cortex and hippocampus, GFAP-positive astrocytes in the hippocampus, and those from both the paternal n-3 N diet and n-3 H diet groups had more MBP-positive myelinated oligodendrocytes in the corpus callosum and hippocampus; whereas females had similar changes only in the OD on area of NSE positive neurons in the cerebral cortex, and MBP-positive myelinated oligodendrocytes in the corpus callosum and hippocampus.
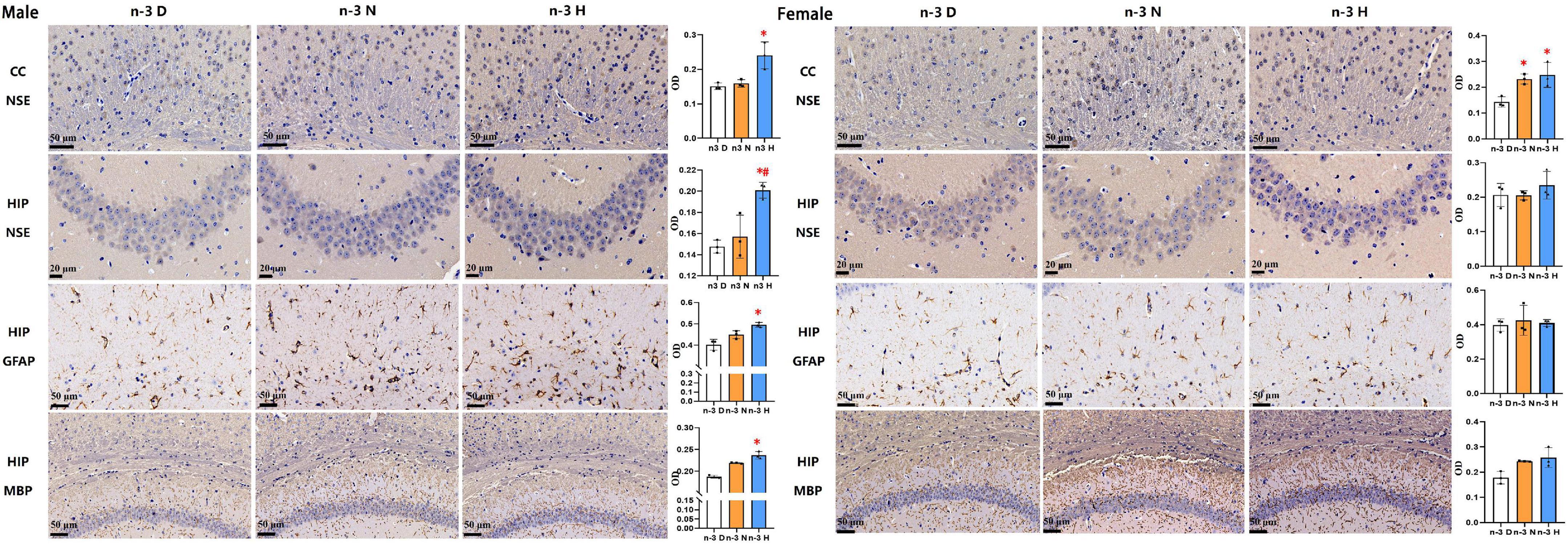
Figure 3. Immunohistochemical analysis in the offspring brains. The paraffin sections of the offspring cerebral cortex (CC) and hippocampus (HIP) were deparaffinized and hydrated, and specifically bound with antibodies for neuron specific enolase (NSE), myelin basic protein (MBP), glial fibrillary acidic protein (GFAP). 3,3-diaminobenzidine (DAB) staining was used to observe changes in neurons in the hippocampus (CA3 region) and cerebral cortex (cingulate), astrocytes in the hippocampus (CA1 region), and myelinated oligodendrocytes in the corpus callosum and hippocampus (CA1 region). Values are means ± SEM; n = 3 per diet intervention on each sex. *Compared to paternal n-3 D diet group, P < 0.05; #Compared to paternal n-3 N diet group, P < 0.05.
The transmission electron microscopy demonstrated that the number of synapses was significantly increased in the hippocampus but not cerebral cortex in males from both the paternal n-3 N diet and n-3 H diet groups, compared with the paternal n-3 D diet group. In females, the synaptic number was increased in the cerebral cortex but not hippocampus in both the paternal n-3 N diet and n-3 H diet groups. No differences were observed in the ultrastructure of mitochondria in offspring hippocampus and cerebral cortex between groups (Figure 4).
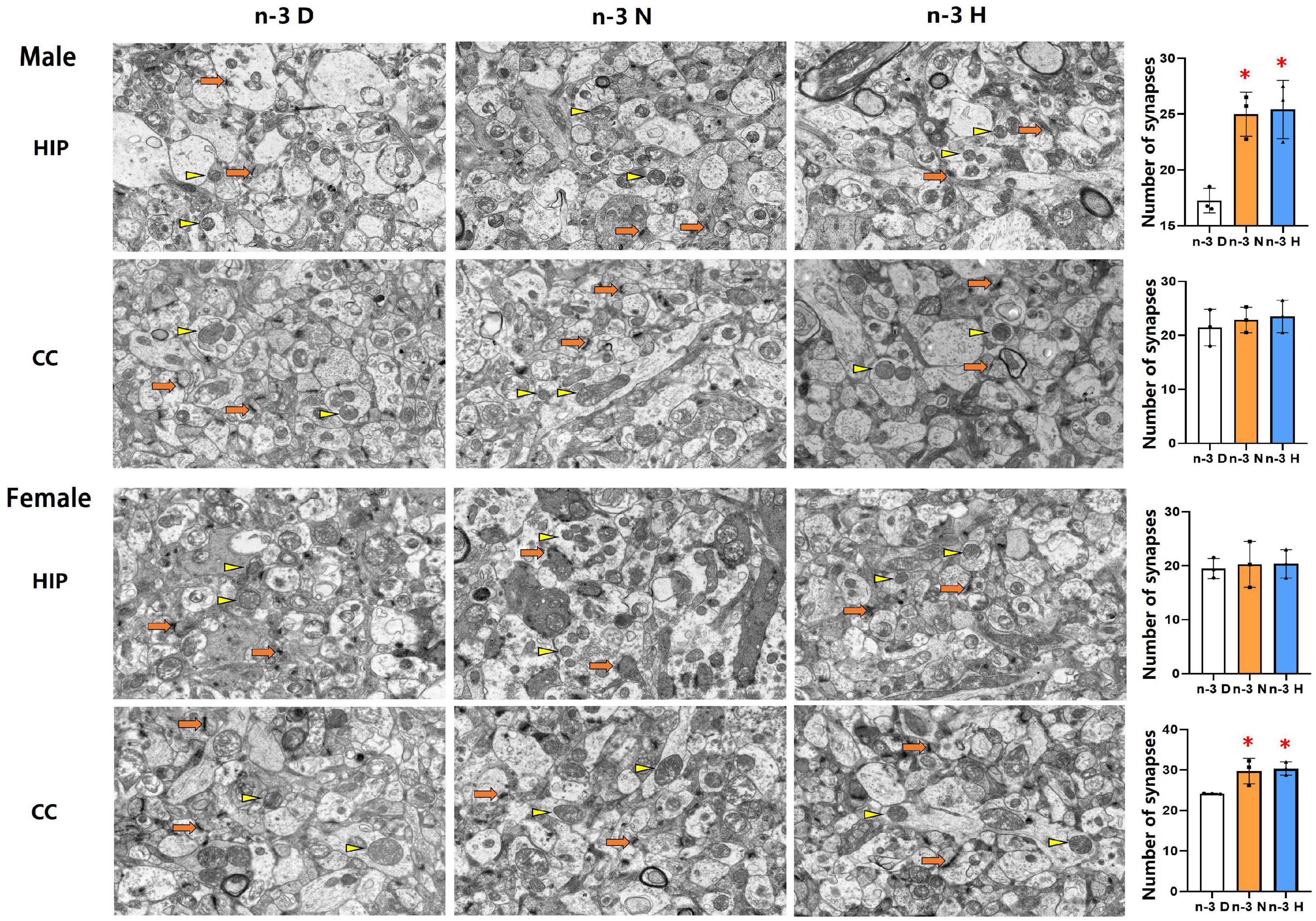
Figure 4. Ultrastructure changes in synapses and mitochondria in offspring cerebral cortex and hippocampus. Electron microscope photographs of the cerebral cortex (CC) and hippocampus (HIP) from offspring mice were taken at magnification (×5,000). Numbers of synapses were calculated based on electron microscope captured image. Arrows and triangles indicated synapses and mitochondria, respectively. Values are means ± SEM; n = 3 per diet intervention on each sex. *Compared to paternal n-3 D diet group, P < 0.05; #Compared to paternal n-3 N diet group, P < 0.05.
Effects of paternal n-3 PUFA supplementation on the expression of genes associated with brain function and mitochondria in offspring
As illustrated in Figure 5, the hippocampus mRNA expression of Gfap, Mbp, Nse, and Bdnf in the male offspring was upregulated by paternal n-3 N diet or n-3 H diet, but only the Mbp expression in female offspring was upregulated by paternal n-3 H diet. Examination on mitochondria showed similar changes between the male and the female offspring, in upregulated expression of genes associated with mitochondria biogenesis (Pgc-1α, CoxI), fusion and fission (Opa1, Drp1), and downregulated expression of genes related to mitochondria autophagy (Pink1) in the hippocampus or cerebral cortex by paternal n-3 N diet or n-3 H diet. Consistently, mtDNA-CN in the hippocampus and cerebral cortex was increased by paternal n-3 N diet or n-3 H diet both in the male and female offspring.
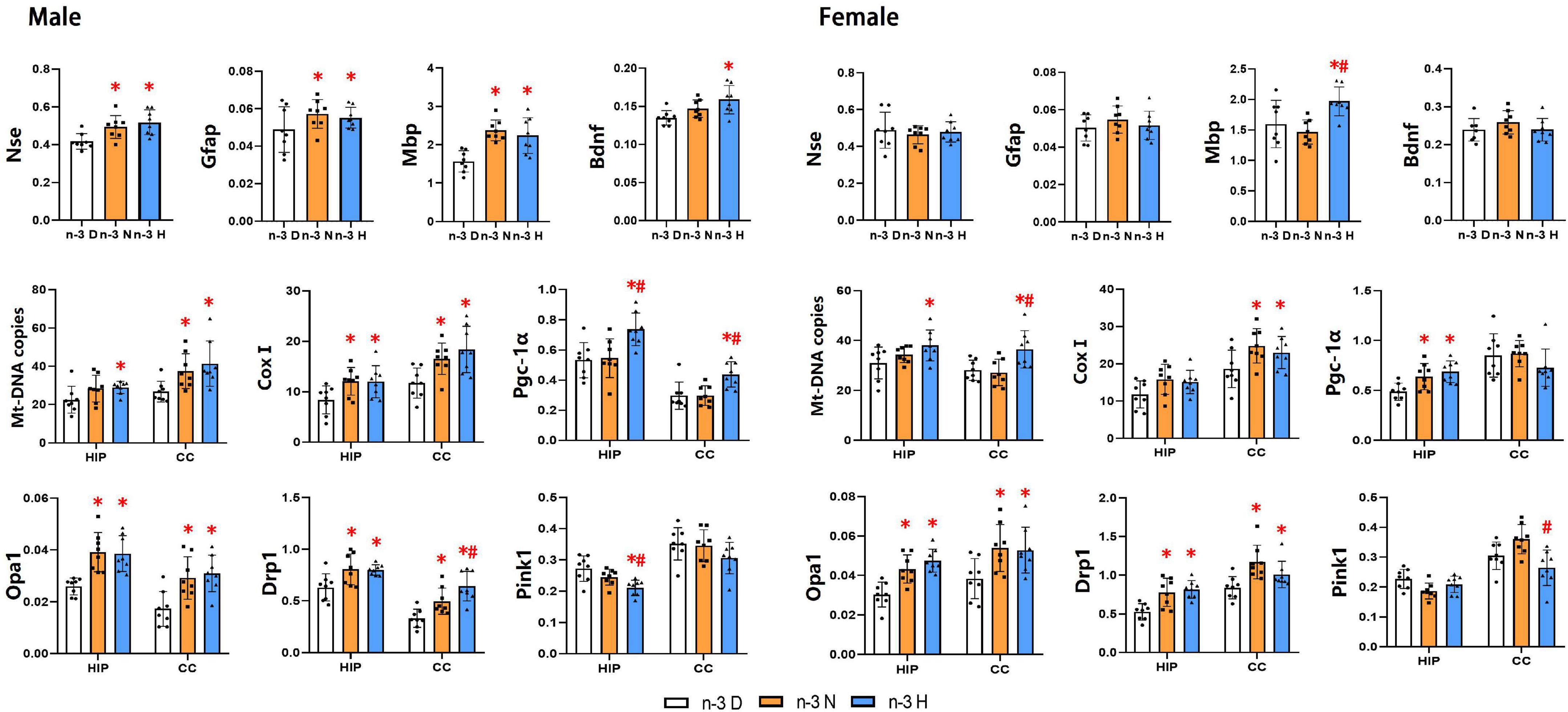
Figure 5. Effects of paternal n-3 PUFA supplementation on the expression of genes associated with brain function and mitochondria in offspring. RT-PCR was used to determine the mRNA expression of Nse, Gfap, Mbp and Bdnf in the hippocampus, and the mRNA expression of mitochondria associated genes (CoxI, Opa1, Drp1, Pink1, and Pgc-1α) and Mt-DNA copies both in the hippocampus (HIP) and cerebral cortex (CC) in offspring mice. The data were normalized to relative mRNA levels using the 2–ΔCT method. Values are means ± SD; n = 8 per diet intervention on each sex. *Compared to paternal n-3 D diet group (or with in the same tissue), P < 0.05; #Compared to paternal n-3 N diet group (or within the tissue), P < 0.05.
Effects of paternal n-3 PUFA supplementation on gene imprinting
A total of nine imprinted genes, which have been considered to be closely associated with brain development and function, were selected for expression analysis (Figure 6). The expression of Zac1, Ube3a, Peg1, Igf2, Peg3, Ndn, Kcnk9 and RasGrf1 showed no differences among the three groups, while the Snrpn was downregulated in mRNA expression by both paternal n-3 N diet and n-3 H diet in testes of the founder mice and their offspring. Therefore, the expression of Snrpn was examined in the offspring brain, and the results indicated that its expression was upregulated in the hippocampus by both paternal n-3 N diet and n-3 H diet either in males or females. In the offspring cerebral cortex, the Snrpn expression was upregulated by both paternal n-3 N diet and n-3 H diet in females, and by paternal n-3 H diet in males.
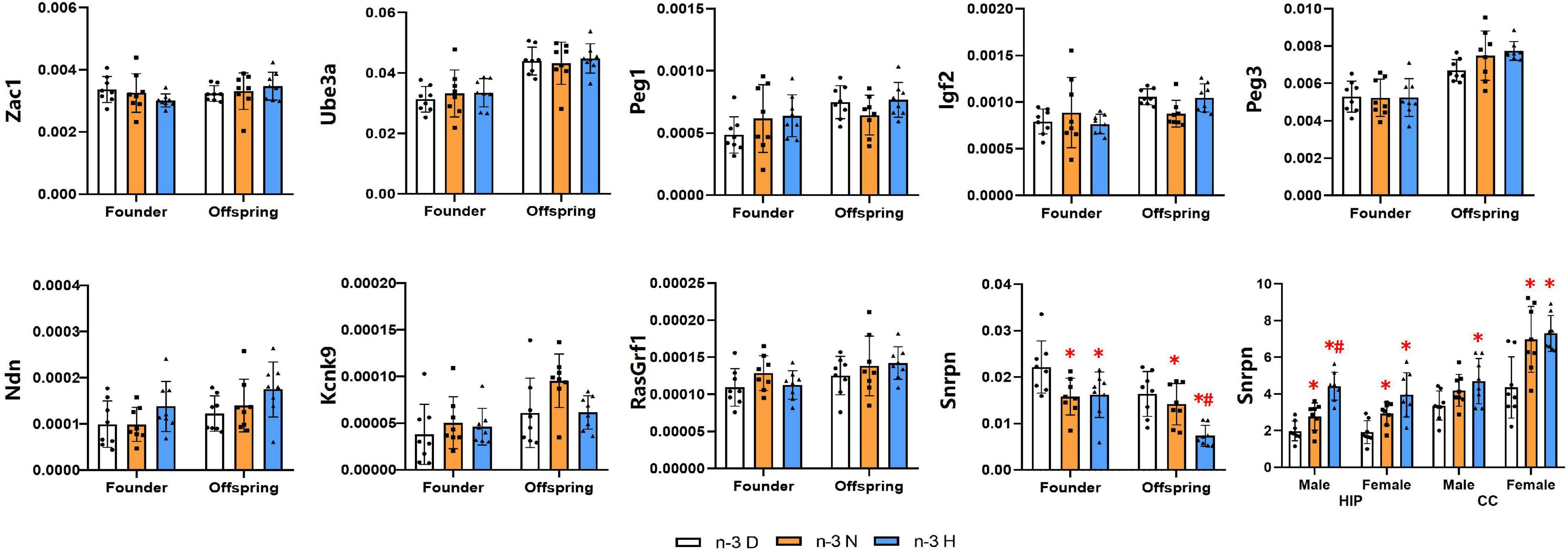
Figure 6. Effects of paternal n-3 PUFA supplementation on the expression of imprinted genes. RT-PCR was used to determine the mRNA expression of Zac1, Ube3a, Peg1, Igf2, Peg3, Ndn, Kcnk9, RasGrf1, and Snrpn in testes of the founders and their offspring, and Snrpn in offspring hippocampus and cerebral cortex. The data were normalized to relative mRNA levels using the 2–ΔCT method. Values are means ± SD; n = 8 per diet intervention on each sex. *Compared to paternal n-3 D diet group within the same generation or within the same sex and tissue, P < 0.05; #Compared to paternal n-3 N diet group within the same generation or within the same sex and tissue, P < 0.05.
DNA methylation analysis showed that the methylation fractions of five CpG sites of the Snrpn DMR1 in testes of the founder were increased by paternal n-3 H diet, and those in testes of the offspring were increased by paternal n-3 N diet or n-3 H diet. In the hippocampus, the methylation fractions of the Snrpn DMR1 were decreased by paternal n-3 H diet in the male offspring, with no changes in the female offspring (Figure 7).
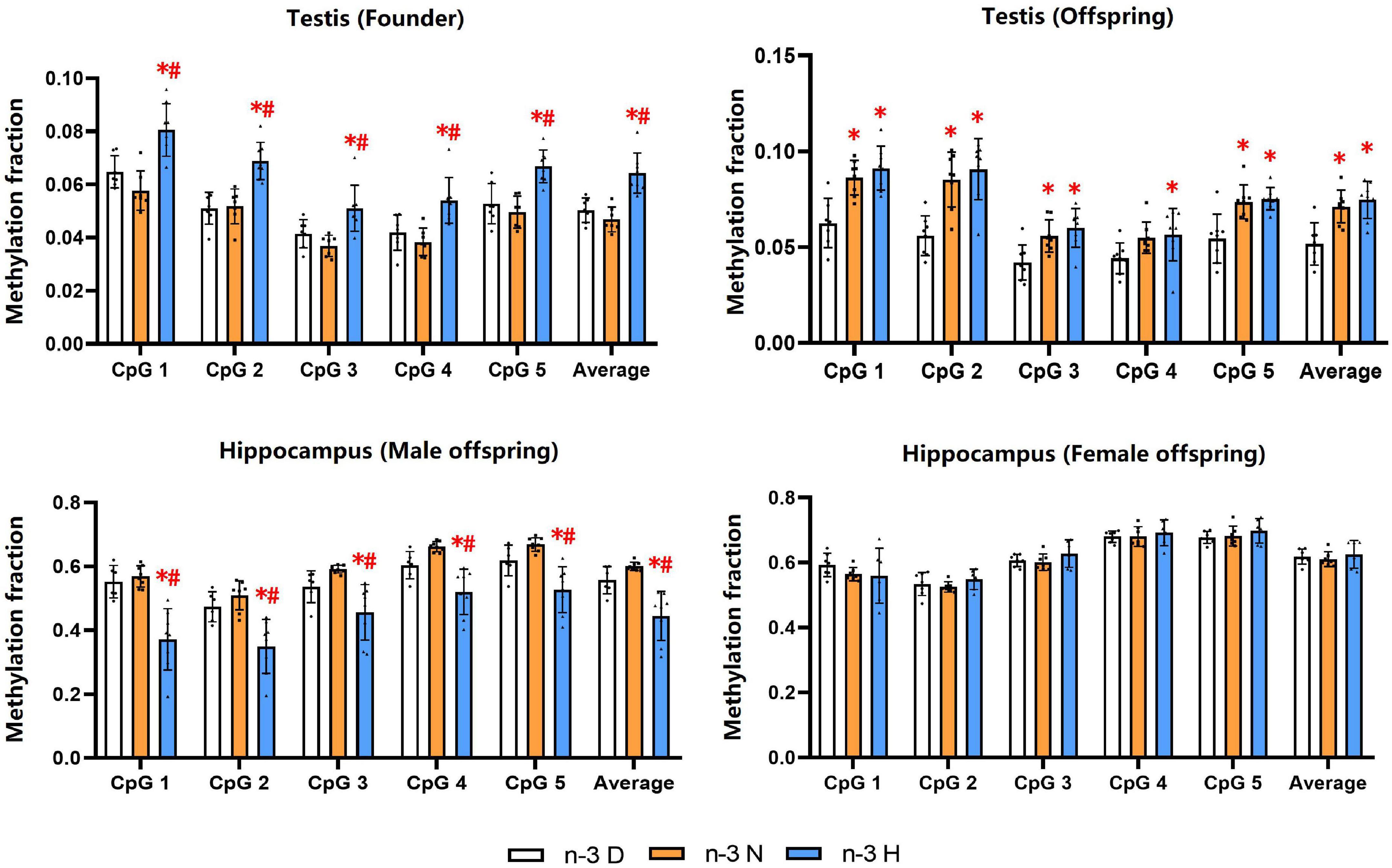
Figure 7. Effects of paternal n-3 PUFA supplementation on DNA methylation of imprinted gene Snrpn. Genomic DNA isolated from testes in both founders and their offspring, and the hippocampus in the offspring was given bisulfite conversion. Converted DNA was amplified by nested PCR, and the PCR products were sequenced directly for examination of CpG methylation in DMR1 of Snrpn. The methylation fraction was calculated from the cytosine and thymine within each CpG dinucleotide (C/C + T). Values are means ± SD; n = 6–7 per diet intervention on each sex. *Compared to paternal n-3 D diet group within the same CpG site, P < 0.05; #Compared to paternal n-3 N diet group within the same CpG site, P < 0.05.
Discussion
A growing body of evidence suggests that the paternal diet plays a crucial role in health and disease in offspring’s adult life through epigenetic modification on sperm (50). However, the effects of paternal nutrition on offspring brain development and function are scarcely reported. In the current study, we found that paternal n-3 PUFA supplementation in preconception reduced anxiety- and depressive-like behavior (OFT, LDT, SPT), improved sociability (TCT), learning and memory (NOR) in the offspring, along with increased synaptic number, upregulated expressions of NSE, GFAP, MBP, BDNF in the hippocampus and cerebral cortex, as well as altered expressions of genes associated with mitochondria biogenesis, fusion, fission and autophagy. Furthermore, the expression of imprinted gene Snrpn was consistently downregulated in testes of the father and their offspring, and was upregulated in the cerebral cortex and hippocampus by paternal n-3 PUFA supplementation, with altered DNA methylation in DMR1 of the Snrpn. Therefore, paternal n-3 PUFA status could impact offspring brain function and histology.
As well known, adequate nutrition in early life, particularly during pregnancy and infancy, is critical in supporting healthy brain development, with long-lasting effects on cognitive, and socio-emotional skills throughout childhood and adulthood (51). Recently, the impact of paternal nutrition prior to conception on offspring brain development and function has been reported in animal models (22). Paternal methyl donor deficiency or enrichment in diets lead to alterations in offspring brain function, including consolidation-conditioned fear memory and anxiety-like behaviors (52), and hippocampal-dependent learning and memory (53). Diet-induced paternal obesity or calorie restriction during pre-conceptional period has demonstrated impairment in hippocampus-dependent learning and memory function (54), and anxiety-like behaviors in the offspring (55). Herein, our results showed that paternal n-3 PUFA supplementation reduced anxiety- and depressive-like behavior, and improved sociability, learning and memory in the offspring. Consistently, increased synaptic number and expressions of NSE, GFAP, MBP in the hippocampus and cerebral cortex in the paternal n-3 N diet and n-3 H diet groups indicated that, like maternal n-3 PUFAs (37), paternal n-3 PUFAs could promote the growth and maturation of neurons, astrocytes and myelin in the offspring.
The hippocampus is a primary region of the brain controlling the formation of memories, mood and learned behaviors. The ability to learn or form a memory requires a neuron to translate a transient signal into gene expression changes that have a long-lasting effect on synapse activity and connectivity (56). The behavioral and neurophysiological changes in offspring mice induced by paternal methyl-donor are associated with altered hippocampal expression of genes including the potassium calcium-activated channel subfamily M regulatory beta subunit 2, methionine adenosyltransferase II alpha, calcium/calmodulin-dependent protein kinase II alpha and protein phosphatase 1 catalytic subunit (52, 53). The cognitive impairment in the offspring mice caused by paternal high fat diet is attributable to the reduced expression of Bdnf (54), which is a key regulator of neural circuit development and function, mediating neuronal differentiation and growth, synapse formation, and dendritic plasticity in the mammalian brain (57). In this study, the mRNA expression of Bdnf was upregulated by paternal n-3 PUFAs in the hippocampus of male offspring, which might contribute to the memory enhancement and the improvement in expressions of Nse, Gfap, Mbp (37). As well, our previous study demonstrated that the appropriate n-3 PUFA intake during pregnancy and lactation epigeneticly affects the expression of Bdnf, and thus is beneficial for neurogenesis and anti-apoptosis in adulthood of the offspring (58).
Mitochondria play a decisive role in brain development, not only providing energy for cell proliferation and differentiation, but also determining neural stem cell differentiation and synaptogenesis (30). The upregulated expression of Pgc-1α, Cox1, Opa1 and Drp1 with downregulated expression of Pink1 by paternal n-3 PUFA supplementation indicated the increased mitochondria biogenesis, dynamics and respiratory function, with reduced autophagy. The better mitochondria function is responsible for the memory enhancement and improvement in the expression of NSE, GFAP and MBP, and conversely the mitochondria dysfunction is a hallmark of many neurological diseases, including autism spectrum disorder, hyperactivity disorder, schizophrenia, Alzheimer’s disease, Parkinson’s disease, etc. (31). Studies on other nutrients demonstrate that paternal high fat intake has significant negative effects on the embryo at a variety of key early developmental stages, with reduced mitochondria membrane potential, resulting in delayed development, reduced placental size and smaller offspring (59).
To note, no differences in the fatty acid profile in offspring brains among the three groups implied that the altered behavior and cognition by paternal n-3 PUFAs are independent of their “direct” action, which is different from maternal n-3 PUFAs. During pregnancy and lactation, maternal n-3 PUFAs are transported to the fetus and infants via the placenta and breast milk and play a directable role in maintaining proper brain development and function (4). The mechanisms by which the paternal nutrition influences the offspring’s health are poorly understood, but emerging evidence suggests that it could be transmitted through the sperm epigenome (DNA methylation, histone modifications and sncRNAs) (22, 50, 60). Paternal lifestyle and exposures to environmental factors may alter the sperm DNA methylation including imprinted genes, and consequently affect both the embryonic developmental programing and the health of future generations (47). Several studies have found that paternal fast food intake and obesity can lead to changes in DNA methylation and expression of Meg3 and Nnat in sperm (61), and Igf2/H19 in the offspring (62, 63). In this study, among the nine imprinted genes selected, just Snrpn was affected by paternal n-3 PUFA supplementation, with increased expression in offspring brains and reduced expression in testes of fathers and their offspring. Correspondently, DNA methylation fractions of the Snrpn DMR1 were increased in testes of fathers and their offspring but reduced in the hippocampus of male offspring. These suggested that the impact of paternal n-3 PUFAs on the offspring brain might be mediated by the imprinting of Snrpn, which has been found to be associated with adult neural stem cell differentiation and positively correlated with cognitive abilities in childhood (64). One mechanistic pathway has recently been identified that proper Snrpn expression directly regulates the expression of nuclear receptor Nr4a1 which is critical for cortical neurodevelopment, and that a disruption in Snrpn expression is linked to developmental brain disorders (65). In addition, the differential expression of brain mitochondrial genes was found in mouse models with partial knockout of the Snrpn promoter, and abnormal mitochondrial number and structure were found in cardiac and skeletal muscle (66). PWS-IC del mice exhibit Prader-Willi syndrome, a neurodevelopmental multifactorial genetic disorder caused by lack of Snrpn expression, including deficits in energy metabolism, behavior, cognition, and structure (67). These findings indicate the regulatory role of Snrpn in mitochondria energy metabolism. Conversely, the prominent epigenetic process, methyl groups provided by S-adenosyl methionine, in mitochondria may affect the methylation of imprinted genes (68). Thus, how Snrpn and mitochondria interact to affect brain development and function needs to be explored.
Interestingly, we found that the effects of paternal n-3 PUFA supplementation on neurobehavioral outcomes and expression of associated genes are sex-specific in the offspring. Specifically, anxiety- and depressive-like behaviors (OFT, LDT, SPT) were reduced and sociability (TCT) was improved both in offspring males and females; whereas, learning and memory (NOR) were improved only in offspring males, with paternal n-3 PUFA supplementation in preconception. Histological findings showed that the number of synapses was increased in both the hippocampus and cerebral cortex in male offspring from the paternal n-3 N diet and n-3 H diet groups, but increased only in the cerebral cortex in female offspring. In keeping with other studies, it has been demonstrated that parental environmental factors including diet, metabolism, and stress, affect the behavior and cognition of offspring differently between males and females. For example, adult female but not male offspring of dams fed the low protein diet exhibited passive, and perhaps maladaptive coping strategies in response to stress, accompanied by a marked reduction in hippocampal 5-HT1A receptor function (69). Nutrient-restricted female offspring showed improved learning, while male offspring showed impaired learning and attentional set shifting and increased impulsivity (70). Chronic consumption of a high linoleic acid diet during pregnancy, lactation and post-weaning period increases depression-like behavior in male, but not female offspring (71). With respect to the pre-conceptional paternal nutrition, as early as 20 years ago, sex-specific, male-line transgenerational responses to paternal nutrition and environment have been found in humans. Early paternal smoking is associated with greater BMI at 9 years in sons, but not daughters, and paternal grandfather’s and grandmother’s food supply was linked to the mortality risk ratios of grandsons and granddaughters, respectively (72, 73). Thereafter, male but not female offspring of fathers fed with a high protein diet exhibited increased insulin sensitivity and decreased glucose induced insulin secretion, with preserved β cell mass and plasticity following metabolic challenge (74). Whereas, when fathers were fed a high fat diet for 10 weeks before mating, female (but not male) offspring had impaired pancreatic β-cell function, with increased bodyweight and glucose intolerance, and reduced insulin secretion (75).
Although the underlying mechanisms for gender differences in parental effects on offspring are not completely known, genetics, epigenetics and gene imprinting, together with the contribution of distinct gonadal steroid hormones and associated inflammatory responses and gene expression, may be involved in this sex dimorphism (76–80). It is acceptable that the sex-specific, male-line transmissions are mediated by the sex chromosomes, X and Y (73), and that paternally expressed genes are generally growth promoting, whereas maternally expressed genes are growth restricting (81). Also, the imprinted gene Dio3 in male pups, while H19 and Xist in female pups, were upregulated by high gestational folic acid supplementation, accompanied by different expressional changes in the candidate autism susceptible gene Auts2 between male and female pups (82). Estrogen has been demonstrated to produce beneficial effects in brain development and function as well as cardioprotective effects, and the advances in understanding the structural, epigenetic and transcriptional mechanisms mediating sexual differentiation of the brain have been reviewed (83–86). It is highlighted that a gene regulatory program activated by estrogen receptor α (Erα) following the perinatal hormone surge, and sustained sex-biased gene expression and chromatin accessibility throughout the postnatal sensitive period, are of importance (86). Regarding estrogen cognitive protective effects, women with high estradiol (E2) show superior spatial reference memory (87). Female mice have a higher preference index in the NOR paradigm (62.3 ± 13.0%) than males (52.7 ± 5.9%), and are resistance to retroactive interference, which is mediated by estrogen signaling involving estrogen receptor α activation and extracellular signal-regulated kinase 1/2 in the dorsal hippocampus (88). In addition, E2 regulates hippocampus-dependent memory by promoting the synthesis of proteins and their degradation mediated by the ubiquitin proteasome system, that support structural changes at hippocampal synapses (89). As well, E2 treatment greatly upregulates the serum levels of Bdnf and transient receptor potential channels 6, the neuronal excitability indicated by an elevation in the thickness of postsynaptic density and the numbers of asymmetric synapses in rat (90). In the current study, compared to males, females in offspring from the paternal n-3 D diet group had higher NOR preference index (63.90 ± 4.30% vs 45.40 ± 3.30%), along with increased number of synapses (19.63 ± 3.42 vs 17.63 ± 3.02) and expression of Bdnf (0.24 ± 0.03 vs 0.14 ± 0.01) in the hippocampus, implying protective effects in females. Further findings showing no changes in these parameters in female offspring with paternal n-3 N diet and n-3 H diet, suggest that estrogen brain protective effects might override or mask any relationship between paternal n-3 PUFAs and offspring cognition.
The higher ratio of n-6/n-3 PUFAs (20–50:1) in modern diets, has been considered to be a risk factor for many chronic diseases (5). Individuals are required to take both series of PUFAs with the highly recommended n-6/n-3 ratio which is 4–5:1 (91). Considering the ratio of n-6/n-3 PUFAs at 1:1 in the Paleolithic diet, we previously investigated the effect of a higher intake of maternal dietary n-3 PUFAs during pregnancy and lactation on offspring and found that dietary n-6/n-3 PUFA ratio at 1–2:1 has optimal neurogenesis and maturation of neurons, astrocytes and myelin in the offspring brain (37, 58). As well, it is reported that diets with a ratio of n-6/n-3 PUFAs at 1:1 can improve the testicular development of boars and rats, and thus may more effectively reduce exogenous oxidative damage in sperm, providing a more favorable environment for sperm survival (92, 93). In the present study, the alteration in some parameters was different between the paternal n-3 N diet and n-3 H diet groups, indicating that paternal dietary n-6/n-3 PUFA ratio at 1:1 prior to conception might be more beneficial for the offspring brain development.
Our data indicate that paternal n-3 PUFAs may have an impact on offspring brain development. However, some limitations of our study should be addressed. In analyzing the impacts of paternal diet and other factors on the resultant offspring, the random effects of the mother and litter size using a random effects regression model were considered by some researchers (94–96). Unfortunately, in the current study, there exist the statistical limitations related to inability to analyze random effects form mothers and litter size due to the animal management practices in our institution, and this factor should be included in our future work. Therefore, it needs to be emphasized that the random effects statistical model is used in order to improve validity and reproducibility of research in developmental programming studies.
In conclusion, paternal pre-conceptional n-3 PUFA supplementation reduced anxiety- and depressive-like behaviors, and improved sociability, learning and memory in offspring, along with alterations in brain structural development and mitochondria, as well as the expression and DMR1 methylation of imprinted-gene Snrpn both in founder mice and their offspring. These data raise the possibility that paternal dietary factors may be relevant causal factors for mental health issues in the subsequent generation.
Data availability statement
The original contributions presented in the study are included in the article/Supplementary material, further inquiries can be directed to the corresponding author.
Ethics statement
The animal study was reviewed and approved by Ethics Committee of the National Institute of Occupational Health and Poison Control, China CDC (No. EAWE-2021-06).
Author contributions
ML performed the experiment, analyzed the data and prepared the manuscript. QS carried out mouse feeding. XJ and WH performed the experiment. PL participated in the statistical analysis. XL and XF participated in designing the research. KQ designed the research and revised the manuscript. All authors read and approved the final manuscript.
Funding
This work was supported by the Research Funds of Reform and Development Budget in Medicine from Beijing Municipal Science and Technology Commission (2021-bjsekyjs to KQ). The funders had no role in study design, data collection, and analysis, interpretation of data, decision to publish, or preparation of the manuscript.
Conflict of interest
The authors declare that the research was conducted in the absence of any commercial or financial relationships that could be construed as a potential conflict of interest.
Publisher’s note
All claims expressed in this article are solely those of the authors and do not necessarily represent those of their affiliated organizations, or those of the publisher, the editors and the reviewers. Any product that may be evaluated in this article, or claim that may be made by its manufacturer, is not guaranteed or endorsed by the publisher.
Supplementary material
The Supplementary Material for this article can be found online at: https://www.frontiersin.org/articles/10.3389/fnut.2022.969848/full#supplementary-material
References
1. Van Dael P. Role of n-3 long-chain polyunsaturated fatty acids in human nutrition and health: review of recent studies and recommendations. Nutr Res Pract. (2021) 15:137–59. doi: 10.4162/nrp.2021.15.2.137
2. Calder PC, Deckelbaum RJ. Editorial: Omega-3 fatty acids: new studies, new data, new questions. Curr Opin Clin Nutr Metab Care. (2021) 24:109–13. doi: 10.1097/MCO.0000000000000726
3. Powell N, Chaudhary S, Zaidi A. it is time for an oil change: polyunsaturated fatty acids and human health. Mo Med. (2021) 118:426–30.
4. Martinat M, Rossitto M, Di Miceli M, Layé S. Perinatal dietary polyunsaturated fatty acids in brain development, role in neurodevelopmental disorders. Nutrients. (2021) 13:1185. doi: 10.3390/nu13041185
5. Simopoulos AP. An Increase in the Omega-6/Omega-3 Fatty Acid Ratio Increases the Risk for Obesity. Nutrients. (2016) 8:128. doi: 10.3390/nu8030128
6. Morgese MG, Trabace L. Maternal malnutrition in the etiopathogenesis of psychiatric diseases: role of polyunsaturated fatty acids. Brain Sci. (2016) 6:24. doi: 10.3390/brainsci6030024
7. Simopoulos AP. Importance of the omega-6/omega-3 balance in health and disease: evolutionary aspects of diet. World Rev Nutr Diet. (2011) 102:10–21. doi: 10.1159/000327785
8. Martins BP, Bandarra NM, Figueiredo-Braga M. The role of marine omega-3 in human neurodevelopment, including Autism Spectrum Disorders and Attention-Deficit/Hyperactivity Disorder – a review. Crit Rev Food Sci Nutr. (2020) 60:1431–46. doi: 10.1080/10408398.2019.1573800
9. Hanson MA, Gluckman PD. Early developmental conditioning of later health and disease: physiology or pathophysiology? Physiol Rev. (2014) 94:1027–76. doi: 10.1152/physrev.00029.2013
10. Hoffman DJ, Powell TL, Barrett ES, Hardy DB. Developmental origins of metabolic diseases. Physiol Rev. (2021) 101:739–95. doi: 10.1152/physrev.00002.2020
11. Wainwright PE. Dietary essential fatty acids and brain function: a developmental perspective on mechanisms. Proc Nutr Soc. (2002) 61:61–9. doi: 10.1079/pns2001130
12. Moriguchi T, Greiner RS, Salem N Jr. Behavioral deficits associated with dietary induction of decreased brain docosahexaenoic acid concentration. J Neurochem. (2000) 75:2563–73. doi: 10.1046/j.1471-4159.2000.0752563.x
13. Luchtman DW, Song C. Cognitive enhancement by omega-3 fatty acids from child-hood to old age: findings from animal and clinical studies. Neuropharmacology. (2013) 64:550–65. doi: 10.1016/j.neuropharm.2012.07.019
14. Colombo J, Carlson SE, Cheatham CL, Shaddy DJ, Kerling EH, Thodosoff JM, et al. Long-term effects of LCPUFA supplementation on childhood cognitive outcomes. Am J Clin Nutr. (2013) 98:403–12. doi: 10.3945/ajcn.112.040766
15. Campoy C, Escolano-Margarit MV, Ramos R, Parrilla-Roure M, Csábi G, Beyer J, et al. Effects of prenatal fish-oil and 5-methyltetrahydrofolate supplementation on cognitive development of children at 6.5 y of age. Am J Clin Nutr. (2011) 94(6 Suppl):1880S–8S. doi: 10.3945/ajcn.110.001107
16. Hurtado JA, Iznaola C, Peña M, Ruíz J, Peña-Quintana L, Kajarabille N, et al. Effects of maternal Ω-3 supplementation on fatty acids and on visual and cognitive development. J Pediatr Gastroenterol Nutr. (2015) 61:472–80. doi: 10.1097/MPG.0000000000000864
17. Makrides M, Gibson RA, McPhee AJ, Yelland L, Quinlivan J, Ryan P. DOMInO Investigative Team. Effect of DHA supplementation during pregnancy on maternal depression and neurodevelopment of young children: a randomized controlled trial. JAMA. (2010) 304:1675–83. doi: 10.1001/jama.2010.1507
18. Makrides M, Gould JF, Gawlik NR, Yelland LN, Smithers LG, Anderson PJ, et al. Four-year follow-up of children born to women in a randomized trial of prenatal DHA supplementation. JAMA. (2014) 311:1802–4. doi: 10.1001/jama.2014.2194
19. Meldrum S, Dunstan JA, Foster JK, Simmer K, Prescott SL. Maternal fish oil supplementation in pregnancy: a 12 year follow-up of a randomised controlled trial. Nutrients. (2015) 7:2061–7. doi: 10.3390/nu7032061
20. Gould JF, Smithers LG, Makrides M. The effect of maternal omega-3 (n-3) LCPUFA supplementation during pregnancy on early childhood cognitive and visual development: a systematic review and meta-analysis of randomized controlled trials. Am J Clin Nutr. (2013) 97:531–44. doi: 10.3945/ajcn.112.045781
21. Soubry A. POHaD: why we should study future fathers. Environ Epigenet. (2018) 4:dvy007. doi: 10.1093/eep/dvy007
22. Dimofski P, Meyre D, Dreumont N, Leininger-Muller B. Consequences of paternal nutrition on offspring health and disease. Nutrients. (2021) 13:2818. doi: 10.3390/nu13082818
23. delBarco-Trillo J, Mateo R, Roldan ER. Differences in the fatty-acid composition of rodent spermatozoa are associated to levels of sperm competition. Biol Open. (2015) 4:466–73. doi: 10.1242/bio.201411288
24. Esmaeili V, Shahverdi AH, Moghadasian MH, Alizadeh AR. Dietary fatty acids affect semen quality: a review. Andrology. (2015) 3:450–61. doi: 10.1111/andr.12024
25. Ferramosca A, Zara V. Diet and Male Fertility: The Impact of Nutrients and Antioxidants on Sperm Energetic Metabolism. Int J Mol Sci. (2022) 23:2542. doi: 10.3390/ijms23052542
26. Martínez-Soto JC, Landeras J, Gadea J. Spermatozoa and seminal plasma fatty acids as predictors of cryopreservation success. Andrology. (2013) 1:365–75. doi: 10.1111/j.2047-2927.2012.00040.x
27. Gao J, Wu H, Cao Y, Liang S, Sun C, Wang P, et al. Maternal DHA supplementation protects rat offspring against impairment of learning and memory following prenatal exposure to valproic acid. J Nutr Biochem. (2016) 35:87–95. doi: 10.1016/j.jnutbio.2016.07.003
28. Basak S, Vilasagaram S, Duttaroy AK. Maternal dietary deficiency of n-3 fatty acids affects metabolic and epigenetic phenotypes of the developing fetus. Prostaglandins Leukot Essent Fatty Acids. (2020) 158:102109. doi: 10.1016/j.plefa.2020.102109
29. Feng Z, Zou X, Jia H, Li X, Zhu Z, Liu X, et al. Maternal docosahexaenoic acid feeding protects against impairment of learning and memory and oxidative stress in prenatally stressed rats: possible role of neuronal mitochondria metabolism. Antioxid Redox Signal. (2012) 16:275–89. doi: 10.1089/ars.2010.3750
30. Khacho M, Harris R, Slack RS. Mitochondria as central regulators of neural stem cell fate and cognitive function. Nat Rev Neurosci. (2019) 20:34–48. doi: 10.1038/s41583-018-0091-3
31. Büeler H. Mitochondrial and autophagic regulation of adult neurogenesis in the healthy and diseased brain. Int J Mol Sci. (2021) 22:3342. doi: 10.3390/ijms22073342
32. Khoshkerdar A, Eryasar E, Morgan HL, Watkins AJ REPRODUCTIVETOXICOLOGY. Impacts of paternal environment and lifestyle on maternal health during pregnancy. Reproduction. (2021) 162:F101–9. doi: 10.1530/REP-20-0605
33. Kopsida E, Mikaelsson MA, Davies W. The role of imprinted genes in mediating susceptibility to neuropsychiatric disorders. Horm Behav. (2011) 59:375–82. doi: 10.1016/j.yhbeh.2010.04.005
34. Perez JD, Rubinstein ND, Dulac C. New perspectives on genomic imprinting, an essential and multifaceted mode of epigenetic control in the developing and adult brain. Annu Rev Neurosci. (2016) 39:347–84. doi: 10.1146/annurev-neuro-061010-113708
35. Varrault A, Dubois E, Le Digarcher A, Bouschet T. Quantifying genomic imprinting at tissue and cell resolution in the brain. Epigenomes. (2020) 4:21. doi: 10.3390/epigenomes4030021
36. Tucci V, Isles AR, Kelsey G, Ferguson-Smith AC, Erice Imprinting Group. Genomic imprinting and physiological processes in mammals. Cell. (2019) 176:952–65. doi: 10.1016/j.cell.2019.01.043
37. Tian C, Fan C, Liu X, Xu F, Qi K. Brain histological changes in young mice submitted to diets with different ratios of n-6/n-3 polyunsaturated fatty acids during maternal pregnancy and lactation. Clin Nutr. (2011) 30:659–67. doi: 10.1016/j.clnu.2011.03.002
38. Arterburn LM, Boswell KD, Koskelo E, Kassner SL, Kelly C, Kyle DJ. A combined subchronic (90-day) toxicity and neurotoxicity study of a single-cell source of docosahexaenoic acid triglyceride (DHASCO oil). Food Chem Toxicol. (2000) 38:35–49. doi: 10.1016/s0278-6915(99)00119-2
39. Atkinson TG, Barker HJ, Meckling-Gill KA. Incorporation of long-chain n-3 fatty acids in tissues and enhanced bone marrow cellularity with docosahexaenoic acid feeding in post-weanling Fischer 344 rats. Lipids. (1997) 32:293–302. doi: 10.1007/s11745-997-0036-x
40. Lloyd-Still JD, Powers CA, Hoffman DR, Boyd-Trull K, Lester LA, Benisek DC, et al. Bioavailability and safety of a high dose of docosahexaenoic acid triacylglycerol of algal origin in cystic fibrosis patients: a randomized, controlled study. Nutrition. (2006) 22:36–46. doi: 10.1016/j.nut.2005.05.006
41. Fuller SJ, Whittingham DG. Effect of cooling mouse spermatozoa to 4 degrees C on fertilization and embryonic development. J Reprod Fertil. (1996) 108:139–45. doi: 10.1530/jrf.0.1080139
42. Heredia L, Torrente M, Colomina MT, Domingo JL. Assessing anxiety in C57BL/6J mice: a pharmacological characterization of the open-field and light/dark tests. J Pharmacol Toxicol Methods. (2014) 69:108–14. doi: 10.1016/j.vascn.2013.12.005
43. Liu MY, Yin CY, Zhu LJ, Zhu XH, Xu C, Luo CX, et al. Sucrose preference test for measurement of stress-induced anhedonia in mice. Nat Protoc. (2018) 13:1686–98. doi: 10.1038/s41596-018-0011-z
44. Liu X, Li X, Xia B, Jin X, Zou Q, Zeng Z, et al. High-fiber diet mitigates maternal obesity-induced cognitive and social dysfunction in the offspring via gut-brain axis. Cell Metab. (2021) 33:923.e–38.e. doi: 10.1016/j.cmet.2021.02.002
45. Antunes M, Biala G. The novel object recognition memory: neurobiology, test procedure, and its modifications. Cogn Process. (2012) 13:93–110. doi: 10.1007/s10339-011-0430-z
46. Pleines UE, Morganti-Kossmann MC, Rancan M, Joller H, Trentz O, Kossmann T. S-100 beta reflects the extent of injury and outcome, whereas neuronal specific enolase is a better indicator of neuroinflammation in patients with severe traumatic brain injury. J Neurotrauma. (2001) 18:491–8. doi: 10.1089/089771501300227297
47. Lazarides E. Intermediate filaments as mechanical integrators of cellular space. Nature. (1980) 283:249–56. doi: 10.1038/283249a0
48. Campagnoni AT, Skoff RP. The pathobiology of myelin mutants reveal novel biological functions of the MBP and PLP genes. Brain Pathol. (2001) 11:74–91. doi: 10.1111/j.1750-3639.2001.tb00383.x
49. Rybka V, Suzuki YJ, Gavrish AS, Dibrova VA, Gychka SG, Shults NV. Transmission electron microscopy study of mitochondria in aging brain synapses. Antioxidants. (2019) 8:171. doi: 10.3390/antiox8060171
50. Champroux A, Cocquet J, Henry-Berger J, Drevet JR, Kocer AA. Decade of exploring the mammalian sperm epigenome: paternal epigenetic and transgenerational inheritance. Front Cell Dev Biol. (2018) 6:50. doi: 10.3389/fcell.2018.00050
51. Cohen Kadosh K, Muhardi L, Parikh P, Basso M, Jan Mohamed HJ, Prawitasari T, et al. Nutritional support of neurodevelopment and cognitive function in infants and young children-an update and novel insights. Nutrients. (2021) 13:199. doi: 10.3390/nu13010199
52. Sahara Y, Matsuzawa D, Ishii D, Fuchida T, Goto T, Sutoh C, et al. Paternal methyl donor deficient diets during development affect male offspring behavior and memory-related gene expression in mice. Dev Psychobiol. (2019) 61:17–28. doi: 10.1002/dev.21801
53. Ryan DP, Henzel KS, Pearson BL, Siwek ME, Papazoglou A, Guo L, et al. A paternal methyl donor-rich diet altered cognitive and neural functions in offspring mice. Mol Psychiatry. (2018) 23:1345–55. doi: 10.1038/mp.2017.53
54. Zhou Y, Zhu H, Wu HY, Jin LY, Chen B, Pang HY, et al. Diet-induced paternal obesity impairs cognitive function in offspring by mediating epigenetic modifications in spermatozoa. Obesity (Silver Spring). (2018) 26:1749–57. doi: 10.1002/oby.22322
55. Govic A, Penman J, Tammer AH, Paolini AG. Paternal calorie restriction prior to conception alters anxiety-like behavior of the adult rat progeny. Psychoneuroendocrinology. (2016) 64:1–11. doi: 10.1016/j.psyneuen.2015.10.020
56. Lagali PS, Corcoran CP, Picketts DJ. Hippocampus development and function: role of epigenetic factors and implications for cognitive disease. Clin Genet. (2010) 78:321–33. doi: 10.1111/j.1399-0004.2010.01503.x
57. Park H, Poo MM. Neurotrophin regulation of neural circuit development and function. Nat Rev Neurosci. (2013) 14:7–23. doi: 10.1038/nrn3379
58. Fan C, Fu H, Dong H, Lu Y, Lu Y, Qi K. Maternal n-3 polyunsaturated fatty acid deprivation during pregnancy and lactation affects neurogenesis and apoptosis in adult offspring: associated with DNA methylation of brain-derived neurotrophic factor transcripts. Nutr Res. (2016) 36:1013–21. doi: 10.1016/j.nutres.2016.06.005
59. Binder NK, Hannan NJ, Gardner DK. Paternal diet-induced obesity retards early mouse embryo development, mitochondrial activity and pregnancy health. PLoS One. (2012) 7:e52304. doi: 10.1371/journal.pone.0052304
60. Bodden C, Hannan AJ, Reichelt AC. Diet-Induced Modification of the Sperm Epigenome Programs Metabolism and Behavior. Trends Endocrinol Metab. (2020) 31:131–49. doi: 10.1016/j.tem.2019.10.005
61. Soubry A, Murphy SK, Vansant G, He Y, Price TM, Hoyo C. Opposing epigenetic signatures in human sperm by intake of fast food versus healthy food. Front Endocrinol. (2021) 12:625204. doi: 10.3389/fendo.2021.625204
62. Costa-Júnior JM, Ferreira SM, Kurauti MA, Bernstein DL, Ruano EG, Kameswaran V, et al. Paternal exercise improves the metabolic health of offspring via epigenetic modulation of the germline. Int J Mol Sci. (2021) 23:1. doi: 10.3390/ijms23010001
63. Wu HY, Cheng Y, Jin LY, Zhou Y, Pang HY, Zhu H, et al. Paternal obesity impairs hepatic gluconeogenesis of offspring by altering Igf2/H19 DNA methylation. Mol Cell Endocrinol. (2021) 529:111264. doi: 10.1016/j.mce.2021.111264
64. Lorgen-Ritchie M, Murray AD, Ferguson-Smith AC, Richards M, Horgan GW, Phillips LH, et al. Imprinting methylation in SNRPN and MEST1 in adult blood predicts cognitive ability. PLoS One. (2019) 14:e0211799. doi: 10.1371/journal.pone.0211799
65. Li H, Zhao P, Xu Q, Shan S, Hu C, Qiu Z, et al. The autism-related gene SNRPN regulates cortical and spine development via controlling nuclear receptor Nr4a1. Sci Rep. (2016) 6:29878. doi: 10.1038/srep29878
66. Yazdi PG, Su H, Ghimbovschi S, Fan W, Coskun PE, Nalbandian A, et al. Differential gene expression reveals mitochondrial dysfunction in an imprinting center deletion mouse model of Prader-Willi syndrome. Clin Transl Sci. (2013) 6:347–55. doi: 10.1111/cts.12083
67. Kummerfeld DM, Raabe CA, Brosius J, Mo D, Skryabin BV, Rozhdestvensky TS. A comprehensive review of genetically engineered mouse models for prader-willi syndrome research. Int J Mol Sci. (2021) 22:3613. doi: 10.3390/ijms22073613
68. Bressan P, Kramer P. Mental health, mitochondria, and the battle of the sexes. Biomedicines. (2021) 9:116. doi: 10.3390/biomedicines9020116
69. Ye W, Pitlock MD, Javors MA, Thompson BJ, Lechleiter JD, Hensler JG. The long-term effect of maternal dietary protein restriction on 5-HT1A receptor function and behavioral responses to stress in adulthood. Behav Brain Res. (2018) 349:116–24. doi: 10.1016/j.bbr.2018.03.038
70. Rodriguez JS, Bartlett TQ, Keenan KE, Nathanielsz PW, Nijland MJ. Sex-dependent cognitive performance in baboon offspring following maternal caloric restriction in pregnancy and lactation. Reprod Sci. (2012) 19:493–504. doi: 10.1177/1933719111424439
71. Yau SY, Yip YSL, Formolo DA, He S, Lee THY, Wen C, et al. Chronic consumption of a high linoleic acid diet during pregnancy, lactation and post-weaning period increases depression-like behavior in male, but not female offspring. Behav Brain Res. (2022) 416:113538. doi: 10.1016/j.bbr.2021.113538
72. Bygren LO, Kaati G, Edvinsson S. Longevity determined by paternal ancestors’ nutrition during their slow growth period. Acta Biotheor. (2001) 49:53–9. doi: 10.1023/a:1010241825519
73. Pembrey ME, Bygren LO, Kaati G, Edvinsson S, Northstone K, Sjöström M, et al. Sex-specific, male-line transgenerational responses in humans. Eur J Hum Genet. (2006) 14:159–66. doi: 10.1038/sj.ejhg.5201538
74. Gong P, Bailbé D, Bianchi L, Pommier G, Liu J, Tolu S, et al. Paternal high-protein diet programs offspring insulin sensitivity in a sex-specific manner. Biomolecules. (2021) 11:751. doi: 10.3390/biom11050751
75. Ng SF, Lin RC, Laybutt DR, Barres R, Owens JA, Morris MJ. Chronic high-fat diet in fathers programs β-cell dysfunction in female rat offspring. Nature. (2010) 467:963–6. doi: 10.1038/nature09491
76. Tobiansky DJ, Kachkovski GV, Enos RT, Schmidt KL, Murphy EA, Floresco SB, et al. Maternal sucrose consumption alters behaviour and steroids in adult rat offspring. J Endocrinol. (2021) 251:161–80. doi: 10.1530/JOE-21-0166
77. Dias-Rocha CP, Almeida MM, Santana EM, Costa JCB, Franco JG, Pazos-Moura CC, et al. Maternal high-fat diet induces sex-specific endocannabinoid system changes in newborn rats and programs adiposity, energy expenditure and food preference in adulthood. J Nutr Biochem. (2018) 51:56–68. doi: 10.1016/j.jnutbio.2017.09.019
78. Wijenayake S, Rahman MF, Lum CMW, De Vega WC, Sasaki A, McGowan PO. Maternal high-fat diet induces sex-specific changes to glucocorticoid and inflammatory signaling in response to corticosterone and lipopolysaccharide challenge in adult rat offspring. J Neuroinflammation. (2020) 17:116. doi: 10.1186/s12974-020-01798-1
79. Nakamura JP, Schroeder A, Gibbons A, Sundram S, Hill RA. Timing of maternal immune activation and sex influence schizophrenia-relevant cognitive constructs and neuregulin and GABAergic pathways. Brain Behav Immun. (2022) 100:70–82. doi: 10.1016/j.bbi.2021.11.006
80. Gallou-Kabani C, Gabory A, Tost J, Karimi M, Mayeur S, Lesage J, et al. Sex- and diet-specific changes of imprinted gene expression and DNA methylation in mouse placenta under a high-fat diet. PLoS One. (2010) 5:e14398. doi: 10.1371/journal.pone.0014398
81. Millership SJ, Van de Pette M, Withers DJ. Genomic imprinting and its effects on postnatal growth and adult metabolism. Cell Mol Life Sci. (2019) 76:4009–21. doi: 10.1007/s00018-019-03197-z
82. Barua S, Kuizon S, Brown WT, Junaid MA. High gestational folic acid supplementation alters expression of imprinted and candidate autism susceptibility genes in a sex-specific manner in mouse offspring. J Mol Neurosci. (2016) 58:277–86. doi: 10.1007/s12031-015-0673-8
83. Knoedler JR, Shah NM. Molecular mechanisms underlying sexual differentiation of the nervous system. Curr Opin Neurobiol. (2018) 53:192–7. doi: 10.1016/j.conb.2018.09.005
84. Gegenhuber B, Tollkuhn J. Sex differences in the epigenome: a cause or consequence of sexual differentiation of the brain? Genes. (2019) 10:432. doi: 10.3390/genes10060432
85. Marchant IC, Chabert S, Martínez-Pinto J, Sotomayor-Zárate R, Ramírez-Barrantes R, Acevedo L, et al. Estrogen, cognitive performance, and functional imaging studies: what are we missing about neuroprotection? Front Cell Neurosci. (2022) 16:866122. doi: 10.3389/fncel.2022.866122
86. Gegenhuber B, Tollkuhn J. Epigenetic mechanisms of brain sexual differentiation. Cold Spring Harb Perspect Biol. (2022) 11:a039099. doi: 10.1101/cshperspect.a039099
87. Patel SA, Frick KM, Newhouse PA, Astur RS. Estradiol effects on spatial memory in women. Behav Brain Res. (2022) 24:113592. doi: 10.1016/j.bbr.2021.113592
88. Rinaudo M, Natale F, La Greca F, Spinelli M, Farsetti A, Paciello F, et al. Hippocampal estrogen signaling mediates sex differences in retroactive interference. Biomedicines. (2022) 10:1387. doi: 10.3390/biomedicines10061387
89. Beamish SB, Frick KMA. Putative role for ubiquitin-proteasome signaling in estrogenic memory regulation. Front Behav Neurosci. (2022) 25:807215. doi: 10.3389/fnbeh.2021.807215
90. Song Q, Huang W, Ye W, Yan H, Wang L, Yang Y, et al. Neuroprotective Effects of Estrogen Through BDNF-Transient Receptor Potential Channels 6 Signaling Pathway in the Hippocampus in a Rat Model of Perimenopausal Depression. Front Aging Neurosci. (2022) 8:869274. doi: 10.3389/fnagi.2022.869274
91. Mariamenatu AH, Abdu EM. Overconsumption of Omega-6 Polyunsaturated Fatty Acids (PUFAs) versus Deficiency of Omega-3 PUFAs in Modern-Day Diets: The Disturbing Factor for Their “Balanced Antagonistic Metabolic Functions” in the Human Body. J Lipids. (2021) 2021:8848161. doi: 10.1155/2021/8848161
92. Lin Y, Cheng X, Mao J, Wu D, Ren B, Xu SY, et al. Effects of different dietary n-6/n-3 polyunsaturated fatty acid ratios on boar reproduction. Lipids Health Dis. (2016) 15:31. doi: 10.1186/s12944-016-0193-8
93. Yan L, Bai XL, Fang ZF, Che LQ, Xu SY, Wu D. Effect of different dietary omega-3/omega-6 fatty acid ratios on reproduction in male rats. Lipids Health Dis. (2013) 12:33. doi: 10.1186/1476-511X-12-33
94. Jiménez JA, Zylka MJ. Controlling litter effects to enhance rigor and reproducibility with rodent models of neurodevelopmental disorders. J Neurodev Disord. (2021) 13:2. doi: 10.1186/s11689-020-09353-y
95. Kwong WY, Osmond C, Fleming TP. Support for Barker hypothesis upheld in rat model of maternal undernutrition during the preimplantation period: application of integrated “random effects’ statistical model. Reprod Biomed Online. (2004) 8:574–6. doi: 10.1016/s1472-6483(10)61105-4
Keywords: n-3 polyunsaturated fatty acids, paternal nutrition, offspring brain, gene imprinting, mouse
Citation: Li M, Shi Q, Jiang X, Liu X, Han W, Fan X, Li P and Qi K (2022) Paternal preconceptional diet enriched with n-3 polyunsaturated fatty acids affects offspring brain function in mice. Front. Nutr. 9:969848. doi: 10.3389/fnut.2022.969848
Received: 15 June 2022; Accepted: 13 October 2022;
Published: 28 October 2022.
Edited by:
Miguel Abraham Velazquez, Newcastle University, United KingdomReviewed by:
Corinne Joffre, INRAE Nouvelle-Aquitaine Bordeaux, FranceMi Mi Tang, Central South University, China
Copyright © 2022 Li, Shi, Jiang, Liu, Han, Fan, Li and Qi. This is an open-access article distributed under the terms of the Creative Commons Attribution License (CC BY). The use, distribution or reproduction in other forums is permitted, provided the original author(s) and the copyright owner(s) are credited and that the original publication in this journal is cited, in accordance with accepted academic practice. No use, distribution or reproduction is permitted which does not comply with these terms.
*Correspondence: Kemin Qi, cWlrZW1pbkBiY2guY29tLmNu