- 1School of Public Health, Sun Yat-sen University, Guangzhou, China
- 2Eastern-Fusion Master Studio of Hezhou, Hezhou, China
- 3Hezhou Research Institute of Longevity Health Science, Hezhou, China
- 4Department of Genetics & Computational Biology, QIMR Berghofer Medical Research Institute, Herston, QLD, Australia
- 5Li Ka Shing Faculty of Medicine, School of Public Health, The University of Hong Kong, Hong Kong, Hong Kong SAR, China
Background: Previous observational studies have found that lower levels of circulating polyunsaturated fatty acids (PUFAs) were associated with a higher risk of sleep apnea (SA). However, the causality of the association remains unclear.
Materials and methods: We used the two-sample Mendelian randomization (MR) study to assess the causal association of omega-3 and omega-6 fatty acids with SA. Single-nucleotide polymorphisms (SNPs) predicting the plasma level of PUFAs at the suggestive genome-wide significance level (p < 5 × 10–6) were selected as instrumental variables (IVs) from the Cohorts for Heart and Aging Research in Genomic Epidemiology (CHARGE) (n = ∼8,000) Consortium. For outcomes, the summary-level statistics of SA were obtained from the latest genome-wide association study (GWAS), which combined five cohorts with a total number of 25,008 SA cases and 172,050 snoring cases (total = 523,366).
Results: We found no association of α-linolenic acid (ALA) [odds ratio (OR) = 1.09 per% changed, 95% confidence interval (CI) 0.67–1.78], eicosapentaenoic acid (EPA) (OR = 0.94, 95% CI 0.88–1.01), docosapentaenoic acid (DPA) (OR = 0.95, 95% CI 0.88–1.02), and docosahexaenoic acid (DHA) (OR = 0.99, 95% CI 0.96–1.02) with the risk of SA using inverse-variance weighted (IVW) method. Moreover, for omega-6 PUFAs, no association between linoleic acid (LA) (OR = 0.98, 95% CI 0.96–1.01), arachidonic acid (AA) (1.00, 95% CI 0.99–1.01), and adrenic acid (AdrA) (0.93, 95% CI 0.71–1.21) with the risk of SA was found. Similarly, no associations of PUFAs with SA were found in single-locus MR analysis.
Conclusion: In the current study, we first found that there is no genetic evidence to support the causal role of omega-3 and omega-6 PUFAs in the risk of SA. From a public health perspective, our findings refute the notion that consumption of foods rich in PUFAs or the use of PUFAs supplementation can reduce the risk of SA.
Introduction
Sleep apnea (SA), a common form of sleep-disordered breathing, is characterized by brief interruptions of breathing during sleep. SA affects almost one billion adults aged 30–69 years worldwide (1) and is linked to a higher risk of cardiovascular diseases (CVDs) (2), type 2 diabetes (3), and Alzheimer’s disease (4). The development of SA involved a higher inflammatory response (5, 6). Therefore, apart from continuous positive airway pressure (CPAP), nutritional supplementation may be a possible alternative approach to decrease the risk of SA, i.e., supplementation with polyunsaturated fatty acids (PUFAs).
As the key components of cellular and intracellular membranes, PUFAs can be classified into omega-3 PUFAs and omega-6 PUFAs. Previous studies showed that PUFAs were associated with lower risks of CVDs (7), type 2 diabetes (8), and autoimmune disorders (9). PUFAs mainly include the plant-derived α-linolenic acid (ALA) and linoleic acid (LA), and seafood-derived eicosapentaenoic acid (EPA) and docosahexaenoic acid (DHA), which were associated with lower immune response (10, 11). Previous epidemiological studies showed that lower levels of circulating omega-3 PUFA were associated with a higher risk of SA (12, 13), suggesting that diet-sourced omega-3 PUFAs may be modifiable targets for SA prevention. Based on these findings and the tolerability and safety of PUFAs (14), it has been speculated that PUFAs supplementation may reduce inflammatory response and decrease the risk of SA, and intervention trials have been suggested (15).
In this situation, in which a rationale exists for studying the role of PUFAs in SA but the evidence is lacking, Mendelian randomization (MR) provides an alternative way of examining causal effects. MR is an instrumental variable (IV) approach that uses genetic variants allocated randomly at conception as IVs and thus is unlikely to be biased by common confounders, such as lifestyle, health status, and socioeconomic positions. Moreover, compared with randomized control trials (RCTs) which estimate effects during a short term, MR tends to reflect the effects of lifelong exposure to PUFAs (16). Previous MR studies showed that higher genetically predicted ALA and LA, and lower EPA and docosapentaenoic acid (DPA), were associated with a lower risk of type 2 diabetes (17). A higher genetically predicted LA was also associated with a lower risk of asthma (9). However, to date we found no MR studies regarding the association between PUFAs and SA. Using genetic variants [i.e., single nucleotide polymorphisms (SNPs)] from the genome-wide association study (GWAS) of PUFAs (18–20), we conducted two-sample MR studies to examine the effect of main omega-3 fatty acids (ALA, EPA, DPA, and DHA) and omega-6 fatty acids [LA, arachidonic acid (AA), and adrenic acid (AdrA)] on SA, using genetic summary statistics from a large multivariate genome-wide association study (GWAS) of SA.
Materials and methods
Study design and data sources
We did a two-sample MR study, using de-identified summary-level data that were publicly available. Information about the data sources and sample sizes used in this study are summarized in the appendix (Supplementary Table 1). An overview of the study design is displayed in Figure 1. Ethical approvals were obtained in all original studies.
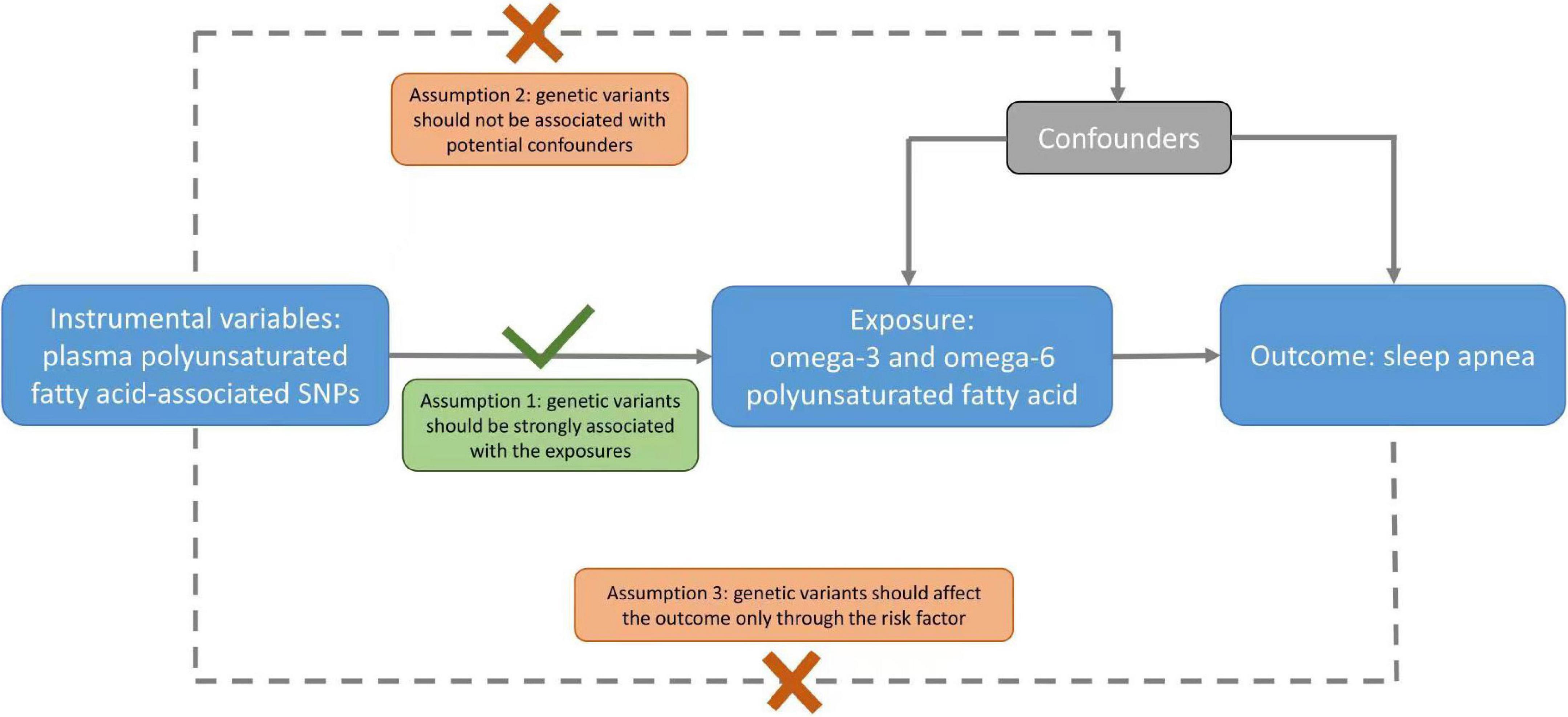
Figure 1. A schematic diagram of the Mendelian randomization (MR) assumptions underpinning an MR analysis of the association of plasma fatty acid levels with sleep apnea (SA). The dashed lines represent the possible violations of the MR assumptions.
Genetic associations with polyunsaturated fatty acids (exposure)
Genetic variants associated with plasma omega-3 and omega-6 PUFAs were obtained from the recent GWAS of plasma fatty acid in European ancestry. Single nucleotide polymorphisms (SNPs) that reach the suggestive significant genome-wide association level (p ≤ 5 × 10–6) and had a minor allele frequency of 0.01 or more were included. In this MR study, four omega-3 (ALA, EPA, DPA, and DHA) and three omega-6 (LA, AA, and AdrA) PUFAs were included. The GWAS of omega-3 (20) and omega-6 (18) PUFAs were from the Cohorts for Heart and Aging Research in Genomic Epidemiology (CHARGE) Consortium (n = 8,866 and 8,631). The unit of omega-3 and omega-6 was the percentage (%) of total fatty acids. Information about DHA and LA-related SNPs was obtained from an up-to-date meta-analysis of GWAS (19).
To date, the best-characterized gene loci for PUFAs are the fatty acid desaturase (FADS) genes, such as FADS1, FADS2, and FADS3. These biologically relevant candidate genes encode the δ-5 and δ-6 desaturases, which are involved in the metabolic conversion of the LA to longer chain omega-6 PUFAs. The SNPs strongly associated with fatty acid were mainly allocated in chromosome 11q12.2 (i.e., in genes of C11orf9/10, FEN1, and FADS), and chromosome 6p24.2 (i.e., ELOVL2). From the meta-analysis of GWAS, the most highly associated SNPs on chromosome 11 explained 3.8% of the variance of ALA, 2.0% of the variance of EPA, and 8.6% of the variance of DPA (20). We further conducted the mechanistically informative analysis using the strongest SNP related to fatty acid biosynthesis in chromosome 11 or 6 for each PUFA, respectively, to minimize the pleiotropic effect (single-locus MR analysis), which method has been widely used in previous studies (17, 21).
Genetic associations with sleep apnea and snoring (outcome)
The genetic associations with SA were obtained from the most recent and largest GWAS of SA and snoring by Campos et al. (22), which used multi-trait analysis of GWAS (MTAG) (23) to boost statistical power, leveraging the high genetic correlations between SA and snoring. The SA multi-trait discovery GWAS combined five cohorts from the United Kingdom (UK Biobank; UKB), Canada (Canadian Longitudinal Study of Aging; CLSA), Australia (Australian Genetics of Depression Study; AGDS), the United States (Partner’s Healthcare Biobank), and Finland genetic research (FinnGen) with a total number of 25,008 SA cases and 172,050 snoring cases (total = 523,366) with replication in an independent sample from 23andMe (total = 1,477,352 and cases = 175,522). Totally, 43 SNPs for SA explained 0.87% variance. For each cohort, SA was coded using either International Classification of Diseases Tenth Revision (ICD-10) codes via primary care records or self-reported diagnostic items via questionnaires (Supplementary material). All cohorts were restricted to European descent individuals with the adjustment for age, sex, batch (where relevant), and genetic ancestry principal components derived from genotype data [individual cohort details have been reported elsewhere (22)]. Since this GWAS paper is currently a pre-print, we used the latest published GWAS for sleep apnea from the FinnGen Study (24), and then repeated the analysis to validate our findings in the sensitivity analysis.
Statistical analysis
We estimated the F-statistic for each SNP as the square of the SNP-exposure association divided by the variance of the SNP-exposure association (25), and we generated the mean F-statistic for exposure (26). Independent variants (r2 < 0.01) were selected using the “clump_data” function (EUR population) of the “MR-Base” R package. In the sensitivity analysis, we replicated the MR analysis using SNPs with a p-value < 5 × 10–8. We obtained MR estimates by meta-analyzing the SNP-specific Wald estimates (effect of SNP on outcome divided by SNP on exposure) using inverse-variance weighted (IVW) with multiplicative random effects, which assumes balanced pleiotropy. The presence of heterogeneity due to pleiotropy was indicated by high Cochran’s Q and I2 statistics. To ensure the same effect allele was used for exposure and outcome for palindromic SNPs (coded A/T or C/G), we aligned them on effect allele frequency and the coding (forward or reverse). In sensitivity analyses, we obtained MR estimates using different methods with different assumptions, including the weighted median (WM) and MR-PRESSO. The WM (of SNP-specific Wald estimates) gives robust estimates if more than 50% of the information is derived from valid SNPs. Mendelian randomization pleiotropy residual sum and outlier (MR-PRESSO) is another way to identify horizontal pleiotropic outliers, and corrects for pleiotropy via outlier removal, if necessary (27). We obtained the empirical p-value for the MR-PRESSO global test via 10,000 simulations and used the outlier corrected estimate if outliers were found. The MR-Egger intercept test is used to statistically check the presence of horizontal pleiotropy. Since body mass index (BMI) could be a confounder in the associations between PUFAs and SA, multivariable MR (MVMR) was conducted to assess the associations of PUFAs with SA after adjustment for BMI (28). To adjust for multiple comparisons, the Bonferroni multiple testing correction was applied, and two-sided p-values of < 0.007 (0.05/7) were considered significant. Power was estimated using the approximation that the sample size for IV analysis to obtain a given power is the sample size for exposure on outcome divided by the r2 for an instrument on exposure (29). The post-power calculation was based on the results of an online tool using several parameters, such as sample size of the outcome GWAS, variance explained by selected SNPs, and expected effect size.1
All statistical analyses were conducted in Stata version 13.1 (StataCorp LP, College Station, TX, United States) and R version 3.6.3 (R Foundation for Statistical Computing, Vienna, Austria) using the “TwoSampleMR,” “MendelianRandomization,” and “MRPRESSO” packages. The current MR study used publicly available summary data and does not require specific ethical approval.
Results
Totally, 6, 17, 12, and 12 SNPs associated with ALA, EPA, DPA, and DHA, respectively, were obtained at suggestive genome-wide significance (p-value < 5 × 10–6) after excluding linkage disequilibrium (r2 < 0.01) with the average F-statistic range from 34 to 95. Similarly, 23, 10, and 5 SNPs associated with LA, AA, and AdrA, respectively, were identified, with the average F-statistic range from 41 to 145. Proxy SNPs were used when there were missing SNPs in the outcome dataset. No SNP was palindromic. Supplementary Tables 2, 3 summarize the information extracted for each SA-related SNP in the current study. Using the single-locus MR analysis, only the strongest SNP associated with fatty acid in chromosomes 11 or 6 were collected to predict the levels of PUFAs, with the average F-statistic range from 104 to 699. Rs174547, rs174546, and rs174550 in FADS1 were used as the genetic instruments of ALA/DPA, DHA, and AdrA, respectively. Rs99780 and rs472031 in FADS2 and FADS3 were used as the genetic instruments of LA and AA, respectively. Finally, rs174538 in C11orf10 was used as the genetic instrument of EPA.
In primary results (Tables 1, 2), we found no association of ALA [odds ratio (OR) = 1.09 per% changed, 95% confidence interval (CI) 0.67–1.78], EPA (OR = 0.94, 95% CI 0.88–1.01), DPA (OR = 0.95, 95% CI 0.88–1.02), and DHA (OR = 0.99, 95% CI 0.96–1.02) with the risk of SA using the IVW method. Moreover, for omega-6 PUFAs, no association between LA (OR = 0.98, 95% CI 0.96–1.01), AA (1.00, 95% CI 0.99–1.01), and AdrA (0.93, 95% CI 0.71–1.21) with the risk of SA was found. Similar results were found in sensitivity analyses using WM and MR-PRESSO and using SNPs with a p-value of < 5 × 10–8 (Supplementary Table 4). The MR-Egger intercept suggested no evidence for directional horizontal pleiotropy (all p-values > 0.05). Supplementary Figures 1,2 show the scatter plots of omega-3 and omega-6 PUFAs with SA in different methods. In sensitivity analysis, no associations of PUFAs with SA were found using the published GWAS from the FinnGen study (Supplementary Tables 5,6). There was no association of ALA and LA with SA found after adjustment for BMI (Supplementary Table 7).
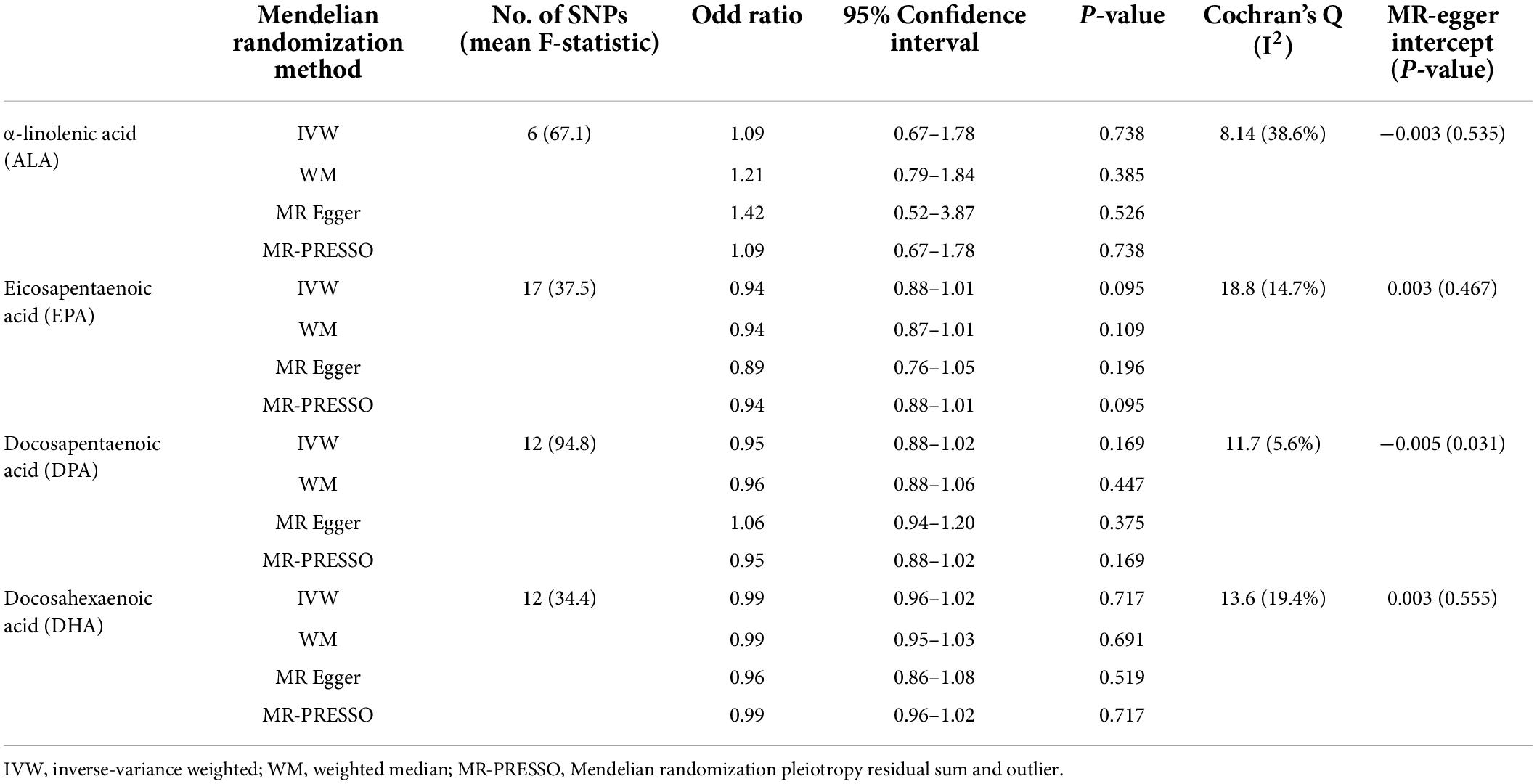
Table 1. Mendelian randomization (MR) estimates of causality between plasma omega-3 polyunsaturated fatty acids (PUFAs) and sleep apnea (SA).
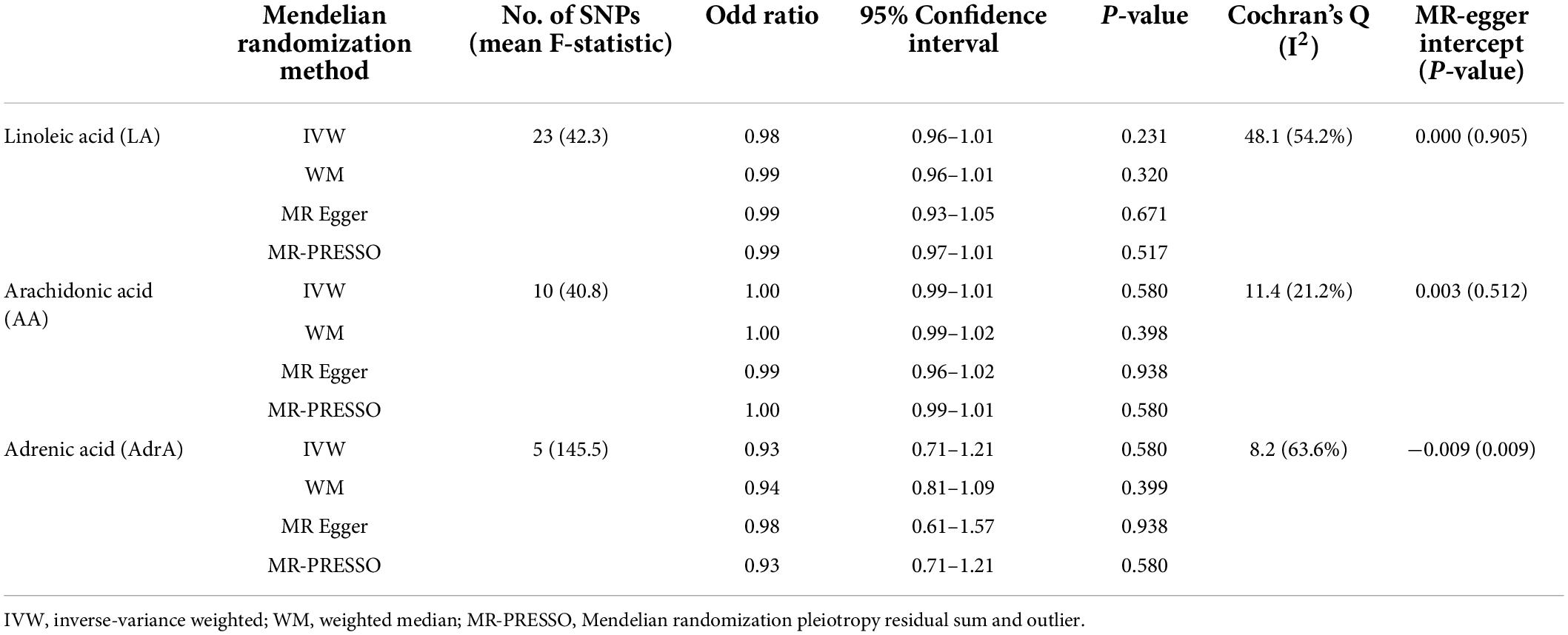
Table 2. Mendelian randomization (MR) estimates of causality between plasma omega-6 polyunsaturated fatty acids and sleep apnea.
The single-locus MR analysis (Table 3) showed similar results to the primary results. A little genetic association of omega-3 and omega-6 PUFAs with SA was found, with the OR ranging from 0.94 to 1.21 (p-values from 0.144 to 0.937).
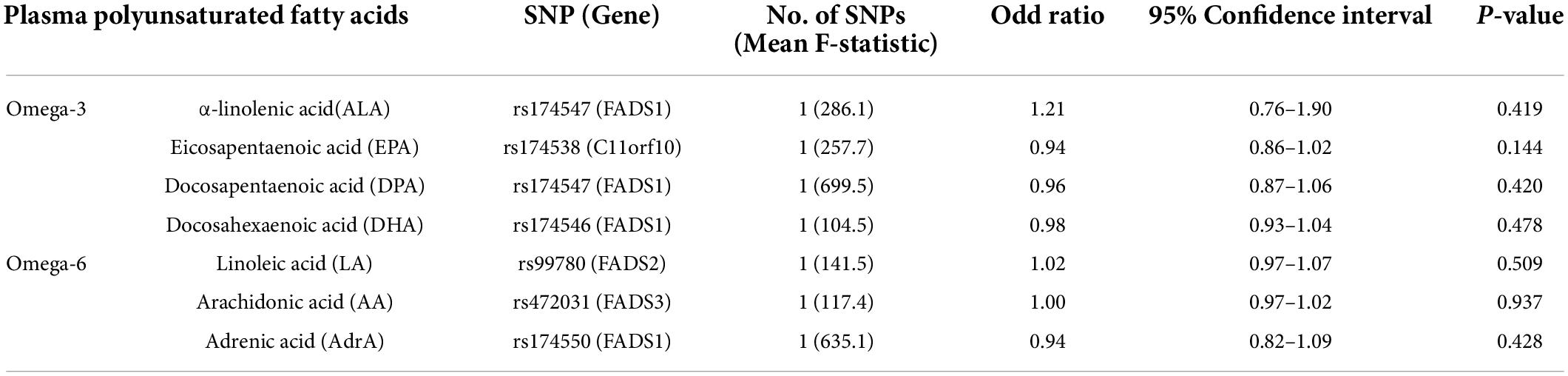
Table 3. Mendelian randomization (MR) estimates of causality between plasma polyunsaturated fatty acids and sleep apnea (FADS, C11orf10, and ELOVL gene loci).
Discussion
Principal findings
Our MR analyses first showed no association between lifelong exposure to plasma omega-3 PUFAs (ALA, EPA, DPA, and EHA) and omega-6 PUFAs (LA, AA, and AdrA) with the risk of SA. Protective effects reported in previous observational studies might be explained by residual confounding.
Comparison with other studies
Results of the present MR study did not support findings from previous observational studies showing that lower circulating omega-3 PUFAs (EPA and DHA) levels were associated with SA severity (12, 13, 30). A randomized, placebo-controlled trial showed that a daily intake of 600 mg omega-3 DHA supplements for 16 weeks improved sleep quality (less sleep disturbed breathing) (31). To date, no RCT assessed the effect of omega-6 PUFAs on SA (32), although better sleep quality was found after omega-6 PUFA supplementation in children with attention deficit hyperactivity disorder (ADHD) (33). Our study adds by clarifying that there is no causal effect of omega-3 and omega-6 PUFAs on SA, which is informative before an RCT and can make the most effective use of scarce resources.
Possible mechanisms
The underlying mechanisms explaining associations between PUFAs and SA are far from clear. One of the proposed mechanisms was an inflammatory response to obstructive sleep apnea (OSA), i.e., mediating by cytokines, such as tumor necrosis factor (TNF-α) (15) and interleukin 6 (IL-6) (34). Animal and human studies have shown that the production of cytokines can be reduced by omega-3 PUFAs (35–37). However, the causal association between cytokines and SA was not evident. Therefore, whether the associations of PUFAs with SA are causal, a reflection of the underlying comorbidities, or merely due to chance, is yet to be confirmed, although this mechanism pathway seems reasonable.
Strengths and limitations
To our knowledge, our study is the first MR study examining the effect of omega-3 and omega-6 PUFAs on the risk of SA. The strengths of the present study included the use of MR to minimize residual confounding and reverse causality in traditional observational studies, and genetic validation of the wide range of PUFAs. Nevertheless, several limitations exist. MR is based on three stringent assumptions, i.e., the genetic instruments are strongly associated with the exposure; no confounders for the associations between the genetic instruments and the outcome; and the genetic instruments are not linked with the outcome other than via the exposure (no pleiotropy). To satisfy these assumptions, we only selected SNPs strongly associated with PUFAs reaching suggestive genome-wide significance, and replicated our findings using the most functionally related SNP in chromosome 11 or 6, including the well-established gene FADS (18). Population stratification might be a confounder in MR studies. However, we only used studies involving people of European descent, with genomic control. Confounding due to population stratification should be minimized. Regarding the potential pleiotropic effects, we conducted sensitivity analyses using multiple methods (i.e., WM, MR-PRESSO, and MVMR) to assess pleiotropy and found no evidence of a pleiotropic effect. Second, the estimates might be biased toward the observational associations if the exposure and outcome data came from the same sample (38). However, the sample of PUFAs GWAS had no overlap with the UK Biobank. Third, the effects of endogenous PUFAs may not be exactly the same as those of PUFAs from dietary intake. However, the essential fatty acids in omega-3 and omega-6 PUFA families, i.e., LA and ALA, cannot be synthesized directly in the human body (39). Serum LA levels are associated with dietary intake of LA (40). Moreover, the use of genetically predicted plasma PUFAs can eliminate measurement error, since the observational studies use one snapshot of measurement rather than lifetime exposure (41). Fourth, since cases of SA and snoring were identified by clinic diagnosis or self-report, misclassification was inevitable in the current study. However, the genetic correlation analyses from our upstream GWAS of SA (22) suggested different diagnostic criteria (i.e., by clinic diagnosis or self-report) have a comparable genetic architecture. After all, ascertaining SA cases using objective measures is difficult for GWAS with a large sample size. Fifth, the possible non-linear associations of PUFAs with SA could not be examined in the present study using summary-level statistics. Further studies using individual-level data are warranted to explore the potential non-linear patterns. Sixth, we could not distinguish the effects of central sleep apnea (CSA) and obstructive sleep apnea (OSA) and thus only examined SA in general, although SA likely represents the effect of OSA given the much higher prevalence of OSA (42).
Conclusion and public health implications
We first found no genetic evidence supporting the causal role of omega-3 and omega-6 PUFAs in the risk of SA. From a public health perspective, our findings refute the notion that consumption of foods rich in PUFAs or the use of PUFAs supplementation can reduce the risk of SA. Further MR studies, especially studies from other populations, providing more objective-diagnosed cases of SA and, ideally, using additional variants as genetic instruments are warranted to replicate the results.
Data availability statement
The exposure data are available on request after approval by AC and MR. The outcome data underlying this article are available in these articles and in their online Supplementary material.
Author contributions
JW, AC, MR, and LX made substantial contributions to the conception and design and interpretation of data. JW and LX analyzed the data and drafted the article. YH, HY, ZL, AC, and MR revised it critically for important intellectual content. LX was guarantor. All authors gave their final approval for the manuscript.
Funding
This work was funded by the China Postdoctoral Science Foundation (2022M713557).
Acknowledgments
We thank the investigators from the United Kingdom (UK Biobank; UKB), Canada (Canadian Longitudinal Study of Aging; CLSA), Australia (Australian Genetics of Depression Study; AGDS), the United States (Partner’s Healthcare Biobank), Finland genetic research (FinnGen), CARDIoGRAMplusC4D, MEGASTROKE, and other GWAS used in the present study.
Conflict of interest
The authors declare that the research was conducted in the absence of any commercial or financial relationships that could be construed as a potential conflict of interest.
Publisher’s note
All claims expressed in this article are solely those of the authors and do not necessarily represent those of their affiliated organizations, or those of the publisher, the editors and the reviewers. Any product that may be evaluated in this article, or claim that may be made by its manufacturer, is not guaranteed or endorsed by the publisher.
Supplementary material
The Supplementary Material for this article can be found online at: https://www.frontiersin.org/articles/10.3389/fnut.2022.956900/full#supplementary-material
Footnotes
References
1. Benjafield AV, Ayas NT, Eastwood PR, Heinzer R, Ip MSM, Morrell MJ, et al. Estimation of the global prevalence and burden of obstructive sleep apnoea: a literature-based analysis. Lancet Respir Med. (2019) 7:687–98. doi: 10.1016/S2213-2600(19)30198-5
2. Wang X, Ouyang Y, Wang Z, Zhao G, Liu L, Bi Y. Obstructive sleep apnea and risk of cardiovascular disease and all-cause mortality: a meta-analysis of prospective cohort studies. Int J Cardiol. (2013) 169:207–14.
3. Huang T, Lin BM, Stampfer MJ, Tworoger SS, Hu FB, Redline S. A population-based study of the bidirectional association between obstructive sleep apnea and type 2 diabetes in three prospective U.S. Cohorts. Diabetes Care. (2018) 41:2111–9. doi: 10.2337/dc18-0675
4. Emamian F, Khazaie H, Tahmasian M, Leschziner GD, Morrell MJ, Hsiung G-YR, et al. The association between obstructive sleep apnea and Alzheimer’s disease: a meta-analysis perspective. Front Aging Neurosci. (2016) 8:78. doi: 10.3389/fnagi.2016.00078
5. Shamsuzzaman AS, Winnicki M, Lanfranchi P, Wolk R, Kara T, Accurso V, et al. Elevated C-reactive protein in patients with obstructive sleep apnea. Circulation. (2002) 105:2462–4.
6. Cao Y, Song Y, Ning P, Zhang L, Wu S, Quan J, et al. Association between tumor necrosis factor alpha and obstructive sleep apnea in adults: a meta-analysis update. BMC Pulm Med. (2020) 20:215. doi: 10.1186/s12890-020-01253-0
7. Zhao JV, Schooling CM. Effect of linoleic acid on ischemic heart disease and its risk factors: a Mendelian randomization study. BMC Med. (2019) 17:61. doi: 10.1186/s12916-019-1293-x
8. Coelho OGL, da Silva BP, Rocha D, Lopes LL, Alfenas RCG. Polyunsaturated fatty acids and type 2 diabetes: impact on the glycemic control mechanism. Crit Rev Food Sci Nutr. (2017) 57:3614–9.
9. Zhao JV, Schooling CM. The role of linoleic acid in asthma and inflammatory markers: a Mendelian randomization study. Am J Clin Nutr. (2019) 110:685–90.
10. Alzoubi MR, Aldomi Al-Domi H. Could omega-3 fatty acids a therapeutic treatment of the immune-metabolic consequence of intermittent hypoxia in obstructive sleep apnea? Diabetes Metab Syndr. (2017) 11:297–304. doi: 10.1016/j.dsx.2016.06.024
11. Tricon S, Burdge GC, Kew S, Banerjee T, Russell JJ, Grimble RF, et al. Effects of cis-9,trans-11 and trans-10,cis-12 conjugated linoleic acid on immune cell function in healthy humans. Am J Clin Nutr. (2004) 80:1626–33.
12. Ladesich JB, Pottala JV, Romaker A, Harris WS. Membrane level of omega-3 docosahexaenoic acid is associated with severity of obstructive sleep apnea. J Clin Sleep Med. (2011) 7:391–6. doi: 10.5664/JCSM.1198
13. Tittus J, Huber MT, Storck K, Köhler A, Köhler JM, von Arnim T, et al. Omega-3 index and obstructive sleep apnea: a cross-sectional study. J Clin Sleep Med. (2017) 13:1131–6. doi: 10.5664/jcsm.6754
14. Cicero AF, Ferroni A, Ertek S. Tolerability and safety of commonly used dietary supplements and nutraceuticals with lipid-lowering effects. Expert Opin Drug Saf. (2012) 11:753–66.
15. Scorza FA, Cavalheiro EA, Scorza CA, Galduróz JC, Tufik S, Andersen ML. Sleep apnea and inflammation – Getting a good night’s sleep with omega-3 supplementation. Front Neurol. (2013) 4:193. doi: 10.3389/fneur.2013.00193
16. Davies NM, Holmes MV, Davey Smith G. Reading Mendelian randomisation studies: a guide, glossary, and checklist for clinicians. BMJ. (2018) 362:k601. doi: 10.1136/bmj.k601
17. Yuan S, Larsson SC. Association of genetic variants related to plasma fatty acids with type 2 diabetes mellitus and glycaemic traits: a Mendelian randomisation study. Diabetologia. (2020) 63:116–23. doi: 10.1007/s00125-019-05019-0
18. Guan W, Steffen BT, Lemaitre RN, Wu JHY, Tanaka T, Manichaikul A, et al. Genome-wide association study of plasma N6 polyunsaturated fatty acids within the cohorts for heart and aging research in genomic epidemiology consortium. Circ Cardiovasc Genet. (2014) 7:321–31. doi: 10.1161/CIRCGENETICS.113.000208
19. Kettunen J, Demirkan A, Würtz P, Draisma HHM, Haller T, Rawal R, et al. Genome-wide study for circulating metabolites identifies 62 loci and reveals novel systemic effects of LPA. Nat Commun. (2016) 7:11122. doi: 10.1038/ncomms11122
20. Lemaitre RN, Tanaka T, Tang W, Manichaikul A, Foy M, Kabagambe EK, et al. Genetic loci associated with plasma phospholipid n-3 fatty acids: a meta-analysis of genome-wide association studies from the CHARGE Consortium. PLoS Genet. (2011) 7:e1002193. doi: 10.1371/journal.pgen.1002193
21. Jones HJ, Borges MC, Carnegie R, Mongan D, Rogers PJ, Lewis SJ, et al. Associations between plasma fatty acid concentrations and schizophrenia: a two-sample Mendelian randomisation study. Lancet Psychiatry. (2021) 8:1062–70. doi: 10.1016/S2215-0366(21)00286-8
22. Campos AI, Ingold N, Huang Y, Mitchell BL, Kho P-F, Han X, et al. Multi-trait genome-wide association study identifies new loci associated with sleep apnoea risk. medRxiv [Preprint]. (2022). doi: 10.1101/2020.09.29.20199893
23. Turley P, Walters RK, Maghzian O, Okbay A, Lee JJ, Fontana MA, et al. Multi-trait analysis of genome-wide association summary statistics using MTAG. Nat Genet. (2018) 50:229–37.
24. Strausz S, Ruotsalainen S, Ollila HM, Karjalainen J, Kiiskinen T, Reeve M, et al. Genetic analysis of obstructive sleep apnoea discovers a strong association with cardiometabolic health. Eur Respir J. (2021). 57:2003091. doi: 10.1183/13993003.03091-2020
25. Bowden J, Del Greco MF, Minelli C, Davey Smith G, Sheehan NA, Thompson JR. Assessing the suitability of summary data for two-sample Mendelian randomization analyses using MR-Egger regression: the role of the I2 statistic. Int J Epidemiol. (2016) 45:1961–74. doi: 10.1093/ije/dyw220
26. Richardson TG, Sanderson E, Palmer TM, Ala-Korpela M, Ference BA, Davey Smith G, et al. Evaluating the relationship between circulating lipoprotein lipids and apolipoproteins with risk of coronary heart disease: a multivariable Mendelian randomisation analysis. PLoS Med. (2020) 17:e1003062. doi: 10.1371/journal.pmed.1003062
27. Verbanck M, Chen CY, Neale B, Do R. Detection of widespread horizontal pleiotropy in causal relationships inferred from Mendelian randomization between complex traits and diseases. Nat Genet. (2018) 50:693–8.
28. Burgess S, Thompson SG. Multivariable Mendelian randomization: the use of pleiotropic genetic variants to estimate causal effects. Am J Epidemiol. (2015) 181:251–60.
29. Freeman G, Cowling BJ, Schooling CM. Power and sample size calculations for Mendelian randomization studies using one genetic instrument. Int J Epidemiol. (2013) 42:1157–63.
30. Nakabayashi K, Jujo K, Saito K, Oka T, Hagiwara N. Evaluation of the association between sleep apnea and polyunsaturated fatty acids profiles in patients after percutaneous coronary intervention. Heart Vessels. (2017) 32:1296–303. doi: 10.1007/s00380-017-1010-7
31. Montgomery P, Burton JR, Sewell RP, Spreckelsen TF, Richardson AJ. Fatty acids and sleep in UK children: subjective and pilot objective sleep results from the DOLAB study–a randomized controlled trial. J Sleep Res. (2014) 23:364–88. doi: 10.1111/jsr.12135
32. Zhao M, Tuo H, Wang S, Zhao L. The effects of dietary nutrition on sleep and sleep disorders. Mediat Inflamm. (2020) 2020:3142874.
33. Yehuda S, Rabinovitz-Shenkar S, Carasso RL. Effects of essential fatty acids in iron deficient and sleep-disturbed attention deficit hyperactivity disorder (ADHD) children. Eur J Clin Nutr. (2011) 65:1167–9. doi: 10.1038/ejcn.2011.80
34. Ünüvar Doğan F, Yosunkaya Ş, Kuzu Okur H, Can Ü. Relationships between obstructive sleep apnea syndrome, continuous positive airway pressure treatment, and inflammatory cytokines. Sleep Disord. (2014) 2014:518920.
35. Meydani SN. Effect of (n-3) polyunsaturated fatty acidson cytokine production and their biologic function. Nutrition. (1996) 12(1 Suppl.):S8–14.
36. Kiecolt-Glaser JK, Belury MA, Andridge R, Malarkey WB, Glaser R. Omega-3 supplementation lowers inflammation and anxiety in medical students: a randomized controlled trial. Brain Behav Immun. (2011) 25:1725–34. doi: 10.1016/j.bbi.2011.07.229
37. Madison AA, Belury MA, Andridge R, Renna ME, Rosie Shrout M, Malarkey WB, et al. Omega-3 supplementation and stress reactivity of cellular aging biomarkers: an ancillary substudy of a randomized, controlled trial in midlife adults. Mol Psychiatry. (2021) 26:3034–42. doi: 10.1038/s41380-021-01077-2
38. Burgess S, Davies NM, Thompson SG. Bias due to participant overlap in two-sample Mendelian randomization. Genet Epidemiol. (2016) 40:597–608.
39. Nagy K, Tiuca ID. Importance of fatty acids in physiopathology of human body: in fatty acids. London: IntechOpen (2017).
40. Ramsden CE, Zamora D, Leelarthaepin B, Majchrzak-Hong SF, Faurot KR, Suchindran CM, et al. Use of dietary linoleic acid for secondary prevention of coronary heart disease and death: evaluation of recovered data from the Sydney diet heart study and updated meta-analysis. BMJ. (2013) 346:e8707. doi: 10.1136/bmj.e8707
41. Carter AR, Gill D, Davies NM, Taylor AE, Tillmann T, Vaucher J, et al. Understanding the consequences of education inequality on cardiovascular disease: Mendelian randomisation study. BMJ. (2019) 365:l1855. doi: 10.1136/bmj.l1855
Keywords: plasma polyunsaturated fatty acid, omega-3, omega-6, sleep apnea, Mendelian randomization
Citation: Wang J, Huang Y, Yang H, Lin Z, Campos AI, Rentería ME and Xu L (2022) Plasma polyunsaturated fatty acid concentrations and sleep apnea risk: A two-sample Mendelian randomization study. Front. Nutr. 9:956900. doi: 10.3389/fnut.2022.956900
Received: 30 May 2022; Accepted: 25 July 2022;
Published: 18 August 2022.
Edited by:
Muzi Na, The Pennsylvania State University (PSU), United StatesReviewed by:
Guannan Bai, Zhejiang University School of Medicine, ChinaZhengyuan Wang, Shanghai Municipal Center for Disease Control and Prevention (SCDC), China
Copyright © 2022 Wang, Huang, Yang, Lin, Campos, Rentería and Xu. This is an open-access article distributed under the terms of the Creative Commons Attribution License (CC BY). The use, distribution or reproduction in other forums is permitted, provided the original author(s) and the copyright owner(s) are credited and that the original publication in this journal is cited, in accordance with accepted academic practice. No use, distribution or reproduction is permitted which does not comply with these terms.
*Correspondence: Lin Xu, xulin27@mail.sysu.edu.cn