- 1Laboratory on Thymus Research, Oswaldo Cruz Institute, Oswaldo Cruz Foundation, Rio de Janeiro, Brazil
- 2Brazilian National Institute of Science and Technology on Neuroimmunomodulation, Oswaldo Cruz Institute, Oswaldo Cruz Foundation, Rio de Janeiro, Brazil
- 3Rio de Janeiro Research Network on Neuroinflammation, Oswaldo Cruz Institute, Oswaldo Cruz Foundation, Rio de Janeiro, Brazil
- 4Laboratory on Leishmaniasis Research, Oswaldo Cruz Institute, Oswaldo Cruz Foundation, Rio de Janeiro, Brazil
- 5Laboratory of Immunology, Reference Center for Lactobacilli Centro de Referencia para Lactobacilos-Consejo Nacional de Investigaciones Científicas y Técnicas (CERELA-CONICET), San Miguel de Tucumán, Argentina
- 6Laboratory of Immunology, Faculty of Biochemistry, Chemistry and Pharmacy, National University of Tucumán, San Miguel de Tucumán, Argentina
- 7School of Pharmacy and Biomedical Sciences, University of Central Lancashire, Preston, United Kingdom
Undernutrition remains a major issue in global health. Low protein-energy consumption, results in stunting, wasting and/or underweight, three deleterious forms of malnutrition that affect roughly 200 million children under the age of five years. Undernutrition compromises the immune system with the generation of various degrees of immunodeficiency, which in turn, renders undernourished individuals more sensitive to acute infections. The severity of various infectious diseases including visceral leishmaniasis (VL), influenza, and tuberculosis is associated with undernutrition. Immunosuppression resulting from protein-energy undernutrition severely impacts primary and secondary lymphoid organs involved in the response to related pathogens. The thymus—a primary lymphoid organ responsible for the generation of T lymphocytes—is particularly compromised by both undernutrition and infectious diseases. In this respect, we will discuss herein various intrathymic cellular and molecular interactions seen in undernutrition alone or in combination with acute infections. Many examples illustrated in studies on humans and experimental animals clearly revealed that protein-related undernutrition causes thymic atrophy, with cortical thymocyte depletion. Moreover, the non-lymphoid microenvironmental compartment of the organ undergoes important changes in thymic epithelial cells, including their secretory products such as hormones and extracellular matrix proteins. Of note, deficiencies in vitamins and trace elements also induce thymic atrophy. Interestingly, among the molecular interactions involved in the control of undernutrition-induced thymic atrophy is a hormonal imbalance with a rise in glucocorticoids and a decrease in leptin serum levels. Undernutrition also yields a negative impact of acute infections upon the thymus, frequently with the intrathymic detection of pathogens or their antigens. For instance, undernourished mice infected with Leishmania infantum (that causes VL) undergo drastic thymic atrophy, with significant reduction in thymocyte numbers, and decreased levels of intrathymic chemokines and cytokines, indicating that both lymphoid and microenvironmental compartments of the organ are affected. Lastly, recent data revealed that some probiotic bacteria or probiotic fermented milks improve the thymus status in a model of malnutrition, thus raising a new field for investigation, namely the thymus-gut connection, indicating that probiotics can be envisioned as a further adjuvant therapy in the control of thymic changes in undernutrition accompanied or not by infection.
Introduction
Despite one of the global health priorities listed in the Sustainable Development Goals (SDGs) by the United Nations is combating hunger and ensuring sustainable food security and proper nutrition (1), malnutrition remains a serious public health problem worldwide. The World Health Organization (WHO) defines malnutrition as the deficiency or excess in the consumption of specific energy and/or nutrients in relation to the needs of an individual, resulting in corresponding pathologies: undernutrition or obesity (2). The lack of macro and micronutrients, and in particular the low consumption of proteins and calories results in four deleterious forms of undernutrition: (i) wasting, (ii) stunting, (iii) underweight, and (iv) micronutrient deficiencies. (i) Wasting is characterized as low weight-for-height, and in children it may be lethal if not appropriately treated; (ii) stunting occurs when the individual presents low height for the respective age, usually due to chronic undernutrition; (iii) underweight is defined as low weight-for-age, and underweighted children may be stunted, wasted or both. Lastly, (iv) micronutrient undernutrition refers to lack of vitamins and minerals that are essential for body functions (2). It is estimated that stunt and wasting affect virtually 200 million children under 5 years of age around the world, whereas in the adult population this number can reach 462 million. On the other hand, around 42 million children globally are overweight or obese (2).
Malnutrition thus constitutes a serious global public health issue, particularly in developing countries. According to a recent report (3) of the Food and Agriculture Organization (FAO) of the United Nations, 720–811 million people in the world suffered from hunger in 2020, increasing by 161 million the number of people who experienced hunger in 2019. The COVID-19 pandemic contributed substantially to this increase. The global assessment of food insecurity and malnutrition for 2020 shows that not only world hunger has increased, but also the prevalence of undernutrition rose by 4% in one single year (3).
Due to the pandemic, the prevalence of different forms of malnutrition has increased worldwide and it is estimated that these effects will be lasting, as already seen in 2021. In fact, the COVID-19 pandemic has widened and worsened inequalities between countries, affecting the livelihoods of an estimated 1.6 billion workers in the formal economy (1). Around 12% of the world’s population suffered from severe food insecurity in 2020. In Latin America and Caribbean region in 2020, 14 million more people were affected by hunger as compared with 2019 (3). Data from the National Survey on Food Insecurity in the Context of the COVID-19 Pandemic in Brazil showed that the country went back 15 years in five, and hunger returned to be a structural problem (4), as depicted in Figure 1.
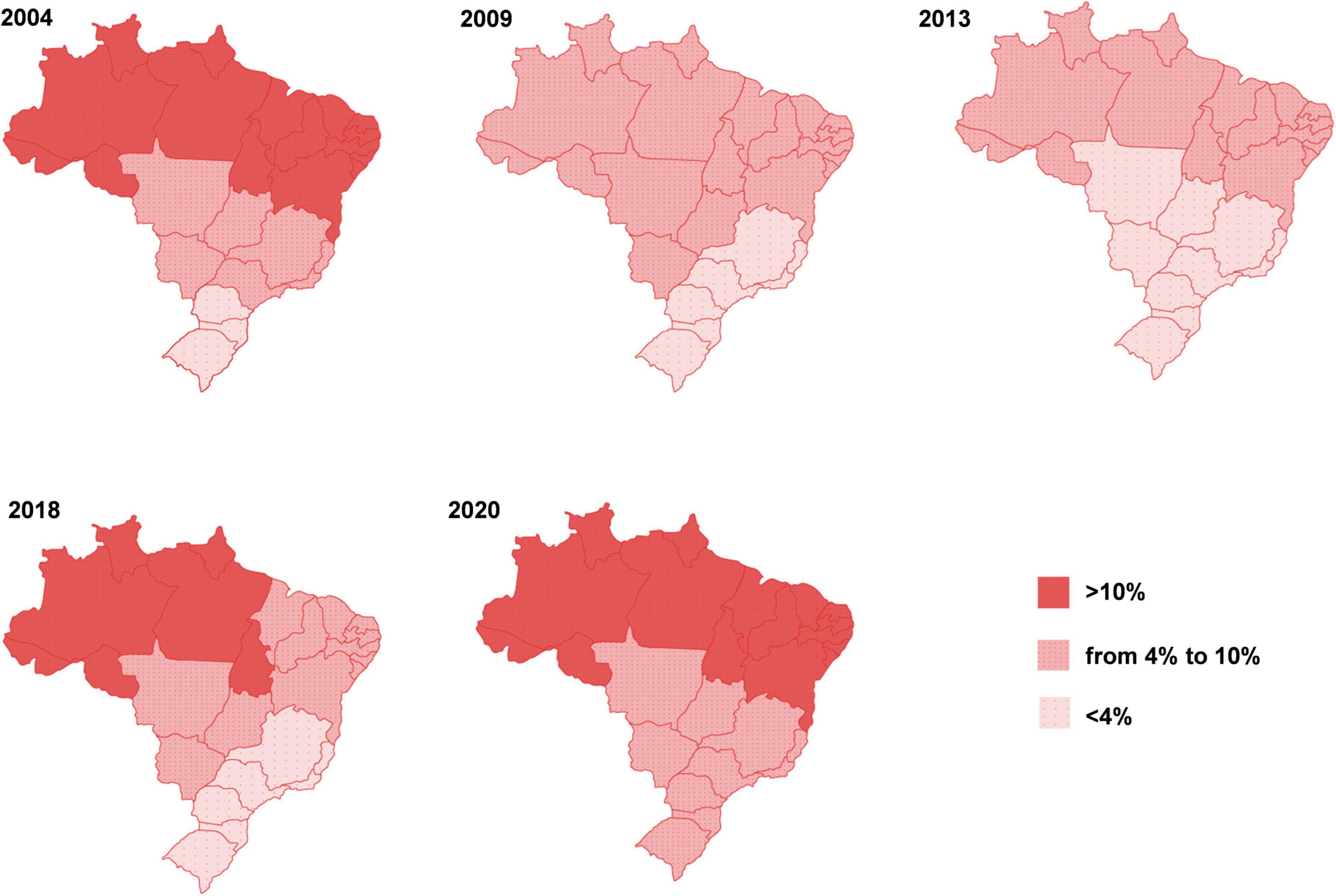
Figure 1. Evolution of hunger in Brazil. The figure shows the percentage of the population affected by severe food insecurity from 2004 to 2020, seen in different macro-regions of the country. Figure reproduced (with permission) from the Report of the National Survey of Food Insecurity in the Context the COVID-19 Pandemic in Brazil published by the Brazilian Research Network of Food and Nutrition Sovereignty and Security (applied herein with permission from Rede PENSSAN, http://olheparaafome.com.br/VIGISAN_Inseguranca_alimentar.pdf).
The decrease in proper food intake results in a series of physiological changes, including: (i) growth restriction; (ii) reduction of fat, muscle, and visceral mass; as well as (iii) reduction in basal metabolic rate and energy expenditure (5). These alterations also comprise biochemical parameters with reduced levels of triiodothyroxine (T3), insulin, insulin-like growth factor-1 (IGF-1) and leptin, among others; together with increased levels of growth hormone (GH) and cortisol (5, 6). Overall, undernutrition induces changes in metabolic, hormonal and glucoregulatory mechanisms (5, 6). Also, undernutrition modulates the intestinal microbiota and dysbiotic events can be observed as a cause and/or consequence of undernutrition, accompanied by local and systemic chronic inflammation (7). Altered nutrient absorption and chronic inflammation seems to be related to the fact that malnourished individuals are more susceptible to various diseases (7–9).
The dynamic relationship between infection, nutrition and immunity is long recognized. Being a systemic disease, undernutrition also affects primary and secondary lymphoid organs, harming the immune system of malnourished individuals (10). Since the immune response depends on cell replication and the synthesis of active protein compounds, undernutrition clearly has a negative impact on immunity (11). Actually, immunosuppression caused by protein-energy undernutrition increases susceptibility to acute infections and leads to the development of more severe forms of disease, whether they are caused by parasites, protozoa, bacteria or viruses.
In the present review we aimed at focusing the effects of undernutrition and infection upon one specific compartment of the immune system, namely the thymus, particularly the changes in the organ involving cellular and molecular interactions. Yet, before going into this point, it seems worthwhile to provide a general, yet concise background of the thymus and the generation of T-lymphocytes.
The thymic microenvironment and intrathymic T-cell differentiation
The thymus is a primary organ in the immune response, where the maturation and differentiation of T cells take place (12). The correct selection and migration of T cells occurs through a series of proliferation and differentiation stages dependent upon receiving instructions from the specialized thymus non-lymphoid microenvironment (13). In mammals, the organ is histologically divided lobules, each one comprising two main regions, namely cortex and medulla, with the cortex being denser in lymphocytes than the medulla. Developing thymocytes in different stages of maturation are specifically located in those regions. For example, immature CD4-CD8- double-negative (DN) and CD4+ CD8+ double-positive (DP) thymocytes are localized in the cortical region of the thymic lobules, whereas more mature CD4+ CD8- or CD4-CD8+ single-positive (SP) thymocytes are placed in the medulla (13). Such specific distribution indicates that thymocyte maturation occurs in parallel with organized and coordinated cell migration (14). In fact, disruption or abnormal cell migration impacts thymocyte development, as seen for example in Chagas disease (15).
Intrathymic thymocyte differentiation and migration, from the entrance of precursor cells to the exit of mature SP cells, is dependent on interactions controlled by the thymic tridimensional network. This is composed by cellular components such as thymic epithelial cells (TEC), thymic dendritic cells (TDC), macrophages and fibroblasts, as well as non-soluble and soluble molecules such as the extracellular matrix (ECM) proteins fibronectin, laminin, type I and IV collagens; cytokines as interleukin (IL)-2, IL-6, IL-7, and IL-22; chemokines as CXCL12, CCL4, and CCL7; hormones as thymosin, thymopoietin, and thymulin; and different typical soluble components of nervous tissues, such as neuropeptides and neurotransmitters (12, 15, 16).
During thymocyte development, cells pass through the positive selection and negative selection events; both being derived from the molecular interactions involving expression and survival signaling by the recognition of the T-cell receptor (TCR), upon interacting with microenvironmental cells, particularly dendritic cells, and epithelial cells. Positive selection can be defined as the process through which TCR-expressing CD4+ CD8+ developing thymocytes are rescued from programmed cell death upon interaction with self-antigens presented to the TCR by molecules of the major histocompatibility complex (MHC) by thymic microenvironmental cells. Positively selected thymocytes still in the cortex of the thymic lobules then move into the boundary of the cortex and medulla of the thymus for the presentation of self-antigens by the medullary thymic epithelial cells for the second time. In the medulla, thymocytes pass through the negative selection. In this case, differentiating cells undergo apoptosis if their TCR interact with high avidity with self-antigens coupled to the MHC class I or class II molecules expressed by microenvironmental cells in the organ (12, 13). Different peripheral tissue antigens (PTAs)—or self-antigens—coupled to MHC molecules are expressed on the membrane of medullary thymic epithelial cells (mTEC). Such expression is regulated by the autoimmune regulator (AIRE) transcription factor and avoids the development of self-antigen reactive cells, therefore preventing autoimmunity (17, 18). Alternatively, some clones that recognize self-antigens with high avidity become regulatory CD4+ CD25+ Foxp3+ T cells (Treg), through a mechanism dependent on the TCR signaling avidity and duration (19, 20).
Positioning of developing thymocytes along with differentiation depend on multiple interactions involving cell-cell, cell-matrix as well as chemokine-mediated interactions. For example, CXCL12 is secreted by TEC, and preferentially attracts immature CD4-CD8- and CD4+ CD8+ cells, by ligation with the receptor CXCR4. The chemokine CCL25 also attracts immature thymocytes, although all thymocyte subsets are responsive, which is in keeping with the fact that its CCR9 receptor is expressed at all stages of murine thymocyte differentiation. Interestingly, CCL19/CCR7 interactions participate in thymocyte exit, illustrating that thymocytes may switch their responses to a given chemokine, tuning their migratory process (21).
Other stimuli can participate in the overall process of intrathymic T-cell development, including hormones. Thymulin is zinc-couple nonapeptide classically defined as a thymic hormone, being produced by TEC. This molecule enhances developing thymocyte proliferation and IL-2 production (22). Interestingly, TECs also produce other thymic hormones as thymopoietin and thymosin-α1 (23), both known to have extrathymic functions, but also to induce progression of thymocyte differentiation.
Overall, we can say that in physiological conditions, at the end of their journey, CD4-SP and CD8-SP thymocytes exit the thymus to populate the peripheral lymphoid organs and participate in adaptive immune responses. The main stages of thymocyte maturation, selective processes and components of the thymic microenvironment are represented in Figure 2.
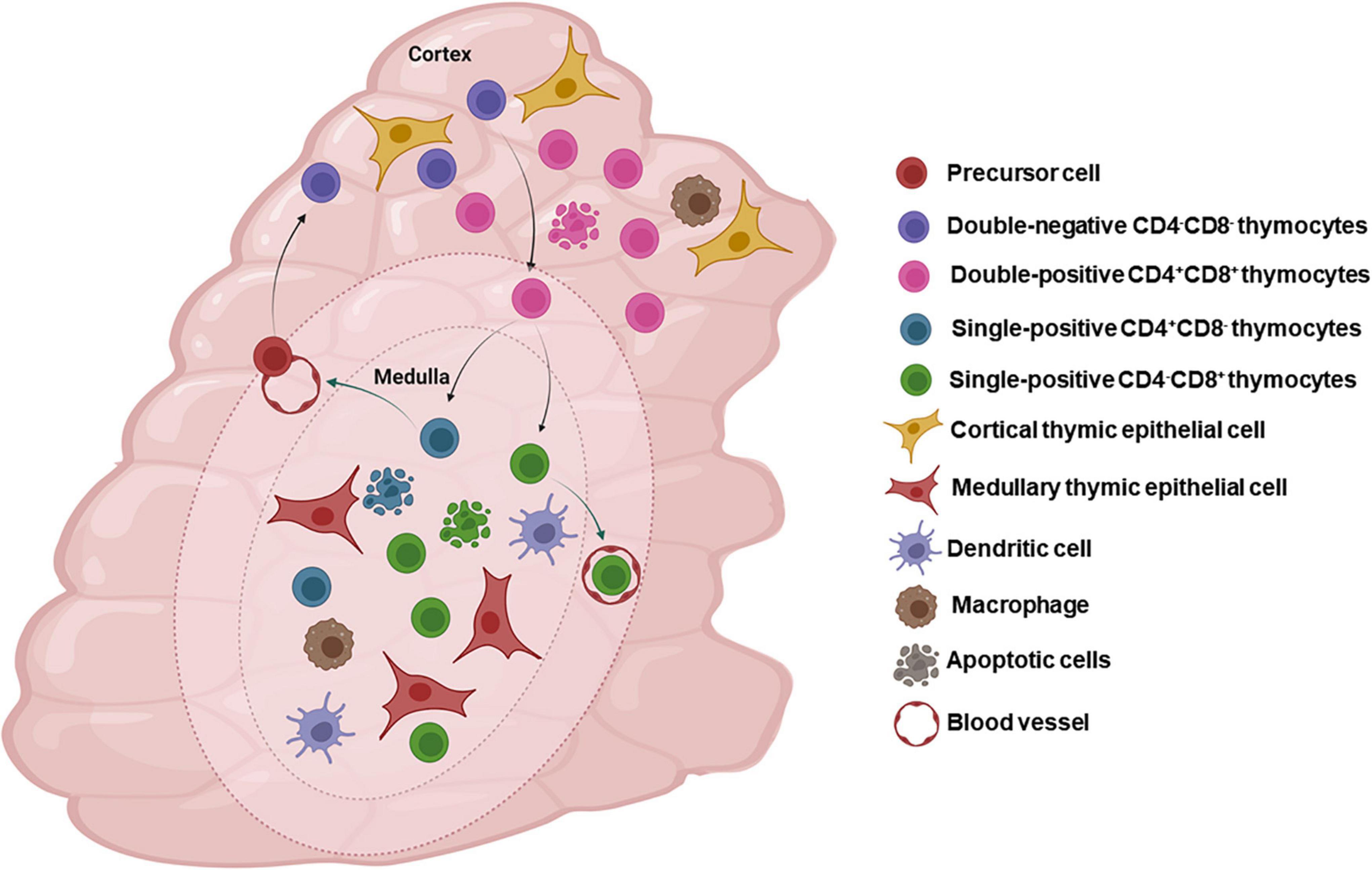
Figure 2. The thymic microenvironment and normal intrathymic T-cell differentiation. The panel schematically depicts in a concise way, the general process of thymocyte differentiation, in the context of the thymic microenvironment. Bone marrow-derived precursors enter the organ through blood vessels in the corticomedullary junction and migrate toward the outer cortex where they proliferate, but do not express the CD3/TCR complexes as well as the accessory molecules CD4 and CD8. There CD4/CD8 double-negative cells (DN) evolve to express TCR and become CD4+CD8+ (double-positive cells) and, under the control of the thymic microenvironment, undergo positive selection, with positively selected thymocytes migrating toward the medulla, where a large majority will die by negative selection, though apoptosis, and are ultimately resorbed within the organ by macrophages. Mature CD4+ or CD8+ single-positive thymocytes will eventually leave the organ to colonize the T-cell regions of peripheral lymphoid organs. Most thymocytes interact with microenvironmental cells in the cortical and medullary regions of the thymic lobules. Figure created with BioRender.com.
Undernutrition causes thymic atrophy, with cortical thymocyte depletion
It is well described that the intestinal homeostasis is maintained by immunomodulation of the gut mucosa. This is promoted by the interaction of endogenous microbiota with, food and a large variety of vitamins and nutrients, generating a proper intestinal immunity, which in turn maintains an adequate systemic immunity, highlighting the importance of the interaction between the gut mucosa immunity and the systemic immune response (24, 25). In parallel, protein-energy undernutrition is also a systemic condition that causes atrophy of lymphoid tissues, and the thymus is one of the most affected organs, with severe thymocyte loss, particularly CD4+ CD8+ cells (26–33). The thymus undergoes atrophy, as seen by histology, with changes in the lymphoid and microenvironmental compartments (26, 28). It was observed that in severely malnourished mice and children the thymic intralobular extracellular matrix containing fibronectin, laminin and type IV collagen increased. The enhancement of these extracellular matrix was correlated with the degree of thymocyte depletion in both humans and experimental models (26–29), although a cause-effect relationship has not been determined so far.
In any case, it is interesting that even in mice developing non-severe undernutrition by food restriction, there is a marked thymic atrophy and cortical thymocyte depletion, due to massive apoptosis, as well as a significant depletion in all the cytokine producing cells assayed (IL-12, IL-4, IL-6, IL-10, TNF-α, IFN-γ), as compared to control animals (30), as summarized in Figure 3.
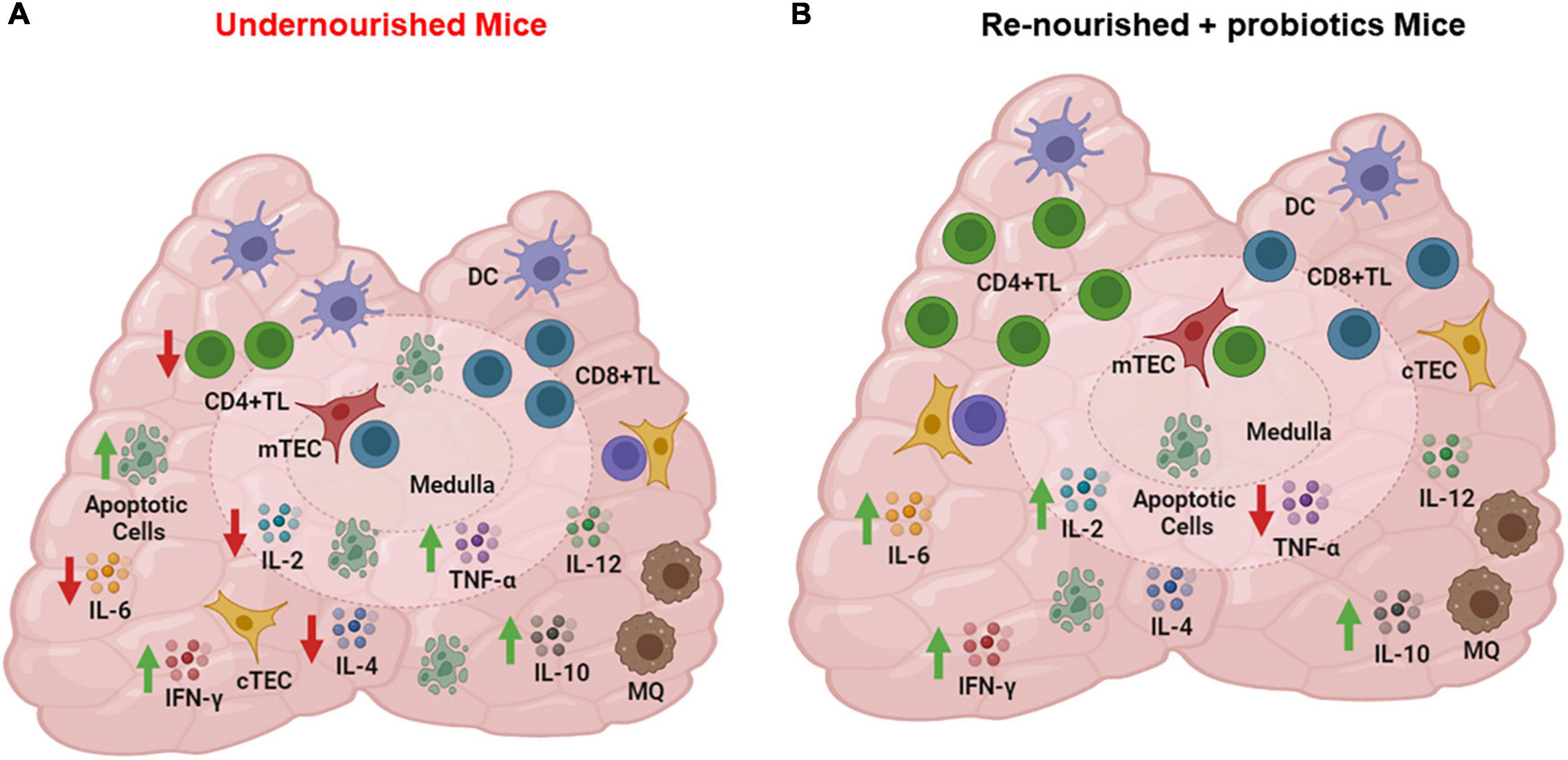
Figure 3. Cytokine producing cells in thymus of undernourished mice. (A) Thymic cells from undernourished mice exhibited a significant reduced production of IL-2, IL-6, and IL-4 (red arrows) and a significant increased production of TNF-α, and IL-10 (green arrows). (B) Thymic cells from renourished mice fed with probiotic fermented milk showed a significant increase in the production of IFN-γ, IL-2, IL-6, and IL-10 (green arrows) and reduction of TNF-α (red arrow). Cytokine production was detected by indirect immunofluorescence assays. Figure created with BioRender.com, based on data from Maldonado Galdeano et al. (30).
Finally, although not detailed herein, it is worthy to mention that deficiencies in vitamins, as well as in micronutrients (zinc, for example) also promote thymic atrophy with cortical thymocyte depletion and changes in the microenvironmental compartment (31). Particularly for the zinc, this should be linked to the fact that this element is important in several reactions in the thymus, being not only necessary to thymulin biological functions but also for promoting regeneration of TECs and T-cell reconstitution (22, 34).
The thymic microenvironment in protein undernutrition and infection
In addition to the lymphoid compartment, the thymic microenvironment is affected in various undernutritional and infectious conditions. Morphological changes in the thymic epithelium from protein-undernourished mice include the decrease in the volume of the epithelial tissue in the cortex and in the medulla of thymic lobules from undernourished mice, as compared to well-nourished control animals (32). By contrast, an increase, of intracytoplasmic accumulations of large, circular, homogeneously electron-dense profiles, rich in free and esterified cholesterol was reported in both in cortical and medullary TEC, of undernourished animals (33). Unfortunately, no data were reported concerning TEC death in this experimental model. In malnourished children the atrophy of the organ is clear with strong diminution of the cortex of the thymic lobules, with concomitant appearance of apoptotic thymocytes, particularly in the CD4+ CD8+ stage of differentiation.
In Trypanosoma cruzi-acutely infected mice, we also demonstrated changes in the expression of medullary and cortical specific TEC markers such as cytokeratins, as compared to controls, together with a general shrinkage of the thymic epithelial network (35). Such phenotypic changes do not prove functional changes of these cells in relation to the interaction with developing thymocytes. However, they did unravel an intrathymic pathology comprising both the cortical and medullary TEC network.
One functional parameter that has been largely evaluated in undernutrition conditions is the thymic hormone production by TEC. It was initially found that protein-undernourished mice exhibited abnormally low levels of circulating thymulin (32, 36), and that such a decrease was also observed in protein-undernourished rats and humans (37). Interestingly, even in human protein undernutrition secondary to anorexia nervosa, low thymulin serum levels were reported (38). Furthermore, decreased thymulin serum levels were reported in mice submitted to diets designed to trigger deficiency in zinc, iron, or vitamins (36, 39, 40). At least regarding zinc deficiency, similar results were found in humans (41). Remarkably, in severe infection conditions thymic endocrine function is also affected. We observed in T. cruzi-infected mice a transient decrease in the serum levels of the thymic hormone thymulin (31). In human HIV infection we and others showed a consistent and long-term diminution of thymulin secretion, in terms of both serum levels and intrathymic contents of the hormone (39–44).
It is noteworthy that the decrease in thymic hormone serum levels seen in undernutrition is not restricted to thymulin, since it was also reported as regards thymopoietin production. Prenatal undernutrition was significantly associated with reduced thymopoietin production in interaction with the duration of exclusive breast-feeding (45). These findings provide support for the importance of fetal and early infant programming of thymic function, and long-term implications for the immune system, and consequently adult disease risk.
As mentioned above, in addition to the abnormalities seen in thymic epithelial cells, the thymus from undernourished children presents a further microenvironmental alteration, namely, an increase in the deposition of ECM proteins. We studied by histological, ultrastructural and immunohistochemical means, thymuses obtained in necropsies from undernourished children. There is a consistent increase in the intralobular ECM-containing network, which could be ascertained histologically by the dense reticulin staining, and immunohistochemically by the higher contents of fibronectin, laminin, and type IV collagen. Importantly, the enhancement of thymic ECM in undernourished individuals positively correlated with the degree of thymocyte depletion (26). This correlation may represent a cause-effect relationship in which the contact of thymocytes with abnormally high amounts of thymic ECM triggers and/or enhances programmed cell death. However, this notion remains hypothetical, demanding experimental demonstration. In any case, it is noteworthy that developing thymocyte do express integrin-type ECM receptors (21), which makes this hypothesis feasible.
Interestingly, similar changes in thymic ECM were observed in glucocorticoid-hormone treated mice and TEC cultures (22), leading to hypothesis that the enhanced ECM deposition seen in undernutrition may be also related to high levels of serum glucocorticoid hormones. Actually, high glucocorticoid levels are seen in undernutrition (46, 47). Such an alteration was also seen in acute infections, as exemplified by experimental Chagas disease (35, 48). In this infection model, changes in ECM were accompanied by alterations in the migratory response of thymocytes, with abnormal export of CD4+CD8+ immature thymocytes, some of them having bypassed the normal negative selection process (48–50). Whether similar cell migration abnormalities exist in undernourished subjects, is to be investigated, although peripheral CD4+CD8+ T cells have been detected in the blood of chagasic patients (51).
Along with the changes in the thymic microenvironment seen in undernutrition conditions, obesity also induces severe alterations in this compartment, as seen in experimental models. One is the db/db mouse that develops a diabetes with obesity, due to a deficit in the expression of leptin receptor, which leads to obesity and type II Diabetes (52). We showed a precocious thymic involution in these animals, with cortical thymocyte depletion as well as changes in the TEC network, as ascertained by ultrastructural changes in these cells and a precocious decrease in the production of the TEC-derived thymulin (53–56). Again, these thymic events may be due to high corticosterone serum levels seen in these mice (57). In any case, these results unravel a thymic alteration in obese animals, which is likely related to an altered adaptive immune response. Whether such changes exist in humans need to be determined. Yet, it is conceivable since during age-dependent thymic atrophy, there is an increase in the numbers of adipose cells in the thymus (58).
Hormonal imbalance underlines thymic changes in protein undernutrition
Besides the alterations above cited, the impact of protein undernutrition in the thymus is accompanied by a hormonal imbalance in the organ. As mentioned above, decrease in thymic hormone production was first described in the 1970 decade, unraveling reduced levels of thymulin in the serum of children with severe undernutrition, in small for gestational age infants, and in thymuses of children who died in various stages of undernutrition (59, 60). In this case, children with the severe forms (marasmus, kwashiorkor, and marasmic kwashiorkor) presented tiny thymuses that contained very low contents of thymulin (37).
Other than thymic hormones, it has been shown that the circulating levels of corticosterone are increased in protein-undernourished mice, as compared to age-matched controls. High corticosterone levels are known to induce thymic atrophy by the depletion of immature thymocytes, which was also observed in protein-undernourished mice (46). Accordingly, we might predict a dysregulation of glucocorticoid control mechanisms.
The HPA axis encompasses the hypothalamus, with the secretion of the neuropeptide CRH (corticotrophin-releasing hormone), which in turn stimulates the adenopituitary gland to secrete ACTH (adrenocorticotrophic hormone) that enhances glucocorticoid secretion by the cortex of the adrenal glands. Physiologically, such axis is self-regulated by negative feed-back with glucocorticoids being able to negatively control the production of both ACTH and CRH. Yet, it has been demonstrated that maternal food restriction during the perinatal period or during lactation disturbs the activity of the HPA axis at weaning, with pups presenting reduced adrenal, thymus and liver weight, and increased circulating free corticosterone levels (61). Disturbances in the HPA axis during protein deprivation were also reported (62). To determine if corticotropin-releasing hormone (CRH) and glucocorticoids were respectively required for hypophagia and catabolism in undernutrition, CRH-deficient mice were subjected to dietary protein deprivation. Interestingly, these animals did not exhibit increased plasma corticosterone as control individuals. In the same vein, CRH deficiency attenuated body and thymus weight loss induced by the restricted diet, suggesting that those effects were dependent on glucocorticoid regulation.
Mild maternal protein deprivation during lactation in rats affects thymic homeostasis in the young progeny, which presents lower body and thymus weights, significant alterations in CD4/CD8-defined T cell subsets and enhanced expression of leptin receptor ObRb in thymocytes. Although alterations in leptin circulating levels were not observed in this study, an increase in leptin signaling response of thymocytes from protein-deprived rats was described, together with a decreased rate of thymocyte spontaneous apoptosis when compared to controls (63). Interestingly, leptin/leptin receptor-deficient animals exhibit an atrophy of lymphoid tissues, particularly the thymus, and such a defect can be reversed by the reposition of the hormone (64, 65). Actually, complementary studies showed that there is a balance between systemic levels of leptin and glucocorticoids, controlling thymic atrophy. It is thus conceivable that in malnutritional states, the imbalance between the levels of leptin and glucocorticoid hormones could be, at least in part, responsible for the thymocyte depletion and consequent thymic atrophy (31). Yet, further studies are necessary to define if such imbalance is also involved in infection-related thymic atrophy.
Undernutrition yields a further negative impact of acute infections upon the thymus
It is known that the thymic atrophy induced by undernutrition produces a negative impact in the immune response against infections (31, 66, 67). In this respect and considering that protein-energy undernutrition can be reversed with an appropriate re-nutrition, it might be possible to recover the functional capacity of the thymus, through adequate food intake. Actually, this is definitely plausible since thymopoiesis is a continuous process along with life, although decreasing with aging. This is important particularly in facing infant malnutrition and infection, telling us that we must develop public policies to extinguish food insecurity and hunger on the planet.
As mentioned above, several findings in humans and mice showed that the thymus is also a target for infection (68, 69), as it has been well described for various types of pathogens, including viruses, bacteria, and parasites, such as T. cruzi, Plasmodium and Leishmania (Table 1). In fact, different infectious agents are able to reach and infect the organ. On this regard, we have recently shown that the thymus of BALB/c mice is a target during experimental infection by Leishmania infantum (70). The presence of the parasite infecting cells of the thymic microenvironment was observed both in well-nourished animals and in malnourished mice. However, it remains to be determined which cell populations are permissive to the parasite infection. Interestingly, it was possible to observe many more intact amastigotes in the undernourished animals than in the well-nourished ones (70). Recent reports have confirmed, using bioluminescence, the presence of the parasite in the thymus of well-nourished BALB/c mice infected with L. donovani (71). These observations demonstrate that the thymus is a target organ during infection by viscerotropic species of Leishmania, including L. infantum and L. donovani. This was further confirmed by the presence of the parasite in the thymus in dogs naturally infected with L. infantum (72). In a broader way, several data show that various parasites as well as viruses and even fungi, can be found within the thymus parenchyma. For example, in respect to Chagas disease, previous work had revealed that both epithelial and non-epithelial thymic microenvironmental cells can be infected by T. cruzi, as ascertained both in vitro and in vivo (68).
As previously mentioned, immunosuppression resulting from protein-energy undernutrition severely impacts primary and secondary lymphoid organs, involved in the response to pathogens, contributing to mortality and morbidity, especially in children (10). In addition, systemic hormonal and metabolic dysfunctions, as well as changes in intestinal barrier function and intestinal microbiota, caused by undernutrition, dramatically increase the susceptibility of individuals to infections, as seen in many different examples, such as visceral leishmaniasis (VL), influenza, dengue, Zika and tuberculosis, among others (10, 67, 140–151). In children aged less than 5 years, undernutrition is an underlying cause of 61% of deaths from diarrhea, 57% of deaths from malaria, 52% of deaths from pneumonia and 45% of deaths from measles (152). Furthermore, it has been estimated that the COVID-19 pandemic will increase childhood mortality from wasting by more than 20% (153). Indeed, recent report already described increased rates of fatal COVID-19 in areas with elevated burden of undernutrition (154).
Another example of the deleterious role of acute infections in undernourished subjects it the study on predictors of mortality in adult patients with influenza infection in Switzerland. This analysis was conducted during four influenza seasons and identified undernutrition as a strong predictor of mortality among those patients (155). Experimental model of protein-energy undernutrition and influenza A virus infection showed that mice fed low-protein diet (2% protein content) exhibited more severe disease than mice fed a control protein diet (18% protein content). Undernourished animals presented higher and sustained virus titers in the lungs, trafficking of inflammatory cells to the lung tissue, and higher virus-induced mortality, when compared to what was seen in control mice. Interestingly, undernourished-infected mice fed with control diet improved virus clearance, as well as recovered protective immunity to viral challenge (67).
It has also been suggested that maternal protein undernutrition increases susceptibility to Zika virus infection, which causes the Congenital Zika Syndrome in children. This syndrome is characterized by multiple neurological, muscular and immune disturbances, secondary to infection in pregnant mothers. In neurological terms, the children may present severe microcephaly, decreased brain tissue with a specific pattern of brain damage, including subcortical calcifications, damage to the back of the eye, with macular scarring and focal retinal pigmentary mottling, congenital contractures, such as clubfoot or arthrogryposis and Hypertonia restricting body movement soon after birth (156). From an experimental perspective, it was showed that pregnant mice subjected to protein undernutrition and infected with Zika virus presented severe alterations of placental structure and embryonic body growth, with offspring displaying a reduction in neurogenesis and postnatal brain size as well as alterations in the expression of genes required for brain development (142). Still regarding arboviruses, experimental models of Dengue virus (DENV) infection have shown that, compared with well-nourished animals, mice fed low protein content diet (5% protein) had a significant reduction in the level of platelets, increased spleen pathology and higher viral titers in the spleen following infection (143). However, studies regarding the association between the nutritional status and dengue infection in humans are controversial; some studies reporting higher risk of dengue shock syndrome or dengue hemorrhagic fever in undernourished children, whereas other studies could not observe these associations (157, 158). Consequently, more data are needed to clarify the influence of nutritional status on dengue infection outcome.
Another controversial association is that observed between malaria and undernutrition. Data from different analyses present conflicting conclusions regarding the potentially protective or exacerbating effects that undernutrition has on Plasmodium spp. infection (159). Because in endemic regions there is a common seasonality of malaria and undernutrition (160), it is difficult to clearly point if one is cause or consequence of the other. Some studies suggested that undernutrition increases the susceptibility of children to infection and/or impairs the individual’s capability to recover from infection (161–163). It has been observed that malnourished children have decreased specific anti-Plasmodium antibody titers when compared to well-nourished children (164). On the other hand, data related to the risk of malaria infection in children with chronic undernutrition revealed a protective effect of undernutrition against infection (165, 166). Several studies in experimental models indicate that animals submitted to a low-protein diet had reduced parasitemia when compared to controls; however, the immune response was also suppressed, and the animals were unable to clear the infection (167, 168). Interestingly, the immunosuppression observed during the deficit in protein consumption makes parasitized animals protected from experimental cerebral malaria (169, 170), a severe form of malaria directly associated with an exacerbated inflammatory response. Notably, it was observed that a brief restriction of food intake prevents neuropathology in an experimental cerebral malaria murine model with P. berghei ANKA (170). One hypothesis that should be investigated is that undernutrition would induce lower inflammatory and adaptive immune response that would partially undermine the autoimmune environment seen in both human and experimental malaria (171).
In general, infection or undernutrition induces similar alterations to lymphoid organs. Independently, undernutrition or acute infectious diseases result in thymic atrophy with drastic reduction of immature CD4+CD8+ double positive (DP) T cells due to increased apoptosis and premature egress of immature thymocytes (29, 36, 68, 77, 92, 172–174). Intrathymic chemokines and extracellular matrix (ECM) components are also altered during pathological conditions of infection or undernutrition. The thymus of T. cruzi-infected mice exhibits increased fibronectin and chemokine deposition, and increased abundance of thymic ECM proteins such as fibronectin, laminin, and type IV collagen has been observed in undernourished children (26, 49). Thymus atrophy with thymocyte depletion, increased intra and inter-lobular connective tissue, and decreased cortico-medullary limits have been also observed in undernourished children (175–177). Indeed, a smaller thymus is a risk factor for mortality and is predictive of decreased immune competence (178). As mentioned above, one of the mechanisms underlining these effects on thymocytes is be related to the hormonal imbalance between the rise in glucocorticoids and the decrease in leptin (50). Yet, other hormonal circuits may be involved as seen in experimental acute Chagas disease, in which prolactin partially reverts the thymic atrophy and the corticosteroid levels (179). Should we emphasize however, that other non-hormone-mediated mechanisms may be involved, and should be investigated.
Consequences of undernutrition and Leishmania infection upon the thymus: Cellular and proteomic approaches
Visceral leishmaniasis (VL) is a neglected disease that frequently afflicts children and malnourished populations in tropical and subtropical regions of the world. The disease is caused by Leishmania infantum or L. donovani parasites, which infect the spleen, liver, bone marrow, and lymph nodes, causing fever, hepatosplenomegaly, and loss of weight. If not properly treated, VL can be fatal (180, 181).
Seminal prospective studies in children who acquired VL revealed that those who had a precondition of moderate to severe undernutrition before infection had 8.7 times greater risk of having classic and severe forms of the disease, whereas well-nourished children did not progress beyond subclinical infections (140). More recent epidemiological surveys revealed that in cases of VL in adults who died, 32.7% of patients had undernutrition as the main comorbidity (182). In Northwest Ethiopia it was observed that roughly 85% children aged under 5 years and 95.5% adults with VL were undernourished (183, 184).
Studies of the relationship between VL and undernutrition in experimental murine models established a murine scale of undernutrition based on weight for age in analogy to the anthropometric classification of human undernutrition. Using this model it was found that undernutrition led to a failure in the barrier function of the lymph nodes in mice infected with L. donovani and, consequently, anticipated the visceralization of the parasite (185), likely due to the reduction of phagocytic cells in the lymph nodes of undernourished animals (186). A reduction in the number of skin-resident dendritic cells (DCs) drained to satellite lymph nodes was also observed, as well as a deregulation in the expression of chemokines and corresponding receptors, involved in the migration of DCs to the lymph nodes (187).
Also, in an undernourished murine model of infection with L. infantum, we observed a drastic reduction in cellularity and changes in the microarchitecture of the spleen and thymus of infected animals, as well as changes in the subpopulations of thymocytes and splenocytes, which were aggravated when the infection was preceded by undernutrition (69, 70, 188, 189). Indeed, undernourished-infected mice exhibited a significant reduction in the cortex:medulla ratio (69).
Aiming at understanding the effects of protein undernutrition in the course of infection and immune response to L. infantum, we established a model of protein undernutrition and infection with L. infantum, which has allowed us to describe cellular and molecular alterations in the thymus, spleen and gut of mice and how they affect the response to the parasite (69, 70, 188–190), using BALB/c mice fed control (14% protein) or low protein (4% protein) isocaloric diets further infected with L. infantum infection. Undernourished-infected mice exhibited a significant reduction of body and thymus weight, showed a significant decrease in CD4+CD8+ (DP) thymocytes, and remarkable alterations in total single positive subpopulations (CD4+ and CD8+) of the organ (69, 70, 188). Those changes were accompanied by reduced systemic levels of leptin and IGF1 and increased corticosterone, all of which have been associated with thymocyte depletion in undernourished individuals (66). Notably, those defects were induced independently by each condition (undernutrition or parasite infection), but the synergy of both exacerbated the observed alterations, accelerating the pathological events seen during infection (69, 70, 188). We further observed a decreased intrathymic abundance of CCL5, CXCL12, CXCL9, and CXCL10 as well as IGF1 in undernourished-infected animals, suggesting altered migration of developing T cells. Nevertheless, in this combined condition thymocytes were able to migrate ex vivo in response to chemotactic stimuli, suggesting that undernutrition may compromise the production of soluble factors inside the thymic microenvironment, altering in vivo thymocyte migration, rather than migratory capability of T cells per se (70).
As mentioned above, the successful production of mature T cells depends on the constant migration of differentiating thymocytes through the thymic microenvironment, and such migration is a process controlled by complex interactions between cell surface molecules, extracellular matrix (ECM) proteins, cytokines, chemokines and hormones (21, 191, 192). We conducted a histopathological study of the tissue organization and using a quantitative mass spectrometry-based proteomics approach, to measure the protein abundance within the thymic interstitial space in undernourished BALB/c mice infected with L. infantum (69). Undernourished-infected animals exhibited a significant reduction of the thymic cortical-medullary ratio and altered the abundance of proteins secreted in the thymic interstitial fluid. Proteomic analyzes revealed that these alterations were accompanied by a significant change in the abundance of soluble factors that are secreted via exosomes into the thymic microenvironment, suggestive of defects in the intrathymic molecular communication mediated by these microvesicles in undernourished animals (69).
Also, early changes in protein abundance were observed in infected animals, and those alterations were exacerbated or annulated when animals were previously undernourished, reinforcing the deleterious role of undernutrition in the response to infection and revealing an unknown role of the thymus during VL (69). Functional analysis of proteomics data showed that molecules involved in cell migration and differentiation such as galectin-1, von Willebrand factor, Rho GDP-dissociation inhibitor 1 and 2, differentiation factor 1 and transgelin-2 were significantly reduced in undernourished-infected mice (69). In addition, we observed an increase in the amounts of proteins involved in β-oxidation of fatty acids as well as in those involved in Krebs’ cycle, both of which suggest a non-proliferative quiescent thymic microenvironment (Figure 4), which in fact was corroborated by the decreased detection of Ki67 proliferation marker in thymocyte subpopulations (69). Together these data compose a scenario where structural and soluble protein factors of the thymic microenvironment are altered by L. infantum infection and worsened by a precedent undernutrition condition.
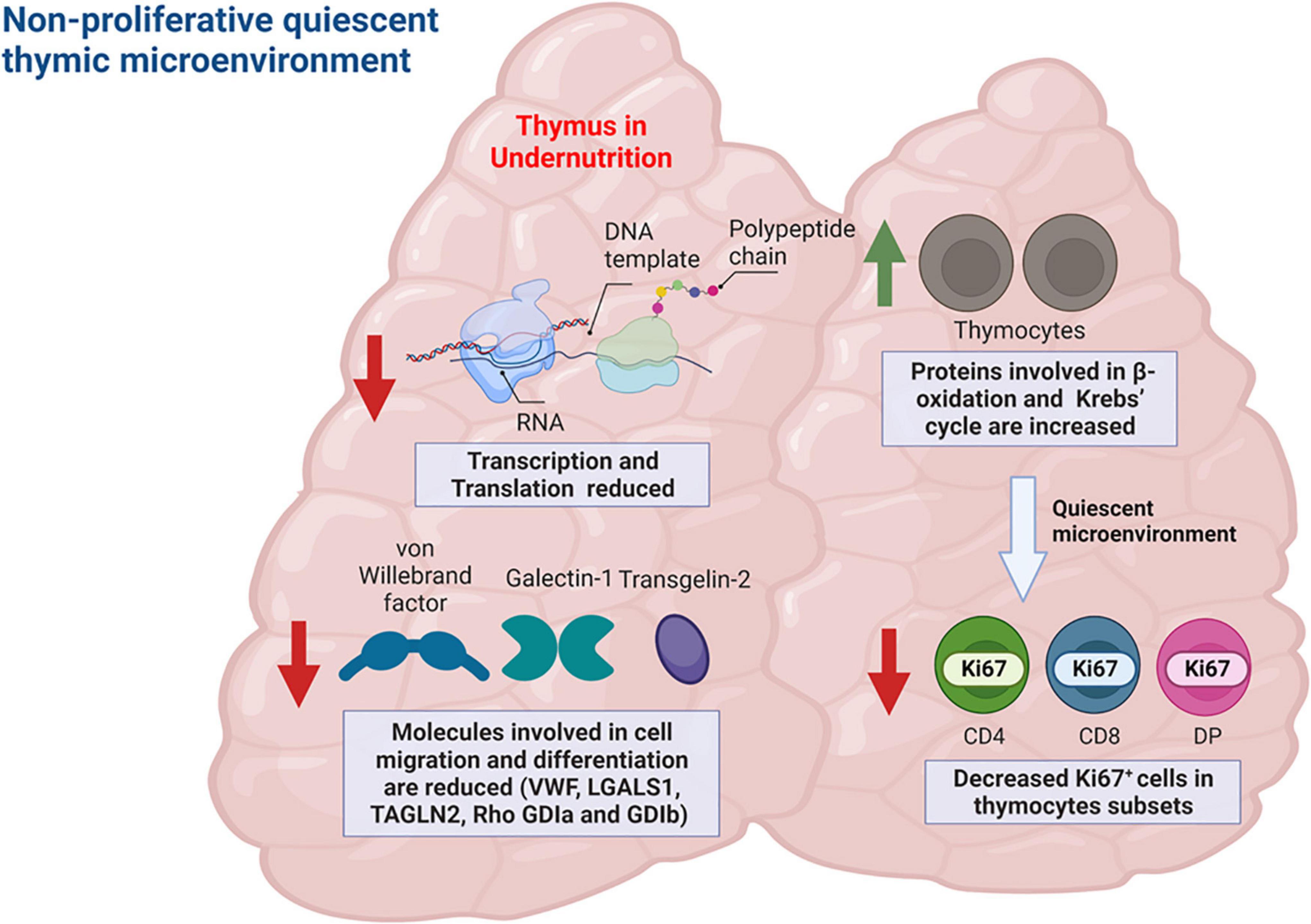
Figure 4. Quantitative proteomics analysis of thymic interstitial fluid of undernourished-infected mice reveals a non-proliferative quiescent thymic microenvironment. Examples of proteins with differential abundance in the undernourished-infected mice relative to the control (well-nourished) mice are represented in the figure. Proteins involved in transcription and translation as well as proteins involved in cell migration and differentiation were reduced in undernourished mice (red arrows). Conversely, proteins involved in fatty acid beta-oxidation and Krebs’ cycle were increased in those animals (green arrow), compatible with a quiescent, non-proliferative metabolic profile. Such profile was corroborated by a decreased percentage of proliferating thymocyte subsets expressing Ki67. Figure created with BioRender.com, based on data from Losada-Barragán et al. (69).
As mentioned above, we detected parasites in the thymus of both well-nourished and undernourished animals infected with L. infantum, but amastigote nests were only observed in mice fed protein restricted diet (70). Furthermore, using qPCR, we observed a significantly higher parasite load in the thymus of undernourished animals when compared to the control mice (193).
Interestingly, T cells generated in the thymus previously infected by Mycobacterium avium are tolerant to the pathogen in the periphery (86). In this line, the persistence of infection and antigenic peptides in the thymus may favor the appearance of pathogen-tolerant T cells, thus contributing to its persistence in other tissues (194). In a similar way, the persistence of L. infantum in the thymus of undernourished animals could favor the emergence of Leishmania-tolerant T cells, impairing the resolution of the infection in the periphery, favoring parasite persistence in the spleen. Such reasoning can also apply for different intrathymic infections.
Probiotics: Potential immunotherapeutic tools for restoring undernutrition related thymic dysfunctions
A large reservoir of microorganisms is found in the gut, reaching a bacterial community that is 10 times more than the number of human eukaryotic cells, coexisting in a symbiotic relationship (195). The role of these microorganisms in the gut physiology and maintaining intestinal homeostasis is indisputable. They participate in the breakdown of food, nutrient absorption, synthesize vitamins, protect us against pathogens, act on the neurodevelopment, and participate in the development and regulation of the immune system (196, 197). The microbiota composition differs from one individual to another, being in direct relation to age, diversity of food consumed, lifestyle, ethnicity, environmental factors and following the use of medicines such as steroids, antibiotics, etc. (198, 199). Among the millions of microorganisms in the human digestive tract, probiotics are found. They can influence the intestinal immune system by several mechanisms including change in the microbiota composition and its function, as well as improvement of the intestinal epithelial barrier (200, 201).
The mechanisms involved in the remote effects of probiotic are poorly understood. Yet, they can balance the microbiota and modulate the expression of pro-inflammatory cytokines. Such an effect occurs through multiple mechanisms, including the modulation and stimulation on MAPK (mitogen-activated protein kinase) pathways, as well as upon the NF-kB transcription factor, especially by inhibiting IkB phosphorylation, thus hindering the transfer of NF-kB (202).
Much less is known about the putative action of probiotics upon the thymus. In a mouse model of non-severe protein undernutrition, we found that the administration of a probiotic fermented milk as a re-nutrition supplementation did improve the thymic microarchitecture, recovering the corticomedullary differentiation in the thymic lobules, in conjunction with a decrease in thymocyte apoptosis. All the structural changes of thymus were accompanied by changes at the functional level, with increase in the numbers of mature and immature thymocytes and enhancement of cytokine production (203).
In a second vein, studies in obese humans and in obese mice, showed a relation between obesity and involution of the thymus gland revealing the important role of nutrients intake on the anatomy and functionality of the thymus (65, 203, 204). The use of a probiotic yogurt as dietary supplementation resulted not only in the control of body weight, serum biochemical parameters, but also in the recovery of the histological structure and thymus weight in obese animals (205). This was confirmed by recent data showing that a probiotic strain L. casei CRL 431 administered in the drinking water to a high-fat diet consuming obese mice, improved the cellularity and functionality of the thymus, with an increase in IL-7 and IL-3 production as seen in Figure 5 (206). These two cytokines are involved in normal intrathymic T-cell development; IL-7 is secreted by TEC and stimulates survival and expansion of the immature thymocytes and increase thymocyte numbers (207) and IL-3, also produced by TECs, is a hematopoietic growth factor that promotes myeloid proliferation (208).
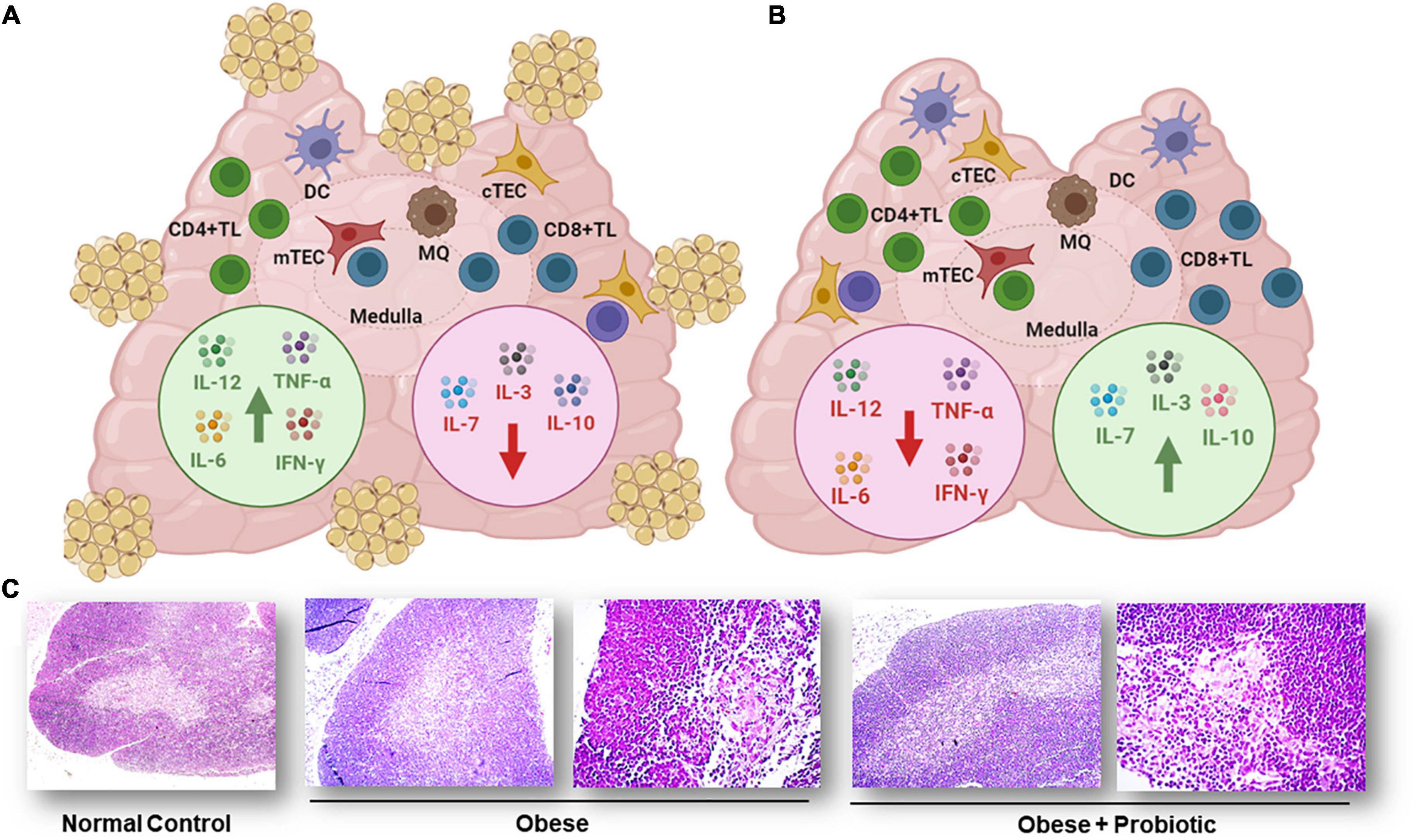
Figure 5. Cytokine production in thymus of obese mouse model. (A) Thymus from obese animals showed adipose deposits covering the tissue, a significant decrease in thymocytes, increased levels of IL-12, IL-6, TNF-α, and IFN-γ (green arrow) and decreased production of IL-3, IL-7, and IL-10 (red arrow). (B) Thymus from obese mice fed probiotic bacteria L. casei CRL 431 did not exhibit visible adipocytes, recovered thymocyte numbers, showed decreased levels of IL-12, IL-6, TNF-α, and IFN-γ (red arrow), and exhibited increased levels of IL-3, IL-7, and IL-10 (green arrow). Cytokines were measured by ELISA in the supernatants of cultured thymocytes. Figure created with BioRender.com, based on data from Balcells et al. (206). (C) Micrographs of thymus sections from normal control and obese mice. Tissue sections from normal control (100×), obese control (100× and 400×) and obese mice feed with probiotic bacteria (100× and 400×) stained with hematoxylin and eosin. Figure modified from Balcells et al. (206).
Concluding remarks and perspectives
As undernutrition remains a major issue in global health, studies on the pathophysiological aspects of this affection, associated or not with infection are yet important, particularly the mechanisms governing dysregulation of the immune response. In this respect, the immunosuppression resulting from protein-energy undernutrition severely impacts primary and secondary lymphoid organs involved in the response to a give pathogen. The thymus is particularly compromised by both undernutrition and infection, with a consistent cortical thymocyte depletion and important changes in thymic epithelial cells. Moreover, undernutrition yields a negative impact upon the thymus in acute infections, frequently with the intrathymic detection of pathogens or their antigens.
The impairment of the thymic cortical area due to undernutrition during acute infections may alter physiological processes crucial for T cell development, such as (i) intrathymic selection of the T cell repertoire (209), (ii) thymocyte proliferation, (iii) adequate migration of developing thymocyte, as well as (iv) changes in the microenvironmental component of the organ, and (v) intrathymic and systemic hormonal circuits (Figure 6), all of which having a deleterious impact in the adaptive immune responses in the periphery. As intrathymic T cell development is a continuous process, it exhibits a certain degree of plasticity, rendering possible the partial (or even total) restoration to normal steady state after a given deleterious stimulus has stopped. Accordingly, it seems plausible that those alterations can be at least partially reverted by dietary interventions, rescuing proper immune responses to infection. However, this remains to be investigated. Addressing whether nutritional rehabilitation combining balanced macronutrients, micronutrients and probiotics can recover the structural and cell differentiation damage caused by undernutrition in the thymus, would allow proposing nutrition-based interventions that would positively impact both the nutritional status of the individuals and their ability to respond adequately to infections.
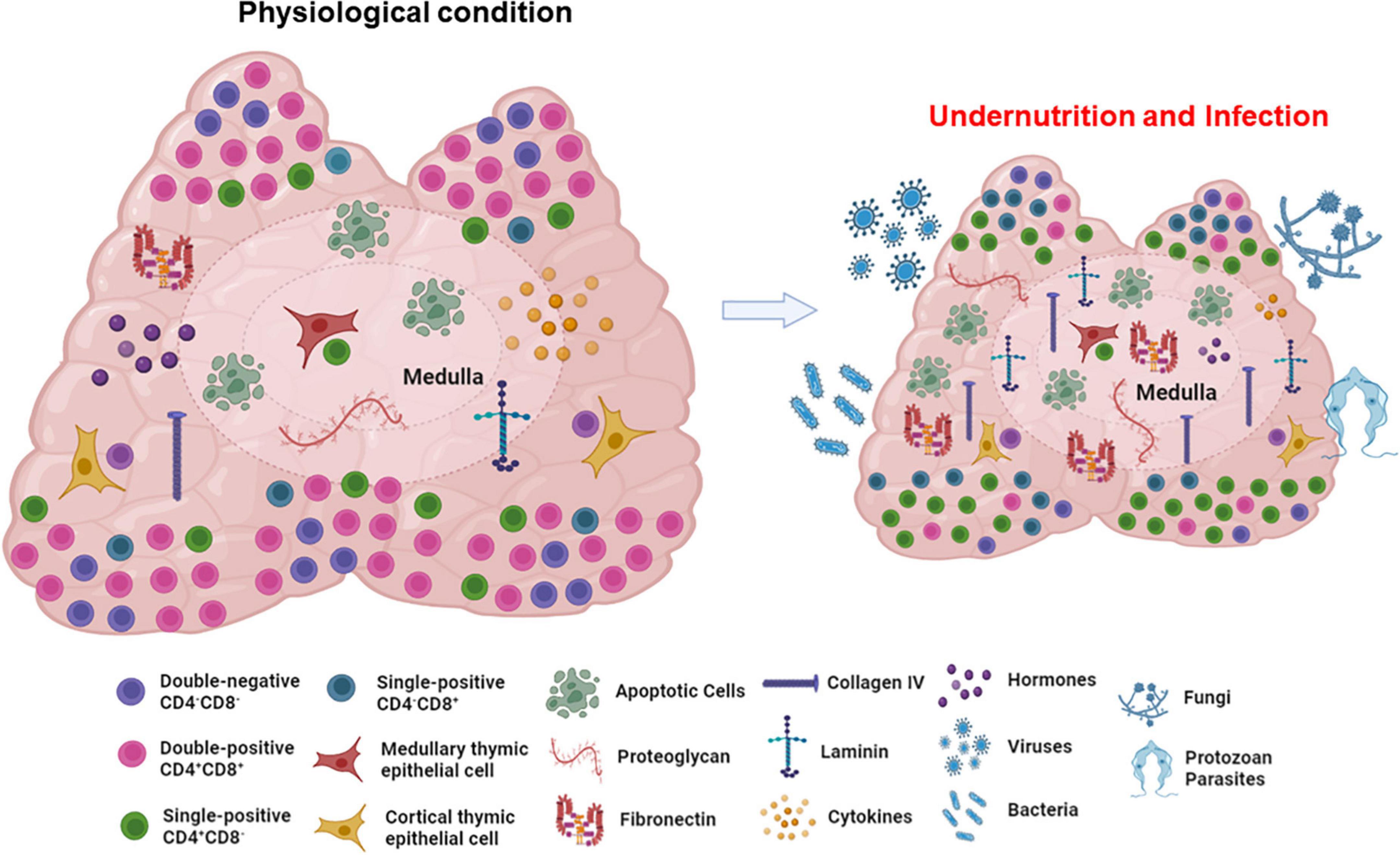
Figure 6. Effects of undernutrition upon the thymic lymphoid and microenvironmental compartments. The scheme clearly shows the atrophy of the thymus in undernutrition conditions, affecting both developing thymocytes and microenvironmental elements of the organ. Importantly, undernourished thymuses are more susceptible to infections and thymic changes are still more pronounced. Figure created with BioRender.com.
Author contributions
WS and PC conceived, wrote, reviewed, and edited the manuscript. JD, CM-G, GP, and DM-d-C wrote the manuscript. All authors contributed to the article and approved the submitted version.
Funding
This work was supported by Fiocruz, CNPq, CAPES, and FAPERJ (Brazil) and the Mercosur Fund for Structural Convergence (FOCEM). It was developed in the frameworks of the Brazilian National Institute of Science and Technology on Neuroimmunomodulation (CNPq) and Rio de Janeiro Research Network on Neuroinflammation (FAPERJ). It was further supported by grants provided by CONICET (Argentina). Fundação de Amparo à Pesquisa do Estado de Rio de Janeiro—FAPERJ (WS: Rio de Janeiro Research Network on Neuroinflammation grant # E-26/211.571/2019; PC: Emergentes grant # E-26/010.002168/2019; and JCNE E-26/203.253/2017; Sediadas grant # E-26/010.101083/2018); FIOCRUZ -PAEF (PC and JD: grant no. IOC-023-FIO-18-2-63); PC is a PQ-fellow of the Conselho Nacional de Desenvolvimento Científico e Tecnológico—CNPq (PQ Process No. 312573/2020-0).
Acknowledgments
This article is dedicated to Juliana de Meis, young scientist from the Laboratory on Thymus Research (Fiocruz, Rio de Janeiro), who passed away on July 16th, 2021, due to COVID-19.
Conflict of interest
The authors declare that the research was conducted in the absence of any commercial or financial relationships that could be construed as a potential conflict of interest.
Publisher’s note
All claims expressed in this article are solely those of the authors and do not necessarily represent those of their affiliated organizations, or those of the publisher, the editors and the reviewers. Any product that may be evaluated in this article, or claim that may be made by its manufacturer, is not guaranteed or endorsed by the publisher.
References
1. United Nations. End Hunger, Achieve Food Security and Improved Nutrition and Promote Sustainable Agriculture. (2021). Available online at: https://sdgs.un.org/goals/goal2 (accessed January 10, 2022).
2. World Health Organization. Malnutrition. (2021). Available online at: https://www.who.int/news-room/fact-sheets/detail/malnutrition (accessed January 10, 2022).
3. Food and Agriculture Organization. The State of Food Security and Nutrition in the World 2021: Transforming Food Systems for Food Security, Improved Nutrition and Affordable Healthy Diets for all. Rome: Food and Agriculture Organization (2021).
4. PENSSAN Network. Relatório Sobre Insegurança Alimentar e Covid-19 no Brasil. (2021). Available online at: http://olheparaafome.com.br/VIGISAN_Inseguranca_alimentar.pdf (accessed January 10, 2022).
5. Dipasquale V, Cucinotta U, Romano C. Acute malnutrition in children: pathophysiology, clinical effects and treatment. Nutrients. (2020) 12:2413. doi: 10.3390/nu12082413
6. Bhutta ZA, Berkley JA, Bandsma RHJ, Kerac M, Trehan I, Briend A. Severe childhood malnutrition. Nat Rev Dis Primers. (2017) 3:17067. doi: 10.1038/nrdp.2017.67
7. Hashimoto T, Perlot T, Rehman A, Trichereau J, Ishiguro H, Paolino M, et al. ACE2 links amino acid malnutrition to microbial ecology and intestinal inflammation. Nature. (2012) 487:477–81. doi: 10.1038/nature11228
8. Bäckhed F, Ley RE, Sonnenburg JL, Peterson DA, Gordon JI. Host-bacterial mutualism in the human intestine. Science. (2005) 307:1915–20. doi: 10.1126/science.1104816
9. Kau AL, Ahern PP, Griffin NW, Goodman AL, Gordon JI. Human nutrition, the gut microbiome and the immune system. Nature. (2011) 474:327–36. doi: 10.1038/nature10213
10. Ibrahim MK, Zambruni M, Melby CL, Melby PC. Impact of childhood malnutrition on host defense and infection. Clin Microbiol Rev. (2017) 30:919–71. doi: 10.1128/CMR.00119-16
11. Schaible UE, Kaufmann SH. Malnutrition and infection: complex mechanisms and global impacts. PLoS Med. (2007) 5:e115. doi: 10.1371/journal.pmed.0040115
12. Ciofani M, Zúñiga-Pflücker JC. The thymus as an inductive site for T lymphopoiesis. Annu Rev Cell Dev Biol. (2007) 23:463–93. doi: 10.1146/annurev.cellbio.23.090506.123547
13. Savino W, Mendes-da-Cruz DA, Lepletier A, Dardenne M. Hormonal control of T-cell development in health and disease. Nat Rev Endocrinol. (2016) 2:77–89. doi: 10.1038/nrendo.2015.168
14. Petrie HT, Zúñiga-Pflücker JC. Zoned out: functional mapping of stromal signaling microenvironments in the thymus. Annu Rev Immunol. (2007) 25:649–79. doi: 10.1146/annurev.immunol.23.021704.115715
15. Pérez AR, de Meis J, Rodriguez-Galan MC, Savino W. The thymus in Chagas disease: molecular interactions involved in abnormal T-cell migration and differentiation. Front Immunol. (2020) 11:1838. doi: 10.3389/fimmu.2020.01838
16. Francelin C, Veneziani LP, Farias ADS, Mendes-da-Cruz DA, Savino W. Neurotransmitters modulate intrathymic T-cell development. Front Cell Dev Biol. (2021) 9:668067. doi: 10.3389/fcell.2021.668067
17. Speck-Hernandez CA, Assis AF, Felicio RF, Cotrim-Sousa L, Pezzi N, Lopes GS, et al. Aire disruption influences the medullary thymic epithelial cell transcriptome and interaction with thymocytes. Front Immunol. (2018) 9:964. doi: 10.3389/fimmu.2018.00964
18. Passos GA, Speck-Hernandez CA, Assis AF, Mendes-da-Cruz DA. Update on Aire and thymic negative selection. Immunology. (2018) 153:10–20. doi: 10.1111/imm.12831
19. Santamaria JC, Borelli A, Irla M. Regulatory T cell heterogeneity in the thymus: impact on their functional activities. Front Immunol. (2021) 12:643153. doi: 10.3389/fimmu.2021.643153
20. Tao Z, Jiang Y, Xia S. Regulation of thymic T regulatory cell differentiation by TECs in health and disease. Scand J Immunol. (2021) 94:e13094. doi: 10.1111/sji.13094
21. Savino W, Mendes-Da-Cruz D, Smaniotto S, Silva-Monteiro E, Villa-Verde DMS. Molecular mechanisms governing thymocyte migration: combined role of chemokines and extracellular matrix. J Leukoc Biol. (2004) 75:951–61. doi: 10.1189/jlb.1003455
22. Savino W, Dardenne M. Neuroendocrine control of thymus physiology. Endocr Rev. (2000) 21:412–43. doi: 10.1210/edrv.21.4.0402
23. Savino W, Dardenne M. Thymic hormone-containing cells VI. Immunohistologic evidence for the simultaneous presence of thymulin, thymopoietin and thymosin alpha 1 in normal and pathological human thymuses. Eur J Immunol. (1984) 14:987–91. doi: 10.1002/eji.1830141105
24. Gorbach SL, Goldin BR. Nutrition and the gastrointestinal microflora. Nutrition Rev. (1992) 50:378–81. doi: 10.1111/j.1753-4887.1992.tb02485.x
25. Mitsuoka T. Bifidobacteria and their role in human health. J Ind Microbiol. (1990) 81:131–6. doi: 10.1007/BF01575871
26. Lyra JS, Madi K, Maeda CT, Savino W. Thymic extracellular matrix in human malnutrition. J Pathol. (1993) 171:231–6. doi: 10.1002/path.1711710312
27. Roux E, Dumont-Girard F, Starobinski M, Siegrist CA, Helg C, Chapuis B, et al. Recovery of immune reactivity after T-cell-depleted bone marrow transplantation depends on thymic activity. Blood. (2000) 96:2299–303.
28. Savino W. The thymus gland is a target in malnutrition. Eur J Clin Nutr. (2002) 56(Suppl. 3):S46–9. doi: 10.1038/sj.ejcn.1601485
29. Ortiz R, Cortés L, Cortés E, Medina H. Malnutrition alters the rates of apoptosis in splenocytes and thymocyte subpopulations of rats. Clin Exp Immunol. (2009) 155:96–106. doi: 10.1111/j.1365-2249.2008.03796.x
30. Maldonado Galdeano C, Novotny Núñez I, de Moreno de LeBlanc A, Carmuega E, Weill R, Perdigón G. Impact of a probiotic fermented milk in the gut ecosystem and in the systemic immunity using a non-severe protein-energy-malnutrition model in mice. BMC Gastroenterol. (2011) 11:64. doi: 10.1186/1471-230X-11-64
31. Savino W, Dardenne M. Nutritional imbalances and infections affect the thymus: consequences on T-cell-mediated immune responses. Proc Nutr Soc. (2010) 69:636–43. doi: 10.1017/S0029665110002545
32. Mittal A, Woodward B, Chandra RK. Involution of thymic epithelium and low serum thymulin bioactivity in weanling mice subjected to severe food intake restriction or severe protein deficiency. Exp Mol Pathol. (1988) 48:226–35. doi: 10.1016/0014-480090059-7
33. Mittal A, Woodward B. Thymic epithelial cells of severely undernourished mice: accumulation of cholesteryl esters and absence of cytoplasmic vacuoles. Proc Soc Exp Biol Med. (1985) 178:385–91. doi: 10.3181/00379727-178-42021
34. Iovino L, Cooper K, deRoos P, Kinsella S, Evandy C, Ugrai T, et al. Activation of the zinc-sensing receptor GPR39 promotes T-cell reconstitution after hematopoietic cell transplant in mice. Blood. (2022) 139:3655–66. doi: 10.1182/blood.2021013950
35. Savino W, Leite-de-Moraes MC, Hontebeyrie-Joskowicz M, Dardenne M. Studies on the thymus in Chagas’ disease. I. Changes in the thymic microenvironment in mice acutely infected with Trypanosoma cruzi. Eur J Immunol. (1989) 19:1727–33. doi: 10.1002/eji.1830190930
36. Chandra RK. Protein-energy malnutrition and immunological responses. J Nutr. (1992) 122(3 Suppl.):597–600. doi: 10.1093/jn/122.suppl_3.597
37. Jambon B, Ziegler O, Maire B, Hutin MF, Parent G, Fall M, et al. Thymulin (facteur thymique serique) and zinc contents of the thymus glands of malnourished children. Am J Clin Nutr. (1988) 48:335–42. doi: 10.1093/ajcn/48.2.335
38. Wade S, Bleiberg F, Mossé A, Lubetzki J, Flavigny H, Chapuis P, et al. Thymulin (Zn-facteur thymique serique) activity in anorexia nervosa patients. Am J Clin Nutr. (1985) 42:275–80. doi: 10.1093/ajcn/42.2.275
39. Kuvibidila S, Dardenne M, Savino W, Lepault F. Influence of iron-deficiency anemia on selected thymus functions in mice: thymulin biological activity, T-cell subsets, and thymocyte proliferation. Am J Clin Nutr. (1990) 51:228–32. doi: 10.1093/ajcn/51.2.228
40. Dardenne M, Savino W, Wade S, Kaiserlian D, Lemonnier D, Bach JF. In vivo and in vitro studies of thymulin in marginally zinc-deficient mice. Eur J Immunol. (1984) 14:454–8. doi: 10.1002/eji.1830140513
41. Prasad AS, Meftah S, Abdallah J, Kaplan J, Brewer GJ, Bach JF, et al. Serum thymulin in human zinc deficiency. J Clin Invest. (1988) 82:1202–10. doi: 10.1172/JCI113717
42. Dardenne M, Bach JF, Safai B. Low serum thymic hormone levels in patients with acquired immunodeficiency syndrome. N Engl J Med. (1983) 309:48–9. doi: 10.1056/NEJM198307073090112
43. Incefy GS, Pahwa S, Pahwa R, Sarngadharan MG, Menez R, Fikrig S. Low circulating thymulin-like activity in children with AIDS and AIDS-related complex. AIDS Res. (1986) 2:109–16. doi: 10.1089/aid.1.1986.2.109
44. Savino W, Dardenne M, Marche C, Trophilme D, Dupuy JM, Pekovic D, et al. Thymic epithelium in AIDS. An immunohistologic study. Am J Pathol. (1986) 122:302–7.
45. McDade TW, Beck MA, Kuzawa CW, Adair LS. Prenatal undernutrition and postnatal growth are associated with adolescent thymic function. J Nutr. (2001) 131:1225–31. doi: 10.1093/jn/131.4.1225
46. Barone KS, O’Brien PC, Stevenson JR. Characterization and mechanisms of thymic atrophy in protein-malnourished mice: role of corticosterone. Cell Immunol. (1993) 148:226–33. doi: 10.1006/cimm.1993.1105
47. Monk JM, Makinen K, Shrum B, Woodward B. Blood corticosterone concentration reaches critical illness levels early during acute malnutrition in the weanling mouse. Exp Biol Med. (2006) 231:264–8. doi: 10.1177/153537020623100304
48. Cotta-de-Almeida V, Bonomo A, Mendes-da-Cruz DA, Riederer I, De Meis J, Lima-Quaresma KR, et al. Trypanosoma cruzi infection modulates intrathymic contents of extracellular matrix ligands and receptors and alters thymocyte migration. Eur J Immunol. (2003) 33:2439–48. doi: 10.1002/eji.200323860
49. Mendes-da-Cruz DA, Silva JS, Cotta-de-Almeida V, Savino W. Altered thymocyte migration during experimental acute Trypanosoma cruzi infection: combined role of fibronectin and the chemokines CXCL12 and CCL4. Eur J Immunol. (2006) 36:1486–93. doi: 10.1002/eji.200535629
50. Lepletier W, Villa-Verde DM, Mendes-da-Cruz DA, Silva-Monteiro E, Perez AR, Aoki Mdel P, et al. Cytokines and cell adhesion receptors in the regulation of immunity to Trypanosoma cruzi. Cytokine Growth Factor Rev. (2007) 18:107–24. doi: 10.1016/j.cytogfr.2007.01.010
51. Morrot A, Terra-Granado E, Pérez AR, Silva-Barbosa SD, Miliæeviæ NM, Farias-de-Oliveira DA, et al. Chagasic thymic atrophy does not affect negative selection but results in the export of activated CD4+CD8+ T cells in severe forms of human disease. PLoS Negl Trop Dis. (2011) 5:e1268. doi: 10.1371/journal.pntd.0001268
52. Alpers CE, Hudkins KL. Mouse models of diabetic nephropathy. Curr Opin Nephrol Hypertens. (2011) 20:278–84. doi: 10.1097/MNH.0b013e3283451901
53. Dardenne M, Savino W, Gastinel LN, Nabarra B, Bach JF. Thymic dysfunction in the mutant diabetic (db/db) mouse. J Immunol. (1983) 130:1195–9.
54. Debray-Sachs M, Dardenne M, Sai P, Savino W, Quiniou MC, Boillot D, et al. Anti-islet immunity and thymic dysfunction in the mutant diabetic C57BL/KsJ db/db mouse. Diabetes. (1983) 32:1048–54. doi: 10.2337/diab.32.11.1048
55. Palmer G, Aurrand-Lions M, Contassot E, Talabot-Ayer D, Ducrest-Gay D, Vesin C, et al. Indirect effects of leptin receptor deficiency on lymphocyte populations and immune response in db/db mice. J Immunol. (2006) 177:2899–907. doi: 10.4049/jimmunol.177.5.2899
56. Nabarra B, Andrianarison I. Thymic reticulum of autoimmune mice. I. Ultrastructural studies of the diabetic (db/db) mouse thymus. Exp Pathol. (1986) 29:45–53. doi: 10.1016/s0232-151380005-6
57. Erickson RL, Browne CA, Lucki I. Hair corticosterone measurement in mouse models of type 1 and type 2 diabetes mellitus. Physiol Behav. (2017) 178:166–71. doi: 10.1016/j.physbeh.2017.01.018
58. Mello Coelho VD, Bunbury A, Rangel LB, Giri B, Weeraratna A, Morin PJ, et al. Fat-storing multilocular cells expressing CCR5 increase in the thymus with advancing age: potential role for CCR5 ligands on the differentiation and migration of preadipocytes. Int J Med Sci. (2009) 7:1–14. doi: 10.7150/ijms.7.1
59. Chandra RK. Serum thymic hormone activity and cell-mediated immunity in healthy neonates, preterm infants, and small-for-gestational age infants. Pediatrics. (1981) 67:407–11.
60. Chandra RK. Serum thymic hormone activity in protein-energy malnutrition. Clin Exp Immunol. (1979) 38:228–30.
61. Léonhardt M, Lesage J, Dufourny L, Dickès-Coopman A, Montel V, Dupouy J-P. Perinatal maternal food restriction induces alterations in hypothalamo-pituitary-adrenal axis activity and in plasma corticosterone-binding globulin capacity of weaning rat pups. Neuroendocrinology. (2002) 75:45–54. doi: 10.1159/000048220
62. Jacobson L. Lower weight loss and food intake in protein-deprived, corticotropin releasing hormone-deficient mice correlate with glucocorticoid insufficiency. Endocrinology. (1999) 140:3543–51. doi: 10.1210/endo.140.8.6910
63. da Silva SV, Salama C, Renovato-Martins M, Helal-Neto E, Citelli M, Savino W, et al. Increased leptin response and inhibition of apoptosis in thymocytes of young rats offspring from protein deprived dams during lactation. PLoS One. (2013) 8:e64220. doi: 10.1371/journal.pone.0064220
64. Farooqi IS, Matarese G, Lord GM, Keogh JM, Lawrence E, Agwu C, et al. Beneficial effects of leptin on obesity, T cell hyporesponsiveness, and neuroendocrine/metabolic dysfunction of human congenital leptin deficiency. J Clin Invest. (2002) 110:1093–103. doi: 10.1172/JCI15693
65. Howard JK, Lord GM, Matarese G, Vendetti S, Ghatei MA, Ritter MA, et al. Leptin protects mice from starvation-induced lymphoid atrophy and increases thymic cellularity in ob/ob mice. J Clin Invest. (1999) 104:1051–9. doi: 10.1172/JCI6762
66. Savino W, Dardenne M, Velloso LA, Dayse Silva-Barbosa S. The thymus is a common target in malnutrition and infection. Br J Nutr. (2007) 98(Suppl. 1):S11–6. doi: 10.1017/S0007114507832880
67. Taylor AK, Cao W, Vora KP, De La Cruz J, Shieh WJ, Zaki SR, et al. Protein energy malnutrition decreases immunity and increases susceptibility to influenza infection in mice. J Infect Dis. (2013) 207:501–10. doi: 10.1093/infdis/jis527
68. Savino W. The thymus is a common target organ in infectious diseases. PLoS Pathog. (2006) 2:e62. doi: 10.1371/journal.ppat.0020062
69. Losada-Barragán M, Umaña-Pérez A, Durães J, Cuervo-Escobar S, Rodríguez-Vega A, Ribeiro-Gomes FL, et al. Thymic microenvironment is modified by malnutrition and Leishmania infantum infection. Front Cell Infect Microbiol. (2019) 9:252. doi: 10.3389/fcimb.2019.00252
70. Losada-Barragán M, Umaña-Pérez A, Cuervo-Escobar S, Berbert LR, Porrozzi R, Morgado FN, et al. Protein malnutrition promotes dysregulation of molecules involved in T cell migration in the thymus of mice infected with Leishmania infantum. Sci Rep. (2017) 7:45991. Erratum Sci Rep. (2017) 7:46809. doi: 10.1038/srep45991
71. Domínguez-Asenjo B, Gutiérrez-Corbo C, Pérez-Pertejo Y, Iborra S, Balaña-Fouce R, Reguera RM. Bioluminescent imaging identifies thymus, as overlooked colonized organ, in a chronic model of Leishmania donovani mouse visceral Leishmaniasis. ACS Infect Dis. (2021) 7:871–83. doi: 10.1021/acsinfecdis.0c00864
72. da Silva AVA, de Souza TL, Figueiredo FB, Mendes AAV Jr, Ferreira LC, Filgueira CPB, et al. Detection of amastigotes and histopathological alterations in the thymus of Leishmania infantum-infected dogs. Immun Inflamm Dis. (2020) 8:127–39. doi: 10.1002/iid3.285
73. Da Costa SC, Calabrese KS, Bauer PG, Savino W, Lagrange PH. Studies of the thymus in Chagas’ disease: III. Colonization of the thymus and other lymphoid organs of adult and newborn mice by Trypanosoma cruzi. Pathol Biol (Paris). (1991) 39:91–7.
74. Cotta-de-Almeida V, Bertho AL, Villa-Verde DM, Savino W. Phenotypic and functional alterations of thymic nurse cells following acute Trypanosoma cruzi infection. Clin Immunol Immunopathol. (1997) 82:125–32. doi: 10.1006/clin.1996.4283
75. Baez NS, Cerbán F, Savid-Frontera C, Hodge DL, Tosello J, Acosta-Rodriguez E, et al. Thymic expression of IL-4 and IL-15 after systemic inflammatory or infectious Th1 disease processes induce the acquisition of “innate” characteristics during CD8+ T cell development. PLoS Pathog. (2019) 15:e1007456. doi: 10.1371/journal.ppat.1007456
76. de Meis J, Morrot A, Farias-de-Oliveira DA, Villa-Verde DM, Savino W. Differential regional immune response in Chagas disease. PLoS Negl Trop Dis. (2009) 3:e417. doi: 10.1371/journal.pntd.0000417
77. Andrade CF, Gameiro J, Nagib PR, Carvalho BO, Talaisys RL, Costa FT, et al. Thymic alterations in Plasmodium berghei-infected mice. Cell Immunol. (2008) 253:1–4. doi: 10.1016/j.cellimm.2008.06.001
78. Francelin C, Paulino LC, Gameiro J, Verinaud L. Effects of Plasmodium berghei on thymus: high levels of apoptosis and premature egress of CD4(+)CD8(+) thymocytes in experimentally infected mice. Immunobiology. (2011) 216:1148–54. doi: 10.1016/j.imbio.2011.03.009
79. Gameiro J, Nagib PR, Andrade CF, Villa-Verde DM, Silva-Barbosa SD, Savino W, et al. Changes in cell migration-related molecules expressed by thymic microenvironment during experimental Plasmodium berghei infection: consequences on thymocyte development. Immunology. (2010) 129:248–56. doi: 10.1111/j.1365-2567.2009.03177.x
80. Huldt G, Gard S, Olovson SG. Effect of Toxoplasma gondii on the thymus. Nature. (1973) 244:301–3. doi: 10.1038/244301a0
81. Kugler DG, Flomerfelt FA, Costa DL, Laky K, Kamenyeva O, Mittelstadt PR, et al. Systemic toxoplasma infection triggers a long-term defect in the generation and function of naive T lymphocytes. J Exp Med. (2016) 213:3041–56. doi: 10.1084/jem.20151636
82. Nobrega C, Cardona P-J, Roque S, Pinto do OP, Appelberg R, Correia-Neves M. The thymus as a target for mycobacterial infections. Microbes Infect. (2007) 9:1521–9. doi: 10.1016/j.micinf.2007.08.006
83. Nobrega C, Nunes-Alves C, Cerqueira-Rodrigues B, Roque S, Barreira-Silva P, Behar SM, et al. T cells home to the thymus and control infection. J Immunol. (2013) 190:1646–58. doi: 10.4049/jimmunol.1202412
84. Reiley WW, Wittmer ST, Ryan LM, Eaton SM, Haynes L, Winslow GM, et al. Maintenance of peripheral T cell responses during Mycobacterium tuberculosis infection. J Immunol. (2012) 189:4451–8. doi: 10.4049/jimmunol.1201153
85. Hühns M, Erbersdobler A, Obliers A, Röpenack P. Identification of HPV types and Mycobacterium tuberculosis complex in historical long-term preserved formalin fixed tissues in different human organs. PLoS One. (2017) 12:e0170353. doi: 10.1371/journal.pone.0170353
86. Nobrega C, Roque S, Nunes-Alves C, Coelho A, Medeiros I, Castro AG, et al. Dissemination of mycobacteria to the thymus renders newly generated T cells tolerant to the invading pathogen. J Immunol. (2010) 184:351–8. doi: 10.4049/jimmunol.0902152
87. Borges M, Barreira-Silva P, Flórido M, Jordan MB, Correia-Neves M, Appelberg R. Molecular and cellular mechanisms of Mycobacterium avium-induced thymic atrophy. J Immunol. (2012) 189:3600–8. doi: 10.4049/jimmunol.1201525
88. Ross EA, Coughlan RE, Flores-Langarica A, Lax S, Nicholson J, Desanti GE, et al. Thymic function is maintained during Salmonella-induced atrophy and recovery. J Immunol. (2012) 189:4266–74. doi: 10.4049/jimmunol.1200070
89. Deobagkar-Lele M, Chacko SK, Victor ES, Kadthur JC, Nandi D. Interferon-γ- and glucocorticoid-mediated pathways synergize to enhance death of CD4(+) CD8(+) thymocytes during Salmonella enterica serovar Typhimurium infection. Immunology. (2013) 138:307–21. doi: 10.1111/imm.12047
90. Chen W, Kuolee R, Austin JW, Shen H, Che Y, Conlan JW. Low dose aerosol infection of mice with virulent type A Francisella tularensis induces severe thymus atrophy and CD4+ CD8+ thymocyte depletion. Microb Pathog. (2005) 39:189–96. doi: 10.1016/j.micpath.2005.08.005
91. Xiang L, Hu X, Zhang J, She J, Li M, Zhou T. Immunodepression induced by influenza A virus (H1N1) in lymphoid organs functions as a pathogenic mechanism. Clin Exp Pharmacol Physiol. (2020) 47:1664–73. doi: 10.1111/1440-1681.13358
92. Liu B, Zhang X, Deng W, Liu J, Li H, Wen M, et al. Severe influenza A(H1N1)pdm09 infection induces thymic atrophy through activating innate CD8(+)CD44(hi) T cells by upregulating IFN-γ. Cell Death Dis. (2014) 5:e1440. doi: 10.1038/cddis.2014.323
93. Fislová T, Gocník M, Sládková T, Durmanová V, Rajcáni J, Varecková E, et al. Multiorgan distribution of human influenza A virus strains observed in a mouse model. Arch Virol. (2009) 154:409–19. doi: 10.1007/s00705-009-0318-8
94. Lamontagne L, Jolicoeur P. Low-virulent mouse hepatitis viruses exhibiting various tropisms in macrophages, T and B cell subpopulations, and thymic stromal cells. Lab Anim Sci. (1994) 44:17–24.
95. Lee S-K, Youn H-Y, Hasegawa A, Nakayama H, Goto N. Apoptotic changes in the thymus of mice infected with mouse hepatitis virus, MHV-2. J Vet Med Sci. (1994) 56:879–82. doi: 10.1292/jvms.56.879
96. Godfraind C, Holmes KV, Coutelier JP. Thymus involution induced by mouse hepatitis virus A59 in BALB/c mice. J Virol. (1995) 69:6541–7. doi: 10.1128/JVI.69.10.6541-6547.1995
97. Meissner EG, Duus KM, Loomis R, D’Agostin R, Su L. HIV-1 replication and pathogenesis in the human thymus. Curr HIV Res. (2003) 1:275–85. doi: 10.2174/1570162033485258
98. Calabrò ML, Zanotto C, Calderazzo F, Crivellaro C, Del Mistro A, De Rossi A, et al. HIV-1 infection of the thymus: evidence for a cytopathic and thymotropic viral variant in vivo. AIDS Res Hum Retroviruses. (1995) 11:11–9. doi: 10.1089/aid.1995.11.11
99. Schmitt N, Nugeyre MT, Scott-Algara D, Cumont MC, Barré-Sinoussi F, Pancino G, et al. Differential susceptibility of human thymic dendritic cell subsets to X4 and R5 HIV-1 infection. AIDS. (2006) 20:533–42. doi: 10.1097/01.aids.0000210607.63138.bc
100. Rozmyslowicz T, Murphy SL, Conover DO, Gaulton GN. HIV-1 infection inhibits cytokine production in human thymic macrophages. Exp Hematol. (2010) 38:1157–66. doi: 10.1016/j.exphem.2010.08.009
101. Stanley SK, McCune JM, Kaneshima H, Justement JS, Sullivan M, Boone E, et al. Human immunodeficiency virus infection of the human thymus and disruption of the thymic microenvironment in the SCID-hu mouse. J Exp Med. (1993) 178:1151–63. doi: 10.1084/jem.178.4.1151
102. Su L, Kaneshima H, Bonyhadi M, Salimi S, Kraft D, Rabin L, et al. HIV-1-induced thymocyte depletion is associated with indirect cytopathogenicity and infection of progenitor cells in vivo. Immunity. (1995) 2:25–36. doi: 10.1016/1074-761390076-4
103. Ho Tsong Fang R, Colantonio AD, Uittenbogaart CH. The role of the thymus in HIV infection: a 10 year perspective. AIDS. (2008) 22:171–84. doi: 10.1097/QAD.0b013e3282f2589b
104. Joshi VV, Oleske JM, Saad S, Gadol C, Connor E, Bobila R, et al. Thymus biopsy in children with acquired immunodeficiency syndrome. Arch Pathol Lab Med. (1986) 110:837–42.
105. Messias CV, Loss-Morais G, Carvalho JB, González MN, Cunha DP, Vasconcelos Z, et al. Zika virus targets the human thymic epithelium. Sci Rep. (2020) 10:1378. doi: 10.1038/s41598-020-58135-y
106. Valdespino-Vázquez MY, Sevilla-Reyes EE, Lira R, Yocupicio-Monroy M, Piten-Isidro E, Boukadida C, et al. Congenital Zika syndrome and extra-central nervous system detection of Zika virus in a pre-term newborn in Mexico. Clin Infect Dis. (2019) 68:903–12. doi: 10.1093/cid/ciy616
107. Halouani A, Jmii H, Bodart G, Michaux H, Renard C, Martens H, et al. Assessment of thymic output dynamics after in utero infection of mice with Coxsackievirus B4. Front Immunol. (2020) 11:481. doi: 10.3389/fimmu.2020.00481
108. Jaïdane H, Halouani A, Jmii H, Elmastour F, Abdelkefi S, Bodart G, et al. In-utero coxsackievirus B4 infection of the mouse thymus. Clin Exp Immunol. (2017) 187:399–407. doi: 10.1111/cei.12893
109. Halouani A, Jmii H, Michaux H, Renard C, Martens H, Pirottin D, et al. Housekeeping gene expression in the fetal and neonatal murine thymus following Coxsackievirus B4 infection. Genes (Basel). (2020) 11:279. doi: 10.3390/genes11030279
110. Brilot F, Geenen V, Hober D, Stoddart CA. Coxsackievirus B4 infection of human fetal thymus cells. J Virol. (2004) 78:9854–61. doi: 10.1128/JVI.78.18.9854-9861.2004
111. Brilot F, Chehadeh W, Charlet-Renard C, Martens H, Geenen V, Hober D. Persistent infection of human thymic epithelial cells by coxsackievirus B4. J Virol. (2002) 76:5260–5. doi: 10.1128/jvi.76.10.5260-5265.2002
112. Jaïdane H, Gharbi J, Lobert PE, Lucas B, Hiar R, M’hadheb MB, et al. Prolonged viral RNA detection in blood and lymphoid tissues from coxsackievirus B4 E2 orally-inoculated Swiss mice. Microbiol Immunol. (2006) 50:971–4. doi: 10.1111/j.1348-0421.2006.tb03874.x
113. Jaïdane H, Gharbi J, Lobert PE, Caloone D, Lucas B, Sané F, et al. Infection of primary cultures of murine splenic and thymic cells with coxsackievirus B4. Microbiol Immunol. (2008) 52:40–6. doi: 10.1111/j.1348-0421.2008.00002.x
114. Brilot F, Jaïdane H, Geenen V, Hober D. Coxsackievirus B4 infection of murine foetal thymus organ cultures. J Med Virol. (2008) 80:659-66. Erratum J Med Virol. (2008) 80:1131. doi: 10.1002/jmv.21016
115. Jaïdane H, Caloone D, Lobert PE, Sane F, Dardenne O, Naquet P, et al. Persistent infection of thymic epithelial cells with coxsackievirus B4 results in decreased expression of type 2 insulin-like growth factor. J Virol. (2012) 86:11151–62. doi: 10.1128/JVI.00726-12
116. Mocarski ES, Bonyhadi M, Salimi S, McCune JM, Kaneshima H. Human cytomegalovirus in a SCID-hu mouse: thymic epithelial cells are prominent targets of viral replication. Proc Natl Acad Sci U.S.A. (1993) 90:104–8. doi: 10.1073/pnas.90.1.104
117. Wainberg MA, Numazaki K, Destephano L, Wong I, Goldman H. Infection of human thymic epithelial cells by human cytomegalovirus and other viruses: effect on secretion of interleukin 1-like activity. Clin Exp Immunol. (1988) 72:415–21.
118. Schwartz JN, Daniels CA, Klintworth GK. Lymphoid cell necrosis, thymic atrophy, and growth retardation in newborn mice inoculated with murine cytomegalovirus. Am J Pathol. (1975) 79:509–22.
119. Numazaki K, Goldman H, Bai XQ, Wong I, Wainberg MA. Effects of infection by HIV-1, cytomegalovirus, and human measles virus on cultured human thymic epithelial cells. Microbiol Immunol. (1989) 33:733–45. doi: 10.1111/j.1348-0421.1989.tb00960.x
120. Auwaerter PG, Kaneshima H, McCune JM, Wiegand G, Griffin DE. Measles virus infection of thymic epithelium in the SCID-hu mouse leads to thymocyte apoptosis. J Virol. (1996) 70:3734–40. doi: 10.1128/JVI.70.6.3734-3740.1996
121. Mrkic B, Odermatt B, Klein MA, Billeter MA, Pavlovic J, Cattaneo R. Lymphatic dissemination and comparative pathology of recombinant measles viruses in genetically modified mice. J Virol. (2000) 74:1364–72. doi: 10.1128/jvi.74.3.1364-1372.2000
122. Takeuchi K, Takeda M, Miyajima N, Ami Y, Nagata N, Suzaki Y, et al. Stringent requirement for the C protein of wild-type measles virus for growth both in vitro and in macaques. J Virol. (2005) 79:7838–44. doi: 10.1128/JVI.79.12.7838-7844.2005
123. Amarilla SP, Gómez-Laguna J, Carrasco L, Rodríguez-Gómez IM, Caridad Y, Ocerín JM, et al. Thymic depletion of lymphocytes is associated with the virulence of PRRSV-1 strains. Vet Microbiol. (2016) 188:47–58. doi: 10.1016/j.vetmic.2016.04.005
124. Wang G, Yu Y, Cai X, Zhou EM, Zimmerman JJ. Effects of PRRSV infection on the porcine thymus. Trends Microbiol. (2020) 28:212–23. doi: 10.1016/j.tim.2019.10.009
125. Elsaesser HJ, Mohtashami M, Osokine I, Snell LM, Cunningham CR, Boukhaled GM, et al. Chronic virus infection drives CD8 T cell-mediated thymic destruction and impaired negative selection. Proc Natl Acad Sci U.S.A. (2020) 117:5420–9. doi: 10.1073/pnas.1913776117
126. Gossmann J, Löhler J, Lehmann-Grube F. Entry of antivirally active T lymphocytes into the thymus of virus-infected mice. J Immunol. (1991) 146:293–7.
127. Yoshimura FK, Wang T, Cankovic M. Sequences between the enhancer and promoter in the long terminal repeat affect murine leukemia virus pathogenicity and replication in the thymus. J Virol. (1999) 73:4890–8. doi: 10.1128/JVI.73.6.4890-4898.1999
128. Yoshimura FK, Luo X. Induction of endoplasmic reticulum stress in thymic lymphocytes by the envelope precursor polyprotein of a murine leukemia virus during the preleukemic period. J Virol. (2007) 81:4374–7. doi: 10.1128/JVI.02292-06
129. Morse SS, Valinsky JE. Mouse thymic virus (MTLV). A mammalian herpesvirus cytolytic for CD4+ (L3T4+) T lymphocytes. J Exp Med. (1989) 169:591–6. doi: 10.1084/jem.169.2.591
130. Patel SJ, Zhao G, Penna VR, Park E, Lauron EJ, Harvey IB, et al. A Murine herpesvirus closely related to ubiquitous human herpesviruses causes T-cell depletion. J Virol. (2017) 91:e02463–16. doi: 10.1128/JVI.02463-16
131. Cavalcante P, Barberis M, Cannone M, Baggi F, Antozzi C, Maggi L, et al. Detection of poliovirus-infected macrophages in thymus of patients with myasthenia gravis. Neurology. (2010) 74:1118–26. doi: 10.1212/WNL.0b013e3181d7d884
132. Cavalcante P, Galbardi B, Franzi S, Marcuzzo S, Barzago C, Bonanno S, et al. Increased expression of Toll-like receptors 7 and 9 in myasthenia gravis thymus characterized by active Epstein-Barr virus infection. Immunobiology. (2016) 221:516–27. doi: 10.1016/j.imbio.2015.12.007
133. Cavalcante P, Serafini B, Rosicarelli B, Maggi L, Barberis M, Antozzi C, et al. Epstein-Barr virus persistence and reactivation in myasthenia gravis thymus. Ann Neurol. (2010) 67:726–38. doi: 10.1002/ana.21902
134. Cavalcante P, Maggi L, Colleoni L, Caldara R, Motta T, Giardina C, et al. Inflammation and epstein-barr virus infection are common features of myasthenia gravis thymus: possible roles in pathogenesis. Autoimmune Dis. (2011) 2011:213092. doi: 10.4061/2011/213092
135. Brito VN, Souto PCS, Cruz-Höfling MA, Ricci LC, Verinaud L. Thymus invasion and atrophy induced by Paracoccidioides brasiliensis in BALB/c mice. Med Mycol. (2003) 41:83–7. doi: 10.1080/mmy.41.2.83.87
136. Alves da Costa T, Di Gangi R, Thomé R, Barreto Felisbino M, Pires Bonfanti A, Lumi Watanabe Ishikawa L, et al. Severe changes in thymic microenvironment in a chronic experimental model of Paracoccidioidomycosis. PLoS One (2016) 11:e0164745. Erratum PLoS One. (2016) 11:e0168810. doi: 10.1371/journal.pone.0168810
137. Di Gangi R, Alves da Costa T, Thomé R, Peron G, Burger E, Verinaud L. Paracoccidioides brasiliensis infection promotes thymic disarrangement and premature egress of mature lymphocytes expressing prohibitive TCRs. BMC Infect Dis. (2016) 16:209. doi: 10.1186/s12879-016-1561-8
138. Sotomayor CE, Rubinstein HR, Cervi L, Riera CM, Masih DT. Immunosuppression in experimental cryptococcosis in rats: induction of thymic suppressor cells. Mycopathologia. (1989) 108:5–10. doi: 10.1007/BF00436777
139. Sotomayor CE, Rubinstein HR, Riera CM, Masih DT. Immunosuppression in experimental cryptococcosis: variation of splenic and thymic populations and expression of class II major histocompatibility complex gene products. Clin Immunol Immunopathol. (1995) 77:19–26. doi: 10.1016/0090-122990132-9
140. Cerf BJ, Jones TC, Badaro R, Sampaio D, Teixeira R, Johnson WD. Malnutrition as a risk factor for severe visceral leishmaniasis. J Infect Dis. (1987) 156:1030–3. doi: 10.1093/infdis/156.6.1030
141. Malafaia G, Serafim TD, Silva ME, Pedrosa ML, Rezende SA. Protein-energy malnutrition decreases immune response to Leishmania chagasi vaccine in BALB/c mice. Parasite Immunol. (2009) 31:41–9. doi: 10.1111/j.1365-3024.2008.01069.x
142. Barbeito-Andrés J, Pezzuto P, Higa LM, Dias AA, Vasconcelos JM, Santos TMP, et al. Congenital Zika syndrome is associated with maternal protein malnutrition. Sci Adv. (2020) 6:eaaw6284. doi: 10.1126/sciadv.aaw6284
143. Chuong C, Bates TA, Akter S, Werre SR, LeRoith T, Weger-Lucarelli J. Nutritional status impacts dengue virus infection in mice. BMC Biol. (2020) 18:106. doi: 10.1186/s12915-020-00828-x
144. Sinha P, Lönnroth K, Bhargava A, Heysell SK, Sarkar S, Salgame P, et al. Food for thought: addressing undernutrition to end tuberculosis. Lancet Infect Dis. (2021) 21:e318–25. doi: 10.1016/S1473-309930792-1
145. Kasai A, Gama P, Alvares EP. Protein restriction inhibits gastric cell proliferation during rat postnatal growth in parallel to ghrelin changes. Nutrition. (2012) 28:707–12. doi: 10.1016/j.nut.2011.10.003
146. Mondal D, Minak J, Alam M, Liu Y, Dai J, Korpe P, et al. Contribution of enteric infection, altered intestinal barrier function, and maternal malnutrition to infant malnutrition in Bangladesh. Clin Infect Dis. (2012) 54:185–92. doi: 10.1093/cid/cir807
147. Black RE, Victora CG, Walker SP, Bhutta ZA, Christian P, de Onis M, et al. Maternal and child undernutrition and overweight in low-income and middle-income countries. Lancet. (2013) 382:427–51. doi: 10.1016/S0140-673660937-X
148. Assa A, Vong L, Pinnell LJ, Avitzur N, Johnson-Henry KC, Sherman PM. Vitamin D deficiency promotes epithelial barrier dysfunction and intestinal inflammation. J Infect Dis. (2014) 210:1296–305. doi: 10.1093/infdis/jiu235
149. David LA, Maurice CF, Carmody RN, Gootenberg DB, Button JE, Wolfe BE, et al. Diet rapidly and reproducibly alters the human gut microbiome. Nature. (2014) 505:559–63. doi: 10.1038/nature12820
150. Subramanian S, Huq S, Yatsunenko T, Haque R, Mahfuz M, Alam MA, et al. Persistent gut microbiota immaturity in malnourished Bangladeshi children. Nature. (2014) 510:417–21. doi: 10.1038/nature13421
151. Bourke CD, Jones KDJ, Prendergast AJ. Current understanding of innate immune cell dysfunction in childhood undernutrition. Front Immunol. (2019) 10:1728. doi: 10.3389/fimmu.2019.01728
152. Bryce J, Boschi-Pinto C, Shibuya K, Black RE, WHO Child Health Epidemiology Reference Group. WHO estimates of the causes of death in children. Lancet. (2005) 365:1147–52. doi: 10.1016/S0140-673671877-8
153. Roberton T, Carter ED, Chou VB, Stegmuller AR, Jackson BD, Tam Y, et al. Early estimates of the indirect effects of the COVID-19 pandemic on maternal and child mortality in low-income and middle-income countries: a modelling study. Lancet Glob Health. (2020) 8:e901–8. doi: 10.1016/S2214-109X30229-1
154. Mertens E, Peñalvo JL. The burden of malnutrition and fatal COVID-19: a global burden of disease analysis. Front Nutr. (2021) 7:619850. doi: 10.3389/fnut.2020.619850
155. Papadimitriou-Olivgeris M, Gkikopoulos N, Wüst M, Ballif A, Simonin V, Maulini M, et al. Predictors of mortality of influenza virus infections in a Swiss Hospital during four influenza seasons: role of quick sequential organ failure assessment. Eur J Intern Med. (2020) 74:86–91. doi: 10.1016/j.ejim.2019.12.022
156. Moore CA, Staples JE, Dobyns WB, Pessoa A, Ventura CV, Fonseca EB, et al. Characterizing the pattern of anomalies in congenital Zika syndrome for pediatric clinicians. JAMA Pediatr. (2017) 171:288–95. doi: 10.1001/jamapediatrics.2016.3982
157. Nguyen TH, Nguyen TL, Lei H-Y, Lin Y-S, Le BL, Huang K-J, et al. Association between sex, nutritional status, severity of dengue hemorrhagic fever, and immune status in infants with dengue hemorrhagic fever. Am J Trop Med Hyg. (2005) 72:370–4.
158. Kalayanarooj S, Nimmannitya S. Is dengue severity related to nutritional status? Southeast Asian J Trop Med Public Health. (2005) 36:378–84.
159. Das D, Grais RF, Okiro EA, Stepniewska K, Mansoor R, van der Kam S, et al. Complex interactions between malaria and malnutrition: a systematic literature review. BMC Med. (2018) 16:186. doi: 10.1186/s12916-018-1177-5
160. Page AL, de Rekeneire N, Sayadi S, Aberrane S, Janssens AC, Rieux C, et al. Infections in children admitted with complicated severe acute malnutrition in Niger. PLoS One. (2013) 8:e68699. doi: 10.1371/journal.pone.0068699
161. Denoeud-Ndam L, Dicko A, Baudin E, Guindo O, Grandesso F, Diawara H, et al. Efficacy of artemether-lumefantrine in relation to drug exposure in children with and without severe acute malnutrition: an open comparative intervention study in Mali and Niger. BMC Med. (2016) 14:167. doi: 10.1186/s12916-016-0716-1
162. Gone T, Lemango F, Eliso E, Yohannes S, Yohannes T. The association between malaria and malnutrition among under-five children in Shashogo district, Southern Ethiopia: a case-control study. Infect Dis Poverty. (2017) 6:9. doi: 10.1186/s40249-016-0221-y
163. Donovan CV, McElroy P, Adair L, Pence BW, Oloo AJ, Lal A, et al. Association of malnutrition with subsequent malaria parasitemia among children younger than three years in Kenya: a secondary data analysis of the Asembo Bay cohort study. Am J Trop Med Hyg. (2021) 104:243–54. doi: 10.4269/ajtmh.20-0002
164. Fillol F, Sarr JB, Boulanger D, Cisse B, Sokhna C, Riveau G, et al. Impact of child malnutrition on the specific anti-Plasmodium falciparum antibody response. Malar J. (2009) 8:116. doi: 10.1186/1475-2875-8-116
165. Alexandre MAA, Benzecry SG, Siqueira AM, Vitor-Silva S, Melo GC, Monteiro WM, et al. The association between nutritional status and malaria in children from a rural community in the Amazonian region: a longitudinal study. PLoS Negl Trop Dis. (2015) 9:e0003743. doi: 10.1371/journal.pntd.0003743
166. Mitangala PN, D’Alessandro U, Donnen P, Hennart P, Porignon D, Bisimwa Balaluka G, et al. Infection palustre et état nutritionnel: résultats d’une cohorte d’enfants âgés de 6 à 59 mois au Kivu en République démocratique du Congo. Rev Epidemiol Sante Publique. (2013) 61:111–20. doi: 10.1016/j.respe.2012.06.404
167. Edirisinghe JS, Fern EB, Targett GA. Resistance to superinfection with Plasmodium berghei in rats fed a protein-free diet. Trans R Soc Trop Med Hyg. (1982) 76:382–6. doi: 10.1016/0035-920390196-1
168. Bhatia A, Vinayak VK. Dietary modulation of malaria infection in rats. Indian J Malariol. (1991) 28:237–42.
169. Hunt NH, Manduci N, Thumwood CM. Amelioration of murine cerebral malaria by dietary restriction. Parasitology. (1993) 107(Pt 5):471–6. doi: 10.1017/s0031182000068049
170. Mejia P, Treviño-Villarreal JH, Hine C, Harputlugil E, Lang S, Calay E, et al. Dietary restriction protects against experimental cerebral malaria via leptin modulation and T-cell mTORC1 suppression. Nat Commun. (2015) 6:6050. doi: 10.1038/ncomms7050
171. Rivera-Correa J, Rodriguez A. Autoimmune anemia in malaria. Trends Parasitol. (2020) 36:91–7. doi: 10.1016/j.pt.2019.12.002
172. Lepletier A, de Almeida L, Santos L, da Silva Sampaio L, Paredes B, González FB, et al. Early double-negative thymocyte export in Trypanosoma cruzi infection is restricted by sphingosine receptors and associated with human chagas disease. PLoS Negl Trop Dis. (2014) 8:e3203. doi: 10.1371/journal.pntd.0003203
173. Farias-de-Oliveira DA, Cotta-de-Almeida V, Villa-Verde DM, Riederer I, Meis JD, Savino W. Fibronectin modulates thymocyte-thymic epithelial cell interactions following Trypanosoma cruzi infection. Mem Inst Oswaldo Cruz. (2013) 108:825–31. doi: 10.1590/0074-0276130071
174. Khanam S, Sharma S, Pathak S. Lethal and nonlethal murine malarial infections differentially affect apoptosis, proliferation, and CD8 expression on thymic T cells. Parasite Immunol. (2015) 37:349–61. doi: 10.1111/pim.12197
175. Smythe PM, Brereton-Stiles GG, Grace HJ, Mafoyane A, Schonland M, Coovadia HM, et al. Thymolymphatic deficiency and depression of cell-mediated immunity in protein-calorie malnutrition. Lancet. (1971) 2:939–43. doi: 10.1016/s0140-673690267-4
176. Schonland M. Depression of immunity in protein-calorie malnutrition: a post-mortem study. J Trop Pediatr Environ Child Health. (1972) 18:217–24. doi: 10.1093/tropej/18.3.217
177. Aref GH, Abdel-Aziz A, Elaraby II, Abdel-Moneim MA, Hebeishy NA, Rahmy AI. A post-mortem study of the thymolymphatic system in protein energy malnutrition. J Trop Med Hyg. (1982) 85:109–14.
178. Garly ML, Trautner SL, Marx C, Danebod K, Nielsen J, Ravn H, et al. Thymus size at 6 months of age and subsequent child mortality. J Pediatr. (2008) 153:683–8, 688.e1–3. doi: 10.1016/j.jpeds.2008.04.069
179. Lepletier A, de Carvalho VF, Rodrigues e Silva PM, Villar S, Pérez AR, Savino W, et al. Trypanosoma cruzi disrupts thymic homeostasis by altering intrathymic and systemic stress-related endocrine circuitries. PLoS Negl Trop Dis. (2013) 7:e2470. doi: 10.1371/journal.pntd.0002470
180. Murray HW, Berman JD, Davies CR, Saravia NG. Advances in leishmaniasis. Lancet. (2005) 366:1561–77. doi: 10.1016/S0140-673667629-5
181. Burza S, Croft SL, Boelaert M. Leishmaniasis. Lancet. (2018) 392:951–70. doi: 10.1016/S0140-673631204-2
182. de Oliveira JM, Fernandes AC, Dorval MEC, Alves TP, Fernandes TD, Oshiro ET, et al. Mortality due to visceral leishmaniasis: clinical and laboratory characteristics. Revista Sociedade Brasileira de Medicina Tropical. (2010) 43:188–93. doi: 10.1590/s0037-86822010000200016
183. Mengesha B, Endris M, Takele Y, Mekonnen K, Tadesse T, Feleke A, et al. Prevalence of malnutrition and associated risk factors among adult visceral leishmaniasis patients in Northwest Ethiopia: a cross sectional study. BMC Res Notes. (2014) 7:75. doi: 10.1186/1756-0500-7-75
184. Diro E, Lynen L, Gebregziabiher B, Assefa A, Lakew W, Belew Z, et al. Clinical aspects of paediatric visceral leishmaniasis in North-west Ethiopia. Trop Med Int Health. (2015) 20:8–16. doi: 10.1111/tmi.12407
185. Anstead GM, Chandrasekar B, Zhao W, Yang J, Perez LE, Melby PC. Malnutrition alters the innate immune response and increases early visceralization following Leishmania donovani infection. Infect Immun. (2001) 69:4709–18. doi: 10.1128/IAI.69.8.4709-4718.2001
186. Ibrahim MK, Barnes JL, Anstead GM, Jimenez F, Travi BL, Peniche AG, et al. The malnutrition-related increase in early visceralization of Leishmania donovani is associated with a reduced number of lymph node phagocytes and altered conduit system flow. PLoS Negl Trop Dis. (2013) 7:e2329. doi: 10.1371/journal.pntd.0002329
187. Ibrahim MK, Barnes JL, Osorio EY, Anstead GM, Jimenez F, Osterholzer JJ, et al. Deficiency of lymph node-resident dendritic cells (DCs) and dysregulation of DC chemoattractants in a malnourished mouse model of Leishmania donovani infection. Infect Immun. (2014) 82:3098–112. doi: 10.1128/IAI.01778-14
188. Cuervo-Escobar S, Losada-Barragán M, Umaña-Pérez A, Porrozzi R, Saboia-Vahia L, Miranda LH, et al. T-cell populations and cytokine expression are impaired in thymus and spleen of protein malnourished BALB/c mice infected with Leishmania infantum. PLoS One. (2014) 9:e114584. doi: 10.1371/journal.pone.0114584
189. Losada-Barragán M, Umaña-Pérez A, Rodriguez-Vega A, Cuervo-Escobar S, Azevedo R, Morgado FN, et al. Proteomic profiling of splenic interstitial fluid of malnourished mice infected with Leishmania infantum reveals defects on cell proliferation and pro-inflammatory response. J Proteomics. (2019) 208:103492. doi: 10.1016/j.jprot.2019.103492
190. Gaitán-Albarracín F, Losada-Barragán M, Pinho N, Azevedo R, Durães J, Arcila-Barrera JS, et al. Malnutrition aggravates alterations observed in the gut structure and immune response of mice infected with Leishmania infantum. Microorganisms. (2021) 9:1270. doi: 10.3390/microorganisms9061270
191. Savino W, Mendes-da-Cruz DA, Silva JS, Dardenne M, Cotta-de-Almeida V. Intrathymic T-cell migration: a combinatorial interplay of extracellular matrix and chemokines? Trends Immunol. (2002) 23:305–13. doi: 10.1016/s1471-490602224-x
192. Savino W, Mendes-da-Cruz DA, Golbert DC, Riederer I, Cotta-de-Almeida V. Laminin-mediated interactions in thymocyte migration and development. Front Immunol. (2015) 6:579. doi: 10.3389/fimmu.2015.00579
193. Durães J. Analysis of Malnutrition Effect on the Thymic Microenvironment of BALB/c Mice Infected With Leishmania (Leishmania) infantum. Ph.D. thesis. Rio de Janeiro: Fundação Oswaldo Cruz (2021).
194. Nunes-Alves C, Nobrega C, Behar SM, Correia-Neves M. Tolerance has its limits: how the thymus copes with infection. Trends Immunol. (2013) 34:502–10. doi: 10.1016/j.it.2013.06.004
195. Gilbert JA, Blaser MJ, Caporaso JG, Jansson JK, Lynch SV, Knight R. Current understanding of the human microbiome. Nat Med. (2018) 24:392–400. doi: 10.1038/nm.4517
196. Unger S, Stintzi A, Shah P, Mack D, O’Connor DL. Gut microbiota of the very-low-birth-weight infant. Pediatr Res. (2015) 77:205–13. doi: 10.1038/pr.2014.162
197. Sordillo JE, Korrick S, Laranjo N, Carey V, Weinstock GM, Gold DR, et al. Association of the infant gut microbiome with early childhood neurodevelopmental outcomes: an ancillary study to the VDAART randomized clinical trial. JAMA Netw Open. (2019) 2:e190905. doi: 10.1001/jamanetworkopen.2019.0905
198. Lynch SV, Pedersen O. The human intestinal microbiome in health and disease. N Engl J Med. (2016) 375:2369–79. doi: 10.1056/NEJMra1600266
199. Rinninella E, Raoul P, Cintoni M, Franceschi F, Miggiano GAD, Gasbarrini A, et al. What is the healthy gut microbiota composition? A changing ecosystem across age, environment, diet, and diseases. Microorganisms. (2019) 7:14. doi: 10.3390/microorganisms7010014
200. Galdeano CM, Perdigón G. Role of viability of probiotic strains in their persistence in the gut and in mucosal immune stimulation. J Appl Microbiol. (2004) 97:673–81. doi: 10.1111/j.1365-2672.2004.02353.x
201. Thomas CM, Versalovic J. Probiotics-host communication: modulation of signaling pathways in the intestine. Gut Microbes. (2010) 1:148–63. doi: 10.4161/gmic.1.3.11712
202. Vincenzi A, Goettert MI, Volken de Souza CF. An evaluation of the effects of probiotics on tumoral necrosis factor (TNF-α) signaling and gene expression. Cytokine Growth Factor Rev. (2021) 57:27–38. doi: 10.1016/j.cytogfr.2020.10.004
203. Yang H, Youm YH, Vandanmagsar B, Rood J, Kumar KG, Butler AA, et al. Obesity accelerates thymic aging. Blood. (2009) 114:3803–12. doi: 10.1182/blood-2009-03-213595
204. Harrington KA, Kennedy DS, Tang B, Hickie C, Phelan E, Torreggiani W, et al. Computed tomographic evaluation of the thymus-does obesity affect thymic fatty involution in a healthy young adult population? Br J Radiol. (2018) 91:20170609. doi: 10.1259/bjr.20170609
205. Balcells F, Mariani C, Weill R, Perdigón G, Maldonado-Galdeano C. Effect of yogurt with or without probiotic addition on body composition changes and immune system in an obese model. J Food Sci Nut. (2017) 3:022. doi: 10.24966/FSN-0176/100022
206. Balcells F, Martínez Monteros MJ, Gómez AL, Cazorla SI, Perdigón G, Maldonado-Galdeano C. Probiotic consumption boosts thymus in obesity and senescence mouse models. Nutrients. (2022) 14:616. doi: 10.3390/nu14030616
207. Hosokawa H, Rothenberg EV. Cytokines, transcription factors, and the initiation of T-cell development. Cold Spring Harb Perspect Biol. (2018) 10:a028621. doi: 10.1101/cshperspect.a028621
208. Dalloul AH, Arock M, Fourcade C, Hatzfeld A, Bertho JM, Debré P, et al. Human thymic epithelial cells produce interleukin-3. Blood. (1991) 77:69–74.
209. Mendes-da-Cruz DA, de Meis J, Cotta-de-Almeida V, Savino W. Experimental Trypanosoma cruzi infection alters the shaping of the central and peripheral T-cell repertoire. Microbes Infect. (2003) 5:825–32. doi: 10.1016/s1286-457900156-4
Keywords: undernutrition, thymus, infectious diseases, visceral leishmaniasis, Chagas disease, probiotics
Citation: Savino W, Durães J, Maldonado-Galdeano C, Perdigon G, Mendes-da-Cruz DA and Cuervo P (2022) Thymus, undernutrition, and infection: Approaching cellular and molecular interactions. Front. Nutr. 9:948488. doi: 10.3389/fnut.2022.948488
Received: 19 May 2022; Accepted: 05 September 2022;
Published: 26 September 2022.
Edited by:
Willem Van Eden, Utrecht University, NetherlandsReviewed by:
Ramona Suharoschi, University of Agricultural Sciences and Veterinary Medicine of Cluj-Napoca, RomaniaBeth Lucas, University of Birmingham, United Kingdom
Copyright © 2022 Savino, Durães, Maldonado-Galdeano, Perdigon, Mendes-da-Cruz and Cuervo. This is an open-access article distributed under the terms of the Creative Commons Attribution License (CC BY). The use, distribution or reproduction in other forums is permitted, provided the original author(s) and the copyright owner(s) are credited and that the original publication in this journal is cited, in accordance with accepted academic practice. No use, distribution or reproduction is permitted which does not comply with these terms.
*Correspondence: Wilson Savino, d2lsc29uLnNhdmlub0BmaW9jcnV6LmJy, c2F2aW5vLndAZ21haWwuY29t; Patricia Cuervo, cGF0cmljaWEuY3VlcnZvQGZpb2NydXouYnI=, cGF0eWNlc2NvYmFyQGdtYWlsLmNvbQ==