- 1I.P. Pavlov Department of Physiology, Institute of Experimental Medicine, Saint Petersburg, Russia
- 2Laboratory of the Molecular Mechanisms of Neuronal Interactions, Institute of Evolutionary Physiology and Biochemistry (RAS), Saint Petersburg, Russia
It is now widely accepted that ketosis (a physiological state characterized by elevated plasma ketone body levels) possesses a wide range of neuroprotective effects. There is a growing interest in the use of ketogenic supplements, including medium-chain triglycerides (MCT), to achieve intermittent ketosis without adhering to a strict ketogenic diet. MCT supplementation is an inexpensive and simple ketogenic intervention, proven to benefit both individuals with normal cognition and those suffering from mild cognitive impairment, Alzheimer's disease, and other cognitive disorders. The commonly accepted paradigm underlying MCT supplementation trials is that the benefits stem from ketogenesis and that MCT supplementation is safe. However, medium-chain fatty acids (MCFAs) may also exert effects in the brain directly. Moreover, MCFAs, long-chain fatty acids, and glucose participate in mutually intertwined metabolic pathways. Therefore, the metabolic effects must be considered if the desired procognitive effects require administering MCT in doses larger than 1 g/kg. This review summarizes currently available research on the procognitive effects of using MCTs as a supplement to regular feed/diet without concomitant reduction of carbohydrate intake and focuses on the revealed mechanisms linked to particular MCT metabolites (ketone bodies, MCFAs), highlighting open questions and potential considerations.
Introduction
It is well established that ketogenic diet (KD) and ketone bodies (KB) exert many neuroprotective effects, such as providing an alternative energy source for the brain cells, modulating neurotransmission, supporting the antioxidant and anti-inflammatory responses. Several extensive reviews have been written on this topic (1–4). Although glucose is the primary energy source in the brain, cerebral glucose metabolism is often reduced in aged individuals and patients with Alzheimer's disease (AD), while the brain ketone body metabolism seems to remain intact (5–7). Therefore, providing the brain with ketone bodies in one way or another has emerged as an approach to support cognitive function—a concept that recently acquired a name: neuroketotherapeutics (8). Historically, the state of ketosis for the sake of harnessing its neuroprotective effects has been achieved through adhering to KD, long known as a treatment of intractable epilepsy (9, 10). Despite its efficacy, the use of KD is limited due to the severity of this dietary regime, which requires an almost complete reduction of dietary carbohydrates to enable ketogenesis from the long-chain fatty acids (LCFA) in the liver. A few alternative strategies have been proposed to achieve ketosis intermittently by adding various ketogenic supplements to a regular diet, including the medium-chain triglycerides (MCTs), as well as the salts and esters of ketone bodies (2, 11).
Medium-chain fatty acids (MCFAs) are saturated fatty acids with a chain length from 6 to 10 carbon atoms. Triglycerides that contain MCFAs are called medium-chain triglycerides. As a consumable product, MCTs are produced from coconut and palm kernel oils, both very rich in MCFAs (primarily, the caprylic (C8) and capric (C10) fatty acids) (12). Because of the difference in chain length, MCFAs and LCFAs have different physical properties (MCFA are soluble in water) and are handled by the cells differently, since many enzymes that use fatty acids as substrates are specific to certain chain lengths. After intestinal absorption, in enterocytes, LCFAs get activated (i.e., fused with CoA to form an acyl-CoA—a form in which fatty acids can enter various metabolic pathways) and esterified, trigger chylomicron formation and get transported via lymph, whereas MCFAs largely avoid activation and are primarily transported directly to the liver through the portal vein. In liver cells, the MCFAs, again, avoid activation in the cytosol and enter mitochondria, where they get activated to undergo β-oxidation to produce acetyl-CoA (13). The activated LCFAs can only cross the mitochondrial membranes with the help of the carnitine transport system. When glucose levels are high, insulin stimulates the acetyl-CoA carboxylase activity leading to an increased production of malonyl-CoA, which, in turn, inhibits the carnitine palmitoyltransferase I (CPT-I), one of the components of the carnitine transport system, thus significantly limiting the amount of LCFAs available for β-oxidation in a healthy, well-fed organism (14, 15). Therefore, in hepatocytes, the MCFAs are rapidly oxidized in the mitochondria, whereas the activated LCFAs are primarily directed toward triglyceride (TG) storage, phospholipid biosynthesis, and excretion in very-low-density lipoprotein (VLDL) particles (13). When enough MCFAs are available, their uncontrolled oxidation may produce amounts of acetyl-CoA, which exceed the capacity of the tricarboxylic acid (TCA) cycle. This acetyl-CoA can be redirected to various metabolic pathways, including ketogenesis in the mitochondria, as well as de novo lipogenesis and cholesterol synthesis in the cytosol. The ketone bodies [acetoacetate (AcAc) and β-hydroxybutyrate (βHB)], produced in the liver, can be transported with blood to other organs, including the brain, where they can be converted back to acetyl-CoA and used in the TCA cycle to produce ATP (14) (Figure 1). LCFAs are ketogenic only under conditions such as starvation and KD (when glucose is low), or diabetes. MCFAs, on the other hand, are ketogenic even in a well-fed state in the presence of carbohydrates (16, 17), which is why they can be used as a ketogenic supplement when added to a regular carbohydrate-rich diet.
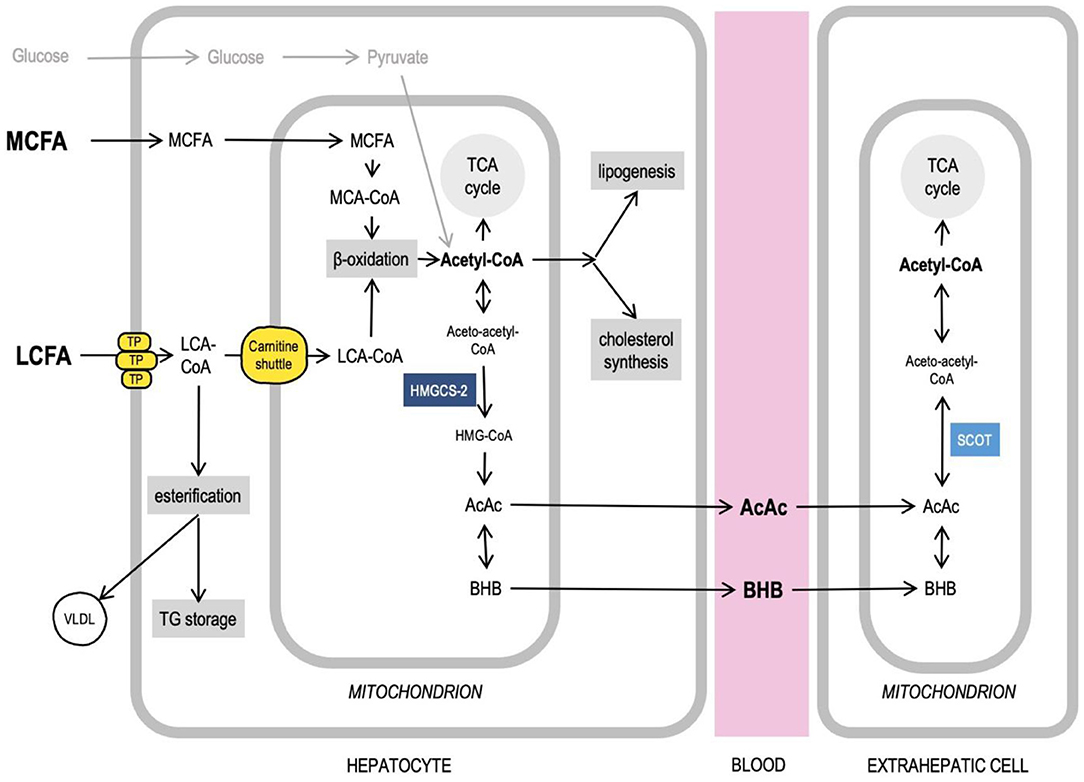
Figure 1. Medium-chain fatty acids, acetyl-CoA, and intersecting liver metabolic pathways. Long-chain fatty acids (LCFA) and medium-chain fatty acids (MCFA) are handled by the cells differently. MCFAs do not require transport proteins (TP) to cross membranes, get activated and undergo oxidation in mitochondria. Acetyl-CoA can feed TCA cycle, ketogenesis, lipogenesis, cholesterol synthesis. Excess LCFAs can be esterified to be stored in liver and excreted as VLDL particles. More details in text. AcAc, acetoacetate; Acetyl-CoA, acetyl-Coenzyme A; βHB, β-hydroxybutyrate; HMG-CoA, β-Hydroxy β-methylglutaryl-Coenzyme A; HMGCS-2, 3-hydroxy-3-methylglutaryl-CoA synthase 2; LCA-CoA, long-chain acyl-Coenzyme A; LCFA, long-chain fatty acids; MCA-CoA, medium-chain acyl-Coenzyme A; MCFA, medium-chain fatty acids; SCOT, Succinyl-CoA:3-ketoacid CoA transferase; TCA cycle, tricarboxylic acid cycle; TG, triglycerides; TP, transport proteins (see details in text); VLDL, very low density lipoprotein.
A growing number of studies have demonstrated that MCT supplementation of a regular diet has a positive effect on cognition, both in healthy individuals and those suffering from mild cognitive impairment (MCI), Alzheimer's disease (AD), and other neurological disorders [for details see review (18)]. Although it is generally assumed that the procognitive effects of MCT supplementation are mediated by KB, and human studies are typically designed in line with this hypothesis, a few animal and in vitro studies have shown that MCFAs may also exert effects in the brain directly, as will be discussed in detail below. The mechanisms of the beneficial effects on cognition are far from being fully understood, and it is often unclear which MCT metabolites and to what extent mediate the effects of MCT supplementation.
Moreover, since MCFAs, LCFAs, and glucose participate in mutually intertwined metabolic pathways, it is important to understand how MCT supplementation of regular diet affects metabolic health in the long term. Doses used in human studies typically lie within 1 g/kg, which is generally considered safe, according to a review of MCT toxicologic properties (19). However, only several studies also monitored cardiometabolic effects alongside cognitive assessment (Table 1), and there exist reports of exceeding the 1 g/kg concentration range until the desired neuroprotective effect has been reached (47). MCTs are cheaper than KB salts and esters and are now widely commercially available. Their consumption is unregulated, and the MCT consumer behavior has not yet become a subject of systematic study.
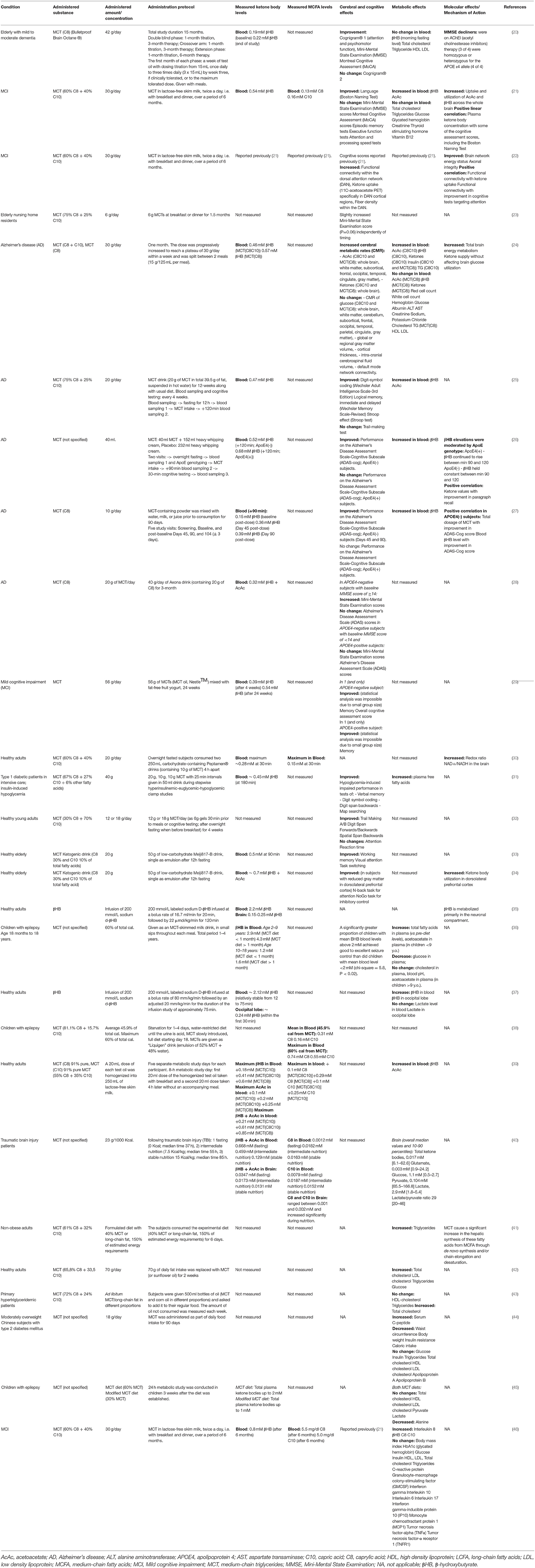
Table 1. Human studies of chronic and acute administration of medium-chain triglycerides (MCT) in healthy subjects and individuals suffering from Alzheimer's disease and Mild Cognitive Impairment.
Below we summarize the available research on the effects, MCT metabolite-specific mechanisms, and metabolic consequences of the MCT supplementation of a regular diet for the purpose of enhancing cognition, highlighting open questions and potential considerations.
Human Studies of MCT Supplementation of Regular Diet
Over the past few years, several human studies explored whether MCT supplementation-induced ketogenesis was sufficient to achieve measurable effects on cognition and brain energy metabolism. In this section, we discuss the studies of MCT supplementation in patients with MCI and AD, and healthy individuals, summarizing the dose, administration protocol, the type of MCFAs used in MCT formulation, major outcomes, and the relationship between the observed effects and the KB plasma levels. The details of the listed human studies can be found in Table 1. For a meta-analysis of MCT supplementation studies in MCI and AD, the reader may be referred to Avgerinos et al. (18).
Studies in Subjects With Mild Cognitive Impairment
In a randomized, double-blind, placebo-controlled crossover study, 6 months followed by another 6 months of open-label extension of MCT (C8) supplementation with up to 42 g a day (25.2 g on average), given with meals, improved some cognitive assessment scores in elderly subjects with mild to moderate dementia (20). In another study in MCI subjects, 6 weeks of MCT supplementation (30 g / day, C8+C10) improved verbal fluency scores compared to placebo (21). Although within the group receiving MCT, the scores in several other cognitive tests have improved compared to the values before the intervention, there was no difference compared to placebo. MCT consumption increased the cerebral metabolism of KB across both cortical and subcortical regions, and the degree of cognitive improvement in some cognitive tests correlated with the brain KB uptake. An analysis of resting-state functional connectivity across eight brain networks in the MCI subjects in this clinical trial was published in a separate paper (22), demonstrating that after MCT supplementation, the connectivity in one of the eight networks, the dorsal attention network (DAN), was 59% higher compared to the placebo group, which was also associated with better scores in some cognitive tests (22). Improved DAN connectivity was associated with increased ketone body uptake and plasma levels post-administration. In a 1.5-month intervention trial, the addition of 6 g of MCT (C8+C10) to either breakfast or dinner was reported to have improved scores in the Mini Mental State Examination test in elderly nursing home residents, although the significance level of this finding was at P=0.06 (23).
Studies in Alzheimer's Disease Patients
A PET study in Alzheimer's Disease (AD) patients demonstrated that 1 month of daily consumption of 30 g of MCT (C8+C10 or C8) led to mild ketonemia and increased total brain energy metabolism due to an increase in ketone body utilization (24). In another open-label placebo-controlled study, although ingestion of a drink containing 20 g of MCT (C8+C10) had no effect on cognition in AD patients, 12 weeks of daily consumption of this drink together with a regular diet led to significant improvements in logical memory tests (25).
Several studies of MCT supplementation in AD patients showed that the intervention efficacy depended on APOE4 status. APOE4 is an allelic variant of Apolipoprotein E, a lipid-binding protein that helps transport fatty acids in the brain between astrocytes and neurons. APOE4 variant disrupts normal fatty acid transport and metabolism in the brain and is a strong genetic factor for late-onset AD (48). Although in vitro βHB has been shown to rescue energy defects associated with the APOE4 isoform (49), several human studies found that APOE4-positive individuals benefited less from ketogenic interventions. For example, a single 38 g oral dose of MCT (95% C8, 5% C10) given after an overnight fast in a low-carb-high-fat drink improved cognitive performance (memory, but not attention) assessed at the peak of the ketonemia in APOE4-negative AD patients, and the degree of improvement correlated with the βHB levels (26). However, although APOE4-positive AD patients in the same study developed even higher levels of βHB and its levels remained high longer than in APOE4-negative patients, however, no cognition-enhancing effect was observed. In a randomized double-blind placebo-controlled study, 3 months of supplementation with 20 g MCT (C8) daily improved cognitive measures in APOE4-negative but not APOE4-positive AD patients (27). In another open-label study in a Japanese population, similarly, 3 months of supplementation with 20 g MCT (C8) improved cognitive assessment scores in APOE4-negative (but not in APOE4-positive) AD patients with higher baseline scores but failed to improve cognition in individuals with more advanced progression of the disease (28). Additionally, in a double-blind randomized placebo-controlled parallel study in individuals diagnosed with MCI with a total number of 4 participants who completed the trial (out of whom 2 received MCT), 24 weeks of MCT supplementation (56 g/day, C8+C10) improved memory and overall AD cognitive assessment scores in one APOE4-negative subject and memory in one APOE4-positive subject (29). The two control subjects in this study demonstrated no memory or overall score improvements. As no statistical analysis was possible due to the small sample size, this report on memory improvement in an APOE4-positive individual should be taken with caution.
Studies in Cognitively Healthy Subjects
The literature on ketogenic supplementation has been primarily concerned with its clinical applications to alleviate some of the symptoms of neurodegenerative and other cognitive diseases. Therefore, the data in healthy subjects is limited. However, it has been demonstrated that a single ingestion of 10 g of MCT (C8+C10) increased the NAD+/NADH redox potential in the brain of healthy volunteers by 18% (30). When intensively treated type 1 diabetic patients with normal cognition received 40 g of MCT (C8+C10) during a controlled period of hypoglycemia, this intervention attenuated the hypoglycemia-induced impairment in cognitive performance (31). Administration of either 12 or 18 g of MCT (C8+C10) for 3 weeks improved cognitive performance in healthy young adults without significant dose-dependent differences (32). Several studies have also demonstrated benefits for elderly individuals not diagnosed with any cognitive impairment. In a double-blind placebo-controlled study, a single ingestion of 20 g of MCT (C8+C10) improved working memory and attention in elderly individuals with normal cognition (33). An fMRI study in healthy elderly individuals found that a single 20 g dose of MCT (C8+C10) improved performance in some cognitive tests, and some of these improvements correlated with an increased rate of KB utilization in the dorsolateral prefrontal cortex (34).
Thus, it appears that the addition of 12–56 g of MCT to a regular diet can lead to significant cognitive improvements, often correlated with the measured elevation of KB. Although the utilization of KB has been reported to double across all brain regions (21), it is unclear why MCT supplementation improves performance in some cognitive tests but not the others. A lower dose of 12 g was sufficient to improve cognition in one study, and a single 10 g dose has significantly increased the NAD+/NADH ratio in the brain. However, very few studies used doses lower than 20 g. Cognitive assessment tests are typically conducted at the peak of the elevated plasma KB levels, based on the assumption that an immediate availability of KB must be required to improve task performance. However, while certain procognitive effects can be observed after a single administration, other effects require continuous chronic administration, suggesting the involvement of different mechanisms. Very few studies measured MCFA concentrations, and none estimated their correlation with cognitive testing results. Most studies focused on aged individuals and those suffering from cognitive decline. It appears that once the cognitive decline has progressed to a certain stage, MCT supplementation becomes less effective. Therefore, MCT supplementation may be recommended as a preventative measure to help sustain cognition before the decline. Healthy younger subjects may benefit from MCT supplementation to the same extent as healthy elderly subjects, if not more. More studies are needed to clarify the effects of MCT supplementation on cognition in healthy young adults.
The Mechanisms of the Procognitive Effects of MCT Supplementation
Most human studies of the procognitive effects of MCT supplementation have been designed to determine whether MCT supplementation can be clinically used to alleviate cognitive deficits or to improve cognitive function (Table 1), and the effects were typically assumed to be mediated by ketone bodies. Several clinical studies in dogs followed this line of investigation and demonstrated that MCT supplementation can be used to reduce the frequency of seizures and improve cognitive performance in dogs (50–54). A number of rodent and in vitro studies revealed that not all the effects of MCT supplementation could be linked to liver ketogenesis and investigated the mechanisms of these effects. In the following section, we review the known mechanisms of MCT supplementation and MCFA action focusing on metabolite-specific effects.
Region and Phenotype-Specificity
Two studies demonstrated that the effect of MCT feeding on brain energy metabolism in specific brain regions was different depending on the presence or absence of stressful conditions.
Brownlow et al. compared the effects of a MCT ketogenic diet (MCT-KD, 27% w/w MCT (C8) with the protein/fat/carbohydrate caloric ratio of 22,5/77/0,5) with a ketogenic supplementation (KS) protocol (MCT (C8) and βHB salts mixture added to regular chow, 10% w/w) (55). This study was meant to assess whether nutritional ketosis could provide cognitive benefits in healthy adult rats with and without accompanying stress (induced by hypoxia). In the MCT-KD-fed animals, βHB stayed chronically elevated at about 1.3 mM, while the KS feeding increased the βHB levels for 4 h post-administration with a subsequent return to baseline. Notably, neither ketogenic treatment affected the βHB concentrations measured in the hippocampus. Conversely, chronic stress reduced the hippocampal βHB concentrations in all animal groups, although this reduction was less pronounced in the MCT-KD group. The finding that the hippocampal βHB level was more affected by stress than ketogenic intervention indicates that the ketone body metabolism likely plays an important role in the hippocampus. Both MCT-KD and, although to a lesser extent, KS significantly upregulated the levels of β-hydroxybutyrate dehydrogenase-1 (BDH1) and acetyl-CoA transferase (ACAT1) in the hippocampus. BDH1 participates in both biosynthesis and utilization of the ketone bodies, while ACAT1 is a mitochondrial enzyme which catalyzes a reversible conversion between acetoacetyl-CoA and 2 molecules of acetyl-CoA—a reaction step in both acetoacetate utilization and the final step of β-oxidation (56, 57). This pattern of enzyme level change in the brain together with the observation that even the MCT-KD had no effect on the brain βHB concentration could potentially be interpreted as a sign of an increase in MCFA β-oxidation in the brain. The hippocampal level of GLUT-1 (endothelial and astrocytic glucose transporter) was lower in the MCT-KD and KS groups compared to control. GLUT-3 (neuronal glucose transporter) hippocampal protein levels were not affected by either ketogenic treatment. Chronic stress reduced GLUT-1 and elevated GLUT-3 levels in the hippocampus, while both ketogenic treatments completely abolished this effect. Finally, both the MCT-KD and KS protocols attenuated the stress-induced decrease of BDNF (brain-derived neurotrophic factor) in the hippocampus (p ≤ 0.05). A similar effect was recently demonstrated in mice, where MCT-KD attenuated the decrease in cortical levels of BDNF induced by a high-fat-high-cholesterol non-ketogenic diet (58).
Hollis and colleagues investigated the effects of 2 weeks of MCT supplementation on anxiety in rats (59). They divided adult male rats into groups based on the anxiety-like behavior (high, normal, and low anxiety). MCT (C8+C10) was mixed into the chow (5% of caloric intake). Under this supplementation protocol, the blood βHB concentration significantly increased from 0,10–0,15 mM to 0,15–0,25 mM. The brain concentration of βHB was not measured. MCT supplementation reduced anxiety in highly anxious animals, but not in animals with normal anxiety levels. The treatment stimulated dominant behavior in animals with varying baseline anxiety level but did not affect depressive-like behavior. MCT supplementation reduced mitochondrial respiration and the Complex I protein levels in the prefrontal cortex (PFC) of the high-anxiety animals to the levels observed in low-anxiety animals. In the same high-anxiety animals, MCT supplementation elevated the intracellular levels of GLUT-1, GLT-1 [also known as EAAT-2, glutamate transporter found in astrocytes and neurons, responsible for glutamate reuptake (60)], and Na+/K+ ATPase subunits (which support the function of GLT-1). No changes were documented in the PFC of low-anxiety animals. Similarly, no changes were found in the Nucleus accumbens of animals of either anxiety level.
The results of these two studies indicate that the MCT supplementation may affect the energy metabolism and neurotransmission in the brain, however the effects may be region- and phenotype-specific. For example, the level of GLUT-1, a transporter required for glucose transport across the blood-brain-barrier, was modulated by the MCT supplementation differently depending on the investigated brain structure and other factors, such as stress or anxiety. More studies are needed to clarify the significance of brain βHB levels under various conditions irrespective of the feeding regime. More studies should also focus on comparing the effects of MCT supplementation among different brain regions.
Differential Effects of C8 and C10
Historically, most MCT studies have been carried out using MCTs containing a mixture of caprylic (C8) and capric (C10) fatty acids. However, the past decade of research has revealed significant differences in the effects of C8 and C10 both in vitro and in vivo.
Hughes et al. incubated neuronal cell line SH-SY5Y cells with either C8, or C10, or βHB for 6 days (61). Only C10 increased the activity of citrate synthase (the enzyme of the first reaction of the TCA cycle), mitochondrial Complex I activity, and catalase activity. Electron microphotographs of the C10-treated cells revealed an increase in number and decrease in the size of mitochondria. Co-incubation with an inhibitor of PPAR-γ (peroxisome proliferator-activated receptor-gamma) abolished the C10 effect on the citrate synthase activity. Therefore, C10 (but not C8 and not the ketone bodies) enhanced energy metabolism and triggered mitochondrial proliferation in this neuronal cell line through interaction with PPAR-γ. This finding is in line with the reports demonstrating that C10 binds with PPAR-γ well, while C8 – very poorly (62). The ability of C10 to activate citrate synthase in a PPAR-γ-mediated manner has also been confirmed in human fibroblasts (63). Additionally, ketogenic diet has been shown to reduce seizure activity in mice via a mechanism involving PPAR-γ (64). PPAR-γ is a ligand-inducible transcription factor expressed in many organs, including the brain, where it is predominantly found in microglia (65). Microglia and astrocytes tend to increase PPAR-γ production during neuroinflammation (66). Saturated fatty acids longer than C8 can act as ligands activating PPAR-γ to trigger transcription of its downstream genes (67). PPAR-γ mediates transcription of antioxidant enzymes such as superoxide dismutase and catalase and inhibits various proinflammatory and inflammatory pathways, including the NF-kB (nuclear factor kappa-light-chain-enhancer of activated B cells) pathway (65, 68, 69). PPAR-γ agonists demonstrated anti-inflammatory properties in various models of neuroinflammatory and neurodegenerative diseases, both in vitro and in vivo (65, 66, 70–73). Therefore, PPAR-γ activation may be one of the neuroprotective mechanisms of the KD and MCT supplementation, which is not directly linked to βHB, but may be acted upon with C10 (Figure 2).
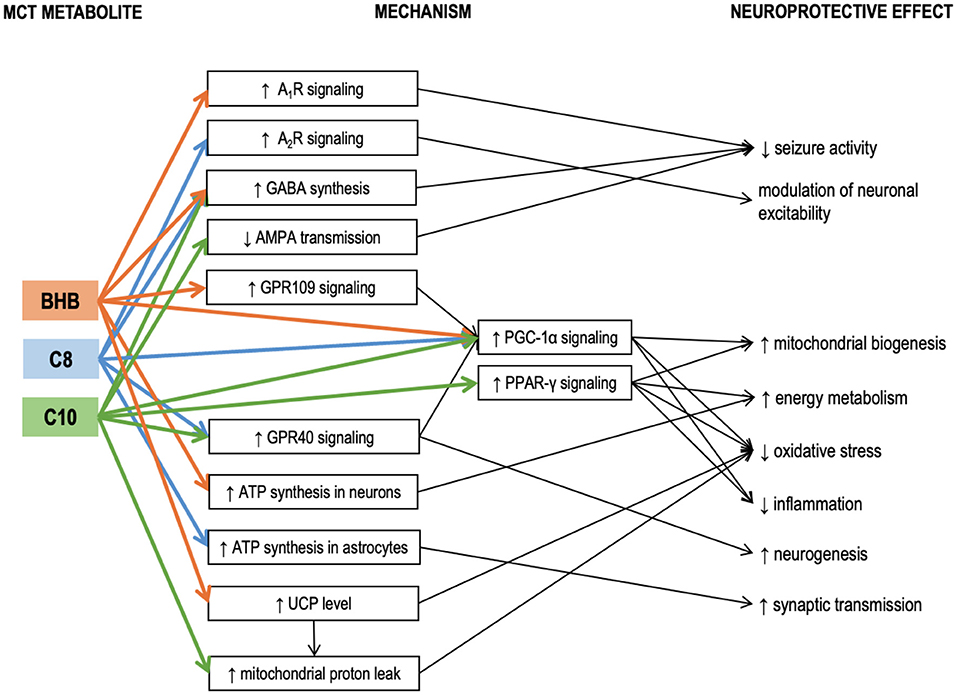
Figure 2. Known mechanisms of the neuroprotective effects of medium-chain triglyceride (MCT) metabolites – caprylic (C8) and capric (C10) medium-chain fatty acids and β-hydroxybutyrate. Details in text. A1R, A2R, adenosine receptors A1 and A2; AMPA, α-amino-3-hydroxy-5-methyl-4-isoxazole propionic acid (AMPA receptor is a subtype of glutamate receptor); βHB, β-hydroxybutyrate; C10, capric acid; C8, caprylic acid; GABA, γ-aminobutyric acid; GPR109, G-protein-coupled receptor 109; GPR40, G-protein-coupled receptor 40; MCT, medium-chain triglycerides; PGC-1α, Peroxisome proliferator-activated receptor γ coactivator 1α; PPAR-γ, Peroxisome proliferator-activated receptor γ; UCP, uncoupling proteins.
Tan et al. compared the effects of chronic administration of MCT (C10) and MCT (C8) in 2 murine models of epilepsy (6Hz test and injection of flurothyl, a GABAA receptor blocker) (74). A diet with 35% calories consumed in the form of MCT (C10) had a significant anticonvulsant effect, while a similar diet with MCT (C8) had no effect. While neither blood nor hippocampal βHB levels were affected by either of the MCT diets, the blood levels of the corresponding MCFAs were elevated. MCT (C10) diet also significantly increased the brain C10 concentration, but no such effect was found in the MCT (C8)-fed animals, suggesting the anticonvulsant effect of the MCT (10) diet could be mediated by the C10 effects in the brain (74). MCT (10) diet increased the plasma antioxidant capacity and upregulated the mRNA expression of several antioxidant enzymes in the hippocampus, including the Hmox1 (heme oxygenase 1). Although Hmox1 transcription is known to be activated by Nrf2 (nuclear factor erythroid 2-related factor 2) (75), which, in turn, may be activated by βHB (76), other antioxidant enzyme genes downstream to Nrf2 were not upregulated, thus further suggesting that βHB was not responsible for the observed effects of the MCT (10) diet. In the same study, when C8 or C10 were added to cultured astrocytes, both MCFAs stimulated basal respiration in mitochondria indicating that astrocytes could oxidize both these MCFAs. However, only C10 increased proton leak. Proton leak, also referred to as uncoupling, is a net movement of protons across the inner mitochondrial membrane not associated with ATP production. Although protein leak decreases ATP production per one mitochondrion, consistent uncoupling has been shown to trigger mitochondrial proliferation and thus a net increase in ATP per cell in the hippocampus, protecting mice against seizures (77). Proton leak also decreases ROS production (78). Therefore, its elevation protects brain cells against oxidative stress (79). A recent study in mouse brain slices likewise confirmed that C8 oxidation was linked to ATP production, while C10 oxidation was linked to increased proton leak (80). Uncoupling proteins (UCPs) are implicated in the mechanism of protein leak (81). Notably, although in the study by Tan et al., C10 stimulated proton leak in cultured astrocytes, no increase in proton leak was registered in the mitochondria isolated from the hippocampus of the MCT-fed animals. Additionally, the MCT (10) diet did not affect the hippocampal expressions of uncoupling protein genes Ucp2, Ucp3, Ucp4, and Ucp5. It is essential to highlight that UCPs are not always conducting protons but are tightly regulated. UCPs are activated by fatty acids (82). C12 and C14 have the strongest stimulating effect on proton transport. C10 has a weaker effect, while C8 and shorter fatty acids are unable to activate UPCs (83). This may explain why several studies have confirmed that C10 but not C8 was able to stimulate protein leak in mitochondrial preparations. It has been demonstrated that ketogenic diet can increase both the levels and the activity of uncoupling proteins in the brain (84). On the other hand, βHB ester consumption has been reported to increase UCP4 and UCP5 protein levels in the brain, accompanied by an increase in brain levels of malonyl-CoA (85). Although the proton leak was not measured in this study, the accumulation of malonyl-CoA should increase fatty acid synthesis and thus activate UCPs. βHB could potentially increase the levels of uncoupling proteins via its effect on peroxisome proliferator-activated receptor-gamma (PPAR-γ) coactivator (PGC)-1α [a transcription coactivator, which controls mitochondrial biogenesis and UCP expression (86, 87)]. Therefore, the lack of ucp upregulation in the study by Tan's group is consistent with the lack of βHB elevation. It is unclear why C10 stimulated the proton leak in vitro but not in vivo. A potential explanation could be that the brain concentration of C10 was not high enough. Further studies are needed to determine whether C10-induced protein leak could be promoted by MCT feeding. To summarize, it appears that βHB can increase the UCP protein expression in the brain, while C10 may potentially activate UCPs to increase the proton leak (Figure 2). These two mechanisms may act together in KD and when taking ketogenic doses of C10-containing MCTs.
MCT consumption has also been linked to PGC-1α in another study, where a single and, to an even greater extent, a repeated intragastric administration of MCT (C8, 0.15 g/kg) reduced the toxic effects of MPTP (1-methyl-4-phenol-1,2,5,6-tetrahydropyridine) in a murine model of Parkinson's disease (88). MPTP is known for its ability to selectively damage dopaminergic neurons. Intraperitoneal injection of MPTP significantly reduced the levels of dopamine and its metabolites in the striatum. MCT administration 1.5 h before the MPTP injection allowed to reduce its toxic effect. Single MCT administration increased striatal mRNA level of PGC-1α. PGC-1α is required for induction of many reactive oxygen species (ROS)-detoxifying enzymes and has been shown to grant neuroprotection against MPTP and kainic acid in other studies (89). A potential mechanism of PGC-1α induction is the activation of GPR109 (G-protein coupled receptor 109, also known as hydroxycarboxylic acid receptor 2, HCA2) by its ligand βHB (90–92). Signaling through GPR109 is involved in some neuroprotective effects of KD and βHB (91). However C8 itself may also increase PGC-1α expression through its interaction with another G protein-coupled receptor GPR40 (also known as free fatty acid receptor 1, FFAR-1), known to be activated by MCFAs [although C8 shows lower affinity compared to C10 (93)]. GPR40 signaling has been associated with neurogenesis (94), potentially participating in signaling cascades involving the phosphorylated-cyclic adenosine monophosphate response element-binding protein (pCREB) and BDNF (95). In vivo administration of MCFA to mice excited a subpopulation of pro-opiomelanocortin (POMC) neurons in the hypothalamus, and this effect was mediated through GPR40 (96). Therefore, βHB and MCFAs could both act to upregulate PGC-1α, triggering antioxidant response and improving cellular energetics through mitochondrial biogenesis (Figure 2).
When various medium-chain fatty acids were compared in their potency to suppress pentylenetetrazol (PTZ) induced epileptiform activity in rat hippocampal slices, C10 was found to be more effective than valproic acid (VPA), a commonly used anti-epileptic drug; C9 (pelargonic fatty acid) was slightly less effective than C10; C8 had no effect (97). In vitro, the anti-seizure effect of C10 could be achieved at 0.1 mM concentration. In the same study, intraperitoneal injection (0.4 g/kg) of C9 (C10 and C8 were not studied) 10 min after the onset of status epilepticus established in awake rats was more effective in suppressing seizures than VPA (97). As valproic acid is a well-known teratogen due to its inhibitory effect on HDAC (histone deacetylases) (98), the authors of the study monitored dose-dependent effects of MCFAs on HDAC activity (97). C8 and C10 had no effect in physiological concentrations (2 mM), although at higher concentrations C10 had a stronger HDAC-inhibitory activity than C8. Therefore, in this model, C10 and C8 were very different in terms of their anticonvulsant activity. In a later study by the same group, C10 but not βHB prevented seizure activity in rat hippocampal slices in two different epilepsy models. The study also revealed that C10 can act as an antagonist of AMPA receptors (99) to suppress seizure activity (Figure 2). The effect of MCT supplementation on AMPA transmission has been confirmed in vivo in a study by our group, where 4 weeks of daily MCT (C8+C10, 2 ml/kg) administration during 6 h of fasting improved spatial and working memory in healthy rats, accompanied by increased expression of the GluN2a- and GluN2b NMDA receptor subunits and the GluA1- and GluA2- AMPA receptor subunits in the medial PFC (100, 101). If C10 inhibits glutamatergic signaling through AMPA receptors, upregulation of the receptor level may be required to sustain glutamatergic transmission necessary for learning.
Wlaz et al. published two similar reports on the effects of single intragastric administrations of C8 (102) and C10 (103) on seizure threshold in several murine epilepsy models assessed in a range of standard tests. C8 (30 mg/kg) was effective against myoclonic and clonic but not tonic seizures induced by PTZ injection, while C10 (30 mg/kg) had no effects in the same tests. Both C8 and C10 (10-30 mg/kg) were effective in the 6-Hz seizure test. Neither C8 nor C10 (30 mg/kg) were effective in the maximal electroshock test. Co-administration of C8 and C10 at therapeutic concentrations had an additive effect, which may be indicative of at least partially different mechanisms of action (102, 103).
One of the mechanisms of the C8 anticonvulsant properties seems to be mediated through adenosine receptors. It is well established that elevated extracellular adenosine reduces seizure activity in several seizure models, and the anticonvulsant action is mediated through A1R adenosine receptors (104, 105). One of the main factors controlling the amount of extracellular adenosine is the adenosine kinase (ADK) (105). KD reduces ADK activity which results in increased extracellular adenosine and suppressed seizure activity (106). Although it has been demonstrated in hippocampal slices that the reduction in excitability can be achieved through ATP/pannexin-1/adenosine/A1R/KATP pathway simply by reducing the glucose concentration in the buffer without any addition of βHB (105), a series of studies in Wistar Albino Glaxo/Rijswijk (WAG/Rij) rats, a genetic model of absence epilepsy, conducted by D'Agostino, Kovacs and colleagues revealed that βHB itself seems to be able to elicit anticonvulsant effects through a mechanisms involving A1R. Using a mixture of ketone salts and medium-chain triglycerides (KSMCT) as the ketogenic supplement (2.5 g/kg/day), they demonstrated that administration of A1R antagonist abolished the anti-seizure effect (i.e., decrease in number of spike-wave discharges – SWD) established by 7 days of KSMCT treatment (107) and dose-dependently decreased or abolished the anxiolytic effects of KSMCT treatment (108). In both studies, KSMCT treatment significantly elevated the blood level of βHB. In another study, they showed that the same KSMCT supplementation protocol alleviated isoflurane-induced anesthesia (immobility), and this effect was mediated through A1R but not A2R receptors (109). This observation was further confirmed in yet another study by the same group, where KEKS food [standard chow with mixed in ketone esters and ketone salts (110)] supplementation decreased the lipopolysaccharide-induced elevation of SWD in the same rat model, while A1R, but not A2AR antagonism abolished the anti-seizure effect of KEKS supplementation (111). In contrast to this series of studies, in a mouse seizure model, 30 mmol/kg (5 g/kg) C8 (administered as free fatty acid) increased the seizure threshold in the 6 Hz psychomotor seizure threshold test, and this effect was blocked by both A1R and A2AR antagonists (112), suggesting that C8 may affect adenosine signaling through a mechanism not involving βHB. The involvement of A2R was further confirmed in another study, where the effects exerted by C8 on POMC neurons were abolished with A2aR and A2bR antagonists (96). Therefore, both KD and ketogenic supplementation of regular diet seem to have neuroprotective effects mediated by A1R and possibly also A2R receptors (Figure 2). It is possible that increased ATP synthesis due to influx of KB or MCFAs in the brain results in elevated extracellular adenosine concentrations, which act upon AR (105, 113), although the exact mechanism is unknown.
Wang and Mitchell compared the effects of 8 weeks of supplementation (5% of caloric intake) with either MCT (C8) or MCT (C10) in aged rats and found differences on both behavioral and biochemical tests (114). The plasma concentrations of MCFAs reached 6,4 μM (C8) and 18,2 μM (C10). Similar to the Tan's group results in mice, the brain level of βHB did not differ among the two MCFA and the LCFA control groups. In behavioral tests, both MCT (C8) and MCT (C10) enhanced social recognition, however only MCT (C10) improved new object recognition. Locomotor activity was decreased in animals in the MCT (C8)-fed group but not in the MCT (C10)-fed group. Protein expression of Ube-3a (ubiquitin protein ligase 3A) was elevated 2-fold in the PFC of the MCT (C10)-fed animals and 4-fold in the PFC of the MCT (C8)-fed animals compared to control. Ube-3a plays an important role in protein degradation and it has been shown that its shortage in the hippocampus of aged rats is associated with memory impairment (115). The mRNA levels of many immediate early genes (IEGs), including Arc, Erg1, Erg2, Junb, Plk3, Nr4a1, and Fosb were reduced in the PFC of the MCT-fed animals. The effects of C8 and C10 containing MCTs were very similar, except for Junb whose level was significantly lower in the MCT (C8)-fed group. As this is one of the first reports on the effects of MCT on neuroplasticity, it is quite difficult to interpret these results. In studies concerning the molecular mechanisms of memory and learning, the expression of IEGs, such as Arc, Erg1, Erg2, Junb, Fosb, is usually measured immediately after learning or an acute stress event. In such experiments, elevated expression of an IEG is interpreted as its involvement in neuroplasticity. Although the role of elevation of decrease of the basal level of IEG mRNA is less clear, some studies have linked increased basal expression of Arc in the hippocampus of aged rats with spatial memory deficits (115). In other words, elevated basal level of IEG expression may signal a reduced sensitivity of the IEG induction in response to incoming stimuli. The observed property of MCTs to decrease basal level of IEGs in PFC requires further research. Wang and Mitchel also measured the levels of the hippocampal growth factors VEGF (vascular endothelial growth factor), GDNF (glial cell-derived neurotrophic factor), and IGF-1 (insulin-like growth factor 1), but found no effect of MCT supplementation (114).
To summarize, a few studies investigated the differential roles of C8 and C10 on brain physiology. C10, but not C8, directly modulates AMPA receptors activity, activates PPAR- γ and facilitates, at least in vitro, the mitochondrial proton leak. The two MCFAs differ in their effects on seizure activity, although the results are controversial for different models and feeding regimes. The mechanism of anticonvulsant effects of C8 may be related to signaling through A2aR and A2bR adenosine receptors. The effects of MCT supplementation on neurotransmission and neuroplasticity-related genes cannot be easily explained by global effects such as increased ATP production or reduced oxidative stress and require more detailed studies. Additionally, more studies should implement experimental designs allowing to distinguish between the effects of βHB and the two MCFAs. The details of the studies related to differential effects of C8 and C10 can be found in Table 3.
MCFA Oxidation and Ketogenesis in the Brain
It is widely accepted that adult mammals' brain uses almost no LCFA (C14-C18) in energy metabolism (121, 122). However, it seems that MCFAs, if administered/present in the diet, may be used by the brain to a far greater extent. The brain is physically protected with the blood-brain barrier (tight junctions between endothelial cells). Therefore, fatty acids must cross cellular membranes to get inside the brain. In the brain, similar to other tissues, LCFA transport across membranes requires various proteins, such as fatty acid transport protein (FATP), fatty acid translocase (FAT)/CD36, plasma membrane-bound fatty acid binding protein (FABPpm), and cytosolic fatty acid-binding protein (FABPc) (123). Only a small amount of LCFAs injected into rat's carotid artery is taken by the brain (124). On the other hand, MCFAs are soluble in water, do not require transport proteins to cross membranes, and predominantly avoid activation in the cytoplasm by acyl-CoA synthases. 94% of C8 and 88% of C10 injected into the carotid artery are taken by the brain (125). When injected directly into CSF, C8 rapidly crosses the blood-brain barrier into the peripheral blood, whereas LCFA transport is limited (96).
Primary cultures of astrocytes from new-born rats oxidize fatty acids (C8, C14, C16) (116, 117). It has been noted that since β-oxidation enzymes are more active in developing brain, the higher-than-expected rates of β-oxidation in some studies could have been an artifact due to the use of cells obtained from a developing brain or stem cells (126). However, β-oxidation is required for neural progenitor cells proliferation in an adult brain's hippocampus (126). Human astrocytes obtained from adult epileptic patients undergoing neurosurgery readily oxidized C16, and fatty acid oxidation was required for neuroprotection following an ischemic stroke in adult mice's hippocampus (118). A recent study in adult mouse brain slices revealed that C8 and C10 were preferentially metabolized and not stored in astrocytes (80). The metabolism of both MCFAs supported glutamine synthesis, which in turn supported neuronal GABA synthesis. In astrocyte preparations, astrocytes oxidized C8 more actively than βHB, while neurons oxidized βHB 3-fold more actively than astrocytes but were unable to oxidize C8 (117). Modeling in a human magnetic resonance study estimated that βHB oxidized is neuronal compartment is approximately 1.85 times more actively than in the astrocytic compartment (35). Therefore, the emerging view is that oxidation of βHB and MCFAs may be largely compartmentalized to neurons and astrocytes, respectively. Consistent with this view, in rat hippocampal slices, βHB but not C8 could support synaptic transmission under hypoglycemic conditions, although C8 added together with βHB was able to improve the rate of recovery of synaptic function after the glucose concentration was restored (31). Therefore, MCFAs and βHB may act in the brain synergistically to support synaptic function. However, contrary to this generalization, a few studies reported that neuron-like cells also were able to oxidize MCFAs. In a human neuronal cell line (originally derived from neuroblastoma), the rate of C8 oxidation was 5-fold greater compared to C10, albeit much lower compared to glucose (119). C8 was also oxidized in a cell line derived from mouse embryonic hypothalamic neuronal primary culture (96). Further studies are required to clarify whether neurons are able to oxidize MCFAs in vivo or ex vivo.
When rats were injected with sodium salt of C8, as much as 20% of the brain's acetyl-CoA was determined to be a product of the injected C8's oxidation (127), although the contribution of the liver ketogenesis to the brain's acetyl-CoA production was not directly accounted for, and the interpretation of the results of this study has been questioned (128). When fatty acids were administered to mice by oral gavage, unlike LCFA, C8 was actively oxidized in the hypothalamus and affected the firing rates of POMC neurons, exhibiting an excitatory effect in some populations and inhibitory in others (96). Therefore, MCFA oxidation has been experimentally confirmed in vivo. Additionally, medium-chain acyl-CoA dehydrogenase (MCAD, an enzyme participating in β-oxidation of both MCFAs and LCFAs when they are shortened to medium-chain length) deficiency, one of the most prevalent disorders of fatty acid oxidation, may lead to accumulation of C8 and C10 in the brain (129). MCAD has been found in both developing and adult rat brain in much greater quantity than LCFA-specific acyl-CoA-dehydrogenases, which may be indicative of the brain's ability to oxidize larger quantities of MCFAs (130). The existence of this disease suggests that a healthy brain may be oxidizing significant amounts of MCFAs. Therefore, although fatty acid oxidation may account for only a small amount of ATP produced in the brain, it may play an important not-yet-understood role in various brain regions.
In addition to being able to carry out β-oxidation, astrocytes can also produce ketone bodies (116, 120, 131–134). Ketogenic diet increases transcription of hmgcs-2 (hydroxy-3-methylglutaryl-CoA synthase 2, the key enzyme of ketogenesis) in the brain (135). Both in the liver and primary astrocyte culture, ketogenesis was more active with C8 as a substrate compared to C16 (120). Although, quantitatively, astrocytes cannot compete with the liver in the quantity of produced ketone bodies, brain ketogenesis may play region-specific regulatory role. For example, astrocytic ketogenesis in the ventromedial hypothalamus is involved in control of food intake in response to a high-fat diet regimen (133).
Therefore, existing data indicate that MCFAs may be oxidized directly in the brain and act as a substrate for ketogenesis in the brain, much more so than LCFAs. These effects of MCFAs in the brain, as well as their relative importance, require further research. Known neuroprotective mechanisms linked to MCT metabolites βHB, C8, and C10 are summarized in Figure 2.
Therapeutic Concentrations of βHB and MCFAs
It is reasonable to assume that if a chemical molecule exerts certain effects, the occurrence of these effects should correlate with the concentration of this molecule. Although the neuroprotective effects of ketogenic diet and MCT supplementation have been predominantly considered as being mediated by βHB, some studies found no correlation between the βHB concentration and the extent of the neuroprotection offered by KD or MCT supplementation, which brought more attention to investigating the direct effects of MCFAs.
In one study, a plasma βHB concentration of 4 mM correlated with the anticonvulsant effect of KD in children (136). In another study, the anticonvulsant effect was found to be “good” or “excellent” when βHB concentration was above 2 mM (36). On the other hand, when C10 was chronically administered to mice in two different seizure models, the anticonvulsant effect did not correlate with βHB concentration (74). MCT added to regular food (9% of daily caloric intake) reduced seizure frequency without significant βHB elevation in dogs (53). In clinical studies of MCT supplementation, the therapeutic effect on cognition was achieved after oral administration of 38 g (26) and 20 g (27) of MCT resulting in the elevation of plasma βHB levels to, correspondingly, 0.43–0.68 mM and 0.30–0.40 mM, which is an order of magnitude less than the therapeutic concentrations reported in the KD studies.
The brain concentrations of βHB are rarely measured in the studies of neuroprotective effects of MCT (Tables 1, 2), however a few studies have looked specifically at the relationship between the plasma and brain concentrations. Intravenous administration of βHB to healthy adult volunteers elevated plasma βHB level from 0.2 mM when fasted in the morning to 2.1 mM following the administration. Using magnetic resonance spectroscopy, βHB concentration in the occipital lobe was estimated as 0.24 mM, about 10-fold lower than in the blood (37). Fasting for 2 and 3 days brought the βHB levels, correspondingly, to 1.7 mM and 3.2 mM in plasma and 0.6 mM and 1.0 mM in the brain, about 3-fold lower than in the blood (37). Although there is about 3-fold difference between the values given by the two studies, starvation is known to increase brain uptake of the KB (143), and ketogenic diet is known to increase the monocarboxylate transporter (required for KB transport) expression in the brain (144), which may explain why the measured values were higher in starved subjects.
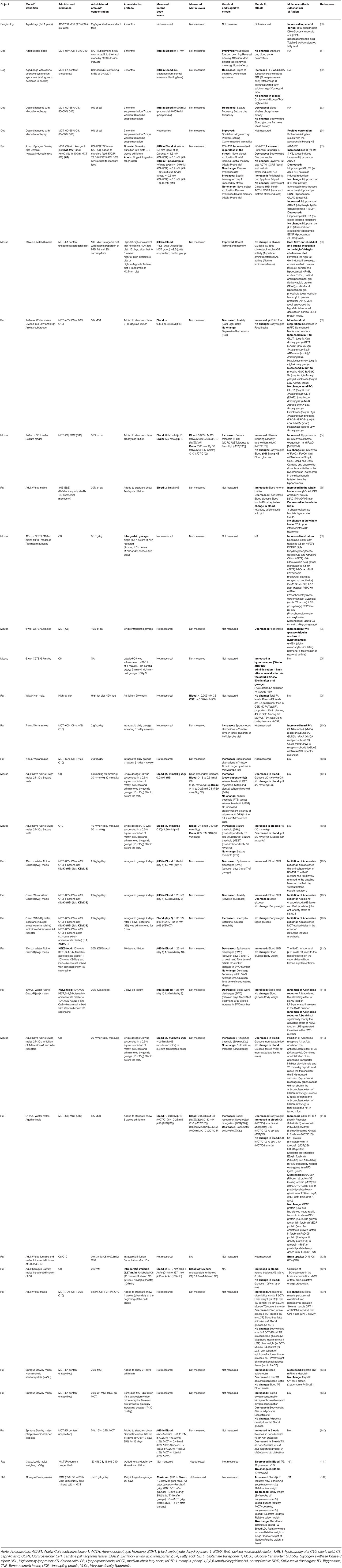
Table 2. Animal studies of chronic and acute administration of medium-chain triglycerides (MCTs) and their metabolites caprylic (C8) and capric (C10) medium-chain fatty acids and β-hydroxybutyrate.
In relation to MCFAs, although a large portion of orally administered MCFAs is metabolized in the liver, the plasma concentrations of MCFAs also become elevated. When children were fed a diet with MCT (C8+C10) providing 46% of caloric intake, the plasma concentrations of C8 and C10 reached 0.31 and 0.16 mM (38). A single oral dose of MCT (C10) or MCT (C8) given to mice resulted in C10 concentrations up to 0.41 mM in plasma and 0.24 mM in the brain (103) and C8 concentrations of 0.51 mM in plasma and 0.25 mM in the brain, the difference between the plasma and the brain being about 2-fold (102). In rats, cerebrospinal fluid (CSF) concentration of FA was measured to be 2.5-fold lower than in blood. However, MCFAs accounted for 1% of all FA in the blood, but 4% in the CSF (96). Therefore, based on the limited available data, it appears that the brain concentration of MCFAs may be roughly estimated to be as high as about half of their plasma concentration. Most in vitro studies of MCFA effects used concentrations (Table 3) comparable to the brain concentrations either measured or estimated based on their plasma concentrations in animal (Table 2) and human (Table 1) studies.
A very insightful metabolic study has recently been conducted by Cunnane's group (39). When 20 ml of MCT were given to healthy volunteers diluted in skim milk, the ketogenic response was 2-fold stronger if the drink was given without an accompanying meal, and the C8 MCT drink was not significantly more ketogenic than the C8+C10 MCT drink. Plasma concentrations of MCFAs (measured as a fraction of total lipids without distinguishing between free and esterified forms) reached almost 0.3 mM (after consuming two 20 ml MCT drinks 4 h apart). When taken together with a meal, MCFA plasma concentrations continued to slowly increase for hours after administration. When given without a meal, C8 MCT administration resulted in a peak with a time profile similar to that of KB, however C8 and C10 plasma concentrations remained elevated without significant peaks after consumption of the C8+C10 and C10 MCT drinks. These results suggest that whatever effects C10 might directly exert in the brain, it does not matter how C10 or C8+C10 are administered. However, if one aims to maximize C8 delivery to the brain, administration of C8 triglyceride without an accompanying meal may be the best strategy.
Another factor to consider is that because most studies are designed to trigger liver ketogenesis with an implicit assumption that the higher the βHB concentration peak, the greater the effect of the intervention, MCT and various ketone supplements (salts and esters of ketone bodies) are typically given in a single dose. Several metabolic studies have demonstrated that smaller MCT doses spread out over the day may result in mild ketonemia and elevated MCFA concentrations. Courchesne-Loyer and colleagues used the following supplementation protocol to determine whether low doses of MCTs could trigger ketonemia: up to 7.5 g MCT were given to healthy volunteers 4 times a day (with meals and before bed) to a total of 30 g/day. Under these conditions, blood βHB was mildly elevated throughout the day reaching up to 0.4 mM, with the daily average increasing from about 0.1 mM pre-intervention to 0.2 mM after 4 weeks of supplementation (17). Bernini and colleagues measured blood and brain concentrations of KB and MCFAs in traumatic brain injury patients who underwent a transition from fasted state to receiving enteral nutrition, either standard or enriched with MCT (23 g/1000 Kcal) (40). There was a positive linear correlation between the blood and the brain KB concentrations. The blood and the brain KB levels decreased during the transition with no differences found between the standard nutrition vs. MCT-enriched nutrition groups. The blood MCFA increased from about 10 μM to 30-40 μM (C8 and C10) when transitioning to MCFA-enriched nutrition. Brain C8 and C10 concentrations also increased significantly. This study shows that when MCTs are mixed with other foods in low quantities and the dose is spread over rather than taken acutely, it does not elevate KB level, however it does increase the blood and brain levels of MCFAs. The effects of non-ketogenic and low-dose MCT supplementation protocols on brain functions have not yet been thoroughly investigated.
To summarize, it has been reported by different research groups in different experimental models that MCFAs exert neuroprotective effects without measurable elevation of βHB levels in the hippocampus (55, 74) or the whole brain (59, 114), while the MCFA concentrations (when they were measured) were elevated in both plasma and the brain. All the above-mentioned studies point out at the incompleteness of the paradigm prevailing in the design of human studies according to which MCT effects are entirely mediated by ketone bodies. Although it is well supported that ketogenesis from MCFA in the liver supplies the brain with alternative fuel with certain procognitive benefits, at this point, it is still an open question to what extent and which beneficial effects of MCFAs are mediated through KB and which through MCFA. A recent review by Lin and colleagues summarized available data on how to maximize the ketogenic potential of MCT ingestion, suggesting that up to 20 g of C8 triglycerides in emulsified form with coffee on empty stomach might be the best strategy (145). However, if the desired effects were mediated by MCFAs rather than KB, an entirely different strategy would be advisable, i.e. taking smaller amounts and mixing MCT with fat and carbohydrate-containing foods to increase the amount of MCFAs in peripheral circulation so that more was available for uptake by the brain.
At this point, there is currently no agreement across the studies on the optimal target therapeutic concentrations of either βHB or MCFAs. Since βHB or MCFAs are involved in multiple metabolic and signaling pathways, and since the effects of MCT supplementation seem to be brain-region- and phenotype-specific, it is possible that different concentrations may be required to achieve different effects in different models and different brain regions. More detailed animal studies are required to better understand the complex effects of MCT metabolites in the brain.
Metabolic Effects of MCT Supplementation
Over the past several decades, a large number of studies have looked at the metabolic effects of MCT consumption either in the form of a high-fat diet or when using MCFAs as a substitute for dietary LCFAs. Although the overall conclusion is that MCFAs in large amounts are far less hepatotoxic than large amounts of LCFAs, one can find many conflicting results. For instance, a MCFA-rich diet compared to a LCFA-rich diet increased (137), decreased (138), or had no effect (139) on TG accumulation in the liver; increased (41, 42, 146, 147), decreased (137, 140, 141), or had no effect (43) on fasting plasma TG concentration; increased (41–43), decreased (44), or had no effect (45) on fasting plasma total cholesterol concentrations (Table 2). The reader may be referred to a recent meta-analysis of differential effects of medium- and long-chain saturated fatty acids on the blood lipid profile (148).
According to a review of toxicological properties of MCT, 1 g/kg dose has been confirmed as safe (19). However, when MCTs are taken for their ketogenic properties, it is reasonable to try and administer a higher dose to achieve higher blood levels of ketone bodies. In one case report, seizures were significantly reduced in a 43-year-old man after supplementing his regular diet with four tablespoons of MCT twice daily (which adds up to about 112 g/day) (47). Large oral doses of MCT may cause osmotic diarrhea, especially when taken on an empty stomach. However, the tolerance is better when taken with meals (27). Since MCFAs, LCFAs, and glucose participate in many intertwined metabolic pathways, one must be cautious when taking increasingly high amounts of MCT without any dietary restrictions. While obesity, insulin resistance, and diabetes are common risk factors for developing AD, all sharing dyslipidemia as a pathological mechanism (149), human clinical trials of MCT effects on cognitive functions generally do not include participants with metabolic disorders (20, 21, 29, 46).
The studies of MCT supplementation of regular diet on markers of metabolic health are still limited. A recent clinical study in patients with MCI receiving 30 g of MCT for 6 weeks assessed a range of cardiometabolic and inflammatory markers and only found a 2.5-fold elevation of IL-8 (46). In one animal study, supplementation of standard feed with MCT in juvenile male rats (10 g/kg) allowed to achieve plasma βHB levels of up to 5 mM for almost 8 h with a parallel almost-2-fold decrease in plasma glucose (142). However, when administered in such a high dose, after 28 days, the liver mass significantly increased while the HDL cholesterol significantly decreased, which raises a concern that MCT added in high amounts to a standard diet without dietary restrictions can be hepatotoxic. It must be noted, however, that, taking into account the rat metabolic rate, the administered dose of 10 g/kg MCT is only 2–5 times higher than the doses used in human clinical trials. In a human study, when a total of 20–30 g of MCT were given in four separate 5–7.5 g doses, no changes were observed in plasma TG and cholesterol concentrations (142). The effects of MCT supplementation will likely be highly variable depending on the constituents of the diet, the timing of meals, and the absence or presence of underlying metabolic disorders. At this moment, the long-term effects of MCT supplementation on cardiovascular risk factors and glucose metabolism are unknown.
It should also be noted that although MCT are ketogenic in the presence of carbohydrates and taking MCT with meals is known to improve gastrointestinal tolerability, administering large MCT doses together with carbohydrates is not advisable. Insulin activates acetyl CoA-carboxylase (150), which increases the cellular concentration of malonyl-CoA, which in turn results in more acetyl-CoA redirected toward de novo lipogenesis, less oxidation of LCFAs and more LCFAs available for esterification to form TG and cholesterol esters. Therefore, to maximize the ketogenic effect of MCT administration and at the same time to minimize the metabolic consequences of taking fats and carbohydrates together in excess, it may be advisable to take MCT after an overnight fast. Alternatively, administering MCT in several small doses spread out throughout the day may also reduce the likelihood of adverse effects in the liver. As mentioned, very few studies to date investigated the neuroprotective effects of this approach (Table 1).
Future Perspectives and Conclusions
The supplementation of a regular diet with MCT is a promising approach to improve cognitive functions in healthy individuals and those suffering from age- or disease-related cognitive impairment. The effects of MCT supplementation on cognitive functions seem to be both phenotype-specific and brain-region-specific. It appears that both ketone bodies and MCFAs directly mediate these effects via partially overlapping and interacting pathways. More research is needed to better understand the underlying mechanisms of these effects. Ideally, more studies should implement experimental designs allowing to distinguish between the effects of βHB and the two MCFAs. For example, chronic MCFA injections into carotid artery could be used to study chronic effects of MCFAs in vivo. Since most studies were conducted with the assumption that the neuroprotective effects of MCT depend on ketogenesis, single doses and larger concentrations were used. Several existing studies point at the possibility that several small doses throughout the day may also offer certain cognition-enhancing benefits. This approach requires further study.
Despite a large amount of research on the effects of MCT ingestion on metabolic health, the consequences of MCT supplementation of a regular diet for the purpose of improving cognition have not been sufficiently investigated, especially when taken at higher doses without reduction of carbohydrate intake. With the increasing popularity of MCT supplementation and growing public awareness of its potential cognition-enhancing effects, more research is needed to clarify the long-term effects of MCT supplementation on cardiovascular and metabolic health, as well as how MCTs are being used outside clinical settings.
Author Contributions
KS and AT conceptualized the article and wrote the original draft. KS prepared the figures. KS, AT, AS, MK, and SA contributed to reviewing and editing of the manuscript. All authors contributed to the article and approved the submitted version.
Funding
This work was supported by Russian Science Foundation, project No. 19-75-10076.
Conflict of Interest
The authors declare that the research was conducted in the absence of any commercial or financial relationships that could be construed as a potential conflict of interest.
Publisher's Note
All claims expressed in this article are solely those of the authors and do not necessarily represent those of their affiliated organizations, or those of the publisher, the editors and the reviewers. Any product that may be evaluated in this article, or claim that may be made by its manufacturer, is not guaranteed or endorsed by the publisher.
References
1. Maalouf M, Rho JM, Mattson MP. The neuroprotective properties of calorie restriction, the ketogenic diet, and ketone bodies. Brain Res Rev. (2009) 59:293–315. doi: 10.1016/j.brainresrev.2008.09.002
2. Kovács Z, D'Agostino DP, Diamond D, Kindy MS, Rogers C, Ari C. Therapeutic potential of exogenous ketone supplement induced ketosis in the treatment of psychiatric disorders: review of current literature. Front Psychiatry. (2019) 10:363. doi: 10.3389/fpsyt.2019.00363
3. Puchalska P, Crawford PA. Multi-dimensional roles of ketone bodies in fuel metabolism, signaling, and therapeutics. Cell Metab. (2017) 25:262–84. doi: 10.1016/j.cmet.2016.12.022
4. Kraeuter A-K, Phillips R, Sarnyai Z. Ketogenic therapy in neurodegenerative and psychiatric disorders: from mice to men. Prog Neuropsychopharmacol Biol Psychiatry. (2020) 101:109913. doi: 10.1016/j.pnpbp.2020.109913
5. Croteau E, Castellano CA, Fortier M, Bocti C, Fulop T, Paquet N, et al. Cross-sectional comparison of brain glucose and ketone metabolism in cognitively healthy older adults, mild cognitive impairment and early Alzheimer's disease. Exp Gerontol. (2018) 107:18–26. doi: 10.1016/j.exger.2017.07.004
6. Castellano C-A, Nugent S, Paquet N, Tremblay S, Bocti C, Lacombe G, et al. Lower brain 18F-fluorodeoxyglucose uptake but normal 11C-acetoacetate metabolism in mild Alzheimer's disease dementia. J Alzheimers Dis. (2015) 43:1343–53. doi: 10.3233/JAD-141074
7. Nugent S, Tremblay S, Chen KW, Ayutyanont N, Roontiva A, Castellano C-A, et al. Brain glucose and acetoacetate metabolism: a comparison of young and older adults. Neurobiol Aging. (2014) 35:1386–95. doi: 10.1016/j.neurobiolaging.2013.11.027
8. Koppel SJ, Swerdlow RH. Neuroketotherapeutics: a modern review of a century-old therapy. Neurochem Int. (2018) 117:114–25. doi: 10.1016/j.neuint.2017.05.019
9. Kinsman SL, Vining EP, Quaskey SA, Mellits D, Freeman JM. Efficacy of the ketogenic diet for intractable seizure disorders: review of 58 cases. Epilepsia. (1992) 33:1132–6. doi: 10.1111/j.1528-1157.1992.tb01770.x
10. Elia M, Klepper J, Leiendecker B, Hartmann H. Ketogenic diets in the treatment of epilepsy. Curr Pharm Des. (2017) 23:5691–701. doi: 10.2174/1381612823666170809101517
11. Camberos-Luna L, Massieu L. Therapeutic strategies for ketosis induction and their potential efficacy for the treatment of acute brain injury and neurodegenerative diseases. Neurochem Int. (2020) 133:104614. doi: 10.1016/j.neuint.2019.104614
12. Babayan VK. Medium-chain triglycerides–their composition, preparation, and application. J Am Oil Chem Soc. (1968) 45:23–5. doi: 10.1007/BF02679040
13. Nguyen P, Leray V, Diez M, Serisier S. Le Bloc'h J, Siliart B, Dumon H. Liver lipid metabolism. J Anim Physiol Anim Nutr (Berl). (2008) 92:272–83. doi: 10.1111/j.1439-0396.2007.00752.x
14. Bach AC, Babayan VK. Medium-chain triglycerides: an update. Am J Clin Nutr. (1982) 36:950–62. doi: 10.1093/ajcn/36.5.950
15. Saggerson D. Malonyl-CoA, a key signaling molecule in mammalian cells. Annu Rev Nutr. (2008) 28:253–72. doi: 10.1146/annurev.nutr.28.061807.155434
16. Seaton TB, Welle SL, Warenko MK, Campbell RG. Thermic effect of medium-chain and long-chain triglycerides in man. Am J Clin Nutr. (1986) 44:630–4. doi: 10.1093/ajcn/44.5.630
17. Courchesne-Loyer A, Fortier M, Tremblay-Mercier J, Chouinard-Watkins R, Roy M, Nugent S, et al. Stimulation of mild, sustained ketonemia by medium-chain triacylglycerols in healthy humans: estimated potential contribution to brain energy metabolism. Nutrition. (2013) 29:635–40. doi: 10.1016/j.nut.2012.09.009
18. Avgerinos KI, Egan JM, Mattson MP, Kapogiannis D. Medium Chain Triglycerides induce mild ketosis and may improve cognition in Alzheimer's disease. A systematic review and meta-analysis of human studies. Ageing Res Rev. (2020) 58:101001. doi: 10.1016/j.arr.2019.101001
19. Traul KA, Driedger A, Ingle DL, Nakhasi D. Review of the toxicologic properties of medium-chain triglycerides. Food Chem Toxicol. (2000) 38:79–98. doi: 10.1016/S0278-6915(99)00106-4
20. Juby AG, Blackburn TE, Mager DR. Use of medium chain triglyceride (MCT) oil in subjects with Alzheimer's disease: a randomized, double-blind, placebo-controlled, crossover study, with an open-label extension. Alzheimers Dement (N Y). (2022) 8:e12259. doi: 10.1002/trc2.12259
21. Fortier M, Castellano C-A, Croteau E, Langlois F, Bocti C, St-Pierre V, et al. A ketogenic drink improves brain energy and some measures of cognition in mild cognitive impairment. Alzheimers Dement. (2019) 15:625–34. doi: 10.1016/j.jalz.2018.12.017
22. Roy M, Edde M, Fortier M, Croteau E, Castellano C-A, St-Pierre V, et al. A ketogenic intervention improves dorsal attention network functional and structural connectivity in mild cognitive impairment. Neurobiol Aging. (2022) 115:77–87. doi: 10.1016/j.neurobiolaging.2022.04.005
23. Abe S, Ezaki O, Suzuki M. Effects of timing of medium-chain triglycerides (8:0 and 10:0) supplementation during the day on muscle mass, function and cognition in frail elderly adults. J Frailty Aging. (2022) 11:100–8. doi: 10.14283/jfa.2021.33
24. Croteau E, Castellano C-A, Richard MA, Fortier M, Nugent S, Lepage M, et al. Ketogenic medium chain triglycerides increase brain energy metabolism in alzheimer's disease. J Alzheimers Dis. (2018) 64:551–61. doi: 10.3233/JAD-180202
25. Ota M, Matsuo J, Ishida I, Takano H, Yokoi Y, Hori H, et al. Effects of a medium-chain triglyceride-based ketogenic formula on cognitive function in patients with mild-to-moderate Alzheimer's disease. Neurosci Lett. (2019) 690:232–6. doi: 10.1016/j.neulet.2018.10.048
26. Reger MA, Henderson ST, Hale C, Cholerton B, Baker LD, Watson GS, et al. Effects of beta-hydroxybutyrate on cognition in memory-impaired adults. Neurobiol Aging. (2004) 25:311–4. doi: 10.1016/S0197-4580(03)00087-3
27. Henderson ST, Vogel JL, Barr LJ, Garvin F, Jones JJ, Costantini LC. Study of the ketogenic agent AC-1202 in mild to moderate Alzheimer's disease: a randomized, double-blind, placebo-controlled, multicenter trial. Nutr Metab (Lond). (2009) 6:31. doi: 10.1186/1743-7075-6-31
28. Ohnuma T, Toda A, Kimoto A, Takebayashi Y, Higashiyama R, Tagata Y, et al. Benefits of use, and tolerance of, medium-chain triglyceride medical food in the management of Japanese patients with Alzheimer's disease: a prospective, open-label pilot study. Clin Interv Aging. (2016) 11:29–36. doi: 10.2147/CIA.S95362
29. Rebello CJ, Keller JN, Liu AG, Johnson WD, Greenway FL. Pilot feasibility and safety study examining the effect of medium chain triglyceride supplementation in subjects with mild cognitive impairment: a randomized controlled trial. BBA Clin. (2015) 3:123–5. doi: 10.1016/j.bbacli.2015.01.001
30. Xin L, Ipek Ö, Beaumont M, Shevlyakova M, Christinat N, Masoodi M, et al. Nutritional ketosis increases NAD+/NADH ratio in healthy human brain: an in vivo study by 31P-MRS. Front Nutr. (2018) 5:62. doi: 10.3389/fnut.2018.00062
31. Page KA, Williamson A, Yu N, McNay EC, Dzuira J, McCrimmon RJ, et al. Medium-chain fatty acids improve cognitive function in intensively treated type 1 diabetic patients and support in vitro synaptic transmission during acute hypoglycemia. Diabetes. (2009) 58:1237–44. doi: 10.2337/db08-1557
32. Ashton JS, Roberts JW, Wakefield CJ, Page RM, MacLaren DPM, Marwood S, et al. The effects of medium chain triglyceride (MCT) supplementation using a C8:C10 ratio of 30:70 on cognitive performance in healthy young adults. Physiol Behav. (2021) 229:113252. doi: 10.1016/j.physbeh.2020.113252
33. Ota M, Matsuo J, Ishida I, Hattori K, Teraishi T, Tonouchi H, et al. Effect of a ketogenic meal on cognitive function in elderly adults: potential for cognitive enhancement. Psychopharmacology (Berl). (2016) 233:3797–802. doi: 10.1007/s00213-016-4414-7
34. Yomogida Y, Matsuo J, Ishida I, Ota M, Nakamura K, Ashida K, et al. An fMRI investigation into the effects of ketogenic medium-chain triglycerides on cognitive function in elderly adults: a pilot study. Nutrients. (2021) 13. doi: 10.3390/nu13072134
35. Pan JW, de Graaf RA, Petersen KF, Shulman GI, Hetherington HP, Rothman DL. [2,4-13 C2]-beta-Hydroxybutyrate metabolism in human brain. J Cereb Blood Flow Metab. (2002) 22:890–8. doi: 10.1097/00004647-200207000-00014
36. Huttenlocher PR. Ketonemia and seizures: metabolic and anticonvulsant effects of two ketogenic diets in childhood epilepsy. Pediatr Res. (1976) 10:536–40. doi: 10.1203/00006450-197605000-00006
37. Pan JW, Telang FW, Lee JH, de Graaf RA, Rothman DL, Stein DT, et al. Measurement of beta-hydroxybutyrate in acute hyperketonemia in human brain. J Neurochem. (2001) 79:539–44. doi: 10.1046/j.1471-4159.2001.00575.x
38. Haidukewych D, Forsythe WI, Sills M. Monitoring octanoic and decanoic acids in plasma from children with intractable epilepsy treated with medium-chain triglyceride diet. Clin Chem. (1982) 28:642–5. doi: 10.1093/clinchem/28.4.642
39. St-Pierre V, Vandenberghe C, Lowry C-M, Fortier M, Castellano C-A, Wagner R, et al. Plasma ketone and medium chain fatty acid response in humans consuming different medium chain triglycerides during a metabolic study day. Front Nutr. (2019) 6:46. doi: 10.3389/fnut.2019.00046
40. Bernini A, Masoodi M, Solari D, Miroz J-P, Carteron L, Christinat N, et al. Modulation of cerebral ketone metabolism following traumatic brain injury in humans. J Cereb Blood Flow Metab. (2020) 40:177–86. doi: 10.1177/0271678X18808947
41. Hill JO, Peters JC, Swift LL, Yang D, Sharp T, Abumrad N, et al. Changes in blood lipids during six days of overfeeding with medium or long chain triglycerides. J Lipid Res. (1990) 31:407–16. doi: 10.1016/S0022-2275(20)43163-3
42. Tholstrup T, Ehnholm C, Jauhiainen M, Petersen M, Høy C-E, Lund P, et al. Effects of medium-chain fatty acids and oleic acid on blood lipids, lipoproteins, glucose, insulin, and lipid transfer protein activities. Am J Clin Nutr. (2004) 79:564–9. doi: 10.1093/ajcn/79.4.564
43. Asakura L, Lottenberg AM, Neves MQ, Nunes VS, Rocha JC, Passarelli M, et al. Dietary medium-chain triacylglycerol prevents the postprandial rise of plasma triacylglycerols but induces hypercholesterolemia in primary hypertriglyceridemic subjects. Am J Clin Nutr. (2000) 71:701–5. doi: 10.1093/ajcn/71.3.701
44. Han JR, Deng B, Sun J, Chen CG, Corkey BE, Kirkland JL, et al. Effects of dietary medium-chain triglyceride on weight loss and insulin sensitivity in a group of moderately overweight free-living type 2 diabetic Chinese subjects. Metab Clin Exp. (2007) 56:985–91. doi: 10.1016/j.metabol.2007.03.005
45. Schwartz RM, Boyes S, Aynsley-Green A. Metabolic effects of three ketogenic diets in the treatment of severe epilepsy. Dev Med Child Neurol. (1989) 31:152–60. doi: 10.1111/j.1469-8749.1989.tb03973.x
46. Myette-Côté É, St-Pierre V, Beaulieu S, Castellano C-A, Fortier M, Plourde M, et al. The effect of a 6-month ketogenic medium-chain triglyceride supplement on plasma cardiometabolic and inflammatory markers in mild cognitive impairment. Prostaglandins Leukot Essent Fatty Acids. (2021) 169:102236. doi: 10.1016/j.plefa.2020.102236
47. Azzam R, Azar NJ. Marked seizure reduction after MCT supplementation. Case Rep Neurol Med. (2013) 2013:809151. doi: 10.1155/2013/809151
48. Qi G, Mi Y, Shi X, Gu H, Brinton RD, Yin F. ApoE4 impairs neuron-astrocyte coupling of fatty acid metabolism. Cell Rep. (2021) 34:108572. doi: 10.1016/j.celrep.2020.108572
49. Garcia C, Tomilov A, Sandoval J, Cortopassi GA. ApoE4 confers mitochondrial substrate oxidation defects that can be bioenergetically compensated by a ketogenic substrate beta-hydroxy butyrate (BHB). Alzheimer's Dement. (2021) 17:e054355. Available at: doi: 10.1002/alz.054369
50. Taha AY, Henderson ST, Burnham WM. Dietary enrichment with medium chain triglycerides (AC-1203) elevates polyunsaturated fatty acids in the parietal cortex of aged dogs: implications for treating age-related cognitive decline. Neurochem Res. (2009) 34:1619–25. doi: 10.1007/s11064-009-9952-5
51. Pan Y, Larson B, Araujo JA, Lau W, de Rivera C, Santana R, et al. Dietary supplementation with medium-chain TAG has long-lasting cognition-enhancing effects in aged dogs. Br J Nutr. (2010) 103:1746–54. doi: 10.1017/S0007114510000097
52. Pan Y, Landsberg G, Mougeot I, Kelly S, Xu H, Bhatnagar S, et al. Efficacy of a therapeutic diet on dogs with signs of cognitive dysfunction syndrome (CDS): a prospective double blinded placebo controlled clinical study. Front Nutr. (2018) 5:127. doi: 10.3389/fnut.2018.00127
53. Berk BA, Law TH, Packer RMA, Wessmann A, Bathen-Nöthen A, Jokinen TS, et al. A multicenter randomized controlled trial of medium-chain triglyceride dietary supplementation on epilepsy in dogs. J Vet Intern Med. (2020) 34:1248–59. doi: 10.1111/jvim.15756
54. Berk BA, Packer RMA, Law TH, Wessmann A, Bathen-Nöthen A, Jokinen TS, et al. Medium-chain triglycerides dietary supplement improves cognitive abilities in canine epilepsy. Epilepsy Behav. (2021) 114:107608. doi: 10.1016/j.yebeh.2020.107608
55. Brownlow ML, Jung SH, Moore RJ, Bechmann N, Jankord R. Nutritional ketosis affects metabolism and behavior in sprague-dawley rats in both control and chronic stress environments. Front Mol Neurosci. (2017) 10:129. doi: 10.3389/fnmol.2017.00129
56. Adeva-Andany MM, Carneiro-Freire N, Seco-Filgueira M, Fernández-Fernández C, Mouriño-Bayolo D. Mitochondrial β-oxidation of saturated fatty acids in humans. Mitochondrion. (2018) 46:73–90. doi: 10.1016/j.mito.2018.02.009
57. Fukao T, Sasai H, Aoyama Y, Otsuka H, Ago Y, Matsumoto H, et al. Recent advances in understanding beta-ketothiolase (mitochondrial acetoacetyl-CoA thiolase, T2) deficiency. J Hum Genet. (2019) 64:99–111. doi: 10.1038/s10038-018-0524-x
58. Lin D-T, Kao N-J, Cross T-WL, Lee W-J, Lin S-H. Effects of ketogenic diet on cognitive functions of mice fed high-fat-high-cholesterol diet. J Nutr Biochem. (2022) 104:108974. doi: 10.1016/j.jnutbio.2022.108974
59. Hollis F, Mitchell ES, Canto C, Wang D, Sandi C. Medium chain triglyceride diet reduces anxiety-like behaviors and enhances social competitiveness in rats. Neuropharmacology. (2018) 138:245–56. doi: 10.1016/j.neuropharm.2018.06.017
60. Rimmele TS, Rosenberg PA. GLT-1: the elusive presynaptic glutamate transporter. Neurochem Int. (2016) 98:19–28. doi: 10.1016/j.neuint.2016.04.010
61. Hughes SD, Kanabus M, Anderson G, Hargreaves IP, Rutherford T, O'Donnell M, et al. The ketogenic diet component decanoic acid increases mitochondrial citrate synthase and complex I activity in neuronal cells. J Neurochem. (2014) 129:426–33. doi: 10.1111/jnc.12646
62. Liberato MV, Nascimento AS, Ayers SD, Lin JZ, Cvoro A, Silveira RL, et al. Medium chain fatty acids are selective peroxisome proliferator activated receptor (PPAR) γ activators and pan-PPAR partial agonists. PLoS ONE. (2012) 7:e36297. doi: 10.1371/journal.pone.0036297
63. Kanabus M, Fassone E, Hughes SD, Bilooei SF, Rutherford T, Donnell MO, et al. The pleiotropic effects of decanoic acid treatment on mitochondrial function in fibroblasts from patients with complex I deficient Leigh syndrome. J Inherit Metab Dis. (2016) 39:415–26. doi: 10.1007/s10545-016-9930-4
64. Simeone TA, Matthews SA, Samson KK, Simeone KA. Regulation of brain PPARgamma2 contributes to ketogenic diet anti-seizure efficacy. Exp Neurol. (2017) 287:54–64. doi: 10.1016/j.expneurol.2016.08.006
65. Heneka MT, Landreth GE. PPARs in the brain. Biochim Biophys Acta. (2007) 1771:1031–45. doi: 10.1016/j.bbalip.2007.04.016
66. Diab A, Deng C, Smith JD, Hussain RZ, Phanavanh B, Lovett-Racke AE, et al. Peroxisome proliferator-activated receptor-gamma agonist 15-deoxy-Delta(12,14)-prostaglandin J(2) ameliorates experimental autoimmune encephalomyelitis. J Immunol. (2002) 168:2508–15. doi: 10.4049/jimmunol.168.5.2508
67. Bocos C, Göttlicher M, Gearing K, Banner C, Enmark E, Teboul M, et al. Fatty acid activation of peroxisome proliferator-activated receptor (PPAR). J Steroid Biochem Mol Biol. (1995) 53:467–73. doi: 10.1016/0960-0760(95)00093-F
68. Ricote M, Li AC, Willson TM, Kelly CJ, Glass CK. The peroxisome proliferator-activated receptor-gamma is a negative regulator of macrophage activation. Nature. (1998) 391:79–82. doi: 10.1038/34178
69. Clark RB, Bishop-Bailey D, Estrada-Hernandez T, Hla T, Puddington L, Padula SJ. The nuclear receptor PPAR gamma and immunoregulation: PPAR gamma mediates inhibition of helper T cell responses. J Immunol. (2000) 164:1364–71. doi: 10.4049/jimmunol.164.3.1364
70. Jiang C, Ting AT, Seed B. PPAR-gamma agonists inhibit production of monocyte inflammatory cytokines. Nature. (1998) 391:82–6. doi: 10.1038/34184
71. Heneka MT, Feinstein DL, Galea E, Gleichmann M, Wüllner U, Klockgether T. Peroxisome proliferator-activated receptor gamma agonists protect cerebellar granule cells from cytokine-induced apoptotic cell death by inhibition of inducible nitric oxide synthase. J Neuroimmunol. (1999) 100:156–68. doi: 10.1016/S0165-5728(99)00192-7
72. Heneka MT, Klockgether T, Feinstein DL. Peroxisome proliferator-activated receptor-gamma ligands reduce neuronal inducible nitric oxide synthase expression and cell death in vivo. J Neurosci. (2000) 20:6862–7. doi: 10.1523/JNEUROSCI.20-18-06862.2000
73. Schütz B, Reimann J, Dumitrescu-Ozimek L, Kappes-Horn K, Landreth GE, Schürmann B, et al. The oral antidiabetic pioglitazone protects from neurodegeneration and amyotrophic lateral sclerosis-like symptoms in superoxide dismutase-G93A transgenic mice. J Neurosci. (2005) 25:7805–12. doi: 10.1523/JNEUROSCI.2038-05.2005
74. Tan KN, Carrasco-Pozo C, McDonald TS, Puchowicz M, Borges K. Tridecanoin is anticonvulsant, antioxidant, and improves mitochondrial function. J Cereb Blood Flow Metab. (2017) 37:2035–48. doi: 10.1177/0271678X16659498
75. Martin D, Rojo AI, Salinas M, Diaz R, Gallardo G, Alam J, et al. Regulation of heme oxygenase-1 expression through the phosphatidylinositol 3-kinase/Akt pathway and the Nrf2 transcription factor in response to the antioxidant phytochemical carnosol. J Biol Chem. (2004) 279:8919–29. doi: 10.1074/jbc.M309660200
76. Xie G, Tian W, Wei T, Liu F. The neuroprotective effects of β-hydroxybutyrate on Aβ-injected rat hippocampus in vivo and in Aβ-treated PC-12 cells in vitro. Free Radic Res. (2015) 49:139–50. doi: 10.3109/10715762.2014.987274
77. Diano S, Matthews RT, Patrylo P, Yang L, Beal MF, Barnstable CJ, et al. Uncoupling protein 2 prevents neuronal death including that occurring during seizures: a mechanism for preconditioning. Endocrinology. (2003) 144:5014–21. doi: 10.1210/en.2003-0667
78. Divakaruni AS, Brand MD. The regulation and physiology of mitochondrial proton leak. Physiology (Bethesda). (2011) 26:192–205. doi: 10.1152/physiol.00046.2010
79. Andrews ZB, Diano S, Horvath TL. Mitochondrial uncoupling proteins in the CNS: in support of function and survival. Nat Rev Neurosci. (2005) 6:829–40. doi: 10.1038/nrn1767
80. Andersen JV, Westi EW, Jakobsen E, Urruticoechea N, Borges K, Aldana BI. Astrocyte metabolism of the medium-chain fatty acids octanoic acid and decanoic acid promotes GABA synthesis in neurons via elevated glutamine supply. Mol Brain. (2021) 14:132. doi: 10.1186/s13041-021-00842-2
81. Echtay KS. Mitochondrial uncoupling proteins–what is their physiological role? Free Radic Biol Med. (2007) 43:1351–71. doi: 10.1016/j.freeradbiomed.2007.08.011
82. Hoang T, Smith MD, Jelokhani-Niaraki M. Toward understanding the mechanism of ion transport activity of neuronal uncoupling proteins UCP2, UCP4, and UCP5. Biochemistry. (2012) 51:4004–14. doi: 10.1021/bi3003378
83. Winkler E, Klingenberg M. Effect of fatty acids on H+ transport activity of the reconstituted uncoupling protein. J Biol Chem. (1994) 269:2508–15. doi: 10.1016/S0021-9258(17)41974-0
84. Sullivan PG, Rippy NA, Dorenbos K, Concepcion RC, Agarwal AK, Rho JM. The ketogenic diet increases mitochondrial uncoupling protein levels and activity. Ann Neurol. (2004) 55:576–80. doi: 10.1002/ana.20062
85. Kashiwaya Y, Pawlosky R, Markis W, King MT, Bergman C, Srivastava S, et al. ketone ester diet increases brain malonyl-CoA and Uncoupling proteins 4 and 5 while decreasing food intake in the normal Wistar Rat. J Biol Chem. (2010) 285:25950–6. doi: 10.1074/jbc.M110.138198
86. Ventura-Clapier R, Garnier A, Veksler V. Transcriptional control of mitochondrial biogenesis: the central role of PGC-1alpha. Cardiovasc Res. (2008) 79:208–17. doi: 10.1093/cvr/cvn098
87. Wu Z, Puigserver P, Andersson U, Zhang C, Adelmant G, Mootha V, et al. Mechanisms controlling mitochondrial biogenesis and respiration through the thermogenic coactivator PGC-1. Cell. (1999) 98:115–24. doi: 10.1016/S0092-8674(00)80611-X
88. Joniec-Maciejak I, Wawer A, Turzyńska D, Sobolewska A, Maciejak P, Szyndler J, et al. Octanoic acid prevents reduction of striatal dopamine in the MPTP mouse model of Parkinson's disease. Pharmacol Rep. (2018) 70:988–92. doi: 10.1016/j.pharep.2018.04.008
89. St-Pierre J, Drori S, Uldry M, Silvaggi JM, Rhee J, Jäger S, et al. Suppression of reactive oxygen species and neurodegeneration by the PGC-1 transcriptional coactivators. Cell. (2006) 127:397–408. doi: 10.1016/j.cell.2006.09.024
90. Norwitz NG, Hu MT, Clarke K. The mechanisms by which the ketone body D-β-hydroxybutyrate may improve the multiple cellular pathologies of Parkinson's disease. Front Nutr. (2019) 6:63. doi: 10.3389/fnut.2019.00063
91. Rahman M, Muhammad S, Khan MA, Chen H, Ridder DA, Müller-Fielitz H, et al. The β-hydroxybutyrate receptor HCA2 activates a neuroprotective subset of macrophages. Nat Commun. (2014) 5:3944. doi: 10.1038/ncomms4944
92. Fu S-P, Wang J-F, Xue W-J, Liu H-M, Liu B, Zeng Y-L, et al. Anti-inflammatory effects of BHBA in both in vivo and in vitro Parkinson's disease models are mediated by GPR109A-dependent mechanisms. J Neuroinflammation. (2015) 12:9. doi: 10.1186/s12974-014-0230-3
93. Pujol JB, Christinat N, Ratinaud Y, Savoia C, Mitchell SE, Dioum EHM. Coordination of GPR40 and ketogenesis signaling by medium chain fatty acids regulates beta cell function. Nutrients. (2018) 10. doi: 10.3390/nu10040473
94. Yonezawa T, Kurata R, Yoshida K, Murayama MA, Cui X, Hasegawa A. Free fatty acids-sensing G protein-coupled receptors in drug targeting and therapeutics. Curr Med Chem. (2013) 20:3855–71. doi: 10.2174/09298673113209990168
95. Boneva NB, Yamashima T. New insights into “GPR40-CREB interaction in adult neurogenesis” specific for primates. Hippocampus. (2012) 22:896–905. doi: 10.1002/hipo.20951
96. Haynes VR, Michael NJ, van den Top M, Zhao F-Y, Brown RD, De Souza D, et al. Neural basis for Octanoic acid regulation of energy balance. Mol Metab. (2020) 34:54–71. doi: 10.1016/j.molmet.2020.01.002
97. Chang P, Terbach N, Plant N, Chen PE, Walker MC, Williams RSB. Seizure control by ketogenic diet-associated medium chain fatty acids. Neuropharmacology. (2013) 69:105–14. doi: 10.1016/j.neuropharm.2012.11.004
98. Gurvich N, Tsygankova OM, Meinkoth JL, Klein PS. Histone deacetylase is a target of valproic acid-mediated cellular differentiation. Cancer Res. (2004) 64:1079–86. doi: 10.1158/0008-5472.CAN-03-0799
99. Chang P, Augustin K, Boddum K, Williams S, Sun M, Terschak JA, et al. Seizure control by decanoic acid through direct AMPA receptor inhibition. Brain. (2016) 139:431–43. doi: 10.1093/brain/awv325
100. Nikitina VA, Krytskaya DU, Schwarz AP, Veniaminova EA, Shavva VS, Apryatin SA, et al. Memory improvement resulting from the medium chain triglyceride treatment is accompanied by elevated cortical NMDA and AMPA receptor gene expression in the rat brain. In: VII Youth School-Conference on Molecular and Cellular Biology of the Institute of Cytology RAS, St Petersburg, Russia, Genes & Cells. Human Stem Cells Institute. (2020) p.169–170.
101. Trofimov AN, Schwarz AP, Krytskaya DU, Shavva VS, Veniaminova EA, Sizoff VV, et al. Mild ketogenic diet as promising approach for cognition enhancement: medium-chain triglyceride supplement improves memory in rats. J Neurochem. (2019) 121. doi: 10.1111/jnc.14776
102. Wlaz P, Socała K, Nieoczym D, Łuszczki JJ, Zarnowska I, Zarnowski T, et al. Anticonvulsant profile of caprylic acid, a main constituent of the medium-chain triglyceride (MCT) ketogenic diet, in mice. Neuropharmacology. (2012) 62:1882–9. doi: 10.1016/j.neuropharm.2011.12.015
103. Wlaz P, Socała K, Nieoczym D, Zarnowski T, Zarnowska I, Czuczwar SJ, et al. Acute anticonvulsant effects of capric acid in seizure tests in mice. Prog Neuropsychopharmacol Biol Psychiatry. (2015) 57:110–6. doi: 10.1016/j.pnpbp.2014.10.013
104. Gouder N, Fritschy J-M, Boison D. Seizure suppression by adenosine A1 receptor activation in a mouse model of pharmacoresistant epilepsy. Epilepsia. (2003) 44:877–85. doi: 10.1046/j.1528-1157.2003.03603.x
105. Ruskin DN, Kawamura M, Masino SA. Adenosine and ketogenic treatments. J Caffeine Adenosine Res. (2020) 10:104–9. doi: 10.1089/caff.2020.0011
106. Masino SA Li T, Theofilas P, Sandau US, Ruskin DN, Fredholm BB, Geiger JD, et al. Ketogenic diet suppresses seizures in mice through adenosine A1 receptors. J Clin Invest. (2011) 121:2679–83. doi: 10.1172/JCI57813
107. Kovács Z, D'Agostino DP, Dobolyi A, Ari C. Adenosine A1 receptor antagonism abolished the anti-seizure effects of exogenous ketone supplementation in wistar albino glaxo rijswijk rats. Front Mol Neurosci. (2017) 10:235. doi: 10.3389/fnmol.2017.00235
108. Kovács Z, D'Agostino DP, Ari C. Anxiolytic effect of exogenous ketone supplementation is abolished by adenosine A1 receptor inhibition in wistar albino glaxo/rijswijk rats. Front Behav Neurosci. (2018) 12:29. doi: 10.3389/fnbeh.2018.00029
109. Kovács Z, Brunner B, D'Agostino DP, Ari C. Inhibition of adenosine A1 receptors abolished the nutritional ketosis-evoked delay in the onset of isoflurane-induced anesthesia in Wistar Albino Glaxo Rijswijk rats. BMC Anesthesiol. (2020) 20:30. doi: 10.1186/s12871-020-0943-z
110. Kovács Z, D'Agostino DP, Diamond DM, Ari C. Exogenous ketone supplementation decreased the lipopolysaccharide-induced increase in absence epileptic activity in wistar albino glaxo rijswijk rats. Front Mol Neurosci. (2019) 12:45. doi: 10.3389/fnmol.2019.00045
111. Brunner B, Ari C, D'Agostino DP, Kovács Z. Adenosine receptors modulate the exogenous ketogenic supplement-evoked alleviating effect on lipopolysaccharide-generated increase in absence epileptic activity in WAG/Rij rats. Nutrients. (2021) 13. doi: 10.3390/nu13114082
112. Socała K, Nieoczym D, Pieróg M, Wlaz P. Role of the adenosine system and glucose restriction in the acute anticonvulsant effect of caprylic acid in the 6 Hz psychomotor seizure test in mice. Prog Neuropsychopharmacol Biol Psychiatry. (2015) 57:44–51. doi: 10.1016/j.pnpbp.2014.10.006
113. Dunwiddie TV, Diao L, Proctor WR. Adenine nucleotides undergo rapid, quantitative conversion to adenosine in the extracellular space in rat hippocampus. J Neurosci. (1997) 17:7673–82. doi: 10.1523/JNEUROSCI.17-20-07673.1997
114. Wang D, Mitchell ES. Cognition and synaptic-plasticity related changes in aged rats supplemented with 8- and 10-carbon medium chain triglycerides. PLoS ONE. (2016) 11:e0160159. doi: 10.1371/journal.pone.0160159
115. Fletcher BR, Hill GS, Long JM, Gallagher M, Shapiro ML, Rapp PR. A fine balance: Regulation of hippocampal Arc/Arg31 transcription, translation and degradation in a rat model of normal cognitive aging. Neurobiol Learn Mem. (2014) 115:58–67. doi: 10.1016/j.nlm.2014.08.007
116. Auestad N, Korsak RA, Morrow JW, Edmond J. Fatty acid oxidation and ketogenesis by astrocytes in primary culture. J Neurochem. (1991) 56:1376–86. doi: 10.1111/j.1471-4159.1991.tb11435.x
117. Edmond J, Robbins RA, Bergstrom JD, Cole RA, de Vellis J. Capacity for substrate utilization in oxidative metabolism by neurons, astrocytes, and oligodendrocytes from developing brain in primary culture. J Neurosci Res. (1987) 18:551–61. doi: 10.1002/jnr.490180407
118. Sayre NL, Sifuentes M, Holstein D, Cheng S-Y, Zhu X, Lechleiter JD. Stimulation of astrocyte fatty acid oxidation by thyroid hormone is protective against ischemic stroke-induced damage. J Cereb Blood Flow Metab. (2017) 37:514–27. doi: 10.1177/0271678X16629153
119. Khabbush A, Orford M, Tsai Y-C, Rutherford T, O'Donnell M, Eaton S, et al. Neuronal decanoic acid oxidation is markedly lower than that of octanoic acid: a mechanistic insight into the medium-chain triglyceride ketogenic diet. Epilepsia. (2017) 58:1423–9. doi: 10.1111/epi.13833
120. Blázquez C, Sánchez C, Daza A, Galve-Roperh I, Guzmán M. The stimulation of ketogenesis by cannabinoids in cultured astrocytes defines carnitine palmitoyltransferase I as a new ceramide-activated enzyme. J Neurochem. (1999) 72:1759–68. doi: 10.1046/j.1471-4159.1999.721759.x
121. Attwell D, Laughlin SB. An energy budget for signaling in the grey matter of the brain. J Cereb Blood Flow Metab. (2001) 21:1133–45. doi: 10.1097/00004647-200110000-00001
122. Speijer D. Oxygen radicals shaping evolution: why fatty acid catabolism leads to peroxisomes while neurons do without it: FADH2/NADH flux ratios determining mitochondrial radical formation were crucial for the eukaryotic invention of peroxisomes and catabolic tissue differentiation. Bioessays. (2011) 33:88–94. doi: 10.1002/bies.201000097
123. Mitchell RW, Hatch GM. Fatty acid transport into the brain: of fatty acid fables and lipid tails. Prostaglandins Leukot Essent Fatty Acids. (2011) 85:293–302. doi: 10.1016/j.plefa.2011.04.007
124. Pardridge WM, Mietus LJ. Palmitate and cholesterol transport through the blood-brain barrier. J Neurochem. (1980) 34:463–6. doi: 10.1111/j.1471-4159.1980.tb06621.x
125. Oldendorf WH. Carrier-mediated blood-brain barrier transport of short-chain monocarboxylic organic acids. Am J Physiol. (1973) 224:1450–3. doi: 10.1152/ajplegacy.1973.224.6.1450
126. Knobloch M, Pilz G-A, Ghesquière B, Kovacs WJ, Wegleiter T, Moore DL, et al. Fatty acid oxidation-dependent metabolic shift regulates adult neural stem cell activity. Cell Rep. (2017) 20:2144–55. doi: 10.1016/j.celrep.2017.08.029
127. Ebert D, Haller RG, Walton ME. Energy contribution of octanoate to intact rat brain metabolism measured by 13C nuclear magnetic resonance spectroscopy. J Neurosci. (2003) 23:5928–35. doi: 10.1523/JNEUROSCI.23-13-05928.2003
128. Schönfeld P, Reiser G. Brain energy metabolism spurns fatty acids as fuel due to their inherent mitotoxicity and potential capacity to unleash neurodegeneration. Neurochem Int. (2017) 109:68–77. doi: 10.1016/j.neuint.2017.03.018
129. Schuck PF, Ferreira GC, Moura AP, Busanello ENB, Tonin AM, Dutra-Filho CS, et al. Medium-chain fatty acids accumulating in MCAD deficiency elicit lipid and protein oxidative damage and decrease non-enzymatic antioxidant defenses in rat brain. Neurochem Int. (2009) 54:519–25. doi: 10.1016/j.neuint.2009.02.009
130. Reichmann H, Maltese WA, DeVivo DC. Enzymes of fatty acid beta-oxidation in developing brain. J Neurochem. (1988) 51:339–44. doi: 10.1111/j.1471-4159.1988.tb01044.x
131. Bixel MG, Hamprecht B. Generation of ketone bodies from leucine by cultured astroglial cells. J Neurochem. (1995) 65:2450–61. doi: 10.1046/j.1471-4159.1995.65062450.x
132. Escartin C, Pierre K, Colin A, Brouillet E, Delzescaux T, Guillermier M, et al. Activation of astrocytes by CNTF induces metabolic plasticity and increases resistance to metabolic insults. J Neurosci. (2007) 27:7094–104. doi: 10.1523/JNEUROSCI.0174-07.2007
133. Le Foll C, Dunn-Meynell AA, Miziorko HM, Levin BE. Regulation of hypothalamic neuronal sensing and food intake by ketone bodies and fatty acids. Diabetes. (2014) 63:1259–69. doi: 10.2337/db13-1090
134. Nonaka Y, Takagi T, Inai M, Nishimura S, Urashima S, Honda K, et al. Lauric acid stimulates ketone body production in the KT-5 astrocyte cell line. J Oleo Sci. (2016) 65:693–9. doi: 10.5650/jos.ess16069
135. Cullingford TE, Eagles DA, Sato H. The ketogenic diet upregulates expression of the gene encoding the key ketogenic enzyme mitochondrial 3-hydroxy-3-methylglutaryl-CoA synthase in rat brain. Epilepsy Res. (2002) 49:99–107. doi: 10.1016/S0920-1211(02)00011-6
136. Gilbert DL, Pyzik PL, Freeman JM. The ketogenic diet: seizure control correlates better with serum beta-hydroxybutyrate than with urine ketones. J Child Neurol. (2000) 15:787–90. doi: 10.1177/088307380001501203
137. Wein S, Wolffram S, Schrezenmeir J, Gasperiková D, Klimes I, Seböková E. Medium-chain fatty acids ameliorate insulin resistance caused by high-fat diets in rats. Diabetes Metab Res Rev. (2009) 25:185–94. doi: 10.1002/dmrr.925
138. Lieber CS, DeCarli LM, Leo MA, Mak KM, Ponomarenko A, Ren C, et al. Beneficial effects versus toxicity of medium-chain triacylglycerols in rats with NASH. J Hepatol. (2008) 48:318–26. doi: 10.1016/j.jhep.2007.09.016
139. Baba N, Bracco EF, Hashim SA. Enhanced thermogenesis and diminished deposition of fat in response to overfeeding with diet containing medium chain triglyceride. Am J Clin Nutr. (1982) 35:678–82. doi: 10.1093/ajcn/35.4.678
140. Edens NK, Friedman MI. Response of normal and diabetic rats to increasing dietary medium-chain triglyceride content. J Nutr. (1984) 114:565–73. doi: 10.1093/jn/114.3.565
141. Jeffery NM, Sanderson P, Newsholme EA, Calder PC. Effects of varying the type of saturated fatty acid in the rat diet upon serum lipid levels and spleen lymphocyte functions. Biochim Biophys Acta. (1997) 1345:223–36. doi: 10.1016/S0005-2760(96)00174-9
142. Kesl SL, Poff AM, Ward NP, Fiorelli TN, Ari C, Van Putten AJ, et al. Effects of exogenous ketone supplementation on blood ketone, glucose, triglyceride, and lipoprotein levels in Sprague-Dawley rats. Nutr Metab (Lond). (2016) 13:9. doi: 10.1186/s12986-016-0069-y
143. Gjedde A, Crone C. Induction processes in blood-brain transfer of ketone bodies during starvation. Am J Physiol. (1975) 229:1165–9. doi: 10.1152/ajplegacy.1975.229.5.1165
144. Leino RL, Gerhart DZ, Duelli R, Enerson BE, Drewes LR. Diet-induced ketosis increases monocarboxylate transporter (MCT1) levels in rat brain. Neurochem Int. (2001) 38:519–27. doi: 10.1016/S0197-0186(00)00102-9
145. Lin T-Y, Liu H-W, Hung T-M. The ketogenic effect of medium-chain triacylglycerides. Front Nutr. (2021) 8:747284. doi: 10.3389/fnut.2021.747284
146. Bray GA, Lee M, Bray TL. Weight gain of rats fed medium-chain triglycerides is less than rats fed long-chain triglycerides. Int J Obes. (1980) 4:27–32.
147. Geelen MJ, Schoots WJ, Bijleveld C, Beynen AC. Dietary medium-chain fatty acids raise and (n-3) polyunsaturated fatty acids lower hepatic triacylglycerol synthesis in rats. J Nutr. (1995) 125:2449–56.
148. Panth N, Abbott KA, Dias CB, Wynne K, Garg ML. Differential effects of medium- and long-chain saturated fatty acids on blood lipid profile: a systematic review and meta-analysis. Am J Clin Nutr. (2018) 108:675–87. doi: 10.1093/ajcn/nqy167
149. Salameh TS, Rhea EM, Banks WA, Hanson AJ. Insulin resistance, dyslipidemia, and apolipoprotein E interactions as mechanisms in cognitive impairment and Alzheimer's disease. Exp Biol Med (Maywood). (2016) 241:1676–83. doi: 10.1177/1535370216660770
Keywords: ketosis, medium-chain fatty acids, medium-chain triglycerides, cardiometabolic health, neuroprotection, procognitive activity, caprylic acid (C8), capric acid (C10)
Citation: Shcherbakova K, Schwarz A, Apryatin S, Karpenko M and Trofimov A (2022) Supplementation of Regular Diet With Medium-Chain Triglycerides for Procognitive Effects: A Narrative Review. Front. Nutr. 9:934497. doi: 10.3389/fnut.2022.934497
Received: 02 May 2022; Accepted: 15 June 2022;
Published: 15 July 2022.
Edited by:
Manohar Garg, The University of Newcastle, AustraliaReviewed by:
Zsolt Kovacs, Eötvös Loránd University, HungaryCristina Miralpeix, INSERM U1215 Neurocentre Magendie, France
Copyright © 2022 Shcherbakova, Schwarz, Apryatin, Karpenko and Trofimov. This is an open-access article distributed under the terms of the Creative Commons Attribution License (CC BY). The use, distribution or reproduction in other forums is permitted, provided the original author(s) and the copyright owner(s) are credited and that the original publication in this journal is cited, in accordance with accepted academic practice. No use, distribution or reproduction is permitted which does not comply with these terms.
*Correspondence: Ksenia Shcherbakova, c2hjaGVyYmFrb3ZhLmtzZW5pYS5qcCYjeDAwMDQwO2dtYWlsLmNvbQ==