- 1Unit of Cardiovascular and Nutritional Epidemiology, Institute of Environmental Medicine, Karolinska Institutet, Stockholm, Sweden
- 2Department of Animal Nutrition and Management, Swedish University of Agricultural Sciences, Uppsala, Sweden
- 3Department of Food Biofunctionality, Institute of Nutritional Sciences, University of Hohenheim, Stuttgart, Germany
- 4Department of Molecular Sciences, Swedish University of Agricultural Sciences, Uppsala, Sweden
- 5Department of Surgical Sciences, Uppsala University, Uppsala, Sweden
- 6Department of Public Health and Clinical Medicine, Nutritional Research. Umeå University, Umeå, Sweden
- 7Division of Food and Nutrition Science, Department of Biology and Biological Engineering, Chalmers University of Technology, Gothenburg, Sweden
Recent studies suggest that a diet rich in sugars significantly affects the gut microbiota. Adverse metabolic effects of sugars may partly be mediated by alterations of gut microbiota and gut health parameters, but experimental evidence is lacking. Therefore, we investigated the effects of high intake of fructose or galactose, with/without fructooligosaccharides (FOS), on gut microbiota composition in rats and explored the association between gut microbiota and low-grade systemic inflammation. Sprague–Dawley rats (n = 6/group) were fed the following isocaloric diets for 12 weeks (% of the dry weight of the sugars or FOS): (1) starch (control), (2) fructose (50%), (3) galactose (50%), (4) starch+FOS (15%) (FOS control), (5) fructose (50%)+FOS (15%), (6) galactose (50%)+FOS (15%), and (7) starch+olive (negative control). Microbiota composition in the large intestinal content was determined by sequencing amplicons from the 16S rRNA gene; 341F and 805R primers were used to generate amplicons from the V3 and V4 regions. Actinobacteria, Verrucomicrobia, Tenericutes, and Cyanobacteria composition differed between diets. Bifidobacterium was significantly higher in all diet groups where FOS was included. Modest associations between gut microbiota and metabolic factors as well as with gut permeability markers were observed, but no associations between gut microbiota and inflammation markers were observed. We found no coherent effect of galactose or fructose on gut microbiota composition. Added FOS increased Bifidobacterium but did not mitigate potential adverse metabolic effects induced by the sugars. However, gut microbiota composition was associated with several metabolic factors and gut permeability markers which warrant further investigations.
Introduction
The gut microbiota in the large intestine of humans and animals comprises more than one trillion microorganisms but is dominated by two major bacterial phyla, Firmicutes and Bacteriodetes (1, 2). Studies have demonstrated that metabolic activity of the gut microbiota is essential in maintaining host homeostasis and health (3, 4). In recent years, probiotic bacteria have received escalating attention, particularly for their beneficial health effects on the host through various mechanisms including modulation of gut microbiota, metabolic effects, and regulation of immune responses (5, 6). Among probiotics, Bifidobacterium and Lactobacillus genera are of major importance due to their functional properties (5). Probiotics have also been studied for anticarcinogenic (7), antipathogenic (8), and anticholesterolemic (9) activities.
Prebiotics represent important factors affecting gut microbiota composition, richness, and activity, and their role in human health (10, 11). Prebiotics are carbohydrates such as dietary fibers including fructooligosaccharides, galactooligosaccharides, and inulin (12). Prebiotics are resistant to digestion in the upper sections of the alimentary tract and undergo fermentation by saccharolytic bacteria such as Bifidobacterium in the intestine (13). Therefore, the change in diet by including prebiotics can result in a significant change in the microbiota after only 24 h (10, 14), although short-term dietary changes are typically transient, long-term dietary changes alter the microbiota composition more robustly (10, 14). Interestingly, studies have suggested alterations in gut microbiota associated with the consumption of sugar and sugar-sweetened beverages in humans, but firm evidence is lacking (15).
Studies in rats have shown that the gut microbiota was significantly affected within a week after feeding simple sugars including a decrease in diversity (16). Common sugars in the diet, such as glucose, fructose, and galactose, are actively absorbed in the small intestine, but the degree of absorption varies with the type of sugar and dose (17, 18). For example, excessive intake of fructose may lead to incompletely digested fructose reaching the colon, where intestinal discomfort and even negative health outcomes due to inappropriate immune response, may result consequently (19, 20). Studies have suggested that high consumption of fructose in the diet could affect the microbial community and lead to an increase in pathogenic bacteria, deterioration of the intestinal barrier function, reduced mucus thickness, and a subsequent increase in translocation of microbiota and increased concentrations of endotoxin in the bloodstream (21, 22). Moreover, consumption of fructose, either in solid or liquid form, has been shown to affect microbiota composition differently (23).
In rodents, chronic intake of galactose is known to cause toxicity due to the accumulation of reactive oxygen species and advanced glycation end products (AGE) (24). Feeding D-galactose to accelerate aging is a well-established animal model (25). In addition, a diet containing 15% galactose has been found to decrease the abundance of Firmicutes, alter the Firmicutes:Bacteroidetes ratio, and decrease the abundance of Clostridium coccoides in rats, compared with diets with 15% glucose or 15% fructose (26). D-galactose-induced aging has been shown to modulate gut microbiota composition significantly at the phylum level in rats (27–29).
A high-fiber diet may provide beneficial health effects through stimulation of the growth of specific gut microbiota (30–33). Prebiotic fiber types, such as fermentable fructooligosaccharides (FOS) and inulin, have consistently been shown to increase the abundance of Bifidobacterium and lactic acid bacteria (LAB) in the large intestine. They have also been shown to suppress the growth of pathogenic bacteria that may result in endotoxemia, and could thereby provide a positive effect on gut barrier integrity and lower sub-clinical systemic inflammatory responses (34, 35). A high intake of fermentable dietary fiber increases the production of short-chain fatty acids (SCFA), whereas reduced production of SCFA has been reported at a low intake of fermentable dietary fiber (36). SCFA, that is, acetate, propionate, and butyrate, modulate the secretion of hormones, insulin sensitivity, and immune responses (37, 38). Low intake of dietary fiber and high intake of sugar are believed to have detrimental effects on microbial diversity and human health (39, 40).
The main hypothesis tested in this study was that a high intake of simple sugars, such as fructose or galactose, causes malabsorption in the small intestine so that excess sugars reach the large intestine, causing unfavorable alterations in gut microbiota composition and activity (12, 41, 42). A second hypothesis tested was that fermentable dietary fiber, such as FOS, counteracts these adverse effects of simple sugars through modulation of the gut microbiota (43). We, therefore, investigated the impact of diets high in fructose or galactose on gut microbiota composition and examined whether simultaneous administration of FOS could mitigate the potential adverse effects of the simple sugars over a 12-week intervention. We also assessed associations between gut microbiota diversity, metabolic factors, and inflammation and gut permeability biomarkers.
Methods
Animals and diets
Samples and data from a previous 12-week rat study, where we investigated the metabolic effects of high sugar diets with and without the addition of FOS, were used for the investigations in the present study (44). The estimated number of animals per group was six, to differentiate an assumed effect of 30% difference (100 vs. 140 units) between treatments and control. A standard deviation of 15 units in 12 comparisons provides a p < 0.001 and power of 0.9. In brief, healthy male Sprague–Dawley rats (n = 90) were purchased from Janvier Labs at age 7 weeks with an initial body weight of 250–274 g and were randomly assigned to one of seven diet groups (n = 12 per group) as follows: (1) fructose, (2) fructose+FOS, (3) galactose, (4) galactose+FOS, (5) starch (control), (6) starch+FOS, and (7) starch+olive (negative control), and one baseline group (n = 6) sacrificed before the commencement of the feeding trial. Six out of 12 rats in each group were sacrificed at the end of week 6 and the other six at the end of week 12. The animal experiment (trial no. V 351/18 BC) was approved by the Regional Council Stuttgart (Baden-Württemberg, Germany) ethics committee. All animal procedures were carried out in accordance with the Federation of European Laboratory Animal Science Association (FELASA) guidelines for the care and use of laboratory animals. The animal studies are reported in accordance and compliance with the ARRIVE guidelines (45).
The experimental diets contained different carbohydrates in isocaloric conditions. The high-carbohydrate diets consisted of 50% fructose or 50% galactose, while the diets with added FOS (inulin-type, DP4-5, MW: 624–679 from chicory root with 95% purity, Boneo GmbH, Germany) contained 15% FOS (all by weight). Starch (native starch, The Carl Roth GmbH+Co.KG, Germany), was used instead of sugars for the control diet and was added to all other diets in varying quantities to adjust total energy intake in order to obtain isocaloric conditions. All diets were prepared with 6% safflower oil as a source of n-6 polyunsaturated fatty acids (PUFA) except for the negative control diet, which used 6% olive oil (low in n-6). Starch+FOS was used as the FOS control diet. The dietary regimen for the groups fed galactose had to be modified due to adverse health effects observed in these rats after 2 weeks of intervention. For the remainder of the study, the rats in the galactose and galactose+FOS groups were given the respective diets for 4 days, followed by 3 days on the starch diet (control). All rats had access to diets and water ad libitum. Body weight and feed intake were recorded weekly. At the end of the respective feeding period, the rats were fasted for 12 h, anesthetized with carbon dioxide gas, and killed by decapitation. Intestinal contents were collected in Eppendorf tubes and immediately stored at −80°C. Blood samples were collected into heparinized monovettes (Monovette, Sarstedt, Germany) at 6 and 12 weeks, and glucose concentrations were measured immediately. Plasma and serum were separated from the blood cells immediately and stored at −80°C after collection. Metabolic factors, inflammation and gut permeability biomarkers, and AGEs were analyzed in plasma samples and the results are reported in our previous study (44), from which data were available for the present study and linked to effects on the gut microbiota.
Gut microbiota analysis
DNA was extracted in singlet samples from the intestinal sample contents of all animals included in the study using QIAamp Fast DNA Stool mini kit (Qiagen, Hilden, Germany) according to the manufacturer's protocol, with the exception that the bacterial cell walls were mechanically disrupted with 0.1 mm zirconium/silica beads (Biospec Products) for 2 x 60 s using a Precellys Evolution device (Bertin Technologies, Montigny-le-Bretonneaux, France).
Amplicons from the V3 and V4 regions of the 16S rRNA gene were generated from the extracted DNA using the primers 341F and 805R. For the PCR reactions, Phusion High-Fidelity PCR Master Mix (New England Biolabs, United States) was used and the PCR products were purified with Qiagen Gel Extraction Kit (Qiagen) and quantified with Qubit 3.0 Fluorometer (Invitrogen, Thermo Fisher Scientific). The final libraries were generated with a NEBNext Ultra DNA Library Prep Kit that incorporated barcodes and adaptors. The amplicons were then sequenced on the Illumina platform at Novogene (Beijing, China).
Bioinformatic analysis
Generated paired-end reads were first assigned to samples based on their unique barcode sequence. Reads were then merged after truncating the barcode and primer sequence using FLASH (v1.2.7, http://ccb.jhu.edu/software/FLASH/) (46). Merged sequences were analyzed using QIIME (v1.7.0) (47, 48). Sequence analysis by clustering of operational taxonomic units (OTUs) was performed using Uparse software (Uparse) (49), with sequences with ≥97% homology assigned to the same OTU. The representative sequences in each OTU were then annotated using the SILVA Database (http://www.arb-silva.de/) for species annotation at each taxonomic rank (50, 51).
Statistical analysis
The statistical analyses were carried out using SAS statistical analysis software (release 9.4; SAS Institute, Cary, NC, United States). The effect of diet on microbiota composition at phylum and genus level was evaluated using one-way analysis of variance (ANOVA) followed by Tukey's multiple comparisons, with differences at 12 weeks defined as the primary outcome. A secondary analysis was carried out to evaluate the effects on 6 weeks if effects of specific diets were found after 12 weeks of intervention. Relative abundance at genus level >5% was used as the cut-off for inclusion in statistical testing. Assumption of normality and homogeneity of variance were assessed using the Shapiro–Wilks test. Data not normally distributed were log-transformed before analysis. All data analyzedusing the generalized linear model are presented as least square (LS) means ± standard error of the mean (SEM), adjusted for baseline values. Values of p < 0.05 were considered statistically significant. Correlations between the most abundant microbial phyla and metabolic factors and inflammation-related markers were assessed by Spearman's rank correlation tests.
Results
Characteristics of energy intake and initial and final body weight of the rats
Data were obtained for 90 rats treated with a high-fructose or high-galactose diet, with or without added FOS, or fed a control diet (starch, starch+FOS, or olive oil control) for 12 weeks (n = 6 rats/group). Energy intake was significantly higher in rats fed fructose than in rats fed starch+FOS. The rats in all intervention groups had an initial body weight of 251–258 g. Body weight after the 12-week intervention was significantly lower in the galactose and galactose+FOS groups compared with the starch (control), starch+olive (negative control), and fructose groups (Table 1).

Table 1. Descriptive characteristics of the rats fed a control diet (starch, starch + FOS, starch+olive) or a high-fructose or high-galactose diet, with and without added fructooligosaccharides (FOS), after 12 weeks of intervention.
Effects of diet on alpha-diversity of the gut microbiota
The baseline richness of bacterial OTUs and Shannon diversity is shown in Figures 1A,B, respectively. Significantly lower diversity was observed in the starch+FOS group compared with all other diet groups after 12 weeks of intervention (Figure 1A). Similarly, Shannon index was significantly lower in the starch+FOS compared with the other diet groups (Figure 1B). At 6 weeks, no similar difference in alpha-diversity was observed (data not shown).
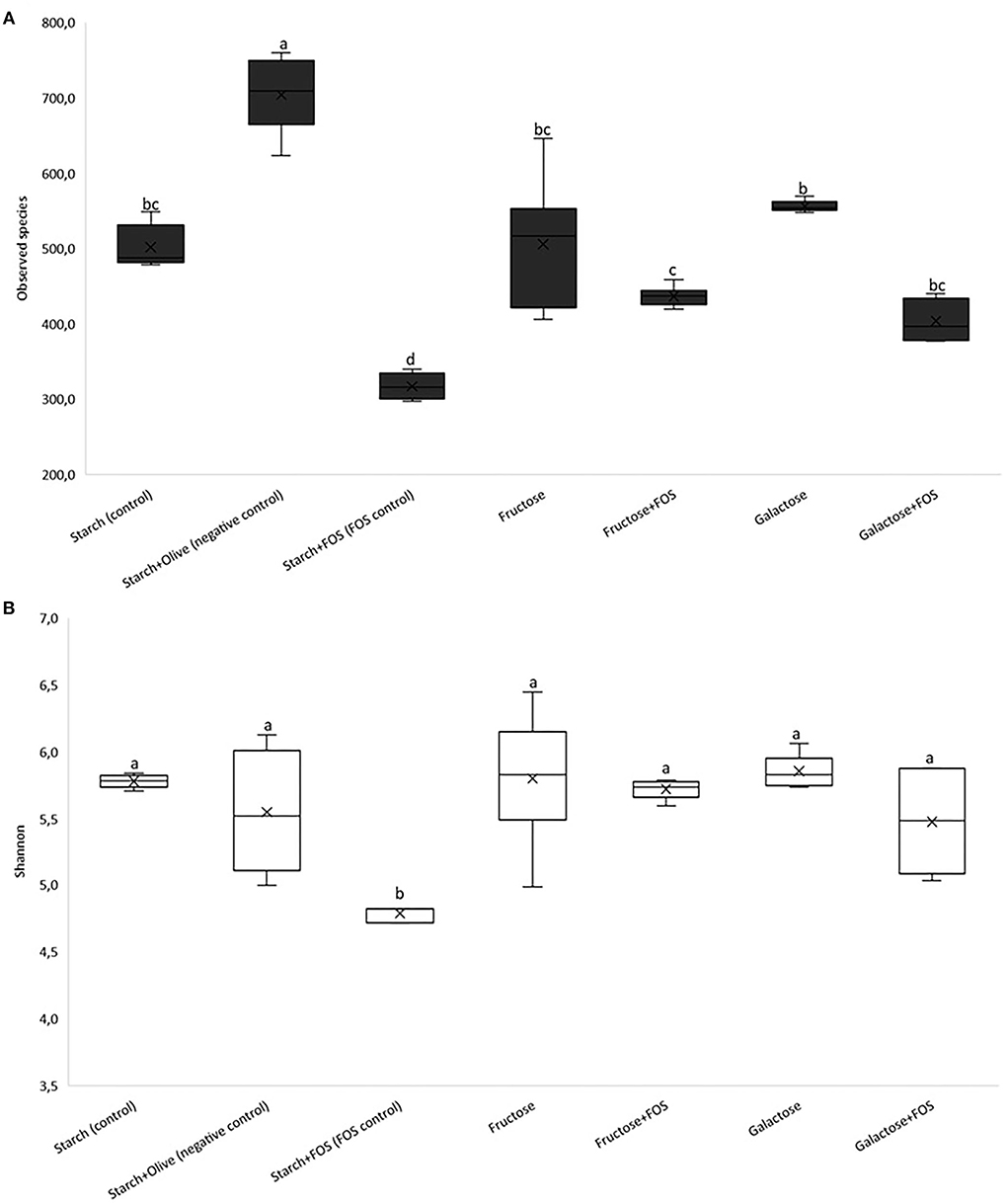
Figure 1. Results of 16S rRNA gene sequence analysis of the gut microbiota community in the large intestine of rats fed a control diet (starch, starch+FOS, starch+olive) or a high-fructose or high-galactose diet, with and without added fructooligosaccharides (FOS), after 12 weeks. Alpha diversity; (A) observed species, (B) Shannon's diversity index. Values shown are LS mean ± SEM of six rats. Groups were assessed by one-way ANOVA followed by Tukey's test. Means with different superscripts (lowercase letters) differ significantly (p < 0.05).
Effects of diet on gut microbiota composition at the phylum and genus levels
Taxonomically, over 300 genera belonging to 22 phyla were identified in the large intestine of the rats, of which 16 genera with relative abundance >5.0% comprised >50% of the variation in microbiota (Figures 2, 3). The microbiota in the large intestine of the rats at baseline was dominated by the phyla Firmicutes (55%), Bacteroidetes (37%), and Proteobacteria (5%) (Figure 2, Table 2).
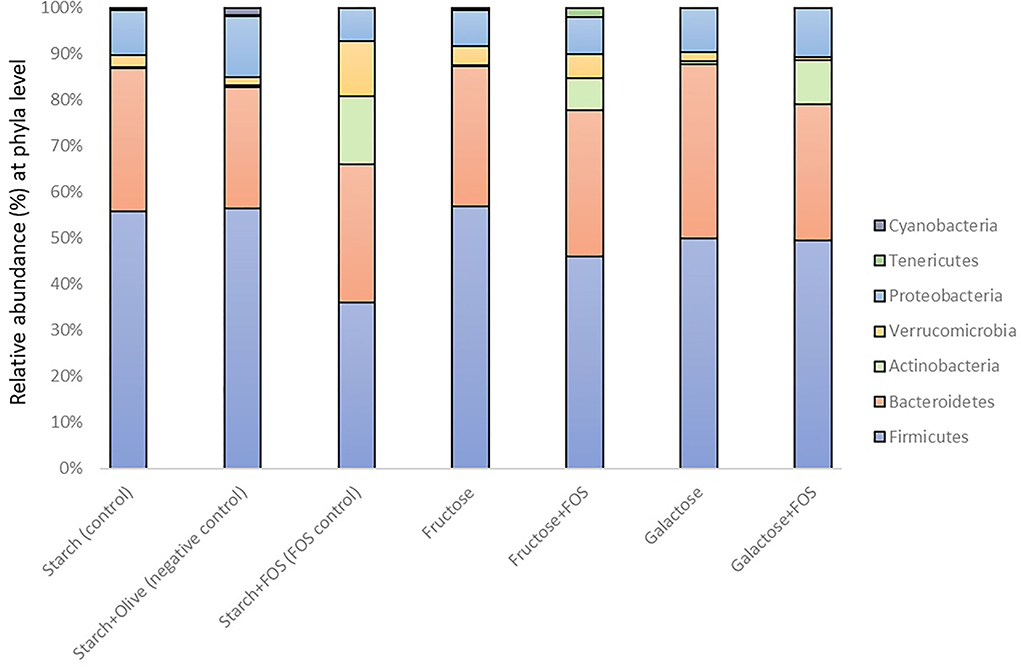
Figure 2. Gut microbiota composition (relative abundance, %) at phylum level in rats fed a control diet (starch, starch+FOS, starch+olive) or a high-fructose or high-galactose diet, with and without added fructooligosaccharides (FOS), after 12 weeks.
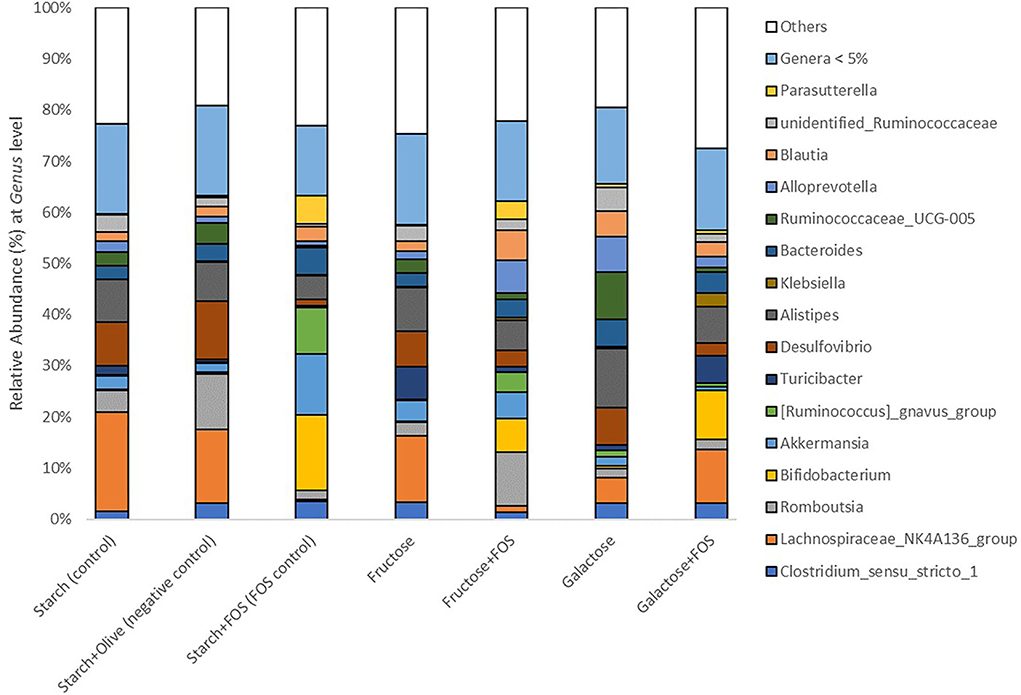
Figure 3. Gut microbiota composition (relative abundance, %) at phylum level in rats fed a control diet (starch, starch+FOS, starch+olive) or a high-fructose or high-galactose diet, with and without added fructooligosaccharides (FOS), after 12 weeks. Only classified genera with relative abundance above 5.0% cut-off level are shown. White bars indicate all genera with mean relative abundance <5.0%.
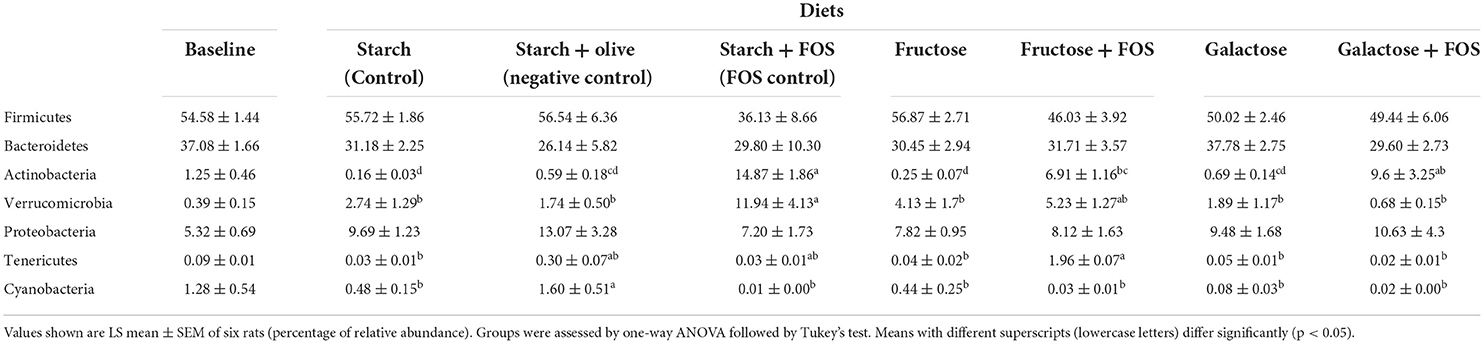
Table 2. Effects of high-carbohydrate diets (fructose and galactose), with and without additional fructooligosaccharides (FOS), on relative abundance (%) of major phyla in the large intestine microbiota of rats after 12 weeks.
In general, a significantly higher relative abundance of Actinobacteria was observed in the starch+FOS and galactose+FOS groups compared with the fructose, galactose, starch (control), and starch+olive (negative control) groups. Tenericutes abundance was significantly higher in the fructose+FOS group than in the starch (control), fructose, galactose, and galactose+FOS groups at 12 weeks of intervention. However, no similar difference in the abundance of Actinobacteria and Tenericutes was observed at 6 weeks (data not shown).
The relative abundance of Verrucomicrobia was significantly higher in the starch+FOS group than in all other diet groups except the fructose+FOS group. A similar difference was observed in the relative abundance of Verrucomicrobia (p < 0.05) at 6 weeks (data not shown).
Cyanobacteria abundance was significantly higher in the starch+olive (negative control) group than in the other diet groups after 12 weeks of intervention (Table 2), but no similar difference was observed at 6 weeks (data not shown). No differences in the effect of diets on microbiota composition were observed for Firmicutes, Bacteroidetes, and Proteobacteria after 12 weeks of intervention.
At the genus level, the relative abundance of Lachnospiraceae_NK4A136_group, Bifidobacterium, Akkermansia, [Ruminococcus]_gnavus_group Desulfovibrio, Klebsiella, Ruminococcaceae_UCG-005, Alloprevotella, unidentified_Ruminococcaceae, and Parasutterella differed significantly between the diets at 12 weeks of intervention (Table 3, Figure 3). The relative abundance of Lachnospiraceae_NK4A136_group was significantly higher in rats fed starch (control), starch+olive (negative control), fructose, and galactose+FOS diets compared with the other diet groups (Table 3, Figure 3). The relative abundance of Bifidobacterium, Akkermansia, and [Ruminococcus]_gnavus_group were significantly higher in rats fed the starch+FOS diet compared to the other diet groups, except for galactose+FOS, where the abundance of Bifidobacterium did not differ significantly from the starch+FOS diet group at 12 weeks (Table 3). However, the relative abundance of Bifidobacterium was significantly higher in the fructose+FOS and galactose groups than in the other diet groups. However, no similar difference was observed in these genera at 6 weeks of intervention (data not shown).
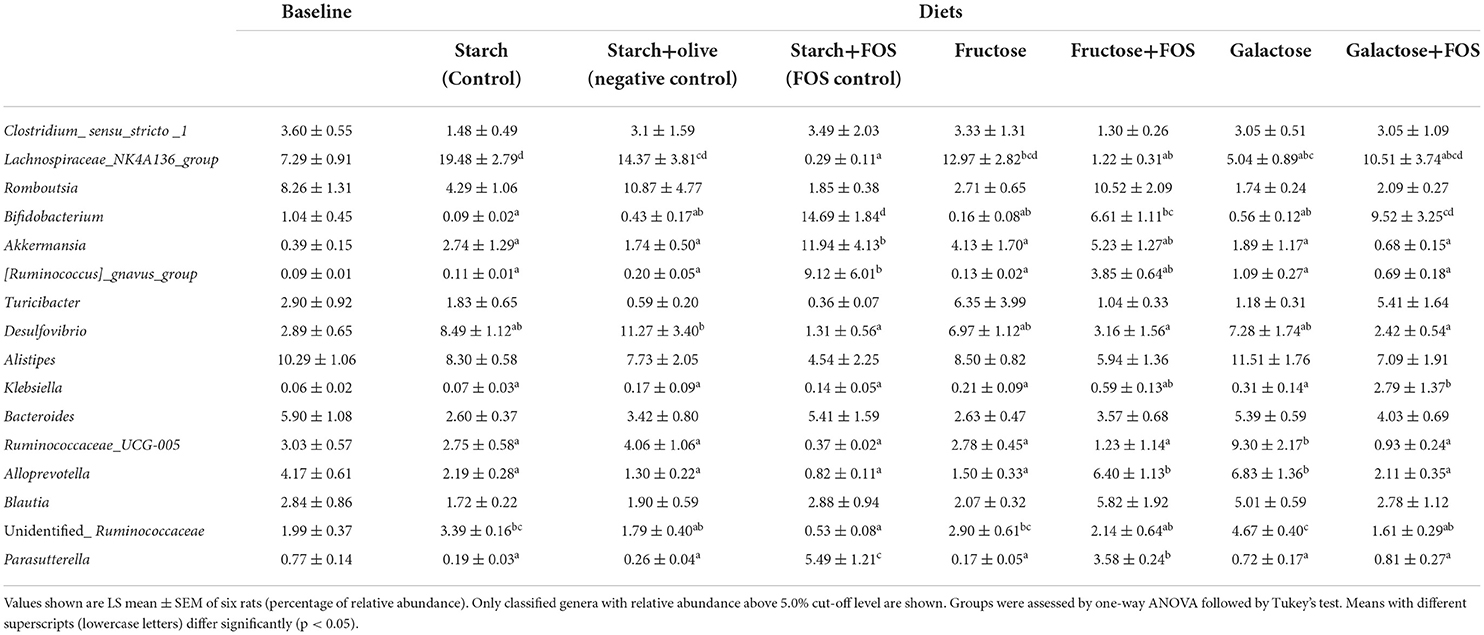
Table 3. Effects of high-carbohydrate diets (fructose and galactose), with and without additional fructooligosaccharides (FOS), on relative abundance (%) of the genus in the large intestine microbiota of rats after 12 weeks.
Moreover, the relative abundance of Desulfovibrio was significantly higher in the starch+olive (negative control) group than other diet groups with FOS added. A significantly higher relative abundance of Klebsiella and Ruminococcaceae_UCG-005 were observed in the galactose+FOS group and galactose, respectively, compared to the other diet groups. At 6 weeks, no similar difference was observed in Klebsiella and Ruminococcaceae_UCG-005 (data not shown).
Alloprevotella abundance was significantly higher in the fructose+FOS and galactose groups than in the other diet groups. The relative abundance of unidentified_Ruminococcaceae was significantly higher in the galactose group than in the other diet groups except for starch (control) and fructose groups. Moreover, Parasutterella abundance was significantly higher in the starch+FOS compared to other diet groups. However, there were no differences in Alloprevotella, unidentified_Ruminococcaceae, and Parasutterella abundance at 6 weeks of intervention (data not shown).
In general, the taxon-based analysis showed marked changes in gut microbiota composition in diets with added FOS, but no obvious changes in groups fed a high-fructose or high-galactose diet without added FOS. The relative abundance of Actinobacteria was higher in all diet groups with added FOS, indicating that FOS significantly stimulated the growth of Actinobacteria. The genus Bifidobacterium was present in higher abundance in diet groups with added FOS than in diet groups without FOS (Figure 4), which contributed most of the increase of Actinobacteria at the phylum level. The relative abundance of Bifidobacterium was significantly higher in the starch+FOS and galactose+FOS groups than in all other diet groups except the fructose+FOS group after 12 weeks. At 6 weeks, a similar pattern of increased abundance of Bifidobacterium (p < 0.05) in rats fed diets with added FOS was observed (data not shown). In summary, the results showed that including FOS in the diet had an important effect on microbiota composition.
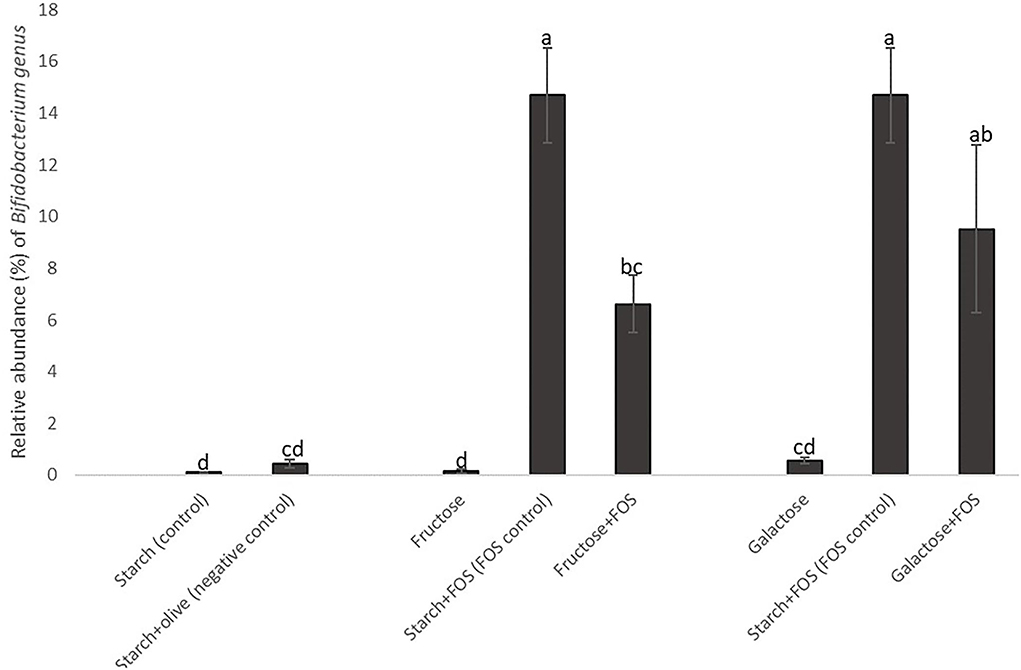
Figure 4. Changes in the relative abundance of the genus Bifidobacterium in the large intestine of rats fed a control diet (starch, starch+FOS, starch+olive) or a high-fructose or high-galactose diet, with and without added fructooligosaccharides (FOS), after 12 weeks. Values shown are LS mean ± SEM of six rats (percentage of relative abundance). Groups were assessed by one-way ANOVA followed by Tukey's test. Means with different superscripts (lowercase letters) differ significantly (p < 0.05).
Association between gut microbiota composition, metabolic factors, and inflammation and gut permeability markers after 12 weeks
In general, modest correlations were observed between the relative abundance of microbial phyla and selected metabolic factors and inflammation and gut permeability markers (Table 4). We found positive correlations between Firmicutes and Cyanobacteria and body weight, whereas Bacteroidetes and Actinobacteria were inversely associated with body weight. The relative abundance of Actinobacteria was inversely associated with endotoxin concentration but positively associated with lysine concentration. Verrucomicrobia composition was inversely associated with Nε-carboxy-methyl-lysine (CML) and pentosidine concentrations. Cyanobacteria composition was positively associated with endotoxin concentration after 12 weeks of intervention (Table 4).
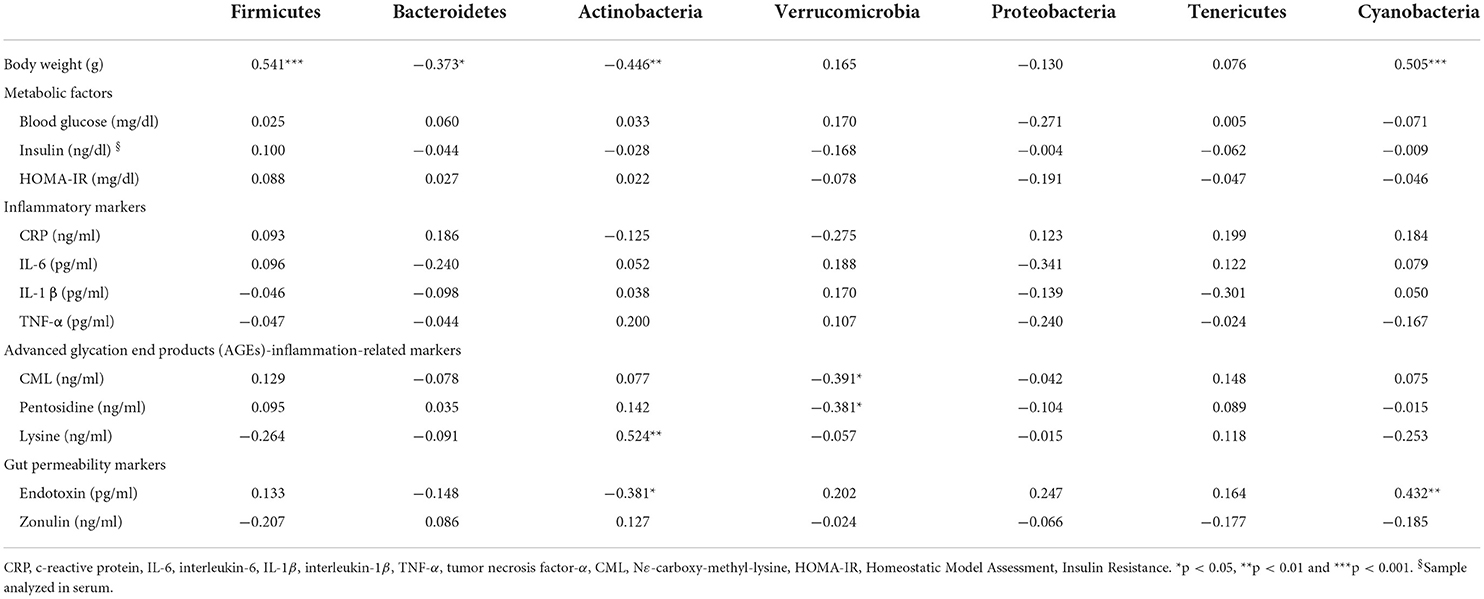
Table 4. Spearman's rank correlation coefficient between gut microbiota composition (phylum level), metabolic factors, and inflammation and gut permeability markers after 12 weeks of intervention.
Scatterplots for the phyla Firmicutes, Bacteroidetes, Actinobacteria, Verrucomicrobia, and Cyanobacteria with selected markers with significant correlations are presented in Supplementary Figure S1.
Discussion
The results in the present study indicated that high-fructose and high-galactose diets did not have any consistent effect on microbiota composition at the phylum level under the conditions evaluated. Only groups treated with FOS showed consistent differences in microbiota composition, in particular, increased abundance of Actinobacteria which was mainly driven by an increase in the relative abundance of Bifidobacterium. However, we found that the gut microbiota was associated with several metabolic factors and biomarkers when data from all diet groups were pooled. To our knowledge, this is the first study to examine the effect on microbiota composition of high fructose and galactose intake, with and without added FOS to alleviate the negative effect of these sugars.
As reported in our previous study (44), the groups fed galactose or galactose+FOS had lower body weight than the other diet groups. This was accompanied by clinical symptoms in rats in these groups, including polyuria and lens opacity after high intake of galactose. Their energy expenditure could have been altered. Several studies have reported similar symptoms after feeding rats with diets containing 50% galactose (52, 53).
Dietary components strongly influence the richness and diversity of gut microbiota (14). The high richness and diverse microbiota have been associated with health benefits such as protection against enteropathogens, and contribute to normal immune function (54–56). To date, there is no uniform definition of a healthy gut microbiota composition, mainly due to large inter-individual variability resulting from differences in, for example, dietary and cultural habits, lifestyle, environment, and antibiotic use (57, 58). On the other hand, many studies have reported skewed microbial composition in several types of diseases. In many cases, this is referred to as dysbiosis, which is commonly associated with a reduction in microbial diversity, a decrease in the abundance of certain families within the order Clostridiales, and an increased abundance of Proteobacteria (39, 59). Increased proportions of Proteobacteria, and in particular, members of the Enterobacteriaceae, have been linked to host susceptibility to infection (39). For instance, it has been shown that dysbiosis shifts the abundance of Proteobacteria, an effect associated with metabolic syndrome and increased risk of diseases such as inflammatory bowel disease and cancers (60). Moreover, several studies have reported that a diet containing FOS significantly modulates species richness and diversity of gut microbiota (34, 43, 61, 62) and that FOS supplementation enhances the growth of unique bacteria and increases diversity (34, 62). In contrast, in our study species richness was significantly lower in starch+FOS than in other diet groups with added FOS after 12 weeks. The effect of diet on gut microbiota diversity was also lower in the starch+FOS group, as indicated by the Shannon index values in Figure 1B.
Several studies have reported that high doses of simple sugars are not cleared by the small intestine, and therefore reach the large intestine and alter gut microbiota composition in a direction associated with metabolic disorders (19, 63, 64). It is difficult to judge whether this is causative or not. High glucose and high fructose intake have been shown to increase Proteobacteria and decrease Bacteroidetes after a 12-week intervention in mice (40, 63). Proteobacteria are Gram-negative bacteria, with lipopolysaccharides (LPS) as structural components of their cell walls, and can rapidly utilize simple sugars (65). Increased growth of Proteobacteria may contribute to increased LPS load, which can alter tight junction proteins and increase intestinal permeability and infiltration of LPS into the bloodstream, inducing the release of cytokines and chemokines (63, 65, 66). Several studies in which D-galactose was used to induce aging in animal models have also reported an increased abundance of Bacteroidetes and lowered abundance of Firmicutes, Actinobacteria, Proteobacteria, and Cyanobacteria (27, 28, 67). However, our study did not show a clear effect of a high-galactose or high-fructose diet on the abundance of Bacteroidetes, Firmicutes, Actinobacteria, Verrucomicrobia, Proteobacteria, Tenericutes, and Cyanobacteria. Stimulating the expression of sodium/glucose transporter-1 (SGLT-1) by SCFAs may increase the absorption of monosaccharides in the small intestine (68). One possible explanation for the lack of effect in our study could be the action of SCFAs in enhancing the absorption of monosaccharides. However, we did not measure the production of SCFA and cannot confirm this suggestion.
Numerous studies have reported beneficial health effects of readily fermentable FOS. FOS intake is widely known to selectively modulate the composition of gut microbiota, especially Actinobacteria (62). Actinobacteria was one of seven major phyla found in rats in this study. Although the abundance of Actinobacteria in the gut is generally low, they play a very important role in human health, including maintenance of gut homeostasis (69). The most prevalent genus in this phylum is Bifidobacterium, which is widely used as a probiotic and has been inversely associated with various pathological conditions such as obesity and diabetes (69, 70). Similar results were observed in our study, where the rats fed diets containing FOS had a significantly higher abundance of Actinobacteria and Bifidobacterium (Figures 3, 4). Bifidobacterium has also been associated with the improvement of gut integrity by enhanced expression of tight junction proteins (66, 71). However, we found no significant association between Bifidobacterium and gut permeability markers (zonulin and endotoxin concentrations) (Supplementary Table S1).
In our study, the starch+FOS diet significantly increased Verrucomicrobia abundance (Figure 2). This phylum is primarily dominated by the genus Akkermansia, which has been studied for its role in the regulation of the immune system, intestinal integrity, peptide secretion, and inflammation (72). Akkermansia muciniphila is involved in the expression of IFNγ-regulated gene and glucose parameters in the gut, and improves glucose metabolism including glucose tolerance and fasting glucose in both animal and human models (73). A higher abundance of A. muciniphila has been linked with healthier metabolic status and improvement in glucose homeostasis and blood lipids (74). However, our results did not support these findings (Supplementary Table S1). In addition, a significantly increased abundance of Ruminococcus gnavus from Firmicutes phyla was also observed in the group fed the starch+FOS diet (Table 3, Figure 3). Diet has shaped the composition of R. gnavus through the metabolism of FOS and degradation of resistant starch in the gut (75, 76). Different combinations of starch and sugars affect the abundance of Ruminococcus_gnavus (76). In a cross-feeding study, non-digestible carbohydrates supplied have modulated inhabiting the mucus niche by R. gnavus (77). Moreover, several studies have demonstrated the roles of R. gnavus in various disease conditions such as multiple myeloma, myelodysplastic, and fecal peritonitis (78, 79).
Recent studies have investigated the association between gut microbiota and various clinical parameters (69, 80, 81). We analyzed the association between seven major phyla (Firmicutes, Bacteroidetes, Proteobacteria, Cyanobacteria, Actinobacteria, Verrucomicrobia, and Tenericutes) and selected metabolic parameters and inflammation and gut permeability markers. The major phylum Firmicutes showed a positive correlation with body weight in this study. Human and animal studies have consistently reported that a high abundance of Firmicutes over Bacteroidetes is associated with a decrease in body weight (81, 82). In contrast, our study demonstrated a positive correlation between Firmicutes and body weight. At the genus level, Bacteroides is beneficial for glucose metabolism through improvements in glucose tolerance and insulin resistance (83, 84). We observed an association between Actinobacteria and body weight, lysine, and endotoxin concentrations (Table 4). A recent study reported a protective effect of Verrucomicrobia against the development of metabolic diseases (72). Our study demonstrated an inverse association between Verrucomicrobia and CML and pentosidine concentrations. In phylogenic analyses, diets high in AGEs have been shown to reduce Verrucomicrobia abundance (85). Cyanobacteria has been reported to have toxicity and pathological effects on human health, including gastrointestinal health and respiratory diseases (86). Various toxics such as endotoxin, hepatotoxin, and neurotoxins produced by Cyanobacteria may affect body organs (86, 87). Our study demonstrated a positive association between Cyanobacteria and body weight and an inverse association between Cyanobacteria abundance and lysine concentration.
The present study had some limitations. First, free fructose and free galactose were used although most sugars are consumed as sucrose and lactose. Second, the use of a high dose of fructose and galactose may have generated a mild toxic effect on the rats. Third, interpretation of the results is more challenging due to modification of the study design as the rats fed the galactose and galactose+FOS demonstrated clinical symptoms and the intervention had to be adapted. Fourth, the present results in a rat model cannot be directly translated into humans. Fifth, the SCFAs profile was not analyzed which could have linked FOS-related gut microbiota composition and activity with metabolic outcomes. However, the present investigation did not include the data on metabolic changes that might relate to the microbiota communities measured. The reason for such exclusion was that there were no substantial effects on metabolic parameters in response to the interventions. Nevertheless, our study also has several strengths. First, the study was large and the effects of different sugars with and without FOS under isocaloric conditions were compared in the same study. Second, by using a rat model, we could evaluate direct intestinal samples to understand the modulation of gut microbiota which is rare in studies of the gut microbiota where fecal samples are typically used and fecal samples do not normally correlate with intestinal samples. Third, in this study, we were able to establish cause and effects between fructose, galactose, FOS, and the gut microbiota and not merely associations as in many other studies.
In summary, we did not find any coherent effect of high intake of galactose or fructose compared with all other diets on gut microbiota composition after 12 weeks. Thus, the data did not support our hypothesis of the direct effects of galactose or fructose on gut microbiota, mediating adverse metabolic effects of these sugars. However, adding FOS to the sugar diets increased the abundance of the genus Bifidobacterium in the phylum Actinobacteria in the rats, supporting our second hypothesis. On pooling the data from the different diet groups, we found modest correlations between major phyla in the gut microbiota and several metabolic factors and inflammation-related markers, confirming the reported link between gut microbiota and cardiometabolic risk factors. Further studies should investigate the impact of gut microbiota activity as measured by different metabolites such as SCFAs, and their relation to cardiometabolic risk factors.
Data availability statement
The datasets presented in this study can be found in online repositories. The names of the repository/repositories and accession number(s) can be found below: https://www.ncbi.nlm.nih.gov/bioproject/PRJNA838460.
Ethics statement
The animal study was reviewed and approved by Regional Council Stuttgart (Baden-Württemberg, Germany) Ethics Committee.
Author contributions
NM designed and conducted the experiments, performed sampling, statistical analysis, and interpretation of results, and wrote the manuscript. JD conducted microbiota analysis, interpreted results, and participated in manuscript writing. JK conducted the experiments. GZ, KM, and AW contributed to designing the experiments and critically reviewed the manuscript. JF was involved in designing the study, conducting the rat trial, interpretation of the results, and manuscript writing. RL formulated the hypotheses and designed the study, contributed to the interpretation of results and manuscript writing, and had overall responsibility for the study. All authors contributed to the article and approved the submitted version.
Funding
This project has received funding from FORMAS under the umbrella of the European Joint Programming Initiative A Healthy Diet for a Healthy Life (JPI HDHL) and of the ERA-NET Cofund HDHL INTIMIC (GA No. 727565 of the EU Horizon 2020 Research and Innovation Programme), Swedish Research Council (Dnr 2017-05840; Formas Dnr 2016-003114), and by a starting grant from Chalmers Foundation. NM was funded by a scholarship from the Ministry of Higher Education Malaysia and Universiti Malaysia Pahang.
Acknowledgments
The authors would like to thank Nadine Sus for the excellent work and arrangement of the rat experiment and BONEO GmbH company for the sponsor of FOS (P95, 93-93%).
Conflict of interest
The authors declare that the research was conducted in the absence of any commercial or financial relationships that could be construed as a potential conflict of interest.
Publisher's note
All claims expressed in this article are solely those of the authors and do not necessarily represent those of their affiliated organizations, or those of the publisher, the editors and the reviewers. Any product that may be evaluated in this article, or claim that may be made by its manufacturer, is not guaranteed or endorsed by the publisher.
Supplementary material
The Supplementary Material for this article can be found online at: https://www.frontiersin.org/articles/10.3389/fnut.2022.922336/full#supplementary-material
References
1. Hillman ET, Lu H, Yao T, Nakatsu CH. Microbial ecology along the gastrointestinal tract. Microbes Environ. (2017) 32:300–13. doi: 10.1264/jsme2.ME17017
2. Barko PC, McMichael MA, Swanson KS, Williams DA. The gastrointestinal microbiome: a review. J Vet Intern Med. (2017) 32:9–25. doi: 10.1111/jvim.14875
3. Ahmadmehrabi S, Tang WHW. Gut microbiome and its role in cardiovascular diseases. Curr Opin Cardiol. (2017) 32:761–6. doi: 10.1097/HCO.0000000000000445
4. Martinez I, Lattimer JM, Hubach KL, Case JA, Yang J, Weber CG, et al. Gut microbiome composition is linked to whole grain-induced immunological improvements. ISME J. (2013) 7:269–80. doi: 10.1038/ismej.2012.104
5. Aureli P, Capurso L, Castellazzi AM, Clerici M, Giovannini M, Morelli L, et al. Probiotics and health: an evidence-based review. Pharmacol Res. (2011) 63:366–76. doi: 10.1016/j.phrs.2011.02.006
6. Haghshenas B, Kiani A, Nami Y. Probiotic potential and safety evaluation of lactic acid bacteria isolated from colostrum. J Biosaf. (2021) 14:37–60.
7. Slizewska K, Markowiak-Kopeć P, Slizewska W. The role of probiotics in cancer prevention. Cancers. (2020) 13:20. doi: 10.3390/cancers13010020
8. Ait Chait Y, Gunenc A, Hosseinian F, Bendali F. Antipathogenic and probiotic potential of Lactobacillus brevis strains newly isolated from Algerian artisanal cheeses. Folia Microbiol (Praha). (2021) 66:429–40. doi: 10.1007/s12223-021-00857-1
9. Michael DR, Davies TS, Moss JWE, Calvente DL, Ramji DP, Marchesi JR, et al. The anti-cholesterolaemic effect of a consortium of probiotics: an acute study in C57BL/6J mice. Sci Rep. (2017) 7:2883. doi: 10.1038/s41598-017-02889-5
10. David LA, Maurice CF, Carmody RN, Gootenberg DB, Button JE, Wolfe BE, et al. Diet rapidly and reproducibly alters the human gut microbiome. Nature. (2014) 505:559–63. doi: 10.1038/nature12820
11. Clemente JC, Ursell LK, Parfrey LW, Knight R. The impact of the gut microbiota on human health: an integrative view. Cell. (2012) 148:1258–70. doi: 10.1016/j.cell.2012.01.035
12. Chassard C, Lacroix C. Carbohydrates and the human gut microbiota. Curr Opin Clin Nutr Metab Care. (2013) 16:453–60. doi: 10.1097/MCO.0b013e3283619e63
13. Markowiak P, Slizewska K. Effects of probiotics, prebiotics, and synbiotics on human health. Nutrients. (2017) 9:1021. doi: 10.3390/nu9091021
14. Conlon MA, Bird AR. The impact of diet and lifestyle on gut microbiota and human health. Nutrients. (2014) 7:17–44. doi: 10.3390/nu7010017
15. Ramne S, Brunkwall L, Ericson U, Gray N, Kuhnle GGC, Nilsson PM, et al. Gut microbiota composition in relation to intake of added sugar, sugar-sweetened beverages and artificially sweetened beverages in the Malmö Offspring Study. Eur J Nutr. (2021) 60:2087–97. doi: 10.1007/s00394-020-02392-0
16. Sen T, Cawthon CR, Ihde BT, Hajnal A, DiLorenzo PM, de La Serre CB, et al. Diet-driven microbiota dysbiosis is associated with vagal remodeling and obesity. Physiol Behav. (2017) 173:305–17. doi: 10.1016/j.physbeh.2017.02.027
17. Cummings JH, Stephen AM. Carbohydrate terminology and classification. Eur J Clin Nutr. (2007) 61(Suppl 1):S5–18. doi: 10.1038/sj.ejcn.1602936
18. Lunn J, Buttriss JL. Carbohydrates and dietary fibre. Nutr Bull. (2007) 32:21–64. doi: 10.1111/j.1467-3010.2007.00616.x
19. Ebert K, Witt H. Fructose malabsorption. Mol Cell Pediat. (2016) 3:10. doi: 10.1186/s40348-016-0035-9
20. Zuo T, Ng SC. The gut microbiota in the pathogenesis and therapeutics of inflammatory bowel disease. Front Microbiol. (2018) 9:2247. doi: 10.3389/fmicb.2018.02247
21. Lambertz J, Weiskirchen S, Landert S, Weiskirchen R. Fructose: a dietary sugar in crosstalk with microbiota contributing to the development and progression of non-alcoholic liver disease. Front Immunol. (2017) 8:1159. doi: 10.3389/fimmu.2017.01159
22. Volynets V, Louis S, Pretz D, Lang L, Ostaff MJ, Wehkamp J, et al. Intestinal barrier function and the gut microbiome are differentially affected in mice fed a western-style diet or drinking water supplemented with fructose. J Nutr. (2017) 147:770–80. doi: 10.3945/jn.116.242859
23. Mastrocola R, Ferrocino I, Liberto E, Chiazza F, Cento AS, Collotta D, et al. Fructose liquid and solid formulations differently affect gut integrity, microbiota composition and related liver toxicity: a comparative in vivo study. J Nutr Biochem. (2018) 55:185–99. doi: 10.1016/j.jnutbio.2018.02.003
24. Qi X, Tester RF. Fructose, galactose and glucose – In health and disease. Clinical Nutrition ESPEN. (2019) 33:18–28. doi: 10.1016/j.clnesp.2019.07.004
25. Sadigh-Eteghad S, Majdi A, McCann SK, Mahmoudi J, Vafaee MS, Macleod MR. D-galactose-induced brain ageing model: a systematic review and meta-analysis on cognitive outcomes and oxidative stress indices. PLoS ONE. (2017) 12:e0184122-e. doi: 10.1371/journal.pone.0184122
26. Stahel P, Kim JJ, Xiao C, Cant JP. Of the milk sugars, galactose, but not prebiotic galacto-oligosaccharide, improves insulin sensitivity in male Sprague-Dawley rats. PLoS One. (2017) 12:e0172260. doi: 10.1371/journal.pone.0172260
27. Zhang H, Li Y, Cui C, Sun T, Han J, Zhang D, et al. Modulation of gut microbiota by dietary supplementation with tuna oil and algae oil alleviates the effects of D-galactose-induced ageing. Appl Microbiol Biotechnol. (2018) 102:2791–801. doi: 10.1007/s00253-018-8775-1
28. Zhang D, Han J, Li Y, Yuan B, Zhou J, Cheong L, et al. Tuna oil alleviates d-galactose induced aging in mice accompanied by modulating gut microbiota and brain protein expression. J Agric Food Chem. (2018) 66:5510–20. doi: 10.1021/acs.jafc.8b00446
29. Li B, Evivie SE, Lu J, Jiao Y, Wang C, Li Z, et al. Lactobacillus helveticus KLDS1.8701 alleviates d-galactose-induced aging by regulating Nrf-2 and gut microbiota in mice. Food Funct. (2018) 9:6586–98. doi: 10.1039/C8FO01768A
30. Hu GX, Chen GR, Xu H, Ge RS, Lin J. Activation of the AMP activated protein kinase by short-chain fatty acids is the main mechanism underlying the beneficial effect of a high fiber diet on the metabolic syndrome. Med Hypotheses. (2010) 74:123–6. doi: 10.1016/j.mehy.2009.07.022
31. Anderson JW, Baird P, Davis RH, Ferreri S, Knudtson M, Koraym A, et al. Health benefits of dietary fiber. Nutr Rev. (2009) 67:188–205. doi: 10.1111/j.1753-4887.2009.00189.x
32. Dahl WJ, Agro NC, Eliasson ÅM, Mialki KL, Olivera JD, Rusch CT, et al. Health benefits of fiber fermentation. J Am Coll Nutr. (2017) 36:127–36. doi: 10.1080/07315724.2016.1188737
33. Pandey KR, Naik SR, Vakil BV. Probiotics, prebiotics and synbiotics: a review. J Food Sci Technol. (2015) 52:7577–87. doi: 10.1007/s13197-015-1921-1
34. Rossi M, Corradini C, Amaretti A, Nicolini M, Pompei A, Zanoni S, et al. Fermentation of fructooligosaccharides and inulin by bifidobacteria: a comparative study of pure and fecal cultures. Appl Environ Microbiol. (2005) 71:6150–8. doi: 10.1128/AEM.71.10.6150-6158.2005
35. Xue L, He J, Gao N, Lu X, Li M, Wu X, et al. Probiotics may delay the progression of nonalcoholic fatty liver disease by restoring the gut microbiota structure and improving intestinal endotoxemia. Sci Rep. (2017) 7:45176. doi: 10.1038/srep45176
36. Koh A, De Vadder F, Kovatcheva-Datchary P, Bäckhed F. From dietary fiber to host physiology: short-chain fatty acids as key bacterial metabolites. Cell. (2016) 165:1332–45. doi: 10.1016/j.cell.2016.05.041
37. Blaak EE. Current metabolic perspective on malnutrition in obesity: towards more subgroup-based nutritional approaches? Proc Nutr Soc. (2020) 79:331–7. doi: 10.1017/S0029665120000117
38. Vinolo MA, Rodrigues HG, Nachbar RT, Curi R. Regulation of inflammation by short chain fatty acids. Nutrients. (2011) 3:858–76. doi: 10.3390/nu3100858
39. Desai MS, Seekatz AM, Koropatkin NM, Kamada N, Hickey CA, Wolter M, et al. A dietary fiber-deprived gut microbiota degrades the colonic mucus barrier and enhances pathogen susceptibility. Cell. (2016) 167:1339–53.e21. doi: 10.1016/j.cell.2016.10.043
40. Satokari R. High intake of sugar and the balance between pro- and anti-inflammatory gut bacteria. Nutrients. (2020) 12:1348. doi: 10.3390/nu12051348
41. Payne AN, Chassard C, Lacroix C. Gut microbial adaptation to dietary consumption of fructose, artificial sweeteners and sugar alcohols: implications for host-microbe interactions contributing to obesity. Obes Rev Off J Int Assoc Study Obesity. (2012) 13:799–809. doi: 10.1111/j.1467-789X.2012.01009.x
42. Li J-M, Yu R, Zhang L-P, Wen S-Y, Wang S-J, Zhang X-Y, et al. Dietary fructose-induced gut dysbiosis promotes mouse hippocampal neuroinflammation: a benefit of short-chain fatty acids. Microbiome. (2019) 7:98. doi: 10.1186/s40168-019-0713-7
43. Chen D, Yang X, Yang J, Lai G, Yong T, Tang X, et al. Prebiotic effect of fructooligosaccharides from morinda officinalis on Alzheimer's disease in rodent models by targeting the microbiota-gut-brain axis. Front Aging Neurosci. (2017) 9:403. doi: 10.3389/fnagi.2017.00403
44. Mhd Omar NA, Frank J, Kruger J, Dal Bello F, Medana C, Collino M, et al. Effects of high intakes of fructose and galactose with or without added fructooligosaccharides on metabolic factors, inflammation and gut integrity in a rat model. Mol Nutr Food Res. (2021) 65: 2001133. doi: 10.1002/mnfr.202001133
45. Kilkenny C, Browne WJ, Cuthill IC, Emerson M, Altman DG. Improving bioscience research reporting: the ARRIVE guidelines for reporting animal research. PLoS Biol. (2010) 8:e1000412. doi: 10.1371/journal.pbio.1000412
46. Magoč T, Salzberg SL. FLASH fast length adjustment of short reads to improve genome assemblies. Bioinformatics. (2011) 27:2957–63. doi: 10.1093/bioinformatics/btr507
47. Caporaso JG, Kuczynski J, Stombaugh J, Bittinger K, Bushman FD, Costello EK, et al. QIIME allows analysis of high-throughput community sequencing data. Nat Methods. (2010) 7:335. doi: 10.1038/nmeth.f.303
48. Bokulich NA, Subramanian S, Faith JJ, Gevers D, Gordon JI, Knight R, et al. Quality-filtering vastly improves diversity estimates from illumina amplicon sequencing. Nat Methods. (2013) 10:57–9. doi: 10.1038/nmeth.2276
49. Edgar RC UPARSE highly accurate OTU sequences from microbial amplicon reads. Nat Methods. (2013) 10:996. doi: 10.1038/nmeth.2604
50. Wang Q, Garrity GM, Tiedje JM, Cole JR. Naive Bayesian classifier for rapid assignment of rRNA sequences into the new bacterial taxonomy. Appl Environ Microbiol. (2007) 73:5261–7. doi: 10.1128/AEM.00062-07
51. Quast C, Pruesse E, Yilmaz P, Gerken J, Schweer T, Yarza P, et al. The SILVA ribosomal RNA gene database project: improved data processing and web-based tools. Nucl Acids Res. (2012) 41:D590–6. doi: 10.1093/nar/gks1219
52. Bank N, Coco M, Aynedjian HS. Galactose feeding causes glomerular hyperperfusion: prevention by aldose reductase inhibition. Am J Physiol. (1989) 256:F994–9. doi: 10.1152/ajprenal.1989.256.6.F994
53. Ji L, Li C, Shen N, Huan Y, Liu Q, Liu S, et al. A simple and stable galactosemic cataract model for rats. Int J Clin Exp Med. (2015) 8:12874–81.
54. Singh RK, Chang H-W, Yan D, Lee KM, Ucmak D, Wong K, et al. Influence of diet on the gut microbiome and implications for human health. J Transl Med. (2017) 15:73. doi: 10.1186/s12967-017-1175-y
55. Candela M, Perna F, Carnevali P, Vitali B, Ciati R, Gionchetti P, et al. Interaction of probiotic Lactobacillus and Bifidobacterium strains with human intestinal epithelial cells: adhesion properties, competition against enteropathogens and modulation of IL-8 production. Int J Food Microbiol. (2008) 125:286–92. doi: 10.1016/j.ijfoodmicro.2008.04.012
56. Olszak T, An D, Zeissig S, Vera MP, Richter J, Franke A, et al. Microbial exposure during early life has persistent effects on natural killer T cell function. Science. (2012) 336:489–93. doi: 10.1126/science.1219328
57. Rinninella E, Cintoni M, Raoul P, Lopetuso LR, Scaldaferri F, Pulcini G, et al. Food components and dietary habits: keys for a healthy gut microbiota composition. Nutrients. (2019) 11:2393. doi: 10.3390/nu11102393
58. Rinninella E, Raoul P, Cintoni M, Franceschi F, Miggiano GAD, Gasbarrini A, et al. What is the healthy gut microbiota composition? A changing ecosystem across age, environment, diet, and diseases. Microorganisms. (2019) 7:14. doi: 10.3390/microorganisms7010014
59. Kashyap PC, Johnson S, Geno DM, Lekatz HR, Lavey C, Alexander JA, et al. A decreased abundance of clostridia characterizes the gut microbiota in eosinophilic esophagitis. Physiol Rep. (2019) 7:e14261. doi: 10.14814/phy2.14261
60. Moran-Ramos S, Lopez-Contreras BE, Canizales-Quinteros S. Gut microbiota in obesity and metabolic abnormalities: a matter of composition or functionality? Arch Med Res. (2017) 48:735–53. doi: 10.1016/j.arcmed.2017.11.003
61. Alles MS, de Roos NM, Bakx JC, van de Lisdonk E, Zock PL, Hautvast JG. Consumption of fructooligosaccharides does not favorably affect blood glucose and serum lipid concentrations in patients with type 2 diabetes. Am J Clin Nutr. (1999) 69:64–9. doi: 10.1093/ajcn/69.1.64
62. Mao B, Gu J, Li D, Cui S, Zhao J, Zhang H, et al. Effects of different doses of fructooligosaccharides (FOS) on the composition of mice fecal microbiota, especially the bifidobacterium composition. Nutrients. (2018) 10:1105. doi: 10.3390/nu10081105
63. Do MH, Lee E, Oh MJ, Kim Y, Park HY. High-glucose or -fructose diet cause changes of the gut microbiota and metabolic disorders in mice without body weight change. Nutrients. (2018) 10:761. doi: 10.3390/nu10060761
64. Kyaw MH, Mayberry JF. Fructose malabsorption: true condition or a variance from normality. J Clin Gastroenterol. (2011) 45:16–21. doi: 10.1097/MCG.0b013e3181eed6bf
65. Mukhopadhya I, Hansen R, El-Omar EM, Hold GL. IBD-what role do proteobacteria play? Nat Rev Gastroenterol Hepatol. (2012) 9:219–30. doi: 10.1038/nrgastro.2012.14
66. Cani PD, Bibiloni R, Knauf C, Waget A, Neyrinck AM, Delzenne NM, et al. Changes in gut microbiota control metabolic endotoxemia-induced inflammation in high-fat diet–induced obesity and diabetes in mice. Diabetes. (2008) 57:1470–81. doi: 10.2337/db07-1403
67. Zhang Z, He S, Cao X, Ye Y, Yang L, Wang J, et al. Potential prebiotic activities of soybean peptides Maillard reaction products on modulating gut microbiota to alleviate aging-related disorders in D-galactose-induced ICR mice. J Funct Foods. (2020) 65:103729. doi: 10.1016/j.jff.2019.103729
68. Hooper LV, Wong MH, Thelin A, Hansson L, Falk PG, Gordon JI. Molecular analysis of commensal host-microbial relationships in the intestine. Science. (2001) 291:881–4. doi: 10.1126/science.291.5505.881
69. Binda C, Lopetuso LR, Rizzatti G, Gibiino G, Cennamo V, Gasbarrini A. Actinobacteria: a relevant minority for the maintenance of gut homeostasis. Digest Liver Dis. (2018) 50:421–8. doi: 10.1016/j.dld.2018.02.012
70. Le TK, Hosaka T, Nguyen TT, Kassu A, Dang TO, Tran HB, et al. Bifidobacterium species lower serum glucose, increase expressions of insulin signaling proteins, and improve adipokine profile in diabetic mice. Biomed Res. (2015) 36:63–70. doi: 10.2220/biomedres.36.63
71. Amar J, Chabo C, Waget A, Klopp P, Vachoux C, Bermúdez-Humarán LG, et al. Intestinal mucosal adherence and translocation of commensal bacteria at the early onset of type 2 diabetes: molecular mechanisms and probiotic treatment. EMBO Mol Med. (2011) 3:559–72. doi: 10.1002/emmm.201100159
72. Everard A, Belzer C, Geurts L, Ouwerkerk JP, Druart C, Bindels LB, et al. Cross-talk between Akkermansia muciniphila and intestinal epithelium controls diet-induced obesity. Proc Natl Acad Sci U S A. (2013) 110:9066–71. doi: 10.1073/pnas.1219451110
73. Greer RL, Dong X, Moraes ACF, Zielke RA, Fernandes GR, Peremyslova E, et al. Akkermansia muciniphila mediates negative effects of IFNγ on glucose metabolism. Nat Commun. (2016) 7:13329. doi: 10.1038/ncomms13329
74. Dao MC, Everard A, Aron-Wisnewsky J, Sokolovska N, Prifti E, Verger EO, et al. Akkermansia muciniphila and improved metabolic health during a dietary intervention in obesity: relationship with gut microbiome richness and ecology. Gut. (2016) 65:426–36. doi: 10.1136/gutjnl-2014-308778
75. Holscher HD. Dietary fiber and prebiotics and the gastrointestinal microbiota. Gut Microbes. (2017) 8:172–84. doi: 10.1080/19490976.2017.1290756
76. Dong J-N, Li S-Z, Chen X, Qin G-X, Wang T, Sun Z, et al. Effects of different combinations of sugar and starch concentrations on ruminal fermentation and bacterial-community composition in vitro. Front Nutr. (2021) 8:727714. doi: 10.3389/fnut.2021.727714
77. Crost EH, Le Gall G, Laverde-Gomez JA, Mukhopadhya I, Flint HJ, Juge N. Mechanistic insights into the cross-feeding of ruminococcus gnavus and ruminococcus bromii on host and dietary carbohydrates. Front Microbiol. (2018) 9:2558. doi: 10.3389/fmicb.2018.02558
78. Gren C, Spiegelhauer MR, Rotbain EC, Ehmsen BK, Kampmann P, Andersen LP. Ruminococcus gnavus bacteraemia in a patient with multiple haematological malignancies. Access Microbiol. (2019) 1:e000048. doi: 10.1099/acmi.0.000048
79. Lefever S, Van Den Bossche D, Van Moerkercke W, D'Hondt M, Alegret Pampols MDC, Struyve M, et al. Ruminococcus gnavus bacteremia, an uncommon presentation of a common member of the human gut microbiota: case report and literature review. Acta Clin Belg. (2019) 74:435–8. doi: 10.1080/17843286.2018.1541045
80. Koliada A, Syzenko G, Moseiko V, Budovska L, Puchkov K, Perederiy V, et al. Association between body mass index and Firmicutes/Bacteroidetes ratio in an adult Ukrainian population. BMC Microbiol. (2017) 17:120. doi: 10.1186/s12866-017-1027-1
81. Million M, Angelakis E, Maraninchi M, Henry M, Giorgi R, Valero R, et al. Correlation between body mass index and gut concentrations of Lactobacillus reuteri, Bifidobacterium animalis, Methanobrevibacter smithii and Escherichia coli. Int J Obes (Lond). (2013) 37:1460–6. doi: 10.1038/ijo.2013.20
82. Sweeney TE, Morton JM. The human gut microbiome: a review of the effect of obesity and surgically induced weight loss. JAMA Surg. (2013) 148:563–9. doi: 10.1001/jamasurg.2013.5
83. Yan X, Feng B, Li P, Tang Z, Wang L. Microflora disturbance during progression of glucose intolerance and effect of sitagliptin: an animal study. J Diabetes Res. (2016) 2016:2093171. doi: 10.1155/2016/2093171
84. Gurung M, Li Z, You H, Rodrigues R, Jump DB, Morgun A, et al. Role of gut microbiota in type 2 diabetes pathophysiology. EBioMedicine. (2020) 51:102590. doi: 10.1016/j.ebiom.2019.11.051
85. Yacoub R, Nugent M, Cai W, Nadkarni GN, Chaves LD, Abyad S, et al. Advanced glycation end products dietary restriction effects on bacterial gut microbiota in peritoneal dialysis patients; a randomized open label controlled trial. PLoS One. (2017) 12:e0184789. doi: 10.1371/journal.pone.0184789
86. Buratti FM, Manganelli M, Vichi S, Stefanelli M, Scardala S, Testai E, et al. Cyanotoxins: producing organisms, occurrence, toxicity, mechanism of action and human health toxicological risk evaluation. Arch Toxicol. (2017) 91:1049–130. doi: 10.1007/s00204-016-1913-6
Keywords: fructose, galactose, fructooligosaccharides, gut microbiota, 16S rRNA
Citation: Mhd Omar NA, Dicksved J, Kruger J, Zamaratskaia G, Michaëlsson K, Wolk A, Frank J and Landberg R (2022) Effect of a diet rich in galactose or fructose, with or without fructooligosaccharides, on gut microbiota composition in rats. Front. Nutr. 9:922336. doi: 10.3389/fnut.2022.922336
Received: 17 April 2022; Accepted: 20 July 2022;
Published: 12 August 2022.
Edited by:
Xingbin Yang, Shaanxi Normal University, ChinaReviewed by:
Babak Haghshenas, Kermanshah University of Medical Sciences, IranMercedes G. López, Instituto Politécnico Nacional de México (CINVESTAV), Mexico
Copyright © 2022 Mhd Omar, Dicksved, Kruger, Zamaratskaia, Michaëlsson, Wolk, Frank and Landberg. This is an open-access article distributed under the terms of the Creative Commons Attribution License (CC BY). The use, distribution or reproduction in other forums is permitted, provided the original author(s) and the copyright owner(s) are credited and that the original publication in this journal is cited, in accordance with accepted academic practice. No use, distribution or reproduction is permitted which does not comply with these terms.
*Correspondence: Nor Adila Mhd Omar, YWRpbGFvQGNoYWxtZXJzLnNl