- 1Shanghai Engineering Research Center of Food Microbiology, School of Health Science and Engineering, University of Shanghai for Science and Technology, Shanghai, China
- 2Hunan Key Laboratory of Bean Products Processing and Safety Control, School of Food and Chemical Engineering, Shaoyang University, Shaoyang, China
- 3Department of Food Science and Technology, School of Agriculture and Biology, Shanghai Jiao Tong University, Shanghai, China
Probiotics have attracted much attention due to their ability to modulate host intestinal microbe, participate in nutrient metabolism or immunomodulatory. Both inflammatory bowel disease (IBD) and bowel cancer are digestive system disease, which have become a global public health problem due to their unclear etiology, difficult to cure, and repeated attacks. Disturbed gut microbiota and abnormal lipid metabolism would increase the risk of intestinal inflammation. However, the link between lipid metabolism, probiotics, and IBD is unclear. In this review, we found that different lipids and their derivatives have different effects on IBD and gut microbes. ω-3 polyunsaturated fatty acids (PUFAs) docosahexaenoic acid, eicosapentaenoic acid, and their derivatives resolvin E1, resolvin D can inhibit oxidative stress and reactive oxygen species activate NFκB and MAPk pathway. While ω-6 PUFAs linoleic acid and arachidonic acid can be derived into leukotrienes and prostaglandins, which will aggravate IBD. Cholesterol can be converted into bile acids to promote lipid absorption and affect microbial survival and colonization. At the same time, it is affected by microbial bile salt hydrolase to regulate blood lipids. Low denstiy lipoprotein (LDL) is easily converted into oxidized LDL, thereby promoting inflammation, while high denstiy lipoprotein (HDL) has the opposite effect. Probiotics compete with intestinal microorganisms for nutrients or ecological sites and thus affect the structure of intestinal microbiota. Moreover, microbial short chain fatty acids, bile salt hydrolase, superoxide dismutase, glutathione, etc. can affect lipid metabolism and IBD. In conclusion, probiotics are directly or indirectly involved in lipids metabolism and their impact on IBD, which provides the possibility to explore the role of probiotics in improving gut health.
Introduction
The intestinal tract is an immune organ for human protection, and it is also an important place for the digestion and absorption of dietary nutrients. Bacterial infection, viral infection, immune imbalance, etc. would induce inflammatory bowel disease (IBD) (1–3). IBD generally does not lead to bowel cancer, but it will increase the incidence of bowel cancer in patients with ulcerative colitis (UC), but without Crohn’s disease (CD) (3, 4). In recent years, the incidence of bowel cancer has increased year by year and shows a younger trend, which is a serious worldwide health problem (5). More and more studies have shown that although the occurrence of bowel cancer is related to genetic factors, it is mainly caused by polluted environment, pathogenic bacteria and toxins, bad dietary and lifestyle (6–9). Intestinal microbe and nutrient metabolism play an important role in controlling host immunity and maintaining intestinal homeostasis (10). However, intestinal dysbiosis and metabolic disorders would promote the occurrence and development of bowel cancer (11, 12). Therefore, gut microbiota and dietary nutrition have always been hotspots in cancer research. For a long time, dietary lipid has been considered as a main dietary risk factor for cancer, and it is closely related to IBD and bowel cancer (12, 13).
Dietary lipid is one of three major nutrients in the human body. It is decomposed into fatty acids, cholesterol and other fat digestion products by the action of various enzymes and bile salts in the small intestine, and then absorbed into the bloodstream through the small intestine wall. In previous studies, it was generally believed that high-fat diet was related to the occurrence of certain tumors, and now it is clearer that this is related to the types of lipids and their metabolites (14). In some animal experiments, it was found that ω-3 polyunsaturated fatty acids (PUFAs) can inhibit the occurrence and development of certain tumors, but the effect of ω-6 PUFAs is the opposite (15, 16). At the same time, body lipid is converted into substances needed by the body under the action of various enzymes to ensure the operation of normal physiological functions. However, lipid metabolites may also affect the body’s health, especially in metabolic disorders or immunodeficiency. For example, the PUFAs metabolites such as leukotrienes (LTs), prostaglandins (PGs), and peroxidized linoleic acid (13-HPODE) would promote the production of inflammatory factors, thereby increasing the risk of IBD and bowel cancer (16, 17). Lipid metabolism is a complex and important biochemical reaction process in the body. It is unknown whether it is affected by gut microbes or leads to changes in the species, quantity, proportion, localization, and biological characteristics of intestinal flora, thereby affecting human health.
With the in-depth study of human gut microbes, it has become a consensus that gut microbes can regulate human physiology and metabolism. Moreover, probiotics have attracted extensive attention due to their beneficial effects on the human body. Probiotics are live microorganisms which, when administered in adequate amounts, confer a health benefit to the host. The published results show that probiotics perform well in promoting nutrient absorption, maintaining intestinal homeostasis, and enhancing immunity (18–22). For example, Lacticaseibacillus casei LC2W can inhibit the colonization of Escherichia coli O157:H7 in vivo and reduce the severity of colitis, and Lactiplantibacillus plantarum AR326 with good adhesion ability can be used as promising probiotics to ameliorate dextran sulfate sodium (DSS) -induced colitis (23–25). That is to say, probiotics play important roles in down-regulating the production of pro-inflammatory cytokines, promoting intestinal epithelial barrier function, increasing the anti-inflammatory response, and contributing to the overall health of the host (21–25). As important health-promoting bacteria, whether probiotics are involved in lipid metabolism and its mechanism is unclear. In this review, we analyze the effects of lipids and their metabolites on IBD and bowel cancer, and summarize the pathways of probiotics regulating microbiota and participating lipid metabolism, in order to exploring the potential of probiotics in regulating host immunity and maintaining intestinal homeostasis.
Inflammatory Bowel Disease and Bowel Cancer Seriously Damage Health
IBD is a chronic disease of the digestive system with unclear etiology, difficult to cure, and repeated attacks. It is accompanied by a variety of complications, which seriously affects the quality of life of patients (26). The incidence of IBD has increased significantly in recent years, and there is a younger trend. To date, the exact causes of IBD have not been fully understood and effective therapeutics are yet to be discovered. With regards to etiology, there are pediatric data, mainly from case-control studies, which suggest that some dietary habits, such as consumption of animal protein, fatty foods, high sugar intake, may contribute to IBD onset (27). Poor dietary habits can lead to changes in intestinal flora, which are inseparable from the occurrence of IBD. Fortunately, oral probiotics Bifidobacterium and Lactobacillus and fecal microbiota transplantation can restore the diversity of patients’ intestinal flora, which has important clinical value for the treatment of IBD (28–30).
As we known, nuclear factor kappa-B (NF-κB) is a hub for pro-inflammatory transcription factors that induce the expression of many pro-inflammatory genes, such as cell adhesion molecules, cyclooxygenase 2 (COX-2), inducible nitric oxide synthase (iNOS), tumor necrosis factor α (TNF-α), nuclear factor-kappa B (NF-kappaB), and interleukin (IL) (31–33). When endotoxin interacts with Toll-like receptor 4 (TLR4), TNF-α interacts with tumor necrosis factor receptor (TNFR), it will activate the κB kinase (IKK) complex of MAPK/EKK kinase, promote the dissociation of phosphorylated NK-beta inhibitors (IKBα) from the IKBα/NF-κB complex and produce NF-κB monomers, which translocate into the nucleus and produce pro-inflammatory factors, such as TNF-α, IL-1β, IL-6, and IFN-γ) and inflammatory markers (iNOS and COX-2) (34, 35). Similarly, lipopolysaccharide (LPS) and TNF-α bind to receptors TLR4 and TNFR, respectively, which can promote the phosphorylation of the transforming growth factor 1 (TAK1), activate the IKBα/NF-κB and MKK4/JUK pathways, and produce inflammation (36). Studies have shown that IBD more than doubles an individual’s lifetime risk of developing bowel cancer, and the risk increases significantly if they have suffered with UC for a sustained period of time (37). In other words, general intestinal inflammation will not cause bowel cancer, but with the increase of UC time, there will be a certain probability rate of developing colon cancer.
Bowel cancer is the most common digestive tract tumor, including colorectal cancer and small bowel cancer. Colorectal cancer is divided into colon cancer and rectal cancer, while small bowel cancer can be divided into duodenal cancer, ileal cancer and jejunum cancer. Moreover, colorectal cancer is more common than small bowel cancer (38). Colorectal cancer is the third most common cancer worldwide, especially in developed countries where an estimated 60% of all cases occur (39). Generally speaking, genetics, inflammation, intestinal adenomas, dietary habits, and carcinogens are all contributing factors to bowel cancer. Gut microbiota participates in many physiological and pathological processes of the host, including food digestion and absorption, substance metabolism, host immunity, and intestinal inflammation (40–43). It is established that physical inactivity, obesity and some dietary factors (red/processed meats, alcohol) are positively associated with colorectal cancer, while healthy lifestyle habits show inverse associations (6, 9, 44). The type and quantity of food ingested are controllable, thereby affecting the supply of nutrients and the composition of microorganisms. Therefore, dietary factor is one of the most important and controllable factors in changing gut microbes (9). Therefore, more and more scientists are beginning to pay attention to the impact of lipids and their metabolites, and gut microbiota especially probiotics on IBD and bowel cancer.
Lipid Metabolism Differentially Affect Inflammatory Bowel Disease and Bowel Cancer
Polyunsaturated Fatty Acids and Cholesterol Relieve Inflammation or Not
Dietary lipids are mainly composed of triglycerides, cholesterol, phospholipids, and glycolipids, which can be digested and absorbed by the body. Lipid metabolism is a complex process, which is affected by many factors. Meanwhile, lipid metabolites will in turn affect the health of the body (Figure 1). Among them, PUFAs and cholesterol have attracted widespread attention due to their association with cardiovascular and cerebrovascular diseases, metabolic diseases, and inflammation. For example, ω-3 PUFAs α linolenic acid can be converted into docosahexaenoic acid (DHA), eicosapentaenoic acid (EPA) and prostaglandin (45). While biologically active derivatives of the ω-6 PUFAs arachidonic acid (AA) include PGs, thromboxane, hydroxyeicosatetraenoic acids and LTs (16, 17, 46, 47). It is reported that dietary supplementation with EPA and DHA decreased low-density lipoprotein cholesterol (LDL-C) synthesis and increased bile acid synthesis and LDL-C clearance by LDL receptor, synergistically with estrogen (48). LTs are potent pre-inflammatory mediators involved in the pathogenesis of inflammation, allergy, asthma, and shock (47).
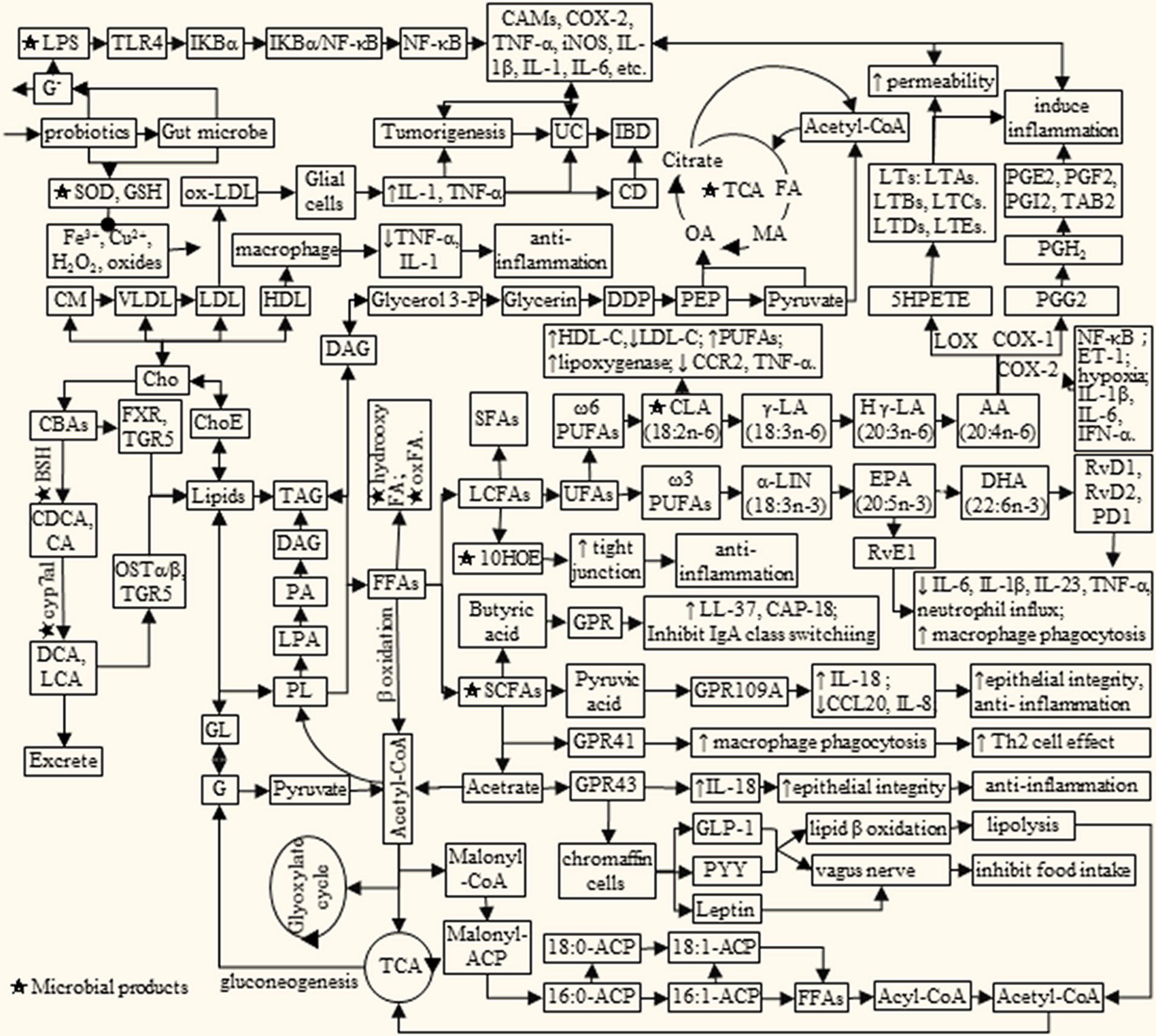
Figure 1. Lipids metabolism interacts with microbial products to influence IBD/cancer. AA, arachidonic acid; BAs, bile acids; BSH, bile salt hydrolase; CA, cholic acid; cAMP, adenosine cyclophosphate; CAMs, cell adhesion molecule; CBAs, conjugated bile acid; CCL20, Chemokine Ligand 20; CD, Crohn’s disease; CDCA, chenodeoxycholic acid; Cho, cholesterol; ChoE, cholesterol ester; CLA, conjugated linoleic acid; CM, chylomicrons; cyp7a1, cholesterol 7α-dehydroxylase; C, concentration of Ca2 +; DDP, dihydroxyacetone phosphate; DCA, deoxycholic acid; DHA, docosahexaenoic acid; EPA, eicosapentaenoic acid; ET-1, endothelin-1; FA, fumaric acid; FFAs, free fat acids; FXR, farnesoid X receptor; G, glucose; Glycerol 3-P, glycerol 3-phosphate; GL, glycosphingolipid; GLP-1, lucagon-like peptide-1; GLP-2, glucagon-like peptide-2; GPR, G protein-coupled receptors; HLD, high density lipoprotein; H γ-LA, homologous γ linolenic acid; IB, D, inflammatory bowel disease; IL, interleukin; LA, linoleic acid; LCA, lithocholic acid; LDL, low density lipoprotein; Le, lenoleic; LNA, linolenic acid; LPS, lipopoly saccharide; LTs, leukotrienes; MA, malic acid; Malonyl-CoA, malonyl coenzyme A; ACP, acyl carrier protein; OA, oxalacetic acid; OSTα/β, organic solutetransport protein α/β heterodimer; PA, pyruvic acid; PD1, protectin D1; PEP, phosphoenolpyruvate; PG, prostaglandin; PL, phospholipids; PYY, peptide tyrosine; RvE1, resolvin E1; RvD, resolvin D; SCFA, short chain fatty acid; TAG, triglyceride; TCA, tricarboxylic acid cycle; TLR4, Toll-likereceptor 4; TGR5, G protein coupled bile acidreceptor; UC, ulcerative colitis; VLDL, very low density lipoprotein; COX2, cyclooxygenase 2; 10HOE, 10-hydroxy-cis-12-otadecenoic acid.
PUFAs is a component of the plasma membrane, which affects the fluidity of the cell membrane and the transmission of cell signals, regulates a variety of enzymes related to lipid metabolism, and participates in the regulation of inflammation and immunity in the body (49–51). For example, activation of phosphatase A2 enables the release of AA from membrane phospholipids, enhances the phosphorylation of NK-IKBα by κB kinase, and promotes inflammation (17, 47). COX-2 plays a key role in the regulation of inflammation by catalyzing the oxygenation of AA to PGs and hydroperoxides (51, 52). However, ω-3 PUFAs exert anti-inflammatory and antioxidant effects by inducing the production of bioactive compounds, such as 17, 18-epoxyeicosatetraenoic acid, resolvin E1 (RvE1), protectin D1, adiponectin, lysis, interleukin 10 (IL-10), interleukin 4 (IL-4), superoxide dismutase (SOD), heme oxygenase-1 (HO-1), and glutathione (GSH) (51–53). DHA can activate TAK1-binding protein 1 through GPR120/β-arrestin2, preventing the activation of IKBα/NF-κB and MKK4/JUK, thereby inhibiting inflammation (49, 50). Moreover, RvE1 is a derivative of EPA, which blocks transient receptor potential vanilloid type -1 and TNF-α signaling and relieves inflammation. Taken together, ω-3 PUFAs can down-regulate endotoxin- and cytokine-induced expression of COX-2 and lipid peroxidase, and inhibit phospholipase activity, thereby reducing AA and its induced eicosanoids content of inflammatory mediators (54).
Blood lipids are the general term for neutral fats and esters in plasma, which are widely present in the human body. Generally speaking, the main components of blood lipids are triglycerides and cholesterol, among which triglycerides are involved in energy metabolism in the human body, while cholesterol is mainly used for the synthesis of cell plasma membrane, steroid hormones and bile acids. We often use total triglycerides, total cholesterol, LDL and high-density lipoprotein (HDL) as the investigation indicators of blood lipids (55). LDL is a lipoprotein particle that carries cholesterol into peripheral tissue cells and can be converted to the oxidized LDL (ox-LDL), which plays a central role in atherosclerosis by acting on multiple cells such as endothelial cells, macrophages, platelets, fibroblasts, and smooth muscle cells through ox-LDL receptor (LOX-1) (56). While ω-3 PUFAs inhibited hepatic endoplasmic reticulum stress induced by feeding of a high-saturated fat diet accompanied by the expression hem agglutinin-like ox-LDL receptor (LOX-1) (57). In contrast, HDL mediates reverse cholesterol transport and is known to be protective against atherosclerosis (58). In addition, HDL mediates anti-inflammatory reprogramming of macrophages via the transcriptional regulator activating transcription factor 3 (ATF3) (59).
Lipid Oxides Exacerbate Inflammation and Tumors
Oxidation is a normal phenomenon in nature. When it occurs in the human body, it is called biological oxidation, which is realized under the catalysis of enzymes. Lipid oxidation generally includes auto- and enzymatic oxidation, which provides energy and produces various metabolites such as lipid peroxides, alcohols, aldehydes, and ketones. It has be reported that the occurrence and development of multiple chronic diseases such as atherosclerosis, inflammation and cellular aging, are closely related to lipid oxides (60–62). As we known, lipid peroxides are only the unstable reaction intermediates, which react with almost all molecules or cells in the human body, destroying DNA, and cellular structures. PUFA is one of the indispensable and easily oxidized fatty acids in our body. Dietary intakes rich in unsaturated fatty acids, have increased dramatically in recent decades. It is suggested that PUFA intake is beneficial to human health, but some lipid peroxides would increase the risk of inflammation and related diseases (53, 57). The final products of lipid peroxidation are reactive aldehydes such as malondialdehyde (MDA) and 4-hydroxynonenal (HNE), which play important pathogenic roles in the aging-related disorders characterized by increases of oxidative stress (63). Recently, Zhang and colleagues demonstrated that the linoleic acid-rich diet had little effect on the severity of colitis in the treated IL-10–/– mice (64). They also found that oxidized linoleic acid had little effect on basal inflammation in mice, but exacerbated chemically induced colitis in mice (65). Moreover, they isolated and characterized epoxyketooctadecenoic acid (EKODE), which was a lipid peroxidation product, and was among the most dramatically increased lipid molecules in the colon of azoxymethane/dextran sodium sulfate-induced colorectal cancer mice, and exacerbated colonic inflammation and colon tumor genesis (66). In addition, most of the excessive LDL in the blood are oxidized and modified lipoproteins, which are retained in the blood vessel wall and involved in the formation of atherosclerosis (58, 60). Therefore, we should try to prevent and reduce the oxidized lipid to avoid the occurrence and development of IBD and bowel cancer.
Lipid Metabolites Interact With Gut Microbiota to Relieve Inflammation
There are many kinds of intestinal microorganisms, which are related to age, body weight, gender, region, and dietary. The structure of the intestinal microbiota is seriously influenced by dietary habits. Therefore, the core dominant microbiota in the human gut is related to the proportions of protein, fat and carbohydrate components in dietary patterns (44, 67). At the same time, microorganisms will in turn act on the lipid metabolism of the host. It is reported that probiotics affect the lipid profile and anthropometric indices in diabetic nephropathy (68). And alterations in the gut microbiota of mice induced by a high-fat diet led to an increase in the proportion of LPS-containing bacteria in the gut (40). Zymomonas mobilis integral fermentation broth, which was incubated at 30°C for 72 h to reach the count of 109 CFU/mL but the metabolites is unclear, is effective in regulating intestinal transit, and the cell-free fermented broth is efficient in reducing cholesterol, LDL and very low density lipoprotein (VLDL) (69). Supplementation with probiotics has been shown to contribute to improving lipid metabolism through bile acid hydrolase (70, 71). And different strains of L. plantarum have different cholesterol-lowering capacities and different influencing factors (72). High-dose composite probiotics from camel milk, which include Lactobacillus kefiranofaciens, L. plantarum, Lactobacillus helveticus, Lactococcus lactis each 1.0 × 1010 CFU/mL and Issatchenkia orientalis 1.0 × 108 CFU/mL, decreased fasting blood glucose and body weight, increased C-peptide, modulated lipid metabolism and improved liver and kidney protected in-jury in db/db mice, which may be related to various probiotics acting through protecting the function of islets and regulating intestinal flora disturbance (41). In other words, lipids and their metabolites interact with the gut microbiota to relieve inflammation.
Probiotics Diversely Involved in Lipid Metabolism to Improve Gut Health
With the in-depth study of the gut microbiota, more and more attention has been paid to probiotics. There are many types of probiotics isolated from various environments, such as Bifidobacterium, Lactobacillus, and Bacteroides, which can be involved in lipid metabolism in direct and indirect ways (7, 8, 42, 73, 74). Therefore, we mainly summarized the probiotics involved in lipid metabolism through cholesterol metabolism, short-chain fatty acids (SCFAs), interaction with gut microbiota to reduce oxidative damage and improve intestinal barrier.
Involved in Cholesterol Metabolism via Bile Acid Cycle
Body cholesterol comes from diet and biosynthesis. Adults can synthesize cholesterol in various tissues except brain tissue, among which the liver and intestinal mucosa are the main sites of synthesis. Cholesterol homeostasis is vital for proper cellular and systemic functions. Disturbed cholesterol balance underlies not only cardiovascular disease but also an increasing number of other diseases such as neurodegenerative diseases and cancers (42, 75–77). Cholesterol can be converted into sterols/oxysterols and bile acids. Among them, bile acids combined with glycine or taurine, and then flow into the intestine through the gallbladder to participate in the emulsification of dietary fat and promote absorption. Conjugated bile acids first generate cholic acid and chenodeoxycholic acid under the action of intestinal bacterial bile acid hydrolase, and then generate 7-deoxycholic acid and lithocholic acid under the action of bile acid 7α dehydroxylase (78–80). Most of the bile acids in the intestine are actively absorbed and passively diffused into the liver through the portal vein, and the rest are excreted in the feces. Several probiotic strains have been extensively studied and their benefits to human health have been widely documented. For example, it has been reported that probiotics, such as Bifidobacterium longum, Lactobacillus acidophilus, L. casei, L. plantarum, exhibit bile salt hydrolases (42, 72–74, 80, 81). For example, L. casei pWQH01, which overexpresses bsh1 from L. plantarum AR113, appear to improve steatosis in vitro in a BSH-dependent manner (81). Lactobacillus gasseri BNR17 can inhibit the secretion of adiponectin and leptin, and reduce adipose tissue, while L. gasseri SBT2055 reduced mesenteric tissue mass, serum leptin levels and adipocyte size in lean Zucker mice (82, 83).
Produce Short Chain Fatty Acids to Participate in Lipid Metabolism
SCFAs are the products of otherwise indigestible fiber-rich diets metabolized by the anaerobic intestinal bacteria in the colon, mainly including formic acid, acetic acid, propionic acid, butyric acid, and valeric acid (19). Acetate is the most abundant and it is used by many gut commensals to produce propionate and butyrate in a growth-promoting cross-feeding process (84). A randomized double-blind show that probiotic L. plantarum IS 10506 supplementation significantly influenced acetate titer, but marginally significant for propionate and butyrate of women with functional constipation in the community of Jakarta (85).
SCFAs regulate lipid metabolism and play a role in immunomodulatory through a variety of ways. Firstly, SCFAs can activate the liver cyclic adenosine monophosphate (cAMP) signaling pathway to enhance the body’s oxidative metabolism, inhibit liver fat production, and thereby improve blood lipid levels. For example, Butyric acid regulates progesterone and estradiol secretion via cAMP signaling pathway in porcine granulosa cells (86). SCFAs can be recognized by G protein coupled receptors (GPRs), such as GPR41 and GPR43, which are expressed in colon, adipose, muscle, and liver tissue. Both GPR41 and GPR43 can reduce the concentration of intracellular cAMP, but GPR43 also increase the concentration of Ca2+, thereby inhibiting the synthesis of cholesterol in the liver to regulate lipid metabolism (87). SCFAs have been shown to ameliorate diseases in animal models of IBD and allergic asthma (88). Asparagus officinalis polysaccharides fermented with L. plantarum NCU116 can modulate the disordered homeostasis of bile acids, which is attributed to the up-regulation of GPR41 and GPR109A as well as intestinal SCFA production (89). Phenyl lactic acid derived from L. plantarum ZJ316 significantly increases the concentrations of propionic acid and butyric acid in the colon, regulates microbiota composition and alleviates Salmonella enterica Typhimurium-induced colitis (90).
Secondly, SCFAs have been shown to stimulate gastrointestinal chromaffin cells to secrete leptin, glucagon-like peptide-1 (GLP-1) and gastrointestinal peptide hormone tyrosine (PYY), thereby activating the afferent pathway of the vagus nerve to inhibits food intake (91–93). SCFAs can be used as a colonocyte energy source in the rat, and their derivatives produced by the digestion of intestinal flora can stimulate the secretion of PYY and GLP-1 via bind to GPR43, and inhibit the emptying of gastric contents (92). The secretion of gastric acid reduces appetite and suppresses eating, thereby alleviating the occurrence of hyperlipidemia. In addition, the combination of SCFAs and GPR43 promotes the secretion of GLP-1, up-regulates the expression of fat β-oxidation genes, promotes lipolysis, and avoids metabolic diseases such as hyperlipidemia caused by fat accumulation (93). Some SCFAs are associated with hepatic lipid metabolism and C-reactive protein concentrations, which may vary with gestational weight. Obesity in pregnancy reduces the amount of SCFAs in the stool, and a decrease in the level of butyrate reduces liver function (94, 95). Sodium propionate and sodium butyrate has been reported to counteract inflammation activation in the central and enteric nervous system (84, 96).
Moreover, SCFAs can also affect lipid metabolism by increasing the host’s sense of satiety and controlling appetite. SCFAs can target fat cells and increase the release of leptin, which has the effect of reducing hunger and inhibiting feeding activities (91, 94). Human milk insulin and leptin are independently associated with beneficial microbial metabolic pathways predicted to increase intestinal barrier function and reduce intestinal inflammation (97). Despite GPR43 being activated by SCFAs, it remains unclear if this receptor plays a role in leptin expression, as this receptor is expressed at low levels in adipocytes (43). While SCFAs stimulated leptin expression by activation of GPR41, which is highly expressed in adipose tissue (29). In animal studies, probiotics interventions to modulate high-fat diet outcomes predominantly caused a decrease in circulating leptin levels and increased SCFA, associated with suppressed weight gain (20). Fecal microbiota transplantation from Kazak individuals with normal glucose tolerance could improve glycolipid disorders by changing the bacterial composition responsible for producing SCFAs and activating the GPR43/GLP-1 pathway in db/db mice (98). In conclusion, probiotics mediate SCFAs production and regulate lipid metabolism in multiple ways.
Regulate Gut Microbiota to and Strengthen Intestinal Barrier
Gut microbes are increasingly linked to human health. Under the influence of certain factors, the intestinal micro-ecosystem is destroyed, resulting in dysbiosis. Gut microbial dysbiosis contribute to obesity, Clostridium difficile infection, autoimmune disorders, IBD, and even bowel cancer (99, 100). Many studies have reported that the intake of exogenous probiotics and their products can improve the intestinal microbiota of animals and humans (42, 43). Probiotics can compete with pathogenic bacteria for space occupation and nutrients, and their metabolites can inhibit the growth of pathogenic bacteria, thus playing a certain protective role in the intestinal tract (23, 24, 90). Probiotics containing B. animalis subsp. Infantis BLI-02, B. breve Bv889, B. bifidum VDD088, B. animalis subsp. lactis CP-9, and L. plantarum PL-02 intervention changed the species, dispersion, and abundance of gut macrobiota and exhibit anti-oxidative activities that regulate oxidative stress and protect cells from oxidative damage in middle aged mice (20). However, the metabolites of opportunistic pathogens would lead to the oxidation of lipid metabolites and promote the development of intestinal inflammation. For example, Aggregatibacter actinomycetemcomitans could markedly promote the oxidation of LDL through oxidative stress involving NADPH oxidase- and myeloperoxidase-derived reactive oxygen species (101). Lipopolysaccharides (LPSs) are cell wall components of Gram-negative bacteria, also called endotoxins. Normally, they are confined to the intestinal lumen and cannot cross the intestinal barrier. However, when the intestinal barrier function is weakened, LPSs can enter the bloodstream and cause systemic inflammation. Elevated concentrations of LPS in circulation may increase risk of atherosclerosis (102). While L. casei has been shown to destroy the biofilm of Proteus mirabilis P14 to avoid damage to the gut barrier (103).
In the food industry, there are some probiotics that can modulate immune response are widely used, such as Lactobacillus johnsonii, Lactobacillus rhamnosus, L. casei, Saccharomyces cerevisiae, Bifidobacterium lactis, and Bifidobacterium animalis. It was reported that Pediococcus pentosaceus CECT 8330 administration protects the DSS-induced colitis and modulates the gut microbial composition and function, immunological profiles, and the gut barrier function (43). Numerous clinical evidence and scientific literature have shown that the use of L. rhamnosus LGG® alone is associated with improved immunity, while the combined use of Bifidobacterium lactis BB-12™, Bifidobacterium infants DSM 33361 and Streptococcus thermophilus TH-4™, significantly reduced necrotizing enterocolitis in preterm infants (29). In addition, probiotics also exhibited strain-specific protective effects in T84 cells challenged with C. difficile-infected fecal water, whereby Saccharomyces boulardii CNCM I-1079 and L. rhamnosus R0011 were most effective in reducing pro-inflammatory cytokine production, while L. rhamnosus GG R0343 along with the two other multi-strain combinations were the least effective (21). Probiotics can reduce inflammation by exerting antioxidant properties. For example, the protective effect of Bifidobacterium infantis BB on allergic mice was correlated with its antioxidative enzyme SOD (104). The study demonstrates that Lactobacillus sakei pro-Bio65 empowers the antioxidant endogenous efficiency of HCE cells, by the upregulation of the glutathione (GSH) content and the enzymatic antioxidant pattern, and concurrently reduces TNF-alpha protein expression (105). Generally speaking, probiotics can regulate gut microbiota, reduce lipid peroxide damage, and strengthen the immune barrier.
Conclusion
IBD and bowel cancer are serious worldwide health problems that are influenced by lipids metabolites and gut microbes. Lipids and their metabolites interact with gut microbiota, which is influenced by supplemental probiotics. The metabolites of triglycerides, cholesterol lipids, and phospholipids can be converted into each other. In this review, we focus on the interconnectedness of triglycerides and cholesterol and their metabolites with gut microbes and IBD. Among them, the derivates of ω-6 PUFAs AA LTs and PGs can promote the production of pro-inflammatory factors such as TNF-α, IL-1β, IL-6, and IFN-γ. While ω-3 PUFAs DHA, EPA and their derivatives RvE1, RvDs, and protectin D would increase the expression of anti-inflammatory factors IL-10 and IL-4. Cholesterol is often present in the blood in the form of VLDL, LDL, and HDL. LDL is easily oxidized to oxLDL and promotes inflammation, but HDL can play an anti-inflammatory function. When UC recurs over a long period of time, it increases the risk of developing bowel cancer. Probiotics such as Bifidobacterium, Lacticaseibacillus, and Lactiplantibacillus, participate in lipid metabolism by regulating intestinal flora, producing SCFAs and BSH. And they produce SOD and GSH, which effectively alleviate oxidative stress damage and inhibit the development of inflammation. There is increasing evidence that probiotics have the potential to improve overall health. However, this research area needs to be thoroughly examined in more clinical studies to further explore the health-promoting effects and mechanisms of probiotics in IBD and bowel cancer. Therefore, it has broad application prospects for the prevention and control of the occurrence and development of intestinal cancer by regulating the intestinal microecology through personalized probiotics.
Author Contributions
TW: conceptualization and writing. GW: resources and review. ZX, HZ, and YW: resources. YX and XS: project administration. LA: funding acquisition. All authors have read and agreed to the published version of the manuscript.
Funding
This research was funded by the National Science Foundation for Distinguished Young Scholars of China (Grant No. 32025029), the Key Research and Development Program of Hunan Province (Grant Nos. 2019TP1028, 2019NK4299, and 2019SK2122), Guizhou Province Traditional Fermented Food Engineering Technology Center (Grant No. 20185251), and the Joint Funds of the Natural Science Foundation of Hunan Province (Grant No. 2329).
Conflict of Interest
The authors declare that the research was conducted in the absence of any commercial or financial relationships that could be construed as a potential conflict of interest.
Publisher’s Note
All claims expressed in this article are solely those of the authors and do not necessarily represent those of their affiliated organizations, or those of the publisher, the editors and the reviewers. Any product that may be evaluated in this article, or claim that may be made by its manufacturer, is not guaranteed or endorsed by the publisher.
References
1. Hong S, Chang S, Hudesman D, Axelrad J. P031 detection of bacterial enteric infection impacts management of outpatients with flares of inflammatory bowel disease. Gastroenterology. (2019) 156:S15. doi: 10.1093/ibd/izy393.036
2. Babayeva GH. Cytomegalovirus and mixed viral infections in patients with inflammatory bowel disease. Gastroenterology. (2019) 53:258–65. doi: 10.1093/bmb/ldp036
3. Bernstein CN, Blanchard JF, Kliewer E, Wajda A. Cancer risk in patients with inflammatory bowel disease: a population-based study. Gastroenterol Clin N. (2015) 91:854–62. doi: 10.3109/00365528909091339
4. Ungaro R, Mehandru S, Allen PB, Peyrin-Biroulet L, Colombel JF. Ulcerative colitis. Lancet. (2017) 389:1756–70. doi: 10.1016/S0140-6736(16)32126-2
5. Bhatia R, Yeoh SW, Vaz K, Studd C, Wilson J, Bell S, et al. Inflammatory bowel disease incidence, prevalence and 12-month initial disease course in Tasmania, Australia. Intern Med J. (2019) 49:150–6. doi: 10.1093/ecco-jcc/jjw148
6. Negrichi S, Taleb S. Hereditary, environmental, and dietary risk factors of colorectal cancer: a case-control study in the Algerian East. Environ Sci Pollut Res Int. (2021) 28:12372–81. doi: 10.1007/s11356-020-10378-y
7. Chung L, Thiele OE, Geis AL, Chan JL, Fu K, DeStefano SC, et al. Bacteroides fragilis Toxin Coordinates a Pro-carcinogenic Inflammatory Cascade via Targeting of Colonic Epithelial Cells. Cell Host Microbe. (2018) 23:203–14. doi: 10.1016/j.chom.2018.01.007
8. Butt J, Jenab M, Werner J, Fedirko V, Weiderpass E, Dahm CC, et al. Association of pre-diagnostic antibody responses to Escherichia coli and Bacteroides fragilis toxin proteins with colorectal cancer in a European cohort. Gut Microbes. (2021) 13:1–14. doi: 10.1080/19490976.2021.1903825
9. Murphy N, Moreno V, Hughes DJ, Vodicka L, Vodicka P, Aglago EK, et al. Lifestyle and dietary environmental factors in colorectal cancer susceptibility. Mol Aspects Med. (2019) 69:2–9. doi: 10.1016/j.mam.2019.06.005
10. Kuhnen A. Genetic and environmental considerations for inflammatory bowel disease. Surg Clin N Am. (2019) 99:1197–207. doi: 10.1016/j.suc.2019.08.014
11. Jackson DN, Theiss AL. Gut bacteria signaling to mitochondria in intestinal inflammation and cancer. Gut Microbes. (2020) 11:285–304. doi: 10.1080/19490976.2019.1592421
12. Bian X, Liu R, Meng Y, Xing D, Xu D, Lu Z. Lipid metabolism and cancer. J Exp Med. (2021) 218:e20201606. doi: 10.1084/jem.20201606
13. Abigail B, Ashley T, Alex RP, Fabio C. Mucosal interactions between genetics, diet, and microbiome in inflammatory bowel disease. Front Immunol. (2016) 7:290. doi: 10.3389/fimmu.2016.00290
14. Karaivazoglou K, Konstantakis C, Tourkochristou E, Assimakopoulos SF, Triantos C. Non-alcoholic fatty liver disease in inflammatory bowel disease patients. Eur J Gastroen Hepat. (2020) 32:903–6. doi: 10.1097/MEG.0000000000001679
15. Mozaffari H, Daneshzad E, Larijani B, Bellissimo N, Azadbakht L. Dietary intake of fish, n-3 polyunsaturated fatty acids, and risk of inflammatory bowel disease: a systematic review and meta-analysis of observational studies. Eur J Nutr. (2019) 59:1–17. doi: 10.1007/s00394-019-01901-0
16. Jacobson K, Calder PC. Role of Omega-6 and Omega-3 Fatty Acids in Inflammatory Bowel Disease. Berlin: Springer International Publishing (2014). p. 75–9.
17. Innes JK, Calder PC. Omega-6 fatty acids and inflammation. Prostaglandins Leukot Essent Fatty Acids. (2018) 132:41–8. doi: 10.1016/j.plefa.2018.03.004
18. Rouxinol-Dias AL, Pinto AR, Janeiro C, Rodrigues D, Moreira M, Dias J, et al. Probiotics for the control of obesity–its effect on weight change. Porto Biomed J. (2016) 1:12–24. doi: 10.1016/j.pbj.2016.03.005
19. Markowiak-Kope P, Liewska K. The effect of probiotics on the production of short chain fatty acids by human intestinal microbiome. Nutrients. (2020) 12:1107. doi: 10.3390/nu12041107
20. Lin WY, Lin JH, Kuo YW, Chiang P, Ho HH. Probiotics and their metabolites reduce oxidative stress in Middle-Aged mice. Curr Microbiol. (2022) 79:104. doi: 10.1007/s00284-022-02783-y
21. Gaisawat MB, Lopez-Escalera S, Macpherson CW, Iskandar MM, Tompkins TA, Kubow S. Probiotics exhibit strain-specific protective effects in T84 cells challenged with Clostridioides difficile-infected fecal water. Front Microbiol. (2022) 12:698638. doi: 10.3389/fmicb.2021.698638
22. Abraham BP, Quigley EMM. Probiotics in inflammatory bowel disease. Gastroenterol Clin N Am. (2017) 46:769–82. doi: 10.1016/j.gtc.2017.08.003
23. Wang G, Zhang Y, Song X, Xia Y, Lai PF, Ai L. Lactobacillus casei LC2W can inhibit the colonization of Escherichia coli O157:H7 in vivo and reduce the severity of colitis. Food Funct. (2019) 10:5843–52. doi: 10.1039/c9fo01390c
24. Wang G, Tang H, Zhang Y, Xiao X, Xia Y, Ai L. The intervention effects of Lactobacillus casei LC2W on Escherichia coli O157:H7-induced mouse colitis. Food Sci Hum Wellness. (2020) 9:289–94. doi: 10.1016/j.fshw.2020.04.008
25. Wang G, Liu Y, Lu Z, Yang Y, Xia Y, Lai PF, et al. The ameliorative effect of a Lactobacillus strain with good adhesion ability against dextran sulfate sodium-induced murine colitis. Food Funct. (2019) 10:397–409. doi: 10.1039/c8fo01453a
26. Barnes EL, Kappelman MD. Editorial: increasing incidence of pediatric inflammatory bowel disease in France: implications for etiology, diagnosis, prognosis, and treatment. Am J Gastroenterol. (2018) 113:273–5. doi: 10.1038/ajg.2017.431
27. Penagini F, Dilillo D, Borsani B, Cococcioni L, Galli E, Bedogni G, et al. Nutrition in pediatric inflammatory bowel disease: from etiology to treatment. A systematic review. Nutrients. (2016) 8:334. doi: 10.3390/nu8060334
28. Zhang X, Tong Y, Lyu X, Wang J, Wang Y, Yang R. Prevention and alleviation of dextran sulfate sodium Salt-Induced inflammatory bowel disease in mice with Bacillus subtilis-Fermented milk via inhibition of the inflammatory responses and regulation of the intestinal flora. Front Microbiol. (2020) 11:622354. doi: 10.3389/fmicb.2020.622354
29. Zheng C, Chen T, Lu J, Wei K, Tian H, Liu W, et al. Adjuvant treatment and molecular mechanism of probiotic compounds in patients with gastric cancer after gastrectomy. Food Funct. (2021) 12:6294–308. doi: 10.1039/d1fo01375k
30. Wang Z, Hua W, Li C, Chang H, Liu R, Ni Y, et al. Protective role of fecal microbiota transplantation on colitis and Colitis-Associated colon cancer in mice is associated with treg cells. Front Microbiol. (2019) 10:2498. doi: 10.3389/fmicb.2019.02498
31. Arnott C, Punniamoorthy G, Tan J, Sadeghipour S, Bursill C, Patel S. The vascular endothelial growth factor inhibitors ranibizumab and aflibercept markedly increase expression of Atherosclerosis-Associated inflammatory mediators on vascular endothelial cells. PLoS One. (2016) 11:e150688. doi: 10.1371/journal.pone.0150688
32. Al-Harbi NO, Imam F, Al-Harbi MM, Ansari MA, Ahmad SF. Dexamethasone attenuates LPS-induced acute lung injury through inhibition of NF-κB, COX-2, and pro-inflammatory mediators. Immunol Invest. (2016) 45:349–69. doi: 10.3109/08820139.2016.1157814
33. Lee H, Liu Z, Yoon CS, Dong L, Ko W, Woo ER, et al. Anti-Neuroinflammatory and Anti-Inflammatory activities of Phenylheptatriyne Isolated from the flowers of Coreopsis lanceolata L. Via NF-kappaB inhibition and HO-1 expression in BV2 and RAW264.7 cells. Int J Mol Sci. (2021) 22:7482. doi: 10.3390/ijms22147482
34. Tang X, Huang G, Zhang T, Li S. Elucidation of colon-protective efficacy of diosgenin in experimental TNBS-induced colitis: inhibition of NF-κB/IkB-α and Bax/Caspase-1 signaling pathways. Biosci Biotech Bioch. (2020) 84:1903–12. doi: 10.1080/09168451.2020.1776590
35. Mcdaniel DK, Eden K, Ringel VM, Allen IC. Emerging roles for noncanonical NF-κB signaling in the modulation of inflammatory bowel disease pathobiology. Inflamm Bowel Dis. (2016) 22:2265–79. doi: 10.1097/MIB.0000000000000858
36. Ruan H, Huang Q, Wan B, Yang M. Curcumin alleviates lipopolysaccharides-induced inflammation and apoptosis in vascular smooth muscle cells via inhibition of the NF-κB and JNK signaling pathways. Inflammopharmacology. (2022) 30:517–25. doi: 10.1007/s10787-021-00912-w
37. Stjärngrim J, Ekbom A, Hammar U, Hultcrantz R, Forsberg AM. Rates and characteristics of postcolonoscopy colorectal cancer in the Swedish IBD population: what are the differences from a non-IBD population? Gut. (2018) 68:1588–96. doi: 10.1136/gutjnl-2018-316651
38. Puccini A, Battaglin F, Lenz HJ. Management of advanced small bowel cancer. Curr Treat Options Oncol. (2018) 19:69. doi: 10.1007/s11864-018-0592-3
39. Farinetti A, Zurlo V, Manenti A, Coppi F, Mattioli AV. Mediterranean diet and colorectal cancer: a systematic review. Nutrition. (2017) 43-44:83–8. doi: 10.1016/j.nut.2017.06.008
40. Yu Y, Raka F, Adeli K. The role of the gut microbiota in lipid and lipoprotein metabolism. J Clin Med. (2019) 8:2227. doi: 10.3390/jcm8122227
41. Manaer T, Yu L, Nabi XH, Dilidaxi D, Liu L, Sailike J. The beneficial effects of the composite probiotics from camel milk on glucose and lipid metabolism, liver and renal function and gut microbiota in db/db mice. BMC Complement Med Ther. (2021) 21:127. doi: 10.1186/s12906-021-03303-4
42. Brandão LR, de Brito Alves JL, da Costa WKA, Ferreira GAH, de Oliveira MP, Gomes da Cruz A, et al. Live and ultrasound-inactivated Lacticaseibacillus casei modulate the intestinal microbiota and improve biochemical and cardiovascular parameters in male rats fed a high-fat diet. Food Funct. (2021) 12:5287–300. doi: 10.1039/d1fo01064f
43. Dong F, Xiao F, Li X, Li Y, Wang Y. Pediococcus pentosaceus CECT 8330 protects DSS-induced colitis and regulates the intestinal microbiota and immune responses in mice. J Transl Med. (2022) 20:33. doi: 10.1186/s12967-022-03235-8
44. Gibiino G, Siena MD, Sbrancia M, Binda C, Fabbri C. Dietary habits and gut microbiota in healthy adults: focusing on the right diet. A systematic review. Int J Mol Sci. (2021) 22:6728. doi: 10.3390/ijms22136728
45. Abdelhamid AS, Brown TJ, Brainard JS, Biswas P, Thorpe GC, Moore HJ, et al. Omega-3 fatty acids for the primary and secondary prevention of cardiovascular disease. Cochrane Database Syst Rev. (2018) 7:CD003177. doi: 10.1002/14651858.CD003177.pub3
46. Calder PC. Omega-3 fatty acids and inflammatory processes: from molecules to man. Biochem Soc Trans. (2017) 45:1105–15. doi: 10.1042/BST20160474
47. Zhou Y, Khan H, Xiao J, Cheang WS. Effects of arachidonic acid metabolites on cardiovascular health and disease. Int J Mol Sci. (2021) 22:12029. doi: 10.3390/ijms222112029
48. Park C, Choi JE, Jin Y, Park Y. Eicosapentaenoic acid and docosahexaenoic acid, but not α-linolenic acid, decreased LDL-cholesterol synergistically with estrogen via regulation of cholesterol synthesis and clearance in ovariectomized rats. Nutr Res. (2019) 66:13–21. doi: 10.1016/j.nutres.2019.03.003
49. Saidi H, Murtaza B, Khan AS, Koceir EA, Hichami A, Khan NA. DHA induces Jurkat T-cell arrest in G2/M phase of cell cycle and modulates the plasma membrane expression of TRPC3/6 channels. Biochimie. (2021) 181:169–75. doi: 10.1016/j.biochi.2020.12.005
50. Chorner Z, Barbeau PA, Castellani L, Wright DC, Chabowski A, Holloway GP. Dietary alpha-linolenic acid supplementation alters skeletal muscle plasma membrane lipid composition, sarcolemmal FAT/CD36 abundance, and palmitate transport rates. Am J Physiol Regul Integr Comp Physiol. (2016) 311:R1234–42. doi: 10.1152/ajpregu.00346.2016
51. Mazzocchi A, De Cosmi V, Rise P, Milani GP, Turolo S, Syren ML, et al. Bioactive compounds in edible oils and their role in oxidative stress and inflammation. Front Physiol. (2021) 12:659551. doi: 10.3389/fphys.2021.659551
52. Saika A, Nagatake T, Kunisawa J. Host- and Microbe-Dependent dietary lipid metabolism in the control of allergy, inflammation, and immunity. Front Nutr. (2019) 6:36. doi: 10.3389/fnut.2019.00036
53. Kleemann R. Krill oil treatment increases distinct PUFAs and oxylipins in adipose tissue and liver and attenuates Obesity-Associated inflammation via direct and indirect mechanisms. Nutrients. (2021) 13:2836. doi: 10.3390/nu13082836
54. De Bus I, Zuilhof H, Witkamp R, Balvers M, Albada B. Novel COX-2 products of n-3 polyunsaturated fatty acid-ethanolamine-conjugates identified in RAW264.7 macrophages. J Lipid Res. (2019) 60:1829–40. doi: 10.1194/jlr.M094235
55. Zhao S, Zhong J, Sun C, Zhang J. Effects of aerobic exercise on TC, HDL-C, LDL-C and TG in patients with hyperlipidemia: a protocol of systematic review and meta-analysis. Medicine. (2021) 100:e25103. doi: 10.1097/MD.0000000000025103
56. Kattoor AJ, Kanuri SH, Mehta JL. Role of Ox-LDL and LOX-1 in atherogenesis. Curr Med Chem. (2019) 26:1693–700. doi: 10.2174/0929867325666180508100950
57. Zhang J, Yang P, Wang H, Huang Q, Liu Z. N-3 PUFAs inhibited hepatic ER stress induced by feeding of a high-saturated fat diet accompanied by the expression LOX-1. J Nutr Biochem. (2020) 88:108481. doi: 10.1016/j.jnutbio.2020.108481
58. Wang HH, Garruti G, Liu M, Portincasa P, Wang DQ. Cholesterol and lipoprotein metabolism and atherosclerosis: recent advances in reverse cholesterol transport. Ann Hepatol. (2017) 16:s27–42. doi: 10.5604/01.3001.0010.5495
59. Labzin LI, Kono H, Seki R, Schmidt SV, Beyer M. High-density lipoprotein mediates anti-inflammatory reprogramming of macrophages via the transcriptional regulator ATF3. Nat Immunol. (2014) 15:152–60. doi: 10.1038/ni.2784
60. Pirillo A, Norata GD, Catapano AL. LOX-1, OxLDL, and atherosclerosis. Mediators Inflamm. (2013) 2013:152786. doi: 10.1155/2013/152786
61. Ghezzi P, Floridi L, Boraschi D, Cuadrado A, Manda G, Levic S, et al. Oxidative stress and inflammation induced by environmental and psychological stressors: a biomarker perspective. Antioxid Redox Signal. (2018) 28:852–72. doi: 10.1089/ars.2017.7147
62. Mihaylova MM, Cheng CW, Cao AQ, Tripathi S, Mana MD, Bauer-Rowe KE, et al. Fasting activates fatty acid oxidation to enhance intestinal stem cell function during homeostasis and aging. Cell Stem Cell. (2018) 22:769–78. doi: 10.1016/j.stem.2018.04.001
63. Barrera G, Pizzimenti S, Daga M, Dianzani C, Arcaro A, Cetrangolo GP, et al. Lipid Peroxidation-Derived aldehydes, 4-Hydroxynonenal and malondialdehyde in Aging-Related disorders. Antioxidants (Basel). (2018) 7:102. doi: 10.3390/antiox7080102
64. Xie M, Yang J, Zhang J, Sherman HL, Zhang G. Effects of linoleic Acid-Rich diet on plasma profiles of eicosanoids and development of colitis in il-10-/- mice. J Agr Food Chem. (2020) 68:7641–7. doi: 10.1021/acs.jafc.0c03024
65. Lei L, Zhang J, Decker EA, Zhang G. Roles of lipid Peroxidation-Derived electrophiles in pathogenesis of colonic inflammation and colon cancer. Front Cell Dev Biol. (2021) 9:665591. doi: 10.3389/fcell.2021.665591
66. Lei L, Yang J, Zhang J, Zhang G. The lipid peroxidation product EKODE exacerbates colonic inflammation and colon tumor genesis. Redox Biol. (2021) 42:101880. doi: 10.1016/j.redox.2021.101880
67. Siracusa F, Schaltenberg N, Villablanca EJ, Huber S, Gagliani N. Dietary habits and intestinal immunity: from food intake to CD4+ TH cells. Front Immunol. (2019) 9:3177. doi: 10.3389/fimmu.2018.03177
68. Moravejolahkami AR, Kermani M, Zehi ZB, Mirenayat S, Mansourian M. The effect of probiotics on lipid profile & anthropometric indices in diabetic nephropathy: a systematic review and meta-analysis of clinical trials. J Diabetes Metab Disord. (2021) 20:893–904.
69. Silva ATDA, Cavalcanti IDL, Fernandes MADL, Coimbra CGDO, Lima GMDS. Effect of Zymomonas mobilis probiotic on cholesterol and its lipoprotein fractions and the intestinal regulation. Clin Nutr. (2020) 39:3750–5. doi: 10.1016/j.clnu.2020.04.002
70. Wang GQ, Huang WL, Xia YJ, Xiong ZQ, Ai LZ. Cholesterol-lowering potentials of Lactobacillus strain overexpression of bile salt hydrolase on high cholesterol diet-induced hypercholesterolemic mice. Food Funct. (2019) 10:1684–95. doi: 10.1039/c8fo02181c
71. Luo J, Yang H, Song BL. Mechanisms and regulation of cholesterol homeostasis. Nat Rev Mol Cell Bio. (2020) 21:225–45. doi: 10.1038/s41580-019-0190-7
72. Wang G, Chen X, Wang L, Zhao L, Xia Y, Ai L. Diverse conditions contribute to the cholesterol-lowering ability of different Lactobacillus plantarum strains. Food Funct. (2021) 12:1079–86. doi: 10.1039/D0FO02073G
73. Jiang J, Wu C, Zhang C, Zhang Q, Yu L, Jx Z, et al. Strain-specific effects of Bifidobacterium longum on hypercholesterolemic rats and potential mechanisms. Int J Mol Sci. (2021) 22:1305. doi: 10.3390/ijms22031305
74. Alaqil AA, Abbas AO, El-Beltagi HS, El-Atty H, Moustafa ES. Dietary supplementation of probiotic Lactobacillus acidophilus modulates cholesterol levels, immune response, and productive performance of laying hens. Animals. (2020) 10:1588. doi: 10.3390/ani10091588
75. Pfrieger FW. Neurodegenerative diseases and cholesterol: seeing the field through the players. Front Aging Neurosci. (2021) 13:766587. doi: 10.3389/fnagi.2021.766587
76. Dai L, Zou L, Meng L, Qiang G, Yan M, Zhang Z. Cholesterol metabolism in neurodegenerative diseases: molecular mechanisms and therapeutic targets. Mol Neurobiol. (2021) 58:2183–201. doi: 10.1007/s12035-020-02232-6
77. Kovac U, Skubic C, Bohinc L, Rozman D, Rezen T. Oxysterols and gastrointestinal cancers around the clock. Front Endocrinol (Lausanne). (2019) 10:483. doi: 10.3389/fendo.2019.00483
78. Ridlon JM, Harris SC, Bhowmik S, Kang DJ, Hylemon PB. Consequences of bile salt biotransformations by intestinal bacteria. Gut Microbes. (2016) 7:22–39. doi: 10.1080/19490976.2015.1127483
79. Wijaya A, Hermann A, Abriouel H, Specht I, Yousif NM, Holzapfel WH, et al. Cloning of the bile salt hydrolase (bsh) gene from Enterococcus faecium FAIR-E 345 and chromosomal location of bsh genes in food enterococci. J Food Prot. (2004) 67:2772–8. doi: 10.4315/0362-028x-67.12.2772
80. Singhal N, Singh NS, Mohanty S, Kumar M, Virdi JS. Rhizospheric Lactobacillus plantarum (Lactiplantibacillus plantarum) strains exhibit bile salt hydrolysis, hypocholestrolemic and probiotic capabilities in vitro. Sci Rep-Uk. (2021) 11:15288. doi: 10.1038/s41598-021-94776-3
81. Huang W, Wang G, Xia Y, Xiong Z, Ai L. Bile salt hydrolase-overexpressing Lactobacillus strains can improve hepatic lipid accumulation in vitro in an NAFLD cell model. Food Nutr Res. (2020) 64:375. doi: 10.29219/fnr.v64.3751
82. Kim J, Yun JM, Mi KK, Kwon O, Cho B. Lactobacillus gasseri BNR17 supplementation reduces the visceral fat accumulation and waist circumference in obese adults: a randomized, Double-Blind, Placebo-Controlled trial. J Med Food. (2018) 21:454–61. doi: 10.1089/jmf.2017.3937
83. Hamad EM, Sato M, Uzu K, Yoshida T, Higashi S, Kawakami H, et al. Milk fermented by Lactobacillus gasseri SBT2055 influences adipocyte size via inhibition of dietary fat absorption in Zucker rats. Br J Nutr. (2009) 101:716–24. doi: 10.1017/S0007114508043808
84. Hu S, Kuwabara R, Haan B, Smink AM, Vos PD. Acetate and butyrate improve β-cell metabolism and mitochondrial respiration under oxidative stress. Int J Mol Sci. (2020) 24:1542. doi: 10.3390/ijms21041542
85. Kusumo PD, Maulahela H, Utari AP, Surono IS, Soebandrio A, Abdullah M. Probiotic Lactobacillus plantarum IS 10506 supplementation increase SCFA of women with functional constipation. Iran J Microbiol. (2019) 11:389–96.
86. Lu N, Li M, Lei H, Jiang X, Tu W, Lu Y, et al. Butyric acid regulates progesterone and estradiol secretion via cAMP signaling pathway in porcine granulosa cells. J Steroid Biochem Mol Biol. (2017) 172:89–97. doi: 10.1016/j.jsbmb.2017.06.004
87. Zhao Y, Chen F, Wu W, Sun M, Bilotta AJ, Yao S, et al. GPR43 mediates microbiota metabolite SCFA regulation of antimicrobial peptide expression in intestinal epithelial cells via activation of mTOR and STAT3. Mucosal Immunol. (2018) 11:752–62. doi: 10.1038/mi.2017.118
88. Sun MM, Wu W, Liu ZJ, Cong Y. Microbiota metabolite short chain fatty acids, GPCR, and inflammatory bowel diseases. J Gastroenterol. (2016) 52:1–8. doi: 10.1007/s00535-016-1242-9
89. Zhang Z, Fan S, Huang D, Xiong T, Nie S, Xie M. Polysaccharides from fermented Asparagus officinalis with Lactobacillus plantarum NCU116 alleviated liver injury via modulation of glutathione homeostasis, bile acid metabolism, and SCFA production. Food Funct. (2020) 11:7681–95. doi: 10.1039/d0fo01435d
90. Zhou Q, Gu R, Xue B, Li P, Gu Q. Phenyl lactic acid alleviates Samonella Typhimurium-induced colitis via regulating microbiota composition, SCFA production and inflammatory responses. Food Funct. (2021) 12:5591–606. doi: 10.1039/d1fo00166c
91. Gabriel FC, Fantuzzi G. The association of short-chain fatty acids and leptin metabolism: a systematic review. Nutr Res. (2019) 72:18–35. doi: 10.1016/j.nutres.2019.08.006
92. Christiansen CB, Gabe M, Svendsen B, Dragsted LO, Rosenkilde MM, Holst JJ. The impact of short-chain fatty acids on GLP-1 and PYY secretion from the isolated perfused rat colon. Am J Physiol Gastrointest Liver Physiol. (2018) 315:G53–65. doi: 10.1152/ajpgi.00346.2017
93. Larraufie P, Martin-Gallausiaux C, Lapaque N, Dore J, Gribble FM, Reimann F, et al. SCFAs strongly stimulate PYY production in human enteroendocrine cells. Sci Rep. (2018) 8:74. doi: 10.1038/s41598-017-18259-0
94. Yonekura S, Senoo T, Kobayashi Y, Yonezawa T, Katoh K, Obara Y. Effects of acetate and butyrate on the expression of leptin and short-form leptin receptor in bovine and rat anterior pituitary cells. Gen Comparat Endocrinol. (2003) 133:165–72. doi: 10.1016/s0016-6480(03)00162-x
95. Zitek M, Celewicz Z, Kikut J, Szczuko M. Implications of SCFAs on the parameters of the lipid and hepatic profile in pregnant women. Nutrients. (2021) 13:1749. doi: 10.3390/nu13061749
96. Han X, Wang Y, Zhang P, Zhu M, Li L, Mao X, et al. Kazak faecal microbiota transplantation induces short-chain fatty acids that promote glucagon-like peptide-1 secretion by regulating gut microbiota in db/db mice. Pharm Biol. (2021) 59:1077–87. doi: 10.1080/13880209.2021.1954667
97. Lemas DJ, Young BE, Baker PN, Tomczik AC, Soderborg TK, Hernandez TL, et al. Alterations in human milk leptin and insulin are associated with early changes in the infant intestinal microbiome. Am J Clin Nutr. (2016) 103:1291–300. doi: 10.3945/ajcn.115.126375
98. Wu Y, Sun Y, Zhang Z, Chen J, Dong G. Effects of peptidoglycan, lipoteichoic acid and lipopolysaccharide on inflammation, proliferation and milk fat synthesis in bovine mammary epithelial cells. Toxins. (2020) 12:497. doi: 10.3390/toxins12080497
99. Jiang JW, Chen XH, Ren Z, Zheng SS. Gut microbial dysbiosis associates hepatocellular carcinoma via the gut-liver axis. Hepatob Pancreat Dis. (2019) 4:2570–82. doi: 10.1021/acsabm.0c01560
100. Van Raay T, Allen-Vercoe E. Microbial interactions and interventions in colorectal cancer. Microbiol Spectrum. (2017) 5:99–130. doi: 10.1128/microbiolspec.BAD-0004-2016
101. Jia R, Kurita-Ochiai T, Oguchi S, Yamamoto M. Periodontal pathogen accelerates lipid peroxidation and atherosclerosis. J Dent Res. (2013) 92:247–52. doi: 10.1177/0022034513475625
102. Verhaar B, Prodan A, Nieuwdorp M, Muller M. Gut microbiota in hypertension and atherosclerosis: a review. Nutrients. (2020) 12:2982. doi: 10.3390/nu12102982
103. Shaaban M, Abd EO, Al-Qaidi B, Ashour HM. Antimicrobial and antibiofilm activities of probiotic Lactobacilli on antibiotic-resistant Proteus mirabilis. Microorganisms. (2020) 8:960. doi: 10.3390/microorganisms8060960
104. Yang B, Luo Y, Liu Z, Yang P, Gui Y. Probiotics SOD inhibited food allergy via downregulation of STAT6-TIM4 signaling on DCs. Mol Immunol. (2018) 103:71–7. doi: 10.1016/j.molimm.2018.09.001
Keywords: inflammatory bowel disease, bowel cancer, lipid metabolism, gut microbiota, probiotics
Citation: Wu T, Wang G, Xiong Z, Xia Y, Song X, Zhang H, Wu Y and Ai L (2022) Probiotics Interact With Lipids Metabolism and Affect Gut Health. Front. Nutr. 9:917043. doi: 10.3389/fnut.2022.917043
Received: 10 April 2022; Accepted: 06 May 2022;
Published: 31 May 2022.
Edited by:
Liang Zhao, Beijing Technology and Business University, ChinaReviewed by:
Feng Zhou, China Agricultural University, ChinaGloria Solano-Aguilar, Agricultural Research Service (USDA), United States
Yanhui Han, University of Massachusetts Amherst, United States
Copyright © 2022 Wu, Wang, Xiong, Xia, Song, Zhang, Wu and Ai. This is an open-access article distributed under the terms of the Creative Commons Attribution License (CC BY). The use, distribution or reproduction in other forums is permitted, provided the original author(s) and the copyright owner(s) are credited and that the original publication in this journal is cited, in accordance with accepted academic practice. No use, distribution or reproduction is permitted which does not comply with these terms.
*Correspondence: Lianzhong Ai, YWlsaWFuemhvbmcxQDEyNi5jb20=
†These authors have contributed equally to this work and share first authorship