- Institute of Lipid Metabolism and Atherosclerosis, Innovative Drug Research Centre, School of Pharmacy, Weifang Medical University, Weifang, China
Cordyceps militaris is a parasitic edible fungus and has been used as tonics for centuries. Polysaccharides are a major water-soluble component of C. militaris. Recently, C. militaris-derived polysaccharides have been given much attention due to their various actions including antioxidant, anti-inflammatory, anti-tumor, anti-hyperlipidemic, anti-diabetic, anti-atherosclerotic, and immunomodulatory effects. These bioactivities are determined by the various structural characteristics of polysaccharides including monosaccharide composition, molecular weight, and glycosidic linkage. The widespread use of advanced analytical analysis tools has greatly improved the elucidation of the structural characteristics of C. militaris-derived polysaccharides. However, the methods for polysaccharide structural characterization and the latest findings related to C. militaris-derived polysaccharides, especially the potential structure-activity relationship, have not been well-summarized in recent reviews of the literature. This review will discuss the methods used in the elucidation of the structure of polysaccharides and structural characteristics as well as the signaling pathways modulated by C. militaris-derived polysaccharides. This article provides information useful for the development of C. militaris-derived polysaccharides as well as for investigating other medicinal polysaccharides.
Introduction
Cordyceps species have been used as medicine, tonics, and food for centuries in many countries (1, 2). Approximately 750 Cordyceps species are mainly distributed in Asia, Europe, and North America (1). Cordyceps militaris (Yong Chong Cao, 蛹虫草) is a well-developed Cordyceps species (3, 4). Pharmacological studies have suggested that artificially cultivated C. militaris is useful against many diseases, especially non-communicable diseases (5–7). Some medicinal and tonic products of C. militaris have been developed and commercialized around the world, especially in Asian countries (3, 8). The annual output value of C. militaris-derived products is estimated to be 10 billion RMB in China (9).
The primary metabolite, polysaccharide, is one of the major water-soluble bioactive components of C. militaris (3, 6). Compared to the secondary metabolites, C. militaris-derived polysaccharides have not been well characterized (1, 2, 4, 10–12). Four years’ ago, Zhang et al. reviewed the extraction, isolation, purification, structural characteristics, and bioactivities of C. militaris-derived polysaccharides (3). The pharmaceutical mechanisms of C. militaris polysaccharides including antioxidant, immunomodulatory, and anti-tumor activities have also been reviewed recently (7). The activity of the polysaccharide is determined by its monosaccharide composition, molecular weight (Mw), glycosidic linkage, and degree of branching. With increasing use of advanced analytical tools, the structural characterization of polysaccharides has improved greatly during the past several years. However, the structural characteristics of these polysaccharides in relation to some bioactivities such as anti-diabetic, and anti-hyperlipidemic, and anti-atherosclerotic effects have not been well summarized. Furthermore, these is a lack of graphic representations that clearly show the reaction processes involved in structural elucidation and the signaling pathways mediated by C. militaris-derived polysaccharides. This is the motivation to review the advances in the methods used for structural elucidation and to further describe the structural characteristics and the signaling pathways that are modulated by C. militaris-derived polysaccharides. In this article, we review the related literature mainly from the year of 2019 to the present that were obtained as search results from PubMed using “C. militaris and polysaccharide” or “mass spectrometry and polysaccharide” as keywords.
Methods for Elucidation of Polysaccharide Structure
Fourier Transform Infrared Spectrometry
Except for chemical methods, Fourier transform infrared (FT-IR) spectrometer is a common and easily available tool to quickly identify polysaccharides. In FT-IR spectrum, a strong U-type band observed at approximately 3,400 cm–1 represents the typical O-H stretching vibration, and the weak bands at around 2,930 cm–1 represent the typical C-H stretching vibration. Furthermore, the strong band presents at approximately 1,050 cm–1 is arisen from the C-O-C glycosidic bond vibration, while the band at around 1,600 and 1,655 cm–1 can be assigned to C = O stretching vibration and the bending vibration of -NH- (-CONH-), respectively (6). The peak at approximately 1,250 cm–1 suggests the presence of sulfate in axial position. Of importance, the bands between 1,000 and 800 cm–1 are useful for determination of α- and β-configurations of glycosyls. For instance, the bands at around 880 cm–1 suggest the potential presence of mannosyl and galactosyl residues in a β-configuration; and the bands at approximately 810 and 850 cm–1 may arise from α-D mannosyl and α-D glucosyl residues, respectively (13–16).
Monosaccharide Composition Determination
To accurately determine monosaccharide composition, a complete acid hydrolysis of polysaccharide is needed. Theoretically, all strong acids can be used to release monosaccharides at a concentration of 2–4 mol/L. Considering the next neutralization step, trifluoroacetic acid (TFA) with a good volatility is the most popular acid because it can be easily removed by a rotary evaporator (17, 18). In brief, approximately 10 mg of polysaccharide dissolved in 1–2 mL of 2.0 mol/L of TFA is put into a 5 mL ampule container, which is sealed and maintained at 110°C for 6 h. Next, neutral monosaccharides can be detected by gas chromatography (GC) after the monosaccharides are converted into acetylated aldononitrile derivatives, however, acidic monosaccharides need to be converted into their corresponding alditols by an appropriate reductant, such as sodium borohydride, before chemical derivatization (14). Compared to GC, high-performance liquid chromatography (HPLC) is more popular because this method can detect all the reducing monosaccharides (neutral, acidic, and basic) after monosaccharide derivatization with 1-phenyl-3-methyl-5-pyrazolone (PMP) (Figure 1A). Moreover, HPLC combined with PMP derivatization can detect more than ten kinds of monosaccharides with a high sensitivity and resolution in a single run (16, 19). Ion chromatography is another method for determining monosaccharide composition without any kind of derivatization. However, some natural monosaccharides, such as galactose/N-acetyl glucosamine or mannose/xylose, have comparably bad resolution on ion chromatography (16). Recently, an HPLC-tandem mass spectrometry (HPLC-MS/MS) method was developed for simultaneous detection of 17 monosaccharides including aldoses, ketoses, amino sugars, and uronic acids, in the multiple reaction monitoring mode after aldononitrile acetate derivatization (20). Additionally, GC-mass spectrometry (GC-MS) can be used for determination of the absolute configuration (D or L) of monosaccharides (21).
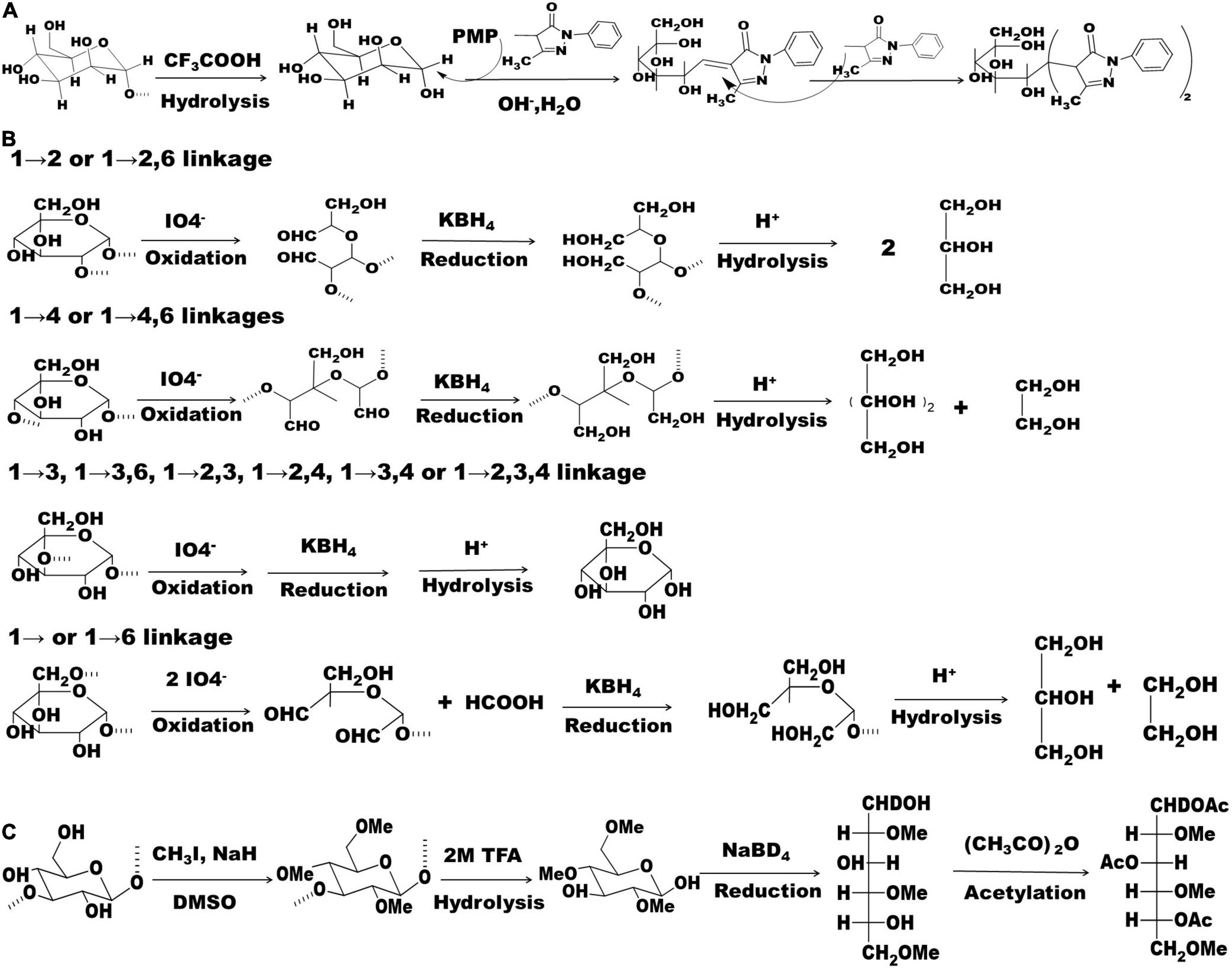
Figure 1. Chemical reactions related to PMP derivatization, periodate oxidation-smith degradation, and methylation. (A) Polysaccharide hydrolysis and monosaccharide derivatization with PMP; (B) chemical reactions of periodate oxidation-Smith degradation; (C) chemical reactions that involved in polysaccharide methylation. PMP: 1-phenyl-3-methyl-5-pyrazolone.
Glycosyl Linkage Determination
Periodate oxidation-Smith degradation is a traditional method used to assess glycosidic linkages (8, 22). Glycosyls with distinct linkages may produce different reaction products (Figure 1B), which can be analyzed by thin layer chromatography and GC. However, it is impossible to assign glycosidic linkage of a polysaccharide that is composed of more than one type of monosaccharide (13). Therefore, this method has been gradually replaced by methylation and nuclear magnetic resonance (NMR) analysis.
Methylation Analysis
Methylation analysis is a classical and putative method used to determine glycosidic linkages (Figure 1C). In brief, approximately 2–3 mg of polysaccharide is needed to perform this assay. During the reaction process, anhydrous conditions and protection with nitrogen are strongly suggested. FT-IR is generally used to evaluate the completeness of methylation. In an FT-IR spectrum, the absence of the U-type peak at approximately 3,400 cm–1 and the significant increase of C-H stretching variation at around 2,930 cm–1 indicate a complete methylation reaction (15, 16). Next, permethylated polysaccharide is completely hydrolyzed as mentioned in the monosaccharide composition analysis above. The resulting hydrolysates are reduced with sodium borodeuteride (NaBD4), and then acetylated with acetic anhydride. To keep in alignment with the database, use of sodium borohydride (NaBH4) as a reductant is not encouraged. The final methylated alditol acetates are generally analyzed using GC-MS (16). The open accessed Complex Carbohydrate Structure Database created by the Complex Carbohydrate Research Center of the University of Geogia1 is generally used to explain the GC-MS data, thereby determining glycosidic linkages. A comparison of the total amount of branched glycosyls with the total amount of glycosyls that derived from the non-reducing end is useful in evaluating the correctness of the methylation analysis. However, polysaccharides with substitutions, such as O-methyl, are not considered in this type of comparison (16). Recently, an ultra-HPLC-MS/MS method was developed for the rapid determination of glycoside linkages of polysaccharide and oligosaccharide in a multiple reaction monitoring mode. The permethylated samples are hydrolyzed and derivatized with PMP before analysis and the resulting linkage profiles can be determined and quantified by the library containing 22 kinds of glycosidic linkages that are built using oligosaccharide standards (23).
Nuclear Magnetic Resonance Analysis
NMR spectroscopy is another powerful tool for determination of glycosidic linkages of carbohydrate. One-dimensional (1D-) (1 H-, and 13C-NMR) and two-dimensional (2D-) NMR experiments, including distortionless enhancement by polarization transfer spectroscopy (DEPT), 1H-1H correlated spectroscopy (COSY), 1H-13C heteronuclear multiple quantum coherence spectroscopy (HMQC), 1H-13C heteronuclear multiple bond correlation spectroscopy (HMBC), total correlation spectroscopy (TOCSY), and nuclear overhauser effect spectroscopy (NOESY), are usually performed for accurately determining glycosidic linkages. In the 1D-NMR spectra, the anomeric signals always display in down-field regions, which could provide useful information for determining distinct glycosyls and even for quantitative analysis of the various glycosyls. However, the rest signals (H2-H6 or C2-C5) of heteropolysaccharides usually overlap with each other in the 1H- and 13C-NMR spectra, which makes it hard for a correct assignment. 2D-NMR experiments play key roles in structural elucidation of polysaccharides. 1H-1H COSY or TOCSY can give valuable information on the associated protons within a sugar ring. In general, the correlation between H1-H3 is easily identified even in the 1H-1H COSY spectrum of a heteropolysaccharide. However, it is difficult to accurately assign the correlation between H3-H6 due to the large degree of overlap of these proton signals in the 1H-NMR spectrum. An HMQC experiment is valuable in determining the direct correlation between protons and carbons, such as H1/C1 or H3/C3. In comparison with 13C-NMR spectrum, DEPT spectrum can provide useful information of O-6 substituted signals, which show inverted peaks at approximately 66 ppm. The long-range couplings (within 3 bonds) between carbon and proton signals in the HMBC spectrum are useful in making up the missing correlations within a sugar ring and even those between the connective glycosyls. Furthermore, NOESY and TOCSY provide complementary information based on bond connectivity. The solvent and relaxation rates of the protons can significantly influence the sensitivity of these correlation signals (24). Collectively, HMBC, NOESY, and TOCSY spectra are important in the construction of glycosyl connections.
Given the complex linkages of glycosyls and heavy overlap of NMR signals, it is virtually impossible to assign all the NMR signals for most of the polysaccharides. However, some NMR signals can be assigned in combination with methylation analysis and using the available literature. Furthermore, NMR data are useful for determining the α- and β-configurations of glycosyls based on carbon-proton spin-coupling constants (25). As for the D-type pyranosyls in the 4C1 conformation, a 1JC1,H1 at ∼170 Hz indicates an α-anomeric sugar configuration, and a 1JC1,H1 at ∼160 Hz suggests a β-anomeric sugar configuration (16). Alternatively, anomeric proton signals in the region of 5.60–4.90 and 4.90–4.30 ppm may be assigned as α- and β-anomers, respectively. It seems that anomeric protons of galactosyl in a β-D configuration does not match the latter rules. However, anomeric carbons in galactosyls in the β-D configuration always show a downfield chemical shift in 13C-NMR spectrum (greater than 104 ppm in general), which is useful to determine the α- and β-configuration of these galactosyls (15, 16). Of importance, an accurate monosaccharide composition analysis is the basis for assignment of glycosyl linkage patterns.
The NMR signals of homopolysaccharides with simple glycosyl linkages are easily assigned. For instance, the structural characteristics of the C. militaris-derived β-D-(1→6)-glucan and the glucan mainly consisted of →4)-α-D-Glcp (1→ glycosyls are elucidated by our lab (14, 15). Furthermore, glycosyls in a specific configuration and linkage pattern usually show similar chemical shifts in NMR spectra. For example, the galactosyls in the β-D-configuration may present as the side chains in C. militaris-derived polysaccharides in the form of →2)-β-D-Galf (1→ and/or β-D-Galf (1→ glycosyls, whose anomeric carbon generally present at approximately 106 ppm in the 13C-NMR spectrum (15). The anomeric signals of →2,6)-α-D-Manp (1→, α-D-Manp (1→, and →6)-α-D-Manp (1→ glycosyls are generally present at approximately 100.5, 102.0, and 98.0 ppm, respectively. Furthermore, O-methyl is also found in C. militaris-derived polysaccharides, and this kind of methyl shows a chemical shift at approximately 54.0 ppm in 13C-NMR spectra (15, 26). The new magnets beyond 1 GHz have greatly enhanced the sensitivity and resolution of the NMR signals, and the 13 C-, 15 N-, and 19F-labeling strategies can further improve the sensitivity and resolution (27). Solid-state NMR spectroscopy in combination with magic angle spinning techniques can provide detailed signals in a non-destructive manner. This method has also been used to determine the structure of polysaccharides in different forms and even in plant and cell walls (28–31). The application of solid-state NMR has been reviewed recently by different teams (32–34).
Mass Spectrometry
Hydrolysis is helpful in elucidating the structure of polysaccharide (Figure 2). Partial hydrolysis with TFA at a concentration of < 0.1 M (such as 0.05 M) is generally used to remove degradable side chains of polysaccharides (35). The released oligosaccharides and the resistant backbone can be further analyzed by methylation and NMR experiments (26). Data obtained before and after partial hydrolysis are usually combined to elucidate the fine structure of a polysaccharide. Enzymatic hydrolysis is another valuable method for determining glycosidic linkages in polysaccharides. Based on enzymatic digestion (such as, α-amylase, β-glucanase, arabinanase, xylanase, galactanase, and pectinase), →4)-α-D-Glcp (1→, →4)-β-D-GlcAp (1→, and →4)-α-D-Galp (1→ glycosyls are found to exist in wild and cultured C. militaris and other species of Cordyceps (17, 18, 36). The saccharide mapping profiles obtained in combination with high performance thin layer chromatography is valuable in distinguishing polysaccharides from different species of Cordyceps (17, 36). Of importance, the hydrolyzed products such as oligosaccharides and low Mw polysaccharides can be further analyzed by mass spectrometry (MS). As reviewed previously, matrix-assisted laser desorption/ionization (MALDI)-MS and HPLC-MS/MS with a high sensitivity are utilized for the structural elucidation and quantification of oligosaccharides and polysaccharides with a low Mw (17, 18, 37).
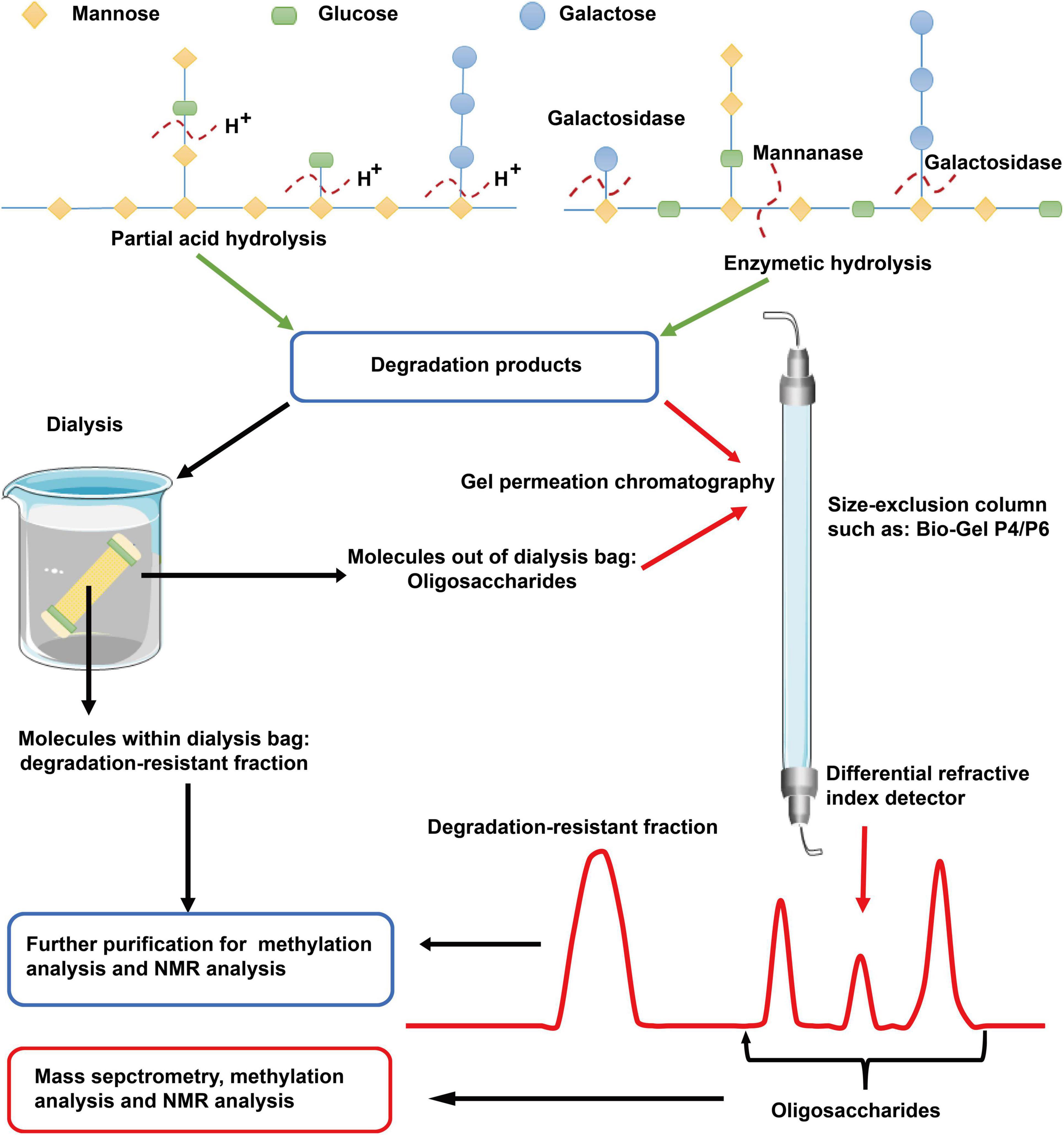
Figure 2. Scheme for elucidation of polysaccharide structure based on degradation. Partial acid hydrolysis with low concentrations of TFA is generally used to remove degradable side chains of polysaccharides. Enzymatic hydrolysis with glycosidase is a more specific method for degradation of glycosyls. The released oligosaccharides and the degradation-resistant fraction can be separated by dialysis using dialysis bags with Mw cutoff < 5.0 kDa or size-exclusion columns such as Bio-Gel P4 or P6. The obtained products can be further analyzed by mass spectrometry, methylation analysis, and NMR experiments. TFA, trifluoroacetic acid; NMR, nuclear magnetic resonance.
The saccharide analysis can be carried out using electrospray ionization MS (ESI-MS) and MALDI-time of flight (MALDI-TOF) MS. Collision-induced dissociation is widely applied for detection of oligosaccharide (38). The ion fragments are informative for explaining the glycosyl linkages of carbohydrates, and the systematic nomenclature rules have been previously documented by Domon and Costello (39). Of note, monosaccharide loss, migration, and rearrangement, during MS analysis is common due to the weak glycosidic bonds. Permethylation is an efficient method to improve the stability of glycans. Hydrophilic interaction chromatography coupled with mass spectrometric detection (HILIC-MS) is also used to sequence carbohydrates (40, 41). Moreover, HPLC-MS was recently used to detect the metabolites of polysaccharides as well as their interaction with proteins (42). Ionization efficiency, instability, and limited sensitivity of molecules with low Mw are the major factors that influence the application of MALDI-TOF mass spectrometry for the analysis of carbohydrates. The compound, 2-hydrazinoquinoline, can react with the reducing end of carbohydrates with high stability and efficiency. This derivatization method can effectively improve the problems of MALDI-TOF mass spectrometry as mentioned above (43). Mass spectrometry imaging is a novel technique used for investigating the distribution of metabolites in plant tissues. For instance, MALDI-TOF MS imaging has been used to reveal the disaccharide distribution in onion bulb tissues and the chemical components of C. sinensis (44, 45). Of note, microarray in combination with MALDI-TOF mass spectrometry was recently developed for the detection of glycosphingolipid glycans (46).
Structural Characteristics of Cordyceps militaris-Derived Polysaccharides
The methods used for cultivation of C. militaris are shown in Figure 3. C. militaris-derived polysaccharides can be obtained from mycelia fermentation (mycelia and fermentation broth) and cultivated fruiting bodies. Fermentation broth can be used to obtain extracellular polysaccharide (exopolysaccharide), while mycelia and fruiting bodies of C. militaris are used to extract intracellular polysaccharide (endo-polysaccharide). The isolation and purification processes for preparation of C. militaris-derived polysaccharides are summarized in Figure 4. Generally, only polysaccharides with high purity are used for the analysis of structural characteristics. Most of the polysaccharides derived from C. militaris are consisted of glucose (Glc), galactose (Gal), and mannose (Man) (3). However, polysaccharides containing other monosaccharides, such as rhamnose, arabinose (Ara), xylose (Xyl), ribose, fucose, galacturonic acid (GalA), glucuronic acid (GlcA), and N-acetyl galactosamine, can also be isolated from C. militaris (3, 18).
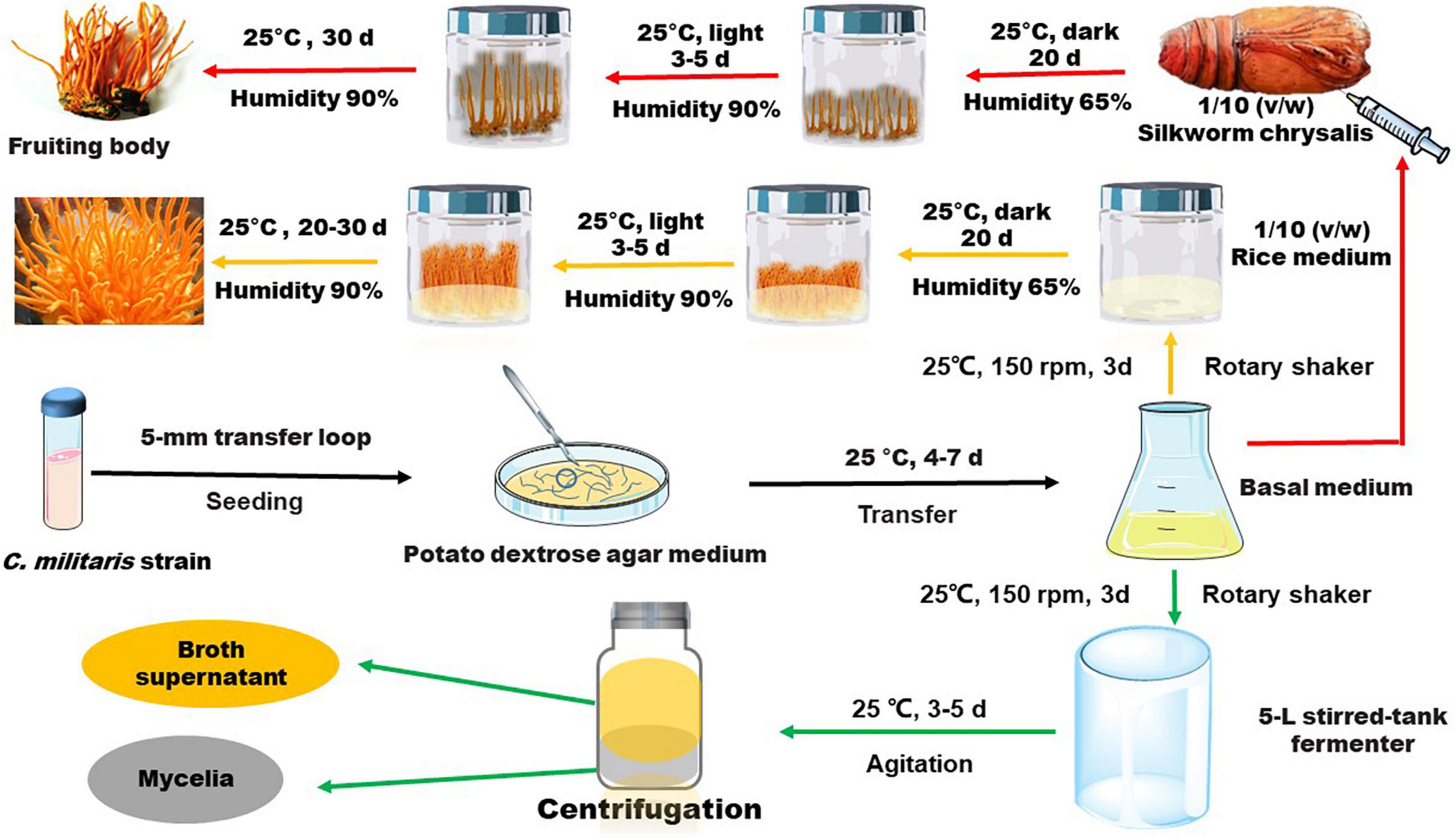
Figure 3. General methods used for fermentation and cultivation of C. militaris. The strain is first cultivated in dishes or test tubes with potato dextrose agar medium, and then it is transferred to liquid medium for a further cultivation. Several days later, the obtained liquid medium is used for an amplified fermentation to obtain mycelia and supernatant through centrifugation. Alternatively, the strain in liquid medium is seeded into solid media (such as rice medium) or be injected into silkworm chrysalis for further incubation to obtain C. militaris fruiting bodies.
Mycelia Fermentation-Derived Polysaccharides
The general fermentation processes are summarized in Figure 3. The fermentation conditions and the proposed mechanisms for biosynthesis of exopolysaccharide in Cordyceps have been reviewed recently by Yang et al. (47). Repeated batch cultivation is reported to enhance exopolysaccharide production of C. militaris (48). UV light irradiation-induced mutagenesis is found to improve production of extracellular and intracellular polysaccharides by more than 120-fold (49, 50). Ionic conditions and pH of the culture media also have important effects on the structural characteristics of polysaccharides. Addition of metal ions is found to influence the production of exopolysaccharides, such as Mg(2+) and Mn(2+) can improve while Ca(2+) and K(+) may reduce the yield (51, 52). Addition of Na2SO4 may induce the presence of sulfate in polysaccharides obtained via fermentation (53). Furthermore, addition of metal ions in the culture medium may result in metal ion-enriched polysaccharides. For instance, addition of FeSO4 solution can promote formation of polysaccharide-iron (III) complexes containing 2.73% of iron (54). The pH of the culture medium is reported to influence gene expression of mycelia, thereby modulating structural characteristics of polysaccharides extracted from fermented mycelia (55). Of note, a weak alkaline (pH 8–9) culture medium is reported to increase production of β-(1→6)-glucan (55). Recently, gene engineering strategies have also been applied to modulate production of C. militaris polysaccharides. For instance, the combined overexpression of phosphoglucomutase and UDP-glucose 6-dehydrogenase genes can increase production of exopolysaccharides by 78.13% compared to that of wild-type strain (56). Furthermore, bacteria in sclerotia have been demonstrated to influence mycelium biomass and metabolites of C. militaris (57). A recent study showed that submerged fermentation with talc microparticles can promote polysaccharide production (58).
Based on the literature, we presumed some chemical structures of fermentation-derived C. militaris polysaccharides as shown in Figure 5. Two purified exopolysaccharides have been extracted from C. militaris strain (CICC 14015), and they have a similar Mw of more than 1,000 kDa. However, their monosaccharide composition and glycosidic linkages are completely different (59, 60). The exopolysaccharide obtained from C. militaris strain (CICC 14014) is different from that of CICC 14015. As reported, this heteropolysaccharide with a lower Mw has →4)-α-D-Glcp (1→ and →4,6)-α-D-Glcp (1→ glycosyls as its main chain (61). Another heteropolysaccharide mainly consisting of →2)-α-D-Manp (1→ and →6)-α-D-Manp (1→ glycosyls is obtained from C. militaris strain (KCTC 6064) (62). Most of the endo-polysaccharides obtained from the fermented mycelia are composed of at least three kinds of monosaccharides. Of note, most of them are mainly composed of glucose and have a backbone consisted of →4)-α-D-Glcp (1→ glycosyls (22, 63–65). The backbone of some heteropolysaccharides is found to be consisted of →2)-α-D-Manp (1→ glycosyls (8).
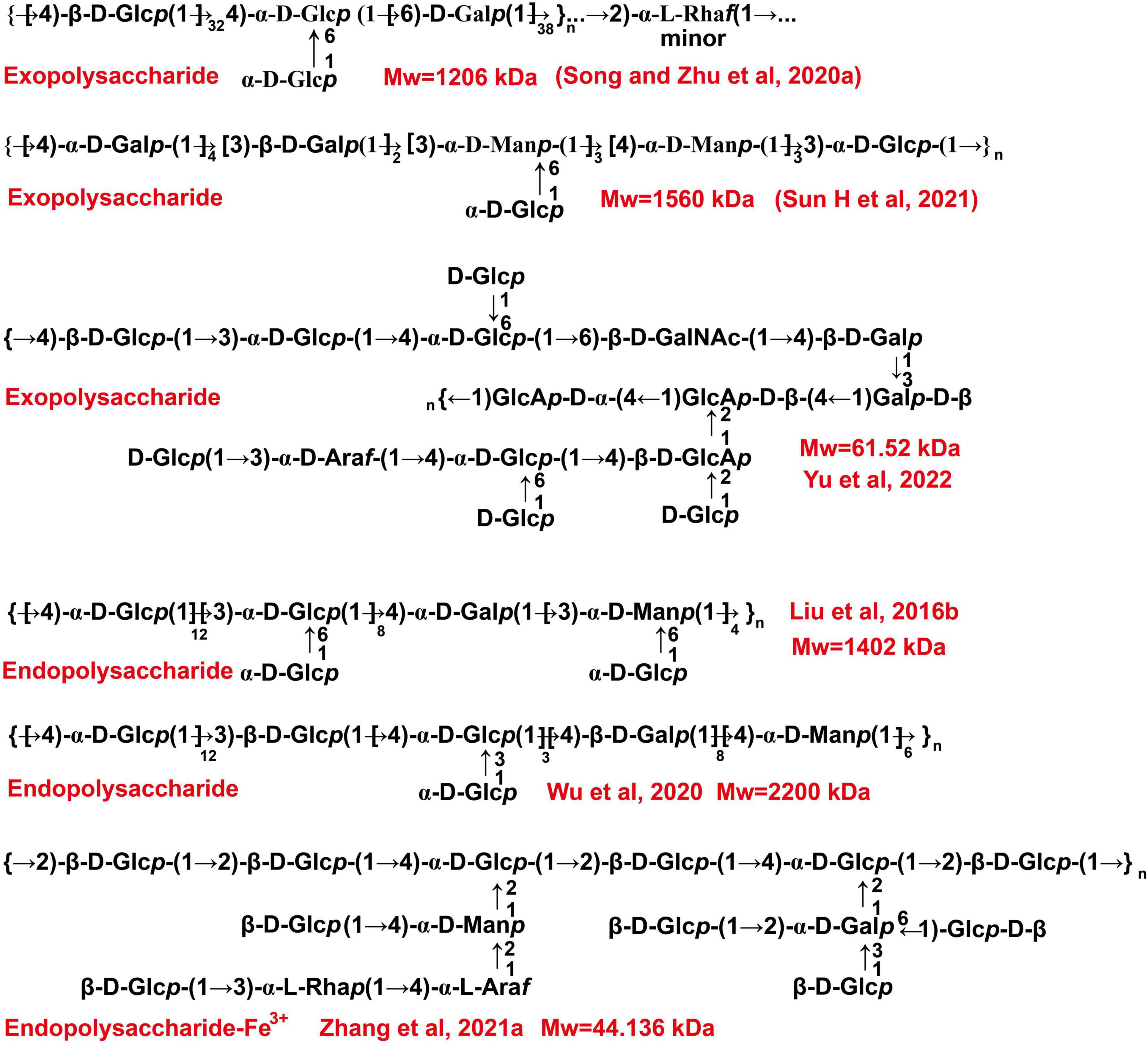
Figure 5. The presumed chemical structures of polysaccharides derived from the fermentation broth of C. militaris.
Structural Characteristics of Polysaccharides Obtained From the Fruiting Body of Cordyceps militaris
The general processes for cultivation of the fruiting body of C. militaris are shown in Figure 3. Many factors, such as light irradiation and intensity, can influence polysaccharide production of the fruiting bodies (50, 66). Spaying biotic elicitors, such as chitosan (1 mg/L), can increase yield of polysaccharide by 1.41-fold (67). Furthermore, culture time also affect the production of polysaccharides. Within 35–45 days, polysaccharide content increases gradually and declines with a prolonged culture time (68). Recently, a comprehensive transcriptomic analysis of C. militaris cultivated on germinated soybeans was carried out by Yoo et al. (69). The gene information obtained from the analysis is useful in modulating the production of metabolites. In this article, we summarize the presumed structures of the C. militaris fruiting body-derived polysaccharides that are provided by the related literature (Figure 6). We also presume some chemical structures of polysaccharides according to the descriptions in the literature as shown in Figure 7.
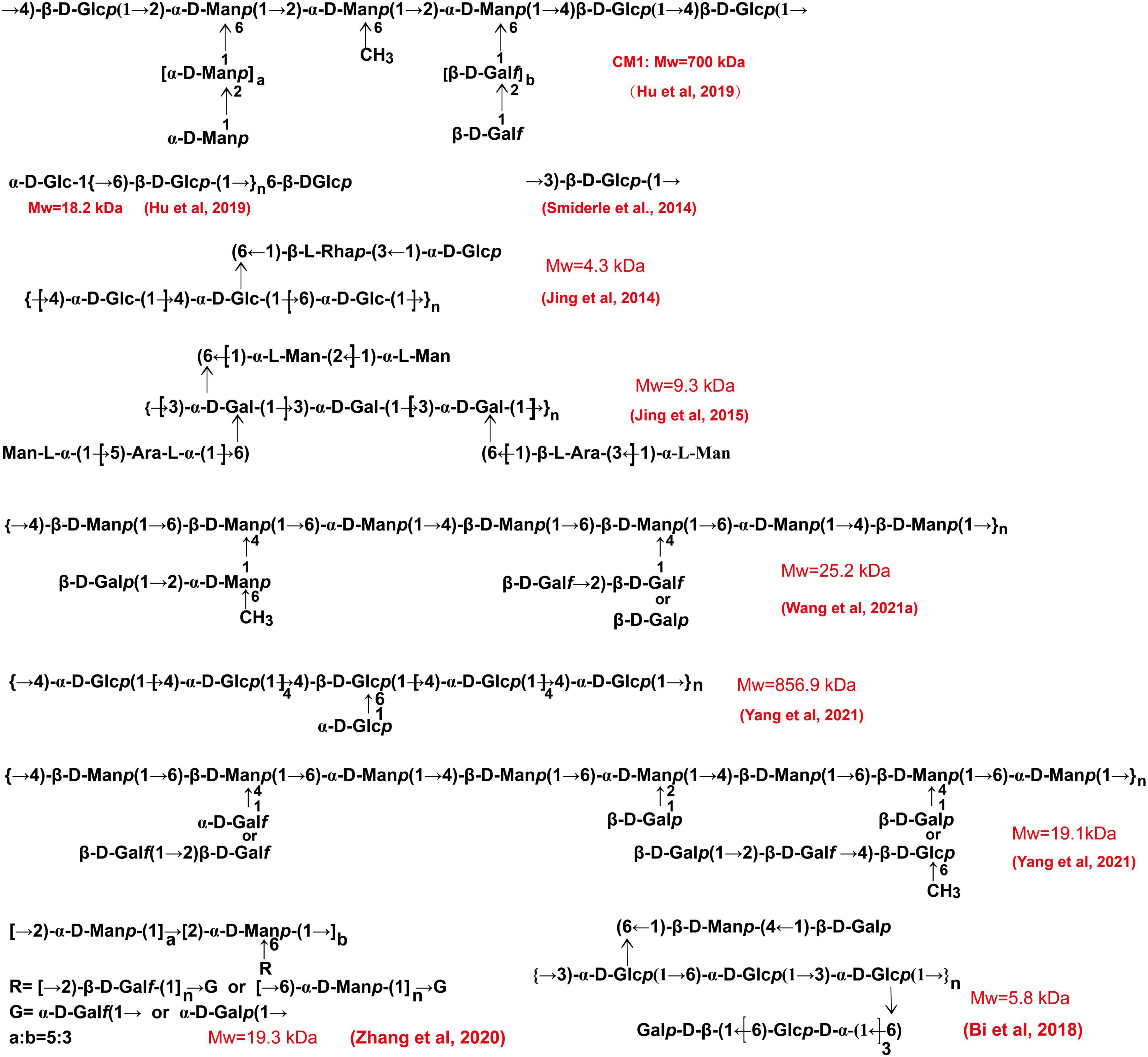
Figure 6. The chemical structures of polysaccharides derived from the fruiting body of C. militaris. These structures are obtained from related literature.
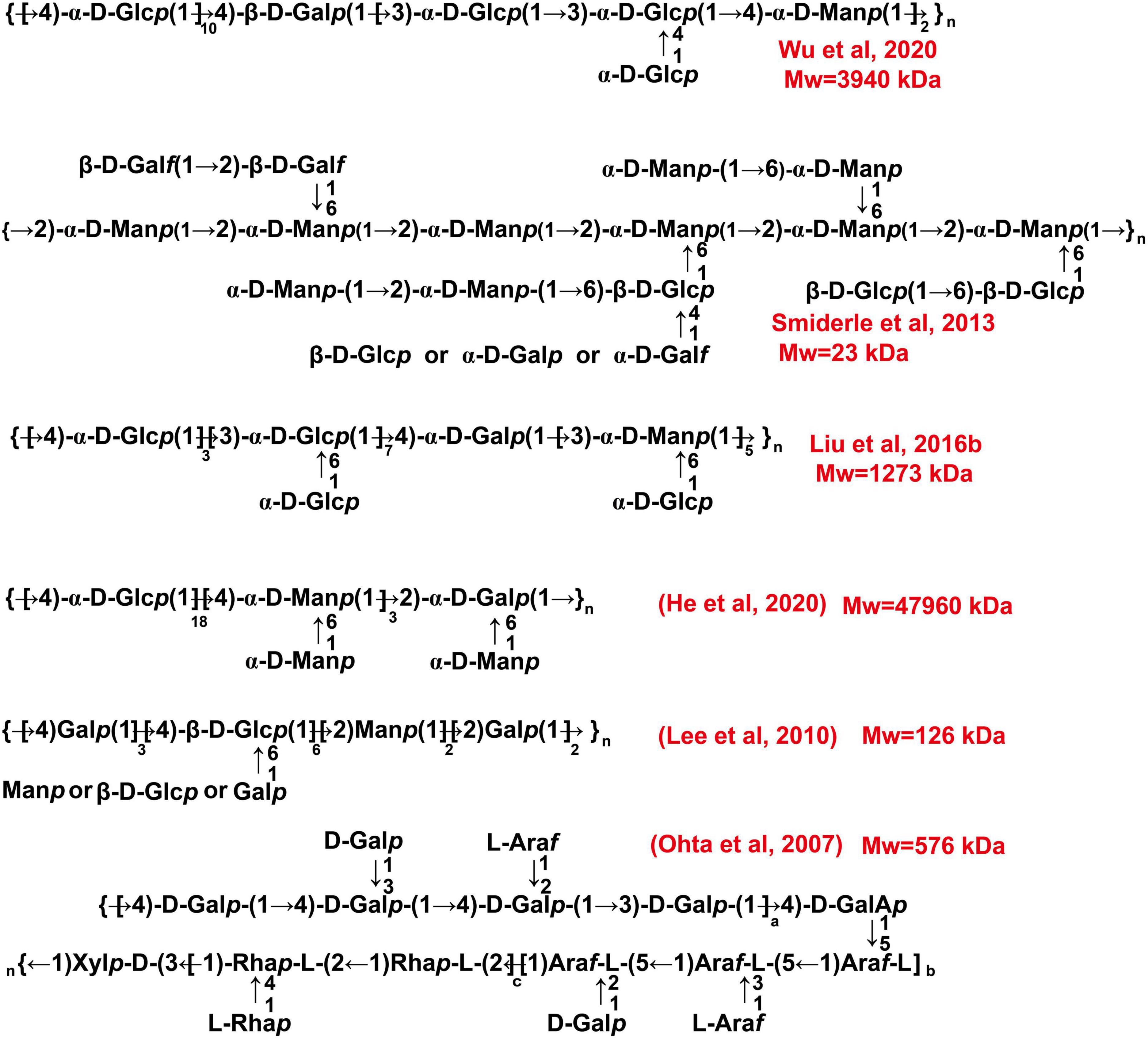
Figure 7. The chemical structures of polysaccharides derived from the fruiting body of C. militaris. We presumed these structures according to the descriptions in the literature.
As Glc is the major component of the cell walls of C. militaris, polysaccharides mainly composed of Glc are the most documented carbohydrate complex. A recent study demonstrated that →4)-α-D-Glcp (1→ linked glucan is specific to Cordyceps caterpillar and can be used as a marker for Cordyceps (70). Indeed, many water-extracted polysaccharides of the fruiting body of C. militaris have →4)-α-D-Glcp (1→ glycosyls as their backbone (22, 62, 64, 71, 72). Furthermore, most of these polysaccharides are heteropolysaccharides. However, several groups isolated glucans from the fruiting body of C. militaris (14, 73). Our group obtained a linear →6)-β-D-Glcp (1→ glucan with a Mw of 18.2 kDa (14). Some heteropolysaccharides are found to contain →4)-β-D-Glcp (1→ and →6)-α-D-Glcp (1→ glycosyls (14, 72). Polysaccharides mainly consisting of →2)-α-D-Manp (1→ glycosyls are also found in the fruiting bodies of C. militaris (3, 19).
The alkaline extracted polysaccharides are also reported. For instance, an alkali-extracted polysaccharide is found to have →2)-α-D-Manp (1→ and →2,6)-α-D-Manp (1→ glycosyls as its backbone (74). The polysaccharide CMPB90-1 obtained via 0.3 mol/L of NaOH is mainly composed of →6)-α-D-Glcp (1→ and →3)-α-D-Glcp (1→ glycosyls (75). Our lab obtained a novel alkali-extracted polysaccharide which mainly composed of →4)-β-D-Manp (1→, →6)-β-D-Manp (1→, and →6)-α-D-Manp (1→ glycosyls (16). Of note, alkaline-extracted (5% KOH) polysaccharides can be further treated with a free-thawing process, and the water insoluble fractions can be further treated with Me2SO to obtain a β-(1→3)-D-glucan (76). Our team obtained a glucan with →4)-α-D-Glcp (1→ and →4,6)-β-D-Glcp (1→ glycosyls as the backbone as shown in Figure 6 (15). Furthermore, one acidic-extractable polysaccharide is found to be consisted of Fuc (1.23%), Ribose (0.57%), Ara (0.29%), Xyl (2.12%), Man (2.73%), Gal (4.66%), and Glc (88.4%) (77).
Bioactivities of Cordyceps militaris-Derived Polysaccharides
The polysaccharides obtained from C. militaris have been demonstrated to have various biological activities, including antioxidant, immunoregulatory, anti-inflammatory, and anti-tumor activities (7, 12). In the following sections, we discuss the potential structure-activity relationship of these polysaccharides. Furthermore, we summarize the hypolipidemic, anti-diabetic, and anti-atherosclerotic functions of C. militaris-derived polysaccharides.
Anti-oxidation
The antioxidant activity and mechanisms of C. militaris-derived polysaccharides have been well documented previously in the literature (3), especially by Gu et al. (7). Here, we discuss the potential structure-activity relationship of these polysaccharides as antioxidants. Xu et al. demonstrated that the monosaccharide composition may significantly influence the antioxidant activity of polysaccharides (78). For instance, polysaccharides from the fruiting body of cultured C. militaris grown on silkworm pupae are reported to have better antioxidant activity than that grown on solid rice medium. The former has more Glc and the latter has more Man (79). The purified polysaccharide primarily consisting of Glc (47.5%), Man (34.3%), Gal (10.8%), and Ara (4.85%) in α-type glycosidic linkage can scavenge DPPH, hydroxyl, and superoxide radicals in vitro (80). The acidic-extractable heteropolysaccharide mostly composed of Glc (88.4%) not only scavenge free radicals in vitro but also improve the activity of antioxidant enzyme in type 2 diabetes mice (77). Furthermore, the mycelia polysaccharide obtained using a weak alkaline (pH 8–9) culture medium is reported to be rich in β-(1→6)-glucan, which has better antioxidant activity than that obtained from weak acidic conditions (pH 5–7) (55). These studies demonstrate that Glc and the β-configuration may enhance the antioxidant activity. Secondly, the ions contained in the polysaccharides may contribute to the antioxidant activity. The polysaccharide-iron (III) composed of →2)-β-D-Glcp (1→ and highly branched →2,4)-α-D-Glcp (1→ glycosyls shows antioxidant activity almost equal to that of vitamin C. This molecule can scavenge DPPH, hydroxyl, and superoxide anion radicals (54). Similarly, addition of sodium selenite can promote production of Se-polysaccharides with a better antioxidant activity than those without Se. Another study demonstrated that the Se-polysaccharide has better antioxidant activity with regard to scavenging free radicals (81). In vivo, Se-polysaccharides can further promote the activity of superoxide dismutase (SOD) (82). Thirdly, the acidic groups may also support the antioxidant activity of these polysaccharides. The acidic polysaccharide mainly consisted of →6) Galp (1→, →4) Glcp (1→, and →4,6) Glcp (1→ glycosyls and GlcA and GalA can improve the activities of glutathione peroxidase and SOD, thereby reducing malondialdehyde concentration (83). Furthermore, sulfation may enhance the free radical-scavenging effect of polysaccharides as revealed in vitro (35). A recent study also indicated that polysaccharides with →2)-α-D-Manp (1→ as the backbone exhibit good antioxidant activity (19). Therefore, glucosyls in β-configuration, →2)-α-D-Manp (1→ linked backbone, metal ions, and acid groups, may contribute to the antioxidant activity of these polysaccharides.
Immune Enhancement
Immune response plays an importance role in host defense system. B-lymphocyte and T-lymphocyte specifically mediates humoral and cellular immunity, respectively. Polysaccharides from C. militaris exhibit mitogenic effects in mouse splenocytes and can promote differentiation of murine T-, B-lymphocytes, and neutrophiles. These polysaccharides also increase IgG function and production of cytokines, such as interleukin (IL)-1β, tumor necrosis factor (TNF)-α, interferon (IFN)-γ, and IL-6 (79, 84, 85). On the cell surface, pattern recognition receptors (PRRs), such as Toll-like receptor (TLR) 2, TLR4, and dectin-1, can trigger different signaling pathways including phosphoinositide-3-kinase/protein kinase B (PKB/AKT), mitogen-activated protein kinase (MAPK), and tyrosine kinases (86, 87). Lipopolysaccharide (LPS) and some polysaccharides can activate these cell surface receptors and exert their immune modulating functions. As recently reviewed by Lee et al. (10), polysaccharides of C. militaris are likely to cause type 1 immunity. They can increase TNF-α secretion in macrophages and enhance NK cell activity. The immune enhancement activity of C. militaris-derived polysaccharides has been recently reviewed by different groups (3, 7), and Phull et al. have reviewed the influence of monosaccharide composition and Mw on the immunomodulatory effect (12). Here, we consider the related signaling pathways as shown in Figure 8 and discuss the potential structure-activity relationship by focusing on glycosidic linkage.
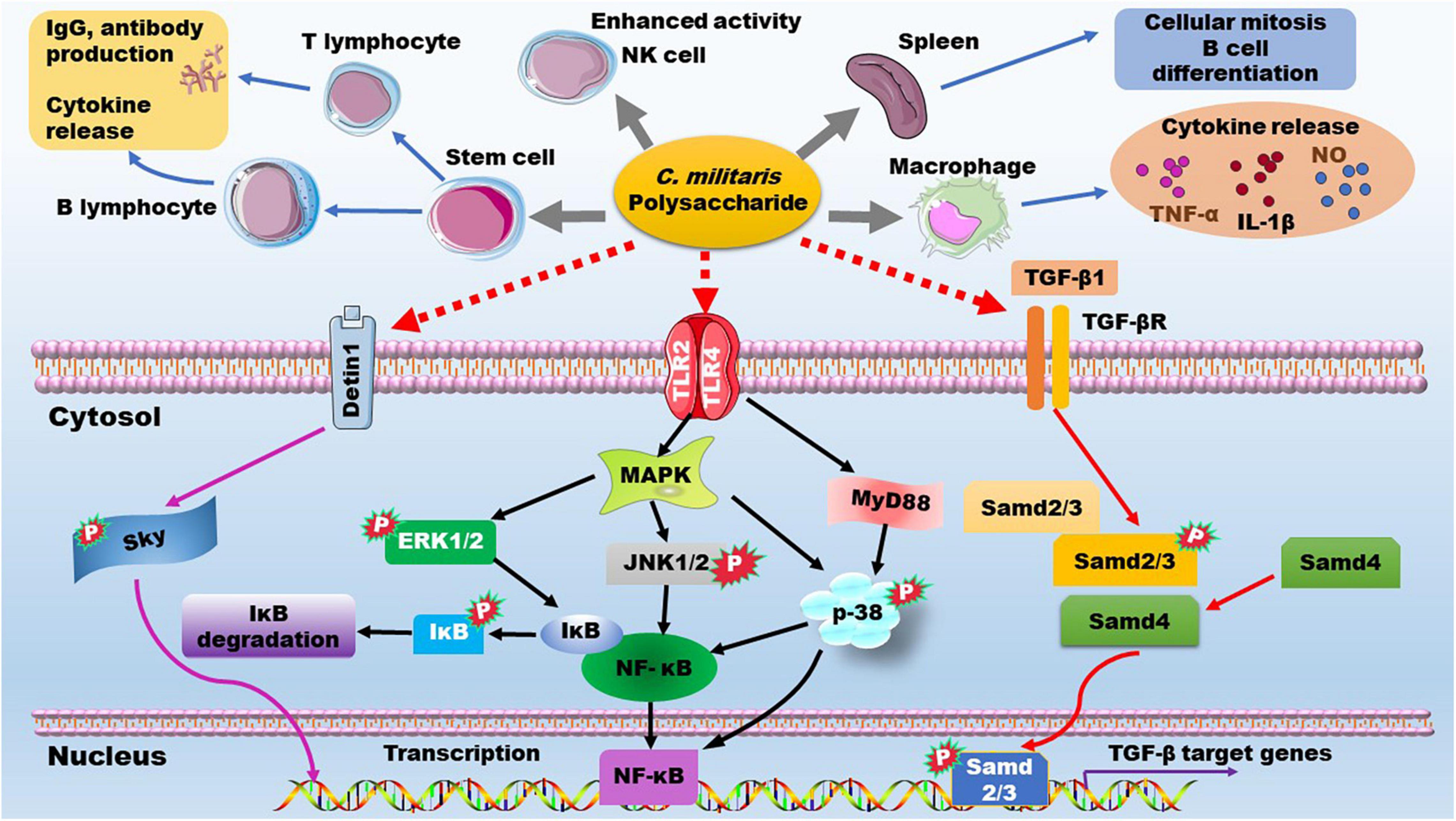
Figure 8. The immune enhancement effects and the underlying mechanisms of C. militaris-derived polysaccharides. ERK, extracellular regulated kinase; IgG, immunoglobulin G; IL, interleukin; JNK, C-Jun Kinase; MAPK, mitogen-activated protein kinase; NF-κB, nuclear factor kappa-B; NK cell, natural killer cell; NO, nitric oxide; PRRs, pattern recognition receptors; TGF-β, transforming growth factor β; TGF-βR, TGR-β receptor; TLR, Toll-like receptor; TNF-α, tumor necrosis factor-α.
C. militaris-derived polysaccharides that are composed of Glc are reported to act on macrophages and increase production of NO, IL-1β, IL-6 in vitro (73). The marker polysaccharide of C. militaris, →4)-α-D-Glcp (1→ linked glucan, can promote production of NO and cytokines in RAW 264.7 macrophages (70). Mechanistically, this polysaccharide is highly selective for the TLR4/MyD88/p38 axis as demonstrated in TLR4-deficient mice (88). The polysaccharide mainly composed of →4)-α-D-Glcp (1→ (∼70%), →4,6)-α-D-Manp (1→, and →2,6)-α-D-Galp (1→ glycosyls could promote macrophage phagocytosis and secretion of NO, TNF-α, and IL-6, via up-regulation of the MAPK/nuclear factor kappa-B (NF-κB) pathway, including phosphorylation of extracellular regulated kinase (ERK), p-38, and C-Jun Kinase (JNK) (71). The acidic exopolysaccharide mainly composed of →4)-α-D-Glcp (1→ can promote cytokine secretion by increasing the phosphorylation of ERK, p-38, and JNK (61). Furthermore, the alkali-extracted polysaccharide CMPB90-1 mainly composed of →6)-α-D-Glcp (1→ and →3)-α-D-Glcp (1→ glycosyls, is reported to improve macrophage M1 polarization through the activation of TLR2/MAPK/NF-κB signaling pathway (75). These data demonstrate that →4)-α-D-Glcp (1→ and →6)-α-D-Glcp (1→ glycosyls play key roles in immune enhancement by C. militaris-derived polysaccharide via the up-regulation of PRRs/MAPK/NF-κB signaling.
Of note, the C. militaris-derived glucogalactomannan, whose backbone is composed of →2) Manp (1→ and →6) Manp (1→ glycosyls, can also interact with PRRs, such as TLR2, TLR4, and dectin-1, and promotes the downstream MAPK/NF-κB signaling pathway, thereby increasing production of NO, reactive oxygen species, and TNF-α, and enhancing phagocytic activity of RAW264.7 macrophages (13, 62, 86). In line with this study, the polysaccharide with →2)-α-D-Manp (1→ as its backbone shows immunomodulatory activity by promoting secretion of inflammatory factors by macrophages and inducing macrophage M1 polarization (19). Furthermore, the arabinogalactan-type polysaccharide obtained from the fruiting body of C. militaris can promote dendritic cell maturation through activation of TLR4, downstream MAPK signaling (phosphorylation of ERK, p38, and JNK) and NF-κB p50/p65, thereby increasing the expression of IL-12, IL-1β, TNF-α, and IFN-α/β (89). The acidic arabinogalactan-type polysaccharide consists of →5)-Araf-(1→, →4)-Galp-(1→, →4)-GalAp-(1→, and Araf (1→ glycosyls can also enhance secretion of inflammatory cytokines and improve production of nitric oxide (NO) by up-regulating the expression of inducible nitric oxide synthase in macrophages (90). Additionally, C. militaris-derived β-glucan can enhance macrophage activation and phagocytosis by increasing protein phosphorylation of Lyn, Syk, and MAPK (91).
Anti-inflammation
The recent review by Phull et al. largely reviewed the immune enhancement activity of C. militaris-derived polysaccharides rather than the anti-inflammatory effects (12). Here, we review the anti-inflammatory activity of these polysaccharides based on an analysis of the literature. Some polysaccharides of C. militaris are found to suppress secretion of eotaxin, IL-4, IL-5, IL-13, and IFN-γ, and reduce serum IgE level, inflammatory cell infiltration, and goblet cell hyperplasia by inhibiting transforming growth factor β1 (TGF-β1) and the phosphorylation of Smad2/3 proteins in ovalbumin challenged asthmatic mice (92). A recent study demonstrated that cordyceps polysaccharide can reduce acute liver injury by promoting hepatocyte proliferation, liver vascular regeneration, and liver tissue repair in line with the upregulation of vascular endothelial growth factor (VEGF), stromal cell-derived factor-1α, proliferating cell nuclear antigen, and signal regulatory protein α1, and the reduction of IL-18 and caspase-1 (93). Se-rich polysaccharides can effectively reduce inflammation by reducing the mRNA expression of TNF-α and IL-6 as well as serum content of LPS-binding proteins in C57BL/6J mice fed a high-fat diet (94). C. militaris-derived β-(1→3)-D-glucan can also inhibit LPS-induced mRNA expression of IL-1β, TNF-α, and cyclooxygenase 2 in THP-1 macrophages and reduce formalin-induced nociceptive response and leukocyte migration (76). Of note, gut microbiota also plays a key role in modulating inflammation. For instance, Akkermansia prefers to ingest polysaccharides as its nutritional source, and A. muciniphila is reported to suppress intestinal inflammation and improve gut barrier function (95). C. militaris-derived polysaccharides can increase the population of Akkermansia and Lachnospiraceae_Eubacterium, and decrease the abundance of Bacteroides, Parabacteroides, and Blautia, thereby suppressing inflammation (96). A recent study demonstrated that C. militaris treatment downregulates the mucosal levels of pro-inflammatory cytokines and upregulates the levels of anti-inflammatory cytokines via inhibiting TLR4/MyD88/NF-κB signaling in pigs. Furthermore, this treatment also modulates gut microbiota and increases the concentrations of acetate and butyrate, thereby improving intestinal barrier function (97). Another study demonstrated that Cordyceps improves inflammation via modulating the abundance of Enterococcus cecorum (98).
Anti-hyperlipidemia and Anti-atherosclerosis
Polysaccharides obtained from mycelia and fruiting body of C. militaris can decrease total cholesterol (TC), triglyceride (TG), and low-density lipoprotein (LDL) cholesterol (LDL-C) levels and increase high density lipoprotein cholesterol (HDL-C) in streptozotocin-induced diabetic mice (99, 100). Crude polysaccharides from the fruiting body of C. militaris can improve reverse cholesterol transport (RCT) in C57BL/6J mice (101). Furthermore, the →6)-β-D-Glcp (1→ linked glucan and the heteropolysaccharide (CM1) primarily consisting of →4)-β-D-Glcp (1→, →2)-α-D-Manp (1→, and →2,6)-α-D-Manp (1→ glycosyls, can improve cholesterol efflux in vitro (14). Recently, studies in our group demonstrated that CM1 can alleviate hyperlipidemia and adipocyte differentiation in LDLR(±) hamsters, whose lipid profiles are similar to human (102). Mechanistically, these polysaccharides are found to up-regulate genes and proteins related to RCT, such as liver X receptors and ATP-binding cassette (ABC) transporters (14, 15, 102). Low-density lipoprotein receptor (LDLR) plays a key role in the clearance of apolipoprotein (apo) B-containing lipoproteins in circulation, and proprotein convertase subtilisin/kexin-type 9 (PCSK9) plays a key role in post-translational degradation of LDLR (103). Our lab obtained a novel alkali-extracted polysaccharide from the fruiting body of C. militaris mainly composed of →4)-β-D-Manp (1→, →6)-β-D-Manp (1→, and →6)-α-D-Manp (1→ glycosyls, which can inhibit PCSK9 secretion in Huh7 cells (16). Additionally, Se-rich polysaccharide from C. militaris can effectively reduce serum TG and LDL-C by 51.5 and 44.1% in C57BL/6J mice, respectively (94). Mechanistically, this molecule can reduce adiponectin levels and decrease gut bacteria, such as Dorea, Lactobacillus, Clostridium, and Ruminococcus, that are negatively associated with obesity. Furthermore, it can increase mucosal beneficial bacteria Akkermansia, and has no effect on the content of short-chain fatty acids (94, 104). A recent study demonstrated that Cordyceps may improve obesity via modulating the abundance of Enterococcus cecorum as well as bile acid metabolism (98). The lipid-lowering mechanisms of these polysaccharides are summarized in Figure 9.
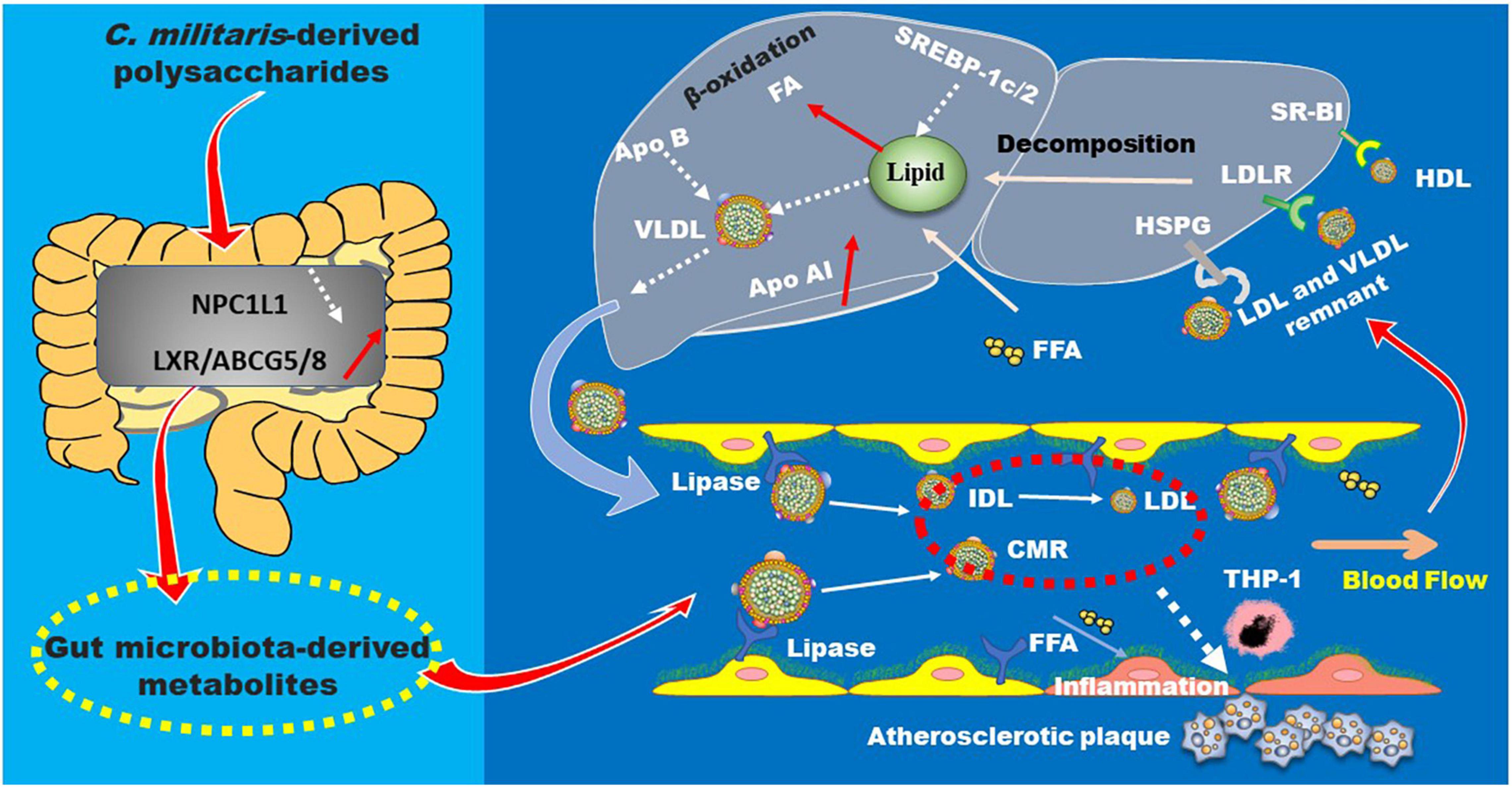
Figure 9. The lipid-lowering mechanisms of C. militaris-derived polysaccharides. These polysaccharides are found to modulate multiple genes and proteins related to lipid absorption and metabolism in the plasma, small intestine, and liver, thereby improving hyperlipidemia and atherosclerosis. ABC, ATP-binding cassette; Apo, apolipoprotein; CRM, chylomicron remnant; FFA, free fatty acid; HSPG, heparan sulfate proteoglycan; IDL, intermediate density lipoprotein; LDL, low-density lipoprotein; LDLR, low-density lipoprotein receptor; LRP1, LDLR-related protein 1; LXRα, liver X receptor α; NPC1L1, Niemann-Pick C1-like protein 1; SR-B1, scavenger receptor B type 1; SREBP, sterol regulatory element-binding protein; VLDL, very low-density lipoprotein.
Given that hyperlipidemia promotes the development of atherosclerosis, our group investigated the anti-atherosclerotic effects of the polysaccharides derived from C. militaris. As demonstrated in apoE(–/–) and LDLR(–/–) mice, the polysaccharide CM1 can significantly decrease atherosclerotic plaque formation in the aorta of these mice. Mechanistically, this molecule can improve the expression of RCT-related genes and proteins in the liver and small intestine of the mice (105). Integrated bioinformatics analysis suggested that these polysaccharides can modulate the expression of hundreds of genes. KEGG and GO enrichment demonstrated that these differentially expressed genes are associated with lipid metabolism, inflammation, oxidation, and shear stress (5). Of importance, these polysaccharides may modulate the lncRNA-miRNA-mRNA axis (6, 85). Additionally, the anti-atherosclerotic effect of these polysaccharides may also be attributed to their effect on the reduction of trimethylamine and oxidized trimethylamine via modulating gut bacteria (100). The mannan core and glycosyls in a β-configuration may play an important role in ameliorating atherosclerosis (105, 106). Of note, the TG-lowering effect of C. militaris-derived polysaccharides is better than their TC-lowering effect (5, 15, 102, 107). It is known that there is a positive correlation between plasma TG and cardiovascular disease (CVD) (108, 109). These data suggest that C. militaris-derived polysaccharides have a potential application in prevention of CVD as TG-lowering compounds.
Anti-diabetic Activity
In streptozotocin-induced diabetic mice, the mycelia polysaccharide increases body weight and thymus index and decreases fasting blood glucose, insulin resistance as well as secretion of pro-inflammatory cytokines (TNF-α and IL-6) and C-reactive protein (99). The purified polysaccharide CBPS-II with a Mw of 1,273 kDa can reduce blood Glc level in streptozotocin-induced diabetic mice (100). The acid-extracted heteropolysaccharide mainly composed of glucose (88.4%) also showed good hypoglycemic effect in type 2 diabetes mice that were induced by a high-fat diet and streptozotocin (77). Of note, the heteropolysaccharide that is primarily composed of →4)-α-D-Glcp (1→, →3,6)-α-D-Manp (1→, and →4)-α-D-Manp (1→ glycosyls can improve glucose tolerance in STZ-induced diabetic mice, and this activity may be related to an inhibitory effect on alpha-glucosidase (59). Several other labs have also demonstrated that polysaccharides from C. militaris can inhibit the activity of alpha-glucosidase (110). It seems that polysaccharides from the fruiting bodies have a better effect on inhibition of alpha-glucosidase than those from fermentation mycelia (65). Furthermore, carboxymethylation and acetylation of these polysaccharides may enhance their inhibitory effects on alpha-glucosidase (111). C. militaris-derived polysaccharides can also protect diabetic nephropathy in mice by regulating cell autophagy (112). Additionally, the anti-hyperglycemic effect of C. militaris-derived polysaccharides can be partially attributed to its effect on promoting Akkermansia, a beneficial bacterium in the gut (96).
Antitumor Activity
The antitumor activity of C. militaris-derived polysaccharides has been summarized by Zhang et al. (3) and Gu et al. (7). Here, we have tried to elucidate the potential structure-activity relationship of these polysaccharides. Moreover, the potential antitumor mechanisms of these polysaccharides are summarized in Figure 10. Polysaccharides obtained from mycelia can attenuate doxorubicin-induced cytotoxic effects during chemotherapy (85). Polysaccharides from mycelia (CMPS-II) seem to have a better effect than that obtained from the fruiting body (CBPS-II). CMPS-II and CBPS-II can up-regulate the expression of apoptosis factors including Caspase-3, Caspase-9, and p53, and down-regulating proliferating cell nuclear antigen (64). These two 1,3-branched-galactomannoglucans with triple-helical chains have similar Mw (1.2–1.4 kDa) and the same backbone of →4)-α-D-Glcp (1→ glycosyls. However, CMPS-II has significantly more Glc than CBPS-II, suggesting that the branched →4)-α-D-Glcp (1→ glycosyls may facilitate the antitumor activity of these polysaccharides. Our team recently demonstrated that the branched glucans primarily consisting of →4)-α-D-Glcp (1→ glycosyls can inhibit the proliferation of tumor cells (113). A previous study also demonstrated that water-extracted C. militaris polysaccharide, largely composed of →4)-α-D-Glcp (1→, →6)-β-D-Glcp (1→, and →4)-β-D-Glcp (1→ glycosyls, inhibits the proliferation of several tumor cell lines in vitro (72). Additionally, a recent study demonstrated that the alkaline-extracted polysaccharides mostly composed of →6)-α-D-Glcp (1→ and →3)-α-D-Glcp (1→ glycosyls shows anti-tumor effects though the modulation of tumor-associated macrophages, which inhibit the killing effect of T lymphocytes on tumor cells through the programmed death lignd-1/programmed death-1 axis (114). Mechanistically, this molecule binds to TLR2, causes release of Ca2+ and activation of p38, AKT, and NF-κB, thereby polarizing tumor-associated macrophages from a tumor-promoting M2 phenotype into a tumor-killing M1 phenotype (114). Furthermore, Se-polysaccharides with a backbone of →4) Glcp (1→ glycosyl exhibit an appreciable antitumor effect in vitro, and the polysaccharides with a lower Mw (65 and 16 kDa) have better activity than the one with a higher Mw of 1,902 kDa (63). The above data indicate that the glucosyls in the α-D-configuration play a key role in the anti-tumor activity of C. militaris-derived polysaccharides.
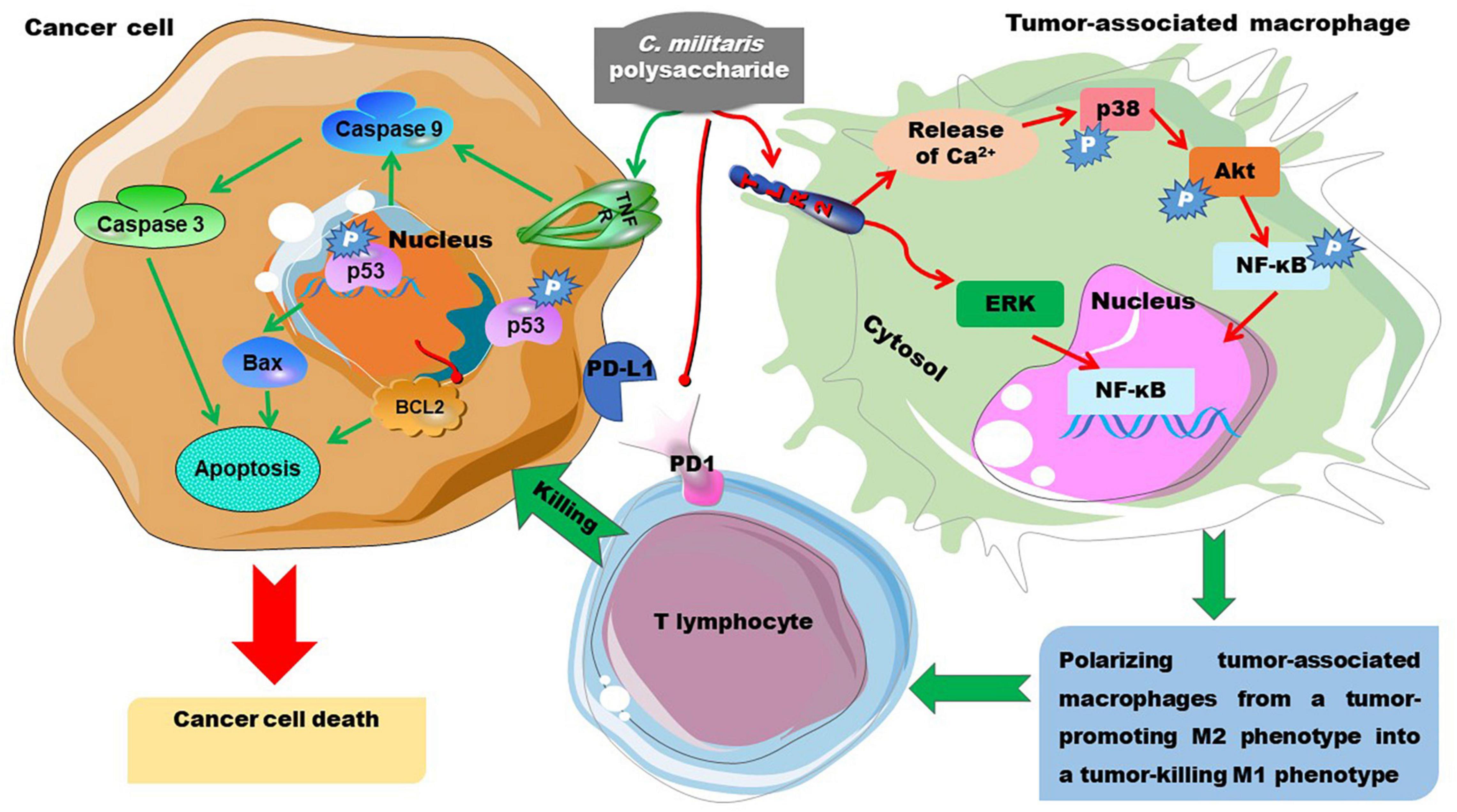
Figure 10. The anti-tumor mechanisms of C. militaris-derived polysaccharides. C. militaris-derived polysaccharides induce cancer cell death by enhancing apoptosis-associated signaling. Alternatively, they polarize tumor-associated macrophages from M2 to M1 phenotype, thereby promoting the cancer cell killing activity of T lymphocytes. AKT/PKB, phosphoinositide-3-kinase/protein kinase B; Bax, B cell leukemia/lymphoma 2-associated X protein; Bcl-2, B cell leukemia/lymphoma 2; ERK, extracellular regulated kinase; NF-κB, nuclear factor kappa-B; PD-1, programmed death-1; PD-L1, programmed death lignd-1.
Others
Addition of C. militaris in diet is reported to improve physical fatigue in mice (115). The crude polysaccharide derived from the fruiting body of C. militaris has good anti-fatigue activity (116). One exopolysaccharide exhibits hypouricemic effect in mice by decreasing urate production and the activity of xanthine oxidase (117). The presence of sulfate and polygalacturonic acids endow C. militaris polysaccharide with anti-angiogenesis activity via reducing the protein expression of VEGF in human umbilical vein endothelial cells (53). A acidic polysaccharide that mainly composed of →6)Galp(1→, →4)Glcp (1→, and →4,6)Glcp(1→ glycosyls can prevent Pb2+-induced liver and kidney toxicity by activating nuclear factor erythroid 2-related factor 2 (Nrf2) signaling pathway and increasing bacterial diversity of gut microbiota (83). Mechanistically, it enhances the protein expression of Nrf2, Kelch-like ECH-associated protein-1, Heme oxygenase, and NAD(P)H: quinone oxidoreductase 1. Furthermore, this polysaccharide promotes the abundance of Ruminococcaceae and reduces the abundance of Lachnospiraceae families; it reduces Roseburia and increases Bacteroides genera (83). The exopolysaccharide Cs-HK1 with a high Mw can protect bifidobacterial cells against antibiotics through physical interactions (118). C. militaris is found to modulate the formation of short-chain fatty acids (97, 119). Additionally, the acidic arabinogalactan-type polysaccharide obtained from mycelia can reduce virus titer of mice infected with influenza A virus (90). C. militaris may also have a potential application for the treatment of mild-to-moderate COVID-19 disease (120).
Comparisons With Other Studies and What Does the Current Work Add to the Existing Knowledge
Recently, the research involving C. militaris-derived polysaccharides has increased rapidly, particularly in their structural characterization and pharmaceutical activities. The pharmaceutical effects of these polysaccharides including antioxidant, immunomodulatory, and anti-tumor activities have been widely reviewed (7, 12). However, the structural characteristics of polysaccharides and the related methods used for elucidating these polysaccharides have not been reviewed in the recent literature. Given that the structure of polysaccharide determines its bioactivity, we describe the methods used for elucidation of polysaccharide structure in this article. Of note, most of the chemical methods are described by presenting the detailed chemical reactions, which are helpful in understanding the principle behind the processes. The development of novel techniques including mass spectrometry are also discussed in this article. Of importance, we review the structural characteristics of C. militaris-derived polysaccharides in the format of presumed chemical structure and have discussed the potential structure-activity relationship. The mechanisms of action of these polysaccharides are presented by constructing the signaling pathways as seen in the figures. Furthermore, the anti-diabetic, anti-hyperlipidemic, anti-atherosclerotic, and gut microbiota modulatory effects that have not been elucidated well in previous reviews in the literature are also summarized in this article. This detailed review focuses on polysaccharide structure and bioactivity and makes it possible to discuss and understand the structure-activity relationship of C. militaris-derived polysaccharides. It is found that different glycosyls and functional groups may play distinct roles in the bioactive functions of these polysaccharides.
Conclusion and Future Perspective
The widespread use of advanced analysis tools such as HPLC, MS, and NMR techniques has greatly improved the structural elucidation of C. militaris-derived polysaccharides. Most of the reported heteropolysaccharides have mannosyls, such as →2)-α-D-Manp (1→, →6)-α-D-Manp (1→, or →6)-β-D-Manp (1→ glycosyls, as their core. The obtained glucans are found to consist of →4)-α-D-Glcp (1→, →6)-α-D-Glcp (1→, or →3)-β-D-Glcp (1→ glycosyls as their backbone. Recently developed novel techniques, such as mass spectrometry imaging and the novel NMR methods, are definitely going to improve our understanding of the structure of C. militaris-derived polysaccharides in the future. C. militaris-derived polysaccharides can modulate multiple signaling pathways and have great potential for use as dietary supplements and health food products for the prevention and treatment of oxidation, inflammation, tumors, immune dysfunction, and metabolic syndrome. The glucosyls in a β-configuration, →2)-α-D-Manp (1→ linked backbone, metal ions, and acid groups, may all contribute to the antioxidant activity of these polysaccharides. The α-D-glucosyls and α-D-mannosyls mainly contribute to the immune enhancing activity, while the β-D linked glycosyls and α-D-mannosyls may facilitate the hypolipidemic and anti-atherosclerotic effects. Additionally, the branched →4)-α-D-Glcp (1→, →6)-α-D-Glcp (1→, and →3)-α-D-Glcp (1→ glycosyls may enable the anti-tumor effect of C. militaris-derived polysaccharides.
The high-frequency degeneration of C. militaris during cultivation has limited the development of the C. militaris industry (9). Gene engineering strategies are expected to further improve the yield of C. militaris-derived polysaccharides. The structural characteristics, including Mw, monosaccharide composition, glycosyl linkage, glycosyl configuration, physicochemical properties, and even the animal models and cultivation conditions of C. militaris may influence their bioactivity. Therefore, standardized procedures are needed to guarantee the quality of C. miliatris-derived polysaccharides. The focus of the standardization procedures should be on cover strain preservation, cultivation conditions, extraction and purification methods, quality control, and impurity detection. The recent studies using purified polysaccharides have greatly improved our understanding of their structure-activity relationships. However, we still do not know the functional groups and/or bioactive domains due to lack of comparative studies. To improve this, researchers may design parallel experiments to evaluate the activity and/or the underlying mechanisms of polysaccharides of interest containing different backbones or differently digested polysaccharides with the same backbone or polysaccharides with different chemical modifications. Furthermore, given the large Mw of these polysaccharides, they may have little change of directly entering the circulation. One possible mechanism is that these molecules can exert their bioactivity via modulating gut microbiota and their metabolites, which can easily penetrate the intestinal barrier and then work within the circulation. Another possibility is that the polysaccharides digested by the gastric acid or gut microbiota with a lower Mw or the corresponding oligosaccharides may be directly absorbed by the intestine, thereby exerting their bioactivities in different organs. The polysaccharides that are resistant to degradation in vivo may either act directly on receptors in the gut or work through mechanisms involving physical binding. These ideas need to be investigated further in future studies.
Author Contributions
MM, W-QY, YL, and Y-LS performed reference collection. MM, W-QY, and YL drew the figures. S-DG wrote and re-edited the manuscript. All authors contributed to the article and approved the submitted version.
Funding
This work was supported by the National Natural Science Foundation of China (81770463 and 82070469).
Conflict of Interest
The authors declare that the research was conducted in the absence of any commercial or financial relationships that could be construed as a potential conflict of interest.
Publisher’s Note
All claims expressed in this article are solely those of the authors and do not necessarily represent those of their affiliated organizations, or those of the publisher, the editors and the reviewers. Any product that may be evaluated in this article, or claim that may be made by its manufacturer, is not guaranteed or endorsed by the publisher.
Abbreviations
ABC, ATP-binding cassette; apo, apolipoprotein; Ara, arabinose; CM1, heteropolysaccharide; COSY, correlated spectroscopy; CVD, cardiovascular disease; DEPT, polarization transfer spectroscopy; ERK, extracellular regulated kinase; ESI-MS, electrospray ionization mass spectrometry; FT-IR, Fourier transform infrared; Gal, galactose; GalA, galacturonic acid; GC, gas chromatography; GC-MS, gas chromatography-mass spectrometry; Glc, glucose; GlcA, glucuronic acid; HDL-C, high density lipoprotein cholesterol; HILIC-MS, Hydrophilic interaction chromatography coupled to mass spectrometric detection; HMBC, heteronuclear multiple bond correlation spectroscopy; HMQC, heteronuclear multiple quantum coherence spectroscopy; HPLC, high-performance liquid chromatography; HPLC-MS/MS, HPLC-tandem mass spectrometry; IFN, interferon; IL, interleukin; JNK, C-Jun Kinase; LDL, low-density lipoprotein; LDL-C, LDL cholesterol; LDLR, Low-density lipoprotein receptor; LPS, Lipopolysaccharide; MALDI, matrix-assisted laser desorption/ionization; MALDI-TOF, MALDI-time of flight; Man, mannose; MAPK, mitogen-activated protein kinase; MS, mass spectrometry; Mw, molecular weight; NF-κB, nuclear factor kappa-B; NMR, nuclear magnetic resonance; NO, nitric oxide; NOESY, nuclear overhauser effect spectroscopy; Nrf2, nuclear factor erythroid 2-related factor 2; PCSK9, proprotein convertase subtilisin/kexin-type 9; PKB/AKT, phosphoinositide-3-kinase/protein kinase B; PMP, 1-phenyl-3-methyl-5-pyrazolone; PRR, pattern recognition receptor; RCT, reverse cholesterol transport; SOD, superoxide dismutase; TC, total cholesterol; TFA, trifluoroacetic acid; TG, triglyceride; TGF-β1, transforming growth factor β1; TLR, Toll-like receptor; TNF, tumor necrosis factor; TOCSY, total correlation spectroscopy; VEGF, vascular endothelial growth factor.
Footnotes
References
1. Olatunji OJ, Tang J, Tola A, Auberon F, Oluwaniyi O, Ouyang Z. The genus Cordyceps: an extensive review of its traditional uses, phytochemistry and pharmacology. Fitoterapia. (2018) 129:293–316. doi: 10.1016/j.fitote.2018.05.010
2. Wu X, Wu T, Huang A, Shen Y, Zhang X, Song W, et al. New insights into the biosynthesis of typical bioactive components in the traditional Chinese medicinal fungus Cordyceps militaris. Front Bioeng Biotechnol. (2021) 9:801721. doi: 10.3389/fbioe.2021.801721
3. Zhang J, Wen C, Duan Y, Zhang H, Ma H. Advance in Cordyceps militaris (Linn) link polysaccharides: isolation, structure, and bioactivities: a review. Int J Biol Macromol. (2019) 132:906–14. doi: 10.1016/j.ijbiomac.2019.04.020
4. Cao C, Yang S, Zhou Z. The potential application of Cordyceps in metabolic-related disorders. Phytother Res. (2020) 34:295–305. doi: 10.1002/ptr.6536
5. Lin P, Yin F, Shen N, Liu N, Zhang BH, Li Y, et al. Integrated bioinformatics analysis of the anti-atherosclerotic mechanisms of the polysaccharide CM1 from Cordyceps militaris. Int J Biol Macromol. (2021) 193:1274–85. doi: 10.1016/j.ijbiomac.2021.10.175
6. Li Y, Miao M, Yin F, Shen N, Yu WQ, Guo SD. The polysaccharide-peptide complex from mushroom Cordyceps militaris ameliorates atherosclerosis by modulating the lncRNA-miRNA-mRNA axis. Food Funct. (2022) 13:3185–97. doi: 10.1039/d1fo03285b
7. Gu C, Zhang D, Zhai W, Zhang H, Wang S, Lv S, et al. Research progress on Cordyceps militaris polysaccharides. Food Biosci. (2022) 45:101503. doi: 10.1016/j.fbio.2021.101503
8. Dong Y, Hu S, Liu C, Meng Q, Song J, Lu J, et al. Purification of polysaccharides from Cordyceps militaris and their anti-hypoxic effect. Mol Med Rep. (2015) 11:1312–7. doi: 10.3892/mmr.2014.2786
9. Lou H, Lin J, Guo L, Wang X, Tian S, Liu C, et al. Advances in research on Cordyceps militaris degeneration. Appl Microbiol Biotechnol. (2019) 103:7835–41. doi: 10.1007/s00253-019-10074-z
10. Lee CT, Huang KS, Shaw JF, Chen JR, Kuo WS, Shen G, et al. Trends in the immunomodulatory effects of Cordyceps militaris: total extracts, polysaccharides and cordycepin. Front Pharmacol. (2020) 11:575704. doi: 10.3389/fphar.2020.575704
11. Jȩdrejko KJ, Lazur J, Muszyńska B. Cordyceps militaris: an overview of its chemical constituents in relation to biological activity. Foods. (2021) 10:2634. doi: 10.3390/foods10112634
12. Phull AR, Ahmed M, Park HJ. Cordyceps militaris as a bio functional food source: pharmacological potential, anti-inflammatory actions and related molecular mechanisms. Microorganisms. (2022) 10:405. doi: 10.3390/microorganisms10020405
13. Luo X, Duan Y, Yang W, Zhang H, Li C, Zhang J. Structural elucidation and immunostimulatory activity of polysaccharide isolated by subcritical water extraction from Cordyceps militaris. Carbohydr Polym. (2017) 157:794–802. doi: 10.1016/j.carbpol.2016.10.066
14. Hu S, Wang J, Li F, Hou P, Yin J, Yang Z, et al. Structural characterisation and cholesterol efflux improving capacity of the novel polysaccharides from Cordyceps militaris. Int J Biol Macromol. (2019) 131:264–72. doi: 10.1016/j.ijbiomac.2019.03.078
15. Yang X, Lin P, Wang J, Liu N, Yin F, Shen N, et al. Purification, characterization and anti-atherosclerotic effects of the polysaccharides from the fruiting body of Cordyceps militaris. Int J Biol Macromol. (2021) 181:890–904. doi: 10.1016/j.ijbiomac.2021.04.083
16. Wang J, Wang Y, Yang X, Lin P, Liu N, Li X, et al. Purification, structural characterization, and PCSK9 secretion inhibitory effect of the novel alkali-extracted polysaccharide from Cordyceps militaris. Int J Biol Macromol. (2021) 179:407–17. doi: 10.1016/j.ijbiomac.2021.02.191
17. Zhao J, Xie J, Li SP. Advanced development in chemical analysis of Cordyceps. J Pharmaceut Biomed Anal. (2014) 87:271–89. doi: 10.1016/j.jpba.2013.04.025
18. Zhao J, Deng Y, Li SP. Advanced analysis of polysaccharides, novel functional components in food and medicine dual purposes Chinese herbs. Trends Analyt Chem. (2017) 96:138–50. doi: 10.1016/j.trac.2017.06.006
19. Zhang Y, Zeng Y, Cui Y, Liu H, Dong C, Sun Y. Structural characterization, antioxidant and immunomodulatory activities of a neutral polysaccharide from Cordyceps militaris cultivated on hull-less barley. Carbohydr Polym. (2020) 235:115969. doi: 10.1016/j.carbpol.2020.115969
20. Li Y, Liang J, Gao JN, Shen Y, Kuang HX, Xia YG. A novel LC-MS/MS method for complete composition analysis of polysaccharides by aldononitrile acetate and multiple reaction monitoring. Carbohydr Polym. (2021) 272:118478. doi: 10.1016/j.carbpol.2021.118478
21. Leontein K, Lindberg B, Lönngren J. Assignment of absolute configuration of sugars by g.l.c. of their acetylated glycosides from chiral alcohols. Carbohydr Res. (1978) 62:359–62. doi: 10.1016/s0008-6215(00)80882-4
22. Liu XC, Zhu ZY, Tang YL, Wang MF, Wang Z, Liu AJ, et al. Structural properties of polysaccharides from cultivated fruit bodies and mycelium of Cordyceps militaris. Carbohydr Polym. (2016) 142:63–72. doi: 10.1016/j.carbpol.2016.01.040
23. Galermo AG, Nandita E, Barboza M, Amicucci MJ, Vo T-TT, Lebrilla CB. Liquid chromatography-tandem mass spectrometry approach for determining glycosidic linkages. Anal Chem. (2018) 90:13073–80. doi: 10.1021/acs.analchem.8b04124
24. Novakovic M, Kupče Ē, Oxenfarth A, Battistel MD, Freedberg DI, Schwalbe H, et al. Sensitivity enhancement of homonuclear multidimensional NMR correlations for labile sites in proteins, polysaccharides, and nucleic acids. Nat Commun. (2020) 11:5317. doi: 10.1038/s41467-020-19108-x
25. Matsumori N, Kaneno D, Murata M, Nakamura H, Tachibana K. Stereochemical determination of acyclic structures based on carbon-proton spin-coupling constants. a method of configuration analysis for natural products. J Org Chem. (1999) 64:866–76. doi: 10.1021/jo981810k
26. Guo S, Mao W, Yan M, Zhao C, Li N, Shan J, et al. Galactomannan with novel structure produced by the coral endophytic fungus Aspergillus ochraceus. Carbohydr Polym. (2014) 105:325–33. doi: 10.1016/j.carbpol.2014.01.079
27. Gimeno A, Valverde P, Ardá A, Jiménez-Barbero J. Glycan structures and their interactions with proteins. a NMR view. Curr Opin Struc Biol. (2020) 62:22–30. doi: 10.1016/j.sbi.2019.11.004
28. Nokab MEHE, van der Wel PCA. Use of solid-state NMR spectroscopy for investigating polysaccharide-based hydrogels: a review. Carbohydr Polym. (2020) 240:116276. doi: 10.1016/j.carbpol.2020.116276
29. Chakraborty A, Deligey F, Quach J, Mentink-Vigier F, Wang P, Wang T. Biomolecular complex viewed by dynamic nuclear polarization solid-state NMR spectroscopy. Biochem Soc Trans. (2020) 48:1089–99. doi: 10.1042/BST20191084
30. Poulhazan A, Dichwella Widanage MC, Muszyński A, Arnold AA, Warschawski DE, Azadi P, et al. Identification and quantification of glycans in whole cells: architecture of microalgal polysaccharides described by solid-state nuclear magnetic resonance. J Am Chem Soc. (2021) 143:19374–88. doi: 10.1021/jacs.1c07429
31. Zhao W, Kirui A, Deligey F, Mentink-Vigier F, Zhou Y, Zhang B, et al. Solid-state NMR of unlabeled plant cell walls: high-resolution structural analysis without isotopic enrichment. Biotechnol Biofuels. (2021) 14:14. doi: 10.1186/s13068-020-01858-x
32. Reif B, Ashbrook AE, Emsley L, Hong M. Solid-state NMR spectroscopy. Nat Rev Methods Primers. (2021) 1:2. doi: 10.1038/s43586-020-00002-1
33. Zhao W, Fernando LD, Kirui A, Deligey F, Wang T. Solid-state NMR of plant and fungal cell walls: a critical review. Solid State Nucl Magn Reson. (2020) 107:101660. doi: 10.1016/j.ssnmr.2020.101660
34. Gao Y, Mortimer JC. Unlocking the architecture of native plant cell walls via solid-state nuclear magnetic resonance. Methods Cell Biol. (2020) 160:121–43. doi: 10.1016/bs.mcb.2020.05.001
35. Jing Y, Zhu J, Liu T, Bi S, Hu X, Chen Z, et al. Structural characterization and biological activities of a novel polysaccharide from cultured Cordyceps militaris and its sulfated derivative. J Agric Food Chem. (2015) 63:3464–71. doi: 10.1021/jf505915t
36. Wu DT, Cheong KL, Wang LY, Lv GP, Ju YJ, Feng K, et al. Characterization and discrimination of polysaccharides from different species of Cordyceps using saccharide mapping based on PACE and HPTLC. Carbohydr Polym. (2014) 103:100–9. doi: 10.1016/j.carbpol.2013.12.034
37. Xiao K, Han Y, Yang H, Lu H, Tian Z. Mass spectrometry-based qualitative and quantitative N-glycomics: an update of 2017-2018. Anal Chim Acta. (2019) 1091:1–22. doi: 10.1016/j.aca.2019.10.007
38. Zhou S, Dong X, Veillon L, Huang Y, Mechref Y. LC-MS/MS analysis of permethylated N-glycans facilitating isomeric characterization. Anal Bioanal Chem. (2017) 409:453–66. doi: 10.1007/s00216-016-9996-8
39. Domon B, Costello CE. A systematic nomenclature for carbohydrate fragmentations in FAB-MS/MS spectra of glycoconjugates. Glycoconjugate J. (1988) 5:397–409. doi: 10.1007/bf01049915
40. Wu J, Wei J, Chopra P, Boons GJ, Lin C, Zaia J. Sequencing heparan sulfate using HILIC LC-NETD-MS/MS. Anal Chem. (2019) 91:11738–46. doi: 10.1021/acs.analchem.9b02313
41. Pismennõi D, Kiritsenko V, Marhivka J, Kütt ML, Vilu R. Development and optimisation of HILIC-LC-MS method for determination of carbohydrates in fermentation samples. Molecules. (2021) 26:3669. doi: 10.3390/molecules26123669
42. Mustafa S, Mobashir M. LC-MS and docking profiling reveals potential difference between the pure and crude fucoidan metabolites. Int J Biol Macromol. (2020) 143:11–29. doi: 10.1016/j.ijbiomac.2019.11.232
43. Lin X, Xiao C, Ling L, Guo L, Guo X. A dual-mode reactive matrix for sensitive and quantitative analysis of carbohydrate by MALDI-TOF MS. Talanta. (2021) 235:122792. doi: 10.1016/j.talanta.2021.122792
44. Zhan L, Huang X, Xue J, Liu H, Xiong C, Wang J, et al. MALDI-TOF/TOF tandem mass spectrometry imaging reveals non-uniform distribution of disaccharide isomers in plant tissues. Food Chem. (2021) 338:127984. doi: 10.1016/j.foodchem.2020.127984
45. Liu QB, Lu JG, Jiang ZH, Zhang W, Li WJ, Qian ZM, et al. In situ chemical profiling and imaging of cultured and natural Cordyceps sinensis by TOF-SIMS. Front Chem. (2022) 10:862007. doi: 10.3389/fchem.2022.862007
46. Du H, Yu H, Yang F, Li Z. Comprehensive analysis of glycosphingolipid glycans by lectin microarrays and MALDI-TOF mass spectrometry. Nat Protoc. (2021) 16:3470–91. doi: 10.1038/s41596-021-00544-y
47. Yang S, Yang X, Zhang H. Extracellular polysaccharide biosynthesis in Cordyceps. Crit Rev Microbiol. (2020) 46:359–80. doi: 10.1080/1040841X.2020.1794788
48. Wang CC, Wu JY, Chang CY, Yu ST, Liu YC. Enhanced exopolysaccharide production by Cordyceps militaris using repeated batch cultivation. J Biosci Bioeng. (2019) 127:499–505. doi: 10.1016/j.jbiosc.2018.09.006
49. Huang SJ, Huang FK, Li YS, Tsai SY. The quality improvement of solid-state fermentation with Cordyceps militaris by UVB irradiation. Food Technol Biotechnol. (2017) 55:445–53. doi: 10.17113/ftb.55.04.17.5235
50. Huang SJ, Huang FK, Purwidyantri A, Rahmandita A, Tsai SY. Effect of pulsed light irradiation on bioactive, nonvolatile components and antioxidant properties of Caterpillar medicinal mushroom Cordyceps militaris (Ascomycetes). Int J Med Mushrooms. (2017) 19:547–60. doi: 10.1615/IntJMedMushrooms.v19.i6.60
51. Cui JD, Zhang YN. Evaluation of metal ions and surfactants effect on cell growth and exopolysaccharide production in two-stage submerged culture of Cordyceps militaris. Appl Biochem Biotechnol. (2012) 168:1394–404. doi: 10.1007/s12010-012-9865-7
52. Lin S, Liu ZQ, Baker PJ, Yi M, Wu H, Xu F, et al. Enhancement of cordyceps polysaccharide production via biosynthetic pathway analysis in Hirsutella sinensis. Int J Bio Macromol. (2016) 92:872–80. doi: 10.1016/j.ijbiomac.2016.08.002
53. Zeng Y, Han Z, Qiu P, Zhou Z, Tang Y, Zhao Y, et al. Salinity-induced anti-Angiogenesis activities and structural changes of the polysaccharides from cultured Cordyceps militaris. PLoS One. (2014) 9:e103880. doi: 10.1371/journal.pone.0103880
54. Zhang X, Zhang X, Gu S, Pan L, Sun H, Gong E, et al. Structure analysis and antioxidant activity of polysaccharide-iron (III) from Cordyceps militaris mycelia. Int J Biol Macromol. (2021) 178:170–9. doi: 10.1016/j.ijbiomac.2021.02.163
55. Liu Y, Li Y, Zhang H, Li C, Zhang Z, Liu A, et al. Polysaccharides from Cordyceps miltaris cultured at different pH: Sugar composition and antioxidant activity. Int J Biol Macromol. (2020) 162:349–58. doi: 10.1016/j.ijbiomac.2020.06.182
56. Wang Y, Yang X, Chen P, Yang S, Zhang H. Homologous overexpression of genes in Cordyceps militaris improves the production of polysaccharides. Food Res Int. (2021) 147:110452. doi: 10.1016/j.foodres.2021.110452
57. Luo L, Zhou J, Xu Z, Guan J, Gao Y, Zou X. Identification and functional analysis of bacteria in sclerotia of Cordyceps militaris. Peer J. (2021) 9:e12511. doi: 10.7717/peerj.12511
58. Tong LL, Wang Y, Yuan L, Liu MZ, Du YH, Mu XY, et al. Enhancement of polysaccharides production using microparticle enhanced technology by Paraisaria dubia. Microb Cell Fact. (2022) 21:12. doi: 10.1186/s12934-021-01733-w
59. Sun H, Yu X, Li T, Zhu Z. Structure and hypoglycemic activity of a novel exopolysaccharide of Cordyceps militaris. Int J Biol Macromol. (2021) 166:496–508. doi: 10.1016/j.ijbiomac.2020.10.207
60. Song Q, Zhu Z. Chemical structure and mechanism of polysaccharide on Pb(2+) tolerance of Cordyceps militaris after Pb(2+) domestication. Int J Biol Macromol. (2020) 165:958–69. doi: 10.1016/j.ijbiomac.2020.09.243
61. Yu Y, Wen Q, Song A, Liu Y, Wang F, Jiang B. Isolation and immune activity of a new acidic Cordyceps militaris exopolysaccharide. Int J Biol Macromol. (2022) 194:706–14. doi: 10.1016/j.ijbiomac.2021.11.115
62. Lee JS, Kwon JS, Won DP, Lee JH, Lee KE, Lee SY, et al. Study of macrophage activation and structural characteristics of purified polysaccharide from the fruiting body of Cordyceps militaris. J Microbiol Biotechnol. (2010) 20:1053–60. doi: 10.4014/jmb.0910.10022
63. Liu F, Zhu ZY, Sun X, Gao H, Zhang YM. The preparation of three selenium-containing Cordyceps militaris polysaccharides: characterization and anti-tumor activities. Int J Biol Macromol. (2017) 99:196–204. doi: 10.1016/j.ijbiomac.2017.02.064
64. Liu XC, Zhu ZY, Liu YL, Sun HQ. Comparisons of the anti-tumor activity of polysaccharides from fermented mycelia and cultivated fruiting bodies of Cordyceps militaris in vitro. Int J Biol Macromol. (2019) 130:307–14. doi: 10.1016/j.ijbiomac.2019.02.155
65. Wu L, Sun H, Hao Y, Zheng X, Song Q, Dai S, et al. Chemical structure and inhibition on alpha-glucosidase of the polysaccharides from Cordyceps militaris with different developmental stages. Int J Biol Macromol. (2020) 148:722–36. doi: 10.1016/j.ijbiomac.2020.01.178
66. Wu CY, Liang ZC, Tseng CY, Hu SH. Effects of illumination pattern during cultivation of fruiting body and bioactive compound production by the Caterpillar medicinal mushroom, Cordyceps militaris (Ascomycetes). Int J Med Mushrooms. (2016) 18:589–97. doi: 10.1615/intjmedmushrooms.v18.i7.40
67. Lin LZ, Wei T, Yin L, Zou Y, Bai WF, Ye ZW, et al. An efficient strategy for enhancement of bioactive compounds in the fruit body of Caterpillar medicinal mushroom, Cordyceps militaris (Ascomycetes), by spraying biotic elicitors. Int J Med Mushrooms. (2020) 22:1161–70. doi: 10.1615/IntJMedMushrooms.2020037155
68. Zhu L, Zhang H, Liu Y, Zhang JS, Gao X, Tang Q. Effect of culture time on the bioactive components in the fruit bodies of Caterpillar mushroom, Cordyceps militaris CM-H0810 (Ascomycetes). Int J Med Mushrooms. (2019) 21:1107–14. doi: 10.1615/IntJMedMushrooms.2019032704
69. Yoo CH, Sadat MA, Kim W, Park TS, Park DK, Choi J. Comprehensive transcriptomic analysis of Cordyceps militaris cultivated on germinated soybeans. Mycobiology. (2022) 50:1–11. doi: 10.1080/12298093.2022.2035906
70. Li LF, But GW, Zhang QW, Liu M, Chen MM, Wen X, et al. A specific and bioactive polysaccharide marker for Cordyceps. Carbohydr Polym. (2021) 269:118343. doi: 10.1016/j.carbpol.2021.118343
71. He BL, Zheng QW, Guo LQ, Huang JY, Yun F, Huang SS, et al. Structural characterization and immune-enhancing activity of a novel high-molecular-weight polysaccharide from Cordyceps militaris. Int J Biol Macromol. (2020) 145:11–20. doi: 10.1016/j.ijbiomac.2019.12.115
72. Jing Y, Cui X, Chen Z, Huang L, Song L, Liu T, et al. Elucidation and biological activities of a new polysaccharide from cultured Cordyceps militaris. Carbohydr Polym. (2014) 102:288–96. doi: 10.1016/j.carbpol.2013.11.061
73. Zhu L, Tang Q, Zhou S, Liu Y, Zhang Z, Gao X, et al. Isolation and purification of a polysaccharide from the caterpillar medicinal mushroom Cordyceps militaris (Ascomycetes) fruit bodies and its immunomodulation of RAW 264.7 macrophages. Int J Med Mushrooms. (2014) 16:247–57. doi: 10.1615/intjmedmushr.v16.i3.50
74. Smiderle FR, Sassaki GL, Van Griensven LJ, Iacomini M. Isolation and chemical characterization of a glucogalactomannan of the medicinal mushroom Cordyceps militaris. Carbohydr Polym. (2013) 97:74–80. doi: 10.1016/j.carbpol.2013.04.049
75. Bi S, Jing Y, Zhou Q, Hu X, Zhu J, Guo Z, et al. Structural elucidation and immunostimulatory activity of a new polysaccharide from Cordyces militaris. Food Funct. (2018) 9:279–93. doi: 10.1039/c7fo01147d
76. Smiderle FR, Baggio CH, Borato DG, Santana-Filho AP, Sassaki GL, Iacomini M, et al. Anti-inflammatory properties of the medicinal mushroom Cordyceps militaris might be related to its linear (13)-beta-D-glucan. PLoS One. (2014) 9:e110266. doi: 10.1371/journal.pone.0110266
77. Zhao H, Lai Q, Zhang J, Huang C, Jia L. Antioxidant and hypoglycemic effects of acidic-extractable polysaccharides from Cordyceps militaris on type 2 diabetes mice. Oxid Med Cell Longev. (2018) 2018:9150807. doi: 10.1155/2018/9150807
78. Xu L, Wang F, Zhang Z, Terry N. Optimization of polysaccharide production from Cordyceps militaris by solid-state fermentation on rice and its antioxidant activities. Foods. (2019) 8:590. doi: 10.3390/foods8110590
79. Wu F, Yan H, Ma X, Jia J, Zhang G, Guo X, et al. Comparison of the structural characterization and biological activity of acidic polysaccharides from Cordyceps militaris cultured with different media. World J Microbiol Biotechnol. (2012) 28:2029–38. doi: 10.1007/s11274-012-1005-6
80. Chen X, Wu G, Huang Z. Structural analysis and antioxidant activities of polysaccharides from cultured Cordyceps militaris. Int J Biol Macromol. (2013) 58:18–22. doi: 10.1016/j.ijbiomac.2013.03.041
81. Zhu ZY, Liu F, Cao H, Sun H, Meng M, Zhang YM. Synthesis, characterization and antioxidant activity of selenium polysaccharide from Cordyceps militaris. Int J Biol Macromol. (2016) 93:1090–9. doi: 10.1016/j.ijbiomac.2016.09.076
82. Dong JZ, Lei C, Ai XR, Wang Y. Selenium enrichment on Cordyceps militaris link and analysis on its main active components. Appl Biochem Biotechnol. (2012) 166:1215–24. doi: 10.1007/s12010-011-9506-6
83. Song Q, Zhu Z. Using Cordyceps militaris extracellular polysaccharides to prevent Pb2+-induced liver and kidney toxicity by activating Nrf2 signals and modulating gut microbiota. Food Funct. (2020) 11:9226–39. doi: 10.1039/d0fo01608j
84. Liu JY, Feng CP, Li X, Chang MC, Meng JL, Xu LJ. Immunomodulatory and antioxidative activity of Cordyceps militaris polysaccharides in mice. Int J Biol Macromol. (2016) 86:594–8. doi: 10.1016/j.ijbiomac.2016.02.009
85. Lin RK, Choong CY, Hsu WH, Tai CJ, Tai CJ. Polysaccharides obtained from mycelia of Cordyceps militaris attenuated doxorubicin-induced cytotoxic effects in chemotherapy. Afr Health Sci. (2019) 19:2156–63. doi: 10.4314/ahs.v19i2.40
86. Lee JS, Kwon DS, Lee KR, Park JM, Ha SJ, Hong EK. Mechanism of macrophage activation induced by polysaccharide from Cordyceps militaris culture broth. Carbohydr Polym. (2015) 120:29–37. doi: 10.1016/j.carbpol.2014.11.059
87. Liu N, Zhang B, Sun Y, Song W, Guo S. Macrophage origin, phenotypic diversity, and modulatory signaling pathways in the atherosclerotic plaque microenvironment. Vessel Plus. (2021) 5:43. doi: 10.20517/2574-1209.2021.25
88. Zhang Q, Liu M, Li L, Chen M, Puno PT, Bao W, et al. Cordyceps polysaccharide marker CCP modulates immune responses via highly selective TLR4/MyD88/p38 axis. Carbohydr Polym. (2021) 271:118443. doi: 10.1016/j.carbpol.2021.118443
89. Kim HS, Kim JY, Kang JS, Kim HM, Kim YO, Hong IP, et al. Cordlan polysaccharide isolated from mushroom Cordyceps militaris induces dendritic cell maturation through toll-like receptor 4 signaling. Food Chem Toxicol. (2010) 48:1926–33. doi: 10.1016/j.fct.2010.04.036
90. Ohta Y, Lee JB, Hayashi K, Fujita A, Park DK, Hayashi T. In vivo anti-influenza virus activity of an immunomodulatory acidic polysaccharide isolated from Cordyceps militaris grown on germinated soybeans. J Agric Food Chem. (2007) 55:10194–9. doi: 10.1021/jf0721287
91. Kwon HK, Jo WR, Park HJ. Immune-enhancing activity of C. militaris fermented with Pediococcus pentosaceus (GRC-ON89A) in CY-induced immunosuppressed model. BMC Complement Altern Med. (2018) 18:75. doi: 10.1186/s12906-018-2133-9
92. Zheng Y, Li L, Cai T. Cordyceps polysaccharide ameliorates airway inflammation in an ovalbumin-induced mouse model of asthma via TGF-beta1/Smad signaling pathway. Respir Physiol Neurobiol. (2020) 276:103412. doi: 10.1016/j.resp.2020.103412
93. Gu L, Yu T, Liu J, Lu Y. Evaluation of the mechanism of cordyceps polysaccharide action on rat acute liver failure. Arch Med Sci. (2020) 16:1218–25. doi: 10.5114/aoms.2020.94236
94. Yu M, Yue J, Hui N, Zhi Y, Hayat K, Yang X, et al. Anti-hyperlipidemia and gut microbiota community regulation effects of Selenium-rich Cordyceps militaris polysaccharides on the high-fat diet-fed mice model. Foods. (2021) 10:2252. doi: 10.3390/foods10102252
95. Thomas LV, Suzuki K, Zhao J. Probiotics: a proactive approach to health. A symposium report. Br J Nutr. (2015) 114:S1–15. doi: 10.1017/S0007114515004043
96. Lee BH, Chen CH, Hsu YY, Chuang PT, Shih MK, Hsu WH. Polysaccharides obtained from Cordyceps militaris alleviate hyperglycemia by regulating gut microbiota in mice fed a high-fat/sucrose diet. Foods. (2021) 10:1870. doi: 10.3390/foods10081870
97. Zheng H, Cao H, Zhang D, Huang J, Li J, Wang S, et al. Cordyceps militaris modulates intestinal barrier function and gut microbiota in a pig model. Front Microbiol. (2022) 13:810230. doi: 10.3389/fmicb.2022.810230
98. Wu GD, Pan A, Zhang X, Cai YY, Wang Q, Huang FQ, et al. Cordyceps improves obesity and its related inflammation via modulation of Enterococcus cecorum abundance and bile acid metabolism. Am J Chin Med. (2022) 10:1–22. doi: 10.1142/S0192415X22500343
99. Liu RM, Dai R, Luo Y, Xiao JH. Glucose-lowering and hypolipidemic activities of polysaccharides from Cordyceps taii in streptozotocin-induced diabetic mice. BMC Complement Altern Med. (2019) 19:230. doi: 10.1186/s12906-019-2646-x
100. Shang XL, Pan LC, Tang Y, Luo Y, Zhu ZY, Sun HQ, et al. (1)H NMR-based metabonomics of the hypoglycemic effect of polysaccharides from Cordyceps militaris on streptozotocin-induced diabetes in mice. Nat Prod Res. (2020) 34:1366–72. doi: 10.1080/14786419.2018.1516216
101. Guo SD, Cui YJ, Wang RZ, Wang RY, Wu WX, Ma T. Separation, purification and primary reverse cholesterol transport study of Cordyceps militaris polysaccharide. Zhongguo Zhong Yao Za Zhi. (2014) 39:3316–20.
102. Yu WQ, Yin F, Shen N, Lin P, Xia B, Li YJ, et al. Polysaccharide CM1 from Cordyceps militaris hinders adipocyte differentiation and alleviates hyperlipidemia in LDLR(+/-) hamsters. Lipids Health Dis. (2021) 20:178. doi: 10.1186/s12944-021-01606-6
103. Guo SD, Xia XD, Gu HM, Zhang DW. Proprotein convertase subtilisin/kexin-type 9 and lipid metabolism. Adv Exp Med Biol. (2020) 1276:137–56. doi: 10.1007/978-981-15-6082-8_9
104. Dao MC, Everard A, Aron-Wisnewsky J, Sokolovska N, Prifti E, Verger EO, et al. Akkermansia muciniphila and improved metabolic health during a dietary intervention in obesity: relationship with gut microbiome richness and ecology. Gut. (2016) 65:426–36. doi: 10.1136/gutjnl-2014-308778
105. Ciecierska A, Drywień ME, Hamulka J, Sadkowski T. Nutraceutical functions of beta-glucans in human nutrition. Rocz Panstw Zakl Hig. (2019) 70:315–24. doi: 10.32394/rpzh.2019.0082
106. Korolenko TA, Bgatova NP, Ovsyukova MV, Shintyapina A, Vetvicka V. Hypolipidemic effects of β-glucans, mannans, and fucoidans: mechanism of action and their prospects for clinical application. Molecules. (2020) 25:1819. doi: 10.3390/molecules25081819
107. Yin F, Lin P, Yu WQ, Shen N, Li Y, Guo SD. The Cordyceps militaris-derived polysaccharide CM1 alleviates atherosclerosis in LDLR(-/-) mice by improving hyperlipidemia. Front Mol Biosci. (2021) 8:783807. doi: 10.3389/fmolb.2021.783807
108. Tokgözoğlu L, Libby P. The dawn of a new era of targeted lipid-lowering therapies. Eur Heart J. (2022) 2022:ehab841. doi: 10.1093/eurheartj/ehab841
109. Crea F. An update on triglyceride-rich lipoprotein and their remnants in atherosclerotic cardiovascular disease. Eur Heart J. (2021) 42:4777–80. doi: 10.1093/eurheartj/ehab844
110. Zhu ZY, Guo MZ, Liu F, Luo Y, Chen L, Meng M, et al. Preparation and inhibition on alpha-d-glucosidase of low molecular weight polysaccharide from Cordyceps militaris. Int J Biol Macromol. (2016) 93:27–33. doi: 10.1016/j.ijbiomac.2016.08.058
111. Ren YY, Sun PP, Ji YP, Wang XT, Dai SH, Zhu ZY. Carboxymethylation and acetylation of the polysaccharide from Cordyceps militaris and their alpha-glucosidase inhibitory activities. Nat Prod Res. (2020) 34:369–77. doi: 10.1080/14786419.2018.1533830
112. Chen DD, Xu R, Zhou JY, Chen JQ, Wang L, Liu XS, et al. Cordyceps militaris polysaccharides exerted protective effects on diabetic nephropathy in mice via regulation of autophagy. Food Funct. (2019) 10:5102–14. doi: 10.1039/c9fo00957d
113. Ji C, Zhang Z, Zhang B, Chen J, Liu R, Song D, et al. Purification, characterization, and in vitro antitumor activity of a novel glucan from the purple sweet potato Ipomoea Batatas (L.) Lam. Carbohydr Polym. (2021) 257:117605. doi: 10.1016/j.carbopol.2020.117605
114. Bi S, Huang W, Chen S, Huang C, Li C, Guo Z, et al. Cordyceps militaris polysaccharide converts immunosuppressive macrophages into M1-like phenotype and activates T lymphocytes by inhibiting the PD-L1/PD-1 axis between TAMs and T lymphocytes. Int J Biol Macromol. (2020) 150:261–80. doi: 10.1016/j.ijbiomac.2020.02.050
115. Zhong L, Zhao L, Yang F, Yang W, Sun Y, Hu Q. Evaluation of anti-fatigue property of the extruded product of cereal grains mixed with Cordyceps militaris on mice. J Int Soc Sports Nutr. (2017) 14:15. doi: 10.1186/s12970-017-0171-1
116. Xu YF. Effect of polysaccharide from Cordyceps militaris (Ascomycetes) on physical fatigue induced by forced swimming. Int J Med Mushrooms. (2016) 18:1083–92. doi: 10.1615/IntJMedMushrooms.v18.i12.30
117. Ma L, Zhang S, Yuan Y, Gao J. Hypouricemic actions of exopolysaccharide produced by Cordyceps militaris in potassium oxonate-induced hyperuricemic mice. Curr Microbiol. (2014) 69:852–7. doi: 10.1007/s00284-014-0666-9
118. Mao YH, Song AX, Wang ZM, Yao ZP, Wu JY. Protection of Bifidobacterial cells against antibiotics by a high molecular weight exopolysaccharide of a medicinal fungus Cs-HK1 through physical interactions. Int J Biol Macromol. (2018) 119:312–9. doi: 10.1016/j.ijbiomac.2018.07.122
119. Omak G, Yilmaz-Ersan L. Effect of Cordyceps militaris on formation of short-chain fatty acids as postbiotic metabolites. Prep Biochem Biotechnol. (2022) 22:1–9. doi: 10.1080/10826068.2022.2033992
Keywords: Cordyceps militaris, polysaccharide, structure-activity relationship, bioactivity, mechanisms of action
Citation: Miao M, Yu W-Q, Li Y, Sun Y-L and Guo S-D (2022) Structural Elucidation and Activities of Cordyceps militaris-Derived Polysaccharides: A Review. Front. Nutr. 9:898674. doi: 10.3389/fnut.2022.898674
Received: 17 March 2022; Accepted: 02 May 2022;
Published: 31 May 2022.
Edited by:
A. M. Abd El-Aty, Cairo University, EgyptReviewed by:
Abdul Rehman Phull, Shah Abdul Latif University, PakistanKebede Taye Desta, National Agrobiodiversity Center, South Korea
Copyright © 2022 Miao, Yu, Li, Sun and Guo. This is an open-access article distributed under the terms of the Creative Commons Attribution License (CC BY). The use, distribution or reproduction in other forums is permitted, provided the original author(s) and the copyright owner(s) are credited and that the original publication in this journal is cited, in accordance with accepted academic practice. No use, distribution or reproduction is permitted which does not comply with these terms.
*Correspondence: Yan-Long Sun, ODQwOTE1NjU3QHFxLmNvbQ==; Shou-Dong Guo, U0QtR1VPQGhvdG1haWwuY29t