- 1Shanghai Key Laboratory of Embryo Original Diseases, The International Peace Maternity and Child Health Hospital, Shanghai Jiao Tong University School of Medicine, Shanghai, China
- 2MOE-Shanghai Key Lab of Children's Environmental Health, Xinhua Hospital, Shanghai Jiao Tong University School of Medicine, Shanghai, China
Vitamin A is essential for brain function, in addition to its important roles in vision, immunity, and reproduction. Previous studies have shown that retinoic acid (RA), the bioactive form of vitamin A, is involved in the regulation of various intracellular responses related to biological rhythms. RA is reported to affect the circadian rhythm by binding to RA receptors, such as receptors in the circadian feedback loops in the mammalian suprachiasmatic nucleus. However, evidence of the impacts of vitamin A deficiency (VAD) on biological rhythms is limited, and most of the related studies were conducted on animals. In this review, we described the physiological functions of biological rhythms and physiological pathways/molecular mechanisms regulating the biological rhythms. We then discussed the current understanding of the associations of VAD with biological rhythm disorders/diseases (sleep disorders, impairments in learning/memory, emotional disorders, and other immune or metabolism diseases) and summarized the currently proposed mechanisms (mainly by retinoid nuclear receptors and related proteins) for the associations. This review may help recognize the role of VAD in biological rhythm disorders and stimulate clinical or epidemiological studies to confirm the findings of related animal studies.
Vitamin A derivatives and signaling
Vitamin A is the first discovered fat-soluble vitamin and is primarily found in animal products or converted from dietary carotenoids in plant products. It is not a single compound but a group of derivatives including retinol, retinal, retinoic acid (RA), and some carotenoids according to different terminal functional groups. In general, vitamin A refers to retinol, while retinoid refers to a general term that includes vitamin A metabolites and compounds and exhibits vitamin A-like biological activity (1). Preformed vitamin A (usually from animal products) and provitamin A (including beta-carotene, usually from plant-derived food) are the two forms of vitamin A in the human diet. After being absorbed in the intestines, these two forms of vitamin A are converted to retinol and then oxidized to form retinal and RA to support the biological functions of vitamin A. The retinyl ester is the storage form of vitamin A in the liver and must be converted to retinol before being utilized, and these vitamin A derivatives are finally metabolized by CYP26 family enzymes (2, 3) (Figure 1). RA has several stereo-isomeric forms, including all-trans RA (ATRA), 13-cis RA, and 9-cis RA, and isomerization occurs under certain conditions (4). Together with its derivatives, vitamin A is involved in regulating diverse life activities, including cell proliferation and differentiation, vision, reproduction, embryogenesis, and immune function (5). Retinoic acid receptors (RARs) and retinoid X receptors (RXRs) are the two families of retinoid nuclear receptors (RNRs), and each family has three identified isotypes, namely, α, β, and γ (6). ATRA binds only to RARs, while 9-cis RA is the main ligand for RXRs and also has a binding affinity for RARs [Until recently, 9-cis RA is reported to be only detected in the pancreas (7, 8)]. RAR-RXR heterodimers form a functional structure that allows them to regulate gene expression. By binding to specific retinoic acid response elements (RAREs), RAR-RXR heterodimers can regulate downstream gene expression (9). When RA binds to RARs, the RAR-RXR dimers release the co-repressor proteins and activate downstream gene expression (10). However, when RA signals are not present, RAR/RXR dimers bind to co-repressors and recruit inhibitors to suppress the expression of downstream genes. Retinoid-related orphan receptors (RORs) belong to the ROR subfamily. Similar to RARs and RXRs in the gene sequence, RORs also have three isotypes, namely, α, β, and γ (11), and the endogenous ligands for RORs have not yet been confirmed. In addition to ATRA, substances confirmed to bind RORs include steroids, terpenoids, polyketides, and cardiac glycosides (12, 13). Since involved in related physiological functions, RORs may be potential targets in the treatment of biological rhythm disorders and related metabolic diseases (11, 14, 15).
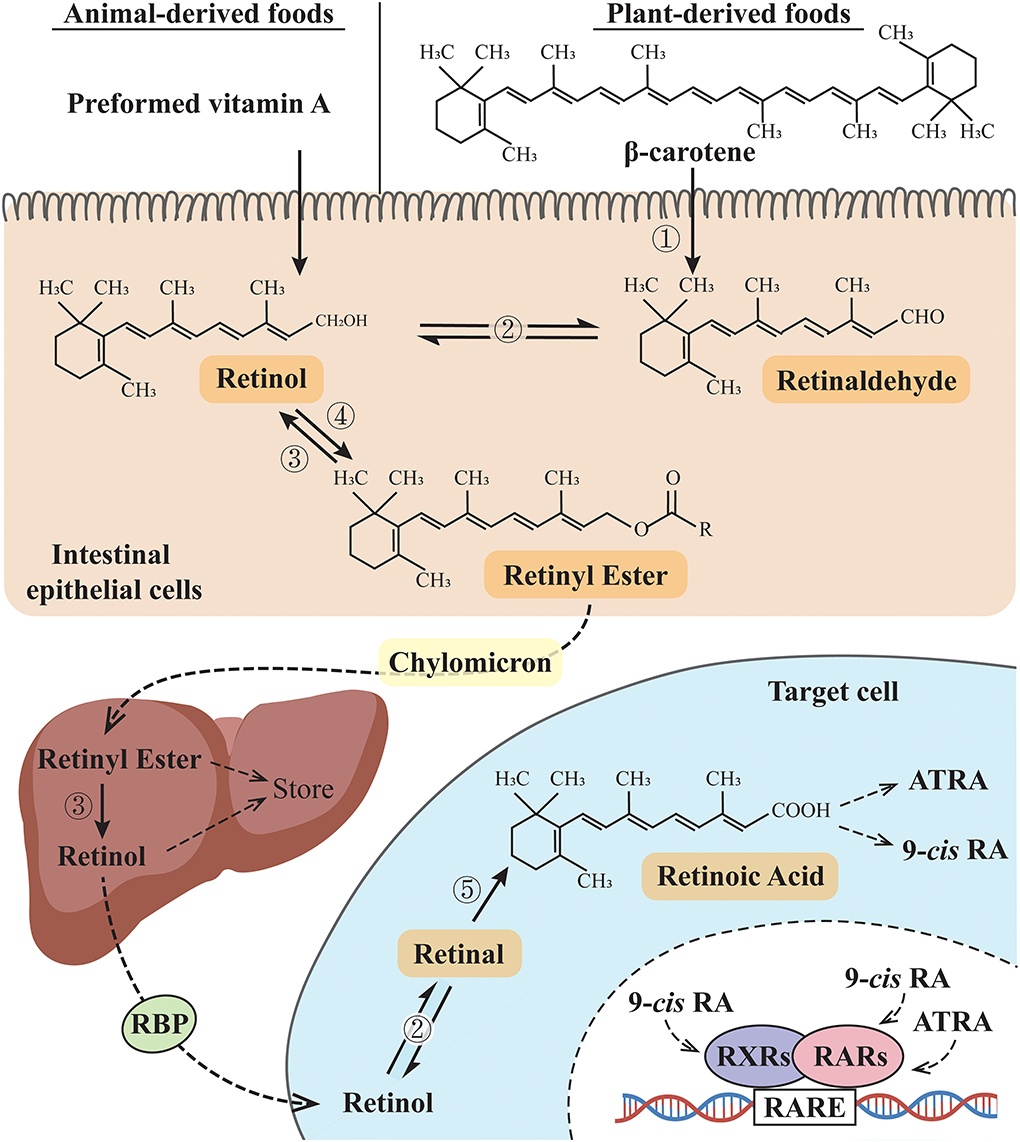
Figure 1. Vitamin A metabolic pathway. ➀ β-Carotene 15,15'-dioxygenases; ➁ Retinol dehydrogenases (RDHs): RDH1, RDH10, and short-chain dehydrogenase/reductase (SDR family) member 9 (DHRS9) (reversible reaction); ➂ retinyl esterase; ➃ lecithin: retinol acyltransferase (LRAT) or acyl-CoA: retinol acyltransferase (ARAT); ➄ retinaldehyde dehydrogenases (RALDH). RBP, retinol-binding protein; 9-cis RA: 9-cis retinoic acid; ATRA, all-trans retinoic acid; RXRs, retinoid X receptors; RARs, retinoic acid receptors; RARE, retinoic acid response elements.
Although vitamin A is present in a variety of foods, vitamin A deficiency (VAD) is still a problem worthy of attention in underdeveloped countries and regions in the world (16, 17). VAD is associated with an abnormal RA signaling pathway (18–20); for instance, the expression of RAR and RXR proteins decreased in rats or mice fed with a VAD diet (21–23). Animal studies also showed that prenatal VAD led to decreased RARα and RARβ expression, while vitamin A supplementation induced increased expression of RARβ (24). However, another animal study reported that in the hippocampus of VAD rats, the RARβ expression decreased, while the RARα expression increased (25).
Physiological functions of biological rhythms
Biological rhythms regulate various physiological, biochemical, and behavioral processes in living organisms. As an invisible “timer”, biological rhythms can influence daily behavioral or physiological cycles, such as the sleep–wake cycle, body temperature, and blood pressure. The circadian rhythm, which is the most well-known biological rhythm, has been widely studied.
In mammals, the circadian system can be divided into the central clock and the peripheral clock. The central clock is controlled by the suprachiasmatic nucleus (SCN) in the anterior part of the hypothalamus directly above the optic chiasm bilateral to the third ventricle (26), producing a central rhythm. The spatiotemporal single-cell analysis revealed eight major cell types (each with a specific pattern of circadian gene expression) and five subtypes of SCN neurons (each with specific combinations of molecules) in the mouse SCN, displaying different circadian rhythmicity and light responsiveness (27). Intercellular coupling mediated by neuropeptides helps produce and maintain synchronous oscillation (28). The peripheral clock is composed of peripheral oscillators located in various peripheral tissues. Interestingly, the peripheral circadian clock contains similar molecular regulation mechanisms as the central circadian clock and is the basis for the normal functioning of various peripheral tissues (29). The central rhythm from the central clock can drive or synchronize the peripheral rhythm through neuro-humoral pathways, while the peripheral rhythm can, in turn, affect and regulate the central rhythm through periodic activities such as daily diet and sleep (30). In addition, the peripheral rhythm retains a certain degree of autonomy (31). As a pacemaker of central rhythm, the SCN can keep its own rhythm. Besides, the SCN can also make responses and changes under the influence of the external environment, such as light, food intake, and social activities, so that individuals can adapt to the external environment (32–34). Furthermore, SCN neurons synthesize GABA, vasopressin, vasoactive intestinal peptides, and other signaling molecules, which directly or indirectly regulate the circadian release of hormones, including melatonin and corticotropin-releasing factor (CRF), to bring the central rhythm information to peripheral tissues. This provides the possibility for the association between biological rhythm dysfunction and related disorders/diseases (35, 36). The coordination and interaction between the central and peripheral rhythms are vital for maintaining normal physiological functions. Disturbances in the central or peripheral rhythm can lead to a wide range of diseases (37, 38).
Roles of vitamin A in regulating biological rhythms
Relationships between vitamin A and biological rhythms
The associations between vitamin A and biological rhythms were shown to occur in different organisms such as insects, birds, and mammals (citations in Table 1). Vitamin A may affect biological rhythms, including the central rhythm produced by the SCN and peripheral cell rhythms. Evidence of the effects of VAD on biological rhythms is limited, and all related studies used nonhuman animals. A recent study on monarch butterflies induced CRISPR/CRISPR-associated protein 9-mediated targeted mutagenesis and found that vitamin A in the brain, rather than in the compound eye, functioned in photoperiod responsiveness and could link the photoperiod cycle with a seasonal response (39). Therefore, the impact on the central rhythm has become a key issue when exploring the effect of vitamin A on biological rhythms. Sherman et al. reported that with the supplementation of ATRA, the amplitude and time phase of the rhythmic gene expression in mouse liver cells were significantly changed (40), suggesting a role of vitamin A signaling in peripheral rhythms. However, Shirai et al. reported that the expression patterns of clock genes in liver cells of VAD mice remained unchanged, indicating that vitamin A may not be essential for maintaining the peripheral rhythm in mammals (41).
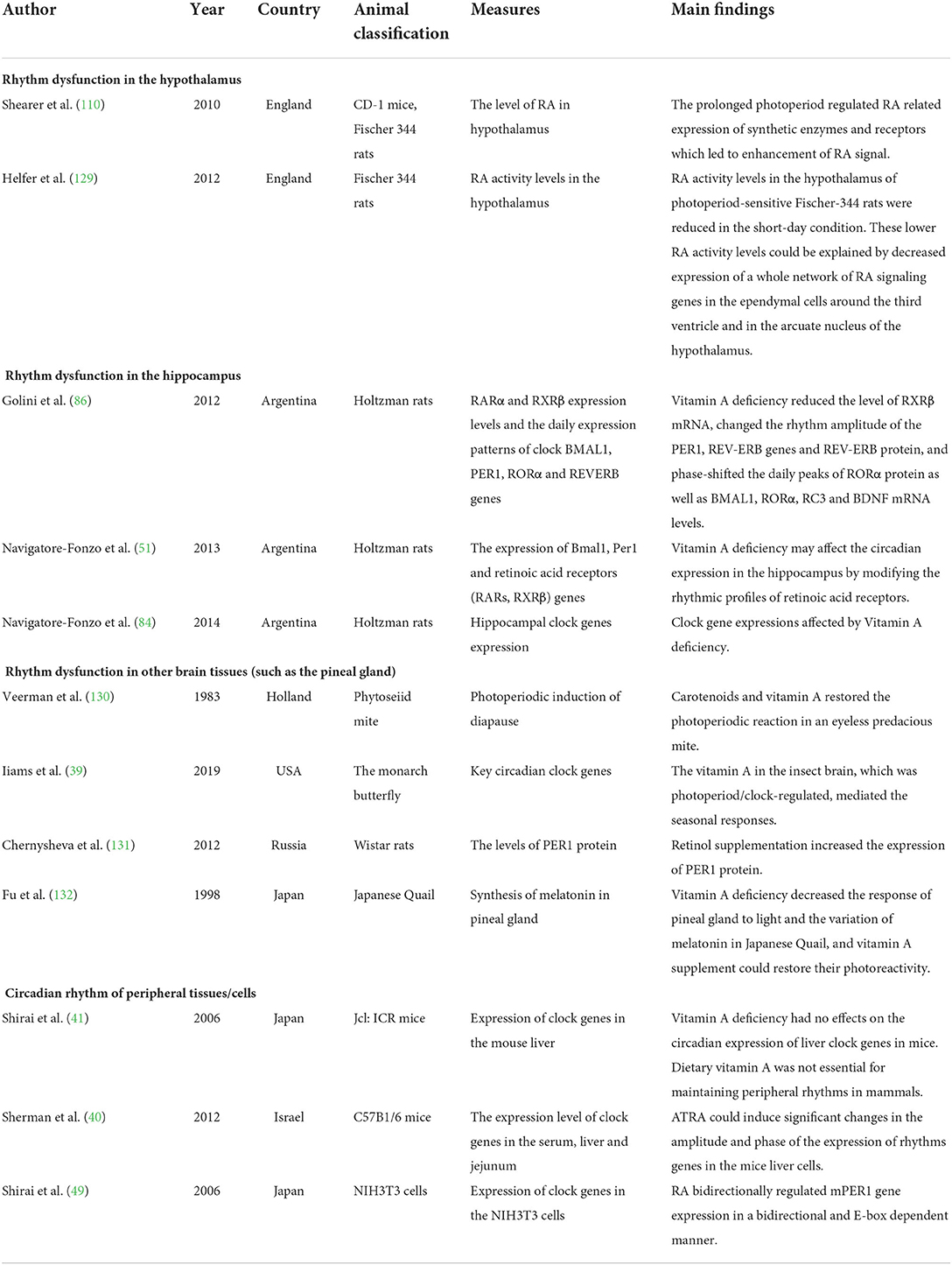
Table 1. Animal studies on the association of vitamin A deficiency with biological rhythm dysfunctions.
Although only animal studies have directly focused on the effects of VAD on biological rhythms, human studies have also explored the possibility of the correlation between vitamin A or carotenoids and biological rhythmicity or chronotype (but not directly on the effects of VAD, as shown in Table 2). Previous human studies reported that lower vitamin A intake levels were associated with disturbed wake–sleep cycles (42), and crocetin (a natural carotenoid compound) supplementation improved sleep time and quality (43–46). However, Asane et al. did not find differences in serum vitamin A levels between the groups with and without insomnia symptoms in adolescent girls (47). Therefore, more human studies are needed to confirm the relationship between VAD and biological rhythm disorders.
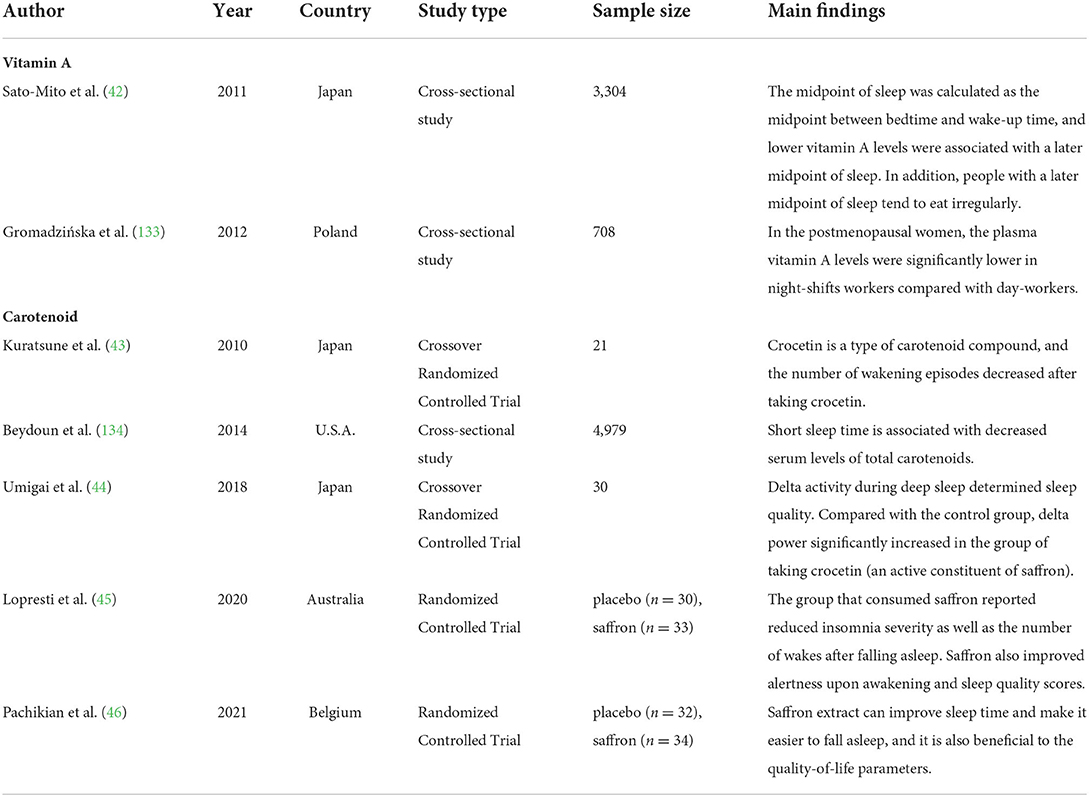
Table 2. Findings of epidemiological studies related to the associations of vitamin A or carotenoid with biological rhythms.
Molecular mechanisms underlying the associations of VAD with biorhythm disorders
Although the exact mechanisms regulating biological rhythms among different species are different, there are some common features.
Dysfunctions of the transcription–translation feedback loops (TTFLs, the molecular basis of biological rhythm regulation) induced by VAD
Various clock genes and TTFLs constitute the molecular basis of biological rhythm regulation in the SCN (48). In mammals, the main molecules involved in the TTFLs include heterodimeric circadian locomotor output cycles kaput (CLOCK), brain and muscle Arnt-like protein 1 (BMAL1), periods (PERs 1, 2, 3), cryptochrome (CRYs 1, 2), RORs (α, β, γ), reverse erythroblastosis virus (REV-ERBs α/β), and RARα/RXRα (40, 49–51). The binding of the E-box and CLOCK/BMAL1 dimers (1) can activate the gene expression of PERs and CRYs, and then the molecules of PERs and CRYs form the PER/CRY complex and inhibit the CLOCK/BMAL1 dimer-dependent gene expression. The feedback loop in which the genes of PERs and CRYs are involved is called the core loop and (2) can also activate the expression of RORs and orphan nuclear receptor REV-ERBs (52–54). The feedback loop in which RORs and REV-ERBs are involved is called the stabilizing loop. In the stabilizing loop, the accumulation of REV-ERBs and RORs has different effects on the expression of the BMAL1 gene, and the accumulation speeds of the REV-ERBs and RORs are also different. Specifically, the accumulation of REV-ERBs is much faster than that of the RORs (55). The RORs and REV-ERBs competitively bind to the ROR response element (RORE) in the upstream promoter region of the BMAL1 gene (11), and the accumulation of REV-ERB molecules can inhibit the expression of the BMAL1 gene, while the accumulated ROR molecules can activate the expression of the BMAL1 gene (49, 50, 56). With the combined effects of these molecules, the expression of the genes related to biological rhythms is therefore regulated at multiple levels (Figure 2).
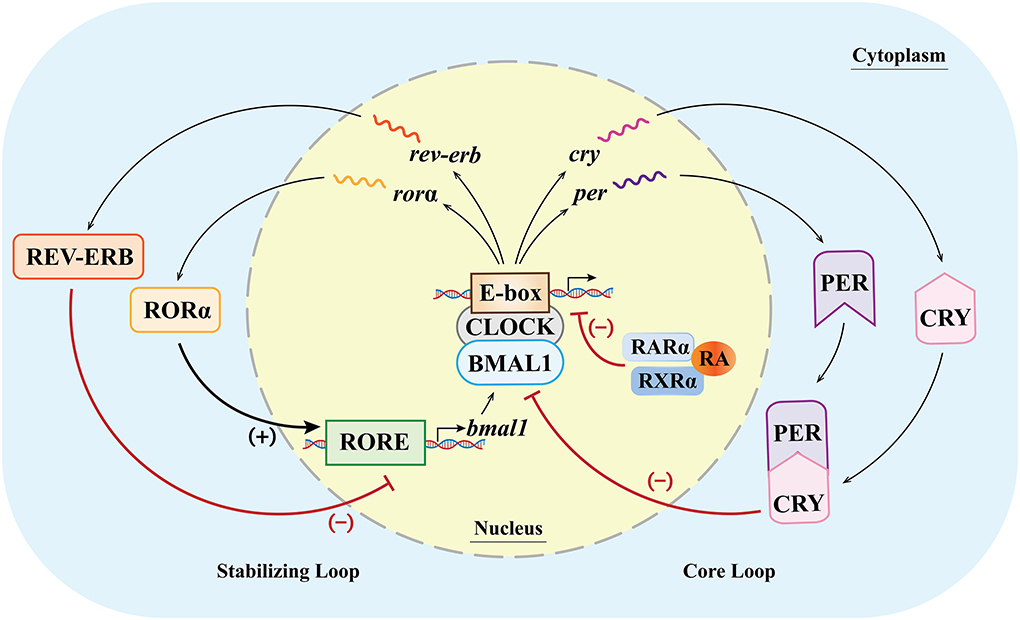
Figure 2. Clock genes and their transcription–translational feedback loops (TTFLs) at the cellular level (inside/outside the nucleus) in mammals. In the core loop, CLOCK and BMAL1 dimers bind to the E-box, promoting the expression of the Per and Cry genes and, in turn, inhibiting the CLOCK/BMAL1-dimer-dependent gene expression. In the stabilizing loop, the combination of the CLOCK/BMAL1 dimer and the E-box can activate the expression of RORα and REV-ERB. The accumulation of the REV-ERB molecule can inhibit the expression of the BMAL1 gene, while the accumulated RORα molecule can activate the expression of the BMAL1 gene (lower case italic characters and single wavy lines indicate mRNA). (+)/ → means activating gene expression; (–)/T means inhibiting gene expression.
Because of multiple factors in the core and stabilizing loops, the duration of periodic change is about 24 h, forming an endogenously driven oscillating biological rhythm (circadian rhythm). Recent studies have shown that numerous cell types in the body can express clock genes (57). For example, the rhythms of smooth muscle cells can be affected by the changes in the PER2 expression induced by RA (58). Therefore, the SCN acts as the primary circadian oscillator, influencing and regulating the rhythms of peripheral cells, and coordinating the central and peripheral rhythms (59).
Although the role of vitamin A in regulating biological rhythms remains unclear, some studies have found that VAD or vitamin A supplementation after deficiency may affect the expression of various biological clock genes through nuclear RA receptors and proteins, which may be the potential mechanisms underlying the effects of VAD on the biological rhythms. Navigatore-Fonzo et al. showed that VAD might affect the transcription and post-transcription processes by affecting the transcriptional regulation of CAT and GPx mediated by RARs or RXRs, or by affecting the formation and functions of the BMAL1/CLOCK heterodimer (60). Animal experiments showed that with or without the presence of RARα, ATRA could significantly downregulate or upregulate the expression of the mouse PER1 gene and other circadian rhythm genes in an E-box-dependent manner (49). The binding of ATRA/9-cis RA to RXRα/RARα prevents the BMAL1/CLOCK heterodimer from activating the transcription of PER2 (58). These studies suggested that vitamin A and its derivatives can bind to RARs and RXRs to inhibit E-box-dependent clock gene expression mediated by the BMAL1/CLOCK dimer, which is the core circadian rhythm regulation pathway in the SCN.
Retinoid-related orphan receptors play an important role in the regulation of the SCN rhythm and are closely related to the RA signaling pathway. Both RORα and RORβ showed a rhythmic expression pattern in the SCN, while RORγ was not detected in the SCN (61). Mice lacking RORα or RORβ showed an abnormal circadian rhythm, while mice lacking RORγ did not show an abnormal rhythm (56, 62, 63). Nevertheless, RORγ has been found in several peripheral organs, such as the liver, and it may play a role in the rhythm of peripheral tissues (64). Stehlin-Gaon et al. found that under certain circumstances, ATRA might reversibly bind to RORβ and activate the related gene expression (65), but whether this finding remained under other circumstances was queried by other studies (66). Considering the important roles of RORα and RORβ in the feedback loops of circadian rhythms and the extensive participation of RORα and RORs in various life activities, it may be favorable to consider the impacts of RORs in the relationship between vitamin A, circadian rhythm, and related diseases/disorders.
Changes in the light signal reception induced by VAD and related circadian rhythm molecules
Vitamin A is essential for the normal physiological functioning of the eyes, and VAD may affect biological rhythms by affecting the reception of light signals. The light cycle is the primary driver that affects biological rhythms (32, 67). The light signals received by the retina are transmitted to the SCN through a process of photoentrainment, whereby light adjusts the central rhythm produced by the SCN to adapt to the external light–dark cycle. The photoentrainment involves a type of intrinsically photosensitive retinal ganglion cells (ipRGCs), whose axons can be sent to the SCN, making the ipRGC a potential photoreceptor for synchronizing biological clock to the environmental day–light cycle (68–70). The retinal and RA are the two main active vitamin A metabolites in the human body. In addition to RA, the retinal formation will also be impaired in the case of VAD. 11-cis retinal is the basic structure of visual pigments, oxidized 11-cis retinal combines with opsin to form rhodopsin in rod cells (71). Evidence indicates that ipRGCs detect light with a vitamin A-based photopigment, melanopsin. Melanopsin is a protein homologous to rhodopsin, using 11-cis retinal as the light-absorbing chromophore. In the vision process, under the light condition, 11-cis retinal is isomerized into all-trans retinal and dissociates with opsin, then reduction of all-trans retinal to all-trans retinol occurs, and all-trans retinol is subsequently transported to the retinal pigment epithelium (RPE). In the RPE, RPE65 protein helps convert all-trans retinal to 11-cis retinal via the enzymatic steps (72). In RPE65-knockout mice, the sensitivity of the ipRGCs is significantly decreased, while the supplementation of exogenous 9-cis retinal, 11-cis retinal, and all-trans retinal can restore the photosensitivity of ipRGCs, indicating that the photosensitivity of the ipRGCs requires vitamin A-based chromophores (69, 73). In the absence of light, oxidized 11-cis retinal is generated again and quickly interacts with opsin to form rhodopsin. Thus, protein conformational changes in a light–dark cycle can convert light signals into electrical signals and transmit the signals to the brain (74). Therefore, retinal may be reduced and even lost in the light–dark cycle. However, the human body cannot synthesize vitamin A by itself, so the supplementation of exogenous vitamin A is needed to ensure the normal circulation process. Therefore, vision loss or night blindness is a significant symptom in VAD cases (75), and a further lack of vitamin A may disturb the entrainment of biological rhythms. In addition, because retinal and RA have different sites of action in the body, their effects on biological rhythms are different, but associations between their effects still remain unknown. In VAD cases, a lack of retinal affects the normal light reception and light entrainment process in the eye, thus affecting biological rhythms, while a lack of RA affects the expression levels of rhythm-related molecules in the SCN and related peripheral tissue cells, leading to rhythm-related disorders. Although targeting different tissues or organs, they seem to exert a comprehensive effect on biological rhythm dysfunctions under VAD.
In zebrafish, interphotoreceptor retinoid-binding protein (IRBP) mediates the transport of retinoids in photoreceptors and RPE cells (76), and IRBP is expressed periodically, making IRBP an intermediary molecule in the effects of vitamin A on biological rhythms (77). In addition, the light cycle is easily associated with the circadian rhythm of melatonin secretion in the pineal gland. The light signals received by the SCN are converted into hormone signals in the pineal gland to regulate the rhythms of the whole body (78). Vitamin A is essential for the normal functioning of the pineal gland, and the circadian synthesis of RA and its signal transduction components occur in the pineal gland (79).
Changes in circadian rhythm molecules in the hippocampus and pineal gland induced by VAD
The pineal gland is a special organ in the association of vitamin A with biological rhythms. In the pineal gland, RA synthesis and RA signal-related genes show circadian changes (80). The pineal gland contains high levels of retinol and retinol-binding protein (81). Animal studies have demonstrated that vitamin A and its analogs may have potential genomic and non-genomic effects on biological rhythm regulation in the pineal gland. The transcription of the arylalkylamine N-acetyltransferase (AANAT), encoding the rhythm-generating melatonin synthetic enzyme, was upregulated under long-term RA intervention (79), and the peaks of the rhythms of the AANAT activity and melatonin level in VAD rats decreased significantly at night (82). RA can rapidly downregulate the phosphorylation of ERK 1/2 in the pineal gland of rats, to quickly regulate the expression of related genes and adapt to the real-time changing biological rhythms via non-genomic effects (79). Vitamin A is necessary for the process of the transmission of rhythmic signals from the SCN to the pineal gland and induces melatonin secretion (83), and the disturbance in this process may be one of the mechanisms underlying the rhythm dysfunction induced by VAD.
As a peripheral rhythm oscillator, the hippocampus has its own rhythm while receiving signals of the central rhythm regulation from the SCN. Animal experiments confirmed that dietary VAD could affect the circadian rhythm and the expression of endogenous rhythm genes related to exercise in the rat hippocampus, changing the circadian rhythm of RAR expression, thereby altering the circadian gene expression rhythms of PER2, CRY1, and CRY2 (84). Another study showed that the circadian expression rhythm patterns of RORα and REV-ERB in the hippocampus of VAD rats changed (85), indicating that vitamin A concentrations had effects on the expression levels of RORα and REV-ERBα in the hippocampus. The underlying mechanism may be that RARE has been found in the regulatory regions of the BMAL1 and RORα, and a decrease in RARs and RXRs caused by VAD may lead to decreased signals acting on RARE; therefore, decreased expression of BMAL1 and RORα may then be induced. In addition, the regulatory regions of BMAL1, CLOCK, PER1, and REV-ERB genes have the RORE element, so the decreased RORα expression caused by VAD may further reduce the expression levels of these genes and ultimately affect the circadian rhythm in the hippocampus (11, 86) (Figure 3).
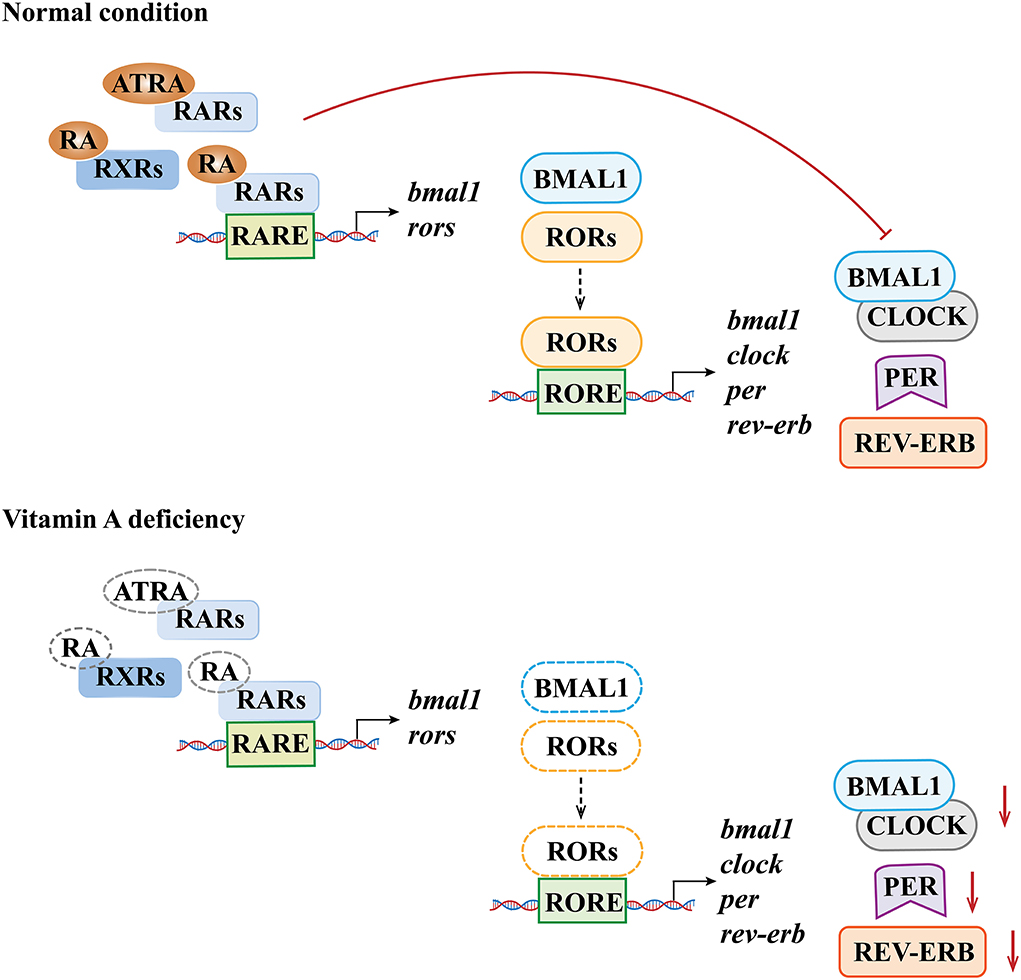
Figure 3. Molecular mechanisms underlying the effects of vitamin A deficiency on biological rhythm molecules. The dotted line denotes the lack of the susbstance.
Associations of VAD with biorhythm dysfunction-related diseases
VAD and sleep disorders
It is well known that biorhythm disorders may present with an abnormal sleep cycle, and evidence suggests that VAD may adversely affect sleep, especially slow wave sleep (SWS) related to photoperiod. The SWS is of great significance for learning, memory, body growth, and repair (87). Under normal circumstances, delta oscillations (0.5–4 Hz) are common in a state of deep sleep and are representative brain waves during the SWS, while theta waves (4–7 Hz) occur in the transition between wake and sleep or lighter stages of sleep. Mice of some strains with fragmented sleep had reduced delta activity and increased theta activity during the SWS. The theta/delta (θ/δ) ratio is used to better quantify the altered EEG pattern. Changes in the relative contribution of the delta rhythm to the SWS or the θ/δ ratio were found to be associated with the changes in the expression levels of RARβ (88). Significant impairments in delta oscillations were also observed in VAD mice (89). Although these studies suggested the potential association between VAD and sleep disorders, the use of vitamin A and its bioactive derivatives in the treatment of sleep disorders caused by biological rhythm dysfunctions warrants future studies.
VAD and learning/memory impairments
Both the rhythm-related genes (BMAL1, PERs, CRYs, and REV-ERBα) and RA receptors (RARα, RARβ, and RARγ) in the hippocampus have endogenous circadian rhythms (51, 60), which is critical for maintaining normal physiological functions of learning and memory (90, 91).
Retinoic acid plays a critical role in regulating neurogenesis, neuronal survival, and synaptic plasticity (92, 93). VAD may cause impairments in synaptic plasticity, learning, and memory in rats (94). Previous studies have demonstrated that RARα antagonists could damage long-term potentiation, indicating that RARα receptors may be involved in synaptic plasticity and the process of learning and memory (95). Prenatal marginal VAD was found to induce decreased RARα mRNA levels in the postnatal hippocampus (96, 97). Brain-derived neurotrophic factor (BDNF) and neurogranin (RC3) are key molecules involved in learning and memory, and BDNF and RC3 genes have rhythmic expression patterns in the hippocampus. A previous study showed that the VAD phase shifted the daily peaks of RC3 and BDNF mRNA levels in the rat hippocampus, affected their daily expression patterns, and might therefore affect the daily cognitive performances in rats (86, 98). Some interesting findings have been reported on crocetin. Crocetin is a natural carotenoid compound (not provitamin A carotenoid) and cannot be converted into vitamin A. However, studies have demonstrated that crocetin can promote the synaptic growth of hippocampal neurons to promote neural plasticity and neuronal communication (99), and plays roles in regulating learning, memory, and sleep. As a kind of carotenoid with a structure similar to that of carotene (the precursor of vitamin A), crocetin and its functions are mentioned here to raise consideration or inspire researchers to explore more possible functions of vitamin A in the future (100).
VAD and emotional disorders/neuroendocrine disorders
The hypothalamus may play an important role in the association of VAD with biological rhythm-related emotional/neuroendocrine disorders. The hypothalamic-pituitary-adrenal (HPA) axis, which is composed of the paraventricular nucleus (PVN) of the hypothalamus, the anterior pituitary gland, and adrenal glands, controls reactions to stress and plays a prominent role in regulating mood and emotional statuses (101). CRF is a hypothalamic hormone and a core driver of the HPA axis (102). With extensive regulatory effects, RA signals can modulate the synthesis of CRF in the hypothalamus, and abnormal CRF signaling induced by VAD may affect the response of the body to stress, which may be associated with the development of anxiety and depression (103, 104). In addition, as a neuropeptide mainly synthesized by the PVN and supraoptic nucleus of the hypothalamus, oxytocin was found to play a role in modulating fear and stress responses (105, 106). RARE has been found in the regulatory region of the oxytocin gene (107); therefore, the decrease in RA signals caused by VAD may lead to decreased oxytocin synthesis, which may have potential associations with the development of anxiety or depression (108). In addition, thyroid hormone and RA had similar synthetic pathways and targets, and VAD may affect the pituitary thyroid axis (109, 110). Specifically, vitamin A is critical for iodine metabolism, and the 9-cis retinoic acid receptor (RXR) is a common heterodimerization partner for the thyroid hormone receptor (T3R). Therefore, VAD may lead to increased thyroid hormone synthesis and thyroid enlargement and aggravate the thyroid dysfunction caused by iodine deficiency (111, 112). These findings suggested a potential association between VAD and emotional/neuroendocrine disorders. However, although both the HPA activity and the oxytocin level in the brain have their own diurnal rhythms (113, 114), circadian rhythm disorders may induce emotional symptoms (115–117), and some clinically approved drugs that are used to treat depression and anxiety were found to play a role in regulating the biological clock (116, 118). Future studies are still warranted to confirm whether biological rhythm dysfunctions play a role in the association of VAD and emotional disorders.
VAD and metabolism/immune dysfunctions/cancers
In addition to regulating the expression of biorhythm genes, RORs also play a crucial role in cell metabolism (lipid and steroid), proliferation, apoptosis, migration, immunity, and many other physiological or pathological processes (119–121). Melatonin, which is a hormone closely related to biological rhythms and mediated by RORα, regulates macrophage polarization, thereby preventing the transformation of atherosclerosis into cardiovascular and cerebrovascular events (122). Moreover, RORα may inhibit the effects of melatonin on testosterone synthesis in mice (123) and inhibit the proliferation, invasion, and migration of liver cancer cells by downregulating the chemokines (124). Since RORs constitute an important part of the molecular mechanism of biorhythm regulation, it can be speculated that RORs may be an intermediate step linking vitamin A, biological rhythms, and various diseases, including but not limited to metabolic syndromes, cardiovascular diseases, cancers, and inflammations (125, 126). Therefore, RORs may be a promising target for the treatment of immune, metabolic, and other biorhythm-related diseases (127, 128).
Conclusion
In this review, we summarized the associations of vitamin A with biological rhythms and the potential impacts of VAD on biorhythm dysfunction, systematically described the potential underlying mechanisms, and provided some novel insights on the possible involvement of VAD in the development of some biorhythm-related diseases/disorders. VAD may affect the photo-response, sleep cycle, learning and memory, cell metabolism, and induce related disorders/diseases relevant to biological rhythm dysfunctions. Vitamin A and related metabolites may bind with their receptors (mainly RARs) to regulate gene expression (shown in the TTFLs), which may be the primary material basis underlying the biological rhythm dysfunctions induced by VAD. The possibility that RA signals may bridge the gap between VAD, circadian rhythm dysfunction, and related clinical disorders/diseases should be considered. However, although RA is the main active metabolite of vitamin A, and VAD is inevitably associated with abnormal RA signaling pathway, there is still a possibility that other factors that affect the functions of RA signaling pathways other than VAD may exist. Thus, the associations of RA signaling pathways with biological-rhythm disorders/diseases may not be exclusively due to VAD, and whether VAD induces related rhythm disorders/diseases via impaired RA signaling pathways warrants confirmation, especially using human studies with randomized controlled trials. More evidence of the influences of VAD on biological rhythms and its detailed mechanisms is warranted, which may be of great significance to clinical outcomes.
Author contributions
XG: investigation and writing—original draft. HW: writing—original draft. JX: conceptualization, project administration, supervision, and writing—review and editing. HH: editing. All authors contributed to the article and approved the submitted version.
Funding
This work was supported by the National Natural Science Foundation of China (81974486 and 81673189), Clinical Research Project of Shanghai Municipal Health Commission (202240392), Shanghai Jiao Tong University School of Medicine (20172016), and Shanghai Sailing Program (21YF1451500).
Conflict of interest
The authors declare that the research was conducted in the absence of any commercial or financial relationships that could be construed as a potential conflict of interest.
Publisher's note
All claims expressed in this article are solely those of the authors and do not necessarily represent those of their affiliated organizations, or those of the publisher, the editors and the reviewers. Any product that may be evaluated in this article, or claim that may be made by its manufacturer, is not guaranteed or endorsed by the publisher.
Abbreviations
AANAT, arylalkylamine N-acetyltransferase; ARAT, acyl-CoA: retinol acyltransferase; ATRA, all-trans retinoic acid; BDNF, Brain-derived neurotrophic factor; BMAL1, brain and muscle Arnt-like protein 1; CLOCK, circadian locomotor output cycles kaput; CRF, corticotropin-releasing factor; CRY, cryptochrome; DHRS9, short-chain dehydrogenase/reductase (SDR family) member 9; HPA, hypothalamic-pituitary-adrenal axis; ipRGCs, intrinsically photosensitive retinal ganglion cells; IRBP, interphotoreceptor retinoid-binding protein; LRAT, lecithin:retinol acyltransferase; PER, Period; PVN, paraventricular nucleus; RA, retinoic acid; RALDH, retinaldehyde dehydrogenases; RARE, retinoic acid response elements; RARs, retinoic acid receptors; RBP, retinol-binding protein; RDHs, retinol dehydrogenases; REV-ERB, reverse erythroblastosis virus; RNRs, retinoid nuclear receptors; RORs, retinoid-related orphan receptors; RORE, ROR response element; RPE, retinal pigment epithelial; RXRs, retinoid X receptors; SCN, suprachiasmatic nucleus; SWS, slow wave sleep; TTFLs, transcription–translation feedback loops; VAD, vitamin A deficiency.
References
1. Wada A. Chemical biology studies using vitamin a analogs. Yakugaku Zasshi. (2021) 141:557–77. doi: 10.1248/yakushi.20-00230
2. Isoherranen N, Zhong G. Biochemical and physiological importance of the Cyp26 retinoic acid hydroxylases. Pharmacol Ther. (2019) 204:107400. doi: 10.1016/j.pharmthera.2019.107400
3. Ransom J, Morgan PJ, McCaffery PJ, Stoney PN. The rhythm of retinoids in the brain. J Neurochem. (2014) 129:366–76. doi: 10.1111/jnc.12620
4. Armstrong JL, Redfern CPF, Veal GJ. 13-Cis retinoic acid and isomerisation in paediatric oncology—is changing shape the key to success? Biochem Pharmacol. (2005) 69:1299–306. doi: 10.1016/j.bcp.2005.02.003
5. Bar-El Dadon S, Reifen R. Vitamin a and the epigenome. Crit Rev Food Sci Nutr. (2017) 57:2404–11. doi: 10.1080/10408398.2015.1060940
6. Pohl E, Tomlinson CWE. Classical pathways of gene regulation by retinoids. Methods Enzymol. (2020) 637:151–73. doi: 10.1016/bs.mie.2020.03.008
7. Kane MA, Folias AE, Pingitore A, Perri M, Obrochta KM, Krois CR, et al. Identification of 9-Cis-retinoic acid as a pancreas-specific autacoid that attenuates glucose-stimulated insulin secretion. Proc Natl Acad Sci U S A. (2010) 107:21884–9. doi: 10.1073/pnas.1008859107
8. Kane MA. Analysis, occurrence, and function of 9-Cis-retinoic acid. Biochim Biophys Acta. (2012) 1821:10–20. doi: 10.1016/j.bbalip.2011.09.012
9. Tafti M, Ghyselinck NB. Functional implication of the vitamin a signaling pathway in the brain. Arch Neurol. (2007) 64:1706–11. doi: 10.1001/archneur.64.12.1706
10. Cunningham TJ, Duester G. Mechanisms of retinoic acid signalling and its roles in organ and limb development. Nat Rev Mol Cell Biol. (2015) 16:110–23. doi: 10.1038/nrm3932
11. Jetten AM. Retinoid-related orphan receptors (Rors): critical roles in development, immunity, circadian rhythm, and cellular metabolism. Nucl Recept Signal. (2009) 7:e003. doi: 10.1621/nrs.07003
12. Ladurner A, Schwarz PF, Dirsch VM. Natural products as modulators of retinoic acid receptor-related orphan receptors (Rors). Nat Prod Rep. (2021) 38:757–81. doi: 10.1039/D0NP00047G
13. Hu X, Wang Y, Hao LY, Liu X, Lesch CA, Sanchez BM, et al. Sterol metabolism controls T(H)17 differentiation by generating endogenous rorγ agonists. Nat Chem Biol. (2015) 11:141–7. doi: 10.1038/nchembio.1714
14. Kojetin DJ, Burris TP. Rev-Erb and Ror nuclear receptors as drug targets. Nat Rev Drug Discov. (2014) 13:197–216. doi: 10.1038/nrd4100
15. Solt LA, Burris TP. Action of Rors and their ligands in (Patho)physiology. Trends Endocrinol Metab. (2012) 23:619–27. doi: 10.1016/j.tem.2012.05.012
16. Stevens GA, Bennett JE, Hennocq Q, Lu Y, De-Regil LM, Rogers L, et al. Trends and mortality effects of vitamin a deficiency in children in 138 low-income and middle-income countries between 1991 and 2013: a pooled analysis of population-based surveys. Lancet Glob Health. (2015) 3:e528–36. doi: 10.1016/S2214-109X(15)00039-X
17. The L. Vitamin a distribution in danger. Lancet. (2018) 391:1866. doi: 10.1016/S0140-6736(18)31035-3
18. Reza Dorosty-Motlagh A, Mohammadzadeh Honarvar N, Sedighiyan M, Abdolahi M. The molecular mechanisms of vitamin a deficiency in multiple sclerosis. J Mol Neurosci. (2016) 60:82–90. doi: 10.1007/s12031-016-0781-0
19. Janesick A, Wu SC, Blumberg B. Retinoic acid signaling and neuronal differentiation. Cell Mol Life Sci. (2015) 72:1559–76. doi: 10.1007/s00018-014-1815-9
20. Timoneda J, Rodríguez-Fernández L, Zaragozá R, Marín MP, Cabezuelo MT, Torres L, et al. Vitamin a deficiency and the lung. Nutrients. (2018) 10:9. doi: 10.3390/nu10091132
21. Husson M, Enderlin V, Alfos S, Féart C, Higueret P, Pallet V. Triiodothyronine administration reverses vitamin a deficiency-related hypo-expression of retinoic acid and triiodothyronine nuclear receptors and of neurogranin in rat brain. Br J Nutr. (2003) 90:191–8. doi: 10.1079/BJN2003877
22. Chen L, Liu Q, Chen X, Li T, Zhou X. Marginal vitamin a deficiency affects the expression levels and localization of retinoic acid receptor and retinoid X receptor in rats meibomian gland. Curr Eye Res. (2019) 44:368–75. doi: 10.1080/02713683.2018.1554154
23. Etchamendy N, Enderlin V, Marighetto A, Pallet V, Higueret P, Jaffard R. Vitamin a deficiency and relational memory deficit in adult mice: relationships with changes in brain retinoid signalling. Behav Brain Res. (2003) 145:37–49. doi: 10.1016/S0166-4328(03)00099-8
24. Tan M, Yang T, Liu H, Xiao L, Li C, Zhu J, et al. Maternal vitamin a deficiency impairs cholinergic and nitrergic neurons, leading to gastrointestinal dysfunction in rat offspring via Rarβ. Life Sci. (2021) 264:118688. doi: 10.1016/j.lfs.2020.118688
25. Bonhomme D, Alfos S, Webster SP, Wolff M, Pallet V, Touyarot K. Vitamin a deficiency impairs contextual fear memory in rats: abnormalities in the glucocorticoid pathway. J Neuroendocrinol. (2019) 31:e12802. doi: 10.1111/jne.12802
26. Andreani TS, Itoh TQ, Yildirim E, Hwangbo DS, Allada R. Genetics of Circadian Rhythms. Sleep Med Clin. (2015) 10:413–21. doi: 10.1016/j.jsmc.2015.08.007
27. Wen S, Ma D, Zhao M, Xie L, Wu Q, Gou L, et al. Spatiotemporal single-cell analysis of gene expression in the mouse suprachiasmatic nucleus. Nat Neurosci. (2020) 23:456–67. doi: 10.1038/s41593-020-0586-x
28. Maywood ES, Reddy AB, Wong GK, O'Neill JS, O'Brien JA, McMahon DG, et al. Synchronization and maintenance of timekeeping in suprachiasmatic circadian clock cells by neuropeptidergic signaling. Curr Biol. (2006) 16:599–605. doi: 10.1016/j.cub.2006.02.023
29. Dibner C, Schibler U, Albrecht U. The mammalian circadian timing system: organization and coordination of central and peripheral clocks. Annu Rev Physiol. (2010) 72:517–49. doi: 10.1146/annurev-physiol-021909-135821
30. Yang X, Lamia KA, Evans RM. Nuclear receptors, metabolism, and the circadian clock. Cold Spring Harb Symp Quant Biol. (2007) 72:387–94. doi: 10.1101/sqb.2007.72.058
31. Koronowski KB, Kinouchi K, Welz PS, Smith JG, Zinna VM, Shi J, et al. Defining the independence of the liver circadian clock. Cell. (2019) 177:1448–62.e14. doi: 10.1016/j.cell.2019.04.025
32. Golombek DA, Rosenstein RE. Physiology of circadian entrainment. Physiol Rev. (2010) 90:1063–102. doi: 10.1152/physrev.00009.2009
33. Potter GD, Cade JE, Grant PJ, Hardie LJ. Nutrition and the circadian system. Br J Nutr. (2016) 116:434–42. doi: 10.1017/S0007114516002117
34. Mei Y, Teng H, Li Z, Zeng C, Li Y, Song W, et al. Restricted feeding resets endogenous circadian rhythm in female mice under constant darkness. Neurosci Bull. (2021) 37:1005–9. doi: 10.1007/s12264-021-00669-w
35. Ono D, Honma KI, Honma S. Gabaergic mechanisms in the suprachiasmatic nucleus that influence circadian rhythm. J Neurochem. (2021) 157:31–41. doi: 10.1111/jnc.15012
36. Miller JEK, Granados-Fuentes D, Wang T, Marpegan L, Holy TE, Herzog ED. Vasoactive intestinal polypeptide mediates circadian rhythms in mammalian olfactory bulb and olfaction. J. Neuroscience. (2014) 34:17. doi: 10.1523/JNEUROSCI.4713-13.2014
37. Huang S, Jiao X, Lu D, Pei X, Qi D, Li Z. Recent advances in modulators of circadian rhythms: an update and perspective. J Enzyme Inhib Med Chem. (2020) 35:1267–86. doi: 10.1080/14756366.2020.1772249
38. Liu Y, Niu L, Liu X, Cheng C, Le W. Recent progress in non-motor features of parkinson's disease with a focus on circadian rhythm dysregulation. Neurosci Bull. (2021) 37:1010–24. doi: 10.1007/s12264-021-00711-x
39. Iiams SE, Lugena AB, Zhang Y, Hayden AN, Merlin C. Photoperiodic and clock regulation of the vitamin a pathway in the brain mediates seasonal responsiveness in the monarch butterfly. Proc Natl Acad Sci U S A. (2019) 116:25214–21. doi: 10.1073/pnas.1913915116
40. Sherman H, Gutman R, Chapnik N, Meylan J, le Coutre J, Froy O. All-trans retinoic acid modifies the expression of clock and disease marker genes. J Nutr Biochem. (2012) 23:209–17. doi: 10.1016/j.jnutbio.2010.11.017
41. Shirai H, Oishi K, Ishida N. Circadian expression of clock genes is maintained in the liver of vitamin a-deficient mice. Neurosci Lett. (2006) 398:69–72. doi: 10.1016/j.neulet.2005.12.055
42. Sato-Mito N, Sasaki S, Murakami K, Okubo H, Takahashi Y, Shibata S, et al. The midpoint of sleep is associated with dietary intake and dietary behavior among young japanese women. Sleep Med. (2011) 12:289–94. doi: 10.1016/j.sleep.2010.09.012
43. Kuratsune H, Umigai N, Takeno R, Kajimoto Y, Nakano T. Effect of crocetin from gardenia jasminoides ellis on sleep: a pilot study. Phytomedicine. (2010) 17:840–3. doi: 10.1016/j.phymed.2010.03.025
44. Umigai N, Takeda R, Mori A. Effect of crocetin on quality of sleep: a randomized, double-blind, placebo-controlled, crossover study. Complement Ther Med. (2018) 41:47–51. doi: 10.1016/j.ctim.2018.09.003
45. Lopresti AL, Smith SJ, Metse AP, Drummond PD. Effects of saffron on sleep quality in healthy adults with self-reported poor sleep: a randomized, double-blind, placebo-controlled trial. J Clin Sleep Med. (2020) 16:937–47. doi: 10.5664/jcsm.8376
46. Pachikian BD, Copine S, Suchareau M, Deldicque L. Effects of saffron extract on sleep quality: a randomized double-blind controlled clinical trial. Nutrients. (2021) 13:5. doi: 10.3390/nu13051473
47. Bahrami A, Khorasanchi Z, Sadeghnia HR, Tayefi M, Avan A, Ferns GA, et al. Depression in adolescent girls: relationship to serum vitamins a and e, immune response to heat shock protein 27 and systemic inflammation. J Affect Disord. (2019) 252:68–73. doi: 10.1016/j.jad.2019.04.048
48. Takahashi JS, Shimomura K, Kumar V. Searching for genes underlying behavior: lessons from circadian rhythms. Science. (2008) 322:909–12. doi: 10.1126/science.1158822
49. Shirai H, Oishi K, Ishida N. Bidirectional clock/bmal1-dependent circadian gene regulation by retinoic acid in vitro. Biochem Biophys Res Commun. (2006) 351:387–91. doi: 10.1016/j.bbrc.2006.10.031
50. Lowrey PL, Takahashi JS. Genetics of circadian rhythms in mammalian model organisms. Adv Genet. (2011) 74:175–230. doi: 10.1016/B978-0-12-387690-4.00006-4
51. Navigatore-Fonzo LS, Golini RL, Ponce IT, Delgado SM, Plateo-Pignatari MG, Gimenez MS, et al. Retinoic acid receptors move in time with the clock in the hippocampus. Effect of a vitamin-a-deficient diet. J Nutr Biochem. (2013) 24:859–67. doi: 10.1016/j.jnutbio.2012.05.006
52. Takahashi JS. Transcriptional architecture of the mammalian circadian clock. Nat Rev Genet. (2017) 18:164–79. doi: 10.1038/nrg.2016.150
53. Weger M, Diotel N, Dorsemans AC, Dickmeis T, Weger BD. Stem cells and the circadian clock. Dev Biol. (2017) 431:111–23. doi: 10.1016/j.ydbio.2017.09.012
54. Chaix A, Zarrinpar A, Panda S. The circadian coordination of cell biology. J Cell Biol. (2016) 215:15–25. doi: 10.1083/jcb.201603076
55. Bell-Pedersen D, Cassone VM, Earnest DJ, Golden SS, Hardin PE, Thomas TL, et al. Circadian rhythms from multiple oscillators: lessons from diverse organisms. Nat Rev Genet. (2005) 6:544–56. doi: 10.1038/nrg1633
56. Akashi M, Takumi T. The orphan nuclear receptor roralpha regulates circadian transcription of the mammalian core-clock bmal1. Nat Struct Mol Biol. (2005) 12:441–8. doi: 10.1038/nsmb925
57. Reilly DF, Westgate EJ, FitzGerald GA. Peripheral circadian clocks in the vasculature. Arterioscler Thromb Vasc Biol. (2007) 27:1694–705. doi: 10.1161/ATVBAHA.107.144923
58. McNamara P, Seo SB, Rudic RD, Sehgal A, Chakravarti D, FitzGerald GA. Regulation of Clock and Mop4 by nuclear hormone receptors in the vasculature: a humoral mechanism to reset a peripheral clock. Cell. (2001) 105:877–89. doi: 10.1016/S0092-8674(01)00401-9
59. Buijs R, Guzman Ruiz M, Méndez-Hernández R, Cortés B. The suprachiasmatic nucleus; a responsive clock regulating homeostasis by daily changing the setpoints of physiological parameters. Autonomic Neurosci. (2019) 218:43–50. doi: 10.1016/j.autneu.2019.02.001
60. Navigatore-Fonzo LS, Golini RS, Delgado SM, Ponce IT, Bonomi MR, Rezza IG, et al. Temporal patterns of lipoperoxidation and antioxidant enzymes are modified in the hippocampus of vitamin a-deficient rats. Hippocampus. (2009) 19:869–80. doi: 10.1002/hipo.20571
61. Ueda HR, Chen W, Adachi A, Wakamatsu H, Hayashi S, Takasugi T, et al. A transcription factor response element for gene expression during circadian night. Nature. (2002) 418:534–9. doi: 10.1038/nature00906
62. Masana MI, Sumaya IC, Becker-Andre M, Dubocovich ML. Behavioral characterization and modulation of circadian rhythms by light and melatonin in C3h/Hen mice homozygous for the rorbeta knockout. Am J Physiol Regul Integr Comp Physiol. (2007) 292:R2357–67. doi: 10.1152/ajpregu.00687.2006
63. Liu AC, Tran HG, Zhang EE, Priest AA, Welsh DK, Kay SA. Redundant function of rev-erbalpha and beta and non-essential role for bmal1 cycling in transcriptional regulation of intracellular circadian rhythms. PLoS Genet. (2008) 4:e1000023. doi: 10.1371/journal.pgen.1000023
64. Guillaumond F, Dardente H, Giguère V, Cermakian N. Differential control of bmal1 circadian transcription by rev-erb and ror nuclear receptors. J Biol Rhythms. (2005) 20:391–403. doi: 10.1177/0748730405277232
65. Stehlin-Gaon C, Willmann D, Zeyer D, Sanglier S, Van Dorsselaer A, Renaud JP, et al. All-trans retinoic acid is a ligand for the orphan nuclear receptor Ror beta. Nat Struct Biol. (2003) 10:820–5. doi: 10.1038/nsb979
66. Laursen KB, Gudas LJ. Combinatorial knockout of Rarα, Rarβ, and Rarγ completely abrogates transcriptional responses to retinoic acid in murine embryonic stem cells. J Biol Chem. (2018) 293:11891–900. doi: 10.1074/jbc.RA118.001951
67. Touitou Y, Reinberg A, Touitou D. Association between light at night, melatonin secretion, sleep deprivation, and the internal clock: health impacts and mechanisms of circadian disruption. Life Sci. (2017) 173:94–106. doi: 10.1016/j.lfs.2017.02.008
68. Berson DM, Dunn FA, Takao M. Phototransduction by retinal ganglion cells that set the circadian clock. Science. (2002) 295:1070–3. doi: 10.1126/science.1067262
69. Fu Y, Zhong H, Wang MH, Luo DG, Liao HW, Maeda H, et al. Intrinsically photosensitive retinal ganglion cells detect light with a vitamin a-based photopigment, melanopsin. Proc Natl Acad Sci U S A. (2005) 102:10339–44. doi: 10.1073/pnas.0501866102
70. Mure LS, Vinberg F, Hanneken A, Panda S. Functional diversity of human intrinsically photosensitive retinal ganglion cells. Science. (2019) 366:1251–5. doi: 10.1126/science.aaz0898
71. Park PS. Rhodopsin oligomerization and aggregation. J Membr Biol. (2019) 252:413–23. doi: 10.1007/s00232-019-00078-1
72. Wolf G. Three vitamins are involved in regulation of the circadian rhythm. Nutr Rev. (2002) 60:257–60. doi: 10.1301/002966402320289386
73. Sexton T, Buhr E, Van Gelder RN. Melanopsin and mechanisms of non-visual ocular photoreception. J Biol Chem. (2012) 287:1649–56. doi: 10.1074/jbc.R111.301226
74. Palczewski K. Chemistry and biology of vision. J Biol Chem. (2012) 287:1612–9. doi: 10.1074/jbc.R111.301150
76. Rajendran RR, Van Niel EE, Stenkamp DL, Cunningham LL, Raymond PA, Gonzalez-Fernandez F. Zebrafish interphotoreceptor retinoid-binding protein: differential circadian expression among cone subtypes. J Exp Biol. (1996) 199:2775–87. doi: 10.1242/jeb.199.12.2775
77. Parker R, Wang JS, Kefalov VJ, Crouch RK. Interphotoreceptor retinoid-binding protein as the physiologically relevant carrier of 11-Cis-retinol in the cone visual cycle. J Neurosci. (2011) 31:4714–9. doi: 10.1523/JNEUROSCI.3722-10.2011
78. Borjigin J, Zhang LS, Calinescu AA. Circadian regulation of pineal gland rhythmicity. Mol Cell Endocrinol. (2012) 349:13–9. doi: 10.1016/j.mce.2011.07.009
79. Ashton A, Stoney PN, Ransom J, McCaffery P. Rhythmic diurnal synthesis and signaling of retinoic acid in the rat pineal gland and its action to rapidly downregulate Erk phosphorylation. Mol Neurobiol. (2018) 55:8219–35. doi: 10.1007/s12035-018-0964-5
80. Bailey MJ, Coon SL, Carter DA, Humphries A, Kim JS, Shi Q, et al. Night/day changes in pineal expression of >600 genes: central role of adrenergic/camp signaling. J Biol Chem. (2009) 284:7606–22. doi: 10.1074/jbc.M808394200
81. Smeland S, Bjerknes T, Malaba L, Eskild W, Norum KR, Blomhoff R. Tissue distribution of the receptor for plasma retinol-binding protein. Biochem J. (1995) 305:419–24. doi: 10.1042/bj3050419
82. Herbert DC, Reiter RJ. Changes in pineal indoleamine metabolism in vitamin a deficient rats. Life Sci. (1985) 37:2515–22. doi: 10.1016/0024-3205(85)90609-5
83. Guillaumond F, Giraudet F, Becquet D, Sage D, Laforge-Anglade G, Bosler O, et al. Vitamin a is a necessary factor for sympathetic-independent rhythmic activation of mitogen-activated protein kinase in the rat pineal gland. Eur J Neurosci. (2005) 21:798–802. doi: 10.1111/j.1460-9568.2005.03901.x
84. Navigatore-Fonzo LS, Delgado SM, Golini RS, Anzulovich AC. Circadian rhythms of locomotor activity and hippocampal clock genes expression are dampened in vitamin a-deficient rats. Nutr Res. (2014) 34:326–35. doi: 10.1016/j.nutres.2014.02.002
85. Golini R, Navigatore-Fonzo L, Randazzo G, Delgado S, Anzulovich AC. Circadian patterns of rora and rev-erb expression are modified in the hippocampus of vitamin a-deficient rats. Biocell. (2015) 39. SUPPL1. A23.
86. Golini RS, Delgado SM, Navigatore Fonzo LS, Ponce IT, Lacoste MG, Anzulovich AC. Daily patterns of clock and cognition-related factors are modified in the hippocampus of vitamin a-deficient rats. Hippocampus. (2012) 22:1720–32. doi: 10.1002/hipo.22007
87. Léger D, Debellemaniere E, Rabat A, Bayon V, Benchenane K, Chennaoui M. Slow-wave sleep: from the cell to the clinic. Sleep Med Rev. (2018) 41:113–32. doi: 10.1016/j.smrv.2018.01.008
88. Maret S, Franken P, Dauvilliers Y, Ghyselinck NB, Chambon P, Tafti M. Retinoic acid signaling affects cortical synchrony during sleep. Science. (2005) 310:111–3. doi: 10.1126/science.1117623
89. Kitaoka K, Hattori A, Chikahisa S, Miyamoto K, Nakaya Y, Sei H. Vitamin a deficiency induces a decrease in eeg delta power during sleep in mice. Brain Res. (2007) 1150:121–30. doi: 10.1016/j.brainres.2007.02.077
90. Jilg A, Lesny S, Peruzki N, Schwegler H, Selbach O, Dehghani F, et al. Temporal dynamics of mouse hippocampal clock gene expression support memory processing. Hippocampus. (2010) 20:377–88. doi: 10.1002/hipo.20637
91. Wang LM, Dragich JM, Kudo T, Odom IH, Welsh DK, O'Dell TJ, et al. Expression of the circadian clock gene period2 in the hippocampus: possible implications for synaptic plasticity and learned behaviour. ASN Neuro. (2009) 1:3. doi: 10.1042/AN20090020
92. Crandall J, Sakai Y, Zhang J, Koul O, Mineur Y, Crusio WE, et al. 13-Cis-Retinoic acid suppresses hippocampal cell division and hippocampal-dependent learning in mice. Proc Natl Acad Sci U S A. (2004) 101:5111–6. doi: 10.1073/pnas.0306336101
93. McCaffery P, Zhang J, Crandall JE. Retinoic acid signaling and function in the adult hippocampus. J Neurobiol. (2006) 66:780–91. doi: 10.1002/neu.20237
94. Olson CR, Mello CV. Significance of vitamin a to brain function, behavior and learning. Mol Nutr Food Res. (2010) 54:489–95. doi: 10.1002/mnfr.200900246
95. Mao CT, Ting-Yu LI. Effects of marginal vitamin a deficiency and vitamin an intervention on neurons at hippocampus in young rats. Acta Nutrimenta Sinica. (2010).
96. Zhang X, Yuan X, Chen L, Wei H, Chen J, Li T. The change in retinoic acid receptor signaling induced by prenatal marginal vitamin a deficiency and its effects on learning and memory. J Nutr Biochem. (2017) 47:75–85. doi: 10.1016/j.jnutbio.2017.05.007
97. Hou N, Ren L, Gong M, Bi Y, Gu Y, Dong Z, et al. Vitamin a deficiency impairs spatial learning and memory: the mechanism of abnormal Cbp-dependent histone acetylation regulated by retinoic acid receptor alpha. Mol Neurobiol. (2015) 51:633–47. doi: 10.1007/s12035-014-8741-6
98. Husson M, Enderlin V, Alfos S, Boucheron C, Pallet V, Higueret P. Expression of neurogranin and neuromodulin is affected in the striatum of vitamin a-deprived rats. Brain Res Mol Brain Res. (2004) 123:7–17. doi: 10.1016/j.molbrainres.2003.12.012
99. Wang X, Jiao X, Liu Z, Li Y. Crocetin potentiates neurite growth in hippocampal neurons and facilitates functional recovery in rats with spinal cord injury. Neurosci Bull. (2017) 33:695–702. doi: 10.1007/s12264-017-0157-7
100. Milani A, Basirnejad M, Shahbazi S, Bolhassani A. Carotenoids: biochemistry, pharmacology and treatment. Br J Pharmacol. (2017) 174:1290–324. doi: 10.1111/bph.13625
101. Miller WL. The hypothalamic-pituitary-adrenal axis: a brief history. Horm Res Paediatr. (2018) 89:212–23. doi: 10.1159/000487755
102. Cai L, Li R, Zhou JN. Chronic all-trans retinoic acid administration induces Crf over-expression accompanied by Avp up-regulation and multiple crf-controlling receptors disturbance in the hypothalamus of rats. Brain Res. (2015) 1601:1–7. doi: 10.1016/j.brainres.2014.12.054
103. Bao AM, Meynen G, Swaab DF. The stress system in depression and neurodegeneration: focus on the human hypothalamus. Brain Res Rev. (2008) 57:531–53. doi: 10.1016/j.brainresrev.2007.04.005
104. Hauger RL, Risbrough V, Brauns O, Dautzenberg FM. Corticotropin releasing factor (Crf) receptor signaling in the central nervous system: new molecular targets. CNS Neurol Disord Drug Targets. (2006) 5:453–79. doi: 10.2174/187152706777950684
105. Viviani D, Charlet A, van den Burg E, Robinet C, Hurni N, Abatis M, et al. Oxytocin selectively gates fear responses through distinct outputs from the central amygdala. Science. (2011) 333:104–7. doi: 10.1126/science.1201043
106. Neumann ID. Involvement of the brain oxytocin system in stress coping: interactions with the hypothalamo-pituitary-adrenal axis. Prog Brain Res. (2002) 139:147–62. doi: 10.1016/S0079-6123(02)39014-9
107. Larcher A, Neculcea J, Chu K, Zingg HH. Effects of retinoic acid and estrogens on oxytocin gene expression in the rat uterus: in vitro and in vivo studies. Mol Cell Endocrinol. (1995) 114:69–76. doi: 10.1016/0303-7207(95)03643-L
108. Lai X, Wu X, Hou N, Liu S, Li Q, Yang T, et al. Vitamin a deficiency induces autistic-like behaviors in rats by regulating the Rarβ-Cd38-oxytocin axis in the hypothalamus. Mol Nutr Food Res. (2018) 62:5. doi: 10.1002/mnfr.201700754
109. Fernández M, Pannella M, Baldassarro VA, Flagelli A, Alastra G, Giardino L, et al. Thyroid hormone signaling in embryonic stem cells: crosstalk with the retinoic acid pathway. Int J Mol Sci. (2020) 21:23. doi: 10.3390/ijms21238945
110. Shearer KD, Goodman TH, Ross AW, Reilly L, Morgan PJ, McCaffery PJ. Photoperiodic regulation of retinoic acid signaling in the hypothalamus. J Neurochem. (2010) 112:246–57. doi: 10.1111/j.1471-4159.2009.06455.x
111. Zimmermann MB. Interactions of vitamin a and iodine deficiencies: effects on the pituitary-thyroid axis. Int J Vitam Nutr Res. (2007) 77:236–40. doi: 10.1024/0300-9831.77.3.236
112. Brossaud J, Pallet V, Corcuff JB. Vitamin A, endocrine tissues and hormones: interplay and interactions. Endocr Connect. (2017) 6:R121–r30. doi: 10.1530/EC-17-0101
113. Parker KJ, Hoffman CL, Hyde SA, Cummings CS, Maestripieri D. Effects of age on cerebrospinal fluid oxytocin levels in free-ranging adult female and infant rhesus macaques. Behav Neurosci. (2010) 124:428–33. doi: 10.1037/a0019576
114. Kalsbeek A, van der Spek R, Lei J, Endert E, Buijs RM, Fliers E. Circadian rhythms in the hypothalamo-pituitary-adrenal (Hpa) axis. Mol Cell Endocrinol. (2012) 349:20–9. doi: 10.1016/j.mce.2011.06.042
115. Chung S, Lee EJ, Yun S, Choe HK, Park SB, Son HJ, et al. Impact of circadian nuclear receptor rev-erbα on midbrain dopamine production and mood regulation. Cell. (2014) 157:858–68. doi: 10.1016/j.cell.2014.03.039
116. Liu C, Chung M. Genetics and epigenetics of circadian rhythms and their potential roles in neuropsychiatric disorders. Neurosci Bull. (2015) 31:141–59. doi: 10.1007/s12264-014-1495-3
117. Liu H, Qiu Z. Overexpression of Mecp2 in the suprachiasmatic nucleus alters circadian rhythm and induces abnormal social behaviors. Neurosci Bull. (2021) 37:1713–7. doi: 10.1007/s12264-021-00746-0
118. Sprouse J, Braselton J, Reynolds L. Fluoxetine modulates the circadian biological clock via phase advances of suprachiasmatic nucleus neuronal firing. Biol Psychiatry. (2006) 60:896–9. doi: 10.1016/j.biopsych.2006.03.003
119. Duez H, Staels B. The nuclear receptors Rev-Erbs and Rors integrate circadian rhythms and metabolism. Diab Vasc Dis Res. (2008) 5:82–8. doi: 10.3132/dvdr.2008.0014
120. Jetten AM, Joo JH. Retinoid-related orphan receptors (Rors): roles in cellular differentiation and development. Adv Dev Biol. (2006) 16:313–55. doi: 10.1016/S1574-3349(06)16010-X
121. Lau P, Fitzsimmons RL, Raichur S, Wang SC, Lechtken A, Muscat GE. The orphan nuclear receptor, roralpha, regulates gene expression that controls lipid metabolism: staggerer (Sg/Sg) mice are resistant to diet-induced obesity. J Biol Chem. (2008) 283:18411–21. doi: 10.1074/jbc.M710526200
122. Ding S, Lin N, Sheng X, Zhao Y, Su Y, Xu L, et al. Melatonin stabilizes rupture-prone vulnerable plaques via regulating macrophage polarization in a nuclear circadian receptor rorα-dependent manner. J Pineal Res. (2019) 67:e12581. doi: 10.1111/jpi.12581
123. Li Z, Zhao J, Liu H, Wang J, Lu W. Melatonin inhibits apoptosis in mouse leydig cells via the retinoic acid-related orphan nuclear receptor P53 pathway. Life Sci. (2020) 246:117431. doi: 10.1016/j.lfs.2020.117431
124. Liu G, Yang ZF, Zhou PY, Zhou C, Guan RY, Sun BY, et al. Ror-A-1 inhibits the proliferation, invasion, and migration of hepatocellular carcinoma mhcc97h via downregulation of chemokine Cxcl5. Cytokine. (2020) 129:155004. doi: 10.1016/j.cyto.2020.155004
125. Conaway HH, Henning P, Lerner UH. Vitamin a metabolism, action, and role in skeletal homeostasis. Endocr Rev. (2013) 34:766–97. doi: 10.1210/er.2012-1071
126. Shearer KD, Stoney PN, Morgan PJ, McCaffery PJ, A. Vitamin for the brain. Trends Neurosci. (2012) 35:733–41. doi: 10.1016/j.tins.2012.08.005
127. Lee JM, Kim H, Baek SH. Unraveling the physiological roles of retinoic acid receptor-related orphan receptor. Exp Mol Med. (2021) 53:1278–86. doi: 10.1038/s12276-021-00679-8
128. Doebelin C, He Y, Campbell S, Nuhant P, Kumar N, Koenig M, et al. Discovery and optimization of a series of sulfonamide inverse agonists for the retinoic acid receptor-related orphan receptor-A. Med Chem. (2019) 15:676–84. doi: 10.2174/1573406415666190222124745
129. Helfer G, Ross AW, Russell L, Thomson LM, Shearer KD, Goodman TH, et al. Photoperiod regulates vitamin a and Wnt/B-catenin signaling in F344 rats. Endocrinology. (2012) 153:815–24. doi: 10.1210/en.2011-1792
130. Veerman A, Overmeer W, Zon A, Boer J, Waard E, Huisman H. Vitamin a is essential for photoperiodic induction of diapause in an eyeless mite. Nature. (1983) 302:248–9. doi: 10.1038/302248a0
131. Chernysheva MP, Romanova IV, Mikhrina AL. Effect of retinol on interaction of the protein period1, oxytocin, and gaba at the prenatal period of formation of the circadian clock-mechanism in rats. Zh Evol Biokhim Fiziol. (2012) 48:481–6. doi: 10.1134/S002209301301012X
132. Fu Z, Kato H, Sugahara K, Kubo T. Vitamin a deficiency reduces the responsiveness of pineal gland to light in japanese quail (Coturnix Japonica). Comp Biochem Physiol A Mol Integr Physiol. (1998) 119:593–8. doi: 10.1016/S1095-6433(97)00471-6
133. Gromadzińska J, Peplonska B, Sobala W, Reszka E, Wasowicz W, Bukowska A, et al. Relationship between intensity of night shift work and antioxidant status in blood of nurses. Int Arch Occup Environ Health. (2013) 86:923–30. doi: 10.1007/s00420-012-0828-7
Keywords: vitamin A, biological rhythm, retinoic acid, retinoic acid nuclear receptor, brain function
Citation: Guo X, Wang H, Xu J and Hua H (2022) Impacts of vitamin A deficiency on biological rhythms: Insights from the literature. Front. Nutr. 9:886244. doi: 10.3389/fnut.2022.886244
Received: 28 February 2022; Accepted: 02 November 2022;
Published: 18 November 2022.
Edited by:
Etienne Challet, Université de Strasbourg, FranceReviewed by:
Mary Ann Asson-Batres, Maine Medical Center Research Institute, United StatesChristopher Olson, Midwestern University, United States
Natalia Kedishvili, University of Alabama at Birmingham, United States
William S. Blaner, Columbia University, United States
Copyright © 2022 Guo, Wang, Xu and Hua. This is an open-access article distributed under the terms of the Creative Commons Attribution License (CC BY). The use, distribution or reproduction in other forums is permitted, provided the original author(s) and the copyright owner(s) are credited and that the original publication in this journal is cited, in accordance with accepted academic practice. No use, distribution or reproduction is permitted which does not comply with these terms.
*Correspondence: Jian Xu, c29uaWEwNjE2QHNqdHUuZWR1LmNu
†These authors have contributed equally to this work and share first authorship