- 1Charles Perkins Centre, The University of Sydney, Sydney, NSW, Australia
- 2School of Medical Sciences, Faculty of Medicine and Health, The University of Sydney, Sydney, NSW, Australia
- 3Sydney Cytometry, The University of Sydney and The Centenary Institute, Sydney, NSW, Australia
Modern industrial practices have transformed the human diet over the last century, increasing the consumption of processed foods. Dietary imbalance of macro- and micro-nutrients and excessive caloric intake represent significant risk factors for various inflammatory disorders. Increased ingestion of food additives, residual contaminants from agricultural practices, food processing, and packaging can also contribute deleteriously to disease development. One common hallmark of inflammatory disorders, such as autoimmunity and allergies, is the defect in anti-inflammatory regulatory T cell (Treg) development and/or function. Treg represent a highly heterogeneous population of immunosuppressive immune cells contributing to peripheral tolerance. Tregs either develop in the thymus from autoreactive thymocytes, or in the periphery, from naïve CD4+ T cells, in response to environmental antigens and cues. Accumulating evidence demonstrates that various dietary factors can directly regulate Treg development. These dietary factors can also indirectly modulate Treg differentiation by altering the gut microbiota composition and thus the production of bacterial metabolites. This review provides an overview of Treg ontogeny, both thymic and peripherally differentiated, and highlights how diet and gut microbiota can regulate Treg development and function.
Introduction
Breakdown of immune tolerance is a feature of many non-communicable diseases. Regulatory T cells (Tregs) are a subset of anti-inflammatory CD4+ T cells defined by their expression of the transcription factor FoxP3 (1). Treg mediate immune tolerance, and their deficiency, or a defect in their function, leads to anomalous immune responses to innocuous food and commensal bacteria-derived antigens, as well as to self-antigens (2, 3). Consequently, this results in various inflammatory disorders, including autoimmunity and allergies. These diseases are commonly associated with a western lifestyle. Diet appears to be one of the most influential environmental factors regulating Treg biology. Macronutrients, micronutrients, caloric content, and food additives were all shown to influence the ontogeny and function of Treg (4, 5). Diet also alters the gut microbiota, subsequently regulating Treg biology (6). Treg have either a central origin and differentiate in the thymus during the T cell selection process, referred to as thymic-derived Treg (tTreg), or a peripheral origin where they differentiate from naïve T cells in response to environmental antigens and cues such as dietary or bacterial metabolites, referred to as peripheral Tregs (pTregs) (7). tTreg and pTreg vary in their ontogeny, are regulated differently, and serve different functions, ensuring their complementary roles in immune tolerance and homeostasis. For example, pTregs are better suppressors of allergic airway diseases than tTregs of the same specificity (8), while tTregs are more efficient at suppressing experimental autoimmune encephalomyelitis (9). The difference in response likely relates to their differential expression of regulatory markers such as CTLA-4, PD-1, and their different capacity for cytokine production. This review will discuss the intrinsic and extrinsic factors that regulate Treg differentiation, highlighting the multiple avenues to promote and maintain Treg through dietary intervention. A glossary of key terms used in this review is presented in Table 1.
TREG Development
Thymic Treg Differentiation
Overview
The thymus is a specialized primary lymphoid organ and is the site of thymopoiesis, a process by which mature and functional T cells are generated. The earliest T cell progenitors are bone marrow-derived hemopoietic stem cells (HSC) that migrate to the thymus through their expression of chemokine receptors CCR9 and CCR7, as well as P-selectin ligand (11). The first step of thymopoiesis is characterized by the generation of the early T lineage progenitors, the earliest T cell lineage-committed HSCs. These progenitors differentiate into CD4−CD8− double-negative and then to CD4+CD8+ double-positive thymocytes, which undergo several developmental checkpoints called positive and negative selection, which occur in different anatomic parts of the thymus (7). These processes ensure the strict selection of thymocytes with functional T-cell receptors (TCR). These TCR bind effectively self-major histocompatibility complex (MHC) but are hyporesponsive to self-antigens. Positive selection occurs within the thymic cortex, where CD4+CD8+ double-positive thymocytes interacting effectively with self-MHC molecules expressed by cortical thymic epithelial cells survive. These cells further differentiate into single positive CD4+CD8− or CD8+CD4− thymocytes. These single positive thymocytes then migrate to the medulla, where they undergo the negative selection, a process eliminating autoreactive thymocytes with high affinity to self-antigens. Self-antigens are presented by various antigen-presenting cells (APCs), particularly medullary thymic epithelial cells (mTECs). Strong TCR signaling to self-antigens leads to clonal deletion by apoptosis, while a small percentage survive to become Tregs. The mechanisms behind the commitment of autoreactive CD4+ T cell toward Tregs differentiation rather than clonal deletion is not fully understood but likely involves multiple factors, including affinity of TCR binding to self-antigen/MHCII complex, duration of TCR signaling, and the phenotype of the APCs presenting the antigens (discussed later) (7). Regardless, TCR interactions with self-antigen appear to be the dominant signal for tTreg induction. It was elegantly demonstrated by utilizing T cells with transgenic TCR of varying affinities to OVA peptide that mice with higher-affinity TCR-bearing T cells have a larger tTreg pool (12). Other factors, such as costimulation and cytokines, support their development and maturation. Engagement of the co-stimulatory molecule CD28 to CD80/CD86 ligand expressed on APCs augmented the TCR signaling pathway to promote tTreg differentiation (13) and survival (14). The presence of CD28 on T cells was correlated with increased diversity of the TCR repertoire of the tTreg pool, possibly by promoting tTreg differentiation from thymocytes expressing infrequent TCRs (14). The pro-survival cytokine IL-2, which signals through CD25 in an autocrine manner (15), was required for early tTreg survival and expansion and later maturation (16). A deficiency of IL-2 could be compensated by other pro-survival cytokines such as IL-7 and/or IL-15 expressed by mTECs (17, 18).
Antigen-Presenting Cells in the Thymus and Their Role in tTreg Induction
mTECs are the main cell subset involved in antigen presentation in the thymus, a process regulated by the transcription factor autoimmune regulator (AIRE). AIRE, along with Fez family zinc finger protein 2 (FEZF2), regulates the expression of tissue-specific antigens (TSAs) for presentation to thymocytes. Together, they coordinate ~60% of the TSAs expressed by mTECs (19). FEZF2 and AIRE control the expression of a mostly distinct set of TSA genes, although almost 40% of TSAs among mTECs were regulated through unknown mechanisms independent of AIRE and FEZF2 (19). This suggests that other transcription factors or potential extrathymic factors regulate the tTreg repertoire.
Apart from mTECs, other APCs can contribute to tTreg differentiation by presenting a distinct repertoire of antigens (20), suggesting that mTEC and other APC have a complementary role in establishing optimal tolerance (21, 22). These APCs include dendritic cells (DCs), plasmacytoid DCs (pDCs), and B cells, which have varying abilities to promote tTreg generation, as reviewed previously (23). Intrathymic-derived CD8α+ conventional type I DCs present thymic-derived antigens acquired by membrane transfer from mTEC (24) but are dispensable for tTreg induction (22). On the other hand, extrathymically-derived SIRPα+ conventional type 2 DC, which can capture peripheral antigens for presentation to thymocyte, were critical for tTreg development (25). Other peripheral APCs can also capture peripheral antigens and egress to the thymus, including PDCA-1+ pDC (25) and gut-derived CX3CR1+ DCs (26). While gut-derived CX3CR1+ DCs have been shown to present microbiota-derived antigens and promote the generation and expansion of microbiota-specific thymic T cells, they did not appear to affect tTreg development (27). Similarly, thymic pDC are weak Treg inducers in vitro (26) and are unlikely to play a role in vivo (26). Altogether, mTECs are the main tTreg inducers, yet more research is needed to confirm the role of other APCs in tTreg development.
Dynamic of tTreg Development at Different Stages of Life
The biology of T cell development fluctuates throughout life. For example, early fetal early thymic progenitors (ETP) are biased toward T cell differentiation (E12–E15), while later waves of ETP (after E16) have myeloid differentiation potential that more closely represented postnatal ETP (28). CD4+ T cells generated in the fetal thymus also produce more cytokines and are skewed toward Th2 differentiation, while adult thymic-derived CD4+ T cells produce fewer cytokines and are skewed toward Th1 cytokine production (29).
Differences in the aging thymic environment also affect tTreg development, with thymic progenitors having a reduced propensity toward Treg differentiation with increasing age (30). This is likely an evolutionary mechanism to maintain a tolerogenic environment during pregnancy and exposure to environmental antigens during early life. The neonatal tTreg pool is also characterized by a greater TCR repertoire diversity and increased expression of FoxP3 and suppressive molecules, such as CTLA-4, compared to adult Tregs (31, 32). The more diverse neonatal tTreg TCR repertoire likely results from the ability of perinatal mTECs to present a more extensive repertoire of TSAs (33). These age-dependent differences suggest a developmental window for the selection of tTregs with specific clonotypes. One example is the development of Padi4-specific tTregs, which was restricted to the neonatal period (34). The inability of the adult thymus to generate Padi4-specific tTregs appears to relate to the presentation of the Padi4 peptide by non-mTEC APCs instead of mTECs, which promotes instead clonal deletion. mTECs are the major tTreg inducer in the neonatal thymus, as other APCs (such as pDC and conventional DC) do not efficiently seed the thymus until later in life (35). Other cells maintain the same function throughout life, such as colonic-derived pDCs. These pDCs are regulated by the neonatal gut microbiota and migrate to the thymus to support PLZF+ innate-like T cell differentiation, with the effect persisting into adulthood (36). These PLZF+ innate-like T cells contribute to the differentiation of thymic Treg with an activated/memory-like phenotype (37).
Peripheral Treg Differentiation
While tTreg and pTreg are characterized by their expression of FoxP3 and their immunosuppressive functions, they differ in their ontogeny. pTreg can arise from naïve CD4+ T cells dependently or independently of peripheral APC. Contrary to tTreg, pTreg differentiation mainly relies on environmental cues, particularly the cytokine tumor growth factor-β (TGF-β) that promotes the upregulation of FoxP3 (38). The commitment to the Treg lineage also depends to a lesser extent on TCR signaling and engagement of co-stimulatory molecules such as CTLA-4 (39). TGF-β promotes the differentiation of Tregs via phosphorylation of Smad3, which then translocates to the nucleus and acts as a transcription factor regulating the expression of Foxp3 (40). Non-cytokine factors can also direct FoxP3 expression and promote de novo pTreg differentiation via epigenetic regulation of the Foxp3 locus. FoxP3 expression is controlled by three major regulatory regions within the FoxP3 locus: the conserved non-coding DNA sequence (CNS) elements CNS1, CNS2, and CNS3. Demethylation of the CNS regions is observed in both tTregs and pTregs, with CNS1 appearing to be critical for pTreg but not tTreg differentiation (41, 42). Similarly, acetylation of histone H3 both at the promoter and CNS regions of the Foxp3 locus promoted pTreg differentiation (43).
Numerous immune and non-immune cells can provide the environmental cues necessary for pTreg differentiation. Among the immune cells, CD103+ DCs, macrophages, regulatory B cells (Bregs), and Treg support pTreg differentiation through their release of TGF-β. Antigen-specific pTregs are induced by TGF-β and retinoic acid produced by gut mucosal CD103+ DC and lung resident macrophages, protecting from food allergies, asthmatic lung inflammation, and airway hyperreactivity, respectively (44). Bregs produce both TGF-β and IL-10 to induce pTregs (45), ensuring allograft tolerance (46). Activated Tregs can also promote the differentiation of Tregs from naïve CD4+ T cells through their surface expression of TGF-β, which activates contact-dependent TGF-β signaling (47). CD103+ DC, gut epithelial and stromal cells are also a source of TGF-β and retinoic acid, supporting pTreg differentiation to maintain oral tolerance (48–50).
Impact of Diet on TREG Development
Diet is a major environmental factor influencing health. A balanced diet consists of 45–65% carbohydrates, 20–35% fat, and 15–25% protein by energy content and sufficient intakes of micronutrients, such as minerals and vitamins. While the role of diet on metabolic health is well-established, an increasing number of studies reveal its impact on the immune system, particularly on Treg differentiation and function. Diet may directly or indirectly affect Treg through changes in the gut microbiota (51). A summary of how different dietary components promote the differentiation of Treg is presented in Figure 1.
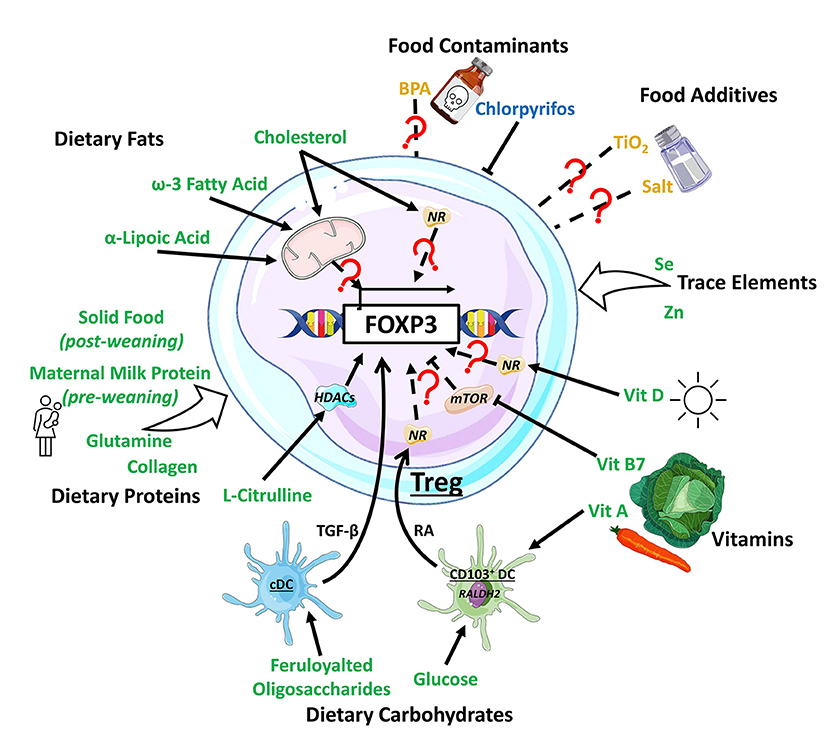
Figure 1. Impact of dietary components on Treg development. Dietary components have varying effect on Treg development, with dietary fats inducing Treg by modulating metabolic activity or through the activation of nuclear receptors (NRs) such as PPARδ. Dietary protein generally support Treg development, with L-citrulline enhancing the histone deacetylase (HDAC) Sirt1 to promote Treg development. Dietary carbohydrates, including feruloylated oligosaccharides and glucose act on conventional dendritic cell (cDC) and CD103+ DC, boosting their production TGF-β and retinoic acid (RA), respectively, to promote Treg. Vitamin (Vit) A is a substrate for RA production required for Treg induction while vitamin B7 and D finetune mTOR-related metabolic signals or bind to NRs to support Treg induction. Dietary trace elements like selenium (Se) and zinc (Zn) are supportive toward Treg development with unknown mechanisms. The food additives TiO2 and salt, and the contaminant biphenol A (BPA) exhibit mixed/complex regulatory patterns toward Treg development while pesticides like chlorpyrifos suppressed Treg development. Dietary components promoting Treg development are colored in green, the ones impeding in blue, and the ones with mixed effect in yellow.
Influence of Diet on tTreg Ontogeny
The role of diet in tTreg induction is not well-established. Nonetheless, two factors related to diet promote tTregs: short-chain fatty acids (SCFA), a by-product of the fermentation of complex carbohydrates by gut bacteria, and dietary cholesterol. The role of SCFA on tTreg ontogeny will be discussed in the gut microbiota section below. Cholesterol, derived from animal products, is prevalent in the Western diet, and supplementation of a standard murine chow with 0.15% cholesterol enhanced TCR responsiveness in CD4+ T cells and increased Treg frequency and suppressive capacity in both central and peripheral compartments (52). The mechanisms behind these effects remain elusive.
On the other hand, dietary salt has been shown to reduce the expansion of thymic tTregs and decrease their suppressive function when mice were fed on a high-salt diet. Dietary salt changed extracellular osmotic pressure, altering cell tonicity by activating the tonicity-responsive enhancer-binding protein and p38 signaling pathway. These changes resulted in the upregulation of serum/glucocorticoid-regulated kinase 1, promoting IFNγ and RORγt expression. As a result, tTregs were repolarized toward Th1 and Th17 lineage. Serum/glucocorticoid-regulated kinase 1 also phosphorylates and inhibits FOXO1 and FOXO3, decreasing FoxP3 expression and thus tTreg differentiation (53). Interestingly, these effects are specific to murine tTreg and not pTreg, with further investigation needed to clarify whether these effects apply to humans.
Influence of Diet on pTreg Ontogeny
Macronutrients and pTreg
Role of Dietary Fats
Dietary fats, such as cholesterol and omega-3 fatty acids, and fat metabolic intermediates, such as α-lipoic acid, increase pTreg populations. The impact of fatty acids and cholesterol on pTreg induction is mostly via metabolic effects, with fatty acid oxidation promoting Treg differentiation. Oral administration of α-lipoic acid in obese mice restored visceral Treg numbers by enhancing naïve T cell oxidative capacity and fatty acid oxidation (54). Similar results were observed in humans, in obese women who received α-lipoic acid supplementation (54). Likewise, in mice treated on a diet containing 0.25% cholesterol and 15% cocoa butter, the accumulation of cholesterol in Treg increased fatty acid oxidation, leading to an increased splenic Treg population (55). Cholesterol also promotes Treg development by activating mammalian target of rapamycin (mTOR) complex 2 and PPARδ signaling (55). Fatty acids may also indirectly induce pTreg differentiation with for example ω-3 docosahexaenoic acid induced production of lipoxin A4 by neutrophils, which increases pTregs (56, 57).
The type and the amount of dietary fat consumed can modulate the pTreg compartment. pTreg are decreased in tissues such as the spleen and white adipose tissues in both high-fat diet (HFD) induced obesity (58) and non-alcoholic steatohepatitis (59) animal models, as well as in a humanized mouse model (60). This was paralleled with low-grade inflammation and metabolic alteration. Similarly, HFD-induced myocardial fibrosis and atherosclerosis in mice were characterized by decreased Tregs in the heart (61) and aorta (62). Dietary fat may also affect pTreg function, as mice fed an HFD containing 35% hydrogenated vegetable oil had decreased IL-10 (60). Whether these Treg number and activity changes are directly due to HFD or are secondary to metabolic alteration is unclear. Changes in the oxidative environment linked to metabolic alteration may directly contribute to decreased Treg. In an HFD-induced non-alcoholic steatohepatitis model, the rise of hepatic oxidative stress and reactive oxygen species triggered Treg apoptosis, increasing liver inflammation (63).
Conversely, isocaloric ketogenic diets consisting of high fat (70–80%) and low carbohydrate (5–10%) content increase naïve T cell fatty acid oxidation, promoting Treg differentiation and IL-10 production (64). To our knowledge, only one study has investigated the impact of a ketogenic diet over 3 weeks on Treg induction in humans (64). Data in mice are inconsistent depending on the model with either an increase in Tregs in a carcinomatous peritonitis model (65) or a decrease in a diabetic obese mouse (66). This difference may be due to the altered metabolic status of the mice.
Role of Dietary Proteins
Dietary proteins are the primary source of food antigens, a key component of pTreg development. Before weaning, the maternal breastmilk proteins constitute the majority of luminal antigens contributing to colonic pTregs generation. Colonic Treg from such origin plays an important role in tolerance maintenance and allergy suppression by dampening the Th2 responses (67, 68). Unlike Tregs in the colon, Tregs in the small intestine lamina propria are induced during weaning when exposure to solid food commences. This population is sparse before weaning, implying breastmilk has a limited contribution to their development. There is, however, a significant boost in their induction upon weaning with a regular chow diet, both in germ-free (GF) or specific-pathogen-free (SPF) mice. Conversely, weaning with an antigen-free diet (deficient in dietary protein antigens) fails to replenish small intestine pTreg. Similar observations have been reported in zebrafish, where pre-exposure to food antigens promoted an intestinal Treg phenotype while lack of prior food antigen exposure did not (69).
Studies centered on the impact of dietary protein on pTreg have primarily focused on specific proteins or amino acids. Cow milk proteins have been implicated in pTreg development, although changes are dependent on the type of protein. Four generations of mice were fed from birth with a nutritionally balanced milk-based diet containing either A1 or A2 beta-casein components. While there were no changes in conventional FoxP3+CD25+ Tregs, the A2-fed cohort had increased non-conventional FoxP3+CD25− Tregs in the spleen (70). Regarding the impact of specific amino acids on Treg development, glutamine supplementation has been the most investigated. A diet in which 25% of casein was replaced by glutamine increased circulating pTregs in both sepsis (71) and DSS-induced colitis mouse model (72), though the mechanism was unresolved. Other amino acids such as collagen and L-citrulline also increased pTreg differentiation in vitro and in vivo, respectively (73). The effect of L-citrulline on naïve T cells was linked to the upregulation of the deacetylase Sirtuin 1 (Sirt1) and downregulation of Smad7, an inhibitor of TGF-β signaling. These changes enhanced Foxp3 transcription and IL-10 production, thus Treg differentiation (73).
Role of Dietary Carbohydrates
Carbohydrates such as feruloylated oligosaccharides and glucose can increase pTreg differentiation (74, 75). Oral administration of 200 or 400 mg/kg/day of feruloylated oligosaccharides increased colonic Tregs in a DSS-induced colitis mouse model, likely through the increased production of TGF-β from DCs (75). The in vitro addition of glucose to isolated small intestine-derived DCs increased RALDH2 activity, leading to higher retinoic acid production and enhanced ability to promote Treg differentiation (74).
Micronutrients and pTreg
Zinc and Selenium
Micronutrients such as zinc and selenium modulate pTreg generation. A zinc-deficient diet significantly decreased splenic pTreg without altering their suppressive function in a mouse model of dermatitis (76). Conversely, a diet enriched in zinc increased pTreg numbers in weaned piglets and adult pigs (77, 78). The mechanism(s) behind zinc's effect on Treg remains elusive, as is whether this effect applies to human Treg. Selenium supplementation in drinking water protected mice from autoimmune thyroiditis (79) and DSS-induced colitis (80) by inducing splenic and colonic lamina propria pTreg, respectively. During DSS-induced colitis, selenium also increased IL-10 production in CD4+ T cells, reducing colonic inflammation. The role of selenium in human Treg biology is under-investigated. Only one study demonstrates that daily selenium supplementation (200 μg/day) for 3 months had no impact on a subset of non-Hodgkin lymphoma patients (81).
Vitamins
Deficiency in vitamins D, B7, and A, has been shown to reduce both the proportion and functionality of pTreg. Vitamin D deficiency reduced splenic Tregs in healthy mice (82) and decreased Treg in sino-nasal tissue during chronic rhinosinusitis (83). Similarly, vitamin B7 deficiency reduced the expression of FoxP3 in murine inguinal lymph nodes and inhibited Treg differentiation in vitro. These effects involved mTOR activation, which inhibited FoxP3 expression in CD4+ T cells while promoting IFNγ and IL-17 (84). Vitamin A is a crucial factor promoting Treg development via its conversion into retinoic acid by the enzyme RALDH2 expressed in CD103+ DC. The absence of vitamin A impairs Treg generation and suppressive function (6).
Food Additives
Food additives are prevalent in modern diets and added to processed food for various reasons, such as increasing shelf-life and improving texture. The whitening agent titanium dioxide (TiO2) is one of the most common food additives (85, 86), and its reported effects on pTreg appear inconsistent. While water supplementation with TiO2 (10 mg/kg body weight/day) for 7 days reduced Tregs in Peyer's patches in rats (87), the addition of TiO2 into food had no impact (88). In mice, the addition of TiO2 to drinking water for 3–4 weeks at either a physiological (10 mg/kg body weight) or high-dose (50 mg/kg/body weight) had no impact on Treg (85). Emulsifiers are used to improve food texture and have been shown to exacerbate obesity and colitis in mice via effects on the gut microbiota (89). Despite the reported pro-inflammatory effect of emulsifiers carboxymethylcellulose or polysorbate-80, their impact on Treg is unknown.
Salt has been used as a preservative for millennia, and its impact on pTreg varies in humans and mice and depends on individuals' health status. In a pilot study of 5 healthy men, 2 week-treatment on a high-salt diet did not alter pTreg numbers (90), while in healthy mice, a high-salt diet reduced bone marrow Treg and impaired their function, as they had decreased ability to produce IL-10 (91). Dietary salt consumption was negatively correlated with circulatory CD69+ Treg and NKG2D+ Treg in rheumatoid arthritis and systemic lupus erythematosus, respectively (92). Decreased salt consumption restored the circulating pTreg pool in these patients (93). On the other hand, high-salt feeding had a negligible effect on pTreg in the spleen, draining lymph nodes and mesenteric lymph nodes in a mouse model of multiple sclerosis (94). Sodium benzoate, another commonly used food preservative, has been shown to induce pTreg by increasing TGF-β via STAT6 dependent mechanisms, protecting mice from experimental autoimmune encephalomyelitis (95).
Food Contaminants
Modern agricultural practices expose food to unwanted contaminants such as pesticides. In mice, intake of 7 mg/kg chlorpyrifos, a widely used organophosphate pesticide, decreased the circulatory Treg pool and suppressed Treg-related gene expression in the spleen (96). While pesticide exposure has extensive links to specific diseases such as cancer (97), their impact on the immune system is understudied. Plastic food packaging is also a significant source of food contamination, particularly packaging containing the synthetic organic chemical bisphenol A (BPA). However, the role of BPA on Treg is inconclusive, with one study finding that mouse exposure to BPA at different developmental stages decreased splenic Tregs in males (98, 99) and another study finding the opposite (100). While not strictly a contaminant, plant cells like any cells, can also produce extracellular vesicles (EV) either under basal conditions or in response to threats. These plant-derived particles have been shown to modulate mammalian cell phenotypes, including immune cells. For instance, plant-derived exosome-like nanoparticles derived from ginger and carrot induced IL-10 production in RAW264.7 macrophage cell line (101) but whether this effect applies to T cells is unknown. Similarly, whether plant-derived EV likely present in food products are immunomodulatory in vivo and affect Treg development remain elusive. Plant-derived EV can modulate bacterial growth (102), and thus, as a result, may alter the gut microbiota composition. As discussed below, changes in gut microbiota may lead to Treg development.
Caloric Restriction
Caloric restriction (CR) has been shown to promote pTreg, protecting mice from experimental autoimmune encephalomyelitis (103, 104) and ischemic stroke (105). By limiting T cell energy availability, CR raises the intracellular ratio of adenosine monophosphate (AMP): adenosine triphosphate. This activates AMP-activated protein kinase, which inhibits mTOR promoting Treg differentiation. CR downregulate acetyl-coenzyme A carboxylase 1, decreasing de novo fatty acid synthesis and biasing naïve T cell differentiation toward Treg rather than Th17 (105). CR also elevates ketone bodies, but this does not contribute to pTreg differentiation, as murine supplementation with ketone ester (a ketone body raising agent) did not affect splenic and intestinal Tregs (106).
Gut Microbiota and TREG Development
Bacteria, viruses, archaea, and fungi colonize the human gastrointestinal tract from birth. Collectively, this community is known as the gut microbiota and has diverse effects on the host. The gut microbiota can influence host metabolic, physiological, and immunological functions and mediate these effects via direct (i.e., membrane components) or indirect (i.e., production of metabolites) mechanisms (107). The overall impact of bacteria-derived metabolites or antigenic components is summarized in Figure 2.
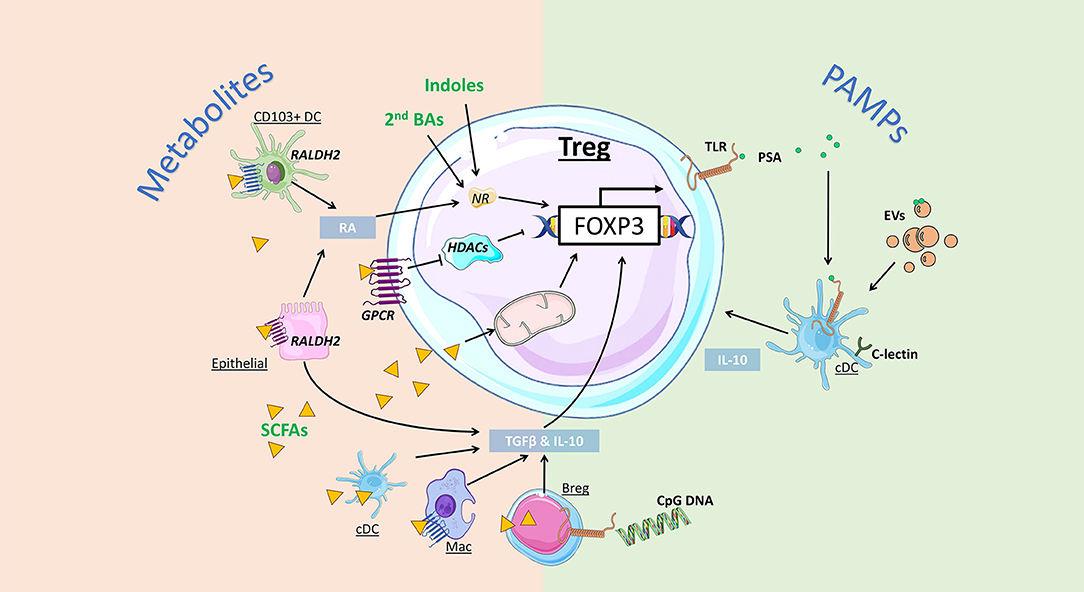
Figure 2. Impact of gut bacteria on regulatory T cell development and function. Microbiota-derived metabolites can regulate Treg development and function via distinct mechanisms. Indoles and secondary bile acids (BAs) can bind to nuclear receptors (NRs) to promote Foxp3 expression and Treg induction. Short-chain fatty acids (SCFAs) can activate G protein-coupled receptors (GPCRs) to promote Treg directly via inhibition of histone deacetylase (HDAC) activity, or indirectly, by enhancing retinal dehydrogenases (RALDH) and retinoic acid (RA) production by CD103+ DCs and epithelial cells. Alternatively, SCFA are directly taken up by cells, including regulatory B cells (Bregs) and conventional DCs (cDCs) to promote expression of Treg-inducing cytokines TGF-β and IL-10. SCFA uptake by T cells can also directly promote Treg induction by increasing mitochondrial activity. Bacterial pathogen associated molecular patterns (PAMPs) can also promote Treg via activation of toll-like receptors (TLR). Bacteroides fragilis-derived polysaccharide A (PSA) promote Treg via TLR signalling on DCs, promoting IL-10 production, as well as Treg-intrinsic TLR2 signals. Extracellular vesicles (EVs) are PAMPs that can bind to TLR and C-lectin expressed by gut DCs and epithelial cells, triggering the release of Treg-inducing cytokines IL-10 and TGF-β.
The intestinal compartment is particularly enriched in Tregs, representing approximately 30% of colonic lamina propria CD4+ T cells (108). Most studies indicate that colonic Tregs are generated de novo through contact with the microbiota (109, 110), with only one study reporting colonic Tregs are thymic-derived (111). Indeed, compared to SPF mice, GF mice have reduced numbers of colonic Tregs (109). Antigenic stimuli from the gut microbiota generate and activate RORγt+ Tregs, a critical subset of colonic Tregs, which are integral to intestinal homeostasis (110). RORγt+ Tregs, which comprise 65% of colonic Tregs, require microbial antigens and are absent in GF conditions (112, 113). RORγt+ Tregs expand during the weaning period when there is an influx of food and microbial antigens. These cells suppress both Th1 and Th2 responses and maintain homeostasis with commensal microbiota (112). A variety of microbes can induce colonic RORγt+ Tregs in GF mice, including bacteria from Clostridia (109), Bacteroides (113), and to a lesser extent, Segmented Filamentous Bacteria (113). As discussed later, other bacterial strains, listed in Table 2, have been reported to influence Treg development through various mechanisms.
Although many studies examine singular mechanisms for Treg induction, it is unlikely that bacteria conform to any one mode of action. For example, 46 strains from the genus Clostridia, derived from Clusters IV and XIVa, induced colonic Tregs in GF mice (109). This was attributed to increased secretion of TGF-β and indoleamine 2,3-dioxygenase (a catabolic enzyme for tryptophan) by colonic intestinal epithelial cells, which induced FoxP3+ Tregs independently of pattern recognition receptor (PRR) signaling. Treg levels were preserved at least 4 months post-induction, and this increased Treg phenotype was vertically and horizontally transmissible to other GF mice along with microbiota composition, indicating that the Clostridia clades could stably induce Tregs. The mechanism behind Clostridia Treg-induction appears multifactorial, dependent on metabolites (SCFA and indole) (136), bacterial antigens, and Treg-promoting cytokines, particularly TGF-β1 in both mouse and human epithelial cell lines (136). The authors also identified Clostridia-specific T cells, suggesting specific antigens contributed to Treg induction (136). Monocolonization of GF mice with single strains of Clostridia, however, was unable to fully recapitulate the Treg-inducing capability of the 17-strain mix, indicating the microbiota cooperatively promoted Tregs through independent mechanisms. Members of Clostridia predominantly ferment dietary fiber or indigestible carbohydrates (particularly polysaccharides) as an energy source; however, some Clostridium species preferentially utilize amino acids/protein as a nutrient source [reviewed in (137)]. Supplementation with different fibers promotes different Clostridia clusters, in distinct GI tract sites, with piglets fed on a diet supplemented with either alfalfa or cellulose for 3 weeks had increased cluster XIVa in the distal and proximal colon, respectively (138). Overall, bacterial species induce Tregs through multiple mechanisms, which can be dramatically affected by diet.
Gut Bacterial Metabolites and PTREG Development
Metabolites
Short-Chain Fatty Acids
SCFAs are by-products of bacterial fermentation of dietary fiber. The three predominant SCFAs, acetate, butyrate, and propionate, ameliorate inflammatory diseases by regulating Treg function and frequency (139–141). SCFAs possess intrinsic histone deacetylase (HDAC) inhibitory properties and directly promote Foxp3 gene expression. They induce pTreg differentiation through the acetylation of histones H3 and H4 within the Foxp3 locus (42, 43, 139–142). Deficiency in GPR43, a receptor for acetate and propionate, counteracted the effect of propionate on the acetylation of histone H3K9 (139). As a result, propionate treatment in GPR43 knockout mice did not increase Treg (139). However, other studies have reported no defect in Treg development in both Gpr43−/− and Gpr41−/− mice (142). SCFAs can also promote Treg differentiation via HDAC-independent mechanisms by altering Treg metabolism. Propionate treatment in multiple sclerosis patients enhanced Treg oxygen consumption rate, altered their mitochondrial morphology, and their improved suppressive functions. These patients had increased proportion of circulating Tregs, which ameliorated disease progression (143).
SCFAs can indirectly upregulate Tregs through their actions on other immune cells. Activation of GPR109A by butyrate in DCs and macrophages increased Aldh1a1 and Il10 expression, improving their ability to induce Treg differentiation and function (144). Moreover, acetate and butyrate treatment in a murine food allergy model increased the number and activity of CD103+ DCs, which enhanced the generation of antigen-specific Tregs and protected against severe anaphylaxis (6). Bregs also promote Tregs via their secretion of TGF-β (145) and IL-10 (146). Acetate can directly induce Bregs via stimulation of the tricarboxylic acid cycle and post-translational acetylation (147, 148). Current literature, however, is fraught with inconsistencies; Daïen et al. (148) found acetate promoted Bregs while butyrate was inhibitory, whereas Zou et al. (147) observed the opposite.
Interestingly, acetate could not induce Tregs in vitro when combined with TGF-β (42) but did upregulate IL-10 production and FoxP3 expression under Th1/Th17-polarizing conditions (142). In mice, acetate upregulated Foxp3 only after induction of allergic airway disease (140). T cell transfer from non-obese diabetic mice fed an acetate-enriched diet could not protect their peers from induction of disease, unlike T cells transferred from their counterparts fed a butyrate-enriched diet (141). Similarly, propionate-mediated amelioration of colitis and candidiasis protection is required prior to FoxP3 induction (139, 149). Therefore, the protective effects of each SCFA may require unique prerequisites which must be considered during experimental design.
Indole
Indole and indole-derivatives are derived from the diet or through bacterial metabolism of the amino acid tryptophan, and these molecules have diverse effects in hosts (150). Diet-derived indoles upregulate Foxp3 and Il10 gene expression via the activation of Aryl hydrocarbon receptors (AhR) (151–153). Moreover, indole-3-carboxaldehyde, derived from commensal bacteria or cruciferous vegetables, increased Il10 transcripts in mice colon (154) and consequently increased the frequency and function of Tregs. Few studies, however, have examined microbially-derived indole and their derivatives on Treg function. Interestingly, butyrate indirectly ameliorated arthritis by promoting tryptophan-digesting bacteria, thereby increasing colonic levels of hydroxyindole-3-acetic acid, increasing Breg function via AhR (155). This highlights the complex interplay between bacterial metabolites and the difficulties of studying these biological products.
Bile Acids
Primary bile acids (BA) are synthesized by the liver, stored in the gallbladder, and secreted into the gut lumen, where they facilitate lipid digestion (156, 157). Most BAs are reabsorbed in the ileum, but 5% escape into the colon, where they undergo biotransformation by the gut microbiota. This involves deconjugation and transformation into secondary BAs, comprising mostly deoxycholic acid (DCA) and lithocholic acid (LCA) in humans (158). BAs have been shown to activate farnesoid X receptor (FXR), vitamin D receptor (VDR), and G protein-coupled bile acid receptor 1 (TGR5) (157, 159). Along with the activation of these receptors, BA metabolites may also upregulate Tregs via epigenetic and metabolic modulations.
3β-hydroxydeoxycholic acid (isoDCA) promotes pTreg differentiation by downregulating the pro-inflammatory profile of DCs (160). It was proposed that isoDCA may function via antagonism of FXR, which contradicts the consensus of BAs as FXR agonists (161). However, the authors did not show direct inhibition of FXR activity by isoDCA. In contrast, Song et al. found that both VDR and FXR deficiency depleted colonic RORγt+ Treg frequency, with VDR being the principal receptor required for BAs to maintain colonic RORγt+ Treg homeostasis (159). Accordingly, VDR deficiency was associated with increased disease severity in murine models of colitis; however, whether this was mediated by BAs or Vitamin D was not examined (159). In contrast, the secondary BA, isoalloLCA, could enhance pTreg differentiation by increasing H3K27 acetylation within the CNS3 region of the Foxp3 locus (162) or by binding to the nuclear hormone receptor NR4A1 (122). Interestingly, enhancement of mitochondrial reactive oxygen species was necessary for isoalloLCA promotion of Tregs (162).
Overall, the BA-mediated induction of Tregs utilizes various mechanisms seemingly unique to each BA. Although studying the effects of individual BAs is common, this is not reflective of the diverse pool of BAs present in hosts. Indeed, Song et al. found supplementation with defined mixes of primary or secondary BAs rescued colonic Treg frequencies while singular BAs did not (159), suggesting that BA induction of Tregs likely requires synergistic activities. Unfortunately, choosing a consistent and defined pool of BAs is difficult, as differences in host diet and microbiota lead to significant individual variation in the BA pool (163).
Fatty Acids
Dietary fatty acids, including polyunsaturated fatty acids such as omega 3 and omega 6 can be processed by gut bacteria to a variety of metabolites. In particular, members of Bifidobacteria can produce conjugated linoleic acid (CLA), which increased murine Treg through PPARγ activation in a murine model of DSS-induced colorectal cancer (164). Dietary supplementation with CLA improved DSS colitis in mice by upregulating colonic IL-10, although Tregs were not directly examined (165). Linoleic acid metabolites can also inhibit Treg development with 12,13-diHOME decreasing pulmonary Tregs via the activation of PPARγ in DC, which in turn decreased their IL-10 secretion in an allergic airway model (166).
Direct Physical Interaction or Indirect
Bacterial Extracellular Vesicles
The activation of host PRRs by microbial ligands present on the bacterial surface, collectively known as pathogen-associated molecular patterns (PAMPs), is a common mechanism of Treg induction. Bacterial PAMPs, such as the membrane components lipopolysaccharide (LPS) and lipoteichoic acid (LTA), can activate TLR4 and TLR2, respectively. The TLR2/MyD88 signaling pathway has been particularly well-studied in the context of Treg induction with a reduction of tTregs and pTregs in TLR2 deficient mice (167) Treg numbers were unaffected in TLR4 deficient mice (168). The most well-known commensal activation of the TLR2 pathway is by the capsular polysaccharide A (PSA) present on Bacteroides fragilis, inducing colonic FoxP3+ Tregs (130). Purified PSA promoted Treg differentiation via the activation of tolerogenic DCs (169). Whether other bacteria such as Akkermansia muciniphila or the defined commensal flora (known as the Altered Schaedler Flora) induce Treg via direct or indirect effects (124, 125) and through PRR activation is still unclear.
Microbial antigens can also regulate Treg biology via Treg-intrinsic signaling. Treg express TLRs, which enables their direct sensing of PAMPs. TLR engagement on Treg regulate their suppressive capacity, likely in a species-specific manner (170). TLR5 activation on human CD25+ Treg increased FoxP3 expression and suppressive activity in vitro (171). TLR2 activation on CD4+ T cells by B. fragilis PSA NCTC9342 induced Treg and augment their suppressive capacity in the absence of APCs (169). These effects are specific for PSA as the synthetic TLR2 ligand Pam3CSK4 did not affect Treg function. Another study identified that hundreds of phylogenetically diverse intestinal bacteria could produce PSA-like capsular polysaccharides (172), and lysates of these strains induced IL-10 secretion and increased CD25+FoxP3+ cells in vitro in human peripheral blood mononuclear cells (172). The list of commensal bacteria able to regulate host immunity is thus far from extensive.
Gut bacterial extracellular vesicles (EV) are nanosized membrane vesicles released constitutively by gram-positive and gram-negative bacteria. EV can cross the intestinal barrier under homeostatic conditions (173, 174) and disseminate to distal organs, including the liver, heart, spleen, kidney, and brain (175). The outer surface of EVs is enriched in bacterial membrane components such as LPS, LTA, peptidoglycan, and various lipoproteins. EV package inside a variety of cargo, including lipids, proteins, carbohydrates, and nucleic acid (174).
Gut bacterial EV can indirectly induce pTreg via DCs. B. fragilis NCTC9343 EVs induced Treg through TLR2-dependent mechanisms on tolerogenic DCs (131) as well as Bifidobacterium bifidum LMG13195 EVs by inducing DCs maturation in vitro (176). In vivo, a 3 day administration of Lactobacillus rhamnosus JB-1 EVs to mice increased proportions of IL-10+ DC and functionally suppressive FoxP3+ Treg in Peyer's patches and mesenteric lymph nodes (177). Induction of tolerogenic IL-10+ DC was dependent on multiple PRRs activation, including TLR2, TLR9, and the C-lectin type receptors Dectin-1 and SignR1, indicating EVs can activate multiple PRRs. L. rhamnosus JB-1 EVs were also enriched in heat shock proteins (HSPs) (177), a highly conserved protein family across species. HSP are critical regulators of Treg via TLR2 (178) with HSPs from E. coli (179), Mycobacterium tuberculosis (180, 181), H. pylori (182), as well as from helminths (183) promoting Treg in vitro. Treg specific for bacterial HSP can also recognize host HSP, which are released during inflammation. These Treg can as a result attenuate inflammatory disorders such as experimental arthritis (184). Furthermore, HSP-specific T cells with suppressive capacity expressed a variety Treg-associated markers such as GITR, CTLA-4, and LAG-3 (184).
Together, these studies demonstrate that EVs promote Treg through various mechanisms including via PAMPs and HSP. Other mechanisms, including small RNAs, which are important in interkingdom communication may also be involved (185).
Gut Bacterial DNA
Bacterial DNA contains a high frequency of unmethylated CpG dinucleotides (CpG), a ligand for TLR9. CpG content differs widely across bacteria (186) resulting in their different ability to stimulate TLR9, with the higher the frequency of unmethylated CpG nucleotides in bacterial DNA, the highest the activation of TLR9 (187). Bacterial CpG is a potent inducer of IL-10+ Breg in vitro in humans (188) and mice (189) via TLR9. In allergy, CpG-TLR9 activation on DC and B cells initiates a Th1 and Treg response that restores immune balance (190). As such, synthetic CpG oligodeoxynucleotides (ODN) have been used therapeutically in the treatment of asthma and allergy (191, 192).
Nucleic acid from gut bacteria is an essential source of TLR9 ligands critical for gut homeostasis, as apical TLR9 signaling in intestinal epithelial cells limits pro-inflammatory signals, and Tlr9−/− mice are more susceptible to colitis (193). Oral administration of synthetic ODN (ID35) derived from L. rhamnosus attenuated colitis (194), and a synthetic ODN derived from Streptococcus thermophilus NCDO 573 (commonly found in fermented milk products) increased IL-10 expression and proportion of CD4+CD25+ Treg in murine splenocytes (195). In humans, TLR9 agonists are used as anti-inflammatory therapy for ulcerative colitis (196) and ODN-primed human pDC induced functionally suppressive CD4+CD25+ Tregs (197).
While unmethylated CpG nucleotides canonically activate TLR9, methylated bacterial DNA has recently emerged as another immunomodulatory factor. DNA isolated from a commensal strain of Bifidobacteria longum subsp. infantis S12 strongly induced CD25highFoxP3+ Tregs in vitro in a dose-dependent manner, whereas DNA isolated from Lactobacillus rhamnosus GG or E. coli strain B had significantly weaker Treg-promoting potential (198). This was attributed to a methylated CpG motif unique to the Bifidobacteria strain. Accordingly, a methylated CpG DNA octamer was more effective than an unmethylated octamer at converting FoxP3+ cells from CD4+ cells in vitro (199). GC-rich motifs are immunosuppressive motifs identified in commensal bacteria (195, 200). Administration of synthetic GC-rich suppressive sequences in mice induced Tregs and protected from DSS-colitis (200).
Overall, the gut microbiota is a rich source of nucleic acids either packaged in EVs or released as extracellular DNA. These nucleic acids have the potential to regulate intestinal Tregs, via TLR9, as well as other mechanisms yet to be identified.
Gut Microbiota and TTREG
While the impact of the gut microbiota on the differentiation of pTreg is well-established, its impact on tTreg is unclear. GF mice have defects in lymphoid and mucosal tissues yet their thymus cellularity and development is typically reported as normal (201). The gut microbiota has recently shown to influence the T cell repertoire by bacterial antigen-specific T cells in the thymus. Bacterial antigens are presented by CX3 DC that migrates from the colon to the thymus. This effect was limited to naïve T cells as tTreg were not impacted (27). While this work shows that gut bacterial antigens can be trafficked to the thymus via migratory DC, it is unknown whether bacterial components can reach the thymus via other mechanisms. tTreg are affected by PAMPs as TLR2 deficient mice had decreased tTreg and pTreg (167) and TLR9 activation in mTEC promotes the recruitment of CD14+ monocyte-derived DCs to the thymus, affecting the negative selection process and tTreg generation (202). Furthermore, bacterial metabolites may also influence tTreg as butyrate has been shown to increase AIRE expression in mTEC ex vivo and promoted tTreg in a fetal thymic organ culture model in a GPR41-dependent manner (203). Similarly, acetate increase AIRE expression in mTEC without affecting tTreg numbers (204). There are evidence showing that bacterial antigens, PAMPs and metabolites reach the thymus where they may affect tTreg development. However, the mechanisms involved, as well as the consequence of gut microbiota alteration on tTreg development remain elusive.
Conclusions and Perspectives
There are multiple means by which Treg differentiation can be regulated by both diet and the gut microbiota. These includes epigenetic changes, alteration to T cell metabolism, and the engagement of host receptors, such as TLRs and AhR. Diet can influence other immune subsets and regulate physiological processes, such as bile acid biology, to regulate Treg biology. The concerted contribution of these pathways may be required for optimal Treg induction and the maintenance of immune tolerance. All aspects of diets (macronutrients, micronutrients, and additives) have been shown to regulate Treg biology to varying extent, suggesting that Treg development is highly responsive to the nutritional status of the host. Indeed, defects in Treg development associated with the adoption of a western diet may underlie the increasing incidence of inflammatory diseases such as autoimmunity, allergies, and inflammatory bowel disease in western countries. Accordingly, dietary intervention or symbiotic (combination of pre- and probiotics) may prove to be a viable strategy in restoring Treg numbers to prevent or treat diseases. It is not clear, however, if any one type of dietary intervention would be effective in restoring Tregs across different disease contexts. T cell development and Treg ontogeny also exhibit high plasticity throughout life, and whether a dietary intervention would be effective at promoting Treg throughout the different life stages remains to be investigated. Maternal nutrition and maternal gut microbiota can also shape the neonatal immune system (205), suggesting that dietary modulation of Treg biology likely begins as early as fetal development. Addressing these questions would allow us to better understand how interventions can be personalized for therapeutic purposes.
Author Contributions
JTan, JTai, and LM wrote, reviewed, and edited the manuscript. DN wrote the manuscript and created the figures. SS and LL wrote the manuscript. All authors contributed to the article and approved the submitted version.
Funding
This project was funded by the Australian Research Council grant APP160100627. DN and JTai are recipients of the Australian Government Research Training Program Scholarship.
Conflict of Interest
The authors declare that the research was conducted in the absence of any commercial or financial relationships that could be construed as a potential conflict of interest.
Publisher's Note
All claims expressed in this article are solely those of the authors and do not necessarily represent those of their affiliated organizations, or those of the publisher, the editors and the reviewers. Any product that may be evaluated in this article, or claim that may be made by its manufacturer, is not guaranteed or endorsed by the publisher.
Acknowledgments
Figures were created using the Servier Medical Art templates, which are licensed under a Creative Commons Attribution 3.0 Unported License; https://smart.servier.com.
References
1. Hori S, Nomura T, Sakaguchi S. Control of regulatory T cell development by the transcription factor Foxp3. Science. (2003) 299:1057–61. doi: 10.1126/science.1079490
2. Stephen-Victor E, Chatila TA. Regulation of oral immune tolerance by the microbiome in food allergy. Curr Opin Immunol. (2019) 60:141. doi: 10.1016/j.coi.2019.06.001
3. Cosovanu C, Neumann C. The many functions of Foxp3+ regulatory T cells in the intestine. Front Immunol. (2020) 11:600973. doi: 10.3389/fimmu.2020.600973
4. Issazadeh-Navikas S, Teimer R, Bockermann R. Influence of dietary components on regulatory T cells. Mol Med. (2012) 18:95. doi: 10.2119/molmed.2011.00311
5. Hornero RA, Hamad I, Côrte-Real B, Kleinewietfeld M. The impact of dietary components on regulatory T cells and disease. Front Immunol. (2020) 11:253. doi: 10.3389/fimmu.2020.00253
6. Tan J, McKenzie C, Vuillermin PJ, Goverse G, Vinuesa CG, Mebius RE, et al. Dietary fiber and bacterial SCFA enhance oral tolerance and protect against food allergy through diverse cellular pathways. Cell Rep. (2016) 15:2809–24. doi: 10.1016/j.celrep.2016.05.047
7. Savage PA, Klawon DEJ, Miller CH. Regulatory T cell development. Annu Rev Immunol. (2020) 38:421–53. doi: 10.1146/annurev-immunol-100219-020937
8. Huang H, Ma Y, Dawicki W, Zhang X, Gordon JR. Comparison of induced versus natural regulatory T cells of the same TCR specificity for induction of tolerance to an environmental antigen. J Immunol. (2013) 191:1136–43. doi: 10.4049/jimmunol.1201899
9. Yadav M, Louvet C, Davini D, Gardner JM, Martinez-Llordella M, Bailey-Bucktrout S, et al. Neuropilin-1 distinguishes natural and inducible regulatory T cells among regulatory T cell subsets in vivo. J Exp Med. (2012) 209:1713–22, S1–19. doi: 10.1084/jem.20120822
10. Cibrián D, Sánchez-Madrid F. CD69: from activation marker to metabolic gatekeeper. Eur J Immunol. (2017) 47:946. doi: 10.1002/eji.201646837
11. Zlotoff DA, Sambandam A, Logan TD, Bell JJ, Schwarz BA, Bhandoola A. CCR7 and CCR9 together recruit hematopoietic progenitors to the adult thymus. Blood. (2010) 115:1897–905. doi: 10.1182/blood-2009-08-237784
12. Lee H-M, Bautista JL, Scott-Browne J, Mohan JF, Hsieh C-S. A broad range of self-reactivity drives thymic regulatory T cell selection to limit responses to self. Immunity. (2012) 37:475–86. doi: 10.1016/j.immuni.2012.07.009
13. Watanabe M, Lu Y, Breen M, Hodes RJ. B7-CD28 co-stimulation modulates central tolerance via thymic clonal deletion and Treg generation through distinct mechanisms. Nat Commun. (2020) 11:6264. doi: 10.1038/s41467-020-20070-x
14. Lio C-WJ, Dodson LF, Deppong CM, Hsieh C-S, Green JM. CD28 facilitates the generation of Foxp3– cytokine responsive regulatory T cell precursors. J Immunol. (2010) 184:6007. doi: 10.4049/jimmunol.1000019
15. Owen DL, Mahmud SA, Vang KB, Kelly RM, Blazar BR, Smith KA, et al. Identification of cellular sources of IL-2 needed for regulatory T cell development and homeostasis. J Immunol. (2018) 200:3926–33. doi: 10.4049/jimmunol.1800097
16. Toomer KH, Lui JB, Altman NH, Ban Y, Chen X, Malek TR. Essential and non-overlapping IL-2Rα-dependent processes for thymic development and peripheral homeostasis of regulatory T cells. Nat Commun. (2019) 10:1037. doi: 10.1038/s41467-019-08960-1
17. Hong C, Luckey M, Park J-H. Intrathymic IL-7: the where, when, and why of IL-7 signaling during T cell development. Semin Immunol. (2012) 24:151. doi: 10.1016/j.smim.2012.02.002
18. Apert C, Romagnoli P, van Meerwijk JPM. IL-2 and IL-15 dependent thymic development of Foxp3-expressing regulatory T lymphocytes. Protein Cell. (2018) 9:322. doi: 10.1007/s13238-017-0425-3
19. Takaba H, Morishita Y, Tomofuji Y, Danks L, Nitta T, Komatsu N, et al. Fezf2 orchestrates a thymic program of self-antigen expression for immune tolerance. Cell. (2015) 163:975–87. doi: 10.1016/j.cell.2015.10.013
20. Perry JSA, Lio C-WJ, Kau AL, Nutsch K, Yang Z, Gordon JI, et al. Distinct contributions of Aire and antigen-presenting-cell subsets to the generation of self-tolerance in the thymus. Immunity. (2014) 41:414–26. doi: 10.1016/j.immuni.2014.08.007
21. Gallegos AM, Bevan MJ. Central tolerance to tissue-specific antigens mediated by direct and indirect antigen presentation. J Exp Med. (2004) 200:1039–49. doi: 10.1084/jem.20041457
22. Herbin O, Bonito AJ, Jeong S, Weinstein EG, Rahman AH, Xiong H, et al. Medullary thymic epithelial cells and CD8α+ dendritic cells coordinately regulate central tolerance but CD8α+ cells are dispensable for thymic regulatory T cell production. J Autoimmun. (2016) 75:141–9. doi: 10.1016/j.jaut.2016.08.002
23. Breed ER, Lee ST, Hogquist KA. Directing T cell fate: how thymic antigen presenting cells coordinate thymocyte selection. Semin Cell Dev Biol. (2018) 84:2. doi: 10.1016/j.semcdb.2017.07.045
24. Perry JSA, Russler-Germain EV, Zhou YW, Purtha W, Cooper ML, Choi J, et al. Scavenger receptor CD36 mediates cell-surface antigen transfer to promote thymic regulatory T cell receptor repertoire development and allo-tolerance. Immunity. (2018) 48:923. doi: 10.1016/j.immuni.2018.04.007
25. Hadeiba H, Lahl K, Edalati A, Oderup C, Habtezion A, Pachynski R, et al. Plasmacytoid dendritic cells transport peripheral antigens to the thymus to promote central tolerance. Immunity. (2012) 36:438–50. doi: 10.1016/j.immuni.2012.01.017
26. Proietto AI, van Dommelen S, Zhou P, Rizzitelli A, D'Amico A, Steptoe RJ, et al. Dendritic cells in the thymus contribute to T-regulatory cell induction. Proc Natl Acad Sci USA. (2008) 105:19869–74. doi: 10.1073/pnas.0810268105
27. Zegarra-Ruiz DF, Kim DV, Norwood K, Kim M, Wu W-JH, Saldana-Morales FB, et al. Thymic development of gut-microbiota-specific T cells. Nature. (2021) 594:413–17. doi: 10.1038/s41586-021-03531-1
28. Ramond C, Berthault C, Burlen-Defranoux O, de Sousa AP, Guy-Grand D, Vieira P, et al. Two waves of distinct hematopoietic progenitor cells colonize the fetal thymus. Nat Immunol. (2014) 15:27–35. doi: 10.1038/ni.2782
29. Adkins B. Peripheral CD4+ lymphocytes derived from fetal versus adult thymic precursors differ phenotypically and functionally. J Immunol. (2003) 171:5157–64. doi: 10.4049/jimmunol.171.10.5157
30. Thiault N, Darrigues J, Adoue V, Gros M, Binet B, Perals C, et al. Peripheral regulatory T lymphocytes recirculating to the thymus suppress the development of their precursors. Nat Immunol. (2015) 16:628–34. doi: 10.1038/ni.3150
31. Motwani K, Peters LD, Vliegen WH, El-Sayed AG, Seay HR, Lopez MC, et al. Human regulatory T cells from umbilical cord blood display increased repertoire diversity and lineage stability relative to adult peripheral blood. Front Immunol. (2020) 11:611. doi: 10.3389/fimmu.2020.00611
32. Hayakawa S, Ohno N, Okada S, Kobayashi M. Significant augmentation of regulatory T cell numbers occurs during the early neonatal period. Clin Exp Immunol. (2017) 190:268–79. doi: 10.1111/cei.13008
33. Yang S, Fujikado N, Kolodin D, Benoist C, Mathis D. Regulatory T cells generated early in life play a distinct role in maintaining self-tolerance. Science. (2015) 348:589. doi: 10.1126/science.aaa7017
34. Stadinski BD, Blevins SJ, Spidale NA, Duke BR, Huseby PG, Stern LJ, et al. A temporal thymic selection switch and ligand binding kinetics constrain neonatal Foxp3+ Treg cell development. Nat Immunol. (2019) 20:1046. doi: 10.1038/s41590-019-0414-1
35. Yang L, Jin R, Lu D, Ge Q. T cell tolerance in early life. Front Immunol. (2020) 11:576261. doi: 10.3389/fimmu.2020.576261
36. Ennamorati M, Vasudevan C, Clerkin K, Halvorsen S, Verma S, Ibrahim S, et al. Intestinal microbes influence development of thymic lymphocytes in early life. Proc Natl Acad Sci USA. (2020) 117:2570–78. doi: 10.1073/pnas.1915047117
37. Kang BH, Park HJ, Park HJ, Lee J-I, Park SH, Jung KC. PLZF+ innate T cells support the TGF-β-dependent generation of activated/memory-like regulatory T cells. Mol Cells. (2016) 39:468. doi: 10.14348/molcells.2016.0004
38. Chen W, Jin W, Hardegen N, Lei K-J, Li L, Marinos N, et al. Conversion of peripheral CD4+CD25- naive T cells to CD4+CD25+ regulatory T cells by TGF-beta induction of transcription factor Foxp3. J Exp Med. (2003) 198:1875–86. doi: 10.1084/jem.20030152
39. Barnes MJ, Griseri T, Johnson AMF, Young W, Powrie F, Izcue A. CTLA-4 promotes Foxp3 induction and regulatory T cell accumulation in the intestinal lamina propria. Mucosal Immunol. (2013) 6:324–34. doi: 10.1038/mi.2012.75
40. Xu L, Kitani A, Strober W. Molecular mechanisms regulating TGF-beta-induced Foxp3 expression. Mucosal Immunol. (2010) 3:230–8. doi: 10.1038/mi.2010.7
41. Colamatteo A, Carbone F, Bruzzaniti S, Galgani M, Fusco C, Maniscalco GT, et al. Molecular mechanisms controlling Foxp3 expression in health and autoimmunity: from epigenetic to post-translational regulation. Front Immunol. (2019) 10:3136. doi: 10.3389/fimmu.2019.03136
42. Arpaia N, Campbell C, Fan X, Dikiy S, van der Veeken J, deRoos P, et al. Metabolites produced by commensal bacteria promote peripheral regulatory T cell generation. Nature. (2013) 504:451–5. doi: 10.1038/nature12726
43. Furusawa Y, Obata Y, Fukuda S, Endo TA, Nakato G, Takahashi D, et al. Commensal microbe-derived butyrate induces the differentiation of colonic regulatory T cells. Nature. (2013) 504:446–50. doi: 10.1038/nature12721
44. Soroosh P, Doherty TA, Duan W, Mehta AK, Choi H, Adams YF, et al. Lung-resident tissue macrophages generate Foxp3+ regulatory T cells and promote airway tolerance. J Exp Med. (2013) 210:775–88. doi: 10.1084/jem.20121849
45. Rosser EC, Mauri C. Regulatory B cells: origin, phenotype, and function. Immunity. (2015) 42:607–12. doi: 10.1016/j.immuni.2015.04.005
46. Lee KM, Stott RT, Zhao G, SooHoo J, Xiong W, Lian MM, et al. TGF-β-producing regulatory B cells induce regulatory T cells and promote transplantation tolerance. Eur J Immunol. (2014) 44:1728. doi: 10.1002/eji.201344062
47. Andersson J, Tran DQ, Pesu M, Davidson TS, Ramsey H, O'Shea JJ, et al. CD4+FoxP3+ regulatory T cells confer infectious tolerance in a TGF-β-dependent manner. J Exp Med. (2008) 205:1975. doi: 10.1084/jem.20080308
48. Bauché D, Marie JC. Transforming growth factor β: a master regulator of the gut microbiota and immune cell interactions. Clin Transl Immunol. (2017) 6:e136. doi: 10.1038/cti.2017.9
49. Goverse G, Molenaar R, Macia L, Tan J, Erkelens MN, Konijn T, et al. Diet-derived short chain fatty acids stimulate intestinal epithelial cells to induce mucosal tolerogenic dendritic cells. J Immunol. (2017) 198:2172–81. doi: 10.4049/jimmunol.1600165
50. Cording S, Wahl B, Kulkarni D, Chopra H, Pezoldt J, Buettner M, et al. The intestinal micro-environment imprints stromal cells to promote efficient Treg induction in gut-draining lymph nodes. Mucosal Immunol. (2014) 7:359–68. doi: 10.1038/mi.2013.54
51. Tan J, Ni D, Ribeiro RV, Pinget GV, Macia L. How changes in the nutritional landscape shape gut immunometabolism. Nutrients. (2021) 13:823. doi: 10.3390/nu13030823
52. Mailer RKW, Gisterå A, Polyzos KA, Ketelhuth DFJ, Hansson GK. Hypercholesterolemia enhances T cell receptor signaling and increases the regulatory T cell population. Sci Rep. (2017) 7:15655. doi: 10.1038/s41598-017-15546-8
53. Hernandez AL, Kitz A, Wu C, Lowther DE, Rodriguez DM, Vudattu N, et al. Sodium chloride inhibits the suppressive function of FOXP3+ regulatory T cells. J Clin Invest. (2015) 125:4212–22. doi: 10.1172/JCI81151
54. Le Garf S, Sibille B, Mothe-Satney I, Eininger C, Fauque P, Murdaca J, et al. Alpha-lipoic acid supplementation increases the efficacy of exercise- and diet-induced obesity treatment and induces immunometabolic changes in female mice and women. FASEB J. (2021) 35:e21312. doi: 10.1096/fj.202001817RR
55. Amersfoort J, Schaftenaar FH, Douna H, van Santbrink PJ, van Puijvelde GHM, Slütter B, et al. Diet-induced dyslipidemia induces metabolic and migratory adaptations in regulatory T cells. Cardiovasc Res. (2021) 117:1309–24. doi: 10.1093/cvr/cvaa208
56. Gao Y, Min K, Zhang Y, Su J, Greenwood M, Gronert K. Female-specific downregulation of tissue polymorphonuclear neutrophils drives impaired regulatory T cell and amplified effector t cell responses in autoimmune dry eye disease. J Immunol. (2015) 195:3086–99. doi: 10.4049/jimmunol.1500610
57. Gao Y, Su J, Zhang Y, Chan A, Sin JH, Wu D, et al. Dietary DHA amplifies LXA4 circuits in tissues and lymph node PMN and is protective in immune-driven dry eye disease. Mucosal Immunol. (2018) 11:1674–83. doi: 10.1038/s41385-018-0070-z
58. Pae M, Baek Y, Lee S, Wu D. Loss of ovarian function in association with a high-fat diet promotes insulin resistance and disturbs adipose tissue immune homeostasis. J Nutr Biochem. (2018) 57:93–102. doi: 10.1016/j.jnutbio.2018.03.011
59. Van Herck MA, Vonghia L, Kwanten WJ, Julé Y, Vanwolleghem T, Ebo DG, et al. Diet reversal and immune modulation show key role for liver and adipose tissue T cells in murine nonalcoholic steatohepatitis. Cell Mol Gastroenterol Hepatol. (2020) 10:467–90. doi: 10.1016/j.jcmgh.2020.04.010
60. Mandaliya DK, Patel S, Seshadri S. The combinatorial effect of acetate and propionate on high-fat diet induced diabetic inflammation or metaflammation and T cell polarization. Inflammation. (2021) 44:68–79. doi: 10.1007/s10753-020-01309-7
61. Hong SK, Choo EH, Ihm SH, Chang KY. High fat diet downregulates regulatory T cells in the myocardium of spontaneous hypertensive rats. Nutr Metab Cardiovasc Dis. (2019) 29:1254–60. doi: 10.1016/j.numecd.2019.08.004
62. Maganto-García E, Tarrio ML, Grabie N, Bu D, Lichtman AH. Dynamic changes in regulatory T cells are linked to levels of diet-induced hypercholesterolemia. Circulation. (2011) 124:185–95. doi: 10.1161/CIRCULATIONAHA.110.006411
63. Ma X, Hua J, Mohamood AR, Hamad ARA, Ravi R, Li Z. A high-fat diet and regulatory T cells influence susceptibility to endotoxin-induced liver injury. Hepatol Baltim Md. (2007) 46:1519–29. doi: 10.1002/hep.21823
64. Hirschberger S, Strauß G, Effinger D, Marstaller X, Ferstl A, Müller MB, et al. Very-low-carbohydrate diet enhances human T-cell immunity through immunometabolic reprogramming. EMBO Mol Med. (2021) 13:e14323. doi: 10.15252/emmm.202114323
65. Watkins A, Fukatsu K, Higashizono K, Watanabe T, Noguchi M, Tominaga E, et al. Influence of a low-carbohydrate high-fat diet on peritoneal inflammation, cancer-associated lymphocytes, and survival in a murine carcinomatous peritonitis model. JPEN J Parenter Enteral Nutr. (2021) 45:1293–301. doi: 10.1002/jpen.2007
66. Tao J, Chen H, Wang Y-J, Qiu J-X, Meng Q-Q, Zou R-J, et al. Ketogenic diet suppressed T-regulatory cells and promoted cardiac fibrosis via reducing mitochondria-associated membranes and inhibiting mitochondrial function. Oxid Med Cell Longev. (2021) 2021:5512322. doi: 10.1155/2021/5512322
67. Knoop KA, McDonald KG, Coughlin PE, Kulkarni DH, Gustafsson JK, Rusconi B, et al. Synchronization of mothers and offspring promotes tolerance and limits allergy. JCI Insight. (2020) 5:137943. doi: 10.1172/jci.insight.137943
68. Knoop KA, McDonald KG, Hsieh C-S, Tarr PI, Newberry RD. Regulatory T cells developing peri-weaning are continually required to restrain Th2 systemic responses later in life. Front Immunol. (2020) 11:603059. doi: 10.3389/fimmu.2020.603059
69. Coronado M, Solis CJ, Hernandez PP, Feijóo CG. Soybean meal-induced intestinal inflammation in zebrafish is T cell-dependent and has a Th17 cytokine profile. Front Immunol. (2019) 10:610. doi: 10.3389/fimmu.2019.00610
70. Chia JSJ, McRae JL, Enjapoori AK, Lefèvre CM, Kukuljan S, Dwyer KM. Dietary cows' milk protein A1 beta-casein increases the incidence of T1D in NOD mice. Nutrients. (2018) 10:E1291. doi: 10.3390/nu10091291
71. Lei C-S, Wu J-M, Lee P-C, Kuo T-C, Chen P-D, Hou Y-C, et al. Antecedent administration of glutamine benefits the homeostasis of CD4+ T cells and attenuates lung injury in mice with gut-derived polymicrobial sepsis. J Parenter Enteral Nutr. (2019) 43:927–36. doi: 10.1002/jpen.1505
72. Hsiung Y-C, Liu J-J, Hou Y-C, Yeh C-L, Yeh S-L. Effects of dietary glutamine on the homeostasis of CD4+ T cells in mice with dextran sulfate sodium-induced acute colitis. PloS ONE. (2014) 9:e84410. doi: 10.1371/journal.pone.0084410
73. Lee Y-C, Su Y-T, Liu T-Y, Tsai C-M, Chang C-H, Yu H-R. L-Arginine and L-citrulline supplementation have different programming effect on regulatory T-cells function of infantile rats. Front Immunol. (2018) 9:2911. doi: 10.3389/fimmu.2018.02911
74. Ko H-J, Hong S-W, Verma R, Jung J, Lee M, Kim N, et al. Dietary glucose consumption promotes RALDH activity in small intestinal CD103+CD11b+ dendritic cells. Front Immunol. (2020) 11:1897. doi: 10.3389/fimmu.2020.01897
75. Xia X, Zhu L, Lei Z, Song Y, Tang F, Yin Z, et al. Feruloylated oligosaccharides alleviate dextran sulfate sodium-induced colitis in vivo. J Agric Food Chem. (2019) 67:9522–31. doi: 10.1021/acs.jafc.9b03647
76. Takahashi H, Nakazawa M, Takahashi K, Aihara M, Minami M, Hirasawa T, et al. Effects of zinc deficient diet on development of atopic dermatitis-like eruptions in DS-Nh mice. J Dermatol Sci. (2008) 50:31–9. doi: 10.1016/j.jdermsci.2007.11.002
77. Kreuzer-Redmer S, Arends D, Schulte JN, Karweina D, Korkuc P, Wöltje N, et al. High dosage of zinc modulates T-cells in a time-dependent manner within porcine gut-associated lymphatic tissue. Br J Nutr. (2018) 120:1349–58. doi: 10.1017/S0007114518002908
78. Kloubert V, Blaabjerg K, Dalgaard TS, Poulsen HD, Rink L, Wessels I. Influence of zinc supplementation on immune parameters in weaned pigs. J Trace Elem Med Biol Organ Soc Miner Trace Elem GMS. (2018) 49:231–40. doi: 10.1016/j.jtemb.2018.01.006
79. Xue H, Wang W, Li Y, Shan Z, Li Y, Teng X, et al. Selenium upregulates CD4(+)CD25(+) regulatory T cells in iodine-induced autoimmune thyroiditis model of NOD.H-2(h4) mice. Endocr J. (2010) 57:595–601. doi: 10.1507/endocrj.K10E-063
80. Sang L-X, Chang B, Zhu J-F, Yang F-L, Li Y, Jiang X-F, et al. Sodium selenite ameliorates dextran sulfate sodium-induced chronic colitis in mice by decreasing Th1, Th17, and γδT and increasing CD4(+)CD25(+) regulatory T-cell responses. World J Gastroenterol. (2017) 23:3850–63. doi: 10.3748/wjg.v23.i21.3850
81. Dehghani M, Shokrgozar N, Ramzi M, Kalani M, Golmoghaddam H, Arandi N. The impact of selenium on regulatory T cell frequency and immune checkpoint receptor expression in patients with diffuse large B cell lymphoma (DLBCL). Cancer Immunol Immunother CII. (2021) 70:2961–9. doi: 10.1007/s00262-021-02889-5
82. An JH, Cho DH, Lee GY, Kang MS, Kim SJ, Han SN. Effects of vitamin D supplementation on CD4+ T cell subsets and mTOR signaling pathway in high-fat-diet-induced obese mice. Nutrients. (2021) 13:796. doi: 10.3390/nu13030796
83. Mulligan JK, Pasquini WN, Carroll WW, Williamson T, Reaves N, Patel KJ, et al. Dietary vitamin D3 deficiency exacerbates sinonasal inflammation and alters local 25(OH)D3 metabolism. PloS ONE. (2017) 12:e0186374. doi: 10.1371/journal.pone.0186374
84. Elahi A, Sabui S, Narasappa NN, Agrawal S, Lambrecht NW, Agrawal A, et al. Biotin deficiency induces Th1- and Th17-mediated proinflammatory responses in human CD4+ T lymphocytes via activation of the mTOR signaling pathway. J Immunol. (2018) 200:2563–70. doi: 10.4049/jimmunol.1701200
85. Pinget G, Tan J, Janac B, Kaakoush NO, Angelatos AS, O'Sullivan J, et al. Impact of the food additive titanium dioxide (E171) on gut microbiota-host interaction. Front Nutr. (2019) 6:57. doi: 10.3389/fnut.2019.00100
86. Ruiz PA, Morón B, Becker HM, Lang S, Atrott K, Spalinger MR, et al. Titanium dioxide nanoparticles exacerbate DSS-induced colitis: role of the NLRP3 inflammasome. Gut. (2017) 66:1216–24. doi: 10.1136/gutjnl-2015-310297
87. Bettini S, Boutet-Robinet E, Cartier C, Coméra C, Gaultier E, Dupuy J, et al. Food-grade TiO2 impairs intestinal and systemic immune homeostasis, initiates preneoplastic lesions and promotes aberrant crypt development in the rat colon. Sci Rep. (2017) 7:40373. doi: 10.1038/srep40373
88. Blevins LK, Crawford RB, Bach A, Rizzo MD, Zhou J, Henriquez JE, et al. Evaluation of immunologic and intestinal effects in rats administered an E 171-containing diet, a food grade titanium dioxide (TiO2). Food Chem Toxicol Int J Publ Br Ind Biol Res Assoc. (2019) 133:110793. doi: 10.1016/j.fct.2019.110793
89. Chassaing B, Koren O, Goodrich J, Poole A, Srinivasan S, Ley RE, et al. Dietary emulsifiers impact the mouse gut microbiota promoting colitis and metabolic syndrome. Nature. (2015) 519:92–96. doi: 10.1038/nature14232
90. Wenstedt EFE, Remmerswaal EBM, van der Bom-Baylon ND, Schrooten EM, Bemelman FJ, Vogt L. The effect of high-salt diet on t-lymphocyte subpopulations in healthy males-a pilot study. J Clin Hypertens. (2020) 22:2152–5. doi: 10.1111/jch.14049
91. Dar HY, Singh A, Shukla P, Anupam R, Mondal RK, Mishra PK, et al. High dietary salt intake correlates with modulated Th17-Treg cell balance resulting in enhanced bone loss and impaired bone-microarchitecture in male mice. Sci Rep. (2018) 8:2503. doi: 10.1038/s41598-018-20896-y
92. Vitales-Noyola M, Layseca-Espinosa E, Baranda L, Abud-Mendoza C, Niño-Moreno P, Monsiváis-Urenda A. Analysis of sodium chloride intake and Treg/Th17 lymphocytes in healthy individuals and patients with rheumatoid arthritis or systemic lupus erythematosus. J Immunol Res. (2018) 2018:9627806. doi: 10.1155/2018/9627806
93. Scrivo R, Massaro L, Barbati C, Vomero M, Ceccarelli F, Spinelli FR, et al. The role of dietary sodium intake on the modulation of T helper 17 cells and regulatory T cells in patients with rheumatoid arthritis and systemic lupus erythematosus. PloS ONE. (2017) 12:e0184449. doi: 10.1371/journal.pone.0184449
94. Krementsov DN, Case LK, Hickey WF, Teuscher C. Exacerbation of autoimmune neuroinflammation by dietary sodium is genetically controlled and sex specific. FASEB J. (2015) 29:3446–57. doi: 10.1096/fj.15-272542
95. Kundu M, Mondal S, Roy A, Martinson JL, Pahan K. Sodium benzoate, a food additive and a metabolite of cinnamon, enriches regulatory T cells via STAT6-mediated upregulation of TGF-β. J Immunol. (2016) 197:3099–110. doi: 10.4049/jimmunol.1501628
96. Huang H-M, Pai M-H, Yeh S-L, Hou Y-C. Dietary exposure to chlorpyrifos inhibits the polarization of regulatory T cells in C57BL/6 mice with dextran sulfate sodium-induced colitis. Arch Toxicol. (2020) 94:141–50. doi: 10.1007/s00204-019-02615-2
97. Varghese JV, Sebastian EM, Iqbal T, Tom AA. Pesticide applicators and cancer: a systematic review. Rev Environ Health. (2021) 36:467–76. doi: 10.1515/reveh-2020-0121
98. Ohshima Y, Yamada A, Tokuriki S, Yasutomi M, Omata N, Mayumi M. Transmaternal exposure to bisphenol a modulates the development of oral tolerance. Pediatr Res. (2007) 62:60–64. doi: 10.1203/PDR.0b013e3180674dae
99. Yan H, Takamoto M, Sugane K. Exposure to Bisphenol A prenatally or in adulthood promotes T(H)2 cytokine production associated with reduction of CD4CD25 regulatory T cells. Environ Health Perspect. (2008) 116:514–19. doi: 10.1289/ehp.10829
100. Malaisé Y, Lencina C, Cartier C, Olier M, Ménard S, Guzylack-Piriou L. Bisphenol A, S or F mother's dermal impregnation impairs offspring immune responses in a dose and sex-specific manner in mice. Sci Rep. (2021) 11:1650. doi: 10.1038/s41598-021-81231-6
101. Mu J, Zhuang X, Wang Q, Jiang H, Deng Z-B, Wang B, et al. Interspecies communication between plant and mouse gut host cells through edible plant derived exosome-like nanoparticles. Mol Nutr Food Res. (2014) 58:1561. doi: 10.1002/mnfr.201300729
102. Urzì O, Raimondo S, Alessandro R. Extracellular vesicles from plants: current knowledge and open questions. Int J Mol Sci. (2021) 22:5366. doi: 10.3390/ijms22105366
103. Choi IY, Piccio L, Childress P, Bollman B, Ghosh A, Brandhorst S, et al. A diet mimicking fasting promotes regeneration and reduces autoimmunity and multiple sclerosis symptoms. Cell Rep. (2016) 15:2136–46. doi: 10.1016/j.celrep.2016.05.009
104. Zeng H, Yang K, Cloer C, Neale G, Vogel P, Chi H. mTORC1 couples immune signals and metabolic programming to establish T(reg)-cell function. Nature. (2013) 499:485–90. doi: 10.1038/nature12297
105. Wang X, Zhou Y, Tang D, Zhu Z, Li Y, Huang T, et al. ACC1 (Acetyl Coenzyme A Carboxylase 1) is a potential immune modulatory target of cerebral ischemic stroke. Stroke. (2019) 50:1869–78. doi: 10.1161/STROKEAHA.119.024564
106. Ang QY, Alexander M, Newman JC, Tian Y, Cai J, Upadhyay V, et al. Ketogenic diets alter the gut microbiome resulting in decreased intestinal Th17 cells. Cell. (2020) 181:1263–75.e16. doi: 10.1016/j.cell.2020.04.027
107. Tan JK, McKenzie C, Mariño E, Macia L, Mackay CR. Metabolite-sensing G protein-coupled receptors-facilitators of diet-related immune regulation. Annu Rev Immunol. (2017) 35:371–402. doi: 10.1146/annurev-immunol-051116-052235
108. Agace WW, McCoy KD. Regionalized development and maintenance of the intestinal adaptive immune landscape. Immunity. (2017) 46:532–48. doi: 10.1016/j.immuni.2017.04.004
109. Atarashi K, Tanoue T, Shima T, Imaoka A, Kuwahara T, Momose Y, et al. Induction of colonic regulatory T cells by indigenous Clostridium species. Science. (2011) 331:337–41. doi: 10.1126/science.1198469
110. Lathrop SK, Bloom SM, Rao SM, Nutsch K, Lio C-W, Santacruz N, et al. Peripheral education of the immune system by colonic commensal microbiota. Nature. (2011) 478:250–4. doi: 10.1038/nature10434
111. Cebula A, Seweryn M, Rempala GA, Pabla SS, McIndoe RA, Denning TL, et al. Thymus-derived regulatory T cells contribute to tolerance to commensal microbiota. Nature. (2013) 497:258–62. doi: 10.1038/nature12079
112. Tanoue T, Atarashi K, Honda K. Development and maintenance of intestinal regulatory T cells. Nat Rev Immunol. (2016) 16:295–309. doi: 10.1038/nri.2016.36
113. Sefik E, Geva-Zatorsky N, Oh S, Konnikova L, Zemmour D, McGuire AM, et al. mucosal immunology. Individual intestinal symbionts induce a distinct population of RORγ+ regulatory T cells. Science. (2015) 349:993–7. doi: 10.1126/science.aaa9420
114. Chen Y, Blaser MJ. Inverse associations of Helicobacter pylori with asthma and allergy. Arch Intern Med. (2007) 167:821–7. doi: 10.1001/archinte.167.8.821
115. Luther J, Dave M, Higgins PDR, Kao JY. Association between Helicobacter pylori infection and inflammatory bowel disease: a meta-analysis and systematic review of the literature. Inflamm Bowel Dis. (2010) 16:1077–84. doi: 10.1002/ibd.21116
116. Owyang SY, Zhang M, El-Zaatari M, Eaton KA, Bishu S, Hou G, et al. Dendritic cell-derived TGF-β mediates the induction of mucosal regulatory T-cell response to Helicobacter infection essential for maintenance of immune tolerance in mice. Helicobacter. (2020) 25:e12763. doi: 10.1111/hel.12763
117. Sun X, Zhang M, El-Zataari M, Owyang SY, Eaton KA, Liu M, et al. TLR2 mediates Helicobacter pylori-induced tolerogenic immune response in mice. PloS ONE. (2013) 8:e74595. doi: 10.1371/journal.pone.0074595
118. Oertli M, Sundquist M, Hitzler I, Engler DB, Arnold IC, Reuter S, et al. DC-derived IL-18 drives Treg differentiation, murine Helicobacter pylori-specific immune tolerance, and asthma protection. J Clin Invest. (2012) 122:1082–96. doi: 10.1172/JCI61029
119. Shin J-H, Chung M-J, Seo J-G. A multistrain probiotic formulation attenuates skin symptoms of atopic dermatitis in a mouse model through the generation of CD4+Foxp3+ T cells. Food Nutr Res. (2016) 60:32550. doi: 10.3402/fnr.v60.32550
120. Kwon H-K, Lee C-G, So J-S, Chae C-S, Hwang J-S, Sahoo A, et al. Generation of regulatory dendritic cells and CD4+Foxp3+ T cells by probiotics administration suppresses immune disorders. Proc Natl Acad Sci USA. (2010) 107:2159–64. doi: 10.1073/pnas.0904055107
121. Kim W-K, Jang YJ, Han DH, Jeon K, Lee C, Han HS, et al. Lactobacillus paracasei KBL382 administration attenuates atopic dermatitis by modulating immune response and gut microbiota. Gut Microbes. (2020) 12:1–4. doi: 10.1080/19490976.2020.1819156
122. Li W, Hang S, Fang Y, Bae S, Zhang Y, Zhang M, et al. A bacterial bile acid metabolite modulates Treg activity through the nuclear hormone receptor NR4A1. Cell Host Microbe. (2021) 29:1366–77.e9. doi: 10.1016/j.chom.2021.07.013
123. Pang W, Jiang Y, Li A, Zhang J, Chen M, Hu L, et al. Bacteroides thetaiotaomicron ameliorates experimental allergic airway inflammation via activation of ICOS+Tregs and inhibition of Th2 response. Front Immunol. (2021) 12:620943. doi: 10.3389/fimmu.2021.620943
124. Geuking MB, Cahenzli J, Lawson MAE, Ng DCK, Slack E, Hapfelmeier S, et al. Intestinal bacterial colonization induces mutualistic regulatory T cell responses. Immunity. (2011) 34:794–806. doi: 10.1016/j.immuni.2011.03.021
125. Zhai R, Xue X, Zhang L, Yang X, Zhao L, Zhang C. Strain-specific anti-inflammatory properties of two akkermansia muciniphila strains on chronic colitis in mice. Front Cell Infect Microbiol. (2019) 9:239. doi: 10.3389/fcimb.2019.00239
126. Shin N-R, Lee J-C, Lee H-Y, Kim M-S, Whon TW, Lee M-S, et al. An increase in the Akkermansia spp. population induced by metformin treatment improves glucose homeostasis in diet-induced obese mice. Gut. (2014) 63:727–35. doi: 10.1136/gutjnl-2012-303839
127. O'Mahony C, Scully P, O'Mahony D, Murphy S, O'Brien F, Lyons A, et al. Commensal-induced regulatory T cells mediate protection against pathogen-stimulated NF-kappaB activation. PLoS Pathog. (2008) 4:e1000112. doi: 10.1371/journal.ppat.1000112
128. Konieczna P, Groeger D, Ziegler M, Frei R, Ferstl R, Shanahan F, et al. Bifidobacterium infantis 35624 administration induces Foxp3 T regulatory cells in human peripheral blood: potential role for myeloid and plasmacytoid dendritic cells. Gut. (2012) 61:354–66. doi: 10.1136/gutjnl-2011-300936
129. Lyons A, O'Mahony D, O'Brien F, MacSharry J, Sheil B, Ceddia M, et al. Bacterial strain-specific induction of Foxp3+ T regulatory cells is protective in murine allergy models. Clin Exp Allergy J Br Soc Allergy Clin Immunol. (2010) 40:811–19. doi: 10.1111/j.1365-2222.2009.03437.x
130. Round JL, Mazmanian SK. Inducible Foxp3+ regulatory T-cell development by a commensal bacterium of the intestinal microbiota. Proc Natl Acad Sci USA. (2010) 107:12204–9. doi: 10.1073/pnas.0909122107
131. Shen Y, Giardino Torchia ML, Lawson GW, Karp CL, Ashwell JD, Mazmanian SK. Outer membrane vesicles of a human commensal mediate immune regulation and disease protection. Cell Host Microbe. (2012) 12:509–20. doi: 10.1016/j.chom.2012.08.004
132. Ochoa-Repáraz J, Mielcarz DW, Ditrio LE, Burroughs AR, Begum-Haque S, Dasgupta S, et al. Central nervous system demyelinating disease protection by the human commensal Bacteroides fragilis depends on polysaccharide A expression. J Immunol. (2010) 185:4101–8. doi: 10.4049/jimmunol.1001443
133. Jang YJ, Kim W-K, Han DH, Lee K, Ko G. Lactobacillus fermentum species ameliorate dextran sulfate sodium-induced colitis by regulating the immune response and altering gut microbiota. Gut Microbes. (2019) 10:696–711. doi: 10.1080/19490976.2019.1589281
134. Rodríguez-Nogales A, Algieri F, Garrido-Mesa J, Vezza T, Utrilla MP, Chueca N, et al. Differential intestinal anti-inflammatory effects of Lactobacillus fermentum and Lactobacillus salivarius in DSS mouse colitis: impact on microRNAs expression and microbiota composition. Mol Nutr Food Res. (2017) 61:1–13. doi: 10.1002/mnfr.201700144
135. Kim W-K, Jang YJ, Han DH, Seo B, Park S, Lee CH, et al. Administration of Lactobacillus fermentum KBL375 causes taxonomic and functional changes in gut microbiota leading to improvement of atopic dermatitis. Front Mol Biosci. (2019) 6:92. doi: 10.3389/fmolb.2019.00092
136. Atarashi K, Tanoue T, Oshima K, Suda W, Nagano Y, Nishikawa H, et al. Treg induction by a rationally selected mixture of Clostridia strains from the human microbiota. Nature. (2013) 500:232–6. doi: 10.1038/nature12331
137. Guo P, Zhang K, Ma X, He P. Clostridium species as probiotics: potentials and challenges. J Anim Sci Biotechnol. (2020) 11:24. doi: 10.1186/s40104-019-0402-1
138. Mu C, Zhang L, He X, Smidt H, Zhu W. Dietary fibres modulate the composition and activity of butyrate-producing bacteria in the large intestine of suckling piglets. Antonie Van Leeuwenhoek. (2017) 110:687–96. doi: 10.1007/s10482-017-0836-4
139. Smith PM, Howitt MR, Panikov N, Michaud M, Gallini CA, Bohlooly-Y M, et al. The microbial metabolites, short-chain fatty acids, regulate colonic treg cell homeostasis. Science. (2013) 341:569–73. doi: 10.1126/science.1241165
140. Thorburn AN, McKenzie CI, Shen S, Stanley D, Macia L, Mason LJ, et al. Evidence that asthma is a developmental origin disease influenced by maternal diet and bacterial metabolites. Nat Commun. (2015) 6:7320. doi: 10.1038/ncomms8320
141. Mariño E, Richards JL, McLeod KH, Stanley D, Yap YA, Knight J, et al. Gut microbial metabolites limit the frequency of autoimmune T cells and protect against type 1 diabetes. Nat Immunol. (2017) 18:552–62. doi: 10.1038/ni.3713
142. Park J, Kim M, Kang SG, Jannasch AH, Cooper B, Patterson J, et al. Short-chain fatty acids induce both effector and regulatory T cells by suppression of histone deacetylases and regulation of the mTOR-S6K pathway. Mucosal Immunol. (2015) 8:80–93. doi: 10.1038/mi.2014.44
143. Duscha A, Gisevius B, Hirschberg S, Yissachar N, Stangl GI, Eilers E, et al. Propionic acid shapes the multiple sclerosis disease course by an immunomodulatory mechanism. Cell. (2020) 180:1067–80.e16. doi: 10.1016/j.cell.2020.02.035
144. Singh N, Gurav A, Sivaprakasam S, Brady E, Padia R, Shi H, et al. Activation of Gpr109a, receptor for niacin and the commensal metabolite butyrate, suppresses colonic inflammation and carcinogenesis. Immunity. (2014) 40:128–39. doi: 10.1016/j.immuni.2013.12.007
145. Shang J, Zha H, Sun Y. Phenotypes, functions, and clinical relevance of regulatory B cells in cancer. Front Immunol. (2020) 11:582657. doi: 10.3389/fimmu.2020.582657
146. Murai M, Turovskaya O, Kim G, Madan R, Karp CL, Cheroutre H, et al. Interleukin 10 acts on regulatory T cells to maintain expression of the transcription factor Foxp3 and suppressive function in mice with colitis. Nat Immunol. (2009) 10:1178–84. doi: 10.1038/ni.1791
147. Zou F, Qiu Y, Huang Y, Zou H, Cheng X, Niu Q, et al. Effects of short-chain fatty acids in inhibiting HDAC and activating p38 MAPK are critical for promoting B10 cell generation and function. Cell Death Dis. (2021) 12:582. doi: 10.1038/s41419-021-03880-9
148. Daïen CI, Tan J, Audo R, Mielle J, Quek LE, Krycer JR, et al. Gut-derived acetate promotes B10 cells with antiinflammatory effects. JCI Insight. (2021) 6:e144156. doi: 10.1172/jci.insight.144156
149. Bhaskaran N, Quigley C, Paw C, Butala S, Schneider E, Pandiyan P. Role of short chain fatty acids in controlling tregs and immunopathology during mucosal infection. Front Microbiol. (2018) 9:1995. doi: 10.3389/fmicb.2018.01995
150. Kumar P, Lee J-H, Lee J. Diverse roles of microbial indole compounds in eukaryotic systems. Biol Rev Camb Philos Soc. (2021) 96:2522–45. doi: 10.1111/brv.12765
151. Rouse M, Singh NP, Nagarkatti PS, Nagarkatti M. Indoles mitigate the development of experimental autoimmune encephalomyelitis by induction of reciprocal differentiation of regulatory T cells and Th17 cells. Br J Pharmacol. (2013) 169:1305–21. doi: 10.1111/bph.12205
152. Kim WH, Lillehoj HS, Min W. Indole treatment alleviates intestinal tissue damage induced by chicken coccidiosis through activation of the aryl hydrocarbon receptor. Front Immunol. (2019) 10:560. doi: 10.3389/fimmu.2019.00560
153. Wojciech L, Tan KSW, Gascoigne NRJ. Taming the sentinels: microbiome-derived metabolites and polarization of T cells. Int J Mol Sci. (2020) 21:E7740. doi: 10.3390/ijms21207740
154. Powell DN, Swimm A, Sonowal R, Bretin A, Gewirtz AT, Jones RM, et al. Indoles from the commensal microbiota act via the AHR and IL-10 to tune the cellular composition of the colonic epithelium during aging. Proc Natl Acad Sci USA. (2020) 117:21519–26. doi: 10.1073/pnas.2003004117
155. Rosser EC, Piper CJM, Matei DE, Blair PA, Rendeiro AF, Orford M, et al. Microbiota-derived metabolites suppress arthritis by amplifying aryl-hydrocarbon receptor activation in regulatory B cells. Cell Metab. (2020) 31:837–51.e10. doi: 10.1016/j.cmet.2020.03.003
156. Zeng H, Umar S, Rust B, Lazarova D, Bordonaro M. Secondary bile acids and short chain fatty acids in the colon: a focus on colonic microbiome, cell proliferation, inflammation, and cancer. Int J Mol Sci. (2019) 20:E1214. doi: 10.3390/ijms20051214
157. Molinero N, Ruiz L, Sánchez B, Margolles A, Delgado S. Intestinal bacteria interplay with bile and cholesterol metabolism: implications on host physiology. Front Physiol. (2019) 10:185. doi: 10.3389/fphys.2019.00185
158. Funabashi M, Grove TL, Wang M, Varma Y, McFadden ME, Brown LC, et al. A metabolic pathway for bile acid dehydroxylation by the gut microbiome. Nature. (2020) 582:566–70. doi: 10.1038/s41586-020-2396-4
159. Song X, Sun X, Oh SF, Wu M, Zhang Y, Zheng W, et al. Microbial bile acid metabolites modulate gut RORγ+ regulatory T cell homeostasis. Nature. (2020) 577:410–15. doi: 10.1038/s41586-019-1865-0
160. Campbell C, McKenney PT, Konstantinovsky D, Isaeva OI, Schizas M, Verter J, et al. Bacterial metabolism of bile acids promotes generation of peripheral regulatory T cells. Nature. (2020) 581:475–9. doi: 10.1038/s41586-020-2193-0
161. Stofan M, Guo GL. Bile acids and FXR: novel targets for liver diseases. Front Med. (2020) 7:544. doi: 10.3389/fmed.2020.00544
162. Hang S, Paik D, Yao L, Kim E, Trinath J, Lu J, et al. Bile acid metabolites control TH17 and Treg cell differentiation. Nature. (2019) 576:143–8. doi: 10.1038/s41586-019-1785-z
163. Heinken A, Ravcheev DA, Baldini F, Heirendt L, Fleming RMT, Thiele I. Systematic assessment of secondary bile acid metabolism in gut microbes reveals distinct metabolic capabilities in inflammatory bowel disease. Microbiome. (2019) 7:75. doi: 10.1186/s40168-019-0689-3
164. Evans NP, Misyak SA, Schmelz EM, Guri AJ, Hontecillas R, Bassaganya-Riera J. Conjugated linoleic acid ameliorates inflammation-induced colorectal cancer in mice through activation of PPARgamma. J Nutr. (2010) 140:515–21. doi: 10.3945/jn.109.115642
165. Yang B, Chen H, Gao H, Wang J, Stanton C, Ross RP, et al. Bifidobacterium breve CCFM683 could ameliorate DSS-induced colitis in mice primarily via conjugated linoleic acid production and gut microbiota modulation. J Funct Foods. (2018) 49:61–72. doi: 10.1016/j.jff.2018.08.014
166. Levan SR, Stamnes KA, Lin DL, Panzer AR, Fukui E, McCauley K, et al. Elevated faecal 12,13-diHOME concentration in neonates at high risk for asthma is produced by gut bacteria and impedes immune tolerance. Nat Microbiol. (2019) 4:1851–61. doi: 10.1038/s41564-019-0498-2
167. Bhaskaran N, Cohen S, Zhang Y, Weinberg A, Pandiyan P. TLR-2 Signaling promotes IL-17A production in CD4+CD25+Foxp3+ regulatory cells during oropharyngeal candidiasis. Pathogens. (2015) 4:90–110. doi: 10.3390/pathogens4010090
168. Netea MG, Sutmuller R, Hermann C, Van der Graaf CAA, Van der Meer JWM, van Krieken JH, et al. Toll-like receptor 2 suppresses immunity against Candida albicans through induction of IL-10 and regulatory T cells. J Immunol. (2004) 172:3712–8. doi: 10.4049/jimmunol.172.6.3712
169. Round JL, Lee SM, Li J, Tran G, Jabri B, Chatila TA, et al. The Toll-like receptor 2 pathway establishes colonization by a commensal of the human microbiota. Science. (2011) 332:974–7. doi: 10.1126/science.1206095
170. Sutmuller RPM, Morgan ME, Netea MG, Grauer O, Adema GJ. Toll-like receptors on regulatory T cells: expanding immune regulation. Trends Immunol. (2006) 27:387–93. doi: 10.1016/j.it.2006.06.005
171. Crellin NK, Garcia RV, Hadisfar O, Allan SE, Steiner TS, Levings MK. Human CD4+ T cells express TLR5 and its ligand flagellin enhances the suppressive capacity and expression of FOXP3 in CD4+CD25+ T regulatory cells. J Immunol. (2005) 175:8051–9. doi: 10.4049/jimmunol.175.12.8051
172. Neff CP, Rhodes ME, Arnolds KL, Collins CB, Donnelly J, Nusbacher N, et al. Diverse intestinal bacteria contain putative zwitterionic capsular polysaccharides with anti-inflammatory properties. Cell Host Microbe. (2016) 20:535–47. doi: 10.1016/j.chom.2016.09.002
173. Stentz R, Carvalho AL, Jones EJ, Carding SR. Fantastic voyage: the journey of intestinal microbiota-derived microvesicles through the body. Biochem Soc Trans. (2018) 46:1021. doi: 10.1042/BST20180114
174. Macia L, Nanan R, Hosseini-Beheshti E, Grau GE. Host- and microbiota-derived extracellular vesicles, immune function, and disease development. Int J Mol Sci. (2019) 21:107. doi: 10.3390/ijms21010107
175. Bittel M, Reichert P, Sarfati I, Dressel A, Leikam S, Uderhardt S, et al. Visualizing transfer of microbial biomolecules by outer membrane vesicles in microbe-host-communication in vivo. J Extracell Vesicles. (2021) 10:e12159. doi: 10.1002/jev2.12159
176. López P, González-Rodríguez I, Sánchez B, Gueimonde M, Margolles A, Suárez A. Treg-inducing membrane vesicles from Bifidobacterium bifidum LMG13195 as potential adjuvants in immunotherapy. Vaccine. (2012) 30:825–9. doi: 10.1016/j.vaccine.2011.11.115
177. Al-Nedawi K, Mian MF, Hossain N, Karimi K, Mao Y-K, Forsythe P, et al. Gut commensal microvesicles reproduce parent bacterial signals to host immune and enteric nervous systems. FASEB J. (2015) 29:684–95. doi: 10.1096/fj.14-259721
178. Brenu EW, Staines DR, Tajouri L, Huth T, Ashton KJ, Marshall-Gradisnik SM. Heat shock proteins and regulatory T cells. Autoimmune Dis. (2013) 2013:813256. doi: 10.1155/2013/813256
179. Ohue R, Hashimoto K, Nakamoto M, Furukawa Y, Masuda T, Kitabatake N, et al. Bacterial heat shock protein 60, GroEL, can induce the conversion of naïve T cells into a CD4 CD25(+) Foxp3-expressing phenotype. J Innate Immun. (2011) 3:605–13. doi: 10.1159/000330786
180. de Wolf C, van der Zee R, den Braber I, Glant T, Maillère B, Favry E, et al. An arthritis-suppressive and treg cell-inducing CD4+ T cell epitope is functional in the context of HLA-restricted T cell responses. Arthritis Rheumatol. (2016) 68:639–47. doi: 10.1002/art.39444
181. Anderton SM, van der Zee R, Prakken B, Noordzij A, van Eden W. Activation of T cells recognizing self 60-kD heat shock protein can protect against experimental arthritis. J Exp Med. (1995) 181:943–52. doi: 10.1084/jem.181.3.943
182. Hsu W-T, Ho S-Y, Jian T-Y, Huang H-N, Lin Y-L, Chen C-H, et al. Helicobacter pylori-derived heat shock protein 60 increases the induction of regulatory T-cells associated with persistent infection. Microb Pathog. (2018) 119:152–61. doi: 10.1016/j.micpath.2018.04.016
183. Wang X, Zhou S, Chi Y, Wen X, Hoellwarth J, He L, et al. CD4+CD25+ Treg induction by an HSP60-derived peptide SJMHE1 from Schistosoma japonicum is TLR2 dependent. Eur J Immunol. (2009) 39:3052–65. doi: 10.1002/eji.200939335
184. Van Herwijnen MJC, Van Der Zee R, Van Eden W, Broere F. Heat shock proteins can be targets of regulatory T cells for therapeutic intervention in rheumatoid arthritis. Int J Hyperth. (2013) 29:448–54. doi: 10.3109/02656736.2013.811546
185. Lee H-J. Microbe-host communication by small RNAs in extracellular vesicles: vehicles for transkingdom RNA transportation. Int J Mol Sci. (2019) 20:1487. doi: 10.3390/ijms20061487
186. Kant R, de Vos WM, Palva A, Satokari R. Immunostimulatory CpG motifs in the genomes of gut bacteria and their role in human health and disease. J Med Microbiol. (2014) 63:293–308. doi: 10.1099/jmm.0.064220-0
187. Dalpke A, Frank J, Peter M, Heeg K. Activation of toll-like receptor 9 by DNA from different bacterial species. Infect Immun. (2006) 74:940–6. doi: 10.1128/IAI.74.2.940-946.2006
188. Gallego-Valle J, Pérez-Fernández VA, Correa-Rocha R, Pion M. Generation of human breg-like phenotype with regulatory function in vitro with bacteria-derived oligodeoxynucleotides. Int J Mol Sci. (2018) 19:E1737. doi: 10.3390/ijms19061737
189. Barr TA, Brown S, Ryan G, Zhao J, Gray D. TLR-mediated stimulation of APC: distinct cytokine responses of B cells and dendritic cells. Eur J Immunol. (2007) 37:3040–53. doi: 10.1002/eji.200636483
190. Fonseca DE, Kline JN. Use of CpG oligonucleotides in treatment of asthma and allergic disease. Adv Drug Deliv Rev. (2009) 61:256–62. doi: 10.1016/j.addr.2008.12.007
191. Sabatel C, Radermecker C, Fievez L, Paulissen G, Chakarov S, Fernandes C, et al. Exposure to bacterial CpG DNA protects from airway allergic inflammation by expanding regulatory lung interstitial macrophages. Immunity. (2017) 46:457–73. doi: 10.1016/j.immuni.2017.02.016
192. Senti G, Johansen P, Haug S, Bull C, Gottschaller C, Müller P, et al. Use of A-type CpG oligodeoxynucleotides as an adjuvant in allergen-specific immunotherapy in humans: a phase I/IIa clinical trial. Clin Exp Allergy J Br Soc Allergy Clin Immunol. (2009) 39:562–70. doi: 10.1111/j.1365-2222.2008.03191.x
193. Lee J, Mo J-H, Katakura K, Alkalay I, Rucker AN, Liu Y-T, et al. Maintenance of colonic homeostasis by distinctive apical TLR9 signalling in intestinal epithelial cells. Nat Cell Biol. (2006) 8:1327–36. doi: 10.1038/ncb1500
194. Shigemori S, Namai F, Ogita T, Sato T, Shimosato T. Oral priming with oligodeoxynucleotide particles from Lactobacillus rhamnosus GG attenuates symptoms of dextran sodium sulfate-induced acute colitis in mice. Anim Sci J Nihon Chikusan Gakkaiho. (2020) 91:e13468. doi: 10.1111/asj.13468
195. Shimosato T, Tohno M, Sato T, Nishimura J, Kawai Y, Saito T, et al. Identification of a potent immunostimulatory oligodeoxynucleotide from Streptococcus thermophilus lacZ. Anim Sci J Nihon Chikusan Gakkaiho. (2009) 80:597–604. doi: 10.1111/j.1740-0929.2009.00680.x
196. Schmitt H, Ulmschneider J, Billmeier U, Vieth M, Scarozza P, Sonnewald S, et al. The TLR9 agonist cobitolimod induces IL10-producing wound healing macrophages and regulatory T cells in ulcerative colitis. J Crohns Colitis. (2020) 14:508–24. doi: 10.1093/ecco-jcc/jjz170
197. Moseman EA, Liang X, Dawson AJ, Panoskaltsis-Mortari A, Krieg AM, Liu Y-J, et al. Human plasmacytoid dendritic cells activated by CpG oligodeoxynucleotides induce the generation of CD4+CD25+ regulatory T cells. J Immunol. (2004) 173:4433–42. doi: 10.4049/jimmunol.173.7.4433
198. Li D, Cheng J, Zhu Z, Catalfamo M, Goerlitz D, Lawless OJ, et al. Treg-inducing capacity of genomic DNA of Bifidobacterium longum subsp. infantis. Allergy Asthma Proc. (2020) 41:372–85. doi: 10.2500/aap.2020.41.200064
199. Lawless OJ, Bellanti JA, Brown ML, Sandberg K, Umans JG, Zhou L, et al. In vitro induction of T regulatory cells by a methylated CpG DNA sequence in humans: potential therapeutic applications in allergic and autoimmune diseases. Allergy Asthma Proc. (2018) 39:143–52. doi: 10.2500/aap.2018.39.4113
200. Bouladoux N, Hall JA, Grainger JR, dos Santos LM, Kann MG, Nagarajan V, et al. Regulatory role of suppressive motifs from commensal DNA. Mucosal Immunol. (2012) 5:623–34. doi: 10.1038/mi.2012.36
201. Kennedy EA, King KY, Baldridge MT. Mouse microbiota models: comparing germ-free mice and antibiotics treatment as tools for modifying gut bacteria. Front Physiol. (2018) 9:1534. doi: 10.3389/fphys.2018.01534
202. Voboril M, Brabec T, Dobeš J, Šplíchalová I, Brezina J, Cepková A, et al. Toll-like receptor signaling in thymic epithelium controls monocyte-derived dendritic cell recruitment and Treg generation. Nat Commun. (2020) 11:2361. doi: 10.1038/s41467-020-16081-3
203. Nakajima A, Kaga N, Nakanishi Y, Ohno H, Miyamoto J, Kimura I, et al. Maternal high fiber diet during pregnancy and lactation influences regulatory T cell differentiation in offspring in mice. J Immunol. (2017) 199:3516–24. doi: 10.4049/jimmunol.1700248
204. Hu M, Eviston D, Hsu P, Mariño E, Chidgey A, Santner-Nanan B, et al. Decreased maternal serum acetate and impaired fetal thymic and regulatory T cell development in preeclampsia. Nat Commun. (2019) 10:3031. doi: 10.1038/s41467-019-10703-1
Keywords: regulatory T cell, tolerance, nutrition, nutritional immunology, gut microbiota, thymopoiesis
Citation: Tan J, Taitz J, Sun SM, Langford L, Ni D and Macia L (2022) Your Regulatory T Cells Are What You Eat: How Diet and Gut Microbiota Affect Regulatory T Cell Development. Front. Nutr. 9:878382. doi: 10.3389/fnut.2022.878382
Received: 17 February 2022; Accepted: 21 March 2022;
Published: 20 April 2022.
Edited by:
Willem Van Eden, Utrecht University, NetherlandsReviewed by:
Barbara Wróblewska, Institute of Animal Reproduction and Food Research (PAS), PolandMartijn Van Herwijnen, Utrecht University, Netherlands
Copyright © 2022 Tan, Taitz, Sun, Langford, Ni and Macia. This is an open-access article distributed under the terms of the Creative Commons Attribution License (CC BY). The use, distribution or reproduction in other forums is permitted, provided the original author(s) and the copyright owner(s) are credited and that the original publication in this journal is cited, in accordance with accepted academic practice. No use, distribution or reproduction is permitted which does not comply with these terms.
*Correspondence: Laurence Macia, laurence.macia@sydney.edu.au