- 1State Key Laboratory of Oncogenes and Related Genes, Center for Single-Cell Omics, School of Public Health, Shanghai Jiao Tong University School of Medicine, Shanghai, China
- 2Shanghai Institute of Nutrition and Health, Chinese Academy of Sciences, Shanghai, China
Apigenin is a flavonoid with antioxidant, anti-inflammatory, and anti-apoptotic activity. In this study, the potential effects of apigenin on cardiometabolic diseases were investigated in vivo and in vitro. Potential signaling networks in different cell types induced by apigenin were identified, suggesting that the molecular mechanisms of apigenin in cardiometabolic diseases vary with cell types. Additionally, the mechanisms of apigenin-induced biological response in different cardiometabolic diseases were analyzed, including obesity, diabetes, hypertension and cardiovascular diseases. This review provides novel insights into the potential role of apigenin in cardiometabolic diseases.
Introduction
Apigenin (4′,5,7-trihydroxyflavone) is named after the genus Apium belonging to family Apiaceae (1). It is widely distributed in vegetables and fruits, such as celery, parsley, oranges and garlic (2), and is also found in herbs such as snow lotus and chamomile (3) (Figure 1A). As a secondary plant metabolite, apigenin is usually stored in plants in a water-soluble glycosylated form (4). Purified apigenin is a yellow powder with a low molecular weight (MW 270.24). It is nearly insoluble in water, moderately soluble in hot alcohol and soluble in dimethyl sulfoxide (DMSO) (5). Pure apigenin is chemically unstable and therefore stored in the dark at −20°C (5).
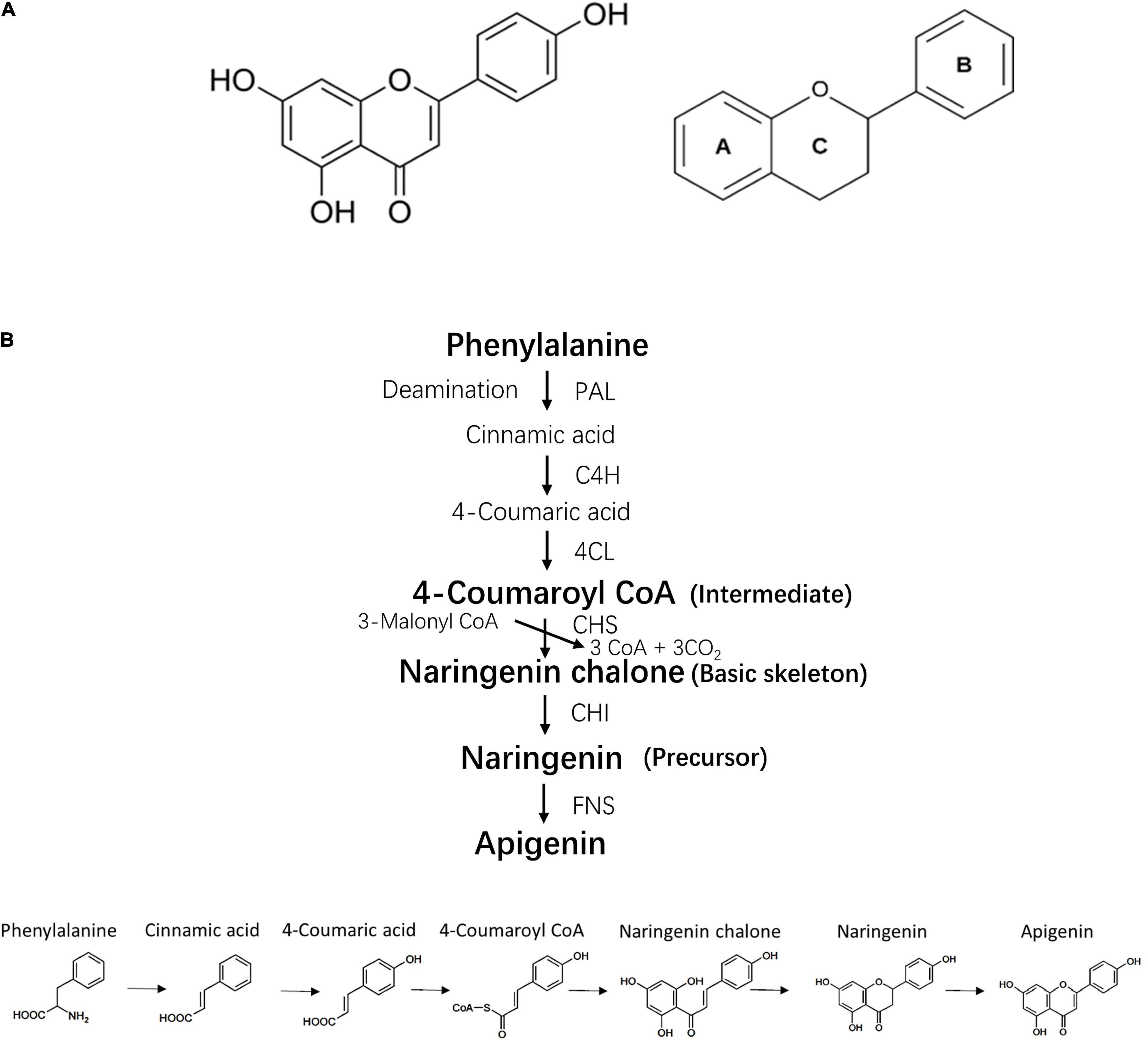
Figure 1. Chemical structure and bio-synthesis of apigenin. (A) Chemical structure of apigenin and its basic skeleton. (B) The process of apigenin bio-synthesis. PAL, phenylalanine ammonia lyase; 4CH, cinnamate 4-hydroxylase; 4CL, 4-coumaroyl CoA ligase; CHS, chalcone synthase; CHI, chalcone isomerase; FNS, flavone synthase.
The biosynthesis of apigenin occurs on the surface of the endoplasmic reticulum and requires four steps including intermediate synthesis, basic skeleton synthesis, precursor synthesis and generation of the apigenin structure (6) (Figure 1B). Current evidence indicates that the bioactivity of apigenin is dependent on its chemical structure, thus the structure-activity relationship of apigenin can be determined by extracting the molecular fragments associated with a specific biological activity. For example, double bonds in the two aromatic rings and hydroxyl groups on C-7 and C-4′ induce the inhibition of α-glucosidase and α-amylase (7). The C- 4′ hydroxyl group in ring B is essential for immunomodulatory properties (8). The hydroxyl radicals at position 5, 7 and 4′ are necessary for Liver X receptor activation (9).
Cardiometabolic disease links the metabolic syndrome disorders (abdominal adiposity, hypertension, dyslipidemia, hyperinsulinemia and glucose intolerance) that are predictive of cardiovascular disease and Type 2 diabetes (T2DM) (10). Recently, apigenin has been found to play a protective role in cardiometabolic diseases in vitro and in vivo. This systematic review summarizes the current perspective.
Protective Roles of Apigenin in Cardiometabolic Diseases
Protective Role of Apigenin in Obesity and Lipid Metabolism
Obesity is attributed to chronic energy imbalance, including excessive energy intake and limited energy expenditure (11, 12). Anti-obesity strategies focus on suppression of energy intake and stimulation of energy expenditure by regulating lipid metabolism, such as inhibiting pancreatic lipase activity and adipocyte differentiation (13). Studies suggest that apigenin controls energy intake by inhibiting appetite and stimulating energy expenditure by regulating lipid metabolism to alleviate obesity.
First, apigenin inhibits obesity by suppressing food consumption. In vitro and in vivo studies confirmed that apigenin upregulates the expression of anorexigenic neuropeptides pro-opiomelanocortin (POMC) and cocaine- and amphetamine-related transcript (CART), resulting in inhibition of food intake. N-29-2 and SH-SY5Y cells transfected with pPOMC-Luc and pCART-Luc vectors were treated with 0.2–5 μM apigenin for 6 h, resulting in upregulation of pPOMC-Luc and pCART-Luc activity. In an in vivo study, 6-week-old C57BL/6J mice were injected (every 24 h) intraperitoneally with 1 or 10 mg/kg of apigenin in a short-term intervention. Male C57BL/6J mice fed with a high-fat diet (HFD) or a standard laboratory chow diet received 0.05% apigenin for 30 days to demonstrate that apigenin reduces food intake and visceral fat over a long-term period (14). POMC and CART neurons found in the retro-chiasmatic area and throughout the rostrocaudal span of the arcuate nucleus (ARC) play a role in appetite control (15). Increasing expression of POMC and CART induces the expression of leptin receptor B (LepRb) to facilitate leptin binding to LepRb, resulting in an anorexic effect and upregulation of insulin receptors to inhibit appetite.
Secondly, apigenin stimulates energy expenditure by regulating lipid metabolism, including adipogenesis, lipolysis, fatty acid oxidation, and cholesterol synthesis. Recent studies indicate that adipose tissues are generally targeted by apigenin eliciting the following effects:
(1) Stimulation of PPARγ signaling. Several studies have demonstrated that apigenin inhibits adipocyte differentiation via STAT3 (the signal transducer and activator of the transcription 3)-CD36-PPARγ (peroxisome proliferator-activated receptor-gamma) axis (16) and AMPK (5′-Adenosine monophosphate-activated protein kinase)/PPARγ axis (17). One study showed that 100 μM apigenin treatment inhibits the differentiation of 3T3-L1 preadipocytes to mature white adipocytes. Mouse models of diet-induced obesity receiving apigenin via subcutaneous injection for 13 days showed that apigenin reduced visceral fat mass (16). Apigenin binds to non-phosphorylated STAT3 to decrease STAT3 phosphorylation and nuclear translocation (18), followed by a decline in the expression of CD36, the downstream target gene involved in fatty acid transport (19). PPARγ is the transcript factor of central ligand-activated transcription factors. It inhibits adipogenesis and controls adipose tissue differentiation to regulate inflammation in obesity. PPARγ expression depends on CD36 expression and therefore apigenin treatment inhibits adipocyte differentiation via downregulation of PPARγ. Other studies reported that apigenin activates the phosphorylation of AMPK (5′-adenosine monophosphate-activated protein kinase) to downregulate adipogenesis via AMPK/PPARγ axis in 3T3-L1 cells treated with 10 μM apigenin for 1 h or 4 days (17) and in HFD mice treated with 200 mg/kg enzyme-treated celery extract (20). AMPK acts as a potential target against adipogenesis (21, 22) and downregulates the expression of PPARγ. The adipogenous genes downstream of PPARγ, such as fatty acid-binding protein 4 and stearoyl-CoA desaturase, are also downregulated, thereby suppressing adipogenesis (17).
(2) Repression of enzyme activity. Guo et al. reported that 0.6 mM apigenin directly inhibits pancreatic lipase activity in vitro (23). Pancreatic lipase catalyzes the conversion of triglycerides to monoglycerides and fatty acids in the intestine. Obesity is alleviated by the suppression of pancreatic lipase, fatty acid synthesis and fat absorption. Gómez-Zorita et al. showed that treatment with 25 μM apigenin decreases the expression of fatty acid synthase (FAS), while increasing the expression of adipose triglyceride lipase (ATGL) in mature adipocytes derived from human mesenchymal stem cells (hMSCs), resulting in reduced adipogenesis (24).
(3) Activation of lipolysis-related genes. Apigenin regulates lipolysis via activation of lipolysis-related genes. In a recent study, 3-week-old HFD mice (C57BL/6J, male) treated with 0.04% apigenin for 12 weeks showed upregulation of lipolysis-related genes in white adipose tissues (WAT), such as FOXO1 (Forkhead Box O1) and SIRT1 (Sirtuin 1) (25).
(4) Induction of fatty acid oxidation. Dietary apigenin induces phosphorylation of AMPK and 1-aminocyclopropane-1-carboxylic acid (ACC) in brown adipose tissues (BAT) to utilize free fatty acids synthesized from white adipose tissues (WAT) (25).
Liver, in addition to adipose tissues, is essential for lipid metabolism. Abnormal lipid metabolism in the liver induced by obesity may cause hepatic steatosis. Apigenin also improves lipid metabolism in the liver to alleviate hepatic steatosis via following mechanisms:
(1) Stimulation of PPARγ signaling. Apigenin modulates PPARγ expression in hepatic lipid metabolism via Nrf2-PPARγ axis. In Hep1-6 cells, apigenin activates nuclear factor erythroid 2-related factor 2 (Nrf2) via translocation into the nucleus to upregulate downstream antioxidant enzymes and downregulate lipid synthesis (26). Activation of Nrf2 by apigenin neutralizes the activation of PPARγ to regulate lipid metabolism in liver (27).
(2) Regulation of SREBP family. Apigenin treatment inhibits lipid homeostasis by the sterol regulatory element-binding protein (SREBP) family. Apigenin significantly decrease lipid accumulation, total intracellular cholesterol (TC), and intracellular triglyceride (TG) levels via the AMPK-SREBP-1/2 (sterol regulatory element-binding protein-1/2) axis in HepG2 cells. Apigenin-induced activation of AMPK downregulates the levels of SREBP-1 and SREBP-2 to reduce the synthesis of cholesterol, fatty acids, and triglycerides in the liver. The inhibition of 3-hydroxy-3-methylglutaryl CoA reductase (HMGCR), which is the downstream target gene of SREBP-1 and FAS, the downstream target gene of SREBP-2 also regulates fatty acid and cholesterol synthesis (28).
(3) Activation of genes related to fatty acid oxidation and cholesterol homeostasis. Other genes related to fatty acid oxidation and cholesterol homeostasis in the liver, such as short/branched-chain acyl-CoA dehydrogenase (ASADSB), enoyl-CoA-hydratase and 3-hydroxyacyl-CoA dehydrogenase (EHHADH), Niemann-Pick type C 2 (NPC2) (29), HMG-CoA reductase (HMG-CoAR), low-density lipoprotein receptor (LDL-R), and cytochrome P450 family 7 subfamily A member 1 (CYP7A1) (30) have been reported to increase with apigenin treatment. In contrast, genes related to lipogenesis, such as PPARγ, lipoprotein lipase (LPL), sterol regulatory element-binding transcription factor 1 (SREBF1), and diacylglycerol O-acyltransferase 2 (DGAT2) were decreased in the liver (29).
Obesity-induced oxidative stress and inflammation also aggravate the symptoms of cardiometabolic diseases, leading to multiple cellular disorders (31, 32). Current studies indicate that apigenin alleviates oxidative stress and inflammation by binding to PPARγ as an agonist to regulate M2 polarization with nuclear factor kappa-light-chain-enhancer of activated B cells (NF-κB) inhibition. Apigenin-induced PPARγ activation blocks p65 nuclear translocation. NF-κB activation is inhibited in adipose tissue macrophages, leading to an increase in M2 macrophage polarization. The anti-inflammatory effects of M2 macrophages alleviate the metabolic disorder caused by obesity-related inflammation. Meanwhile, cytokines such as IL-6, IL-1β, and TNF-α are suppressed by the inhibition of NF-κB signaling (33) following apigenin treatment. The protective effect of apigenin on adipocyte browning in the inflammatory environment is also mediated via p65/NF-κB pathway. The inflammatory environment suppresses adipocyte browning to reduce lipid metabolism (34). Apigenin suppresses p65 translocation into the nucleus to inhibit NF-κB activation and inflammatory markers in adipocytes to attenuate inflammation and suppress adipocyte browning (25, 35). Apigenin also plays a protective role in the inhibition of white-to-brown adipose tissue differentiation. The inflammation induced via activation of uncoupling protein 1 and PGE2 receptor 4 (EP4) activates the cyclooxygenase 2 (COX2)/prostaglandin E2 (PGE2) axis, resulting in conversion of white to brown adipose tissue to generate heat by excessive energy expenditure (36).
Studies reported that obesity leads to many health complications. First, obesity has been associated with gastrointestinal disorders, such as gastroesophageal reflux disease, irritable bowel syndrome, and dyspepsia (37, 38). Colon inflammation adversely affects enteric motor function, leading to gastrointestinal disorders (39). Apigenin reduces the levels of malondialdehyde (MDA), interleukin-6 (IL-6), and interleukin-1β (IL-1β), as well as eosinophil infiltration in colon tissue to alleviate inflammation. Further, apigenin regulates inducible nitric oxide synthase (iNOS) expression and substance P (SP) levels in high-fat-diet (HFD)-fed obese mice (40). SP is a neurotransmitter that stimulates the contraction of various intestinal tissues and is a neurokinin receptor 1 (41). Obesity induces the expression of SP leading to enhanced tachykinergic transmission in the enteric nervous system, resulting in abnormal colonic motor function. The suppression of SP by apigenin attenuates enteric motor dysfunctions (40). NO produced by iNOS may trigger inflammation and play a role in enteric nitrergic pathways (42). The downregulation of iNOS by apigenin attenuates inflammation and enteric motor dysfunction. The regulation of gut bacteria by apigenin also prevents colonic dysfunction in mice via modulation of NOD-like receptor family pyrin domain containing 6 (Nlrp6) (43). Nlrp6 is highly expressed in the intestine. Nlrp6 deficiency may lead to proliferation of Prevotellaceae, the gut bacteria found in patients with bowel diseases (44), by promoting Nlrp6 inflammasome, IL-18 secretion, and regulation of gut bacterial homeostasis. Further, apigenin improves intestinal dysbiosis via augmentation of Akkermansia and Incertae Sedis along with reduction of Faecalibaculum and Dubosiella at the genus level (45). Obesity has also been associated with sarcopenia (46). Obesity-induced muscle atrophy also contributes to impaired glucose and lipid homeostasis, proinflammatory responses, and inflammation-induced mitochondrial dysfunction (47). Apigenin ameliorates skeletal muscle atrophy by enhancing mitochondrial function in an obese mouse model exposed to HFD and in C2C12 cells. Apigenin treatment upregulated mitochondria-related genes, including peroxisome proliferator-activated receptor-γ coactivator-1α (PGC1α), mt-TFAM (transcription factor of PGC1α), cytochrome C, and somatic cytochrome C (CyCs) following the activation of AMPK. Such upregulation is essential for initiation of mitochondrial biogenesis and improved mitochondrial function alleviate obesity-induced skeletal muscle atrophy (48, 49).
In summary, apigenin alleviates obesity and its complications via a variety of mechanisms including inhibition of appetite, glucose signaling pathways and lipid metabolism. It also regulates the intestinal microbiome, enhances mitochondrial function and attenuates inflammation and oxidative stress. The aforementioned experimental approaches and mechanisms underlying the effects of apigenin on obesity are listed in Table 1. Dosages and duration of apigenin treatment in vivo and in vitro are also listed in Table 1.
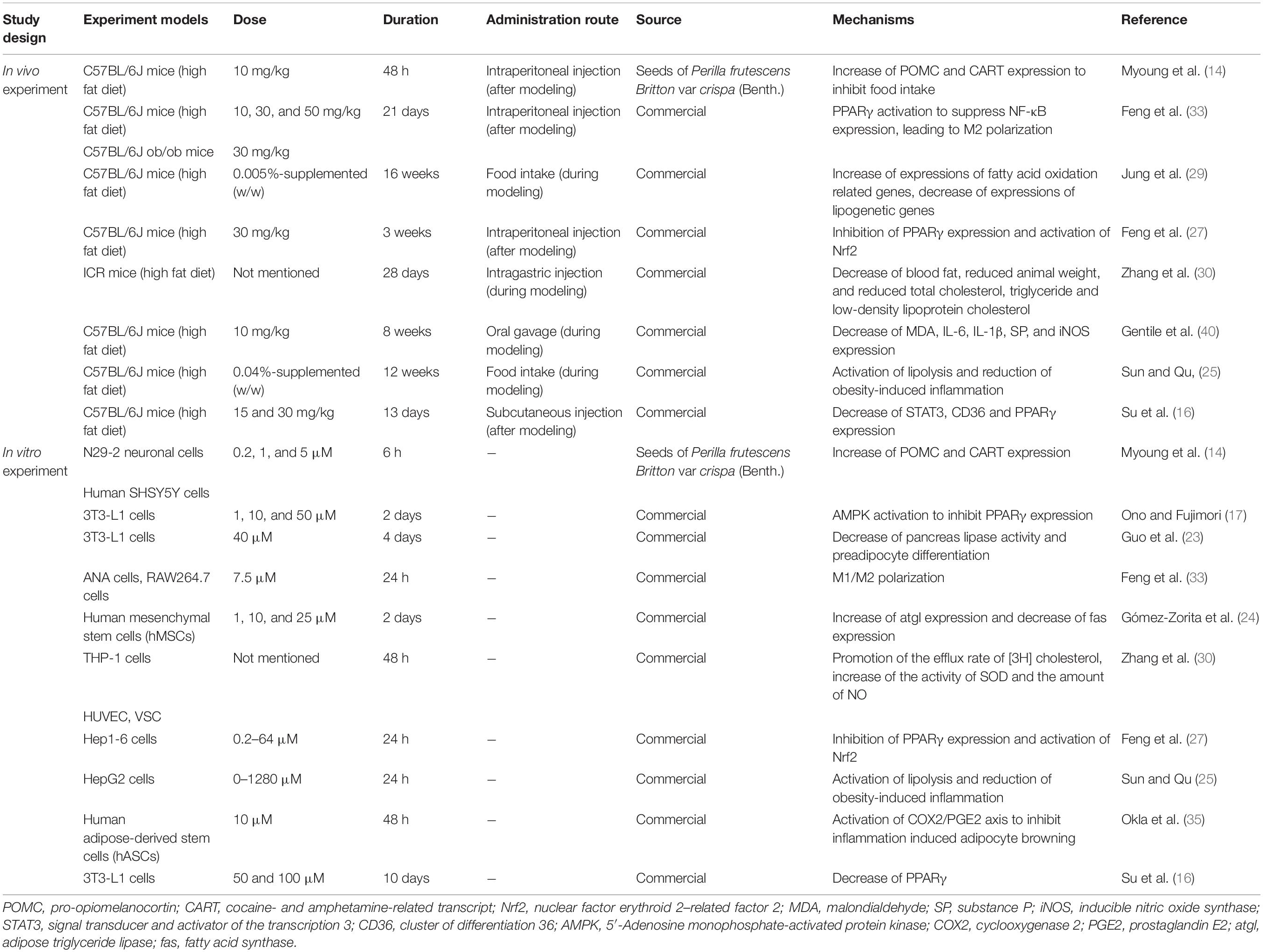
Table 1. Experiment designs and effects of apigenin on obesity and lipid metabolism (in vivo and in vitro).
Protective Role of Apigenin in Diabetes
Diabetes also plays an important role in cardiometabolic disease (50–52). Several studies investigating the effects of apigenin on type 2 diabetes mellitus (T2DM) report decreased insulin resistance, reduced abnormal glycolipid metabolism, and alleviation of oxidative stress (53, 54).
Insulin resistance plays a significant role in the pathophysiology of T2DM (55). Insulin resistance adversely affects glycometabolism in insulin-targeted organs and tissues (54). Abnormal glycolipid metabolism is a typical clinical manifestation in patients with T2DM (56). Apigenin alleviates insulin resistance and glycolipid metabolic disorders via following mechanisms:
(1) Inhibition of insulin receptor kinase. Apigenin inhibits tyrosine nitration of the insulin receptor kinase domain leading to alleviation of insulin resistance. Tyrosine nitration of IRβ (intracellular β subunits of the insulin receptor) may lead to decreased tyrosine phosphorylation, resulting in impaired insulin signal transduction in HFD mice (57). In vitro studies showed that apigenin decreases the Cu2+-catalyzed insulin receptor kinase domain fragment KK-1 and inhibits the formation of 3,3′-dityrosine (58).
(2) Regulation of miRNAs. Apigenin regulates miRNAs, which are associated with insulin resistance and glucose homeostasis. In vitro experiments involving Huh7 cells and in vivo studies investigating miR103 transgenic mice validates apigenin-mediated inhibition of the phosphorylation of transactivating response RNA-binding proteins (TRBP). Additionally, miRNA-generating complexes inhibited, leading to suppression of precursor miRNA103 maturation expressed in liver and fat, resulting in insulin resistance and impaired glucose metabolism and homeostasis (59, 60). Thus, apigenin-induced suppression of miRNA103 alleviates glucose intolerance (60).
(3) Upregulation of GLUT4/AMPK signaling. Apigenin extracted from Sophora davidii (Franch.) promotes glucose transporter 4 (GLUT4) expression and activates AMPK phosphorylation in L6 cells and insulin target tissues in KK-Ay mice (61). In insulin target tissues such as liver and fat, the upregulation of GLUT4 and the activation of AMPK facilitates glucose utilization to ameliorate insulin resistance (62, 63).
(4) Inhibition ofα-amylase. Several studies reported that apigenin decreases the inhibition of α-amylase in Kunming mice, thus reducing the digestion of dietary carbohydrates (64). The digestive enzyme α-amylase hydrolyzes dietary carbohydrates into disaccharides and polysaccharides (65). Inhibition of the digestion of dietary carbohydrates delays glucose absorption and blocks the progression of T2DM. Therefore, the inhibition of α-amylase by apigenin ameliorates T2DM (64).
Oxidative stress also triggers β-cell dysfunction, impaired glucose tolerance, and insulin resistance (66). The production of reactive oxygen species (ROS) in oxidative stress exacerbates the progression of T2DM and related complications. Apigenin treatment mitigates oxidative stress and intracellular ROS production via following mechanisms:
(1) Decreased ROS production. Apigenin pre-treatment of streptozocin (STZ)-treated RINm5F pancreatic β cells ameliorates STZ-induced intracellular ROS production, as well as DNA damage, lipid peroxidation, and apoptosis. Apigenin pre-treatment upregulates the expression of antioxidant enzymes, such as superoxide dismutase (SOD), catalase (CAT), and glutathione peroxidase (GSH-Px) in RINm5F pancreatic β cells and diabetic rats (67). SOD catalyzes the conversion of superoxide radicals (O2–) to molecular oxygen (O2) and hydrogen peroxide (H2O2), resulting in a protective effect against ROS in cells (68). Catalase is a highly specific enzyme that catalyzes the decomposition of hydrogen peroxide into water and molecular oxygen (69). GSH-Px is a cytosolic enzyme that catalyzes the reduction of hydrogen peroxide and lipid peroxides by glutathione, releasing water, oxygen, and alcohol (70). These three enzymes are indispensable in defending against free radicals (71).
(2) Inhibition of AGE. Apigenin inhibits the formation of advanced glycation end products (AGEs) and thereby alleviates oxidative stress (72). AGE-mediated damage leads to altered protein structure and functions via cross-linking between molecules via the receptor for AGEs (RAGE). AGEs increase ROS formation and damage anti-oxidant systems (73). Apigenin treatment of human blood plasma proteins in vitro reduced the levels of AGEs (72).
(3) Regulation of Keap1-Nrf2 signaling. The anti-oxidant function of apigenin is mediated via the Kelch-like ECH-associated protein 1 (Keap1)-Nrf2 axis targeting liver tissues to alleviate oxidative stress (74). Nrf2 is a primary transcription factor interacting with the anti-oxidant response element (ARE) to regulate antioxidant protein expression. Keap1 is the specific repressor of Nrf2, which acts as an adaptor protein of the Cullin3-based ubiquitin E3 ligase complex to facilitate the ubiquitination and subsequent proteolysis of Nrf2 (74), acting as a sensor of oxidative stress (75, 76). Apigenin occupies the Nrf2-binding site to prevent the binding between Keap1 and Nrf2 and thereby promotes nuclear translocation of Nrf2, thus facilitating its anti-oxidant function (74).
In addition, persistent inflammation leads to pathogenesis of diabetes (77). Apigenin significantly prevents mitogen-activated protein kinase activation (MAPK) from inhibiting inflammation (NF-κB-TNF-α axis) and apoptosis (increased expression of Bcl-2 and decreased Bax and caspase-3) in diabetic rats (78).
Currently, the apigenin-mediated regulation of blood glucose homeostasis can be summarized as follows: regulating the key enzymes and improving oxidative stress as well as inflammation. A detailed summary of the studies discussed above and the proposed mechanisms of apigenin-mediated effects in diabetes are presented in Table 2. Dosages and duration of apigenin treatments in vivo and in vitro are also listed in Table 2.
Protective Role of Apigenin in Hypertension
Hypertension plays a central role in cardiometabolic diseases (79), which is prevalent in almost 80% of patients with metabolic syndrome (80). Recent studies reported that apigenin improves hypertension via attenuation of oxidative stress and recovery of mitochondrial dysfunction.
Apigenin plays a protective role in hypertension by alleviating oxidative stress. Apigenin can significantly restore normal blood pressure and reverse renal damage in cyclosporine-induced hypertensive Sprague-Dawley rats by decreasing lipid hydroperoxides and increasing anti-oxidant levels (81). Apigenin also controls elevated blood pressure in N-nitro-L-arginine methylester-induced hypertensive Sprague-Dawley rats by improving NO bioavailability, attenuating oxidative stress, and reducing vascular damage (82).
Apigenin also regulates pulmonary hypertension (PH). Mitochondrial dysfunction plays a vital role in PH, it may lead to the imbalance of ion homeostasis and downregulation of enzymes in apoptosis (83). Apigenin activates mitochondria-dependent apoptosis via hypoxia-inducible factor 1α (HIF-1α)-KV1.5 channel pathway. The inhibition of HIF-1α by apigenin upregulates the expression of KV1.5 channels to restore mitochondrial function, thereby attenuating PH (84).
Apigenin has also been reported to diminish the complications induced by hypertension, such as renal damage and fibrosis due to abnormal collagen accumulation in kidneys (85). Apigenin significantly attenuated hypertension and renal fibrosis in deoxycorticosterone acetate (DOCA)-salt-induced hypertensive rats (86). Apigenin activates transient receptor potential vanilloid 4 (TRPV4), a non-selective cation channel widely expressed in the kidney. Ca2+ influx is then promoted in vascular endothelium and smooth muscle to induce vasodilation (87) and activation of the AMPK/SIRT1 signaling pathway to inhibit the TGF-β1 and Smad-2/3 signaling pathway (Sma and Mad proteins from Caenorhabditis elegans and Drosophila, respectively). This inhibition stimulates cellular transformation into fibroblasts and increases the synthesis of matrix proteins to induce renal fibrosis (86, 88, 89). Thus, apigenin alleviates renal fibrosis and structural and functional damage.
Current evidence suggests that apigenin decreases blood pressure mainly via improved NO bioactivity and oxidative stress, regulation of apoptosis-related mitochondrial genes and promotion of vasodilation in vascular endothelium. Experimental studies and mechanisms of action involving apigenin in hypertension are listed in Table 3. Experimental dosages and durations of apigenin treatment in vivo and in vitro are listed in Table 3.
Protective Role of Apigenin in Cardiovascular Diseases
Apigenin prevents cardiovascular diseases via antioxidant and anti-apoptotic mechanisms in vascular endothelial cells and cardiomyocytes.
Vascular endothelial dysfunction is a major mediator in cardiovascular diseases (90). Abnormal glucose metabolism and oxidative stress in vascular endothelial cells may lead to vascular endothelial dysfunction. Several studies have discussed the ameliorative effect of apigenin on endothelial dysfunction. First, apigenin increases apelin expression to rescue endothelial dysfunction. Apelin is an endogenous ligand for the G-protein-coupled APJ receptor expressed in the cardiovascular system. It increases glucose uptake and SOD activity, reversing the impaired glucose metabolism and homeostasis and the severe oxidative stress in human endothelial cells (91–94). Oxidative stress in endothelial cells leads to endothelial dysfunction and angiogenesis (92). The suppression of apelin in human endothelial cells can be reversed by apigenin treatment. Second, apigenin inhibits NF-κB-associated signaling pathways and suppresses intercellular adhesion molecule-1 (ICAM-1) expression in vascular endothelial dysfunction. ICAM-1 is a cell surface receptor that binds lymphocyte function-associated antigen 1 (LFA-1), mediating the interaction between keratinocytes and leukocytes (95). ICAM-1 plays an essential role in controlling abnormal inflammatory infiltration, adhesion, and migration (96). Apigenin inhibits NF-κB activation to improve NO production and SOD activity in endothelial cells and suppress ICAM-1 expression in human vascular endothelial cells (HUVECs) (97). Third, apigenin inactivates the PI3K (phosphoinositide-3-kinase) Akt (protein kinase B) axis in HUVECs during vascular endothelial dysfunction. The PI3K/Akt axis is an essential pathway in the pathogenesis of cardiovascular complications in T2DM (98). Apigenin treatment inhibited the phosphorylation of Akt-residues Ser473 and Thr308 to prevent vascular endothelial dysfunction (99). Finally, apigenin decreased ROS and improved NO levels to alleviate vascular endothelial dysfunction induced by mitochondria-dependent apoptosis via inhibition of protein kinase C βII (PKCβII) phosphorylation. PKCβII promotes oxidative stress, ROS production and mitochondria-dependent apoptosis in vascular endothelial dysfunction (100, 101). Apigenin treatment upregulated the expression of the anti-apoptotic gene, B-cell lymphoma-2 (Bcl-2), while the pro-apoptotic gene, Bcl-2 associated X (Bax), was downregulated, resulting in attenuation of mitochondria-dependent apoptosis in endothelial cells (102).
Further, cardiac hypertrophy is another manifestation of cardiovascular diseases (103). Current evidence suggests that abnormal glycolipid metabolism and overexpression of HIF-1α in cardiac cells causes cardiac hypertrophy (104). In vivo and in vitro experimental data suggest that apigenin alleviates cardiac hypertrophy via suppression of HIF-1α, thereby reversing the expression of PPARα/γ and target genes including glycerol-3-phosphate acyltransferase (GPAT), glucose transporter 4 (GLUT-4), carnitine palmitoyltransferase 1 (CPT-1) and pyruvate dehydrogenase kinase 4 (PDK-4). Downregulation of GLUT4 and upregulation of PDK-4 can inhibit excessive glucose intake and oxidation, preventing abnormal glucose metabolism. Downregulation of GPAT and upregulation of CPT-1 decreases the rate of triglyceride synthesis and augments fatty acid oxidation, thereby improving lipid metabolism (105, 106). Thus, the hypoxic myocardial energy utilization (107–110) can be reversed.
In summary, apigenin can ameliorate cardiovascular diseases via reduction of oxidative stress and mitochondria-dependent apoptosis in vascular endothelial cells as well as regulation of glucose and lipid metabolism in cardiomyocytes. The experimental studies and mechanisms of action involving apigenin in hypertension are presented in Table 4. Experimental dosages and duration of apigenin treatment in vivo and in vitro are also listed in Table 4.
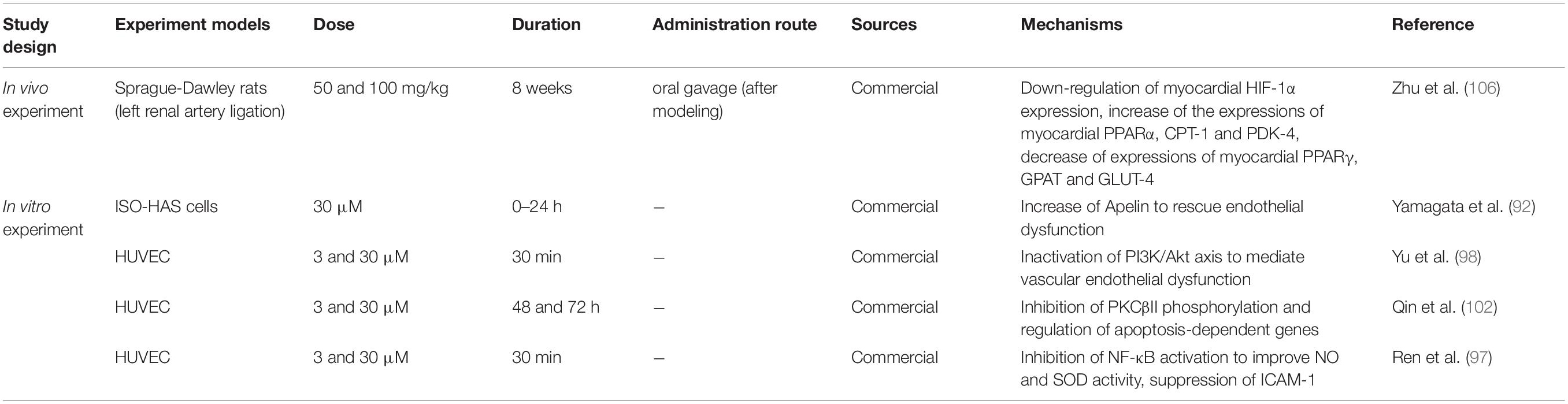
Table 4. Experiment designs and effects of apigenin on cardiovascular diseases (in vivo and in vitro).
Apigenin Analogs and Their Effects on Alleviating Cardiometabolic Diseases
Apigenin analogs are derived from the basic flavonoid skeleton via hydroxyl group substitution, glycosylation, hydroxylation, and methylation (111, 112). In plants, apigenin is stored as glycosides such as apigenin 7-O-apioglucoside in celery and parsley (113, 114) and apigenin 8-C-glucoside isolated from bamboo leaves (115). Several apigenin analogs carry the basic flavonoid skeleton similar to apigenin and exhibit biological activity in cardiometabolic diseases.
(1) Apiin. Apiin (apigenin 7-O-apioglucoside) is derived from celery and exhibits anti-adipogenic and anti-obesity effects in HFD mice via the AMPK/PPARγ axis (20), similar to apigenin. Apiin also alleviates insulin resistance in HFD mice via downregulation of glucogenic genes, PEPCK (phosphoenolpyruvate carboxykinase) and G6Pase (glucose-6-phosphatase) in the liver, and promotion of glycogen synthesis via inhibition of glycogen synthase phosphorylation and induction of GSK3β (glycogen synthase kinase3β) phosphorylation (116).
(2) Apigetrin. Apigetrin (apigenin 7-glucoside) ameliorated pancreatic β cell damage via reduction of endoplasmic reticulum (ER) stress in RINm5F cells via the regulation of ER stress biomarkers, such as upregulation of CCAAT/enhancer-binding protein homologous protein (C/EBP), induction of spliced X-box binding protein 1 (XBP1), phosphorylation of protein kinase RNA-like ER kinase (PERK) and eukaryotic initiation factor 2α (eIF2alpha), and cleavage of caspase-12 (117).
(3) Vitexin. Vitexin (apigenin 8-C-glucoside) regulates lipid metabolism via AMPK-mediated pathway in 3T3-L1 cells (118) in vitro and the liver of HFD mice (119) in vivo to alleviate obesity and non-alcoholic fatty liver disease. Vitexin also protects pancreatic β-cells via inhibition of high mobility group box 1 (HMGB1) (120), which is released from damaged pancreatic β-cells and induces inflammation in LPS (lipopolysaccharide)-induced rats and LPS-treated INS-cells.
(4) Acacetin. Acacetin (4′-methoxy 5,7-dihydroxyflavone) suppress adipogenesis in 3T3-L1 cells and HFD mice via upregulation of SIRT1 expression and AMPK phosphorylation (121). Acacetin also increases glucose uptake by enhancing GLUT4 translocation to the plasma membrane via the CaMKII-AMPK pathway by increasing intracellular calcium concentrations in L6 and HepG2 cells (122). In addition to regulating glycometabolism, acacetin alleviates endothelial dysfunction in insulin-resistant rats by inhibiting the release of inflammatory factors, such as NF-κB and IL-1β, and improving vasodilatory function via the estrogen signaling pathway (123).
(5) Apigenin 7, 4′-dimethyl ether. Apigenin 7, 4′-dimethyl ether (ADE) enhances glucose uptake in L6 cells and inhibits α-glucosidase enzyme (124), which releases glucose to form glycolipid and glycopeptide via hydrolyzation of α-glycosidic bonds from the non-reducing ends of oligosaccharide substrates and transfer of free glucose residues to another carbohydrate substrate.
(6) 8-(6″-umbelliferyl)-apigenin. 8-(6″-umbelliferyl)-apigenin promotes glucose uptake in 3T3-L1 cells, indicating improved glucose consumption (125).
Potential Signaling Pathways Mediated by Apigenin for Amelioration of Cardiometabolic Disease in Different Cell Types
In summary, the potential signaling pathways mediated by apigenin resulting in alleviation of cardiometabolic diseases in different cell types are illustrated in Figures 2A–E. Apigenin alleviates cardiometabolic diseases mainly by regulating glycolipid metabolism, oxidative stress, and oxidative stress-induced inflammation and apoptosis. Notably, apigenin plays contrasting roles in different types of cells. Apigenin acts as an agonist of PPARγ in adipose tissue macrophages. PPARγ binds to p65 to inhibit nuclear translocation to block NF-κB signaling pathway resulting in attenuation of inflammation (Figure 2A) (33). However, apigenin inhibits PPARγ expression in adipocytes, hepatocytes, and cardiomyocytes by acting as an antagonist (Figures 2B–D).
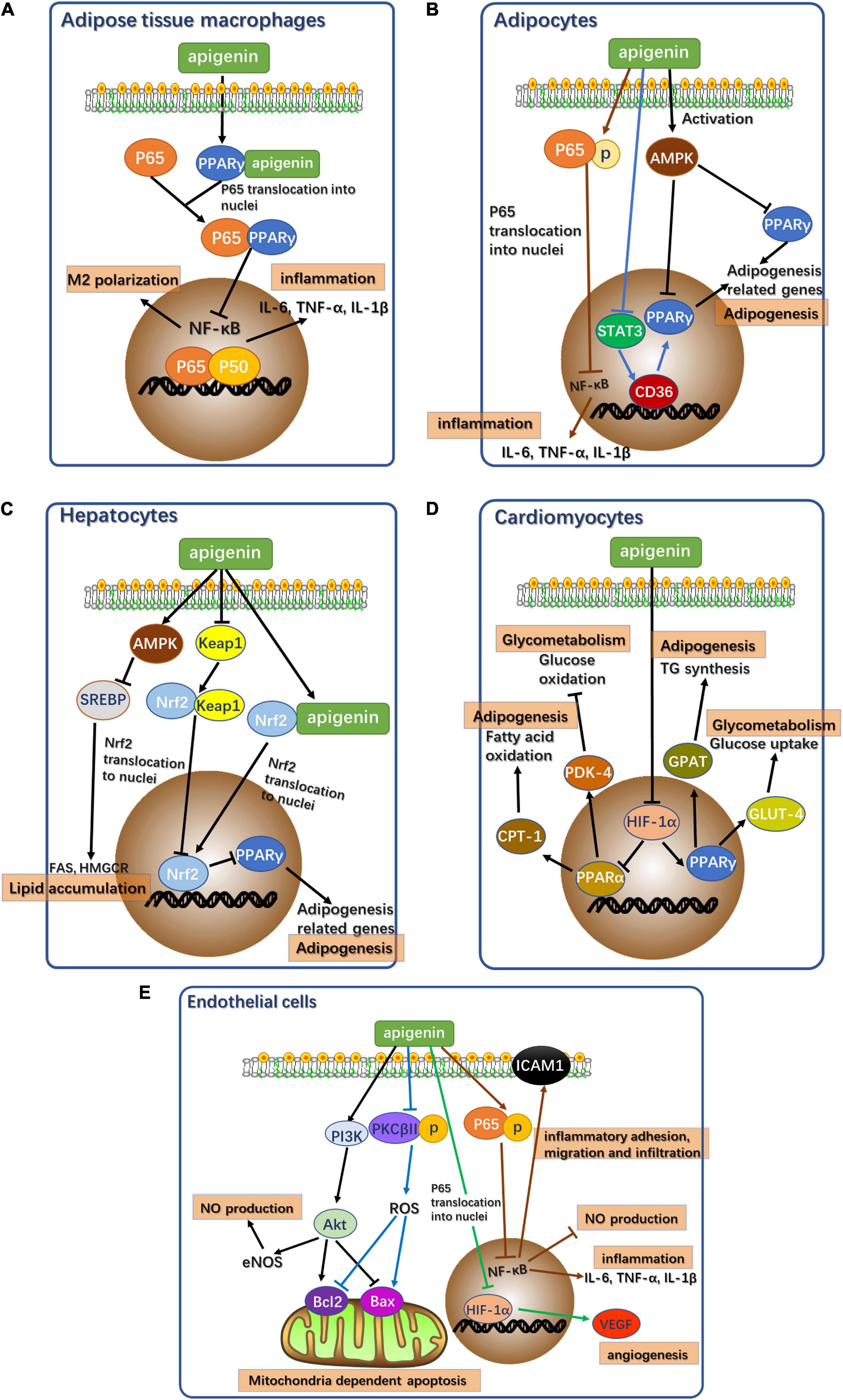
Figure 2. Potential signaling pathways of apigenin affecting cardiometabolic diseases in different types of cells. (A) Potential signaling pathways of apigenin affecting cardiometabolic diseases in adipose tissue macrophages. p65: RelA, NF-κB component. P50: p50 NF-κB component. (B) Potential signaling pathways of apigenin affecting cardiometabolic diseases in adipocytes. AMPK, AMP-activated protein kinase. p65: RelA, NF-κB component. STAT3, Signal transducer and activator of transcription 3. (C) Potential signaling pathways of apigenin affecting cardiometabolic diseases in hepatocytes. AMPK, AMP-activated protein kinase. SREBP, sterol regulatory element-binding proteins. FAS, fatty acid synthase. HMGCR, 3-Hydroxy-3-Methylglutaryl-Coenzyme A Reductase. Keap1, Kelch like ECH associated protein 1. Nrf2, NF-E2-related factor 2. (D) Potential signaling pathways of apigenin affecting cardiometabolic diseases in cardiomyocytes. HIF-1α, hypoxia inducible factor 1 subunit alpha; CPT1, carnitine palmitoyl transferase I; PDK4, pyruvate dehydrogenase kinase 4; GPAT, glycerol-3-phosphate acyltransferase; GLUT4, glucose transporter type 4. (E) Potential signaling pathways of apigenin affecting cardiometabolic diseases in endothelial cells. PI3K, Phosphoinositide 3-kinase; Akt, protein kinase B; eNOS, endothelial nitric oxide synthase; PKCβII, protein kinase C subunit β II; ROS, reactive oxygen species; Bcl-2, B-cell lymphoma-2; Bax, Bcl2 associated X; HIF-1α, hypoxia inducible factor 1 subunit alpha; VEGF, vascular endothelial growth factor; p65, RelA, NF-κB component.
Apigenin in Adipocytes
In adipocytes, apigenin acts as a functional regulator of lipid metabolism to reduce fat accumulation. Apigenin downregulates PPARγ expression by inhibiting the STAT3/CD36 axis (16) and the activation of AMPK (17). Apigenin directly induces p65 phosphorylation to prevent its nuclear translocation to ensure continued inhibition of NF-κB signaling in the absence of PPARγ as a mediator (25, 35).
Apigenin in Hepatocytes
Since the liver is an important site of energy metabolism, apigenin is a potential mediator of glycolipid metabolism in hepatocytes. Apigenin acts as a PPARγ antagonist via direct activation of Nrf2 and indirect activation of Nrf2 via the Keap1-Nrf2 pathway (126). Additionally, apigenin activates AMPK to inhibit SREBP-1 and SPEBP-2 to regulate hepatic fatty acid oxidation and cholesterol synthesis (28).
Apigenin in Cardiomyocytes
Apigenin treatment of cardiomyocytes regulates glucose and lipid metabolism to maintain normal cellular function. HIF-1α activation via apigenin regulates the PPAR family, leading to the appropriate regulation of downstream target genes related to glycolipid metabolism. Apigenin suppresses PPARγ expression via the activation of HIF-1α as an antagonist (105, 106). Meanwhile, the upregulation of HIF-1α following apigenin treatment increases PPARα expression (105, 106).
Apigenin in Endothelial Cells
Apigenin plays a protective role in vascular endothelial dysfunction by regulating several signaling pathways in endothelial cells to alleviate oxidative stress, inflammation, and mitochondria-dependent apoptosis. The inhibition of the NF-κB signaling pathway with apigenin treatment also ameliorates inflammatory response in endothelial cells and increases NO production (97). NF-κB inhibition also suppresses the expression of ICAM-1 to improve abnormal inflammatory adhesion, migration, and infiltration, resulting in the alleviation of vascular endothelial dysfunction (97). In addition to NF-κB pathways, apigenin also activates the PI3K-Akt pathway and inhibits PKCβII activation by reducing oxidative stress and oxidative stress-related apoptosis in mitochondria (102). The expression of anti-apoptotic gene, Bcl-2, and the pro-apoptotic gene, Bax, in these two pathways reduces abnormal apoptosis. The activation of the PI3K-Akt pathway also promotes eNOS activity to restore NO levels and thereby attenuates oxidative stress (102). Apigenin treatment also mitigates angiogenesis induced by inflammation. HIF-1α inhibition by apigenin directly reduces the expression of vascular endothelial growth factor (VEGF) in angiogenesis, thus alleviating the angiogenesis induced by vascular dysfunction (127).
Conclusion and Perspectives
A review of studies investigating apigenin suggests critical biological mechanisms, including reducing oxidative stress and oxidative stress-induced inflammation and apoptosis, and improving glycolipid metabolism. Figure 3 summarizes the potential signaling pathways of apigenin underlying the protection against cardiometabolic diseases.
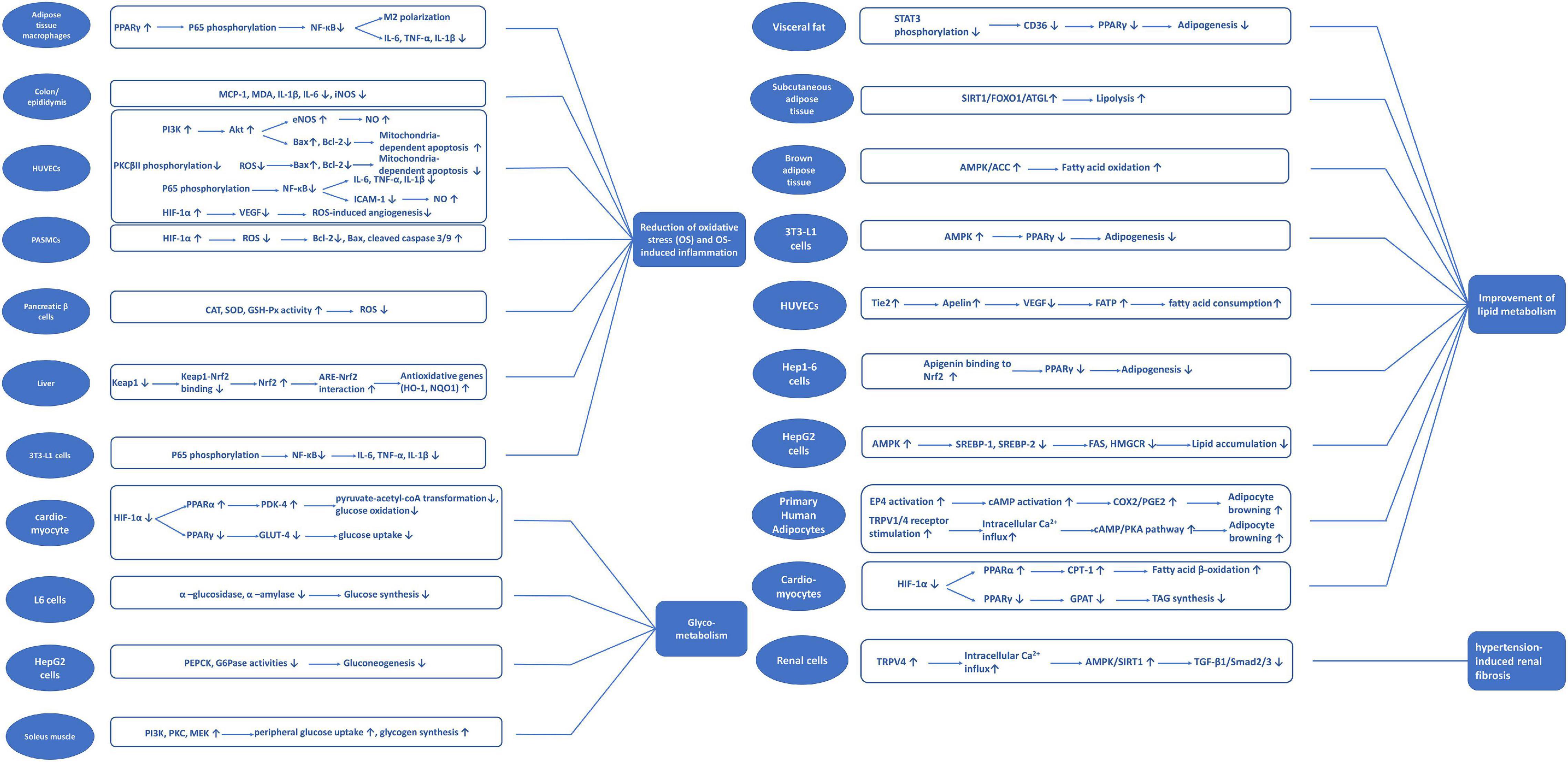
Figure 3. Potential signaling pathways classified with different mechanisms of apigenin on cardiometabolic diseases.
The molecular structure of apigenin suggests poor water solubility, chemical instability and moderate permeability, which prevent maximum bioavailability. Therefore, new delivery and design strategies have been formulated including the development of apigenin glycosides and acylated derivatives to enhance water solubility (128, 129). Apigenin-AuNP complex can be developed at room temperature at pH 10 to enhance the stability of apigenin in the body (130). Nano-apigenin using poly (lactic-co-glycolide) (PLGA) can also improve the bioactivity of apigenin (131). Pharmacokinetic and pharmacodynamic profiles of apigenin in rats and mice have been studied. The peak plasma concentration Cmax and the time to reach the peak plasma concentration Tmax were 1.07 ng/mL and 1 h, respectively, and the area under the concentration-time curve (AUC0–24) was 3.9 ng h/mL in mice (132). However, the bioavailability of apigenin in humans is still unknown. Further studies are needed to confirm the bioavailability and safety profile in humans.
In summary, the extensive review and validation of in vitro and in vivo evidence suggests that apigenin is a natural compound that can be used to protect against cardiometabolic diseases. Environment-wide association studies (EWAS) also indicate that apigenin is one of the protective factors in cardiovascular diseases at the population level (133). Further studies are required to establish the optimum dose of apigenin in alleviating cardiometabolic diseases in humans, developing a novel approach for clinical management of the disease.
Author Contributions
XL designed this review, helped with writing and revising of the manuscript, and provided critical feedback. YX contributed to collecting and screening the literature, as well as summarizing the data, and then composed and revised the manuscript. HW reviewed the manuscript. All authors were involved in final approval of the submitted version.
Funding
This study was supported by grants from the National Nature Science Foundation (81803215 and 81630086); three-year Action Program of Shanghai Municipality for Strengthening the Construction of Public Health System (GWV-10.2-YQ34); the National Key R&D Program of China (2018YFC2000700); the Key Research Program (ZDRW-ZS-2017-1) of the Chinese Academy of Sciences; innovative research team of high-level local Universities in Shanghai; Medicine and Engineering Interdisciplinary Research Fund of Shanghai Jiao Tong University (YG2020YQ06).
Conflict of Interest
The authors declare that the research was conducted in the absence of any commercial or financial relationships that could be construed as a potential conflict of interest.
Publisher’s Note
All claims expressed in this article are solely those of the authors and do not necessarily represent those of their affiliated organizations, or those of the publisher, the editors and the reviewers. Any product that may be evaluated in this article, or claim that may be made by its manufacturer, is not guaranteed or endorsed by the publisher.
References
1. Sung B, Chung HY, Kim ND. Role of apigenin in cancer prevention via the induction of apoptosis and autophagy. J Cancer Prev. (2016) 21:216–26. doi: 10.15430/JCP.2016.21.4.216
2. Madunić J, Madunić IV, Gajski G, Popić J, Garaj-Vrhovac V. Apigenin: a dietary flavonoid with diverse anticancer properties. Cancer Lett. (2018) 413:11–22. doi: 10.1016/j.canlet.2017.10.041
3. Huang WY, Cai YZ, Zhang Y. Natural phenolic compounds from medicinal herbs and dietary plants: potential use for cancer prevention. Nutr Cancer. (2010) 62:1–20. doi: 10.1080/01635580903191585
4. Svehlikova V, Bennett RN, Mellon FA, Needs PW, Piacente S, Kroon PA, et al. Isolation, identification and stability of acylated derivatives of apigenin 7-O-glucoside from chamomile (Chamomilla recutita L. Rauschert). Phytochemistry. (2004) 65:2323–32. doi: 10.1016/j.phytochem.2004.07.011
5. Saldanha E, Pai RJ, George T, D’Souza S, Adnan M, Pais M, et al. Health effects of various dietary agents and phytochemicals (Therapy of acute pancreatitis). In: Grumezescu AM, Holban AM editors. Therapeutic, Probiotic, and Unconventional Foods. Cambridge, MA: Academic Press (2018). p. 303–14. doi: 10.1016/b978-0-12-814625-5.00016-9
6. Seo YJ, Kim BS, Chun SY, Park YK, Kang KS, Kwon TG. Apoptotic effects of genistein, biochanin-A and apigenin on LNCaP and PC-3 cells by p21 through transcriptional inhibition of polo-like kinase-1. J Korean Med Sci. (2011) 26:1489–94. doi: 10.3346/jkms.2011.26.11.1489
7. Li K, Yao F, Xue Q, Fan H, Yang L, Li X, et al. Inhibitory effects against α-glucosidase and α-amylase of the flavonoids-rich extract from Scutellaria baicalensis shoots and interpretation of structure-activity relationship of its eight flavonoids by a refined assign-score method. Chem Cent J. (2018) 12:82. doi: 10.1186/s13065-018-0445-y
8. Kilani-Jaziri S, Mustapha N, Mokdad-Bzeouich I, El Gueder D, Ghedira K, Ghedira-Chekir L. Flavones induce immunomodulatory and anti-inflammatory effects by activating cellular anti-oxidant activity: a structure-activity relationship study. Tumour Biol. (2016) 37:6571–9. doi: 10.1007/s13277-015-4541-5
9. Fouache A, Zabaiou N, De Joussineau C, Morel L, Silvente-Poirot S, Namsi A, et al. Flavonoids differentially modulate liver X receptors activity-structure-function relationship analysis. J Steroid Biochem Mol Biol. (2019) 190:173–82. doi: 10.1016/j.jsbmb.2019.03.028
10. Pescatello LS, VanHeest JL. Physical activity mediates a healthier body weight in the presence of obesity. Br J Sports Med. (2000) 34:86–93. doi: 10.1136/bjsm.34.2.86
13. Yun JW. Possible anti-obesity therapeutics from nature–a review. Phytochemistry. (2010) 71:1625–41. doi: 10.1016/j.phytochem.2010.07.011
14. Myoung HJ, Kim G, Nam KW. Apigenin isolated from the seeds of Perilla frutescens britton var crispa (Benth.) inhibits food intake in C57BL/6J mice. Arch Pharm Res. (2010) 33:1741–6. doi: 10.1007/s12272-010-1105-5
15. Hill JW. Gene expression and the control of food intake by hypothalamic POMC/CART neurons. Open Neuroendocrinol J. (2010) 3:21–7.
16. Su T, Huang C, Yang C, Jiang T, Su J, Chen M, et al. Apigenin inhibits STAT3/CD36 signaling axis and reduces visceral obesity. Pharmacol Res. (2020) 152:104586. doi: 10.1016/j.phrs.2019.104586
17. Ono M, Fujimori K. Antiadipogenic effect of dietary apigenin through activation of AMPK in 3T3-L1 cells. J Agric Food Chem. (2011) 59:13346–52. doi: 10.1021/jf203490a
18. Hu T, Yeh JE, Pinello L, Jacob J, Chakravarthy S, Yuan G-C, et al. Impact of the N-Terminal domain of STAT3 in STAT3-dependent transcriptional activity. Mol Cell Biol. (2015) 35:3284–300. doi: 10.1128/mcb.00060-15
19. Rozovski U, Harris DM, Li P, Liu Z, Jain P, Ferrajoli A, et al. STAT3-activated CD36 facilitates fatty acid uptake in chronic lymphocytic leukemia cells. Oncotarget. (2018) 9:21268–80. doi: 10.18632/oncotarget.25066
20. Cho BO, Che DN, Shin JY, Kang HJ, Kim JH, Jang SI. Anti-obesity effects of enzyme-treated celery extract in mice fed with high-fat diet. J Food Biochem. (2020) 44:e13105. doi: 10.1111/jfbc.13105
21. Wandee J, Prawan A, Senggunprai L, Kongpetch S, Tusskorn O, Kukongviriyapan V. Metformin enhances cisplatin induced inhibition of cholangiocarcinoma cells via AMPK-mTOR pathway. Life Sci. (2018) 207:172–83. doi: 10.1016/j.lfs.2018.05.046
22. Grempler R, Wolff M, Simon E, Schmid R, Eisele C, Rieber K, et al. Discovery and translation of a target engagement marker for AMP-activated protein kinase (AMPK). PLoS One. (2018) 13:e0197849. doi: 10.1371/journal.pone.0197849
23. Guo X, Liu J, Cai S, Wang O, Ji B. Synergistic interactions of apigenin, naringin, quercetin and emodin on inhibition of 3T3-L1 preadipocyte differentiation and pancreas lipase activity. Obes Res Clin Pract. (2016) 10:327–39. doi: 10.1016/j.orcp.2015.08.004
24. Gómez-Zorita S, Lasa A, Abendaño N, Fernández-Quintela A, Mosqueda-Solís A, Garcia-Sobreviela MP, et al. Phenolic compounds apigenin, hesperidin and kaempferol reduce in vitro lipid accumulation in human adipocytes. J Transl Med. (2017) 15:237. doi: 10.1186/s12967-017-1343-0
25. Sun Y-S, Qu W. Dietary apigenin promotes lipid catabolism, thermogenesis, and browning in adipose tissues of HFD-Fed mice. Food Chem Toxicol. (2019) 133:110780. doi: 10.1016/j.fct.2019.110780
26. Bollong MJ, Lee G, Coukos JS, Yun H, Zambaldo C, Chang JW, et al. A metabolite-derived protein modification integrates glycolysis with KEAP1-NRF2 signalling. Nature. (2018) 562:600–4. doi: 10.1038/s41586-018-0622-0
27. Feng X, Yu W, Li X, Zhou F, Zhang W, Shen Q, et al. Apigenin, a modulator of PPARγ, attenuates HFD-induced NAFLD by regulating hepatocyte lipid metabolism and oxidative stress via Nrf2 activation. Biochem Pharmacol. (2017) 136:136–49. doi: 10.1016/j.bcp.2017.04.014
28. Lu J, Meng Z, Cheng B, Liu M, Tao S, Guan S. Apigenin reduces the excessive accumulation of lipids induced by palmitic acid via the AMPK signaling pathway in HepG2 cells. Exp Ther Med. (2019) 18:2965–71. doi: 10.3892/etm.2019.7905
29. Jung UJ, Cho Y-Y, Choi M-S. Apigenin ameliorates dyslipidemia, hepatic steatosis and insulin resistance by modulating metabolic and transcriptional profiles in the liver of high-fat diet-induced obese mice. Nutrients. (2016) 8:305. doi: 10.3390/nu8050305
30. Zhang K, Song W, Li D, Jin X. Apigenin in the regulation of cholesterol metabolism and protection of blood vessels. Exp Ther Med. (2017) 13:1719–24. doi: 10.3892/etm.2017.4165
31. Leisegang K, Henkel R, Agarwal A. Obesity and metabolic syndrome associated with systemic inflammation and the impact on the male reproductive system. Am J Reprod Immunol. (2019) 82:e13178. doi: 10.1111/aji.13178
32. Lauridsen C. From oxidative stress to inflammation: redox balance and immune system. Poult Sci. (2019) 98:4240–6. doi: 10.3382/ps/pey407
33. Feng X, Weng D, Zhou F, Owen YD, Qin H, Zhao J, et al. Activation of PPARgamma by a natural flavonoid modulator, apigenin ameliorates obesity-related inflammation via regulation of macrophage polarization. EBioMedicine. (2016) 9:61–76. doi: 10.1016/j.ebiom.2016.06.017
34. Villarroya F, Cereijo R, Gavaldà-Navarro A, Villarroya J, Giralt M. Inflammation of brown/beige adipose tissues in obesity and metabolic disease. J Intern Med. (2018) 284:492–504. doi: 10.1111/joim.12803
35. Okla M, Al Madani JO, Chung S, Alfayez M. Apigenin reverses interleukin-1β-Induced suppression of adipocyte browning via COX2/PGE2 signaling pathway in human adipocytes. Mol Nutr Food Res. (2020) 64:e1900925. doi: 10.1002/mnfr.201900925
36. García-Alonso V, Clària J. Prostaglandin E2 signals white-to-brown adipogenic differentiation. Adipocyte. (2014) 3:290–6. doi: 10.4161/adip.29993
37. Fujimoto A, Hoteya S, Iizuka T, Ogawa O, Mitani T, Kuroki Y, et al. Obesity and gastrointestinal diseases. Gastroenterol Res Pract. (2013) 2013:760574.
38. Ho W, Spiegel BMR. The relationship between obesity and functional gastrointestinal disorders: causation, association, or neither? Gastroenterol Hepatol. (2008) 4:572–8.
39. Volarevic V, Zdravkovic N, Harrell CR, Arsenijevic N, Fellabaum C, Djonov V, et al. Galectin-3 regulates indoleamine-2,3-dioxygenase-dependent cross-talk between colon-infiltrating dendritic cells and T regulatory cells and may represent a valuable biomarker for monitoring the progression of ulcerative colitis. Cells. (2019) 8:709. doi: 10.3390/cells8070709
40. Gentile D, Fornai M, Colucci R, Pellegrini C, Tirotta E, Benvenuti L, et al. The flavonoid compound apigenin prevents colonic inflammation and motor dysfunctions associated with high fat diet-induced obesity. PLoS One. (2018) 13:e0195502. doi: 10.1371/journal.pone.0195502
41. Navratilova E, Porreca F. Substance P and inflammatory pain: getting it wrong and right simultaneously. Neuron. (2019) 101:353–5. doi: 10.1016/j.neuron.2019.01.034
42. Sung T-S, La J-H, Kim T-W, Yang I-S. Alteration of nitrergic neuromuscular transmission as a result of acute experimental colitis in rat. J Vet Sci. (2006) 7:143–50. doi: 10.4142/jvs.2006.7.2.143
43. Radulovic K, Normand S, Rehman A, Delanoye-Crespin A, Chatagnon J, Delacre M, et al. A dietary flavone confers communicable protection against colitis through NLRP6 signaling independently of inflammasome activation. Mucosal Immunol. (2018) 11:811–9. doi: 10.1038/mi.2017.87
44. Elinav E, Strowig T, Kau AL, Henao-Mejia J, Thaiss CA, Booth CJ, et al. NLRP6 inflammasome regulates colonic microbial ecology and risk for colitis. Cell. (2011) 145:745–57. doi: 10.1016/j.cell.2011.04.022
45. Qiao Y, Zhang Z, Zhai Y, Yan X, Zhou W, Liu H, et al. Apigenin alleviates obesity-associated metabolic syndrome by regulating the composition of the gut microbiome. Front Microbiol. (2022) 12:805827. doi: 10.3389/fmicb.2021.805827
46. Lipina C, Hundal HS. Lipid modulation of skeletal muscle mass and function. J Cachexia Sarcopenia Muscle. (2017) 8:190–201. doi: 10.1002/jcsm.12144
47. Perry BD, Caldow MK, Brennan-Speranza TC, Sbaraglia M, Jerums G, Garnham A, et al. Muscle atrophy in patients with Type 2 diabetes mellitus: roles of inflammatory pathways, physical activity and exercise. Exerc Immunol Rev. (2016) 22:94–109.
48. Choi WH, Son HJ, Jang YJ, Ahn J, Jung CH, Ha TY. Apigenin ameliorates the obesity-induced skeletal muscle atrophy by attenuating mitochondrial dysfunction in the muscle of obese mice. Mol Nutr Food Res. (2017) 61:1700218. doi: 10.1002/mnfr.201700218
49. Deng X, Zhang S, Wu J, Sun X, Shen Z, Dong J, et al. Promotion of mitochondrial biogenesis via activation of AMPK-PGC1α signaling pathway by ginger (Zingiber officinale Roscoe) extract, and its major active component 6-Gingerol. J Food Sci. (2019) 84:2101–11. doi: 10.1111/1750-3841.14723
50. Hernandez AF, Green JB, Janmohamed S, D’Agostino RB Sr, Granger CB, Jones NP, et al. Albiglutide and cardiovascular outcomes in patients with type 2 diabetes and cardiovascular disease (Harmony Outcomes): a double-blind, randomised placebo-controlled trial. Lancet. (2018) 392:1519–29. doi: 10.1016/S0140-6736(18)32261-X
51. van Sloten TT, Sedaghat S, Carnethon MR, Launer LJ, Stehouwer CDA. Cerebral microvascular complications of type 2 diabetes: stroke, cognitive dysfunction, and depression. Lancet Diabetes Endocrinol. (2020) 8:325–36. doi: 10.1016/S2213-8587(19)30405-X
52. Douros A, Filion KB, Yin H, Yu OH, Etminan M, Udell JA, et al. Glucagon-like peptide 1 receptor agonists and the risk of incident diabetic retinopathy. Diabetes Care. (2018) 41:2330. doi: 10.2337/dc17-2280
53. Taylor R. Type 2 diabetes: etiology and reversibility. Diabetes Care. (2013) 36:1047–55. doi: 10.2337/dc12-1805
54. Petersen MC, Shulman GI. Mechanisms of insulin action and insulin resistance. Physiol Rev. (2018) 98:2133–223.
55. Sampath Kumar A, Maiya AG, Shastry BA, Vaishali K, Ravishankar N, Hazari A, et al. Exercise and insulin resistance in type 2 diabetes mellitus: a systematic review and meta-analysis. Ann Phys Rehabil Med. (2019) 62:98–103. doi: 10.1016/j.rehab.2018.11.001
56. Perry RJ, Samuel VT, Petersen KF, Shulman GI. The role of hepatic lipids in hepatic insulin resistance and type 2 diabetes. Nature. (2014) 510:84–91. doi: 10.1038/nature13478
57. Charbonneau A, Marette A. Inducible nitric oxide synthase induction underlies lipid-induced hepatic insulin resistance in mice: potential role of tyrosine nitration of insulin signaling proteins. Diabetes. (2010) 59:861–71. doi: 10.2337/db09-1238
58. Fang X, Gao W, Yang Z, Gao Z, Li H. Dual Anti-/prooxidant behaviors of flavonoids pertaining to Cu(II)-catalyzed tyrosine nitration of the insulin receptor kinase domain in an antidiabetic study. J Agric Food Chem. (2020) 68:6202–11. doi: 10.1021/acs.jafc.0c01676
59. Xu Q, Li Y, Shang Y-F, Wang H-L, Yao M-X. miRNA-103: molecular link between insulin resistance and nonalcoholic fatty liver disease. World J Gastroenterol. (2015) 21:511–6. doi: 10.3748/wjg.v21.i2.511
60. Ohno M, Shibata C, Kishikawa T, Yoshikawa T, Takata A, Kojima K, et al. The flavonoid apigenin improves glucose tolerance through inhibition of microRNA maturation in miRNA103 transgenic mice. Sci Rep. (2013) 3:2553. doi: 10.1038/srep02553
61. Huang Y, Hao J, Tian D, Wen Y, Zhao P, Chen H, et al. Antidiabetic activity of a flavonoid-rich extract from sophora davidii (Franch.) Skeels in KK-Ay mice via activation of AMP-activated protein kinase. Front Pharmacol. (2018) 9:760. doi: 10.3389/fphar.2018.00760
62. Alam F, Islam MA, Khalil MI, Gan SH. Metabolic control of type 2 diabetes by targeting the GLUT4 glucose transporter: intervention approaches. Curr Pharm Des. (2016) 22:3034–49. doi: 10.2174/1381612822666160307145801
63. Madhavi YV, Gaikwad N, Yerra VG, Kalvala AK, Nanduri S, Kumar A. Targeting AMPK in diabetes and diabetic complications: energy homeostasis, autophagy and mitochondrial health. Curr Med Chem. (2019) 26:5207–29. doi: 10.2174/0929867325666180406120051
64. Zhang BW, Li X, Sun WL, Xing Y, Xiu ZL, Zhuang CL, et al. Dietary flavonoids and acarbose synergistically inhibit α-Glucosidase and lower postprandial blood glucose. J Agric Food Chem. (2017) 65:8319–30. doi: 10.1021/acs.jafc.7b02531
65. Derosa G, Maffioli P. α-Glucosidase inhibitors and their use in clinical practice. Arch Med Sci. (2012) 8:899–906. doi: 10.5114/aoms.2012.31621
66. Wright E Jr, Scism-Bacon JL, Glass LC. Oxidative stress in type 2 diabetes: the role of fasting and postprandial glycaemia. Int J Clin Pract. (2006) 60:308–14. doi: 10.1111/j.1368-5031.2006.00825.x
67. Mao XY, Yu J, Liu ZQ, Zhou HH. Apigenin attenuates diabetes-associated cognitive decline in rats via suppressing oxidative stress and nitric oxide synthase pathway. Int J Clin Exp Med. (2015) 8:15506–13.
68. Matsuda S, Nakagawa Y, Kitagishi Y, Nakanishi A, Murai T. Reactive oxygen species, superoxide dimutases, and PTEN-p53-AKT-MDM2 signaling loop network in mesenchymal stem/stromal cells regulation. Cells. (2018) 7:36. doi: 10.3390/cells7050036
69. Lee JN, Dutta RK, Maharjan Y, Liu Z-Q, Lim J-Y, Kim S-J, et al. Catalase inhibition induces pexophagy through ROS accumulation. Biochem Biophys Res Commun. (2018) 501:696–702. doi: 10.1016/j.bbrc.2018.05.050
70. Deveci HA, Akyuva Y, Nur G, Nazıroğlu M. Alpha lipoic acid attenuates hypoxia-induced apoptosis, inflammation and mitochondrial oxidative stress via inhibition of TRPA1 channel in human glioblastoma cell line. Biomed Pharmacother. (2019) 111:292–304. doi: 10.1016/j.biopha.2018.12.077
71. Wang N, Yi WJ, Tan L, Zhang JH, Xu J, Chen Y, et al. Apigenin attenuates streptozotocin-induced pancreatic β cell damage by its protective effects on cellular antioxidant defense. In Vitro Cell Dev Biol Anim. (2017) 53:554–63. doi: 10.1007/s11626-017-0135-4
72. Liu L, Xie Y, Song Z, Shang S, Chen X. Influence of dietary flavonoids on the glycation of plasma proteins. Mol Biosyst. (2012) 8:2183–7. doi: 10.1039/c2mb25038a
73. Nowotny K, Jung T, Höhn A, Weber D, Grune T. Advanced glycation end products and oxidative stress in type 2 diabetes mellitus. Biomolecules. (2015) 5:194–222. doi: 10.3390/biom5010194
74. Abed DA, Lee S, Hu L. Discovery of disubstituted xylylene derivatives as small molecule direct inhibitors of Keap1-Nrf2 protein-protein interaction. Bioorganic Med Chem. (2020) 28:115343. doi: 10.1016/j.bmc.2020.115343
75. Li L, Leung PS. Pancreatic cancer, pancreatitis, and oxidative stress. In: Gracia-Sancho J, Salvadó J editors. Gastrointestinal Tissue. Cambridge, MA: Academic Press (2017). p. 173–86. doi: 10.1016/b978-0-12-805377-5.00012-6
76. Covas G, Marinho HS, Cyrne L, Antunes F. Activation of Nrf2 by H2O2: de novo synthesis versus nuclear translocation. In: Cadenas E, Packer L editors. Methods in Enzymology. Cambridge, MA: Academic Press (2013). p. 157–71. doi: 10.1016/B978-0-12-405881-1.00009-4
77. Pedersen BK. Anti-inflammatory effects of exercise: role in diabetes and cardiovascular disease. Eur J Clin Invest. (2017) 47:600–11. doi: 10.1111/eci.12781
78. Malik S, Suchal K, Khan SI, Bhatia J, Kishore K, Dinda AK, et al. Apigenin ameliorates streptozotocin-induced diabetic nephropathy in rats via MAPK-NF-κB-TNF-α and TGF-β1-MAPK-fibronectin pathways. Am J Physiol Renal Physiol. (2017) 313:F414–22. doi: 10.1152/ajprenal.00393.2016
79. Tasic I, Lovic D. Hypertension and cardiometabolic disease. Front Biosci (Schol Ed). (2018) 10:166–74. doi: 10.2741/s506
80. Katsimardou A, Imprialos K, Stavropoulos K, Sachinidis A, Doumas M, Athyros V. Hypertension in metabolic syndrome: novel insights. Curr Hypertens Rev. (2020) 16:12–8. doi: 10.2174/1573402115666190415161813
81. Haleagrahara N, Chakravarthi S, Bangra Kulur A, Yee TM. Plant flavone apigenin protects against cyclosporine-induced histological and biochemical changes in the kidney in rats. Biomed Prev Nutr. (2014) 4:589–93. doi: 10.1016/j.bionut.2014.07.006
82. Paredes MD, Romecín P, Atucha NM, O’Valle F, Castillo J, Ortiz MC, et al. Beneficial effects of different flavonoids on vascular and renal function in L-NAME hypertensive rats. Nutrients. (2018) 10:484. doi: 10.3390/nu10040484
83. Krick S, Platoshyn O, Sweeney M, Kim H, Yuan JX. Activation of K+ channels induces apoptosis in vascular smooth muscle cells. Am J Physiol Cell Physiol. (2001) 280:C970–9. doi: 10.1152/ajpcell.2001.280.4.C970
84. He Y, Fang X, Shi J, Li X, Xie M, Liu X. Apigenin attenuates pulmonary hypertension by inducing mitochondria-dependent apoptosis of PASMCs via inhibiting the hypoxia inducible factor 1α–KV1.5 channel pathway. Chem Biol Interact. (2020) 317:108942. doi: 10.1016/j.cbi.2020.108942
85. Simonini M, Casanova P, Citterio L, Messaggio E, Lanzani C, Manunta P. Endogenous ouabain and related genes in the translation from hypertension to renal diseases. Int J Mol Sci. (2018) 19:1948. doi: 10.3390/ijms19071948
86. Wei X, Gao P, Pu Y, Li Q, Yang T, Zhang H, et al. Activation of TRPV4 by dietary apigenin antagonizes renal fibrosis in deoxycorticosterone acetate (DOCA)-salt-induced hypertension. Clin Sci (Lond). (2017) 131:567–81. doi: 10.1042/CS20160780
87. Shen J, Tu L, Chen D, Tan T, Wang Y, Wang S. TRPV4 channels stimulate Ca2+-induced Ca2+ release in mouse neurons and trigger endoplasmic reticulum stress after intracerebral hemorrhage. Brain Res Bull. (2019) 146:143–52. doi: 10.1016/j.brainresbull.2018.11.024
88. Grafe I, Alexander S, Peterson JR, Snider TN, Levi B, Lee B, et al. TGF-β family signaling in mesenchymal differentiation. Cold Spring Harbor Perspect Biol. (2018) 10:a022202. doi: 10.1101/cshperspect.a022202
89. Thakur S, Viswanadhapalli S, Kopp JB, Shi Q, Barnes JL, Block K, et al. Activation of AMP-activated protein kinase prevents TGF-β1-induced epithelial-mesenchymal transition and myofibroblast activation. Am J Pathol. (2015) 185:2168–80. doi: 10.1016/j.ajpath.2015.04.014
90. Knapp M, Tu X, Wu R. Vascular endothelial dysfunction, a major mediator in diabetic cardiomyopathy. Acta Pharmacol Sin. (2019) 40:1–8. doi: 10.1038/s41401-018-0042-6
91. Kakizawa S. Apelin. In: Takei Y, Ando H, Tsutsui K editors. Handbook of Hormones. Cambridge, MA: Academic Press (2016). p. e31–3.
92. Yamagata K, Tagawa C, Matsufuji H, Chino M. Dietary apigenin regulates high glucose and hypoxic reoxygenation-induced reductions in apelin expression in human endothelial cells. J Nutr Biochem. (2012) 23:929–36. doi: 10.1016/j.jnutbio.2011.04.019
93. Attané C, Daviaud D, Dray C, Dusaulcy R, Masseboeuf M, Prévot D, et al. Apelin stimulates glucose uptake but not lipolysis in human adipose tissue ex vivo. J Mol Endocrinol. (2011) 46:21–8. doi: 10.1677/JME-10-0105
94. Zhao Y, Li Y, Li Z, Xu B, Chen P, Yang X. Superoxide anions modulate the performance of apelin in the paraventricular nucleus on sympathetic activity and blood pressure in spontaneously hypertensive rats. Peptides. (2019) 121:170051. doi: 10.1016/j.peptides.2018.12.005
95. Meng D, Qin Y, Lu N, Fang K, Hu Y, Tian Z, et al. Kupffer cells promote the differentiation of adult liver hematopoietic stem and progenitor cells into lymphocytes via ICAM-1 and LFA-1 interaction. Stem Cells Int. (2019) 2019:4848279. doi: 10.1155/2019/4848279
96. Ueki S, Nishikawa J, Fukuchi M, Konno Y, Takeda M, Moritoki Y, et al. ICAM-1 upregulation is not required for retinoic acid-induced human eosinophil survival. Immunol Lett. (2018) 196:68–73. doi: 10.1016/j.imlet.2018.01.013
97. Ren B, Qin W, Wu F, Wang S, Pan C, Wang L, et al. Apigenin and naringenin regulate glucose and lipid metabolism, and ameliorate vascular dysfunction in type 2 diabetic rats. Eur J Pharmacol. (2016) 773:13–23. doi: 10.1016/j.ejphar.2016.01.002
98. Yu W, Sun H, Zha W, Cui W, Xu L, Min Q, et al. Apigenin attenuates adriamycin-induced cardiomyocyte apoptosis via the PI3K/AKT/mTOR pathway. Evid Based Complement Alternat Med. (2017) 2017:2590676. doi: 10.1155/2017/2590676
99. Dirimanov S, Högger P. Screening of inhibitory effects of polyphenols on akt-phosphorylation in endothelial cells and determination of structure-activity features. Biomolecules. (2019) 9:219. doi: 10.3390/biom9060219
100. Zhu X, Liu Y, Huang H, Zhang Y, Huang S, Zhou W, et al. PKCβII-induced upregulation of PGP9.5 and VEGF in postoperative persistent pain in rats. J Pain Res. (2018) 11:2095–106. doi: 10.2147/JPR.S144852
101. Joo HK, Lee YR, Choi S, Park MS, Kang G, Kim CS, et al. Protein kinase C beta II upregulates intercellular adhesion molecule-1 via mitochondrial activation in cultured endothelial cells. Korean J Physiol Pharmacol. (2017) 21:377–84. doi: 10.4196/kjpp.2017.21.4.377
102. Qin W, Ren B, Wang S, Liang S, He B, Shi X, et al. Apigenin and naringenin ameliorate PKCβII-associated endothelial dysfunction via regulating ROS/caspase-3 and NO pathway in endothelial cells exposed to high glucose. Vascul Pharmacol. (2016) 85:39–49. doi: 10.1016/j.vph.2016.07.006
103. Lin X, Yang P, Reece EA, Yang P. Pregestational type 2 diabetes mellitus induces cardiac hypertrophy in the murine embryo through cardiac remodeling and fibrosis. Am J Obstet Gynecol. (2017) 217:216.e1–13. doi: 10.1016/j.ajog.2017.04.008
104. Bilu C, Einat H, Barak O, Zimmet P, Vishnevskia-Dai V, Govrin A, et al. Linking type 2 diabetes mellitus, cardiac hypertrophy and depression in a diurnal animal model. Sci Rep. (2019) 9:11865. doi: 10.1038/s41598-019-48326-7
105. Zhu ZY, Wang F, Jia CH, Xie ML. Apigenin-induced HIF-1α inhibitory effect improves abnormal glucolipid metabolism in Ang II/hypoxia-stimulated or HIF-1α-overexpressed H9c2 cells. Phytomedicine. (2019) 62:152713. doi: 10.1016/j.phymed.2018.10.010
106. Zhu ZY, Gao T, Huang Y, Xue J, Xie ML. Apigenin ameliorates hypertension-induced cardiac hypertrophy and down-regulates cardiac hypoxia inducible factor-lα in rats. Food Funct. (2016) 7:1992–8. doi: 10.1039/c5fo01464f
107. Huang Y, Lei L, Liu D, Jovin I, Russell R, Johnson RS, et al. Normal glucose uptake in the brain and heart requires an endothelial cell-specific HIF-1α-dependent function. Proc Natl Acad Sci U S A. (2012) 109:17478–83. doi: 10.1073/pnas.1209281109
108. Cerychova R, Pavlinkova G. HIF-1, metabolism, and diabetes in the embryonic and adult heart. Front Endocrinol. (2018) 9:460. doi: 10.3389/fendo.2018.00460
109. Krishnan J, Suter M, Windak R, Krebs T, Felley A, Montessuit C, et al. Activation of a HIF1α-PPARγ axis underlies the integration of glycolytic and lipid anabolic pathways in pathologic cardiac hypertrophy. Cell Metab. (2009) 9:512–24. doi: 10.1016/j.cmet.2009.05.005
110. Cole MA, Abd Jamil AH, Heather LC, Murray AJ, Sutton ER, Slingo M, et al. On the pivotal role of PPARα in adaptation of the heart to hypoxia and why fat in the diet increases hypoxic injury. FASEB J. (2016) 30:2684–97. doi: 10.1096/fj.201500094R
111. Lu J, Ni Z, Shen H, Tu Z, Liu H, Lu R, et al. The non-additive contribution of hydroxyl substituents to Akt kinase–apigenin affinity. Mol Simulation. (2014) 41:653–62. doi: 10.1080/08927022.2014.913099
112. Wang Y, Chen S, Yu O. Metabolic engineering of flavonoids in plants and microorganisms. Appl Microbiol Biotechnol. (2011) 91:949–56. doi: 10.1007/s00253-011-3449-2
113. Farzaei MH, Abbasabadi Z, Ardekani MR, Rahimi R, Farzaei F. Parsley: a review of ethnopharmacology, phytochemistry and biological activities. J Tradit Chin Med. (2013) 33:815–26. doi: 10.1016/s0254-6272(14)60018-2
114. Liu G, Zhuang L, Song D, Lu C, Xu X. Isolation, purification, and identification of the main phenolic compounds from leaves of celery (Apium graveolens L. var. dulce Mill./Pers.). J Sep Sci. (2017) 40:472–9. doi: 10.1002/jssc.201600995
115. Zhang Y, Jiao J, Liu C, Wu X, Zhang Y. Isolation and purification of four flavone C-glycosides from antioxidant of bamboo leaves by macroporous resin column chromatography and preparative high-performance liquid chromatography. Food Chem. (2008) 107:1326–36.
116. Li T, Chang R, Zhang H, Du M, Mao X. Water extract of potentilla discolor bunge improves hepatic glucose homeostasis by regulating gluconeogenesis and glycogen synthesis in high-fat diet and streptozotocin-induced type 2 diabetic mice. Front Nutr. (2020) 7:161. doi: 10.3389/fnut.2020.00161
117. Zhang R, Shi J, Wang T, Qiu X, Liu R, Li Y, et al. Apigetrin ameliorates streptozotocin-induced pancreatic β-cell damages via attenuating endoplasmic reticulum stress. In Vitro Cell Dev Biol Anim. (2020) 56:622–34. doi: 10.1007/s11626-020-00478-x
118. Peng Y, Sun Q, Xu W, He Y, Jin W, Yuan L, et al. Vitexin ameliorates high fat diet-induced obesity in male C57BL/6J mice via the AMPKα-mediated pathway. Food Funct. (2019) 10:1940–7. doi: 10.1039/c9fo00148d
119. Inamdar S, Joshi A, Malik S, Boppana R, Ghaskadbi S. Vitexin alleviates non-alcoholic fatty liver disease by activating AMPK in high fat diet fed mice. Biochem Biophys Res Commun. (2019) 519:106–12. doi: 10.1016/j.bbrc.2019.08.139
120. Wang F, Yin J, Ma Y, Jiang H, Li Y. Vitexin alleviates lipopolysaccharide-induced islet cell injury by inhibiting HMGB1 release. Mol Med Rep. (2017) 15:1079–86. doi: 10.3892/mmr.2017.6114
121. Liou CJ, Wu SJ, Chen LC, Yeh KW, Chen CY, Huang WC. Acacetin from traditionally used Saussurea involucrata Kar. et Kir. suppressed adipogenesis in 3T3-L1 adipocytes and attenuated lipid accumulation in obese mice. Front Pharmacol. (2017) 8:589. doi: 10.3389/fphar.2017.00589
122. Kwon E-B, Kang M-J, Ryu HW, Lee S, Lee J-W, Lee MK, et al. Acacetin enhances glucose uptake through insulin-independent GLUT4 translocation in L6 myotubes. Phytomedicine. (2020) 68:153178. doi: 10.1016/j.phymed.2020.153178
123. Wei Y, Yuan P, Zhang Q, Fu Y, Hou Y, Gao L, et al. Acacetin improves endothelial dysfunction and aortic fibrosis in insulin-resistant SHR rats by estrogen receptors. Mol Biol Rep. (2020) 47:6899–918. doi: 10.1007/s11033-020-05746-3
124. Krishna MS, Joy B, Sundaresan A. Effect on oxidative stress, glucose uptake level and lipid droplet content by Apigenin 7, 4’-dimethyl ether isolated from Piper longum L. J Food Sci Technol. (2015) 52:3561–70. doi: 10.1007/s13197-014-1387-6
125. Pan G, Zhao L, Xiao N, Yang K, Ma Y, Zhao X, et al. Total synthesis of 8-(6″-umbelliferyl)-apigenin and its analogs as anti-diabetic reagents. Eur J Med Chem. (2016) 122:674–83. doi: 10.1016/j.ejmech.2016.07.015
126. Yang M, Jiang ZH, Li CG, Zhu YJ, Li Z, Tang YZ, et al. Apigenin prevents metabolic syndrome in high-fructose diet-fed mice by Keap1-Nrf2 pathway. Biomed Pharmacother. (2018) 105:1283–90. doi: 10.1016/j.biopha.2018.06.108
127. Osada M, Imaoka S, Funae Y. Apigenin suppresses the expression of VEGF, an important factor for angiogenesis, in endothelial cells via degradation of HIF-1α protein. FEBS Lett. (2004) 575:59–63. doi: 10.1016/j.febslet.2004.08.036
128. Hollman PC, Katan MB. Health effects and bioavailability of dietary flavonols. Free Radic Res. (1999) 31:S75–80.
129. Ross JA, Kasum CM. Dietary flavonoids: bioavailability, metabolic effects, and safety. Ann Rev Nutr. (2002) 22:19–34. doi: 10.1146/annurev.nutr.22.111401.144957
130. Llevot A, Astruc D. Applications of vectorized gold nanoparticles to the diagnosis and therapy of cancer. Chem Soc Rev. (2012) 41:242–57. doi: 10.1039/c1cs15080d
131. Das S, Das J, Samadder A, Paul A, Khuda-Bukhsh AR. Strategic formulation of apigenin-loaded PLGA nanoparticles for intracellular trafficking, DNA targeting and improved therapeutic effects in skin melanoma in vitro. Toxicol Lett. (2013) 223:124–38. doi: 10.1016/j.toxlet.2013.09.012
132. Cheruvu HS, Yadav NK, Valicherla GR, Arya RK, Hussain Z, Sharma C, et al. LC-MS/MS method for the simultaneous quantification of luteolin, wedelolactone and apigenin in mice plasma using hansen solubility parameters for liquid-liquid extraction: application to pharmacokinetics of Eclipta alba chloroform fraction. J Chromatogr B Analyt Technol Biomed Life Sci. (2018) 108:76–86. doi: 10.1016/j.jchromb.2018.01.035
133. Milanlouei S, Menichetti G, Li Y, Loscalzo J, Willett WC, Barabási AL. A systematic comprehensive longitudinal evaluation of dietary factors associated with acute myocardial infarction and fatal coronary heart disease. Nat Commun. (2020) 11:6074. doi: 10.1038/s41467-020-19888-2
Keywords: apigenin, flavonoid, cardiometabolic disease, metabolic syndrome, signaling pathways
Citation: Xu Y, Li X and Wang H (2022) Protective Roles of Apigenin Against Cardiometabolic Diseases: A Systematic Review. Front. Nutr. 9:875826. doi: 10.3389/fnut.2022.875826
Received: 14 February 2022; Accepted: 14 March 2022;
Published: 15 April 2022.
Edited by:
Shi-Jun Yue, Shaanxi University of Chinese Medicine, ChinaCopyright © 2022 Xu, Li and Wang. This is an open-access article distributed under the terms of the Creative Commons Attribution License (CC BY). The use, distribution or reproduction in other forums is permitted, provided the original author(s) and the copyright owner(s) are credited and that the original publication in this journal is cited, in accordance with accepted academic practice. No use, distribution or reproduction is permitted which does not comply with these terms.
*Correspondence: Xue Li, eW9sYW5kYWRhbGVlQG91dGxvb2suY29t; Hui Wang, aHVpd2FuZ0BzaHNtdS5lZHUuY24=