- National Research Council Canada, Aquatic and Crop Resource Development Research Centre, Halifax, NS, Canada
The fisheries and aquaculture industries are some of the major economic sectors in the world. However, these industries generate significant amounts of wastes that need to be properly managed to avoid serious health and environmental issues. Recent advances in marine waste valorization indicate that fish waste biomass represents an abundant source of high-value biomolecules including enzymes, functional proteins, bioactive peptides, and omega-3 rich oils. Enzyme-assisted processes, for the recovery of these value-added biomolecules, have gained interest over chemical-based processes due to their cost-effectiveness as well as their green and eco-friendly aspects. Currently, the majority of commercially available proteases that are used to recover value-added compounds from fisheries and aquaculture wastes are mesophilic and/or thermophilic that require significant energy input and can lead to unfavorable reactions (i.e., oxidation). Cold-adapted proteases extracted from cold-water fish species, on the other hand, are active at low temperatures but unstable at higher temperatures which makes them interesting from both environmental and economic points of view by upcycling fish waste as well as by offering substantial energy savings. This review provides a general overview of cold-adapted proteolytic enzymes from cold-water fish species and highlights the opportunities they offer in the valorization of fisheries and aquaculture wastes.
Introduction
The fisheries and aquaculture industries represent some of the major economic sectors in the world. A recent report by the Food and Agricultural Organization (FAO) indicated that the total world fisheries and aquaculture production (fish, crustaceans, and mollusks) reached 177.8 million tons in 2019 and generated USD 406 billion in terms of total first sale value (1). However, 50–70% of this global fisheries and aquaculture production is regarded as waste and is discarded either at sea or in landfills (2–4).
Organic wastes generated from food, aquaculture and fisheries industries represent a serious global issue due to their economic, social and environmental impacts (5). In addition, these wastes can, to a certain degree, contribute to climate change through greenhouse gas (GHG) emissions when dumped in landfills. For instance, according to a report by Bogner et al. (6), methane emission from landfills and wastewater jointly accounted for nearly 90% of waste sector emissions, which translated to about 18% of annual global anthropogenic methane emissions (6). Waste valorization, as a mitigation approach, has a positive impact on climate change, which is mainly attributable to the reduction in GHG emissions due to recycling and waste minimization as well as energy recovery from the waste (7). Several studies indicated that fisheries and aquaculture wastes can be considered as potential sources of biomolecules including enzymes, functional proteins, bioactive peptides, omega-3 rich oils and polysaccharides such as chitin (8–12). Current industrial processes for the extraction of these biomolecules are unsustainable as they are heavily based on the use of harsh chemical treatments and/or costly enzymatic reactions which consume significant amounts of water and energy and generate large volumes of effluent that needs treatment before discharge (13, 14). The majority of commercially available enzymes that are used to recover value-added compounds from fish waste have optimal activities between 50 and 80°C. This temperature range poses a serious safety risk (i.e., growth of pathogenic micro-organisms) and unfavorable reactions (i.e., oxidation). By contrast, when used at lower temperatures, their enzyme activity is dramatically reduced. Cold-adapted enzymes, on the other hand, are active between 0 and 30°C but unstable at temperatures higher than 50°C (15).
Fish viscera represent an abundant by-product of fisheries and aquaculture industries and typically account for 5–10% of the fish body weight (16). Fish viscera comprise a number of digestive enzymes including acidic (i.e., pepsin) and alkaline proteases (i.e., trypsin, chymotrypsin, and elastase) that are widely used in bioprocessing (16). In addition, proteases present in the viscera of cold-water fish species have been reported to possess cold-adapted properties (17–19). Therefore, using fish-derived cold-adapted proteases to upcycle fisheries and aquaculture wastes can represent a sustainable approach for the conversion of wastes into value-added products which would utilize the wastes completely and eliminate their negative environmental impact. The aim of this review is to provide a general overview of cold-adapted proteolytic enzymes from cold-water fish species and to highlight the opportunities they offer in the valorization of fisheries and aquaculture wastes.
Enzyme Cold Adaptation
The mechanisms of cold adaptation are complex; however, one common characteristic of all cold-adapted enzymes is their high molecular flexibility compared with their mesophilic and thermophilic counterparts, which show low activity and are structurally rigid at low temperatures (20–22). The increased molecular flexibility, which is required to compensate for the low working temperature, has been proposed as the main structural feature of cold-adapted enzymes (23) and is currently the most widely accepted theory for cold adaptation (24). However, there is a debate whether the flexibility of cold-adapted enzymes is a global flexibility (i.e., flexibility throughout the enzyme structure) or a local flexibility (i.e., distinct regions in the enzyme structure) (22). Other features observed with cold-adapted enzymes include (i) a smaller number of hydrogen bonds, (ii) structures that are less densely packed, (iii) higher surface hydrophilicity, as well as (iv) an increased number of methionine residues (21).
Current evidences also indicate that the high specific activity of cold-adapted enzymes is most of the time associated with a low thermo-stability (23). In this regard and compared to mesophilic and thermophilic homologs, cold-adapted enzymes have a lower thermal stability (in order to prevent freezing) and exhibit a lower enthalpy and a more negative entropy of activation (25). Additionally, several cold-adapted proteases have been reported to be more susceptible to auto-digestion compared to their mesophilic analogs – a feature that has been associated with their increased catalytic efficiency and decreased thermal stability (21, 26).
Cold-Adapted Proteases From Cold-Water Fish Species
Proteases, the enzymes that catalyze the hydrolysis of peptide bonds, are the major group of industrial enzymes, accounting for more than 60% of the global enzyme market (27, 28). Proteases are divided into endopeptidases and exopeptidases depending on their cleavage points within the peptide chain. Based on the reactive groups at the active site, proteases can further be categorized into serine-, cysteine-, aspartic-, or metallo-peptidases. So far, serine peptidases are the most extensively investigated and characterized protease subgroup (29).
To date, little research has been conducted on fish-derived cold-adapted proteolytic enzymes. Perhaps, the sensitivity of cold-adapted enzymes to autolysis, thermal inactivation as well as molecular aggregation could explain the limited studies in this area (21). The available studies on cold-adapted fish derived proteolytic enzymes mainly focused on a small number of acidic (i.e., pepsin) and alkaline (i.e., trypsin, chymotrypsin, and elastase) proteases. These acidic and alkaline proteolytic enzymes, which represent about 5% of the total fish mass (28), are the major digestive proteolytic enzymes in fish viscera and are found in the stomach and pyloric ceca/small intestine, respectively.
Acidic Proteases
Pepsin, which belongs to the aspartic endopeptidase family (Table 1), is the major acidic protease in fisheries and aquaculture wastes. Pepsin has been extracted and characterized from a number of cold-water fish species (Table 1), including Arctic capelin (18), Greenland cod (30), Polar cod (19), Atlantic cod (18, 31, 32), Chum salmon (33), and rainbow trout (34, 35).
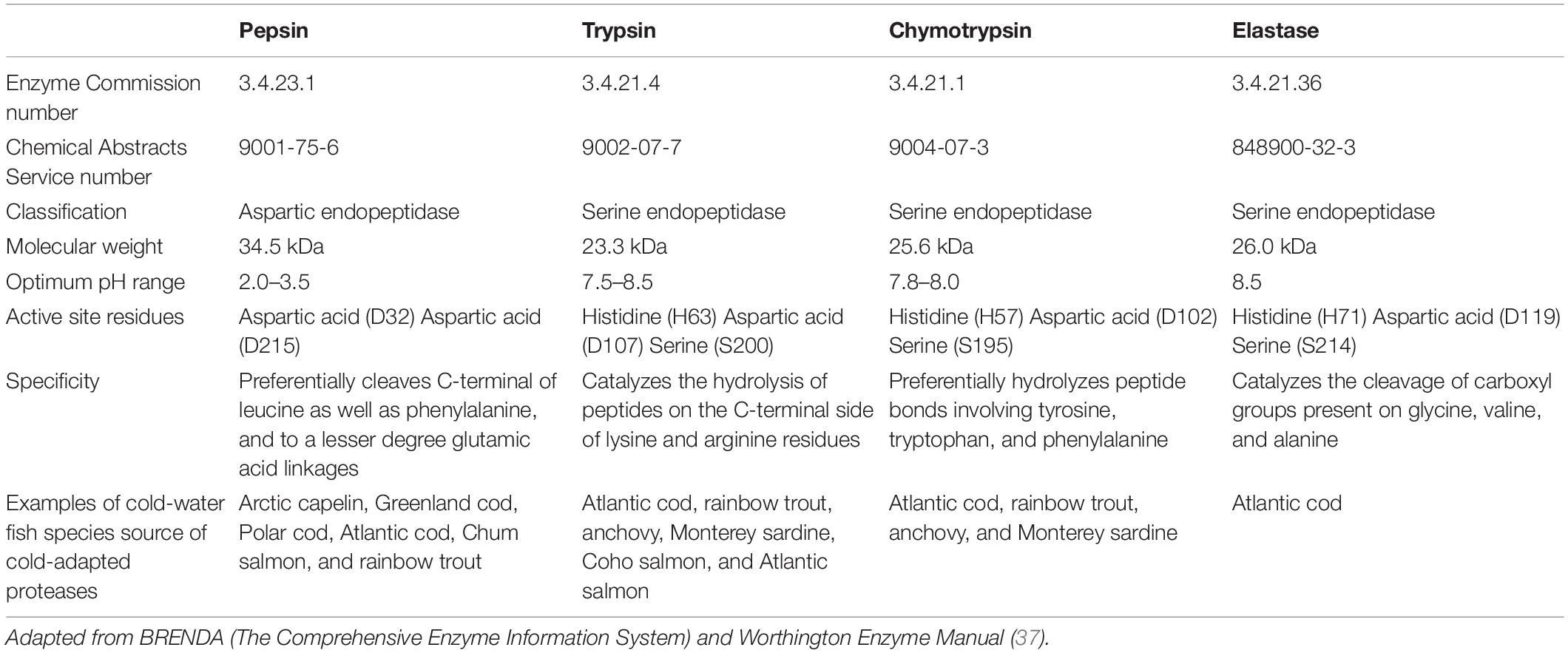
Table 1. General characteristics of major digestive proteases present in fisheries and aquaculture wastes.
Fish-derived pepsin has been utilized in various applications including protein hydrolysis, collagen extraction and as a rennet substitute in addition to being useful for some therapeutic purposes (36). The use of fish-derived cold-adapted pepsin to treat fisheries and aquaculture wastes could represent a low-cost and safe waste treatment approach since processing the organic biomass at low temperatures avoids possible side effects (i.e., oxidation) while the low pH conditions reduce the risk of spoilage as acidic pH inhibits most pathogens (14).
Alkaline Proteases
Trypsin, chymotrypsin, and elastase, which all belong to the serine-protease family, are the main alkaline proteases in fisheries and aquaculture wastes. The general characteristics of these three proteases are summarized in Table 1.
Cold-adapted serine proteases have been isolated and characterized from a number of cold-water fish species (Table 1). For example, cold-adapted trypsin, chymotrypsin and elastase were extracted from Atlantic cod (38–44). Trypsin and chymotrypsin were isolated from rainbow trout (45, 46) as well as anchovy (47–49). Other studies focused on the recovery of trypsin and chymotrypsin from Monterey sardine (50–54). When it comes to salmonids, trypsin with cold-adapted properties was extracted from Coho salmon (55) and Atlantic salmon (56–58).
These three serine proteases (trypsin, chymotrypsin, and elastase), which are found in the pancreatic juices and are highly active in the pyloric ceca and small intestine of fish, have been used in several industrial applications due to their high specificity as well as stability and activity under severe unfavorable conditions, such as alkaline pH and in the presence of surfactants or oxidizing agents (59). Some examples of industrial applications of cold-adapted alkaline proteases comprise the production of a seafood flavoring hydrolyzate from crustaceans and as a key ingredient in natural skin care products (21) in addition to being applied in biomedical research (60).
Typical Enzyme Recovery Processes
Enzyme isolation and purification are complex processes, and generally rely on various techniques that are sequentially applied to achieve the required purity levels (61). Among the methods that have been developed for this purpose, precipitation, aqueous two-phase partitioning and membrane fractionation have been the most commonly used.
Precipitation
Proteins, including enzymes, can be precipitated through the disruption of their conformations, which can be achieved through pH shift, ionic strength and/or the addition of water-miscible solvents. Shifting the pH of the medium can either cause protonation of certain groups in the protein (by decreasing the pH), dissociation of protons (by increasing the pH), or charge neutralization (i.e., net electrical charge of the protein in solution is canceled through an equal number of opposite charges) (62). The latter state refers to the isoelectric point in which the protein has the lowest solubility. Precipitation at isoelectric point is widely utilized to recover proteins (63–68), however, it is not usually applied for enzyme isolation due to irreversible denaturation which is the greatest disadvantage of isoelectric point precipitation (69).
The disruption of protein (or enzyme) macro-structures can also be achieved through the addition of significantly high amounts of salt (such as sodium chloride, ammonium sulfate, and sodium sulfate) or through the addition of organic solvents, such as methanol, ethanol, butanol and acetone (62). The large amounts of salt cause protein precipitation through the “salting out” effect. This approach can be used to recover enzymes. However, the salts need to be removed through desalting processes such as dialysis, gel filtration or ion exchange chromatography.
Precipitation through the addition of water-miscible solvents is generally attributed to the competition of protein and the solvent’s polar groups with water (62). If solvent-based precipitation techniques are chosen for enzyme recovery, care has to be taken to extract the enzymes at low temperatures as organic solvents are well known to denature enzymes even at cold temperatures (70).
Partition Through Aqueous Two-Phase Systems
Aqueous two-phase partitioning is a one-step, liquid–liquid purification approach based on affinity partitioning and is generally achieved by mixing polymer/polymer (typically polyethylene glycol or dextran) or polymer/salt (such as phosphate, sulfate, or citrate) with water (71). In general, polymer/salt based aqueous two-phase systems (ATPS) are preferred due to their lower costs and lower viscosity compared to polymer/polymer based ATPS (72).
In downstream processing, ATPS represents the initial isolation and purification step, which is achieved by the partial elimination of impurities and the reduction of the working volume (73). The simplicity and selectivity of the ATPS process combined with the low cost and the non-toxic nature of phase forming compounds make the large-scale implementation of ATPS possible (72).
Aqueous two-phase systems has been reported to efficiently recover enzymes from fish wastes such as farmed giant catfish viscera (74, 75) and hybrid catfish viscera (76). Despite the promising potential of ATPS to purify enzymes instead of the conventional salt precipitation techniques, some challenges associated with ATPS remain. Firstly, the enzymes still need to be separated from the phase forming reagents after the partition is completed. Secondly, ATPS in industrial settings uses large quantities of chemicals and therefore, sustainable approaches to reuse and/or recycle these chemicals are still needed. Lastly, the use of inorganic salts creates both disposal and environmental issues therefore, new ATPS processes based on biodegradable and/or volatile reagents as alternate to inorganic salts need to developed and optimized (72).
Membrane Fractionation
Membrane ultrafiltration is a well-established separation process which allows for both purification and concentration (77, 78). Ultrafiltration has routinely been used for the industrial separation and purification of enzymes produced through fermentation (79). The main advantages of ultrafiltration compared to conventional enzyme separation systems is the improved activity recovery (79) as well as the minimum denaturation and/or degradation of bio-products (since ultrafiltration operates under relatively gentle conditions in terms of temperature and pressure) (80). The major disadvantage of ultrafiltration, however, is the membrane clogging due to the precipitates formed by the final product (81).
Despite the advantages of ultrafiltration, only a few research studies have investigated this membrane-based process for the isolation and separation of fish proteolytic enzymes (82, 83). The majority of studies focused on applying ultrafiltration for the fractionation of fish protein hydrolyzates into bioactive peptide fractions.
Potential Industrial Applications of Fish-Derived Cold-Adapted Proteases
Several potential biotechnological and industrial applications have been suggested for cold-adapted enzymes which have been extensively reviewed elsewhere (22, 23, 26). Among the potential applications, two major industries have been suggested for cold-adapted proteases; laundry detergents and food industries. For instance, in the laundry detergent industry, cold-adapted alkaline proteases, which are active at low temperatures and high pHs, can be used as detergent additives instead of chemical agents or mesophilic proteases that are easily denatured at those harsh conditions (84). In the food industry and due to their high catalytic activity at low temperatures and their instability at elevated temperatures (85), cold-adapted proteases can be used:
(i) In the production of fish protein hydrolyzates and flavoring compounds. In this respect, cold-adapted proteases have been reported to produce fish protein hydrolyzates with acceptable odor in addition to reducing the bitterness and favoring the release of small-size peptides with flavor-forming characteristics (86),
(ii) In the extraction of target ingredients (such as pigments and fish oils). For example, cold-adapted proteases have been shown to enhance the recovery of pigments (i.e., carotene and astaxanthin) from shellfish wastes (87, 88),
(iii) As meat tenderizing agents (89), and
(iV) As rennet substitutes (89).
Other potential industries where cold-adapted fish-derived proteases can find applications include the leather and textile industries [in which cold-adapted proteases can replace harmful chemical reagents (90)] as well as the environmental remediation industry, especially for the digestion of protein-rich solid wastes and liquid effluents in cold weather conditions (91).
Despite the numerous possible applications of cold-adapted enzymes, only a small number have been tested in a real industrial setting. For instance, β-galactosidase, methylesterase, polygalacturonase, and glycogen branching enzyme have been assessed, respectively, in the hydrolysis of lactose present in milk, in fruit firming, in juice processing, and in bread production (85). So far, no large-scale trials have been performed for any suggested industrial applications related to cold-adapted proteases.
Opportunities of Upcycling Fisheries and Aquaculture Wastes Using Fish-Derived Cold-Adapted Proteases
The high specific activity at low temperatures combined with the decreased thermal stability of cold-adapted fish-derived proteases are highly sought after characteristics in various industrial applications (89, 92). It is well known that processing at low temperatures reduces and/or eliminates undesirable chemical reactions, such as oxidation. When cold-adapted acidic proteases are used, the low pH value of the medium significantly lowers the risk of spoilage since acidic conditions inhibit the proliferation of most pathogens (14). Considering these advantageous characteristics, a closed-loop, cost-effective and environmentally sustainable process for upcycling fisheries and aquaculture wastes using cold-water fish-derived cold-adapted proteases can be proposed (as depicted in Figure 1). In this process, cold-adapted endogenous proteolytic enzymes recovered from viscera of cold-water fish species can be utilized as natural biocatalysts to obtain fish hydrolyzates from fish wastes through enzymatic hydrolysis at low temperatures. The produced fish hydrolyzates can further be separated into a number of value-added products such bioactive peptides and omega-3 rich lipids (Figure 1). The bone residues obtained at the end of the hydrolysis (Figure 1) can be converted into either gelatin, which is a multifunctional proteins ingredient in the food industry (93–95) or hydroxyapatite, which is a bioactive and biocompatible product that has a wide range of medical, dental and agricultural applications (96).
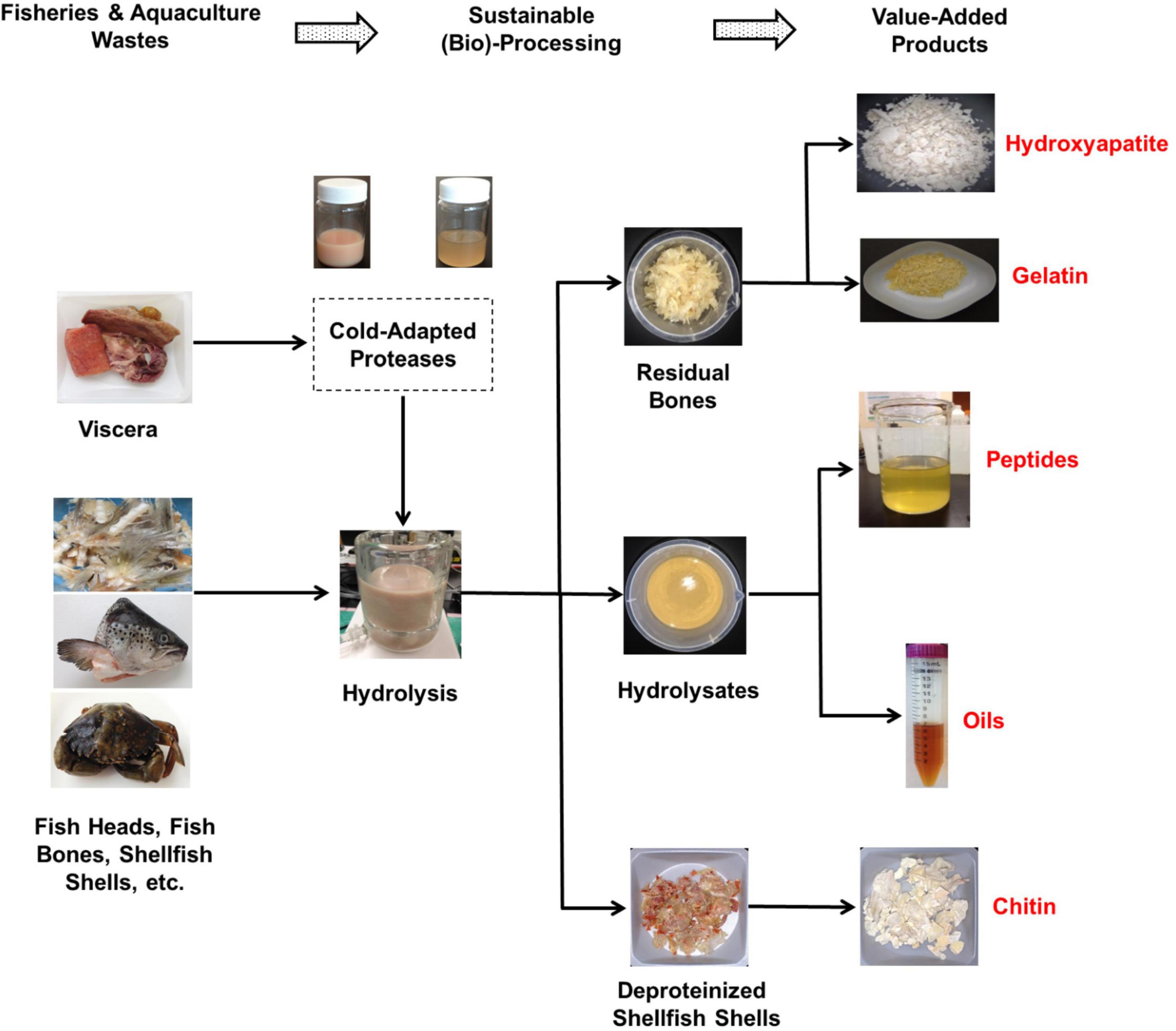
Figure 1. A closed-loop, cost-effective, and environmentally sustainable process for upcycling fisheries and aquaculture wastes using fish- and/or shellfish-derived cold-adapted proteases.
Shrimps, crabs, and lobsters are popular seafood around the world (12). The processing of these shellfish generates significant amounts of wastes, in the order of 40–50% of the total mass (97). The proposed process can also be applied in the valorization of crustacean wastes (86, 98). In this regard, shellfish wastes can be hydrolyzed using cold-adapted proteases from cold-water fish species (and/or crustaceans). The enzymatic hydrolysis process for shellfish wastes produces protein hydrolyzates, oils and deproteinized shells (Figure 1). The shells can further be processed into chitin (and/or chitosan) which is a biocompatible, non-toxic and biodegradable polymer with a range of usable applications (11).
Conclusion and Future Perspective
Research and development on seafood waste valorization has been increasing in the last few years. However, the industrial and commercial implementations of the developed seafood waste valorization approaches are limited in comparison to the large number of research studies that have been carried out and the patents that have been awarded. Currently, rendering seafood waste into fishmeal is still the preferred valorization route, with an annual production of about 5 million tons (99, 100). The present review shows that the recovery of cold-adapted proteases from cold-water fish species can open new opportunities for the development of sustainable, efficient, and cost-effective processes for the valorization of fisheries and aquaculture wastes into more valuable biomolecules (i.e., proteins, peptides, oils, polysaccharides, and hydroxyapatite) compared to fishmeal. However, the availability and variability of fish wastes, remain the most challenging issues for the commercial scale success of this process.
The future perspective of this research topic should center on (i) assessing whether the efficiency of fish-derived cold-adapted proteases in recovering value added products exceeds that of commercial proteases, which could in turn justify and increase the chances for the large-scale implementation of the process, (ii) the isolation and purification of the most promising cold-adapted fish-derived protease using eco-friendly, economic and yet effective extraction processes (such as ATPS) and (iii) the production of the most promising cold-adapted fish-derived protease using genetic engineering and recombinant expression tools.
Author Contributions
The author confirms being the sole contributor of this work and has approved it for publication.
Funding
This work was internally funded by the National Research Council Canada (NRC) Oceans Program.
Conflict of Interest
The author declares that the research was conducted in the absence of any commercial or financial relationships that could be construed as a potential conflict of interest.
Publisher’s Note
All claims expressed in this article are solely those of the authors and do not necessarily represent those of their affiliated organizations, or those of the publisher, the editors and the reviewers. Any product that may be evaluated in this article, or claim that may be made by its manufacturer, is not guaranteed or endorsed by the publisher.
Acknowledgments
Financial support by the National Research Council Canada (NRC) Oceans Program was gratefully acknowledged. The review by Sean Tibbetts was highly appreciated. This is ACRD publication no. 58305.
References
1. FAO. FAO Yearbook. Fishery and Aquaculture Statistics 2019/FAO annuaire. Statistiques des pêches et de l’aquaculture 2019/FAO Anuario. Estadísticas de Pesca y Acuicultura 2019. Rome: FAO (2021). doi: 10.4060/cb7874
2. Olsen RL, Toppe J, Karunasagar I. Challenges and realistic opportunities in the use of by-products from processing of fish and shellfish. Trends Food Sci Technol. (2014) 36:144–51. doi: 10.1016/j.tifs.2014.01.007
3. Turid R, Ivar S, Rasa S, Rustad T, Storrø I, Slizyte R. Possibilities for the utilisation of marine by-products. Int J Food Sci Technol. (2011) 46:2001–14.
4. Rustad T, Storrø I, Slizyte R. Possibilities for the utilisation of marine by-products. Int J Food Sci Technol. (2011) 46:2001–14. doi: 10.1111/j.1365-2621.2011.02736.x
5. Abeliotis K, Lasaridi K, Costarelli V, Chroni C. The implications of food waste generation on climate change: the case of greece. Sustain Product Consump. (2015) 3:8–14. doi: 10.1016/j.spc.2015.06.006
6. Bogner J, Pipatti R, Hashimoto S, Diaz C, Mareckova K, Diaz L, et al. Mitigation of global greenhouse gas emissions from waste: conclusions and strategies from the Intergovernmental Panel on Climate Change (IPCC) Fourth Assessment Report. Working Group III (Mitigation). Waste Manag Res. (2008) 26:11–32. doi: 10.1177/0734242X07088433
7. Ackerman F. Waste management and climate change. Local Environ. (2000) 5:223–9. doi: 10.1080/13549830050009373
8. Saleh NE, Wassef EA, Abdel-Mohsen HH. Sustainable fish and seafood production and processing. Sustainable Fish Production and Processing. Cambridge, MA: Academic Press (2022). p. 259–91. doi: 10.1016/b978-0-12-824296-4.00002-5
9. Khiari Z, Rico D, Martin-Diana AB, Barry-Ryan C. Structure elucidation of ACE-inhibitory and antithrombotic peptides isolated from mackerel skin gelatine hydrolysates. J Sci Food Agricult. (2014) 94:1663–71. doi: 10.1002/jsfa.6476
10. Olivieri A, Rico D, Khiari Z, Henehan G, O’Sullivan J, Tipton K. From caffeine to fish waste: amine compounds present in food and drugs and their interactions with primary amine oxidase. J Neur Transmis. (2011) 118:1079–89. doi: 10.1007/s00702-011-0611-z
11. Pati S, Jena P, Shahimi S, Nelson BR, Acharya D, Dash BP, et al. Characterization dataset for pre- and post-irradiated shrimp waste chitosan. Data Brief. (2020) 32:106081. doi: 10.1016/j.dib.2020.106081
12. Ghosh S, Sarkar T, Pati S, Kari ZA, Edinur HA, Chakraborty R. Novel bioactive compounds from marine sources as a tool for functional food development. Front Mar Sci. (2022) 9:832957. doi: 10.3389/fmars.2022.832957
13. Vázquez JA, Rodríguez-Amado I, Montemayor MI, Fraguas J, del González MP, Murado MA. Chondroitin sulfate, hyaluronic acid and chitin/chitosan production using marine waste sources: characteristics, applications and eco-friendly processes: a review. Mar Drugs. (2013) 11:747–74. doi: 10.3390/md11030747
14. Khiari Z, Mason B. Comparative dynamics of fish by-catch hydrolysis through chemical and microbial methods. LWT. (2018) 97:135–43. doi: 10.1016/j.lwt.2018.06.032
15. Wang G, Gao Y, Hu B, Lu X, Liu X, Jiao B. A novel cold-adapted β-galactosidase isolated from Halomonas sp. S62: gene cloning, purification and enzymatic characterization. World J Microbiol Biotechnol. (2013) 29:1473–80. doi: 10.1007/s11274-013-1311-7
16. Ketnawa S, Benjakul S, Martínez-Alvarez O, Rawdkuen S. Three-phase partitioning and proteins hydrolysis patterns of alkaline proteases derived from fish viscera. Separat Purificat Technol. (2014) 132:174–81. doi: 10.1016/j.seppur.2014.05.006
17. de Vecchi S, Coppes Z. Marine fish digestive proteases - relevance to food industry and the south-west atlantic region - a review. J Food Biochem. (1996) 20:193–214. doi: 10.1111/j.1745-4514.1996.tb00551.x
18. Gildberg A. Purification and characterization of pepsins from the arctic fish capelin (Mallotus villosus). Comparat Biochem Physiol Part A Physiol. (1983) 75:337–42. doi: 10.1016/0300-9629(83)90090-7
19. Arunchalam K, Haard NF. Isolation and characterization of pepsin from polar cod (Boreogadus saida). Comparat Biochem Physiol Part B Biochem. (1985) 80:467–73. doi: 10.1016/0305-0491(85)90274-3
20. Závodszky P, Kardos J, Svingor Á, Petsko GA. Adjustment of conformational flexibility is a key event in the thermal adaptation of proteins. Proc Natl Acad Sci USA. (1998) 95:7406–11. doi: 10.1073/pnas.95.13.7406
21. Gudmundsdóttir Á, Pálsdóttir HM. Atlantic cod trypsins: from basic research to practical applications. Mar Biotechnol. (2005) 7:77–88. doi: 10.1007/s10126-004-0061-9
22. Siddiqui KS, Cavicchioli R. Cold-adapted enzymes. Ann Rev Biochem. (2006) 75:403–33. doi: 10.1146/annurev.biochem.75.103004.142723
23. Gerday C, Aittaleb M, Bentahir M, Chessa JP, Claverie P, Collins T, et al. Cold-adapted enzymes: from fundamentals to biotechnology. Trends Biotechnol. (2000) 18:103–7. doi: 10.1016/S0167-7799(99)01413-4
24. Smalås AO, Leiros H-KS, Os V, Willassen NP. Cold adapted enzymes. Biotechnol Ann Rev. (2000) 6:1–57. doi: 10.1016/S1387-2656(00)06018-X
25. Bjelic S, Brandsdal BO, Åqvist J. Cold adaptation of enzyme reaction rates. Biochemistry. (2008) 47:10049–57. doi: 10.1021/bi801177k
26. Feller G. Psychrophilic enzymes: from folding to function and biotechnology. Scientifica. (2013) 2013:1–28. doi: 10.1155/2013/512840
27. Ben Rebah F, Miled N. Fish processing wastes for microbial enzyme production: a review. 3 Biotech. (2013) 3:255–65. doi: 10.1007/s13205-012-0099-8
28. Espósito TS, Amaral IPG, Buarque DS, Oliveira GB, Carvalho LB, Bezerra RS. Fish processing waste as a source of alkaline proteases for laundry detergent. Food Chem. (2009) 112:125–30. doi: 10.1016/j.foodchem.2008.05.049
29. Grzonka Z, Jankowska E, Kasprzykowski F, Kasprzykowska R, Łankiewicz L, Wiczk W, et al. Structural studies of cysteine proteases and their inhibitors. Acta Biochim Polon. (2001) 48:1–20. doi: 10.18388/abp.2001_5108
30. Squires EJ, Haard NF, Feltham LAW. Gastric proteases of the Greenland cod Gadus ogac. I. Isolation and kinetic properties. Biochem Cell Biol. (1986) 64:205–14. doi: 10.1139/o86-030
31. Gildberg A, Olsen RL, Bjarnason JB Characteristics and Composition of Pepsins From Atlantic Cod. Structure and Function of the Aspartic Proteinases. Boston, MA: Springer (1991). p. 107–10. doi: 10.1007/978-1-4684-6012-4_12
32. Gildberg A, Olsen RL, Bjarnason JB. Catalytic properties and chemical composition of pepsins from Atlantic cod (Gadus morhua). Comparat Biochem Physiol Part B Biochem. (1990) 96:323–30. doi: 10.1016/0305-0491(90)90382-4
33. Sánchez-Chiang L, Cisternas E, Ponce O. Partial purification of pepsins from adult and juvenile salmon fish Oncorhynchus keta. Effect of NaCl on proteolytic activities. Comparat Biochem Physiol Part B Biochem. (1987) 87:793–7. doi: 10.1016/0305-0491(87)90390-7
34. Twining SS, Alexander PA, Huibregtse K, Glick DM. A pepsinogen from rainbow trout. Comparat Biochem Physiol Part B Biochem. (1983) 75:109–12. doi: 10.1016/0305-0491(83)90046-9
35. Wald M, Rehbein H, Beermann C, Bußmann B, Schwarz K. Purification and characterization of pepsinogen and pepsin from the stomach of rainbow trout (Oncorhynchus mykiss). Eur Food Res Technol. (2016) 242:1925–35. doi: 10.1007/s00217-016-2692-2
36. Zhao L, Budge SM, Ghaly AE, Brooks MS. Extraction, purification and characterization of fish pepsin: a critical review. J Food Proces Technol. (2011) 2:1–14. doi: 10.4172/2157-7110.1000126
37. Worthington K, Worthington V. Worthington Enzyme Manual. Lakewood, NJ: Worthington Biochemical Corporation (2011).
38. Stefansson B, Sandholt GB, Gudmundsdottir Á Elucidation of different cold-adapted Atlantic cod (Gadus morhua) trypsin X isoenzymes. Biochim Biophys Acta Prot Proteom. (2017) 1865:11–9. doi: 10.1016/j.bbapap.2016.10.005
39. Asgeirsson B, Mäntylä E, Bjarnason JB. Isolation, characterization and utilization of psychrophilic proteinases from Atlantic cod. Bioorganic Chemistry in Healthcare and Technology. NATO ASI Series (Series A: Life Sciences). Boston, MA: Springer (1991). p. 299–302. doi: 10.1007/978-1-4684-1354-0_36
40. Leth-Larsen R, Ásgeirsson B, Thórólfsson M, Nørregaard-Madsen M, Højrup P. Structure of chymotrypsin variant B from Atlantic cod, Gadus morhua. Biochim Biophys Acta Prot Struct Mol Enzymol. (1996) 1297:49–56. doi: 10.1016/0167-4838(96)00088-X
41. Ásgeirsson B, Leth-Larsen R, Thórólfsson M, Nedertoft MM, Højrup P. The third, serine proteinase with chymotrypsin specificity isolated from Atlantic cod (Gadus morhua) is a type-II elastase. Eur J Biochem. (1998) 255:638–46. doi: 10.1046/j.1432-1327.1998.2550638.x
42. Ásgeirsson B, Bjarnason JB. Properties of elastase from Atlantic cod a cold-adapted proteinase. Biochim Biophys Acta (BBA) Prot Struct Mol. (1993) 1164:91–100. doi: 10.1016/0167-4838(93)90116-9
43. Ásgeirsson B, Bjarnason JB. Structural and kinetic properties of chymotrypsin from Atlantic cod (Gadus morhua). Comparison with bovine chymotrypsin. Comparat Biochem Physiol Part B Biochem. (1991) 99:327–35. doi: 10.1016/0305-0491(91)90050-N
44. Stefansson B, Helgadóttir L, Olafsdottir S, Gudmundsdottir Á, Bjarnason JB. Characterization of cold-adapted Atlantic cod (Gadus morhua) trypsin I – Kinetic parameters, autolysis and thermal stability. Comparat Biochem Physiol B Biochem Mol Biol. (2010) 155:186–94. doi: 10.1016/j.cbpb.2009.11.004
45. Kristjánsson MM. Purification and characterization of trypsin from the pyloric caeca of rainbow trout (Oncorhynchus mykiss). J Agricult Food Chem. (1991) 39:1738–42. doi: 10.1021/jf00010a009
46. Kristjánsson MM, Nielsen HH Purification and characterization of two chymotrypsin-like proteases from the pyloric caeca of rainbow trout (Oncorhynchus mykiss). Comparat Biochem Physiol Part B Biochem. (1992) 101:247–53. doi: 10.1016/0305-0491(92)90187-V
47. Heu MS, Kim HR, Pyeun JH Comparison of trypsin and chymotrypsin from the viscera of anchovy, Engraulis japonica. Comp Biochem Physiol B. (1995) 112:557–67. doi: 10.1016/0305-0491(95)00111-5
48. Choi YJ, Heu MS, Kim HR, Pyeun JH. Properties of proteases responsible for degradation of muscle proteins during anchovy sauce fermentation. Developments in Food Science. Elsevier (2004). p. 425–39. doi: 10.1016/S0167-4501(04)80042-6
49. Martínez A, Olsen RL, Serra JL. Purification and characterization of two trypsin-like enzymes from the digestive tract of anchovy Engraulis encrasicholus. Comparat Biochem Physiol Part B Biochem. (1988) 91:677–84. doi: 10.1016/0305-0491(88)90191-5
50. Castillo-Yañez FJ, Pacheco-Aguilar R, Lugo-Sanchez ME, Garcia-Sanchez G, Quintero-Reyes IE. Biochemical characterization of an isoform of chymotrypsin from the viscera of Monterey sardine (Sardinops sagax caerulea), and comparison with bovine chymotrypsin. Food Chem. (2009) 112:634–9. doi: 10.1016/j.foodchem.2008.06.023
51. Castillo-Yáñez FJ, Pacheco-Aguilar R, García-Carreño FL, de los Ángeles Navarrete-Del Toro M, López MF. Purification and biochemical characterization of chymotrypsin from the viscera of Monterey sardine (Sardinops sagax caeruleus). Food Chem. (2006) 99:252–9. doi: 10.1016/j.foodchem.2005.06.052
52. Castillo-Yáñez FJ, Pacheco-Aguilar R, García-Carreño FL, Navarrete-Del Toro MDLÁ. Isolation and characterization of trypsin from pyloric caeca of Monterey sardine Sardinops sagax caerulea. Comparat Biochem Physiol B Biochem Mol Biol. (2005) 140:91–8. doi: 10.1016/j.cbpc.2004.09.031
53. Arvizu-Flores AA, Quintero-Reyes IE, Felix-Lopez M, Islas-Osuna MA, Yepiz-Plascencia G, Pacheco-Aguilar R, et al. Thermodynamic activation and structural analysis of trypsin I from Monterey sardine (Sardinops sagax caerulea). Food Chem. (2012) 133:898–904. doi: 10.1016/j.foodchem.2012.01.111
54. Carretas-Valdez MI, Moreno-Cordova EN, Ibarra-Hernandez BG, Cinco-Moroyoqui FJ, Castillo-Yañez FJ, Casas-Flores S, et al. Characterization of the trypsin-III from Monterey sardine (Sardinops caeruleus): insights on the cold-adaptation from the A236N mutant. Int J Biol Macromol. (2020) 164:898–904. doi: 10.1016/j.ijbiomac.2020.08.136
55. Haard NF, Dimes LE, Arndt RE, Dong FM. Estimation of protein digestibility-IV. Digestive proteinases from the pyloric caeca of Coho salmon (Oncorhynchus kisutch) fed diets containing soybean meal. Comparat Biochem Physiol B Biochem Mol Biol. (1996) 115:533–40. doi: 10.1016/S0305-0491(96)00189-7
56. Schrøder HK, Willassen NP, Smalås AO. Structure of a non-psychrophilic trypsin from a cold-adapted fish species. Acta Crystallogr Sec D Biol Crystallogr. (1998) 54:780–98. doi: 10.1107/S0907444997018611
57. Male R, Lorens JB, Smalås AO, Torrissen KR. Molecular cloning and characterization of anionic and cationic variants of trypsin from atlantic salmon. Eur J Biochem. (1995) 232:677–85. doi: 10.1111/j.1432-1033.1995.677zz.x
58. Torrissen KR, Male R, Nævdal G. Trypsin isozymes in Atlantic salmon, Salmo salar L.: studies of heredity, egg quality and effect on growth of three different populations. Aquacult Res. (1993) 24:407–15. doi: 10.1111/j.1365-2109.1993.tb00564.x
59. Ktari N, Ben Khaled H, Nasri R, Jellouli K, Ghorbel S, Nasri M. Trypsin from zebra blenny (Salaria basilisca) viscera: purification, characterisation and potential application as a detergent additive. Food Chem. (2012) 130:467–74. doi: 10.1016/j.foodchem.2011.07.015
60. Gudmundsdóttir Á, Hilmarsson H, Stefansson B Potential use of Atlantic cod trypsin in biomedicine. BioMed Res Int. (2013) 2013:749078. doi: 10.1155/2013/749078
61. Ramos OL, Malcata FX 3.48 – Food-Grade Enzymes. Comprehensive Biotechnology. Amsterdam: Elsevier (2017). p. 587–03. doi: 10.1016/B978-0-12-809633-8.09173-1
62. Englard S, Seifter S. Precipitation techniques. Methods in Enzymology. Academic Press (1990). p. 285–300. doi: 10.1016/0076-6879(90)82024-V
63. Khiari Z, Kelloway S, Mason B. Turning invasive green crab (Carcinus maenas) into opportunity: recovery of chitin and protein isolate through isoelectric solubilization/precipitation. Waste Biomass Valoriz. (2020) 11:133–42. doi: 10.1007/s12649-018-0398-3
64. Khiari Z, Ndagijimana M, Betti M Low molecular weight bioactive peptides derived from the enzymatic hydrolysis of collagen after isoelectric solubilization/precipitation process of turkey by-products. Poult. Sci. (2014) 93:2347–62. doi: 10.3382/ps.2014-03953
65. Khiari Z, Gonzalez-Gonzalez CR. Advances in food protein biotechnology. Progress in Food Biotechnology. (2018). p. 1–49. doi: 10.2174/9781681087412118040003
66. Khiari Z, Pietrasik Z, Gaudette NJ, Betti M. Poultry protein isolate prepared using an acid solubilization/precipitation extraction influences the microstructure, the functionality and the consumer acceptability of a processed meat product. Food Struct. (2014) 2:49–60. doi: 10.1016/j.foostr.2014.08.002
67. Du L, Khiari Z, Pietrasik Z, Betti M. Physicochemical and functional properties of gelatins extracted from turkey and chicken heads. Poultry Sci. (2013) 92:2463–74. doi: 10.3382/ps.2013-03161
68. Khiari Z, Omana DA, Pietrasik Z, Betti M Evaluation of poultry protein isolate as a food ingredient: physicochemical properties and sensory characteristics of marinated chicken breasts. J Food Sci. (2013) 78:S1069–75. doi: 10.1111/1750-3841.12167
69. Novák P, Havlíček V 4 – Protein extraction and precipitation. Proteomic Profiling and Analytical Chemistry. Amsterdam: Elsevier (2016). p. 51–62. doi: 10.1016/B978-0-444-63688-1.00004-5
70. Scopes RK Separation by precipitation. In: Protein Purification: Principles and Practice. New York, NY: Springer-Verlag (1987). p. 41–71. doi: 10.1007/978-1-4757-1957-4
71. Asenjo JA, Andrews BA Aqueous two-phase systems for protein separation: a perspective. J Chromatogr A. (2011) 1218:8826–35. doi: 10.1016/j.chroma.2011.06.051
72. Raja S, Murty VR, Thivaharan V, Rajasekar V, Ramesh V. Aqueous two phase systems for the recovery of biomolecules – a review. Sci Technol. (2011) 1:7–16. doi: 10.5923/j.scit.20110101.02
73. Leong YK, Koroh FE, Show PL, Lan JCW, Loh HS. Optimisation of extractive bioconversion for green polymer via aqueous two-phase system. Chem Eng Transact. (2015) 45:1495–500. doi: 10.3303/CET1545250
74. Ketnawa S, Benjakul S, Ling TC, Martínez-Alvarez O, Rawdkuen S. Enhanced recovery of alkaline protease from fish viscera by phase partitioning and its application. Chem Cent J. (2013) 7:1–9. doi: 10.1186/1752-153X-7-79
75. Vannabun A, Ketnawa S, Phongthai S, Benjakul S, Rawdkuen S. Characterization of acid and alkaline proteases from viscera of farmed giant catfish. Food Biosci. (2014) 6:9–16. doi: 10.1016/j.fbio.2014.01.001
76. Klomklao S, Benjakul S, Kishimura H, Osako K, Tanaka M. Effect of salts and polyethylene glycols on the partitioning and recovery of trypsin from hybrid catfish viscera in aqueous two-phase systems. J Food Biochem. (2010) 34:730–47. doi: 10.1111/j.1745-4514.2009.00311.x
77. Matsuura T. Synthetic Membranes and Membrane Separation Processes. Boca Raton, FL: CRC press (2020). p. 1–476. doi: 10.1201/9781003068037
78. Swennen K, Courtin CM, Van der Bruggen B, Vandecasteele C, Delcour JA Ultrafiltration and ethanol precipitation for isolation of arabinoxylooligosaccharides with different structures. Carbohydr Polym. (2005) 62:283–92. doi: 10.1016/j.carbpol.2005.08.001
79. Aptel P, Clifton M. Ultrafiltration. Synthetic Membranes: Science, Engineering and Applications. Dordrecht: Springer Netherlands (1986). p. 249–305. doi: 10.1007/978-94-009-4712-2_10
80. Cui Z Protein separation using ultrafiltration – an example of multi-scale complex systems. China Particuol. (2005) 3:343–8. doi: 10.1016/S1672-2515(07)60213-9
81. Vijayaraghavan P, Raj SRF, Vincent SGP. Industrial enzymes: recovery and purification challenges. Agro-Industrial Wastes as Feedstock for Enzyme Production: Apply and Exploit the Emerging and Valuable Use Options of Waste Biomass. Academic Press (2016). p. 95–110. doi: 10.1016/B978-0-12-802392-1.00004-6
82. Gildberg A. Recovery of proteinases and protein hydrolysates from fish viscera. Bioresour Technol. (1992) 39:271–6. doi: 10.1016/0960-8524(92)90216-K
83. Li Z, Youravong W, H-Kittikun A. Separation of proteases from yellowfin tuna spleen by ultrafiltration. Bioresour Technol. (2006) 97:2364–70. doi: 10.1016/j.biortech.2005.10.019
84. Al-Ghanayem AA, Joseph B. Current prospective in using cold-active enzymes as eco-friendly detergent additive. Appl Microbiol Biotechnol. (2020) 104:2871–82. doi: 10.1007/s00253-020-10429-x
85. Santiago M, Ramírez-Sarmiento CA, Zamora RA, Parra LP Discovery, molecular mechanisms, and industrial applications of cold-active enzymes. Front Microbiol. (2016) 7:1408. doi: 10.3389/fmicb.2016.01408
86. Mathew GM, Huang CC, Sindhu R, Binod P, Pandey A. Enzymes in seafood processing. Value-Addition in Food Products and Processing Through Enzyme Technology. Elsevier (2022). p. 189–204. doi: 10.1016/B978-0-323-89929-1.00034-2
87. Bougatef A. Trypsins from fish processing waste: characteristics and biotechnological applications – comprehensive review. J Clean Product. (2013) 57:257–65. doi: 10.1016/j.jclepro.2013.06.005
88. de la Fuente B, Tornos A, Príncep A, Lorenzo JM, Pateiro M, Berrada H, et al. Scaling-up processes: patents and commercial applications. Adv Food Nutr Res. (2020) 92:187–223. doi: 10.1016/bs.afnr.2019.12.003
89. Shahidi F, Janak Kamil YVA. Enzymes from fish and aquatic invertebrates and their application in the food industry. Trends Food Sci Technol. (2001) 12:435–64. doi: 10.1016/S0924-2244(02)00021-3
90. Ramkumar A, Sivakumar N, Victor R. Fish waste-potential low cost substrate for bacterial protease production: a brief review. Open Biotechnol J. (2016) 10:335–41. doi: 10.2174/1874070701610010335
91. Furhan J. Adaptation, production, and biotechnological potential of cold-adapted proteases from psychrophiles and psychrotrophs: recent overview. J Genet Eng Biotechnol. (2020) 18:36. doi: 10.1186/s43141-020-00053-7
92. Ferraro V, Cruz IB, Jorge RF, Malcata FX, Pintado ME, Castro PML. Valorisation of natural extracts from marine source focused on marine by-products: a review. Food Res Int. (2010) 43:2221–33. doi: 10.1016/j.foodres.2010.07.034
93. Khiari Z, Rico D, Martin-Diana AB, Barry-Ryan C. Characterization of blue whiting skin gelatines extracted after pretreatment with different organic acids. J Aquat Food Product Technol. (2015) 24:546–55. doi: 10.1080/10498850.2013.791904
94. Khiari Z, Rico D, Martin-Diana AB, Barry-Ryan C. Comparison between gelatines extracted from mackerel and blue whiting bones after different pre-treatments. Food Chem. (2013) 139:347–54. doi: 10.1016/j.foodchem.2013.01.017
95. Khiari Z, Rico D, Martin-Diana AB, Barry-Ryan C. Valorization of fish by-products: rheological, textural and microstructural properties of mackerel skin gelatins. J Mat Cycles Waste Manag. (2017) 19:180–91. doi: 10.1007/s10163-015-0399-2
96. Amaechi BT, AbdulAzees PA, Alshareif DO, Shehata MA, Lima PP, Abdollahi A, et al. Comparative efficacy of a hydroxyapatite and a fluoride toothpaste for prevention and remineralization of dental caries in children. BDJ Open. (2019) 5:1–9. doi: 10.1038/s41405-019-0026-8
97. Muthu M, Gopal J, Chun S, Devadoss AJP, Hasan N, Sivanesan I. Crustacean waste-derived chitosan: antioxidant properties and future perspective. Antioxidants. (2021) 10:228. doi: 10.3390/antiox10020228
98. Fernandes P. Enzymes in fish and seafood processing. Front Bioeng Biotechnol. (2016) 4:59. doi: 10.3389/fbioe.2016.00059
99. Jannathulla R, Rajaram V, Kalanjiam R, Ambasankar K, Muralidhar M, Dayal JS. Fishmeal availability in the scenarios of climate change: inevitability of fishmeal replacement in aquafeeds and approaches for the utilization of plant protein sources. Aquacult Res. (2019) 50:3493–506. doi: 10.1111/are.14324
Keywords: seafood waste, cold-adapted enzymes, proteases, upcycling, sustainable valorization processes
Citation: Khiari Z (2022) Sustainable Upcycling of Fisheries and Aquaculture Wastes Using Fish-Derived Cold-Adapted Proteases. Front. Nutr. 9:875697. doi: 10.3389/fnut.2022.875697
Received: 14 February 2022; Accepted: 09 March 2022;
Published: 07 April 2022.
Edited by:
Nilesh Prakash Nirmal, Mahidol University, ThailandReviewed by:
Tanmay Sarkar, West Bengal State Council of Technical Education, IndiaSiddhartha Pati, NatNov Bioscience Pvt. Ltd., India
Kaushik Kumar Bharadwaj, Gauhati University, India
Copyright © 2022 Khiari. This is an open-access article distributed under the terms of the Creative Commons Attribution License (CC BY). The use, distribution or reproduction in other forums is permitted, provided the original author(s) and the copyright owner(s) are credited and that the original publication in this journal is cited, in accordance with accepted academic practice. No use, distribution or reproduction is permitted which does not comply with these terms.
*Correspondence: Zied Khiari, WmllZC5LaGlhcmlAbnJjLWNucmMuZ2MuY2E=