- 1Food Safety Research Center (Salt), Semnan University of Medical Sciences, Semnan, Iran
- 2Department of Food Safety and Hygiene, School of Health, Fasa University of Medical Sciences, Fasa, Iran
- 3Department of Clinical Nutrition, Faculty of Nutrition Sciences and Food Technology, National Nutrition and Food Technology Research Institute, Shahid Beheshti University of Medical Sciences, Tehran, Iran
- 4Department of Food Science and Technology, Faculty of Nutrition Sciences and Food Technology, National Nutrition and Food Technology Research Institute, Shahid Beheshti University of Medical Sciences, Tehran, Iran
- 5Department of Food Technology Research, Faculty of Nutrition Sciences and Food Technology, National Nutrition and Food Technology Research Institute, Shahid Beheshti University of Medical Sciences, Tehran, Iran
The production of safe and healthy foodstuffs is considered as one of the most important challenges in the food industry, and achieving this important goal is impossible without using various processes and preservatives. However, recently, there has been a growing concern about the use of chemical preservatives and attention has been focused on minimal process and/or free of chemical preservatives in food products. Therefore, researchers and food manufacturers have been induced to utilize natural-based preservatives such as antimicrobial enzymes in their production. Lactoperoxidase, as an example of antimicrobial enzymes, is the second most abundant natural enzyme in the milk and due to its wide range of antibacterial activities, it could be potentially applied as a natural preservative in various food products. On the other hand, due to the diffusion of lactoperoxidase into the whole food matrix and its interaction and/or neutralization with food components, the direct use of lactoperoxidase in food can sometimes be restricted. In this regard, lactoperoxidase can be used as a part of packaging material, especially edible and coating, to keep its antimicrobial properties to extend food shelf-life and food safety maintenance. Therefore, this study aims to review various antimicrobial enzymes and introduce lactoperoxidase as a natural antimicrobial enzyme, its antimicrobial properties, and its functionality in combination with an edible film to extend the shelf-life of food products.
Introduction
Food safety (the opposite of food risk) is defined as the absence of chemical, microbial, or physical hazards in consuming foods (1). Microbial spoilage and foodborne pathogens are of great concern for the industry, authorization agencies, the health sector, and the consumer. They can lead to food deterioration and foodborne diseases, and even death in the population, in addition to restricting food shelf-life, worldwide losing production, and a public trust in the food industry (1–5). This deterioration is caused mainly by Listeria monocytogenes, Staphylococcus aureus, Bacillus cereus, and Escherichia coli through the consumption of most foods, including dairy products, meat products (especially poultry), fresh fruit and vegetable produces, and sea foods as well (6). They are frequently present in food products mainly due to post-processing recontamination during cold storage and handling (7–9). To this end, there is an imperative need to apply approaches to control the noted microbial growth in the foods (10–13).
Traditionally, several methods, including cooling, freezing, pasteurization, canning, modified atmosphere packaging (MAP), and the utilization of chemical preservatives in different fresh food products, are ascertained, which mean that the shelf-life of foods is increased and the growth of microorganisms causing human health risks is prevented (9). However, several disadvantages like high cost, a possible injury to food quality, and a potential negative impact of synthetic preservatives on human health have been observed in the last few decades (14).
In recent years, consumers have a tendency to use natural bio-preservatives (antimicrobial compounds) due to new concerns about the safety of synthetic preservatives (15–19). Today, researchers have focused on using antimicrobial agents as an efficient approach to decrease or postpone the growth and proliferation of spoilage and pathogenic microorganisms in different foodstuffs, in addition to preserve nutritional quality and increase the shelf-life of food products (2, 20–22). In this regard, organic acids, natural herbal extracts especially essential oils, bacteriocins like lacticin, nisin, and pediocin, and antimicrobial enzymes such as lysozyme, chitinase, glucose oxidase, and lactoperoxidase system (LPOS) have been considered as suitable natural antimicrobial compounds for the conservation of various foodstuffs (9, 23–25). Nevertheless, a direct use of these compounds (by dipping, dusting, and spraying) has some restrictions because the active agents could be neutralized, vaporized, or diffused rapidly into the whole food matrix and possibly react with food components (3, 26). Consequently, their antimicrobial activity against spoilage and pathogenic microorganisms may decrease during storage time and subsequently restrict their application in the food industry (3, 14).
Recently, the development of packaging (plastic or edible films and coatings) for the entrapment of antimicrobial compounds (as a kind of active packaging) could be a promising alternative approach to extend food shelf-life and food safety maintenance without affecting food's sensory characteristics (10). In addition to the effectiveness of active packaging as a good barrier to gas (oxygen and carbon dioxide), moisture, and solute migration, films and coatings could successfully decrease the microbial growth in food products by reducing the diffusion amount of antimicrobial compounds from coating and film materials into the food medium through a controlled release of antimicrobial agents (14). Therefore, lower contents of antimicrobial agents would be required in edible coatings and films to provide food conservation compared to a direct application (14). However, because of the consumers' health concerns related to the use of plastic packaging materials, there is an urgent need to use edible or biodegradable films or coating (23). Thus, antimicrobial bio-packaging received a growing interest in the food packaging industry due to their higher acceptance by the increasing “natural,” “healthier,” and “higher-quality” food concepts along with the need for an extended shelf-life (27, 28).
Edible films and coatings can be produced from diverse natural constituents, such as polysaccharides (alginate, chitosan, carrageenan, pectin, and cellulose derivatives), proteins (whey protein isolate, zein, and gelatin), and lipids (waxes) alone or in combination, taken from renewable agricultural sources or food processing wastes (14, 29–31). Several types of research have proven the ability of films and coatings containing different natural antimicrobial compounds to maintain the quality and to intensify the safety of a wide range of food products, especially highly perishable foodstuffs like fruits, vegetables, and meat products (7, 8, 24, 26, 32–34).
The LPOS is a natural antimicrobial enzyme system in milk, tears, and saliva (35–40). This system contains three principal elements: (1) lactoperoxidase enzyme (LPO), (2) hydrogen peroxide (H2O2), and (3) thiocyanate (SCN). LPO catalyzes the oxidation of SCN− ion, creating oxidizing products like hypothiocyanite (OSCN−) and hypothiocyanous acid (HOSCN), with the aid of H2O2 (6, 7). An antimicrobial activity of the LPOS is supposed to arise from the oxidation of sulfhydryl (SH) groups present in microbial proteins and enzymes via these intermediate oxidizing products leading to a change in cellular functions, such as membrane integrity, passage systems, and metabolic enzymes and subsequent death of the cells (6, 15, 27, 41). It is well-known that this system has bacteriostatic and bactericidal activities against Gram-positive and Gram-negative foodborne organisms, respectively (16). Furthermore, it shows antifungal and antiviral performances (23).
The incorporation of the LPOS, generally recognized as a safe (GRAS) compound, into different kinds of edible coatings and films to control the food spoilage originated from pathogenic microorganisms has been investigated in recent years (1, 5, 15, 28, 42). However, to the best of our knowledge, there is no review regarding the antimicrobial activity of films and coatings containing LPOS in different food products. Therefore, the principal objectives of this review are to discuss the antimicrobial enzymes, physicochemical, and antimicrobial properties of the LPOS. Moreover, this study reviews the potential use of films and coatings as polymeric matrices for LPOS incorporation into food products to preserve food quality.
Antimicrobial Enzyme
There are two main purposes associated with food preservation including decreasing the prevalence of foodborne diseases resulting from food pathogens and stopping or postponing microbial spoilage. The latter causes the food unpleasant but not essentially unsafe for consumers (43–46). Concerns about chemical preservatives increased the tendency to natural antimicrobial compounds extracted from animals, plants, and microorganisms (47).
Enzymes are highly specific and efficient in minor concentrations and are working in mild temperature and pH conditions. Furthermore, they react quickly, making them a cost-effective approach in food processing. Enzymes have been experientially consumed since very old ages. They are mainly extracted from natural sources (i.e., plants, animals, and microbial sources) and may be simply inactivated following the occurrence of an appropriate transformation. So, they are considered to be natural and safe food components that are more desirable than chemical compounds, as food-processing agents, by producers and consumers. Regarding the enormous number of enzymes successfully employed in the food industry (as biological catalysts for speeding up chemical reactions like hydrolysis, synthesis, and biocatalysis), it is reasonable to respect the potential of enzymes in the food preservation sector (43). Antimicrobial enzymes are widespread in the environment and play an important role in protecting livelihood invasion by several microorganisms, particularly bacteria and fungi (48). Recently, they are achieving distinct attention as novel and unique agents in food preservation due to antimicrobial and antibiofilm activity (49). They can extend the shelf-life of food products by inhibiting the growth of spoilage microorganisms through several mechanisms, involving decreased necessary nutrient for microbial growth, the production of bacteriostatic or bactericidal substances, and damaging cell wall and/or the inactivation of a vital enzyme in microorganisms (50). In this respect, there are several natural antimicrobial enzyme systems in animal products, such as milk and eggs like LPOS, and lysozyme, which act as a defense system against different kinds of pathogenic microorganisms, by damaging an essential compound or creating a compound that is toxic for them (51–53).
The most promising antimicrobial enzymes are shown in Figure 1. As depicted in this figure, there are two categories of antimicrobial enzymes, i.e., the hydrolases and the oxidoreductases. It is worthy to mention that the microorganisms' cell walls are the substrates for the hydrolases and their destruction could inactivate the cells. Given the significant differences in the cell wall morphology of bacteria and fungi, the potential substrates for these kinds of enzymes differ for these two classes of microorganisms. Therefore, they are classified as bacteriolytic enzymes and antifungal enzymes. The cell wall of bacteria is mainly composed of peptidoglycan (a carbohydrate backbone containing N-acetyl-D-glucosamine and N-acetylmuramic acid linked by peptide bonds), which is effective in the firmness of the bacterial cell. Cell wall deprivation by bacteriolytic enzymes may lead to cell lysis due to the osmotic pressure inside the cell. It is worthy to note that Gram-negative bacteria are less sensitive to these enzymes than Gram-positive bacteria due to the performance of the outer membrane present in the Gram-negative bacteria as an obstacle to infusion. The fungal cell wall is mainly composed of chitin, a polysaccharide created by N-acetyl-D-glucosamine and glucosidic acid linkages. Therefore, antifungal enzymes, which are effective on this structure, are possibly applicable against several foodborne fungi, similar to bacteriolytic enzymes (43). Lytic enzymes are usually classified into two groups, namely, proteases and polysaccharide hydrolases. N-acetylhexosaminidases (like Lysozyme), N-acetylmuramyl-L-alanine amidases, and endopeptidases are the main examples of this group varying in construction, target substrate, reaction process, and several physicochemical characteristics (54). Generally, the combination of these two types of hydrolyzing enzymes achieves a better antimicrobial function (54).
Unlike hydrolases, oxidoreductases, including glucose oxidase and LPOS, show their influence by the creation of cytotoxic components, which can damage key elements (especially proteins) in the microorganisms cells (43). This group is classified into two distinct categories: (1) H2O2-producing enzymes (oxidases) and (2) H2O2-responsive enzymes (peroxidases). Oxidases, like glucose oxidase, generate H2O2 in the absence of catalase or peroxidases, which could be lethal due to the toxicity of generated H2O2 and the consumption of oxygen necessary for aerobic microorganisms (50). H2O2 kills microbial cells via the peroxidation and degradation of the cell membrane and the prevention of protein and DNA synthesis. It is reported that low levels of H2O2 (up to 3%) are efficient as antimicrobial compounds against different kinds of food spoilage microorganisms and food borne pathogens (54, 55). H2O2-responsive enzymes mainly include haloperoxidases, myeloperoxidase (MPO), and LPO, present in several plants, animals, and humans. They use H2O2 as a substrate to oxidize halides (such as I2, Cl2, and Br2) or pseudohalide (like thiocyanate) to more strong antimicrobial agents (like OSCN− in the case of LPOS) (54, 56). It is worthwhile to mention that the combination of the LPOS and glucose oxidase could also be a useful approach. In such conditions, glucose oxidase consumes glucose to produce H2O2 needed by the LPOS to oxidize thiocyanate (54). Table 1 shows the sources, mechanisms of action, and the microorganisms affected by several antimicrobial enzymes having potential food applications.
Despite several advantages of antimicrobial enzymes, the main disadvantage is sensitivity at different conditions like temperatures, salt, and pH, which could result in enzyme denaturation and inhibiting their activity (52). Therefore, the preservation of enzymes activity is essential for achieving the advantages of antimicrobial enzymes (43). Recently, the incorporation of antimicrobial enzymes, especially the LPOS and lysozymes, in active packagings, like cellulose, gelatin, and chitosan edible coatings and films, is a promising method to retain the catalytic activity of enzymes to inhibit food spoilage microorganisms (52, 54, 57, 58). It is worthy of mentioning that a few antimicrobial enzymes, including lysozyme and the LPOS, are listed as GRAS and approved for incorporation into food products (14).
Lactoperoxidase System
The LPOS is a natural antibacterial system in milk and human saliva. This system is made of three components, including lactoperoxidase (LPO), H2O2, and thiocyanate (SCN−) and it is only active when all of these three compounds exist together (49, 59, 60). H2O2 and SCN− originate from hepatic and cellular metabolism (61, 62). In this system, the oxidation of thiocyanate by H2O2 is catalyzed by LPO. It results in the appearance of intermediate products such as hypothiocyanate ion, sulfurdicyanide (HO SCN), and cyanosulfurous acid (HO SCN) with an antimicrobial activity against bacteria, fungi, and viruses (63, 64).
Lactoperoxidase
Oxidative enzymes such as peroxidases exert an antimicrobial and antibiofilm activity by utilizing H2O2 to oxidize isocyanate and halides to stronger antimicrobial agents against invasive microorganisms (54, 65–67). Mammalian peroxidases differ from plant peroxidases in size, amino acid composition, the nature of the prosthetic group, and its binding to the protein. Mammalian peroxidases consist of ~576–738 amino acids with a covalently bound heme moiety, whereas there are nearly 300 amino acids with a non-covalently bound heme moiety in plant peroxidases (59). Lactoperoxidase, MPO, eosinophil peroxidase (EPO), and thyroid peroxidase (TPO) can be named as the family of mammalian heme-containing peroxidase enzymes (68).
Lactoperoxidase enzyme (EC 1.11.1.7), a heme-containing chain glycoprotein and as a member of the mammalian peroxidase family, is a natural enzyme secreted by mammalian glands and found in tears, saliva, and milk (41, 69). It is synthesized in the infants' gastrointestinal tract. It is also the enzyme that is synthesized by the mammary gland in the highest amount to protect the glands against pathogens (68, 70).
Peroxidases secreted in the lacrimal, salivary, and mammary glands are chemically and immunologically similar (71). LPO was named so because it was first isolated from milk in a crystalline form. Due to the similarity of mammalian peroxidase, other animal and human peroxidases are mostly called lactoperoxidase (68, 72). LPO is the second most abundant natural enzyme present in milk and has a great role in milk preservation, especially, in places where there is no possibility or impossibility of rapid cooling or refrigeration of milk after obtaining it (68, 73). LPO exhibits a bactericidal effect on Gram-negative and Gram-positive bacteria and antifungal and antiviral properties (2). Due to its wide range of antibacterial activities, LPO was investigated for its potential role as a natural preservative in foods and milk and in medicine (49, 74, 75).
Lactoperoxidase enzyme, a glycoprotein with 8–10% carbohydrate, consists of a single peptide chain containing 612 amino acids with a molecular weight of ~78 kDa (49, 76, 77). Bovine LPO is a basic protein with a high isoelectric pH value of 9.2, and human LPO is moderately cationic with an isoelectric pH value of 7.5 (71). At least ten fractions of LPO are recognized, and there is no considerable difference in the activity of various LPO fractions (49).
Protoporphyrin IX as the heme group is present in the catalytic center of the LPO and covalently bound to the polypeptide chain through a disulfide bridge, indicating the absence of free thiol groups present in the enzyme molecule (78–80). As a part of the heme group, the iron compound constitutes 0.07% of the LPO. Molecular conformation and structural integrity of the protein are stabilized by the calcium ion that is tightly bound to LPO (71, 78). As LPO is one of the most stable enzymes against heat, it is usually utilized as an indicator of pasteurization process efficiency (81). Furthermore, it is stable to the acidity up to pH 3 and proteolytic action of gastric juice. However, LPO is inactivated by light in the presence of riboflavin and oxygen or by the overgrowth of microorganisms. Furthermore, an excess of H2O2 causes an irreversible inactivation of LPO (64, 71).
The Thiocyanate Ion (SCN−)
The thiocyanate anion is ubiquitously distributed in tissues and secretions of mammals. It is present in various glands and organs such as thyroid, salivary, and mammary glands and their secretion as well as the kidney and stomach and in biological fluids, including lymph, plasma, cerebral, cervical, spinal, and synovial fluids (72, 82, 83). Various factors such as eating and smoking habits of man affect the concentration of SCN− in humans. Furthermore, the content of SCN− in bovine milk depends on the breed, species, diet, health of udder, season, and geographic regions (71, 84). The content of SCN− present in animal metabolism is largely dependent on the food supplied, as two major dietary sources such as glucosinolates and cyanogenic glycosides resulting in a higher amount of SCN− (84).
It has been reported by Fweja et al. that 1–35 mg of thiocyanate per liter is in the fresh milk, which is not always adequate to activate the LPOS (85). Therefore, as determined by the Codex Alimentarius Commission (CAC), ~10 mg of SCN− per liter should be added to raw milk to exogenously activate the system (86). On the other hand, it has been reported that human saliva and human gastric juices contain 50–300 and 40–50 ppm of thiocyanate, respectively, which are much more than the values (15 ppm) necessary for the activation of the LPOS (49, 87). The excess SCN− in the body has shown to exert toxic effects and interferes with iodine metabolism causing goiters (64). However, clinical experiments have demonstrated that the treated milk with thiocyanate does not reach the limits needed to affect thyroid function (84, 88). Iodine metabolism is interfered when the thiocyanate concentration is more than 20 ppm in the human plasma (89).
Hydrogen Peroxide
Hydrogen peroxide is the third component of the LPOS, and it is not generally found in raw milk (64, 90). Polymorphonuclear leucocytes may endogenously produce this component in the process of phagocytosis. H2O2 can also be produced by numerous microorganisms such as Lactobacilli, Streptococci, and Lactococci under aerobic conditions in adequate amounts to activate the LPOS (49, 91, 92). H2O2 generator systems such as sodium percarbonate and glucose oxidase can also produce H2O2 (71). As mentioned previously, H2O2 is naturally found in raw milk at very low concentrations. Therefore, it is necessary to add H2O2 exogenously to activate LPO (93, 94). H2O2 is very toxic to mammalian cells, but the cells are protected against toxicity when H2O2 is utilized at a low level in the presence of LPO and SCN− (64, 88). Based on the criteria set by the CAC, the addition of 8.5 ppm of H2O2 is necessary for an exogenous activity of the LPOS (86), while for preserving milk using only H2O2, nearly 500–800 ppm of this component are required (64). It has been indicated that the application of glucose oxidase/glucose and xanthine oxidase/hypoxanthine as H2O2-producing systems may result in a more efficient antimicrobial LPOS than the addition of H2O2 only (71, 95).
Modes Of Action And An Antimicrobial Spectrum Of The LPOS
As mentioned before, LPO participates in the peroxidation of thiocyanate and some halides such as I2, Br2 to produce some intermediate components that kill or prevent the growth of various microorganisms. The peroxidative reactions are complex, and they depend on the content of H2O2, the presence or absence of endogenous electron donors, and the occurrence of various pathways (49, 59, 91).
In the first step of an enzymatic mechanism, an initiation reaction of the resting LPO (Fe+3) to its ground state using H2O2 occurs. Following this reaction, propagation reactions take place. These reactions involve converting the ground state of LPO into the so-called compound I state by a reaction with H2O2. At low concentrations of SCN− (<3 μM) and halide, compound I reacts with existing one-electron donors such as proteins and peptides, to create compound II that is consistently reduced to the ground state at a low rate. Compound II may react with excess H2O2 (>5 mM) and form compound III resulting in ferrylperoxidase adduct and an irreversible inactivation of LPO. Compound I created from the reaction of peroxidase with H2O2 participates in the oxidation of SCN− and halides (49, 59, 71, 91). The oxidation of SCN− results in the formation of short-lived oxidation products that participate in an antimicrobial activity of the LPOS. OSCN− is the main intermediate oxidation product of SCN−. Furthermore, based on the reaction conditions, other short-lived intermediates such as thiocyanogen [(SCN)2], cyanogen thiocyanate (NC-SCN), cyanosulfurous acid (HO2SCN), and cyanosulfuric acid (HO3SCN) may be formed (49, 96, 97). OSCN− is in equilibrium with HOSCN and at the pH value of 5.3 that LPO shows the maximum activity, the amount of these two forms are equal (71, 98). Both forms show an antimicrobial activity; however, the acid form is more bactericidal. Uncharged HOSCN has more solubility in the non-polar media and can more efficiently pass through the cell membrane (59, 99). On the other hand, the basic form of OSCN− is more stable than its acid form. The stability of OSCN− is affected by various factors such as the presence or absence of LPO, metal ions (Fe, Ni, Cu, Mn, etc.), light, pH, glycerol, and ammonium sulfate (49, 59, 100).
An antimicrobial activity of the LPOS occurs through the oxidation of SH groups of microbial enzymes and other proteins with HOSCN and OSCN− (Figure 2). Reducing agents containing SH groups such as cysteine, glutathione, dithiothreitol, mercapto-ethanol, and sodium hydrosulfite can prevent an antimicrobial activity of the LPOS either by scavenging thiocyanate ions or by a direct interaction with the heme group of the enzyme (49, 59, 71, 91).
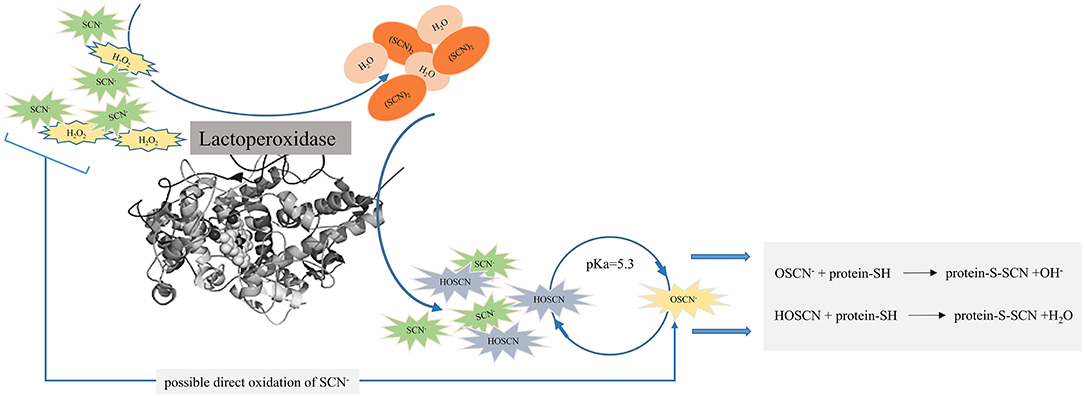
Figure 2. Antimicrobial activity of lactoperoxidase enzyme (LPO) by the oxidation of sulfhydryl (SH) groups of microbial enzymes and other proteins with hypothiocyanous acid (HOSCN) and hypothiocyanite (OSCN−).
The oxidation of the SH group of proteins alters proteins and enzymes such as glycolysis, respiration, and nutrient transport. Furthermore, the cell wall structure is damaged by the oxidation of the SH group, which increases its permeability and the release of important nutrients and cell components such as ions, amino acids, and peptides, inhibiting the growth of microorganisms or the occurrence of cell death (59, 70, 72, 101).
The LPOS can exert a bacteriostatic and/or bactericidal activity against various sensitive microorganisms, such as bacteria, fungi, and viruses. Based on the test medium, the type of electron donor, temperature, pH, type of microorganism, cell density and incubation time, the mechanisms of inhibitory effects of the LPOS vary from oxidative killing to the blocking of glycolytic pathways, or interfering with cytopathic effects (59, 64, 102–104).
Various groups of bacteria show a different sensitivity to the LPOS. Due to the more sensitivity of Gram-negative bacteria, the LPOS exerts a bacteriostatic effect, whereas Gram-positive bacteria are more resistant; therefore, the system has an inhibitory action only on the growth of these microorganisms (70, 102). It has been indicated that Gram-negative and catalytic organisms such as coliforms, Salmonella, Shigella, and Pseudomonas are not only prevented by LPOS but also based on the condition of media such as cell density, pH, time, and temperature of incubation, they might be killed when H2O2 is provided exogenously (49, 105). Gram-positive, catalase-negative bacteria, such as streptococci and lactobacilli are only inhibited by the LPOS (88, 106). These differences in LPOS sensitivity can be attributed to the different structures, functions, and properties of the cell wall (64, 101).
Applications Of The Lpos In Films And Coatings
The use of edible films and coatings containing antimicrobial agents is an environmentally friendly approach for extending the shelf-life of food products. Antimicrobial edible films are preferred to direct the application of antimicrobial agents due to the controlled diffusion of these agents to food surfaces (107). Several compounds have been used as antimicrobial compounds in edible films and coatings, such as organic acids, plant extracts, bacteriocins, and enzymes (108). Table 2 represents the selected publications on the application of the LPOS in edible films and coatings.
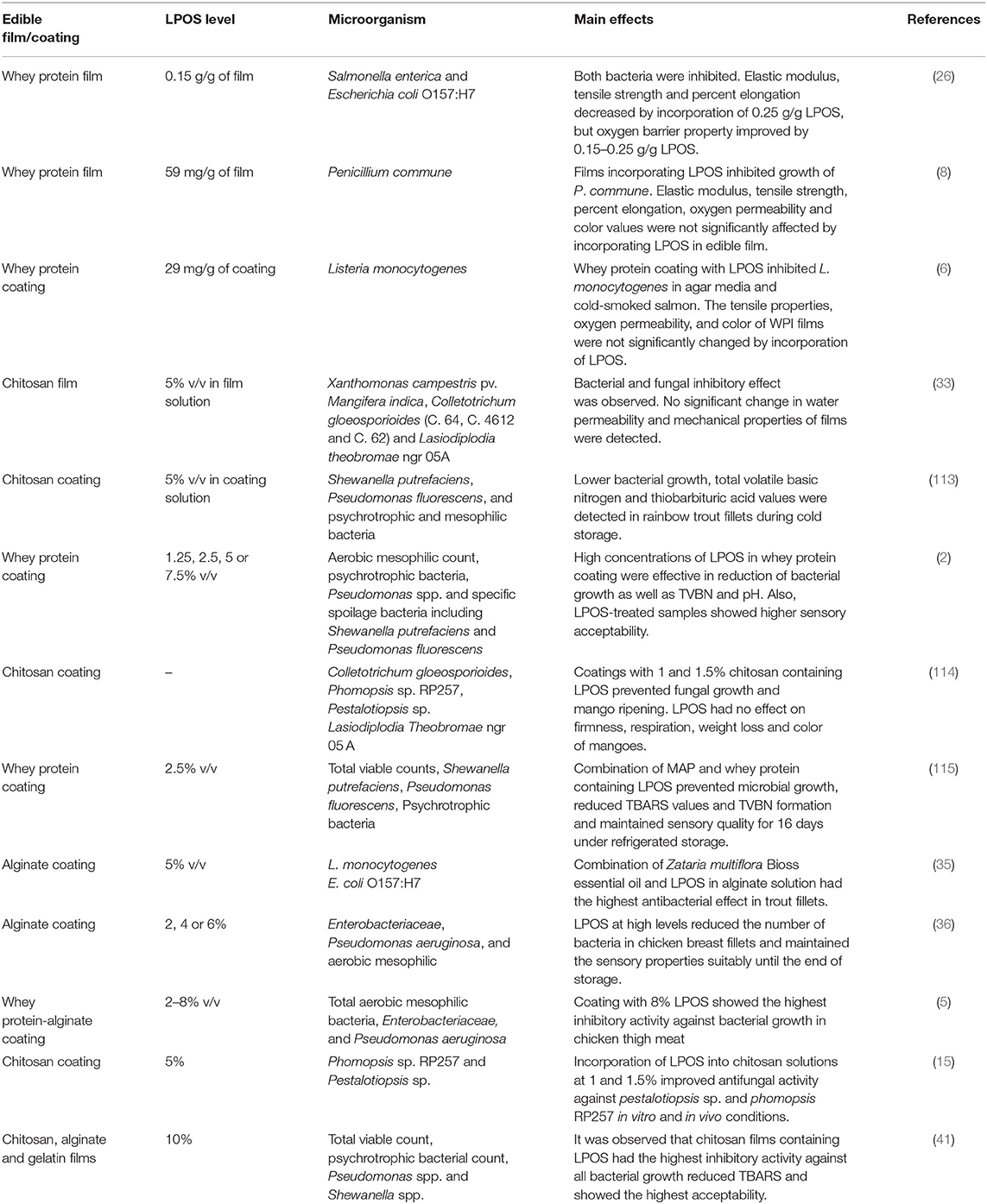
Table 2. Selected publications on the application of lactoperoxidase system (LPOS) in different edible films and coatings.
Min et al. (26) investigated the antimicrobial effect of whey protein films containing LPOS (0.15 g/g) against Salmonella enterica and E. coli O157:H7. They observed that the films inhibited S. enterica and E. coli O157:H7 (4 log CFU/cm2) and were inoculated either onto agar before placing the film disc or onto the top of the film disc. In a similar study, a whey protein isolate film containing 29 mg/g LPOS prevented 4.2 CFU/cm2 of L. monocytogenes inoculated on agar media. The application of LPOS-whey protein isolate coating (with 40 mg LPOS/g coating) in cold-smoked salmon decreased to near 3 and 1 log CFU/g of L. monocytogenes and total aerobic microorganisms, respectively. Also, whey protein coating containing LPOS inhibited L. monocytogenes in smoked salmon at 4 and 10°C for 35 and 14 days, respectively (6). The difference between an inhibitory activity of the LPOS in agar media and smoked salmon was attributed to a higher aw of agar media that simplified the diffusion of oxidizing products from the film and a higher level of SH compounds in smoked salmon, which showed an interaction with oxidizing agents and decreased LPOS efficiency.
In a study by Lee and Min (109), defatted soybean meal was used to prepare film containing LPOS (1, 2, 3, 4, 5, and 10% w/w), which showed an inhibitory effect against Salmonella typhimurium DT 104 at the levels of 5% and 10% regardless of the inoculation level.
In another study by Min et al. (7), the LPOS at different levels (0, 3, 5, or 7% w/w) was incorporated into whey protein isolate coating and applied for roasted turkey and showed 3 and 2 log CFU/g reduction of S. enterica and E. coli O157:H7, respectively. In addition, turkey meat was inoculated with S. enterica (6.0 log CFU/g) or E. coli O157:H7 (5.6 log CFU/g), coated with whey protein containing LPOS (5 and 3% w/w) and stored at 4 and 10°C for 42 days. The results indicated an inhibitory effect against both bacteria at both temperatures during storage and the inhibition was more remarkable when the coating was formed on the surface of the turkey before inoculation than when the coating was formed on the inoculated surface.
Yener et al. (27) evaluated an antimicrobial activity of the LPOS incorporated in alginate films on E. coli (NRRL B-3008), Listeria innocua (NRRL B-33314), and Pseudomonas fluorescens (NRRLB-253) in the presence of different levels of H2O2 (0.2, 0.4, or 0.8 mM) and KSCN (1, 2 or 4 mM). It was indicated that the level of H2O2 and KSCN affected an antimicrobial activity of the LPOS. All bacteria were inhibited by using the LPOS along with 0.4 or 0.8 mM H2O2 and 4 mM KSCN during a 6-h incubation. Also, by applying 0.8 mM H2O2 and 4 mM KSCN, the LPOS showed an inhibitory effect against L. innocua and P. fluorescens for a 24-h incubation period. The highest and lowest resistant bacteria to the LPOS were E. coli and P. fluorescens, respectively.
The antimicrobial effects of chitosan films containing LPOS (5% v/v) on Xanthomonas campestris pv. Mangifera indica, Colletotrichum gloeosporioides (C. 64, C. 4612, and C. 62), and Lasiodiplodia theobromae ngr 05A were investigated. The results showed that chitosan at the levels of 1 and 1.5% containing LPOS with or without iodine completely inhibited X. campestris pv. M. indica. It was stated that, compared to Gram-negative bacteria, Gram-positive bacteria had a lower resistance to LPOS without iodine. In the case of molds, 1 and 1.5% chitosan incorporated by LPOS with or without iodine inhibited C. gloeosporioides C64 and L. theobromae, whereas C. gloeosporioides C4612 was susceptible to iodine and C62 was resistant (33).
Lactoperoxidase system (5% v/v) was added to chitosan coating, which was applied for the shelf-life extension of the rainbow trout. The results indicated an inhibitory activity against Shewanella putrefaciens, P. fluorescens, and psychrotrophic and mesophilic bacteria during a 16-day storage. Regarding the production of total volatile basic nitrogen (TVBN) and thiobarbituric acid values, lower levels were detected in LPOS-coated chitosan (113). In a similar study, Shokri et al. (2) investigated the effect of different LPOS concentrations (1.25, 2.5, 5, or 7.5%) incorporated into whey protein coating as an antimicrobial strategy for preserving the rainbow trout during a 16-day storage at 4°C. Samples treated with 7.5% LPOS showed a lower mesophilic aerobic count, psychrotrophic bacteria, Pseudomonas spp., and specific spoilage bacteria, including S. putrefaciens and P. fluorescens in fish filets. Total volatile basic nitrogen values were below the upper limit of acceptability for the treated samples with 2.5%, 5%, and 7.5% LPOS. pH values of LPOS-treated samples, except at 1.25% level, were lower than control samples. Regarding sensory properties, high LPOS concentrations had greater acceptability. An inhibitory effect of the LPOS against bacteria was ascribed to the presence of OSCN− and HOSCN that oxidized the SH groups in the structure of enzymes and other proteins.
Cissé et al. (114) studied the effect of chitosan coating (with 0.5, 1, or 1.5% chitosan) with or without LPOS on the postharvest quality of mango. It was reported that coatings with 1 and 1.5% chitosan containing LPOS prevented fungal growth and mango ripening. However, the firmness, respiration, weight loss, and color of mangoes were not affected by the LPOS but were influenced by the chitosan level.
In a study by Rostami et al. (115), the effect of whey protein coating containing LPOS in combination with MAP on the microbiological, chemical, and sensory properties of Pike-Perch filets was determined during a 16-day of storage. It was demonstrated that whey protein coating + LPOS + MAP had the lowest bacterial count and S. putrefaciens and P. fluorescens and the total viable count as well as psychrotrophic bacteria remained below 7 log CFU/g at the end of storage. The highest and the lowest TVBN values were detected in whey protein coating and coating + LPOS + MAP, respectively. The TVBN level in samples coated with whey protein, whey protein + LPOS, and whey protein + MAP was higher than the acceptable limit of 30–35 mg N/100 g. It was noted that the use of MAP in combination with the LPOS reduced fat oxidation and consequently thiobarbituric acid reactive substances due to the decreased oxygen level. Regarding pH, samples coated with whey protein + LPOS and whey protein + LPOS showed a lower pH increase due to lower bacterial growth. Also, a sensory analysis was indicative of higher acceptability of samples coated with whey protein + LPOS + MAP during a 16-day storage. It was highlighted that in MAP packaging, replacing the oxygen with nitrogen decreased the growth of aerobic bacteria during the logarithmic phase, and the lag phase was prolonged by carbon dioxide. Similarly, Shokri and Ehsani (4) assessed the effect of whey protein coating containing 2.5% LPOS and 1.5 or 3% α-tocopherol on the quality characteristics of Pike-Perch filets during a 16-day storage at refrigeration temperature. The results demonstrated that whey protein-LPOS-coated samples had a lower bacterial count, TVBN and pH, and a higher overall acceptability. Regarding the TBA value, treatments containing only α-tocopherol had lower values than samples containing both α-tocopherol and LPOS. It was concluded that a combination of α-tocopherol and the LPOS could exhibit an antibacterial and antioxidant activity for the shelf-life extension of Pike-Perch filets during cold storage. It was also noted that α-tocopherol diminished an antimicrobial activity of the LPOS through the inhibition of SH group oxidation and the formation of disulfide bonds.
Sharifi et al. (35) studied an antimicrobial effect of alginate coating containing Zataria multiflora Bioss essential oil (0.5 and 1% w/v) and the LPOS (5% v/v) individually and in combination against L. monocytogenes and E. coli O157:H7 in trout filets during a 16-day storage. The lowest bacterial count was observed in samples coated with alginate solution containing 0.5% and 1% essential oil, and the LPOS was indicative of a synergistic effect of these two antimicrobial agents.
Yousefi et al. (36) investigated the effect of alginate coating with the different levels of LPOS (2, 4, or 6%) on the quality and sensory properties of chicken breast filets during a 16-day cold storage. The results showed that the pH of chicken breast filets coated with alginate-LPOS coating was lower than control samples. However, increasing the LPOS level led to an increase in pH until day 12 due to the production of H2O and the reduction of H+ concentration. Alginate-LPOS coating decreased TVBN formation in chicken filets. Chicken breast filets coated with alginate-LPOS at levels of 4% and 6% had lower Enterobacteriaceae, Pseudomonas aeruginosa, and aerobic mesophilic bacteria. Also, coated samples with 6% LPOS had the highest overall acceptability. The antimicrobial activity of coating containing LPOS was attributed to the interaction of OSCN− and HOSCN with SH groups of proteins in the cell membrane and the release of cell components. Also, it has been reported that an uptake of glucose, purines, and pyrimidines and the production of proteins, DNA, and RNA were prevented.
In a study by Molayi et al. (5), whey protein-alginate coatings with lactoperoxidase (2, 4, 6, or 8% v/v) were applied for the control of total aerobic mesophilic bacteria, Enterobacteriaceae, and P. aeruginosa in chicken thigh meat. It was observed that increasing the level of LPOS enhanced an antibacterial activity and in this regard, coating with 8% v/v LPOS showed the highest activity. It was mentioned that the LPOS in the coating required 2 days for a complete activation against Enterobacteriaceae. Also, the highest inhibitory effect of the LPOS against aerobic mesophilic bacteria occurred after 4 days.
Lactoperoxidase was added at different levels (1.25, 2.5, 5, and 7.5%) to whey protein coating and its effect on the microbial, chemical, and sensory properties of shrimp was investigated and stored at the refrigerated temperature for 16 days. It was revealed that the LPOS at the level of 7.5% significantly decreased mesophilic aerobic bacteria, psychrotrophic bacteria, Pseudomonas spp., P. fluorescens, and S. purefaciens in shrimp samples. Coating of shrimp with whey protein and different levels of LPOS decreased TVBN formation, which remained below the unacceptable limit even after a 16-day storage. LPOS-coated samples (5 and 7.5%) showed the highest scores in different sensory parameters (9).
Saravani et al. (116) investigated the effect of whey protein coating containing LPOS (5% v/v) and Bunium persicum essential oil (0.5% v/v) on the microbial and chemical characteristics of Gouda cheese during a 90-day storage. It was indicated that bacteria number was constant in all essential oil and essential oil-LPOS-coated cheese samples during a 90-day storage. However, LABs and Enterobacter spp. were less susceptible to the antibacterial effects of LPOS. In addition, the occurrence of lipid oxidation decreased in cheese samples coated with whey protein-essential oil and whey protein-essential oil-LPOS. It was suggested to utilize LPOS with essential oil to achieve an antibacterial activity for both Gram-negative and Gram-positive bacteria. In a similar study, the LPOS (5% v/v) and B. persicum essential oil (0.5 or 1% w/v) were incorporated into alginate coating and its effect on L. monocytogenes in chicken breast filets was evaluated during a 20-day storage at 4°C. The lowest count of L. monocytogenes was obtained in the coated samples containing LPOS + 1% essential oil (1). It has been declared that the degree of susceptibility of bacteria was dependent on aerobiosis/anaerobiosis and growth phase and generally Gram-negative catalase-positive bacteria such as Pseudomonas spp. and coliforms are killed, but Gram-positive and catalase-negative bacteria, such as streptococci or lactobacilli, are inhibited by LPOS. In this regard, the LPOS is generally used along with other preservation methods.
The effect of alginate coating containing LPOS (5%), Z. multiflora essential oil (0.5%, 1%), or both on microbial and chemical characteristics of rainbow trout filets has been investigated during a 16-day storage. The results revealed that the combination of essential oil (1%) and LPOS (5%) was efficient in reducing mesophilic viable counts, psychrotrophic bacteria, S. putrefaciens, and P. fluorescens as well as the control of pH increase and the reduction of total volatile nitrogen. Also, the use of the LOPS individually was more effective than Z. multiflora essential oil alone (69).
In a study by Ehsani et al. (41), different active films including chitosan, alginate, and gelatin containing LPOS or sage essential oil were prepared, which were utilized for wrapping fish burgers and stored at 4°C for 20 days. Total viable count, psychrotrophic bacterial count, Pseudomonas spp. and Shewanella spp., TBARS, pH, and the sensory properties of the samples were determined during refrigeration. It was observed that chitosan films containing LPOS had the highest inhibitory activity against all bacterial growth. The underlying mechanisms included synergistic interactions between the components, the prevention of biochemical pathways and enzymes, and an interaction with cell wall components and pore formation in the cell wall. Also, chitosan films with LOPS reduced TBARS and showed the highest acceptability.
Mohamed et al. (15) prepared chitosan coating containing LPOS and LPOS with iodine (LPOSI) and evaluated their antifungal activity against Phomopsis sp. RP257 and Pestalotiopsis sp. isolated from mango. It was indicated that in vitro condition, Pestalotiopsis sp. was inhibited by chitosan alone at the levels of 1 and 1.5% and incorporation of the LPOS and LPOSI to 0.5% chitosan resulted in an increase of inhibition from 26 to 93%. In the case of Phomopsis sp. RP257, chitosan films with the LPOS and LPOSI were effective, especially at 1 and 1.5% chitosan levels. Similarly, it was stated that chitosan films completely prevented Pestalotiopsis sp. in mango at 1 and 1.5% while Phomopsis sp. RP257 inhibited 50% at the mentioned levels. Also, LPOS addition improved an antifungal activity.
Conclusion
Recently, general trend has emerged that consumers are concerned about the safety of chemical preservatives utilized in food. Hence, extensive and integrated efforts have been carried out to discover natural-based preservatives that are able to prevent microbial growth in food and improve the safety and shelf-life of food products. This study revealed that lactoperoxidase as a natural antimicrobial enzyme in the LPOS could be potentially applied as a replacement for chemical preservatives to improve the quality and shelf-life of food products. An antimicrobial activity of LPO is supposed to arise from the oxidation of SH groups present in microbial proteins and enzymes via these intermediate oxidizing products leading to a change in cellular functions, such as membrane integrity, passage systems, and metabolic enzymes and subsequent death of the cells. This system has bacteriostatic and bactericidal activities against Gram-positive and Gram-negative foodborne microorganisms, respectively. Additionally, it shows antifungal and antiviral performances. However, a direct incorporation of LPOS in food matrices could lead to the reduction of its activity as a consequence of an interaction with food components and its utilization in edible films and coatings. Conducted researches in respect of films and coatings containing LPOS showed an antimicrobial activity and improved food product shelf-life and in some cases, reduced chemical spoilage in food products without a negative impact on sensory properties. Nevertheless, the types of films and coating with LPOS were limited to alginate, chitosan, gelatin, and whey protein and further researches are required to examine the antimicrobial effects of the different types of edible films and coatings incorporating LPOS. Additionally, an antimicrobial effect of the LPOS in combination with other natural antimicrobial agents and hurdle technology can be a subject of future researches.
Author Contributions
This study was designed by NK. The manuscript was written by MY, NK, AN, MS, and AM. MY and NK critically revised the manuscript. All authors listed have approved the manuscript for publication, contributed to this article, and approved the submitted version.
Conflict of Interest
The authors declare that the research was conducted in the absence of any commercial or financial relationships that could be construed as a potential conflict of interest.
Publisher's Note
All claims expressed in this article are solely those of the authors and do not necessarily represent those of their affiliated organizations, or those of the publisher, the editors and the reviewers. Any product that may be evaluated in this article, or claim that may be made by its manufacturer, is not guaranteed or endorsed by the publisher.
References
1. Didar H, Khanzadi S, Azizzadeh M. Effects of alginate coating incorporated with Bunium persicum essential oil and lactoperoxidase system on inoculated Listeria monocytogenes in chicken breast fillets. J Health Sci Technol. (2018) 2:2–8. doi: 10.32592/JHST.2018.2.1.102
2. Shokri S, Ehsani A, Jasour MS. Efficacy of lactoperoxidase system-whey protein coating on shelf-life extension of rainbow trout fillets during cold storage (4 C). Food Bioprocess Technol. (2015) 8:54–62. doi: 10.1007/s11947-014-1378-7
3. Li B, Kennedy J, Peng J, Yie X, Xie B. Preparation and performance evaluation of glucomannan–chitosan–nisin ternary antimicrobial blend film. Carbohydr Polym. (2006) 65:488–94. doi: 10.1016/j.carbpol.2006.02.006
4. Shokri S, Ehsani A. Efficacy of whey protein coating incorporated with lactoperoxidase and α-tocopherol in shelf life extension of Pike-Perch fillets during refrigeration. LWT-Food Sci Technol. (2017) 85:225–31. doi: 10.1016/j.lwt.2017.07.026
5. Molayi R, Ehsani A, Yousefi M. The antibacterial effect of whey protein–alginate coating incorporated with the lactoperoxidase system on chicken thigh meat. Food Sci Nutr. (2018) 6:878–83. doi: 10.1002/fsn3.634
6. Min S, Harris LJ, Krochta JM. Listeria monocytogenes inhibition by whey protein films and coatings incorporating the lactoperoxidase system. J Food Sci. (2005) 70:m317–24. doi: 10.1111/j.1365-2621.2005.tb11474.x
7. Min S, Harris LJ, Krochta JM. Inhibition of Salmonella enterica and Escherichia coli O157: H7 on roasted turkey by edible whey protein coatings incorporating the lactoperoxidase system. J Food Prot. (2006) 69:784–93. doi: 10.4315/0362-028X-69.4.784
8. Min S, Krochta JM. Inhibition of Penicillium commune by edible whey protein films incorporating lactoferrin, lacto-ferrin hydrolysate, and lactoperoxidase systems. J Food Sci. (2005) 70:M87–94. doi: 10.1111/j.1365-2621.2005.tb07108.x
9. Farshidi M, Yousefi M, Ehsani A. The combined effects of lactoperoxidase system and whey protein coating on microbial, chemical, textural, and sensory quality of shrimp (Penaeus merguiensis) during cold storage (4±1 C). Food Sci Nutr. (2018) 6:1378–86. doi: 10.1002/fsn3.669
10. Sofi S, Singh J, Rafiq S, Ashraf U, Dar B, Nayik GA, et al. comprehensive review on antimicrobial packaging and its use in food packaging. Curr Nutr Food Sci. (2018) 14:305–12. doi: 10.2174/1573401313666170609095732
11. Cakmak KC, Gülçin I. Anticholinergic and antioxidant activities of usnic acid-An activity-structure insight. Toxicol Rep. (2019) 6:1273–80. doi: 10.1016/j.toxrep.2019.11.003
12. Gulcin I. Antioxidants and antioxidant methods: an updated overview. Arch Toxicol. (2020) 94:651–715. doi: 10.1007/s00204-020-02689-3
13. Gülçin I. Antioxidant activity of food constituents: an overview. Arch Toxicol. (2012) 86:345–91. doi: 10.1007/s00204-011-0774-2
14. Aloui H, Khwaldia K. Natural antimicrobial edible coatings for microbial safety and food quality enhancement. Comprehens Rev Food Sci Food Saf. (2016) 15:1080–103. doi: 10.1111/1541-4337.12226
15. Mohamed C., Elise Ng, Etienne TV, Loiseau G, Montet D. Antifungal activity of edible coating made from chitosan and lactoperoxidase system against Phomopsis sp RP257 and Pestalotiopsis sp isolated from mango. J Food Saf. (2020) 40:e12785. doi: 10.1111/jfs.12785
16. Elliot R, McLay J, Kennedy M, Simmonds R. Inhibition of foodborne bacteria by the lactoperoxidase system in a beef cube system. Int J Food Microbiol. (2004) 91:73–81. doi: 10.1016/S0168-1605(03)00366-0
17. Yousefi M, Khorshidian N, Hosseini H. Potential application of essential oils for mitigation of Listeria monocytogenes in meat and poultry products. Front Nutr. (2020) 7:577287. doi: 10.3389/fnut.2020.577287
18. Khorshidian N, Yousefi M, Khanniri E, Mortazavian AM. Potential application of essential oils as antimicrobial preservatives in cheese. Innov Food Sci Emerg Technol. (2018) 45:62–72. doi: 10.1016/j.ifset.2017.09.020
19. Ferreira S, Sanchez G, Alves MJ, Fraqueza MJ. Editorial: natural compounds in food safety and preservation. Front Nutr. (2021) 8:759594. doi: 10.3389/fnut.2021.759594
20. Khorshidian N, Khanniri E, Mohammadi M, Mortazavian AM, Yousefi M. Antibacterial activity of pediocin and pediocin-producing bacteria against Listeria monocytogenes in meat products. Front Microbiol. (2021) 12:709959. doi: 10.3389/fmicb.2021.709959
21. Polat Yemiş G, Delaquis P. Natural compounds with antibacterial activity against Cronobacter spp. in powdered infant formula: a review. Front Nutr. (2020) 7:595964. doi: 10.3389/fnut.2020.595964
22. Pilevar Z, Hosseini H, Abdollahzadeh E, Shojaee-Aliabadi S, Tajedin E, Yousefi M, et al. Effect of Zataria multiflora Boiss. Essential oil, time, and temperature on the expression of Listeria monocytogenes virulence genes in broth and minced rainbow trout. Food Control. (2020) 109:106863. doi: 10.1016/j.foodcont.2019.106863
23. Mecitoglu Ç, Yemenicioglu A. Partial purification and preparation of bovine lactoperoxidase and characterization of kinetic properties of its immobilized form incorporated into cross-linked alginate films. Food Chem. (2007) 104:726–33. doi: 10.1016/j.foodchem.2006.11.074
24. Min S, Krochta JM, Rumsey TR. Diffusion of thiocyanate and hypothiocyanite in whey protein films incorporating the lactoperoxidase system. J Food Eng. (2007) 80:1116–24. doi: 10.1016/j.jfoodeng.2006.09.003
25. Barroug S, Chaple S, Bourke P. Combination of natural compounds with novel non-thermal technologies for poultry products: a review. Front Nutr. (2021) 8:628723. doi: 10.3389/fnut.2021.628723
26. Min S, Harris LJ, Krochta JM. Antimicrobial effects of lactoferrin, lysozyme, and the lactoperoxidase system and edible whey protein films incorporating the lactoperoxidase system against Salmonella enterica and Escherichia coli O157: H7. J Food Sci. (2005) 70:m332–8. doi: 10.1111/j.1365-2621.2005.tb11476.x
27. Yener FY, Korel F, Yemenicioglu A. Antimicrobial activity of lactoperoxidase system incorporated into cross-linked alginate films. J Food Sci. (2009) 74:M73–9. doi: 10.1111/j.1750-3841.2009.01057.x
28. Yildirim S, Röcker B, Pettersen MK, Nilsen-Nygaard J, Ayhan Z, Rutkaite R, et al. Active packaging applications for food. Comprehens Rev Food Sci Food Saf. (2018) 17:165–99. doi: 10.1111/1541-4337.12322
29. Abdollahzadeh E, Nematollahi A, Hosseini H. Composition of antimicrobial edible films and methods for assessing their antimicrobial activity: a review. Trends Food Sci Technol. (2021) 110:291–303. doi: 10.1016/j.tifs.2021.01.084
30. Bagheripoor N, Khoshgozaran-Abras S, Sohrabvandi S, Khorshidian N, Mortazavian AM, MollaKhalili N, et al. Application of active edible coatings to improve the shelf-life of cheese. Food Sci Technol Res. (2018) 24:949–62. doi: 10.3136/fstr.24.949
31. Huang Q, Wan C, Zhang Y, Chen C, Chen J. Gum arabic edible coating reduces postharvest decay and alleviates nutritional quality deterioration of ponkan fruit during cold storage. Front Nutr. (2021) 8:717596. doi: 10.3389/fnut.2021.717596
32. Langroodi AM, Nematollahi A, Sayadi M. Chitosan coating incorporated with grape seed extract and Origanum vulgare essential oil: an active packaging for turkey meat preservation. J Food Meas Character. (2021) 15:2790–804. doi: 10.1007/s11694-021-00867-0
33. Mohamed C, Clementine KA, Didier M, Gérard L, Noëlle D-CM. Antimicrobial and physical properties of edible chitosan films enhanced by lactoperoxidase system. Food Hydrocoll. (2013) 30:576–80. doi: 10.1016/j.foodhyd.2012.07.018
34. Liu W, Wang Q, Mei J, Xie J. Shelf-life extension of refrigerated turbot (Scophthalmus maximus) by using weakly acidic electrolyzed water and active coatings containing daphnetin emulsions. Front Nutr. (2021) 8:696212. doi: 10.3389/fnut.2021.696212
35. Sharifi F, Khanzadi S, Hashemi M, Azizzadeh M. Control of Listeria monocytogenes and Escherichia coli O157: H7 inoculated on fish fillets using alginate coating containing lactoperoxidase system and Zataria multiflora boiss essential oil. J Aquat Food Product Technol. (2017) 26:1014–21. doi: 10.1080/10498850.2017.1375057
36. Yousefi M, Farshidi M, Ehsani A. Effects of lactoperoxidase system-alginate coating on chemical, microbial, and sensory properties of chicken breast fillets during cold storage. J Food Saf. (2018) 38:e12449. doi: 10.1111/jfs.12449
37. Sisecioglu M, Cankaya M, Gulcin I, Ozdemir H. The inhibitory effect of propofol on bovine lactoperoxidase. Protein Pept Lett. (2009) 16:46–9. doi: 10.2174/092986609787049394
38. Sişecioglu M, Çankaya M, Gülçin I, Özdemir H. Interactions of melatonin and serotonin with lactoperoxidase enzyme. J Enzyme Inhib Med Chem. (2010) 25:779–83. doi: 10.3109/14756360903425239
39. Sisecioglu M, Gulcin I, Cankaya M, Atasever A, Ozdemir H. The effects of norepinephrine on lactoperoxidase enzyme (LPO). Sci Res Essays. (2010) 5:1351–6.
40. Sisecioglu M, Kirecci E, Cankaya M, Ozdemir H, Gulcin I, Atasever A. The prohibitive effect of lactoperoxidase system (LPS) on some pathogen fungi and bacteria. African J Pharm Pharmacol. (2010) 4:671–7.
41. Ehsani A, Hashemi M, Afshari A, Aminzare M, Raeisi M, Zeinali T. Effect of different types of active biodegradable films containing lactoperoxidase system or sage essential oil on the shelf life of fish burger during refrigerated storage. LWT. (2020) 117:108633. doi: 10.1016/j.lwt.2019.108633
42. Ehsani A, Hashemi M, Aminzare M, Raeisi M, Afshari A, Mirza Alizadeh A, et al. Comparative evaluation of edible films impregnated with sage essential oil or lactoperoxidase system: impact on chemical and sensory quality of carp burgers. J Food Proc Preserv. (2019) 43:e14070. doi: 10.1111/jfpp.14070
43. Fuglsang CC, Johansen C, Christgau S, Adler-Nissen J. Antimicrobial enzymes: applications and future potential in the food industry. Trends Food Sci Technol. (1995) 6:390–6. doi: 10.1016/S0924-2244(00)89217-1
44. Gülçin I, Oktay M, Kireçci E. Küfrevioglu ÖI. Screening of antioxidant and antimicrobial activities of anise (Pimpinella anisum L) seed extracts. Food Chem. (2003) 83:371–82. doi: 10.1016/S0308-8146(03)00098-0
45. Gulcin I, Tel AZ, Kirecci E. Antioxidant, antimicrobial, antifungal, and antiradical activities of Cyclotrichium niveum (Boiss.) Manden and Scheng. Int J Food Prop. (2008) 11:450–71 HPLC/MS/MS. doi: 10.1080/10942910701567364
46. Tohma H, Köksal E, Kiliç Ö, Alan Y, Yilmaz MA, Gülçin I, et al. RP-HPLC/MS/MS analysis of the phenolic compounds, antioxidant and antimicrobial activities of Salvia L. species. Antioxidants. (2016) 5:38. doi: 10.3390/antiox5040038
47. Hansen EH, Albertsen L, Schäfer T, Johansen C, Frisvad JC, Molin S, et al. Curvularia haloperoxidase: antimicrobial activity and potential application as a surface disinfectant. Appl Environ Microbiol. (2003) 69:4611–7. doi: 10.1128/AEM.69.8.4611-4617.2003
48. Thallinger B, Argirova M, Lesseva M, Ludwig R, Sygmund C, Schlick A, et al. Preventing microbial colonisation of catheters: antimicrobial and antibiofilm activities of cellobiose dehydrogenase. Int J Antimicrob Agents. (2014) 44:402–8. doi: 10.1016/j.ijantimicag.2014.06.016
49. Seifu E, Buys EM, Donkin E. Significance of the lactoperoxidase system in the dairy industry and its potential applications: a review. Trends Food Sci Technol. (2005) 16:137–54. doi: 10.1016/j.tifs.2004.11.002
50. James J, Simpson BK, Marshall MR. Application of enzymes in food processing. Crit Rev Food Sci Nutr. (1996) 36:437–63. doi: 10.1080/10408399609527735
51. Juneja VK, Dwivedi HP, Yan X. Novel natural food antimicrobials. Annu Rev Food Sci Technol. (2012) 3:381–403. doi: 10.1146/annurev-food-022811-101241
52. Alonso-González M, Corral-González A, Felix M, Romero A, Martín-Alfonso J. Developing active poly (vinyl alcohol)-based membranes with encapsulated antimicrobial enzymes via electrospinning for food packaging. Int J Biol Macromol. (2020) 162:913–21. doi: 10.1016/j.ijbiomac.2020.06.217
53. Jooyandeh H, Aberoumand A, Nasehi B. Application of lactoperoxidase system in fish and food products: a review. Am Eurasian J Agric Environ Sci. (2011) 10:89–96.
54. Thallinger B, Prasetyo EN, Nyanhongo GS, Guebitz GM. Antimicrobial enzymes: an emerging strategy to fight microbes and microbial biofilms. Biotechnol J. (2013) 8:97–109. doi: 10.1002/biot.201200313
55. Vartiainen J, Rättö M, Paulussen S. Antimicrobial activity of glucose oxidase-immobilized plasma-activated polypropylene films. Packaging Technol Sci Int J. (2005) 18:243–51. doi: 10.1002/pts.695
56. Köksal Z, Alim Z. Lactoperoxidase, an antimicrobial enzyme, is inhibited by some indazoles. Drug Chem Toxicol. (2020) 43:22–6. doi: 10.1080/01480545.2018.1488861
57. Bastarrachea LJ, Wong DE, Roman MJ, Lin Z, Goddard JM. Active packaging coatings. Coatings. (2015) 5:771–91. doi: 10.3390/coatings5040771
58. Anastas PT, Rodriguez A, de Winter TM, Coish P, Zimmerman JB. A review of immobilization techniques to improve the stability and bioactivity of lysozyme. Green Chem Lett Rev. (2021) 14:302–38. doi: 10.1080/17518253.2021.1890840
59. Bafort F, Parisi O, Perraudin JP, Jijakli MH. Mode of Action of lactoperoxidase as related to its antimicrobial activity: a review. Enzyme Res. (2014) 2014:517164. doi: 10.1155/2014/517164
60. Wolfson LM, Sumner SS. Antibacterial activity of the lactoperoxidase system: a review. J Food Prot. (1993) 56:887–92. doi: 10.4315/0362-028X-56.10.887
61. Golmohamadi A, Morra MJ, Popova I, Nindo CI. Optimizing the use of Sinapis alba seed meal extracts as a source of thiocyanate (SCN–) for the lactoperoxidase system. LWT - Food Sci Technol. (2016) 72:416–22. doi: 10.1016/j.lwt.2016.05.007
62. Tayefi-Nasrabadi H. Hoseinpour-fayzi MA, Mohasseli M. Effect of heat treatment on lactoperoxidase activity in camel milk: a comparison with bovine lactoperoxidase. Small Ruminant Res. (2011) 99:187–90. doi: 10.1016/j.smallrumres.2011.04.007
63. Al-Baarri ANm, Damayanti NT, Legowo AM, Tekiner IH, Hayakawa S. Enhanced antibacterial activity of lactoperoxidase–thiocyanate–hydrogen peroxide system in reduced-lactose milk whey. Int J Food Sci. (2019) 2019:8013402. doi: 10.1155/2019/8013402
64. Silva E, Oliveira J, Silva Y, Urbano S, Sales D, Moraes E, et al. Lactoperoxidase system in the dairy industry: challenges and opportunities. Czech J Food Sci. (2020) 38:337–46. doi: 10.17221/103/2020-CJFS
65. Atasever A, Ozdemir H, Gulcin I, Kufrevioglu OI. One-step purification of lactoperoxidase from bovine milk by affinity chromatography. Food Chem. (2013) 136:864–70. doi: 10.1016/j.foodchem.2012.08.072
66. Sisecioglu M, Gülçin I, Çankaya M, Ozdemir H. The inhibitory effects of l-adrenaline on lactoperoxidase enzyme purified from bovine milk. Int J Food Prop. (2012) 15:1190–9. doi: 10.1080/10942912.2010.511924
67. Sisecioglu M, Uguz M, Cankaya M, Özdemir H, Gülçin I. Effects of ceftazidime pentahydrate, prednisolone, amikacin sulfate, ceftriaxone sodium and teicoplanin on bovine milk lactoperoxidase activity. Int J Pharmacol. (2011) 7:79–83. doi: 10.3923/ijp.2011.79.83
68. Sharma S, Singh AK, Kaushik S, Sinha M, Singh RP, Sharma P, et al. Lactoperoxidase: structural insights into the function, ligand binding and inhibition. Int J Biochem Mol Biol. (2013) 4:108–28.
69. Barkhori Mehni S, Khanzadi S, Hashemi M, Azizzadeh M. The effect of sodium alginate coating incorporated with lactoperoxidase system and Zataria multiflora boiss essential oil on shelf life extension of rainbow trout fillets during refrigeration. Iranian J Chem Chem Eng. (2019) 38:1163–172. doi: 10.30492/ijcce.2019.30763
70. Jafary F, Kashanian S, Samsam Sharieat SZ. Purification, immobilization, and characterization of bovine lactoperoxidase. Int J Food Prop. (2013) 16:905–16. doi: 10.1080/10942912.2011.566400
71. Kussendrager KD, van Hooijdonk ACM. Lactoperoxidase: physico-chemical properties, occurrence, mechanism of action and applications. Br J Nutr. (2000) 84:19–25. doi: 10.1017/S0007114500002208
72. Reiter B, Härnulv G. Lactoperoxidase antibacterial system: natural occurrence, biological functions and practical applications. J Food Prot. (1984) 47:724–32. doi: 10.4315/0362-028X-47.9.724
73. Ndambi O, Kamga P, Imele H, Mendi S, Fonteh F. Effects of milk preservation using the lactoperoxidase system on processed yoghurt and cheese quality. African J Food Agric Nutr Dev. (2008) 8:358–74. doi: 10.4314/ajfand.v8i3.19198
74. Lara-Aguilar S, Alcaine SD. Lactose oxidase: a novel activator of the lactoperoxidase system in milk for improved shelf life. J Dairy Sci. (2019) 102:1933–42. doi: 10.3168/jds.2018-15537
75. Al-Baarri ANm, Legowo AM, Arum SK, Hayakawa S. Extending shelf life of Indonesian soft milk cheese (Dangke) by lactoperoxidase system and lysozyme. Int J Food Sci. (2018) 2018:4305395. doi: 10.1155/2018/4305395
76. Ozdemir H, Aygul I, Küfrevioglu OI. Purification of lactoperoxidase from bovine milk and investigation of the kinetic properties. Prep Biochem Biotechnol. (2001) 31:125–34. doi: 10.1081/PB-100103378
77. Cals MM Mailliart P, Brignon G, Anglade P, Dumas BR. Primary structure of bovine lactoperoxidase, a fourth member of a mammalian heme peroxidase family. Eur J Biochem. (1991) 198:733–9. doi: 10.1111/j.1432-1033.1991.tb16073.x
78. Booth KS, Kimura S, Lee HC, Ikeda-Saito M, Caughey WS. Bovine myeloperoxidase and lactoperoxidase each contain a high affinity site for calcium. Biochem Biophys Res Commun. (1989) 160:897–902. doi: 10.1016/0006-291X(89)92519-9
79. Koksal Z, Gulcin I, Ozdemir H. An important milk enzyme: lactoperoxidase. In: Gigli I, editor. Milk Proteins-from Structure to Biological Properties and Health Aspects. Rijeka: InTech Open (2016). p. 141–15.
80. Niaz B, Saeed F, Ahmed A, Imran M, Maan AA, Khan MK, et al. Lactoferrin (LF): A natural antimicrobial protein. Int J Food Prop. (2019) 22:1626–41. doi: 10.1080/10942912.2019.1666137
81. Dumitraşcu L, Stănciuc N, Stanciu S, Râpeanu G. Thermal inactivation of lactoperoxidase in goat, sheep and bovine milk – a comparative kinetic and thermodynamic study. J Food Eng. (2012) 113:47–52. doi: 10.1016/j.jfoodeng.2012.05.028
82. Narkowicz S, Jaszczak E, Polkowska Z, Kiełbratowska B, Kotłowska A, Namieśnik J. Determination of thiocyanate as a biomarker of tobacco smoke constituents in selected biological materials of human origin. Biomed Chromatogr. (2018) 32:e4111. doi: 10.1002/bmc.4111
83. Wijkstrom-Frei C, El-Chemaly S, Ali-Rachedi R, Gerson C, Cobas MA, Forteza R, et al. Lactoperoxidase and human airway host defense. Am J Respir Cell Mol Biol. (2003) 29:206–12. doi: 10.1165/rcmb.2002-0152OC
84. Yong L, Wang Y, Yang D, Liu Z, Abernethy G, Li J. Investigation of concentration of thiocyanate ion in raw cow's milk from China, New Zealand and the Netherlands. Food Chem. (2017) 215:61–6. doi: 10.1016/j.foodchem.2016.07.130
85. Fweja LWT, Lewis MJ, Grandison AS. Challenge testing the lactoperoxidase system against a range of bacteria using different activation agents. J Dairy Sci. (2008) 91:2566–74. doi: 10.3168/jds.2007-0322
86. Codex Alimentarius Commission. Report of the Twenty-Seventh Session of the Codex Alimentarius Commission. Codex Alimentarius Commission, 28 June – 3 July, 2004. Geneva, Switzerland, Document No 04/27/41 (2004).
87. Bjorck L. The lactoperoxidase/thiocyanate/hydrogen peroxide system as a temporary preservative for raw milk in developing countries. Milchwissenschaft. (1979) 34:726–29.
88. Jooyandeh H, Aberoumand A, Nasehi B. Application of lactoperoxidase system in fish and food products: a review. Am. Eurasian J Agric Environ Sci. (2011) 10:89–96.
89. World Health Organization. Benefits and Potential Risks of the Lactoperoxidase System of Raw Milk Preservation: Report of an FAO (2006).
90. Animal Production and Health Division. Manual on the Use of the LP-System in Milk Handling and Preservation. Food & Agriculture Organization (1999).
91. Wit dJ, Van Hooydonk A. Structure, functions and applications of lactoperoxidase in natural antimicrobial systems. Netherlands Milk Dairy J. (1996) 50:227–44.
92. Wolfson LM, Sumner SS. Antibacterial activity of the lactoperoxidase system against Salmonella typhimurium in trypticase soy broth in the presence and absence of a heat treatment. J Food Prot. (1994) 57:365–8. doi: 10.4315/0362-028X-57.5.365
93. Arefin S, Sarker AH, Islam MA. Harun-ur-Rashid, Islam N. Use of Hydrogen Peroxide (H2O2) in raw cow's milk preservation. J Adv Vet Anim Res. (2017) 4:371–7. doi: 10.5455/javar.2017.d236
94. Özer B, Grandison A, Robinson R, Atamer M. Effects of lactoperoxidase and hydrogen peroxide on rheological properties of yoghurt. J Dairy Res. (2003) 70:227–32. doi: 10.1017/S0022029903006149
95. Reiter B, Perraudin J. Lactoperoxidase: Biological Functions in Peroxidases in Chemistry and Biology. Everse J, Everse, KE, Grisham MB, editors. Vol. 1. Boca Raton, FL: CRC Press (1991).
96. Al-Baarri AN, Ogawa M, Hayakawa S. Application of lactoperoxidase system using bovine whey and the effect of storage condition on lactoperoxidase activity. Int J Dairy Sci. (2011) 6:72–8. doi: 10.3923/ijds.2011.72.78
97. Ghibaudi E, Laurenti E. Unraveling the catalytic mechanism of lactoperoxidase and myeloperoxidase: a reflection on some controversial features. Eur J Biochem. (2003) 270:4403–12. doi: 10.1046/j.1432-1033.2003.03849.x
98. Pruitt KM, Kamau DN. The lactoperoxidase system of bovine and human milk. In: Robinson DS, Eskin NAM, editors. Oxidative Enzymes in Foods. London: Elsevier Applied Science (1991). p. 133–74.
99. Ashby MT. Hypothiocyanite. Adv Inorg Chem. (2012) 64:263–303. doi: 10.1016/B978-0-12-396462-5.00008-8
100. Thomas EL. Products of Lactoperoxidase-Catalyzed Oxidation of Thiocyanate and Halides. New York, NY: Marcel Dekker (1985).
101. Sarr D, Tóth E, Gingerich A, Rada B. Antimicrobial actions of dual oxidases and lactoperoxidase. J Microbiol. (2018) 56:373–86. doi: 10.1007/s12275-018-7545-1
102. Almehdar HA, El-Fakharany EM, Uversky VN, Redwan EM. Disorder in milk proteins: structure, functional disorder, and biocidal potentials of lactoperoxidase. Curr Prot Peptide Sci. (2015) 16:352–65. doi: 10.2174/1389203716666150316114956
103. Sousa SG, Santos MD, Fidalgo LG, Delgadillo I, Saraiva JA. Effect of thermal pasteurisation and high-pressure processing on immunoglobulin content and lysozyme and lactoperoxidase activity in human colostrum. Food Chem. (2014) 151:79–85. doi: 10.1016/j.foodchem.2013.11.024
104. Al-Baarri AN, Ogawa M, Hayakawa S. Scale-up studies on immobilization of lactoperoxidase using milk whey for producing antimicrobial agent. J Indonesian Trop Anim Agric. (2010) 35:185–91. doi: 10.14710/jitaa.35.3.185-191
105. Björck L, Rosen C, Marshall V, Reiter B. Antibacterial activity of the lactoperoxidase system in milk against pseudomonads and other gram-negative bacteria. Appl Microbiol. (1975) 30:199–204. doi: 10.1128/am.30.2.199-204.1975
106. Marks N, Grandison A, Lewis M. Challenge testing of the lactoperoxidase system in pasteurized milk. J Appl Microbiol. (2001) 91:735–41. doi: 10.1046/j.1365-2672.2001.01435.x
107. Benbettaïeb N, Debeaufort F, Karbowiak T. Bioactive edible films for food applications: mechanisms of antimicrobial and antioxidant activity. Crit Rev Food Sci Nutr. (2019) 59:3431–55. doi: 10.1080/10408398.2018.1494132
108. Campos CA, Gerschenson LN, Flores SK. Development of edible films and coatings with antimicrobial activity. Food Bioprocess Technol. (2011) 4:849–75. doi: 10.1007/s11947-010-0434-1
109. Lee H, Min SC. Antimicrobial edible defatted soybean meal-based films incorporating the lactoperoxidase system. LWT Food Sci Technol. (2013) 54:42–50. doi: 10.1016/j.lwt.2013.05.012
110. Ozturk G, German JB, de Moura Bell JM. Effects of industrial heat treatments on the kinetics of inactivation of antimicrobial bovine milk xanthine oxidase. NPJ Sci Food. (2019) 3:1–7. doi: 10.1038/s41538-019-0046-8
111. Yeroslavsky G, Girshevitz O, Foster-Frey J, Donovan DM, Rahimipour S. Antibacterial and antibiofilm surfaces through polydopamine-assisted immobilization of lysostaphin as an antibacterial enzyme. Langmuir. (2015) 31:1064–73. doi: 10.1021/la503911m
112. Cynthya M, Prabhawathi V, Mukesh D. Papain immobilized polyurethane film as antimicrobial food package. World Acad Sci Eng Technol Int J Bioeng Life Sci. (2014) 8:1367–70. doi: 10.5281/zenodo.1097287
113. Jasour MS, Ehsani A, Mehryar L, Naghibi SS. Chitosan coating incorporated with the lactoperoxidase system: an active edible coating for fish preservation. J Sci Food Agric. (2015) 95:1373–8. doi: 10.1002/jsfa.6838
114. Cissé M, Polidori J, Montet D, Loiseau G, Ducamp-Collin MN. Preservation of mango quality by using functional chitosan-lactoperoxidase systems coatings. Postharvest Biol Technol. (2015) 101:10–4. doi: 10.1016/j.postharvbio.2014.11.003
115. Rostami H, Abbaszadeh S, Shokri S. Combined effects of lactoperoxidase system-whey protein coating and modified atmosphere packaging on the microbiological, chemical and sensory attributes of Pike-Perch fillets. J Food Sci Technol. (2017) 54:3243–50. doi: 10.1007/s13197-017-2767-5
Keywords: lactoperoxidase, film, coating, antimicrobial, enzyme, preservation
Citation: Yousefi M, Nematollahi A, Shadnoush M, Mortazavian AM and Khorshidian N (2022) Antimicrobial Activity of Films and Coatings Containing Lactoperoxidase System: A Review. Front. Nutr. 9:828065. doi: 10.3389/fnut.2022.828065
Received: 02 December 2021; Accepted: 31 January 2022;
Published: 04 March 2022.
Edited by:
Ahmet Yemenicioglu, Izmir Institute of Technology, TurkeyReviewed by:
Dilhun Keriman Arserim Ucar, Bingöl University, TurkeyIlhami Gulcin, Atatürk University, Turkey
Çigdem Mecitoglu Güçbilmez, Bahri Dagdas International Agricultural Research Institute (BDUTAE), Turkey
Copyright © 2022 Yousefi, Nematollahi, Shadnoush, Mortazavian and Khorshidian. This is an open-access article distributed under the terms of the Creative Commons Attribution License (CC BY). The use, distribution or reproduction in other forums is permitted, provided the original author(s) and the copyright owner(s) are credited and that the original publication in this journal is cited, in accordance with accepted academic practice. No use, distribution or reproduction is permitted which does not comply with these terms.
*Correspondence: Nasim Khorshidian, bmtob3JzaGlkaWFuQHNibXUuYWMuaXI=