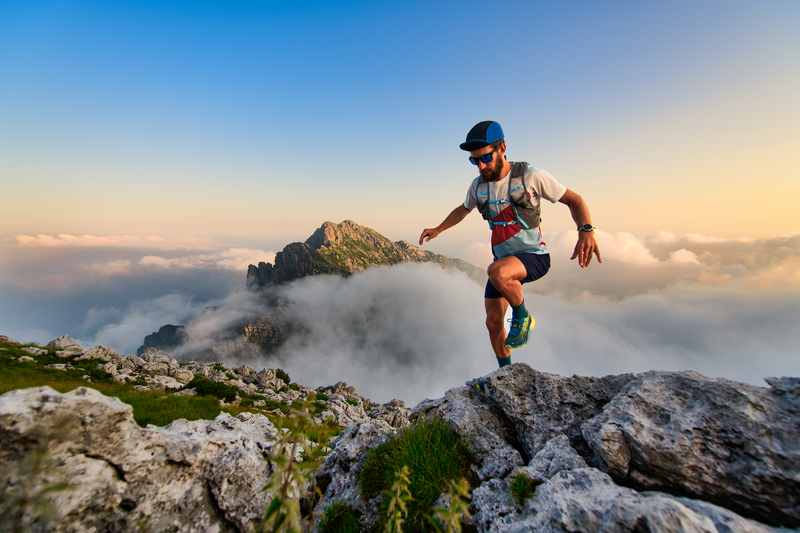
94% of researchers rate our articles as excellent or good
Learn more about the work of our research integrity team to safeguard the quality of each article we publish.
Find out more
ORIGINAL RESEARCH article
Front. Nutr. , 13 June 2022
Sec. Food Chemistry
Volume 9 - 2022 | https://doi.org/10.3389/fnut.2022.808351
This article is part of the Research Topic Food Protein-based Colloids: Structure, Digestion, and Nutrients Delivery View all 12 articles
Proteins and minerals in infant formula not only serve as nutrients, but also have important effects on the physical and chemical stability of emulsions. In this study, calcium carbonate (0 or 9.08 mM) and potassium chloride (0 or 15.96 mM), as representatives of divalent and monovalent minerals, were added to 1,3-dioleoyl-2-palmitoylglycerol (OPO) emulsions in different ratios (10:0, 9:1, 6:4, 5:5, and 0:10) of whey protein isolate (WPI) and sodium caseinate (CN). The influence of proteins and minerals on emulsion stability was investigated by analyzing particle size, zeta potential, creaming index, rheological properties, storage stability, and lipid oxidation. 1,3-dioleoyl-2-palmitoylglycerol (OPO) emulsions could be destabilized by adding Ca2+, as shown by the increase in particle size index, creaming index, and the decrease in zeta potential magnitude. Divalent ions could affect the electrostatic interactions between lipid droplets and the interactive effects of ion surface adsorption. In addition, the effect of different protein ratios on the physical stability of emulsions was not significant under the same ion-type conditions. In terms of chemical stability, higher oxidized values were found in emulsions stabilized with only CN than in those containing WPI. Our study showed that protein ratios and minerals played an important role in the stability of OPO emulsions, which might provide a reference for the development and utilization of liquid infant formula.
In the first 6 months of life, breast milk from healthy and well-nourished mothers is regarded as the best food for infants (1). In addition, it contains nutrients that aid in the protection and maturation of the infant intestine (2). Breast milk contains 3–5% fat, 0.8–0.9% protein, 6.9–7.2% carbohydrate, and 0.2% minerals, and other physiologically active components (IgA, bifidus factor, etc.) (3). Among the macronutrients, breast milk lipids are the primary source of energy and vital nutrients for the newborn. In terms of the composition of breast milk fat, triglycerides account for 98% of the fat content and oleic acid, palmitic acid, and linoleic acid are the three most abundant fatty acids, followed by stearic and myristic acids, where 70% of palmitic acid is esterified at the sn-2 position and unsaturated fatty acids (oleic acid, linoleic acid, etc.) are located at the sn-1,3 position. The unique composition and distribution of fatty acids leads to a representative human milk fat named 1,3-dioleoyl-2-palmitoylglycerol (OPO) (4, 5).
Human milk fat substitutes refer to the triglyceride mixture synthesized by modern enzymatic modification technology. It modifies animal and vegetable fats according to the nutritional composition, fatty acid composition, and fat distribution of human milk (6, 7). It is intended to meet the nutritional needs of infants and their growth and development. Carnielli et al. provided solid evidence that breastfed newborns absorbed palmitic acid at the sn-2 position (8). López-Lópe et al. compared the total fatty acid compositions with the sn-2 positional fatty acid compositions of colostrum, transitional milk, mature milk, and infant formula. They found that infant formula containing sn-2 positional palmitic acid is more easily absorbed than regular formula (9). Koo et al. demonstrated that palmitic acid in the sn-2 position of infant formula had a physiological function, including enhancing calcium absorption in the small intestine and decreasing bone mass (10). OPO, as a triglyceride typical of breast fat, influences infant digestion and absorption, making OPO a value-added ingredient in commercial powder infant milk.
Currently, liquid infant formula is a potential popular product because it is easier to drink and has brighter packaging that appeals to infants and young children. For the development of liquid infant formula products, the physical and chemical stability of these products are just as crucial as their nutritional content. The emulsifier has a significant impact on emulsion stability. As main milk proteins, whey protein (WP) and casein are important emulsifiers in liquid infant formula products, which have also been widely used in the food industry (11). The ratio of WP to casein in breast milk varied depending on the stage of breastfeeding, for example, it could be as high as 9:1 in early lactation and subsequently decline to 5:5 in late lactation. An overall ratio of roughly 6:4 is used in most infant formula products. Milk proteins adsorb at the interface between the two phases of the oil-in-water (O/W) system, forming a protective layer on the surface of the lipid droplet before and after homogenization and inhibiting interfacial tension to maintain emulsions. It is well known that WP and casein have different properties, which have an impact on the physical and chemical stability of liquid infant formula.
Minerals in infant formula have a significant impact on the physical and chemical stability of emulsions, in addition to the complex fat structure and protein composition. Minerals boost the ionic strength in the aqueous phase, lowering the electrostatic repulsion between droplets and promoting phase separation (12–15). Some minerals bind to oppositely charged groups on the surface of emulsion droplets, decreasing their zeta potential and thus reducing electrostatic repulsion (16). In several commercial emulsions, calcium-induced flocculation and creaming have been identified as the sources of long-term destabilization (17).
Previous studies have shown that the creaming stability of emulsions with 0.5% protein decreased, while the emulsion with 3% protein was not affected by the addition of CaCl2 to 0.5% (18, 19). In this study, an OPO emulsion was prepared to imitate liquid infant formula. We investigated the effect of a series of protein ratios (10:0, 9:1, 6:4, 5:5, and 0:10) and ion types on the physicochemical properties of the OPO emulsion. The ratios were selected based on the content of WP and casein in breast milk throughout the lactation period and the combined effect of monovalent (represented by K+) and divalent (represented by Ca2+) ions in infant formula milk powder. This study may provide a theoretical basis for the physical stability of liquid infant formula.
1, 3-dioleic-2-palmitate triglyceride-type human milk fat substitutes (triglycerides > 98%) were kindly donated by Renzhichu Technology Group Ltd. (Jiangxi, China), which would be used directly for producing infant formula. Provon® whey protein isolates (WPI, purity 90%) were purchased from Glanbia Nutritionals Ltd. (Ireland). Sodium caseinate (CN, BR, from bovine milk) was purchased from Macklin Biochemical Co., Ltd. (Shanghai, China). Other solvents and reagents were of analytical grade.
Oil-in-water emulsions were prepared according to previous reports with simple modifications. Briefly, an aqueous solution consisting of WPI: CN (1% w/v in total) and phosphate buffered saline (PBS, 0.01 M, pH 7.0) was stirred for 5 h to ensure complete dissolution of the protein. After storing the emulsion at 4°C overnight, Ca2+ and K+concentrations (9.08 and 15.96 mM) were added once the protein dispersion had returned to room temperature. The solutions were stirred for 10 min using a DS-101S magnetic stirring apparatus (Yarong Instrument Ltd., Zhengzhou, China) to ensure complete dissolution of the salts. To make a fresh emulsion, 10% (v/v) of OPO human milk fat substitutes were mixed with sufficient dissolution at 12,000 rpm for 3 min, homogenized 2 times at 30 MPa, and loaded with sodium azide (0.02% w/v) as an antibacterial agent. The pH of the emulsion was adjusted to 7.0 with 1 M NaOH and/or 1 M HCl for analysis.
The particle size distributions and zeta potential of infant formula emulsion samples were measured using a nano-ZSE laser particle size analyzer (Malvern Instruments Ltd., England) according to dynamic light scattering. Infant formula (×100 dilution) emulsion samples were prepared to avoid multiple scattering effects, and the absorption of 0.01 was used to calculate particle size distributions as described previously (20). Each sample was tested three times, and the data are presented as average ± standard deviation (SD).
The infant formula emulsion sample (pH 7.0) was transferred to a test tube [internal diameter (Φ) = 20 mm, height (H) = 500 mm], and then stored at room temperature for 4 months. After storage, several samples were separated into a thin “cream” layer at the top and a transparent “serum” layer at the bottom (21). The creaming destabilization kinetics was evaluated by measuring creaming index as the percentage of serum layer height (HS) from the total emulsion height (HT) (22).
Rheological properties were carried out in a DHR-2 rheometer (TA Instruments Ltd., New Castle, DE, USA). The temperature was maintained at 25 ± 0.5°C. The apparent viscosity of the emulsion sample was measured upon shear rate ramp-up from 5 to 100 s−1 according to the modified method as described previously (23). The exposed surfaces of the samples were covered with a thin layer of silicone oil to prevent dehydration. The shear rate was then recorded as the shear stress was increased.
The infant formula emulsion sample (2 ml) was poured into a cuvette and then stored at room temperature for 40 days, and 5 μl of each sample was taken out at the time intervals of 0, 8, 16, 24, 32, and 40 days for analysis. The physical stability of emulsions during storage was characterized by regular sampling and measuring their average particle size and zeta potential.
The method for characterizing the oxidation stability of oil is based on the method of Qiu et al. (24) and slightly modified. The emulsion sample was placed in an oven at 60°C, and the samples were taken out at different time periods (0, 10, 20, 30, and 40 days) for the analysis of oxidation state.
Primary oxidation product hydrogen peroxide value (POV) was measured according to the report of Zeng et al. (25) with slight modifications. Briefly, 1 ml of the emulsion sample was vortexed vigorously three times with 5 ml of isooctane: isopropanol (2:1 v/v) followed by centrifugation for 5 min at 2,800 × g (FA-45-6-30 rotor, centrifuge 5804R, Eppendorf AG, Hamburg, Germany). Then, 0.2 ml of the isooctane: isopropanol extract was mixed with 4.8 ml of methanol: butanol (2:1 v/v). This mixture was then reacted with 20 μl of 3.94 M potassium thiocyanate and 20 μl of ferrous iron solution. The mixture was allowed to react for 20 min at room temperature in the dark before measuring the absorbance of the sample at 510 nm using a TU-1900 spectrophotometer (Baiqi Biotechnology Ltd., Jinan, China). The concentration of hydroperoxides was determined based on a standard curve of hydroperoxide.
Thiobarbituric acid reactive substances (TBARS) were measured according to Xu et al. (26). The infant formula emulsion sample (0.6 ml) was combined with 4.0 ml of TBA (thiobarbituric acid) solution (prepared by mixing 15 g of trichloroacetic acid, 0.375 g of TBA, 1.76 ml of 12 M HCl, and 82.9 ml of H2O) in test tubes and placed in a boiling water bath for 30 min. The tubes were cooled to room temperature for 10 min and then centrifuged at 860 × g (2,500 rpm) for 20 min followed by measuring the absorbance at 532 nm. TBARS were calculated from a standard curve prepared with 1,1,3,3-tetraethoxypropane.
The results are presented as means ± SD using SPSS 18.0 software (IBM Corporation, NY, USA) and Origin 85 software (Microsoft Corporation, Redmond, WA, USA). The statistical analysis was performed by one-way ANOVA followed by Duncan's test at p < 0.05.
Particle size distribution in the OPO emulsion samples is illustrated in Figure 1. In Figure 1A, the emulsion in the control (without ions) with a higher WPI ratio (10:0 and 9:1) was characterized with larger particles and a tail peak in peak distribution. The result indicated that a number of original droplets had accumulated. Emulsions with increasing CN ratios (6:4, 5:5, and 0:10) showed a single peak distribution and a tight particle size distribution, indicating a good symmetry dispersion. Compared with the control, the particle size distribution of the ion groups showed a bimodal distribution. The size distribution was control group < K+ group < Ca2+ group, indicating that the large particles in emulsions were gradually increased and formed by droplet aggregation.
Figure 1. Particle size distribution of control (A), containing K+ (B), and Ca2+ (C) of 1,3-dioleoyl-2-palmitoylglycerol (OPO) emulsions (10% v/v OPO human milk fat substitutes) with different ratios of whey protein isolate (WPI) and caseinate (CN; 10:0, 9:1, 6:4, 5:5, 0:10, 1% w/v in total) as emulsifiers.
The zeta potential values of emulsions with various WPI and CN ratios are shown in Figure 2. The zeta potential amplitude of the control samples (without ionic addition) decreased as the CN concentration in emulsions increased. When adding K+ and Ca2+ into emulsions, the absolute zeta potential value did not change significantly with increasing CN ratio. Under the conditions of different or same protein ratios, the absolute zeta potential value was K+ group > Ca2+ group and the change in the absolute zeta potential value was Ca2+ group > K+ group > control group.
Figure 2. Zeta potential of fresh OPO emulsions (10% v/v OPO human milk fat substitutes) with different ratios of WPI and CN (10:0, 9:1, 6:4, 5:5, 0:10, 1% w/v in total) as emulsifiers. The results are expressed as mean ± SD (n = 3). Different letters (a–j) indicate significant differences at the 0.05 level.
According to Table 1, the impacts of different protein ratios and mineral ions in emulsions did not show layering behavior when emulsions were placed at room temperature for 20 days, except the 6:4 and 5:5 (WPI:CN) emulsions after adding K+ and Ca2+, which showed a slight alteration (7.92 ± 0.71, 13.59 ± 0.43, 10.79 ± 2.76, and 7.13 ± 1.24%, respectively). However, varying degrees of cream layer separation were found from 20 to 120 days. Emulsions emulsified by WPI were stable, whereas emulsions with K+ and Ca2+ showed the most obvious layering phenomenon (81.69 ± 1.84 and 82.02 ± 6.44%). It is shown that the overabundance of unabsorbed biopolymers resulted in higher droplet accumulation, larger particles, and more pronounced stratification behavior after longer storage time.
Table 1. The creaming index of fresh 1,3-dioleoyl-2-palmitoylglycerol (OPO) emulsions (10% v/v OPO human milk fat substitutes and whey protein isolate (WPI): CN/10:0, 9:1, 6:4, 5:5, 0:10, 1% w/v in total) for 120 days.
The rheological behavior of the OPO emulsion system was examined as a function of different protein ratios and ionic strength. In particular, the flow behavior of the emulsion sample was investigated taking into account the dependence of the shear stress on the sample viscosity. The effect of different protein ratios and ionic strength on the rheological properties of emulsions was examined (Figures 3A–D). The flocculated emulsions exhibited strong shear thinning behavior (Figure 3). Viscosity decreased with increasing shear rate. When the shear rate was in the range of 5–10 s−1, the viscosity of each emulsion group decreased rapidly as shear stress increased. Then, the viscosity tended to be consistent within the shear rate of 20–100 s−1. Compared with Figure 3A, the viscosity of the K+ and Ca2+ groups was higher than the control group in the range of 5–10 s−1. This difference could be attributed to factors such as flocculated volume fraction of the droplets, flocculation size, and spatial distribution or intensity of interaction between flocculated droplets (27). Different protein ratios were found to be insensitive to changes in emulsion viscosity.
Figure 3. Shear stress and viscosity relationships of OPO emulsions (10% v/v OPO human milk fat substitutes) at different protein ratios (WPI:CN/10:0, 9:1, 6:4, 5:5 and 0:10, 1% w/v in total; (A) either in the presence of K+ (B) or Ca2+ (C). (D) Comparison of the shear stress and viscosity relationship of OPO emulsions for WPI:CN/6:4 (control) with K+ or Ca2+.
To understand the relationship between shear stress and viscosity of emulsions by the addition of K+ and Ca2+, only the ratio of 6:4 (WPI:CN) was chosen for viscosity analysis. Figure 3D shows that the viscosity of the 6:4 emulsion without salt ions is lower than that of the emulsion with K+ and Ca2+, which was Ca2+ group > K+ group > control group in the range of 5–20 s−1.
The storage stability (particle size and zeta potential) of emulsions after 40 days is shown in Table 2. The results showed that the emulsion particle size gradually increased with storage time. It was also demonstrated that the absolute value of the zeta potential gradually decreased as the emulsion storage time increased. In detail, the particle size of emulsions without ions and with K+ or Ca2+ were 355–460, 426–507, and 424–514 nm, respectively, after storage for 40 days. In addition, the zeta potential in the control, K+, and Ca2+ groups was −41.53~-51.27, −39.53~-52.33, and −29.67~-36.10 mV, respectively, after storage for 40 days. According to the analysis with or without K+ and Ca2+, the trend of the physical stability of emulsions during storage decreased in the following order: control group > K+ group > Ca2+ group.
Table 2. Effects of K+ and Ca2+ on storage stability (particle size and zeta potential) in different OPO emulsions (10% v/v OPO human milk fat substitutes and WPI: CN/10:0, 9:1, 6:4, 5:5, 0:10, 1% w/v in total).
In this study, the rate of lipid oxidation in different emulsions containing K+ or Ca2+ was measured. All samples were kept at 60°C for 40 days to accelerate lipid oxidation. To investigate the rate of lipid oxidation, primary and secondary lipid oxidation products were measured as POV and TBARS (Figure 4), respectively. The results showed that the rate of lipid oxidation increased at first and then decreased as the storage time was prolonged. Due to its more compact structure and lack of phosphate groups, WPI might limit its iron-binding ability and thus promote oxidation (28). In addition, the relative oxidation value of emulsions with CN alone as the emulsifier was greater than that of other emulsions with varied ratios of WPI, based on the POV and TBARS content in Figure 4. The higher oxidation rate might be because the affinity of phosphate groups of casein molecules for iron was better than that of carboxylic acid groups of other proteins (29). In the 30-day oxidation stage, the POV content at different protein ratios (10:0, 9:1, 6:4, 5:5, and 0:10) without and with K+ and Ca2+ was 3.39–9.39, 10.73–13.52, and 11.41–15.18 μg/ml and the TBARS content was 0.05–0.16, 0.06–0.35, and 0.08–0.56 μg/ml, respectively. These data indicated that K+ and Ca2+ can promote the oxidation of emulsions, possibly because these ions destabilize the physical state of emulsions as mentioned above.
Figure 4. The peroxidevalue (POV) and thiobarbituric acid reactive substances (TBARS) of control, containing K+ (A–C) and containing Ca2+ (a–c) in OPO emulsions (10% v/v OPO human milk fat substitutes and WPI: CN/10:0, 9:1, 6:4, 5:5, 0:10, 1% w/v in total) as emulsifiers.
Whey protein isolate and CN are typical protein-type emulsifiers, which can exert their emulsification and adsorption properties in emulsions and are improved by electrostatic or three-dimensional repulsive forces to maintain emulsion stability (30). CN, characterized by its disordered structure and hydrophobic properties, possesses greater adsorption at the oil–water interface than a tiny, compact, and globular WPI (31).
As represented in Figures 1B,C, K+ and Ca2+ binding may induce the aggregation of emulsion droplets as salt ions reduce the repulsive force between droplets (32). However, the particle size distribution peak of the samples shifted toward larger particle size with the addition of potassium ions, except for the ratio 5:5 in Figure 1B. According to Keowmaneechai et al., surfactants such as casein to replace the WP absorbed from the droplet surface, the aggregation induced by metal ions was found to be almost reversible (33). In addition, the homogenized proteins were exposed to more calcium binding sites, which might accelerate particle aggregation (34).
Therefore, compared with K+, emulsions with Ca2+ produce extensive droplet aggregation resulting in an unstable emulsion. The absolute value of the zeta potential was the highest when the proportion of CN was the highest, as shown in Figure 2. Amino acid side chains from protein dissociation are more conducive to induce electrostatic repulsion to enhance emulsion stability when the pH (the pH of emulsions is seven) is further away from the emulsifier's isoelectric point (35, 36). At pH values above the isoelectric point, protein molecules have more –COO− groups than –NH3+ groups, so protein molecules present more negatively charged groups. It has been reported that Ca2+ can bind to the two main proteins of whey protein through the free carboxylic groups of aspartic and glutamic acids (35, 37). Therefore, Ca2+ could combine with the –COO− groups to reduce the negative charge on the surface of emulsion droplets in this study. In the absence of K+, emulsions might lead to a less extensive diffuse double layer, interdroplet repulsion, and eventually droplet aggregation (38). The effect of ion valence on emulsion zeta potential in this study indicated that multivalent ions in the emulsion were more effective than monovalent ions combined with countercharge in the interaction effects of electrostatic shielding and ion surface adsorption. Our results were consistent with the conclusions of previous studies (39).
Creaming index is an important indicator to measure the degree of aggregation of lipid droplets in the emulsion. Lipid droplet network layout, structural rearrangement, and final phase separation kinetics are the critical aspects, which impact emulsion instability, and depletion flocculation is one of the main causes (40–42). Emulsions with a high creaming index are generally unstable (16). According to Srinivasan et al., a high CN emulsion content resulted in low stability due to depletion flocculation generated by the unabsorbed CN in the aqueous phase (43). Our results showed that the addition of K+ and Ca2+ plays an important role in emulsion stability. The electrostatic repulsion between the droplets was gradually shielded by the anti-ions around the droplets, and the small particulate matter adsorbed on each other, so that the large particles aggregated and the fat floated upward with the addition of K+ and Ca2+ (44). In addition, the effects of K+ and Ca2+ on creaming index might be gradually increasing particle size and making droplet aggregation to result in flocculation with storage days. A higher degree of flocculation is preferable to a higher degree of creaming in emulsions (45). Creaming instability measurements in the absence of K+ and Ca2+ support the result of particle size and zeta potential measurements.
The rheological property is also a key factor to consider in O/W emulsion stability. Previous research showed that the floccules created in the emulsion trapped water molecules in the two oil–water phases. This increased the effective volume fraction of particles and promoted the flocculated emulsion to have a higher viscosity than the unflocculated emulsion (45, 46). Our results indicated that the Ca2+- containing emulsion has a higher viscosity, as shown in Figure 3D. It would be expected that Ca2+ could promote the flocculation of droplets to a greater extent and increase its strength due to electrostatic screen interactions, reduce droplet charge, and act as ionic bridges, while potassium ion could only play a role of electrostatic screen interactions, with weak strength and low emulsion viscosity (33).
Emulsion destabilization is often due to various physical phenomena. As shown in Table 2, the emulsion particle size in each group increased to different degrees and the Ca2+ group had the highest particle size on day 40 of the storage period. Previous studies have indicated that flocculation, rather than coalescence, is the cause of droplet aggregation of emulsions with calcium (19) and potassium (47). Flocculation occurs because the metal ions reduce the electrostatic repulsion between the droplets, thus these droplets get closer. According to Ray and Rousseau, droplet aggregation caused by electrostatic action is blocked when the absolute value of the zeta potential of the emulsion is higher than 30 mV (58) (48). In contrast, the absolute value of the zeta potential of a calcium ion was 29.67 ± 0.40, which is the smallest among the three groups. This result showed that the emulsion of adding Ca2+ would be unstable, which was consistent with the previous results. The emulsion particle size and zeta potential during the 40 days of study indicated that the storage stability of each emulsion is different due to factors such as protein ratios and ionic strength, which is consistent with the results reported by Ye (49). The above results indicated that K+ and Ca2+ affect the long-term stability of OPO emulsions, which may have significant effects on the formulation of potassium- and calcium-enhanced emulsion-based products with a long expected shelf-life.
In addition to physical properties, lipid oxidation is a major issue in food storage and consumption, influencing the flavor, odor, and color of food (11). Our results showed that lipid oxidation might be caused by the different structures of WPI and CN, enabling their affinity for iron to be different. However, during the oxidation process, the principal product, hydroperoxide, dissociates into secondary oxidation products, such as malondialdehyde and hexanal, resulting in a decrease in the oxidation content of POV (50).
As shown in Figures 4B-b,C-c, our results indicated that both POV and TBARS values increase after adding K+ and Ca2+ into the emulsion, which can promote the oxidation of emulsions enabling emulsions to be unstable. The results were the same as particle size and creaming index.
We chose the different ratios of WPI and CN to better simulate the different stages of breastfeeding, and added OPO human milk fat substitute, which was conducive to the digestion and absorption of breast milk by infants. Furthermore, minerals are also essential, and K+ and Ca2+ account for a high proportion of minerals in infant formula. Thus, macronutrients and micronutrients complement each other. However, this infant formula was not ideal, which might be related to the concentration of added minerals. Therefore, the effect of varying mineral ion concentrations on the physicochemical properties of the OPO emulsion must be thoroughly explored to prepare a stable liquid infant formula. In addition, from a nutritional point of view, in vitro gastrointestinal digestion would be ideal, and could be further investigated.
This study demonstrates the impact of protein ratios and mineral ions on the stability of OPO emulsions as mimics of a liquid infant formula. Changes in WPI to CN ratios had a significant impact on the zeta potential but not on particle size. The addition of Ca2+ increased the average particle size and decreased the zeta potential. It caused emulsion instability, possibly because Ca2+ reduced the charge of the droplets and acted as an ionic bridge by shielding the interaction between static electricity and ions. These interactions promoted flocculation of the droplets and subsequently failed to prevent oil oxidation.
The raw data supporting the conclusions of this article will be made available by the authors, without undue reservation.
QW finished the conceptualization, set the methodology, and wrote the original draft. YX finished the methodology and analyzed the data with the software. YL finished the investigation and validation. FQ guided the methodology and supervised the results. GM and XZ participated in project administration, acquired funding, and reviewed and edited this manuscript. All authors have read and accepted the published version of this manuscript.
The authors declare that the research was conducted in the absence of any commercial or financial relationships that could be construed as a potential conflict of interest.
All claims expressed in this article are solely those of the authors and do not necessarily represent those of their affiliated organizations, or those of the publisher, the editors and the reviewers. Any product that may be evaluated in this article, or claim that may be made by its manufacturer, is not guaranteed or endorsed by the publisher.
This research was funded by the National Natural Science Foundation of China (Nos. 31901614, 31660470).
1. Walker A. Breast milk as the gold standard for protective nutrients. J Pediatr. (2010) 156:S3–7. doi: 10.1016/j.jpeds.2009.11.021
2. He S, Liu G, Zhu X. Human breast milk-derived exosomes may help maintain intestinal epithelial barrier integrity. Pediatr Res. (2021) 90:366–72. doi: 10.1038/s41390-021-01449-y
3. Demmelmair H, Koletzko B. Lipids in human milk. Best practice & research. Clin Endocrinol Metab. (2018) 32:57–68. doi: 10.1016/j.beem.2017.11.002
4. Qin XL, Zhong JF, Wang YH, Yang B, Lan DM, Wang FH. 1,3-Dioleoyl-2-palmitoylglycerol-rich human milk fat substitutes: Production, purification, characterization and modeling of the formulation. Eur J Lipid Sci Technol. (2014) 116:282–90. doi: 10.1002/ejlt.201300343
5. Wei W, Feng Y, Zhang X, Cao X, Feng, F. Synthesis of structured lipid 1,3-dioleoyl-2-palmitoylglycerol in both solvent and solvent-free system. LWT-Food Sci. Technol. (2015) 60:1187–94. doi: 10.1016/j.lwt.2014.09.013
6. Wei W, Jin Q, Wang X. Human milk fat substitutes: past achievements and current trends. Prog Lipid Res. (2019) 74:69–86. doi: 10.1016/j.plipres.2019.02.001
7. Ilyasoglu H, Gultekin-Ozguven M, Ozcelik, B. Production of human milk fat substitute with medium-chain fatty acids by lipase-catalyzed acidolysis: optimization by response surface methodology. LWT-Food Sci Technol. (2011) 44:999–1004. doi: 10.1016/j.lwt.2010.11.027
8. Carnielli VP, Luijendijk I, Vangoudoever JB, Sulkers EJ, Sauer P. Structural position and amount of palmitic acid in infant formulas: effects on fat, fatty acid, and mineral balance. Pediatr Res. (1994) 36:10–10. doi: 10.1203/00006450-199407000-00047
9. López-López A, López-Sabater MC, Campoy-Folgoso C, Rivero-Urgell M, Castellote-Bargalló AI. Fatty acid and sn-2 fatty acid composition in human milk from Granada (Spain) and in infant formulas. Eur J Clin Nutr. (2002) 56:1242–54. doi: 10.1038/sj.ejcn.1601470
10. Koo WW, Hockman EM, Dow M. Palm olein in the fat blend of infant formulas: effect on the intestinal absorption of calcium and fat, and bone mineralization. J Am Coll Nutr. (2006) 25:117–22. doi: 10.1080/07315724.2006.10719521
11. Ries D, Ye A, Haisman D, Singh, H. Antioxidant properties of caseins and whey proteins in model oil-in-water emulsions. Int Dairy J. (2010) 20:72–8. doi: 10.1016/j.idairyj.2009.09.001
12. Ji J, Zhang J, Chen J, Wang Y, Dong N, Hu C, et al. Preparation and stabilization of emulsions stabilized by mixed sodium caseinate and soy protein isolate. Food Hydrocoll. (2015) 51:156–65. doi: 10.1016/j.foodhyd.2015.05.013
13. Liang Y, Gillies G, Patel H, Matia-Merino L, Ye A, Golding M. Physical stability, microstructure and rheology of sodium-caseinate-stabilized emulsions as influenced by protein concentration and non-adsorbing polysaccharides. Food Hydrocoll. (2014) 36:245–55. doi: 10.1016/j.foodhyd.2013.10.006
14. Yerramilli M, Ghosh S. Long-term stability of sodium caseinate-stabilized nanoemulsions. J Food Sci Technol. (2017) 54:82–92. doi: 10.1007/s13197-016-2438-y
15. Zembyla M, Murray BS, Radford SJ, Sarkar A. Water-in-oil Pickering emulsions stabilized by an interfacial complex of water-insoluble polyphenol crystals and protein. J Colloid Interf Sci. (2019) 548:88–99. doi: 10.1016/j.jcis.2019.04.010
16. Abdolmaleki K, Mohammadifar MA, Mohammadi R, Fadavi G, Meybodi NM. The effect of pH and salt on the stability and physicochemical properties of oil-in-water emulsions prepared with gum tragacanth. Carbohydr Polym. (2016) 140:342–8. doi: 10.1016/j.carbpol.2015.12.081
17. Erxleben SW, Pelan E, Wolf B. Effect of ethanol on the stability of sodium caseinate stabilised emulsions. Food Hydrocoll. (2021) 121:107058. doi: 10.1016/j.foodhyd.2021.107058
18. Ye A, Singh H. Interfacial composition and stability of sodium caseinate emulsions as influenced by calcium ions. Food Hydrocoll. (2001) 15:195–207. doi: 10.1016/S0268-005X(00)00065-5
19. Ye A, Singh H. Influence of calcium chloride addition on the properties of emulsions stabilized by whey protein concentrate. Food Hydrocoll. (2000) 14:337–46. doi: 10.1016/S0268-005X(00)00010-2
20. Qian C, Decker EA, Xiao H, McClements DJ. Physical and chemical stability of β-carotene-enriched nanoemulsions: Influence of pH, ionic strength, temperature, and emulsifier type. Food Chem. (2012) 132:1221–9. doi: 10.1016/j.foodchem.2011.11.091
21. Ravindran S, Williams MAK, Ward RL, Gillies G. Understanding how the properties of whey protein stabilized emulsions depend on pH, ionic strength and calcium concentration, by mapping environmental conditions to zeta potential. Food Hydrocoll. (2018) 79:572–8. doi: 10.1016/j.foodhyd.2017.12.003
22. Owens C, Griffin K, Khouryieh H, Williams K. Creaming and oxidative stability of fish oil-in-water emulsions stabilized by whey protein-xanthan-locust bean complexes: impact of pH. Food Chem. (2018) 239:314–22. doi: 10.1016/j.foodchem.2017.06.096
23. Castel V, Rubiolo AC, Carrara CR. Droplet size distribution, rheological behavior and stability of corn oil emulsions stabilized by a novel hydrocolloid (Brea gum) compared with gum arabic. Food Hydrocoll. (2017) 63:170–7. doi: 10.1016/j.foodhyd.2016.08.039
24. Qiu C, Zhao M, Decker EA, McClements DJ. Influence of protein type on oxidation and digestibility of fish oil-in-water emulsions: gliadin, caseinate, and whey protein. Food Chem. (2015) 175:249–57. doi: 10.1016/j.foodchem.2014.11.112
25. Zeng T, Wu ZL, Zhu JY, Yin SW, Tang CH, Wu LY, et al. Development of antioxidant Pickering high internal phase emulsions (HIPEs) stabilized by protein/polysaccharide hybrid particles as potential alternative for PHOs. Food Chem. (2017) 231:122–30. doi: 10.1016/j.foodchem.2017.03.116
26. Xu X, Liu W, Luo L, Liu C, McClements DJ. Influence of anionic polysaccharides on the physical and oxidative stability of hydrolyzed rice glutelin emulsions: impact of polysaccharide type and pH. Food Hydrocoll. (2017) 72:185–94. doi: 10.1016/j.foodhyd.2017.05.018
28. Guzun-Cojocaru T, Koev C, Yordanov M, Karbowiak T, Cases E, Cayot P. Oxidative stability of oil-in-water emulsions containing iron chelates: Transfer of iron from chelates to milk proteins at interface. Food Chem. (2011) 125:326–33. doi: 10.1016/j.foodchem.2010.08.004
29. Sugiarto M, Ye A, Singh H. Characterisation of binding of iron to sodium caseinate and whey protein isolate. Food Chem. (2009) 114:1007–13. doi: 10.1016/j.foodchem.2008.10.062
30. Kellerby SS, McClements DJ, Decker EA. Role of proteins in oil-in-water emulsions on the stability of lipid hydroperoxides. J Agric Food Chem. (2006) 54:7879–84. doi: 10.1021/jf061340s
31. Hu M, McClements DJ, Decker EA. Impact of whey protein emulsifiers on the oxidative stability of salmon oil-in-water emulsions. J Agric Food Chem. (2003) 51:1435–9. doi: 10.1021/jf0203794
32. Shao Y, Tang CH. Characteristics and oxidative stability of soy protein-stabilized oil-in-water emulsions: influence of ionic strength and heat pretreatment. Food Hydrocoll. (2014) 37:149–58. doi: 10.1016/j.foodhyd.2013.10.030
33. Keowmaneechai E, McClements DJ. Effect of CaCl2 and KCl on physiochemical properties of model nutritional beverages based on whey protein stabilized oil-in-water emulsions. J Food Sci. (2002) 67:665–671. doi: 10.1111/j.1365-2621.2002.tb10657.x
34. Radford SJ, Dickinson E, Golding M. Stability and rheology of emulsions containing sodium caseinate: combined effects of ionic calcium and alcohol. J Colloid Interface Sci. (2004) 274:673–86. doi: 10.1016/j.jcis.2003.12.045
35. Pappas CP, Rothwell J. The effects of heating, alone or in the presence of calcium or lactose, on calcium binding to milk proteins. Food Chem. (1991) 42:183–201. doi: 10.1016/0308-8146(91)90033-K
36. Walker HW, Grant SB. Influence of surface charge and particle size on the stabilization of colloidal particles by model polyelectrolytes. Coll Surf A. (1998) 135:123–33. doi: 10.1016/S0927-7757(97)00226-4
37. Baumy JJ, Brule G. Binding of bivalent cations to a-lactalbumin and b-lactoglobulin: effect of pH and ionic strength. Lait. (1988) 68:33–48. doi: 10.1051/lait:198813
38. Kulmyrzaev AA, Schubert H. Influence of KCl on the physicochemical properties of whey protein stabilized emulsions. Food Hydrocoll. (2004) 18:13–9. doi: 10.1016/S0268-005X(03)00037-7
39. Shao P, Ma H, Zhu J, Qiu Q. Impact of ionic strength on physicochemical stability of o/w emulsions stabilized by Ulva fasciata polysaccharide. Food Hydrocoll. (2017) 69:202–9. doi: 10.1016/j.foodhyd.2017.01.039
40. Cuevas-Bernardino JC, Lobato-Calleros C, Román-Guerrero A, Alvarez-Ramirez J, Vernon-Carter EJ. Physicochemical characterisation of hawthorn pectins and their performing in stabilising oil-in-water emulsions. React Funct Polym. (2016) 103:63–71. doi: 10.1016/j.reactfunctpolym.2016.03.024
41. Hu HY, Xing LJ, Hu YY, Qiao CL, Wu T, Zhou GH, et al. Effects of regenerated cellulose on oil-in-water emulsions stabilized by sodium caseinate. Food Hydrocoll. (2016) 52:38–46. doi: 10.1016/j.foodhyd.2015.06.019
42. Liang Y, Gillies G, Matia-Merino L, Ye A, Patel H, Golding M. Structure and stability of sodium-caseinate-stabilized oil-in-water emulsions as influenced by heat treatment. Food Hydrocoll. (2016) 66:307–17. doi: 10.1016/j.foodhyd.2016.11.041
43. Srinivasan M, Singh H, Munro PA. The effect of sodium chloride on the formation and stability of sodium caseinate emulsions. Food Hydrocoll. (2000) 14:497–507. doi: 10.1016/S0268-005X(00)00030-8
44. Xiao J, Wang X, Gonzalez AP, Huang QR. Kafirin nanoparticlesstabilized Pickering emulsions: Microstructure and rheological properties. Food Hydrocoll. (2016) 54:30e3943. doi: 10.1016/j.foodhyd.2015.09.008
45. Sriprablom J, Luangpituksa P, Wongkongkatep J, Pongtharangkul T, Suphantharika M. Influence of pH and ionic strength on the physical and rheological properties and stability of whey protein stabilized o/w emulsions containing xanthan gum. J Food Eng. (2019) 242:141–52. doi: 10.1016/j.jfoodeng.2018.08.031
46. Dickinson E. Properties of emulsions stabilized with milk proteins: overview of some recent developments. J Dairy Sci. (1997) 80:2607–19. doi: 10.3168/jds.S0022-0302(97)76218-0
47. Hunt JA, Dalgleish DG. The effect of the presence of KCl on the adsorption behavior of whey protein and caseinate in oil-in-water emulsions. Food Hydrocoll. (1996) 10:159–65. doi: 10.1016/S0268-005X(96)80030-0
48. Ray M, Rousseau D. Stabilization of oil-in-water emulsions using mixtures of denatured soy whey proteins and soluble soybean polysaccharides. Food Res Int. (2013) 52:298–307. doi: 10.1016/j.foodres.2013.03.008
49. Ye A. Interfacial composition and stability of emulsions made with mixtures of commercial sodium caseinate and whey protein concentrate. Food Chem. (2008) 110:946–52. doi: 10.1016/j.foodchem.2008.02.091
Keywords: calcium, potassium, OPO emulsion, stability, lipid oxidation
Citation: Wang Q, Xu Y, Liu Y, Qian F, Mu G and Zhu X (2022) Effects of Proteins and Mineral Ions on the Physicochemical Properties of 1,3-Dioleoyl-2-Palmitoylglycerol Emulsion to Mimic a Liquid Infant Formula. Front. Nutr. 9:808351. doi: 10.3389/fnut.2022.808351
Received: 03 November 2021; Accepted: 29 April 2022;
Published: 13 June 2022.
Edited by:
Weilin Liu, Zhejiang Gongshang University, ChinaReviewed by:
Aiqian Ye, Massey University, New ZealandCopyright © 2022 Wang, Xu, Liu, Qian, Mu and Zhu. This is an open-access article distributed under the terms of the Creative Commons Attribution License (CC BY). The use, distribution or reproduction in other forums is permitted, provided the original author(s) and the copyright owner(s) are credited and that the original publication in this journal is cited, in accordance with accepted academic practice. No use, distribution or reproduction is permitted which does not comply with these terms.
*Correspondence: Guangqing Mu, R3VhbmdxaW5nTXVAMTYzLmNvbQ==; Xuemei Zhu, emh1eHVlbWVpMjAwNUBhbGl5dW4uY29t
†These authors have contributed equally to this work and share first authorship
Disclaimer: All claims expressed in this article are solely those of the authors and do not necessarily represent those of their affiliated organizations, or those of the publisher, the editors and the reviewers. Any product that may be evaluated in this article or claim that may be made by its manufacturer is not guaranteed or endorsed by the publisher.
Research integrity at Frontiers
Learn more about the work of our research integrity team to safeguard the quality of each article we publish.