- 1Department of Biological Sciences, College of Bioscience and Biotechnology, Hunan Agricultural University, Changsha, China
- 2Lab of Food Function and Nutrigenomics, College of Food Science and Technology, Hunan Agricultural University, Changsha, China
Metabolic syndrome (MS) is a metabolic disorder that arises from the increasing prevalence of obesity. The pathophysiology seems to be largely attributable to the imbalance of lipid and glucose metabolism, redox signaling pathways, and gut microbiota. The increased syndromes, such as type 2 diabetes and cardiovascular disease demands natural therapeutic attention for those at high risk. Vine tea, as a traditional medicinal and edible resource rich in flavonoids, especially for dihydromyricetin (DHM), exhibits promising health benefits on the intervention of MS, but the specific molecular mechanism has not been systematically elucidated. The present article aims to summarize the regulatory effects and biological targets of vine tea or DHM on MS, and analyze the underlying potential molecular mechanisms in cells, animals, and humans, mainly by regulating the redox associated signaling pathways, such as Nrf2, NF-κB, PI3K/IRS2/AKT, AMPK-PGC1α-SIRT1, SIRT3 pathways, and the crosstalk among them, and by targeting several key biomarkers. Moreover, vine tea extract or DHM has a positive impact on the modulation of intestinal microecology by upregulating the ratio of Firmicutes/Bacteroidetes (F/B) and increasing the relative abundance of Akkermansia muciniphila. Therefore, this review updated the latest important theoretical basis and molecular evidence for the development and application of vine tea in dietary functional products or drugs against MS and also imputed the future perspectives to clarify the deep mechanism among vine tea or DHM, redox associated signaling pathways, and gut microbiota.
Introduction
Vine tea, also known as “Mei” tea, is the tender stem and leaves of Ampelopsis grossedentata, a plant of the Vitis family. Vine tea is commonly used as traditional medicine or functional tea by Tujia and Zhuang nationality in Hunan, Hubei, Guizhou, Jiangxi, and other provinces (1). Vine tea is containing abundant flavonoids, polysaccharides, alkaloids, other polyphenols, and active ingredients, thus presenting multiple biofunctions. The content of flavonoids in vine tea can reach about 45% (m/m), so it is called as “King of flavonoid-contained plant” (2). The flavonoids, separated and identified by Zhang et al. (3) from vine tea, are mainly dihydromyricetin (DHM), myricetin-3-O-beta-D-galactopyranoside, myricetin, and myricitrin, of which the most abundant is DHM, with the concentration of higher than 35% (m/m) (4).
In detail, this review aims to summarize the current state of vine tea research in metabolic syndrome (MS) and the recent studies in humans. The literature search took place in the PubMed, Web of Science, and CNKI databases by using the following queries: vine tea and metabolic syndrome, vine tea and diabetes, vine tea and obesity, vine tea and intestinal microorganism, Ampelopsis grossedentata and metabolic syndrome, Ampelopsis grossedentata and diabetes, Ampelopsis grossedentata and obesity, Ampelopsis grossedentata and intestinal microorganism, Dihydromyricetin and metabolic syndrome, Dihydromyricetin and diabetes, Dihydromyricetin and obesity, Dihydromyricetin and intestinal microorganism. The results were screened based on their titles, abstracts, and full-text availability, according to our inclusion criteria: MS, diabetes and obesity cell, animal or clinical studies evaluating vine tea or DHM. All non-English-related titles were excluded from the present review. Filter limits, such as text availability, article type, and publication date were not applied.
MS and Vine Tea
Metabolic syndrome refers to the pathological disorders of protein, fat, carbohydrate, and other substances in the human body, which can cause a complex metabolic disorder syndrome and result in cardiovascular diseases and diabetes (5). The clinical symptoms of MS are hyperlipidemia, hyperglycemia, and central-obesity, which may induce cardiac, cerebral infarction, and arteriosclerosis in severe cases (6). The pathophysiology is probably largely attributable to insulin resistance with the excessive flux of fatty acids implicated and the proinflammatory state (7). The development of MS may associate with the change of gut microbes (8). According to the WHO statistics, about 17.9 million people die of cardiovascular diseases worldwide every year, while about 422 million patients with diabetes worldwide and 1.6 million people die each year from diabetes and its complications. In 2019, there were more than 400 million patients with dyslipidemia and 116.4 million diabetics in China. However, the current treatment of MS is mainly through drug counseling, which cannot delay the process of arteriosclerosis and prevent the occurrence of cardio-cerebral infarction (9).
A large number of studies have shown that the Chinese traditional characteristic substances, such as hawthorn (10), Platycodon grandiflorum (11), chrysanthemum (12), and other common dual-purpose substances rich in flavonoids are of great significance in intervening metabolic disorders. In recent years, studies have found that flavonoids in vine tea not only have anti-inflammatory and bactericidal (13), anti-tumor (14), anti-oxidation (15), and other biofunctions, but also have significant effects in reducing blood lipid (16), blood glucose (17), protecting the cardiovascular system, reducing whole blood and plasma viscosity, expanding blood vessels, and increasing blood flow velocity (18). The modulation effects of flavonoids from vine tea on MS may indeed be influenced by gut microbiota. Downregulation of the ratio of Firmicutes and Bacteroidetes (F/B) in the human gastrointestinal tract was observed to alleviate metabolic disorders. Studies have shown that the F/B value is upregulated in obese patients (19). The level of glucagon-like peptide 1 (GLP-1) can be increased with the decrease of F/B values in insulin resistant mice (20). These results suggest that vine tea and its extracts can interfere with MS by regulating the abundance and composition of gut microbes.
Thus, this article documented the digestion, absorption, and metabolism of vine tea and its main functional component DHM in the human body, and their intervention effects on abnormal glucose and lipid metabolism and the potential molecular mechanism in animals and human studies were also summarized, aiming to provide a reliable theoretical basis for in-depth research and development of vine tea and DHM in functional food and clinical application of human body.
DHM From Vine Tea and Its Extraction
Dihydromyricetin, with a molecular weight of 320.25, is a white needle crystal, easily soluble in hot water, hot ethanol and acetone, slightly soluble in ethanol and methanol, very slightly soluble in ethyl acetate, insoluble in chloroform and petroleum ether. As a typical flavonoid compound, DHM has two chiral centers, so it is possible to obtain four potential enantiomers (21), which are vulnerable to environmental pH value and have poor stability. DHM is more stable under neutral and weakly acidic conditions (22). In addition, DHM produces an irreversible oxidation reaction when the ambient temperature exceeds 100°C (23). In fact, metal ions (such as Fe3+, Al3+, and Cu2+) affect the chemical stability of DHM (24).
Dihydromyricetin, as the highest content of flavonoids in vine tea, shows obvious differences from regions. Chang proposed that in Guangxi province, DHM content in young leaves of vine tea in Guilin and Cenxi was 3~15 times higher than that in Nanning (25). Zheng et al. had extracted DHM from vine tea from Hubei, Fujian, Yunnan, Guangxi, and Hunan in different ways, and the results showed that the extraction amount of DHM in vine tea from Yunnan province was the highest, which was 1.5 times that of Hubei province (26). In addition, different extraction methods were important factors. Studies showed that the DHM content in vine tea is about 21.86% under the conditions of 90°C, the solid-liquid ratio of 1:20 with the extraction time of 120 min (27). Under the conditions of 45–48°C, the solid-liquid ratio of 25 ml/g with the extraction time of 27–28 min, the content of DHM in ethanol extract is about 31.56% (28). Thus, it is very important to show the origin of vine tea while it was used to develop functional products and the extraction methods matter.
Digestion, Absorption, Metabolism, and Toxicity of DHM
When the administration of DHM, most of the prototype compounds and metabolites are excreted from the body through feces, while a small number of prototype compounds are absorbed through the small intestine, enter the blood circulation through the portal vein and reach to the liver, which further generates phase II metabolites through glucuronidation, sulfation, and methylation, absorbed by our body or excreted with urine. The oral bioavailability of DHM is quite low because of the occurrence of a large number of biotransformation reactions in the gastrointestinal tract and blood (29). The peak concentration (Cmax) of DHM was only reached 21.63 ± 3.62 ng/ml after 2.67 h treatment with an oral dose of 20 mg/kg. The half-life is 3.70 ± 0.99 h, and the resident time is 5.98 ± 0.58 h (30). In addition, DHM is easily eliminated and degraded by methylation, sulfide, and reduction reactions after entering the blood (31). In the rat model, the Cmax was 165.67 ± 16.35 ng/ml, the half-life was 2.05 ± 0.52 h, and the residence time was only 2.62 ± 0.36 h after intravenous injection of 2 mg/kg DHM. The bioavailability of DHM in the oral group and the intravenous group was only 4.02% (30). When the blood concentration is 100 ng/ml, the cell contact concentration is about 0.3 μM, which is much lower than the actual cell treatment concentration (0.01–300 μM) (Table 1). This also explained why oral DHM products were not effective. Meanwhile, DHM solution is easy to be oxidized and acylated, limiting its application in functional food and clinical medicine (38).
Structure modification of DHM was regarded as an effective way to improve its stability and functionality. DHM esterification, when formed by the reaction with acetic anhydride, increased its solubility in peanut oil by 4~6 times as well as its antioxidant activity (39). DHM reacts with perchloric acid and acetic anhydride to form DHM acetylated derivatives. Pharmacokinetic data showed that the half-life of DHM acetylated derivatives was two times that of DHM monomer, and the residence time in vivo was longer. The apparent volume of distribution (Vd) showed that the acetylated derivatives had a wider distribution area in vivo and higher stability (40). In addition, the water solubility of DHM glycosides increased 89 times and the Browning resistance increased 14.5 times (41). DHM can increase its solubility in water from 0.74 to 53.64 mg/ml by forming an inclusion complex with hydroxypropyl-β-cyclodextrin, and the thermal decomposition reaction of DHM can also be delayed by forming inclusion (42).
Vine tea had no obvious toxic side effects on rats, mice, rabbits, and domestic dogs, which could be used as a non-toxic food raw material. The administration dose at 250 mg/kg to mice (43), at 400 mg/kg to broilers (44), at 150 mg/kg to domestic dogs (45), and intragastric administration of 93 mg/kg DHM to rabbits have no obvious toxicological side effects on body weight, food intake, blood physiological and biochemical indicators, organ weight, histopathological indicators, and skin allergy indicators (46). Thus, vine tea/DHM was regarded as safe with multiple functionalities.
Application of Vine Tea and Its Functional Components in Medicine and Food
Vine tea and DHM related products have gathered the attention of the scientific and industrial community due to their various possible applications. The health food of vine tea approved by China's Food and Drug Administration, such as “Kubao Zhishukang Capsule (12.7 g total flavonoids/100 g)” and “Jinqi vine tea (3.5 g total flavonoids/100 g)” which have the effect on lowering blood lipid, and “Xihuang Bazhen tea” which was found to enhance the immune function and protect the function of chemical liver injury. According to the document of “announcement on three new food raw materials such as the leaves of vine tea” (No. 16, 2013) adopted by the national health and Family Planning Commission, vine tea is legal to use as raw material to develop common food (47). In addition, the current food production principles (SC) classification of vine tea support it to be used as common food or common food base material to develop into “tea and related products.” All these enable a steadily growing of bag brewed vine tea (48), compound vine-tuo tea (49), “Jinhua vine tea (50),” and vine tea related drinks (51). In addition, products, such as camellia noodles (52), camellia gummy candy, and camellia jelly have been put into the market (53, 54). Although several rattan tea products have been developed, research on their safety and function is still not clear.
The Potential Molecular Mechanisms of Vine Tea and DHM Underlying the Interventional Effect on MS in Cell and Animal Models
Metabolic syndrome is a common metabolic disorder defined by a constellation of interconnected physiological, biochemical, clinical, and metabolic factors, which directly increases the risk of cardiovascular disease, type 2 diabetes mellitus (T2DM), and cause mortality, seriously threatening human health and quality of life (55). Due to modern patterns of life style, such as surplus energy intake and sedentary life habits, the incidence of MS is increasing and tends to be prevalent in young people. Vine tea and its main functional component DHM were used to treat obesity model rats (10–500 mg/kg/day for 6–24 weeks) and diabetes model rats (30–500 mg/kg/day for 2–16 weeks) and inflammatory model mice (57.5–500 mg/kg/day for 1–120 days), as shown in Table 2, mainly through the targeting redox signaling pathways, such as Nrf2 antioxidant pathway, NF-κB inflammatory pathway, PI3K/IRS2/AKT insulin resistance pathway, and AMPK-PGC1α-SIRT1, SIRT3 energy metabolism related pathway.
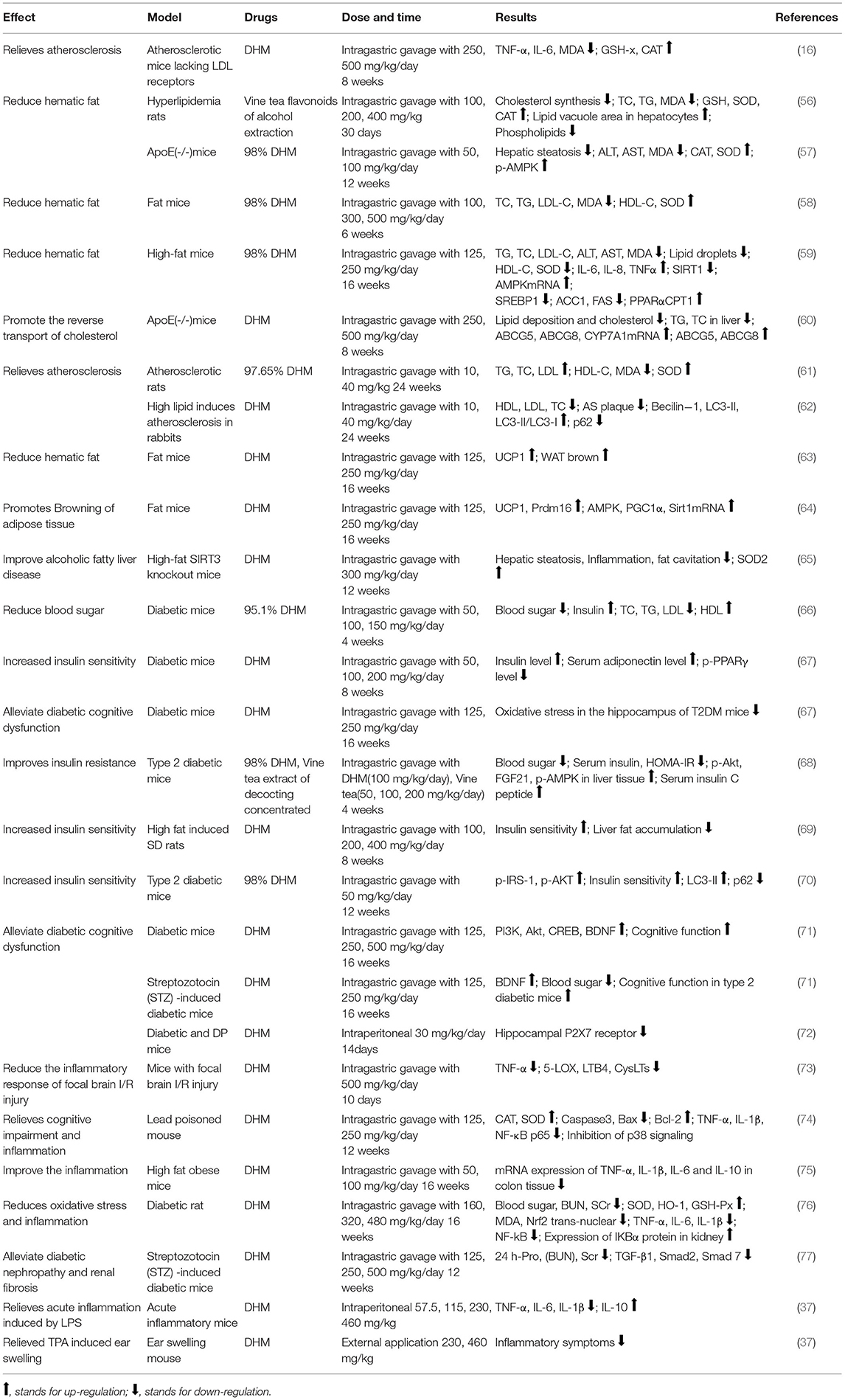
Table 2. The effect and molecular mechanism of dihydromyricetin (DHM) on metabolic syndrome (MS) in animals.
Regulation on Blood Lipid
Vine tea and DHM play an important role in reducing blood lipid and blood glucose. DHM can significantly inhibit the biosynthesis of intracellular cholesterol in the high-fat model, decrease the levels of triglyceride (TG), total cholesterol (TC), and low-density lipoprotein (LDL-C), and increase the levels of high-density lipoprotein (HDL-C), superoxide dismutase (SOD), and catalase (CAT). Moreover, vine tea and DHM can lower the expression of alanine transaminase (ALT) and aspartate aminotransferase (AST) and reduce the level of malondialdehyde (MDA) (56, 57). In hepatocytes of obese mice treated with DHM, the lipid droplet status was significantly improved, the fat vacuole area was reduced, the expression of liver lipid anabolism-related genes (SREBP1, ACC1, and FAS) was decreased, and the expressions of liver lipid metabolism related genes (PPARα and CPT1) were upregulated (58). In high-fat ApoE (-/-) mice, DHM showed an effect on lowering the hepatic steatosis, regulating the expression of antioxidant enzymes, inhibiting the expression of adenylate phosphorylated protein kinase (p-AMPK) in liver tissue to inhibit hepatocyte apoptosis, protect liver tissue, alleviate liver injury, and improve the metabolic abnormalities in high-fat mice (59). In addition, DHM significantly reduced the lipid deposition and cholesterol content in mouse peritoneal macrophages, increase the expressions of ABCG5, ABCG8, and CYP7A1mRNA, promote the decomposition and excrement of cholesterol in the liver, and increases the protein expressions of ABCG5 and ABCG8 in the small intestine in mice (60). In atherosclerosis (AS) model, the mRNA expression of platelet phospholipase A2 of group IIA (sPLA2-IIA) was significantly decreased, the expressions of Beclin-1 and LC3-II were significantly increased, and the expression of p62 was significantly downregulated; while diffuse uplift in thoracic aorta wall, the proportion of AS plaque area and the degree of common carotid artery lesions were all reduced (61, 62). In high-fat obese mice, DHM reduced the size of shoulder adipose tissue cells and increased the expressions of UCP1 and Prdm16 in white adipose tissue (WAT) (63). The mRNA and protein expressions of adenylate activated protein kinase (AMPK), peroxisome proliferation-activated receptor γ (PGC1α), and silent message regulator (Sirt1) were increased in subscapular adipose tissue of mice. It is speculated that DHM can promote adipocyte brown-resistance to obesity through the AMPK-PGC1α-SIRT1 pathway (64). In addition, DHM-induced activation of SIRT3 can promote the expression of mitochondrial respiratory chain enzyme complex in hepatocytes, increase SOD-2-mediated mitochondrial antioxidant capacity, and inhibit oxidative stress in hepatocytes. These results suggested that DHM may inhibit the oxidative stress in hepatocytes by upregulating SIRT3 expression by activating the AMPK/PGC1α pathway and promoting mitochondrial translocation (65).
Regulation on Blood Glucose and Insulin Resistance
In diabetic models, DHM decrease the contents of TC, TG, and LDL-C while HDL-C and serum adiponectin were enhanced (66). Insulin resistance is commonly occurring in patients with diabetes and other metabolic disorders. Proinflammatory factor tumor necrosis factor-α (TNF-α) can inhibit serine phosphorylation of insulin receptor substrate-1 (IRS-1) by reducing the level of guanylate kinase related protein 42, thereby inhibiting the downstream glycogen synthesis and glucose transporter 4 (GLUT-4) activity to cause insulin resistance (78). At the same time, it can also induce hepatic steatosis by activating sterol regulatory element binding protein-1c (SREBP-1c), an important transcription factor of lipid synthesis gene expression, resulting in increased fatty acid synthesis and excessive accumulation of triglycerides (79). The treatment with DHM showed more intact islet tissue and increased β cells and insulin level, with the decrease of blood glucose. The expression levels of p-Akt, p-IRS-1, and p-AMPK in liver tissue were significantly increased, and insulin sensitivity was improved (67–69). In the cell model, it can also reduce the blood glucose level, upregulate the ratio of Bax/Bcl-2, the expression of GLUT4 and Akt2, and downregulate the phosphorylation of peroxisome proliferator- activated receptor γ (PPAR γ) and ERK to improve the insulin sensitivity (32–35).
Autophagy is a type of programmed apoptosis, which can regulate the renewal of new and old cells, and plays an important role in maintaining the intracellular homeostasis in response to intracellular stress (80). The expression of autophagy related genes (ATG14, RB1CC1/FIP200, GABARAPL1, and WIPI1) and protein LC3BII and ATG5 in skeletal muscle in patients with T2DM following in severe insulin resistance are downregulated (81). Impaired autophagy will further aggravate the metabolic disorder associated with diabetes in insulin target tissues (such as liver, skeletal muscle, and adipose tissue) and pancreatic β cells (82). In mouse skeletal muscle tissue, DHM can upregulate the expression of autophagy factor LC3-II and downregulate the expression of p62, and the number of autophagosomes after induction depends on SIRT3 regulation, suggesting that DHM may regulate SIRT3 induced autophagy in skeletal muscle through AMPK-PGC1α pathway to improve the insulin sensitivity (70).
Dihydromyricetin upregulated the expression of autophagy factor LC3-II and downregulated the expression of p62 in skeletal muscle, and the number of autophagosomes after induction was dependent on SIRT3 regulation, suggesting that DHM may improve the insulin sensitivity by regulating SIRT3-induced autophagy in skeletal muscle through AMPK-PGC1α pathway (70). It is well known that patients with diabetes are associated with many complications, among which neurodegenerative diseases, such as Alzheimer's disease (AD) have a great impact on the lives of patients and their families. In mice with T2DM, DHM inhibits oxidative stress in the hippocampus (66), increases the protein expression of phosphatidylinositol 3-kinase (PI3K), Akt, and brain-derived neurotrophic factor (BDNF) in the hippocampus, and stimulate improvement of cognitive dysfunction in T2DM mice (71). The expression of the P2X7 receptor, a family of ATP-gated ions, was downregulated in the hippocampus of mice, and diabetic neuropathic pain (DNP) and depression (DP) symptoms were both significantly improved (72).
Regulation on Redox and Inflammatory Associated Signaling Pathways
The development of obesity and type 2 diabetes is closely related to mitochondrial dysfunction and oxidative stress. Ectopic lipid deposition results from insulin resistance, causing mitochondrial function reduction and oxidative imbalance. Oxidative stress is able to destroy the liver antioxidant enzyme system, resulting in hepatocyte injury and abnormal liver function (83, 84). From the point of antioxidant enzyme, DHM can upregulate the levels of SOD and CAT (56, 57). In addition, Liang found that DHM could alleviate vascular wall thickening, endothelial roughness, and media smooth muscle fiber hyperplasia in rats (61). DHM significantly reduces serum C-reactive protein (CRP), serum TNF-α, and interleukin (IL-1) levels in mice with focal brain I/R injury (73). DHM can downregulate the expressions of apoptotic protease 3 (Caspase3), Bcl-2 family protein (Bax), and upregulate the expression of Bcl-2 protein in an inflammatory mice model. DHM decreased the level of TNF-α and interleukin-1 β (IL-1β), followed by the decreased translocation of nuclear transcription factor NF-κB and inhibition of MAPK family protein p38. It is suggested that DHM may alleviate oxidative stress and apoptosis through the MAPK pathway (36, 74, 75). DHM reduced the nuclear factor erythroid-2-related factor 2 (Nrf2) nuclear translocation, upregulated downstream heme oxygenase (HO-1) expression in diabetic rats (76). Downregulation of NF-κB expression, pro-inflammatory factor interleukin-6 (IL-6), and interleukin-8 (IL-8) levels, and upregulation of anti-inflammatory factor interleukin-10 (IL-10) by DHM treatment were also observed (37, 77).
Regulation on Intestinal Microbiota
Gut microbiota plays a crucial role in human health. The alteration of the composition of gut microbiotas is related to nutrient acquisition and energy regulation. Studies have shown that human intestinal flora can produce DHM metabolites in vitro and confirmed that DHM can be reduced and dehydroxylated to form metabolites under the action of intestinal microbes (24). Furthermore, DHM can regulate the composition of intestinal microflora by changing the richness and diversity of intestinal microflora (85). The ratio of F/B was significantly reduced by DHM in the gut microbes, and its decrease is positively correlated with the reduction of the obesity risk (86). Xie et al. confirmed that vine tea extract could reduce the proportion of F/B and increase the relative abundance of Akkermansia ruminoccoccus in high-fat diet mice (87). It inhibits the reproduction of Enterobacteriaceae, interferes with the relative abundance of intestinal microorganisms, prevents the imbalance of gut microbes, and improves non-alcoholic fatty liver disease (NAFLD). Multiple studies have shown that the relative abundance of Bacteroides, Ruminoccoccus, and Akkermansia were significantly changed in patients with NAFLD, and the serum endotoxin was increased, which induced the occurrence of insulin resistance and intestinal inflammation (88). Moreover, the decreased expression of ZO-1 in the inner wall of the small intestine leads to changes in intestinal permeability and a large number of enterogenic bacteria and metabolites enter the blood and the liver tissue, increasing the burden of the liver and leading to hepatotoxicity (89). DHM restores the ratio imbalance between Firmicutes and Bacteroidetes induced by HFD and upregulates the relative abundance of Akkermansia and Prevotella. Additionally, DHM significantly reduces the serum endotoxin level and increases the level of ZO-1 protein in intestinal tissue (87).
Despite the evidence indicating the interaction between DHM and gut microbes, the current studies focus on the effect of DHM on gut flora and the biotransformation of DHM under gut microbiota. The specific gut microbes responsible for the metabolism of DHM are still unknown. At present, 8 kinds of DHM metabolites in the urine and fecal of rats have been isolated and identified, but no follow-up investigations have been conducted (29), and the effects of DHM on the intestinal immunity, intestinal function, and inflammatory development after changing the composition of gut microbes have not been fully clarified (90).
Interventional Effects of Vine Tea and DHM on Human MS
According to our literature search strategy, there are only two clinical studies published on vine tea or DHM against human MS up to date (Table 3). Ran divided the 80 patients with T2DM into vine tea group (970 mg/day, DHM) group and placebo group for a month in a double-blind clinical trial to monitor the blood glucose, insulin, blood lipids, and renal function indexes (91). Compared with the placebo, the levels of fasting blood glucose, glycated albumin, bladder C, and RBP-4 were significantly decreased in the vine tea group (91). The limitations of this first clinical research are the small popularity especially on elder people and the lack of experiment design on people who only received insulin or metformin. TNF-α, fibroblast growth factor 21 (FGF21), and insulin homeostasis assessment index were significantly reduced in patients treated with DHM (300 mg/day) in NAFLD (92). However, the parameters were only determined at the end of the intervention as it is of great importance to know the changes over time. The clinical research of DHM was very limited as one challenge is the low bioavailability of DHM. The other reason is the “dose-time-toxicity-functionality” of DHM, which is still unknown.
Discussion and Conclusions
Dihydromyricetin, as the main functional compound of vine tea, is a plant resource with homologous medicinal and food characteristics in China with multiple bio-functions. Vine tea and DHM showed effects on regulating MS and their molecular pathways, such as Nrf2/ARE, NF-κB/JNK, AMPK/PGC1α/Sirt1 in obesity and diabetes models in animal models (Figure 1). It would be interesting to discuss the intervention mechanism of MS by combining the oxidative stress and inflammatory pathway with insulin resistance and adipose cell browning in the future.
However, vine tea species should be clarified when used as raw material for functional food development due to the huge variation of DHM content among the species. Additionally, the poor water solubility of DHM leads to its low membrane permeability and bioavailability, which limited the wide application of vine tea. Therefore, improving the bioavailability of DHM by using molecular modification technology and encapsulation technology is the crucial step for the deep development of vine tea and its related products. Interestingly, the existing data of animal models showed that the cell contact concentration of DHM was much lower than the treatment concentration in the cell model, which might be one of the reasons for the poor results obtained from animal experiments. The effect of DHM with high purity of 98% on animal MS was not as good as that of vine tea extract with 60% of DHM, which may be related to the complex form of DHM in less pure extracts. Micro-nano systems, such as polysaccharides, modified starch, and Chinese medicine decocting may protect the biological activity and structural stability of flavonoids. Thus, forming a complex or using an encapsulation technique would be a promising way to improve the bioavailability of DHM.
In addition, considering the different doses ranging from 10 to 500 mg/kg/day were applied in different animal models, it could be very interesting in the future to narrow down these differences and establish a safe range of DHM intake for humans. Sufficient data regarding of “dose-time-toxicity-function” of DHM from the long-term investigation will bring light to its application. In regards to the published articles, there were a lot of studies that had no purity results, which should be mentioned for future study. Although the interaction mechanism of gut microbiota and DHM has growing attraction in recent times (Figure 2), there is still a lot of work remaining to reveal the effects of DHM on the species structure and richness of gut microbiota and the intervention mechanism of its metabolites on MS, especially on whether these metabolites were absorbed in the blood and enter to the body circulation.
In the future, we can focus on DHM's highly enriched vine tea species, and improve the bioavailability of DHM. The deeper molecular mechanism, analyzed by multiomics to find the potential targeted protein and gene, as well as the biomarker of DHM on blood and gut were worth studying. Furthermore, more human clinical trials should be conducted to verify its function. Considering the availability of vine tea resources and its excellent health promoting effects, it would be very beneficial to have more vine tea related products entering foods, medicines, and clinical use.
Author Contributions
XZ, YS, CL, and HZ wrote the first draft of the manuscript. YS, SQ, CZ, CL, and MS wrote sections of the manuscript. All authors contributed to manuscript revision, read, and approved the submitted version.
Funding
This study was partially supported by the National Key Research and Development Program of China (2019YFC1604903), the Natural Science Foundation of China (31101268), and the Natural Science Foundation of Hunan Province (2019JJ40121 and 2019JJ40132).
Conflict of Interest
The authors declare that the research was conducted in the absence of any commercial or financial relationships that could be construed as a potential conflict of interest.
Publisher's Note
All claims expressed in this article are solely those of the authors and do not necessarily represent those of their affiliated organizations, or those of the publisher, the editors and the reviewers. Any product that may be evaluated in this article, or claim that may be made by its manufacturer, is not guaranteed or endorsed by the publisher.
References
1. Ran JY, Fang JG, Xie XJ, Xiong W, Wang WQ. Scientific research on herbal resource of vine tea. Zhong Cao Yao. (2016) 47:3728–35. doi: 10.7501/j.issn.0253-2670.2016.20.028
2. Li JC, Li SY, Wang Y, Wang WN. Predictive analysis on chemical composition, pharmacological effects and quality marker (Q-marker) of ampelopsis grossedentata. J Southwest Minzu Univ (Nat Sci Ed). (2021) 47:254–66. doi: 10.11920/xnmdzk.2021.03.005
3. Zhang YS, Zhang QY, Wang B, Li LY, Zhao YY. Chemical constituents from ampelopsis grossedentata. J Chin Pharm Sci. (2006) 15:211–4.
4. Wang YF, Le Y, Sun D, Zhang SK, Zhu YJ, Xu P. Supercritical carbon dioxide extraction of bioactive compounds from ampelopsis grossedentata stems: process optimization and antioxidant activity. Int J Mol Sci. (2011) 12:6856–70. doi: 10.3390/ijms12106856
5. Weinstock RS, Drews KL, Caprio S, Leibel NI, McKay SV, Zeitler PS, et al. Metabolic syndrome is common and persistent in youth-onset type 2 diabetes. Obesity. (2015) 23:1357–61. doi: 10.1002/oby.21120
6. Liu LY, Liu AX, Wang LL, XU L. Epidemiological investigation of metabolic syndrome in the elderly and study on preventive measures. J Prev Med Public Health. (2021) 32:107–10. doi: 10.3969/j.issn.1006-2483.2021.03.025
7. Eckel RH, Grundy SM, Zimmet PZ. The metabolic syndrome. Lancet. (2005) 365:1415–28. doi: 10.1016/S0140-6736(05)66378-7
8. Vijay Kumar M, Aitken JD, Carvalho FA, Cullender TC, Mwangi S, Srinivasan S, et al. Metabolic syndrome and altered gut microbiota in mice lacking toll-like receptor 5. Science. (2010) 328:228–31. doi: 10.1126/science.1179721
9. Cao XM, Ying FB. Clinical efficacy of edaravone, vinpocetine and shuxuetong in the treatment of acute cerebral infarction and its influence on hemorheology. Chin J Gerontology. (2012) 32:4515–6. doi: 10.3969/j.issn.1005-9202.2012.20.079
10. Song M, Cao BX. Effects of hawthorn on lipid metabolism and lipase in mice with hyperlipidemia. Chin J Mod Drug Appl. (2012) 6:119–20. doi: 10.3969/j.issn.1673-9523.2012.20.102
11. Gao YF, Chen C, Zhang HX, Liang GC. Effects of platycodins on the experimental hyperlipidemia in rats. Zhong Cao Yao. (2000) 31:46–7. doi: 10.3321/j.issn:0253-2670.2000.10.025
12. Abulati A, Xuemei F, Xinmin M, Lan Y. Effects of flavonoids of coreopsis tinctoria nutt. On energy metabolism disorder of high glucose and high fat-induced cell model. Chin J Inform Tradit Chin Med. (2020) 27:35–41. doi: 10.3969/j.issn.1005-5304.201908139
13. Liang HY, He KK, Li T, Cui SM, Tang M, Kang SY, et al. Mechanism and antibacterial activity of vine tea extract and dihydromyricetin against Staphylococcus aureus. Sci Rep. (2020) 10:21416. doi: 10.1038/s41598-020-78379-y
14. Zhang Z, Zhang H, Chen S, Xu Y, Yao A, Liao Q, et al. Dihydromyricetin induces mitochondria-mediated apoptosis in HepG2 cells through down-regulation of the Akt/Bad pathway. Nutr Res. (2017) 38:27–33. doi: 10.1016/j.nutres.2017.01.003
15. Chen Y, Luo HQ, Sun LL, Xu MT, Yu J, Liu LL, et al. Dihydromyricetin attenuates myocardial hypertrophy induced by transverse aortic constriction via oxidative stress inhibition and SIRT3 pathway enhancement. Int J Mol Sci. (2018) 19:2592. doi: 10.3390/ijms19092592
16. Liu TT, Zeng Y, Tang K, Chen X, Zhang W, Xu XL. Dihydromyricetin ameliorates atherosclerosis in LDL receptor deficient mice. Atherosclerosis. (2017) 262:39–50. doi: 10.1016/j.atherosclerosis.2017.05.003
17. He JD, Zhang JP, Dong LJ, Dang XF, Wang L, Cheng L, et al. Dihydromyricetin attenuates metabolic syndrome and improves insulin sensitivity by upregulating insulin receptor substrate-1 (Y612) tyrosine phosphorylation in db/db mice. Diabetes Metab Syndr Obes. (2019) 12:2237–49. doi: 10.2147/DMSO.S218487
18. Yang L, Zheng C. Optimization of the technology of extracting dihydromyricetin from ampelopsis by orthogonal experimental design. Adv Mater Res. (2012) 1914:1709–14. doi: 10.4028/www.scientific.net/AMR.550-553.1709
19. Ley RE, Turnbaugh PJ, Klein S, Gordon JI. Human gut microbes associated with obesity. Nat. (2006) 444:1022–3. doi: 10.1038/4441022a
20. Greenhill C. Firmicutes and bacteroidetes involved in insulin resistance by mediating levels of glucagon-like peptide 1. Nat Rev Endocrinol. (2015) 11:254. doi: 10.1038/nrendo.2015.40
21. Wang C, Xiong W, Reddy Perumalla S, Fang J, Calvin Sun C. Solid-state characterization of optically pure (+) dihydromyricetin extracted from ampelopsis grossedentata leaves. Int J Pharm. (2016) 511:245–52. doi: 10.1016/j.ijpharm.2016.07.018
22. He GX, Pei G, Li B, Ou YW. Studies on stability of dihydromyricetin. Chin New Drugs J. (2007) 16:1888–90.
23. Tong Q, Hou X, Fang J, Wang W, Xiong W, Liu X, et al. Determination of dihydromyricetin in rat plasma by LC–MS/MS and its application to a pharmacokinetic study. J Pharm Biomed Anal. (2015) 114:455–61. doi: 10.1016/j.jpba.2015.06.030
24. Liu D, Mao Y, Ding L, Zeng XA. Dihydromyricetin: a review on identification and quantification methods, biological activities, chemical stability, metabolism and approaches to enhance its bioavailability. Trends Food Sci Technol. (2019) 91:586–97. doi: 10.1016/j.tifs.2019.07.038
25. Chang JF, Qin JP, Diao Y, Huang ZP, Liu PF. Determination of contents of dihydromyricetin, rutin and myricetin in ampelopsis grossedentata stems by HPLC. Chin J Mod Appl Pharm. (2017) 34:1154–7. doi: 10.13748/j.cnki.issn1007-7693.2017.08.018
26. Zheng L, Gao SW, Liu PP, Zheng PC, Gong Zzm. Study on the dihydromyricetin in Ampelopsis grossedentata. Hubei Agri Sci. (2020) 59:133–134+140. doi: 10.14088/j.cnki.issn0439-8114.2020.24.030
27. Xiong W, Wang HB, Li XH, Hu JW, Fu JP. Study on synchronous extraction of dihydromyricetin and polysaccharide from ampelopsis grossedentata by hot water method. Shengwu Huagong. (2015) 1:5–6+11.
28. Li F. Extraction of Antioxidants From Rattan Tea and Evaluation the Bioactivities of Dihydromyricetin. Zhenjiang: Jiangsu University (2017).
29. Li F. Quality Evaluation of Ampelopsis grossedentata and Metabolic-Related Study of Its Bioactive Ingredient Dihydromyricetin. Wuhan: Huazhong University of Science and Technology. (2018).
30. Liu L, Yin X, Wang X, Li X. Determination of dihydromyricetin in rat plasma by LC-MS/MS and its application to a pharmacokinetic study. Pharm Biol. (2017) 55:657–62. doi: 10.1080/13880209.2016.1266669
31. Fan L, Tong Q, Dong W, Yang G, Hou X, Xiong W, et al. Tissue distribution, excretion, and metabolic profile of dihydromyricetin, a flavonoid from vine tea (Ampelopsis grossedentata) after oral administration in rats. J Agri Food Chem. (2017) 65:4597–604. doi: 10.1021/acs.jafc.7b01155
32. Liu YX, Pan XQ, Hu Z. Effect of dihydromyricetin against H[[sb]]2[[/s]]O[[sb]]2[[/s]] induced injury in human umbilical vein endothelial cell. J Wenzhou Med Univ. (2014) 4:34–37. doi: 10.3969/j.issn.2095-9400.2014.04.007
33. Wan XY, Gao LH, Feng GH, Zhang Y, Liu X, Li L. Effects of dihydromyricetin on insulin resistance in 3 T3-L1 adipocytes and its mechanism. Chin Pharm Bull. (2014) (11):1580–5. doi: 10.3969/j.issn.1001-1978.2014.11.020
34. Pan HM, Jiang BP, Le L, Xiao W, Xu LJ, Xiao PG. Effects of main compounds from ampelosis grossedentata on oxidative stress in insulin-resistant HepG2 cells. Chin J Mod Med. (2015) 17:267–71. doi: 10.13313/j.issn.1673-4890.2015.3.019
35. Liu L, Zhou M, Lang H, Zhou Y, Mi M. Dihydromyricetin enhances glucose uptake by inhibition of MEK/ERK pathway and consequent down-regulation of phosphorylation of PPARγ in 3T3-L1 cells. J Cell Mol Med. (2017) 18:580–6. doi: 10.1111/jcmm.13403
36. Lu HJ, Zhu ZM, Chen WZ, He JQ, Yang SS, Zhang KF, et al. Dihydromyricetin inhibits high glucose induced pc12 cells apoptosis by down-regulating JNK pathway. Prog Biochem Biophys. (2018) 45:663–71. doi: 10.16476/j.pibb.2017.0477
37. Hou XL. Dihydromyricetin Prevents Atherosclerosis by Protecting Endothelial Cells and Inhibiting Inflammatory Reaction and the Underlying Mechanisms. Wuhan: Huazhong University of Science&Technology. (2015).
38. Wang C, Tong Q, Hou X, Hu S, Fang J, Sun CC. Enhancing bioavailability of dihydromyricetin through inhibiting precipitation of soluble cocrystals by a crystallization inhibitor. Cryst Growth Des. (2016) 16:5030–9. doi: 10.1021/acs.cgd.6b00591
39. Guo QQ, Zeng JH, Lu Y, Shu XG. Effects of solubility, thermal stability and antioxidant properties of acylating dihydromyricetin. Adv Mater Res. (2013) 791–793:101–5. doi: 10.4028/www.scientific.net/AMR.791-793.101
40. Deng Y, Xiao YS, Xin L, Zhang BK, Yan M, Tan SL, et al. Pharmacokinetics of myricetin, dihydromyricetin from ampelopsis grossedentata and its acetylated derivatives in SD rats. Chin J Hosp Pharm. (2019) 39:2266–72. doi: 10.13286/j.cnki.chinhosppharmacyj.2019.22.04
41. Woo HJ, Kang HK, Nguyen TTH, Kim GE, Kim YM, Park JS, et al. Synthesis and characterization of ampelopsin glucosides using dextransucrase from leuconostoc mesenteroides B-1299CB4: glucosylation enhancing physicochemical properties. Enzyme Micro Tech. (2012) 51:311–8. doi: 10.1016/j.enzmictec.2012.07.014
42. Liu B, Ma Y, Yuan C, Su C, Hu L, Wang J. Characterization, stability and antioxidant activity of the inclusion complex of dihydromyricetin with hydroxypropyl-β-cyclodextrin. J Food Biochem. (2012) 36:634–41. doi: 10.1111/j.1745-4514.2011.00577.x
43. Wang ZH, Luo JD, Lyu HJ, Tian YR, He JQ, Feng SD, et al. Dihydromyricin inhibited hepatic lipid deposition induced by high-fat diet in obese mice by activating SIRT1-AMPK pathway. Chin Pharm Bull. (2021) 37:107–13. doi: 10.3969/j.issn.1001-1978.2021.01.017
44. Guo H, Xie P, Zhang M, Zheng S, Feng J. Effect of short-term feeding of dihydromyricetin on the intestinal mucosal morphology recovery and immune function of broilers. Chin J Veter Med. (2010) 46:19–22. doi: 10.3969/j.issn.0529-6005.2010.04.006
45. Chen LF, Dong QQ, Zhang Q. Effects of dihydromyricetin on recurrent oral ulcers of rabbits. China J Chin Mater Med. (2008) (02):65–9. doi: 10.3321/j.issn:1001-5302.2008.02.018
46. Ou XH, Ye Y. Study on the pharmacokinetics of dihydromyricetin in rabbits. J Anhui Agri Sci. (2012) 40:8941–2. doi: 10.13989/j.cnki.0517-6611.2012.16.051
47. Three foods including vitis gracilis are listed as national new food raw materials. Food Ind. (2014) 6–6.
48. Cheng Y. Study on the process of the teabag of ampelopsis grossedentata. J Hunan Environ-Biol Polytech. (2005) 11:344–6. doi: 10.3969/j.issn.1671-6361.2005.04.013
49. Chen X, Zhao WJ. Research of sense quality of compound tea of Ampelopsis grossedentatu (Hand-Mazz)wt wang and tuo tea. J Mod Agric Sci. (2013) 000:257–9. doi: 10.3969/j.issn.1007-5739.2013.22.161
50. Cai ZA, Liu SC, Liu XJ, Huang JN, Zhou L, Zhu Q. Study of the growth of eurotium cristatum fungi on some kinds of tea and plant materials. J Food Sci. (2010) 30:263–8. doi: 10.3969/j.issn.1000-369X.2010.04.005
51. Zheng C, Zeng JH, Gu CQ, Wei XC. Preparation of health ampelopsis grossedentata drinks. J Guangzhou Univ (Nal Sci Ed). (2008) 7:34–9. doi: 10.3969/j.issn.1671-4229.2008.06.008
52. Li GF, Xu ZH, Liu QQ, Li JX. Study on processing technology of vine tea noodles. Cereals Oils. (2017) 30:17–20. doi: 10.3969/j.issn.1008-9578.2017.11.006
53. Liu YY, Zhang JH, Liu QQ, Xie Y. Optimization of formula for hovenia dulcis-ampelopsis grossedentata soft candies. The Food Ind. (2018) 039:61–5.
54. Yan Z. Preparation of healthy ampelopsis grossedentata jelly. Food Sci Tech. (2002) (07):22–3. doi: 10.13684/j.cnki.spkj.2002.07.008
55. Kaur J. A comprehensive review on metabolic syndrome. Cardiol Res Pract. (2014) 2014:943162. doi: 10.1155/2014/943162
56. Zuo XM, Liu Q, Liao FF, Fu M, Li SH, Xiao Q. Study on the lipid-lowering effect of total flavonoids from ampelopsis grossedentata on hyperlipidemic rats. Agri Sci Tech. (2019) 6:18–22. doi: 10.16175/j.cnki.1009-4229.2020.01.006
57. Liu H. Protective effects and mechanism of dihydromyricetin on non-alcoholic fatty liver disease in ApoE-/-mice. Chin Tradit Patent Med. (2017) 39:2448–53. doi: 10.3969/j.issn.1001-1528.2017.12.002
58. Chen YQ, Ni DJ, Cheng Q, Huang HB, Meng Y, Wu MC. Study on the hypolipidemic effect of flavones and dihydromyricetin from Tengcha. J Food Sci. (2007) 27:221–5. doi: 10.18261/ISSN1504-3037-2007-03-07
59. Xu T. Dihydromyrecetin Improves Liver Fat Deposition and Mechanism in Obese Mice Induced by High Fat Diet. Hengyang: University of South China (2019).
60. Zhu JP, Wei XY, Xu XL. Effect of dihydromyricetin on reverse cholesterol transport in high-fat feeding ApoE-/-mice. Chin Pharm Bull. (2018) 34:1610–6. doi: 10.3969/j.issn.1001-1978.2018.11.026
61. Liang ZD, Zeng XB, Wei GN, He F, Lyu JH, Su H, et al. Effects of dihydromyricetin on blood lipid metabolism and antioxidation in atherosclerosis rats. Herald Med. (2015) 34:710–3. doi: 10.3870/yydb.2015.06.002
62. Chen L, Zhou J, Liao HY, Liao HQ, Zhang T, Zhong HJ. Effect of dihydromyricetin on expression of autophagy related protein in atherosclerosis rabbits. J Clinic Cardiol. (2016) 32:624–9. doi: 10.13201/j.issn.1001-1439.2016.06.021
63. Luo JD, Wu D, Lyu HJ, He JQ, Yang SS, Feng SD, et al. Effects of dihydromyricetin on high fat diet induced obesity in mice and its mechanism. Chin J Appl Physiol. (2020) 36:6–11. doi: 10.12047/j.cjap.5851.2020.002
64. Lyv HJ, Luo JD, Wu D, Xu T, He JQ, Yang SS, et al. Dihydromyricetin promoted browning of subscapular adipose tissues in obese mice fed with high-fat diet via activating AMPK-PGC1?-Sirt1 signaling pathway. Chin Pharm Bull. (2019) 35:1687–92. doi: 10.3969/j.issn.1001-1978.2019.12.013
65. Zeng X, Yang J, Hu O, Huang J, Ran L, Chen M, et al. Dihydromyricetin ameliorates nonalcoholic fatty liver disease by improving mitochondrial respiratory capacity and redox homeostasis through modulation of SIRT3 signaling. Antioxid Redox Sign. (2018) 30:163–83. doi: 10.1089/ars.2017.7172
66. Ling H, Zhu Z, Yang J, He J, Yang S, Wu D, et al. Dihydromyricetin improves type 2 diabetes-induced cognitive impairment via suppressing oxidative stress and enhancing brain-derived neurotrophic factor-mediated neuroprotection in mice. Acta Bioch Bioph Sin. (2018) 50:298–306. doi: 10.1093/abbs/gmy003
67. Liu L, Wan J, Lang H, Si M, Zhu J, Zhou Y, et al. Dihydromyricetin delays the onset of hyperglycemia and ameliorates insulin resistance without excessive weight gain in Zucker diabetic fatty rats. Mol Cell Endocrinol. (2017) 439:105–15. doi: 10.1016/j.mce.2016.10.028
68. Wu Y, Wu B, Wan J, Zhang Y, Zhu JD, Zhou Y, et al. Rattan tea extracts improve insulin resistance in type 2 diabetes rats. J Third Mil Med Univ. (2015) 37:454–8. doi: 10.16016/j.1000-5404.201409087
69. Le L, Jiang B, Wan W, Zhai W, Xu L, Hu K, et al. Metabolomics reveals the protective of Dihydromyricetin on glucose homeostasis by enhancing insulin sensitivity. Sci Rep. (2016) 6:36184. doi: 10.1038/srep36184
70. Shi L, Zhang T, Zhou Y, Zeng X, Ran L, Zhang Q, et al. Dihydromyricetin improves skeletal muscle insulin sensitivity by inducing autophagy via the AMPK-PGC-1α-Sirt3 signaling pathway. Endocrine. (2015) 50:378–89. doi: 10.1007/s12020-015-0599-5
71. Zhu ZM, Yang JH, Yang SS, He JQ, Zhang KF, Feng SD, et al. The effects of dihydromyricetin on cognitive dysfunction in type 2 diabetes mice. Chin J Appl Physiol. (2017) 33:323–8. doi: 10.12047/j.cjap.5478.2017.079
72. Guan S, Shen Y, Ge H, Xiong W, He L, Liu L, et al. Dihydromyricetin alleviates diabetic neuropathic pain and depression comorbidity symptoms by inhibiting P2X(7) receptor. Front Psychiatry. (2019) 10:770. doi: 10.3389/fpsyt.2019.00770
73. Deng Z, Chen J, Huang Z, Huang X, Pang Y, Guo Z, et al. Dihydromyricetin reduces inflammatory reaction induced by focal cerebral ischemia-reperfusion injury in mice. J Apoplexy Nerv Dis. (2016) 33:973–5. doi: 10.19845/j.cnki.zfysjjbzz.2016.11.003
74. Liu CM, Yang W, Ma JQ, Yang HX, Feng ZJ, Sun JM, et al. Dihydromyricetin inhibits lead-induced cognitive impairments and inflammation by the adenosine 5′-monophosphate-activated protein kinase pathway in mice. J Agric Food Chem. (2018) 66:7975–82. doi: 10.1021/acs.jafc.8b02433
75. Wang XL, Kang C, Zhou QC, Gong XH, Gu YY, Mi MT. Effect of Dihydromyricetin on the Expression of Inflammatory Factors in Colon Tissue of Obese Mice Induced by High Fat Diet. Nutrition medicine development forum and military nutrition medicine conference, Hohhot, China (2017).
76. Liu X. Role of Inflammation and Oxidative Stress in the Renoprotective Effects of Dihydromyricetin in Type 2 Diabetis Mellitus Rats. Jinan: Shandong University (2018).
77. Liu ZL, Zhang JD, Peng W, Wu SZ, Xiao H. The effect of dihydromyricetin on renal fibrosis in diabetic nephropathy rats. J Gannan Medic Univ. (2019) 39:541–5. doi: 10.3969/j.issn.1001-5779.2019.06.001
78. Ando Y, Shinozawa Y, Iijima Y, Yu B-C, Sone M, Ooi Y, et al. Tumor necrosis factor (TNF)-α-induced repression of GKAP42 protein levels through cGMP-dependent kinase (cGK)-Iα causes insulin resistance in 3T3-L1 adipocytes. J Biol Chem. (2015) 290:5881–92. doi: 10.1074/jbc.M114.624759
79. You M, Matsumoto M, Pacold CM, Cho WK, Crabb DW. The role of AMP-activated protein kinase in the action of ethanol in the liver. Gastroenterology. (2004) 127:1798–808. doi: 10.1053/j.gastro.2004.09.049
80. Aman Y, Schmauck-Medina T, Hansen M, Morimoto RI, Simon AK, Bjedov I, et al. Autophagy in healthy aging and disease. Nat Aging. (2021) 1:634–50. doi: 10.1038/s43587-021-00098-4
81. Møller AB, Kampmann U, Hedegaard J, Thorsen K, Nordentoft I, Vendelbo MH, et al. Altered gene expression and repressed markers of autophagy in skeletal muscle of insulin resistant patients with type 2 diabetes. Sci Rep. (2017) 7:43775. doi: 10.1038/srep43775
82. Kitada M, Koya D. Autophagy in metabolic disease and ageing. Nat Rev Endocrinol. (2021) 17:647–61. doi: 10.1038/s41574-021-00551-9
83. Sangwung P, Petersen KF, Shulman GI, Knowles JW. Mitochondrial dysfunction, insulin resistance, and potential genetic implications. Endocrinology. (2020) 161:bqaa017. doi: 10.1210/endocr/bqaa017
84. Gonzalez Franquesa A, Patti ME. Insulin resistance and mitochondrial dysfunction. Adv Exp Med Biol. (2017) 982:465–520. doi: 10.1007/978-3-319-55330-6_25
85. Li F, Zhao X, Tong Q, Zhou X, Jing C, Wei X, et al. Interactions of dihydromyricetin, a flavonoid from vine tea (Ampelopsis grossedentata) with gut microbiota. J Food Sci. (2018) 83:1444–53. doi: 10.1111/1750-3841.14128
86. Chen G, Xie M, Wan P, Chen D, Ye H, Chen L, et al. Digestion under saliva, simulated gastric and small intestinal conditions and fermentation in vitro by human intestinal microbiota of polysaccharides from Fuzhuan brick tea. Food Chem. (2018) 244:331–319. doi: 10.1016/j.foodchem.2017.10.074
87. Xie K, He X, Chen K, Sakao K, Hou DX. Ameliorative effects and molecular mechanisms of vine tea on Western diet-induced NAFLD. Food Funct. (2020) 11:5976–91. doi: 10.1039/D0FO00795A
88. Miele L, Valenza V, La Torre G, Montalto M, Cammarota G, Ricci R, et al. Increased intestinal permeability and tight junction alterations in nonalcoholic fatty liver disease. Hepatology. (2009) 49:1877–87. doi: 10.1002/hep.22848
89. Festi D, Schiumerini R, Eusebi LH, Marasco G, Taddia M, Colecchia A. Gut microbiota and metabolic syndrome. World J Gastroenterol. (2014) 20:16079–94. doi: 10.3748/wjg.v20.i43.16079
90. Harte AL, da Silva NF, Creely SJ, McGee KC, Billyard T, Youssef-Elabd EM, et al. Elevated endotoxin levels in non-alcoholic fatty liver disease. J Inflamm-Lond. (2010) 7:15. doi: 10.1186/1476-9255-7-15
91. Ran L, Wang X, Lang H, Xu J, Wang J, Liu H, et al. Ampelopsis grossedentata supplementation effectively ameliorates the glycemic control in patients with type 2 diabetes mellitus. Eur J Clin Nutr. (2019) 73:776–82. doi: 10.1038/s41430-018-0282-z
Keywords: dihydromyricetin, metabolic syndrome, redox signaling pathway, gut microbiota, biotransformation
Citation: Zhou X, Song Y, Zeng C, Zhang H, Lv C, Shi M and Qin S (2022) Molecular Mechanism Underlying the Regulatory Effect of Vine Tea on Metabolic Syndrome by Targeting Redox Balance and Gut Microbiota. Front. Nutr. 9:802015. doi: 10.3389/fnut.2022.802015
Received: 26 October 2021; Accepted: 17 January 2022;
Published: 17 February 2022.
Edited by:
Hongkui Wei, Huazhong Agricultural University, ChinaReviewed by:
Uma Devi Palanisamy, Monash University Malaysia, MalaysiaMarta Llansola, Principe Felipe Research Center (CIPF), Spain
Xu-Dong Zhou, Hunan University of Chinese Medicine, China
Copyright © 2022 Zhou, Song, Zeng, Zhang, Lv, Shi and Qin. This is an open-access article distributed under the terms of the Creative Commons Attribution License (CC BY). The use, distribution or reproduction in other forums is permitted, provided the original author(s) and the copyright owner(s) are credited and that the original publication in this journal is cited, in accordance with accepted academic practice. No use, distribution or reproduction is permitted which does not comply with these terms.
*Correspondence: Meng Shi, c2hpbWVuZ0BodW5hdS5lZHUuY24=; Si Qin, cWluc2ltYW5AaHVuYXUuZWR1LmNu
†These authors have contributed equally to this work and share first authorship