- 1Institute of Food Science and Nutrition, University of Sargodha, Sargodha, Pakistan
- 2Institute of Chemistry, University of Sargodha, Sargodha, Pakistan
- 3School of Food Science and Engineering, South China University of Technology, Guangzhou, China
- 4Department of Food Science and Technology, Muhammad Nawaz Shareef (MNS) University of Agriculture, Multan, Pakistan
- 5Food Microbiology and Biotechnology Laboratory, North Carolina Agricultural and Technical State University, Greensboro, NC, United States
- 6Campus Straubing for Biotechnology and Sustainability, Technical University of Munich, Straubing, Germany
- 7German Institute of Food Technologies (DIL e.V.), Quakenbrück, Germany
Background: In recent years, researchers have focused on functional ingredients, functional foods, and nutraceuticals due to the rapidly increasing interest in bioactive components, especially in bioactive peptides. Dairy proteins are a rich and balanced source of amino acids and their derived bioactive peptides, which possess biological and physiological properties. In the dairy industry, microbial fermentation and enzymatic hydrolysis are promising methods for producing bioactive peptides because of their rapid efficiency, and mild reaction conditions. However, these methods utilize less raw material, take long reaction time, result in low yields, and low activity products when used alone, which pose industry to seek for novel methods as pretreatments to increase the yield of bioactive peptides.
Scope and Approach: This review emphasizes the production of peptides from the dairy proteins and discusses the potential use of novel technologies as pretreatments to conventional methods of bioactive peptides production from dairy proteins, including the mechanisms of novel technologies along with respective examples of use, advantages, limitations, and challenges to each technology.
Key Findings and Conclusion: Noteworthily, hydrolysis of dairy proteins liberate wide-range of peptides that possess remarkable biological functions to maintain human health. Novel technologies in the dairy industry such as ultrasound-assisted processing (UAP), microwave-assisted processing (MAP), and high pressure processing (HPP) are innovative and environmentally friendly. Generally, novel technologies are less effectual compared to conventional methods, therefore used in combination with fermentation and enzymatic hydrolysis, and are promising pretreatments to modify peptides’ profile, improve the yields, and high liberation of bioactive peptides as compared to conventional technologies. UAP is an innovative and most efficient technology as its mechanical effects and cavitation change the protein conformation, increase the biological activities of enzymes, and enhance enzymatic hydrolysis reaction rate.
Highlights
- Novel technologies are innovative, environmentally friendly, and promising pretreatments.
- Mechanisms and applications of novel technologies as pretreatments have been discussed.
- Novel technologies coupled with conventional methods are energy efficient and result high extraction yield and rate have been reviewed.
- Potential bioactivity and functions of dairy proteins have been discussed.
- Ultrasound assisted processing showed most efficient applications in dairy industry have been outlined.
Introduction
Bioactive peptides are specific peptide motifs of 2–20 amino acids embedded in parent proteins that possess the ability to alter or influence metabolic activities in the human body because of their particular fragments in proteins (1, 2). Bioactive peptides offer several biological functionalities such as free radicals inhibition, thrombosis inhibition, and immunity improvement (3). There are two main methods of bioactive peptides production by microbial fermentation and enzymatic hydrolysis of proteins. A wide range of bioactive peptides can be produced using different cleavage specificities of the proteolytic enzymes (4). Usually, bioactive peptides consist of less than 20 amino acids and 10 kDa molecular weight. Their functionalities also depend upon the sequence of amino acids, their compositions, and molecular weights (5). Milk proteins contain several peptides that exert strong biological properties and are widely studied as a source of bioactive peptides (6, 7). Many studies have reported the availability of bioactive peptides in milk, fermented dairy products, and various types of cheese (8–10). Milk derived bioactive peptides are associated with many health beneficial effects, including immunomodulation, antithrombotic activity, antihypertension, antimicrobial activity, and opiate activity (11, 12).
The bioactive peptides are separated, identified, and purified by employing high-performance liquid chromatography (HPLC) (5). However, characterization of peptides is carried out by the protein hydrolyzed fractionation method (13) and functional properties of peptides are assessed by the amino acid composition of the bioactive peptide (14). For fractionation, the ultrafiltration membrane system is the preliminary step to separate the required molecular weight fractions from hydrolyzates (15). Figure 1 illustrates and summarizes the process of bioactive peptides production from dairy proteins, including source preparation, extraction, and hydrolysis of protein (denaturation), fractionation of desired peptides through gel-filtration chromatography (GFC), their purification by HPLC, and identification through liquid chromatography-mass spectrometry (LC-MS/MS).
The dairy industry relies on microbial fermentation and enzymatic hydrolysis to produce bioactive peptides, which alone give low yields of peptides. Various novel technologies are evolving, coupled with conventional methods to generate high yields of bioactive peptides from dairy proteins quickly and at a low cost. Figure 2 illustrates and summarizes the conventional and green novel technologies employed in the dairy industry to produce bioactive peptides. Ultrasound, microwave (16) and high-pressure processing (17) are the efficient, novel, green technologies, but these are emerging technologies with attention to dairy industry, and their promising effects have been entirely understood when employed as pretreatments. Ultrasound waves break, weaken, or clean the electrostatic and hydrophobic interactions of milk proteins through shear forces and cavitation and bring conformational changes in proteins (18, 19). Microwave heating has many benefits like easy operation, less processing, and high efficient energy, making it suitable in continuous food processing (20). High-pressure processing (HPP) is a potential technique used as a pre-treatment method to release bioactive peptides by enhancing the enzymatic digestibility of proteins due to conformational changes in proteins that influence their functional properties boosting their digestibility. It has also been applied to milk and milk products (21, 22).
This review emphasizes the production of peptides from dairy proteins and discusses the potential use of novel technologies in context to conventional methods of bioactive peptides production from dairy proteins, including the mechanisms and their respective examples of use, advantages, limitations, and challenges to each technology.
Milk and Fermented Dairy Products: Source of Bioactive Peptides
Milk and dairy products comprise various essential nutrients such as bioactive agents (antioxidants), minerals, omega-3 fatty acids, linoleic acid, oleic acid, and vitamins, making them nutritious foodstuff (23). Oxidative stress and damage to the body can be prevented by consuming antioxidant-rich foods (24). Milk and its products are a well-known source of antioxidants as they contain: significant amounts of daidzein polyphenolic metabolites, antioxidative enzymes, i.e., glutathione peroxidase, catalase, superoxide dismutase, and sulfur-containing amino acids, i.e., carotenoids, vitamins A and E, cysteine, and methionine (25). Generally, bovine milk protein is comprised of lactoferrin, caseins, immunoglobulins, beta-lactoglobulin (β-LG), alpha-lactalbumin (α-LA), fractions of protease-peptide, and some whey proteins (transferrin and serum albumin) as main fractions (26). Figure 3 shows the major bioactive components of milk with biological properties.
Milk contains various useful molecules encompassing bioactive peptides (27, 28). Dietary proteins contain bioactive peptides in them, which are naturally found inactive in parent protein sequences and liberated only during food processing or gastrointestinal digestion. Peptides work as regulatory compounds with hormone-like activity after liberation. In dairy, milk proteins are the potent source of bioactive peptides which exert various biological functions, i.e., antioxidant, antimicrobial, anticancer, and anti-hypertensive factors (29, 30). As cited in Table 1, many researchers have assessed the biological activities of bioactive peptides from various milk sources, including camel and bovine casein hydrolyzates (31), buffalo casein (32), camel whey protein hydrolyzate (33), camel milk lactoferrin (34), goat milk (35), yak milk (36), goat milk (37), buffalo milk (38), skim milk (6) camel milk (39), whey protein hydrolyzate (40), UHT treated milk (41), and milk and dairy products (42) by using various microorganisms and microbial enzymes for proteolysis. The bioactive peptides are liberated during gastrointestinal digestion (in vivo), milk products’ manufacturing, and proteolysis (in vitro).
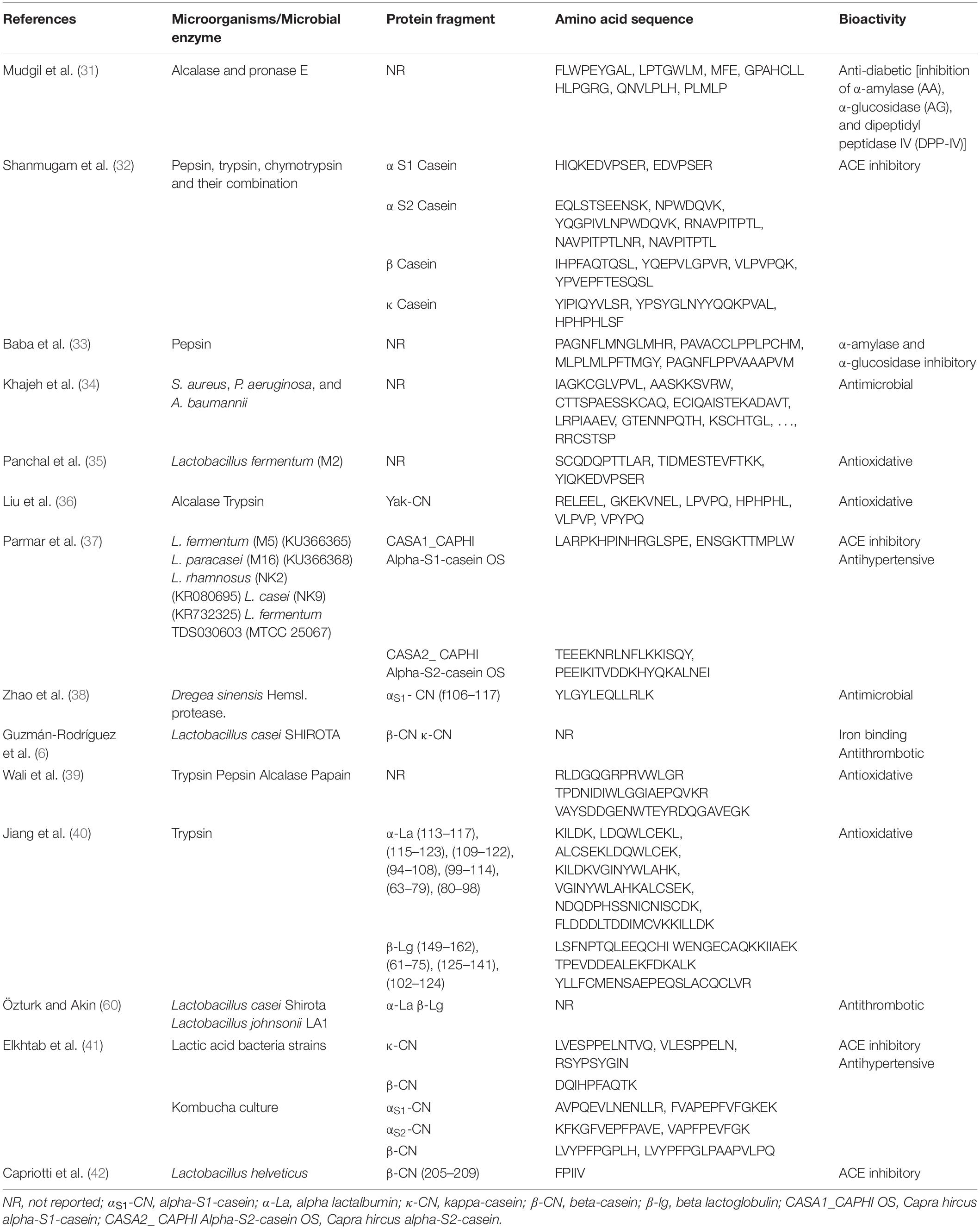
Table 1. Bioactive peptides released from milk proteins by various microorganisms and microbial enzymes.
Reportedly, fermented milk products contain phosphor-peptides, ACE-inhibitory peptides, and casomorphins (43). Bovine α-lactalbumin and β- casein have shown bioactive peptide sequences like LDQW, INYW, and NSLP, FP, HQP, respectively (44, 45). Another in vitro study revealed two antioxidative peptide sequences KVLPVPEK and AVPYPQR, by following milk casein hydrolysis (46). However, digestion and fermentation of goat milk can also release antioxidative peptide sequences like EALEKFDK and EALEKFDK (47, 48).
Cheese is a widely used fermented milk product. Many studies have reported that cheese is a vital source of wide-range of biologically active substances such as proteins and all essential amino acids (except cysteine and methionine), minerals, vitamins, and short-chain fatty acids (49, 50). Cheese contains bioactive compounds with biological activities such as peptides, conjugated linoleic acid (CLA), exopolysaccharides, γ-aminobutyric acid (GABA), vitamins, and organic acids, and fatty acids. According to in vitro and in vivo studies, these bioactive compounds may have antiproliferative, antimicrobial, and antioxidant activities and inhibit ACE (angiotensin-converting enzyme) (51, 52). As shown in Table 2, many bioactive peptides have been identified in different fermented dairy products, such as Iranian ultrafiltered white cheese (53), fermented milk (54), cultured dairy product (55), Hard cow milk cheese (56), fermented casein (57), Prato cheese (58), fermented whey proteins (59), commercial fermented milk (60), goat milk Tulum cheese and cow milk Tulum cheese (61), cow and buffalo cheddar cheeses (62), fermented milk (Lassi) (63), yogurt (64), symbiotic yogurt (65), and curd and whey (66).
Conventional Methods of Production
In the dairy industry, the conventional methods for producing bioactive peptides are microbial fermentation and enzymatic hydrolysis, summarized in Table 1.
Microbial Fermentation
Fermentation is a primeval preservation method that utilizes lactic acid bacteria proteolytic systems as an efficient approach to produce bioactive peptides from food. Generally, lactic acid bacteria fermentation is carried out both naturally and under controlled conditions, which improve technological and nutritional properties of food and ultimately develops texture and flavor in them (67, 68). Usually, the milk fermentation is carried out by Lactobacillus strains; till now, most known bioactive peptides have been isolated through milk cultures (27). Because different milk sources (cow, buffalo, goat, yak, camel, or mare) have distinctive proteins, different bioactive peptides are produced on hydrolysis of casein and whey proteins usage of the same Lactobacillus strain (69). Lactic acid bacteria fulfill their need for essential and growth-promoting amino acids from milk proteins as a primary source (70). Microbial fermentation is an efficient and economical method to produce peptides (71) which is extensively employed to functionalize milk products and byproducts in the dairy industry (72, 73). During the manufacturing of fermented dairy products starter and non-starter, bacteria can produce bioactive peptides because of the high proteolytic activities exerted by dairy starter cultures (74). Ueno et al. (75) and Phelan et al. (76) utilized L. helveticus CM4 to produce an endopeptidase that possessed the ability to produce antihypertensive peptides by using synthetic pro-peptides as a substrate. Also, lactic acid bacteria have helped achieve multifunctional bioactive peptides (77, 78). Zanutto-Elgui et al. (79) have reported the production of bioactive peptides having antioxidant and antimicrobial activity from goat and bovine milk by using the proteolytic activity of Aspergillus flavipes and Aspergillus oryzae enzymes.
Microbial fermentation is comparatively economical than the enzymatic method for bioactive peptides production. Microbial fermentation method applications have some industrial limitations as they yield low peptide production and lack specificness of peptide generation (69).
Enzymatic Hydrolysis
Enzymatic hydrolysis is a reliable, efficient, and the principal method to hydrolyze whole proteins for the production of bioactive peptides under the mild condition of enzyme activity, substrate concentration, hydrolysis time, temperature, and pH. These peptides exert anti-inflammatory, opioid, immunomodulatory, anticancer, antioxidant, antithrombotic, and antihypertensive activities (67, 80). The results of the efficiency of enzymatic hydrolysis mostly depend on two factors: the primary structure of parent protein (substrate) and specificity of the enzyme applied (81). Animal, plant (neutrase, thermolysin, ficin, pronase, flavourzyme, and papain), microbial, and digestive (chymotrypsin, trypsin, and pepsin) origin enzymes have been used to hydrolyze the large sequence peptides into small sequence peptides having 500–1,800 kDa molecular weights and 2–20 amino acid units (82, 83). In vitro studies have shown that the parent milk proteins undergo hydrolysis with pancreatic proteinases (mostly trypsin) and liberate most known biological peptides. Though, endoprotease combinations (proline-specific endopeptidase, carboxypeptidase, elastase, pancreatin, thermolysin, pepsin, and chymotrypsin) are also reported to produce bioactive peptides (84, 85). Furthermore, intact protein molecules can be hydrolyzed by combining enzymes like Thermolysin, Trypsin, Pancreatin™, Chymosin, and Alcalae™ to produce bioactive peptides (76). Combinations of carboxypeptidase, elastase, chymotrypsin, trypsin, and pepsin have been used to liberate various α-lactalbumin and β-lactoglobulin corresponding fractions and ACE-inhibitory peptides having different IC50 values (84–86). Liu et al. (36) isolated the antioxidative peptides (RELEEL) from the yak casein hydrolyzate using the combination of alcalase and trypsin digestion. Abdel-Hamid et al. (87) subjected buffalo skimmed milk to hydrolysis using papain, pepsin, trypsin, and isolated known and novel ACE inhibitory antioxidative peptides. Wali et al. (39) used a combination of trypsin, pepsin, alcalase, and papain to hydrolyze the Bactrian camel milk and isolated three novel antioxidant peptides.
Enzymatic hydrolysis has certain shortcomings, such as higher cost to produce pure bioactive peptides, casein coagulation on heating, and bitterness, therefore, choice of enzymatic hydrolysis conditions must be taken into account before application (88, 89).
Novel Processing Technologies
The novel processing technologies such as ultrasound-assisted processing (UAP), microwave-assisted processing (MAP), high-pressure processing (HPP), pulsed electric field processing (PEF), subcritical water processing (SWP), and ohmic heating relies on physical processes to improve the degree of hydrolysis during bioactive peptides production (90, 91). However, following applications of UAP, MAP, and HPP as pretreatments have been found in the dairy industry to prepare bioactive peptides.
Ultrasound-Assisted Processing
Ultrasound-assisted processing is a novel, eco-friendly, and non-thermal physical technology that involves >20 kHz frequency of sound waves to produce peptides (91, 92). In ultrasound treatment, acoustic cavitation, acoustic streaming, and mechanical vibrations are produced on the passage of ultrasound waves through a medium. Acoustic streaming can allow and improve the transfer of mass through a medium. The mechanical vibrations can change solid particle size and structure (93). In a liquid medium, ultrasound treatment follows the cavitation process in which pre-existing micro-bubbles expand and contract. However, during these oscillations, bubbles keep growing until they reach their resonance size range and then collapse violently in case of transient/inertial cavitation (94, 95). In transient cavitation, physical shearing, high-pressure and extreme localized temperatures (2,000–5,000 K) are produced on collapsing of increased sized bubbles (within few acoustic cycles) into fragments at low ultrasound frequency. However, stable cavitation results in relatively mild streaming effects on collapsing of the little increased bubbles (over a large number of acoustic cycles) at higher frequencies. Cavitation also owns the ability to induce chemical changes along with physical effects. When cavitation is applied to an aqueous medium, a highly reactive radical is formed inside the bubble (on reaction of gas molecules and water vapor reaction) due to the availability of generated localized high temperature. The ultrasound cavitation chemical effects are visible at 300–500 kHz frequencies and physical effects are visible at 20 kHz frequency (94). Protein structures undergo conformational changes by ultrasound processing, such as acoustic cavitation, forces of chemical and physical effects (96, 97).
As presented in Table 3, recently UAP has been employed as pretreatment for various milk proteins hydrolysis, including whey proteins (98), caprine milk protein (99), fresh milk (100), cheddar cheese (101), whey protein isolate (102), whey protein (103), and milk protein concentrate (104, 105). UAP in combination with enzymatic hydrolysis has been employed for various proteins, i.e., eggshell membrane (106), egg white (107), and isolated oat protein (108). Ulug et al. (91) reported that the application of UAP is carried out in combination with enzymatic hydrolysis, to increase the production of bioactive peptide, as UAP alone cannot break the peptidic bond. Ultrasound pretreatment enhance the enzymes accessability into the peptide bonds of foods that results in the increased release of bioactive peptides. Basically, ultrasound processing generates the acoustic forces that increase the available surface area for enzyme protein interactions by reducing the size of the fat globules that get covered with whey proteins and casein micelles, ultimately, increases the access of proteolytic enzymes to the proteins (109, 110). Wu et al. (111) in their study on the thermodynamic properties of whey protein hydrolyzed by alcalase with ultrasonic pretreatment reported that the hydrolyzates showed significantly increased ACE inhibitory and immunomodulatory activities when the whey protein enzymatic hydrolysis was assisted by the ultrasound. Sonication pretreatment induces the whey protein unfolding, increased free sulfhydryl content, and conformational changes with increased β-sheets and β-turns formation (111). Similar study exhibited that ultrasound-assisted pretreatment combined with low purity enzymes show the increased hydrolysis rate that may be due to changes in free sulfhydryl clusters and disulfide bond (112), hydrophobic protein content, and surface hydrophobicity (113). Lorenzetti et al. (102) reported that ultrasound pretreatment before hydrolysis of whey protein isolate could help to develop the economic ingredients for the dairy industry.
UAP application is beneficial to reduce the disadvantages resulting from hydrolysis by conventional enzymes, i.e., long-time hydrolysis and low conversion rate (114). Generally, UAP equipment requires fewer installations, low maintenance, around 85% energy efficiency, and cost between €10,000 and 200,000 (115). Undeniably, UAP is one of the novel and most preferable techniques for producing bioactive peptides due to numerous advantages such as faster start-up, extraction selectivity, high process control, reduced temperature and time, and faster mass and energy transfer (116).
Microwave-Assisted Processing
Microwaves encompass electromagnetic radiation of 300 MHz–300 GHz range (117). Microwave energy follows molecular interactions (ionic conduction and dipolar rotation mechanisms) as a medium transportation mode. On applying electromagnetic field, charged colloidal molecules migrate and flow through a stationary medium in ionic conduction and led to resistance in the solution, which produces thermal energy. On the other hand, dipole rearrangement occurs on electromagnetic fields in dipolar rotation (118).
Microwave treatment is carried out in the food processing ovens in which an alternating electric field is used to generate the microwaves having 2.45 GHz frequency and <1 cm wavelength typically. These microwaves do not cause breakage of covalent bonds because of their non-ionizing radiation nature (119, 120) but, these can either induce thermal or non-thermal changes in the milk. Microwaves generate heat by friction that results from the oscillation of molecules as dipoles of water try to align their arrangements under the influence of microwave field. So, thermal effects are resulted from the generation of localized heat due to friction of molecules, on the other hand, non-thermal effects (accelerated protein unfolding rate) alone arise from the rearrangement of molecules in milk (120).
Microwave-assisted processing has been employed for various milk proteins hydrolysis including cheddar cheese (101), bovine whey proteins (121), milk protein concentrate (105), bovine serum albumin (122), and bovine whey protein concentrate (123) as cited in Table 4. MAP is one of the most preferred alternative technologies to conventional heat processing methods as it enhances functional properties, extends shelf life, and improves microbial safety of food products (124, 125). In their study, Izquierdo and coworkers found that MAP could make proteins specific sites potentially available to proteolytic enzymes by continuous protein molecules unfolding and rearrangement (123). In a study, the surface plasmon resonance sensing method was used to investigate the unfolding of protein by employing MAP at 2.45 GHz. The results showed that at the same temperature, MAP heating has a higher impact on the unfolding and denaturation of a bovine crystalline than conventional heating (126).
Microwave is the most extensively studied and world-widely popular method in both academics and food processing industry due to high heating rates which eventually lead to a clean environment of work, easy operation, low processing time, and low maintenance requirements (127, 128). In the food industry, MAP has extensive applications to extract bioactive compounds from plant materials. During extraction, MAP is used to facilitate quick heating of solvent to separate analytes and matrix. Many studies have been reported to show the efficient production of bioactive peptides from MAP as pretreatment combined with proteolytic enzymes by accelerating the rapid hydrolysis of protein into peptides and producing more coverage of sequence (129). Before proteolytic hydrolysis, cleavage sites of proteins are probably exposed by microwave radiations that cause a change in protease cleavage sites (130).
Generally, in contrast to conventional methods, MAP offers several benefits: reproducibility, reduced processing time, hydrolysis efficiency, cost-effectiveness, convenience, and simple handling, making it one of the most preferred methods (131).
High Hydrostatic Pressure Processing
High-pressure processing (HPP) is a green, novel, and non-thermal technology that encompasses the application of 100–1000 MPa pressure, with or without treatment of heat primarily for the deactivation of pathogenic microorganisms along with molds, yeast, and vegetative bacteria, enhancing nutritional and functional properties of food products in the food industry. Depending on the food type, HPP treatment duration varies between 0 and 30 min (132, 133). Also, both treatment duration and pressure-transmitting fluid, and adiabatic heating result in a 3–9°C increase of temperature per 100 MPa (134). This technology has advantages over other technologies due to low to moderate temperature and causing the least damage to the bioactive compounds. HPP involves the combination of pressure and heat, resulting in conformational changes of protein and biological, chemical, and physical changes in food compounds (135).
High-pressure processing can be carried out in three different modes like semi-continuous, continuous, and batch. Batch HPP is an efficient and simple mode. The pressure chamber is filled with a prepacked sample and sealed, the air in the pressure chamber is replaced by pouring water, and then pressure is built until the desired point is achieved. After a particular time chamber is depressurized. Finally, processed food is taken out. On the other hand, continuous/dynamic HPP (136) involves utilizing a moving piston to push the food through a narrow gap (137). While in the case of semi-continuous HPP, the flow of liquid is introduced and contained in the same chamber at constant pressure for a specific time, after that, processed liquid food is stored in sterile tanks (138).
High-pressure processing has been employed for various milk proteins hydrolysis including whey protein concentrate (139, 140), cheddar cheese (101), bovine whey protein beta-lactoglobulin (141), whey protein isolate (142), cheddar cheese (22), beta-lactoglobulin (143), and bovine whey proteins (144) as cited in Table 5. Relatively, HPP is a well-developed technology that has many applications to milk and cheese (21, 22, 145). Munir et al. (101) reported the increased ACE Inhibitory activity HPP treated milk cheese and indicated that HPP results in efficient bioactive peptides liberation and proteolysis by imparting change in indigenous milk enzymes structures by subjecting more active sites for protein reaction (22, 146). Various studies have been reported in which the patterns of native and pressure-treated proteins have been compared. Indeed, Maynard and coworkers found that under pressurization tryptic β-LG hydrolysis generated a low concentration of intermediate hydrolysis peptides (147). On the other hand, Knudsen and coworkers reported the application of HPP at the beginning step of tryptic β-LG hydrolysis that generated an increased amount of high molecular weight peptides and hydrophobic peptides (148).
In the food industry, high-pressure processing is well known as a clean method compared to conventional methods as it offers numerous advantages such as homogeneous and constant pressurization at ambient temperatures, utilize less energy due to maintenance of constant pressure when reached absolute pressure, quick pressurization, and de-pressurization, reduced processing time, and it’s throughout applications irrespective of shape or size in the food system (149–151). However, the applications of this technology have certain limitations such as batch operation and costly infrastructure around 0.6–4 M US dollars accounting for 75–80% of the investment as the initial investment (152, 153). HPP has limited effects on covalent bond cleavage and production of bioactive peptides alone, therefore, it’s employed in combination with enzymatic hydrolysis to denature protein and improved access to sites of enzyme cleavage to get efficient and increased production process of bioactive peptides (91).
Future Outlook
Numerous studies on the identification and evaluation of in vitro bioactivity of peptides from protein hydrolyzates of several sources of protein suggest that novel technologies should be employed to isolate novel ingredients to prepare novel functional foods. But, the application of novel technologies is an emerging field of rising significance in the dairy industry as, till now, there are minimal studies on the improvement of fermentation/enzymatic hydrolysis using UA, MA, and HPP as pretreatments to produce bioactive peptides while fermentation/enzymatic hydrolysis are promising conventional methods to generate peptides at industrial level. Thus, fermentation/enzymatic hydrolysis of dairy proteins treated with ultrasound, microwave, and high-pressure is possible to generate improved bioactive peptides at a lower cost and short time compared to only conventional applications methods.
In the dairy industry, mostly milk is used as a medium in novel technologies. So, there is a gap in understanding that either the treatment of novel technologies enhances or alters the fermentation/enzymatic hydrolysis in whole milk, fermented milk, yogurt, cheese, and other dairy products. The synergistic effect of possible novel technologies can be investigated to understand the liberation of bioactive peptides at a low cost and short time. For instance, microwave heating and ultrasound waves/HPP pressure combination could be tested to explore the effect of heat treatment and high frequency/pressure on the release of bioactive peptides from dairy proteins. As several studies reported that the applications of these novel technologies could generate lower-cost ingredients with a higher content of available amino acids for the dairy industry.
Future studies are expected to establish the actual applications of novel technologies by investigating the maximum potential of these processing technologies to comprehend their possible specificities in the proteins’ cleavage, generate novel, and known bioactive peptides, effects on specificness, and modification of amino acids in dairy proteins.
Conclusion
It is noteworthy that milk protein hydrolysis liberates a wide variety of bioactive peptides that possess remarkable biological functions to maintain human health. The knowledge of bioactive peptides from milk and other dairy proteins and their health benefits increases with each passing day. It’s also opening new doors to exciting offers such as novel functional foods that can help manage and prevent several chronic diseases including cardiovascular diseases, diabetes, hypertension, cancer, etc.
Although the dairy industry is slow in embracing novel technologies but reported studies to depict that UAP, MAP, and HPP are innovative, environmentally friendly, and promising pretreatments to modify the profile of peptides, improve the yields of peptides, and higher liberation of bioactive peptides as compared to conventional processing technologies. Novel technologies require sustainable, environment-friendly, and highly specialized cost equipment and workers to operate the equipment. These novel processing technologies are coupled with conventional methods for unfolding, denaturing, or aggregating the milk proteins by breaking down weak molecular interactions with less or no effect on covalent bonds. The influence of pretreatments is intensified by fermentation/enzymatic hydrolysis, which results in a higher amount of liberated low molecular weight bioactive peptides, enhanced hydrolysis, and increased proteolysis of dairy proteins which ultimately increases their bioactivity. Many studies have reported the isolation of novel bioactive peptides from dairy proteins after employing novel technologies as pretreatments.
Ultrasound-assisted processing is an innovative and most efficient technology as it offers easy control, simple operation, mild operating conditions, the ability to achieve industrial amplification and production, and effective influence of auxiliary enzymatic hydrolysis. Its mechanical effects and cavitation change the protein conformation, increase the biological functionalities of enzymes, and enhance the reaction rate of enzymatic hydrolysis. Though novel technologies are innovative, environmentally friendly, and promising pretreatments, their trend is increasing and acquisitioning momentum to produce bioactive peptides.
Author Contributions
MAM and SI: writing original draft. IH, MMANR, AR, and MSM: reviewing and editing. SI and MMANR: conceptualization and methodology. MAM, MMANR, and SAI: supervision and project administration. SAS: visualization and data curation. SAI: funding acquisition. All authors contributed to the article and approved the submitted version.
Funding
This work was funded in part by the USDA/NIFA through the Agricultural Research Program at North Carolina Agricultural and Technical State University (Evans-Allen Program, project number NC.X-291-5-15-170-1) and by an 1890 Capacity Building Program Grant (No. 2020-38821-31113/project accession No. 021765). SI would like to acknowledge the support of the Agricultural Research Station at North Carolina Agricultural and Technical State University (Greensboro, NC, United States). This research was funded, in part, by grants (Project Nos. NC.X337-5-21-170-1 and NC.X341-5-21-170-1) from the National Institute of Food and Agriculture (NIFA).
Author Disclaimer
The contents are solely the responsibility of the authors and do not necessarily represent the official views of NIFA.
Conflict of Interest
The authors declare that the research was conducted in the absence of any commercial or financial relationships that could be construed as a potential conflict of interest.
Publisher’s Note
All claims expressed in this article are solely those of the authors and do not necessarily represent those of their affiliated organizations, or those of the publisher, the editors and the reviewers. Any product that may be evaluated in this article, or claim that may be made by its manufacturer, is not guaranteed or endorsed by the publisher.
References
1. Nagpal R, Behare P, Rana R, Kumar A, Kumar M, Arora S, et al. Bioactive peptides derived from milk proteins and their health beneficial potentials: an update. Food Funct. (2011) 2:18–27. doi: 10.1039/c0fo00016g
2. Phelan M, Kerins D. The potential role of milk-derived peptides in cardiovascular disease. Food Funct. (2011) 2:153–67. doi: 10.1039/c1fo10017c
3. Shazly AB, He Z, El-Aziz MA, Zeng M, Zhang S, Qin F, et al. Fractionation and identification of novel antioxidant peptides from buffalo and bovine casein hydrolysates. Food Chem. (2017) 232:753–62. doi: 10.1016/j.foodchem.2017.04.071
4. Zhang Y, Zhang H, Wang L, Guo X, Qi X, Qian H. Influence of the degree of hydrolysis (DH) on antioxidant properties and radical-scavenging activities of peanut peptides prepared from fermented peanut meal. Eur Food Res Technol. (2011) 232:941–50. doi: 10.1007/s00217-011-1466-0
5. Singh BP, Vij S, Hati S. Functional significance of bioactive peptides derived from soybean. Peptides. (2014) 54:171–9. doi: 10.1016/j.peptides.2014.01.022
6. Guzmán-Rodríguez F, Gómez-Ruizy L, Rodríguez-Serrano G, Alatorre-Santamaría S, García-Garibay M, Cruz-Guerrero WA. Iron binding and antithrombotic peptides released during the fermentation of milk by Lactobacillus casei shirota. Rev Mex Ing Quim. (2019) 18:1161–6. doi: 10.24275/uam/izt/dcbi/revmexingquim/2019v18n3/Guzman
7. Toldrá F, Reig M, Aristoy MC, Mora L. Generation of bioactive peptides during food processing. Food Chem. (2018) 267:395–404. doi: 10.1016/j.foodchem.2017.06.119
8. Soleymanzadeh N, Mirdamadi S, Mirzaei M, Kianirad M. Novel β-casein derived antioxidant and ACE-inhibitory active peptide from camel milk fermented by Leuconostoc lactis PTCC1899: identification and molecular docking. Int Dairy J. (2019) 97:201–8. doi: 10.1016/j.idairyj.2019.05.012
9. Sultan S, Huma N, Butt MS, Aleem M, Abbas M. Therapeutic potential of dairy bioactive peptides: a contemporary perspective. Crit Rev Food Sci Nutr. (2018) 58:105–15. doi: 10.1080/10408398.2015.1136590
10. Taha S, El Abd M, De Gobba C, Abdel-Hamid M, Khalil E, Hassan D. Antioxidant and antibacterial activities of bioactive peptides in buffalo’s yoghurt fermented with different starter cultures. Food Sci Biotechnol. (2017) 26:1325–32. doi: 10.1007/s10068-017-0160-9
11. Pescuma M, Hébert EM, Rabesona H, Drouet M, Choiset Y, Haertlé T, et al. Proteolytic action of Lactobacillus delbrueckii subsp. bulgaricus CRL 656 reduces antigenic response to bovine β-lactoglobulin. Food Chem. (2011) 127:487–92. doi: 10.1016/j.foodchem.2011.01.029
12. Stelwagen K, Carpenter E, Haigh B, Hodgkinson A, Wheeler TT. Immune components of bovine colostrum and milk1. J Anim Sci. (2009) 87:3–9. doi: 10.2527/jas.2008-1377
13. Mahdi C, Untari H, Padaga MC. Identification and characterization of bioactive peptides of fermented goat milk as A sources of antioxidant as a therapeutic natural product. IOP Conf Ser. (2018) 299:012014. doi: 10.1088/1757-899X/299/1/012014
14. Kitts D, Weiler K. Bioactive proteins and peptides from food sources. applications of bioprocesses used in isolation and recovery. Curr Pharm Des. (2003) 9:1309–23. doi: 10.2174/1381612033454883
15. Roblet C, Amiot J, Lavigne C, Marette A, Lessard M, Jean J, et al. Screening of in vitro bioactivities of a soy protein hydrolysate separated by hollow fiber and spiral-wound ultrafiltration membranes. Food Res Int. (2012) 46:237–49. doi: 10.1016/j.foodres.2011.11.014
16. Atuonwu JC, Leadley C, Bosman A, Tassou SA, Lopez-Quiroga E, Fryer PJ. Comparative assessment of innovative and conventional food preservation technologies: process energy performance and greenhouse gas emissions. Innov Food Sci Emerg Technol. (2018) 50:174–87. doi: 10.1016/j.ifset.2018.09.008
17. Misra NN, Koubaa M, Roohinejad S, Juliano P, Alpas H, Inácio RS, et al. Landmarks in the historical development of twenty first century food processing technologies. Food Res Int. (2017) 97:318–39. doi: 10.1016/j.foodres.2017.05.001
18. Madadlou A, Sheehan D, Emam-Djomeh Z, Mousavi ME. Ultrasound-assisted generation of ACE-inhibitory peptides from casein hydrolyzed with nanoencapsulated protease. J Sci Food Agric. (2011) 91:2112–6. doi: 10.1002/jsfa.4438
19. Nguyen NHA, Anema SG. Effect of ultrasonication on the properties of skim milk used in the formation of acid gels. Innov Food Sci Emerg Technol. (2010) 11:616–22. doi: 10.1016/j.ifset.2010.05.006
20. Martins CPC, Cavalcanti RN, Couto SM, Moraes J, Esmerino EA, Silva MC, et al. Microwave processing: current background and effects on the physicochemical and microbiological aspects of dairy products. Compr Rev Food Sci Food Saf. (2019) 18:67–83. doi: 10.1111/1541-4337.12409
21. Delgado-Martínez FJ, Carrapiso AI, Contador R, Ramírez MR. Volatile compounds and sensory changes after high pressure processing of mature “Torta del Casar” (raw ewe’s milk cheese) during refrigerated storage. Innov Food Sci Emerg Technol. (2019) 52:34–41. doi: 10.1016/j.ifset.2018.11.004
22. Voigt DD, Chevalier F, Donaghy JA, Patterson MF, Qian MC, Kelly AL. Effect of high-pressure treatment of milk for cheese manufacture on proteolysis, lipolysis, texture and functionality of cheddar cheese during ripening. Innov Food Sci Emerg Technol. (2012) 13:23–30. doi: 10.1016/j.ifset.2011.10.004
23. Saxelin M, Korpela R, Mäyrä-Mäkinen A. Introduction: classifying functional dairy products. In: Mattila-Sandholm T, Saarela M editors. Functional Dairy Products. (Sawston: Woodhead Publishing) (2003). p. 1–16. doi: 10.1533/9781855736917.1
24. Wang YC, Yu RC, Chou CC. Antioxidative activities of soymilk fermented with lactic acid bacteria and bifidobacteria. Food Microbiol. (2006) 23:128–35. doi: 10.1016/j.fm.2005.01.020
25. Usta B, Yılmaz-Ersan L. Antioxidant enzymes of milk and their biological effects. Ziraat Fakültesi Derg Uludag ? Üniversitesi. (2013) 27: 123–30.
26. Punia H, Tokas J, Malik A, Sangwan S, Baloda S, Singh N, et al. Identification and detection of bioactive peptides in milk and dairy products: remarks about agro-foods. Molecules. (2020) 25:3328. doi: 10.3390/molecules25153328
27. Dziuba B, Dziuba M. Milk proteins-derived bioactive peptides in dairy products: molecular, biological and methodological aspects. Acta Sci Pol Technol. Aliment. (2014) 13:5–25. doi: 10.17306/j.afs.2014.1.1
28. Shioda S, Nakamachi T. PACAP as a neuroprotective factor in ischemic neuronal injuries. Peptides. (2015) 72:202–7. doi: 10.1016/j.peptides.2015.08.006
29. Egger L, Ménard O. Update on bioactive peptides after milk and cheese digestion. Curr Opin Food Sci. (2017) 14:116–21. doi: 10.1016/j.cofs.2017.03.003
30. Sánchez A, Vázquez A. Bioactive peptides: a review. Food Qual Saf. (2017) 1:29–46. doi: 10.1093/fqsafe/fyx006
31. Mudgil P, Kamal H, Priya Kilari B, Mohd Salim MAS, Gan CY, Maqsood S. Simulated gastrointestinal digestion of camel and bovine casein hydrolysates: identification and characterization of novel anti-diabetic bioactive peptides. Food Chem. (2021) 353:129374. doi: 10.1016/J.FOODCHEM.2021.129374
32. Shanmugam VP, Kapila S, Kemgang TS, Reddi S, Kapila R, Muthukumar S, et al. Isolation and characterization of angiotensin converting enzyme inhibitory peptide from buffalo casein. Int J Pept Res Ther. (2021) 27:1481–91. doi: 10.1007/S10989-021-10185-0/TABLES/3
33. Baba WN, Mudgil P, Kamal H, Kilari BP, Gan CY, Maqsood S. Identification and characterization of novel α-amylase and α-glucosidase inhibitory peptides from camel whey proteins. J Dairy Sci. (2021) 104:1364–77. doi: 10.3168/JDS.2020-19271
34. Khajeh E, Jamshidian-Mojaver M, Naeemipour M, Farzin H. The identification of a novel peptide derived from lactoferrin isolated from camel milk with potential antimicrobial activity. Iran J Med Microbiol. (2021) 15:302–16. doi: 10.30699/IJMM.15.3.302
35. Panchal GK, Das S, Sakure A, Singh BP, Hati S. Production and characterization of antioxidative peptides during lactic fermentation of goat milk. J Food Process Preserv. (2021) 45:e15992. doi: 10.1111/JFPP.15992
36. Liu Q, Yang M, Zhao B, Yang F. Isolation of antioxidant peptides from yak casein hydrolysate. RSC Adv. (2020) 10:19844–51. doi: 10.1039/d0ra02644a
37. Parmar H, Hati S, Panchal G, Sakure AA. Purification and production of novel angiotensin i-converting enzyme (ACE) inhibitory bioactive peptides derived from fermented goat milk. Int J Pept Res Ther. (2020) 26:997–1011. doi: 10.1007/s10989-019-09902-7
38. Zhao Q, Shi Y, Wang X, Huang A. Characterization of a novel antimicrobial peptide from buffalo casein hydrolysate based on live bacteria adsorption. J Dairy Sci. (2020) 103: 11116–11128.
39. Wali A, Yanhua G, Ishimov U, Yili A, Aisa HA, Salikhov S. Isolation and identification of three novel antioxidant peptides from the bactrian camel milk hydrolysates. Int J Pept Res Ther. (2019) 26:1–10.
40. Jiang B, Na J, Wang L, Li D, Liu C, Feng Z. Separation and enrichment of antioxidant peptides from whey protein isolate hydrolysate by aqueous two-phase extraction and aqueous two-phase flotation. Foods. (2019) 8:34. doi: 10.3390/foods8010034
41. Elkhtab E, El-Alfy M, Shenana M, Mohamed A, Yousef AE. New potentially antihypertensive peptides liberated in milk during fermentation with selected lactic acid bacteria and kombucha cultures. J Dairy Sci. (2017) 100:9508–20. doi: 10.3168/jds.2017-13150
42. Capriotti AL, Cavaliere C, Piovesana S, Samperi R, Laganà A. Recent trends in the analysis of bioactive peptides in milk and dairy products. Anal Bioanal Chem. (2016) 408:2677–85. doi: 10.1007/s00216-016-9303-8
43. Korhonen H. Milk-derived bioactive peptides: from science to applications. J Funct Foods. (2009) 1:177–87. doi: 10.1016/j.jff.2009.01.007
44. O’Keeffe MB, Fitzgerald RJ. Identification of short peptide sequences in complex milk protein hydrolysates. Food Chem. (2015) 184:140–6. doi: 10.1016/j.foodchem.2015.03.077
45. Sadat L, Cakir-Kiefer C, N’Negue MA, Gaillard JL, Girardet JM, Miclo L. Isolation and identification of antioxidative peptides from bovine α-lactalbumin. Int Dairy J. (2011) 21:214–21. doi: 10.1016/j.idairyj.2010.11.011
46. Tonolo F, Folda A, Cesaro L, Scalcon V, Marin O, Ferro S, et al. Milk-derived bioactive peptides exhibit antioxidant activity through the keap1-Nrf2 signaling pathway. J Funct Foods. (2020) 64:103696. doi: 10.1016/j.jff.2019.103696
47. Ahmed AS, El-Bassiony T, Elmalt LM, Ibrahim HR. Identification of potent antioxidant bioactive peptides from goat milk proteins. Food Res Int. (2015) 74:80–8. doi: 10.1016/j.foodres.2015.04.032
48. Zhang Y, Shen Y, Zhang H, Wang L, Zhang H, Qian H, et al. Isolation, purification and identification of two antioxidant peptides from water hyacinth leaf protein hydrolysates (WHLPH). Eur Food Res Technol. (2018) 244:83–96. doi: 10.1007/s00217-017-2941-z
49. Diana M, Rafecas M, Arco C, Quílez J. Free amino acid profile of Spanish artisanal cheeses: Importance of gamma-aminobutyric acid (GABA) and ornithine content. J Food Compost Anal. (2014) 35:94–100. doi: 10.1016/j.jfca.2014.06.007
50. Walther B, Schmid A, Sieber R, Wehrmüller K. Cheese in nutrition and health. Dairy Sci Technol. (2008) 88:389–405. doi: 10.1051/dst:2008012
51. Geurts L, Everard A, Le Ruyet P, Delzenne NM, Cani PD. Ripened dairy products differentially affect hepatic lipid content and adipose tissue oxidative stress markers in obese and type 2 diabetic mice. J Agric Food Chem. (2012) 60:2063–8. doi: 10.1021/jf204916x
52. Sprong RC, Schonewille AJ, van der Meer R. Dietary cheese whey protein protects rats against mild dextran sulfate sodium-induced colitis: role of mucin and microbiota. J Dairy Sci. (2010) 93:1364–71. doi: 10.3168/jds.2009-2397
53. Yousefi L, Habibi Najafi MB, Edalatian Dovom MR, Mortazavian AM. Production of angiotensin-converting enzyme inhibitory peptides in Iranian ultrafiltered white cheese prepared with Lactobacillus brevis KX572382. Int J Food Sci Technol. (2020) 56:14891. doi: 10.1111/ijfs.14891
54. Kim S, Lim SD. Separation and purification of lipase inhibitory peptide from fermented milk by Lactobacillus plantarum Q180. Food Sci Anim Resour. (2020) 40:87–95. doi: 10.5851/kosfa.2019.e87
55. Mullaiselvan I, Kanagaraj V, Sekar S, Dharmar B, Sambandan R. Studies on immunomodulatory effect of casein phospho peptide isolated from cultured dairy product. Int Res J Pure Appl Chem. (2020) 21:28–40. doi: 10.9734/irjpac/2020/v21i2330300
56. Timón ML, Andrés AI, Otte J, Petrón MJ. Antioxidant peptides (<3 kDa) identified on hard cow milk cheese with rennet from different origin. Food Res Int. (2019) 120:643–9. doi: 10.1016/j.foodres.2018.11.019
57. Fan M, Guo T, Li W, Chen J, Li F, Wang C, et al. Isolation and identification of novel casein-derived bioactive peptides and potential functions in fermented casein with Lactobacillus helveticus. Food Sci Hum Wellness. (2019) 8:156–76. doi: 10.1016/j.fshw.2019.03.010
58. Baptista DP, Galli BD, Cavalheiro FG, Negrão F, Eberlin MN, Gigante ML. Lactobacillus helveticus LH-B02 favours the release of bioactive peptide during prato cheese ripening. Int Dairy J. (2018) 87:75–83. doi: 10.1016/j.idairyj.2018.08.001
59. Daliri EBM, Lee BH, Park BJ, Kim SH, Oh DH. Antihypertensive peptides from whey proteins fermented by lactic acid bacteria. Food Sci Biotechnol. (2018) 27:1781–9. doi: 10.1007/s10068-018-0423-0
60. Pérez-Escalante E, Jaimez-Ordaz J, Castañeda-Ovando A, Contreras-López E, Añorve-Morga J, González-Olivares LG. Antithrombotic activity of milk protein hydrolysates by lactic acid bacteria isolated from commercial fermented milks. Braz Arch Biol Technol. (2018) 61:18180132. doi: 10.1590/1678-4324-2018180132
61. Özturk Hl, Akin N. Comparison of some functionalities of water soluble peptides derived from Turkish cow and goat milk tulum cheeses during ripening. Food Sci Technol. (2017) 38:38.
62. Rafiq S, Huma N, Pasha I, Shahid M, Xiao H. Angiotensin-converting enzyme-inhibitory and antithrombotic activities of soluble peptide extracts from buffalo and cow milk cheddar cheeses. Int J Dairy Technol. (2017) 70:380–8. doi: 10.1111/1471-0307.12373
63. Padghan PV, Mann B, Sharma R, Bajaj R, Saini P. Production of angiotensin-I-converting-enzyme-inhibitory peptides in fermented milks (Lassi) fermented by Lactobacillus acidophillus with consideration of incubation period and simmering treatment. Int J Pept Res Ther. (2017) 23:69–79. doi: 10.1007/s10989-016-9540-x
64. Jin Y, Yu Y, Qi Y, Wang F, Yan J, Zou H. Peptide profiling and the bioactivity character of yogurt in the simulated gastrointestinal digestion. J Proteom. (2016) 141:24–46. doi: 10.1016/j.jprot.2016.04.010
65. Sah BNP, Vasiljevic T, McKechnie S, Donkor ON. Antioxidant peptides isolated from synbiotic yoghurt exhibit antiproliferative activities against HT-29 colon cancer cells. Int Dairy J. (2016) 63:99–106. doi: 10.1016/j.idairyj.2016.08.003
66. Dabarera M, Athiththan L, Perera R. Antihypertensive peptides from curd. Ayu. (2015) 36:214. doi: 10.4103/0974-8520.175534
67. Abd El-Salam MH, El-Shibiny S. Preparation, properties, and uses of enzymatic milk protein hydrolysates. Crit Rev Food Sci Nutr. (2017) 57:1119–32. doi: 10.1080/10408398.2014.899200
68. Savijoki K, Ingmer H, Varmanen P. Proteolytic systems of lactic acid bacteria. Appl Microbiol Biotechnol. (2006) 71:394–406. doi: 10.1007/s00253-006-0427-1
69. Raveschot C, Cudennec B, Coutte F, Flahaut C, Fremont M, Drider D, et al. Production of bioactive peptides by Lactobacillus species: from gene to application. Front Microbiol. (2018) 9:2354. doi: 10.3389/fmicb.2018.02354
70. Juillard V, Laan H, Kunji ERS, Jeronimus-Stratingh CM, Bruins AP, Konings WN. The extracellular P(I)-type proteinase of Lactococcus lactis hydrolyzes β-casein into more than one hundred different oligopeptides. J Bacteriol. (1995) 177:3472–8. doi: 10.1128/jb.177.12.3472-3478.1995
72. Hafeez Z, Cakir-Kiefer C, Roux E, Perrin C, Miclo L, Dary-Mourot A. Strategies of producing bioactive peptides from milk proteins to functionalize fermented milk products. Food Res Int. (2014) 63:71–80. doi: 10.1016/j.foodres.2014.06.002
73. Pihlanto A. Lactic fermentation and bioactive peptides. In: M Kongo editor. Lactic Acid Bacteria—R & D for Food, Health and Livestock Purposes. (Rijeka: In Tech Prepress) (2013). p. 309–32.
74. Ashar MN, Chand R. Antihypertensive peptides purified from milks fermented with Lactobacillus delbrueckii ssp. bulgaricus. Milchwissenschaft. (2004) 59:14–7.
75. Ueno K, Mizuno S, Yamamoto N. Purification and characterization of an endopeptidase that has an important role in the carboxyl terminal processing of antihypertensive peptides in Lactobacillus helveticus CM4. Lett Appl Microbiol. (2004) 39:313–8. doi: 10.1111/j.1472-765X.2004.01560.x
76. Phelan M, Aherne A, FitzGerald RJ, O’Brien NM. Casein-derived bioactive peptides: biological effects, industrial uses, safety aspects and regulatory status. Int Dairy J. (2009) 19:643–54. doi: 10.1016/j.idairyj.2009.06.001
77. Aguilar-Toalá JE, Santiago-López L, Peres CM, Peres C, Garcia HS, Vallejo-Cordoba B, et al. Assessment of multifunctional activity of bioactive peptides derived from fermented milk by specific Lactobacillus plantarum strains. J Dairy Sci. (2017) 100:65–75. doi: 10.3168/jds.2016-11846
78. Elfahri KR, Donkor ON, Vasiljevic T. Potential of novel Lactobacillus helveticus strains and their cell wall bound proteases to release physiologically active peptides from milk proteins. Int Dairy J. (2014) 38:37–46. doi: 10.1016/j.idairyj.2014.03.010
79. Zanutto-Elgui MR, Vieira JCS, Prado DZD, Buzalaf MAR, Padilha PM, Elgui de Oliveira D, et al. Production of milk peptides with antimicrobial and antioxidant properties through fungal proteases. Food Chem. (2019) 278:823–31. doi: 10.1016/j.foodchem.2018.11.119
80. Castro RJS, De Sato HH. Comparison and synergistic effects of intact proteins and their hydrolysates on the functional properties and antioxidant activities in a simultaneous process of enzymatic hydrolysis. Food Bioprod Process. (2014) 92:80–8. doi: 10.1016/j.fbp.2013.07.004
81. Otte J, Shalaby SM, Zakora M, Pripp AH, El-Shabrawy SA. Angiotensin-converting enzyme inhibitory activity of milk protein hydrolysates: effect of substrate, enzyme and time of hydrolysis. Int Dairy J. (2007) 17:488–503. doi: 10.1016/j.idairyj.2006.05.011
82. Daud NA, Babji AS, Yusop SM. Effects of enzymatic hydrolysis on the antioxidative and antihypertensive activities from red tilapia fish protein. J Nutr Food Sci. (2015) 5:387. doi: 10.4172/2155-9600.1000387
83. Luo Y, Pan K, Zhong Q. Physical, chemical and biochemical properties of casein hydrolyzed by three proteases: partial characterizations. Food Chem. (2014) 155:146–54. doi: 10.1016/j.foodchem.2014.01.048
84. Mullally MM, Meisel H, Fitzgerald RJ. Angiotensin-I-converting enzyme inhibitory activities of gastric and pancreatic proteinase digests of whey proteins. Int Dairy J. (1997) 7:299–303. doi: 10.1016/S0958-6946(97)00018-6
85. Pihlanto-Leppälä A, Koskinen P, Phlola K, Tupasela T, Korhonen H. Angiotensin I-converting enzyme inhibitory properties of whey protein digests: concentration and characterization of active peptides. J Dairy Res. (2000) 67:53–64. doi: 10.1017/S0022029999003982
86. McDonagh D, FitzGerald RJ. Production of caseinophosphopeptides (CPPs) from sodium caseinate using a range of commercial protease preparations. Int Dairy J. (1998) 8:39–45. doi: 10.1016/S0958-6946(98)00019-3
87. Abdel-Hamid M, Otte J, De Gobba C, Osman A, Hamad E. Angiotensin I-converting enzyme inhibitory activity and antioxidant capacity of bioactive peptides derived from enzymatic hydrolysis of buffalo milk proteins. Int Dairy J. (2017) 66:91–8. doi: 10.1016/j.idairyj.2016.11.006
88. Liu Q, Kong B, Xiong YL, Xia X. Antioxidant activity and functional properties of porcine plasma protein hydrolysate as influenced by the degree of hydrolysis. Food Chem. (2010) 118:403–10. doi: 10.1016/J.FOODCHEM.2009.05.013
89. Tavano OL. Protein hydrolysis using proteases: an important tool for food biotechnology. J Mol Catal B Enzym. (2013) 90:1–11. doi: 10.1016/j.molcatb.2013.01.011
90. Bamdad F, Bark S, Kwon CH, Suh J-W, Sunwoo H. Anti-inflammatory and antioxidant properties of peptides released from β-lactoglobulin by high hydrostatic pressure-assisted enzymatic hydrolysis. Molecules. (2017) 22:949. doi: 10.3390/molecules22060949
91. Ulug SK, Jahandideh F, Wu J. Novel technologies for the production of bioactive peptides. Trends Food Sci Technol. (2021) 108:27–39. doi: 10.1016/j.tifs.2020.12.002
92. Cárcel JA, García-Pérez JV, Benedito J, Mulet A. Food process innovation through new technologies: use of ultrasound. J Food Eng. (2012) 110:200–7. doi: 10.1016/j.jfoodeng.2011.05.038
93. Tho P, Manasseh R, Ooi A. Cavitation microstreaming patterns in single and multiple bubble systems. J Fluid Mech. (2007) 576:191–233. doi: 10.1017/S0022112006004393
94. Ashokkumar M, Mason T. Kirk-Othmer Encyclopedia of Chemical Technology. Sonochemistry. Hoboken, NJ: John Wiley & Sons (2007). p. 1–34.
95. Yasui K. Influence of ultrasonic frequency on multibubble sonoluminescence. J Acoust Soc Am. (2002) 112:1405–13. doi: 10.1121/1.1502898
96. Chandrasekaran S, Ramanathan S, Basak T. Microwave food processing-A review. Food Res Int. (2013) 52:243–61. doi: 10.1016/j.foodres.2013.02.033
97. Dabbour M, He R, Mintah B, Golly MK, Ma H. Ultrasound pretreatment of sunflower protein: impact on enzymolysis, ACE-inhibition activity, and structure characterization. J Food Process Preserv. (2020) 44:e14398. doi: 10.1111/jfpp.14398
98. Abadía-García L, Castaño-Tostado E, Cardador-Martínez A, Martín-Del-campo ST, Amaya-Llano SL. Production of ACE inhibitory peptides from whey proteins modified by high intensity ultrasound using bromelain. Foods. (2021) 10:102099. doi: 10.3390/FOODS10092099
99. Koirala S, Prathumpai W, Anal AK. Effect of ultrasonication pretreatment followed by enzymatic hydrolysis of caprine milk proteins and on antioxidant and angiotensin converting enzyme (ACE) inhibitory activity of peptides thus produced. Int Dairy J. (2021) 118:105026. doi: 10.1016/J.IDAIRYJ.2021.105026
100. Cui P, Yang X, Liang Q, Huang S, Lu F, owusu J, et al. Ultrasound-assisted preparation of ACE inhibitory peptide from milk protein and establishment of its in-situ real-time infrared monitoring model. Ultrason Sonochem. (2020) 62:104859. doi: 10.1016/j.ultsonch.2019.104859
101. Munir M, Nadeem M, Mahmood Qureshi T, Gamlath CJ, Martin GJO, Hemar Y, et al. Effect of sonication, microwaves and high-pressure processing on ACE-inhibitory activity and antioxidant potential of cheddar cheese during ripening. Ultrason Sonochem. (2020) 67:105140. doi: 10.1016/j.ultsonch.2020.105140
102. Lorenzetti A, Penha FM, Cunha Petrus JC, Rezzadori K. Low purity enzymes and ultrasound pretreatment applied to partially hydrolyze whey protein. Food Biosci. (2020) 38:100784. doi: 10.1016/j.fbio.2020.100784
103. Abadía-García L, Castaño-Tostado E, Ozimek L, Romero-Gómez S, Ozuna C, Amaya-Llano SL. Impact of ultrasound pretreatment on whey protein hydrolysis by vegetable proteases. Innov Food Sci Emerg Technol. (2016) 37:84–90. doi: 10.1016/j.ifset.2016.08.010
104. Uluko H, Li H, Cui W, Zhang S, Liu L, Chen J, et al. Response surface optimization of angiotensin converting enzyme inhibition of milk protein concentrate hydrolysates in vitro after ultrasound pretreatment. Innov Food Sci Emerg Technol. (2013) 20:133–9. doi: 10.1016/j.ifset.2013.08.012
105. Uluko H, Zhang S, Liu L, Tsakama M, Lu J, Lv J. Effects of thermal, microwave, and ultrasound pretreatments on antioxidative capacity of enzymatic milk protein concentrate hydrolysates. J Funct Foods. (2015) 18:1138–46. doi: 10.1016/j.jff.2014.11.024
106. Jain S, Anal AK. Optimization of extraction of functional protein hydrolysates from chicken egg shell membrane (ESM) by ultrasonic assisted extraction (UAE) and enzymatic hydrolysis. Food Sci Technol. (2016) 69:295–302. doi: 10.1016/j.lwt.2016.01.057
107. Jovanović JR, Stefanović AB, Šekuljica NŽ, Tanasković SMJ, Dojčinović MB, Bugarski BM, et al. Ultrasound pretreatment as an useful tool to enhance egg white protein hydrolysis: kinetics, reaction model, and thermodinamics. J Food Sci. (2016) 81:C2664–75. doi: 10.1111/1750-3841.13503
108. Wang B, Atungulu GG, Khir R, Geng J, Ma H, Li Y, et al. Ultrasonic treatment effect on enzymolysis kinetics and activities of ACE-inhibitory peptides from oat-isolated protein. Food Biophys. (2015) 10:244–52. doi: 10.1007/s11483-014-9375-y
109. Bermúdez-Aguirre D, Mawson R, Barbosa-Cánovas GV. Microstructure of fat globules in whole milk after thermosonication treatment. J Food Sci. (2008) 73:E325–32. doi: 10.1111/J.1750-3841.2008.00875.X
110. Leong TSH, Walter V, Gamlath CJ, Yang M, Martin GJO, Ashokkumar M. Functionalised dairy streams: tailoring protein functionality using sonication and heating. Ultrason Sonochem. (2018) 48:499–508. doi: 10.1016/j.ultsonch.2018.07.010
111. Wu Q, Zhang X, Jia J, Kuang C, Yang H. Effect of ultrasonic pretreatment on whey protein hydrolysis by alcalase: thermodynamic parameters, physicochemical properties and bioactivities. Process Biochem. (2018) 67:46–54. doi: 10.1016/J.PROCBIO.2018.02.007
112. Zhou C, Ma H, Yu X, Liu B, Yagoub AEGA, Pan Z. Pretreatment of defatted wheat germ proteins (by-products of flour mill industry) using ultrasonic horn and bath reactors: Effect on structure and preparation of ACE-inhibitory peptides. Ultrason Sonochem. (2013) 20:1390–400. doi: 10.1016/j.ultsonch.2013.04.005
113. Yang X, Li Y, Li S, Oladejo AO, Wang Y, Huang S, et al. Effects of multi-frequency ultrasound pretreatment under low power density on the enzymolysis and the structure characterization of defatted wheat germ protein. Ultrason Sonochem. (2017) 38:410–20. doi: 10.1016/j.ultsonch.2017.03.001
114. Qu W, Ma H, Jia J, He R, Luo L, Pan Z. Enzymolysis kinetics and activities of ACE inhibitory peptides from wheat germ protein prepared with SFP ultrasound-assisted processing. Ultrason Sonochem. (2012) 19:1021–6. doi: 10.1016/j.ultsonch.2012.02.006
115. Patist A, Bates D. Industrial Applications of High power Ultrasonics. In Food Engineering Series. Berlin: Springer (2011). p. 599–616. doi: 10.1007/978-1-4419-7472-3_24
116. Chemat F, Zill-E-Huma, Khan MK. Applications of ultrasound in food technology: processing, preservation and extraction. Ultrason Sonochem. (2011) 18:813–35. doi: 10.1016/j.ultsonch.2010.11.023
117. Barba FJ, Zhu Z, Koubaa M, Sant’Ana AS, Orlien V. Green alternative methods for the extraction of antioxidant bioactive compounds from winery wastes and by-products: a review. Trends Food Sci Technol. (2016) 49:96–109. doi: 10.1016/j.tifs.2016.01.006
118. Kingston HM, Jassie LB. Introduction to Microwave Sample Preparation. Washington, DC: American Chemical Society (1988). doi: 10.1021/ac00179a733
119. Anantheswaran RC, Ramaswamy HS. Bacterial Destruction and Enzyme Inactivation During Microwave Heating. In Food Science and Technology. New York, NY: Marcel Dekker (2001). p. 191–214.
120. Gomaa A. An Investigation of Effects of Microwave Treatment on the Structure, Enzymatic Hydrolysis, and Nutraceutical Properties of-Lactoglobulin. Montreal: McGill University (2010).
121. El Mecherfi KE, Curet S, Lupi R, Larré C, Rouaud O, Choiset Y, et al. Combined microwave processing and enzymatic proteolysis of bovine whey proteins: the impact on bovine β-lactoglobulin allergenicity. J Food Sci Technol. (2019) 56:177–86. doi: 10.1007/s13197-018-3471-9
122. Chen Z, Li Y, Lin S, Wei M, Du F, Ruan G. Development of continuous microwave-assisted protein digestion with immobilized enzyme. Biochem Biophys Res Commun. (2014) 445:491–6. doi: 10.1016/j.bbrc.2014.02.025
123. Izquierdo FJ, Peñas E, Baeza ML, Gomez R. Effects of combined microwave and enzymatic treatments on the hydrolysis and immunoreactivity of dairy whey proteins. Int Dairy J. (2008) 18:918–22. doi: 10.1016/j.idairyj.2008.01.005
124. Hashemi SMB, Gholamhosseinpour A, Niakousari M. Application of microwave and ohmic heating for pasteurization of cantaloupe juice: microbial inactivation and chemical properties. J Sci Food Agric. (2019) 99:4276–86. doi: 10.1002/jsfa.9660
125. Tewari G, Juneja V. Advances in Thermal and Non-Thermal Food Preservation. Ames, IA: Blackwell Publishing (2008).
126. George DF, Bilek MM, McKenzie DR. Non-thermal effects in the microwave induced unfolding of proteins observed by chaperone binding. Bioelectromagnetics. (2008) 29:324–30. doi: 10.1002/bem.20382
127. Tang J. Unlocking potentials of microwaves for food safety and quality. J Food Sci. (2015) 80:E1776–93. doi: 10.1111/1750-3841.12959
128. Vadivambal R, Jayas DS. Non-uniform temperature distribution during microwave heating of food materials-A review. Food and Bioprocess Technology. (2010) 3:161–71. doi: 10.1007/s11947-008-0136-0
129. Zhong H, Marcus SL, Li L. Microwave-assisted acid hydrolysis of proteins combined with liquid chromatography MALDI MS/MS for protein identification. J Am Soc Mass Spectrom. (2005) 16:471–81. doi: 10.1016/j.jasms.2004.12.017
130. Li Y, Li J, Lin SJ, Yang ZS, Jin HX. Preparation of antioxidant peptide by microwave- assisted hydrolysis of collagen and its protective effect against H2O2-induced damage of RAW264.7 cells. Mar Drugs. (2019) 17:642. doi: 10.3390/md17110642
131. Sparr Eskilsson C, Björklund E. Analytical-scale microwave-assisted extraction. J Chromatogr. (2000) 902:227–50. doi: 10.1016/S0021-9673(00)00921-3
132. Ghasemkhani N, Akbarian M, Morshedi A, Poursharif Z, Aghamohammadi B, Moayedi F. Microbiological effects of high pressure processing on foodnet Microbiological effects of high pressure processing on food. J Bio. (2014) 4:133–45.
133. Naderi N, House JD, Pouliot Y, Doyen A. Effects of high hydrostatic pressure processing on hen egg compounds and egg products. Compr Rev Food Sci Food Saf. (2017) 16:707–20. doi: 10.1111/1541-4337.12273
134. Balasubramaniam VM, Ting EY, Stewart CM, Robbins JA. Recommended laboratory practices for conducting high-pressure microbial inactivation experiments. Innov Food Sci Emerg Technol. (2004) 5:299–306. doi: 10.1016/j.ifset.2004.04.001
135. Messens W, Van Camp J, Huyghebaert A. The use of high pressure to modify the functionality of food proteins. Trends Food Sci Technol. (1997) 8:107–12. doi: 10.1016/S0924-2244(97)01015-7
136. Oliveira MMD, Augusto PED, Cruz AG, Da, Cristianini M. Effect of dynamic high pressure on milk fermentation kinetics and rheological properties of probiotic fermented milk. Innov Food Sci Emerg Technol. (2014) 26:67–75. doi: 10.1016/j.ifset.2014.05.013
137. Singh RP, Yousef AE. Technical Elements of New and Emerging Non-Thermal Food Technologies. Rome: FAO (2001).
138. Balasubramaniam V, Barbosa-Cánovas GV, Lelieveld H. High Pressure Processing of Food. Food Engineering Series. New York, NY: Springer (2016). p. 39–65.
139. Landim AP, Matsubara NK, da Silva-Santos JE, Mellinger-Silva C, Rosenthal A. Application of preliminary high-pressure processing for improving bioactive characteristics and reducing antigenicity of whey protein hydrolysates. Food Sci Technol Int. (2021) 0:10820132211022106. doi: 10.1177/10820132211022106
140. Paula A, Landim M, Hidalgo Chávez DW, Santos Da Rosa J, Mellinger-Silva C, Rosenthal A. Effect of high hydrostatic pressure on the antioxidant capacity and peptic hydrolysis of whey proteins. Ciênc Rural. (2021) 51:2021. doi: 10.1590/0103-8478CR20200560
141. Boukil A, Suwal S, Chamberland J, Pouliot Y, Doyen A. Ultrafiltration performance and recovery of bioactive peptides after fractionation of tryptic hydrolysate generated from pressure-treated B -lactoglobulin. J Membr Sci. (2018) 556:42–53. doi: 10.1016/j.memsci.2018.03.079
142. Piccolomini A, Iskandar M, Lands L, Kubow S. High hydrostatic pressure pre-treatment of whey proteins enhances whey protein hydrolysate inhibition of xidative stress and IL-8 secretion in intestinal epithelial cells. Food Nutr Res. (2012) 56:17549. doi: 10.3402/fnr.v56i0.17549
143. Chicón R, López-Fandiño R, Quirós A, Belloque J. Changes in chymotrypsin hydrolysis of β-lactoglobulin A induced by high hydrostatic pressure. J Agric Food Chem. (2006) 54:2333–41. doi: 10.1021/jf051983s
144. Peñas E, Préstamo G, Luisa Baeza M, Martínez-Molero MI, Gomez R. Effects of combined high pressure and enzymatic treatments on the hydrolysis and immunoreactivity of dairy whey proteins. Int Dairy J. (2006) 16:831–9. doi: 10.1016/j.idairyj.2005.08.009
145. Huppertz T, Fox PF, Kelly AL. Properties of casein micelles in high pressure-treated bovine milk. Food Chem. (2004) 87:103–10. doi: 10.1016/j.foodchem.2003.10.025
146. Juan B, Barron LJR, Ferragut V, Trujillo AJ. Effects of high pressure treatment on volatile profile during ripening of ewe milk cheese. J Dairy Sci. (2007) 90:124–35. doi: 10.3168/jds.S0022-0302(07)72614-0
147. Maynard F, Weingand A, Hau J, Jost R. Effect of high-pressure treatment on the tryptic hydrolysis of bovine β-lactoglobulin AB. Int Dairy J. (1998) 8:125–33. doi: 10.1016/S0958-6946(98)00030-2
148. Knudsen JC, Otte J, Olsen K, Skibsted LH. Effect of high hydrostatic pressure on the conformation of β-lactoglobulin A as assessed by proteolytic peptide profiling. Int Dairy J. (2002) 12:791–803. doi: 10.1016/S0958-6946(02)00078-X
149. Farr D. High pressure technology in the food industry. Trends Food Sci Technol. (1990) 1:14–6. doi: 10.1016/0924-2244(90)90004-I
150. Knorr D. Hydrostatic pressure treatment of food: microbiology. In: GW Gould editor. New Methods of Food Preservation. (Boston, MA: Springer) (1995). p. 159–75. doi: 10.1007/978-1-4615-2105-1_8
151. Koutchma T. Adapting High Hydrostatic Pressure (HPP) for Food Processing Operations. Cambridge, MA: Academic Press (2014).
152. Balasubramaniam VM, Martínez-Monteagudo SI, Gupta R. Principles and application of high pressure–based technologies in the food industry. Ann Rev Food Sci Technol. (2015) 6:435–62. doi: 10.1146/annurev-food-022814-015539
Keywords: dairy proteins, bioactive peptides production, green technologies, ultrasound-assisted extraction, fermentation, enzymatic hydrolysis
Citation: Murtaza MA, Irfan S, Hafiz I, Ranjha MMAN, Rahaman A, Murtaza MS, Ibrahim SA and Siddiqui SA (2022) Conventional and Novel Technologies in the Production of Dairy Bioactive Peptides. Front. Nutr. 9:780151. doi: 10.3389/fnut.2022.780151
Received: 20 September 2021; Accepted: 05 April 2022;
Published: 26 May 2022.
Edited by:
Blanca Hernandez-Ledesma, Spanish National Research Council (CSIC), SpainReviewed by:
Jorge Welti-Chanes, Instituto de Tecnología y Educación Superior de Monterrey (ITESM), MexicoDominic Agyei, University of Otago, New Zealand
Abdel-Moneim Eid Abdel-Moneim, Egyptian Atomic Energy Authority, Egypt
Waseem Khalid, Government College University, Faisalabad, Pakistan
Copyright © 2022 Murtaza, Irfan, Hafiz, Ranjha, Rahaman, Murtaza, Ibrahim and Siddiqui. This is an open-access article distributed under the terms of the Creative Commons Attribution License (CC BY). The use, distribution or reproduction in other forums is permitted, provided the original author(s) and the copyright owner(s) are credited and that the original publication in this journal is cited, in accordance with accepted academic practice. No use, distribution or reproduction is permitted which does not comply with these terms.
*Correspondence: Mian Anjum Murtaza, YW5qdW0ubXVydGF6YUB1b3MuZWR1LnBr; Muhammad Modassar A. N. Ranjha, TW9kYXNzYXJSYW5qaGFAZ21haWwuY29t; Salam A. Ibrahim, aWJyYWgwMDFAbmNhdC5lZHU=