- 1School of Food and Wine, Ningxia University, Yinchuan, China
- 2National R & D Center for Mutton Processing, Yinchuan, China
Tenderness is a key attribute of meat quality that affects consumers’ willingness to purchase meat. Changes in the physiological environment of skeletal muscles following slaughter can disrupt the balance of redox homeostasis and may lead to cell death. Excessive accumulation of reactive oxygen species (ROS) in the myocytes causes DNA damage and activates poly ADP-ribose polymerase 1 (PARP1), which is involved in different intracellular metabolic pathways and is known to affect muscle tenderness during post-slaughter maturation. There is an urgent requirement to summarize the related research findings. Thus, this paper reviews the current research on the protein structure of PARP1 and its metabolism and activation, outlines the mechanisms underlying the function of PARP1 in regulating muscle tenderness through cysteine protease 3 (Caspase-3), oxidative stress, heat shock proteins (HSPs), and energy metabolism. In addition, we describe the mechanisms of PARP1 in apoptosis and necrosis pathways to provide a theoretical reference for enhancing the mature technology of post-mortem muscle tenderization.
1 Introduction
Tenderness is an important indicator to evaluate the edible value of meat. Surveys have reported that consumers have a strong desire to buy meat with better tenderness. Muscle includes muscle fibers, intermuscular fat and connective tissue (1). Its structure and complex relationship are the material basis of tenderness. Myofibrillar protein hydrolyzed by endogenous enzymes in muscle fibers is the most important factor affecting meat tenderness (2). Calpain can catalyze the degradation of myofibrils, improve the tenderness of postmortem muscles, and enhance meat quality. Huang et al. (3) used Calpain inhibitor to study its effect on chicken breast tenderness confirming that Calpain catalyzed myogenic fiber degradation is the primary reason for increased muscle tenderness and meat maturation after slaughter. During postmortem maturation, muscle triggers a series of cascade reactions due to environmental changes. Mitochondria in myocytes not only respond to ATP depletion in myocytes by accelerating the glycolytic process but also release different regulatory factors to induce apoptosis and regulate muscle tenderness. These factors include cytochrome c (cyt-c) (4), endonuclease G (Endo G), and apoptosis inducing factor (AIF) (5). At the same time, mitochondria are damaged, the body produces too much ROS, and the body is in a state of oxidative stress due to imbalance in oxygen radical metabolism in response to environmental change (6).
Poly ADP-ribose polymerase 1 is a DNA repair enzyme that has been implicated in DNA damage repair, apoptosis, necrosis, chromosome modification, and transcription (7). It can interact with Calpain and Caspase to catalyze the degradation of myogenic fibronectin, which in turn regulates muscle tenderness. However, the influence of PARP1 on meat tenderness and its related mechanism are poorly known. Therefore, this paper reviews the molecular structure characteristics and metabolic mechanism of PARP1, and summarizes the related mechanism of PARP1 in postmortem muscles participating in the pathway of apoptosis and necrosis and affecting muscle tenderness. The paper further focuses on the skeletal protein degradation and apoptosis processes to provide directions for postmortem muscle tenderization research.
2 PARP family and PARP1 activity
2.1 PARP family
Poly ADP-ribose polymerase is a family of poly ADP-ribose polymerases with 17 members identified that are largely distributed in the nucleus of eukaryotic cells, and responsible for catalyzing ADP-ribose modification in cells (8). The current family members, namely, PARP1, PARP2, and PARP3 are DNA-dependent and their common tryptophan-glycine-arginine (WGR) structural domain is a major regulator of catalytic activity, which can interact with damaged DNA to repair it in a timely manner (9). PARP1 is the most abundant and well-studied protease in the PARP family, which plays a significant role in apoptosis and DNA repair. PARP2 is responsible for base excision and single-strand break repair in DNA; it can bind specifically to damaged DNA end gaps and form a catalytic conformation. Chen et al. (10) demonstrated that PARP2 synthesizes a new poly-ADP ribose chain through the N-terminus with PARP1 located at the site of DNA damage. The N-terminal binding domain of PARP3 consists of only 40 amino acids and has a partial PARP1 N-terminal binding domain function; the WGR structural can bind to the DNA and transmits the information to the C-terminal structural domain (11). Vyas et al. (12) and Rulten et al. (13), respectively, demonstrated that PARP3 can modify the target proteins via the mono ADP-ribose (MAR) activity, which is different from the poly-ADP-ribose (PAR) activity of PARP1 and PARP2.
2.2 Structure of PARP1
Poly ADP-ribose polymerase 1, 1,014 amino acids long, consists of an N-terminal DNA-binding domain (DBD), automodification domain (AMD), and C-terminal catalytic domain (CAT), as shown in Figure 1. The DBD domain contains three zinc finger (Zn) structures, one nuclear localization signal (NLS), and an aspartate-glutamate-valine-aspartic acid (DEVD) structural related to apoptosis (14). Eustermann et al. (15) reported that Zn1 and Zn2 of the DBD domain can specifically recognize DNA damage gaps by binding to the 5′ and 3′ ends, respectively, which are distributed on both sides of DNA break sites, whereas Zn3 links the structural domains to activate the target protein. Zhou verified that NLS can recognize the Caspase cleavage site at DNA strand breaks and localize PARP1 in the nucleus. Subsequently, the two zinc finger structures can bind to and repair the DNA damage site (16). The AMD structural domain is adjacent to the WGR structural and contains a breast cancer type 1 (BRCT) structural that catalyzes PARP1-mediated synthesis of poly-ADP ribose chains. The CAT structural domain, a crucial region for linking NAD+ and catalyzing PAR synthesis, consists of the α-helical subdomain (HD) and ADP-ribosyl transferase (ART) subdomain, which contains a nicotinamide adenine dinucleotide (NAD+) binding site and a PAR catalytic site (17, 18). Rudolph et al. (19) reported that WGR, a core component of the CAT structural domain in PARP1, can interact with DNA, Zn1, Zn3, and CAT to form an inter-regional network linking the damaged DNA to the CAT structural domain. Furthermore, the Arginine (Arg) 591 site of the WGR structural domain can interact with the HD structural domain of PARP1.
2.3 PARP1 metabolism and activation
2.3.1 PARP1 metabolism
Metabolism of intracellular PARP1 occurs via PAR metabolism, including the formation and degradation of PAR multimers. In the absence of DNA damage, PARP1 activity is very low. In the presence of DNA damage, PARP1 is activated and its activity increases more than 500-fold. Excessive activation of PARP1 can produce an abundance of PAR, thereby inducing the release of AIF in mitochondria after polymerization (20). Gibson and Kraus (21) suggested that PARP1 synthesized by PARP1 in the nucleus could serve as a scaffold for DNA repair and recruit DNA repair proteins to the damaged site.
Poly ADP-ribose polymerase 1 cleaves the substrate NAD+ to ADP ribose and niacinamide, further catalyzes ADP ribose transfer, and polymerizes to glutamate residues of nuclear proteins, thus synthesizing large homopolymer PARs with more than 200 PARs and high branching (22). Buch-Larsen et al. (23) reported that PARP1 covalently binds adenosine diphosphate-ribose units via lipid exchange reactions to active modification sites of glutamate (Glu) and serine (Ser) residues, including Glu 488 and 491 and Ser 499, 507, and 519, which are subsequently and repeatedly catalyzed by PARP1 to form PAR chains on PARP1 itself or target proteins (Figure 2). PAR is degraded rapidly after synthesis by poly ADP-ribose glycohydrolase (PARG), whereas the binding activity of PARP1 to DNA is reactivated after PAR degradation.
2.3.2 PARP1 activation
The Zn or WGR structure in PARP1 protein binds to DNA to activate PARP1, which in turn participates in DNA repair. The activation of PARP1 is primarily caused by DNA damage. Intracellular oxidative stress can precipitate mitochondria to produce excessive reactive oxygen species (ROS, H2O2, NO, etc.) to induce DNA damage, and subsequently activate PARP1 with negative feedback. In the presence of slight DNA damage, PARP1 activation will timely repair the DNA break. In the presence of moderate damage, intracellular Caspase-3 and Caspase-7 would cleave PARP1 into PARP1-89 kDa and PARP1-24 kDa fragments to initiate cell apoptosis. However, when DNA is severely damaged, PARP1 will be overactivated and use the excess of intracellular NAD+, resulting in NAD+ depletion, reduced ATP levels, and eventually leading to cell necrosis (24). The activation of PARP1 accelerates the depletion of intracellular energy, decreases the ATP content, reduces NAD+ content, and alters the internal environment to accelerate cell death (25). In addition, the activation degree of PARP1 determines whether cells undergo apoptosis or necrosis by altering the levels of intracellular ATP (26).
3 PARP1 mediates cell death
Apoptosis and necrosis are the major death modes of postmortem myocytes, which are inter convertible and share similar characteristics. Cao et al. (27) observed that muscle cells of postmortem beef displayed necrosis characteristics such as vitrification of muscle fibers, lax cell nucleus and cytoplasm, and disappearance of the nucleus and cytoplasm, proving that apoptosis and necrosis of muscle cells coexisted. Similarly, Degterev et al. (28) believe that apoptosis and necrosis are two extreme types of cell death, and the two modes of death can be transformed into each other, and there will be coexistence of apoptosis and necrosis characteristics. Intracellular enzymes exist in cell apoptosis and necrosis and affect muscle tenderization by acting on cytoskeleton proteins.
3.1 PARP1 mediates apoptosis
After animal slaughter, the physiological activity of muscle tissue did not stop immediately. Under ischemia, hypoxia, and nutrient disruption apoptosis inducing factors can trigger myocytes to initiate the apoptotic program via coordinated control of multiple genes (29). As shown in Figure 3, the main cell-initiated apoptotic pathways include the exogenous death receptor pathway, endogenous mitochondrial pathway, PARP1 can all be involved in the onset of apoptosis through these pathways (30).
The death receptor pathway is activated by the cleavage of specific substrates by apoptotic effector enzymes. Death receptors on the cell surface bind to death ligands to recruit the Fas-associating protein with a novel death domain (FADD), which further binds to Caspase-8 precursors and activates Caspase-8 by FADD, which further binds to Caspase-8 precursor and activates Caspase-8 by forming a death-inducing signaling complex (DISC) (31). Activated Caspase-8, in turn, activates downstream Caspase-3, Caspase-6, and Caspase-7 through a cascade reaction, thus completing the apoptotic process (32). PARP1 is a substrate of Caspase, which is cleaved by Caspase-3 and Caspase-7 into PARP1 fragments (24 kDa N-terminal and 89 kDa C-terminal) in the Zn3 region, thereby losing PARP1 activity, which can retain ATP for subsequent energy consumption during apoptosis (33). Using DNA fragmentation, Lu et al. (34) demonstrated that Caspase-3 in the activated state cleaves PARP1 at specific sites into a binding structural domain (24 kDa) and a catalytic structural domain (89 kDa), leading to PARP1 inactivation. The 24 kDa PARP1 fragment is associated with DNA damage, which can bind to DNA ports and prevent the binding of intact PARP1 and DNA damage, ensuring that repair proteins are not recruited to chromosomes, resulting in DNA strand repair, and finally, complete apoptosis mediated by Caspase-3 (35). Mortusewicz et al. (36) reported that PARP1 recruited at the site of DNA damage could be cleaved, dissociating the 89 kDa fragment after cleavage and retaining the 24 kDa fragment at the site of DNA damage. The 89 kDa PARP1 fragment does not contain the DBD structural domain, and damaged DNA cannot activate it, thereby reducing intracellular energy consumption and providing sufficient energy to support apoptosis. In addition, the 89 kDa fragment catalyzes ADP ribosylation on RNA polymerase III (Pol III) complexes, activating innate immune responses and promoting apoptosis (37). Qin (38) used a high glucose-induced oxidative stress model to activate PARP1 and then inhibited PARP1 activity by ABT888. Moreover, Qin (38) confirmed that PARP1 reduced high glucose-induced cardiomyocyte apoptosis by activating the IGF-1R/Akt pathway. In conclusion, PARP1 participates in the occurrence of cell apoptosis by producing Caspase-3-mediated cleavage products of 24 kDa and 89 kDa fragments.
The mitochondrial pathway initiates in the mitochondria and is usually activated in response to injury or stress in cells within the body. When the mitochondria receive apoptotic signals, the mitochondrial membrane permeability transition pore (MPTP) is opened. Apoptotic factors, such as Cyt-c, AIF, and Endo G, are released from the mitochondria into the cytoplasm. Cyt-c released into the cytoplasm oligomerizes with apoptosis protease-activating factor (Apaf-1), leading to the conformational change in Apaf-1 to generate a heptameric apoptotic complex, which activates the apoptosis-initiating enzyme Caspase-9, thereby activating the downstream apoptosis effector enzymes Caspase-3 and Caspase-7 and initiating the apoptotic cascade response (39). However, AIF and Endo G can act directly on the nucleus, constituting another apoptotic pathway independent of apoptotic enzymes (40). Chen et al. (5) stated that gallic acid can induce apoptosis by AIF and Endo G released from the mitochondrial pathway in NCI-H292 cells. During apoptosis in the mitochondrial pathway, AIF mediates the onset of non-Caspase-dependent apoptosis by recruiting downstream nucleases that interact with cyclophilin A (Cyp A) to form active nucleases causing DNA damage by cleavage (41). After the DNA is cleaved by AIF, PARP1 functions as a protease to repair the damaged DNA and prevent chromosome shrinkage and DNA fragmentation. When large amounts of DNA are damaged, PARP1 fragments interact with AIF in the cytoplasm through the PAR polymer. PARP1 activation mediates AIF-dependent apoptosis, causing it to translocate from the mitochondria to the nucleus where apoptosis occurs. Sun et al. (42) reported that ionizing radiation can induce the death of HepG2 cells, thus releasing AIF from the mitochondria and transferring it to the nucleus by ionizing radiation, causing DNA breakage and leading to cell apoptosis. This finding indicates that ionizing radiation can induce HepG2 cell apoptosis through the AIF pathway. Yu et al. (43) demonstrated that AIF enters the mitochondria through the N-terminal mitochondrial localization signal, which is truncated by Calpain and cathepsin into a 57 kDa fragment and released into the cytoplasm in response to the death signal.
When the endoplasmic reticulum is strongly stimulated, the number of error proteins or unfolded proteins in the endoplasmic reticulum rapidly increases, beyond the range that the endoplasmic reticulum can handle, thereby disrupting the internal homeostasis and causing apoptosis (44). Calcium depletion is the primary cause of apoptosis induced by endoplasmic reticulum stress (ERS). When ERS occurs, a large amount of Ca2+ in the ER enters the cytosol, and activates Calpain and Caspase-12, further activating the downstream effector Caspase-3, ultimately leading to apoptosis (45). In the absence of DNA damage, the release of Ca2+ from the inositol triphosphate (IP3) receptor in the ER activates PARP1. Homburg et al. (46) studied nerve cells and contractile cardiomyocytes and reported that the release of intracellular stored Ca2+ could mediate PARP1 activation. After activation, PARP1 synthesizes excessive PAR to consume energy in cells. Caspase can timely bind to the active site of PARP1 and cleave it into fragments to destroy the PARP1 activity and provide energy for the subsequent occurrence of apoptosis.
3.2 PARP1 mediates necrosis
Regulatory cell necrosis is characterized by increased membrane permeability, irregular changes in the appearance of certain cells, cell membrane fragmentation, cell contents leakage, and severe inflammatory reaction (47). Cell necrosis includes programmed necrosis, iron necrosis, and cyclophilin D-dependent necrosis, Parthanatos et al. (48). Among them, Parthanatos occurs mediated by PARP1, where cells lose their integrity, phospholipid bilayer exfoliates, nucleus shrinks, mitochondrial depolarization and chromatin agglutination occurs, and the DNA is broken into fragments of approximately 50 kb, in a Caspase-independent manner.
The hallmarks of the occurrence of Parthanatos include excessive activation of PARP1, PAR accumulation, and AIF nuclear translocation. Oxidative stress can cause DNA damage, and PARP1, a DNA damage receptor, is over-activated causing the occurrence of Parthanatos (49, 50). As shown in Figure 4, PARP1 is rapidly activated after the occurrence of DNA damage, and PAR polymer accumulates in the cells and consumes a large amount of NAD+, thus inhibiting the activity of the mitochondrial oxidative respiratory chain complex enzyme, blocking the tricarboxylic acid cycle pathway, impairing mitochondrial energy metabolism, and inducing the release of AIF from the mitochondria and its transfer to the nucleus (51–53). After AIF enters the nucleus, the large DNA fragment is degraded to a 50 kb fragment. In the mode of death of Parthanatos, AIF can be combined with macrophage migration inhibitory factor (MIF) in the cytoplasm, and this complex then enters the nucleus (54). MIF can perform nuclease function to degrade 3′ ssDNA, the intermediate product in the repair process of double-strand break (DSB), leading to the failure of DNA damage repair, resulting in nuclear shrinkage, chromatin agglutination, and DNA cleavage into small fragments (43). Park et al. (55) reported that the knockout of the MIF gene protected the neurons from damage caused by necrosis, further confirming the indispensable importance of MIF in the occurrence of Parthanatos. Baritaud et al. (56) reported that AIF enters the nucleus and binds to histone H2AX and the nucleic acid endonuclease CypA, leading to the formation of DNA degradation complexes to cause chromatin agglutination and promote DNA breakage. Andrabi et al. (57) demonstrated that PARP1 fragments interact with AIF in the cytoplasm through PAR polymers. Park et al. (58) reported that β-Lapachone induced non-caspase-dependent death of hepatocytes, with significantly enhanced activity of PARP1. Simultaneously, nuclear translocation of AIF was observed. The analysis showed that β-Lapachone activated the PARP1 activity and promoted the emergence of AIF, which in turn induced hepatocyte necrosis.
4 PARP1 regulates the tenderization mechanism of postmortem muscles
Poly ADP-ribose polymerase 1, as a protease, can affect the structure of cytoskeleton proteins by acting on endogenous enzymes through cell apoptosis and cell necrosis, and can directly or indirectly participate in the muscle tenderization mechanism. D’Alessandro et al. (59) performed an integrated proteomics, interactomics and metabolomics analysis of Longissimus dorsi tender and tough meat samples from Chianina beef cattle. Indicating that tenderness was related to apoptosis through increase of HSPs and PARP fragment, that’s because oxidative stress promoted meat tenderness and elicited heat shock protein responses, which in turn triggered apoptosis-like cascades along with PARP fragmentation. At present, the regulatory mechanism of muscle tenderness involves Caspase, Calpain endogenous enzymes, and energy metabolism.
4.1 Tenderness is influenced by the regulation of cytoskeletal protein degradation
Improvement in the post-slaughter tenderness of the meat is primarily attributed to the degradation of myogenic fibrous proteins, especially structural and cytoskeletal proteins (60). Proteins highly associated with meat tenderness largely include myosin (Titin), concomitant actin (Nebulin), and troponin-T (Troponin-T) (61). At present, there are many enzymes related to the degradation of cytoskeletal proteins, including Calpain, cathepsin, and apoptotic enzymes, whose hydrolysis of cytoskeletal proteins is closely related to the improvement of postmortem muscle tenderness (62, 63).
Caspase-3 is a terminal factor in the apoptotic cascade that affects muscle tenderization by disrupting the myofibrillar structure and degrading cytoskeletal proteins. Huang et al. (64) reported that Caspase-3 inhibitors inhibited the degradation of myofibrillar proteins in skeletal muscle cells, indirectly proving that Caspase-3 was involved in the degradation of myofibrillar proteins. Activated Caspase-3, an important protease, is involved in postmortem meat tenderization and has been implicated in myofibrillar degradation during muscle maturation. Huang et al. (65) incubated myofibrillar proteins of beef skeletal muscles with recombinant Caspase-3 in vitro and found that numerous myofibrillar protein degradation including titin, nebulin, troponin-T etc. Indicating the involvement of Caspase-3 in protein hydrolysis in muscles. PARP1 is a marker of hydrolytic skeletal muscle protein that is specifically recognized by Caspase-3 and cleaved into 89 kDa and 24 kDa fragments. It regulates the process of the death receptor pathway in apoptosis (66, 67). Cao et al. (68) reported that caspases cleave the PARP1 of 113 kDa to 24 kDa PARP1, which is also generated by the breakdown of PARP1 substrates of caspases. Huang et al. (69) reported that Caspase-3 activation sheared PARP1 protein, accelerated the apoptosis of muscle cells in duck meat, promoted the degradation of myofibrillar proteins, and improved the tenderness of duck meat. Kemp et al. (70, 71) studied the protein hydrolysis in the skeletal muscles of pork after slaughter and reported that Caspase-3 cleaves PARP1 protein and degrades the cytoskeleton, which plays a key function in pork tenderization. Zhang (72) incubated dairy goat meat with calcium chloride and tea polyphenols during post-slaughter maturation and found that these significantly increased the protease activities of PARP1 and Caspase-3 in myocytes, resulting in increased degradation of interstitial line proteins. This finding suggested that apoptosis inducers can regulate muscle tenderness by promoting apoptosis in skeletal muscle cells (72). Saccà et al. (73) used the degradation pattern of PARP1 to assess the aging process in bovine muscles confirming that apoptotic processes occur in Longissimus lumborum (LL) and Infraspinatus (IS) during the early postmortem period and that may contribute to cell degradation of skeletal proteins. In conclusion, PARP1 is activated by caspase-3 cleavage to accelerate skeletal protein degradation (Figure 5A), thereby positively affecting the post-slaughter muscle tenderization of livestock.
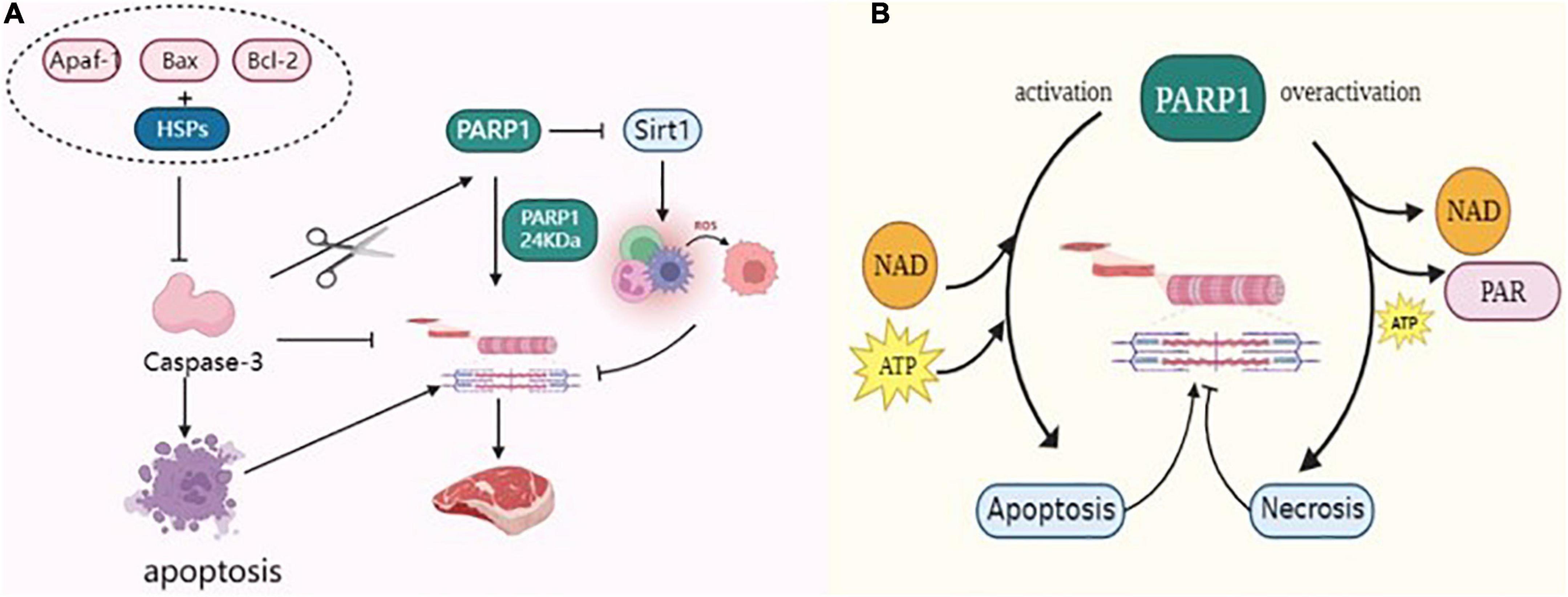
Figure 5. (A) PARP1 through Caspase-3, Sirt1, and HSPs regulates the tenderization mechanism of postmortem muscle. (B) PARP1 through energy metabolism regulates the tenderization mechanism of postmortem muscle.
Postmortem environmental changes result in excessive production of ROS in myocytes, such that the antioxidant defense system becomes insufficient to combat ROS, resulting in an imbalance between the oxidative and antioxidant systems and causing oxidative stress in the myocytes (74). Oxidative stress mediates protein oxidation, weakens the activity of proteolytic enzymes, and causes cross-linking and polymerization between protein molecules, thereby affecting the degradation of skeletal proteins, which is detrimental to the tenderization of muscles (75). Calpain degrades skeletal proteins, destroys the ultrastructure of muscle fibers, weakens the Z-line such that it disappears, causes fragmentation of myofibrils, and accelerates muscle tenderness. Carlin et al. (76) used Calpain to incubate porcine myofibrils supplemented with H2O2 and reported that oxidation changed the structure of myosin and actin and reduced the proteolytic activity of Calpain. Chen et al. (77) reported that packaging porcine dorsal longissimus muscle with high oxygen during storage enhanced the hydrophobicity of the protein surface, increased the carbonyl content, and enhanced protein oxidation. Protein oxidation inhibited the activity of μ-Calpain in the muscles, thereby delaying the degradation of skeletal proteins such as troponin-T and intercalary line proteins in pork. Silent information regulator 1 (Sirt1) enhances mitochondrial biogenesis, upregulates the activities of antioxidant enzymes superoxide dismutase (SOD) and succinate dehydrogenase (SDH) to play an antioxidant role and increases the expression of Sirt1, thus inhibiting the accumulation of ROS in myocytes (78). In contrast, excessive activation of PARP1 inhibits the expression of Sirt1, reduces the activity of mitochondrial complex I, inhibits the function of nicotinamide adenine dinucleotide (NADH) oxidase, induces the uncoupling of mitochondrial electron transport chain, induces the generation of superoxide anion radical (O2-–), thus aggravating the oxidative stress in myocytes (79, 80). Cantó et al. (81) reported that over-activation of PARP1 can deplete large amounts of NAD+, thereby reducing intracellular NAD+ content to 20–30%, which in turn inhibits Sirt1 function and leads to increased levels of intracellular oxidative stress. Zhang et al. (82) enhanced the ubiquitination of PARP1 in mouse cells and found that the oxidative stress of cells was reduced following PARP1 proteasome degradation. As shown in Figure 5A, the overexpression of PARP1 inhibited Sirt1 activity, aggravated oxidative stress in myocytes, weakened the ability of proteolytic enzymes to degrade skeletal proteins, and reduced meat tenderness. Therefore, muscle tenderness can be improved by regulating PARP1 activity during postmortem maturation.
4.2 Tenderness is influenced by the regulation of apoptotic processes
Apoptosis enzyme belongs to cysteine protease and is mainly involved in cell apoptosis, which plays an important role in the transformation of muscle into meat. Therefore, the process of cell apoptosis is closely related to the tenderness of meat.
Heat shock proteins (HSPs) are a class of anti-apoptotic chaperone proteins, also known as heat stress proteins, that regulate the mitochondrial apoptotic pathway and can interact with apoptotic bodies (Apaf-1, Bax, Bcl-2, etc.), thereby preventing the activation of Caspase-3 and inhibiting the degradation of interstitial line proteins, and are molecular markers of meat tenderness (83, 84). As shown in Figure 5A, HSPs can bind to Caspase-3 to reduce its activity, which in turn affects the apoptotic process of cells. Ding et al. (85) reported that HSP27 binds to Caspase-3 during beef ripening and reduces the activity of Caspase-3, thereby inhibiting the degradation of myogenic fibrous proteins and thus hindering muscle tenderization. Balan et al. (86) observed the degradation of HSP27 in post-slaughter beef and reported that its degradation significantly correlated with the degradation of interstitial line proteins and troponin-T, and hypothesized that HSP27 degradation enhanced the hydrolysis of myogenic fibronectin by endogenous enzymes. Further studies demonstrated that HSPs not only bind the myofibrillar protein to act as a substitute for μ-Calpain, competitively inhibit μ-Calpain activity, and protect the integrity of myofibrils by reducing the enzymatic hydrolysis of myofibrillar protein, but also bind to the substrate site of Caspase-3 to inhibit its activity, followed by inhibiting the degradation of skeleton proteins (87). On the contrary, PARP1 is the activation substrate of Caspase-3, which can activate Caspase-3 and subsequently cleave PARP1 such that it loses its biological activity. Activated Caspase-3 can accelerate apoptosis and promote the degradation of skeletal proteins, which ameliorates muscle tenderness. Therefore, PARP1 can compete with HSPs for the Caspase-3 substrate site, promote the activation of Caspase-3, accelerate the degradation of myofibrillar protein, and facilitate the tenderization of postmortem muscles.
The ATP content in cells can affect cell apoptosis or necrosis, which is the crucial factor determining the cell death mode. Apoptosis requires energy consumption; ATP is required for the activation of Apaf-1 and Caspase precursors generated in the mitochondrial pathway (88). When energy is exhausted, apoptosis is inhibited, energy dependent life processes in myocytes stop, cell membranes are disrupted, and cytoplasm leaks out, leading to cell necrosis. Mitochondria are central regulators of apoptosis and provide sites for metabolic pathways such as oxidative phosphorylation, tricarboxylic acid cycle, energy conversion, and calcium ion storage under normal conditions (89, 90). After animal slaughter, the disruption of communication between muscle tissue and the outside world leads to tissue ischemia and hypoxia, which then leads to excessive ROS production in the metabolic processes of muscle cells. This cascade affects the functions of skeletal muscle mitochondria and damages the DNA structure in the cells, thus activating PARP1. The level of PARP1 activity is indirectly related to the mode of cell death, thus delaying or promoting muscle tenderization. The occurrence of necrosis is related to the decrease of intracellular NAD+ concentration. Excessive activation of PARP1 decreases NAD+ concentration, which is the primary factor causing the abnormal energy metabolism of cells. Simultaneously, PARP1 consumes a large amount of ATP while generating the PAR polymer, thus turning the cell death process from apoptosis to necrosis, which is not conducive to the tenderization of meat. However, NAD+ is a cofactor of glyceraldehyde 3-phosphate dehydrogenase (GAPDH), which participates in redox reactions. In addition, its depletion prevents ATP production by glycolysis in postmortem muscles. Chen et al. (91) used an acyl-CoA-binding domain containing 3 (ACBD3) to activate PARP1 and reported that a large amount of PAR was synthesized in the cytoplasm, which, in turn, reduced the intracellular NAD+ content. The introduction of mutant genes on the DEVD structural of PARP1 generates the mutant PARP1 that cannot be cleaved. The mutant PARP1 protein loses PAR activity and does not consume intracellular ATP and NAD+ (92). As shown in Figure 5B, PARP1 consumes large amounts of NAD+ and ATP, depleting intracellular energy and leading to cell necrosis, which in turn inhibits the activity of apoptosis enzymes. Caspase affects the tenderization of postmortem muscles, and inhibition of its activity delays the degradation of myogenic fibronectin in myocytes, which is negatively correlated with meat tenderness and is detrimental to post-slaughter meat tenderization (93).
5 Conclusion
This review details the structure and metabolic mechanism of PARP1, discusses the mechanism by which PARP1 mediates apoptosis and necrosis, and analyzes the potential mechanism by which it regulates muscle from the perspective of its ability to be activated by affecting oxidative stress and energy metabolism, get activated by Caspase-3 cleavage, and influence the competition for substrate sites by HSPs. PARP1 as a DNA damage receptor, in the presence of moderate DNA damage, PARP1 activation and participates in apoptosis through death receptors, mitochondria, and endoplasmic reticulum. In the presence of severe DNA damage it mediates the Parthanatos cell necrosis pathway. During the conversion of livestock from muscle to meat after slaughter, the mode of cell death affects the tenderness of the meat. Apoptosis activates Caspase-3, which destroys the structural integrity of muscle fibers and improves muscle tenderness, whereas cell necrosis exacerbates the accumulation of ROS and inhibits the activity of apoptotic enzymes, which is detrimental to post-slaughter meat tenderness.
We believe that although studies on regulating meat tenderness by regulating PARP1 are scarce, PARP1 can provide novel ideas for subsequent muscle quality research. In the future, multiomics analyses such as metabolomics, transcriptomics, and proteomics can be used to study the link between PARP1 and meat tenderness. In addition, new ideas can be explored to improve meat tenderness after slaughter. The aim is to provide a theoretical reference for the development of high-quality and safe meat production technology.
Author contributions
YL: conceptualization, project administration, and funding acquisition. RLi, YH, JW, QZ, XC, LH, and JZ: resources. RLi: writing—original draft preparation. YL and RLu: writing—review and editing. All authors read and agreed to the published version of the manuscript.
Funding
This study was funded by the National Natural Science Foundation of China (32202135), the Ningxia Natural Science Foundation of Ningxia Hui Autonomous Region (2021AAC03098), the Key Research and Development Program of Ningxia Hui Autonomous Region (Talent Introduction Program) (2020BEB04023), and the Ningxia Youth Science and Technology Talent Support Project Ningxia Natural Science Foundation.
Conflict of interest
The authors declare that the research was conducted in the absence of any commercial or financial relationships that could be construed as a potential conflict of interest.
Publisher’s note
All claims expressed in this article are solely those of the authors and do not necessarily represent those of their affiliated organizations, or those of the publisher, the editors and the reviewers. Any product that may be evaluated in this article, or claim that may be made by its manufacturer, is not guaranteed or endorsed by the publisher.
References
1. Holman B, van de Ven R, Mao Y, Coombs C, Hopkins D. Using instrumental (Cie and Reflectance) measures to predict consumers’ acceptance of beef colour. Meat Sci. (2017). 127:57–62. doi: 10.1016/j.meatsci.2017.01.005
2. Wang T, Feng X, Li L, Luo J, Liu X, Zheng J, et al. Effects of quercetin on tenderness, apoptotic and autophagy signalling in chickens during post-mortem ageing. Food Chem. (2022) 383:132409. doi: 10.1016/j.foodchem.2022.132409
3. Huang J, Zhao L, Yang J, Zhang B, Xu X, Chen K, et al. The effect of μ/M-calpain on protein degradation of chicken breast meat. J Food Sci. (2019) 84:1054–9. doi: 10.1111/1750-3841.14596
4. Chen L, Chai Y, Luo J, Wang J, Liu X, Wang T, et al. Apoptotic changes and myofibrils degradation in post-mortem chicken muscles by ultrasonic processing. LWT. (2021) 142:110985. doi: 10.1016/j.lwt.2021.110985
5. Chen C, Shih Y, Yeh M, Liao N, Chung H, Liu K, et al. Ursolic acid induces apoptotic cell death through aif and endo G release through a mitochondria-dependent pathway in Nci-H292 human lung cancer cells in vitro. In Vivo (Athens Greece). (2019) 33:383–91. doi: 10.21873/invivo.11485
6. Chen C, Guo Z, Shi X, Guo Y, Ma G, Ma J, et al. H O -Induced oxidative stress improves meat tenderness by accelerating glycolysis via hypoxia-inducible factor-1α signaling pathway in postmortem bovine muscle. Food Chem. (2022) 16:100466. doi: 10.1016/j.fochx.2022.100466
7. Kumar V, Kumar A, Mir K, Yadav V, Chauhan S. Pleiotropic role of Parp1: an overview. 3 Biotech. (2022) 12:3. doi: 10.1007/s13205-021-03038-6
8. Langelier M, Eisemann T, Riccio A, Pascal J. Parp family enzymes: regulation and catalysis of the poly(Adp-Ribose) posttranslational modification. Curr Opin Struct Biol. (2018) 53:187–98. doi: 10.1016/j.sbi.2018.11.002
9. Li X, Erden O, Li L, Ye Q, Wilson A, Du W. Binding to Wgr domain by salidroside activates Parp1 and protects hematopoietic stem cells from oxidative stress. Antioxid Redox Signal. (2014) 20:1853–65. doi: 10.1089/ars.2013.5600
10. Chen Q, Kassab M, Dantzer F, Yu X. Parp2 mediates branched poly adp-ribosylation in response to DNA damage. Nat Commun. (2018) 9:3233. doi: 10.1038/s41467-018-05588-5
11. Eustermann S, Videler H, Yang J, Cole P, Gruszka D, Veprintsev D, et al. The DNA-binding domain of human Parp-1 interacts with DNA single-strand breaks as a monomer through its second zinc finger. J Mol Biol. (2011) 407:149–70. doi: 10.1016/j.jmb.2011.01.034
12. Vyas S, Matic I, Uchima L, Rood J, Zaja R, Hay R, et al. Family-wide analysis of poly(Adp-Ribose) polymerase activity. Nat Commun. (2014) 5:4426. doi: 10.1038/ncomms5426
13. Rulten S, Fisher A, Robert I, Zuma M, Rouleau M, Ju L, et al. Parp-3 and aplf function together to accelerate nonhomologous end-joining. Mol Cell. (2011) 41:33–45. doi: 10.1016/j.molcel.2010.12.006
14. Liu L, Li J, Ke Y, Zeng X, Gao J, Ba X, et al. The key players of parthanatos: opportunities for targeting multiple levels in the therapy of parthanatos-based pathogenesis. Cell Mol Life Sci. (2022) 79:60. doi: 10.1007/s00018-021-04109-w
15. Eustermann S, Wu W, Langelier M, Yang J, Easton L, Riccio A, et al. Structural basis of detection and signaling of DNA single-strand breaks by human Parp-1. Mol Cell. (2015) 60:742–54. doi: 10.1016/j.molcel.2015.10.032
16. Lampart A, Sluzalska K, Czyrek A, Szerszen A, Otlewski J, Wiedlocha A, et al. Nuclear localization sequence of Fgf1 is not required for its intracellular anti-apoptotic activity in differentiated cells. Cells. (2022) 11:522. doi: 10.3390/cells11030522
17. Márton J, Fodor T, Nagy L, Vida A, Kis G, Brunyánszki A, et al. Parp10 (Artd10) modulates mitochondrial function. PLoS One. (2018) 13:e0187789. doi: 10.1371/journal.pone.0187789
18. Hottiger M, Hassa P, Lüscher B, Schüler H, Koch-Nolte F. Toward a unified nomenclature for mammalian adp-ribosyltransferases. Trends Biochem Sci. (2010) 35:208–19. doi: 10.1016/j.tibs.2009.12.003
19. Rudolph J, Muthurajan U, Palacio M, Mahadevan J, Roberts G, Erbse A, et al. The Brct domain of Parp1 binds intact DNA and mediates intrastrand transfer. Mol Cell. (2021) 81:4994–5006.e5. doi: 10.1016/j.molcel.2021.11.014
20. Regdon Z, Robaszkiewicz A, Kovács K, Rygielska Ż, Hegedűs C, Bodoor K, et al. Lps protects macrophages from aif-independent parthanatos by downregulation of Parp1 expression, induction of Sod2 expression, and a metabolic shift to aerobic glycolysis. Free Radic Biol Med. (2019) 131:184–96. doi: 10.1016/j.freeradbiomed.2018.11.034
21. Gibson B, Kraus W. New insights into the molecular and cellular functions of poly(Adp-Ribose) and parps. Nat Rev Mol Cell Biol. (2012) 13:411–24. doi: 10.1038/nrm3376
22. Amé J, Spenlehauer C, de Murcia G. The parp superfamily. BioEssays. (2004) 26:882–93. doi: 10.1002/bies.20085
23. Buch-Larsen S, Hendriks I, Lodge J, Rykær M, Furtwängler B, Shishkova E, et al. Mapping physiological adp-ribosylation using activated ion electron transfer dissociation. Cell Rep. (2020) 32:108176. doi: 10.1016/j.celrep.2020.108176
24. Herrmann G, Russell W, Garg N, Yin Y. Poly(Adp-Ribose) polymerase 1 regulates mitochondrial DNA repair in an Nad-dependent manner. J Biol Chem. (2021) 296:100309. doi: 10.1016/j.jbc.2021.100309
25. Gottschalk A, Timinszky G, Kong S, Jin J, Cai Y, Swanson S, et al. Poly(Adp-Ribosyl)ation directs recruitment and activation of an atp-dependent chromatin remodeler. Proc Natl Acad Sci USA. (2009) 106:13770–4. doi: 10.1073/pnas.0906920106
26. Bouchard V, Rouleau M, Poirier G. Parp-1, a determinant of cell survival in response to DNA damage. Exp Hematol. (2003) 31:446–54. doi: 10.1016/s0301-472x(03)00083-3
27. Cao J, Sun W, Zhou G, Xu X, Peng Z, Hu Z. Morphological and biochemical assessment of apoptosis in different skeletal muscles of bulls during conditioning. J Anim Sci. (2010) 88:3439–44. doi: 10.2527/jas.2009-2412
28. Degterev A, Huang Z, Boyce M, Li Y, Jagtap P, Mizushima N, et al. Chemical inhibitor of nonapoptotic cell death with therapeutic potential for ischemic brain injury. Nat Chem Biol. (2005) 1:112–9. doi: 10.1038/nchembio711
29. Musumeci G, Imbesi R, Szychlinska M, Castrogiovanni P. Apoptosis and skeletal muscle in aging. Open J Apoptosis. (2015) 4:41–6. doi: 10.4236/ojapo.2015.42004
30. Gupta S, Gollapudi S. Susceptibility of naïve and subsets of memory T cells to apoptosis via multiple signaling pathways. Autoimmun Rev. (2007) 6:476–81. doi: 10.1016/j.autrev.2007.02.005
31. Flusberg D, Sorger P. Surviving apoptosis: life-death signaling in single cells. Trends Cell Biol. (2015) 25:446–58. doi: 10.1016/j.tcb.2015.03.003
32. Salvesen G, Dixit V. Caspases: intracellular signaling by proteolysis. Cell. (1997) 91:443–6. doi: 10.1016/s0092-8674(00)80430-4
33. Mashimo M, Bu X, Aoyama K, Kato J, Ishiwata-Endo H, Stevens L, et al. Parp1 inhibition alleviates injury in Arh3-deficient mice and human cells. JCI Insight. (2019) 4:e124519. doi: 10.1172/jci.insight.124519
34. Lu A, Abo R, Ren Y, Gui B, Mo J, Blackwell D, et al. Enabling drug discovery for the parp protein family through the detection of mono-adp-ribosylation. Biochem Pharmacol. (2019) 167:97–106. doi: 10.1016/j.bcp.2019.05.007
35. Mashimo M, Onishi M, Uno A, Tanimichi A, Nobeyama A, Mori M, et al. The 89-Kda Parp1 cleavage fragment serves as a cytoplasmic par carrier to induce aif-mediated apoptosis. J Biol Chem. (2021) 296:100046. doi: 10.1074/jbc.RA120.014479
36. Mortusewicz O, Amé J, Schreiber V, Leonhardt H. Feedback-regulated poly(Adp-Ribosyl)ation by Parp-1 is required for rapid response to DNA damage in living cells. Nucleic Acids Res. (2007) 35:7665–75. doi: 10.1093/nar/gkm933
37. Chen Q, Ma K, Liu X, Chen S, Li P, Yu Y, et al. Truncated Parp1 mediates adp-ribosylation of rna polymerase iii for apoptosis. Cell Discov. (2022) 8:3. doi: 10.1038/s41421-021-00355-1
38. Qin W, Wei S, Wang X, Wang J, Wang W, Liu F, et al. Poly(Adp-Ribose) polymerase 1 inhibition protects against low shear stress induced inflammation. Biochim Biophys Acta. (2013) 1833:59–68. doi: 10.1016/j.bbamcr.2012.10.013
39. Wang H, Zhu J, Jiang L, Shan B, Xiao P, Ai J, et al. Mechanism of heshouwuyin inhibiting the Cyt C/Apaf-1/Caspase-9/Caspase-3 pathway in spermatogenic cell apoptosis. BMC Complement Med Ther. (2020) 20:180. doi: 10.1186/s12906-020-02904-9
40. Bu Q, Wang J, Zheng Y, Zou Y, Wei M. Mgl Induces nuclear translocation of endog and aif in caspase-independent T cell death. Cell Mol Biol Lett. (2015) 20:816–24. doi: 10.1515/cmble-2015-0051
41. Candé C, Vahsen N, Kouranti I, Schmitt E, Daugas E, Spahr C, et al. Aif and cyclophilin a cooperate in apoptosis-associated chromatinolysis. Oncogene. (2004) 23:1514–21. doi: 10.1038/sj.onc.1207279
42. Sun H, Yang S, Li J, Zhang Y, Gao D, Zhao S. Caspase-independent cell death mediated by apoptosis-inducing factor (Aif) nuclear translocation is involved in ionizing radiation induced Hepg2 cell death. Biochem Biophys Res Commun. (2016) 472:137–43. doi: 10.1016/j.bbrc.2016.02.082
43. Yu S, Wang Y, Frydenlund D, Ottersen O, Dawson V, Dawson T. Outer mitochondrial membrane localization of apoptosis-inducing factor: mechanistic implications for release. ASN Neuro. (2009) 1:e00021. doi: 10.1042/an20090046
44. Huang F, Wei Q, Li X, Liu C, Zhang C. Research progress on mechanisms of apoptosis to postmortem tenderization in muscle. Sci Agric Sin. (2021) 54:2192–202.
45. Wang L, Han L, Ma X, Yu Q, Zhao S. Effect of mitochondrial apoptotic activation through the mitochondrial membrane permeability transition pore on yak meat tenderness during postmortem aging. Food Chem. (2017) 234:323–31. doi: 10.1016/j.foodchem.2017.04.185
46. Homburg S, Visochek L, Moran N, Dantzer F, Priel E, Asculai E, et al. A fast signal-induced activation of poly(Adp-Ribose) polymerase: a novel downstream target of phospholipase C. J Cell Biol. (2000) 150:293–307. doi: 10.1083/jcb.150.2.293
47. D’Arcy M. Cell death: a review of the major forms of apoptosis, necrosis and autophagy. Cell Biol Int. (2019) 43:582–92. doi: 10.1002/cbin.11137
48. Jiang H, Yang Y, Zhang Y, Xie Z, Zhao X, Sun Y, et al. The dual role of poly(Adp-Ribose) polymerase-1 in modulating parthanatos and autophagy under oxidative stress in rat cochlear marginal cells of the stria vascularis. Redox Biol. (2018) 14:361–70. doi: 10.1016/j.redox.2017.10.002
49. Yang X, Zhang N, Yang Y, Xu T, An S. Recent progress in parthanatos—a new form of cell death. Chine J Cell Biol. (2021) 43:8. doi: 10.11844/cjcb.2021.01.0006
50. Fatokun A, Dawson V, Dawson T. Parthanatos: mitochondrial-linked mechanisms and therapeutic opportunities. Br J Pharmacol. (2014) 171:2000–16. doi: 10.1111/bph.12416
51. Herceg Z, Wang Z. Functions of poly(Adp-Ribose) polymerase (Parp) in DNA repair, genomic integrity and cell death. Mutat Res. (2001) 477:97–110. doi: 10.1016/s0027-5107(01)00111-7
52. Harraz M, Dawson T, Dawson V. Advances in neuronal cell death 2007. Stroke. (2008) 39:286–8. doi: 10.1161/strokeaha.107.511857
53. Mondal A, Roberge J, Gilleran J, Peng Y, Jia D, Akel M, et al. Bone morphogenetic protein inhibitors and mitochondria targeting agents synergistically induce apoptosis-inducing factor (Aif) caspase-independent cell death in lung cancer cells. Cell Commun Signal. (2022) 20:99. doi: 10.1186/s12964-022-00905-4
54. Wang Y, An R, Umanah G, Park H, Nambiar K, Eacker S, et al. A nuclease that mediates cell death induced by DNA damage and poly(Adp-Ribose) polymerase-1. Science (New York NY). (2016) 354:aad6872. doi: 10.1126/science.aad6872
55. Park H, Kam T, Dawson T, Dawson V. Poly (Adp-Ribose) (Par)-dependent cell death in neurodegenerative diseases. Int Rev Cell Mol Biol. (2020) 353:1–29. doi: 10.1016/bs.ircmb.2019.12.009
56. Baritaud M, Boujrad H, Lorenzo H, Krantic S, Susin S. Histone H2ax: the missing link in aif-mediated caspase-independent programmed necrosis. Cell Cycle (Georgetown Tex). (2010) 9:3166–73. doi: 10.4161/cc.9.16.12887
57. Andrabi S, Kim N, Yu S, Wang H, Koh D, Sasaki M, et al. Poly(Adp-Ribose) (Par) polymer is a death signal. Proc Natl Acad Sci USA. (2006) 103:18308–13. doi: 10.1073/pnas.0606526103
58. Park E, Min K, Lee T, Yoo Y, Kim Y, Kwon T. B -Lapachone induces programmed necrosis through the Rip1-parp-aif-dependent pathway in human hepatocellular carcinoma Sk-Hep1 cells. Cell Death Dis. (2014) 5:e1230. doi: 10.1038/cddis.2014.202
59. D’Alessandro A, Marrocco C, Rinalducci S, Mirasole C, Failla S, Zolla L. Chianina beef tenderness investigated through integrated omics. J Proteomics. (2012) 75:4381–98. doi: 10.1016/j.jprot.2012.03.052
60. Huff Lonergan E, Zhang W, Lonergan S. Biochemistry of postmortem muscle — lessons on mechanisms of meat tenderization. Meat Sci. (2010) 86:184–95. doi: 10.1016/j.meatsci.2010.05.004
61. Feng Y, Zhang S, Sun B, Xie P, Wen K, Xu C. Changes in physical meat traits, protein solubility, and the microstructure of different beef muscles during post-mortem aging. Foods (Basel Switzerland). (2020) 9:806. doi: 10.3390/foods9060806
62. Bhat Z, Morton J, Mason S, Bekhit A. Role of calpain system in meat tenderness: a review. Food Sci Hum Wellness. (2018) 7:196–204. doi: 10.1016/j.fshw.2018.08.002
63. Carlson K, Prusa K, Fedler C, Steadham E, Outhouse A, King D, et al. Postmortem protein degradation is a key contributor to fresh pork loin tenderness. J Anim Sci. (2017) 95:1574–86. doi: 10.2527/jas.2016.1032
64. Huang M, Huang F, Xue M, Xu X, Zhou G. The effect of active caspase-3 on degradation of chicken myofibrillar proteins and structure of myofibrils. Food Chem. (2011) 128:22–7. doi: 10.1016/j.foodchem.2011.02.062
65. Huang F, Huang M, Zhou G, Xu X, Xue M. In vitro proteolysis of myofibrillar proteins from beef skeletal muscle by caspase-3 and caspase-6. J Agric Food Chem. (2011) 59:9658–63. doi: 10.1021/jf202129r
66. Martire S, Fuso A, Rotili D, Tempera I, Giordano C, De Zottis I, et al. Parp-1 modulates amyloid beta peptide-induced neuronal damage. PLoS One. (2013) 8:e72169. doi: 10.1371/journal.pone.0072169
67. Kaufmann S, Desnoyers S, Ottaviano Y, Davidson N, Poirier G. Specific proteolytic cleavage of poly(Adp-Ribose) polymerase: an early marker of chemotherapy-induced apoptosis. Cancer Res. (1993) 53:3976–85.
68. Cao J, Ou C, Zou Y, Ye K, Zhang Q, Khan M, et al. Activation of caspase-3 and its correlation with shear force in bovine skeletal muscles during postmortem conditioning. J Anim Sci. (2013) 91:4547–52. doi: 10.2527/jas.2013-6469
69. Huang W, Cao J, Wang D, Xu W, Zhang M. Impact of caspase-3 activation on the tenderness of duck skeletal muscle during postmortem conditioning. Sci Agric Sin. (2012) 45:1372–9. doi: 10.3864/j.issn.0578-1752.2012.07.015
70. Kemp C, Bardsley R, Parr T. Changes in caspase activity during the postmortem conditioning period and its relationship to shear force in porcine longissimus muscle. J Anim Sci. (2006) 84:2841–6. doi: 10.2527/jas.2006-163
71. Kemp C, Parr T, Bardsley R, Buttery P. Comparison of the relative expression of caspase isoforms in different porcine skeletal muscles. Meat Sci. (2006) 73:426–31. doi: 10.1016/j.meatsci.2005.12.009
72. Zhang S. The Mechanism of Apoptosis Inducers on Caspase-3 Activation Pathway During Matutation of Lamb. Master’s Thesis. Yangling: Northwest A&F University (2008).
73. Saccà E, Pizzutti N, Corazzin M, Lippe G, Piasentier E. Assessment of calpain and caspase systems activities during ageing of two bovine muscles by degradation patterns of αii spectrin and Parp-1. Anim Sci J Nihon Chikusan Gakkaiho. (2016) 87:462–6. doi: 10.1111/asj.12473
74. Wu J, Yang C, Yang M, Liang Z, Wu Y, Kong X, et al. The role of Er stress and Atp/Ampk in oxidative stress meditated hepatotoxicity induced by citrinin. Ecotoxicol Environ Saf. (2022) 237:113531. doi: 10.1016/j.ecoenv.2022.113531
75. Lametsch R, Lonergan S, Huff-Lonergan E. Disulfide bond within Mu-calpain active site inhibits activity and autolysis. Biochim Biophys Acta. (2008) 1784:1215–21. doi: 10.1016/j.bbapap.2008.04.018
76. Carlin K, Huff-Lonergan E, Rowe L, Lonergan S. Effect of oxidation, Ph, and ionic strength on calpastatin inhibition of Mu- and M-calpain. J Anim Sci. (2006) 84:925–37. doi: 10.2527/2006.844925x
77. Chen L, Zhou G, Zhang W. Effects of high oxygen packaging on tenderness and water holding capacity of pork through protein oxidation. Food Bioproc Technol. (2015) 8:2287–97. doi: 10.1007/s11947-015-1566-0
78. Zhou Y, Li K, Liu L, Li S. Microrna-132 promotes oxidative stress-induced pyroptosis by targeting sirtuin 1 in myocardial ischaemia-reperfusion injury. Int J Mol Med. (2020) 45:1942–50. doi: 10.3892/ijmm.2020.4557
79. Zhang F, Lau S, Monks TJA. Dual role for poly(Adp-Ribose) polymerase-1 during caspase-dependent apoptosis. Toxicol Sci. (2012) 128:103–14. doi: 10.1093/toxsci/kfs142
80. Magyar K, Deres L, Eros K, Bruszt K, Seress L, Hamar J, et al. A quinazoline-derivative compound with parp inhibitory effect suppresses hypertension-induced vascular alterations in spontaneously hypertensive rats. Biochim Biophys Acta. (2014) 1842:935–44. doi: 10.1016/j.bbadis.2014.03.008
81. Cantó C, Menzies K, Auwerx J. Nad(+) metabolism and the control of energy homeostasis: a balancing act between mitochondria and the nucleus. Cell Metab. (2015) 22:31–53. doi: 10.1016/j.cmet.2015.05.023
82. Zhang N, Zhang Y, Wu B, Wu S, You S, Lu S, et al. Deacetylation-dependent regulation of Parp1 by Sirt2 dictates ubiquitination of Parp1 in oxidative stress-induced vascular injury. Redox Biol. (2021) 47:102141. doi: 10.1016/j.redox.2021.102141
83. Kong F, Wang H, Guo J, Peng M, Ji H, Yang H, et al. Hsp70 suppresses apoptosis of Brl cells by regulating the expression of Bcl-2, cytochrome C, and caspase 8/3. In Vitro Cell Dev Biol Anim. (2016) 52:568–75. doi: 10.1007/s11626-016-0005-5
84. Malheiros J, Enríquez-Valencia C, da Silva Duran B, de Paula T, Curi R, de Vasconcelos Silva J, et al. Association of Cast2, Hsp90aa1, Dnaja1 and Hspb1 genes with meat tenderness in nellore cattle. Meat Sci. (2018) 138:49–52. doi: 10.1016/j.meatsci.2018.01.003
85. Ding Z, Huang F, Zhang C, Zhang L, Sun H, Zhang H. Effect of heat shock protein 27 on thein vitrodegradation of myofibrils by caspase-3 and M−calpain. Int J Food Sci Technol. (2017) 53:121–8. doi: 10.1111/ijfs.13565
86. Balan P, Kim Y, Blijenburg R. Small heat shock protein degradation could be an indicator of the extent of myofibrillar protein degradation. Meat Sci. (2014) 97:220–2. doi: 10.1016/j.meatsci.2014.01.019
87. Lomiwes D, Hurst S, Dobbie P, Frost D, Hurst R, Young O, et al. The protection of bovine skeletal myofibrils from proteolytic damage post mortem by small heat shock proteins. Meat Sci. (2014) 97:548–57. doi: 10.1016/j.meatsci.2014.03.016
88. Katoh I, Sato S, Fukunishi N, Yoshida H, Imai T, Kurata S. Apaf-1-deficient fog mouse cell apoptosis involves hypo-polarization of the mitochondrial inner membrane, Atp depletion and citrate accumulation. Cell Res. (2008) 18:1210–9. doi: 10.1038/cr.2008.87
89. Zhang J, Lautar S, Huang S, Ramsey C, Cheung A, Li J. Gpi 6150 prevents H O cytotoxicity by inhibiting poly(Adp-Ribose) polymerase. Biochem Biophys Res Commun. (2000) 278:590–8. doi: 10.1006/bbrc.2000.3816
90. Liu Y, Li Z, Zhang L, Wang D, Liu Y. Phenotypic plasticity of vascular smooth muscle cells in vascular calcification: role of mitochondria. Front Cardiovasc Med. (2022) 9:972836. doi: 10.3389/fcvm.2022.972836
91. Chen Y, Bang S, Park S, Shi H, Kim S. Acyl-Coa-binding domain containing 3 modulates Nad+ metabolism through activating poly(Adp-Ribose) polymerase 1. Biochem J. (2015) 469:189–98. doi: 10.1042/bj20141487
92. Herceg Z, Wang Z. Failure of poly(Adp-Ribose) polymerase cleavage by caspases leads to induction of necrosis and enhanced apoptosis. Mol Cell Biol. (1999) 19:5124–33. doi: 10.1128/mcb.19.7.5124
Keywords: PARP1, apoptosis and necrosis pathway, Caspase-3, tenderization, energy metabolism
Citation: Li R, Luo R, Luo Y, Hou Y, Wang J, Zhang Q, Chen X, Hu L and Zhou J (2022) Biological function, mediate cell death pathway and their potential regulated mechanisms for post-mortem muscle tenderization of PARP1: A review. Front. Nutr. 9:1093939. doi: 10.3389/fnut.2022.1093939
Received: 09 November 2022; Accepted: 30 November 2022;
Published: 15 December 2022.
Edited by:
Jinxuan Cao, Beijing Technology and Business University, ChinaCopyright © 2022 Li, Luo, Luo, Hou, Wang, Zhang, Chen, Hu and Zhou. This is an open-access article distributed under the terms of the Creative Commons Attribution License (CC BY). The use, distribution or reproduction in other forums is permitted, provided the original author(s) and the copyright owner(s) are credited and that the original publication in this journal is cited, in accordance with accepted academic practice. No use, distribution or reproduction is permitted which does not comply with these terms.
*Correspondence: Yulong Luo, MTgyNDcxMjA2MDlAMTYzLmNvbQ==