- 1GenPhySE, Université de Toulouse, INRAE, ENVT, Castanet-Tolosan, France
- 2Centre of Nutrition and Food Sciences, Queensland Alliance for Agriculture and Food Innovation, The University of Queensland, Brisbane, QLD, Australia
- 3METEX ANIMAL NUTRITION, Paris, France
- 4Centre for Animal Science, Queensland Alliance for Agriculture and Food Innovation, The University of Queensland, Brisbane, QLD, Australia
- 5Department of Animal Sciences and Aquatic Ecology, Faculty of Bioscience Engineering, Ghent University, Ghent, Belgium
Prebiotics are dietary substrates which promote host health when utilized by desirable intestinal bacteria. The most commonly used prebiotics are non-digestible oligosaccharides but the prebiotic properties of other types of nutrients such as polyphenols are emerging. Here, we review recent evidence showing that amino acids (AA) could function as a novel class of prebiotics based on: (i) the modulation of gut microbiota composition, (ii) the use by selective intestinal bacteria and the transformation into bioactive metabolites and (iii) the positive impact on host health. The capacity of intestinal bacteria to metabolize individual AA is species or strain specific and this property is an opportunity to favor the growth of beneficial bacteria while constraining the development of pathogens. In addition, the chemical diversity of AA leads to the production of multiple bacterial metabolites with broad biological activities that could mediate their prebiotic properties. In this context, we introduce the concept of “Aminobiotics,” which refers to the functional role of some AA as prebiotics. We also present studies that revealed synergistic effects of the co-administration of AA with probiotic bacteria, indicating that AA can be used to design novel symbiotics. Finally, we discuss the difficulty to bring free AA to the distal gut microbiota and we propose potential solutions such as the use of delivery systems including encapsulation to bypass absorption in the small intestine. Future studies will need to further identify individual AA, dose and mode of administration to optimize prebiotic effects for the benefit of human and animal health.
Introduction
The gastrointestinal tract is colonized by a complex microbial community composed of hundreds species of bacteria, fungi, protozoa, and yeasts, collectively referred to as the gut microbiota (1–3). In humans like in other monogastric animals, the gut microbiota has major physiological functions for the host, including resistance against colonization by pathogens, degradation of undigested proteins and complex carbohydrates, regulation of nutrient absorption, metabolism, and immunity among others. Disruption of the gut microbiota balance (dysbiosis) has been linked to numerous human diseases such as inflammatory bowel disease, obesity, cancer, diabetes, and autism (4). In farm animals, dysbiosis has also been associated with impairment of the gut development and nutrient absorption, infection by enteric pathogens, inflammation and, ultimately, reduced performance, health, and welfare (2, 4, 5). Diet constitutes the main environmental factor able to modulate the gut microbiota composition and function (6, 7). Dietary constituents may provide competitive advantages to selected microorganisms according to their metabolic requirements and capacities. Complex carbohydrates derived from plants are the main nutrients affecting the gut microbiota and dietary intervention targeting intestinal bacteria have mainly used fermentable fibers, leading to the concept of prebiotics (7).
The term prebiotic was initially defined in 1995 by Gibson and Roberfroid as “a non-digestible food ingredient that beneficially affects the host by selectively stimulating the growth and/or activity of one or a limited number of bacteria already resident in the colon, and thus attempt to improve host health” (8). This definition of prebiotics applied mostly to non-digestible oligosaccharides such as inulin-type fructans and fructo- or galacto-oligosaccarides that promote the growth of Bifidobacterium and Lactobacillus spp. associated with protection against pathogens and beneficial immunomodulatory and metabolic effects (8, 9). Based on recent advances in the field of gut microbiota, the definition of prebiotics was updated in 2017 by the International Scientific Association for Probiotics and Prebiotics (ISAPP) as “a substrate that is selectively utilized by host microorganisms conferring a health benefit” (10). This expanded definition of prebiotics possibly includes non-carbohydrate substances. For instance, polyphenols are now recognized as a novel class of prebiotics since they are able to provide a health benefit to the host through a modulation of the gut microbiota composition and/or activity (11).
Although less studied than non-digestible carbohydrates and polyphenols, dietary proteins and amino acids (AA) can also influence host health through the regulation of immunity, gut barrier function, oxidative stress but also microbiota composition and its production of bioactive metabolites (12, 13). Thus, this review highlights the potential utilization of AA as a novel class of prebiotics. The structural and chemical diversity of AA represent an opportunity for targeting a broader range of gut bacteria than standard prebiotics by targeting their metabolic requirements/capacities. Moreover, AA are precursors of a broad range of bioactive bacterial metabolites, much more diverse than those derived from saccharides (14). To explore the concept of AA as prebiotics, we first review briefly the metabolism of AA by intestinal bacteria followed by a detailed description of the effects of dietary AA supplementation on intestinal bacteria, metabolites, and the consequences for the host. We also present the potential health benefit of AA supplementation co-administered with beneficial live bacteria (i.e., probiotics). Finally, we discuss technological strategies that may be required to deliver free AA to gut bacteria bypassing the proximal small intestine.
Amino acid requirements and avoidance in bacterial populations
Host and microbiota-derived proteases hydrolyze dietary and endogenous (host or microorganism-derived) proteins in the intestinal lumen into peptides and AA that can be used by gut bacteria after uptake (14). Available AA can be used by intestinal bacteria for protein synthesis or as carbon and energy sources (15). Luminal AA of dietary and endogenous origin are the main constituents of bacterial protein in the pig ileum, indicating it is likely that de novo synthesis of AA by the intestinal bacteria of the foregut of non-ruminants is limited (16). The capacity to synthetize AA differs greatly across bacterial taxa (14). For instance, Escherichia coli encodes genes for the biosynthesis of all 20 α-AA while Lactobacillus has limited capacity for AA biosynthesis and thus relies on the uptake of extracellular AA (14, 17). It has been proposed that some commensal and pathogenic gut bacteria might have lost biosynthetic pathways for AA due to the high availability of AA in the gut environment (18). It is also important to consider that the autotrophy for AA in gut bacteria can be strain dependent (14). In addition to exogeneous AA, some species of intestinal bacteria such as E. coli, use ammonia as the preferred nitrogen source (19). Intestinal bacteria utilize AA in a species-dependent manner, as demonstrated in bacteria derived from the pig microbiota (20, 21). For example, in vitro incubation with 14C-labeled AA demonstrated that intestinal E. coli and Klebsiella spp. and Streptococcus spp. have differential utilization of AA for protein synthesis (20). The bacterial intracellular AA composition also seems to be species specific compatible with the concept of different AA requirements (22). Interestingly, the saccharolytic pathogen Campylobacter jejuni uses a limited range of AA (serine, aspartate, asparagine, glutamate, glutamine, and proline) (23). Further to the roles of protein synthesis and energy source, luminal AA also play an important signaling role in the gut ecosystem, which may have significant impact on the development of enteric pathogens (24). The sensing of arginine by Enterohemorrhagic E. coli (EHEC) induces the expression of virulence genes (25). In contrast, the presence of some AA in the medium can impair growth of specific bacteria strains, such as high concentrations of valine and leucine inhibiting E. coli growth (26). Overall, the bacterial use of exogenous AA can be classified as nutritionally essential (as a protein building block), non-essential (as energy source), preferred, or avoided/toxic for the growth of specific bacterial species as shown in Table 1.
Metabolite release from amino acid catabolism by intestinal bacteria
The microbial catabolism of AA may impact the host intestinal lumen caused by the release of multiple metabolites resulting from a combination of deamination, decarboxylation, or desulfurization reactions (12). The deamination of AA releases ammonia which may negatively impair mitochondrial respiration, resulting in decreased cell proliferation and barrier function in the intestinal epithelium (27, 28). In addition, the degradation of cysteine by the gut microbiota releases hydrogen sulfide which can reduce mitochondrial respiration and increase inflammation when present at high concentration (29). Microbial catabolism of tyrosine releases p-cresol which can induce DNA damages and reduce mitochondrial respiration in intestinal epithelial cells and has been implicated in renal, cardiovascular and neurological disorders (30). Imidazole propionate, a bacterial metabolite derived from histidine, was shown to disrupt insulin signaling and was implicated in diabetes (31). Based on these observations, AA fermentation (also called putrefaction) has often been considered detrimental for health (32).
In contrast, other bacterial metabolites derived from AA were shown to have beneficial effects for host health. Deamination and decarboxylation of glutamate, threonine, alanine, lysine, glycine, and aspartate produces short-chain fatty acids (SCFA; acetate, propionate, and butyrate) which have protective effects against infection and enhance the gut barrier function and immunity (12). The microbial catabolism of branched-chain AA (BCAA) releases branched chain fatty acids (isovalerate, isobutyrate, isocaproate, and 2-methylbutyrate) that can serve as an energy substrate for epithelial cells and promote the barrier function in vitro (12, 13, 33). Valerate, a bacterial metabolite derived from proline but also from propionate and ethanol, directly inhibits the growth of Clostridioides difficile but not of commensal bacteria (34). Decarboxylation of arginine and lysine produces the polyamines agmatine, spermine, and cadaverine, respectively (35). These metabolites influence mitochondrial function, epithelial proliferation, gut barrier function, and have trophic effects on the developing gut (35). However, the specific effects of gut microbiota-derived polyamines has not been clearly distinguished from those derived from the diet or from host cells and detrimental effects of polyamines have also been described (36). The histidine-derived metabolite histamine reduced the secretion of pro-inflammatory cytokines ex vivo (37). The gut microbiota also produces numerous catabolites from tryptophan, including indole, indole-3-propionate, indole-3-aldehyde (38). These bacterial metabolites have protective effects for the gut barrier since they reduce epithelial permeability and inflammation (38, 39) and can also contribute to the effects of the gut microbiota on the brain (40). Interestingly, neurotransmitters (GABA, histamine, serotonin, dopamine) which may have an important role in the gut-brain axis, can also be produced by the gut microbiota through AA catabolism (14). Thus, many AA-derived bacterial metabolites have protective effect on host health.
In summary, AA are used by intestinal bacteria in a species (or strain) specific manner, which highlights the potential of specific AA used as prebiotics for promoting the selective growth of beneficial over pathogenic gut microbes. Moreover, stimulating the production of protective AA-derived metabolites by the gut microbiota has the potential to mediate beneficial effects of AA used as prebiotics on host health.
Dietary amino acids as modulators of the gut microbiota and consequences for host health
Modification of dietary protein intake would be the most straightforward approach to modify AA supply to the gut microbiota. Increasing protein intake results in a larger amount of dietary protein reaching the distal part of the gut where the microbiota is mostly located (14, 41, 42). The digestibility and AA profiles of dietary protein also influences the availability of AA for the gut microbiota. In general, plant are less digestible than animal proteins and the biological value of the AA composition is commonly higher in the latter compared to the first (43). Modifying dietary protein intake in terms of quality and quantity can also change AA availability for the gut microbiota, which might have both detrimental and beneficial consequences for health (44, 45). It has been suggested in the literature that casein or whey protein, particularly rich in BCAA, could protect against obesity and modulate microbiota in humans and rodents. Similarly, lean seafood or meat with high amounts of aromatic acids, glycine, and taurine could be associated with increased energy expenditure, anti-obesogenic effect, and modulation of microbiota (44). It is also well described in the literature that high-protein intake has been associated with negative effects on gut health, such as inflammatory bowel disease in humans and postweaning diarrhea in piglets (46). In contrast, reducing crude protein level in piglet’s diets has been associated with reduced diarrhea score as reported in a recent meta-analysis (47). As discussed above, pathogenic, and commensal bacteria utilize AA in a specific manner and detrimental or protective metabolites are produced from AA by the gut microbiota. Thus, changing protein intake does not facilitate a targeted supply of AA to the gut microbiota to promote health. Alternatively, dietary supplementation with specific AA is a targeted approach with potential beneficial consequences for host health through a selective growth promotion of beneficial bacteria and through the production of protective metabolites.
Accumulating evidence is showing that dietary supplementation with free AA can modulate the microbiota composition and activity both in vitro (33) and in vivo (Tables 2–4). This is associated with consequences for the host as reported in mice and humans (Table 2), pigs (Table 3), and chickens (Table 4). Most studies reveal an effect of AA supplementation on the gut microbiota diversity or composition. Thus, the comparison between studies presented in Tables 2–4 does not reveal general trends regarding the effects of AA supplementation on the gut microbiota. The divergence of the results obtained after AA supplementation can be explained by multiple differences between studies including the AA tested, host species, gut segment, dose, and duration of AA supply and exposition or not to an inflammatory or infectious challenge. It is interesting to notice that some effects of the AA supplementations were observed in the large intestine, despite that free-AA are anticipated to be absorbed mostly in the upper part of the intestine. It can be hypothesized that a high level of free-AA might temporally overwhelm the absorptive capacity of the small intestine. A surprising observation is that no linear dose-dependent effects were observed on the microbiota in studies testing the same AA at different level of supplementation (Table 3). Thus, further studies are clearly needed to define more clearly what are the effects of AA supplementation on the gut microbiota. This future work should help determine which AA have the most favorable effects on the gut microbiota and what is the effective level of supplementation. In addition, only few studies presented in Tables 2–4 have investigated the effects of AA supplementation on the production of AA-derived metabolites, the latter being potentially more predictable than the effects on the composition of the microbiota due to functional redundancy (i.e., different bacterial communities can express similar metabolic capabilities).
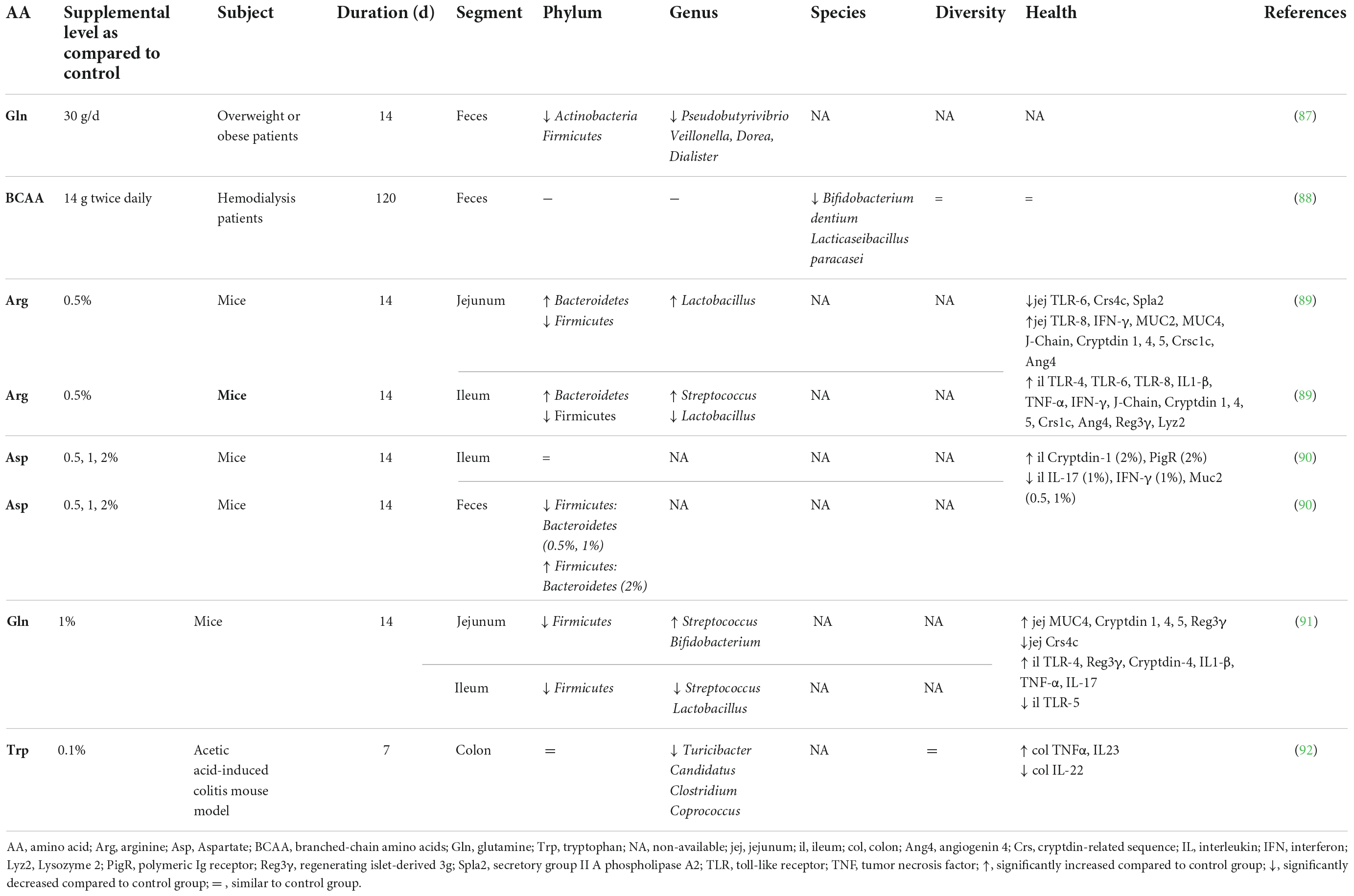
Table 2. Effect of free amino acid supplementation on microbiota and health outcomes in humans and mice.
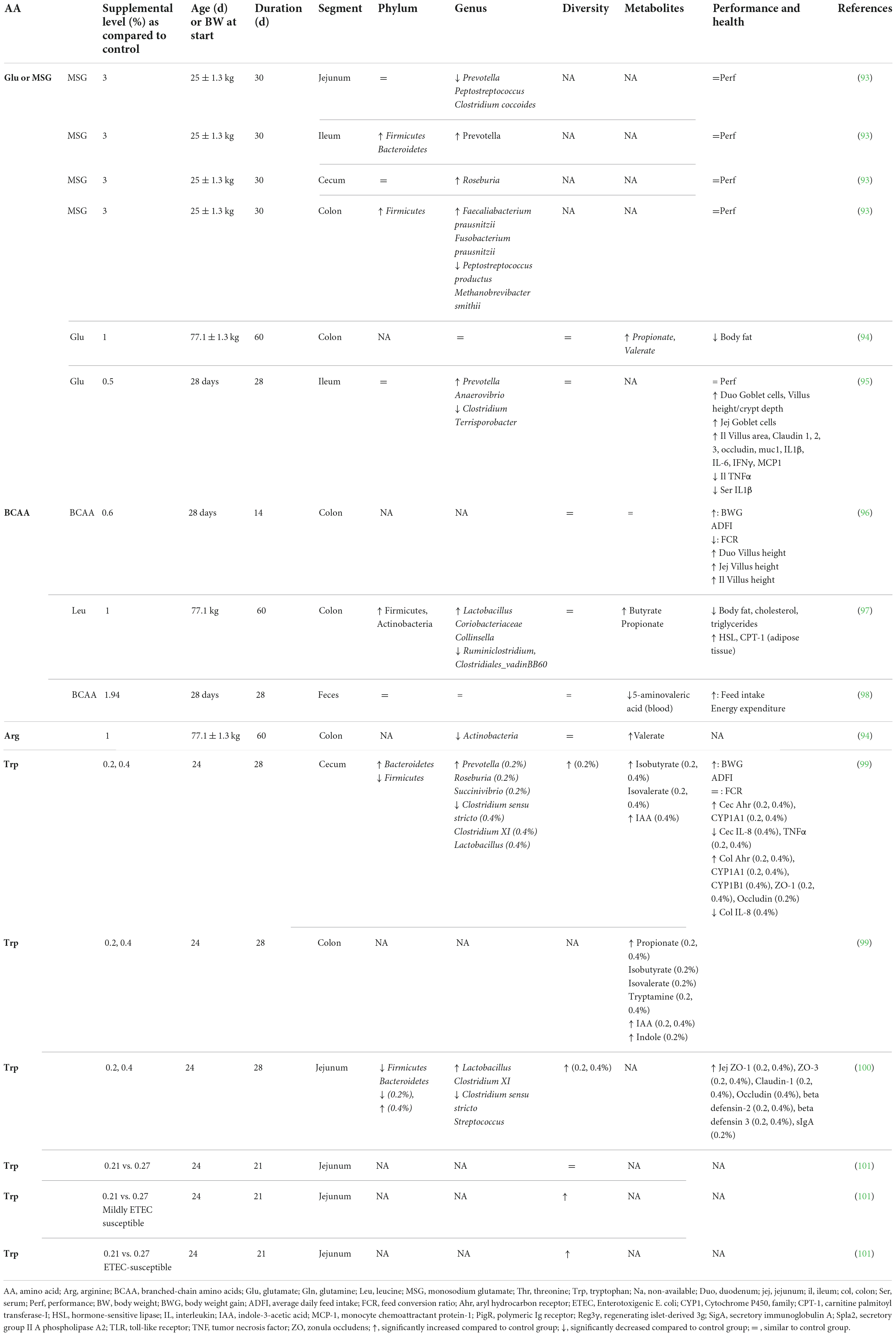
Table 3. Effect of free amino acid supplementation on microbiota, performance and health outcomes in pigs.
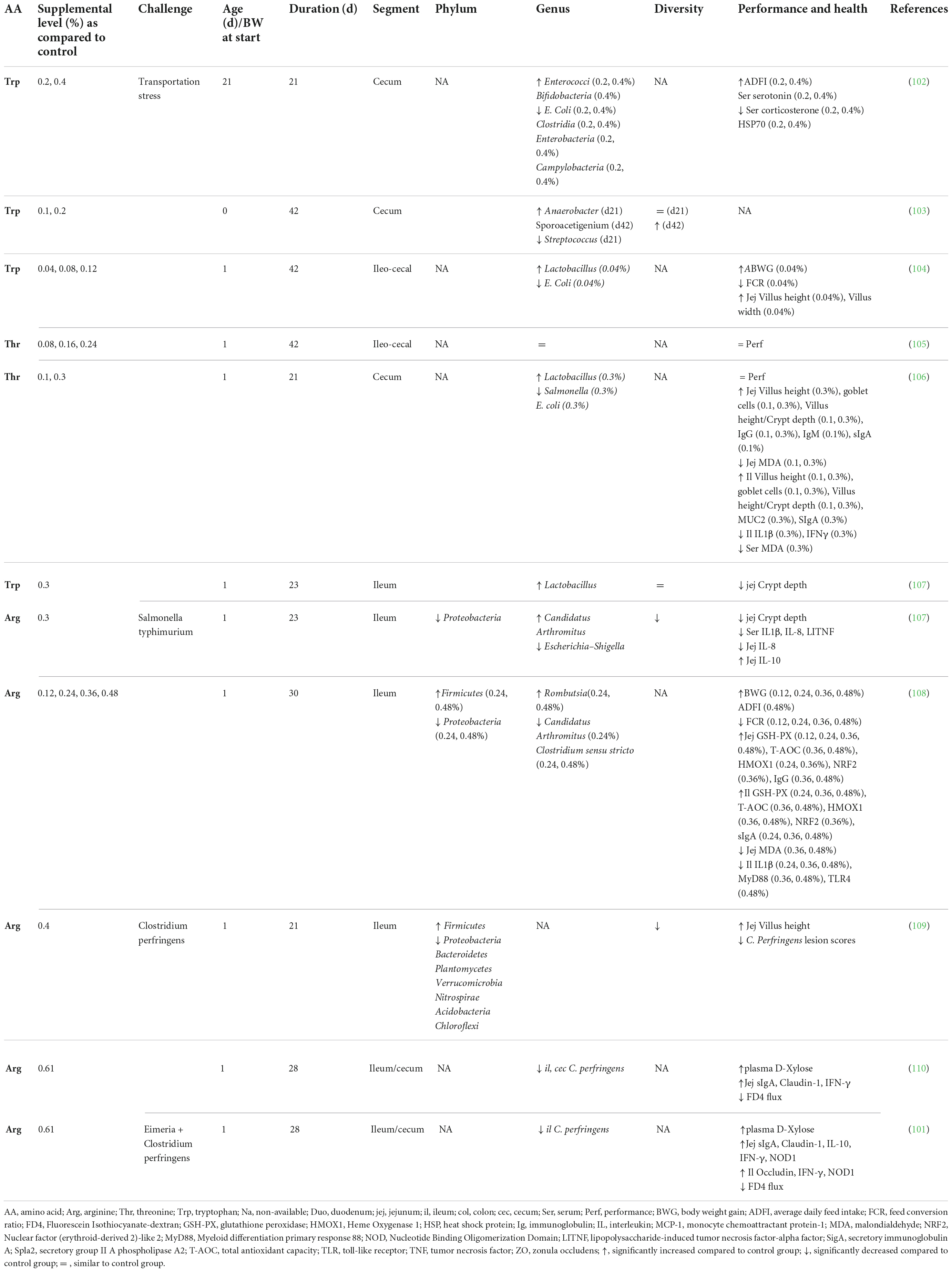
Table 4. Effect of free amino acid supplementation on microbiota, performance and health outcomes in chickens.
The evaluation of host parameters focused on intestinal barrier function, immunity and metabolism revealed modulations associated with the modification of the gut microbiota induced by AA. However, it is not clear yet if these modifications at the gut level are beneficial for the host, which is a requirement to fulfill the definition of prebiotics. In farm animals, the effects of AA supplementation on the microbiota were associated with either no or beneficial effects on growth performance. An important perspective will be to demonstrate that the modulation of the gut microbiota by AA is directly involved in the effect observed at the host level.
Combination between amino acids and probiotics (symbiotic approach)
The potential synergistic effect of AA supplementation with probiotics has been investigated. Probiotics are “live microorganisms which when administered in adequate amounts confer a health benefit on the host” (46). Combining AA and probiotics corresponds with the concept of “symbiotic” which is “a mixture comprising live microorganisms and substrate(s) selectively utilized by host microorganisms that confers a health benefit on the host” (48). One potential mechanism is that AA could promote the survival of the exogenous microbial species (i.e., the probiotic) in addition of being used as substrates for their growth. For instance, the catabolism of glutamine has been shown to improve the acid tolerance of Lactobacillus (49, 50). Therefore, the association of glutamine and the probiotic bacteria L. plantarum was expected to increase its survivability and thereby improve its positive effect on gut health. Accordingly, the authors showed that the combination of glutamine (1–4 mM) and L. plantarum decreased the translocation of E. coli in weanling rabbit ileal loops (51). They observed a synergistic effect indicating that the provision of the two compounds (AA and probiotics) together was more efficient than the anticipated sum of effects resulting from each compound alone. Other studies investigated the effects of the combination of arginine and Lactobacillus in rats with acute liver injury. The authors showed that the co-administration of L. plantarum and arginine reduced the hepatocellular necrosis and inflammatory cell infiltration, whereas the effect of individual administration of probiotics or arginine alone had lower effects than the two together (52). The potential mechanism of this synergistic effect may involve the metabolization of arginine by L. plantarum into polyamines, nitric oxide or its utilization as an energy source. Another study showed that a supplementation with L. plantarum and arginine 10 days before an LPS-challenge induced a synergistic reduction of liver damage and inflammation (53). The authors hypothesized that probiotics could direct arginine toward polyamines rather than NO synthesis by decreasing inflammation level and promoting cell proliferation and healing in the liver (53). Altogether, these results suggest that symbiotics composed of AA and probiotics have the potential to promote health to a larger extent than the simple sum of both dietary supplements alone. Multiple combination of probiotics and AA should be tested in future studies, notably by selecting the most promising association based on the capacity of the probiotic strain to metabolize specific AA (Table 1).
Discussion
Based on the data presented above, it appears AA supplementation can modulate the gut microbiota composition, its metabolic activity and these effects can be associated with benefits for the host. Thus, we consider that dietary AA supplements could fulfill the requirements to be considered as a novel class of prebiotics, the “Aminobiotics.” Additional studies are still required to support this promising concept since important questions remain open.
First, most of the doses of AA supplementation used in the literature are relatively high, raising the question of whether the observed effects are linked to direct effects of AA on the microbiota or to indirect effects mediated by the host (i.e., after absorption), or a combination of both. For example, it has been shown that AA supplementation can increase the expression of intestinal β-defensin (an endogenous small cationic polypeptide that functions as a broad-spectrum antimicrobial) by blocking nuclear factor kappa-B (NF-κB) and MAPK inflammatory signaling pathways and activating the mammalian target of rapamycin signaling pathway (mTOR) (54, 55). Similarly, several AA have been reported to modulate the secretion of immunoglobulin A (IgA) in the intestine (56, 57) which could in turn affect the gut microbiota. Therefore, it is very likely that dietary AA affect gut microbial composition both directly (i.e., as a substrate) and indirectly (i.e., through modulation of host factors). Second, only nine AA out of the 20 proteinogenic ones have been tested and they were tested only a limited number of times which urges to be cautious when drawing conclusions. Third, microbiota analysis was not always the main endpoint of the studies which can also generate some biases.
Another challenge will be to develop strategies to supply AA reaching the lower gut were most of the microbiota develops. Indeed, when provided in a free form, AA are rapidly absorbed in the proximal small intestine (58). In contrast, the microbiota density is higher in the distal part of the digestive tract, mostly in the ileum, caecum, and colon (59). One potential strategy to circumvent this lack of space/time synchrony between dietary free AA release and microbiota, would be to delay the release of specific free AA by using protective delivery systems such as fat matrix encapsulation. The encapsulation of AA with probiotics may hypothetically optimize their use by the target communities of bacteria. Another possibility to deliver AA to the gut microbiota would be to combine AA with polyphenols that escape absorption in the small intestine. AA bound to polyphenols might potentially reach the colon and be degraded by the gut microbiota. A recent study showed that the supplementation of piglets with a mix of 0.1% AA (L-arginine, L-leucine, L-valine, L-isoleucine, L-cystine) and 100 ppm grape polyphenols increased the concentration in the caecum of bacterial metabolites derived from AA (e.g., isovalerate and 2-methylbutyrate) (49). Moreover, it is important to consider that the intestinal AA absorption capacity by the host is region dependent. Concurrent with higher expression of brush-border exopeptidases in the distal part of the small intestine (60), the absorption capacity for free AA by enterocytes is the highest in mid- to lower small intestine (61, 62). The latter is mediated by a complex system of brush-border Na+ dependent and independent transporters with considerable overlap and competition between AA (63). In addition, differences in rate of absorption between AA are noticeable (61). Therefore, any strategy to supply free AA to location-specific microbiota should be carefully designed.
AA supplementation can also be associated with deleterious effects on the gut microbiota. For example, in poultry, it has been reported that the consumption of diets high in glycine such as fish meal or gelatin are associated with increased populations of pathogenic C. perfringens (64). In line with these results, a study by Dahiya et al. reported that birds fed glycine at high levels (34.3 or 47.7 g/kg) in an encapsulated form to slowly release the AA along the entire length of the gut exhibited a higher number of C. perfringens and a lower number of Lactobacillus in the ileum compared to birds fed low levels of encapsulated glycine (7.6 or 21.0 g/kg). This higher colonization was associated with higher intestinal lesions and reduced performance (65). This reinforces the importance of carefully selecting both the AA supplement type and dose to modulate microbiota to deliver a healthy outcome.
Conclusion
Dietary supplementations with free AA modulate the microbiota composition and metabolic activity in association with consequences for host health. These properties indicate that AA have the potential to be used as a novel class of prebiotics (“Aminobiotics”). The successful utilization of AA as prebiotics to selectively nourish gut microbiota still requires to (i) select the most appropriate AA and dose of supplementation, (ii) develop strategies to deliver the desired AA profile to the microbiota, and (iii) demonstrate that the modulation of the microbiota by AA is directly involved in benefits for host health. Overall, utilization of AA as prebiotics, alone or in combination with probiotics, will expand the nutritional tools available to target the gut microbiota for human and animal health.
Data availability statement
The original contributions presented in this study are included in the article/supplementary material, further inquiries can be directed to the corresponding author.
Author contributions
TC-D, WL, ER, JM, and MB contributed to the conception and structure of the manuscript. TC-D, MB, and CT created and organized the tables. TC-D wrote the first draft of the manuscript. TC-D, WL, ER, JM, CT, and MB contributed to manuscript writing and revision. All authors approved the final version for submission.
Conflict of interest
The authors declare that the research was conducted in the absence of any commercial or financial relationships that could be construed as a potential conflict of interest.
Publisher’s note
All claims expressed in this article are solely those of the authors and do not necessarily represent those of their affiliated organizations, or those of the publisher, the editors and the reviewers. Any product that may be evaluated in this article, or claim that may be made by its manufacturer, is not guaranteed or endorsed by the publisher.
References
1. Park H, Yeo S, Arellano K, Kim H, Holzapfel W. Role of the gut microbiota in health and disease. In: Di Gioia D, Biavati B editors. Probiotics and prebiotics in animal health and food safety. Cham: Springer International Publishing (2018). p. 35–62. doi: 10.1007/978-3-319-71950-4_2
2. Patil Y, Gooneratne R, Ju X. Interactions between host and gut microbiota in domestic pigs: a review. Gut Microbes. (2020) 11:310–34. doi: 10.1080/19490976.2019.1690363
3. Lawley T, Walker A. Intestinal colonization resistance. Immunology. (2013) 138:1–11. doi: 10.1111/j.1365-2567.2012.03616.x
4. DeGruttola A, Low D, Mizoguchi A, Mizoguchi E. Current understanding of dysbiosis in disease in human and animal models. Inflamm Bowel Dis. (2016) 22:1137–50. doi: 10.1097/MIB.0000000000000750
5. Ducatelle R, Eeckhaut V, Haesebrouck F, Immerseel F. A review on prebiotics and probiotics for the control of dysbiosis: present status and future perspectives. Animal. (2015) 9:43–8. doi: 10.1017/S1751731114002584
6. Redondo-Useros N, Nova E, González-Zancada N, Díaz L, Gómez-Martínez S, Marcos A. Microbiota and lifestyle: a special focus on diet. Nutrients. (2020) 12:1776.
7. Zmora N, Suez J, Elinav E. You are what you eat: diet, health and the gut microbiota. Nat Rev Gastroenterol Hepatol. (2019) 16:35–56. doi: 10.1038/s41575-018-0061-2
8. Gibson G, Roberfroid M. Dietary modulation of the human colonic microbiota: introducing the concept of prebiotics. J Nutr. (1995) 125:1401–12. doi: 10.1093/jn/125.6.1401
9. Delzenne N, Olivares M, Neyrinck A, Beaumont M, Kjølbæk L, Larsen T, et al. Nutritional interest of dietary fiber and prebiotics in obesity: lessons from the MyNewGut consortium. Clin Nutr Edinb Scotl. (2020) 39:414–24. doi: 10.1016/j.clnu.2019.03.002
10. Gibson G, Hutkins R, Sanders M, Prescott S, Reimer R, Salminen S, et al. Expert consensus document: the international scientific association for probiotics and prebiotics (ISAPP) consensus statement on the definition and scope of prebiotics. Nat Rev Gastroenterol Hepatol. (2017) 14:491–502.
11. Rodríguez-Daza M, Pulido-Mateos E, Lupien-Meilleur J, Guyonnet D, Desjardins Y, Roy D. Polyphenol-mediated gut microbiota modulation: toward prebiotics and further. Front Nutr. (2021) 8:689456. doi: 10.3389/fnut.2021.689456
12. Beaumont M, Blachier F. Amino acids in intestinal physiology and health. Adv Exp Med Biol. (2020) 1265:1–20. doi: 10.1007/978-3-030-45328-2_1
13. Chalvon-Demersay T, Luise D, Le Floc’h N, Tesseraud S, Lambert W, Bosi P, et al. Functional Amino acids in pigs and chickens: implication for gut health. Front Vet Sci. (2021) 8:663727. doi: 10.3389/fvets.2021.663727
14. Portune K, Beaumont M, Davila A, Tomé D, Blachier F, Sanz Y. Gut microbiota role in dietary protein metabolism and health-related outcomes: the two sides of the coin. Trends Food Sci Technol. (2016) 57:213–32.
15. Reese A, Pereira F, Schintlmeister A, Berry D, Wagner M, Hale L, et al. Microbial nitrogen limitation in the mammalian large intestine. Nat Microbiol. (2018) 3:1441–50. doi: 10.1038/s41564-018-0267-7
16. Libao-Mercado A, Zhu C, Cant J, Lapierre H, Thibault J, Sève B, et al. Dietary and endogenous amino acids are the main contributors to microbial protein in the upper gut of normally nourished pigs. J Nutr. (2009) 139:1088–94. doi: 10.3945/jn.108.103267
17. Davila A, Blachier F, Gotteland M, Andriamihaja M, Benetti P, Sanz Y, et al. Intestinal luminal nitrogen metabolism: role of the gut microbiota and consequences for the host. Pharmacol Res. (2013) 68:95–107.
18. Yu X, Walker D, Liu Y, Zhang L. Amino acid biosynthesis deficiency in bacteria associated with human and animal hosts. Infect Genet Evol. (2009) 9:514–7. doi: 10.1016/j.meegid.2009.02.002
19. Reitzer L. Nitrogen assimilation and global regulation in Escherichia coli. Annu Rev Microbiol. (2003) 57:155–76.
20. Dai Z, Zhang J, Wu G, Zhu W. Utilization of amino acids by bacteria from the pig small intestine. Amino Acids. (2010) 39:1201–15. doi: 10.1007/s00726-010-0556-9
21. Dai Z, Li X, Xi P, Zhang J, Wu G, Zhu W. Metabolism of select amino acids in bacteria from the pig small intestine. Amino Acids. (2012) 42:1597–608.
22. Dai Z, Wu G, Zhu W. Amino acid metabolism in intestinal bacteria: links between gut ecology and host health. Front Biosci Landmark Ed. (2011) 16:1768–86. doi: 10.2741/3820
23. Guccione E, Del Rocio Leon-Kempis M, Pearson B, Hitchin E, Mulholland F, Van Diemen P, et al. Amino acid-dependent growth of Campylobacter jejuni: key roles for aspartase (AspA) under microaerobic and oxygen-limited conditions and identification of AspB (Cj0762), essential for growth on glutamate. Mol Microbiol. (2008) 69:77–93. doi: 10.1111/j.1365-2958.2008.06263.x
24. Pifer R, Russell R, Kumar A, Curtis M, Sperandio V. Redox, amino acid, and fatty acid metabolism intersect with bacterial virulence in the gut. Proc Natl Acad Sci USA. (2018) 115:E10712–9. doi: 10.1073/pnas.1813451115
25. Menezes-Garcia Z, Kumar A, Zhu W, Winter S, Sperandio V. L-Arginine sensing regulates virulence gene expression and disease progression in enteric pathogens. Proc Natl Acad Sci USA. (2020) 117:12387–93. doi: 10.1073/pnas.1919683117
26. Yang Y, Pollard A, Höfler C, Poschet G, Wirtz M, Hell R, et al. Relation between chemotaxis and consumption of amino acids in bacteria. Mol Microbiol. (2015) 96:1272–82.
27. Andriamihaja M, Davila A, Eklou-Lawson M, Petit N, Delpal S, Allek F, et al. Colon luminal content and epithelial cell morphology are markedly modified in rats fed with a high-protein diet. Am J Physiol Gastrointest Liver Physiol. (2010) 299:G1030–7.
28. Tudela C, Boudry C, Stumpff F, Aschenbach J, Vahjen W, Zentek J, et al. Down-regulation of monocarboxylate transporter 1 (MCT1) gene expression in the colon of piglets is linked to bacterial protein fermentation and pro-inflammatory cytokine-mediated signalling. Br J Nutr. (2015) 113:610–7. doi: 10.1017/S0007114514004231
29. Blachier F, Beaumont M, Kim E. Cysteine-derived hydrogen sulfide and gut health: a matter of endogenous or bacterial origin. Curr Opin Clin Nutr Metab Care. (2019) 22:68–75. doi: 10.1097/MCO.0000000000000526
30. Blachier F, Andriamihaja M. Effects of the L-tyrosine-derived bacterial metabolite p-cresol on colonic and peripheral cells. Amino Acids. (2022) 54:325–38. doi: 10.1007/s00726-021-03064-x
31. Koh A, Molinaro A, Ståhlman M, Khan M, Schmidt C, Mannerås-Holm L, et al. Microbially produced imidazole propionate impairs insulin signaling through mTORC1. Cell. (2018) 175:947.e–61.e. doi: 10.1016/j.cell.2018.09.055
32. Windey K, De Preter V, Verbeke K. Relevance of protein fermentation to gut health. Mol Nutr Food Res. (2012) 56:184–96.
33. Van den Abbeele P, Ghyselinck J, Marzorati M, Koch A, Lambert W, Michiels J, et al. The effect of amino acids on production of SCFA and bCFA by members of the porcine colonic microbiota. Microorganisms. (2022) 10:762. doi: 10.3390/microorganisms10040762
34. McDonald J, Mullish B, Pechlivanis A, Liu Z, Brignardello J, Kao D, et al. Inhibiting growth of clostridioides difficile by restoring valerate, produced by the intestinal microbiota. Gastroenterology. (2018) 155:1495–507.e15. doi: 10.1053/j.gastro.2018.07.014
35. Bekebrede A, Keijer J, Gerrits W, Boer V. The molecular and physiological effects of protein-derived polyamines in the intestine. Nutrients. (2020) 12:E197. doi: 10.3390/nu12010197
36. Grosheva I, Zheng D, Levy M, Polansky O, Lichtenstein A, Golani O, et al. High-throughput screen identifies host and microbiota regulators of intestinal barrier function. Gastroenterology. (2020) 159:1807–23. doi: 10.1053/j.gastro.2020.07.003
37. Levy M, Thaiss C, Zeevi D, Dohnalová L, Zilberman-Schapira G, Mahdi J, et al. Microbiota-modulated metabolites shape the intestinal microenvironment by regulating NLRP6 inflammasome signaling. Cell. (2015) 163:1428–43. doi: 10.1016/j.cell.2015.10.048
38. Roager H, Licht T. Microbial tryptophan catabolites in health and disease. Nat Commun. (2018) 9:3294.
39. Bansal T, Alaniz R, Wood T, Jayaraman A. The bacterial signal indole increases epithelial-cell tight-junction resistance and attenuates indicators of inflammation. Proc Natl Acad Sci USA. (2010) 107:228–33. doi: 10.1073/pnas.0906112107
40. Gao K, Mu C, Farzi A, Zhu W. Tryptophan metabolism: a link between the gut microbiota and brain. Adv Nutr Bethesda Md. (2020) 11:709–23.
41. Gibson J, Sladen G, Dawson A. Protein absorption and ammonia production: the effects of dietary protein and removal of the colon. Br J Nutr. (1976) 35:61–5. doi: 10.1079/bjn19760009
42. Heo J, Kim J, Yoo J, Pluske JR. A between-experiment analysis of relationships linking dietary protein intake and post-weaning diarrhea in weanling pigs under conditions of experimental infection with an enterotoxigenic strain of Escherichia coli. Anim Sci J Nihon Chikusan Gakkaiho. (2015) 86:286–93. doi: 10.1111/asj.12275
43. Beaumont M, Portune K, Steuer N, Lan A, Cerrudo V, Audebert M, et al. Quantity and source of dietary protein influence metabolite production by gut microbiota and rectal mucosa gene expression: a randomized, parallel, double-blind trial in overweight humans. Am J Clin Nutr. (2017) 106:1005–19. doi: 10.3945/ajcn.117.158816
44. Madsen L, Myrmel L, Fjære E, Liaset B, Kristiansen K. Links between dietary protein sources, the gut microbiota, and obesity. Front Physiol. (2017) 8:1047. doi: 10.3389/fphys.2017.01047
45. Blachier F, Beaumont M, Portune K, Steuer N, Lan A, Audebert M, et al. High-protein diets for weight management: interactions with the intestinal microbiota and consequences for gut health. a position paper by the my new gut study group. Clin Nutr Edinb Scotl. (2019) 38:1012–22.
46. Hill C, Guarner F, Reid G, Gibson G, Merenstein D, Pot B, et al. The international scientific association for probiotics and prebiotics consensus statement on the scope and appropriate use of the term probiotic. Nat Rev Gastroenterol Hepatol. (2014) 11:506–14.
47. Luise D, Chalvon-Demersay T, Lambert W, Bosi P, Trevisi P. Meta-analysis to evaluate the impact of the reduction of dietary crude protein on the gut health of post-weaning pigs. Ital J Anim Sci. (2021) 20:1386–97.
48. Swanson K, Gibson G, Hutkins R, Reimer R, Reid G, Verbeke K, et al. The international scientific association for probiotics and prebiotics (ISAPP) consensus statement on the definition and scope of synbiotics. Nat Rev Gastroenterol Hepatol. (2020) 17:687–701. doi: 10.1038/s41575-020-0344-2
49. Vermeulen N, Gänzle M, Vogel R. Glutamine deamidation by cereal-associated lactic acid bacteria. J Appl Microbiol. (2007) 103:1197–205. doi: 10.1111/j.1365-2672.2007.03333.x
50. Teixeira J, Seeras A, Sanchez-Maldonado A, Zhang C, Su M, Gänzle M. Glutamine, glutamate, and arginine-based acid resistance in Lactobacillus reuteri. Food Microbiol. (2014) 42:172–80. doi: 10.1016/j.fm.2014.03.015
51. Panigrahi, P. Oral gram(+) bacteria and glutamine composition for prevention and/or treatment of gastro-intestinal dysfunctions including inflammation in the gastro-intestinal tract, neonatal necrotizing enterocolitis (nec) and bacterial sepsis [Internet]. US20040096427A1. (2004). Available online at: https://patents.google.com/patent/US20040096427A1/en (accessed December 13, 2021).
52. Adawi D, Kasravi F, Molin G, Jeppsson B. Effect of lactobacillus supplementation with and without arginine on liver damage and bacterial translocation in an acute liver injury model in the rat. Hepatol Baltim Md. (1997) 25:642–7. doi: 10.1002/hep.510250325
53. Rishi P, Bharrhan S, Singh G, Kaur I. Effect of Lactobacillus plantarum and L-arginine against endotoxin-induced liver injury in a rat model. Life Sci. (2011) 89:847–53. doi: 10.1016/j.lfs.2011.09.007
54. Sherman H, Chapnik N, Froy O. Albumin and amino acids upregulate the expression of human beta-defensin 1. Mol Immunol. (2006) 43:1617–23. doi: 10.1016/j.molimm.2005.09.013
55. Ren M, Zhang S, Liu X, Li S, Mao X, Zeng X, et al. different lipopolysaccharide branched-chain amino acids modulate porcine intestinal endogenous β-defensin expression through the Sirt1/ERK/90RSK pathway. J Agric Food Chem. (2016) 64:3371–9. doi: 10.1021/acs.jafc.6b00968
56. Ren M, Zhang S, Zeng X, Liu H, Qiao S. branched-chain amino acids are beneficial to maintain growth performance and intestinal immune-related function in weaned piglets fed protein restricted diet. Asian Australas J Anim Sci. (2015) 28:1742–50. doi: 10.5713/ajas.14.0131
57. Xing S, Zhang B, Lin M, Zhou P, Li J, Zhang L, et al. Effects of alanyl-glutamine supplementation on the small intestinal mucosa barrier in weaned piglets. Asian Australas J Anim Sci. (2017) 30:236–45. doi: 10.5713/ajas.16.0077
58. Eugenio F, van Milgen J, Duperray J, Sergheraert R, Le Floc’h N. Feeding intact proteins, peptides, or free amino acids to monogastric farm animals. Amino Acids. (2022) 54:157–68.
59. Blachier F, Andriamihaja M, Kong X. Fate of undigested proteins in the pig large intestine: what impact on the large intestine epithelium? Anim Nutr. (2021) 9:110–8. doi: 10.1016/j.aninu.2021.08.001
60. Fan M, Stoll B, Jiang R, Burrin D. Enterocyte digestive enzyme activity along the crypt-villus and longitudinal axes in the neonatal pig small intestine. J Anim Sci. (2001) 79:371–81. doi: 10.2527/2001.792371x
61. Buraczewska L. Absorption of amino acids in different parts of the small intestine in growing pigs. III. Absorption of constituents of protein hydrolysates. Acta Physiol Pol. (1981) 32:569–84.
62. Gaudichon C, Mahé S, Benamouzig R, Luengo C, Fouillet H, Daré S, et al. Net postprandial utilization of [15N]-labeled milk protein nitrogen is influenced by diet composition in humans. J Nutr. (1999) 129:890–5. doi: 10.1093/jn/129.4.890
63. Bröer S. Amino acid transport across mammalian intestinal and renal epithelia. Physiol Rev. (2008) 88:249–86.
64. Drew M, Syed N, Goldade B, Laarveld B, Van Kessel A. Effects of dietary protein source and level on intestinal populations of clostridium perfringens in broiler chickens. Poult Sci. (2004) 83:414–20.
65. Dahiya J, Hoehler D, Van Kessel A, Drew M. Dietary encapsulated glycine influences clostridium perfringens and Lactobacilli growth in the gastrointestinal tract of broiler chickens. J Nutr. (2007) 137:1408–14. doi: 10.1093/jn/137.6.1408
66. Rogosa M. Acidaminococcus gen. n., Acidaminococcus fermentans sp. n., anaerobic gram-negative diplococci using amino acids as the sole energy source for growth. J Bacteriol. (1969) 98:756–66. doi: 10.1128/jb.98.2.756-766.1969
67. Allison M, Baetz A, Wiegel J. Alternative pathways for biosynthesis of leucine and other amino acids in Bacteroides ruminicola and Bacteroides fragilis. Appl Environ Microbiol. (1984) 48:1111–7. doi: 10.1128/aem.48.6.1111-1117.1984
68. Fonknechten N, Chaussonnerie S, Tricot S, Lajus A, Andreesen J, Perchat N, et al. Clostridium sticklandii, a specialist in amino acid degradation:revisiting its metabolism through its genome sequence. BMC Genomics. (2010) 11:555. doi: 10.1186/1471-2164-11-555
70. Dunn M, Shankman S, Camien M, Block H. The amino acid requirements of twenty-three lactic acid bacteria. J Biol Chem. (1947) 168:1–22. doi: 10.1007/s00705-006-0851-7
71. Wallace R. Catabolism of amino acids by Megasphaera elsdenii LC1. Appl Environ Microbiol. (1986) 51:1141–3. doi: 10.1128/aem.51.5.1141-1143.1986
72. Chen G, Russell J. Transport of glutamine by Streptococcus bovis and conversion of glutamine to pyroglutamic acid and ammonia. J Bacteriol. (1989) 171:2981–5. doi: 10.1128/jb.171.6.2981-2985.1989
73. Liao S, Poonpairoj P, Ko K, Takatuska Y, Yamaguchi Y, Abe N, et al. Occurrence of agmatine pathway for putrescine synthesis in Selenomonas ruminatium. Biosci Biotechnol Biochem. (2008) 72:445–55. doi: 10.1271/bbb.70550
74. Guccione E, Leon-Kempis M, del R, Pearson B, Hitchin E, Mulholland F, et al. Amino acid-dependent growth of Campylobacter jejuni: key roles for aspartase (AspA) under microaerobic and oxygen-limited conditions and identification of AspB (Cj0762), essential for growth on glutamate. Mol Microbiol. (2008) 69:77–93.
75. Neumann-Schaal M, Hofmann J, Will S, Schomburg D. Time-resolved amino acid uptake of Clostridium difficile 630Δerm and concomitant fermentation product and toxin formation. BMC Microbiol. (2015) 15:281. doi: 10.1186/s12866-015-0614-2
76. Sivolodskii E. Application of the profiles of amino acid utilization as the sole carbon and nitrogen sources for pseudomonad taxonomy. Microbiology. (2009) 78:711. doi: 10.1134/S0026261709060071
77. Selvarasu S, Ow D, Lee S, Lee M, Oh S, Karimi I, et al. Characterizing Escherichia coli DH5alpha growth and metabolism in a complex medium using genome-scale flux analysis. Biotechnol Bioeng. (2009) 102:923–34. doi: 10.1002/bit.22119
78. Loesche W, Gibbons R. Amino acid fermentation by Fusobacterium nucleatum. Arch Oral Biol. (1968) 13:191–202.
79. Potrykus J, White R, Bearne S. Proteomic investigation of amino acid catabolism in the indigenous gut anaerobe Fusobacterium varium. Proteomics. (2008) 8:2691–703. doi: 10.1002/pmic.200700437
80. Jablonski P, Jaworski M, Hovde CJ. A minimal medium for growth of Pasteurella multocida. FEMS Microbiol Lett. (1996) 140:165–9.
81. Chen G, Russell J. Fermentation of peptides and amino acids by a monensin-sensitive ruminal Peptostreptococcus. Appl Environ Microbiol. (1988) 54:2742–9. doi: 10.1128/aem.54.11.2742-2749.1988
82. Kwan G, Plagenz B, Cowles K, Pisithkul T, Amador-Noguez D, Barak J. Few differences in metabolic network use found between Salmonella enterica colonization of plants and typhoidal mice. Front Microbiol. (2018) 9:695. doi: 10.3389/fmicb.2018.00695
83. Zhu Y, Weiss E, Otto M, Fey P, Smeltzer M, Somerville G. Staphylococcus aureus biofilm metabolism and the influence of arginine on polysaccharide intercellular adhesin synthesis, biofilm formation, and pathogenesis. Infect Immun. (2007) 75:4219–26. doi: 10.1128/IAI.00509-07
84. Somerville G, Proctor R. At the crossroads of bacterial metabolism and virulence factor synthesis in Staphylococci. Microbiol Mol Biol Rev. (2009) 73:233–48. doi: 10.1128/MMBR.00005-09
85. Onoue Y, Mori M. Amino acid requirements for the growth and enterotoxin production by Staphylococcus aureus in chemically defined media. Int J Food Microbiol. (1997) 36:77–82.
86. Lopes J, Cruz F. Chemically defined media for growing anaerobic bacteria of the genus Veillonella. Antonie Van Leeuwenhoek. (1976) 42:411–20. doi: 10.1007/BF00410172
87. de Souza A, Zambom A, Abboud K, Reis S, Tannihão F, Guadagnini D, et al. Oral supplementation with L-glutamine alters gut microbiota of obese and overweight adults: a pilot study. Nutr Burbank Los Angel Cty Calif. (2015) 31:884–9. doi: 10.1016/j.nut.2015.01.004
88. Genton L, Pruijm M, Teta D, Bassi I, Cani P, Gaïa N, et al. Gut barrier and microbiota changes with glycine and branched-chain amino acid supplementation in chronic haemodialysis patients. J Cachexia Sarcopenia Muscle. (2021) 12:1527–39. doi: 10.1002/jcsm.12781
89. Ren W, Chen S, Yin J, Duan J, Li T, Liu G, et al. Dietary arginine supplementation of mice alters the microbial population and activates intestinal innate immunity. J Nutr. (2014) 144:988–95. doi: 10.3945/jn.114.192120
90. Bin P, Liu S, Chen S, Zeng Z, Huang R, Yin Y, et al. The effect of aspartate supplementation on the microbial composition and innate immunity on mice. Amino Acids. (2017) 49:2045–51. doi: 10.1007/s00726-017-2467-5
91. Ren W, Duan J, Yin J, Liu G, Cao Z, Xiong X, et al. Dietary L-glutamine supplementation modulates microbial community and activates innate immunity in the mouse intestine. Amino Acids. (2014) 46:2403–13. doi: 10.1007/s00726-014-1793-0
92. Chen S, Wang M, Yin L, Ren W, Bin P, Xia Y, et al. Effects of dietary tryptophan supplementation in the acetic acid-induced colitis mouse model. Food Funct. (2018) 9:4143–52. doi: 10.1039/c8fo01025k
93. Feng Z, Li T, Wu L, Xiao D, Blachier F, Yin Y. Monosodium L-glutamate and dietary fat differently modify the composition of the intestinal microbiota in growing pigs. Obes Facts. (2015) 8:87–100. doi: 10.1159/000380889
94. Hu C, Li F, Duan Y, Yin Y, Kong X. Glutamic acid supplementation reduces body fat weight in finishing pigs when provided solely or in combination with arginine and it is associated with colonic propionate and butyrate concentrations. Food Funct. (2019) 10:4693–704.
95. Kyoung H, Lee J, Cho J, Choe J, Kang J, Lee H, et al. Dietary glutamic acid modulates immune responses and gut health of weaned pigs. Anim Open Access J MDPI. (2021) 11:504. doi: 10.3390/ani11020504
96. Zhang S, Qiao S, Ren M, Zeng X, Ma X, Wu Z, et al. Supplementation with branched-chain amino acids to a low-protein diet regulates intestinal expression of amino acid and peptide transporters in weanling pigs. Amino Acids. (2013) 45:1191–205. doi: 10.1007/s00726-013-1577-y
97. Hu C, Li F, Duan Y, Yin Y, Kong X. Dietary supplementation with leucine or in combination with arginine decreases body fat weight and alters gut microbiota composition in finishing pigs. Front Microbiol. (2019) 10:1767. doi: 10.3389/fmicb.2019.01767
98. Spring S, Premathilake H, Bradway C, Shili C, DeSilva U, Carter S, et al. Effect of very low-protein diets supplemented with branched-chain amino acids on energy balance, plasma metabolomics and fecal microbiome of pigs. Sci Rep. (2020) 10:15859. doi: 10.1038/s41598-020-72816-8
99. Liang H, Dai Z, Liu N, Ji Y, Chen J, Zhang Y, et al. Dietary L-tryptophan modulates the structural and functional composition of the intestinal microbiome in weaned piglets. Front Microbiol. (2018) 9:1736. doi: 10.3389/fmicb.2018.01736
100. Liang H, Dai Z, Kou J, Sun K, Chen J, Yang Y, et al. Dietary L-tryptophan supplementation enhances the intestinal mucosal barrier function in weaned piglets: implication of tryptophan-metabolizing microbiota. Int J Mol Sci. (2018) 20:20. doi: 10.3390/ijms20010020
101. Messori S, Trevisi P, Simongiovanni A, Priori D, Bosi P. Effect of susceptibility to enterotoxigenic Escherichia coli F4 and of dietary tryptophan on gut microbiota diversity observed in healthy young pigs. Vet Microbiol. (2013) 162:173–9. doi: 10.1016/j.vetmic.2012.09.001
102. Bello A, Idrus Z, Meng G, Awad E, Farjam A. Gut microbiota and transportation stress response affected by tryptophan supplementation in broiler chickens. Ital J Anim Sci. (2018) 17:107–13.
103. Kumar S, Adhikari P, Oakley B, Kim W. Changes in cecum microbial community in response to total sulfur amino acid (TSAA: DL-methionine) in antibiotic-free and supplemented poultry birds. Poult Sci. (2019) 98:5809–19. doi: 10.3382/ps/pez380
104. Kolbadinejad A, Rezaeipour V. Efficacy of ajwain (Trachyspermum ammi L.) seed at graded levels of dietary threonine on growth performance, serum metabolites, intestinal morphology and microbial population in broiler chickens. J Anim Physiol Anim Nutr. (2020) 104:1333–42. doi: 10.1111/jpn.13357
105. Eftekhari A, Rezaeipour V, Abdullahpour R. Effects of acidified drinking water on performance, carcass, immune response, jejunum morphology, and microbiota activity of broiler chickens fed diets containing graded levels of threonine. Livest Sci. (2015) 180:158–63.
106. Chen Y, Cheng Y, Li X, Yang W, Wen C, Zhuang S, et al. Effects of threonine supplementation on the growth performance, immunity, oxidative status, intestinal integrity, and barrier function of broilers at the early age. Poult Sci. (2017) 96:405–13. doi: 10.3382/ps/pew240
107. Zhang B, Li G, Shahid M, Gan L, Fan H, Lv Z, et al. Dietary L-arginine supplementation ameliorates inflammatory response and alters gut microbiota composition in broiler chickens infected with Salmonella enterica serovar Typhimurium. Poult Sci. (2020) 99:1862–74. doi: 10.1016/j.psj.2019.10.049
108. Ruan D, Fouad A, Fan Q, Huo X, Kuang Z, Wang H, et al. Dietary L-arginine supplementation enhances growth performance, intestinal antioxidative capacity, immunity and modulates gut microbiota in yellow-feathered chickens. Poult Sci. (2020) 99:6935–45. doi: 10.1016/j.psj.2020.09.042
109. Zhang B, Lv Z, Li Z, Wang W, Li G, Guo Y. Dietary L-arginine supplementation alleviates the intestinal injury and modulates the gut microbiota in broiler chickens challenged by Clostridium perfringens. Front Microbiol. (2018) 9:1716. doi: 10.3389/fmicb.2018.01716
Keywords: prebiotics, amino acids, aminobiotics, gut microbiota, gut health
Citation: Beaumont M, Roura E, Lambert W, Turni C, Michiels J and Chalvon-Demersay T (2022) Selective nourishing of gut microbiota with amino acids: A novel prebiotic approach? Front. Nutr. 9:1066898. doi: 10.3389/fnut.2022.1066898
Received: 11 October 2022; Accepted: 29 November 2022;
Published: 19 December 2022.
Edited by:
Hani El-Nezami, The University of Hong Kong, Hong Kong SAR, ChinaReviewed by:
Yao-Tsung Yeh, Fooyin University, TaiwanZhoujin Tan, Hunan University of Chinese Medicine, China
Copyright © 2022 Beaumont, Roura, Lambert, Turni, Michiels and Chalvon-Demersay. This is an open-access article distributed under the terms of the Creative Commons Attribution License (CC BY). The use, distribution or reproduction in other forums is permitted, provided the original author(s) and the copyright owner(s) are credited and that the original publication in this journal is cited, in accordance with accepted academic practice. No use, distribution or reproduction is permitted which does not comply with these terms.
*Correspondence: Tristan Chalvon-Demersay, dHJpc3Rhbi5jaGFsdm9uLWRlbWVyc2F5QG1ldGV4LW5vb3Zpc3RhZ28uY29t