- 1Department of Endocrinology and Metabolism, Affiliated Hospital of Jiangsu University, Zhenjiang, Jiangsu, China
- 2Department of Endocrinology and Metabolism, The First People’s Hospital of Lianyungang, Lianyungang, Jiangsu, China
- 3Department of Endocrinology, Taicang Hospital of Traditional Chinese Medicine, Taicang, Jiangsu, China
Except for improving glycemic control, liraglutide, one of the glucagon-like peptide-1 receptor agonists, has exerted promising therapeutic effects for dyslipidemia. It has been proved that gut microbiota plays a dramatic role in regulating lipid metabolism. This study aims to explore whether liraglutide could improve dyslipidemia by modulating the gut microbiota in mice fed a high-fat diet (HFD). The C57BL/6 mice were fed a HFD to establish an animal model of dyslipidemia, and then administered with liraglutide or normal saline (NS) for 12 weeks. Indices of glucolipid metabolism were evaluated. Gut microbiota of the mice was analyzed by 16S rRNA gene sequencing. Compared with HFD group, liraglutide significantly alleviated weight, total cholesterol (TC) and low-density lipoprotein cholesterol (LDL) levels, meanwhile elevating high-density lipoprotein cholesterol (HDL) levels (all p < 0.05). The gut microbiota analysis revealed that liraglutide greatly reduced the relative abundance of Firmicutes and augmented that of Bacteroidetes, with a concomitant drop in the Firmicutes/Bacteroidetes ratio. Meanwhile, liraglutide dramatically changed the overall composition, promoted the growth of beneficial microbes (Akkermansia, Lactobacillus, Parabacteroides, Oscillospira, etc.), and inhibited the growth of harmful microbes (AF12, Shigella, Proteobacteria, Xenorhabdus, etc.). Especially, the relative abundance of Akkermansia increased the most after liraglutide treatment. Correlation analysis suggested that TC and LDL were positively correlated with some harmful bacteria, and negatively associated with beneficial bacteria. This study confirmed that liraglutide had a certain therapeutic effect on dyslipidemia in HFD-fed mice and could regulate the composition of the gut microbiota associated with lipid metabolism, especially Akkermansia. Thus, affecting gut microbiota might be a potential mechanism of liraglutide in attenuating dyslipidemia.
Introduction
Dyslipidemia, including the elevation of serum total cholesterol (TC), total triglycerides (TG), and low-density lipoprotein cholesterol (LDL) as well as relative reduction of high-density lipoprotein cholesterol (HDL), is one of the worldwide prevalent health hazards. In China, the prevalence of dyslipidemia has exceeded 43%, according to 2019 statistics (1). Increasing evidences have emphasized that lipid metabolic disturbance, especially the imbalance of serum LDL and HDL levels, acts as a major risk factor for atherosclerotic cardiovascular disease (ASCVD), including hypertension, myocardial infarction, stroke, and sudden cardiac death (2–4). Thus, early detection and treatment of dyslipidemia are imperative to reduce cardiovascular events.
The widely used lipid-lowering drugs such as statins, fibrates, bile acid sequestrates and niacin, have shown great potential in preventing and treating dyslipidemia (5). Many synthetic anti-obesity medications have also been used to improve dyslipidemia, as obesity is an important risk for dyslipidemia; however, they all exert undesirable adverse reactions such as myopathy, pancreatitis (6), and transaminase elevations, as well as treatment resistance, which may hinder medication compliance (7, 8). Therefore, any treatment that safely lowers lipid levels is in great demand for the management of dyslipidemia and obesity.
Glucagon-like peptide-1 (GLP-1) is a gastric-derived anorexigenic peptide produced by intestinal enteroendocrine L cells mainly upon fat and carbohydrate intake. Once secreted, GLP-1 will be rapidly degraded by dipeptidyl peptidase-4 (DPP-4), therefore its half-life is only a few minutes (9). Liraglutide, one of the glucagon-like peptide-1 receptor agonists (GLP-1RAs), shares 97% of the amino acid sequence with endogenous human GLP-1, but has a prolonged half-life of 13 h because of its resistance to DPP-4 degradation. Although liraglutide mainly improves blood glucose levels, recent data has also revealed it has a beneficial effect on cardiovascular outcomes (10, 11), by modulating other risk factors, such as dyslipidemia (12), blood pressure (13), and endothelial dysfunction (14). Liraglutide is also the first GLP-1RA approved for treating obesity in both type 2 diabetes mellitus (T2DM) and non-T2DM patients (15), because it has the advantage that its weight loss and lipid-lowering efficacy is independent of hypoglycemic mechanisms.
Additionally, gut microbiota has been reported to play a crucial role in the maintenance and establishment of human health (16). A lager number of studies have shown that altered gut microbiota composition has been associated with dyslipidemia (3, 17) and cardiometabolic diseases (18). Increasing the abundance of some beneficial bacteria could improve dyslipidemia. Therefore, modulating gut microbiota may be a beneficial strategy to prevent the development of dyslipidemia (19). On the other hand, there is evidence that GLP-1 may change the composition of gut microbiota by influencing the biological function of intestinal epithelium (20). Further, GLP-1RAs are known to affect the intestinal environment and change the gut microbiota (21, 22). For example, liraglutide was reported to control glucose-induced insulin secretion partly through influencing the gut microbiota and the intestinal immune system (23). Its beneficial effect on weight loss via modulating the structure of gut microbiota was also confirmed in both diabetic obese and simple obese rodents (22, 24). Therefore, we speculated that liraglutide might also be able to improve dyslipidemia by modulating the gut microbiota.
To this aim, we treated high-fat diet (HFD)-fed mice with liraglutide or vehicle (saline solution) for 12 weeks. At the end of the experiment, 16S rRNA gene sequencing was used to analyze the gut microbiota of the mice. The change of gut microbiota contributed to the beneficial effects of liraglutide against dyslipidemia, which might serve as a potential therapeutic target for dyslipidemia.
Materials and methods
Animals and the experimental design
A total of 24 male C57BL/6 mice (4 weeks old), provided by the Laboratory Animal Research Center of Jiangsu University (Zhenjiang, China), were randomly assigned to two groups: normal control group (NC, n = 8) and HFD group (n = 16). These mice were singly housed in a specific-pathogen-free (22 ± 2°C) environment with a 12/12-h light/dark cycle and could free access to a standard laboratory diet and water. After 1 week of adaptation, the NC group was administered a standard diet, whereas the HFD group was administered a HFD (37% carbohydrate, 18% protein, and 45% fat) for 12 weeks to induce dyslipidemia. Then, the HFD group was further randomly subdivided into the HFD group (n = 8) and the HL group (HFD + liraglutide, n = 8). The HL group received a daily subcutaneous injected with liraglutide (Victoza, Novo Nordisk, Denmark, 0.2 mg/kg body weight), while the NC and HFD groups were injected with an equal volume of NS for another 12 weeks. Fasting body weights were monitored every 2 weeks. After overnight fasting, all the mice were sacrificed at the 24th week, and the blood and fecal samples were collected, which were stored at−80°C until the next analysis. The day before sacrifice, all mice were fasted overnight and the intraperitoneal insulin tolerance test (IPITT) was carried out.
Biochemical analysis
Fasting blood glucose (FBG) was measured by chemiluminescence method, and automatic biochemical analyzer was used to detect lipid profile. All animal experiments were performed according to protocols (UJS-IACUC-2020032535) approved by the Animal Research Committee at the Institute of Laboratory Animals, Jiangsu University Medical College.
Fecal DNA extraction and 16S rRNA gene sequencing
Microbial DNA of fecal samples was extracted by using the Quant-iT PicoGreen dsDNA Assay Kit (Invitrogen, Carlsbad, CA, USA), which was then used as the template to amplify the V3 and V4 hypervariable regions of ribosomal 16S rRNA genes by PCR (25 cycles, Initial denaturation 98°C for 2 min→Denaturation 98°C for 15 s→Annealing 55°C for 30 s →Extension 72°C for 30 s→Final extension 72°C for 5 min). The specific forward primer was 338F 5′-ACTCCTACGGGAGGCAGCA-3′ and the reverse primer was 806R 5′-GGACTACHVGGGTWTCTAAT-3′. All PCR were performed in triplicate with 25 μl reaction mixture containing 5 μl of 5× reaction Buffer, 5 μl of 5× GC Buffer, 2 μl of 2.5 mM dNTPs, 1 μl of each primer (10 μM), 0.25 μl of Q5 DNA Polymerase, and 2 μl of template DNA. The amplified PCR products were extracted on 1.20% agarose gels and purified. 16S rRNA gene sequencing was performed using the Illumina NovaSeq-PE250 platform at Shanghai Personal Biotechnology Co., Ltd. (Shanghai, China) to obtain 2 × 480 bp paired-end reads.
Statistical analysis
Data analyses were conducted with SPSS version 26.0 (SPSS Inc., Chicago, IL, USA). Data were presented as means ± standard error of the mean (SEM), and statistical significance among the three groups was analyzed by one-way analysis of variance (ANOVA). The raw data obtained by sequencing were stored in FASTQ format and performed with QIIME 2 (2019.4) (25). Sequences were then quality filtered, denoised, merged, and chimera removed using the DADA2 plugin (26). Amplicon sequence variants (ASVs) were aligned with mafft (27) and used to construct a phylogeny with fasttree2 (28). Alpha-diversity metrics (Chao1, Observed species, Shannon, and Simpson), and beta diversity metrics [weighted UniFrac (29), unweighted UniFrac (30), Jaccard distance, and Bray–Curtis dissimilarity] were estimated using the diversity plugin to reflect community richness and diversity. Principal coordinates analysis (PCoA) and principal components analysis (PCA) were then performed based on the matrix of beta diversity distance to study the differences between different microbial communities. Relative abundance of bacteria was assessed by the linear discriminant analysis effect size (LEfSe). Spearman’s correlation analysis was performed to explore relationship between metabolic parameters and gut microbiota composition. The area under the curve (AUC) of blood glucose in IPITT was calculated. Statistical difference was set as p < 0.05 value.
Results
Liraglutide suppressed body weight gain and ameliorated lipid profile
The body weights of mice in all the three groups showed an overall tendency to increase as the experiment went on. After the injection of liraglutide, significant weight loss was observed within the first 2 weeks. During the period of administration, the body weights of the HL group were basically close to those of NC group (p > 0.05), while the average body weight of the HFD group was the highest among the three groups (p < 0.05). At the end of experiment, the weights of the HL group were decreased obviously (Figure 1A, p < 0.05).
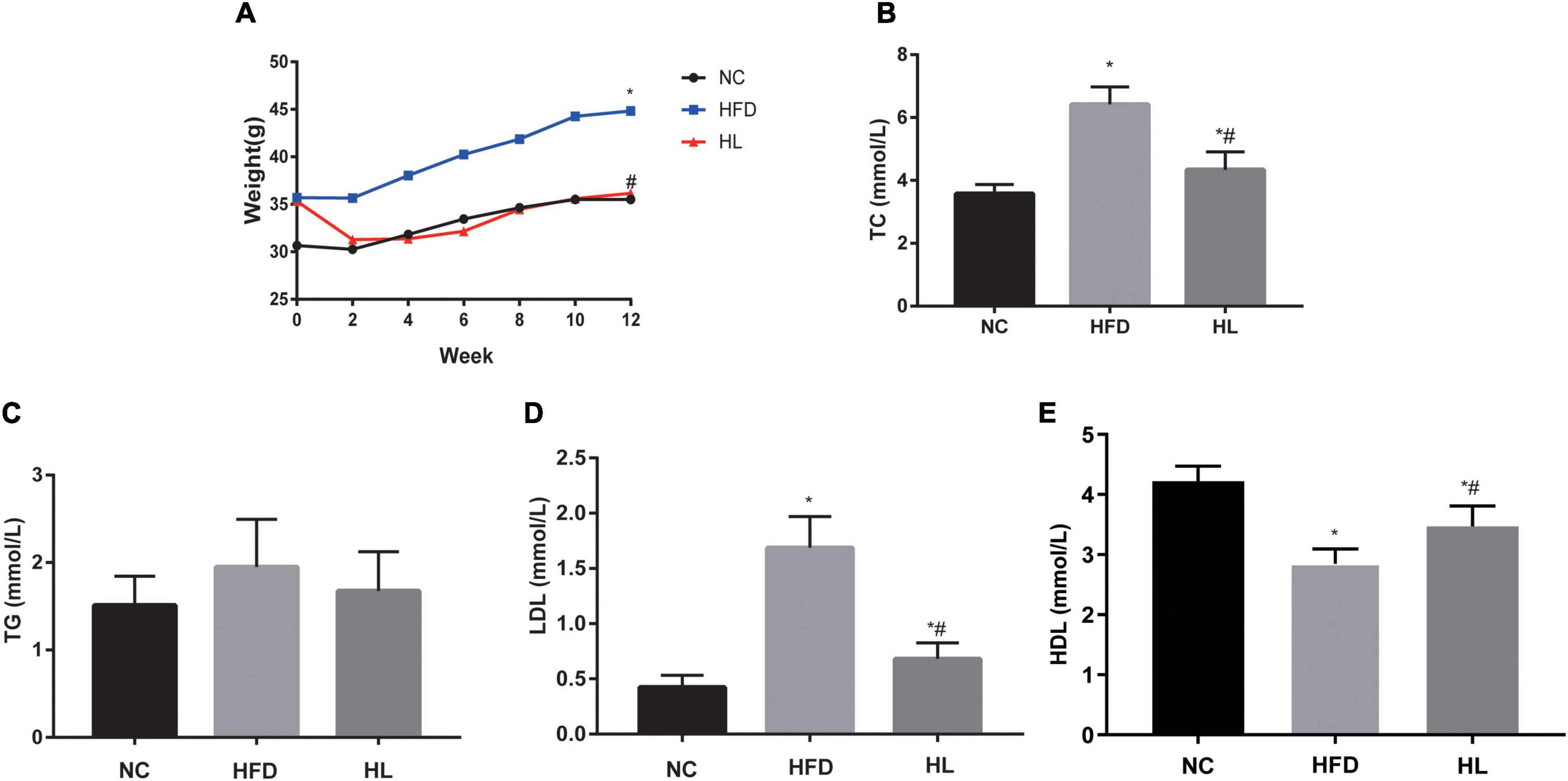
Figure 1. Liraglutide attenuated weight gain and blood lipid profile. (A) Body weight. (B) Total cholesterol (TC) levels. (C) Triglyceride (TG) levels. (D) Low-density lipoprotein (LDL) cholesterol levels. (E) High-density lipoprotein (HDL) cholesterol levels. Data are presented as the mean ± SEM. *p < 0.05 vs. NC group; #p < 0.05 vs. HFD group.
The key biochemical parameters of serum were monitored to explore the impact of liraglutide on dyslipidemia. Compared with the HFD group, supplementation with liraglutide dramatically attenuated the lipid profile levels, including TC, TG and LDL (Figures 1B–D). Especially, TC and LDL levels in HL group were statistically significant compared with the other two groups (Figures 1B,D, p < 0.05). Contrarily, HDL levels were decreased greatly in HFD group, but increased dramatically after liraglutide treatment (Figure 1E, p < 0.05).
Liraglutide improved insulin sensitivity
It could be clearly observed that the average FBG level of the HFD group was generally higher than those of the NC and HL groups, with statistically significant difference between the HFD group and the NC group (Figure 2A, p < 0.05). There was no difference of FBG levels between the HL group and the NC group (Figure 2A, p > 0.05), indicating that liraglutide could attenuate body weight gain and improve dyslipidemia without increasing the risk of hypoglycemia. The results of IPITT showed that compared with the HFD group, liraglutide apparently improved the 5-point blood glucose levels (Figure 2B, p < 0.05). Although the blood glucose level of the HL group at 0 min was slightly higher than that of the NC group, the blood glucose levels at 30, 60, 90, and 120 min after intraperitoneal insulin administration were lower than those in the NC group. Meanwhile, the AUC of blood glucose levels was the lowest in the HL group (Figure 2C, p < 0.05). These results demonstrated that liraglutide could greatly increase insulin sensitivity in HFD-fed mice.
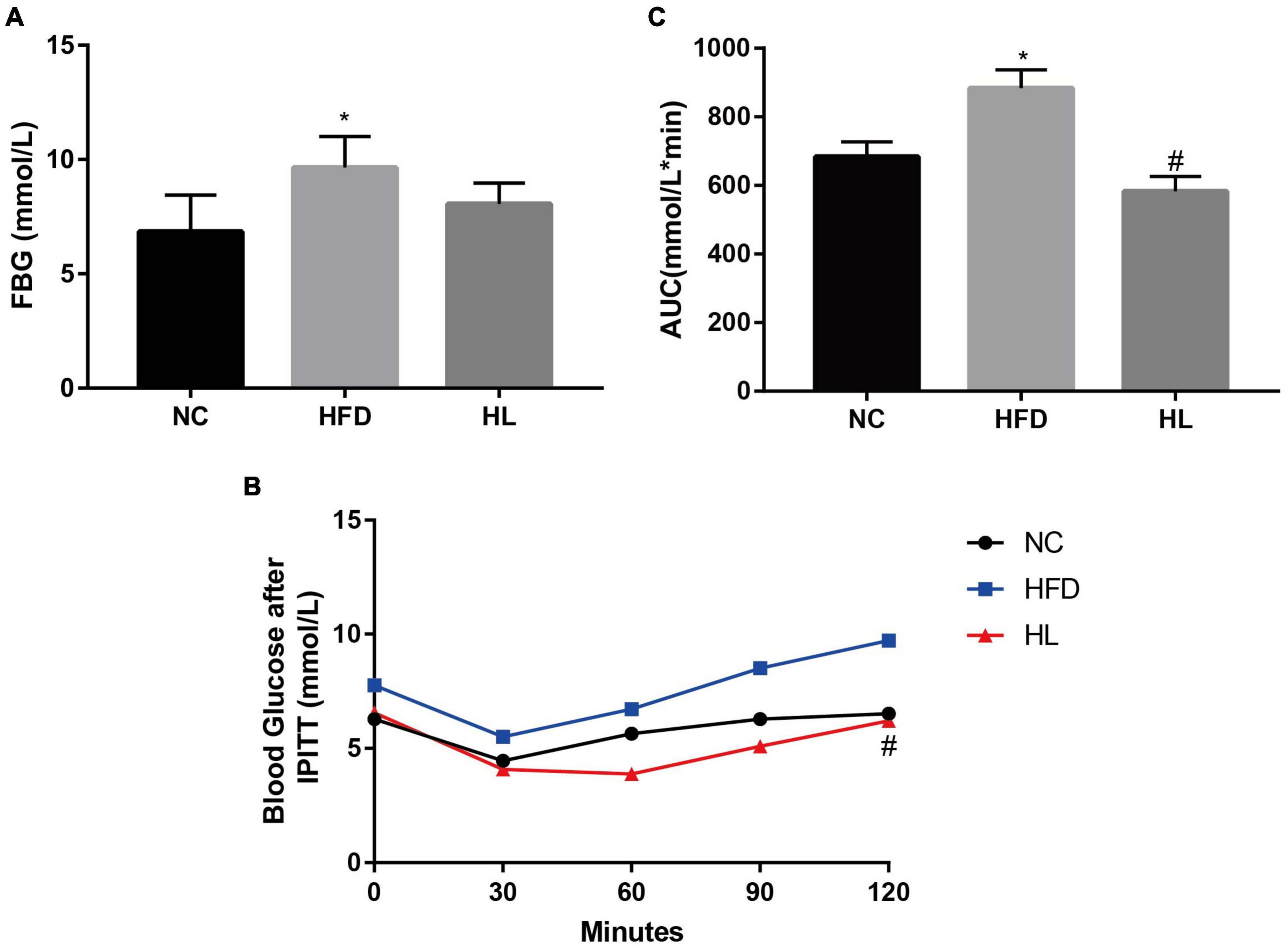
Figure 2. Liraglutide reduced FBG and improved insulin sensitivity in HFD-fed mice. (A) Fasting blood glucose (FBG) levels. (B) Blood glucose after intraperitoneal insulin tolerance test (IPITT). (C) The area under the curve (AUC) of IPITT. Data are presented as the mean ± SEM. *p < 0.05 vs. NC group; #p < 0.05 vs. HFD group.
Liraglutide altered the structure of gut microbiota in high-fat diet-fed mice
The PCoA and the PCA plots levels suggested greatly different structural patterns between the three groups (Figures 3A,B). It was noteworthy that the dots of HL group were close to the NC group, revealing that after liraglutide invention, the diversity of the gut microbiota was similar with that of the NC group. This means that liraglutide treatment could partially reversed the changes in the gut microbiota caused by HFD in mice.
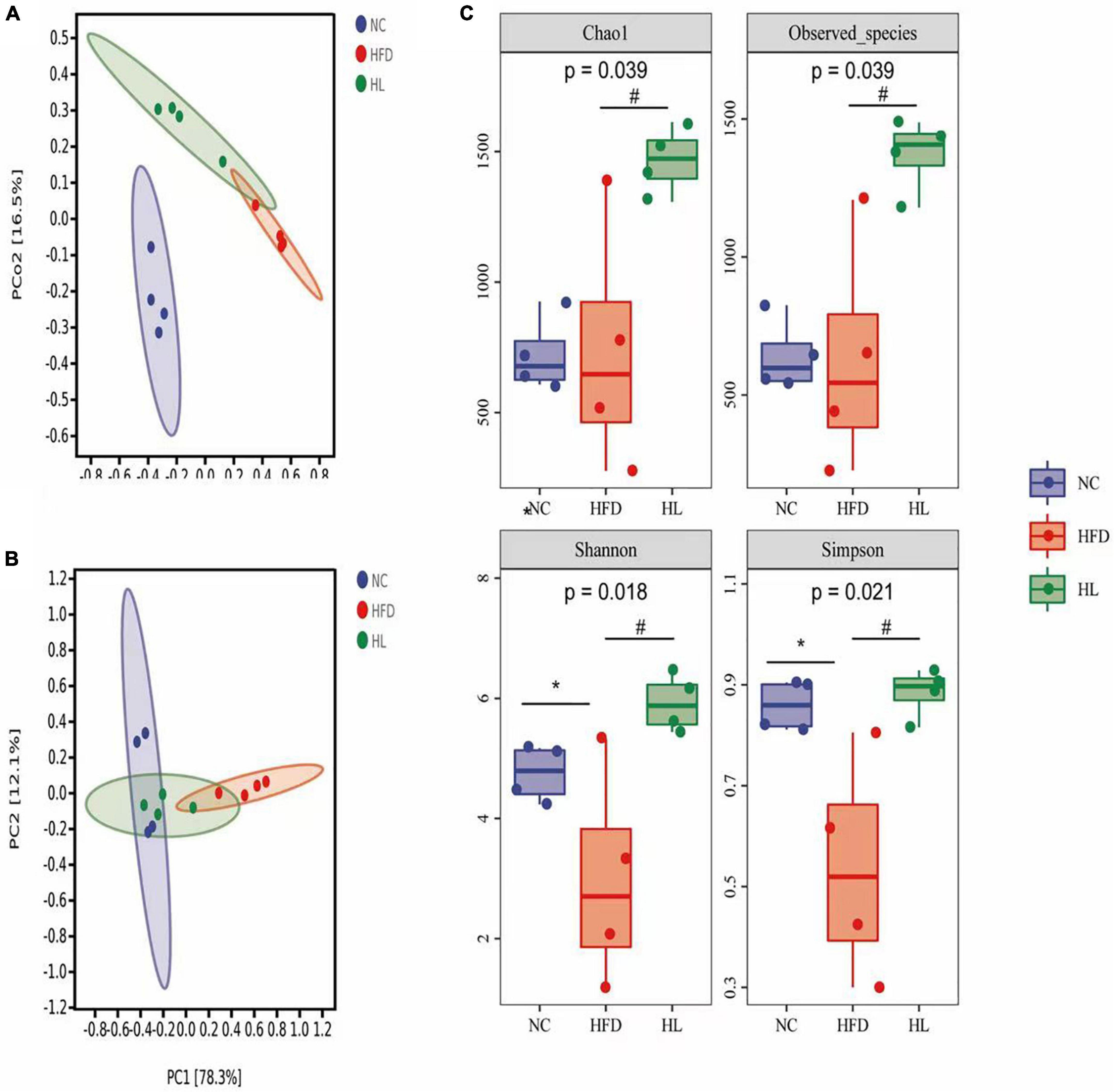
Figure 3. Liraglutide altered the overall structure of gut microbiota in HFD-fed mice. (A) Principal coordinates analysis (PCoA). (B) Principal components analysis (PCA). (C) Alpha-diversity metrics (Chao1, Observed_species, Shannon, and Simpson). Data are presented as the mean ± SEM. *p < 0.05 vs. NC group; #p < 0.05 vs. HFD group.
As illustrated in Figure 3C, Chao1 and Observed species in the HL group were significantly higher than those of the HFD group (p < 0.05). Compared with the NC group, Shannon and Simpson indices in the HFD group were also dropped, while the values of the HL group were significantly increased (p < 0.05). This indicated that liraglutide treatment could contribute to an increase in both diversity and richness of the gut microbiota.
Liraglutide improved the microbial composition in high-fat diet-fed mice
At the phylum level (Figure 4A), high-fat feeding obviously augmented the relative abundance of Firmicutes and reduced the relative abundance of Bacteroidetes, thus the ratio of Firmicutes to Bacteroidetes (F/B) increased accordingly. Inversely, liraglutide administration significantly reversed the relative abundance of Firmicutes and Bacteroidetes, with a concomitant drop in the F/B ratio. At the genus level (Figure 4B), the abundance of Akkermansia, Lactobacillus, Parabacteroides, Oscillospira, Sutterella, and Allobaculum were suppressed by HFD, while their growth was promoted by liraglutide. Especially, Akkermansia was significantly enriched in the HL group. Further, at the species level, Akkermansia muciniphila had the most variable proportion of the whole species, which was 25.13% in the NC group, 1.90% in the HFD group, and 33.18% in the HL group. This result also showed that liraglutide greatly augmented the abundance of A. muciniphila (Figure 4C).
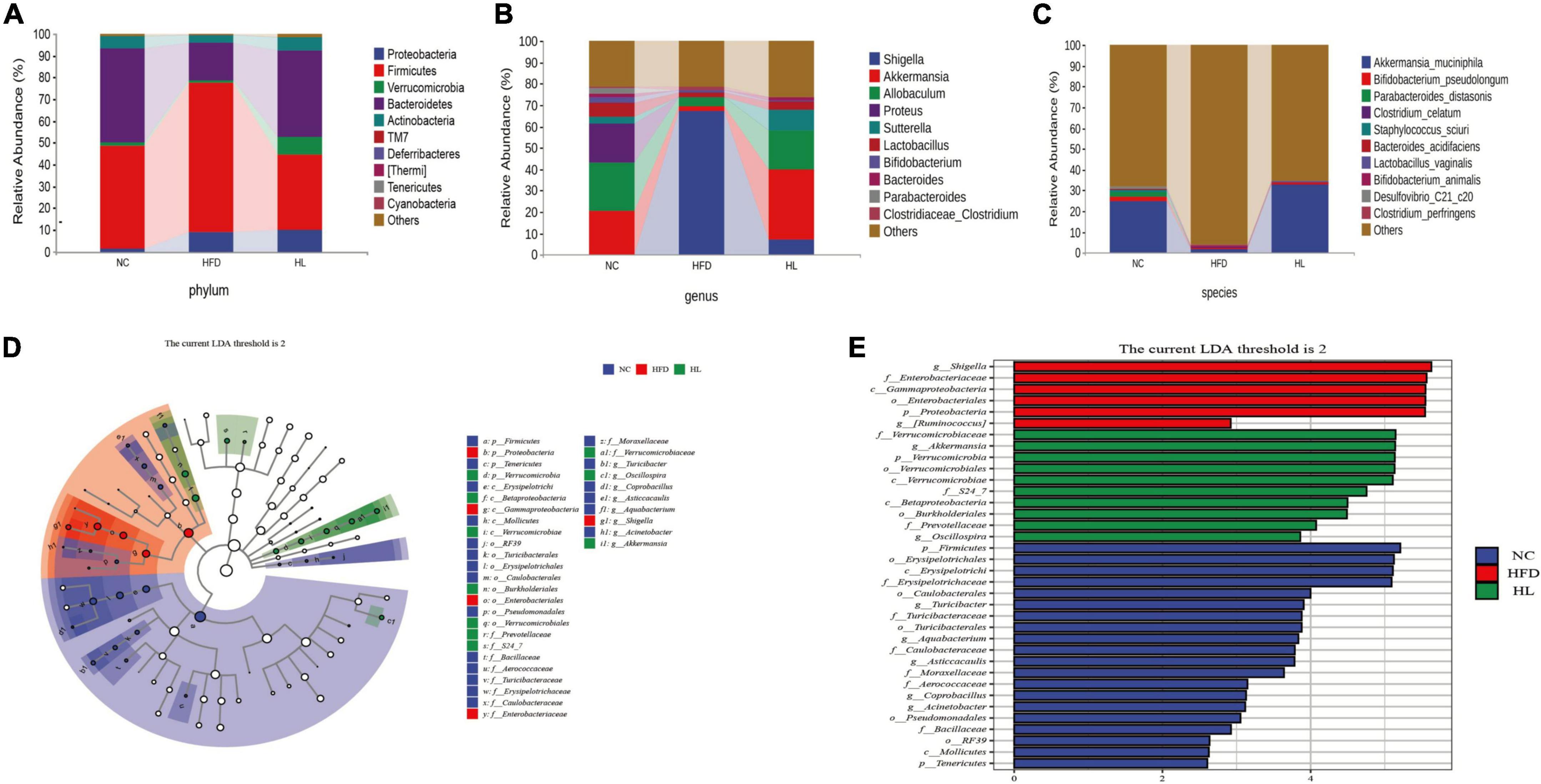
Figure 4. Liraglutide changed the composition of gut microbiota in HFD-fed mice. (A) Composition of gut microbiota at the phylum level. (B) Composition of gut microbiota at the genus level. (C) Composition of gut microbiota at the species level. (D) Linear discriminant analysis effect size (LEfSe) cladogram. (E) Linear discriminant analysis effect size (LEfSe) histogram.
Additionally, the LEfSe method was applied to explore the biomarkers of dyslipidemia in gut microbiota (Figures 4D,E). In total, there were 20, 6, and 10 obviously different OTUs in NC, HFD, and HL group, respectively (Figure 4E). Cladogram analysis also supported the different levels of labeled taxa obtained from the LEfSe in the experimental groups (Figure 4D). After liraglutide treatment, the HL group was enriched in Verrucomicrobia at the phylum level, Verrucomicrobiae and Betaproteobacteria at the class level, Verrucomicrobiales and Burkholderiales at order level, Verrucomicrobiaceae and Prevotellaceae at the family level, and Akkermansia at the genus level. It is worth noting that Akkermansia belongs to the family Verrucomicrobiaceae, and further to the order Verrucomicrobiales, class Verrucomicrobiae, and phylum Verrucomicrobia.
Relationship between gut microbiota composition and metabolic parameters
In order to comprehensively analyze the correlation between metabolic parameters and the composition of intestinal microflora, Spearman’s correlation analysis was performed. As shown in Figure 5, AF12, Shigella, and Xenorhabdus were positively correlated with weight and lipid profile, while Bifidobacterium, Adlercreutzia, Allobaculum, and Aquabacterium were found to be negatively related to weight and lipid profile (p < 0.05). Besides, Akkermansia was negatively associated with TC and LDL (p < 0.05). Interestingly, these negatively related strains were enriched in the intestine of mice in HL group, but deficient in HFD group. Thus, it could be supposed that these related microbiota stains might be essential factors for the beneficial effect of liraglutide on dyslipidemia.
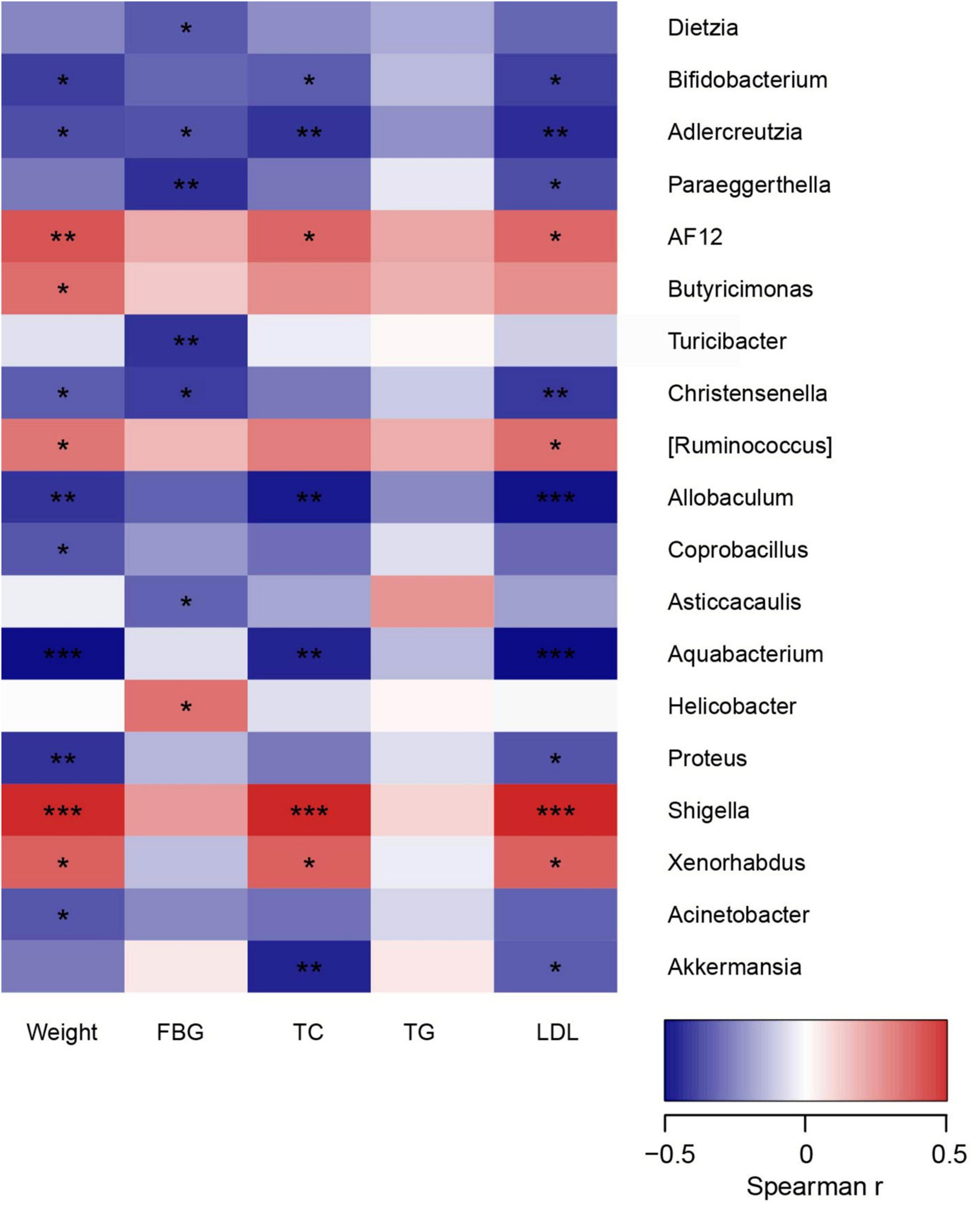
Figure 5. The relationship between microbiota composition and metabolic parameters. *p < 0.05, **p < 0.01, and ***p < 0.001.
Discussion
The pathophysiology of dyslipidemia is very complex, which has been only partially elucidated. In this study, liraglutide treatment could effectively attenuate body weight and lipid profile levels in mice with HFD-induced dyslipidemia. Meanwhile, liraglutide mainly exerted the hypolipidemic effect by modulating the composition of gut microbiota, especially by increasing the abundance of Akkermansia.
There are tens of thousands of microorganisms colonizing the human intestinal tract, which can help to break down the indigestible food. Previous studies have revealed that the gut microbiota accounts for 30% of the energy absorption of the host (31, 32). Besides, it carries out varieties of metabolic activities in the body of the host and produces a range of metabolites which further affect human’s health (33). The relationship between the gut microbiota and metabolic diseases, especially obesity, is one of the main foci of research in recent years. Emerging evidence has clarified that gut microbiota can regulate the lipid metabolism, which is closely associated with dyslipidemia (17, 19, 34).
In recent decades, the gut microbiota, dominated by Bacteroidetes and Firmicutes, has been reported to have a critical impact on the development of obesity (19, 34). Firmicutes/Bacteroidetes (F/B) ratio is also commonly used to reflect the changes in microbial structure at the phylum level and is used as a marker to measure overweight and obesity (35). The HFD can significantly decrease the relative abundance of Bacteroides and increase the abundance of Firmicutes (34), resulting in gut microbiota dysbiosis. The dysbiosis, in turn, further disrupts the normal lipid metabolism and then causes dyslipidemia, forming a vicious cycle. Many researches demonstrate that a higher F/B ratio has a positive correlation with obesity in obese mice and patients (34) (36). When obese subjects reduced their intake of HFD and lost weight, the proportion of Bacteroidetes increased miraculously (37). Similar results were also confirmed in ob/ob mice and HFD-induced obese (DIO) mice (38, 39). Our study revealed that the abundance of Firmicutes increased and Bacteroides decreased at the phylum level in HFD group, which was similar with the above results. After liraglutide intervention, the proportion of Firmicutes was dramatically reduced, while the relative abundance of Bacteroides was greatly augmented. The ratio of F/B was then accordingly decreased after liraglutide treatment.
To further evaluate precise changes in the gut microbiota, we analyzed the microbiota differences at other taxonomic levels. Compared with the NC group, HFD reduced the abundance of Akkermansia, Lactobacillus, Parabacteroides, Oscillospira, Sutterella, and Allobaculum, etc. After 12 weeks of liraglutide administration, the relative abundance of these genera was dramatically increased, especially the proportion of Akkermansia having the greatest change. Many studies have shown that the above strains are negatively related to obesity, and they are also referred to as thin bacteria (40, 41). For example, the well-known Lactobacillus genus is typically one of the top three probiotics, and its abundance is inversely related to the growth of body weight and fat mass (42). Kim et al. found that long-term oral administration of Lactobacillus gasseri BNR17 contributed to the reduction of visceral fat in obese adults (43); Jang et al. elucidated that oral administration of a variety of Lactobacillus inhibited HFD-induced NF-κB activation and increased the activation of AMP-activated protein kinase and SIRT-1 expression in liver, so as to reduce obesity and relieve inflammatory symptoms (44). Oscillospira is another beneficial bacterium, which is strongly correlated with leanness and obesity, as well as human health (34). The abundance of Oscillospira was discovered to be decreased significantly in patients with non-alcoholic steatohepatitis, which had a negative correlation with BMI and inflammatory indicators (45). In addition, numerous evidences demonstrate that Oscillospira plays a critical role in many metabolic activities that are associated with dyslipidemia and obesity (34) (46). Paracobacteria has far-ranging cholic acid conversion functions, hydrolyzing kinds of bound bile acids and converting them to secondary bile acids (such as ursodeoxycholic acid and lithocholic acid). These secondary bile acids play a role in improving lipid metabolism disorders via activating intestinal FXR signaling pathway (47).
As mentioned above, the proportion of Akkermansia in the HL group enriched the most. When analyzing the difference between species at different taxonomic levels, we found that Verrucomicrobia, Verrucomicrobiaes, Verrucomicrobiaceae, and Akkermansia in the feces of HL group mice had relatively high LDA values, suggesting that these taxa might be used as the marker species. In the further analysis of the level of microflora, the result showed that A. muciniphila had the largest change in the proportion of the whole species, which was nearly 18 times of HFD group. What is more, A. muciniphila belongs to the genus Akkermansia, which further belongs to family Verrucomicrobiaceae, order Verrucomicrobiales, class Verrucomicrobiae, and phylum Verrucomicrobia. Therefore, A. muciniphila might play a key role in the effects of weight loss and lipid-lowering mediated by liraglutide. Akkermansia, a strictly anaerobic Gram-negative enteric bacterium first isolated and proposed by Derrien et al. in 2004 from human feces, is non-motile and usually found in 1–4% abundance in the gut (48). Akkermansia has the specific function of degrading intestinal epithelial mucins and can use mucins as sole carbon and nitrogen sources for growth, with the main metabolites being oligosaccharides and short chain fatty acids (49). Although Akkermansia is associated with metabolic diseases and beneficial effects have been reported in host metabolism, its molecular mechanism has not been identified. Some researchers have found that Akkermansia can reduce the expression of genes involved in fatty acid synthesis and transport in both liver and muscle, alleviate endoplasmic reticulum stress, and thus improve lipid accumulation and metabolic disorders (50). Akkermansia was also found to increase thermogenesis and GLP-1 secretion in HFD- fed C57BL/6 mice by inducing uncoupling protein 1 in brown adipose tissue and GLP-1 secretion throughout the body (51). In this study, Akkermansia was reported to be negatively correlated with TC and LDL. Thus, we speculated that Akkermansia might be a key specie to promote the reduction of lipid levels, and liraglutide might reduce hyperlipidemia in HFD-fed mice by enriching the abundance of Akkermansia.
In the current study, liraglutide not only promoted the growth of beneficial bacteria, but also greatly inhibited the growth of harmful bacteria such as AF12, Shigella, Proteobacteria, etc. Spearman correlation analysis showed that these genera were also positively correlated with body weight and lipid profile. AF12 is a poorly investigated taxon, which was reported to be enriched in obese mice (52). Shigella is a kind of Gram-negative pumilobacter, which is the typical intestinal pathogen of human bacterial dysentery. All strains of Shigella have strong endotoxin, like lipopolysaccharide (LPS), which can combine with toll-like receptor 4 to activate a variety of cell signaling pathways, induce chronic subclinical inflammation, and make the intestinal wall permeability increased, thus promoting the absorption of toxins. Then, those toxins act on the central nervous system and cardiovascular system, causing a series of clinical toxemia symptoms, such as fever, mental disturbance, and even toxic shock (53). Data from different sources indicate that the increase of circulating LPS level helps to explain why the relative abundance of Shigella is significantly increased in both obese rodents and humans (54, 55). Additionally, as a potential diagnostic biomarker of gut microbiota dysbiosis, the increased abundance of Proteobacteria was reported to be correlated with metabolic disorders (52, 56). The abundance of these harmful bacteria was significantly decreased by liraglutide, indicating its potential effect on preventing dyslipidemia and the related metabolic endotoxemia.
Another interesting finding in this study was that liraglutide increased the abundance of Sutterella, which was negatively associated with inflammation (57). Contrarily, the prevalence of Proteobacteria was markedly attenuated by liraglutide, as the increased relative abundance of Proteobacteria was found to be positively related to inflammation (56). Accumulating evidence suggests that inflammation is a critical and reversible mechanism by which obesity promotes the progression of the inflammatory diseases such as T2DM, NAFLD, dyslipidemia, and various types of cancer (58, 59). Inflammation induced by HFD is a contributing factor to metabolic disorders, and local hyperlipidemia is also speculated to be strongly associated with adipocyte death, altered adipose tissue function, and chronic low-grade inflammation (60). However, to clarify whether liraglutide breaks the link between inflammation and dyslipidemia through regulating related gut microbiota, we need to do further experiments.
The innovation of this study is that we put forward that the growth of Akkermansia might be closely related to liraglutide mediating weight loss and lipid reduction. However, whether transplantation of the gut microbiota of mice after liraglutide intervention into HFD-fed mice could also display similar lipid-lowing function needs to be further explored. Another limitation is that this study only conducted a single dosage of liraglutide and did not explore the suitable dose range and potential therapeutic relevance, which is incomplete. Thus, we need further studies to do so verify this phenomenon.
Conclusion
In summary, liraglutide could prevent HFD-induced dyslipidemia, and mainly exerted the hypolipidemic effect by modifying the structure and composition of gut microbiota in HFD-fed mice. As the relative abundance of Akkermansia increased the most after liraglutide treatment, we speculated that Akkermansia might play a key role in liraglutide’s lipid-lowering effect. To confirm this hypothesis, further investigations are needed.
Data availability statement
The original contributions presented in the study are publicly available. This data can be found here: https://figshare.com/s/ebdc21b93632da6a0387.
Ethics statement
This animal study was reviewed and approved by the Biomedical Research Ethics Committee of Affiliated Hospital of Jiangsu University, Zhenjiang, China.
Author contributions
LZ and GY conceived and designed the study. LZ and YQ performed the analysis, prepared the figures, and drafted the manuscript. YQ, PZ, ZZ, XD, and XW performed the experiment and collected the data. LY, DW, and GY conceived the study and participated in its design and coordination. All authors discussed the results and approved the final manuscript.
Funding
This study was supported in part by grants from the National Natural Science Foundation of China (82000809, 81870548, and 81570721), the Social Development Project of Jiangsu Province (BE2018692), the Natural Science Foundation of Jiangsu Province, China (BK20191222), and the Social Development Project of Zhenjiang City (SH2019041), Jiangsu Province.
Conflict of interest
The authors declare that the research was conducted in the absence of any commercial or financial relationships that could be construed as a potential conflict of interest.
Publisher’s note
All claims expressed in this article are solely those of the authors and do not necessarily represent those of their affiliated organizations, or those of the publisher, the editors and the reviewers. Any product that may be evaluated in this article, or claim that may be made by its manufacturer, is not guaranteed or endorsed by the publisher.
Abbreviations
GLP-1RA, glucagon-like peptide-1 receptor agonists; HFD, high-fat diet; FBG, fasting blood glucose; TG, triglycerides; TC, total cholesterol; LDL, low-density lipoprotein; T2DM, type 2 diabetes mellitus; AUC, area under the curve; IPITT, intraperitoneal insulin tolerance test.
References
1. Opoku S, Gan Y, Fu W, Chen D, Addo-Yobo E, Trofimovitch D, et al. Prevalence and risk factors for dyslipidemia among adults in rural and urban China: findings from the China National Stroke Screening and prevention project (CNSSPP). BMC Public Health. (2019) 19:1500. doi: 10.1186/s12889-019-7827-5
2. Karr S. Epidemiology and management of hyperlipidemia. Am J Manag Care. (2017) 23(9 Suppl.):S139–48.
3. Jia X, Xu W, Zhang L, Li X, Wang R, Wu S. Impact of gut microbiota and microbiota-related metabolites on hyperlipidemia. Front Cell Infect Microbiol. (2021) 11:634780. doi: 10.3389/fcimb.2021.634780
4. Ferhatbegovic L, Mrsic D, Kusljugic S, Pojskic B. LDL-C: The Only Causal Risk Factor for ASCVD. Why Is It Still Overlooked and Underestimated? Curr Atheroscler Rep. (2022) 24:635–42. doi: 10.1007/s11883-022-01037-3
5. Tong X, Xu J, Lian F, Yu X, Zhao Y, Xu L, et al. Structural alteration of gut microbiota during the amelioration of human type 2 diabetes with hyperlipidemia by metformin and a traditional chinese herbal formula: a multicenter, randomized, open label clinical trial. mBio. (2018) 9:e2392-17. doi: 10.1128/mBio.02392-17
6. Sadighara M, Amirsheardost Z, Minaiyan M, Hajhashemi V, Naserzadeh P, Salimi A, et al. Toxicity of atorvastatin on pancreas mitochondria: a justification for increased risk of diabetes mellitus. Basic Clin Pharmacol Toxicol. (2017) 120:131–7. doi: 10.1111/bcpt.12656
7. Wilkinson MJ, Laffin LJ, Davidson MH. Overcoming toxicity and side-effects of lipid-lowering therapies. Best Pract Res Clin Endocrinol Metab. (2014) 28:439–52. doi: 10.1016/j.beem.2014.01.006
8. Rauf A, Akram M, Anwar H, Daniyal M, Munir N, Bawazeer S, et al. Therapeutic potential of herbal medicine for the management of hyperlipidemia: latest updates. Environ Sci Pollut Res Int. (2022) 29:40281–301. doi: 10.1007/s11356-022-19733-7
9. Drucker DJ, Habener JF, Holst JJ. Discovery, characterization, and clinical development of the glucagon-like peptides. J Clin Invest. (2017) 127:4217–27. doi: 10.1172/JCI97233
10. Marso SP, Daniels GH, Brown-Frandsen K, Kristensen P, Mann JF, Nauck MA, et al. Liraglutide and Cardiovascular Outcomes in Type 2 Diabetes. N Engl J Med. (2016) 375:311–22. doi: 10.1056/NEJMoa1603827
11. Alruwaili H, Dehestani B, le Roux CW. Clinical Impact of Liraglutide as a Treatment of Obesity. Clin Pharmacol. (2021) 13:53–60. doi: 10.2147/CPAA.S276085
12. Wu YR, Shi XY, Ma CY, Zhang Y, Xu RX, Li JJ. Liraglutide improves lipid metabolism by enhancing cholesterol efflux associated with ABCA1 and ERK1/2 pathway. Cardiovasc Diabetol. (2019) 18:146. doi: 10.1186/s12933-019-0954-6
13. Iorga RA, Bacalbasa N, Carsote M, Bratu OG, Stanescu AMA, Bungau S, et al. Metabolic and cardiovascular benefits of GLP-1 agonists, besides the hypoglycemic effect (Review). Exp Ther Med. (2020) 20:2396–400. doi: 10.3892/etm.2020.8714
14. Ahmed HM, Khraishah H, Cho L. Cardioprotective anti-hyperglycaemic medications: a review of clinical trials. Eur Heart J. (2018) 39:2368–75. doi: 10.1093/eurheartj/ehx668
15. Astrup A, Rossner S, Van Gaal L, Rissanen A, Niskanen L, Al Hakim M, et al. Effects of liraglutide in the treatment of obesity: a randomised, double-blind, placebo-controlled study. Lancet. (2009) 374:1606–16. doi: 10.1016/S0140-6736(09)61375-1
16. Chen X, D’Souza R, Hong ST. The role of gut microbiota in the gut-brain axis: current challenges and perspectives. Protein Cell. (2013) 4:403–14. doi: 10.1007/s13238-013-3017-x
17. Deng X, Ma J, Song M, Jin Y, Ji C, Ge W, et al. Effects of products designed to modulate the gut microbiota on hyperlipidaemia. Eur J Nutr. (2019) 58:2713–29. doi: 10.1007/s00394-018-1821-z
18. Chan YK, Brar MS, Kirjavainen PV, Chen Y, Peng J, Li D, et al. High fat diet induced atherosclerosis is accompanied with low colonic bacterial diversity and altered abundances that correlates with plaque size, plasma A-FABP and cholesterol: a pilot study of high fat diet and its intervention with Lactobacillus rhamnosus GG (LGG) or telmisartan in ApoE(-/-) mice. BMC Microbiol. (2016) 16:264. doi: 10.1186/s12866-016-0883-4
19. Lu Y, Wan H, Wu Y, Yang J, Yu L, He Y, et al. Naoxintong capsule alternates gut microbiota and prevents hyperlipidemia in high-fat-diet fed rats. Front Pharmacol. (2022) 13:843409. doi: 10.3389/fphar.2022.843409
20. Hwang I, Park YJ, Kim YR, Kim YN, Ka S, Lee HY, et al. Alteration of gut microbiota by vancomycin and bacitracin improves insulin resistance via glucagon-like peptide 1 in diet-induced obesity. FASEB J. (2015) 29:2397–411. doi: 10.1096/fj.14-265983
21. Zhang Q, Xiao X, Zheng J, Li M, Yu M, Ping F, et al. Featured article: Structure moderation of gut microbiota in liraglutide-treated diabetic male rats. Exp Biol Med. (2018) 243:34–44. doi: 10.1177/1535370217743765
22. Zhao L, Chen Y, Xia F, Abudukerimu B, Zhang W, Guo Y, et al. A Glucagon-Like Peptide-1 receptor agonist lowers weight by modulating the structure of gut microbiota. Front Endocrinol. (2018) 9:233. doi: 10.3389/fendo.2018.00233
23. Charpentier J, Briand F, Lelouvier B, Servant F, Azalbert V, Puel A, et al. Liraglutide targets the gut microbiota and the intestinal immune system to regulate insulin secretion. Acta Diabetol. (2021) 58:881–97. doi: 10.1007/s00592-020-01657-8
24. Claus SP. Will gut microbiota help design the next generation of GLP-1-Based Therapies for Type 2 Diabetes? Cell Metab. (2017) 26:6–7. doi: 10.1016/j.cmet.2017.06.009
25. Bolyen E, Rideout JR, Dillon MR, Bokulich NA, Abnet CC, Al-Ghalith GA, et al. Reproducible, interactive, scalable and extensible microbiome data science using QIIME 2. Nat Biotechnol. (2019) 37:852–7. doi: 10.1038/s41587-019-0209-9
26. Callahan BJ, McMurdie PJ, Rosen MJ, Han AW, Johnson AJ, Holmes SP. DADA2: High-resolution sample inference from Illumina amplicon data. Nat Methods. (2016) 13:581–3. doi: 10.1038/nmeth.3869
27. Katoh K, Misawa K, Kuma K, Miyata T. MAFFT: a novel method for rapid multiple sequence alignment based on fast Fourier transform. Nucleic Acids Res. (2002) 30:3059–66. doi: 10.1093/nar/gkf436
28. Price MN, Dehal PS, Arkin AP. FastTree 2–approximately maximum-likelihood trees for large alignments. PLoS One. (2010) 5:e9490. doi: 10.1371/journal.pone.0009490
29. Lozupone CA, Hamady M, Kelley ST, Knight R. Quantitative and qualitative beta diversity measures lead to different insights into factors that structure microbial communities. Appl Environ Microbiol. (2007) 73:1576–85. doi: 10.1128/AEM.01996-06
30. Lozupone C, Knight R. UniFrac: a new phylogenetic method for comparing microbial communities. Appl Environ Microbiol. (2005) 71:8228–35. doi: 10.1128/AEM.71.12.8228-8235.2005
31. Cani PD, Van Hul M, Lefort C, Depommier C, Rastelli M, Everard A. Microbial regulation of organismal energy homeostasis. Nat Metab. (2019) 1:34–46. doi: 10.1038/s42255-018-0017-4
32. Jang HR, Park HJ, Kang D, Chung H, Nam MH, Lee Y, et al. A protective mechanism of probiotic Lactobacillus against hepatic steatosis via reducing host intestinal fatty acid absorption. Exp Mol Med. (2019) 51:1–14. doi: 10.1038/s12276-019-0293-4
33. Fan Y, Pedersen O. Gut microbiota in human metabolic health and disease. Nat Rev Microbiol. (2021) 19:55–71. doi: 10.1038/s41579-020-0433-9
34. Ji J, Zhang S, Yuan M, Zhang M, Tang L, Wang P, et al. Fermented rosa roxburghii tratt juice alleviates high-fat diet-induced hyperlipidemia in rats by modulating gut microbiota and metabolites. Front Pharmacol. (2022) 13:883629. doi: 10.3389/fphar.2022.883629
35. Sweeney TE, Morton JM. The human gut microbiome: a review of the effect of obesity and surgically induced weight loss. JAMA Surg. (2013) 148:563–9. doi: 10.1001/jamasurg.2013.5
36. Xiao L, Sonne SB, Feng Q, Chen N, Xia Z, Li X, et al. High-fat feeding rather than obesity drives taxonomical and functional changes in the gut microbiota in mice. Microbiome. (2017) 5:43. doi: 10.1186/s40168-017-0258-6
37. Sommer F, Backhed F. The gut microbiota–masters of host development and physiology. Nat Rev Microbiol. (2013) 11:227–38. doi: 10.1038/nrmicro2974
38. Caricilli AM, Picardi PK, de Abreu LL, Ueno M, Prada PO, Ropelle ER, et al. Gut microbiota is a key modulator of insulin resistance in TLR 2 knockout mice. PLoS Biol. (2011) 9:e1001212. doi: 10.1371/journal.pbio.1001212
39. Mujico JR, Baccan GC, Gheorghe A, Diaz LE, Marcos A. Changes in gut microbiota due to supplemented fatty acids in diet-induced obese mice. Br J Nutr. (2013) 110:711–20. doi: 10.1017/S0007114512005612
40. Schneeberger M, Everard A, Gomez-Valades AG, Matamoros S, Ramirez S, Delzenne NM, et al. Akkermansia muciniphila inversely correlates with the onset of inflammation, altered adipose tissue metabolism and metabolic disorders during obesity in mice. Sci Rep. (2015) 5:16643. doi: 10.1038/srep16643
41. Wang K, Liao M, Zhou N, Bao L, Ma K, Zheng Z, et al. Parabacteroides distasonis Alleviates Obesity and Metabolic Dysfunctions via Production of Succinate and Secondary Bile Acids. Cell Rep. (2019) 26:222–35e5. doi: 10.1016/j.celrep.2018.12.028
42. Lecomte V, Kaakoush NO, Maloney CA, Raipuria M, Huinao KD, Mitchell HM, et al. Changes in gut microbiota in rats fed a high fat diet correlate with obesity-associated metabolic parameters. PLoS One. (2015) 10:e0126931. doi: 10.1371/journal.pone.0126931
43. Kim J, Yun JM, Kim MK, Kwon O, Cho B. Lactobacillus gasseri BNR17 Supplementation Reduces the Visceral Fat Accumulation and Waist Circumference in Obese Adults: A Randomized Double-Blind, Placebo-Controlled Trial. J Med Food. (2018) 21:454–61. doi: 10.1089/jmf.2017.3937
44. Jang HM, Han SK, Kim JK, Oh SJ, Jang HB, Kim DH. Lactobacillus sakei Alleviates High-Fat-Diet-Induced Obesity and Anxiety in Mice by Inducing AMPK Activation and SIRT1 Expression and Inhibiting Gut Microbiota-Mediated NF-kappaB Activation. Mol Nutr Food Res. (2019) 63:e1800978. doi: 10.1002/mnfr.201800978
45. Petersen C, Bell R, Klag KA, Lee SH, Soto R, Ghazaryan A, et al. T cell-mediated regulation of the microbiota protects against obesity. Science. (2019) 365:eaat9351. doi: 10.1126/science.aat9351
46. Yang J, Li Y, Wen Z, Liu W, Meng L, Huang H. Oscillospira - a candidate for the next-generation probiotics. Gut Microbes. (2021) 13:1987783. doi: 10.1080/19490976.2021.1987783
47. de Boer JF, Bloks VW, Verkade E, Heiner-Fokkema MR, Kuipers F. New insights in the multiple roles of bile acids and their signaling pathways in metabolic control. Curr Opin Lipidol. (2018) 29:194–202. doi: 10.1097/MOL.0000000000000508
48. Derrien M, Collado MC, Ben-Amor K, Salminen S, de Vos WM. The Mucin degrader Akkermansia muciniphila is an abundant resident of the human intestinal tract. Appl Environ Microbiol. (2008) 74:1646–8. doi: 10.1128/AEM.01226-07
49. Belzer C, de Vos WM. Microbes inside–from diversity to function: the case of Akkermansia. ISME J. (2012) 6:1449–58. doi: 10.1038/ismej.2012.6
50. Zhang L, Qin Q, Liu M, Zhang X, He F, Wang G. Akkermansia muciniphila can reduce the damage of gluco/lipotoxicity, oxidative stress and inflammation, and normalize intestine microbiota in streptozotocin-induced diabetic rats. Pathog Dis. (2018) 76:fty028. doi: 10.1093/femspd/fty028
51. Yoon HS, Cho CH, Yun MS, Jang SJ, You HJ, Kim JH, et al. Akkermansia muciniphila secretes a glucagon-like peptide-1-inducing protein that improves glucose homeostasis and ameliorates metabolic disease in mice. Nat Microbiol.. (2021) 6:563–73. doi: 10.1038/s41564-021-00880-5
52. Li J, Pang B, Shao D, Jiang C, Hu X, Shi J. Artemisia sphaerocephala Krasch polysaccharide mediates lipid metabolism and metabolic endotoxaemia in associated with the modulation of gut microbiota in diet-induced obese mice. Int J Biol Macromol. (2020) 147:1008–17. doi: 10.1016/j.ijbiomac.2019.10.069
53. Medzhitov R, Horng T. Transcriptional control of the inflammatory response. Nat Rev Immunol. (2009) 9:692–703. doi: 10.1038/nri2634
54. Oliveira AG, Carvalho BM, Tobar N, Ropelle ER, Pauli JR, Bagarolli RA, et al. Physical exercise reduces circulating lipopolysaccharide and TLR4 activation and improves insulin signaling in tissues of DIO rats. Diabetes. (2011) 60:784–96. doi: 10.2337/db09-1907
55. Saad MJ, Santos A, Prada PO. Linking gut microbiota and inflammation to obesity and insulin resistance. Physiology. (2016) 31:283–93. doi: 10.1152/physiol.00041.2015
56. Shin NR, Whon TW, Bae JW. Proteobacteria: microbial signature of dysbiosis in gut microbiota. Trends Biotechnol. (2015) 33:496–503. doi: 10.1016/j.tibtech.2015.06.011
57. Wang C, Zhang H, Liu H, Zhang H, Bao Y, Di J, et al. The genus Sutterella is a potential contributor to glucose metabolism improvement after Roux-en-Y gastric bypass surgery in T2D. Diabetes Res Clin Pract. (2020) 162:108116. doi: 10.1016/j.diabres.2020.108116
58. Deng T, Lyon CJ, Bergin S, Caligiuri MA, Hsueh WA. Obesity, Inflammation, and Cancer. Annu Rev Pathol. (2016) 11:421–49. doi: 10.1146/annurev-pathol-012615-044359
59. Karczewski J, Sledzinska E, Baturo A, Jonczyk I, Maleszko A, Samborski P, et al. Obesity and inflammation. Eur Cytokine Netw. (2018) 29:83–94. doi: 10.1684/ecn.2018.0415
Keywords: Akkermansia, dyslipidemia, glucagon-like peptide-1 receptor agonist, liraglutide, gut microbiota
Citation: Zhao L, Qiu Y, Zhang P, Wu X, Zhao Z, Deng X, Yang L, Wang D and Yuan G (2022) Gut microbiota mediates positive effects of liraglutide on dyslipidemia in mice fed a high-fat diet. Front. Nutr. 9:1048693. doi: 10.3389/fnut.2022.1048693
Received: 12 October 2022; Accepted: 14 November 2022;
Published: 29 December 2022.
Edited by:
Lei Zhou, Guangxi University, ChinaReviewed by:
Kun Li, Nanjing Agricultural University, ChinaFei Liu, Nanjing Agricultural University, China
Copyright © 2022 Zhao, Qiu, Zhang, Wu, Zhao, Deng, Yang, Wang and Yuan. This is an open-access article distributed under the terms of the Creative Commons Attribution License (CC BY). The use, distribution or reproduction in other forums is permitted, provided the original author(s) and the copyright owner(s) are credited and that the original publication in this journal is cited, in accordance with accepted academic practice. No use, distribution or reproduction is permitted which does not comply with these terms.
*Correspondence: Li Zhao, emhhb2xpODZAdWpzLmVkdS5jbg==; Guoyue Yuan, eXVhbmd1b3l1ZUB1anMuZWR1LmNu
†These authors have contributed equally to this work and share first authorship