- 1Anhui Province Key Laboratory of Pollutant Sensitive Materials and Environmental Remediation, School of Life Sciences Huaibei Normal University, Huaibei, China
- 2R&D Center of Anhui Kouzi Distillery Co., Ltd, Huaibei, China
- 3Key Laboratory of Se-enriched Products Development and Quality Control, Ministry of Agriculture and Rural Affairs, Ankang, China
- 4National-Local Joint Engineering Laboratory of Se-enriched Food Development, Ankang R&D Center for Se-enriched Products, Ankang, China
The fermentation medium of a newly identified Cordyceps cicadae S1 was optimized by response surface methodology, with the optimal medium containing sucrose (80 g/L), yeast powder (60 g/L), KH2PO4 (5 g/L), MgSO4·7H2O (1 g/L) and Na2SeO3 (0. 1 g/L). Under these conditions, the extracellular polysaccharide yield was 8.09 g/L. A novel selenium-enriched polysaccharide (PACI-1) was isolated from Cordyceps cicadae, purified and identified as a homofructose polysaccharide with a low average molecular weight of 9.95 × 103 Da. The fine structure of PACI-1 was analyzed using NMR, CD, and AFM. Additionally, the in vitro antioxidant results showed that the PACI-1 had stronger antioxidant capacity than natural polysaccharides. These results provided a candidate strain for producing selenium polysaccharide and a new polysaccharide from C. cicadae, which showed good antioxidant activity.
Introduction
Cordyceps cicadae, which belongs to the Clavicipitaceous family and Paecilomyces genus, is an entomogenous fungus (1). This medicinal fungus produces numerous primary metabolites in its fermentation liquid, including polysaccharides, proteins, nucleotides, adenosine, and ergosterol (2–4). Polysaccharides, the most abundant component in C. cicadae fermentation broth, have good antioxidant activity (5). Polysaccharides have been investigated to fulfill the purpose of raising their uses for antioxidant activity with removing free radicals (6, 7), thereby reducing the risk of ischaemic and cardiovascular diseases, Alzheimer's disease, cancer, Parkinson's disease etc. (8).
Selenium is an essential trace element for humans and animals. It can only be obtained from food and cannot be synthesized independently by the body. The daily recommended intake for adults is 60 μg (9). Selenium is a cofactor of more than 30 enzymes and a key component of selenoproteins. Selenium affects the functions of several body systems, including the central nervous system, endocrine system, immune system, and cardiovascular system (10). In the body, organic selenium compounds enhance immunity, exert anti-aging effects, prevent cardiovascular and cerebrovascular diseases, and inhibit cancer cell metastasis (11, 12). Today, billions of people worldwide live in selenium-deficient areas, mainly in China, New Zealand, and Europe (13). Accordingly, there has been increasing research attention on selenium compounds. Because inorganic selenium compounds have the disadvantages of high toxicity and unstable biological activity (14), obtaining high-yield organic selenium compounds is a research hotspot.
Selenium polysaccharides are a good selenium supplement as they combine the biological activities of selenium and polysaccharides. It has been reported that fungi can use inorganic selenium sources in the culture medium to convert exopolysaccharides into selenium-enriched exopolysaccharides in the process of liquid fermentation (15). For example, Alvandi added Na2SeO3 to the liquid fermentation medium of Fomes fomentarius to produce extracellular selenium polysaccharide. The modified extracellular selenium polysaccharide showed significant antioxidant activity and good antibacterial activity (16). In the present study, the medicinal fungus C. cicadae was taken as the research subject. Sodium selenite was added to its fermentation medium and the biotransformation method was used to synthesize selenium polysaccharide with the aim of obtaining a high-yield organic selenium compound. The experiment was divided into two stages. First, the components of the selenium-containing C. cicadae medium were optimized by response surface methodology to obtain the maximum yield of selenium polysaccharide. Second, the polysaccharide in the fermentation medium was isolated and purified and its structure was determined by high-performance liquid chromatography (HPLC), atomic force microscopy (AFM), Fourier-transform infrared spectroscopy (FT-IR), circular dichroism (CD), and nuclear magnetic resonance spectroscopy (NMR). Furthermore, its biological activity was studied, and the relationship between its structure and biological activity was preliminarily analyzed.
Materials and methods
Materials
The Cordyceps cicadae S1 strain was screened and collected in the laboratory. Potato dextrose agar (PDA) was purchased from Best Biotechnology Co., Ltd. (Hangzhou, China); 1,1-diphenyl-2-pyridyl hydrazide (DPPH) and 2,2'-diazo-bis-3-ethylbenzothiazoline-6-sulphonic acid (ABTS) were purchased from Zhiji Biotechnology Co., Ltd. (Shanghai, China). The dialysis membranes, DEAE-52 cellulose anion exchange column, and Sephadex G-100 were purchased from Shanghai Qite Analytical Instrument Co., Ltd. (Shanghai, China). Other chemical reagents and solvents were of analytical grade and provided by Sinopharm Chemical Reagent Co., Ltd. (Shanghai, China).
Identification of strains and determination of optimal medium conditions
Identification of strains
The S1 strain was isolated from Cordyceps cicadae in the laboratory and inoculated into PDA medium by the plate scribing method. C. cicadae was cultured in a 28°C incubator for 5 days and the colony morphology was observed. DNA extraction, PCR amplification, fragment purification, and sequencing were conducted as previously reported (17). The obtained sequences were compared by BLAST and the phylogenetic tree was constructed using MEGA-X. The phylogenetic relationship between the isolated strains and the near-source strains registered in the GenBank database was analyzed.
Microbial cultivation
After 5 days of culture, the strain was used as the seed and inoculated into the seed culture medium (glucose 50 g/L, maltose 50 g/L, yeast powder 3 g/L, K2HPO4 3 g/L, MgSO4·7H2O 0.2 g/L and pH 5.5), then cultured at 28°C with shaking (180 rpm) for 3 days. The cultured seed was inoculated into the liquid fermentation medium (fructose 20 g/L, yeast powder 20 g/L, K2HPO4 1 g/L, MgSO4·7H2O 3 g/L, sodium selenite 0.05 g/L) at 10% (v/v). The liquid fermentation was carried out at 28°C and 180 rpm for 3 days.
Extraction and characterization of polysaccharides
The polysaccharide in the fermentation broth was extracted according to the method published by Qiao et al. (18). The carbohydrate content was determined by the phenol–sulfuric acid method with glucose as the standard. (19).
One-factor-at-a-time experiments
The fructose (carbon source) in the initial fermentation medium was successively replaced with glucose, maltose, lactose, and sucrose. The yeast powder (nitrogen source) in the initial fermentation medium was successively replaced with potassium nitrate, ammonium sulfate, beef extract, and peptone. The initial concentrations of both the carbon and nitrogen sources were 20 g/L. The carbon source and nitrogen source were tested at 20, 40, 60, 80, and 100 g/L. The concentration of MgSO4·7H2O was tested at 0, 1, 2, and 3 g/L. The concentration of K2HPO4 was tested at 0, 1, 3, 5, 7, and 9 g/L. The concentration of sodium selenite was tested at 0, 0.025, 0.050, 0.075, 0.10, and 0.125 g/L. Subsequently, the seed liquid was inoculated into the designed fermentation medium and cultured for 3 d under each set of fermentation conditions. Determination of the best condition for each tested parameters using the content of extracellular polysaccharide as an indicator.
Plackett-Burman (P-B) design
In the single-factor test, the influences of the concentration of sucrose, yeast powder, dipotassium hydrogen phosphate, potassium ions and magnesium ions were determined. Positive (+1) and negative (−1) levels of these five influencing factors were trialed, with the positive level of each factor being twice the negative level. The Plackett-Burman test was created in Minitab17 and repeated three times to determine the significance of each factor on the culture conditions (20).
Box-Behnken design
Based on the results of the P-B experiment, taking sucrose (X1), yeast powder (X2), and K2HPO4 (X3) as the independent variables and the yield of C. cicadae as response value (Y), response surface optimisation modeling was performed. The design of each factor level is shown in Table 1.
Extraction and purification of extracellular polysaccharide from C. cicadae S1
The method of Xu et al. (21) was used with modification. 1,000 mL of C. cicadae fermentation broth was concentrated to 200 mL at 55°C using a rotary evaporator (Yarong re-2000b, Shanghai, China), then precipitated with absolute ethanol (600 mL) at 4°C for 12 h and centrifuged at 4,000 rpm for 10 min to collect the precipitate. The precipitate was dissolved in 100 mL of ultrapure water and the protein was removed from the solution using Sevag reagent (1-butanol/trichloromethane, 1:4 v/v). The remaining polysaccharide solution was dialysed using a 3,500 Da dialysis bag for 48 h and then precipitated with three times the volume of absolute ethanol for 12 h. The precipitate was freeze-dried in a freeze dryer (BoYiKang FD-1A-50, Beijing, China) to obtain the crude polysaccharide (PACI).
PACI was redissolved in pure water to a final concentration of 0.1 g/mL and then purified using a DEAE-52 column (2.6 × 30 cm) which was equilibrated with pure water. Then, 3 mL of the PACI solution was loaded onto the column, which was eluted with pure water and a step gradient of 0.1 to 0.3 M sodium chloride at a flow rate of 0.5 mL/min. Eluent (6 mL) was automatically collected in each tube. Trace detection was performed using the phenol-sulfuric acid method. PACI was divided into two peaks and the main fractions of each peak were freeze-dried to obtain a solid powder. A solution of the main fractions was purified using a Sephadex G-100 column (2.6 × 50 cm) with pure water as the eluent at a flow rate of 0.5 mL/min. Then, the main fraction was filtered using 8,000 Da molecular mass membranes to desalt. The quantity of polysaccharide was determined by the phenol-sulfuric acid method. After freeze-drying, one fraction (PACI-1) was obtained.
Structural analysis of PACI-1
Molecular weight (Mw) determination
The uniformity and molecular weight of PACI-1 were determined by HPLC (Agilent 1260 series, Agilent Technologies, USA) with an evaporative light-scattering detector (ELSD) and ultrahydrogel 250 column (7.8 × 300 nm, Waters Corp., USA). The injection volume was 20 μL of 2 mg/mL sample solution, the mobile phase was ultrapure water, the flow rate was 1 mL/min, and the column temperature was 35°C. The T-series dextran standard was used to construct the standard curve, which was used to determine the molecular weight of PACI-1 (3).
Monosaccharide composition analysis
The monosaccharide composition of PACI-1 was determined by HPLC with PMP pre-column derivatisation, as previously reported (22). PACI-1 (10 mg) was hydrolysed with 2 M trifluoroacetic acid solution at 120°C for 6 h, and the excess trifluoroacetic acid solution was removed by a rotary evaporator. At 70°C, the obtained hydrolysate was derived with 0.5 M PMP in methanol and 0.3 M aqueous NaOH solution for 30 min, then neutralized with 0.3 M HCl. The derivatives were separated by HPLC equipped with a ZORBAX eclipse XDB-C18 column (4.6 × 250 mm) and a DAD detector. The column temperature was 30°C, the mobile phase was acetonitrile and ammonium acetate, and the flow rate was 1.0 mL/min.
FT-IR spectroscopy
Dried PACI-1 (1 mg) was mixed with 100 mg of dried KBr and pressed into a disk. FT-IR spectra were recorded in the range of 4,000–500 cm−1 (Spectrum 100 FT-IR, Thermo Fisher Scientific, USA) (23).
Circular dichroism (CD)
The CD spectrum (J-815, JASCO, Japan) of 0.5 mg/mL polysaccharide was recorded with a 1 cm path length. The CD spectrum accumulated at a rate of 50 nm/min from 190 to 400 nm. The bandwidth was 2.5 nm in this range (24).
Molecular morphology observation by AFM
Atomic force microscopy (AFM) was employed to observe the molecular morphology of PACI-1. PACI-1 was dissolved in pure water (1 × 10−3 mg/mL), dried on freshly cleaved mica in a dryer for 2 h and then observed using AFM (Hitachi High-Tech company, China) (25).
NMR spectroscopy
The PACI-1 (20 mg) were re-dissolved in 0.5 mL D2O, and then trans- ferred into 5 mm NMR tube for testing. The analysis was performed using an NMR analyzer. (VANCE-600, Bruker Inc., Rheinstetten, Germany)(23).
Antioxidant activity of PACI-1
DPPH scavenging activity
The DPPH radical scavenging ability of PACI-1 was determined using the method of Sharma et al. (26) with modification. 2 mL DPPH was added to PACI-1 solutions (2 mL) of different concentrations (2, 4, 6, 8, 10 mg/mL). After reaction in the dark for 30 min, the absorbance value (Aj) was measured at 517 nm. The DPPH free radical solution was replaced with absolute ethanol to determine Ai and the PACI-1 solution was replaced with absolute ethanol to determine Ac. Ascorbic acid was used as the positive control. Each test was performed in triplicate. The equation to calculate DPPH radical scavenging capacity is:
ABTS scavenging activity
The ABTS radical scavenging activity of PACI-1 was determined according to the method of Zeng (8). ABTS (4 mL) and potassium persulphate solution were added to PACI-1 sample solutions (400 μL) of different concentrations (1, 2, 4, 6, 8, 10 mg/mL). The mixture was shaken evenly and allowed to react at room temperature for 6 min. Then, the absorbance was measured at 734 nm to determine A0. Distilled water was used as the blank and ascorbic acid was used as the positive control to determine A1. Each test was performed in triplicate.
The equation to calculate ABTS radical scavenging capacity is:
Statistical analysis
All results are expressed as the mean value of at least three replicates ± standard deviation (S.D.). The data obtained were subjected to One-way analysis of variance (ANOVA) using IBM SPSS Statistics 21. A p-value of < 0.05 was considered statistically significant.
Results
Morphological identification
On PDA medium, the colonies were round with neat edges. They were flocculent in the early stages and powdery in the later stages. The colonies were light yellow on the front and the back. Aerial hyphae and vegetative hyphae were present (Figure 1). Based on morphology, the strain was preliminarily identified as mold.
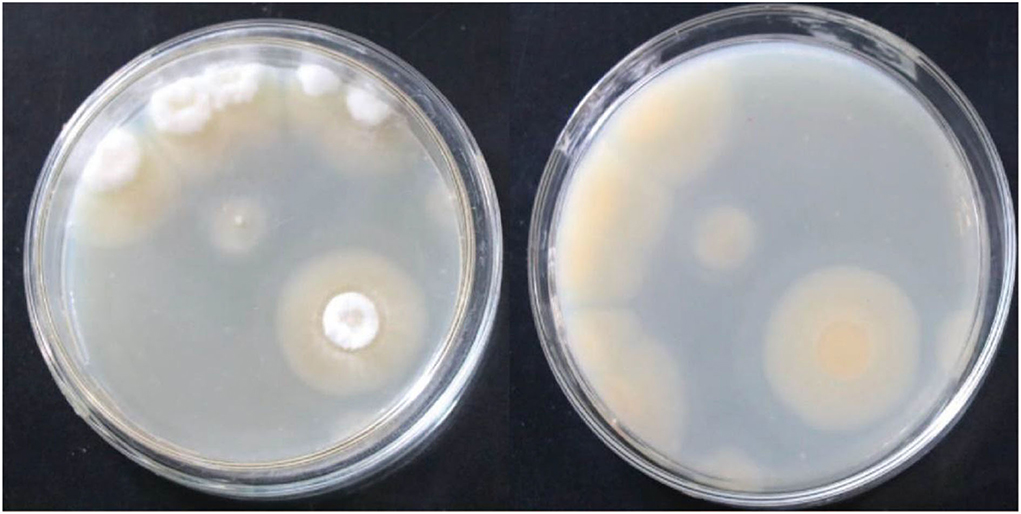
Figure 1. Frontal and reverse colony morphology of the strain S1 after culturing on a PDA medium plate at 30°C for 144 h.
Molecular systematics and phylogenetic analysis
A phylogenetic tree of the S1 sequence was constructed (Figure 2). The sequence for strain S1 (Genbank Accession no. MW188645) had the highest similarity with C. cicadae strain minfu13 (99.82%). Therefore, the strain should be classified as C. cicadae. In combination with the morphology and molecular systematics results, it was determined that the strain was C. cicadae and it was named S1.
Single-factor test results
Figures 3A–G shows that when the concentration of sodium selenite was 0.1 g/L, the maximum yield of extracellular polysaccharides in C. cicadae was 3.97 g/L. As the concentration of sodium selenite increased, the polysaccharide yield decreased; therefore, the optimum concentration of sodium selenite was 100 mg/L. Among the five different carbon sources selected, when the carbon source was sucrose, the yield of polysaccharide was the highest (2.95 g/L); this was significantly higher than with the other four carbon sources. Sucrose is composed of the monosaccharides glucose and fructose, both of which are hexoses and can participate directly in the glycolysis and pentose phosphate pathways in the fermentation process of C. cicadae. This improved the carbon conversion efficiency of the reaction process and thereby improved the yield of extracellular polysaccharides (27). However, a further increase in the sucrose concentration inhibited the growth of bacteria. Too much sugar increased bacterial respiration and decreased dissolved oxygen in the fermentation broth, resulting in insufficient oxygen to meet the metabolic demands of the bacteria and affecting the synthesis of metabolites, including polysaccharides (28). Therefore, the optimum carbon source concentration was 8%.
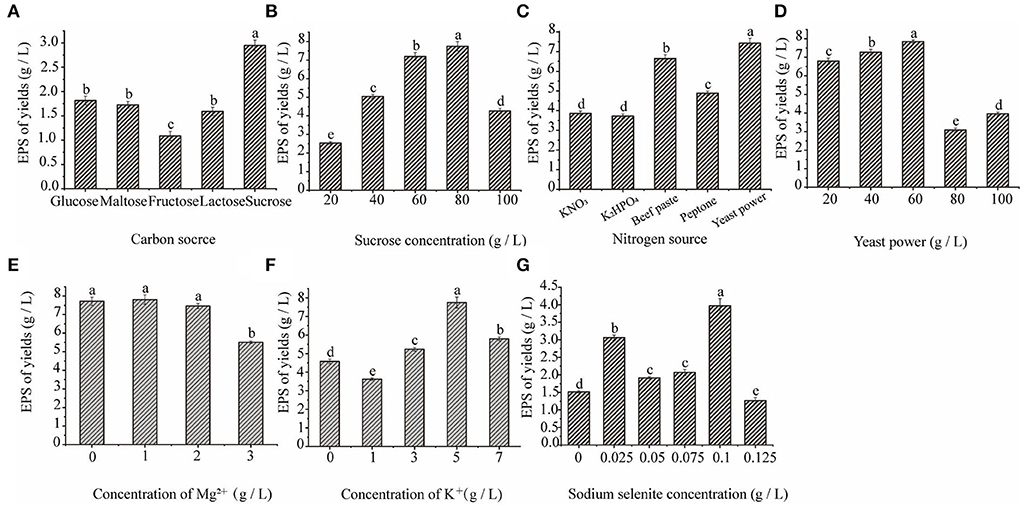
Figure 3. The effect of carbon source (A), sucrose concentration (B), nitrogen source (C), yeast powder concentration (D), magnesium ion concentration (E), potassium ion concentration (F), and sodium selenite concentration (G) on C. cicadae polysaccharide yield.
Among the five different nitrogen sources, the highest yield of polysaccharide was 7.42 g/L after the addition of yeast powder, which was significantly higher than with the other nitrogen sources. Yeast powder is an organic nitrogen source, which is more conducive to improving the yield of medicinal fungal polysaccharides than inorganic nitrogen sources. Organic nitrogen sources are rich in proteins, polypeptides, free amino acids, and a small amount of fat, trace elements, and auxin, which are conducive to the growth of bacteria and the synthesis of various metabolites (29). When the concentration of yeast powder was 60 g/L, the maximum yield of polysaccharide was 7.85 g/L. The reason of yeast powder as the best nitrogen source may be that it can provide the necessary factors for microbial growth and can affect those enzyme activities involving the biosynthesis pathway of polysaccharide. However, as the concentration of yeast powder increased, the yield of polysaccharide decreased. This may have been because the higher concentrations of the nitrogen source led to the accumulation of nitrogen in the later stages of fermentation, which inhibited the synthesis of polysaccharides. Therefore, the optimum concentration of the nitrogen source was 60 g/L.
The addition of an appropriate amount of Mg2+ and K+ to the culture medium significantly promoted the yield of extracellular polysaccharide. Figures 3F,G show that when the concentration of Mg2+ was 1 g/L, the concentration of extracellular polysaccharide was the highest (7.81 g/L). As the Mg2+ concentration increased, the yield of extracellular polysaccharide decreased. Therefore, the optimum Mg2+ concentration was 1 g/L. K+ significantly promoted the production of extracellular polysaccharide by C. cicadae and the polysaccharide yield was the highest (7.75 g/L) when the concentration of K2HPO4 was 5 g/L. Metal ions can function as enzyme activators during the growth and polysaccharide production of C. cicadae, which is beneficial to bacterial growth and metabolism. However, high concentrations of Mg2+ and K+ are toxic to C. cicadae cells and negatively affect their growth and development.
In summary, the single-factor results for the C. cicadae liquid fermentation medium determined that the optimal conditions are as follows: 0.1 g/L sodium selenite, 80 g/L sucrose, 60 g/L yeast powder, 1 g/L MgSO4·7H2O, and 5 g/L K2 HPO4.
Plackett Burman test results
The Plackett Burman test was used to determine the three factors that had the greatest impact on the polysaccharide yield from the liquid fermentation of C. cicadae. The test results are shown in Supplementary Table S1. Yeast powder and sucrose had a significant effect on the polysaccharide yield (P < 0.01), as did dipotassium hydrogen phosphate (P < 0.05). The order of significance was X2 (yeast powder) > X1 (sucrose) > X3 (K2HPO4). Therefore, the three factors with significant influence—yeast powder (X2), sucrose (X1) and K2HPO4 (X3)—were selected as the model factors for the subsequent response surface analysis.
Response surface analysis
The response surface analysis results of the interaction among sucrose, yeast powder, and potassium hydrogen phosphate are shown in Supplementary Table S2. Minitab17 was used to analyse the results and a linear equation between the polysaccharide content (Y) and sucrose (X1), yeast powder (X2) and K2HPO4 (X3) was obtained: Y = 8.354-−0.019 X1 + 0.017 X2 + 0.047 X3−2.387 X X1−1.931 X X2−1.654 X X3−0.168 X X2−0.174 X X3 + 0.303 X X3(R2 = 0.9724).
A variance analysis was conducted to determine the reliability of this equation, with the results shown in Supplementary Table S3. The model value of F = 19.60 (P < 0.01) indicated that the model had a significant impact on the test results. The mismatch term of F = 0.28 (P > 0.05) had no significant impact on the test results. In the primary term, factor X2 had the greatest influence on the test results and the F values were in the order X2 > X1 > X3. X12, X22 and X32 in the secondary term had a significant impact, while no factors in the interactive term had a significant impact, indicating that there was no interaction between the factors. The adjusted model R2 value of 0.9228 indicated that 92.28% of the experimental results could be explained by the model.
This model was applied to predict the optimal medium composition. Taking one of the three factors as the central value and observing the three-dimensional response diagram of the remaining two factors, the influence of each factor on the response value was analyzed. The results are shown in Figures 4A–C. According to the Minitab17 results, when Y was maximal (8.3540 g/L), X1 = 0, X2 = 0.0101, X3 = 0.0101, sucrose = 79.9936 g/L, yeast powder = 60.0058 g/L, and K2HPO4 = 5.0153 g/L. For practical considerations, these values were revised to 80 g/L sucrose, 60 g/L yeast powder, and 5 g/L K2HPO4 for subsequent experiments. The average experimental yield of C. cicadae polysaccharide was 8.0942 g/L, which differed by only 3.1% from the predicted value. Therefore, this model has practical reference value.
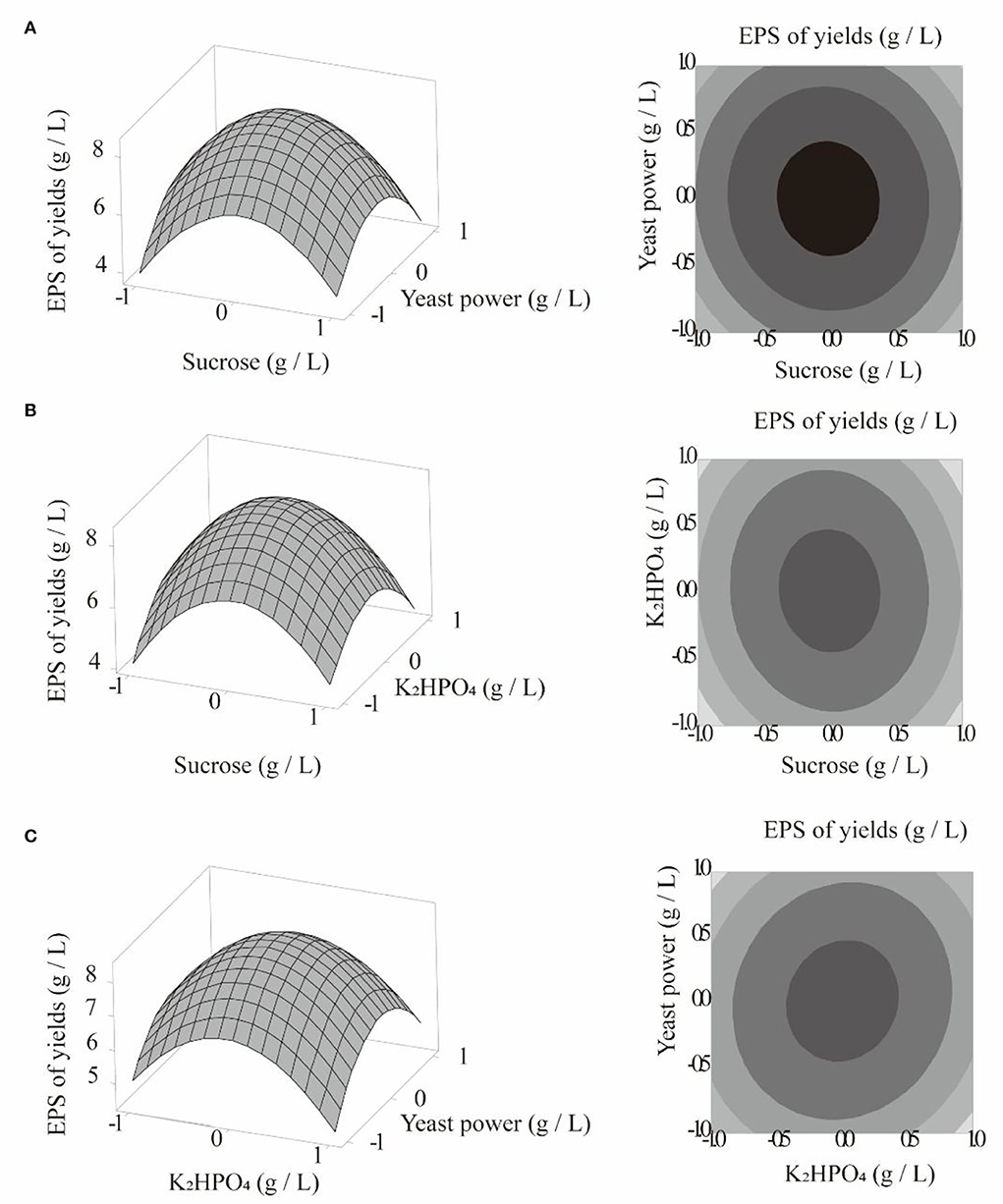
Figure 4. Curved surface plot and curved graph: polysaccharide yield, sucrose, and yeast powder (A); polysaccharide yield, dipotassium hydrogen phosphate, and sucrose (B); polysaccharide yield, dipotassium hydrogen phosphate, and yeast powder (C).
Purification and separation of PACI
After extraction of the crude polysaccharides from the fermentation broth by alcohol precipitation, PACI was separated using a DEAE-52 cellulose column and Sephadex G-100 gel column. According to the Figure 5A, there were three main fractions existed in it at the beginning of the purification process. The two fractions were then concentrated and named PACI-1 and PACI-2, respectively. After drying, the amount of PACI-2 was very small so was not studied further.
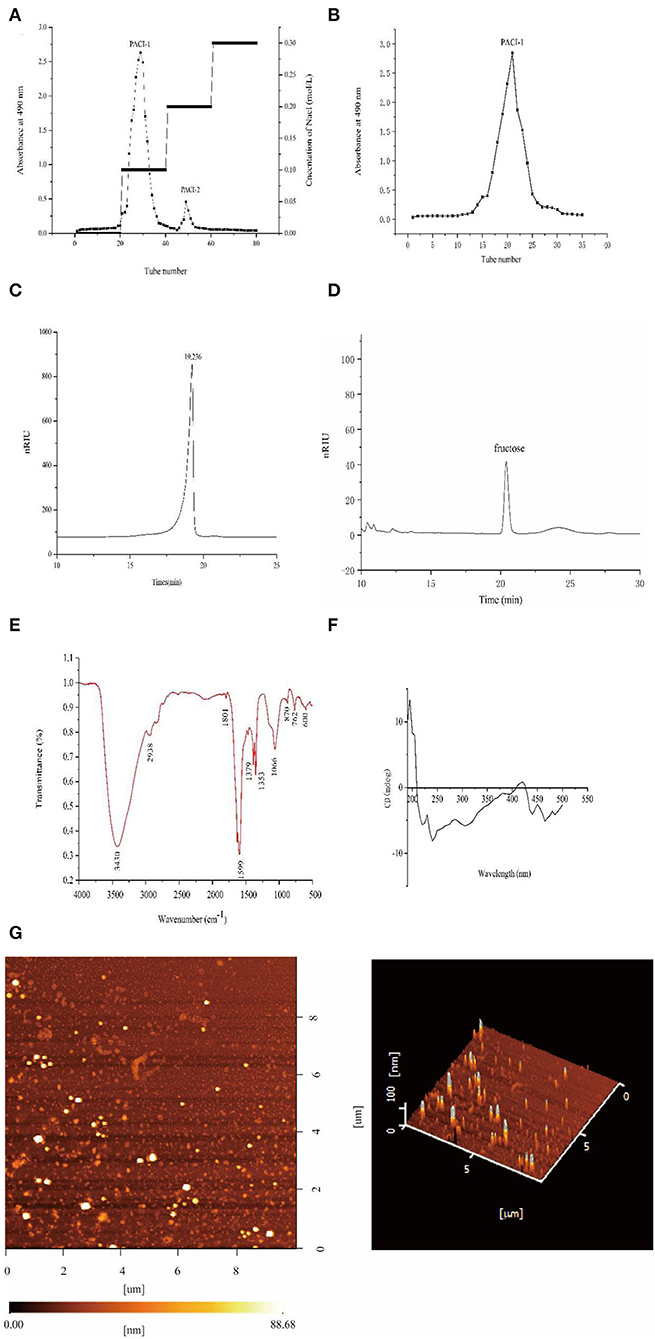
Figure 5. Structural analysis of PACI-1. DEAE-52 anion exchange elution curve (A). G-100 gel elution curve (B). High-performance liquid chromatography spectrum (C). Monosaccharide composition (D). IR spectrum (E). CD spectrum (F). AFM images of PACI-1 (G).
Molecular weight and monosaccharide composition
The average molecular weight of PACI-1 was determined by HPLC. The HPLC spectrum contained a single symmetrical peak (Figure 5C), which indicated that the polysaccharide PACI-1 obtained after separation and purification had high purity and homogeneity. The calibration curve obtained from the dextran standard was log Mw = −0.2422 X + 8.7833 (R2 = 0.9974). According to calculations based on the standard curve, the molecular weight of PACI-1 was 9.95 × 103 Da. PACI-1 was determined to be a homopolysaccharide composed of fructose (Figure 5D).
Infrared spectroscopy
The FT-IR spectrum identified the following structural characteristics of the polysaccharide (Figure 5E). The peak from 3,500 to 3,100cm−1 corresponded to the stretching vibrations of O-H and C-H, which are characteristic absorption peaks of sugars. The peak at 3,430 cm−1 indicated that the polysaccharide had obvious intermolecular hydrogen bonding (30). The peaks between 3,000 and 2,800 cm−1 corresponded to the stretching vibrations of carbohydrate C-H moieties and the absorption peak at 2,938 cm−1 corresponded to stretching vibrations of C-H (31). The absorption peak at 1,379 cm−1 corresponded to the deformation of =CH2 and the peak at 1,353 cm−1 corresponded to C-H bending vibrations. The absorption peak at 1,066 cm−1 may have been due to the overlap of ring vibrations with the tensile vibrations of the C-OH side group and vibrations of the C-O-C glycosidic bond, indicating the presence of pyranose (32). Additionally, the stretching vibration of the pyran skeleton was also detected at 600 cm−1 and the peak at 870 cm−1 indicated the presence of β-glycosidic bonds (33).
Circular dichroism
The CD spectrum of PACI-1 comprised a large positive peak at 195 nm with a positive cotton effect, which was due to the presence of C-O and O-H groups in the polysaccharide molecule (Figure 5F). There was a negative cotton effect at 240 nm. The presence of both positive and negative cotton peaks between 190 and 250 nm was indicative of molecular asymmetry; that is, PACI-1 could readily form curls, folds, and three-strand spiral structures in aqueous solution (34).
Atomic force microscopy
AFM images were obtained to determine the surface morphology and roughness of PACI-1. In AFM, as the height is not affected by the “widening effect,” only the length and width change at different measurement positions. Therefore, the height can be used as a reference to determine the true diameters of molecules. The height of PACI-1 was about 88.68 nm, much higher than 15–50 nm, indicating that PACI-1 had a three-strand helical structure (35). This was consistent with the CD results. Additionally, the height of PACI-1 was much higher than that of monosaccharide chains without selenium modification (0.1–1.0 nm) (36).
As seen in Figure 5E, PACI-1 had an irregular polymer particle morphology. No irregular linear molecular chain conformations were observed. This phenomenon indicated that there was cross-linking of the PACI-1 polysaccharide chains to form polymer particles. This may have been due to van der Waals forces between the polysaccharide chains or hydrogen bond interactions between the molecules, causing the polysaccharide chains to intertwine and form a spherical structure (34).
NMR spectroscopy
1H NMR can be used to determine the configuration of glycosidic bonds in polysaccharides (Figure 6). Generally, the α proton signal of α-type glycosidic bonds is at 5–6 ppm and the β proton signal of β-type glycosidic bonds is at 3–5 ppm (37). The 1H NMR spectrum contained a peak at 4.89 ppm, indicating that PACI-1 may contain β-type glycosidic bonds. Additionally, the main heterocephalic carbon proton signal of CPA-1 was at 3–4 ppm, indicating that PACI-1 mainly contained β-configured pyranoside bonds. This conclusion was the same as that obtained using infrared spectroscopy.
In vitro antioxidant activity of PACI-1
The scavenging ability of PACI-1 for DPPH and ABTS free radicals was studied with Vitamin C as the positive control. The DPPH radical scavenging ability of PACI-1 is shown in Figure 7A. The free radical scavenging ability of PACI-1 increased as the polysaccharide concentration increased. At 8 mg/mL, the DPPH scavenging rate of PACI-1 reached 56.46%, which was higher than that of FVR-1 (47.38%), a natural polysaccharide component from Flammulina velutipes (38). As shown in Figure 7B, the scavenging ability of PACI-1 for ABTS free radicals had an obvious concentration dependence as the polysaccharide concentration increased. The IC50 value for the scavenging of ABTS free radicals by PACI-1 was 8.45 mg/mL, which was greater than the ABTS radical scavenging ability of the natural polysaccharide PKP-E-1-1 isolated from Pinus koraiensis (IC50 = 1.52 × 103 mg/mL) (33). These results confirmed the antioxidant capacity of PACI-1, which increased as the concentration of PACI-1 increased.
Discussion
After optimizing the medium formula, the amount of polysaccharide of 8.09 g/L produced by C. cicadae liquid fermentation was 642.20% higher than that without optimisation (1.09 g/L). This increase in polysaccharide yield was much higher than that obtained by Yang and Xu (21.70 and 50%) (39). Additionally, the optimized result was 1.41 times that obtained by Wang using shaking flask fermentation (5.71g/L) (40), and 16 times that obtained by Wang(486.16 ± 19.60 mg/L) (41). In the present study, after optimizing the components of the liquid medium, not only was the improvement in polysaccharide yield greater than those reported in other publications, but the yield was also relatively high. Therefore, this preliminary work provided an excellent approach to improving the polysaccharide yield.
The FT-IR absorption peaks near 605 cm−1 (Se=O), 760 cm−1 (C–O–Se), and 1610 cm−1 (O–Se–O) indicated that the selenite group underwent substitution on the polysaccharide (40). The FT-IR spectrum of polysaccharide component PACI-1 contained an absorption peak at 762 cm−1, which corresponded to Se-O-C stretching vibrations (42, 43). These changes indicated that PACI-1 had been selenised.
The chromatographic analysis revealed PACI-1 to be a homopolysaccharide composed of fructose with an average molecular weight of 9.945 × 103 Da. As a comparison, the molecular weights of polysaccharides JCH-1 and JCH-2, purified from Isaria cicadae Miquel, were 3.09 × 104 and 5.55 × 105 Da (21), respectively, while the molecular weight of heteropolysaccharide (IPS1) from Paecilomyces cicadae was 2.40 × 106 Da (44). Chen determined that the molecular weight of the extracellular polysaccharide W-CBP50 II in fermentation broth was 9.97 × 104 Da (45). PACI-1, with its lower molecular weight of <1 × 104 Da, would be classified as a low-molecular weight polysaccharide (33). The differences in the molecular weights of these polysaccharides may be related to the different sources, extraction methods, and purification steps for polysaccharides obtained from the fermentation liquids of different strains. Alternatively, the lower molecular weight of PACI-1 may have been caused by the addition of inorganic selenium (6, 46).
PACI-1 was determined to be a homopolysaccharide composed of fructose, which was inconsistent with previous experimental results (47). It was previously reported that the polysaccharide of C. cicadae mycelium was a heteropolysaccharide, with glucose, mannose, and galactose as the main components. Table 2 shows that the polysaccharides extracted from C. cicadae have been mainly heteropolysaccharides, while there are few reports in which the extracted polysaccharide was a homopolysaccharide (51). Therefore, this separation and purification study provides the first report on selenium-enriched syn-fructose polysaccharide.
The antioxidant experiment conducted in this study showed that the selenium-enriched polysaccharide PACI-1 had a certain antioxidant capacity, which was higher than that of natural polysaccharides but lower than that of other selenium-enriched polysaccharides (52). It has been reported that low-molecular weight polysaccharides have high antioxidant activity. Therefore, the lower antioxidant activity of natural polysaccharide components FVRP-1 and PKP-E-1-1 compared to PACI-1 may have been due to their higher average molecular weights of 29,930 and 1.09 × 104 Da, respectively. The antioxidant activity of polysaccharides is not related to just one factor but several (45), including the degree of branching, connections, conformation, and other factors (31). Therefore, the complex relationship between the polysaccharide structure and antioxidant activity needs further exploration and research to elucidate the reasons underpinning the activity of PACI-1.
Conclusion
In this study, the components of the liquid fermentation medium of strain S1 were optimized. The response surface results identified the optimal medium ratio at which the greatest yield of extracellular polysaccharide was obtained (8.09 g/L). A selenium source was added to the fermentation medium of strain S1 to prepare the selenium polysaccharide and the extracellular polysaccharide in the fermentation broth was studied. The structure of component PACI-1 was analyzed by HPLC, FT-IR, CD, AFM, and NMR. The results showed that PACI-1 had an average molecular weight of 9.945 × 103 Da in selenium- and fructose-enriched medium, and a three-strand helical-spherical structure in aqueous solution. Additionally, the antioxidant activity of PACI-1 was between those of the natural polysaccharides and selenium polysaccharides. However, the complete structure-activity relationship of PACI-1 remains unclear. Future studies will further explore the relationship between the bioactivity and structure of extracellular polysaccharides from C. cicadae.
Data availability statement
The original contributions presented in the study are included in the article/Supplementary material, further inquiries can be directed to the corresponding author.
Author contributions
WZ: conceptualization, methodology, software, investigation, formal analysis, and writing—original draft. JX: data curation and writing—original draft. HL: visualization and investigation. YZ: resources. LC: Supervision. SSh: software. SSo: validation. HZh: visualization. TD: data analysis. HZe and QX: conceptualization, funding acquisition, resources, and supervision. All authors contributed to the article and approved the submitted version.
Funding
This work was financially supported by the Open Project Program from Key Laboratory of Se-enriched Products Development and Quality Control, Ministry of Agriculture/ National-Local Joint Engineering Laboratory of Se-enriched Food Development (Grant No. Se-2021C08), Major science and technology projects in Huaibei (Grant No. HK2021016), and Industry university research cooperation project of Anhui Kouzi Distillery Co., Ltd. and Huaibei Normal University (Grant No. KZJY-001), National University Student Innovation and Entrepreneurship Project (Grant No. 202210373018).
Conflict of interest
Authors JX, HL, YZ, LC, SS, and QX were employed by Anhui Kouzi Distillery Co., Ltd.
The remaining authors declare that the research was conducted in the absence of any commercial or financial relationships that could be construed as a potential conflict of interest.
Publisher's note
All claims expressed in this article are solely those of the authors and do not necessarily represent those of their affiliated organizations, or those of the publisher, the editors and the reviewers. Any product that may be evaluated in this article, or claim that may be made by its manufacturer, is not guaranteed or endorsed by the publisher.
Supplementary material
The Supplementary Material for this article can be found online at: https://www.frontiersin.org/articles/10.3389/fnut.2022.1032289/full#supplementary-material
References
1. Sun Y, Kmonickova E, Han R, Zhou W, Yang K, Lu H, et al. Comprehensive evaluation of wild Cordyceps cicadae from different geographical origins by TOPSIS method based on the macroscopic infrared spectroscopy (IR) fingerprint. Spectrochim Acta A Mol Biomol Spectrosc. (2019) 214:252–60. doi: 10.1016/j.saa.2019.02.031
2. Ren X, He L, Cheng J, Chang J. Optimization of the solid-state fermentation and properties of a polysaccharide from Paecilomyces cicadae (Miquel) Samson and its antioxidant activities in vitro. Plos ONE. (2014) 9:e87578. doi: 10.1371/journal.pone.0087578
3. Cai Z-N, Li W, Mehmood S, Pan W-J, Wang Y, Meng F-J, et al. Structural characterization, in vitro and in vivo antioxidant activities of a heteropolysaccharide from the fruiting bodies of Morchella esculenta. Carbohydr Polym. (2018) 195:29–38. doi: 10.1016/j.carbpol.2018.04.069
4. Ke B-J, Lee C-L. Investigation on the fermentation for high adenosine and N6-(2-hydroxyethyl)-adenosine (HEA) productions of Cordyceps cicadae. New Biotechnol. (2016) 33:S206–7. doi: 10.1016/j.nbt.2016.06.1433
5. Ji X, Guo J, Pan F, Kuang F, Chen H, Guo X. Liu Y. Structural elucidation and antioxidant activities of a neutral polysaccharide from arecanut (Areca catechu L). Front Nutr. (2022) 9:853115. doi: 10.3389/fnut.2022.853115
6. Mohanta B, Sen DJ, Mahanti B, Nayak AK. Antioxidant potential of herbal polysaccharides: An overview on recent researches. Sens Int. (2022) 3:100158. doi: 10.1016/j.sintl.2022.100158
7. Ji X, Guo J, Ding D, Gao J, Hao L, Guo X, et al. Structural characterization and antioxidant activity of a novel high-molecular-weight polysaccharide from Ziziphus Jujuba cv. Muzao Food Measure. (2022) 16:2191–200. doi: 10.1007/s11694-022-01288-3
8. Huawei Z, Jie Q, Xin Z, Dayong X, Minghua X, Feng L, Jianfan S, Xuan J, Dai C. Optimization of submerged and solid state culture conditions for Monascus pigment production and characterization of its composition and antioxidant activity. Pigm Resin Technol. (2018) 48. doi: 10.1108/PRT-05-2018-0046
9. Kipp AP, Strohm D, Brigelius-Flohé R, Schomburg L, Bechthold A, Leschik-Bonnet E, et al. Revised reference values for selenium intake. J Trace Elem Med Biol. (2015) 32:195–9. doi: 10.1016/j.jtemb.2015.07.005
10. Beckett GJ, Arthur JR. Selenium and endocrine systems. J Endocrinol. (2005) 184:455–65. doi: 10.1677/joe.1.05971
11. Rayman MP. The importance of selenium to human health. Lancet. (2000) 356:233–41. doi: 10.1016/S0140-6736(00)02490-9
12. Yang W, Huang G, Chen F, Huang H. Extraction/synthesis and biological activities of selenopolysaccharide. Trends Food Sci Technol. (2021) 109:211–8. doi: 10.1016/j.tifs.2021.01.028
13. Chen N, Zhao C, Zhang T. Selenium transformation and selenium-rich foods. Food Biosci. (2021) 40:100875. doi: 10.1016/j.fbio.2020.100875
14. Brown KM, Arthur JR. Selenium, selenoproteins and human health: a review. Public Health Nutr. (2001) 4:593–9. doi: 10.1079/PHN2001143
15. El-Bayoumy K, Sinha R. Mechanisms of mammary cancer chemoprevention by organoselenium compounds. Mutat Res. (2004) 551:181–97. doi: 10.1016/j.mrfmmm.2004.02.023
16. Alvandi H, Hatamian-Zarmi A, Hosseinzadeh BE, Mokhtari-Hosseini ZB, Langer E, Aghajani H. Improving the biological properties of Fomes fomentarius MG835861 exopolysaccharide by bioincorporating selenium into its structure. Carbohydrate Polymer Technol Appl. (2021) 2:100159. doi: 10.1016/j.carpta.2021.100159
17. Kapoor N, Lokesh G, Sanjai S. Secondary structure prediction of ITS rRNA region and molecular phylogeny: an integrated approach for the precise speciation of Muscodor specie. Ann Microbiol. (2018) 68:763–72. doi: 10.1007/s13213-018-1381-8
18. Qiao J, Shuai Y, Zeng X, Xu D, Rao S, Zeng H, et al. Comparison of chemical compositions, bioactive ingredients, and in vitro antitumor activity of four products of cordyceps (ascomycetes) strains from China. Int J Med Mushrooms. (2019) 21:331–42. doi: 10.1615/IntJMedMushrooms.2019030329
19. Samkumar A, Karppinen K, Dhakal B, Martinussen I. Jaakola L. Insights into sugar metabolism during bilberry (Vaccinium myrtillus L) fruit development. Physiol Plantarum. (2022) 174:e13657. doi: 10.1111/ppl.13657
20. Stylianou E, Pateraki C, Ladakis D, Vlysidis A, Koutinas A. Optimization of fermentation medium for succinic acid production using Basfia succiniciproducens. Environ Technol Inno. (2021) 24:101914. doi: 10.1016/j.eti.2021.101914
21. Xu Z, Yan X, Song Z, Li W, Zhao W, Ma H, et al. Two heteropolysaccharides from Isaria cicadae Miquel differ in composition and potentially immunomodulatory activity. Int J Biol Macromol. (2018) 117:610–6. doi: 10.1016/j.ijbiomac.2018.05.164
22. Huo J, Lei M, Li F, Hou J, Wu W. Structural characterization of a polysaccharide from gastrodia elata and its bioactivity on gut microbiota. Molecules. (2021) 26:4443. doi: 10.3390/molecules26154443
23. Wu Q, Luo M, Yao X, Yu L. Purification, structural characterization, and antioxidant activity of the COP-W1 polysaccharide from Codonopsis tangshen Oliv. Carbohydrate Polymers 236:116020. doi: 10.1016/j.carbpol.2020.116020
24. Li Q, Wang W, Zhu Y, Chen Y, Zhang W, Yu P, et al. Structural elucidation and antioxidant activity a novel Se-polysaccharide from Se-enriched Grifola frondosa. Carbohydr Polym. (2017) 161:42–52. doi: 10.1016/j.carbpol.2016.12.041
25. Liu M, Gong Z, Liu H, Wang J, Wang D, Yang Y, et al. Structural characterization and anti-tumor activity in vitro of a water-soluble polysaccharide from dark brick tea. Int J Biol Macromol. (2022) 205:615–25. doi: 10.1016/j.ijbiomac.2022.02.089
26. Sharma OP, Bhat TK. DPPH antioxidant assay revisited. Food Chem. (2009) 113:1202–5. doi: 10.1016/j.foodchem.2008.08.008
27. Xu C, Yu J, Zhao S, Wu S, He P, Jia X, et al. Effect of carbon source on production, characterization and bioactivity of exopolysaccharide produced by Phellinus vaninii Ljup. An Acad Bras Cienc. (2017) 89:2033–41. doi: 10.1590/0001-3765201720150786
28. Qiang L, Yumei L, Sheng H, Yingzi L, Dongxue S, Dake H, et al. Optimization of fermentation conditions and properties of an exopolysaccharide from Klebsiella sp. H-207 and application in adsorption of hexavalent chromium. Plos ONE. (2013) 8:e53542. doi: 10.1371/journal.pone.0053542
29. Wang C-C, Wu J-Y, Chang C-Y, Yu S-T, Liu Y-C. Enhanced exopolysaccharide production by Cordyceps militaris using repeated batch cultivation. J Biosci Bioeng. (2019) 127:499–505. doi: 10.1016/j.jbiosc.2018.09.006
30. Wang F, Ye S, Ding Y, Ma Z, Zhao Q, Zang M, et al. Research on structure and antioxidant activity of polysaccharides from Ginkgo biloba leaves. J Mol Struct. (2022) 1252:132185. doi: 10.1016/j.molstruc.2021.132185
31. Hu S, Hu W, Li Y, Li S, Tian H, Lu A, et al. Construction and structure-activity mechanism of polysaccharide nano-selenium carrier. Carbohydr Polym. (2020) 236:116052. doi: 10.1016/j.carbpol.2020.116052
32. Nie C, Zhu P, Ma S, Wang M, Hu Y. Purification, characterization and immunomodulatory activity of polysaccharides from stem lettuce. Carbohydrate Polymers. (2018) 188:236–242. doi: 10.1016/j.carbpol.2018.02.009
33. Li F, Wei Y, Liang L, Huang L, Yu G, Li Q, et al. novel low-molecular-mass pumpkin polysaccharide: Structural characterization, antioxidant activity, and hypoglycemic potential. Carbohydr Polym. (2021) 251:117090. doi: 10.1016/j.carbpol.2020.117090
34. Tan M, Zhao Q, Zhao B. Physicochemical properties, structural characterization and biological activities of polysaccharides from quinoa (Chenopodium quinoa Willd) seeds. Int J Biol Macromol. (2021) 193:1635–44. doi: 10.1016/j.ijbiomac.2021.10.226
35. Wang K, Wang J, Li Q, Zhang Q, You R, Cheng Y, et al. Structural differences and conformational characterization of five bioactive polysaccharides from Lentinus edodes. Food Res Int. (2014) 62:223–32. doi: 10.1016/j.foodres.2014.02.047
36. Shen S, Jia S, Wu Y, Yan R, Lin Y-H, Zhao D, et al. Effect of culture conditions on the physicochemical properties and antioxidant activities of polysaccharides from Nostoc flagelliforme. Carbohydr Polym. (2018) 198:426–33. doi: 10.1016/j.carbpol.2018.06.111
37. Wang L, Li X, Wang B. Synthesis, characterization and antioxidant activity of selenium modified polysaccharides from Hohenbuehelia serotina. Int J Biol Macromol. (2018) 120:1362–8. doi: 10.1016/j.ijbiomac.2018.09.139
38. Liu Y, Zhang B, Ibrahim SA, Gao S-S, Yang H, Huang W. Purification, characterization and antioxidant activity of polysaccharides from Flammulina velutipes residue. Carbohydr Polym. (2016) 145:71–7. doi: 10.1016/j.carbpol.2016.03.020
39. Yang S, Jin L, Ren X, Lu J, Meng Q. Optimization of fermentation process of Cordyceps militaris and antitumor activities of polysaccharides in vitro. J Food Drug Anal. (2014) 22:468–476. doi: 10.1016/j.jfda.2014.01.028
40. Wang Z, Cai T, He X. Characterization, sulfated modification and bioactivity of a novel polysaccharide from Millettia dielsiana. Int J Biol Macromol. (2018) S0141813017334803. doi: 10.1016/j.ijbiomac.2018.05.147
41. Wang L-Y, Cheong K-L, Wu D-T, Meng L-Z, Zhao J, Li S-P. Fermentation optimization for the production of bioactive polysaccharides from Cordyceps sinensis fungus UM01. Int J Biol Macromol. (2015) 79:180–5. doi: 10.1016/j.ijbiomac.2015.04.040
42. Qiu S, Chen J, Chen X, Fan Q, Zhang C, Wang D, et al. Optimization of selenylation conditions for lycium barbarum polysaccharide based on antioxidant activity. Carbohydr Polym. (2014) 103:148–53. doi: 10.1016/j.carbpol.2013.12.032
43. Liu F, Zhu Z-Y, Sun X, Gao H, Zhang Y-M. The preparation of three selenium-containing Cordyceps militaris polysaccharides: Characterization and anti-tumor activities. Int J Biol Macromol. (2017) 99:196–204. doi: 10.1016/j.ijbiomac.2017.02.064
44. Tian J, Wang X, Zhang X, Zhang C, Chen X, Dong M, et al. Isolation, structural characterization and neuroprotective activity of exopolysaccharide from Paecilomyces cicada TJJ1213. Int J Biol Macromol. (2021) 183:1034–46. doi: 10.1016/j.ijbiomac.2021.05.047
45. Chen X, Wu G, Huang Z. Structural analysis and antioxidant activities of polysaccharides from cultured Cordyceps militaris. Int J Biol Macromol. (2013) 58:18–22. doi: 10.1016/j.ijbiomac.2013.03.041
46. Ji X, Cheng Y, Tian J, Zhang S, Jing Y, Shi M. Structural characterization of polysaccharide from jujube (Ziziphus jujuba Mill) fruit. Chem Biol Technol Agric. (2021) 8:54. doi: 10.1186/s40538-021-00255-2
47. Olatunji OJ, Feng Y, Olatunji OO, Tang J, Wei Y, Ouyang Z, et al. Polysaccharides purified from Cordyceps cicadae protects PC12 cells against glutamate-induced oxidative damage. Carbohydr Polym. (2016) 153:187–95. doi: 10.1016/j.carbpol.2016.06.108
48. Tian J, Zhang C, Wang X, Rui X, Zhang Q, Chen X, et al. Structural characterization and immunomodulatory activity of intracellular polysaccharide from the mycelium of Paecilomyces cicadae TJJ1213. Food Res Int. (2021) 147:110515. doi: 10.1016/j.foodres.2021.110515
49. Xiang A, Li W, Zhao Y, Ju H, Xu S, Zhao S, et al. Yuan Y. Purification, characterization and antioxidant activity of selenium-containing polysaccharides from pennycress (Thlaspi arvense L). Carbohydr Res. (2022) 512:108498. doi: 10.1016/j.carres.2021.108498
50. Sun H, Zhu Z, Tang Y, Ren Y, Song Q, Tang Y, et al. Structural characterization and antitumor activity of a novel Se-polysaccharide from selenium-enriched Cordyceps gunnii. Food Funct. (2018) 9:2744–54. doi: 10.1039/C8FO00027A
51. Yin J-Y, Chen H-H, Lin H-X, Xie M-Y, Nie S-P. Structural features of alkaline extracted polysaccharide from the seeds of Plantago asiatica L. and its rheological properties. Molecules. (2016) 21:E1181. doi: 10.3390/molecules21091181
Keywords: Cordyceps cicadae, selenium-enriched, polysaccharide, response surface optimization, antioxidation activity
Citation: Zhuansun W, Xu J, Liu H, Zhao Y, Chen L, Shan S, Song S, Zhang H, Dong T, Zeng H and Xu Q (2022) Optimisation of the production of a selenium-enriched polysaccharide from Cordyceps cicadae S1 and its structure and antioxidant activity. Front. Nutr. 9:1032289. doi: 10.3389/fnut.2022.1032289
Received: 30 August 2022; Accepted: 28 September 2022;
Published: 20 October 2022.
Edited by:
Junxiang Zhu, Qingdao Agricultural University, ChinaReviewed by:
Kai Yang, Zhejiang University of Technology, ChinaXiaolong Ji, Zhengzhou University of Light Industry, China
Copyright © 2022 Zhuansun, Xu, Liu, Zhao, Chen, Shan, Song, Zhang, Dong, Zeng and Xu. This is an open-access article distributed under the terms of the Creative Commons Attribution License (CC BY). The use, distribution or reproduction in other forums is permitted, provided the original author(s) and the copyright owner(s) are credited and that the original publication in this journal is cited, in accordance with accepted academic practice. No use, distribution or reproduction is permitted which does not comply with these terms.
*Correspondence: Huawei Zeng, SHVhd2VpemVuZ0AxNjMuY29t; Qinxiang Xu, eHVxaW54aWFuZ0Brb3V6aS5jbg==