- 1School of Marine and Biological Engineering, Yancheng Teachers’ University, Yancheng, China
- 2Department of Basic Medical Science, Jiangsu Vocational College of Medicine, Yancheng, China
- 3College of Food Science and Technology, Nanjing Agricultural University, Nanjing, China
The yeast mannoproteins (MPs), a major component of yeast cell walls with large exploration potentiality, have been attracting increasing attention due to their beneficial effects. However, the information about the anti-obesogenic activity of MPs is still limited. Thus, the effects of MPs on the high-fat diet (HFD)-induced obesity and dysbiosis of gut microbiota were investigated in this work. The results showed that MPs could significantly attenuate the HFD-induced higher body weight, fat accumulation, liver steatosis, and damage. Simultaneously, the inflammation in HFD-induced mice was also ameliorated by MPs. The pyrosequencing analysis showed that intervention by MPs could lead to an obvious change in the structure of gut microbiota. Furthermore, the prevention of obesity by MPs is highly linked to the promotion of Parabacteroides distasonis (increased from 0.39 ± 0.12% to 2.10 ± 0.20%) and inhibition of Lactobacillus (decreased from 19.99 ± 3.94% to 2.68 ± 0.77%). Moreover, the increased level of acetate (increased from 3.28 ± 0.22 mmol/g to 7.84 ± 0.96 mmol/g) and activation of G protein-coupled receptors (GPRs) by MPs may also contribute to the prevention of obesity. Thus, our preliminary findings revealed that MPs from yeast could be explored as potential prebiotics to modulate the gut microbiota and prevent HFD-induced obesity.
Introduction
Obesity, recognized as a disease with serious morbidity and increased mortality, has dramatically spread throughout the developed and developing countries due to a shift to diets, including addictive and/or high-calorie foods and lack of exercise (1, 2). In the past four decades, the prevalence of obesity and overweight has nearly tripled worldwide, which has become a growing public health challenge of the twenty-first century (3). Furthermore, obesity may lead to an increased risk of other obesity-related metabolic disorders, such as non-alcoholic fatty liver disease (NAFLD), type 2 diabetes (T2DM), and cardiovascular diseases (CDs) (4, 5). Obesity is a chronic and progressive process with multi-factorial factors and complex interactions, including physiological, sociopolitical, behavioral, and environmental factors (3, 6). Although the molecular mechanism for obesity is still not fully understood, obesity essentially represents a long-term positive imbalance between energy intake and energy expenditure, thereby increasing body fat (7). Pharmaceutical drugs, such as orlistat, could prevent, and treat obesity, however, its adverse effects, including acute kidney injury, subacute liver failure, and gastrointestinal adverse effects, block its further application (8). Thus, a potential novel therapeutic strategy for the prevention and treatment of obesity is still highly needed.
In recent decades, the effect of the gut microbiota on obesity has attracted much attention due to its key role in calorie harvest, energy homeostasis, and regulation of fat storage (9, 10). Recently and more strikingly, the experiments using germ-free mice and fecal microbiota transplantation have demonstrated the causality between the gut microbiota and the development of obesity (11, 12). More specifically, some key beneficial gut microbiota responsible for the prevention of obesity, such as Akkermansia muciniphila (13), Parabacteroides distasonis (14), and Dysosmobacter welbionis (15), and pathogenic bacteria which could promote obesity, such as Erysipelatoclostridium Ramosum (16) and Enterobacter cloacae B29 (17), have been identified, separated, and verified at the species level. The gut microbiota is expected to be a novel therapeutic target for the prevention and treatment of obesity. Thus, a series of microbiota-targeted diets are presented and evaluated with the growing public awareness of the gut microbiota (18, 19). Thereinto, dietary polysaccharides, which served as potential prebiotics, have recently emerged with the growing public awareness of their probiotic effect on gut microbiota (20).
Yeast is an important food resource used for fermentation in the food industry, and a large amount of yeast by-products is available every year (21). The yeast cell wall is mainly composed of β-glucan (60%) and mannoproteins (MPs, 40%), making them a potential source for providing functional ingredients (22). Nowadays, yeast by-products are mainly processed into animal feed or used to produce β-glucan (22, 23). β-Glucan from yeast has been widely investigated, whereas MPs from yeast attract much less attention. MPs are highly glycosylated proteins with molecular weights ranging from 20 to 200 kDa, containing 80–90% of carbohydrates and 5–20% of protein, and the potential structure of MPs has been reported in previous work (22, 24, 25). In recent years, the MPs have attracted more and more attention due to their alleged health-promoting functions, such as stimulation of angiogenesis, immunoactivities, and antineoplastic activities (25–27). However, the effects of MPs on obesity and gut microbiota are still unknown. Thus, the aim of the present work was to evaluate the potential anti-obesogenic effect of MPs on a high-fat diet (HFD)-induced obesity. Furthermore, the role of gut microbiota in the prevention of obesity by MPs was also investigated.
Materials and methods
Materials
The MPs from Saccharomyces cerevisiae were kindly provided by Angel Nutritech Co., Ltd. (China). The MPs were prepared according to the previous work with some modifications (25, 26). Briefly, after being sieved and purified, the slurry of S. cerevisiae cells was mixed with 3% sodium chloride, and the solution was incubated at 55°C for 24 h with agitation at 120 rpm/min. The residual autolyzed cells were obtained by centrifugation at 5,000 g for 10 min, and the MPs were extracted by water at 121°C for 4 h. The supernatant was collected and mixed with a triple volume of absolute alcohol. After keeping at 4°C for one night, the precipitated MPs were collected and deproteinized using the trichloroacetic acid method. The obtained solution was further mixed with a triple volume of absolute alcohol. The precipitate was collected, dissolved in distilled water, and further separated by Sepharose CL-4B to obtain the purified MPs. The contents of carbohydrates and protein were 86.3 ± 2.37% and 14.6 ± 1.45%, respectively. The molecular weight of MPs was 78 kD. The mice diets, including D12450J with 10 kcal% fat and D12492 with 60 kcal% fat, were purchased from Research Diets, Inc. (New Brunswick, NJ, USA).
Mice and diets
Six-week-old C57BL/6 male mice (n = 24, Shanghai SLAC Laboratory Animal Co., Ltd., Shanghai, China) were bred in the Animal Center of Nanjing Agricultural University (SYXK < Jiangsu > 2011-0037). All animal experimental protocols in this work were approved by the Institutional Animal Ethics Committee of the Experimental Animal Center of Nanjing Agricultural University. The mice were housed in specific pathogen-free (SPF) animal rooms under a 12-h dark-light cycle with ad libitum access to food and water. After an accommodation period of 1 week, mice were randomly divided into three groups (n = 8 per group), and fed for 10 weeks with a normal-chow diet (D12450J, coded as ND group), HFD (D12492, coded as HFD group), and HFD plus daily yeast MPs with a dosage of 400 mg/kg of body weight (coded as HFD-MP). The dosage of MPs in this work was chosen according to the previous work (23). Mice were supplemented daily with 0.2 mL of water in the ND and HFD groups and 0.2 mL of MPs solution (400 mg of MP was dissolved in 10 mL of sterilized water) in the HFD-MP group by intragastric gavage. The body weight and food intake were assessed on a weekly basis. After overnight fasting, mice were anesthetized using carbon dioxide and then euthanized by cardiac puncture at the end of 10 weeks. The blood was drawn in tubes containing EDTA and centrifuged at 4,000 g to obtain plasma. The adipose tissue and liver were obtained and weighed. A part of the epididymis fat and liver samples were fixed with a 4% of paraformaldehyde solution. After embedding in paraffin, the epididymis fat and liver samples were sectioned and stained with hematoxylin and eosin (H&E stain). Then, the slices were observed under a light microscope.
Biochemical analysis
The plasma levels of triglycerides (TGs), low-density lipoprotein cholesterol (LDL-C), total cholesterol (TC), fasting plasma glucose, and alanine transaminase (ALT) were detected by a commercial kit according to the manufacturer’s instructions. The plasma interleukin-1β (IL-1β), IL-10, IL-6, and tumor necrosis factor-alpha (TNF-α) levels were evaluated by commercial ELISA kits from Neobioscience Biological Technology Co., Ltd. (Shenzhen, China).
Gut microbiota analysis by 16S rRNA gene sequencing
The genomic DNA was extracted from the feces of the mice using the QiAamp DNA Stool Mini Kit (no. 51504, Qiagen, Germany). The V3-V4 region was amplified from purified DNA with the primers 341F (CCTACGGGNGGCWGCAG) and 805R (GACTACHVGGGTATCTAATCC). Sequencing was performed at an Illumina MiSeq platform by DeepBiome Co., Ltd. (Jinan, China) based on the manufacturer’s guidelines to obtain the raw fastq files. The quality filtering of data was carried out using Trimmomatic (version 0.36). The paired reads were merged by USEARCH (version 11.2.64)1 using the default parameters. The zero-radius operational taxonomic unit (ZOTU) was obtained using USEARCH. The bioinformatic analysis was performed by a previously reported method (28).
Short-chain fatty acid analyses
The levels of short-chain fatty acids (SCFAs) in mice cecal contents, including acetic, propionic, and n-butyric, were analyzed by gas chromatography (GC, 6890 N, Agilent) equipped with flame ionization detector (FID) and HP-INNOWAX capillary column (30 m × 0.25 mm × 0.25 μm, Agilent) using 2-ethylbutyric acid as internal standard (29). Briefly, the distilled water was added to cecal contents at a ratio of 1:5 (w/v). After centrifugation, the samples were mixed with internal standard solution (0.3 mg/mL of 2-ethylbutyric acid prepared in 0.2 mol/L of HCl solution) in equal volumes to obtain the solutions for GC analysis. The conditions of GC analysis were described in the previous work (29).
Ribonucleic acid extraction and quantification of gene expression
The total ribonucleic acid (RNA) in liver tissue was extracted by TaKaRa MiniBEST Universal RNA Extraction Kit (TaKaRa Bio. Inc., Beijing, China). The RNA was diluted and reverse-transcribed to cDNA by PrimeScript RT Master Mix (TaKaRa) after quantifying by using NanoDrop 2000 Spectrophotometer (Thermo Fisher Scientific Inc., Waltham, MA, USA). Then, the cDNA was used for RT-qPCR analysis using SYBR Green Master Mix on a QuantStudio 6 Flex (Thermo Fisher Scientific Inc.). The glyceraldehyde-3-phosphate dehydrogenase (GAPDH) was used as a housekeeping gene, and the mRNA expression was calculated using the 2–ΔΔCt method. The specific primers used are summarized in Supplementary Table 1.
Statistical analysis
The outliers in this work were checked by GraphPad Prism 9.3.1 based on the Grubbs test (San Diego, CA, USA). The data were presented as mean ± SEM. The normality of all data was checked by SPSS 22 software (IBM) according to Shapiro–Wilk test. If the data for multiple-group comparisons had normal distribution, the statistical significance was performed by SPSS 22 software (IBM) using the one-way ANOVA procedure followed by the Tukey test; otherwise, the statistical significance was calculated using the Mann–Whitney test. The relationship between the parameters of obesity and gut microbiota was analyzed by Spearman’s correlation analysis using SPSS 22 software using the data of all samples in ND, HFD, and HFD-MP groups (24 samples). All results were considered statistically significant at p < 0.05.
Results
Mannoproteins treatment ameliorated obesity and liver steatosis in high-fat diet-induced obese mice
As shown in Figure 1, HFD significantly resulted in the obesity of mice by increasing the body weight, promoting the accumulation of white adipose tissue, and inducing hepatic lipid accumulation and steatosis. MP treatment could significantly decrease body weight gain from the sixth week until the end of this work. Compared with the HFD group, the MP-treated mice showed reduced body weight, and accumulation of epididymal, mesentery, and subcutaneous fat tissues (Figures 1A–F). Furthermore, MP intervention could significantly ameliorate the steatosis and damage to the liver. as indicated by decreased levels of ALT in plasma (Figure 1H) and H&E staining of liver tissue (Figures 1K,L). It was observed that HFD could induce extensive liver injury, increased fatty vesicles, and inflammatory cell infiltration, which was significantly reversed by MP intervention. However, MPs showed a limited effect on the accumulation of perirenal fat (Figure 1E) and liver weight (Figure 1G). As shown in Supplementary Figure 1, the food intake and energy intake of the HFD-MP group showed no significant difference from that of the HFD group. Thus, the attenuation of obesity by MP treatment was not due to the reduction in food consumption.
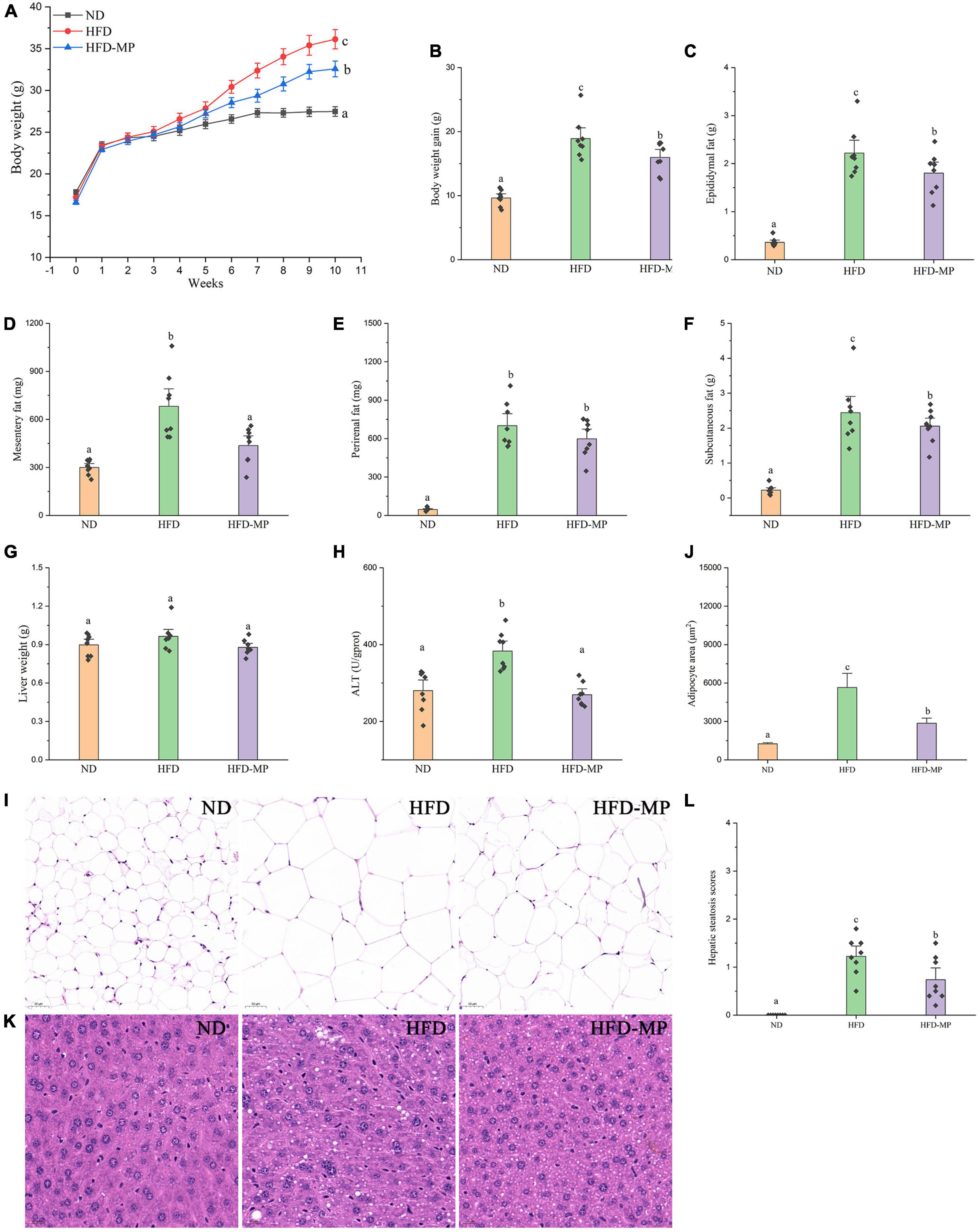
Figure 1. Impact of HFD and MP intervention on body features of mice. (A) Body weight, (B) body weight gain, weights of (C) epididymal, (D) mesentery, (E) perirenal, (F) subcutaneous fat pads, (G) liver weight, (H) plasma alanine transaminase (ALT) level, epididymal adipocyte sections after H&E staining (I), quantification of adipocyte area by ImageJ software (J), liver (K) sections after H&E staining, and hepatic steatosis scores (L). The data are represented as the mean ± SEM. Statistical differences were carried out by one-way ANOVA followed by Turkey’s test, p < 0.05 indicates significant differences.
Mannoproteins treatment improved hyperlipidemia, decreased plasma glucose, and ameliorated systemic inflammation in high-fat diet-induced obese mice
High-fat diet could significantly increase the level of glucose in plasma, which was reduced after administration of MPs (Figure 2A). HFD usually leads to hyperlipidemia, and thereby increases the potential risk for metabolism-related diseases. Thus, the plasma levels of TC, TG, and LDL-C were also evaluated, and the results showed that high concentrations of TC, TG, and LDL-C induced by HFD were largely reduced by treatment with MPs (Figures 2B–D). Thereinto, the levels of TG and LDL-C in the HFD-MP group showed no significant difference from those in the ND group (p > 0.05), suggesting the superior action of MPs for the prevention of hyperlipidemia. Obesity is closely related to chronic low-grade inflammation (30). The pro-inflammatory cytokines in plasma, including TNF-α, IL-1β, IL-6, and IL-10, were detected to evaluate the anti-inflammation effect of MPs in HFD-induced obese mice (Figure 3). It was found that HFD could significantly increase the plasma levels of IL-1β and IL-6, but showed a limited effect on the content of TNF-α and IL-10. MP treatment could reverse the level of IL-6, which showed no significant difference from that in the ND group. However, MP intervention could not change the levels of TNF-α, IL-1β, and IL-10. Furthermore, the effect of MPs on the relative mRNA expression levels of TNF-α, IL-1β, and IL-6 in the liver was investigated (Supplementary Figure 2). It was found that HFD could increase the relative mRNA expression levels of TNF-α, IL-1β, and IL-6, whereas MP treatment could significantly downregulate the mRNA expression levels of TNF-α, IL-1β, and IL-6 (p < 0.05).
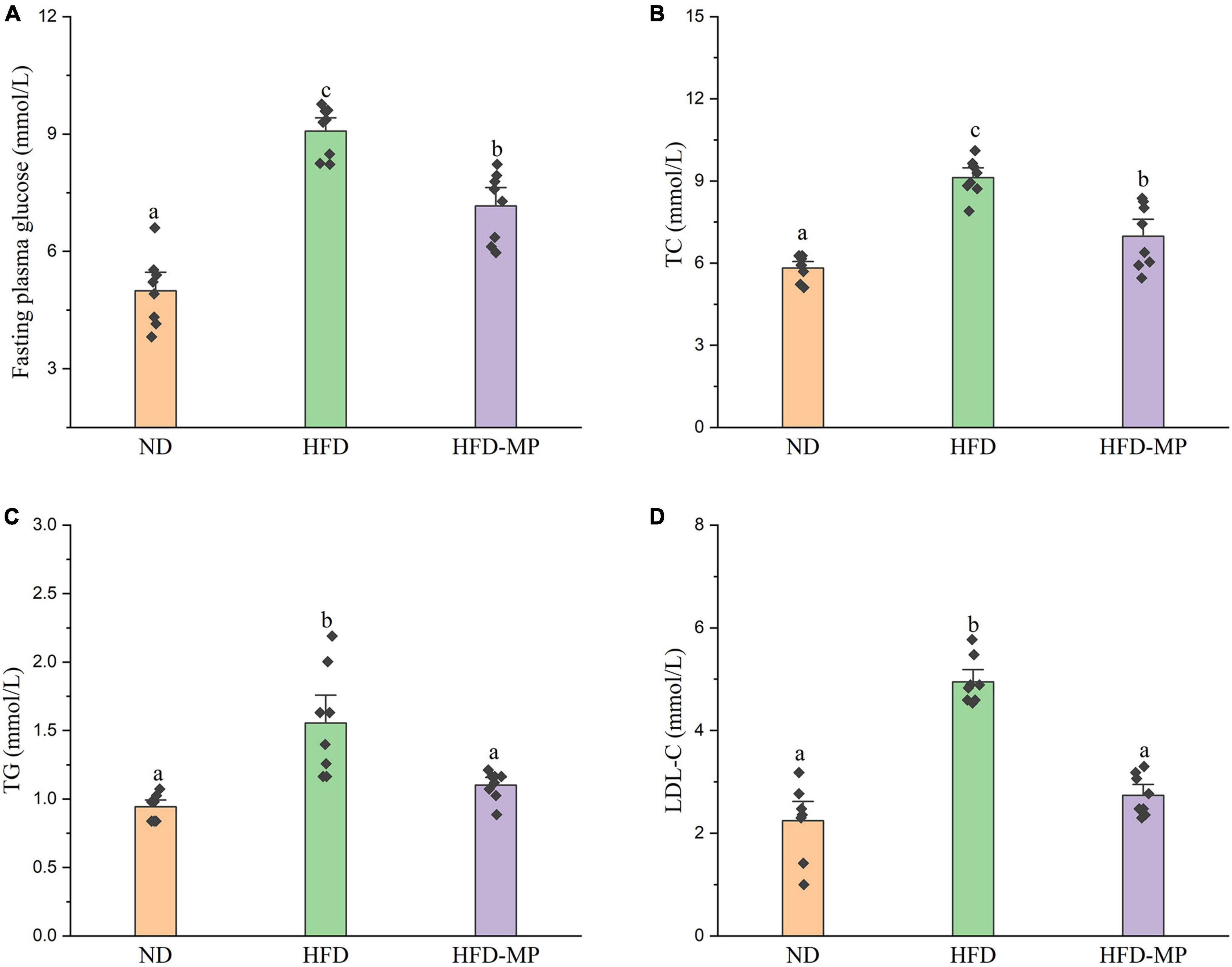
Figure 2. MP intervention reduced HFD-induced high levels of (A) fasting plasma glucose, (B) TC, (C) TG, and (D) LDL-C in plasma. The data are represented as the mean ± SEM. Statistical differences were carried out by one-way ANOVA followed by Turkey’s test, p < 0.05 indicates significant differences.
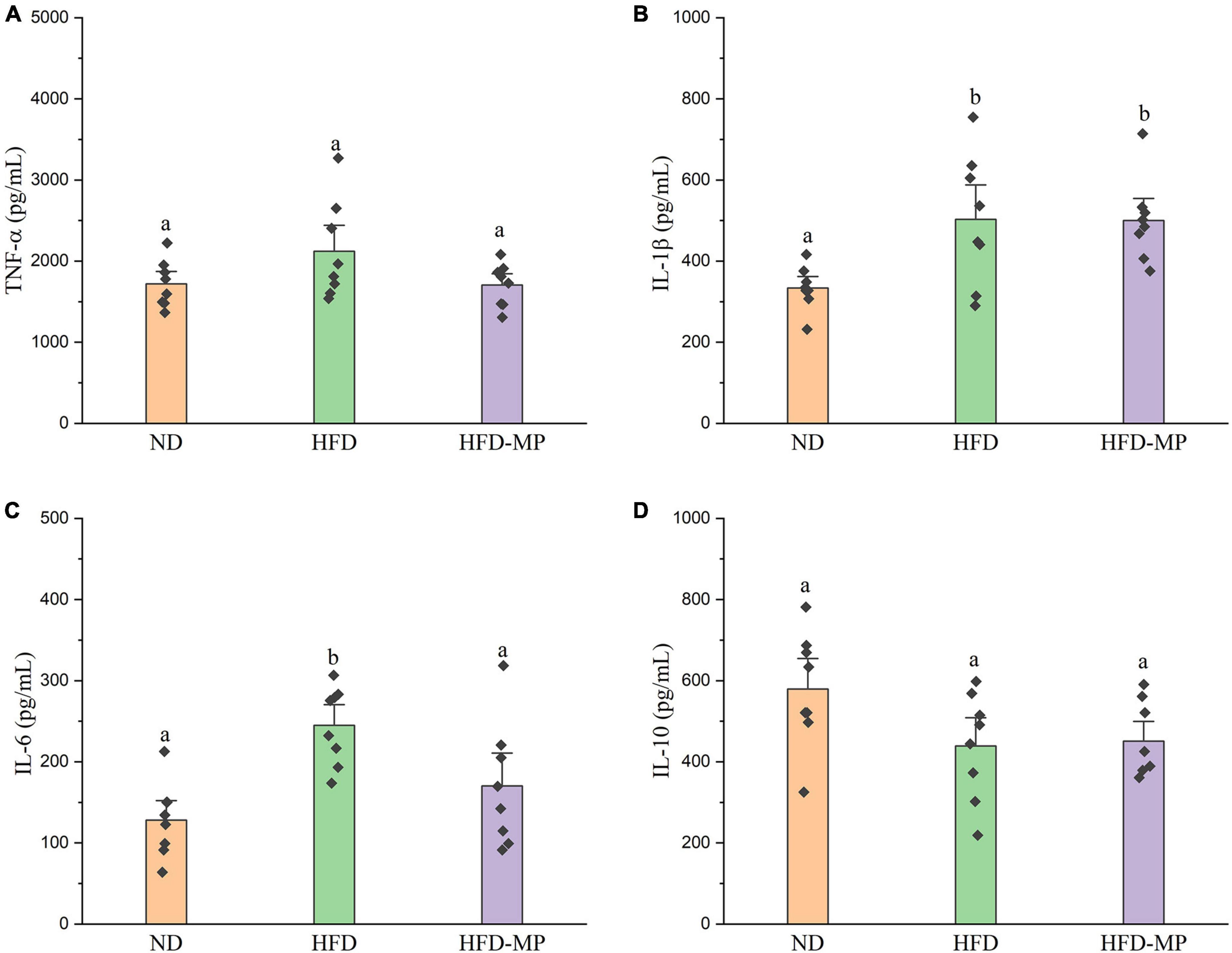
Figure 3. MP intervention attenuated HFD-induced chronic inflammation, including (A) TNF-α, (B) IL-1β, (C) IL-6, and (D) IL-10. The data are represented as the mean ± SEM. Statistical differences were carried out by one-way ANOVA followed by Tukey’s test, p < 0.05 indicates significant differences.
Mannoproteins attenuated high-fat diet-induced dysbiosis of gut microbiota
An increasing number of studies have demonstrated that gut microbiota is related to the etiology of obesity and obesity-related complications (31). Thus, it was hypothesized that the gut microbiota was a potential target responsible for the prevention of obesity by MPs. In the present work, the high-throughput sequencing technology was used to systematically analyze the changes in gut microbiota after supplementation with MPs. The Chao1, Richness, Simpson, and Shannon indexes were calculated to quantify the alpha-diversity of gut microbiota, as shown in Supplementary Figure 3. HFD could significantly reduce the Chao1 and Richness indexes. Furthermore, Simpson and Shannon indexes in the HFD group also showed increased or decreased trends compared with those in the ND group with no significant difference. As expected, MPs could reverse these changes of alpha-diversity induced by HFD, by increasing the Chao1, Richness, and Shannon indexes, and decreasing the Simpson index.
Diet plays an important role in shaping the structure and composition of gut microbiota (32). Principal coordinate analysis (PCoA) was first carried out to visualize the differences in the structure of gut microbiota after HFD and MP treatments. It was found that ND and HFD groups could be clearly distinguished on the basis of the results of PCoA (Figure 4A). Compared with the HFD group, significant separation was also observed after MP treatment, suggesting that MP intervention could change the HFD-treated structure of gut microbiota. Furthermore, principal component analysis (PCA) and hierarchical cluster analysis largely agreed with the result of PCoA (Figures 4B,C). Interestingly, based on the PC1 (41.43%) value in the result of PCA, MPs could lead to a significant shift in the gut microbiota from the HFD group toward the ND group. Thus, MPs significantly modulated the HFD-induced dysbiosis of gut microbiota back to health status. At the phylum level, the gut microbiota of ND, HFD, and HFD-MP groups was all mainly composed of Firmicutes and Bacteroidetes (Figure 5A), which was consistent with the previous works (33, 34). Differently, HFD could significantly increase the relative abundance of Firmicutes and decrease the level of Bacteroidetes compared with the ND group, thereby significantly enhancing the ratio of Firmicutes to Bacteroidetes (Figures 5B–D). MPs could reverse this change induced by HFD treatment, by increasing the relative abundance of Bacteroidetes and decreasing the level of Firmicutes. Furthermore, the ratio of Firmicutes to Bacteroidetes in the HFD-MP group showed no significant difference compared to that observed in the ND group.
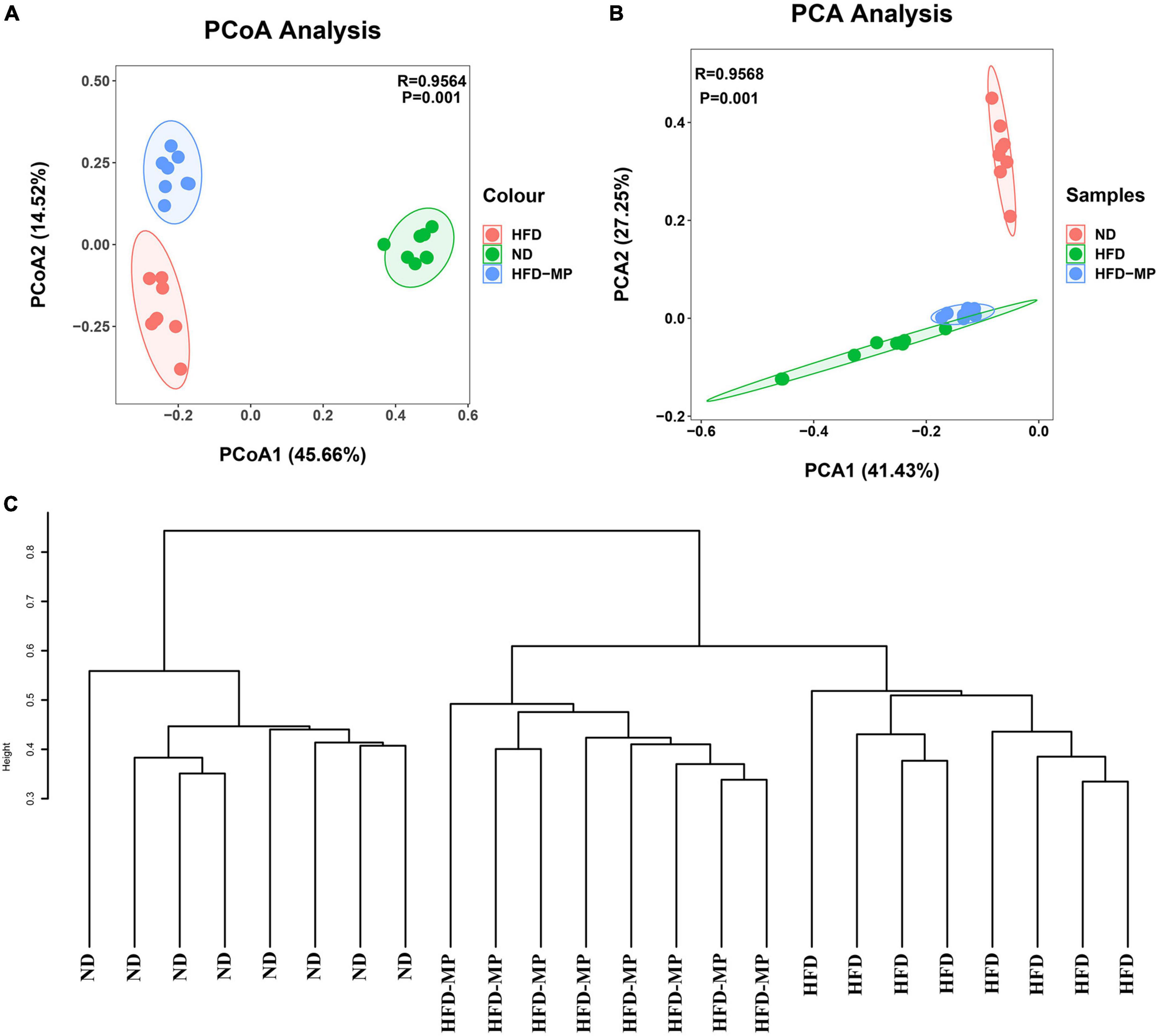
Figure 4. MPs attenuated HFD-induced dysbiosis of gut microbiota evaluated by beta-diversity analyses, including (A) PCoA, (B) PCA, and (C) hierarchical cluster analysis.
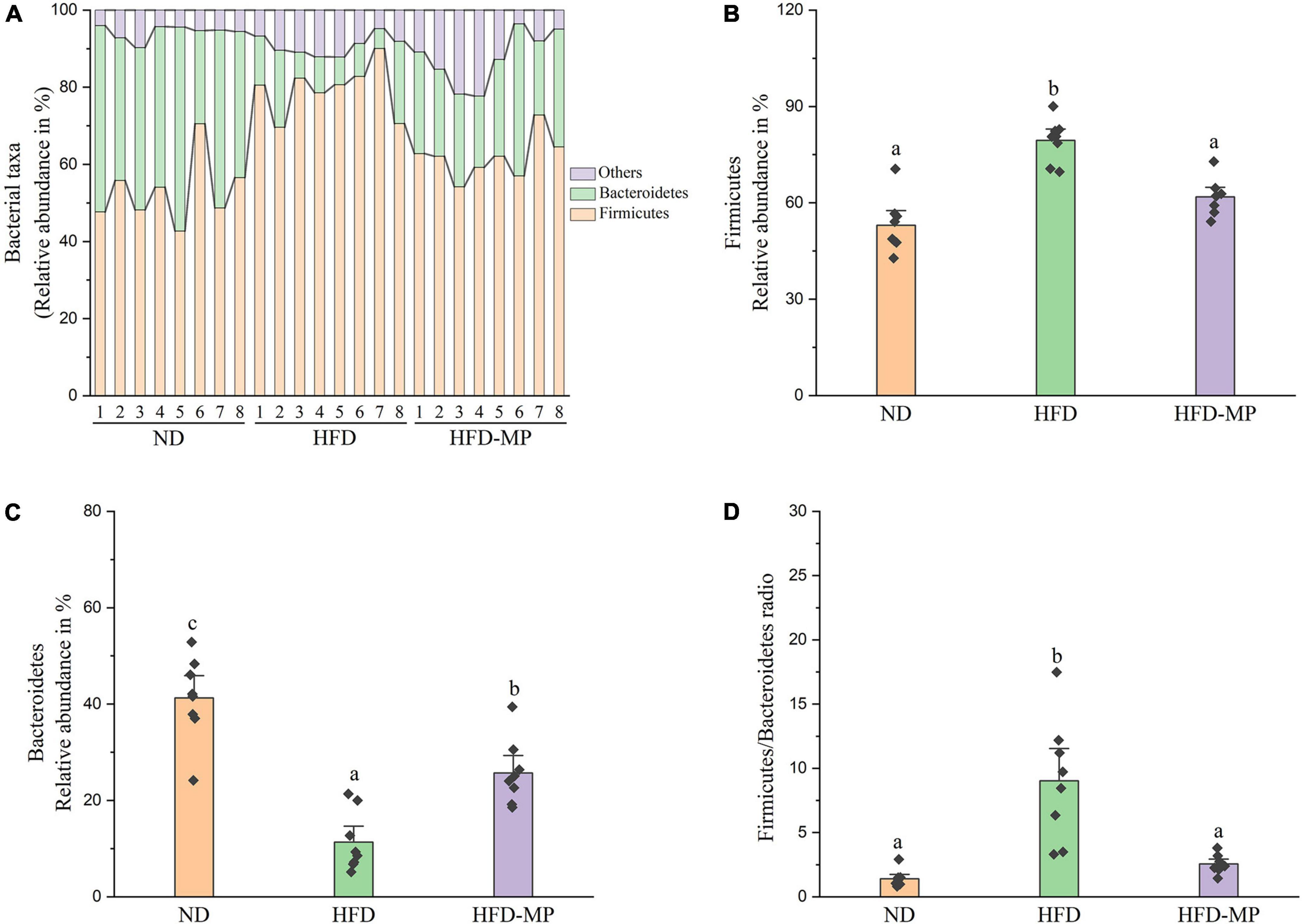
Figure 5. MPs modulate the HFD-disrupted gut microbiota composition at the phylum level. (A) Bacterial taxonomic profiling and relative abundances of (B) Firmicutes, (C) Bacteroidetes, and (D) the ratio of Firmicutes to Bacteroidetes. The data are represented as the mean ± SEM. Statistical differences were carried out by one-way ANOVA followed by Tukey’s test, p < 0.05 indicates significant differences.
The gut microbiota at the family level was comparatively analyzed in this work (Figure 6). The result showed that HFD treatment could decrease the relative abundance of Porphyromonadaceae and increase the levels of Lactobacillaceae, Ruminococcaceae, Rikenellaceae, and Desulfovibrionaceae. Compared with the HFD group, MP intervention could significantly increase the relative abundance of Bacteroidaceae, Ruminococcaceae, and Rikenellaceae, and decrease the level of Lactobacillaceae. The gut microbiota at the genus level was also analyzed, as shown in Table 1. Most strikingly, the relative abundance of Lactobacillus was increased from 3.94 ± 1.30% to 19.99 ± 3.94% after HFD treatment, which was then decreased to 2.68 ± 0.77% by MP intervention. Likewise, HFD resulted in a significant decrease in the level of Parabacteroides, which was reversed by MP treatment. Thus, the modulated effect of MPs on the relative abundance of Lactobacillus and Parabacteroides may play an important role in the prevention of HFD-induced obesity. Furthermore, MPs could also increase the relative abundance of Alistipes, Bacteroides, and Mucispirillum compared with that in the ND and HFD groups. Then, the relationship between the changed gut microbiota at the genus level by MP and phenotypical changes in obesity was analyzed by Spearman correlation, as shown in Supplementary Figure 4. It was found that Parabacteroides and Alistipes showed a significant correlation with obesity.
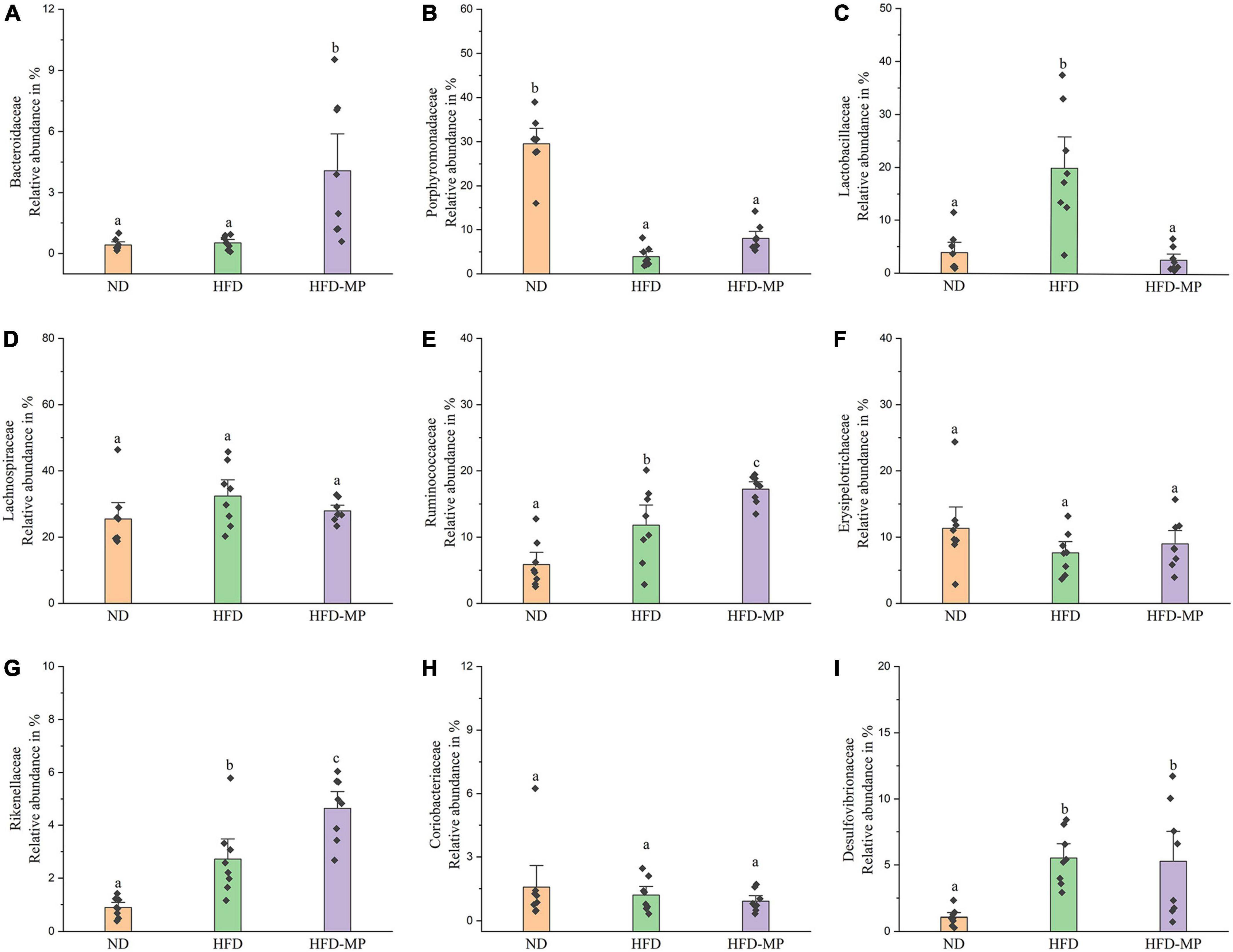
Figure 6. The comparative analysis of nine main families of the gut microbiota, including (A) Bacteroidaceae, (B) Porphyromonadaceae, (C) Lactobacillaceae, (D) Lachnospiraceae, (E) Ruminococcaceae, (F) Erysipelotrichaceae, (G) Rikenellaceae, (H) Coriobacteriaceae, and (I) Desulfovibrionaceae. The data are represented as the mean ± SEM. Statistical differences were carried out by one-way ANOVA followed by Tukey’s test, p < 0.05 indicates significant differences.
The different gut microbiota at the same genus level may show different responses after treatment with MPs, thus the gut microbiota at the ZOTU level was analyzed to further excavate the key gut microbiota contributing to the prevention of obesity. The ZOTUs with a relative abundance of more than 0.1% were used to proceed with further analysis. As shown in Figure 7, HFD and MPs could significantly change 62 ZOTUs compared with the ND group. HFD could lead to 41 changed ZOTUs, including increasing 23 ZOTUs and decreasing 18 ZOTUs. Thereinto, nine ZOTUs were found to be significantly reversed by MP intervention. Then, the relationship between the relative abundance of these reversed ZOTUs and phenotypical changes of obesity was analyzed by Spearman correlation (Figure 8), and the result showed that seven ZOTUs were positively corrected with obesity, and two ZOTUs were negatively associated with obesity. Thereinto, two ZOTUs (ZOTU2 and ZOTU14) belonging to Lactobacillus and two ZOTUs (ZOTU43 and ZOTU108) belonging to Parabacteroides distasonis may play a key role in the prevention of obesity.
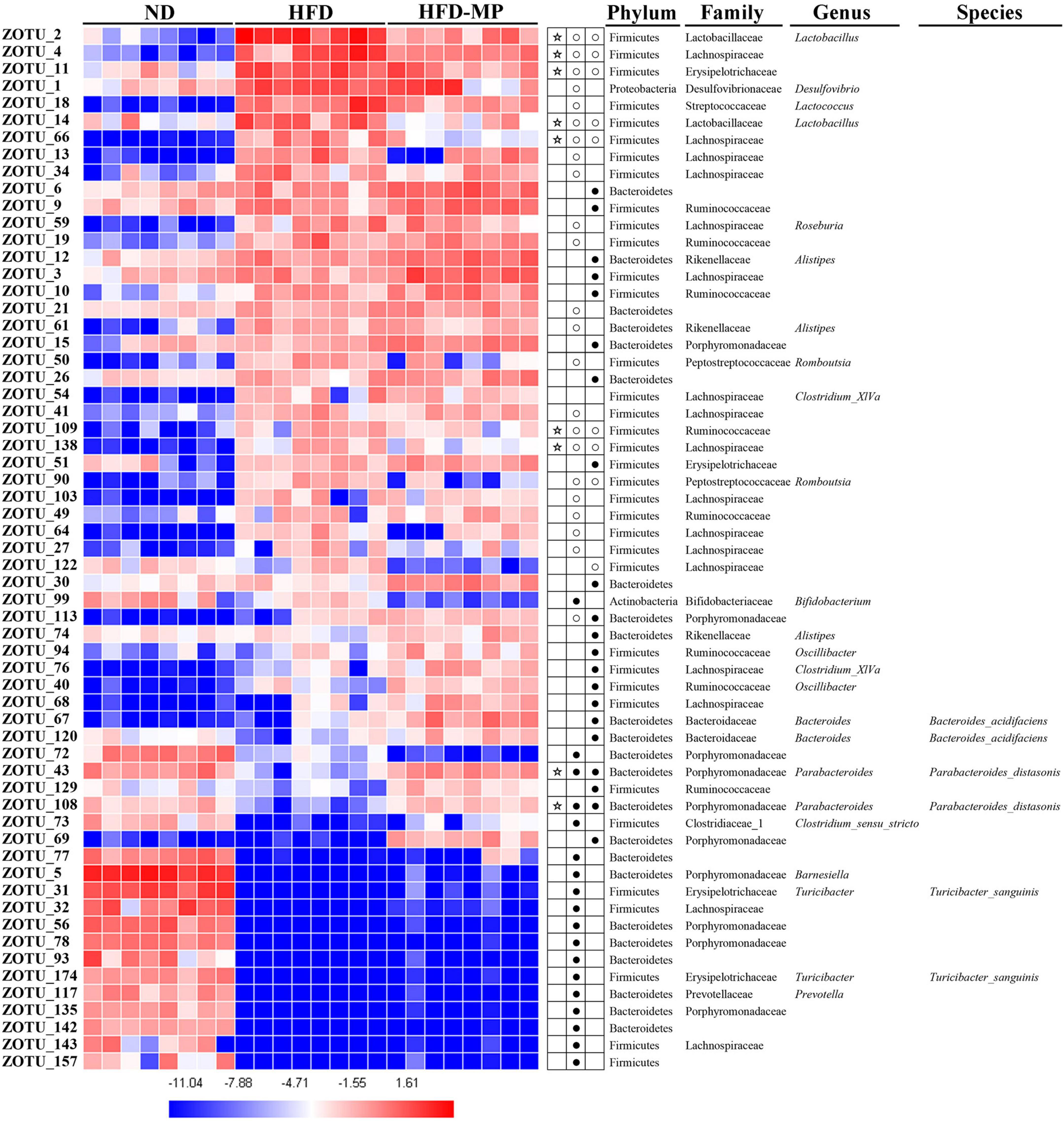
Figure 7. Gut microbiota composition was altered by HFD or MP intervention at the ZOTUs levels. Heatmap shows the relative abundance of 62 ZOTUs (ln transformed). The dots (●) and circles (○) showed the more or less relative abundances of ZOTUs in ND or MPs groups compared with the HFD group. The star (☆) represented ZOTUs changed by HFD but were reversed after treatment with MPs. Statistical differences were carried out by one-way ANOVA followed by Tukey’s test, p < 0.05 indicates significant differences.
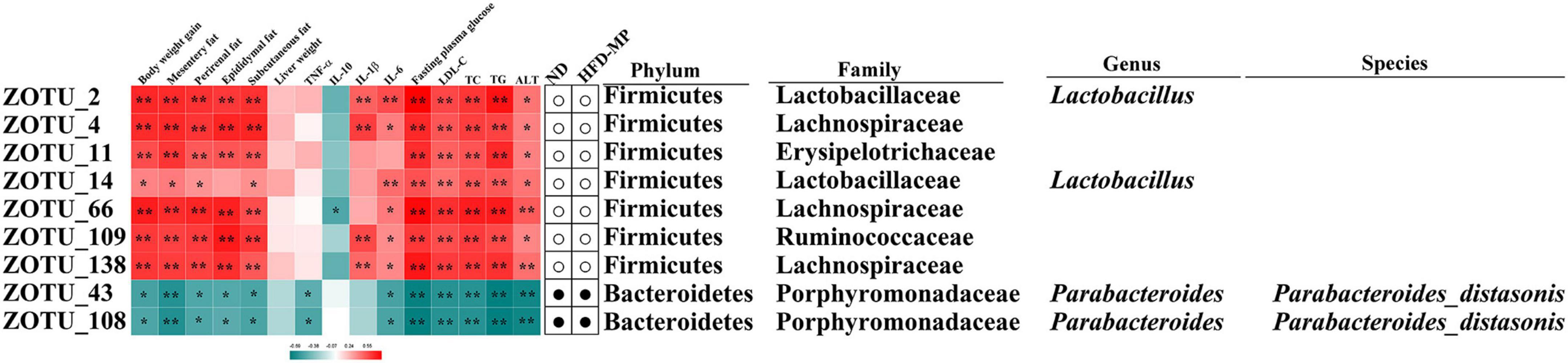
Figure 8. Nine ZOTUs reversed by MP intervention were significantly correlated with features of obesity. The heatmap shows the R-value of Spearman’s correlation between the relative abundance of ZOTUs and features of obesity. The dots (correlation between the more or less relative abundance of ZOTUs in the ND or MP groups compared with the HFD group. * and **Show the significant associations (p < 0.05 and p < 0.01, respectively) based on Spearman’s correlation analysis.
Mannoproteins increased the content of acetic acid in high-fat diet-fed mice
The SCFAs are the main metabolites produced by the fermentation of polysaccharides by gut microbiota, which are speculated to play an important role in the biological activities of polysaccharides (35). Thereinto, acetic acid, propionic acid, and butyric acid are the most abundant SCFAs in the human body and colon, whereas other SCFAs, such as formate, valerate, and caproate, are minor end products in the colon (36). Thus, the levels of acetate, propionate, and butyrate were measured in different groups, and the result showed that HFD significantly reduced the levels of acetate, propionate, and butyrate in the cecal contents of mice (Supplementary Figure 5). The MP intervention could lead to a significant increase in the level of acetic acid from 3.28 ± 0.22 mmol/g to 7.84 ± 0.96 mmol/g; however, MPs showed a limited effect on the content of propionic acid and butyric acid. Furthermore, the effect of MPs on the mRNA expression of G protein-coupled receptors (GPRs), including GPR43 and GPR41, in the liver was investigated to further verify the key role of SCFAs in the attenuation of obesity (Supplementary Figure 6). The result showed that HFD treatment significantly reduced the mRNA expression of GPR41 and GPR43, whereas MP intervention could upregulate the expression of GPR41 and GPR43.
Discussion
Obesity has become a leading public health problem with pandemic proportions and can further increase the rates of complications, such as cardiovascular disease and type 2 diabetes (T2D) (37). An increasing number of studies have demonstrated that gut microbiota populations are sensitive to genetic, environmental, and diet influences, and hence can directly or indirectly affect the energy balance and energy stores (12). Thus, the gut microbiota is expected as a promising target for the prevention and treatment of obesity (38). Polysaccharides, serving as a superior prebiotic, could modulate the gut microbiota by selectively stimulating the growth of beneficial bacteria and inhibiting the harmful microbiota, thereby improving host health (39). Furthermore, polysaccharides could also modulate the metabolism of probiotics (40). MP is one of the most important components of yeast cell walls. The previous work has shown that MPs could stimulate angiogenesis (27) and had immunoactivities and antineoplastic activities (26). However, the effects of MPs on obesity and gut microbiota are still unknown. Here, the HFD-induced obesity mice model was used to investigate the potential anti-obesogenic effect of MPs. Over 10 weeks of treatment, HFD could significantly induce obesity in the mice model. As expected, MPs significantly prevented HFD-induced body weight gain, fat accumulation, and liver steatosis. Furthermore, the levels of glucose, TC, TG, and LDL-C in plasma were also ameliorated by the treatment with MP. Thus, the results in the present work demonstrated that MP intervention could reduce obesity and metabolic disorders in HFD-fed mice. However, it would be more convincing if the dose setting or positive control was involved in this work.
It has been reported that HFD could damage the gut integrity and lead to a leaky gut, and the endotoxin lipopolysaccharide (LPS) released from Gram-negative bacteria in the gut enter the bloodstream, thereby leading to metabolic inflammation in obese mice (30). Thus, HFD-induced obesity is usually associated with chronic, low-grade inflammation. In the present work, IL-1β, and IL-6 levels were significantly enhanced after HFD treatment, and MPs could significantly reduce the level of IL-6, which showed no significant difference from those in the ND group. The decrease in the pro-inflammatory cytokines by the dietary polysaccharides contributing to the prevention of metabolic diseases has been widely reported. For example, an insoluble polysaccharide from the sclerotium of Poria cocos could reduce the plasmatic TNF-α in ob/ob mice (41). Likewise, the polysaccharides isolated from Hirsutella sinensis decreased the serum levels of the pro-inflammatory cytokines IL-1β and TNF-α in the HFD-fed mice (42). Thus, the improvement of metabolic disorders by MPs might be related to the suppression of chronic inflammation.
The accumulating evidence has demonstrated that dietary habit, especially a HFD, could lead to dysbiosis of gut microbiota, which might thereby lead to some pathologic conditions of obesity and obesity-related complications. Recently, some foods or food additives, such as processed foods (43), dietary emulsifiers (44), and artificial sweeteners (45), could promote metabolic diseases by disordering the gut microbiota. Alpha-diversity, including Chao1, Richness, Simpson, and Shannon indexes, could reflect the diversity and richness of bacteria (46). In this work, HFD could significantly affect the structure and composition of gut microbiota, which is evidenced by decreasing alpha-diversity and changing beta-diversity indexes. The reports in animal and clinical studies showed that decreased alpha-diversity and richness values were observed in obese subjects and animals (28, 47, 48). MP treatment could not only increase the alpha-diversity, including Chao1, Richness, Simpson, and Shannon indexes, but could also change the structure of gut microbiota from the HFD group toward the ND group based on beta-diversity.
The result at the phylum level indicated that the gut microbiota was dominated by Firmicutes and Bacteroidetes. The ratio of Firmicutes to Bacteroidetes has been reported to relate to metabolic diseases, and high levels of Firmicutes and low levels of Bacteroidetes were observed in obese humans and animals (49–51). Thus, the decrease in the ratio of Firmicutes to Bacteroidetes may contribute to the prevention of obesity and metabolic diseases. A decrease in the ratio of Firmicutes to Bacteroidetes by anti-obesogenic candidates was widely reported (52, 53). In this work, a similar trend toward a decreased ratio of Firmicutes to Bacteroidetes was obtained after MP treatment, which may contribute to the prevention of obesity by MPs. At the family level, the MPs induced increased levels of Bacteroidaceae, Ruminococcaceae, and Rikenellaceae. Therefore, the relative abundance of Bacteroidaceae has been reported to negatively link to obesity (54, 55). Furthermore, the level of Bacteroidaceae is determined by SCFA-producing bacteria (56), which could be regarded as a positive outcome predictor of individual weight loss (57). Lactobacillaceae was usually considered as beneficial bacteria in the gut for the prevention of obesity (58, 59). On the other hand, some reports showed that Lactobacillaceae has a positive relationship with obesity (60, 61). The different bacteria in the same family may play different roles in the metabolic phenotype of obesity, thus the gut microbiota was also analyzed at the genus or ZOTU levels.
It was found that Lactobacillus, belonging to Lactobacillaceae, and Parabacteroides, belonging to Porphyromonadaceae, were significantly reversed by MP treatment. Furthermore, Alistipes (belonging to Rikenellaceae) which was increased by MPs showed a significant correlation with obesity. To further identify species-level phylotypes or specific bacterial taxa contributing to the prevention of obesity by MPs, the gut microbiota was analyzed at the ZOTU level. Lactobacillus (ZOTU2 and ZOTU14), which was positively corrected with obesity, and P. distasonis (ZOTU43 and ZOTU108), which showed negative relation to obesity, were significantly reversed after MP intervention. P. distasonis, regarded as one of the 18 core members in the human gut microbiota, plays an important role in human health (62). The lower level of P. distasonis has been observed in patients and animals with metabolic diseases (63, 64). Furthermore, the alleviation of obesity and obesity-related dysfunctions by P. distasonis has been reported, which was due to the generation of succinate and secondary bile acids in the gut (14). Moreover, the other health-promoting functions of P. distasonis, such as blocking colon tumor formation (65) and alleviating colitis (66), have also been widely reported. Thus, P. distasonis has been regarded as potential probiotic for improving our health (67). The previous work showed that polysaccharides, such as inulin, could promote the proliferation of P. distasonis and thereby improve human health (68). In this work, the MPs could also increase the level of P. distasonis in HFD-induced obese mice contributing to the prevention of obesity. Lactobacillus showed a particularly interesting role in this work. It is well known that lots of species in the genus Lactobacillus are probiotic bacteria (69, 70), which can reduce the risk of metabolic diseases. On the other hand, a report showed that some species belonging to Lactobacillus, such as Lactobacillus reuteri, were positively associated with obesity, while others were related to normal weight (71). Recently, a systematic review of randomized controlled clinical trials summarized the effect of Lactobacillus on obesity, and it was found that the beneficial or detrimental effects of Lactobacillus on obesity are strain-dependent (72). Thus, the prevention of obesity by MPs might be related to the inhibition of Lactobacillus. Unfortunately, the species for Lactobacillus in this work could not be identified by sequencing, which should be further investigated.
SCFAs, the key metabolites produced by gut microbiota, play an important role in improving colonic and systemic health (35), which could help to explain why and how the changes in gut microbiota contribute to human health and diseases (73). A growing amount of evidence suggests that SCFAs could enter into the bloodstream, and thereby affect the tissues and organs beyond the gut (74). Therefore, the specific species, diversity, and absolute amount of gut microbiota play a key role in the production of SCFAs (75). The diet intervention could alter either the bacterial species or the bacterial biosynthetic enzymes, thereby leading to alterations in microbial SCFA production (76). A potential strategy based on the modulation of gut microbiota by prebiotics has been presented to stimulate the production of SCFAs, thereby preventing diseases and improving human health (77, 78). Thus, we suspected that the SCFAs would be changed due to the modulation of gut microbiota by MP, which thereby contributes to the prevention of obesity. In this work, MPs could increase the level of acetate decreased by HFD treatment, whereas they showed limited effects on the contents of propionate and butyrate. Furthermore, the mRNA expression of GPR41 and GPR43 in the liver was significantly upregulated by MPs, suggesting that SCFAs played a key role in the prevention of obesity by MPs. A lot of reports have shown that acetate administration could reduce body weight, decrease hepatic fat accumulation, and improve insulin sensitivity in HFD-fed mice (79, 80). Likewise, SCFA intervention studies in humans also further demonstrated that consumption of acetate could significantly reduce the body weight of patients with obesity (81). SCFAs could activate GPR41 and GPR43 to improve immune responses, and the activation of GPR41/43 could further modulate the levels of pro-inflammatory factors. It has been reported that polysaccharides-derived SCFAs could significantly reduce the level of pro-inflammatory factors, such as LPS in the blood. Furthermore,SCFA might also directly reverse LPS-induced inflammation (75, 82). In addition, acetate can significantly regulate the levels of DNA methylation at the host miR-378a promoter, which also contribute to the improvement of obesity and glucose intolerance (83). It has been reported that Parabacteroides could utilize polysaccharides with its glycoside hydrolase, and further produced acetate to affect host health (84, 85). It is expected to be a potential strategy to increase the level of acetate by prebiotics, thereby preventing and treating obesity. Thus, the increased level of acetate by MPs may also contribute to the prevention of obesity in this work. In addition to SCFAs, the other metabolites were not measured in the present study, which could be further investigated by metabolomics in our next work (86).
Conclusion
In conclusion, the HFD-induced obese mice model was used to investigate the potential anti-obesogenic effect of MPs and its potential mechanism. The result showed that MPs significantly attenuated HFD-induced obesity. MPs could not only increase the alpha-diversity of gut microbiota, but also change the structure of gut microbiota from the HFD group to the ND group. Furthermore, harmful Lactobacillus and probiotic P. distasonis may be potential key gut microbiota responsible for the prevention of obesity by MPs. This preliminary research showed promise for the efficacy of MPs in the prevention of HFD-induced obesity, thus MPs were expected to serve as a functional food for the improvement of human health.
Data availability statement
The original contributions presented in this study are publicly available. This data can be found here: Genome Sequence Archive in the BIG Data Center Chinese Academy of Sciences (https://ngdc.cncb.ac.cn/gsa/) with BioProject number of PRJCA009982 under accession codes of CRA007210.
Ethics statement
All animal experiment protocols were approved by the Institutional Animal Ethics Committee of Experimental Animal Center of Nanjing Agricultural University.
Author contributions
XL, JSW, JLW, GC, and XZ contributed to the conception and design of the study. YK organized the database. DC performed the statistical analysis. XL wrote the first draft of the manuscript. GC and XZ wrote sections of the manuscript. All authors contributed to manuscript revision, read, and approved the submitted version.
Funding
This study was supported by the Angel Nutritech Nutrition Fund (AF2019002), the Research on Open project of Jiangsu Key Laboratory for Bioresources of Saline Soils (JKLBS2020014), and the Priority Academic Program Development of Jiangsu Higher Education Institutions.
Conflict of interest
The authors declare that the research was conducted in the absence of any commercial or financial relationships that could be construed as a potential conflict of interest.
Publisher’s note
All claims expressed in this article are solely those of the authors and do not necessarily represent those of their affiliated organizations, or those of the publisher, the editors and the reviewers. Any product that may be evaluated in this article, or claim that may be made by its manufacturer, is not guaranteed or endorsed by the publisher.
Supplementary material
The Supplementary Material for this article can be found online at: https://www.frontiersin.org/articles/10.3389/fnut.2022.1019344/full#supplementary-material
Footnotes
References
1. Lingvay I, Sumithran P, Cohen RV, le Roux CW. Obesity management as a primary treatment goal for type 2 diabetes: time to reframe the conversation. Lancet. (2022) 399:394–405. doi: 10.1016/S0140-6736(21)01919-X
2. SantaCruz-Calvo S, Bharath L, Pugh G, SantaCruz-Calvo L, Lenin RR, Lutshumba J, et al. Adaptive immune cells shape obesity-associated type 2 diabetes mellitus and less prominent comorbidities. Nat Rev Endocrinol. (2022) 18:23–42. doi: 10.1038/s41574-021-00575-1
3. Malik VS, Hu FB. The role of sugar-sweetened beverages in the global epidemics of obesity and chronic diseases. Nat Rev Endocrinol. (2022) 18:205–18. doi: 10.1038/s41574-021-00627-6
4. Xu Z, Jiang W, Huang W, Lin Y, Chan FKL, Ng SC. Gut microbiota in patients with obesity and metabolic disorders - a systematic review. Genes Nutr. (2022) 17:2. doi: 10.1186/s12263-021-00703-6
5. Shi Q, Wang Y, Hao Q, Vandvik PO, Guyatt G, Li J, et al. Pharmacotherapy for adults with overweight and obesity: a systematic review and network meta-analysis of randomised controlled trials. Lancet. (2022) 399:259–69. doi: 10.1016/S0140-6736(21)01640-8
6. Xu X, Zhao C, Li B, Tang G, Shang A, Gan R, et al. Effects and mechanisms of tea on obesity. Crit Rev Food Sci. (2021):1–18. Online ahead of print. doi: 10.1080/10408398.2021.1992748
7. Gérard P. Gut microbiota and obesity. Cell Mol Life Sci. (2016) 73:147–62. doi: 10.1007/s00018-015-2061-5
8. Martel J, Ojcius DM, Chang C, Lin C, Lu C, Ko Y, et al. Anti-obesogenic and antidiabetic effects of plants and mushrooms. Nat Rev Endocrinol. (2017) 13:149–60. doi: 10.1038/nrendo.2016.142
9. de Clercq NC, Groen AK, Romijn JA, Nieuwdorp M. Gut microbiota in obesity and undernutrition. Adv Nutr. (2016) 7:1080–9. doi: 10.3945/an.116.012914
10. Lynch SV, Pedersen O. The human intestinal microbiome in health and disease. N Engl J Med. (2016) 375:2369–79. doi: 10.1056/NEJMra1600266
11. Zhao L. The gut microbiota and obesity: from correlation to causality. Nat Rev Microbiol. (2013) 11:639–47. doi: 10.1038/nrmicro3089
12. Rosenbaum M, Knight R, Leibel RL. The gut microbiota in human energy homeostasis and obesity. Trends Endocrinol Metab. (2015) 26:493–501. doi: 10.1016/j.tem.2015.07.002
13. Yoon HS, Cho CH, Yun MS, Jang SJ, You HJ, Kim J, et al. Akkermansia muciniphila secretes a glucagon-like peptide-1-inducing protein that improves glucose homeostasis and ameliorates metabolic disease in mice. Nat Microbiol. (2021) 6:563–73. doi: 10.1038/s41564-021-00880-5
14. Wang K, Liao M, Zhou N, Bao L, Ma K, Zheng Z, et al. Parabacteroides distasonis alleviates obesity and metabolic dysfunctions via production of succinate and secondary bile acids. Cell Rep. (2019) 26:222–35. doi: 10.1016/j.celrep.2018.12.028
15. Le Roy T, Moens De Hase E, Van Hul M, Paquot A, Pelicaen R, Régnier M, et al. Dysosmobacter welbionis is a newly isolated human commensal bacterium preventing diet-induced obesity and metabolic disorders in mice. Gut. (2022) 71:534–43. doi: 10.1136/gutjnl-2020-323778
16. Woting A, Pfeiffer N, Loh G, Klaus S, Blaut M. Clostridium ramosum promotes high-fat diet-induced obesity in gnotobiotic mouse models. mBio. (2014) 5:e01530–14. doi: 10.1128/mBio.01530-14
17. Fei N, Bruneau A, Zhang X, Wang R, Wang J, Rabot S, et al. Endotoxin producers overgrowing in human gut microbiota as the causative agents for nonalcoholic fatty liver disease. mBio. (2020) 11:e03263–19. doi: 10.1128/mBio.03263-19
18. Gentile CL, Weir TL. The gut microbiota at the intersection of diet and human health. Science. (2018) 362:776–80. doi: 10.1126/science.aau5812
19. Gimeno RE, Briere DA, Seeley RJ. Leveraging the gut to treat metabolic disease. Cell Metab. (2020) 31:679–98. doi: 10.1016/j.cmet.2020.02.014
20. Makki K, Deehan EC, Walter J, Bäckhed F. The impact of dietary fiber on gut microbiota in host health and disease. Cell Host Microbe. (2018) 23:705–15. doi: 10.1016/j.chom.2018.05.012
21. Li J, Karboune S. Characterization of the composition and the techno-functional properties of mannoproteins from Saccharomyces cerevisiae yeast cell walls. Food Chem. (2019) 297:124867. doi: 10.1016/j.foodchem.2019.05.141
22. Li J, Karboune S, Sedman J, Ismail A. Characterization of the structural properties of mannoproteins isolated from selected yeast-based products upon the enzymatic treatment. LWT Food Sci Technol. (2020) 131:109596. doi: 10.1016/j.lwt.2020.109596
23. Chen G, Chen D, Zhou W, Peng Y, Chen C, Shen W, et al. Improvement of metabolic syndrome in high-fat diet-induced mice by yeast β-Glucan is linked to inhibited proliferation of Lactobacillus and Lactococcus in gut microbiota. J Agric Food Chem. (2021) 69:7581–92. doi: 10.1021/acs.jafc.1c00866
24. Wu J, Guan Y, Zhong Q. Yeast mannoproteins improve thermal stability of anthocyanins at pH 7.0. Food Chem. (2015) 172:121–8. doi: 10.1016/j.foodchem.2014.09.059
25. Liu H, Liu L, Hui H, Wang Q. Structural characterization and antineoplastic activity of Saccharomyces cerevisiae mannoprotein. Int J Food Prop. (2015) 18:359–71. doi: 10.1080/10942912.2013.819364
26. Liu H, Wang Q, He Y. Immunoactivities and antineoplastic activities of Saccharomyces cerevisiae mannoprotein. Carbohyd Polym. (2011) 83:1690–5. doi: 10.1016/j.carbpol.2010.10.026
27. Yoon BH, Lee SM, Chang H, Ha CH. Mannoproteins from Saccharomyces cerevisiae stimulate angiogenesis by promoting the akt-eNOS signaling pathway in endothelial cells. Biochem Bioph Res Commun. (2019) 519:767–72. doi: 10.1016/j.bbrc.2019.09.069
28. Chen G, Xie M, Wan P, Chen D, Dai Z, Ye H, et al. Fuzhuan brick tea polysaccharides attenuate metabolic syndrome in High-Fat diet induced mice in association with modulation in the gut microbiota. J Agric Food Chem. (2018) 66:2783–95. doi: 10.1021/acs.jafc.8b00296
29. Chen G, Xie M, Wan P, Chen D, Ye H, Chen L, et al. Digestion under saliva, simulated gastric and small intestinal conditions and fermentation in vitro by human intestinal microbiota of polysaccharides from Fuzhuan brick tea. Food Chem. (2018) 244:331–9. doi: 10.1016/j.foodchem.2017.10.074
30. Chang C, Lin C, Lu C, Martel J, Ko Y, Ojcius DM, et al. Ganoderma lucidum reduces obesity in mice by modulating the composition of the gut microbiota. Nat Commun. (2015) 6:7489. doi: 10.1038/ncomms8489
31. Canfora EE, Meex RCR, Venema K, Blaak EE. Gut microbial metabolites in obesity, NAFLD and T2DM. Nat Rev Endocrinol. (2019) 15:261–73. doi: 10.1038/s41574-019-0156-z
32. Choi BSY, Daniel N, Houde VP, Ouellette A, Marcotte B, Varin TV, et al. Feeding diversified protein sources exacerbates hepatic insulin resistance via increased gut microbial branched-chain fatty acids and mTORC1 signaling in obese mice. Nat Commun. (2021) 12:3377. doi: 10.1038/s41467-021-23782-w
33. Chen G, Xie M, Dai Z, Wan P, Ye H, Zeng X, et al. Kudingcha and fuzhuan brick tea prevent obesity and modulate gut microbiota in High-Fat diet fed mice. Mol Nutr Food Res. (2018) 62:1700485. doi: 10.1002/mnfr.201700485
34. Zhang X, Chen J, Yi K, Peng L, Xie J, Gou X, et al. Phlorizin ameliorates obesity-associated endotoxemia and insulin resistance in high-fat diet-fed mice by targeting the gut microbiota and intestinal barrier integrity. Gut Microbes. (2020) 12:1842990. doi: 10.1080/19490976.2020.1842990
35. Koh A, De Vadder F, Kovatcheva-Datchary P, Bäckhed F. From dietary fiber to host physiology: short-chain fatty acids as key bacterial metabolites. Cell. (2016) 165:1332–45. doi: 10.1016/j.cell.2016.05.041
36. Dalile B, Van Oudenhove L, Vervliet B, Verbeke K. The role of short-chain fatty acids in microbiota–gut–brain communication. Nat Rev Gastroenterol Hepat. (2019) 16:461–78. doi: 10.1038/s41575-019-0157-3
37. López-Almela I, Romaní-Pérez M, Bullich-Vilarrubias C, Benítez-Páez A, Gómez Del Pulgar EM, Francés R, et al. Bacteroides uniformis combined with fiber amplifies metabolic and immune benefits in obese mice. Gut Microbes. (2021) 13:1865706. doi: 10.1080/19490976.2020.1865706
38. Clemente JC, Ursell LK, Parfrey LW, Knight R. The impact of the gut microbiota on human health: an integrative view. Cell. (2012) 148:1258–70. doi: 10.1016/j.cell.2012.01.035
39. Verspreet J, Damen B, Broekaert WF, Verbeke K, Delcour JA, Courtin CM. A critical look at prebiotics within the dietary fiber concept. Annu Rev Food Sci Technol. (2016) 7:167–90. doi: 10.1146/annurev-food-081315-032749
40. Le Y, Yang H. Xanthan gum modified fish gelatin and binary culture modulates the metabolism of probiotics in fermented milk mainly via amino acid metabolism pathways. Food Res. Int. (2022) 161:111844. doi: 10.1016/j.foodres.2022.111844
41. Sun S, Wang K, Ma K, Bao L, Liu H. An insoluble polysaccharide from the sclerotium of Poria cocos improves hyperglycemia, hyperlipidemia and hepatic steatosis in ob/ob mice via modulation of gut microbiota. Chin J Nat Med. (2019) 17:3–14. doi: 10.1016/S1875-5364(19)30003-2
42. Wu T, Lin C, Chang C, Lin T, Martel J, Ko Y, et al. Gut commensal Parabacteroides goldsteinii plays a predominant role in the anti-obesity effects of polysaccharides isolated from Hirsutella sinensis. Gut. (2019) 68:248–62. doi: 10.1136/gutjnl-2017-315458
43. Snelson M, Tan SM, Clarke RE, de Pasquale C, Thallas-Bonke V, Nguyen T, et al. Processed foods drive intestinal barrier permeability and microvascular diseases. Sci Adv. (2021) 7:eabe4841. doi: 10.1126/sciadv.abe4841
44. Chassaing B, Koren O, Goodrich JK, Poole AC, Srinivasan S, Ley RE, et al. Dietary emulsifiers impact the mouse gut microbiota promoting colitis and metabolic syndrome. Nature. (2015) 519:92–6. doi: 10.1038/nature14232
45. Suez J, Korem T, Zeevi D, Zilberman-Schapira G, Thaiss CA, Maza O, et al. Artificial sweeteners induce glucose intolerance by altering the gut microbiota. Nature. (2014) 514:181–6. doi: 10.1038/nature13793
46. Zhao X, Chen L, Wongmaneepratip W, He Y, Zhao L, Yang H. Effect of vacuum impregnated fish gelatin and grape seed extract on moisture state, microbiota composition, and quality of chilled seabass fillets. Food Chem. (2021) 354:129581. doi: 10.1016/j.foodchem.2021.129581
47. Zhernakova A, Kurilshikov A, Bonder MJ, Tigchelaar EF, Schirmer M, Vatanen T, et al. Population-based metagenomics analysis reveals markers for gut microbiome composition and diversity. Science. (2016) 352:565–9. doi: 10.1126/science.aad3369
48. Sommer F, Bäckhed F. The gut microbiota - masters of host development and physiology. Nat Rev Microbiol. (2013) 11:227–38. doi: 10.1038/nrmicro2974
49. Turnbaugh PJ, Bäckhed F, Fulton L, Gordon JI. Diet-Induced obesity is linked to marked but reversible alterations in the mouse distal gut microbiome. Cell Host Microbe. (2008) 3:213–23. doi: 10.1016/j.chom.2008.02.015
50. Zeng S, Li S, Xiao P, Cai Y, Chu C, Chen B, et al. Citrus polymethoxyflavones attenuate metabolic syndrome by regulating gut microbiome and amino acid metabolism. Sci Adv. (2020) 6:eaax6208. doi: 10.1126/sciadv.aax6208
51. Rom O, Liu Y, Liu Z, Zhao Y, Wu J, Ghrayeb A, et al. Glycine-based treatment ameliorates NAFLD by modulating fatty acid oxidation, glutathione synthesis, and the gut microbiome. Sci Transl Med. (2020) 12:eaaz2841. doi: 10.1126/scitranslmed.aaz2841
52. Hong Y, Sheng L, Zhong J, Tao X, Zhu W, Ma J, et al. Desulfovibrio vulgaris, a potent acetic acid-producing bacterium, attenuates nonalcoholic fatty liver disease in mice. Gut Microbes. (2021) 13:1930874. doi: 10.1080/19490976.2021.1930874
53. Guo J, Han X, Tan H, Huang W, You Y, Zhan J. Blueberry extract improves obesity through regulation of the gut microbiota and bile acids via pathways involving FXR and TGR5. iScience. (2019) 19:676–90. doi: 10.1016/j.isci.2019.08.020
54. Wang Z, Lam K, Hu J, Ge S, Zhou A, Zheng B, et al. Chlorogenic acid alleviates obesity and modulates gut microbiota in high-fat-fed mice. Food Sci Nutr. (2019) 7:579–88. doi: 10.1002/fsn3.868
55. Zhang C, Wu W, Li X, Xin X, Liu D. Daily supplementation with fresh angelica keiskei juice alleviates High-Fat Diet-Induced obesity in mice by modulating gut microbiota composition. Mol Nutr Food Res. (2019) 63:1900248. doi: 10.1002/mnfr.201900248
56. Wu J, Liu Y, Dou Z, Wu T, Liu R, Sui W, et al. Black garlic melanoidins prevent obesity, reduce serum LPS levels and modulate the gut microbiota composition in high-fat diet-induced obese C57BL/6J mice. Food Funct. (2020) 11:9585–98. doi: 10.1039/D0FO02379E
57. Zhang S, Wu P, Tian Y, Liu B, Huang L, Liu Z, et al. Gut microbiota serves a predictable outcome of short-term low-carbohydrate diet (LCD) intervention for patients with obesity. Microbiol Spectr. (2021) 9:e0022321. doi: 10.1128/Spectrum.00223-21
58. Saeb A, Grundmann SM, Gessner DK, Schuchardt S, Most E, Wen G, et al. Feeding of cuticles from Tenebrio molitor larvae modulates the gut microbiota and attenuates hepatic steatosis in obese Zucker rats. Food Funct. (2022) 13:1421–36. doi: 10.1039/D1FO03920B
59. Ojo BA, O’Hara C, Wu L, El-Rassi GD, Ritchey JW, Chowanadisai W, et al. Wheat germ supplementation increases lactobacillaceae and promotes an anti-inflammatory gut milieu in C57BL/6 mice fed a High-Fat, High-Sucrose diet. J Nutr. (2019) 149:1107–15. doi: 10.1093/jn/nxz061
60. Ye J, Zhao Y, Chen X, Zhou H, Yang Y, Zhang X, et al. Pu-erh tea ameliorates obesity and modulates gut microbiota in high fat diet fed mice. Food Res Int. (2021) 144:110360. doi: 10.1016/j.foodres.2021.110360
61. Zeng H, Larson KJ, Cheng W, Bukowski MR, Safratowich BD, Liu Z, et al. Advanced liver steatosis accompanies an increase in hepatic inflammation, colonic, secondary bile acids and Lactobacillaceae/Lachnospiraceae bacteria in C57BL/6 mice fed a high-fat diet. J Nutr Biochem. (2020) 78:108336. doi: 10.1016/j.jnutbio.2019.108336
62. Falony G, Joossens M, Vieira-Silva S, Wang J, Darzi Y, Faust K, et al. Population-level analysis of gut microbiome variation. Science. (2016) 352:560–4. doi: 10.1126/science.aad3503
63. Yang J, Wei H, Zhou Y, Szeto C, Li C, Lin Y, et al. High-Fat diet promotes colorectal tumorigenesis through modulating gut microbiota and metabolites. Gastroenterology. (2022) 162:135–49. doi: 10.1053/j.gastro.2021.08.041
64. Del Chierico F, Nobili V, Vernocchi P, Russo A, De Stefanis C, Gnani D, et al. Gut microbiota profiling of pediatric nonalcoholic fatty liver disease and obese patients unveiled by an integrated meta-omics-based approach. Hepatology. (2017) 65:451–64. doi: 10.1002/hep.28572
65. Koh GY, Kane A, Lee K, Xu Q, Wu X, Roper J, et al. Parabacteroides distasonis attenuates toll-like receptor 4 signaling and Akt activation and blocks colon tumor formation in high-fat diet-fed azoxymethane-treated mice. Int J Cancer. (2018) 143:1797–805. doi: 10.1002/ijc.31559
66. Cuffaro B, Assohoun ALW, Boutillier D, Súkeníková L, Desramaut J, Boudebbouze S, et al. In vitro characterization of gut Microbiota-Derived commensal strains: selection of Parabacteroides distasonis strains alleviating TNBS-Induced colitis in mice. Cells. (2020) 9:2104. doi: 10.3390/cells9092104
67. Ezeji JC, Sarikonda DK, Hopperton A, Erkkila HL, Cohen DE, Martinez SP, et al. Parabacteroides distasonis: intriguing aerotolerant gut anaerobe with emerging antimicrobial resistance and pathogenic and probiotic roles in human health. Gut Microbes. (2021) 13:1922241. doi: 10.1080/19490976.2021.1922241
68. Kiewiet MBG, Elderman ME, El Aidy S, Burgerhof JGM, Visser H, Vaughan EE, et al. Flexibility of gut microbiota in ageing individuals during dietary fiber Long-Chain inulin intake. Mol Nutr Food Res. (2021) 65:2000390. doi: 10.1002/mnfr.202000390
69. Wang M, Zhang B, Hu J, Nie S, Xiong T, Xie M. Intervention of five strains of Lactobacillus on obesity in mice induced by high-fat diet. J Funct Foods. (2020) 72:104078. doi: 10.1016/j.jff.2020.104078
70. Long X, Zeng X, Tan F, Yi R, Pan Y, Zhou X, et al. Lactobacillus plantarum KFY04 prevents obesity in mice through the PPAR pathway and alleviates oxidative damage and inflammation. Food Funct. (2020) 11:5460–72. doi: 10.1039/D0FO00519C
71. Million M, Maraninchi M, Henry M, Armougom F, Richet H, Carrieri P, et al. Obesity-associated gut microbiota is enriched in Lactobacillus reuteri and depleted in Bifidobacterium animalis and Methanobrevibacter smithii. Int J Obes. (2012) 36:817–25. doi: 10.1038/ijo.2011.153
72. Crovesy L, Ostrowski M, Ferreira DMTP, Rosado EL, Soares-Mota M. Effect of Lactobacillus on body weight and body fat in overweight subjects: a systematic review of randomized controlled clinical trials. Int J Obes. (2017) 41:1607–14. doi: 10.1038/ijo.2017.161
73. van der Hee B, Wells JM. Microbial regulation of host physiology by short-chain fatty acids. Trends Microbiol. (2021) 29:700–12. doi: 10.1016/j.tim.2021.02.001
74. Sanders ME, Merenstein DJ, Reid G, Gibson GR, Rastall RA. Probiotics and prebiotics in intestinal health and disease: from biology to the clinic. Nat. Rev. Gastroenterol. Hepatol. (2019) 16:605–16. doi: 10.1038/s41575-019-0173-3
75. Canfora EE, Jocken JW, Blaak EE. Short-chain fatty acids in control of body weight and insulin sensitivity. Nat. Rev. Endocrinol. (2015) 11:577–91. doi: 10.1038/nrendo.2015.128
76. Zhang LS, Davies SS. Microbial metabolism of dietary components to bioactive metabolites: opportunities for new therapeutic interventions. Genome Med. (2016) 8:46. doi: 10.1186/s13073-016-0296-x
77. Lavelle A, Sokol H. Gut microbiota-derived metabolites as key actors in inflammatory bowel disease. Nat. Rev. Gastroenterol. Hepatol. (2020) 17:223–37. doi: 10.1038/s41575-019-0258-z
78. Sonnenburg ED, Sonnenburg JL. Starving our microbial self: the deleterious consequences of a diet deficient in microbiota-accessible carbohydrates. Cell Metab. (2014) 20:779–86. doi: 10.1016/j.cmet.2014.07.003
79. den Besten G, Bleeker A, Gerding A, van Eunen K, Havinga R, van Dijk TH, et al. Short-Chain fatty acids protect against High-Fat diet–induced obesity via a PPARγ-Dependent switch from lipogenesis to fat oxidation. Diabetes. (2015) 64:2398–408. doi: 10.2337/db14-1213
80. Kondo T, Kishi M, Fushimi T, Kaga T. Acetic acid upregulates the expression of genes for fatty acid oxidation enzymes in liver to suppress body fat accumulation. J Agric Food Chem. (2009) 57:5982–6. doi: 10.1021/jf900470c
81. Kondo T, Kishi M, Fushimi T, Ugajin S, Kaga T. Vinegar intake reduces body weight, body fat mass, and serum triglyceride levels in obese japanese subjects. Biosci Biotechnol Biochem. (2009) 73:1837–43. doi: 10.1271/bbb.90231
82. Fernández J, Redondo-Blanco S, Gutiérrez-del-Río I, Miguélez EM, Villar CJ, Lombo F. Colon microbiota fermentation of dietary prebiotics towards short-chain fatty acids and their roles as anti-inflammatory and antitumour agents: a review. J Funct Foods. (2016) 25:511–22. doi: 10.1016/j.jff.2016.06.032
83. Du J, Zhang P, Luo J, Shen L, Zhang S, Gu H, et al. Dietary betaine prevents obesity through gut microbiota-drived microRNA-378a family. Gut Microbes. (2021) 13:1862612. doi: 10.1080/19490976.2020.1862612
84. Liu Z, Zhang Y, Ai C, Wen C, Dong X, Sun X, et al. Gut microbiota response to sulfated sea cucumber polysaccharides in a differential manner using an in vitro fermentation model. Food Res Int. (2021) 148:110562. doi: 10.1016/j.foodres.2021.110562
85. Lei Y, Tang L, Liu S, Hu S, Wu L, Liu Y, et al. Parabacteroides produces acetate to alleviate heparanase-exacerbated acute pancreatitis through reducing neutrophil infiltration. Microbiome. (2021) 9:115. doi: 10.1186/s40168-021-01065-2
Keywords: yeast, mannoproteins, obesity, gut microbiota, Parabacteroides distasonis, Lactobacillus
Citation: Li X, Wu J, Kang Y, Chen D, Chen G, Zeng X and Wang J (2022) Yeast mannoproteins are expected to be a novel potential functional food for attenuation of obesity and modulation of gut microbiota. Front. Nutr. 9:1019344. doi: 10.3389/fnut.2022.1019344
Received: 15 August 2022; Accepted: 21 September 2022;
Published: 14 October 2022.
Edited by:
Bin Liang, Ludong University, ChinaReviewed by:
Yan Jin, Tianjin University of Science and Technology, ChinaSimin Feng, Zhejiang University of Technology, China
Hongshun Yang, National University of Singapore, Singapore
Copyright © 2022 Li, Wu, Kang, Chen, Chen, Zeng and Wang. This is an open-access article distributed under the terms of the Creative Commons Attribution License (CC BY). The use, distribution or reproduction in other forums is permitted, provided the original author(s) and the copyright owner(s) are credited and that the original publication in this journal is cited, in accordance with accepted academic practice. No use, distribution or reproduction is permitted which does not comply with these terms.
*Correspondence: Guijie Chen, Z3VpamllY2hlbkBuamF1LmVkdS5jbg==; Xiaoxiong Zeng, emVuZ3h4QG5qYXUuZWR1LmNu