- 1Laboratory of Aquatic Animal Nutrition and Feed, College of Fisheries, Guangdong Ocean University, Zhanjiang, Guangdong, China
- 2Aquatic Animals Precision Nutrition and High Efficiency Feed Engineering Research Center of Guangdong Province, Zhanjiang, Guangdong, China
- 3Key Laboratory of Aquatic, Livestock and Poultry Feed Science and Technology in South China, Ministry of Agriculture, Zhanjiang, Guangdong, China
The substitution of high-level soy meals for fish meal (FM) generally leads to fish enteritis, accompanied by significant variations in gut flora. Relevant studies have pointed out a close relationship between tryptophan metabolism mediated by gut flora and vertebrate inflammatory bowel disease. Present study examines the role of tryptophan metabolism and gut flora profile in fish enteritis caused by different soybean meals. The 960 groupers were randomly assigned into 4 groups (n = 4), which including: (1) FM (the control group, fed with 50% FM feed), (2) SBM40 (replacing 40% FM with soybean meal), (3) SPC40 (replacing 40% FM with soybean protein concentrate), and (4) FSBM40 (replacing 40% FM with fermented soybean meal). Under average temperature and natural light, the groupers were cultivated with feeds of iso-nitrogen and iso-lipid for 10 weeks. The results showed that soybean meal feeds at all experimental levels had negative effects on fish gut physiology and growth performance. Typical enteritis features and fluctuations of immune system occur, which can be observed in the enzyme activities of total superoxide dismutase and lysozyme and in the contents of immunoglobulin M, complement 3 and complement 4. 16SrDNA high-throughput sequencing indicated that it greatly influenced the gut flora with the abundance of maleficent bacteria, like Vibrio, amplified with increasing dietary soybean meals. According to the “3 + 2” full-length transcriptome sequencing, soy meals at the three experimental levels inhibited the key gene expressions of tryptophan metabolic pathway in fish gut, however, there are some differences in the types of key genes that are inhibited. The canonical correlation analysis showed that the changes in key gene expressions in tryptophan metabolic pathway had a positive correlation with the expressions of pro-inflammatory genes (P < 0.05) and negatively correlated with the expression of anti-inflammatory genes (P < 0.05). It is speculated from this study that tryptophan metabolism is closely related to fish soy meal-related enteritis, and the abnormal tryptophan metabolism caused by intestinal flora imbalance may play an important role. In the future research, we can further study the tolerance of fish to soy meals feed from two aspects of tryptophan metabolism and intestinal flora changes.
Introduction
In recent years, the total amount of aquaculture has continued to increase, but the fish meal (FM) output almost remained unchanged. Since FM presents to be a key source of protein for aquaculture, aquaculture farmers urgently need new and sustainable alternatives for it (1). Thus, attention has been paid to plant materials during the procedure. Among them, soy products are considered one of the most promising FM substitutes because of their high yield and relatively balanced amino acid composition (2). However, soy products with high substitution levels, especially soybean meal (SBM), are easy to cause gut mucosal damage and enteritis in fish, which seriously endangers the gut health of fish, and limits its extensive application in aquaculture industry.
According to former research, the influence of SBM on fish enteritis varies with its anti-nutritional factors (ANFs), including phytates, protease inhibitors, saponins, lectins, phytosterols, and oligosaccharides (3). The histological characteristics of SBM induced enteritis (SBMIE) in fish include infiltration of different inflammatory cells, the swelling submucosa and lamina propria, the shortening of mucosal fold, and a decrease in absorption vacuoles of gut epithelial cell (4). It has been found that high-level SBM has negative impact on the gut health of a variety of fish, such as zebrafish, carp, rainbow trout, and Atlantic salmon (5–7). Fish SBMIE mainly occurs in the hindgut, a crucial part of protein absorption by endocytosis and is more susceptible to food stimulation, causing intestinal diseases (8). In order to reduce the antigen content, the SBM is often processed into different product types.
In aquatic feed, soy products used mainly include SBM, soybean protein concentrate (SPC), as well as fermented soybean meal (FSBM). SBM, a by-product of soybean oil extraction, contained various ANFs mentioned above. SPC is a product made from soy by extracting oil and low molecular weight soluble protein components by alcohol. Compared with SBM, SPC removed most ANFs and is a good plant protein source in the fish and shrimp diet (9). However, the solubility of SPC extracted by the alcohol method is decreased, and the nitrogen solubility index is reduced to about 10%, which limits its application (10). FSBM refers to a protein material prepared by fermentation of SBM by microorganisms. Fermentation of microorganism significantly purifies the content of ANFs in SBM, and decomposes soybean protein into small molecular proteins, small peptides, and amino acids, thus improving the digestion and absorption abilities (11). However, at present, due to the different fermentation technology, there are also many problems in FSBM, such as the lack of strict standards and specifications, and its product quality is still uneven (12).
Previous studies on fish SBMIE mostly focused on the influence of ANFs in SBM on gut health. But researchers found that the role of ANFs is complex, such as fish enteritis induced by soy saponins may be related to the basal protein source (13); or dose-dependent and independent of the basal protein source (14); or it is a secondary effect on the interference characteristics of cell membrane, and the SBMIE is mainly induced by external antigens (15). According to existing research, no clear results about the pathogenic mechanism of fish SBMIE have been made, but researchers generally believe that the gut flora play an important role during this process (16–18).
Tryptophan is an essential amino acid. In recent years, lysine and methionine have been widely used in formula diets, making tryptophan the main limiting amino acid in diet (19). In aquaculture, researchers found that tryptophan can promote the growth and gut development of carp and shrimp, regulate the gut microecological balance, and improve the survival rate (SR) of groupers (20–22). The related studies have shown the benefits of tryptophan on the gut health of aquaculture objects, but there are few reports on the fish SBMIE. However, in mammals, the relationship between tryptophan metabolism and human inflammatory bowel disease has become a research hotspot in recent years (23, 24). In present study, we also found that different soy meal substitution for FM at high levels, resulting in abnormal tryptophan metabolism in the gut tract of pearl gentian grouper.
Pearl gentian grouper is a typical marine carnivorous fish and is the important cultivated varieties in China and many places in the world. Its meat is tender, grows fast, has strong disease resistance, obvious hybridization advantages, and good market price. It can also be used as an ornamental fish, and has a broad market prospect. This study analysed the effects of different soy meals with high substitution levels on the growth physiology and gut tryptophan metabolism pathway of pearl gentian grouper, and preliminary analysed the gut flora profile variations. Present research offers a theoretical basis for the study of gut health problems caused by the substitution for FM with high level of plant proteins.
Materials and methods
Experimental diets
The experimental diets and their chemical compositions are shown in Table 1. The red FM, produced by Corporación Pesquera Inca S.A.C. at Bayovar Plant in Peru, contains 72.53% crude protein. The SBM (48.92% crude protein) and SPC (70.72% crude protein) were purchased from Zhanjiang Haibao Feed (Zhanjiang, China). The FSBM, containing 60.75% crude protein, was purchased from Xijie Foshan Co. Ltd. (Foshan, China). Four experimental diets of iso-lipid (about 10% total lipid) and iso-nitrogen (about 50% crude protein) were used by SBM, SPC, and FSBM to substitute 40% of FM protein; these diets were designated as FM (the control group), SBM40, SPC40, and FSBM40, respectively. To achieve amino acid balance, the experimental feeds were mixed with methionine and lysine (25). The specific preparation and storage methods of the four kinds of feeds were explained as below: first, the researchers ground the raw materials into powder, then screened it with 60-mesh sieves and weighed the following formula, before using the sequential expansion method to mix the micro constituents evenly. Second, the researchers added lipids and deionised water into it and then stirred it to get a fine mixture. Afterward, the feeds were air-dried to 10% humidity using pelletisers with diameters of 2.0 and 3.0 mm, respectively. The experimental diets were then conserved in sealed plastic bags at −20°C (26).
Feeding experiment design
The healthy juvenile groupers, which weighed about 9 g, were bought from a commercial hatchery in Zhanjiang City, Guangdong, China. Before the experiment, 960 groupers were given 1 week to adapt to the experimental conditions, after which they were offered nothing to eat for a day. The researchers then anaesthetised the groupers with eugenol and divided them into four groups. According to size, fishes were randomly placed in 1,000 L cylindrical glass tanks. Every tank contained 60 fish. The experimental feeds were offered from 8:00 a.m. to 4:00 p.m. every day for 10 weeks, with four replicates. The uneaten feeds were siphoned to calculate the weight of the intake feeds, and the feed consumption ratio was determined (27). The feeding experiment was conducted within inner cultural systems belonging to the Marine Biological Research Base of Zhanjiang City (Guangdong, China). To ensure saturated oxygen concentration, air stones were used to continuously inflate air into containers. The experimental environment in containers involved a natural light cycle and a temperature of 29 ± 1°C. The concentrations of ammonia and nitrate were both lower than 0.03 mg L–1, and the dissolved oxygen involved in this study was ≥7 mg L–1. Within the first 14 days, 60% of the water in each container was exchanged every day, and after that, nearly 100% of the water was exchanged every day.
Sample collection
The study was supported by the Expert Committee of the Fisheries College of Guangdong Ocean University. The research methods followed all applicable regulations and guidelines. Before sampling, the studied groupers were starved for 24 h. Then, they were anaesthetised with eugenol (1:10,000). After counting and weighing all fish in each tank, their specific growth rate (SGR), weight gain rate (WGR), hepatosomatic index (HSI), SR, and feed conversion ratio (FCR) were determined. To determine the enzyme activity, the researchers randomly selected six groupers from each water tank, drew blood from their tail veins, and stored it at 4°C for a night, before using a centrifuge at 3,500 rpm for 10 min to obtain serum. The blood was then stored at −80°C, preparing for analysing the enzyme activity. The hindgut tissues were excised, and any mesenteric and adipose tissues were removed. Hindgut samples were cut into small pieces and put in test tubes filled with RNAlater at 4°C for a night before being stored at −80°C for gene expression analysis.
For gut flora and transcriptome sequencing, the researchers randomly chose eight groupers. The hindgut tissue samples were obtained as mentioned above and placed in cryopreservation tubes, then directly put into liquid nitrogen, half of which was employed for 16S high-throughput sequencing, while the rest for transcriptome sequencing.
Histological observation of enteritis
After the diet experiment, three fish were randomly chosen from every container. The DI sample was stored in 4% paraformaldehyde universal tissue fixative, which belongs to the Servicebio Technology Company of Wuhan, from China, for 24 h before being H&E stained. The stained sections were observed and photographed under 10 × 10 times microscopically (Olympus CKX41 microscope, Tokyo, Japan).
Analysis of physiological indexes
In the present study, the researchers employed the BCA method to analyse the gut and serum samples (Beyotime Biotechnology Co., Ltd., Shanghai, China).
The enzyme activities of lysozyme (LYS) in serum and total superoxide dismutase (T-SOD) in hindgut were measured by fish ELISA kits, which were then employed to determine immunoglobulin M (IgM) content, complement 3 (C3) content, and complement 4 (C4) content in hindgut tissues. The testing kits were products of Shanghai Jianglai Biotechnology Co., Ltd. (Shanghai, China). The methods were performed according to the operating instructions in detail.
Immune-related gene expressions
TRIzol kits (Invitrogen, Carlsbad, CA, USA) were applied to test the total RNA of the hindgut tissues. An Evo M-MLV reverse transcription kit (Takara, Japan) was employed to prepare qualified RNA samples to cDNA. They were then stored at −20°C for use. The primers were designed by Shenggong Bioengineering Co., Ltd. (Shanghai, China). The researchers obtained the template sequences of these primers from the sequencing of the “3 + 2” full-length transcriptome of the hindgut tissues of the studied groupers within the lab. The original data were recorded in the NCBI Sequential Read Archive (SRA) with accession numbers PRJNA664623 and PRJNA66441. Primers carried out by Primer Premier 5.0 software for pro-inflammatory genes were interleukin (IL)1β, IL12, IL17, IL32, and tumour necrosis factor (TNF)α, and anti-inflammatory genes were IL4, IL5, IL10, and transforming growth factor (TGF)β1 (Table 2). The β-actin is presented to be the internal reference gene. The gene expressions were examined with RT-qPCR (Mastercycler ep realplex, Eppendorf, Germany). The PCR reaction environment were 95°C for 2 min with 1 cycle, 95°C for 15 s, 60°C for 10 s and 72°C for 20 s with 40 cycles, respectively. The target gene expressions were calculated using the 2–ΔΔ Ct method.
16S high-throughput analysis of intestinal flora
According to the operation instructions, E.Z.N.A.™ Kit (Omega Bio-Tek, Norcross, GA, USA) was applied to extract the total RNA from the DI flora. Gene Denovo Co., Ltd. (Guangzhou, China) assisted in sequence detection and data analysis. The original data were recorded in the NCBI SRA, with the accession number PRJNA666309. The Supplementary File included specified analysis procedures.
Transcriptome sequencing and KEGG annotation
Through the PacBio Sequel and Illumina HiSeq™ 4000 platforms, the “3 + 2” full-length transcriptome sequencing was carried out. Gene Denovo Co., Ltd. (Guangzhou, China) completed the sequencing process. The original data of the sequencing are stored as mentioned above. The gene expressions of | log2FC| > 1 and P < 0.05 were classified as differential genes (DEGs). The researchers put the DEGs gene into the KEGG database and carried out an in-depth analysis of the significantly activated signal pathways, which were relevant to signal transduction, infectious diseases and immune diseases/systems (P < 0.05).
Validation of the tryptophan metabolism-related genes by RT-qPCR
To validate the accuracy of the “3 + 2” full-length transcriptome data, 10 DEGs from the tryptophan metabolism pathway were chosen for RT-qPCR: AO, DDC, IDO2, AOX1, ALDH, DAO1, KYN, KAT1, CYP1A1, and LAAO. The primer templates were the same as those mentioned above, and the primers are presented in Table 3.
The β-actin is presented to be the internal control gene. QRT-PCR (Mastercycler ep realplex in Eppendorf, Germany) helped in detecting gene expression. The reaction environment of PCR and analysing approaches were identical, as mentioned above.
Correlation analysis
To explore the correlation between downstream inflammatory genes and tryptophan metabolism, the crucial DEGs in the signal pathway of tryptophan metabolism were studied using canonical correlation analysis (CCA) with the inflammatory genes in internal tissue in the SBM40, SPC40, and FSBM40 groups, respectively. The key DEGs selected in the tryptophan metabolism signalling pathway include AO, DDC, IDO2, AOX1, ALDH, DAO1, KYN, KAT1, CYP1A1, and LAAO; the inflammatory genes include pro-inflammatory genes (IL1β, TNFα, and IκBα) and anti-inflammatory- related genes (IL10 and TGFβ1) in the hindgut tissues.
Statistics
With the help of free online omicshare tools, the CCA method was carried out to process the original data1. After homogeneity variance tests by SPSS 22.0 (SPSS Inc., Chicago, IL, USA), the results were studied with one-way ANOVA and are shown as the mean ± SD, with P < 0.05 indicating a statistically significant difference.
Results
Growth performance
As indicated in Table 4, the WGR and SGR of the experimental groups were lower than that of the FM, which served as the control group (P < 0.05). Among the experimental groups, there was little difference in WGR and SGR (P > 0.05). On the contrary, FCR increased significantly in each experimental group (P < 0.05). Among the experimental groups, there was little difference in FCR (P > 0.05). In addition, there was little difference in HSI and SR among the groups (P > 0.05).
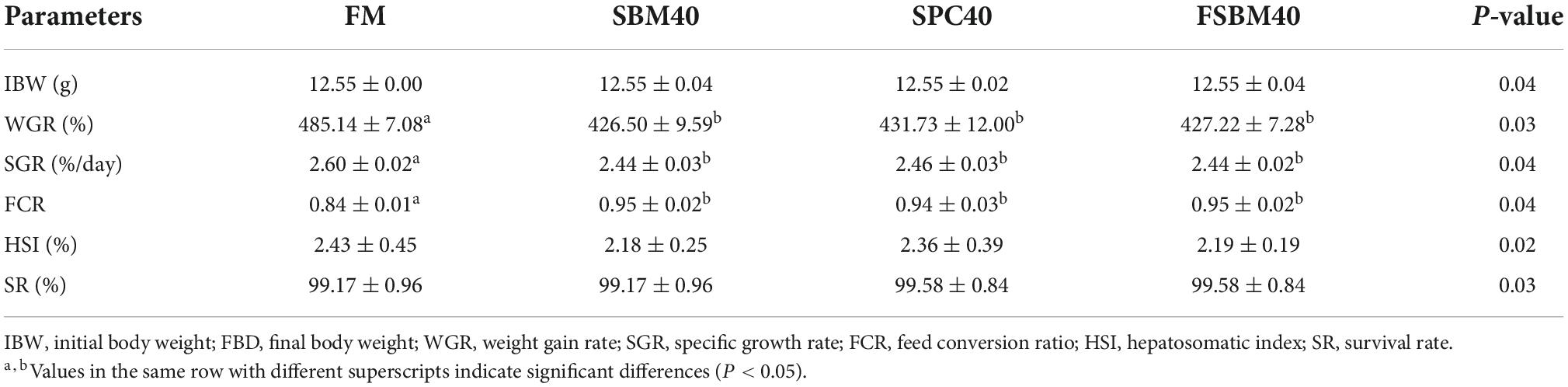
Table 4. Effect of different soy meals at 40% substitution level for fish meal on the growth of grouper (n = 4).
Histological observations
The H&E staining and semi-quantitative analysis showed that the experimental levels of SBM, SPC and FSBM all caused enteritis, and they presented significant distinctions compared to the control group (P < 0.05), among which the SBM40 and FSBM40 groups were more serious, and enteritis induced by SPC40 was significantly lighter than the SBM40 and FSBM40 groups (P < 0.05) (Figure 1 and Table 5).
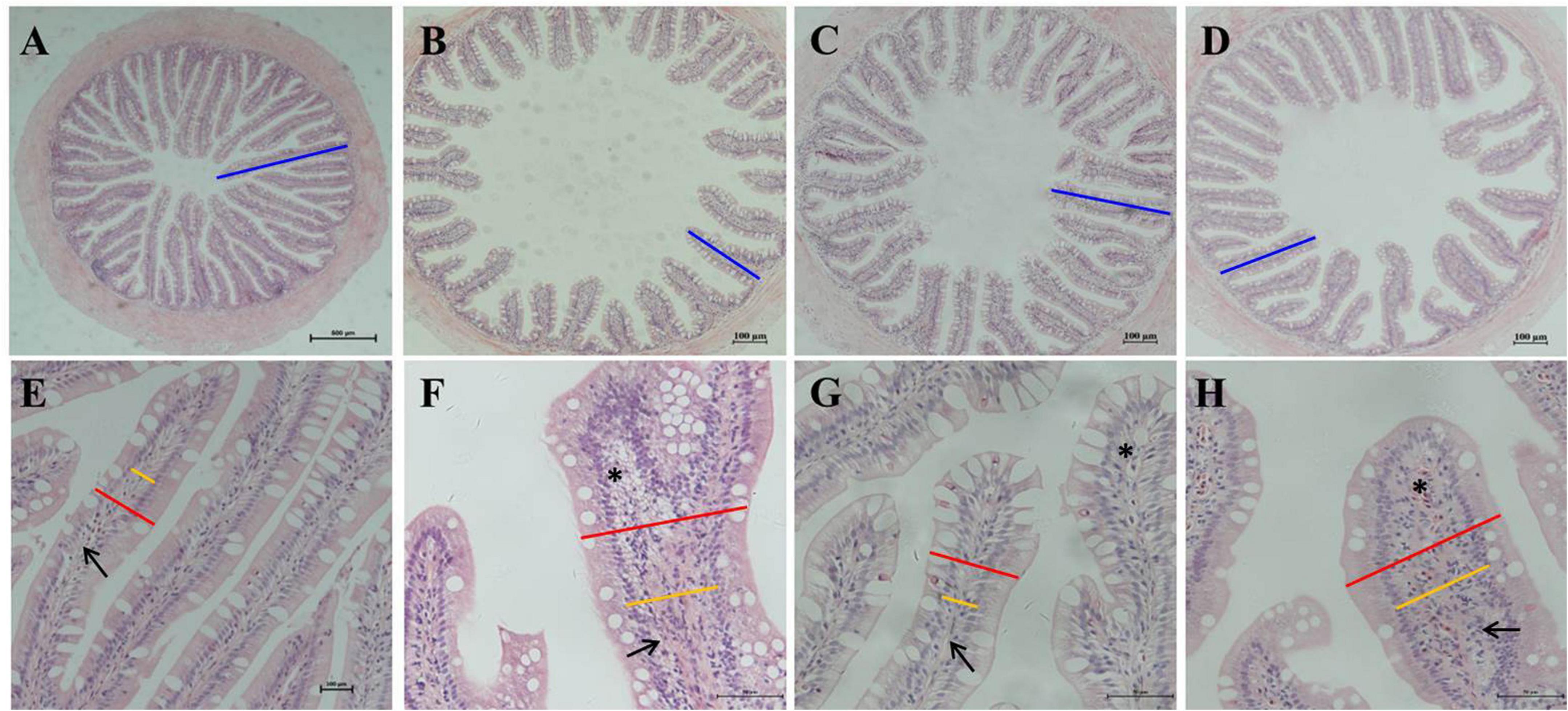
Figure 1. Hematoxylin-eosin staining in the hindgut of pearl gentian grouper. (A–D) Reduction and fusion of mucosal folds in SBM40, SPC40, and FSBM40 groups. (E–H) Increased width of lamina propria and inflammatory cell infiltration SBM40, SPC40, and FSBM40 groups. (A,E) Fish meal control group; (B,F) 40% SBM substitution for fish meal group; (C,G) 40% SPC substitution for fish meal group; (D,H) 40% FSBM substitution for fish meal group. Blue bar, the height of plica; red bar, the width of plica; yellow bar, the width of lamina propria; arrow, lamina propria; asterisk, inflammatory infiltration.
Physiological indexes determination
As exhibited in Table 6, IgM content, C3 content, and C4 content were all significantly reduced in hindgut tissues of all soy meal substitution groups (P < 0.05), while the T-SOD and LYS enzyme activities increased substantially in all soy meal substitution groups (P < 0.05).
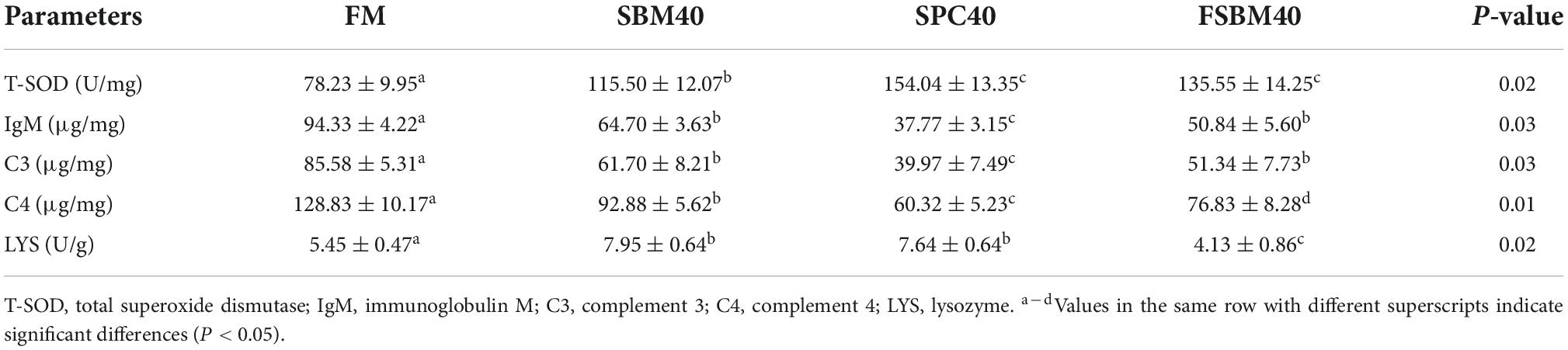
Table 6. Effect of different soy meals at 40% substitution levels for fish meal on the enzyme activities of pearl gentian grouper (n = 3).
Immune-related gene expressions
As presented in Table 7, except for the gene expressions of p65, which significantly decreased (P < 0.05) and there was a little difference in IκBα (P > 0.05) in the SPC40 group, the pro-inflammatory gene expressions of IL1β, IL8, TNFα, p65, and IκBα significantly increased in all soy meal substitution groups (P < 0.05). The anti-inflammatory-related gene expressions of IL5, IL10, and TGFβ1 were reduced considerably in all substitution groups (P < 0.05).
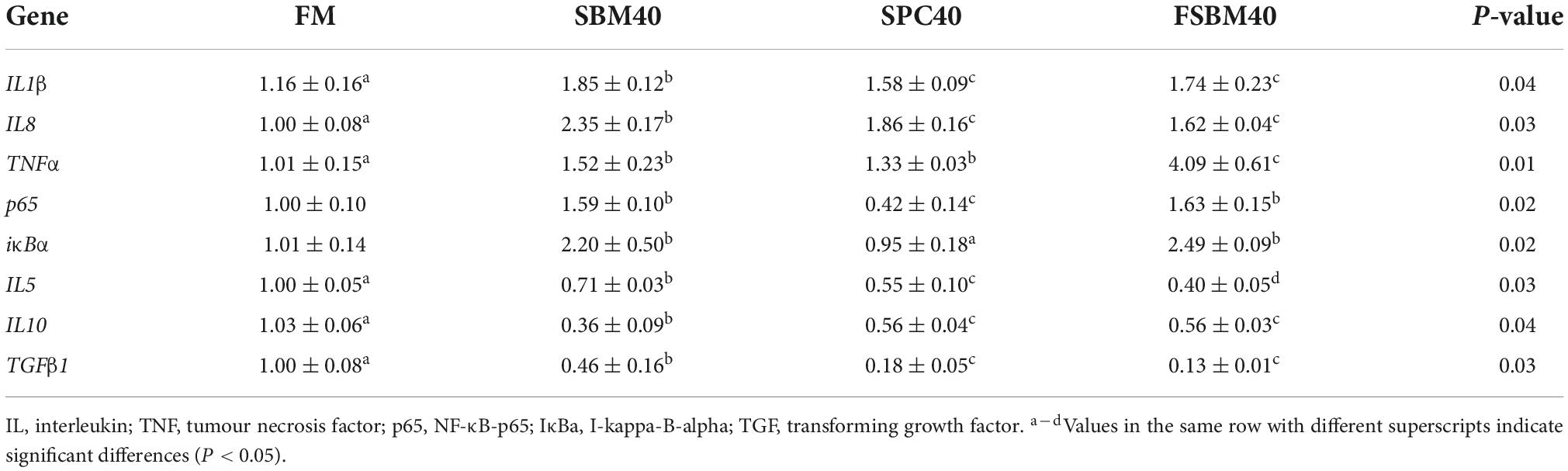
Table 7. Effect of different soy meals at 40% substitution levels for fish meal on the inflammatory-related gene expressions in hindgut of pearl gentian grouper (n = 3).
Sample diversity analysis of 16S sequencing
From the 12 samples, there were 983,965 original reads. The average amount of original reads of the FM, SBM40, SPC40, and FSBM40 groups were 79,339.67 ± 4,821.81, 86,036.67 ± 790.22, 81,344.00 ± 4,753.11 and 81,268.00 ± 4,575.00, respectively. After clustering into identical operational taxonomic units (OTUs) by sequences with similarity ≥97%, the researchers gained 899 OTUs. The average number of OTUs in the FM, SBM40, SPC40, and FSBM40 groups were 175.00 ± 32.79, 608.67 ± 56.84, 368.00 ± 42.58, and 717.33 ± 156.62, respectively. Except for a small significant difference between SBM40 and FSBM40 (P > 0.05), there were significant differences among the FM and SPC40 groups (P < 0.05). A Venn plot showed that 133 OTUs were among the groups (Figure 2A). The PCoA analysis revealed that different experimental groups could be clustered well, indicating that our samples were reasonably processed (Figure 2B).
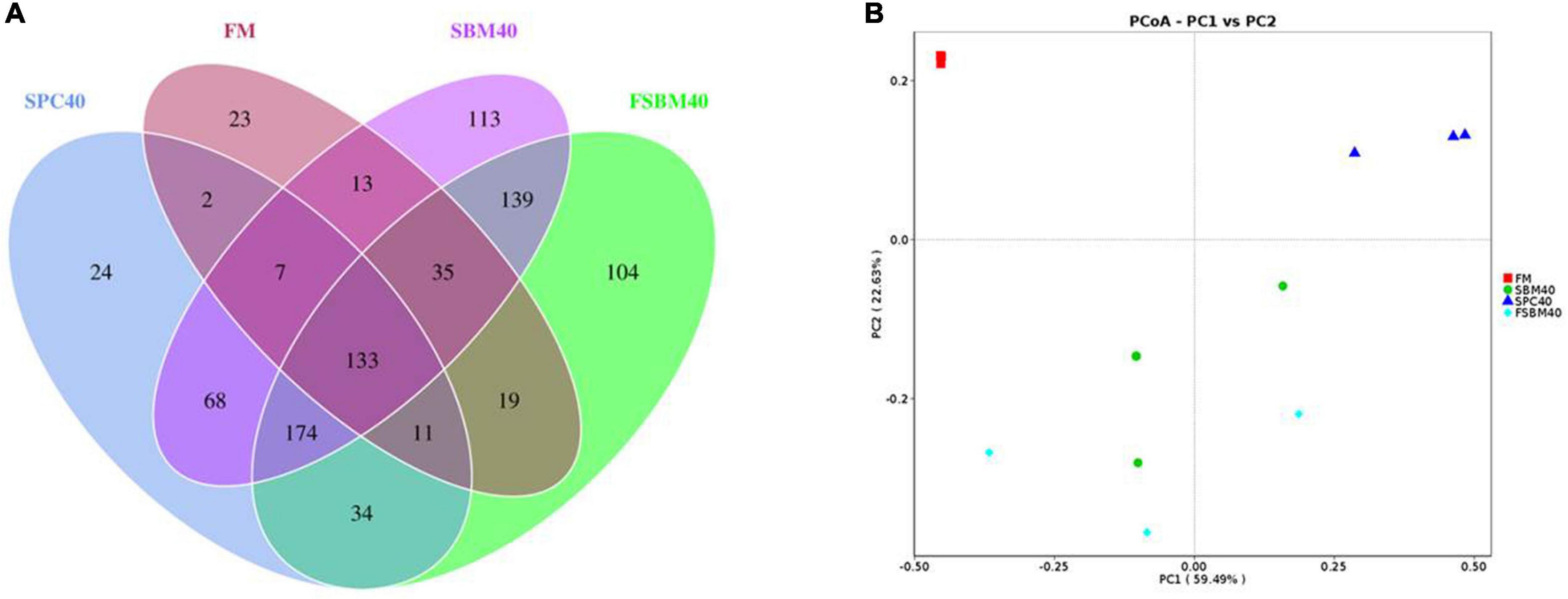
Figure 2. Venn plot (A) and PCoA (B) analysis of OTU by high-throughput sequencing after replacing fish meal with different soy meals in pearl gentian grouper (n = 3).
Comparison of gut flora composition and abundance
The relatively abundant top six species at the phylum level are ranked as follows: (1) Proteobacteria, (2) Firmicutes, (3) Bacteroidetes, (4) Acidobacteria, (5) Actinobacteria, and (6) Cyanobacteria. The abundance of Proteobacteria significantly decreased in all soy meal substitution groups (P < 0.05), while the abundances of Firmicutes, Bacteroidetes, and Actinobacteria had a significant increase in all soy meal substitution groups (P < 0.05). There was little difference in acidobacteria abundance between the SBM40 group and the SPC40 groups, but it significantly increased in the FSBM40 group (Figures 3A,B).
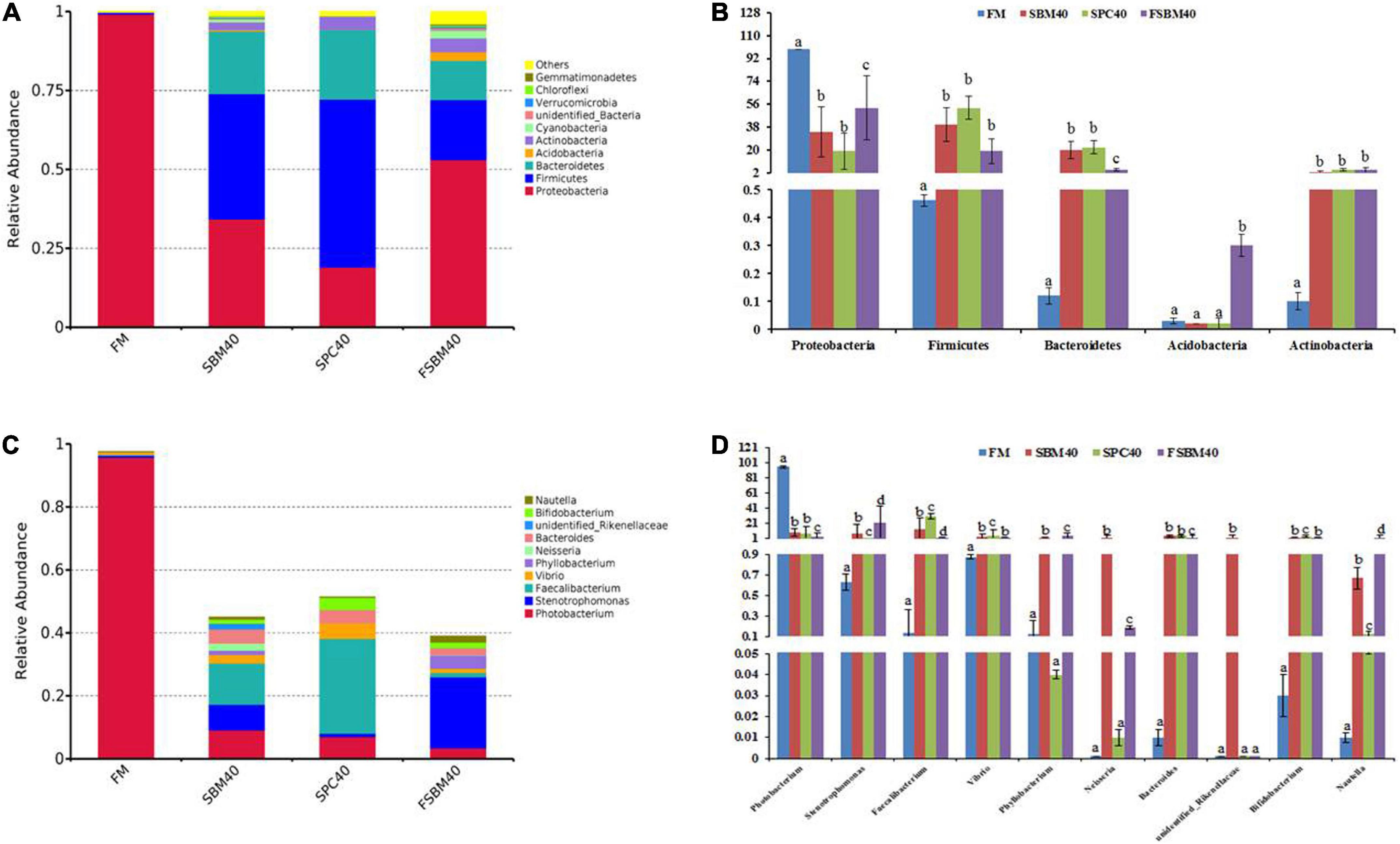
Figure 3. The gut flora composition and abundance of different soy meals substitution for fish meal in pearl gentian grouper [(A,B) phylum level and (C,D) genus level]. (A) Rarefaction curve; (B) rank abundance; (C) species accumulation boxplot. Different letters distributed on the column represented significant variances between the groups at P < 0.05 (n = 3).
The relatively abundant top six species at the genus level are ranked as follows: (1) Photobacterium, (2) Stenotrophomonas, (3) Vibrio, (4) Phyllobacterium, (5) Neisseria, and (6) Bacteroides. The abundance of Photobacterium experienced a significant decrease in all soy meal substitution groups (P < 0.05). The abundances of Phyllobacterium and Neisseria had little difference in the SPC40 group (P > 0.05), and the abundance of unidentified_Rikenellaceae had little difference between the SPC40 and FSBM40 groups (P > 0.05). The abundances of the left species significantly increased in all soy meal substitution groups (P < 0.05; Figures 3C,D).
Statistics and KEGG enrichment of differential genes
Compared to the FM group, there were 554 co-contained DEGs in the SBM40, SPC40, and FSBM40 groups, named profile A. The numbers of unique DEGs were 1,003, 2,254, and 1,656, named profile B, profile C, and profile D, respectively (Figure 4).
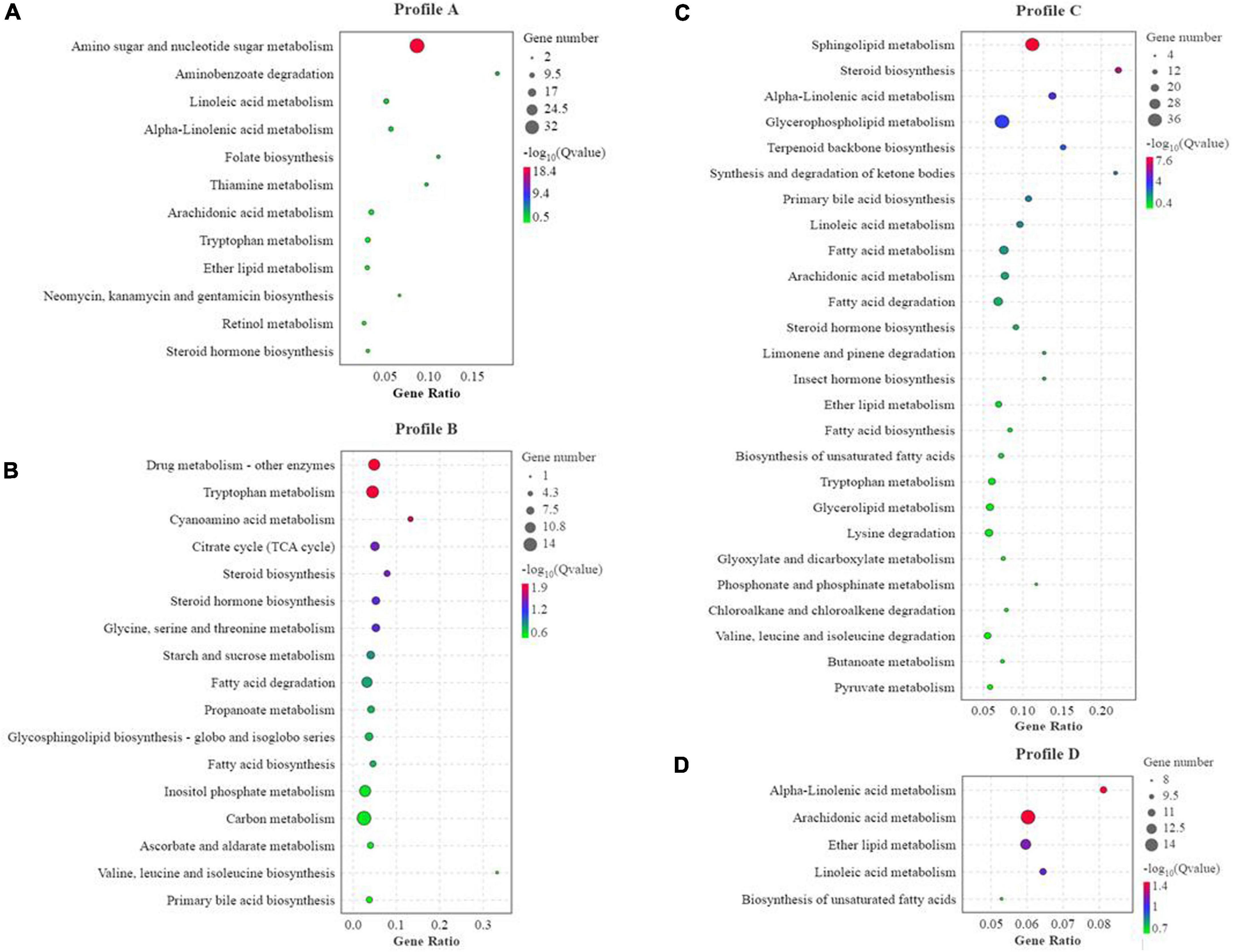
Figure 4. KEGG enrichment analysis of differential genes of different soy meals substitute for fish meal in hindgut of pearl gentian grouper (n = 4). (A) KEGG enrichment of co-contained DEGs in SBM40, SPC40, and FSBM40 group related to nutrition metabolism; (B) KEGG enrichment of unique DEGs in SBM40 group related to nutrition metabolism; (C) KEGG enrichment of unique DEGs in SPC40 group related to nutrition metabolism; (D) KEGG enrichment of unique DEGs in FSBM40 group related to nutrition metabolism (n = 4).
In profile A, the enrichment result showed that there were 238 pathways, of which 30 pathways indicated significant enrichment (P < 0.05). Among these pathways, there were 60 pathways relevant to nutrition metabolism, of which 12 pathways indicated significant enrichment (P < 0.05). Thus, within all the significant enrichment pathways, 40% (12/30) significant enrichment pathways were related to nutrition metabolism (Figure 4A). In profile B, the enrichment result showed that there were 297 pathways, of which 51 were significantly enriched (P < 0.05). Among these pathways, 81 pathways were relevant to nutrition metabolism, of which 17 pathways indicated significant enrichment (P < 0.05). In other words, within all the significant enrichment pathways, 33.33% (17/51) pathways were related to nutrition metabolism (Figure 4B). In profile C, the enrichment result showed 320 pathways, of which 35 were significantly enriched (P < 0.05). Among them, 98 pathways were relevant to nutrition metabolism, of which 26 indicated a significant enrichment (P < 0.05). Hence, within all the significant enrichment pathways, 74.3% (26/35) significant enrichment pathways were related to nutrition metabolism (Figure 4C). In profile D, the enrichment result showed that 305 pathways, of which 38 were significantly enriched (P < 0.05). Among them, 75 pathways were related to nutrition metabolism, of which six pathways indicated significant enrichment (P < 0.05). Thus, within all the significant enrichment pathways, 13.2% (5/38) were related to nutrition metabolism (Figure 4D). The tryptophan metabolism pathway was significantly affected in the experiments of all three soy meal substitutions for FM (P < 0.05).
Validation of the tryptophan metabolism pathway by RT-qPCR
In general, the results of RT-qPCR corresponded to the results of transcriptome sequencing data, which confirmed the accuracy of RNA-seq (Figure 5). Therefore, RNA-seq in this study can provide a relatively valuable reference for further analysis. The results of the RT-qPCR showed that the key genes selected from the tryptophan metabolism pathway in different soy meal substitution groups were generally significantly inhibited (P < 0.05). However, there were some differences in the types of key genes of the tryptophan metabolism pathway affected in different treatment groups (Figure 6).
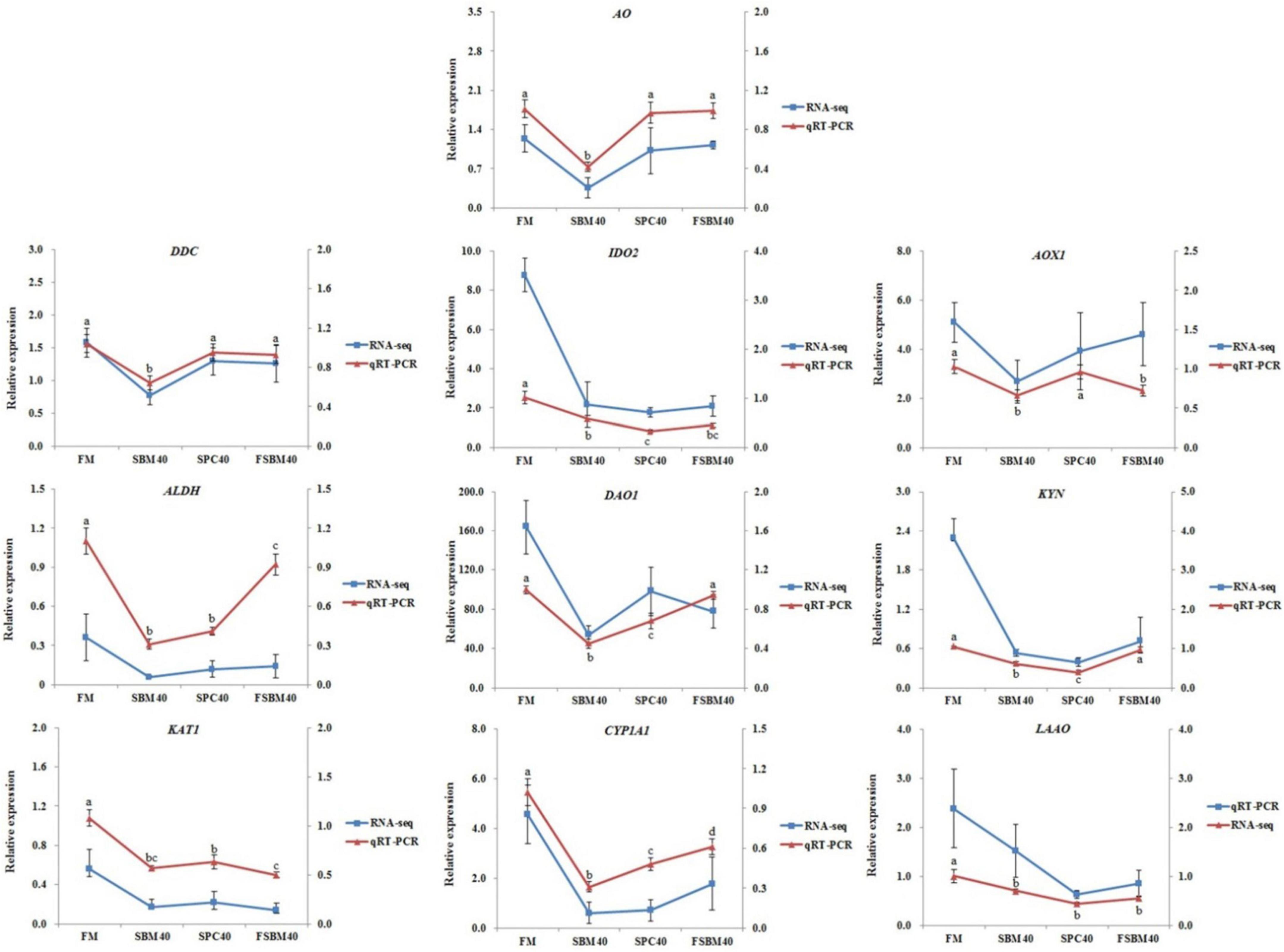
Figure 5. The contrast of RNA-seq and RT-qPCR results and the expressions of the key genes in tryptophan metabolism pathway in hindgut were chosen to confirm the precision of “3 + 2” transcriptome sequencing. The relative expression degree in RNA-seq analysis was counted by FPKM value. Different letters distributed on the broken line represented significant variances between the groups at P < 0.05 (n = 4).
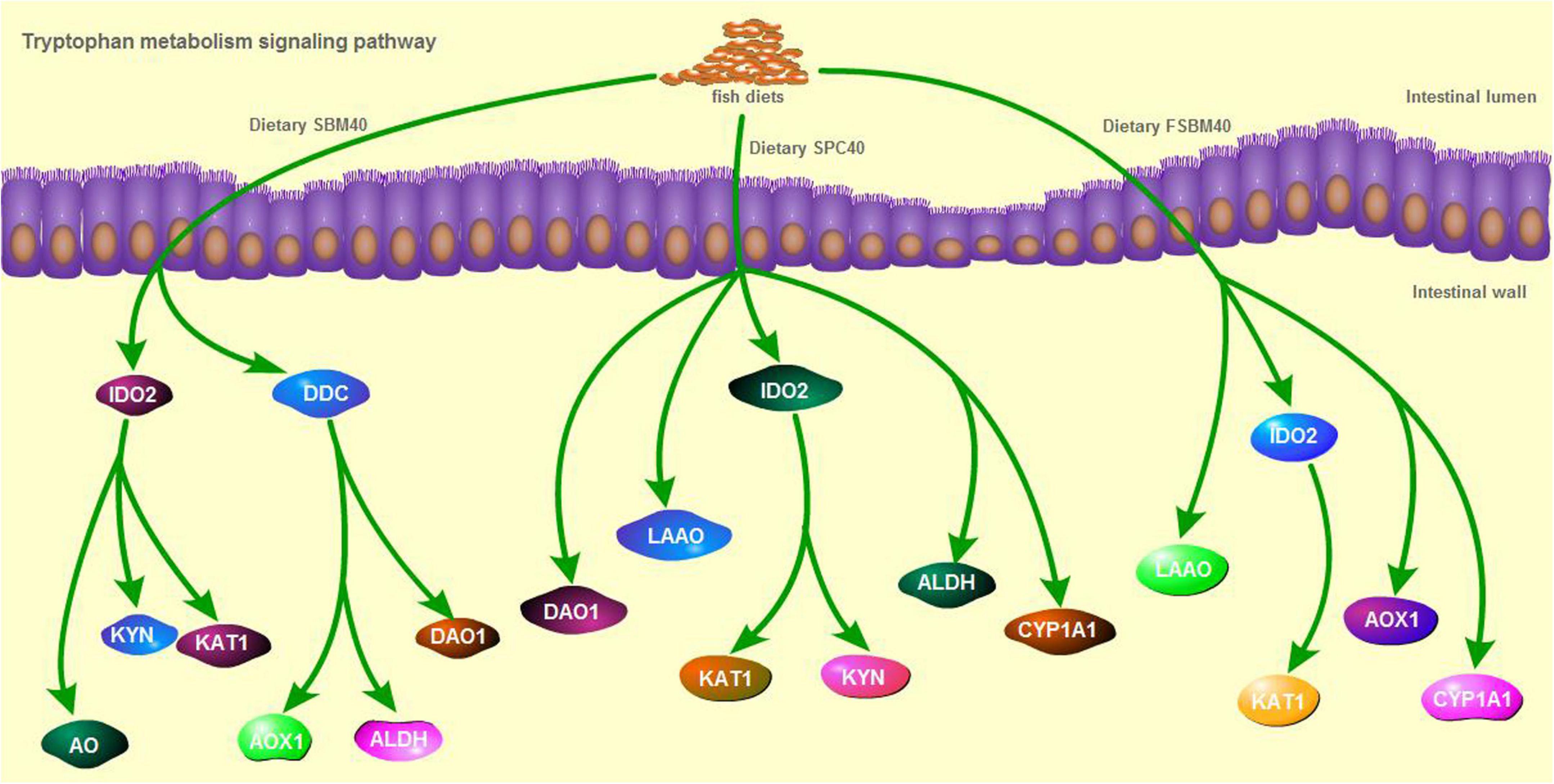
Figure 6. The key genes inhibited in tryptophan metabolism pathway of different soy meals induced enteritis in pearl gentian grouper.
Correlation analysis
Figure 7 shows the correlations between the significant genes in the inflammatory genes and tryptophan metabolism pathways using the Envfit test. The results show that with increases in SBM, the key genes inhibited in tryptophan metabolism pathways were generally significantly correlated with the enteritis-related inflammatory genes (P < 0.05, Supplementary Tables 1–3). Thus, it had a negative correlation with the anti-inflammatory gene and a positive correlation with the pro-inflammatory gene.
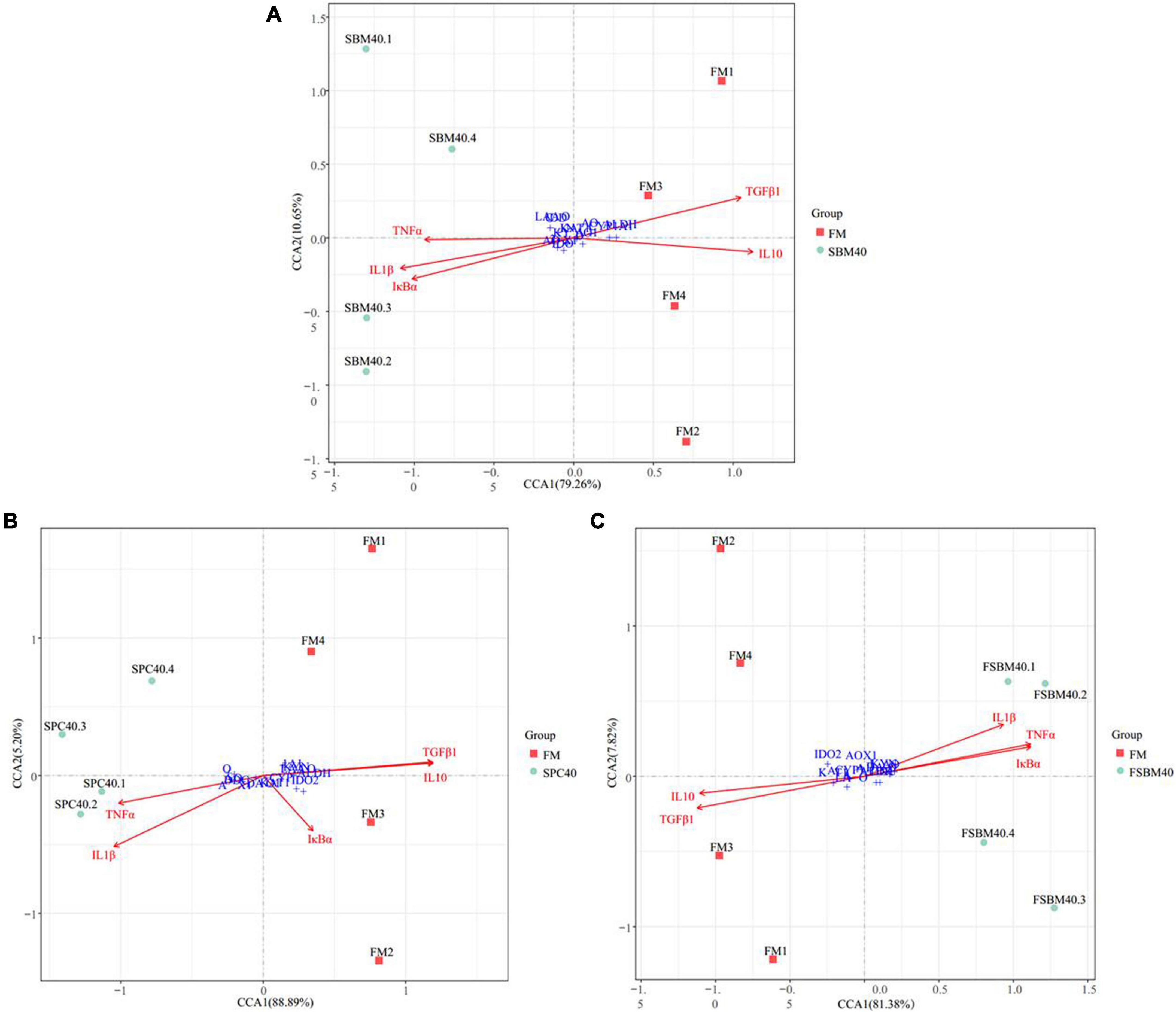
Figure 7. The canonical correlation analysis (CCA) between the key genes inhibited in tryptophan metabolism pathway and the inflammatory genes of different soy meals induced enteritis in groupers (n = 4). In CCA plot, the arrows represent explanatory variables and the points represent response variables. The lengths of the arrows represent the strength of the influence of the explanatory variable on the response variable; the longer lengths indicate greater influences. The angles between the arrows and coordinate axis represent the correlation between the explanatory variable and coordinate axis; smaller angles indicate stronger correlation. A sample point located in the same direction as the arrow indicates that the changes in explanatory and response variables are positively correlated, while in the opposite direction which indicates a negative correlation. The value in the coordinate axis label in the plot represents the interpretation proportion of the explanatory variables combination and the response variable variation. FM, fish meal control group; SBM40, 40% SBM substitution for FM; SPC40, 40% SPC substitution for FM; FSBM40, 40% FSBM substitution for FM.
Discussion
The present study exhibited that different soy meals (SBM, SPC, and FSBM) substitution for FM at the experimental level obviously lowered the growth performance, gut physiology and immune state, and gut flora composition. Studies on Epinephelus coioides (initial weight of 84 ± 2.5 g) found that 20% SBM substitution for FM (60% basal FM) presented optimal growth performance (10). Studies on the brown-marbled grouper (initial weight of 6.1 ± 0.7 g) showed that using 20–50% SBM to substitute for FM (50% basal FM) was unlikely to lower growth performance, but it significantly decreased at a 60% substitution level (12). SPC with substitution levels lower than 40% presented a significant reduction in the WGR, SGR, and FCR of turbot and Japanese flounder (28, 29). The optimal amount of FSBM in the hybrid tilapia diet substitution for FM is 34.2% (30), and FSBM in channel catfish diet can substitute 100% FM, but 25% is best (31). The present study found that WGR and SGR at a high level of substitution (40%) were significantly lower than in the FM control group. A previous study indicated that the soy protein replacing FM in fish growth physiology may be related to the ANFs it contains (3). SBM, SPC, and FSBM, as three soy protein sources with different processing forms, have different content and types of ANFs. The unpublished feed test results in the present research showed that alcohol-soluble ANFs in SPC were basically completely removed, such as soybean isoflavones and (7S and 11S) and conglycinin. Although FSBM was fermented, compared with the SBM diet, the content of soybean isoflavones and (7S and 11S) conglycinin and other ANFs showed little change. However, compared with SBM and FSBM, the pearl gentian grouper fed with SPC feed did not show obvious advantages. It is speculated that ANFs may not be the only main influencing factor, but they may also be the influence of other unknown antigens or non-alcohol-soluble ANFs (such as phytic acid). FSBM did not improve the growth performance of pearl gentian groper, which may be due to its high content of ANFs or palatability problems (32). Previous studies on E. coicoiaes found that adding more than 14% FSBM to the diet would significantly reduce WGR and SGR, and the most suitable amount of FSBM to replace white FM (52% of basal FM) was 10% (10).
The present research also indicated that for pearl gentian groupers, the experimental level of soy proteins also had a significant impact on their gut enzyme activity. The LYS plays an important role in non-specific immunity in fish and IgM for specific immunity. Both C3 and C4 are crucial for activating their complement systems (33). In the research, all the soy protein sources with a 40% substitution level reduced the contents of C3, C4, and IgM, in which the addition of soy proteins may lead to the impaired gut immune system of pearl gentian groupers. This is also reflected in immune-related genes, such as inflammatory-related genes. In addition, previous research confirmed that the occurrence of enteritis in fish always goes with down-regulation of anti-inflammatory genes and up-regulation of pro-inflammatory genes (34). The present experiment found similar results.
Our previous analysis of the gut immune system of SBMIE pearl gentian groupers found that among the signal pathways significantly activated in the gut, 55.17% were related to intestinal immunity, contagious disease, and signal transduction; in the signal pathways greatly inhibited in the gut, nutrient metabolism-related pathways accounted for 67.44% (35). The results showed that for pearl gentian groupers, the production of SBMIE is likely to be the result of the combined action of immune and nutritional imbalance. Combined with this study, we found different gut responses of SBMIE pearl gentian groupers that ate SPC and FSBM. After eating SPC, grouper mainly showed that the pathways relevant to intestinal nutrient metabolism were restrained in general (74.3%, 26/35), while after eating FSBM, the pathways related to immunity, contagious disease, and signal transduction were generally inhibited (60.5%, 23/38). Among them, the tryptophan metabolism pathway is significantly inhibited in the SBMIE pearl gentian groupers caused by the studied soy protein sources in the present study. According to existing research, tryptophan, as a communication medium between gut flora and the host, generates many tryptophan metabolites under the action of a series of endogenous enzymes or biological metabolisms, and its metabolites regulate various physiological processes of the host to varying degrees. Tryptophan deficiency directly leads to the reduction of metabolites, which is closely related to gut imbalance, and even induced IBD (36).
Tryptophan is the only amino acid in animals that combines with serum protein through covalent bonds. It is widely involved in the synthesis of proteins and nucleic acids, and it can enter the liver through the hepatic portal vein and regulate protein deposition and metabolism. Insufficient tryptophan content will reduce the absorption and utilisation rate of protein and immune function of livestock and poultry and increase the susceptibility of livestock and poultry to disease (37, 38). There are three main pathways of tryptophan catabolism: the kynurenine pathway, 5-hydroxytryptamine (5-HT) pathway and the gut flora pathway. The kynurenine pathway is the most important metabolic pathway through which about 95% of tryptophan is metabolised (39, 40). This pathway occurs in three parts of the body, which, in order of degree, are the liver, brain, and small intestine. The rate-limiting enzymes are TDO and –2,3–dioxygenase IDO, respectively (41). Tryptophan is catalysed by a series of enzymes, such as TDO/IDO to produce a variety of metabolites, such as N-formyl kynurenine, kynurenine, 3-hydroxykynurenine, kynurenic acid, and picolinic acid, which are finally decomposed into CO2 and ATP (42). After being absorbed by the body, these metabolites can regulate intestinal barrier immunity in a variety of ways. Second, 1–2% of tryptophan intake is converted through the 5-HT pathway, mainly in the gastrointestinal tract, of which 90% occurs in enterochromaffin cells and 10% in gut neurons, and the rate-limiting enzymes are tryptophan hydroxylase (43). 5-HT not only regulates the immune activity of the body, but it also plays a key role as a neurotransmitter in the brain-gut axis, which is a bidirectional system that connects the gastrointestinal tract with the central nervous system (44). In addition, 4–6% of tryptophan can be directly metabolised by intestinal flora into ligands of aromatic hydrocarbon receptor (AhR), including indole and indole derivatives (45, 46). In terrestrial animal studies, it is pointed out that AhR can bind to its ligand through TLR-NF-κB signalling pathway, and then it regulates the expression of downstream target genes. For example, studies in mammals have found that a tryptophan-rich diet can activate the expression of the AhR gene and subsequently increase the expression of the IL22 gene in the colon (47). AhR is a negative regulator of IL17-mediated signal transduction and plays an important role in resisting pathogens of immune diseases (48). IL10 is an effective anti-inflammatory cytokine that can inhibit the production of pro-inflammatory mediators. IL10 signals through IL10 receptors, which act on various cell types, such as intestinal epithelial cells, where IL10 receptors are associated with the development and maintenance of barrier function (49). In fish SBMIE, NF-κB signalling pathway presents to be quite conservative, and similar results were obtained in our previous analysis of pearl gentian groupers enteritis induced by SBM and FSBM (35). In this experiment, through CCA analysis, we found that there was a significant correlation between the key genes of the tryptophan metabolism pathway and enteritis induced by three soy protein sources of pearl gentian grouper, which also suggested that abnormal tryptophan metabolism in fish soy enteritis deserves special attention.
Some studies have pointed out that the gut flora variation will lead to a change in tryptophan metabolism and then affect the intestinal physiological function (50). Similarly, tryptophan deficiency in feed can also change the composition of gut flora and damage intestinal immune function (51). Previous research has pointed out that the tryptophan content in SBM is generally equivalent to that in FM (∼0.65%), while the tryptophan content in SPC and FSBM is higher than that in FM (52). However, the three kinds of soy protein feeds in this research have led to the inhibition of tryptophan metabolism, which is likely to be closely related to gut flora variation. The three soy protein sources at this experimental level significantly influenced the intestinal flora of pearl gentian groupers. In mammalian studies, it was found that the abundance changes in Bacteroides, Bacteroides, Bifidobacterium, Fusobacterium, Lactobacillus, and Faecalibacterium (53–55) were significantly correlated with the degree of IBD. Similarly, the abundance changes in some species at the level of gut flora were also found in the SBMIE pearl gentian grouper, but the change trend was opposite. Previous research pointed out that under pathological state, the OTU abundance variations of mammalian gut flora at the level of the phylum or genus run contrary to those of fish sometimes (56–58). At present, the analysis of intestinal flora in this experiment is only at the genus level, and it should be further studied at the species level in the future.
Conclusion
This study found that the tryptophan metabolic pathway has an important impact on the enteritis of pearl gentian groupers induced by soy protein, during which gut flora may play a crucial role. However, a more in-depth analysis should be further improved in future work. Technically, targeted metabonomics can be used to analyse the changes of tryptophan metabolites, such as indoles and indole derivatives produced by gut flora metabolism; using metagenomics to analyse the species of tryptophan metabolising flora in the gut at the species level, as a potential probiotic additive for repairing fish soy-induced enteritis. The research offers a theoretical reference for the development of new feed additives to prevent soy-induced enteritis in fish and has important economic and ecological benefits.
Data availability statement
The datasets presented in this study can be found in online repositories. The names of the repository/repositories and accession number(s) can be found in the article/Supplementary material.
Ethics statement
All experimental methods were approved by the Ethics Review Board of Guangdong Ocean University. All of the procedures were performed in accordance with the relevant guidelines and regulations.
Author contributions
WZ and AP were engaged in the whole experiment and formulated the draft of this manuscript. BT designed the experiment and composed and amended the draft critically. YX and YL took part in the tests. RX amended the first draft. HZ and QY completed the data analysis. JD and SC approved the final version. All authors contributed to the article and approved the submitted version.
Funding
This research was supported by the National Key R&D Program of China (2019YFD0900200), the National Natural Science Foundation of China (NSFC 31772864), and the China Agriculture Research System of MOF and MARA (CARS-47).
Acknowledgments
We are grateful to the Key Laboratory of Aquatic, Livestock and Poultry Feed Science and Technology in South China, Ministry of Agriculture, for providing technical assistances.
Conflict of interest
The authors declare that the research was conducted in the absence of any commercial or financial relationships that could be construed as a potential conflict of interest.
Publisher’s note
All claims expressed in this article are solely those of the authors and do not necessarily represent those of their affiliated organizations, or those of the publisher, the editors and the reviewers. Any product that may be evaluated in this article, or claim that may be made by its manufacturer, is not guaranteed or endorsed by the publisher.
Supplementary material
The Supplementary Material for this article can be found online at: https://www.frontiersin.org/articles/10.3389/fnut.2022.1014502/full#supplementary-material
Footnotes
References
1. Mohan K, Rajan D, Muralisankar T, Ganesan A, Sathishkumar P, Revathi N. Use of black soldier fly (Hermetia illucens L.) larvae meal in aquafeeds for a sustainable aquaculture industry: a review of past and future needs. Aquaculture. (2022) 553:738095. doi: 10.1016/j.aquaculture.2022.738095
2. Watson A, Casu F, Bearden D, Yost J, Denson M, Gaylord T, et al. Investigation of graded levels of soybean meal diets for red drum, Sciaenops ocellatus, using quantitative PCR derived biomarkers. Comp Biochem Physiol Part D Genomics Proteomics. (2019) 29:274–85. doi: 10.1016/j.cbd.2019.01.002
3. Krogdahl Å, Penn M, Thorsen J, Refstie S, Bakkle A. Important antinutrients in plant feedstuffs for aquaculture: an update on recent findings regarding responses in salmonids. Aquac Res. (2010) 41:333–44. doi: 10.1111/j.1365-2109.2009.02426.x
4. Sahlmann C, Sutherland B, Kortner T, Koop B, Krogdahl Å, Bakkle A. Early response of gene expression in the distal intestine of Atlantic salmon (Salmo salar L.) during the development of soybean meal induced enteritis. Fish Shellfish Immunol. (2013) 34:599–609. doi: 10.1016/j.fsi.2012.11.031
5. Yamamoto T, Goto T, Kine Y, Endo Y, Kitaoka Y, Sugita T, et al. Effect of an alcohol extract from a defatted soybean meal supplemented with a casein-based semi-purified diet on the biliary bile status and intestinal conditions in rainbow trout Oncorhynchus mykiss (Walbaum). Aquac Res. (2008) 39:986–94. doi: 10.1111/j.1365-2109.2008.01969.x
6. Urán P, Gonçalves A, Taverne-Thiele J, Schrama J, Rombout J. Soybean meal induces intestinal inflammation in common carp (Cyprinus carpio L.). Fish Shellfish Immunol. (2008) 25:751–60. doi: 10.1016/j.fsi.2008.02.013
7. Fuentes-Appelgren P, Opazo R, Barros L, Feijoó C, Romero J. Effect of the dietary inclusion of soybean components on the innate immune system in zebrafish. Zebrafish. (2014) 11:41–9. doi: 10.1089/zeb.2013.0934
8. Bakke-Mckellep A, Press C, Baeverfjord G, Krogdahl Å, Landsverk T. Changes in immune and enzyme histochemical phenotypes of cells in the intestinal mucosa of Atlantic salmon, Salmo salar L. with soybean meal-induced enteritis. J Fish Dis. (2000) 23:115–27. doi: 10.1046/j.1365-2761.2000.00218.x
9. Ai Q, Xie X. The nutrition of Silurus meridionalis: effects of different levels of dietary soybean protein on growth. Acta Hydrobiol Sin. (2002) 26:57–65.
10. An M, Fan Z, Wang Q, Sun J, Xu D, Guo Y, et al. Effects of substituting fish meal with soybean meal on growth, digestion and antioxidant capacity of Epinephelus coicoiaes. Jiangsu Agric Sci. (2018) 46:128–32. doi: 10.15889/j.issn.1002-1302.2018.16.032
11. Zhou W, Yan Y, Yang H, Sun Y. Effects of soybean meal partially replacing fish meal on growth, digestive enzyme activity and immune function of Epinephelus coioides. Feed Res. (2014) 19:61–4. doi: 10.13557/j.cnki.issn1002-2813.2014.19.041
12. Faudzi N, Yong A, Shapawi R, Senoo S, Biswas A, Takii K. Soy protein concentrate as an alternative in replacement of fish meal in the feeds of hybrid grouper, brown-marbled grouper (Epinephelus fuscoguttatus) × giant grouper (E. lanceolatus) juvenile. Aquac Res. (2017) 49:431–41. doi: 10.1111/are.13474
13. Krogdahl Å, Bakke A. Antinutrients. In: C Lee editor. Dietary Nutrients, Additives and Fish Health. Hoboken, NJ: Wiley Blackwell (2015). p. 211–35.
14. Kortner T, Skugor S, Penn M, Mydland L, Djordjevic B, Hillestad M, et al. Dietary soyasaponin supplementation to pea protein concentrate reveals nutrigenomic interactions underlying enteropathy in Atlantic salmon (Salmo salar). BMC Vet Res. (2012) 8:101. doi: 10.1186/1746-6148-8-101
15. Knudsen D, Jutfelt F, Sundh H, Sundell K, Koppe W, Frøkiaer H. Dietary soya saponins increase gut permeability and play a key role in the onset of soya bean-induced enteritis in Atlantic salmon (Salmo salar L.). Br J Nutr. (2008) 100:120–9. doi: 10.1017/S0007114507886338
16. Gu M, Bai N, Xu B, Xu X, Jia Q, Zhang Z. Protective effect of glutamine and arginine against soybean mealinduced enteritis in the juvenile turbot (Scophthalmus maximus). Fish Shellfish Immunol. (2017) 70:95–105. doi: 10.1016/j.fsi.2017.08.048
17. Hu Y, Zhang J, Xue J, Chu W, Hu Y. Effects of dietary soy isoflavone and soy saponin on growth performance, intestinal structure, intestinal immunity and gut microbiota community on rice field eel (Monopterus albus). Aquaculture. (2021) 537:736506. doi: 10.1016/j.aquaculture.2021.736506
18. Gu M, Pan S, Li Q, Qi Z, Bai N. Protective effects of glutamine against soy saponins-induced enteritis, tight junction disruption, oxidative damage and autophagy in the intestine of Scophthalmus maximus L. Fish Shellfish Immunol. (2021) 114:49–57. doi: 10.1016/j.fsi.2021.04.013
19. Sun M, Ma N, He T, Johnston L, Ma X. Tryptophan (Trp) modulates gut homeostasis via aryl hydrocarbon receptor (AhR). Crit Rev Food Sci. (2020) 60:1760–8. doi: 10.1080/10408398.2019.1598334
20. Tang L. The Study of the Effects Tryptophan on Digestive and Absorbtive Capacity, Disease Resistant, and the TOR Expression in Organs and Tissue in Jian carp (Cyprinus carpio var. Jian). Yaan: Sichuan Agriculture University (2010).
21. Ma Y. Effects of Tryptophan in Two Protein Levels Diets on Feed Intake, Growth Performance, and Immunity of Litopenaeus vannamei. Hubei: Huazhong Agricultural University (2016).
22. Hseu J, Lu F, Su H, Wang L, Tsai C, Hwang P. Effect of exogenous tryptophan on cannibalism, survival and growth in juvenile grouper, Epinephelus coioides. Aquaculture. (2003) 218:251–63. doi: 10.1016/S0044-8486(02)00503-3
23. Agus A, Planchais J, Soko H. Gut microbiota regulation of tryptophan metabolism in health and disease. Cell Host Microbe. (2018) 23:716.
24. Fan Y, Pedersen O. Gut microbiota in human metabolic health and disease. Nat Rev Microbiol. (2020) 19:55–71. doi: 10.1038/s41579-020-0433-9
25. Miao S, Zhao C, Zhu J, Hu J, Dong X, Sun L. Dietary soybean meal affects intestinal homoeostasis by altering the microbiota, morphology and inflammatory cytokine gene expression in northern snakehead. Sci Rep. (2018) 8:113–23. doi: 10.1038/s41598-017-18430-7
26. Zhang W, Tan B, Ye G, Wang J, Dong X, Yang Q, et al. Identification of potential biomarkers for soybean meal-induced enteritis in juvenile pearl gentian grouper, Epinephelus fuscoguttatus♀×Epinephelus lanceolatus♂. Aquaculture. (2019) 512:734337. doi: 10.1016/j.aquaculture.2019.734337
27. Zhang W, Tan B, Deng J, Yang Q, Chi S, Pang A, et al. PRR-mediated immune response and intestinal flora profile in soybean meal induced enteritis of pearl gentian groupers, Epinephelus fuscoguttatus ♀× Epinephelus lanceolatus ♂. Front Immunol. (2022) 13:814479. doi: 10.3389/fimmu.2022.814479
28. Liu X, Ai Q, Mai K, Liu F, Xu W. Effects of replacing fish meal with soy protein concentrate on feed intake and growth of turbot (Scophthalmus maximus). J Fish China. (2014) 38:91–8. doi: 10.3724/SP.J.1231.2014.48852
29. Deng J, Mai K, Ai Q, Zhang W, Wang X, Xu W, et al. Effects of replacing fish meal with soy protein concentrate on feed intake and growth of juvenile Japanese flounder, Paralichthys olivaceus. Aquaculture. (2006) 258:503–13. doi: 10.1016/j.aquaculture.2006.04.004
30. Cheng C, Liu Y. Study on fermented soybean meal instead of fish meal in hybrid tilapia feed. Guangdong Feed. (2004) 13:26–7.
31. Li H, Huang F, Hu B, Zhou Y, Zhang L. Effects of replacement of fish meal with fermented soybean in the diet channel catfish (Ictalurus punctutus) on growth performance and apparent digestibility of feed. Freshw Fish. (2007) 37:41–4.
32. Shen W, Matsui T. Intestinal absorption of small peptides: a review. Int J Food Sci Tech. (2019) 54:1942–8. doi: 10.1111/ijfs.14048
33. Uribe C, Folch H, Enriquez R, Moran G. Innate and adaptive immunity in teleost fish: a review. Vet Med Czech. (2011) 56:486–503. doi: 10.17221/3294-VETMED
34. Lepage P, Seksik P, Sutren M, Cochetière M, Jian R, Marteau P, et al. Biodiversity of the mucosa-associated microbiota is stable along the distal digestive tract in healthy individuals and patients with IBD. Inflamm Bowel Dis. (2005) 11:473–80. doi: 10.1097/01.mib.0000159662.62651.06
35. Zhang W. Study on the Differential Mechanism of Intestinal Mucosal Barrier Damage in Epinephelus fuscoguttatus♀×E. lanceolatus♂ Caused by Three Kinds of Soybean Protein. Zhanjiang: Guangdong Ocean University (2020).
36. Cui C, Li Y, Yang H, Jin W, Wu J, Han C, et al. Research progress of tryptophan metabolism and inflammatory bowel disease. Med Recapitulate. (2021) 27:2726–30.
37. Floc’h N, Otten W, Merlot E. Tryptophan metabolism, from nutrition to potential therapeutic applications. Amino Acids. (2011) 41:1195–205. doi: 10.1007/s00726-010-0752-7
38. Wu G. Amino acids: metabolism, functions, and nutrition. Amino Acids. (2009) 37:1–17. doi: 10.1007/s00726-009-0269-0
39. Kaszaki J, Érces D, Gabriella V, Szabó A, Vécsei L, Boros M. Kynurenines and intestinal neurotransmission: the role of N-methyl-D-aspartate receptors. J Neural Transm. (2012) 119:211–23. doi: 10.1007/s00702-011-0658-x
40. Agus A, Planchais J, Sokol H. Gut microbiota regulation of tryptophan metabolism in health and disease. Cell Host Microbe. (2018) 23:716–24. doi: 10.1016/j.chom.2018.05.003
41. Ebrahimi A, Kardar G, Toolabi L, Ghanbari H, Sadroddiny E. Inducible expression of indoleamine 2,3-dioxygenase attenuates acute rejection of tissue-engineered lung allografts in rats. Gene. (2016) 576:412–20. doi: 10.1016/j.gene.2015.10.054
42. Platten M, Nollen E, Röhrig U, Fallarino F, Opitz C. Tryptophan metabolism as a common therapeutic target in cancer, neurodegeneration and beyond. Nat Rev Drug Discov. (2019) 18:379–401. doi: 10.1038/s41573-019-0016-5
43. Qin H, Wong H, Zang K, Li X, Bian Z. Enterochromaffin cell hyperplasia in the gut: factors, mechanism and therapeutic clues. Life Sci. (2019) 239:116886. doi: 10.1016/j.lfs.2019.116886
44. O’Mahony S, Clarke G, Borre Y, Dinan T, Cryan J. Serotonin, tryptophan metabolism and the brain-gut-microbiome axis. Behav Brain Res. (2015) 277:32–48. doi: 10.1016/j.bbr.2014.07.027
45. Wang G, Huang S, Wang Y, Cai S, Yu H, Liu H, et al. Bridging intestinal immunity and gut microbiota by metabolites. Cell Mol Life Sci. (2019) 76:3917–37. doi: 10.1007/s00018-019-03190-6
46. Huć T, Nowinski A, Drapala A, Konopelski P, Ufnal M. Indole and indoxyl sulfate, gut bacteria metabolites of tryptophan, change arterial blood pressure via peripheral and central mechanisms in rats. Pharmacol Res. (2018) 130:172–9. doi: 10.1016/j.phrs.2017.12.025
47. Comai S, Bertazzo A, Brughera M, Crotti S. Tryptophan in health and disease. Adv Clin Chem. (2020) 95:165–218. doi: 10.1016/bs.acc.2019.08.005
48. Vyhlídalová B, Krasulová K, Pečinková P, Marcalíková A, Vrzal R, Zemánková L, et al. Gut microbial catabolites of tryptophan are ligands and agonists of the aryl hydrocarbon receptor: a detailed characterization. Int J Mol Sci. (2020) 21:2614. doi: 10.3390/ijms21072614
49. Perez L, Kempski J, Mcgee M, Pelzcar P, Agalioto T, Giannou A, et al. TGF-βsignaling in Th17 cells promotes IL-22 production and colitis-associated colon cancer. Nat Commun. (2020) 11:2608. doi: 10.1038/s41467-020-16363-w
50. Golubeva A, Joyce S, Moloney G, Burokas A, Sherwin E, Arboleya S, et al. Microbiota-related changes in bile acid&tryptophan metabolism are associated with gastrointestinal dysfunction in a mouse model of autism. Ebiomedicine. (2017) 24:166–78. doi: 10.1016/j.ebiom.2017.09.020
51. Hashimoto T, Perlot T, Rehman A, Trichereau J, Ishiguro H, Paolino M, et al. ACE2 links amino acid malnutrition to microbial ecology andintestinal inflammation. Nature. (2012) 487:477–81. doi: 10.1038/nature11228
52. National Research Council [NRC].Nutrient Requirements of Fish and Shrimp. Washington, DC: The National Academic Press (2011). p. 401.
53. Frank D, Amand A, Feldman R, Boedeker E, Harpaz N, Pace N. Molecular-phylogenetic characterization of microbial community imbalances in human inflammatory bowel diseases. Proc Natl Acad Sci USA. (2007) 104:13780–5. doi: 10.1073/pnas.0706625104
54. Nemoto H, Kataoka K, Ishikawa H, Ikata K, Arimochi H, Iwasaki T, et al. Reduced diversity and imbalance of fecal microbiota in patients with ulcerative colitis. Dig Dis Sci. (2012) 57:2955–64. doi: 10.1007/s10620-012-2236-y
55. Kostic A, Xavier R, Gevers D. The microbiome in inflammatory bowel disease: current status and the future ahead. Gastroenterology. (2014) 146:1489–99. doi: 10.1053/j.gastro.2014.02.009
56. Jing G, Kang X, Liu H, Gang L, Bai M, Li T, et al. Impact of the gut microbiota on intestinal immunity mediated by tryptophan metabolism. Front Cell Infect Microbiol. (2018) 8:13. doi: 10.3389/fcimb.2018.00013
57. Lin L, Zhang J. Role of intestinal microbiota and metabolites on gut homeostasis and human diseases. BMC Immunol. (2017) 18:2. doi: 10.1186/s12865-016-0187-3
Keywords: Epinephelus fuscoguttatus♀ × E. lanceolatus♂, soy meal, gut flora, tryptophan metabolism, enteritis
Citation: Zhang W, Pang A, Tan B, Xin Y, Liu Y, Xie R, Zhang H, Yang Q, Deng J and Chi S (2022) Tryptophan metabolism and gut flora profile in different soybean protein induced enteritis of pearl gentian groupers. Front. Nutr. 9:1014502. doi: 10.3389/fnut.2022.1014502
Received: 08 August 2022; Accepted: 18 November 2022;
Published: 19 December 2022.
Edited by:
Nicola Fiotti, University of Trieste, ItalyReviewed by:
Ishtiyaq Ahmad, University of Kashmir, IndiaVincenzo Parrino, The University of Messina, Italy
Copyright © 2022 Zhang, Pang, Tan, Xin, Liu, Xie, Zhang, Yang, Deng and Chi. This is an open-access article distributed under the terms of the Creative Commons Attribution License (CC BY). The use, distribution or reproduction in other forums is permitted, provided the original author(s) and the copyright owner(s) are credited and that the original publication in this journal is cited, in accordance with accepted academic practice. No use, distribution or reproduction is permitted which does not comply with these terms.
*Correspondence: Beiping Tan, YnB0YW5AMTI2LmNvbQ==
†These authors have contributed equally to this work