- 1School of Food Science and Engineering, Guangdong Provincial Key Laboratory of Intelligent Food Manufacturing, Foshan University, Foshan, China
- 2College of Food Science and Technology and College of Life Sciences, Northwest University, Xi'an, China
- 3School of Food Science and Engineering, South China University of Technology, Guangzhou, China
- 4National Institute of Food Science and Technology, University of Agriculture, Faisalabad, Pakistan
The bactericidal effect of dielectric barrier discharge-atmospheric cold plasma (DBD-ACP, 20, and 30 kV) against Alicyclobacillus acidoterrestris on the saline solution and apple juice was investigated. Results show that DBD-ACP is effective for the inactivation of A. acidoterrestris by causing significant changes in cell membrane permeability and bacterial morphology. The effect of culture temperatures on the resistance of A. acidoterrestris to DBD-ACP was also studied. A. acidoterrestris cells grown at 25°C had the lowest resistance but it was gradually increased as the culture temperature was increased (25–45°C) (p < 0.05). Moreover, results from Fourier transform infrared spectroscopy (FT-IR) and Gas Chromatography-Mass Spectrometer (GC-MS) analysis showed that the increase in the culture temperature can gradually cause the decreased level of cyclohexaneundecanoic acid in the cell membrane of A. acidoterrestris (p < 0.05). In contrast, cyclopentaneundecanoic acid, palmitic acid, and stearic acid showed an increasing trend in which the fluidity of the bacterial cell membrane decreased. This study shows a specific correlation between the resistance of A. acidoterrestris and the fatty acid composition of the cell membrane to DBD-ACP.
Introduction
Alicyclobacillus acidoterrestris is a kind of spore-producing acidophilic heat-resistant bacteria. Many studies have shown that this bacterium is one of the main reasons for causing the spoilage of various fruit juices such as apple juice, orange juice, and grape juice (1–3). The fruit juice contaminated with A. acidoterrestris did not show obvious rancidity or swelling at the initial stage. However, if its metabolites, (2, 6-dibromophenol, and 2, 6-dibromophenol) produced by A. acidoterrestris cells can cause quality issues by increasing turbidity as well as formation of white precipitates in the juice. These losses can cause problems for fruit juices by causing huge financial losses (4). Keeping in mind the potential risk to the quality of fruit and its beverages, A. acidoterrestris has been proposed as a reference microorganism for quality control in the juice industry. Heat pasteurization is the most common method in fruit juice processing. However, due to the acidophilic and heat-resistant characteristics of A. acidoterrestris cells, it is difficult to inactivate by heat pasteurization. Moreover, thermal processing could easily cause flavor components and heat-sensitive nutrients such as vitamins that significantly damage fruit juice (5). Therefore, A. acidoterrestris is an actual problem that urgently needs to be solved in fruit juice processing. It is essential to seek a safe, reliable, and effective means to kill A. acidoterrestris with less influence on fruit juice components.
Nonthermal plasma technology is an aggregate formed by atoms, electrons, charged particles, free radicals, and ultraviolet photons generated by ionized or partially ionized gases (6–8). Cold plasm can inactivate various microbial vegetative cells in food at low temperatures (generally lower than 40°C) and short time, but it has little effect on the quality of the food itself. So it has been considered as a potential technology for sterilization of various types of food (9). Studies have shown that many factors including gas composition, voltage and treatment time, and food characteristics affect the efficiency of cold plasma sterilization. Additionally, the inherent characteristics, including cell structure and intrinsic protective mechanisms of microorganisms and environmental factors, are also essential factors (10, 11). Temperature and pH are two of the common factors that can induce changes in the resistance of bacteria to decontamination processing, including plasma, which in turn affects sterilization efficiency (7, 12–14). However, from the results reported so far, the studies about temperature-mediated bacterial tolerance to plasma are obscure and insufficient (14, 15). Because of the potential hazard of A. acidoterrestris in the juice industry, it is of great significance to fully understand the bactericidal effect and influencing factors of non-thermal plasma on A. acidoterrestris to promote the application of this technology in juice sterilization. Therefore, this work is mainly aimed to investigate the bactericidal effect and mechanism for the inactivation of A. acidoterrestris cells by dielectric barrier discharge-atmospheric cold plasma (DBD-ACP). The changes in cell membrane permeability and morphology of A. acidoterrestris cells before and after DBD-ACP treatment were evaluated by an inverted fluorescence microscope, intracellular leakage contents, and scanning electron microscope. The effects of growth temperatures on the resistance of A. acidoterrestris cells to DBD-ACP were also studied based on cell membrane fluidity and membrane fatty acid composition from Fourier transform infrared spectroscopy (FT-IR) and gas-mass spectrometry. This work is expected to provide insight into the inactivation properties, mechanism, and resistance under various growth temperatures of A. acidoterrestris cells induced by DBD-ACP.
Materials and methods
Bacterial cultures
The bacterial strain of A. acidoterrestris ATCC 49025 was purchased as a lyophilized culture from the Microbial Culture Collection Center of Guangdong Institute of Microbiology (Guangzhou, China). A. acidoterrestris cells were revived by transferring the lyophilized culture to an AAM liquid medium (pH 4.0, per liter of deionized water containing 0.2 g ammonia sulfate, 0.25 g calcium chloride, 0.5 g magnesium sulfate, 2.0 g yeast extract, 5.0 g glucose, and 3.0 g monopotassium phosphate) and incubated overnight at 45°C in a shaker (200 rpm). A loopful of the culture of A. acidoterrestris cells suspension was inoculated onto cooled AAM agar medium and incubated at 45°C for 36 h. Then a single colony was transferred to a 500 mL-conical flask containing 200 mL of sterile AAM liquid medium and incubated to a late log phase of growth at 45°C. Pre-cultured A. acidoterrestris cells were transferred to a fresh AAM liquid medium (200 mL, OD 600 nm ≈ 0.10) for incubation at 25, 35, 45 and 55 °C.
DBD-plasma treatment
The cultured A. acidoterrestris was harvested by centrifugation at 4,000 × g for 10 min at 4°C. The collected pellet was washed with sterile water thrice and resuspended into sterilized saline water or apple juice (JinLiuYuan, not from concentration). It was prepared to be treated with DBD-ACP (CTP-2000K plasma equipment, equipped with DBD-50 reactor, Nanjing Suman Co., Ltd. Nanjing, China) at 20 kV and 30 kV for 0, 0.5, 1.0, 1.5 and 2.0 min, where the distance between the upper plate and liquid surface was 4 mm, and the frequency was 1.0 kHz. The treated bacterial solution was serially diluted, and the spread plate was counted, and cultured at 45°C for 36–48.0 h to detect the total number of colonies (CFU/mL). The lethality was analyzed according to the change in the number of colonies before (N0) and after (N) DBD-ACP treatment. Each treatment was repeated three times and the experimental results were averaged.
Cell membrane permeability of A. Acidoterrestris
A. acidoterrestris cells were subjected to DBD-ACP treatment under 30 kV for 0, 0.5, 1.0 and 2.0 min. After DBD-ACP treatment, 10 μL of A. acidoterrestris cells suspension was added to 990 μL of sterilized saline water containing 3.0 μL of propidium iodide (PI, 1 mg mL−1, Beijing Soleibao Technology Co., Ltd., Beijing, China) and incubated darkly at room temperature for 20 min. The red fluorescence of A. acidoterrestris cells from PI staining was observed by an inverted fluorescence microscope (Nikon Eclipse Ti2-A, Nikon Instruments Co. LTD., Tokyo, Japan). Additionally, membrane permeabilization was also evaluated by measuring the leakage of nucleic acids including DNA and RNA, and proteins from A. acidoterrestris cells using a NanoDrop spectrophotometer (ND-2000, Thermo Fisher Scientific, Massachusetts, USA) to record the absorbance at 260 nm and 280 nm according to the method reported by Cai et al. (16).
Scanning electron microscopy
Bacterial sample preparation for morphological observation was carried out regarding the previous method (17). A. acidoterrestris cells were collected by centrifuging at 4,000 × g for 5.0 min and then treated with 2.5% (v/v) glutaraldehyde in PBS buffer were stored overnight at 4°C. After centrifugation, the bacterial samples were dehydrated by gradient using 30–100% ethanol solution. The dehydrated cells were treated with tert-butanol twice. The samples were dropped on silver paper for vacuum freeze-drying, and the morphological changes of A. acidoterrestris cells could be observed after spraying gold.
Cell membrane fatty acid extraction and analysis
Fatty acid composition of A. acidoterrestris cells was detected using the method previously reported by Pan et al. (18). After membrane fatty acid extraction and methylation, the fatty acid profiles were detected by gas chromatography-mass spectrometry (GC-MS) (8890B−7000D, Agilent Technologies, Palo Alto, California, USA) by matching the mass spectra with the mass spectral library 2016 of the National Institute of Standards and Technology (NIST, Gaithersburg, Maryland, USA) and the retention times in the bacterial acid methyl ester (BAME) mix solution (analytical standard, Sigma-Supelco, Bellefonte, PA, USA).
Fourier transform infrared spectroscopy analysis
The lyophilized A. acidoterrestris cells were analyzed by infrared spectrum on Bruker Vetex70 FT-IR spectrometer (Bruker, Germany). The spectrum collection range was 600–4,000 cm−1 and the resolution was 4 cm−1. The spectral data is smoothed, normalized and converted to a second derivative to improve peak resolution using the Savtitzky-Golay algorithm (19).
Statistical analyses
Statistical analysis was performed using OriginPro 8.0 (Origin Lab, Northampton, MA, USA) in triplicate with three independent experiments and results expressed as means ± SD. Analysis of variance (ANOVA) followed by Tukey's test was carried out using SPSS 22.0 software (IBM, NY, USA), and values were considered significantly different if p < 0.05.
Results and discussion
Inactivation of A. Acidoterrestris cells by DBD-ACP
The inactivation efficacy of DBD-ACP under various treatment voltage and time against A. acidoterrestris cells in 0.85% sterile saline solution and apple juice is shown in Figure 1. For A. acidoterrestris cells in 0.85% sterile saline solution, 0.5 and 1.2-log reductions occurred for the exposure of DBD-ACP at 20 kV for 0.5 and 1 min and increased to approximately 1.9- and 2.5-log reductions for 1.5 and 2 min. Comparatively, DBD-ACP exhibited a much stronger bactericidal effect on A. acidoterrestris cells at 30 kV, which induces the bacterial populations was inactivated by approximately 1.2, 2.1, 3.4 and 4.9-log reductions with the same treatment time.
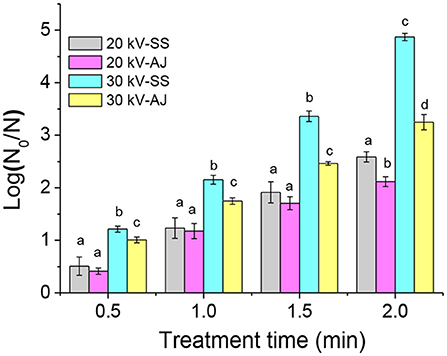
Figure 1. Inactivation of A. acidoterrestris by DBD-plasma at 20 kV and 30 kV on apple juice (AJ) and 0.85% sterile saline solution (SS). Different letters (a–d) on the top of the bars denote significant differences (p < 0.05).
A similar trend of DBD-ACP inactivation was detected for A. acidoterrestris cells in apple juice. However, DBD-ACP has a relatively lower inactivation against A. acidoterrestris cells in apple juice than in sterile saline solution. A similar work conducted by Mahnot et al. (20), found that S. enterica serovar Typhimurium in distilled water and the inactivation (>5 log-reduction) was observed much higher than in other simulating tender coconut water. Moreover, they also suggested that the presence of phosphate and Mg2+ ions reduced the inactivation of S. enterica serovar Typhimurium. Therefore, it could be inferred that the presence of nutrients and ions in apple juice may help to repair and recover A. acidoterrestris cells after DBD-ACP treatment.
Membrane permeability of A. Acidoterrestris cells
The cell membrane permeability of A. acidoterrestris cells after DBD-ACP treatment was observed by staining with PI, which incorporates genomic DNA by emitting red fluorescence if the bacterial cell membrane is permeable (21). As shown in Figure 2A, A. acidoterrestris cells without DBD-ACP treatment did not show much red fluorescence. Whereas the DBD-ACP treated A. acidoterrestris was stained with PI to emit higher degrees of red fluorescence with increasing time (Figures 2B–D). These results indicated that the plasma-treated A. acidoterrestris cell membrane was damaged, resulting in an increased permeability.
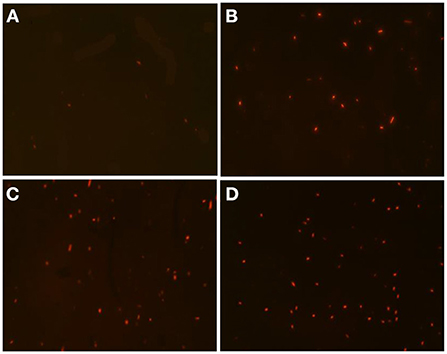
Figure 2. The PI staining A. acidoterrestris cells treated by DBD-plasma at 30 kV for 0 (A), 0.5 min (B), 1.0 min (C), and 2.0 min (D), respectively.
The cell membrane permeability induced by DBD-ACP may increase the leakage of cytoplasmic contents, including nucleic acids and proteins. Thus, the leakage of cytoplasmic contents of A. acidoterrestris cells was further determined in this study. The results of protein leakage of the DBD-ACP treated and untreated bacteria are shown in Figure 3A. The protein content in the untreated bacteria group was about 0.25 mg/L. However, this leakage of protein increases to 1.61 and 2.72 mg/L after being treated by DBD-ACP at 20 and 30 kV for 2.0 min. The nucleic acid concentration of A. acidoterrestris cells with or without DBD-ACP treatment presented a similar behavior (Figure 3B). As compared to untreated cells, the nucleic acid concentration was increased from 4.12 and 3.98 ng/μL to 34.31 and 50.42 ng/μL for A. acidoterrestris cells treated by DBD-ACP at 20 and 30 kV for 2.0 min, respectively. These results were consistent with the results obtained by PI staining with an inverted fluorescence microscope. Qian et al. (2022) found that the leakage of nucleic acids and proteins of L. monocytogenes and S. enteritidis induced reached the maximum content by cold plasma at 90 and 150 s, respectively (22). Olatunde et al. (23) reported a loss in cell membrane integrity of bacteria, including L. monocytogenes, S. aureus, P. aeruginosa, E. coli, and V. parahaemolyticus, which was measured by the increased conductivity and DNA content in supernatant induced by DBD-ACP treatment. In conclusion, the changes in membrane permeability that causes the formation of reversible permeabilization in bacterial cells were responsible for the lethal damage of A. acidoterrestris after DBD-ACP treatment.
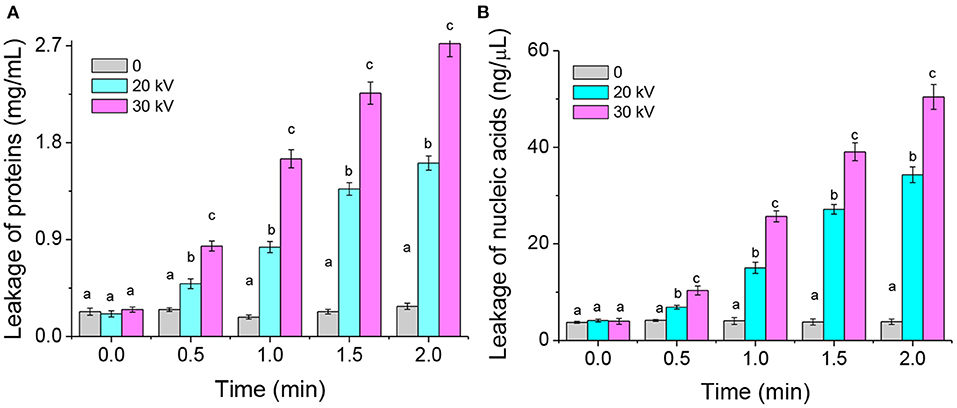
Figure 3. Leakage of proteins (A) and nucleic acids (B) from A. acidoterrestris cells induced by DBD-ACP. Different letters (a-d) on the top of the bars denote significant differences (p < 0.05).
Morphological changes of A. Acidoterrestris cells induced by DBD-ACP
Figures 4A–D show the cell morphological changes of A. acidoterrestris cells before and after DBD-ACP treatment at the voltage of 30 kV for 0.5, 1.0 and 2.0 min. Results showed that the untreated A. acidoterrestris cells showed a short rod-like shape with a smooth surface, while the DBD-ACP treated cells showed wrinkles, shrinkage, and deformation on the surface. These results suggested that A. acidoterrestris cells exhibit significant changes in their morphology with the increasing treatment. Such cell morphological deformations have been observed in E. coli, L. monocytogenes, and S. enteritidis after cold plasma treatment (24–26). Thus, it could be inferred that the lethal effect of A. acidoterrestris cell could be attributed to its destructive effects on the bacterial membrane with the leakage of cytoplasmic components by reactive oxygen species (ROS), particularly O3 and atomic oxygen generated during the processing of DBD-ACP treatment.
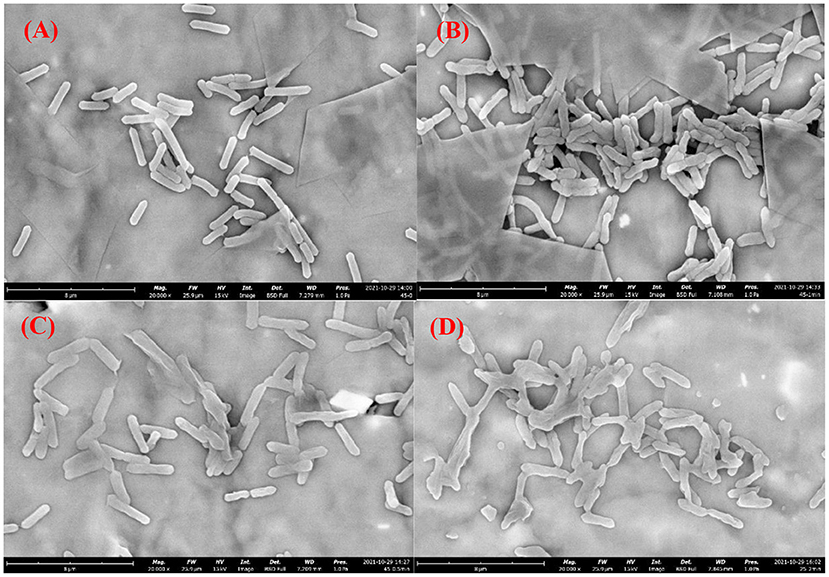
Figure 4. Morphological images of Alicyclobacillus acidoterrestris cells growth at 45°C exposure to DBD-plasma at 30 kV for 0 (A), 0.5 (B), 1.0 (C) and 2.0 min (D), respectively.
Effect of growth temperature on the resistance of A. Acidoterrestris to DBD-ACP
The temperature, pH, and other growth conditions play a vital role in bacterial resistance against inactivation technologies such as thermal, acidic, high-pressure, and pulsed electric field treatments. After being grown at 25, 35, 45, and 55°C to the late-logarithmic phase, A. acidoterrestris cells were collected and re-suspended on different systems (sterilized saline solution and apple juice) for DBD-ACP treatment. As shown in Figure 5, the inactivation of A. acidoterrestris cells increases with the increasing treatment voltage and time. On the sterilized saline solution, the inactivation levels of A. acidoterrestris cells cultivated at 25°C that increased from 1.8 to 3.8 log-reduction and from 1.9 to 6.7 log-reduction for DBD-ACP at 20 kV and 30 kV with treatment time from 0.5 to 2.0 min. Bacterial cells at different growth temperatures exhibited different resistance to DBD-ACP treatment. Among them, A. acidoterrestris cells grown at 45°C were found to be the most resistant, followed by at 35°C. Interestingly, the resistance of A. acidoterrestris cells at 25 and 55°C were similar (p > 0.05), and more sensitive to DBD-ACP than at 45 and 35°C no matter on the sterilized saline solution (Figures 5A,B) and apple juice (Figures 5C,D).
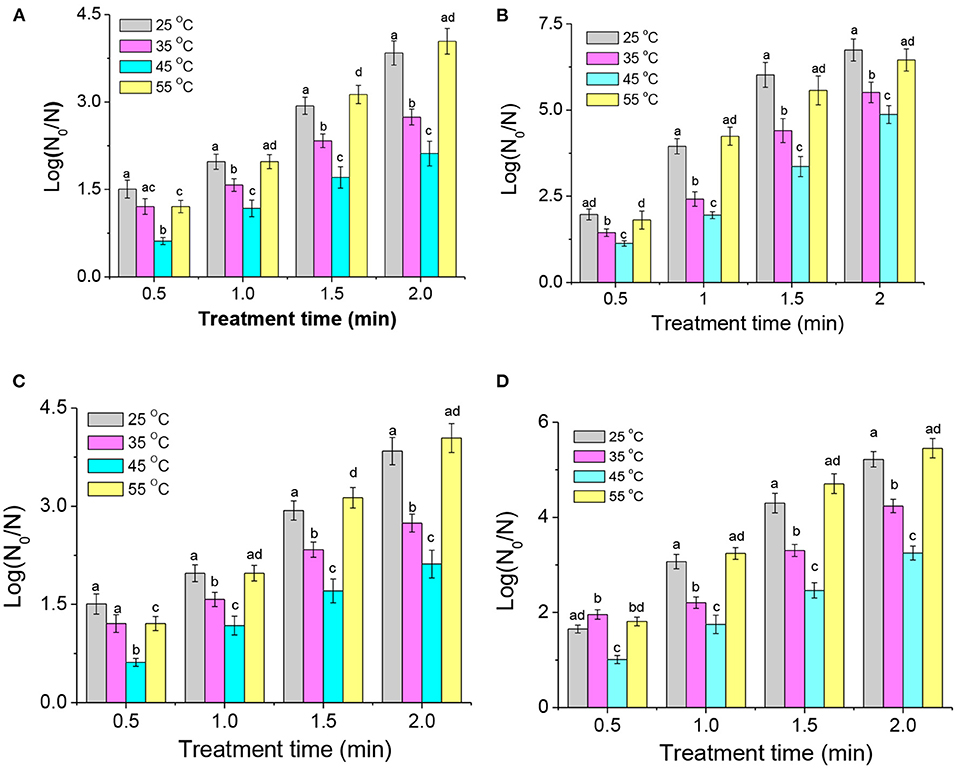
Figure 5. The effect of growth temperatures on the resistance of A. acidoterrestris cells on the sterile saline solution to DBA-ACP under 20 kV (A) and 30 kV (B), and apple juice with 20 kV (C) and 30 kV (D) for different treatment times. Different letters (a–d) on the top of the bars denote significant differences (p < 0.05).
For the sterilized saline solution, DBD-ACP treatment at the voltage of 20 kV with 1.0 min resulted in a 2.3 and 2.4 log-reduction (p>0.05) of A. acidoterrestris cultivated at 25 and 55°C, respectively. In contrast, the degree of inactivation of A. acidoterrestris was significantly lower by 1.6 log-reduction and (1.0 logs) (p < 0.05) for being cultivated at 35°C and 45°C, respectively. As the treatment time increased to 2.0 min, the inactivation of A. acidoterrestris cultured at different temperatures increased significantly. However, the inactivation behavior of A. acidoterrestris by DBD-ACP treatment was still similar. Specifically, the inactivation of A. acidoterrestris grown at 55°C was the highest that reaching to 5.3 log-reduction after DBD-ACP treatment, followed by A. acidoterrestris cultured at 25°C (4.8 log-reductions), and the inactivation rate was significantly decreased to 3.4 and 2.6 log-reduction for cells grown at 35 and 45°C, respectively (p < 0.05). Additionally, when the treatment voltage was 30 kV with the same treatment time of 2.0 min (Figure 5B), then the inactivation of A. acidoterrestris cultured at 25 and 55°C reached 6.7 and 6.4 log-reduction, respectively. The amount of inactivation at 35 and 45°C was significantly reduced (p < 0.05) to 5.5 and 4.9 log-reduction, respectively. The effect of growth temperatures on A. acidoterrestris cells exposed to DBD-ACP treatment with apple juice has similar results. For example, A. acidoterrestris cells grown at 25 and 55°C, DBD-ACP induced 5.2 and 5.4 log-reductions at 30 kV for 2 min, while A. acidoterrestris cells cultivated at 35 and 45°C resulted in 4.2 and 3.2 log reductions of viability, respectively. These results suggest that the resistance of A. acidoterrestris to DBD-ACP gradually increased with the increase of culture temperature in the range of 25 ~ 45°C expect 55°C. The above results concerning the effect of growth temperature on plasma inactivation are inconsistent with the resistance data reported by Pan et al. (27) revealing that the inactivation of L. monocytogenes cultivated at 10°C was found to be the most resistant to plasma exposure. Additionally, Fernandez et al. (15) found that the effect of growth temperatures (20, 25, 37, and 45°C) on the inactivation efficiency of Salmonella enterica serovar Typhimurium by cold plasma was insignificant (15). This inconsistency may be due to the different bacteria owning various adaptive mechanisms to temperature, resulting in different consequences regarding the effect of growth temperature on the resistance to DBD-ACP. However, the present study was similar to the literature showing that bacteria such as E. coli, L. monocytogenes, and S. aureus inoculated at low cultured temperatures showed high sentences to thermal or some nonthermal processing techniques including pulsed electrical field, high-pressure processing and atmosphere uniform glow discharge plasma (13, 14, 28). For example, Kayes et al. (14) found that E. coli, S. aureus, and B. subtilis cultured at 35°C were more tolerant to air plasma than bacteria cultured at 10°C (14).
Analysis of the cell membrane fluidity
The distinct difference in resistance to DBD-ACP may be responsible for the alterations of cell membrane fluidity and fatty acid composition of A. acidoterrestris at the different growth temperatures. The cellular membrane is one of the main targets being attacked by ROS and free radicals induced by cold plasma. As membrane fluidity is distinctive for the exchange of nutrients, ions, and regulatory molecules of cells, which directly affects the permeation of ROS into bacterial cells (7, 29). Therefore, the membrane fluidity was worth investigating.
FT-IR is a sensitive, non-destructive technique commonly used to detect changes in bacterial cell composition (30, 31). In Figure 6A, the FT-IR spectra of A. acidoterrestris cultured at different temperatures showed some typical characteristic peaks: 1,070 cm−1 and 1,234 cm−1 are typical P = O asymmetric and symmetric stretching vibration peaks, representing the main chain of nucleic acid and phosphodiester, respectively; while 1,399 cm−1 is the asymmetric and symmetric deformation of CH3− and CH2− of the protein; 1,542 cm−1 is the N-H characteristic vibration peak of protein amide II band; 1,655 cm−1 is the absorption peak near 1 is mainly the vibration peak of protein amide I, which is assigned to C=O stretching vibration; 2,928 cm−1 is the asymmetric stretching vibration peak of ω-cyclic fatty acid acyl chain υsCH2−. The above results in agreement with the previous study (32), indicating that the basic structure of the bacteria cultured at different temperatures is consistent. The differences in the infrared spectra exhibited by A. acidoterrestris at different temperatures are not noticeable.
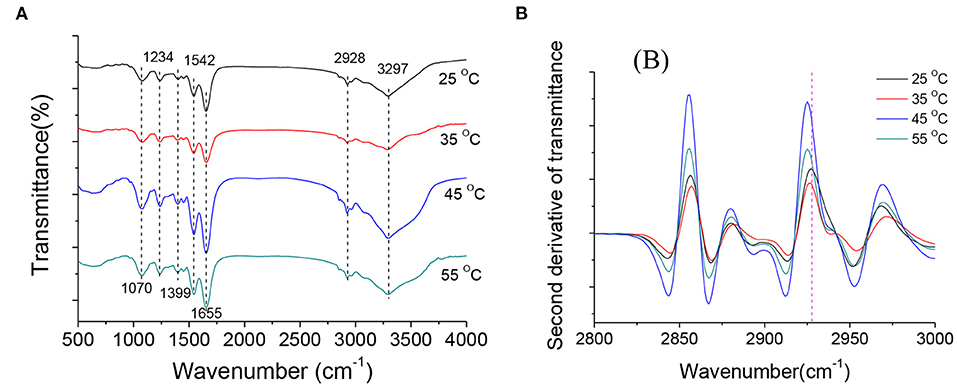
Figure 6. Raw FT-IR spectra of A. acidoterrestris at late log phase under different growth temperatures (A) and calculated second derivatives of FT-IR spectra in the spectral range of 2,800–3,000 cm−1 (B).
To further study the changes in fatty acid and fluidity of the cell membrane of A. acidoterrestris at different temperatures. In this study, the 2,800 ~ 3,000 cm−1 infrared spectral data were processed by the second derivative to enhance spectral resolution. It can be seen from Figure 6B that the spectra of this band at different culture temperatures show specific differences, indicating that different culture temperatures have certain effects on the composition of fatty acids in the cell membrane of A. acidoterrestris. According to the asymmetric stretching vibration of ω-cyclic fatty acid acyl chain υsCH2− near 2,928 cm−1, the changes in cell membrane fluidity at different temperatures were analyzed, and it was found that when the culture temperature increased from 25 to 55°C, the peak frequency from 2,927 cm−1 drops to 2,923 cm−1. Previous studies suggested that the peak frequency of fatty acid acyl chain υsCH2- is related to cell membrane fluidity. It is generally believed that the larger the value, the greater the fluidity of the cell membrane, and vice versa (19). Thus, it can be inferred that the membrane fluidity of A. acidoterrestris cells decreased gradually with the increase in culture temperature.
Membranes fatty acid analysis of A. Acidoterrestris Cells
Previously, studies have shown that bacteria at different temperatures and pH alter membrane fatty acid composition in order to maintain a proper cell membrane fluidity and to ensure the normal functioning of membrane physiology (33, 34). For example, the saturated fatty acid content in Shewanella putrefaciens cell decreased with the decrease of incubation temperature (30, 10 and 4°C), while the content of palmitoleic acid (C16:1), lauric acid (C12:0), and myristic acid (C14:0) increased (33). Similarly, E. coli cells were apt to increase the proportions of saturated fatty acids at the expense of unsaturated fatty acids, as reported by Liu et al. (28), who found that the proportion of unsaturated fatty acids decreased from 51.63 to 29.58% as the growth temperature increased from 15 to 45°C. In a recent study, A. acidoterrestris was found to increase the level of cyclic fatty acids with the expense of saturated fatty acids to improve the acid-tolerance capability (3).
The membrane fatty acid composition of the cell of A. acidoterrestris cultured at different temperatures is shown in Table 1. There are five main types of fatty acids detected, namely myristic acid (C14:0), palmitic acid (C16:0), stearic acid (C18:0), cyclohexaneundecanoic acid (ω-cyclohexyl C17:0) and cyclopentanetridecanoic acid (ω-cyclopentane C18:0), which has good consistency with the results obtained by Zhao et al. (3). In this study, A. acidoterrestris as an acidophilic heat-resistant bacterium and ω-cyclohexyl C17:0 and ω-cyclopentane C18:0 accounted for more than 80%, which is good in consistence with previous studies that ω-cyclic fatty acids appear in the cell membrane of acidophilic, heat-resistant bacteria, in which the proportion could reach 60 to 90% (35, 36). The fatty acid components including C16:0, C18:0, ω-cyclohexyl C17:0, and ω-cyclopentane C18:0 showed a significant change under various culture temperatures. Among them, the relative content of ω-cyclohexyl C17:0 was the highest when the culture temperature was 25°C, accounting for 81.9%. With the increase in culture temperature (35-55°C), the proportion of ω-cyclohexyl C17:0 was gradually decreased, accounting for 72.37, 59.23, and 49.86%, respectively (p < 0.05). In contrast, the level of ω-cyclopentane C18:0 showed an upward trend, increasing from 10.83 to 32.21% with an increased temperature of 25 to 55°C, respectively. In a study, as compared with straight-chain fatty acids, cyclic fatty acids can reduce the trans-gauche isomerization of lipid chains, thereby increasing the order degree of lipid chains and the area of lipid layers, and reducing the fluidity of cell membranes (37, 38). However, a study has shown that the loose packing of ω-alicyclic fatty acids in the cell membrane is in the disorder of lipid tails increasing membrane fluidity, and the larger the ring size (3- to 7-membered cycloalkyl) in ω-alicyclic fatty acids, the disorder degree is greater (36). On the other hand, the relative proportion of C16:0 and C18:0 also increased, i.e., from 4.36, 2.43% at 25°C and to 11.35 and 5.85%, at 55°C. Generally, straight-chain fatty acids, including C16:0 and C18:0 are neatly arranged in the cell membrane, and the stacking is good and tight. Therefore, the higher the proportion of straight-chain fatty acids in the cell membrane, the lower the fluidity of the cell membrane (17, 39, 40). Thus, it could be inferred that the decreased cell membrane fluidity with the increased culture temperature of A. acidoterrestris cells are the results of an increase of C16:0, C18:0, and ω-cyclopentane C18:0.
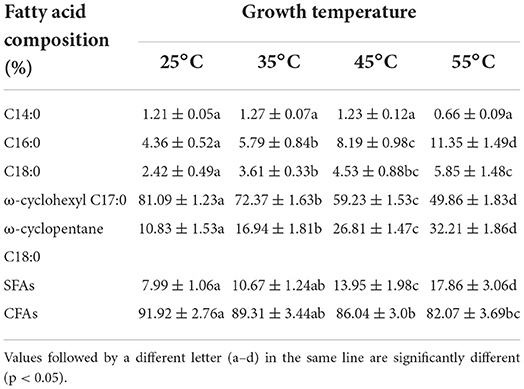
Table 1. The proportions of primary membrane fatty acid composition of A. acidoterrestris under different growth temperatures.
Implication for the resistance of A. Acidoterrestris cells with membrane fatty acid profile
The stacked loose ω-alicyclic fatty acids can protect acidophilus from high temperature and low pH conditions by forming a dynamic barrier that restricts lipid diffusion and H+ transmembrane diffusion (36). However, different from the mechanism of high temperature and low acid action, various reactive oxygen species and reactive nitrogen components such as ozone, hydroxyl radicals, and singlet oxygen radicals generated by plasma treatment, which will damage the function of fatty acids, induce lipids, especially the peroxidation of unsaturated fatty acids even destroys the cell membrane and leads to cell lysis and bacterial death (25, 41). In addition, the free radicals generated by plasma treatment can also cross the cell membrane and enter the cytoplasm to attack the biological macromolecules such as nucleic acids (25). In this study, A. acidoterrestris as an acidophilic heat-resistant bacteria, and ω-cyclohexyl C17:0 and ω-cyclopentane C18:0 accounted for more than 80%. With the increase in culture temperature (25–55°C), the total content of straight-chain fatty acids in the cell membrane of A. acidoterrestris increased gradually from 8.0 to 17.86% (p <0.05). In contrast, the content of cyclic fatty acids decreased from 92.92 to 82.07%. It can be inferred that loosely stacked ω-alicyclic fatty acids (especially ω-cyclohexyl C17:0) may be more conducive to the accumulation of oxygen free radicals and other reactive components in the cell membrane, that not only cause damage to the cell membrane but also increases oxygen free radicals and other components entry and cause cell death. In contrast, the well-arranged, packed, tight straight-chained fatty acids may prevent the entry of these harmful substances to a certain extent. Therefore, the decrease of cyclic fatty acid content and the increase of straight-chain fatty acids caused by the increase in culture temperature may be the reason for the increase in plasma tolerance of A. acidoterrestris in the culture temperature range of 25 to 45°C. It is worth mentioning that the content of straight-chain fatty acids in the cell membrane of A. acidoterrestris was the highest when cultured at 55°C, but these cells are highly sensitive to DBD-ACP treatment. These results may be related to the aerobic metabolism of A. acidoterrestris was improved at higher temperatures (55°C). Reportedly, the generation of intracellular reactive oxygen species by aerobic metabolism was increased with environment temperature (42). Therefore, it could speculate that a growth temperature of 55°C might cause remarkable effects on the plasma-mediated accumulated abundance of intracellular ROS inducing bacteria to be more sensitive to DBD-ACP treatment (7, 43).
Conclusions
The inactivation of DBD-ACP on A. acidoterrestris and effects of growth temperatures on their resistance was investigated. Results show that DBD-ACP is effective in the inactivation of A. acidoterrestris by causing a significant increase in cell membrane permeability with leakage of cytoplasmic contents and changes in bacterial morphology. A. acidoterrestris cells at different growth temperatures (25, 35 45 and 55°C) exhibited different resistance to DBD-ACP treatment. A. acidoterrestris cells grown at 45°C were found to be the most resistant, followed by at 35°C, 25°C and 55°C. The growth temperatures of A. acidoterrestris induced noticeable modifications of membrane fatty acid profile and fluidity during cultivation. For example, the most abundant fatty acids, i.e. ω-cyclohexyl C17:0 and ω-cyclopentane C18:0, changed from 81.09% to 49.86% and 10.83% to 32.21% at the growth temperatures of 25 to 55°C. Growth temperature-mediated alterations in fatty acid profile and membrane fluidity of A. acidoterrestris were associated with its viability to DBD plasma exposure and a significant increase in resistance with increased growth temperatures from 25 to 45°C. It is worth mentioning that A. acidoterrestris cells grown at 55°C are highly sensitive to DBD-ACP treatment. These results may be related to the growth temperature of 55°C causing remarkable effects on the plasma-mediated accumulated abundance of intracellular ROS inducing bacteria to be more sensitive to DBD-ACP treatment. In conclusion, this study demonstrated DBD-ACP is a promising technology in inactivating A. acidoterrestris, but it is worth noting growth temperature plays a role in the resistance of A. acidoterrestris to DBD-ACP, suggesting a potential way to improve the sterilization efficiency in practical application by adjusting the preconditioning temperature. However, more investigations are still needed to further understand how A. acidoterrestris regulate membrane fatty acid and fluidity based on the genetics and metabolomics in response to various temperature.
Data availability statement
The original contributions presented in the study are included in the article/supplementary material, further inquiries can be directed to the corresponding authors.
Author contributions
L-HW: conceptualization, methodology, software, investigation, and writing—original draft. LC: methodology, validation, formal analysis, visualization, and data curation. SZ and RA: writing—review & editing. YH: resources, methodology, and supervision. X-AZ: resources, writing—review & editing, supervision, and data curation. All authors contributed to the article and approved the submitted version.
Funding
Support for this research by the Project of Science and Technology Department of Shaanxi Province (2021JQ-448), the Key Laboratory Project of Guangdong Province (2022B1212010015), and the National Natural Science Foundation of China (3210160758) are all gratefully acknowledged.
Conflict of interest
The authors declare that the research was conducted in the absence of any commercial or financial relationships that could be construed as a potential conflict of interest.
Publisher's note
All claims expressed in this article are solely those of the authors and do not necessarily represent those of their affiliated organizations, or those of the publisher, the editors and the reviewers. Any product that may be evaluated in this article, or claim that may be made by its manufacturer, is not guaranteed or endorsed by the publisher.
References
1. Van Luong TS, Moir C, Chandry PS, Pinfold T, Olivier S, Broussolle V, et al. Combined high pressure and heat treatment effectively disintegrates spore membranes and inactivates Alicyclobacillus acidoterrestris spores in acidic fruit juice beverage. Innovative Food Sci Emerg Technol. (2020) 66:102523. doi: 10.1016/j.ifset.2020.102523
2. Feng X, He C, Jiao L, Liang X, Zhao R, Guo Y. Analysis of differential expression proteins reveals the key pathway in response to heat stress in Alicyclobacillus acidoterrestris DSM 3922T. Food Microbiol. (2019) 80:77–84. doi: 10.1016/j.fm.2019.01.003
3. Zhao N, Zhang J, Qi Y, Xu J, Wei X, Fan M. New insights into thermo-acidophilic properties of Alicyclobacillus acidoterrestris after acid adaptation. Food Microbiol. (2021) 94:103657. doi: 10.1016/j.fm.2020.103657
4. Pornpukdeewattana S, Jindaprasert A, Massa S. Alicyclobacillus spoilage and control-a review. Crit Rev Food Sci Nutr. (2020) 60:108–22. doi: 10.1080/10408398.2018.1516190
5. Guo L, Azam SR, Guo Y, Liu D, Ma H. Germicidal efficacy of the pulsed magnetic field against pathogens and spoilage microorganisms in food processing: an overview. Food Control. (2021) 136:108496. doi: 10.1016/j.foodcont.2021.108496
6. Roobab U, Chacha JS, Abida A, Rashid S, Muhammad Madni G, Lorenzo J M, et al. Emerging trends for nonthermal decontamination of raw and processed meat: ozonation, high-hydrostatic pressure and cold plasma. Foods. (2022) 11:2173. doi: 10.3390/foods11152173
7. Asghar A, Rashid MH, Ahmed W, Roobab U, Inam-ur-Raheem M, Shahid A, et al. An in-depth review of novel cold plasma technology for fresh-cut produce. J Food Process Pres. (2022) 7:e16560. doi: 10.1111/jfpp.16560
8. Mahnot NK, Mahanta CL, Farkas BE, Keener KM, Misra N. Atmospheric cold plasma inactivation of Escherichia coli and Listeria monocytogenes in tender coconut water: Inoculation and accelerated shelf-life studies. Food Control. (2019) 106:106678. doi: 10.1016/j.foodcont.2019.06.004
9. Charoux CM, Free L, Hinds LM, Vijayaraghavan RK, Daniels S, O'Donnell CP, et al. Effect of non-thermal plasma technology on microbial inactivation and total phenolic content of a model liquid food system and black pepper grains. LWT-Food Sci Technol. (2020) 118:108716. doi: 10.1016/j.lwt.2019.108716
10. Umair M, Jabbar S, Ayub Z, Aadil RM, Abid M, Zhang J, et al. Recent advances in plasma technology: Influence of atmospheric cold plasma on spore inactivation. Food Rev Int. (2021) 1:1–23. doi: 10.1080/87559129.2021.1888972
11. Liao X, Liu D, Xiang Q, Ahn J, Chen S, Ye X, et al. Inactivation mechanisms of non-thermal plasma on microbes: a review. Food Control. (2017) 75:83–91. doi: 10.1016/j.foodcont.2016.12.021
12. Wang LH, Wang MS, Zeng XA, Liu ZW. Temperature-mediated variations in cellular membrane fatty acid composition of Staphylococcus aureus in resistance to pulsed electric fields. Biochim Biophys Acta, Biomembr. (2016) 1858:1791–800. doi: 10.1016/j.bbamem.2016.05.003
13. Hayman MM, Anantheswaran RC, Knabel SJ. The effects of growth temperature and growth phase on the inactivation of Listeria monocytogenes in whole milk subject to high pressure processing. Int J Food Microbiol. (2007) 115:220–6. doi: 10.1016/j.ijfoodmicro.2006.10.019
14. Kayes MM, Critzer FJ, Kelly-Wintenberg K, Roth JR, Montie TC, Golden DA. Inactivation of foodborne pathogens using a one atmosphere uniform glow discharge plasma. Foodborne Pathog Dis. (2007) 4:50–9. doi: 10.1089/fpd.2006.62
15. Fernandez A, Noriega E, Thompson A. Inactivation of Salmonella enterica serovar Typhimurium on fresh produce by cold atmospheric gas plasma technology. Food Microbiol. (2013) 33:24–9. doi: 10.1016/j.fm.2012.08.007
16. Cai R, Zhang M, Cui L, Yuan Y, Yang Y, Wang Z, et al. Antibacterial activity and mechanism of thymol against Alicyclobacillus acidoterrestris vegetative cells and spores. LWT-Food Sci Technol. (2019) 105:377–84. doi: 10.1016/j.lwt.2019.01.066
17. Wang L-H, Wen Q-H, Zeng X-A, Han Z, Brennan CS. Influence of naringenin adaptation and shock on resistance of Staphylococcus aureus and Escherichia coli to pulsed electric fields. LWT-Food Sci Technol. (2019) 107:308–17. doi: 10.1016/j.lwt.2019.03.029
18. Pang D, Huang Z, Li Q, Wang E, Liao S, Li E, et al. Antibacterial Mechanism of Cinnamaldehyde: modulation of biosynthesis of Phosphatidylethanolamine and Phosphatidylglycerol in Staphylococcus aureus and Escherichia coli. J Agric Food Chem. (2021) 69:13628–36. doi: 10.1021/acs.jafc.1c04977
19. Alvarez-Ordóñez A, Halisch J, Prieto M. Changes in Fourier transform infrared spectra of Salmonella enterica serovars Typhimurium and Enteritidis after adaptation to stressful growth conditions. Int J Food Microbiol. (2010) 142:97–105. doi: 10.1016/j.ijfoodmicro.2010.06.008
20. Mahnot NK, Mahanta CL, Keener KM, Misra N. Strategy to achieve a 5-log Salmonella inactivation in tender coconut water using high voltage atmospheric cold plasma (HVACP). Food Chem. (2019) 284:303–11. doi: 10.1016/j.foodchem.2019.01.084
21. Wang L-H, Pyatkovskyy T, Yousef A, Zeng X-A, Sastry SK. Mechanism of Bacillus subtilis spore inactivation induced by moderate electric fields. Innovative Food Sci Emerg Technol. (2020) 62:102349. doi: 10.1016/j.ifset.2020.102349
22. Qian J, Ma L, Yan W, Zhuang H, Huang M, Zhang J, et al. Inactivation kinetics and cell envelope damages of foodborne pathogens Listeria monocytogenes and Salmonella Enteritidis treated with cold plasma. Food Microbiol. (2022) 101:103891. doi: 10.1016/j.fm.2021.103891
23. Olatunde OO, Benjakul S, Vongkamjan K. Dielectric barrier discharge cold atmospheric plasma: bacterial inactivation mechanism. J Food Saf. (2019) 39:12705. doi: 10.1111/jfs.12705
24. Ziuzina D, Patil S, Cullen PJ, Keener K, Bourke P. Atmospheric cold plasma inactivation of Escherichia coli, Salmonella enterica serovar Typhimurium and Listeria monocytogenes inoculated on fresh produce. Food Microbiol. (2014) 42:109–16. doi: 10.1016/j.fm.2014.02.007
25. Jin T, Dai C, Xu Y, Chen Y, Xu Q, Wu Z. Applying cold atmospheric plasma to preserve the postharvest qualities of winter jujube (Zizyphus jujuba Mill. cv. Dongzao) during cold storage. Front Nutr. (2022) 9:934841. doi: 10.3389/fnut.2022.934841
26. Bourke P, Ziuzina D, Han L, Cullen P, Gilmore BF. Microbiological interactions with cold plasma. J Appl Microbiol. (2017) 123:308–24. doi: 10.1111/jam.13429
27. Pan Y-Y, Zhang Y, Cheng J-H, Sun D-W. Inactivation of Listeria monocytogenes at various growth temperatures by ultrasound pretreatment and cold plasma. LWT-Food Sci Technol. (2020) 118:108635. doi: 10.1016/j.lwt.2019.108635
28. Liu Z-W, Zeng X-A, Ngadi M, Han Z. Effect of cell membrane fatty acid composition of Escherichia coli on the resistance to pulsed electric field (PEF) treatment. LWT-Food Sci Technol. (2017) 76:18–25. doi: 10.1016/j.lwt.2016.10.019
29. Hong S-H, Szili EJ, Jenkins ATA, Short RD. Ionized gas (plasma) delivery of reactive oxygen species (ROS) into artificial cells. J Phys D: Appl Phys. (2014) 47:362001. doi: 10.1088/0022-3727/47/36/362001
30. Al-Holy MA, Lin M, Cavinato AG, Rasco BA. The use of Fourier transform infrared spectroscopy to differentiate Escherichia coli O157: H7 from other bacteria inoculated into apple juice. Food Microbiol. (2006) 23:162–8. doi: 10.1016/j.fm.2005.01.017
31. Campos J, Sousa C, Mourão J, Lopes J, Antunes P, Peixe L. Discrimination of non-typhoid Salmonella serogroups and serotypes by Fourier Transform Infrared Spectroscopy: A comprehensive analysis. Int J Food Microbiol. (2018) 285:34–41. doi: 10.1016/j.ijfoodmicro.2018.07.005
32. Al-Qadiri HM, Lin M, Cavinato AG, Rasco BA. Fourier transform infrared spectroscopy, detection and identification of Escherichia coli O157: H7 and Alicyclobacillus strains in apple juice. Int J Food Microbiol. (2006) 111:73–80. doi: 10.1016/j.ijfoodmicro.2006.05.004
33. Yang S-P, Xie J, Cheng Y, Zhang Z, Zhao Y, Qian Y-F. Response of Shewanella putrefaciens to low temperature regulated by membrane fluidity and fatty acid metabolism. LWT-Food Sci Technol. (2020) 117:108638. doi: 10.1016/j.lwt.2019.108638
34. Cebrián G, Condón S, Mañas P. Heat resistance, membrane fluidity and sublethal damage in Staphylococcus aureus cells grown at different temperatures. Int J Food Microbiol. (2019) 289:49–56. doi: 10.1016/j.ijfoodmicro.2018.09.002
35. Huertas J-P, Ros-Chumillas M, Garre A, Fernández PS, Aznar A, Iguaz A, et al. Impact of heating rates on alicyclobacillus acidoterrestris heat resistance under non-isothermal treatments and use of mathematical modelling to optimize orange juice processing. Foods. (2021) 10:1496. doi: 10.3390/foods10071496
36. Poger D, Mark AE. Effect of ring size in ω-alicyclic fatty acids on the structural and dynamical properties associated with fluidity in lipid bilayers. Langmuir. (2015) 31:11574–82. doi: 10.1021/acs.langmuir.5b02635
37. Oshima M, Ariga T. Omega-cyclohexyl fatty acids in acidophilic thermophilic bacteria. Studies on their presence, structure, and biosynthesis using precursors labeled with stable isotopes and radioisotopes. J Biol Chem. (1975) 250:63–78. doi: 10.1016/S0021-9258(19)41026-0
38. Kannenberg E, Blume A, Poralla K. Properties of ω-cyclohexane fatty acids in membranes. FEBS Lett. (1984) 172:331–44. doi: 10.1016/0014-5793(84)81151-5
39. Najjar MB, Chikindas M, Montville TJ. Changes in Listeria monocytogenes membrane fluidity in response to temperature stress. Appl Environ Microbiol. (2007) 73:6429–35. doi: 10.1128/AEM.00980-07
40. Boudjemaa R, Cabriel C, Dubois-Brissonnet F, Bourg N, Dupuis G, Gruss A, et al. Impact of bacterial membrane fatty acid composition on the failure of daptomycin to kill Staphylococcus aureus. Antimicrob Agents Chemother. (2018) 62:e00023–18. doi: 10.1128/AAC.00023-18
41. Korachi M, Gurol C, Aslan N. Atmospheric plasma discharge sterilization effects on whole cell fatty acid profiles of Escherichia coli and Staphylococcus aureus. J Electrostat. (2010) 68:508–12. doi: 10.1016/j.elstat.2010.06.014
42. Schulte PM. The effects of temperature on aerobic metabolism: towards a mechanistic understanding of the responses of ectotherms to a changing environment. J Exp Biol. (2015) 218:1856–66. doi: 10.1242/jeb.118851
Keywords: Alicyclobacillus acidoterrestris, cold plasma, scanning electron microscopy, FT-IR, GC-MS
Citation: Wang L-H, Chen L, Zhao S, Huang Y, Zeng X-A and Aadil RM (2022) Inactivation efficacy and mechanisms of atmospheric cold plasma on Alicyclobacillus acidoterrestris: Insight into the influence of growth temperature on survival. Front. Nutr. 9:1012901. doi: 10.3389/fnut.2022.1012901
Received: 06 August 2022; Accepted: 15 August 2022;
Published: 15 September 2022.
Edited by:
Debao Niu, Guangxi University, ChinaReviewed by:
Suyun Lin, Jiangxi Agricultural University, ChinaIbrahim Khalifa, Huazhong Agricultural University, China
Copyright © 2022 Wang, Chen, Zhao, Huang, Zeng and Aadil. This is an open-access article distributed under the terms of the Creative Commons Attribution License (CC BY). The use, distribution or reproduction in other forums is permitted, provided the original author(s) and the copyright owner(s) are credited and that the original publication in this journal is cited, in accordance with accepted academic practice. No use, distribution or reproduction is permitted which does not comply with these terms.
*Correspondence: Yanyan Huang, aHVhbmdfeWFueWFuQGZvc3UuZWR1LmNu; Xin-An Zeng, eGF6ZW5nQHNjdXQuZWR1LmNu