- 1Key Laboratory of Systems Health Science of Zhejiang Province, School of Life Science, Hangzhou Institute for Advanced Study, University of Chinese Academy of Sciences, Hangzhou, China
- 2College of Pharmacy, Hunan University of Chinese Medicine, Changsha, China
Gut microbiota is increasingly recognized to affect host health and disease, including ischemic stroke (IS). Here, we systematically review the current understanding linking gut microbiota as well as the associated metabolites to the pathogenesis of IS (e.g., oxidative stress, apoptosis, and neuroinflammation). Of relevance, we highlight that the implications of gut microbiota-dependent intervention could be harnessed in orchestrating IS.
Introduction
Stroke ranks among the top three fatal diseases in developed countries and has even more lethality in developing countries (1, 2). The stroke is classified to ischemic stroke (IS) and hemorrhagic stroke. The IS is responsible for 87% of stroke (3). Except for population growth and aging, non-negligible risk factors such as high body mass index, environmental particulate pollution, high fasting blood glucose, high systolic blood pressure, alcohol consumption, low physical activity, renal insufficiency, and high temperature also exacerbate the prevalence of IS (4). Clinically, thrombolysis is an early treatment for IS. Recombinant tissue plasminogen activator (rtPA), an effective thrombolytic drug, is the only Food and Drug Administration (FDA)-approved medicine for acute IS. However, the bleeding risk and short treatment window (3–4.5 h after onset) of rtPA limits its clinical use (5). Considering that postischemic neuroprotective therapy can prolong the time window of thrombolytic treatment, it is urgent to develop neuroprotective strategies to protect brain cells from ischemia and reperfusion injury (6–8).
Gut microbiota enables bidirectional communication between the gut and the brain through the “microbial-gut-brain axis”, associated with immune, endocrine, and neuromodulatory mechanisms (9, 10). Cytokines and chemokines produced in the brain could be released into the systemic circulation after IS, potentially impacting gut microbiota imbalance. Clinical studies showed that the diversity of gut microbiota in the IS population is significantly reduced, among which Escherichia, Megamonas, Bacillus, Bifidobacterium, and Ruminococcus are increased significantly, while Parabacteroides, Ekmanella, Prevotella, and Faecalibacterium burden are decreased in IS population compared to the healthy people (11, 12). Likewise, Yamashiro et al. studied the intestinal microbiota of 41 IS patients and 40 healthy people and confirmed the significant differences of gut microbiota between them. In the middle cerebral artery occlusion (MCAO) mouse model, the gut microbiota could cross the intestinal barrier and migrate to various organs through the blood to induce inflammatory responses (13, 14). These findings suggest that gut microbiota might be used as a neuroprotective strategy for IS (15, 16). Interestingly, studies on animal experiments demonstrated that fecal microbiota transplantation from healthy donors could restore the gut microbiota imbalance caused by IS and consequently improved neural function (17). Moreover, gut microbial metabolites were found to regulate the IS process. For example, trimethylamine oxide (TMAO), a metabolite of Gammaproteobacteria, can promote atherosclerosis and cerebral vascular embolism by affecting cholesterol reverse transport and metabolism (18). The immunogenic endotoxin lipopolysaccharide (LPS) may promote neuroinflammation by inducing the migration of peripheral immune cells to the brain (19). Short-chain fatty acids (SCFAs) can reduce the severity of neuroinflammation, protect brain tissue, and reduce neuronal damage in IS patients (17).
This review aims to elucidate the mechanism of gut microbiota in the pathological process of IS and provide new ideas for IS treatment.
IS pathogenesis and microbiota
IS is associated with the interruption of cerebral blood flow induced by thrombotic or embolic occlusion of a cerebral artery. The pathological change of brain cell damage in IS is a dynamic developmental process of complex spatiotemporal cascade reactions in the penumbra area involving oxidative stress, inflammation, and apoptosis (20–22). Acute reduction or deprivation of cerebral blood flow limits glucose and oxygen access to brain tissue, and the limitation of glucose and oxygen reduces adenosine triphosphate (ATP) production and further induces bioenergetic failure, acidotoxicity, and excitotoxicity in brain cells (23). ATP consumption also causes the depolarization of neuronal plasma membrane and dysfunction of ATP-dependent ion pumps, further disrupting sodium, potassium, and calcium (Ca) ionic balance and leading to anoxic depolarization in neurons and glial cells (23, 24). Neuronal cell bioenergetic failure and depolarization lead to cellular sodium and Ca2+ accumulation, inducing mitochondria dysfunction and catabolic enzyme activation to promote reactive oxygen species (ROS) and oxidative intermediates generation, resulting in endogenous redox disbalance and oxidative severe stress damage (cell apoptosis and necrosis) (25). Furthermore, complex triggers such as bioenergetic failure, ROS, and cell necrosis initiate brain cell immune response characterized by the activation of glial cells, the infiltration of monocytes/macrophage and leukocytes, and the production of pro-inflammatory cytokines and chemokines (26) (Figure 1).
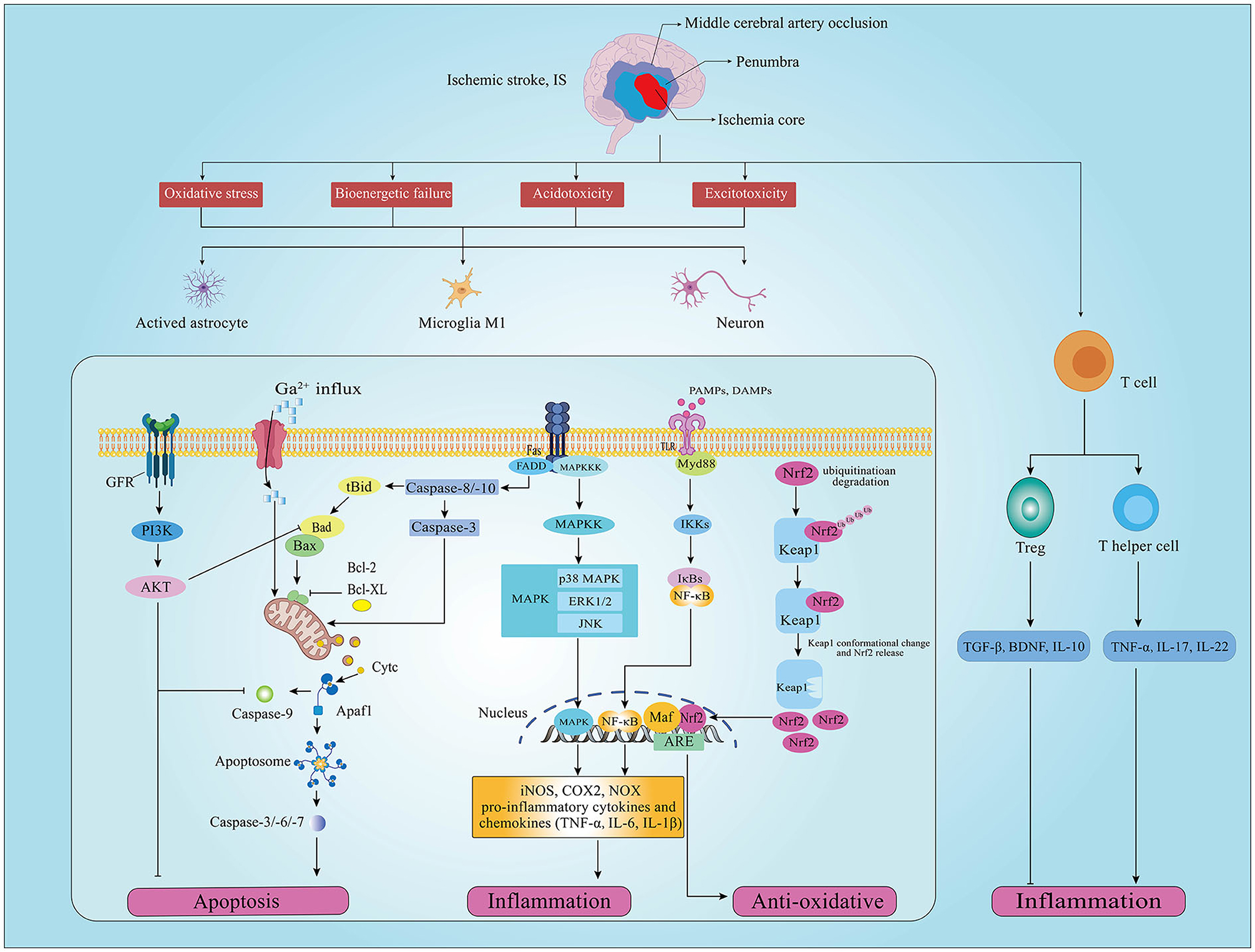
Figure 1. Schematic diagram of oxidative stress, apoptosis, and inflammation signaling pathways involved in ischemic stroke (IS). Oxidative stress, inflammation and apoptosis occur in neurons of the penumbra after IS, which are regulated by different upstream pathways. (GFR, growth factor receptor; PI3K, phosphatidylinositol 3-kinase; AKT, protein kinase B; Bad, Bcl2 associated agonist of cell death; Bcl-2, B-cell lymphoma-2; Bcl-XL, B-cell lymphoma-extra large; Bax, Bcl-2-associated X; Cytc, cytochrome c; Apafl, apoptotic protease activating factor-1; TGF-β, transforming growth factor beta; BDNF, brain-derived neurotrophic factor; Keapl, Kelch-like ECH-associated protein 1; Nrf2, nuclear factor E2 related factor 2; Ub, ubiquitination; ARE, adenylate Uridylate-rich element; Maf, macrophage activating factor; TLR4, toll-like receptor 4; Myd88, myeloid differentiation primary response 88; Ikks, I-κB kinase; NF-κB, nuclear factor kappa B; IL-1β, interleukin 1 beta; MAPK, mitogen-activated protein kinases; JNK, c-Jun N-terminal kinase; ERK, extracellular regulated protein kinases; iNOS, inducible NO synthase; COX2, cyclooxygenase 2, COX2; NOX, nitrogen oxide; TNF-α, tumor necrosis factor alpha; Treg, regulatory T cell).
Numerous studies have reported the role of gut microbiota in oxidative stress, inflammation, and apoptosis (Figure 2), indicating its potential protective effects in IS (27–30).
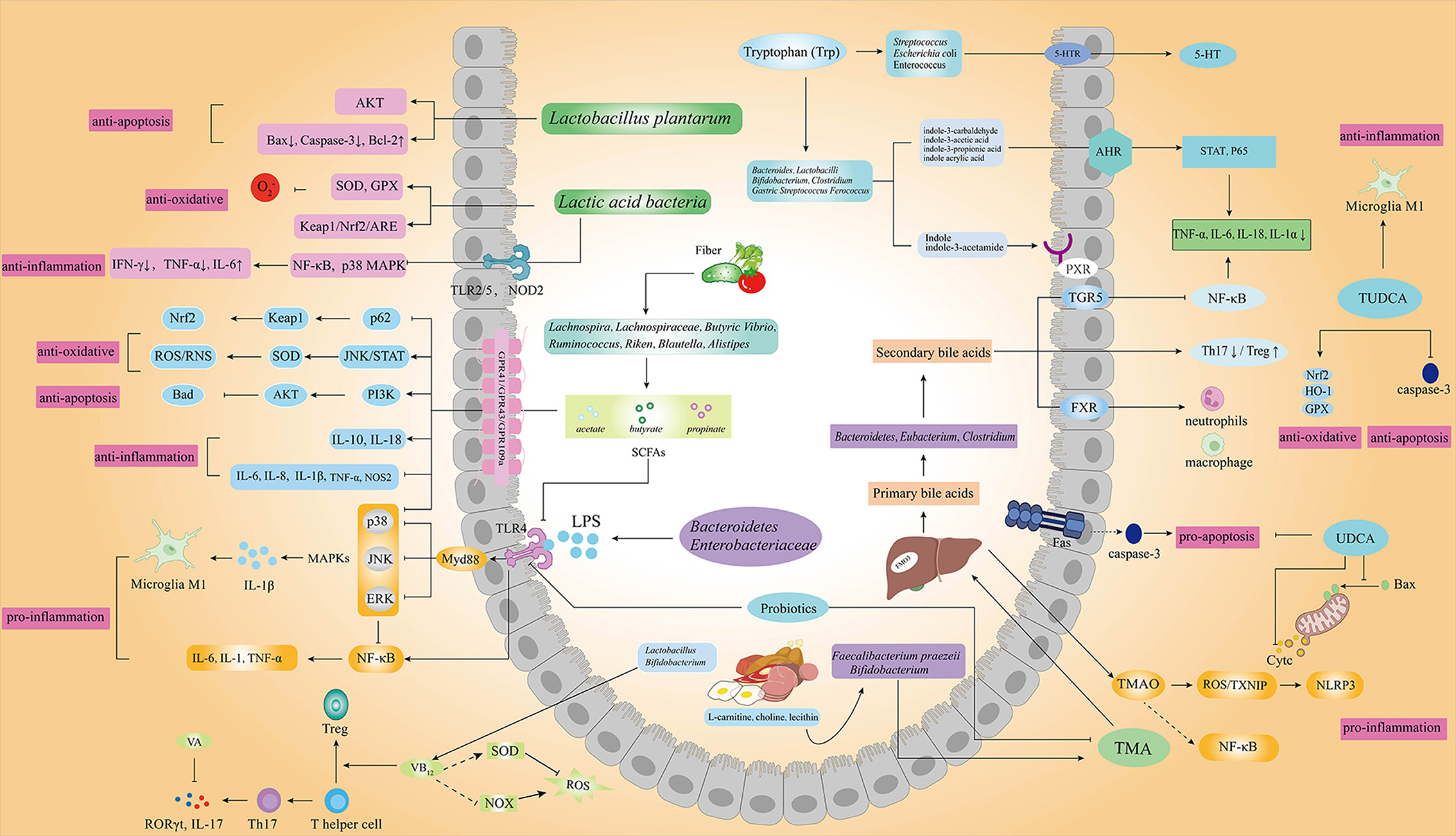
Figure 2. Gut microbiota and its metabolic products associated with IS. Gut microbiota and the metabolism of SCFAs, tryptophan, bile acids, TMAO, LPS, and vitamins play important roles in oxidative stress, inflammation, and apoptosis of IS through different signaling pathways. (AKT, protein kinase B; Bax, Bcl2-associated X; Bcl-2, B-cell lymphoma-2; SOD, superoxide dismutase; GPX, glutathione peroxidase; Keapl, kelch-like ECH-associated protein 1; Nrf2, nuclear factor E2 related factor 2; Ub, ubiquitination; ARE, adenylate uridylate-rich element; IFN-γ, interferon gamma; IL-6, interleukin 6; IL-17, interleukin 17; IL-18, interleukin 18; IL-1α, interleukin 1 alpha; Treg, regulatory T cell; Th17, helper T 17 cell; RORγt, retinoic acid related orphan nuclear receptor γt; IL-10, interleukin 10; iNOS, inducible NO synthase; COX2, cyclooxygenase 2; NOX, nitrogen oxide; NOS2, nitric oxide synthase 2; GPR43/GPR41, G protein-coupled receptors 43/41; MAPK, mitogen-activated protein kinases; JNK, c-Jun N-terminal kinase; ERK, extracellular regulated protein kinases; TMA, trimethylamine; TMAO, trimethylamine oxide; TXNIP, thioredoxin-interacting protein; FMO3, flavin-containing monooxygenase 3; FXR, farnesoid X receptor; TNF-α, tumor necrosis factor alpha; PI3K, phosphatidylinositol 3-kinase; STAT, signal transducer and activator of transcription; TUDCA, tauroursodeoxycholic acid; UDCA, ursodeoxycholic acid; HO-1, heme oxygenase-1; PXR, pregnane X receptors; AHR, aryl hydrocarbon receptors; 5-HT, 5-hydroxytryptamine; 5-HTR, 5-hydroxytryptamine receptor).
Oxidative stress and microbiota
Oxidative stress (OS) induced by the excess generation of ROS attacks cellular lipids, proteins, and nucleic acid, causing cell apoptosis, death, or even necrosis (25). OS also promotes the release of cytochrome C (Cytc) and the activation of astrocytes and microglia, inducing early inflammation and cell apoptosis (31). Of note, the transcription factor NF-E2-related factor (Nrf2)/antioxidant response element (ARE) signaling pathway is recognized as the central player in the antioxidant system (32). By binding to its inhibitor Kelch-like epichlorohydrin-associated protein-1 (Keap1) under normal conditions, Nrf2 mainly exists in the cytoplasm as an inactive state but would be rapidly degraded following ubiquitin protease activation, maintaining the regular and low transcriptional activity of Nrf2 (33). When free sulfhydryl groups (i.e., Nrf2 or electrophilic stress sensors) are oxidized by ROS, Nrf2 is activated and translocated into the nucleus to interact with AREs, resulting in the gene expression of phase II antioxidants and detoxification enzymes (Figure 1).
The gut microbiota can affect the ROS level. Both commensal and pathogenic bacteria in the gut can alter intracellular ROS by modulating mitochondrial activity, which may be related to Keap1/Nrf2/ARE signaling pathway (34, 35) (Figure 1). For example, some Lactobacilli can stimulate cells to produce ROS through specific membrane components or secreted factors (36); in addition, LPS from gram-negative bacteria can increase the sensitivity of neurons to OS (37). Indeed, IS and microbiota dysbiosis often occurs simultaneously in patients and animal models (38, 39). Interestingly, ROS generated in IS stimulates IL-6 and other inflammatory factors production by activating the nuclear factor κB (NF-κB) pathway, indirectly increasing the abundance of facultative anaerobic bacteria (mainly Enterobacteriaceae) and promoting microbiota dysbiosis (40, 41). These studies suggest that targeting gut microbiota is an effective method to regulate OS and further prevent IS.
Apoptosis and microbiota
Neurons and glial cells in the penumbra undergo apoptosis in the hours to days following IS (42). Apoptosis is an ATP-dependent programmed cell death characterized by the early degradation of DNA and intact cell membrane without cellular contents efflux. Ca2+ overload and mitochondrial dysfunction in IS are the main inducers of neuronal apoptosis, which consist of two principal pathways: the endogenous apoptosis (or mitochondrial) pathway and the exogenous apoptosis (or death receptor) pathways (43). The endogenous apoptosis pathway is induced by the release of key apoptosis-related proteins in mitochondria, and the exogenous apoptosis pathway is triggered by the combination of surface death receptors and their ligands (43) (Figure 1).
Apoptosis stimuli such as Ca2+ overload and ROS mediate endogenous apoptosis. Excessive Ca2+ ions influx at the pre-synaptic terminal causes uncontrolled glutamate release to the synaptic terminal, activating ionotropic N-methyl-d-aspartate receptors (NMDAR) and resulting in Ca2+ further influx (44). Abnormal aggregation of Ca2+ leads to calpain activation to split the Bcl-2 interaction domain (BID) into truncated BID (tBID), together with caspase-8 cleavage. tBID interacts with the Bad-Bax for opening the mitochondrial transition pore (MTP) and promoting the release of mitochondrial Cytc, endonuclease G, and apoptosis-inducing factors (AIFs) (45, 46). Cytc binds to the apoptotic protease activating factor 1 (Apaf-1) and pro-caspase-9 to form apoptosomes, activating caspase-9 and inducing downstream effector caspase-3. Activation of caspase-3 leads to mitochondrial membrane permeabilization, chromatin condensation, DNA fragmentation, and ultimately cell death (47). Bax, Bad, and BH3 protein polymers can bind on the outer membrane of mitochondria to promote MTP formation, while Bcl-2 family inhibitors could inhibit MTP formation by competitively binding with Bax and Bad (48). The exogenous apoptosis pathway is activated by the binding of cell surface death receptors such as TNF receptor 1 (TNFR-1), Fas receptor (FasR), p75 neurotrophin receptor (p75NTR), and their corresponding ligands (49). The combination of legends, such as TNF-α and Fas ligand (FasL), and death receptors leads to the activation of caspase-8 and caspase-10, which in turn activate downstream effector, finally leading to neuronal death (50, 51). Although reversing the necrotic neurons in the infarcted area is difficult, targeted interfering upstream apoptosis signals is a potential neuroprotective strategy to rescue the damaged neurons (52) (Figure 1).
Gut microbiota is reported to regulate the apoptosis of multiple cells from the intestine, kidney, and brain (53, 54). Liu et al. demonstrated that the intestinal probiotic Clostridium butyricum could phosphorylate protein kinase B (AKT) by regulating the protein expression levels of brain-derived neurotrophic factor (BDNF), Bcl-2, and Bax to prevent neuronal apoptosis in cerebral I/R injury diabetic mice (55). SCFAs are found reduced in IS mice model, within which the butyric acid showed the highest negative correlation with IS parameter (56). Ursodeoxycholic acid (UDCA) and taurine deoxycholic acid (TUDCA) could be produced by microbiota and are also reported to play important roles in regulating neuronal apoptosis (57, 58). Collectively, these studies indicate that gut microbiota could be a potential strategy for targeting apoptosis in IS therapy.
Inflammation and microbiota
The activation of inflammatory cells, like microglia, astrocyte, and T cell, is the hallmark of the inflammatory response in IS (59). After IS onset, insufficient oxygen, glucose, and energy production result in the accumulation of some toxic metabolites, such as excitotoxic products, acidic metabolites, and inflammatory mediators, and cause extensive neuron and glial cell death. These dead cells induce innate immune inflammatory responses by releasing damage-associated molecular patterns (DAMPs) [e.g., ATP, high mobility histone B (HMGB1), and hypoxia-inducible factor 1α (HIF-1α)] (23). Indeed, microglia activation releases danger signals to activate the innate immune response in lesions, promoting tumor necrosis factor-alpha (TNF-α), interleukin-1β (IL-1β), interleukin-6 (IL-6), and other potentially cytotoxic molecules such as prostaglandins, ROS, and nitric oxide (NO) (60). Activated astrocytes also release inflammatory factors, such as inducible nitric oxide synthase (iNOS), cyclooxygenase-2 (COX2), and NADPH oxidase (NOX) (61). Cytokines produced by microglia and astrocytes play critical roles in IS pathology (62). Proinflammatory cytokines (e.g., TNF-α, IL-1β, and IL-6) and chemokines exacerbate brain damage, while anti-inflammatory cytokines [e.g., IL-10 and transforming growth factor beta (TGF-β)] are neuroprotective (63, 64) (Figure 1).
Toll-like receptors (TLRs) expressed in astrocytes and microglias play essential roles in DAMPs/pathogen-associated molecular pattern (PAMPs) recognization and inflammatory responses (65). For example, TLR4 activated by DAMPs through the downstream myeloid differentiation factor 88 (MyD88)-dependent pathway can mediate NF-κB translocation to the nucleus for initiating the transcription of inflammatory cytokines and the following inflammatory response cascades, thereby inducing vascular damage (66). Mitogen-activated protein kinases (MAPKs) is also the critical signaling molecules associated with inflammatory responses by regulating multiple extracellular kinases, including extracellular signal-regulated protein kinase (ERK)1/2, p38MAP Kinase (p38), c-Jun N-terminal kinases (JNK) (67). MAPK signaling pathway affects the balance of pro-inflammatory and anti-inflammatory responses by regulating the production of TNF-α, IL-1, IL-6, and IL-12 (68). Moreover, T cells are recruited to the injured brain tissue within the first days after stroke and play a decisive role in secondary neuroinflammation in IS (69–71). The helper T (Th) cell subpopulations have differential effects on IS outcomes. Pro-inflammatory such as Th1 and Th17 promote inflammatory damage, worsing IS outcomes, whereas regulatory T cells (Tregs) have been shown to suppress an excess neuroinflammatory reaction to brain injury (72, 73) (Figure 1). Therefore, it could effectively alleviate the inflammation injury of IS by targeting the inflammation-related cells and cytokines.
Gut microbiota could affect the central neuron system (CNS) and brain function by interacting with the immune system (74). IS is associated with decreased SCFAs and SCFAs-producing bacteria such as Bacteroidaceae, Ruminococcaceae, and Faecalibacterium and also associated with increased fecal valeric acid, which is positively correlated with inflammatory marker levels (e.g., high-sensitivity C-reactive protein and white blood cell counts) (38, 75, 76). Numerous studies have demonstrated gut microbiota is a key regulator of IS-related immune cells (77, 78). For example, Group B Streptococcus and Listeria monocytogenes could stimulate the differentiation of effector T cell, increase T cell infiltration to the brain, and disrupt the integrity of the blood-brain barrier (BBB), consequently inducing neuroimmune inflammatory responses (79). The gut microbiota is also associated with inflammation-associated mediators. Germ-free (GF) mice recolonized with microbiota from IS mice showed higher IL-17 and IFN-γ expression and Th17 cell infiltration in hemispheres in the context of IS, than those received the health mouse microbiota (15). Moreover, in the experimental IS mouse model, antibiotics-induced “GF” mice show moderate brain injury than those mice with normal microbiota (80). Together, it is reasonable that gut microbiota may be a key target for the effective management and treatment of IS therapy.
Microbiota, microbial metabolites, and IS
Probiotics
Probiotics such as Lactobacillus, Bacillus subtilis, and Bifidobacterium have positive effects on anti-oxidative, immune modulation, and pathogen antagonism (81). Probiotic fermented milk showed significant antioxidant capacity in vitro and vivo as a dietary source (82, 83). Lactic acid bacteria (LAB) could be autolyzed under stress, such as low pH conditions, and release intracellular antioxidant substances in vitro (84). In vivo, LAB like Lactobacillus plantarum was reported to have salutary effects against exogenous insults via the Nrf2/ARE signaling pathway in mice and drosophila (85). Cheon et al. found that LAB KU200793 in fermented foods showed high antioxidant activity and protected human neuroblastoma cell SH-SY5Y from 1-methyl-4-phenyl pyridine-induced damage (86). The modulatory role of probiotics in immunity and inflammation has long been investigated, although the precise mechanisms are still not fully understood (87, 88).
In terms of apoptosis, Sirin, S. et al. found that Bulgarian bacterium B3 and Lactobacillus plantarum GD2 protected amyloid beta-induced mitochondrial dysfunction SH-SY5Y cells from apoptosis (89). In vivo experiments showed that probiotic preparations (Lactobacillus helveticus R0052 and Bifidobacterium longum R0175) attenuated LPS-induced apoptosis in rat hippocampus through the gut-brain axis by increasing Bcl-2 and inhibiting Bax and caspase-3 (90). Probiotic cocktail, including bifidobacteria, lactobacillus, laccoccus, and yeast, showed anti-apoptosis function by activating the AKT signaling pathway of the hippocampus (91). The evidence supports the beneficial effect of probiotics on IS therapy.
Moreover, LAB may trigger natural killer T cells to promote IFN-γ (92) and alleviates intestinal inflammation by regulating the NF-κB and p38 MAPK pathway mediated by TLR2/TLR5 receptors (93) and NOD2 receptors (94). The mechanism might be associated with LAB-secreted polypeptide, which could inhibit I-κB phosphorylation and the process of NF-κB translocation to the nucleus (95). Studies have also demonstrated the role of microbiota in neuronal function, such as neurogenesis and neuroinflammation (96). Indeed, Lactobacillus plantarum showed significant anti-inflammation activity by decreasing IFN-γ, TNF-α, and BBB permeability and increasing IL-10 (97, 98). Other probiotics, such as Faecalibacterium prausnitzii, also had anti-inflammatory functions in depression-like and anxiety-like behavior rat model (99) (Figure 2).
Short-chain fatty acids
SCFAs are gut microbiota metabolites that could serve as key signaling molecules in the gut-brain communication (100) and can control neurodevelopment, neurotransmitters, and microglia activation (101, 102). Acetic acid, propionic acid, and butyric acid account for more than 95% of intestinal SCFAs and play important roles in oxidative stress, immune inflammation, and neuronal apoptosis (103). SCFAs, especially butyrate, can regulate oxidative stress. In healthy humans, administration of butyrate at physiological concentrations has been shown to increase the antioxidant glutathione and reduce ROS production (104, 105). Wang et al. showed that sodium butyrate supplementation relieves cerebral ischemia-reperfusion injury via increasing superoxide dismutase expression in mice, which was related to the JNK/STAT pathway (106). Also, sodium butyrate could improve brain damage in mice by reducing the oxidative stress of neurons via the Keap1/Nrf2/HO-1 pathway (99). Butyrate-producing Clostridium butyricum resists oxidative damage by regulating the p62-Keap1-Nrf2 signaling pathway (107).
The regulatory role of SCFAs on cell apoptosis mainly focuses on the intestinal cell (108–110). Some studies reveal the anti-apoptosis role of SCFAs in IS. For example, butyrate was reported against neuronal apoptosis via up-regulating histone H3 acetylation, heat shock protein 70 (HSP70), and p-Akt and down-regulating p53 in ischemic brain tissue (111, 112). SCFAs play important roles in regulating neuroinflammation and microglia and could reduce inflammation after IS (113). Oral administration of SCFAs can repair the defective microglia, promote the activation of the anti-inflammatory phenotype of microglia, and increase the expression of tight junction protein to enhance the structural integrity of BBB while reduce the harmful substances invasion of the brain (114, 115). Acetate reduces LPS-induced glial cell activation by activating GPR43 and inhibiting IL-1β expression and MAPK phosphorylation in rats with neuroinflammation (116, 117). Patiala et al. found that sodium butyrate down-regulates pro-inflammatory mediators TNF-α and NOS2 and up-regulates the expression of anti-inflammatory mediator IL-10 in the MCAO mice (118) (Figure 2).
Tryptophan and its derivatives
The level of serum L-Trp in IS patients is increased significantly, suggesting that Trp may be a potential biomarker of IS (119). Wang et al. reported that Trp supplementation affected immunity by regulating the balance of anti-inflammatory cytokines and pro-inflammatory cytokines and signal transducer and activator of transcription 3 (STAT3) and p65 signal transduction (120). 5-hydroxytryptamine (5-HT), the vital metabolite of Trp, serves as the junction of the gut-brain microbiota axis and the suppressor of inflammation (121). Also, Trp can produce indole and indole derivatives (e.g., indole-3-propionic acid), the ligands of pregnane X receptors (PXR) and aryl hydrocarbon receptors (AHR), via the gut microbial, such as Lactobacillus, Clostridium, and Peptostreptococcus (122–125). Rothhammer et al. found that indole-3-acetic acid can regulate the inflammatory response of the central nervous system, which might be associated with inhibiting the secretion of pro-inflammatory factors such as TNF-α, IL-1β, and monocyte chemoattractant protein-1 (MCP-1) from macrophages (126, 127). Indole-3-acetaldehyde produced by Lactobacillus reuteri can increase the expression level of IL-22 (127) (Figure 2). In conclusion, the function of Trp metabolism for regulating inflammation may provide a therapeutic solution for IS.
Bile acids
BA metabolites are one of the most widely metabolites in host-microbe interactions and may play critical roles in the oxidative stress, apoptosis, and inflammation of IS. Gut microbiota regulates BA synthesis by regulating enzyme Cholesterol 7α-hydroxylase (CYP7A1), CYP7B1, and CYP27A1 and in turn, BA could re-modulate gut microbiota by promoting BA metabolism-microbiota growth and prevent the other microbes (18). For example, Clostridium affects the metabolism of BA, inhibits the activation of farnesoid X receptor (FXR), and promotes inflammation (128).
Indeed, a 20-year prospective follow-up study showed decreased BA excretion functioned as an independent risk factor for IS incidence and mortality (129). A clinical study found that total BAs levels were inversely associated with 3-month mortality in patients with acute IS (130). The reason may be partly associated with immunity because the imbalance of Th17/Treg was reported to aggravate IS (131), while BAs could control the host immune response by directly regulating the balance of the Th17/Treg (132, 133). Studies have shown that gut microbiota could affect the occurrence and development of IS via metabolizing primary BAs produced by the liver to secondary BAs by Bacteroides, Eubacterium, and Clostridium spp. (134, 135). In recent years, Nature has reported that UDCA, an intestinal bacteria-derived secondary bile acid, showed anti-oxidative activity by reducing the production of ROS and reactive nitrogen production and maintaining the level of GSH (136). UDCA was also reported had an anti-apoptotic activates by maintaining mitochondrial membrane integrity through preventing the transfer of apoptotic Bax from the cytoplasm to mitochondria, the release of Cytc, and the subsequent activation of caspase (57, 137, 138). Furthermore, UDCA could also prevent apoptosis through the Fas-L-induced death receptor pathway (139). TUDCA, a taurine conjugate of UDCA that could pass the BBB (140, 141), was reported to inhibit oxidative stress by enhancing the expression of Nrf2, the antioxidant enzymes heme oxygenase-1(HO-1), and glutathione peroxidase (GPx) (142). TUDCA also plays an anti-inflammatory role by inhibiting the activation of glial cells (143). Rodrigues reported that TUDCA could significantly increase the level of brain bile acid, inhibit mitochondrial swelling and caspase-3 expression, reduce infarct area, and improve neural function (144). Hence, TUDCA showed a neuroprotective effect against apoptosis in the MCAO animal model; the mechanism may be associated with activating PI3K dependent Bad signaling pathway and inhibiting E2F-1/p53/Bax pathway (145–148) (Figure 2). In conclusion, targeting BAs could also be one of the neuroprotective strategies of IS.
Choline metabolites
Choline metabolites mainly include trimethylamine (TMA), dimethylamine, methylamine, dimethylglycine, and other substances. After being absorbed into the blood, TMA is rapidly oxidized to TMAO by liver flavin-containing monooxygenase-3 (FMO-3) or other flavin monooxygenases (FMOs) (149). TMAO could also be produced by microbiota such as Faecalibacterium praezeii and Bifidobacterium (150). Studies showed that TMAO could accelerate the process of atherosclerosis by enhancing platelet aggregation, inflammation, and oxidative stress (151, 152). Li et al. found that a high concentration of TMAO could promote the expression of TNF-α, IL-1β, and superoxide, inhibit the expression of endothelial nitric oxide synthase (eNOS), and cause vascular inflammation and oxidative stress (153). Mechanistically, TMAO induces oxidative stress and inflammation response via the ROS/thioredoxin-interacting protein (TXNIP)/ NOD-, LRR- and pyrin domain-containing protein 3 (NLRP3) signaling pathway (154, 155). Besides, TMAO induces foam cell formation through MAPK/JNK pathway for promoting arterial occlusion (156). Glial scarring and astrogliosis models are widely used to mimic the clinical conditions of the human stroke (157). Recently, it has been demonstrated that TMAO promoted reactive astrogliosis and glial scarring by interacting with the Smad ubiquitination-related factor 2 (Smurf2)/Activity-like kinase 5 (ALK5) pathway, resulting in severer nerve injury after IS (158). Reducing TMAO and its precursor TMA is an important strategy to prevent IS. Some studies have shown that probiotics could reduce plasma TMAO levels and alleviate related diseases. Qiu et al. found Enterobacter aerogenes reduced serum TMAO and cecal TMA levels in mice with a high-choline diet (159). And they subsequently found that Lactobacillus plantarum could modulate cecal TMA or serum TMAO levels by affecting gut microbiota but not the expression of liver FMO3, which could stimulate the reverse transport of cholesterol against inflammation, finally alleviating atherosclerosis caused by TMAO (160) (Figure 2). These studies indicate promising strategies for preventing and treating IS by reducing the intake of choline-rich diet or using probiotics to inhibit the formation of TMAO.
Lipopolysaccharide
LPS, also known as endotoxin, is present in the outer membrane of Gram-negative bacteria, such as Bacteroidetes and Enterobacteriaceae (161–163). Bacteroides account for approximately 50% of the human gut microbiota (164) and produce a considerable amount of LPS (165, 166). Lehr HA et al. found LPS increased atherosclerosis in hypercholesterolemic and decreased atherosclerosis and plaque in mice lacking TLR4 (167). Clinical research shows that LPS increases the risk of IS, and elevated plasma LPS levels are associated with poorer short-term outcomes of acute IS patients (168). Indeed, LPS significantly increased the infarct volume in MCAO mice, partially via TLR4/NF-κB pathway, resulting in the production of pro-inflammatory cytokines such as TNF-α, IL-1, and IL-6 (169). LPS aggravated brain damage, while increasing Lactobacillus, Bifidobacteria, and butyric acid-producing bacteria were reported to inhibit TLR4 signaling, reduce plasma LPS level, as well as and maintain T cell abundance (for example, reducing Th1 and Th17 cells while increasing Treg cells) (Figure 2).
Vitamins and related compounds
Vitamins, including vitamin A (VA), vitamin B (VB), vitamin K (VK), vitamin H (VH), folic acid, thiamine, and riboflavin, are essential micronutrients that cannot be synthesized in mammals and must be absorbed through the intestine. The gut microbiota is a great source of crucial vitamins. Lactobacillus and Bifidobacterium are inseparable from VB, VK, and folic acid synthesis (170). VA was reported to regulate intestinal immune response and can maintain gut microbiota homeostasis (171, 172). Studies have shown that VA supplementation can relieve arterial occlusion, which is related to the role of VA in reducing IL-17 and RORγt gene expression and Th17 cells (173, 174). As an essential cofactor for energy production, VB directly participates in the tricarboxylic acid cycle of cells, promotes ATP production, and provides energy for exercise. VB12 is an essential cofactor in amino acid and nucleotide biosynthesis in gut microbiota. It protects against IS by scavenging free radicals to inhibit oxidation-induced damage and resist oxidation neuroinflammation and apoptosis (175, 176). VB12 can also change gut immunity by promoting the differentiation of Th cells to Treg, which is crucial in reducing post-stroke neuroinflammation (177). Patients with VB12 deficiency showed lower levels of Tregs, while VB12 supplementation decreased the inflammatory circulating cytokine profiles (177). Furthermore, VB12 promotes SCFA-producing commensal bacteria in the gut (178), indicating it might reduce toxic neuroinflammation after IS by promoting gut SCFA (Figure 2). Therefore, in addition to its potential to prevent IS, VB12 is also a therapeutic factor in the acute phase of IS.
Conclusion and future perspectives
In this review, we highlight the role of the gut microbiota and metabolites related to IS. IS results from complex pathophysiological processes, such as oxidative stress, neuroinflammation, and apoptosis. Although several drugs are available to prevent the cascade of events that lead to ischemic injury, limited effective neuroprotective drugs are currently available to improve patient outcomes. In recent years, studies about the relationship between gut microbiota and neurological diseases have become a popular subject, indicating that gut microbiota could also play a vital role in the occurrence, development, and prognosis of IS. Gut microbiota can modulate oxidative stress, inflammation, and apoptosis-related signaling pathways through its constituents (e.g., LPS, peptidoglycan) and metabolites (e.g., SCFAs and BAs). Further, therapies targeting gut microbiota and their metabolites might be promising strategies to prevent or treat IS.
Author contributions
JL wrote the manuscript. JL, JW, and SC designed the review. JW, YL, and SC revised the manuscript. All authors contributed to the article and approved the submitted version.
Funding
This study was supported by the Research Funds of Hangzhou Institute for Advanced Study, University of Chinese Academy of Science (B04006C010006 and B04006C018007).
Conflict of interest
The authors declare that the research was conducted in the absence of any commercial or financial relationships that could be construed as a potential conflict of interest.
Publisher's note
All claims expressed in this article are solely those of the authors and do not necessarily represent those of their affiliated organizations, or those of the publisher, the editors and the reviewers. Any product that may be evaluated in this article, or claim that may be made by its manufacturer, is not guaranteed or endorsed by the publisher.
References
1. Collaborators GBDRF. Global burden of 87 risk factors in 204 countries and territories, 1990-2019: a systematic analysis for the Global Burden of Disease Study 2019. Lancet. (2020) 396:1223–49. doi: 10.1016/S0140-6736(20)30752-2
2. Krishnamurthi RV, Ikeda T, Feigin VL. Global, regional and country-specific burden of ischaemic stroke, intracerebral haemorrhage and subarachnoid haemorrhage: a systematic analysis of the Global Burden of Disease Study 2017. Neuroepidemiology. (2020) 54:171–9. doi: 10.1159/000506396
3. Andrabi SS, Parvez S, Tabassum H. Ischemic stroke and mitochondria: mechanisms and targets. Protoplasma. (2020) 257:335–43. doi: 10.1007/s00709-019-01439-2
4. Collaborators GBDV. Five insights from the Global Burden of Disease Study 2019. Lancet. (2020) 396:1135–59.
5. Prabhakaran S, Ruff I, Bernstein RA. Acute stroke intervention: a systematic review. JAMA. (2015) 313:1451–62. doi: 10.1001/jama.2015.3058
6. Yang Y, Li Q, Ahmad F, Shuaib A. Survival and histological evaluation of therapeutic window of post-ischemia treatment with magnesium sulfate in embolic stroke model of rat. Neurosci Lett. (2000) 285:119–22. doi: 10.1016/S0304-3940(00)01048-X
7. Furuichi Y, Katsuta K, Maeda M, Ueyama N, Moriguchi A, Matsuoka N, et al. Neuroprotective action of tacrolimus (FK506) in focal and global cerebral ischemia in rodents: dose dependency, therapeutic time window and long-term efficacy. Brain Res. (2003) 965:137–45. doi: 10.1016/S0006-8993(02)04151-3
8. Murata Y, Rosell A, Scannevin RH, Rhodes KJ, Wang X, Lo EH. Extension of the thrombolytic time window with minocycline in experimental stroke. Stroke. (2008) 39:3372–7. doi: 10.1161/STROKEAHA.108.514026
9. Bruce-Keller AJ, Salbaum JM, Berthoud HR. Harnessing gut microbes for mental health: getting from here to there. Biol Psychiatry. (2018) 83:214–23. doi: 10.1016/j.biopsych.2017.08.014
10. Hooks KB, Konsman JP, O'malley MA. Microbiota-gut-brain research: a critical analysis. Behav Brain Sci. (2018) 42:e60. doi: 10.1017/S0140525X18002133
11. Ji W, Zhu Y, Kan P, Cai Y, Wang Z, Wu Z, et al. Analysis of intestinal microbial communities of cerebral infarction and ischemia patients based on high throughput sequencing technology and glucose and lipid metabolism. Mol Med Rep. (2017) 16:5413–7. doi: 10.3892/mmr.2017.7227
12. Yamashiro K, Tanaka R, Urabe T, Ueno Y, Yamashiro Y, Nomoto K, et al. Gut dysbiosis is associated with metabolism and systemic inflammation in patients with ischemic stroke. PLoS ONE. (2017) 12:e0171521. doi: 10.1371/journal.pone.0171521
13. Caso JR, Hurtado O, Pereira MP, García-Bueno B, Menchén L, Alou L, et al. Colonic bacterial translocation as a possible factor in stress-worsening experimental stroke outcome. Am J Physiol Regul Integr Comp Physiol. (2009) 296:R979–985. doi: 10.1152/ajpregu.90825.2008
14. Tascilar N, Irkorucu O, Tascilar O, Comert F, Eroglu O, Bahadir B, et al. Bacterial translocation in experimental stroke: what happens to the gut barrier? Bratisl Lek Listy. (2010) 111:194–9.
15. Singh V, Roth S, Llovera G, Sadler R, Garzetti D, Stecher B, et al. Microbiota dysbiosis controls the neuroinflammatory response after stroke. J Neurosci. (2016) 36:7428–40. doi: 10.1523/JNEUROSCI.1114-16.2016
16. Spychala MS, Venna VR, Jandzinski M, Doran SJ, Durgan DJ, Ganesh BP, et al. Age-related changes in the gut microbiota influence systemic inflammation and stroke outcome. Ann Neurol. (2018) 84:23–36. doi: 10.1002/ana.25250
17. Lee J, D'aigle J, Atadja L, Quaicoe V, Honarpisheh P, Ganesh BP, et al. Gut microbiota-derived short-chain fatty acids promote poststroke recovery in aged mice. Circ Res. (2020) 127:453–465. doi: 10.1161/CIRCRESAHA.119.316448
18. Wahlstrom A, Sayin SI, Marschall HU, Backhed F. Intestinal crosstalk between bile acids and microbiota and its impact on host metabolism. Cell Metab. (2016) 24:41–50. doi: 10.1016/j.cmet.2016.05.005
19. Lukiw WJ, Cong L, Jaber V, Zhao Y. Microbiome-derived Lipopolysaccharide (LPS) selectively inhibits Neurofilament Light Chain (NF-L) gene expression in Human Neuronal-Glial (HNG) cells in primary culture. Front Neurosci. (2018) 12:896. doi: 10.3389/fnins.2018.00896
20. Hachinski V, Donnan GA, Gorelick PB, Hacke W, Cramer SC, Kaste M, et al. Stroke: working toward a prioritized world agenda. Cerebrovasc Dis. (2010) 30:127–47. doi: 10.1159/000315099
21. Xu S, Lu J, Shao A, Zhang JH, Zhang J. Glial cells: role of the immune response in ischemic stroke. Front Immunol. (2020) 11:294. doi: 10.3389/fimmu.2020.00294
22. Ponsaerts L, Alders L, Schepers M, De Oliveira RMW, Prickaerts J, Vanmierlo T, et al. Neuroinflammation in ischemic stroke: inhibition of cAMP-specific Phosphodiesterases (PDEs) to the rescue. Biomedicines. (2021) 9:703. doi: 10.3390/biomedicines9070703
23. Fann DY-W, Lee S-Y, Manzanero S, Chunduri P, Sobey CG, Arumugam TV. Pathogenesis of acute stroke and the role of inflammasomes. Ageing Res Rev. (2013) 12:941−66. doi: 10.1016/j.arr.2013.09.004
24. White SH, Brisson CD, Andrew RD. Examining protection from anoxic depolarization by the drugs dibucaine and carbetapentane using whole cell recording from CA1 neurons. J Neurophysiol. (2012) 107:2083–95. doi: 10.1152/jn.00701.2011
25. Fukuyama N, Takizawa S, Ishida H, Hoshiai K, Shinohara Y, Nakazawa H. Peroxynitrite formation in focal cerebral ischemia-reperfusion in rats occurs predominantly in the peri-infarct region. J Cereb Blood Flow Metab. (1998) 18:123–9. doi: 10.1097/00004647-199802000-00001
26. Qin C, Yang S, Chu Y-H, Zhang H, Pang X-W, Chen L, et al. Correction To: Signaling pathways involved in ischemic stroke: molecular mechanisms and therapeutic interventions. Signal Transduct Target Ther. (2022) 7:278. doi: 10.1038/s41392-022-01129-1
27. Marciano F, Vajro P. Oxidative Stress and Gut Microbiota * *Conflict of interest: None. In: Gastrointestinal Tissue. Amsterdam: Elsevier (2017).
28. Westfall S, Lomis N, Kahouli I, Dia SY, Singh SP, Prakash S. Microbiome, probiotics and neurodegenerative diseases: deciphering the gut brain axis. Cell Mol Life Sci CMLS. (2017) 74:3769–87. doi: 10.1007/s00018-017-2550-9
29. Sun L, Jia H, Li J, Yu M, Yang Y, Tian D, et al. Cecal gut microbiota and metabolites might contribute to the severity of acute myocardial ischemia by impacting the intestinal permeability, oxidative stress, and energy metabolism. Front Microbiol. (2019) 10:1745. doi: 10.3389/fmicb.2019.01745
30. Wang K, Wan Z, Ou A, Liang X, Guo X, Zhang Z, et al. Monofloral honey from a medical plant, Prunella Vulgaris, protected against dextran sulfate sodium-induced ulcerative colitis via modulating gut microbial populations in rats. Food Funct. (2019) 10:3828–38. doi: 10.1039/C9FO00460B
31. Zhao M, Zhu P, Fujino M, Zhuang J, Guo H, Sheikh I, et al. Oxidative stress in hypoxic-ischemic encephalopathy: molecular mechanisms and therapeutic strategies. Int J Mol Sci. (2016) 17. doi: 10.3390/ijms17122078
32. Tu W, Wang H, Li S, Liu Q, Sha H. The anti-inflammatory and anti-oxidant mechanisms of the Keap1/Nrf2/ARE signaling pathway in chronic diseases. Aging Dis. (2019) 10:637–51. doi: 10.14336/AD.2018.0513
33. Zenkov NK, Menshchikova EB, Tkachev VO. Keap1/Nrf2/ARE redox-sensitive signaling system as a pharmacological target. Biochemistry. (2013) 78:19–36. doi: 10.1134/S0006297913010033
34. Jin W, Wang HD, Hu ZG, Yan W, Chen G, Yin HX. Transcription factor Nrf2 plays a pivotal role in protection against traumatic brain injury-induced acute intestinal mucosal injury in mice. J Surg Res. (2009) 157:251–60. doi: 10.1016/j.jss.2008.08.003
35. Zorov DBPlotnikov EY, Silachev DN, Zorova LD, Pevzner IBZorov SD, et al. Microbiota and mitobiota. Putting an equal sign between mitochondria and bacteria. Biochemistry. (2014) 79:1017–31. doi: 10.1134/S0006297914100046
36. Jones RM, Mercante JW, Neish AS. Reactive oxygen production induced by the gut microbiota: pharmacotherapeutic implications. Curr Med Chem. (2012) 19:1519–29. doi: 10.2174/092986712799828283
37. Cobley JN, Fiorello ML, Bailey DM. 13 reasons why the brain is susceptible to oxidative stress. Redox Biol. (2018) 15:490–503. doi: 10.1016/j.redox.2018.01.008
38. Sun H, Gu M, Li Z, Chen X, Zhou J. Gut microbiota dysbiosis in acute ischemic stroke associated with 3-month unfavorable outcome. Front Neurol. (2021) 12:799222. doi: 10.3389/fneur.2021.799222
39. Chidambaram SB, Rathipriya AG, Mahalakshmi AM, Sharma S, Hediyal TA, Ray B, et al. The influence of gut dysbiosis in the pathogenesis and management of ischemic stroke. Cells. (2022) 11:1239. doi: 10.3390/cells11071239
40. Ershler WB. A gripping reality: oxidative stress, inflammation, and the pathway to frailty. J Appl Physiol. (2007) 103:3–5. doi: 10.1152/japplphysiol.00375.2007
41. Winter SE, Lopez CA, Bäumler AJ. The dynamics of gut-associated microbial communities during inflammation. EMBO Rep. (2013) 14:319–27. doi: 10.1038/embor.2013.27
42. Uzdensky AB. Apoptosis regulation in the penumbra after ischemic stroke: expression of pro- and antiapoptotic proteins. Apoptosis. (2019) 24:687–702. doi: 10.1007/s10495-019-01556-6
43. Sekerdag E, Solaroglu I, Gursoy-Ozdemir Y. Cell death mechanisms in stroke and novel molecular and cellular treatment options. Curr Neuropharmacol. (2018) 16:1396–415. doi: 10.2174/1570159X16666180302115544
44. Han Y, Yuan M, Guo YS, Shen XY, Gao ZK, Bi X. Mechanism of endoplasmic reticulum stress in cerebral ischemia. Front Cell Neurosci. (2021) 15:704334. doi: 10.3389/fncel.2021.704334
45. Culmsee C, Zhu C, Landshamer S, Becattini B, Wagner E, Pellecchia M, et al. Apoptosis-inducing factor triggered by poly(ADP-ribose) polymerase and Bid mediates neuronal cell death after oxygen-glucose deprivation and focal cerebral ischemia. J Neurosci. (2005) 25:10262–72. doi: 10.1523/JNEUROSCI.2818-05.2005
46. Nikoletopoulou V, Markaki M, Palikaras K, Tavernarakis N. Crosstalk between apoptosis, necrosis and autophagy. Biochim Biophys Acta. (2013) 1833:3448–59. doi: 10.1016/j.bbamcr.2013.06.001
47. Datta A, Sarmah D, Mounica L, Kaur H, Kesharwani R, Verma G, et al. Cell death pathways in ischemic stroke and targeted pharmacotherapy. Transl Stroke Res. (2020) 11:1185–202. doi: 10.1007/s12975-020-00806-z
48. Peña-Blanco A, García-Sáez AJ. Bax, Bak and beyond - mitochondrial performance in apoptosis. FEBS J. (2018) 285:416–31. doi: 10.1111/febs.14186
49. Rodríguez-Yáñez M, Castillo J. Role of inflammatory markers in brain ischemia. Curr Opin Neurol. (2008) 21:353–7. doi: 10.1097/WCO.0b013e3282ffafbf
50. Namura S, Zhu J, Fink K, Endres M, Srinivasan A, Tomaselli KJ, et al. Activation and cleavage of caspase-3 in apoptosis induced by experimental cerebral ischemia. J Neurosci. (1998) 18:3659–68. doi: 10.1523/JNEUROSCI.18-10-03659.1998
51. Kim JY, Barua S, Huang MY, Park J, Yenari MA, Lee JE. Heat shock protein 70 (HSP70) induction: chaperonotherapy for neuroprotection after brain injury. Cells. (2020) 9. doi: 10.3390/cells9092020
52. Yuan J. Neuroprotective strategies targeting apoptotic and necrotic cell death for stroke. Apoptosis. (2009) 14:469–77. doi: 10.1007/s10495-008-0304-8
53. Chen P, Chen F, Lei J, Wang G, Zhou B. The gut microbiota metabolite Urolithin B improves cognitive deficits by inhibiting Cyt C-mediated apoptosis and promoting the survival of neurons through the PI3K pathway in aging mice. Front Pharmacol. (2021) 12:768097. doi: 10.3389/fphar.2021.768097
54. Saiki H, Okano Y, Yasuma T, Toda M, Takeshita A, Abdel-Hamid AM, et al. A microbiome-derived peptide induces apoptosis of cells from different tissues. Cells. (2021) 10. doi: 10.3390/cells10112885
55. Liu J, Sun J, Wang F, Yu X, Ling Z, Li H, et al. Neuroprotective effects of clostridium butyricum against vascular dementia in mice via metabolic butyrate. Biomed Res Int. (2015) 2015:412946. doi: 10.1155/2015/412946
56. Chen R, Xu Y, Wu P, Zhou H, Lasanajak Y, Fang Y, et al. Transplantation of fecal microbiota rich in short chain fatty acids and butyric acid treat cerebral ischemic stroke by regulating gut microbiota. Pharmacol Res. (2019) 148:104403. doi: 10.1016/j.phrs.2019.104403
57. Rodrigues CM, Ma X, Linehan-Stieers C, Fan G, Kren BT, Steer CJ. Ursodeoxycholic acid prevents cytochrome c release in apoptosis by inhibiting mitochondrial membrane depolarization and channel formation. Cell Death Differ. (1999) 6:842–54. doi: 10.1038/sj.cdd.4400560
58. Rodrigues CM, Stieers CL, Keene CD, Ma X, Kren BT, Low WC, et al. Tauroursodeoxycholic acid partially prevents apoptosis induced by 3-nitropropionic acid: evidence for a mitochondrial pathway independent of the permeability transition. J Neurochem. (2000) 75:2368–79. doi: 10.1046/j.1471-4159.2000.0752368.x
59. Li Q, Barres BA. Microglia and macrophages in brain homeostasis and disease. Nat Rev Immunol. (2018) 18:225–42. doi: 10.1038/nri.2017.125
60. Colonna M, Butovsky O. Microglia function in the central nervous system during health and neurodegeneration. Annu Rev Immunol. (2017) 35:441–68. doi: 10.1146/annurev-immunol-051116-052358
61. Liu M, Xu Z, Wang L, Zhang L, Liu Y, Cao J, et al. Cottonseed oil alleviates ischemic stroke injury by inhibiting the inflammatory activation of microglia and astrocyte. J Neuroinflammation. (2020) 17:270. doi: 10.1186/s12974-020-01946-7
62. Shi K, Tian DC, Li ZG, Ducruet AF, Lawton MT, Shi FD. Global brain inflammation in stroke. Lancet Neurol. (2019) 18:1058–66. doi: 10.1016/S1474-4422(19)30078-X
63. Dinarello CA. Overview of the IL-1 family in innate inflammation and acquired immunity. Immunol Rev. (2018) 281:8–27. doi: 10.1111/imr.12621
64. Tabares-Guevara JH, Villa-Pulgarin JA, Hernandez JC. Atherosclerosis: immunopathogenesis and strategies for immunotherapy. Immunotherapy. (2021) 13:1231–44. doi: 10.2217/imt-2021-0009
65. Fitzgerald KA, Kagan JC. Toll-like receptors and the control of immunity. Cell. (2020) 180:1044–66. doi: 10.1016/j.cell.2020.02.041
66. Prakash P, Kulkarni PP, Lentz SR, Chauhan AK. Cellular fibronectin containing extra domain A promotes arterial thrombosis in mice through platelet Toll-like receptor 4. Blood. (2015) 125:3164–72. doi: 10.1182/blood-2014-10-608653
67. Jeong JH, Jang S, Jung BJ, Jang KS, Kim BG, Chung DK, et al. Differential immune-stimulatory effects of LTAs from different lactic acid bacteria via MAPK signaling pathway in RAW 264.7 cells. Immunobiology. (2015) 220:460–6. doi: 10.1016/j.imbio.2014.11.002
68. Schindler JF, Monahan JB, Smith WG. p38 pathway kinases as anti-inflammatory drug targets. J Dent Res. (2007) 86:800–11. doi: 10.1177/154405910708600902
69. Gelderblom M, Leypoldt F, Steinbach K, Behrens D, Choe CU, Siler DA, et al. Temporal and spatial dynamics of cerebral immune cell accumulation in stroke. Stroke. (2009) 40:1849–57. doi: 10.1161/STROKEAHA.108.534503
70. Vogelgesang A, May VE, Grunwald U, Bakkeboe M, Langner S, Wallaschofski H, et al. Functional status of peripheral blood T-cells in ischemic stroke patients. PLoS ONE. (2010) 5:e8718. doi: 10.1371/journal.pone.0008718
71. Schwartz M, Raposo C. Protective autoimmunity: a unifying model for the immune network involved in CNS repair. Neuroscientist. (2014) 20:343–58. doi: 10.1177/1073858413516799
72. Shichita T, Sugiyama Y, Ooboshi H, Sugimori H, Nakagawa R, Takada I, et al. Pivotal role of cerebral interleukin-17-producing gammadeltaT cells in the delayed phase of ischemic brain injury. Nat Med. (2009) 15:946–50. doi: 10.1038/nm.1999
73. Gelderblom M, Weymar A, Bernreuther C, Velden J, Arunachalam P, Steinbach K, et al. Neutralization of the IL-17 axis diminishes neutrophil invasion and protects from ischemic stroke. Blood. (2012) 120:3793–802. doi: 10.1182/blood-2012-02-412726
74. Stilling RM, Dinan TG, Cryan JF. Microbial genes, brain and behaviour - epigenetic regulation of the gut-brain axis. Genes Brain Behav. (2014) 13:69–86. doi: 10.1111/gbb.12109
75. Qadis AQ, Goya S, Yatsu M, Yoshida YU, Ichijo T, Sato S. Effects of a bacteria-based probiotic on subpopulations of peripheral leukocytes and their cytokine mRNA expression in calves. J Vet Med Sci. (2014) 76:189–95. doi: 10.1292/jvms.13-0370
76. Tan C, Wu Q, Wang H, Gao X, Xu R, Cui Z, et al. Dysbiosis of gut microbiota and short-chain fatty acids in acute ischemic stroke and the subsequent risk for poor functional outcomes. JPEN J Parenter Enteral Nutr. (2021) 45:518–29. doi: 10.1002/jpen.1861
77. Arpaia N, Campbell C, Fan X, Dikiy S, Van Der Veeken J, Deroos P, et al. Metabolites produced by commensal bacteria promote peripheral regulatory T-cell generation. Nature. (2013) 504:451–5. doi: 10.1038/nature12726
78. Zhou W, Liesz A, Bauer H, Sommer C, Lahrmann B, Valous N, et al. Postischemic brain infiltration of leukocyte subpopulations differs among murine permanent and transient focal cerebral ischemia models. Brain Pathol. (2013) 23:34–44. doi: 10.1111/j.1750-3639.2012.00614.x
79. Logsdon AF, Erickson MA, Rhea EM, Salameh TS, Banks WA. Gut reactions: How the blood-brain barrier connects the microbiome and the brain. Exp Biol Med. (2018) 243:159–65. doi: 10.1177/1535370217743766
80. Benakis C, Brea D, Caballero S, Faraco G, Moore J, Murphy M, et al. Commensal microbiota affects ischemic stroke outcome by regulating intestinal γδ T cells. Nat Med. (2016) 22:516–23. doi: 10.1038/nm.4068
81. Suez J, Zmora N, Segal E, Elinav E. The pros, cons, and many unknowns of probiotics. Nat Med. (2019) 25:716–29. doi: 10.1038/s41591-019-0439-x
82. Sharma R, Kapila R, Kapasiya M, Saliganti V, Dass G, Kapila S. Dietary supplementation of milk fermented with probiotic Lactobacillus fermentum enhances systemic immune response and antioxidant capacity in aging mice. Nutr Res. (2014) 34:968–81. doi: 10.1016/j.nutres.2014.09.006
83. Fardet A, Rock E. In vitro and in vivo antioxidant potential of milks, yoghurts, fermented milks and cheeses: a narrative review of evidence. Nutr Res Rev. (2018) 31:52–70. doi: 10.1017/S0954422417000191
84. Crow VL, Coolbear T, Gopal PK, Martley FG, Mckay LL, Riepe H. The role of autolysis of lactic acid bacteria in the ripening of cheese. Int Dairy J. (1995) 5:855–75. doi: 10.1016/0958-6946(95)00036-4
85. Jones RM, Desai C, Darby TM, Luo L, Wolfarth AA, Scharer CD, et al. Lactobacilli Modulate Epithelial Cytoprotection through the Nrf2 Pathway. Cell Rep. (2015) 12:1217–25. doi: 10.1016/j.celrep.2015.07.042
86. Cheon MJ, Lim SM, Lee NK, Paik HD. Probiotic properties and neuroprotective effects of Lactobacillus buchneri KU200793 Isolated from Korean fermented foods. Int J Mol Sci. (2020) 21:1227. doi: 10.3390/ijms21041227
87. Doenyas C. Gut microbiota, inflammation, and probiotics on neural development in autism spectrum disorder. Neuroscience. (2018) 374:271–86. doi: 10.1016/j.neuroscience.2018.01.060
88. Cristofori F, Dargenio VN, Dargenio C, Miniello VL, Barone M, Francavilla R. Anti-inflammatory and immunomodulatory effects of probiotics in gut inflammation: a door to the body. Front Immunol. (2021) 12:578386. doi: 10.3389/fimmu.2021.578386
89. Sirin S, Aslim B. Characterization of lactic acid bacteria derived exopolysaccharides for use as a defined neuroprotective agent against amyloid beta(1-42)-induced apoptosis in SH-SY5Y cells. Sci Rep. (2020) 10:8124. doi: 10.1038/s41598-020-65147-1
90. Mohammadi G, Dargahi L, Naserpour T, Mirzanejad Y, Alizadeh SA, Peymani A, et al. Probiotic mixture of Lactobacillus helveticus R0052 and Bifidobacterium longum R0175 attenuates hippocampal apoptosis induced by lipopolysaccharide in rats. Int Microbiol. (2019) 22:317–23. doi: 10.1007/s10123-018-00051-3
91. Xu N, Fan W, Zhou X, Liu Y, Ma P, Qi S, et al. Probiotics decrease depressive behaviors induced by constipation via activating the AKT signaling pathway. Metab Brain Dis. (2018) 33:1625–33. doi: 10.1007/s11011-018-0269-4
92. Fink LN, Zeuthen LH, Christensen HR, Morandi B, Frokiaer H, Ferlazzo G. Distinct gut-derived lactic acid bacteria elicit divergent dendritic cell-mediated NK cell responses. Int Immunol. (2007) 19:1319–27. doi: 10.1093/intimm/dxm103
93. Vizoso Pinto MG, Rodriguez Gómez M, Seifert S, Watzl B, Holzapfel WH, Franz CM. Lactobacilli stimulate the innate immune response and modulate the TLR expression of HT29 intestinal epithelial cells in vitro. Int J Food Microbiol. (2009) 133:86–93. doi: 10.1016/j.ijfoodmicro.2009.05.013
94. Macho Fernandez E, Valenti V, Rockel C, Hermann C, Pot B, Boneca IG, et al. Anti-inflammatory capacity of selected lactobacilli in experimental colitis is driven by NOD2-mediated recognition of a specific peptidoglycan-derived muropeptide. Gut. (2011) 60:1050–9. doi: 10.1136/gut.2010.232918
95. Chon H, Choi B, Jeong G, Lee E, Lee S. Suppression of proinflammatory cytokine production by specific metabolites of Lactobacillus plantarum 10hk2 via inhibiting NF-κB and p38 MAPK expressions. Comp Immunol Microbiol Infect Dis. (2010) 33:e41–49. doi: 10.1016/j.cimid.2009.11.002
96. Sherwin E, Sandhu KV, Dinan TG, Cryan JF. May the force be with you: the light and dark sides of the microbiota–gut–brain axis in neuropsychiatry. CNS Drugs. (2016) 30:1019–41. doi: 10.1007/s40263-016-0370-3
97. Dhaliwal J, Singh DP, Singh S, Pinnaka AK, Boparai RK, Bishnoi M, et al. Lactobacillus plantarum MTCC 9510 supplementation protects from chronic unpredictable and sleep deprivation-induced behaviour, biochemical and selected gut microbial aberrations in mice. J Appl Microbiol. (2018) 125:257–69. doi: 10.1111/jam.13765
98. Chong Chong HX, Yusoff NAA, Hor YY, Lew LC, Jaafar MH, Choi SB, et al. (2019). Lactobacillus plantarum DR7 alleviates stress and anxiety in adults: a randomised, double-blind, placebo-controlled study. Benef Microbes. (2019) 10:355–73. doi: 10.3920/BM2018.0135
99. Hao Z, Wang W, Guo R, Liu H. Faecalibacterium prausnitzii (ATCC 27766) has preventive and therapeutic effects on chronic unpredictable mild stress-induced depression-like and anxiety-like behavior in rats. Psychoneuroendocrinology. (2019) 104:132–42. doi: 10.1016/j.psyneuen.2019.02.025
100. O'mahony SM, Clarke G, Borre YE, Dinan TG, Cryan JF. Serotonin, tryptophan metabolism and the brain-gut-microbiome axis. Behav Brain Res. (2015) 277:32–48. doi: 10.1016/j.bbr.2014.07.027
101. Yissachar N, Zhou Y, Ung L, Lai NY, Mohan JF, Ehrlicher A, et al. An intestinal organ culture system uncovers a role for the nervous system in microbe-immune crosstalk. Cell. (2017) 168:1135–48.e1112. doi: 10.1016/j.cell.2017.02.009
102. Jameson KG, Hsiao EY. Linking the gut microbiota to a brain neurotransmitter. Trends Neurosci. (2018) 41:413–4. doi: 10.1016/j.tins.2018.04.001
103. Chambers ES, Morrison DJ, Frost G. Control of appetite and energy intake by SCFA: what are the potential underlying mechanisms? Proc Nutr Soc. (2015) 74:328–36. doi: 10.1017/S0029665114001657
104. Hamer HM, Jonkers DM, Bast A, Vanhoutvin SA, Fischer MA, Kodde A, et al. Butyrate modulates oxidative stress in the colonic mucosa of healthy humans. Clin Nutr. (2009) 28:88–93. doi: 10.1016/j.clnu.2008.11.002
105. Sacco P, Decleva E, Tentor F, Menegazzi R, Borgogna M, Paoletti S, et al. Butyrate-loaded chitosan/hyaluronan nanoparticles: a suitable tool for sustained inhibition of ROS release by activated neutrophils. Macromol Biosci. (2017) 17:1700214. doi: 10.1002/mabi.201700214
106. Wang RX, Li S, Sui X. Sodium butyrate relieves cerebral ischemia-reperfusion injury in mice by inhibiting JNK/STAT pathway. Eur Rev Med Pharmacol Sci. (2019) 23:1762–9. doi: 10.26355/eurrev_201902_17138
107. Li H, Shang Z, Liu X, Qiao Y, Wang K, Qiao J. Clostridium butyricum alleviates enterotoxigenic escherichia coli K88-induced oxidative damage through regulating the p62-Keap1-Nrf2 signaling pathway and remodeling the cecal microbial community. Front Immunol. (2021) 12:771826. doi: 10.3389/fimmu.2021.771826
108. Tang Y, Chen Y, Jiang H, Nie D. Short-chain fatty acids induced autophagy serves as an adaptive strategy for retarding mitochondria-mediated apoptotic cell death. Cell Death Differ. (2011) 18:602–18. doi: 10.1038/cdd.2010.117
109. Donohoe DR, Holley D, Collins LB, Montgomery SA, Whitmore AC, Hillhouse A, et al. A gnotobiotic mouse model demonstrates that dietary fiber protects against colorectal tumorigenesis in a microbiota- and butyrate-dependent manner. Cancer Discov. (2014) 4:1387–97. doi: 10.1158/2159-8290.CD-14-0501
110. Tsugawa H, Kabe Y, Kanai A, Sugiura Y, Hida S, Taniguchi S, et al. Short-chain fatty acids bind to apoptosis-associated speck-like protein to activate inflammasome complex to prevent Salmonella infection. PLoS Biol. (2020) 18:e3000813. doi: 10.1371/journal.pbio.3000813
111. Li D, Bai X, Jiang Y, Cheng Y. Butyrate alleviates PTZ-induced mitochondrial dysfunction, oxidative stress and neuron apoptosis in mice via Keap1/Nrf2/HO-1 pathway. Brain Res Bull. (2021) 168:25–35. doi: 10.1016/j.brainresbull.2020.12.009
112. Zhou Z, Xu N, Matei N, Mcbride DW, Ding Y, Liang H, et al. Sodium butyrate attenuated neuronal apoptosis via GPR41/Gβγ/PI3K/Akt pathway after MCAO in rats. J Cereb Blood Flow Metab. (2021) 41:267–81. doi: 10.1177/0271678X20910533
113. Maslowski KM, Vieira AT, Ng A, Kranich J, Sierro F, Yu D, et al. Regulation of inflammatory responses by gut microbiota and chemoattractant receptor GPR43. Nature. (2009) 461:1282–6. doi: 10.1038/nature08530
114. Erny D, Hrabe De Angelis AL, Jaitin D, Wieghofer P, Staszewski O, David E, et al. Host microbiota constantly control maturation and function of microglia in the CNS. Nat Neurosci. (2015) 18:965–77. doi: 10.1038/nn.4030
115. Garcia-Gutierrez E, Narbad A, Rodríguez JM. Autism spectrum disorder associated with gut microbiota at immune, metabolomic, and neuroactive level. Front Neurosci. (2020) 14:578666. doi: 10.3389/fnins.2020.578666
116. Smith MD, Bhatt DP, Geiger JD, Rosenberger TA. Acetate supplementation modulates brain adenosine metabolizing enzymes and adenosine A2A receptor levels in rats subjected to neuroinflammation. J Neuroinflammation. (2014) 11:99. doi: 10.1186/1742-2094-11-99
117. Kobayashi M, Mikami D, Kimura H, Kamiyama K, Morikawa Y, Yokoi S, et al. Short-chain fatty acids, GPR41 and GPR43 ligands, inhibit TNF-α-induced MCP-1 expression by modulating p38 and JNK signaling pathways in human renal cortical epithelial cells. Biochem Biophys Res Commun. (2017) 486:499–505. doi: 10.1016/j.bbrc.2017.03.071
118. Patnala R, Arumugam TV, Gupta N, Dheen ST. HDAC inhibitor sodium butyrate-mediated epigenetic regulation enhances neuroprotective function of microglia during ischemic stroke. Mol Neurobiol. (2017) 54:6391–411. doi: 10.1007/s12035-016-0149-z
119. Khan A, Shin MS, Jee SH, Park YH. Global metabolomics analysis of serum from humans at risk of thrombotic stroke. Analyst. (2020) 145:1695–705. doi: 10.1039/C9AN02032B
120. Wang B, Sun S, Liu M, Chen H, Liu N, Wu Z, et al. Dietary L-tryptophan regulates colonic serotonin homeostasis in mice with dextran sodium sulfate-induced colitis. J Nutr. (2020) 150:1966–76. doi: 10.1093/jn/nxaa129
121. Mclean PG, Borman RA, Lee K. 5-HT in the enteric nervous system: gut function and neuropharmacology. Trends Neurosci. (2007) 30:9–13. doi: 10.1016/j.tins.2006.11.002
122. Nyangale EP, Mottram DS, Gibson GR. Gut microbial activity, implications for health and disease: the potential role of metabolite analysis. J Proteome Res. (2012) 11:5573–85. doi: 10.1021/pr300637d
123. Davila AM, Blachier F, Gotteland M, Andriamihaja M, Benetti PH, Sanz Y, et al. Intestinal luminal nitrogen metabolism: role of the gut microbiota and consequences for the host. Pharmacol Res. (2013) 68:95–107. doi: 10.1016/j.phrs.2012.11.005
124. Marsland BJ. Regulating inflammation with microbial metabolites. Nat Med. (2016) 22:581–3. doi: 10.1038/nm.4117
125. Dodd D, Spitzer MH, Van Treuren W, Merrill BD, Hryckowian AJ, Higginbottom SK, et al. A gut bacterial pathway metabolizes aromatic amino acids into nine circulating metabolites. Nature. (2017) 551:648–52. doi: 10.1038/nature24661
126. Rothhammer V, Mascanfroni ID, Bunse L, Takenaka MC, Kenison JE, Mayo L, et al. Type I interferons and microbial metabolites of tryptophan modulate astrocyte activity and central nervous system inflammation via the aryl hydrocarbon receptor. Nat Med. (2016) 22:586–97. doi: 10.1038/nm.4106
127. Nicolas GR, Chang PV. Deciphering the chemical lexicon of host-gut microbiota interactions. Trends Pharmacol Sci. (2019) 40:430–45. doi: 10.1016/j.tips.2019.04.006
128. Theriot CM, Bowman AA, Young VB. Antibiotic-induced alterations of the gut microbiota alter secondary bile acid production and allow for clostridium difficile spore germination and outgrowth in the large intestine. mSphere. (2016) 1. doi: 10.1128/mSphere.00045-15
129. Charach G, Karniel E, Novikov I, Galin L, Vons S, Grosskopf I, et al. Reduced bile acid excretion is an independent risk factor for stroke and mortality: a prospective follow-up study. Atherosclerosis. (2020) 293:79–85. doi: 10.1016/j.atherosclerosis.2019.12.010
130. Huang L, Xu G, Zhang R, Wang Y, Ji J, Long F, et al. Increased admission serum total bile acids can be associated with decreased 3-month mortality in patients with acute ischemic stroke. Lipids Health Dis. (2022) 21:15. doi: 10.1186/s12944-021-01620-8
131. Deng P, Wang L, Zhang Q, Chen S, Zhang Y, Xu H, et al. Therapeutic potential of a combination of electroacupuncture and human iPSC-derived small extracellular vesicles for ischemic stroke. Cells. (2022) 11:820. doi: 10.3390/cells11050820
132. Hang S, Paik D, Yao L, Kim E, Trinath J, Lu J, et al. Bile acid metabolites control T(H)17 and T(reg) cell differentiation. Nature. (2019) 576:143–8. doi: 10.1038/s41586-019-1785-z
133. Paik D, Yao L, Zhang Y, Bae S, D'agostino GD, Zhang M, et al. Human gut bacteria produce TH17-modulating bile acid metabolites. Nature. (2022) 603:907–12. doi: 10.1038/s41586-022-04480-z
134. Franco P, Porru E, Fiori J, Gioiello A, Cerra B, Roda G, et al. Identification and quantification of oxo-bile acids in human faeces with liquid chromatography-mass spectrometry: a potent tool for human gut acidic sterolbiome studies. J Chromatogr A. (2019) 1585:70–81. doi: 10.1016/j.chroma.2018.11.038
135. Guzior DV, Quinn RA. Review: microbial transformations of human bile acids. Microbiome. (2021) 9:140. doi: 10.1186/s40168-021-01101-1
136. Chun HS, Low WC. Ursodeoxycholic acid suppresses mitochondria-dependent programmed cell death induced by sodium nitroprusside in SH-SY5Y cells. Toxicology. (2012) 292:105–12. doi: 10.1016/j.tox.2011.11.020
137. Rodrigues CM, Fan G, Ma X, Kren BT, Steer CJ. A novel role for ursodeoxycholic acid in inhibiting apoptosis by modulating mitochondrial membrane perturbation. J Clin Invest. (1998) 101:2790–9. doi: 10.1172/JCI1325
138. Rodrigues CM, Fan G, Wong PY, Kren BT, Steer CJ. Ursodeoxycholic acid may inhibit deoxycholic acid-induced apoptosis by modulating mitochondrial transmembrane potential and reactive oxygen species production. Mol Med. (1998) 4:165–78. doi: 10.1007/BF03401914
139. Azzaroli F, Mehal W, Soroka CJ, Wang L, Lee J, Crispe IN, et al. Ursodeoxycholic acid diminishes Fas-ligand-induced apoptosis in mouse hepatocytes. Hepatology. (2002) 36:49–54. doi: 10.1053/jhep.2002.34511
140. Cortez L, Sim V. The therapeutic potential of chemical chaperones in protein folding diseases. Prion. (2014) 8:197–202. doi: 10.4161/pri.28938
141. Vang S, Longley K, Steer CJ, Low WC. The unexpected uses of Urso- and tauroursodeoxycholic acid in the treatment of non-liver diseases. Glob Adv Health Med. (2014) 3:58–69. doi: 10.7453/gahmj.2014.017
142. Yanguas-Casás N, Barreda-Manso MA, Nieto-Sampedro M, Romero-Ramírez L. TUDCA: an agonist of the bile acid receptor GPBAR1/TGR5 with anti-inflammatory effects in microglial cells. J Cell Physiol. (2017) 232:2231–45. doi: 10.1002/jcp.25742
143. Yanguas-Casás N, Barreda-Manso MA, Nieto-Sampedro M, Romero-Ramírez L. Tauroursodeoxycholic acid reduces glial cell activation in an animal model of acute neuroinflammation. J Neuroinflammation. (2014) 11:50. doi: 10.1186/1742-2094-11-50
144. Rodrigues CM, Spellman SR, Solá S, Grande AWLinehan-Stieers C, Low WC, et al. Neuroprotection by a bile acid in an acute stroke model in the rat. J Cereb Blood Flow Metab. (2002) 22:463–71. doi: 10.1097/00004647-200204000-00010
145. Sola S, Castro RE, Laires PA, Steer CJ, Rodrigues CM. Tauroursodeoxycholic acid prevents amyloid-beta peptide-induced neuronal death via a phosphatidylinositol 3-kinase-dependent signaling pathway. Mol Med. (2003) 9:226–34. doi: 10.2119/2003-00042.Rodrigues
146. Castro RE, Sola S, Ramalho RM, Steer CJ, Rodrigues CM. The bile acid tauroursodeoxycholic acid modulates phosphorylation and translocation of bad via phosphatidylinositol 3-kinase in glutamate-induced apoptosis of rat cortical neurons. J Pharmacol Exp Ther. (2004) 311:845–52. doi: 10.1124/jpet.104.070532
147. Ramalho RM, Ribeiro PS, Sola S, Castro RE, Steer CJ, Rodrigues CM. Inhibition of the E2F-1/p53/Bax pathway by tauroursodeoxycholic acid in amyloid beta-peptide-induced apoptosis of PC12 cells. J Neurochem. (2004) 90:567–75. doi: 10.1111/j.1471-4159.2004.02517.x
148. Kusaczuk M. Tauroursodeoxycholate-bile acid with chaperoning activity: molecular and cellular effects and therapeutic perspectives. Cells. (2019) 8:1471. doi: 10.3390/cells8121471
149. Schugar RC, Willard B, Wang Z, Brown JM. Postprandial gut microbiota-driven choline metabolism links dietary cues to adipose tissue dysfunction. Adipocyte. (2018) 7:49–56. doi: 10.1080/21623945.2017.1398295
150. Nicholson JK, Holmes E, Kinross J, Burcelin R, Gibson G, Jia W, et al. Host-gut microbiota metabolic interactions. Science. (2012) 336:1262–7. doi: 10.1126/science.1223813
151. Zhu W, Gregory JC, Org E, Buffa JA, Gupta N, Wang Z, et al. Gut microbial metabolite TMAO enhances platelet hyperreactivity and thrombosis risk. Cell. (2016) 165:111–24. doi: 10.1016/j.cell.2016.02.011
152. Zhu W, Wang Z, Tang WHW, Hazen SL. Gut microbe-generated trimethylamine N-Oxide from dietary choline is prothrombotic in subjects. Circulation. (2017) 135:1671–3. doi: 10.1161/CIRCULATIONAHA.116.025338
153. Li T, Chen Y, Gua C, Li X. Elevated circulating trimethylamine N-Oxide levels contribute to endothelial dysfunction in aged rats through vascular inflammation and oxidative stress. Front Physiol. (2017) 8:350. doi: 10.3389/fphys.2017.00350
154. Ding C, Zhao Y, Shi X, Zhang N, Zu G, Li Z, et al. New insights into salvianolic acid A action: Regulation of the TXNIP/NLRP3 and TXNIP/ChREBP pathways ameliorates HFD-induced NAFLD in rats. Sci Rep. (2016) 6:28734. doi: 10.1038/srep28734
155. Paramel Varghese G, Folkersen L, Strawbridge RJ, Halvorsen B, Yndestad A, Ranheim T, et al. NLRP3 inflammasome expression and activation in human atherosclerosis. J Am Heart Assoc. (2016) 5:e003031. doi: 10.1161/JAHA.115.003031
156. Geng J, Yang C, Wang B, Zhang X, Hu T, Gu Y, et al. Trimethylamine N-oxide promotes atherosclerosis via CD36-dependent MAPK/JNK pathway. Biomed Pharmacother. (2018) 97:941–7. doi: 10.1016/j.biopha.2017.11.016
157. Hermann DM, Popa-Wagner A, Kleinschnitz C, Doeppner TR. Animal models of ischemic stroke and their impact on drug discovery. Expert Opin Drug Discov. (2019) 14:315–26. doi: 10.1080/17460441.2019.1573984
158. Su H, Fan S, Zhang L, Qi H. TMAO aggregates neurological damage following ischemic stroke by promoting reactive astrocytosis and glial scar formation via the Smurf2/ALK5 axis. Front Cell Neurosci. (2021) 15:569424. doi: 10.3389/fncel.2021.569424
159. Qiu L, Yang D, Tao X, Yu J, Xiong H, Wei H. Enterobacter aerogenes ZDY01 attenuates choline-induced trimethylamine N-Oxide levels by remodeling gut microbiota in mice. J Microbiol Biotechnol. (2017) 27:1491–9. doi: 10.4014/jmb.1703.03039
160. Qiu L, Tao X, Xiong H, Yu J, Wei H. Lactobacillus plantarum ZDY04 exhibits a strain-specific property of lowering TMAO via the modulation of gut microbiota in mice. Food Funct. (2018) 9:4299–309. doi: 10.1039/C8FO00349A
161. Lindberg AA, Weintraub A, Zähringer U, Rietschel ET. Structure-activity relationships in lipopolysaccharides of Bacteroides fragilis. Rev Infect Dis. (1990) 12 Suppl 2:S133–141. doi: 10.1093/clinids/12.Supplement_2.S133
162. Weiss J. Bactericidal/permeability-increasing protein (BPI) and lipopolysaccharide-binding protein (LBP): structure, function and regulation in host defence against Gram-negative bacteria. Biochem Soc Trans. (2003) 31:785–90. doi: 10.1042/bst0310785
163. Siebler J, Galle PR, Weber MM. The gut-liver-axis: endotoxemia, inflammation, insulin resistance and NASH. J Hepatol. (2008) 48:1032–4. doi: 10.1016/j.jhep.2008.03.007
164. Wu GD, Compher C, Chen EZ, Smith SA, Shah RD, Bittinger K, et al. Comparative metabolomics in vegans and omnivores reveal constraints on diet-dependent gut microbiota metabolite production. Gut. (2016) 65:63–72. doi: 10.1136/gutjnl-2014-308209
165. Quigley EMM. Microbiota-brain-gut axis and neurodegenerative diseases. Curr Neurol Neurosci Rep. (2017) 17:94. doi: 10.1007/s11910-017-0802-6
166. Zhao Y, Jaber V, Lukiw WJ. Secretory products of the human GI tract microbiome and their potential impact on Alzheimer's disease (AD): detection of lipopolysaccharide (LPS) in AD hippocampus. Front Cell Infect Microbiol. (2017) 7:318. doi: 10.3389/fcimb.2017.00318
167. Lehr HA, Sagban TA, Ihling C, Zähringer U, Hungerer KD, Blumrich M, et al. Immunopathogenesis of atherosclerosis: endotoxin accelerates atherosclerosis in rabbits on hypercholesterolemic diet. Circulation. (2001) 104:914–20. doi: 10.1161/hc3401.093153
168. Klimiec E, Pera J, Chrzanowska-Wasko J, Golenia A, Slowik A, Dziedzic T. Plasma endotoxin activity rises during ischemic stroke and is associated with worse short-term outcome. J Neuroimmunol. (2016) 297:76–80. doi: 10.1016/j.jneuroim.2016.05.006
169. Doll DN, Engler-Chiurazzi EB, Lewis SE, Hu H, Kerr AE, Ren X, et al. Lipopolysaccharide exacerbates infarct size and results in worsened post-stroke behavioral outcomes. Behav Brain Funct. (2015) 11:32. doi: 10.1186/s12993-015-0077-5
170. Engevik MA, Morra CN, Röth D, Engevik K, Spinler JK, Devaraj S, et al. Microbial metabolic capacity for intestinal folate production and modulation of host folate receptors. Front Microbiol. (2019) 10:2305. doi: 10.3389/fmicb.2019.02305
171. Cantorna MT, Snyder L, Arora J. Vitamin A and vitamin D regulate the microbial complexity, barrier function, and the mucosal immune responses to ensure intestinal homeostasis. Crit Rev Biochem Mol Biol. (2019) 54:184–92. doi: 10.1080/10409238.2019.1611734
172. Pham VT, Dold S, Rehman A, Bird JK, Steinert RE. Vitamins, the gut microbiome and gastrointestinal health in humans. Nutr Res. (2021) 95:35–53. doi: 10.1016/j.nutres.2021.09.001
173. Mohammadzadeh Honarvar N, Harirchian MH, Koohdani F, Siassi F, Abdolahi M, Bitarafan S, et al. The effect of vitamin A supplementation on retinoic acid-related orphan receptor γt (RORγt) and interleukin-17 (IL-17) gene expression in Avonex-treated multiple sclerotic patients. J Mol Neurosci. (2013) 51:749–53. doi: 10.1007/s12031-013-0058-9
174. Mottaghi A, Ebrahimof S, Angoorani P, Saboor-Yaraghi AA. Vitamin A supplementation reduces IL-17 and RORc gene expression in atherosclerotic patients. Scand J Immunol. (2014) 80:151–7. doi: 10.1111/sji.12190
175. Spence JD, Bang H, Chambless LE, Stampfer MJ. Vitamin intervention for stroke prevention trial: an efficacy analysis. Stroke. (2005) 36:2404–9. doi: 10.1161/01.STR.0000185929.38534.f3
176. De Queiroz KB, Cavalcante-Silva V, Lopes FL, Rocha GA, D'almeida V, Coimbra RS. Vitamin B(12) is neuroprotective in experimental pneumococcal meningitis through modulation of hippocampal DNA methylation. J Neuroinflammation. (2020) 17:96. doi: 10.1186/s12974-020-01763-y
177. Boran P, Yildirim S, Karakoc-Aydiner E, Ogulur I, Ozen A, Haklar G, et al. Vitamin B12 deficiency among asymptomatic healthy infants: its impact on the immune system. Minerva Pediatr. (2021) 73:59–66. doi: 10.23736/S2724-5276.16.04274-X
Keywords: gut microbiota, microbial metabolites, ischemic stroke, oxidative stress, inflammation, apoptosis
Citation: Long J, Wang J, Li Y and Chen S (2022) Gut microbiota in ischemic stroke: Where we stand and challenges ahead. Front. Nutr. 9:1008514. doi: 10.3389/fnut.2022.1008514
Received: 31 July 2022; Accepted: 07 November 2022;
Published: 01 December 2022.
Edited by:
Hui Han, Chinese Academy of Sciences (CAS), ChinaReviewed by:
Guangtian Cao, China Jiliang University, ChinaKai Wang, Chinese Academy of Agricultural Sciences (CAAS), China
Copyright © 2022 Long, Wang, Li and Chen. This is an open-access article distributed under the terms of the Creative Commons Attribution License (CC BY). The use, distribution or reproduction in other forums is permitted, provided the original author(s) and the copyright owner(s) are credited and that the original publication in this journal is cited, in accordance with accepted academic practice. No use, distribution or reproduction is permitted which does not comply with these terms.
*Correspondence: Shuai Chen, Y2hlbnNodWFpQG1haWwuY29t
†These authors have contributed equally to this work