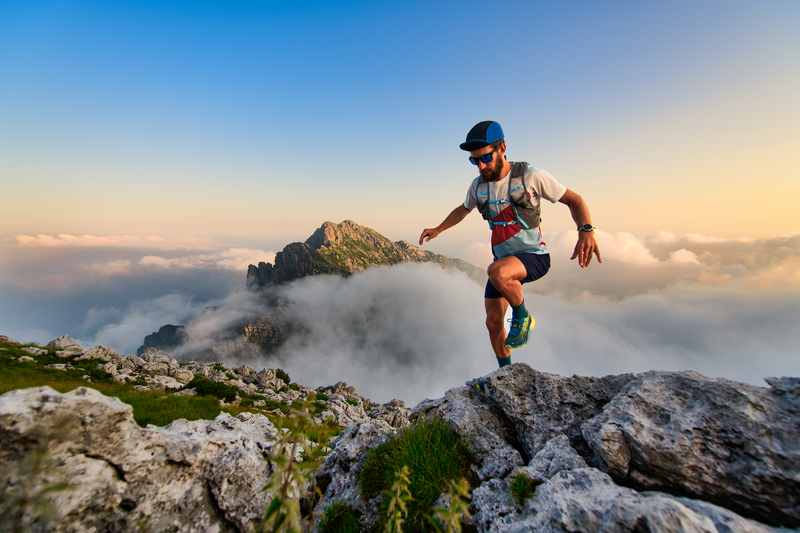
95% of researchers rate our articles as excellent or good
Learn more about the work of our research integrity team to safeguard the quality of each article we publish.
Find out more
REVIEW article
Front. Nutr. , 19 October 2022
Sec. Nutrition and Metabolism
Volume 9 - 2022 | https://doi.org/10.3389/fnut.2022.1002574
This article is part of the Research Topic The 4th NACAN Summit Proceedings: Nutrition and Food for a Healthy Life View all 11 articles
Sphingolipids are common structural components of cell membranes and are crucial for cell functions in physiological and pathophysiological conditions. Sphingomyelin and its metabolites, such as sphingoid bases, ceramide, ceramide-1-phosphate, and sphingosine-1-phosphate, play signaling roles in the regulation of human health. The diverse structures of sphingolipids elicit various functions in cellular membranes and signal transduction, which may affect cell growth, differentiation, apoptosis, and maintain biological activities. As nutrients, dietary sphingomyelin and its metabolites have wide applications in the food and pharmaceutical industry. In this review, we summarized the distribution, classifications, structures, digestion, absorption and metabolic pathways of sphingolipids, and discussed the nutritional functioning of sphingomyelin in chronic metabolic diseases. The possible implications of dietary sphingomyelin in the modern food preparations including dairy products and infant formula, skin improvement, delivery system and oil organogels are also evaluated. The production of endogenous sphingomyelin is linked to pathological changes in obesity, diabetes, and atherosclerosis. However, dietary supplementations of sphingomyelin and its metabolites have been shown to maintain cholesterol homeostasis and lipid metabolism, and to prevent or treat these diseases. This seemly paradoxical phenomenon shows that dietary sphingomyelin and its metabolites are candidates for food additives and functional food development for the prevention and treatment of chronic metabolic diseases in humans.
Phospholipids act as the main component of biological membranes, emulsifier and surfactant. There are two types of phospholipids. The first one is glycerophospholipids with a glycerol backbone such as phosphatidylcholine, phosphatidylethanolamine, phosphatidylserine, phosphatidylglycerol, phosphatidylinositol, cardiolipin, plasmalogen, phosphatidic acid and platelet activating factor (1). The other one is sphingolipids with sphingosine, the second largest group of membrane lipids. Sphingolipids such as ceramides, sphingomyelin, and glycosphingolipids have a polar head group and two non-polar tails (a long hydrocarbon fatty acyl group and a sphingoid base backbone) (2). Glycerophospholipids are important amphiphilic substances due to the presence of a hydrophilic head composed of substituent groups linked by phosphoric acid and a hydrophobic tail composed of fatty acyl groups. Currently, the LIPID MAPS database includes more than 4900 known sphingolipids, which are commonly found in all viruses, prokaryotes and eukaryotes (3). Sphingolipids are mainly found in membranes of cells, where the hydrophobic tail of phospholipids and other membrane lipids such as cholesterol are embedded. Both plasma and organelle membranes contain sphingomyelin, which distributes symmetrically throughout the membrane’s bilayer. Contrarily, sphingolipids that are glycosylated (glycosphingolipids) are often arranged asymmetrically with their saccharide moieties facing the extracellular environment (4). Since the discovery in the 1880s (5), sphingolipids have been located on cell membranes, lipoproteins and other lipid-rich tissue structures, and are considered very important for maintaining membrane integrity, lipid raft formation, cell metabolism and signal transduction, cell growth, differentiation and apoptosis (6, 7). In foods, sphingolipids are present in dairy products, egg, meat product and soybeans, and some fruits and vegetables in small amounts (8).
The de novo synthesis of sphingolipids begins with the formation of ceramides, which is derived from serine and palmitate in a process that consists of condensation, reduction, acylation, and desaturation in the endoplasmic reticulum (ER). Then, ceramides are transported to the Golgi apparatus for the formations of sphingomyelin, ceramide-1-phosphate (C1P), inositol phosphorylceramides and glycosphingolipids (9–11). Sphingomyelin is one of the most important sphingolipids in animal tissues. Sphingomyelin from sources varies in sphingosine [long-chain bases (LCBs)] and fatty acyl groups (12). Sphingomyelin co-exists with phosphatidylcholine on the outer leaflet of the cell membrane. The dietary sphingomyelin is digested by intestinal alkaline SMase (Alk-SMase) and neutral ceramidase (N-CDase), and eventually hydrolyzed to ceramides, phosphorylcholine, sphingosine and fatty acids in the small intestine (3, 13). Unlike sphingomyelin and ceramides, sphingosine can be absorbed intact into intestinal mucosal cells and converted to sphingosine-1-phosphate (S1P), ceramides, sphingomyelin and glycosphingolipids, which are transported with chylomicrons into the lymph circulation and then the blood circulation, and newborn high-density lipoproteins (HDL) into the blood circulation (3, 13–15). The sphingomyelin metabolism and its plasma level are also affected by the types of fatty acids in the diet, vitamin B6, vitamin C, vitamin D, and vitamin K (16–23). The endogenous sphingomyelin is involved in adipose tissue function, obesity, diabetes and atherosclerosis related pathology (24–27), while exogenous dietary sphingomyelin may be beneficial for regulating cholesterol homeostasis and lipid metabolism, and for the prevention and treatment of chronic metabolic diseases (28–32). Due to the nutritional functions of dietary sphingomyelin and its metabolites, it has broad application prospects in food industry.
When Johann Ludwig Wilhelm Thudichum isolated sphingolipids from the nerve tissues in 1880s, he named the molecule “sphingosine” after the Greek mythological creature sphinx, the beast of ancient Egyptian mythology that loved to present many enigmas to the inquirers (5). The LIPID MAPS Lipid Classification System classifies lipids into eight categories, fatty acyls, glycerolipids, glycerophospholipids, sphingolipids, sterol lipids, prenol lipids, saccharolipids, and polyketides. As shown in Table 1, sphingolipids have the most structural diversity and are subdivided into sphingoid bases, ceramides, phosphosphingolipids, phosphonosphingolipids, neutral glycosphingolipids, acidic glycosphingolipids, basic glycosphingolipids, amphoteric glycosphingolipids, arspidenosphingolipids and other sphingolipids (2). Sphingoid bases that include sphingosines, sphinganines, phytosphingosines, sphingoid base homologs and variants (C14, C16, and C17), sphingoid base S1P, lysosphingomyelins and lyso(glyco)sphingolipids, N-methylated sphingoid bases and sphingoid base analogs are the backbone of sphingolipids. A ceramide is generated when a fatty acyl group is linked to the sphingoid bases through an amide-linkage. There are ceramides, dihydroceramides, phytoceramides, acylceramides and its phosphate derivative C1P. Phosphosphingolipids such as sphingomyelins, ceramide phosphoethanolamines and ceramide phosphoinositols, are formed when the head group such as phosphocholine is linked to the ceramide via a phosphodiester linkage. In addition, simple and complex glycosphingolipids can be formed when mono or polysaccharides are attached to ceramide via glycosidic bonds such as in cerebrosides and gangliosides, respectively. Based on the polar parts, glycosphingolipids can be classified into three categories neutral, acidic, alkaline and amphoteric glycosphingolipids.
Studies have identified more than 600 lipid species from 23 lipids classes and more than 2,300 novel features (33). The sphingoid base backbone is synthesized de novo from serine and a fatty acid. After a fatty acyl chain is added to the amine group of sphingoid bases, sphingosines, sphinganine and phytosphingosine are transformed to ceramide, dihydroceramide, and phytoceramide, respectively. The attachment of hydrophilic head groups to the OH-group of C-1 of the ceramide yields phosphosphingolipids, phosphonosphingolipids, glycosphingolipids, and other species, including protein adducts (34). Naturally derived sphingoid bases vary in alkyl chain length and branching, the level of saturation, the number of hydroxyl groups and other characteristics (35–37). The variations of sphingosine bases, fatty acyl and hydrophilic head groups together make them the most diverse and complex lipid class. As shown in Table 1, sphingosine has a double bond at the C-4 position, and sphinganine and phytosphingosine are fully saturated, while phytosphingosine has an extra hydroxyl group. The abbreviations “d” and “t” seen in the shorthand nomenclature in Table 1 is to indicate the number of hydroxyl groups followed by the number of carbon atoms in the backbone and the number of double bonds in the fatty acyl group, while “d” is for the two (di-) hydroxyls group and “t” (tri-) for the additional hydroxyl group (38). So, sphingosine, sphinganine and phytosphingosine are designated as d18:1, d18:0, and t18:0, respectively. In addition, sphingoid base also includes the 1-phosphates, lysosphingolipids and N-methyl derivatives. The fatty acyl groups of ceramides are typically saturated or monounsaturated with various chain length from 14 to 36 carbons, while ceramides with C16, C18, and C24 are the most common ones in mammalian cells (2, 39, 40). When head groups (phosphate, phosphocholine or carbohydrate) are attached to ceramides at the C1-hydroxyl position, more complex sphingolipids such as C1P, phosphosphingolipids or glycosphingolipids are created (39). N-acylation of sphingosine creates dihydroceramides with C14 to C26 or in rare cases even up to C36 fatty acyl groups. The saturated or monounsaturated fatty acyl groups can be modified with an α and one additional hydroxylation. Sphingomyelin is composed of a sphingosine, a fatty acyl group and a phosphorylcholine head group (41). Its overall structure is roughly equivalent to replacing glycerol and a fatty acyl group in glycerophospholipid with sphingosine.
Glycosphingolipids can be divided into four sub-classes according to the types of carbohydrates and additional substituents, including neutral glycosphingolipids, acidic glycosphingolipids, basic glycosphingolipids and amphoteric glycosphingolipids (2). Neutral glycosphingolipids, also known as cerebrosides, contain one or more uncharged sugars, such as glucose (Glc), galactose (Gal) or N-acetylgalactosamine (GalNAc), N-acetylglucosamine (GlcNAc) and fucose. Acidic glycosphingolipids contain ionized groups adjoined to neutral or charged carbohydrates such as sialic acid to form sulfoglycosphingolipids, glucuronosphingolipids and gangliosides. Gangliosides contain negatively charged sialic acids (N-acetylneuraminic acid or N-glycolylneuraminic acid), and are named and classified according to the number of sialic acid residues attached (M/D/T representing gangliosides containing 1, 2, or 3 sialic acids, respectively) and inner sugar moieties such as Glc, Gal or GalNAc connected to ceramides. For example, the subscripts 1, 2, 3, 4 of gangliosides usually can be expressed as: 1 is Gal-GalNAc-Gal-Glc-ceramide, 2 is GalNAc-Gal-Glc-ceramide, 3 is Gal-Glc-ceramide and 4 is Gal-ceramide (42). Basic glycosphingolipids refer only to psychosine or galactosylsphingosine that contains Gal residue, while psychosine sulfate belongs to amphoteric glycosphingolipids. Last, sphingolipids can be covalently attached to proteins to form adducts such as β-hydroxyceramides and inositol-phosphoceramides, which can be found on surface proteins of skin cells and membrane anchor proteins of fungi (43, 44). In cells, ceramides the building blocks of all sphingolipids, can be synthesized de novo from palmitoyl-CoA and serine or sphingosine and a fatty acyl CoA. Sphingomyelin contains phosphate, but not the glycerol backbone that glycerophospholipids have. When a glucosyl or a galactosyl group is attached to the C1 of ceramides, cerebrosides are formed. Galactocerebrosides are used to form sulfatides, whereas glucocerebrosides are used to synthesize gangliosides, globosides, and other related compounds (45).
The contents of sphingolipids in foods vary from low in fruits and some vegetables to high in dairy products, egg and soybeans (8). Table 2 summarizes the distribution and contents of sphingolipids in common foods. Since most sphingolipid research results have been focused on their structures, we could not collect contents of all sphingolipids in food. Based on the reported sphingolipid contents in nmol/g, the estimates in mg/100 g were converted using an average molecular weight for sphingosines of 299 g/mol, sphinganines of 301 g/mol, ceramides of 587 g/mol, sphingomyelin of 751 g/mol, and cerebroside of 779 g/mol. The first three sphingoid bases, sphingosines, sphinganine and phytosphingosine, are usually found in mammals, plants and fungi, and sphingosine (d18:1) is the most abundant of these three in mammalian cells (39, 46). In addition, substantial amounts of C20 and minor amounts of C12 to C22 as well as 1-deoxy and 1-deoxymethyl LCBs can also be found in animal products (47, 48). Phytosphingosines and their derivatives are usually found in plants to form plant sphingolipids, such as phytosphingosine (t18:0) and phytosphingosines 8-enine (t18:1) in potatoes and sweet potatoes, 9-Methyl-4, 8-sphingadienine (d19:2) and 2-Amino-4, 8, 10-octadecatriene-1, 3-diol (d18:3) in rice, mushroom and sea cucumber (49, 50). Many fungi also methylate the alkyl chain at C-9 to produce C19 bases, whereas Saccharomyces cerevisiae only produces sphingosine with predominant d18:0, t18:0, and some d20:0, t20:0 (51). Free sphingosine and LCBs are only present in cells at very low levels. Most of them can be phosphorylated to form S1P and other phosphorylated sphingosine bases.
Ceramides are widely distributed in mammals, especially in the skin (52). Sphingomyelins are high in animal-derived foods, such as aquatic products (2–10% of total phospholipids), meat products (5–10% of total phospholipids), eggs (approximately 1.5% of total phospholipids), and dairy products (approximately 25% of total phospholipids). Bioactive complex lipids are very abundant in milk and can be used as the main raw material for obtaining sphingomyelin and gangliosides (53). Insects mainly contain ceramide phosphate ethanolamine, and fungi have ceramide phosphate inositol and mannose-containing head group, and not found in most plants except legumes and nuts (2, 54). Soybeans are a good source of the plant-derived sphingolipids such as full-fat soy flakes (55). The types of fatty acids in sphingomyelins are different in foods. Taking eggs and milk for example, sphingomyelins derived from eggs have higher percentage of short-chain saturated fatty acids (SFAs) than that from milk (56). Although the content of glycosphingolipids in foods are lower than that of sphingomyelins, they are widely distributed in cereal, legumes and nuts, livestock and poultry meat, aquatic products, egg, milk and dairy products, vegetables, and fruits, as shown in Table 2. The sphingolipids in vegetables and fruits are relatively lower than other foods, mainly containing cerebrosides or glycosyl inositol phosphate ceramides (GIPCs) which can connect complex sugar chains (57, 58). Glucosylceramide-based sphingolipids, which have the basic structure of the tetrasaccharide ceramide series, are the main glycosphingolipids in plants, whereas some mannosylceramide series also exist (59). In invertebrates and vertebrates, different saccharides and their derivatives are added to the first sugar moiety of cerebroside, which is mainly glucose, to form hundreds of gangliosides with different head group structures. In addition, vertebrates also have a series of glycolipids containing galactose (60).
In mammals, about 40 enzymes catalyze de novo synthesis, catabolism, recycling and interconversion of sphingolipids and products derived from them as summarized in Figure 1. The de novo synthesis of sphingolipids begins with the ceramide production. In general, 3-ketodihydrosphinganine is formed after the rate-limiting enzyme serine palmitoyltransferase (SPT) complex condenses palmitoyl-CoA and serine in a reaction that requires pyridoxal phosphate, NADPH, and Mn2+ (61, 62), and then reduced by 3-ketodihydrosphingosine reductase to generate sphinganine (47, 61). The mutations of the genes encoding SPTLC1 and SPTLC2, the key subunits of SPT, induce a permanent shift in the substrate specificity from L-serine to L-alanine and L-glycine, which results in the formations of atypical and neurotoxic metabolites, the 1-deoxy-sphingolipids and 1-deoxymethyl sphingoid bases, respectively, and their acylated ceramides (63). Besides, the recently discovered SPTLC3 prefers myristic acid to palmitic acid (64) and may use stearic acid as a fatty acid substrate (65). SPT can also use alanine and glycine, as well as myristate and stearate, to produce a large amount of sphingosine bases. After that, the sphinganine is acylated by one of six ceramide synthases (CerS1-6) to form dihydroceramide (66), which is desaturated by dihydroceramide desaturase to generate ceramide (67). The other fatty acyl moiety in sphingolipids is long chain fatty acids (LCFAs) with 18–26 carbons, which are formed by a family of elongases extending myristic acid and palmitic acid in the ER (68). These variations in LCBs are combined with different acyl groups by the actions of CerS1-6, which makes ceramides a family of closely related but distinct molecules with different functions owing to variations in LCFA length and hydroxylation and desaturation of the LCB and LCFA components (69).
Figure 1. Intracellular metabolism and transport of sphingolipids in mammalian cells. The de novo synthesis of sphingolipids initiates with the synthesis of ceramide (Cer) through condensation by SPT complex utilizing palmitoyl-CoA and serine, which is followed by reduction, acylation, and desaturation on the cytosolic leaflet of the ER. Fatty acids with 12–16 carbons are extended by a family of elongase enzymes to form long-chain fatty acids (LCFAs, C18-C26) in the ER, which are used in the synthesis of Cer. Cers are transported to the Golgi apparatus through vesicular transport, and non-vesicular transport via the Cer transport protein (CERT). A variety of head groups are added to Cer to form complex sphingolipids in the Golgi apparatus such as sphingomyelin (SM), ceramide-1-phosphate (CIP), and glucosylceramide (GlcCer) by sphingomyelin synthase 1/2 (SMS1/2), ceramide kinase (CerK) and glucosylceramide synthase (GCS), respectively. The GlcCer is then translocated into the lumen of the Golgi apparatus via a membrane-bound transporter and further transformed into cerebrosides and gangliosides by different enzymes. C1P is transferred from the Golgi apparatus to plasma membrane via non-vesicular translocation of C1P transfer protein (CPTP). The SM, cerebrosides and gangliosides are delivered to the plasma membrane via vesicular trafficking, and then SM is metabolized to Cer, sphingosine (So) and sphingosine-1-phosphate (S1P). Cer is deacylated by ceramidase to generate So, and then phosphorylated by sphingosine kinase (SoK) to produce S1P in the lysosome and also in plasma membrane. The S1P lyase cleaves S1P into ethanolamine phosphate and fatty aldehydes, the latter are further metabolized and reduced to fatty acyl-CoA. All synthetic reactions to produce complex sphingolipids from Cer are reversible, such as SM, C1P, and GlcCer are hydrolyzed by sphingomyelinase (SMase), C1P phosphatase and glucosylceramidase, respectively. S1P is dephosphorylate by S1P phosphatase (S1PP) to get So, which is acylated by ceramide synthases (CerS) to form Cers. In the lysosome, SM, cerebrosides and gangliosides are metabolized by each hydrolase to produce Cer, and then degraded by acid ceramidase to generate sphingosine. In addition, Cers can also be acylated at the 1-OH position to form acylceramides. ER, Endoplasmic reticulum; SPT, serine palmitoyltransferase; CerS, ceramide synthase; CERT, ceramide transfer protein; Cer, ceramide; LCFAs, long chain fatty acids; LCBs, long chain bases; CerK, ceramide kinase; C1P, ceramide-1-phosphate; SMS1/2, sphingomyelin synthase; SMases, Sphingomyelinase; CerS, ceramide synthase; So, sphingosine; SoK, sphingosine kinase; S1P, sphingosine-1-phosphate; S1PP, sphingosine-1-phosphate phosphatase; GlcCer, glucosylceramide; GCS, glucosylceramide synthase; FAPP2, phosphatidylinositol-4-phosphate adaptor protein 2.
Ceramides are transported to the Golgi apparatus via vesicular and non-vesicular mechanisms (11). The latter mechanism via a ceramide transport protein (CERT) to assemble into sphingomyelin only exists in mammals (70), as shown in Figure 1. A variety of head groups are added to ceramide to form complex sphingolipids in the Golgi apparatus such as sphingomyelin, CIP, inositol phosphorylceramides and glycosphingolipids. The sphingomyelin synthase 1/2 (SMS1/2) enzymes located in the luminal side of the Golgi membrane transfer a phosphocholine headgroup from phosphatidylcholine to ceramide yielding diacylglycerol and sphingomyelin (71). According to Mitsutake and Igarashi, sphingomyelin synthase SMS2 on the plasma membrane dynamically regulates the activity of lipid microdomains and mice lacking SMS2 are resistant to the effects of a high-fat diet (HFD) on body weight, glucose intolerance, and fatty liver (72). Galactosylceramide (GalCer) and glucosylceramide (GlcCer) are synthesized by ceramide galactosyltransferase (CGT) and glucosylceramide synthase (GCS) transferring the monosaccharides from UDP-galactose and UDP-glucose to ceramide at the cytoplasmic side of the Golgi membrane, respectively (61, 73). The GlcCer is then translocated into the lumen of the Golgi via a membrane-bound transporter and further transformed to lactosylceramide (LacCer), which is synthesized by LacCer synthase transferring galactose from UDP-galactose to GlcCer (41, 74). The LacCer acts as a common backbone to produce more complex glycosphingolipids by various enzymes (41). Phosphatidylinositol-4-phosphate adaptor protein 2 (FAPP2) can transfer GlcCer among Golgi networks and couple it specifically to the synthesis of cerebrosides instead of anionic gangliosides (75). The sphingomyelin, cerebrosides and gangliosides are delivered to the plasma membrane via vesicular trafficking (76). Sphingomyelin and glycosphingolipids are delivered to the plasma membrane via vesicular transport, while C1P is transferred from the Golgi to other compartments such as the plasma membrane via non-vesicular translocation activity of C1P transfer protein (CPTP). On the cell membrane, sphingomyelin can be metabolized to ceramide on the outer leaflet of the membrane, where it is hydrolyzed to sphingosine by ceramidase, and metabolized to S1P by sphingosine kinase (SoK) (77).
Ceramides can be phosphorylated by ceramide kinase (CerK) to generate C1P, which can be dephosphorylated by a C1P phosphatase (78). Ceramides can also be acylated at the 1-OH position to form 1-O-acyl-ceramides (79). In addition, recent studies have shown that the ω-acylation of ceramides forms three-chained ceramides in the skin, thereby regulating epithelial permeability and skin barrier function (80). Ceramides can also be deacylated to generate sphingosine by ceramidase, and then phosphorylated by SoK to produce S1P in the lysosome and on plasma membrane (8). The S1P on the plasma membrane is flipped through the plasma membrane and transported to exocytoplasmic leaflets by the spinster homolog 2 (SPNS2), where it interacts with its receptors (81). The S1P lyase cleaves S1P (or dihydro S1P) into ethanolamine phosphate and fatty aldehydes, the latter are further metabolized and reduced to form acyl-CoA (76). All synthetic reactions to produce complex sphingolipids from ceramides are reversible. For instance, sphingomyelin, C1P and GlcCer are hydrolyzed by sphingomyelinase (SMase), C1P phosphatase and glucosylceramidase, respectively. This pathway may rapidly increase intracellular ceramide levels, while S1P catabolism is irreversible (82). S1P is catalyzed by S1P phosphatase (S1PP) and S1P lyase to dephosphorylate to sphingosine or irreversibly cleave to ethanolamine-1-phosphate and hexadecenal, respectively (83). The sphingolipids may be recirculated through endocytosis and transported from the endosome to the lysosome. In the lysosome, sphingomyelin, cerebrosides and gangliosides are metabolized to ceramides by hydrolases, and the ceramides are then degraded by acid ceramidase to generate sphingosine. Due to its positive charge, sphingosine is able to leave the lysosome and moves among the membranes such as ER membrane to be recycled (84). The sphingolipids have diverse biological activities in a receptor-dependent or -independent manner. Ceramide and sphingosines are mainly involved in cell apoptosis (85, 86), while S1P and C1P are related to cell survival (87), suggesting their antagonistic effects in some cases. Since ceramide and diacylglycerol may have opposite effects on cell proliferation and survival, SMS1/2 probably play an important role in regulating cellular fate. Therefore, the enzymes involved in these metabolic pathways maintain the homeostasis of bioactive sphingolipid metabolites.
Many prospective studies have shown that different dietary patterns affect de novo sphingolipids synthesis, which in turn alters sphingolipid metabolism in human. The feeding of a HFD stimulates de novo synthesis of sphingolipids and alter sphingolipid metabolism in cells and tissues, which is associated systemic insulin resistance and imbalance of lipid accumulation, thereby exacerbating obesity-related diseases. HFD enhances de novo synthesis and turnover of sphingolipids through the salvage pathway, which promotes the synthesis and accumulation of ceramides, more specifically long chain ceramide species in skeletal muscle, plasma and liver tissues (88–90). Intake of SFAs enriched HFD can upregulate genes involved in ceramide synthesis (16), and promote releases of inflammatory cytokines such as tumor necrosis factor-α (TNF-α) and interleukin-1β (IL-1β) by inhibiting protein kinase B (Akt/PKB) (91). In addition, HFD can also increase the levels of sphingomyelin and S1P in the liver, skeletal muscle, adipose tissue, and cardiovascular tissue (89). Plant foods-based Mediterranean diet (92) has been negatively associated with the risks of cardiovascular diseases (CVDs) (93). This is associated with decrease in the plasma ceramide concentration. This may be attributed to the high ratio of monounsaturated fatty acids (MUFAs) to SFAs in the Mediterranean diet, which can inhibit the SFAs-induced accumulation of ceramides (17). In contrast, EPA (C20:5, n-3) and DHA (C22:6, n-3) decrease the expressions of genes involved in ceramide synthesis and ceramide content in the skeletal muscle and liver in rodents (88). Vitamins B6, C, D, and K have been shown to regulate sphingolipids metabolism (18–23). S1P lyase is a vitamin B6-dependent enzyme that can degrade S1P in the last step of sphingolipid metabolism. A genetic disease called SPL dysfunction syndrome (SPLIS) is caused by mutations in SGPL1, which encodes sphingosine phosphorylase (SPL). SPLIS patients exhibit lymphopenia, nephropathy, adrenal insufficiency, and/or neurological deficits. Vitamin B6 supplementation increases S1P abundance and activity levels, and decreases sphingolipids in the SPLIS patients (18). In addition, SPT responsible for 3-ketodihydrosphinganine synthesis is also a vitamin B6-dependent enzyme (19). One study has shown that the treatment with 1.2 mM calcium and 50 μg/mL vitamin C can significantly stimulate the content of ceramide in human keratinocytes (20). There may also be a relationship between the vitamin D intake and endogenous sphingolipids concentration in humans. Vitamin D supplementation can change levels of long-chain ceramides in the circulation and related sphingolipids metabolism, especially the increased levels of ceramide (C18) and dihydroceramide (C18) in subjects with type 2 diabetes (T2DM) (21). γ-tocotrienol, a form of vitamin E, can increase the levels of dihydroceramide and sphinganine but does not affect ceramide and sphingosine in cells (22). Vitamin K can regulate sphingolipids metabolism in the nervous system (23). However, more in vivo studies are needed to understand how vitamins regulate sphingolipid metabolism.
The digestion and utilization of dietary sphingolipids starts in the jejunum section of the small intestine. The typical plasma membrane of mammalian cells contains about 20% cholesterol, 15–20% sphingomyelin, and 5% glycolipids of the total lipids, whereas the small intestinal brush border contains about 10% cholesterol, 5% sphingomyelin and over 30% glycolipids of the total lipids (7, 94). Bouhours and Guignard detected 750 nmol of sphingomyelin, 160 nmol of free ceramide, and 390 nmol of glucosylceramide from 1 mL of isolated rat intestinal epithelial cells (95). The levels of these sphingolipids change with the differentiation of rat epithelial cells and the development of the intestine (96, 97). Humans normally consume 2–8 g/day of phospholipids from the Western diet, accounting for 1–10% of total daily fat intake (98). Among them, sphingomyelin is about 0.3–0.4 g/day, which is mainly derived from fish, meat, milk and egg products. After digestion, about 3–6 g/day of phospholipids enter the blood through lipoproteins, of which sphingomyelin accounts for about 7%. In addition, sphingomyelin in human bile accounts for about 2% of total phospholipids, and about 0.1–0.2 g/day mainly palmitoyl and stearoyl species is delivered to the intestines (14).
Figure 2 shows the digestion and absorption of dietary sphingomyelin. After the dietary lipids are digested in gastrointestinal tract, triglyceride (TG) is hydrolyzed into free fatty acids, n-2 monoglycerides and a small amount of diacylglycerides by pancreatic lipase. Cholesterol esters are hydrolyzed by cholesterol esterase to form free cholesterol and fatty acids. Glycerophospholipids are cleaved by pancreatic phospholipase at n-1 or n-2 position to produce lysophospholipids and free fatty acids. Sphingomyelin is hydrolyzed to ceramide and phosphorylcholine by Alk-SMase in the intestinal mucosa under the optimal pH of 9.0 (99). Alk-SMase is produced by the liver and released into the jejunum vie bile, and is activated in the presence of bile salts and trypsin (3). Alk-SMase, with the hundred times of hydrolysis capacity higher than that of neutral SMase and acidic SMase, is strictly bile salt dependent and effectively stimulated by taurocholate and taurochenodeoxycholate (14, 100). Most sphingomyelin and its hydrolysate ceramides could not be absorbed intact and contribute to the chylomicron and plasma sphingomyelin pools (14). Ceramides are further hydrolyzed into sphingosine and fatty acids by intestinal N-CDase and bile salt-stimulated lipase (BSSL), while sphingosine could be absorbed into intestinal mucosal cells and rapidly metabolized in the enterocytes (13). BSSL from pancreatic juice plays a key role in the digestion of TG and hydrolysis of the amide bond of ceramide between sphingosine and fatty acyl group. The activity of BSSL depends on bile salts with the optimal pH 8.5. It is worth noting that there is almost no ceramide formation in the proximal intestine where BSSL is most active (101, 102). All the digested products and bile acid (BA) can be assembled into micelles, which assist in crossing the unstirred water layer for entering the enterocytes.
Figure 2. Pathways for the digestion and absorption of dietary sphingomyelin. The digestion of dietary sphingomyelin (SM) in the small intestinal lumen begins with the decomposition of SM to ceramide by Alk-SMase. Ceramide is further hydrolyzed into sphingosine (So) and fatty acids by N-CDase and BSSL, then the So is absorbed intact into the enterocytes. In the enterocytes, part of So comes from the de novo synthesis of palmitic acid and serine, and part of it comes from intestinal absorption. When the So is absorbed into the enterocytes, only a small amount of free So is transported with chylomicrons to the lymph and blood. Most of the So is catalyzed by sphingosine kinase (SoK) to generate sphingosine-1-phosphate (S1P), and then degraded to ethanolamine phosphate and hexadecenal by S1P lyase, or to a less extend dephosphorylated to new So by S1P phosphatase (S1PP) and then to form ceramides and sphingomyelin. Hexadecenal will be converted to palmitic acid and then esterified into triglycerides (TG), which can be incorporated into chylomicrons for transport. FA, fatty acid; So, sphingosine; S1P, sphingosine-1-phosphate; Cer, ceramide; GlcCer, glucosylceramide; SM, sphingomyelin; SMases, Sphingomyelinase; Alk-SMase, alkaline SMase; N-CDase, neutral ceramidase; BSSL, bile salt-stimulated lipase; SPT, serine palmitoyltransferase; CerS, ceramide synthase; SMS1/2, sphingomyelin synthase 1/2; CerS, ceramide synthase; SoK, sphingosine kinase; S1PP, sphingosine-1-phosphate phosphatase; TG, triglycerides; GCS, glucosylceramide synthase.
When sphingosine is absorbed into the enterocytes, only a small amount of free sphingosine is incorporated into chylomicrons to be transported in the lymph and then, the blood. Most of the sphingosine receives a phosphate to generate S1P in the enterocytes, a process catalyzed by SoK. S1P is mainly degraded to ethanolamine phosphate and hexadecenal by S1P lyase, or to a less extend dephosphorylated to new sphingosine by S1PP and then to form ceramides and sphingomyelin. Fatty acids can be esterified into TG, and loaded with phospholipids and cholesterol esters onto chylomicrons, which enter the blood through lymphatic system (3, 14). Interestingly, the sphingosine first can be dephosphorylated by Sok to form S1P and secondly phosphorylated by S1PP to generate the new sphingosine, then acylated by CerS to form ceramide and more complex sphingolipids such as sphingomyelin and glycosphingolipids, which can be then incorporated into chylomicrons in the enterocytes for the release into the lymph. Dephosphorylation and then phosphorylation are prerequisites for the synthesis of sphingolipids by exogenous sphingosine in the enterocytes (13–15). In addition, dietary glycosphingolipids such as glucosylceramides, cerebrosides and gangliosides are degraded to produce ceramides by a series of enzymes such as glucosidase, galactosidase, glucosylceramidase, galactosylceramidase (3, 41). In the enterocytes, ceramides can also be synthesized from dietary palmitic acid and serine as shown in Figure 1.
Lipoproteins can be divided into chylomicrons, very low-density lipoproteins (VLDL), intermediate density lipoproteins (IDL), and low-density lipoproteins (LDL) and HDL according to their densities. HDL are further categorized into HDL1, HDL2, HDL3 based on their densities. HDL1 only appears after taking a high-cholesterol diet. Abnormal lipoprotein metabolism is associated with arteriosclerosis, diabetes, obesity and tumor occurrence (103). The concentrations of more than 200 sphingolipids in plasma lipoproteins vary. Sphingomyelin, LacCer, hexosylceramide, ceramides, S1P and dhS1P, C1P, sphingosine and dihydrosphingosine, are constituted 87.7, 5.8, 3.4, 2.8, 0.22, 0.15, and 0.005% of total sphingolipids in the human blood, respectively (104). Sphingolipids in lipoproteins can be secreted with apolipoprotein B (apoB) or apoA-I, and can be transferred from one lipoprotein to another. In addition, the complex sphingolipids in the lipoproteins can be modified from other existing sphingolipids, or decomposed into other sphingolipid components, such as ceramides, S1P, and Glc-ceramide (103). The human plasma contains 416 mg/ml sphingomyelin, which accounts for about 20% of total plasma phospholipids (104, 105) and 87.7% of total plasma sphingolipids (104, 105). The C16 and C24:1-sphingomyelin are the major species in human plasma. Approximately 3.15, 32.85, 34.1, and 29.9% plasma sphingomyelin are present in VLDL, LDL, HDL2, and HDL3, respectively. The particle concentrations of these four lipoproteins in human plasma are 0.073, 1.514, 8.35, and 22.1 nmol/ml, and their molecular weights are 10.0 × 106, 2.0 × 106, 4.0 × 105, and 2.0 × 105, and percentages of protein composition are 10, 20, 40, and 55%, respectively (104). However, the amount of sphingomyelin has been significantly reduced in HDL and increased in LDL and VLDL in women with insulin-dependent diabetes (106). Approximately 75% of plasma sphingomyelin is present in LDL and VLDL, and about 25–37% is present in HDL, showing that the decrease of sphingomyelin, phosphatidylcholine and lysophosphatidylcholine may be potentially atherogenic. The reduction of phospholipid content in HDL may weaken its ability to promote the outflow of cholesterol from cells, and inhibit the transfer of cholesterol esters from HDL to larger lipoproteins containing apoB (106). Approximately 8.73, 39.94, 28.74, and 22.59% plasma ceramides are present in VLDL, LDL, HDL2, and HDL3, respectively. The prominent ceramide is C24-ceramide in human plasma. About 1.33, 3.73, 16.3, and 78.64% plasma S1P are present in VLDL, LDL, HDL2, and HDL3, respectively. The ratios of sphingomyelin/ceramides in VLDL, LDL, HDL2, and HDL3 are 22.3:1, 50.9:1, 72.9:1, and 78.9:1,whereas the ratios of ceramides/S1P in those lipoproteins are 49.8:1, 80.7:1, 13.4:1, and 2.2:1, respectively (104). In addition, more than 50 species of complex glycosphingolipids account for about 9–10% of plasma sphingolipids, of which the most abundant ones are GlcCer and LacCer, accounting for about 8–14, 46–60, and 28–44% in VLDL, LDL, and HDL, respectively (103, 104).
Sphingomyelin formed in the enterocytes enters the body via chylomicrons as shown in Figure 3. The absorption of sphingosine leads to the formation of sphingomyelin and ceramides, which are incorporated into pre-chylomicron with TG, free cholesterol, cholesterol esters and other phospholipids under the action of microsomal triglyceride transfer protein (MTP) with apoB-48 in enterocytes. The pre-chylomicron then fuses with the lipid droplets to form chylomicron, which is secreted into the lymph circulation and then the blood circulation. TG in chylomicron is hydrolyzed into free fatty acids by lipoprotein lipase (LPL), which leads to the formation of chylomicron remnants in the circulation (14, 103, 107). A part of sphingomyelin may also associate with apoA-I in the Golgi and be assembled into nascent HDL that is then secreted to the plasma and is responsible for reverse cholesterol transport to move cholesterol from extrahepatic tissues to the liver. New born HDL is mainly synthesized in the liver and can also be synthesized in the small intestine (14). The blood of healthy people mainly contains HDL2 and HDL3. ApoA-I interacts with the ATP binding cassette (ABC) transporters family and accepts sphingomyelin and glycosphingolipids to form pre-β HDL. Then lecithin–cholesterol acyltransferase (LCAT) converts free cholesterol to cholesterol esters in pre-β HDL, which move to the core of the newborn HDL and migrate with the lipoprotein particle in the blood to form mature HDL2 and HDL3 (103). In vitro experiments show that ABCA1, ABCA7, or ABCG1 expressed in HEK293 cells incubated with apoA-I efflux sphingomyelin (108–110). There are few studies on the transport of glycosphingolipids. Unlike sphingomyelin and ceramides, glycosphingolipids cannot be transported to apoB-containing lipoproteins by MTP. Instead, it may be assembled into HDL under the action of two possible transporters ABCA12 and ABCC1. Studies have shown that ABCC1 and ABCA12 are involved in the transport of glucosylceramide in vivo, but it is unclear whether its effluxes glucosylceramide to HDL or apoA1, or plays a role in plasma glycosphingolipid transport or metabolic disorders (103, 111, 112). Therefore, more in vivo evidence is needed to show that these transporters in basal levels play a role in sphingomyelin release, since their overexpression may limit the fluidity of membrane, which is conducive to outflow. As for ceramides, how they are involved in the assembly of HDL is still unknown. Beside in the enterocytes, ABC transporters on the plasma membrane of macrophages, liver or other peripheral tissues can efflux sphingomyelin to HDL, apolipoprotein such as apoA-I, albumin or other receptors (103). Phospholipid transfer protein (PLTP) can maintain particle stability during the wide range of lipoprotein particle modifications in plasma. It can transfer sphingomyelin, ceramides, S1P and TG from VLDL/LDL to HDL (14). However, it is not clear whether PLTP can transfer GluCer, GalCer or other complex sphingolipids. On the plasma membrane, synthesis of sphingomyelin by SMS2 from ceramides can contribute to HDL sphingomyelin, degradation of sphingomyelin by SMase may also increase HDL ceramides (103). In addition, S1P from the plasma membrane of peripheral tissues such as erythrocytes and platelets, can be delivered to HDL with apoM and apoA-I via Spinster2 (Spns2) (103, 113). How other sphingolipids components are incorporated into lipoprotein particles is unclear so far, and more research is needed to evaluate these and other possibilities.
Figure 3. Transport of dietary sphingomyelin from enterocytes to plasma in lipoproteins. Most sphingomyelin (SM) and ceramide (Cer) can be reformed from sphingosine (So) in enterocytes, which will be transferred to the nucleation site with triglyceride (TG), free cholesterol (FC), cholesterol esters (CE) and other phospholipids (PLs) under the action of MTP and incorporated onto apolipoprotein B-48 (apoB-48) to form the original lipoprotein. The original lipoprotein then fuses with the lipid droplets to form chylomicron, which is secreted into the lymph and blood. TG in chylomicrons is hydrolyzed into free fatty acids by lipoprotein lipase (LPL), which leads to the formation of chylomicron residues in the circulation. Apolipoprotein A-I (apoA-I) interacts with the ATP binding cassette (ABC) transporters family and accepts SM and glycosphingolipids to form pre-β HDL. Then lecithin–cholesterol acyltransferase (LCAT) converts FC to CE in pre-β HDL, which moves to the core of the newborn HDL and is secreted into the blood to form mature HDL2 and HDL3. In the plasma membrane, synthesis of SM by SMS2 from Cer can contribute to SM in HDL, and degradation of SM by SMase may also increase Cer in HDL. S1P from the plasma membrane of peripheral tissues such as erythrocytes and platelets, is delivered to HDL with apolipoprotein M (apoM) and apoA-I via Spinster2 (Spns2). In addition, SM, Cer, S1P and TG are transferred from VLDL/LDL to HDL via phospholipid transfer protein (PLTP). FA, fatty acid; So, sphingosine; S1P, sphingosine-1-phosphate; Cer, ceramide; SM, sphingomyelin; PLs, phospholipids; CE, cholesterol esters; FC, free cholesterol; TG, triglyceride; apoB, apolipoprotein B; apoA-I, apolipoprotein A-I; apoM, apolipoprotein M; MTP, microsomal triglyceride transfer protein; LPL, lipoprotein lipase; GLs, glycosphingolipids; LCAT, lecithin–cholesterol acyltransferase; ABC transporters, ATP binding cassette transporters; SMases, Sphingomyelinase; SMS2, sphingomyelin synthase 2; PLTP, phospholipid transfer protein; Spns2, Spinster2.
Metabolic syndrome (MetS) has become a global public health issue. It is the aggregate of pathological conditions in which proteins, fats, carbohydrates and other substances are metabolized abnormally, leading to central and abdominal obesity, insulin resistance, atherogenic dyslipidemia and systemic hypertension (114). Genetic defects of genes involved in metabolism may also lead to MetS. Additionally, dysfunctions of the liver or pancreas might result in MetS (115). Individual therapy of hypertriglyceridemia, hyperglycemia, and hypertension, as well as dietary restriction and frequent exercise, have been suggested as treatments for MetS. The dietary sphingomyelin and its metabolites may play an important role in regulating cholesterol homeostasis and lipid metabolism, and alleviating the symptoms of obesity, diabetes and atherosclerosis as shown in Table 3. Previous studies have shown that dietary sphingomyelin and its metabolites can significantly modify the plasma and hepatic cholesterol and TG metabolism in rats (116, 117) and inhibits the absorption of cholesterol both in Caco-2 cells and animal studies (118–120). Interestingly, sphingomyelin from milk is more effective in inhibiting cholesterol absorption than that from eggs, which may be attributed to the higher degree of saturation and longer chain length of fatty acyl group in milk sphingomyelin (56). Natural phospholipids, especially sphingomyelin, have high affinity for cholesterol to slow the rate of luminal hydrolysis, micellar solubilization, and transfer of micellar lipids to the enterocytes (121). We have found that the ability of egg sphingomyelin to inhibit cholesterol absorption, and simulate cholesterol transport at a higher rate than phosphatidylcholine in the Caco-2 monolayer. This is mediated by downregulation of the Niemann-Pick-Like Protein 1 (NPC1L1) mRNA, which is a key player in cholesterol absorption (122). A randomized crossover study shows for the first time that diets enriched with milk sphingomyelin, increased serum HDL cholesterol levels, but did not affect the serum TG, total cholesterol (TC), non-HDL cholesterol levels, also cholesterol absorption and cholesterol fractional synthesis rate in ten healthy adult males and females (123). The milk sphingomyelin also could regulate intestinal permeability and affect the intestinal tight junction protein expression through increasing the IL-8 secretion in Caco-2/TC7 cells, which may provide a clue to the protective role of obesity or leaky gut diseases (124). Sphingosine treatment significantly reduces cholesterol absorption in Caco-2 and HT-29-D4 cells (125). Due to the differences of sphingoid bases and fatty acid compositions, dietary sphingomyelin can improve lipids absorption and metabolism, probably via micellar solubilization, affinity to the hydrolases, or regulation of key proteins involved in cholesterol absorption.
Table 3. Effects of dietary sphingomyelin and its metabolites on human physiology and chronic metabolic diseases.
Compared with other phospholipids, sphingomyelin has a larger interface area and stronger hydrogen bond formation capability, which is essential for the interactions between sphingomyelin and other lipids in the cell membrane (54). The important intermolecular hydrogen bond is formed by the 2-NH or choline nitrogen of sphingomyelin and the hydroxyl group of cholesterol. The phosphate oxygen and the 3-OH among sphingomyelin molecules may also form intermolecular hydrogen bonds and a network, thereby interfering with the release of cholesterol from these lipid complexes (126). Nilsson et al. (127) found that about 25% of dietary sphingomyelin can reach to the colon, in the form of 10% undegraded sphingomyelin, 30–90% ceramides and 3–6% free sphingosine. Dietary sphingomyelin in the colon may have the potential for reducing the development of aortic root plaque (128), affecting the gut microbiota such as bifidobacterial (129), neutralizing the intestinal inflammation caused by lipopolysaccharide (LPS) and improving in the intestinal barrier function attenuated by Western diet (130). Based on these, we hypothesize that dietary sphingomyelin inhibits the intestinal cholesterol absorption pathways, as shown in Figure 4. (i) Cell-independent effects. The strong hydrogen bonding or interaction between sphingomyelin and cholesterol causes the molecules in the micelles to pack more tightly, thereby slowing down the release of cholesterol in the micelles and inhibiting the absorption and transport of cholesterol in the enterocytes. Sphingomyelin may reduce the solubility of cholesterol in micelles, thereby reducing the thermodynamic movement of free cholesterol that is available to be absorbed by enterocytes. (ii) Cell-dependent effects. Sphingomyelin inhibits cholesterol absorption by reducing the expression of NPC1L1 in the enterocytes. In vitro studies have shown that LCBs such as sphingosine compete with palmitic acid for absorption through an acyl-CoA synthetase-dependent mechanism (54). Acyl-CoA synthetases convert intracellular long-chain fatty acids to their acyl-CoA, which promotes their retention in the cells, and in turn, facilitates their transport. (iii) Intestinal remodeling role. Sphingomyelin may improve the distribution and abundance of gut microbiota, prevent LPS from being transported to the mesenteric lymphatic circulation, or modulate its BA and short-chain fatty acid (SCFA) pathways to inhibit cholesterol absorption and transport.
Figure 4. Hypothesis that dietary sphingomyelin inhibits the intestinal cholesterol absorption pathways. Alk-SMase, alkaline SMase; SREBP-2, sterol regulatory element-binding protein-2; NPC1L1, Niemann-Pick-Like Protein 1; N-CDase, neutral ceramidase; LPS, lipopolysaccharide; BA, bile acid; TGR5, Takeda G protein-coupled receptor 5; FXR, farnesoid X receptor; SCFA, short-chain fatty acids.
Obesity, the excess accumulation of body fat, has become a major public health issue worldwide. Overweight and obesity rates were respectively 34.3 and 16.4% between 2015 and 2019 in adults based on Chinese criteria (131). The sphingomyelin and its metabolites play a role in lipogenesis (132). Endogenous sphingomyelin is involved in adipose tissue functions and obesity-related pathology (24, 25). Exogenous dietary sphingomyelin may be beneficial for obesity prevention and treatment (28, 129, 133, 134). The sphingomyelin purified from chicken skin could significantly decrease hepatic lipid, plasma non-HDL cholesterol and insulin levels via improvement of adiponectin signaling such as adiponectin receptor 2 (Adipor2), peroxisome proliferator activated receptor alpha (Ppara), pyruvate dehydrogenase kinase 4 (Pdk4), and stearoyl CoA desaturase (Scd1) in Zucker rats fed a HFD (133). Dietary supplementation of sphingomyelin from egg yolk lowered the hepatic lipid content in mice fed a HFD, and intestinal cholesterol absorption content via inactivation of the LXR-SREBP-1c pathway, and increased fecal lipid and cholesterol output (28). Furthermore, dietary sphingomyelin from milk and egg yolk attenuated the HFD-induced hepatic steatosis via regulating the lipid absorption and metabolism, and lowered blood LPS via bifidogenic effects and alterations in distal gut microbiota (129, 134). Milk polar lipids containing 25% sphingomyelin may also affect gut physiology and the abundance of metabolically relevant bacteria in mice fed a HFD such as Bifidobacterium spp., Akkermansia muciniphila and Lactobacillus reuteri, which are associated with the fecal excretion of lipids and bile salts (135). In addition, the milk lipid concentrated-butter serum (LC-BS) and its main lipid fractions including ceramides and sphingomyelin can decrease TG and cholesterol levels in the liver and plasma of obese KK-Ay mice (136). These results indicate that dietary supplementation of sphingomyelin and ceramides may prevent fatty liver and hypercholesterolemia, and attenuate obesity.
Insulin resistance in peripheral tissues is one characteristic for the occurrence and development of T2DM. Mitochondrial dysfunctions lead to the accumulation of lipid-derived metabolites such as ceramides, which may increase the risk of diabetes (137). Endogenous ceramides can cause T2DM and its chronic complications through a variety of ways. Therefore, either inhibition of ceramide de novo synthesis to enhance the mitochondrial functions in HFD-fed and db/db mice (26), or enhancement of the ceramide degradation to form S1P is a potential method to reduce ceramide level and attenuate lipotoxicity associated with it (138). For example, promoting ceramide degradation may reduce the synthesis of harmful lipids and promote synthesis of those beneficial ones such as S1P (138). The treatment with exogenous S1P significantly increases non-glucose stimulated insulin secretion in isolated mouse islets and HIT-T 15 cells via the activation of phospholipase C-Ca2+ system (139). The extracellular S1P can also attenuate the cytokine-induced apoptosis in pancreatic β cell through acting on its receptors, suggesting that S1P in the blood has potential protective effects in vivo (140). Milk sphingomyelin supplementation significantly increased fecal lipids, but lowered serum non-HDL cholesterol, hepatic TC and neutral lipids via regulating the mRNA levels of related genes for lipid metabolism in obese KK-Ay mice (29). Recently, treatments with exogenous glucosylceramides and ceramides from sea cucumber improve glucose tolerance and alleviate insulin resistance in high-fructose-diet-fed SD rats via upregulating the IRS/PI3K/Akt signaling pathway (141).
Atherosclerotic dyslipidemia, related to insulin resistance and visceral obesity, are important risk factors for CVDs (142). In the development of atherosclerosis, the endogenous sphingomyelin on the arterial wall can be degraded into ceramide by SMase, while the increase in ceramide content will promote the aggregation of lipoproteins (103). Studies have found that the content of ceramides in atherosclerotic LDL is 10–50 times of the normal level (143), and the endogenous sphingomyelin level in the plasma is correlated with atherosclerosis, which has been implicated as a risk factor for coronary artery disease (27). Therefore, the rate-limiting enzymes for the de novo synthesis of ceramides and sphingomyelin such as SPT andSMS1/2 may be targets for pharmacological intervention to prevent or treat atherosclerosis. The SMS2 and ApoE double knockout (KO) mice have lower sphingomyelin levels in lipoproteins, and contents of sphingomyelin, ceramides, free cholesterol, and cholesteryl ester contents in the brachiocephalic artery (144). Endogenous sphingomyelin synthesis promotes atherosclerosis. However, dietary sphingomyelin appears to prevent atherosclerosis by inhibiting cholesterol absorption (30, 118–120), modifying the plasma and hepatic cholesterol and TG metabolism (116, 117), and influencing lipoprotein formation and mucosal growth in the gut (14). Dietary sphingomyelin from egg yolk does not affect circulating sphingomyelin levels or increase atherosclerosis in apoE–/– mice fed a HFD, but it is anti-atherogenic in apoE–/– mice fed a normal chow diet, which may be related to sphingomyelin-mediated alterations in gut flora, a topic that needs further investigation (30). Dietary sphingomyelin from egg yolk reduced aortic neutral lipid accumulation in aortic root lipid accumulation of apoE–/– mice fed a HFD, without affecting serum or hepatic lipids, as well as hepatic gene expression. Furthermore, there was a modest modulation of gut microbiota in apoE–/– mice fed a HFD supplemented with sphingomyelin from egg yolk. It is worth noting that the mass ratio of sphingomyelin to cholesterol is 1:2 in the diet, which is similar to the ratio in egg yolk, indicating that egg yolk food matrix may have health benefits and/or counteract effects of dietary cholesterol (128).
Dietary sphingomyelin from milk also lowered atherogenic lipoprotein cholesterol, reduced inflammation and attenuated atherosclerosis development in LDLr–/– mice fed a diet supplemented with anhydrous milk fat (31). Milk fat globule membrane (MFGM) comprises a core of TG surrounded by a structural membrane composed of phospholipids, cholesterol, proteins, and glycoproteins (145, 146). While compared with other animal-derived phospholipids containing lower than 5% sphingomyelin or plant-derived phospholipids without sphingomyelin, MFGM extracts have received widespread attention as a potential nutrient, especially their natural phospholipid composition (98, 147). A multi-center double-blind crossover study containing 58 overweight postmenopausal women (ages 56–62) shows that diets enriched with milk phospholipids can improve the cardiometabolic health by decreasing levels of blood lipids associated cardiometabolic risks and cholesterol absorption involving in specific interactions in the small intestine, and increasing cholesterol-ileal efflux and fecal loss of coprostanol without affecting the major bacterial phyla of gut microbiota and fecal SCFAs (32). In addition, diets enriched with milk phospholipids decreased the levels of blood ceramide (C24:1) and sphingomyelin (C16:1, C18:1) species to improve atherosclerosis, and increased total ceramides in feces (148).
Urbanization and the general lack of time have shortened the nursing period despite great attempts to support breastfeeding for newborns. Only 35% of infants between the ages of birth and 6 months are breastfed globally (149). Substantial research has been conducted to study the potential application of MFGM in dairy products and infant formula (150–153). A recent review critically analyzed preclinical and clinical research data involving MFGM and its bioactive components in connection to the formation of gut microbiota, infection resistance, cognitive development, and infant metabolism (151). MFGM is highly organized and made up of a variety of elements, including polar lipids (phospholipids and glycosphingolipids), apolar lipids (cholesterol and cerebrosides) and specialized proteins (mostly glycoproteins) (154). Among them, sphingomyelin accounts for over 25% of milk phospholipids (145, 146). Sphingomyelin is an essential component of the milk phospholipid fraction owing to its high concentration of the MFGM and positive impacts on human health, including brain development in babies and protection of neonates from bacterial infections (155). Interestingly, long-chain SFAs (C16:0, C22:0, C23:0, and C24:0) with high melting points make up around 97% of all MFGM sphingomyelins. Rarely, milk sphingomyelins include C23:0 fatty acids in amounts of 17% or higher (154). This distinguishing feature of milk sphingomyelins points to a distinct, most likely a biological, function much beyond its straightforward structural function in the MFGM. Albi et al. (152) hypothesized that the sphingomyelin in human breast milk is important for regulating gene expressions and forming myelin sheets to assist the neural development. Data of clinical and mechanistic preclinical studies indicate that full-fat dairy diets reduce cardiometabolic risk through boosting gut health, decreasing inflammation, and regulating dyslipidemia. These cardiometabolic advantages at the gut and systemic levels are attributed, at least in part, to milk polar lipids produced from the phospholipid- and sphingolipid-rich MFGM, which is more abundant in full-fat dairy milk (153). Deficiency of choline, a metabolite of sphingomyelin, may contribute to the decreased gain of lean body mass and pulmonary and neurocognitive development of premature newborns despite receiving enough macronutrients and gaining body weight (156). At present, there are 3,939 patented inventions involving applications of sphingomyelin in infant formula milk worldwide.1 As brain development occurs between 0 and 3 years of age, these findings suggest a novel intervention strategy to include sphingomyelin into newborn formulae to create products equivalent to breast feeding. Consequently, more patented inventions relate to infant formulae containing large lipid globules and/or those coated with sphingomyelin such as United States Patent No. 11389403 are developed to support infant growth trajectories or physical development during the first year of life more similar to those infants fed the breast milk (157). The synthetic composition described in United States Patent No. 11297872 includes sphingomyelin, choline, CIP, and other glycosphingolipids, as well as metabolic precursors and metabolites in formula milk. This composition may be used to support or optimize de novo myelination, as well as brain structure, brain connectivity, intellectual potential, cognitive potential, learning potential, and cognitive enjoyment in a formula fed subject (158). The creation of a lipid formulation that resembles the human milk fat composition would be a big step for the baby formula business and would allow to produce high-tech formulas for newborns.
Any significant ultraviolet (UV) exposure sets off an inflammatory reaction in the epidermis, causing the skin to become dry and rough and compromising its barrier function (159). Photoaging, or prolonged exposure to UV radiation, affects both the dermal and epidermal layers of the skin, causing laxity, thickness, wrinkling, and changes in pigmentation (159, 160). Reactive oxygen species (ROS) are produced when skin is exposed to UV light. The oxidative damages due to the ROS production in keratinocytes and fibroblasts are reduced by protective and detoxifying non-enzymatic and enzymatic antioxidant molecules (161). Numerous dietary supplements have been shown to improve skin elasticity and health, including ascorbic acid, tocopherols, carotenoids, and polyphenols, which can be taken orally to prevent skin damages from UV radiation (159, 162, 163). The data of several animal research and clinical trials have shown the benefits of consumption of food-derived sphingomyelin and its’ metabolites on skin health, which include those derived from plants, animals, and marine species (164–171). In comparison to control mice, the supplementation of sphingomyelin (146 mg/kg body weight/day) considerably reduced covalently bound ω-hydroxy ceramides and significantly attenuated an increase in transepidermal water loss (TEWL) (165). Dietary sphingomyelin seems to further improve skin condition even under normal circumstances. Using hairless mice, Haruta-Ono et al. found that dietary milk sphingomyelin markedly decreased TEWL and stratum corneum hydration on epidermal conditions in the sphingomyelin-fed animals (166–168). Dietary soy sauce Lees ceramide, which was more efficient than maize glucosylceramide, also dramatically improved epidermal barrier function and reduced TEWL in normal hairless mice (169). Evidence from clinical trials suggests that regular, long-term orally supplementation with sphingomyelin may improve skin health (170, 171). Oral sphingomyelin supplementation dramatically improved volunteers’ perceptions of their skin’s condition, including the moisture of their face skin and the appearance of wrinkles around their eyes, as well as the hydration of the skin at the heel and the flexibility of the skin under the eye (170). Sphingomyelin, glucosylceramide, and lactosylceramide were the key components of the milk sphingolipid-enriched fraction cream, which helped the skin’s ability to retain water and maintain the barrier function (171). Experimental results suggest that the primary sphingoid bases in mammals, sphingosine and sphinganine from dietary sphingomyelin, may be partially reused in epidermal sphingolipids (168). Although the mechanism underlying skin condition enhancement is still not fully understood, previous studies have postulated some potential explanations. The fact that supplementary sphingomyelin reduced the covalently bound ω-hydroxy ceramides brought on by a single dose of UVB radiation is one reason for the mitigation of skin barrier deficiencies (165, 172). Dietary sphingolipids regulate the expressions of genes related to the development of the stratum corneum, which relates the production of the cornified envelope and tight junction proteins (173, 174). Another potential mechanism for the improvement of the skin barrier caused by dietary sphingomyelin is the reduction of the inflammatory response in the skin. According to Oba et al., in hairless mouse skin exposed to a single dose of UVB, the oral administration of milk sphingomyelin dramatically decreased the mRNA levels of genes linked to acute inflammation, such as thymic stromal lymphopoietin (TSLP), IL-1β, and IL-6 (165). The levels of thymus activation-regulated chemokine, and TSLP mRNA in hairless mice fed a magnesium-deficient diet were also markedly reduced by dietary milk sphingomyelin (172). Due to the functions of sphingomyelin and its metabolite ceramide to promote skin elasticity and health, there are more than 20,000 patents related to an effective ingredient of a skin beautifier and can be further blended with a food or feed to provide protection. Taking the United States Patent No. 20110065670 as an example, it relates to a meal or drink that improves the appearance of the skin as well as a skin beautifier that contains the useful element sphingomyelin and offers beauty benefits such skin moisturizing, skin beautification, wrinkle prevention, and skin roughness prevention (175).
Vesicles, which include smaller vesicles, liposome, and exosomes with a diameter ranging from 30 to 100 nm, are biological entities encased by a membrane comprised of one or more lipid bilayers (176). Over the years, lipid vesicles have been regarded as effective medication delivery and carrier systems for bioactive compounds. Therefore, they are extensively employed in many different applications, including aesthetic, medicinal, therapeutic applications and growing food industry. Hydrophobic compounds can be solubilized inside of an aqueous vesicle since the vesicle is surrounded by a phospholipid bilayer (unilamellar vesicles or liposomes) or several concentric bilayers (multilamellar vesicles) (177). Lipid vesicles made of glycerophospholipids like soya lecithin or milk polar lipids, or model systems like dipalmitoyl-phosphatidylcholine, have been the primary focus of research for their potential use in the food industry (178, 179). Sphingomyelin molecules, the most abundant type of sphingolipid in biological membranes, perform structural functions and preferentially bind with cholesterol to create organized regions termed lipid rafts (126, 180). According to research by Morshed et al., lutein may be solubilized into the bilayers of egg-sphingomyelin vesicles, known as sphingosomes. This work opens up possibilities for the development of functional foods and drinks that are enriched with lutein and that have health benefits (177). The most thoroughly researched antimicrobial lipids are free fatty acids, monoglycerides, cholesteryl ester, sphingolipids, and others. Nano-sized lipid-based carriers may provide a tool of drug delivery for these antimicrobial agents, as they increase lipid solubility and dispersion in aqueous formulations. Additionally, nanocarriers may overcome drug resistance. Zhang et al. discussed several types of antimicrobial lipids in nanocarriers for the delivery of their activities. CAL02, a potential infection-controlling liposome composed of cholesterol and sphingomyelin, has been discussed as a novel anti-infection strategy, indicating that the antibacterial functions of antimicrobial lipids are worth to be investigated further (181). Sphingomyelin nanosystems are the promising form of carriers with potential for the combination of various types of medications (182–184). Bouzo et al. (182) encapsulated the anti-cancer drug etoposide in the sphingomyelin nanosystems, which mediated an antiproliferative response by interacting with colorectal cancer cells expressing the guanylyl cyclase receptor. Nagachinta et al. (183) designed sphingomyelin-based biocompatible nanosystems for intracellular delivery of microRNAs to colorectal cancer cells. Jatal et al. (184) revealed for the first time the possibility of combining sphingomyelin nanosystems with the 4N1Ks peptide for the development of novel senolytic cancer treatments. Therefore, sphingomyelin-based vesicles can be used to develop combination therapies. In addition, some inventions relate to the controlled delivery of different pharmaceutical agents (185–187). WIPO Patent No. WO/2001/041732 relates to compositions including sphingomyelins and methods for the intranasal delivery of active agents to the brain by means of neural pathways (188). WO/2022/047234 relates to devices and methods for the treatment of middle ear and/or inner ear disorders, and applicable to the treatment of other diseases in the human body. This kit includes sphingomyelins, stearyl, palmitoyl, tricosanyl sphingomyelins, ceramides, stearyl, palmitoyl ceramides, glycosphingolipids for delivering a drug formulation to treat diseases (189). The pharmaceutical agent protected by United States Patent No. 9186366 contains sphingomyelin and acts as a sialomucin secretion promoter, an antiallergic agent, an antioxidant, an infection defense agent, a hair growth agent, a therapeutic agent for demyelinating disease, an ant-pigmentation agent, an anti-inflammatory agent, or an agent that improves learning ability (190). As mentioned, sphingomyelin plays a role in cell structure, signaling, differentiation and functions. It is important to conduct more in in vitro and in vivo studies to assess the effectiveness of sphingomyelin vesicles in fortified foods.
In food applications, the demand for plastic fats from plants and animals such as margarine and shortening is on the rise. However, the contents of SFAs and trans fatty acids (TFAs) are relatively high in the plastic fats, which has been linked to a number of negative health effects, including altered lipoprotein profiles and a higher risk of MetS and CVDs (191, 192). This has promoted the research of oil organogels instead of traditional plastic fats in the food industry. The organogelation of liquid vegetable oils has become a hot spot in the research of food-specific oils with the purpose of reducing the content of SFAs and TFAs and increasing the intake of MUFA and polyunsaturated fatty acids (PUFA) in our diets (193). Organogelation of liquid vegetable oil is to fix or confine the liquid vegetable oil in a thermally reversible network to obtain a three-dimensional supramolecular network and liquid oil co-exist structure system, so that it has specific structural and functional properties which is called oil organogels (194). Oil organogels are structural oils constructed by applying less than 5% oleogelators to liquid oil including vegetable waxes, fatty acids, hydroxylated fatty acids, fatty alcohols, fatty acid–fatty alcohol mixtures (stearic acid and stearyl alcohol), fatty esters, monoglycerides, diacylglycerides, phospholipids, ceramides, sorbitan monostearate, phytosterols, sterols (γ-oryzanol and β-sitosterol), tocopherol mixtures, polyphenols mixtures, lecithin–sorbitan tristearate mixtures (193–200). According to Rogers et al. (194), the architecture of mixed ceramide networks can be easily tailored to closely resemble colloidal fat crystal networks, such as translucent ceramide-canola oil gels with 2% synthetically pure ceramide, or opaque enzymatically hydrolyzed egg yolk sphingomyelin-canola oil gels with 7% crude extracts. Rogers et al. found that 2% N-acetyl-D-erythro-sphingosine (C2) and other short-chain ceramides were able to form edible oils like canola oil into self-assembling organogels without the addition of SFAs and TFAs. The viabilities of colon, prostate, ovarian, and leukemia cell lines were reduced by C2 ceramide, but not C18 ceramide (196). Due to the obvious side chain characteristics, the melting of the organogelator can be adjusted according to the application to obtain functional properties. Sphingomyelin-derived ceramides obtained from dairy products, eggs, and soybeans are mostly long-chain ceramides. The high melting temperature of ceramides might be beneficial since they provide thermal stability to systems such as chocolate. However, their application as food-grade oleogelators is limited due to their extremely crystalline fibril structures and high melting points. Guo et al. added phosphatidylcholine to the mixed gel system of ceramides, and optimized the gel performance by changing the molar ratio of the components and the gel formation temperature (197–199). Generally, the effect of multi-component systems of oleogelators is often better than that of a single-component, and the gel performance can be optimized by changing the ratios of the components. In addition, some inventions relate to oleogelators by adding sphingomyelin and its metabolites in foods, beverages, nutraceuticals, pharmaceuticals, pet foods, or animal feeds (201–203). Ceramides have limited applications in food-grade oleogelators due to their crystalline fibril structures and high melting points, but a variety of sphingomyelin and derivatives can be used for the food-grade oleogelators. Future study may be conducted on the functions of monomers and multi-component compounds in oil organogels.
In this review, we summarized the content, structures, digestion, absorption, and metabolism of sphingolipids, and discussed the nutritional functioning of sphingomyelin in chronic metabolic diseases and the possible implications in food industry. Sphingolipids are a diverse category of chemically complicated molecules that are present in the brain, plasma, skin, and various foods including dairy, eggs, and soybeans. There is a need for a more comprehensive assessment of the varieties of sphingolipids in foods since certain diets may include adequate concentrations of atypical or typical sphingolipids that may have beneficial or detrimental impacts on human health. Many metabolites of sphingomyelin and derivatives are candidates for food-grade material, such as sphingoid bases, ceramides, C1P, whose structural formula are shown in Table 1. Sphingomyelin and ceramide are hardly absorbed in the small intestine. They can only be degraded into sphingosine to enter the circulation and be utilized by the body. Meanwhile, sphingoid bases, ceramides and sphingomyelin can also be interconverted, with ceramides being the central molecule. Ceramides can be desaturated to generate dihydroceramide, phosphorylated to form C1P and sphingomyelin, deacylated to generate sphingosine and then phosphorylated to produce S1P. Sphingomyelin is hydrolyzed by SMase to generate phosphorylcholine and ceramides, which in turn can be synthesized to C1P under the action of CerK. Phosphatidylcholine, sphingomyelin, and C1P are very similar in structure, with two long hydrophobic hydrocarbon chains and a polar head group. These characteristics of structure, absorption and metabolism can allow sphingomyelin and its metabolites to have wide applications in the food and pharmaceutical industry.
Clearly, dietary sphingomyelin benefits human health. Dietary sphingomyelin and its metabolites and derivatives have great potentials for clinical application in chronic diseases, cognitive development and intestinal health. Table 3 summarizes the ways by which dietary sphingomyelin may be effective in chronic metabolic diseases, including inhibiting cholesterol absorption, modifying the plasma hepatic cholesterol and TG metabolism, influencing lipoprotein formation and mucosal growth in the gut, improving glucose tolerance and alleviating of insulin resistance, and modulating gut microbiota community. In addition, sphingolipids are prevalent in the brain, where they generate cell-type-specific profiles that vary throughout development, aging, and pathological brain changes. Bioactive metabolic intermediates of gangliosides and sphingomyelin, especially ceramides and S1P, have emerged as crucial players in the preservation of brain health (204). The related papers mentioned in this review provide important references for the design of dietary sphingomyelin in the future.
When compared to other nutrients, dietary sphingolipids have drawn less attention and remain to be fully understood. Since no indication of sphingomyelin deficiency has been observed, it is not considered an essential nutrient. Many hypotheses have been proposed to explain the functional mechanism of sphingomyelin and its metabolites. However, many questions how dietary sphingomyelin and its metabolites influence a variety of intricate cell functions still remain unanswered. Future investigations are needed to determine the fate of the exogenously added sphingomyelin, and differentiate the roles of sphingomyelin itself and its metabolites. Especially, endogenous sphingomyelin is involved in pathological changes in obesity, diabetes and atherosclerosis; however, exogenous dietary sphingomyelin and its metabolites have been shown to maintain cholesterol homeostasis and lipid metabolism, as well as the prevention or treatment of chronic metabolic diseases. This seemingly paradoxical phenomenon needs to be further verified by rigorous clinical trials and its mechanism should be explored in more depth. Understanding of the underlying molecular mechanisms provide novel intervention strategies and target for the prevention and treatment of a variety of human illnesses. In general, the exciting but unexplained phenomenon shows that dietary sphingomyelin and its metabolites are candidates for food additives in food industry or natural medicines for metabolic related disease prevention.
FY searched the publications, summarized the data in tables, drew figures, discussed the literature, and wrote the review. GC modified the review. Both authors read and approved the final version of this review.
We gratefully acknowledge the financial support from the Scientific Research Project of Hubei Provincial Health Commission (WJ2021Q050) and the Scientific Research Project of Hubei Provincial Department of Education (Q20202009).
The authors declare that the research was conducted in the absence of any commercial or financial relationships that could be construed as a potential conflict of interest.
All claims expressed in this article are solely those of the authors and do not necessarily represent those of their affiliated organizations, or those of the publisher, the editors and the reviewers. Any product that may be evaluated in this article, or claim that may be made by its manufacturer, is not guaranteed or endorsed by the publisher.
1. Lagace TA, Ridgway ND. The role of phospholipids in the biological activity and structure of the endoplasmic reticulum. Biochim Biophys Acta. (2013) 1833:2499–510. doi: 10.1016/j.bbamcr.2013.05.018
2. Fahy E, Subramaniam S, Brown HA, Glass CK, Merrill AH Jr, Murphy RC, et al. A comprehensive classification system for lipids. J Lipid Res. (2005) 46:839–61. doi: 10.1194/jlr.E400004-JLR200
3. Li W, Belwal T, Li L, Xu Y, Liu J, Zou L, et al. Sphingolipids in foodstuff: compositions, distribution, digestion, metabolism and health effects - A comprehensive review. Food Res Int. (2021) 147:110566. doi: 10.1016/j.foodres.2021.110566
4. Merrill AH. Chapter 13 - Sphingolipids. In: DE Vance, JE Vance editors. Biochemistry of Lipids, Lipoproteins and Membranes. San Diego, CA: Elsevier (2008). p. 363–97.
5. Nature Chemical Biology. Unlocking the lipid labyrinth. Nat Chem Biol. (2010) 6:471. doi: 10.1038/nchembio.404
6. Duan R-D. Physiological functions and clinical implications of sphingolipids in the gut. J Digest Dis. (2011) 12:60–70. doi: 10.1111/j.1751-2980.2011.00481.x
7. Danielsen EM, Hansen GH. Lipid raft organization and function in brush borders of epithelial cells. Mol Membr Biol. (2006) 23:71–9. doi: 10.1080/09687860500445604
8. Vesper H, Schmelz EM, Nikolova-Karakashian MN, Dillehay DL, Lynch DV, Merrill AH Jr. Sphingolipids in food and the emerging importance of sphingolipids to nutrition. J Nutr. (1999) 129:1239–50. doi: 10.1093/jn/129.7.1239
9. Mandon EC, Ehses I, Rother J, van Echten G, Sandhoff K. Subcellular localization and membrane topology of serine palmitoyltransferase, 3-dehydrosphinganine reductase, and sphinganine N-acyltransferase in mouse liver. J Biol Chem. (1992) 267:11144–8.
10. Michel C, van Echten-Deckert G. Conversion of dihydroceramide to ceramide occurs at the cytosolic face of the endoplasmic reticulum. FEBS Lett. (1997) 416:153–5. doi: 10.1016/S0014-5793(97)01187-3
11. Kajiwara K, Ikeda A, Aguilera-Romero A, Castillon GA, Kagiwada S, Hanada K, et al. Osh proteins regulate COPII-mediated vesicular transport of ceramide from the endoplasmic reticulum in budding yeast. J Cell Sci. (2014) 127(Pt. 2):376–87. doi: 10.1242/jcs.132001
12. Barenholz Y, Gatt S. Chapter 4 Sphingomyelin: metabolism, chemical synthesis, chemical and physical properties. In: Hawthorne JN, Ansell GB editors. New Comprehensive Biochemistry. (Vol. 4), Amsterdam: Elsevier (1982). p. 129–77.
13. Ohlsson L, Hertervig E, Jönsson BA, Duan RD, Nyberg L, Svernlöv R, et al. Sphingolipids in human ileostomy content after meals containing milk sphingomyelin. Am J Clin Nutr. (2010) 91:672–8. doi: 10.3945/ajcn.2009.28311
14. Nilsson A, Duan RD. Absorption and lipoprotein transport of sphingomyelin. J Lipid Res. (2006) 47:154–71. doi: 10.1194/jlr.M500357-JLR200
15. Schmelz EM, Crall KJ, Larocque R, Dillehay DL, Merrill AH Jr. Uptake and metabolism of sphingolipids in isolated intestinal loops of mice. J Nutr. (1994) 124:702–12. doi: 10.1093/jn/124.5.702
16. Chocian G, Chabowski A, żendzian-Piotrowska M, Harasim E, Łukaszuk B, Górski J. High fat diet induces ceramide and sphingomyelin formation in rat’s liver nuclei. Mol Cell Biochem. (2010) 340:125–31. doi: 10.1007/s11010-010-0409-6
17. Wang DD, Toledo E, Hruby A, Rosner BA, Willett WC, Sun Q, et al. Plasma ceramides, mediterranean diet, and incident cardiovascular disease in the PREDIMED trial (prevención con dieta mediterránea). Circulation. (2017) 135:2028–40. doi: 10.1161/circulationaha.116.024261
18. Zhao P, Liu ID, Hodgin JB, Benke PI, Selva J, Torta F, et al. Responsiveness of sphingosine phosphate lyase insufficiency syndrome to vitamin B6 cofactor supplementation. J Inherit Metab Dis. (2020) 43:1131–42. doi: 10.1002/jimd.12238
19. Beattie AE, Clarke DJ, Wadsworth JM, Lowther J, Sin HL, Campopiano DJ. Reconstitution of the pyridoxal 5′-phosphate (PLP) dependent enzyme serine palmitoyltransferase (SPT) with pyridoxal reveals a crucial role for the phosphate during catalysis. Chem Commun. (2013) 49:7058–60. doi: 10.1039/c3cc43001d
20. Kim KP, Shin KO, Park K, Yun HJ, Mann S, Lee YM, et al. Vitamin C stimulates epidermal ceramide production by regulating its metabolic enzymes. Biomol Ther. (2015) 23:525–30. doi: 10.4062/biomolther.2015.044
21. Koch A, Grammatikos G, Trautmann S, Schreiber Y, Thomas D, Bruns F, et al. Vitamin D supplementation enhances C18(dihydro)ceramide levels in type 2 diabetes patients. Int J Mol Sci. (2017) 18:1532. doi: 10.3390/ijms18071532
22. Jiang Q, Rao X, Kim CY, Freiser H, Zhang Q, Jiang Z, et al. Gamma-tocotrienol induces apoptosis and autophagy in prostate cancer cells by increasing intracellular dihydrosphingosine and dihydroceramide. Int J Cancer. (2012) 130:685–93. doi: 10.1002/ijc.26054
23. Fischbeck A, Krüger M, Blaas N, Humpf H-U. Analysis of sphingomyelin in meat based on hydrophilic interaction liquid chromatography coupled to electrospray ionization-tandem mass spectrometry (HILIC-HPLC-ESI-MS/MS). J Agric Food Chem. (2009) 57:9469–74. doi: 10.1021/jf9025376
24. Walls SM Jr., Attle SJ, Brulte GB, Walls ML, Finley KD, Chatfield DA, et al. Identification of sphingolipid metabolites that induce obesity via misregulation of appetite, caloric intake and fat storage in Drosophila. PLoS Genet. (2013) 9:e1003970. doi: 10.1371/journal.pgen.1003970
25. Le Barz M, Boulet MM, Calzada C, Cheillan D, Michalski M-C. Alterations of endogenous sphingolipid metabolism in cardiometabolic diseases: towards novel therapeutic approaches. Biochimie. (2020) 169:133–43. doi: 10.1016/j.biochi.2019.10.003
26. Ussher JR, Koves TR, Cadete VJ, Zhang L, Jaswal JS, Swyrd SJ, et al. Inhibition of de novo ceramide synthesis reverses diet-induced insulin resistance and enhances whole-body oxygen consumption. Diabetes. (2010) 59:2453–64. doi: 10.2337/db09-1293
27. Jiang XC, Paultre F, Pearson TA, Reed RG, Francis CK, Lin M, et al. Plasma sphingomyelin level as a risk factor for coronary artery disease. Arterioscler Thromb Vasc Biol. (2000) 20:2614–8. doi: 10.1161/01.atv.20.12.2614
28. Chung RW, Kamili A, Tandy S, Weir JM, Gaire R, Wong G, et al. Dietary sphingomyelin lowers hepatic lipid levels and inhibits intestinal cholesterol absorption in high-fat-fed mice. PLoS One. (2013) 8:e55949. doi: 10.1371/journal.pone.0055949
29. Yamauchi I, Uemura M, Hosokawa M, Iwashima-Suzuki A, Shiota M, Miyashita K. The dietary effect of milk sphingomyelin on the lipid metabolism of obese/diabetic KK-A(y) mice and wild-type C57BL/6J mice. Food Funct. (2016) 7:3854–67. doi: 10.1039/c6fo00274a
30. Chung RWS, Wang Z, Bursill CA, Wu BJ, Barter PJ, Rye KA. Effect of long-term dietary sphingomyelin supplementation on atherosclerosis in mice. PLoS One. (2017) 12:e0189523. doi: 10.1371/journal.pone.0189523
31. Millar CL, Jiang C, Norris GH, Garcia C, Seibel S, Anto L, et al. Cow’s milk polar lipids reduce atherogenic lipoprotein cholesterol, modulate gut microbiota and attenuate atherosclerosis development in LDL-receptor knockout mice fed a Western-type diet. J Nutr Biochem. (2020) 79:108351. doi: 10.1016/j.jnutbio.2020.108351
32. Vors C, Joumard-Cubizolles L, Lecomte M, Combe E, Ouchchane L, Drai J, et al. Milk polar lipids reduce lipid cardiovascular risk factors in overweight postmenopausal women: towards a gut sphingomyelin-cholesterol interplay. Gut. (2020) 69:487–501. doi: 10.1136/gutjnl-2018-318155
33. Yu D, Rupasinghe TWT, Boughton BA, Natera SHA, Hill CB, Tarazona P, et al. A high-resolution HPLC-QqTOF platform using parallel reaction monitoring for in-depth lipid discovery and rapid profiling. Anal Chim Acta. (2018) 1026:87–100. doi: 10.1016/j.aca.2018.03.062
34. Wang X, Wang Y, Xu J, Xue C. Sphingolipids in food and their critical roles in human health. Crit Rev Food Sci Nutr. (2021) 61:462–91. doi: 10.1080/10408398.2020.1736510
35. Karlsson KA. On the chemistry and occurrence of sphingolipid long-chain bases. Chem Phys Lipids. (1970) 5:6–43. doi: 10.1016/0009-3084(70)90008-3
37. Stewart ME, Downing DT. Free sphingosines of human skin include 6-hydroxysphingosine and unusually long-chain dihydrosphingosines. J Invest Dermatol. (1995) 105:613–8. doi: 10.1111/1523-1747.ep12323736
38. Pruett ST, Bushnev A, Hagedorn K, Adiga M, Haynes CA, Sullards MC, et al. Biodiversity of sphingoid bases (“sphingosines”) and related amino alcohols. J Lipid Res. (2008) 49:1621–39. doi: 10.1194/jlr.R800012-JLR200
39. Merrill AH Jr. Sphingolipid and glycosphingolipid metabolic pathways in the era of sphingolipidomics. Chem Rev. (2011) 111:6387–422. doi: 10.1021/cr2002917
40. Maula T, Al Sazzad MA, Slotte JP. Influence of hydroxylation, chain length, and chain unsaturation on bilayer properties of ceramides. Biophys J. (2015) 109:1639–51. doi: 10.1016/j.bpj.2015.08.040
41. Norris GH, Blesso CN. Dietary and endogenous sphingolipid metabolism in chronic inflammation. Nutrients. (2017) 9:1180. doi: 10.3390/nu9111180
42. Cutillo G, Saariaho AH, Meri S. Physiology of gangliosides and the role of antiganglioside antibodies in human diseases. Cell Mol Immunol. (2020) 17:313–22. doi: 10.1038/s41423-020-0388-9
43. Simenel C, Coddeville B, Delepierre M, Latge JP, Fontaine T. Glycosylinositolphosphoceramides in Aspergillus fumigatus. Glycobiology. (2008) 18:84–96. doi: 10.1093/glycob/cwm122
44. Nemes Z, Marekov LN, Fesus L, Steinert PM. A novel function for transglutaminase 1: attachment of long-chain omega-hydroxyceramides to involucrin by ester bond formation. Proc Natl Acad Sci U.S.A. (1999) 96:8402–7. doi: 10.1073/pnas.96.15.8402
45. Engelking LR. Chapter 59 - Sphingolipids. Third ed. In: LR Engelking editor. Textbook of Veterinary Physiological Chemistry. Boston, MA: Academic Press (2015). p. 378–83. doi: 10.1016/j.jnutbio.2018.02.015
46. Kolter T. A view on sphingolipids and disease. Chem Phys Lipids. (2011) 164:590–606. doi: 10.1016/j.chemphyslip.2011.04.013
47. Han X, Jiang X. A review of lipidomic technologies applicable to sphingolipidomics and their relevant applications. Eur J Lipid Sci Technol. (2009) 111:39–52. doi: 10.1002/ejlt.200800117
48. Penno A, Reilly MM, Houlden H, Laurá M, Rentsch K, Niederkofler V, et al. Hereditary sensory neuropathy type 1 is caused by the accumulation of two neurotoxic sphingolipids. J Biol Chem. (2010) 285:11178–87. doi: 10.1074/jbc.M109.092973
49. Lynch DV, Dunn TM. An introduction to plant sphingolipids and a review of recent advances in understanding their metabolism and function. New Phytol. (2004) 161:677–702. doi: 10.1111/j.1469-8137.2004.00992.x
50. Sugawara T, Aida K, Duan J, Hirata T. Analysis of glucosylceramides from various sources by liquid chromatography-ion trap mass spectrometry. J Oleo Sci. (2010) 59:387–94. doi: 10.5650/jos.59.387
51. Ternes P, Sperling P, Albrecht S, Franke S, Cregg JM, Warnecke D, et al. Identification of fungal sphingolipid C9-methyltransferases by phylogenetic profiling. J Biol Chem. (2006) 281:5582–92. doi: 10.1074/jbc.M512864200
52. Hellgren LI. Occurrence of bioactive sphingolipids in meat and fish products. Eur J Lipid Sci Technol. (2001) 103:661–7. doi: 10.1002/1438-9312(200110)103:103.0.CO;2-8
53. Hellgren LI, Nordby P. Chapter 17 - Bioactive lipids in dairy fat. In: Watson RR, Collier RJ, Preedy VR editors. Dairy in Human Health and Disease Across the Lifespan. Cambridge, MA: Academic Press (2017). p. 233–7.
54. Norris GH, Milard M, Michalski MC, Blesso CN. Protective properties of milk sphingomyelin against dysfunctional lipid metabolism, gut dysbiosis, and inflammation. J Nutr Biochem. (2019) 73:108224. doi: 10.1016/j.jnutbio.2019.108224
55. Ahn EH, Schroeder JJ. Bioactive sphingolipids are constituents of soy and dairy products. J Food Sci. (2002) 67:522–4. doi: 10.1111/j.1365-2621.2002.tb10630.x
56. Blesso CN. Egg phospholipids and cardiovascular health. Nutrients. (2015) 7:2731–47. doi: 10.3390/nu7042731
57. Blaas N, Humpf H-U. Structural profiling and quantitation of glycosyl Inositol phosphoceramides in plants with fourier transform mass spectrometry. J Agric Food Chem. (2013) 61:4257–69. doi: 10.1021/jf4001499
58. Pata MO, Hannun YA, Ng CK. Plant sphingolipids: decoding the enigma of the Sphinx. New Phytol. (2010) 185:611–30. doi: 10.1111/j.1469-8137.2009.03123.x
59. Sperling P, Heinz E. Plant sphingolipids: structural diversity, biosynthesis, first genes and functions. Biochim Biophys Acta. (2003) 1632:1–15. doi: 10.1016/s1388-1981(03)00033-7
60. Hannich JT, Umebayashi K, Riezman H. Distribution and functions of sterols and sphingolipids. Cold Spring Harb Perspect Biol. (2011) 3:a004762. doi: 10.1101/cshperspect.a004762
61. Gault CR, Obeid LM, Hannun YA. An overview of sphingolipid metabolism: from synthesis to breakdown. Adv Exp Med Biol. (2010) 688:1–23. doi: 10.1007/978-1-4419-6741-1_1
62. Ha CE, Bhagavan NV. Chapter 16 - Lipids II: phospholipids, glycosphingolipids, and cholesterol. Third ed. In: Ha CE, Bhagavan NV editors. Essentials of Medical Biochemistry. San Diego, CA: Academic Press (2023). p. 355–83.
63. Bode H, Bourquin F, Suriyanarayanan S, Wei Y, Alecu I, Othman A, et al. HSAN1 mutations in serine palmitoyltransferase reveal a close structure-function-phenotype relationship. Hum Mol Genet. (2016) 25:853–65. doi: 10.1093/hmg/ddv611
64. Hornemann T, Penno A, Rütti MF, Ernst D, Kivrak-Pfiffner F, Rohrer L, et al. The SPTLC3 subunit of serine palmitoyltransferase generates short chain sphingoid bases. J Biol Chem. (2009) 284:26322–30. doi: 10.1074/jbc.M109.023192
65. Harmon JM, Bacikova D, Gable K, Gupta SD, Han G, Sengupta N, et al. Topological and functional characterization of the ssSPTs, small activating subunits of serine palmitoyltransferase. J Biol Chem. (2013) 288:10144–53. doi: 10.1074/jbc.M113.451526
66. Levy M, Futerman AH. Mammalian ceramide synthases. IUBMB Life. (2010) 62:347–56. doi: 10.1002/iub.319
67. Geeraert L, Mannaerts GP, van Veldhoven PP. Conversion of dihydroceramide into ceramide: involvement of a desaturase. Biochem J. (1997) 327 (Pt. 1):125–32. doi: 10.1042/bj3270125
68. Sassa T, Kihara A. Metabolism of very long-chain Fatty acids: genes and pathophysiology. Biomol Ther. (2014) 22:83–92. doi: 10.4062/biomolther.2014.017
69. Cingolani F, Futerman AH, Casas J. Ceramide synthases in biomedical research. Chem Phys Lipids. (2016) 197:25–32. doi: 10.1016/j.chemphyslip.2015.07.026
70. Hanada K, Kumagai K, Yasuda S, Miura Y, Kawano M, Fukasawa M, et al. Molecular machinery for non-vesicular trafficking of ceramide. Nature. (2003) 426:803–9. doi: 10.1038/nature02188
71. Tafesse FG, Ternes P, Holthuis JC. The multigenic sphingomyelin synthase family. J Biol Chem. (2006) 281:29421–5. doi: 10.1074/jbc.R600021200
72. Mitsutake S, Igarashi Y. Chapter Twelve - Sphingolipids in lipid microdomains and obesity. In: G Litwack editor. Vitamins & Hormones. (Vol. 91), Cambridge, MA: Academic Press (2013). p. 271–84.
73. Jeckel D, Karrenbauer A, Burger KN, van Meer G, Wieland F. Glucosylceramide is synthesized at the cytosolic surface of various Golgi subfractions. J Cell Biol. (1992) 117:259–67. doi: 10.1083/jcb.117.2.259
74. Chatterjee S, Castiglione E. UDPgalactose:glucosylceramide beta 1—-4-galactosyltransferase activity in human proximal tubular cells from normal and familial hypercholesterolemic homozygotes. Biochim Biophys Acta. (1987) 923:136–42. doi: 10.1016/0304-4165(87)90136-x
75. D’Angelo G, Polishchuk E, Di Tullio G, Santoro M, Di Campli A, Godi A, et al. Glycosphingolipid synthesis requires FAPP2 transfer of glucosylceramide. Nature. (2007) 449:62–7. doi: 10.1038/nature06097
76. Hannun YA, Obeid LM. Sphingolipids and their metabolism in physiology and disease. Nat Rev Mol Cell Biol. (2018) 19:175–91. doi: 10.1038/nrm.2017.107
77. Bartke N, Hannun YA. Bioactive sphingolipids: metabolism and function. J Lipid Res. (2009) 50(Suppl.):S91–6. doi: 10.1194/jlr.R800080-JLR200
78. Rovina P, Schanzer A, Graf C, Mechtcheriakova D, Jaritz M, Bornancin F. Subcellular localization of ceramide kinase and ceramide kinase-like protein requires interplay of their Pleckstrin Homology domain-containing N-terminal regions together with C-terminal domains. Biochim Biophys Acta. (2009) 1791:1023–30. doi: 10.1016/j.bbalip.2009.05.009
79. Ferreira NS, Engelsby H, Neess D, Kelly SL, Volpert G, Merrill AH, et al. Regulation of very-long acyl chain ceramide synthesis by acyl-CoA-binding protein. J Biol Chem. (2017) 292:7588–97. doi: 10.1074/jbc.M117.785345
80. Grond S, Eichmann TO, Dubrac S, Kolb D, Schmuth M, Fischer J, et al. PNPLA1 deficiency in mice and humans leads to a defect in the synthesis of Omega-O-Acylceramides. J Invest Dermatol. (2017) 137:394–402. doi: 10.1016/j.jid.2016.08.036
81. Nagahashi M, Takabe K, Terracina KP, Soma D, Hirose Y, Kobayashi T, et al. Sphingosine-1-phosphate transporters as targets for cancer therapy. Biomed Res Int. (2014) 2014:651727. doi: 10.1155/2014/651727
82. Kim YM, Park TS, Kim SG. The role of sphingolipids in drug metabolism and transport. Expert Opin Drug Metab Toxicol. (2013) 9:319–31. doi: 10.1517/17425255.2013.748749
83. Johnson KR, Johnson KY, Becker KP, Bielawski J, Mao C, Obeid LM. Role of human sphingosine-1-phosphate phosphatase 1 in the regulation of intra- and extracellular sphingosine-1-phosphate levels and cell viability. J Biol Chem. (2003) 278:34541–7. doi: 10.1074/jbc.M301741200
84. Riboni L, Bassi R, Caminiti A, Prinetti A, Viani P, Tettamanti G. Metabolic fate of exogenous sphingosine in neuroblastoma neuro2A cells. Dose-dependence and biological effects. Ann N Y Acad Sci. (1998) 845:46–56. doi: 10.1111/j.1749-6632.1998.tb09661.x
85. Hetz CA, Hunn M, Rojas P, Torres V, Leyton L, Quest AF. Caspase-dependent initiation of apoptosis and necrosis by the Fas receptor in lymphoid cells: onset of necrosis is associated with delayed ceramide increase. J Cell Sci. (2002) 115(Pt. 23):4671–83. doi: 10.1242/jcs.00153
86. Cuvillier O. Sphingosine in apoptosis signaling. Biochim Biophys Acta. (2002) 1585:153–62. doi: 10.1016/s1388-1981(02)00336-0
87. Cowart LA. Sphingolipids: players in the pathology of metabolic disease. Trends Endocrinol Metab. (2009) 20:34–42. doi: 10.1016/j.tem.2008.09.004
88. Blachnio-Zabielska AU, Chacinska M, Vendelbo MH, Zabielski P. The crucial role of C18-Cer in fat-induced skeletal muscle insulin resistance. Cell Physiol Biochem. (2016) 40:1207–20. doi: 10.1159/000453174
89. Choi S, Snider AJ. Sphingolipids in high fat diet and obesity-related diseases. Mediators Inflamm. (2015) 2015:520618. doi: 10.1155/2015/520618
90. Kahle M, Schäfer A, Seelig A, Schultheiß J, Wu M, Aichler M, et al. High fat diet-induced modifications in membrane lipid and mitochondrial-membrane protein signatures precede the development of hepatic insulin resistance in mice. Mol Metab. (2015) 4:39–50. doi: 10.1016/j.molmet.2014.11.004
91. Gomez-Muñoz A, Presa N, Gomez-Larrauri A, Rivera I-G, Trueba M, Ordoñez M. Control of inflammatory responses by ceramide, sphingosine 1-phosphate and ceramide 1-phosphate. Prog Lipid Res. (2016) 61:51–62. doi: 10.1016/j.plipres.2015.09.002
92. Trichopoulou A. Traditional Mediterranean diet and longevity in the elderly: a review. Public Health Nutr. (2004) 7:943–7. doi: 10.1079/PHN2004558
93. Estruch R, Ros E, Salas-Salvadó J, Covas MI, Corella D, Arós F, et al. Primary prevention of cardiovascular disease with a mediterranean diet supplemented with extra-virgin olive oil or nuts. N Engl J Med. (2018) 378:e34. doi: 10.1056/NEJMoa1800389
94. Jiang XC, Li Z. Sphingolipids and cholesterol. Adv Exp Med Biol. (2022) 1372:1–14. doi: 10.1007/978-981-19-0394-6_1
95. Bouhours JF, Guignard H. Free ceramide, sphingomyelin, and glucosylceramide of isolated rat intestinal cells. J Lipid Res. (1979) 20:879–907.
96. Breimer ME, Hansson GC, Karlsson KA, Leffler H. Studies on differentiating epithelial cells of rat small intestine. Alterations in the lipophilic part of glycosphingolipids during cell migration from crypt villus tip. Biochim Biophys Acta. (1982) 710:415–27. doi: 10.1016/0005-2760(82)90125-4
97. Bouhours JF, Bouhours D, Hansson GC. Developmental changes of glycosphingolipid composition of epithelia of rat digestive tract. Adv Lipid Res. (1993) 26:353–72.
98. Cohn JS, Kamili A, Wat E, Chung RW, Tandy S. Dietary phospholipids and intestinal cholesterol absorption. Nutrients. (2010) 2:116–27. doi: 10.3390/nu2020116
99. Norris GH, Blesso CN. Dietary sphingolipids: potential for management of dyslipidemia and nonalcoholic fatty liver disease. Nutr Rev. (2017) 75:274–85. doi: 10.1093/nutrit/nux004
100. Minamioka H, Imai H. Sphingoid long-chain base composition of glucosylceramides in Fabaceae: a phylogenetic interpretation of Fabeae. J Plant Res. (2009) 122:415. doi: 10.1007/s10265-009-0227-7
101. Nilsson Å, Duan RD, Ohlsson L. Digestion and absorption of milk phospholipids in newborns and adults. Front Nutr. (2021) 8:724006. doi: 10.3389/fnut.2021.724006
102. Nyberg L, Farooqi A, Bläckberg L, Duan RD, Nilsson A, Hernell O. Digestion of ceramide by human milk bile salt-stimulated lipase. J Pediatr Gastroenterol Nutr. (1998) 27:560–7. doi: 10.1097/00005176-199811000-00013
103. Iqbal J, Walsh MT, Hammad SM, Hussain MM. Sphingolipids and lipoproteins in health and metabolic disorders. Trends Endocrinol Metab. (2017) 28:506–18. doi: 10.1016/j.tem.2017.03.005
104. Hammad SM, Pierce JS, Soodavar F, Smith KJ, Al Gadban MM, Rembiesa B, et al. Blood sphingolipidomics in healthy humans: impact of sample collection methodology. J Lipid Res. (2010) 51:3074–87. doi: 10.1194/jlr.D008532
105. Dougherty RM, Galli C, Ferro-Luzzi A, Iacono JM. Lipid and phospholipid fatty acid composition of plasma, red blood cells, and platelets and how they are affected by dietary lipids: a study of normal subjects from Italy, Finland, and the USA. Am J Clin Nutr. (1987) 45:443–55. doi: 10.1093/ajcn/45.2.443
106. Bagdade JD, Subbaiah PV. Abnormal high-density lipoprotein composition in women with insulin-dependent diabetes. J Lab Clin Med. (1989) 113:235–40.
107. Illingworth DR. Lipoprotein metabolism. Am J Kidney Dis. (1993) 22:90–7. doi: 10.1016/s0272-6386(12)70173-7
108. Wang N, Lan D, Gerbod-Giannone M, Linsel-Nitschke P, Jehle AW, Chen W, et al. ATP-binding cassette transporter A7 (ABCA7) binds apolipoprotein A-I and mediates cellular phospholipid but not cholesterol efflux. J Biol Chem. (2003) 278:42906–12. doi: 10.1074/jbc.M307831200
109. Kobayashi A, Takanezawa Y, Hirata T, Shimizu Y, Misasa K, Kioka N, et al. Efflux of sphingomyelin, cholesterol, and phosphatidylcholine by ABCG1. J Lipid Res. (2006) 47:1791–802. doi: 10.1194/jlr.M500546-JLR200
110. Hotta N, Abe-Dohmae S, Taguchi R, Yokoyama S. Preferential incorporation of shorter and less unsaturated acyl phospholipids into high density lipoprotein-like particles in the ABCA1- and ABCA7-mediated biogenesis with apoA-I. Chem Phys Lipids. (2015) 187:1–9. doi: 10.1016/j.chemphyslip.2015.01.005
111. Raggers RJ, van Helvoort A, Evers R, van Meer G. The human multidrug resistance protein MRP1 translocates sphingolipid analogs across the plasma membrane. J Cell Sci. (1999) 112:415–22. doi: 10.1242/jcs.112.3.415
112. Ishibashi Y, Kohyama-Koganeya A, Hirabayashi Y. New insights on glucosylated lipids: metabolism and functions. Biochim Biophys Acta. (2013) 1831:1475–85. doi: 10.1016/j.bbalip.2013.06.001
113. Hammad SM. Blood sphingolipids in homeostasis and pathobiology. Adv Exp Med Biol. (2011) 721:57–66. doi: 10.1007/978-1-4614-0650-1_4
114. McCracken E, Monaghan M, Sreenivasan S. Pathophysiology of the metabolic syndrome. Clin Dermatol. (2018) 36:14–20. doi: 10.1016/j.clindermatol.2017.09.004
115. Zakir F, Mohapatra S, Farooq U, Mirza MA, Iqbal Z. Chapter 1 - Introduction to metabolic disorders. In: Dureja H, Murthy SN, Wich PR, Dua K editors. Drug Delivery Systems for Metabolic Disorders. Cambridge, MA: Academic Press (2022). p. 1–20.
116. Imaizumi K, Tominaga A, Sato M, Sugano M. Effects of dietary sphingolipids on levels of serum and liver lipids in rats. Nutr Res. (1992) 12:543–8. doi: 10.1016/S0271-5317(05)80024-7
117. Kobayashi T, Shimizugawa T, Osakabe T, Watanabe S, Okuyama H. A long-term feeding of sphingolipids affected the levels of plasma cholesterol and hepatic triacylglycerol but not tissue phospholipids and sphingolipids. Nutr Res. (1997) 17:111–4. doi: 10.1016/S0271-5317(96)00237-0
118. Nyberg L, Duan RD, Nilsson A. A mutual inhibitory effect on absorption of sphingomyelin and cholesterol. J Nutr Biochem. (2000) 11:244–9. doi: 10.1016/s0955-2863(00)00069-3
119. Eckhardt ER, Wang DQ, Donovan JM, Carey MC. Dietary sphingomyelin suppresses intestinal cholesterol absorption by decreasing thermodynamic activity of cholesterol monomers. Gastroenterology. (2002) 122:948–56. doi: 10.1053/gast.2002.32539
120. Noh SK, Koo SI. Egg sphingomyelin lowers the lymphatic absorption of cholesterol and alpha-tocopherol in rats. J Nutr. (2003) 133:3571–6. doi: 10.1093/jn/133.11.3571
121. Noh SK, Koo SI. Milk sphingomyelin is more effective than egg sphingomyelin in inhibiting intestinal absorption of cholesterol and fat in rats. J Nutr. (2004) 134:2611–6. doi: 10.1093/jn/134.10.2611
122. Yang F, Chen G, Ma M, Qiu N, Zhu L, Li J. Egg-yolk sphingomyelin and phosphatidylcholine attenuate cholesterol absorption in Caco-2 cells. Lipids. (2018) 53:217–33. doi: 10.1002/lipd.12018
123. Ramprasath VR, Jones PJ, Buckley DD, Woollett LA, Heubi JE. Effect of dietary sphingomyelin on absorption and fractional synthetic rate of cholesterol and serum lipid profile in humans. Lipids Health Dis. (2013) 12:125. doi: 10.1186/1476-511x-12-125
124. Milard M, Penhoat A, Durand A, Buisson C, Loizon E, Meugnier E, et al. Acute effects of milk polar lipids on intestinal tight junction expression: towards an impact of sphingomyelin through the regulation of IL-8 secretion? J Nutr Biochem. (2019) 65:128–38. doi: 10.1016/j.jnutbio.2018.12.007
125. Garmy N, Taïeb N, Yahi N, Fantini J. Interaction of cholesterol with sphingosine: physicochemical characterization and impact on intestinal absorption. J Lipid Res. (2005) 46:36–45. doi: 10.1194/jlr.M400199-JLR200
126. Slotte JP. The importance of hydrogen bonding in sphingomyelin’s membrane interactions with co-lipids. Biochim Biophys Acta. (2016) 1858:304–10. doi: 10.1016/j.bbamem.2015.12.008
127. Nilsson A. Metabolism of sphingomyelin in the intestinal tract of the rat. Biochim Biophys Acta. (1968) 164:575–84. doi: 10.1016/0005-2760(68)90187-2
128. Millar CL, Norris GH, Vitols A, Garcia C, Seibel S, Anto L, et al. Dietary egg sphingomyelin prevents aortic root plaque accumulation in apolipoprotein-E knockout mice. Nutrients. (2019) 11:1124. doi: 10.3390/nu11051124
129. Norris GH, Jiang C, Ryan J, Porter CM, Blesso CN. Milk sphingomyelin improves lipid metabolism and alters gut microbiota in high fat diet-fed mice. J Nutr Biochem. (2016) 30:93–101. doi: 10.1016/j.jnutbio.2015.12.003
130. Norris GH, Porter CM, Jiang C, Blesso CN. Dietary milk sphingomyelin reduces systemic inflammation in diet-Induced obese mice and inhibits LPS activity in macrophages. Beverages. (2017) 3:37.
131. Pan XF, Wang L, Pan A. Epidemiology and determinants of obesity in China. Lancet Diabetes Endocrinol. (2021) 9:373–92. doi: 10.1016/s2213-8587(21)00045-0
132. Lambert JM, Anderson AK, Cowart LA. Sphingolipids in adipose tissue: what’s tipping the scale? Adv Biol Regul. (2018) 70:19–30. doi: 10.1016/j.jbior.2018.10.002
133. Yunoki K, Renaguli M, Kinoshita M, Matsuyama H, Mawatari S, Fujino T, et al. Dietary sphingolipids ameliorate disorders of lipid metabolism in Zucker fatty rats. J Agric Food Chem. (2010) 58:7030–5. doi: 10.1021/jf100722f
134. Norris GH, Porter CM, Jiang C, Millar CL, Blesso CN. Dietary sphingomyelin attenuates hepatic steatosis and adipose tissue inflammation in high-fat-diet-induced obese mice. J Nutr Biochem. (2017) 40:36–43. doi: 10.1016/j.jnutbio.2016.09.017
135. Milard M, Laugerette F, Durand A, Buisson C, Meugnier E, Loizon E, et al. Milk polar lipids in a high-fat diet can prevent body weight gain: modulated abundance of gut bacteria in relation with fecal loss of specific fatty acids. Mol Nutr Food Res. (2019) 63:1801078. doi: 10.1002/mnfr.201801078
136. Watanabe S, Takahashi T, Tanaka L, Haruta Y, Shiota M, Hosokawa M, et al. The effect of milk polar lipids separated from butter serum on the lipid levels in the liver and the plasma of obese-model mouse (KK-Ay). J Funct Foods. (2011) 3:313–20. doi: 10.1016/j.jff.2011.06.002
137. Ross JS, Russo SB, Chavis GC, Cowart LA. Sphingolipid regulators of cellular dysfunction in Type 2 diabetes mellitus: a systems overview. Clin Lipidol. (2014) 9:553–69.
138. Bellini L, Campana M, Mahfouz R, Carlier A, Véret J, Magnan C, et al. Targeting sphingolipid metabolism in the treatment of obesity/type 2 diabetes. Expert Opin Ther Targets. (2015) 19:1037–50. doi: 10.1517/14728222.2015.1028359
139. Shimizu H, Okajima F, Kimura T, Ohtani K-I, Tsuchiya T, Takahashi H, et al. Sphingosine 1-phosphate stimulates insulin secretion in HIT-T 15 cells and mouse islets. Endocrine J. (2000) 47:261–9. doi: 10.1507/endocrj.47.261
140. Laychock SG, Sessanna SM, Lin MH, Mastrandrea LD. Sphingosine 1-phosphate affects cytokine-induced apoptosis in rat pancreatic islet beta-cells. Endocrinology. (2006) 147:4705–12. doi: 10.1210/en.2006-0456
141. Yang JY, Zhang TT, Dong Z, Shi HH, Xu J, Mao XZ, et al. Dietary supplementation with exogenous sea-cucumber-derived ceramides and glucosylceramides alleviates insulin resistance in high-fructose-diet-fed rats by upregulating the IRS/PI3K/Akt signaling pathway. J Agric Food Chem. (2021) 69:9178–87. doi: 10.1021/acs.jafc.0c06831
142. Huang PL. A comprehensive definition for metabolic syndrome. Dis Model Mech. (2009) 2:231–7. doi: 10.1242/dmm.001180
143. Ichi I, Nakahara K, Miyashita Y, Hidaka A, Kutsukake S, Inoue K, et al. Association of ceramides in human plasma with risk factors of atherosclerosis. Lipids. (2006) 41:859–63. doi: 10.1007/s11745-006-5041-6
144. Fan Y, Shi F, Liu J, Dong J, Bui HH, Peake DA, et al. Selective reduction in the sphingomyelin content of atherogenic lipoproteins inhibits their retention in murine aortas and the subsequent development of atherosclerosis. Arterioscler Thromb Vasc Biol. (2010) 30:2114–20. doi: 10.1161/atvbaha.110.213363
145. Bourlieu C, Michalski MC. Structure-function relationship of the milk fat globule. Curr Opin Clin Nutr Metab Care. (2015) 18:118–27. doi: 10.1097/mco.0000000000000138
146. Bourlieu C, Cheillan D, Blot M, Daira P, Trauchessec M, Ruet S, et al. Polar lipid composition of bioactive dairy co-products buttermilk and butterserum: emphasis on sphingolipid and ceramide isoforms. Food Chem. (2018) 240:67–74. doi: 10.1016/j.foodchem.2017.07.091
147. Jiménez-Flores R, Brisson G. The milk fat globule membrane as an ingredient: why, how, when? Dairy Sci Technol. (2008) 88:5–18. doi: 10.1051/dst:2007005
148. Le Barz M, Vors C, Combe E, Joumard-Cubizolles L, Lecomte M, Joffre F, et al. Milk polar lipids favorably alter circulating and intestinal ceramide and sphingomyelin species in postmenopausal women. JCI Insight. (2021) 6:e146161. doi: 10.1172/jci.insight.146161
149. Zhao J, Zhao Y, Du M, Binns CW, Lee AH. Maternal education and breastfeeding practices in China: a systematic review and meta-analysis. Midwifery. (2017) 50:62–71. doi: 10.1016/j.midw.2017.03.011
150. Silva RCD, Colleran HL, Ibrahim SA. Milk fat globule membrane in infant nutrition: a dairy industry perspective. J Dairy Res. (2021) 88:105–16. doi: 10.1017/s0022029921000224
151. Brink LR, Lönnerdal B. Milk fat globule membrane: the role of its various components in infant health and development. J Nutr Biochem. (2020) 85:108465. doi: 10.1016/j.jnutbio.2020.108465
152. Albi E, Arcuri C, Kobayashi T, Tomishige N, Cas MD, Paroni R, et al. Sphingomyelin in human breast milk might be essential for the hippocampus maturation. Front Biosci. (2022) 27:247. doi: 10.31083/j.fbl2708247
153. Bruno RS, Pokala A, Torres-Gonzalez M, Blesso CN. Cardiometabolic health benefits of dairy-milk polar lipids. Nutr Rev. (2021) 79(Suppl. 2):16–35. doi: 10.1093/nutrit/nuab085
154. Dewettinck K, Rombaut R, Thienpont N, Le TT, Messens K, Van Camp J. Nutritional and technological aspects of milk fat globule membrane material. Int Dairy J. (2008) 18:436–57. doi: 10.1016/j.idairyj.2007.10.014
155. Bourlieu C, Bouzerzour K, Ferret-Bernard S, Bourgot CL, Chever S, Ménard O, et al. Infant formula interface and fat source impact on neonatal digestion and gut microbiota. Eur J Lipid Sci Technol. (2015) 117:1500–12. doi: 10.1002/ejlt.201500025
156. Bernhard W, Poets CF, Franz AR. Choline and choline-related nutrients in regular and preterm infant growth. Eur J Nutr. (2019) 58:931–45. doi: 10.1007/s00394-018-1834-7
157. Van Der BEM. Abrahamse-berkeveld M, Acton DS, Stefanie S. Infant Formula with Special Lipid Architecture for Promoting Healthy Growth. United States Patent 11389403. Washington, DC: U.S. Patent and Trademark Office (2022).
158. Schneider Nora HJ, Sean D, Tamas B, Jonathan O. Nutritional Composition and Infant Formula for Promoting De Novo Myelination. United States Patent 11297872. Washington, DC: U.S. Patent and Trademark Office (2022).
159. Morifuji M. The beneficial role of functional food components in mitigating ultraviolet-induced skin damage. Exp Dermatol. (2019) 28(Suppl. 1):28–31. doi: 10.1111/exd.13825
160. Imokawa G. Recent advances in characterizing biological mechanisms underlying UV-induced wrinkles: a pivotal role of fibrobrast-derived elastase. Arch Dermatol Res. (2008) 300(Suppl. 1):S7–20. doi: 10.1007/s00403-007-0798-x
161. Erden Inal M, Kahraman A, Köken T. Beneficial effects of quercetin on oxidative stress induced by ultraviolet A. Clin Exp Dermatol. (2001) 26:536–9. doi: 10.1046/j.1365-2230.2001.00884.x
162. Svobodová A, Psotová J, Walterová D. Natural phenolics in the prevention of UV-induced skin damage. A review. Biomed Pap Med Fac Univ Palacky Olomouc Czech Repub. (2003) 147:137–45.
163. Sugawara T. Sphingolipids as functional food components: benefits in skin improvement and disease prevention. J Agric Food Chem. (2022) 70:9597–609. doi: 10.1021/acs.jafc.2c01731
164. Tessema EN, Gebre-Mariam T, Neubert RHH, Wohlrab J. Potential applications of phyto-derived ceramides in improving epidermal barrier function. Skin Pharmacol Physiol. (2017) 30:115–38. doi: 10.1159/000464337
165. Oba C, Morifuji M, Ichikawa S, Ito K, Kawahata K, Yamaji T, et al. Dietary milk sphingomyelin prevents disruption of skin barrier function in hairless mice after UV-B irradiation. PLoS One. (2015) 10:e0136377. doi: 10.1371/journal.pone.0136377
166. Haruta-Ono Y, Ueno H, Ueda N, Kato K, Yoshioka T. Investigation into the dosage of dietary sphingomyelin concentrate in relation to the improvement of epidermal function in hairless mice. Anim Sci J. (2012) 83:178–83. doi: 10.1111/j.1740-0929.2011.00940.x
167. Haruta Y, Kato K, Yoshioka T. Dietary phospholipid concentrate from bovine milk improves epidermal function in hairless mice. Biosci Biotechnol Biochem. (2008) 72:2151–7. doi: 10.1271/bbb.80212
168. Haruta-Ono Y, Setoguchi S, Ueno HM, Higurashi S, Ueda N, Kato K, et al. Orally administered sphingomyelin in bovine milk is incorporated into skin sphingolipids and is involved in the water-holding capacity of hairless mice. J Dermatol Sci. (2012) 68:56–62. doi: 10.1016/j.jdermsci.2012.07.006
169. Ohta K, Hiraki S, Miyanabe M, Ueki T, Manabe Y, Sugawara T. Dietary ceramide prepared from soy sauce lees improves skin barrier function in hairless mice. J Oleo Sci. (2021) 70:1325–34. doi: 10.5650/jos.ess21128
170. Higurashi S, Haruta-Ono Y, Urazono H, Kobayashi T, Kadooka Y. Improvement of skin condition by oral supplementation with sphingomyelin-containing milk phospholipids in a double-blind, placebo-controlled, randomized trial. J Dairy Sci. (2015) 98:6706–12. doi: 10.3168/jds.2015-9529
171. Lee K, Kim S, Kim A, Suh HJ, Hong KB. Sphingolipid identification and skin barrier recovery capacity of a milk sphingolipid-enriched fraction (MSEF) from buttermilk powder. Int J Cosmet Sci. (2020) 42:270–6. doi: 10.1111/ics.12612
172. Morifuji M, Oba C, Ichikawa S, Ito K, Kawahata K, Asami Y, et al. A novel mechanism for improvement of dry skin by dietary milk phospholipids: effect on epidermal covalently bound ceramides and skin inflammation in hairless mice. J Dermatol Sci. (2015) 78:224–31. doi: 10.1016/j.jdermsci.2015.02.017
173. Ideta R, Sakuta T, Nakano Y, Uchiyama T. Orally administered glucosylceramide improves the skin barrier function by upregulating genes associated with the tight junction and cornified envelope formation. Biosci Biotechnol Biochem. (2011) 75:1516–23. doi: 10.1271/bbb.110215
174. Hasegawa T, Shimada H, Uchiyama T, Ueda O, Nakashima M, Matsuoka Y. Dietary glucosylceramide enhances cornified envelope formation via transglutaminase expression and involucrin production. Lipids. (2011) 46:529–35. doi: 10.1007/s11745-011-3546-0
175. Kato Ken UN, Susumu M, Toshimitsu Y. Skin Beautifie. United States Patent 20110065670. Washington, DC: U.S. Patent and Trademark Office (2011).
176. Morshed A, Karawdeniya BI, Bandara Y, Kim MJ, Dutta P. Mechanical characterization of vesicles and cells: a review. Electrophoresis. (2020) 41:449–70. doi: 10.1002/elps.201900362
177. Lopez C, Mériadec C, David-Briand E, Dupont A, Bizien T, Artzner F, et al. Loading of lutein in egg-sphingomyelin vesicles as lipid carriers: thermotropic phase behaviour, structure of sphingosome membranes and lutein crystals. Food Res Int. (2020) 138(Pt. A):109770. doi: 10.1016/j.foodres.2020.109770
178. Singh H. The milk fat globule membrane—A biophysical system for food applications. Curr Opin Coll Interface Sci. (2006) 11:154–63. doi: 10.1016/j.cocis.2005.11.002
179. Xia F, Hu D, Jin H, Zhao Y, Liang J. Preparation of lutein proliposomes by supercritical anti-solvent technique. Food Hydrocoll. (2012) 26:456–63. doi: 10.1016/j.foodhyd.2010.11.014
180. Lopez C, Madec M-N, Jimenez-Flores R. Lipid rafts in the bovine milk fat globule membrane revealed by the lateral segregation of phospholipids and heterogeneous distribution of glycoproteins. Food Chem. (2010) 120:22–33. doi: 10.1016/j.foodchem.2009.09.065
181. Zhang Q, Wu W, Zhang J, Xia X. Antimicrobial lipids in nano-carriers for antibacterial delivery. J Drug Target. (2020) 28:271–81. doi: 10.1080/1061186x.2019.1681434
182. Bouzo BL, Lores S, Jatal R, Alijas S, Alonso MJ, Conejos-Sánchez I, et al. Sphingomyelin nanosystems loaded with uroguanylin and etoposide for treating metastatic colorectal cancer. Sci Rep. (2021) 11:17213. doi: 10.1038/s41598-021-96578-z
183. Nagachinta S, Bouzo BL, Vazquez-Rios AJ, Lopez R, Fuente M. Sphingomyelin-based nanosystems (SNs) for the development of anticancer miRNA therapeutics. Pharmaceutics. (2020) 12:189. doi: 10.3390/pharmaceutics12020189
184. Jatal R, Mendes Saraiva S, Vázquez-Vázquez C, Lelievre E, Coqueret O, López-López R, et al. Sphingomyelin nanosystems decorated with TSP-1 derived peptide targeting senescent cells. Int J Pharm. (2022) 617:121618. doi: 10.1016/j.ijpharm.2022.121618
185. Fahmy Tarek MSE, Flavell Richard A, Jason P, Alyssa S. Vehicles for Controlled Delivery of Different Pharmaceutical Agents. WIPO Patent WO/2013/155487. Geneva: WIPO (2013).
186. Veldman Robert JVBWJ, Marcel V, Gerben K. Pharmaceutical Formulations Employing Short-Chain Sphingolipids and Their Use. United States Patent 7915227. Washington, DC: U.S. Patent and Trademark Office (2011).
187. Kulkarni VS. Sphingolipid-Containing Cationic Liposomes for Topical Delivery of Bioactive Material. WIPO Patent WO/2001/042424. Geneva: WIPO (2001).
188. Gore SL. Compositions and Methods for Intranasal Delivery of Active Agents to the Brain. WIPO Patent WO/2001/041732. Geneva: WIPO (2001).
189. Pavlichenko Ida AJ, Haritosh P, Michael A, Cathy Z. Drug Combination Kits and Methods of Drug Delivery. WIPO Patent WO/2022/047234. Geneva: WIPO (2022).
190. Kato Ken MS, Leo T, Hiroshi U, Noriko U, Yuko H, Toshimitsu Y. Medicine, Food and Drink or Feed Containing Sphingomyelin. United States Patent 9186366. Washington, DC: U.S. Patent and Trademark Office (2015).
191. Liu AG, Ford NA, Hu FB, Zelman KM, Mozaffarian D, Kris-Etherton PM. A healthy approach to dietary fats: understanding the science and taking action to reduce consumer confusion. Nutr J. (2017) 16:53. doi: 10.1186/s12937-017-0271-4
192. Zhu Y, Bo Y, Liu Y. Dietary total fat, fatty acids intake, and risk of cardiovascular disease: a dose-response meta-analysis of cohort studies. Lipids Health Dis. (2019) 18:91. doi: 10.1186/s12944-019-1035-2
193. Pehlivanoğlu H, Demirci M, Toker OS, Konar N, Karasu S, Sagdic O. Oleogels, a promising structured oil for decreasing saturated fatty acid concentrations: production and food-based applications. Crit Rev Food Sci Nutr. (2018) 58:1330–41. doi: 10.1080/10408398.2016.1256866
194. Rogers MA, Wright AJ, Marangoni AG. Oil organogels: the fat of the future? Soft Matter. (2009) 5:1594–6. doi: 10.1039/B822008P
195. Puscas A, Muresan V, Socaciu C, Muste S. Oleogels in food: a review ofcurrent and potential applications. Foods. (2020) 9:70. doi: 10.3390/foods9010070
196. Rogers MA, Spagnuolo PA, Wang TM, Angka L. A potential bioactive hard-stock fat replacer comprised of a molecular gel. Food Sci Nutr. (2017) 5:579–87. doi: 10.1002/fsn3.433
197. Guo S, Song M, He X, Yang F, Cao Y, Rogers M, et al. Water-induced self-assembly of mixed gelator system (ceramide and lecithin) for edible oil structuring. Food Funct. (2019) 10:3923–33. doi: 10.1039/c9fo00473d
198. Guo S, Song M, Gao X, Dong L, Hou T, Lin X, et al. Assembly pattern of multicomponent supramolecular oleogel composed of ceramide and lecithin in sunflower oil: self-assembly or self-sorting? Food Funct. (2020) 11:7651–60. doi: 10.1039/d0fo00635a
199. Guo S, Lv M, Chen Y, Hou T, Zhang Y, Huang Z, et al. Engineering water-induced ceramide/lecithin oleogels: understanding the influence of water added upon pre- and post-nucleation. Food Funct. (2020) 11:2048–57. doi: 10.1039/c9fo02540e
200. Temkov M, Mure?an V. Tailoring the structure of lipids, oleogels and fat replacers by different approaches for solving the trans-fat issue-a review. Foods. (2021) 10:1376. doi: 10.3390/foods10061376
201. Savin Gabriela CV, Jin-mi J, Raffaele M, Martin L. Oil Gel. United States Patent 8796342. Washington, DC: U.S. Patent and Trademark Office (2014).
202. Thomas D. Water-Based Emulsifier Wax Gels. United States Patent 20040110851. Washington, DC: U.S. Patent and Trademark Office (2004).
203. Thomas D. Emulgator-Wachs-Gele auf Wasserbasis. German Patent DE10255554. München: The German Patent and Trade Mark Office (2004).
204. van Kruining D, Luo Q, van Echten-Deckert G, Mielke MM, Bowman A, Ellis S, et al. Sphingolipids as prognostic biomarkers of neurodegeneration, neuroinflammation, and psychiatric diseases and their emerging role in lipidomic investigation methods. Adv Drug Deliv Rev. (2020) 159:232–44. doi: 10.1016/j.addr.2020.04.009
205. Sugawara T, Miyazawa T. Separation and determination of glycolipids from edible plant sources by high-performance liquid chromatography and evaporative light-scattering detection. Lipids. (1999) 34:1231–7. doi: 10.1007/s11745-999-0476-3
206. Wang L, Wang T, Fehr WR. HPLC quantification of sphingolipids in soybeans with modified palmitate content. J Agric Food Chem. (2006) 54:7422–8. doi: 10.1021/jf061624c
207. Gutierrez E, Wang T, Fehr WR. Quantification of sphingolipids in soybeans. J Am Oil Chem Soc. (2004) 81:737–42. doi: 10.1007/s11746-004-0971-y
208. Gutierrez E, Wang T. Effect of processing on sphingolipid content in soybean products. J Am Oil Chem Soc. (2004) 81:971–7. doi: 10.1007/s11746-004-1009-1
209. Sullards MC, Merrill AH Jr. Analysis of sphingosine 1-phosphate, ceramides, and other bioactive sphingolipids by high-performance liquid chromatography-tandem mass spectrometry. Sci STKE. (2001) 2001:pl1. doi: 10.1126/stke.2001.67.pl1
210. Fang F, Ho C-T, Sang S, Rosen RT. Determination of sphingolipids in nuts and seeds by a single quadrupole liquid chromatography–mass spectrometry method. J Food Lipids. (2005) 12:327–43. doi: 10.1111/j.1745-4522.2005.00028.x
211. Pham PH, Duffy T-L, Dmytrash AL, Lien VW, Thomson AB, Clandinin MT. Estimate of dietary ganglioside intake in a group of healthy Edmontonians based on selected foods. J Food Compos Anal. (2011) 24:1032–7. doi: 10.1016/j.jfca.2011.01.011
212. Fong BY, Ma L, Khor GL, van der Does Y, Rowan A, McJarrow P, et al. Ganglioside composition in beef, chicken, pork, and fish determined using liquid chromatography–high-resolution mass spectrometry. J Agric Food Chem. (2016) 64:6295–305. doi: 10.1021/acs.jafc.6b02200
213. Wang X, Zhang H, Song Y, Cong P, Li Z, Xu J, et al. Comparative lipid profile analysis of four fish species by ultraperformance liquid chromatography coupled with quadrupole time-of-flight mass spectrometry. J Agric Food Chem. (2019) 67:9423–31. doi: 10.1021/acs.jafc.9b03303
214. Li H, Song Y, Zhang H, Wang X, Cong P, Xu J, et al. Comparative lipid profile of four edible shellfishes by UPLC-Triple TOF-MS/MS. Food Chem. (2020) 310:125947. doi: 10.1016/j.foodchem.2019.125947
215. Duan J, Sugawara T, Hirata T. Rapid quantitative analysis of sphingolipids in seafood using HPLC with evaporative light-scattering detection: its application in tissue distribution of sphingolipids in fish. J Oleo Sci. (2010) 59:509–13. doi: 10.5650/jos.59.509
216. Zhao Y-Y, Xiong Y, Curtis JM. Measurement of phospholipids by hydrophilic interaction liquid chromatography coupled to tandem mass spectrometry: the determination of choline containing compounds in foods. J Chromatogr A. (2011) 1218:5470–9. doi: 10.1016/j.chroma.2011.06.025
217. Liu Z, Li C, Pryce J, Rochfort S. Comprehensive characterization of bovine milk lipids: phospholipids, sphingolipids, glycolipids, and ceramides. J Agric Food Chem. (2020) 68:6726–38. doi: 10.1021/acs.jafc.0c01604
Keywords: sphingolipids, sphingomyelin, structure, metabolism, digestion, chronic metabolic diseases, applications
Citation: Yang F and Chen G (2022) The nutritional functions of dietary sphingomyelin and its applications in food. Front. Nutr. 9:1002574. doi: 10.3389/fnut.2022.1002574
Received: 25 July 2022; Accepted: 26 September 2022;
Published: 19 October 2022.
Edited by:
Fanbin Kong, University of Georgia, United StatesReviewed by:
Xuebing Xu, Wilmar (Shanghai) Biotechnology Research & Development Center Co., Ltd., ChinaCopyright © 2022 Yang and Chen. This is an open-access article distributed under the terms of the Creative Commons Attribution License (CC BY). The use, distribution or reproduction in other forums is permitted, provided the original author(s) and the copyright owner(s) are credited and that the original publication in this journal is cited, in accordance with accepted academic practice. No use, distribution or reproduction is permitted which does not comply with these terms.
*Correspondence: Fang Yang, ZmFuZ3k1MjFAaGJ0Y20uZWR1LmNu
Disclaimer: All claims expressed in this article are solely those of the authors and do not necessarily represent those of their affiliated organizations, or those of the publisher, the editors and the reviewers. Any product that may be evaluated in this article or claim that may be made by its manufacturer is not guaranteed or endorsed by the publisher.
Research integrity at Frontiers
Learn more about the work of our research integrity team to safeguard the quality of each article we publish.