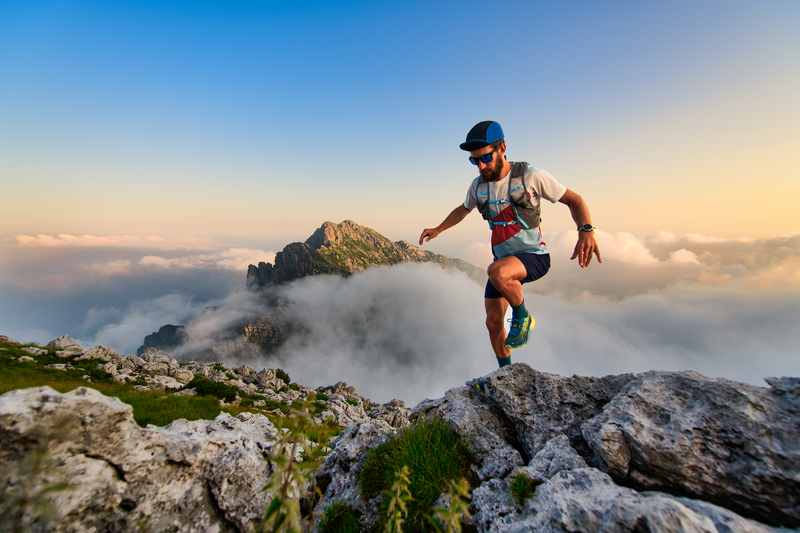
95% of researchers rate our articles as excellent or good
Learn more about the work of our research integrity team to safeguard the quality of each article we publish.
Find out more
ORIGINAL RESEARCH article
Front. Nutr. , 12 October 2022
Sec. Nutrition and Microbes
Volume 9 - 2022 | https://doi.org/10.3389/fnut.2022.1000829
This article is part of the Research Topic Xenobiotics from Diet and Health: Impact on Microbiome View all 5 articles
Objectives: Although xenobiotics derived from food processing may cause modifications in the composition of the gut microbiota (GM) evidence is scarce. The aim of this study is to evaluate the impact of potential dietary carcinogens as heterocyclic amines (HAs), polycyclic aromatic hydrocarbons (PAHs), nitrates, nitrites, nitroso compounds and acrylamide, in combination to fibers (poly)phenols on the GM composition in a group of materially deprived subjects.
Study design: Transversal observational study in a sample of 19 subjects recipients of Red Cross food aid. Dietary information was recorded by means of 3 non-consecutive 24 h recalls. Questions focused on the type of cooking and the extent of cooking and roasting were included. Information on potential carcinogens was mainly obtained from the European Prospective Investigation into Cancer and Nutrition (EPIC) and Computerized Heterocyclic Amines Resource for Research in Epidemiology of Disease (CHARRED) Carcinogen Databases. Microbial composition was determined by 16S ribosomal RNA gene sequencing in fecal samples.
Results: Higher levels of Lachnospiraceae and Eggerthellaceae families were found in individuals consuming less than 50 ng/day of 2-amino-3,8 dimethylimidazo (4,5,f) quinoxaline (MeIQx) (considered as lower risk dose for colorectal adenoma) while those consuming more than 40 ng/day of 2-amino-1-methyl-6-phenylimidazo (4,5,b) pyridine (PhIP) (higher risk for colorectal adenoma) showed lower relative abundance of Muribaculaceae and greater presence of Streptococcaceae and Eubacterium coprostanoligenes group.
Conclusion: The associations identified between diet and processing by-products on GM in this study could be used as potential targets for the designing of dietary interventions tailored to this collective.
Gut microbiota (GM) is the set of microorganisms, including bacteria, archaea, viruses, and some unicellular eukaryotes, which inhabit the digestive tract, the colon being the area most densely populated (1). In adults living in developed countries, the most abundant intestinal microorganisms are bacteria belonging to the phyla Bacteroidota, Bacillota (former Firmicutes), Actinobacteriota, Pseudomonadota (former Proteobacteria), and Verrucomicrobiota in a lesser proportion (2–4). In the last years, there was increasing scientific evidence supporting the critical role of the GM in the maintenance of gut homeostasis and in the prevention of different non-communicable diseases, particularly metabolism-related conditions, and several types of cancers (4). From environmental factors, diet plays a fundamental role in shaping the composition and activity of the GM and, thus, determines the inter-relationship between the gut microbiome and the host (5). In humans, the transition from the ancestral diet to the current westernized pattern, represented by a high presence of fats, sugars, animal proteins and processed foods, has shown to impact on GM composition and activity, by means of the reduction in the abundance of certain microorganisms such as Prevotella or Xylanobacter with capacity to degrade cellular wall components from plants as cellulose and xylan (6) and by a drastic decrease in microbial diversity (6–8). This dietary change may be particularly striking in socially disadvantaged groups where an increase in consumption of processed foods high in fat, sugar or salt has been detected (9), as well as a decrease in the intake of fresh products, such as fruit and vegetables or fish (10). Apart from the nutritional aspects, modern diets have led to an increased intake of processed food. Xenobiotic is a general term used to define “chemical substances that are foreign to animal life” including vegetable derived compounds, drugs or additives (11). Within this broad term, the International Agency of Research in Cancer (IARC) provides a particular category for those substances which exhibit demonstrated cytotoxic and genotoxic character (12). In this sense, heterocyclic amines (HAs) and polycyclic aromatic hydrocarbons (PAHs) are generated during high-temperature cooking by grilling, barbecuing, or frying processes, being their concentration in the food directly proportional to the exposure time and temperature (13, 14). Some authors have proposed that these substances may lead to modifications of the GM composition and functions, with influence in host homeostasis (15, 16), although scientific evidence in this field is still scarce (17). The GM also plays a pivotal role as producer and transformer of xenobiotics to activated derivatives, and/or as attenuator of the toxic action of these compounds by diverse mechanisms (18). Some lactic acid bacteria and other microorganisms can bind or metabolize xenobiotics, contributing either to their sequestration and excretion in feces, or to their transformation into less toxic compounds (19, 20) whereas sometimes the GM can metabolize xenobiotics and transform them into derived molecules with enhanced toxicity (17). Some gastrointestinal microbes can generate toxic compounds themselves, as is the case of the colibactin produced by Escherichia coli group B (21) or enterotoxins formed by Bacteroides fragilis, among others (22). The modification of the toxicity of some dietary xenobiotics can also occur through interactions between the GM and host-detoxification mechanisms. This mainly involves impairment of the activity of hepatic enzymes from the cytochrome P450 complex that participates in the hepatic phase I of detoxification (23) and the reactivation of deactivated glucuronic acid-conjugated compounds during the phase II of detoxification in the liver, by the activity of microbial β-glucuronidases harbored by some intestinal enterobacteria and by members of Clostridium and Bacteroides genera (24). It is also worth mentioning that some members of the GM can metabolize dietary (poly)phenols, promoting the increase of their biological health benefits, as it is the case of the transformation of ellagitannins into urolithins by members of Clostridium leptum group and Bacteroides/Prevotella (25) and the conversion of lignans into enterolignans, process in which can participate some Clostridium, Bacteroides, Peptostreptococcus and Eggerthella strains, among others (26). Dietary fiber can also act as a sequestrating agent of some toxic dietary compounds, decreasing intestinal toxicity (27). Based on this evidence, the main objective of this pilot study was to analyze the associations between diet and GM, with special emphasis on bioactive compounds and xenobiotics derived from food processing, in a group of materially deprived subjects with a diet rich in processed foods.
The MESAS (Economic, Healthy, and Sustainable Menus) pilot project corresponds to an educational and dietary program in deprived human groups addressed to recipients of food aid from the Red Cross of Asturias (North of Spain). The aid received usually consists of basic non-perishable foodstuffs packs. Thus, in order to offer didactic and dietary tools adapted to these individuals and to facilitate the acquisition of fresh food for the achievement of a balanced and healthy diet, the aim of the MESAS project is to identify the main dietary targets in this group. The data presented in this work are relative to the basal status of the sample studied in which twenty adult subjects with non- declared pathologies were randomly selected among those receiving food aid from Alimerka Foundation provided by the non-profit organization Spanish Red Cross. Inclusion criteria were not to be diagnosed with any chronic condition and not to have consumed antibiotics in the last month. The subjects participating in the aid program were randomly recruited and informed of the objectives of the study, being those interested in participating invited to a personal interview in which the purpose of the project and the required involvement were explained. The volunteers who agreed to participate signed an informed consent form. From the population sample, only those for which both dietary and GM information was available were included in the present study (n = 19).
The whole procedure and methodology of this project was approved by the Ethics Committee of the Hospital Universitario Central de Asturias (CEImPA2021.307). The procedures were performed in accordance with the fundamental principles set out in the Declaration of Helsinki, the Oviedo Bioethics Convention, and the Council of Europe Convention on Human Rights and Biomedicine, as well as in Spanish legislation on Bioethics. Directive 95/46/EC of the European Parliament and the Council of October 1995, on the protection of individuals regarding the processing of personal data and on the free movement of such data was strictly followed.
Information regarding the dietary intake of the participants was collected by means of a one unique personalized interview, of no more than 30 min of duration, through three non-consecutive 24 h recalls. At the same interview, participants were scheduled for blood collection and were given the fecal sample collection bottle. Specific questions about cooking habits (boiled, fried, grilled, baked/broiled, or barbecued) and the degree of doneness or toasting in the case of meats, fried potatoes, or toasted bread (undercooked, medium, well-done, very well-done) were included. In order to standardize this point, photographs of the different cooking temperatures, in which the degree of browning increased progressively, were developed specifically for this study. Additionally, complementary questions such as which part of the food was consumed (breast or thigh in the case of chicken) or the possible consumption and/or cooking of the skin (cooking with skin and eating the skin; cooking with skin, but not consuming it; and cooking without skin) were incorporated to improve the quality of the information. The classification of the food into groups was carried out according to the Centre for Higher Education in Nutrition and Dietetics (CESNID) criteria. Food composition tables of CESNID (28) and the United States Department of Agriculture (USDA) (29) were used to transform food consumption into energy and macronutrients intake. The fiber and phenolic content of the foods was extracted from Marlett and Cheung tables (30) and the Phenol Explorer 3.6 (31). For each dietary compound, the five major food sources were identified.
The nutritional analysis of the sample was carried out based on food consumption per individual. Information on HAs, PAHs, nitrates, and nitrites was mainly obtained from the European Prospective Investigation into Cancer and Nutrition (EPIC) Carcinogen Database (32). The EPIC database compiles information from 139 references regarding the content of these compounds per 100 g of food in more than 200 food items. The food composition table is classified according to the preservation method, cooking method, degree of browning, and temperature (32). For those foods or culinary preparations not included in the EPIC database, information was completed with the Computerized Heterocyclic Amines Resource for Research in Epidemiology of Disease (CHARRED) database (33) in the case of HAs and benzo (a) pyrene (B(a)P), and the European Food Safety Authority (EFSA) data in the case of nitrates (34). Acrylamide content was provided by the U.S. Food and Drug Administration (FDA) composition tables (35) and other external reference sources have been used when necessary for acrylamide (36–38), HAs (39), total PAHs (40) and nitrosamines (41–44).
Height (m) and weight (kg) were taken by standardized protocols (45) and Body Mass Index (BMI) was calculated using the formula: weight/(height)2. Subjects were classified as normal weighted (18.5–24.9 kg/m2), overweighted (25.0–29.9 kg/m2), and obese (≥ 30.0 kg/m2), based on the Spanish Society for the Study of Obesity (SEEDO) criteria (46). The percentage of body fat was estimated through bioelectrical impedance in a calibrated TANITA equipment (Tanita Corporation of America, Inc., Arlington Heights, IL, USA). Waist and hip circumferences were measured with an inelastic and extensible tape according to standard criteria (47) and waist-hip ratio was calculated as the ratio of waist circumference over the hip circumference.
Fasting blood samples were drawn by venipuncture and collected in separate tubes for serum and plasma. Samples were kept on ice and centrifuged (1,000 x g, 15 min) within 2–4 h after collection. Plasma and serum aliquots were stored at –20°C until analysis were performed. From blood samples, biochemical analysis of fasting plasma glucose, cholesterol, high- and low-density lipoproteins (HDL and LDL) and triglycerides were determined by using an automated biochemical autoanalyzer in external laboratories.
Fecal samples were collected within ± 24 h of blood collection in sterile containers supplied to each volunteer along with the instructions for sample collection. Samples were frozen within a period no longer than two hours from deposition and stored at −20°C until analysis. At lab, fecal samples were weighted, diluted 1/10 (p/v) in sterile PBS solution and homogenized at full speed (260 rpm) in a LabBlender 400 stomacher (Seward Medical, London, UK) for 3 min. Samples were centrifuged (13 000 rpm, 15 min, 4°C) and then, supernatant, and bacterial pellet were separated. From the pellet obtained, DNA was extracted in accordance with the Q Protocol for DNA extraction defined by the International Human Microbiome Standards Consortium (48) using QIAamp Fast DNA Stool Mini Kit (Qiagen, Sussex, UK). Quantification of extracted/purified DNA and 260/280 ratio was performed using Take3 Micro-Volume plate and Gen5 microplate reader (BioTek Instrument Inc., Winooski, VT, USA). DNA was finally kept frozen at –80°C until analysis.
Variable region V3-V4 of bacterial 16S rRNA genes present in each fecal community was amplified by PCR and the resulting amplicons were sequenced on an Illumina NovaSeq 6000 platform instrument. Following sequencing of the library, the obtained individual sequence reads were filtered to remove low quality sequences. All Illumina quality-approved, trimmed, and filtered data were exported, and the information was integrated in order to generate de novo 16S rRNA Operational Taxonomic Units (OTUs) with ≥97% sequence homology using Uparse software (Uparse v7.0.1090) (49). All reads were classified to the lowest possible taxonomic rank using Quantitative Insights Into Microbial Ecology (QIIME) and a reference dataset from the SILVA 138 database (50). The whole procedure of sequencing and annotation was undergone at Novogene Bioinformatics Technology Co., Ltd.
Results were analyzed using the IBM SPSS software version 25.0 (IBM SPSS, Inc., Chicago, IL, USA). Goodness of fit to the normal distribution was checked by means of the Kolmogorov-Smirnov test. As normality of the variables was not achieved, non-parametric tests were used. Overall, categorical variables were summarized as number and percentage (n (%)) and continuous variables as median and percentiles 25 and 75 (P25 – P75). Spearman correlation and stepwise regression analysis (adjusted for BMI, age and energy intake) were conducted. Heatmaps were generated using ClustVis web tool (51) and logarithmic Linear Discriminant Analysis (LDA) Effect Size (LEfSe) within the Galaxy web application (52). LefSe graphs were created for the xenobiotic compounds, derived from food processing, showing statistically significant results in Spearman correlations and stepwise regression analysis conducted and for which a risk threshold was available in the literature.
A general description of the main general lifestyle, anthropometric and clinical history characteristics of the population under study is shown in Table 1. The volunteers were mostly women under 50 years living in a family unit of 3 or 4 members. Regarding lifestyle and anthropometric characteristics, about half of the sample reported less than 6 hours of sleep per day and a BMI ≥ 30 kg/m2.
A brief description of the food intake in the sample is presented in Table 2. The median energy daily intake is approximately 1,500 kcal, being meat and derivates above the maximum recommended level of 100 g/day. Also, the daily intake of red meat and processed meats were below the upper limits of 100 g/day and 50 g/day recommended, respectively, by the World Cancer Research Fund International (WCRF) (53). No ethanol consumption has been reported in the sample.
The major food sources of xenobiotics, fibers and (poly)phenols in the sample are shown in Table 3. The HA 2-amino-3,8 dimethylimidazol (4,5, f) quinoxaline (MeIQx) is provided by chicken, breast, pork and minced meat. Insoluble dietary fiber derives from white bread, potato, and pasta, among others. In addition, coffee has been identified as one of the major contributors to (poly)phenol and phenolic acids consumption (Table 3).
The GM diversity determined by the Shannon index and richness measured as Observed species were 6.13 and 706, respectively (Figures 1A,B). At the phylum level, Bacillota was the most abundant, followed by Actinobacteriota, Bacteroidota, and Pseudomonadota (Figure 1C). At the family level, the most abundant was Lachnospiraceae, followed by Bifidobacteriaceae, Ruminococcaceae, Prevotellaceae and then Coriobacteriaceae (Figure 1D). GM relative abundances at the phylum level, disaggregated by individual evidenced a global increase of Bacteroidota at the expenses of the decrease of Bacillota from individuals MESAS11 to MESAS19, (with the exception of individual MESAS12) in contrast to an increase of Actinobacteriota at the expenses of the reduction of Bacillota in the remaining individuals (Figure 1E).
Figure 1. Diversity indices and GM relative abundance composition. Box plot of Shannon index (A) and Observed species index (B). GM profile at phylum (C) and family level (D); only taxa showing mean relative abundances higher than 1% are shown. GM main phyla relative abundance distribution in the sample of study disaggregated by individual (E).
In order to look for dietary, anthropometric, biochemical or lifestyle factors that could be related with variations in the two most abundant intestinal microbial phyla (Bacillota and Actinobacteriota) in the sample, individuals were clustered in two groups according to the Bacillota/Actinobacteriota abundance ratio: group 1 (ratio ≥2) and group 2 (ratio <2). Individuals in the group 2 presented a significantly higher consumption of red meat and significantly higher fasting glucose levels as well as a total/HDL-cholesterol atherogenic index higher than individuals from the group 1, without reaching risk threshold values of 110 mg/dL and 4.5 defined for these blood parameters, respectively (Table 4). When comparing the GM between both groups of individuals, several taxa displayed significant differences (Table 4). Remarkably, the only significant variation within the Actinobacteriota phylum was found for the family Bifidobacteriaceae and the genus Bifidobacterium, which had twice the relative abundances in the group that consumed more red meat than individuals who consumed less red meat. No other anthropometric, biochemical, lifestyle or GM parameters displayed significant differences between these two subgroups in the sample (data not shown).
Table 4. Dietary and microbiota differences according to microbiota profile distribution of main phyla in the sample of study.
Associations of dietary components with GM are shown at the phylum (Figure 2A) and family level (Figure 2B). Pseudomonadota and Verrucomicrobiota are the phyla showing the most significant direct correlations with xenobiotics (HAs, total PAHs, and nitrates). Verrucomicrobiota is also significantly associated with some fibers and (poly)phenols, as well as Euryarchaeota and Bacteroidota. These phyla are inversely correlated with starch, (poly)phenols and phenolic acids and lignans, respectively. At the family level (Figure 2B), 2-amino-3,4,8 trimethylimidazo (4,5,f) quinoxaline (DiMeIQx) and 2-amino-1-methyl-6-phenylimidazo (4,5,b) pyridine (PhIP) are directly associated with Streptococcaceae, this family being inversely correlated with the nitrosamine N-Nitrosopiperidine (NPIP). From (poly)phenols, inverse associations were detected for some of them with Clostridia UCG-014, Prevotellaceae and Ruminococcaceae while starch is directly associated with Lachnospiraceae and Eggerthellaceae. Based on these results, a stepwise regression analysis was conducted for the major microbial phyla and dietary sources, potential carcinogens, and (poly)phenol, controlled by age, BMI and energy intake (Table 5). The results obtained revealed that the associations represented in Figure 2 remained after adjusting for energy intake and BMI. It is also remarkable that in most cases dietary sources are not the main variables explaining the correlations found but the specific dietary component instead is sufficient by itself to explain at least for the minimum coefficient of the association.
Figure 2. Spearman correlations representation between the most abundant bacterial phyla (A) or families (B) (rows) and consumption of xenobiotics and bioactive compounds derived from the diet in the sample (columns). (*), (**) p – value < 0.05 and 0.01, respectively. Only taxa showing mean relative abundances higher than 1% are shown. MeIQx, 2-amino-3,8 dimethylimidazo (4,5,f) quinoxaline; DiMeIQx, 2-amino-3,4,8 trimethylimidazo (4,5,f) quinoxaline; PhIP, 2-amino-1-methyl-6-phenylimidazo (4,5,b) pyridine; B(a)P, benzo (a) pyrene; DiB(a)A, dibenzo (a) anthracene; Total PAH, total polycyclic aromatic hydrocarbons; NDMA, N-nitrosodimethylamine; NPIP, N-Nitrosopiperidine; NPYR, N-Nitrosopyrrolidine; (I), insoluble; (S), soluble.
Table 5. Analysis of variables accounting for significant correlations found between microbiota and the intake of xenobiotics, fibers and (poly)phenols, at the phylum level.
Since xenobiotic intake can vary widely between individuals, a LEfSe analysis was conducted to detect differences in GM profiles between individuals consuming xenobiotics below or over the risk doses described in the literature. PhIP and MeIQx were the only carcinogenic compounds showing significant associations with specific taxa of the GM both by Spearman correlation and by stepwise regression analysis for which risk daily consumption doses were reached in the sample. In the case of MeIQx (Figure 3A), higher levels of Lachnospiraceae and Eggerthellaceae families were found in individuals consuming less than 50 ng/day (lower risk for colorectal adenoma) (54) while those consuming more than 40 ng/day of PhIP (higher risk for colorectal adenoma) (54) showed lower relative abundance of Muribaculaceae and greater presence of Streptococcaceae and Eubacterium coprostanoligenes group (Figure 3B).
Figure 3. LEfSe analysis of microbial taxa differentially altered as a function of safety intake thresholds for some xenobiotics. (A) MeIQx at levels below (n = 11) or over (n = 8) the risk dose (50 ng/day) (54) and (B) PhIP at levels below (n = 12) or over (n = 7) the risk dose (40 ng/day) (54). Only families with relative abundance of at least 1% in at least two samples were considered in the analysis.
In developed countries, foods with solid science-based evidence on their benefits for health, such as fruit, vegetables, whole grains, and fish, usually have a relatively high cost. As a result, vulnerable at-risk groups often adopt diets that are far from the Mediterranean dietary standard (9), increasing the risk long-term to suffer non-communicable diseases, such as diabetes, hypertension, hyperlipidemia and obesity (55, 56). To the best of our knowledge, this is the first study examining the potential impact of the diet of a socially vulnerable population on the composition of the GM, providing a more in-depth analysis into the HAs, PAHs, nitrates, nitrites, nitroso compounds and acrylamide resulting from food processing. In spite of this, it is interesting to note that in our sample those individuals consuming significantly higher amounts of red meat also displayed significantly higher levels of microorganisms from the genus Bifidobacterium than individuals with lower intake of meat. Members of the genus Bifidobacterium are considered beneficial for health (57). Lower intestinal Bifidobacterium levels have been generally associated with higher consumption of red meat and animal meat (58) in the context of high fat and high calorie westernized diets, with high consumption of meat. However, the population analyzed in the present study are socially disadvantaged individuals whose daily intake of red meat did not exceeded the maximum recommended (53). In this regard, lower levels of fecal Bifidobacterium have been also reported in vegetarian individuals with respect to omnivores (59).
Our results evidence that the intake of some (poly)phenol, fibers and xenobiotics derived from food processing were associated with the GM composition, with a differential impact as depending on the microbial groups. It seems plausible that the consumption of processed foods, as well as fast cooking techniques, may lead to a higher intake of carcinogens in this sample. In this regard, only 42% and 37% of the sample had a MeIQx or PhIP intake above the recommended values (50 ng/day and 40 ng/day, respectively) (54). The average daily intakes of nitrate (54 mg) and acrylamide (10 μg) were within the normal limits, with no volunteers exceeding threshold levels (3.70 and 0.17 mg/kg/day, respectively) (60, 61). When comparing the levels of xenobiotics intake obtained in the present work with those of other studies in the general population at the same geographical location, a higher consumption of MeIQx and lower of phenolic compounds and fibers was observed in our sample (62). This is consistent with the lower consumption of fruit, vegetables, and plant-based foods in this human group.
Net effects exerted by dietary xenobiotics on the GM are dependent on their intake levels, the diet considered globally, and the interactions between these compounds and the GM (18). Indeed, microorganisms from the GM can present different degrees of sensitivity/resistance to dietary xenobiotics. Some members of the GM can bind toxics, contributing to their elimination with feces, and others can metabolize toxics, directly or through microbial metabolic interactions, resulting in new derived compounds with higher or lower toxicity. In this way, some of the variations in relative abundances of gut microbial taxa studied in the present work may be related with the microbial fitness exhibited against some of the potential carcinogens, fibers and (poly)phenols. The effect of each toxic compound could be enhanced or attenuated by other dietary components, which can result in associations of xenobiotics and/or bioactive compounds with the GM that vary depending on the characteristics of the GM itself, dietary habits and lifestyle. Therefore, although causality cannot be established from this type of research, it is necessary to highlight the difficulty in evaluating the impact of dietary components in human populations. In this sense, food groups, such as vegetables, which are sources of nitrites but also of (poly)phenols and fiber may have a different association with the GM than those compounds derived from cooked meat. For some dietary phenolics and fibers it has been demonstrated experimentally that they can counteract, total or partially, the effects of potentially harmful xenobiotics, even avoiding their formation during cooking, thus decreasing the potential toxicity of foreign chemicals to the organism (63–65). These associations between the GM and xenobiotics have been demonstrated by in vitro and in vivo systems. For instance, the formation of quinone-derived compounds was prevented from (poly)phenols of green tea in the presence of N-nitrosamine by the action of gut microbiome (66). Likewise, dietary wheat bran was shown to attenuate chronic cadmium toxicity in rats (67) and mulberry and dandelion water extracts were shown to prevent alcohol-induced steatosis and alleviate gut microbiome dysbiosis in rats (68). However, as our sample presented an intake of the HA MeIQx higher than the recommended levels and a low intake of phenolic compounds, the potential protective effect of dietary (poly)phenols would be presumably lower than in a population with higher intake of fruits and vegetables. Nevertheless, the long-term effect of the interaction between dietary bioactive compounds such as (poly)phenols and fibers from vegetables and xenobiotics derived from food processing, as HAs and PAHs, at the intestinal level and their effect on the GM remains largely unknown.
At the phylum taxonomic level, associations of several xenobiotics (mainly HAs) with the GM seem to have an opposite direction to that of several (poly)phenols whereas other potentially harmful xenobiotics display associations similar to dietary compounds derived from plants. This is the case of total PAH, dibenzo (a) anthracene (DiB(a)A) and starch, all of them showing negative association with Verrucomicrobiota and Euryarchaeota and positive correlation with the phylum Bacillota. In addition, both Pseudomonadota and Verrucomicrobiota are directly correlated with some HAs (MeIQx, DiMeIQx, and PhIP) and nitrates. Verrucomicrobiota is also significantly associated with some bioactive dietary components, as well as Euryarchaeota and Bacteroidota. Thus, these three phyla (and the family Prevotellaceae within the phylum Bacteroidota) are inversely correlated with starch, (poly)phenols and phenolic acids, and lignans, respectively. It has been generally reported that the intake of dietary sources rich in (poly)phenols and/or fiber can shape the GM by promoting the abundance of beneficial bacteria and inhibiting some pathogenic microbial groups (69–71) whereas food chemicals can disrupt human GM and impact negatively the intestinal homeostasis (72). Studies focusing on the association between xenobiotics and GM are still scarce and data available are mainly from in vitro models and in vivo murine models. In this regard, Kim and Hur (73) found during in vitro simulated human digestion, that the mutagenicity of HAs was drastically reduced in the presence of enterobacteria, Escherichia coli and Lactobacillus sakei. Ribière et al. (74) evidenced in a murine model that oral exposure to B(a)P induced an increase in the relative abundance of Bacteroidaceae, Porphyromonadaceae and Paraprevotellaceae and decreased Lactobacillaceae and Verrucomicrobioaceae families. Furthermore, the genus Bifidobacterium and families Coriobacteriaceae, Rikenellaceae and Desulfovibrionaceae increased in the presence of this xenobiotic derived from food processing.
Consistent with some of the associations found for HAs and the most abundant intestinal microbial taxa, we evidenced differentially higher abundance of the genus Streptococcus and members of the Eubacterium coprostanoligenes group in the GM of individuals with daily intake of PhIP in doses considered at higher risk. In contrast, individuals with lower intake of this xenobiotic displayed differentially higher abundance of the family Muribaculaceae. Differentially higher abundance of Eggerthellaceae and Lachnospiraceae was found in those individuals with daily intake of MeIQx below the doses considered at risk. Eubacterium coprostanoligenes has been related with the maintenance of intestinal mucosal barrier function (75), so that their differentially higher levels in individuals with higher intake of PhIP may be interpreted as a reinforcement of the protection of the intestinal mucosa against moderately high intake of this xenobiotic. In contrast, Lachnospiraceae tends to be differentially reduced in pathological states (76–78) whereas Muribaculaceae, a recently described family, has been related with long-term health effects (79, 80).
Interpreting the findings on the relationship between xenobiotics and GM obtained in our human sample is challenging. The scientific literature currently available generally describes positive association of the genus Akkermansia (phylum Verrucomicrobiota) with dietary resistant starch (81), which is apparently contradictory with our results. These differences could be partially related to the inverse relationship between the levels of Clostridia and Lachnospirales (Bacillota phylum) and Eggerthellaceae (Actinobacteriota phylum) and MeIQx intake, as well as to the existence of some key species in the degradation of resistant starch, such as Ruminococcus bromii (82). The previously reported negative association between resveratrol with the intestinal microbial genus Methanobrevibacter (Euryarchaeota phylum) in humans is according to our results and could be related with the complex crosstalk among the (poly)phenols consumption, intestinal permeability and GM composition (83). The inverse association between the relative abundance of Prevotellaceae (phylum Bacteroidota) and lignans could be related with some other positive association found by us at the family level for other members of the GM with lignans, as it is the case of Peptostreptococcaceae and Coriobacteriaceae (genus Eggerthella), two groups of microorganisms with strains able to participate in the metabolization of lignans (26). Other positive and negative associations found in the present work between xenobiotics (toxic and bioactive compounds) and GM may be due to changes in the relative abundance of the different microbial taxa at a variable extent, depending on their interaction with dietary xenobiotics. Our work suggests a comparatively higher potential carcinogens exposure and a lower consumption of protective bioactive compounds in the healthy vulnerable population under study with respect to other groups at the same geographical location (62). This was accompanied by differential intestinal microbial altered profiles in those individuals with intake of certain xenobiotics over the risk threshold, which can potentially increase the risk of long-term non-communicable diseases. Comparing dietary habits of African American volunteers (a population presenting increased incidence and mortality by colorectal cancer) and Caucasian Americans evidenced higher intake of HAs and decreased intake of vitamins, including vitamin D in the first group, which was correlated with differences in GM composition (84). Groups of economically and socially vulnerable individuals may be susceptible for early basic nutritional interventions to improve their nutritional and GM profile if these results will be confirmed in future studies.
The results obtained point to a possible association between potential carcinogens in the diet and the composition of the GM in subjects with a low socioeconomic level, despite the limited sample size of this work. However, when extrapolating the results, it should be taken into account the proportion of gender in the sample and the high BMI, both factors that could influence the composition of the GM. If confirmed in future studies, these data would serve to evidence the need for strategies aimed at nutritional intervention in these groups for the promotion of health.
The raw data presented in the study are deposited in the NCBI BioProject repository, accession number: PRJNA870886.
The studies involving human participants were reviewed and approved by Ethics Committee of the Hospital Universitario Central de Asturias (CEImPA2021.307). The patients/participants provided their written informed consent to participate in this study.
SG and CR-G designed the study. SG and AZ recruited the participants. AZ performed the nutritional assessment and statistical analysis. SA, SR-S, MG-M, NS, AN, and MG contributed and assisted to methodology and analytical tools. SG, CR-G, and AZ drafted the manuscript. All authors have read and agreed to the published version of the manuscript.
This work received support from Alimerka Foundation, the project RTI2018-098288-B-I00 financed by MCIN/AEI/10.13039/501100011033/FEDER, “Una manera de hacer Europa” and by the project AYUD/2021/50981 from the Principality of Asturias, to support the activity of research groups. AZ was the recipient of a predoctoral contract and NS of a postdoctoral contract (Convocatoria intramural 2021) funded by FINBA (Spain). SR-S was the recipient of a predoctoral contract (Severo Ochoa 2021-BP20-012) funded by the Plan Regional de Investigación, Principality of Asturias. MG-M was the recipient of a predoctoral FPU contract (FPU18/03393) funded by the Ministry of Universities (Spain). SA was the recipient of a postdoctoral Juan de la Cierva contract (Ministry of Science and Innovation, Ref. IJCI-2017-32156).
We thank Fundación Alimerka, Spanish Red Cross and all the participants involved in the study.
The authors declare that the research was conducted in the absence of any commercial or financial relationships that could be construed as a potential conflict of interest.
All claims expressed in this article are solely those of the authors and do not necessarily represent those of their affiliated organizations, or those of the publisher, the editors and the reviewers. Any product that may be evaluated in this article, or claim that may be made by its manufacturer, is not guaranteed or endorsed by the publisher.
1. Eslami M, Sadrifar S, Karbalaei M, Keikha M, Kobyliak NM, Yousefi B. Importance of the microbiota inhibitory mechanism on the Warburg effect in colorectal cancer cells. J Gastrointest Cancer. (2020) 51:738–47. doi: 10.1007/s12029-019-00329-3
2. Huttenhower C, Gevers D, Knight R, Abubucker S, Badger JH, Chinwalla AT, et al. Structure, function and diversity of the healthy human microbiome. Nature. (2012) 486:207–14. doi: 10.1038/nature11234
3. Latorre-Pérez A, Hernández M, Iglesias JR, Morán J, Pascual J, Porcar M, et al. The Spanish gut microbiome reveals links between microorganisms and Mediterranean diet. Sci Rep. (2021) 11:21602. doi: 10.1038/s41598-021-01002-1
4. Singh AK, Cabral C, Kumar R, Ganguly R, Rana HK, Gupta A, et al. Beneficial effects of dietary polyphenols on gut microbiota and strategies to improve delivery efficiency. Nutrients. (2019) 11:2216. doi: 10.3390/nu11092216
5. Johnson AJ, Vangay P, Al-Ghalith GA, Hillmann BM, Ward TL, Shields-Cutler RR, et al. Daily sampling reveals personalized diet-microbiome associations in humans. Cell Host Microbe. (2019) 25:789–802. doi: 10.1016/j.chom.2019.05.005
6. De Filippo C, Cavalieri D, Di Paola M, Ramazzotti M, Poullet JB, Massart S, et al. Impact of diet in shaping gut microbiota revealed by a comparative study in children from Europe and rural Africa. Proc Natl Acad Sci USA. (2010) 107:14691–6. doi: 10.1073/pnas.1005963107
7. Schnorr SL, Candela M, Rampelli S, Centanni M, Consolandi C, Basaglia G, et al. Gut microbiome of the Hadza hunter-gatherers. Nat Commun. (2014) 5:3654. doi: 10.1038/ncomms4654
8. Yatsunenko T, Rey FE, Manary MJ, Trehan I, Dominguez-Bello MG, Contreras M, et al. Human gut microbiome viewed across age and geography. Nature. (2012) 486:222–7. doi: 10.1038/nature11053
9. National Health Service (NHS) Health and Social Care Information Centre.Health survey for England 2004: The health of minority ethnic groups-headline tables. London: National Statistics (2004). p. 1–59.
10. Roux C, Le Couedic P, Durand-Gasselin S, Luquet F-M. Consumption patterns and food attitudes of a sample of 657 low-income people in France. Food Policy. (2000) 25:91–103. doi: 10.1016/S0306-9192(99)00066-4
11. Patterson AD, Gonzalez FJ, Idle JR. Xenobiotic metabolism: a view through the metabolometer. Chem Res Toxicol. (2010) 23:851–60. doi: 10.1021/tx100020p
12. International Agency for Research on Cancer (IARC) Working Group. IARC Monographs on the evaluation of carcinogenic risks to humans. Lancet Oncol. (2018) 114:1599–600. doi: 10.1016/S1470-2045(15)00444-1
13. Chiavarini M, Bertarelli G, Minelli L, Fabiani R. Dietary intake of meat cooking-related mutagens (HCAs) and risk of colorectal adenoma and cancer: A systematic review and meta-analysis. Nutrients. (2017) 9:514. doi: 10.3390/nu9050514
14. Miller PE, Lazarus P, Lesko SM, Cross AJ, Sinha R, Laio J, et al. Meat-related compounds and colorectal cancer risk by anatomical subsite. Nutr Cancer. (2013) 65:202–26. doi: 10.1080/01635581.2013.756534
15. Roca-Saavedra P, Mendez-Vilabrille V, Miranda JM, Nebot C, Cardelle-Cobas A, Franco CM, et al. Food additives, contaminants and other minor components: effects on human gut microbiota—a review. J Physiol Biochem. (2018) 74:69–83. doi: 10.1007/s13105-017-0564-2
16. Jin Y, Wu S, Zeng Z, Fu Z. Effects of environmental pollutants on gut microbiota. Environ Pollut. (2017) 222:1–9. doi: 10.1016/j.envpol.2016.11.045
17. Zhang Z, Li D. Thermal processing of food reduces gut microbiota diversity of the host and triggers adaptation of the microbiota: evidence from two vertebrates. Microbiome (2018) 6:99. doi: 10.1186/s40168-018-0471-y
18. Nogacka AM, Gómez-Martín M, Suárez A, González-Bernardo O, de los Reyes-Gavilán CG, González S. Xenobiotics formed during food processing: their relation with the intestinal microbiota and colorectal cancer. Int J Mol Sci. (2019) 20:2051. doi: 10.3390/ijms20082051
19. Orrhage K, Sillerstr E, Gustafsson J-A, Nord C, Rafter J. Binding of mutagenic heterocyclic amines by intestinal and lactic acid bacteria. Mutat Res. (1994) 311:239–48. doi: 10.1016/0027-5107(94)90182-1
20. Zhang J, Empl MT, Schwab C, Fekry MI, Engels C, Schneider M, et al. Gut microbial transformation of the dietary imidazoquinoxaline mutagen MeIQx reduces its cytotoxic and mutagenic potency. Toxicol Sci. (2017) 159:266–76. doi: 10.1093/toxsci/kfx132
21. Cougnoux A, Dalmasso G, Martinez R, Buc E, Delmas J, Gibold L, et al. Bacterial genotoxin colibactin promotes colon tumour growth by inducing a senescence-associated secretory phenotype. Gut. (2014) 63:1932–42. doi: 10.1136/gutjnl-2013-305257
22. Druzhinin VG, Matskova LV, Fucic A. Induction and modulation of genotoxicity by the bacteriome in mammals. Mutat Res Rev Mutat Res. (2018) 776:70–7. doi: 10.1016/j.mrrev.2018.04.002
23. Do KN, Fink LN, Jensen TE, Gautier L, Parlesak A. TLR2 controls intestinal carcinogen detoxication by CYP1A1. PLoS One. (2012) 7:e32309. doi: 10.1371/journal.pone.0032309
24. Dashnyam P, Mudududdla R, Hsieh TJ, Lin TC, Lin HY, Chen PY, et al. β-Glucuronidases of opportunistic bacteria are the major contributors to xenobiotic-induced toxicity in the gut. Sci Rep. (2018) 8:16372. doi: 10.1038/s41598-018-34678-z
25. García-Villalba R, Beltrán D, Espín JC, Selma MV, Tomás-Barberán FA. Time course production of urolithins from ellagic acid by human gut microbiota. J Agric Food Chem. (2013) 61:8797–806. doi: 10.1021/jf402498b
26. Clavel T, Borrmann D, Braune A, Doré J, Blaut M. Occurrence and activity of human intestinal bacteria involved in the conversion of dietary lignans. Anaerobe. (2006) 12:140–7. doi: 10.1016/j.anaerobe.2005.11.002
27. Ferguson LR, Harris PJ, Kestell P, Zhu S, Munday R, Munday CM. Comparative effects in rats of intact wheat bran and two wheat bran fractions on the disposition of the mutagen 2-amino-3-methylimidazo[4,5-f]quinoline. Mutat Res - Fundam Mol Mech Mutagen. (2011) 716:59–65. doi: 10.1016/j.mrfmmm.2011.08.005
28. Farran A, Zamora R, Cervera P. Tablas de composición de alimentos del Centro de Enseñanza Superior en Nutrición y Dietética (CESNID). Guaynabo: Mc Graw Hill Interamericana (2004).
29. United States Department of Agriculture (USDA).Food Composition Databases. (2004). Available online at: https://fdc.nal.usda.gov/download-datasets.html (accessed November 29, 2021).
30. Marlett J, Cheung T. Database and quick methods of assessing typical dietary fiber intakes using data for 228 commonly consumed foods. J Am Diet Assoc. (1997) 1151:1139–48. doi: 10.1016/S0002-8223(97)00275-7
31. Neveu V, Perez-Jiménez J, Vos F, Crespy V, du Chaffaut L, Mennen L, et al. Phenol-Explorer: an online comprehensive database on polyphenol contents in foods. Database (Oxford). (2010) 2010:ba024. doi: 10.1093/database/bap024
32. Jakszyn P, Ibáñez R, Pera G, Agudo A, García-Closas R, Amiano P, et al. Food content of potential carcinogens. Barcelona: Catalan Institute of Oncology (2004).
33. National Institute of Health (NIH).CHARRED: Computerized Heterocyclic Amines Resource for Research in Epidemiology of Disease. (2006). Available online at: https://dceg.cancer.gov/tools/design/charred (accessed September 21, 2021).
34. European Food Safety Authority (Efsa). Opinion of the scientific panel on contaminants in the food chain on a request from the European commission to perform a scientific risk assessment on nitrate in vegetables. EFSA J. (2008) 689:1–79. doi: 10.2903/j.efsa.2008.689
35. Food and Drug Administration (FDA).Survey data on acrylamide in food. Data from. (2015). Available online at: https://www.fda.gov/food/chemical-contaminants-food/survey-data-acrylamide-food (accessed September 21, 2021).
36. Svensson K, Abramsson L, Becker W, Glynn A, Hellenäs KE, Lind Y, et al. Dietary intake of acrylamide in Sweden. Food Chem Toxicol. (2003) 41:1581–6. doi: 10.1016/S0278-6915(03)00188-1
37. Hellenäs K-E, Fohgelberg P, Fäger U, Busk L, Zetterberg A, Ionescu C, et al. Acrylamide in Swedish food - targeted sampling 2011 and 2012. Uppsala: Swedish Food Agency (2013). p. 1–26.
38. Konings EJM, Baars AJ, van Klaveren JD, Spanjer MC, Rensen PM, Hiemstra M, et al. Acrylamide exposure from foods of the Dutch population and an assessment of the consequent risks. Food Chem Toxicol. (2003) 41:1569–79. doi: 10.1016/S0278-6915(03)00187-X
39. Falcó G, Domingo JL, Llobet JM, Teixidó A, Casas C, Müller L. Polycyclic aromatic hydrocarbons in foods: human exposure through the diet in Catalonia. Spain. J Food Prot. (2003) 66:2325–31. doi: 10.4315/0362-028X-66.12.2325
40. Palacios Colón L, Rascón AJ, Ballesteros E. Trace-level determination of polycyclic aromatic hydrocarbons in dairy products available in Spanish supermarkets by semi-automated solid-phase extraction and gas chromatography–mass spectrometry detection. Foods. (2022) 11:1–15. doi: 10.3390/foods11050713
41. De Mey E, De Klerck K, De Maere H, Dewulf L, Derdelinckx G, Peeters MC, et al. The occurrence of N-nitrosamines, residual nitrite and biogenic amines in commercial dry fermented sausages and evaluation of their occasional relation. Meat Sci. (2014) 96:821–8. doi: 10.1016/j.meatsci.2013.09.010
42. Campillo N, Viñas P, Martínez-Castillo N, Hernández-Córdoba M. Determination of volatile nitrosamines in meat products by microwave-assisted extraction and dispersive liquid-liquid microextraction coupled to gas chromatography-mass spectrometry. J Chromatogr A. (2011) 1218:1815–21. doi: 10.1016/j.chroma.2011.02.010
43. Lee HS. Literature compilation of volatile N-nitrosamines in processed meat and poultry products - an update. Food Addit Contam - Chem Anal Control Expo Risk Assess. (2019) 36:1491–500. doi: 10.1080/19440049.2019.1649472
44. Park JE, Seo JE, Lee JY, Kwon H. Distribution of seven N-nitrosamines in food. Toxicol Res. (2015) 31:279–88. doi: 10.5487/TR.2015.31.3.279
45. Fernández-Navarro T, Díaz I, Gutiérrez-Díaz I, Rodríguez-Carrio J, Suárez A, de los Reyes-Gavilán CG, et al. Exploring the interactions between serum free fatty acids and fecal microbiota in obesity through a machine learning algorithm. Food Res Int. (2019) 121:533–41. doi: 10.1016/j.foodres.2018.12.009
46. Sociedad Española para el Estudio de la Obesidad (Seedo). Consenso SEEDO 2000 para la evaluación del sobrepeso y la obesidad y el establecimiento de criterios de intervención terapéutica. Med Clin. (2000) 115:587–97. doi: 10.1016/S0025-7753(00)71632-0
47. Visscher T, Seidell JC, Molarius A, van der Kuip D, Hofman A, Witteman J. A comparison of body mass index, waist-hip ratio and waist circumference as predictors of all-cause mortality among the elderly: the Rotterdam study. Int J Obes. (2001) 25:1730–5. doi: 10.1038/sj.ijo.0801787
48. Dore, J, Ehrlich SD, Levenez F, Pelletier E, Alberti A, Bertrand L, et al. Standard Operating Procedure for Fecal Samples DNA Extraction. Protocol Q IHMS Consortium. IHMS_SOP 06 V2. (2015).
49. Edgar RC. UPARSE: highly accurate OTU sequences from microbial amplicon reads. Nat Methods. (2013) 10:996–8. doi: 10.1038/nmeth.2604
50. Wang Q, Garrity GM, Tiedje JM, Cole JR. Naïve bayesian classifier for rapid assignment of rRNA sequences into the new bacterial taxonomy. Appl Environ Microbiol. (2007) 73:5261–7. doi: 10.1128/AEM.00062-07
51. Metsalu T, Vilo J. ClustVis: A web tool for visualizing clustering of multivariate data using principal component analysis and heatmap. Nucleic Acids Res. (2015) 43:W566–70. doi: 10.1093/nar/gkv468
52. Afgan E, Baker D, Batut B, van den Beek M, Bouvier D, Ech M, et al. The Galaxy platform for accessible, reproducible and collaborative biomedical analyses: 2018 update. Nucleic Acids Res. (2018) 46:W537–44. doi: 10.1093/nar/gky379
53. World Cancer Research Fund International (WCRF)/American Institute for Cancer Research (AICR).Diet, nutrition, physical activity and colorectal cancer. Continuous Update Project Expert Report. London: World Cancer Research Fund International (2018). p. 1–62.
54. Martínez Gongora V, Matthes KL, Castaño PR, Linseisen J, Rohrmann S. Dietary heterocyclic amine intake and colorectal adenoma risk: a systematic review and meta-analysis. Cancer Epidemiol Biomark Prev. (2019) 28:99–109. doi: 10.1158/1055-9965.EPI-17-1017
55. Hart CL, Gruer L, Watt GCM. Cause specific mortality, social position, and obesity among women who had never smoked: 28 year cohort study. BMJ. (2011) 342:d3785. doi: 10.1136/bmj.d3785
56. Mullachery PH, Vela E, Cleries M, Comin-Colet J, Nasir K, Diez Roux AV, et al. Inequalities by income in the prevalence of cardiovascular disease and its risk factors in the adult population of Catalonia. J Am Heart Assoc. (2022) 11:e026587. doi: 10.1161/JAHA.122.026587
57. Tojo R, Suárez A, Clemente MG, de Los Reyes-Gavilán CG, Margolles A, Gueimonde M, et al. Intestinal microbiota in health and disease: role of bifidobacteria in gut homeostasis. World J Gastroenterol. (2014) 20:15163–76. doi: 10.3748/wjg.v20.i41.15163
58. Zhang M, Zou X, Zhao D, Zhao F, Li C. Pork meat proteins alter gut microbiota and lipid metabolism genes in the colon of adaptive immune-deficient mice. Mol Nutr Food Res. (2020) 64:e1901105. doi: 10.1002/mnfr.201901105
59. Matijašić BB, Obermajer T, Lipoglavšek L, Grabnar I, Avguštin G, Rogelj I. Association of dietary type with fecal microbiota in vegetarians and omnivores in Slovenia. Eur J Nutr. (2014) 53:1051–64. doi: 10.1007/s00394-013-0607-6
60. Mortensen A, Aguilar F, Crebelli R, Di Domenico A, Dusemund B, Frutos M, et al. Re-evaluation of sodium nitrate (E 251) and potassium nitrate (E 252) as food additives. EFSA J. (2017) 15:1–123. doi: 10.2903/j.efsa.2017.4787
61. Agencia Española de Seguridad Alimentaria y Nutrición (AESAN).Acrilamida. (2020). Available online at: http://www.aesan.gob.es/AECOSAN/web/seguridad_alimentaria/subdetalle/acrilamida.htm (accessed September 1, 2021).
62. Zapico A, Ruiz-Saavedra S, Gómez-Martín M, de los Reyes-Gavilán CG, González S. Pilot study for the dietary assessment of xenobiotics derived from food processing in an adult Spanish sample. Foods. (2022) 11:1–19. doi: 10.3390/foods11030470
63. Yu Z, Xu M, Santana-Rios G, Shen R, Izquierdo-Pulido M, Williams DE, et al. A comparison of whole wheat, refined wheat and wheat bran as inhibitors of heterocyclic amines in the Salmonella mutagenicity assay and in the rat colonic aberrant crypt focus assay. Food Chem Toxicol. (2001) 39:655–65. doi: 10.1016/s0278-6915(01)00012-6
64. Friedman M, Zhu L, Feinstein Y, Ravishankar S. Carvacrol facilitates heat-induced inactivation of Escherichia coli O157: H7 and inhibits formation of heterocyclic amines in grilled ground beef patties. J Agric Food Chem. (2009) 57:1848–53. doi: 10.1021/jf8022657
65. Rounds L, Havens CM, Feinstein Y, Friedman M, Ravishankar S. Concentration-dependent inhibition of Escherichia coli O157: H7 and heterocyclic amines in heated ground beef patties by apple and olive extracts, onion powder and clove bud oil. Meat Sci. (2013) 94:461–7. doi: 10.1016/j.meatsci.2013.03.010
66. Farag MA, Shakour ZTA, Elmassry MM, Donia MS. Metabolites profiling reveals gut microbiome-mediated biotransformation of green tea polyphenols in the presence of N-nitrosamine as pro-oxidant. Food Chem. (2022) 371:131147. doi: 10.1016/j.foodchem.2021.131147
67. Li Y, Liu K, Shen J, Liu Y. Wheat bran intake can attenuate chronic cadmium toxicity in mice gut microbiota. Food Funct. (2016) 7:3524–30. doi: 10.1039/c6fo00233a
68. Park S, Kim DS, Wu X, J Yi Q. Mulberry and dandelion water extracts prevent alcohol-induced steatosis with alleviating gut microbiome dysbiosis. Exp Biol Med. (2018) 243:882–94. doi: 10.1177/1535370218789068
69. Ma G, Chen Y. Polyphenol supplementation benefits human health via gut microbiota: a systematic review via meta-analysis. J Funct Foods. (2020) 66:103829. doi: 10.1016/j.jff.2020.103829
70. Loo YT, Howell K, Chan M, Zhang P, Ng K. Modulation of the human gut microbiota by phenolics and phenolic fiber-rich foods. Compr Rev Food Sci Food Saf. (2020) 19:1268–98. doi: 10.1111/1541-4337.12563
71. Fang F, Junejo SA, Wang K, Yang X, Yuan Y, Zhang B. Fibre matrices for enhanced gut health: a mini review. Int J Food Sci Technol. (2022):1–7. doi: 10.1111/ijfs.15702
72. Defois C, Ratel J, Garrait G, Denis S, Le Goff O, Talvas J, et al. Food chemicals disrupt human gut microbiota activity and impact intestinal homeostasis as revealed by in vitro systems. Sci Rep. (2018) 8:11006. doi: 10.1038/s41598-018-29376-9
73. Kim HS, Hur SJ. Changes in the mutagenicity of heterocyclic amines, nitrite, and N-nitroso compound in pork patties during in vitro human digestion. LWT Food Sci Technol. (2018) 92:47–53. doi: 10.1016/j.lwt.2018.01.079
74. Ribière C, Peyret P, Parisot N, Darcha C, Déchelotte PJ, Barnich N, et al. Oral exposure to environmental pollutant benzo[a]pyrene impacts the intestinal epithelium and induces gut microbial shifts in murine model. Sci Rep. (2016) 6:31027. doi: 10.1038/srep31027
75. Bai D, Sun T, Zhao J, Du J, Bu X, Cao W, et al. Oroxylin A maintains the colonic mucus barrier to reduce disease susceptibility by reconstituting a dietary fiber-deprived gut microbiota. Cancer Lett. (2021) 515:73–85. doi: 10.1016/j.canlet.2021.05.018
76. Pietrucci D, Cerroni R, Unida V, Farcomeni A, Pierantozzi M, Mercuri NB, et al. Dysbiosis of gut microbiota in a selected population of Parkinson’s patients. Parkinsonism Relat Disord. (2019) 65:124–30. doi: 10.1016/j.parkreldis.2019.06.003
77. Dou X, Gao N, Yan D, Shan A. Sodium butyrate alleviates mouse colitis by regulating gut microbiota dysbiosis. Animals. (2020) 10:1154. doi: 10.3390/ani10071154
78. Clos-Garcia M, Garcia K, Alonso C, Iruarrizaga-Lejarreta M, D’amato M, Crespo A, et al. Integrative analysis of fecal metagenomics and metabolomics in colorectal cancer. Cancers (Basel). (2020) 12:1142. doi: 10.3390/cancers12051142
79. Cao W, Chin Y, Chen X, Mi Y, Xue C, Wang Y, et al. The role of gut microbiota in the resistance to obesity in mice fed a high fat diet. Int J Food Sci Nutr. (2020) 71:453–63. doi: 10.1080/09637486.2019.1686608
80. Sibai M, Altuntaş E, Ylldlrlm B, Öztürk G, Ylldlrlm S, Demircan T. Microbiome and longevity: high abundance of longevity-linked Muribaculaceae in the gut of the long-living rodent Spalax leucodon. OMICS. (2020) 24:592–601. doi: 10.1089/omi.2020.0116
81. Liu Y, Chandran Matheyambath A, Ivusic Polic I, LaPointe G. Differential fermentation of raw and processed high-amylose and waxy maize starches in the Simulator of the Human Intestinal Microbial Ecosystem (SHIME®). J Funct Foods. (2021) 86:1–14. doi: 10.1016/j.jff.2021.104735
82. Ze X, Duncan SH, Louis P, Flint HJ. Ruminococcus bromii is a keystone species for the degradation of resistant starch in the human colon. ISME J. (2012) 6:1535–43. doi: 10.1038/ismej.2012.4
83. Peron G, Gargari G, Meroño T, Miñarro A, Lozano EV, Escuder PC, et al. Crosstalk among intestinal barrier, gut microbiota and serum metabolome after a polyphenol-rich diet in older subjects with “leaky gut”: The MaPLE trial. Clin Nutr. (2021) 40:5288–97. doi: 10.1016/j.clnu.2021.08.027
Keywords: xenobiotic, microbiota, sustainable diet, fiber, Mediterranean diet, meat
Citation: Zapico A, Arboleya S, Ruiz-Saavedra S, Gómez-Martín M, Salazar N, Nogacka AM, Gueimonde M, de los Reyes-Gavilán CG and González S (2022) Dietary xenobiotics, (poly)phenols and fibers: Exploring associations with gut microbiota in socially vulnerable individuals. Front. Nutr. 9:1000829. doi: 10.3389/fnut.2022.1000829
Received: 22 July 2022; Accepted: 19 September 2022;
Published: 12 October 2022.
Edited by:
Kai Wang, Chinese Academy of Agricultural Sciences (CAAS), ChinaReviewed by:
Maria Carolina Rodriguez Daza, Wageningen University and Research, NetherlandsCopyright © 2022 Zapico, Arboleya, Ruiz-Saavedra, Gómez-Martín, Salazar, Nogacka, Gueimonde, de los Reyes-Gavilán and González. This is an open-access article distributed under the terms of the Creative Commons Attribution License (CC BY). The use, distribution or reproduction in other forums is permitted, provided the original author(s) and the copyright owner(s) are credited and that the original publication in this journal is cited, in accordance with accepted academic practice. No use, distribution or reproduction is permitted which does not comply with these terms.
*Correspondence: Clara G. de los Reyes-Gavilán, Z3JleWVzX2dhdmlsYW5AaXBsYS5jc2ljLmVz; Sonia González, c29uaWFnc29sYXJlc0B1bmlvdmkuZXM=
Disclaimer: All claims expressed in this article are solely those of the authors and do not necessarily represent those of their affiliated organizations, or those of the publisher, the editors and the reviewers. Any product that may be evaluated in this article or claim that may be made by its manufacturer is not guaranteed or endorsed by the publisher.
Research integrity at Frontiers
Learn more about the work of our research integrity team to safeguard the quality of each article we publish.