- 1College of Horticulture, Nanjing Agricultural University, Nanjing, China
- 2Institute of Horticulture, Zhejiang Academy of Agricultural Sciences, Hangzhou, China
- 3College of Electrical Engineering, Nanjing Vocational University of Industry Technology, Nanjing, China
- 4Agricultural College, Liaocheng University, Liaocheng, China
To determine the effect of different temperature on strawberry after harvest, physiological indicator analysis and proteomics analysis were conducted on ripened strawberry (“Sweet Charlie”) fruit stored at 4, 23, and 37°C for 10 or 20 days. Results showed that 4°C maintained a better visual quality of strawberry, and the weight loss and firmness remained stable within 3 days. Low temperature negatively affected anthocyanin but positively affected soluble sugars. Though anthocyanin content was higher with increasing temperature, anthocyanin synthesis related proteins were downregulated. Higher indole-acetic acid (IAA) content in seeds and lower abscisic acid (ABA) content were found in berry at 4°C. Antioxidant related proteins were upregulated during storage, showing a significant up-regulation of peroxidase (POD) at 4°C, and ascorbate-glutathione (AsA-GSH) cycle related proteins and heat shock proteins (HSPs) at 37°C. In addition, overexpressed sugar phosphate/phosphate translocator, 1-aminocyclopropane-1-carboxylate oxidase, and aquaporin PIP2-2 had a positive effect in response to low temperature stress for containing higher protopectin content and POD activity.
Introduction
Strawberry (Fragaria × ananassa Duch.) is one of the most important globally cultivated fruit crops. Strawberry fruit is widely consumed not only for its flavor and appearance but also for its medicinal value, such as eyesight protection, improved blood circulation (1), and anticarcinogenic activity (2). Unfortunately, strawberries are perishable and susceptible to mechanical injury and physiological deterioration, which cause serious economic loss. Too high or too low storage temperature after harvest will have an important impact on the internal and external quality of strawberry fruit (3). At present, some researchers have made in-depth studies on the mechanism of low-temperature storage of strawberry after harvest (4, 5). The evaluation of strawberry quality for the market is focused on visual and internal characteristics, such as color, hardness, acidity, sweetness, and aroma (6). With the development of economy, more, and more people are concerned about the nutritional quality of strawberries. Strawberries are good sources of natural antioxidants and its antioxidant activity are positively correlated with anthocyanin or total phenol content (7, 8). In addition to its antioxidant activity, anthocyanins are also important to fruit color during the ripening of strawberry fruit, grapes, and cherries (3, 9, 10). Strawberry aroma is one of the most popular fruit flavors, and the flavor strawberries is mainly determined by a complex mixture of esters, aldehydes, alcohols, and sulfur compounds (5, 11). Among the identified aromatic compounds, esters, and furanones are consider the main aroma determinants in fresh strawberry.
Changes of physiological and molecular occurred in plant in response to low or high temperature have been extensively studied (5, 12–14). HSPs are stress proteins with anti-stress effects, which can be expressed in large quantities under stress such as high temperature, drought, peroxidation, and heavy metals (15). Soluble sugars, amino acids, organic acids are important compatible substances, which played crucial role in the resistance of plants to environmental changes (16, 17). The richer the osmotic adjustment substances, the better the adaptability to the environment. Softening of fruit is closely related to the disassembly of primary cell wall and middle lamella structures and the decrease of soluble pectin (18). Studies have shown that the changes in the composition and structure of the cell wall are caused by the coordinated action of hydrolytic enzymes (19).
Temperature regulation is the most important environmental factor for extending the shelf-life of strawberry fruit. Cordenunsi et al. (20) reported that the quality of strawberry fruit was maintained better at 6°C for 6 days than at 16 and 25°C, and low temperature inhibited the accumulation of anthocyanins and vitamin C, but promoted soluble sugars. Higher storage temperatures increase respiration rates and shorten storage life, resulting in fruit quality loss (5, 21, 22). Strawberry fruit stored in different relative humidity (RH) environments at 0.5, 10, and 20°C for 4 days were studied by Shin et al. (22), who found that firmness and soluble solid concentrations decreased at higher storage temperature, while anthocyanin concentrations were increased rapidly at 20°C as the fruit ripened.
Proteomics is a kind of discipline that studies the composition of cell proteins and the various activity characteristics of proteins (23, 24). Compared with genome, proteomics can provide a direct basis for explaining the essence of life phenomena. In proteomics, Mass Spectrometry (MS) technology is the core content of its research, mainly to realize its molecular identification, modification, and the interaction mechanism between various molecules through the correct determination of the mass of the protein molecule (25). At present, researchers are devoted to the study of the biological characteristics of fruits during the pre-harvest and post-harvest storage processes (26). However, there are not many research data on the proteomics of fruit changes (27, 28).
The objective of our study was to investigate the response mechanism of strawberry fruit harvested at the red ripe stage to different temperatures. Although the effect of temperature on the quality of strawberry has been studied by some researchers (5, 9, 22); however, not all of the physiological quality data were assessed at the same time, and limited research has been conducted on protein expression. In addition, previous studies have shown that the response temperature of strawberry heat shock protein is generally 30–37°C (29). Under low temperature storage conditions, strawberry fruits could maintain acceptable quality for up to 7 days, but for high temperature stress, the time is much shorter (5). Therefore, strawberry fruits treated at 4°C, 23°C (simulated room temperature), and 37°C (heat shock temperature) on day 3, 7, 10, and/or 20 were collected for determination.
Materials and Methods
Plant Material and Growth Conditions
Octoploid strawberry (Fragaria × ananassa “Sweet Charlie”) were grown in a greenhouse under standard cultivation conditions (20–25°C, relative humidity of 70–85%, 14 h/10 h light/dark cycles) during spring seasons from 2018 to 2020. According to our previous research, we divide strawberry development into 7 stages [SG (Small green, 7 days after anthesis), LG (Large green, 12 days after anthesis), DG (De greening, 16 days after anthesis), WT (White, 20 days after anthesis), IR (Initial red, 23 days after anthesis), PR (Partial red, 25 days after anthesis), and FR (Full red, 28 days after anthesis)] (30). The FR (Full red, 28 days after anthesis) fruit were collected and kept in a 4°C cold room (low temperature, LT) or 37°C incubator (high temperature, HT) for 10 and/or 20 d. The control check (CK) was kept in a 23°C greenhouse (room temperature, RT) at a relative humidity of 70–85%.There are three repetitions for per treatment, and 28 fruits for per replication. Ten fruits were collected on day 3, 7, 10, and/or 20, respectively, and then the seeds and berries were separated with tweezers. Some were used for physical evaluation, and some were frozen in liquid nitrogen for subsequent experiments. Twenty fruits with seeds removed on day 5 were collected for protein expression determination.
Weight Loss, Firmness, and Color
Fruit weights were recorded, and the percentage weight loss from harvest was calculated. Firmness was measured by a puncture test on each fruit with intact skin using a Force Five pressure tester (Model FDV-30, Wagner Instruments, Greenwich, CT, USA) fitted with a 7.9 mm diameter flathead probe. Surface color of 10 individual fruit per treatment was measured with a hand-held precise color reader (model WR-10; China).
Anthocyanins Determination
Total anthocyanins were determined using the pH differential method (31). Absorbance was measured in a METASH spectrophotometry (METASH UV-5100) at 520 and 700 nm in buffers at pH 1.0 and 4.5, using A = (A520 – A700)pH 1.0 – (A520 – A700)pH 4.5 with molar extinction coefficients of Cyanidin-3-glucoside (29,600) for strawberry fruit juice. The samples used for anthocyanin components measurement were collected on day 5. Anthocyanin components were determined by liquid chromatography-mass spectrometry (LC-MS), with the peak area used to evaluate the content of each component (31).
Soluble Sugars, Organic Acids, Hormones, and Cell Wall Components Determinations
0.5 g fruit were ground in liquid nitrogen and mixed with 1.5 ml 80% ethanol, 85°C for 30 min, and centrifuge at 12,000 g for 10 min. Aspirate the supernatant and repeat the above step. Mix the supernatant together and freeze-dry. Fifteen milliliters ultrapure water was used to dissolve lyophilized precipitate and filtered the solution with 0.22 water-filter for the measurement of soluble sugars and organic acids. Soluble sugar and organic acid contents were determined using high-performance liquid chromatography (HPLC) (31, 32).
Hormone contents were determined using the enzyme-linked immunosorbent assay (ELISA) method (32, 33). Protopectin, soluble pectin, cellulose, and hemicellulose were determined using related kits (Solarbio Life Sciences, Beijing, China). The determination of pectin used galacturonic acid as a standard curve, and the absorbance was measured at 530 nm. The determination of cellulose uses glucose as a standard curve. Results were showed as g of per 1,000 g of fresh weight. All measurements were repeated three times.
Determination of Aroma Compounds
Strawberry fruit (3 g) were ground in liquid nitrogen and mixed with 3 ml saturated NaCl and 2 μl octanol (81.8 mg/L) as an internal standard. Gas chromatography-mass spectrometry (GC-MS) was performed according to the method of Zheng et al. (34). Samples were equilibrated for 10 min at 50°C and then extracted for 30 min with fiber coating divinylbenzene/carboxen/polydimethylsiloxane (DVB/CAR/PDMS) with 50/30-μm thickness. And then, the aroma components were detected with GC system coupled with MS instrument.
Determination of Amino Acid and Antioxidant Enzyme Activity
Sample of 1 × 10−5 kg were added to 0.003 L of 0.02 mol·L−1 hydrochloric acid and extracted with sonication for 30 min (minutes). The samples were then extracted overnight at 4°C and centrifuged to obtain the supernatant. One milliliter of the supernatant was mixed with 0.001 L of 4% sulfosalicylic acid and centrifuged to get the supernatant. The supernatant was filtered with a 0.22 μm water-based membrane, and then an automatic amino acid analyzer (L-8900, Hitachi, Japan) was used to determine the amino acid content.
One gram of sample was added to 0.01 L of 0.1 mol·L−1 phosphate buffer (pH 7.0), ground into a homogenate, and then centrifuged at 4,000 g for 15 min. The supernatant was used for superoxide dismutase (SOD) and POD determination. Three biological repetitions were conducted. The activity of POD was determined using the guaiacol method (35), and the activity of SOD was determined by the nitro blue tetrazolium (NBT) photochemical reduction method (36).
Strawberry Fruit Microscopy and Staining
Strawberry fruit paraffin sections were performed as Han et al. (37). Strawberry fruit at different temperatures and times were cut into approximately 5-mm3 pieces and fixed in formalin–acetic acid–alcohol (FAA) solution (5% formaldehyde, 5% glacial acetic acid, and 63% ethyl alcohol) at 4°C for 1 week. The strawberry pieces were dehydrated in an ethanol series solution and then embedded in paraffin. Sections were cut to a thickness of 10 μm using a microtome (Leica RM2255, Germany) and stained by 1% (w/v) Toluidine Blue O. After staining, the sections were observed using a microscopy imaging system (Olympus, Tokyo, Japan).
Protein Extraction and Tandem Mass Tags Proteomic
The sample was ground by liquid nitrogen into a powder. After that, four volumes of phenol extraction buffer (1% protease inhibitor and 1% phosphatase inhibitor) were added to the powder and ultrasonically lysed. An equal volume of Tris was added to equilibrate the phenol and centrifuged at 5,500 g for 10 min at 4°C, and five volumes of 0.1 mol·L−1 ammonium acetate/methanol were added to the supernatant for precipitation overnight. The protein precipitate was washed with methanol and acetone. Finally, the protein was redissolved in 8 mol·L−1 urea, and the protein concentration was determined with a BCA kit according to the manufacturer's instructions. After trypsin digestion, the peptides were desalted by a Strata X C18 SPE column (Phenomenex) and vacuum-dried. The peptides were reconstituted in 0.5 mol·L−1 TEAB and processed according to the manufacturer's protocol for the Tandem Mass Tags (TMT) kit.
LC-MS/MS Analysis
The peptides were dissolved in the mobile phase A of liquid chromatography [0.1% (v/v) formic acid aqueous solution] and separated using an EASY-nLC 1000 ultra-performance liquid system. Solvent A was an aqueous solution containing 0.1% formic acid and 2% acetonitrile; solvent B was an aqueous solution containing 0.1% formic acid and 90% acetonitrile. The liquid gradient settings were 0–38 min, 9–26% B; 38–52 min, 26–35% B; 52–56 min, 35–80% B; and 56–60 min, 80% B. The flow was maintained at 500 nL/min.
The peptides were subjected to an NSI source followed by tandem mass spectrometry (MS/MS) in a Q Exactive™ Plus (Thermo) coupled online to the ultra-performance liquid chromatograph (UPLC). The electrospray voltage applied was 2.0 kV. Peptide precursor ions and their secondary fragments were detected and analyzed using a high-resolution Orbitrap. The scanning range of the primary mass spectrum was set to 400–1,500 m/z (mass-to-charge ratio), and the scanning resolution was set to 70,000; the scanning range of the secondary mass spectrum was set to a fixed starting point of 100 m/z, and the secondary scanning resolution was set to 35,000. Automatic gain control (AGC) was set at 5E4. The signal threshold was set to 38,000 ions/s, the maximum injection time was set to 130 ms, and the dynamic rejection time of the tandem mass spectrometry scan was set to 30 s.
Bioinformatic Analysis of Proteins
Gene Ontology (GO) annotation proteome was derived from the UniProt-GOA database (http://www.ebi.ac.uk/GOA/). Firstly, converting identified protein ID to UniProt ID and then mapping to GO IDs by protein ID. If some identified proteins were not annotated by UniProt-GOA database, the InterProScan soft would be used to annotated protein's GO functional based on protein sequence alignment method. Then proteins were classified by Gene Ontology annotation based on three categories: biological process, cellular component and molecular function. Kyoto Encyclopedia of Genes and Genomes (KEGG) database was used to annotate protein pathway. Firstly, using KEGG online service tools KAAS to annotated protein's KEGG database description. Then mapping the annotation result on the KEGG pathway database using KEGG online service tools KEGG mapper. All differentially expressed protein database accession or sequence were searched against the STRING database version 10.1 for protein-protein interactions. Only interactions between the proteins belonging to the searched data set were selected, thereby excluding external candidates. STRING defines a metric called “confidence score” to define interaction confidence; we fetched all interactions that had a confidence score ≥0.7 (high confidence).
RNA Extraction and Quantitative Real-Time (qRT)-PCR
Total RNA was extracted from the strawberry using Quick RNA Isolation Kit (Hua Yue Yang, Beijing China). The cDNA was reverse transcribed according to HifairTM II 1st Strand cDNA Synthesis SuperMix Kit for qRT-PCR (gDNA digester plus) (Yeasen, Shanghai, China). Specific primers were designed using online software Primer 3 Plus (http://primer3.ut.ee/; Supplementary Table 1). For different transcripts, NCBI Gene (https://www.ncbi.nlm.nih.gov) was used for comparing the difference of sequences between different transcripts, and then designed specific primers using the different part of the transcript sequence. TaKaRa SYBR Premix Ex Taq™ II (Takara, Dalian, China) was used to perform qPCR on ABI prism 7900 Real-Time PCR system (Applied Biosystems, USA). The thermal cycles were set as follows: 95°C for 2 min for pre-incubation, 40 cycles of 95°C for 10 s and 58°C for 30 s for amplification. The relative gene expression levels were calculated by the 2−ΔΔCt method. The strawberry gene Actin was used as the reference.
Gene Transient Expression in Strawberry
Transient expression in strawberry was conducted according to Zheng et al. (38). The constructed vectors FaP1-GFP, FaP2-GFP, and FaP3-GFP were transfected into Agrobacterium (strain EHA105), following which the strawberry fruit were infected with pCAMBIA-1302 as CK at the large green stage (14 days after anthesis). Seven days later, the anthocyanin content, soluble sugar and organic acid content, and cell wall component content were determined. The antioxidant enzyme activities of POD and SOD were determined, and volatile organic compounds were determined using -GC-MS.
Statistical Analysis
The statistics were analyzed using statistical analysis of variance (ANOVA) SPSS statistics 17.0 (SPSS Inc., Chicago, IL, USA), TB tools v1.072 (39), and Origin Pro 9 (Origin Inc., Northampton, MA, USA). All experiments were performed at least three replicates. Experimental data were presented as means ± standard deviation (SD). Duncan post-hoc test (P <0.05) was performed to test the existence of statistical differences between different treatments.
Results
Effect of Temperature Regimes on Strawberry Fruit Quality
To determine the the physical index changes of strawberry in response to different temperatures, fruit color, anthocyanin content, fruit sugar and organic acid content, amino acid content, and plant hormone content were measured on day 3, 7, and 10 at 4, 23, and 37°C storage temperature treatments. The results showed that the fruit color developed into a dark red and lost its glossiness with prolonged storage time, and an increase in temperature resulted in more severe discoloration (Figure 1A).
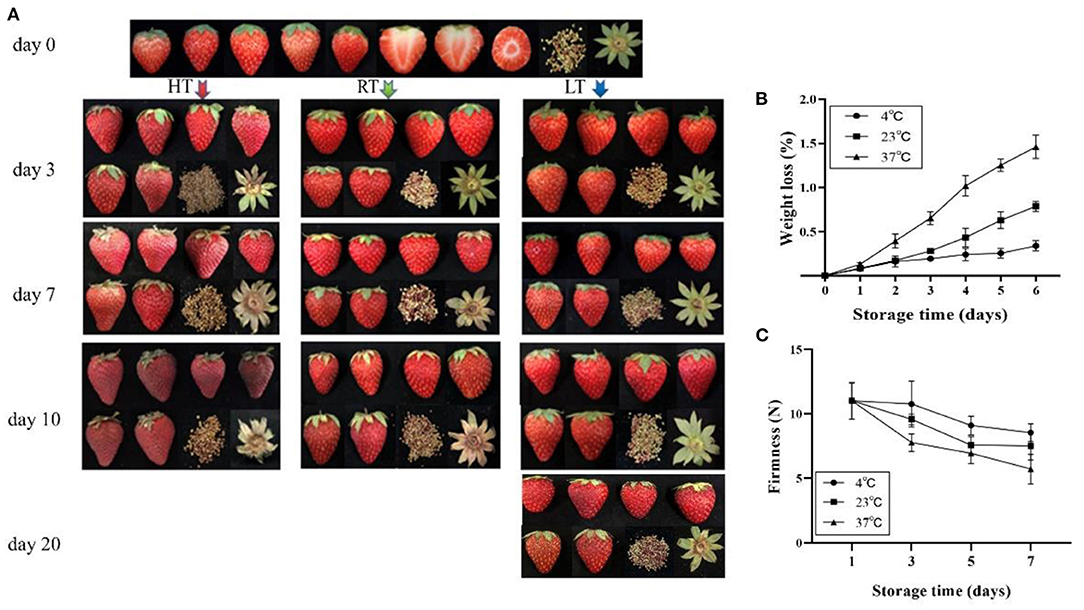
Figure 1. Overall quality of strawberry at 4, 23, and 37°C. (A) Appearance of fruit; (B) weight loss; and (C) firmness change of fruit. The fruit color developed into a dark red and lost its glossiness over time and temperature. Fruit stored at 4°C maintained a better quality and firmness, but 37°C accelerated fruit weight loss 1 day later. LT, RT, and HT represent the storage temperature of 4, 23, and 37°C, respectively. Each value represents the mean of three replicates. Error bar stands for standard deviation (SD) and date are expressed as means ± SD (n = 3, three replications).
Fruit Weight Loss, Firmness, and Color
Weight loss from the berries increased over time (Figure 1B) and was affected by storage temperature. Among the three temperature, weight losses were similar on day 1, but by day 5 were 0.24 and 0.63% at 4 and 23°C, respectively; and by day 4 were 1.05% at 37°C. Firmness was affected by temperature and decreased over time (Figure 1C). At 4°C, firmness decreased by 2.21% for the first 3 days, while decreased by 29.39% at 37°C. Lightness (L* value) and chroma (C* value) were changes over time (Table 1). At 4°C, lightness of the strawberry fruit was not affected by storage time but significantly declined with higher storage temperatures. Chroma increased slightly at 4°C, but decreased at higher temperature (23 and 37°C).
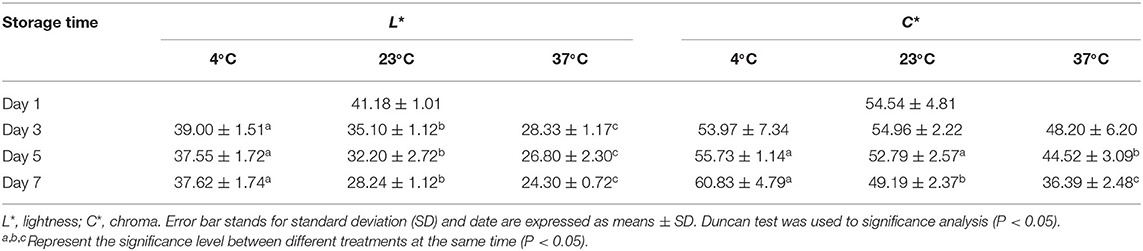
Table 1. Lightness and chroma value of strawberry in fruit stored for up to 1, 3, 5, and 7 days at 4, 23, and 37°C.
Anthocyanins
Furthermore, higher temperatures resulted in greater anthocyanin accumulation (Figure 2A), among which, more cyanidin 3-galactoside, cyanidin, pelargonidin, cyaniding 3,5-diglucoside, and luteolin were accumulated under high temperature (Table 2; Supplementary Table 2). Soluble sugar and organic acid of strawberry were affected by temperature and days. Patterns of change were similar between 4 and 23°C, but more fructose, glucose, and sucrose were accumulated at 4°C (Figures 2B–D). Overall, high temperature promoted the accumulation of organic acid (Figures 2E–H).
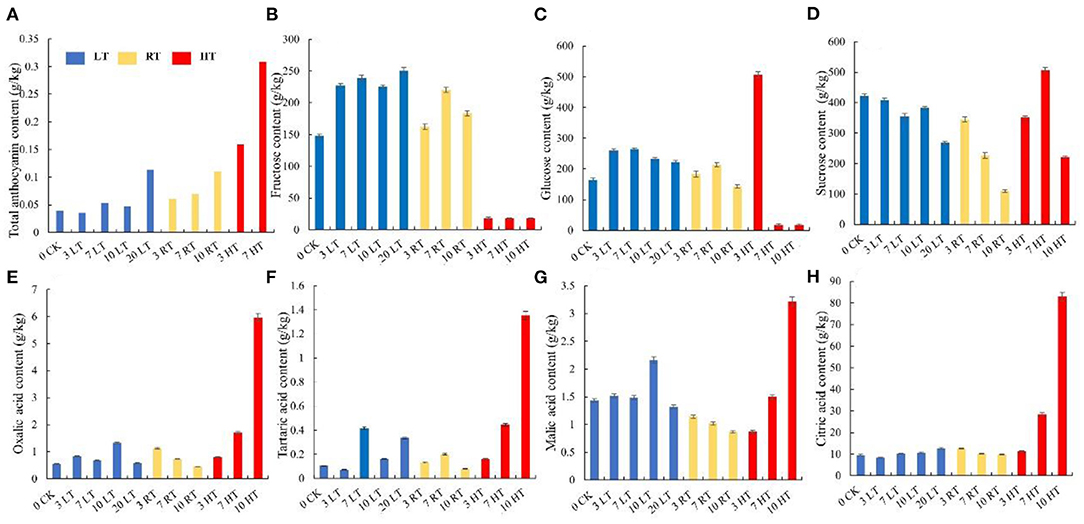
Figure 2. Total anthocyanin, sugars, and organic acids content in strawberry at 4, 23, and 37°C. Total anthocyanin content was higher at higher temperature (A); higher soluble sugars contents and lower organic acids were measured at 4°C, while the situation was opposite 37°C (B–H). LT, RT, and HT represent the storage temperature of 4, 23, and 37°C, respectively. Each value represents the mean of three replicates. Error bar stands for standard deviation (SD) and date are expressed as means ± SD.
Amino Acid and Hormones
The content of amino acid was also affected by storage time and temperature (Supplementary Figure 1). Generally, temperature has a greater influence on the amino acids in strawberry berry. Among the 17 amino acids, the proportion of alanine was increased at higher temperature. Leucine and isoleucine were higher at 37°C than at 4 and 23°C. In addition, threonine content increased at 4°C, while γ-aminobutyric acid increased at 37°C. IAA, ABA, methyl jasmonate, zeatin, and brassinosteroid are important hormones in regulating plant growth and development and stress resistance (40). Our results showed that five hormones were all induced by 37°C treatment, and more IAA was detected in the seed and less ABA was detected in the berry at 4°C (Supplementary Figure 2).
Volatile Organic Compounds
To determine the effect of temperature on aroma, the component of volatile organic compounds in strawberry were measured. In strawberries, alcohols, aldehydes, esters, alkanes, acids, aromatic benzenoids, ketone, and alkenes were the main components. Among them, esters were markedly affected by storage time and temperature (Supplementary Figure 3; Supplementary Table 3). Hexanoic acid ethyl ester, hexanoic acid methyl ester, and ethyl acetate were the compounds most affected by high temperature. Their levels increased rapidly in the first 3 days at 37°C, then declined. Octanoic acid ethyl ester showed increased with time at 4 and 23°C, while at 37°C, its level was rapidly increased and then declined. Acetic acid hexyl ester decreased with time in all three temperatures. The content of alcohols was higher at low temperature than at high temperature. Linalool content showed a low level at 37°C. Aldehydes and acids were less in strawberry fruit and among the detected aldehydes, 2-hexenal and hexanal were decreased with time and almost not detected at 23 and 37°C 3 days later. Ketones showed low level at 4°C, but significantly increased with storage temperature. The content of 4-methoxy-2,5-dimethyl-3(2H)-furanone was low at 4°C, while at 37°C, it accounted for 44.26% on day 10.
Cell Structure and Cell Wall Composition
To further investigate the effects of temperature on cell tissue, paraffin sections, and cell wall composition were measured. As shown in Figure 3A, cell structure was destroyed under high temperature from the day 3, and the higher the temperature, the severe the damage. Meanwhile, the change in the width and area of the epidermal cells under the same field of view was measured, which showed that the biggest change was at HT and then at RT (Figure 3B). The number of epidermal cells at low temperature remained basically unchanged between day 3 and 7, while at RT and HT, the number of cells was increased by 4 and 6.3 times, respectively (Figure 3B). The cell wall composition was greatly affected by high temperature, showed high content from day 3 (Supplementary Figure 4). The content of hemicellulose, cellulose and soluble pectin remained similar for the first 7 days. The content of protopectin was higher at 23°C than 4°C from day 3.
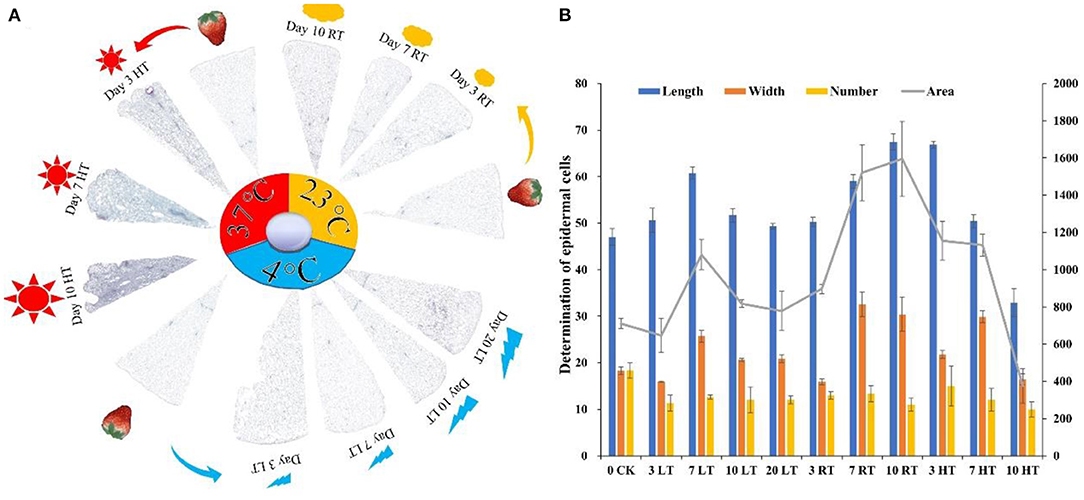
Figure 3. Paraffin section (A) and determination of epidermal cells (B) of strawberry structure at 4, 23, and 37°C. Four degree Celsius contained a good tissue structure, while destroyed at 37°C (A); the number, length, and width of epidermal cells were deceased at 37°C. The tissue fills the entire imaging field of view to ensure that the background light of each photo is consistent (n = 3). LT, RT, and HT represent the storage temperature of 4, 23, and 37°C, respectively. Each value represents the mean of three replicates. Error bar stands for standard deviation (SD) and date are expressed as means ± SD.
Proteomics Profile in Response to Different Temperatures
We used TMT proteomics to identify and quantify the induced proteins under various storage temperature conditions. The data analysis indicated that the total identified proteins were 6,657 and 5,595 of them were quantifiable. The number of differentially expressed proteins (DEPs) was summarized in a heatmap based on Pearson's correlation coefficient (Figure 4A). A total of 1,138 DEPs was identified in HT vs. RT, including 490 upregulated and 648 downregulated DEPs; LT vs. RT consisted of 177 upregulated and 180 downregulated DEPs; and LT vs. HT had 772 upregulated and 506 downregulated DEPs (Figure 4B).
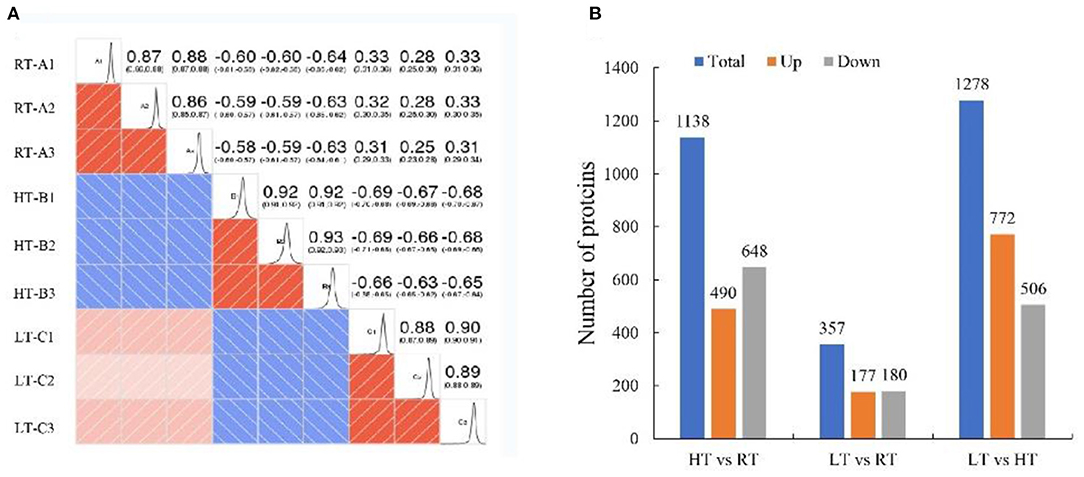
Figure 4. Proteomic analysis of samples at 4, 23, and 37°C. (A) Pearson correlation coefficient. (B) Number of differentially expressed proteins. LT, RT, and HT represent the storage temperature of 4, 23, and 37°C, respectively.
Functional Enrichment Analysis of DEPs
The associations of proteins with specific biological processes were identified and were divided into three major groups, including cellular component (CC, 1970), molecular function (MF, 5211), and biological process (BP, 5693) (Figure 5A). In HT vs. RT, the detected enriched proteins included CC (256), MF (877), and BP (895). The most enriched proteins in MF were binding (414) followed by catalytic activity (389); in CC, cell (97) and organelle (60) were highly enriched, and in the BP category, metabolic process showed the highest number of proteins (333) followed by cellular process (222) (Figures 5A,B). In LT vs. RT, MF, BP, and CC were enriched with 293, 322, and 94 proteins, respectively, and most of the proteins were enriched in biological process (Figures 5A,C). In LT vs. HT, the enriched proteins included 269 in CC, 986 in MF, and 947 in BP (Figures 5A,D).
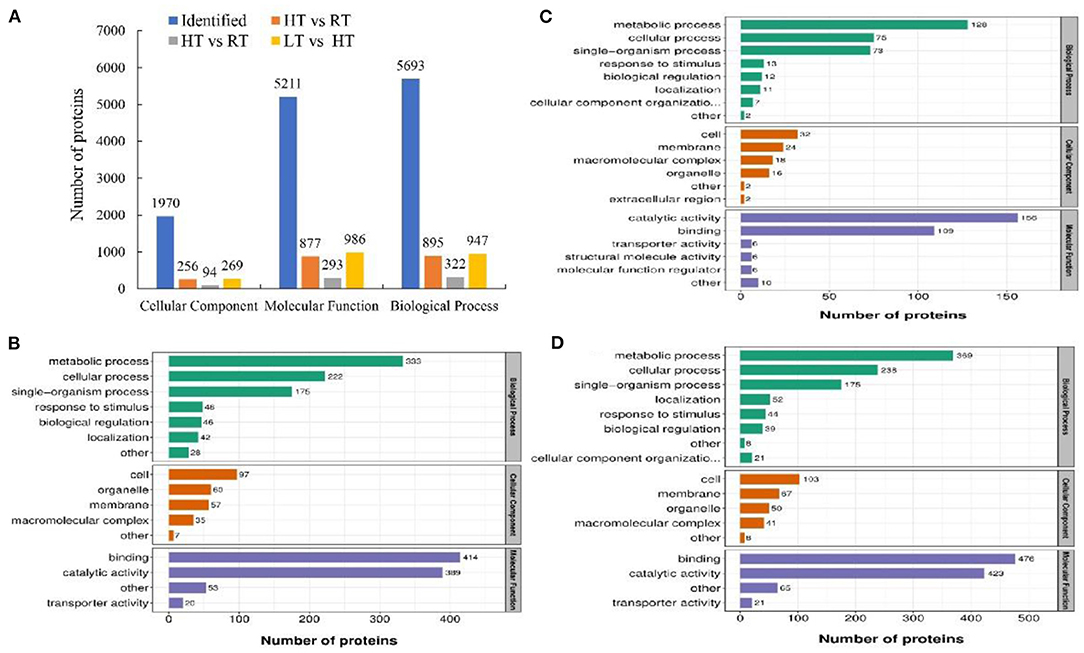
Figure 5. Number of enriched proteins and GO classification involved in specific biological processes in different comparisons at 4, 23, and 37°C. (A) Number of identified proteins and significantly enriched proteins with P < 0.05 involved in three categories including biological process, cellular component and molecular function. (B–D) Number of different GO terms in three categories. In (B–D) green columns represented the categorization of biological process, orange columns represented the categorization of cellular component, and blue columns represented the categorization of molecular function. (B) HT vs. RT; C: LT vs. RT; D: LT vs. HT. LT, RT, and HT represent the storage temperature of 4, 23, and 37°C, respectively.
To investigate the role of proteins in metabolic pathways, we analyzed the DEPs according to KEGG enrichment analysis (Supplementary Figure 5). Glutathione metabolism and cutin, suberin, and wax biosynthesis pathways were upregulated in HT vs. RT, while phenylpropanoid biosynthesis, flavonoid biosynthesis, and starch and sucrose metabolism downregulated. Compared with the RT, photosynthesis and photosynthesis-antenna proteins were upregulated under LT condition, while glycolysis/gluconeogenesis, and biosynthesis of secondary metabolites pathways were downregulated. Additionally, In the LT vs. HT, photosynthesis, phenylpropanoid biosynthesis, and starch and sucrose metabolism were upregulated, while glutathione metabolism, and ascorbate and aldarate metabolism were downregulated. In addition, the protein interaction networks for all listed samples were determined, and protein–protein interaction (PPI) maps were generated with the closely interacting proteins (Supplementary Figure 6; Supplementary Table 4). A great number of proteins interacted with heat shock protein.
Analysis of Proteins in Phenylpropanoid Biosynthetic Pathway
Phenylpropanoid biosynthetic is one of the important ways for plants to produce secondary metabolites, such as flavonoids, lignin (41). Twenty-five DEPs involved in phenylpropanoid biosynthesis under different condition were identified (Figure 6; Table 3; Supplementary Tables 5–7). Compared with at 23°C condition, 23 and 17 DEPs were identified at 37 and 4°C, respectively. Most of them were downregulated, including phenylalanine ammonia-lyase (PAL), 4-coumarate—CoA ligase (4CL), dihydroflavonol 4-reductase, leucoanthocyanidin dioxygenase, and cinnamoyl-CoA reductase, while only two POD proteins were upregulated at 4°C. Eighteen DEPs were found in LT vs. HT, and all of them were upregulated except for two caffeic acid 3-O-methyltransferase (COMT).
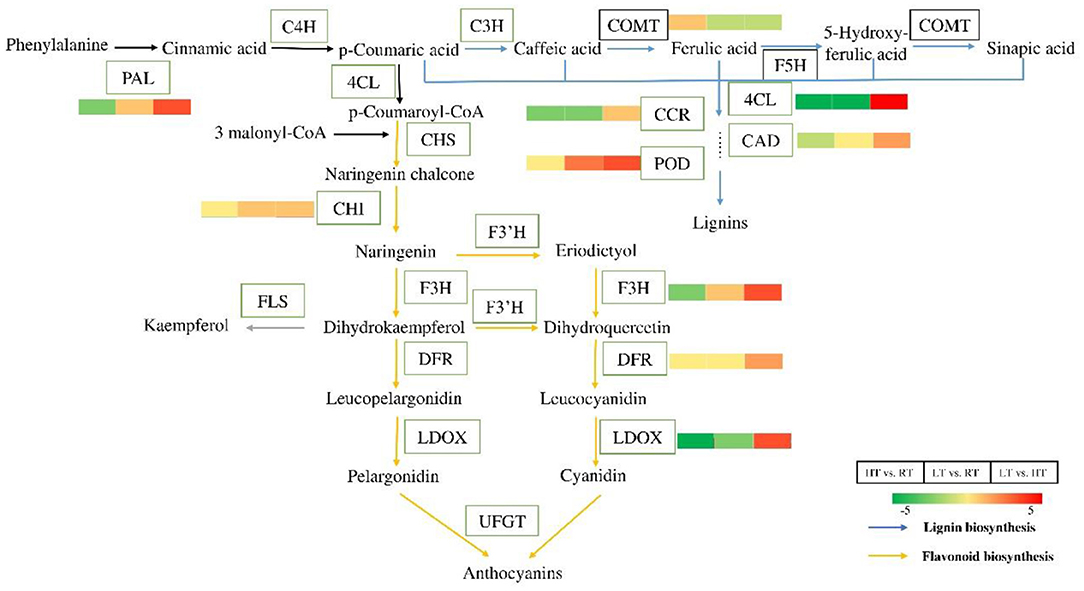
Figure 6. Differential proteins in the process of phenylpropane biosynthesis at 4, 23, and 37°C. Flavonoids and lignin are important secondary metabolite of the phenylpropane pathway. Almost all the proteins were downregulated in flavonoids and lignin biosynthesis at 4 and 37°C. But the related proteins were upregulated at 4°C compared to at 37°C. The color bars represent the number of up-regulated or down-regulated protein. The greener the color, the more the down-regulated proteins, and the redder the color, the more the up-regulated proteins. PAL, phenylalanine ammonia-lyase; C4H, coumarate 3-hydroxylase; 4CL, 4-coumarate—CoA ligase; CHS, chalcone synthase; CHI, chalcone—flavonone isomerase; F3H, flavanone 3-hydroxylase; F3′H, flavonoid-3′-hydroxylase; DFR, dihydroflavonol 4-reductase; LDOX, leucoanthocyanidin dioxygenase; UFGT, UDP-glycose flavonoid glycosyltransferase; FLS, flavonol synthase; C3H, coumarate 3-hydroxylase; COMT, caffeic acid 3-O-methyltransferase; F5H, ferulate-5-hydroxylase; CCR, cinnamoyl-CoA reductase; CAD, cinnamyl alcohol dehydrogenase; POD, peroxidase.
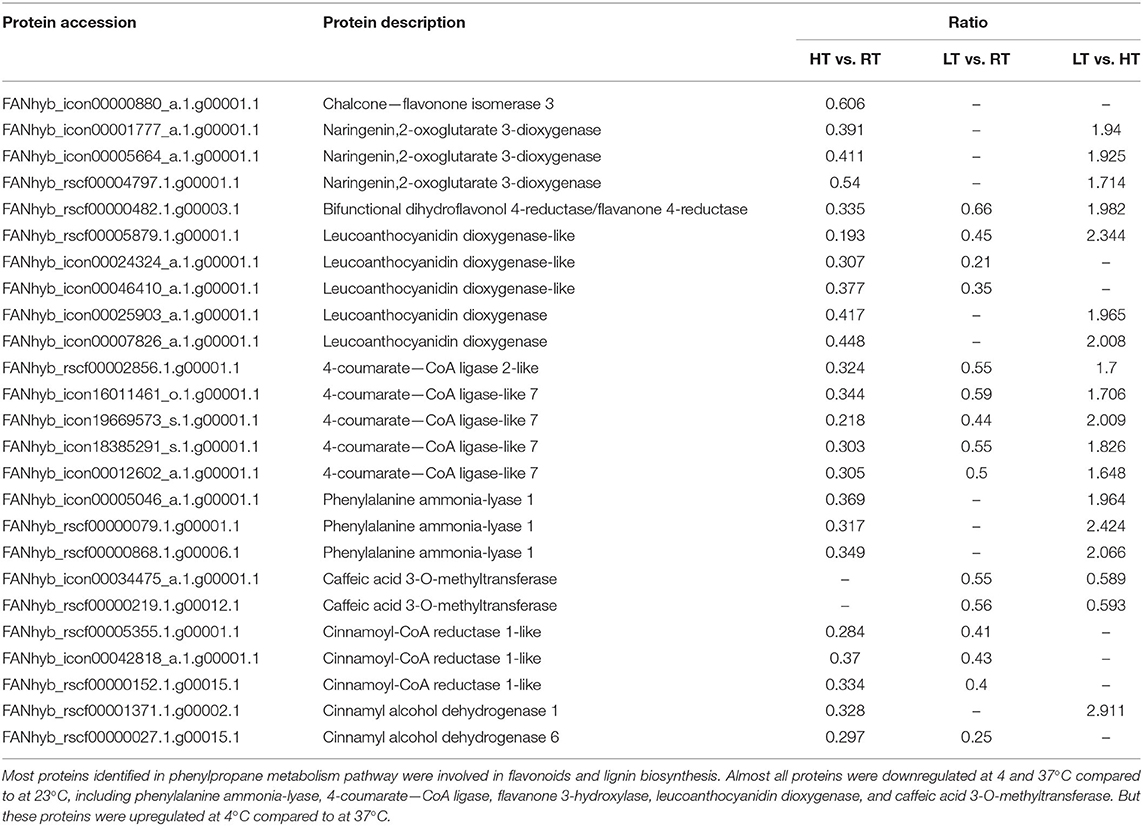
Table 3. Differentially expressed proteins involved in phenylpropane metabolism processes in strawberry at 4, 23, and 37°C.
Analysis of ROS-Related DEPs
The redox-related DEPs under different temperatures are presented in the Supplementary Table 4. At 37°C, the expression of TPR repeat-containing thioredoxin TDX and one POD protein was induced. Only a single catalase (CAT) protein was identified and upregulated by the high temperature treatment. High temperatures triggered antioxidant enzymes as well as AsA-GSHcycle enzymes. Monodehydroascorbate reductase (MDAR) and three ascorbate peroxidase (APX) proteins were identified, and all four proteins were upregulated. Seven glutathione S-transferase (GST) proteins were found, six of which were upregulated, and a single GST protein was down-regulated (Table 4). In addition, there were nine DEPs (three upregulated and six downregulated) that were modulated at 4°C, including two thioredoxin-like proteins (one upregulated and one downregulated), one downregulated phospholipid hydroperoxide glutathione peroxidase, two upregulated PODs, one downregulated APX, and three downregulated GST proteins. In LT vs. HT, 21 DEPs (3 upregulated and 18 downregulated) were identified. The downregulated proteins included three thioredoxin-like proteins, one CAT, one MDAR and six APX and six GST proteins. The three upregulated proteins were POD protein (Table 4).
Analysis of the HSP Family
HSPs are stress-responsive proteins, that can be expressed in large quantities under adversity. High temperature induced the expression of HSPs in the different comparisons, including high molecular weight HSPs and low molecular weight HSPs (Table 5; Supplementary Tables 5–7). All of the DEPs were upregulated in HT vs. RT, except heat shock cognate 70 kDa protein-like (FANhyb_rscf00001174.1g00004.1). In contrast, the same proteins showed the opposite expression pattern (upregulated) in LT vs. HT (Table 5; Supplementary Table 6). However, the differentially expressed protein in LT vs. RT was not found, which indicated that 4°C treatment could not cause any damage to the strawberry fruit.
Analysis of Cell Wall Degrading Enzymes
In response to high temperature at 37°C, two polygalacturonase (PG), three beta-galactosidase (β-Gal) isoforms, and one pectinesterase (PE) were downregulated compared with 23°C. Five xyloglucan endotransglucosylase/hydrolase (XTH) proteins were identified, and three of them were downregulated. Expansin-A10 was downregulated (Table 6; Supplementary Tables 5–7). Furthermore, PG expression was induced at 4°C and four β-Gal proteins were found as compared to 23°C. Among the four β-Gal proteins, three of them were upregulated, while one was downregulated. Four PE proteins were found, and two of them were downregulated, while the other two were upregulated. Two XTH DEPs were identified, and one of them was downregulated, while the other one was upregulated. Expansin-A10 showed down-regulated (Table 6). In the comparison of LT vs. HT, one PG protein and five β-Gal proteins were upregulated, and four PE proteins were identified, two of which were downregulated, while the other two were upregulated. Three XTH proteins and one expansin (EXP) protein were found, all of which were upregulated (Table 6).
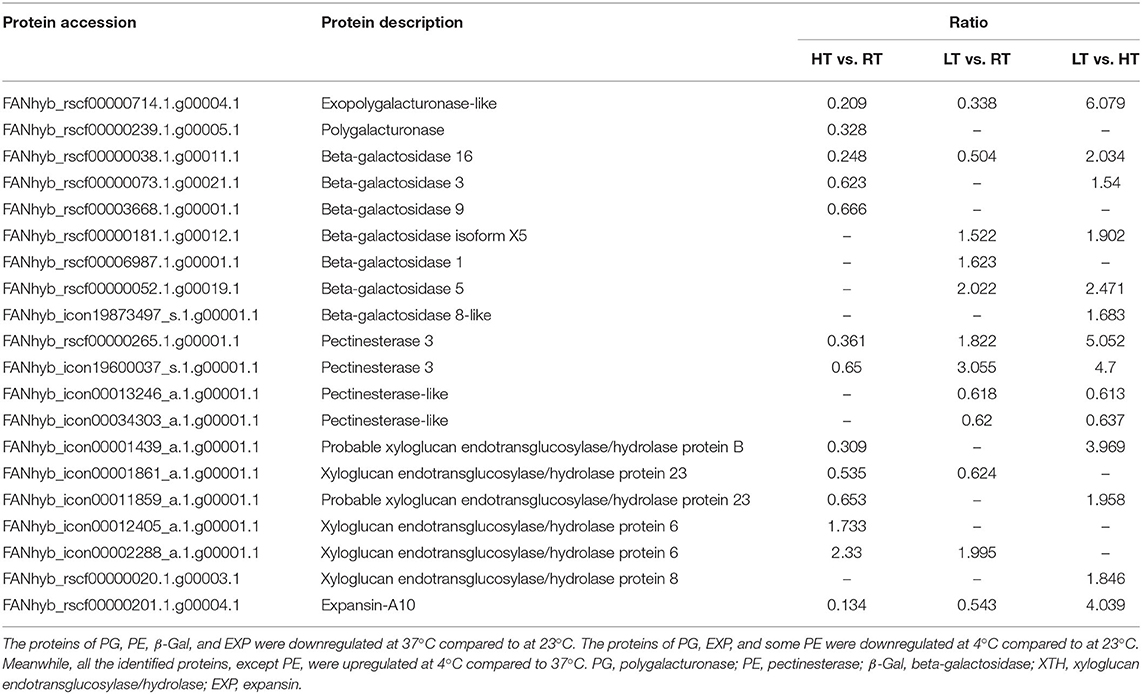
Table 6. Differentially expressed proteins involved in the cell wall degradation in strawberry at 4, 23, and 37°C.
Verification of DEPs via qPCR
The proteins involved in anthocyanins metabolic pathway and cell wall degradation, including FaPAL, FaCHS, FaF3H, FaCHI, FaDFR, FaANS, FaPG, FaPE, FaCEL, FaPL, Faβ-GAL, and FaEXP, were further confirmed by qRT-PCR. As shown in Supplementary Figure 7, the expression levels of some genes related to anthocyanin synthesis were upregulated on day 3 and then downregulated on day 7. Most of the genes expressed at 4°C were decreased for the first 7 days, and then increased on day 10, ultimately decreasing on day 20. Under the storage temperature of 23°C, a large percentage of genes was decreased with the increasing storage time. In addition, some gene expression levels were enhanced on day 3 after 37°C treatment, such as FaCHS, FaCHI2, FaF3H, FaDFR2, and FaGST. Furthermore, the expression level of cell wall degradation related genes was decreased at 4°C during the first 7 days and then increased on day 10 (Supplementary Figure 7). For example, the expression level of the FaPG, FaPL, FaCEL, Faβ-GAL, and FaEXP2 genes showed a similar trend. At high temperature, the genes expression levels of FaPG, FaPL, FaCEL, and FaEXP were downregulated with storage time, and FaPE maintained a higher expression level on day 7. Meanwhile, FaCEL, FaEXP1, and FaEXP2 were kept higher expression level on day 3. FaPE, FaXEP1, and FaEXP5 were upregulated at 23°C, and Faβ-GAL maintained a higher expression level during storage (Supplementary Figure 7). Overall, the trend of gene expression was consistent with the results of the TMT data.
Function Analysis of Proteins Selected From TMT
To investigate the function of proteins under temperature stress, we selected three proteins from TMT, including sugar phosphate/phosphate translocator (P1; GenBank Accession No. XM_004291415.2), 1-aminocyclopropane-1-carboxylate oxidase (P2; XM_004304031.2), and aquaporin PIP2-2 (P3; XM_004291529.2), and performed a BLAST search in the NCBI library. The results showed that all three proteins increased fruit color at 23°C from the 4th day after overexpression and delayed fruit browning at 4°C (Figure 7A). The total anthocyanin content was increased at 23°C after protein overexpression; however, it decreased at 37°C (Figure 7B). The P1 protein promoted sugar and citric acid accumulation at 4°C, but decreased the sugar and organic acid contents at 23 and 37°C; and both the P2 and P3 proteins decreased the sugar content and organic acid content at different temperatures (Figure 8).
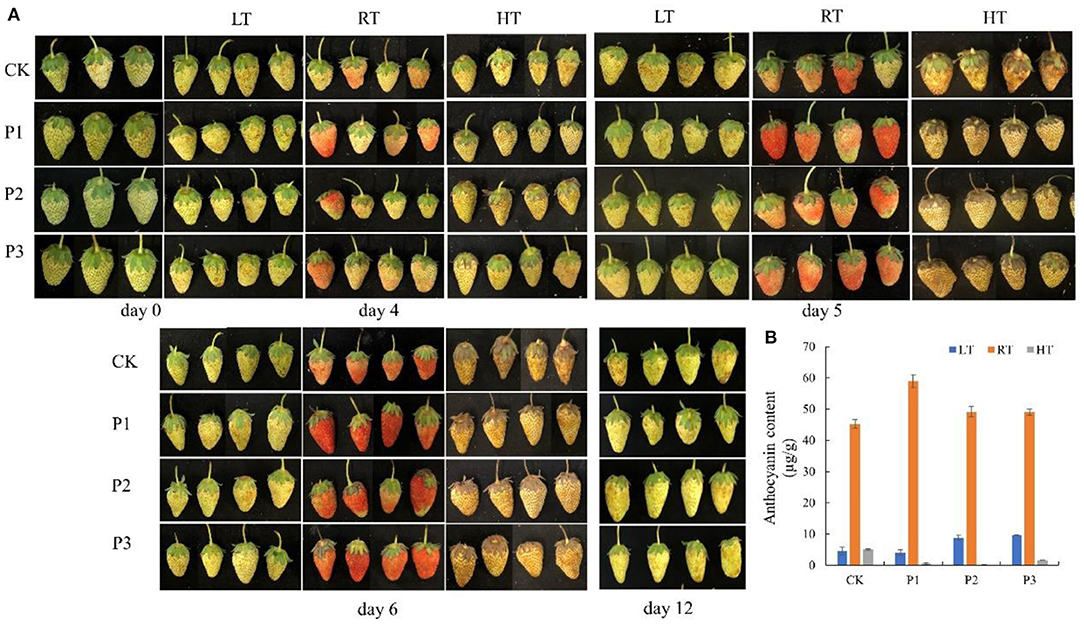
Figure 7. Overexpression of proteins in strawberry at 4, 23, and 37°C. All three proteins significantly promoted fruit coloring at 23°C from the 4th day after overexpression and delayed fruit browning at 4°C (A); total anthocyanin content was significantly increased at 23°C after protein overexpression, and P2 and P3 increased the anthocyanin content at 4°C (B). P1, sugar phosphate/phosphate translocator; P2, 1-aminocyclopropane-1-carboxylate oxidase; P3, aquaporin PIP2-2. LT, RT, and HT represent the storage temperature of 4, 23, and 37°C, respectively. Each value represents the mean of three replicates. Error bar stands for standard deviation (SD) and date are expressed as means ± SD.
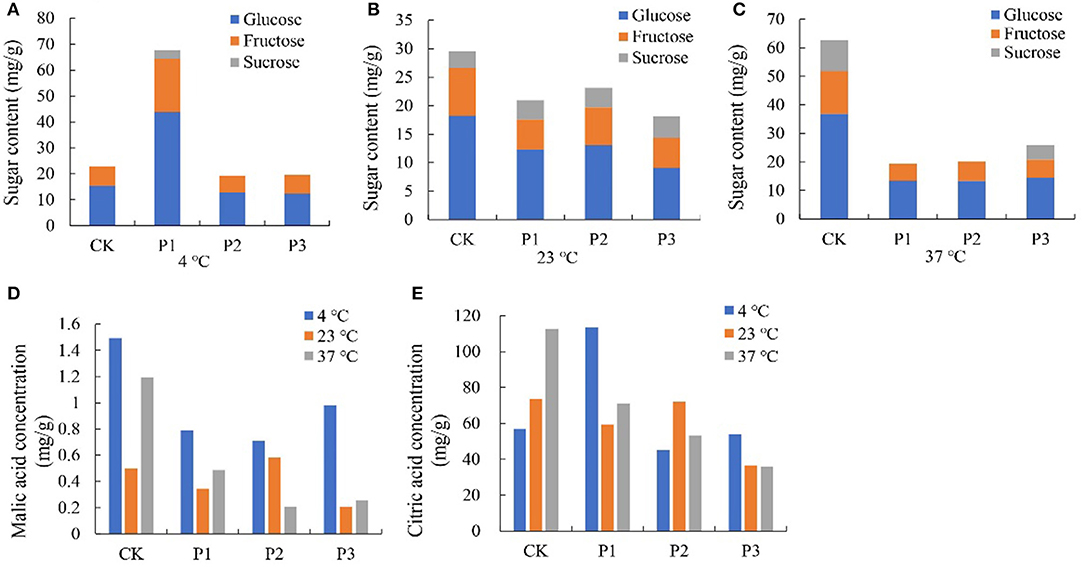
Figure 8. Effect of different overexpressed proteins on sugars and organic acids content in strawberry at 4°C, 23°C and 37°C. (A–C) The sugar content of strawberry at different storage temperatures; (D,E) The malic and citric acid content of strawberry at different storage temperatures. P1 protein promoted sugar and citric acid accumulation at 4°C, but decreased the sugar and organic acid content at 23°C and 37°C. Both the P2 and P3 proteins decreased the sugar content and organic acid content at three temperatures. P1, P2 and P3 reprented proteins of sugar phosphate/phosphate translocator; 1-aminocyclopropane-1-carboxylate oxidase and aquaporin PIP2-2, respectively.
Protein Overexpression Altered Aromatic Components
At 4°C storage temperature, 2-hexenal, diazirine, hexanal, and trichloromethane were the main aromatic components, and acetic acid ethenyl ester was only induced by P3, which accounted for 64.89% of the total volatiles. Esters and aldehydes were the main aromatic at 23°C, including 2-hexenal, 2-hexen-1-ol, acetate, (Z)-, acetic acid, methyl ester, and hexanal, but the main aromatic components were not changed. Furthermore, the P1 and P3 proteins also induced more aromatic components at 4 and 23°C, respectively, while the P2 protein induced more aromatic components at 37°C. Moreover, the P1 and P2 proteins induced greater trichloromethane, and toluene content. In addition, trichloromethane, and hexanal were increased by P3. 1-Hexanol and ethyl acetate were not detected in the strawberries under high temperature treatment (Supplementary Figure 8; Supplementary Table 8).
Protein Overexpression Altered Cell Wall Component Content and Antioxidant Enzyme Activities
Cell wall component contents and antioxidant enzyme activities were assessed to further evaluate the function of P1, P2, and P3 proteins on fruit quality. The results showed that all three proteins increased the hemicellulose content at 23°C but decreased it at 37°C in comparison with the control (Supplementary Figure 9A). The P1 and P3 proteins decreased the cellulose content at 4 and 37°C, whereas the P3 protein increased the cellulose content at 23°C (Supplementary Figure 9B). In addition, the soluble pectin content was decreased after protein overexpression, and the protopectin content at 37°C was increased by P2 (Supplementary Figures 9C,D). Furthermore, the three proteins increased SOD activity at 4 and 37°C, while P3 protein activity was increased at 23°C. All three proteins increased the POD activity at 4 and 23°C, but decreased its activity at 37°C (Supplementary Figures 9E,F).
Protein Overexpression Ripening Associated Gene Expression
The expression of FaPAL and FaCHS was upregulated at low temperatures, while the expression of FaCHI2, FaANS, FaUFGT, and FaGST expression was downregulated (Supplementary Figure 10). In addition, FaCHI1, FaDFR1, and FaDFR2 were upregulated by P1, and FaF3H was upregulated by P3. FaPE and FaEXP were downregulated by all three proteins, except for FaPE and FaXEP1, which were upregulated by P1. The expression of FaPG, FaPL, and FaCEL was decreased by the P1 protein, but increased by the P2 protein. The P3 protein had no effect on FaPG and FaCEL, but did upregulated Faβ-GAL.
Most genes, including FaCHS, FaCHI, FaF3H, and FaGST were downregulated with the overexpression of the three proteins at 23°C compared with the control (Supplementary Figure 10). P1 upregulated FaPAL, FaDFR, and FaUFGT expression, and only FaDFR1 was upregulated by the P2 protein. In addition, FaPE, FaPL, Faβ-GAL, and FaEXP2 were downregulated by all three proteins, while FaEXP1 and FaEXP5 were upregulated. FaPG and FaCEL were also downregulated by the P2 and P3 proteins.
The gene expression results at 37°C were similar to the results of the three proteins (Supplementary Figure 10). FaPLA, FaCHS, FaDFR1, FaUFGT, and FaGST were upregulated, while FaCHI1, FaF3H, FaDFR2, and FaANS were downregulated by the three proteins. Most cell wall-related genes were upregulated by the three proteins, such as FaPG, FaPL, FaCEL, and FaEXP, and only FaPE was downregulated. As for Faβ-GAL, the expression was upregulated by P1, which was opposite to that of the P2 protein.
Discussion
The appearance of fruit is the most important index for evaluating strawberry quality. Previous studies focus on the effect of low temperature on fruit quality (5, 13, 42). However, few reports have examined the effect of long-term high temperature storage on fruit quality and the response mechanism. Therefore, this study examined the influence of 4, 23, and 37°C storage temperatures to fruit quality and explored the mechanism of fruit response to different temperatures at the proteome level.
Storage Temperature Affected the Quality of Strawberry After Harvest
Storage at 4°C resulted in better visual quality than at 23°C, which was better than at 37°C (Figure 1A). An important result of loss of visual quality was the onset of water loss (Figure 1B). These results were similar to those of Shin et al. (22) who found that lower temperature maintained a better fruit quality and decreased the rate of fruit water loss. The fruit, stored at 4 and 23°C, remained relatively stable red color, but at 37°C, color changed to dark red (Figure 1A). The change of anthocyanin content was consistent with fruit color (Figure 2A). Higher storage temperature result in greater anthocyanin accumulation (5, 13, 20).
Decrease of firmness and loss of fruit peel lightness are common processes during the senescence of strawberry fruit (43). In our study, firmness and lightness kept a higher level at 4°C but significantly decreased at 37°C (Figure 1C; Table 1). Harker et al. (44) found that firmness of fruit is related to a number of cellular characteristics, including adhesion between neighboring cells, cell fragility, and internal turgor pressure. The maintenance of hardness at low temperature might be related to a better cell structure (Figure 3).
The special aroma of strawberry fruit is composed of a variety of volatile organic compounds in a certain proportion. The different content of one or several aroma components may lead to different odors. Over 360 volatile organic compounds have been identified from strawberries (45), but only a few volatiles (primarily methyl and ethyl esters) were the main contributors to strawberry aroma (5). In our study, higher level esters were induced by higher storage temperature (46), while lower level aldehydes were detected at the same time (Supplementary Figure 1; Supplementary Table 3). The amino acid metabolism pathway is an important way promoting the synthesis of aroma. In our study, the increased proportion of alanine, leucine, and isoleucine might this be the main reason for the formation of esters compounds at higher temperature (47).
Sugars and organic acids are important indexes for evaluating strawberry fruit quality and flavor (22). Good strawberry flavor is resulted in high sugar and relatively high acid content. Study have shown that glucose and fructose were the major sugars in strawberry fruit, which accounted for more than 65% of total soluble solids (48). In our study, fructose and glucose contents on day 7 and 10 were markedly lower at 37°C than lower temperature, while acid contents were higher 3 days later at 37°C (Figure 2). Increased respiration rates are likely to be the cause of a lower sugar content at elevated temperatures (5). In addition, sugars and acids, as osmotic regulators, also participated in the stress resistance process of plants (49).
Effect of Storage Temperature on Fruit Cell Wall Degradation and Phenylalanine Metabolism
The protein involved in fruit softening is mainly by inducing cell wall polysaccharide degradation or cell wall loosening (50, 51). PG is an enzyme involved in degradation of the pectic fraction of cell walls, but its action is not sufficient to promote fruit softening alone (51, 52). Other proteins, such as EXPs, may be involved in the process by inducing loosening of fruit cell walls, they have variety differences in strawberries (52). Despite the complexity of fruit softening and the presumably high number of proteins involved, most proteins identified in our study were downregulated at 37°C (Table 6). The identification of these proteins involved the loss of firmness could be related to the expression of related genes under different stress times.
Phenylpropane metabolism is an important way to produce plant secondary metabolites, which contribute to plant responses toward biotic and abiotic stimuli (53, 54). Flavonoids are one of the important branches of plant phenylpropane metabolism, which play an important role in the interaction between plants and environment (54, 55). CHS and F3H have been characterized as rate-limiting enzymes in flavonoid pathway, and studies have shown that they were strongly upregulated in strawberry at 2-d cold treatment (4). Though these proteins were identified downregulated in response to cold and heat stress, related gene expression increased on day 3 and then decreased, suggesting that these proteins were induced under short-term stress. LDOX is a key enzyme at the end of the anthocyanin synthesis pathway (55). In our study, the abundance of LDOX protein decreased at 37°C, but the content of anthocyanin increased in response to heat stress. Though strawberry is a non-climactic fruit, previous study has confirmed that respiration functioned up-stream of the ethylene-dependent signaling in the regulation of anthocyanin synthesis and high temperature can enhance the respiratory rate and ethylene production (56). We speculate that, on the one hand, high temperature could enhance proteins expression by increasing respiration and ethylene production. However, on the other hand, high temperature could also directly reduce protein expression in the fruit. Additional studies are needed to clarify this supposition.
Temperature Treatment Induced the Antioxidant System and HSPs in Strawberry Fruit
Plant contain components of enzymic and non-enzymic antioxidants which play an important role in regulating level of reactive oxygen species (ROS) in response to abiotic stress (57). In this study, POD was up-regulated during LT storage, while down-regulated during HT storage (Figure 6). PODs are enzymes that typically catalyze peroxide. Dangcham et al. (58) found that POD activity could induced by low temperature in mangosteen pericarp during storage. In addition, POD is required in the final step of lignin biosynthesis, and lignin content is associated with pericarp hardening (58, 59). The higher hardness of strawberries at 4°C, in our study, might be related to high POD activity (Figure 1C). Here, we found that the abundance of catalase isozyme 1 was significantly increased at HT compared to other storage temperatures (Table 4). CAT mainly removes hydrogen peroxide (H2O2) produced in the process of mitochondrial electron transport, β-fatty acid oxidation, and photorespiration to prevent active oxygen free radicals from harming plants (59, 60). Here, the higher abundance of CAT protein at HT storage found in this study suggested an important role in for this enzyme in cellular redox sensing under HT stress (Table 4).
Ascorbate-glutathione (AsA-GSH) cycle is one of the most important plant antioxidant systems (25). APX is one of the most important enzymes associated with the AsA-GSH cycle, and its over-expression in tomato confers tolerance to chilling stresses (61). Interestingly, the abundance of APX protein, in this study, was increased at HT, while decreased at LT. These results were consistent with Ergin et al. (62), who found that the activities of APX and CAT in both heat tolerant and heat sensitive cultivars increased with high temperatures. It is suggested that APX played a crucial role in HT stress.
HSPs, as molecular chaperones, have an important part in maintaining protein stability under stress conditions, such as drought, heat, heavy metal, and oxygen deprivation (62, 63). As shown from the TMT analysis, HSP70, HSP83, HSP90-1, and small HSPs were strongly upregulated by 37°C (Table 5). It has been reported that plant such as potato, Arabidopsis, and strawberry begin to synthesize HSP when they exposed to heat stress (64, 65). Although some studies have shown that some HSPs can be induced by cold, in this study, HSPs protein were only identified at HT. The difference of these proteins indicated that the accumulation of HSP might be related to cultivars and treatment temperatures (4).
Selected P1, P2, and P3 Proteins Improved Fruit Stress Resistance
P1 belongs to the triose-phosphate transporter (TPT) family, which plays a crucial role in photosynthesis (63, 66). A previous study also confirmed that TPT is essential for the survival to Plasmodium berghei (67). In this study, the P1 protein enhanced the concentration of sugar, citric acid, and protopectin at 4°C, as well as POD and SOD activities, which suggested that P1 played an important role in improving the antioxidant activity of the fruit and delaying the reduction of fruit flavors. However, the contents of sugars, organic acids, and cell wall components were reduced by the P1 protein under 37°C, which indicated that high temperature had a negative effect on maintaining fruit quality substances.
P2 is one of the key enzymes in ethylene synthesis (68), and a previous study confirmed that the expression of the P2 gene was upregulated in plants subjected to environmental stress (69). In our study, the contents of sugars and organic acids were decreased by P2 at different storage temperatures, while the protopectin content and SOD activity were promoted. Meantime, the POD activity at lower temperature was also induced (Figure 8; Supplementary Figure 9). These results suggested that the P2 protein had a positive response to different temperatures.
P3 is a subclass of the smallest aquaporin family located on the endoplasmic reticulum membrane (70). Xu et al. (71) found that TaTIP2;2 may be a negative regulator of abiotic stress by the heterologous expression of TaTIP2;2 in Arabidopsis thaliana. Our study results showed that the P3 protein decreased sugar, organic acid, and cellulose contents at 37°C, and the sugar and organic acids were similar between at 4°C and the control, while 4°C enhanced the content of hemicellulose and POD activity (Figure 8; Supplementary Figure 9). These results indicated that the P3 protein has a negative effect on fruit quality at high temperature, but plays an important role in low temperature stress.
In summary, the data presented in this study indicated that storage temperature significantly affected strawberry anthocyanin, aroma compounds, cell structure, sugars, organic acids, and overall quality. New details information is presented on the effect of storage temperature on strawberry anthocyanins, aroma, and antioxidant capacity from the perspective of proteomics. These resulted suggested that the overall quality was better maintained at 4°C, but ester aroma compounds and antioxidant capacity were enhanced at higher temperature (23 and 37°C). Though the proteins involved in anthocyanin synthesis were downregulated, anthocyanin content was enhanced.
Data Availability Statement
The mass spectrometry proteomics data have been deposited to the ProteomeXchange Consortium via the PRIDE partner repository with the dataset identifier PXD030184.
Author Contributions
TZ and JL conducted sample treatment, data analysis, and drafted the manuscript. TD and ZS helped with data analysis. HJ participated in the design of the study. TP revised the manuscript. HJ and JF designed and coordinated this study and revised the manuscript. All authors have read and approved the final manuscript.
Funding
This study was supported by the National Key Research and Development Project (No. 2018YFD1000200), National Natural Science Foundation of China (Nos. 31872938 and 31872047), Jiangsu Excellent Youth Fund Project (No. BK20180076), Jiangsu Natural Science Foundation (No. iBK20201176), Natural Science Research Project of Colleges and Universities in Jiangsu (No. 20KJD210001), and Jiangsu Independent Innovation of Agricultural Science and Technology (No. CX(19)3088).
Conflict of Interest
The authors declare that the research was conducted in the absence of any commercial or financial relationships that could be construed as a potential conflict of interest.
Publisher's Note
All claims expressed in this article are solely those of the authors and do not necessarily represent those of their affiliated organizations, or those of the publisher, the editors and the reviewers. Any product that may be evaluated in this article, or claim that may be made by its manufacturer, is not guaranteed or endorsed by the publisher.
Acknowledgments
We would like to express our gratitude to Central Laboratory of College of Horticulture, Nanjing Agricultural University. We thank Shaoyan Lin from the State Key Laboratory of Crop Genetics and Germplasm Enhancement for helping me analyze GC-MS data.
Supplementary Material
The Supplementary Material for this article can be found online at: https://www.frontiersin.org/articles/10.3389/fnut.2021.812666/full#supplementary-material
Supplementary Figure 1. Amino acid content in seed and berry of strawberry at 4, 23, and 37°C.
Supplementary Figure 2. Hormone concentration in seed and berry at 4, 23, and 37°C.
Supplementary Figure 3. Strawberry aroma components mass spectrum at 4, 23, and 37°C.
Supplementary Figure 4. Cell wall component content of strawberry at 4, 23, and 37°C.
Supplementary Figure 5. Differentially expressed proteins (DEPs) in KEGG pathways at 4, 23, and 37°C.
Supplementary Figure 6. Protein interaction network in different metabolism pathways at 4, 23, and 37°C.
Supplementary Figure 7. Relative gene expression level in strawberry at 4, 23, and 37°C.
Supplementary Figure 8. Strawberry aroma components mass spectrum after proteins overexpression at 4, 23, and 37°C.
Supplementary Figure 9. Cell wall component content and antioxidant enzyme activity after proteins overexpression at 4, 23, and 37°C.
Supplementary Figure 10. Effect of proteins overexpression on relative gene expression level at 4, 23, and 37°C.
Supplementary Table 1. Primers used for qRT-PCR on strawberry.
Supplementary Table 2. Chromatogram of anthocyanin content in strawberry berry and seed.
Supplementary Table 3. Aroma content in strawberry under different storage temperature.
Supplementary Table 4. Differentially expressed protein involved in interaction network.
Supplementary Table 5. Differentially expressed proteins in HT vs. RT.
Supplementary Table 6. Differentially expressed proteins in LT vs. RT.
Supplementary Table 7. Differentially expressed proteins in LT vs. HT.
Supplementary Table 8. Aroma content after protein overexpression.
Abbreviations
APX, ascorbate peroxidase; CAT, catalase; EXP, expansin; GST, glutathione S-transferase; HSPs, heat shock proteins; HT, high temperature (37°C); LT, low temperature (4°C); PAL, phenylalanine ammonia-lyase; P1, sugar phosphate/phosphate translocator; P2, 1-aminocyclopropane-1-carboxylate oxidase; P3, aquaporin PIP2-2; PG, polygalacturonase; RT, room temperature (23°C); 4CL, 4-Coumarate—CoA ligase; XTH, xyloglucan endotransglucosylase/hydrolase; β-Gal, beta-galactosidase.
References
1. Naemura A, Mitani T, Ijiri Y, Tamura Y, Yamashita T, Okimura M, et al. Anti-thrombotic effect of strawberries. Blood Coagul Fibrinolysis. (2005) 16:501–9. doi: 10.1097/01.mbc.0000184737.50594.a8
2. Wang SY, Feng RT, Lu YJ, Bowman L, Ding M. Inhibitory effect on activator protein-1, nuclear factor-kappaB, and cell transformation by extracts of strawberries (Fragaria × ananassa Duch.). J Agric Food Chem. (2005) 53:4187–93. doi: 10.1021/jf0478049
3. Zhang LP, Wang L, Zeng XG, Chen RX, Yang SZ, Pan SY. Comparative transcriptome analysis reveals fruit discoloration mechanisms in postharvest strawberries in response to high ambient temperature. Food Chem X. (2019) 2:100025. doi: 10.1016/j.fochx.2019.100025
4. Koehler G, Wilson RC, Goodpaster JV, Sønsteby A, Lai X, Witzmann FA, et al. Proteomic study of low-temperature responses in strawberry cultivars (Fragaria × ananassa) that differ in cold tolerance. Plant Physiol. (2012) 159:1787–805. doi: 10.1104/pp.112.198267
5. Ayala-Zavala JF, Wang SY, Wang CY, Gonzalez-Aguilar GA. Effect of storage temperatures on antioxidant capacity and aroma compounds in strawberry fruit. LWT Food Sci Technol. (2004) 37:687–95. doi: 10.1016/j.lwt.2004.03.002
6. Azodanlou R, Darbellay C, Luisier JL, Villettaz JC, Amado R. Quality assessment of strawberries (Fragaria species). J Agric Food Chem. (2003) 51:715–21. doi: 10.1021/jf0200467
7. Wang SY, Lin HS. Antioxidant activity in fruit and leaves of blackberry, raspberry, and strawberry varies with cultivar and developmental stage. J Agric Food Chem. (2000) 48:140–6. doi: 10.1021/jf9908345
8. Meyers KJ, Watkins CB, Pritts MP, Liu RH. Antioxidant and antiproliferative activities of strawberries. J Agric Food Chem. (2003) 51:6887–92. doi: 10.1021/jf034506n
9. Wang H, Cao GH, Prior RL. Oxygen radical absorbing capacity of anthocyanins. J Agric Food Chem. (1997) 45:304–9. doi: 10.1021/jf960421t
10. Given NK, Venis MA, Grierson D. Phenylalanine ammonia-lyase activity and anthocyanin synthesis in ripening strawberry fruit. J Plant Physiol. (1988) 133:25–30. doi: 10.1016/S0176-1617(88)80079-8
11. Bood K, Zabetakis I. The biosynthesis of strawberry flavor (II): biosynthetic and molecular biology studies. J Food Sci. (2002) 67:2–8. doi: 10.1111/j.1365-2621.2002.tb11349.x
12. Zhu J, Dong CH, Zhu JK. Interplay between cold-responsive gene regulation, metabolism and RNA processing during plant cold acclimation. Curr Opin Plant Biol. (2007) 10:190–295. doi: 10.1016/j.pbi.2007.04.010
13. Kalt W, Forney CH, Martin A, Prior RL. Antioxidant capacity, vitamin C, phenolics, and anthocyanins after fresh storage of small fruit. J Agric Food Chem. (1999) 47:4638–44. doi: 10.1021/jf990266t
14. Guy C, Kaplan F, Kopka J, Selbig J, Hincha DK. Metabolomics of temperature stress. Physiol Plant. (2008) 132:220–35. doi: 10.1111/j.1399-3054.2007.00999.x
15. Wang WX, Vinocur B, Shoseyov O, Altman A. Role of plant heat-shock proteins and molecular chaperones in the abiotic stress response. Trends Plant Sci. (2004) 9:244–52. doi: 10.1016/j.tplants.2004.03.006
16. Kaplan F, Kopka J, Haskell DW, Zhao W, Schiller KC, Gatzke N, et al. Exploring the temperature-stress metabolome of Arabidopsis. Plant Physiol. (2004) 136:4159–68. doi: 10.1104/pp.104.052142
17. Xu S, Li JL, Zhang XQ, Wei H, Cui LJ. Effects of heat acclimation pretreatment on changes of membrane lipid peroxidation, antioxidant metabolites, and ultrastructure of chloroplasts in two cool-season turfgrass species under heat stress. Environ Exp Bot. (2006) 56:274–85. doi: 10.1016/j.envexpbot.2005.03.002
18. Giongo L, Poncetta P, Loretti P, Costa F. Texture profiling of blueberries (Vaccinium spp.) during fruit development, ripening and storage. Postharvest Biol Technol. (2013) 76:34–9. doi: 10.1016/j.postharvbio.2012.09.004
19. Deng Y, Wu Y, Li Y. Changes in firmness, cell wall composition and cell wall hydrolases of grapes stored in high oxygen atmospheres. Food Res Int. (2005) 38:769–76. doi: 10.1016/j.foodres.2005.03.003
20. Cordenunsi BR, Genovese MI, Nascimento JRO, Hassimotto NMA, Santos RJ, Lajolo FM. Effects of temperature on the chemical composition and antioxidant activity of three strawberry cultivars. Food Chem. (2005) 91:113–21. doi: 10.1016/j.foodchem.2004.05.054
21. Nunes MCN, Brecht JK, Morais A, Sargent SA. Controlling temperature and water loss to maintain ascorbic acid levels in strawberries during postharvest handling. J Food Sci. (1998) 63:1033–6. doi: 10.1111/j.1365-2621.1998.tb15848.x
22. Shin Y, Liu RH, Nock JF, Holliday D, Watkins CB. Temperature and relative humidity effects on quality, total ascorbic acid, phenolics and flavonoid concentrations, and antioxidant activity of strawberry. Postharvest Biol Tec. (2007) 45:349–57. doi: 10.1016/j.postharvbio.2007.03.007
23. Wasinger VC, Cordwell SJ, Cerpa-Poljak A, Yan JX, Gooley AA, Wilkins MR, et al. Progress with gene-product mapping of the Mollicutes: Mycoplasma genitalium. Electrophoresis. (1995) 16:1090–4. doi: 10.1002/elps.11501601185
24. Aebersold R, Mann M. Mass spectrometry-based proteomics. Nature. (2003) 422:198–207. doi: 10.1038/nature01511
25. Rocco M, D'Ambrosio C, Manns MP, Trautwein C. Proteomic analysis of tomato fruits from two ecotypes during ripening. Proteomics. (2006) 6:3781–91. doi: 10.1002/pmic.200600128
26. Yun Z, Jin S, Ding YD, Wang Z, Gao HJ, Pan ZY, et al. Comparative transcriptomics and proteomics analysis of citrus fruit, to improve understanding of the effect of low temperature on maintaining fruit quality during lengthy post-harvest storage. J Exp Bot. (2012) 63:2873–93. doi: 10.1093/jxb/err390
27. Pan ZY, Liu Q, Yun Z, Guan R, Zeng WF, Xu Q, et al. Comparative proteomics of a lycopene-accumulating mutant reveals the important role of oxidative stress on carotenogenesis in sweet orange (Citrus sinensis [L.] Osbeck). Proteomics. (2009) 9:5455–70. doi: 10.1002/pmic.200900092
28. Liao WY, Lin LF, Jheng JL, Wang CC, Yang JH, Chou ML. Identification of heat shock transcription factor genes involved in thermotolerance of octoploid cultivated strawberry. Int J Mol Sci. (2016) 17:2130. doi: 10.3390/ijms17122130
29. Cheng GW, Breen PJ. Activity of phenylalanine ammonia-lyase (PAL) and concentrations of anthocyanins and phenolics in developing strawberry fruit. J Am Soc Hortic Sci. (1991) 116:865–9. doi: 10.21273/JASHS.116.5.865
30. Lv JH, Pang QQ, Chen X, Li T, Fang JG, Lin SY, et al. Transcriptome analysis of strawberry fruit in response to exogenous arginine. Planta. (2020) 252:82. doi: 10.1007/s00425-020-03489-w
31. Zhang K, Liu ZJ, Guan L, Zheng T, Jiu ST, Zhu XD, et al. Changes of anthocyanin component biosynthesis in 'Summer Black' grape berries after the red flesh mutation occurred. J Agric Food Chem. (2018) 66:9209–18. doi: 10.1021/acs.jafc.8b02150
32. Wang Y, Li B, Du M, Eneji E, Wang B, Duan L, et al. Mechanism of phytohormone involvement in feedback regulation of cotton leaf senescence induced by potassium deficiency. J Exp Bot. (2012) 63:5887–901. doi: 10.1093/jxb/ers238
33. Garrido-Bigotes A, Figueroa PM, Figueroa CR. Jasmonate metabolism and its relationship with abscisic acid during strawberry fruit development and ripening. Plant Growth Regul. (2018) 37:101–13. doi: 10.1007/s00344-017-9710-x
34. Zheng T, Zhang SH, Leng XP, Sadeghnezhad E, Li T, Pervaiz T, et al. Profiling analysis of volatile and non-volatile compounds in Vitis Vinifera berries (cv. Chardonnay) and spontaneous bud mutation. Front Nutr. (2021) 8:715528. doi: 10.3389/fnut.2021.715528
35. Pan X, Welti R, Wang X. Quantitative analysis of major plant hormones in crude plant extracts by high-performance liquid chromatography–mass spectrometry. Nat Protocols. (2010) 5:986–92. doi: 10.1038/nprot.2010.37
36. Giannopolitis CN, Ries SK. Superoxide dismutase I. Occurrence in higher plants. Plant Physiol. (1977) 59:309–14. doi: 10.1104/pp.59.2.309
37. Han Y, Ban Q, Li H, Hou Y, Jin N, Han S, et al. DkXTH8, a novel xyloglucan endotransglucosylase/hydrolase in persimmon, alters cell wall structure and promotes leaf senescence and fruit postharvest softening. Sci Rep. (2016) 6:39155. doi: 10.1038/srep39155
38. Zheng T, Zhang K, Sadeghnezhad E, Jiu ST, Zhu XD, Dong TY, et al. Chitinase family genes in grape differentially expressed in a manner specific to fruit species in response to Botrytis cinerea. Mol Biol Rep. (2020) 47:7349–63. doi: 10.1007/s11033-020-05791-y
39. Chen C, Chen H, Zhang Y, Thomas HR, Frank MH, He Y, et al. TBtools: an integrative toolkit developed for interactive analyses of big biological data. Mol Plant. (2020) 13:1194–202. doi: 10.1016/j.molp.2020.06.009
40. Chen JX, Mao LC, Lu WJ, Ying TJ, Luo ZS. Transcriptome profiling of postharvest strawberry fruit in response to exogenous auxin and abscisic acid. Planta. (2016) 243:183–97. doi: 10.1007/s00425-015-2402-5
42. Castelló ML, Fito PJ, Chiralt A. Effect of osmotic dehydration and vacuum impregnation on respiration rate of cut strawberries. LWT Food Sci Technol. (2006) 39:1171–9. doi: 10.1016/j.lwt.2005.07.001
43. Shin Y, Ryu JA, Liu RH, Nock JF, Watkins CB. Harvest maturity, storage temperature and relative humidity affect fruit quality, antioxidant contents and activity, and inhibition of cell proliferation of strawberry fruit. Postharvest Biol Tec. (2008) 49:201–9. doi: 10.1016/j.postharvbio.2008.02.008
44. Harker FR, Redgwell RJ, Hallett IC, Murray SH, Carter G. Texture of fresh fruit. Hort Rev. (1997) 20:121–224. doi: 10.1002/9780470650646.ch2
45. Forney CF, Kalt W, McDonald JE, Jordan MA. Changes in strawberry fruit quality during ripening on and off the plant. Acta Hortic. (1998) 464:506. doi: 10.17660/ActaHortic.1998.464.98
46. Pérez AG, Olías R, Luaces P, Sanz C. Biosynthesis of strawberry aroma compounds through amino acid metabolism. J Agric Food Chem. (2002) 50:4037–42. doi: 10.1021/jf011465r
47. Wang SY, Camp MJ. Temperatures after bloom affect plant growth and fruit quality of strawberry. Sci Hortic. (2000) 85:183–99. doi: 10.1016/S0304-4238(99)00143-0
48. Rontein D, Basset G, Hanson AD. Metabolic engineering of osmoprotectant accumulation in plants. Metab Eng. (2002) 4:49–56. doi: 10.1006/mben.2001.0208
49. Gu TT, Jia SF, Huang XR, Wang H, Fu WM. Transcriptome and hormone analyses provide insights into hormonal regulation in strawberry ripening. Planta. (2019) 250:145–62. doi: 10.1007/s00425-019-03155-w
50. Carpita NC, Gibeaut DM. Structural models of primary cell walls in flowering plants: consistency of molecular structure with the physical properties of the walls during growth. Plant J. (1993) 3:1–30.
51. Villarreal NM, Rosli HG, Martínez GA, Civello PM. Polygalacturonase activity and expression of related genes during ripening of strawberry cultivars with contrasting fruit firmness. Postharvest Biol Tec. (2008) 47:141–50. doi: 10.1016/j.postharvbio.2007.06.011
52. Hadfield KA, Bennett AB. Polygalacturonases: many genes in search of a function. Plant Physiol. (1998) 117:337–43. doi: 10.1104/pp.117.2.337
53. Camera SL, Gouzerh G, Dhondt S, Hoffmann L, Frittig B, Legrand M, et al. Metabolic reprogramming in plant innate immunity: the contributions of phenylpropanoid and oxylipin pathways. Immunol Rev. (2004) 198:267–84. doi: 10.1111/j.0105-2896.2004.0129.x
54. Weisshaar B, Jenkins GI. Phenylpropanoid biosynthesis and its regulation. Curr Opin Plant Biol. (1998) 1:251–7. doi: 10.1016/S1369-5266(98)80113-1
55. Niu J, Zhang G, Zhang W, Goltsev V, Sun S, Wang J, et al. Anthocyanin concentration depends on the counterbalance between its synthesis and degradation in plum fruit at high temperature. Sci Rep. (2017) 7:7684. doi: 10.1038/s41598-017-07896-0
56. Abrahams S, Lee E, Walker AR, Tanner GJ, Larkin PJ, Ashton AR. The Arabidopsis TDS4 gene encodes leucoanthocyanidin dioxygenase (LDOX) and is essential for proanthocyanidin synthesis and vacuole development. Plant J. (2003) 35:624–36. doi: 10.1046/j.1365-313X.2003.01834.x
57. Apel K, Hirt H. Reactive oxygen species: metabolism, oxidative stress, and signal transduction. Annu Rev Plant Biol. (2004) 55:373–99. doi: 10.1146/annurev.arplant.55.031903.141701
58. Dangcham S, Bowen J, Ferguson IB, Ketsaa S. Effect of temperature and low oxygen on pericarp hardening of mangosteen fruit stored at low temperature. Postharvest Biol Technol. (2008) 50:37–44. doi: 10.1016/j.postharvbio.2008.02.005
59. Cai C, Xu CJ, Shan LL, Zhou CH, Zhang WS, Ferguson I, et al. Low temperature conditioning reduces postharvest chilling injury in loquat fruit. Postharvest Biol Technol. (2006) 41:252–9. doi: 10.1016/j.postharvbio.2006.04.015
60. Yang T, Poovaiah BW. Hydrogen peroxide homeostasis: activation of plant catalase by calcium. Proc Natl Acad Sci USA. (2002) 99:4097–102. doi: 10.1073/pnas.052564899
61. Wang Y, Wisniewski M, Meilan R, Cui M, Webb R, Fuchigami L. Overexpression of cytosolic ascorbate peroxidase in tomato confers tolerance to chilling and salt stress. J Am Soc Hortic Sci. (2005) 130:167–73. doi: 10.21273/JASHS.130.2.167
62. Ergin S, Gülen H, Kesici M, Turhan E, Ipek A, Köksal N. Effects of high temperature stress on enzymatic and nonenzymatic antioxidants and proteins in strawberry plants. Turk J Agric For. (2016) 40:908–17. doi: 10.3906/tar-1606-144
63. Xie H, Yang DH, Yao H, Bai G, Zhang YH, Xiao BG. iTRAQ-based quantitative proteomic analysis reveals proteomic changes in leaves of cultivated tobacco (Nicotiana tabacum) in response to drought stress. Biochem Bioph Res Commun. (2016) 469:768–75. doi: 10.1016/j.bbrc.2015.11.133
64. Savic J, Dragicevic I, Pantelic D, Oljaca J, Momcilovic I. Expression of small heat shock proteins and heat tolerance in potato (Solanum tuberosum L.). Arch Biol Sci. (2012) 64:135–44. doi: 10.2298/ABS1201135S
65. Zhong L, Zhou W, Wang H, Ding S, Lu Q, Wen X, et al. Chloroplast small heat shock protein HSP21 interacts with plastid nucleoid protein pTAC5 and is essential for chloroplast development in Arabidopsis under heat stress. Plant Cell. (2013) 25:2925–43. doi: 10.1105/tpc.113.111229
66. Lee Y, Nishizawa T, Takemoto M, Kumazaki K, Yamashita K, Hirata K, et al. Structure of the triose-phosphate/phosphate translocator reveals the basis of substrate specificity. Nat Plants. (2017) 3:825–32. doi: 10.1038/s41477-017-0022-8
67. Banerjee T, Jaijyan DK, Surolia N, Singh AP, Surolia A. Apicoplast triose phosphate transporter (TPT) gene knockout is lethal for Plasmodium. Mol Biochem Parasit. (2012) 186:44–50. doi: 10.1016/j.molbiopara.2012.09.008
68. Mathooko FM. Regulation of ethylene biosynthesis in higher plants by carbon dioxide. Postharvest Biol Tec. (1996) 7:1–26. doi: 10.1016/0925-5214(95)00026-7
69. Barry CS, Blume B, Bouzayen M, Cooper W, Hamilton AJ, Grierson D. Differential expression of the 1-aminocyclopropane-1-carboxylate oxidase gene family of tomato. Plant J. (1996) 9:525–35. doi: 10.1046/j.1365-313X.1996.09040525.x
70. Bienert GP, Chaumont F. Plant aquaporins: roles in water homeostasis, nutrition, and signaling processes. Trans Pumps Plant Signal. (2011) 7:3–36. doi: 10.1007/978-3-642-14369-4_1
Keywords: Fragaria × ananassa, post-harvest fruit quality, storage temperature, proteomic profiling, protein function
Citation: Lv J, Zheng T, Song Z, Pervaiz T, Dong T, Zhang Y, Jia H and Fang J (2022) Strawberry Proteome Responses to Controlled Hot and Cold Stress Partly Mimic Post-harvest Storage Temperature Effects on Fruit Quality. Front. Nutr. 8:812666. doi: 10.3389/fnut.2021.812666
Received: 10 November 2021; Accepted: 30 December 2021;
Published: 15 February 2022.
Edited by:
Haroon Khan, Abdul Wali Khan University Mardan, PakistanReviewed by:
Sajid Ali, Bahauddin Zakariya University, PakistanHammad Ullah, University of Naples Federico II, Italy
Copyright © 2022 Lv, Zheng, Song, Pervaiz, Dong, Zhang, Jia and Fang. This is an open-access article distributed under the terms of the Creative Commons Attribution License (CC BY). The use, distribution or reproduction in other forums is permitted, provided the original author(s) and the copyright owner(s) are credited and that the original publication in this journal is cited, in accordance with accepted academic practice. No use, distribution or reproduction is permitted which does not comply with these terms.
*Correspondence: Haifeng Jia, amlhaGFpZmVuZ0BuamF1LmVkdS5jbg==
†These authors have contributed equally to this work