- 1Horticultural Sciences Department, University of Florida, Gainesville, FL, United States
- 2Plant Pathology Department, University of Florida, Gainesville, FL, United States
- 3Tropical Research and Education Center, University of Florida, Homestead, FL, United States
In recent decades, increasing attention has been paid to food safety and organic horticulture. Thus, people are looking for natural products to manage plant diseases, pests, and weeds. Essential oils (EOs) or EO-based products are potentially promising candidates for biocontrol agents due to their safe, bioactive, biodegradable, ecologically, and economically viable properties. Born of necessity or commercial interest to satisfy market demand for natural products, this emerging technology is highly anticipated, but its application has been limited without the benefit of a thorough analysis of the scientific evidence on efficacy, scope, and mechanism of action. This review covers the uses of EOs as broad-spectrum biocontrol agents in both preharvest and postharvest systems. The known functions of EOs in suppressing fungi, bacteria, viruses, pests, and weeds are briefly summarized. Related results and possible modes of action from recent research are listed. The weaknesses of applying EOs are also discussed, such as high volatility and low stability, low water solubility, strong influence on organoleptic properties, and phytotoxic effects. Therefore, EO formulations and methods of incorporation to enhance the strengths and compensate for the shortages are outlined. This review also concludes with research directions needed to better understand and fully evaluate EOs and provides an outlook on the prospects for future applications of EOs in organic horticulture production.
Introduction
Plant essential oils (EOs) are natural, complex, volatile aromatic, hydrophobic, oily liquids composed of multiple related compounds synthesized in aromatic plants as secondary metabolites (1). Aromatic plants have been appreciated and used for their aromatic and medicinal properties since ancient times. Some aromatic herbs, such as thyme (Thymus vulgaris), savory (Satureja hortensis), cinnamon (Cinnamomum verum), cumin (Cuminum cyminum), rosemary (Salvia rosmarinus), and clove (Syzygium aromaticum) are used worldwide as seasonings to enrich food flavors (2). In addition, many perfumes and fragrances use the fragrant oil of aromatic plants as their main ingredients, for instance, lavender oil, rosemary oil, lemongrass oil, and mint oil, etc. Recently, EOs have also been used as the major therapeutic agents for aroma and massage therapy due to their antiseptic and skin permeability properties. Inhalation, topical application to the skin, massage, and bath are the major methods used in aromatherapy. Aromatherapy utilizes various EOs to treat mental stress and anxiety, as well as numerous other ailments like depression, indigestion, headache and migraine, insomnia, muscular pain, respiratory problems, skin ailments, and swollen joints (2). In a review of the pharmaceutical and therapeutic potential of EOs, Edris (3) summarized published reports of EOs that have shown potential to improve immunity, enhance energy and mental clarity, suppress cancer, and prevent cardiovascular diseases, cholesterol, and diabetes.
Conventional fungicides can cause potential ecotoxicological risks and be hazardous to a wide range of non-target organisms in aquatic systems because they impact basic biological processes that are not unique to fungi (4). The increasing need to control plant pathogens and arthropod pests in organic fruit production promotes the search for safe and effective compounds from natural sources, especially plant-derived compounds. Essential oils have potential in insecticidal, anti-bacterial, anti-fungal, and anti-viral functions as they effectively destroy several pests and pathogens due to the actions of various functional groups such as alcohols, aldehydes, phenolics, terpenes, ketones, and other antimicrobial compounds (1, 5). However, most EOs still need to be handled cautiously and following labeling recommendations given for each situation because EOs may be phytotoxic and cytotoxic at high concentrations (6, 7).
The efficacy of conventional treatments and EO treatments have been compared in some studies. In a study by Zaka et al. (8), four plant essential oils, two plant extracts, two herbicides, and two insecticides were tested against Tribolium confusum, a stored grain insect pest. The results suggested that even though the two conventional insecticides, abamectin and cypermethrin, caused higher mortalities in a shorter time, the EOs also showed promising results. Neem EO and citrus plant extract also killed adults of T. confusum quicker compared to other treatments. EOs can be better alternatives to highly toxic and hazardous chemicals for stored grain pests management. In another study by Khaliq et al. (9), five EOs (Calotropis procera, Azadirachta indica, Eucalyptus camaldulensis, Datura stramonium and Nicotiana tabacum) and a conventional fumigant (phosphine gas) were tested at various concentrations individually and synergistically against red flour beetle (Tribolium castaneum). The EO of N. tabacum (15%) and phosphine gas (500 ppm) caused the highest mortality. The highest synergistic toxic effect were observed for 15% N. tabacum and A. indica EOs with 500 ppm phosphine gas combinations. The EOs presented promise as alternatives or synergists to improve the efficacy of conventional insecticides. Antibiotics and the EOs may act synergistically by affecting multiple targets, physicochemical interactions, and inhibiting antibacterial-resistance mechanisms. With a better understanding of the mechanisms underpinning synergism, it may be possible to create safe combinations to reduce antibiotic use (10).
Essential oil composition is mainly determined by genetic, climatic, geographical, and seasonal factors (11). Main antioxidant bioactive compounds of several of the most commonly used EOs are shown in Figure 1. Furthermore, most of the terpenoids and phenols found in EOs have generally fewer toxic effects on plants and mammals than synthetic chemicals (13, 14). The function of EOs in plants is antimicrobial, antioxidant, and insecticidal defense, moreover, their strong flavor makes plants less palatable for herbivores (15, 16).
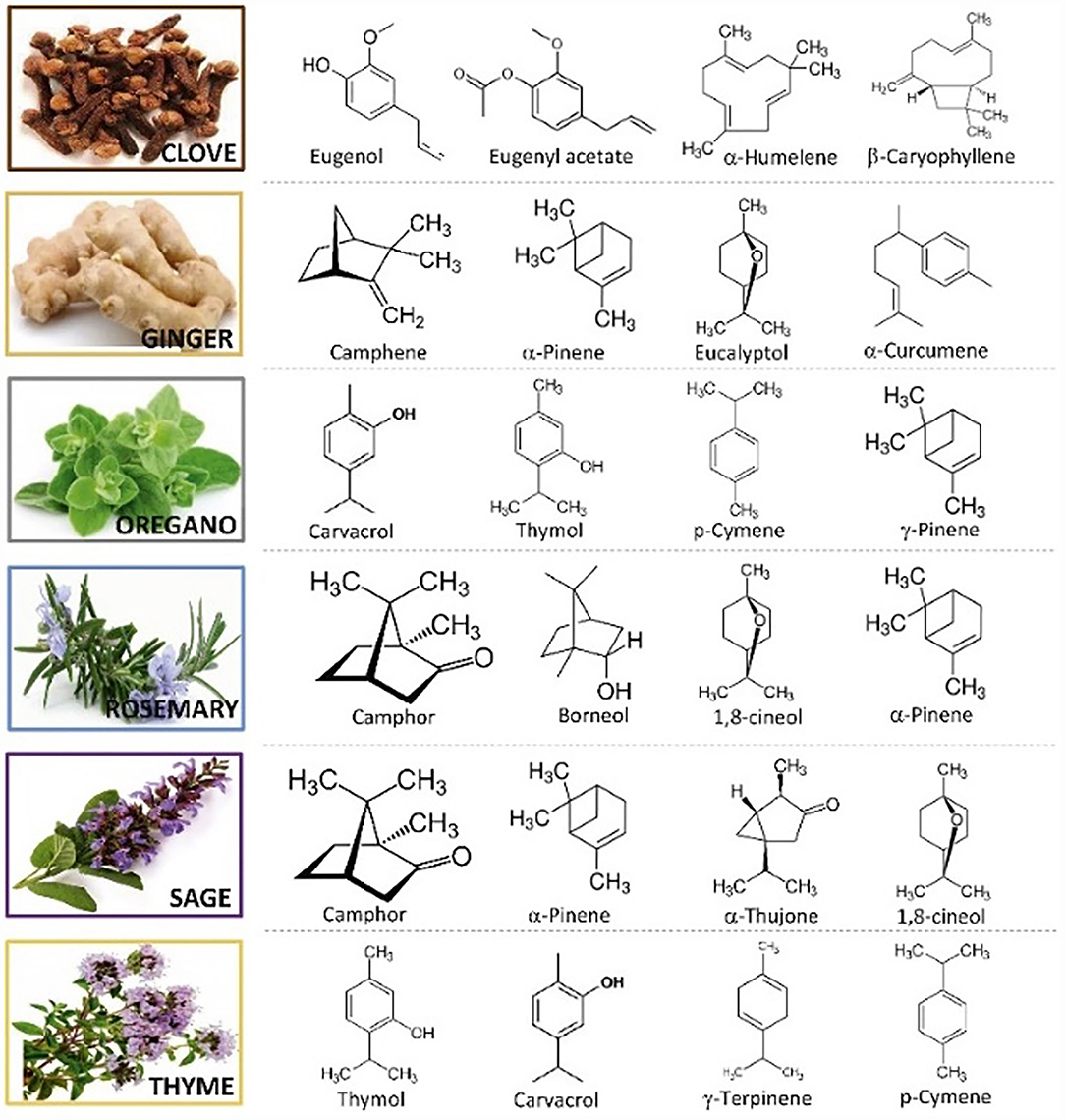
Figure 1. Main antioxidant bioactive compounds found in essential oils (12).
Various techniques are used for the manufacture and extraction of EOs from plant materials. Traditional methods include cold compression and distillation, along with solvent extraction methods such as maceration, enfleurage, fermentation, percolation, hydro diffusion, and gravity extraction (17, 18). Other promising techniques have been recently applied, such as solvent-free microwave extraction (19), ultrasonic-microwave assisted extraction (20), supercritical fluid extraction (21), subcritical water extraction (22), and ohmic heating-assisted extraction (23). The purpose of these methods is to remove and concentrate the oils from the plant tissue in a pure form. Overall, distillation is the most commonly used method (15), including hydro distillation (24), steam distillation (25), solar distillation (26), and molecular distillation, an extremely low pressure distillation process (27). CO2 supercritical fluid extraction is the most efficient method (28). It uses CO2 under very high pressure as the solvent; the solvent and extractant separate when the pressure is released.
Many EOs are approved as food additives and fragrance ingredients and are labeled Generally Recognized as Safe (GRAS) in the USA by the Food and Drug Administration (FDA) in CFR - Code of Federal Regulations Title 21 subpart A - General Provisions Sec. 182.20 Essential oils, oleoresins (solvent-free), and natural extractives (https://www.accessdata.fda.gov/scripts/cdrh/cfdocs/cfcfr/CFRSearch.cfm?fr=182.20). Therefore, utilization of EOs as natural preservatives to extend food shelf-life is gaining interest in the food industry (12). Results of studies regarding the use of EOs as antimicrobial agents suggest that EOs can be promising alternatives to synthetic preservatives for postharvest fruits and vegetables (29–31). However, possible negative effects of EOs, such as organoleptic changes (especially smell and taste) and phytotoxicity effects on fresh produce, are sources of concern among handlers and consumers (32).
Horticultural systems of food, fiber, and medicinal crops are complex, multiscale networks inclusive of the entire production, consumption, and post-consumption cycles of thousands of commodities worldwide. Within these systems, the methods of horticultural crop production are influenced by climate, market infrastructure, accessibility to quality farm inputs, regulations, ethical and societal norms, and cultural traditions and values. Organic farming, initially a response to develop alternative approaches to an increasing reliance on synthetic inputs (33) has matured to a global industry whose 2019 market value was estimated at 106.4 billion euros (34). Any farmer may employ the principles of organic farming, which emphasize soil health, animal welfare, and pest prevention, but they may be held accountable to financial penalties if they market their products as organic without the legal validation from a regulatory body. Of the 187 countries reporting adoption of organic practices, 108 have regulatory programs that define and enforce standards of production, processing, and marketing (34).
In the USA, the National Organic Program (NOP) is housed in the Department of Agriculture's (USDA) Agricultural Marketing Service and enforced by USDA accredited agencies (35). The NOP certifies production systems. In part, producers must demonstrate adoption of integrated soil and pest management strategies that conserve natural resources, employ cultural and ecological strategies to manage pests, and use formulated pesticides for pathogens, insects, and weeds only when necessary. Inputs used for crop production are deemed compliant; and raw materials used in inputs must meet the criteria described in the Organic Foods Production Act of 1990 and must be approved by the farmer's certification agency before they are used on the farm to ensure compliance is maintained. In general, synthetic materials are prohibited but there are some exceptions. A current list of all prohibited and approved raw materials allowed for use in organic systems, including those used in food processing, cosmetics, is maintained on the National List (36). Compliant materials and inputs, including formulated pesticides, are readily recognized by farmers if they bear the NOP EPA label or elect to pay a fee for validation of compliance by the Organic Materials Review Institute (OMRI) to use the OMRI-Listed® label.
Compliant pesticides must also satisfy regulatory criteria established by the US Environmental Protection Agency (EPA). Most active and inert ingredients used in compliant pesticides are considered “minimum risk” by the EPA when used according to labeled instructions. Biopesticides are defined by the National Pesticide Information Center as “made of living things, or come from living things, or found in nature” and include three types: microbes, natural substances such as EOs, and plant-incorporated protectants or PIPs, which are genes and proteins introduced to plants via genetic engineering and thus are prohibited by the NOP. Biopesticides made of or from microbes or natural substances have been used in organic farming for decades, but not all commercially-available biopesticides are compliant with the NOP.
The current review aims to provide an overview of the latest studies that have investigated the efficacy of preharvest and postharvest EO applications to manage diseases, arthropod pests, and weeds of horticultural crops. We summarize some of the reasons why EOs are still not in widespread use, list the modes of action of EO products, and outline common EO application methods used today in the food industry. Finally, we highlight gaps in research-based knowledge that should guide the future directions of efforts to increase EO utilization in regulated and non-regulated organic horticulture systems globally.
Properties of EOs as Broad-spectrum Anti-microbial Agents
Disease Management
Fungicides are indispensable to global food security as plant diseases are a prime constraint on agricultural and horticultural production (37). Fungicide use on fruits and vegetables accounts for more than 35% of the global pesticide market; while fungicides account for <10% of the total mass of pesticides used in the United States (4). Conventional growers rely on application of synthetic fungicides to mitigate infections and control diseases properly (38). However, abuses of synthetic compounds have resulted in more health hazards and environmental pollution, as well as promoting resistant biotypes of fungal pathogens (39). Currently, a major goal of organic agriculture is to search for alternative disease control methods and concentrate on reaching profitable markets and extending shelf life without compromising product quality and causing environmental pollution (14).
Antifungal Properties
Over 19,000 phytopathogenic fungi are known to cause diseases in agricultural and horticultural crops globally (40). Fungal pathogens are the most prevalent among all pathogens and are major causes of quality deterioration of fruits and vegetables. Fungal diseases, which are mainly caused by Fusarium, Aspergillus, and Penicillium spp., typically cause decay, accelerated ripening, and in some cases an accumulation of mycotoxins (41, 42). Phytopathogenic fungi play a critical role in the profitability, quality, and quantity of fresh products. They are responsible for nearly 30% of all crop diseases and cause quality losses worth billions of dollars worldwide each year (43, 44).
Antifungal properties of EOs against phytopathogenic fungi have been reported in numerous studies. The fungicidal assays of EOs include different methods with either in vitro or in vivo assessments. The results are mostly expressed as half maximal inhibitory concentration (IC50), minimal inhibitory concentration (MIC), minimum fungicidal concentration (MFC), and zone of inhibition (ZOI) (43). Some commonly studied aspects are mycelial growth inhibition, conidial germination, dry hyphal mass weight, germ tube elongation, and hyphal morphology observation by scanning electron microscopy (SEM) and transmission electron microscopy (TEM).
Essential oils extracted from plants have different effects on various phytopathogenic fungi (Table 1). It was observed on Alternaria alternata-inoculated tomato leaves that cinnamon oil and origanum oil vapors reduced necrotic lesions, delayed conidial germination and germ-tube elongation but fennel oil and thyme oil vapors did not. However, these four EOs showed similar antifungal activities against the in vitro mycelial growth of A. alternata in dose-dependent manners (49). Peppermint oil showed the greatest influence on charcoal rot (Macrophomina phaseolina) infection in geranium (Pelargonium graveolens), followed by basil oil, while marjoram oil had no effect on the pathogen's growth (111). An in vivo postharvest study revealed cardamon (1,000 μL L−1) and citronella (750 μL L−1) EOs significantly inhibited Colletotrichum sp. and Lasiodiplodia sp. growth, which cause anthracnose and stem-end rot of papaya, respectively (95). In addition, it was demonstrated that applying each EO or its major constituent resulted in slightly different responses. Thyme oil activity is closely related to its major constituent, thymol. However, the direct application of thymol resulted in a delayed early A. alternata infection process, while direct thyme oil only caused a delay in the infection process. Thyme oil from leaves and thymol had MICs of 500 and 250 μg mL−1 against A. alternata, respectively; while a commercial fungicide Nativo® had a MIC of 1,250 μg mL−1 under the same conditions (52). Vapor contact treatment had stronger antifungal activity than direct contact treatment. The EO of Origanum vulgare, as well as two of its primary components, thymol and carvacrol, exhibited strong antifungal activity against Botrytis cinerea. From an in vitro study, using direct contact assays, thymol (17.56 mg L−1) had the lowest EC50 against B. cinerea mycelial growth, followed by carvacrol (26.22 mg L−1) and the EO (52.92 mg L−1). In vapor contact assays, the EC50 values were substantially lower than those in direct contact assays, with thymol (0.94 mg L−1) having the lowest EC50 value, followed by carvacrol (1.61 mg L−1), and the EO (16.44 mg L−1). In vapor contact assays, the EO exhibited an MIC of 31.25 mg L−1 against B. cinerea spore germination and an MFC of 62.5 mg L−1. Thymol and carvacrol had identical MIC and MFC values of 7.81 and 15.63 mg L−1, respectively. From an in vivo study, compared to the control, EO vapor contact treatment reduced the deterioration of cherry tomatoes by 70.44% at 62.5 mg L−1. Fruit decay was reduced by 96.39% at a concentration of 250 mg L−1. Thymol and carvacrol at 62.5 mg L−1 suppressed the development of B. cinerea lesions by 94.13 and 95.37%, respectively, whereas they both completely suppressed the development of B. cinerea at 125 mg L−1 (85).
The mechanisms of EO antifungal activity could be: (1) Cell wall and membrane disruption leading to cell membrane permeability change and leakage of cell cytoplasm. The ultrastructure analysis demonstrated that thymol, major constituent of thyme oil, acted at the cellular level against fungi by disrupting cell wall and plasma membrane with subsequent cytoplasm disorder (52). The results from SEM and TEM revealed that EO-damaged hyphae cell membranes and changed the cell membrane permeability, leading to the changes in the cytoplasm components, such as reducing soluble sugars, proteins, and ergosterol (86). Another study using SEM and TEM confirmed that mint oil could disrupt cell walls and destroy the ultrastructure of hyphae and conidia, resulting in cellular nucleic acids and proteins leakage and marked shriveling and crinkling of the hyphae and conidia (84). (2) Influence cell energy metabolism pathway. In one in vitro and in vivo study on EOs against Aspergillus niger it was further reported that EOs could probably inhibit glycolysis, which in turn influenced cell energy metabolism of fungal pathogens (68). In another study, it was shown that EOs can disrupt the integrity of plasma membranes and cause mitochondrial dysfunction, inducing metabolic stagnation in fungi. Moreover, EOs can modulate mycotoxin gene expression in the aflatoxin biosynthesis pathway on Aspergillus flavus (61). (3) Defense dysfunction. Essential oils could destroy the normal morphology and activities of cell wall and membrane and cause defense dysfunction against stress response. Analyses of multiple metabolic pathways illustrated that spore development, membrane permeability, oxidative stress, and amino acid metabolism were all disturbed (64). (4) Accumulation of ROS. It was reported that EOs could stimulate accumulation of ROS in mycelia and spores and cause a rapid increase in intracellular reactive oxygen species levels (113). In other research, it was shown that intracellular ROS generated by EOs damaged cell membranes and this might have caused pathogen cell death (124). (5) Anti-aflatoxigenic effect. Thyme EO significantly reduced the aflatoxin B1 (AFB1) production of Aspergillus flavus in vitro. This anti-aflatoxigenic property was attributed to the down-regulation of the secondary metabolism gene laeA and to the modulation of hydrolase gene expression involved in fungal colonization and establishment (62). Rosemary EO could reduce the production of ergosterol and the biomass of mycelium, and inhibit the production of aflatoxins B1 and B2, indicating that the antiaflatoxigenic effect of rosemary EO is independent of its antifungal effect and is likely due to its direct action upon toxin biosynthesis (63). (6) Regulate specific gene expression in the host. The expression of the pathogenesis-related (PR) gene PR-8 in apple was induced by 2.5-fold by EOs compared to untreated inoculated fruit, which suggested that EOs induced resistance against pathogens through the priming of defense responses (87).
Antibacterial Properties
Bacteria causing diseases on plants also have a considerable economic impact. Plant pathogenic bacteria survive in diverse environments, both in plants, as pathogens, and outside their hosts as saprophytes. About 350 bacteria, which are pathovars or subspecies belonging to the phyla Proteobacteria, Actinobacteria, and Firmicutes, are known to be phytopathogenic [(158); Table 2].
The antibacterial activities of EO are mostly expressed in MIC, minimal bactericidal concentration (MBC), and ZOI. Regarding in vitro antibacterial activities tests, overall Gram-positive organisms are more susceptible to EO compared with Gram-negative organisms, which is due to the structure of the cellular membrane. It was reported that the susceptibility of Gram-positive bacteria was observed to be greater than that of Gram-negative bacteria when treated with EO distilled from Citrus medica (159), Ocimum basilicum (160), Mentha spicata (161), Nepeta ucrainica (162), Zanthoxylum schinifolium (163), and Zingiber officinale (164).
The antibacterial activity of basil (Ocimum ciliatum) EO, as a new source of methyl chavicol, was tested against ten important phytopathogenic bacteria. The antibacterial test results indicated that the EO had antibacterial activity against all of the bacteria tested. The most vulnerable bacterium was Brenneria nigrifluens, while the most resistant bacterium was Pseudomonas tolaasii, based on the ZOI values. Moreover, the EO had the lowest MIC values against Ralstonia solanacearum and the lowest MBC was found to have the strongest bactericidal property against the Xanthomonas citri (137). Eleven EOs were screened for antibacterial activities and abilities to influence the growth and virulence factors of Erwinia amylovora, causing fire blight. According to ZOI values, Foeniculum vulgare and Pimpinella anisum EOs showed strong antibacterial activity and Artemisia aucheri and Heracleum persicum EOs had moderate antibacterial activity. The other seven EOs did not show substantial growth inhibition but could reduce the production of virulence factors in E. amylovora at non-lethal concentrations. Both A. aucheri and F. vulgare EOs indicated the highest bacteriostatic (MBC 15.6 μg/mL) and bactericidal (MIC 7.8 μg/mL) activities. In contrast, Citrus sinensis and Citrus aurantifolia exhibited minimal bacteriostatic (MBC 250 μg/mL) and bactericidal (MIC 250 μg/ mL) activities. From an in vivo study, EOs of Apium graveolens (celery seed) and Curcuma longa (turmeric) demonstrated the greatest reduction in the impact of E. amylovora virulence factors. They reduced disease progression of E. amylovora on immature pear fruit by 41.71 and 30.17%, and disease progression in pear seedling shoots by 26.9 and 16.7%, respectively (144).
The mechanisms of EO antibacterial activities have been widely studied. In a recent study, the mechanisms of antibacterial activity of finger citron essential oil were investigated by observing changes of bacteria morphology according to scanning electron microscopy, time-kill analysis, and permeability of cell and membrane integrity. Morphology of Escherichia coli and Staphylococcus aureus were changed and damaged more seriously with higher concentration and longer exposure time to finger citron EO. It significantly suppressed the growth rate of surviving bacteria and led to lysis of the cell wall, intracellular ingredient leakage, including small ions, nucleic acids, and proteins, and finally cell death (159). Cinnamon EO was reported to cause the leakage of small electrolytes, rapidly increasing the electric conductivity of S. aureus and E. coli within the first few hours of exposure, decreasing the bacterial metabolic activity 3–5-fold. Furthermore, the concentration of proteins and nucleic acids in cell suspension also increased with increased cinnamon EO (165). Under the transmission electron microscope, Cinnamomum longepaniculatum leaf EO decreased cell size, and ruptured the cell walls and cell membranes of treated bacteria. Moreover, nucleoplasm was reduced and gathered onto the side, which might be attributed to its hydrophobicity (166). In addition, as a result of post-contact effects, cell constituents release assays, and ultrastructural analysis revealed that the loss of integrity of the cell membranes and vital intracellular constituents could be one of the mechanisms of action of the green huajiao (Zanthoxylum schinifolium) EO against selected foodborne pathogens (163). Electron microscopy observation indicated that fennel seeds EO disrupt membrane integrity, according to the leakage of electrolytes and the losses of protein and sugar contents of targeted bacteria (163). In other research, it was shown that the nanoemulsion amplified the antibacterial activity of Thymus daenensis EO against E. coli by increasing the EO ability to disrupt cell membrane integrity. The results were investigated by measuring potassium, protein, and nucleic acid leakage from the cells, and by electron microscopy (167). Litsea cubeba EO can inhibit the respiratory metabolism, the hexose monophosphate pathway and its key enzyme (glucose-6-phosphate dehydrogenase) of methicillin-resistant Staphylococcus aureus. Moreover, citral, the main component of Litsea cubeba EO, could further form chimeras with DNA of methicillin-resistant Staphylococcus aureus to inhibit its biological activity (168).
In summary, EO antibacterial mechanisms might include loss of integrity of the cell walls and membranes, leakage of electrolytes, loss of intracellular constituents, increase of bacteria electrical conductivity and nucleic acid concentration in cell suspension, and inhibition of respiratory metabolism decrease bacterial metabolic activity.
Arthropod Pest Management
Indiscriminate use of conventional synthetic chemical insecticides has caused different types of environmental and toxicological problems, such as environmental pollution, toxicity to non-targeted organisms, and development of pesticide resistance. Therefore, it is important to identify more biodegradable alternatives that are less persistent in the environment than chemical insecticides (169). Interest in the potential use of natural and botanical products for pest management, such as EOs or their derivatives, has grown dramatically in the past two decades due to their lower mammalian toxicity and faster environmental degradation (170–172).
EOs have antimicrobial or insecticidal properties that can protect plants from herbivores and microorganisms. In line with the known synergistic effects of complex EO mixtures, the knockdown and mortality rates, and biocidal activity against some adult dipteran insects increased by up to 100% for certain EO mixtures compared to individual EOs (173). Another study indicated that citral can be synergistic to limonene and geranyl acetate when their concentrations increase in the mixture. Moreover, the binary mixture of the two major constituents of lemongrass oil, citral and limonene, displayed synergistic cytotoxicity on an ovarian cell line of the cabbage looper (174). However, another comparison of toxicity and deterrent activity with artificial blends as binary mixtures revealed that synergism was not a generalized phenomenon and both species and blend specific variations can occur (175).
Essential oils are believed to interfere with basic metabolic, biochemical, physiological and behavioral functions of insects. However, little is known about their complete modes of action (169, 176). The mono- and sesquiterpenoid constituents of EOs are fast-acting neurotoxins in insects and related arthropods, possibly interacting with multiple types of receptors in their nervous system (171). These compounds also display potentially important sublethal behavioral effects on arthropods, such as repellence; oviposition deterrence; ovicidal, larvicidal pupicidal and antifeedant effects (169). Different EOs may work via different mechanisms. The acute toxicity and feeding deterrent activity of ten common EOs against three postharvest stored-grain pests (Sitophilus oryzae, Tribolium castaneum, and Rhyzopertha dominica) were evaluated (175). Thymol, carvacrol, eugenol, and trans-anethole showed differential species-specific toxicity, and acted as acute toxins rather than feeding deterrents. However, linalool was a general feeding deterrent against all three species. The antifeedant activity could be due to physiological toxicity rather than interaction with gustatory receptors. The authors further reported combined toxic and antifeedant effects for various combinations of anethole, carvacrol, or linalool. Moreover, the observed decreased beetle mortality, but increased feeding deterrence, implied that the physiological toxicity induced by acute toxins synergized with the deterrent activity of a compound in a mixture, but the dose may not be sufficient to kill (175).
Essential oils have also been used as insect attractants in pest control programs. Coriandrum sativum and Nerium indicum EOs were strong attractants of both adults and nymphs of Cyrtorhinus lividipennis, a major predator of the rice planthopper (177); Thus, these OEs can be used in augmentative biological control against rice pests. In addition, EOs are used as lures for detecting and monitoring invasive ambrosia beetles (178, 179).
Essential oils were shown to control preharvest and postharvest phytophagous insects during their development, growth, and adult emergence (169). As of this writing, screening, discovery, and demonstration of bioactivity have been reported in numerous studies of various EOs against various insect pests (180). Ebadollahi et al. (181) summarized in their review paper that the main components in the EOs extracted from the Lamiaceae plant family exhibit insecticidal effects. For instance, terpinen-4-ol displayed contact and fumigant toxicity against adults of Cimex lectularius (182), α-pinene showed fumigant and contact toxicities and repellency against adults of T. castaneum (183), terpinolene demonstrated larvicidal and pupicidal activity against Culex quinquefasciatus (184), and α-terpineol exhibited fumigant toxicity against the adults of Sitophilus granaries (185). In addition, Stepanycheva et al. (186) reported that the EOs obtained from Mentha pulegium and Thymus mastichina showed acute toxicity effects against western flower thrips (Frankliniella occidentalis Perg.) by fumigation.
EOs have also been used against pestiferous mites and nematodes. Lemongrass (Cymbopogon citratus) oil showed promising miticidal activity and ovicidal effects against Sarcoptes scabiei (187). Besides, Ozdemir and Gozel (188) tested 10 EOs and discovered Lavandula officinalis, Artemisia absinthium, Piper nigrum, Citrus bergamia and Mentha arvensis have the most nematicidal effects against the root-knot nematode Meloidogyne incognita. Other than the commonly seen EOs, some novel EOs have also shown pesticidal properties. The EOs from Cuminum cyminum and Pimpinella anisum were toxic to the agricultural pests, Myzus persicae and Spodoptera littoralis, to the common housefly Musca domestica and to the lymphatic filariasis and Zika virus vector Culex quinquefasciatus, without affecting non-target invertebrates (189). The EO of Eugenia uniflora is toxic to the bronze bug, Thaumastocoris peregrinus, and selective to its parasitoid, Cleruchoides noackae (190).
Even though there have been thousands of studies conducted in which the bioactivities of EOs and their derivatives against insects were documented, most of those studies are limited to laboratory conditions and are on the R&D phase instead of the product development end. Therefore, only a handful of EO-based pesticides have been successfully commercialized, lagging far behind relative to the extensive scientific literature devoted to the area. Table 3 lists some of the current commercial EO-based insecticides/miticides.
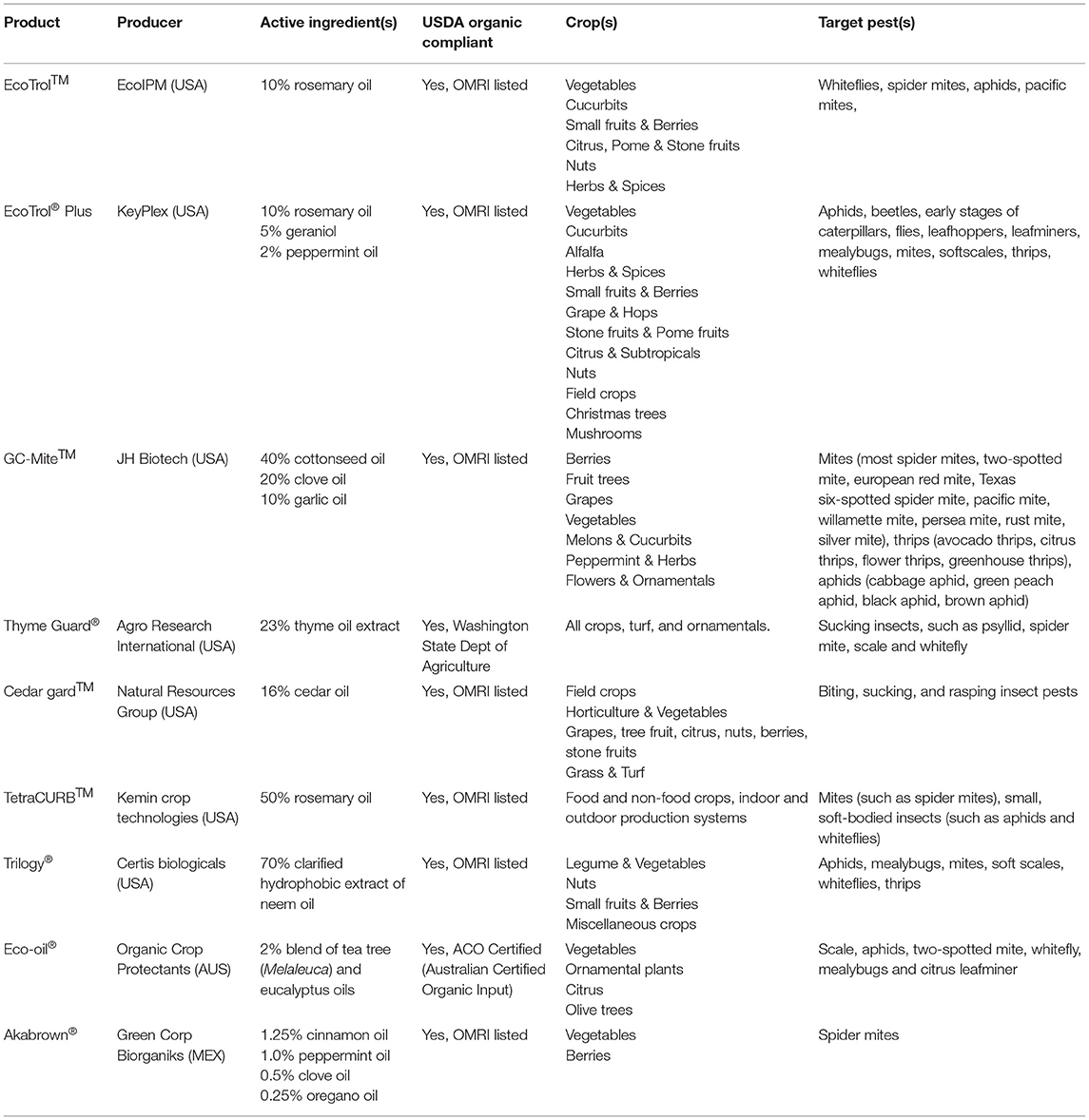
Table 3. Currently available commercially formulated insecticides/miticides based on plant essential oils.
Isman (171) pointed out that commercial development of EO-based bioinsecticides can follow several different pathways, producing products with active ingredients including (1) a mixture of EOs; (2) a single EO, or a single terpenoid constituent; (3) a blend of synthetically produced terpenoids emulating a plant EO; and (4) non-natural blends of terpenoids obtained from different plant sources. Meanwhile, there are notable challenges for the commercialization of EO-based pesticides: (1) stability of EOs in storage and transport and persistence in field application; (2) residual action and efficacy after application; (3) phytotoxicity on crop and ornamental plants (191).
Formulation can be a potential method to improve EO-based pesticide performance. Formulation technologies, which involve using appropriate volumes of solvents and emulsifiers to make aqueous emulsions, can enhance the stability of non-polar EO constituents, thus increasing their efficacy and persistence, slowing down release, and preventing rapid evaporation. Nanoformulation technologies, including nano-emulsification and nanoencapsulation, are expected to improve EO chemical activity and persistence, and enable penetration into insect tissues, thus enhancing their insecticidal activity (192–194). In addition, almost any oil can be phytotoxic if applied as an aqueous emulsion at concentrations exceeding 2%, and in some cases, the phytotoxic concentration can be as low as 1%. Formulation is also a highly promising way to mitigate or eliminate phytotoxicity.
Three core constraints determining the potential for EO-based biopesticide production are (1) availability and consistency of the source plant, (2) cost, and (3) regulatory approval (171). The price largely depends on the availability of the EO materials. Widely used oils, such as for the fragrance and flavoring industries, produced on a massive scale can reduce the price level. In contrast, producing rarely used oils will result in extremely high prices for pest management products. One of the least expensive EOs is orange oil. The fruit peel used for extraction is a waste product of the orange juice industry, and the oil can be produced by cold pressing rather than hydrodistillation (191). Many EOs and some pure EO constituents are exempt from USA federal regulations on pesticides because of their minimal risk to human, animal, and environmental health (171, 191). While these low toxicity products are more likely to be allowed for use in organic systems, each product must be evaluated for compliance to the NOP federal rule prior to use in USA organic systems.
Overall, EO-based pesticides appear to be safer alternatives to conventional synthetic chemical insecticides in integrated pest management. They display toxic, repellent, and antifeedant activity against various insect and mite species. However, the commercialization of EO-based pesticides faces some challenges, including achieving necessary stability, persistence, efficacy, and mitigating phytotoxicity. Nanoformulation is a promising approach to improve the performance of EO-based pesticides. Further studies are required to develop novel formulations that would increase efficacy with lower costs.
Weed Management
Weeds can interfere with the productivity of horticultural crops, reducing the yield and quality of fresh and processed products. Since the 1940s, when synthetic herbicides were discovered, their application has become the primary technique utilized for weed management (43). Conventional synthetic herbicides are water soluble, polar, and heat stable; therefore it is difficult to diminish and reduce the lethality (195). That causes evolution of herbicide-resistant weed biotypes, and soil and water pollution, which may eventually adversely influence humans. Thus, more selection of safe, efficient, and cost-effective organic herbicides, featuring diverse classes of compounds and having dissimilar mechanisms of action, are urgently needed.
The study of plant-plant interactions via the release of secondary metabolites could be a start for discovering of new substances with herbicidal activity (196). Essential oils and their constituents, primarily terpenoids, are good candidates as alternatives to synthetic herbicides. Utilization of EOs in weed management is mainly due to their allelochemical compounds (197). The term allelopathy refers to a plant-plant interaction, whereby allelochemicals released by one plant influence the physiological and biochemical processes of other surrounding plants directly or indirectly (198). Allelochemicals are a broad category of complex compounds that include, but are not limited to, EOs, carbohydrates, amino acids, salicylates, alkaloids, phenolics, flavonoids, jasmonates, momilactones, hydroxamic acids, brassinosteroids, and glucosinolates (199).
Generally, both monoterpenoids and sesquiterpenoids contribute to the phytotoxic and allelopathic effects of EOs (200, 201). However, their herbicidal activity often seems to be selective (202). For instance, at all doses tested, Cistus ladanifer EO totally inhibited Amaranthus hybridus germination and nearly stopped Conyza canadensis and Parietaria judaica germination. The EO had less impact on Portulaca oleracea, only limiting its germination at higher dosages tested. It had no effect on the germination of Chenopodium album. The EO displayed high phytotoxic activity in terms of seedling length, and it was effective at all concentrations tested (203). Whatever effect an active EO component has against one target species will not always be maintained against another species, even if they are in the same family or genus (202). This is a critical characteristic because herbicides require activity specificity. As a result, the primary task would be to discover the most effective chemicals for the various target weed species. Moreover, these compounds can function independently in certain situations, but also synergistically or antagonistically in others. Therefore, the nature of the interaction cannot be predicted in general based on the individual chemicals operating alone (202).
The phytotoxic and herbicidal potential of EOs against weeds has been extensively studied (Table 4). Essential oils have been investigated for their potential impact on seed germination rate and physiological growth. Rosemary EO, rich in 1,8-cineole, was found to significantly increase the amounts of proline and the relative membrane permeability of leaves, as well as strongly inhibit the germination of seeds and the growth of two weed species, amaranth weed (Amaranthus retroflexus) and radish (Rhaphanus sativus) (199). Citrus aurantiifolia EO and its major constituents, limonene (~41%) and citral (~28%), were demonstrated to affect mitotic activity and induce chromosomal abnormalities in three grassy agricultural weeds [Avena fatua, Echinochloa crus-galli, and Phalaris minor; (235)]. Citral was shown to be the most toxic followed by C. aurantiifolia oil and limonene through phytotoxicity and cytotoxicity assays. However, not all EOs display an herbicidal effect. For example, Achillea gypsicola EO was shown to be ineffective against the germination of Chenopodium album and Rumex crispus seeds (236). Moreover, EO from Pinus radiata was found to exhibit an herbicidal effect that was significantly more effective on dicots than monocots (222). The herbicidal potential of Thymbra capitata, Mentha × piperita, and Santolina chamaecyparissus EOs were evaluated on Avena fatua, Echinochloa crus-galli, Portulaca oleracea, and Amaranthus retroflexus. Both Thymbra capitata and Mentha × piperita EOs showed a broad spectrum of activity, with Thymbra capitata at the highest doses applied (12 μL mL−1) killing plants of all weed species except for Portulaca oleracea at 90%. Mentha × piperita at the highest dose (20 μL mL−1) completely controlled Avena fatua and Amaranthus retroflexus plants but displayed 90 and 40% efficacy on Portulaca oleracea and Echinochloa crus-galli, respectively. Although Santolina chamaecyparissus EO was less active than the other EOs, it demonstrated an excellent selective activity, being highly effective against A. retroflexus, showing 90% efficacy at the highest dose, 20 μL mL−1 (230).
Regarding phytotoxic effects of EO, visible symptoms such as inhibition of germination and seedling development, along with necrosis and chlorosis (237), or leaf burning (238), were previously reported to be related to the following mechanisms of action (43, 239).
(1) Essential oils and their pure components induce oxidative damage and loss of membrane integrity by generation of ROS, causing ion or electrolyte leakage, membrane depolarization, cuticular wax interruption, stomata clogging, and epidermal cell shrinkage. Citronellol was reported to inhibit root and shoot growth of Triticum aestivum by ROS-mediated membrane disruption. ROS production could result in lipid peroxidation, membrane damage, and solute leakage (240). As a consequence of the disruption of the membrane integrity, Citronellal-treated weed leaves showed cuticular wax interruption, stomata clogging, epidermal cell shrinkage, and rapid electrolyte leakage (241).
(2) Inhibition of DNA synthesis and mitosis. It is widely believed that EO phytotoxic effects are mediated by suppression of DNA synthesis, interfering with mitotic activity, or disrupting surrounding membranes of mitochondria and nuclei in some organelles such as mitochondria (242). The compound 1,8-cineole, the major component of rosemary and mentha EOs, was suggested to inhibit DNA synthesis and mitotic activity in both cell nuclei and organelles in root apical meristem of Brassica campestris (243, 244).
(3) Reduction of cellular and mitochondrial respiration. Most of the fundamental cellular activities, including cell division, ion and solute transportation across membranes, and synthesis of molecules such as membrane lipids, chlorophyll, proteins, and nucleic acids, require a source of metabolic energy. Mitochondrial respiration supplies ATP to support these processes (239). Abrahim et al. (245) confirmed that α-pinene, a major component of rosemary EO, strongly impaired mitochondrial energy metabolism and inhibited ATP production of maize seedlings by uncoupling of oxidative phosphorylation and inhibition of electron transfer. They further illustrated that α-pinene can inhibit or completely suppress mitochondrial respiration depending on its dose.
(4) Inhibition of photosynthesis. It was demonstrated that the EOs from Cymbopogon nardus and Eucalyptus citriodora as well as pure citronellal reduced chlorophyll content and total protein content in crabgrass (Digitaria horizontalis) and burrgrass (Cenchrus echinatus) by more than 80 and 90%, respectively (201). Origanum vulgare EO was confirmed to negatively affect nitrogen assimilation into glutamine, causing excessive accumulation of toxic ammonia in leaf cells as well as oxidative stress. Afterward, a series of events that suppress the effectiveness or efficiency of PSII, producing oxidative stress and, ultimately, a significant decrease in plant growth and development, followed by leaf necrosis and plant death (246).
(5) Microtubule polymerization. Chaimovitsh et al. (247) indicated that both limonene and citral could disrupt microtubules and that limonene could also cause membrane leakage. Limonene displayed dual capacity in terms of microtubules and membrane functionality.
(6) Proline accumulation and lipid peroxidation. In plants, proline functions as a mediator of osmotic adjustment and plasma membrane integrity and protection. Its accumulation could be related to the increase of protein hydrolysis caused by stress (248). The phytotoxicity of various EOs on weeds can be determined by the expression and accumulation of oxidative stress products, such as proline and lipid peroxidase. Khare et al. (211) found mentha EO emulsion injures weeds by disturbing the membrane integrity and inducing oxidative stress to the weed as indicated by relatively higher levels of electrolyte leakage, proline and lipid peroxidase. In general, EOs inhibit electron flow in mitochondria, resulting in an increase in the generation of ROS, which promotes lipid peroxidation. It is possible that membrane breakdown might result in lipid release inside the cytoplasm of targeted cells, because fatty acids and other lipids are known to be structural components of membranes. The liberated lipids in the cytoplasm might then become the target of oxidative activity (211). Cymbopogon citratus EO also demonstrated strong phytotoxic activity against barnyard grass (Echinochloa crus-galli) by increasing relative electrolyte leakage and lipid peroxidase activity (249).
Essential oils are promising candidates for the development of novel bio-herbicides due to their strong phytotoxic activity. Currently available EO-based commercial organic herbicides are listed in Table 5. The primary challenges are (1) developing appropriate formulations to minimize their high volatility and optimize effectiveness while permitting field application. So far, most of the tests are done in a small scale under laboratory conditions. (2) determining their modes of action. Because EOs are a complex mixture of biologically active molecules capable of affecting multiple targets at the same time, they might be useful in preventing the formation of resistant weeds. Combining the classical analytical techniques with new -omics approaches, such as genomics, transcriptomics, proteomics, and metabolomics, would speed up discovering new mechanisms of action (196).
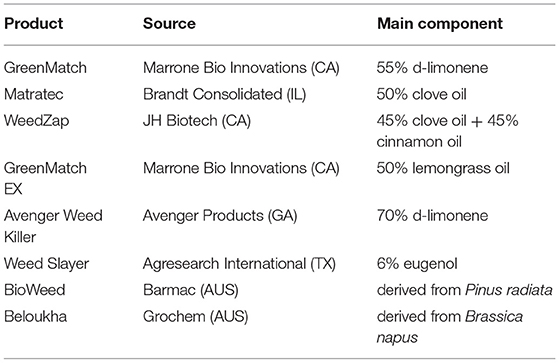
Table 5. Currently available commercially formulated organic herbicides based on essential oils or plant extracts (196, 250).
In the future, a better understanding of mechanisms of action would promote the development of bio-herbicides, especially focusing on the potential synergism among single molecules. Therefore, it may help to reduce the herbicides application doses, avoid herbicide resistance in weeds, and hit multiple targets simultaneously.
Essential Oil Formulations and Applications in the Food Industry
Despite proven efficacy of EOs, they still do not enjoy widespread application due to their high volatility, low stability, low water solubility, composition variability, a strong influence on organoleptic properties, and phytotoxic effects. Essential oils are also very sensitive to light radiation, especially UV, and elevated temperature, which could cause oxidation, isomerization, polymerization, dehydrogenation, and eventually degradation (251). The degradation may alter the biological properties of the EO as well as exerting potent toxicity due to the presence of alteration compounds (43). Due to these limitations, many EOs are not suitable for use in their raw form (252). To break through these limitations and improve the efficiency and persistence of EOs, formulation techniques applied to EOs has a bright and promising future. A product formulation is a homogeneous and stable combination of an active ingredient and inactive materials as additives that involve specialized processing of the product to improve its biological qualities, durability, and stability (253). Formulations are commonly used for pesticides and herbicides. A recent study demonstrated that polydopamine microcapsules templated by Pickering emulsions stabilized by cinnamoyl chloride modified cellulose nanocrystals for EO encapsulation. The EO (turpentine) functioned as a botanical pesticide and a solvent for the herbicide. The system improved active ingredients encapsulation efficiency, exhibited excellent multi-active ingredients encapsulation, adhesive and UV resistance properties, and controlled release of active compound (254). Therefore, formulation technique should be applicable to EOs in the food industry to achieve comparable advantages (43). In this section, non-compliant and compliant raw materials, processing methods, and formulations (products) are discussed to allow for a more complete presentation of essential oils and their applications.
Emulsification
An emulsion is defined as a combination of two immiscible liquids, containing spherical droplets as the dispersed phase and the addition of surfactant as the continuous phase (255). Emulsions are primarily classified based on their particle size and kinetic stability as macroemulsions (or coarse emulsions), microemulsions, and nanoemulsions (256). Macroemulsions are opaque and are thermodynamically metastable and susceptible to breakdown. Microemulsions are transparent and thermodynamically stable, but their stability is affected by slight environmental condition variations such as composition and temperatures. Nanoemulsions are thermodynamically metastable as phase separation occurs over time, however, they have kinetic stability because there is no gravitational separation and droplet aggregation due to the attractive force between the tiny sized droplets is minimal (255, 257).
Emulsion-based delivery systems can be formulated with food-grade ingredients to disseminate EOs to areas where microorganisms grow and proliferate (258). The nanoemulsions are more suitable than microemulsions or free EOs for these applications because their kinetic stability is not affected by physical and chemical variations, such as temperature and pH. Thus, they need fewer surfactants for preparation and are more cost-effective (255, 259). Furthermore, because of their subcellular size and improved diffusion, nanoemulsions can improve product physicochemical stability and reduce the influence on the organoleptic characteristics of foods while increasing bioactivity (259).
Edible coatings and films based on nanoemulsions of EOs have been investigated to prolong fresh produce shelf life. Several main types of matrices were usually used as the base to create EO nanoemulsions for use as edible coatings. These matrices have included starch, chitosan, sodium alginate, hydroxypropyl methylcellulose, and carnauba wax (260). As EOs are emulsified, they have been found to have higher stability and lower influence on food sensory qualities, and reduced interaction with other food matrix ingredients, while delivering enhanced biological activity due to the increased surface area and small droplet size (261). It was also demonstrated that for a film-forming formulation the addition of lemongrass EO emulsions by emulsification with two emulsifiers (Tween 80/pectin) to glycerol-plasticized cassava starch resulted in both EO and emulsifier phases showing good interaction and compatibility. Emulsification caused crucial changes in the active starch films, such as improved colorimetric attributes, thermal stability, moisture barrier properties, and mechanical properties (increasing extensibility, resistance, and stiffness). This film-forming formulation further increases the potential application of EO in the food packaging industry (262).
To summarize, nanoemulsion-based delivery systems could be a suitable method for developing EO products with higher kinetic stability and bioactivity, less organoleptic impact, and more cost-effectiveness than free EOs.
Encapsulation
Essential oils include components that are extremely sensitive to volatilization, and chemical changes due to oxygen, light, humidity, and heat exposure (263). Hence, EO encapsulation has been widely utilized to protect those sensitive compounds from undesired conditions, and improve their activity duration and functional performance (264). Moreover, encapsulation increases EO solubility, and provides targeted delivery and controlled release of EOs (265). The process of enveloping a particle or molecule of interest with a coating or constructing a functional barrier between a bioactive core and wall material in order to minimize physical and chemical interactions between the core and the outside molecules is referred to as EO encapsulation (266). Generally, polymeric particles (50), liposomes (267), and solid lipid nanoparticles (268) have been used as wall materials to protect the EOs from degradation.
Polymeric particles such as methacrylate polymer (269), poly(D,L-lactic-co-glycolic acid) (PLGA) (270), poly-ε-caprolactone (PCL) (271), poly (lactic acid) (PLA) (272), poly (L-lactide-co-ε-caprolactone)/silk fibroin (PLCL/SF) (273), β-cyclodextrin (274), cellulose nanofibrils (275), alginate (276), starch (277), chitosan (278), gum arabic, maltodextrin, and inulin (279), were commonly used as wall materials individually or as a mixture. For EO encapsulation in polymeric particles, spray drying, coacervation, nanoprecipitation, and rapid expansion of supercritical solutions (RESS) are widely used technologies (263).
Spray drying is a popular method of producing microparticles since it is a simple, rapid, and reproducible technique that produces stable final products with lower cost and allows continuous industry-scale production (280–282). In this process, the mixture solution of the active ingredient and the encapsulating materials are fed to the spray-dryer and atomized by hot gas, resulting in extremely rapid water evaporation and, as a result, quasi-instantaneous entrapment of the EO in a rapid-formed crust (43, 283).
Coacervation is defined as the phase separation of one or many hydrocolloids from the initial colloidal solution (284). One phase is rich in polymer and is known as the coacervate phase, while the other does not contain polymer and is known as the equilibrium solution. Coacervation techniques can be simple or complex depending on the number of polymers used. There is just one polymer in simple coacervation, but complex coacervation involves the interaction of two oppositely charged colloids (263).
Nanoprecipitation, also known as solvent displacement or interfacial deposition, was invented by Fessi et al. (285). Two miscible phases are involved: an organic phase (the solvent) and an aqueous phase (the non-solvent). This method is suitable for encapsulating hydrophobic compounds such as EOs. For EO encapsulation by nanoprecipitation, the polymer and the EO are solubilized in an organic solvent, then added to water under moderate magnetic stirring, which causes the interfacial deposition of a polymer after the organic solvent has been displaced. Afterward, the organic solvent is evaporated with a rotavapor to form the nanoparticles suspension in water (286).
The RESS procedure has recently been regarded as an effective method for producing free-solvent particles with consistent morphology and size distribution (287). Supercritical carbon dioxide (ScCO2) is considered to be a promising supercritical fluid for use in RESS because it is non-toxic, inflammable, and cheap, with a low critical temperature (31.3 °C) and pressure (73.8 bar) (288).
Liposomes are colloidal, vesicular structures composed of one or more phospholipid bilayers that define one or more aqueous compartments surrounded by a lipid membrane. Phospholipids are amphiphilic molecules that spontaneously self-assemble in aqueous environments (263). These liposomes form sphere-like shells and encapsulate hydrophilic compounds (such as EOs) as well as lipophilic or even amphiphilic molecules in the inner aqueous phase with oil-soluble substances in the lipid bilayer membrane (289). Encapsulation of EOs in liposomes often uses thin film hydration, reverse phase evaporation, and supercritical fluid methods (263). The thin film method, first developed by Bangham et al. (290), is one of the most widely used and simplest techniques for formulating of liposomes. However, due to low production capacity, the presence of organic solvent residues in the final product, and heterogeneous size distribution, this technique has limited commercial applicability (291). Several approaches are used to homogenize and reduce size of liposomes formed by the thin film hydration method, such as sonication, extrusion, and freeze-thaw (263). The extrusion refers to multiple times passage of heterogeneous sized particles via a track-etched polycarbonate membrane with holes of varying sizes, including hot-melt extrusion, melt injection extrusion process, co-extrusion, and electrostatic extrusion (292). Freeze-thaw cycles increase interactions between the lipid film and the EO to incorporate and display high encapsulation efficiency (293). The process of reverse phase evaporation involves mixing a phospholipid organic phase with the lipophilic active substances in an aqueous phase to form oil-in-water emulsion. Then the organic solvent is evaporated, yielding large unilamellar vesicles (294).
Two methods for supercritical fluid technology are modified RESS and particles from gas saturated solutions (PGSS)-drying of emulsion (263). In the process of modified RESS, liposomal materials and EO are dissolved in a supercritical CO2/ethanol solvent, and the solution is then sprayed into a buffer solution using a coaxial nozzle to create a liposome suspension (295). The process of PGSS was to create encapsulated particles by saturating the suspension of the bioactive chemical and the wall material with CO2 at a proper pressure and temperature to lower the viscosity. At atmospheric pressure, the vaporization and expansion of CO2 are triggered through a nozzle, which creates an intense cooling effect, resulting in forming a very fine and dry powder (296).
Solid lipid nanoparticles (SLNs) are nanocarriers containing lipids or lipid-like molecules, solid at room temperature. Compared to other colloidal carriers, liquid lipid is replaced by solid lipid in SLNs. The use of solid lipid rather than liquid lipid is advantageous since it has been demonstrated to enhance control over the release kinetics of encapsulated chemicals and the stability of integrated chemically-sensitive lipophilic components (297). Solid lipid nanoparticles can improve the stability and solubility of EOs in water, avoid the use of organic solvents, display high drug payload and no biotoxicity of the carrier, increase the bioavailability of entrapped bioactives, provide controlled release of EOs, and favor large scale production. However, SLNs also demonstrate some disadvantages, such as high pressure-induced drug degradation, lipid crystallization and drug incorporation, and unpredictable gelation phenomena (298). Solid lipid nanoparticles are mainly prepared by high pressure homogenization or micro emulsification (297).
For the preservation of fruits and vegetables, encapsulation methods have been extensively researched. Protein/polysaccharides were usually used as wall materials of EO microcapsules, such as β-cyclodextrin, pectin, chitosan, starch–gellan, alginate, sodium alginate, gelatin, and carboxymethyl cellulose (299). In a recent study, lemongrass EO-containing poly(lactic acid) nanocapsules were made to achieve long-term thermal stability of the EO. Compared to the apples treated with non-encapsulated lemongrass EO, the fruit treated with encapsulated EO showed three times smaller bitter rot lesions (272). López-Gómez et al. (300) tested the effect of different sized active packages (including β-cyclodextrin-EOs inclusion complex) on the quality of grapes, nectarines, and lettuces, representing berry fruit, stone fruit, and leafy vegetables. The EOs–β-cyclodextrin inclusion complex was dissolved in water-diluted lacquer, then sprayed on all internal surfaces of the packages. They found active cardboard packages with greater active surface better preserved quality of grapes, nectarines and lettuce, for which the sensory quality was acceptable after 30, 25, and 14 days, respectively.
To sum up, encapsulation could be an effective formulation method, which could address some of the drawbacks associated with the usage of EOs in their raw form. In particular, encapsulation protects EOs from light, air, and humidity, allows targeted delivery and controlled release of EOs, boosts EO solubility and bioactivities, and reduces degradation during storage, such as through oxidation or volatilization.
Edible Coatings
Edible coatings have attracted great attention among various postharvest packaging technologies due to their cost-effectiveness, environmentally friendly characteristics, and ability to carry bioactive substances. An edible coating is defined as a thin layer of edible material on a food surface, which can be digested by the human body or are biodegradable in the environment, used as a barrier to protect the product from physical damage and chemical reactions (301, 302). So far, edible coatings are widely used on whole or fresh-cut fruits and vegetables to prolong their shelf life and maintain their sensory qualities.
Different kinds of EOs, such as ginger (303), oregano (304), cinnamon (305), lemongrass (306), and lavender (307) have been incorporated into edible coating as anti-microbial agents. Direct application of EOs to fresh commodity surfaces is limited due to their instability, hydrophobicity, and organoleptic effect. However, emulsification and encapsulation of EOs that is compatible with coating systems can maintain the bioactivity, control the release, increase the effective rate, minimize the organoleptic impact, and increase the contact area and duration on food surfaces (308).
The name, EO-edible coatings, refers to coatings in which a thin layer of a mixture of EOs and biological polymers that are able to carry EOs are used to create a food coating. Three types of biological polymers are commonly used for EO-edible coatings: polysaccharides, proteins, and lipids (301). Natural gum, chitosan, starch, pectin, and alginate are commonly used polysaccharides for EO-edible coatings. The protein-based coatings typically contain gluten, collagen, zein, casein, or whey proteins. The lipid-based coatings mainly include wax, acyl glycerol, and fatty acids (301). In addition, composite coatings refer to the combination of water colloids and lipids, which could provide multiple benefits (309, 310). Four manufacturing methods of EO-edible coatings are: (1) dipping (for thick and uniform materials), (2) spraying (for thin and uniform materials), (3) spreading (for medium thickness and a little uniform materials), and (4) thin film hydration (for poor uniformity of liposome particle size materials) (301).
In brief, EOs encapsulated in edible coating have better stability and show good fresh-keeping potential. Further research should keep exploring novel combinations of basic materials and EOs for coatings that can be used on a variety of food items, which are safe, economic, and effective.
Future Prospects
Overall, EOs demonstrate antimicrobial, pesticidal, and herbicidal effects in both in vitro and in vivo studies. They display great potential in organic product cultivation and food preservation. However, most of the studies that have been conducted are limited to laboratory conditions and in-field analysis of product efficacy is needed. Currently, the number of commercially available biocontrol products using EOs as active components is limited. The reasons could be (1) Their effectiveness is selective. Their efficacies are variable among different pathogen species, sometimes even within the same pathogen species but different strains. (2) EOs can be a double-edged sword. They may display anti-fungal effects and phytotoxicity effects at the same time. It would be hard to find a proper application concentration for every crop at every developmental stage that can totally suppress the pathogens without damaging the plants. 3 Our understanding of the anti-pathogen, pesticidal, and herbicidal effects of EOs and their components is still developing. More work is needed to elucidate their modes of actions, cost-effectiveness, and potential impacts on non-targeted species. There is enormous potential for selecting plants to produce EOs with enhanced efficacy, such as blending EOs to achieve synergistic effects or a broader spectrum of action and formulated with other materials to extend residual effects. As people are more concerned about human and environmental health and are willing to pay more for organic commodities, theoretical and practical studies of EOs should continue simultaneously and more focus should be placed on the development, extension, and market entry of newly formulated EO products.
In the food industry, EOs have great potential to be used as organic food preservatives. Nanoemulsions of EOs have been found to enhance quality and shelf life of fresh commodities. Encapsulation should be used to mask the strong organoleptic impact of EOs while maintaining their preservative effects. Nevertheless, the pungent odor of EOs will remain a big problem. Some valuable research topics can include methods to minimize the odor of EOs or discover effective EO combinations in which the unique EO odor is compatible with or enhances the original food flavor. Furthermore, since EOs are relatively expensive, the development of economic, simple, and stable EO extraction techniques on an industrial scale would support product innovation and expand adoption.
Author Contributions
YC, JB, and AS conceived and designed the structure of the manuscript. YC conducted the literature review and primarily wrote the manuscript. JB, AS, DC, PH, and DT reviewed and edited the manuscript and contributed additional literature and writing. JB and AS revised the manuscript. All authors contributed to the article and approved the submitted version.
Funding
This work was supported by award 2020-51300-32181 from the USDA National Institute of Food and Agriculture, Organic Agriculture Research and Extension Initiative program.
Conflict of Interest
The authors declare that the research was conducted in the absence of any commercial or financial relationships that could be construed as a potential conflict of interest.
Publisher's Note
All claims expressed in this article are solely those of the authors and do not necessarily represent those of their affiliated organizations, or those of the publisher, the editors and the reviewers. Any product that may be evaluated in this article, or claim that may be made by its manufacturer, is not guaranteed or endorsed by the publisher.
Acknowledgments
The authors acknowledge financial support from the USDA NIFA Organic Agriculture Research and Extension Initiative program, which has provided funding to support YC's Ph.D. research assistantship and tuition.
References
1. Swamy MK, Akhtar MS, Sinniah UR. Antimicrobial properties of plant essential oils against human pathogens and their mode of action: an updated review. Evid Based Comp Altern Med. (2016) 2016:21. doi: 10.1155/2016/3012462
2. Ali B, Al-Wabel NA, Shams S, Ahamad A, Khan SA, Anwar F. Essential oils used in aromatherapy: a systemic review. Asian Pac J Trop Biomed. (2015) 5:601–11. doi: 10.1016/j.apjtb.2015.05.007
3. Edris AE. Pharmaceutical and therapeutic potentials of essential oils and their individual volatile constituents: a review. Phytotherapy Res. (2007) 21:308–23. doi: 10.1002/ptr.2072
4. Zubrod JP, Bundschuh M, Arts G, Brühl CA, Imfeld G, Knäbel A, et al. Fungicides: an overlooked pesticide class? Environ Sci Technol. (2019) 53:3347–65. doi: 10.1021/acs.est.8b04392
5. Sartorelli P, Marquioreto AD, Amaral-Baroli A, Lima MEL, Moreno PRH. Chemical composition and antimicrobial activity of the essential oils from two species of eucalyptus. Phytotherapy Res. (2007) 21:231–3. doi: 10.1002/ptr.2051
6. Lopez-Reyes JG, Spadaro D, Prelle A, Garibaldi A, Gullino ML. Efficacy of plant essential oils on postharvest control of rots caused by fungi on different stone fruits in vivo. J Food Prot. (2013) 76:631–9. doi: 10.4315/0362-028X.JFP-12-342
7. Sinha S, Jothiramajayam M, Ghosh M, Mukherjee A. Evaluation of toxicity of essential oils palmarosa, citronella, lemongrass and vetiver in human lymphocytes. Food Chem Toxicol. (2014) 68:71–7. doi: 10.1016/j.fct.2014.02.036
8. Zaka SM, Iqbal N, Saeed Q, Akrem A, Batool M, Khan AA, et al. Toxic effects of some insecticides, herbicides, and plant essential oils against Tribolium confusum Jacquelin du val (Insecta: Coleoptera: Tenebrionidae). Saudi J Biol Sci. (2019) 26:1767–71. doi: 10.1016/j.sjbs.2018.05.012
9. Khaliq A, Ullah MI, Afzal M, Ali S, Sajjad A, Ahmad A, et al. Management of Tribolium castaneum using synergism between conventional fumigant and plant essential oils. Int J Trop Insect Sci. (2020) 40:781–8. doi: 10.1007/s42690-020-00131-w
10. Langeveld WT, Veldhuizen EJ, Burt SA. Synergy between essential oil components and antibiotics: a review. Crit Rev Microbiol. (2014) 40:76–94. doi: 10.3109/1040841X.2013.763219
11. Van Vuuren S. Antimicrobial activity of South African medicinal plants. J Ethnopharmacol. (2008) 119:462–72. doi: 10.1016/j.jep.2008.05.038
12. Pateiro M, Barba FJ, Domínguez R, Sant'Ana AS, Khaneghah AM, Gavahian M, et al. Essential oils as natural additives to prevent oxidation reactions in meat and meat products: a review. Food Res Int. (2018) 113:156–66. doi: 10.1016/j.foodres.2018.07.014
13. Abdollahi A, Hassani A, Ghosta Y, Meshkatalsadat MH, Shabani R. Screening of antifungal properties of essential oils extracted from sweet basil, fennel, summer savory and thyme against postharvest phytopathogenic fungi. J Food Saf. (2011) 31:350–6. doi: 10.1111/j.1745-4565.2011.00306.x
14. Palou L, Ali A, Fallik E, Romanazzi G. GRAS, plant-and animal-derived compounds as alternatives to conventional fungicides for the control of postharvest diseases of fresh horticultural produce. Postharvest Biol Technol. (2016) 122:41–52. doi: 10.1016/j.postharvbio.2016.04.017
16. Pandey AK, Singh P. The genus Artemisia: a 2012–2017 literature review on chemical composition, antimicrobial, insecticidal and antioxidant activities of essential oils. Medicines. (2017) 4:68. doi: 10.3390/medicines4030068
17. Vinatoru M, Mason T, Calinescu I. Ultrasonically assisted extraction (UAE) and microwave assisted extraction (MAE) of functional compounds from plant materials. Trends Anal Chem. (2017) 97:159–78. doi: 10.1016/j.trac.2017.09.002
18. Zin MM, Anucha CB, Bánvölgyi S. Recovery of phytochemicals via electromagnetic irradiation (microwave-assisted-extraction): Betalain and phenolic compounds in perspective. Foods. (2020) 9:918. doi: 10.3390/foods9070918
19. Yingngam B, Brantner A, Treichler M, Brugger N, Navabhatra A, Nakonrat P. Optimization of the eco-friendly solvent-free microwave extraction of Limnophila aromatica essential oil. Ind Crops Prod. (2021) 165:113443. doi: 10.1016/j.indcrop.2021.113443
20. Wang Y, Li R, Jiang ZT, Tan J, Tang SH, Li TT, et al. Green and solvent-free simultaneous ultrasonic-microwave assisted extraction of essential oil from white and black peppers. Ind Crops Prod. (2018) 114:164–72. doi: 10.1016/j.indcrop.2018.02.002
21. Ahangari H, King JW, Ehsani A, Yousefi M. Supercritical fluid extraction of seed oils—A short review of current trends. Trends Food Sci Technol. (2021) 111:249–60. doi: 10.1016/j.tifs.2021.02.066
22. Samadi M, Zainal Abidin Z, Yoshida H, Yunus R, Awang Biak D, Lee C, et al. Subcritical water extraction of essential oil from Aquilaria malaccensis leaves. Sep Sci Technol. (2020) 55:2779–98. doi: 10.1080/01496395.2019.1650768
23. Tunç MT, Koca I. Ohmic heating assisted hydrodistillation of clove essential oil. Ind Crops Prod. (2019) 141:111763. doi: 10.1016/j.indcrop.2019.111763
24. Dao T, Tran T, Nhan N, Quyen N, Tien L, Anh T, et al. Optimization of essential oil yield from Vietnamese green pepper (Piper nigrum) using hydro-distillation method. In: IOP Conference Series: Materials Science and Engineering. London: IOP Publishing (2020). p. 022039.
25. Kaya DA, Ghica MV, Dănilă E, Öztürk S, Türkmen M, Albu Kaya MG, et al. Selection of optimal operating conditions for extraction of Myrtus Communis L. essential oil by the steam distillation method. Molecules. (2020) 25:2399. doi: 10.3390/molecules25102399
26. Afzal A, Munir A, Ghafoor A, Alvarado JL. Development of hybrid solar distillation system for essential oil extraction. Renew Energy. (2017) 113:22–9. doi: 10.1016/j.renene.2017.05.027
27. Deng W, Liu K, Cao S, Sun J, Zhong B, Chun J. Chemical composition, antimicrobial, antioxidant, and antiproliferative properties of grapefruit essential oil prepared by molecular distillation. Molecules. (2020) 25:217. doi: 10.3390/molecules25010217
28. Ranjitha J, Vijiyalakshmi S. Facile methods for the extraction of essential oil from the plant species-a review. Int J Pharm Sci Res. (2014) 5:1107–15. doi: 10.13040/IJPSR.0975-8232
29. Chen C, Cai N, Chen J, Wan C. Clove essential oil as an alternative approach to control postharvest blue mold caused by Penicillium italicum in citrus fruit. Biomolecules. (2019) 9:197. doi: 10.3390/biom9050197
30. Shah S, Hashmi MS, Qazi IM, Durrani Y, Sarkhosh A, Hussain I, et al. Pre-storage chitosan-thyme oil coating control anthracnose in mango fruit. Sci Hortic. (2021) 284:110139. doi: 10.1016/j.scienta.2021.110139
31. Taghavi T, Kim C, Rahemi A. Role of natural volatiles and essential oils in extending shelf life and controlling postharvest microorganisms of small fruits. Microorganisms. (2018) 6:104. doi: 10.3390/microorganisms6040104
32. Spréa RM, Fernandes Â, Calhelha RC, Pereira C, Pires TC, Alves MJ, et al. Chemical and bioactive characterization of the aromatic plant Levisticum officinale WDJ Koch: a comprehensive study. Food Funct. (2020) 11:1292–303. doi: 10.1039/C9FO02841B
33. Treadwell DD, McKinney D, Creamer NG. From philosophy to science: a brief history of organic horticulture in the United States. HortScience. (2003) 38:1009–14. doi: 10.21273/HORTSCI.38.5.1009
34. International Federation of Organic Agriculture Movements (IFOAM). Annual report 2020: Reflect, reunite, revitalize: Organics International. (2020). Available online at: https://www.ifoam.bio/about-us/annual-reports (accessed September 27, 2021).
35. United States Department of Agriculture. National organic program: Final rule. Codified at 7 C.F.R., part 205. (2000). Available online at: https://www.ecfr.gov/current/title-7/subtitle-B/chapter-I/subchapter-M/part-205?toc=1 (accessed September 1, 2021).
36. United States Department of Agriculture. National organic program: National list of allowed and prohibited substances. Codified at 7 C.F.R., part 205.600. (2021). Available online at: https://www.ecfr.gov/current/title-7/part-205/subpart-g (accessed September 1, 2021).
37. O'Brien PA. Biological control of plant diseases. Aust Plant Pathol. (2017) 46:293–304. doi: 10.1007/s13313-017-0481-4
39. Gupta S, Dikshit A. Biopesticides: an ecofriendly approach for pest control. J Biopesticides. (2010) 3:186.
40. Jain A, Sarsaiya S, Wu Q, Lu Y, Shi J. A review of plant leaf fungal diseases and its environment speciation. Bioengineered. (2019) 10:409–24. doi: 10.1080/21655979.2019.1649520
41. Reverberi M, Ricelli A, Zjalic S, Fabbri AA, Fanelli C. Natural functions of mycotoxins and control of their biosynthesis in fungi. Appl Microbiol Biotechnol. (2010) 87:899–911. doi: 10.1007/s00253-010-2657-5
42. Tripathi P, Dubey N. Exploitation of natural products as an alternative strategy to control postharvest fungal rotting of fruit and vegetables. Postharvest Biol Technol. (2004) 32:235–45. doi: 10.1016/j.postharvbio.2003.11.005
43. Raveau R, Fontaine J, Lounès-Hadj Sahraoui A. Essential oils as potential alternative biocontrol products against plant pathogens and weeds: a review. Foods. (2020) 9:365. doi: 10.3390/foods9030365
44. Shuping D, Eloff JN. The use of plants to protect plants and food against fungal pathogens: a review. Afr J Trad Comp Altern Med. (2017) 14:120–7. doi: 10.21010/ajtcam.v14i4.14
45. Chen Q, Xu S, Wu T, Guo J, Sha S, Zheng X, et al. Effect of citronella essential oil on the inhibition of postharvest Alternaria alternata in cherry tomato. J Sci Food Agric. (2014) 94:2441–7. doi: 10.1002/jsfa.6576
46. Soylu EM, Kose F. Antifungal activities of essential oils against citrus black rot disease agent Alternaria alternata. J Essential Oil Bear Plants. (2015) 18:894–903. doi: 10.1080/0972060X.2014.895158
47. Xu S, Ni Z, Ma L, Zheng X. Control of Alternaria rot of cherry tomatoes by food-grade Laurus nobilis essential oil microemulsion. J Food Saf. (2017) 37:e12286. doi: 10.1111/jfs.12286
48. França K, Silva T, Cardoso T, Ugulino A, Rodrigues A, de Mendonça Júnior A. In vitro effect of essential oil of peppermint (Mentha x piperita L.) on the mycelial growth of Alternaria alternata. J Exp Agric Int. (2018) 26:1–7. doi: 10.9734/JEAI/2018/44243
49. Hong JK, Jo YS, Ryoo DH, Jung JH, Kwon HJ, Lee YH, et al. Alternaria spots in tomato leaves differently delayed by four plant essential oil vapours. Res Plant Dis. (2018) 24:292–301. doi: 10.5423/RPD.2018.24.4.292
50. Castro JC, Endo EH, de Souza MR, Zanqueta EB, Polonio JC, Pamphile JA, et al. Bioactivity of essential oils in the control of Alternaria alternata in dragon fruit (Hylocereus undatus Haw.). Ind Crops Prod. (2017) 97:101–9. doi: 10.1016/j.indcrop.2016.12.007
51. Perveen K, Bokhari NA. Management of Alternaria leaf blight in tomato plants by mentha essential oil. Plant Protect Sci. (2020) 56:191–6. doi: 10.17221/100/2019-PPS
52. Perina FJ, Amaral DC, Fernandes RS, Labory CR, Teixeira GA, Alves E. Thymus vulgaris essential oil and thymol against Alternaria alternata (Fr.) Keissler: effects on growth, viability, early infection and cellular mode of action. Pest Manag Sci. (2015) 71:1371–8. doi: 10.1002/ps.3933
53. Mehdizadeh L, Taheri P, Pirbalouti AG, Moghaddam M. Phytotoxicity and antifungal properties of the essential oil from the Juniperus polycarpos var. turcomanica (B. Fedsch.) RP Adams leaves. Physiol Mol Biol Plants. (2020) 26:759. doi: 10.1007/s12298-020-00776-4
54. Geng H, Yu X, Lu A, Cao H, Zhou B, Zhou L, et al. Extraction, chemical composition, and antifungal activity of essential oil of bitter almond. Int J Mol Sci. (2016) 17:1421. doi: 10.3390/ijms17091421
55. Yazdanpanah L, Mohamadi N. Antifungal activity of Satureja hortensis L. essential oil against Alternaria citri. Eur J Exp Biol. (2014) 4:399–403.
56. Huang X, Chen S-y, Zhang Y, Wang Y-h, Zhang X, Bi Z-y, et al. Chemical composition and antifungal activity of essential oils from three Artemisia species against Alternaria solani. J Essential Oil Bear Plants. (2019) 22:1581–92. doi: 10.1080/0972060X.2019.1708812
57. Tomazoni EZ, Pansera MR, Pauletti GF, Moura S, Ribeiro RT, Schwambach J. In vitro antifungal activity of four chemotypes of Lippia alba (Verbenaceae) essential oils against Alternaria solani (Pleosporeaceae) isolates. Anais Acad Bras Ciências. (2016) 88:999–1010. doi: 10.1590/0001-3765201620150019
58. Rahmatzai N, Zaitoun AA, Madkour MH, Ahmady A, Hazim Z, Mousa M. In vitro and in vivo antifungal activity of botanical oils against Alternaria solani causing early blight of tomato. Int J Biosci. (2017) 10:91–9. doi: 10.12692/ijb/10.1.91-99
59. Muy-Rangel MD, Osuna-Valle JR, García-Estrada RS, Martín-Hernández S, Quintana-Obregón EA. In vitro antifungal activity of garlic essential oil (Allium sativum L.) against Alternaria tenuissima. Rev Mexicana Fitopatol. (2018) 36:162–71. doi: 10.18781/R.MEX.FIT.1708-3
60. Kataria D, Chahal K, Kumar A, Singh R. Chemical characterization of essential oil and extracts of Daucus carota seeds and their antifungal activity against wheat fungi. Allelopathy J. (2017) 42:123–34. doi: 10.26651/2017-42-1-1110
61. Hu Y, Zhang J, Kong W, Zhao G, Yang M. Mechanisms of antifungal and anti-aflatoxigenic properties of essential oil derived from turmeric (Curcuma longa L.) on Aspergillus flavus. Food Chem. (2017) 220:1–8. doi: 10.1016/j.foodchem.2016.09.179
62. Oliveira RC, Carvajal-Moreno M, Correa B, Rojo-Callejas F. Cellular, physiological and molecular approaches to investigate the antifungal and anti-aflatoxigenic effects of thyme essential oil on Aspergillus flavus. Food Chem. (2020) 315:126096. doi: 10.1016/j.foodchem.2019.126096
63. da Silva Bomfim N, Kohiyama CY, Nakasugi LP, Nerilo SB, Mossini SAG, Romoli JCZ, et al. Antifungal and antiaflatoxigenic activity of rosemary essential oil (Rosmarinus officinalis L.) against Aspergillus flavus. Food Addit Contam Part A. (2020) 37:153–61. doi: 10.1080/19440049.2019.1678771
64. Hu Z, Yuan K, Zhou Q, Lu C, Du L, Liu F. Mechanism of antifungal activity of Perilla frutescens essential oil against Aspergillus flavus by transcriptomic analysis. Food Control. (2021) 123:107703. doi: 10.1016/j.foodcont.2020.107703
65. Khaldi A, Meddah B, Moussaoui A, Sonnet P. Anti-mycotoxin effect and antifungal properties of essential oil from Ammodaucus leucotrichus Coss. & Dur. on Aspergillus flavus and Aspergillus ochraceus. J Essential Oil Bear Plants. (2017) 20:36–44. doi: 10.1080/0972060X.2017.1282840
66. Davari M, Ezazi R. Chemical composition and antifungal activity of the essential oil of Zhumeria majdae, Heracleum persicum and Eucalyptus sp. against some important phytopathogenic fungi. J Mycol Med. (2017) 27:463–8. doi: 10.1016/j.mycmed.2017.06.001
67. Kang Y, ZhenYang H, KeXin C, Chen L, LiHui D. Activity and mechanism of perilla essential oil in inhibiting Aspergillus glaucus. Shipin Kexue Food Sci. (2020) 41:63–9. doi: 10.7506/spkx1002-6630-20191103-022
68. Jahani M, Pira M, Aminifard MH. Antifungal effects of essential oils against Aspergillus niger in vitro and in vivo on pomegranate (Punica granatum) fruits. Sci Hortic. (2020) 264:109188. doi: 10.1016/j.scienta.2020.109188
69. Baghloul F, Mansori R, Djahoudi A. In vitro antifungal effect of Rosmarinus officinalis essential oil on Aspergillus niger. Natl J Physiol Pharm Pharmacol. (2017) 7:285–9. doi: 10.5455/njppp.2017.7.7021513102016
70. Muñoz Castellanos L, Amaya Olivas N, Ayala-Soto J, De La O Contreras CM, Zermeño Ortega M, Sandoval Salas F, et al. In vitro and in vivo antifungal activity of clove (Eugenia caryophyllata) and pepper (Piper nigrum L.) essential oils and functional extracts against Fusarium oxysporum and Aspergillus niger in tomato (Solanum lycopersicum L.). Int J Microbiol. (2020) 2020:8. doi: 10.1155/2020/1702037
71. Soares C, Morales H, Faria J, Figueiredo A, Pedro L, Venâncio A. Inhibitory effect of essential oils on growth and on aflatoxins production by Aspergillus parasiticus. World Mycotoxin J. (2016) 9:525–34. doi: 10.3920/WMJ2015.1987
72. Kocić-Tanackov S, Dimić G, Mojović L, Gvozdanović-Varga J, Djukić-Vuković A, Tomović V, et al. Antifungal activity of the onion (Allium cepa L.) essential oil against Aspergillus, Fusarium and Penicillium species isolated from food. J Food Process Preserv. (2017) 41:e13050. doi: 10.1111/jfpp.13050
73. Božik M, Císarová M, Tančinová D, Kourimská L, Hleba L, Klouček P. Selected essential oil vapours inhibit growth of Aspergillus spp. in oats with improved consumer acceptability. Ind Crops Prod. (2017) 98:146–52. doi: 10.1016/j.indcrop.2016.11.044
74. Bisht D, Saroj A, Durgapal A, Chanotiya C, Samad A. Inhibitory effect of cinnamon (Cinnamomum tamala (Buch.-Ham.). T. Nees & Eberm.). essential oil and its aldehyde constituents on growth and spore germination of phytopathogenic fungi. Trends Phytochem Res. (2021) 5:62–70. doi: 10.30495/TPR.2021.1914085.1184
75. Mourão DD, De Souza MR, Dos Reis JV, Ferreira TP, Osorio PR, Dos Santos ER, et al. Fungistatic activity of essential oils for the control of Bipolaris leaf spot in maize. J Med Plants Res. (2019) 13:280–7. doi: 10.5897/JMPR2019.6781
76. Perboni LT, Agostinetto D, Farias CRJD, Chaves FC, Garcia JR. Antifungal activity of Heteranthera reniformis extracts against Bipolaris oryzae. Rev Caatinga. (2021) 34:339–49. doi: 10.1590/1983-21252021v34n210rc
77. Silva W, Moreira-Nuñez V, Gaviria-Hernandéz V, Gonçalves V, Azambuja R, de Farias C. Fungitoxicity of plant extracts and essential oil of rosemary in mycelial growth and sporulation of Bipolaris oryzae. agistra. (2017) 29:257–65.
78. Elsherbiny EA, Safwat NA, Elaasser MM. Fungitoxicity of organic extracts of Ocimum basilicum on growth and morphogenesis of Bipolaris species (teleomorph Cochliobolus). J Appl Microbiol. (2017) 123:841–52. doi: 10.1111/jam.13543
79. Yangui I, Zouaoui Boutiti M, Boussaid M, Messaoud C. Essential oils of Myrtaceae Species growing wild in Tunisia: Chemical variability and antifungal activity against Biscogniauxia mediterranea, the causative agent of charcoal canker. Chem Biodivers. (2017) 14:e1700058. doi: 10.1002/cbdv.201700058
80. de Oliveira Filho JG, da Cruz Silva G, de Aguiar AC, Cipriano L, de Azeredo HMC, Junior SB, et al. Chemical composition and antifungal activity of essential oils and their combinations against Botrytis cinerea in strawberries. J Food Meas Character. (2021) 15:1815–25. doi: 10.1007/s11694-020-00765-x
81. Reang SP, Mishra J, Prasad R. In vitro antifungal activities of five plant essential oils against Botrytis cinerea causing gray mold of orange. J Pharmacogn Phytochem. (2020) 9:1046–8. doi: 10.20546/ijcmas.2020.906.222
82. Yue Q, Shao X, Wei Y, Jiang S, Xu F, Wang H, et al. Optimized preparation of tea tree oil complexation and their antifungal activity against Botrytis cinerea. Postharvest Biol Technol. (2020) 162:111114. doi: 10.1016/j.postharvbio.2019.111114
83. Rguez S, Slimene IB, Abid G, Hammemi M, Kefi A, Elkahoui S, et al. Tetraclinis articulata essential oil reduces Botrytis cinerea infections on tomato. Sci Hortic. (2020) 266:109291. doi: 10.1016/j.scienta.2020.109291
84. Xueuan R, Dandan S, Zhuo L, Qingjun K. Effect of mint oil against Botrytis cinerea on table grapes and its possible mechanism of action. Eur J Plant Pathol. (2018) 151:321–8. doi: 10.1007/s10658-017-1375-6
85. Zhao Y, Yang YH, Ye M, Wang KB, Fan LM, Su FW. Chemical composition and antifungal activity of essential oil from Origanum vulgare against Botrytis cinerea. Food Chem. (2021) 365:130506. doi: 10.1016/j.foodchem.2021.130506
86. Wang L, Hu W, Deng J, Liu X, Zhou J, Li X. Antibacterial activity of Litsea cubeba essential oil and its mechanism against Botrytis cinerea. RSC Adv. (2019) 9:28987–95. doi: 10.1039/C9RA05338G
87. Banani H, Olivieri L, Santoro K, Garibaldi A, Gullino ML, Spadaro D. Thyme and savory essential oil efficacy and induction of resistance against Botrytis cinerea through priming of defense responses in apple. Foods. (2018) 7:11. doi: 10.3390/foods7020011
88. Tahmasebi M, Golmohammadi A, Nematollahzadeh A, Davari M, Chamani E. Control of nectarine fruits postharvest fungal rots caused by Botrytis cinerea and Rhizopus stolonifer via some essential oils. J Food Sci Technol. (2020) 57:1647–55. doi: 10.1007/s13197-019-04197-4
89. Duduk N, Markovic T, Vasic M, Duduk B, Vico I, Obradovic A. Antifungal activity of three essential oils against Colletotrichum acutatum, the causal agent of strawberry anthracnose. J Essential Oil Bear Plants. (2015) 18:529–37. doi: 10.1080/0972060X.2015.1004120
90. He J, Wu D, Zhang Q, Chen H, Li H, Han Q, et al. Efficacy and mechanism of cinnamon essential oil on inhibition of Colletotrichum acutatum isolated from ‘Hongyang'kiwifruit. Front Microbiol. (2018) 9:1288. doi: 10.3389/fmicb.2018.01288
91. Morkeliune A, Rasiukevičiute N, Šernaite L, Valiuškaite A. The use of essential oils from thyme, sage and peppermint against Colletotrichum acutatum. Plants. (2021) 10:114. doi: 10.3390/plants10010114
92. Chávez-Magdaleno M, Luque-Alcaraz A, Gutiérrez-Martínez P, Cortez-Rocha M, Burgos-Hernández A, Lizardi-Mendoza J, et al. Effect of chitosan-pepper tree (Schinus molle) essential oil biocomposites on the growth kinetics, viability and membrane integrity of Colletotrichum gloeosporioides. Rev Mex Ingeniería Química. (2018) 17:29–45. doi: 10.24275/uam/izt/dcbi/revmexingquim/2018v17n1/Chavez
93. Khaledi N, Hassani F. Antifungal activity of Bunium persicum essential oil and its constituents on growth and pathogenesis of Colletotrichum lindemuthianum. J Plant Protect Res. (2018) 58:431–41. doi: 10.24425/jppr.2018.124646
94. Vilaplana R, Pazmiño L, Valencia-Chamorro S. Control of anthracnose, caused by Colletotrichum musae, on postharvest organic banana by thyme oil. Postharvest Biol Technol. (2018) 138:56–63. doi: 10.1016/j.postharvbio.2017.12.008
95. Samithri Y, Karunanayake K, Kulasinghe A. In vitro study of selected essential oils against Colletotrichum sp. and Lasiodiplodia sp. causing postharvest diseases in papaya. Ceylon J Sci. (2020) 49:389–96. doi: 10.4038/cjs.v49i5.7806
96. Kumar A, Suravajhala R, Bhagat M. Bioactive potential of Cedrus deodara (Roxb) Loud essential oil (bark) against Curvularia lunata and molecular docking studies. SN Appl Sci. (2020) 2:1–9. doi: 10.1007/s42452-020-2837-6
97. Mourão DD, Ferreira de Souza Pereira T, Souza DJ, Chagas Júnior AF, Dalcin MS, Veloso RA, et al. Essential oil of Cymbopogon citratus on the control of the Curvularia leaf spot disease on maize. Medicines. (2017) 4:62. doi: 10.3390/medicines4030062
98. Chacón C, Bojórquez-Quintal E, Caamal-Chan G, Ruíz-Valdiviezo VM, Montes-Molina JA, Garrido-Ramírez ER, et al. In vitro antifungal activity and chemical composition of Piper auritum kunth essential oil against Fusarium oxysporum and Fusarium equiseti. Agronomy. (2021) 11:1098. doi: 10.3390/agronomy11061098
99. Ferreira FMD, Hirooka EY, Ferreira FD, Silva MV, Mossini SAG, Machinski M Jr. Effect of Zingiber officinale Roscoe essential oil in fungus control and deoxynivalenol production of Fusarium graminearum Schwabe in vitro. Food Addit Contam Part A. (2018) 35:2168–74. doi: 10.1080/19440049.2018.1520397
100. Luchesi LA, Paulus D, Busso C, Frata MT, Oliveira JB. Chemical composition, antifungal and antioxidant activity of essential oils from Baccharis dracunculifolia and Pogostemon cablin against Fusarium graminearum. Nat Prod Res. (2020) 1–4. doi: 10.1080/14786419.2020.1802267
101. Sharma A, Rajendran S, Srivastava A, Sharma S, Kundu B. Antifungal activities of selected essential oils against Fusarium oxysporum f. sp. lycopersici 1322: with emphasis on Syzygium aromaticum essential oil. J Biosci Bioeng. (2017) 123:308–13. doi: 10.1016/j.jbiosc.2016.09.011
102. Eke P, Adamou S, Fokom R, Nya VD, Fokou PVT, Wakam LN, et al. Arbuscular mycorrhizal fungi alter antifungal potential of lemongrass essential oil against Fusarium solani, causing root rot in common bean (Phaseolus vulgaris L.). Heliyon. (2020) 6:e05737. doi: 10.1016/j.heliyon.2020.e05737
103. Rachitha P, Krupashree K, Jayashree G, Gopalan N, Khanum F. Growth inhibition and morphological alteration of Fusarium sporotrichioides by Mentha piperita essential oil. Pharmacognosy Res. (2017) 9:74. doi: 10.4103/0974-8490.199771
104. Gakuubi MM, Maina AW, Wagacha JM. Antifungal activity of essential oil of Eucalyptus camaldulensis dehnh. against selected Fusarium spp. Int J Microbiol. (2017) 2017:7. doi: 10.1155/2017/8761610
105. da Silva Bomfim N, Nakassugi LP, Oliveira JFP, Kohiyama CY, Mossini SAG, Grespan R, et al. Antifungal activity and inhibition of fumonisin production by Rosmarinus officinalis L. essential oil in Fusarium verticillioides (Sacc.) Nirenberg. Food Chem. (2015) 166:330–6. doi: 10.1016/j.foodchem.2014.06.019
106. Avanço GB, Ferreira FD, Bomfim NS, Peralta RM, Brugnari T, Mallmann CA, et al. Curcuma longa L. essential oil composition, antioxidant effect, and effect on Fusarium verticillioides and fumonisin production. Food Control. (2017) 73:806–13. doi: 10.1016/j.foodcont.2016.09.032
107. Ren X, Xu Z, Deng R, Huang L, Zheng R, Kong Q. Peppermint essential oil suppresses geotrichum citri-aurantii growth by destructing the cell structure, internal homeostasis, cell cycle. J Agric Food Chem. (2021) 69:7786–97. doi: 10.1021/acs.jafc.1c02918
108. de Araújo IG, da Silva Franca KR, de Figueredo FM, de Mendonça Júnior AF, dos Santos Rodrigues APM, Cardoso TAL. Antifungal activity of Melaleuca essential oil against Lasiodiplodia theobromae in maize seeds. Int J Curr Microbiol App Sci. (2019) 8:1736–46. doi: 10.20546/ijcmas.2019.808.205
109. Ugulino ALN, Mendonça Júnior A, Rodrigues A, Santos AB, França KS, Cardoso TAL, et al. Inhibition effect of vegetable oils on the mycelial growth of Macrophomina phaseolina (Tassi.). Goid. J Agric Sci. (2018) 10:49–56. doi: 10.5539/jas.v10n6p49
110. El-Shoraky FS, Shala A. Antifungal activity of spearmint and peppermint essential oils against macrophomina root rot of cotton. J Plant Protect Pathol. (2018) 9:775–81. doi: 10.21608/jppp.2018.44061
111. Ghazi AA, Attia EA, Rashed NM. Management of charcoal rot (Macrophomina phaseolina) infection in geranium (Pelargonium graveolens L.) using biocontrol agents and essential oils. Environ Biodiversity Soil Sec. (2018) 2:131–42. doi: 10.21608/jenvbs.2018.6399.1039
112. Xiong X, Zhang L, Li X, Zeng Q, Deng R, Ren X, et al. Antifungal mechanisms of lavender essential oil in the inhibition of rot disease caused by Monilinia fructicola in postharvest flat peaches. Can J Microbiol. (2021) 67:724–36. doi: 10.1139/cjm-2020-0484
113. Xu Y, Wei J, Wei Y, Han P, Dai K, Zou X, et al. Tea tree oil controls brown rot in peaches by damaging the cell membrane of Monilinia fructicola. Postharvest Biol Technol. (2021) 175:111474. doi: 10.1016/j.postharvbio.2021.111474
114. Kalajdžić J, Mili C B, Stankov A, Petreš M, Grahovac M, Vukotić J, et al. Potential of wild oregano essential oil in control of Monilinia rot on stored sweet cherry fruits. Rom Biotechnol Lett. (2021) 26:2779–87. doi: 10.25083/rbl/26.4/2779-2787
115. He S, Ren X, Lu Y, Zhang Y, Wang Y, Sun L. Microemulsification of clove essential oil improves its in vitro and in vivo control of Penicillium digitatum. Food Control. (2016) 65:106–11. doi: 10.1016/j.foodcont.2016.01.020
116. Wang Y, Bian W, Ren X, Song X, He S. Microencapsulation of clove essential oil improves its antifungal activity against Penicillium digitatum in vitro and green mould on Navel oranges. J Horticult Sci Biotechnol. (2018) 93:159–66. doi: 10.1080/14620316.2017.1345332
117. Trabelsi D, Hamdane AM, Said MB, Abdrrabba M. Chemical composition and antifungal activity of essential oils from flowers, leaves and peels of Tunisian Citrus aurantium against Penicillium digitatum and Penicillium italicum. J Essential Oil Bear Plants. (2016) 19:1660–74. doi: 10.1080/0972060X.2016.1141069
118. Mani López E, Valle Vargas GP, Palou E, López Malo A. Penicillium expansum inhibition on bread by lemongrass essential oil in vapor phase. J Food Prot. (2018) 81:467–71. doi: 10.4315/0362-028X.JFP-17-315
119. Li WR, Shi QS, Liang Q, Huang XM, Chen YB. Antifungal effect and mechanism of garlic oil on Penicillium funiculosum. Appl Microbiol Biotechnol. (2014) 98:8337–46. doi: 10.1007/s00253-014-5919-9
120. Chidi F, Bouhoudan A, Khaddor M. Antifungal effect of the tea tree essential oil (Melaleuca alternifolia) against Penicillium griseofulvum and Penicillium verrucosum. J King Saud Univ Sci. (2020) 32:2041–5. doi: 10.1016/j.jksus.2020.02.012
121. Debonne E, Van Bockstaele F, De Leyn I, Devlieghere F, Eeckhout M. Validation of in-vitro antifungal activity of thyme essential oil on Aspergillus niger and Penicillium paneum through application in par-baked wheat and sourdough bread. LWT. (2018) 87:368–78. doi: 10.1016/j.lwt.2017.09.007
122. Hong Z, Talib KM, Mujtaba KG, Dabin H, Yahya F, Congying Z, et al. Antifungal potential of cinnamon essential oils against Phytophthora colocasiae causing taro leaf blight. Chem Biol Technol Agric. (2021) 8:1–9. doi: 10.1186/s40538-021-00238-3
123. Wang Y, Wei K, Han X, Zhao D, Zheng Y, Chao J, et al. The antifungal effect of garlic essential oil on Phytophthora nicotianae and the inhibitory component involved. Biomolecules. (2019) 9:632. doi: 10.3390/biom9100632
124. Kim JE, Lee JE, Huh MJ, Lee SC, Seo SM, Kwon JH, et al. Fumigant antifungal activity via reactive oxygen species of Thymus vulgaris and Satureja hortensis essential oils and constituents against Raffaelea quercus-mongolicae and Rhizoctonia solani. Biomolecules. (2019) 9:561. doi: 10.3390/biom9100561
125. Saroj A, Chanotiya C, Maurya R, Pragadheesh V, Yadav A, Samad A. Antifungal action of Lippia alba essential oil in Rhizoctonia solani disease management. SN Appl Sci. (2019) 1:1–12. doi: 10.1007/s42452-019-1207-8
126. Oliveira J, Parisi M, Baggio J, Silva P, Paviani B, Spoto M, et al. Control of Rhizopus stolonifer in strawberries by the combination of essential oil with carboxymethylcellulose. Int J Food Microbiol. (2019) 292:150–8. doi: 10.1016/j.ijfoodmicro.2018.12.014
127. Rezende JL, Fernandes CC, Costa AOM, Santos LS, Vicente F, Sperandio EM, et al. Antifungal potential of essential oils from two varieties of Citrus sinensis (lima orange and bahia navel orange) in postharvest control of Rhizopus stolonifer (Ehrenb.: Fr.) Vuill. Food Sci Technol. (2020) 40:405–9. doi: 10.1590/fst.30519
128. Ma BX, Ban XQ, He JS, Huang B, Zeng H, Tian J, et al. Antifungal activity of Ziziphora clinopodioides Lam. essential oil against Sclerotinia sclerotiorum on rapeseed plants (Brassica campestris L.). Crop Protect. (2016) 89:289–95. doi: 10.1016/j.cropro.2016.07.003
129. Valadares ACF, Alves CCF, Alves JM, De Deus IP, de Oliveira JG, Dos Santos TCL, et al. Essential oils from Piper aduncum inflorescences and leaves: chemical composition and antifungal activity against Sclerotinia sclerotiorum. Anais Acad Bras Ciências. (2018) 90:2691–9. doi: 10.1590/0001-3765201820180033
130. Silva FFA d., Alves CCF, Oliveira JG d., Vieira TM, et al. Chemical constituents of essential oil from Murraya paniculata leaves and its application to in vitro biological control of the fungus Sclerotinia sclerotiorum. Food Sci Technol. (2019) 39:413–7. doi: 10.1590/fst.20218
131. Türkmen M, Kara M, Maral H, Soylu S. Determination of chemical component of essential oil of Origanum dubium plants grown at different altitudes and antifungal activity against Sclerotinia sclerotiorum. J Food Process Preserv. (2021) e15787. doi: 10.1111/jfpp.15787
132. Varo A, Mulero-Aparicio A, Adem M, Roca L, Raya-Ortega M, López-Escudero F, et al. Screening water extracts and essential oils from Mediterranean plants against Verticillium dahliae in olive. Crop Prot. (2017) 92:168–75. doi: 10.1016/j.cropro.2016.10.018
133. Zheng J, Liu T, Guo Z, Zhang L, Mao L, Zhang Y, et al. Fumigation and contact activities of 18 plant essential oils on Villosiclava virens, the pathogenic fungus of rice false smut. Sci Rep. (2019) 9:1–10. doi: 10.1038/s41598-019-43433-x
134. Kotan R, Dadasoglu F, Karagoz K, Cakir A, Ozer H, Kordali S, et al. Antibacterial activity of the essential oil and extracts of Satureja hortensis against plant pathogenic bacteria and their potential use as seed disinfectants. Sci Hortic. (2013) 153:34–41. doi: 10.1016/j.scienta.2013.01.027
135. Orzali L, Valente MT, Scala V, Loreti S, Pucci N. Antibacterial activity of essential oils and Trametes versicolor extract against Clavibacter michiganensis subsp. michiganensis and Ralstonia solanacearum for seed treatment and development of a rapid in vivo assay. Antibiotics. (2020) 9:628. doi: 10.3390/antibiotics9090628
136. Soltan H, Ahmed S, Emam D. Comparative antibacterial activity of garlic essential oil extracted by hydro-distillation and diethyl ether extraction methods on four pathogenic bacteria. Adv Plant Agric Res. (2016) 4:0013.0012. doi: 10.15406/apar.2016.04.00132
137. Moghaddam M, Alymanesh MR, Mehdizadeh L, Mirzaei H, Pirbalouti AG. Chemical composition and antibacterial activity of essential oil of Ocimum ciliatum, as a new source of methyl chavicol, against ten phytopathogens. Ind Crops Prod. (2014) 59:144–8. doi: 10.1016/j.indcrop.2014.05.006
138. Purnavab S, Ketabchi S, Rowshan V. Chemical composition and antibacterial activity of methanolic extract and essential oil of Iranian Teucrium polium against some of phytobacteria. Nat Prod Res. (2015) 29:1376–9. doi: 10.1080/14786419.2014.1000320
139. Behiry SI, El-Hefny M, Salem MZ. Toxicity effects of Eriocephalus africanus L. leaf essential oil against some molecularly identified phytopathogenic bacterial strains. Nat Prod Res. (2020) 34:3394–8. doi: 10.1080/14786419.2019.1566824
140. Lee JE, Jung M, Lee SC, Huh MJ, Seo SM, Park IK. Antibacterial mode of action of trans-cinnamaldehyde derived from cinnamon bark (Cinnamomum verum) essential oil against Agrobacterium tumefaciens. Pesticide Biochem Physiol. (2020) 165:104546. doi: 10.1016/j.pestbp.2020.02.012
141. Ashmawy NA, Al Farraj DA, Salem MZ, Elshikh MS, Al-Kufaidy R, Alshammari MK, et al. Potential impacts of Pinus halepensis Miller trees as a source of phytochemical compounds: antibacterial activity of the cones essential oil and n-butanol extract. Agroforestry Syst. (2020) 94:1403–13. doi: 10.1007/s10457-018-0324-5
142. Zefzoufi M, Smaili A, Fdil R, Rifai LA, Faize L, Koussa T, et al. Composition of essential oil of Moroccan Dysphania ambrosioides and its antimicrobial activity against bacterial and fungal phytopathogens. J Plant Pathol. (2020) 102:47–58. doi: 10.1007/s42161-019-00371-x
143. Ashmawy NA, Behiry SI, Al-Huqail AA, Ali HM, Salem MZ. Bioactivity of selected phenolic acids and hexane extracts from Bougainvilla spectabilis and Citharexylum spinosum on the growth of pectobacterium carotovorum and Dickeya solani Bacteria: an opportunity to save the environment. Processes. (2020) 8:482. doi: 10.3390/pr8040482
144. Akhlaghi M, Tarighi S, Taheri P. Effects of plant essential oils on growth and virulence factors of Erwinia amylovora. J Plant Pathol. (2020) 102:409–19. doi: 10.1007/s42161-019-00446-9
145. Al-Ani RA, Adhab MA, Nawar HH. Antibacterial activity of clove, cinnamon, and datura extracts against Erwinia carotovora subsp. atroseptica causative agent of black stem and soft rot on potato. J Med Plants Res. (2012) 6:1891–5. doi: 10.5897/JMPR11.1272
146. Simirgiotis MJ, Burton D, Parra F, López J, Muñoz P, Escobar H, et al. Antioxidant and antibacterial capacities of origanum vulgare l. Essential oil from the arid Andean region of Chile and its chemical characterization by GC-MS. Metabolites. (2020) 10:414. doi: 10.3390/metabo10100414
147. Vasconcelos NG, Queiroz JH, Silva KE, Vasconcelos PC, Croda J, Simionatto S, et al. Synergistic effects of Cinnamomum cassia L. essential oil in combination with polymyxin B against carbapenemase-producing Klebsiella pneumoniae and Serratia marcescens. PLoS ONE. (2020) 15:e0236505. doi: 10.1371/journal.pone.0236505
148. Pereira V, Dias C, Vasconcelos M, Rosa E, Saavedra M. Antibacterial activity and synergistic effects between Eucalyptus globulus leaf residues (essential oils and extracts) and antibiotics against several isolates of respiratory tract infections (Pseudomonas aeruginosa). Ind Crops Prod. (2014) 52:1–7. doi: 10.1016/j.indcrop.2013.09.032
149. El-Hosseiny L, El-Shenawy M, Haroun M, Abdullah F. Comparative evaluation of the inhibitory effect of some essential oils with antibiotics against Pseudomonas aeruginosa. Int J Antibiot. (2014) 2014:5. doi: 10.1155/2014/586252
150. Bozkurt IA, Soylu S, Merve K, Soylu EM. Chemical composition and antibacterial activity of essential oils isolated from medicinal plants against gall forming plant pathogenic bacterial disease agents. Kahramanmaraş Sütçü Imam Üniversitesi Tarim ve Doga Dergisi. (2020) 23:1474–82. doi: 10.18016/ksutarimdoga.vi.723544
151. Scavo A, Pandino G, Restuccia C, Parafati L, Cirvilleri G, Mauromicale G. Antimicrobial activity of cultivated cardoon (Cynara cardunculus L. var. altilis DC.) leaf extracts against bacterial species of agricultural and food interest. Ind Crops Prod. (2019) 129:206–11. doi: 10.1016/j.indcrop.2018.12.005
152. Mohamed AA, Behiry SI, Younes HA, Ashmawy NA, Salem MZ, Márquez-Molina O, et al. Antibacterial activity of three essential oils and some monoterpenes against Ralstonia solanacearum phylotype II isolated from potato. Microb Pathog. (2019) 135:103604. doi: 10.1016/j.micpath.2019.103604
153. Tu QB, Wang PY, Sheng S, Xu Y, Wang JZ, You S, et al. Microencapsulation and antimicrobial activity of plant essential oil against Ralstonia solanacearum. Waste Biomass Valorization. (2020) 11:5273–82. doi: 10.1007/s12649-020-00987-6
154. Villada-Ramos J, Aguillón-Osma J, Soto-Rueda E, Loango-Chamorro N. Evaluation of antibacterial activity of extract essential oil of Tagetes patula L. In vitro against Ralstonia solanacearum biovar 2. Arch Phytopathol Plant Protect. (2021) 54:17–8, 1484–1500. doi: 10.1080/03235408.2021.1916185
155. Vanti GL, Kurjogi M, Basavesha K, Teradal NL, Masaphy S, Nargund VB. Synthesis and antibacterial activity of solanum torvum mediated silver nanoparticle against Xxanthomonas axonopodis pv. punicae and Ralstonia solanacearum. J Biotechnol. (2020) 309:20–8. doi: 10.1016/j.jbiotec.2019.12.009
156. Bagy HMK, Abo-Elyousr KA. Antibacterial activity of some essential oils on bacterial spot disease of tomato plant caused by Xanthomonas axonopodis pv. vesicatoria. Int J Phytopathol. (2019) 8:53–61. doi: 10.33687/phytopath.008.02.2967
157. Mirzaei-Najafgholi H, Tarighi S, Golmohammadi M, Taheri P. The effect of citrus essential oils and their constituents on growth of Xanthomonas citri subsp. citri. Molecules. (2017) 22:591. doi: 10.3390/molecules22040591
158. Leonard S, Hommais F, Nasser W, Reverchon S. Plant–phytopathogen interactions: bacterial responses to environmental and plant stimuli. Environ Microbiol. (2017) 19:1689–716. doi: 10.1111/1462-2920.13611
159. Li Z-H, Cai M, Liu Y-S, Sun P-L, Luo S-L. Antibacterial activity and mechanisms of essential oil from Citrus medica L. var. sarcodactylis. Molecules. (2019) 24:1577. doi: 10.3390/molecules24081577
160. Al Abbasy DW, Pathare N, Al-Sabahi JN, Khan SA. Chemical composition and antibacterial activity of essential oil isolated from Omani basil (Ocimum basilicum Linn.). Asian Pac J Trop Dis. (2015) 5:645–9. doi: 10.1016/S2222-1808(15)60905-7
161. Shahbazi Y. Chemical composition and in vitro antibacterial activity of Mentha spicata essential oil against common food-borne pathogenic bacteria. J Pathog. (2015) 2015:5. doi: 10.1155/2015/916305
162. Shakeri A, Khakdan F, Soheili V, Sahebkar A, Rassam G, Asili J. Chemical composition, antibacterial activity, and cytotoxicity of essential oil from Nepeta ucrainica L. spp. kopetdaghensis. Ind Crops Prod. (2014) 58:315–21. doi: 10.1016/j.indcrop.2014.04.009
163. Diao W-R, Hu Q-P, Feng S-S, Li WQ, Xu J-G. Chemical composition and antibacterial activity of the essential oil from green huajiao (Zanthoxylum schinifolium) against selected foodborne pathogens. J Agric Food Chem. (2013) 61:6044–9. doi: 10.1021/jf4007856
164. Mesomo MC, Corazza ML, Ndiaye PM, Dalla Santa OR, Cardozo L, de Paula Scheer A. Supercritical CO2 extracts and essential oil of ginger (Zingiber officinale R.): chemical composition and antibacterial activity. J Supercrit Fluids. (2013) 80:44–9. doi: 10.1016/j.supflu.2013.03.031
165. Zhang Y, Liu X, Wang Y, Jiang P, Quek S. Antibacterial activity and mechanism of cinnamon essential oil against Escherichia coli and Staphylococcus aureus. Food Control. (2016) 59:282–9. doi: 10.1016/j.foodcont.2015.05.032
166. Li L, Li Z-W, Yin Z-Q, Wei Q, Jia R-Y, Zhou L-J, et al. Antibacterial activity of leaf essential oil and its constituents from Cinnamomum longepaniculatum. Int J Clin Exp Med. (2014) 7:1721.
167. Moghimi R, Ghaderi L, Rafati H, Aliahmadi A, McClements DJ. Superior antibacterial activity of nanoemulsion of Thymus daenensis essential oil against E. coli. Food Chem. (2016) 194:410–5. doi: 10.1016/j.foodchem.2015.07.139
168. Hu W, Li C, Dai J, Cui H, Lin L. Antibacterial activity and mechanism of Litsea cubeba essential oil against methicillin-resistant Staphylococcus aureus (MRSA). Ind Crops Prod. (2019) 130:34–41. doi: 10.1016/j.indcrop.2018.12.078
169. Dey D, Gupta M. Use of essential oils for insect pest management-a review. Innovat Farm. (2016) 1:21–9.
170. Isman MB. Plant essential oils for pest and disease management. Crop Prot. (2000) 19:603–8. doi: 10.1016/S0261-2194(00)00079-X
171. Isman MB. Commercial development of plant essential oils and their constituents as active ingredients in bioinsecticides. Phytochem Rev. (2020) 19:235–41. doi: 10.1007/s11101-019-09653-9
172. Robu V, Covaci G, Popescu IM. The use of essential oils in organic farming. Res J Agric Sci. (2015) 47:134.
173. Soonwera M, Sittichok S. Adulticidal activities of Cymbopogon citratus (Stapf.) and Eucalyptus globulus (Labill.) essential oils and of their synergistic combinations against Aedes aegypti (L.), Aedes albopictus (Skuse), and Musca domestica (L.). Environ Sci Pollut Res. (2020) 27:20201–14. doi: 10.1007/s11356-020-08529-2
174. Tak J-H, Jovel E, Isman MB. Synergistic interactions among the major constituents of lemongrass essential oil against larvae and an ovarian cell line of the cabbage looper, Trichoplusia ni. J Pest Sci. (2017) 90:735–44. doi: 10.1007/s10340-016-0827-7
175. Kanda D, Kaur S, Koul O. A comparative study of monoterpenoids and phenylpropanoids from essential oils against stored grain insects: acute toxins or feeding deterrents. J Pest Sci. (2017) 90:531–45. doi: 10.1007/s10340-016-0800-5
176. Ganassi S, Cascone P, Di Domenico C, Pistillo M, Formisano G, Giorgini M, et al. Electrophysiological and behavioural response of Philaenus spumarius to essential oils and aromatic plants. Sci Rep. (2020) 10:1. doi: 10.1038/s41598-020-59835-1
177. Liu S, Zhao J, Hamada C, Cai W, Khan M, Zou Y, et al. Identification of attractants from plant essential oils for Cyrtorhinus lividipennis, an important predator of rice planthoppers. J Pest Sci. (2019) 92:769–80. doi: 10.1007/s10340-018-1054-1
178. Kendra PE, Owens D, Montgomery WS, Narvaez TI, Bauchan GR, Schnell EQ, et al. α-Copaene is an attractant, synergistic with quercivorol, for improved detection of Euwallacea nr. fornicatus (Coleoptera: Curculionidae: Scolytinae). PLoS ONE. (2017) 12:e0179416. doi: 10.1371/journal.pone.0179416
179. Kendra PE, Tabanca N, Montgomery WS, Niogret J, Owens D, Carrillo D. Essential oils as lures for invasive ambrosia beetles. In: Handbook of Essential Oils. Boca Raton, FL: CRC Press (2020). p. 497–515.
180. Raguso RA. Functions of essential oils and natural volatiles in plant-insect interactions. In: Handbook of Essential Oils. Boca Raton, FL: CRC Press (2020). p. 481–96.
181. Ebadollahi A, Ziaee M, Palla F. Essential oils extracted from different species of the Lamiaceae plant family as prospective bioagents against several detrimental pests. Molecules. (2020) 25:1556. doi: 10.3390/molecules25071556
182. Gaire S, Scharf ME, Gondhalekar AD. Toxicity and neurophysiological impacts of plant essential oil components on bed bugs (Cimicidae: Hemiptera). Sci Rep. (2019) 9:1–12. doi: 10.1038/s41598-019-40275-5
183. Saad MM, El-Deeb DA, Abdelgaleil SA. Insecticidal potential and repellent and biochemical effects of phenylpropenes and monoterpenes on the red flour beetle, Tribolium castaneum Herbst. Environ Sci Pollut Res. (2019) 26:6801–10. doi: 10.1007/s11356-019-04151-z
184. Andrade-Ochoa S, Correa-Basurto J, Rodríguez-Valdez L, Sánchez-Torres L, Nogueda-Torres B, Nevárez-Moorillón G. In vitro and in silico studies of terpenes, terpenoids and related compounds with larvicidal and pupaecidal activity against Culex quinquefasciatus Say (Diptera: Culicidae). Chem Cent J. (2018) 12:1–21. doi: 10.1186/s13065-018-0425-2
185. Kordali S, Usanmaz A, Bayrak N, Çakir A. Fumigation of volatile monoterpenes and aromatic compounds against adults of Sitophilus granarius (L.) (Coleoptera: Curculionidae). Rec Nat Prod. (2017) 11:362–73.
186. Stepanycheva E, Petrova M, Chermenskaya T, Pavela R. Fumigant effect of essential oils on mortality and fertility of thrips Frankliniella occidentalis Perg. Environ Sci Pollut Res. (2019) 26:30885–92. doi: 10.1007/s11356-019-06239-y
187. Li M, Liu B, Bernigaud C, Fischer K, Guillot J, Fang F. Lemongrass (Cymbopogon citratus) oil: a promising miticidal and ovicidal agent against Sarcoptes scabiei. PLoS Neglect Trop Dis. (2020) 14:e0008225. doi: 10.1371/journal.pntd.0008225
188. Ozdemir E, Gozel U. Efficiency of some plant essential oils on root-knot nematode Meloidogyne incognita. J Agric Sci Technol A. (2017) 7:178–83. doi: 10.17265/2161-6256/2017.03.005
189. Benelli G, Pavela R, Petrelli R, Cappellacci L, Canale A, Senthil-Nathan S, et al. Not just popular spices! Essential oils from Cuminum cyminum and Pimpinella anisum are toxic to insect pests and vectors without affecting non-target invertebrates. Ind Crops Prod. (2018) 124:236–43. doi: 10.1016/j.indcrop.2018.07.048
190. Stenger LD, Abati R, Pawlak IG, Varpechoski GO, de Souza Vismara E, Barbosa LR, et al. Toxicity of essential oil of Eugenia uniflora (L.) to Thaumastocoris peregrinus (Hemiptera: Thaumastocoridae) and selectivity to the parasitoid Cleruchoides noackae (Lin & Hubert) (Hymenoptera: Mymaridae). Crop Protect. (2021) 147:105693. doi: 10.1016/j.cropro.2021.105693
191. Isman MB. Pesticides based on plant essential oils: Phytochemical and practical considerations. In: Medicinal and Aromatic Crops: Production, Phytochemistry, and Utilization. Washington, DC: ACS Publications (2016). p. 13–26.
192. Ibrahim SS. Essential oil nanoformulations as a novel method for insect pest control in horticulture. In: Horticultural Crops. London: IntechOpen (2019). p. 195–209.
193. Mohafrash SM, Fallatah SA, Farag SM, Mossa AT. Mentha spicata essential oil nanoformulation and its larvicidal application against Culex pipiens and Musca domestica. Ind Crops Prod. (2020) 157:112944. doi: 10.1016/j.indcrop.2020.112944
194. Ya-Ali P, Yarahmadi F, Mehrnia MA. Efficacies of two nano-formulations of Tasmanian blue gum essential oil to control Callosobruchus maculatus. J Econ Entomol. (2020) 113:1555–62. doi: 10.1093/jee/toaa069
195. Rashid B, Husnain T, Riazuddin S. Herbicides and pesticides as potential pollutants: a global problem. In: Plant Adaptation and Phytoremediation. Dordrecht: Springer (2010). p. 427–47.
196. Verdeguer M, Sánchez-Moreiras AM, Araniti F. Phytotoxic effects and mechanism of action of essential oils and terpenoids. Plants. (2020) 9:1571. doi: 10.3390/plants9111571
197. Dudai N, Poljakoff-Mayber A, Mayer A, Putievsky E, Lerner H. Essential oils as allelochemicals and their potential use as bioherbicides. J Chem Ecol. (1999) 25:1079–89. doi: 10.1023/A:1020881825669
198. Thomas B, Murphy DJ, Murray BG. Encyclopedia of Applied Plant Sciences. Waltham, MA: Academic Press (2016).
199. Alipour M, Saharkhiz MJ, Niakousari M, Damyeh MS. Phytotoxicity of encapsulated essential oil of rosemary on germination and morphophysiological features of amaranth and radish seedlings. Sci Hortic. (2019) 243:131–9. doi: 10.1016/j.scienta.2018.08.023
200. Martino LD, Formisano C, Mancini E, Feo VD, Piozzi F, Rigano D, et al. Chemical composition and phytotoxic effects of essential oils from four Teucrium species. Nat Prod Commun. (2010) 5:1934578X1000501230. doi: 10.1177/1934578X1000501230
201. Ootani MA, dos Reis MR, Cangussu ASR, Capone A, Fidelis RR, Oliveira W, et al. Phytotoxic effects of essential oils in controlling weed species Digitaria horizontalis and Cenchrus echinatus. Biocatal Agric Biotechnol. (2017) 12:59–65. doi: 10.1016/j.bcab.2017.08.016
202. Vokou D, Douvli P, Blionis GJ, Halley JM. Effects of monoterpenoids, acting alone or in pairs, on seed germination and subsequent seedling growth. J Chem Ecol. (2003) 29:2281–301. doi: 10.1023/A:1026274430898
203. Verdeguer M, Blázquez MA, Boira H. Chemical composition and herbicidal activity of the essential oil from a Cistus ladanifer L. population from Spain. Nat Prod Res. (2012) 26:1602–9. doi: 10.1080/14786419.2011.592835
204. Han C, Shao H, Zhou S, Mei Y, Cheng Z, Huang L, et al. Chemical composition and phytotoxicity of essential oil from invasive plant, Ambrosia artemisiifolia L. Ecotoxicol Environ Saf. (2021) 211:111879. doi: 10.1016/j.ecoenv.2020.111879
205. Pouresmaeil M, Nojadeh MS, Movafeghi A, Maggi F. Exploring the bio-control efficacy of Artemisia fragrans essential oil on the perennial weed Convolvulus arvensis: inhibitory effects on the photosynthetic machinery and induction of oxidative stress. Ind Crops Prod. (2020) 155:112785. doi: 10.1016/j.indcrop.2020.112785
206. Dias M, Nozari R, Santarém E. Herbicidal activity of natural compounds from Baccharis spp. on the germination and seedlings growth of Lactuca sativa and Bidens pilosa. Allelopathy J. (2017) 42:21–36. doi: 10.26651/2017-42-1-1103
207. Synowiec A, Możdżeń K, Krajewska A, Landi M, Araniti F. Carum carvi L. essential oil: a promising candidate for botanical herbicide against Echinochloa crus-galli (L.) P. Beauv. in maize cultivation. Ind Crops Prod. (2019) 140:111652. doi: 10.1016/j.indcrop.2019.111652
208. Poonpaiboonpipat T, Krumsri R, Kato-Noguchi H. Allelopathic and herbicidal effects of crude extract from Chromolaena odorata (L.) RM King and H. Rob. on Echinochloa crus-galli and Amaranthus viridis. Plants. (2021) 10:1609. doi: 10.3390/plants10081609
209. Gurgel ESC, de Oliveira MS, Souza MC, da Silva SG, de Mendonca MS, da Silva Souza Filho AP. Chemical compositions and herbicidal (phytotoxic) activity of essential oils of three Copaifera species (Leguminosae-Caesalpinoideae) from Amazon-Brazil. Ind Crops Prod. (2019) 142:111850. doi: 10.1016/j.indcrop.2019.111850
210. Merad N, Andreu V, Chaib S, de Carvalho Augusto R, Duval D, Bertrand C, et al. Essential oils from two apiaceae species as potential agents in organic crops protection. Antibiotics. (2021) 10:636. doi: 10.3390/antibiotics10060636
211. Khare P, Srivastava S, Nigam N, Singh AK, Singh S. Impact of essential oils of E. citriodora, O. basilicum and M. arvensis on three different weeds and soil microbial activities. Environ Technol Innovat. (2019) 14:100343. doi: 10.1016/j.eti.2019.100343
212. Pinto M, Soares C, Martins M, Sousa B, Valente I, Pereira R, et al. Herbicidal effects and cellular targets of aqueous extracts from young Eucalyptus globulus Labill leaves. Plants. (2021) 10:1159. doi: 10.3390/plants10061159
213. Kaur P, Gupta S, Kaur K, Kaur N, Kumar R, Bhullar MS. Nanoemulsion of Foeniculum vulgare essential oil: a propitious striver against weeds of Triticum aestivum. Ind Crops Prod. (2021) 168:113601. doi: 10.1016/j.indcrop.2021.113601
214. Ibáñez MD, Blázquez MA. Phytotoxic effects of commercial Eucalyptus citriodora, Lavandula angustifolia, and Pinus sylvestris essential oils on weeds, crops, invasive species. Molecules. (2019) 24:2847. doi: 10.3390/molecules24152847
215. Kong Q, Zhou L, Wang X, Luo S, Li J, Xiao H, et al. Chemical composition and allelopathic effect of essential oil of Litsea pungens. Agronomy. (2021) 11:1115. doi: 10.3390/agronomy11061115
216. Singh N, Singh HP, Batish DR, Kohli RK, Yadav SS. Chemical characterization, phytotoxic, and cytotoxic activities of essential oil of Mentha longifolia. Environ Sci Pollut Res. (2020) 27:13512–23. doi: 10.1007/s11356-020-07823-3
217. Ricci D, Epifano F, Fraternale D. The essential oil of Monarda didyma L(Lamiaceae) exerts phytotoxic activity in vitro against various weed seed. Molecules. (2017) 22:222. doi: 10.3390/molecules22020222
218. Bozok F. Herbicidal activity of Nepeta flavida essential oil. J Essential Oil Bear Plants. (2018) 21:1687–93. doi: 10.1080/0972060X.2019.1577183
219. Sarić-Krsmanović M, Gajić Umiljendić J, Radivojević L, Šantrić L, Potočnik I, Durović-Pejčev R. Bio-herbicidal effects of five essential oils on germination and early seedling growth of velvetleaf (Abutilon theophrasti Medik.). J Environ Sci Health Part B. (2019) 54:247–51. doi: 10.1080/03601234.2018.1550309
220. Frabboni L, Tarantino A, Petruzzi F, Disciglio G. Bio-herbicidal effects of oregano and rosemary essential oils on chamomile (Matricaria chamomilla L.) crop in organic farming system. Agronomy. (2019) 9:475. doi: 10.3390/agronomy9090475
221. El-Rokiek KG, Ibrahim ME, El-Din SAS, El-Sawi SA. Using anise (Pimpinella anisum L.) essential oils as natural herbicide. J Mater Environ Sci. (2020) 11:1689–98.
222. Ismail A, Habiba K, Yassine M, Mohsen H, Bassem J, Lamia H. Essential oils of Tunisian Pinus radiata D. Don, chemical composition and study of their herbicidal activity. Viet J Chem. (2021) 59:247–52. doi: 10.1002/vjch.202000103
223. Andriana Y, Xuan TD, Quy TN, Tran H.-D., Le -T, et al. Biological activities and chemical constituents of essential oils from Piper cubeba Bojer and Piper nigrum L. Molecules. (2019) 24:1876. doi: 10.3390/molecules24101876
224. Dahiya S, Batish DR, Singh HP. Pogostemon benghalensis essential oil inhibited the weed growth via causing oxidative damage. Braz J Botany. (2020) 43:447–57. doi: 10.1007/s40415-020-00613-8
225. Bozhuyuk AU. Herbicidal activity and chemical composition of two essential oils on seed germinations and seedling growths of three weed species. J Essential Oil Bear Plants. (2020) 23:821–31. doi: 10.1080/0972060X.2020.1828178
226. Maccioni A, Santo A, Falconieri D, Piras A, Farris E, Maxia A, et al. Phytotoxic effects of Salvia rosmarinus essential oil on Acacia saligna seedling growth. Flora. (2020) 269:151639. doi: 10.1016/j.flora.2020.151639
227. Hazrati H, Saharkhiz MJ, Niakousari M, Moein M. Natural herbicide activity of Satureja hortensis L. essential oil nanoemulsion on the seed germination and morphophysiological features of two important weed species. Ecotoxicol Environ Saf. (2017) 142:423–30. doi: 10.1016/j.ecoenv.2017.04.041
228. Laosinwattana C, Wichittrakarn P, Teerarak M. Chemical composition and herbicidal action of essential oil from Tagetes erecta L. leaves. Ind Crops Prod. (2018) 126:129–34. doi: 10.1016/j.indcrop.2018.10.013
229. Verdeguer M, Torres-Pagan N, Muñoz M, Jouini A, García-Plasencia S, Chinchilla P, et al. Herbicidal activity of Thymbra capitata (L.) Cav. essential oil. Molecules. (2020) 25:2832. doi: 10.3390/molecules25122832
230. Jouini A, Verdeguer M, Pinton S, Araniti F, Palazzolo E, Badalucco L, et al. Potential effects of essential oils extracted from Mediterranean aromatic plants on target weeds and soil microorganisms. Plants. (2020) 9:1289. doi: 10.3390/plants9101289
231. Ulukanli Z, Cenet M, Ince H, Yilmaztekin M. Antimicrobial and herbicidal activities of the essential oil from the Mediterranean Thymus eigii. J Essential Oil Bear Plants. (2018) 21:214–22. doi: 10.1080/0972060X.2017.1327824
232. Ghasemi G, Alirezalu A, Ghosta Y, Jarrahi A, Safavi SA, Abbas-Mohammadi M, et al. Composition, antifungal, phytotoxic, and insecticidal activities of thymus kotschyanus essential oil. Molecules. (2020) 25:1152. doi: 10.3390/molecules25051152
233. Zhou S, Han C, Zhang C, Kuchkarova N, Wei C, Zhang C, et al. Allelopathic, phytotoxic, and insecticidal effects of Thymus proximus Serg. essential oil and its major constituents. Front Plant Sci. (2021) 12:689875. doi: 10.3389/fpls.2021.689875
234. Onaran A, Yilar M. Antifungal and herbicidal activity of Trachystemon orientalis (L.) G. Don against some plant pathogenic fungi and Cuscuta campestris Yunck. Igdir Üniversitesi Fen Bilimleri Enstitüsü Dergisi. (2018) 8:37433. doi: 10.21597/jist.407807
235. Fagodia SK, Singh HP, Batish DR, Kohli RK. Phytotoxicity and cytotoxicity of Citrus aurantiifolia essential oil and its major constituents: Limonene and citral. Ind Crops Prod. (2017) 108:708–15. doi: 10.1016/j.indcrop.2017.07.005
236. Kordali S, Cakir A, Akcin TA, Mete E, Akcin A, Aydin T, et al. Antifungal and herbicidal properties of essential oils and n-hexane extracts of Achillea gypsicola Hub-Mor. and Achillea biebersteinii Afan(Asteraceae). Ind Crops Prod. (2009) 29:562–70. doi: 10.1016/j.indcrop.2008.11.002
237. Almeida de Sousa MA, Mesquita MLR, Orlanda JFF, Catunda FEA. Chemical composition and phytotoxic activity of 'Lippia origanoides' essential oil on weeds. Aust J Crop Sci. (2020) 14:3015–24. doi: 10.21475/ajcs.20.14.03.p2595
238. Tworkoski T. Herbicide effects of essential oils. Weed Sci. (2002) 50:425–31. doi: 10.1614/0043-1745(2002)050[0425:HEOEO]2.0.CO;2
239. Amri I, Hamrouni L, Hanana M, Jamoussi B. Reviews on phytotoxic effects of essential oils and their individual components: news approach for weeds management. Int J Appl Biol Pharm Technol. (2013) 4:96–114.
240. Kaur S, Rana S, Singh HP, Batish DR, Kohli RK. Citronellol disrupts membrane integrity by inducing free radical generation. Zeitschrift Naturforschung C. (2011) 66:260–6. doi: 10.1515/znc-2011-5-609
241. Singh HP, Batish DR, Kaur S, Kohli RK, Arora K. Phytotoxicity of the volatile monoterpene citronellal against some weeds. Zeitschrift Naturforschung C. (2006) 61:334–40. doi: 10.1515/znc-2006-5-606
242. Hanana M, Mansour MB, Algabr M, Amri I, Gargouri S, Romane A, et al. Potential use of essential oils from four Tunisian species of Lamiaceae: biological alternative for fungal and weed control. Rec Nat Prod. (2017) 11:258–69.
243. Koitabashi R, Suzuki T, Kawazu T, Sakai A, Kuroiwa H, Kuroiwa T. 1, 8-Cineole inhibits root growth and DNA synthesis in the root apical meristem of Brassica campestris L. J Plant Res. (1997) 110:1–6. doi: 10.1007/BF02506836
244. Nishida N, Tamotsu S, Nagata N, Saito C, Sakai A. Allelopathic effects of volatile monoterpenoids produced by Salvia leucophylla: inhibition of cell proliferation and DNA synthesis in the root apical meristem of Brassica campestris seedlings. J Chem Ecol. (2005) 31:1187–203. doi: 10.1007/s10886-005-4256-y
245. Abrahim D, Francischini AC, Pergo EM, Kelmer-Bracht AM, Ishii-Iwamoto EL. Effects of α-pinene on the mitochondrial respiration of maize seedlings. Plant Physiol Biochem. (2003) 41:985–91. doi: 10.1016/j.plaphy.2003.07.003
246. Araniti F, Landi M, Lupini A, Sunseri F, Guidi L, Abenavoli M. Origanum vulgare essential oils inhibit glutamate and aspartate metabolism altering the photorespiratory pathway in Arabidopsis thaliana seedlings. J Plant Physiol. (2018) 231:297–309. doi: 10.1016/j.jplph.2018.10.006
247. Chaimovitsh D, Shachter A, Abu-Abied M, Rubin B, Sadot E, Dudai N. Herbicidal activity of monoterpenes is associated with disruption of microtubule functionality and membrane integrity. Weed Sci. (2017) 65:19–30. doi: 10.1614/WS-D-16-00044.1
248. Singh S, Tiwari S. Responses of plants to herbicides: recent advances and future prospectives. Plant Life Under Changing Environ. (2020) 237–50. doi: 10.1016/B978-0-12-818204-8.00011-4
249. Poonpaiboonpipat T, Pangnakorn U, Suvunnamek U, Teerarak M, Charoenying P, Laosinwattana C. Phytotoxic effects of essential oil from Cymbopogon citratus and its physiological mechanisms on barnyardgrass (Echinochloa crus-galli). Ind Crops Prod. (2013) 41:403–7. doi: 10.1016/j.indcrop.2012.04.057
250. Hasan M, Mokhtar AS, Rosli AM, Hamdan H, Motmainna M, Ahmad-Hamdani MS. Weed control efficacy and crop-weed selectivity of a new bioherbicide WeedLock. Agronomy. (2021) 11:1488. doi: 10.3390/agronomy11081488
251. Turek C, Stintzing FC. Stability of essential oils: a review. Comp Rev Food Sci Food Saf. (2013) 12:40–53. doi: 10.1111/1541-4337.12006
252. Pascual-Villalobos MJ, Guirao P, Díaz-Baños FG, Cantó-Tejero M, Villora G. Oil in water nanoemulsion formulations of botanical active substances. In: Nano-Biopesticides Today and Future Perspectives. Elsevier (2019). p. 223–47.
253. Libs E, Salim E. Formulation of essential oil pesticides technology and their application. Agri Res Tech. (2017) 9:555759. doi: 10.19080/ARTOAJ.2017.09.555759
254. Tang C, Li Y, Pun J, Osman ASM, Tam KC. Polydopamine microcapsules from cellulose nanocrystal stabilized pickering emulsions for essential oil and pesticide encapsulation. Colloids Surf A Physicochem Eng Aspects. (2019) 570:403–13. doi: 10.1016/j.colsurfa.2019.03.049
255. Aswathanarayan JB, Vittal RR. Nanoemulsions and their potential applications in food industry. Front Sustain Food Syst. (2019) 3:95. doi: 10.3389/fsufs.2019.00095
256. Komaiko JS, McClements DJ. Formation of food-grade nanoemulsions using low-energy preparation methods: a review of available methods. Comp Rev Food Sci Food Saf. (2016) 15:331–52. doi: 10.1111/1541-4337.12189
257. McClements DJ, Rao J. Food-grade nanoemulsions: formulation, fabrication, properties, performance, biological fate, potential toxicity. Crit Rev Food Sci Nutr. (2011) 51:285–330. doi: 10.1080/10408398.2011.559558
258. Donsi F, Annunziata M, Vincensi M, Ferrari G. Design of nanoemulsion-based delivery systems of natural antimicrobials: effect of the emulsifier. J Biotechnol. (2012) 159:342–50. doi: 10.1016/j.jbiotec.2011.07.001
259. Donsì F, Ferrari G. Essential oil nanoemulsions as antimicrobial agents in food. J Biotechnol. (2016) 233:106–20. doi: 10.1016/j.jbiotec.2016.07.005
260. de Oliveira Filho JG, Miranda M, Ferreira MD, Plotto A. Nanoemulsions as edible coatings: a potential strategy for fresh fruits and vegetables preservation. Foods. (2021) 10:2438. doi: 10.3390/foods10102438
261. Tripathi AD, Sharma R, Agarwal A, Haleem DR. Nanoemulsions based edible coatings with potential food applications. Int J Biobased Plast. (2021) 3:112–25. doi: 10.1080/24759651.2021.1875615
262. Mendes J, Norcino L, Martins H, Manrich A, Otoni C, Carvalho E, et al. Correlating emulsion characteristics with the properties of active starch films loaded with lemongrass essential oil. Food Hydrocoll. (2020) 100:105428. doi: 10.1016/j.foodhyd.2019.105428
263. El Asbahani A, Miladi K, Badri W, Sala M, Addi EA, Casabianca H, et al. Essential oils: from extraction to encapsulation. Int J Pharm. (2015) 483:220–43. doi: 10.1016/j.ijpharm.2014.12.069
264. Majeed H, Bian YY, Ali B, Jamil A, Majeed U, Khan QF, et al. Essential oil encapsulations: uses, procedures, and trends. RSC Adv. (2015) 5:58449–63. doi: 10.1039/C5RA06556A
265. Amiri S, Rahimi A. Poly (ε-caprolactone) electrospun nanofibers containing cinnamon essential oil nanocapsules: a promising technique for controlled release and high solubility. J Ind Text. (2019) 48:1527–44. doi: 10.1177/1528083718764911
266. Kalemba D, Kunicka A. Antibacterial and antifungal properties of essential oils. Curr Med Chem. (2003) 10:813–29. doi: 10.2174/0929867033457719
267. Hammoud Z, Gharib R, Fourmentin S, Elaissari A, Greige-Gerges H. New findings on the incorporation of essential oil components into liposomes composed of lipoid S100 and cholesterol. Int J Pharm. (2019) 561:161–70. doi: 10.1016/j.ijpharm.2019.02.022
268. Fazly Bazzaz B, Khameneh B, Namazi N, Iranshahi M, Davoodi D, Golmohammadzadeh S. Solid lipid nanoparticles carrying Eugenia caryophyllata essential oil: the novel nanoparticulate systems with broad-spectrum antimicrobial activity. Lett Appl Microbiol. (2018) 66:506–13. doi: 10.1111/lam.12886
269. Froiio F, Ginot L, Paolino D, Lebaz N, Bentaher A, Fessi H, et al. Essential oils-loaded polymer particles: preparation, characterization and antimicrobial property. Polymers. (2019) 11:1017. doi: 10.3390/polym11061017
270. Almeida KB, Ramos AS, Nunes JB, Silva BO, Ferraz ER, Fernandes AS, et al. PLGA nanoparticles optimized by Box-Behnken for efficient encapsulation of therapeutic Cymbopogon citratus essential oil. Colloids Surf B Biointerfaces. (2019) 181:935–42. doi: 10.1016/j.colsurfb.2019.06.010
271. Jummes B, Sganzerla WG, da Rosa CG, Noronha CM, Nunes MR, Bertoldi FC, et al. Antioxidant and antimicrobial poly-ε-caprolactone nanoparticles loaded with Cymbopogon martinii essential oil. Biocatal Agric Biotechnol. (2020) 23:101499. doi: 10.1016/j.bcab.2020.101499
272. Antonioli G, Fontanella G, Echeverrigaray S, Delamare APL, Pauletti GF, Barcellos T. Poly (lactic acid) nanocapsules containing lemongrass essential oil for postharvest decay control: in vitro and in vivo evaluation against phytopathogenic fungi. Food Chem. (2020) 326:126997. doi: 10.1016/j.foodchem.2020.126997
273. Nadeem M, Bhutto MA, Yu F, Xie X, El-Hamshary H, El-Faham A, et al. Physico-chemical and biological evaluation of PLCL/SF nanofibers loaded with oregano essential oil. Pharmaceutics. (2019) 11:386. doi: 10.3390/pharmaceutics11080386
274. Huang H, Huang C, Yin C, Khan MR, Zhao H, Xu Y, et al. Preparation and characterization of β-cyclodextrin–oregano essential oil microcapsule and its effect on storage behavior of purple yam. J Sci Food Agric. (2020) 100:4849–57. doi: 10.1002/jsfa.10545
275. de Souza HJB, Botrel DA, de Barros Fernandes RV, Borges SV, Campelo Felix PH, Viana LC, et al. Hygroscopic, structural, and thermal properties of essential oil microparticles of sweet orange added with cellulose nanofibrils. J Food Process Preserv. (2020) 44:e14365. doi: 10.1111/jfpp.14365
276. Paris M, Ramírez-Corona N, Palou E, López-Malo A. Modelling release mechanisms of cinnamon (Cinnamomum zeylanicum) essential oil encapsulated in alginate beads during vapor-phase application. J Food Eng. (2020) 282:110024. doi: 10.1016/j.jfoodeng.2020.110024
277. Li L, Zhang W, Peng J, Xue B, Liu Z, Luo Z, et al. A novel shell material—Highland barley starch for microencapsulation of cinnamon essential oil with different preparation methods. Materials. (2020) 13:1192. doi: 10.3390/ma13051192
278. Arabpoor B, Yousefi S, Weisany W, Ghasemlou M. Multifunctional coating composed of Eryngium campestre L. essential oil encapsulated in nano-chitosan to prolong the shelf-life of fresh cherry fruits. Food Hydrocolloids. (2021) 111:106394. doi: 10.1016/j.foodhyd.2020.106394
279. Shahidi Noghabi M, Molaveisi M. Microencapsulation optimization of cinnamon essential oil in the matrices of gum Arabic, maltodextrin, and inulin by spray-drying using mixture design. J Food Process Eng. (2020) 43:e13341. doi: 10.1111/jfpe.13341
280. Aguiar MCS, Fernandes JB, Forim MR. Evaluation of the microencapsulation of orange essential oil in biopolymers by using a spray-drying process. Sci Rep. (2020) 10:1–11. doi: 10.1038/s41598-020-68823-4
281. Estevinho BN, Rocha F, Santos L, Alves A. Microencapsulation with chitosan by spray drying for industry applications–A review. Trends Food Sci Technol. (2013) 31:138–55. doi: 10.1016/j.tifs.2013.04.001
282. Shishir MRI, Chen W. Trends of spray drying: a critical review on drying of fruit and vegetable juices. Trends Food Sci Technol. (2017) 65:49–67. doi: 10.1016/j.tifs.2017.05.006
283. Goncalves A, Estevinho BN, Rocha F. Design and characterization of controlled-release vitamin A microparticles prepared by a spray-drying process. Powder Technol. (2017) 305:411–7. doi: 10.1016/j.powtec.2016.10.010
284. Manaf M, Subuki I, Jai J, Raslan R, Mustapa A. Encapsulation of volatile citronella essential oil by coacervation: efficiency and release study. In: IOP Conference Series: Materials Science and Engineering IOP Publishing. (2018). 012072 p.
285. Fessi H, Puisieux F, Devissaguet JP, Ammoury N, Benita S. Nanocapsule formation by interfacial polymer deposition following solvent displacement. Int J Pharm. (1989) 55:R1–4. doi: 10.1016/0378-5173(89)90281-0
286. Lammari N, Louaer O, Meniai AH, Elaissari A. Encapsulation of essential oils via nanoprecipitation process: overview, progress, challenges and prospects. Pharmaceutics. (2020) 12:431. doi: 10.3390/pharmaceutics12050431
287. Gomes MT, Santana ÁL, Santos DT, Meireles MA. Trends on the rapid expansion of supercritical solutions process applied to food and non-food industries. Recent Pat Food Nutr Agric. (2019) 10:82–92. doi: 10.2174/2212798410666180925160459
288. Jiao Z, Wang X, Han S, Zha X, Xia J. Preparation of vitamin C liposomes by rapid expansion of supercritical solution process: experiments and optimization. J Drug Deliv Sci Technol. (2019) 51:1–6. doi: 10.1016/j.jddst.2019.02.015
289. Hasan MM, Hasan M, Mondal JC, Al Hasan M, Talukder S, Rashid HA. Liposomes: an advance tools for novel drug delivery system. Pharma Innovation J. (2017) 6:304–11.
290. Bangham A, De Gier J, Greville G. Osmotic properties and water permeability of phospholipid liquid crystals. Chem Phys Lipids. (1967) 1:225–46. doi: 10.1016/0009-3084(67)90030-8
291. Varona S, Martin A, Cocero MAJ. Liposomal incorporation of lavandin essential oil by a thin-film hydration method and by particles from gas-saturated solutions. J Ind Eng Chem Res. (2011) 50:2088–97. doi: 10.1021/ie102016r
292. Bamidele OP, Emmambux MN. Encapsulation of bioactive compounds by “extrusion” technologies: a review. Crit Rev Food Sci Nutr. (2020) 61:3100–18. doi: 10.1080/10408398.2020.1793724
293. Colletier J-P, Chaize B, Winterhalter M, Fournier D. Protein encapsulation in liposomes: efficiency depends on interactions between protein and phospholipid bilayer. BMC Biotechnol. (2002) 2:1–8. doi: 10.1186/1472-6750-2-9
294. Szoka F, Papahadjopoulos D. Procedure for preparation of liposomes with large internal aqueous space and high capture by reverse-phase evaporation. Proc Nat Acad Sci USA. (1978) 75:4194–8. doi: 10.1073/pnas.75.9.4194
295. Wen Z, Liu B, Zheng Z, You X, Pu Y, Li Q. Preparation of liposomes entrapping essential oil from Atractylodes macrocephala Koidz by modified RESS technique. Chem Eng Res Design. (2010) 88:1102–7. doi: 10.1016/j.cherd.2010.01.020
296. Yun J.-H., Lee H.-Y., Asaduzzaman A, Chun -S, et al. Micronization and characterization of squid lecithin/polyethylene glycol composite using particles from gas saturated solutions (PGSS) process. J Ind Eng Chem. (2013) 19:686–91. doi: 10.1016/j.jiec.2012.10.005
297. Yadav N, Khatak S, Sara US. Solid lipid nanoparticles-a review. Int J Appl Pharm. (2013) 5:8–18.
298. Mehnert W, Mäder K. Solid lipid nanoparticles: production, characterization and applications. Adv Drug Deliv Rev. (2012) 64:83–101. doi: 10.1016/j.addr.2012.09.021
299. Guo Q, Du G, Jia H, Fan Q, Wang Z, Gao Z, et al. Essential oils encapsulated by biopolymers as antimicrobials in fruits and vegetables: a review. Food Biosci. (2021) 44:101367. doi: 10.1016/j.fbio.2021.101367
300. López–Gómez A, Ros–Chumillas M, Buendía-Moreno L, Martínez–Hernández GB. Active cardboard packaging with encapsulated essential oils for enhancing the shelf life of fruit and vegetables. Front Nutr. (2020) 7:559978. doi: 10.3389/fnut.2020.559978
301. Ju J, Xie Y, Guo Y, Cheng Y, Qian H, Yao W. Application of edible coating with essential oil in food preservation. Crit Rev Food Sci Nutr. (2019) 59:2467–80. doi: 10.1080/10408398.2018.1456402
302. Paidari S, Zamindar N, Tahergorabi R, Kargar M, Ezzati S, Musavi SH. Edible coating and films as promising packaging: A mini review. J Food Meas Charact. (2021) 15:4205–14. doi: 10.1007/s11694-021-00979-7
303. Noori S, Zeynali F, Almasi H. Antimicrobial and antioxidant efficiency of nanoemulsion-based edible coating containing ginger (Zingiber officinale) essential oil and its effect on safety and quality attributes of chicken breast fillets. Food Control. (2018) 84:312–20. doi: 10.1016/j.foodcont.2017.08.015
304. Xiong Y, Li S, Warner RD, Fang Z. Effect of oregano essential oil and resveratrol nanoemulsion loaded pectin edible coating on the preservation of pork loin in modified atmosphere packaging. Food Control. (2020) 114:107226. doi: 10.1016/j.foodcont.2020.107226
305. Murmu SB, Mishra HN. The effect of edible coating based on Arabic gum, sodium caseinate and essential oil of cinnamon and lemon grass on guava. Food Chem. (2018) 245:820–8. doi: 10.1016/j.foodchem.2017.11.104
306. Yousuf B, Srivastava AK. Flaxseed gum in combination with lemongrass essential oil as an effective edible coating for ready-to-eat pomegranate arils. Int J Biol Macromol. (2017) 104:1030–8. doi: 10.1016/j.ijbiomac.2017.07.025
307. Heydari S, Jooyandeh H, Alizadeh Behbahani B, Noshad M. The impact of Qodume Shirazi seed mucilage-based edible coating containing lavender essential oil on the quality enhancement and shelf life improvement of fresh ostrich meat: an experimental and modeling study. Food Sci Nutr. (2020) 8:6497–512. doi: 10.1002/fsn3.1940
308. Buranasuksombat U, Kwon YJ, Turner M, Bhandari B. Influence of emulsion droplet size on antimicrobial properties. Food Sci Biotechnol. (2011) 20:793–800. doi: 10.1007/s10068-011-0110-x
309. Bhagath Y, Manjula K. Influence of composite edible coating systems on preservation of fresh meat cuts and products: a brief review on their trends and applications. Int Food Res J. (2019) 26:377–92.
Keywords: bactericidal, disease management, formulation, fungicidal, herbicidal, insecticidal, pest management, phytotoxic
Citation: Chang Y, Harmon PF, Treadwell DD, Carrillo D, Sarkhosh A and Brecht JK (2022) Biocontrol Potential of Essential Oils in Organic Horticulture Systems: From Farm to Fork. Front. Nutr. 8:805138. doi: 10.3389/fnut.2021.805138
Received: 29 October 2021; Accepted: 20 December 2021;
Published: 13 January 2022.
Edited by:
Chunpeng Wan, Jiangxi Agricultural University, ChinaReviewed by:
Dan Li, National University of Singapore, SingaporeHsin-Bai Yin, Agricultural Research Service (USDA), United States
Copyright © 2022 Chang, Harmon, Treadwell, Carrillo, Sarkhosh and Brecht. This is an open-access article distributed under the terms of the Creative Commons Attribution License (CC BY). The use, distribution or reproduction in other forums is permitted, provided the original author(s) and the copyright owner(s) are credited and that the original publication in this journal is cited, in accordance with accepted academic practice. No use, distribution or reproduction is permitted which does not comply with these terms.
*Correspondence: Jeffrey K. Brecht, amticmVjaHRAdWZsLmVkdQ==